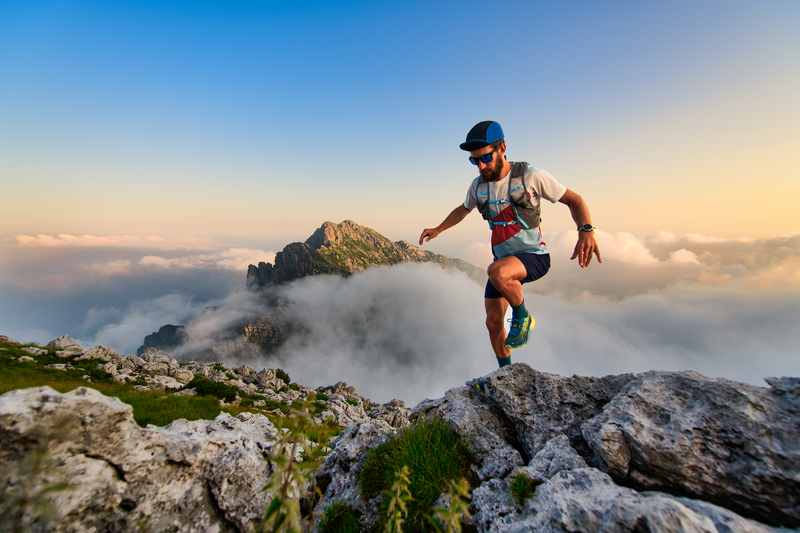
95% of researchers rate our articles as excellent or good
Learn more about the work of our research integrity team to safeguard the quality of each article we publish.
Find out more
MINI REVIEW article
Front. Mol. Neurosci. , 15 June 2022
Sec. Brain Disease Mechanisms
Volume 15 - 2022 | https://doi.org/10.3389/fnmol.2022.931704
This article is part of the Research Topic RNA at a Breaking Point? Cytoplasmic Cleavage and other Post-Transcriptional RNA Processing in Neurodevelopment and Disease View all 18 articles
Central nervous system (CNS) disorders, such as ischemic stroke, Alzheimer’s disease, Parkinson’s disease, spinal cord injury, glioma, and epilepsy, involve oxidative stress and neuronal apoptosis, often leading to long-term disability or death. Emerging studies suggest that oxidative stress may induce epigenetic modifications that contribute to CNS disorders. Non-coding RNAs are epigenetic regulators involved in CNS disorders and have attracted extensive attention. Long non-coding RNAs (lncRNAs) are non-coding RNAs more than 200 nucleotides long and have no protein-coding function. However, these molecules exert regulatory functions at the transcriptional, post-transcriptional, and epigenetic levels. However, the major role of lncRNAs in the pathophysiology of CNS disorders, especially related to oxidative stress, remains unclear. Here, we review the molecular functions of lncRNAs in oxidative stress and highlight lncRNAs that exert positive or negative roles in oxidation/antioxidant systems. This review provides novel insights into the therapeutic potential of lncRNAs that mediate oxidative stress in CNS disorders.
Central nervous system (CNS) disorders, such as acute ischemic stroke (AIS), Alzheimer’s disease (AD), Parkinson’s disease (PD), spinal cord injury (SCI), glioma, and epilepsy, usually lead to serious clinical consequences, long-term disability, or death (Xu et al., 2021). Several pathological processes involved in CNS disorders, including neuroinflammation, mitochondrial dysfunction, apoptosis, oxidative stress, and autophagy result in impaired CNS structure and dysfunction (Anderson et al., 2016; Khoshnam et al., 2017). In these pathological processes, oxidative stress plays a pivotal role in each disease (Scannevin et al., 2012). Oxidative stress refers to a pathological state in which free radicals in the body exceed its antioxidant capacity. Due to the redox imbalance, excessive reactive oxygen species (ROS) and reactive nitrogen (RNS) are generated, leading to Fenton reactions occurring via the action of metal ions to form hydroxyl radicals (⋅OH) (Sies, 2015; Sinha and Dabla, 2015). Excessive ROS induces lipid peroxidation and DNA, RNA, and protein oxidation, leading to neuronal dysfunction and death (Chen et al., 2011; Ouyang et al., 2015). Previous studies suggest that patients with higher concentrations of lipid peroxidation mediators have worse prognosis in CNS disorders, such as in AIS and AD (Klimiuk et al., 2019; Maciejczyk et al., 2020). Therefore, considering that hyperactive oxidative stress responses arise after the occurrence of CNS disorders, finding effective strategies to modulate oxidative stress in the CNS is important for restricting oxidative injuries and protecting neurological function (Costa et al., 2016).
Emerging studies suggest that oxidative stress may induce epigenetic modifications that ultimately lead to CNS disorders (Zhao et al., 2016). Non-coding RNAs have attracted extensive attention as epigenetic regulators involved in CNS disorders (Wu and Kuo, 2020). Long non-coding RNAs (LncRNAs) are a class of RNAs more than 200 nucleotides long but have no protein-coding function. These molecules were initially considered as a transcription by-product without important biological functions. However, numerous studies found that lncRNAs serve as an important “medium” in cells (Hombach and Kretz, 2016). LncRNAs are involved in the regulation of cell proliferation, differentiation, the cell cycle, and apoptosis at the transcriptional, post-transcriptional, and epigenetic levels. Furthermore, a few annotated lncRNAs play an important role in oxidative stress-related diseases and CNS disorders (Chu et al., 2022). For instance, elevated levels of the lncRNA rhabdomyosarcoma 2 related transcript (RMST) augment AIS by reducing microRNA (miR)-221-3p-mediated regulation of phosphoinositide-3-kinase regulatory subunit 1 (PIK3R1) and activating the transforming growth factor-β (TGF-β) pathway (Li et al., 2022). In contrast, knocking down lncRNA Gm11974 attenuates neuronal injury in AIS by modulating the miR-122-5p/semaphorin 3A (SEMA3A) signaling pathway (Yang et al., 2021). These results reveal that lncRNAs exert a positive or negative role in oxidative stress responses and suggest that lncRNAs may be key molecules involved in oxidative stress.
Despite recent advances, the role of lncRNAs and their downstream regulatory networks in regulating oxidative stress remains unclear. In this review, we collect existing evidence and discuss the characteristics of lncRNAs and their involvement in the oxidative/antioxidant system in different CNS disorders including AIS, neurodegenerative diseases, traumatic diseases, epilepsy, and glioma. Moreover, we discuss the potential molecular mechanisms involved in the regulation of oxidative stress, which may provide new insights into potential therapeutic lncRNA targets in CNS disorders that mediate oxidative stress.
Oxidative stress occurs when the physiological balance between oxidants and antioxidants is disrupted, which shifts the balance to favor oxidants and results in potential damage to the body (Sies et al., 2017). Oxidative stress and the related inflammatory responses, autophagy and apoptosis, are key factors involved in CNS disorders (Hybertson et al., 2011). ROS are continuously produced by all aerobic organisms through both enzymatic and non-enzymatic reactions. The most common ROS include superoxide anion radicals (O2⋅–), hydroxyl radicals (⋅OH), hydrogen peroxide (H2O2), nitric oxide (NO), and nitrite peroxide (ONOO–). In humans, the major ROS sources are mitochondria and various ROS-producing enzymes, including nicotinamide adenine dinucleotide phosphate (NADPH) oxidase (NOX), xanthine oxidase (XO), nitric oxide synthase (NOS), and myeloperoxidase (MPO) (Pizzino et al., 2017; Jakubczyk et al., 2020). The CNS has a relatively poor antioxidant defense due to its high oxygen consumption and high polyunsaturated fatty acid levels. At the physiological level, neurons are repaired through their own antioxidant defense system, which prevents neuronal oxidative damage (Salim, 2017). When excessive ROS production exceeds the repair capacity of the endogenous antioxidant system, biological macromolecules (such as lipids, proteins, and nucleic acids) undergo oxidative damage and can even activate apoptosis. Endogenous antioxidant enzymes such as superoxide dismutase (SOD), catalase (CAT) and glutathione peroxidase (GPx), and non-enzymatic antioxidants such as glutathione, ubiquinone, and ascorbic acid (vitamin C) help maintain cellular redox homeostasis (Figure 1; Rodrigo et al., 2013; Salim, 2017).
Among the antioxidant defense mechanisms, nuclear factor-erythroid related factor-2 (Nrf2) is a master regulator of transcriptional activation in antioxidant effects and can balance ROS production. Under oxidative stress, Nrf2 dissociates from Kelch-like Ech-associated protein 1 (Keap1) and translocates into the nucleus, where it binds to antioxidant response elements (ARE) (Silva-Palacios et al., 2018), thereby activating downstream antioxidant defense enzymes such as NAD(P)H quinone dehydrogenase 1 (NQO1), heme oxygenase 1 (HO-1), glutathione S-transferase (GST), and enzymes involved in glutathione synthesis and metabolism (γ-glutamyl cysteine synthetase) (Yamamoto et al., 2018; Baird and Yamamoto, 2020). Unfortunately, decreased antioxidant proteins have been found in many CNS disorders. Recent findings on the association of lncRNAs with oxidative stress may provide new ideas for explaining this phenomenon.
LncRNAs account for 80–90% of all ncRNAs. Compared with other ncRNAs such as microRNAs (miRs) and circular RNAs, lncRNAs have longer sequences, more complex spatial structures, and more diverse and complex mechanisms involved in the regulation of gene expression (Esteller, 2011). LncRNAs generally have similar characteristics to protein-coding genes, but tend to contain only one intron and have a low tendency for co-transcriptional splicing. Similar to mRNA, lncRNAs are alternately spliced and are primarily transcribed by RNA polymerase II, with about half having 5′-Cap and 3′-polyadenosine structures. LncRNAs also have a special secondary structure that provides several protein and DNA/RNA binding sites (Wei et al., 2018). Thus, lncRNAs regulate gene expression in various ways: (i) by interfering with transcription factor binding to target genes; (ii) interacting with small RNA; (iii) binding to proteins and acting as ribonuclein scaffolds; (iv) binding to chromatin to regulate chromatin remodeling; (v) and binding mRNA and affecting translation, shearing, and degradation (Shi et al., 2013; Deniz and Erman, 2017). LncRNAs have differences in size, molecular partners, and mechanism of action. According to their relative position and host protein-coding genes, lncRNAs can be divided into exons, introns, overlapping lncRNAs, and intergenic lncRNAs (Figure 2; Tan et al., 2021).
