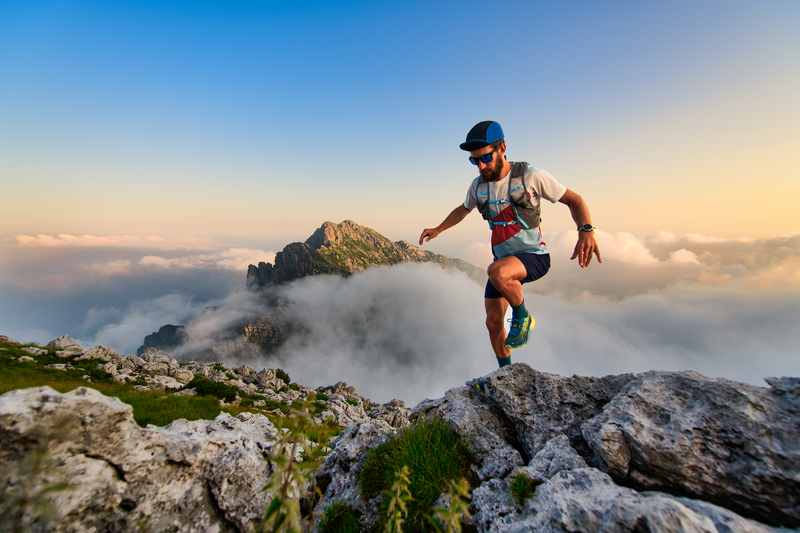
95% of researchers rate our articles as excellent or good
Learn more about the work of our research integrity team to safeguard the quality of each article we publish.
Find out more
MINI REVIEW article
Front. Mol. Neurosci. , 11 August 2022
Sec. Methods and Model Organisms
Volume 15 - 2022 | https://doi.org/10.3389/fnmol.2022.855786
This article is part of the Research Topic Zebrafish as a Tool for Neurosciences: Evolutionary Conservation and Translational Relevance View all 7 articles
The variety in the display of animals’ cognition, emotions, and behaviors, typical of humans, has its roots within the anterior-most part of the brain: the forebrain, giving rise to the neocortex in mammals. Our understanding of cellular and molecular events instructing the development of this domain and its multiple adaptations within the vertebrate lineage has progressed in the last decade. Expanding and detailing the available knowledge on regionalization, progenitors’ behavior and functional sophistication of the forebrain derivatives is also key to generating informative models to improve our characterization of heterogeneous and mechanistically unexplored cortical malformations. Classical and emerging mammalian models are irreplaceable to accurately elucidate mechanisms of stem cells expansion and impairments of cortex development. Nevertheless, alternative systems, allowing a considerable reduction of the burden associated with animal experimentation, are gaining popularity to dissect basic strategies of neural stem cells biology and morphogenesis in health and disease and to speed up preclinical drug testing. Teleost vertebrates such as zebrafish, showing conserved core programs of forebrain development, together with patients-derived in vitro 2D and 3D models, recapitulating more accurately human neurogenesis, are now accepted within translational workflows spanning from genetic analysis to functional investigation. Here, we review the current knowledge of common and divergent mechanisms shaping the forebrain in vertebrates, and causing cortical malformations in humans. We next address the utility, benefits and limitations of whole-brain/organism-based fish models or neuronal ensembles in vitro for translational research to unravel key genes and pathological mechanisms involved in neurodevelopmental diseases.
Since the early comparative studies in animal models (i.e., mouse, chick, and fish), developmental biologists have shed light on the precise choreography of genetically controlled events that shape the vertebrate brain in different domains along the AP (antero-posterior) and D-V (dorso-ventral) axes. These events produce the conserved organization of the forebrain anteriorly, morphologically and functionally distinguished in different domains (Puelles, 1995; Puelles et al., 2000). The pallium originates from the dorsal division of the anterior most part of the forebrain (telencephalon) and gives rise to the neocortex in mammals (Haines and Mihailoff, 2018); responsible for the high computation capacity beyond sensory-motor integration, such as language and abstract thinking skills.
Within the developing pallium, a precise balance and timing of signaling drive neural fate commitment and patterning, as well as the rate of progenitor pools’ expansion (level 1), migratory behavior and differentiation (level 2), and the establishment of connectivity patterns (level 3). Fine changes in local patterning schemes and precursor cells’ behavior across evolution underlie the array of diversity in vertebrate cognitive capabilities and the formation and expansion of the multilayered cytoarchitecture of the mammalian cortex, responsible for high-order functions typical of humans (Striedter, 2005; Van Essen et al., 2018; Stepien et al., 2021). From a comparative point of view, mechanisms of cortex development at the level of repertoire of stem cells and proliferation strategies vary drastically even within closely related species of vertebrates and underly size and folding variation. The molecular roots of these differences are intensely studied by employing lissencephalic (harboring smooth brain) and gyrencephalic mammalians (showing superficial foldings and therefore expansion of the neocortex) (Lui et al., 2011; Dehay et al., 2015; Florio et al., 2015, 2016; Kalebic et al., 2019; Stepien et al., 2021). Furthermore, the presence of a homologous domain giving rise to the neocortex within the pallium territory in non-mammalian vertebrates is equally debated and little consensus exists to this day (Northcutt, 1995; Wullimann and Mueller, 2004; Medina and Abellán, 2009; Nieuwenhuys, 2009). Nevertheless, the basic core of orderly molecular and cellular processes governing anatomical and functional compartmentalization (bauplan) of the forebrain and fundamental principle of neurogenesis are conserved within the vertebrate lineage (Bachy et al., 2002; Puelles and Rubenstein, 2003; Wilson and Houart, 2004; Medina et al., 2005). Interestingly, specific cortical high-order cognitive abilities have also been mapped in teleosts (Portavella et al., 2002; Salas et al., 2003; von Trotha et al., 2014; Messina et al., 2022) and ancestral features can also be found in invertebrates (Williams et al., 2004; Cavallin et al., 2018).
The continuous identification of new genetic lesions affecting these different levels of neurogenesis and resulting in heterogeneous neurodevelopmental disorders with malformation of cortical development (MCD) highlights the importance of these processes during cortex formation. Improving our knowledge of the specific biology and genetics of pallium patterning, regionalization, precursor behavior and cell type specification and its variation on the main vertebrate theme can potentially impact our ability to model, understand and manage MCD. Indeed, these heterogeneous conditions can manifest with various degrees of microcephaly, lissencephaly and cortical dysplasia, originating from impaired proliferation, migration and/or white matter and circuits establishment. They are often associated with cognitive impairments and with at least 40% of non-treatable epileptic conditions (Guerrini and Dobyns, 2014; Barkovich et al., 2015; Represa, 2019). The clinical variability and genetic heterogeneity, as well as the poor knowledge on the underlying patho-mechanisms of the newly described conditions, often make these pediatric disorders not accurately managed. To this aim, non-primate mammalian systems such as rodents are undoubtedly providing an enormous help. Nevertheless, human-specific traits are often not successfully recapitulated in these models (especially related to folding formation) (Wong and Roper, 2016). On the other hand, more recently, the genetically accessible ferrets, gyrencephalic models with an expanded neocortex, are becoming popular to gain insights into neocortex expansion and to model specific traits which characterize human cortex development and disease (Hutsler et al., 2005; Martínez-Cerdeño et al., 2012; Florio et al., 2015, 2016; Fernández et al., 2016; Johnson et al., 2018; Kalebic et al., 2019; Pinson et al., 2019).
Nevertheless, given the current acceleration in the identification of candidate genetic lesions in previously undiagnosed MCD conditions, scientists are exploring alternative systems. These should (i) be more readily implementable than mammalian species in healthcare-oriented institutes, (ii) offer possibilities for fast candidate gene variants validation serving differential diagnosis, while (iii) parallelly allow the search of basic patho-mechanisms, and (iv) via methodologies which reduce the overall burden related to animals experimentation. To this aim, teleost fish can be advantageous (Figure 1 and Table 1). They represent the largest clade of vertebrates and, together with chick and mice, have long served as genetic and developmental models to study the principles of neural induction, and for the search of evolutionary paths to plasticity and adaptation of pallium formation and function within vertebrates (Salas et al., 2003; Menuet et al., 2007; Alié et al., 2018; Dunlap et al., 2019). Indeed, homologous neuronal ensembles, basal circuits and neurogenic modes within the pallium of teleost fish are being identified (Fotowat et al., 2019; Porter and Mueller, 2020). On this ground, and because of a number of factors determining its practical usefulness in developmental genetics and disease modeling, the small and transparent teleost fish zebrafish is effectively used. In many instances, the use of fish can replace less ethically acceptable methodologies required for brain analysis in mammalian embryos and young adults. Along these lines, more teleost models (such as medaka) are recently joining the forces and proving to be crucial to dissect multiple mechanisms of pallium expansion, vertebrate brain growth and rare brain diseases (Indrieri et al., 2013; Peluso et al., 2013; Kirchmaier et al., 2015; Dunlap et al., 2019; Coolen et al., 2020; Nakanishi et al., 2021).
