- 1Laboratório de Engenharia Tecidual e Imunofarmacologia, Instituto Gonçalo Moniz, Fundação Oswaldo Cruz (IGM-FIOCRUZ/BA), Salvador, Brazil
- 2Instituto SENAI de Sistemas Avançados de Saúde (CIMATEC ISI-SAS), Centro Universitário SENAI/CIMATEC, Salvador, Brazil
- 3Laboratório de Neurobiologia Celular e Molecular, Instituto de Biofísica Carlos Chagas Filho, Universidade Federal do Rio de Janeiro, Rio de Janeiro, Brazil
- 4Programa Redes de Pesquisa em Saúde no Estado do Rio de Janeiro, Rio de Janeiro, Brazil
- 5Instituto de Ciências Biomédicas, Universidade Federal do Rio de Janeiro, Rio de Janeiro, Brazil
- 6Programa Redes de Pesquisa em Nanotecnologia no Estado do Rio de Janeiro, Rio de Janeiro, Brazil
- 7Department of Laboratory Medicine, Karolinska Institute, Stockholm, Sweden
- 8Laboratory of Cellular Therapy, Division of Oncology, University of Modena and Reggio Emilia (UNIMORE), Modena, Italy
Neurological disorders include a wide spectrum of clinical conditions affecting the central and peripheral nervous systems. For these conditions, which affect hundreds of millions of people worldwide, generally limited or no treatments are available, and cell-based therapies have been intensively investigated in preclinical and clinical studies. Among the available cell types, mesenchymal stem/stromal cells (MSCs) have been widely studied but as yet no cell-based treatment exists for neurological disease. We review current knowledge of the therapeutic potential of MSC-based therapies for neurological diseases, as well as possible mechanisms of action that may be explored to hasten the development of new and effective treatments. We also discuss the challenges for culture conditions, quality control, and the development of potency tests, aiming to generate more efficient cell therapy products for neurological disorders.
Introduction
Mesenchymal stem cells (MSCs) are among the major cell types used in regenerative medicine and represent a promising therapeutic tool for several presently incurable neurological disorders. First found in bone marrow (Friedenstein et al., 1970), MSCs can be isolated from almost any adult tissue, including fat, peripheral blood, muscle, skin, and teeth, in addition to birth-associated tissues such as umbilical cord (Wharton’s jelly and cord blood), amnion, and placenta (Berebichez-Fridman and Montero-Olvera, 2018). MSCs comprise a heterogeneous population of fibroblast-like multipotent and self-renewing cells. Regardless of the source or harvest and expansion methods, MSCs must meet three minimum criteria to ascertain their equivalence and stemness: (I) plastic adherence under standard culture conditions; (II) expression of CD105, CD73, and CD90, and lack of expression of CD45, CD34, CD14/CD11b, CD79α/CD19, and HLA-DR surface markers; and (III) ability to differentiate into osteoblasts, adipocytes, and chondroblasts (Dominici et al., 2006).
Despite these common defining characteristics, MSCs have source-dependent differences that influence their applicability. Currently, bone marrow (BM) is the most widely used source of MSCs for clinical trials, followed by the umbilical cord (UC), and adipose tissue (AT; Kabat et al., 2020). BM-MSCs have long been considered the gold standard in cell therapy, with very well-characterized properties. They are easily obtained by BM aspiration from iliac crests, allowing autologous transplantation, which reduces the risk of immunological rejection. However, the harvesting procedure, in addition to being painful, has a low cell yield that declines with increasing donor age, as do the cell lifespan, proliferative capacity, and differentiation potential (Zaim et al., 2012). In recent years, AT has been reported to be a richer and more practical source of autologous MSCs in terms of availability, abundance, and accessibility compared to BM, but the sampling procedure is technically invasive (Chu et al., 2019). Birth-related tissues such as UC have great advantages over other sources, as they can be readily collected with no pain or risk to either mother or child and are usually discarded. MSCs derived from these tissues are considered more primitive according to the expression profile of cell surface markers (Conconi et al., 2011) and have intermediate features between embryonic and adult stem cells, with multilineage differentiation potential, rapid proliferation rate, low senescence, and hypoimmunogenicity, which allows safe allogeneic transplantation (El Omar et al., 2014; Nagamura-Inoue and Mukai, 2016). Furthermore, UC tissue provides large quantities of harvestable MSCs, which can be long-term cryopreserved for future cultivation (Vangsness et al., 2015).
The therapeutic potential of MSCs is attributed to their homing property, multilineage differentiation, and paracrine function. MSCs can migrate toward injured tissues, engraft, and differentiate into functional cells (Fu et al., 2019). However, rather than cellular replacement, MSCs contribute to tissue repair mainly by the paracrine action of their secretome, which comprises a wide range of immunomodulatory, angiogenic, antiapoptotic, and growth factors, supporting cell survival and tissue regeneration (Teixeira and Salgado, 2020). These features of MSCs make them promising for the treatment of neurological diseases by modulating the inflammatory and inhibitory milieu of injured/degenerating nervous tissue (Figure 1).
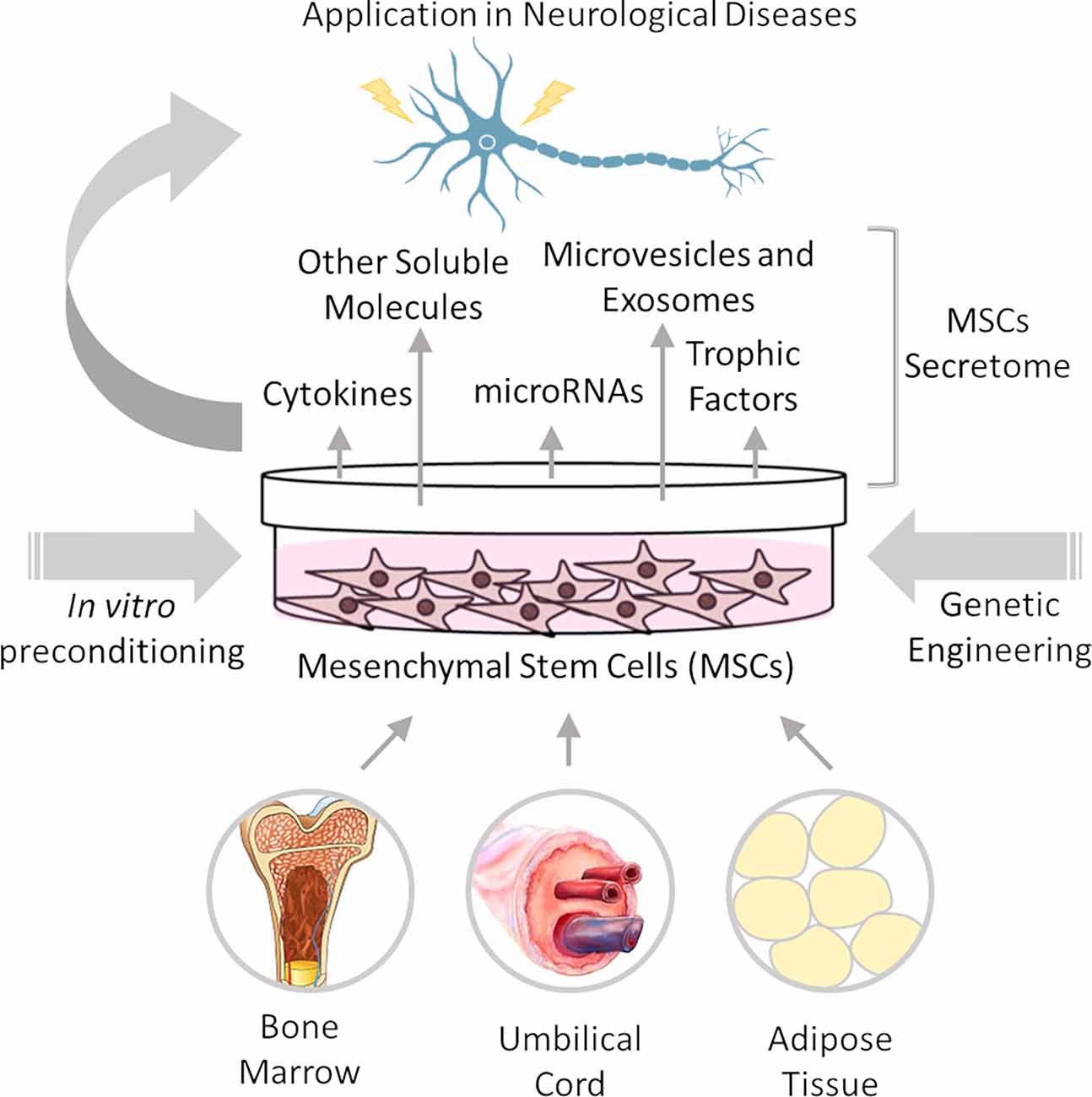
Figure 1. Mesenchymal stem cell therapy for neurological diseases. Mesenchymal stem/stromal cells (MSCs) can be isolated from several adult and perinatal tissues, including bone marrow, umbilical cord, and adipose tissue. MSC neuroprotective actions are based on their paracrine action through secretion of cytokines, trophic factors, and microRNAs, among other molecules, which are released directly into the extracellular space or packaged in microvesicles and exosomes. MSC efficacy can be improved in vitro prior to transplantation, by different preconditioning methods and/or genetic engineering to increase the production or release of specific factors.
In this review, we discuss the methods currently employed to foster the development of more efficient cell therapy products, and also provide an overview of current research on therapeutic applications of MSCs in neurological disorders.
MSC-Derived Exosomes and Extracellular Vesicles (Evs)
Although the transplantation of MCSs has yielded promising results in several preclinical and clinical studies, the risks of transplantation of cells that are extensively manipulated during culturing, transport, and storage persist. Furthermore, little is known about the long-term behavior of transplanted cells. The use of cell-derived products that reproduce the effects of cell therapy could allay these concerns. Thus, extracellular vesicles (Evs) derived from MSCs emerge as an alternative for therapy. To date, 74 studies related to Evs are reported as completed, are listed in clinicaltrials.org, and have posted their results and shown the safety of using Evs (NCT04281901, NCT04491240, NCT04491240; Shi et al., 2021). Evs are bilayer lipid-coated structures containing intracellular contents that can be classified by their origin and size. Exosomes are vesicles 40–150 nm in diameter that are generated by endosomes; microvesicles are vesicles 100–1,000 nm in diameter, generated from the budding of the plasma membrane (Lindoso et al., 2017). Other forms of Evs are apoptotic bodies and excretion vesicles, but in the present context of MSC mechanisms, we will refer to both exosomes and microvesicles as Evs. These Evs carry proteins, bioactive lipids, and coding and non-coding RNA and can modify the physiology of the cell target. As MSCs are very weakly immunogenic, their Evs also have low immunogenicity, which makes them safe for transplantation (Zhu et al., 2017; Montaner-Tarbes et al., 2018; Saleh et al., 2019; Rodrigues et al., 2021).
MSC-derived Evs can be identified by their affinity to certain molecules. Thus, exosomes have an affinity to cholera toxin B, which binds to the GM1 ganglioside, while annexin V and shiga toxin B bind to phosphatidylserine and globotriaosylceramide, and their biogenesis is related to the cytoplasm and nucleus, respectively (Lai and Lim, 2019). The lipid composition of Evs can define their origins and activity. The concentration of the lipid dilysocardiolipin can affect the delivery activity of Evs (Haraszti et al., 2019). Other lipids include ceramides, sphingomyelins, phosphatidylcholines, phosphatidylethanolamines, phosphatidylserines, phosphatidylinositols, eicosanoids, and cholesterol (Le Saux et al., 2020). Moreover, Evs can carry lipid mediators with immunomodulatory effects, such as prostaglandin E2 and resolvins (Holopainen et al., 2019).
Proteins Related to MSC-Derived Evs
Proteins associated with MSC-derived Evs include antigens (e.g., CD9, CD63, and CD81), adhesion molecules, and surface receptors (e.g., PDGFRB, EGFR, and PLAUR; Kim H. S. et al., 2012). Adhesion molecules such as the RAB family are important to regulate the docking and fusion of Evs (Kim H. S. et al., 2012). MSC-derived Evs carry proteins that can sustain cellular homeostasis. In addition to typically extracellular proteins and membrane-associated proteins, Evs can transport typically intracellular proteins or even organelles, such as mitochondria (Morrison et al., 2017; Zhang Z. et al., 2020). Antioxidant enzymes such as catalase can be found in BM (Godoy et al., 2017) and Wharton’s jelly (WJ), and MSC-derived Evs have been shown to prevent oxidative stress in hippocampal neurons (Bodart-Santos et al., 2019; Puig-Pijuan et al., 2020). Inflammation can be modulated by EV-associated proteins such as CD73, TGF-β, and PTX3 (Alvarez et al., 2018; Crain et al., 2019; Kim et al., 2020). Furthermore, proteins found in AT-MSC-derived Evs are related to the MAPK, VEGF, and Jak-Stat pathways (Xing et al., 2020).
The protein composition of MSC-derived Evs can be modified by their culture conditions. Human BM-MSCs cultured with 1% O2 in a serum-deprived medium produced Evs with elevated levels of transporters, peptidases, receptors, G-coupled receptors, and ion channels (Yuan et al., 2019). Priming endometrial MSCs with interferon-γ (IFN-γ) increased immunomodulatory proteins in their Evs (Marinaro et al., 2019). Serum deprivation modified the composition of UC, AT, or BM-MSC-derived Evs for 24 h in order to improve the delivery of exosomes, but not their microvesicles, to target neurons (Haraszti et al., 2019). Hypoxia also changed the proteomic profile of Evs in MSCs, with an increase in proteins related to growth factors and a decrease in proteins related to oxidative metabolism (Gessner et al., 2021; Gregorius et al., 2021). A 3D culture using CellHesion® VP increased EV production by MSCs and changed their protein profile, with an increase in proteins related to immune response (Kim E. S. et al., 2021). Priming of MSCs with toll-like receptor III agonist poly(I:C) upregulated EV proteins related to immune response, among other processes (Pierce and Kurata, 2021). Exosomes derived from human AT-MSCs stimulated by LPS increased in angiogenic potential (Wu et al., 2021). Preconditioning of MSCs with IFN-γ induced release of Evs containing annexin-1, lactotransferrin, and aminopeptidase N (Takeuchi et al., 2021). Priming of MSCs with melatonin increased ubiquitin-specific protease 29 in their Evs, which promoted increased microglia activation to the M2 phenotype and resulted in better recovery of motor behavior (Liu W. et al., 2021). Another molecule capable of enhancing the release of EVs and their therapeutic effect is metformin (Liao et al., 2021).
miRNAs Related to MSC-Derived Evs
Many groups have shown that MSCs release miRNA-containing Evs that modulate molecular pathways in the target cell. miRNAs are non-coding RNAs approximately 22 nucleotides long, and are involved in post-transcriptional regulation of gene expression (Asgarpour et al., 2020). The importance of miRNA in the therapeutic effects of MSC-derived Evs is evidenced by inhibiting miRNA maturation or function. Knockdown of argonaute-2, a key protein for miRNA function, abolishes the protective effects of MSC-derived Evs in a model of optic nerve injury (Mead and Tomarev, 2017). The miRNA content of BM-MSC-derived Evsproved to be important for the neuroprotective effect in a rat model of chemobrain (El-Derany and Noureldein, 2021). Certain specific miRNAs were reported to have a pivotal role in the MSC-derived Ev effect. Endometrial MSCs primed with IFN-y 18 significantly altered miRNAs (Marinaro et al., 2019). Evs derived from hydrogen sulfide-primed BM-MSCs had an intensified therapeutic effect in a model of hypoxia-ischemia through miR-7b-5p activity (Chu et al., 2020). BM-MSCs transfer mir-29b-3p to neurons through Evs, preventing hypoxic-ischemic injury (Hou et al., 2020). Inhibition of mir-21-5p in BM-MSC-derived Evs attenuated the functional recovery of rats subjected to a spinal cord injury (Zhou et al., 2019). Overexpression of CDKN2B, a downstream target for mir-106b, reversed the effect of mir106b-containing MSC-derived Evs on a mouse model of Parkinson’s disease (Bai et al., 2021). Interestingly, the overexpression of miRNAs in MSCs may be reflected in their respective Evs. Mir-29b-3p is accumulated in Evs derived from BM-MSCs overexpressing this miRNA, and transfection with mir-29b-3p inhibitor downregulated it in respective Evs (Hou et al., 2020). Transfection of BM-MSCs with the mir-17-92 cluster enriched their Evs and improved the tissue and functional behavior of rats subjected to a brain-injury model (Zhang Y. et al., 2021). Silencing mir-29c-3p also reduced the effect of BM-MSC-derived Evs in an AD model (Sha et al., 2021). The miR-125a found in BM-MSCs promotes M2 macrophage polarization, which was attenuated by the knockdown of this miRNA (Chang et al., 2021). Transfection of AT-MSCs with miR-22 mimics resulted in a mir-22 enriched pool of Evs and reduced inflammation in a mouse model of AD (Zhai et al., 2021). Also, Evs of MSCs transfected with miR-26a-5p and miR-221-3p mimic, respectively, attenuated ischemia/reperfusion injury and stroke (Ai et al., 2021; Cheng et al., 2021). A gain- and loss-of-function study showed that mir-26a is important for the neuroprotective effect of Evs from AT-MSCs in a model of ischemia/reperfusion (Hou et al., 2021). A recent study showed that viral transduction of MSCs may impact their miRNA cargo in Evs (Zubkova et al., 2021). Priming MSCs with conditioned medium of activated microglia changed the miRNAs of Evs to a more immune-regulatory profile, which resulted in inhibition of microglial and astrocyte activation and better behavioral function (Markoutsa et al., 2022). Other stimuli can alter the miRNA content in MSC-derived Evs, such as exposure to glioblastoma-derived microvesicles (Garnier et al., 2022).
Long Noncoding RNAs Related to MSC-Derived Evs
Other noncoding RNAs found in MSC-derived Evs are long noncoding RNAs (lncRNAs) and circular RNAs (circRNAs). To date, little is known about the roles of circRNAs and lncRNAs in the therapeutic effect of MSC-derived Evs, especially for neurodegenerative diseases. CircRNAs have been shown to regulate gene expression by interacting with RNA-binding proteins and functioning as miRNA sponges (Hansen et al., 2013; Zang et al., 2020). Recently, transfection of AT-MSCs with cicrAkap7 proved to enhance the neuroprotective effect of their Evs in a mouse model of ischemic injury (Xu et al., 2020). CircRNAs have also been implicated in the therapeutic effect of Evs in several models, by regulating angiogenesis (Zhang J. et al., 2021) and reducing pyroptose and cytotoxicity (Cao et al., 2020; Yan et al., 2020). LncRNAs are the most abundant class of non-coding RNAs, with >200 nucleotides (Policarpo et al., 2021). In MSC-derived Evs, the lncRNA SNHG7 showed protective potential in a model of diabetic retinopathy (Cao et al., 2021).
In vivo Delivery of MSC-Derived Evs
Treatment with Evs can be performed by either local or systemic delivery, depending on the target and the injury model. Because of their very small size, Evs can be easily systemically injected without the risk of embolism. BM-MSC-derived Evs can accumulate in the infarcted hemisphere after injection into the tail vein in rats in a stroke model (Moon et al., 2019). Homing of Evs to injured organs may be favored by their expression of chemokine receptors such as CXCR4 (Kim S. J. et al., 2012). Furthermore, Evs can cross the blood-brain barrier, although this mechanism is not fully understood (Matsumoto et al., 2017). Other organs can uptake Evs, as human MSC-derived Evs were found in liver, spleen, and BM 6 h after intravenous injection (Wen et al., 2019). MSC-derived Evs injected intraocularly (Mead and Tomarev, 2017) or systemically (Seyedrazizadeh et al., 2020) can protect retinal ganglion cells. Recently it was shown that endometrial MSC-derived Evs were neuroprotective in a hippocampal injury model after intranasal administration (Leon-Moreno et al., 2020). Intranasally administered human BM-MSC-derived Evs can reach the intact or injured forebrain (Kodali et al., 2019). Systemic or intranasal administration of AT or BM-MSC-derived Evs protect neurons and decrease the memory deficit in mice with AD (Leon-Moreno et al., 2020; Losurdo et al., 2020). Furthermore, Evs from BM, as well as UC-MSCs inhibit apoptosis and improve functional recovery in rodents subjected to brain ischemia (Han et al., 2020; Seifali et al., 2020; Xin et al., 2020). BM-MSC-derived Evs systemically injected in rats subjected to subarachnoid hemorrhage protected neurons from apoptosis (Gao et al., 2020).
MSC Modifications
MSC Preconditioning
Pretreatment With Hypoxic Conditions
In order to enhance the therapeutic potential of MSCs, to improve their proliferative and survival rate, which is important considering the need for a large number of cells for transplants, or to improve the secretion of factors related to neuroprotection or reduction of inflammation, studies have evaluated the effects of pre-treatment of MSCs, using different approaches (Omid Sadatpoor et al., 2020).
In recent decades, studies have evaluated the effects of hypoxic conditions on the biological characteristics of MSCs. Oxygen concentration is an important factor in the function of stem cells (Simon and Keith, 2008). An atmosphere of 1%–5% O2 shows effects on MSCs compared to normoxic conditions (20%) in relation to proliferation, differentiation, migration capacity, and metabolism (Grayson et al., 2006; Potier et al., 2007; Rosová et al., 2008; Hung et al., 2012; Ejtehadifar et al., 2015; Kakudo et al., 2015; Elabd et al., 2018). In a model of intracerebral hemorrhage, olfactory mucosa MSCs under hypoxia alleviated cellular senescence, with a 1.2-fold decrease in the percentage of β-galactosidase-positive cells compared to cells under normoxia. Additionally, an enhancement of autophagy promoted by the increase in miR-326 expression could be seen in MSCs under hypoxia (Liu J. et al., 2021). Moreover, when cultured under 5% O2, significant increases in proliferation of MSCs at day 5 and in the expression levels of markers such as C-Myc and Nestin were observed. A reduction in the number of β-galactosidase-positive cells was observed in all MSCs under hypoxia compared to those in normoxia, demonstrating that hypoxic conditions affect not only the proliferative potential of MSCs but also the senescence process, which could interfere in the biological activity of the cells, thus preventing the desired effects after transplantation (Kwon et al., 2017). In another study, an atmosphere of 2% O2 affected the paracrine effects of aged BM-MSCs in an in vitro model of ischemic stroke. The conditioned medium of aged BM-MSCs under hypoxia showed an increase in VEGF levels from 2 to 5–3 to 6 ng/ml, demonstrating that the treatment was able to achieve the therapeutic level of above 1 ng/ml. Therefore, hypoxia treatment of MSCs seems to contribute to the neuroprotective effects of these cells after ischemic stroke. Interestingly, the cells used in the study were from patients more than 70 years old, which indicates that the treatment could also contribute to restoring the beneficial effects of cells derived from elderly donors since the age of the donors affects the biological characteristics of MSCs (Zhang et al., 2019a).
Pretreatment With Growth Factor and Cytokines
Preconditioning with growth factors, cytokines, and other biomolecules has been used to simulate the target microenvironment of MSCs after transplantation, in order to promote the prior adaptation of cells and secretion of important molecules (Hu and Li, 2018).
The immunomodulatory activity of MSCs is widely discussed as one of the most prominent roles of these cells. However, other than being beneficial to modulate the inflammation site, the secretion of growth factors and cytokines also has an autocrine effect on MSC behavior, interfering with their proliferation and differentiation (Eom et al., 2014). As a strategy to enhance those autocrine effects, it is possible to manipulate MSC behavior in vitro by adding certain growth factors and cytokines to the culture, increasing cell survival, migration, and cytokine secretion (Liu et al., 2011). Bone morphogenetic protein (BMP)-3, fibroblast growth factor (FGF), vascular endothelial growth factor (VEGF), and epidermal growth factor (EGF) are the best factors to pretreat the MSCs to increase cell proliferation and survival (Rodrigues et al., 2010). Other than optimizing culture conditions, growth factors and cytokines can be used to induce MSC differentiation toward neural identity in vitro; some studies have demonstrated transdifferentiation of MSCs into neural progenitor cells, using EGF and bFGF together with neural induction factors such as N-2 and B-27 (Peng et al., 2019).
Another study using an in vitro model of LPS-induced neuroinflammation managed to reduce the secretion of IL-6 and TNF-α by 35% and 41%, respectively, in addition to a 13-fold increase in the secretion of IL-10 by microglial cell line BV2 after incubation in conditioned medium of MSCs preconditioned with the cytokine IL-1. This anti-inflammatory effect was related to the increased secretion of G-CSF in the supernatant of the MSCs against the pro-inflammatory environment generated by preconditioning with IL-1 (Redondo-Castro et al., 2017). Likewise, in the model of periventricular leukomalacia induced in neonatal rats, treatment with human UC-MSCs preconditioned with IFN-γ increased the preservation of tissue myelin basic protein (MBP) by about 18%, while treatment with control MSCs reached only 2.5% protein preservation, both compared to the untreated group. Genetic analysis of cells after preconditioning revealed an increase in the expression of TGS-6 and IDO, proteins with anti-inflammatory functions, which may explain the results (Morioka et al., 2017).
