- 1Department of Psychiatry and Psychotherapy Medical University of Vienna, Vienna, Austria
- 2Department of Rehabilitation Sciences, The Hong Kong Polytechnic University, Hong Kong, Hong Kong SAR, China
- 3Department of Biomedical Imaging and Image-guided Therapy, High Field MR Centre, Medical University of Vienna, Vienna, Austria
- 4Department of Molecular Neuroscience, Center for Brain Research, Medical University of Vienna, Vienna, Austria
Background: Theta burst stimulation (TBS) belongs to one of the biological antidepressant treatment options. When applied bilaterally, excitatory intermittent TBS (iTBS) is commonly targeted to the left and inhibitory continuous TBS (cTBS) to the right dorsolateral prefrontal cortex. TBS was shown to influence neurotransmitter systems, while iTBS is thought to interfere with glutamatergic circuits and cTBS to mediate GABAergic neurotransmission.
Objectives: We aimed to expand insights into the therapeutic effects of TBS on the GABAergic and glutamatergic system utilizing 3D-multivoxel magnetic resonance spectroscopy imaging (MRSI) in combination with a novel surface-based MRSI analysis approach to investigate changes of cortical neurotransmitter levels in patients with treatment-resistant depression (TRD).
Methods: Twelve TRD patients (five females, mean age ± SD = 35 ± 11 years) completed paired MRSI measurements, using a GABA-edited 3D-multivoxel MEGA-LASER sequence, before and after 3 weeks of bilateral TBS treatment. Changes in cortical distributions of GABA+/tNAA (GABA+macromolecules relative to total N-acetylaspartate) and Glx/tNAA (Glx = mixed signal of glutamate and glutamine), were investigated in a surface-based region-of-interest (ROI) analysis approach.
Results: ANCOVAs revealed a significant increase in Glx/tNAA ratios in the left caudal middle frontal area (pcorr. = 0.046, F = 13.292), an area targeted by iTBS treatment. Whereas, contralateral treatment with cTBS evoked no alterations in glutamate or GABA concentrations.
Conclusion: This study demonstrates surface-based adaptions in the stimulation area to the glutamate metabolism after excitatory iTBS but not after cTBS, using a novel surface-based analysis of 3D-MRSI data. The reported impact of facilitatory iTBS on glutamatergic neurotransmission provides further insight into the neurobiological effects of TBS in TRD.
Introduction
Major depressive disorder (MDD) represents a severe psychiatric disease affecting up to 3.8% of the population worldwide and has risen further during the last years (COVID-19 Mental Disorders Collaborators, 2021). Several treatment options of pharmacological [e.g., selective serotonin reuptake inhibitors (SSRIs) or ketamine] or non-pharmacological, biological interventions [i.e., transcranial magnetic stimulation (TMS) or electroconvulsive therapy (ECT)] are currently available. Modifications of neurotransmitter systems are key aspects of the antidepressant actions of different interventions in order to restore GABAergic or glutamatergic function (Kalueff and Nutt, 2007; Sanacora et al., 2012). Several studies have shown SSRIs or ketamine to affect a variety of neurotransmitter systems including the serotonergic (Spindelegger et al., 2009; Hahn et al., 2010; Lanzenberger et al., 2012), GABAergic (Sanacora et al., 2002; Brennan et al., 2017; Silberbauer et al., 2020) or the glutamatergic system (Rowland et al., 2005; Taylor et al., 2008; Spurny et al., 2021). Moreover, certain antidepressants directly interfere with the glutamatergic or GABAergic system. The N-methyl-D-aspartate (NMDA) receptor antagonist ketamine is a treatment option for use in TRD patients, leading to rapid symptom reductions (Kasper et al., 2021; McIntyre et al., 2021). Although ketamine is targeting the glutamatergic system, adaptions in GABA levels could be reported (Silberbauer et al., 2020). According to a recent study, the clinical efficacy in treatment resistant depression (TRD) of rTMS does not differ from ketamine (Mikellides et al., 2021). Hence, both the glutamatergic and GABAergic systems are promising targets for the treatment of TRD.
While the biological binding sites and downstream effects of pharmacological interventions are abundantly studied, this is oftentimes less clear for non-pharmacological, biological treatments. Since TMS constitutes a biological treatment approach with few side effects, it finds broad acceptance in patients, especially suffering from treatment resistant depression (TRD). When two different pharmacological treatment trials fail to significantly improve clinical symptoms, MDD is commonly classified as TRD, although this definition varies between studies (Gaynes et al., 2020). In a meta-analysis of 29 randomized, double-blind and sham-controlled trials, Berlim et al. (2014) demonstrated response and remission rates of 29% and 19% of subjects with major depression receiving excitatory high-frequency (≥ 5 Hz) TMS.
Previous imaging studies reported diverse effects of TMS on different morphological and physiological parameters. Stimulation of the dorsolateral prefrontal cortex (DLPFC) was reported to affect functional connectivity between the PFC and cingulate regions (Baeken et al., 2014; Salomons et al., 2014). Similar to pharmacological treatments, TMS was shown to evoke changes in neurotransmitter systems in both animal and human studies. Two 1H-MRS studies found correlations between glutamate levels in the motor cortex and excitability with TMS (Stagg et al., 2009; Tremblay et al., 2013). In disease, a study by Pogarell et al. (2006) revealed adaptions in the dopaminergic system in MDD patients following repetitive TMS (rTMS) treatment. Furthermore, an investigation by Lewis et al. (2016) reported changes in cortical excitability in patients suffering from MDD in the primary motor cortex and the ACC (Lewis et al., 2016). Dubin et al. (2016) were one of the first to investigate the therapeutic effect of TMS on neurotransmitter distribution using MRS, showing elevated GABA levels. However, effects on the glutamatergic system in MDD are less conclusive and similar to GABA limited to a handful of studies. While Dubin and colleagues reported no effects on glutamate in the PFC, a different approach revealed elevations in the glutamate/glutamine (Glu/Gln) ratio after TMS treatment in MDD patients (Croarkin et al., 2016; Dubin et al., 2016).
In addition to rTMS, the high frequency form theta burst stimulation (TBS), utilizing frequencies of 50 Hz, showed promising results in MDD. For the treatment of depression, bihemispheric TBS is typically applied using excitatory intermittent (iTBS) or inhibitory continuous TBS (cTBS) to the DLPFC, since the DLPFC was shown to provide a suitable target for TBS to treat TRD (George et al., 1995). However, especially TBS impacts on neurotransmitter levels are understudied. Both the GABAergic and glutamatergic system seems to be involved in underlying neurobiological mechanisms of iTBS and cTBS, respectively. Thereby, it is speculated that iTBS produces long-term potentiation (LTP) effects by affecting NMDA receptor related Ca2+ influx. On the other hand, cTBS seems to activate interneural inhibitory pathways leading to long-term depression (LTD)-like effects (Huang et al., 2011). Moreover, the involvement of glutamatergic neurotransmission was demonstrated when ketamine drastically reduced iTBS effects in rats (Labedi et al., 2014). Nevertheless, both TBS-induced LTP and LTD are thought to be accompanied by presynaptic changes in GABA release (Larson and Munkacsy, 2015; Li et al., 2019).
Previous MRS studies have reported inconclusive results, trying to replicate these preclinical findings in humans. Iwabuchi et al. (2017) showed reduced GABA/Glx levels in the DLPFC and ACC after iTBS to the left DLPFC (Iwabuchi et al., 2017). Moreover, increased GABA concentrations in the PCC after iTBS to the left inferior parietal lobe could be revealed (Vidal-Pineiro et al., 2015). In addition, iTBS was described to increase the N100 amplitude (a marker for GABA-mediated inhibition), while cTBS reduced this amplitude in the cerebellum of healthy individuals (Harrington and Hammond-Tooke, 2015). On the other hand, cTBS, applied to the motor cortex, was reported to increase GABA concentrations while no effects were shown on glutamate (Stagg et al., 2009) suggesting an enhancement of interneural circuits. Based on the evidence of preclinical and clinical studies in animals and humans, Li et al. (2019) proposed a model to explain differential aftermaths of intermittent and continuous TBS on GABA and glutamate. This model suggests iTBS inhibits GABAergic interneurons, which in consequence leads to reduced inhibition of glutamatergic pyramidal cells, while continuous bursts of cTBS might increase the inhibitory activities of interneurons resulting in higher GABA concentrations.