Figure 1. Schematic illustration of the key features of in vivo zebrafish and in vitro iPSCs and COs models. The relative increase in structural and functional complexity of the forebrain and the timing to obtain mature neuronal populations are indicated by the y and x axes, respectively. BioRender (BioRender.com) and Illustrator (Adobe) were used to generate the illustration. iPSCs, induced pluripotent stem cells; COs, cerebral organoids.
Table 1. Glossary of the main terms employed in this review, including a brief description of the key models and processes discussed here.
A different and complementary approach, quickly gaining popularity and reducing further the burden on animal models, is the establishment of induced pluripotent stem cells (iPSCs) directly from patients’ bioptic material and iPSCs-derived cerebral organoids (COs). The latter are becoming attractive in vitro alternatives in conjunction to the in vivo models to recapitulate species (i.e. human)-specific traits of early neurogenesis, differentiation, connectivity and even cytoarchitecture at small scale (Krefft et al., 2021; Zilova et al., 2021; Figure 1 and Table 1). Direct comparisons of COs development derived from different primates, mammals or other vertebrates are also becoming supportive tools for our understanding of the mechanisms underlying neocortex expansion (Li et al., 2017b; Kanton et al., 2019; Pollen et al., 2019). This review provides an updated overview of the key developmental steps and precursor cells that build the mammalian cortex. We next summarize representative examples of known genetic alterations impacting various molecular and cellular processes and leading to cortical malformations as the cause of intellectual disability and epilepsy. In the context of classifying and dissecting the causes of an increasing number of undiagnosed cortical malformations discovered via patients’ genomic screening, we next examine the possible value of the alternative and complementary fish and dish-based models to the in vivo mammalian systems. We discuss fruitful examples showing case their usefulness in contributing dissecting the fundamentals of neuronal precursors’ behavior and the underlying mechanisms in healthy and diseased brains. We specifically outline the limitations and challenges derived, for example, from the differences in pallium developmental processes and cell type repertoire (teleosts) or from the minimal tissue complexity and lack of whole-organismal context (organoids).
Since the experiments of Spemann and Mangold in the early 20th century, developmental biologists used animal models to demonstrate that brain development is achieved by tightly controlled gene expression schemes that generate specific, recognizable local fingerprints. These events depend on the ability of cells in the early embryological fields to respond to varying doses of inducing and inhibiting molecules (morphogens) secreted by neighboring cells, which sequentially restrict the fate of each previously homogeneous domain, leading to patterning, regionalization, and cell type specification. These events step up the global brain architecture and its functional sub-specialization in different domains, and are globally conserved in evolution (Rallu et al., 2002; De Robertis, 2009). Understanding these processes has represented the ground research for flourishing comparative studies in developmental biology over the last decades that inspired various innovative experimental strategies. As a result, human genetic and brain diseases modeling in vivo have been improved and the breakthrough of organoids – including COs- is revolutionizing the study of the healthy and diseased mammalian brain.
In vertebrate embryos, parallelly to the neural plate folding, signaling activated by retinoic acid, FGF, Wnt and Wnt inhibitors pattern the A-P (anterior-posterior) territories, while a fine-tuning of Bmp, Shh, and Wnt signals from axial mesodermal and non-neural ectodermal cells contribute to define instead the D-V (dorso-ventral) embryo axis (Wilson and Rubenstein, 2000; Houart et al., 2002; Rallu et al., 2002; Wilson and Houart, 2004). In this context, the establishment of the forebrain (Table 1) at the anterior bulge of the developing brain occurs already by the end of gastrulation, via a fine signaling balance of the different morphogens secreted from the organizer and the surrounding tissues (Rubenstein and Beachy, 1998; Stern, 2001; Wilson and Houart, 2004). A nested grid of highly conserved transcription factors is therefore generated and defines the boundaries between the different domains within the forebrain (Fernandez et al., 1998; Puelles et al., 2000). Key signaling events worth mentioning include the activation of Wnt inhibitors, e.g., Dickkopf-1 (Mukhopadhyay et al., 2001), Cerberus (Bouwmeester et al., 1996; Piccolo et al., 1999) and the secreted frizzled-related protein Sfrp3, (Kawano and Kypta, 2003) which counteract “caudalizing factors” and are essential for the vertebrate head formation. Crucial is also the modulatory activity of Fgf8 which globally sets the telencephalon aside from the neighboring diencephalon and contributes to its patterning (Shanmugalingam et al., 2000; Yamaguchi, 2001; Houart et al., 2002). This is practically orchestrated by Bf-1 (Tao and Lai, 1992; Dou et al., 1999; Kitagawa et al., 2004), Emx, and Dlx genes (Fernandez et al., 1998; Puelles et al., 2000). The Emx/Pax6 + dorsal pallium region of the telencephalon gives rise to the cortex, the medial pallium to the hippocampus and the ventral domain to the amygdala. On the ventral side of the telencephalon, the Dlx + subpallium is defined from where the basal ganglia and the hypothalamus originates. Posteriorly to the telencephalon, the diencephalic domain produces the thalamus and pretectum (Rubenstein et al., 1994; Rubenstein and Beachy, 1998; Puelles et al., 2000; Wilson and Rubenstein, 2000; Houart et al., 2002; Wilson and Houart, 2004; Cavodeassi and Houart, 2012).
The development and plasticity of the neocortex, originating from the dorsal pallium in mammals as a late evolutionary innovation (Striedter, 2005; Kelava et al., 2013; Namba et al., 2019) depends on the response of progenitor cells to the above mentioned modulatory signalings via a precise and stereotypical spatial-temporal logic of events. These ultimately control the correct proliferation rate of the initial pool of stem cells, the movement of the newborn neurons to their destination, their maturation in a number of different neuronal cell types and the establishment of their final connectivity pattern. From here, the resulting neural circuitries are distinctively wired, serving functional specialization for higher functions. Each of these events is especially critical to obtain the laminar arrangement of the mammalian pallium (Rakic, 2009a; Rakic et al., 2009b; Fietz et al., 2010; Salomoni and Calegari, 2010; Taverna et al., 2014; Subramanian et al., 2020), ultimately formed by circa 80% of excitatory neurons and the rest of inhibitory interneurons moving from the ventral telencephalon (Parnavelas et al., 2000; Lodato and Arlotta, 2015; Laclef and Métin, 2018). Briefly, in the region lining on the brain ventricle of the developing mammalian cortex (ventricular zone, VZ) the founder neuroepithelial cells with their characteristic apico-basal polarity along the radial axis undergo various rounds of symmetric cell division (“proliferative division,” Table 1). These events produce two new progenitor cells from one and therefore contribute to expanding the pool of progenitor cells. Apical and basal (or outer) (a, b RG cells, respectively) originated from these founder cells and expressing characteristic markers of glia cells (Götz and Huttner, 2005; Kriegstein and Alvarez-Buylla, 2009) maintain the apico-basal polarity and divide mainly by asymmetric cell division (“neurogenic division,” Table 1). This ensures the birth of new RG cells, serving self-renewal purposes (and thereby keeping the pool of stem cells), and differentiating neurons or various types of committed basal progenitors (BP), such as intermediate progenitor cells (IPCs). These cells migrate basally to the subventricular zone (SVZ) and produce neurons only after additional rounds of cell divisions (Noctor et al., 2001, 2004; Kriegstein and Götz, 2003; Haubensak et al., 2004; Kriegstein and Alvarez-Buylla, 2009; Uzquiano et al., 2018; Table 1). A great deal of variability exists across different mammalian species with respect to the fine details regarding the arsenal of progenitors’ cells, their morphology, and behavior, as well as the proliferation potential and the neurogenesis length (Betizeau et al., 2013; Kalebic et al., 2018, 2019; Stepien et al., 2020, 2021).
