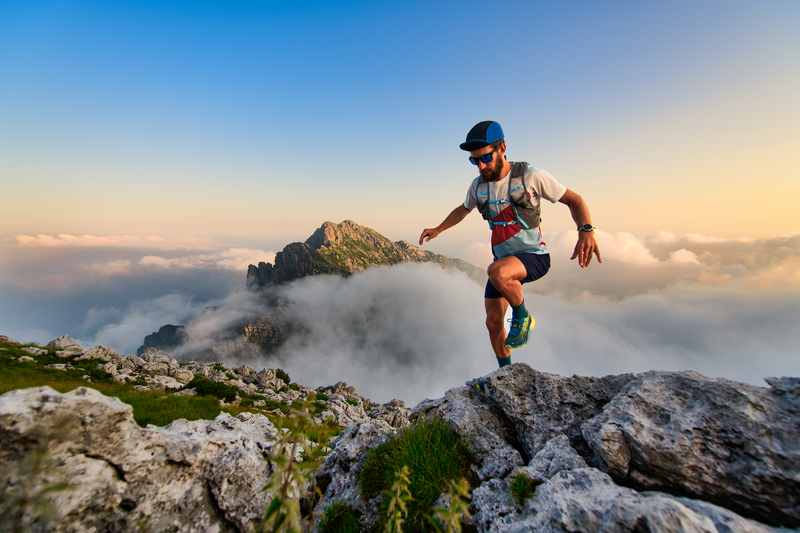
95% of researchers rate our articles as excellent or good
Learn more about the work of our research integrity team to safeguard the quality of each article we publish.
Find out more
REVIEW article
Front. Mol. Neurosci. , 13 October 2022
Sec. Methods and Model Organisms
Volume 15 - 2022 | https://doi.org/10.3389/fnmol.2022.940484
This article is part of the Research Topic Using Zebrafish to Investigate Normal Development and Function of the Nervous System: How nervous system disorders occur and testing of potential therapeutic approaches View all 8 articles
The zebrafish is increasingly recognized as a model organism for translational research into human neuropathology. The zebrafish brain exhibits fundamental resemblance with human neuroanatomical and neurochemical pathways, and hallmarks of human brain pathology such as protein aggregation, neuronal degeneration and activation of glial cells, for example, can be modeled and recapitulated in the fish central nervous system. Genetic manipulation, imaging, and drug screening are areas where zebrafish excel with the ease of introducing mutations and transgenes, the expression of fluorescent markers that can be detected in vivo in the transparent larval stages overtime, and simple treatment of large numbers of fish larvae at once followed by automated screening and imaging. In this review, we summarize how zebrafish have successfully been employed to model human neurodegenerative diseases such as Parkinson’s disease, Alzheimer’s disease, amyotrophic lateral sclerosis, and Huntington’s disease. We discuss advantages and disadvantages of choosing zebrafish as a model for these neurodegenerative conditions.
The zebrafish (Danio rerio) is a tropical freshwater teleost fish that is part of the Cyprinidae family that was introduced into biological research by George Streisinger in the 1970s. He selected zebrafish for three reasons: the generation of large numbers of embryos accessible from the one-cell-stage, the optical clarity of the developing fish, and a diploid genome allowing for genetic studies. Several large scale forward genetic screens by Nobel Prize laureate Christiane Nüsslein-Volhard and others firmly established the zebrafish as an ideal model system for developmental biology (Mullins et al., 1994; Haffter et al., 1996; Amsterdam et al., 1999; Draper et al., 2004). Today, the zebrafish is gaining traction for the study of human diseases. Here, we focus on the use and usefulness of the zebrafish model to study human neurodegenerative diseases such as Parkinson’s disease (PD), Alzheimer’s disease (AD), amyotrophic lateral sclerosis/Lou Gehrig’s disease (ALS), and Huntington’s disease (HD).
Zebrafish development is conveniently fast. Zebrafish are sexually mature by 12 weeks of age and can produce several hundred embryos each week (Lawrence et al., 2012) which develop ex utero, allowing for experimental manipulation as well as observation from the one-cell stage. All major organs and the central nervous system (CNS) are fully functional by 72 h post-fertilization (hpf) (Fulwiler and Gilbert, 1991; Kimmel et al., 1995; Blader and Strähle, 2000; Schmidt et al., 2013). The zebrafish brain shows structural and functional similarities in its anatomy with the mammalian brain, as well as corresponding neural circuitry including most major neurochemical signal transduction pathways (Fulwiler and Gilbert, 1991; Blader and Strähle, 2000; Postlethwait et al., 2000; Howe et al., 2013; Schmidt et al., 2013; Figures 1A,A′). The neurotransmitter system is conserved in the zebrafish from early in development, sharing clear similarities with the mammalian systems such as dopaminergic cell clusters in the olfactory bulb and hypothalamus, and producing neurotransmitters such as dopamine (DA), serotonin (5-HT), acetylcholine (ACh), histamine (HA), glutamate and GABA (Kaslin and Panula, 2001; Panula et al., 2006; Wasel and Freeman, 2020).
Genetically, the zebrafish shares a high level of conservation with humans. A comprehensive sequencing study demonstrated that zebrafish share at least one ortholog with over 70% of all human genes (Howe et al., 2013) including many of the risk genes identified in various human neurodegenerative diseases. Among these are genes implicated in the pathology of PD (SNCA, PINK1, LRRK2, and Parkin), familial AD (PSEN1 and PSEN2), ALS (SOD1, TARDBP, C9orf72, and FUS), and HD (Huntingtin).
One of the major advantages of the zebrafish is their optical transparency throughout development (Figure 1B). This allows researchers to perform high resolution live in vivo imaging of the entire CNS (Figure 1C) without the need for small invasive optical windows commonly used in traditional mammalian research models (Godinho, 2011; Cong et al., 2017). The uncomplicated expression of transgenes with the binary UAS/Gal4 system allows for labeling of cells and structures as well as expression of human genes in all, specific or single neurons, for example (Kawakami et al., 2016). Human genes carrying disease-causing mutations can be easily expressed using this method to assess their impact on neuronal health. Additionally, advances in the CRISPR/Cas9 technology have greatly fine-tuned the tools of gene editing to introduce mutations and transgenes into the fish genome (Sager et al., 2010; Kimura et al., 2014; Liu et al., 2017; Rissone et al., 2020). Importantly, acute injection of highly active synthetic RNA Oligo CRISPR guide RNAs drastically speeds up the process as phenotypes can be assessed in the F0 generation and later confirmed by stable mutants (Keatinge et al., 2021). Injection of mRNA or DNA plasmids into the one-cell stage provides an uncomplicated and quick method to transiently express any gene/protein of interest or introduce new DNA into the embryo’s genome. State of the art imaging allows researchers to observe the migration of neuronal precursors, the movements of microglia, or neuronal cell death for example, in the intact animal and in real time (Formella et al., 2018). A single motor neuron can be labeled and followed during outgrowth, death, before and after injury, and in disease conditions (Kabashi et al., 2011; Zelenchuk and Brusés, 2011; Asakawa et al., 2021). The development of the fluorescent Ca2+ reporter GCaMP in the mid 1990s added another major tool for neurobiological studies by making neuronal activity imageable, and GCaMP was quickly adapted in zebrafish research (Dreosti and Lagnado, 2011; Akerboom et al., 2012; Kettunen, 2012). Neuronal activity of a single or cluster of neurons or indeed whole brain activity can now be monitored at rest or in the freely swimming larval fish (Ahrens et al., 2013; Bianco and Engert, 2015; Bergmann et al., 2018). Compared to electrophysiological recordings, optical monitoring of neuronal function allows for unprecedented spatial resolution as the fish offers optical access to the entire CNS to investigate both abnormal single cell as well as population behavior. Recently, another tool for understanding brain-wide neuronal dynamics was added by tracing a map from over 2,000 labeled neurons of the underlying circuit architecture of the larval zebrafish brain, building an interactive atlas at cellular resolution (Kunst et al., 2019). With the arrival of optogenetics, the activity of specific neural cells can now not only be monitored but also induced or silenced to study their downstream influence (Boyden, 2011; Wyart and Bene, 2011). Optogenetics can also modulate the multimerization status of a protein in vivo through external light illumination and thus be used to examine the dynamics and consequences of aggregate formation, a hallmark of many neurodegenerative diseases. This technique has for example been used to investigate TDP-34 aggregation in zebrafish motor neurons (Asakawa et al., 2020). The most recent development of a family of fluorescent biosensors called genetically encoded death indicators (GEDI) keep zebrafish at the forefront of neurodegenerative research. GEDIs detect a stage where a neuron is irreversibly committed to degeneration providing an earlier and more acute demarcation of the moment of death in a degenerating neuron than previously possible. Importantly, this is possible in vivo and in an un-anaesthetized animal (Linsley et al., 2021).
Figure 1. (A,A′) Schematic illustration of the monoaminergic localities and anatomy of the zebrafish brain. The monoaminergic system is well developed in the zebrafish, with brain specific regions of neuronal clusters for the different neurotransmitters, dopamine (yellow), noradrenaline (red), serotonin (green), acetylcholine (purple), and histamine (blue). (A) The brain is developed and CNS fully functional in the larval zebrafish from as early as 3 dpf. (A′) The schematic lateral view of the adult zebrafish brain shows a well-defined and complex architecture. Schematic representation and annotations have been adapted from (A) (Alunni et al., 2013) and (B) Wullimann et al. (2011), Parker et al. (2013). AC, ansulate commissure; AP, area postrema; Cb, cerebellum; CC, crista cerebellaris; Di, diencephalon; Epi, epiphysis; Fb, forebrain; FL, facial lobe; Ha, habenula; Hb, hindbrain; Hyp, hypothalamus; LC, locus coeruleus; Mb, midbrain; Mes, mesencephalon; MO, medulla oblongata; MT, mesencephalic tegmentum; NC, nucleus commisuralis; OB, olfactory bulb; OC, optic chiasma; Pa, pallium; PoR, preoptic region; Pt, pretectum; PT, posterior tuberculum; Rhomb, rhombencephalon; RN, raphé nuclei; SC, spinal cord; Spa, subpallium; T, thalamus; Tec, tectum; Tel, telencephalon; VT, ventral thalamus; VL, vagal lobe. (B) The zebrafish is optically transparent during the first week of development. Lines for mutations in genes controlling pigment cell formation (nacrew2/w2, roya9/a9) as well as melanin production (albb4/b4) have been generated allowing for non-invasive in vivo imaging. Images in (B) have been taken and adapted from Antinucci and Hindges (2016) gp. (C) Examples of neuronal cell types imaged in zebrafish larvae. Neurons and processes in the forebrain [Dlx: GFP (green), tubulin (red) scale bar 100 μm]. Lateral view of a zebrafish embryo: Monica Folgueira and Steve Wilson, Wellcome collection, microglia (mpeg:mCherry) scale bar 10 μm, active spinal neuron (GCaMP7s) scale bar 5 μm, motor neurons (nefma:Kalt4UAS Scarlett) scale bar 20 μm, single spinal neuron [mTFP1(cyan), H2a-mCherry (red)], scale bar 5 μm.
Despite all these advantages, embryonic fish and the larval stages are not always an ideal model for human neurodegenerative diseases with their adult onset, or even old age. As in mouse models, zebrafish may also not show the same pathological consequences upon gene deletion or mutation as in humans.
Here, we summarize how the zebrafish model has contributed to research into the pathogenesis of four human neurodegenerative diseases (PD, AD, ALS, and HD) and discuss on the background of recent advances in gene editing and manipulation of neuronal activity, how zebrafish can be a powerful model to investigate human neurodegenerative diseases.
Parkinson’s disease (PD) is one of the most common neurodegenerative diseases with an annual estimated global incidence rate of between 5 and 35 per 100,000 individuals. PD is characterized by the combined loss of dopaminergic neurons in the substantia nigra, and intracellular α-synuclein accumulation in neurons of various brain regions (Lewy pathology) (Poewe et al., 2017). While PD has traditionally been perceived as a movement disorder, several non-motor- symptoms including autonomic dysfunction, cognitive deficits, as well as mood disorders are now recognized (Poewe et al., 2017). In the pathogenesis of PD, age is the most important contributing factor. While onset is generally rare before the age of 50, incidence rates increase with each increasing decade. Furthermore, life expectancy has been shown to significantly decline as PD progresses, with a mortality rate twice as high as the average within 10 years of disease onset (Pinter et al., 2015). As with most neurodegenerative diseases, the majority of PD cases are sporadic, with familial PD only accounting for around 5–10% of all cases. Genetically, mutations in synuclein α, P-TEN induced kinase 1 (PINK1), Leucine Rich Repeat Kinase 2 (LRRK2) and the E3 Ubiquitin Protein Ligase Parkin. Environmental factors such as frequent exposures to harmful chemicals such as pesticides or previous brain contusions in individuals also play a substantial role in developing PD (Bretaud et al., 2004). Caffeine and nicotine intake, however, have been associated with a decreased risk of PD. While current treatments have effectively increased quality of life and life expectancy in patients, PD remains incurable.
Of the plethora of different neurodegenerative diseases and movement disorders, PD is by far one of the most established in the zebrafish model and its promise as a PD animal model for the development of therapeutics has been highlighted in a recent review (Razali et al., 2021). High conservation of PD-related genes and sensitivity to PD risk-related drugs in zebrafish have allowed for the generation of various genetic and transgenic, as well as chemically induced, models of PD (Tables 1, 2). Despite the absence of dopaminergic neurons in the zebrafish midbrain, a functional homolog of the mammalian substantia nigra is the diencephalic dopaminergic cluster within the posterior tuberculum (Rink and Wullimann, 2001), and the serotonergic and histaminergic systems present in the zebrafish show high resemblance to the mammalian system (Kaslin and Panula, 2001; Wang et al., 2006). While unable to recapitulate the full spectrum of the disease, the zebrafish model may be particularly useful to study PD-related hypokinetic syndromes. As will be presented below, phenotypic hallmarks mimicking bradykinesia in PD patients can be achieved in the zebrafish following induced dopaminergic cell aberrations.
One of the most recognizable PD-associated genes in humans is the SNCA gene encoding α-synuclein. α-synuclein mutations are linked to early onset familial PD, and α-synuclein aggregation in Lewy bodies is associated with sporadic cases of PD (Meade et al., 2019). Although the physiological function of α-synuclein is still unclear, it is believed to be responsible for regulating the synaptic transmission process (Marques and Outeiro, 2012; Burré, 2015). However, in pathological conditions, misfolded α-synuclein proteins form aggregates, which contribute to the formation of Lewy bodies (LBs) in neurons (Mahul-Mellier et al., 2020).
Synucleins are a family of neuronal proteins consisting of α-, β-, and γ-synuclein. While the zebrafish does not express an ortholog of the human α-synuclein gene, the β-, and γ-synucleins can be found in the zebrafish genome and are expressed in three isoforms. sncb, sncg1, and sncg2 that encode for β-, γ 1-, and γ2-synucleins, respectively, which seem to compensate for the absence of α-synuclein. Importantly, zebrafish and human synucleins share high sequence similarity, with the zebrafish γ1-synuclein functionally most similar to human α-synuclein (Sun and Gilter, 2008). Milanese et al. demonstrated that knockdown of both zebrafish β- and γ1-synucleins resulted in severe impairment of the differentiation of dopaminergic neurons and the development of the dopamine system (Milanese et al., 2012). This in turn led to hypokinetic motor behavior such as reduced spontaneous swim activity, indicating that zebrafish β- and γ1-synuclein proteins are required for movement regulation and dopamine homeostasis (Toni and Cioni, 2015; Vaz et al., 2018; Robea et al., 2020). Both motor and dopaminergic deficits could be rescued by exogenous expression of human α-synuclein (Milanese et al., 2012). In line with the ability of human α-synuclein to revert phenotypes caused by the loss of β- and γ1-synucleins in the zebrafish, Prabhudesai et al. (2012) generated a model to overexpress human α-synuclein in the zebrafish embryo. While evidence for LBs formation typical in human PD pathology remains to be determined, accumulation of α-synuclein induced significant neurotoxicity in the developing embryo leading to widespread neuronal cell death, morphological deformities, as well as rapid mortality. Further studies found that over-expression and aggregation of human α-synuclein protein in zebrafish larvae led to reduced mitochondrial activity and increased presence of reactive oxygen species (ROS), which led to neuronal apoptosis and cell death (O’Donnell et al., 2014; Robea et al., 2020).
The PTEN-induced kinase 1 (PINK1) gene that encodes for PINK1 protein plays a critical role in regulating oxidative stress and protecting neurons against mitochondrial dysfunctions (Gonçalves and Morais, 2021). Mutations in the PINK1 gene leading to reduced PINK1 protein translation is a major cause to autosomal recessive early onset PD. In a pioneering study investigating loss-of-function of PINK1 in zebrafish, Anichtchik et al. observed significant morphological abnormalities and phenotypes including axonal tract deformations, impaired escape responses, and increased mortality following PINK1 knockdown by morpholino (MO) injection. Importantly, Acridine Orange assays and oxidative probes demonstrated increased neuronal cell death and accumulation of ROS, reflecting the increased susceptibility of neurons to oxidative insults and cell death following damage of cellular mitochondria (Anichtchik et al., 2008). This was a significant observation as previous investigations of PINK1 knockout (KO) mice did not reveal dopaminergic cell loss (Kitada et al., 2007). In slight discrepancy, Xi et al. observed severely disorganized patterning of the dopaminergic neurons but no increased cell loss in PINK1 morphants. The authors did, however, observe loss of balance, retarded responses to tactile stimuli, as well as significantly reduced swim distance and speed following PINK1 knockdown (Xi et al., 2010). Another study using morpholino-mediated knockdown of PINK1 showed a decrease in TH (tyrosine hydroxylase)-positive dopaminergic neurons in specific regions within the diencephalon, which was exacerbated when PINK1 morphants were exposed to the neurotoxin 1-methyl-4-phenyl-1,2,3,6-tetrahydropyridine (MPTP) that also caused motor deficits (Sallinen et al., 2010). To investigate the functional role of PINK1 on downstream genes and pathways, Priyadarshini et al. (2013) carried out a microarray analysis and showed that expression of 177 genes was significantly altered following PINK1 depletion. Downstream pathways affected included processes of mitochondrial function, as well as TGF-β signaling, with the most impacted pathway found to be hypoxia-induced factor (HIF) signaling. ROS expression levels were significantly increased in the PINK1 morphants providing further evidence that PINK1 loss-of-function leads to increased oxidative stress and an inflammatory environment (Priyadarshini et al., 2013). Subsequently, stable PINK1 null (pink1–/–) mutant zebrafish were generated that showed persistent loss of dopaminergic neurons in the absence of severe morphological abnormalities (Flinn et al., 2014). In addition to mitochondrial impairment and dysfunction following PINK1 KO, Flinn et al. observed significant microgliosis in the pink1–/– mutants. This was a significant observation as it provided the first insight on the impact on immune cells in the context of a zebrafish PD model.