However, due to the inconclusive evidence of the potential of TBS to restore disrupted GABAergic and glutamatergic neurotransmission in TRD subjects, further research is needed. Since previous findings of MRS studies were restricted to a limited number of locations by the use of single-voxel sequences, we aimed to extend our understanding by applying a 3D-multivoxel MRSI approach to cover a range of cortical regions, involved in the pathophysiology of MDD. Due to the cortical stimulation method of TBS, changes within these regions are of high interest. Hence, we applied a novel surface-based analysis approach to multi-voxel MRS data, based on a similar method used in PET imaging (Greve et al., 2014). We investigated adaptions in cortical GABA+/tNAA (GABA+ = a combination of GABA and macromolecules; tNAA = total N-acetylaspartate) and Glx/tNAA (Glx = combined signal of glutamate and glutamine) after 3 weeks of iTBS to left and cTBS treatment to the right DLPFC in a cohort of TRD patients.
Methods
Study Design
All study patients underwent 3 weeks of TBS treatment at the Department of Psychiatry and Psychotherapy at the Medical University of Vienna, Austria. MRSI measurements were conducted within 2 weeks prior to and after the TBS treatment period (see Figure 1). This study was approved by the Ethics Committee of the Medical University of Vienna (EK 1761/2015) and is part of a larger clinical trial with multimodal neuroimaging (ClinicalTrials.gov Identifier: NCT02810717).
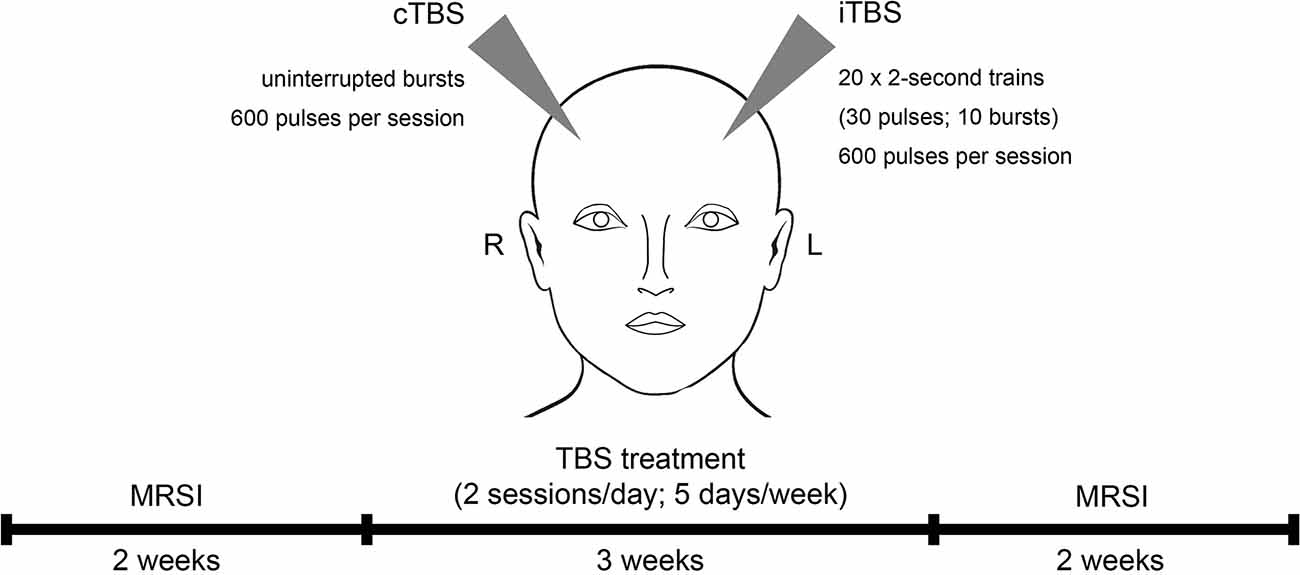
Figure 1. Study design and treatment regime. Study subjects received theta burst stimulation (TBS) over 3 weeks including two stimulations a day for 5 days per week. TBS sessions comprised intermittent TBS (iTBS) to the left and continuous TBS (cTBS) to the right dorsolateral prefrontal cortex. Magnetic resonance spectroscopy imaging (MRSI) measurements were conducted within 2 weeks before and after the treatment period.
Participants
Twelve TRD patients (five females, mean age ± SD = 35 ± 11 years) with a DSM-4 diagnosis of single or recurrent MDD were included in our analysis. TRD was defined as an insufficient response to two treatment trials in adequate dosage and time (>4 weeks) according to the criteria set by the GSRD group (Group for the studies of Resistant Depression; Bartova et al., 2019). Moreover, participants were included if they had a HAMD-17 total score of ≥18, a Clinical Global Impression Scale score of ≥4 and a stable treatment regime of 4 weeks prior to the study inclusion, which remained unchanged during the study participation. Exclusion criteria included psychotic symptoms, severe internal illnesses within the last 5 years, neurological diseases or brain injuries, substance abuse left handedness, or any contraindications to TMS treatment and MRI.
Transcranial Magnetic Stimulation
Over the course of three weeks, patients received intermittent (stimulating) TBS (iTBS) to the left DLPFC and continuous (inhibiting) TBS (cTBS) to the right DLPFC. iTBS consisted of 2-s trains (30 pulses; 10 bursts) repeated 20 times (600 pulses per session). cTBS comprised uninterrupted bursts of 600 pulses per session (see Figure 1). The TBS protocol was performed similar to Huang et al. (2005) (3-pulse 50-Hz bursts delivered at 5 Hz) by using a MagPro magnetic stimulator (MagVenture, Denmark K) and a figure-of-eight shaped cool coil (Cool-B70). Daily treatment (5 days per week) included two TBS sessions, separated by 1 h. Within each session, bilateral treatment with iTBS and cTBS was conducted, starting in randomized order, which reversed for consecutive sessions (Li et al., 2014). The stimulation area (DLPFC) was defined in Montreal Neurological Institute (MNI) space [coordinates: (−38, +44, +26)—left DLPFC; (+38, +44, +26)—right DLPFC], using neuro-navigation (LOCALITE® TMS Navigator Germany), based on individual structural MRIs of each participant (Hecht, 2010). Stimulation intensity was based on 120% of the individual resting motor threshold (Ge et al., 2017).
Magnetic Resonance Spectroscopy
MRI measurements were performed on a 3 Tesla MAGNETOM Prisma Siemens MR Scanner using a 64-channel head coil. For an accurate volume of interest (VOI)-placement and surface extraction, 3D T1-weighted anatomical images were acquired via an MPRAGE sequence (208 slices, 288 × 288 matrix size, voxel size 1.15 × 1.15 × 0.85 mm3) with GRAPPA acceleration. For MRS, a constant-density, spiral-encoded, 3D-MRSI sequence with MEGA-LASER editing (Bogner et al., 2014) was used with a VOI = 110 × 120 × 45 mm3 and field of view (FOV) = 160 × 160 × 160 mm3. The acquired matrix size of 10 × 10 × 10 (approx. 4 cm3 voxel size) was interpolated to a 16 × 16 × 16 matrix (approx. 1 cm3 voxel size) during spectral processing steps. Since the VOI was placed close to the skull to cover cortical regions, tissue saturation slabs (25 mm thickness, sat. delta frequency: −3.5 ppm) were used to suppress signals from subcutaneous lipids (see Figure 2). Siemens advanced shimming procedure with manual adjustments was used. During the EDIT-ON acquisition, MEGA-editing pulses (60 Hz Gaussian pulses of 14.8 ms duration) were set to 1.9 ppm, editing the coupled 4CH2 triplet of GABA resonating at 3.02 ppm. Twenty-four acquisition-weighted averages and two-step phase cycling were employed for 3D-MRSI, resulting in a total scan time of 17:23 min.
