- Department of Neurology, Tongji Hospital, Tongji Medical College, Huazhong University of Science and Technology, Wuhan, China
The blood–brain barrier (BBB) acts as a physical and biochemical barrier that plays a fundamental role in regulating the blood-to-brain influx of endogenous and exogenous components and maintaining the homeostatic microenvironment of the central nervous system (CNS). Acute stroke leads to BBB disruption, blood substances extravasation into the brain parenchyma, and the consequence of brain edema formation with neurological impairment afterward. Caspase-1, one of the evolutionary conserved families of cysteine proteases, which is upregulated in acute stroke, mainly mediates pyroptosis and compromises BBB integrity via lytic cellular death and inflammatory cytokines release. Nowadays, targeting caspase-1 has been proven to be effective in decreasing the occurrence of hemorrhagic transformation (HT) and in attenuating brain edema and secondary damages during acute stroke. However, the underlying interactions among caspase-1, BBB, and stroke still remain ill-defined. Hence, in this review, we are concerned about the roles of caspase-1 activation and its associated mechanisms in stroke-induced BBB damage, aiming at providing insights into the significance of caspase-1 inhibition on stroke treatment in the near future.
Introduction
Stroke is the second-leading cause of death and affects more than 13.7 million individuals per year worldwide1. About 70% of incident strokes are ischemic (9.5 million) and the rest are intracerebral hemorrhage (ICH) or subarachnoid hemorrhage (SAH) (Phipps and Cronin, 2020). Specifically, in the United States, approximately 7.9 million individuals experience a stroke, of which 87% (690,000) are ischemic, and approximately 2 million individuals experience a transient ischemic attack each year (Kleindorfer et al., 2021). Although various animal experiments for novel therapeutic drugs were promising yet, efforts were made to preserve the blood–brain barrier (BBB) integrity and minimize unfavorable outcomes in acute stroke. BBB disruption in stroke, which was critical in the pathophysiology underlying stroke and stroke-related secondary damage, draws much attention nowadays.
The BBB, a structure of tightly sealed endothelial cells (ECs) located at the luminal surface of cerebral vasculature (Sweeney et al., 2019), is composed of ECs, pericyte, astrocyte, and extracellular matrix (ECM) and is supported by neurons and vessels. Importantly, that barrier guards the central nervous system (CNS) homeostasis and regulates molecular movement between blood and brain (Begley and Brightman, 2003; Abbott et al., 2010). During acute stroke, BBB can be easily impaired via mechanical stretch (Liu et al., 2016a), oxidative stress (Yang et al., 2017), inflammation (Jansson et al., 2014), and metabolites (Savage et al., 2012), permitting a large inflow of hematogenous fluid containing blood-borne cells, chemicals, and fluid extravasate into brain parenchyma, giving rise to brain edema (Stokum et al., 2016; Xiao et al., 2020) and subsequent neurological impairment (Sweeney et al., 2019). Unfavorable events, such as hemorrhagic transformation (HT) (Terruso et al., 2009) and hematoma expansion (Keep et al., 2014; Sawada et al., 2017), can also be attributed to BBB dysfunction during the ischemia and hemorrhagic stroke, respectively.
Caspases are an evolutionary conserved family of cysteine proteases that are centrally involved in regulating multiple patterns of programmed cell death (Van Opdenbosch and Lamkanfi, 2019), among which apoptosis and pyroptosis contribute to BBB dysfunction the most in stroke. In contrast to the classical non-lytic apoptotic cell death, caspase-1 mainly mediates the major lytic cell death mode, namely pyroptosis, with pore formation on the cellular membrane and inflammatory cytokines release (Van Opdenbosch and Lamkanfi, 2019). Caspase-1 has been previously confirmed to mediate BBB disruption in the CNS, specifically critical in ECs’ injury and repair of the BBB (Israelov et al., 2020). As specific caspase-1 inhibitors have been confirmed to alleviate BBB deterioration since 2010 (Wu et al., 2010), targeting caspase-1 in stroke contributes to a promising end with a better functional outcome (Wu et al., 2010; Lin et al., 2018; Liang et al., 2019, 2020; Li et al., 2020b; Zhang et al., 2020), milder cerebral edema (Wu et al., 2010; Lin et al., 2018; Liang et al., 2019; Li et al., 2020b; Zhang et al., 2020), and lower occurrence of HT (Ismael et al., 2018; Chen et al., 2021a). However, the mechanisms about caspase-1 inhibition on stroke-induced BBB damage remain ill-defined yet. Thus, we will review the roles of caspase-1 on BBB in acute stroke through the priming, activation, and effects, aiming at elucidating potential therapeutic targets via a better understanding of the function and regulation of caspase-1 during the acute stroke.
Caspase-1 and Caspase-1-Dependent Main Effects
Caspase-1 was the first caspase reported as a protease in 1989 (Black et al., 1989) and encoded by the sequence of 11q22.1 in humans and 9A1 in mice (McIlwain et al., 2013), widely expressed among multiple organs such as spleen, liver, intestine, and brain (Stienstra et al., 2011; Kumaresan et al., 2016; Heneka et al., 2018). Caspase-1 is generally situated in the cytosol as an inactive zymogen procaspase-1 after translation and is activated proteolytically via the protein complexes “inflammasomes” for proteolytic activation (Lamkanfi and Dixit, 2009). Prototypical inflammasome is a heterologous oligomeric protein complex comprising of a sensor protein from some subfamilies of pattern recognition receptors, a caspase-1 family protease, and mostly an apoptosis speck-like protein (ASC), which couples the previous two together. Various sensor proteins, comprising of several members of the intracellular nucleotide-binding domain and leucine-rich-repeat containing (NLR) family namely NLRP1b, NLRP3, NLRC4, and NLRP6, the HIN200 family member absent in melanoma 2 (AIM2), and the TRIM family member Pyrin (Van Opdenbosch and Lamkanfi, 2019), were activated via diverse signals and distinguished the inflammasomes from each other. The most popular one NLRP3 (also termed as cryopyrin or Nalp3) is highly expressed in astrocytes, ECs, neurons, and microglia in CNS (Cassel and Sutterwala, 2010; Liu et al., 2013). Procaspase-1 harbors an N-terminal caspase activation and recruitment domain CARD, an internal large domain (p20), and a short C-terminal domain (p10), with three domains separated by linker sequences. After inflammasome activation, procaspase-1 is transformed into caspase-1 with a tetramer linked by two symmetrically arranged p20/p10 dimers (Figure 1).
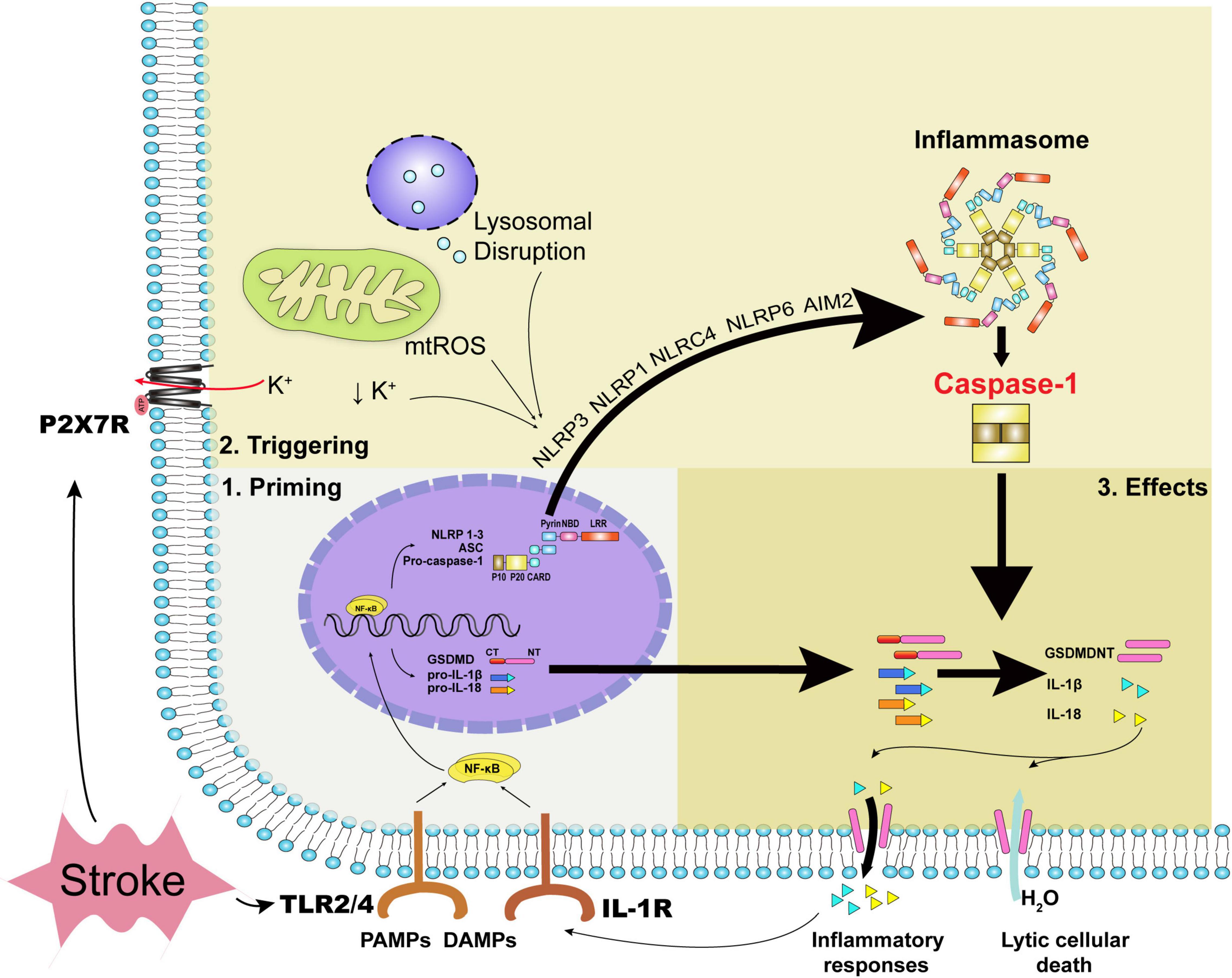
Figure 1. The priming process, triggering process, and executive effects of caspase-1. Acute stroke primarily leads to oxidative stress, disturbance of metabolites, and mechanical stress. DAMPs, inflammatory cytokines together with PAMPs can stimulate the priming process with the transcription of inflammasomes’ constituents and executive components such as pro-IL-1β, pro-IL-18, and GSDMD. During acute stroke, decreased intracellular potassium concentration, oxidative stress, and lysosomal disruption trigger NLRP3 inflammasome and caspase-1 activation, while dsDNA the form of cytosolic DNA activates caspase-1 via AIM2 inflammasome. Specifically, mechanical stress due to hematoma or edema compression can regulate ATP-gated ion channel P2X7R with potassium efflux. Caspase-1 activation leads to the formation of GSDMDNT and IL-1β or IL-18, yielding pore formation and inflammatory responses ultimately. DAMPs indicate damage-associated molecular patterns; PAMPs, pathogen associated molecular patterns; NLRP, NOD-like receptor family pyrin domain-containing; NLRC, NOD-like receptor family CARD domain containing; mtROS, mitochondrial reactive oxygen species; AIM2, absent in melanoma 2; TLR, toll-like receptor; GSDMD, gasdermin D; IL, interleukin; GSDMDNT, N-terminal fragment of gasdermin D.
Two steps, the priming and triggering processes, were essential for caspase-1 activation, respectively. The priming process indicates the expression of inflammasome composites and inflammatory cytokines. Pathogen-associated molecular patterns (PAMPs), damage-associated molecular patterns (DAMPs), and inflammatory cytokines stimulate Toll-like receptors (TLRs), and interleukin (IL)-1R evokes nuclear factor kappa B (NF-κB) expression, along with the expression of NLRP1-3, ASC, pro-caspase-1, gasdermin D (GSDMD), pro-IL-1β, pro-IL-18, etc., subsequently (Van Opdenbosch and Lamkanfi, 2019). PAMPs refer to pathogens and related products such as lipopolysaccharide, while DAMPs refer to the immunostimulatory molecular patterns in sterile injury (Chen and Nunez, 2010). After the priming process, the assembly and triggering processes of inflammasomes subsequently proceed via specific cytoplasmic pattern recognition receptors, sensing that the ligands are either the same or different as the previous priming signal (Rathinam and Fitzgerald, 2016). For instance, the commonly discussed NLRP3 can be triggered mainly by mitochondrial reactive oxygen species (ROS), decreased intracellular potassium concentration, lysosomal destabilization, and the release of lysosomal cathepsins (Kelley et al., 2019). AIM2 can be triggered by double-strain DNA (dsDNA) released from dead cells (Li et al., 2020c). The triggering signals for NLRP1b, NLRC4, and NLRP6 inflammasomes in sterile inflammation during acute stroke were possible exogenous and endogenous substances, which were not clearly defined (Abulafia et al., 2009; Poh et al., 2019; Zhang et al., 2020). Apoptotic pathway can also interact with caspase-1 as the apoptotic effector caspase-8 has been found to associate with the NLRP3 inflammasome and caspase-1 activation (Sarhan et al., 2018).
After inflammasomes and caspase-1 activation, caspase-1-mediated pyroptosis ensues with abrupt cellular death and inflammatory cytokines release. Pyroptosis occurs mostly in phagocytes, such as microglial cells, but also in neurons, astrocytes, and ECs in the CNS. Lytic cell death is accomplished via the pore-forming N-terminal fragment of GSDMD (GSDMDNT), which is usually linked to a carboxy-terminal inhibitory domain (Liu et al., 2016b; Sborgi et al., 2016) that is kept in an autoinhibitory state and released from GSDMD via caspase-1-mediated cleavage (Ding et al., 2016). With the size of cellular pore approximately 10–20 nm (Ding et al., 2016; Sborgi et al., 2016), water is permeable and culminates in cell swelling and lysis (Evavold et al., 2018). Unlike the explosive rupture in necrosis, morphology underlying pyroptosis manifests as cellular swelling, flattening of the cytoplasm due to plasma membrane leakage, and lots of bubble-like protrusions appearing on the surface of the cellular membrane before its rupture (Chen et al., 2016). Moreover, increased permeability can contribute to the flux of ions with a decreased intracellular potassium concentration, further triggering canonical NLRP3 inflammasome and caspase-1 activation (Ruhl and Broz, 2015). Caspase-1 activation can increase the cleavage of multiple enzymatic substrates, including not only the classical cytokines IL-1β and IL-18, but also cytokines IL-1F7b and IL-33, a plasma membrane Ca2+-ATPase (PMCA2), and calpastatin, the endogenous calpain inhibitor (Winkler and Rosen-Wolff, 2015). As with other cytoplasmic molecules such as intracellular potassium and DAMPs, especially HMGB1 and ATP (Sun et al., 2020), the mature IL-1β and IL-18, which have a diameter of 4.5 nm (Ding et al., 2016) and secreted in glial cells as well as ECs, are secreted into extracellular spaces depending upon GSDMD-dependent membrane permeability and initiate inflammatory process subsequently (Tapia et al., 2019). In particular, the release of DAMPs can contribute to caspase-1 activation in a vicious cycle (Savage et al., 2012). It is noteworthy to mention that non-canonical inflammasome pathway can also be activated in the stimulus of PAMPs, mediating GSDMD-dependent membrane pore formation and intracellular potassium efflux via caspase-4/5 (caspase-11 in mice) without the cleavage of pro-IL-1β/pro-IL-18 (Van Opdenbosch and Lamkanfi, 2019). Moreover, IL-1β release can also be facilitated via the absence of pyroptosis (Evavold et al., 2018) independently of plasma membrane pore formation (Karmakar et al., 2020).