In a more targeted method, MSCs conditioned with serum from stroke victims were more effective in promoting functional gains and reducing the Modified Neurological Severity Score (mNSS) of animals with induced ischemic stroke, compared with groups treated with cells stimulated by serum from healthy donors or FBS. Analysis of the gene expression of the treated cells showed an increase in the expression of 88 proteins in relation to the controls, including important factors related to cellular communication and signal transduction, such as FGF, VEGF, EGF, BNDF, and MMP-9 (Moon et al., 2018). Preconditioning of MSCs with TGF-β was able to increase homing of MSCs carrying a lethal gene to treat a model of glioblastoma in mice. Conditioning with TGF-β increased expression of the CXCR4 receptor in MSCs, resulting in an increase in the efficiency of these cells in delivering the therapeutic gene, and consequently a 50% reduction of the tumor volume in relation to the group treated with unstimulated MSCs (Li et al., 2019). In a model of multiple sclerosis in mice, treatment with preconditioned MSCs with SDF-1α also promoted an increase in CXCR4 expression in transplanted cells (about six times higher than the levels expressed by unconditioned MSCs), which led to a significant reduction in apoptosis of these cells, thus promoting greater myelination and improving the spatial learning and memory of treated animals compared to control groups (Beigi Boroujeni et al., 2020). Taken together, these data suggest that preconditioning with cytokines and growth factors is an effective method to promote increased expression of receptors and molecules of interest in MSCs, which can improve their therapeutic potential for different neurological diseases.
Pretreatment With Pharmacological Agents and Bioactive Molecules
Despite the recognized potential of MSCs to promote neuroprotection and tissue repair in CNS lesions and diseases via biomolecule secretion (Uccelli et al., 2011), some extrinsic factors, such as the source of achievement, donor characteristics, and culture conditions can interfere with their secretory activity (Hagmann et al., 2013; Elahi et al., 2016; Heathman et al., 2016). Therefore, in vitro preconditioning of MSCs with a variety of active substances, ranging from inorganic compounds to pharmacological agents, has been explored to increase or standardize the therapeutic potential of MSCs.
Preconditioning with pharmacological agents has been used to optimize the secretory profile of MSCs for application in different models. Linares et al. (2016) investigated the effect of preconditioning BM-MSCs with a combination of mood-stabilizing drugs, lithium chloride, and valproic acid, for the treatment of a Huntington’s disease model in rats. This preconditioning resulted in an increase in the expression of genes involved with trophic effects, stress response, antioxidants, and anti-apoptosis, among others, which contributed to an increase of approximately 60% in the survival of the treated cells after the graft, compared to unconditioned MSCs, and contributed to a better functional performance for the group treated with preconditioned cells. In another study, the conditioned medium of AT-MSCs preconditioned with the iron chelator deferoxamine (DFX) was effective in promoting neuroprotection and apoptosis reduction in a culture of DRG neurons challenged by hyperglycemic insult. The reduced rate of apoptosis (1.6% for preconditioned MSCs vs. 3.5% for control MSCs) was related to increased expression of genes involved in neuroprotection, such as GDNF, NGF, and NT3 (Oses et al., 2017). The functional performance of rats in a stroke model due to middle cerebral artery occlusion (MCAO) increased after treatment with BM-MSCs that were preconditioned with sodium hydrosulfide (NaHS). This result was accompanied by a reduction of approximately 13.5% of the area affected by the infarction after treatment with MSCs induced with NaHS compared to the untreated group, due to the increased expression and secretion of VEGF and BDNF in MSCs (Zhang et al., 2016).
MSCs in Three-Dimensional Cultures
An innovation of tissue engineering, a three-dimensional culture of MSCs such as spheroid culture or 3D culture using biomaterials such as scaffolds and hydrogels may confer improvements in MSC properties compared to two-dimensional culture methods.
3D MSC cultures show increased rates of cell proliferation, viability, and survival compared to 2D cultures (Baraniak and McDevitt, 2012; Alimperti et al., 2014; Murphy et al., 2014), in addition to maintaining their multilineage differentiation potential, favoring their use in cartilage and bone-repair models (Murphy et al., 2014; Occhetta et al., 2015; Yan and Wu, 2020). On the other hand, in the case of 3D MSC cultures with biomaterials, physical characteristics related to the surrounding environment, such as topography, surface tension, and matrix rigidity are able to define the degree of differentiation and the fate of MSCs, without the need for additional biochemical signals. For example, the use of soft, elastic, or rigid matrices can direct MSC differentiation into neural cells, myocytes, or osteoblasts, respectively (Wang et al., 2010; Wen et al., 2014).
The immunomodulatory properties of MSC are also regulated by 3D culture. Several studies using cultures of MSC spheroids derived from different sources demonstrated that this configuration was more effective in promoting the secretion of immunosuppressive factors such as PGE2, TSG-6, and STC-1 (Bartosh et al., 2013), in addition to decreasing the proliferation of immune cells (Miceli et al., 2019) and inducing macrophage polarization for an anti-inflammatory profile (Ylöstalo et al., 2012; Vallés et al., 2015). Similar results were obtained from 3D MSC cultures in hydrogels, with increased secretion of PGE2 and TSG-6, in addition to HGF (Papadimitropoulos et al., 2014; Follin et al., 2015).
MSC spheroids also showed advantages compared to two-dimensional culture, in the secretion of angiogenic factors such as HGF, PDGF, TGF-β, VEGF, FGF1, GRO-α, SDF-1, and EGF, and were able to induce increases in tube formation and capillary maturity (Park et al., 2014). The secretome of MSC grown in scaffolds was more efficient in promoting healing in in vitro models of corneal wounds, compared to the secretome obtained from a 2D culture. This result was related to higher levels of HGF and ICAM-1 present in the secretome of a 3D culture compared to the concentrations produced by the monolayer (Carter et al., 2019). The difficulty of gas exchange and the gradient of cytokines created within the 3D cultures are related to the increased expression of HIF-1 and HGF, explaining their greater regenerative potential (Bhang et al., 2011).
Genetically Modified MSCs
As discussed above, many of the regenerative and protective effects evoked by MSC therapy in CNS are due to a paracrine action, mediated by trophic factors, cytokines, and other molecules secreted by these cells. Genetic engineering of MSCs to overexpress and/or secrete specific molecules of interest in the damaged tissue is a valuable tool to enhance their therapeutic potential (Nolta, 2016). An increase in the efficacy of MSC therapy also implies a reduction in the number of cells required per dose and consequently lowers the cost of large-scale cell production. Several virus-based approaches have been used for genetic engineering of MSCs, including retrovirus, lentivirus, and adenovirus, each of which has advantages and limitations. Recently, an efficient non-viral process using a cationic polymer for MSC programming has been described (Ho et al., 2020). Other approaches, such as plasmid transfection, can also be used to modify MSCs, but viral vectors are still the most widely used tool for genetic engineering of MSCs in preclinical trials, as in the majority of studies described in this section. Therefore, numerous MSC lines overexpressing several trophic factors have been developed and tested in models of neurological diseases in recent years (for a detailed review of engineered MSCs, different modification methods, and applications in regenerative medicine, see Damasceno et al., 2020). Neurotrophic factors, such as nerve growth factor (NGF), brain-derived neurotrophic factor (BDNF), and neurotrophin 3 (NT-3) and 4 (NT-4), are a family of soluble proteins involved in a plethora of functions but mainly related to neuronal survival. Mouse BM-MSCs overexpressing BDNF were tested in a model of chronic retinal degeneration. Intravitreal injection of BDNF-MSCs promoted a reduction of apoptotic markers in the retina, resulting in long-term neuroprotection of photoreceptors and better functional outcomes in electroretinography analysis. In all parameters, BDNF-MSCs induced better results than non-modified MSCs, suggesting that genetic engineering improved the therapeutic efficacy of the MSCs (Lejkowska et al., 2019). Intravitreal injection of erythropoietin-expressing MSCs also increased photoreceptor neuroprotection in a model of sodium iodate-induced retinal degeneration, as shown by electroretinography (Koh et al., 2021). Neuroinflammation is another key aspect of several neurodegenerative conditions. Intracerebral injection of BM-MSCs overexpressing interleukin IL-13 in cuprizone-induced multiple-sclerosis mice induces anti-inflammatory microglial activation and reduces demyelination and oligodendrocyte loss, effects not achieved with naïve MSC therapy (Le Blon et al., 2013).
An important aspect regarding the efficacy of cell therapy is that the MSC secretome can be directly modulated by the often-unfavorable host environment (Wang et al., 2014). Therefore, genetic modification can also be used to improve MSC survival, homing, and grafting after transplantation. In a mouse model of acute spinal cord injury, BM-MSCs overexpressing human insulin-like growth factor 1 (hIGF1-MSCs) have increased survival after transplantation in relation to naïve MSCs. Therapy with hIGF1-MSCs reduced demyelination and promoted more-robust functional recovery than naïve MSCs (Allahdadi et al., 2019). Human MSCs overexpressing fibroblast growth factor (FGF) by plasmid transfection also induced motor improvement in a spinal cord-injury model; the proposed mechanism of this effect was through increased differentiation of endogenous neural stem cells (Huang F. et al., 2021). Although systemic circulation and interaction with other cell types can be important for the activation of MSCs, an increase in the number of cells that reach and remain in the injury site can result in optimized therapeutic effects. Several reports in murine models have indicated that a few hours after systemic transplantation, MSCs tend to accumulate in the lungs, and very few cells reach the brain or the spinal cord (Dos Santos Ramalho et al., 2019; Mello et al., 2020). MSCs overexpressing FGF increased their ability to migrate toward the lesion site after intracerebroventricular injection in a mouse model of traumatic brain injury, although the study did not assess differences in functional outcomes (Shahror et al., 2019). Although overexpression of integrin α4, a protein related to transendothelial migration, did not increase homing of rat MSCs in a model of stroke, it reduced cell aggregation and cerebral embolism, increasing the safety of therapy (Cui et al., 2017). Human UC-MSCs overexpressing the chemokine CCL2 by plasmid transfection showed increased migration to the injury site in rats submitted to middle cerebral arterial occlusion, a classic model of stroke. Moreover, in the acute phase of lesion, CCL2-MSC-treated animals showed a significant reduction in lesion volume and improved functional outcome in comparison to subjects treated with non-modified MSCs (Lee et al., 2020). Similarly, a previous study using the same model showed that MSCs overexpressing CCR2, the CCL2 receptor, had increased migration rates to the injury site and induced improvement in behavioral tests, due to the preservation of the blood-brain barrier integrity (Huang et al., 2018). Huang Y. et al. (2021) recently showed that small interfering RNA-mediated ablation of CUEDC2, a novel protein related to cancer and oxidative stress, in rat MSCs increased the efficacy of cell therapy in reducing the infarcted area after stroke induction. Finally, human MSCs that have been genetically modified are already being tested in clinical trials, and some of these studies are discussed in this review.
MSC Therapy in Neurological Diseases
In the following sections, we briefly discuss the most recent studies regarding the safety, efficacy, and mechanism of action of MSC-based therapy in several neurological conditions, including amyotrophic lateral sclerosis, glaucoma, stroke, spinal cord injury, and autism. Most of the preclinical data described here were obtained from tests on animals, usually rats and mice, submitted to allogeneic or xenogeneic transplantation of human MSCs. Lesioned and transgenic rodent models have been essential not only for understanding the etiology and pathophysiology of these diseases, but also for evaluating the efficacy of different therapeutic approaches regarding MSC source, dosage, delivery route, and timing, as well as the possible adverse effects, providing remarkable insight into clinical translation of MSC transplantation to human patients.
Amyotrophic Lateral Sclerosis
Amyotrophic lateral sclerosis (ALS) is a neurodegenerative disease that mainly affects the motor neurons (MN), leading to progressive muscle atrophy, paralysis, and death, usually 3–5 years after onset. The incidence of ALS is relatively constant worldwide, with a rate of 1–2 cases per 100,000 inhabitants (Brown and Al-Chalabi, 2017). Most ALS cases are idiopathic, termed sporadic ALS. Approximately 5%–10% of ALS cases are familial. Variations have been observed in approximately 120 genes that are associated with a risk of ALS, such as C9ORF72, SOD1, TARDBP, FUS, and VAPB, among others (Brown and Al-Chalabi, 2017). The discovery of genes associated with familial ALS allowed the development of animal models to study ALS (Philips and Rothstein, 2015), and more recently, the generation of patient-induced pluripotent cells has also contributed to understanding the diversity and complexity of ALS (Vasques et al., 2020).
It is not known exactly what process triggers the degeneration of MN. ALS is considered a multifactorial disease, where different mechanisms seem to be involved in its development. Some of the proposed hypotheses include protein aggregation, glutamatergic excitotoxicity, glial cell toxicity, neuroinflammation, oxidative stress, mitochondrial dysfunction, lack of growth factors, and dysfunction in axonal transport, among others (Mejzini et al., 2019).
Currently, there are no effective treatments for ALS. Many groups have been testing cell therapy as an alternative, especially with MSCs. Different routes of administration, doses, and injection times have been tested. BM is the main source of MSCs tested in animal models of ALS. These cells, derived from humans or rodents, have shown promising results, delaying disease progression and increasing animal survival (Zhao et al., 2007; Zhang et al., 2009; Kim et al., 2010; Forostyak et al., 2011, 2014; Uccelli et al., 2012; Zhou et al., 2013; Řehořová et al., 2019). As an alternative, some groups have tested BM mononuclear cells (BMMCs), a heterogeneous population of cells that contain a small percentage of both MSCs and hematopoietic stem cells. Preclinical studies using BMMCs have shown modest positive results; in some cases, the treatment delayed disease progression but with no change in survival (Gubert et al., 2016, 2019; Martínez-Muriana et al., 2020). Other sources of MSCs, such as AT and UC, have also been tested in ALS models (Marconi et al., 2013; Kook et al., 2020; Ciervo et al., 2021). Both showed some positive results, although more studies using these sources are important to compare the efficacy of these sources.
Although most preclinical studies using MSCs for ALS have shown positive results, this appears to vary according to the dose and injection site. Habisch and co-workers (2007) showed that 105 human BM-MSCs injected intrathecally in SOD1-G93A mice had no effect on animal survival and delayed disease progression only in females (Habisch et al., 2007). Zhou and co-workers, using the same animal lineage, showed that a single intrathecal injection with a dose 5× higher of human BM-MSCs had a modest effect on motor performance and survival, but multiple injections resulted in a positive outcome (Zhang et al., 2009; Zhou et al., 2013). Testing different doses (104, 2 × 105, or 106), Kim et al. (2010) showed that only the higher doses produced a positive outcome, especially 106 human BM-MSCs. After intrathecal injections, few or no cells were found in the regions most affected by the disease (Zhang et al., 2009; Forostyak et al., 2014). Despite that, using multiple injections, intrathecal cell therapy modulates neuroinflammation, as observed by the reduction of microgliosis and astrogliosis as well as decreased expression of iNOS and TNF-α (Zhou et al., 2013).
As an alternative to the intrathecal route, some groups have tested intraventricular injections. However, this route has not shown promising results. Transplantation of 2.5 × 105 UC-MSCs protected MN and decreased neuroinflammation but had no effect on disease progression and animal survival (Sironi et al., 2017). In addition, lateral ventricle injection of human BM-MSCs had a negative effect on both motor performance and survival (Bursch et al., 2019). The authors attributed these results to an increase in microgliosis.
In order to directly access the site of MN degeneration, some groups have tested intraspinal injections. Vercelli and co-workers showed that 105 human BM-MSCs injected into the lumbar spinal cord slowed disease progression (Vercelli et al., 2008). Using the same route, our group showed that BMMCs could also affect motor performance, but only when the cells were administered in the pre-symptomatic phase (Gubert et al., 2016). In agreement with these results, Bursch and coworkers showed an effect on disease progression but with no change in animal survival after repeated intraspinal injections of human BM-MSCs (Bursch et al., 2019).
In ALS, muscle atrophy results from the loss of neuromuscular junctions (NMJ). Considering that it is necessary not only to protect the motor neurons but to keep them functional, some groups have tested intramuscular injections of MSCs. Intramuscular injections of MSCs overexpressing GDNF for three consecutive weeks preserved NMJ, slowed disease progression, and increased animal survival (Suzuki et al., 2008). Recently, Kook et al. (2020) showed that repeated intramuscular injections (gastrocnemius muscle) performed once a week from the 12th week of life also had positive effects on motor performance and survival. They attributed these results to modulation of the iNOS pathway and a reduction in levels of intracellular reactive oxygen species (ROS) in the muscle (Kook et al., 2020).
In ALS, the immune system has been increasingly studied. Many research groups have tested intravenous injection as an alternative route. In addition to being a less invasive and easy to administer route, studies using this approach have shown positive results, such as a reduction in disease progression and an increase in animal survival (Zhang et al., 2007; Uccelli et al., 2012). Interestingly, these effects were observed even when the cells were administered after the onset of symptoms. As mechanisms, the authors observed a reduction in the numbers of microglia and astrocytes, as well as a reduction of IL-β and TNF-α expression (Uccelli et al., 2012).
Each of the routes mentioned above could affect different components of ALS. Therefore, many groups have proposed combining routes of administration to achieve a better outcome. Forostyak and co-workers reported positive effects on disease progression and survival after intravenous and intraspinal administrations at the onset of the disease (Forostyak et al., 2011). The outcome improved when intrathecal and intramuscular injections were combined, rather than when using the routes individually (Řehořová et al., 2019). The injections were administered three times, at onset and after 14 and 28 days. The authors reported neuroprotection, modulation in necroptosis pathways, and reduction of NF-κB and TNF-α expression.
Clinical trials with MSCs in ALS patients are underway. Most of the groups have used autologous MSCs and demonstrated that these cells are safe (detailed information regarding MSC-based clinical trials in ALS in recent years is summarized in Table 1). A meta-analysis study analyzing data for 152 patients treated with MSCs indicated a transitory effect of these cells after intrathecal administration (Morata-Tarifa et al., 2021). One study showed that patients with ALS who received intrathecal autologous transplants could be divided into two groups: responsive and non-responsive to treatment. In the responsive group, MSCs had higher VEGF expression than MSCs in the non-responsive group (Kim et al., 2014). This demonstrates that there are differences between MSCs, justifying the search for a source that can generate more-homogeneous cells with a higher capacity to express trophic factors and cytokines. In line with this idea, a randomized placebo-controlled phase 3 test was performed with about 189 patients. Autologous BM-MSCs modified to secrete higher levels of neurotrophic factors (NurOwn®) were injected intrathecally three times (at 8-week intervals). The therapy was safe and, although it did not reach the designated primary end-point, analysis of a subgroup of patients that were at a less severe stage of the disease revealed a significant preservation of function compared to the placebo (Cudkowicz et al., 2022). Therefore, MSC therapy for ALS seems promising, but considering the enormous heterogeneity of the patients, larger clinical trials are needed.
Glaucoma and Retinal Lesions
Vision impairments affect the economy and quality of life and even increase the risk of death. It is estimated that 2.2 billion people worldwide have some visual impairment, and approximately 36 million of them are blind (Bourne et al., 2017; Williams et al., 2020). Cataract is the most common cause of blindness but is reversible in most cases (Williams et al., 2020). The leading cause of irreversible blindness, glaucoma, affects about 64 million people worldwide, of whom 6.9 million have some vision impairment or are blind (Williams et al., 2020). Glaucoma is characterized by degeneration of the optic nerve and death of retinal ganglion cells (RGCs), leading to progressive loss of vision. RGCs die mostly by apoptosis and, like other neurons, cannot be replaced. Furthermore, until now no clinical treatment exists to sustain RGC survival and promote the regeneration of axons to their central targets. Cell therapy is an alternative approach that has yielded promising results. BM cells were shown to provide long-term protection and RGC regeneration and synaptic reconnection in the superior colliculus in an optic-nerve crush model (Zaverucha-do-Valle et al., 2011, 2014; Mesentier-Louro et al., 2014, 2019). These were the first studies to report RGC reconnection resulting from cell therapy. Moreover, BM-MSCs were also shown to be neuroprotective in models of ocular hypertension (Emre et al., 2015; Mead et al., 2016). Although UC-MSCs were reported to have neuroprotective effects on RGCs subjected to an optic-nerve injury (Nascimento-Dos-Santos et al., 2019; Wang Y. et al., 2021), the effect was transient. In contrast, da Silva-Junior et al. (2021) recently reported that human WJ-MSCs could protect RGCs for a relatively long period and promote axonal regeneration to brain targets after intravitreal injection in an optic-nerve crush model. Millán-Rivero and colleagues (2018) showed that human UC-derived-MSCs protected RGCs and some integrated into the retina (Millán-Rivero et al., 2018). The same was reported with BM-MSCs (Li et al., 2009). These findings contrast with other reports that MSCs injected intravitreally did not integrate into the rat retina (Hill et al., 2009), and even after 18 weeks post-injection, the majority of BM-MSCs remained in the vitreous body (Mesentier-Louro et al., 2014). Importantly, a recent study indicated that care with the type of transplantation of cells (syngeneic, allogeneic, or xenogeneic) may impact the results, since MSCs from different donor species can exert different effects (Norte-Munoz et al., 2021).
Diabetic retinopathy is a complication of diabetes that results in damage to retinal blood vessels, and is expected to affect about one-third of diabetics by 2045 (Williams et al., 2020). To date, diabetic retinopathy is treated by laser coagulation, anti-VEGF, or glucocorticoid drugs; however, these methods have serious side effects and do not ensure the preservation of vision (Gaddam et al., 2019). Systemic treatment with BM-MSCs proved to be safe in patients with diabetic retinopathy (Gu et al., 2018). Intravitreally injected BM-MSCs integrated into the inner retina and improved the electrophysiological retinal function of the retina in rats in a model of diabetic retinopathy (Çerman et al., 2016). Systemic or local injection of UC-MSCs attenuated angiogenesis and inflammation in the retina in a model of diabetic retinopathy (Yu et al., 2020; Zhao et al., 2020). Overexpression of LIF in MSCs was shown to increase the neuroprotective effect in diabetic retinopathy (Chen et al., 2021).
Optic neuritis is an inflammatory demyelinating disorder of the optic nerve, often related to multiple sclerosis (Redler and Levy, 2020). Intraperitoneal injection of human BM-MSCs decreased RGC death and improved their function in a mouse model of multiple sclerosis (Gramlich et al., 2020). BM-MSCs administered intravenously proved to be safe for patients with neuromyelitis spectrum disorder and improved neurological disability, with recovery in neural structures in the optic nerve and retina for up 2 years after treatment (Fu et al., 2016). Another study reported positive effects after follow up for 18 months of treatment with UC-MSCs in patients with neuromyelitis optica (Lu et al., 2012).
Many clinical trials with MSCs for optical disease are registered on ClinicalTrials.org (Table 2). Studies using MSCs have reported safety and effectiveness in the treatment of patients with optic-nerve injuries (Kahraman and Oner, 2020; Sung et al., 2020). One study investigated 29 patients with optic atrophy, who were followed up to 1 year after suprachoroid UC-MSC implantation and showed improvement in visual parameters (Kahraman and Oner, 2020). Intravitreal injection of BM-MSCs in patients with retinitis pigmentosa resulted in visual improvement (Weiss and Levy, 2018; Tuekprakhon et al., 2021). Nevertheless, there is much concern about the clinical use of MSCs, since some reports described visual impairment in patients after intraocular injection of BM-MSCs (Kasetty et al., 2020). However, MSC-derived Evs are an alternative to avoid the presence of cells in the vitreous body. Mathew et al. (2021) reported the distribution of Evs on the retina in vitro and in vivo. MSC-derived Evs were rapidly cleared from the vitreous body and endocytosed by astrocytes, microglia, and neurons in a dose-dependent manner (Mathew et al., 2021). Evs from embryonic MSCs were shown to protect RGCs for at least 60 days and promote regeneration in an optic-nerve crush model (Seyedrazizadeh et al., 2020). MSC-derived Evs were shown to be neuroprotective in in vitro models of diabetic retinopathy (Beltramo et al., 2014). Evs were also reported to protect RGCs in a model of optic-nerve injury (Mead et al., 2016; Pan et al., 2019). In contrast, the angiogenic potential of MSC-derived Evs was reported to induce diabetes-like features of diabetic retinopathy in vitro (Beltramo et al., 2014). However, Evs have been shown to have protective effects on the retina in models of diabetic retinopathy (Fu et al., 2021; Li W. et al., 2021; Xu et al., 2021). Therefore, it is necessary to investigate which Ev components are undesirable for this treatment and if it is possible to direct MSCs to deliver Evs with appropriate molecules to enhance their effect.