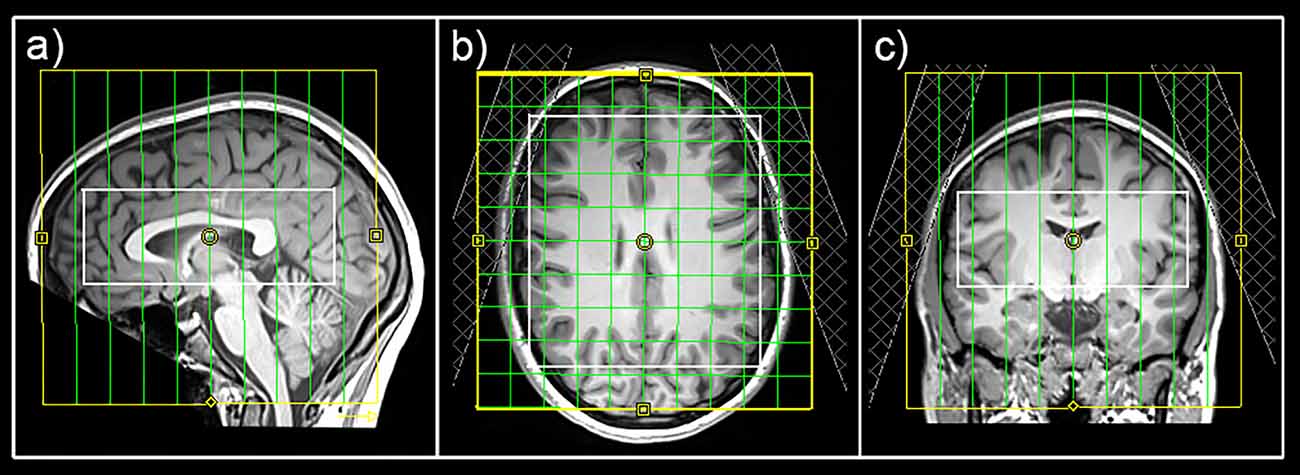
Figure 2. Placement of the field of view (yellow), volume of interest (white), and tissue separation slaps in sagittal (A), horizontal (B), and coronal (C) views.
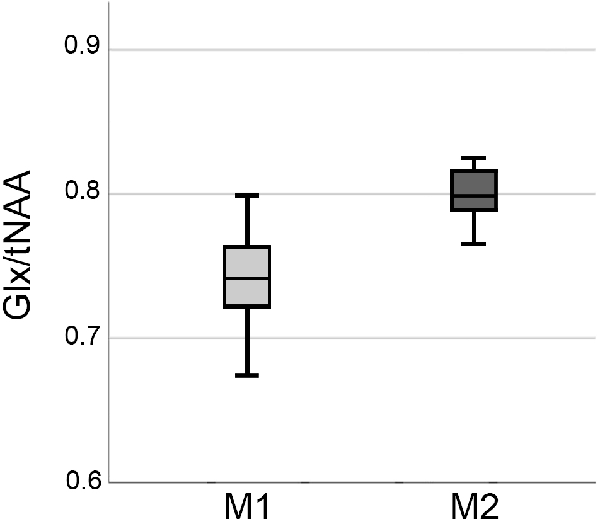
Figure 3. Boxplots showing elevations in Glx/tNAA ratio in the right caudal middle frontal area before (M1) and after the treatment period (M2). Glx, combined measure of glutamate and glutamine; tNAA, total N-acetylaspartate.
An in-house software tool using MATLAB (R2013a, MathWorks, Natick, MA, USA), Bash (4.2.25, Free Software Foundation, Boston, MA, USA), MINC (2.0, MINC Tools, McConnell Brain Imaging Center, Montreal, QC, Canada) and LCModel software (6.3-1, S. Provencher, LCModel, Oakville, ON, Canada) was used for the quantification of all spectra within the VOI (Spurny et al., 2019). A simulated basis set was created using the GAMMA library for the difference spectrum [containing GABA+, Glx, and tNAA among others (Hnilicova et al., 2016)]. An exemplary spectrum is shown in Supplementary Figure 1. Cramér–Rao lower bounds (CRLB) thresholds were set at 30% and spectra were visually inspected.
Surface-Based MRSI Analysis
For surface-based quantification, metabolic maps of GABA+, Glx, and tNAA were interpolated to the resolution of anatomical images and ratio maps of GABA+/tNAA and Glx/tNAA were calculated. Ratios to tNAA were favored over total creatine (tCr), since changes in tCr after rTMS treatment were previously reported (Grohn et al., 2019). FreeSurfer 6.01 was used for the surface-based analysis approach of MRSI data. Previous investigations have successfully shown cortical analysis approaches of metabolic maps in FreeSurfer using positron emission tomography (PET) data (Greve et al., 2014). Hence, this analysis was based on previous reports. Individual ratio maps of single subjects were spatially normalized by projecting onto the standard surface (fsaverage) using the tkregister2 command. All vertices of individual surfaces were assigned to the corresponding region-of-interest (ROI) using the Desikan atlas (Desikan et al., 2006). The following ROIs were included in the analysis: superiorfrontal, rostral middle frontal, caudal middle frontal, pars opercularis, and precentral for both Glx/tNAA and GABA+/tNAA and additionally pars triangularis, postcentral, paracentral, posterior cingulate, and caudal anterior cingulate for Glx/tNAA ratios only, due to insufficient data quality in GABA+ maps. Furthermore, each surface was filtered by removing vertices that did not pass the CRLB threshold or laid above twice the standard variation within its respective brain region. After filtering steps, all remaining vertices were averaged within each ROI. Group-wise comparisons between measurements were done with calculated mean cortical neurotransmitter ratios within ROIs of each subject.
Statistical Analyses
Statistical analyses were performed using SPSS Statistics (v26.0, 2010, SPSS, Inc., an IBM Company, Chicago, United States of America). Two-tailed paired t-tests were conducted to test for differences in HAM-D measures before and after the treatment period (p < 0.025). Univariate analyses of covariance (ANCOVAs) including sex and age as covariates were performed for each ROI and neurotransmitter ratio independently, to test for differences between measurements. Sidak correction was applied to correct for multiple comparisons (ROIs * neurotransmitter ratios, Table 1). Residuals were tested for normal distribution using the Kolmogorov-Smirnoff test. Moreover, post hoc Spearman correlation analyses between changes in neurotransmitter ratios and changes in HAM-D scores were performed in ROIs showing significant adaptions in Glx/tNAA and GABA+/tNAA ratios. Again, Sidak correction was applied to correct for multiple comparisons.
Results
All 12 TRD patients (five female, mean age ± SD = 35 ± 11 years) completed both MRSI measurements. Detailed stable pharmacological treatment of the patient cohort can be found in Supplementary Table 1. HAM-D measures showed significant reductions after the treatment period (19.9 ± 2.8 before treatment to 12 ± 6.8 post treatment (mean ± SD), p = 0.002) with a response rate of 33% (HAM-D reductions ≥ 50%) and remission rate of 25% (HAM-D < 7) of the TRD patients.
Due to insufficient data quality of GABA+ maps in the right superiofrontal area, this ROI had to be excluded resulting in a total of 29 ROIs in the final analysis (see Table 1). Hence, results from ANCOVAs were corrected for 29 comparisons using the Sidak correction method.
ANCOVAs revealed a significant difference in Glx/tNAA ratios in the left caudal middle frontal area (pcorr. = 0.046, F = 13.292), an area targeted by (excitatory) iTBS treatment. Boxplots illustrating mean Glx/tNAA ratios before and after the treatment are shown in Figure 3. No changes in GABA+/tNAA ratios could be detected in any ROI investigated. Although a cluster of elevated GABA+/tNAA ratios can be seen in the right caudal middle frontal area (see Figure 4D), an area targeted with inhibitory cTBS, changes within this area did not reach statistical significance.