Importantly, the developing cortex of lissencephalic models, such as mice, have little bRG cells which divide both symmetrically and asymmetrically (Wang et al., 2011) and are instead mostly populated by IPCs-type of cells which have limited proliferative potential (Haubensak et al., 2004; Noctor et al., 2004). On the other hand, gyrencephalic animals like humans have evolved mechanisms to generate foldings which increase the overall surface and therefore host more neural cells in a limited space (Zilles et al., 2013). In these animals, the BP of the SVZ can sustain multiple rounds of divisions to generate other precursor cells before dividing into neurons (Götz and Huttner, 2005; Lui et al., 2011; Florio and Huttner, 2014). Indeed, the increase in the number of the BP outer RG cells (oRG) originating from aRG, and their proliferative potential has been proposed as a contributor for the increase in cortex size (Nonaka-Kinoshita et al., 2013; Fernández et al., 2016), but it does not seem to be sufficient, given the presence of lissencephalic animals with a high number of BP (Kelava et al., 2013; Stepien et al., 2021). Seminal works in the past years have demonstrated that a variety of morphology exists among BP cells even in the same species and that this instead might be a key factor determining neocortical expansion (Betizeau et al., 2013; Kalebic et al., 2019). The different BP morphotypes can vary from cells with monopolar to multipolar processes and it appears as if the increase in the number of processes boosts the proliferative capability, most likely by making the BP cells more available to external signaling. This mechanism might represent one of the major cellular features deciding cortex size expansion and neocortex appearance (Lui et al., 2011; Kalebic et al., 2019; Subramanian et al., 2020). Sustained gliogenesis in the outer SVZ has been implicated in the expansion of the neuropile, and therefore, the neocortex convolutions in primates (Rash et al., 2019). Of note, the genetic bases of such peculiarity of precursor cell types for neocortex expansion are mapped using gyrencephalic models (Florio et al., 2015, 2016; Cárdenas and Borrell, 2020; Heide et al., 2020; Stepien et al., 2021). At the signaling level, a number of pro-proliferative molecules from -and interacting with-the ECM niche of the progenitor pool, likely involving Pi3K-AkT, mTOR, and MAPK signaling pathways, is being recognized as a crucial trigger for the distinctive proliferative capacity of BP cells as well as for their positioning in the developing cortex (Sheppard and Pearlman, 1997; Fietz et al., 2012; Moreno-Layseca and Streuli, 2014; Cavallin et al., 2018; Heinzen et al., 2018; Long et al., 2018; Kalebic et al., 2019; Subramanian et al., 2020; Kyrousi et al., 2021).
Next, the basal projections from progenitor cells allow newborn neurons and glia produced by asymmetric division to migrate radially to the destination in the cortical plate (CP) by somal translocation or active locomotion (Sheppard and Pearlman, 1997; Nadarajah et al., 2001). The first migrating neurons populate the deep layer of the forming cortex, while newborn ones are located more superficially (Rakic, 1974; Buchsbaum and Cappello, 2019). Again, fine signaling is required for the different phases of migration, with Reelin being the most relevant to control different phases of this migratory activity (Trommsdorff et al., 1999; Kuo et al., 2005; Hirota and Nakajima, 2017). To complete cortex formation, inhibitory interneurons from the ventral telencephalon (the transient ganglionic eminences in humans) migrate long-range moving tangentially to the RG fibers to reach their final correct position and connect with other neurons in the CP (Lavdas et al., 1999; Bellion et al., 2005; Miyoshi et al., 2010; Faux et al., 2012; Barber and Pierani, 2016; Table 1). Their peculiar saltatory migration behavior, characterized by pauses in between fast periods of movement, is obtained via microtubules-dependent nuclear translocation (Bellion et al., 2005; Silva et al., 2018). This mechanism seems to be essential to control the stream of migratory cells populating the cortex and to even influence neurogenesis in the cortex (Silva et al., 2018). Lastly, cortical neurogenesis is nearly completed when gliogenesis starts, the remaining RG cells differentiate into astroglia, and the pool of progenitors is extinguished (Noctor et al., 2008; Ohtsuka and Kageyama, 2019).
The high complexity and specialization of the molecular and cellular events governing neocortex development and function in space and time make them particularly vulnerable to genetic alterations. A large group of malformations of cortical development (MCD) has been described which are characterized by various defects at the level of brain size (decreased or increased), final position of neurons, and formation of cortical layers and foldings (Toi et al., 2009; Guarnieri et al., 2018; Romero et al., 2018; Severino et al., 2020; Subramanian et al., 2020). Different conditions have been classically categorized based on the underlying perturbed cellular event, namely: alteration in the proliferative state of the precursors (a), migratory defects (b), or neural connectivity and circuits formations (c) (Table 2). For instance, microcephaly (reduced brain size) is mainly associated with unbalanced proliferation and/or apoptosis of the neuronal progenitors. Aberrant migratory behavior across developing cortical layers results in ectopic neurons located in the periventricular and subcortical region, disorganization and loss of cortical folding or lamination lead to gyrification defects and result in various heterotopias, polymicrogyria, lissencephaly and dysplasia (Guerrini and Dobyns, 2014). The latest acceleration in the use of genomic sequencing in undiagnosed patients reveals high heterogeneity in the genetic and cellular mechanisms of MCD, which often show shared cellular and morphological features, challenging the boundaries of the original definitions. Deepening our knowledge of the underlying mechanisms of the newly described conditions is particularly relevant to improving the functional classification of these disorders often associated with severe cognitive impairments (Guerrini and Dobyns, 2014) and untreatable epilepsy (Represa, 2019). Many disease-causing mutations have already been identified, which affect the function of key proteins involved in cytoskeleton, chromosomes arrangement machinery, organelle stability and bio-trafficking (Table 2). The fine spatial and temporal balance of these events in the developing pallium controls neurogenesis, migration, and connectivity. Of note, the sustained activity of signaling pathways involved is relevant for cortical malformations (Hong et al., 2000; Hevner, 2015; Jansen et al., 2015; Ossola and Kalebic, 2021; Table 2). We summarize the main features and known alterations of a subset of these conditions. For a more detailed classification, please refer to Table 2 and (Woods, 2004; Guerrini and Parrini, 2010; Barkovich et al., 2015; Watrin et al., 2015; Passemard et al., 2017; Buchsbaum and Cappello, 2019).
Table 2. MCD classification and representative examples of MCD-causing genes as well as IPSCs, COs and zebrafish models: the table summarizes the clinical classification and the disease mechanisms underlying different forms of MCD.
Microcephaly is a rare clinical trait defined by the reduced head and brain size, with an occipital-frontal circumference often below the 3rd percentile. It can occur at birth (primary), or postnatally (secondary), as an isolated condition or in combination with other clinical features, i.e., developmental delay, epilepsy, and CNS malformations (Abdel-Salam et al., 2000; Woods, 2004; Marino et al., 2020; Becerra-Solano et al., 2021), as well as intellectual disability (Marino et al., 2020). Aside from environmental factors and viral infections, genetic mutations represent the most frequent underlying cause (Table 2). Indeed, many mutations in conserved genes have been already identified, which negatively impact progenitors’ expansion as well as migration of newborn neurons. Proteins directly controlling microtubules dynamics (Chakraborti et al., 2016) (e.g., KIF14, TBCD) (Filges et al., 2014; Flex et al., 2016), centrosome biogenesis (e.g., STIL) and centrosome proteins regulating microtubules and spindle orientation (e.g., ASPM) (Darvish et al., 2010) are often involved. The involvement of factors controlling chromatin organization (e.g., MCPH1) (Darvish et al., 2010) as well as altered kinase activity (e.g., PTEN) (Dhaliwal et al., 2021) and abnormal substrate phosphorylation of phosphates (e.g., PPP1R15B, (Kernohan et al., 2015) have also been described. The resulting alteration of mitotic spindle formation and orientation, cell cycle, as well as proper chromosome segregation during mitosis often generates a shift between symmetric versus asymmetric cell division, depleting the pool of progenitor cells and causing apoptosis of early precursors. A different group of genes causing microcephaly and linked functionally to Golgi homeostasis and trafficking is also emerging. For instance, secondary microcephaly combined with periventricular heterotopia was linked to impaired Golgi assembly and trafficking affecting membrane proteins, proliferation and cell migration (e.g., ARFGEF2, Banne et al., 2013). Furthermore, fragmented Golgi has also been recently associated with a neurodevelopmental condition showing microcephaly and caused by mutations in the new disease-gene ARF3, encoding a small GTPase involved in post-Golgi trafficking (Fasano et al., 2021). More recently, loss of function of WDR81 which impairs endosomal trafficking of pro-proliferative signal EGFR was functionally linked to microcephaly (Carpentieri et al., 2022).