Figure 2. lncRNAs are classified into four categories by their location relative to neighboring protein-coding genes. (A) Exon lncRNAs, (B) intron lncRNAs, (C) overlapping lncRNAs, and (D) intergenic lncRNAs.
There is no clear, unified standard for lncRNA classification. Indeed, lncRNA can be basically divided into the following categories based on their relative positions with encoding genes: intergenic lncRNA (lincRNA), sense lncRNA, antisense lncRNA, untranslated lncRNA, promoter-related lncRNA (pancRNA), introns lncRNA (intronic RNA), and enhancing lncRNA. The position of the lncRNA in the genome often determines its regulating mechanism and related functions (Chen et al., 2021a). In addition, lncRNAs are roughly classified into four categories according to their roles: signal molecules, decoy molecules, guide molecules, and scaffold molecules (Figure 3; Wang and Chang, 2011). As signal molecules, lncRNAs participate in the conduction of some signal pathways. Some lncRNAs regulate the transcription of downstream genes and reflect their spatiotemporal expression. As decoy molecules, lncRNAs can combine with and remove some transcription factors to regulate gene expression. As guide molecules, lncRNAs can recruit cis- or trans- genes for chromatin modification enzymes. As scaffold molecules, lncRNAs can bind various proteins to form complexes and modify histones on chromatin (Dahariya et al., 2019; He et al., 2021).
Figure 3. lncRNA mechanisms of action. (A) As signaling molecules, lncRNAs convey specific cell development and spatiotemporal information to regulate corresponding gene expression. (B) As decoy molecules, lncRNAs sequester target molecules, which inhibits downstream functions. (C) As guide molecules, lncRNAs recruit chromatin modification complexes to target genes in either cis or trans. (D) As scaffold molecules, lncRNAs form functional complexes to participate in histone modification and/or stabilize nuclear structures.
The functions of lncRNAs are well known and numerous abnormal lncRNAs are observed in CNS disorders. However, research on the mechanism and possible consequences of abnormal lncRNAs in CNS disorders remains limited, especially for CNS disorders involving oxidative stress. In this review, we specifically focus on how lncRNAs regulate oxidative stress in CNS disorders (Table 1). By mediating oxidative stress, lncRNAs may provide new potential targets for the treatment of CNS disorders.
Table 1. The molecular targets, downstream pathways and oxidative stress regulation of lncRNAs in central nervous system (CNS) disorders.
During AIS, the sudden reduction or interruption of glucose and oxygen supply rapidly disturbs energy metabolism in brain tissue, which leads to the generation of abundant ROS and oxidative intermediates, resulting in severe oxidative damage in a short period of time (Wang et al., 2018a). In early AIS, cellular metabolism shifts to anaerobic glycolysis, resulting in decreased NADPH, increased O2–, and dysregulated neuronal energy metabolism (Burmistrova et al., 2019). This also causes neuronal ion channels to malfunction and cell membranes to depolarize, leading to excessive release of excitatory transmitters and subsequent excitatory toxicity (Amantea and Bagetta, 2017). Excessive glutamate release causes Na+–Ca2+ exchanger (NCX) dysfunction and mitochondrial depolarization, resulting in calcium overload and increased ROS production (Shi et al., 2018). Additionally, phospholipase is activated, which leads to polyunsaturated fatty acid (PUFA) release and ROS generation as PUFAs are metabolized into inflammatory mediators such as prostaglandins (Chuang et al., 2015; Figure 4).
Figure 4. The pathways involved in oxidative stress and in the formation of reactive oxygen species in the different pathologies of central nervous system disorders.
Nuclear paraspeckle assembly transcript 1 (NEAT1) is a lncRNA that is dysregulated in various human cancers (Li et al., 2021a). NEAT1 plays a protective role in oxygen-glucose deprivation/reoxygenation (OGD/R)-activated brain microvascular endothelial cells (Zhou et al., 2019). As an activator of the antioxidant pathway, NEAT1 overexpression stabilizes Mfn2 mRNA by recruiting Nova, thereby increasing Mfn2 expression and alleviating ischemia-reperfusion-induced oxidative stress and apoptosis via the Mfn2/Sirt3 pathway (Zhou et al., 2022).
The lncRNA rhabdomyosarcoma 2-associated transcript (RMST) has pivotal roles in regulating AIS through multiple pathophysiological mechanisms, including oxidative stress. In OGD/R-induced AIS, increased RMST was observed. Downregulating RMST ameliorated increased MDA and ROS while decreasing SOD and NO levels. The effect of RMST downregulation abrogates OGD/R-triggered oxidative stress, likely by downregulating SEMA3A expression via sponging miR-377 (Zhao et al., 2021).
Previous studies reported that the lncRNA AK139328 is associated with ischemia/reperfusion injury (IRI) in various organs (Chen et al., 2015; Yu et al., 2018). AK139328 is increased in PC12 cells activated by OGD/R. However, AK139328 silencing decreases ROS production and upregulates endothelial nitric oxide synthase (eNOS) protein expression, which suggests that knocking down AK139328 may alleviate OGD/R-induced oxidative stress in PC12 cells (Liu et al., 2021).
OGD could upregulate lncRNA small nucleolar RNA host gene 14 (SNHG14) and downregulate its derived miR-199b in BV2 cells. SNHG14-derived miR-199b targets the 3′ UTR of aquaporin 4 (AQP4) to increase SOD activity and markedly decrease MDA levels (Liu et al., 2021).
MACC1-AS1 is the antisense lncRNA of MACC1 and has been identified as an oncogene in multiple cancers (Zhao et al., 2018; Guo et al., 2020). miR-6867-5p suppresses the proliferation of endometriosis (Park et al., 2019); MACC1-AS1, the endogenous competitor of miR-6867-5p, exerts anti-apoptosis effects, maintains cell barrier function, and reduces anti-oxidative stress in hypoxia-induced human brain microvascular endothelial cells (HBMECs) under hypoxic conditions by regulating miR-6867-5p/TWIST1 (Yan et al., 2020).
Opa-interacting protein 5 antisense RNA 1 (OIP5-AS1) is involved in the development of multiple human cancers (Deng et al., 2018; Wang et al., 2019). OIP5-AS1 could also function through miRNAs. Overexpressing OIP5-AS1 attenuates oxidative stress and inflammation in a middle cerebral artery occlusion/reperfusion (MCAO/R) rat model, possibly via antioxidant functions activated by targeting miR-186-5p to increase C1q/TNF-related protein 3 (CTRP3) and Nrf2 (Chen et al., 2021b). Similarly, neuroprotective effects were observed from the lncRNA CCAAT enhancer binding protein α antisense RNA 1 (CEBPA-AS1).
OGD/R upregulates CEBPA-AS1 expression in SH-SY5Y cells, while CEBPA-AS1 silencing antagonizes the effects of OGD/R on oxidative stress by decreasing ROS levels and increasing SOD and GSH levels. These results indicate that CEBPA-AS1 knockdown reduces OGD/R-induced oxidative stress in neurons (Di et al., 2021).
The lncRNA potassium voltage-gated channel subfamily Q member 1 opposite strand 1 (KCNQ1OT1) aggravates oxidative stresses and inflammation during hypoxia. KCNQ1OT1 could target miRNAs to alter oxidative stress. For example, KCNQ1OT1 is upregulated in blood samples from patients with AIS. In OGD/R model PC12 cells, the cells were protected from oxidative stress injury. KCNQ1OT1 might target miR-140-3p to enhance hypoxia-inducible factor-1α (HIF-1α) expression (Yi et al., 2020).
The lncRNA ZFAS1 is significantly downregulated in patients with AIS. Mechanistically, lncRNA ZFAS1 acts as a “sponge” for miR-582-3p, which upregulates nitric oxide synthase 3 (NOS3) expression and associated antioxidant functions (Zhang and Zhang, 2020).
LncRNA small nucleolar RNA host gene16 (SNHG16) has been well-documented for oncogenic properties in various malignancies (Gong et al., 2020). X-linked inhibitor-of-apoptosis protein (XIAP) suppresses neurological dysfunction and neuronal apoptosis, thereby relating to preconditioning treatment for cerebral I/R injury (Wang et al., 2021b). Overexpressing SNHG16 enhances cell proliferation and inhibits apoptosis. SNHG16 might enhance XIAP expression by sponging miR-421 to attenuate cell inflammation and oxidative stress in an OGD/R-induced SK-N-SH cell model (Cao et al., 2022).
The lncRNA growth-arrest-specific transcript 5 (GAS5) is widely reported as a tumor suppressor gene (Saussez et al., 1998). Suppressing GAS5 exerts anti-oxidative stress effects in MCAO rats, downregulates GAS5-impaired NOS activity, reduces MDA and protein carbonyl content, and enhances SOD, CAT, and glutathione peroxidase (GSH-Px) activities. Additionally, GAS5 increases cell viability and decreases apoptosis in OGD/R-induced PC12 cells and MCAO rats (Wu et al., 2021).
Previous studies indicate that some antioxidants (CAT and SOD) and pro-oxidants (LDH, MDA, and NO) could be potential endogenous targets for stroke therapy (Chen et al., 2011). In MCAO mice, silencing lncRNA Gm11974 contributes to the recovery of neurological function. In addition, depleting Gm11974 suppresses neuronal apoptosis in OGD-stimulated N2a cells, These neuroprotective effects may occur because Gm11974 silencing increases SOD and CAT activity while decreasing MDA, LDH, and NO levels (Yang et al., 2021).