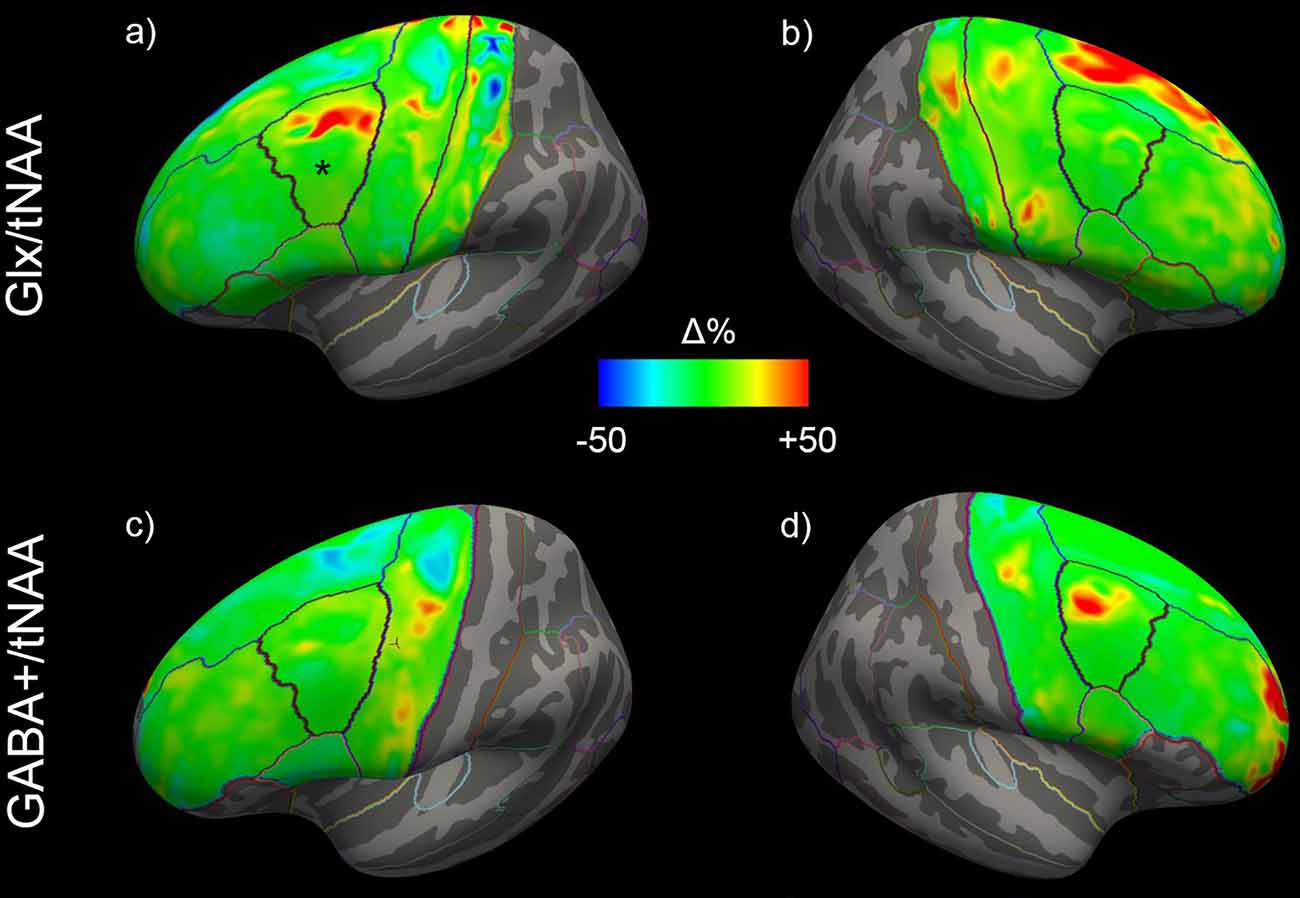
Figure 4. Mean changes of Glx/tNAA and GABA+/tNAA ratios of the left (A,C) and right (B,D) hemisphere across all study participants. The left caudal middle frontal area in (A), showing significant changes, is marked with a *. Glx, combined measure of glutamate and glutamine; tNAA, total N-acetylaspartate; GABA+, a combination of GABA and macromolecules.
Changes in cortical Glx/tNAA and GABA+/tNAA are depicted in Figure 4. Moreover, distributions of Glx/tNAA and GABA+/tNAA before and after the treatment are shown in Figure 5 and Supplementary Figure 2.
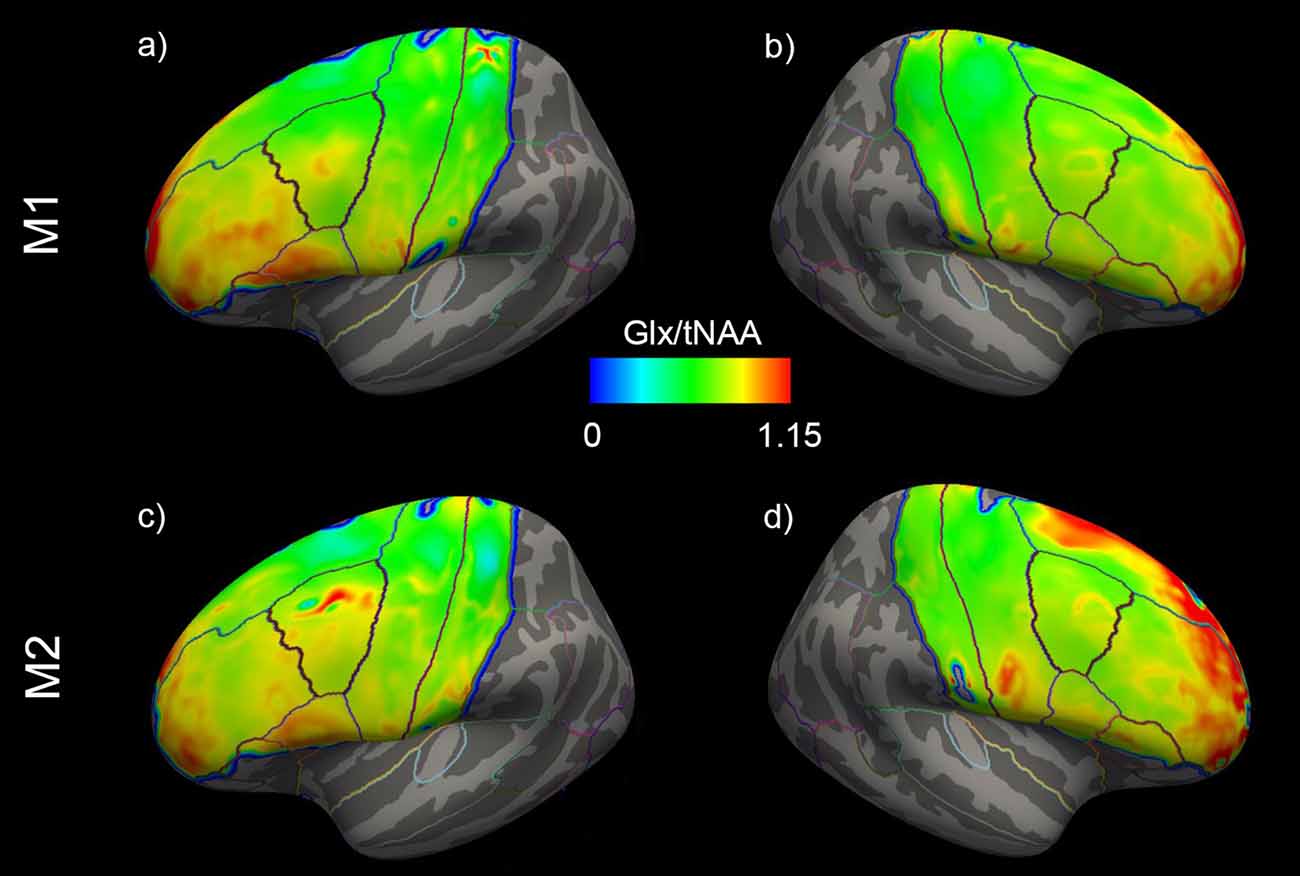
Figure 5. Mean distribution of Glx/tNAA ratios before (=M1) and after (=M2) the treatment period of the left (A,C) and right (B,D) hemispheres. Glx, combined measure of glutamate and glutamine; Tnaa, total N-acetylaspartate.