Impaired migration of newborn neurons from the ventricle to the CP along the RG scaffold causes different forms of neuronal migration disorders (NMDs). This is a group of highly heterogeneous conditions, which can show mixed features, including microcephaly or other cerebral malformations (Sheen et al., 2006; Lui et al., 2011; Buchsbaum and Cappello, 2019; Table 2). Among NMDs, lissencephaly (also known as “smooth brain”) refers to a large number of heterogeneous conditions, mostly characterized by an aberrant and reduced cerebral folding, in combination with other cerebral malformations; while gray matter heterotopia specifically refers to abnormally located neuronal clusters (Watrin et al., 2015; Di Donato et al., 2017; Buchsbaum and Cappello, 2019; Buchsbaum et al., 2020; Koenig et al., 2021). Furthermore, neuronal migration errors (Guerrini and Parrini, 2010) or aberrant proliferation of glia precursors (Rash et al., 2019) also lead to polymicrogyria, characterized by architectural anomalies and cytological variations involving the number of cortical convolutions. Abnormal focal migration of the newborn neurons causing impaired cortical lamination has also been linked to focal cortical dysplasia, which is among the most frequent causes of refractory epilepsy in children (Tassi et al., 2002; Guerrini and Dobyns, 2014). As previously discussed, interneurons are essential for correct cortex formation and to develop healthy connectivity and signal transmission (André et al., 2010). Impaired behavior during their long migratory tour from the ventral telencephalon to the cortex and their arborization can cause a disorganized lamination with impaired circuits activity within the cortex, leading to epilepsy and cognitive dysfunction (Pancoast et al., 2005; Carabalona et al., 2012; Nakagawa et al., 2017; Buchsbaum and Cappello, 2019; Subramanian et al., 2020). Furthermore, studies exist that functionally correlate the alterations in interneurons’ location and connectivity patterns to seizures occurrence in cells derived from patients with focal cortical dysplasia (Calcagnotto et al., 2005; Cepeda et al., 2005; Subramanian et al., 2020).
At the molecular level, these conditions are associated with mutations in genes encoding proteins involved in microtubules dynamics and transport cytoskeleton-cell matrix interactions, e.g., LIS1, DCX, KIF2A, TUB1A, (Chakraborti et al., 2016), MAP1B, (Heinzen et al., 2018), ARX, FLNA (Parrini et al., 2006), ARFGEF2, (Sheen et al., 2004). Mutations in these genes hinder neuronal polarity and cause migratory impairment and unbalanced proliferative potential of the precursor cells (Carabalona et al., 2012; Buchsbaum and Cappello, 2019). In addition, impaired signaling, caused by mutations in key molecules of the mTOR, FGF and REELIN signaling and ECM components, can impair proliferation, migratory behavior or neurites formation during cortex development leading to severe forms of MCD. Among these, lissencephaly (Hong et al., 2000) and polymicrogyria besides characteristic forms of muscular dystrophy (Devisme et al., 2012) and polymicrogyria (Godfrey et al., 2007).
As discussed, new disease genes and candidate pathogenic variants involved in MCD are continuously described, which may underlie the poorly unexplored heterogeneity in the clinical, genetic, and mechanistic aspects of cortical malformations. Yet, our little understanding challenges the utility of the existing disease classification and the development of tailored therapies. Classically, mouse models have provided -and continue to provide- considerable experimental evidence into the fundamental mechanisms of forebrain development and cortex formation in health and disease (Buchsbaum and Cappello, 2019; Reinert et al., 2021). For instance, mutant studies in mice models have predicted the involvement of Reelin signaling in complex forms of cortical malformation before the actual discovery of patients harboring mutations in genes belonging to the signaling cascade (D’Arcangelo et al., 1995; Trommsdorff et al., 1999; Hong et al., 2000). On the other side, successful models for deciphering the mechanisms underlying differences in neocortex size are primates, and more recently ferrets, gyrencephalic mammals, which harbor an expanded neocortex likewise humans and for which in utero genetic manipulation of embryonic brain is now possible (Hutsler et al., 2005; Florio et al., 2015, 2016; Gilardi and Kalebic, 2021). These models are particularly beneficial for recapitulating specific patho/physiological traits of MCD, especially when addressing cortical growth and folding defects, which were not previously observed in mammals with smooth cortex such as mice (Masuda et al., 2015; Kalebic et al., 2019; Pinson et al., 2019).
The acceleration of large patients’ sequencing efforts made possible by advances and increased affordability of the NGS (next-generation sequencing) on one side and the consensus on minimizing the animal distress associated with the invasive nature of experimentation in these large animals, quest for possible alternative models. These might be used for fast and large-scale functional validation of variants of unknown impact. Ideally, valid model systems successfully complementing the established and emerging mammalian ones should: (i) be rapidly implementable and manageable in medical research institutes, (ii) bring to a fast validation of the candidate genetic variants, (iii) while reducing the reliance on invasive animal procedures and still (iv) allowing scientific and translational gains in terms of mechanistic understanding of the disease. In this sense, despite not harboring the multi-layered cortex and given the conservation of fundamental mechanisms, the arsenal of genetic, imaging, and behavioral tools, teleost fish (Figure 1 and Table 1) are actively being used. Many studies now provide a critical comparison of telencephalon development and the specific neurogenic programs in teleosts. This knowledge is beneficial for data interpretation, given the increased use of these models (especially zebrafish) for validating MCD-causing mutations and deciphering the underlying mechanism (Table 2).
Parallelly, a different in vitro approach is also gaining increasing popularity, which is the use of iPSCs and COs derived from patients’ fibroblasts (Figure 1 and Table 1). Despite many drawbacks associated with the in vitro nature of the system, these models are useful to directly assess certain mechanisms of human cortex neurogenesis in health and disease or to draw hypotheses that can help strategize in vivo experiments. This section examines the ground similarities and differences between teleost and mammalian pallium and the use of zebrafish, iPSCs and COs for brain development and disease, highlighting representative examples. We discuss the main benefits and limitations, which still need to be overcome to improve the translational value of these models.
The genetic and molecular paradigms of the ground patterning and regionalization of the forebrain are conserved within various vertebrates (Puelles et al., 2000; Wilson and Houart, 2004; Medina et al., 2005; Murakami et al., 2005) and even in more distantly amniotes vertebrates, i.e., teleost fish (Heisenberg et al., 1996; Fernandez et al., 1998; Shinya et al., 2001; Puelles and Rubenstein, 2003; Kitagawa et al., 2004; Ganz et al., 2012). These processes have deep roots in evolution such that the molecular topography of a “paleopallium” can be traced back even to basal Bilateria (Medina and Abellán, 2009; Tomer et al., 2010).
