- 1Laboratory for Molecular Biodiscovery, KU Leuven, Leuven, Belgium
- 2Department of Neuropathology, Amsterdam Neuroscience, Amsterdam UMC, University of Amsterdam, Amsterdam, Netherlands
- 3Department of Clinical and Experimental Epilepsy, UCL Queen Square Institute of Neurology, London, United Kingdom
- 4Chalfont Centre for Epilepsy, Chalfont St Peter, United Kingdom
- 5Department of Medicinal Chemistry, Faculty of Pharmacy, Jagiellonian University Medical College, Kraków, Poland
- 6UCB Pharma, Braine-l’Alleud, Belgium
Synaptic vesicle glycoprotein 2A (SV2A) regulates action potential-dependent neurotransmitter release and is commonly known as the primary binding site of an approved anti-epileptic drug, levetiracetam. Although several rodent knockout models have demonstrated the importance of SV2A for functional neurotransmission, its precise physiological function and role in epilepsy pathophysiology remains to be elucidated. Here, we present a novel sv2a knockout model in zebrafish, a vertebrate with complementary advantages to rodents. We demonstrated that 6 days post fertilization homozygous sv2a–/– mutant zebrafish larvae, but not sv2a+/– and sv2a+/+ larvae, displayed locomotor hyperactivity and spontaneous epileptiform discharges, however, no major brain malformations could be observed. A partial rescue of this epileptiform brain activity could be observed after treatment with two commonly used anti-epileptic drugs, valproic acid and, surprisingly, levetiracetam. This observation indicated that additional targets, besides Sv2a, maybe are involved in the protective effects of levetiracetam against epileptic seizures. Furthermore, a transcriptome analysis provided insights into the neuropathological processes underlying the observed epileptic phenotype. While gene expression profiling revealed only one differentially expressed gene (DEG) between wildtype and sv2a+/– larvae, there were 4386 and 3535 DEGs between wildtype and sv2a–/–, and sv2a+/– and sv2a–/– larvae, respectively. Pathway and gene ontology (GO) enrichment analysis between wildtype and sv2a–/– larvae revealed several pathways and GO terms enriched amongst up- and down-regulated genes, including MAPK signaling, synaptic vesicle cycle, and extracellular matrix organization, all known to be involved in epileptogenesis and epilepsy. Importantly, we used the Connectivity map database to identify compounds with opposing gene signatures compared to the one observed in sv2a–/– larvae, to finally rescue the epileptic phenotype. Two out of three selected compounds rescued electrographic discharges in sv2a–/– larvae, while negative controls did not. Taken together, our results demonstrate that sv2a deficiency leads to increased seizure vulnerability and provide valuable insight into the functional importance of sv2a in the brain in general. Furthermore, we provided evidence that the concept of connectivity mapping represents an attractive and powerful approach in the discovery of novel compounds against epilepsy.
Introduction
Epilepsy, affecting individuals of all ages regardless of gender or socio-economic status, is a chronic brain disease characterized by an ongoing liability to unprovoked recurrent epileptic seizures due to excessive, hypersynchronous discharges of neurons in the brain (Stafstrom and Carmant, 2015). Epilepsy can be a result of an insult to the brain such as injury, stroke, central nervous system (CNS) infections, and vascular or congenital brain malformations (Engel, 2001; Singh and Trevick, 2016), or can be a consequence of a genetic variant in one or more proteins that control brain excitability. Although methods such as surgery, vagus nerve stimulation, and dietary changes have been employed to treat epilepsy, anti-epileptic drugs (AEDs) are the most commonly used treatment approach. Current marketed AEDs consist of a variety of structural classes acting through a range of different mechanisms, for instance by modulation of voltage-gated ion channels, glutamate-mediated excitatory neurotransmission, and GABA-mediated inhibitory pathways (Löscher, 2017). In contrast, levetiracetam primarily exerts its antiseizure activity by selectively binding to synaptic vesicle glycoprotein 2A (SV2A) in the brain (Loscher et al., 2016). Levetiracetam is a first-line AED clinically used for focal, myoclonic, and generalized tonic-clonic seizures. Additionally, it has been used in an off-label manner for the treatment of status epilepticus (SE) and other non-epileptic conditions such as movement disorders and neuropathic pain (Abou-Khalil, 2008; Crepeau and Treiman, 2010; Meehan et al., 2011), as well as it could possibly be effective in patients with Alzheimer’s disease to improve cognitive symptoms (Kong et al., 2021; Vossel et al., 2021).
The SV2 family is well conserved among vertebrates (Chang and Sudhof, 2009) and in mammals consists of SV2A, SV2B, and SV2C (Sills, 2010). All members are integral membrane proteins composed of a highly N-glycosylated 80 kDa backbone comprised of 12 transmembrane (TM) helices organized in 2 TM domains, two large loops (cytoplasmic and intravesicular) differing among the isoforms, and N- and C-terminal cytoplasmic sequences (Bartholome et al., 2017). Multiple N-glycosylation sites appear to be essential for proper folding and correct trafficking of SV2 in the synapses (Scott and Panin, 2014). SV2 isoforms are not neurotransmitter-specific and have varying distribution in the brain (Bartholome et al., 2017) with SV2A being the most abundant and ubiquitously expressed in almost all the neurons, and also found in neuroendocrine cells and neuromuscular junctions (Crèvecœur et al., 2013). SV2A functions as a synaptic vesicle glycoprotein essential for neurotransmitter release. Specifically, SV2A modulates the readily available synaptic vesicle pool and synaptic vesicle size (Xu and Bajjalieh, 2001). It is also involved in calcium-dependent neurotransmitter exocytosis by regulating the expression and trafficking of a synaptic vesicle calcium sensor protein, synaptogamin (Yao et al., 2010), and contributing to a maturation step of primed vesicles rendering them competent for release (Nowack et al., 2010).
Nevertheless, the precise physiological role of SV2A remains to be elucidated. Studies in animal models demonstrated that SV2A loss-of-function caused premature mortality and severe epilepsy. SV2A knockout mice appeared normal at birth, but exhibited spontaneous fatal seizures after 1–2 weeks, without any obvious abnormalities in the brain or synapse morphology, followed by death within 3 weeks (Crowder et al., 1999; Janz et al., 1999; Menten-Dedoyart et al., 2016). Partial SV2A deficiency in SV2A heterozygous mice did not cause spontaneous seizure activity, however, it enhanced drug-induced seizure vulnerability and accelerated epileptogenesis (Kaminski et al., 2009). Similarly, homozygous rats carrying an SV2A-targeted missense mutation exhibited normal development but were susceptible to PTZ induced-seizures and kindling development (Tokudome et al., 2016). Additionally, a mutation in SV2A caused photosensitive reflex epilepsy in the Fepi chicken model (Douaud et al., 2011). Furthermore, electrophysiological studies on hippocampal slices from wildtype and SV2A knockout mice indicated that deletion of SV2A had more of a suppressive effect on the frequency and amplitude of inhibitory postsynaptic currents (IPSC) than on excitatory currents, likely resulting in seizure onset and epilepsy (Bartholome et al., 2017). A decreased SV2A expression was also found in rodent models of temporal lobe epilepsy (TLE), further supporting the hypothesis that SV2A deficiency may contribute to epileptogenic progression and seizure initiation (Gorter et al., 2006; De Smedt et al., 2007). Dysfunction of SV2A impaired the synaptic GABA release by reducing the synaptogamin 1 levels, highlighting the imbalance between excitatory and inhibitory neurotransmission in modulating the epileptogenic processes (Crowder et al., 1999; Tokudome et al., 2016).
In humans, only three variants have been reported to link SV2A to epilepsy. A homozygous R383Q missense mutation in SV2A gene resulted in infantile-onset intractable epilepsy, involuntary movements, microcephaly, and developmental delay (Serajee and Huq, 2015). Moreover, recently also rare heterozygous variants (R570C and G660R) were found in two infantile patients with drug-resistant epilepsy with myoclonic and generalized clonic-tonic seizures (Wang et al., 2019; Calame et al., 2021). Treatment with levetiracetam did not alleviate the patients’ seizures or surprisingly even worsened the epileptic symptoms until treatment discontinuation (Wang et al., 2019; Calame et al., 2021). Furthermore, clinical studies investigated the importance of SV2A in normal brain functioning and its pathogenic potential in the case of genetic disorders (Bartholome et al., 2017). For instance, reduced SV2A expression was observed in epileptic foci resected from patients with drug-resistant TLE (Feng et al., 2009), TLE with hippocampal sclerosis (van Vliet et al., 2009), focal cortical dysplasia, and tuberous sclerosis complex (Toering et al., 2009).
Although traditional drug discovery strategies, including either molecular (target-based) or empirical (phenotypical) approaches, have led to the development of about 30 AEDs with diverse molecular targets, there are still many challenges in the pharmacological treatment of epilepsy. Recent advances in network-oriented approaches and “omics” technologies have led to a range of novel computational strategies being orthogonal to current concepts of drug discovery (Musa et al., 2018). One of these emerging approaches is called ‘connectivity mapping’ whereby potential compounds are judged not by their binding affinity to a particular target or reversion of a certain phenotype, but by their ability to induce a transcriptional response opposite to the one underpinning the disease state (Lamb et al., 2006). For this, large reference perturbation databases (e.g., CMap and LINCS) containing transcriptome profiles of dozens of cultivated cell lines treated with thousands of chemical compounds were created, that can be queried by researchers worldwide. Since the first introduction of the concept and CMap database in 2006, several successful applications have been reported in distinct disease areas (Ishimatsu-Tsuji et al., 2010; Li H. et al., 2020; Scott et al., 2020). For instance, recently connectivity mapping identified metformin, nifedipine, and pyrantel tartrate as candidate anti-epileptics, which was confirmed in a larval zebrafish seizure model (Brueggeman et al., 2019).
Zebrafish are lower vertebrates that possess many advantages over classical rodent models including high genetic and physiological homology to humans, high fecundity, external fertilization, transparency through early larval stages that enables powerful imaging techniques, and ease of various genetic manipulations (Hortopan et al., 2010; Copmans et al., 2017). Due to these attributes, they have successfully been deployed in large-scale, systematic drug discovery screens to identify small molecules that can suppress disease phenotypes (MacRae and Peterson, 2015; Rennekamp and Peterson, 2015; Patton et al., 2021). Importantly, zebrafish are an established model for studying epilepsy disorders (Copmans et al., 2017; Yaksi et al., 2021). The development of mutant zebrafish models in recent translational research has allowed rapid functional determination of candidate epilepsy genes (Baraban et al., 2013; Samarut et al., 2018; Swaminathan et al., 2018; Siekierska et al., 2019).
In the current study, we explored the role of SV2A in the brain development and pathophysiology of epilepsy using an in vivo zebrafish model. To that end, we disrupted the sv2a function in zebrafish by generating a knockout line using CRISPR/Cas9 technology. Our results demonstrated that sv2a deficiency leads to premature death, hyperactivity, and severe spontaneous epileptiform discharges. Pharmacological validation of the sv2a model demonstrated a partial rescue of the epileptiform discharges after treatment with levetiracetam, indicating that other mechanisms, in addition to Sv2a, are likely to be involved. Moreover, transcriptome analysis identified dysregulated genes in several pathways essential for correct brain development and involved in epileptogenesis. Furthermore, we successfully applied connectivity mapping to identify compounds that reverted the epileptic phenotype of sv2a–/– larvae. Altogether, our results provide causative factors for SV2A loss-of-function epileptogenesis and indicate promising alternative directions in the discovery of AEDs.
Materials and Methods
Zebrafish Husbandry
Adult zebrafish (Danio rerio) were maintained in a UV-sterilized recirculating system equipped with a mechanical and biological filtration unit and kept under a 14/10 h light/dark cycle at the temperature of 27–28°C and a pH of 6.8–7.5. Water quality was monitored for pH, temperature, conductivity, ammonia, nitrite (SL1000 Portable Parallel Analyzer, Hach Instruments, United States), and nitrate levels (Tetra, Melle, Germany). Zebrafish were fed three times per day with flake food (TetraMin, Tetra, Germany) and Artemia (brine shrimp). Embryos were obtained via natural spawning and were kept in petri dishes (92 × 16 mm, Sarstedt, Nümbrecht, Germany) at 28°C in a Peltier-cooled incubator (IPP 260, Memmert, Schwabach, Germany) in Danieau’s medium (1.5 mM HEPES, pH 7.2, 17.4 mM NaCl, 0.21 mM KCl, 0.12 mM MgSO4, 0.18 mM Ca(NO3)2 and 0.6 μM methylene blue). All zebrafish experiments were approved by the Ethics Committee of the KU Leuven (P023/2017 and P027/2019) and by the Belgian Federal Department of Public Health, Food Safety, and Environment (LA1210199).
Establishing sv2a Knockout Zebrafish Line Using CRISPR/Cas9 Method
Single-guide RNA (sgRNA) targeting the antisense strand of zebrafish sv2a exon 2 (5′-GTGGACCCTCTACTTTGTGC(GGT)-3′) was designed and synthesized by GeneArt® (Thermo Fisher Scientific). sv2a sgRNA mRNA was transcribed using MegaScript T7 Kit (Ambion), DNase treated (TURBO DNase, Life Technologies) and was purified with MEGAclear Transcription Clean-Up Kit (Ambion). Cas9 nuclease mRNA was purchased from GeneArt (Invitrogen). Next, a mix of 150 pg Cas9 mRNA and 7 pg sv2a sgRNA in 1 nl volume was co-injected into early one-cell stage wildtype embryos of AB line using a Femtojet 4i pressure microinjector (Eppendorf) and a M3301R Manual Micromanipulator (WPI). The injected embryos were raised till adulthood. To identify founders with germline transmitted mutation and high rate of indel mutations, each of the F0 CRISPR/Cas9-injected adults was outcrossed with a wildtype. F1 offspring was collected, individually lysed to obtain genomic DNA for subsequent PCR amplification with primers flanking the potential mutated target site, screened by Sanger sequencing (LGC Genomics, Germany), and analyzed with SeqMan Pro software (DNASTAR, Lasergene). F1 generation embryos of the selected F0 founder were raised to adulthood, fin clipped and Sanger sequenced. Individuals carrying the same mutation (1 bp insertion of G at CRISPR site) were identified and pooled together. All experiments were performed on F2 generation adults.