The most common cause of autosomal late onset PD, mutations in leucine-rich repeat kinase 2 (LRRK2) that encodes the LRRK2 protein account for 5–13% of familial PD and 1–5% of sporadic PD (Drolet et al., 2011). Under physiological conditions, LRRK2 regulates kinase activity important for controlled protein degradation and immune response (Li et al., 2014). In the context of PD, LRRK2 function has been implicated in pathologic α-synuclein aggregation and the internalization and attenuation of its pathological propagation by vigilant microglia (Rui et al., 2018). Furthermore, PD patients with LRRK2 mutations show dysregulated kinase activity leading to increased ROS production and proinflammatory responses resulting ultimately in dopaminergic cell death (Li et al., 2014). Initial studies modeling LRRK2 deficiency in the zebrafish using MO knockdowns reported slightly contradicting findings on dopaminergic neuronal loss as well as locomotor deficits (Sheng et al., 2010; Ren et al., 2011; Prabhudesai et al., 2016). However, the recent generation of a genetic zebrafish LRRK2 deletion by CRISPR/Cas9 confirmed the finding of increased apoptotic cell death within the brain parenchyma, albeit not specific to neurons (Suzzi et al., 2017). Furthermore, LRRK2 mutants showed reduced mitosis and proliferation leading to impaired neurogenesis and neuronal regeneration, as well as locomotor deficits and impaired swimming patterns similar to PD-related bradykinesia (Suzzi et al., 2017).
A leading cause of autosomal recessive early onset PD arises from mutations in the E3 ubiquitin protein ligase Parkin, which regulates the ubiquitination of proteins required for dopaminergic cell survival (Pickrell and Youle, 2015) as well as mitochondrial processes (Kamienieva et al., 2021). Mitochondrial and metabolic dysfunction following the loss-of-function of Parkin has been shown to play a critical role in increased stress and reduced cell survival (Müller-Rischart et al., 2013). Phenotypes caused by the knockdown of Parkin have been shown to mirror that of PINK1 deficiency in zebrafish, such as mitochondrial impairment and loss of dopaminergic neurons. Flinn et al. demonstrated for the first time that Parkin knockdown led to impaired mitochondrial complex I activity and reduced energy metabolism, as well as significantly reduced numbers of diencephalic dopaminergic neurons that were significantly more susceptible to the toxic effects of the mitochondrial neurotoxin MPTP. Cell loss was mostly restricted to the posterior tuberculum in zebrafish, an area anatomically homologous to the human substantia nigra (Flinn et al., 2009). However, a replicative study with Parkin knockdown to 50–60% neither recapitulated the loss of diencephalic dopaminergic neurons nor alterations in mitochondrial integrity, and the authors did not observe any morphological abnormalities or motor deficits in the morphant larvae (Fett et al., 2010). This discrepancy may result from different levels of remaining functional Parkin due to different knockdown methods. Nevertheless, Parkin-deficient larvae were found to be more sensitive to proteotoxic stress inducers, such as heat shock, leading to increased cellular apoptosis (Fett et al., 2010).
Other genetic zebrafish models of PD include MO injections against the genes Park 7 and Park 15, encoding DJ-1 and F-box only protein 7 (FBXO7). Patients with loss-of-function of the human PARK7 gene that encodes DJ-1 present with early onset autosomal recessive PD (Bonifati et al., 2003). Zebrafish DJ-1 shares more than 80% conservation with human DJ-1 and is expressed throughout all dopaminergic neurons in the brain (Bai et al., 2006). While the knockdown of DJ-1 by itself did not lead to loss of dopaminergic neurons, DJ-1 morphants showed increased expression of apoptosis regulator genes such as p53 and Bax, and were more susceptible to oxidative stress and neurotoxicity (Bretaud et al., 2007). Knockdown of the FBXO7 ortholog (encoding PARK15) led to developmental abnormalities and deformations, significant loss of dopaminergic neurons, and behavioral as well as locomotor dysfunctions (Zhao et al., 2012).
In addition to the various genetic models of PD, several methods have been adopted to chemically induce neurotoxicity and the pathological hallmarks of PD in zebrafish (Table 2). One of the most common models is the administration of the toxin MPTP. In humans, MPTP exposure was found to induce dopaminergic neuronal cell loss and motor symptoms reminiscent of PD (Smeyne and Jackson-Lewis, 2005). Larval zebrafish were also found to be susceptible to MPTP treatment, resulting in the specific reduction of diencephalic dopaminergic neurons and significantly reduced locomotor activity (Bretaud et al., 2004). Several follow-up studies have since supported the use of MPTP to induce dopaminergic neuronal cell loss (Lam et al., 2005; McKinley et al., 2005; Wen et al., 2008; Sallinen et al., 2009) as well as locomotor deficits (Lam et al., 2005; Sallinen et al., 2009; Babu et al., 2016) in zebrafish. Using an enhancer trap transgenic fish line for the vesicular monoamine transporter 2 (Vmat2) gene, Wen et al. (2008) performed the first in vivo study to demonstrate selective loss of dopaminergic neurons following MPTP exposure. Subsequent studies in the field have adopted the zebrafish MPTP model in studies aiming to develop PD therapeutics. Investigating the potential of a platinum nanoparticle using leaf extract of a plant (BmE-PtNP), Nellore et al. (2013) showed that BmE-PtNPs were capable of ameliorating oxidative stress, rescuing both dopaminergic loss and motor deficits in MPTP-treated zebrafish larvae. Similarly, cyclopentylamino carboxymethylthiazolylindole (NecroX) compounds like the necrosis inhibitor-5 (NecroX-5) (Liu et al., 2015), as well as the iridoid glycoside loganin (Yao et al., 2017) exhibited neuroprotective potentials.
Furthermore, 6-OHDA administration in zebrafish resulted in selective loss of TH-positive dopaminergic neurons within days of application (Zhang et al., 2015, 2016; Cronin and Grealy, 2017), as well as locomotor impairments including reduced swim time and distance, and anxiety-related behavior (Feng et al., 2014; Zhang et al., 2015; Benvenutti et al., 2018). Direct intraventricular injection of 6-OHDA into the ventral diencephalon of adult fish also resulted in significant ablation of diencephalic dopaminergic neurons and locomotor deficits reminiscent of PD-related bradykinesia (Vijayanathan et al., 2017). However, significant recovery of neurons was observed within 30 days post-lesion.
Finally, to investigate the link between pesticides and PD, Lulla et al. (2016) used the pesticide ziram and demonstrated a γ1-synuclein-dependent mechanism leading to dopaminergic cell loss in zebrafish. While overexpression of γ1-synuclein resulted in intracytoplasmic neuronal aggregates and neurotoxicity, γ1-synuclein knockdown rescued the dopaminergic neuronal loss and motor impairments induced by ziram treatment (Lulla et al., 2016). Rotenone treatment of zebrafish embryos resulted in developmental retardation and deformities (Melo et al., 2015; Byrnes et al., 2018) accompanied by increased oxidative stress, cellular apoptosis and loss of dopaminergic neurons (Khotimah et al., 2015; Martel et al., 2015). In line with that, rotenone treatment induced locomotor deficits including markedly decreased motility and swim speed (Khotimah et al., 2015; Wang et al., 2017). Furthermore, using the light/dark box test approach, Wang et al. (2017) observed that zebrafish treated with rotenone spent more time in the light and showed longer latencies to enter the dark area, mirroring signs of anxiety and depression that are present in some PD patients. Even low doses of the pesticide paraquat impaired neurogenesis of dopaminergic neurons and caused motor and behavioral impairments in zebrafish embryos (Nellore and Nandita, 2015) along with increased expression of oxidative stress and apoptosis pathway components (Wang et al., 2016, 2018). Using a transgenic reporter line for macrophages, Wang et al. observed significant macrophage recruitment and migration following treatment with paraquat, providing evidence of immune cell reactivity in the paraquat-induced model of PD (Wang et al., 2016). Interestingly, experiments carried out by different groups on adult zebrafish using repeated intraperitoneal applications of paraquat resulted in conflicting results regarding motor deficits, anxiety and aggression (Bortolotto et al., 2014; Nunes et al., 2017).
Alzheimer’s disease (AD) is a chronic neurodegenerative disorder and the most frequent cause of dementia. AD is typically characterized by the presence of extracellular β-amyloid (Aβ) deposits generated from cleaved amyloid precursor protein (APP), as well as intracellular neurofibrillary tangles (NFTs) made up of aggregated hyperphosphorylated tau proteins. The disease leads to gradual hippocampal and parietal brain atrophy (Frisoni et al., 2010). GWAS (genome-wide association studies) have identified several high-risk loci genes that are involved in the regulation of immune responses (Lambert et al., 2013; dos Santos et al., 2017; McQuade and Blurton-Jones, 2019) suggesting a potential role for microglia in AD pathogenesis. Interestingly, Aβ plaques can be found in the brain even before the onset of cognitive decline. On the other hand, NFTs have been associated with neurodegeneration, cell death, and cognitive dysfunction (Bondi et al., 2017; Cass, 2017; DeTure and Dickson, 2019). As evidenced from recent PET imaging studies and meta-analyses of published biomarker data, a strong association between total tau levels in both cerebrospinal fluid and blood with cognitive impairment in AD patients has been detected (Hampel et al., 2018; Wattmo et al., 2020).
The first zebrafish tau model transiently expressing human tau protein in neurons showed tau hyperphosphorylation and accumulation in neuronal cell bodies and produced a cytoskeletal disruption that closely resembled the NFTs seen in human disease (Tomasiewicz et al., 2002; Table 3). Subsequently, stable zebrafish tau transgenic lines for the expression of human tau (111) or human mutant tau (Paquet et al., 2009) within the CNS were generated, which achieved significantly higher levels of tau expression and resulted in NFT-like tau accumulations. The success of these early tau transgenic models was validated by in vivo imaging that demonstrated rapid hyperphosphorylation and aggregation of tau as well as neuronal cell death (Paquet et al., 2009).
In a recent study, Lopez et al. (2017) generated a zebrafish line that expressed mutant A152T human tau pan-neuronally leading to increased cellular apoptosis, neurodegeneration, and impaired locomotor behavior in response to stimuli. Interestingly, despite effectively recapitulating the classical hallmarks of AD pathology, tau hyperphosphorylation and NFT formation were only observed in the spinal cord and not in the brain of the larval zebrafish (Tomasiewicz et al., 2002; Paquet et al., 2009; Fett et al., 2010; Lopez et al., 2017). Expression and hyperphosphorylation of mutant human P301L-tau in neuronal cells of adult fish did not result in neurodegeneration, nor formation of NFTs in the brain (Cosacak et al., 2017).
The ‘amyloid hypothesis’ of AD was first proposed in the 1980s and posits that the neurotoxic build-up and deposition of Aβ aggregates is a major factor in AD pathogenesis (Glenner and Wong, 1984; Hardy and Allsop, 1991; Selkoe, 1991). However, it has since been demonstrated that Aβ accumulation in the brain is independent of the age at onset and severity of AD (Edison et al., 2007; Li et al., 2008; Fagan et al., 2009). Nonetheless, Aβ has a crucial role in AD pathology. In the zebrafish, Aβ plays a physiological role in the maintenance of healthy cerebrovascular functioning as increased levels of Aβ have been associated with aberrant cerebrovascular branching in the developing hindbrain (Cameron et al., 2012; Luna et al., 2013). Likewise, MO-mediated knockdown of APP levels and blocking of Aβ production in zebrafish larvae led to significant cerebrovascular defects (Luna et al., 2013). Since then, cerebroventricular microinjections of synthetic Aβ peptides have been adopted as the most direct approach to model AD pathology in the zebrafish to mirror Aβ aggregation, tau protein phosphorylation, and induced toxicity (Nery et al., 2014; Bhattarai et al., 2016, 2017; Table 4). Direct hindbrain ventricular injections of human Aβ finally recapitulated all the hallmarks of AD such as rapid formation of β-sheet aggregations, increased neuronal toxicity and cell death, as well as adverse motor functioning (Nery et al., 2014; Bhattarai et al., 2016). Interestingly, lithium chloride treatment reduced tau phosphorylation and ameliorated the motor deficits in the injected zebrafish (Nery et al., 2014).
However, zebrafish Aβ models also exhibited some phenotypes atypical of clinical AD progression. For example, Aβ overexpression increased neuronal progenitor plasticity and proliferation, and enhanced neurogenesis in the adult zebrafish brain via interleukin (IL)-4 secretion by neurons and activated microglia (Bhattarai et al., 2016, 2017). Interestingly, progenitor proliferation was preserved but neurogenesis diminished with aging (Nery et al., 2014). In a recent follow-up study by the same group, single-cell transcriptomic data demonstrated that IL-4 regulates serotonin production and downstream regulation of brain-derived neurotrophic factor (BDNF) expression leading to increased plasticity and proliferation of neural stem cells in the adult zebrafish brain (Bhattarai et al., 2020).
Another major focus in zebrafish AD research involves the Presenilin (PSEN) genes that have been implicated in the hereditary forms of AD (Strooper, 2007; Veugelen et al., 2016; Wong et al., 2020). PSEN is part of the γ-secretase complex involved in regulating cellular proliferation, and PSEN mutations have been suggested to play a role in the generation of plaque-building Aβ peptides (Haapasalo and Kovacs, 2011; Veugelen et al., 2016). During disease pathology, AD-related PSEN mutations have been shown to accelerate the catalytic proteolysis of APP by γ-secretase, resulting in the increased production of longer, amyloidogenic Aβ peptides (Kabir et al., 2020). Zebrafish carry two orthologs of the two different PSEN genes (PSEN1 and PSEN2) found in the human genome and these are ubiquitously expressed throughout development (Leimer et al., 1999; Groth et al., 2002). Early in vitro work found that human PSEN1 could be replaced by zebrafish Psen1, maintaining the efficacy of generating Aβ from APP (Leimer et al., 1999). While complete Psen1 KO (Psen1–/–) was found to be lethal in mice, psen1–/– zebrafish were viable and did not exhibit gross morphological defects when compared to wild type zebrafish (Sundvik et al., 2013). Intriguingly, MO-mediated knockdown of Psen2 led to significant apoptosis and neuronal loss in zebrafish (Campbell et al., 2006), while Psen2–/– mice only exhibited a weak phenotype (Herreman et al., 1999). Impaired Notch signaling could be recapitulated following the loss-of-function of either of both PSEN genes in the zebrafish (Nornes et al., 2003, 2008, 2009; Campbell et al., 2006; Sundvik et al., 2013). Notch signaling is often affected in AD pathology, and regulates the histamine system in the brain and the development of histaminergic neurons (Zlomuzica et al., 2016).
One of the most widely used methods to pharmacologically induce an AD-like phenotype is through okadaic acid (OKA) application. OKA is a polyether C38 fatty acid toxin and a potent and selective inhibitor of the protein phosphatases 1 (PP1) and 2A (PP2A) (Kamat et al., 2014; Kamat and Nath, 2015). OKA-induced inhibition of these protein phosphatases, in particular PP2A, has previously been shown to cause tau hyperphosphorylation and NFT formation, as well as other hallmarks of AD pathology both in vitro and in vivo (Hensley et al., 2010a; Zhang and Simpkins, 2010; Kamat et al., 2012a,b, 2013, 2014; Kamat and Nath, 2015). Exposure of zebrafish to OKA resulted in tau hyperphosphorylation, as well as deposition of Aβ and formation of senile plaques (Nada et al., 2016). Furthermore, exposure to OKA induced learning and memory deficits in zebrafish as revealed by the pre-treatment learning and post-treatment memory test developed by Williams et al. (2002), Administration of lanthionine ketimine-5-ethyl ester, shown to elicit neurotrophic and neuroprotective properties in murine models (Hensley et al., 2010a,b) led to reduced cell apoptosis, increased BDNF production and rescue of the learning deficits of OKA-treated fish (Koehler et al., 2018). The efficacies of glycogen synthase kinase 3β (GSK3β) inhibitors have also been tested in zebrafish AD models. GSK3β is a serine/threonine protein kinase that has been involved in AD pathogenesis. Its overexpression in zebrafish is associated with increased Aβ production, tau hyperphosphorylation, neuronal cell death, reactive gliosis, and cognitive impairments (Hooper et al., 2008; Llorens-Marítin et al., 2014; Jaworski et al., 2019; Toral-Rios et al., 2020). Supporting an earlier in vivo study, a recent investigation of the selective GSK3β inhibitor, TDZD-8, in zebrafish revealed that the reduced GSK3β activity was associated with reduced tau phosphorylation and mortality rates. Importantly, treatment with TDZD-8 also rescued OKA-induced cognitive deficits (Paquet et al., 2009; Koehler et al., 2019).
Amyotrophic lateral sclerosis also known as motor neuron disease (MND) or Lou Gehrig’s disease, is a progressive neurodegenerative disease of the human motor system (Kiernan et al., 2011; Brown and Al-Chalabi, 2017). ALS is relatively rare with incidence rates of only 2–3 per 100,000 individuals worldwide, and remains an incurable, fatal disorder. Characterized by loss of primary motor neurons in the brain and spinal cord, ALS leads to progressive spasticity and muscle atrophy, dysarthria and dysphagia, and ultimately paralysis and death (Loeffler et al., 2016; Grad et al., 2017; Matsubara et al., 2019). ALS is categorized into two forms, sporadic ALS that makes up approximately 90% of all cases, and familial ALS (Kiernan et al., 2011; Brown and Al-Chalabi, 2017). More than 30 different genes have been associated with familial ALS (Hardiman et al., 2017). Of these, mutations in four genes are the most prevalent and contribute to up to 70% of all familial ALS cases (Hardiman et al., 2017). They comprise the mutations in SOD1 (superoxide dismutase 1), TARDBP (encoding TAR DNA-binding protein-43 [TDP-43]), C9orf72 (open reading frame 72 on chromosome 9), and FUS (Fused in Sarcoma) (Al-Chalabi et al., 2017; Roggenbuck et al., 2017).