Teleost fish models are a successful radiation of vertebrates and account for at least half of existing ones. They have populated developmental biology and neurobiology laboratories since the first studies on body plan formation and neural induction. These fish have been instrumental in establishing the first embryological events patterning the vertebrate telencephalon (e.g., see Houart et al., 1998; Kitagawa et al., 2004; Hashiguchi and Mullins, 2013), and complementing findings in mouse and other species. However, it should be noted that, conversely to mammals, teleost fish, show a “non-laminar” pallium structure that does not generate a bona fide cortex (Ito and Yamamoto, 2009). Instead of forming by invaginating cells as in mammals, the teleostean pallium domain is generated by an eversion process, whose underlying mechanisms are still under debate. This process ultimately generates a medial T-shaped ventricle and two lobes, in net contrast with the overall mature morphology of the mammalian counterpart (Wullimann et al., 1996; Wullimann and Mueller, 2004; Nieuwenhuys, 2009; Yamamoto, 2009). To this date, there is a lack of a solid conclusion on the existence of homologous forebrain and pallium sub-domains between teleosts and mammals. Hence, the teleostean pallium has long been considered rather “simple” because of these morphological differences and the uncertainty on assigning homology. This has precluded teleost models for a long time from further exploitation in neuroscience and brain disease modeling and limits the use and data interpretation of teleost fish in the field of MCD. Nevertheless, it is largely accepted by a wide community that, to a certain extend, an embryological origin, anatomical and functional homology exists between mammalian and teleost forebrain (Wullimann et al., 1996; Wullimann and Mueller, 2004; Nieuwenhuys, 2009; Yamamoto, 2009). Anatomical and functional components homologous to a number of pallial domains which originated from the dorsal portion of the telencephalon have already been proposed. In the course of the last decade, researchers have found in teleost fish regions homologous to the amygdala driving motivated behavior (von Trotha et al., 2014; Porter and Mueller, 2020), a domain resembling the hippocampus, which mediates memory and spatial information (Portavella et al., 2002; Salas et al., 2003; Rodríguez et al., 2021) and one responsible for cortex-type of high cognitive functions, such as logical and inference to determine social status (Grosenick et al., 2007) or numerosity tuning (Messina et al., 2022). On the other side, differences and plastic changes of the brain and pallium development at the level of morphology, connectivity and function exist across Bilateria and within vertebrates (Northcutt, 1995). One could say that the pallium itself underwent multiple events of sophistication and diversification in aquatic and land vertebrates, likely due to the elaboration of early stem cells independently from allometric relationships (Striedter, 2005; Noreikiene et al., 2015; Montgomery et al., 2016). Such phenotypic plasticity independently of other brain regions is manifest in teleosts, which results in considerable variations in the forebrain topology, neuroanatomy, and functional organization, likely underlying their ability to adapt to a variety of environments (Ito et al., 2007; Sylvester et al., 2013). Therefore, it should be mentioned that teleosts also represent an experimental advantage to showcase fundamental principles on how brain modularity and diversification within and across species is obtained. As shown indeed, this can occur by genetic changes (adaptation), which trigger mosaic responses in basal and shared neurogenic programs and thereby underly anatomical changes (Rétaux et al., 2013; Hall and Tropepe, 2020), as also demonstrated within mammals (Florio et al., 2015, 2016; Kalebic et al., 2018, 2019; Stepien et al., 2021). These changes can affect early patterning and sub-domain fate decision, therefore proportioning brain regions via directly modulating, the timing of local neurogenesis, cell cycle state and migratory behavior. Examples of the relevance of these shifts for forebrain plasticity in domain size and function were accumulated from comparative studies in teleosts. For instance, even small heterochronic and heterotopic variations in Shh and Fgf signaling doses can determine morphological and functional changes in the development of various forebrain domains in different teleosts. Namely, the expansion of Shh secreted from the midline was causatively linked to the expansion of the diencephalic domain (i.e., of the ventral hypothalamic region) in cave-dwelling cavefish as compared to the surface conspecifics adapted to surface. Of note, species differences also at the level of neuropeptidergic cell clusters (e.g., an increase in orexigenic NPY or Hcrt producing cells), controlling behavioral adaptations such as locomotion likely for foraging needs, were sustained in cave-adapted fish (Menuet et al., 2007; Alié et al., 2018). Again, evidence exists also in sand and rock-dwelling cichlids that similar variations in competing Shh and Wnt signaling expand or restrict the dorsal telencephalon (pallium). Specifically, an early function of Wnt inhibitors in development produces a larger pallium in sand dwellers fish as compared to the rock counterparts. In the latter on the other side expansion of Shh signaling and blocking Wnt inhibitors produce an extended subpallium. The potential modulatory effect of changes in these signalings on telencephalon development was further demonstrated by manipulating them in zebrafish (Rétaux et al., 2013; Sylvester et al., 2013).
When considering teleost fish as neurodevelopmental and disease models, one should also consider that the enrichment of progenitor cell types, their proliferative potential, the migratory routes of the newborn neurons resulting in the peculiar laminar organization of the neocortex are distinctive features of mammals, further elaborated in primates and in some gyrencephalic animals. Nevertheless, despite the differences discussed before, conservation of the founder precursor cells and the core mechanisms of neurogenesis exists in teleost telencephalon, as well as the functional competence derived from it (Portavella et al., 2002; Salas et al., 2003; Wilson and Houart, 2004; Nieuwenhuys, 2009; Rakic, 2009a; von Trotha et al., 2014; Porter and Mueller, 2020). Specifically, when it comes to the compendium of cell types, RG cells with proliferative potentials exist in various teleost’s brain regions, including the telencephalon. Debate exists on whether radial migration occurs during pallium development in these fish. Interestingly, (Mueller et al., 2011) described proliferative (Brdu +) cells migrating from the medial proliferative area of the dorsal telencephalon to the lateral and posterior area of the pial surface in developing zebrafish forebrain between 3 and 8 days post-fertilization. Similarly, photoconversion experiments in zebrafish provide evidence of a migratory cell population moving from the proliferative VZ of the developing pallium to the lateral wall during expansion of the telencephalic domain between 2 and 5 days of development (Folgueira et al., 2012). However, this behavior was not confirmed by later birth-dating experiments (Furlan et al., 2017); while findings of potentially migratory RG-like cells have been recently proposed also in cichlids (Mack et al., 2021). Noteworthily, conversely to mammalian species, teleost RG cells conserve the ability to proliferate and give rise to newborn neurons that integrate into existing circuits, in various neurogenic areas of the brain also in adulthood and in response to injury and disease. This feature defines the distinctive regenerative potential of the teleosts brain (Zupanc and Clint, 2003; Strobl-Mazzulla et al., 2010; Baumgart et al., 2012; Lange et al., 2020) and was also described for other non-mammalian vertebrates (García-Verdugo et al., 2002). Of note, the use of transgenic lines in zebrafish allowed also the real time visualization of migratory interneurons from the developing ventral to the dorsal telencephalon (Mione et al., 2008), resembling the saltatory migratory behavior observed in mammals and other vertebrates (Bellion et al., 2005; Laclef and Métin, 2018). In addition, other newborn neurons migrating out of the proliferative zones have been found in various sites of the adult zebrafish brain. Tangential migration of interneurons from ventral telencephalon was documented, which resembles rodent and tetrapods rostral migratory stream populating the anterior-most part of the telencephalon, the olfactory bulb. Altogether the available data seem to support a possible conserved mechanism of dual origin and migratory behaviors contributing to telencephalon cell types, and highlight zebrafish as an interesting model to study and visualize the mechanisms of these processes not invasively (Doetsch et al., 1997; Byrd and Brunjes, 2001; Grandel et al., 2006; Alvarez-Buylla et al., 2008; Mione et al., 2008; Ganz et al., 2012). Recent work demonstrates also the utility of alternative teleost models (such as killifish) besides zebrafish to dissect the impact of divergent and adaptive mechanisms at the level of precursor cells and neurogenesis and their function in sustaining pallium growth in vertebrates (Coolen et al., 2020).
Teleost fish are successful vertebrate models for developmental neurogenetics to study mechanisms of vertebrate brain plasticity, development, and function, as well as human brain diseases. Zebrafish, the most famous member of this clade, is increasingly becoming part of interdisciplinary research efforts that aim to establish fast causal links between newly discovered gene variants in undiagnosed patients and heterogeneous MCD. Zebrafish models of different classes of MCD (i.e., tubulinopathies, golgipathies, and dystroglycanopathies) affecting cortex formation in humans have already contributed to our understanding of pathogenic variants and mechanisms involved in the disease (Table 2).
Numerous are the features and benefits that favor the use of zebrafish in neurodevelopmental biology and disease modeling (listed in Table 3). In detail, this fresh water fish is a small laboratory pet, originally collected from Asia and already employed early on in large mutagenesis screens to map gene function during vertebrate development (Amsterdam et al., 1999), similarly to the other famous teleost from rice fields, medaka (Loosli et al., 2000). Reaching only few mm in adulthood, zebrafish can be relatively easy to handle. It usually adapts well to laboratory raring conditions, for which high-standard - and continuously updating guidelines- are available (Aleström et al., 2020). Conversely to rodents and other large mammalian models, fertilized zebrafish eggs develop fast and externally from the mother. This allows to collect and analyze embryos without animal suffering. Furthermore, given the fast embryogenesis, one can follow the entire process of gastrulation, embryo/brain patterning and regionalization from anterior to posterior within hours after the fertilization event, from 4 to 5 hours up to 5–6 days of development. At this stage small fish reach the status of an independent feeding larva with a fully functional nervous system. In addition, under the right housing and raring conditions, these fish become sexually mature and can reproduce in less than 6 months generating large offspring (between 200 and 300 embryos per clutch). Compared to other mammalian systems, these features are extremely useful when generating transgenic and mutant lines. A stable line in zebrafish can be produced in less than a year. Such high fecundity also allows a sufficient sample size for statistical analysis, again minimizing additional adults’ use for breeding. Compared to mice models, this feature enables easier screening and selection of the animals carrying the desired mutant and transgenic alleles and reduces line generation and maintenance costs. Transient transgenesis to mark a few cell types and dynamics are also widely used in zebrafish, which allows analysis of transgenic animals even within few days from the microinjections performed to obtain the genetic modification (Kawakami, 2005).