Genotyping of the Larvae Using High Resolution Melting Analysis
To extract genomic DNA, a fin clip of a larva was placed in a separate tube with 20 μl of 50 mM NaOH and heated at 95°C for 10 min, followed by neutralization with 100 mM Tris HCl (pH 8.0) (1/10 volume). Lysed samples were genotyped by performing a PCR reaction with Precision Melt Supermix for high resolution melting (HRM) analysis (Bio-Rad #172-5112) and sv2a-specific primers (Supplementary Table 1) in a CFX96 touch RT-PCR detection system (Bio-Rad) using Hardshell® Low Profile Thin-wall 96-well skirted PCR plates (Bio-Rad). Curves were analyzed using the Precision Melt Analysis™ Software (Bio-Rad). Genotypes of the individual larvae clustering together were confirmed by Sanger sequencing (LGC Genomics, Germany) and were analyzed using SeqMan software (DNASTAR, Lasergene).
Survival Assay and Morphology
Larvae were cultured in Danieau’s medium and scored daily up to 10 days post fertilization (dpf) for any morphological or behavioral abnormalities (e.g., pericardial edema, head, eye, or jaw malformations, bent spine, and lack of touch response) and lethality. The larvae were photographed using a stereomicroscope (Leica MZ10 F) equipped with a digital camera (DFC310 FX) and Leica Application Suite software (version 3.6.0). Dead larvae were immediately collected for genotyping as described before.
Locomotor Activity Assessment
6 dpf zebrafish larvae were transferred individually into a 96-well plate containing 100 μl Danieau’s medium per well. After positioning the plate inside a DanioVision box (Noldus, Netherlands), larval behavior was recorded for 10 min in the light after 30 min habituation period in the dark. Ethovision XT16 (Noldus) was used to quantify locomotor behavior as total velocity (mm/s).
Non-invasive Local Field Potential Recordings
Electrographic brain activity of 5 and 6 dpf larvae was assessed by non-invasive local field potential (LFP) recordings from the optic tectum as described previously (Heylen et al., 2021). A blunt glass electrode (soda lime glass, Hilgenberg, Germany) pulled with DMZ Universal Puller (Zeitz, Germany) to an opening of approximately 15–20 microns, connected to a high-impedance amplifier, was filled with artificial cerebrospinal fluid (124 mM NaCl, 2 mM KCl, 2 mM MgSO4, 2 mM CaCl2, 1.25 mM KH2PO4, 26 mM NaHCO3 and 10 mM glucose) and positioned on the skin above the optic tectum of a larva embedded in 2% low-melting point agarose (Thermo Fisher Scientific). The differential signal between the recording electrode and the reference electrode was amplified 10,000 times by EXT-02F/2 extracellular amplifier (NPI Electronic), band pass filtered at 3–300 Hz and digitized at 2 kHz via a PCI-6251 interface (National Instruments) with WinEDR (John Dempster, University of Strathclyde). A HumBug noise eliminator (Quest Scientific) was used to remove 50–60 Hz noise. Spontaneous discharges were considered as epileptiform events when their amplitudes exceeded three times the baseline and lasted for at least 100 ms. Each recording lasted for 10 min and was visualized with Clampfit 10.2 software (Molecular Devices Corporation). In order to quantify the signal from epileptiform brain discharges, we employed a specifically developed software (Li J. et al., 2020). In brief, LFP recordings were examined by Welch’s power spectral density (PSD) analysis, using 100 ms long windows extracted with a Hamming window and 80% overlap. The window length was chosen considering that an epileptiform discharge may last as short as 50–100 ms. Subsequently, the average spectral power in consecutive 10 Hz frequency bands (i.e., 0–10 Hz, 10–20 Hz, 140–150 Hz) was computed. The magnitude of the average PSD values gives an indication about the number of epileptiform discharges throughout the recordings in each frequency band.
Pharmacological Evaluation
Sodium valproate, levetiracetam, CAY-10415, digoxin, and calmidazolium HCl were purchased from Sigma. Alvocidib, AS-605240, and PD-98059 were purchased from Selleckchem. All compounds were dissolved in dimethyl sulfoxide (DMSO) and kept at −20°C. For experiments, stock solutions were diluted in Danieau’s medium to achieve a final DMSO concentration of 1% v/v. As a vehicle (VHC) control 1% DMSO in Danieau’s medium was used. Before performing pharmacological experiments, maximum tolerated concentration (MTC) of the compounds was determined as described previously (Zhang et al., 2017): sodium valproate 500 μM, levetiracetam 7 mM, alvocidib 2 μM, PD-98059 12 μM, AS-605240 800 nM, CAY-10415 8 μM, digoxin 4 μM, and calmidazolium HCl 1 μM. In brief, 12 larvae of 5 dpf were transferred individually into a 96-well plate containing 100 μl volume per well and exposed to a certain concentration (twofold dilution series, starting from the highest soluble concentration). After 22-h exposure, the following parameters were investigated: touch response, morphology, posture, edema, signs of necrosis, presence of swim bladder and heartbeat. The MTC was defined as the highest soluble concentration at which no larvae died nor showed signs of toxicity or locomotor impairment in comparison to VHC-treated control larvae. For pharmacological evaluation, larvae were treated with VHC or compounds at their respective MTC 22 h prior to an experiment.
Hematoxylin and Eosin Staining
Hematoxylin and eosin staining was performed on 6 dpf larvae as described previously (Siekierska et al., 2019; Partoens et al., 2021). Larvae were anesthetized in 0.008% tricaine and fin clipped for genotyping. Subsequently, they were fixed in 4% paraformaldehyde (PFA) and kept in 70% ethanol. At least four larvae per genotype were embedded in 1% agarose (Invitrogen) in 1x TAE buffer (Thermo Fisher Scientific). A customized mold was utilized to align zebrafish larvae and produce agarose blocks with identically distributed wells of the same depth. Agarose blocks were put in an enclosed automated tissue processor (Shandon Excelsior ES, Thermo Fisher Scientific) for overnight dehydration and embedded in paraffin. Larval heads were sectioned using a HM 325 manual rotary microtome (Thermo Fisher Scientific) at a thickness of 5 μm and sections were applied to microscope slides. Slides were stained with hematoxylin and eosin using Varistain™ Gemini ES Automated Slide Stainer (Thermo Fisher Scientific) according to laboratory protocols. The resulting sections were imaged at 20× and 40× magnification in SPOT 5.1 software (SPOT Imaging) by a SPOT-RT3 camera mounted on a Leica microscope. Four equivalent sections were selected for each genotype, and hematoxylin-positive stained nuclei were counted using QuPath (0.1.2) software to support size difference. The results were expressed as average hematoxylin-positive stained nuclei within a selected brain area.
RNA Sequencing
For RNA sequencing, 6 dpf zebrafish larvae were anesthetized and decapitated using a razor blade. 10 heads were pooled in quadruplicate per genotype and RNA was extracted as described below. RNA concentration and purity were determined spectrophotometrically using the NanoDrop ND-1000 (NanoDrop Technologies) and RNA integrity was assessed using a Bioanalyser 2100 (Agilent). A total of 500 ng of total RNA per sample was used as input for sample preparation. Library preparation and sequencing were completed by VIB nucleomics core.1 TruSeq RNA-Seq sample preparation kit was used to prepare sequencing library of mRNA in accordance with the manufacturer guidelines. This library was subjected to single-end sequencing on a NextSeq500 v2 High75 flow-cell. Sequencing data are available in the ArrayExpress database2 under accession number E-MTAB-11505.
Bioinformatic Analysis
Read quality was assessed using ShortRead 1.36.1 package from Bioconductor3 (Morgan et al., 2009). Low quality ends (<Q20) and adapters (at least 10 nt overlap and 90% match) were trimmed using FastX 0.0.14 (Hannon, 2010) and cutadapt 1.15 (Martin, 2011), respectively. If any of the reads dropped below 35 nt in length they were excluded. Poly-A reads (more than 90% of the bases equaling A), ambiguous reads and low quality reads (more than 50% of the bases < Q25) were removed with FastX 0.0.14 and ShortRead 1.36.1. Using bowtie 2.3.3.1 (Langmead and Salzberg, 2012), reads that aligned to phix illumina were identified and removed. Reads were aligned to the zebrafish reference genome, GRCz11, using STAR 2.5.2b (Dobin et al., 2013). The aligned reads were then passed to samtools 1.5 (Li et al., 2009) for quality filtering, sorting and indexing. Quantification of expression levels was performed with featureCounts 1.5.3 (Liao et al., 2014). The unnormalized RNA count matrix was passed on to the R package DESeq2 (Love et al., 2014). Genes were considered expressed if it had 1 counts-per-million in at least one condition. Gene expression changes with a Benjamini-Hochberg adjusted p-value < 0.05 were considered statistically significant.
Gene Ontology and Pathway Enrichment Analysis
The Gene Ontology (GO) knowledgebase (Ashburner et al., 2000) and Kyoto Encyclopedia of Genes and Genome (KEGG) (Kanehisa and Goto, 2000) for zebrafish were queried to test DEGs for gene ontology and pathway enrichment, respectively, using the Enrichr package (Chen et al., 2013). Enriched GO term lists containing the top 10 terms amongst molecular function, cellular component and biological process were processed using the ‘Enrichment Map’ plugin for Cytoscape4 to create a visual representation of the GO enrichment (Isserlin et al., 2014).
RNA Extraction and RT-qPCR Analysis
Total RNA was extracted using TRIzol reagent (Invitrogen), followed by phenol-chlorophorm extraction and isopropanol precipitation. After ethanol washes, the RNA pellet was air-dried, dissolved in nuclease-free water (Fermentas) and treated with RNase-free DNase (Roche) to remove possible genomic DNA contamination. Reverse transcription of 2 μg of total RNA to single-stranded cDNA was performed using random primers and SuperScript III Reverse Transcriptase (Invitrogen) according to the manufacturer’s instructions. Next, the generated cDNA was diluted (1:20) and amplified using gene specific primers (Supplementary Table 1) and 2x SsoAdvanced Universal SYBR Green Supermix (Bio-Rad) in HardShell® Low-Profile Thin-Wall 96-Well Skirted PCR Plates (Bio-Rad) on CFX96 Touch Real-Time PCR Detection System (Bio-Rad) under cycling conditions according to the manufacturer’s protocol. The relative expression levels were quantified using the comparative Cq method (ΔΔCq) with CFX Maestro software (Bio-Rad). Transcripts were normalized against 18s housekeeping gene that was experimentally determined to have the most stable expression in our reaction conditions.
Connectivity Map Analysis
Connectivity map (CMap) uses cellular responses to genetic and chemical perturbations to connect diseases with genes underlying them and therapeutics to treat them (Lamb et al., 2006). After converting zebrafish identifiers to their human orthologs using BioMart (Durinck et al., 2009), the top 50 up- and top 50 down-regulated genes in sv2a–/– zebrafish sorted by Wald statistic were fed into the CMap query application. These were then compared to the CMap library containing over one and a half million gene expression signatures derived from the L1000 platform, a high-throughput gene expression assay, to identify compounds reversing the entered disease expression profile. Each compound was assigned a connectivity score between −100 and 100 for the supplied list: while a positive score indicates a similarity between a given compound signature and that of the query, a negative score indicates that the two signatures are opposing. Compounds targeting master regulators of cellular homeostasis and proliferation, such as “DNA synthesis,” “RNA synthesis,” “tRNA synthetase,” “RNA polymerase,” and “protein synthesis,” were not considered for further investigation. Based on availability, three compounds with opposing gene signatures (scores < −90), together with three compounds with highly similar or non-matching signatures (scores > 90 or equaling 0, negative controls) were selected for further testing, as described previously (see Pharmacological evaluation).
Statistical Analysis
Unpaired Student’s t-test was used to compare means between two groups; for three or more groups one-way or two-way ANOVA followed by Dunnett’s or Sidak’s multiple comparison test, respectively, was used. Log-rank (Mantel-Cox) test was used for Kaplan-Meier curves analysis. Outliers were identified using the ROUT method (Q = 1%). Statistical analyses were performed using GraphPad Prism 9. Significance of all statistical comparisons was set at p < 0.05.
Results
CRISPR/Cas9-Mediated Generation of sv2a Loss-of-Function in Zebrafish
Zebrafish sv2a gene is the only ortholog of the human SV2A gene. It displays 79.6% overall amino acid identity with the human protein and all important ligand (levetiracetam) binding residues are conserved (Supplementary Figure 1A; Lee et al., 2015). qPCR analysis of sv2a expression during 1-7 dpf in wildtype zebrafish larvae revealed that sv2a was already detectable at first day of embryonic development and its expression gradually increased reaching a plateau at 3-4 dpf (Figure 1A). In addition, the affinity of levetiracetam for the zebrafish Sv2a protein was evaluated in vitro and is in line with values reported for recombinant and native human and rat SV2A (Supplementary Figure 1B; Gillard et al., 2006; Gillard et al., 2011; Wood et al., 2020). Therefore, zebrafish is a relevant in vivo model to investigate the function of SV2A. To generate a knockout zebrafish model with loss of sv2a function, we targeted early exon 1 of the sv2a transcript (ENSDART00000172232.2), located in the synaptogamin-binding domain (Figure 1B). We simultaneously injected sv2a-specific sgRNA targeting exon 2 and Cas9 mRNA into single-cell zebrafish embryos. We selected a positive founder carrying a 1-bp (G) insertion, which results in a frameshift of the sv2a transcript leading to a premature stop codon at position 186 (Figure 1B). We confirmed the mutated genomic sequence by Sanger sequencing and HRM assay (Figure 1C). The selected founder was outcrossed with a wildtype adult zebrafish and F1 generation offspring bearing the frameshift mutation was pulled together to generate F2 offspring, which was used to perform the experiments. Via RT-qPCR, we confirmed that there was a reduction of sv2a mRNA by 80% in sv2a–/– larvae at 6 dpf and approximately half of the transcript present in sv2a+/– larvae (Figure 1D), proving the efficiency of the knockout likely leading to a full loss of Sv2a function in homozygous zebrafish.