No significant correlations could be found between changes in HAM-D scores and changes in neurotransmitter ratios after correction for multiple comparisons in any ROI investigated.
Discussion
Here we report elevated Glx/tNAA ratios in the left caudal middle frontal area after 3 weeks of TBS treatment in TRD patients using a surface-based MRSI analysis approach. Significant increases of Glx/tNAA were found in the left caudal middle frontal area after iTBS, while Glx/tNAA in the corresponding right area remained unchanged after cTBS. No changes in GABA+/tNAA ratios were revealed across the investigated cortical regions. Similar to previous surface-based PET analysis approaches (Greve et al., 2014), the proposed surface-based investigations of MRSI data provide a suitable tool when adaptions in neurotransmitter levels of cortical regions are expected, i.e., by utilizing cortical stimulation methods. Moreover, following the treatment, TRD patients experienced a marked reduction of HAM-D scores, a response rate of ~33%, and a remission rate of ~25%, which seems promising when compared to the 13.7% remission rate of the equivalent TRD patient collective in the third treatment step of the STAR*D study (Rush et al., 2006). Hence, the impact of the stimulation on neurotransmitters gives further insight into the neurobiological effects of TBS.
A dysregulation of glutamate, glutamine, and GABA metabolism in MDD could be previously shown (Croarkin et al., 2011; Sanacora et al., 2012; Abdallah et al., 2014). Moreover, rTMS was assumed to directly influence these neurotransmitter systems. In line with Croarkin et al. (2016), showing elevated glutamine/glutamate ratios in the anterior cingulate cortex and DLPFC, we found an increase in Glx/tNAA levels in the stimulation area of iTBS. Prior studies suggested modulation of the glutamatergic system after rTMS demonstrating higher glutamate levels in both preclinical (Yue et al., 2009) and clinical studies (Michael et al., 2003). Moreover, Luborzewski et al. (2007) were able to show a link between clinical effectiveness of rTMS treatment to the DLPFC and glutamate elevations through rTMS therapy. On a neurobiological level, rTMS is thought to alter synaptic connections and thereby affect long-term potentiation (Fitzgerald et al., 2006). Hence, cTBS is suggested to lower synaptic strength, while iTBS leads to opposite effects in both GABAergic and glutamatergic cells (Huang et al., 2005, 2007). These findings highlight the importance of glutamate and its receptors to the physiological TBS response in the human brain (Huang et al., 2007; Ishikawa et al., 2007; Li et al., 2019). As proposed in the model by Li and colleagues, describing the neurophysiological effects of TBS treatment, the stimulating or facilitatory iTBS is thought to be accompanied by inhibition of GABAergic interneurons via feedforward inhibition leading to decreased suppression of glutamatergic cells (Li et al., 2019). In consideration of this model, our results demonstrate increased Glx/tNAA ratios in the stimulation area of iTBS. Hence, the decreased suppression of glutamatergic cells seems to be reflected in increased Glx/tNAA content, while the inhibition of interneurons was not reflected in altered GABA+/tNAA ratios.
On the other hand, inhibitory cTBS is speculated to activate the I-1 pathway as well as leading to long-term depression by prolonged Ca2+ increases and thereby slowly increasing the activity of GABAergic interneurons (Li et al., 2019). This was supported by increased GABA levels detected after cTBS to the motor cortex, without changes in glutamate being observed (Stagg et al., 2009). Moreover, several studies implicated a contribution of GABAergic neurotransmission in TBS-evoked plasticity (Larson and Munkacsy, 2015). Findings that cTBS lead to decreased numbers in calbindin interneurons (Suppa et al., 2016) or a report of a modulation of different classes of interneurons after both cTBS and iTBS (Labedi et al., 2014) adds to the importance of the interneural network in TBS mechanisms. Interestingly, increases in GABA concentrations could be shown after both iTBS and cTBS when some clinical studies reported elevated GABA levels or an influence on the marker for GABAergic inhibition after iTBS treatment in patients (Harrington and Hammond-Tooke, 2015; Vidal-Pineiro et al., 2015; Dubin et al., 2016), while cTBS was also shown to increase GABA concentrations in the motor cortex of healthy individuals (Stagg et al., 2009). However, the attribution of GABAergic interneurons could not be reflected in alterations in total GABA+ content in the scope of our study. Although, there seems to be a cluster with GABA+/tNAA increases in the stimulation area of cTBS, our data did not reach statistical significance within this ROI. Hence, in contrast to previous MRS studies of patient cohorts, we could not show GABA alterations after iTBS (Harrington and Hammond-Tooke, 2015; Dubin et al., 2016). Reasons for the absence of significant changes in GABA+/tNAA content can be manifold. While the use of GABA-edited MRSI provides the basis for the quantification of both excitatory and inhibitory neurotransmitters, the GABA signal is prone to artifacts. Both motion artifacts, due to the long measurement time, as well as voxel blurring, resulting from the rather big voxel sizes and spatial interpolations in the post-processing steps, leading to confounding effects on spatial specificity, have potentially attributed to the lack of significant changes in GABA+ ratios. Moreover, considering the voxel sizes, changes in GABA+/tNAA may have been too subtle, in regard to the derived SNR, to lead to significant results in this sample size. In addition, it has to be considered that here a combined signal of GABA and macromolecules, mainly containing lipids and methyl and methylene resonances of proteins (Behar and Ogino, 1993; Povazan et al., 2015), (=GABA+) was quantified. Although macromolecule content is thought to be stable (Cudalbu et al., 2021), the influence of TBS treatment on macromolecule levels cannot be excluded. Hence, further studies are needed to clarify the influence of TBS treatment in TRD on GABAergic neurotransmission.
While TRD patients showed significant reductions in HAM-D scores, the correlation analyses conducted revealed no significant relationships between changes in HAM-D scores and neurotransmitter ratios in the stimulation area. However, a correlation between clinical effectiveness and changes in neurotransmitter levels, as reported in Luborzewski et al. (2007) may be more distinct in bigger sample sizes. Nevertheless, the absence of the speculated relationship in this study suggests that changes in Glx/tNAA levels in the stimulation area of iTBS may attribute but are not solely responsible for reductions in HAM-D scores.
This surface-based analysis approach for MRSI data provides a suitable method when changes in cortical neurotransmitter concentrations are of interest. Based on previous surface-based PET analysis approaches (Greve et al., 2014), analysis of cortical metabolites can be done using cortex-based atlases in individual subjects (Desikan et al., 2006). However, an appropriate MRSI sequence with reliable signal suppression in lipid-rich areas is required. In the course of this study, tissue separation slabs were used to cancel signals of lipid-rich regions (see Figure 2). An effect of lipid suppression on spectral quality is demonstrated in Supplementary Figure 3. Moreover, this method allows the quantification of several cortical regions simultaneously, which discriminates these investigations from previous MRS studies focusing on the effects of rTMS treatment using single voxel approaches in very selected brain regions.
Some limitations of this study need to be mentioned. Due to the previously discussed reasons, available data of GABA+/tNAA ratios was limited to a restricted number of cortical brain regions compared to derived Glx maps. In line with most MRSI studies conducted at 3T, we quantified the combined signal of glutamate and glutamine (Glx), due to overlapping peaks of both compounds. Hence, changes in the combined Glx measures cannot be clearly attributed to either metabolite. Therefore, future approaches may use higher field strengths to allow a distinct quantification of glutamate and glutamine. Moreover, MRSI does not allow distinguishing between intra- and extracellular neurotransmitter content. All TRD patients included in these analyses had stable treatment regimens of at least 4 weeks prior to the study inclusion, which remained unchanged in the course of this study. However, an attribution of pharmacological interventions in the derived GABA+ and Glx concentrations cannot be excluded. Moreover, we could not include a group receiving sham treatment due to the limited number of available TRD patients undergoing MRSI.