The transparent bodies of zebrafish larvae allow for non-invasive visualization of motor neurons, from somas to their neuromuscular synapses, as well as their circuitry. This and the availability of genome editing, optogenetics and ease of drug screening facilitate functional analyses of ALS-associated proteins and consequential motor neuron degeneration with subcellular resolution (Asakawa et al., 2021).
The genes most commonly associated with ALS show high conservation in zebrafish with sequence identity of over 70% for SOD1 (Da Costa et al., 2014), TARDBP (Kabashi et al., 2011), C9orf72 (Iyer et al., 2018), and FUS (Morrice et al., 2020). Stable mutant zebrafish lines have been established for all four of these genes (Tables 5–8), and all effectively recapitulate the various hallmarks observed in ALS.
Mutations in SOD1 are a major cause of familial ALS, and one of the most extensively studied. Interestingly, SOD1 expression is ubiquitous and despite efforts to ascertain its role, it is still unclear how mutant SOD1 leads to selective death of motor neurons. Evidence presented thus far suggests that pathology arises from gain-of-function mutations of SOD1 in ALS. As summarized in Table 5, overexpression of human mutant SOD1 in zebrafish resulted in significantly impaired axonal outgrowth and limited branching, as well as impaired swimming capabilities. In one of the first studies, transient overexpression of human G93A-SOD1 caused axon pathology, degeneration of the neuromuscular junctions, and motor neuron loss (Sakowski et al., 2012). Similarly, zebrafish larvae injected with mutant human SOD1 mRNA showed significant axonopathy and displayed impaired movements during behavioral tests when compared to controls injected with wild type SOD1 mRNA (Robinson et al., 2019). These phenotypes were confirmed in various stable transgenic zebrafish models of mutant SOD1 (Ramesh et al., 2010; Sakowski et al., 2012; Benedetti et al., 2016).
TARDBP encodes the RNA/DNA-binding protein TDP-43 which functions in RNA processing and metabolism. TARDBP mutations result in accumulation of mutant TDP-43 in inclusion bodies in over 90% of all ALS cases. Also present in frontotemporal lobe dementia (FTLD), ubiquitinated and hyperphosphorylated TDP-43 C-terminal fragments accumulate in neurons and glia in brains of FLTD patients, but interestingly not in the spinal cord (Berning and Walker, 2019).
Zebrafish transgenic for human mutant TARDBP exhibit hallmarks of ALS pathogenesis such as abnormalities in motor axon formation and branching, and motor functioning (Table 6). Testing the effects of three separate TARDBP mutations (A315T, A382T, and G348C) found in ALS patients, Kabashi et al. (2010) showed for the first time that mutant TDP-43 expression in the zebrafish led to abnormal motor axon development, motor neuron defects and toxicity, as well as severe deficits of motor function. In a follow-up study, Laird et al. (2010) demonstrated that overexpression of proganulin, null mutations of which cause TDP-43 accumulation in FTLD, rescued motor neuron degeneration in the TDP43-A315T zebrafish line. Zebrafish express both independent orthologs of TARDBP (tardbp and tardbpl) throughout development, and single deletion of one ortholog is compensated for by increased expression of a splice variant of the other (Schmid et al., 2013). Therefore, homozygous double mutants (tardbp–/–; tardbpl–/–) were generated to establish the impact of TDP-43 loss-of-function (Schmid et al., 2013; Bose et al., 2019). tardbp–/–; tardbpl–/– zebrafish exhibited mispatterned vasculature, impaired outgrowth of spinal motor neuron axons, and smaller muscle fibers with disorganized assembly of myofibrils (Schmid et al., 2013). Expression of Filamin C was also upregulated in the tardbp–/–; tardbpl–/– double mutants. Filamin C is an actin cross-linking protein found in smooth muscle cells surrounding the brain vasculature, and analysis of Filamin C expression in frontal cortex of FTLD-TDP patients confirmed its upregulation as part of TDP-43 mediated pathology (Schmid et al., 2013). Further analyses also found aberrant quantal transmission and perturbed synapse architecture at the NMJ in the double mutants and highlighted different sensitivity to therapeutic drugs in partial vs. complete loss of allelic functions of TDP-43 (Bose et al., 2019).
Mutations in the FUS gene cause an aggressive, sometimes juvenile-onset motor neuron disease (Lattante et al., 2013). FUS is an RNA-binding protein with structural and functional similarity to TDP-43, and mis-localization of FUS from the nucleus into the cytoplasm leads to FUS aggregation in inclusion bodies similar to TDP-43 inclusions in motor neurons. As with the other ALS-associated genes, FUS has been suggested to contribute to disease pathogenesis through either a loss-of-function in the nucleus or gain-of-function after its mis-localization to the cytoplasm. As summarized in Table 7, several zebrafish models with genetic manipulation of FUS have been developed. The earlier studies employed antisense MOs to knockdown FUS, resulting in morphant larvae with shortened motor axons, aberrant branching and marked abnormality in motor behavior (Kabashi et al., 2011; Armstrong and Drapeau, 2013). In the same studies, the authors also investigated the possible toxic gain-of-function of FUS by overexpression of the human gene carrying the ALS-causing R521H mutation. This resulted in significant axonopathy, increased motor neuron excitability, NMJ abnormalities, as well as impaired motor function (Kabashi et al., 2011; Armstrong and Drapeau, 2013). To investigate the mechanism by which mutations in FUS cause motor neuron degeneration, Yu et al. examined the role of U1 snRNP (Yu et al., 2015), a small nuclear ribonucleoprotein that had been shown to be the most abundant factor interacting with FUS in previous in vitro assays (Yamazaki et al., 2012; Groen et al., 2013). They found that FUS is essential for the interaction between U1 snRNP and RNA Polymerase II (RNAP II) during splicing, thus physically and functionally coupling transcription to splicing (Yu et al., 2015). MO-mediated knockdown of U1 snRNP in zebrafish led to the development of significantly truncated motor axons in the developing zebrafish larvae (Yu et al., 2015). Confirming that the pathological impact of FUS mutations seems to lie in its impaired function or mislocalized gain of function, full deletion of zebrafish FUS employing CRISPR/Cas9 resulted neither in visible defects of axonal length or branching, nor any abnormality of swimming behavior (Lebedeva et al., 2016). The absence of a phenotype typical for ALS upon FUS deletion in zebrafish is in line with previous findings in FUS knockout mouse models that displayed a range of phenotypes atypical of ALS (Hicks et al., 2000; Kino et al., 2015).
Expansions of the G4C2 (GGGGCC) hexanucleotide repeats in the C9orf72 gene have been identified as one of the most common genetic causes of ALS (Stepto et al., 2014; Balendra and Isaacs, 2018). While healthy individuals commonly have up to 30 G4C2 repeats, ALS patients carry versions of 70 or more G4C2 repeat expansions. While expansion mutations of C9orf72 are mostly responsible for the detrimental effects on motor neuron degeneration, loss of function resulting from C9orf72 haploinsufficiency has also been suggested to induce neurodegeneration.
Various mutant, deletion and transgenic C9orf72 zebrafish models have since been generated to investigate the functional role of C9orf72 in ALS pathogenesis (Table 8). Multi-fold G4C2 repeats were engineered to achieve neurotoxicity and induce disease. In one of the early studies, increased apoptotic cell death was observed in embryos expressing 72× G4C2 repeats compared to age-matched embryos with 38× G4C2 repeats (Lee et al., 2013). In line with data suggesting correlating increase in neurotoxicity with numbers of G4C2 repeats, it was found that injection of 89× G4C2 repeats at the 1-cell -stage led to severe toxicity and mortality by 7 dpf (Shaw et al., 2018). Expression of 10 or fewer G4C2 repeats did not have any negative impact on axon physiology (Swinnen et al., 2018). The zebrafish models with multiple G4C2 expansions displayed hallmarks of ALS such as muscle atrophy, motor axon abnormalities, motor neuron loss, as well as behavioral and locomotor deficits.
The first in vivo C9orf72 loss-of-function model was developed via a MO-induced knockdown leading to signs of motor neuron degeneration with axonopathy phenotypes and abnormal motor behavior (Ciura et al., 2013). Recent studies mimicking C9orf72 loss of function involve the generation of novel transgenic lines which include the specific deletion of the functional DENN domain of C9orf72 (Yeh et al., 2018) as well as a miRNA-based gene-silencing approach to achieve loss of over 50% C9orf72 function (C9-miR) (Butti et al., 2020). The reduced C9orf72 expression resulted in aberrant axonal growth, increased neuronal apoptosis and muscle atrophy, as well as and locomotor deficits when compared to age-matched controls.
Furthermore, mislocalization of TDP-43 from the nucleus to the cytoplasm was observed in the C9-miR zebrafish, recapitulating another classical neuropathological hallmark of ALS (Butti et al., 2020).
Besides genetic causes, extrinsic factors such as the exposure to environmental neurotoxins have been associated with ALS etiology (Zarei et al., 2015). Bisphenol A (BPA) is widely used in the production of polycarbonate plastics and adversely affects hormonal and metabolic pathways (Vandenberg et al., 2007; Seachrist et al., 2016). Morrice et al. (2018) tested the effects of synthetic estrogen BPA on zebrafish and found that BPA led to reduced motor axon length and branching as well as loss of neuromuscular junction integrity. They further noted increased numbers of apoptotic motor neurons and the presence of activated microglia.
Huntington’s disease (HD) is an autosomal-dominant inheritable neurodegenerative disorder that results from a CAG repeat extension in exon 1 of the huntingtin gene (HTT), which translates into a long polyQ repeat in the huntingtin protein. The length of these polyQ repeats correlate with the age of onset, penetrance and severity of the disease (Langbehn et al., 2010). HD has a prevalence of around 10 per 100,000 individuals globally, with the age of onset, penetrance, and severity of the disease largely dependent on the number of CAG/polyQ repeats present in the huntingtin gene. Mutant HTT (mHTT) fragments are very unstable as a consequence of the long polyQ repeat and forms aggregates that are localized in intranuclear inclusions, a hallmark of HD pathology (Squitieri et al., 1994; Lee et al., 2012; Semaka et al., 2013). Impaired proteostasis of mHTT result in toxic effects leading to neuronal death of GABAergic medium spiny neurons in the striatum and in neuronal loss in the cortex and other brain regions (Eidelberg and Surmeier, 2011; Ehrlich, 2012; Estrada-Sánchez and Rebec, 2013; Garret et al., 2018; Hsu et al., 2018). Despite having been first described by George Huntington over a century ago, the disease remains incurable. No treatment options are currently available to prevent or slow down the progression of HD, with the average patient succumbing to the disease within 20 years from onset of disease symptoms including behavioral, cognitive, as well as motor dysfunctions (Bates et al., 2015; McColgan and Tabrizi, 2018).
To identify the physiological function of Htt, various groups have addressed the impact of htt depletion on early development in the zebrafish (Table 9). The zebrafish homolog of human HTT encodes a protein of 3,121 amino acids with 70% identity to mammalian HTT, but only 4 glutamines compared to 7 in mice and up to 35 in humans (Karlovich et al., 1998). As in humans, HTT is ubiquitously expressed in the zebrafish brain and is crucial for the formation of telencephalic progenitor cells as well as pre-placodal cells (Lumsden et al., 2007; Henshall et al., 2009). The zebrafish telencephalon has been proposed to be the anatomical equivalence of the mammalian striatum (Rink and Wullimann, 2002). Moreover, the loss of placode-derived tissue including olfactory and lateral line sensory neurons in the zebrafish is consistent with clinical observations of progressive olfactory abnormalities in individuals with HD (Mitchell et al., 2005; Laroche et al., 2020).
Focusing on regions of HTT expression throughout the CNS, Henshall et al. investigated how loss of HTT affected different brain regions in the zebrafish (Henshall et al., 2009). Inhibition of HTT mRNA translation impaired the formation of the anterior-most region of the neural plate, evidenced by reduced expression of genes characteristically expressed within that region (six1, dlx3b, and emx3). The anterior neural plate is important for the induction of forebrain structures such as the pre-placodal cells and telencephalic precursors (Whitlock and Westerfield, 2000; Andoniadou and Martinez-Barbera, 2013; Schmidt et al., 2013). In an independent follow-up study, Lo Sardo et al. investigated HTT function in neural tube formation. In concurrent experiments with htt-null mouse embryonic stem cells and HTT MO depleted zebrafish embryos, they found that HTT is essential for homotypic interactions between neuroepithelial cells (Lo Sardo et al., 2012). Inhibition of HTT translation resulted in impaired rosette formation and neurulation, similar to N-Cadherin ablation (Lele et al., 2002). HTT was also found to be required for proper expression and distribution of the apical marker ZO-1, the impairment of which resulted in mispositioned cells in the diencephalic neural tube and cellular aggregates in the brain ventricles at 24 h post fertilization (Lo Sardo et al., 2012). Furthermore, hTT morphants exhibited altered ventricular space and reduced cephalic regions compared with controls. Interestingly, as in N-Cadherin mutants (Lele et al., 2002), the impact of HTT depletion was restricted to alar regions in the forebrain, with cells remaining organized in more basal positions.
Knockdown of HTT also led to decreased hemoglobin in the blood, increased erythroid and ubiquitous transferrin receptor transcript levels, as well as exhausted maternal iron stores in the yolk (Lumsden et al., 2007). In line with iron deficiency and dysregulation of iron metabolism observed in human HD pathology (Morrison and Nevin, 1994), the results suggested that HTT acts downstream of transferrin receptor -mediated endocytosis of iron, thus implicating its role in the release of iron from endocytic compartments into the cytosol.
Brain-derived neurotrophic factor (BDNF) is a major contributor in neural development including formation, differentiation, and survival of neurons (Cohen-Cory et al., 2010; Kowiański et al., 2018). Wild type HTT increases the expression of bdnf at the transcriptional level. In the brain, HTT retains the transcription factor REST (repressor element 1 silencing transcription factor) in the cytoplasm, thereby reducing its repressor function of REST-regulated gene transcription and in turn facilitating BDNF expression (Gopalakrishnan, 2009). Post-mortem samples of HD brain revealed translocation of REST into the nucleus, leading to reduced transcription of BDNF (Zuccato et al., 2003, 2007). Similar to what has been seen in mouse models (Zuccato et al., 2001; Cattaneo et al., 2005) the effect of HTT knockdown in zebrafish causes reduced expression levels of BDNF to about 50% (Diekmann et al., 2009). This caused a high rate of neuronal apoptotic cell death and small eyes and head, enlargement of brain ventricles and later lower jaw abnormalities with missing branchial arches. All these observed phenotypes caused by knock down of zHTT could be rescued following treatment with recombinant BDNF administered through the embryo medium. Similarly, Henshall et al. found the defects in sensory nerve cell numbers of the lateral line descending from placodal cells they observed in HTT morphant embryos were rescued following treatment with exogenous BDNF (Henshall et al., 2009). By combining virtual screening for compounds that disrupt the REST transcription repressor complex and zebrafish in vivo experiments, Conforti et al. (2013) identified quinolone-like compound 91 (C91) as being able to interfere with the REST transcriptional machinery, thereby rescuing BDNF mRNA expression in zHTT morphants as well as embryos transiently expressing human N-terminal mHTT.
The accumulation of mHTT in neurons indicates that protein quality control is compromised. The C-terminus of Hsc70-interacting protein (CHIP) plays a crucial role in ensuring the proper folding and conformation of proteins, linking molecular chaperones with the ubiquitin-protease system by acting as both co-chaperone and ubiquitin ligase (McDonough and Patterson, 2003). The co-expression of CHIP with either a generic polyQ-fragment or pathogenic fragment of human HTT led to increased solubility and hence reduced aggregation of the mHTT protein in zebrafish (Miller et al., 2005). Furthermore, CHIP expression rescued the formation of inclusions in neurons and toxicity in mouse primary neuron cultures as well as zebrafish embryos (Miller et al., 2005). However, the apoptotic neuronal cells observed in mHTT expressing embryos were not the cells showing insoluble inclusion bodies of aggregated mHTT (Schiffer et al., 2007; Diekmann et al., 2009). In fact, soluble oligomers were highly interactive with RNA-binding proteins as well as proteins functioning in ribosome biogenesis, translation, transcription, and vesicle transport, whereas insoluble inclusions were less interactive and associated strongly with protein quality control components (Kim et al., 2016).
Exploiting the advantage of the zebrafish model for chemical screens, Schiffer et al. tested several compounds to inhibit polyQ aggregation of mutant HTT. Two anti-prion compounds of the N′-benzylidene-benzohydrazide class were identified that successfully inhibited polyQ aggregation in zebrafish embryos expressing mHTT with a 102Q (Schiffer et al., 2007). Williams et al. used the rhodopsin promoter to express an EGFP tagged mHTT containing a 71Q expansion in the zebrafish eye. This resulted in the accumulation of mHTT aggregates in the embryonic zebrafish retina, leading to loss of rhodopsin expression and consequential rod photoreceptor degeneration (Williams et al., 2008). In addition, the group performed an in vitro chemical screen to identify novel enhancers of autophagy-inducing pathways independent of mTOR (mammalian target of rapamycin). Autophagy is a major clearance mechanism for intracellular protein aggregates which can be upregulated via the administration of the mTOR inhibitor rapamycin (Ravikumar et al., 2004). Treatment of embryos expressing mHTT (71Q) with various drug candidates that all targeted different components of an mTOR-independent autophagy pathway (verapamil, calpastatin, clonidine, and 2′5′ddA), reduced the extend of mHTT aggregation in the retina significantly and substantially enhanced rhodopsin expression compared to untreated fish (Williams et al., 2008).
