- Laboratory of Neuropathology and Neuroscience, Graduate School of Pharmaceutical Sciences, The University of Tokyo, Tokyo, Japan
Alzheimer’s disease (AD) is a neurodegenerative disorder characterized by memory loss and personality changes, eventually leading to dementia. The pathological hallmarks of AD are senile plaques and neurofibrillary tangles, which comprise abnormally aggregated β-amyloid peptide (Aβ) and hyperphosphorylated tau protein. To develop preventive, diagnostic, and therapeutic strategies for AD, it is essential to establish animal models that recapitulate the pathophysiological process of AD. In this review, we will summarize the advantages and limitations of various mouse models of AD, including transgenic, knock-in, and injection models based on Aβ and tau. We will also discuss other mouse models based on neuroinflammation because recent genetic studies have suggested that microglia are crucial in the pathogenesis of AD. Although each mouse model has its advantages and disadvantages, further research on AD pathobiology will lead to the establishment of more accurate mouse models, and accelerate the development of innovative therapeutics.
Introduction
Dementia is defined clinically as a decline in memory and impaired thinking ability, which are two domains of cognition. Alzheimer’s disease (AD) is the most common cause of dementia (Knopman et al., 2021). More than 55 million people have dementia worldwide, and the prevalence of dementia is expected to reach 78 million people by 20301. Most AD patients (>99%) have the sporadic form of AD, whereas very few patients (<1%) have familial AD (FAD), which is inherited in an autosomal dominant manner. AD is characterized neuropathologically by two types of depositions; i.e., β-amyloid plaques and neurofibrillary tangles. β-amyloid plaques are deposited extracellularly, and consist mainly of β-amyloid protein (Aβ). Aβ is produced from its precursor, known as an amyloid precursor protein (APP), via sequential cleavage by the β- and γ-secretases (Figure 1A). Neurofibrillary tangles are intraneuronal aggregates comprised of hyperphosphorylated forms of the microtubule-binding protein tau. Longitudinal studies of biomarker changes in AD patients have demonstrated that Aβ deposition occurs first, followed by the accumulation of tau pathology (Bateman et al., 2012; Fagan et al., 2014). However, these pathologies do not act in isolation, and rather form a continuum (Jack et al., 2018). The deposition of Aβ and tau is spatially and temporally associated with the progression of AD.
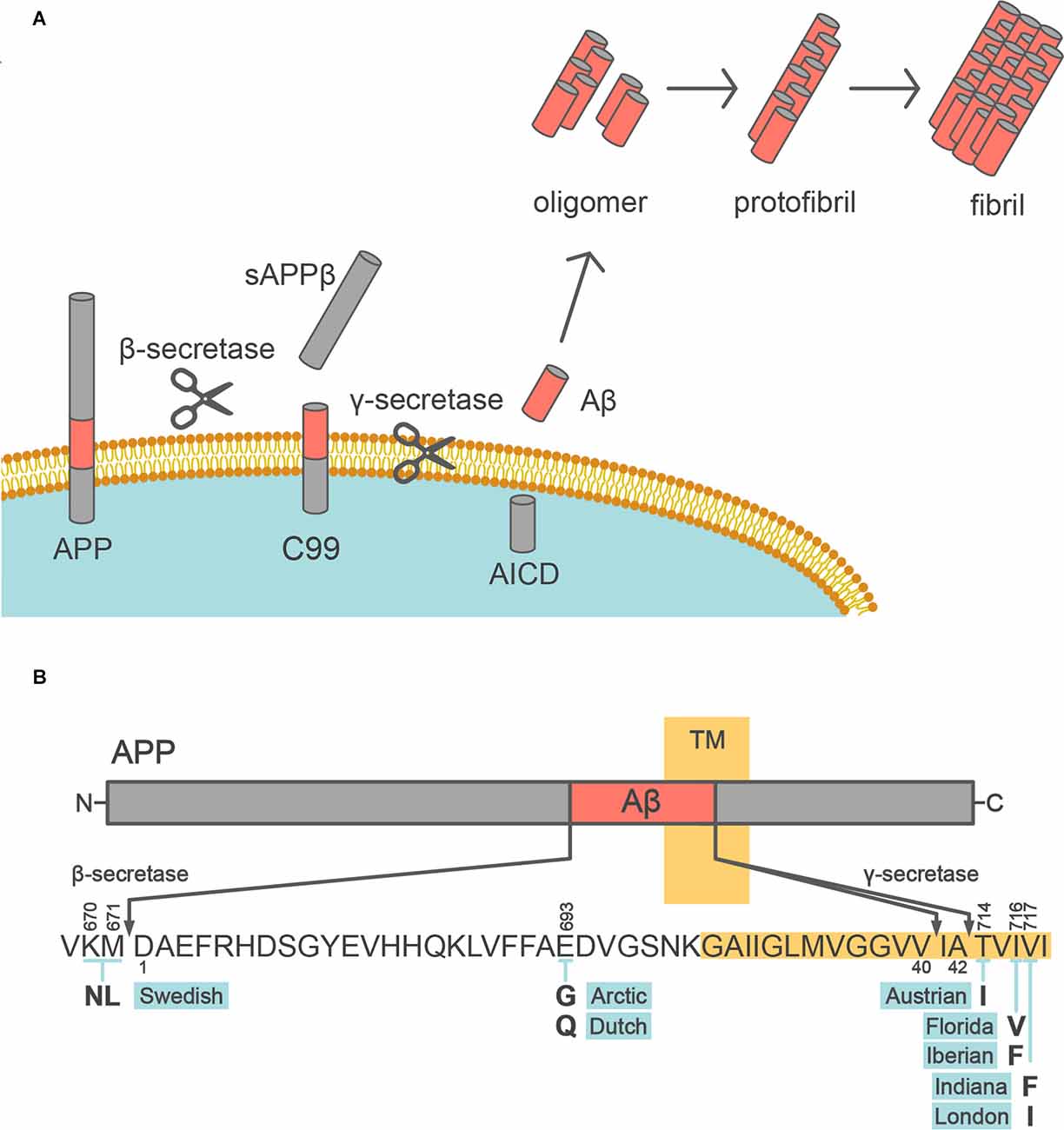
Figure 1. Aβ production from APP. (A) Schematic depiction of the Aβ production pathway. APP is sequentially cleaved by β- and γ-secretases to release Aβ into the extracellular space. Aβ monomer is prone to aggregate, forming oligomers, protofibrils, and fibrils. (B) Diagram of the APP (above) and sequence of Aβ (below). The transmembrane segment (TM) is highlighted in yellow. The bold letters below the Aβ sequence indicate the missense FAD mutations within the Aβ sequence. The above and below the number of the Aβ sequence follow the numbering of the longest isoform of APP and Aβ amino acid sequence, respectively.
Amyloid-β Peptide in the Pathogenesis of Alzheimer’s Disease
Aβ was first isolated from the cerebrovascular amyloid of AD patients in 1984 (Glenner and Wong, 2012) and recognized as the main component of senile plaque. Aβ spontaneously aggregates into β-sheet structures in the form of higher-order oligomers, protofibrils, and fibrils. Numerous studies on human AD biomarkers have demonstrated that the cerebral Aβ deposition begins many years before other AD-associated changes [i.e., increased tau in the cerebrospinal fluid (CSF), decreased cerebral glucose metabolism, brain atrophy, and clinical dementia]. Aβ pathology is considered to occur in stereotypical spatial patterns or topographies throughout the disease course (Thal et al., 2002), whereas recent studies demonstrated that FAD mutation types affect the patterns of Aβ accumulation (Chhatwal et al., 2022).
Aβ is produced by the two-step cleavage of APP by β-secretase and γ-secretase (Kikuchi et al., 2017; Figure 1A). First, APP is processed within the ectodomain by β-site APP-cleaving enzyme 1 (BACE1) as the β-secretase, yielding secreted sAPPβ and the C-terminal fragment C99. Then, C99 is subjected to intramembrane proteolysis by γ-secretase, which releases Aβ. BACE1 is a single-span membrane-anchored aspartic protease, which is mainly expressed in neurons. γ-Secretase is a complex of four protein subunits, namely, presenilin 1 (PSEN1) or PSEN2, nicastrin, presenilin enhancer 2, and anterior pharynx-defective 1 (Takasugi et al., 2003). γ-Secretase generates heterogeneity in the C-terminal length of Aβ, by primarily producing Aβ40 and Aβ42. Aβ40, which is a 40 amino acid peptide, is the most common form of Aβ in humans. Aβ42 differs from Aβ40 only in that it has two extra amino acid residues at the C-terminus (isoleucine-alanine) but is the most toxic and aggregation-prone Aβ species and is the main species deposited in the brains of AD patients (Jarrett et al., 1993; Iwatsubo et al., 1994; Figure 1B). On the other hand, a large variety of N-terminally truncated or elongated Aβ peptides, known as Aβ-related peptides, have been detected in human brains, CSF, and plasma, with the development of mass spectrometry techniques (Wang et al., 1996; Moore et al., 2012; Kaneko et al., 2014). Although the physiological functions of these peptides, including Aβ, remain incompletely understood, the combination of APP669–711 [a.k.a. Aβ (−3)–40, an Aβ-related peptide], Aβ42, and Aβ40 in human plasma can be a surrogate biomarker of cerebral Aβ accumulation (Kaneko et al., 2014; Nakamura et al., 2018).
Tau in the Neurodegenerative Diseases
Tau was first described as a major component of neurofibrillary tangles (NFTs) in 1986 (Grundke-Iqbal et al., 1986; Kosik et al., 1986; Nukina and Ihara, 1986). Tau pathology spreads throughout the AD brain in a stereotypical pattern across neural circuits throughout the brain (Braak and Braak, 1991; Jack et al., 2018). The progression of tau pathology is associated with neuronal death (Gómez-Isla et al., 1997). NFTs consist of abundant intracellular paired helical filaments (PHF) whose main constituent is tau (Figure 2A). Aggregated tau is deposited in a variety of neurodegenerative diseases, not only AD, and these diseases are collectively referred to as tauopathy. The tau protein is encoded by the MAPT gene on chromosome 17, which contains 16 exons. Based on its function, the tau protein is divided into the following four domains: N-terminal domain, a proline-rich domain, microtubule-binding repeat domain, and C-terminal domain, and is alternatively spliced at the N-terminal domain and microtubule-binding repeat domain to form six isoforms (0N3R, 0N4R, 1N3R, 1N4R, 2N3R, and 2N4R; Figure 2B). Depending on the presence of the repeat domain, tau proteins become either 3R or 4R tau. In the adult human brain, both 3R and 4R tau are expressed at approximately equal levels, but the 3R/4R tau ratio is altered in patients with most of the tauopathies. Tau proteins undergo several different post-translational modifications, including acetylation, glycosylation, nitration, O-GlcNAcylation, phosphorylation, SUMOylation, truncation, and ubiquitination (Wesseling et al., 2020). Tau can be phosphorylated at 85 different residues, and abnormally hyperphosphorylated tau (p-tau) is a key component of neurofibrillary tangles. Some phosphorylation sites of tau (threonine residues at 181, 217, and 231) are specifically phosphorylated in AD patients, and CSF and plasma tau are useful biomarkers of AD pathology (Barthélemy et al., 2020; Janelidze et al., 2020).