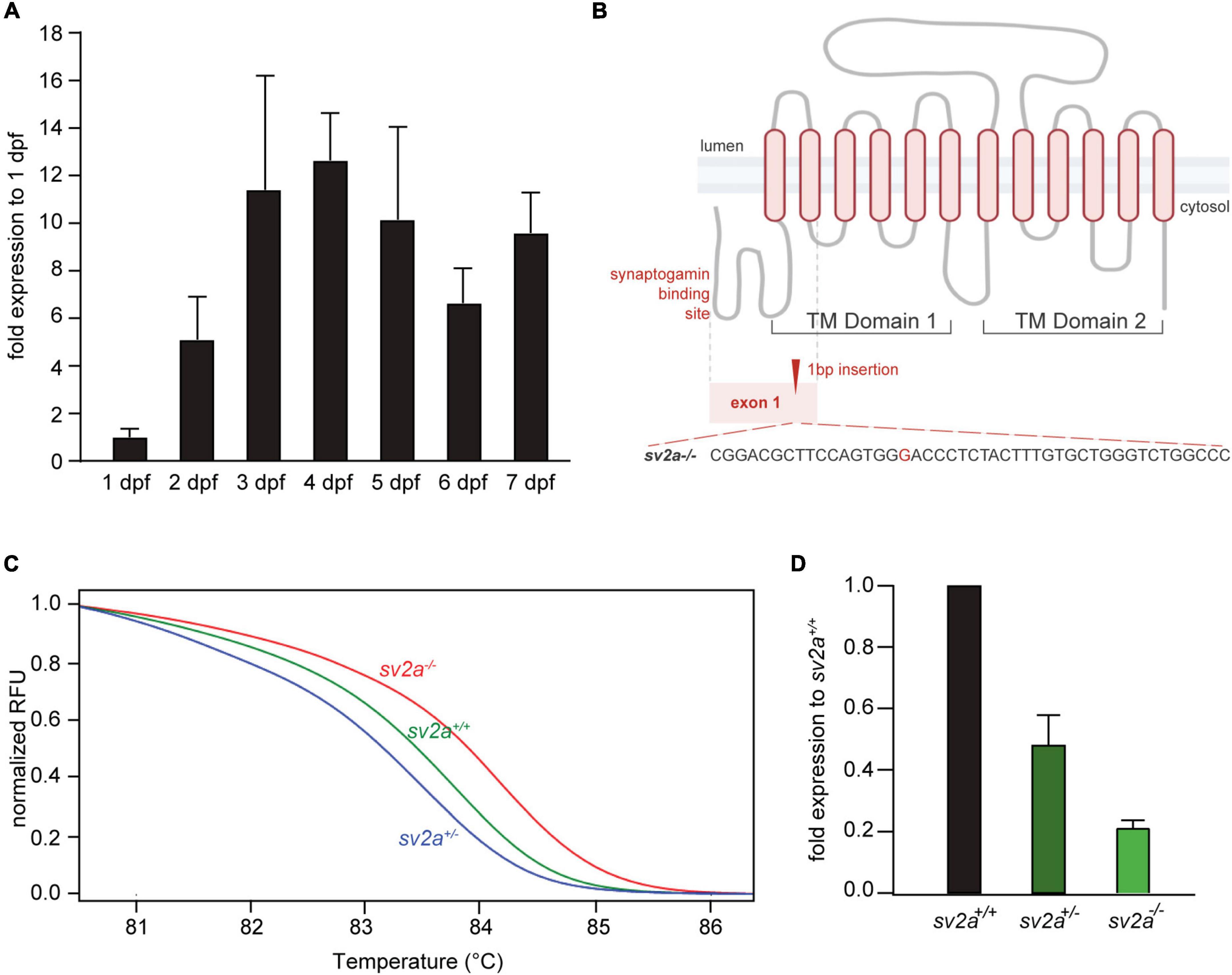
Figure 1. sv2a expression in developing zebrafish larvae (1-7 dpf) and CRISPR-mediated sv2a knockout in zebrafish. (A) qPCR analysis of sv2a mRNA levels in wildtype larvae normalized to 18s and represented as the fold expression to 1 dpf. Values are reported as mean ± SD of three independent experiments. (B) Schematic representation of the SV2A protein with the two distinct transmembrane (TM) domains and the cytosolic synaptogamin binding site. Gray dashed line indicates exon 1 encoding synaptogamin binding domain and the first two TM helices. Red dashed line magnifies the region with the 1 nucleotide insertion (G) at the target site in exon 1. (C) High resolution melting (HRM) curve analysis discriminates sv2a+/+, sv2a+/–, and sv2a–/– zebrafish larvae at 6 dpf. (D) qPCR analysis of sv2a mRNA levels in sv2a+/+, sv2a+/–, and sv2a–/– larvae at 6 dpf normalized to 18s and represented as the fold expression to sv2a+/+ larvae. Values are reported as the mean ± SD of three independent experiments.
sv2a Loss-of-Function Leads to Early Lethality in Zebrafish Embryos
Homozygous sv2a–/– mutant larvae had a normal appearance without major morphological abnormalities. The majority of the larvae at 6 dpf displayed some minor defects such as lack of swim bladder, slight curvature on the body axis, and, interestingly, darker pigmentation (Figure 2A). Heterozygous sv2a+/– larvae were morphologically similar to their wildtype siblings (Figure 2A). We also did not find any significant difference in body length between the different genotypes (Figure 2B, data shown at 6 dpf). The survival analysis demonstrated that sv2a–/– larvae died prematurely between 8 and 10 dpf (Figure 2C), whereas their wildtype and heterozygous siblings could be raised until adulthood. Subsequently, we conducted behavioral tracking to quantify the individual larval movement as total velocity (mm/s). Interestingly, sv2a–/– larvae were hyperactive in comparison to sv2a+/– and sv2a+/+ larvae (Figure 2D). Of note, other parameters, including total activity (%), total mobility (%) and total distance moved (mm), were assessed as well and showed a similar elevation in sv2a–/– larvae as observed for total velocity (mm/s) (data not shown).
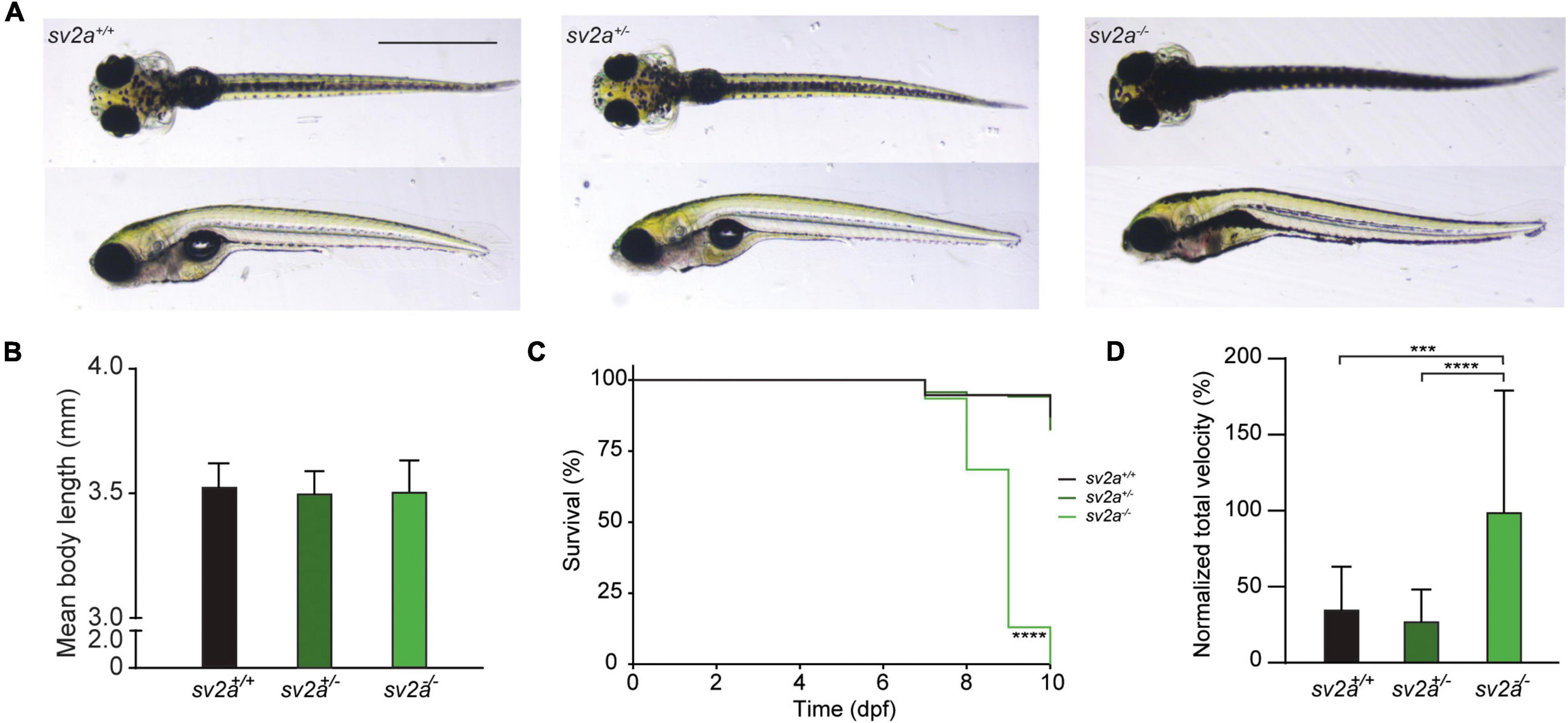
Figure 2. Morphological, survival and behavioral analysis of sv2a knockout zebrafish. (A) Representative images of sv2a+/+, sv2a+/–, and sv2a –/– zebrafish larvae at 6 dpf. Scale bar 1 mm. (B) Comparison of mean body length of sv2a+/+ (n = 8), sv2a+/– (n = 8), and sv2a –/– (n = 8) zebrafish larvae at 6 dpf (mean ± SD). (C) Kaplan-Meier curve demonstrating survival of sv2a+/+ (n = 94), sv2a+/– (n = 208), and sv2a–/– (n = 92) zebrafish larvae. Significant larval death in sv2a–/– larvae can be observed in a time-dependent manner. Statistical analysis was performed using Log-rank (Mantel-Cox) test (****p < 0.0001). (D) Locomotor activity of sv2a+/+ (n = 15), sv2a+/– (n = 53), and sv2a–/– (n = 40) zebrafish larvae at 6 dpf. Data were assessed over the total tracking period of 10 min, normalized to sv2a–/– larvae, and expressed as normalized total velocity (%) (mean ± SD). Statistical analysis was performed by one-way ANOVA followed by Dunnett’s multiple comparison (***p < 0.001, ****p < 0.0001). Data were collected from four independent experiments.
sv2a Knockout Zebrafish Larvae Display Spontaneous Electrographic Seizures
To investigate whether sv2a knockout resulted in abnormal brain activity, we recorded LFP on larval optic tecta of 6 dpf sv2a–/–, sv2a+/–, and sv2a+/+ larvae. Epileptiform events consisted of polyspiking bursts with amplitudes equal to or exceeding threefold the baseline (Figure 3A). To quantify these events, we performed a PSD analysis by computing average power in consecutive 10 Hz frequency bands ranging from 1 to 150 Hz and normalized against sv2a+/+ larvae (Figure 3B). sv2a–/– larvae had a significantly higher PSD within the 20 and 50 Hz frequency range (Figure 3B), confirming the frequency band characterizing polyspiking discharges (Schubert et al., 2014). PSDs, plotted as mean PSD per condition over the 20–50 Hz region (Figure 3C), confirmed that there was a significant increase (p < 0.05) in the PSD values for sv2a–/– larvae in comparison to sv2a+/– and sv2a+/+ siblings. The morphological, behavioral, and epileptic phenotype of sv2a–/– CRISPR was fully recapitulated and confirmed in the sv2a morphants generated by an antisense morpholino knockdown (Supplementary Figure 2).
