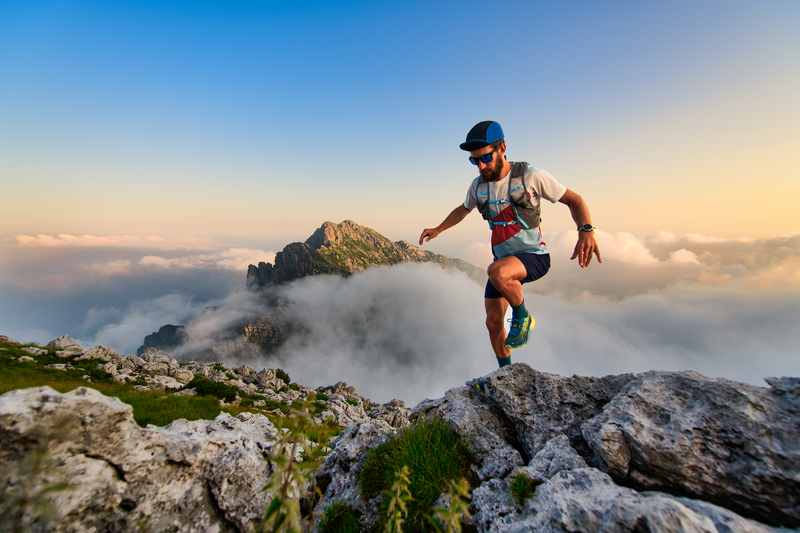
95% of researchers rate our articles as excellent or good
Learn more about the work of our research integrity team to safeguard the quality of each article we publish.
Find out more
MINI REVIEW article
Front. Mol. Neurosci. , 15 July 2022
Sec. Brain Disease Mechanisms
Volume 15 - 2022 | https://doi.org/10.3389/fnmol.2022.932497
This article is part of the Research Topic Implications of Neurotrophins and Their Receptors in the Pathophysiology and Treatments of Depression and Addiction View all 6 articles
This article reviews the current progress in our understanding of the mechanisms by which growth factors, including brain-derived neurotrophic factor (BDNF) and vascular endothelial growth factor (VEGF), and select neurotrophin-regulated gene products, such as VGF (non-acronymic) and VGF-derived neuropeptides, function in the central nervous system (CNS) to modulate neuropsychiatric and neurodegenerative disorders, with a discussion of the possible therapeutic applications of these growth factors to major depressive disorder (MDD) and Alzheimer’s disease (AD). BDNF and VEGF levels are generally decreased regionally in the brains of MDD subjects and in preclinical animal models of depression, changes that are associated with neuronal atrophy and reduced neurogenesis, and are reversed by conventional monoaminergic and novel ketamine-like antidepressants. Downstream of neurotrophins and their receptors, VGF was identified as a nerve growth factor (NGF)- and BDNF-inducible secreted protein and neuropeptide precursor that is produced and trafficked throughout the CNS, where its expression is greatly influenced by neuronal activity and exercise, and where several VGF-derived peptides modulate neuronal activity, function, proliferation, differentiation, and survival. Moreover, levels of VGF are reduced in the CSF of AD subjects, where it has been repetitively identified as a disease biomarker, and in the hippocampi of subjects with MDD, suggesting possible shared mechanisms by which reduced levels of VGF and other proteins that are similarly regulated by neurotrophin signaling pathways contribute to and potentially drive the pathogenesis and progression of co-morbid neuropsychiatric and neurodegenerative disorders, particularly MDD and AD, opening possible therapeutic windows.
Major depressive disorder (MDD) is among the most prevalent psychiatric disorders across the world with a high economic and psychological burden. According to the World Mental Health (WMH) survey (Otte et al., 2016), depression has an average annual prevalence of 6%, with 20% of the world’s population fulfilling the criteria for MDD once in their lifetime, and the economic burden of depression in the United States estimated to be $83.1 billion in 2000 (Cohen and Sclar, 2012) and increased from $236.6 billion to $326.2 billion between 2010 and 2018 (Greenberg et al., 2021). Females are at a two-fold higher risk than males, after puberty, of developing MDD, although it can affect either sex at any age, with a median age of onset of 25 years, peaking from mid-late adolescence until the early 40 s (Otte et al., 2016).
The oldest and still currently employed treatment option for MDD is monoamine therapy, based on the hypothesized interaction of monoamines norepinephrine and serotonin that modulates this condition (Hirschfeld, 2000). Widely used MDD therapeutics include selective serotonin and norepinephrine uptake inhibitors (SSRIs and SNRIs), which increase synaptic serotonin or norepinephrine levels, respectively, resembling treatment with monoamine oxidase inhibitors (MAOIs), although SSRIs and SNRIs are more widely used and have significantly lower rates of relapse than MAOI therapy (Clevenger et al., 2018). Although the treatment rates for MDD have increased, and different treatments have been introduced, patient response rates have remained relatively low (Cohen and Sclar, 2012), and treatment-resistant depression is common, encouraging the search for novel antidepressant treatment strategies that result from a better understanding of disease pathophysiology.
Dysregulation and loss of neuronal density in the hippocampus during chronic stress are associated with MDD and may be responsible for symptoms, such as depressed mood, anhedonia, and low energy or fatigue, which are thought to result from induced changes in intracellular signaling, gene modification, neuronal function, and connectivity, in the limbic brain regions that regulate mood and drive (Wong and Licinio, 2001; Duric and Duman, 2013). Chronic stress, aging, and depressive mood reduce neurogenesis in the hippocampus and increase neuronal apoptosis in the rodent cerebral cortex (Bachis et al., 2008). Currently used antidepressants generally positively affect neurotransmitter signaling in the limbic system, rescuing neuronal loss and the deficits in neurogenesis and intracellular signaling that are associated with anhedonia and low mood (Warner-Schmidt and Duman, 2006; Rantamaki and Castren, 2008; Duric and Duman, 2013). This suggests a potentially shared mechanism for antidepressant actions that neurotrophic growth factors and downstream proteins they regulate could therapeutically target. Although not explained by common genetic variants (Gibson et al., 2017), major depression is frequently comorbid with Alzheimer’s disease (AD), and both conditions have significant heritable components and shared dysfunction in neuroinflammation, oxidative stress, cellular signaling, and neurotransmission (Martin-Sanchez et al., 2021). Given the evidence of common pathways and associated changes in MDD and AD, genome-wide association and multiscale causal network studies have sought to uncover molecular signatures and genetic variations shared between AD and MDD, indicative of potential comorbidity (Sullivan et al., 2012; Porcelli et al., 2013; Beckmann et al., 2020; Martin-Sanchez et al., 2021).
Neurotrophic factors and their signaling pathways have been proposed to be major targets responsible for the efficacy of antidepressant therapy (Duman, 2004, 2005; Schmidt et al., 2008). These growth factors regulate peripheral nervous system (PNS) and CNS neuronal generation, proliferation, differentiation, and survival, and include the classical neurotrophin family, specifically nerve growth factor (NGF), brain-derived neurotrophic factor (BDNF), neurotrophin-3 (NT-3), neurotrophin-4 (NT-4), and cytokine family members, such as vascular endothelial growth factor (VEGF) (Nagahara and Tuszynski, 2011) (Figure 1).
Figure 1. Antidepressant efficacy of therapeutics, delivered to CNS via intracortical, intrahippocampal, intracerebroventricular, and intranasal routes, that target neurotrophin signaling pathways. Panels (A,B) depict intracerebroventricular (icv), intracortical, or intrahippocampal infusions of neuropeptide TLQP-62 or AAV-VGF into rodent brain, while panel (C) depicts intranasal administration of small molecule agonists, including the TrkB agonist LM22A-4, and neuropeptides, such as TLQP-62, for delivery of pharmacotherapeutics to the CNS. Proposed diffusion pathways of agents delivered intranasally, bypassing the blood-brain-barrier (BBB), for distal delivery via the CSF and the perivascular spaces around cranial nerves, to the various brain regions shown in panel (D). In panel (E), the antidepressant-like actions of TLQP-62 are shown to be mediated through an unknown receptor (in gray), leading to activation of known glutamatergic signaling pathways, including NMDAR and mGluR5 (Thakker-Varia et al., 2014), the BDNF/TrkB signaling cascade (Jiang et al., 2019a), and calcium channels which activate GluR1 (Jiang et al., 2019a). Other BDNF mimetic/agonists, including LM22A-4 (Fletcher et al., 2021) and 7,8-DHF (Wurzelmann et al., 2017), have shown antidepressant efficacy via TrkB activation. Additionally, vascular endothelial growth factor (VEGF) is shown to induce antidepressant effects via PI3K/PKB/mTORC1 pathways that activate CREB. Activated CREB increases BDNF and VGF transcription, leading to increased BDNF and VGF translation and secretion, and stimulation of BDNF/TrkB signaling – an autoregulatory loop.