Besides the ease in management and the speed of breeding and development, many other unequaled factors contribute to springboard zebrafish for neurobiology. Transparent embryos (White et al., 2008; Antinucci and Hindges, 2016) eliminate the need for invasive procedures to manipulate and observe live events during embryogenesis, impossible in mammalian systems. Together with the embryo clarity, the continuous improvements of imaging tools of high-precision now facilitate further the investigation of brain development and function and allow to image even whole adult nervous system without invasive methodologies (Ahrens et al., 2013; Deán-Ben et al., 2016; Cong et al., 2017; Symvoulidis et al., 2017; Hontani et al., 2022). These features, in combination with an arsenal of transgenic lines marking cells from various growing brain territories of the forebrain (which reaches less than 300 μm in young larvae), i.e., main neuronal progenitors such as RG as well as differentiated telencephalic neurons (Bernardos and Raymond, 2006; Mione et al., 2008; Lal et al., 2018) permit to trace proliferative, neurogenic and migratory behaviors; potentially in the whole-brain (Mione et al., 2008; Novorol et al., 2013). Tools to image and quantify sub-cellular dynamics relevant to nervous system development and function are also used (Vacaru et al., 2014; Bercier et al., 2019; Asakawa et al., 2020; Lauri et al., 2021). Furthermore, study design, as well as results interpretation in zebrafish, are further supported by up to date databases, and methods for mapping molecular, cellular, and morpho-anatomical aspects of brain development available for zebrafish (Randlett et al., 2015; Gupta et al., 2018; Kunst et al., 2019).
In addition, the presence of genes in zebrafish which are orthologous to their human counterparts (Howe et al., 2013) is key for the generation of genetic models of diseases. Of note, the presence of more than one gene in zebrafish which is orthologous to a single human gene (genetic redundancy) - and which likely evolved different functions in the fish- is often beneficial to overcome the lethality observed by knocking out the single orthologous gene in mice (Kurahashi et al., 2005; Pei and Strauss, 2013). Furthermore, mutants deriving from large genetic screenings are available in zebrafish and for other laboratory teleosts, which help elucidate signaling, molecules and mechanisms involved forebrain patterning and regionalization (Schier et al., 1996; Loosli et al., 2000; Kitagawa et al., 2004). Adding to this, nowadays the generation of disease models in zebrafish is facilitated and accelerated by cutting-edge CRISPR-Cas methods (Hwang et al., 2013; Ablain et al., 2015; Varshney et al., 2015). This method can reach such high efficiency in this small freshwater fish to even allow to bypass the generation of stable genetically modified lines. These can be expensive, laborious, time-consuming and even prohibitive for some research groups. Therefore, while having the limitations of mosaic and transient models, the analysis of gene function in embryos, larvae, and adults of the F0 generation (named “crispants”), which can resemble null mutants, is permitting valid functional analyses in large settings (Hoshijima et al., 2019; Kroll et al., 2021; Masselink, 2021). The speed by which data are generated in this way is not currently achievable in mice models.
Moreover, advanced genome editing techniques with nucleotide precision recently adapted for zebrafish (Rosello et al., 2021) could even allow the generation of patient-specific mutants in the near future. It should also be added that a set of well-established behavioral assays is well characterized to monitor and classify cognitive tasks and epileptogenic responses in young zebrafish larvae (Orger et al., 2004; Levin and Cerutti, 2009), together with the use of in vivo reporters for brain activity and of electrophysiology protocols to record spontaneous epileptic events in mutants (Rosch et al., 2018; Yaksi et al., 2021). The establishment of reporter lines for many intracellular events, as diverse as calcium fluxes (Akerboom et al., 2012), pERK activity (Wong et al., 2019) or GTPases-mediated dynamics (Lauri et al., 2021) as well as of optogenetics (Simmich et al., 2012; Asakawa et al., 2020) are ideal for interrogating brain development and activity in healthy and disease fish models (Turrini et al., 2017; Asakawa et al., 2020).
Lastly, because a blood-brain barrier is fully formed only in relatively old zebrafish larvae, the uptake of CNS-directed drugs in the embryos and young larvae is possible via intraperitoneal injection or directly by dissolving the desired compound in the raring water (Quiñonez-Silvero et al., 2020). This possibility, together with the high availability of embryos per single fertilization event, reduces experimental times and cost for large screening and more invasive procedures associated with mammalian models; making zebrafish ideal for this purpose even in company-based settings (Ali et al., 2012; Chakraborti et al., 2016). In this context, it is worth considering that according to the EU Directive 2010/63/EU on animal experiments, early free-feeding embryos and larvae are classified as equivalent to in vitro systems. Thereby, even the costs, time and administrative load associated with the lengthy process to obtain authorization for animal experiments is easily reduced when working with zebrafish.
When applied to newly discovered and unknown MCD, these distinctive features can serve to get the first insights into the pathogenic mechanisms of disease and strategize large animal experiments. Of note, this scheme is in full agreement with the 3R principle (Sneddon et al., 2017), which advocates the use of alternative experimental models to reduce the overall animal number and burden associated with in vivo experiments, i.e., disease modeling.
Clear limitations need to be recognized when using zebrafish to model human brain malformations (Table 3). As discussed, we currently have limited knowledge on the complexity, developmental and functional potential of teleosts and zebrafish forebrain, which does not account for all the constituent progenitor cell types of the human neocortex and does not develop a laminated cerebral cortex. From here, an obvious limitation exists when dissecting disease mechanisms underlying cortical folding defects, which could underly ad hoc processes only present in gyrencephalic vertebrates. Clearly, to better design and interpret zebrafish MCD models that are generated, more thorough comparative investigations are needed. Furthermore, the occurrence of neurogenic activity in the adult fish brain and regeneration upon injury can help us to gain insights of translational value into possible triggers of neuronal regeneration in vertebrates (Strobl-Mazzulla et al., 2010; März et al., 2011; Schmidt et al., 2013; Barbosa et al., 2015). However, the specific regenerative mechanisms in place might alter the phenotype obtained in fish models of brain disease. Moreover, the presence of more than one orthologous gene for some human candidate genes potentially involved in disease might make it necessary to establish a double gene manipulation (e.g., double knockdown approach) to achieve a valid model recapitulating the human disease. While -as discussed- the large number of embryos and larvae available per experiment is ideal for statistical evaluation of the results, little inbreeding in zebrafish lines can underly a high variability in the response of individual animals to genetic manipulations and behavioral assays (Fitzgerald et al., 2019). On the other hand, the use of medaka is ideal for genetic studies, as inbreed, fertile and vital lines have been established in this fish (Murata et al., 2019) and, more recently, also in zebrafish (LaFave et al., 2014).
Even with these limitations in mind, it is clear that the combination of a set of specific features lacking in larger animal models makes zebrafish an extremely resourceful model system. This explains the popularity of zebrafish in translational research to (1) establish fast quantitative platforms to screen and investigate the increasing number of candidate pathogenic variants, (2) perform an initial investigation of the underlying mechanisms of disease, and (3) examine potential therapeutic targets. Indeed, from a translational point of view, zebrafish models are useful to validate previously undiscovered rare neurodevelopmental diseases within integrated functional genomics pipelines. Here, different pathogenic variants are first identified via NGS screening in patients, their differential impact on cellular and molecular events is often assessed in in vitro systems. Zebrafish is widely used to map the effect of these variants on embryogenesis and neural development and to connect back to the clinical features observed in humans.