The Activation of Caspase-1 in Acute Stroke
The blood–brain barrier can be impaired via mechanical stretching (Liu et al., 2016a), oxidative stress (Yang et al., 2017), inflammation (Jansson et al., 2014), and metabolites (Savage et al., 2012) in both ischemic stroke (Wu et al., 2016; Hirsch et al., 2021) and hemorrhagic stroke (Wu et al., 2010; Gan et al., 2021). Interestingly, caspase-1 can mediate those processes to some extent by driving detrimental effects on BBB in acute stroke. Therefore, we will first review the priming and triggering processes of caspase-1 during a stroke in this part.
The Activation of Caspase-1 in Ischemic Stroke
Although various models have been developed for ischemic stroke, such as transient middle cerebral artery occlusion (MCAO; Li et al., 2019, 2020c), permanent MCAO (Dong et al., 2013; Liang et al., 2020), embolic MCAO (Ishrat et al., 2015), and photothrombotic stroke (Li et al., 2020b) etc., caspase-1 expression can be enhanced in the acute phase of all models above. For 2-h transient MCAO in mice, NLRP3 and caspase-1 expression are significantly upregulated at 12 h, peaking at 24 h, and remaining elevated for more than 48 h in a time-dependent manner (Yang et al., 2014); while for permanent MCAO in mice, NLRP3 and caspase-1 expression are detected in ischemic penumbra within 24 h, peaking at 3 days and remaining elevated for 7 days after stroke (Barrington et al., 2017; Liang et al., 2020). Two successive phases, ischemia and reperfusion, probably occur during ischemic stroke. In the ischemic phase, cerebral blood flow is reduced, resulting in a deficient supply of glucose and oxygen (Sandoval and Witt, 2008). As glucose and oxygen are essential to maintain an adequate supply of ATP to guarantee physiological cellular function and maintain normal ion gradients (Abdullahi et al., 2018), oxidative phosphorylation is discontinued (Hata et al., 2000), and ion transporters on ECs are unable to function with maintaining Na+–K+–ATPase and Ca2+–ATPase due to lack of energy. Thus, in the ischemic phase, decreased intracellular K+ concentration together with calcium overload (Abdullahi et al., 2018) mainly elicit the triggering process via metabolites dysregulation. When the reperfusion phase follows, blood flow starts to recover, potentially beneficial for neuronal survival, but oxidative stress can ensue first with NLRP3 and caspase-1 activation (Ishrat et al., 2015). DsDNA released from dead cells can drive inflammasomes AIM2 activation (Li et al., 2020c). Due to increased permeability, ECM degeneration, and angiogenesis mediated by BBB dysfunction (Sandoval and Witt, 2008), vasogenic and inflammatory edema formed contribute to mechanical strains and modulate triggering of NLRP3 via P2X7 receptors (Albalawi et al., 2017). Other inflammasomes such as NLRP1 (Fann et al., 2013), NLRC4 (Poh et al., 2019), and NLRP6 (Zhang et al., 2020) have also been implicated in the response of acute stroke with the triggering process shown in Figure 1.
The Activation of Caspase-1 in Hemorrhagic Stroke
For hemorrhagic stroke, NLRP3 upregulation, caspase-1 activation, and IL-1β release occur as early as 3 h post-ICH and intensify gradually in the area around the hematoma from 1 to 5 days (Cheng et al., 2017; Yao et al., 2017). Similarly, two phases occur after a hemorrhagic stroke, as (1) the initial mechanical compression damage induced by hematomas and (2) the secondary injury characterized by excitotoxicity, inflammation, and oxidative stress.
During the initial compression by hematoma, mechanical strain-induced P2X7 receptors can activate NLRP3 inflammasomes and caspase-1 (Albalawi et al., 2017). Ischemic events can also be prevalent among hemorrhagic stroke (Prabhakaran et al., 2010; Topkoru et al., 2017), with a similar caspase-1 activation process as described above. Moreover, the breakdown of blood products was more unique in ICH. A large amount of ATP released by RBC lysis also triggered the P2X7R/NLRP3 inflammasome activation, as previously discussed (Chen et al., 2013; Feng et al., 2015). Products of hematoma, such as hemolysate degradation (hemoglobin, iron, or heme), erythrocyte thrombin, and fibrinogen, enable the priming and triggering pathways (Keep et al., 2014; Tso and Macdonald, 2014). Hemoglobin activates the TLR2/TLR4 heterodimer and promotes oxidative stress (Urday et al., 2015). Similarly, heme (Dutra et al., 2014) and iron (Mori et al., 2001) can both generate mitochondrial ROS, activate NLRP3/caspase-1 pathway, and lead to BBB hyperpermeability and cerebral edema, specifically on ECs, which can be alleviated by iron chelator deferoxamine (Lee et al., 2010). Other products, such as fibrinogen as DAMPs (Rosin and Okusa, 2011) and thrombin as the ROS contributor (Ye et al., 2017), also promote caspase-1 activation in hemorrhagic stroke.
Other Potential Factors Related to Caspase-1 Activation Process
Meanwhile, despite the direct activation of caspase-1, several comorbidities along with stroke can exacerbate caspase-1 activation toward unfavorable outcomes. Hyperglycemia or diabetics has shown the most eminent effects on stroke so far. Diabetes can amplify NLRP3 and caspase-1 activation in rats after ischemia (Ward et al., 2019), which can mediate the facilitation of tPA-induced BBB damage (Ismael et al., 2020, 2021; Chen et al., 2021a) and the tendency of HT in ischemic stroke (Ismael et al., 2020). Hyperglycemia induces superimposed effects on caspase-1 in stroke, as specific NLRP3 inhibitor MCC950 showed stronger alleviation of BBB destructions in hyperglycemic stroke than stroke without hyperglycemia (Hong et al., 2018). Whether superimposed effects exist among caspase-1 and other risk factors on BBB disruption in stroke, such as hypertension, hyperlipidemia, as well as aging (Jiang et al., 2018), deserves further research.
Caspase-1-Dependent Effects on BBB Dysfunction in Acute Stroke
Accumulating evidence have shown caspase-1 activation plays pivotal roles in exacerbating cerebral edema (Wu et al., 2010; Lin et al., 2018; Liang et al., 2019; Li et al., 2020b; Zhang et al., 2020) and increasing the occurrence of HT (Ismael et al., 2018; Chen et al., 2021a) via loosening BBB integrity (Liang et al., 2020). Caspase-1 mainly mediates effects on pyroptosis via pore formation, inflammatory cytokines release and other routes such as apoptosis as well. Considering that caspase-1 can be expressed on BBB, in this part, we will focus on caspase-1-dependent effects on BBB structures during a stroke. Meanwhile, since the effects share resembling characteristics in various patterns of ischemic and hemorrhagic stroke (Wu et al., 2010; Lin et al., 2018; Li et al., 2019, 2020b; Liang et al., 2019, 2020), caspase-1-dependent effects on BBB will be concerned with stroke events as a whole (Figure 2).
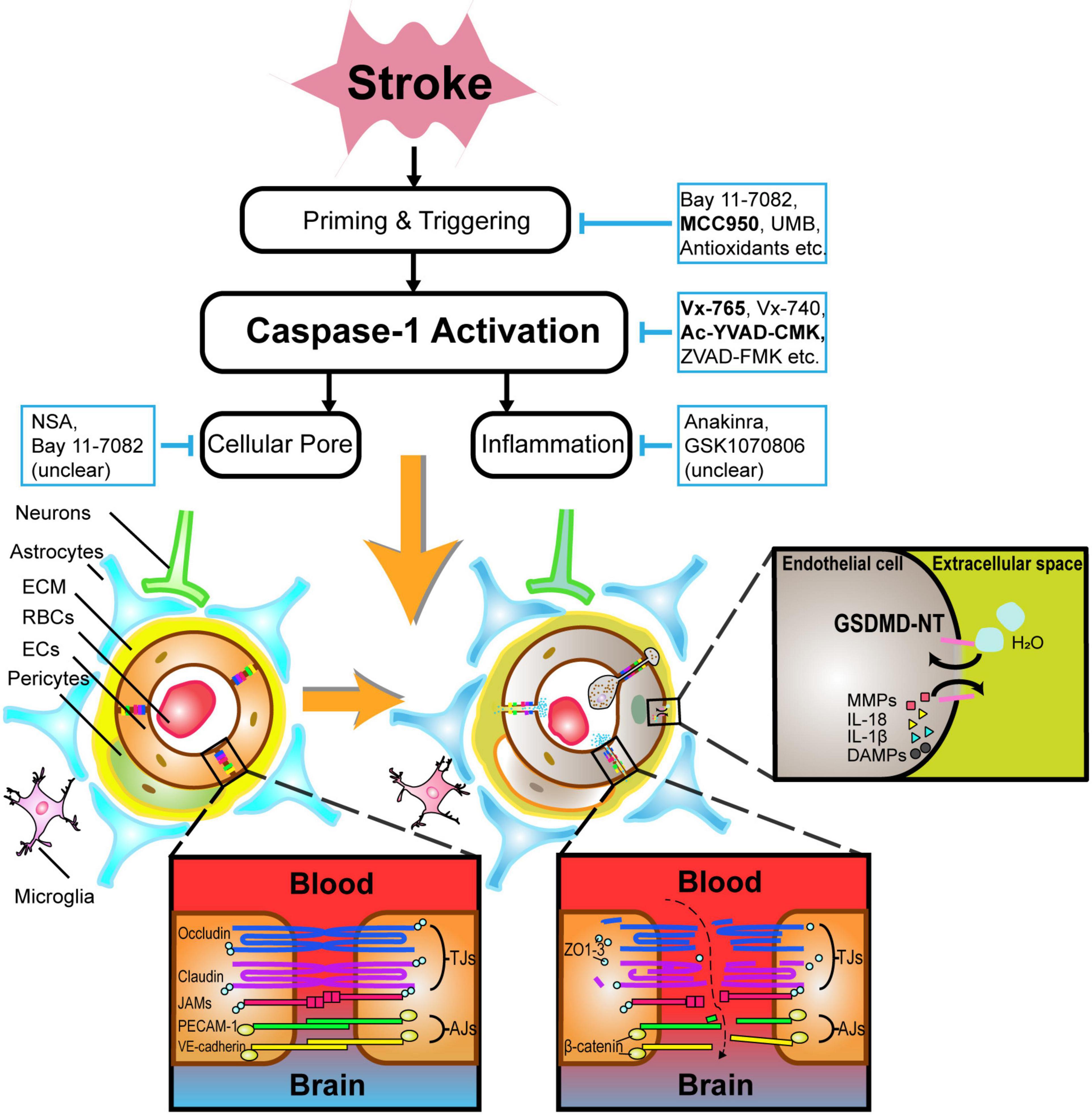
Figure 2. An illustration of the pathophysiology underlying caspase-1-mediated blood–brain barrier injuries and potential drugs targeting caspase-1-associated pathways in acute stroke. After caspase-1 activation, not only BBB components such as endothelial cells and astrocytes undergo lytic cellular death, but BBB supporting structures such as neurons and microglia are subject to pyroptosis likewise. In particular, the phosphorylation of tight junction proteins, particularly occludins and claudins, contribute to BBB hyperpermeability. GSDMDNT-mediated pore formation brings about the exchange of ions and water molecules together with inflammatory cytokines, cumulating in cellular swelling and lysis. Caspase-1-related neutrophils and lymphocytes transmigration also drive BBB disintegration to some extent. Alternatively, selective inhibitors of caspase-1-associated pathways such as Vx-765, Ac-YVAD-CMK, MCC950, etc., are promising in alleviating BBB injuries during acute stroke. ECM indicates extracellular matrix; RBCs, red blood cells; ECs, endothelial cells; IL, interleukin; DAMPs, damage-associated molecular patterns; MMPs, matrix metalloproteinases; GSDMDNT, N-terminal fragment of gasdermin D.
Caspase-1-Dependent Cellular Death on Blood–Brain Barrier in Acute Stroke
Caspase-1-dependent formation of plasma-membrane pores mediating lytic cell death occur mostly in glial cells, pericytes, ECs, and neurons (Zhang et al., 2016; Yang et al., 2017), as shown in Figure 2.
Microvascular ECs, which possess adherens junctions and tight junctions (TJs) proteins such as zonula occludens (ZO)-1 and occludins, are crucial for blocking the recruitment of peripheral leukocytes and preventing paracellular transport of multiple hydrophilic compounds across the BBB (Yang et al., 2013). Claudins (primarily claudin-5) and occludins are major transmembrane TJ proteins, phosphoproteins with four transmembrane domains spanning the intercellular cleft homotypically binding to proteins on adjacent ECs, and with cytoplasmic terminals binding to many cytoplasmic proteins including ZO-1, ZO-2, ZO-3, and cingulin, which are linked to actin cytoskeleton and maintain the structural and functional integrity (Stamatovic et al., 2016). Adherens junctions also have transmembrane proteins, cadherins, that bind to adjacent ECs and cytoplasmic plaque proteins β- or γ-catenin on the cytoplasmic domains forming the cadherin–catenin complex, which is also linked to actin cytoskeleton (Stamatovic et al., 2016). Pyroptosis in acute stroke can drive TJ proteins, adherens junction proteins, and actin cytoskeleton dysfunction on ECs, leading to their death eventually (Cao et al., 2016; Liang et al., 2020). Apart from structure destruction, posttranslational protein modification on TJs, namely, occludin, claudin-5, and ZO-1 can further increase BBB permeability via different kinases on distinct residues (Krizbai and Deli, 2003; Gonzalez-Mariscal et al., 2008) potentially via the Rho/Rho-associated protein kinase (Beckers et al., 2010), protein kinase C (Dorfel and Huber, 2012), and mitogen-activated protein kinase signaling pathways (Fujibe et al., 2004). Furthermore, ECs can also produce matrix metalloproteinases (MMPs), which potently degrade TJ and ECM structures after the lysis of ECs (Reuter et al., 2013).