Cyclic AMP response element binding protein (CREB), a leucine zipper transcription factor, inhibits ROS production and inhibits severe ischemic injury by upregulating brain-derived neurotrophic factor (BDNF) and Bcl-2 (Kitagawa, 2007). The role of lncRNA AK046177 has not been elucidated, though in MCAO rats, inhibiting AK046177 expression significantly alleviates I/R- or OGD/R-mediated neuronal injury. This neuroprotective effect after cerebral ischemia injury occurs via AK046177 inhibition, while increases cAMP synthesis, promotes CREB expression and phosphorylation, stimulates Nrf2 activation, and attenuates I/R- or OGD/R- mediated injury (Wang et al., 2020a).
The lncRNA SNHG7 is downregulated in OGD-treated neurons. SNHG7 expression reduces OGD-induced cell damage. Increased SNHG7 expression reverses N2a cell viability during OGD, promotes SOD and CAT activity, and decreases OGD-triggered MDA and ROS production. FGF9, a target of miR-134-5p, represses OGD/R-induced cell damage by promoting cell viability and repressing cell apoptosis (Gao et al., 2020). SNHG7 overexpression protects against OGD-induced neuronal damage by modulating cytotoxicity, cell viability, apoptosis, and oxidative stress. The underlying molecular mechanism is that SNHG7 stimulates FGF9 expression by associating with miR-134-5p (Sun et al., 2021).
In summary, multiple lncRNAs are involved in the pathogenesis of AIS through oxidative stress. Regulating lncRNAs expression has neuroprotective effects in AIS cells and animal models. In addition, most of these lncRNAs regulate the expression of their downstream mRNAs by sponging their target miRNAs, which regulates oxidative stress (Zhang et al., 2020c). These recent studies provide a new mechanism for lncRNAs to participate in AIS pathogenesis and suggest potential novel therapeutic strategies for AIS.
Oxidative stress plays an important role in AD pathogenesis. Excessive oxidative stress causes lipid peroxidation, protein nitrification, and nucleic acid destruction, which affects the synaptic capacity of neurons and even leads to apoptosis (Jiang et al., 2016a). When amyloid (Aβ) is irreversibly deposited in brain tissue, oxygen free radicals are produced, which causes oxidative stress, resulting in neuronal dysfunction, metabolic disorder, and a significant decline in learning, cognition, and memory (Cheignon et al., 2018). Aβ activates N-methyl-D-aspartic acid receptors to promote oxygen free radical production, thereby inducing neuronal damage (Shelat et al., 2008). In vitro cultured neurons exposed to Aβ have increased lipid peroxidation and hydrogen peroxide levels. Antioxidants may inhibit Aβ aggregation (Meske et al., 2008). In addition, Aβ can inactivate antioxidant enzymes, which induces ROS generation and forms a positive feedback pathway to aggravate oxidative stress in the nervous system (Dessalew et al., 2007). Moreover, oxidative stress activates glycogen synthetic kinase (GSK-3), which is an isomer of Tau protein kinase. Therefore, oxidative stress also promotes Tau protein phosphorylation, resulting in neurological impairment (Rönnemaa et al., 2008).
LncRNAs may antagonize Aβ neurotoxicity through various mechanisms, including antioxidant activity. In hippocampal neurons, Aβ25-35 treatment induces oxidative stress, which is indicated by significantly decreased SOD and GSH-Px activity and increased MDA levels. XIST knockdown alleviates the effects of Aβ25-35 treatment on SOD, GSH-Px, and MDA levels. Studies investigating the underlying mechanism by which XIST functions suggest that XIST exerts regulatory functions by binding to miR-132 and upregulating its expression. Importantly, miR-132 has been reported to play a neuroprotective role against oxidative stress (Wong et al., 2013; Wang et al., 2018b).
Aβ25-35 upregulates H19 and downregulates miR-129 in PC12 cells. Further, silencing H19 and upregulating miR-129 improves cell viability and represses apoptosis in PC12 cells stimulated by Aβ25-35 in AD. This protective effect was partly achieved by two ncRNAs, elevated SOD and CAT expression, and decreased MDA expression (Zhang et al., 2021).
The lncRNA BDNF-AS, a natural antisense transcript to BDNF, negatively modulates BDNF in vitro and in vivo (Modarresi et al., 2012). In Aβ25-35-induced PC12 cells, ROS production is significantly increased, MDA activity is substantially elevated, and SOD and CAT activity are dramatically decreased. Silencing BDNF-AS reverses the Aβ25-35 induced oxidative stress response, suggesting that BDNF-AS may participate in AD development and progression via oxidative stress (Guo et al., 2018). A previous study suggests that BDNF protects neurons against 3-nitropropionic acid-induced oxidative stress by increasing sestrin2 expression and decreasing ROS formation (Wu et al., 2016). Therefore, silencing BDNF-AS may exert anti-oxidative effects by increasing BDNF expression.
Wilms’ tumor suppressor (WT1) is highly expressed in AD and promote apoptosis, which leads to neurological failure (Lovell et al., 2003). The lncRNA WT1-AS, a natural antisense transcript to WT1, negatively modulates WT1. In Aβ25-35-induced SH-SY5Y cells, WT1-AS is downregulated. In contrast, overexpressing WT1-AS significantly decreases ROS, MDA, and LDH levels in SH-SY5Y cells, while SOD and GSH-Px are markedly elevated. miR-375, which is a target of WT1, targets the 3′ UTR of SIX4. Overexpressing WT1-AS inhibits miR-375 expression by suppressing WT1, which prevents AD occurrence and development (Wang et al., 2020b).
LncRNA taurine upregulated gene 1 (TUG1) functions by regulating miRNA expression. TUG1 silencing strengthens antioxidant ability and depresses neuronal apoptosis in an Aβ25-35-induced AD mouse model. Further, TUG1 might perform antioxidant functions by targeting miR-15a to inhibit Rho-associated protein kinase 1 (ROCK1) (Li et al., 2020).
Although several studies confirm that oxidative stress is an important mechanism of AD pathogenesis, the number of studies on lncRNAs that regulate oxidative stress during AD pathogenesis is limited. In Aβ25-35-induced AD cells and animal models, XIST, H19, BDNF-As, WT1-As, and TUG1 regulate oxidative stress by modulating target miRNA or via other pathways. By investigating the regulatory mechanism of these lncRNAs, we will further understand the role of lncRNAs in AD pathogenesis and identify potential therapeutic strategies for AD.
Parkinson’s disease is a progressive age-related neurodegenerative disease (Andrei Surguchov, 2022). The main pathological features of PD are the loss of dopaminergic neurons in the substantia nigra dense region and the formation of Lewy bodies (Samii et al., 2004). The main clinical manifestations of PD are static tremor, myotonia, bradykinesia, and abnormal posture and gait. These symptoms are often accompanied by non-motor symptoms such as anxiety, depression, and cognitive decline (Khoo et al., 2013; Lopiano et al., 2016). The pathogenesis of PD is still unclear. Recent studies have confirmed that oxidative stress plays an important role in PD occurrence and development (Gaki and Papavassiliou, 2014). Oxidative stress damages neurons through free radicals. Substantia nigra dopaminergic neurons are particularly susceptible to oxidative stress (Rodriguez-Pallares et al., 2012). ROS target mitochondria, resulting in dysfunction and reduced energy production. Dopaminergic neurons in the substantia nigra have super-long unmyelinated axons and high energy consumption (Pissadaki and Bolam, 2013). In patients with PD, substantia nigra dopamine neurons under oxidative stress have energy demands that exceeds the energy supply, which eventually kills the damaged neurons. In the remaining dopaminergic neurons, cellular metabolism is accelerated due to compensatory dopamine synthesis, which produces more free radicals and further increases oxidative stress (Dias et al., 2013). Furthermore, oxidative deamination of dopamine by monoamine oxidase (MAO) produces H2O2 as a by-product, while enzymatic oxidation of the electron-rich catechin portion of dopamine by tyrosinase, cycloxase, and other enzymes produces O2– (Muñoz et al., 2012). Spontaneous dopamine oxidation can also occur through interactions with unstable iron and other biomaterials, thereby producing ROS (H2O2, O2–, and OH) (Sun et al., 2018). Considering the significant role of oxidative stress in PD pathogenesis, ameliorating oxidative stress by regulating lncRNAs can deepen our understanding of PD pathogenesis and elucidate new treatments.
LncRNA myocardial infarction-associated transcript (MIAT) was originally isolated as a candidate gene for myocardial infarction. The over-expanding role of MIAT in various human diseases has been recently revealed, including PD (Shen et al., 2021). MIAT is highly expressed in 1-methyl-4-phenyl-1,2,3,6-tetrahydropyridine (MPTP)-treated mice and 1-methyl-4-phenylpyridinium ion (MPP +)-treated cells. Downregulating MIAT promotes SOD and GSH production, but inhibits MDA in MPP + -treated cells. Blocking MIAT increases cell viability and inhibits cell apoptosis. Additionally, MIAT enhances Nrf2 expression by modulating its target, miR-221-3p (Lang et al., 2022).
The lncRNA non-coding RNA activated by DNA damage (NORAD) is a highly conserved lncRNA that is necessary for genome stability. Dysregulated NORAD is present in various cancer types (Soghli et al., 2021). In MPP + -induced neuroblastoma cells, reduced NORAD expression is observed. Interestingly, NORAD overexpression relieves cytotoxicity and inflammatory responses of neuroblastoma cells after MPP + treatment. NORAD upregulation also inhibits MPP + -induced LDH increase, reduces increased ROS activity, and suppresses SOD activity in SK-N-SH and SK-N-AS cells. The inhibition of oxidative stress by NORAD upregulation is partly achieved by regulating the miR-204-5p/solute carrier family 5-member 3 (SLC5A3) axis (Zhou et al., 2020).