As previously reported in HD BAC transgenic mice, an N-terminal 17 (N17) amino acid fragment of HTT adjacent to the polyQ expansion domain regulates protein stability, toxicity, and sub-cellular localization (Gu et al., 2015). Investigating the role of N17 in zebrafish, Veldman et al. (2015) generated a novel, inducible line that expressed either a deleted N17 domain coupled with 97Q expansion (mHTT-ΔN17-exon1) or intact N17 and 97Q expansion alone (mHTT-exon1). The mHTT-ΔN17-exon1 embryos showed robust and rapidly progressive movement disorders, reminiscent of that seen in other animal models and human patients while the mHTT-exon1 embryos displayed a delayed onset and slower progression of movement deficits. Importantly, accumulation of mutant HTT was observed in neurons expressing the combined N17 deletion and 97Q expansion, but not in neurons expressing the 97Q expansion construct alone (Veldman et al., 2015). Recently, a CRISPR/Cas9-induced Htt deletion was performed in zebrafish (Sidik et al., 2020), and in contrast to previous MO studies, and rodent models whereby homozygous HTT knockout is embryonic lethal at pre-gastrulation stage (Nasir et al., 1995; Zeitlin et al., 1995), these zebrafish were viable into adulthood but showed slow growth, smaller body size and poor fitness. Thus, further investigation is needed to establish the function of wild type Htt during development and the pathogenic role of the poly-Q expansion in zebrafish.
The zebrafish model is widely used to study various aspects of the CNS, from development and physiological function to degeneration and disease. We have highlighted many advantages, but we also want to address areas where zebrafish models show limitations.
Many of the studies summarized here, especially those dating 10 or more years back, have used MO to knock down mRNA or block protein expression. In general, the advantage of gene knockdown by MO injection has been the quick generation of larval fish depleted of proteins of interest but some of the produced phenotypes were caused by unspecific side effects. In recent years, many studies have dealt with the discrepancies between phenotypes of morphants and mutations leading to gene deletion or premature stop codons (Kok et al., 2015; Morcos et al., 2015; Stainier et al., 2015; Zimmer et al., 2019). MOs have been shown to induce off-target effects that were mostly morphological deformities, while engineered gene mutations generating nonsense-mRNAs were found to activate nonsense-mediated decay machinery and induce genetic compensation, masking the null mutant phenotype (Kok et al., 2015; El-Brolosy et al., 2019). Another obvious difficulty concerning the use of MOs to model human disease is their transient nature, as MOs only last up to 6 days of early development. The argument is often raised against the use of zebrafish that analysis is usually confined to the early transparent larval stages and not adult fish, even if the CNS is fully formed at 3dpf. However, adult fish can be employed for analysis, as demonstrated by Vijayanathan et al. (2017) who injected the neurotoxin 6-OHDA into the ventral diencephalon resulting in significant ablation of diencephalic dopaminergic neurons and locomotor deficits reminiscent of PD-related bradykinesia. Although zebrafish may intuitively not be the first choice to model late onset human disease and degenerative pathologies due to aging, we highlight in this article that many aspects of neurodegeneration can be successfully modeled and investigated in zebrafish larvae. Also, results from gene function analyses in early developmental stages may present clues about the predisposition for certain pathologies and the premanifest stages of disease. With the advent of CRISPR/Cas gene editing in zebrafish, the limitations of MO use have been widely overcome. In addition, the development and availability of pigmentation mutants, such as the casper (nacrew2/w2; roya9/a9) and crystal (nacrew2/w2; albb4/b4; roya9/a9) mutants, may be of use to future investigations that require in vivo imaging in adult zebrafish (Antinucci and Hindges, 2016).
Motor dysfunction is a hallmark of many neurodegenerative disorders and is often used as a readout for disease progression. To observe and analyze motor function in zebrafish, imaging and tracking software have been developed to determine locomotor dysfunction. However, most studies have evaluated motor deficits through either total locomotor activity (swim duration, swim velocity, swim distance) or response time toward a tactile-evoked escape response. These tests may not be a true reflection of the more subtle muscular and movement disorders resulting from neurodegeneration. For example, it is difficult to establish balance or movement coordination in the zebrafish model, whereas it is straightforward in quadruped rodent models. Similarly, while anxiety and fatigue tests, as well as gait analyses have been routinely carried out in rodents, those assays have been more difficult to establish in the zebrafish model. Therefore, a need remains for the design of behavioral assays in zebrafish that more accurately mirror the repertoire of progressive motor disorders in neurodegenerative disorders.
The ability to maintain large stock numbers of zebrafish at relatively low cost provides laboratories a cost-effective alternative to the breeding of rodents and other mammalian models (Aleström et al., 2019). The zebrafish can provide researchers with a platform for rapid high throughput readouts with large sample sizes which is particularly beneficial in studies involving clinical drug discovery as hundreds of samples can be placed into 96-well plates for simultaneous chemical screening (Letamendia et al., 2012; Mathias et al., 2012; Figure 2). Recent advances in microscopy facilitate high throughput bulk imaging in combination with compound screens in zebrafish larvae in vivo, such as the VAST (Vertebrate Automated Screening Technology) BioImager system developed for the zebrafish (Figure 2; Pulak, 2016; Guo et al., 2017; Early et al., 2018). However, while studies have demonstrated the efficacy of drug testing in the zebrafish to ameliorate phenotypes in the various models of neurodegenerative disorders, the method of drug administration is not entirely controllable. Treatment is usually carried out through the application of the respective drugs into the swimming media, and while experimental concentrations can be calculated, it remains difficult to accurately determine the amount that is absorbed by the zebrafish. Moreover, it remains to be established if zebrafish metabolize and excrete drugs in the same fashion as mammals. Therefore, these issues may present a caveat when translating results into human clinical application.
Figure 2. Drug screening and automated imaging as well as analysis of zebrafish larvae. The VAST system is a fully validated, highly accurate integrity screening system that provides a method of screening large population numbers rapidly. Automated imaging and analysis pipelines ensure unbiased and fast data generation. VAST Bioimager photo provided by Dr. Jason Early (SmFzb24uRWFybHlAZWQuYWMudWs=).
Research into PD pathology has already integrated the zebrafish model well (Figure 3). Transgenic expression of human α-synuclein and knockdown of genes carrying mutations in PD patients (PINK1, LRRK2, Parkin, DJ-1, and FBXO7) have recapitulated the classic symptoms of the disease such as loss of dopaminergic neurons, neuroinflammation, locomotor and behavioral dysfunctions. Similarly, neurotoxin and pesticide exposure (MPTP, 6-OHDA, ziram, rotenone, and paraquat) resulted in loss of dopaminergic neurons as well as locomotor impairments. The zebrafish model thus presents a platform to investigate mechanisms of aggregate formation and consequences of gene mutation or pesticide exposure in the CNS. Several studies also included behavioral tests to measure depression, anxiety, social interaction aversion and aggression, features that are present in many PD patients. However, the results were quite variable and there is a need to establish better behavioral screens for the psychiatric symptoms of PD in the zebrafish model.
Figure 3. Schematic overview of zebrafish model compared to human disease condition. Human disease condition on the left compared to zebrafish phenotypes on the right.
Many recent studies have employed zebrafish to model AD, and despite successful recapitulation of Aβ-sheet aggregation, increased neuronal toxicity and death, modeling AD in the zebrafish has thus far been limited (Figure 3). Deletions of the PSEN genes were viable and did not exhibit morphological or neurological defects. Tau phosphorylation and NFTs formation were successfully induced, but only appeared in the spinal cord and not in the brain of the larval zebrafish. Aβ aggregation could only be observed when human Aβ was injected into the fish brain, but not from endogenous protein. Additionally, Aβ overexpression induced phenotypes atypical of clinical AD, such as increased neuronal progenitor plasticity and proliferation, and enhanced neurogenesis. However, treatment of fish larvae with the known protein phosphatase inhibitor OKA successfully induced tau hyperphosphorylation, as well as deposition of Aβ, formation of senile plaques, and induced learning and memory deficits in zebrafish, mimicking the classical hallmarks of AD. With the number of AD cases expected to continually rise over the coming years, the need for drug discoveries is imperative. Thus, recent efforts have focused on the advantages of the zebrafish as a cost-effective and rapid in vivo pharmacological model for AD drug discovery. In this context, the use of CRISPR/Cas gene editing may help to establish better zebrafish models of AD.
While ALS research has been typically carried out in rodent models, data obtained in the zebrafish thus far is showing great promise for modeling ALS (Figure 3). Zebrafish mutants of FUS, TDP-34, C9orf72, and SOD1 all displayed hallmarks of ALS such as muscle atrophy, motor axon abnormalities, motor neuron loss, as well as behavioral and motor deficits. Importantly, zebrafish studies have greatly advanced our understanding of the molecular mechanisms involved in ALS pathogenesis. For example, analysis of tardbp null fish revealed that the actin cross-linking protein Filamin C was upregulated as part of TDP-43 mediated pathogenesis, a fact later confirmed in the analysis of human samples. Investigation into FUS function in zebrafish revealed that FUS is essential for the interaction between U1 snRNP and RNA Polymerase II (RNAP II) during splicing, thus physically and functionally coupling transcription to splicing (250). BPA treatment of zebrafish not only induced motor axonopathy and loss of neuromuscular junction integrity, but was also associated with increased numbers of apoptotic motor neurons and the presence of activated microglia. Importantly, the zebrafish may prove to be particularly useful when disease phenotypes fail to manifest in classical mammalian models. For example, while knockdown of C9orf72 in zebrafish led to typical symptoms of ALS, previous studies in rodent models failed to show adverse effects of C9orf72 deficiency on neural health (Lagier-Tourenne et al., 2013; Burberry et al., 2016). Finally, the excellent accessibility for imaging and optogenetics allows for real time analysis of developing axon pathology and motor neuron death overtime in zebrafish.
In comparison to other disease models, not many studies have been carried out to explore HD pathology in the zebrafish. The overexpression of human mHTT, and different Htt knockdown analyses showed increased apoptosis and neuronal cell death in brain regions ortholog to those affected in HD patients (like the striatum), and disturbed neural tube formation. Importantly, zebrafish are viable in the absence of Huntingtin in contrast to mice, which has helped to better understand the neurodevelopmental aspects of HD. Studies in zebrafish revealed that impaired HTT function in early development could have limiting effects on precursor cell numbers of neurons affected by the loss of normal HTT in later development. Other studies identified REST as a molecular target of HTT in regulating BDNF expression and strikingly, found that the soluble mutant forms of HTT mRNA and protein were the toxic components causing cell death. Two anti-prion compounds were identified that successfully inhibited poly-Q aggregation. Finally, zebrafish transgenic for a truncated form of mHTT (mHTT-ΔN17) displayed a rapidly progressive movement disorder, reminiscent of that seen in other animal models and human patients (Figure 3).
To find new therapeutics for neurodegenerative diseases, the blood brain barrier constitutes a major hinderance for drugs to reach their targets in the brain. Nanotechnology is becoming a promising field of research for brain drug delivery using nanosized particles. Here the zebrafish is also proving an advantageous model for assessing blood brain barrier permeability with respect to novel neuro-specific technologies. Zebrafish provide an excellent model to test nanoparticle biocompatibility and toxicity (Chakraborty et al., 2016; White et al., 2019), and recent studies show success in treatment with nanoparticles to provide neuroprotection from Aβ induced toxicity as seen in AD (Saleem et al., 2022).
In summary, current data encourage the use of zebrafish as a model for neurodegenerative diseases. While we have described some of the limitations, the zebrafish presents a rapid, cost effective, and highly practical platform compared to classical mammalian models. Recent analyses employing cutting edge technology such as optogenetics have provided new insights into processes such as aggregate formation and the downstream consequences on a molecular level. Moreover, new tools such as very early cell death reporters make zebrafish an asset in neurodegeneration research. The plethora of cellular fluorescent reporters and cutting-edge imaging techniques, combined with the accessibility for drug testing, underscores the potential of zebrafish for the development of therapies for human neurodegenerative diseases.
KC, AK, DS, and JP contributed to the conceptualization and writing – review and editing. DS and JP contributed to the resources, supervision, and project administration. KC and AK contributed to the writing – original draft and visualization. JP contributed to the funding acquisition. All authors contributed to the article and approved the submitted version.
This work was supported in part by funding from the MRC/UK DRI (Momentum and Programme Awards to JP).
We thank Dr. Rafael Almeida and Dr. Jenea Bin for providing confocal images of single cells in zebrafish larvae (Figure 1).
The authors declare that the research was conducted in the absence of any commercial or financial relationships that could be construed as a potential conflict of interest.
All claims expressed in this article are solely those of the authors and do not necessarily represent those of their affiliated organizations, or those of the publisher, the editors and the reviewers. Any product that may be evaluated in this article, or claim that may be made by its manufacturer, is not guaranteed or endorsed by the publisher.