Stroke
Stroke is the second leading cause of death and the main cause of long-term disability, significantly reducing the patient’s quality of life and representing an important economic burden. Ischemic stroke is characterized by an interruption in blood flow to the brain, generally due to a blood clot, causing oxygen and glucose deprivation. The affected brain tissue can be divided into the core area, where neural death occurs by necrosis, and the penumbra area, in which neural cells are metabolically impaired but still able to recover if the blood flow is rapidly restored. Ischemic strokes are the most prevalent, accounting for 85% of cases, and the mortality rate is around 15% (Ganesh et al., 2016). The current FDA-approved treatment is the thrombolytic drug tissue plasminogen activator if administered within 4.5 h after onset of symptoms. After this time window, surgical removal of the clot is the remaining option. In hemorrhagic stroke, a vessel rupture allows extravasation of blood to the brain parenchyma, subarachnoid or intraventricular spaces. This bleed causes a primary lesion, inducing death of neural cells due to oxygen and glucose deprivation and mechanical pressure from blood. Blood metabolites trigger a secondary inflammatory response, contributing to an increase in the initial injury (Zheng et al., 2016). Although less frequent, the hemorrhagic-stroke mortality rate is around 50%, comprising 60% of stroke-related deaths (Yang et al., 2017). To date, the treatment options available for hemorrhagic stroke are limited to cranial decompression surgery, indicated in only a few cases; blood-pressure control; and life-support measures (Fogarty Mack, 2014).
In recent years, several preclinical and clinical studies have evaluated the therapeutic potential of MSC therapy in ischemic and hemorrhagic stroke (for reviews fully dedicated to MSC therapy in stroke, see Turnbull et al., 2019 and Li J. et al., 2021). In a model of focal ischemic stroke induced by thermocoagulation of blood vessels, our group demonstrated that in the acute phase of the insult, only one-tenth of the dose of BM-MSCs is needed to induce the same sensory-motor recovery as bone marrow-derived mononuclear cell therapy (de Vasconcelos dos Santos et al., 2010). A commonly used animal model of ischemic stroke is the middle cerebral artery occlusion (MCAO). Rats submitted to MCAO and treated with 3 × 106 BM-MSCs injected systemically showed better results in neurological severity scores (NSS) 72 h after surgery, and lesion volume was also reduced in relation to the vehicle group. Interestingly, Nogo-A and its receptor, NgR, were downregulated in the ischemic core in the BM-MSC-treated group. Nogo-A inhibits central axon growth after lesion and inhibits neuronal repair after ischemia (Zhang J. et al., 2020). Intra-arterial injection of 5 × 105 rat BM-MSCs 24 h after MCAO also induced a reduction in the infarct area and improvement of motor function. BM-MSC-derived mitochondria were detected in host cells post-transplantation, which was correlated with an increase in angiogenesis in the peri-infarcted area (Liu et al., 2019). Using the MCAO model, a recent report demonstrated that rat BM-MSCs pretreated with IFN-γ (aMSCγ) injected intravenously in the acute phase of the lesion-induced a better outcome than naïve MSCs. Although both aMSCγ- and naïve MSC-treated groups displayed similar results regarding motor function improvement, NSS, lesion volume reduction, and microgliosis attenuation in relation to the vehicle group, aMSCγ therapy also induced the proliferation of neural progenitor cells and oligodendrogenesis in the ipsilateral subventricular zone (Tobin et al., 2020). BM-MSCs expressing the BDNF receptor TrkB (BM-MSC-TrkB) directly injected into the penumbra area 5 days after MCAO, in combination with electroacupuncture therapy, improved motor function in the rotarod test 15 days after the lesion. BM-MSC-TrkB had higher survival rates post-transplantation and increased migratory ability to the lesion site in relation to naïve MSCs (Ahn et al., 2019). BM-MSC transplantation also improved neurological outcomes and reduced the infarcted area after MCAO in spontaneously hypertensive rats (Liu et al., 2022). It has recently been proposed that the beneficial effects of BM-MSC therapy in rats submitted to MCAO could be related to the regulation of the gut microbiome (Zhao et al., 2021). In a chronic model of ischemic stroke, 8 weeks after MCAO induction, three weekly injections of MSCs promoted a better functional recovery than a single injection, an effect that seems to be correlated to increased preservation of corpus callosum fibers (Takemura et al., 2021).
Human-derived MSCs also exert important neuroprotective effects in rats submitted to MCAO. Stereotaxic injection of 1 × 107 human BM-MSCs into the cerebral cortex 3 days after injury-induced functional preservation in relation to the vehicle, up to 4 weeks post-lesion. Therapy also decreased the infarcted area and increased the expression of neural markers, such as βIII-Tubulin and GFAP (Xie et al., 2019). Combined therapy of human BM-MSCs with minocycline, a broad-spectrum antibiotic that inhibits microglial activation, resulted in better effects than isolated therapies. The group that received both therapies— 1.5 × 106 cells injected intravenously 72 h after MCAO and 5 g/ml minocycline injected intraperitoneally daily for 2 weeks post-surgery—had improved NSS results and better motor outcome 21 days after the lesion, as well as a reduced infarcted area (Cho and Jeun, 2018). Culture methods also can improve the efficacy of MSC therapy. Three-dimensional culture of human BM-MSCs increased the persistence of injected cells around the injury site and improved the functional outcome in rats submitted to MCAO in relation to a standard monolayer culture (Yuan et al., 2019). Donor age is another important determinant of the regenerative capacity of human MSCs. Human BM-MSCs from older donors secrete less BDNF in vitro than younger MSCs. Rats submitted to MCAO treated with younger human BM-MSCs showed better functional recovery than the group treated with older human BM-MSCs (Yamaguchi et al., 2018).
Our group has recently demonstrated that intravenous injection of 3 × 106 human WJ-MSCs reduced the hematoma volume in rats submitted to moderate intracerebral hemorrhage (ICH) by intrastriatal collagenase injection. Animals treated with human WJ-MSCs showed no significant functional difference in relation to the sham group in the elevated body swing test 8 days after lesion, differently from vehicle-treated animals, which displayed an increased percentage of swings to the contralateral side of the lesion (Mello et al., 2020). Human placenta-derived MSC therapy also reduced mortality from 50% to 8% in relation to the vehicle, when injected via tail vein in the acute phase of moderate collagenase-induced ICH. Hematoma volume was also significantly reduced in animals that received cell therapy, as well as brain edema, ventricular enlargement, and neuronal death (Choi et al., 2018). Similarly, rat AT-MSCs injected directly into the peri-lesioned area 48 h after collagenase injection in mice reduced the brain edema, improving the functional outcome. These positive effects were attributed to a reduction in apoptosis in the hematoma area and diminished expression of pro-inflammatory markers and aquaporin-4, a water channel found in astrocytes also associated with inflammation and edema formation (Zhang et al., 2019b). Intraventricular injection of BM-MSCs exerted similar neuroprotective effects in a rat model of ICH due to autologous blood injection: functional improvement along with reduced levels of IL-1α, IL-6, and IFN-γ (Huang et al., 2019).
MSC-derived products have already been tested in stroke models. Intranasal injection of human AT-MSC-derived Evs 24 h after a thermocoagulation ischemic lesion-induced a reduction in infarct volume and re-stabilization of vascularization in the peri-infarct area, culminating in behavioral improvement (Rohden et al., 2021). Recently, Zhao et al. (2020) showed that exosomes isolated from rat BM-MSCs overexpressing microRNA-223-3p injected into the tail vein 24 h and 14 days after MCAO induced an improvement in NSS, in relation to naïve-BM-MSC exosomes, but both groups were significantly different from the vehicle. The infarct area was also reduced in all exosome-treated groups, especially in microRNA-223-3p subjects. Interestingly, exosomes from rat BM-MSCs overexpressing microRNA-133b, related to functional recovery in spinal cord injury and cerebral ischemia, reduced apoptosis and the number of degenerating neurons in a rat model of hemorrhagic stroke (Shen et al., 2018). A previous study also indicated that knocking-down microRNA-133b in MSCs and their exosomes reduced the therapeutic potential of cell therapy after MCAO. Alternatively, MSCs overexpressing microRNA-133b induced a significant increase in functional recovery in relation to naïve MSCs, emphasizing the importance of this specific molecule in the beneficial effects of MSC therapy in ischemic stroke (Xin et al., 2013).
Several clinical trials using human MSCs in stroke have been conducted in recent years. In February 2022, 31 clinical trials using MSCs from different sources in stroke were registered at clinicaltrials.gov, most of them using ischemic patients. Several trials have shown that different doses of MSCs, injected through different delivery routes, are safe, and some have indicated a certain degree of efficacy (detailed information regarding active MSC-based clinical trials in stroke in recent years is summarized in Table 3). A phase I/II trial demonstrated the safety of intravenous transplantation of allogeneic BM-MSCs from a single donor, cultured under low oxygen (5%) conditions (ischemia-tolerant MSCs). The dose of 1.5 million cells/kg was used in 21 patients in phase II after it was considered safe in the prior phase in five individuals. The behavioral end points suggest significant neurological improvement after 12 months of follow-up (Levy et al., 2019). Different doses of allogeneic BM-MSCs genetically modified to express the Notch-1 receptor intracellular domain injected directly into the residual lesion area in chronic ischemic-stroke patients were also considered safe, although about 20% of the subjects showed adverse effects, more likely due to the surgical procedure than to the cell therapy itself. An improvement in clinical outcome after 12 and 24 months of follow-up, which correlated with a reduced lesion volume, was also suggested (Steinberg et al., 2016, 2018). Autologous BM-MSCs have been tested in a cohort of 16 subacute ischemic-stroke patients, where different cell doses, ranging from 10 × 107–30 × 107 and injected intravenously, were shown to be safe. An indication of functional improvement was detected (Jaillard et al., 2020). A recent post hoc analysis suggests that younger patient age and reduced time after the ischemic injury to start the therapy were the main factors related to lower limb recovery after autologous MSC transplantation in ischemic stroke patients (Chang et al., 2021). There is currently only one active trial using hemorrhagic-stroke patients. The purpose of this study is to evaluate the safety and feasibility of different doses of allogeneic BM-MSCs, injected intravenously or intraventricularly in the acute phase of hemorrhagic stroke. No results are presently available (NCT03371329). Therefore, despite the encouraging results described above, further studies are necessary to validate the efficacy of MSC therapy, especially in hemorrhagic stroke.
Spinal Cord Injury
Spinal cord injury (SCI) is a devastating neurological disorder that compromises sensory, motor, and autonomic functions, resulting in paraplegia or tetraplegia. The pathophysiology of SCI is temporally divided into acute (<48 h), subacute (2–14 days), intermediate (2 weeks to 6 months), and chronic (>6 months) phases and includes a primary injury that usually arises from compression, contusion, or transection of the spinal cord, and a secondary injury cascade that exacerbates the initial damage, causing even greater loss of neurological function (Ahuja et al., 2017). The current treatment for SCI includes surgical decompression, steroid administration, and rehabilitation therapies, which provide poor outcomes. In recent years, MSC transplantation has yielded encouraging results in preclinical and clinical research.
Most preclinical studies use MSCs derived from BM, AT, and UC in acute/subacute SCI models, while few studies have evaluated the efficacy of cell therapy in models of chronic SCI, which is more clinically relevant. Transplantation of BM-MSC sheets in acute and subacute SCI rat models reduced infiltration of inflammatory cells and the area of the injury lesion, promoted axonal regeneration, and attenuated glial scar formation, one of the major limiting factors to functional recovery after SCI (Okuda et al., 2017; Yamazaki et al., 2021). Basso, Beattie, and Bresnahan (BBB) scores showed improved locomotor function in sheet groups. Intravenously or intraperitoneally injected BM-MSCs were able to migrate toward the lesion site, where they survived up to 8 weeks and increased local expression of trophic factors such as BDNF, NGF, and NT3. Better tissue preservation with enhanced fiber sparing and/or regeneration was observed, in addition to the motor and sensory recovery (Ramalho et al., 2018; Dos Santos Ramalho et al., 2019). The benefits of BM-MSCs in acute SCI models were even greater when the cells were transplanted into biomaterial scaffolds. Better motor function recovery, neuroprotection, axonal regeneration, and reduced cavitation were observed 8 weeks after implantation of a nanofibrous silk-fibroin scaffold seeded with 5 × 105 BM-MSCs (Wang X. H. et al., 2021) or an NGF persistent-delivery scaffold seeded with 1 × 105 cells (Ji et al., 2021). Even better outcomes were achieved when BM-MSCs were differentiated in vitro into nerve cells prior to transfer onto scaffolds. In a chronic SCI model, intravenous infusion of BM-MSCs promoted neovascularization and improved the integrity of the blood-spinal cord barrier (BSCB). Axonal regeneration and remyelination process were observed, resulting in significant locomotor recovery (Morita et al., 2016).
AT-MSCs also exert positive effects on rats with SCI. AT-MSCs embedded in a fibrin matrix reduced microglial infiltration and astroglial activation as well as upregulated the expression of angiogenic and anti-apoptotic proteins, contributing to less cavitation, tissue retention, and motor-function recovery (Mukhamedshina et al., 2018). Human AT-MSCs transplanted into an acute compressive SCI model induced angiogenesis in the perilesional area and recruited resident pericytes through secretion of paracrine factors, improving BSCB integrity and contributing to the maturation of newly formed blood vessels. Revascularization reduced astrogliosis, promoted neuronal recovery, and correlated with functional improvement (Menezes et al., 2020).
The above-mentioned advantages regarding methods to obtain UC-MSCs and their properties have encouraged their use in the treatment of SCI. In different subacute SCI mouse models, injection of human UC-MSCs into the center of the injury area decreased the local levels of several proinflammatory cytokines such as TNF-α, IFN-γ, IL-6, and IL-7, in addition to promoting the transition of macrophages to the M2 type, attenuating the local inflammatory response and resulting in tissue repair and motor-function recovery (Bao et al., 2018; Wu et al., 2020). Differences in the ability to inhibit the inflammatory response between human UC-MSCs from different donors are determinants of their effectiveness in the recovery of mice after SCI (Zhu et al., 2022). Intrathecal transplantation of human UC-MSCs in rats with subacute SCI promoted regeneration and remyelination, as well as reduced astrogliosis, decreased cavity and glial scar formation, and supported functional recovery (Yang et al., 2020; Cao et al., 2022). The application of WJ-MSCs has also been promising. Intrathecal transplantation of WJ-MSCs following SCI improved neurological function in rats by inhibiting the inflammasome complex (Mohamadi et al., 2019), and their regenerative effect has been shown to be dose-dependent and potentiated by repeated application (Krupa et al., 2018).
In recent years, MSC-derived Evs have been extensively explored as a potential therapeutic strategy for SCI. Both MSC-derived exosomes and small extracellular vesicles are effective in promoting recovery of locomotor function in rodent SCI animal models, mainly by dampening the post-traumatic inflammatory process. The anti-inflammatory effect of BM-MSC-Evs has been shown to be mediated, through different mechanisms, by inhibition of the TLR4/NF-κB signaling pathway (Fan et al., 2021; Nie and Jiang, 2021). Additionally, MSC-Ev infusion promoted macrophage polarization toward the M2-phenotype (Chang et al., 2021; Nakazaki et al., 2021) and improved their phagocytic ability to clear myelin debris, creating a favorable environment for axonal regeneration (Sheng et al., 2021). Greater BSCB integrity was also observed, due to a reduction in pericyte pryoptosis, which was essential for tissue preservation and neuroprotection (Zhou et al., 2022).
Several clinical trials have supported the feasibility and safety of transplantation of MSCs in treating patients with SCI (Satti et al., 2016; Larocca et al., 2017; Albu et al., 2021). However, the trials have not well replicated the motor-function improvement reported in preclinical studies, showing efficacy mainly in sensory recovery. Intrathecal administration of autologous BM-MSCs in patients with SCI promoted variable improvement of sensitivity, motor and sexual functions, spasticity, sphincter control, and urodynamic parameters, regardless of the level or degree of injury (Vaquero et al., 2016, 2017, 2018). Recently, results from a phase 2 study supported the safety of intravenous infusion of autologous BM-MSCs performed in 13 patients approximately 50 days after SCI. The subjects showed improvements in neurological functions and quality of life 6 months after the intervention (Honmou et al., 2021). Combined intrathecal transplantation of these cells with autologous Schwann cells has also promoted improvements, especially in the sensation of bladder and rectal filling (Oraee-Yazdani et al., 2021). Few clinical studies have evaluated the efficacy of AT-MSCs in SCI treatment. Hur and collaborators performed autologous AT-MSC transplantation in patients with complete or incomplete SCI, but only mild improvements in neurological function were achieved (Hur et al., 2016). A preliminary report of an ongoing phase I study conducted at the Mayo Clinic (NCT03308565) using autologous AT-MSCs described promising outcomes and no adverse events (Bydon et al., 2020). Impressive results have been described regarding the efficacy of UC-MSC transplantation in patients with SCI. In a phase I trial with two patients with acute complete injuries, human UC-MSCs loaded on collagen scaffolds (NeuroRegen scaffolds) transplanted into the injury site promoted recovery of the bowel and bladder sensory function and significant motor improvement. Six months post-surgery, one patient started to walk voluntarily with the support of a brace and the other could raise his leg and move his toes (Xiao et al., 2018). The results of long-term follow-up of these and other enrolled subjects showed that three patients with an acute complete injury who received human UC-MSCs recovered the ability to walk and the remaining four patients had significant improvements in sensory and motor functions (Tang et al., 2022). Key completed and ongoing clinical trials of SCI using MSCs are summarized in Table 4.
Autism
Autism was first described in 1930 as a clinical disorder that affects social interaction and communication (Kanner, 1943). Currently, it comprises a series of disorders that are described as Autistic Spectrum Disorders (ASD). ASDs encompass a spectrum of neurological disorders related to an abnormal neurodevelopment process. These disorders affect individuals early in life, with clinical symptoms manifesting until the age of 5 years (Lord et al., 2006). The diagnosis, which is performed by clinical evaluation, follows international standards determined by the standards described in DSM-5 (Diagnostic and Statistical Manual of Mental Disorders) and takes into account deficiencies in social interaction, communication, limited interest, and repetitive behavior (Lord et al., 2006).
According to the Autism and Developmental Disabilities Monitoring (ADDM) Network and the Centers for Disease Control and Prevention (CDC), responsible for surveillance of ASD in the United States, about 1 in 54 children has an autism spectrum disorder, with an approximately fourfold higher incidence in males (Maenner et al., 2020). The number of patients diagnosed with autism has increased exponentially, which may be related both to an increase in the number of affected individuals and to an increase in previously undetermined diagnoses that are now included within the ASDs.
As ASDs include a diversity of disorders, there is wide heterogeneity both in the severity of symptoms and in the molecular mechanisms that lead to the development of ASD (Geschwind and Levitt, 2007). In most cases, ASDs are associated with genetic factors; more than 100 conditions have been directly associated with these disorders, with dozens of genes described as susceptibility factors (Betancur, 2011). The genetic changes described so far are related to transcriptional control, chromatin remodeling, protein synthesis, cell metabolism, function, and development of synapses (Thapar and Rutter, 2021). Several environmental risk factors are also involved in the development of ASD, and the complex interaction between genetic and environmental factors might determine the impact on neurodevelopment and the severity of neural-cognitive impairments. Among the physiological impairments, a critical one is neuroinflammation (Bilbo et al., 2018), and abnormal activation of microglia has been reported in the brain of ASD patients (Vargas et al., 2005; Morgan et al., 2012; Lee et al., 2017). While in a healthy environment, microglia are associated with synaptic organization and brain repair, when abnormally activated, microglia leads to inflammation and damage (Conti et al., 2020), as observed in degenerative neurological diseases such as Alzheimer’s (Wang et al., 2015) and Traumatic Brain Injury (Hernandez-Ontiveros et al., 2013). Other than microglia, immune system molecules such as TGFβ, IL-1, and LIF are important for brain development because they are directly involved in the normal formation of synaptic neural circuits and cell differentiation, and the imbalance in the expressions of these molecules may lead to devastating consequences (Deverman and Patterson, 2009).
Currently, none of the diseases within the spectrum has a cure; treatments include therapies to assist the interaction of these individuals with society, behavioral therapies, and the use of drugs to treat associated symptoms such as seizures (Eissa et al., 2018). However, the role of inflammation in some of the disorders in the autistic spectrum opens a possibility for the use of immunomodulation as a strategy for the development of new therapies (Marchezan et al., 2018). In light of this, MSCs emerge as a promising tool, since these cells have the ability to migrate to inflammation sites and modulate the immune response through the paracrine secretion of several anti-inflammatory, pro-survival, and neurotrophic factors, and are also able to engraft into the neural network (Siniscalco et al., 2018). Animal models available for studies of ASD include BTBR T+tf/J, a mouse strain that shows impairment in communication, deficits in social interaction, repetitive behaviors, and anxiety, mimicking the main symptoms observed in ASD patients (Chao et al., 2018). Studies with these mice suggested an improvement in neurogenesis, specifically in the hippocampal area, and in social behavior in animals treated with MSCs, where an amelioration of repetitive behavior, a decrease in cognitive rigidity, and in the autism severity composite score as a whole were observed (Segal-Gavish et al., 2016). Another study with MSCs pretreated with hbFGF, PDGF-AA, and Heregulin β1 to induce higher secretion of neurotrophic factors, also found an improvement in mouse social behavior, communication through vocalization, and reduction in repetitive behavior and cognitive rigidity (Perets et al., 2017).
MSCs were rapidly included in clinical studies of ASD. To date, three studies conducted in three different countries have been completed, and demonstrated the safety of using MSCs in ASD patients (Table 5). The preferred source of MSCs in the ASD clinical trials was UC since these cells are weakly immunogenic and powerfully immunosuppressive (Nagamura-Inoue and He, 2014). The first clinical study, published in 2013 (Lv et al., 2013) performed a trial using mononuclear cells and UC-MSCs. They separated 37 patients into three groups: 14 received only mononuclear cells, nine received both mononuclear and UC-MSCs, and 14 served as a control group; all groups received rehabilitation therapy. The study found functional and subjective improvements in patients of both groups treated with cells, assessed through the change in scores on the Childhood Autism Rating Scale (CARS) and Aberrant Behavior Checklist (ABC). In 2019, another group performed a phase I-II clinical trial using only UC-MSCs to treat ASD patients and evaluated the effects on cytokine levels (Riordan et al., 2019). Twenty participants (children around 10 years of age) received four intravenous infusions of 9 × 106 cells over the course of 1 week, three times (infusions in weeks 1, 25, and 37), and were followed for up to 89 weeks after the first injection. The investigators opted for multiple UC-MSC injections based on their clinical observations that the effect of MSC infusion decays 3–6 months after the cell administration. The authors observed this tendency even after multiple injections in a group of patients, who showed slight improvement but then regressed to the initial scores in CARS. Another set of patients, however, was able to sustain the improvement in test scores, in both behavioral (CARS) and cytokine scores [macrophage-derived chemokine (MDC) and thymus, and activation-regulated chemokine (TARC)]. However, the positive results for this small group were not statistically significant. Nevertheless, this study helped to support the safety of repeated, periodic administration of UC-MSCs in a long-term treatment in children with ASD (this article was retracted due to ethical/financial issues, but the clinical trial data are valid and available). The third and most recently published clinical trial with UC-MSCs in ASD is a phase I clinical study with cord-tissue mesenchymal stromal cells (hCT-MSC) administered intravenously, 2 × 106 cells/kg, to 12 young ASD children (2–11 years old; Sun et al., 2020). This study did not find an improvement in any behavioral parameter, but focused on the safety of the treatment, showing that HLA antibodies were generated against some of the cell lines, suggesting that it is important to evaluate if this could affect the treatment. Despite the presence of anti-HLA antibodies, the study demonstrated the safety of transplanting hCT-MSCs. This group has planned three additional clinical studies to investigate the effect of hCT-MSC transplantation in ASD adults (18–35 years of age; NCT04484077), toddlers (18–48 months; NCT04294290), and a larger study with 164 children (4–11 years), presently in the recruitment stage (NCT04089579).