In addition to their major role in neuronal survival and function during development and in adulthood, growth factors, such as BDNF exert important pro-survival and functional effects in models of neurological, neuropsychiatric, and neurodegenerative disorders (Nagahara and Tuszynski, 2011), mediated in many cases through changes in gene expression (Lindholm et al., 1994). Studies of postmortem human brain tissues have revealed reduced BDNF mRNA and protein levels in the limbic regions of the brain, including the hippocampus, prefrontal cortex (PFC), and amygdala of depressed patients and suicide subjects (Dwivedi et al., 2003; Karege et al., 2005). In animal models, a significant reduction of BDNF expression in the dentate gyrus of the hippocampus in rats exposed to chronic restraint stress has been reported (Smith et al., 1995; Nowacka and Obuchowicz, 2013). However, in the ventral tegmental area (VTA)-nucleus acumbens (NAc) limbic projections, increased BDNF release is associated with increased susceptibility to pro-depressive behavior (Berton et al., 2006; Shirayama et al., 2015), while in models of chronic and sub-chronic variable stress (SCVS), reduced BDNF expression in hippocampal dentate gyrus and NAc was reported (Rasmusson et al., 2002; Nowacka and Obuchowicz, 2013; Brancato et al., 2017).
The human BDNF Val66Met gene polymorphism is the most widely studied variation with a single nucleotide polymorphism (SNP) at nucleotide 196 leading to a substitution of methionine (Met) for valine (Val) at codon 66, Val66Met, which interferes with BDNF trafficking, sorting, and secretion, and is associated with cognitive deficits (Egan et al., 2003; Chen et al., 2008; Chiaruttini et al., 2009; Nowacka and Obuchowicz, 2013). Meta-analysis pooling of gene association studies of human subjects with the Val66Met BDNF SNP showed an association with depression in late life only. However, the Val66Met SNP (1) failed to predict MDD risk, (2) was correlated with MDD in males only, and (McCullough et al., 2000) had increased chronicity of MDD with no association with disease recurrence (Verhagen et al., 2010; Gyekis et al., 2013; Lee et al., 2013; Tsang et al., 2017; Youssef et al., 2018). Meta-analysis further indicated that the BDNF Met allele carriers had an increased antidepressant response compared with Val/Val homozygotes in the Asian population (Zou et al., 2010; Kishi et al., 2017), whereas, another study revealed varied responses to a different conventional antidepressant in Val/Val homozygotes and Met carriers in depressed Caucasian subjects (Colle et al., 2015b). Perturbed BDNF expression has therefore been linked to depressive disorders (Alder et al., 2003; Dwivedi et al., 2003; Duman, 2004; Chen et al., 2008; Colle et al., 2015a; Bai et al., 2016; Jiang et al., 2019b).
Several classes of antidepressant drugs, including MAOIs, SSRIs, tricyclic antidepressants (TCAs), and SNRIs, increase BDNF expression in the brain in healthy rodents. Rapid-acting antidepressants, such as ketamine increase TrkB phosphorylation in the rat hippocampus (Garcia et al., 2008). Utilizing selective transcription and translation inhibitors, the antidepressant efficacy of ketamine and other NMDA receptor antagonists was found to require a robust increase in BDNF translation but not transcription (Garcia et al., 2008; Bjorkholm and Monteggia, 2016). BDNF protein levels are also differentially expressed in the brain following acute and chronic antidepressant treatment. Repeated application of electroconvulsive shock (ECS), but not a single application, produced 40–100% increases in BDNF levels in the hippocampus, cortex, amygdala, and brainstem; however, chronic but not acute treatment with antidepressants, such as desipramine (TCA), fluoxetine (SSRI), and phenelzine (MAOI), increased BDNF protein levels in the frontal cortex (10–30%) but not in the hippocampus, olfactory bulb, amygdala and brainstem (Balu et al., 2008).
Brain-derived neurotrophic factor (BDNF), however, lacks suitable pharmacokinetics for systemic administration due to its short plasma half-life and poor blood brain barrier (BBB) penetration. Consequently, BDNF mimetics with better pharmacokinetics have been administered that target the tropomyosin-like receptor kinase B (TrkB) receptor for BDNF. The TrkB agonist LM22A has been intranasally administered to rodents (Massa et al., 2010), showing promising results by activating TrkB, PI3K-Akt, and Ras-MAPK pathways, which play a critical role in MDD (Reichardt, 2006; Dietz et al., 2009; Duman and Aghajanian, 2012; Lu et al., 2014). Interestingly, the levels of BDNF and its receptor TrkB are inversely related; increased TrkB expression accompanies decreased BDNF expression in a repeated immobilization stress model and the forced swim test (Nibuya et al., 1999; Shi et al., 2010). Expression and activation of brain BDNF/TrkB pathways are also highly region- and circuit-specific. For example, a single bilateral infusion of TrkB agonist 7,8-Dihydroxyflavone (7,8-DHF), but not the TrkB antagonist ANA-12, into the infralimbic part of the medial prefrontal cortex (mPFC), dentate gyrus (Levi et al., 2004) and CA3 region exerted antidepressant effects in learned helplessness (LH) rat model, while the effect was absent following infusion of 7,8-DHF into the prelimbic region of the PFC (Shirayama et al., 2015; Zhang et al., 2015). In contrast, bilateral infusion of ANA-12 but not 7,8-DHF, into NAc exerted an antidepressant-like effect in LH rats (Shirayama et al., 2015). Furthermore, intraperitoneal (IP) injection of 7,8-DHF or ANA-12 also led to antidepressant and pro-depressant responses, respectively (Zhang et al., 2015). While BDNF/TrkB activation has antidepressant efficacy, proBDNF binding to its receptor p75NTR has the opposite effect; proBDNF/p75NTR signaling is upregulated, and BDNF/TrkB signaling is downregulated by chronic mild unpredictable stress in rats (Bai et al., 2016).
The antidepressant efficacies of ketamine and desipramine are reduced by gene ablation of BDNF and vascular endothelial growth factor (VEGF), respectively (Warner-Schmidt and Duman, 2006; Lin et al., 2015). VEGF is a potent mitogen and survival factor for endothelial cells and neurons, and additionally modulates synaptic transmission in the adult hippocampus and subventricular zone (Fabel et al., 2003; McCloskey et al., 2005), neuroprotection (Feng et al., 2011), and hippocampal neurogenesis (Fournier and Duman, 2012), consistent with a potential role in MDD. The VEGF family consists of six members: VEGF (A-E) and placental-derived growth factor (P1DF). VEGF-A is spliced into four variants, with VEGF120 and VEGF164 known to be the predominant brain variants (Warner-Schmidt and Duman, 2006). Furthermore, in addition to its regulation by antidepressants, VEGF is also modulated by exercise, a potent stimulator of neurogenesis and neurotrophic growth factor expression (Fabel et al., 2003; Warner-Schmidt and Duman, 2006; Ploughman, 2008). Nevertheless, some studies have found no correlation between BDNF and VEGF levels with aerobic exercise in depressed patients (Krogh et al., 2014). Currently, exercise is thought to affect executive functioning by (1) increasing oxygen saturation and angiogenesis in brain areas crucial for task performance, (2) increasing neurotransmitter levels, and (McCullough et al., 2000) upregulating neurotrophic growth factors, such as BDNF (Duman and Monteggia, 2006; Ploughman, 2008). Given the evidence that exercise stimulates brain angiogenesis and increases oxygen saturation, implicating a role for VEGF, it is interesting that Fabel et al. (2003) reported that blockade of peripheral VEGF signaling in a rat exercise model reduced CNS neurogenesis, and that removal of VEGF-antagonist increased neurogenesis, suggesting that exercise-induced neurogenesis requires peripheral VEGF signaling. The biological effects of VEGF are transduced by two receptor tyrosine kinases, fetal liver kinase (Flk-1 or VEGFR2) and fms-like tyrosine kinase (Flt-1), and by a family of putative coreceptors called neuropilins (NRPs) (Warner-Schmidt and Duman, 2006). Therefore, pharmacological modulation of VEGF receptors could have efficacy in MDD, consistent with the recent finding that neuronal VEGF-VEGFR2 (Flk-1) pathways play a key role in the rapid antidepressant actions of ketamine (Deyama et al., 2019b). Recent studies further demonstrate that antidepressant actions of VEGF in mPFC are blocked by neutralizing anti-BDNF antiserum, while antidepressant actions of BDNF in mPFC are blocked by VEGF ablation or neutralizing anti-VEGF antiserum (Deyama et al., 2019a; Duman et al., 2021). VEGF, therefore, functions in complex interplay with BDNF in the mechanism of action of ketamine. Nevertheless, the neuropathological effects of VEGF in MDD and other neuropsychiatric conditions, including schizophrenia require additional investigation (Tsang et al., 2017; Pu et al., 2020).