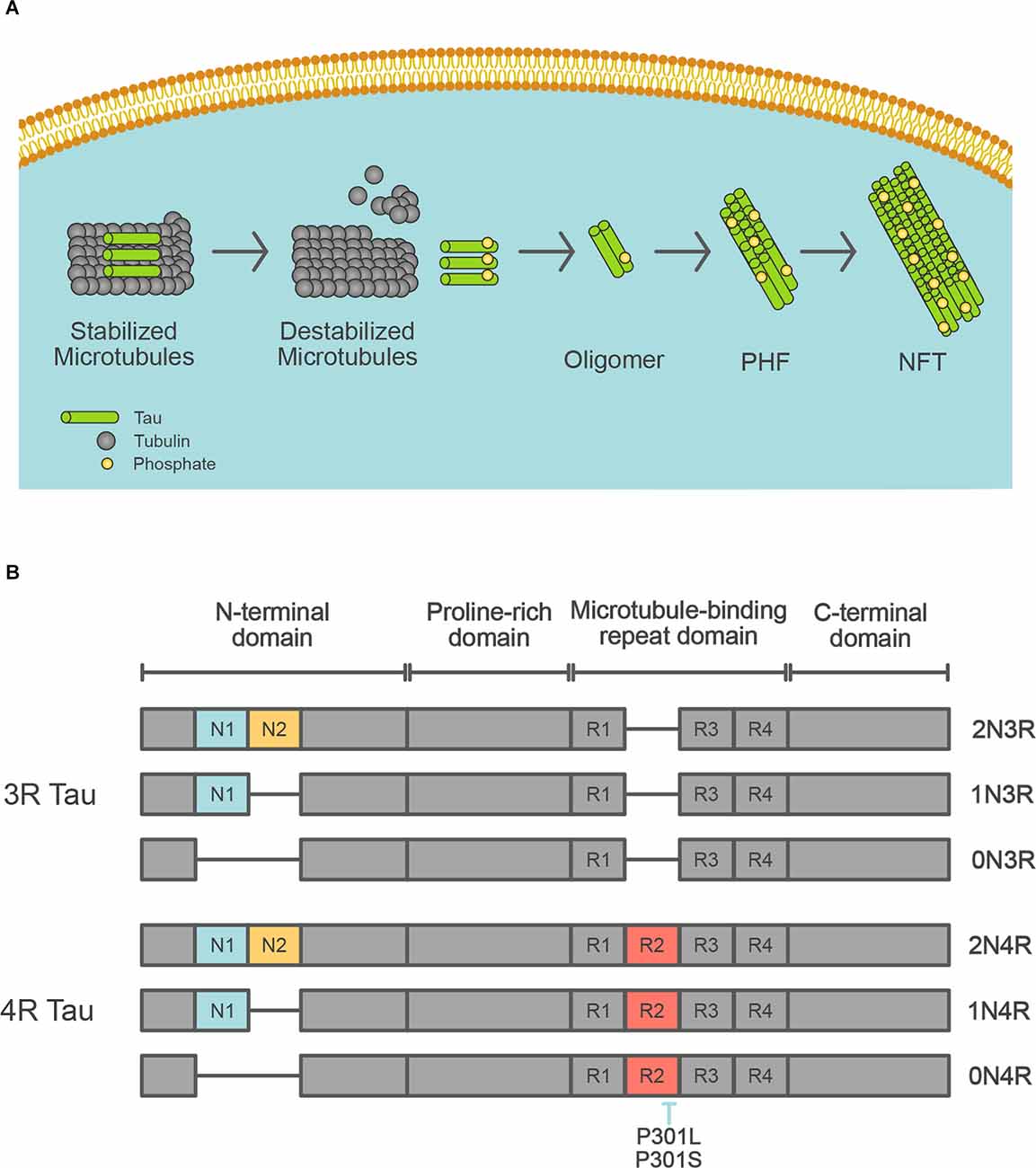
Figure 2. Tau isoforms in the human brain. (A) Schematic depiction of the formation of neurofibrillary tangles. Tau protein control stabilization of microtubules by kinases. Phosphorylated tau leads to microtubule disassembly. Irregular hyperphosphorylation of tau proteins results in the generation of insoluble tau oligomers, which then accumulate to form PHF, then NFT. (B) Schematic diagram of tau isoforms that are expressed in the adult human brain. MAPT gene encodes tau protein. The six tau isoforms are generated by splicing of exons 2, 3, and/or 10 that encode for N1 (blue), N2 (yellow), and R2 (red), respectively. The inclusion of exon 10 results in the generation of mRNA encoding 4R tau. The location of missense MAPT mutations utilized in the transgenic mice is shown.
Gene Mutations Linked to Familial Alzheimer’s Disease
As Down syndrome, in which patients demonstrate the same neuropathological changes as AD patients (i.e., senile plaques and neurofibrillary tangles), is caused by a trisomy of chromosome 21, which is where APP is located, the association between the APP gene and AD has attracted much attention. The first FAD mutation identified as a cause of autosomal-dominant AD was in the APP gene, and mutations were subsequently identified in PSEN1 and PSEN2 (Goate et al., 1991; Levy-Lahad et al., 1995; Rogaev et al., 1995; Sherrington et al., 1995). In addition to point mutations, an increased copy number of the APP gene also causes AD (Rovelet-Lecrux et al., 2006). No FAD mutations have been found in the MAPT gene, but mutations in MAPT cause familial frontotemporal dementia (FTD) and several other tauopathies (Iqbal et al., 2016; Figure 2B). The most common FTD mutations are P301L and P301S, which lead to tau aggregation. Based on these mutations, mouse models with aggregated tau pathology have been generated. The major FAD mutations are summarized in Tables 1–3, with a full and more updated version available on the Alzforum website2.
FAD mutations in APP are named according to the region of origin of the affected family, and mainly occur at its two cleavage sites, leading to Aβ overproduction or increased aggregation (Figure 1B). The Swedish mutation (K670N/M671L) is at the β-secretase cleavage site, and results in increased β-secretase mediated cleavage leading to enhanced production of both Aβ40 and Aβ42. The Indiana (V717F), London (V717I), and other mutations at the γ-cleavage sites promote the production of Aβ42, which is more toxic than Aβ40. APP mutations within Aβ, such as the Arctic (E693G) and Dutch (E693Q) mutations, increase Aβ aggregation and promote the formation of stable oligomers and protofibrils. Moreover, almost all PSEN1 and PSEN2 mutations in FAD cause an increase in the Aβ42/Aβ40 ratio (Borchelt et al., 1996; Duff et al., 1996; Citron et al., 1997; Tomita et al., 1997; Table 2). Finally, a protective variant of APP (A673T) against AD and age-associated cognitive decline was identified (Jonsson et al., 2012). Of note, this variant reduced β-secretase cleavage and total Aβ production, supporting the notion that brain Aβ deposition is a central mechanism in the pathogenesis of AD (Hardy and Selkoe, 2002; Selkoe and Hardy, 2016; Karran and De Strooper, 2022).
Aβ Deposited Mouse Models Based on APP Mutations
Wild-type mice do not develop senile plaques or neurofibrillary tangles even in old age, and hence cannot be used as model animals for AD. Based on the genetic findings that almost all FAD-linked mutations are associated with alterations of Aβ production/aggregation, advances in genetic engineering technology have enabled the development of model mice using the APP and PSEN1 genes (Tables Table 1; 2). In the previous few decades, several model mice with cerebral Aβ deposition have been developed by the overexpression or knock-in of the human APP gene carrying FAD-linked mutations. In particular, some model mice have been widely utilized in many studies to clarify the pathological process of AD, and to test the therapeutic effects of potential interventions ( Table 3).
PDAPP Mice
PDAPP was the first AD mouse model that was found to have Aβ deposition and expresses the human APP gene with the Indiana mutation (V717F) driven by the platelet-derived growth factor (PDGF)-β promoter (Games et al., 1995). PDAPP mice begin to develop human Aβ deposits in the cerebral cortex at approximately 6–9 months of age, together with increased gliosis in an age-dependent manner. Synaptic and dendritic densities are reduced in the molecular layer of the hippocampal dentate gyrus by 8 months of age. By 3 months of age, PDAPP mice show memory deficits, which also progress in an age-dependent manner.
Tg2576 Mice
Tg2576 is one of the most widely used AD mouse models, which overexpresses the human APP gene with the Swedish mutation (KM670/671NL) under the hamster prion promoter (Hsiao et al., 1996). Tg2576 mice show amyloid plaque formation from 11 to 13 months of age, and microglial activation in or around plaques in all the regions of the neocortex and hippocampus from 10 to 16 months of age. Although no neuronal loss has been detected, some memory functions such as spatial alternation and spatial reference learning are found to be impaired by 10 months of age (Frautschy et al., 1998).
APP23 Mice
APP23 mice express the human APP gene with the Swedish mutation (KM670/671NL) under the human Thy1 promoter (Sturchler-Pierrat et al., 1997). APP23 mice develop Aβ deposition in the brain starting from 6 months of age and these deposits increase with age in size and number, leading to the occupation of a substantial area in the neocortex and hippocampus by 24 months of age. A massive glial response can be detected in brain areas with Aβ plaques. Neuronal loss adjacent to Aβ deposition is apparent in 12-month-old mice. Cognitive decline in this model starts from 3 months of age, preceding amyloid deposition (Van Dam et al., 2003).
J20 Mice
J20 mice overexpress the human APP gene with two FAD-linked mutations, the Swedish mutation (KM670/671NL) and the Indiana mutation (V717F), under the PDGF-β promoter (Mucke et al., 2000). J20 mice develop diffuse amyloid deposition at 5–7 months of age and high hippocampal plaque loads at 8–10 months of age. An increased number of activated astrocytes and microglia are observed in the hippocampus at 6–9 months of age (Wright et al., 2013). Preceding amyloid deposition, neuronal loss and some cognitive deficits appear in this model.