Clinical trials have so far shown the safety of using MSCs in ASD patients, and a promising, but still incipient, the potential for improving the condition of the patients. Another aspect of these studies is the enormous quantity of cells that are necessarily specific for each patient, which could limit the widespread use of the therapy. Considering those aspects, new preclinical studies have shown that ASD individuals could benefit from the MSC effects without the need to manufacture such a large quantity of cells from different donors, or other issues such as immunogenicity and tumor formation, by using exosomes instead. Exosomes might be isolated from the expansion of a single donor MSC and used allogeneically to treat multiple patients (Mendt et al., 2019). The Perets group, who demonstrated the efficacy of BM-MSCs to improve autistic-like behavior in BTBR T+tf/J (BTBR) mice in 2017, has shown that administration of MSC-exosomes had similar effects, increasing male-to-male social interaction and reducing repetitive behaviors, as well as female vocalizations and maternal behaviors (Perets et al., 2018). Moreover, exosomes could be administered as a non-invasive intranasal treatment, loaded with gold nanoparticles that are able to cross the blood-brain barrier and preserve all their properties; this would make the treatment appealing commercially and clinically, especially considering the unstable behavior of ASD patients (Geffen et al., 2020; Perets et al., 2020). Independently, another group performed a similar experiment, with intranasal administration of exosomes, and demonstrated improvement in mouse social behavior; reduction of the pro-inflammatory cytokines IL-1b, IL-6, and TNFα; and increase of the anti-inflammatory cytokine IL-10 in the brains of treated mice, suggesting that exosomes have anti-inflammatory and neuroprotective roles in the autistic brain (Liang et al., 2020). Further studies are required to evaluate the efficacy of MSC exosome therapy and unravel the molecular pathways involved in the effector mechanisms.
Additional information regarding current MSC-based clinical trials in other neurological disorders such as multiple sclerosis, Alzheimer’s, Parkinson’s, and Huntington’s diseases is summarized in Table 6. All clinical trials reported here were accessed on clinicaltrial.gov between January and March 2022.
Quality Control of MSCs for Neurological Diseases
Quality control refers to examining whether the characteristics of a product meet a series of pre-specified criteria. Critical quality attributes such as differentiation, proliferation, genomic stability, sterility, and functionality depend on the cell type, sources of origin, and the purpose of the research. Quality controls for MSC culturing and release testing must be appropriately quantified and characterized, to ensure that the manufacturing process is robust and consistently produces MSCs with identical properties from one batch to the next.
With several clinical trials involving MSCs currently underway, there is a critical need to develop standards that can be applied to processing methods and to establish a consensus on assays for both MSC processing control and MSC product release.
Since the advent of clinical translation of MSCs, assays, and test protocols have been required by regulatory agencies to evaluate the sterility, safety, viability, identity, purity, stability, and potency of the cell product to be administered to patients. Additionally, GMP is concerned with both production and quality control. The U.S. Food and Drug Administration (FDA) and the European Medicines Agency (EMA) have similar requirements. As defined, GMP guidelines cover not only the actual physical process of making the cell product but also the quality assurance that the product is produced under conditions that are consistent, safe, and effective for their intended use.
In the United States, guidelines for cell-based therapeutics are regulated by the FDA (U. S. Department of Health and Human Services Food and Drug Administration, Center for Biologics Evaluation and Research, 2001) and are included in the drug manufacturing regulations as described in Title 21 of the Code of Federal Regulations (CFR), including the use of human tissue and cell products (21CFR1271). In Europe, the European Medicines Agency (EMA) produces the Guideline on Human Cell-based Medicinal Products (EMEA/CHMP/410869/2006—European Medicines Agency, 2008). The same approach has been adopted by MHRA in the UK (Medicines and Healthcare Products Regulatory Agency, 2015). According to these regulatory bodies, hMSCs should be characterized based on their most critical attributes, according to the legal requirements previously established by their guidelines and which are summarized in Table 7.
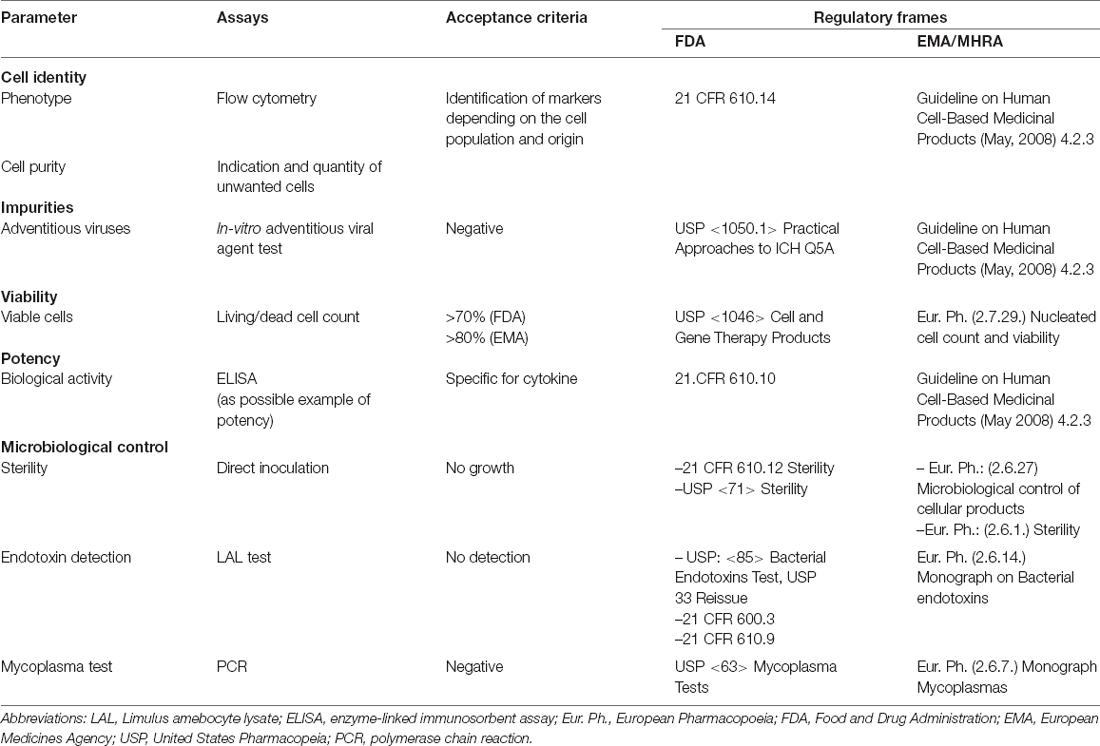
Table 7. Release specifications for hMSC-based products according to GMP standards of the two major regulatory authorities.
For each step in a process, whether it involves cell isolation or enrichment, in vitro culture, genetic modification, or final product fill-and-finish, the overall approach should be to reduce the risk of contamination of the product, establish documentation to verify that the entire process is correctly performed, and minimize variability in the process while maintaining the salient characteristics and function of the cells of interest. To demonstrate process control and monitor variability, assays should be developed to determine the cell phenotype, genotype, and/or function at the critical steps of the process. The final product should be tested for identity, safety (viral), purity, potency, sterility, endotoxin, and mycoplasma.
To date, one MSC-based product has been approved for neurological diseases. This is Lenzumestrocel (Neuronata-R® Inj), produced by Corestem Inc., a SouthKorean biotech company. Corestem launched Lenzumestrocel for amyotrophic lateral sclerosis, and the product was approved as an orphan drug for the treatment of ALS by the Ministry of Food and Drug Safety (MFDS) in South Korea in 2014. Lenzumestrocel is based on autologous BM-derived MSCs that are isolated and mixed with cerebrospinal fluid collected from the patient, to be administered as the final product by intrathecal injection. The first Lenzumestrocel injection takes place 4 weeks after the first bone-marrow extraction, followed by a second injection 4 weeks later. The proposed mechanism of action is based on several effects that can prevent motor-neuron death and slow the progression of ALS, such as an action on regulatory T lymphocytes, a neuroprotective effect by the expression of growth factors, and an anti-inflammatory effect due to microglial cells (Oh et al., 2015, 2018). More specifically, for Lenzumestrocel release, the manufacturers adopted the following criteria: viability (94%), sterility (0% contaminants), identity (CD29, CD44, CD73, CD105, CD34, and CD45), and as potency, the level of VEGF measured in pg per 104 cells (Oh et al., 2018). These criteria are not dissimilar to the requirements within the FDA and EMA regulatory frames.
For MSC-based products, cell sources and isolation processes vary widely, and it is best to address any concerns early in development. Among the available assays to characterize MSCs, cell phenotyping by multiparametric flow cytometry has been most often used to identify and enumerate cell subsets (Robb et al., 2019).
While a consensus on MSC identity has matured, it remains challenging to establish assays to measure and predict the mechanisms of action of MSCs in neurological disorders, the so-called potency assay. This is the measure of activity using a suitably quantitative biological assay and is based on the product attribute(s) associated with the relevant biological properties. A correlation between the expected clinical response and the activity in the biological assay should be established in pharmacodynamic or clinical studies (Rudge and Nims, 2017). While mandatory for more-advanced phase III studies, this is an aspect to consider during early clinical investigations (phase I/II).
The definition of potency particularly applied to MSCs for neurological diseases, is a critical aspect of quality testing (Bravery et al., 2013; Galipeau et al., 2016). Several aspects must be considered, starting from the likelihood that a single assay may not provide an adequate definition of MSC potency for neurological diseases where cells may have complex mechanisms of action. In addition, MSCs can have multiple active ingredients and/or multiple biological activities, which may be influenced by host-related microenvironmental signals. Finally, a single biological assay may not be quantitative or be insufficiently robust to define the properties of MSCs. Therefore, investigators should make efforts to translate the biological hypothesis, driving early research on assays that may quantify, for example, MSC pro-angiogenic, anti-apoptotic, or anti-inflammatory properties (Jiao et al., 2011) to be progressively validated within preclinical in vivo and clinical developments.
While protocols for quality testing of MSC products are now available, such as immunophenotyping, microbial sterility, endotoxin, and mycoplasma testing, and karyology, better-defined tests of potency and clinical efficacy are required. This is a concerning aspect in the cell-therapy field, calling for the development of international standards. This endeavor will not only benefit all discoveries related to MSC biology but will firmly establish the promising MSC therapeutic profile for still-lethal neurological diseases.
Conclusion
MSC-based cell therapies are among the strategies most often used for the treatment of neurological disorders in preclinical studies, because of the several advantages provided by these cells, such as an increase in cell survival and proliferation, neuroprotection and regeneration, immunomodulation, as well as a delay of disease progression in some cases. Additionally, the potential of MSCs could be enhanced by genetic modification of these cells, aiming toward overexpression of factors involved in cellular regeneration, or by pre-treatment of MSCs under different culture conditions. Besides, due to their secretory capacity, cell-free approaches using MSC-derived exosomes and extracellular vesicles emerge as another possible strategy. In addition, although some results are not entirely promising, clinical studies have also shown positive effects such as safety, tolerability, and functional improvements after transplantation of MSCs. However, further studies might aid in developing better strategies to obtain larger quantities of healthy cells for use in cell therapies and to reduce the variability of results due to the biological characteristics of MSCs.
Author Contributions
MS, MD, and RM-O conceived the main topic ideas and planned the manuscript. RG, JV, GLAS, FG, TS, AS-J, GS, and DS wrote subsections of the manuscript and prepared tables. RG edited the manuscript. JV prepared the figure. All authors contributed to the article and approved the submitted version.
Funding
This work was supported by grants and fellowships from the Departamento de Ciência e Tecnologia (DECIT/MS) do Ministério da Saúde, Conselho Nacional de Desenvolvimento Científico e Tecnológico (CNPq), Instituto Nacional de Ciência e Tecnologia em Medicina Regenerativa, Fundação de Amparo à Pesquisa do Estado do Rio de Janeiro (FAPERJ), Coordenação de Aperfeiçoamento de Pessoal de Nível Superior (CAPES).
Conflict of Interest
The authors declare that the research was conducted in the absence of any commercial or financial relationships that could be construed as a potential conflict of interest.
Publisher’s Note
All claims expressed in this article are solely those of the authors and do not necessarily represent those of their affiliated organizations, or those of the publisher, the editors and the reviewers. Any product that may be evaluated in this article, or claim that may be made by its manufacturer, is not guaranteed or endorsed by the publisher.
Acknowledgments
We thank Janet W. Reid (JWR Associates, New York, NY, USA) for revising the manuscript.
References
Ahn, S. M., Kim, Y. R., Shin, Y. I., Ha, K. T., Lee, S. Y., Shin, H. K., et al. (2019). Therapeutic potential of a combination of electroacupuncture and TrkB-expressing mesenchymal stem cells for ischemic stroke. Mol. Neurobiol. 56, 157–173. doi: 10.1007/s12035-018-1067-z
Ahuja, C. S., Wilson, J. R., Nori, S., Kotter, M. R. N., Druschel, C., Curt, A., et al. (2017). Traumatic spinal cord injury. Nat. Rev. Dis. Primers 3:17018. doi: 10.1038/nrdp.2017.18
Ai, Z., Cheng, C., Zhou, L., Yin, S., Wang, L., and Liu, Y. (2021). Bone marrow mesenchymal stem cells-derived extracellular vesicles carrying microRNA-221-3p protect against ischemic stroke via ATF3. Brain Res. Bull. 172, 220–228. doi: 10.1016/j.brainresbull.2021.04.022
Albu, S., Kumru, H., Coll, R., Vives, J., Vallés, M., Benito-Penalva, J., et al. (2021). Clinical effects of intrathecal administration of expanded Wharton jelly mesenchymal stromal cells in patients with chronic complete spinal cord injury: a randomized controlled study. Cytotherapy 24, S1465–S3249. doi: 10.1016/j.jcyt.2020.08.008
Alghwiri, A. A., Jamali, F., Aldughmi, M., Khalil, H., Al-Sharman, A., Alhattab, D., et al. (2020). The effect of stem cell therapy and comprehensive physical therapy in motor and non-motor symptoms in patients with multiple sclerosis: a comparative study. Medicine (Baltimore) 99:e21646. doi: 10.1097/MD.0000000000021646
Alimperti, S., Lei, P., Wen, Y., Tian, J., Campbell, A. M., and Andreadis, S. T. (2014). Serum-free spheroid suspension culture maintains mesenchymal stem cell proliferation and differentiation potential. Biotechnol. Prog. 30, 974–983. doi: 10.1002/btpr.1904
Allahdadi, K. J., de Santana, T. A., Santos, G. C., Azevedo, C. M., Mota, R. A., Nonaka, C. K., et al. (2019). IGF-1 overexpression improves mesenchymal stem cell survival and promotes neurological recovery after spinal cord injury. Stem Cell Res. Ther. 10:146. doi: 10.1186/s13287-019-1223-z
Alvarez, V., Sanchez-Margallo, F. M., Macias-Garcia, B., Gomez-Serrano, M., Jorge, I., Vazquez, J., et al. (2018). The immunomodulatory activity of extracellular vesicles derived from endometrial mesenchymal stem cells on CD4+ T cells is partially mediated by TGFbeta. J. Tissue Eng. Regen. Med. 12, 2088–2098. doi: 10.1002/term.2743
Anbari, F., Khalili, M. A., Bahrami, A. R., Khoradmehr, A., Sadeghian, F., Fesahat, F., et al. (2014). Intravenous transplantation of bone marrow mesenchymal stem cells promotes neural regeneration after traumatic brain injury. Neural Regen. Res. 9, 919–923. doi: 10.4103/1673-5374.133133
Asgarpour, K., Shojaei, Z., Amiri, F., Ai, J., Mahjoubin-Tehran, M., Ghasemi, F., et al. (2020). Exosomal microRNAs derived from mesenchymal stem cells: cell-to-cell messages. Cell Commun. Signal. 18:149. doi: 10.1186/s12964-020-00650-6
Bai, X., Dong, Q., Zhao, L., Yao, Y., and Wang, B. (2021). microRNA-106b-containing extracellular vesicles affect autophagy of neurons by regulating CDKN2B in Parkinson’s disease. Neurosci. Lett. 760:136094. doi: 10.1016/j.neulet.2021.136094
Bao, C. S., Li, X. L., Liu, L., Wang, B., Yang, F. B., and Chen, L. G. (2018). Transplantation of Human umbilical cord mesenchymal stem cells promotes functional recovery after spinal cord injury by blocking the expression of IL-7. Eur. Rev. Med. Pharmacol. Sci. 22, 6436–6447. doi: 10.26355/eurrev_201810_16056
Baraniak, P. R., and McDevitt, T. C. (2012). Scaffold-free culture of mesenchymal stem cell spheroids in suspension preserves multilineage potential. Cell Tissue Res. 347, 701–711. doi: 10.1007/s00441-011-1215-5
Barczewska, M., Grudniak, M., Maksymowicz, S., Siwek, T., Oldak, T., Jezierska-Wozniak, K., et al. (2019). Safety of intrathecal injection of Wharton’s jelly-derived mesenchymal stem cells in amyotrophic lateral sclerosis therapy. Neural Regen. Res. 14, 313–318. doi: 10.4103/1673-5374.243723
Bartosh, T. J., Ylöstalo, J. H., Bazhanov, N., Kuhlman, J., and Prockop, D. J. (2013). Dynamic compaction of human mesenchymal stem/precursor cells into spheres self-activates caspase-dependent IL1 signaling to enhance secretion of modulators of inflammation and immunity (PGE2, TSG6 and STC1). Stem Cells 31, 2443–2456. doi: 10.1002/stem.1499
Beltramo, E., Lopatina, T., Berrone, E., Mazzeo, A., Iavello, A., Camussi, G., et al. (2014). Extracellular vesicles derived from mesenchymal stem cells induce features of diabetic retinopathy in vitro. Acta Diabetol. 51, 1055–1064. doi: 10.1007/s00592-014-0672-1
Beigi Boroujeni, F., Pasbakhsh, P., Mortezaee, K., Pirhajati, V., Alizadeh, R., Aryanpour, R., et al. (2020). Intranasal delivery of SDF-1α-preconditioned bone marrow mesenchymal cells improves remyelination in the cuprizone-induced mouse model of multiple sclerosis. Cell Biol. Int. 44, 499–511. doi: 10.1002/cbin.11250
Berebichez-Fridman, R., and Montero-Olvera, P. R. (2018). Sources and clinical applications of mesenchymal stem cells: state-of-the-art review. Sultan Qaboos Univ. Med. J. 18, e264–e277. doi: 10.18295/squmj.2018.18.03.002
Betancur, C. (2011). Etiological heterogeneity in autism spectrum disorders: more than 100 genetic and genomic disorders and still counting. Brain Res. 1380, 42–77. doi: 10.1016/j.brainres.2010.11.078
Bhang, S. H., Cho, S. W., La, W. G., Lee, T. J., Yang, H. S., Sun, A. Y., et al. (2011). Angiogenesis in ischemic tissue produced by spheroid grafting of human adipose-derived stromal cells. Biomaterials 32, 2734–2747. doi: 10.1016/j.biomaterials.2010.12.035
Bhasin, A., Srivastava, M., Bhatia, R., Mohanty, S., Kumaran, S., and Bose, S. (2012). Autologous intravenous mononuclear stem cell therapy in chronic ischemic stroke. J. Stem Cells Regen. Med. 8, 181–189. doi: 10.46582/jsrm.0803011
Bilbo, S. D., Block, C. L., Bolton, J. L., Hanamsagar, R., and Tran, P. K. (2018). Beyond infection-maternal immune activation by environmental factors, microglial development and relevance for autism spectrum disorders. Exp. Neurol. 299, 241–251. doi: 10.1016/j.expneurol.2017.07.002
Bodart-Santos, V., De Carvalho, L. R. P., De Godoy, M. A., Batista, A. F., Saraiva, L. M., Lima, L. G., et al. (2019). Extracellular vesicles derived from human Wharton’s jelly mesenchymal stem cells protect hippocampal neurons from oxidative stress and synapse damage induced by amyloid-β oligomers. Stem Cell Res. Ther. 10:332. doi: 10.1186/s13287-019-1432-5
Boika, A., Aleinikava, N., Chyzhyk, V., Zafranskaya, M., Nizheharodava, D., and Ponomarev, V. (2020). Mesenchymal stem cells in Parkinson’s disease: motor and nonmotor symptoms in the early posttransplant period. Surg. Neurol. Int. 11:380. doi: 10.25259/SNI_233_2020
Bourne, R., Flaxman, S. R., Braithwaite, T., Cicinelli, M. V., Das, A., Jonas, J. B., et al. (2017). Magnitude, temporal trends and projections of the global prevalence of blindness and distance and near vision impairment: a systematic review and meta-analysis. Lancet Glob Health 5, e888–e897. doi: 10.1016/S2214-109X(17)30293-0
Bravery, C. A., Carmen, J., Fong, T., Oprea, W., Hoogendoorn, K. H., Woda, J., et al. (2013). Potency assay development for cellular therapy products: an ISCT review of the requirements and experiences in the industry. Cytotherapy 15, 9–19. doi: 10.1016/j.jcyt.2012.10.008
Brown, R. H., and Al-Chalabi, A. (2017). Amyotrophic lateral sclerosis. N. Engl. J. Med. 377, 162–172. doi: 10.1056/NEJMra1603471
Bursch, F., Rath, K. J., Sarikidi, A., Boselt, S., Kefalakes, E., Osmanovic, A., et al. (2019). Analysis of the therapeutic potential of different administration routes and frequencies of human mesenchymal stromal cells in the SOD1(G93A) mouse model of amyotrophic lateral sclerosis. J. Tissue Eng. Regen. Med. 13, 649–663. doi: 10.1002/term.2846
Bydon, M., Dietz, A. B., Goncalves, S., Moinuddin, F. M., Alvi, M. A., Goyal, A., et al. (2020). CELLTOP clinical trial: first report from a phase 1 trial of autologous adipose tissue-derived mesenchymal stem cells in the treatment of paralysis due to traumatic spinal cord injury. Mayo Clin. Proc. 95, 406–414. doi: 10.1016/j.mayocp.2019.10.008
Canesi, M., Giordano, R., Lazzari, L., Isalberti, M., Isaias, I. U., Benti, R., et al. (2016). Finding a new therapeutic approach for no-option Parkinsonisms: mesenchymal stromal cells for progressive supranuclear palsy. J. Transl. Med. 14:127. doi: 10.1186/s12967-016-0880-2
Cao, T. T., Chen, H., Pang, M., Xu, S. S., Wen, H. Q., Liu, B., et al. (2022). Dose optimization of intrathecal administration of human umbilical cord mesenchymal stem cells for the treatment of subacute incomplete spinal cord injury. Neural Regen. Res. 17, 1785–1794. doi: 10.4103/1673-5374.332151
Cao, G., Meng, X., Han, X., and Li, J. (2020). Exosomes derived from circRNA Rtn4-modified BMSCs attenuate TNF-α-induced cytotoxicity and apoptosis in murine MC3T3–E1 cells by sponging miR-146a. Biosci. Rep. 40:BSR20193436. doi: 10.1042/BSR20193436
Cao, X., Xue, L. D., Di, Y., Li, T., Tian, Y. J., and Song, Y. (2021). MSC-derived exosomal lncRNA SNHG7 suppresses endothelial-mesenchymal transition and tube formation in diabetic retinopathy via miR-34a-5p/XBP1 axis. Life Sci. 272:119232. doi: 10.1016/j.lfs.2021.119232
Carter, K., Lee, H. J., Na, K. S., Fernandes-Cunha, G. M., Blanco, I. J., Djalilian, A., et al. (2019). Characterizing the impact of 2D and 3D culture conditions on the therapeutic effects of human mesenchymal stem cell secretome on corneal wound healing in vitro and ex vivo. Acta Biomater. 99, 247–257. doi: 10.1016/j.actbio.2019.09.022
Çerman, E., Akkoç, T., Eraslan, M., Şahin, Ö., Özkara, S., Vardar Aker, F., et al. (2016). Retinal electrophysiological effects of intravitreal bone marrow derived mesenchymal stem cells in streptozotocin induced diabetic rats. PLoS One 11:e0156495. doi: 10.1371/journal.pone.0156495
Chang, Q., Hao, Y., Wang, Y., Zhou, Y., Zhuo, H., and Zhao, G. (2021). Bone marrow mesenchymal stem cell-derived exosomal microRNA-125a promotes M2 macrophage polarization in spinal cord injury by downregulating IRF5. Brain Res. Bull. 170, 199–210. doi: 10.1016/j.brainresbull.2021.02.015
Chang, W. H., Lee, J., Chung, J. W., Kim, Y. H., Bang, O. Y., and The Starting-Collaborators (2021). Probable factors associated with response to mesenchymal stem cell therapy in stroke patients: a post hoc analysis of the STARTING-2 trial. J. Pers. Med. 11:1137. doi: 10.3390/jpm11111137