Astrocytes belong to the neuron–glia system, and are the most abundant cell type in the CNS. Astrocytes provide many fundamental functions, including BBB formation, BBB structural support, the regulation of blood flow, etc. (Rossi, 2015), with water channel aquaporin 4 highly expressed on astrocytic endfeet, critically regulating water flux between the blood and brain (Nagelhus and Ottersen, 2013). In acute stroke, astrocytes transformed into reactive emerging roles in endogenous neuroprotection and repair (Koizumi et al., 2018). However, astrocytes still undergo the pyroptosis process (Li et al., 2020b; Zhang et al., 2020), with astrocytes swelling compressing vessels exacerbating vascular hypoperfusion (Sykova, 2001). The disruption of astrocytes together with aquaporin 4 unpolarization occurs in stroke subsequently, which can be reversed via targeting caspase-1, especially among the diabetics (Ward et al., 2019). Moreover, pyroptosis contributes to unregulated patterns among astrocytes and potential detrimental injury to CNS, as unregulated process induces brain edema, forms a compact glial scar, aggravates inflammation, and generates a toxic microenvironment for the components of CNS (Choudhury and Ding, 2016).
Pericytes belong to a perivascular cell type that encapsulates the microvasculature of the brain and spinal cord, forms lock and socket junctions with ECs, and contributes significantly to the maintenance of the BBB (Bennett and Kim, 2021). Pericytes can be impaired in neurovascular pathology, particularly in acute stroke (Bennett and Kim, 2021). The encapsulation rate of pericytes, which is crucial for the maintenance of microvasculature and is usually decreased during a stroke, can be elevated via caspase-1 inhibition (Liang et al., 2020).
Other cells, especially microglia and neurons, may also undergo pyroptosis and modulate BBB dysfunction. Microglia are mononuclear phagocytes, the main resident immune cells within the CNS, representing up to 10% of the total cell amount of the brain (Soulet and Rivest, 2008), which respond to sharp change in brain homeostasis and remove damaged cells via phagocytosis the first. Two distinct phenotypes of microglia have been documented (1) M1 microglia, the classically activated one, is considered to play a proinflammatory role in releasing inflammatory cytokines, including tumor necrosis factor-α, IL-1β, IL-6, IL-18, IL-23, inducible nitric oxide synthase, MMP-9, and MMP-3 (Yenari et al., 2010; Ransohoff and Brown, 2012), and (2) M2 microglia, the alternatively activated phenotype, is critical for neurogenesis, angiogenesis, and anti-inflammation by producing IL-10, growth factor including transforming growth factor β, brain-derived neurotrophic factor, and vascular endothelial growth factor (Ponomarev et al., 2013). In acute stroke, microglia show functionally distinct phenotypes according to the location and the different phases of stroke (Faustino et al., 2011). Caspase-1 activation contributes to increased classically-activated (proinflammatory) M1-type microglia polarization and reduced the number of (anti-inflammatory) M2-type cells surrounding the hematoma (Franco and Fernandez-Suarez, 2015; Hu et al., 2015; Lin et al., 2018), with a huge amount of M1 polarization induced by an increase in IL-1β secretion after hemorrhagic stroke (Zhang et al., 2017b). Similar phenomena of shifting microglia polarization can also be observed in transient ischemic mice (Li et al., 2019). Pore formation on the membrane facilitates the release of intracellular inflammatory factors from microglia, along with robust inflammation in the acute phase of ischemic stroke, leading to irreversible injury. Neurons, a crucial part in neurovascular unit supporting the BBB function, were highly susceptible to cause scattered dead neurons in the core of ischemic area (Lipton, 1999). Other than the necrotic process, pyroptosis still leads to neurons death to some degree, thereby contributing to the denervation of BBB and poor recovery of BBB (Kaplan et al., 2020). Conversely, the release cytosolic dsDNA induced by neurons leads to the triggering of AIM2 in a vicious cycle and drives a secondary immune response, including glial activation, release of cytokines and chemokines (Guruswamy and ElAli, 2017), and recruitment of peripheral immune cells (Kleinschnitz et al., 2013; Mracsko et al., 2014).
Caspase-1-Dependent Release of Proinflammatory Cytokines on Blood–Brain Barrier in Acute Stroke
IL-1β, commonly produced by astrocytes and microglia in CNS, is one of the most extensively explored cytokines in pyroptosis during stroke (Blamire et al., 2000). Brain- and blood-derived IL-1 are both able to culminate in BBB disintegration in a transient model of focal cerebral ischemia (Denes et al., 2013) via the protein kinase C-theta in human brain microvascular ECs (Rigor et al., 2012). Treatment with IL-1 receptor antagonist (IL-1Ra) or overexpression of IL-1Ra using an adenoviral approach has shown a dramatical reduction of BBB injury following ischemic stroke in rodents (Betz et al., 1995; Garcia et al., 1995; Pradillo et al., 2012, 2017). The underlying pathophysiology of IL-1β on caspase-1-mediated BBB dysfunction can be as follows: (1) IL-1β directly interacts with IL-1R in microglia (Holmes et al., 2003) and upregulates the priming process; (2) IL-1 affects astrocytes and microglial functions via promoting the proliferation (Herx and Yong, 2001) and release of cytokines and chemokines (Parker et al., 2002; Andre et al., 2005), as well as the activation of MMP-9 (Thornton et al., 2008); (3) IL-1β can induce chemokines CXCL1 (KC), CXCL2 (MIP-2), CXCL12 (SDF-1), and adhesion molecules (Israelov et al., 2020) from astrocytes and ECs along with the release of inflammatory factors, the infiltration of leukocytes and T-cells (Blamire et al., 2000; Fogal and Hewett, 2008; Sobowale et al., 2016), and the excretion neutrophil-derived MMPs (McColl et al., 2007, 2008) to the injury site (Sedgwick et al., 2000) in Figure 2.
IL-18, originally discovered as an IFN-inducing factor, modulates IFN-production from IL-18R expressing Th1 and NK cells in the periphery (Nakanishi et al., 2001; Nakanishi, 2018). In CNS, IL-18 can be upregulated by neurons, astrocytes, and microglia following lipopolysaccharide stimulation or treatment with IFN-γ (Alboni et al., 2010). Although IL-18 was shown to mediate inflammatory response resulting in loss of appetite, sleep dysregulation, and several neurodegenerative diseases (Alboni et al., 2010), caspase-1 mediated IL-18 on BBB in stroke deserves further exploration.
Interaction of Caspase-1 With Other Programmed Cell Death Pathways on Blood–Brain Barrier in Acute Stroke
The apoptotic pathway, which is functionally mediated by initiator (caspases 8, 9, and 10) (Behrouzifar et al., 2018; Israelov et al., 2020) and effector (caspases 3, 6, and 7) (Liu et al., 2017) caspases, can also mediate BBB dysfunction with increased hyperpermeability. Interestingly, the apoptotic pathway can partially interact with caspase-1. To some extent, caspase-8 can regulate the priming and activation of the canonical and non-canonical inflammasome pathways and modulate proIL-1β maturation (Van Opdenbosch and Lamkanfi, 2019). Caspase-1 activation has also shown the ability to induce apoptosis in GSDMD-deficient macrophages by engaging Bid-caspase-9-caspase-3 axis through the intrinsic apoptosis pathway (Tsuchiya et al., 2019). It is noteworthy mentioning that NLRP3 and AIM2 agonists can also induce caspase-8-mediated apoptosis (Pierini et al., 2012; Sagulenko et al., 2013) in caspase-1-deficient macrophages. However, fewer protective effects on BBB were found via caspase-8 or caspase-9 inhibition than caspase-1 inhibition (Israelov et al., 2020). Thus, targeting caspase-1 and related pathway has been suggested to be more effective in maintaining the integrity of BBB.
Therapeutic Targets of Caspase-1 and Its Associated Pathways
Targeting caspase-1 and its associated pathways has been suggested as an efficient method to alleviate BBB damage throughout acute stroke. We will review potentially effective drugs that target caspase-1 and promote protective effects on BBB in acute stroke in the following part, as listed in Table 1.
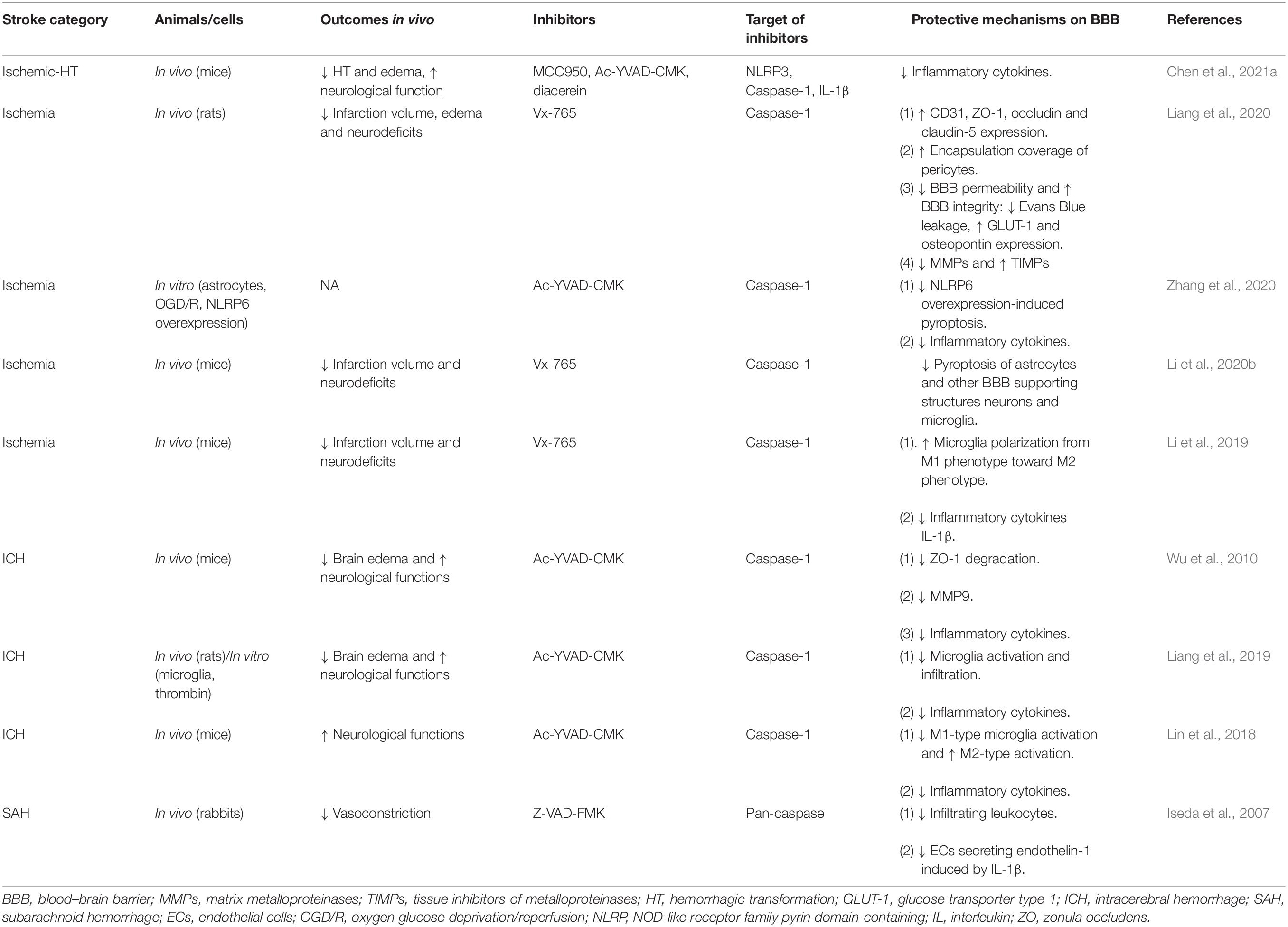
Table 1. The drugs preserving blood-brain barrier integrity via direct caspase-1 inhibition in acute stroke.
Caspase-1 Inhibitors
VX-740 (Pralnacasan) and VX-765 (Belnacasan), two analog peptidomimetic inhibitors of caspase-1 are converted rapidly to their active forms, VRT-18858 and VRT-043198 respectively, under the action of plasma and liver esterases (Rudolphi et al., 2003; Wannamaker et al., 2007). Ac-YVAD-CMK, as another specific and irreversible inhibitor of caspase-1, is a tetrapeptide sequence based on the target sequence of caspase-1 in pro-IL-1β (Garcia-Calvo et al., 1998). Except for specific caspase-1 inhibitors, pan-caspase inhibitors such as Z-VAD-FMK (Iseda et al., 2007; Lin et al., 2016) and Q-VD-OPh (Caserta et al., 2003), which inhibit the activation of some but not all caspases, have been applied to cell death researches, including pyroptosis and apoptosis. Vx-765 (Liang et al., 2020) and Ac-YVAD-CMK (Wu et al., 2010) have been commonly used in the protection of BBB in acute stroke. Liang et al. (2020) reported that Vx-765 ameliorated the BBB leakage during acute stroke with increased expression of ZO-1, occludin, and claudin-5; enhanced encapsulation rate of pericytes; downregulated proinflammatory mediators MMPs; and upregulated tissue inhibitors of metalloproteinases. Similarly, in ICH, Ac-YVAD-CMK showed similar protective effects on TJs with ZO-1 maintenance and inflammatory pathways with decreased IL-1β and MMP-9 (Wu et al., 2010). Less edema formation (Wu et al., 2010; Lin et al., 2018; Liang et al., 2019) and less occurrence of HT (Chen et al., 2021a) were common in the application of Vx-765 and Ac-YVAD-CMK (Wu et al., 2010), while upregulating the levels of endothelial markers CD31 and TJs (Liang et al., 2020) and reducing the phosphorylation of tight junction (Liang et al., 2020) were prominent with the usage of Vx-765. Lin et al. (2018) also suggested the potential role of microglia in mediating BBB dysfunction as Ac-YVAD-CMK reduces M1-type microglia polarization and increases the number of M2-type cells surrounding the hematoma.
Despite direct inhibition of caspase-1, thalidomide is an effective anti-inflammatory drug that significantly inhibits the activity of caspase-1, but its application is limited because of its strong teratogenic activity (Keller et al., 2009). Ritonavir, once a protease inhibitor used to treat HIV, was later found to effectively reduce the level of IL-18 in mouse pancreatic cancer by suppressing caspase-1 activation (Kast, 2008) with the potential application in stroke.