TgCRND8 Mice
TgCRND8 mice overexpress the human APP gene harboring two FAD-linked mutations, the Swedish mutation (KM670/671NL) and the Indiana mutation (V717F), under the hamster prion promoter (Chishti et al., 2001). Deposition of Aβ in this mouse model starts from 3 months of age and becomes more extensive with age. Activated microglia appear accompanied by amyloid plaque accumulation at 3 months of age, shortly followed by a robust astrocytic response (Dudal et al., 2004). The acquisition and learning reversal of spatial information are impaired by 3 months of age.
PS2APP Mice
PS2APP mice were created by crossing two single transgenic models, APPswe mice and PS2 (N141I) mice (Richards et al., 2003). This model overexpresses the human APP gene carrying the Swedish mutation (KM670/671NL) and the human PSEN2 gene with N141I mutation under Thy1.2 promoter and mouse prion promoter, respectively. Amyloid plaques begin to appear in the subiculum and frontolateral cortices at 9 months of age, spreading to not only most of the neocortex, hippocampal formation, and amygdala but also to thalamic and pontine nuclei by 13–17 months. Gliosis is observed around Aβ plaques at 9 months. Cognitive functions are impaired in 8-month-old mice.
APPswe/PSEN1dE9 (APP/PS1) Mice
APPswe/PSEN1dE9 mice were developed by coinjecting two vectors driven by the mouse prion promoter. One vector encodes the APP gene harboring the Swedish (KM670/671NL) mutation and the other encodes the FAD-linked PSEN1 gene without exon 9 (dE9; Jankowsky et al., 2004). APPswe/PSEN1dE9 mice show Aβ deposition from 6 months of age and it becomes abundant in the hippocampus and cortex by 9 months. These deposits are surrounded by reactive astrocytes (Kamphuis et al., 2012). Performance in the Morris water maze is impaired at 12 months of age (Lalonde et al., 2005).
Tg-ArcSwe Mice
Tg-ArcSwe mice overexpress the human APP gene harboring two FAD-linked mutations, the Arctic mutation (E693G) and the Swedish mutation (KM670/671NL), driven by Thy1 promoter (Lord et al., 2006). Extracellular amyloid deposition is observed at 5–6 months of age and becomes quite frequent in the cerebral cortex, subiculum, and hippocampus at 9 months. These amyloid plaques are surrounded by marked astrogliosis. Spatial learning performance is impaired in 4-month-old mice (Lord et al., 2009). Neuronal loss was not detected in this mouse model.
5xFAD Mice
This mouse model was generated by coexpressing the following five FAD mutations in APP/PS1 double-transgenic mice: the Swedish (KM670/671NL), Florida (I716V), and London (V717I) mutations in the APP gene, and the M146L (A > C) and L286V mutations in the PSEN1 gene, causing the accelerated formation of Aβ plaques and very high cerebral Aβ42 levels (Oakley et al., 2006). Aβ plaques in the brain appear at 2 months of age and increase in an age-dependent manner, becoming saturated by 6–9 months of age, together with robust gliosis. Regarding cognitive impairment, spatial working memory in the Y-maze, associative memory in the trace fear conditioning test, and spatial memory in the Morris water maze are all impaired by 6 months of age.
A7 Mice
A7 mice overexpress APP harboring the Swedish (KM670/671NL) and Austrian (T714I) mutations under the control of the Thy1.2 promoter (Yamada et al., 2009). A7 mice demonstrate progressive amyloid deposition in the cerebral cortex from 9 to 12 months. Other pathologies than Aβ deposition have not been reported so far.
AppNL-G-F Knock-In Mice
AppNL-G-F knock-in mice were developed to increase the amount of Aβ42 without overexpressing the human APP gene. The Aβ sequence was humanized, and the Swedish (KM670/671NL), Arctic (E693G), and Iberian (I716F) mutations were introduced into the App mouse gene. Cortical Aβ deposition begins by 2 months, and is almost saturated together with robust gliosis by 7 months (Saito et al., 2014). Performance on the spatial alternation task, learning ability, and spatial memory retention is impaired by 6 months of age. AppNL-G-F knock-in mice does not show any neuronal loss.
Tauopathy Mouse Models Based on Mapt Mutations Or Seed Injection
Although familial mutations in the MAPT gene have not been identified in FAD patients, the identification of familial FTD-linked mutations has facilitated the development of tauopathy mouse models (Table 4). There are broadly two types of tauopathy model mice, i.e., genetically modified models, and tau seed-injection models. These models are diverse regarding the genetic engineering method (transgenic or knock-in), expressed isoforms of tau (0N4R, 1N4R, etc.), tau expression level, and brain regions in which the introduced tau is highly expressed (Table 5).
JNPL3 Mice
JNPL3 mice were the first mouse model established to carry a MAPT mutation (Lewis et al., 2000). They express human 0N4R tau with the P301L mutation, driven by the mouse prion protein promoter. This model is particularly characterized by strong motor and behavioral deficits, which may be caused by neuropathological changes in the spinal cord. At 4.5 months, they show tangle pathology in the diencephalon, brainstem, cerebellar nuclei, and spinal cord, as well as motor impairment. Neuronal loss occurs from 10 months of age, particularly in the spinal cord. Astrogliosis in the brainstem, diencephalon, and basal telencephalon is seen from 10 months of age.
PS19 Mice
PS19 mice express human 1N4R tau with the P301S mutation, driven by the mouse prion promoter (Yoshiyama et al., 2007). PS19 mice are characterized by early synapse loss and microglial activation before neurofibrillary tangle pathology and neuronal loss. PS19 mice develop microgliosis as early as 3 months of age. Neurofibrillary tangle-like inclusions are seen from 6 months, and are widespread throughout the neocortex, amygdala, hippocampus, brain stem, and spinal cord. Neuronal loss and brain atrophy are seen by 8 months, principally in the hippocampus and also spreading to the neocortex and entorhinal cortex. Astrogliosis is robustly increased at 6-months old compared with at 3 months old. Motor deficits, such as clasping and limb retraction when lifted by the tail are seen, followed by limb weakness. These deficits progress to paralysis at 7–10 months, and about 80% of PS19 mice die by 12 months of age (Yoshiyama et al., 2007).
rTg4510 Mice
rTg4510 mice express human 0N4R tau with the P301L mutation, and are unique in that transgene expression can be regulated (Santacruz et al., 2005). These mice carry the mutant MAPT gene downstream of a tetracycline operon-responsive element, and a tetracycline-controlled transactivator is expressed under the control of the calcium/calmodulin-dependent protein kinase II alpha (CaMKIIα) promoter. The transgene expression is inactivated by the administration of the tetracycline analog doxycycline. The expression level of this mutant human tau is 13-fold higher than that of the endogenous mouse protein. However, the transgenes disrupt six endogenous mouse genes, including Fibroblast Growth Factor 14 which might affect the neuropathological and neurodegenerative phenotypes observed in rTg4510 mice (Gamache et al., 2019). Argyrophilic tangle-like inclusions are observed in the cortex and hippocampus by 5.5 months (Santacruz et al., 2005). Neuronal loss starts from 5.5 months in CA1 hippocampal neurons, and gross atrophy of the forebrain was seen by 10 months of age. Retention of spatial memory is impaired from 2.5 to 4 months of age. Notably, neuronal death ceases and the decline in cognitive function is arrested or even reversed following transgene suppression with dox (Santacruz et al., 2005).
3xTg Mice
These mice were established by the coinjection of two AD-associated human genes carrying FAD-linked mutations [i.e., APP with the Swedish mutation (KM670/671NL), and MAPT with the P301L mutation] into the embryo of homozygous Psen1 M146V knock-in mice (Oddo et al., 2003). The expression of both transgenes is regulated by the mouse Thy1.2 promoter. These mice develop progressive neuropathology, including intracellular and extracellular Aβ deposits and conformationally altered phosphorylated tau aggregates.
Human MAPT Knock-In Mice
These mice carry the humanized WT MAPT gene, instead of the endogenous murine MAPT gene (Saito et al., 2019). They express all six isoforms of human tau, with the level of 4R mRNA being approximately 70% of that of 3R mRNA. AppNL-G-F/MAPT double knock-in mice lack neurofibrillary tangles and do not show neurodegeneration, although they develop amyloid plaques and Aβ-associated pathologies. Notably, tau humanization accelerates the cell-to-cell propagation of AD patient-brain derived tau both in the absence and presence of Aβ deposition.
Seed Injection Models
The first seed injection model was established in 2009 (Clavaguera et al., 2009), and transmission and diffusion of the tau were demonstrated. Seed injection models are created by injecting brain lysates from the brains of AD patients or model mice, or recombinant tau into mouse brains. In these models, the spreading of pathological tau occurs via synaptic connections rather than between proximal cells (Ahmed et al., 2014). Seed injection models identified several important elements that affect tau pathology, including age, Aβ, oligomerization, or splicing isoform of tau (Jackson et al., 2016; He et al., 2018; Hosokawa et al., 2021; Nies et al., 2021).
Mouse Models Modified TREM2 Signaling
Triggering receptor expressed on myeloid cells 2 (TREM2), which is expressed in myeloid cells including microglia in the brain, is an AD risk gene and the R47H variant shows a robust odds ratio (Guerreiro et al., 2013; Jonsson et al., 2013) and is implicated in the development of late-onset AD (Ulland and Colonna, 2018). Microglia have been shown to respond to Aβ accumulation and neurodegenerative lesions, progressively acquiring a unique transcriptional and functional signature (Keren-Shaul et al., 2017; Krasemann et al., 2017). These microglia attenuate the progression of neurodegeneration in some mouse models, but inappropriate microglial activation accelerates neurodegenerative disease in other models. The loss of TREM2 in APP model mice was found to facilitate the accumulation and spread of tau (Yuan et al., 2016; Leyns et al., 2019), suggesting the important role of TREM2 in the pathological connection between Aβ and tau. Note that marked species-specific differences in TREM2-mediated signaling have been identified, and hence further investigation of the TREM2 pathway in various species is required in the future (Zhou et al., 2020).
Preclinical Analyses of Potential AD Therapies Using Model Mice
The safety and efficacy of any drug must be confirmed using animal models in a preclinical study before it proceeds to clinical studies. As described above, there are various types of AD mouse models, and the model that is used for a preclinical study has a substantial effect on the outcomes.