Chao, O. Y., Yunger, R., and Yang, Y. M. (2018). Behavioral assessments of BTBR T+Itpr3tf/J mice by tests of object attention and elevated open platform: implications for an animal model of psychiatric comorbidity in autism. Behav. Brain Res. 347, 140–147. doi: 10.1016/j.bbr.2018.03.014
Chen, S. N., Xu, Z. G., Ma, Y. X., Chen, S., He, G. H., Han, M., et al. (2021). Protective effect of LIF-huMSCs on the retina of diabetic model rats. Int. J. Ophthalmol. 14, 1508–1517. doi: 10.18240/ijo.2021.10.06
Cheng, C., Chen, X., Wang, Y., Cheng, W., Zuo, X., Tang, W., et al. (2021). MSCs-derived exosomes attenuate ischemia-reperfusion brain injury and inhibit microglia apoptosis might via exosomal miR-26a-5p mediated suppression of CDK6. Mol. Med. 27:67. doi: 10.1186/s10020-021-00324-0
Cho, D. Y., and Jeun, S. S. (2018). Combination therapy of human bone marrow-derived mesenchymal stem cells and minocycline improves neuronal function in a rat middle cerebral artery occlusion model. Stem Cell Res. Ther. 9:309. doi: 10.1186/s13287-018-1011-1
Choi, B. Y., Kim, O. J., Min, S. H., Jeong, J. H., Suh, S. W., and Chung, T. N. (2018). Human placenta-derived mesenchymal stem cells reduce mortality and hematoma size in a rat intracerebral hemorrhage model in an acute phase. Stem Cells Int. 2018:1658195. doi: 10.1155/2018/1658195
Chu, X., Liu, D., Li, T., Ke, H., Xin, D., Wang, S., et al. (2020). Hydrogen sulfide-modified extracellular vesicles from mesenchymal stem cells for treatment of hypoxic-ischemic brain injury. J. Control. Release 328, 13–27. doi: 10.1016/j.jconrel.2020.08.037
Chu, D. T., Nguyen Thi Phuong, T., Tien, N., Tran, D. K., Minh, L. B., Thanh, V. V., et al. (2019). Adipose tissue stem cells for therapy: an update on the progress of isolation, culture, storage and clinical application. J. Clin. Med. 8:917. doi: 10.3390/jcm8070917
Ciervo, Y., Gatto, N., Allen, C., Grierson, A., Ferraiuolo, L., Mead, R. J., et al. (2021). Adipose-derived stem cells protect motor neurons and reduce glial activation in both in vitro and in vivo models of ALS. Mol. Ther. Methods Clin. Dev. 21, 413–433. doi: 10.1016/j.omtm.2021.03.017
Cohen, J. A., Imrey, P. B., Planchon, S. M., Bermel, R. A., Fisher, E., Fox, R. J., et al. (2018). Pilot trial of intravenous autologous culture-expanded mesenchymal stem cell transplantation in multiple sclerosis. Mult. Scler. 24, 501–511. doi: 10.1177/1352458517703802
Conconi, M. T., Di Liddo, R., Tommasini, M., Calore, C., and Parnigotto, P. P. (2011). Phenotype and differentiation potential of stromal populations obtained from various zones of human umbilical cord: an overview. Open Tissue Eng. Regen. Med. J. 4, 6–20. doi: 10.2174/1875043501104010006
Connick, P., Kolappan, M., Crawley, C., Webber, D. J., Patani, R., Michell, A. W., et al. (2012). Autologous mesenchymal stem cells for the treatment of secondary progressive multiple sclerosis: an open-label phase 2a proof-of-concept study. Lancet Neurol. 11, 150–156. doi: 10.1016/S1474-4422(11)70305-2
Connick, P., Kolappan, M., Patani, R., Scott, M. A., Crawley, C., He, X. L., et al. (2011). The mesenchymal stem cells in multiple sclerosis (MSCIMS) trial protocol and baseline cohort characteristics: an open-label pre-test: post-test study with blinded outcome assessments. Trials 12:62. doi: 10.1186/1745-6215-12-62
Conti, P., Lauritano, D., Caraffa, A., Gallenga, C. E., Kritas, S. K., Ronconi, G., et al. (2020). Microglia and mast cells generate proinflammatory cytokines in the brain and worsen inflammatory state: suppressor effect of il-37. Eur. J. Pharmacol. 875:173035. doi: 10.1016/j.ejphar.2020.173035
Crain, S. K., Robinson, S. R., Thane, K. E., Davis, A. M., Meola, D. M., Barton, B. A., et al. (2019). Extracellular vesicles from wharton’s jelly mesenchymal stem cells suppress CD4 expressing T cells through transforming growth factor beta and adenosine signaling in a canine model. Stem Cells Dev. 28, 212–226. doi: 10.1089/scd.2018.0097
Cudkowicz, M. E., Lindborg, S. R., Goyal, N. A., Miller, R. G., Burford, M. J., Berry, J. D., et al. (2022). A randomized placebo-controlled phase 3 study of mesenchymal stem cells induced to secrete high levels of neurotrophic factors in amyotrophic lateral sclerosis. Muscle Nerve 65, 291–302. doi: 10.1002/mus.27472
Cui, L. L., Nitzsche, F., Pryazhnikov, E., Tibeykina, M., Tolppanen, L., Rytkönen, J., et al. (2017). Integrin α4 overexpression on rat mesenchymal stem cells enhances transmigration and reduces cerebral embolism after intracarotid injection. Stroke 48, 2895–2900. doi: 10.1161/STROKEAHA.117.017809
Damasceno, P. K. F., de Santana, T. A., Santos, G. C., Orge, I. D., Silva, D. N., Albuquerque, J. F., et al. (2020). Genetic engineering as a strategy to improve the therapeutic efficacy of mesenchymal stem/stromal cells in regenerative medicine. Front. Cell Dev. Biol. 8:737. doi: 10.3389/fcell.2020.00737
de Celis-Ruiz, E., Fuentes, B., Moniche, F., Montaner, J., Borobia, A. M., Gutiérrez-Fernández, M., et al. (2021). Allogeneic adipose tissue-derived mesenchymal stem cells in ischaemic stroke (AMASCIS-02): a phase IIb, multicentre, double-blind, placebo-controlled clinical trial protocol. BMJ Open 11:e051790. doi: 10.1136/bmjopen-2021-051790
da Silva-Junior, A. J., Mesentier-Louro, L. A., Nascimento-Dos-Santos, G., Teixeira-Pinheiro, L. C., Vasques, J. F., Chimeli-Ormonde, L., et al. (2021). Human mesenchymal stem cell therapy promotes retinal ganglion cell survival and target reconnection after optic nerve crush in adult rats. Stem Cell Res. Ther. 12:69. doi: 10.1186/s13287-020-02130-7
Deverman, B. E., and Patterson, P. H. (2009). Cytokines and CNS development. Neuron 64, 61–78. doi: 10.1016/j.neuron.2009.09.002
Dominici, M., Le Blanc, K., Mueller, I., Slaper-Cortenbach, I., Marini, F., Krause, D., et al. (2006). Minimal criteria for defining multipotent mesenchymal stromal cells. the international society for cellular therapy position statement. Cytotherapy 8, 315–317. doi: 10.1080/14653240600855905
Dos Santos Ramalho, B., Marques Pestana, F., Andrade Prins, C., Soares Dos Santos Cardoso, F., Rufino Cavalcante, D., Augusto Lopes de Souza, S., et al. (2019). Effects of different doses of mesenchymal stem cells on functional recovery after compressive spinal-cord injury in mice. Neuroscience 400, 17–32. doi: 10.1016/j.neuroscience.2018.12.005
Eissa, N., Al-Houqani, M., Sadeq, A., Ojha, S. K., Sasse, A., and Sadek, B. (2018). Current enlightenment about etiology and pharmacological treatment of autism spectrum disorder. Front. Neurosci. 12:304. doi: 10.3389/fnins.2018.00304
Ejtehadifar, M., Shamsasenjan, K., Movassaghpour, A., Akbarzadehlaleh, P., Dehdilani, N., Abbasi, P., et al. (2015). The effect of hypoxia on mesenchymal stem cell biology. Adv. Pharm. Bull. 5:2. doi: 10.15171/apb.2015.021
El Omar, R., Beroud, J., Stoltz, J. F., Menu, P., Velot, E., and Decot, V. (2014). Umbilical cord mesenchymal stem cells: the new gold standard for mesenchymal stem cell-based therapies. Tissue Eng. Part B Rev. 20, 523–544. doi: 10.1089/ten.TEB.2013.0664
Elabd, C., Ichim, T. E., Miller, K., Anneling, A., Grinstein, V., Vargas, V., et al. (2018). Comparing atmospheric and hypoxic cultured mesenchymal stem cell transcriptome: implication for stem cell therapies targeting intervertebral discs. J. Transl. Med. 16:1. doi: 10.1186/s12967-018-1601-9
Elahi, K. C., Klein, G., Avci-Adali, M., Sievert, K. D., MacNeil, S., and Aicher, W. K. (2016). Human mesenchymal stromal cells from different sources diverge in their expression of cell surface proteins and display distinct differentiation patterns. Stem Cells Int. 2016:5646384. doi: 10.1155/2016/5646384
El-Derany, M. O., and Noureldein, M. H. (2021). Bone marrow mesenchymal stem cells and their derived exosomes resolve doxorubicin-induced chemobrain: critical role of their miRNA cargo. Stem Cell Res. Ther. 12:322. doi: 10.1186/s13287-021-02384-9
Emre, E., Yüksel, N., Duruksu, G., Pirhan, D., Subaşi, C., Erman, G., et al. (2015). Neuroprotective effects of intravitreally transplanted adipose tissue and bone marrow-derived mesenchymal stem cells in an experimental ocular hypertension model. Cytotherapy 17, 543–559. doi: 10.1016/j.jcyt.2014.12.005
Eom, Y. W., Oh, J. E., Lee, J. I., Baik, S. K., Rhee, K. J., Shin, H. C., et al. (2014). The role of growth factors in maintenance of stemness in bone marrow-derived mesenchymal stem cells. Biochem. Biophys. Res. Commun. 445, 16–22. doi: 10.1016/j.bbrc.2014.01.084
European Medicines Agency (2008). Guideline on Human Cell-Based Medicinal Products. Available online at: https://www.ema.europa.eu/en/documents/scientific-guideline/guideline-human-cell-based-medicinal-products_en.pdf. Accessed February 23, 2022.
Fan, L., Dong, J., He, X., Zhang, C., and Zhang, T. (2021). Bone marrow mesenchymal stem cells-derived exosomes reduce apoptosis and inflammatory response during spinal cord injury by inhibiting the TLR4/MyD88/NF-κB signaling pathway. Hum. Exp. Toxicol. 40, 1612–1623. doi: 10.1177/09603271211003311
Fogarty Mack, P. (2014). Intracranial haemorrhage: therapeutic interventions and anaesthetic management. Br. J. Anaesth. 113, ii17–ii25. doi: 10.1093/bja/aeu397
Follin, B., Juhl, M., Cohen, S., Pedersen, A. E., Gad, M., Kastrup, J., et al. (2015). Human adipose-derived stromal cells in a clinically applicable injectable alginate hydrogel: phenotypic and immunomodulatory evaluation. Cytotherapy 17, 1104–1118. doi: 10.1016/j.jcyt.2015.04.008
Forostyak, S., Homola, A., Turnovcova, K., Svitil, P., Jendelova, P., and Sykova, E. (2014). Intrathecal delivery of mesenchymal stromal cells protects the structure of altered perineuronal nets in SOD1 rats and amends the course of ALS. Stem Cells 32, 3163–3172. doi: 10.1002/stem.1812
Forostyak, S., Jendelova, P., Kapcalova, M., Arboleda, D., and Sykova, E. (2011). Mesenchymal stromal cells prolong the lifespan in a rat model of amyotrophic lateral sclerosis. Cytotherapy 13, 1036–1046. doi: 10.3109/14653249.2011.592521
Friedenstein, A. J., Chailakhjan, R. K., and Lalykina, K. S. (1970). The development of fibroblast colonies in monolayer cultures of guinea-pig bone marrow and spleen cells. Cell Tissue Kinet. 3, 393–403. doi: 10.1111/j.1365-2184.1970.tb00347.x
Fu, Y., Gao, X., He, G. H., Chen, S., Gu, Z. H., Zhang, Y. L., et al. (2021). Protective effects of umbilical cord mesenchymal stem cell exosomes in a diabetic rat model through live retinal imaging. Int. J. Ophthalmol. 14, 1828–1833. doi: 10.18240/ijo.2021.12.04
Fu, X., Liu, G., Halim, A., Ju, Y., Luo, Q., and Song, A. G. (2019). Mesenchymal stem cell migration and tissue repair. Cells 8:784. doi: 10.3390/cells8080784
Fu, Y., Yan, Y., Qi, Y., Yang, L., Li, T., Zhang, N., et al. (2016). Impact of autologous mesenchymal stem cell infusion on neuromyelitis optica spectrum disorder: a pilot, 2-year observational study. CNS Neurosci. Ther. 22, 677–685. doi: 10.1111/cns.12559
Gaddam, S., Periasamy, R., and Gangaraju, R. (2019). Adult stem cell therapeutics in diabetic retinopathy. Int. J. Mol. Sci. 20:4876. doi: 10.3390/ijms20194876
Galipeau, J., Krampera, M., Barrett, J., Dazzi, F., Deans, R. J., DeBruijn, J., et al. (2016). International Society for Cellular Therapy perspective on immune functional assays for mesenchymal stromal cells as potency release criterion for advanced phase clinical trials. Cytotherapy 18, 151–159. doi: 10.1016/j.jcyt.2015.11.008
Ganesh, A., Lindsay, P., Fang, J., Kapral, M. K., Côté, R., Joiner, I., et al. (2016). Integrated systems of stroke care and reduction in 30-day mortality: a retrospective analysis. Neurology 86, 898–904. doi: 10.1212/WNL.0000000000002443
Gao, X., Xiong, Y., Li, Q., Han, M., Shan, D., Yang, G., et al. (2020). Extracellular vesicle-mediated transfer of miR-21-5p from mesenchymal stromal cells to neurons alleviates early brain injury to improve cognitive function via the PTEN/Akt pathway after subarachnoid hemorrhage. Cell Death Dis. 11:363. doi: 10.1038/s41419-020-2530-0
Garnier, D., Ratcliffe, E., Briand, J., Cartron, P. F., Oliver, L., and Vallette, F. M. (2022). The Activation of mesenchymal stem cells by glioblastoma microvesicles alters their exosomal secretion of miR-100-5p, miR-9-5p and let-7d-5p. Biomedicines 10:112. doi: 10.3390/biomedicines10010112
Geffen, Y., Perets, N., Horev, R., Yudin, D., Oron, O., Elliott, E., et al. (2020). Exosomes derived from adipose mesenchymal stem cells: a potential non-invasive intranasal treatment for autism. Cytotherapy 22:S49. doi: 10.1016/j.jcyt.2020.03.059
Geschwind, D. H., and Levitt, P. (2007). Autism spectrum disorders: developmental disconnection syndromes. Curr. Opin. Neurobiol. 17, 103–111. doi: 10.1016/j.conb.2007.01.009
Gessner, A., Koch, B., Klann, K., Fuhrmann, D. C., Farmand, S., Schubert, R., et al. (2021). Characterization of extracellular vesicles from preconditioned human adipose-derived stromal/stem cells. Int. J. Mol. Sci. 22:2873. doi: 10.3390/ijms22062873
Giordano, R., Canesi, M., Isalberti, M., Isaias, I. U., Montemurro, T., Vigano, M., et al. (2014). Autologous mesenchymal stem cell therapy for progressive supranuclear palsy: translation into a phase I controlled, randomized clinical study. J. Transl. Med. 12:14. doi: 10.1186/1479-5876-12-14
Giordano, R., Canesi, M., Isalberti, M., Marfia, G., Campanella, R., Vincenti, D., et al. (2021). Safety and effectiveness of cell therapy in neurodegenerative diseases: take-home messages from a pilot feasibility phase I study of progressive supranuclear palsy. Front. Neurosci. 15:723227. doi: 10.3389/fnins.2021.723227
Godoy, M. A., Saraiva, L. M., Carvalho, L. R. P., Vasconcelos-Dos-Santos, A., Beiral, H. J. V., Ramos, A. B., et al. (2017). Mesenchymal stem cells and cell-derived extracellular vesicles protect hippocampal neurons from oxidative stress and synapse damage induced by amyloid-beta oligomers. J. Biol. Chem. 293, 1957–1975. doi: 10.1074/jbc.M117.807180
Gramlich, O. W., Brown, A. J., Godwin, C. R., Chimenti, M. S., Boland, L. K., Ankrum, J. A., et al. (2020). Systemic mesenchymal stem cell treatment mitigates structural and functional retinal ganglion cell degeneration in a mouse model of multiple sclerosis. Transl. Vis. Sci. Technol. 9:16. doi: 10.1167/tvst.9.8.16
Grayson, W. L., Zhao, F., Izadpanah, R., Bunnell, B., and Ma, T. (2006). Effects of hypoxia on human mesenchymal stem cell expansion and plasticity in 3D constructs. J. Cell Physiol. 207:2. doi: 10.1002/jcp.20571
Gregorius, J., Wang, C., Stambouli, O., Hussner, T., Qi, Y., Tertel, T., et al. (2021). Small extracellular vesicles obtained from hypoxic mesenchymal stromal cells have unique characteristics that promote cerebral angiogenesis, brain remodeling and neurological recovery after focal cerebral ischemia in mice. Basic Res. Cardiol. 116:40. doi: 10.1007/s00395-021-00881-9
Gu, X., Yu, X., Zhao, C., Duan, P., Zhao, T., Liu, Y., et al. (2018). Efficacy and safety of autologous bone marrow mesenchymal stem cell transplantation in patients with diabetic retinopathy. Cell. Physiol. Biochem. 49, 40–52. doi: 10.1159/000492838
Gubert, F., Bonacossa-Pereira, I., Decotelli, A. B., Furtado, M., Vasconcelos-Dos-Santos, A., Mendez-Otero, R., et al. (2019). Bone-marrow mononuclear cell therapy in a mouse model of amyotrophic lateral sclerosis: functional outcomes from different administration routes. Brain Res. 1712, 73–81. doi: 10.1016/j.brainres.2019.02.003
Gubert, F., Decotelli, A. B., Bonacossa-Pereira, I., Figueiredo, F. R., Zaverucha-Do-Valle, C., Tovar-Moll, F., et al. (2016). Intraspinal bone-marrow cell therapy at pre- and symptomatic phases in a mouse model of amyotrophic lateral sclerosis. Stem Cell Res. Ther. 7:41. doi: 10.1186/s13287-016-0293-4
Habisch, H. J., Janowski, M., Binder, D., Kuzma-Kozakiewicz, M., Widmann, A., Habich, A., et al. (2007). Intrathecal application of neuroectodermally converted stem cells into a mouse model of ALS: limited intraparenchymal migration and survival narrows therapeutic effects. J. Neural Transm. (Vienna) 114, 1395–1406. doi: 10.1007/s00702-007-0748-y
Hagmann, S., Moradi, B., Frank, S., Dreher, T., Kämmerer, P. W., Richter, W., et al. (2013). Different culture media affect growth characteristics, surface marker distribution and chondrogenic differentiation of human bone marrow-derived mesenchymal stromal cells. BMC Musculoskelet. Disord. 14:223. doi: 10.1186/1471-2474-14-223
Han, M., Cao, Y., Xue, H., Chu, X., Li, T., Xin, D., et al. (2020). Neuroprotective effect of mesenchymal stromal cell-derived extracellular vesicles against cerebral ischemia-reperfusion-induced neural functional injury: a pivotal role for AMPK and JAK2/STAT3/NF-κB signaling pathway modulation. Drug Des. Devel. Ther. 14, 2865–2876. doi: 10.2147/DDDT.S248892
Hansen, T. B., Jensen, T. I., Clausen, B. H., Bramsen, J. B., Finsen, B., Damgaard, C. K., et al. (2013). Natural RNA circles function as efficient microRNA sponges. Nature 495, 384–388. doi: 10.1038/nature11993
Haraszti, R. A., Miller, R., Dubuke, M. L., Rockwell, H. E., Coles, A. H., Sapp, E., et al. (2019). Serum deprivation of mesenchymal stem cells improves exosome activity and alters lipid and protein composition. iScience 16, 230–241. doi: 10.1016/j.isci.2019.05.029
Harris, V. K., Stark, J., Vyshkina, T., Blackshear, L., Joo, G., Stefanova, V., et al. (2018). Phase I trial of intrathecal mesenchymal stem cell-derived neural progenitors in progressive multiple sclerosis. EBioMedicine 29, 23–30. doi: 10.1016/j.ebiom.2018.02.002
Heathman, T. R., Rafiq, Q. A., Chan, A. K., Coopman, K., Nienow, A. W., Kara, B., et al. (2016). Characterization of human mesenchymal stem cells from multiple donors and the implications for large scale bioprocess development. Biochem. Eng. J. 108, 14–23. doi: 10.1016/j.bej.2015.06.018
Hernandez-Ontiveros, D. G., Tajiri, N., Acosta, S., Giunta, B., Tan, J., and Borlongan, C. V. (2013). Microglia activation as a biomarker for traumatic brain injury. Front. Neurol. 4:30. doi: 10.3389/fneur.2013.00030
Ho, Y. K., Woo, J. Y., Tu, G. X. E., Deng, L. W., and Too, H. P. (2020). A highly efficient non-viral process for programming mesenchymal stem cells for gene directed enzyme prodrug cancer therapy. Sci. Rep. 10:14257. doi: 10.1038/s41598-020-71224-2
Holopainen, M., Colas, R. A., Valkonen, S., Tigistu-Sahle, F., Hyvarinen, K., Mazzacuva, F., et al. (2019). Polyunsaturated fatty acids modify the extracellular vesicle membranes and increase the production of proresolving lipid mediators of human mesenchymal stromal cells. Biochim. Biophys. Acta Mol. Cell Biol. Lipids 1864, 1350–1362. doi: 10.1016/j.bbalip.2019.06.010
Honmou, O., Yamashita, T., Morita, T., Oshigiri, T., Hirota, R., Iyama, S., et al. (2021). Intravenous infusion of auto serum-expanded autologous mesenchymal stem cells in spinal cord injury patients: 13 case series. Clin. Neurol. Neurosurg. 203:106565. doi: 10.1016/j.clineuro.2021.106641
Hou, Z., Chen, J., Yang, H., Hu, X., and Yang, F. (2021). microRNA-26a shuttled by extracellular vesicles secreted from adipose-derived mesenchymal stem cells reduce neuronal damage through KLF9-mediated regulation of TRAF2/KLF2 axis. Adipocyte 10, 378–393. doi: 10.1080/21623945.2021.1938829
Hou, K., Li, G., Zhao, J., Xu, B., Zhang, Y., Yu, J., et al. (2020). Bone mesenchymal stem cell-derived exosomal microRNA-29b-3p prevents hypoxic-ischemic injury in rat brain by activating the PTEN-mediated Akt signaling pathway. J. Neuroinflammation 17:46. doi: 10.1186/s12974-020-1725-8
Hill, A. J., Zwart, I., Tam, H. H., Chan, J., Navarrete, C., Jen, L. S., et al. (2009). Human umbilical cord blood-derived mesenchymal stem cells do not differentiate into neural cell types or integrate into the retina after intravitreal grafting in neonatal rats. Stem Cells Dev. 18, 399–409. doi: 10.1089/scd.2008.0084
Hu, C., and Li, L. (2018). Preconditioning influences mesenchymal stem cell properties in vitro and in vivo. J. Cell Mol. Med. 22, 1428–1442. doi: 10.1111/jcmm.13492
Huang, F., Gao, T., Wang, W., Wang, L., Xie, Y., Tai, C., et al. (2021). Engineered basic fibroblast growth factor-overexpressing human umbilical cord-derived mesenchymal stem cells improve the proliferation and neuronal differentiation of endogenous neural stem cells and functional recovery of spinal cord injury by activating the PI3K-Akt-GSK-3β signaling pathway. Stem Cell Res. Ther. 12:468. doi: 10.1186/s13287-021-02537-w
Huang, P., Freeman, W. D., Edenfield, B. H., Brott, T. G., Meschia, J. F., and Zubair, A. C. (2019). Safety and efficacy of intraventricular delivery of bone marrow-derived mesenchymal stem cells in hemorrhagic stroke model. Sci. Rep. 9:5674. doi: 10.1038/s41598-019-42182-1
Huang, Y., Wang, J., Cai, J., Qiu, Y., Zheng, H., Lai, X., et al. (2018). Targeted homing of CCR2-overexpressing mesenchymal stromal cells to ischemic brain enhances post-stroke recovery partially through PRDX4-mediated blood-brain barrier preservation. Theranostics 8, 5929–5944. doi: 10.7150/thno.28029
Huang, Y., Xiao, X., Xiao, H., Hu, Z., and Tan, F. (2021). CUEDC2 ablation enhances the efficacy of mesenchymal stem cells in ameliorating cerebral ischemia/reperfusion insult. Aging (Albany NY) 13, 4335–4356. doi: 10.18632/aging.202394
Hung, S. P., Ho, J. H., Shih, Y. R. V., Lo, T., and Lee, O. K. (2012). Hypoxia promotes proliferation and osteogenic differentiation potentials of human mesenchymal stem cells. J. Orthop. Res. 30, 260–266. doi: 10.1002/jor.21517
Hur, J. W., Cho, T. H., Park, D. H., Lee, J. B., Park, J. Y., and Chung, Y. G. (2016). Intrathecal transplantation of autologous adipose-derived mesenchymal stem cells for treating spinal cord injury: a human trial. J. Spinal Cord Med. 39, 655–664. doi: 10.1179/2045772315Y.0000000048
Jaillard, A., Hommel, M., Moisan, A., Zeffiro, T. A., Favre-Wiki, I. M., Barbieux-Guillot, M., et al. (2020). Autologous mesenchymal stem cells improve motor recovery in subacute ischemic stroke: a randomized clinical trial. Transl. Stroke Res. 11, 910–923. doi: 10.1007/s12975-020-00787-z
Jamali, F., Aldughmi, M., Khasawneh, M. W., Dahbour, S., Salameh, A. A., and Awidi, A. (2021). A new tool for safety evaluation and a combination of measures for efficacy assessment of cotransplanting human allogenic neuronal stem cells and mesenchymal stem cells for the treatment of Parkinson disease: protocol for an interventional study. JMIR Res. Protoc. 10:e29695. doi: 10.2196/29695
Ji, H., Gu, J., Song, X., Bao, J., Peng, X., Xie, L., et al. (2021). A nerve growth factor persistent delivery scaffold seeded with neurally differentiated bone marrow mesenchymal stem cells promoted the functional recovery of spinal cord injury in rats. Am. J. Transl. Res. 13, 2127–2142.