In MPTP-induced PD rat models, silencing RMST also has anti-oxidative stress effects. Silencing RMST could reduce inflammatory responses and suppress neuron apoptosis in the substantia nigra of PD rats. In addition, silencing RMST increases SOD, CAT, and GSH-Px activity, reduces NOS activity, and decreases MDA and NO content. These results suggest that RMST could be neuroprotective against dopamine neurons injury caused by oxidative stress (Ma et al., 2021).
miR-205-5p plays a protective role during PD. Upregulation miR-205-5p inhibits the expression of leucine-rich repeat kinase 2 (LRRK2) and prevents neurite outgrowth defects induced by the R1441G LRRK2 mutation, which indicates a major role of miR-205-5p in neuron survival (Cho et al., 2013). AL049437 is a “sponge” or an endogenous competitor of miR-205-5p. Silencing AL049437 promotes the protective function of miR-205-5p, suppresses apoptosis of SH-SY5Y cells, and reduces oxidative stress (Zhang et al., 2020b).
As an activator of the antioxidant pathway, overexpression of MALAT1 was observed in MPTP-treated PD mice. MALAT1 contributes to inflammasome activation in microglial cells, which triggers neuronal injury by interacting with enhancer of zeste homolog 2 (EZH2) to regulate Nrf2-mediated antioxidative responses. Reducing MALAT1 increases antioxidant capacity and attenuates oxidative stress damage (Cai et al., 2020).
Long non-coding RNA-p21 (lnc-p21) regulates mRNA translation, gene expression, protein stability, and p53-dependent apoptosis (Hall et al., 2015). Lnc-p21 is upregulated in PD and remains overexpressed during PD progression (Kraus et al., 2017). In human neuroblastoma SH-SY5Y cells treated with MPP +, lnc-p21 is highly expressed. Knocking down lnc-p21 mitigates MPP +-induced oxidative stress and neuroinflammation, as evidenced by decreased ROS generation, increased SOD activity, and decreased interleukin 6 (IL-6), tumor necrosis factor α (TNF-α) and IL-1β levels. TRPM2 is activated by oxidative stress, resulting in elevated intracellular Ca2+ concentrations (Nazıroğlu, 2011). Mechanistically, lnc-p21 knockdown exerts anti-oxidative function by downregulating TRPM2 expression via sponging miR-625, the target of lnc-p21 (Ding et al., 2019).
Gene microarray analysis revealed decreased lncRNA-T199678 expression in an exogenous α-synuclein-induced SH-SY5Y cellular model of PD (Lin et al., 2018). In α-synuclein-induced SH-SY5Y cellular PD models, ROS levels are significantly increased, while overexpressing lncRNA-T199678 reverses intracellular oxidative stress induced by exogenous α-synuclein. miR-101-3p is a potential target for lncRNA-T199678 and binds with α-synuclein at a specific 3′ UTR binding site. Therefore, overexpressing lncRNA-T199678 inhibits α-synuclein-induced neuronal damage via regulating intracellular oxidative stress, cell cycle dysfunction, and apoptosis by targeting miR-101-3p (Bu et al., 2020).
In vitro and in vivo studies of PD suggest that lncRNAs regulate miRNA participation in oxidative stress through endogenous competition mechanisms and directly regulate SOD and LDH levels. Moreover, Nrf2 is a main target of lncRNA regulation. Therefore, lncRNAs may be involved in regulating oxidative stress in PD through various mechanisms and may become a potential target for PD treatment.
Spinal cord injury is a common CNS disorder that is characterized by different degrees of sensorimotor dysfunction, which often leads to paraplegia, quadriplegia, and other pathological changes that seriously affect the quality of life in a patient (James et al., 2019). Oxidative stress plays an important role in SCI pathogenesis. Previous studies showed that abundant ROS are generated immediately after SCI, which can induce oxidative stress not neutralized in time. More importantly, oxidative stress is involved in secondary events, such as excitatory toxicity, inflammatory responses, and neuronal and glial apoptosis (Fatima et al., 2015). Taking effective measures to prevent or decrease oxidative stress after injury is an effective measure to intervene in SCI. For each link of free radical chain reactions, an appropriate inhibitor or free radical scavenger can achieve effective anti-oxidation (Kikuchi et al., 2013). At present, various antioxidants have been studied. Some results suggest that lncRNAs may affect oxidative stress through various mechanisms and play a therapeutic role in SCI.
LncRNA cancer susceptibility candidate 9 (CASC9) has been extensively studied in various cancers and functions as an oncogene in bladder cancer and esophageal squamous cell carcinoma (Huo et al., 2020; Zhao et al., 2020). Lipopolysaccharide (LPS) downregulates CASC9 and upregulates its derived miR-383-5p in LPS-induced PC12 cells. CASC9-derived miR-383-5p targets the 3′ UTR of LDHA to downregulate Nrf2 and HO-1 proteins. CASC9 also exert functions through miRNAs. Overexpressing CASC9 attenuates oxidative stress and inflammation in an SCI rat model. This antioxidant function may occur by targeting miR-383-5p to inhibit Nrf2/HO-1 signaling (Guan and Wang, 2021).
lncRNA growth arrest specific transcript 5 (GAS5) regulates the development of some CNS disorders. For instance, GAS5 was identified as a potential tumor suppressor in glioma (Zhao et al., 2015). Overexpressing GAS5 facilitates cell apoptosis induced by OGD in MCAO mouse models by reducing mitogen activated protein kinase 4 (MAP4K4) expression (Deng et al., 2020). The CELF2/VAV1 pathway might coordinate with GAS5 to enhance OGD-triggered oxidative stress and cell injury. GAS5 knockdown downregulates VAV1 expression by recruiting CELF2 to the coding region of VAV1 mRNA, which mitigates SCI by reducing oxidative stress and caspase-3 activity in rat models (Wang et al., 2021a).
LncRNA tectonic family member 2 (TCTN2) is a functional RNA that is involved in autophagy in neurons, thereby modulating neuronal apoptosis and improving SCI (Ren et al., 2019). Insulin-like growth factor 1 receptor (IGF1R) has an active role in neural stem cell-mediated motor recovery after spinal cord transection (Hwang et al., 2018). In the spinal cord of SCI model rats and LPS-stimulated PC12 cells, increased miR-329-3p levels are observed. Inhibiting miR-329-3p reverses LPS-induced neuronal apoptosis, oxidative stress, and inflammation by upregulating IGF1R. miR-329-3p is a target of TCTN2. Thus, exosomes derived from TCTN2-modified mesenchymal stem cells may improve SCI via the miR-329-3p/IGF1R axis (Liu et al., 2022).
LncRNA SOX2 overlapping transcript (SOX2OT) is linked to the development of multiple human cancers (Zhang et al., 2016; Zhan et al., 2020). Neurod1 is involved in SCI-stimulated oxidative stress and inflammatory damage (Fu et al., 2018). SOX2OT was identified as a miR-331-3p sponge that positively regulates Neurod1 expression. SOX2OT knockdown ameliorates LPS-mediated inflammation, improves cell viability, reduces apoptosis, and inhibits oxidative stress in PC12 cells by modulating the miR-331-3p/Neurod1 axis and activating Janus kinase signaling (Li et al., 2021b).
Oxidative stress is closely related to cancer cell survival and the development of glioma (Gilbert et al., 2014). Excessive ROS levels produced by oxidative stress can affect cell function and change gene expression, which may promote glioma progression (Kim et al., 2014). LncRNA H19 encodes a 2.3-kb long transcript and is a carcinogenic lncRNA in several cancers, including glioma (Jiang et al., 2016b). Overexpressing H19 in gliomas can contribute to malignant transformation, promote tumor proliferation, invasion, infiltration, and chemoresistance (Jia et al., 2016). In glioma cell models, H19 levels are induced by oxidative stress and are increased in U251 and LN229 cells. Meanwhile, overexpressing H19 in U251 and LN229 cells causes temozolomide resistance, which indicates that H19 confers temozolomide resistance to glioma cells (Duan et al., 2018).
Epilepsy is a disease in which epigenetic mechanisms play an important role in the pathogenesis (Surguchov et al., 2017). Temporal lobe epilepsy (TLE) is a common CNS disorder that is characterized by recurrent seizures (Chen et al., 2016). LncRNA maternally expressed gene 3 (MEG3) is a well-known tumor suppressor gene (Wang et al., 2012). In TLE rat models, MEG3 expression is downregulated along with high MDA content and decreased SOD activity. Upregulating MEG3 enhances cell viability, reverses oxidative stress, and inhibits apoptosis through activating the PI3K/AKT/mTOR pathway in TLE. These findings may contribute to developing new therapeutic targets for epilepsy (Zhang et al., 2020a).
The cellular and molecular changes underlying CNS injuries provide a wealth of potential biomarkers and therapeutic targets. Oxidative stress plays an important role in the occurrence and development of CNS disorders. In addition, secondary pathological damage caused by oxidative stress, including neuroinflammatory responses, mitochondrial damage, and increased apoptosis, are the main cause of exacerbated CNS disorders. Therefore, regulating oxidative stress in CNS disorders may help alleviate neurological damage and provide a new therapeutic strategy for CNS disorders.
In recent years, accumulating studies show that lncRNAs, which were originally considered “junk” and “noise,” are widely involved in multiple human diseases, including CNS disorders and are associated with oxidative stress. We demonstrate that many lncRNAs regulate oxidative stress by interacting with miRNAs, thereby regulating SOD activity, MDA expression levels, and other functions. In addition, some lncRNAs directly regulate antioxidant pathways (such as Nrf2/HO-1 signaling) to regulate the pathogenesis of CNS disorders. These oxidative stress-related lncRNAs may be potential key biomarkers and therapeutic targets of CNS disorders (Figure 5). Further, these lncRNAs may provide a novel strategy for disease diagnosis and treatment. Future studies will elucidate the precise mechanisms by which lncRNAs regulate oxidative stress in CNS disorders.
Figure 5. Roles and functions of lncRNAs in regulating oxidative stress during central nervous system disorders.
YZ and XX wrote the manuscript. YZ produced the figures. XX edited and revised the review. Both authors have read and approved the final manuscript.
The authors declare that the research was conducted in the absence of any commercial or financial relationships that could be construed as a potential conflict of interest.