Caspase-1 Upstream Pathways Inhibition
Inhibitors of the Priming Process
After the addition of a specific inhibitor of NF-κB (Bay 11-7082), the priming of NLRP3 inflammasome and caspase-1 was dose-dependently reduced (Bauernfeind et al., 2009). Dong et al. (2013) reported that parthenolide, via the downregulation NF-κB, phospho-p38 mitogen-activated protein kinase, and caspase-1 expressions, has shown the amelioration of BBB permeability and brain damage from infarction in rats with permanent MCAO. A20/tumor necrosis factor-α-induced protein 3 is a key molecule that inhibits NF-κB pathway activation by ubiquitination and exhibits significant anti-inflammatory effects (Wertz et al., 2004), and D-series of resolve in can upregulate A20 expression and inhibit NF-κB activation to produce anti-inflammatory effects and minimize the effects of NLRP3 (Voet et al., 2018; Wei et al., 2020). Dexmedetomidine, a highly selective adrenergic α2 receptor agonist through suppressing the TLR4/NF-κB pathway, can downregulate the expression levels of NLRP3, ASC, caspase-1, and IL-1β in ICH (Song and Zhang, 2019), and upregulate the expression of TJ proteins in SAH mice (Yin et al., 2018). Theaflavin has also shown anti-inflammatory effects targeting TLR4/NF-κB p65 signaling pathway to protect BBB integrity in rat ICH model (Fu et al., 2018). Moreover, directly attenuating the stability and expression levels of NLRP3 mRNA via conserved microRNA-223 (Yang et al., 2015), microRNA-668 inhibitors (He and Zhang, 2020), or small interfering RNA (Yao et al., 2017) can also acquire similar effects on decreasing NLRP3 expression.
Inhibitors of the Triggering Process
Although the inhibition of NLRP3 (Chen et al., 2021a), NLRC4 (Gan et al., 2021), AIM2 (Li et al., 2020c), or NLRP6 (Meng et al., 2019) inflammasomes could all show the protective roles on BBB in acute stroke, multiple evidence have focused on NLRP3 due to its association with endogenous stimulus (Cao et al., 2016; Ward et al., 2019; Palomino-Antolin et al., 2021). MCC950 (also known as CP-456773 and CRID3) is the selective inhibitor of NLRP3 inflammasome by blocking ATP hydrolysis of NLRP3 by directly binding to the NACHT domain (Ren et al., 2018; Coll et al., 2019). Ward et al. (2019) confirmed that MCC950 maintained the vascular integrity, attenuated brain edema, improved neurological outcome and cognitive function after stroke. Blockade of NLRP3 via MCC950 in both early-phase and post-reperfusion phase among transient ischemic models protects the mice from I/R injury by mitigating inflammation and stabilizing the BBB, particularly in a dose-dependent manner (Franke et al., 2021; Palomino-Antolin et al., 2021). Similar protective effects of MCC950 on BBB have also been documented by Ren et al. (2018) in ICH.
As discussed previously, targeting NLRP3 inflammasomes that activate the process via decreased K+, mitochondrial ROS, and lysosomal disruption can also be effective in maintaining BBB structure. In ICH rats, blocking P2X7R via small interfering RNA, which can be a promising therapeutic method, can minimize the efflux of potassium concentration, attenuate inflammatory progression and brain damage via downregulating mechanical effects on NLRP3 (Feng et al., 2015). Thioredoxin-interacting protein (TXNIP), which is required for the activation of NLRP3 inflammasome (Zhou et al., 2010; Ishrat et al., 2015), is an endogenous inhibitor of the thioredoxin system, a major cellular thiol-reducing and antioxidant system (Watanabe et al., 2010). Umbelliferone (Wang et al., 2015), ruscogenin (Cao et al., 2016), ketogenic diets (Guo et al., 2018), and EGb761 (Du et al., 2020) treatment also suppressed NLRP3 inflammasome activation via attenuating the TXNIP/NLRP3 pathway and potentially upregulating the expression of TJ proteins (Cao et al., 2016). Through suppressing the endoplasmic reticulum AMPK/TXNIP/NLRP3 signaling pathway, Xu et al. (2019b) reported that exogenous apelin-13 significantly preserved BBB integrity, attenuated brain edema, and improved long-term spatial learning and memory abilities after SAH. The activation of peroxisome proliferator-activated γ receptor, such as pioglitazone (Xia et al., 2018) and medioresinol (Wang et al., 2021b), has less mitochondrial ROS production on brain microvascular ECs (Wang et al., 2021b). Other antioxidants which inhibit oxidative stress-induced NLRP3 via NADPH are NOX inhibitor apocynin (Qin et al., 2017), mPTP inhibitor (TRO19622) and mitochondrial ROS scavenger (Mito-TEMPO)(Ma et al., 2014), Nrf2 pathway promotor isoliquiritigenin (Zeng et al., 2017), and hydrogen inhalation (Zhuang et al., 2019) or hydrogen-rich saline (Shao et al., 2016), all preserve BBB function, in particular ECs (Zhuang et al., 2019) during acute stroke. As spleen tyrosine kinase-dependent ROS production can similarly activate NLRP3 inflammasomes, the facts that TREM-1 facilitates the recruitment of spleen tyrosine kinase and microglial pyroptosis can be blocked by specific TREM-1 inhibitor LP17 in experimental SAH (Xu et al., 2021a). Meanwhile, NEK7 was an essential protein that acts downstream of potassium efflux to mediate NLRP3 inflammasome assembly and activation (He et al., 2016), and NEK7 small interfering RNA injection also reversed BBB opening and microglia accumulation in SAH mice (Li et al., 2020a). Other non-specific drugs such as minocycline (Li et al., 2016; Lu et al., 2016), melatonin (Dong et al., 2016), resveratrol (Zhang et al., 2017a), chrysophanol (Zhang et al., 2014), glibenclamide (Xu et al., 2019a), and sinomenine (Qiu et al., 2016) have been reported to alleviate cerebral edema or early brain injury upon modulating the NLRP3 inflammasomes. Moreover, Zhang et al. (2019) reported that as a lysosomal stabilizing agent, dexamethasone and TAK1 inhibitor 5Z-7-oxozeaenol (Xu et al., 2021b), can prevent lysosomal membrane permeability and caspase-1 activation in ECs during nicotine-induced ECs disruption, despite not validated on the cerebral vasculature yet.
Inhibition of other canonical inflammasomes such as AIM2 (Li et al., 2020c), NLRP6 (Meng et al., 2019), or NLRC4 (Gan et al., 2021), can potentially preserve BBB in acute stroke as well. The reduction of AIM2 inflammasome and caspase-1 expression via cyclic GMP–AMP synthase antagonist A151 (Li et al., 2020c), NLRP6 reduction via NLRP6 siRNA (Meng et al., 2019), and restraining NLRC4 activation via RGS2 (Gan et al., 2021) all show the attenuation of cerebral edema.
Caspase-1 Downstream Pathways Inhibition
Gasdermin D Inhibitors
Necrosulfonamide has also been identified as a chemical inhibitor of GSDMD, which directly bonds with GSDMD and inhibits GSDMDNT oligomerization (Rathkey et al., 2018). Bay 11-7082, a previously identified NF-κB inhibitor, has been reported to potently inhibit pyroptosis through interfering GSDMD pore formation and IL-1β secretion, with its extensive suppression of inflammasomes priming, specks formation as well as inflammatory caspases activation (Wang et al., 2021a). Due to the lack of specificity, the toxicity of Bay 11-7082 in cells and tissue causes its restricted application (Nandhu et al., 2017). Disulfiram is also a GSDMD inhibitor, covalently modifying cysteine 191/192 in GSDMD while not interfering with other members of gasdermin family, which abrogates the process of pore formation with normal intervening IL-1β and GSDMD processing (Hu et al., 2020). Nevertheless, little evidence was about its protective effects on BBB.
Proinflammatory Biomarkers Inhibitors
The specific inhibitors of IL-1β, such as diacerein, have also been proven to alleviate brain edema, minimize HT, and improve neurological outcome in ischemic stroke rats (Chen et al., 2021a). Anakinra, a recombinant IL-1Ra, prevents IL-1 signaling with the validation of its role in chronic inflammatory disease (Cavalli and Dinarello, 2018) and the risk of serious infection (Galloway et al., 2011). Canakinumab is also a fully human monoclonal anti-IL-1β antibody directed against human IL-1β. Alternatively, GSK1070806, a neutralizing humanized monoclonal antibody, and Tadekinig alfa, a recombinant human IL-18 binding protein, are both involved in capturing bioactive IL-18 away from its receptor. The roles of monoclonal antibodies so far mainly focused on neutralizing proinflammatory cytokines in autoinflammatory diseases or potentially life-threatening infections (Van Opdenbosch and Lamkanfi, 2019); however, the values of monoclonal antibodies for cytokines on BBB protection in acute stroke also deserve further evaluation.
Non-specific Therapeutic Targets
Moreover, there were some therapeutic methods with less specific target but can interfere with caspase-1 in BBB protection. Hypothermia could reverse the expression NLRP3, cleaved caspase-1, and GSDMDNT together with increased autophagy in diabetic rats with permanent MCAO (Tu et al., 2019), and rescue the BBB biomarkers such as the increment of ZO-1 and claudin-5 (Tu et al., 2019). Chen et al. (2021b) reported that atorvastatin markedly increased survival rate, attenuated brain water content, downregulated the protein expression of NLRP1, cleaved caspase-1, IL-1β, and IL-18 with less early brain injury in SAH. Other promising drugs, such as HSYA together with Lex (Tan et al., 2020), etc., are also capable of decreasing cerebral edema via suppressing pyroptosis process to some degree.
Prospectives and Conclusion
Caspase-1 can be primed, triggered, and activated during acute stroke, and can participate in BBB dysfunction due to stroke-related oxidative stress, metabolic dysfunction, and mechanical stress. Targeting caspase-1 is effective in maintaining BBB integrity as less brain swelling, fewer occurrences of HT, and better functional recovery. Considering the caspase-1 activation to be a hub, which is converged by various triggering pathways to promote diverse programmed death patterns, namely, pyroptosis and apoptosis, it should be notably indispensable and potent during a stroke. In detail, the underlying pathophysiology about BBB impairment in stroke such as protein phosphorylation, breakdown of tight junction proteins, lytic cellular death upon BBB, and supporting structures as well as neutrophils and lymphocytes transcytosis, all can be reversed by selective caspase-1 inhibition in some extent. Our review sheds light on caspase-1 as a promising and crucial target throughout stroke intervention in the coming future.
So far, caspase-1 knock-out mice appear fertile and healthy (Kuida et al., 1995). In the clinical trials, Vx-765 is also safe in resistant-epilepsy treatment, but less effective in resistant-epilepsy treatment during a Phase IIb study, while Vx-740 is safe and significantly reducing joint symptoms and inflammation in patients with rheumatoid arthritis in Phase I/IIa studies but confirmed potential liver toxicity after long-term administration in animal studies (Kudelova et al., 2015). Thus, targeting caspase-1 should be safe and practical in humans. Although stroke and stroke-related comorbidities can lead to enhanced caspase-1 activity, there is little clinical evidence about targeting caspase-1 in stroke or stroke-associated comorbidities nowadays. Future researches about caspase-1 on BBB dysfunction in stroke will progress on, including (1) the interactions of caspase-1 with other programmed death pathways such as ferroptosis potentially linked by oxidative stress, (2) more prompt targets along the pathways of caspase-1-mediated BBB dysfunction, (3) the roles of targeting caspase-1 in hematoma expansion given that hematoma expansion may associate with BBB dysfunction, (4) clinical application of caspase-1 as biomarkers in predicting adverse events such as HT, and (5) the time-window, effectiveness, and dosage of drugs targeting caspase-1 in clinical practice.
Author Contributions
LL and SZ led and conceptualized the study. XY, GS, QL, and YF searched for documents about this topic. XY and SH reviewed and sorted those collected documents. XY and LL wrote the first draft. SZ reviewed and edited the final draft. All authors contributed to the article and approved the submitted version.
Funding
This work was financially supported by General Projects of Health Commission of Hubei Province (Grant No. WJ2019M121), Hubei Technological Innovation Special Fund (CN) (Grant No. 2019ACA132), and the Fundamental Research Funds for the Central Universities (Grant No. 2019kfyXKJC075).
Conflict of Interest
The authors declare that the research was conducted in the absence of any commercial or financial relationships that could be construed as a potential conflict of interest.
Publisher’s Note
All claims expressed in this article are solely those of the authors and do not necessarily represent those of their affiliated organizations, or those of the publisher, the editors and the reviewers. Any product that may be evaluated in this article, or claim that may be made by its manufacturer, is not guaranteed or endorsed by the publisher.
Acknowledgments
We appreciate all the participants in our study.
Abbreviations
BBB, blood–brain barrier; ECs, endothelial cells; ECM, extracellular matrix; CNS, central nervous system; HT, hemorrhagic transformation; ASC, apoptosis-associated speck-like protein containing a caspase recruitment domain; NLR, nucleotide-binding domain leucine-rich repeat containing gene family; AIM2, absent in melanoma 2; PAMPs, pathogen-associated molecular patterns; DAMPs, damage-associated molecular patterns; TLRs, toll-like receptors; IL, interleukin; NF- κ B, nuclear factor kappa B; GSDMD, gasdermin D; GSDMDNT, N-terminal fragment of GSDMD; ROS, reactive oxygen species; MCAO, middle cerebral artery occlusion; dsDNA, double-strain DNA; TJs, tight junctions; ZO, zonula occludens; ROCK, Rho/Rho-associated protein kinase; MMPs, matrix metalloproteases; ICH, intracerebral hemorrhage; SAH, subarachnoid hemorrhage; TXNIP, thioredoxin-interacting protein; IL-1Ra, IL-1 receptor antagonist.