Brain-derived neurotrophic factor (BDNF) and TrkB play crucial roles in cognition, memory, and depression (Duman and Monteggia, 2006; Rantamaki and Castren, 2008; Nagahara and Tuszynski, 2011), driven at least in part by downstream effectors, including VGF (non-acronymic) (Bonni et al., 1995; Alder et al., 2003). VGF is a neurosecretory protein that was first identified (Levi et al., 1985) as a nerve growth factor (NGF)-regulated transcript in rat pheochromocytoma cells (PC12), and was independently identified by the Wagner and Salton labs (Cho et al., 1989; Salton et al., 1991), and belongs to the granin family (Levi et al., 1985; Salton et al., 2000; Bartolomucci et al., 2011). VGF mRNA is widely expressed in the PNS and CNS, abundantly in the hypothalamus, consistent with known function in metabolic regulation and energy balance (Snyder and Salton, 1998; Hahm et al., 1999, 2002), and in the hippocampus, in line with important roles for VGF in memory and depression-like behavior (Bozdagi et al., 2008; Lin et al., 2015, 2021; Li et al., 2017; Jiang et al., 2019a). VGF undergoes proteolytic cleavage by prohormone convertases and proteases in the regulated secretory pathway to produce at least 12 VGF-derived peptides (Levi et al., 2004; Quinn et al., 2021). VGF-derived peptides have been reported in several CSF proteomic studies to be potential biomarkers of neuropsychiatric and neurodegenerative disorders, including MDD and AD (Bartolomucci et al., 2010; Llano et al., 2019; van Steenoven et al., 2020; Quinn et al., 2021), consistent with possible functional roles for VGF in disease pathophysiology. Indeed, Vgf gene ablation impacts memory and hippocampal function (Bozdagi et al., 2008; Lin et al., 2015), and infusion of VGF-derived peptide TLQP-62 into the brain improves memory (Lin et al., 2015; Li et al., 2017). VGF expression in the hippocampus is decreased in mouse models of depression-like behavior and in postmortem samples of hippocampus and Brodmann area 25 from both medicated and unmedicated male and female patients with MDD compared to controls (Jiang et al., 2018, 2019a), while expression is increased by exercise and chronic antidepressant treatment, including fluoxetine and ketamine (Hunsberger et al., 2007; Thakker-Varia et al., 2007; Jiang et al., 2018, 2019a). VGF overexpression in mouse vmPFC via infusion of AAV-VGF rescued behavioral deficits induced by chronic restraint stress (Jiang et al., 2019a). Furthermore, ketamine and desipramine antidepressant efficacies were reduced by targeted VGF ablation in the hippocampus and/or vmPFC (Jiang et al., 2018, 2019a), reminiscent of the reduction in antidepressant efficacy of voluntary exercise that was observed in germline VGF-deficient mice (Hunsberger et al., 2007). Taken together, these studies support a critical role of the neurotrophin-regulated gene Vgf in depressive behavior and antidepressant efficacy.
Administration of the C-terminal VGF-derived peptide TLQP-62 (by convention named by its N-terminal 4 amino acids and length) to the hippocampus or vmPFC has antidepressant efficacy that was shown to be BDNF-dependent and increases pTrkB and pCREB levels (Lin et al., 2014, 2015). TLQP-62 dependence on BDNF/TrkB was determined utilizing conditional BDNF knockout mice (Jiang et al., 2018, 2019a), targeted shRNA approaches (Li et al., 2017), and BDNF/TrkB inhibitors (Lv et al., 2018). Additional experiments implicated downstream activation of mTORC1, reduced bicaudal C homolog 1 (BICC1) signaling, and increased levels of synaptic GluA1 and pGluA1, following antidepressant TLQP-62 infusion into the hippocampus or PFC (Jiang et al., 2018, 2019a,b; Lv et al., 2018). TLQP-62 infused icv (intra-cerebroventricular) or into the hippocampus improved contextual fear memory or lipopolysaccharide (LPS)-induced memory dysfunction, and either activated BDNF/TrkB pathways or was shown to require BDNF expression (Figure 1) (Lin et al., 2015; Li et al., 2017), consistent with a general mechanism underlying VGF actions in the brain. A proposed autoregulatory feedback model suggests that increased BDNF/TrkB signaling results in Vgf gene induction and the production of VGF-derived peptides, including procognitive and antidepressant TLQP-62, which both stimulates and requires BDNF/TrkB signaling for efficacy (Jiang et al., 2019a). Moreover, in neuronal cell cultures, pTrkB levels were significantly increased by 24 h of TLQP-62 treatment, which in addition increased neurogenesis and the proliferation of neural stem cells, and increased synaptic plasticity through actions on glutamatergic receptors, including mGluR5 and NMDA receptors (Thakker-Varia et al., 2014). Taken together, these studies suggest an important role for VGF and VGF-derived peptides as critical mediators of memory and depression-like behavior, and that novel VGF therapeutics may provide new approaches to MDD.
The progressive neurodegenerative disorder Alzheimer’s disease (AD) is the most common form of dementia, affecting 50-75% of patients, and is reaching pandemic levels (Blennow et al., 2006), while Major Depressive Disorder (MDD) is a debilitating mental illness characterized by high prevalence and resistance to treatment. Both AD and MDD have devastating public health impacts, and comorbidity is frequent but not explained by common genetic variants (Gibson et al., 2017) and could in part be driven by overlapping pathophysiologic mechanisms and signaling networks (Figure 2). BDNF protects against neurodegeneration through signaling pathways that activate the cyclic AMP response element-binding protein (CREB) (Figure 1), increasing hippocampal neurogenesis, controlling the amyloidogenic pathway and Aβ production, and regulating downstream gene expression in the hippocampus (Matrone et al., 2008; Blurton-Jones et al., 2009; Caccamo et al., 2010). VEGF and BDNF pathways play critical, interdependent roles in the antidepressant efficacy of ketamine in MDD as noted above, while in early-onset and late-onset AD, VEGF-A expression in the brain and vasculature undergoes complex, stage-dependent dysregulation (Ali et al., 2022). In the APP/PS1 AD mouse model, intraperitoneal administration of neutralizing anti-VEGF-A antibodies ameliorated capillary stalling, normalized BBB permeability, and increased cerebral blood flow (CBF) (capillary stalling and reduced CBF are associated with cognitive impairment in AD patients) (Ali et al., 2022). On the other hand, delivery of VEGF-A to the brains of mouse AD models partially rescued vascular loss, reduced amyloid plaque load, and rescued impaired memory (Religa et al., 2013; Garcia et al., 2014) as did intraperitoneal VEGF administration (Wang et al., 2011). VEGF-A signaling in the brain may, therefore, be impaired in AD (Jefferies et al., 2013; Ali et al., 2022), much as it appears to be in MDD, suggesting a potential mechanism underlying disease comorbidity.
Figure 2. Schematic of the Comorbidity of Major Depressive Disorder (MDD) and Alzheimer’s disease (AD), disorders that share pathophysiology, disease mechanisms, and potentially treatments. MDD and AD are often comorbid, in many cases sharing a number of pathophysiological features that are summarized in this figure. Cognitive disorders are a core feature of both MDD and AD, often referred to in MDD as pseudodementia or dementia of depression. Cognitive symptoms (particularly memory) in MDD can be reversible with treatment, or more commonly can persist following improvement or remission of depressive symptoms, progressing to overt dementia and AD (Rodrigues et al., 2014; Perini et al., 2019). Immune and glucocorticoid dysfunction are common to AD and MDD, leading to neuronal atrophy, as depicted. Expression of neurotrophic growth factors, including BDNF, VEGF, and VGF, and neuropeptides, such as TLQP-62, is also reduced in both AD and MDD. Treatment with antidepressants and increased exercise increase the levels of neurotrophins in MDD, helping to rescue neuronal function. In AD, exercise and future novel therapeutics may be similarly utilized to increase levels of these neurotrophins and select downstream gene products (VGF), halting the progression of neurodegenerative disease.
Among hundreds of genes involved in AD, the BDNF-regulated VGF gene was identified as a key network driver of AD pathogenesis and progression, and as a potential therapeutic target (Tasaki et al., 2018; Beckmann et al., 2020). VGF levels are consistently decreased in brain tissue and CSF samples of patients with AD (Ruetschi et al., 2005; Zhao et al., 2008; Bartolomucci et al., 2010; Cocco et al., 2010; Asano et al., 2011; Jahn et al., 2011; Wijte et al., 2012; Hendrickson et al., 2015; Holtta et al., 2015; Spellman et al., 2015) and postmortem brain tissue samples from depressed male and female subjects (Jiang et al., 2018, 2019a) as compared with controls. VGF levels were also reduced in patients with MCI only in those who then progressed to AD, further indicating the potential role of VGF as a biomarker in dementia (Jahn et al., 2011; Llano et al., 2019). Importantly, overexpression of VGF in preclinical 5xFAD mouse AD models, via germline overexpression, AAV-mediated VGF delivery, or long-term icv TLQP-62 or TLQP-21 peptide administration, reduced amyloid load, phospho-tau levels, synaptic pathology, dystrophic neurite damage, astrogliosis, microgliosis, and memory impairment, and rescued neurogenesis and LTD deficits (Beckmann et al., 2020; El Gaamouch et al., 2020). Thus, VGF is perhaps one of a limited number of proteins that are down-regulated in both AD and MDD.