Secretase Inhibitors and Modulators
The development of secretase inhibitors has been attempted, aiming to treat AD by reducing Aβ production. In the development of semagacestat, the first γ-secretase inhibitor tested in a phase III clinical trial, pharmacodynamics studies were performed using PDAPP mice (Panza et al., 2010). However, semagacestat caused irreversible symptoms, such as the worsening of cognitive function, skin cancer, and infections in PDAPP mice, leading to the suspension of the phase III trial and the further development of this potential drug (Doody et al., 2013). Furthermore, Tg2576 mice were utilized in the development as well as comparison of other γ-secretase inhibitors, such as avagacestat (Mitani et al., 2012). However, phase II trials of avagacestat were discontinued owing to gastrointestinal and dermatological adverse events that occurred in these mice (Coric et al., 2015).
BACE1, which is responsible for the N-terminal processing of Aβ, has been investigated as a therapeutic target molecule. Various BACE1 inhibitors, such as verubecestat, umibecestat, and atabecestat have been developed, and their efficacy and pharmacodynamics have been investigated in animal models (Kennedy et al., 2016; Neumann et al., 2018; Koriyama et al., 2021). However, none of them have shown therapeutic effects in clinical trials (Egan et al., 2018, 2019; Novak et al., 2020). Although secretase inhibitors may have therapeutic potential as a maintenance therapy after plaque removal or as a preventive drug (McDade et al., 2021), to date, none of the BACE1 inhibitors have passed clinical trials and become available as therapeutic agents.
Immunotherapies Targeting Amyloid-β Peptide
The use of anti-Aβ antibodies to prevent the formation of Aβ plaques has been investigated since the 1990s. In 1996, it was shown that two monoclonal antibodies, AMY-33 and 6F/3D, inhibit Aβ aggregation in vitro, indicating the possibility that monoclonal antibodies that prevent Aβ aggregation may exist in vivo (Solomon et al., 1996). Since then, Schenk et al. showed that immunization of PDAPP mice with human Aβ42 prevented the formation of Aβ plaques, neuritic dystrophy, and astrogliosis, and markedly reduced the extent and progression of AD pathology (Schenk et al., 1999). In addition, peripherally administered antibodies against Aβ were found to enter the central nervous system and significantly trigger the clearance of Aβ accumulation in PDAPP mice (Bard et al., 2000). Moreover, it was reported that immunization with Aβ reduced amyloid plaque formation and also prevented cognitive deficits in Aβ deposited mouse models (Janus et al., 2000; Morgan et al., 2000). These preclinical results prompted researchers to consider anti-Aβ immunotherapy as a potential treatment of AD. Pre-aggregated synthetic Aβ42 (AN-1792) combined with the immunogenic adjuvant QS-21, the first active immunotherapy strategy for AD, has entered clinical trials. However, 6% of the patients treated with AN-1792 developed symptoms of meningoencephalitis in the phase IIa trial initiated in 2001, and the trial was discontinued in 2003 (Gilman et al., 2005). Nicoll et al. reported that the vaccinated patients showed very few Aβ plaques in the brain parenchyma, and small Aβ aggregates were found associated with microglia (Nicoll et al., 2003).
The activation of passive immunity by the administration of anti-Aβ monoclonal antibodies has also been investigated (Wilcock et al., 2004). Passive immunotherapy has several advantages, such as the ability to control the number of antibodies and to prevent the occurrence of unexpected effects caused by high antibody specificity. At present, lecanemab, gantenerumab, donanemab, and aducanumab have reached the final stage of development. All of these potential drugs have undergone preclinical studies using mouse models. Lecanemab is an anti-protofibril antibody, and is a humanized version of the mouse antibody mAb158. The administration of mAb158 to aged Tg-ArcSwe mice reduced Aβ protofibrils without changing insoluble Aβ levels (Tucker et al., 2015). Gantenerumab recognizes the N-terminal and central regions of Aβ. Gantenerumab bound to cerebral Aβ and enhanced the removal of small Aβ plaques by recruiting microglia, and prevented new plaque formation in PS2APP mice (Bohrmann et al., 2012). Donanemab is an antibody against Aβp3-x, which is a pyroglutamate form of Aβ, and aggregates early in the deposition cascade (Saido et al., 1995). Donanemab is derived from the murine antibody mE8-IgG2, and was shown to bind specifically to Aβ plaques and to remove existing plaques in aged PDAPP mice (DeMattos et al., 2012). Aducanumab is a human monoclonal antibody that binds aggregated forms of Aβ, but not Aβ monomers. Aducanumab bound the cerebral Aβ deposits and reduced Aβ burden in a dose-dependent manner when administered to aged Tg2576 and APP23 mice (Sevigny et al., 2016; Uhlmann et al., 2020). These findings suggest that Aβ deposited mouse models based on APP mutations are useful for the development of anti-Aβ deposition therapies, although the effects of these therapies on human cognitive function remain controversial.
Immunotherapies Targeting Tau
Antibodies bind extracellular molecules and do not penetrate the cell membrane. Thus, tau had not initially been considered as a target molecule for immunotherapy. However, after the identification of extracellular tau (eTau), which is involved in the propagation of tau pathology in model mice (Yamada, 2017), researchers have considered immunotherapy against tau as a potential treatment for AD. As in Aβ, many tau-targeting drugs aim for the clearance of tau. Anti-tau antibodies can be classified by their epitopes; i.e., antibodies against pan-tau, p-tau, and tau with a pathological conformation (Congdon and Sigurdsson, 2018). Semorinemab was the first anti-tau antibody to be developed, which binds to the N-terminus of all six isoforms of human tau, and both monomeric and oligomeric eTau. Treatment for 13 weeks with semorinemab dose-dependently reduced brain pathology in tau P301L transgenic mice (expressing human tau P301L under the Thy1 promoter; Ayalon et al., 2021). Gosuranemab also targets the N-terminus of eTau. Gosuranemab neutralized the toxicity of eTau in rTg4510 mice (Sopko et al., 2020). However, these antibodies did not improve outcomes in clinical trials, probably owing to the inability of tau N-terminus-targeting antibodies to inhibit the spread of tau in humans. The JNJ-63733657 antibody targets the microtubule-binding region of tau, and has a high affinity for p-tau. This antibody is thought to target aggregated tau more potently than antibodies targeting the N-terminus. Zagotenemab is a humanized anti-tau antibody derived from the MCI-1 antibody that specifically targets soluble tau aggregates. The peripheral administration of MCI-1 substantially reduced tau pathology in the JNPL3 mouse model (Chai et al., 2011). However, the development of zagotenemab has been halted because of its lack of efficacy in humans. The lack of success of anti-tau antibodies in clinical trials may be owing to differences in the mechanisms of tau pathology propagation in model mice and humans, or because the approach of targeting eTau may not be effective against the tauopathies.
Antisense Oligonucleotides
In recent years, with the development of modified nucleic acids and drug delivery technologies, antisense nucleotides (ASOs) have been used as drugs for the treatment of neurodegenerative diseases. BIIB080 was the first ASO targeting tau expression to enter clinical trials. This ASO inhibits the translation of tau mRNA into protein, and treatment of PS19 model mice with BIIB080 led to the prevention of tau pathology, accompanied by the prevention of hippocampal volume loss, neuronal death, and behavioral deficits (DeVos et al., 2017).
Inhibitors of Post-translational Modifications of Tau
Post-translational modifications of tau, including hyperphosphorylation and acetylation, interfere with the binding of tauto microtubules, and promote tau misfolding. Thus, targeting any of these modifications, alone or in combination, has the potential to prevent tau aggregation and restore its normal function. Memantine, which is a phosphatase modifier that enhances PP2A (Protein phosphatase 2) activity, improves cognition, and reduces tau pathology in model mice (Chohan et al., 2006). Kinase inhibitors, such as CDK5 inhibitors, attenuated pathology, and neurodegeneration in tauopathy mice (Malhotra et al., 2021). An acetyltransferase inhibitor was shown to rescue tau-induced memory deficits and to prevent hippocampal atrophy in PS19 mice (Min et al., 2015). However, to date, no therapeutic reagent against the post-translational modifications of tau has shown beneficial effects in clinical trials.
Photo-Oxygenation System as a Potential Treatment for AD
The challenge of immunotherapy using anti-Aβ antibodies is that they do not easily permeate the blood-brain barrier. To overcome this problem, the development of low-molecular-weight therapeutic drugs has been promoted. Photocatalysts activated by light irradiation only when they bind β-sheet structures of amyloid have been developed (Taniguchi et al., 2016; Ni et al., 2018; Suzuki et al., 2019). This photo-oxygenation system successfully inhibited the aggregation of recombinant Aβ and tau in vitro. In addition, photo-oxygenation treatment of AppNL-G-F mice decreased cerebral Aβ levels, and facilitated microglial clearance in vivo (Nagashima et al., 2021; Ozawa et al., 2021). It is expected that this new, and innovative amyloid-targeting system will greatly influence the establishment of therapeutic interventions for neurodegenerative diseases in the future (Ikeda et al., 2021).
Identification of Biomarkers of AD
The Aβ-targeting therapies described above are most effective when they are initiated early in the course of AD because cerebral Aβ deposition is the earliest pathology of AD. Thus, the development of biomarkers that can detect an increase in brain Aβ accumulation before the onset of dementia is needed. Common AD biomarkers include Aβ and tau levels in the CSF (Arai et al., 1995; Motter et al., 1995; Kanai et al., 1998). However, the time lag between CSF collection and autopsy in patients has been a big challenge in understanding the association between the progression of brain pathology and biomarker alterations. Thus, model mice have been utilized to analyze the association between biomarkers and brain pathology. A correlation between the decrease in CSF Aβ level and the increase in brain Aβ level has been observed in various mouse models (Kawarabayashi et al., 2001; Maia et al., 2013). In addition, CSF tau levels were increased in a mouse model with Aβ deposition in the brain (Maia et al., 2013). Recently, the novel plasma biomarker APP669–711/Aβ42, which detects the presence of Aβ accumulation in the brain with high sensitivity and specificity was established (Nakamura et al., 2018). Changes in this plasma biomarker have been reproduced in APP/PS1 model (Matsuzaki, Yokoyama, and Tomita, unpublished observations). The analysis of biomarkers in AD mouse models provides a valuable opportunity to clarify the biological mechanism of the biomarkers identified in AD patients, and supports the validity of further biomarker studies in vivo.