Footnotes
References
Abbott, N. J., Patabendige, A. A., Dolman, D. E., Yusof, S. R., and Begley, D. J. (2010). Structure and function of the blood-brain barrier. Neurobiol. Dis. 37, 13–25. doi: 10.1016/j.nbd.2009.07.030
Abdullahi, W., Tripathi, D., and Ronaldson, P. T. (2018). Blood-brain barrier dysfunction in ischemic stroke: targeting tight junctions and transporters for vascular protection. Am. J. Physiol. Cell Physiol. 315, C343–C356. doi: 10.1152/ajpcell.00095.2018
Abulafia, D. P., de Rivero Vaccari, J. P., Lozano, J. D., Lotocki, G., Keane, R. W., and Dietrich, W. D. (2009). Inhibition of the inflammasome complex reduces the inflammatory response after thromboembolic stroke in mice. J. Cereb. Blood Flow Metab. 29, 534–544. doi: 10.1038/jcbfm.2008.143
Albalawi, F., Lu, W., Beckel, J. M., Lim, J. C., McCaughey, S. A., and Mitchell, C. H. (2017). The P2X7 Receptor Primes IL-1beta and the NLRP3 Inflammasome in Astrocytes Exposed to Mechanical Strain. Front. Cell Neurosci. 11:227. doi: 10.3389/fncel.2017.00227
Alboni, S., Cervia, D., Sugama, S., and Conti, B. (2010). Interleukin 18 in the CNS. J. Neuroinflammation 7:9. doi: 10.1186/1742-2094-7-9
Andre, R., Pinteaux, E., Kimber, I., and Rothwell, N. J. (2005). Differential actions of IL-1 alpha and IL-1 beta in glial cells share common IL-1 signalling pathways. Neuroreport 16, 153–157. doi: 10.1097/00001756-200502080-00017
Barrington, J., Lemarchand, E., and Allan, S. M. (2017). A brain in flame; do inflammasomes and pyroptosis influence stroke pathology? Brain Pathol. 27, 205–212. doi: 10.1111/bpa.12476
Bauernfeind, F. G., Horvath, G., Stutz, A., Alnemri, E. S., MacDonald, K., Speert, D., et al. (2009). Cutting edge: NF-kappaB activating pattern recognition and cytokine receptors license NLRP3 inflammasome activation by regulating NLRP3 expression. J. Immunol. 183, 787–791. doi: 10.4049/jimmunol.0901363
Beckers, C. M., van Hinsbergh, V. W., and van Nieuw Amerongen, G. P. (2010). Driving Rho GTPase activity in endothelial cells regulates barrier integrity. Thromb. Haemost. 103, 40–55. doi: 10.1160/TH09-06-0403
Begley, D. J., and Brightman, M. W. (2003). Structural and functional aspects of the blood-brain barrier. Prog. Drug Res. 61, 39–78. doi: 10.1007/978-3-0348-8049-7_2
Behrouzifar, S., Vakili, A., Bandegi, A. R., and Kokhaei, P. (2018). Neuroprotective nature of adipokine resistin in the early stages of focal cerebral ischemia in a stroke mouse model. Neurochem. Int. 114, 99–107. doi: 10.1016/j.neuint.2018.02.001
Bennett, H. C., and Kim, Y. (2021). Pericytes Across the Lifetime in the Central Nervous System. Front. Cell Neurosci. 15:627291. doi: 10.3389/fncel.2021.627291
Betz, A. L., Yang, G. Y., and Davidson, B. L. (1995). Attenuation of stroke size in rats using an adenoviral vector to induce overexpression of interleukin-1 receptor antagonist in brain. J. Cereb. Blood Flow Metab. 15, 547–551. doi: 10.1038/jcbfm.1995.68
Black, R. A., Kronheim, S. R., Merriam, J. E., March, C. J., and Hopp, T. P. (1989). A pre-aspartate-specific protease from human leukocytes that cleaves pro-interleukin-1 beta. J. Biol. Chem. 264, 5323–5326.
Blamire, A. M., Anthony, D. C., Rajagopalan, B., Sibson, N. R., Perry, V. H., and Styles, P. (2000). Interleukin-1beta -induced changes in blood-brain barrier permeability, apparent diffusion coefficient, and cerebral blood volume in the rat brain: a magnetic resonance study. J. Neurosci. 20, 8153–8159.
Cao, G., Jiang, N., Hu, Y., Zhang, Y., Wang, G., Yin, M., et al. (2016). Ruscogenin Attenuates Cerebral Ischemia-Induced Blood-Brain Barrier Dysfunction by Suppressing TXNIP/NLRP3 Inflammasome Activation and the MAPK Pathway. Int. J. Mol. Sci. 17:ijms17091418. doi: 10.3390/ijms17091418
Caserta, T. M., Smith, A. N., Gultice, A. D., Reedy, M. A., and Brown, T. L. (2003). Q-VD-OPh, a broad spectrum caspase inhibitor with potent antiapoptotic properties. Apoptosis 8, 345–352. doi: 10.1023/a:1024116916932
Cassel, S. L., and Sutterwala, F. S. (2010). Sterile inflammatory responses mediated by the NLRP3 inflammasome. Eur. J. Immunol. 40, 607–611. doi: 10.1002/eji.200940207
Cavalli, G., and Dinarello, C. A. (2018). Anakinra Therapy for Non-cancer Inflammatory Diseases. Front. Pharmacol. 9:1157. doi: 10.3389/fphar.2018.01157
Chen, G. Y., and Nunez, G. (2010). Sterile inflammation: sensing and reacting to damage. Nat. Rev. Immunol. 10, 826–837. doi: 10.1038/nri2873
Chen, H., Guan, B., Chen, S., Yang, D., and Shen, J. (2021a). Peroxynitrite activates NLRP3 inflammasome and contributes to hemorrhagic transformation and poor outcome in ischemic stroke with hyperglycemia. Free Radic. Biol. Med. 165, 171–183. doi: 10.1016/j.freeradbiomed.2021.01.030
Chen, J., Zhang, C., Yan, T., Yang, L., Wang, Y., Shi, Z., et al. (2021b). Atorvastatin ameliorates early brain injury after subarachnoid hemorrhage via inhibition of pyroptosis and neuroinflammation. J. Cell Physiol. 236, 6920–6931. doi: 10.1002/jcp.30351
Chen, S., Ma, Q., Krafft, P. R., Hu, Q., Rolland, W. II, Sherchan, P., et al. (2013). P2X7R/cryopyrin inflammasome axis inhibition reduces neuroinflammation after SAH. Neurobiol. Dis. 58, 296–307. doi: 10.1016/j.nbd.2013.06.011
Chen, X., He, W. T., Hu, L., Li, J., Fang, Y., Wang, X., et al. (2016). Pyroptosis is driven by non-selective gasdermin-D pore and its morphology is different from MLKL channel-mediated necroptosis. Cell Res. 26, 1007–1020. doi: 10.1038/cr.2016.100
Cheng, Y., Wei, Y., Yang, W., Song, Y., Shang, H., Cai, Y., et al. (2017). Cordycepin confers neuroprotection in mice models of intracerebral hemorrhage via suppressing NLRP3 inflammasome activation. Metab. Brain Dis. 32, 1133–1145. doi: 10.1007/s11011-017-0003-7
Choudhury, G. R., and Ding, S. (2016). Reactive astrocytes and therapeutic potential in focal ischemic stroke. Neurobiol. Dis. 85, 234–244. doi: 10.1016/j.nbd.2015.05.003
Coll, R. C., Hill, J. R., Day, C. J., Zamoshnikova, A., Boucher, D., Massey, N. L., et al. (2019). MCC950 directly targets the NLRP3 ATP-hydrolysis motif for inflammasome inhibition. Nat. Chem. Biol. 15, 556–559. doi: 10.1038/s41589-019-0277-7
Denes, A., Wilkinson, F., Bigger, B., Chu, M., Rothwell, N. J., and Allan, S. M. (2013). Central and haematopoietic interleukin-1 both contribute to ischaemic brain injury in mice. Dis. Model Mech. 6, 1043–1048. doi: 10.1242/dmm.011601
Ding, J., Wang, K., Liu, W., She, Y., Sun, Q., Shi, J., et al. (2016). Pore-forming activity and structural autoinhibition of the gasdermin family. Nature 535, 111–116. doi: 10.1038/nature18590
Dong, L., Qiao, H., Zhang, X., Zhang, X., Wang, C., Wang, L., et al. (2013). Parthenolide is neuroprotective in rat experimental stroke model: downregulating NF-kappaB, phospho-p38MAPK, and caspase-1 and ameliorating BBB permeability. Mediators Inflamm. 2013:370804. doi: 10.1155/2013/370804
Dong, Y., Fan, C., Hu, W., Jiang, S., Ma, Z., Yan, X., et al. (2016). Melatonin attenuated early brain injury induced by subarachnoid hemorrhage via regulating NLRP3 inflammasome and apoptosis signaling. J. Pineal Res. 60, 253–262. doi: 10.1111/jpi.12300
Dorfel, M. J., and Huber, O. (2012). A phosphorylation hotspot within the occludin C-terminal domain. Ann. N Y. Acad. Sci. 1257, 38–44. doi: 10.1111/j.1749-6632.2012.06536.x
Du, C., Xi, C., Wu, C., Sha, J., Zhang, J., and Li, C. (2020). Ginkgo biloba extract protects early brain injury after subarachnoid hemorrhage via inhibiting thioredoxin interacting protein/NLRP3 signaling pathway. Iran. J. Basic Med. Sci. 23, 1340–1345. doi: 10.22038/ijbms.2020.42834.10090
Dutra, F. F., Alves, L. S., Rodrigues, D., Fernandez, P. L., de Oliveira, R. B., Golenbock, D. T., et al. (2014). Hemolysis-induced lethality involves inflammasome activation by heme. Proc. Natl. Acad. Sci. U S A. 111, E4110–E4118. doi: 10.1073/pnas.1405023111
Evavold, C. L., Ruan, J., Tan, Y., Xia, S., Wu, H., and Kagan, J. C. (2018). The Pore-Forming Protein Gasdermin D Regulates Interleukin-1 Secretion from Living Macrophages. Immunity 48:e36. doi: 10.1016/j.immuni.2017.11.013
Fann, D. Y., Lee, S. Y., Manzanero, S., Tang, S. C., Gelderblom, M., Chunduri, P., et al. (2013). Intravenous immunoglobulin suppresses NLRP1 and NLRP3 inflammasome-mediated neuronal death in ischemic stroke. Cell Death Dis. 4:e790. doi: 10.1038/cddis.2013.326
Faustino, J. V., Wang, X., Johnson, C. E., Klibanov, A., Derugin, N., Wendland, M. F., et al. (2011). Microglial cells contribute to endogenous brain defenses after acute neonatal focal stroke. J. Neurosci. 31, 12992–13001. doi: 10.1523/JNEUROSCI.2102-11.2011
Feng, L., Chen, Y., Ding, R., Fu, Z., Yang, S., Deng, X., et al. (2015). P2X7R blockade prevents NLRP3 inflammasome activation and brain injury in a rat model of intracerebral hemorrhage: involvement of peroxynitrite. J. Neuroinflamm. 12:190. doi: 10.1186/s12974-015-0409-2
Fogal, B., and Hewett, S. J. (2008). Interleukin-1beta: a bridge between inflammation and excitotoxicity? J. Neurochem. 106, 1–23. doi: 10.1111/j.1471-4159.2008.05315.x
Franco, R., and Fernandez-Suarez, D. (2015). Alternatively activated microglia and macrophages in the central nervous system. Prog. Neurobiol. 131, 65–86. doi: 10.1016/j.pneurobio.2015.05.003
Franke, M., Bieber, M., Kraft, P., Weber, A. N. R., Stoll, G., and Schuhmann, M. K. (2021). The NLRP3 inflammasome drives inflammation in ischemia/reperfusion injury after transient middle cerebral artery occlusion in mice. Brain Behav. Immun. 92, 223–233. doi: 10.1016/j.bbi.2020.12.009
Fu, G., Wang, H., Cai, Y., Zhao, H., and Fu, W. (2018). Theaflavin alleviates inflammatory response and brain injury induced by cerebral hemorrhage via inhibiting the nuclear transcription factor kappa beta-related pathway in rats. Drug Des. Devel. Ther. 12, 1609–1619. doi: 10.2147/DDDT.S164324
Fujibe, M., Chiba, H., Kojima, T., Soma, T., Wada, T., Yamashita, T., et al. (2004). Thr203 of claudin-1, a putative phosphorylation site for MAP kinase, is required to promote the barrier function of tight junctions. Exp. Cell Res. 295, 36–47. doi: 10.1016/j.yexcr.2003.12.014
Galloway, J. B., Hyrich, K. L., Mercer, L. K., Dixon, W. G., Watson, K. D., Lunt, M., et al. (2011). The risk of serious infections in patients receiving anakinra for rheumatoid arthritis: results from the British Society for Rheumatology Biologics Register. Rheumatology 50, 1341–1342. doi: 10.1093/rheumatology/ker146
Gan, H., Zhang, L., Chen, H., Xiao, H., Wang, L., Zhai, X., et al. (2021). The pivotal role of the NLRC4 inflammasome in neuroinflammation after intracerebral hemorrhage in rats. Exp. Mol. Med. 53, 1807–1818. doi: 10.1038/s12276-021-00702-y
Garcia, J. H., Liu, K. F., and Relton, J. K. (1995). Interleukin-1 receptor antagonist decreases the number of necrotic neurons in rats with middle cerebral artery occlusion. Am. J. Pathol. 147, 1477–1486.