Jiao, J., Milwid, J. M., Yarmush, M. L., and Parekkadan, B. (2011). A mesenchymal stem cell potency assay. Methods Mol. Biol. 677, 221–231. doi: 10.1007/978-1-60761-869-0_16
Kabat, M., Bobkov, I., Kumar, S., and Grumet, M. (2020). Trends in mesenchymal stem cell clinical trials 2004–2018: is efficacy optimal in a narrow dose range. Stem Cells Transl. Med. 9, 17–27. doi: 10.1002/sctm.19-0202
Kahraman, N. S., and Oner, A. (2020). Umbilical cord-derived mesenchymal stem cell implantation in patients with optic atrophy. Eur. J. Ophthalmol. 31, 3463–3470. doi: 10.1177/1120672120977824
Kakudo, N., Morimoto, N., Ogawa, T., Taketani, S., and Kusumoto, K. (2015). Hypoxia enhances proliferation of human adipose-derived stem cells via HIF-1 activation. PLoS One 10:e0139890. doi: 10.1371/journal.pone.0139890
Karussis, D., Karageorgiou, C., Vaknin-Dembinsky, A., Gowda-Kurkalli, B., Gomori, J. M., Kassis, I., et al. (2010). Safety and immunological effects of mesenchymal stem cell transplantation in patients with multiple sclerosis and amyotrophic lateral sclerosis. Arch. Neurol. 67, 1187–1194. doi: 10.1001/archneurol.2010.248
Kasetty, M. A., Hedges, T. R., 3rd, Witkin, A. J. (2020). Bilateral epiretinal membrane formation following intravitreal injections of autologous mesenchymal stem cells. Retin. Cases Brief Rep. . [Online ahead of print]. doi: 10.1097/ICB.0000000000001032
Kim, H. J., Cho, K. R., Jang, H., Lee, N. K., Jung, Y. H., Kim, J. P., et al. (2021). Intracerebroventricular injection of human umbilical cord blood mesenchymal stem cells in patients with Alzheimer’s disease dementia: a phase I clinical trial. Alzheimers Res. Ther. 13:154. doi: 10.1186/s13195-021-00897-2
Kim, H. S., Choi, D. Y., Yun, S. J., Choi, S. M., Kang, J. W., Jung, J. W., et al. (2012). Proteomic analysis of microvesicles derived from human mesenchymal stem cells. J. Proteome Res. 11, 839–849. doi: 10.1021/pr200682z
Kim, E. S., Kida, K., Mok, J., Seong, Y., Jo, S. Y., Kanaki, T., et al. (2021). Cellhesion VP enhances the immunomodulating potential of human mesenchymal stem cell-derived extracellular vesicles. Biomaterials 271:120742. doi: 10.1016/j.biomaterials.2021.120742
Kim, H., Kim, H. Y., Choi, M. R., Hwang, S., Nam, K. H., Kim, H. C., et al. (2010). Dose-dependent efficacy of ALS-human mesenchymal stem cells transplantation into cisterna magna in SOD1–G93A ALS mice. Neurosci. Lett. 468, 190–194. doi: 10.1016/j.neulet.2009.10.074
Kim, H. Y., Kim, H., Oh, K. W., Oh, S. I., Koh, S. H., Baik, W., et al. (2014). Biological markers of mesenchymal stromal cells as predictors of response to autologous stem cell transplantation in patients with amyotrophic lateral sclerosis: an investigator-initiated trial and in vivo study. Stem Cells 32, 2724–2731. doi: 10.1002/stem.1770
Kim, H., Lee, M. J., Bae, E. H., Ryu, J. S., Kaur, G., Kim, H. J., et al. (2020). Comprehensive molecular profiles of functionally effective MSC-derived extracellular vesicles in immunomodulation. Mol. Ther. 28, 1628–1644. doi: 10.1016/j.ymthe.2020.04.020
Kim, S. J., Moon, G. J., Cho, Y. H., Kang, H. Y., Hyung, N. K., Kim, D., et al. (2012). Circulating mesenchymal stem cells microparticles in patients with cerebrovascular disease. PLoS One 7:e37036. doi: 10.1371/journal.pone.0037036
Kodali, M., Castro, O. W., Kim, D. K., Thomas, A., Shuai, B., Attaluri, S., et al. (2019). Intranasally administered human msc-derived extracellular vesicles pervasively incorporate into neurons and microglia in both intact and status epilepticus injured forebrain. Int. J. Mol. Sci. 21:181. doi: 10.3390/ijms21010181
Koh, A. E., Alsaeedi, H. A., Rashid, M., Lam, C., Harun, M., Ng, M. H., et al. (2021). Corrigendum: transplanted erythropoietin-expressing mesenchymal stem cells promote pro-survival gene expression and protect photoreceptors from sodium iodate-induced cytotoxicity in a retinal degeneration model. Front. Cell Dev. Biol. 9:764504. doi: 10.3389/fcell.2021.764504
Kook, M. G., Lee, S., Shin, N., Kong, D., Kim, D. H., Kim, M. S., et al. (2020). Repeated intramuscular transplantations of hUCB-MSCs improves motor function and survival in the SOD1 G93A mice through activation of AMPK. Sci. Rep. 10:1572. doi: 10.1038/s41598-020-58221-1
Krupa, P., Vackova, I., Ruzicka, J., Zaviskova, K., Dubisova, J., Koci, Z., et al. (2018). The effect of human mesenchymal stem cells derived from wharton’s jelly in spinal cord injury treatment is dose-dependent and can be facilitated by repeated application. Int. J. Mol. Sci. 19:1503. doi: 10.3390/ijms19051503
Kuzma-Kozakiewicz, M., Marchel, A., Kaminska, A., Gawel, M., Sznajder, J., Figiel-Dabrowska, A., et al. (2018). Intraspinal transplantation of the adipose tissue-derived regenerative cells in amyotrophic lateral sclerosis in accordance with the current experts’ recommendations: choosing optimal monitoring tools. Stem Cells Int. 2018:4392017. doi: 10.1155/2018/4392017
Kwon, S. Y., Chun, S. Y., Ha, Y. S., Kim, D. H., Kim, J., Song, P. H., et al. (2017). Hypoxia enhances cell properties of human mesenchymal stem cells. Tissue Eng. Regen. Med. 14:5. doi: 10.1007/s13770-017-0068-8
Lai, R. C., and Lim, S. K. (2019). Membrane lipids define small extracellular vesicle subtypes secreted by mesenchymal stromal cells. J. Lipid Res. 60, 318–322. doi: 10.1194/jlr.R087411
Larocca, T. F., Macêdo, C. T., Souza, B. S. F., Andrade-Souza, Y. M., Villarreal, C. F., Matos, A. C., et al. (2017). Image-guided percutaneous intralesional administration of mesenchymal stromal cells in subjects with chronic complete spinal cord injury: a pilot study. Cytotherapy 19, 1189–1196. doi: 10.1016/j.jcyt.2017.06.006
Le Blon, D., Guglielmetti, C., Hoornaert, C., Quarta, A., Daans, J., Dooley, D., et al. (2013). Intracerebral transplantation of interleukin 13-producing mesenchymal stem cells limits microgliosis, oligodendrocyte loss and demyelination in the cuprizone mouse model. J. Neuroinflammation 13:288. doi: 10.1186/s12974-016-0756-7
Le Saux, S., Aarrass, H., Lai-Kee-Him, J., Bron, P., Armengaud, J., Miotello, G., et al. (2020). Post-production modifications of murine mesenchymal stem cell (mMSC) derived extracellular vesicles (EVs) and impact on their cellular interaction. Biomaterials 231:119675. doi: 10.1016/j.biomaterials.2019.119675
Lee, A. S., Azmitia, E. C., and Whitaker-Azmitia, P. M. (2017). Developmental microglial priming in postmortem autism spectrum disorder temporal cortex. Brain Behav. Immun. 62, 193–202. doi: 10.1016/j.bbi.2017.01.019
Lee, J., Chang, W. H., Chung, J. W., Kim, S. J., Kim, S. K., Lee, J. S., et al. (2022). Efficacy of intravenous mesenchymal stem cells for motor recovery after ischemic stroke: a neuroimaging study. Stroke 53, 20–28. doi: 10.1161/STROKEAHA.121.034505
Lee, S., Kim, O. J., Lee, K. O., Jung, H., Oh, S. H., and Kim, N. K. (2020). Enhancing the therapeutic potential of ccl 2-overexpressing mesenchymal stem cells in acute stroke. Int. J. Mol. Sci. 21:7795. doi: 10.3390/ijms21207795
Lejkowska, R., Kawa, M. P., Pius-Sadowska, E., Rogińska, D., Łuczkowska, K., Machaliński, B., et al. (2019). Preclinical evaluation of long-term neuroprotective effects of BDNF-engineered mesenchymal stromal cells as intravitreal therapy for chronic retinal degeneration in Rd6 mutant mice. Int. J. Mol. Sci. 20:777. doi: 10.3390/ijms20030777
Leon-Moreno, L. C., Castaneda-Arellano, R., Aguilar-Garcia, I. G., Desentis-Desentis, M. F., Torres-Anguiano, E., Gutierrez-Almeida, C. E., et al. (2020). Kinematic changes in a mouse model of penetrating hippocampal injury and their recovery after intranasal administration of endometrial mesenchymal stem cell-derived extracellular vesicles. Front. Cell Neurosci. 14:579162. doi: 10.3389/fncel.2020.579162
Levy, M. L., Crawford, J. R., Dib, N., Verkh, L., Tankovich, N., and Cramer, S. C. (2019). Phase I/II study of safety and preliminary efficacy of intravenous allogeneic mesenchymal stem cells in chronic stroke. Stroke 50, 2835–2841. doi: 10.1161/STROKEAHA.119.026318
Li, W., Jin, L. Y., Cui, Y. B., and Xie, N. (2021). Human umbilical cord mesenchymal stem cells-derived exosomal microRNA-17-3p ameliorates inflammatory reaction and antioxidant injury of mice with diabetic retinopathy via targeting STAT1. Int. Immunopharmacol. 90:107010. doi: 10.1016/j.intimp.2020.107010
Li, N., Li, X. R., and Yuan, J. Q. (2009). Effects of bone-marrow mesenchymal stem cells transplanted into vitreous cavity of rat injured by ischemia/reperfusion. Graefes Arch. Clin. Exp. Ophthalmol. 247, 503–514. doi: 10.1007/s00417-008-1009-y
Li, M., Zeng, L., Liu, S., Dangelmajer, S., Kahlert, U. D., Huang, H., et al. (2019). Transforming growth factor-β promotes homing and therapeutic efficacy of human mesenchymal stem cells to glioblastoma. J. Neuropathol. Exp. Neurol. 78, 315–325. doi: 10.1093/jnen/nlz016
Li, J., Zhang, Q., Wang, W., Lin, F., Wang, S., and Zhao, J. (2021). Mesenchymal stem cell therapy for ischemic stroke: a look into treatment mechanism and therapeutic potential. J. Neurol. 268, 4095–4107. doi: 10.1007/s00415-020-10138-5
Liang, Y., Duan, L., Xu, X., Li, X., Liu, M., Chen, H., et al. (2020). Mesenchymal stem cell-derived exosomes for treatment of autism spectrum disorder. ACS Appl. Bio Mater. 3, 6384–6393. doi: 10.1021/acsabm.0c00831
Liao, Z., Li, S., Lu, S., Liu, H., Li, G., Ma, L., et al. (2021). Metformin facilitates mesenchymal stem cell-derived extracellular nanovesicles release and optimizes therapeutic efficacy in intervertebral disc degeneration. Biomaterials 274:120850. doi: 10.1016/j.biomaterials.2021.120850
Linares, G. R., Chiu, C. T., Scheuing, L., Leng, Y., Liao, H. M., Maric, D., et al. (2016). Preconditioning mesenchymal stem cells with the mood stabilizers lithium and valproic acid enhances therapeutic efficacy in a mouse model of Huntington’s disease. Exp. Neurol. 281, 81–92. doi: 10.1016/j.expneurol.2016.04.003
Lindoso, R. S., Collino, F., and Vieyra, A. (2017). Extracellular vesicles as regulators of tumor fate: crosstalk among cancer stem cells, tumor cells and mesenchymal stem cells. Stem Cell Investig. 4:75. doi: 10.21037/sci.2017.08.08
Liu, X., Duan, B., Cheng, Z., Jia, X., Mao, L., Fu, H., et al. (2011). Sdf-1/cxcr4 axis modulates bone marrow mesenchymal stem cell apoptosis, migration and cytokine secretion. Protein Cell 2, 845–854. doi: 10.1007/s13238-011-1097-z
Liu, K., Guo, L., Zhou, Z., Pan, M., and Yan, C. (2019). Mesenchymal stem cells transfer mitochondria into cerebral microvasculature and promote recovery from ischemic stroke. Microvasc. Res. 123, 74–80. doi: 10.1016/j.mvr.2019.01.001
Liu, J., He, J., Ge, L., Xiao, H., Huang, Y., Zeng, L., et al. (2021). Hypoxic preconditioning rejuvenates mesenchymal stem cells and enhances neuroprotection following intracerebral hemorrhage via the miR-326-mediated autophagy. Stem Cell Res. Ther. 12:1. doi: 10.1186/s13287-021-02480-w
Liu, W., Tang, P., Wang, J., Ye, W., Ge, X., Rong, Y., et al. (2021). Extracellular vesicles derived from melatonin-preconditioned mesenchymal stem cells containing USP29 repair traumatic spinal cord injury by stabilizing NRF2. J. Pineal Res. 71:e12769. doi: 10.1111/jpi.12769
Liu, Y., Zhao, Y., Min, Y., Guo, K., Chen, Y., Huang, Z., et al. (2022). Effects and mechanisms of bone marrow mesenchymal stem cell transplantation for treatment of ischemic stroke in hypertensive rats. Int. J. Stem Cells 15, 217–226. doi: 10.15283/ijsc21136
Llufriu, S., Sepulveda, M., Blanco, Y., Marin, P., Moreno, B., Berenguer, J., et al. (2014). Randomized placebo-controlled phase II trial of autologous mesenchymal stem cells in multiple sclerosis. PLoS One 9:e113936. doi: 10.1371/journal.pone.0113936
Lord, C., Risi, S., DiLavore, P. S., Shulman, C., Thurm, A., and Pickles, A. (2006). Autism from 2 to 9 years of age. Arch. Gen. Psychiatry 63, 694–701. doi: 10.1001/archpsyc.63.6.694
Losurdo, M., Pedrazzoli, M., D’Agostino, C., Elia, C. A., Massenzio, F., Lonati, E., et al. (2020). Intranasal delivery of mesenchymal stem cell-derived extracellular vesicles exerts immunomodulatory and neuroprotective effects in a 3xTg model of Alzheimer’s disease. Stem Cells Transl. Med. 9, 1068–1084. doi: 10.1002/sctm.19-0327
Lu, Z., Ye, D., Qian, L., Zhu, L., Wang, C., Guan, D., et al. (2012). Human umbilical cord mesenchymal stem cell therapy on neuromyelitis optica. Curr. Neurovasc. Res. 9, 250–255. doi: 10.2174/156720212803530708
Lv, Y. T., Zhang, Y., Liu, M., Qiuwaxi, J. N., Ashwood, P., Cho, S. C., et al. (2013). Transplantation of human cord blood mononuclear cells and umbilical cord-derived mesenchymal stem cells in autism. J. Transl. Med. 11:196. doi: 10.1186/1479-5876-11-196
Maenner, M. J., Shaw, K. A., Baio, J., Washington, A., Patrick, M., DiRienzo, M., et al. (2020). Prevalence of autism spectrum disorder among children aged 8 years - autism and developmental disabilities monitoring network, 11 sites, United States, 2016. MMWR Surveill. Summ. 69, 1–12. doi: 10.15585/mmwr.ss6904a1
Marchezan, J., dos Santos, E. G. A. W., Deckmann, I., and dos Santos Riesgo, R. (2018). Immunological dysfunction in autism spectrum disorder: a potential target for therapy. Neuroimmunomodulation 25, 300–319. doi: 10.1159/000492225
Marconi, S., Bonaconsa, M., Scambi, I., Squintani, G. M., Rui, W., Turano, E., et al. (2013). Systemic treatment with adipose-derived mesenchymal stem cells ameliorates clinical and pathological features in the amyotrophic lateral sclerosis murine model. Neuroscience 248, 333–343. doi: 10.1016/j.neuroscience.2013.05.034
Marinaro, F., Gomez-Serrano, M., Jorge, I., Silla-Castro, J. C., Vazquez, J., Sanchez-Margallo, F. M., et al. (2019). Unraveling the molecular signature of extracellular vesicles from endometrial-derived mesenchymal stem cells: potential modulatory effects and therapeutic applications. Front. Bioeng. Biotechnol. 7:431. doi: 10.3389/fbioe.2019.00431
Markoutsa, E., Mayilsamy, K., Gulick, D., Mohapatra, S. S., and Mohapatra, S. (2022). Extracellular vesicles derived from inflammatory-educated stem cells reverse brain inflammation—implication of miRNAs. Mol Ther. 30, 816–830. doi: 10.1016/j.ymthe.2021.08.008
Martínez-Muriana, A., Pastor, D., Mancuso, R., Rando, A., Osta, R., Martínez, S., et al. (2020). Combined intramuscular and intraspinal transplant of bone marrow cells improves neuromuscular function in the SOD1 G93A mice. Stem Cell Res. Ther. 11:53. doi: 10.1186/s13287-020-1573-6
Mathew, B., Torres, L. A., Gamboa Acha, L., Tran, S., Liu, A., Patel, R., et al. (2021). Uptake and distribution of administered bone marrow mesenchymal stem cell extracellular vesicles in retina. Cells 10:730. doi: 10.3390/cells10040730
Matsumoto, J., Stewart, T., Banks, W. A., and Zhang, J. (2017). The transport mechanism of extracellular vesicles at the blood-brain barrier. Curr. Pharm. Des. 23, 6206–6214. doi: 10.2174/1381612823666170913164738
Mead, B., Hill, L. J., Blanch, R. J., Ward, K., Logan, A., Berry, M., et al. (2016). Mesenchymal stromal cell-mediated neuroprotection and functional preservation of retinal ganglion cells in a rodent model of glaucoma. Cytotherapy 18, 487–496. doi: 10.1016/j.jcyt.2015.12.002
Mead, B., and Tomarev, S. (2017). Bone marrow-derived mesenchymal stem cells-derived exosomes promote survival of retinal ganglion cells through miRNA-dependent mechanisms. Stem Cells Transl. Med. 6, 1273–1285. doi: 10.1002/sctm.16-0428
Medicines and Healthcare Products Regulatory Agency (2015). Guidance Advanced Therapy Medicinal Products: Regulation and Licensing Available online at: https://www.gov.uk/guidance/advanced-therapy-medicinal-products-regulation-and-licensing. Accessed February 23, 2022.