Concluding Remarks and Future Prospectives
Elucidation of the molecular pathogenesis of AD based on genetic and biochemical analyses has established Aβ and tau as the two main pathogenic factors. In this regard, the use of genetically engineered mice, as well as mice injected with patient brain-derived taufibrils, has made substantial contributions. These mice have also played important roles in the development of therapeutic strategies and diagnostic biomarkers. While an AD mouse model in which the deposition of both Aβ and tau occurs in a similar manner to that observed in sporadic AD patients has not yet been established, cats, dogs, wolverine, and nonhuman primates spontaneously develop age-dependent AD-like brain pathologies (Nakamura et al., 1995; Roertgen et al., 1996; Vite and Head, 2014). The use of nonhuman primates is especially essential to assess the efficacy and safety of therapeutics for AD (Gandy et al., 2004; Lemere et al., 2004; Britschgi et al., 2009), but it has ethical issues due to their high resemblance. To secure and maintain facilities for breeding and housing is also costly difficult, but conversely, mouse models do not have these disadvantages. We should maximize the advantages of each animal model by clarifying the mechanism in detail with mouse models where genetic intervention is easier and validating them with nonhuman primates, and should minimize animal suffering or discomfort by applying 3Rs principle (Replacement, Reduction, and Refinement). Moreover, further understanding of the pathological process of Aβ and tau deposition, as well as its reproduction in the animals will assist in the development of more accurate disease models, which will narrow the gap between preclinical studies and clinical trials.
Author Contributions
MY, HK, and LT wrote the draft and revised it. TT was involved in discussing, drafting, and editing the manuscript. All authors contributed to the article and approved the submitted version.
Funding
This work was supported in part by a Grant-in-Aid for Scientific Research (A) [19H01015 to TT] and JSPS Fellows [22J14778 to MY] from the Japan Society for the Promotion of Science; Strategic Research Program for Brain Sciences from the Japan Agency for Medical Research and Development [JP20dm0207073 to TT]; Moonshot R&D—MILLENNIA Program from Japan Science and Technology Agency [JPMJMS2024 to TT].
Conflict of Interest
The authors declare that the research was conducted in the absence of any commercial or financial relationships that could be construed as a potential conflict of interest.
Publisher’s Note
All claims expressed in this article are solely those of the authors and do not necessarily represent those of their affiliated organizations, or those of the publisher, the editors and the reviewers. Any product that may be evaluated in this article, or claim that may be made by its manufacturer, is not guaranteed or endorsed by the publisher.
Acknowledgments
We would like to thank our current and previous laboratory members for helpful discussions.
Footnotes
References
Ahmed, Z., Cooper, J., Murray, T. K., Garn, K., McNaughton, E., Clarke, H., et al. (2014). A novel in vivo model of tau propagation with rapid and progressive neurofibrillary tangle pathology: the pattern of spread is determined by connectivity, not proximity. Acta Neuropathol. 127, 667–683. doi: 10.1007/s00401-014-1254-6
Arai, H., Terajima, M., Miura, M., Higuchi, S., Muramatsu, T., Machida, N., et al. (1995). Tau in cerebrospinal fluid: a potential diagnostic marker in Alzheimer’s disease. Ann. Neurol. 38, 649–652. doi: 10.1002/ana.410380414
Ayalon, G., Lee, S. H., Adolfsson, O., Foo-Atkins, C., Atwal, J. K., Blendstrup, M., et al. (2021). Antibody semorinemab reduces tau pathology in a transgenic mouse model and engages tau in patients with Alzheimer’s disease. Sci. Transl. Med. 13:eabb2639. doi: 10.1126/scitranslmed.abb2639
Bard, F., Cannon, C., Barbour, R., Burke, R. L., Games, D., Grajeda, H., et al. (2000). Peripherally administered antibodies against amyloid β-peptide enter the central nervous system and reduce pathology in a mouse model of Alzheimer’s disease. Nat. Med. 6, 916–919. doi: 10.1038/78682
Barthélemy, N. R., Horie, K., Sato, C., and Bateman, R. J. (2020). Blood plasma phosphorylated-tau isoforms track CNS change in Alzheimer’s disease. J. Exp. Med. 217:e20200861. doi: 10.1084/jem.20200861
Bateman, R. J., Xiong, C., Benzinger, T. L. S., Fagan, A. M., Goate, A., Fox, N. C., et al. (2012). Clinical and biomarker changes in dominantly inherited Alzheimer’s disease. N. Engl. J. Med. 367, 795–804. doi: 10.1056/NEJMoa1202753
Bohrmann, B., Baumann, K., Benz, J., Gerber, F., Huber, W., Knoflach, F., et al. (2012). Gantenerumab: a novel human anti-Aβ antibody demonstrates sustained cerebral amyloid-β binding and elicits cell-mediated removal of human amyloid-β. J. Alzheimers. Dis. 28, 49–69. doi: 10.3233/JAD-2011-110977
Borchelt, D. R., Thinakaran, G., Eckman, C. B., Lee, M. K., Davenport, F., Ratovitsky, T., et al. (1996). Familial Alzheimer’s disease-linked presenilin 1 variants elevate Aβ1-421-40 ratio in vitro and in vivo. Neuron 17, 1005–1013. doi: 10.1016/s0896-6273(00)80230-5
Braak, H., and Braak, E. (1991). Neuropathological stageing of Alzheimer-related changes. Acta Neuropathol. 82, 239–259. doi: 10.1007/BF00308809
Britschgi, M., Olin, C. E., Johns, H. T., Takeda-Uchimura, Y., Lemieux, M. C., Rufibach, K., et al. (2009). Neuroprotective natural antibodies to assemblies of amyloidogenic peptides decrease with normal aging and advancing Alzheimer’s disease. Proc. Natl. Acad. Sci. U S A 106, 12145–12150. doi: 10.1073/pnas.0904866106
Chai, X., Wu, S., Murray, T. K., Kinley, R., Cella, C. V., Sims, H., et al. (2011). Passive immunization with anti-Tau antibodies in two transgenic models: reduction of Tau pathology and delay of disease progression. J. Biol. Chem. 286, 34457–34467. doi: 10.1074/jbc.M111.229633
Chhatwal, J. P., Schultz, S. A., McDade, E., Schultz, A. P., Liu, L., Hanseeuw, B. J., et al. (2022). Variant-dependent heterogeneity in amyloid β burden in autosomal dominant Alzheimer’s disease: cross-sectional and longitudinal analyses of an observational study. Lancet. Neurol. 21, 140–152. doi: 10.1016/S1474-4422(21)00375-6
Chishti, M. A., Yang, D. S., Janus, C., Phinney, A. L., Horne, P., Pearson, J., et al. (2001). Early-onset amyloid deposition and cognitive deficits in transgenic mice expressing a double mutant form of amyloid precursor protein 695. J. Biol. Chem. 276, 21562–21570. doi: 10.1074/jbc.M100710200
Chohan, M. O., Khatoon, S., Iqbal, I. G., and Iqbal, K. (2006). Involvement of I2PP2A in the abnormal hyperphosphorylation of tau and its reversal by Memantine. FEBS Lett. 580, 3973–3979. doi: 10.1016/j.febslet.2006.06.021
Citron, M., Westaway, D., Xia, W., Carlson, G., Diehl, T., Levesque, G., et al. (1997). Mutant presenilins of Alzheimer’s disease increase production of 42-residue amyloid β-protein in both transfected cells and transgenic mice. Nat. Med. 3, 67–72. doi: 10.1038/nm0197-67
Clavaguera, F., Bolmont, T., Crowther, R. A., Abramowski, D., Frank, S., Probst, A., et al. (2009). Transmission and spreading of tauopathy in transgenic mouse brain. Nat. Cell Biol. 11, 909–913. doi: 10.1038/ncb1901
Congdon, E. E., and Sigurdsson, E. M. (2018). Tau-targeting therapies for Alzheimer’s disease. Nat. Rev. Neurol. 14, 399–415. doi: 10.1038/s41582-018-0013-z
Coric, V., Salloway, S., Van Dyck, C. H., Dubois, B., Andreasen, N., Brody, M., et al. (2015). Targeting prodromal Alzheimer’s disease with avagacestat: a randomized clinical trial. JAMA Neurol. 72, 1324–1333. doi: 10.1001/jamaneurol.2015.0607
DeMattos, R. B., Lu, J., Tang, Y., Racke, M. M., DeLong, C. A., Tzaferis, J. A., et al. (2012). A plaque-specific antibody clears existing β-amyloid plaques in Alzheimer’s disease mice. Neuron 76, 908–920. doi: 10.1016/j.neuron.2012.10.029
DeVos, S. L., Miller, R. L., Schoch, K. M., Holmes, B. B., Kebodeaux, C. S., Wegener, A. J., et al. (2017). Tau reduction prevents neuronal loss and reverses pathological tau deposition and seeding in mice with tauopathy. Sci. Transl. Med. 9:eaag0481. doi: 10.1126/scitranslmed.aag0481
Doody, R. S., Raman, R., Farlow, M., Iwatsubo, T., Vellas, B., Joffe, S., et al. (2013). A Phase 3 trial of semagacestat for treatment of Alzheimer’s disease. N. Engl. J. Med. 369, 341–350. doi: 10.1056/NEJMoa1210951
Dudal, S., Krzywkowski, P., Paquette, J., Morissette, C., Lacombe, D., Tremblay, P., et al. (2004). Inflammation occurs early during the Aβ deposition process in TgCRND8 mice. Neurobiol. Aging 25, 861–871. doi: 10.1016/j.neurobiolaging.2003.08.008
Duff, K., Eckman, C., Zehr, C., Yu, X., Prada, C.-M., Perez-tur, J., et al. (1996). Increased amyloid-β42(43) in brains of mice expressing mutant presenilin 1. Nature 383, 710–713. doi: 10.1038/383710a0
Egan, M. F., Kost, J., Tariot, P. N., Aisen, P. S., Cummings, J. L., Vellas, B., et al. (2018). Randomized trial of verubecestat for mild-to-moderate Alzheimer’s disease. N. Engl. J. Med. 378, 1691–1703. doi: 10.1056/NEJMoa1706441
Egan, M. F., Kost, J., Voss, T., Mukai, Y., Aisen, P. S., Cummings, J. L., et al. (2019). Randomized trial of verubecestat for prodromal Alzheimer’s disease. N. Engl. J. Med. 380, 1408–1420. doi: 10.1056/NEJMoa1812840
Fagan, A. M., Xiong, C., Jasielec, M. S., Bateman, R. J., Goate, A. M., Benzinger, T. L. S., et al. (2014). Longitudinal change in CSF biomarkers in autosomal-dominant Alzheimer’s disease. Sci. Transl. Med. 6:226ra30. doi: 10.1126/scitranslmed.3007901
Frautschy, S. A., Yang, F., Irrizarry, M., Hyman, B., Saido, T. C., Hsiao, K., et al. (1998). Microglial response to amyloid plaques in APPsw transgenic mice. Am. J. Pathol. 152, 307–317.