Conclusion
This study demonstrates a significant increase in Glx/tNAA ratios in the stimulation area of excitatory iTBS treatment of TRD patients. Our findings suggest changes in glutamate metabolism, following excitatory iTBS, to be mediated by reduced inhibition of pyramidal cell, while neurotransmitter concentrations remained stable after inhibitory cTBS on the contralateral hemisphere. These results may help to contribute to a better understanding of the neurobiological implications of TBS in TRD patients.
Data Availability Statement
The datasets presented in this article are not readily available due to data protection laws. Processed data is available from the authors upon reasonable request. Requests to access the datasets should be directed to RL, rupert.lanzenberger@meduniwien.ac.at.
Ethics Statement
The studies involving human participants were reviewed and approved by Ethics Committee of the Medical University of Vienna (EK 1761/2015). The patients/participants provided their written informed consent to participate in this study.
Author Contributions
BS-D wrote the manuscript, performed MRSI measurements together with MR, and analyzed the data under the supervision of WB. GG and JU conducted TBS stimulations and were responsible for medical support together with TV and PB-M. WB provided technical support. GK, AH, RL, and SK were responsible for the conceptualization of the study. All authors were involved in interpretation of the data, critically reviewed the manuscript and approved the final content of the manuscript.
Funding
This research was funded in whole, or in part by the Austrian Science Fund (FWF; KLI 551, to SK) and grant number P 30701 to WB, the Medical Imaging Cluster of the Medical University of Vienna, and by the grant “Interdisciplinary translational brain research cluster (ITHC) with highfield MR” from the Federal Ministry of Science, Research and Economy (BMWFW), Austria. For the purpose of open access, the author has applied a CC BY public copyright license to any Author Accepted Manuscript version arising from this submission. MR is a recipient of a DOC Fellowship of the Austrian Academy of Sciences.
Conflict of Interest
In the past 3 years SK has received grant/research support from Lundbeck; he has served as a consultant or on advisory boards for Angelini, Biogen, Esai, Janssen, IQVIA, Lundbeck, Mylan, Recordati, Sage and Schwabe; and he has served on speaker bureaus for Abbott, Angelini, Aspen Farmaceutica S.A., Biogen, Janssen, Lundbeck, Recordati, Sage, Sanofi, Schwabe, Servier, Sun Pharma and Vifor. Without any relevance to this work, RL declares that he received travel grants and/or conference speaker honoraria within the last 3 years from Bruker BioSpin MR and Heel, and has served as a consultant for Ono Pharmaceutical. He received investigator-initiated research funding from Siemens Healthcare regarding clinical research using PET/MR. He is a shareholder of the start-up company BM Health GmbH since 2019. GK declares that he received conference speaker honorarium from Roche, AOP Orphan and Pfizer. TV has served on speaker bureaus for Jansen.
The remaining authors declare that the research was conducted in the absence of any commercial or financial relationships that could be construed as a potential conflict of interest.
Publisher’s Note
All claims expressed in this article are solely those of the authors and do not necessarily represent those of their affiliated organizations, or those of the publisher, the editors and the reviewers. Any product that may be evaluated in this article, or claim that may be made by its manufacturer, is not guaranteed or endorsed by the publisher.
Acknowledgments
We would like to express our gratitude towards Jonathan Downar for his assistance in the conceptualization of the study. We would further like to thank Richard Frey, Gregor Gryglewski, Marius Hienert, Marie Spies, Christoph Kraus, Alexander Kautzky, Arkadiusz Komorowski, Paul Michenthaler and the diploma students of the Neuroimaging Labs (NIL) for medical support. Moreover, we would like to express our gratitude towards Sebastian Ganger, Philipp Moser and Eva Heckova for technical support, and all additional staff of our department involved in the realization of this research.
Supplementary Material
The Supplementary Material for this article can be found online at: https://www.frontiersin.org/articles/10.3389/fnmol.2022.913274/full#supplementary-material.
Footnotes
References
Abdallah, C. G., Jiang, L., De Feyter, H. M., Fasula, M., Krystal, J. H., Rothman, D. L., et al. (2014). Glutamate metabolism in major depressive disorder. Am. J. Psychiatry 171, 1320–1327. doi: 10.1176/appi.ajp.2014.14010067
Baeken, C., Marinazzo, D., Wu, G. R., Van Schuerbeek, P., De Mey, J., Marchetti, I., et al. (2014). Accelerated HF-rTMS in treatment-resistant unipolar depression: insights from subgenual anterior cingulate functional connectivity. World J. Biol. Psychiatry 15, 286–297. doi: 10.3109/15622975.2013.872295
Bartova, L., Dold, M., Kautzky, A., Fabbri, C., Spies, M., Serretti, A., et al. (2019). Results of the european group for the study of resistant depression (GSRD) - basis for further research and clinical practice. World J. Biol. Psychiatry 20, 427–448. doi: 10.1080/15622975.2019.1635270
Behar, K. L., and Ogino, T. (1993). Characterization of macromolecule resonances in the 1H NMR spectrum of rat brain. Magn. Reson. Med. 30, 38–44. doi: 10.1002/mrm.1910300107
Berlim, M. T., van den Eynde, F., Tovar-Perdomo, S., and Daskalakis, Z. J. (2014). Response, remission and drop-out rates following high-frequency repetitive transcranial magnetic stimulation (rTMS) for treating major depression: a systematic review and meta-analysis of randomized, double-blind and sham-controlled trials. Psychol. Med. 44, 225–239. doi: 10.1017/S0033291713000512
Bogner, W., Gagoski, B., Hess, A. T., Bhat, H., Tisdall, M. D., van der Kouwe, A. J., et al. (2014). 3D GABA imaging with real-time motion correction, shim update and reacquisition of adiabatic spiral MRSI. Neuroimage 103, 290–302. doi: 10.1016/j.neuroimage.2014.09.032
Brennan, B. P., Admon, R., Perriello, C., LaFlamme, E. M., Athey, A. J., Pizzagalli, D. A., et al. (2017). Acute change in anterior cingulate cortex GABA, but not glutamine/glutamate, mediates antidepressant response to citalopram. Psychiatry Res. Neuroimag. 269, 9–16. doi: 10.1016/j.pscychresns.2017.08.009
COVID-19 Mental Disorders Collaborators (2021). Global prevalence and burden of depressive and anxiety disorders in 204 countries and territories in 2020 due to the COVID-19 pandemic. Lancet 398, 1700–1712. doi: 10.1016/S0140-6736(21)02143-7
Croarkin, P. E., Levinson, A. J., and Daskalakis, Z. J. (2011). Evidence for GABAergic inhibitory deficits in major depressive disorder. Neurosci. Biobehav. Rev. 35, 818–825. doi: 10.1016/j.neubiorev.2010.10.002