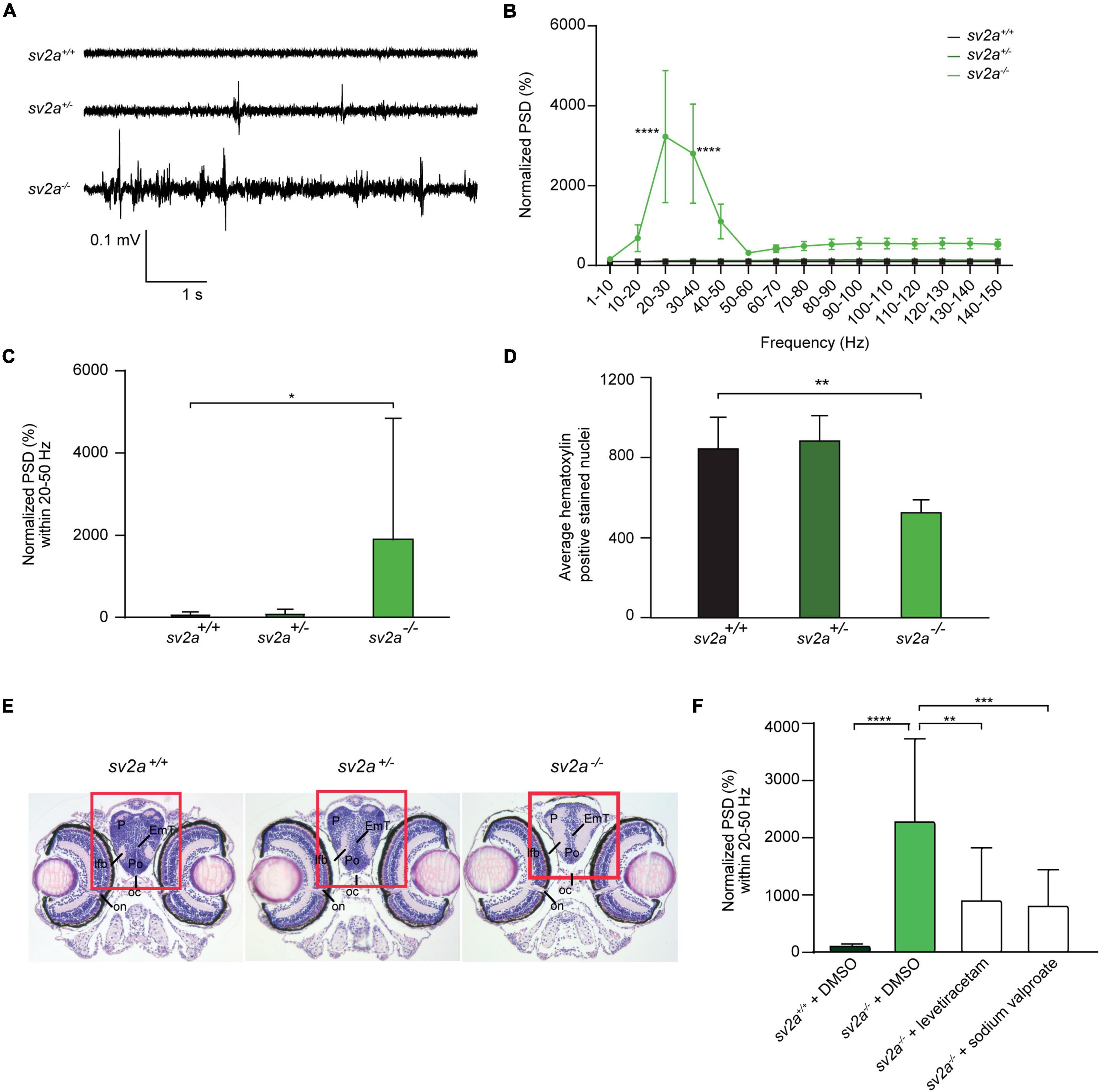
Figure 3. Spontaneous electrographic seizures and brain malformation in sv2a knockout zebrafish. (A) Representative local field potential (LFP) recordings of sv2a+/+, sv2a+/–, and sv2a –/– zebrafish larvae at 6 dpf. (B) Power spectral density (PSD) analysis of sv2a+/+ (n = 9), sv2a+/– (n = 11), and sv2a –/– (n = 10) zebrafish larvae at 6 dpf. A significant increase in PSD values was observed in sv2a–/– larvae compared to sv2a+/+ larvae. Results were normalized to sv2a+/+ larvae as 100%. Statistical analysis was performed using two-way ANOVA with Sidak’s multiple comparisons test (****p < 0.0001 compared to sv2a+/+). (C) PSD values (mean ± SD) plotted per condition over the 20–50 Hz region. Statistical analysis was performed using one-way ANOVA followed by Dunnett’s multiple comparison (*p < 0.05). (D) Hematoxylin-positive stained nuclei (mean ± SD) counted using QuPath (0.1.2) software. The region with significant loss of hematoxylin-positive stained nuclei was highlighted in red. The results are expressed as mean ± SD of 4 equivalent sections per genotype. Statistical analysis was performed using one-way ANOVA followed by Dunnett’s multiple comparison (**p < 0.01). (E) Hematoxylin and eosin staining of paraffin-embedded coronary sections from the forebrain of sv2a+/+, sv2a+/–, and sv2a –/– zebrafish larvae at 6 dpf. Scale bar 100 μm. oc, optic chiasm; on, optic nerve; Po, preoptic region; P, pallium; lfb, lateral forebrain bundle. (F) Effect of levetiracetam and sodium valproate on locomotor behavior and epileptiform brain activity in 6 dpf sv2a–/– zebrafish larvae. PSD values (mean ± SD) from LFP recordings at 6 dpf plotted per condition over the 20-50 Hz region for sv2a+/+ and sv2a–/– larvae after incubation with levetiracetam and valproic acid at their MTC or DMSO control at 5 dpf for 22 h. Number of larvae per condition: n = 9–35. Statistical analysis was performed using ordinary one-way ANOVA. **p < 0.01, ***p < 0.001, ****p < 0.0001.
To determine whether 6 dpf sv2a–/– larvae showed differences in the brain organization, a histological analysis was performed by hematoxylin and eosin staining. The optic nerve was chosen as a reference point to obtain comparable sections per genotype. We found that there were significantly less hematoxylin-positive stained nuclei detected in the pallium (midbrain) of sv2a–/– larvae than in those of their sv2a+/– and sv2a+/+ siblings (Figure 3D). However, additional examination of the brain showed no gross morphological abnormalities in sv2a–/– brains. As shown in Figure 3E, on the example of the telencephalic section of sv2a-deficient zebrafish larva, there were no gross structural changes, developmental aberrations, or differences in the formation of characteristic brain areas including the telencephalon, pallium, preoptic region, and thalamic structures.
Effects of Anti-epileptic Drugs on Seizures of sv2a Knockout Zebrafish
We then tested anti-seizure effects of sodium valproate and levetiracetam, commonly used AEDs in epilepsy treatment, in the LFP assay on 6 dpf sv2a–/– mutant larvae. Noteworthy, SV2A is the distinct binding site for levetiracetam (Lynch et al., 2004). Our results demonstrated that sodium valproate substantially reduced epileptiform brain activity (Figure 3F) to 35% (p < 0.001). Surprisingly, treatment with levetiracetam also showed a significant effect on the mean PSD values by reducing the epileptiform events to 40% (p < 0.01) in sv2a–/– larvae. This strongly suggests that other targets besides SV2A must be involved in levetiracetam’s mechanism of action for seizure suppression.
Characterization of sv2a–/– Larval Transcriptome Reveals That sv2a Knockout Alters Gene Expression in Developing Larval Brains
To obtain mechanistic insights into the consequence of the sv2a absence in developing larval brains, RNA sequencing was performed on the heads of sv2a–/–, sv2a+/–, and sv2a+/+ 6 dpf larvae. Approximately 37.4 million single-end reads were produced per sample. After quality assessment and filtering, over 37.1 million remained, of which 92.6% aligned to the zebrafish reference genome GRCz11. Overall, 28,484 genes were expressed in at least one of the three genotypes. While the majority of expressed genes was protein-coding (85.9%), long non-coding RNAs (6.5%), non-coding RNAs (3.7%), and pseudogenes (0.8%) were detected as well. The remaining percentages of expressed genes categorized to a variety of other RNAs species (Supplementary Table 2).
Both principal component analysis (PCA) and t-distributed stochastic neighborhood embedding (t-SNE) of the gene expression profiles revealed that two divergent groups could be distinguished (Supplementary Figures 3A,B), which was confirmed by a Spearman’s correlation matrix of the gene expression showing that the sv2a–/– samples clustered separately from their sv2a+/– and sv2a+/+ siblings (Figure 4A). Further reflecting this grouping, analysis of the gene expression profiles of sv2a+/+ and sv2a+/– samples revealed only one differentially expressed gene (DEG), namely sv2a. In contrast, between sv2a+/+ and sv2a–/–, and sv2a+/– and sv2a–/–, there were 4386 and 3535 DEGs, respectively (Figure 4B and Supplementary Figure 3C). Of the 4386 DEGs between sv2a+/+ and sv2a–/–, 1833 (135 with absolute fold change > 2) were up- and 2553 (990 with absolute fold change > 2) were down-regulated in sv2a–/– larvae. Top ten up- and down-regulated protein-coding genes sorted by Wald statistic are listed in Table 1 (complete list in Supplementary Table 2). To validate the RNA-seq data, a set of five up- and five down-regulated genes, including gonadotropin-releasing hormone 3 (gnrh3), FOS like 1, AP-1 transcription factor subunit a (fosl1a), v-fos FBJ murine osteosarcoma viral oncogene homolog Ab (fosab), activating transcription factor 3 (atf3), transmembrane protein 176l.3a (tmem176l.3a), ATP-binding cassette, sub-family G (WHITE), member 2b (abcg2b), four and a half LIM domains 5 (fhl5), fatty acid binding protein 1b, tandem duplicate 1 (fabp1b.1), nuclear receptor subfamily 0, group B, member 2a (nr0b2a) and apolipoprotein Da, duplicate 1 (apoda.1), was selected for RT-qPCR analysis in a cohort of three sv2a–/– and three sv2a+/+ samples. Significant differences in the expression levels were observed for the up-regulated genes fosl1a and atf3, and down-regulated genes abcg2b, fhl5, fabp1b.1, nr0b2a, and apoda.1. A non-significant up-regulated trend was detected for gnrh3, fosab, and tmem176l.3a. In conclusion, the experimental expression pattern of these up- and downregulated genes was in line with the results obtained by RNA sequencing (Supplementary Figure 3D).
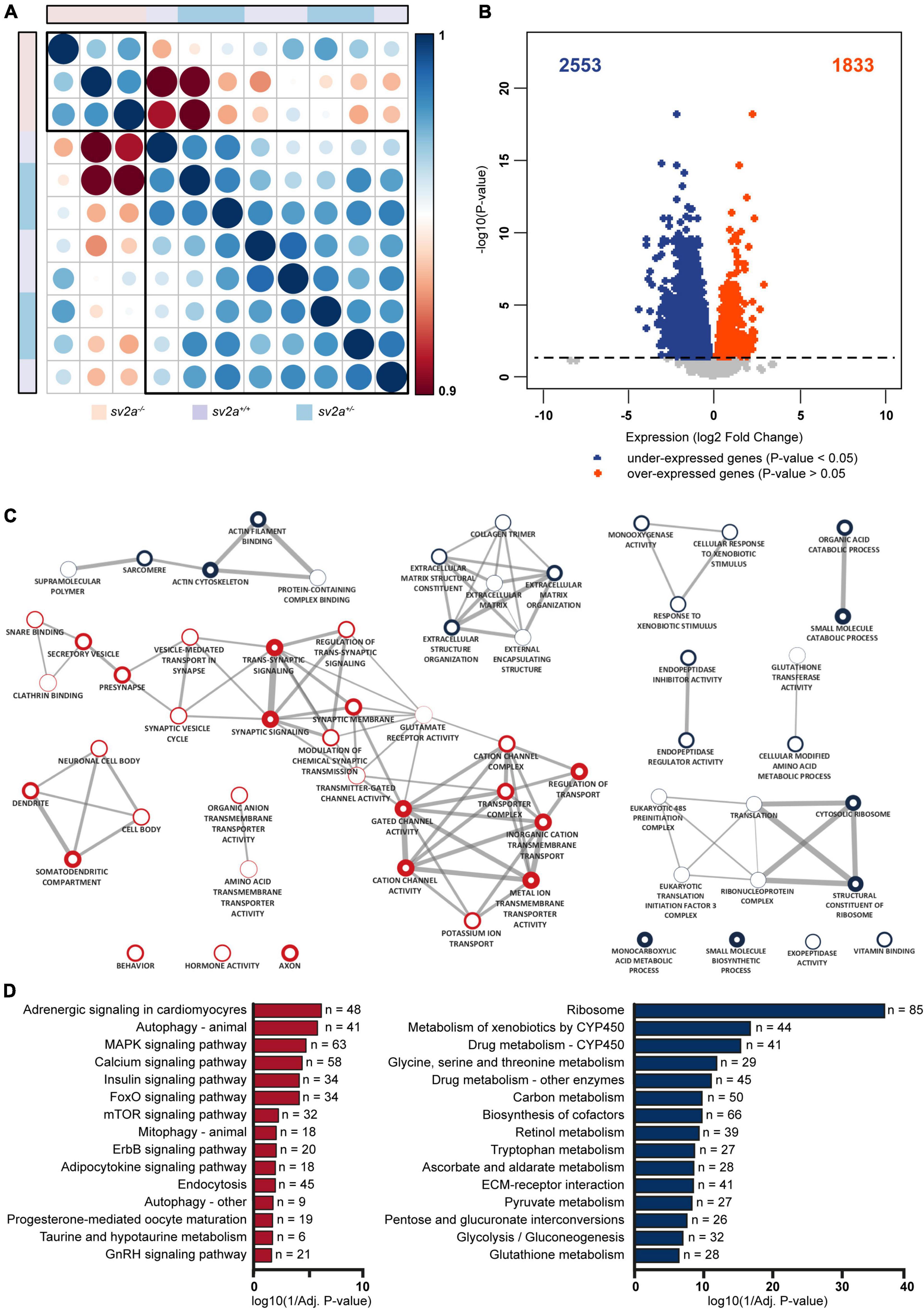
Figure 4. Transcriptome analysis and gene ontology (GO) enrichment map of sv2a–/– larvae. (A) Spearman’s rank correlation matrix of the RNA-Seq data showing separate clustering of sv2a–/– samples from sv2a+/+ and sv2a+/– samples. Scale bar indicates the strength of the correlation, with 1 indicating a strong positive correlation (dark blue) and 0.9 indicating weak correlation (dark red) between samples. Areas of the circles show absolute values of corresponding correlation coefficients. Samples of different genotypes are indicated in distinct colors codes. (B) Volcano plot showing the differentially expressed genes (DEG) (padj < 0.05) between sv2a–/– and sv2a+/+ larvae. Of the 4386 DEGs, 2553 were found to be down- (blue) and 1833 to be up-regulated (red) in sv2a–/– larvae compared to sv2a+/+. (C) GO enrichment map from up- and down-regulated genes between sv2a+/+ and sv2a–/– zebrafish larvae. Each node represents a different GO term, the red and blue outside of nodes indicate enrichment in up- or down-regulated genes, respectively. The larger the node the greater the number of genes in the enriched GO term. Connecting lines indicate common genes shared between nodes, the thicker the line the more genes in common. (D) Enriched pathways from differentially expressed genes between sv2a–/– and sv2a+/+ zebrafish larvae. Pathways enriched amongst up-regulated genes are indicated in red, pathways enriched amongst down-regulated genes are indicated in blue. The x-axis represents the log10 (1/p-value), n indicates the number of genes appearing in each category.