Mejzini, R., Flynn, L. L., Pitout, I. L., Fletcher, S., Wilton, S. D., Akkari, P. A., et al. (2019). ALS genetics, mechanisms and therapeutics: where are we now? Front. Neurosci. 13:1310. doi: 10.3389/fnins.2019.01310
Mello, T. G., Rosado-de-Castro, P. H., Campos, R. M. P., Vasques, J. F., Rangel-Junior, W. S., Mattos, R. S. A. R., et al. (2020). Intravenous human umbilical cord-derived mesenchymal stromal cell administration in models of moderate and severe intracerebral hemorrhage. Stem Cells Dev. 29, 586–598. doi: 10.1089/scd.2019.0176
Mendt, M., Rezvani, K., and Shpall, E. (2019). Mesenchymal stem cell-derived exosomes for clinical use. Bone Marrow Transplant. 54, 789–792. doi: 10.1038/s41409-019-0616-z
Menezes, K., Rosa, B. G., Freitas, C., da Cruz, A. S., de Siqueira Santos, R., Nascimento, M. A., et al. (2020). Human mesenchymal stromal/stem cells recruit resident pericytes and induce blood vessels maturation to repair experimental spinal cord injury in rats. Sci. Rep. 10:19604. doi: 10.1038/s41598-020-76290-0
Mesentier-Louro, L. A., Teixeira-Pinheiro, L. C., Gubert, F., Vasques, J. F., Silva-Junior, A. J., Chimeli-Ormonde, L., et al. (2019). Long-term neuronal survival, regeneration, and transient target reconnection after optic nerve crush and mesenchymal stem cell transplantation. Stem Cell Res. Ther. 10:121. doi: 10.1186/s13287-019-1226-9
Mesentier-Louro, L. A., Zaverucha-do-Valle, C., da Silva-Junior, A. J., Nascimento-Dos-Santos, G., Gubert, F., de Figueirêdo, A. B., et al. (2014). Distribution of mesenchymal stem cells and effects on neuronal survival and axon regeneration after optic nerve crush and cell therapy. PLoS One 9:e110722. doi: 10.1371/journal.pone.0110722
Miceli, V., Pampalone, M., Vella, S., Carreca, A. P., Amico, G., and Conaldi, P. G. (2019). Comparison of immunosuppressive and angiogenic properties of human amnion-derived mesenchymal stem cells between 2D and 3D culture systems. Stem Cells Int. 2019:7486279. doi: 10.1155/2019/7486279
Millán-Rivero, J. E., Nadal-Nicolás, F. M., García-Bernal, D., Sobrado-Calvo, P., Blanquer, M., Moraleda, J., et al. (2018). Human Wharton’s jelly mesenchymal stem cells protect axotomized rat retinal ganglion cells via secretion of anti-inflammatory and neurotrophic factors. Sci. Rep. 8:16299. doi: 10.1038/s41598-018-34527-z
Mohamadi, Y., Noori Moghahi, S. M. H., Mousavi, M., Borhani-Haghighi, M., Abolhassani, F., Kashani, I. R., et al. (2019). Intrathecal transplantation of Wharton’s jelly mesenchymal stem cells suppresses the NLRP1 inflammasome in the rat model of spinal cord injury. J. Chem. Neuroanat. 97, 1–8. doi: 10.1016/j.jchemneu.2019.01.011
Moon, G. J., Cho, Y. H., Kim, D. H., Sung, J. H., Son, J. P., Kim, S., et al. (2018). Serum-mediated activation of bone Marrow-derived mesenchymal stem cells in ischemic stroke patients: a novel preconditioning method. Cell Transplant. 27:3. doi: 10.1177/0963689718755404
Moon, G. J., Sung, J. H., Kim, D. H., Kim, E. H., Cho, Y. H., Son, J. P., et al. (2019). Application of mesenchymal stem cell-derived extracellular vesicles for stroke: biodistribution and microRNA study. Transl. Stroke Res. 10, 509–521. doi: 10.1007/s12975-018-0668-1
Montaner-Tarbes, S., Novell, E., Tarancón, V., Borrás, F. E., Montoya, M., Fraile, L., et al. (2018). Targeted-pig trial on safety and immunogenicity of serum-derived extracellular vesicles enriched fractions obtained from porcine respiratory and reproductive virus infections. Sci. Rep. 8:17487. doi: 10.1038/s41598-018-36141-5
Morata-Tarifa, C., Azkona, G., Glass, J., Mazzini, L., and Sanchez-Pernaute, R. (2021). Looking backward to move forward: a meta-analysis of stem cell therapy in amyotrophic lateral sclerosis. NPJ Regen. Med. 6:20. doi: 10.1038/s41536-021-00131-5
Morgan, J. T., Chana, G., Abramson, I., Semendeferi, K., Courchesne, E., Everall, I. P., et al. (2012). Abnormal microglial-neuronal spatial organization in the dorsolateral prefrontal cortex in autism. Brain Res. 1456, 72–81. doi: 10.1016/j.brainres.2012.03.036
Morioka, C., Komaki, M., Taki, A., Honda, I., Yokoyama, N., Iwasaki, K., et al. (2017). Neuroprotective effects of human umbilical cord-derived mesenchymal stem cells on periventricular leukomalacia-like brain injury in neonatal rats. Inflamm. Regen. 37:1. doi: 10.1186/s41232-016-0032-3
Morita, T., Sasaki, M., Kataoka-Sasaki, Y., Nakazaki, M., Nagahama, H., Oka, S., et al. (2016). Intravenous infusion of mesenchymal stem cells promotes functional recovery in a model of chronic spinal cord injury. Neuroscience 335, 221–231. doi: 10.1016/j.neuroscience.2016.08.037
Morrison, T. J., Jackson, M. V., Cunningham, E. K., Kissenpfennig, A., McAuley, D. F., O’Kane, C. M., et al. (2017). Mesenchymal stromal cells modulate macrophages in clinically relevant lung injury models by extracellular vesicle mitochondrial transfer. Am. J. Respir. Crit. Care Med. 196, 1275–1286. doi: 10.1164/rccm.201701-0170OC
Mukhamedshina, Y. O., Akhmetzyanova, E. R., Kostennikov, A. A., Zakirova, E. Y., Galieva, L. R., Garanina, E. E., et al. (2018). Adipose-derived mesenchymal stem cell application combined with fibrin matrix promotes structural and functional recovery following spinal cord injury in rats. Front. Pharmacol. 9:343. doi: 10.3389/fphar.2018.00343
Murphy, K. C., Fang, S. Y., and Leach, J. K. (2014). Human mesenchymal stem cell spheroids in fibrin hydrogels exhibit improved cell survival and potential for bone healing. Cell Tissue Res. 357, 91–99. doi: 10.1007/s00441-014-1830-z
Nabavi, S. M., Arab, L., Jarooghi, N., Bolurieh, T., Abbasi, F., Mardpour, S., et al. (2019). Safety, feasibility of intravenous and intrathecal injection of autologous bone marrow derived mesenchymal stromal cells in patients with amyotrophic lateral sclerosis: an open label phase i clinical trial. Cell J. 20, 592–598. doi: 10.22074/cellj.2019.5370
Nagamura-Inoue, T., and He, H. (2014). Umbilical cord-derived mesenchymal stem cells: their advantages and potential clinical utility. World J. Stem Cells 6, 195–202. doi: 10.4252/wjsc.v6.i2.195
Nagamura-Inoue, T., and Mukai, T. (2016). Umbilical cord is a rich source of mesenchymal stromal cells for cell therapy. Curr. Stem Cell Res. Ther. 11, 634–642. doi: 10.2174/1574888x10666151026115017
Nakazaki, M., Morita, T., Lankford, K. L., Askenase, P. W., and Kocsis, J. D. (2021). Small extracellular vesicles released by infused mesenchymal stromal cells target M2 macrophages and promote TGF-β upregulation, microvascular stabilization and functional recovery in a rodent model of severe spinal cord injury. J. Extracell. Vesicles 10:e12137. doi: 10.1002/jev2.12137
Nascimento-Dos-Santos, G., Teixeira-Pinheiro, L. C., Da Silva-Junior, A. J., Carvalho, L. R. P., Mesentier-Louro, L. A., Hauswirth, W. W., et al. (2019). Effects of a combinatorial treatment with gene and cell therapy on retinal ganglion cell survival and axonal outgrowth after optic nerve injury. Gene Ther. 27, 27–39. doi: 10.1038/s41434-019-0089-0
Nie, H., and Jiang, Z. (2021). Bone mesenchymal stem cell-derived extracellular vesicles deliver microRNA-23b to alleviate spinal cord injury by targeting toll-like receptor TLR4 and inhibiting NF-κB pathway activation. Bioengineered 12, 8157–8172. doi: 10.1080/21655979.2021.1977562
Nolta, J. A. (2016). “Next-generation” mesenchymal stem or stromal cells for the in vivo delivery of bioactive factors: progressing toward the clinic. Transfusion 56, S15–S17. doi: 10.1111/trf.13564
Norte-Munoz, M., Lucas-Ruiz, F., Gallego-Ortega, A., Garcia-Bernal, D., Valiente-Soriano, F. J., De La Villa, P., et al. (2021). Neuroprotection and axonal regeneration induced by bone marrow mesenchymal stromal cells depend on the type of transplant.. Front. Cell Dev. Biol. 9:772223. doi: 10.3389/fcell.2021.772223
Occhetta, P., Centola, M., Tonnarelli, B., Redaelli, A., Martin, I., and Rasponi, M. (2015). High-throughput microfluidic platform for 3D cultures of mesenchymal stem cells, towards engineering developmental processes. Sci. Rep. 5, 1–12. doi: 10.1038/srep10288
Oh, K. W., Moon, C., Kim, H. Y., Oh, S. I., Park, J., Lee, J. H., et al. (2015). Phase I trial of repeated intrathecal autologous bone marrow-derived mesenchymal stromal cells in amyotrophic lateral sclerosis. Stem Cells Transl. Med. 4, 590–597. doi: 10.5966/sctm.2014-0212
Oh, K. W., Noh, M. Y., Kwon, M. S., Kim, H. Y., Oh, S. I., Park, J., et al. (2018). Repeated intrathecal mesenchymal stem cells for amyotrophic lateral sclerosis. Ann. Neurol. 84, 361–373. doi: 10.1002/ana.25302
Okuda, A., Horii-Hayashi, N., Sasagawa, T., Shimizu, T., Shigematsu, H., Iwata, E., et al. (2017). Bone marrow stromal cell sheets may promote axonal regeneration and functional recovery with suppression of glial scar formation after spinal cord transection injury in rats. J. Neurosurg. Spine 26, 388–395. doi: 10.3171/2016.8.SPINE16250
Omid Sadatpoor, S., Salehi, Z., Rahban, D., and Salimi, A. (2020). Manipulated mesenchymal stem cells applications in neurodegenerative diseases. Int. J. Stem Cells 13:1. doi: 10.15283/ijsc19031
Oraee-Yazdani, S., Akhlaghpasand, M., Golmohammadi, M., Hafizi, M., Zomorrod, M. S., Kabir, N. M., et al. (2021). Combining cell therapy with human autologous Schwann cell and bone marrow-derived mesenchymal stem cell in patients with subacute complete spinal cord injury: safety considerations and possible outcomes. Stem Cell Res. Ther. 12:445. doi: 10.1186/s13287-021-02515-2
Oses, C., Olivares, B., Ezquer, M., Acosta, C., Bosch, P., Donoso, M., et al. (2017). Preconditioning of adipose tissue-derived mesenchymal stem cells with deferoxamine increases the production of pro-angiogenic, neuroprotective and anti-inflammatory factors: potential application in the treatment of diabetic neuropathy. PLoS One 12:e0178011. doi: 10.1371/journal.pone.0178011
Özmert, E., and Arslan, U. (2020a). Management of retinitis pigmentosa by Wharton’s jelly-derived mesenchymal stem cells: prospective analysis of 1-year results. Stem Cell Res. Ther. 11:353. doi: 10.1186/s13287-020-01870-w
Özmert, E., and Arslan, U. (2020b). Management of retinitis pigmentosa by Wharton’s jelly derived mesenchymal stem cells: preliminary clinical results. Stem Cell Res. Ther. 11:25. doi: 10.1186/s13287-020-1549-6
Pan, D., Chang, X., Xu, M., Zhang, M., Zhang, S., Wang, Y., et al. (2019). UMSC-derived exosomes promote retinal ganglion cells survival in a rat model of optic nerve crush. J. Chem. Neuroanat. 96, 134–139. doi: 10.1016/j.jchemneu.2019.01.006
Papadimitropoulos, A., Piccinini, E., Brachat, S., Braccini, A., Wendt, D., Barbero, A., et al. (2014). Expansion of human mesenchymal stromal cells from fresh bone marrow in a 3D scaffold-based system under direct perfusion. PLoS One 9:e102359. doi: 10.1371/journal.pone.0102359
Park, I. S., Rhie, J. W., and Kim, S. H. (2014). A novel three-dimensional adipose-derived stem cell cluster for vascular regeneration in ischemic tissue. Cytotherapy 16, 508–522. doi: 10.1016/j.jcyt.2013.08.011
Peng, C., Li, Y., Lu, L., Zhu, J., Li, H., Hu, J., et al. (2019). Efficient one-step induction of human umbilical cord-derived mesenchymal stem cells (UC-MSCs) produces MSC-derived neurospheres (MSC-NS) with unique transcriptional profile and enhanced neurogenic and angiogenic secretomes. Stem Cells Int. 2019:9208173. doi: 10.1155/2019/9208173
Perets, N., Hertz, S., London, M., and Offen, D. (2018). Intranasal administration of exosomes derived from mesenchymal stem cells ameliorates autistic-like behaviors of BTBR mice. Mol. Autism 9, 1–12. doi: 10.1186/s13229-018-0240-6
Perets, N., Oron, O., Herman, S., Elliott, E., and Offen, D. (2020). Exosomes derived from mesenchymal stem cells improved core symptoms of genetically modified mice model of autism shank3b. Mol. Autism 11:65. doi: 10.1186/s13229-020-00366-x
Perets, N., Segal-Gavish, H., Gothelf, Y., Barzilay, R., Barhum, Y., Abramov, N., et al. (2017). Long term beneficial effect of neurotrophic factors-secreting mesenchymal stem cells transplantation in the BTBR mouse model of autism. Behav. Brain Res. 331, 254–260. doi: 10.1016/j.bbr.2017.03.047
Petrou, P., Gothelf, Y., Argov, Z., Gotkine, M., Levy, Y. S., Kassis, I., et al. (2016). Safety and clinical effects of mesenchymal stem cells secreting neurotrophic factor transplantation in patients with amyotrophic lateral sclerosis: results of phase 1/2 and 2a clinical trials. JAMA Neurol. 73, 337–344. doi: 10.1001/jamaneurol.2015.4321
Petrou, P., Kassis, I., Yaghmour, N. E., Ginzberg, A., and Karussis, D. (2021a). A phase II clinical trial with repeated intrathecal injections of autologous mesenchymal stem cells in patients with amyotrophic lateral sclerosis. Front. Biosci. (Landmark Ed.) 26, 693–706. doi: 10.52586/4980
Petrou, P., Kassis, I., Ginzberg, A., Halimi, M., Yaghmour, N., Abramsky, O., et al. (2021b). Long-term clinical and immunological effects of repeated mesenchymal stem cell injections in patients with progressive forms of multiple sclerosis. Front. Neurol. 12:639315. doi: 10.3389/fneur.2021.639315
Philips, T., and Rothstein, J. D. (2015). Rodent models of amyotrophic lateral sclerosis. Curr. Protoc. Pharmacol. 69:5.67.61–65.67.21. doi: 10.1002/0471141755.ph0567s69
Pierce, L. M., and Kurata, W. E. (2021). Priming With toll-like receptor 3 agonist poly(I:C) enhances content of innate immune defense proteins but not microRNAs in human mesenchymal stem cell-derived extracellular vesicles. Front. Cell Dev. Biol. 9:676356. doi: 10.3389/fcell.2021.676356
Policarpo, R., Sierksma, A., De Strooper, B., and d’Ydewalle, C. (2021). From junk to function: LncRNAs in CNS health and disease. Front. Mol. Neurosci. 14:714768. doi: 10.3389/fnmol.2021.714768
Potier, E., Ferreira, E., Andriamanalijaona, R., Pujol, J. P., Oudina, K., Logeart-Avramoglou, D., et al. (2007). Hypoxia affects mesenchymal stromal cell osteogenic differentiation and angiogenic factor expression. Bone 40:4. doi: 10.1016/j.bone.2006.11.024
Puig-Pijuan, T., De Godoy, M. A., Pinheiro Carvalho, L. R., Bodart-Santos, V., Lindoso, R. S., Pimentel-Coelho, P. M., et al. (2020). Human Wharton’s jelly mesenchymal stem cells protect neural cells from oxidative stress through paracrine mechanisms. Future Sci. OA. 6:FSO627. doi: 10.2144/fsoa-2020-0036
Ramalho, B. S., Almeida, F. M., Sales, C. M., de Lima, S., and Martinez, A. M. B. (2018). Injection of bone marrow mesenchymal stem cells by intravenous or intraperitoneal routes is a viable alternative to spinal cord injury treatment in mice. Neural Regen. Res. 13, 1046–1053. doi: 10.4103/1673-5374.233448
Redler, Y., and Levy, M. (2020). Rodent models of optic neuritis. Front. Neurol. 11:580951. doi: 10.3389/fneur.2020.580951
Redondo-Castro, E., Cunningham, C., Miller, J., Martuscelli, L., Aoulad-Ali, S., Rothwell, N. J., et al. (2017). Interleukin-1 primes human mesenchymal stem cells towards an anti-inflammatory and pro-trophic phenotype in vitro. Stem Cell Res. Ther. 8:1. doi: 10.1186/s13287-017-0531-4
Řehořová, M., Vargová, I., Forostyak, S., Vacková, I., Turnovcová, K., Kupcová Skalníková, H., et al. (2019). A combination of intrathecal and intramuscular application of human mesenchymal stem cells partly reduces the activation of necroptosis in the spinal cord of SOD1(G93A) rats. Stem Cells Transl. Med. 8, 535–547. doi: 10.1002/sctm.18-0223
Riordan, N. H., Hincapié, M. L., Morales, I., Fernández, G., Allen, N., Leu, C., et al. (2019). Allogeneic human umbilical cord mesenchymal stem cells for the treatment of autism spectrum disorder in children: safety profile and effect on cytokine levels. Stem Cells Transl. Med. 8, 1008–1016. doi: 10.1002/sctm.19-0010
Riordan, N. H., Morales, I., Fernández, G., Allen, N., Fearnot, N. E., Leckrone, M. E., et al. (2018). Clinical feasibility of umbilical cord tissue-derived mesenchymal stem cells in the treatment of multiple sclerosis. J. Transl. Med. 16:57. doi: 10.1186/s12967-018-1433-7
Robb, K. P., Fitzgerald, J. C., Barry, F., and Viswanathan, S. (2019). Mesenchymal stromal cell therapy: progress in manufacturing and assessments of potency. Cytotherapy 21, 289–306. doi: 10.1016/j.jcyt.2018.10.014
Rodrigues, S. C., Cardoso, R., Gomes, C. F., Duarte, F. V., Freire, P. C., Neves, R., et al. (2021). Toxicological profile of umbilical cord blood-derived small extracellular vesicles. Membranes (Basel) 11:647. doi: 10.3390/membranes11090647
Rodrigues, M., Griffith, L. G., and Wells, A. (2010). Growth factor regulation of proliferation and survival of multipotential stromal cells. Stem Cell Res. Ther. 1:32. doi: 10.1186/scrt32
Rohden, F., Teixeira, L. V., Bernardi, L. P., Ferreira, P., Colombo, M., Teixeira, G. R., et al. (2021). Functional recovery caused by human adipose tissue mesenchymal stem cell-derived extracellular vesicles administered 24 h after stroke in rats. Int. J. Mol. Sci. 22:12860. doi: 10.3390/ijms222312860
Rosová, I., Dao, M., Capoccia, B., Link, D., and Nolta, J. A. (2008). Hypoxic preconditioning results in increased motility and improved therapeutic potential of human mesenchymal stem cells. Stem Cells 26:8. doi: 10.1634/stemcells.2007-1104
Rudge, S. R., and Nims, R. W. (2017). “ICH Q6B specifications: test procedures and acceptance criteria for biotechnological/biological products,” in ICH Quality Guidelines: An Implementation Guide, (Hoboken, NJ: John Wiley and Sons), 467–486.
Saleh, A. F., Lázaro-Ibáñez, E., Forsgard, M. A., Shatnyeva, O., Osteikoetxea, X., Karlsson, F., et al. (2019). Extracellular vesicles induce minimal hepatotoxicity and immunogenicity. Nanoscale 11, 6990–7001. doi: 10.1039/c8nr08720b
Satti, H. S., Waheed, A., Ahmed, P., Ahmed, K., Akram, Z., Aziz, T., et al. (2016). Autologous mesenchymal stromal cell transplantation for spinal cord injury: a phase I pilot study. Cytotherapy 18, 518–522. doi: 10.1016/j.jcyt.2016.01.004
Segal-Gavish, H., Karvat, G., Barak, N., Barzilay, R., Ganz, J., Edry, L., et al. (2016). Mesenchymal stem cell transplantation promotes neurogenesis and ameliorates autism related behaviors in BTBR mice. Autism Res. 9, 17–32. doi: 10.1002/aur.1530
Seifali, E., Hassanzadeh, G., Mahdavipour, M., Mortezaee, K., Moini, A., Satarian, L., et al. (2020). Extracellular vesicles derived from human umbilical cord perivascular cells improve functional recovery in brain ischemic rat via the inhibition of apoptosis. Iran. Biomed. J. 24, 347–360. doi: 10.29252/ibj.24.6.342
Seyedrazizadeh, S. Z., Poosti, S., Nazari, A., Alikhani, M., Shekari, F., Pakdel, F., et al. (2020). Extracellular vesicles derived from human ES-MSCs protect retinal ganglion cells and preserve retinal function in a rodent model of optic nerve injury. Stem Cell Res. Ther. 11:203. doi: 10.1186/s13287-020-01702-x
Sha, S., Shen, X., Cao, Y., and Qu, L. (2021). Mesenchymal stem cells-derived extracellular vesicles ameliorate Alzheimer’s disease in rat models via the microRNA-29c-3p/BACE1 axis and the Wnt/beta-catenin pathway. Aging (Albany NY) 13, 15285–15306. doi: 10.18632/aging.203088
Shahror, R. A., Ali, A. A. A., Wu, C. C., Chiang, Y. H., and Chen, K. Y. (2019). Enhanced homing of mesenchymal stem cells overexpressing fibroblast growth factor 21 to injury site in a mouse model of traumatic brain injury. Int. J. Mol. Sci. 11:2624. doi: 10.3390/ijms20112624
Shen, H., Yao, X., Li, H., Li, X., Zhang, T., and Sun, Q. (2018). Role of exosomes derived from miR-133b modified mscs in an experimental rat model of intracerebral hemorrhage. J. Mol. Neurosci. 64, 421–430. doi: 10.1007/s12031-018-1041-2
Sheng, X., Zhao, J., Li, M., Xu, Y., Zhou, Y., Xu, J., et al. (2021). Bone marrow mesenchymal stem cell-derived exosomes accelerate functional recovery after spinal cord injury by promoting the phagocytosis of macrophages to clean myelin debris. Front. Cell Dev. Biol. 9:772205. doi: 10.3389/fcell.2021.772205
Shi, J., Zhao, Y. C., Niu, Z. F., Fan, H. J., Hou, S. K., Guo, X. Q., et al. (2021). Mesenchymal stem cell-derived small extracellular vesicles in the treatment of human diseases: progress and prospect. World J. Stem Cells 13, 49–63. doi: 10.4252/wjsc.v13.i1.49
Simon, M. C., and Keith, B. (2008). The role of oxygen availability in embryonic development and stem cell function. Nat. Rev. Mol. Cell Biol. 9:4. doi: 10.1038/nrm2354
Siniscalco, D., Kannan, S., Semprún-Hernández, N., Eshraghi, A. A., Brigida, A. L., and Antonucci, N. (2018). Stem cell therapy in autism: recent insights. Stem Cells Cloning 11, 55–67. doi: 10.2147/SCCAA.S155410
Sironi, F., Vallarola, A., Violatto, M. B., Talamini, L., Freschi, M., De Gioia, R., et al. (2017). Multiple intracerebroventricular injections of human umbilical cord mesenchymal stem cells delay motor neurons loss but not disease progression of SOD1G93A mice. Stem Cell Res. 25, 166–178. doi: 10.1016/j.scr.2017.11.005
Steinberg, G. K., Kondziolka, D., Wechsler, L. R., Lunsford, L. D., Coburn, M. L., Billigen, J. B., et al. (2016). Clinical outcomes of transplanted modified bone marrow-derived mesenchymal stem cells in stroke: a phase 1/2a study. Stroke 47, 1817–1824. doi: 10.1161/STROKEAHA.116.012995
Steinberg, G. K., Kondziolka, D., Wechsler, L. R., Lunsford, L. D., Kim, A. S., Johnson, J. N., et al. (2018). Two-year safety and clinical outcomes in chronic ischemic stroke patients after implantation of modified bone marrow-derived mesenchymal stem cells (SB623): a phase 1/2a study. J. Neurosurg. 23, 1–11. doi: 10.3171/2018.5.JNS173147
Sun, J. M., Dawson, G., Franz, L., Howard, J., McLaughlin, C., Kistler, B., et al. (2020). Infusion of human umbilical cord tissue mesenchymal stromal cells in children with autism spectrum disorder. Stem Cells Transl. Med. 9, 1137–1146. doi: 10.1002/sctm.19-0434
Sung, Y., Lee, S. M., Park, M., Choi, H. J., Kang, S., Choi, B. I., et al. (2020). Treatment of traumatic optic neuropathy using human placenta-derived mesenchymal stem cells in asian patients. Regen. Med. 15, 2163–2179. doi: 10.2217/rme-2020-0044
Suzuki, M., McHugh, J., Tork, C., Shelley, B., Hayes, A., Bellantuono, I., et al. (2008). Direct muscle delivery of GDNF with human mesenchymal stem cells improves motor neuron survival and function in a rat model of familial ALS. Mol. Ther. 16, 2002–2010. doi: 10.1038/mt.2008.197
Sykova, E., Rychmach, P., Drahoradova, I., Konradova, S., Ruzickova, K., Vorisek, I., et al. (2017). Transplantation of mesenchymal stromal cells in patients with amyotrophic lateral sclerosis: results of phase i/iia clinical trial. Cell Transplant. 26, 647–658. doi: 10.3727/096368916X693716
Takemura, M., Sasaki, M., Kataoka-Sasaki, Y., Kiyose, R., Nagahama, H., Oka, S., et al. (2021). Repeated intravenous infusion of mesenchymal stem cells for enhanced functional recovery in a rat model of chronic cerebral ischemia. J. Neurosurg. 1 . [Online ahead of print]. doi: 10.3171/2021.8.JNS21687
Takeuchi, S., Tsuchiya, A., Iwasawa, T., Nojiri, S., Watanabe, T., Ogawa, M., et al. (2021). Small extracellular vesicles derived from interferon-gamma pre-conditioned mesenchymal stromal cells effectively treat liver fibrosis. NPJ Regen. Med. 6:19. doi: 10.1038/s41536-021-00132-4