Croarkin, P. E., Nakonezny, P. A., Wall, C. A., Murphy, L. L., Sampson, S. M., Frye, M. A., et al. (2016). Transcranial magnetic stimulation potentiates glutamatergic neurotransmission in depressed adolescents. Psychiatry Res. Neuroimag. 247, 25–33. doi: 10.1016/j.pscychresns.2015.11.005
Cudalbu, C., Behar, K. L., Bhattacharyya, P. K., Bogner, W., Borbath, T., de Graaf, R. A., et al. (2021). Contribution of macromolecules to brain (1) H MR spectra: experts’ consensus recommendations. NMR Biomed. 34:e4393. doi: 10.1002/nbm.4393
Desikan, R. S., Segonne, F., Fischl, B., Quinn, B. T., Dickerson, B. C., Blacker, D., et al. (2006). An automated labeling system for subdividing the human cerebral cortex on MRI scans into gyral based regions of interest. Neuroimage 31, 968–980. doi: 10.1016/j.neuroimage.2006.01.021
Dubin, M. J., Mao, X., Banerjee, S., Goodman, Z., Lapidus, K. A., Kang, G., et al. (2016). Elevated prefrontal cortex GABA in patients with major depressive disorder after TMS treatment measured with proton magnetic resonance spectroscopy. J. Psychiatry Neurosci. 41, E37–E45. doi: 10.1503/jpn.150223
Fitzgerald, P. B., Fountain, S., and Daskalakis, Z. J. (2006). A comprehensive review of the effects of rTMS on motor cortical excitability and inhibition. Clin. Neurophysiol. 117, 2584–2596. doi: 10.1016/j.clinph.2006.06.712
Gaynes, B. N., Lux, L., Gartlehner, G., Asher, G., Forman-Hoffman, V., Green, J., et al. (2020). Defining treatment-resistant depression. Depress. Anxiety 37, 134–145. doi: 10.1002/da.22968
Ge, R., Blumberger, D. M., Downar, J., Daskalakis, Z. J., Dipinto, A. A., Tham, J. C. W., et al. (2017). Abnormal functional connectivity within resting-state networks is related to rTMS-based therapy effects of treatment resistant depression: a pilot study. J. Affect. Disord. 218, 75–81. doi: 10.1016/j.jad.2017.04.060
George, M. S., Wassermann, E. M., Williams, W. A., Callahan, A., Ketter, T. A., Basser, P., et al. (1995). Daily repetitive transcranial magnetic stimulation (rTMS) improves mood in depression. Neuroreport 6, 1853–1856. doi: 10.1097/00001756-199510020-00008
Greve, D. N., Svarer, C., Fisher, P. M., Feng, L., Hansen, A. E., Baare, W., et al. (2014). Cortical surface-based analysis reduces bias and variance in kinetic modeling of brain PET data. Neuroimage 92, 225–236. doi: 10.1016/j.neuroimage.2013.12.021
Grohn, H., Gillick, B. T., Tkac, I., Bednarik, P., Mascali, D., Deelchand, D. K., et al. (2019). Influence of repetitive transcranial magnetic stimulation on human neurochemistry and functional connectivity: a pilot MRI/MRS study at 7 T. Front. Neurosci. 13:1260. doi: 10.3389/fnins.2019.01260
Hahn, A., Lanzenberger, R., Wadsak, W., Spindelegger, C., Moser, U., Mien, L. K., et al. (2010). Escitalopram enhances the association of serotonin-1A autoreceptors to heteroreceptors in anxiety disorders. J. Neurosci. 30, 14482–14489. doi: 10.1523/JNEUROSCI.2409-10.2010
Harrington, A., and Hammond-Tooke, G. D. (2015). Theta burst stimulation of the cerebellum modifies the TMS-evoked N100 potential, a marker of GABA inhibition. PLos One 10:e0141284. doi: 10.1371/journal.pone.0141284
Hecht, D. (2010). Depression and the hyperactive right-hemisphere. Neurosci. Res. 68, 77–87. doi: 10.1016/j.neures.2010.06.013
Hnilicova, P., Povazan, M., Strasser, B., Andronesi, O. C., Gajdosik, M., Dydak, U., et al. (2016). Spatial variability and reproducibility of GABA-edited MEGA-LASER 3D-MRSI in the brain at 3 T. NMR Biomed. 29, 1656–1665. doi: 10.1002/nbm.3613
Huang, Y. Z., Chen, R. S., Rothwell, J. C., and Wen, H. Y. (2007). The after-effect of human theta burst stimulation is NMDA receptor dependent. Clin. Neurophysiol. 118, 1028–1032. doi: 10.1016/j.clinph.2007.01.021
Huang, Y. Z., Edwards, M. J., Rounis, E., Bhatia, K. P., and Rothwell, J. C. (2005). Theta burst stimulation of the human motor cortex. Neuron 45, 201–206. doi: 10.1016/j.neuron.2004.12.033
Huang, Y. Z., Rothwell, J. C., Chen, R. S., Lu, C. S., and Chuang, W. L. (2011). The theoretical model of theta burst form of repetitive transcranial magnetic stimulation. Clin. Neurophysiol. 122, 1011–1018. doi: 10.1016/j.clinph.2010.08.016
Ishikawa, S., Matsunaga, K., Nakanishi, R., Kawahira, K., Murayama, N., Tsuji, S., et al. (2007). Effect of theta burst stimulation over the human sensorimotor cortex on motor and somatosensory evoked potentials. Clin. Neurophysiol. 118, 1033–1043. doi: 10.1016/j.clinph.2007.02.003
Iwabuchi, S. J., Raschke, F., Auer, D. P., Liddle, P. F., Lankappa, S. T., Palaniyappan, L., et al. (2017). Targeted transcranial theta-burst stimulation alters fronto-insular network and prefrontal GABA. Neuroimage 146, 395–403. doi: 10.1016/j.neuroimage.2016.09.043
Kalueff, A. V., and Nutt, D. J. (2007). Role of GABA in anxiety and depression. Depress. Anxiety 24, 495–517. doi: 10.1002/da.20262
Kasper, S., Cubala, W. J., Fagiolini, A., Ramos-Quiroga, J. A., and Souery, D. (2021). Practical recommendations for the management of treatment-resistant depression with esketamine nasal spray therapy: basic science, evidence-based knowledge and expert guidance. World J. Biol. Psychiatry 22, 468–482. doi: 10.1080/15622975.2020.1836399
Labedi, A., Benali, A., Mix, A., Neubacher, U., and Funke, K. (2014). Modulation of inhibitory activity markers by intermittent theta-burst stimulation in rat cortex is NMDA-receptor dependent. Brain Stimul. 7, 394–400. doi: 10.1016/j.brs.2014.02.010
Lanzenberger, R., Kranz, G. S., Haeusler, D., Akimova, E., Savli, M., Hahn, A., et al. (2012). Prediction of SSRI treatment response in major depression based on serotonin transporter interplay between median raphe nucleus and projection areas. Neuroimage 63, 874–881. doi: 10.1016/j.neuroimage.2012.07.023
Larson, J., and Munkacsy, E. (2015). Theta-burst LTP. Brain Res. 1621, 38–50. doi: 10.1016/j.brainres.2014.10.034
Lewis, C. P., Port, J. D., Frye, M. A., Vande Voort, J. L., Ameis, S. H., Husain, M. M., et al. (2016). An exploratory study of spectroscopic glutamatergic correlates of cortical excitability in depressed adolescents. Front. Neural Circuits 10:98. doi: 10.3389/fncir.2016.00098
Li, C. T., Chen, M. H., Juan, C. H., Huang, H. H., Chen, L. F., Hsieh, J. C., et al. (2014). Efficacy of prefrontal theta-burst stimulation in refractory depression: a randomized sham-controlled study. Brain 137, 2088–2098. doi: 10.1093/brain/awu109
Li, C. T., Huang, Y. Z., Bai, Y. M., Tsai, S. J., Su, T. P., Cheng, C. M., et al. (2019). Critical role of glutamatergic and GABAergic neurotransmission in the central mechanisms of theta-burst stimulation. Hum. Brain Mapp. 40, 2001–2009. doi: 10.1002/hbm.24485
Luborzewski, A., Schubert, F., Seifert, F., Danker-Hopfe, H., Brakemeier, E. L., Schlattmann, P., et al. (2007). Metabolic alterations in the dorsolateral prefrontal cortex after treatment with high-frequency repetitive transcranial magnetic stimulation in patients with unipolar major depression. J. Psychiatr. Res. 41, 606–615. doi: 10.1016/j.jpsychires.2006.02.003