Ahrens, M. B., Orger, M. B., Robson, D. N., Li, J. M., and Keller, P. J. (2013). Whole-brain functional imaging at cellular resolution using light-sheet microscopy. Nat. Methods 10, 413–420. doi: 10.1038/nmeth.2434
Akerboom, J., Chen, T.-W., Wardill, T. J., Tian, L., Marvin, J. S., Mutlu, S., et al. (2012). Optimization of a GCaMP Calcium Indicator for Neural Activity Imaging. J. Neurosci. 32, 13819–13840. doi: 10.1523/JNEUROSCI.2601-12.2012
Al-Chalabi, A., van den Berg, L. H., and Veldink, J. (2017). Gene discovery in amyotrophic lateral sclerosis: implications for clinical management. Nat. Rev. Neurol. 13, 96–104. doi: 10.1038/nrneurol.2016.182
Aleström, P., D’Angelo, L., Midtlyng, P. J., Schorderet, D. F., Schulte-Merker, S., Sohm, F., et al. (2019). Zebrafish: housing and husbandry recommendations. Lab. Anim. 54, 213–224. doi: 10.1177/0023677219869037
Alunni, A., Coolen, M., Foucher, I., and Bally-Cuif, L. (2013). Patterning and cell type specification in the developing CNS and PNS. II Generation neuronal diversity. Amsterdam: Elsevier Science, 645–677. doi: 10.1016/b978-0-12-397265-1.00069-1
Amsterdam, A., Burgess, S., Golling, G., Chen, W., Sun, Z., Townsend, K., et al. (1999). A large-scale insertional mutagenesis screen in zebrafish. Gene Dev. 13, 2713–2724. doi: 10.1101/gad.13.20.2713
Andoniadou, C. L., and Martinez-Barbera, J. P. (2013). Developmental mechanisms directing early anterior forebrain specification in vertebrates. Cell. Mol. Life Sci. 70, 3739–3752. doi: 10.1007/s00018-013-1269-5
Anichtchik, O., Diekmann, H., Fleming, A., Roach, A., Goldsmith, P., and Rubinsztein, D. C. (2008). Loss of PINK1 Function Affects Development and Results in Neurodegeneration in Zebrafish. J. Neurosci. 28, 8199–8207. doi: 10.1523/JNEUROSCI.0979-08.2008
Antinucci, P., and Hindges, R. (2016). A crystal-clear zebrafish for in vivo imaging. Sci. Rep. 6:29490. doi: 10.1038/srep29490
Armstrong, G. A. B., and Drapeau, P. (2013). Loss and gain of FUS function impair neuromuscular synaptic transmission in a genetic model of ALS. Hum. Mol. Genet. 22, 4282–4292. doi: 10.1093/hmg/ddt278
Armstrong, G. A. B., Liao, M., You, Z., Lissouba, A., Chen, B. E., and Drapeau, P. (2016). Homology directed knockin of point mutations in the zebrafish tardbp and fus genes in ALS using the CRISPR/Cas9 system. PLoS One 11:e0150188. doi: 10.1371/journal.pone.0150188
Asakawa, K., Handa, H., and Kawakami, K. (2020). Optogenetic modulation of TDP-43 oligomerization accelerates ALS-related pathologies in the spinal motor neurons. Nat. Commun. 11:1004. doi: 10.1038/s41467-020-14815-x
Asakawa, K., Handa, H., and Kawakami, K. (2021). Illuminating ALS Motor Neurons With Optogenetics in Zebrafish. Front. Cell. Dev. Biol. 9:640414. doi: 10.3389/fcell.2021.640414
Babu, N. S., Murthy, C. L. N., Kakara, S., Sharma, R., Swamy, C. V. B., and Idris, M. M. (2016). 1-Methyl-4-phenyl-1,2,3,6-tetrahydropyridine induced Parkinson’s disease in zebrafish. Proteomics 16, 1407–1420. doi: 10.1002/pmic.201500291
Bai, Q., Garver, J. A., Hukriede, N. A., and Burton, E. A. (2007). Generation of a transgenic zebrafish model of Tauopathy using a novel promoter element derived from the zebrafish eno2 gene. Nucleic Acids Res. 35, 6501–6516. doi: 10.1093/nar/gkm608
Bai, Q., Mullett, S. J., Garver, J. A., Hinkle, D. A., and Burton, E. A. (2006). Zebrafish DJ-1 is evolutionarily conserved and expressed in dopaminergic neurons. Brain Res. 1113, 33–44. doi: 10.1016/j.brainres.2006.07.057
Balendra, R., and Isaacs, A. M. (2018). C9orf72-mediated ALS and FTD: multiple pathways to disease. Nat. Rev. Neurol. 14, 544–558. doi: 10.1038/s41582-018-0047-2
Bates, G. P., Dorsey, R., Gusella, J. F., Hayden, M. R., Kay, C., Leavitt, B. R., et al. (2015). Huntington disease. Nat. Rev. Dis. Primers 1:15005. doi: 10.1038/nrdp.2015.5
Benedetti, L., Ghilardi, A., Rottoli, E., Maglie, M. D., Prosperi, L., Perego, C., et al. (2016). INaP selective inhibition reverts precocious inter- and motorneurons hyperexcitability in the Sod1-G93R zebrafish ALS model. Sci. Rep. 6:24515. doi: 10.1038/srep24515
Benvenutti, R., Marcon, M., Reis, C. G., Nery, L. R., Miguel, C., Herrmann, A. P., et al. (2018). N-acetylcysteine protects against motor, optomotor and morphological deficits induced by 6-OHDA in zebrafish larvae. Peerj 6:e4957. doi: 10.7717/peerj.4957
Bergmann, K., Santoscoy, P. M., Lygdas, K., Nikolaeva, Y., MacDonald, R. B., Cunliffe, V. T., et al. (2018). Imaging Neuronal Activity in the Optic Tectum of Late Stage Larval Zebrafish. J. Dev. Biol. 6:6. doi: 10.3390/jdb6010006
Berning, B. A., and Walker, A. K. (2019). The Pathobiology of TDP-43 C-Terminal Fragments in ALS and FTLD. Front. Neurosci. 13:335. doi: 10.3389/fnins.2019.00335
Bhattarai, P., Cosacak, M. I., Mashkaryan, V., Demir, S., Popova, S. D., Govindarajan, N., et al. (2020). Neuron-glia interaction through Serotonin-BDNF-NGFR axis enables regenerative neurogenesis in Alzheimer’s model of adult zebrafish brain. PLoS Biol. 18:e3000585. doi: 10.1371/journal.pbio.3000585
Bhattarai, P., Thomas, A. K., Cosacak, M. I., Papadimitriou, C., Mashkaryan, V., Froc, C., et al. (2016). IL4/STAT6 Signaling Activates Neural Stem Cell Proliferation and Neurogenesis upon Amyloid-β42 Aggregation in Adult Zebrafish Brain. Cell Rep. 17, 941–948. doi: 10.1016/j.celrep.2016.09.075
Bhattarai, P., Thomas, A. K., Zhang, Y., and Kizil, C. (2017). The effects of aging on Amyloid-β42-induced neurodegeneration and regeneration in adult zebrafish brain. Neurogenesis 4:e1322666. doi: 10.1080/23262133.2017.1322666
Bianco, I. H., and Engert, F. (2015). Visuomotor Transformations Underlying Hunting Behavior in Zebrafish. Curr. Biol. 25, 831–846. doi: 10.1016/j.cub.2015.01.042
Blader, P., and Strähle, U. (2000). Zebrafish developmental genetics and central nervous system development. Hum. Mol. Genet. 9, 945–951. doi: 10.1093/hmg/9.6.945
Bondi, M. W., Edmonds, E. C., and Salmon, D. P. (2017). Alzheimer’s Disease: past, Present, and Future. J. Int. Neuropsych. Soc. 23, 818–831. doi: 10.1017/S135561771700100X
Bonifati, V., Rizzu, P., van Baren, M. J., Schaap, O., Breedveld, G. J., Krieger, E., et al. (2003). Mutations in the DJ-1 Gene Associated with Autosomal Recessive Early-Onset Parkinsonism. Science 299, 256–259. doi: 10.1126/science.1077209
Bortolotto, J. W., Cognato, G. P., Christoff, R. R., Roesler, L. N., Leite, C. E., Kist, L. W., et al. (2014). Long-term exposure to paraquat alters behavioral parameters and dopamine levels in adult zebrafish (Danio Rerio). Zebrafish 11, 142–153. doi: 10.1089/zeb.2013.0923
Bose, P., Armstrong, G. A. B., and Drapeau, P. (2019). Neuromuscular junction abnormalities in a zebrafish loss-of-function model of TDP-43. J. Neurophysiol. 121, 285–297. doi: 10.1152/jn.00265.2018
Boyden, E. S. (2011). A history of optogenetics: the development of tools for controlling brain circuits with light. F1000 Biol. Rep. 3:11. doi: 10.3410/B3-11
Bretaud, S., Allen, C., Ingham, P. W., and Bandmann, O. (2007). p53-dependent neuronal cell death in a DJ-1-deficient zebrafish model of Parkinson’s disease. J. Neurochem. 100, 1626–1635. doi: 10.1111/j.1471-4159.2006.04291.x
Bretaud, S., Lee, S., and Guo, S. (2004). Sensitivity of zebrafish to environmental toxins implicated in Parkinson’s disease. Neurotoxicol. Teratol. 26, 857–864. doi: 10.1016/j.ntt.2004.06.014
Brown, R. H., and Al-Chalabi, A. (2017). Amyotrophic Lateral Sclerosis. N. Engl. J. Med. 377, 162–172. doi: 10.1056/NEJMra1603471
Burberry, A., Suzuki, N., Wang, J.-Y., Moccia, R., Mordes, D. A., Stewart, M. H., et al. (2016). Loss-of-function mutations in the C9ORF72 mouse ortholog cause fatal autoimmune disease. Sci. Transl. Med. 8:347ra93. doi: 10.1126/scitranslmed.aaf6038
Burré, J. (2015). The Synaptic Function of α-Synuclein. J. Park. Dis. 5, 699–713. doi: 10.3233/JPD-150642
Butti, Z., Pan, Y. E., Giacomotto, J., and Patten, K. S. (2020). Reduced C9orf72 function leads to defective synaptic vesicle release and neuromuscular dysfunction in zebrafish. Commun. Biol. 4:792. doi: 10.21203/rs.3.rs-49118/v1
Byrnes, J., Ganetzky, R., Lightfoot, R., Tzeng, M., Nakamaru-Ogiso, E., Seiler, C., et al. (2018). Pharmacologic modeling of primary mitochondrial respiratory chain dysfunction in zebrafish. Neurochem. Int. 117, 23–34. doi: 10.1016/j.neuint.2017.07.008
Cameron, D. J., Galvin, C., Alkam, T., Sidhu, H., Ellison, J., Luna, S., et al. (2012). Alzheimer’s-Related Peptide Amyloid-β Plays a Conserved Role in Angiogenesis. PLoS One 7:e39598. doi: 10.1371/journal.pone.0039598
Campbell, W. A., Yang, H., Zetterberg, H., Baulac, S., Sears, J. A., Liu, T., et al. (2006). Zebrafish lacking Alzheimer presenilin enhancer 2 (Pen-2) demonstrate excessive p53-dependent apoptosis and neuronal loss. J. Neurochem. 96, 1423–1440. doi: 10.1111/j.1471-4159.2006.03648.x
Cass, S. P. (2017). Alzheimer’s Disease and Exercise. Curr. Sport Med. Rep. 16, 19–22. doi: 10.1249/JSR.0000000000000332
Cattaneo, E., Zuccato, C., and Tartari, M. (2005). Normal huntingtin function: an alternative approach to Huntington’s disease. Nat. Rev. Neurosci. 6, 919–930. doi: 10.1038/nrn1806
Chakraborty, C., Sharma, A. R., Sharma, G., and Lee, S.-S. (2016). Zebrafish: a complete animal model to enumerate the nanoparticle toxicity. J. Nanobiotechnol. 14:65. doi: 10.1186/s12951-016-0217-6
Ciura, S., Lattante, S., Ber, I. L., Latouche, M., Tostivint, H., Brice, A., et al. (2013). Loss of function of C9orf72 causes motor deficits in a zebrafish model of amyotrophic lateral sclerosis. Ann. Neurol. 74, 180–187. doi: 10.1002/ana.23946
Cohen-Cory, S., Kidane, A. H., Shirkey, N. J., and Marshak, S. (2010). Brain-derived neurotrophic factor and the development of structural neuronal connectivity. Dev. Neurobiol. 70, 271–288. doi: 10.1002/dneu.20774
Conforti, P., Zuccato, C., Gaudenzi, G., Ieraci, A., Camnasio, S., Buckley, N. J., et al. (2013). Binding of the repressor complex REST-mSIN3b by small molecules restores neuronal gene transcription in Huntington’s disease models. J. Neurochem. 127, 22–35. doi: 10.1111/jnc.12348
Cong, L., Wang, Z., Chai, Y., Hang, W., Shang, C., Yang, W., et al. (2017). Rapid whole brain imaging of neural activity in freely behaving larval zebrafish (Danio rerio). eLife 6:e28158. doi: 10.7554/eLife.28158.035
Cosacak, M. I., Bhattarai, P., Bocova, L., Dzewas, T., Mashkaryan, V., Papadimitriou, C., et al. (2017). Human TAUP301L overexpression results in TAU hyperphosphorylation without neurofibrillary tangles in adult zebrafish brain. Sci. Rep. 7:12959. doi: 10.1038/s41598-017-13311-5
Cronin, A., and Grealy, M. (2017). Neuroprotective and Neuro-restorative Effects of Minocycline and Rasagiline in a Zebrafish 6-Hydroxydopamine Model of Parkinson’s Disease. Neuroscience 367, 34–46. doi: 10.1016/j.neuroscience.2017.10.018
Da Costa, M. M., Allen, C. E., Higginbottom, A., Ramesh, T., Shaw, P. J., and McDermott, C. J. (2014). A new zebrafish model produced by TILLING of SOD1-related amyotrophic lateral sclerosis replicates key features of the disease and represents a tool for in vivo therapeutic screening. Dis. Model Mech. 7, 73–81. doi: 10.1242/dmm.012013
DeTure, M. A., and Dickson, D. W. (2019). The neuropathological diagnosis of Alzheimer’s disease. Mol. Neurodegener. 14:32. doi: 10.1186/s13024-019-0333-5
Diekmann, H., Anichtchik, O., Fleming, A., Futter, M., Goldsmith, P., Roach, A., et al. (2009). Decreased BDNF Levels Are a Major Contributor to the Embryonic Phenotype of Huntingtin Knockdown Zebrafish. J. Neurosci. 29, 1343–1349. doi: 10.1523/JNEUROSCI.6039-08.2009
dos Santos, L. R., Pimassoni, L. H. S., Sena, G. G. S., Camporez, D., Belcavello, L., Trancozo, M., et al. (2017). Validating GWAS Variants from Microglial Genes Implicated in Alzheimer’s Disease. J. Mol. Neurosci. 62, 215–221. doi: 10.1007/s12031-017-0928-7
Draper, B. W., McCallum, C. M., Stout, J. L., Slade, A. J., and Moens, C. B. (2004). A High-Throughput Method for Identifying N-Ethyl-N-Nitrosourea (ENU)-Induced Point Mutations in Zebrafish. Methods Cell. Biol. 77, 91–112. doi: 10.1016/S0091-679X(04)77005-3
Dreosti, E., and Lagnado, L. (2011). Optical reporters of synaptic activity in neural circuits. Exp. Physiol. 96, 4–12. doi: 10.1113/expphysiol.2009.051953
Drolet, R. E., Sanders, J. M., and Kern, J. T. (2011). Leucine-rich repeat kinase 2 (LRRK2) cellular biology: a review of recent advances in identifying physiological substrates and cellular functions. J. Neurogenet. 25, 140–151. doi: 10.3109/01677063.2011.627072
Early, J. J., Marshall-Phelps, K. L., Williamson, J. M., Swire, M., Kamadurai, H., Muskavitch, M., et al. (2018). An automated high-resolution in vivo screen in zebrafish to identify chemical regulators of myelination. eLife 7:e35136. doi: 10.7554/eLife.35136.032
Edison, P., Archer, H. A., Hinz, R., Hammers, A., Pavese, N., Tai, Y. F., et al. (2007). Amyloid, hypometabolism, and cognition in Alzheimer disease. Neurology 68, 501–508. doi: 10.1212/01.wnl.0000244749.20056.d4
Ehrlich, M. E. (2012). Huntington’s disease and the striatal medium spiny neuron: cell-autonomous and non-cell-autonomous mechanisms of disease. Neurotherapeutics 9, 270–284. doi: 10.1007/s13311-012-0112-2
Eidelberg, D., and Surmeier, D. J. (2011). Brain networks in Huntington disease. J. Clin. Invest. 121, 484–492. doi: 10.1172/JCI45646
El-Brolosy, M. A., Kontarakis, Z., Rossi, A., Kuenne, C., Günther, S., Fukuda, N., et al. (2019). Genetic compensation triggered by mutant mRNA degradation. Nature 568, 193–197. doi: 10.1038/s41586-019-1064-z
Estrada-Sánchez, A. M., and Rebec, G. V. (2013). Role of cerebral cortex in the neuropathology of Huntington’s disease. Front. Neural Circuit 7:19. doi: 10.3389/fncir.2013.00019
Fagan, A. M., Mintun, M. A., Shah, A. R., Aldea, P., Roe, C. M., Mach, R. H., et al. (2009). Cerebrospinal fluid tau and ptau181 increase with cortical amyloid deposition in cognitively normal individuals: implications for future clinical trials of Alzheimer’s disease. EMBO Mol. Med. 1, 371–380. doi: 10.1002/emmm.200900048
Feng, C.-W., Wen, Z.-H., Huang, S.-Y., Hung, H.-C., Chen, C.-H., Yang, S.-N., et al. (2014). Effects of 6-Hydroxydopamine Exposure on Motor Activity and Biochemical Expression in Zebrafish (Danio Rerio) Larvae. Zebrafish 11, 227–239. doi: 10.1089/zeb.2013.0950
Fett, M. E., Pilsl, A., Paquet, D., van Bebber, F., Haass, C., Tatzelt, J., et al. (2010). Parkin Is Protective against Proteotoxic Stress in a Transgenic Zebrafish Model. PLoS One 5:e11783. doi: 10.1371/journal.pone.0011783
Flinn, L. J., Keatinge, M., Bretaud, S., Mortiboys, H., Matsui, H., Felice, E. D., et al. (2014). TigarB causes mitochondrial dysfunction and neuronal loss in PINK1 deficiency. Ann. Neurol. 74, 837–847. doi: 10.1002/ana.23999
Flinn, L., Mortiboys, H., Volkmann, K., Köster, R. W., Ingham, P. W., and Bandmann, O. (2009). Complex I deficiency and dopaminergic neuronal cell loss in parkin-deficient zebrafish (Danio rerio). Brain 132, 1613–1623. doi: 10.1093/brain/awp108
Formella, I., Svahn, A. J., Radford, R. A. W., Don, E. K., Cole, N. J., Hogan, A., et al. (2018). Real-time visualization of oxidative stress-mediated neurodegeneration of individual spinal motor neurons in vivo. Redox Biol. 19, 226–234. doi: 10.1016/j.redox.2018.08.011
Frisoni, G. B., Fox, N. C., Jack, C. R., Scheltens, P., and Thompson, P. M. (2010). The clinical use of structural MRI in Alzheimer disease. Nat. Rev. Neurol. 6, 67–77. doi: 10.1038/nrneurol.2009.215
Fulwiler, C., and Gilbert, W. (1991). Zebrafish embryology and neural development. Curr. Opin. Cell Biol. 3, 988–991. doi: 10.1016/0955-0674(91)90118-I