To this date, numerous examples exist of functional validations of candidate pathogenic variants in neurodevelopmental diseases involving multi-modal functional genomics approaches (Sakai et al., 2018; Lauri et al., 2021). For instance, recent patients’ genomic sequencing, in vitro and in vivo zebrafish modeling was carried out in a single study to assess the pathogenicity of newly discovered mutations in the aminoacyl-tRNA synthetase-encoding VAR2 gene. Mutations in this gene lead to microcephaly, intellectual disability, and epilepsy (Siekierska et al., 2019). A var2 knock-out zebrafish model was generated within this integrated framework, and demonstrated the causal link of loss of function mutations with major features of the neurodevelopmental disorder observed in patients, even at the level of neurobehavioral traits. Indeed, specific assays could be used to score the phenotypes in zebrafish larvae. In addition, the assessment of the complementing potential of various mutations in this zebrafish model was helpful in differentiating the pathogenic contributions of the variants and to highlight the underlying genetic mechanism in the vertebrate embryo (Siekierska et al., 2019). Many zebrafish models have proven their utility in validating genes and variants affecting proteins participating in various intracellular processes and to model aspects of MCD diseases, directly complementing mammalian models. An extensive account is reported in Table 2. As an example, a recent genome-wide and exome screening identified a truncated mutation with loss of function of MAP11 (microtubule associated protein 11) as cause of recessive primary microcephaly. While the role in mitotic spindle dynamics and proliferation was proven in vitro, the pathogenicity of the loss of function generating microcephaly was demonstrated using zebrafish mutants, that complemented and validated the finding (Perez et al., 2019). Thanks to these integrated approaches, overlooked genes and mechanisms causing MCD are emerging. For instance, recently, we identified a previously unknown Golgipathy involving dominant mutations in the new disease gene encoding for the small GTPase ARF3. We demonstrated the validity of using zebrafish to recapitulate the microcephaly observed in patients and to validate the disruptive effect on the Golgi stability in vitro and in embryos (Fasano et al., 2021). Dedicated studies assessing and differentiating the mechanisms of previously discovered genetic lesions using zebrafish models also exist. For example, mutant and morpholino-induced knock-down of a number of genes involved in primary microcephaly have been described, which recapitulated the basic centrosome defects. The data also proved an increased rate of proliferative and dividing cells failing to progress through prometaphase in the neuroepithelium of developing zebrafish (Novorol et al., 2013; Table 2). It is worth mentioning that, thanks to fish in vivo models, intracellular trafficking and ECM deposition defects were demonstrated to be the cause of a heterogeneous group of dystroglycanopathies leading to dystrophies associated with retinal defects and lissencephaly (Lin et al., 2011; Stevens et al., 2013), while the embryo lethality observed in the corresponding mouse model of these diseases had previously hindered their experimental usefulness (Kurahashi et al., 2005). This exemplifies further the complementary use of zebrafish in the field.
Besides microcephaly, even more complex aberrations at the level of white matter formation and connectivity, as well as telencephalon cell positioning and global organization seen in patients with TSC, can be modeled and studied in zebrafish tsc2 mutants. This can be done at the cellular and neurobehavioral level (i.e., assessing epileptogenic output) (Kim et al., 2011b; Scheldeman et al., 2017; Kedra et al., 2020), and indicates deep conservation of the fundamental processes of telencephalon ontogeny and function in vertebrates, and the usefulness of zebrafish in modeling MCD.
Generally, examples exist also of direct use of zebrafish embryo models of rare (neuro)developmental diseases in the search of possible candidate drugs to counteract impaired signaling cascades [such as Ras/MAPK (Bobone et al., 2021)] or ameliorating seizures. (Baraban et al., 2013; Dinday and Baraban, 2015) performed an extensive screening to identify antiepileptic molecules effective on zebrafish model of Dravet Syndrome. (Griffin et al., 2017) took further advantage of the model, showing case of a in vivo, rapid path toward the off-label employment of lorcaserin. This FDA-approved compound antagonizing serotonin signaling pathway was transferred from preclinical discovery to potential clinical treatments of Dravet syndrome. From here, the compassionate use of commercial Belviq® is now approved for a small population of children affected by the syndrome, who successfully exhibit a reduction of generalized tonic-clonic events.
To fully exploit the unique features of zebrafish (and more generally teleosts) for functional validation and classification of rapidly emerging heterogeneous MCD in humans, experimental designs, questions, and data interpretation in these models should be carefully confined by the limitations discussed. In translational workflows dedicated to solving the underlying biology of MCD in vivo zebrafish models should be complementary to other feasible alternatives closer to large mammals and humans (Figure 1). Of particular interest in this sense and complementing the popularity of gyrencephalic mammals, is the establishment of in vitro human models of neural proliferation and differentiation using induced pluripotent stem cells (iPSCs), as well as iPSCs-derived COs for better recapitulating the structural and functional complexity of the human brain (Figure 1 and Table 1). These advances have been possible thanks to the studies of the early 20th century on neural induction from tissues of animal models and capitalize on the cell-autonomous competence of progenitor stem cells, especially in organizing anterior neural tissue (Lancaster et al., 2013; Paşca et al., 2015). iPSCs can be obtained from somatic cells by induction of known factors that reprogram the adult tissue toward different cell/tissue fate, including cortical neurons. COs have been more recently established (Lancaster et al., 2013) as 3D-self-oganizing cerebral tissue cultures derived from stem cells (i.e., iPSCs) and can be useful to study all the different processes of cortex formation, including interneurons migration (Lancaster et al., 2013).
iPSCs can be an informative and renewable source of cells to model human and mammalian neurogenesis. Their use already helps reduce the number of animals and the possible distress caused by studying cortical development and function in mammals. Furthermore, a precious source of information derives from iPSCs directly obtained from residual bioptic material of rare patients. In addition, among the main advantages of using iPSCs to obtain simplified models of neural networks (Table 3), one should note: (1) the easy accessibility to all cell types obtained, (2) the relatively fast timing of circa 50–60 days to obtain mature neurons, and (3) the possibility to analyze cell morphology and dynamics with relatively user-friendly tools (i.e., imaging tools and softwares). The procedure required to obtain cells to generate iPSCs is only minimally invasive in humans and animals and the use of iPSCs replaces the need to employ directly human embryonic stem cells, for which bioethical concerns exist. Another advantage is the optimization of gene modifications, which include the CRISPR/Cas approaches and facilitates the study of gene function (Ben Jehuda et al., 2018). Moreover, patients-derived clones, which are also genetically modified for therapeutic purposed, can be stored via biobanking and available at modern healthcare institutes for safe autologous transplantation, and regenerative and personalized approaches (Huang et al., 2019; Palechor-Ceron et al., 2019).
Nevertheless, these cells are in a 2D environment that hardly recapitulates the elaborated, multi-layered architecture of the human brain. They might show a low programming efficiency and can be very expensive to obtain. 3D systems, such as embryoid bodies (EB) first and cerebral organoid models (COs) later, are obtained via different protocols (Chiaradia and Lancaster, 2020) and they mostly rely on the ability of self-organization of stem cells in rosettes and on the stochastic cell differentiation within a homogeneous group of cells (Weiss and Taylor, 1960). Potentially, COs generated from normal or patient-derived iPSCs can better recapitulate the human cell type compendium in the cortex (Eze et al., 2021) (Table 3) and the impact of genetic mutations on human cortical neurogenesis as compared to mice models (Bershteyn et al., 2017; Iefremova et al., 2017; Li et al., 2017a; Kyrousi et al., 2021). Compared to iPSCs, COs can mimic the different specifications of the CNS (such as forebrain organoids). Developmental tissue organization along the axes (including the presence of VZ and of multiple layers) and the birth of various cellular identities at different stages of neurogenesis and differentiation such as RG, IPs, excitatory and inhibitory neurons and glia in defined temporal sequences in COs broadly match those observed in the human fetus (Shi et al., 2012). Furthermore, neuronal ensembles developing COs that self-organize establish connectivity in a three-dimensional setting and subdomains identity (Lancaster et al., 2013). Over a period of more than 9 months, relatively mature features, such as the presence of dendritic spines and active neuronal networks, have been documented in COs by a high-throughput single-cell transcriptional profiling method and extracellular recordings with high-density silicon microelectrodes (Quadrato et al., 2017). Moreover, neurons within the established COs are electrically active (Lancaster et al., 2013; Lancaster and Knoblich, 2014). The spontaneous Ca2 + spikes observed under baseline conditions increase upon stimulation with glutamate and decrease by inhibiting action potential, which demonstrates their dependence upon neural activity (Lancaster et al., 2013; Lancaster and Knoblich, 2014).