The activity-dependent neuroprotective protein (ADNP), which plays a key role in neurogenesis and is essential for brain formation, is another protein that is decreased in postmortem AD brain samples. Accumulating mosaic somatic mutations in the ADNP and other genes in the brains of patients with AD have been proposed to contribute to disease progression, including impaired cognition, increased amyloid plaque burden, and tauopathy (Park et al., 2019; Gozes, 2021; Ivashko-Pachima et al., 2021). Low-level brain somatic mutations in glutamate and dopamine receptor signaling pathway genes have similarly been identified in neuropsychiatric diseases, specifically schizophrenia (Gozes, 2021; Kim et al., 2021). ADNP additionally functions in the pathophysiology of schizophrenia via a key regulatory role in autophagy, which when it is dysregulated also contributes to AD pathogenesis (Merenlender-Wagner et al., 2014, 2015; Sragovich et al., 2017). Recently identified ADNP interactions with SHANK3 (Ivashko-Pachima et al., 2022) and SIRT1 (Hadar et al., 2021) may provide critical new targets for understanding the role that ADNP plays in neuropsychiatric, neurodevelopmental, and neurodegenerative disease. Furthermore, the smallest active snippet of ADNP, an eight-amino-acid peptide NAP (NAPVSIPQ), protected against ADNP deficiencies in autism patients with Helsmoortel-Van Der Aa syndrome (Merenlender-Wagner et al., 2014; Sragovich et al., 2017; Gozes, 2021; Ivashko-Pachima et al., 2021). Therefore, therapeutic overexpression or CNS administration of these ADNP- or VGF-derived peptides have the potential to prevent the progression of comorbid neuropsychiatric and neurodegenerative diseases.
Neurotrophic growth factors and their regulated gene products, such as VGF, could regulate comorbid MDD and AD via shared actions on neurogenesis or synaptic plasticity. Neurogenesis is a dynamic process that is known to be regulated by various external stimuli, including physiological, pathological, and pharmacological changes, that also regulate hippocampal synaptogenesis (Duman, 2005; Thakker-Varia et al., 2014). These stimuli include exercise, enriched environment, antidepressants, and intrinsic factors that include neurotrophic growth factors, such as BDNF, VEGF, VGF, and VGF-derived neuropeptides, like TLQP-62 (Salton et al., 2000; Duman, 2005; Dranovsky and Hen, 2006; Thakker-Varia et al., 2014). Several clinical studies have linked depression with decreased hippocampal volume associated with decreased pyramidal neuronal arborization (Sapolsky, 2000; Sheline, 2000), and perhaps novel peptides like TLQP-62 could find therapeutic utility if they were able to restore neuronal loss and rescue hippocampal atrophy. However, clinical evidence is very limited, and therefore additional studies are required to understand the process of neuronal loss in patients suffering from depression (Sapolsky, 2000; Czeh and Lucassen, 2007).
In pre-clinical studies, TLQP-62 infused intrahippocampally in rodents has antidepressant efficacy, and VGF overexpression or icv peptide administration reduces AD-related phenotypes in 5xFAD mice. However, an extension of these findings to clinical trials that assess novel therapeutics in human subjects, involving either CNS gene therapy (AAV-VGF) or intranasal peptide administration, still has significant technical hurdles to overcome. In this regard, it is somewhat sobering that rescue of reduced levels of BDNF in the hippocampus and entorhinal cortex by sustained BDNF gene delivery via viral vectors, in subjects with AD, did not have significant clinical efficacy (Connor et al., 1997; Hock et al., 2000; Nagahara and Tuszynski, 2011), although it does encourage the search for novel, alternative therapeutics that activate these pathways and may have better clinical results.
RJ and SS wrote the manuscript. Both authors contributed to the article and approved the submitted version.
Research in the authors’ laboratory was supported by grants from the NIH and Cure Alzheimer’s Fund.
The authors declare that the research was conducted in the absence of any commercial or financial relationships that could be construed as a potential conflict of interest.
All claims expressed in this article are solely those of the authors and do not necessarily represent those of their affiliated organizations, or those of the publisher, the editors and the reviewers. Any product that may be evaluated in this article, or claim that may be made by its manufacturer, is not guaranteed or endorsed by the publisher.
We acknowledge the contributions of Dr. Allen L. Pan and members of the Salton lab for helpful comments on this manuscript, and thank the Mount Sinai Instructional Technology Group for their assistance with the figure illustrations.
Alder, J., Thakker-Varia, S., Bangasser, D. A., Kuroiwa, M., Plummer, M. R., Shors, T. J., et al. (2003). Brain-derived neurotrophic factor-induced gene expression reveals novel actions of VGF in hippocampal synaptic plasticity. J. Neurosci. 23, 10800–10808.
Ali, M., Falkenhain, K., Njiru, B. N., Murtaza-Ali, M., Ruiz-Uribe, N. E., Haft-Javaherian, M., et al. (2022). VEGF signalling causes stalls in brain capillaries and reduces cerebral blood flow in Alzheimer’s mice. Brain 145, 1449–1463. doi: 10.1093/brain/awab387
Asano, T., Koizumi, S., Takagi, A., Hatori, T., Kuwabara, K., Fujino, O., et al. (2011). Identification of a novel biomarker candidate, a 4.8-kDa peptide fragment from a neurosecretory protein VGF precursor, by proteomic analysis of cerebrospinal fluid from children with acute encephalopathy using SELDI-TOF-MS. BMC Neurol. 11:101. doi: 10.1186/1471-2377-11-101
Bachis, A., Cruz, M. I., Nosheny, R. L., and Mocchetti, I. (2008). Chronic unpredictable stress promotes neuronal apoptosis in the cerebral cortex. Neurosci. Lett. 442, 104–108. doi: 10.1016/j.neulet.2008.06.081
Bai, Y. Y., Ruan, C. S., Yang, C. R., Li, J. Y., Kang, Z. L., Zhou, L., et al. (2016). ProBDNF Signaling Regulates Depression-Like Behaviors in Rodents under Chronic Stress. Neuropsychopharmacology 41, 2882–2892. doi: 10.1038/npp.2016.100
Balu, D. T., Hoshaw, B. A., Malberg, J. E., Rosenzweig-Lipson, S., Schechter, L. E., and Lucki, I. (2008). Differential regulation of central BDNF protein levels by antidepressant and non-antidepressant drug treatments. Brain Res. 1211, 37–43. doi: 10.1016/j.brainres.2008.03.023
Bartolomucci, A., Pasinetti, G. M., and Salton, S. R. (2010). Granins as disease-biomarkers: translational potential for psychiatric and neurological disorders. Neuroscience 170, 289–297. doi: 10.1016/j.neuroscience.2010.06.057
Bartolomucci, A., Possenti, R., Mahata, S. K., Fischer-Colbrie, R., Loh, Y. P., and Salton, S. R. (2011). The extended granin family: structure, function, and biomedical implications. Endocr. Rev. 32, 755–797. doi: 10.1210/er.2010-0027
Beckmann, N. D., Lin, W. J., Wang, M., Cohain, A. T., Charney, A. W., Wang, P., et al. (2020). Multiscale causal networks identify VGF as a key regulator of Alzheimer’s disease. Nat. Commun. 11:3942. doi: 10.1038/s41467-020-17405-z
Berton, O., McClung, C. A., Dileone, R. J., Krishnan, V., Renthal, W., Russo, S. J., et al. (2006). Essential role of BDNF in the mesolimbic dopamine pathway in social defeat stress. Science 311, 864–868. doi: 10.1126/science.1120972
Bjorkholm, C., and Monteggia, L. M. (2016). BDNF – a key transducer of antidepressant effects. Neuropharmacology 102, 72–79. doi: 10.1016/j.neuropharm.2015.10.034
Blennow, K., de Leon, M. J., and Zetterberg, H. (2006). Alzheimer’s disease. Lancet 368, 387–403. doi: 10.1016/S0140-6736(06)69113-7
Blurton-Jones, M., Kitazawa, M., Martinez-Coria, H., Castello, N. A., Muller, F. J., Loring, J. F., et al. (2009). Neural stem cells improve cognition via BDNF in a transgenic model of Alzheimer disease. Proc. Natl. Acad. Sci. U.S.A. 106, 13594–13599. doi: 10.1073/pnas.0901402106
Bonni, A., Ginty, D. D., Dudek, H., and Greenberg, M. E. (1995). Serine 133-phosphorylated CREB induces transcription via a cooperative mechanism that may confer specificity to neurotrophin signals. Mol. Cell. Neurosci. 6, 168–183. doi: 10.1006/mcne.1995.1015
Bozdagi, O., Rich, E., Tronel, S., Sadahiro, M., Patterson, K., Shapiro, M. L., et al. (2008). The neurotrophin-inducible gene Vgf regulates hippocampal function and behavior through a brain-derived neurotrophic factor-dependent mechanism. J. Neurosci. 28, 9857–9869. doi: 10.1523/JNEUROSCI.3145-08.2008
Brancato, A., Bregman, D., Ahn, H. F., Pfau, M. L., Menard, C., Cannizzaro, C., et al. (2017). Sub-chronic variable stress induces sex-specific effects on glutamatergic synapses in the nucleus accumbens. Neuroscience 350, 180–189. doi: 10.1016/j.neuroscience.2017.03.014
Caccamo, A., Maldonado, M. A., Bokov, A. F., Majumder, S., and Oddo, S. (2010). CBP gene transfer increases BDNF levels and ameliorates learning and memory deficits in a mouse model of Alzheimer’s disease. Proc. Natl. Acad. Sci. U.S.A. 107, 22687–22692. doi: 10.1073/pnas.1012851108
Chen, Z. Y., Bath, K., McEwen, B., Hempstead, B., and Lee, F. (2008). Impact of genetic variant BDNF (Val66Met) on brain structure and function. Novartis Found. Symp. 289, 180–188. doi: 10.1002/9780470751251.ch14