To understand how zebrafish larvae lacking sv2a compare with other previously established zebrafish epilepsy models, the sv2a–/– transcriptome was compared to the transcriptomes of five zebrafish epilepsy models: gabra1 (Samarut et al., 2018) recapitulating idiopathic generalized epilepsy, depdc5 (Swaminathan et al., 2018) and tsc2 (Scheldeman et al., 2017) modeling mTORopathies, scn1lab (unpublished data) recapitulating Dravet syndrome, and kainic acid-injected (unpublished data), a chemically-induced larval epilepsy model. Compared to the other genetic epilepsy models, sv2a–/– zebrafish displayed a considerably greater number of DEGs. In addition, none of the five models demonstrated a substantial overlap of DEGs with the sv2a–/– model (Supplementary Figure 3E). These observations indicate that the mechanisms involved in the sv2a loss-of-function zebrafish epilepsy model at least partly differ from those observed in the other zebrafish epilepsy models and therefore highlight the uniqueness of this model.
GO enrichment analysis demonstrated a substantial amount of GO terms enriched in both the up- and down-regulated genes between sv2a–/– and wildtype zebrafish larvae (Supplementary Table 3). Enrichment Map analysis of the top 10 GO terms (Biological Process, Molecular Function or Cellular Component) amongst up-regulated genes revealed one major cluster containing terms related to synapses and ion channels and one smaller cluster containing terms related to neurons (Figure 4C). For the down-regulated genes there were four smaller clusters identified, including one that contained terms related to extracellular matrix (ECM) and another with terms related to translation (Figure 4C). Organic anion transport was the only common enriched GO term shared by the up- and down-regulated gene lists. The larger number of down-regulated genes compared to up-regulated genes was reflected in the total number of significantly enriched GO terms (p-value < 0.05), which was larger amongst down-regulated genes (202 terms amongst down-regulated genes versus 165 amongst up-regulated genes).
The pathway analysis identified 20 enriched pathways (p-value < 0.05) from the up-regulated genes (Supplementary Table 3). Three interesting pathways amongst the top 10 of this list were MAPK signaling, calcium signaling and mTOR signaling (Figure 4D). Amongst the down-regulated genes there were 40 enriched pathways (p-value < 0.05) (Figure 4D and Supplementary Table 3) and noteworthy pathways from this list were glycolysis/gluconeogenesis and ECM-receptor interaction.
Connectivity Mapping Correctly Predicts Therapeutic Compounds Capable of Reverting the sv2a–/– Epileptic Phenotype
In order to explore the potential of connectivity mapping for the discovery of novel anti-epileptic drugs, we employed the CMap database to identify substances with gene signatures opposed the one observed in sv2a–/– zebrafish larvae (Figure 5A). To this end, a list of up- and down-regulated genes selected by Wald statistic was used to query the library (see materials and methods for more details). Twenty-one compounds were found to have highly opposing scores (<−90), of which three were selected for further testing (Table 2). The overall greatest negative connectivity score was found for PD-98059, a potent mitogen-activated protein kinase (MEK) inhibitor (Dudley et al., 1995), being very valuable in the elucidation of the MAPK signaling pathway in different immune responses (Guha et al., 2001; Reiling et al., 2001). Additionally, we selected alvocidib (formerly flavopiridol), a cyclin-dependent kinase (CDK) inhibitor developed for the treatment of acute myeloid leukemia (Zeidner and Karp, 2015) and AS-605240, a potent and selective phosphoinositide 3-kinase (PI3K) gamma inhibitor that never progressed into clinical development but is still commonly used for in vitro research purposes. We also included several negative controls: compounds that theoretically should not interfere with or worsen the sv2a–/– disease phenotype (CAY-10415, and calmidazolium HCl and digoxin, respectively) (Table 2).
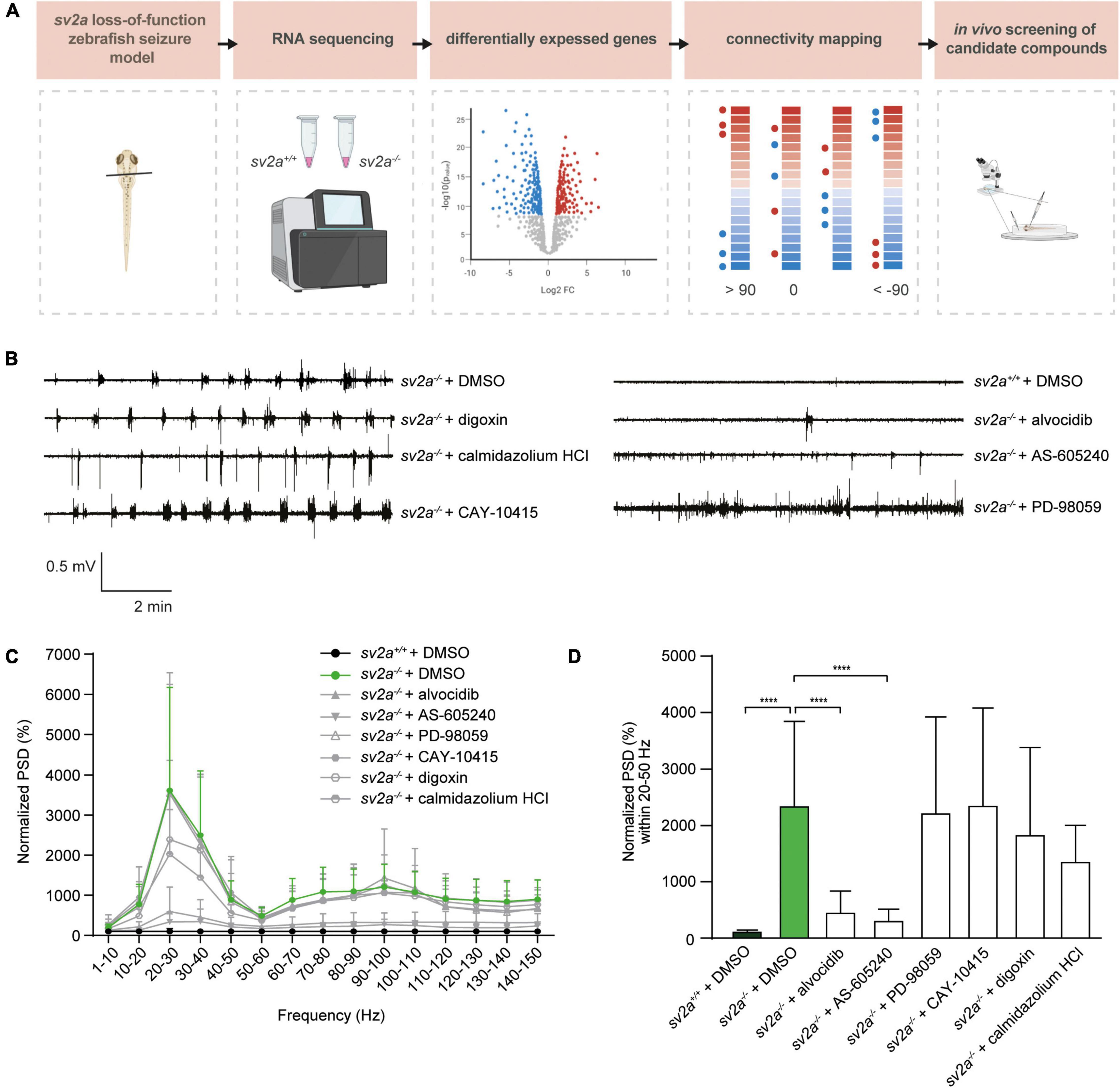
Figure 5. Connectivity mapping in sv2a–/– zebrafish larvae. (A) Overview of the connectivity mapping workflow. Heads of sv2a–/– and sv2a+/+ zebrafish larvae were sampled and RNA was extracted. After RNA sequencing and differential expression analysis, top 50 up- and down-regulated genes in sv2a–/– larvae (compared to sv2a+/+) were selected based on Wald statistic and compared to gene expression profiles of compounds in the CMap database in order to identify compounds reversing the differential expression signature (scores < −90). Three of these compounds (alvocidib, AS-605240, PD-98059) and three negative controls (scores > 90; digoxin, calmidazolium HCl, or equaling zero; CAY-10415) were tested in the sv2a loss-of-function in LFP recordings. (B) Representative LFP recordings of sv2a–/– and sv2a+/+ larvae after incubation with selected CMap compounds at their MTCs or DMSO control at 5 dpf for 22 h. (C) PSD analysis of sv2a–/– and sv2a+/+ larvae after incubation of selected CMap compounds at their MTCs or DMSO control at 5 dpf for 22 h. Results were normalized to sv2a+/+ larvae as 100%. Number of larvae per condition: n = 9–27. (D) Power spectral density (PSD) values (mean ± SD) from LFP recordings at 6 dpf plotted per condition over the 20-50 Hz region for sv2a–/– and sv2a+/+ larvae after incubation of selected CMap compounds at their MTCs or DMSO control at 5 dpf for 22 h. Number of larvae per condition: n = 9–27. Data are presented as mean ± SD. Statistical analysis was performed using ordinary one-way ANOVA. ***p < 0.001, ****p < 0.0001.
5 dpf sv2a–/– zebrafish larvae were treated with the selected compounds at their MTCs for 22 h (Figures 5B–D). At 6 dpf, LFP recordings were performed to determine if the identified compounds were able to decrease epileptiform brain activity. Interestingly, two out of three compounds with opposing gene signatures (alvocidib and AS-605240) were able to significantly decrease epileptiform brain discharges in sv2a–/– zebrafish larvae, while all three negative controls did not significantly affect the PSD values. Since incubation in separate wells for 22 h resulted in a loss of the observed hyperactivity in sv2a–/– larvae, we were not able to investigate behavioral effects of these compounds using the automated high-throughput tracking system.
Discussion
In the current study, we report the generation and characterization of a novel sv2a knockout zebrafish model using CRISPR/Cas9 and the application of connectivity mapping in finding novel drug candidates against epilepsy. To summarize, the absence of sv2a in knockout larvae resulted in premature death, locomotor hyperactivity, and severe spontaneous electrographic seizures. Meanwhile, their sv2a+/– and sv2a+/+ siblings developed into fertile adults and did not show any abnormalities in their behavior or brain activity. Therefore, the knockout larvae recapitulate phenotypic features typically observed in the SV2A null mice models (Crowder et al., 1999; Janz et al., 1999; Kaminski et al., 2009; Menten-Dedoyart et al., 2016) as these mice failed to grow, developed severe seizures, and resulted in early death. On the contrary, partial SV2A protein deficiency in heterozygous mice did not have any impact on the lifespan or basic sensimotor functions (Kaminski et al., 2009; Lamberty et al., 2009). Even though Sv2a is essential for survival and proper functioning of the nervous system, no gross brain malformations or differences in the formation of characteristic brain areas could be observed in sv2a–/– larvae. These findings again are in line with those of SV2A knockout mice, where the overall brain architecture, including the cerebral cortex, hippocampus, and thalamus, was similar to wildtype animals (Crowder et al., 1999; Janz et al., 1999), indicating that deletion of sv2a does not lead to developmental changes in brain structure. Loss of sv2a expression in sv2a–/– larvae resulted, however, in a substantial decrease of cell nuclei in the pallium region, but the underlying cause of this neuronal loss remains to be elucidated. Therefore, the epileptic phenotype is likely an effect of the modulation of neurotransmitter levels at the synapses.
It is well known that the GABA excitatory to inhibitory switch (E-I switch) is a hallmark of the developing nervous system (Ben-Ari et al., 1989; Ben-Ari et al., 2007; Zhang et al., 2010; Momose-Sato et al., 2012; Lysenko et al., 2018; Kang et al., 2019). In a previous study a rapid increase of SV2A expression in the CA1 region of mouse hippocampus was observed at P7, which is the time point when the GABA E-I switch is completed (Valeeva et al., 2013). This might explain why the absence of SV2A is becoming dominant on GABAergic inhibition only after P7, which is precisely the age when SV2A knockout mice start to have seizures (Crowder et al., 1999). In zebrafish, the period between 2 and 4 dpf has been proven to be critical for the E–I switch of GABAergic action (Zhang et al., 2010). Interestingly, we found that the sv2a expression gradually increased and stabilized around 4 dpf. In fact, epileptiform brain activity could already be detected in 5 dpf sv2a–/– larvae (Supplementary Figure 4), which is in line with SV2A knockout mice studies. However, since in zebrafish the major organs including the brain are more developed after 5 dpf (Garcia et al., 2016), therefore we employed more mature 6 dpf larvae to study Sv2a function.
Unexpectedly, epileptiform activity in sv2a–/– larvae could be attenuated by treatment with levetiracetam, even though there was nearly a complete absence of its presumed target, as confirmed by low levels of sv2a via RT-qPCR. Previous studies have demonstrated decreased anticonvulsant efficacy of levetiracetam observed in SV2A heterozygous 6-Hz mice model, which was consistent with less binding sites available (Kaminski et al., 2009). Moreover, it has been previously shown that SV2A expression was reduced during epileptogenesis and in the chronic epileptic phase in rats, and throughout the hippocampus of TLE patients (van Vliet et al., 2009). It has not been investigated, however, whether this affected the effectiveness of levetiracetam. Interestingly and in line with our results, SV2A-caused photosensitive reflex epilepsy chicken model was sensitive to levetiracetam since the drug (partially) rescued the epileptic phenotype, although the levels of SV2A were shown to be very low in homozygous animals (Douaud et al., 2011). Hence the unanticipated finding that levetiracetam exerted antiseizure activity in sv2a–/– larvae, inconsistent with the absence of its presumed target, strongly suggests that levetiracetam may act (at least partly) on other target(s) than Sv2a in order to exert its antiepileptic activity.