Gamache, J., Benzow, K., Forster, C., Kemper, L., Hlynialuk, C., Furrow, E., et al. (2019). Factors other than hTau overexpression that contribute to tauopathy-like phenotype in rTg4510 mice. Nat. Commun. 10:2479. doi: 10.1038/s41467-019-10428-1
Games, D., Adams, D., Alessandrini, R., Barbour, R., Borthelette, P., Blackwell, C., et al. (1995). Alzheimer-type neuropathology in transgenic mice overexpressing V717F β-amyloid precursor protein. Nature 373, 523–527. doi: 10.1038/373523a0
Gandy, S., DeMattos, R. B., Lemere, C. A., Heppner, F. L., Leverone, J., Aguzzi, A., et al. (2004). Alzheimer’s Aβ vaccination of rhesus monkeys (Macaca mulatta). Mech. Ageing Dev. 125, 149–151. doi: 10.1016/j.mad.2003.12.002
Gilman, S., Koller, M., Black, R. S., Jenkins, L., Griffith, S. G., Fox, N. C., et al. (2005). Clinical effects of Aβ immunization (AN1792) in patients with AD in an interrupted trial. Neurology 64, 1553–1562. doi: 10.1212/01.WNL.0000159740.16984.3C
Glenner, G. G., and Wong, C. W. (2012). Alzheimer’s disease: initial report of the purification and characterization of a novel cerebrovascular amyloid protein. 1984. Biochem. Biophys. Res. Commun. 425, 534–539. doi: 10.1016/j.bbrc.2012.08.020
Goate, A., Chartier-Harlin, M. C., Mullan, M., Brown, J., Crawford, F., Fidani, L., et al. (1991). Segregation of a missense mutation in the amyloid precursor protein gene with familial Alzheimer’s disease. Nature 349, 704–706. doi: 10.1038/349704a0
Gómez-Isla, T., Hollister, R., West, H., Mui, S., Growdon, J. H., Petersen, R. C., et al. (1997). Neuronal loss correlates with but exceeds neurofibrillary tangles in Alzheimer’s disease. Ann. Neurol. 41, 17–24. doi: 10.1002/ana.410410106
Grundke-Iqbal, I., Iqbal, K., Quinlan, M., Tung, Y. C., Zaidi, M. S., and Wisniewski, H. M. (1986). Microtubule-associated protein tau. A component of Alzheimer paired helical filaments. J. Biol. Chem. 261, 6084–6089.
Guerreiro, R., Wojtas, A., Bras, J., Carrasquillo, M., Rogaeva, E., Majounie, E., et al. (2013). TREM2 variants in Alzheimer’s disease. N. Engl. J. Med. 368, 117–127. doi: 10.1056/NEJMoa1211851
Hardy, J., and Selkoe, D. J. (2002). The amyloid hypothesis of Alzheimer’s disease: progress and problems on the road to therapeutics. Science 297, 353–356. doi: 10.1126/science.1072994
He, Z., Guo, J. L., McBride, J. D., Narasimhan, S., Kim, H., Changolkar, L., et al. (2018). Amyloid-β plaques enhance Alzheimer’s brain tau-seeded pathologies by facilitating neuritic plaque tau aggregation. Nat. Med. 24, 29–38. doi: 10.1038/nm.4443
Hosokawa, M., Masuda-Suzukake, M., Shitara, H., Shimozawa, A., Suzuki, G., Kondo, H., et al. (2021). Development of a novel tau propagation mouse model endogenously expressing 3 and 4 repeat tau isoforms. Brain 145, 349–361. doi: 10.1093/brain/awab289
Hsiao, K., Chapman, P., Nilsen, S., Eckman, C., Harigaya, Y., Younkin, S., et al. (1996). Correlative memory deficits, Aβ elevation and amyloid plaques in transgenic mice. Science 274, 99–102. doi: 10.1126/science.274.5284.99
Ikeda, T., Hori, Y., Sohma, Y., Kanai, M., and Tomita, T. (2021). Photo-oxygenation: an innovative new therapeutic approach against amyloidoses. Adv. Exp. Med. Biol. 1339, 415–422. doi: 10.1007/978-3-030-78787-5_52
Iqbal, K., Liu, F., and Gong, C. X. (2016). Tau and neurodegenerative disease: the story so far. Nat. Rev. Neurol. 12, 15–27. doi: 10.1038/nrneurol.2015.225
Iwatsubo, T., Odaka, A., Suzuki, N., Mizusawa, H., Nukina, N., and Ihara, Y. (1994). Visualization of Aβ42(43) and Aβ40 in senile plaques with end-specific Aβ monoclonals: evidence that an initially deposited species is Aβ4243. Neuron 13, 45–53. doi: 10.1016/0896-6273(94)90458-8
Jack, C. R., Bennett, D. A., Blennow, K., Carrillo, M. C., Dunn, B., Haeberlein, S. B., et al. (2018). NIA-AA research framework: toward a biological definition of Alzheimer’s disease. Alzheimer’s Dement. 14, 535–562. doi: 10.1016/j.jalz.2018.02.018
Jackson, S. J., Kerridge, C., Cooper, J., Cavallini, A., Falcon, B., Cella, C. V., et al. (2016). Short fibrils constitute the major species of seed-competent tau in the brains of mice transgenic for human P301S Tau. J. Neurosci. 36, 762–772. doi: 10.1523/JNEUROSCI.3542-15.2016
Janelidze, S., Stomrud, E., Smith, R., Palmqvist, S., Mattsson, N., Airey, D. C., et al. (2020). Cerebrospinal fluid p-tau217 performs better than p-tau181 as a biomarker of Alzheimer’s disease. Nat. Commun. 11:1683. doi: 10.1038/s41467-020-15436-0
Jankowsky, J. L., Fadale, D. J., Anderson, J., Xu, G. M., Gonzales, V., Jenkins, N. A., et al. (2004). Mutant presenilins specifically elevate the levels of the 42 residue β-amyloid peptide in vivo: evidence for augmentation of a 42-specific γ secretase. Hum. Mol. Genet. 13, 159–170. doi: 10.1093/hmg/ddh019
Janus, C., Pearson, J., McLaurin, J. A., Mathews, P. M., Jiang, Y., Schmidt, S. D., et al. (2000). Aβ peptide immunization reduces behavioural impairment and plaques in a model of Alzheimer’s disease. Nature 408, 979–982. doi: 10.1038/35050110
Jarrett, J. T., Berger, E. P., and Lansbury, P. T. (1993). The carboxy terminus of the β amyloid protein is critical for the seeding of amyloid formation: implications for the pathogenesis of Alzheimer’s disease. Biochemistry 32, 4693–4697. doi: 10.1021/bi00069a001
Jonsson, T., Atwal, J. K., Steinberg, S., Snaedal, J., Jonsson, P. V., Bjornsson, S., et al. (2012). A mutation in APP protects against Alzheimer’s disease and age-related cognitive decline. Nature 488, 96–99. doi: 10.1038/nature11283
Jonsson, T., Stefansson, H., Steinberg, S., Jonsdottir, I., Jonsson, P. V., Snaedal, J., et al. (2013). Variant of TREM2 associated with the risk of Alzheimer’s disease. N. Engl. J. Med. 368, 107–116. doi: 10.1056/NEJMoa1211103
Kamphuis, W., Mamber, C., Moeton, M., Kooijman, L., Sluijs, J. A., Jansen, A. H. P., et al. (2012). GFAP isoforms in adult mouse brain with a focus on neurogenic astrocytes and reactive astrogliosis in mouse models of Alzheimer’s disease. PLoS One 7:e42823. doi: 10.1371/journal.pone.0042823
Kanai, M., Matsubara, E., Isoe, K., Urakami, K., Nakashima, K., Arai, H., et al. (1998). Longitudinal study of cerebrospinal fluid levels of tau, Aβ1–40 and Aβ1–42(43) in Alzheimer’s disease: a study in Japan. Ann. Neurol. 44, 17–26. doi: 10.1002/ana.410440108
Kaneko, N., Nakamura, A., Washimi, Y., Kato, T., Sakurai, T., Arahata, Y., et al. (2014). Novel plasma biomarker surrogating cerebral amyloid deposition. Proc. Jpn. Acad. Ser. B Phys. Biol. Sci. 90, 353–364. doi: 10.2183/pjab.90.353
Karran, E., and De Strooper, B. (2022). The amyloid hypothesis in Alzheimer’s disease: new insights from new therapeutics. Nat. Rev. Drug Discov. 21, 306–318. doi: 10.1038/s41573-022-00391-w
Kawarabayashi, T., Younkin, L. H., Saido, T. C., Shoji, M., Ashe, K. H., and Younkin, S. G. (2001). Age-dependent changes in brain, CSF and plasma amyloid β protein in the Tg2576 transgenic mouse model of Alzheimer’s disease. J. Neurosci. 21, 372–381. doi: 10.1523/JNEUROSCI.21-02-00372.2001
Kennedy, M. E., Stamford, A. W., Chen, X., Cox, K., Cumming, J. N., Dockendorf, M. F., et al. (2016). The BACE1 inhibitor verubecestat (MK-8931) reduces CNS β-amyloid in animal models and in Alzheimer’s disease patients. Sci. Transl. Med. 8:363ra150. doi: 10.1126/scitranslmed.aad9704
Keren-Shaul, H., Spinrad, A., Weiner, A., Matcovitch-Natan, O., Dvir-Szternfeld, R., Ulland, T. K., et al. (2017). A unique microglia type associated with restricting development of Alzheimer’s disease. Cell 169, 1276–1290.e17. doi: 10.1016/j.cell.2017.05.018
Kikuchi, K., Kidana, K., Tatebe, T., and Tomita, T. (2017). Dysregulated metabolism of the Amyloid-β protein and therapeutic approaches in Alzheimer’s disease. J. Cell. Biochem. 118, 4183–4190. doi: 10.1002/jcb.26129
Knopman, D. S., Amieva, H., Petersen, R. C., Chételat, G., Holtzman, D. M., Hyman, B. T., et al. (2021). Alzheimer’s disease. Nat. Rev. Dis. Prim. 7:33. doi: 10.1038/s41572-021-00269-y
Koriyama, Y., Hori, A., Ito, H., Yonezawa, S., Baba, Y., Tanimoto, N., et al. (2021). Discovery of atabecestat (JNJ-54861911): a thiazine-based β-amyloid precursor protein cleaving enzyme 1 inhibitor advanced to the phase 2b/3 EARLY clinical trial. J. Med. Chem. 64, 1873–1888. doi: 10.1021/acs.jmedchem.0c01917
Kosik, K. S., Joachim, C. L., and Selkoe, D. J. (1986). Microtubule-associated protein tau (tau) is a major antigenic component of paired helical filaments in Alzheimer’s disease. Proc. Natl. Acad. Sci. U S A 83, 4044–4048. doi: 10.1073/pnas.83.11.4044