Chiaruttini, C., Vicario, A., Li, Z., Baj, G., Braiuca, P., Wu, Y., et al. (2009). Dendritic trafficking of BDNF mRNA is mediated by translin and blocked by the G196A (Val66Met) mutation. Proc. Natl. Acad. Sci. U.S.A. 106, 16481–16486. doi: 10.1073/pnas.0902833106
Cho, K. O., Skarnes, W. C., Minsk, B., Palmieri, S., Jackson-Grusby, L., and Wagner, J. A. (1989). Nerve growth factor regulates gene expression by several distinct mechanisms. Mol. Cell. Biol. 9, 135–143. doi: 10.1128/mcb.9.1.135-143.1989
Clevenger, S. S., Malhotra, D., Dang, J., Vanle, B., and IsHak, W. W. (2018). The role of selective serotonin reuptake inhibitors in preventing relapse of major depressive disorder. Ther. Adv. Psychopharmacol. 8, 49–58. doi: 10.1177/2045125317737264
Cocco, C., D’Amato, F., Noli, B., Ledda, A., Brancia, C., Bongioanni, P., et al. (2010). Distribution of VGF peptides in the human cortex and their selective changes in Parkinson’s and Alzheimer’s diseases. J. Anat. 217, 683–693. doi: 10.1111/j.1469-7580.2010.01309.x
Cohen, L. J., and Sclar, D. A. (2012). Issues in adherence to treatment with monoamine oxidase inhibitors and the rate of treatment failure. J. Clin. Psychiatry 73, 31–36. doi: 10.4088/JCP.11096su1c.05
Colle, R., Gressier, F., Verstuyft, C., Deflesselle, E., Lepine, J. P., Ferreri, F., et al. (2015b). Brain-derived neurotrophic factor Val66Met polymorphism and 6-month antidepressant remission in depressed Caucasian patients. J. Affect. Disord. 175, 233–240. doi: 10.1016/j.jad.2015.01.013
Colle, R., Deflesselle, E., Martin, S., David, D. J., Hardy, P., Taranu, A., et al. (2015a). BDNF/TRKB/P75NTR polymorphisms and their consequences on antidepressant efficacy in depressed patients. Pharmacogenomics 16, 997–1013. doi: 10.2217/pgs.15.56
Connor, B., Young, D., Yan, Q., Faull, R. L., Synek, B., and Dragunow, M. (1997). Brain-derived neurotrophic factor is reduced in Alzheimer’s disease. Brain Res. Mol. Brain Res. 49, 71–81. doi: 10.1016/s0169-328x(97)00125-3
Czeh, B., and Lucassen, P. J. (2007). What causes the hippocampal volume decrease in depression? Are neurogenesis, glial changes and apoptosis implicated? Eur. Arch. Psychiatry Clin. Neurosci. 257, 250–260. doi: 10.1007/s00406-007-0728-0
Deyama, S., Bang, E., Wohleb, E. S., Li, X. Y., Kato, T., Gerhard, D. M., et al. (2019b). Role of Neuronal VEGF Signaling in the Prefrontal Cortex in the Rapid Antidepressant Effects of Ketamine. Am. J. Psychiatry 176, 388–400. doi: 10.1176/appi.ajp.2018.17121368
Deyama, S., Bang, E., Kato, T., Li, X. Y., and Duman, R. S. (2019a). Neurotrophic and Antidepressant Actions of Brain-Derived Neurotrophic Factor Require Vascular Endothelial Growth Factor. Biol. Psychiatry 86, 143–152. doi: 10.1016/j.biopsych.2018.12.014
Dietz, D. M., Dietz, K. C., Nestler, E. J., and Russo, S. J. (2009). Molecular mechanisms of psychostimulant-induced structural plasticity. Pharmacopsychiatry 42, S69–S78. doi: 10.1055/s-0029-1202847
Dranovsky, A., and Hen, R. (2006). Hippocampal neurogenesis: regulation by stress and antidepressants. Biol. Psychiatry 59, 1136–1143. doi: 10.1016/j.biopsych.2006.03.082
Duman, R. S. (2004). Role of neurotrophic factors in the etiology and treatment of mood disorders. Neuromolecular Med. 5, 11–25. doi: 10.1385/NMM:5:1:011
Duman, R. S. (2005). Neurotrophic factors and regulation of mood: role of exercise, diet and metabolism. Neurobiol. Aging 26, 88–93. doi: 10.1016/j.neurobiolaging.2005.08.018
Duman, R. S., and Aghajanian, G. K. (2012). Synaptic dysfunction in depression: potential therapeutic targets. Science 338, 68–72. doi: 10.1126/science.1222939
Duman, R. S., Deyama, S., and Fogaca, M. V. (2021). Role of BDNF in the pathophysiology and treatment of depression: activity-dependent effects distinguish rapid-acting antidepressants. Eur. J. Neurosci. 53, 126–139. doi: 10.1111/ejn.14630
Duman, R. S., and Monteggia, L. M. (2006). A neurotrophic model for stress-related mood disorders. Biol. Psychiatry 59, 1116–1127. doi: 10.1016/j.biopsych.2006.02.013
Duric, V., and Duman, R. S. (2013). Depression and treatment response: dynamic interplay of signaling pathways and altered neural processes. Cell. Mol. Life Sci. 70, 39–53. doi: 10.1007/s00018-012-1020-7
Dwivedi, Y., Rizavi, H. S., Conley, R. R., Roberts, R. C., Tamminga, C. A., and Pandey, G. N. (2003). Altered gene expression of brain-derived neurotrophic factor and receptor tyrosine kinase B in postmortem brain of suicide subjects. Arch. Gen. Psychiatry 60, 804–815. doi: 10.1001/archpsyc.60.8.804
Egan, M. F., Kojima, M., Callicott, J. H., Goldberg, T. E., Kolachana, B. S., Bertolino, A., et al. (2003). The BDNF val66met polymorphism affects activity-dependent secretion of BDNF and human memory and hippocampal function. Cell 112, 257–269. doi: 10.1016/s0092-8674(03)00035-7
El Gaamouch, F., Audrain, M., Lin, W. J., Beckmann, N., Jiang, C., Hariharan, S., et al. (2020). VGF-derived peptide TLQP-21 modulates microglial function through C3aR1 signaling pathways and reduces neuropathology in 5xFAD mice. Mol. Neurodegener. 15:4. doi: 10.1186/s13024-020-0357-x
Fabel, K., Fabel, K., Tam, B., Kaufer, D., Baiker, A., Simmons, N., et al. (2003). VEGF is necessary for exercise-induced adult hippocampal neurogenesis. Eur. J. Neurosci. 18, 2803–2812. doi: 10.1111/j.1460-9568.2003.03041.x
Feng, Y., Rhodes, P. G., and Bhatt, A. J. (2011). Dexamethasone pre-treatment protects brain against hypoxic-ischemic injury partially through up-regulation of vascular endothelial growth factor A in neonatal rats. Neuroscience 179, 223–232. doi: 10.1016/j.neuroscience.2011.01.050
Fletcher, J. L., Dill, L. K., Wood, R. J., Wang, S., Robertson, K., Murray, S. S., et al. (2021). Acute treatment with TrkB agonist LM22A-4 confers neuroprotection and preserves myelin integrity in a mouse model of pediatric traumatic brain injury. Exp. Neurol. 339:113652. doi: 10.1016/j.expneurol.2021.113652
Fournier, N. M., and Duman, R. S. (2012). Role of vascular endothelial growth factor in adult hippocampal neurogenesis: implications for the pathophysiology and treatment of depression. Behav. Brain Res. 227, 440–449. doi: 10.1016/j.bbr.2011.04.022
Garcia, K. O., Ornellas, F. L., Martin, P. K., Patti, C. L., Mello, L. E., Frussa-Filho, R., et al. (2014). Therapeutic effects of the transplantation of VEGF overexpressing bone marrow mesenchymal stem cells in the hippocampus of murine model of Alzheimer’s disease. Front. Aging Neurosci. 6:30. doi: 10.3389/fnagi.2014.00030
Garcia, L. S., Comim, C. M., Valvassori, S. S., Reus, G. Z., Barbosa, L. M., Andreazza, A. C., et al. (2008). Acute administration of ketamine induces antidepressant-like effects in the forced swimming test and increases BDNF levels in the rat hippocampus. Prog. Neuropsychopharmacol. Biol. Psychiatry 32, 140–144. doi: 10.1016/j.pnpbp.2007.07.027
Gibson, J., Russ, T. C., Adams, M. J., Clarke, T. K., Howard, D. M., Hall, L. S., et al. (2017). Assessing the presence of shared genetic architecture between Alzheimer’s disease and major depressive disorder using genome-wide association data. Transl. Psychiatry 7:e1094. doi: 10.1038/tp.2017.49
Gozes, I. (2021). A Different Outlook at Psychiatric and Neurological Diseases: brain Somatic Mutations Are Implicated in Schizophrenia. Biol. Psychiatry 90, 6–8. doi: 10.1016/j.biopsych.2021.04.013
Greenberg, P. E., Fournier, A. A., Sisitsky, T., Simes, M., Berman, R., Koenigsberg, S. H., et al. (2021). The Economic Burden of Adults with Major Depressive Disorder in the United States (2010 and 2018). Pharmacoeconomics 39, 653–665. doi: 10.1007/s40273-021-01019-4
Gyekis, J. P., Yu, W., Dong, S., Wang, H., Qian, J., Kota, P., et al. (2013). No association of genetic variants in BDNF with major depression: a meta- and gene-based analysis. Am. J. Med. Genet. B Neuropsychiatr. Genet. 162B, 61–70. doi: 10.1002/ajmg.b.32122
Hadar, A., Kapitansky, O., Ganaiem, M., Sragovich, S., Lobyntseva, A., Giladi, E., et al. (2021). Introducing ADNP and SIRT1 as new partners regulating microtubules and histone methylation. Mol. Psychiatry 26, 6550–6561. doi: 10.1038/s41380-021-01143-9
Hahm, S., Fekete, C., Mizuno, T. M., Windsor, J., Yan, H., Boozer, C. N., et al. (2002). VGF is required for obesity induced by diet, gold thioglucose treatment, and agouti and is differentially regulated in pro-opiomelanocortin- and neuropeptide Y-containing arcuate neurons in response to fasting. J. Neurosci. 22, 6929–6938. doi: 10.1523/JNEUROSCI.22-16-06929.2002
Hahm, S., Mizuno, T. M., Wu, T. J., Wisor, J. P., Priest, C. A., Kozak, C. A., et al. (1999). Targeted deletion of the Vgf gene indicates that the encoded secretory peptide precursor plays a novel role in the regulation of energy balance. Neuron 23, 537–548. doi: 10.1016/s0896-6273(00)80806-5
Hendrickson, R. C., Lee, A. Y., Song, Q., Liaw, A., Wiener, M., Paweletz, C. P., et al. (2015). High Resolution Discovery Proteomics Reveals Candidate Disease Progression Markers of Alzheimer’s Disease in Human Cerebrospinal Fluid. PLoS One 10:e0135365. doi: 10.1371/journal.pone.0135365
Hirschfeld, R. M. (2000). History and evolution of the monoamine hypothesis of depression. J. Clin. Psychiatry 61, 4–6.