Tang, F., Tang, J., Zhao, Y., Zhang, J., Xiao, Z., Chen, B., et al. (2022). Long-term clinical observation of patients with acute and chronic complete spinal cord injury after transplantation of NeuroRegen scaffold. Sci. China Life Sci. 65, 909–926. doi: 10.1007/s11427-021-1985-5
Teixeira, F. G., and Salgado, A. J. (2020). Mesenchymal stem cells secretome: current trends and future challenges. Neural Regen. Res. 15, 75–77. doi: 10.4103/1673-5374.264455
Thapar, A., and Rutter, M. (2021). Genetic advances in autism. J. Autism Dev. Disord. 51, 4321–4332. doi: 10.1007/s10803-020-04685-z
Tobin, M. K., Stephen, T. K. L., Lopez, K. L., Pergande, M. R., Bartholomew, A. M., Cologna, S. M., et al. (2020). Activated mesenchymal stem cells induce recovery following stroke via regulation of inflammation and oligodendrogenesis. J. Am. Heart Assoc. 9:e013583. doi: 10.1161/JAHA.119.013583
Tuekprakhon, A., Sangkitporn, S., Trinavarat, A., Pawestri, A. R., Vamvanij, V., Ruangchainikom, M., et al. (2021). Intravitreal autologous mesenchymal stem cell transplantation: a non-randomized phase I clinical trial in patients with retinitis pigmentosa. Stem Cell Res. Ther. 12:52. doi: 10.1186/s13287-020-02122-7
Turnbull, M. T., Zubair, A. C., Meschia, J. F., and Freeman, W. D. (2019). Mesenchymal stem cells for hemorrhagic stroke: status of preclinical and clinical research. NPJ Regen. Med. 4:10. doi: 10.1038/s41536-019-0073-8
U. S. Department of Health and Human Services Food and Drug Administration, Center for Biologics Evaluation and Research (2001). Guidance for human somatic cell therapy and gene therapy. Hum. Gene Ther. 12, 303–314. doi: 10.1089/10430340150218431
Uccelli, A., Milanese, M., Principato, M. C., Morando, S., Bonifacino, T., Vergani, L., et al. (2012). Intravenous mesenchymal stem cells improve survival and motor function in experimental amyotrophic lateral sclerosis. Mol. Med. 18, 794–804. doi: 10.2119/molmed.2011.00498
Uccelli, A., Laroni, A., Brundin, L., Clanet, M., Fernandez, O., Nabavi, S. M., et al. (2019). MEsenchymal StEm cells for Multiple Sclerosis (MESEMS): a randomized, double blind, cross-over phase I/II clinical trial with autologous mesenchymal stem cells for the therapy of multiple sclerosis. Trials 20:263. doi: 10.1186/s13063-019-3346-z
Uccelli, A., Laroni, A., and Freedman, M. S. (2011). Mesenchymal stem cells for the treatment of multiple sclerosis and other neurological diseases. Lancet Neurol. 10:7. doi: 10.1016/S1474-4422(11)70121-1
Vallés, G., Bensiamar, F., Crespo, L., Arruebo, M., Vilaboa, N., and Saldana, L. (2015). Topographical cues regulate the crosstalk between MSCs and macrophages. Biomaterials 37, 124–133. doi: 10.1016/j.biomaterials.2014.10.028
Vangsness, C. T. Jr., Sternberg, H., and Harris, L. (2015). Umbilical cord tissue offers the greatest number of harvestable mesenchymal stem cells for research and clinical application: a literature review of different harvest sites. Arthroscopy 31, 1836–1843. doi: 10.1016/j.arthro.2015.03.014
Vaquero, J., Zurita, M., Rico, M. A., Aguayo, C., Bonilla, C., Marin, E., et al. (2018). Intrathecal administration of autologous mesenchymal stromal cells for spinal cord injury: safety and efficacy of the 100/3 guideline. Cytotherapy 20, 806–819. doi: 10.1016/j.jcyt.2018.03.032
Vaquero, J., Zurita, M., Rico, M. A., Bonilla, C., Aguayo, C., Fernández, C., et al. (2017). Repeated subarachnoid administrations of autologous mesenchymal stromal cells supported in autologous plasma improve quality of life in patients suffering incomplete spinal cord injury. Cytotherapy 19, 349–359. doi: 10.1016/j.jcyt.2016.12.002
Vaquero, J., Zurita, M., Rico, M. A., Bonilla, C., Aguayo, C., Montilla, J., et al. (2016). An approach to personalized cell therapy in chronic complete paraplegia: the Puerta de Hierro phase I/II clinical trial. Cytotherapy 18, 1025–1036. doi: 10.1016/j.jcyt.2016.05.003
Vargas, D. L., Nascimbene, C., Krishnan, C., Zimmerman, A. W., and Pardo, C. A. (2005). Neuroglial activation and neuroinflammation in the brain of patients with autism. Ann. Neurol. 57, 67–81. doi: 10.1002/ana.20315
de Vasconcelos dos Santos, A., da Costa Reis, J., Diaz Paredes, B., Moraes, L., Giraldi-Guimarães, A., Mendez-Otero, R, et al. (2010). Therapeutic window for treatment of cortical ischemia with bone marrow-derived cells in rats. Brain Res. 1306, 149–158. doi: 10.1016/j.brainres.2009.09.094
Vasques, J. F., Mendez-Otero, R., and Gubert, F. (2020). Modeling ALS using iPSCs: is it possible to reproduce the phenotypic variations observed in patients in vitro? Regen. Med. 15:1919. doi: 10.2217/rme-2020-0067
Vercelli, A., Mereuta, O. M., Garbossa, D., Muraca, G., Mareschi, K., Rustichelli, D., et al. (2008). Human mesenchymal stem cell transplantation extends survival, improves motor performance and decreases neuroinflammation in mouse model of amyotrophic lateral sclerosis. Neurobiol. Dis. 31, 395–405. doi: 10.1016/j.nbd.2008.05.016
Wang, Y., Chen, X., Cao, W., and Shi, Y. (2014). Plasticity of mesenchymal stem cells in immunomodulation: pathological and therapeutic implications. Nat. Immunol. 15, 1009–1016. doi: 10.1038/ni.3002
Wang, L. S., Chung, J. E., Chan, P. P. Y., and Kurisawa, M. (2010). Injectable biodegradable hydrogels with tunable mechanical properties for the stimulation of neurogenesic differentiation of human mesenchymal stem cells in 3D culture. Biomaterials 31, 1148–1157. doi: 10.1016/j.biomaterials.2009.10.042
Wang, Y., Lv, J., Huang, C., Li, X., Chen, Y., Wu, W., et al. (2021). Human umbilical cord-mesenchymal stem cells survive and migrate within the vitreous cavity and ameliorate retinal damage in a novel rat model of chronic glaucoma. Stem Cells Int. 2021:8852517. doi: 10.1155/2021/8852517
Wang, W. Y., Tan, M. S., Yu, J. T., and Tan, L. (2015). Role of pro-inflammatory cytokines released from microglia in Alzheimer’s disease. Ann. Transl. Med. 3:136. doi: 10.3978/j.issn.2305-5839.2015.03.49
Wang, X. H., Tang, X. C., Li, X., Qin, J. Z., Zhong, W. T., Wu, P., et al. (2021). Implantation of nanofibrous silk scaffolds seeded with bone marrow stromal cells promotes spinal cord regeneration (6686 words). Artif. Cells Nanomed. Biotechnol. 49, 699–708. doi: 10.1080/21691401.2021.2013250
Weiss, J. N., Benes, S. C., and Levy, S. (2016a). Stem Cell Ophthalmology Treatment Study (SCOTS): improvement in serpiginous choroidopathy following autologous bone marrow derived stem cell treatment. Neural Regen. Res. 11, 1512–1516. doi: 10.4103/1673-5374.191229
Weiss, J. N., Levy, S., and Benes, S. C. (2016b). Stem Cell Ophthalmology Treatment Study (SCOTS): bone marrow-derived stem cells in the treatment of Leber’s hereditary optic neuropathy. Neural Regen. Res. 11, 1685–1694. doi: 10.4103/1673-5374.193251
Weiss, J. N., Levy, S., and Benes, S. C. (2015a). Stem Cell Ophthalmology Treatment Study (SCOTS) for retinal and optic nerve diseases: a case report of improvement in relapsing auto-immune optic neuropathy. Neural Regen. Res. 10, 1507–1515. doi: 10.4103/1673-5374.165525
Weiss, J. N., Levy, S., and Malkin, A. (2015b). Stem cell ophthalmology treatment study (SCOTS) for retinal and optic nerve diseases: a preliminary report. Neural Regen. Res. 10, 982–988. doi: 10.4103/1673-5374.158365
Weiss, J. N., Levy, S., and Benes, S. C. (2017). Stem cell ophthalmology treatment study: bone marrow derived stem cells in the treatment of non-arteritic ischemic optic neuropathy (NAION). Stem Cell Investig. 4:94. doi: 10.21037/sci.2017.11.05
Weiss, J. N., and Levy, S. (2016). Neurologic stem cell treatment study (NEST) using bone marrow derived stem cells for the treatment of neurological disorders and injuries: study protocol for a nonrandomized efficacy trial. Clin. Trials Degener. Dis. 1, 176–180. doi: 10.4103/2468-5658.196984
Weiss, J. N., and Levy, S. (2018). Stem cell ophthalmology treatment study: bone marrow derived stem cells in the treatment of retinitis pigmentosa. Stem Cell Investig. 5:18. doi: 10.21037/sci.2018.04.02
Weiss, J. N., and Levy, S. (2019). Dynamic light scattering spectroscopy of the retina-a non-invasive quantitative technique to objectively document visual improvement following ocular stem cell treatment. Stem Cell Investig. 6:8. doi: 10.21037/sci.2019.03.01
Weiss, J. N., and Levy, S. (2020). Stem cell ophthalmology treatment study (SCOTS): bone marrow-derived stem cells in the treatment of age-related macular degeneration. Medicines (Basel) 7:16. doi: 10.3390/medicines7040016
Weiss, J. N., and Levy, S. (2021). Stem cell ophthalmology treatment study (SCOTS): bone marrow-derived stem cells in the treatment of Stargardt disease. Medicines (Basel) 8:10. doi: 10.3390/medicines8020010
Wen, S., Dooner, M., Papa, E., Del Tatto, M., Pereira, M., Borgovan, T., et al. (2019). Biodistribution of mesenchymal stem cell-derived extracellular vesicles in a radiation injury bone marrow murine model. Int. J. Mol. Sci. 20:5468. doi: 10.3390/ijms20215468
Wen, J. H., Vincent, L. G., Fuhrmann, A., Choi, Y. S., Hribar, K. C., Taylor-Weiner, H., et al. (2014). Interplay of matrix stiffness and protein tethering in stem cell differentiation. Nat. Mat. 13, 979–987. doi: 10.1038/nmat4051
Williams, L. B., Prakalapakorn, S. G., Ansari, Z., and Goldhardt, R. (2020). Impact and trends in global ophthalmology. Curr. Ophthalmol. Rep. 8, 136–143. . [Online ahead of print]. doi: 10.1007/s40135-020-00245-x
Wu, S. C., Kuo, P. J., Rau, C. S., Huang, L. H., Lin, C. W., Wu, Y. C., et al. (2021). Increased angiogenesis by exosomes secreted by adipose-derived stem cells upon lipopolysaccharide stimulation. Int. J. Mol. Sci. 22:8877. doi: 10.3390/ijms22168877
Wu, L. L., Pan, X. M., Chen, H. H., Fu, X. Y., Jiang, J., and Ding, M. X. (2020). Repairing and analgesic effects of umbilical cord mesenchymal stem cell transplantation in mice with spinal cord injury. Biomed. Res. Int. 2020:7650354. doi: 10.1155/2020/7650354
Xiao, Z., Tang, F., Zhao, Y., Han, G., Yin, N., Li, X., et al. (2018). Significant improvement of acute complete spinal cord injury patients diagnosed by a combined criteria implanted with neuroregen scaffolds and mesenchymal stem cells. Cell Transplant. 27, 907–915. doi: 10.1177/0963689718766279
Xie, P., Deng, M., Sun, Q. G., Ma, Y. G., Zhou, Y., Ming, J. H., et al. (2019). Therapeutic effect of transplantation of human bone marrow-derived mesenchymal stem cells on neuron regeneration in a rat model of middle cerebral artery occlusion. Mol. Med. Rep. 20, 3065–3074. doi: 10.3892/mmr.2019.10536
Xin, D., Li, T., Chu, X., Ke, H., Yu, Z., Cao, L., et al. (2020). Mesenchymal stromal cell-derived extracellular vesicles modulate microglia/macrophage polarization and protect the brain against hypoxia-ischemic injury in neonatal mice by targeting delivery of miR-21a-5p. Acta Biomater. 113, 597–613. doi: 10.1016/j.actbio.2020.06.037
Xin, H., Li, Y., Liu, Z., Wang, X., Shang, X., Cui, Y., et al. (2013). MiR-133b promotes neural plasticity and functional recovery after treatment of stroke with multipotent mesenchymal stromal cells in rats via transfer of exosome-enriched extracellular particles. Stem Cells 31, 2737–2746. doi: 10.1002/stem.1409
Xing, X., Han, S., Cheng, G., Ni, Y., Li, Z., and Li, Z. (2020). Proteomic analysis of exosomes from adipose-derived mesenchymal stem cells: a novel therapeutic strategy for tissue injury. Biomed. Res. Int. 2020:6094562. doi: 10.1155/2020/6094562
Xu, L., Ji, H., Jiang, Y., Cai, L., Lai, X., Wu, F., et al. (2020). Exosomes derived from circakap7-modified adipose-derived mesenchymal stem cells protect against cerebral ischemic injury. Front. Cell Dev. Biol. 8:569977. doi: 10.3389/fcell.2020.569977
Xu, Z., Tian, N., Li, S., Li, K., Guo, H., Zhang, H., et al. (2021). Extracellular vesicles secreted from mesenchymal stem cells exert anti-apoptotic and anti-inflammatory effects via transmitting microRNA-18b in rats with diabetic retinopathy. Int. Immunopharmacol. 101:108234. doi: 10.1016/j.intimp.2021.108234
Yamaguchi, S., Horie, N., Satoh, K., Ishikawa, T., Mori, T., Maeda, H., et al. (2018). Age of donor of human mesenchymal stem cells affects structural and functional recovery after cell therapy following ischaemic stroke. J. Cereb. Blood Flow Metab. 38, 1199–1212. doi: 10.1177/0271678X17731964
Yamazaki, K., Kawabori, M., Seki, T., Takamiya, S., Konno, K., Watanabe, M., et al. (2021). Mesenchymal stem cell sheet promotes functional recovery and palliates neuropathic pain in a subacute spinal cord injury model. Stem Cells Int. 2021:9964877. doi: 10.1155/2021/9964877
Yan, L., and Wu, X. (2020). Exosomes produced from 3D cultures of umbilical cord mesenchymal stem cells in a hollow-fiber bioreactor show improved osteochondral regeneration activity. Cell Biol. Toxicol. 36, 165–178. doi: 10.1007/s10565-019-09504-5
Yan, B., Zhang, Y., Liang, C., Liu, B., Ding, F., Wang, Y., et al. (2020). Stem cell-derived exosomes prevent pyroptosis and repair ischemic muscle injury through a novel exosome/circHIPK3/ FOXO3a pathway. Theranostics 10, 6728–6742. doi: 10.7150/thno.42259
Yang, Y., Cao, T. T., Tian, Z. M., Gao, H., Wen, H. Q., Pang, M., et al. (2020). Subarachnoid transplantation of human umbilical cord mesenchymal stem cell in rodent model with subacute incomplete spinal cord injury: preclinical safety and efficacy study. Exp. Cell Res. 395:112184. doi: 10.1016/j.yexcr.2020.112184
Yang, Y., Pang, M., Du, C., Liu, Z. Y., Chen, Z. H., Wang, N. X., et al. (2021). Repeated subarachnoid administrations of allogeneic human umbilical cord mesenchymal stem cells for spinal cord injury: a phase 1/2 pilot study. Cytotherapy 23, 57–64. doi: 10.1016/j.jcyt.2020.09.012
Yang, Q., Tong, X., Schieb, L., Vaughan, A., Gillespie, C., Wiltz, J. L., et al. (2017). Vital signs: recent trends in stroke death rates - United States, 2000–2015. Morb. Mortal. Wkly. Rep. 66, 933–939. doi: 10.15585/mmwr.mm6635e1
Ylöstalo, J. H., Bartosh, T. J., Coble, K., and Prockop, D. J. (2012). Human mesenchymal stem/stromal cells cultured as spheroids are self-activated to produce prostaglandin E2 that directs stimulated macrophages into an anti-inflammatory phenotype. Stem Cells 30, 2283–2296. doi: 10.1002/stem.1191
Yu, C., Yang, K., Meng, X., Cao, B., and Wang, F. (2020). Downregulation of long noncoding RNA MIAT in the retina of diabetic rats with tail-vein injection of human umbilical-cord mesenchymal stem cells. Int. J. Med. Sci. 17, 591–598. doi: 10.7150/ijms.38078
Yuan, O., Lin, C., Wagner, J., Archard, J. A., Deng, P., Halmai, J., et al. (2019). Exosomes derived from human primed mesenchymal stem cells induce mitosis and potentiate growth factor secretion. Stem Cells Dev. 28, 398–409. doi: 10.1089/scd.2018.0200
Yuan, X., Rosenberg, J. T., Liu, Y., Grant, S. C., and Ma, T. (2019). Aggregation of human mesenchymal stem cells enhances survival and efficacy in stroke treatment. Cytotherapy 21, 1033–1048. doi: 10.1016/j.jcyt.2019.04.055
Zaim, M., Karaman, S., Cetin, G., and Isik, S. (2012). Donor age and long-term culture affect differentiation and proliferation of human bone marrow mesenchymal stem cells. Ann. Hematol. 91, 1175–1186. doi: 10.1007/s00277-012-1438-x
Zang, J., Lu, D., and Xu, A. (2020). The interaction of circRNAs and RNA binding proteins: an important part of circRNA maintenance and function. J. Neurosci. Res. 98, 87–97. doi: 10.1002/jnr.24356
Zaverucha-do-Valle, C., Gubert, F., Bargas-Rega, M., Coronel, J. L., Mesentier-Louro, L. A., Mencalha, A., et al. (2011). Bone marrow mononuclear cells increase retinal ganglion cell survival and axon regeneration in the adult rat. Cell Transplant. 20, 391–406. doi: 10.3727/096368910X524764
Zaverucha-do-Valle, C., Mesentier-Louro, L., Gubert, F., Mortari, N., Padilha, A. B., Paredes, B. D., et al. (2014). Sustained effect of bone marrow mononuclear cell therapy in axonal regeneration in a model of optic nerve crush. Brain Res. 1587, 54–68. doi: 10.1016/j.brainres.2014.08.070
Zhai, L., Shen, H., Sheng, Y., and Guan, Q. (2021). ADMSC Exo-MicroRNA-22 improve neurological function and neuroinflammation in mice with Alzheimer’s disease. J. Cell Mol. Med. 25, 7513–7523. doi: 10.1111/jcmm.16787
Zhang, Y., Ma, L., Su, Y., Su, L., Lan, X., Wu, D., et al. (2019a). Hypoxia conditioning enhances neuroprotective effects of aged human bone marrow mesenchymal stem cell-derived conditioned medium against cerebral ischemia in vitro. Brain Res. 1725:146432. doi: 10.1016/j.brainres.2019.146432
Zhang, Y., Deng, H., Hu, Y., Pan, C., Wu, G., Li, Q., et al. (2019b). Adipose-derived mesenchymal stem cells stereotactic transplantation alleviate brain edema from intracerebral hemorrhage. J. Cell Biochem. 120, 14372–14382. doi: 10.1002/jcb.28693
Zhang, A., Jia, Z., Guo, X., and Yang, T. (2007). Aldosterone induces epithelial-mesenchymal transition via ROS of mitochondrial origin. Am. J. Physiol. Renal Physiol. 293, F723–731. doi: 10.1152/ajprenal.00480.2006
Zhang, J., Li, Z., Liu, W., Zeng, W., Duan, C., and He, X. (2020). Effects of bone marrow mesenchymal stem cells transplantation on the recovery of neurological functions and the expression of Nogo-A, NgR, Rhoa and ROCK in rats with experimentally-induced convalescent cerebral ischemia. Ann. Transl. Med. 8:390. doi: 10.21037/atm.2020.03.144
Zhang, Q., Liu, S., Li, T., Yuan, L., Liu, H., Wang, X., et al. (2016). Preconditioning of bone marrow mesenchymal stem cells with hydrogen sulfide improves their therapeutic potential. Oncotarget 7, 58089–58104. doi: 10.18632/oncotarget.11166
Zhang, Z., Sheng, H., Liao, L., Xu, C., Zhang, A., Yang, Y., et al. (2020). Mesenchymal stem cell-conditioned medium improves mitochondrial dysfunction and suppresses apoptosis in okadaic acid-treated SH-SY5Y cells by extracellular vesicle mitochondrial transfer. J. Alzheimers Dis. 78, 1161–1176. doi: 10.3233/JAD-200686
Zhang, Z., Wang, X., and Wang, S. (2008). Isolation and characterization of mesenchymal stem cells derived from bone marrow of patients with Parkinson’s disease. In vitro Cell Dev. Biol. Anim. 44, 169–177. doi: 10.1007/s11626-008-9093-1
Zhang, Y., Zhang, Y., Chopp, M., Pang, H., Zhang, Z. G., Mahmood, A., et al. (2021). MiR-17–92 cluster-enriched exosomes derived from human bone marrow mesenchymal stromal cells improve tissue and functional recovery in rats after traumatic brain injury. J. Neurotrauma 38, 1535–1550. doi: 10.1089/neu.2020.7575
Zhang, J., Zhang, Y., Ma, Y., Luo, L., Chu, M., Zhang, Z., et al. (2021). Therapeutic potential of exosomal circRNA derived from synovial mesenchymal cells via targeting circEDIL3/miR-485-3p/PIAS3/STAT3/VEGF functional module in rheumatoid arthritis. Int. J. Nanomedicine 16, 7977–7994. doi: 10.2147/IJN.S333465
Zhang, C., Zhou, C., Teng, J. J., Zhao, R. L., Song, Y. Q., and Zhang, C. (2009). Multiple administrations of human marrow stromal cells through cerebrospinal fluid prolong survival in a transgenic mouse model of amyotrophic lateral sclerosis. Cytotherapy 11, 299–306. doi: 10.1080/14653240902806986
Zhao, Y., Gan, Y., Xu, G., Hua, K., and Liu, D. (2020). Exosomes from MSCs overexpressing microRNA-223-3p attenuate cerebral ischemia through inhibiting microglial M1 polarization mediated inflammation. Life Sci. 260:118403. doi: 10.1016/j.lfs.2020.118403
Zhao, L. N., Ma, S. W., Xiao, J., Yang, L. J., Xu, S. X., and Zhao, L. (2021). Bone marrow mesenchymal stem cell therapy regulates gut microbiota to improve post-stroke neurological function recovery in rats. World J. Stem Cells 13, 1905–1917. doi: 10.4252/wjsc.v13.i12.1905
Zhao, C. P., Zhang, C., Zhou, S. N., Xie, Y. M., Wang, Y. H., Huang, H., et al. (2007). Human mesenchymal stromal cells ameliorate the phenotype of SOD1–G93A ALS mice. Cytotherapy 9, 414–426. doi: 10.1080/14653240701376413
Zheng, H., Chen, C., Zhang, J., and Hu, Z. (2016). Mechanism and therapy of brain edema after intracerebral hemorrhage. Cerebrovasc. Dis. 42, 155–169. doi: 10.1159/000445170
Zhou, X., Chu, X., Yuan, H., Qiu, J., Zhao, C., Xin, D., et al. (2019). Mesenchymal stem cell derived EVs mediate neuroprotection after spinal cord injury in rats via the microRNA-21-5p/FasL gene axis. Biomed. Pharmacother. 115:108818. doi: 10.1016/j.biopha.2019.108818
Zhou, Y., Wen, L. L., Li, Y. F., Wu, K. M., Duan, R. R., Yao, Y. B., et al. (2022). Exosomes derived from bone marrow mesenchymal stem cells protect the injured spinal cord by inhibiting pericyte pyroptosis. Neural Regen. Res. 17, 194–202. doi: 10.4103/1673-5374.314323
Zhou, C., Zhang, C., Zhao, R., Chi, S., Ge, P., and Zhang, C. (2013). Human marrow stromal cells reduce microglial activation to protect motor neurons in a transgenic mouse model of amyotrophic lateral sclerosis. J. Neuroinflammation 10:52. doi: 10.1186/1742-2094-10-52
Zhu, X., Wang, Z., Sun, Y. E., Liu, Y., Wu, Z., Ma, B., et al. (2022). Neuroprotective effects of human umbilical cord-derived mesenchymal stem cells from different donors on spinal cord injury in mice. Front. Cell Neurosci. 15:768711. doi: 10.3389/fncel.2021.768711
Zhu, X., Badawi, M., Pomeroy, S., Sutaria, D. S., Xie, Z., Baek, A., et al. (2017). Comprehensive toxicity and immunogenicity studies reveal minimal effects in mice following sustained dosing of extracellular vesicles derived from HEK293T cells. J. Extracell. Vesicles 6:1324730. doi: 10.1080/20013078.2017.1324730
Keywords: mesenchymal stem cells, extracellular vesicles, regenerative medicine, cell therapy, neurological diseases, neuroprotection
Citation: Soares MBP, Gonçalves RGJ, Vasques JF, da Silva-Junior AJ, Gubert F, Santos GC, de Santana TA, Sampaio GLA, Silva DN, Dominici M and Mendez-Otero R (2022) Current Status of Mesenchymal Stem/Stromal Cells for Treatment of Neurological Diseases. Front. Mol. Neurosci. 15:883378. doi: 10.3389/fnmol.2022.883378
Received: 25 February 2022; Accepted: 19 May 2022;
Published: 16 June 2022.
Edited by:
Johannes Boltze, University of Warwick, United KingdomReviewed by:
Anna Andrzejewska, Mossakowski Medical Research Centre (PAN), PolandDominik Egger, University of Natural Resources and Life Sciences Vienna, Austria
Copyright © 2022 Soares, Gonçalves, Vasques, da Silva-Junior, Gubert, Santos, de Santana, Sampaio, Silva, Dominici and Mendez-Otero. This is an open-access article distributed under the terms of the Creative Commons Attribution License (CC BY). The use, distribution or reproduction in other forums is permitted, provided the original author(s) and the copyright owner(s) are credited and that the original publication in this journal is cited, in accordance with accepted academic practice. No use, distribution or reproduction is permitted which does not comply with these terms.
*Correspondence: Renata G. J. Gonçalves, cmVuYXRhLmd1ZWRlc0BiaW9mLnVmcmouYnI=
† These authors have contributed equally to this work and share first authorship
‡ These authors share senior authorship