Although levetiracetam is a broad-spectrum AED with neuroprotective properties and is widely used to treat focal onset and generalized seizures, the exact detail of the mechanism of action of levetiracetam is not clear. Unquestionably, levetiracetam binds to SV2A to modulate neurotransmitter release including GABA and glutamate (Lynch et al., 2004), which supports the evidence that this mechanism is important for the anticonvulsant properties of the drug (Kaminski et al., 2009). Nevertheless, levetiracetam (unlike its more potent analog brivaracetam) has only moderate affinity to SV2A and possesses several other putative pharmacologic mechanisms of action (Klitgaard et al., 2016). It has been demonstrated that levetiracetam inhibited presynaptic calcium channels through an intracellular pathway (Vogl et al., 2012) and also acted as an AMPA receptor antagonist (Carunchio et al., 2007; Vogl et al., 2012; Steinhoff and Staack, 2019). These pathways could contribute to the anticonvulsant effects of levetiracetam by reducing neuronal excitability. Moreover, it was shown that the major metabolite of levetiracetam inhibited histone deacetylase and induced histone hyperacetylation in human cells (Eyal et al., 2004). Modulation of chromatin structure through histone modifications is an important regulator of gene transcription in the brain and altered histone acetylation seems to contribute to changes in gene expression associated with epilepsy and epileptogenic processes (Citraro et al., 2017). Additional investigations have also revealed that levetiracetam could induce embryonic neurogenesis in vitro by increased cell proliferation and differentiation of rat embryonic neural stem cells, mainly through an NMDA receptor-mediated mechanism (Alavi et al., 2021). Interestingly, activated microglia, that maintain immune homeostasis in the brain, contribute to the changes occurring during epileptic processes (Victor and Tsirka, 2020). Levetiracetam inhibited microglial activation by suppressing excess microglial phagocytosis during epileptogenesis (Itoh et al., 2019), which might prevent the occurrence of spontaneous recurrent seizures. More recently, it has been demonstrated that levetiracetam significantly suppressed the inflammatory reactions in microglial cells that did not express SV2A, and FosL1, activator protein 1(AP-1) transcription factor subunit, was identified as the most likely target of levetiracetam responsible for this anti-inflammatory mechanism (Niidome et al., 2021). All the above-described effects may contribute to the unique pharmacological profile of levetiracetam, suggesting that binding to SV2A might not represent its primary mechanism of action. Alternatively, as our CRISPR knockout did not result in a complete loss of sv2a mRNA and because of the absence of a seizure phenotype in sv2a+/– zebrafish larvae and studies in SV2A heterozygous mice showing that full restoration is not required for phenotype recovery, we cannot exclude that the observed effects of levetiracetam are resulting from its action on residual Sv2a protein levels. Unfortunately, due to the lack of zebrafish-specific and cross-reacting antibodies it was not possible to further investigate this hypothesis.
To gain more insight into the molecular basis underlying the observed epileptic phenotype in sv2a knockout larvae, we further investigated differences between the distinct genotypes at the transcriptome level, which is to the best of our knowledge the first report of a transcriptome study in a sv2a deleterious animal model. Large perturbations of the sv2a–/– larval transcriptome were observed when compared to their wildtype siblings. Many of the DEGs were involved in synapses, ion channels, neurons, and ECM, demonstrating that our sv2a loss-of-function zebrafish model mimics aspects of human epilepsy (Okamoto et al., 2010; Winden et al., 2011; Pitkanen et al., 2015; Engel and Pitkanen, 2020). For example, although molecular mechanisms of epilepsy are not well understood and differ amongst distinct patient populations, dysregulation of proteins determining excitability, including ion channels and GABA receptors, are commonly observed (Snowball and Schorge, 2015; Akyuz et al., 2021). Additionally, it is known that ECM are linked to synaptic reorganization and are often seen in epileptogenic zones in patients (Leite and Peixoto-Santos, 2021). Other interesting pathways enriched amongst differentially expressed genes in sv2a–/– larvae were MAPK-, mTOR-, and calcium signaling and their involvement in epileptogenesis has been previously confirmed (Steinlein, 2014; Pernice et al., 2016; Scheldeman et al., 2017). Despite the observation that our sv2a knockout model shares mechanisms common for epilepsy, a comparison with the transcriptome profiles of five other established larval zebrafish epilepsy models did not show any large overlap of DEGs with the other models. This finding implies that the mechanisms involved in the sv2a loss-of-function model at least partly differ from those observed in the other zebrafish epilepsy models, highlighting not only the novelty and uniqueness of our model, but also its potential use for drug screening to find AEDs with novel mechanisms of action.
Accordingly, we applied the concept of connectivity mapping as a novel drug discovery strategy in our sv2a zebrafish model. We demonstrated that two predicted candidates, AS-605240 and alvocidib, were able to significantly decrease epileptiform brain discharges in the sv2a–/– larvae. AS-605240 is an effective PI3Kγ inhibitor (Ksionda et al., 2018). The PI3K/Akt/mTOR pathway is essential for intracellular signaling, regulating neuronal survival, growth, and plasticity (Switon et al., 2017) and, importantly, is known to be overactivated after epileptogenic brain injury in animal models as well as in patients with acquired epilepsies (Vezzani, 2012). Alvocidib on the other hand, is known to inhibit CDK (Zeidner and Karp, 2015). Interestingly, it was shown that CDK5 plays important neuronal function and is crucial for homeostatic synaptic plasticity. Moreover, association between chronic loss of CDK5 and presence of seizures has been reported in other animal epilepsy models (Dixit et al., 2017). Although PI3K and CDK are plausible anti-epileptic targets of the identified candidates, further elucidation of the mechanism of action of AS-605240 and alvocidib is recommended. Overall, the results achieved in this explorative study clearly show that connectivity mapping using zebrafish transcriptome data as an input is reliable and effective, as we experimentally confirmed two out of three positively as well as three out of three negatively predicted therapeutic candidates. In addition, we demonstrated that preceding a traditional phenotypical drug screen with a network-based computational analysis tremendously improves observed hit rates and therefore reduces time and cost spent on a typical drug screening. However, one drawback of the connectivity mapping strategy is its tendency to be very variable, since no consensus input dataset neither threshold connectivity value exists. For future research, a more defined design and fixed methodology might increase reproducibility and less bias in the promising field of network-based drug screening. In this context, it is also important to note that the concept of connectivity mapping, which relies on ameliorating an observed disease phenotype by reversing a dysregulated gene expression pattern, differs from the traditional drug discovery concept that aims at finding (ant)agonists that functionally act on protein-based targets. As such, one cannot exclude the possibility that drugs that are not identified in the CMap analysis, and thus do not reverse the dysregulated gene expression profile, have disease-ameliorating effects.
Collectively, we demonstrated that the absence of sv2a resulted in an increased seizure susceptibility and provided important insight into the functional relevance of sv2a in the brain in general. Additionally, since sv2a–/– larvae, lacking the presumed target of levetiracetam, were responsive to this AED, the model is a great asset to the field for investigating its putative targets. In addition, given the fact that the sv2a–/– differentially expressed gene dataset did not show large overlap with datasets of other larval epilepsy models, our sv2a loss-of-function zebrafish model could be of great value in the search for anti-epileptics with novel mechanisms of action. Specifically, we supplied evidence that the concept of connectivity mapping represents an attractive and powerful strategy in the discovery of novel AEDs.
Data Availability Statement
The datasets presented in this study can be found in online repositories. The names of the repository/repositories and accession number(s) can be found in the article/Supplementary Material.
Ethics Statement
The animal study was reviewed and approved by Ethics Committee of the KU Leuven (P023/2017 and P027/2019) and by the Belgian Federal Department of Public Health, Food Safety, and Environment (LA1210199).
Author Contributions
YZ, LH, MP, MG, and AS performed the zebrafish experiments and analyzed the data. LH, JM, and PG analyzed the transcriptome data. YZ, LH, and AS designed the experiments. PW and RK conceived the study. PW and AS supervised the study. YZ, LH, PW, and AS wrote the manuscript. All authors contributed to the article and approved the submitted version.
Funding
This work was supported by the Fonds Wetenschappelijk Onderzoek (1S56820N to LH and 1S84418N to MP) and KU Leuven (C32/18/067 to AS). YZ was supported by a student scholarship funded by the UCB.
Conflict of Interest
RK, PG, and MG were employed at UCB Pharma at the time this study was performed.
The remaining authors declare that the research was conducted in the absence of any commercial or financial relationships that could be construed as a potential conflict of interest.
Publisher’s Note
All claims expressed in this article are solely those of the authors and do not necessarily represent those of their affiliated organizations, or those of the publisher, the editors and the reviewers. Any product that may be evaluated in this article, or claim that may be made by its manufacturer, is not guaranteed or endorsed by the publisher.
Acknowledgments
RNA sequencing and data production was performed by VIB Nucleomics Core (www.nucleomics.be). Figures 1B, 5A were partly created using BioRender. We thank Kevin Longin for help with the experiments.
Supplementary Material
The Supplementary Material for this article can be found online at: https://www.frontiersin.org/articles/10.3389/fnmol.2022.881933/full#supplementary-material
Supplementary Table 1 | Overview qPCR and HRM primers.
Supplementary Table 2 | DEG list.
Supplementary Table 3 | Enriched GO terms and KEGG pathways.
Footnotes
- ^ www.nucleomics.be
- ^ http://www.ebi.ac.uk/arrayexpress
- ^ http://www.bioconductor.org
- ^ http://www.cytoscape.org/
References
Abou-Khalil, B. (2008). Levetiracetam in the treatment of epilepsy. Neuropsychiatr. Dis. Treat. 4, 507–523.
Akyuz, E., Polat, A. K., Eroglu, E., Kullu, I., Angelopoulou, E., and Paudel, Y. N. (2021). Revisiting the role of neurotransmitters in epilepsy: an updated review. Life Sci. 265:118826. doi: 10.1016/j.lfs.2020.118826
Alavi, M. S., Negah, S. S., Ghorbani, A., Hosseini, A., and Sadeghnia, H. R. (2021). Levetiracetam promoted rat embryonic neurogenesis via NMDA receptor-mediated mechanism in vitro. Life Sci. 284:119923. doi: 10.1016/j.lfs.2021.119923
Ashburner, M., Ball, C. A., Blake, J. A., Botstein, D., Butler, H., Cherry, J. M., et al. (2000). Gene ontology: tool for the unification of biology. The gene ontology consortium. Nat. Genet. 25, 25–29. doi: 10.1038/75556
Baraban, S. C., Dinday, M. T., and Hortopan, G. A. (2013). Drug screening in Scn1a zebrafish mutant identifies clemizole as a potential Dravet syndrome treatment. Nat. Commun. 4:2410. doi: 10.1038/ncomms3410
Bartholome, O., Van Den Ackerveken, P., Sánchez Gil, J., De La Brassinne Bonardeaux, O., Leprince, P., Franzen, R., et al. (2017). Puzzling out synaptic vesicle 2 family members functions. Front. Mol. Neurosci. 10:148. doi: 10.3389/fnmol.2017.00148
Ben-Ari, Y., Cherubini, E., Corradetti, R., and Gaiarsa, J. L. (1989). Giant synaptic potentials in immature rat CA3 hippocampal neurones. J. Physiol. 416, 303–325. doi: 10.1113/jphysiol.1989.sp017762
Ben-Ari, Y., Gaiarsa, J. L., Tyzio, R., and Khazipov, R. (2007). GABA: a pioneer transmitter that excites immature neurons and generates primitive oscillations. Physiol. Rev. 87, 1215–1284. doi: 10.1152/physrev.00017.2006
Brueggeman, L., Sturgeon, M. L., Martin, R. M., Grossbach, A. J., Nagahama, Y., Zhang, A., et al. (2019). Drug repositioning in epilepsy reveals novel antiseizure candidates. Ann. Clin. Transl. Neurol. 6, 295–309. doi: 10.1002/acn3.703
Calame, D. G., Herman, I., and Riviello, J. J. (2021). A de novo heterozygous rare variant in SV2A causes epilepsy and levetiracetam-induced drug-resistant status epilepticus. Epilepsy Behav. Rep. 15:100425. doi: 10.1016/j.ebr.2020.100425
Carunchio, I., Pieri, M., Ciotti, M. T., Albo, F., and Zona, C. (2007). Modulation of AMPA receptors in cultured cortical neurons induced by the antiepileptic drug levetiracetam. Epilepsia 48, 654–662. doi: 10.1111/j.1528-1167.2006.00973.x
Chang, W. P., and Sudhof, T. C. (2009). SV2 renders primed synaptic vesicles competent for Ca2+ -induced exocytosis. J. Neurosci. 29, 883–897. doi: 10.1523/jneurosci.4521-08.2009
Chen, E. Y., Tan, C. M., Kou, Y., Duan, Q., Wang, Z., Meirelles, G. V., et al. (2013). Enrichr: interactive and collaborative HTML5 gene list enrichment analysis tool. BMC Bioinformat. 14:128. doi: 10.1186/1471-2105-14-128
Citraro, R., Leo, A., Santoro, M., D’agostino, G., Constanti, A., and Russo, E. (2017). Role of Histone Deacetylases (HDACs) in epilepsy and epileptogenesis. Curr. Pharm. Des. 23, 5546–5562. doi: 10.2174/1381612823666171024130001
Copmans, D., Siekierska, A., and De Witte, P. A. M. (2017). “Chapter 26 - zebrafish models of epilepsy and epileptic seizures A2 - Pitkänen, Asla,” in Models of Seizures and Epilepsy, 2nd Edn, eds P. S. Buckmaster, A. S. Galanopoulou, and S. L. Moshé (Cambridge, MA: Academic Press), 369–384.