Hock, C., Heese, K., Hulette, C., Rosenberg, C., and Otten, U. (2000). Region-specific neurotrophin imbalances in Alzheimer disease: decreased levels of brain-derived neurotrophic factor and increased levels of nerve growth factor in hippocampus and cortical areas. Arch. Neurol. 57, 846–851. doi: 10.1001/archneur.57.6.846
Holtta, M., Minthon, L., Hansson, O., Holmen-Larsson, J., Pike, I., Ward, M., et al. (2015). An integrated workflow for multiplex CSF proteomics and peptidomics-identification of candidate cerebrospinal fluid biomarkers of Alzheimer’s disease. J. Proteome Res. 14, 654–663. doi: 10.1021/pr501076j
Hunsberger, J. G., Newton, S. S., Bennett, A. H., Duman, C. H., Russell, D. S., Salton, S. R., et al. (2007). Antidepressant actions of the exercise-regulated gene VGF. Nat. Med. 13, 1476–1482. doi: 10.1038/nm1669
Ivashko-Pachima, Y., Ganaiem, M., Ben-Horin-Hazak, I., Lobyntseva, A., Bellaiche, N., Fischer, I., et al. (2022). SH3- and actin-binding domains connect ADNP and SHANK3, revealing a fundamental shared mechanism underlying autism. Mol. Psychiatry [Epub ahead of print]. doi: 10.1038/s41380-022-01603-w
Ivashko-Pachima, Y., Hadar, A., Grigg, I., Korenkova, V., Kapitansky, O., Karmon, G., et al. (2021). Discovery of autism/intellectual disability somatic mutations in Alzheimer’s brains: mutated ADNP cytoskeletal impairments and repair as a case study. Mol. Psychiatry 26, 1619–1633. doi: 10.1038/s41380-019-0563-5
Jahn, H., Wittke, S., Zurbig, P., Raedler, T. J., Arlt, S., Kellmann, M., et al. (2011). Peptide fingerprinting of Alzheimer’s disease in cerebrospinal fluid: identification and prospective evaluation of new synaptic biomarkers. PLoS One 6:e26540. doi: 10.1371/journal.pone.0026540
Jefferies, W. A., Price, K. A., Biron, K. E., Fenninger, F., Pfeifer, C. G., and Dickstein, D. L. (2013). Adjusting the compass: new insights into the role of angiogenesis in Alzheimer’s disease. Alzheimers Res. Ther. 5:64. doi: 10.1186/alzrt230
Jiang, C., Lin, W. J., and Salton, S. R. (2019b). Role of a VGF/BDNF/TrkB Autoregulatory Feedback Loop in Rapid-Acting Antidepressant Efficacy. J. Mol. Neurosci. 68, 504–509. doi: 10.1007/s12031-018-1124-0
Jiang, C., Lin, W. J., Labonte, B., Tamminga, C. A., Turecki, G., Nestler, E. J., et al. (2019a). VGF and its C-terminal peptide TLQP-62 in ventromedial prefrontal cortex regulate depression-related behaviors and the response to ketamine. Neuropsychopharmacology 44, 971–981. doi: 10.1038/s41386-018-0277-4
Jiang, C., Lin, W. J., Sadahiro, M., Labonte, B., Menard, C., Pfau, M. L., et al. (2018). VGF function in depression and antidepressant efficacy. Mol. Psychiatry 23, 1632–1642. doi: 10.1038/mp.2017.233
Karege, F., Vaudan, G., Schwald, M., Perroud, N., and La Harpe, R. (2005). Neurotrophin levels in postmortem brains of suicide victims and the effects of antemortem diagnosis and psychotropic drugs. Brain Res. Mol. Brain Res. 136, 29–37. doi: 10.1016/j.molbrainres.2004.12.020
Kim, M. H., Kim, I. B., Lee, J., Cha, D. H., Park, S. M., Kim, J. H., et al. (2021). Low-Level Brain Somatic Mutations Are Implicated in Schizophrenia. Biol. Psychiatry 90, 35–46. doi: 10.1016/j.biopsych.2021.01.014
Kishi, T., Yoshimura, R., Ikuta, T., and Iwata, N. (2017). Brain-Derived Neurotrophic Factor and Major Depressive Disorder: evidence from Meta-Analyses. Front. Psychiatry 8:308. doi: 10.3389/fpsyt.2017.00308
Krogh, J., Rostrup, E., Thomsen, C., Elfving, B., Videbech, P., and Nordentoft, M. (2014). The effect of exercise on hippocampal volume and neurotrophines in patients with major depression–a randomized clinical trial. J. Affect. Disord. 165, 24–30. doi: 10.1016/j.jad.2014.04.041
Lee, Y., Lim, S. W., Kim, S. Y., Chung, J. W., Kim, J., Myung, W., et al. (2013). Association between the BDNF Val66Met Polymorphism and Chronicity of Depression. Psychiatry Investig. 10, 56–61. doi: 10.4306/pi.2013.10.1.56
Levi, A., Eldridge, J. D., and Paterson, B. M. (1985). Molecular cloning of a gene sequence regulated by nerve growth factor. Science 229, 393–395. doi: 10.1126/science.3839317
Levi, A., Ferri, G. L., Watson, E., Possenti, R., and Salton, S. R. (2004). Processing, distribution, and function of VGF, a neuronal and endocrine peptide precursor. Cell. Mol. Neurobiol. 24, 517–533. doi: 10.1023/b:cemn.0000023627.79947.22
Li, C., Li, M., Yu, H., Shen, X., Wang, J., Sun, X., et al. (2017). Neuropeptide VGF C-Terminal Peptide TLQP-62 Alleviates Lipopolysaccharide-Induced Memory Deficits and Anxiety-like and Depression-like Behaviors in Mice: the Role of BDNF/TrkB Signaling. ACS Chem. Neurosci. 8, 2005–2018. doi: 10.1021/acschemneuro.7b00154
Lin, P., Wang, C., Xu, B., Gao, S., Guo, J., Zhao, X., et al. (2014). The VGF-derived peptide TLQP62 produces antidepressant-like effects in mice via the BDNF/TrkB/CREB signaling pathway. Pharmacol. Biochem. Behav. 120, 140–148. doi: 10.1016/j.pbb.2014.03.003
Lin, W. J., Jiang, C., Sadahiro, M., Bozdagi, O., Vulchanova, L., Alberini, C. M., et al. (2015). VGF and Its C-Terminal Peptide TLQP-62 Regulate Memory Formation in Hippocampus via a BDNF-TrkB-Dependent Mechanism. J. Neurosci. 35, 10343–10356. doi: 10.1523/JNEUROSCI.0584-15.2015
Lin, W. J., Zhao, Y., Li, Z., Zheng, S., Zou, J. L., Warren, N. A., et al. (2021). An increase in VGF expression through a rapid, transcription-independent, autofeedback mechanism improves cognitive function. Transl. Psychiatry 11:383. doi: 10.1038/s41398-021-01489-2
Lindholm, D., Castren, E., Berzaghi, M., Blochl, A., and Thoenen, H. (1994). Activity-dependent and hormonal regulation of neurotrophin mRNA levels in the brain–implications for neuronal plasticity. J. Neurobiol. 25, 1362–1372. doi: 10.1002/neu.480251105
Llano, D. A., Devanarayan, P., Devanarayan, V., and Alzheimer’s Disease Neuroimaging, I. (2019). VGF in Cerebrospinal Fluid Combined With Conventional Biomarkers Enhances Prediction of Conversion From MCI to AD. Alzheimer Dis. Assoc. Disord. 33, 307–314. doi: 10.1097/WAD.0000000000000328
Lu, Y., Wang, C., Xue, Z., Li, C., Zhang, J., Zhao, X., et al. (2014). PI3K/AKT/mTOR signaling-mediated neuropeptide VGF in the hippocampus of mice is involved in the rapid onset antidepressant-like effects of GLYX-13. Int. J. Neuropsychopharmacol. 18:yu110. doi: 10.1093/ijnp/pyu110
Lv, D., Chen, Y., Shen, M., Liu, X., Zhang, Y., Xu, J., et al. (2018). Mechanisms underlying the rapid-acting antidepressant-like effects of neuropeptide VGF (non-acronymic) C-terminal peptide TLQP-62. Neuropharmacology 143, 317–326. doi: 10.1016/j.neuropharm.2018.09.046
Martin-Sanchez, A., Pinero, J., Nonell, L., Arnal, M., Ribe, E. M., Nevado-Holgado, A., et al. (2021). Comorbidity between Alzheimer’s disease and major depression: a behavioural and transcriptomic characterization study in mice. Alzheimers Res. Ther. 13:73. doi: 10.1186/s13195-021-00810-x
Massa, S. M., Yang, T., Xie, Y., Shi, J., Bilgen, M., Joyce, J. N., et al. (2010). Small molecule BDNF mimetics activate TrkB signaling and prevent neuronal degeneration in rodents. J. Clin. Invest. 120, 1774–1785. doi: 10.1172/JCI41356