Garret, M., Du, Z., Chazalon, M., Cho, Y. H., and Baufreton, J. (2018). Alteration of GABAergic neurotransmission in Huntington’s disease. CNS Neurosci. Ther. 24, 292–300. doi: 10.1111/cns.12826
Glenner, G. G., and Wong, C. W. (1984). Alzheimer’s disease and Down’s syndrome: sharing of a unique cerebrovascular amyloid fibril protein. Biochem. Biophys. Res. Commun. 122, 1131–1135. doi: 10.1016/0006-291X(84)91209-9
Godinho, L. (2011). Live Imaging of Zebrafish Development. Cold Spring Harb. Protoc. 2011, 770–777. doi: 10.1101/pdb.prot5647
Gonçalves, F. B., and Morais, V. A. (2021). PINK1: a Bridge between Mitochondria and Parkinson’s Disease. Life 11:371. doi: 10.3390/life11050371
Gopalakrishnan, V. (2009). REST and the RESTless: in stem cells and beyond. Futur Neurol. 4, 317–329. doi: 10.2217/fnl.09.1
Grad, L. I., Rouleau, G. A., Ravits, J., and Cashman, N. R. (2017). Clinical Spectrum of Amyotrophic Lateral Sclerosis (ALS). CSH Perspect. Med. 7:a024117. doi: 10.1101/cshperspect.a024117
Groen, E. J. N., Fumoto, K., Blokhuis, A. M., Engelen-Lee, J., Zhou, Y., van den Heuvel, D. M. A., et al. (2013). ALS-associated mutations in FUS disrupt the axonal distribution and function of SMN. Hum. Mol. Genet. 22, 3690–3704. doi: 10.1093/hmg/ddt222
Groth, C., Nornes, S., McCarty, R., Tamme, R., and Lardelli, M. (2002). Identification of a second presenilin gene in zebrafish with similarity to the human Alzheimer’s disease gene presenilin2. Dev. Genes Evol. 212, 486–490. doi: 10.1007/s00427-002-0269-5
Gu, X., Cantle, J. P., Greiner, E. R., Lee, C. Y. D., Barth, A. M., Gao, F., et al. (2015). N17 Modifies Mutant Huntingtin Nuclear Pathogenesis and Severity of Disease in HD BAC Transgenic Mice. Neuron 85, 726–741. doi: 10.1016/j.neuron.2015.01.008
Guo, Y., Veneman, W. J., Spaink, H. P., and Verbeek, F. J. (2017). Three-dimensional reconstruction and measurements of zebrafish larvae from high-throughput axial-view in vivo imaging. Biomed. Opt. Express 8:2611. doi: 10.1364/BOE.8.002611
Haapasalo, A., and Kovacs, D. M. (2011). The Many Substrates of Presenilin/γ-Secretase. J. Alzheimers Dis. 25, 3–28. doi: 10.3233/JAD-2011-101065
Haffter, P., Granato, M., Brand, M., Mullins, M. C., Hammerschmidt, M., Kane, D. A., et al. (1996). The identification of genes with unique and essential functions in the development of the zebrafish, Danio rerio. Development 123, 1–36. doi: 10.1242/dev.123.1.1
Hampel, H., O’Bryant, S. E., Molinuevo, J. L., Zetterberg, H., Masters, C. L., Lista, S., et al. (2018). Blood-based biomarkers for Alzheimer disease: mapping the road to the clinic. Nat. Rev. Neurol. 14, 639–652. doi: 10.1038/s41582-018-0079-7
Hardiman, O., Al-Chalabi, A., Chio, A., Corr, E. M., Logroscino, G., Robberecht, W., et al. (2017). Amyotrophic lateral sclerosis. Nat. Rev. Dis. Primers 3:17071. doi: 10.1038/nrdp.2017.71
Hardy, J., and Allsop, D. (1991). Amyloid deposition as the central event in the aetiology of Alzheimer’s disease. Trends Pharmacol. Sci. 12, 383–388. doi: 10.1016/0165-6147(91)90609-V
Henshall, T. L., Tucker, B., Lumsden, A. L., Nornes, S., Lardelli, M. T., and Richards, R. I. (2009). Selective neuronal requirement for huntingtin in the developing zebrafish. Hum. Mol. Genet. 18, 4830–4842. doi: 10.1093/hmg/ddp455
Hensley, K., Christov, A., Kamat, S., Zhang, X. C., Jackson, K. W., Snow, S., et al. (2010a). Proteomic Identification of Binding Partners for the Brain Metabolite Lanthionine Ketimine (LK) and Documentation of LK Effects on Microglia and Motoneuron Cell Cultures. J. Neurosci. 30, 2979–2988. doi: 10.1523/JNEUROSCI.5247-09.2010
Hensley, K., Venkova, K., and Christov, A. (2010b). Emerging Biological Importance of Central Nervous System Lanthionines. Molecules 15, 5581–5594. doi: 10.3390/molecules15085581
Herreman, A., Hartmann, D., Annaert, W., Saftig, P., Craessaerts, K., Serneels, L., et al. (1999). Presenilin 2 deficiency causes a mild pulmonary phenotype and no changes in amyloid precursor protein processing but enhances the embryonic lethal phenotype of presenilin 1 deficiency. Proc. Natl. Acad. Sci. U.S.A. 96, 11872–11877. doi: 10.1073/pnas.96.21.11872
Hewamadduma, C. A. A., Grierson, A. J., Ma, T. P., Pan, L., Moens, C. B., Ingham, P. W., et al. (2013). Tardbpl splicing rescues motor neuron and axonal development in a mutant tardbp zebrafish. Hum. Mol. Genet. 22, 2376–2386. doi: 10.1093/hmg/ddt082
Hicks, G. G., Singh, N., Nashabi, A., Mai, S., Bozek, G., Klewes, L., et al. (2000). Fus deficiency in mice results in defective B-lymphocyte development and activation, high levels of chromosomal instability and perinatal death. Nat. Genet. 24, 175–179. doi: 10.1038/72842
Hooper, C., Killick, R., and Lovestone, S. (2008). The GSK3 hypothesis of Alzheimer’s disease. J. Neurochem. 104, 1433–1439. doi: 10.1111/j.1471-4159.2007.05194.x
Howe, K., Clark, M. D., Torroja, C. F., Torrance, J., Berthelot, C., Muffato, M., et al. (2013). The zebrafish reference genome sequence and its relationship to the human genome. Nature 496, 498–503. doi: 10.1038/nature12111
Hsu, Y.-T., Chang, Y.-G., and Chern, Y. (2018). Insights into GABAAergic system alteration in Huntington’s disease. Open Biol. 8:180165. doi: 10.1098/rsob.180165
Iyer, S., Subramanian, V., and Acharya, K. R. (2018). C9orf72, a protein associated with amyotrophic lateral sclerosis (ALS) is a guanine nucleotide exchange factor. Peerj 6:e5815. doi: 10.7717/peerj.5815
Jaworski, T., Banach-Kasper, E., and Gralec, K. (2019). GSK-3β at the Intersection of Neuronal Plasticity and Neurodegeneration. Neural Plast. 2019, 1–14. doi: 10.1155/2019/4209475
Kabashi, E., Bercier, V., Lissouba, A., Liao, M., Brustein, E., Rouleau, G. A., et al. (2011). FUS and TARDBP but Not SOD1 Interact in Genetic Models of Amyotrophic Lateral Sclerosis. PLoS Genet. 7:e1002214. doi: 10.1371/journal.pgen.1002214
Kabashi, E., Lin, L., Tradewell, M. L., Dion, P. A., Bercier, V., Bourgouin, P., et al. (2010). Gain and loss of function of ALS-related mutations of TARDBP (TDP-43) cause motor deficits in vivo. Hum. Mol. Genet. 19, 671–683. doi: 10.1093/hmg/ddp534
Kabir, T., Uddin, S., Setu, J. R., Ashraf, G. M., Bin-Jumah, M. N., and Abdel-Daim, M. M. (2020). Exploring the Role of PSEN Mutations in the Pathogenesis of Alzheimer’s Disease. Neurotox. Res. 38, 833–849. doi: 10.1007/s12640-020-00232-x
Kamat, P. K., and Nath, C. (2015). Okadaic acid: a tool to study regulatory mechanisms for neurodegeneration and regeneration in Alzheimer’s disease. Neural Regen. Res. 10, 365–367. doi: 10.4103/1673-5374.153679
Kamat, P. K., Rai, S., and Nath, C. (2013). Okadaic acid induced neurotoxicity: an emerging tool to study Alzheimer’s disease pathology. Neurotoxicology 37, 163–172. doi: 10.1016/j.neuro.2013.05.002
Kamat, P. K., Rai, S., Swarnkar, S., Shukla, R., and Nath, C. (2014). Molecular and cellular Mechanism of Okadaic Acid (OKA)-induced neurotoxicity: a novel tool for alzheimer’s disease therapeutic application. Mol. Neurobiol. 50, 852–865. doi: 10.1007/s12035-014-8699-4
Kamat, P. K., Tota, S., Rai, S., Shukla, R., Ali, S., Najmi, A. K., et al. (2012a). Okadaic acid induced neurotoxicity leads to central cholinergic dysfunction in rats. Eur. J. Pharmacol. 690, 90–98. doi: 10.1016/j.ejphar.2012.06.006
Kamat, P. K., Tota, S., Rai, S., Swarnkar, S., Shukla, R., and Nath, C. (2012b). A study on neuroinflammatory marker in brain areas of okadaic acid (ICV) induced memory impaired rats. Life Sci. 90, 713–720. doi: 10.1016/j.lfs.2012.03.012
Kamienieva, I., Duszyński, J., and Szczepanowska, J. (2021). Multitasking guardian of mitochondrial quality: parkin function and Parkinson’s disease. Transl. Neurodegener. 10:5. doi: 10.1186/s40035-020-00229-8
Karlovich, C. A., John, R. M., Ramirez, L., Stainier, D. Y. R., and Myers, R. M. (1998). Characterization of the Huntington’s disease (HD) gene homolog in the zebrafish Danio rerio. Gene 217, 117–125. doi: 10.1016/S0378-1119(98)00342-4
Kaslin, J., and Panula, P. (2001). Comparative anatomy of the histaminergic and other aminergic systems in zebrafish (Danio rerio). J. Comp. Neurol. 440, 342–377. doi: 10.1002/cne.1390
Kawakami, K., Asakawa, K., Hibi, M., Itoh, M., Muto, A., and Wada, H. (2016). Chapter Three Gal4 Driver Transgenic Zebrafish Powerful Tools to Study Developmental Biology, Organogenesis, and Neuroscience. Adv. Genet. 95, 65–87. doi: 10.1016/bs.adgen.2016.04.002
Keatinge, M., Tsarouchas, T. M., Munir, T., Porter, N. J., Larraz, J., Gianni, D., et al. (2021). CRISPR gRNA phenotypic screening in zebrafish reveals pro-regenerative genes in spinal cord injury. PLoS Genet 17:e1009515. doi: 10.1371/journal.pgen.1009515
Kettunen, P. (2012). Calcium Signaling. Adv. Exp. Med. Biol. 740, 1039–1071. doi: 10.1007/978-94-007-2888-2_48
Khotimah, H., Sumitro, M. A., and Widodo, M. A. (2015). Zebrafish Parkinson’s Model: rotenone decrease motility, Dopamine, and increase α-synuclein Aggregation and Apoptosis of Zebrafish Brain. Int. J. PharmTech Res. 8, 614–621.
Kiernan, M. C., Vucic, S., Cheah, B. C., Turner, M. R., Eisen, A., Hardiman, O., et al. (2011). Amyotrophic lateral sclerosis. Lancet 377, 942–955. doi: 10.1016/S0140-6736(10)61156-7
Kim, Y. E., Hosp, F., Frottin, F., Ge, H., Mann, M., Hayer-Hartl, M., et al. (2016). Soluble Oligomers of PolyQ-Expanded Huntingtin Target a Multiplicity of Key Cellular Factors. Mol. Cell 63, 951–964. doi: 10.1016/j.molcel.2016.07.022
Kimmel, C. B., Ballard, W. W., Kimmel, S. R., Ullmann, B., and Schilling, T. F. (1995). Stages of embryonic development of the zebrafish. Dev. Dyn. 203, 253–310. doi: 10.1002/aja.1002030302
Kimura, Y., Hisano, Y., Kawahara, A., and Higashijima, S. (2014). Efficient generation of knock-in transgenic zebrafish carrying reporter/driver genes by CRISPR/Cas9-mediated genome engineering. Sci. Rep. 4:6545. doi: 10.1038/srep06545
Kino, Y., Washizu, C., Kurosawa, M., Yamada, M., Miyazaki, H., Akagi, T., et al. (2015). FUS/TLS deficiency causes behavioral and pathological abnormalities distinct from amyotrophic lateral sclerosis. Acta Neuropathol. Commun. 3:24. doi: 10.1186/s40478-015-0202-6
Kitada, T., Pisani, A., Porter, D. R., Yamaguchi, H., Tscherter, A., Martella, G., et al. (2007). Impaired dopamine release and synaptic plasticity in the striatum of PINK1-deficient mice. Proc. Natl. Acad. Sci. U.S.A. 104, 11441–11446. doi: 10.1073/pnas.0702717104
Koehler, D., Shah, Z. A., and Williams, F. E. (2019). The GSK3β inhibitor, TDZD-8, rescues cognition in a zebrafish model of okadaic acid-induced Alzheimer’s disease. Neurochem. Int. 122, 31–37. doi: 10.1016/j.neuint.2018.10.022
Koehler, D., Shah, Z. A., Hensley, K., and Williams, F. E. (2018). Lanthionine ketimine-5-ethyl ester provides neuroprotection in a zebrafish model of okadaic acid-induced Alzheimer’s disease. Neurochem. Int. 115, 61–68. doi: 10.1016/j.neuint.2018.02.002
Kok, F. O., Shin, M., Ni, C.-W., Gupta, A., Grosse, A. S., van Impel, A., et al. (2015). Reverse Genetic Screening Reveals Poor Correlation between Morpholino-Induced and Mutant Phenotypes in Zebrafish. Dev. Cell 32, 97–108. doi: 10.1016/j.devcel.2014.11.018
Kowiański, P., Lietzau, G., Czuba, E., Waśkow, M., Steliga, A., and Moryś, J. (2018). BDNF: a key factor with multipotent impact on brain signaling and synaptic plasticity. Cell. Mol. Neurobiol. 38, 579–593. doi: 10.1007/s10571-017-0510-4
Kunst, M., Laurell, E., Mokayes, N., Kramer, A., Kubo, F., Fernandes, A. M., et al. (2019). A Cellular-Resolution Atlas of the Larval Zebrafish Brain. Neuron 103, 21–38.e5. doi: 10.1016/j.neuron.2019.04.034
Lagier-Tourenne, C., Baughn, M., Rigo, F., Sun, S., Liu, P., Li, H.-R., et al. (2013). Targeted degradation of sense and antisense C9orf72 RNA foci as therapy for ALS and frontotemporal degeneration. Proc. Natl. Acad. Sci. U.S.A. 110, E4530–E4539. doi: 10.1073/pnas.1318835110
Laird, A. S., Hoecke, A. V., Muynck, L. D., Timmers, M., Bosch, L. V. D., Damme, P. V., et al. (2010). Progranulin is Neurotrophic In Vivo and Protects against a Mutant TDP-43 Induced Axonopathy. PLoS One 5:e13368. doi: 10.1371/journal.pone.0013368
Lam, C. S., Korzh, V., and Strahle, U. (2005). Zebrafish embryos are susceptible to the dopaminergic neurotoxin MPTP. Eur. J. Neurosci. 21, 1758–1762. doi: 10.1111/j.1460-9568.2005.03988.x
Lambert, J.-C., Ibrahim-Verbaas, C. A., Harold, D., Naj, A. C., Sims, R., Bellenguez, C., et al. (2013). Meta-analysis of 74,046 individuals identifies 11 new susceptibility loci for Alzheimer’s disease. Nat. Genet. 45, 1452–1458. doi: 10.1038/ng.2802
Langbehn, D. R., Hayden, M. R., Paulsen, J. S., and the PREDICT-HD Investigators of the Huntington Study Group (2010). CAG-repeat length and the age of onset in Huntington disease (HD): a review and validation study of statistical approaches. Am. J. Med. Genet. Part B Neuropsychiatr. Genet. 153, 397–408. doi: 10.1002/ajmg.b.30992
Laroche, M., Lessard-Beaudoin, M., Garcia-Miralles, M., Kreidy, C., Peachey, E., Leavitt, B. R., et al. (2020). Early deficits in olfaction are associated with structural and molecular alterations in the olfactory system of a huntington disease mouse model. Hum. Mol. Genet. 29, 2134–2147. doi: 10.1093/hmg/ddaa099
Lattante, S., Rouleau, G. A., and Kabashi, E. (2013). TARDBP and FUS Mutations associated with amyotrophic lateral sclerosis: summary and update. Hum. Mutat. 34, 812–826. doi: 10.1002/humu.22319
Lawrence, C., Adatto, I., Best, J., James, A., and Maloney, K. (2012). Generation time of zebrafish (Danio rerio) and medakas (Oryzias latipes) housed in the same aquaculture facility. Lab. Anim. 41, 158–165. doi: 10.1038/laban0612-158
Lebedeva, S., de Domingues, A. M. J., Butter, F., and Ketting, R. F. (2016). Characterization of genetic loss-of-function of Fus in zebrafish. RNA Biol. 14, 29–35. doi: 10.1080/15476286.2016.1256532
Lee, J.-M., Ramos, E. M., Lee, J.-H., Gillis, T., Mysore, J. S., Hayden, M. R., et al. (2012). CAG repeat expansion in Huntington disease determines age at onset in a fully dominant fashion. Neurology 78, 690–695. doi: 10.1212/WNL.0b013e318249f683
Lee, Y.-B., Chen, H.-J., Peres, J. N., Gomez-Deza, J., Attig, J., Štalekar, M., et al. (2013). Hexanucleotide Repeats in ALS/FTD Form Length-Dependent RNA Foci, Sequester RNA Binding Proteins, and Are Neurotoxic. Cell Rep. 5, 1178–1186. doi: 10.1016/j.celrep.2013.10.049
Leimer, U., Lun, K., Romig, H., Walter, J., Grünberg, J., Brand, M., et al. (1999). Zebrafish (Danio rerio) Presenilin Promotes Aberrant Amyloid β-Peptide Production and Requires a Critical Aspartate Residue for Its Function in Amyloidogenesis †. Biochemistry 38, 13602–13609. doi: 10.1021/bi991453n
Lele, Z., Folchert, A., Concha, M., Rauch, G.-J., Geisler, R., Rosa, F., et al. (2002). parachute / n-cadherin is required for morphogenesis and maintained integrity of the zebrafish neural tube. Development 129, 3281–3294. doi: 10.1242/dev.129.14.3281
Lemmens, R., Hoecke, A. V., Hersmus, N., Geelen, V., D’Hollander, I., Thijs, V., et al. (2007). Overexpression of mutant superoxide dismutase 1 causes a motor axonopathy in the zebrafish. Hum. Mol. Genet. 16, 2359–2365. doi: 10.1093/hmg/ddm193
Letamendia, A., Quevedo, C., Ibarbia, I., Virto, J. M., Holgado, O., Diez, M., et al. (2012). Development and Validation of an Automated High-Throughput System for Zebrafish In Vivo Screenings. PLoS One 7:e36690. doi: 10.1371/journal.pone.0036690
Li, H., Wetten, S., Li, L., St Jean, P. L., Upmanyu, R., Surh, L., et al. (2008). Candidate Single-Nucleotide Polymorphisms From a Genomewide Association Study of Alzheimer Disease. Arch Neurol. 65, 45–53. doi: 10.1001/archneurol.2007.3