McIntyre, R. S., Rosenblat, J. D., Nemeroff, C. B., Sanacora, G., Murrough, J. W., Berk, M., et al. (2021). Synthesizing the evidence for ketamine and esketamine in treatment-resistant depression: an international expert opinion on the available evidence and implementation. Am. J. Psychiatry 178, 383–399. doi: 10.1176/appi.ajp.2020.20081251
Michael, N., Gosling, M., Reutemann, M., Kersting, A., Heindel, W., Arolt, V., et al. (2003). Metabolic changes after repetitive transcranial magnetic stimulation (rTMS) of the left prefrontal cortex: a sham-controlled proton magnetic resonance spectroscopy (H-1 MRS) study of healthy brain. Eur. J. Neurosci. 17, 2462–2468. doi: 10.1046/j.1460-9568.2003.02683.x
Mikellides, G., Michael, P., Psalta, L., Schuhmann, T., and Sack, A. T. (2021). A retrospective naturalistic study comparing the efficacy of ketamine and repetitive transcranial magnetic stimulation for treatment-resistant depression. Front. Psychiatry 12:784830. doi: 10.3389/fpsyt.2021.784830
Pogarell, O., Koch, W., Popperl, G., Tatsch, K., Jakob, F., Zwanzger, P., et al. (2006). Striatal dopamine release after prefrontal repetitive transcranial magnetic stimulation in major depression: preliminary results of a dynamic [123I] IBZM SPECT study. J. Psychiatr. Res. 40, 307–314. doi: 10.1016/j.jpsychires.2005.09.001
Povazan, M., Hangel, G., Strasser, B., Gruber, S., Chmelik, M., Trattnig, S., et al. (2015). Mapping of brain macromolecules and their use for spectral processing of (1)H-MRSI data with an ultra-short acquisition delay at 7 T. Neuroimage 121, 126–135. doi: 10.1016/j.neuroimage.2015.07.042
Rowland, L. M., Bustillo, J. R., Mullins, P. G., Jung, R. E., Lenroot, R., Landgraf, E., et al. (2005). Effects of ketamine on anterior cingulate glutamate metabolism in healthy humans: a 4-T proton MRS study. Am. J. Psychiatry 162, 394–396. doi: 10.1176/appi.ajp.162.2.394
Rush, A. J., Trivedi, M. H., Wisniewski, S. R., Nierenberg, A. A., Stewart, J. W., Warden, D., et al. (2006). Acute and longer-term outcomes in depressed outpatients requiring one or several treatment steps: a STAR*D report. Am. J. Psychiatry 163, 1905–1917. doi: 10.1176/ajp.2006.163.11.1905
Salomons, T. V., Dunlop, K., Kennedy, S. H., Flint, A., Geraci, J., Giacobbe, P., et al. (2014). Resting-state cortico-thalamic-striatal connectivity predicts response to dorsomedial prefrontal rTMS in major depressive disorder. Neuropsychopharmacology 39, 488–498. doi: 10.1038/npp.2013.222
Sanacora, G., Mason, G. F., Rothman, D. L., and Krystal, J. H. (2002). Increased occipital cortex GABA concentrations in depressed patients after therapy with selective serotonin reuptake inhibitors. Am. J. Psychiatry 159, 663–665. doi: 10.1176/appi.ajp.159.4.663
Sanacora, G., Treccani, G., and Popoli, M. (2012). Towards a glutamate hypothesis of depression: an emerging frontier of neuropsychopharmacology for mood disorders. Neuropharmacology 62, 63–77. doi: 10.1016/j.neuropharm.2011.07.036
Silberbauer, L. R., Spurny, B., Handschuh, P., Klobl, M., Bednarik, P., Reiter, B., et al. (2020). Effect of ketamine on limbic gaba and glutamate: a human in vivo multivoxel magnetic resonance spectroscopy study. Front. Psychiatry 11:549903. doi: 10.3389/fpsyt.2020.549903
Spindelegger, C., Lanzenberger, R., Wadsak, W., Mien, L. K., Stein, P., Mitterhauser, M., et al. (2009). Influence of escitalopram treatment on 5-HT 1A receptor binding in limbic regions in patients with anxiety disorders. Mol. Psychiatry 14, 1040–1050. doi: 10.1038/mp.2008.35
Spurny, B., Heckova, E., Seiger, R., Moser, P., Klobl, M., Vanicek, T., et al. (2019). Automated ROI-based labeling for multi-voxel magnetic resonance spectroscopy data using freeSurfer. Front. Mol. Neurosci. 12:28. doi: 10.3389/fnmol.2019.00028
Spurny, B., Vanicek, T., Seiger, R., Reed, M. B., Klobl, M., Ritter, V., et al. (2021). Effects of SSRI treatment on GABA and glutamate levels in an associative relearning paradigm. Neuroimage 232:117913. doi: 10.1016/j.neuroimage.2021.117913
Stagg, C. J., Wylezinska, M., Matthews, P. M., Johansen-Berg, H., Jezzard, P., Rothwell, J. C., et al. (2009). Neurochemical effects of theta burst stimulation as assessed by magnetic resonance spectroscopy. J. Neurophysiol. 101, 2872–2877. doi: 10.1152/jn.91060.2008
Suppa, A., Huang, Y. Z., Funke, K., Ridding, M. C., Cheeran, B., Di Lazzaro, V., et al. (2016). Ten years of theta burst stimulation in humans: established knowledge, unknowns and prospects. Brain Stimul. 9, 323–335. doi: 10.1016/j.brs.2016.01.006
Taylor, M., Murphy, S. E., Selvaraj, S., Wylezinkska, M., Jezzard, P., Cowen, P. J., et al. (2008). Differential effects of citalopram and reboxetine on cortical Glx measured with proton MR spectroscopy. J. Psychopharmacol. 22, 473–476. doi: 10.1177/0269881107081510
Tremblay, S., Beaule, V., Proulx, S., de Beaumont, L., Marjanska, M., Doyon, J., et al. (2013). Relationship between transcranial magnetic stimulation measures of intracortical inhibition and spectroscopy measures of GABA and glutamate+glutamine. J. Neurophysiol. 109, 1343–1349. doi: 10.1152/jn.00704.2012
Vidal-Pineiro, D., Martin-Trias, P., Falcon, C., Bargallo, N., Clemente, I. C., Valls-Sole, J., et al. (2015). Neurochemical modulation in posteromedial default-mode network cortex induced by transcranial magnetic stimulation. Brain Stimul. 8, 937–944. doi: 10.1016/j.brs.2015.04.005
Keywords: TBS, MRS, GABA, glutamate, depression, TMS, TRD
Citation: Spurny-Dworak B, Godbersen GM, Reed MB, Unterholzner J, Vanicek T, Baldinger-Melich P, Hahn A, Kranz GS, Bogner W, Lanzenberger R and Kasper S (2022) The Impact of Theta-Burst Stimulation on Cortical GABA and Glutamate in Treatment-Resistant Depression: A Surface-Based MRSI Analysis Approach. Front. Mol. Neurosci. 15:913274. doi: 10.3389/fnmol.2022.913274
Received: 05 April 2022; Accepted: 07 June 2022;
Published: 13 July 2022.
Edited by:
Boldizsar Czeh, University of Pécs, HungaryReviewed by:
Lindsay M. Oberman, National Institute of Mental Health (NIH), United StatesAlexander Gussew, University Hospital in Halle, Germany
Copyright © 2022 Spurny-Dworak, Godbersen, Reed, Unterholzner, Vanicek, Baldinger-Melich, Hahn, Kranz, Bogner, Lanzenberger and Kasper. This is an open-access article distributed under the terms of the Creative Commons Attribution License (CC BY). The use, distribution or reproduction in other forums is permitted, provided the original author(s) and the copyright owner(s) are credited and that the original publication in this journal is cited, in accordance with accepted academic practice. No use, distribution or reproduction is permitted which does not comply with these terms.
*Correspondence: Rupert Lanzenberger, cnVwZXJ0LmxhbnplbmJlcmdlckBtZWR1bml3aWVuLmFjLmF0; Siegfried Kasper, c2llZ2ZyaWVkLmthc3BlckBtZWR1bml3aWVuLmFjLmF0