Matrone, C., Ciotti, M. T., Mercanti, D., Marolda, R., and Calissano, P. (2008). NGF and BDNF signaling control amyloidogenic route and Abeta production in hippocampal neurons. Proc. Natl. Acad. Sci. U.S.A. 105, 13139–13144. doi: 10.1073/pnas.0806133105
McCloskey, D. P., Croll, S. D., and Scharfman, H. E. (2005). Depression of synaptic transmission by vascular endothelial growth factor in adult rat hippocampus and evidence for increased efficacy after chronic seizures. J. Neurosci. 25, 8889–8897. doi: 10.1523/JNEUROSCI.2577-05.2005
McCullough, J. P. Jr., Klein, D. N., Keller, M. B., Holzer, C. E. III, Davis, S. M., Kornstein, S. G., et al. (2000). Comparison of DSM-III-R chronic major depression and major depression superimposed on dysthymia (double depression): validity of the distinction. J. Abnorm. Psychol. 109, 419–427.
Merenlender-Wagner, A., Malishkevich, A., Shemer, Z., Udawela, M., Gibbons, A., Scarr, E., et al. (2015). Autophagy has a key role in the pathophysiology of schizophrenia. Mol. Psychiatry 20, 126–132. doi: 10.1038/mp.2013.174
Merenlender-Wagner, A., Shemer, Z., Touloumi, O., Lagoudaki, R., Giladi, E., Andrieux, A., et al. (2014). New horizons in schizophrenia treatment: autophagy protection is coupled with behavioral improvements in a mouse model of schizophrenia. Autophagy 10, 2324–2332. doi: 10.4161/15548627.2014.984274
Nagahara, A. H., and Tuszynski, M. H. (2011). Potential therapeutic uses of BDNF in neurological and psychiatric disorders. Nat. Rev. Drug Discov. 10, 209–219. doi: 10.1038/nrd3366
Nibuya, M., Takahashi, M., Russell, D. S., and Duman, R. S. (1999). Repeated stress increases catalytic TrkB mRNA in rat hippocampus. Neurosci. Lett. 267, 81–84. doi: 10.1016/s0304-3940(99)00335-3
Nowacka, M., and Obuchowicz, E. (2013). BDNF and VEGF in the pathogenesis of stress-induced affective diseases: an insight from experimental studies. Pharmacol. Rep. 65, 535–546. doi: 10.1016/s1734-1140(13)71031-4
Otte, C., Gold, S. M., Penninx, B. W., Pariante, C. M., Etkin, A., Fava, M., et al. (2016). Major depressive disorder. Nat. Rev. Dis. Primers 2:16065. doi: 10.1038/nrdp.2016.65
Park, J. S., Lee, J., Jung, E. S., Kim, M. H., Kim, I. B., Son, H., et al. (2019). Brain somatic mutations observed in Alzheimer’s disease associated with aging and dysregulation of tau phosphorylation. Nat. Commun. 10:3090. doi: 10.1038/s41467-019-11000-7
Perini, G., Cotta Ramusino, M., Sinforiani, E., Bernini, S., Petrachi, R., and Costa, A. (2019). Cognitive impairment in depression: recent advances and novel treatments. Neuropsychiatr. Dis. Treat. 15, 1249–1258. doi: 10.2147/NDT.S199746
Ploughman, M. (2008). Exercise is brain food: the effects of physical activity on cognitive function. Dev. Neurorehabil. 11, 236–240. doi: 10.1080/17518420801997007
Porcelli, S., Salfi, R., Politis, A., Atti, A. R., Albani, D., Chierchia, A., et al. (2013). Association between Sirtuin 2 gene rs10410544 polymorphism and depression in Alzheimer’s disease in two independent European samples. J. Neural. Transm. 120, 1709–1715. doi: 10.1007/s00702-013-1045-6
Pu, J., Liu, Y., Gui, S., Tian, L., Xu, S., Song, X., et al. (2020). Vascular endothelial growth factor in major depressive disorder, schizophrenia, and bipolar disorder: a network meta-analysis. Psychiatry Res. 292, 113319. doi: 10.1016/j.psychres.2020.113319
Quinn, J. P., Kandigian, S. E., Trombetta, B. A., Arnold, S. E., and Carlyle, B. C. (2021). VGF as a biomarker and therapeutic target in neurodegenerative and psychiatric diseases. Brain Commun. 3:fcab261. doi: 10.1093/braincomms/fcab261
Rantamaki, T., and Castren, E. (2008). Targeting TrkB neurotrophin receptor to treat depression. Expert Opin. Ther. Targets 12, 705–715. doi: 10.1517/14728222.12.6.705
Rasmusson, A. M., Shi, L., and Duman, R. (2002). Downregulation of BDNF mRNA in the hippocampal dentate gyrus after re-exposure to cues previously associated with footshock. Neuropsychopharmacology 27, 133–142. doi: 10.1016/S0893-133X(02)00286-5
Reichardt, L. F. (2006). Neurotrophin-regulated signalling pathways. Philos. Trans. R. Soc. Lond. B Biol. Sci. 361, 1545–1564. doi: 10.1098/rstb.2006.1894
Religa, P., Cao, R., Religa, D., Xue, Y., Bogdanovic, N., Westaway, D., et al. (2013). VEGF significantly restores impaired memory behavior in Alzheimer’s mice by improvement of vascular survival. Sci. Rep. 3:2053. doi: 10.1038/srep02053
Rodrigues, R., Petersen, R. B., and Perry, G. (2014). Parallels between major depressive disorder and Alzheimer’s disease: role of oxidative stress and genetic vulnerability. Cell. Mol. Neurobiol. 34, 925–949. doi: 10.1007/s10571-014-0074-5
Ruetschi, U., Zetterberg, H., Podust, V. N., Gottfries, J., Li, S., Hviid Simonsen, A., et al. (2005). Identification of CSF biomarkers for frontotemporal dementia using SELDI-TOF. Exp. Neurol. 196, 273–281. doi: 10.1016/j.expneurol.2005.08.002
Salton, S. R., Ferri, G. L., Hahm, S., Snyder, S. E., Wilson, A. J., Possenti, R., et al. (2000). VGF: a novel role for this neuronal and neuroendocrine polypeptide in the regulation of energy balance. Front. Neuroendocrinol. 21:199–219. doi: 10.1006/frne.2000.0199
Salton, S. R., Fischberg, D. J., and Dong, K. W. (1991). Structure of the gene encoding VGF, a nervous system-specific mRNA that is rapidly and selectively induced by nerve growth factor in PC12 cells. Mol. Cell. Biol. 11, 2335–2349. doi: 10.1128/mcb.11.5.2335-2349.1991
Sapolsky, R. M. (2000). The possibility of neurotoxicity in the hippocampus in major depression: a primer on neuron death. Biol. Psychiatry 48, 755–765. doi: 10.1016/s0006-3223(00)00971-9
Schmidt, H. D., Banasr, M., and Duman, R. S. (2008). Future Antidepressant Targets: neurotrophic Factors and Related Signaling Cascades. Drug Discov. Today Ther. Strateg. 5, 151–156. doi: 10.1016/j.ddstr.2008.10.003
Sheline, Y. I. (2000). 3D MRI studies of neuroanatomic changes in unipolar major depression: the role of stress and medical comorbidity. Biol. Psychiatry 48, 791–800. doi: 10.1016/s0006-3223(00)00994-x
Shi, S. S., Shao, S. H., Yuan, B. P., Pan, F., and Li, Z. L. (2010). Acute stress and chronic stress change brain-derived neurotrophic factor (BDNF) and tyrosine kinase-coupled receptor (TrkB) expression in both young and aged rat hippocampus. Yonsei Med. J. 51, 661–671. doi: 10.3349/ymj.2010.51.5.661
Shirayama, Y., Yang, C., Zhang, J. C., Ren, Q., Yao, W., and Hashimoto, K. (2015). Alterations in brain-derived neurotrophic factor (BDNF) and its precursor proBDNF in the brain regions of a learned helplessness rat model and the antidepressant effects of a TrkB agonist and antagonist. Eur. Neuropsychopharmacol. 25, 2449–2458. doi: 10.1016/j.euroneuro.2015.09.002
Smith, M. A., Makino, S., Kvetnansky, R., and Post, R. M. (1995). Stress and glucocorticoids affect the expression of brain-derived neurotrophic factor and neurotrophin-3 mRNAs in the hippocampus. J. Neurosci. 15, 1768–1777. doi: 10.1523/JNEUROSCI.15-03-01768.1995
Snyder, S. E., and Salton, S. R. (1998). Expression of VGF mRNA in the adult rat central nervous system. J. Comp. Neurol. 394, 91–105.