All claims expressed in this article are solely those of the authors and do not necessarily represent those of their affiliated organizations, or those of the publisher, the editors and the reviewers. Any product that may be evaluated in this article, or claim that may be made by its manufacturer, is not guaranteed or endorsed by the publisher.
Amantea, D., and Bagetta, G. (2017). Excitatory and inhibitory amino acid neurotransmitters in stroke: from neurotoxicity to ischemic tolerance. Curr. Opin. Pharmacol. 35, 111–119. doi: 10.1016/j.coph.2017.07.014
Anderson, M. A., Burda, J. E., Ren, Y., Ao, Y., O’shea, T. M., Kawaguchi, R., et al. (2016). Astrocyte scar formation aids central nervous system axon regeneration. Nature 532, 195–200. doi: 10.1038/nature17623
Andrei Surguchov (2022). “Biomarkers in Parkinson’s disease,” in Neurodegenerative Diseases Biomarkers, eds P. V. Peplow, B. Martinez, and T. A. Gennarelli (Berlin: Springer), 155–180. doi: 10.1016/j.nicl.2017.09.009
Baird, L., and Yamamoto, M. (2020). The molecular mechanisms regulating the KEAP1-NRF2 Pathway. Mol. Cell. Biol. 40:e00099–20. doi: 10.1128/MCB.00099-20
Bu, L. L., Xie, Y. Y., Lin, D. Y., Chen, Y., Jing, X. N., Liang, Y. R., et al. (2020). LncRNA-T199678 mitigates α-synuclein-induced dopaminergic neuron injury via miR-101-3p. Front. Aging Neurosci. 12:599246. doi: 10.3389/fnagi.2020.599246
Burmistrova, O., Olias-Arjona, A., Lapresa, R., Jimenez-Blasco, D., Eremeeva, T., Shishov, D., et al. (2019). Targeting PFKFB3 alleviates cerebral ischemia-reperfusion injury in mice. Sci. Rep. 9:11670. doi: 10.1038/s41598-019-48196-z
Cai, L. J., Tu, L., Huang, X. M., Huang, J., Qiu, N., Xie, G. H., et al. (2020). LncRNA MALAT1 facilitates inflammasome activation via epigenetic suppression of Nrf2 in Parkinson’s disease. Mol. Brain 13, 130. doi: 10.1186/s13041-020-00656-8
Cao, X., Ma, J., and Li, S. (2022). Mechanism of lncRNA SNHG16 in oxidative stress and inflammation in oxygen-glucose deprivation and reoxygenation-induced SK-N-SH cells. Bioengineered 13, 5021–5034. doi: 10.1080/21655979.2022.2026861
Cheignon, C., Tomas, M., Bonnefont-Rousselot, D., Faller, P., Hureau, C., and Collin, F. (2018). Oxidative stress and the amyloid beta peptide in Alzheimer’s disease. Redox Biol. 14, 450–464. doi: 10.1016/j.redox.2017.10.014
Chen, H., Yoshioka, H., Kim, G. S., Jung, J. E., Okami, N., Sakata, H., et al. (2011). Oxidative stress in ischemic brain damage: mechanisms of cell death and potential molecular targets for neuroprotection. Antioxid. Redox Signal. 14, 1505–1517. doi: 10.1089/ars.2010.3576
Chen, J., Liu, X. M., Yue, X., and Chen, S. Z. (2016). The clinical efficacy and safety of levetiracetam add-on therapy for child refractory epilepsy. Eur. Rev. Med. Pharmacol. Sci. 20, 2689–2694.
Chen, Y., Li, Z., Chen, X., and Zhang, S. (2021a). Long non-coding RNAs: from disease code to drug role. Acta Pharm Sin B 11, 340–354. doi: 10.1016/j.apsb.2020.10.001
Chen, Y., Liu, W., Chen, M., Sun, Q., Chen, H., and Li, Y. (2021b). Up-regulating lncRNA OIP5-AS1 protects neuron injury against cerebral hypoxia-ischemia induced inflammation and oxidative stress in microglia/macrophage through activating CTRP3 via sponging miR-186-5p. Int. Immunopharmacol. 92:107339. doi: 10.1016/j.intimp.2020.107339
Chen, Z., Luo, Y., Yang, W., Ding, L., Wang, J., Tu, J., et al. (2015). Comparison analysis of dysregulated LncRNA profile in mouse plasma and liver after hepatic ischemia/reperfusion injury. PLoS One 10:e0133462. doi: 10.1371/journal.pone.0133462
Cho, H. J., Liu, G., Jin, S. M., Parisiadou, L., Xie, C., Yu, J., et al. (2013). MicroRNA-205 regulates the expression of Parkinson’s disease-related leucine-rich repeat kinase 2 protein. Hum. Mol. Genet. 22, 608–620. doi: 10.1093/hmg/dds470
Chu, P. M., Yu, C. C., Tsai, K. L., and Hsieh, P. L. (2022). Regulation of oxidative stress by long non-coding RNAs in vascular complications of diabetes. Life (Basel) 12, 274. doi: 10.3390/life12020274
Chuang, D. Y., Simonyi, A., Kotzbauer, P. T., Gu, Z., and Sun, G. Y. (2015). Cytosolic phospholipase A2 plays a crucial role in ROS/NO signaling during microglial activation through the lipoxygenase pathway. J. Neuroinflamm. 12, 199. doi: 10.1186/s12974-015-0419-0
Costa, L. G., Garrick, J. M., Roquè, P. J., and Pellacani, C. (2016). Mechanisms of neuroprotection by quercetin: counteracting oxidative stress and more. Oxid. Med. Cell. Longev. 2016, 2986796. doi: 10.1155/2016/2986796
Dahariya, S., Paddibhatla, I., Kumar, S., Raghuwanshi, S., Pallepati, A., and Gutti, R. K. (2019). Long non-coding RNA: classification, biogenesis and functions in blood cells. Mol. Immunol. 112, 82–92. doi: 10.1016/j.molimm.2019.04.011
Deng, J., Deng, H., Liu, C., Liang, Y., and Wang, S. (2018). Long non-coding RNA OIP5-AS1 functions as an oncogene in lung adenocarcinoma through targeting miR-448/Bcl-2. Biomed. Pharmacother. 98, 102–110. doi: 10.1016/j.biopha.2017.12.031
Deng, Y., Chen, D., Gao, F., Lv, H., Zhang, G., Sun, X., et al. (2020). Silencing of long non-coding RNA GAS5 suppresses neuron cell apoptosis and nerve injury in ischemic stroke through inhibiting DNMT3B-dependent MAP4K4 methylation. Transl. Stroke Res. 11, 950–966. doi: 10.1007/s12975-019-00770-3
Deniz, E., and Erman, B. (2017). Long noncoding RNA (lincRNA), a new paradigm in gene expression control. Funct. Integr. Genomics 17, 135–143. doi: 10.1007/s10142-016-0524-x
Dessalew, N., Patel, D. S., and Bharatam, P. V. (2007). 3D-QSAR and molecular docking studies on pyrazolopyrimidine derivatives as glycogen synthase kinase-3beta inhibitors. J. Mol. Graph. Model. 25, 885–895. doi: 10.1016/j.jmgm.2006.08.009
Di, G., Yang, X., Cheng, F., Liu, H., and Xu, M. (2021). CEBPA-AS1 knockdown alleviates oxygen-glucose deprivation/reperfusion-induced neuron cell damage by the MicroRNA 24-3p/BOK axis. Mol. Cell. Biol. 41, e0006521. doi: 10.1128/MCB.00065-21
Dias, V., Junn, E., and Mouradian, M. M. (2013). The role of oxidative stress in Parkinson’s disease. J. Parkinsons Dis. 3, 461–491.
Ding, X. M., Zhao, L. J., Qiao, H. Y., Wu, S. L., and Wang, X. H. (2019). Long non-coding RNA-p21 regulates MPP(+)-induced neuronal injury by targeting miR-625 and derepressing TRPM2 in SH-SY5Y cells. Chem. Biol. Interact. 307, 73–81. doi: 10.1016/j.cbi.2019.04.017
Duan, S., Li, M., Wang, Z., Wang, L., and Liu, Y. (2018). H19 induced by oxidative stress confers temozolomide resistance in human glioma cells via activating NF-κB signaling. Onco Targets Ther. 11, 6395–6404.