Li, J.-Q., Tan, L., and Yu, J.-T. (2014). The role of the LRRK2 gene in Parkinsonism. Mol. Neurodegener. 9:47. doi: 10.1186/1750-1326-9-47
Linsley, J. W., Shah, K., Castello, N., Chan, M., Haddad, D., Doric, Z., et al. (2021). Genetically encoded cell-death indicators (GEDI) to detect an early irreversible commitment to neurodegeneration. Nat. Commun. 12:5284. doi: 10.1038/s41467-021-25549-9
Lissouba, A., Liao, M., Kabashi, E., and Drapeau, P. (2018). Transcriptomic analysis of zebrafish TDP-43 transgenic lines. Front. Mol. Neurosci. 11:463. doi: 10.3389/fnmol.2018.00463
Liu, J., Zhou, Y., Qi, X., Chen, J., Chen, W., Qiu, G., et al. (2017). CRISPR/Cas9 in zebrafish: an efficient combination for human genetic diseases modeling. Hum. Genet. 136, 1–12. doi: 10.1007/s00439-016-1739-6
Liu, J.-C., Koppula, S., Huh, S.-J., Park, P.-J., Kim, C.-G., Lee, C.-J., et al. (2015). Necrosis inhibitor-5 (NecroX-5), attenuates MPTP-induced motor deficits in a zebrafish model of Parkinson’s disease. Genes Genomics 37, 1073–1079. doi: 10.1007/s13258-015-0364-4
Llorens-Marítin, M., Jurado, J., Hernández, F., and Ávila, J. (2014). GSK-3β, a pivotal kinase in Alzheimer disease. Front. Mol. Neurosci. 7:46. doi: 10.3389/fnmol.2014.00046
Lo Sardo, V., Zuccato, C., Gaudenzi, G., Vitali, B., Ramos, C., Tartari, M., et al. (2012). An evolutionary recent neuroepithelial cell adhesion function of huntingtin implicates ADAM10-Ncadherin. Nat. Neurosci. 15, 713–721. doi: 10.1038/nn.3080
Loeffler, J., Picchiarelli, G., Dupuis, L., and Aguilar, J. G. D. (2016). The Role of Skeletal Muscle in Amyotrophic Lateral Sclerosis. Brain Pathol. 26, 227–236. doi: 10.1111/bpa.12350
Lopez, A., Lee, S. E., Wojta, K., Ramos, E. M., Klein, E., Chen, J., et al. (2017). A152T tau allele causes neurodegeneration that can be ameliorated in a zebrafish model by autophagy induction. Brain 140, 1128–1146. doi: 10.1093/brain/awx005
Lulla, A., Barnhill, L., Bitan, G., Ivanova, M. I., Nguyen, B., O’Donnell, K., et al. (2016). Neurotoxicity of the Parkinson Disease-Associated Pesticide Ziram Is Synuclein-Dependent in Zebrafish Embryos. Environ. Health Perspect. 124, 1766–1775. doi: 10.1289/EHP141
Lumsden, A. L., Henshall, T. L., Dayan, S., Lardelli, M. T., and Richards, R. I. (2007). Huntingtin-deficient zebrafish exhibit defects in iron utilization and development. Hum. Mol. Genet. 16, 1905–1920. doi: 10.1093/hmg/ddm138
Luna, S., Cameron, D. J., and Ethell, D. W. (2013). Amyloid-β and app deficiencies cause severe cerebrovascular defects: important work for an old villain. PLoS One 8:e75052. doi: 10.1371/journal.pone.0075052
Mahul-Mellier, A.-L., Burtscher, J., Maharjan, N., Weerens, L., Croisier, M., Kuttler, F., et al. (2020). The process of Lewy body formation, rather than simply α-synuclein fibrillization, is one of the major drivers of neurodegeneration. Proc. Natl. Acad. Sci. U.S.A. 117, 4971–4982. doi: 10.1073/pnas.1913904117
Marques, O., and Outeiro, T. F. (2012). Alpha-synuclein: from secretion to dysfunction and death. Cell Death Dis. 3:e350. doi: 10.1038/cddis.2012.94
Martel, S., Keow, J. Y., and Ekker, M. (2015). Rotenone Neurotoxicity Causes Dopamine Neuron Loss in Zebrafish. Univ. Ott. J. Med. 5, 16–21. doi: 10.18192/uojm.v5i2.1413
Mathias, J. R., Saxena, M. T., and Mumm, J. S. (2012). Advances in zebrafish chemical screening technologies. Future Med. Chem. 4, 1811–1822. doi: 10.4155/fmc.12.115
Matsubara, T., Oda, M., Takahashi, T., Watanabe, C., Tachiyama, Y., Morino, H., et al. (2019). Amyotrophic lateral sclerosis of long clinical course clinically presenting with progressive muscular atrophy. Neuropathology 39, 47–53. doi: 10.1111/neup.12523
McColgan, P., and Tabrizi, S. J. (2018). Huntington’s disease: a clinical review. Eur. J. Neurol. 25, 24–34. doi: 10.1111/ene.13413
McDonough, H., and Patterson, C. (2003). CHIP: a link between the chaperone and proteasome systems. Cell Stress Chaperon 8, 303–308. doi: 10.1379/1466-1268(2003)008<0303:CALBTC>2.0.CO;2
McKinley, E. T., Baranowski, T. C., Blavo, D. O., Cato, C., Doan, T. N., and Rubinstein, A. L. (2005). Neuroprotection of MPTP-induced toxicity in zebrafish dopaminergic neurons. Mol. Brain Res. 141, 128–137. doi: 10.1016/j.molbrainres.2005.08.014
McQuade, A., and Blurton-Jones, M. (2019). Microglia in alzheimer’s disease: exploring how genetics and phenotype influence risk. J. Mol. Biol. 431, 1805–1817. doi: 10.1016/j.jmb.2019.01.045
Meade, R. M., Fairlie, D. P., and Mason, J. M. (2019). Alpha-synuclein structure and Parkinson’s disease – lessons and emerging principles. Mol. Neurodegener. 14:29. doi: 10.1186/s13024-019-0329-1
Melo, K. M., Oliveira, R., Grisolia, C. K., Domingues, I., Pieczarka, J. C., Filho, J., et al. (2015). Short-term exposure to low doses of rotenone induces developmental, biochemical, behavioral, and histological changes in fish. Environ. Sci. Pollut. Res. 22, 13926–13938. doi: 10.1007/s11356-015-4596-2
Milanese, C., Sager, J. J., Bai, Q., Farrell, T. C., Cannon, J. R., Greenamyre, J. T., et al. (2012). Hypokinesia and Reduced Dopamine Levels in Zebrafish Lacking β- and γ1-Synucleins. J. Biol. Chem. 287, 2971–2983. doi: 10.1074/jbc.M111.308312
Miller, V. M., Nelson, R. F., Gouvion, C. M., Williams, A., Rodriguez-Lebron, E., Harper, S. Q., et al. (2005). CHIP Suppresses Polyglutamine Aggregation and Toxicity In Vitro and In Vivo. J. Neurosci. 25, 9152–9161. doi: 10.1523/JNEUROSCI.3001-05.2005
Mitchell, I. J., Heims, H., Neville, E. A., and Rickards, H. (2005). Huntingtons Disease Patients Show Impaired Perception of Disgust in the Gustatory and Olfactory Modalities. J. Neuropsychiatry Clin. Neurosci. 17, 119–121. doi: 10.1176/jnp.17.1.119
Morcos, P. A., Vincent, A. C., and Moulton, J. D. (2015). Gene Editing Versus Morphants. Zebrafish 12, 319–319. doi: 10.1089/zeb.2015.1114
Morrice, J. R., Gregory-Evans, C. Y., and Shaw, C. A. (2018). Modeling Environmentally-Induced Motor Neuron Degeneration in Zebrafish. Sci. Rep. 8:4890. doi: 10.1038/s41598-018-23018-w
Morrice, J. R., Gregory-Evans, C. Y., and Shaw, C. A. (2020). Investigating microglia during motor neuron degeneration using a zebrafish model. Micron 133:102852. doi: 10.1016/j.micron.2020.102852
Morrison, P. J., and Nevin, N. C. (1994). Serum iron, total iron binding capacity and ferritin in early huntington disease patients. Ir. J. Med. Sci. 163, 236–237. doi: 10.1007/BF02943258
Müller-Rischart, A. K., Pilsl, A., Beaudette, P., Patra, M., Hadian, K., Funke, M., et al. (2013). The E3 Ligase Parkin Maintains Mitochondrial Integrity by Increasing Linear Ubiquitination of NEMO. Mol. Cell 49, 908–921. doi: 10.1016/j.molcel.2013.01.036
Mullins, M. C., Hammerschmidt, M., Haffter, P., and Nüsslein-Volhard, C. (1994). Large-scale mutagenesis in the zebrafish: in search of genes controlling development in a vertebrate. Curr. Biol. 4, 189–202. doi: 10.1016/S0960-9822(00)00048-8
Nada, S. E., Williams, F. E., and Shah, Z. A. (2016). Development of a Novel and Robust Pharmacological Model of Okadaic Acid-induced Alzheimer’s Disease in Zebrafish. CNS Neurol. Disord. Drug Targets 15, 86–94. doi: 10.2174/1871527314666150821105602
Nasir, J., Floresco, S. B., O’Kusky, J. R., Diewert, V. M., Richman, J. M., Zeisler, J., et al. (1995). Targeted disruption of the Huntington’s disease gene results in embryonic lethality and behavioral and morphological changes in heterozygotes. Cell 81, 811–823. doi: 10.1016/0092-8674(95)90542-1
Nellore, J., and Nandita, P (2015). Paraquat exposure induces behavioral deficits in larval zebrafish during the window of dopamine neurogenesis. Toxicol. Rep. 2, 950–956. doi: 10.1016/j.toxrep.2015.06.007
Nellore, J., Pauline, C., and Amarnath, K. (2013). Bacopa monnieri Phytochemicals Mediated Synthesis of Platinum Nanoparticles and Its Neurorescue Effect on 1-Methyl 4-Phenyl 1,2,3,6 Tetrahydropyridine-Induced Experimental Parkinsonism in Zebrafish. J. Neurodegener. Dis. 2013:972391. doi: 10.1155/2013/972391
Nery, L. R., Eltz, N. S., Hackman, C., Fonseca, R., Altenhofen, S., Guerra, H. N., et al. (2014). Brain Intraventricular Injection of Amyloid-β in Zebrafish Embryo Impairs Cognition and Increases Tau Phosphorylation, Effects Reversed by Lithium. PLoS One 9:e105862. doi: 10.1371/journal.pone.0105862
Newman, M., Wilson, L., Camp, E., Verdile, G., Martins, R., and Lardelli, M. (2010). A zebrafish melanophore model of amyloid β toxicity. Zebrafish 7, 155–159. doi: 10.1089/zeb.2009.0628
Nornes, S., Groth, C., Camp, E., Ey, P., and Lardelli, M. (2003). Developmental control of Presenilin1 expression, endoproteolysis, and interaction in zebrafish embryos. Exp. Cell Res. 289, 124–132. doi: 10.1016/S0014-4827(03)00257-X
Nornes, S., Newman, M., Verdile, G., Wells, S., Stoick-Cooper, C. L., Tucker, B., et al. (2008). Interference with splicing of Presenilin transcripts has potent dominant negative effects on Presenilin activity. Hum. Mol. Genet. 17, 402–412. doi: 10.1093/hmg/ddm317
Nornes, S., Newman, M., Wells, S., Verdile, G., Martins, R. N., and Lardelli, M. (2009). Independent and cooperative action of Psen2 with Psen1 in zebrafish embryos. Exp. Cell Res. 315, 2791–2801. doi: 10.1016/j.yexcr.2009.06.023
Nunes, M. E., Müller, T. E., Braga, M. M., Fontana, B. D., Quadros, V. A., Marins, A., et al. (2017). Chronic Treatment with Paraquat Induces Brain Injury, Changes in Antioxidant Defenses System, and Modulates Behavioral Functions in Zebrafish. Mol. Neurobiol. 54, 3925–3934. doi: 10.1007/s12035-016-9919-x
O’Donnell, K. C., Lulla, A., Stahl, M. C., Wheat, N. D., Bronstein, J. M., and Sagasti, A. (2014). Axon degeneration and PGC-1α-mediated protection in a zebrafish model of α-synuclein toxicity. Dis. Model Mech. 7, 571–582. doi: 10.1242/dmm.013185
Ohki, Y., Wenninger-Weinzierl, A., Hruscha, A., Asakawa, K., Kawakami, K., Haass, C., et al. (2017). Glycine-alanine dipeptide repeat protein contributes to toxicity in a zebrafish model of C9orf72 associated neurodegeneration. Mol. Neurodegener. 12:6. doi: 10.1186/s13024-016-0146-8
Panula, P., Sallinen, V., Sundvik, M., Kolehmainen, J., Torkko, V., Tiittula, A., et al. (2006). Modulatory neurotransmitter systems and behavior: towards zebrafish models of neurodegenerative diseases. Zebrafish 3, 235–247. doi: 10.1089/zeb.2006.3.235
Parker, M. O., Brock, A. J., Walton, R. T., and Brennan, C. H. (2013). The role of zebrafish (Danio rerio) in dissecting the genetics and neural circuits of executive function. Front. Neural Circuit 7:63. doi: 10.3389/fncir.2013.00063
Paquet, D., Bhat, R., Sydow, A., Mandelkow, E.-M., Berg, S., Hellberg, S., et al. (2009). A zebrafish model of tauopathy allows in vivo imaging of neuronal cell death and drug evaluation. J. Clin. Invest. 119, 1382–1395. doi: 10.1172/JCI37537
Pickrell, A. M., and Youle, R. J. (2015). The Roles of PINK1, Parkin, and Mitochondrial Fidelity in Parkinson’s Disease. Neuron 85, 257–273. doi: 10.1016/j.neuron.2014.12.007
Pinter, B., Diem-Zangerl, A., Wenning, G. K., Scherfler, C., Oberaigner, W., Seppi, K., et al. (2015). Mortality in Parkinson’s disease: a 38-year follow-up study. Mov. Disord. 30, 266–269. doi: 10.1002/mds.26060
Poewe, W., Seppi, K., Tanner, C. M., Halliday, G. M., Brundin, P., Volkmann, J., et al. (2017). Parkinson disease. Nat. Rev. Dis. Primers 3:17013. doi: 10.1038/nrdp.2017.13
Postlethwait, J. H., Woods, I. G., Ngo-Hazelett, P., Yan, Y.-L., Kelly, P. D., Chu, F., et al. (2000). Zebrafish Comparative Genomics and the Origins of Vertebrate Chromosomes. Genome Res. 10, 1890–1902. doi: 10.1101/gr.164800
Prabhudesai, S., Bensabeur, F. Z., Abdullah, R., Basak, I., Baez, S., Alves, G., et al. (2016). LRRK2 knockdown in zebrafish causes developmental defects, neuronal loss, and synuclein aggregation. J. Neurosci. Res. 94, 717–735. doi: 10.1002/jnr.23754
Prabhudesai, S., Sinha, S., Attar, A., Kotagiri, A., Fitzmaurice, A. G., Lakshmanan, R., et al. (2012). A Novel “Molecular Tweezer” Inhibitor of α-Synuclein Neurotoxicity in Vitro and in Vivo. Neurotherapeutics 9, 464–476. doi: 10.1007/s13311-012-0105-1
Priyadarshini, M., Tuimala, J., Chen, Y. C., and Panula, P. (2013). A zebrafish model of PINK1 deficiency reveals key pathway dysfunction including HIF signaling. Neurobiol. Dis. 54, 127–138. doi: 10.1016/j.nbd.2013.02.002
Pulak, R. (2016). Tools for automating the imaging of zebrafish larvae. Methods 96, 118–126. doi: 10.1016/j.ymeth.2015.11.021
Ramesh, T., Lyon, A. N., Pineda, R. H., Wang, C., Janssen, P. M. L., Canan, B. D., et al. (2010). A genetic model of amyotrophic lateral sclerosis in zebrafish displays phenotypic hallmarks of motoneuron disease. Dis. Model Mech. 3, 652–662. doi: 10.1242/dmm.005538
Ravikumar, B., Vacher, C., Berger, Z., Davies, J. E., Luo, S., Oroz, L. G., et al. (2004). Inhibition of mTOR induces autophagy and reduces toxicity of polyglutamine expansions in fly and mouse models of Huntington disease. Nat. Genet. 36, 585–595. doi: 10.1038/ng1362
Razali, K., Othman, N., Nasir, M. H. M., Doolaanea, A. A., Kumar, J., Ibrahim, W. N., et al. (2021). The promise of the zebrafish model for parkinson’s disease: today’s science and tomorrow’s treatment. Front. Genet. 12:655550. doi: 10.3389/fgene.2021.655550
Ren, G., Xin, S., Li, S., Zhong, H., and Lin, S. (2011). Disruption of LRRK2 Does Not Cause Specific Loss of Dopaminergic Neurons in Zebrafish. PLoS One 6:e20630. doi: 10.1371/journal.pone.0020630
Rink, E., and Wullimann, M. F. (2001). The teleostean (zebrafish) dopaminergic system ascending to the subpallium (striatum) is located in the basal diencephalon (posterior tuberculum). Brain Res. 889, 316–330. doi: 10.1016/S0006-8993(00)03174-7
Rink, E., and Wullimann, M. F. (2002). Connections of the ventral telencephalon and tyrosine hydroxylase distribution in the zebrafish brain (Danio rerio) lead to identification of an ascending dopaminergic system in a teleost. Brain Res. Bull. 57, 385–387. doi: 10.1016/S0361-9230(01)00696-7
Rissone, A., Ledin, J., and Burgess, S. M. (2020). “Targeted Editing of Zebrafish Genes to Understand Gene Function and Human Disease Pathology,” in The Zebrafish in Biomedical Research, eds S. Cartner, J. Eisen, S. Farmer, K. Guillemin, M. Kent, and G. Sanders (Amsterdam: Elsevier), 637–647. doi: 10.1016/B978-0-12-812431-4.00049-X
Robea, M.-A., Balmus, I.-M., Ciobica, A., Strungaru, S., Plavan, G., Gorgan, L. D., et al. (2020). Parkinson’s disease-induced zebrafish models: focussing on oxidative stress implications and sleep processes. Oxid. Med. Cell. Longev. 2020, 1–15. doi: 10.1155/2020/1370837
Robinson, K. J., Yuan, K. C., Don, E. K., Hogan, A. L., Winnick, C. G., Tym, M. C., et al. (2019). Motor neuron abnormalities correlate with impaired movement in zebrafish that express mutant superoxide dismutase 1. Zebrafish 16, 8–14. doi: 10.1089/zeb.2018.1588
Roggenbuck, J., Quick, A., and Kolb, S. J. (2017). Genetic testing and genetic counseling for amyotrophic lateral sclerosis: an update for clinicians. Genet. Med. 19, 267–274. doi: 10.1038/gim.2016.107
Rui, Q., Ni, H., Li, D., Gao, R., and Chen, G. (2018). The Role of LRRK2 in Neurodegeneration of Parkinson Disease. Curr. Neuropharmacol. 16, 1348–1357. doi: 10.2174/1570159X16666180222165418
Sager, J. J., Bai, Q., and Burton, E. A. (2010). Transgenic zebrafish models of neurodegenerative diseases. Brain Struct. Funct. 214, 285–302. doi: 10.1007/s00429-009-0237-1