Krasemann, S., Madore, C., Cialic, R., Baufeld, C., Calcagno, N., El Fatimy, R., et al. (2017). The TREM2-APOE pathway drives the transcriptional phenotype of dysfunctional microglia in neurodegenerative diseases. Immunity 47, 566–581.e9. doi: 10.1016/j.immuni.2017.08.008
Lalonde, R., Kim, H. D., Maxwell, J. A., and Fukuchi, K. (2005). Exploratory activity and spatial learning in 12-month-old APP(695)SWE/co+PS1/DeltaE9 mice with amyloid plaques. Neurosci. Lett. 390, 87–92. doi: 10.1016/j.neulet.2005.08.028
Lemere, C. A., Beierschmitt, A., Iglesias, M., Spooner, E. T., Bloom, J. K., Leverone, J. F., et al. (2004). Alzheimer’s disease aβ vaccine reduces central nervous system aβ levels in a non-human primate, the Caribbean vervet. Am. J. Pathol. 165, 283–297. doi: 10.1016/s0002-9440(10)63296-8
Levy-Lahad, E., Wasco, W., Poorkaj, P., Romano, D. M., Oshima, J., Pettingell, W. H., et al. (1995). Candidate gene for the chromosome 1 familial Alzheimer’s disease locus. Science (80-) 269, 973–977. doi: 10.1126/science.7638622
Lewis, J., McGowan, E., Rockwood, J., Melrose, H., Nacharaju, P., Van Slegtenhorst, M., et al. (2000). Neurofibrillary tangles, amyotrophy and progressive motor disturbance in mice expressing mutant (P301L) tau protein. Nat. Genet. 25, 402–405. doi: 10.1038/78078
Leyns, C. E. G., Gratuze, M., Narasimhan, S., Jain, N., Koscal, L. J., Jiang, H., et al. (2019). TREM2 function impedes tau seeding in neuritic plaques. Nat. Neurosci. 22, 1217–1222. doi: 10.1038/s41593-019-0433-0
Lord, A., Gumucio, A., Englund, H., Sehlin, D., Sundquist, V. S., Söderberg, L., et al. (2009). An amyloid-β protofibril-selective antibody prevents amyloid formation in a mouse model of Alzheimer’s disease. Neurobiol. Dis. 36, 425–434. doi: 10.1016/j.nbd.2009.08.007
Lord, A., Kalimo, H., Eckman, C., Zhang, X. Q., Lannfelt, L., and Nilsson, L. N. G. (2006). The arctic Alzheimer mutation facilitates early intraneuronal Aβ aggregation and senile plaque formation in transgenic mice. Neurobiol. Aging 27, 67–77. doi: 10.1016/j.neurobiolaging.2004.12.007
Maia, L. F., Kaeser, S. A., Reichwald, J., Hruscha, M., Martus, P., Staufenbiel, M., et al. (2013). Changes in amyloid-β and tau in the cerebrospinal fluid of transgenic mice overexpressing amyloid precursor protein. Sci. Transl. Med. 5:194re2. doi: 10.1126/scitranslmed.3006446
Malhotra, N., Gupta, R., and Kumar, P. (2021). Pharmacological relevance of CDK inhibitors in Alzheimer’s disease. Neurochem. Int. 148:105115. doi: 10.1016/j.neuint.2021.105115
McDade, E., Voytyuk, I., Aisen, P., Bateman, R. J., Carrillo, M. C., De Strooper, B., et al. (2021). The case for low-level BACE1 inhibition for the prevention of Alzheimer’s disease. Nat. Rev. Neurol. 17, 703–714. doi: 10.1038/s41582-021-00545-1
Min, S. W., Chen, X., Tracy, T. E., Li, Y., Zhou, Y., Wang, C., et al. (2015). Critical role of acetylation in tau-mediated neurodegeneration and cognitive deficits. Nat. Med. 21, 1154–1162. doi: 10.1038/nm.3951
Mitani, Y., Yarimizu, J., Saita, K., Uchino, H., Akashiba, H., Shitaka, Y., et al. (2012). Differential effects between γ-secretase inhibitors and modulators on cognitive function in amyloid precursor protein-transgenic and nontransgenic mice. J. Neurosci. 32, 2037–2050. doi: 10.1523/JNEUROSCI.4264-11.2012
Moore, B. D., Chakrabarty, P., Levites, Y., Kukar, T. L., Baine, A.-M., Moroni, T., et al. (2012). Overlapping profiles of Aβ peptides in the Alzheimer’s disease and pathological aging brains. Alzheimers. Res. Ther. 4:18. doi: 10.1186/alzrt121
Morgan, D., Diamond, D. M., Gottschall, P. E., Ugen, K. E., Dickey, C., Hardy, J., et al. (2000). Aβ peptide vaccination prevents memory loss in an animal model of Alzheimer’s disease. Nature 408, 982–985. doi: 10.1038/35050116
Motter, R., Vigo-Pelfrey, C., Kholodenko, D., Barbour, R., Johnson-Wood, K., Galasko, D., et al. (1995). Reduction of β-amyloid peptide42 in the cerebrospinal fluid of patients with Alzheimer’s disease. Ann. Neurol. 38, 643–648. doi: 10.1002/ana.410380413
Mucke, L., Masliah, E., Yu, G. Q., Mallory, M., Rockenstein, E. M., Tatsuno, G., et al. (2000). High-level neuronal expression of Aβ1–42 in wild-type human amyloid protein precursor transgenic mice: synaptotoxicity without plaque formation. J. Neurosci. 20, 4050–4058. doi: 10.1523/JNEUROSCI.20-11-04050.2000
Nagashima, N., Ozawa, S., Furuta, M., Oi, M., Hori, Y., Tomita, T., et al. (2021). Catalytic photooxygenation degrades brain Aβ in vivo. Sci. Adv. 7:eabc9750. doi: 10.1126/sciadv.abc9750
Nakamura, A., Kaneko, N., Villemagne, V. L. V. L. V. L., Kato, T., Doecke, J., Doré, V., et al. (2018). High performance plasma amyloid-β biomarkers for Alzheimer’s disease. Nature 554, 249–254. doi: 10.1038/nature25456
Nakamura, S., Tamaoka, A., Sawamura, N., Shoji, S., Nakayama, H., Ono, F., et al. (1995). Carboxyl end-specific monoclonal antibodies to amyloid β protein (Aβ) subtypes (Aβ40 and Aβ42(43)) differentiate Aβ in senile plaques and amyloid angiopathy in brains of aged cynomolgus monkeys. Neurosci. Lett. 201, 151–154. doi: 10.1016/0304-3940(95)12160-9
Neumann, U., Ufer, M., Jacobson, L. H., Rouzade-Dominguez, M., Huledal, G., Kolly, C., et al. (2018). The BACE-1 inhibitor CNP520 for prevention trials in Alzheimer’s disease. EMBO Mol. Med. 10:e9316. doi: 10.15252/emmm.201809316
Ni, J., Taniguchi, A., Ozawa, S., Hori, Y., Kuninobu, Y., Saito, T., et al. (2018). Near-infrared photoactivatable oxygenation catalysts of amyloid peptide. Chem 4, 807–820. doi: 10.1016/j.chempr.2018.02.008
Nicoll, J. A. R., Wilkinson, D., Holmes, C., Steart, P., Markham, H., and Weller, R. O. (2003). Neuropathology of human Alzheimer’s disease after immunization with amyloid-β peptide: a case report. Nat. Med. 9, 448–452. doi: 10.1038/nm840
Nies, S. H., Takahashi, H., Herber, C. S., Huttner, A., Chase, A., and Strittmatter, S. M. (2021). Spreading of Alzheimer tau seeds is enhanced by aging and template matching with limited impact of amyloid-β. J. Biol. Chem. 297:101159. doi: 10.1016/j.jbc.2021.101159
Novak, G., Streffer, J. R., Timmers, M., Henley, D., Brashear, H. R., Bogert, J., et al. (2020). Long-term safety and tolerability of atabecestat (JNJ-54861911), an oral BACE1 inhibitor, in early Alzheimer’s disease spectrum patients: a randomized, double-blind, placebo-controlled study and a two-period extension study. Alzheimers Res. Ther. 12. doi: 10.1186/s13195-020-00614-5
Nukina, N., and Ihara, Y. (1986). One of the antigenic determinants of paired helical filaments is related to tau protein. J. Biochem. 99, 1541–1544. doi: 10.1093/oxfordjournals.jbchem.a135625
Oakley, H., Cole, S. L., Logan, S., Maus, E., Shao, P., Craft, J., et al. (2006). Intraneuronal β-amyloid aggregates, neurodegeneration and neuron loss in transgenic mice with five familial Alzheimer’s disease mutations: potential factors in amyloid plaque formation. J. Neurosci. 26, 10129–10140. doi: 10.1523/JNEUROSCI.1202-06.2006
Oddo, S., Caccamo, A., Shepherd, J. D., Murphy, M. P., Golde, T. E., Kayed, R., et al. (2003). Triple-transgenic model of Alzheimer’s disease with plaques and tangles: intracellular Aβ and synaptic dysfunction. Neuron 39, 409–421. doi: 10.1016/s0896-6273(03)00434-3
Ozawa, S., Hori, Y., Shimizu, Y., Taniguchi, A., Suzuki, T., Wang, W., et al. (2021). Photo-oxygenation by a biocompatible catalyst reduces amyloid-β levels in Alzheimer’s disease mice. Brain 144, 1884–1897. doi: 10.1093/brain/awab058
Panza, F., Frisardi, V., Imbimbo, B. P., Capurso, C., Logroscino, G., Sancarlo, D., et al. (2010). Review: γ-Secretase inhibitors for the treatment of Alzheimer’s disease: the current state. CNS Neurosci. Ther. 16, 272–284. doi: 10.1111/j.1755-5949.2010.00164.x
Richards, J. G., Higgins, G. A., Ouagazzal, A. M., Ozmen, L., Kew, J. N. C., Bohrmann, B., et al. (2003). PS2APP transgenic mice, coexpressing hPS2mut and hAPPswe, show age-related cognitive deficits associated with discrete brain amyloid deposition and inflammation. J. Neurosci. 23, 8989–9003. doi: 10.1523/JNEUROSCI.23-26-08989.2003
Roertgen, K. E., Parisi, J. E., Clark, H. B., Barnes, D. L., O’Brien, T. D., and Johnson, K. H. (1996). Aβ-associated cerebral angiopathy and senile plaques with neurofibrillary tangles and cerebral hemorrhage in an aged wolverine (Gulo gulo). Neurobiol. Aging 17, 243–247. doi: 10.1016/0197-4580(95)02069-1