Fatima, G., Sharma, V. P., Das, S. K., and Mahdi, A. A. (2015). Oxidative stress and antioxidative parameters in patients with spinal cord injury: implications in the pathogenesis of disease. Spinal Cord 53, 3–6. doi: 10.1038/sc.2014.178
Fu, X., Shen, Y., Wang, W., and Li, X. (2018). MiR-30a-5p ameliorates spinal cord injury-induced inflammatory responses and oxidative stress by targeting neurod 1 through MAPK/ERK signalling. Clin. Exp. Pharmacol. Physiol. 45, 68–74. doi: 10.1111/1440-1681.12856
Gaki, G. S., and Papavassiliou, A. G. (2014). Oxidative stress-induced signaling pathways implicated in the pathogenesis of Parkinson’s disease. Neuromolecular Med. 16, 217–230. doi: 10.1007/s12017-014-8294-x
Gao, X. Z., Ma, R. H., and Zhang, Z. X. (2020). miR-339 promotes hypoxia-induced neuronal apoptosis and impairs cell viability by targeting FGF9/CACNG2 and mediating MAPK pathway in ischemic stroke. Front. Neurol. 11:436. doi: 10.3389/fneur.2020.00436
Gilbert, M. R., Liu, Y., Neltner, J., Pu, H., Morris, A., Sunkara, M., et al. (2014). Autophagy and oxidative stress in gliomas with IDH1 mutations. Acta Neuropathol. 127, 221–233. doi: 10.1007/s00401-013-1194-6
Gong, C. Y., Tang, R., Nan, W., Zhou, K. S., and Zhang, H. H. (2020). Role of SNHG16 in human cancer. Clin. Chim. Acta 503, 175–180. doi: 10.1016/j.cca.2019.12.023
Guan, C., and Wang, Y. (2021). LncRNA CASC9 attenuates lactate dehydrogenase-mediated oxidative stress and inflammation in spinal cord injury via sponging miR-383-5p. Inflammation 44, 923–933. doi: 10.1007/s10753-020-01387-7
Guo, C. C., Jiao, C. H., and Gao, Z. M. (2018). Silencing of LncRNA BDNF-AS attenuates Aβ(25-35)-induced neurotoxicity in PC12 cells by suppressing cell apoptosis and oxidative stress. Neurol. Res. 40, 795–804. doi: 10.1080/01616412.2018.1480921
Guo, Y., Zhong, J., Wu, F., and Zhan, Z. (2020). Long noncoding RNA MACC1-AS1 promotes the stemness of hepatocellular carcinoma cells by antagonizing miR-145. J. Int. Med. Res. 48, 300060520920411. doi: 10.1177/0300060520920411
Hall, J. R., Messenger, Z. J., Tam, H. W., Phillips, S. L., Recio, L., and Smart, R. C. (2015). Long noncoding RNA lincRNA-p21 is the major mediator of UVB-induced and p53-dependent apoptosis in keratinocytes. Cell Death Dis. 6, e1700. doi: 10.1038/cddis.2015.67
He, C., Wang, K., Gao, Y., Wang, C., Li, L., Liao, Y., et al. (2021). Roles of noncoding RNA in reproduction. Front. Genet. 12:777510. doi: 10.3389/fgene.2021.777510
Hombach, S., and Kretz, M. (2016). Non-coding RNAs: classification, biology and functioning. Adv. Exp. Med. Biol. 937, 3–17. doi: 10.1007/978-3-319-42059-2_1
Huo, W., Tan, D., and Chen, Q. (2020). CASC9 facilitates cell proliferation in bladder cancer by regulating CBX2 expression. Nephron 144, 388–399. doi: 10.1159/000507828
Hwang, D. H., Park, H. H., Shin, H. Y., Cui, Y., and Kim, B. G. (2018). Insulin-like growth factor-1 receptor dictates beneficial effects of treadmill training by regulating survival and migration of neural stem cell grafts in the injured spinal cord. Exp. Neurobiol. 27, 489–507. doi: 10.5607/en.2018.27.6.489
Hybertson, B. M., Gao, B., Bose, S. K., and Mccord, J. M. (2011). Oxidative stress in health and disease: the therapeutic potential of Nrf2 activation. Mol. Aspects Med. 32, 234–246. doi: 10.1016/j.mam.2011.10.006
James, S. L., Theadom, A., Ellenbogen, R. G., Bannick, M. S., Montjoy-Venning, W., Lucchesi, L. R., et al. (2019). Global, regional, and national burden of traumatic brain injury and spinal cord injury, 1990-2016: a systematic analysis for the global burden of disease study 2016. Lancet Neurol. 18, 56–87. doi: 10.1016/S1474-4422(18)30415-0
Jakubczyk, K., Dec, K., Kałduñska, J., Kawczuga, D., Kochman, J., and Janda, K. (2020). Reactive oxygen species - sources, functions, oxidative damage. Pol. Merkur. Lekarski. 48, 124–127.
Jia, P., Cai, H., Liu, X., Chen, J., Ma, J., Wang, P., et al. (2016). Long non-coding RNA H19 regulates glioma angiogenesis and the biological behavior of glioma-associated endothelial cells by inhibiting microRNA-29a. Cancer Lett. 381, 359–369. doi: 10.1016/j.canlet.2016.08.009
Jiang, T., Sun, Q., and Chen, S. (2016a). Oxidative stress: a major pathogenesis and potential therapeutic target of antioxidative agents in Parkinson’s disease and Alzheimer’s disease. Prog. Neurobiol. 147, 1–19. doi: 10.1016/j.pneurobio.2016.07.005
Jiang, X., Yan, Y., Hu, M., Chen, X., Wang, Y., Dai, Y., et al. (2016b). Increased level of H19 long noncoding RNA promotes invasion, angiogenesis, and stemness of glioblastoma cells. J. Neurosurg. 124, 129–136.
Khoo, T. K., Yarnall, A. J., Duncan, G. W., Coleman, S., O’brien, J. T., Brooks, D. J., et al. (2013). The spectrum of nonmotor symptoms in early Parkinson disease. Neurology 80, 276–281. doi: 10.1212/WNL.0b013e31827deb74
Khoshnam, S. E., Winlow, W., Farzaneh, M., Farbood, Y., and Moghaddam, H. F. (2017). Pathogenic mechanisms following ischemic stroke. Neurol. Sci. 38, 1167–1186. doi: 10.1007/s10072-017-2938-1
Kikuchi, K., Tancharoen, S., Takeshige, N., Yoshitomi, M., Morioka, M., Murai, Y., et al. (2013). The efficacy of edaravone (radicut), a free radical scavenger, for cardiovascular disease. Int. J. Mol. Sci. 14, 13909–13930. doi: 10.3390/ijms140713909
Kim, S. H., Kwon, C. H., and Nakano, I. (2014). Detoxification of oxidative stress in glioma stem cells: mechanism, clinical relevance, and therapeutic development. J. Neurosci. Res. 92, 1419–1424. doi: 10.1002/jnr.23431
Kitagawa, K. (2007). CREB and cAMP response element-mediated gene expression in the ischemic brain. FEBS J. 274, 3210–3217. doi: 10.1111/j.1742-4658.2007.05890.x
Klimiuk, A., Maciejczyk, M., Choromańska, M., Fejfer, K., Waszkiewicz, N., and Zalewska, A. (2019). Salivary redox biomarkers in different stages of dementia severity. J. Clin. Med. 8, 840. doi: 10.3390/jcm8060840
Kraus, T. F. J., Haider, M., Spanner, J., Steinmaurer, M., Dietinger, V., and Kretzschmar, H. A. (2017). Altered long noncoding RNA expression precedes the course of Parkinson’s disease-a preliminary report. Mol. Neurobiol. 54, 2869–2877. doi: 10.1007/s12035-016-9854-x
Lang, Y., Zhang, H., Yu, H., Li, Y., Liu, X., and Li, M. (2022). Long non-coding RNA myocardial infarction-associated transcript promotes 1-Methyl-4-phenylpyridinium ion-induced neuronal inflammation and oxidative stress in Parkinson’s disease through regulating microRNA-221-3p/transforming growth factor/nuclear factor E2-related factor 2 axis. Bioengineered 13, 930–940. doi: 10.1080/21655979.2021.2015527
Li, J., Wang, N., Nie, H., Wang, S., Jiang, T., Ma, X., et al. (2022). Long non-coding RNA RMST worsens ischemic stroke via MicroRNA-221-3p/PIK3R1/TGF-β Signaling pathway. Mol. Neurobiol. 59, 2808–2821. doi: 10.1007/s12035-021-02632-2
Li, K., Yao, T., Zhang, Y., Li, W., and Wang, Z. (2021a). NEAT1 as a competing endogenous RNA in tumorigenesis of various cancers: role, mechanism and therapeutic potential. Int. J. Biol. Sci. 17, 3428–3440. doi: 10.7150/ijbs.62728
Li, R., Li, X., Huang, Y., Qiu, H., Li, L., and Bi, Z. (2021b). LncRNA SOX2OT knockdown alleviates lipopolysaccharide-induced damage of PC12 cells by regulating miR-331-3p/Neurod1 axis. World Neurosurg. 147, e293–e305. doi: 10.1016/j.wneu.2020.12.049
Li, X., Wang, S. W., Li, X. L., Yu, F. Y., and Cong, H. M. (2020). Knockdown of long non-coding RNA TUG1 depresses apoptosis of hippocampal neurons in Alzheimer’s disease by elevating microRNA-15a and repressing ROCK1 expression. Inflamm. Res. 69, 897–910. doi: 10.1007/s00011-020-01364-8
Lin, D., Liang, Y., Jing, X., Chen, Y., Lei, M., Zeng, Z., et al. (2018). Microarray analysis of an synthetic α-synuclein induced cellular model reveals the expression profile of long non-coding RNA in Parkinson’s disease. Brain Res. 1678, 384–396. doi: 10.1016/j.brainres.2017.11.007
Liu, J., Lin, M., Qiao, F., and Zhang, C. (2022). Exosomes derived from lncRNA TCTN2-modified mesenchymal stem cells improve spinal cord injury by miR-329-3p/IGF1R axis. J. Mol. Neurosci. 72, 482–495. doi: 10.1007/s12031-021-01914-7
Liu, L., Zheng, B., and Wang, Z. (2021). Protective effects of the knockdown of lncRNA AK139328 against oxygen glucose deprivation/reoxygenation-induced injury in PC12 cells. Mol. Med. Rep. 24, 621. doi: 10.3892/mmr.2021.12260
Lopiano, L., Modugno, N., Marano, P., Sensi, M., Meco, G., Cannas, A., et al. (2016). Motor outcomes in patients with advanced Parkinson’s disease treated with levodopa/carbidopa intestinal gel in Italy: an interim analysis from the GREENFIELD observational study. Neurol. Sci. 37, 1785–1792. doi: 10.1007/s10072-016-2664-0