Garcia-Calvo, M., Peterson, E. P., Leiting, B., Ruel, R., Nicholson, D. W., and Thornberry, N. A. (1998). Inhibition of human caspases by peptide-based and macromolecular inhibitors. J. Biol. Chem. 273, 32608–32613. doi: 10.1074/jbc.273.49.32608
Gonzalez-Mariscal, L., Tapia, R., and Chamorro, D. (2008). Crosstalk of tight junction components with signaling pathways. Biochim. Biophys. Acta 1778, 729–756. doi: 10.1016/j.bbamem.2007.08.018
Guo, M., Wang, X., Zhao, Y., Yang, Q., Ding, H., Dong, Q., et al. (2018). Ketogenic Diet Improves Brain Ischemic Tolerance and Inhibits NLRP3 Inflammasome Activation by Preventing Drp1-Mediated Mitochondrial Fission and Endoplasmic Reticulum Stress. Front. Mol. Neurosci. 11:86. doi: 10.3389/fnmol.2018.00086
Guruswamy, R., and ElAli, A. (2017). Complex Roles of Microglial Cells in Ischemic Stroke Pathobiology: New Insights and Future Directions. Int. J. Mol. Sci. 18:ijms18030496. doi: 10.3390/ijms18030496
Hata, R., Maeda, K., Hermann, D., Mies, G., and Hossmann, K. A. (2000). Evolution of brain infarction after transient focal cerebral ischemia in mice. J. Cereb. Blood Flow Metab. 20, 937–946. doi: 10.1097/00004647-200006000-00006
He, J., and Zhang, X. (2020). miR-668 inhibitor attenuates mitochondrial membrane potential and protects against neuronal apoptosis in cerebral ischemic stroke. Folia Neuropathol. 58, 22–29. doi: 10.5114/fn.2020.94003
He, Y., Zeng, M. Y., Yang, D., Motro, B., and Nunez, G. (2016). NEK7 is an essential mediator of NLRP3 activation downstream of potassium efflux. Nature 530, 354–357. doi: 10.1038/nature16959
Heneka, M. T., McManus, R. M., and Latz, E. (2018). Inflammasome signalling in brain function and neurodegenerative disease. Nat. Rev. Neurosci. 19, 610–621. doi: 10.1038/s41583-018-0055-7
Herx, L. M., and Yong, V. W. (2001). Interleukin-1 beta is required for the early evolution of reactive astrogliosis following CNS lesion. J. Neuropathol. Exp. Neurol. 60, 961–971. doi: 10.1093/jnen/60.10.961
Hirsch, Y., Geraghty, J. R., Katz, E. A., and Testai, F. D. (2021). Inflammasome Caspase-1 Activity is Elevated in Cerebrospinal Fluid After Aneurysmal Subarachnoid Hemorrhage and Predicts Functional Outcome. Neurocrit. Care 34, 889–898. doi: 10.1007/s12028-020-01113-z
Holmes, C., El-Okl, M., Williams, A. L., Cunningham, C., Wilcockson, D., and Perry, V. H. (2003). Systemic infection, interleukin 1beta, and cognitive decline in Alzheimer’s disease. J. Neurol. Neurosurg. Psychiatry 74, 788–789. doi: 10.1136/jnnp.74.6.788
Hong, P., Li, F. X., Gu, R. N., Fang, Y. Y., Lai, L. Y., Wang, Y. W., et al. (2018). Inhibition of NLRP3 Inflammasome Ameliorates Cerebral Ischemia-Reperfusion Injury in Diabetic Mice. Neural Plast. 2018:9163521. doi: 10.1155/2018/9163521
Hu, J. J., Liu, X., Xia, S., Zhang, Z., Zhang, Y., Zhao, J., et al. (2020). FDA-approved disulfiram inhibits pyroptosis by blocking gasdermin D pore formation. Nat. Immunol. 21, 736–745. doi: 10.1038/s41590-020-0669-6
Hu, X., Leak, R. K., Shi, Y., Suenaga, J., Gao, Y., Zheng, P., et al. (2015). Microglial and macrophage polarization-new prospects for brain repair. Nat. Rev. Neurol. 11, 56–64. doi: 10.1038/nrneurol.2014.207
Iseda, K., Ono, S., Onoda, K., Satoh, M., Manabe, H., Nishiguchi, M., et al. (2007). Antivasospastic and antiinflammatory effects of caspase inhibitor in experimental subarachnoid hemorrhage. J. Neurosurg. 107, 128–135. doi: 10.3171/JNS-07/07/0128
Ishrat, T., Mohamed, I. N., Pillai, B., Soliman, S., Fouda, A. Y., Ergul, A., et al. (2015). Thioredoxin-interacting protein: a novel target for neuroprotection in experimental thromboembolic stroke in mice. Mol. Neurobiol. 51, 766–778. doi: 10.1007/s12035-014-8766-x
Ismael, S., Nasoohi, S., Yoo, A., Ahmed, H. A., and Ishrat, T. (2020). Tissue Plasminogen Activator Promotes TXNIP-NLRP3 Inflammasome Activation after Hyperglycemic Stroke in Mice. Mol. Neurobiol. 57, 2495–2508. doi: 10.1007/s12035-020-01893-7
Ismael, S., Nasoohi, S., Yoo, A., Mirzahosseini, G., Ahmed, H. A., and Ishrat, T. (2021). Verapamil as an Adjunct Therapy to Reduce tPA Toxicity in Hyperglycemic Stroke: Implication of TXNIP/NLRP3 Inflammasome. Mol. Neurobiol. 58, 3792–3804. doi: 10.1007/s12035-021-02384-z
Ismael, S., Zhao, L., Nasoohi, S., and Ishrat, T. (2018). Inhibition of the NLRP3-inflammasome as a potential approach for neuroprotection after stroke. Sci. Rep. 8:5971. doi: 10.1038/s41598-018-24350-x
Israelov, H., Ravid, O., Atrakchi, D., Rand, D., Elhaik, S., Bresler, Y., et al. (2020). Caspase-1 has a critical role in blood-brain barrier injury and its inhibition contributes to multifaceted repair. J. Neuroinflamm. 17:267. doi: 10.1186/s12974-020-01927-w
Jansson, D., Rustenhoven, J., Feng, S., Hurley, D., Oldfield, R. L., Bergin, P. S., et al. (2014). A role for human brain pericytes in neuroinflammation. J. Neuroinflamm. 11:104. doi: 10.1186/1742-2094-11-104
Jiang, X., Andjelkovic, A. V., Zhu, L., Yang, T., Bennett, M. V. L., Chen, J., et al. (2018). Blood-brain barrier dysfunction and recovery after ischemic stroke. Prog. Neurobiol. 163-164, 144–171. doi: 10.1016/j.pneurobio.2017.10.001
Kaplan, L., Chow, B. W., and Gu, C. (2020). Neuronal regulation of the blood-brain barrier and neurovascular coupling. Nat. Rev. Neurosci. 21, 416–432. doi: 10.1038/s41583-020-0322-2
Karmakar, M., Minns, M., Greenberg, E. N., Diaz-Aponte, J., Pestonjamasp, K., Johnson, J. L., et al. (2020). N-GSDMD trafficking to neutrophil organelles facilitates IL-1beta release independently of plasma membrane pores and pyroptosis. Nat. Commun. 11:2212. doi: 10.1038/s41467-020-16043-9
Kast, R. E. (2008). Ritonavir and disulfiram may be synergistic in lowering active interleukin-18 levels in acute pancreatitis, and thereby hasten recovery. JOP 9, 350–353.
Keep, R. F., Zhou, N., Xiang, J., Andjelkovic, A. V., Hua, Y., and Xi, G. (2014). Vascular disruption and blood-brain barrier dysfunction in intracerebral hemorrhage. Fluids Barriers CNS 11:18. doi: 10.1186/2045-8118-11-18
Keller, M., Sollberger, G., and Beer, H. D. (2009). Thalidomide inhibits activation of caspase-1. J. Immunol. 183, 5593–5599. doi: 10.4049/jimmunol.0900476
Kelley, N., Jeltema, D., Duan, Y., and He, Y. (2019). The NLRP3 Inflammasome: An Overview of Mechanisms of Activation and Regulation. Int. J. Mol. Sci. 20:ijms20133328. doi: 10.3390/ijms20133328
Kleindorfer, D. O., Towfighi, A., Chaturvedi, S., Cockroft, K. M., Gutierrez, J., Lombardi-Hill, D., et al. (2021). 2021 Guideline for the Prevention of Stroke in Patients With Stroke and Transient Ischemic Attack: A Guideline From the American Heart Association/American Stroke Association. Stroke 52, e364–e467. doi: 10.1161/STR.0000000000000375
Kleinschnitz, C., Kraft, P., Dreykluft, A., Hagedorn, I., Gobel, K., Schuhmann, M. K., et al. (2013). Regulatory T cells are strong promoters of acute ischemic stroke in mice by inducing dysfunction of the cerebral microvasculature. Blood 121, 679–691. doi: 10.1182/blood-2012-04-426734
Koizumi, S., Hirayama, Y., and Morizawa, Y. M. (2018). New roles of reactive astrocytes in the brain; an organizer of cerebral ischemia. Neurochem. Int. 119, 107–114. doi: 10.1016/j.neuint.2018.01.007
Krizbai, I. A., and Deli, M. A. (2003). Signalling pathways regulating the tight junction permeability in the blood-brain barrier. Cell Mol. Biol. 49, 23–31.
Kudelova, J., Fleischmannova, J., Adamova, E., and Matalova, E. (2015). PHARMACOLOGICAL CASPASE INHIBITORS: RESEARCH TOWARDS THERAPEUTIC PERSPECTIVES. J. Physiol. Pharmacol. 66, 473–482.
Kuida, K., Lippke, J. A., Ku, G., Harding, M. W., Livingston, D. J., Su, M. S., et al. (1995). Altered cytokine export and apoptosis in mice deficient in interleukin-1 beta converting enzyme. Science 267, 2000–2003. doi: 10.1126/science.7535475
Kumaresan, V., Ravichandran, G., Nizam, F., Dhayanithi, N. B., Arasu, M. V., Al-Dhabi, N. A., et al. (2016). Multifunctional murrel caspase 1, 2, 3, 8 and 9: Conservation, uniqueness and their pathogen-induced expression pattern. Fish Shellfish Immunol. 49, 493–504. doi: 10.1016/j.fsi.2016.01.008
Lamkanfi, M., and Dixit, V. M. (2009). The inflammasomes. PLoS Pathog. 5:e1000510. doi: 10.1371/journal.ppat.1000510
Lee, J. Y., Keep, R. F., He, Y., Sagher, O., Hua, Y., and Xi, G. (2010). Hemoglobin and iron handling in brain after subarachnoid hemorrhage and the effect of deferoxamine on early brain injury. J. Cereb. Blood Flow Metab. 30, 1793–1803. doi: 10.1038/jcbfm.2010.137
Li, J., Hao, J. H., Yao, D., Li, R., Li, X. F., Yu, Z. Y., et al. (2020b). Caspase-1 inhibition prevents neuronal death by targeting the canonical inflammasome pathway of pyroptosis in a murine model of cerebral ischemia. CNS Neurosci. Ther. 26, 925–939. doi: 10.1111/cns.13384
Li, Q., Cao, Y., Dang, C., Han, B., Han, R., Ma, H., et al. (2020c). Inhibition of double-strand DNA-sensing cGAS ameliorates brain injury after ischemic stroke. EMBO Mol. Med. 12:e11002. doi: 10.15252/emmm.201911002
Li, G., Dong, Y., Liu, D., Zou, Z., Hao, G., Gao, X., et al. (2020a). NEK7 Coordinates Rapid Neuroinflammation After Subarachnoid Hemorrhage in Mice. Front. Neurol. 11:551. doi: 10.3389/fneur.2020.00551
Li, J., Chen, J., Mo, H., Chen, J., Qian, C., Yan, F., et al. (2016). Minocycline Protects Against NLRP3 Inflammasome-Induced Inflammation and P53-Associated Apoptosis in Early Brain Injury After Subarachnoid Hemorrhage. Mol. Neurobiol. 53, 2668–2678. doi: 10.1007/s12035-015-9318-8
Li, Q., Dai, Z., Cao, Y., and Wang, L. (2019). Caspase-1 inhibition mediates neuroprotection in experimental stroke by polarizing M2 microglia/macrophage and suppressing NF-κB activation. Biochem. Biophys. Res. Commun. 513, 479–485. doi: 10.1016/j.bbrc.2019.03.202
Liang, H., Sun, Y., Gao, A., Zhang, N., Jia, Y., Yang, S., et al. (2019). Ac-YVAD-cmk improves neurological function by inhibiting caspase-1-mediated inflammatory response in the intracerebral hemorrhage of rats. Int. Immunopharmacol. 75:105771. doi: 10.1016/j.intimp.2019.105771
Liang, Y., Song, P., Chen, W., Xie, X., Luo, R., Su, J., et al. (2020). Inhibition of Caspase-1 Ameliorates Ischemia-Associated Blood-Brain Barrier Dysfunction and Integrity by Suppressing Pyroptosis Activation. Front. Cell Neurosci. 14:540669. doi: 10.3389/fncel.2020.540669
Lin, Q. R., Li, C. G., Zha, Q. B., Xu, L. H., Pan, H., Zhao, G. X., et al. (2016). Gossypol induces pyroptosis in mouse macrophages via a non-canonical inflammasome pathway. Toxicol. Appl. Pharmacol. 292, 56–64. doi: 10.1016/j.taap.2015.12.027
Lin, X., Ye, H., Siaw-Debrah, F., Pan, S., He, Z., Ni, H., et al. (2018). AC-YVAD-CMK Inhibits Pyroptosis and Improves Functional Outcome after Intracerebral Hemorrhage. Biomed. Res. Int. 2018:3706047. doi: 10.1155/2018/3706047
Lipton, P. (1999). Ischemic cell death in brain neurons. Physiol. Rev. 79, 1431–1568. doi: 10.1152/physrev.1999.79.4.1431
Liu, C., Wu, C., Yang, Q., Gao, J., Li, L., Yang, D., et al. (2016a). Macrophages Mediate the Repair of Brain Vascular Rupture through Direct Physical Adhesion and Mechanical Traction. Immunity 44, 1162–1176. doi: 10.1016/j.immuni.2016.03.008
Liu, R., Zhong, X., Zeng, J., Huang, Z., Li, X., Xiao, H., et al. (2017). 3’-Daidzein sulfonate sodium inhibits neuronal apoptosis induced by cerebral ischemia-reperfusion. Int. J. Mol. Med. 39, 1021–1028. doi: 10.3892/ijmm.2017.2915
Liu, S. B., Mi, W. L., and Wang, Y. Q. (2013). Research progress on the NLRP3 inflammasome and its role in the central nervous system. Neurosci. Bull. 29, 779–787. doi: 10.1007/s12264-013-1328-9
Liu, X., Zhang, Z., Ruan, J., Pan, Y., Magupalli, V. G., Wu, H., et al. (2016b). Inflammasome-activated gasdermin D causes pyroptosis by forming membrane pores. Nature 535, 153–158. doi: 10.1038/nature18629
Lu, Y., Xiao, G., and Luo, W. (2016). Minocycline Suppresses NLRP3 Inflammasome Activation in Experimental Ischemic Stroke. Neuroimmunomodulation 23, 230–238. doi: 10.1159/000452172