Sakowski, S. A., Lunn, J. S., Busta, A. S., Oh, S. S., Zamora-Berridi, G., Palmer, M., et al. (2012). Neuromuscular effects of G93A-SOD1 expression in zebrafish. Mol. Neurodegener. 7, 44–44. doi: 10.1186/1750-1326-7-44
Saleem, S., Banerjee, R., and Kannan, R. R. (2022). Chrysin-Loaded Chitosan Nanoparticle-Mediated Neuroprotection in Aβ1–42-Induced Neurodegenerative Conditions in Zebrafish. ACS Chem. Neurosci. 13, 2017–2034. doi: 10.1021/acschemneuro.2c00240
Sallinen, V., Kolehmainen, J., Priyadarshini, M., Toleikyte, G., Chen, Y.-C., and Panula, P. (2010). Dopaminergic cell damage and vulnerability to MPTP in Pink1 knockdown zebrafish. Neurobiol. Dis. 40, 93–101. doi: 10.1016/j.nbd.2010.06.001
Sallinen, V., Torkko, V., Sundvik, M., Reenilä, I., Khrustalyov, D., Kaslin, J., et al. (2009). MPTP and MPP+ target specific aminergic cell populations in larval zebrafish. J. Neurochem. 108, 719–731. doi: 10.1111/j.1471-4159.2008.05793.x
Schiffer, N. W., Broadley, S. A., Hirschberger, T., Tavan, P., Kretzschmar, H. A., Giese, A., et al. (2007). Identification of Anti-prion Compounds as Efficient Inhibitors of Polyglutamine Protein Aggregation in a Zebrafish Model. J. Biol. Chem. 282, 9195–9203. doi: 10.1074/jbc.M607865200
Schmid, B., Hruscha, A., Hogl, S., Banzhaf-Strathmann, J., Strecker, K., van der Zee, J., et al. (2013). Loss of ALS-associated TDP-43 in zebrafish causes muscle degeneration, vascular dysfunction, and reduced motor neuron axon outgrowth. Proc. Natl. Acad. Sci. U.S.A. 110, 4986–4991. doi: 10.1073/pnas.1218311110
Schmidt, R., Strähle, U., and Scholpp, S. (2013). Neurogenesis in zebrafish – from embryo to adult. Neural Dev. 8:3. doi: 10.1186/1749-8104-8-3
Seachrist, D. D., Bonk, K. W., Ho, S.-M., Prins, G. S., Soto, A. M., and Keri, R. A. (2016). A review of the carcinogenic potential of bisphenol A. Reprod. Toxicol. 59, 167–182. doi: 10.1016/j.reprotox.2015.09.006
Selkoe, D. J. (1991). The molecular pathology of Alzheimer’s disease. Neuron 6, 487–498. doi: 10.1016/0896-6273(91)90052-2
Semaka, A., Kay, C., Doty, C., Collins, J. A., Bijlsma, E. K., Richards, F., et al. (2013). CAG size-specific risk estimates for intermediate allele repeat instability in Huntington disease. J. Med. Genet. 50:696. doi: 10.1136/jmedgenet-2013-101796
Shaw, M. P., Higginbottom, A., McGown, A., Castelli, L. M., James, E., Hautbergue, G. M., et al. (2018). Stable transgenic C9orf72 zebrafish model key aspects of the ALS/FTD phenotype and reveal novel pathological features. Acta Neuropathol. Commun. 6:125. doi: 10.1186/s40478-018-0629-7
Sheng, D., Qu, D., Kwok, K. H. H., Ng, S. S., Lim, A. Y. M., Aw, S. S., et al. (2010). Deletion of the WD40 Domain of LRRK2 in Zebrafish Causes Parkinsonism-Like Loss of Neurons and Locomotive Defect. PLoS Genet. 6:e1000914. doi: 10.1371/journal.pgen.1000914
Sidik, H., Ang, C. J., and Pouladi, M. A. (2020). Huntingtin confers fitness but is not embryonically essential in zebrafish development. Dev. Biol. 458, 98–105. doi: 10.1016/j.ydbio.2019.10.037
Smeyne, R. J., and Jackson-Lewis, V. (2005). The MPTP model of Parkinson’s disease. Mol. Brain Res. 134, 57–66. doi: 10.1016/j.molbrainres.2004.09.017
Squitieri, F., Andrew, S. E., Goldberg, Y. P., Kremer, B., Spence, N., Zelsler, J., et al. (1994). DNA haplotype analysis of Huntington disease reveals clues to the origins and mechanisms of CAG expansion and reasons for geographic variations of prevalence. Hum. Mol. Genet. 3, 2103–2114. doi: 10.1093/hmg/3.12.2103
Stainier, D. Y. R., Kontarakis, Z., and Rossi, A. (2015). Making Sense of Anti-Sense Data. Dev. Cell 32, 7–8. doi: 10.1016/j.devcel.2014.12.012
Stepto, A., Gallo, J.-M., Shaw, C. E., and Hirth, F. (2014). Modelling C9ORF72 hexanucleotide repeat expansion in amyotrophic lateral sclerosis and frontotemporal dementia. Acta Neuropathol. 127, 377–389. doi: 10.1007/s00401-013-1235-1
Strooper, B. D. (2007). Loss-of-function presenilin mutations in Alzheimer disease. EMBO Rep. 8, 141–146. doi: 10.1038/sj.embor.7400897
Sun, Z., and Gilter, A. D. (2008). Discovery and characterization of three novel synuclein genes in zebrafish. Dev. Dyn. 237, 2490–2495. doi: 10.1002/dvdy.21569
Sundvik, M., Chen, Y.-C., and Panula, P. (2013). Presenilin1 Regulates Histamine Neuron Development and Behavior in Zebrafish, Danio rerio. J. Neurosci. 33, 1589–1597. doi: 10.1523/JNEUROSCI.1802-12.2013
Suzzi, S., Ahrendt, R., Hans, S., Semenova, S. A., Bilican, S., Sayed, S., et al. (2017). Loss of lrrk2 impairs dopamine catabolism, cell proliferation, and neuronal regeneration in the zebrafish brain. biorxiv [Preprint]. doi: 10.1101/140608
Suzzi, S., Ahrendt, R., Hans, S., Semenova, S. A., Chekuru, A., Wirsching, P., et al. (2021). Deletion of lrrk2 causes early developmental abnormalities and age-dependent increase of monoamine catabolism in the zebrafish brain. PLoS Genet. 17:e1009794. doi: 10.1371/journal.pgen.1009794
Swinnen, B., Bento-Abreu, A., Gendron, T. F., Boeynaems, S., Bogaert, E., Nuyts, R., et al. (2018). A zebrafish model for C9orf72 ALS reveals RNA toxicity as a pathogenic mechanism. Acta Neuropathol. 135, 427–443. doi: 10.1007/s00401-017-1796-5
Tomasiewicz, H. G., Flaherty, D. B., Soria, J. P., and Wood, J. G. (2002). Transgenic zebrafish model of neurodegeneration. J. Neurosci. Res. 70, 734–745. doi: 10.1002/jnr.10451
Toni, M., and Cioni, C. (2015). Fish Synucleins: an Update. Mar. Drugs 13, 6665–6686. doi: 10.3390/md13116665
Toral-Rios, D., Pichardo-Rojas, P. S., Alonso-Vanegas, M., and Campos-Peña, V. (2020). GSK3β and Tau Protein in Alzheimer’s Disease and Epilepsy. Front. Cell. Neurosci. 14:19. doi: 10.3389/fncel.2020.00019
Vandenberg, L. N., Hauser, R., Marcus, M., Olea, N., and Welshons, W. V. (2007). Human exposure to bisphenol A (BPA). Reprod. Toxicol. 24, 139–177. doi: 10.1016/j.reprotox.2007.07.010
Vaz, R. L., Outeiro, T. F., and Ferreira, J. J. (2018). Zebrafish as an animal model for drug discovery in Parkinson’s disease and other movement disorders: a systematic review. Front. Neurol. 9:347. doi: 10.3389/fneur.2018.00347
Veldman, M. B., Rios-Galdamez, Y., Lu, X.-H., Gu, X., Qin, W., Li, S., et al. (2015). The N17 domain mitigates nuclear toxicity in a novel zebrafish Huntington’s disease model. Mol. Neurodegener. 10:67. doi: 10.1186/s13024-015-0063-2
Veugelen, S., Saito, T., Saido, T. C., Chávez-Gutiérrez, L., and De Strooper, B. (2016). Familial Alzheimer’s Disease Mutations in Presenilin Generate Amyloidogenic Aβ Peptide Seeds. Neuron 90, 410–416. doi: 10.1016/j.neuron.2016.03.010
Vijayanathan, Y., Lim, F. T., Lim, S. M., Long, C. M., Tan, M. P., Majeed, A. B. A., et al. (2017). 6-OHDA-Lesioned Adult Zebrafish as a Useful Parkinson’s Disease Model for Dopaminergic Neuroregeneration. Neurotox. Res. 32, 496–508. doi: 10.1007/s12640-017-9778-x
Wang, Q., Liu, S., Hu, D., Wang, Z., Wang, L., Wu, T., et al. (2016). Identification of apoptosis and macrophage migration events in paraquat-induced oxidative stress using a zebrafish model. Life Sci. 157, 116–124. doi: 10.1016/j.lfs.2016.06.009
Wang, X. H., Souders, C. L., Zhao, Y. H., and Martyniuk, C. J. (2018). Paraquat affects mitochondrial bioenergetics, dopamine system expression, and locomotor activity in zebrafish (Danio rerio). Chemosphere 191, 106–117. doi: 10.1016/j.chemosphere.2017.10.032
Wang, Y., Liu, W., Yang, J., Wang, F., Sima, Y., Zhong, Z., et al. (2017). Parkinson’s disease-like motor and non-motor symptoms in rotenone-treated zebrafish. Neurotoxicology 58, 103–109. doi: 10.1016/j.neuro.2016.11.006
Wang, Y., Takai, R., Yoshioka, H., and Shirabe, K. (2006). Characterization and Expression of Serotonin Transporter Genes in Zebrafish. Tohoku J. Exp. Med. 208, 267–274. doi: 10.1620/tjem.208.267
Wasel, O., and Freeman, J. L. (2020). Chemical and Genetic Zebrafish Models to Define Mechanisms of and Treatments for Dopaminergic Neurodegeneration. Int. J. Mol. Sci. 21:5981. doi: 10.3390/ijms21175981
Wattmo, C., Blennow, K., and Hansson, O. (2020). Cerebro-spinal fluid biomarker levels: phosphorylated tau (T) and total tau (N) as markers for rate of progression in Alzheimer’s disease. BMC Neurol. 20:10. doi: 10.1186/s12883-019-1591-0
Wen, L., Wei, W., Gu, W., Huang, P., Ren, X., Zhang, Z., et al. (2008). Visualization of monoaminergic neurons and neurotoxicity of MPTP in live transgenic zebrafish. Dev. Biol. 314, 84–92. doi: 10.1016/j.ydbio.2007.11.012
White, D. T., Saxena, M. T., and Mumm, J. S. (2019). Let’s get small (and smaller): combining zebrafish and nanomedicine to advance neuroregenerative therapeutics. Adv. Drug Deliv. Rev. 148, 344–359. doi: 10.1016/j.addr.2019.01.011
Whitlock, K. E., and Westerfield, M. (2000). The olfactory placodes of the zebrafish form by convergence of cellular fields at the edge of the neural plate. Development 127, 3645–3653. doi: 10.1242/dev.127.17.3645
Williams, A., Sarkar, S., Cuddon, P., Ttofi, E. K., Saiki, S., Siddiqi, F. H., et al. (2008). Novel targets for Huntington’s disease in an mTOR-independent autophagy pathway. Nat. Chem. Biol. 4, 295–305. doi: 10.1038/nchembio.79
Williams, F. E., White, D., and Messer, W. S. (2002). A simple spatial alternation task for assessing memory function in zebrafish. Behav. Process. 58, 125–132. doi: 10.1016/S0376-6357(02)00025-6
Wong, T. H., Seelaar, H., Melhem, S., Rozemuller, A. J. M., and van Swieten, J. C. (2020). Genetic screening in early-onset Alzheimer’s disease identified three novel presenilin mutations. Neurobiol. Aging 86, 201.e9–201.e14. doi: 10.1016/j.neurobiolaging.2019.01.015
Wullimann, M. F., Mueller, T., Distel, M., Babaryka, A., Grothe, B., and Köster, R. W. (2011). The long adventurous journey of rhombic lip cells in jawed vertebrates: A comparative developmental analysis. Front. Neuroanat. 5:27. doi: 10.3389/fnana.2011.00027
Wyart, C., and Bene, F. D. (2011). Let there be light: zebrafish neurobiology and the optogenetic revolution. Rev. Neurosci. 22, 121–130. doi: 10.1515/rns.2011.013
Xi, Y., Ryan, J., Noble, S., Yu, M., Yilbas, A. E., and Ekker, M. (2010). Impaired dopaminergic neuron development and locomotor function in zebrafish with loss of pink1 function. Eur. J. Neurosci. 31, 623–633. doi: 10.1111/j.1460-9568.2010.07091.x
Yamazaki, T., Chen, S., Yu, Y., Yan, B., Haertlein, T. C., Carrasco, M. A., et al. (2012). Protein Interactions Link the Motor Neuron Diseases ALS and SMA. Cell Rep. 2, 799–806. doi: 10.1016/j.celrep.2012.08.025
Yao, L., Peng, S., Xu, Y., Lin, S. L., Li, Y., Liu, C., et al. (2017). Unexpected Neuroprotective Effects of Loganin on 1-Methyl-4-Phenyl-1,2,3,6-Tetrahydropyridine-Induced Neurotoxicity and Cell Death in Zebrafish. J. Cell. Biochem. 118, 615–628. doi: 10.1002/jcb.25749
Yeh, T.-H., Liu, H.-F., Li, Y.-W., Lu, C.-S., Shih, H.-Y., Chiu, C.-C., et al. (2018). C9orf72 is essential for neurodevelopment and motility mediated by Cyclin G1. Exp. Neurol. 304, 114–124. doi: 10.1016/j.expneurol.2018.03.002
Yu, Y., Chi, B., Xia, W., Gangopadhyay, J., Yamazaki, T., Winkelbauer-Hurt, M. E., et al. (2015). U1 snRNP is mislocalized in ALS patient fibroblasts bearing NLS mutations in FUS and is required for motor neuron outgrowth in zebrafish. Nucleic Acids Res. 43, 3208–3218. doi: 10.1093/nar/gkv157
Zarei, S., Carr, K., Reiley, L., Diaz, K., Guerra, O., Altamirano, P. F., et al. (2015). A comprehensive review of amyotrophic lateral sclerosis. Surg. Neurol. Int. 6:171. doi: 10.4103/2152-7806.169561
Zeitlin, S., Liu, J.-P., Chapman, D. L., Papaioannou, V. E., and Efstratiadis, A. (1995). Increased apoptosis and early embryonic lethality in mice nullizygous for the Huntington’s disease gene homologue. Nat. Genet. 11, 155–163. doi: 10.1038/ng1095-155
Zelenchuk, T. A., and Brusés, J. L. (2011). In Vivo labeling of zebrafish motor neurons using an mnx1 enhancer and Gal4/UAS. Genesis 49, 546–554. doi: 10.1002/dvg.20766
Zhang, C., Li, C., Chen, S., Li, Z., Jia, X., Wang, K., et al. (2016). Berberine protects against 6-OHDA-induced neurotoxicity in PC12 cells and zebrafish through hormetic mechanisms involving PI3K/AKT/Bcl-2 and Nrf2/HO-1 pathways. Redox Biol. 11, 1–11. doi: 10.1016/j.redox.2016.10.019
Zhang, L. Q., Sa, F., Chong, C. M., Wang, Y., Zhou, Z. Y., Chang, R. C. C., et al. (2015). Schisantherin A protects against 6-OHDA-induced dopaminergic neuron damage in zebrafish and cytotoxicity in SH-SY5Y cells through the ROS/NO and AKT/GSK3β pathways. J. Ethnopharmacol. 170, 8–15. doi: 10.1016/j.jep.2015.04.040
Zhang, Z., and Simpkins, J. W. (2010). Okadaic acid induces tau phosphorylation in SH-SY5Y cells in an estrogen-preventable manner. Brain Res. 1345, 176–181. doi: 10.1016/j.brainres.2010.04.074
Zhao, T., der Linde, H. Z., Severijnen, L.-A., Oostra, B. A., Willemsen, R., and Bonifati, V. (2012). Dopaminergic Neuronal Loss and Dopamine-Dependent Locomotor Defects in Fbxo7-Deficient Zebrafish. PLoS One 7:e48911. doi: 10.1371/journal.pone.0048911
Zimmer, A. M., Pan, Y. K., Chandrapalan, T., Kwong, R. W. M., and Perry, S. F. (2019). Loss-of-function approaches in comparative physiology: is there a future for knockdown experiments in the era of genome editing? J. Exp. Biol. 222:jeb175737. doi: 10.1242/jeb.175737
Zlomuzica, A., Dere, D., Binder, S., Silva, M. A. D. S., Huston, J. P., and Dere, E. (2016). Neuronal histamine and cognitive symptoms in Alzheimer’s disease. Neuropharmacology 106, 135–145. doi: 10.1016/j.neuropharm.2015.05.007
Zuccato, C., Belyaev, N., Conforti, P., Ooi, L., Tartari, M., Papadimou, E., et al. (2007). Widespread Disruption of Repressor Element-1 Silencing Transcription Factor/Neuron-Restrictive Silencer Factor Occupancy at Its Target Genes in Huntington’s Disease. J. Neurosci. 27, 6972–6983. doi: 10.1523/JNEUROSCI.4278-06.2007
Zuccato, C., Ciammola, A., Rigamonti, D., Leavitt, B. R., Goffredo, D., Conti, L., et al. (2001). Loss of Huntingtin-Mediated BDNF Gene Transcription in Huntington’s Disease. Science 293, 493–498. doi: 10.1126/science.1059581
Keywords: zebrafish, Alzheimer’s disease, multiple sclerosis, Parkinson’s disease, amyotrophic lateral sclerosis (ALS), Huntington’s disease (HD)
Citation: Chia K, Klingseisen A, Sieger D and Priller J (2022) Zebrafish as a model organism for neurodegenerative disease. Front. Mol. Neurosci. 15:940484. doi: 10.3389/fnmol.2022.940484
Received: 10 May 2022; Accepted: 01 July 2022;
Published: 13 October 2022.
Edited by:
Wietske van der Ent, University of Oslo, NorwayReviewed by:
Suraiya Saleem, Indian Institute of Technology Madras, IndiaCopyright © 2022 Chia, Klingseisen, Sieger and Priller. This is an open-access article distributed under the terms of the Creative Commons Attribution License (CC BY). The use, distribution or reproduction in other forums is permitted, provided the original author(s) and the copyright owner(s) are credited and that the original publication in this journal is cited, in accordance with accepted academic practice. No use, distribution or reproduction is permitted which does not comply with these terms.
*Correspondence: Dirk Sieger, ZGlyay5zaWVnZXJAZWQuYWMudWs=; Josef Priller, am9zZWYucHJpbGxlckBlZC5hYy51aw==
Disclaimer: All claims expressed in this article are solely those of the authors and do not necessarily represent those of their affiliated organizations, or those of the publisher, the editors and the reviewers. Any product that may be evaluated in this article or claim that may be made by its manufacturer is not guaranteed or endorsed by the publisher.
Research integrity at Frontiers
Learn more about the work of our research integrity team to safeguard the quality of each article we publish.