Lovell, M. A., Xie, C., Xiong, S., and Markesbery, W. R. (2003). Wilms’ tumor suppressor (WT1) is a mediator of neuronal degeneration associated with the pathogenesis of Alzheimer:s disease. Brain Res. 983, 84–96. doi: 10.1016/s0006-8993(03)03032-4
Ma, X., Wang, Y., Yin, H., Hua, L., Zhang, X., Xiao, J., et al. (2021). Down-regulated long non-coding RNA RMST ameliorates dopaminergic neuron damage in Parkinson’s disease rats via regulation of TLR/NF-κB signaling pathway. Brain Res. Bull. 174, 22–30. doi: 10.1016/j.brainresbull.2021.04.026
Maciejczyk, M., Zalewska, A., and Gerreth, A. K. (2020). Salivary redox biomarkers in selected neurodegenerative diseases. J. Clin. Med. 9:97. doi: 10.3390/jcm9020497
Meske, V., Albert, F., and Ohm, T. G. (2008). Coupling of mammalian target of rapamycin with phosphoinositide 3-kinase signaling pathway regulates protein phosphatase 2A– and glycogen synthase kinase-3 -dependent phosphorylation of Tau. J. Biol. Chem. 283, 100–109. doi: 10.1074/jbc.M704292200
Modarresi, F., Faghihi, M. A., Lopez-Toledano, M. A., Fatemi, R. P., Magistri, M., Brothers, S. P., et al. (2012). Inhibition of natural antisense transcripts in vivo results in gene-specific transcriptional upregulation. Nat. Biotechnol. 30, 453–459. doi: 10.1038/nbt.2158
Muñoz, P., Huenchuguala, S., Paris, I., and Segura-Aguilar, J. (2012). Dopamine oxidation and autophagy. Parkinsons Dis. 2012:920953. doi: 10.1155/2012/920953
Nazıroğlu, M. (2011). TRPM2 cation channels, oxidative stress and neurological diseases: where are we now? Neurochem. Res. 36, 355–366. doi: 10.1007/s11064-010-0347-4
Ouyang, Y. B., Stary, C. M., White, R. E., and Giffard, R. G. (2015). The use of microRNAs to modulate redox and immune response to stroke. Antioxid. Redox Signal. 22, 187–202. doi: 10.1089/ars.2013.5757
Park, S., Lim, W., Bazer, F. W., Whang, K. Y., and Song, G. (2019). Quercetin inhibits proliferation of endometriosis regulating cyclin D1 and its target microRNAs in vitro and in vivo. J. Nutr. Biochem. 63, 87–100. doi: 10.1016/j.jnutbio.2018.09.024
Pissadaki, E. K., and Bolam, J. P. (2013). The energy cost of action potential propagation in dopamine neurons: clues to susceptibility in Parkinson’s disease. Front. Comput. Neurosci. 7:13. doi: 10.3389/fncom.2013.00013
Pizzino, G., Irrera, N., Cucinotta, M., Pallio, G., Mannino, F., Arcoraci, V., et al. (2017). Oxidative stress: harms and benefits for human health. Oxid. Med. Cell. Longev. 2017, 8416763. doi: 10.1155/2017/8416763
Ren, X. D., Wan, C. X., and Niu, Y. L. (2019). Overexpression of lncRNA TCTN2 protects neurons from apoptosis by enhancing cell autophagy in spinal cord injury. FEBS Open Bio 9, 1223–1231. doi: 10.1002/2211-5463.12651
Rodrigo, R., Fernández-Gajardo, R., Gutiérrez, R., Matamala, J. M., Carrasco, R., Miranda-Merchak, A., et al. (2013). Oxidative stress and pathophysiology of ischemic stroke: novel therapeutic opportunities. CNS Neurol. Disord. Drug Targets 12, 698–714. doi: 10.2174/1871527311312050015
Rodriguez-Pallares, J., Parga, J. A., Joglar, B., Guerra, M. J., and Labandeira-Garcia, J. L. (2012). Mitochondrial ATP-sensitive potassium channels enhance angiotensin-induced oxidative damage and dopaminergic neuron degeneration. Relevance for aging-associated susceptibility to Parkinson’s disease. Age (Dordr) 34, 863–880. doi: 10.1007/s11357-011-9284-7
Rönnemaa, E., Zethelius, B., Sundelöf, J., Sundström, J., Degerman-Gunnarsson, M., Berne, C., et al. (2008). Impaired insulin secretion increases the risk of Alzheimer disease. Neurology 71, 1065–1071. doi: 10.1212/01.wnl.0000310646.32212.3a
Salim, S. (2017). Oxidative stress and the central nervous system. J. Pharmacol. Exp. Ther. 360, 201–205.
Saussez, S., Marchant, H., Nagy, N., Decaestecker, C., Hassid, S., Jortay, A., et al. (1998). Quantitative glycohistochemistry defines new prognostic markers for cancers of the oral cavity. Cancer 82, 252–260.
Scannevin, R. H., Chollate, S., Jung, M. Y., Shackett, M., Patel, H., Bista, P., et al. (2012). Fumarates promote cytoprotection of central nervous system cells against oxidative stress via the nuclear factor (erythroid-derived 2)-like 2 pathway. J. Pharmacol. Exp. Ther. 341, 274–284. doi: 10.1124/jpet.111.190132
Shelat, P. B., Chalimoniuk, M., Wang, J. H., Strosznajder, J. B., Lee, J. C., Sun, A. Y., et al. (2008). Amyloid beta peptide and NMDA induce ROS from NADPH oxidase and AA release from cytosolic phospholipase A2 in cortical neurons. J. Neurochem. 106, 45–55. doi: 10.1111/j.1471-4159.2008.05347.x
Shen, Y., Cui, X., Hu, Y., Zhang, Z., and Zhang, Z. (2021). LncRNA-MIAT regulates the growth of SHSY5Y cells by regulating the miR-34-5p-SYT1 axis and exerts a neuroprotective effect in a mouse model of Parkinson’s disease. Am. J. Transl. Res. 13, 9993–10013.
Shi, M., Cao, L., Cao, X., Zhu, M., Zhang, X., Wu, Z., et al. (2018). DR-region of Na(+)/K(+) ATPase is a target to treat excitotoxicity and stroke. Cell Death Dis. 10:6. doi: 10.1038/s41419-018-1230-5
Shi, X., Sun, M., Liu, H., Yao, Y., and Song, Y. (2013). Long non-coding RNAs: a new Frontier in the study of human diseases. Cancer Lett. 339, 159–166. doi: 10.1016/j.canlet.2013.06.013
Sies, H. (2015). Oxidative stress: a concept in redox biology and medicine. Redox Biol. 4, 180–183. doi: 10.1016/j.redox.2015.01.002
Silva-Palacios, A., Ostolga-Chavarría, M., Zazueta, C., and Königsberg, M. (2018). Nrf2: Molecular and epigenetic regulation during aging. Ageing Res. Rev. 47, 31–40. doi: 10.1016/j.arr.2018.06.003
Sinha, N., and Dabla, P. K. (2015). Oxidative stress and antioxidants in hypertension-a current review. Curr. Hypertens. Rev. 11, 132–142. doi: 10.2174/1573402111666150529130922
Soghli, N., Yousefi, T., Abolghasemi, M., and Qujeq, D. (2021). NORAD, a critical long non-coding RNA in human cancers. Life Sci. 264:118665. doi: 10.1016/j.lfs.2020.118665
Sun, W., Sun, L., Sun, X., and Ma, S. (2021). Long non-coding RNA SNHG7 upregulates FGF9 to alleviate oxygen and glucose deprivation-induced neuron cell injury in a miR-134-5p-dependent manner. Metab. Brain Dis. 36, 2483–2494.
Sun, Y., Pham, A. N., Hare, D. J., and Waite, T. D. (2018). Kinetic modeling of pH-dependent oxidation of dopamine by iron and its relevance to Parkinson’s disease. Front. Neurosci. 12:859. doi: 10.3389/fnins.2018.00859
Surguchov, A., Surgucheva, I., Sharma, M., Sharma, R., and Singh, V. (2017). Pore-forming proteins as mediators of novel epigenetic mechanism of epilepsy. Front. Neurol. 8:3. doi: 10.3389/fneur.2017.00003
Tan, X., Liu, Y., Liu, Y., Zhang, T., and Cong, S. (2021). Dysregulation of long non-coding RNAs and their mechanisms in Huntington’s disease. J. Neurosci. Res. 99, 2074–2090. doi: 10.1002/jnr.24825
Wang, C., Wan, H., Wang, Q., Sun, H., Sun, Y., Wang, K., et al. (2020a). Safflor Yellow B Attenuates Ischemic Brain Injury via Downregulation of Long Noncoding AK046177 and Inhibition of MicroRNA-134 Expression in Rats. Oxid. Med. Cell Longev. 2020:4586839. doi: 10.1155/2020/4586839
Wang, Q., Ge, X., Zhang, J., and Chen, L. (2020b). Effect of lncRNA WT1-AS regulating WT1 on oxidative stress injury and apoptosis of neurons in Alzheimer’s disease via inhibition of the miR-375/SIX4 axis. Aging (Albany NY) 12, 23974–23995. doi: 10.18632/aging.104079
Wang, S. D., Fu, Y. Y., Han, X. Y., Yong, Z. J., Li, Q., Hu, Z., et al. (2021b). Hyperbaric oxygen preconditioning protects against cerebral ischemia/reperfusion injury by inhibiting mitochondrial apoptosis and energy metabolism disturbance. Neurochem. Res. 46, 866–877. doi: 10.1007/s11064-020-03219-4
Wang, D., Xu, X., Pan, J., Zhao, S., Li, Y., Wang, Z., et al. (2021a). GAS5 knockdown alleviates spinal cord injury by reducing VAV1 expression via RNA binding protein CELF2. Sci. Rep. 11:3628. doi: 10.1038/s41598-021-83145-9
Wang, K. C., and Chang, H. Y. (2011). Molecular mechanisms of long noncoding RNAs. Mol. Cell 43, 904–914.
Wang, L. W., Li, X. B., Liu, Z., Zhao, L. H., Wang, Y., and Yue, L. (2019). Long non-coding RNA OIP5-AS1 promotes proliferation of gastric cancer cells by targeting miR-641. Eur. Rev. Med. Pharmacol. Sci. 23, 10776–10784. doi: 10.26355/eurrev_201912_19780
Wang, P., Ren, Z., and Sun, P. (2012). Overexpression of the long non-coding RNA MEG3 impairs in vitro glioma cell proliferation. J. Cell Biochem. 113, 1868–1874. doi: 10.1002/jcb.24055
Wang, S. W., Liu, Z., and Shi, Z. S. (2018a). Non-coding RNA in acute ischemic stroke: mechanisms, biomarkers and therapeutic targets. Cell Transplant 27, 1763–1777. doi: 10.1177/0963689718806818
Wang, X., Wang, C., Geng, C., and Zhao, K. (2018b). LncRNA XIST knockdown attenuates Aβ(25-35)-induced toxicity, oxidative stress, and apoptosis in primary cultured rat hippocampal neurons by targeting miR-132. Int. J. Clin. Exp. Pathol. 11, 3915–3924.