Ma, Q., Chen, S., Hu, Q., Feng, H., Zhang, J. H., and Tang, J. (2014). NLRP3 inflammasome contributes to inflammation after intracerebral hemorrhage. Ann. Neurol. 75, 209–219. doi: 10.1002/ana.24070
McColl, B. W., Rothwell, N. J., and Allan, S. M. (2007). Systemic inflammatory stimulus potentiates the acute phase and CXC chemokine responses to experimental stroke and exacerbates brain damage via interleukin-1- and neutrophil-dependent mechanisms. J. Neurosci. 27, 4403–4412. doi: 10.1523/JNEUROSCI.5376-06.2007
McColl, B. W., Rothwell, N. J., and Allan, S. M. (2008). Systemic inflammation alters the kinetics of cerebrovascular tight junction disruption after experimental stroke in mice. J. Neurosci. 28, 9451–9462. doi: 10.1523/JNEUROSCI.2674-08.2008
McIlwain, D. R., Berger, T., and Mak, T. W. (2013). Caspase functions in cell death and disease. Cold Spring Harb. Perspect. Biol. 5:a008656. doi: 10.1101/cshperspect.a008656
Meng, C., Zhang, J., Zhang, L., Wang, Y., Li, Z., and Zhao, J. (2019). Effects of NLRP6 in Cerebral Ischemia/Reperfusion (I/R) Injury in Rats. J. Mol. Neurosci. 69, 411–418. doi: 10.1007/s12031-019-01370-4
Mori, T., Nagata, K., Town, T., Tan, J., Matsui, T., and Asano, T. (2001). Intracisternal increase of superoxide anion production in a canine subarachnoid hemorrhage model. Stroke 32, 636–642. doi: 10.1161/01.str.32.3.636
Mracsko, E., Javidi, E., Na, S. Y., Kahn, A., Liesz, A., and Veltkamp, R. (2014). Leukocyte invasion of the brain after experimental intracerebral hemorrhage in mice. Stroke 45, 2107–2114. doi: 10.1161/STROKEAHA.114.005801
Nagelhus, E. A., and Ottersen, O. P. (2013). Physiological roles of aquaporin-4 in brain. Physiol. Rev. 93, 1543–1562. doi: 10.1152/physrev.00011.2013
Nakanishi, K. (2018). Unique Action of Interleukin-18 on T Cells and Other Immune Cells. Front. Immunol. 9:763. doi: 10.3389/fimmu.2018.00763
Nakanishi, K., Yoshimoto, T., Tsutsui, H., and Okamura, H. (2001). Interleukin-18 regulates both Th1 and Th2 responses. Annu. Rev. Immunol. 19, 423–474. doi: 10.1146/annurev.immunol.19.1.423
Nandhu, M. S., Kwiatkowska, A., Bhaskaran, V., Hayes, J., Hu, B., and Viapiano, M. S. (2017). Tumor-derived fibulin-3 activates pro-invasive NF-kappaB signaling in glioblastoma cells and their microenvironment. Oncogene 36, 4875–4886. doi: 10.1038/onc.2017.109
Palomino-Antolin, A., Narros-Fernandez, P., Farre-Alins, V., Sevilla-Montero, J., Decouty-Perez, C., Lopez-Rodriguez, A. B., et al. (2021). Time-dependent dual effect of NLRP3 inflammasome in brain ischemia. Br. J. Pharmacol. 2021:15732. doi: 10.1111/bph.15732
Parker, L. C., Luheshi, G. N., Rothwell, N. J., and Pinteaux, E. (2002). IL-1 beta signalling in glial cells in wildtype and IL-1RI deficient mice. Br. J. Pharmacol. 136, 312–320. doi: 10.1038/sj.bjp.0704715
Phipps, M. S., and Cronin, C. A. (2020). Management of acute ischemic stroke. BMJ 368:l6983. doi: 10.1136/bmj.l6983
Pierini, R., Juruj, C., Perret, M., Jones, C. L., Mangeot, P., Weiss, D. S., et al. (2012). AIM2/ASC triggers caspase-8-dependent apoptosis in Francisella-infected caspase-1-deficient macrophages. Cell Death Differ. 19, 1709–1721. doi: 10.1038/cdd.2012.51
Poh, L., Kang, S. W., Baik, S. H., Ng, G. Y. Q., She, D. T., Balaganapathy, P., et al. (2019). Evidence that NLRC4 inflammasome mediates apoptotic and pyroptotic microglial death following ischemic stroke. Brain Behav. Immun. 75, 34–47. doi: 10.1016/j.bbi.2018.09.001
Ponomarev, E. D., Veremeyko, T., and Weiner, H. L. (2013). MicroRNAs are universal regulators of differentiation, activation, and polarization of microglia and macrophages in normal and diseased CNS. Glia 61, 91–103. doi: 10.1002/glia.22363
Prabhakaran, S., Gupta, R., Ouyang, B., John, S., Temes, R. E., Mohammad, Y., et al. (2010). Acute brain infarcts after spontaneous intracerebral hemorrhage: a diffusion-weighted imaging study. Stroke 41, 89–94. doi: 10.1161/STROKEAHA.109.566257
Pradillo, J. M., Denes, A., Greenhalgh, A. D., Boutin, H., Drake, C., McColl, B. W., et al. (2012). Delayed administration of interleukin-1 receptor antagonist reduces ischemic brain damage and inflammation in comorbid rats. J. Cereb. Blood Flow Metab. 32, 1810–1819. doi: 10.1038/jcbfm.2012.101
Pradillo, J. M., Murray, K. N., Coutts, G. A., Moraga, A., Oroz-Gonjar, F., Boutin, H., et al. (2017). Reparative effects of interleukin-1 receptor antagonist in young and aged/co-morbid rodents after cerebral ischemia. Brain Behav. Immun. 61, 117–126. doi: 10.1016/j.bbi.2016.11.013
Qin, Y. Y., Li, M., Feng, X., Wang, J., Cao, L., Shen, X. K., et al. (2017). Combined NADPH and the NOX inhibitor apocynin provides greater anti-inflammatory and neuroprotective effects in a mouse model of stroke. Free Radic. Biol. Med. 104, 333–345. doi: 10.1016/j.freeradbiomed.2017.01.034
Qiu, J., Wang, M., Zhang, J., Cai, Q., Lu, D., Li, Y., et al. (2016). The neuroprotection of Sinomenine against ischemic stroke in mice by suppressing NLRP3 inflammasome via AMPK signaling. Int. Immunopharmacol. 40, 492–500. doi: 10.1016/j.intimp.2016.09.024
Ransohoff, R. M., and Brown, M. A. (2012). Innate immunity in the central nervous system. J. Clin. Invest. 122, 1164–1171. doi: 10.1172/JCI58644
Rathinam, V. A., and Fitzgerald, K. A. (2016). Inflammasome Complexes: Emerging Mechanisms and Effector Functions. Cell 165, 792–800. doi: 10.1016/j.cell.2016.03.046
Rathkey, J. K., Zhao, J., Liu, Z., Chen, Y., Yang, J., Kondolf, H. C., et al. (2018). Chemical disruption of the pyroptotic pore-forming protein gasdermin D inhibits inflammatory cell death and sepsis. Sci. Immunol. 3:aat2738. doi: 10.1126/sciimmunol.aat2738
Ren, H., Kong, Y., Liu, Z., Zang, D., Yang, X., Wood, K., et al. (2018). Selective NLRP3 (Pyrin Domain-Containing Protein 3) Inflammasome Inhibitor Reduces Brain Injury After Intracerebral Hemorrhage. Stroke 49, 184–192. doi: 10.1161/STROKEAHA.117.018904
Reuter, B., Rodemer, C., Grudzenski, S., Couraud, P. O., Weksler, B., Romero, I. A., et al. (2013). Temporal profile of matrix metalloproteinases and their inhibitors in a human endothelial cell culture model of cerebral ischemia. Cerebrovasc. Dis. 35, 514–520. doi: 10.1159/000350731
Rigor, R. R., Beard, R. S. Jr., Litovka, O. P., and Yuan, S. Y. (2012). Interleukin-1beta-induced barrier dysfunction is signaled through PKC-theta in human brain microvascular endothelium. Am. J. Physiol. Cell Physiol. 302, C1513–C1522. doi: 10.1152/ajpcell.00371.2011
Rosin, D. L., and Okusa, M. D. (2011). Dangers within: DAMP responses to damage and cell death in kidney disease. J. Am. Soc. Nephrol. 22, 416–425. doi: 10.1681/ASN.2010040430
Rossi, D. (2015). Astrocyte physiopathology: At the crossroads of intercellular networking, inflammation and cell death. Prog. Neurobiol. 130, 86–120. doi: 10.1016/j.pneurobio.2015.04.003
Rudolphi, K., Gerwin, N., Verzijl, N., van der Kraan, P., and van den Berg, W. (2003). Pralnacasan, an inhibitor of interleukin-1beta converting enzyme, reduces joint damage in two murine models of osteoarthritis. Osteoarthritis Cartilage 11, 738–746. doi: 10.1016/s1063-4584(03)00153-5
Ruhl, S., and Broz, P. (2015). Caspase-11 activates a canonical NLRP3 inflammasome by promoting K(+) efflux. Eur. J. Immunol. 45, 2927–2936. doi: 10.1002/eji.201545772
Sagulenko, V., Thygesen, S. J., Sester, D. P., Idris, A., Cridland, J. A., Vajjhala, P. R., et al. (2013). AIM2 and NLRP3 inflammasomes activate both apoptotic and pyroptotic death pathways via ASC. Cell Death Differ. 20, 1149–1160. doi: 10.1038/cdd.2013.37
Sandoval, K. E., and Witt, K. A. (2008). Blood-brain barrier tight junction permeability and ischemic stroke. Neurobiol. Dis. 32, 200–219. doi: 10.1016/j.nbd.2008.08.005
Sarhan, J., Liu, B. C., Muendlein, H. I., Li, P., Nilson, R., Tang, A. Y., et al. (2018). Caspase-8 induces cleavage of gasdermin D to elicit pyroptosis during Yersinia infection. Proc. Natl. Acad. Sci. U S A. 115, E10888–E10897. doi: 10.1073/pnas.1809548115
Savage, C. D., Lopez-Castejon, G., Denes, A., and Brough, D. (2012). NLRP3-Inflammasome Activating DAMPs Stimulate an Inflammatory Response in Glia in the Absence of Priming Which Contributes to Brain Inflammation after Injury. Front. Immunol. 3:288. doi: 10.3389/fimmu.2012.00288
Sawada, S., Ono, Y., Egashira, Y., Takagi, T., Tsuruma, K., Shimazawa, M., et al. (2017). In Models of Intracerebral Hemorrhage, Rivaroxaban is Superior to Warfarin to Limit Blood Brain Barrier Disruption and Hematoma Expansion. Curr. Neurovasc. Res. 14, 96–103. doi: 10.2174/1567202613666161216150835
Sborgi, L., Ruhl, S., Mulvihill, E., Pipercevic, J., Heilig, R., Stahlberg, H., et al. (2016). GSDMD membrane pore formation constitutes the mechanism of pyroptotic cell death. EMBO J. 35, 1766–1778. doi: 10.15252/embj.201694696
Sedgwick, J. D., Riminton, D. S., Cyster, J. G., and Korner, H. (2000). Tumor necrosis factor: a master-regulator of leukocyte movement. Immunol. Today 21, 110–113. doi: 10.1016/s0167-5699(99)01573-x
Shao, A., Wu, H., Hong, Y., Tu, S., Sun, X., Wu, Q., et al. (2016). Hydrogen-Rich Saline Attenuated Subarachnoid Hemorrhage-Induced Early Brain Injury in Rats by Suppressing Inflammatory Response: Possible Involvement of NF-kappaB Pathway and NLRP3 Inflammasome. Mol. Neurobiol. 53, 3462–3476. doi: 10.1007/s12035-015-9242-y
Sobowale, O. A., Parry-Jones, A. R., Smith, C. J., Tyrrell, P. J., Rothwell, N. J., and Allan, S. M. (2016). Interleukin-1 in Stroke: From Bench to Bedside. Stroke 47, 2160–2167. doi: 10.1161/STROKEAHA.115.010001
Song, H. L., and Zhang, S. B. (2019). Therapeutic effect of dexmedetomidine on intracerebral hemorrhage via regulating NLRP3. Eur. Rev. Med. Pharmacol. Sci. 23, 2612–2619. doi: 10.26355/eurrev_201903_17411
Soulet, D., and Rivest, S. (2008). Microglia. Curr. Biol. 18, R506–R508. doi: 10.1016/j.cub.2008.04.047
Stamatovic, S. M., Johnson, A. M., Keep, R. F., and Andjelkovic, A. V. (2016). Junctional proteins of the blood-brain barrier: New insights into function and dysfunction. Tissue Barriers 4:e1154641. doi: 10.1080/21688370.2016.1154641
Stienstra, R., van Diepen, J. A., Tack, C. J., Zaki, M. H., van de Veerdonk, F. L., Perera, D., et al. (2011). Inflammasome is a central player in the induction of obesity and insulin resistance. Proc. Natl. Acad. Sci. U S A. 108, 15324–15329. doi: 10.1073/pnas.1100255108
Stokum, J. A., Gerzanich, V., and Simard, J. M. (2016). Molecular pathophysiology of cerebral edema. J. Cereb. Blood Flow Metab. 36, 513–538. doi: 10.1177/0271678X15617172
Sun, Z., Nyanzu, M., Yang, S., Zhu, X., Wang, K., Ru, J., et al. (2020). VX765 Attenuates Pyroptosis and HMGB1/TLR4/NF-kappaB Pathways to Improve Functional Outcomes in TBI Mice. Oxid. Med. Cell Longev. 2020:7879629. doi: 10.1155/2020/7879629
Sweeney, M. D., Zhao, Z., Montagne, A., Nelson, A. R., and Zlokovic, B. V. (2019). Blood-Brain Barrier: From Physiology to Disease and Back. Physiol. Rev. 99, 21–78. doi: 10.1152/physrev.00050.2017
Sykova, E. (2001). Glial diffusion barriers during aging and pathological states. Prog. Brain Res. 132, 339–363. doi: 10.1016/S0079-6123(01)32087-3
Tan, L., Wang, Y., Jiang, Y., Wang, R., Zu, J., and Tan, R. (2020). Hydroxysafflor Yellow A Together with Blood-Brain Barrier Regulator Lexiscan for Cerebral Ischemia Reperfusion Injury Treatment. ACS Omega 5, 19151–19164. doi: 10.1021/acsomega.0c02502
Tapia, V. S., Daniels, M. J. D., Palazon-Riquelme, P., Dewhurst, M., Luheshi, N. M., Rivers-Auty, J., et al. (2019). The three cytokines IL-1beta, IL-18, and IL-1alpha share related but distinct secretory routes. J. Biol. Chem. 294, 8325–8335. doi: 10.1074/jbc.RA119.008009
Terruso, V., D’Amelio, M., Di Benedetto, N., Lupo, I., Saia, V., Famoso, G., et al. (2009). Frequency and determinants for hemorrhagic transformation of cerebral infarction. Neuroepidemiology 33, 261–265. doi: 10.1159/000229781