Crepeau, A. Z., and Treiman, D. M. (2010). Levetiracetam: a comprehensive review. Expert Rev. Neurother. 10, 159–171. doi: 10.1586/ern.10.5
Crèvecœur, J., Foerch, P., Doupagne, M., Thielen, C., Vandenplas, C., Moonen, G., et al. (2013). Expression of SV2 isoforms during rodent brain development. BMC Neurosci. 14:87. doi: 10.1186/1471-2202-14-87
Crowder, K. M., Gunther, J. M., Jones, T. A., Hale, B. D., Zhang, H. Z., Peterson, M. R., et al. (1999). Abnormal neurotransmission in mice lacking synaptic vesicle protein 2A (SV2A). Proc. Natl. Acad. Sci. U. S. A. 96, 15268–15273. doi: 10.1073/pnas.96.26.15268
De Smedt, T., Raedt, R., Vonck, K., and Boon, P. (2007). Levetiracetam: the profile of a novel anticonvulsant drug-part I: preclinical data. CNS Drug Rev. 13, 43–56. doi: 10.1111/j.1527-3458.2007.00004.x
Dixit, A. B., Banerjee, J., Tripathi, M., Sarkar, C., and Chandra, P. S. (2017). Synaptic roles of cyclin-dependent kinase 5 & its implications in epilepsy. Indian J. Med. Res. 145, 179–188. doi: 10.4103/ijmr.IJMR_1249_14
Dobin, A., Davis, C. A., Schlesinger, F., Drenkow, J., Zaleski, C., Jha, S., et al. (2013). STAR: ultrafast universal RNA-seq aligner. Bioinformatics 29, 15–21. doi: 10.1093/bioinformatics/bts635
Douaud, M., Feve, K., Pituello, F., Gourichon, D., Boitard, S., Leguern, E., et al. (2011). Epilepsy caused by an abnormal alternative splicing with dosage effect of the SV2A gene in a chicken model. PLoS One 6:e26932. doi: 10.1371/journal.pone.0026932
Dudley, D. T., Pang, L., Decker, S. J., Bridges, A. J., and Saltiel, A. R. (1995). A synthetic inhibitor of the mitogen-activated protein kinase cascade. Proc. Natl. Acad. Sci. U. S. A. 92, 7686–7689. doi: 10.1073/pnas.92.17.7686
Durinck, S., Spellman, P. T., Birney, E., and Huber, W. (2009). Mapping identifiers for the integration of genomic datasets with the R/Bioconductor package biomaRt. Nat. Protoc. 4, 1184–1191. doi: 10.1038/nprot.2009.97
Engel, J. Jr., and Pitkanen, A. (2020). Biomarkers for epileptogenesis and its treatment. Neuropharmacology 167:107735. doi: 10.1016/j.neuropharm.2019.107735
Engel, J. (2001). A proposed diagnostic scheme for people with epileptic seizures and with epilepsy: report of the ILAE task force on classification and terminology. Epilepsia 42, 796–803. doi: 10.1046/j.1528-1157.2001.10401.x
Eyal, S., Yagen, B., Sobol, E., Altschuler, Y., Shmuel, M., and Bialer, M. (2004). The activity of antiepileptic drugs as histone deacetylase inhibitors. Epilepsia 45, 737–744. doi: 10.1111/j.0013-9580.2004.00104.x
Feng, G., Xiao, F., Lu, Y., Huang, Z., Yuan, J., Xiao, Z., et al. (2009). Down-regulation synaptic vesicle protein 2A in the anterior temporal neocortex of patients with intractable epilepsy. J. Mol. Neurosci. 39, 354–359. doi: 10.1007/s12031-009-9288-2
Garcia, G. R., Noyes, P. D., and Tanguay, R. L. (2016). Advancements in zebrafish applications for 21st century toxicology. Pharmacol. Ther. 161, 11–21. doi: 10.1016/j.pharmthera.2016.03.009
Gillard, M., Chatelain, P., and Fuks, B. (2006). Binding characteristics of levetiracetam to synaptic vesicle protein 2A (SV2A) in human brain and in CHO cells expressing the human recombinant protein. Eur. J. Pharmacol. 536, 102–108. doi: 10.1016/j.ejphar.2006.02.022
Gillard, M., Fuks, B., Leclercq, K., and Matagne, A. (2011). Binding characteristics of brivaracetam, a selective, high affinity SV2A ligand in rat, mouse and human brain: relationship to anti-convulsant properties. Eur. J. Pharmacol. 664, 36–44. doi: 10.1016/j.ejphar.2011.04.064
Gorter, J. A., Van Vliet, E. A., Aronica, E., Breit, T., Rauwerda, H., Lopes Da Silva, F. H., et al. (2006). Potential new antiepileptogenic targets indicated by microarray analysis in a rat model for temporal lobe epilepsy. J. Neurosci. 26, 11083–11110. doi: 10.1523/jneurosci.2766-06.2006
Guha, M., O’connell, M. A., Pawlinski, R., Hollis, A., Mcgovern, P., Yan, S. F., et al. (2001). Lipopolysaccharide activation of the MEK-ERK1/2 pathway in human monocytic cells mediates tissue factor and tumor necrosis factor alpha expression by inducing Elk-1 phosphorylation and Egr-1 expression. Blood 98, 1429–1439. doi: 10.1182/blood.v98.5.1429
Hannon, G. J. (2010). Fastx-toolkit. Available online at: http://hannonlab.cshl.edu/fastx_toolkit/ [accessed on February 02, 2010].
Heylen, L., Pham, D. H., De Meulemeester, A. S., Samarut, E., Skiba, A., Copmans, D., et al. (2021). Pericardial injection of kainic acid induces a chronic epileptic state in larval Zebrafish. Front. Mol. Neurosci. 14:753936. doi: 10.3389/fnmol.2021.753936
Hortopan, G. A., Dinday, M. T., and Baraban, S. C. (2010). Zebrafish as a model for studying genetic aspects of epilepsy. Dis. Model. Mech. 3, 144–148. doi: 10.1242/dmm.002139
Ishimatsu-Tsuji, Y., Soma, T., and Kishimoto, J. (2010). Identification of novel hair-growth inducers by means of connectivity mapping. FASEB J. 24, 1489–1496. doi: 10.1096/fj.09-145292
Isserlin, R., Merico, D., Voisin, V., and Bader, G. D. (2014). Enrichment Map - a Cytoscape app to visualize and explore OMICs pathway enrichment results. F1000Res 3:141. doi: 10.12688/f1000research.4536.1
Itoh, K., Taniguchi, R., Matsuo, T., Oguro, A., Vogel, C. F. A., Yamazaki, T., et al. (2019). Suppressive effects of levetiracetam on neuroinflammation and phagocytic microglia: a comparative study of levetiracetam, valproate and carbamazepine. Neurosci. Lett. 708:134363. doi: 10.1016/j.neulet.2019.134363
Janz, R., Goda, Y., Geppert, M., Missler, M., and Sudhof, T. C. (1999). SV2A and SV2B function as redundant Ca2+ regulators in neurotransmitter release. Neuron 24, 1003–1016. doi: 10.1016/s0896-6273(00)81046-6
Kaminski, R. M., Gillard, M., Leclercq, K., Hanon, E., Lorent, G., Dassesse, D., et al. (2009). Proepileptic phenotype of SV2A-deficient mice is associated with reduced anticonvulsant efficacy of levetiracetam. Epilepsia 50, 1729–1740. doi: 10.1111/j.1528-1167.2009.02089.x
Kanehisa, M., and Goto, S. (2000). KEGG: kyoto encyclopedia of genes and genomes. Nucleic Acids Res. 28, 27–30. doi: 10.1093/nar/28.1.27
Kang, E., Song, J., Lin, Y., Park, J., Lee, J. H., Hussani, Q., et al. (2019). Interplay between a mental disorder risk gene and developmental polarity switch of GABA action leads to excitation-inhibition imbalance. Cell Rep. 28:e1413. doi: 10.1016/j.celrep.2019.07.024
Klitgaard, H., Matagne, A., Nicolas, J. M., Gillard, M., Lamberty, Y., De Ryck, M., et al. (2016). Brivaracetam: rationale for discovery and preclinical profile of a selective SV2A ligand for epilepsy treatment. Epilepsia 57, 538–548. doi: 10.1111/epi.13340
Kong, Y., Huang, L., Li, W., Liu, X., Zhou, Y., Liu, C., et al. (2021). The synaptic vesicle protein 2A interacts with key pathogenic factors in Alzheimer’s disease: implications for treatment. Front. Cell Dev. Biol. 9:609908. doi: 10.3389/fcell.2021.609908
Ksionda, O., Mues, M., Wandler, A. M., Donker, L., Tenhagen, M., Jun, J., et al. (2018). Comprehensive analysis of T cell leukemia signals reveals heterogeneity in the PI3 kinase-Akt pathway and limitations of PI3 kinase inhibitors as monotherapy. PLoS One 13:e0193849. doi: 10.1371/journal.pone.0193849
Lamb, J., Crawford, E. D., Peck, D., Modell, J. W., Blat, I. C., Wrobel, M. J., et al. (2006). The Connectivity Map: using gene-expression signatures to connect small molecules, genes, and disease. Science 313, 1929–1935. doi: 10.1126/science.1132939
Lamberty, Y., Detrait, E., Leclercq, K., Michel, A., and De Ryck, M. (2009). Behavioural phenotyping reveals anxiety-like features of SV2A deficient mice. Behav. Brain Res. 198, 329–333. doi: 10.1016/j.bbr.2008.11.005
Langmead, B., and Salzberg, S. L. (2012). Fast gapped-read alignment with Bowtie 2. Nat. Methods 9, 357–359. doi: 10.1038/nmeth.1923
Lee, J., Daniels, V., Sands, Z. A., Lebon, F., Shi, J., and Biggin, P. C. (2015). Exploring the interaction of SV2A with racetams using homology modelling, molecular dynamics and site-directed mutagenesis. PLoS One 10:e0116589. doi: 10.1371/journal.pone.0116589
Leite, J. P., and Peixoto-Santos, J. E. (2021). Glia and extracellular matrix molecules: What are their importance for the electrographic and MRI changes in the epileptogenic zone? Epilepsy Behav. 121:106542. doi: 10.1016/j.yebeh.2019.106542
Li, H., Handsaker, B., Wysoker, A., Fennell, T., Ruan, J., Homer, N., et al. (2009). The sequence alignment/map format and SAMtools. Bioinformatics 25, 2078–2079. doi: 10.1093/bioinformatics/btp352
Li, H., Shi, X., Jiang, H., Kang, J., Yu, M., Li, Q., et al. (2020). CMap analysis identifies Atractyloside as a potential drug candidate for type 2 diabetes based on integration of metabolomics and transcriptomics. J. Cell Mol. Med. 24, 7417–7426. doi: 10.1111/jcmm.15357
Li, J., Copmans, D., Partoens, M., Hunyadi, B., Luyten, W., and De Witte, P. (2020). Zebrafish-based screening of antiseizure plants used in traditional chinese medicine: magnolia officinalis extract and its constituents magnolol and honokiol exhibit potent anticonvulsant activity in a therapy-resistant epilepsy model. ACS Chem. Neurosci. 11, 730–742. doi: 10.1021/acschemneuro.9b00610
Liao, Y., Smyth, G. K., and Shi, W. (2014). featureCounts: an efficient general purpose program for assigning sequence reads to genomic features. Bioinformatics 30, 923–930. doi: 10.1093/bioinformatics/btt656
Löscher, W. (2017). Animal models of seizures and epilepsy: past, present, and future role for the discovery of antiseizure drugs. Neurochem. Res. 42, 1873–1888. doi: 10.1007/s11064-017-2222-z
Loscher, W., Gillard, M., Sands, Z. A., Kaminski, R. M., and Klitgaard, H. (2016). Synaptic vesicle glycoprotein 2A ligands in the treatment of epilepsy and beyond. CNS Drugs 30, 1055–1077. doi: 10.1007/s40263-016-0384-x
Love, M. I., Huber, W., and Anders, S. (2014). Moderated estimation of fold change and dispersion for RNA-seq data with DESeq2. Genome Biol. 15:550. doi: 10.1186/s13059-014-0550-8
Lynch, B. A., Lambeng, N., Nocka, K., Kensel-Hammes, P., Bajjalieh, S. M., Matagne, A., et al. (2004). The synaptic vesicle protein SV2A is the binding site for the antiepileptic drug levetiracetam. Proc. Natl. Acad. Sci. U. S. A. 101, 9861–9866. doi: 10.1073/pnas.0308208101
Lysenko, L. V., Kim, J., Madamba, F., Tyrtyshnaia, A. A., Ruparelia, A., and Kleschevnikov, A. M. (2018). Developmental excitatory-to-inhibitory GABA polarity switch is delayed in Ts65Dn mice, a genetic model of Down syndrome. Neurobiol. Dis. 115, 1–8. doi: 10.1016/j.nbd.2018.03.005
MacRae, C. A., and Peterson, R. T. (2015). Zebrafish as tools for drug discovery. Nat. Rev. Drug Discov. 14, 721–731. doi: 10.1038/nrd4627
Martin, M. (2011). Cutadapt removes adapter sequences from high-throughput sequencing reads. EMBnet J. 17, 10–12. doi: 10.14806/ej.17.1.200
Meehan, A. L., Yang, X., Mcadams, B. D., Yuan, L., and Rothman, S. M. (2011). A new mechanism for antiepileptic drug action: vesicular entry may mediate the effects of levetiracetam. J. Neurophysiol. 106, 1227–1239. doi: 10.1152/jn.00279.2011