Wei, C. W., Luo, T., Zou, S. S., and Wu, A. S. (2018). The role of long noncoding RNAs in central nervous system and neurodegenerative diseases. Front. Behav. Neurosci. 12:175. doi: 10.3389/fnbeh.2018.00175
Wong, H. K., Veremeyko, T., Patel, N., Lemere, C. A., Walsh, D. M., Esau, C., et al. (2013). De-repression of FOXO3a death axis by microRNA-132 and -212 causes neuronal apoptosis in Alzheimer’s disease. Hum. Mol. Genet. 22, 3077–3092. doi: 10.1093/hmg/ddt164
Wu, C. L., Chen, S. D., Yin, J. H., Hwang, C. S., and Yang, D. I. (2016). Nuclear factor-kappaB-dependent sestrin2 induction mediates the antioxidant effects of BDNF against mitochondrial inhibition in rat cortical neurons. Mol. Neurobiol. 53, 4126–4142. doi: 10.1007/s12035-015-9357-1
Wu, R., Yun, Q., Zhang, J., and Bao, J. (2021). Long non-coding RNA GAS5 retards neural functional recovery in cerebral ischemic stroke through modulation of the microRNA-455-5p/PTEN axis. Brain Res. Bull. 167, 80–88. doi: 10.1016/j.brainresbull.2020.12.002
Wu, Y. Y., and Kuo, H. C. (2020). Functional roles and networks of non-coding RNAs in the pathogenesis of neurodegenerative diseases. J. Biomed. Sci. 27:49. doi: 10.1186/s12929-020-00636-z
Xu, J., Zheng, Y., Wang, L., Liu, Y., Wang, X., Li, Y., et al. (2021). miR-124: a promising therapeutic target for central nervous system injuries and diseases. Cell. Mol. Neurobiol. doi: 10.1007/s10571-021-01091-6 [Epub ahead of print].
Yamamoto, M., Kensler, T. W., and Motohashi, H. (2018). The KEAP1-NRF2 system: a thiol-based sensor-effector apparatus for maintaining redox homeostasis. Physiol. Rev. 98, 1169–1203. doi: 10.1152/physrev.00023.2017
Yan, G., Zhao, H., and Hong, X. (2020). LncRNA MACC1-AS1 attenuates microvascular endothelial cell injury and promotes angiogenesis under hypoxic conditions via modulating miR-6867-5p/TWIST1 in human brain microvascular endothelial cells. Ann. Transl. Med. 8:876. doi: 10.21037/atm-20-4915
Yang, L., Wang, L., Wang, J., and Liu, P. (2021). Long non-coding RNA Gm11974 aggravates oxygen-glucose deprivation-induced injury via miR-122-5p/SEMA3A axis in ischaemic stroke. Metab. Brain Dis. 36, 2059–2069. doi: 10.1007/s11011-021-00792-7
Yi, M., Li, Y., Wang, D., Zhang, Q., Yang, L., and Yang, C. (2020). KCNQ1OT1 exacerbates ischemia-reperfusion injury through targeted inhibition of miR-140-3P. Inflammation 43, 1832–1845.
Yu, S. Y., Dong, B., Fang, Z. F., Hu, X. Q., Tang, L., and Zhou, S. H. (2018). Knockdown of lncRNA AK139328 alleviates myocardial ischaemia/reperfusion injury in diabetic mice via modulating miR-204-3p and inhibiting autophagy. J. Cell. Mol. Med. 22, 4886–4898. doi: 10.1111/jcmm.13754
Zhan, Y., Chen, Z., He, S., Gong, Y., He, A., Li, Y., et al. (2020). Long non-coding RNA SOX2OT promotes the stemness phenotype of bladder cancer cells by modulating SOX2. Mol. Cancer 19:25. doi: 10.1186/s12943-020-1143-7
Zhang, R., Wang, J., Liu, B., Wang, W., Fan, X., Zheng, B., et al. (2020c). Differentially expressed lncRNAs, miRNAs and mRNAs with associated ceRNA networks in a mouse model of myocardial ischemia/reperfusion injury. Mol. Med. Rep. 22, 2487–2495. doi: 10.3892/mmr.2020.11300
Zhang, L., Wang, J., Liu, Q., Xiao, Z., and Dai, Q. (2020b). Knockdown of long non-coding RNA AL049437 mitigates MPP+-induced neuronal injury in SH-SY5Y cells via the microRNA-205-5p/MAPK1 axis. Neurotoxicology 78, 29–35. doi: 10.1016/j.neuro.2020.02.004
Zhang, H., Tao, J., Zhang, S., and Lv, X. (2020a). LncRNA MEG3 reduces hippocampal neuron apoptosis via the PI3K/AKT/mTOR pathway in a rat model of temporal lobe epilepsy. Neuropsychiatr. Dis. Treat. 16, 2519–2528. doi: 10.2147/NDT.S270614
Zhang, Y. Y., Bao, H. L., Dong, L. X., Liu, Y., Zhang, G. W., and An, F. M. (2021). Silenced lncRNA H19 and up-regulated microRNA-129 accelerates viability and restrains apoptosis of PC12 cells induced by Aβ(25-35) in a cellular model of Alzheimer’s disease. Cell. Cycle 20, 112–125. doi: 10.1080/15384101.2020.1863681
Zhang, Y., and Zhang, Y. (2020). lncRNA ZFAS1 improves neuronal injury and inhibits inflammation, oxidative stress, and apoptosis by sponging mir-582 and upregulating NOS3 expression in cerebral ischemia/reperfusion injury. Inflammation 43, 1337–1350. doi: 10.1007/s10753-020-01212-1
Zhang, Y., Yang, R., Lian, J., and Xu, H. (2016). LncRNA Sox2ot overexpression serves as a poor prognostic biomarker in gastric cancer. Am J. Transl. Res. 8, 5035–5043.
Zhao, H., Han, Z., Ji, X., and Luo, Y. (2016). Epigenetic regulation of oxidative stress in ischemic stroke. Aging Dis. 7, 295–306. doi: 10.14336/AD.2015.1009
Zhao, L., Zhang, M., Yan, F., and Cong, Y. (2021). Knockdown of RMST impedes neuronal apoptosis and oxidative stress in OGD/R-induced ischemic stroke via depending on the miR-377/SEMA3A signal network. Neurochem. Res. 46, 584–594. doi: 10.1007/s11064-020-03194-w
Zhao, W., Chen, T., and Zhao, Y. (2020). Upregulated lncRNA CASC9 contributes to progression of non-small cell lung cancer through inhibition of miR-335-3p and activation S100A14 expression. Onco Targets Ther. 13, 6027–6036. doi: 10.2147/OTT.S249973
Zhao, X., Wang, P., Liu, J., Zheng, J., Liu, Y., Chen, J., et al. (2015). Gas5 exerts tumor-suppressive functions in human glioma cells by targeting miR-222. Mol. Ther. 23, 1899–1911. doi: 10.1038/mt.2015.170
Zhao, Y., Liu, Y., Lin, L., Huang, Q., He, W., Zhang, S., et al. (2018). The lncRNA MACC1-AS1 promotes gastric cancer cell metabolic plasticity via AMPK/Lin28 mediated mRNA stability of MACC1. Mol. Cancer 17:69. doi: 10.1186/s12943-018-0820-2
Zhou, S., Zhang, D., Guo, J., Chen, Z., Chen, Y., and Zhang, J. (2020). Long non-coding RNA NORAD functions as a microRNA-204-5p sponge to repress the progression of Parkinson’s disease in vitro by increasing the solute carrier family 5 member 3 expression. IUBMB Life 72, 2045–2055. doi: 10.1002/iub.2344
Zhou, Z. W., Ren, X., Zheng, L. J., Li, A. P., and Zhou, W. S. (2022). LncRNA NEAT1 ameliorate ischemic stroke via promoting Mfn2 expression through binding to Nova and activates Sirt3. Metab. Brain Dis. 37, 653–664. doi: 10.1007/s11011-021-00895-1
Zhou, Z. W., Zheng, L. J., Ren, X., Li, A. P., and Zhou, W. S. (2019). LncRNA NEAT1 facilitates survival and angiogenesis in oxygen-glucose deprivation (OGD)-induced brain microvascular endothelial cells (BMECs) via targeting miR-377 and upregulating SIRT1, VEGFA, and BCL-XL. Brain Res. 1707, 90–98. doi: 10.1016/j.brainres.2018.10.031
Keywords: long non-coding RNAs, oxidative stress, therapeutic target, central nervous system, pathogenesis
Citation: Xu X and Zhang Y (2022) Regulation of Oxidative Stress by Long Non-coding RNAs in Central Nervous System Disorders. Front. Mol. Neurosci. 15:931704. doi: 10.3389/fnmol.2022.931704
Received: 29 April 2022; Accepted: 24 May 2022;
Published: 15 June 2022.
Edited by:
Andrei Surguchov, University of Kansas Medical Center, United StatesReviewed by:
Irina G. Sourgoutcheva, University of Kansas Medical Center, United StatesCopyright © 2022 Xu and Zhang. This is an open-access article distributed under the terms of the Creative Commons Attribution License (CC BY). The use, distribution or reproduction in other forums is permitted, provided the original author(s) and the copyright owner(s) are credited and that the original publication in this journal is cited, in accordance with accepted academic practice. No use, distribution or reproduction is permitted which does not comply with these terms.
*Correspondence: Yi Zhang, Y211emhhbmd5aUAxNjMuY29t
Disclaimer: All claims expressed in this article are solely those of the authors and do not necessarily represent those of their affiliated organizations, or those of the publisher, the editors and the reviewers. Any product that may be evaluated in this article or claim that may be made by its manufacturer is not guaranteed or endorsed by the publisher.
Research integrity at Frontiers
Learn more about the work of our research integrity team to safeguard the quality of each article we publish.