Thornton, P., Pinteaux, E., Allan, S. M., and Rothwell, N. J. (2008). Matrix metalloproteinase-9 and urokinase plasminogen activator mediate interleukin-1-induced neurotoxicity. Mol. Cell Neurosci. 37, 135–142. doi: 10.1016/j.mcn.2007.09.002
Topkoru, B., Egemen, E., Solaroglu, I., and Zhang, J. H. (2017). Early Brain Injury or Vasospasm? An Overview of Common Mechanisms. Curr. Drug Targets 18, 1424–1429. doi: 10.2174/1389450117666160905112923
Tso, M. K., and Macdonald, R. L. (2014). Subarachnoid hemorrhage: a review of experimental studies on the microcirculation and the neurovascular unit. Transl. Stroke Res. 5, 174–189. doi: 10.1007/s12975-014-0323-4
Tsuchiya, K., Nakajima, S., Hosojima, S., Thi Nguyen, D., Hattori, T., and Manh, et al. (2019). Caspase-1 initiates apoptosis in the absence of gasdermin D. Nat. Commun. 10:2091. doi: 10.1038/s41467-019-09753-2
Tu, Y., Guo, C., Song, F., Huo, Y., Geng, Y., Guo, M., et al. (2019). Mild hypothermia alleviates diabetes aggravated cerebral ischemic injury via activating autophagy and inhibiting pyroptosis. Brain Res. Bull. 150, 1–12. doi: 10.1016/j.brainresbull.2019.05.003
Urday, S., Kimberly, W. T., Beslow, L. A., Vortmeyer, A. O., Selim, M. H., Rosand, J., et al. (2015). Targeting secondary injury in intracerebral haemorrhage–perihaematomal oedema. Nat. Rev. Neurol. 11, 111–122. doi: 10.1038/nrneurol.2014.264
Van Opdenbosch, N., and Lamkanfi, M. (2019). Caspases in Cell Death, Inflammation, and Disease. Immunity 50, 1352–1364. doi: 10.1016/j.immuni.2019.05.020
Voet, S., Mc Guire, C., Hagemeyer, N., Martens, A., Schroeder, A., Wieghofer, P., et al. (2018). A20 critically controls microglia activation and inhibits inflammasome-dependent neuroinflammation. Nat. Commun. 9:2036. doi: 10.1038/s41467-018-04376-5
Wang, Y., Guan, X., Gao, C. L., Ruan, W., Zhao, S., Kai, G., et al. (2021b). Medioresinol as a novel PGC-1alpha activator prevents pyroptosis of endothelial cells in ischemic stroke through PPARalpha-GOT1 axis. Pharmacol. Res. 169:105640. doi: 10.1016/j.phrs.2021.105640
Wang, J., Yao, J., Liu, Y., and Huang, L. (2021a). Targeting the gasdermin D as a strategy for ischemic stroke therapy. Biochem. Pharmacol. 188:114585. doi: 10.1016/j.bcp.2021.114585
Wang, X., Li, R., Wang, X., Fu, Q., and Ma, S. (2015). Umbelliferone ameliorates cerebral ischemia-reperfusion injury via upregulating the PPAR gamma expression and suppressing TXNIP/NLRP3 inflammasome. Neurosci. Lett. 600, 182–187. doi: 10.1016/j.neulet.2015.06.016
Wannamaker, W., Davies, R., Namchuk, M., Pollard, J., Ford, P., Ku, G., et al. (2007). (S)-1-((S)-2-{[1-(4-amino-3-chloro-phenyl)-methanoyl]-amino}-3,3-dimethyl-butanoy l)-pyrrolidine-2-carboxylic acid ((2R,3S)-2-ethoxy-5-oxo-tetrahydro-furan-3-yl)-amide (VX-765), an orally available selective interleukin (IL)-converting enzyme/caspase-1 inhibitor, exhibits potent anti-inflammatory activities by inhibiting the release of IL-1beta and IL-18. J. Pharmacol. Exp. Ther. 321, 509–516. doi: 10.1124/jpet.106.111344
Ward, R., Li, W., Abdul, Y., Jackson, L., Dong, G., Jamil, S., et al. (2019). NLRP3 inflammasome inhibition with MCC950 improves diabetes-mediated cognitive impairment and vasoneuronal remodeling after ischemia. Pharmacol. Res. 142, 237–250. doi: 10.1016/j.phrs.2019.01.035
Watanabe, R., Nakamura, H., Masutani, H., and Yodoi, J. (2010). Anti-oxidative, anti-cancer and anti-inflammatory actions by thioredoxin 1 and thioredoxin-binding protein-2. Pharmacol. Ther. 127, 261–270. doi: 10.1016/j.pharmthera.2010.04.004
Wei, C., Guo, S., Liu, W., Jin, F., Wei, B., Fan, H., et al. (2020). Resolvin D1 ameliorates Inflammation-Mediated Blood-Brain Barrier Disruption After Subarachnoid Hemorrhage in rats by Modulating A20 and NLRP3 Inflammasome. Front. Pharmacol. 11:610734. doi: 10.3389/fphar.2020.610734
Wertz, I. E., O’Rourke, K. M., Zhou, H., Eby, M., Aravind, L., Seshagiri, S., et al. (2004). De-ubiquitination and ubiquitin ligase domains of A20 downregulate NF-kappaB signalling. Nature 430, 694–699. doi: 10.1038/nature02794
Winkler, S., and Rosen-Wolff, A. (2015). Caspase-1: an integral regulator of innate immunity. Semin. Immunopathol. 37, 419–427. doi: 10.1007/s00281-015-0494-4
Wu, B., Ma, Q., Khatibi, N., Chen, W., Sozen, T., Cheng, O., et al. (2010). Ac-YVAD-CMK Decreases Blood-Brain Barrier Degradation by Inhibiting Caspase-1 Activation of Interleukin-1beta in Intracerebral Hemorrhage Mouse Model. Transl. Stroke Res. 1, 57–64. doi: 10.1007/s12975-009-0002-z
Wu, Q., Wang, X. L., Yu, Q., Pan, H., Zhang, X. S., Zhang, Q. R., et al. (2016). Inflammasome Proteins in Cerebrospinal Fluid of Patients with Subarachnoid Hemorrhage are Biomarkers of Early Brain Injury and Functional Outcome. World Neurosurg. 94, 472–479. doi: 10.1016/j.wneu.2016.07.039
Xia, P., Pan, Y., Zhang, F., Wang, N., Wang, E., Guo, Q., et al. (2018). Pioglitazone Confers Neuroprotection Against Ischemia-Induced Pyroptosis due to its Inhibitory Effects on HMGB-1/RAGE and Rac1/ROS Pathway by Activating PPAR. Cell Physiol. Biochem. 45, 2351–2368. doi: 10.1159/000488183
Xiao, M., Xiao, Z. J., Yang, B., Lan, Z., and Fang, F. (2020). Blood-Brain Barrier: More Contributor to Disruption of Central Nervous System Homeostasis Than Victim in Neurological Disorders. Front. Neurosci. 14:764. doi: 10.3389/fnins.2020.00764
Xu, W., Li, T., Gao, L., Zheng, J., Yan, J., Zhang, J., et al. (2019b). Apelin-13/APJ system attenuates early brain injury via suppression of endoplasmic reticulum stress-associated TXNIP/NLRP3 inflammasome activation and oxidative stress in a AMPK-dependent manner after subarachnoid hemorrhage in rats. J. Neuroinflamm. 16:247. doi: 10.1186/s12974-019-1620-3
Xu, F., Shen, G., Su, Z., He, Z., and Yuan, L. (2019a). Glibenclamide ameliorates the disrupted blood-brain barrier in experimental intracerebral hemorrhage by inhibiting the activation of NLRP3 inflammasome. Brain Behav. 9:e01254. doi: 10.1002/brb3.1254
Xu, P., Hong, Y., Xie, Y., Yuan, K., Li, J., Sun, R., et al. (2021a). TREM-1 Exacerbates Neuroinflammatory Injury via NLRP3 Inflammasome-Mediated Pyroptosis in Experimental Subarachnoid Hemorrhage. Transl. Stroke Res. 12, 643–659. doi: 10.1007/s12975-020-00840-x
Xu, P., Tao, C., Zhu, Y., Wang, G., Kong, L., Li, W., et al. (2021b). TAK1 mediates neuronal pyroptosis in early brain injury after subarachnoid hemorrhage. J. Neuroinflamm. 18:188. doi: 10.1186/s12974-021-02226-8
Yang, F., Wang, Z., Wei, X., Han, H., Meng, X., Zhang, Y., et al. (2014). NLRP3 deficiency ameliorates neurovascular damage in experimental ischemic stroke. J. Cereb. Blood Flow Metab. 34, 660–667. doi: 10.1038/jcbfm.2013.242
Yang, G., Qian, C., Wang, N., Lin, C., Wang, Y., Wang, G., et al. (2017). Tetramethylpyrazine Protects Against Oxygen-Glucose Deprivation-Induced Brain Microvascular Endothelial Cells Injury via Rho/Rho-kinase Signaling Pathway. Cell Mol. Neurobiol. 37, 619–633. doi: 10.1007/s10571-016-0398-4
Yang, Y., Thompson, J. F., Taheri, S., Salayandia, V. M., McAvoy, T. A., Hill, J. W., et al. (2013). Early inhibition of MMP activity in ischemic rat brain promotes expression of tight junction proteins and angiogenesis during recovery. J. Cereb. Blood Flow Metab. 33, 1104–1114. doi: 10.1038/jcbfm.2013.56
Yang, Z., Zhong, L., Xian, R., and Yuan, B. (2015). MicroRNA-223 regulates inflammation and brain injury via feedback to NLRP3 inflammasome after intracerebral hemorrhage. Mol. Immunol. 65, 267–276. doi: 10.1016/j.molimm.2014.12.018
Yao, S. T., Cao, F., Chen, J. L., Chen, W., Fan, R. M., Li, G., et al. (2017). NLRP3 is Required for Complement-Mediated Caspase-1 and IL-1beta Activation in ICH. J. Mol. Neurosci. 61, 385–395. doi: 10.1007/s12031-016-0874-9
Ye, X., Zuo, D., Yu, L., Zhang, L., Tang, J., Cui, C., et al. (2017). ROS/TXNIP pathway contributes to thrombin induced NLRP3 inflammasome activation and cell apoptosis in microglia. Biochem. Biophys. Res. Commun. 485, 499–505. doi: 10.1016/j.bbrc.2017.02.019
Yenari, M. A., Kauppinen, T. M., and Swanson, R. A. (2010). Microglial activation in stroke: therapeutic targets. Neurotherapeutics 7, 378–391. doi: 10.1016/j.nurt.2010.07.005
Yin, D., Zhou, S., Xu, X., Gao, W., Li, F., Ma, Y., et al. (2018). Dexmedetomidine attenuated early brain injury in rats with subarachnoid haemorrhage by suppressing the inflammatory response: The TLR4/NF-kappaB pathway and the NLRP3 inflammasome may be involved in the mechanism. Brain Res. 1698, 1–10. doi: 10.1016/j.brainres.2018.05.040
Zeng, J., Chen, Y., Ding, R., Feng, L., Fu, Z., Yang, S., et al. (2017). Isoliquiritigenin alleviates early brain injury after experimental intracerebral hemorrhage via suppressing ROS- and/or NF-kappaB-mediated NLRP3 inflammasome activation by promoting Nrf2 antioxidant pathway. J. Neuroinflamm. 14:119. doi: 10.1186/s12974-017-0895-5
Zhang, J., Jiang, N., Zhang, L., Meng, C., Zhao, J., and Wu, J. (2020). NLRP6 expressed in astrocytes aggravates neurons injury after OGD/R through activating the inflammasome and inducing pyroptosis. Int. Immunopharmacol. 80:106183. doi: 10.1016/j.intimp.2019.106183
Zhang, N., Zhang, X., Liu, X., Wang, H., Xue, J., Yu, J., et al. (2014). Chrysophanol inhibits NALP3 inflammasome activation and ameliorates cerebral ischemia/reperfusion in mice. Mediat. Inflamm. 2014:370530. doi: 10.1155/2014/370530
Zhang, Z., Zhang, Z., Lu, H., Yang, Q., Wu, H., and Wang, J. (2017b). Microglial Polarization and Inflammatory Mediators After Intracerebral Hemorrhage. Mol. Neurobiol. 54, 1874–1886. doi: 10.1007/s12035-016-9785-6
Zhang, X., Wu, Q., Zhang, Q., Lu, Y., Liu, J., Li, W., et al. (2017a). Resveratrol Attenuates Early Brain Injury after Experimental Subarachnoid Hemorrhage via Inhibition of NLRP3 Inflammasome Activation. Front. Neurosci. 11:611. doi: 10.3389/fnins.2017.00611
Zhang, Y., Chen, Y., Zhang, Y., Li, P. L., and Li, X. (2019). Contribution of cathepsin B-dependent Nlrp3 inflammasome activation to nicotine-induced endothelial barrier dysfunction. Eur. J. Pharmacol. 865:172795. doi: 10.1016/j.ejphar.2019.172795
Zhang, Y., Wang, T., Yang, K., Xu, J., Ren, L., Li, W., et al. (2016). Cerebral Microvascular Endothelial Cell Apoptosis after Ischemia: Role of Enolase-Phosphatase 1 Activation and Aci-Reductone Dioxygenase 1 Translocation. Front. Mol. Neurosci. 9:79. doi: 10.3389/fnmol.2016.00079
Zhou, R., Tardivel, A., Thorens, B., Choi, I., and Tschopp, J. (2010). Thioredoxin-interacting protein links oxidative stress to inflammasome activation. Nat. Immunol. 11, 136–140. doi: 10.1038/ni.1831
Keywords: caspase-1, ischemic stroke, hemorrhagic stroke, blood-brain barrier, pyroptosis, cerebral edema, hemorrhagic transformation
Citation: Ye X, Song G, Huang S, Liang Q, Fang Y, Lian L and Zhu S (2022) Caspase-1: A Promising Target for Preserving Blood–Brain Barrier Integrity in Acute Stroke. Front. Mol. Neurosci. 15:856372. doi: 10.3389/fnmol.2022.856372
Received: 17 January 2022; Accepted: 18 February 2022;
Published: 18 March 2022.
Edited by:
Yi Yang, First Affiliated Hospital of Jilin University, ChinaReviewed by:
Samaneh Maysami, Keele University, United KingdomPatrick T. Ronaldson, The University of Arizona, United States
Copyright © 2022 Ye, Song, Huang, Liang, Fang, Lian and Zhu. This is an open-access article distributed under the terms of the Creative Commons Attribution License (CC BY). The use, distribution or reproduction in other forums is permitted, provided the original author(s) and the copyright owner(s) are credited and that the original publication in this journal is cited, in accordance with accepted academic practice. No use, distribution or reproduction is permitted which does not comply with these terms.
*Correspondence: Lifei Lian, bGxmMjAwMEAxMjYuY29t; Suiqiang Zhu, emh1c3VpcWlhbmdAMTYzLmNvbQ==
†These authors have contributed equally to this work and share last authorship