Menten-Dedoyart, C., Serrano Navacerrada, M. E., Bartholome, O., Sanchez Gil, J., Neirinckx, V., Wislet, S., et al. (2016). Development and validation of a new mouse model to investigate the role of SV2A in epilepsy. PLoS One 11:e0166525. doi: 10.1371/journal.pone.0166525
Momose-Sato, Y., Nakamori, T., and Sato, K. (2012). Pharmacological mechanisms underlying switching from the large-scale depolarization wave to segregated activity in the mouse central nervous system. Eur. J. Neurosci. 35, 1242–1252. doi: 10.1111/j.1460-9568.2012.08040.x
Morgan, M., Anders, S., Lawrence, M., Aboyoun, P., Pages, H., and Gentleman, R. (2009). ShortRead: a bioconductor package for input, quality assessment and exploration of high-throughput sequence data. Bioinformatics 25, 2607–2608. doi: 10.1093/bioinformatics/btp450
Musa, A., Ghoraie, L. S., Zhang, S. D., Glazko, G., Yli-Harja, O., Dehmer, M., et al. (2018). A review of connectivity map and computational approaches in pharmacogenomics. Brief Bioinform. 19, 506–523. doi: 10.1093/bib/bbw112
Niidome, K., Taniguchi, R., Yamazaki, T., Tsuji, M., Itoh, K., and Ishihara, Y. (2021). FosL1 is a novel target of levetiracetam for suppressing the microglial inflammatory reaction. Int. J. Mol. Sci. 22:10962. doi: 10.3390/ijms222010962
Nowack, A., Yao, J., Custer, K. L., and Bajjalieh, S. M. (2010). SV2 regulates neurotransmitter release via multiple mechanisms. Am. J. Physiol. Cell Physiol. 299, C960–C967. doi: 10.1152/ajpcell.00259.2010
Okamoto, O. K., Janjoppi, L., Bonone, F. M., Pansani, A. P., Da Silva, A. V., Scorza, F. A., et al. (2010). Whole transcriptome analysis of the hippocampus: toward a molecular portrait of epileptogenesis. BMC Genomics 11:230. doi: 10.1186/1471-2164-11-230
Partoens, M., De Meulemeester, A. S., Giong, H. K., Pham, D. H., Lee, J. S., De Witte, P. A., et al. (2021). Modeling neurodevelopmental disorders and epilepsy caused by loss of function of kif2a in Zebrafish. eNeuro 8:ENEURO.0055-21.2021. doi: 10.1523/ENEURO.0055-21.2021
Patton, E. E., Zon, L. I., and Langenau, D. M. (2021). Zebrafish disease models in drug discovery: from preclinical modelling to clinical trials. Nat. Rev. Drug Discov. 20, 611–628. doi: 10.1038/s41573-021-00210-8
Pernice, H. F., Schieweck, R., Kiebler, M. A., and Popper, B. (2016). mTOR and MAPK: from localized translation control to epilepsy. BMC Neurosci. 17:73. doi: 10.1186/s12868-016-0308-1
Pitkanen, A., Lukasiuk, K., Dudek, F. E., and Staley, K. J. (2015). Epileptogenesis. Cold Spring Harb. Perspect. Med. 5:a022822. doi: 10.1101/cshperspect.a022822
Reiling, N., Blumenthal, A., Flad, H. D., Ernst, M., and Ehlers, S. (2001). Mycobacteria-induced TNF-alpha and IL-10 formation by human macrophages is differentially regulated at the level of mitogen-activated protein kinase activity. J. Immunol. 167, 3339–3345. doi: 10.4049/jimmunol.167.6.3339
Rennekamp, A. J., and Peterson, R. T. (2015). 15 years of zebrafish chemical screening. Curr. Opin. Chem. Biol. 24, 58–70. doi: 10.1016/j.cbpa.2014.10.025
Samarut, E., Swaminathan, A., Riche, R., Liao, M., Hassan-Abdi, R., Renault, S., et al. (2018). gamma-Aminobutyric acid receptor alpha 1 subunit loss of function causes genetic generalized epilepsy by impairing inhibitory network neurodevelopment. Epilepsia 59, 2061–2074. doi: 10.1111/epi.14576
Scheldeman, C., Mills, J. D., Siekierska, A., Serra, I., Copmans, D., Iyer, A. M., et al. (2017). mTOR-related neuropathology in mutant tsc2 zebrafish: phenotypic, transcriptomic and pharmacological analysis. Neurobiol. Dis. 108, 225–237. doi: 10.1016/j.nbd.2017.09.004
Schubert, J., Siekierska, A., Langlois, M., May, P., Huneau, C., Becker, F., et al. (2014). Mutations in STX1B, encoding a presynaptic protein, cause fever-associated epilepsy syndromes. Nat. Genet. 46, 1327–1332. doi: 10.1038/ng.3130
Scott, A. T., Weitz, M., Breheny, P. J., Ear, P. H., Darbro, B., Brown, B. J., et al. (2020). Gene expression signatures identify novel therapeutics for metastatic pancreatic neuroendocrine tumors. Clin. Cancer Res. 26, 2011–2021. doi: 10.1158/1078-0432.CCR-19-2884
Scott, H., and Panin, V. M. (2014). The role of protein N-glycosylation in neural transmission. Glycobiology 24, 407–417. doi: 10.1093/glycob/cwu015
Serajee, F. J., and Huq, A. M. (2015). Homozygous mutation in synaptic vesicle glycoprotein 2A gene results in intractable epilepsy, involuntary movements, microcephaly, and developmental and growth retardation. Pediatr. Neurol. 52, 642–646.e1. doi: 10.1016/j.pediatrneurol.2015.02.011
Siekierska, A., Stamberger, H., Deconinck, T., Oprescu, S. N., Partoens, M., Zhang, Y., et al. (2019). Biallelic VARS variants cause developmental encephalopathy with microcephaly that is recapitulated in vars knockout zebrafish. Nat. Commun. 10:708. doi: 10.1038/s41467-018-07953-w
Sills, G. J. (2010). SV2A in epilepsy: the plot thickens. Epilepsy Curr. 10, 47–49. doi: 10.1111/j.1535-7511.2009.01351.x
Singh, A., and Trevick, S. (2016). The epidemiology of global epilepsy. Neurol. Clin. 34, 837–847. doi: 10.1016/j.ncl.2016.06.015
Snowball, A., and Schorge, S. (2015). Changing channels in pain and epilepsy: exploiting ion channel gene therapy for disorders of neuronal hyperexcitability. FEBS Lett. 589, 1620–1634. doi: 10.1016/j.febslet.2015.05.004
Stafstrom, C. E., and Carmant, L. (2015). Seizures and epilepsy: an overview for neuroscientists. Cold Spring Harb. Perspect. Med. 5:a022426. doi: 10.1101/cshperspect.a022426
Steinhoff, B. J., and Staack, A. M. (2019). Levetiracetam and brivaracetam: a review of evidence from clinical trials and clinical experience. Ther. Adv. Neurol. Disord. 12:1756286419873518. doi: 10.1177/1756286419873518
Steinlein, O. K. (2014). Calcium signaling and epilepsy. Cell Tissue Res. 357, 385–393. doi: 10.1007/s00441-014-1849-1
Swaminathan, A., Hassan-Abdi, R., Renault, S., Siekierska, A., Riche, R., Liao, M., et al. (2018). Non-canonical mTOR-independent Role of DEPDC5 in Regulating GABAergic network development. Curr. Biol. 28:e1925. doi: 10.1016/j.cub.2018.04.061
Switon, K., Kotulska, K., Janusz-Kaminska, A., Zmorzynska, J., and Jaworski, J. (2017). Molecular neurobiology of mTOR. Neuroscience 341, 112–153. doi: 10.1016/j.neuroscience.2016.11.017
Toering, S. T., Boer, K., De Groot, M., Troost, D., Heimans, J. J., Spliet, W. G., et al. (2009). Expression patterns of synaptic vesicle protein 2A in focal cortical dysplasia and TSC-cortical tubers. Epilepsia 50, 1409–1418. doi: 10.1111/j.1528-1167.2008.01955.x
Tokudome, K., Okumura, T., Shimizu, S., Mashimo, T., Takizawa, A., Serikawa, T., et al. (2016). Synaptic vesicle glycoprotein 2A (SV2A) regulates kindling epileptogenesis via GABAergic neurotransmission. Sci. Rep. 6:27420. doi: 10.1038/srep27420
Valeeva, G., Valiullina, F., and Khazipov, R. (2013). Excitatory actions of GABA in the intact neonatal rodent hippocampus in vitro. Front. Cell. Neurosci. 7:20. doi: 10.3389/fncel.2013.00020
van Vliet, E. A., Aronica, E., Redeker, S., Boer, K., and Gorter, J. A. (2009). Decreased expression of synaptic vesicle protein 2A, the binding site for levetiracetam, during epileptogenesis and chronic epilepsy. Epilepsia 50, 422–433. doi: 10.1111/j.1528-1167.2008.01727.x
Vezzani, A. (2012). Before epilepsy unfolds: finding the epileptogenesis switch. Nat. Med. 18, 1626–1627. doi: 10.1038/nm.2982
Victor, T. R., and Tsirka, S. E. (2020). Microglial contributions to aberrant neurogenesis and pathophysiology of epilepsy. Neuroimmunol. Neuroinflamm. 7, 234–247. doi: 10.20517/2347-8659.2020.02
Vogl, C., Mochida, S., Wolff, C., Whalley, B. J., and Stephens, G. J. (2012). The synaptic vesicle glycoprotein 2A ligand levetiracetam inhibits presynaptic Ca2+ channels through an intracellular pathway. Mol. Pharmacol. 82, 199–208. doi: 10.1124/mol.111.076687
Vossel, K., Ranasinghe, K. G., Beagle, A. J., La, A., Ah Pook, K., Castro, M., et al. (2021). Effect of levetiracetam on cognition in patients with alzheimer disease with and without epileptiform activity: a randomized clinical trial. JAMA Neurol. 78, 1345–1354. doi: 10.1001/jamaneurol.2021.3310
Wang, D., Zhou, Q., Ren, L., Lin, Y., Gao, L., Du, J., et al. (2019). Levetiracetam-induced a new seizure type in a girl with a novel SV2A gene mutation. Clin. Neurol. Neurosurg. 181, 64–66. doi: 10.1016/j.clineuro.2019.03.020
Winden, K. D., Karsten, S. L., Bragin, A., Kudo, L. C., Gehman, L., Ruidera, J., et al. (2011). A systems level, functional genomics analysis of chronic epilepsy. PLoS One 6:e20763. doi: 10.1371/journal.pone.0020763
Wood, M., Daniels, V., Provins, L., Wolff, C., Kaminski, R. M., and Gillard, M. (2020). Pharmacological profile of the novel antiepileptic drug candidate padsevonil: interactions with synaptic vesicle 2 proteins and the GABAA receptor. J. Pharmacol. Exp. Ther. 372, 1–10. doi: 10.1124/jpet.119.261149
Xu, T., and Bajjalieh, S. M. (2001). SV2 modulates the size of the readily releasable pool of secretory vesicles. Nat. Cell Biol. 3, 691–698. doi: 10.1038/35087000
Yaksi, E., Jamali, A., Diaz Verdugo, C., and Jurisch-Yaksi, N. (2021). Past, present and future of zebrafish in epilepsy research. FEBS J. 288, 7243–7255. doi: 10.1111/febs.15694
Yao, J., Nowack, A., Kensel-Hammes, P., Gardner, R. G., and Bajjalieh, S. M. (2010). Cotrafficking of SV2 and synaptotagmin at the synapse. J. Neurosci. 30, 5569–5578. doi: 10.1523/JNEUROSCI.4781-09.2010
Zeidner, J. F., and Karp, J. E. (2015). Clinical activity of alvocidib (flavopiridol) in acute myeloid leukemia. Leuk. Res. 39, 1312–1318. doi: 10.1016/j.leukres.2015.10.010
Zhang, R. W., Wei, H. P., Xia, Y. M., and Du, J. L. (2010). Development of light response and GABAergic excitation-to-inhibition switch in zebrafish retinal ganglion cells. J. Physiol. 588, 2557–2569. doi: 10.1113/jphysiol.2010.187088
Keywords: zebrafish, SV2A, levetiracetam, epilepsy, connectivity mapping (CMap)
Citation: Zhang Y, Heylen L, Partoens M, Mills JD, Kaminski RM, Godard P, Gillard M, de Witte PAM and Siekierska A (2022) Connectivity Mapping Using a Novel sv2a Loss-of-Function Zebrafish Epilepsy Model as a Powerful Strategy for Anti-epileptic Drug Discovery. Front. Mol. Neurosci. 15:881933. doi: 10.3389/fnmol.2022.881933
Received: 23 February 2022; Accepted: 08 April 2022;
Published: 24 May 2022.
Edited by:
Claire Russell, Royal Veterinary College (RVC), United KingdomReviewed by:
Fahad Mahmood, Queen Elizabeth Hospital Birmingham, United KingdomRichard Ewald Rosch, King’s College London, United Kingdom
Copyright © 2022 Zhang, Heylen, Partoens, Mills, Kaminski, Godard, Gillard, de Witte and Siekierska. This is an open-access article distributed under the terms of the Creative Commons Attribution License (CC BY). The use, distribution or reproduction in other forums is permitted, provided the original author(s) and the copyright owner(s) are credited and that the original publication in this journal is cited, in accordance with accepted academic practice. No use, distribution or reproduction is permitted which does not comply with these terms.
*Correspondence: Peter A. M. de Witte, cGV0ZXIuZGV3aXR0ZUBrdWxldXZlbi5iZQ==; Aleksandra Siekierska, b2xhLnNpZWtpZXJza2FAa3VsZXV2ZW4uYmU=
†These authors have contributed equally to this work and share first authorship