Rogaev, E. I., Sherrington, R., Rogaeva, E. A., Levesque, G., Ikeda, M., Liang, Y., et al. (1995). Familial Alzheimer’s disease in kindreds with missense mutations in a gene on chromosome 1 related to the Alzheimer’s disease type 3 gene. Nature 376, 775–778. doi: 10.1038/376775a0
Rovelet-Lecrux, A., Hannequin, D., Raux, G., Le Meur, N., Laquerrière, A., Vital, A., et al. (2006). APP locus duplication causes autosomal dominant early-onset Alzheimer’s disease with cerebral amyloid angiopathy. Nat. Genet. 38, 24–26. doi: 10.1038/ng1718
Saido, T. C., Iwatsubo, T., Mann, D. M. A., Shimada, H., Ihara, Y., and Kawashima, S. (1995). Dominant and differential deposition of distinct β-amyloid peptide species, AβN3(pE), in senile plaques. Neuron 14, 457–466. doi: 10.1016/0896-6273(95)90301-1
Saito, T., Matsuba, Y., Mihira, N., Takano, J., Nilsson, P., Itohara, S., et al. (2014). Single app knock-in mouse models of Alzheimer’s disease. Nat. Neurosci. 17, 661–663. doi: 10.1038/nn.3697
Saito, T., Mihira, N., Matsuba, Y., Sasaguri, H., Hashimoto, S., Narasimhan, S., et al. (2019). Humanization of the entire murine Mapt gene provides a murine model of pathological human tau propagation. J. Biol. Chem. 294, 12754–12765. doi: 10.1074/jbc.RA119.009487
Santacruz, K., Lewis, J., Spires, T., Paulson, J., Kotilinek, L., Ingelsson, M., et al. (2005). Tau suppression in a neurodegenerative mouse model improves memory function. Science 309, 476–481. doi: 10.1126/science.1113694
Schenk, D., Barbour, R., Dunn, W., Gordon, G., Grajeda, H., Guldo, T., et al. (1999). Immunization with amyloid-β attenuates Alzheimer disease-like pathology in the PDAPP mouse. Nature 400, 173–177. doi: 10.1038/22124
Selkoe, D. J., and Hardy, J. (2016). The amyloid hypothesis of Alzheimer’s disease at 25 years. EMBO Mol. Med. 8, 595–608. doi: 10.15252/emmm.201606210
Sevigny, J., Chiao, P., Bussière, T., Weinreb, P. H., Williams, L., Maier, M., et al. (2016). The antibody aducanumab reduces Aβ plaques in Alzheimer’s disease. Nature 537, 50–56. doi: 10.1038/nature19323
Sherrington, R., Rogaev, E., Liang, Y., Rogaeva, E., Lebasque, G., Ikeda, M., et al. (1995). Cloning of a gene bearing missense mutations in early-onset familial Alzheimer’s disease. Nature 375, 754–760. doi: 10.1038/375754a0
Solomon, B., Koppel, R., Hanan, E., and Katzav, T. (1996). Monoclonal antibodies inhibit in vitro fibrillar aggregation of the Alzheimer β-amyloid peptide. Proc. Natl. Acad. Sci. USA 93, 452–455. doi: 10.1073/pnas.93.1.452
Sopko, R., Golonzhka, O., Arndt, J., Quan, C., Czerkowicz, J., Cameron, A., et al. (2020). Characterization of tau binding by gosuranemab. Neurobiol. Dis. 146:105120. doi: 10.1016/j.nbd.2020.105120
Sturchler-Pierrat, C., Abramowski, D., Duke, M., Wiederhold, K. H., Mistl, C., Rothacher, S., et al. (1997). Two amyloid precursor protein transgenic mouse models with Alzheimer disease-like pathology. Proc. Natl. Acad. Sci. USA 94, 13287–13292. doi: 10.1073/pnas.94.24.13287
Suzuki, T., Hori, Y., Sawazaki, T., Shimizu, Y., Nemoto, Y. Y., Taniguchi, A., et al. (2019). Photo-oxygenation inhibits tau amyloid formation. Chem. Commun. 55, 6165–6168. doi: 10.1039/c9cc01728c
Takasugi, N., Tomita, T., Hayashi, I., Tsuruoka, M., Niimura, M., Takahashi, Y., et al. (2003). The role of presenilin cofactors in the γ-secratase complex. Nature 422, 438–441. doi: 10.1038/nature01506
Taniguchi, A., Shimizu, Y., Oisaki, K., Sohma, Y., and Kanai, M. (2016). Switchable photooxygenation catalysts that sense higher-order amyloid structures. Nat. Chem. 8, 974–982. doi: 10.1038/nchem.2550
Thal, D. R., Rüb, U., Orantes, M., and Braak, H. (2002). Phases of Aβ-deposition in the human brain and its relevance for the development of AD. Neurology 58, 1791–1800. doi: 10.1212/wnl.58.12.1791
Tomita, T., Maruyama, K., Saido, T. C. T. C., Kume, H., Shinozaki, K., Tokuhiro, S., et al. (1997). The presenilin 2 mutation (N141I) linked to familial Alzheimer’s disease (Volga German families) increases the secretion of amyloid β protein ending at the 42nd (or 43rd) residue. Proc. Natl. Acad. Sci. U S A 94, 2025–2030. doi: 10.1073/pnas.94.5.2025
Tucker, S., Möller, C., Tegerstedt, K., Lord, A., Laudon, H., Sjödahl, J., et al. (2015). The murine version of BAN2401 (mAb158) selectively reduces amyloid-β protofibrils in brain and cerebrospinal fluid of tg-ArcSwe mice. J. Alzheimers Dis. 43, 575–588. doi: 10.3233/JAD-140741
Uhlmann, R. E., Rother, C., Rasmussen, J., Schelle, J., Bergmann, C., Ullrich Gavilanes, E. M., et al. (2020). Acute targeting of pre-amyloid seeds in transgenic mice reduces Alzheimer-like pathology later in life. Nat. Neurosci. 23, 1580–1588. doi: 10.1038/s41593-020-00737-w
Ulland, T. K., and Colonna, M. (2018). TREM2 - a key player in microglial biology and Alzheimer’s disease. Nat. Rev. Neurol. 14, 667–675. doi: 10.1038/s41582-018-0072-1
Van Dam, D., D’Hooge, R., Staufenbiel, M., Van Ginneken, C., Van Meir, F., and De Deyn, P. P. (2003). Age-dependent cognitive decline in the APP23 model precedes amyloid deposition. Eur. J. Neurosci. 17, 388–396. doi: 10.1046/j.1460-9568.2003.02444.x
Vite, C. H., and Head, E. (2014). Aging in the canine and feline brain. Vet. Clin. North Am. Small Anim. Pract. 44, 1113–1129. doi: 10.1016/j.cvsm.2014.07.008
Wang, R., Sweeney, D., Gandy, S. E., and Sisodia, S. S. (1996). The profile of soluble amyloid β protein in cultured cell media. J. Biol. Chem. 271, 31894–31902. doi: 10.1074/jbc.271.50.31894
Wesseling, H., Mair, W., Kumar, M., Schlaffner, C. N., Tang, S., Beerepoot, P., et al. (2020). Tau PTM profiles identify patient heterogeneity and stages of Alzheimer’s disease. Cell 183, 1699–1713.e13. doi: 10.1016/j.cell.2020.10.029
Wilcock, D. M., Rojiani, A., Rosenthal, A., Levkowitz, G., Subbarao, S., Alamed, J., et al. (2004). Passive amyloid immunotherapy clears amyloid and transiently activates microglia in a transgenic mouse model of amyloid deposition. J. Neurosci. 24, 6144–6151. doi: 10.1523/JNEUROSCI.1090-04.2004
Wright, A. L., Zinn, R., Hohensinn, B., Konen, L. M., Beynon, S. B., Tan, R. P., et al. (2013). Neuroinflammation and neuronal loss precede Aβ plaque deposition in the hAPP-J20 mouse model of Alzheimer’s disease. PLoS One 8:e59586. doi: 10.1371/journal.pone.0059586
Yamada, K. (2017). Extracellular tau and its potential role in the propagation of tau pathology. Front. Neurosci. 11:667. doi: 10.3389/fnins.2017.00667
Yamada, K., Yabuki, C., Seubert, P., Schenk, D., Hori, Y., Ohtsuki, S., et al. (2009). Aβ immunotherapy: Intracerebral sequestration of Aβ by an anti-Aβ monoclonal antibody 266 with high affinity to soluble Aβ. J. Neurosci. 29, 11393–11398. doi: 10.1523/JNEUROSCI.2021-09.2009
Yoshiyama, Y., Higuchi, M., Zhang, B., Huang, S. M., Iwata, N., Saido, T. C. C., et al. (2007). Synapse loss and microglial activation precede tangles in a P301S tauopathy mouse model. Neuron 53, 337–351. doi: 10.1016/j.neuron.2007.01.010
Yuan, P., Condello, C., Keene, C. D., Wang, Y., Bird, T. D., Paul, S. M., et al. (2016). TREM2 haplodeficiency in mice and humans impairs the microglia barrier function leading to decreased amyloid compaction and severe axonal dystrophy. Neuron 90, 724–739. doi: 10.1016/j.neuron.2016.05.003
Keywords: Alzheimer’s disease, β-amyloid, tau, therapeutics, mouse model
Citation: Yokoyama M, Kobayashi H, Tatsumi L and Tomita T (2022) Mouse Models of Alzheimer’s Disease. Front. Mol. Neurosci. 15:912995. doi: 10.3389/fnmol.2022.912995
Received: 05 April 2022; Accepted: 23 May 2022;
Published: 21 June 2022.
Edited by:
Stephan C. F. Neuhauss, University of Zurich, SwitzerlandReviewed by:
Elva Diaz, University of California, Davis, United StatesKoji Yamanaka, Nagoya University, Japan
Copyright © 2022 Yokoyama, Kobayashi, Tatsumi and Tomita. This is an open-access article distributed under the terms of the Creative Commons Attribution License (CC BY). The use, distribution or reproduction in other forums is permitted, provided the original author(s) and the copyright owner(s) are credited and that the original publication in this journal is cited, in accordance with accepted academic practice. No use, distribution or reproduction is permitted which does not comply with these terms.
*Correspondence: Taisuke Tomita, taisuke@mol.f.u-tokyo.ac.jp orcid.org/0000-0002-0075-5943
† These authors have contributed equally to this work