Spellman, D. S., Wildsmith, K. R., Honigberg, L. A., Tuefferd, M., Baker, D., Raghavan, N., et al. (2015). Development and evaluation of a multiplexed mass spectrometry based assay for measuring candidate peptide biomarkers in Alzheimer’s Disease Neuroimaging Initiative (ADNI) CSF. Proteomics Clin. Appl. 9, 715–731. doi: 10.1002/prca.201400178
Sragovich, S., Merenlender-Wagner, A., and Gozes, I. (2017). ADNP Plays a Key Role in Autophagy: from Autism to Schizophrenia and Alzheimer’s Disease. Bioessays 39:1700054. doi: 10.1002/bies.201700054
Sullivan, P. F., Daly, M. J., and O’Donovan, M. (2012). Genetic architectures of psychiatric disorders: the emerging picture and its implications. Nat. Rev. Genet. 13, 537–551. doi: 10.1038/nrg3240
Tasaki, S., Gaiteri, C., Mostafavi, S., De Jager, P. L., and Bennett, D. A. (2018). The Molecular and Neuropathological Consequences of Genetic Risk for Alzheimer’s Dementia. Front. Neurosci. 12:699. doi: 10.3389/fnins.2018.00699
Thakker-Varia, S., Behnke, J., Doobin, D., Dalal, V., Thakkar, K., Khadim, F., et al. (2014). VGF (TLQP-62)-induced neurogenesis targets early phase neural progenitor cells in the adult hippocampus and requires glutamate and BDNF signaling. Stem Cell Res. 12, 762–777. doi: 10.1016/j.scr.2014.03.005
Thakker-Varia, S., Krol, J. J., Nettleton, J., Bilimoria, P. M., Bangasser, D. A., Shors, T. J., et al. (2007). The neuropeptide VGF produces antidepressant-like behavioral effects and enhances proliferation in the hippocampus. J. Neurosci. 27, 12156–12167. doi: 10.1523/JNEUROSCI.1898-07.2007
Tsang, R. S., Mather, K. A., Sachdev, P. S., and Reppermund, S. (2017). Systematic review and meta-analysis of genetic studies of late-life depression. Neurosci. Biobehav. Rev. 75, 129–139. doi: 10.1016/j.neubiorev.2017.01.028
van Steenoven, I., Koel-Simmelink, M. J. A., Vergouw, L. J. M., Tijms, B. M., Piersma, S. R., Pham, T. V., et al. (2020). Identification of novel cerebrospinal fluid biomarker candidates for dementia with Lewy bodies: a proteomic approach. Mol. Neurodegener. 15:36. doi: 10.1186/s13024-020-00388-2
Verhagen, M., van der Meij, A., van Deurzen, P. A., Janzing, J. G., Arias-Vasquez, A., Buitelaar, J. K., et al. (2010). Meta-analysis of the BDNF Val66Met polymorphism in major depressive disorder: effects of gender and ethnicity. Mol. Psychiatry 15, 260–271. doi: 10.1038/mp.2008.109
Wang, P., Xie, Z. H., Guo, Y. J., Zhao, C. P., Jiang, H., Song, Y., et al. (2011). VEGF-induced angiogenesis ameliorates the memory impairment in APP transgenic mouse model of Alzheimer’s disease. Biochem. Biophys. Res. Commun. 411, 620–626. doi: 10.1016/j.bbrc.2011.07.003
Warner-Schmidt, J. L., and Duman, R. S. (2006). Hippocampal neurogenesis: opposing effects of stress and antidepressant treatment. Hippocampus 16, 239–249. doi: 10.1002/hipo.20156
Wijte, D., McDonnell, L. A., Balog, C. I., Bossers, K., Deelder, A. M., Swaab, D. F., et al. (2012). A novel peptidomics approach to detect markers of Alzheimer’s disease in cerebrospinal fluid. Methods 56, 500–507. doi: 10.1016/j.ymeth.2012.03.018
Wong, M. L., and Licinio, J. (2001). Research and treatment approaches to depression. Nat. Rev. Neurosci. 2, 343–351. doi: 10.1038/35072566
Wurzelmann, M., Romeika, J., and Sun, D. (2017). Therapeutic potential of brain-derived neurotrophic factor (BDNF) and a small molecular mimics of BDNF for traumatic brain injury. Neural Regen. Res. 12, 7–12. doi: 10.4103/1673-5374.198964
Youssef, M. M., Underwood, M. D., Huang, Y. Y., Hsiung, S. C., Liu, Y., Simpson, N. R., et al. (2018). Association of BDNF Val66Met Polymorphism and Brain BDNF Levels with Major Depression and Suicide. Int. J. Neuropsychopharmacol. 21, 528–538. doi: 10.1093/ijnp/pyy008
Zhang, J. C., Yao, W., Dong, C., Yang, C., Ren, Q., Ma, M., et al. (2015). Comparison of ketamine, 7,8-dihydroxyflavone, and ANA-12 antidepressant effects in the social defeat stress model of depression. Psychopharmacology 232, 4325–4335. doi: 10.1007/s00213-015-4062-3
Zhao, Z., Lange, D. J., Ho, L., Bonini, S., Shao, B., Salton, S. R., et al. (2008). Vgf is a novel biomarker associated with muscle weakness in amyotrophic lateral sclerosis (ALS), with a potential role in disease pathogenesis. Int. J. Med. Sci. 5, 92–99. doi: 10.7150/ijms.5.92
Keywords: Alzheimer’s disease, TLQP-62, TrkB, VGF, MDD (major depressive disorder), BDNF (brain derived neurotrophic factor), VEGF – vascular endothelial growth factor, ADNP (activity dependent neuroprotective protein)
Citation: Joshi R and Salton SRJ (2022) Neurotrophin Crosstalk in the Etiology and Treatment of Neuropsychiatric and Neurodegenerative Disease. Front. Mol. Neurosci. 15:932497. doi: 10.3389/fnmol.2022.932497
Received: 29 April 2022; Accepted: 23 June 2022;
Published: 15 July 2022.
Edited by:
Satoshi Deyama, Kanazawa University, JapanReviewed by:
Illana Gozes, Tel Aviv University, IsraelCopyright © 2022 Joshi and Salton. This is an open-access article distributed under the terms of the Creative Commons Attribution License (CC BY). The use, distribution or reproduction in other forums is permitted, provided the original author(s) and the copyright owner(s) are credited and that the original publication in this journal is cited, in accordance with accepted academic practice. No use, distribution or reproduction is permitted which does not comply with these terms.
*Correspondence: Stephen R. J. Salton, c3RlcGhlbi5zYWx0b25AbXNzbS5lZHU=
Disclaimer: All claims expressed in this article are solely those of the authors and do not necessarily represent those of their affiliated organizations, or those of the publisher, the editors and the reviewers. Any product that may be evaluated in this article or claim that may be made by its manufacturer is not guaranteed or endorsed by the publisher.
Research integrity at Frontiers
Learn more about the work of our research integrity team to safeguard the quality of each article we publish.