Comparative iPSCs and COs preparations are also useful to understand the ontogeny of species-specific similarities and differences in proliferative potential, cortex size and complexity, underlying the variability in cognitive specialization across taxa (Espuny-Camacho et al., 2013; Mora-Bermúdez et al., 2016). For instance, the timing of cortical progenitor proliferation and cell-type specification is most likely based on genetic control in different species, as evinced by experiments with mixed progenitor cell culture assays between and within species (human and macaque) (Otani et al., 2016). From this study, species-specific programs regulating the modality of progenitor expansion also emerge, which ultimately determines differences in the cortex size between various primates and mice (Otani et al., 2016). Comparative COs studies also show that protracted neurogenesis might be a driving factor of human neocortex expansion (Espuny-Camacho et al., 2013; Stepien et al., 2021). This kind of information could be the ground to interpret various available models of human MCD (Li et al., 2017b).
Given the increase in the use of COs in biomedical science, one should also consider that, notwithstanding these key features, at present, the extent to which brain organoids recapitulate the cellular diversity, regional complexity, and circuit functionality of the brain remains poorly addressed. COs lack macroscale patterning, microglia, and healthy vascularization. In addition, they are limited in the degree of neuronal connectivity and relationship with other tissues (Table 3). Efforts are being made to better characterize the features of COs compared to the mammalian cortex, and new methods are being developed to counteract these significant limitations. Notably, the first COs have been obtained by self-patterning, but most recently, region-specific brain organoids were derived using extrinsic signals known to pattern brain regions during embryogenesis (Susaimanickam et al., 2022). By modulating the concentrations of the morphogens, researchers obtained COs of the hippocampus (Paşca et al., 2015; Sakaguchi et al., 2015) or midbrain (Qian et al., 2016). Further, by modulating Sonic Hedgehog levels it was possible to pattern forebrain organoids into dorsal and ventral mid-domains (Cederquist et al., 2019) and addition of BDNF, GDNF and ascorbic acid can lead to differentiation toward the midbrain and brainstem identity (Eura et al., 2020). Of note, (Pellegrini et al., 2020) recently established choroid plexus-forming organoids producing cerebrospinal fluid, a source of developmental factors to sustain COs maintenance. The availability of this CNS barrier in vitro also holds promises to study drug delivery into the human brain (Pellegrini et al., 2020). To achieve a higher and more physiological complexity, in recent studies, human microglia has been xenotransplanted into COs, leading to an acceleration of the synchronized oscillatory network activity (Popova et al., 2021), while vascularized brain organoids start to be engineered to more faithfully recapitulate human condition (Cakir et al., 2019). On the other hand, in vivo organoid transplantation can provide a better organismal context, such as supplying inductive microglia and interaction with other cell types.
In addition, it is worth considering the potential threat of the high variability existing between different preparation of COs, which raises concern about the validity of the conclusion drawn with respect to human brain development and disease (Quadrato et al., 2016, 2017; Velasco et al., 2019). Nevertheless, single-cell RNA sequencing from various cells of different COs preparations show that they can have relatively mature features, including spontaneously active neuronal networks (Quadrato et al., 2017). COs can potentially reproduce the complexity of the central nervous system developed in the embryo in terms of the richness of the cell types produced with a variability that would also be observed in vivo (Velasco et al., 2019). Single-cell RNA analysis generating an atlas of early human brain development recently demonstrated that human specific cortical progenitors not found in mice models are present in COs, however they display low fidelity to neuroepithelial and early radial glia cell types, but improves as neurogenesis progresses (Eze et al., 2021). Moreover, (Ziffra et al., 2021) demonstrated a limited contribution of epigenetics to the patterns of cell type diversity and cell fate specification of COs as compared to human cortex. Lastly, the poor control over the self-organization and differentiation mechanisms resulting in heterogeneous preparations and inter-lab variability is being addressed by a chemical and physical engineering methods that better control and advance in vitro cortical programming and morphogenesis. For instance, a sequence of exposure to Wnt inhibitors first and to Wnt molecules later seems to efficiently guide dorsal forebrain commitment (Chiaradia and Lancaster, 2020). Furthermore, microfluidic devices are being engineered to reproduce morphogen gradients quantitatively (Garreta et al., 2021). Moreover, multi-domains cerebral tissues grown on a chip have been established that show functional interconnectivity and can model epileptic discharges more accurately (Saberi et al., 2022). More biotechnological efforts in this direction are indeed necessary to provide features able to unveil the fidelity and robustness of COs as a model for cortical development.
A considerable amount of neurodevelopmental disorders has been modeled by region-specific brain organoids, i.e., microcephaly, lissencephaly, Rett syndrome, schizophrenia, autism spectrum disorder, Pelizaeus-Merzbacher disease, Timothy and Prader-willi syndrome. These models demonstrated their usefulness in unveiling patho-mechanisms and potential therapeutic opportunities (Lu et al., 2022; Susaimanickam et al., 2022). Another relevant example of how COs can capture clinically relevant features originating from altered cerebrum development and function is the study on Autism Spectrum Disorder (ASD) (Paulsen et al., 2022). Here, COs were used to identify developmental abnormalities resulting from haploinsufficiency in three ASD risk genes from different donors and to evaluate phenotypic convergence (Paulsen et al., 2022). Other studies where brain organoids were useful to understand the molecular basis of human interneuron migration have been performed by Birey and colleagues (Birey et al., 2022) by using assembloids obtained from the integration of cortical and ventral forebrain organoids and unveiling details of cortical interneuron migration defects in Timothy syndrome. Besides the zebrafish approaches discussed (Table 2), iPSCs and COs developed from patients with MCD are already contributing to the understanding of specific diseased cortical networks (Table 2). For example, neuronal 3D cultures from a patient exhibiting primary microcephaly due to ASPM mutation more faithfully recapitulated the cerebral size reduction seen in the patient as compared to animal models (Pulvers et al., 2010). This model also showed characteristic neurogenic, structural, and network activity defects (Li et al., 2017a). Similarly, COs from patients with CDK5RAP2 mutations affecting centrosomes dynamics recapitulated the microcephaly which was difficult to analyze in mice models and provided valuable insights into the disease mechanism underlying altered neurogenesis (Lancaster et al., 2013). Furthermore, IPSCs-derived neurons and forebrain organoids with LIS1 mutations exhibited proliferative defects, resembling also the zebrafish model (Iefremova et al., 2017); while the molecular mechanisms underlying focal dysplasia and tuberous sclerosis due to mutations in TSC1 and TSC2 have been studied in 2D and 3D cortical systems from human brain (Marinowic et al., 2017; Blair et al., 2018). Complementing the models available in zebrafish (Kedra et al., 2020) (Table 2), these studies showed an induced mTOR signaling and sustained gliogenesis as major contributors to the disease (Marinowic et al., 2017; Blair et al., 2018). Moreover, COs models of how migratory defects impact the correct formation of the cortex are also available (Buchsbaum et al., 2020).
From a more translational point of view, the use of brain organoids as model systems for drug development has been largely proposed (Lancaster et al., 2013; Li et al., 2017b; Klaus et al., 2019; Costamagna et al., 2021; Matsui and Shinozawa, 2021; Xu et al., 2021; Lu et al., 2022; Susaimanickam et al., 2022). Nageshappa et al. (Nageshappa et al., 2016) demonstrated the use of cortical neurons derived from human iPSCs-based model of MECP2 duplication for the identification of the histone deacetylase inhibitor, the epigenetic modifier NCH-51, as a potential clinical candidate to rescue the altered neuronal phenotype characterized by increased activity frequency, synchronized bursts, and more active synapses found in MECP2 patients. CDLK5-deficient human organoids showing increased frequency and synchrony of spikes (neuron hyperexcitability), a hallmark of the intractable early-onset epilepsy affecting pediatric patients were employed for drug screening application. The calcium-imaging screening in the model identified promising compounds of different classes (inhibitor of muscarine receptors, Notch inhibitor, GSK3 inhibitor and hyperpolarization-activated cyclic nucleotide-gated channel blocker) as potential therapeutic target for pediatric patients with defective CDLK5 (Shcheglovitov and Peterson, 2021).
The ontogeny, morphogenesis, and structural/functional identity of the forebrain domains, and especially of the pallium, from which the cortex and neocortex originated, arose via divergent paths in vertebrates which likely represent key events for multiple adaption strategies throughout evolution. Clearly, the neocortex and its expansion are only visible in a subset of mammals. Its radial construction is achieved via a delicate series of events and precursor cell types, whose proliferative potential and neurogenic timing most likely shapes the differences observed in size and complexity across mammals. The alteration of the fine-tuning of these processes leads to rare conditions with heterogeneous causes and severe clinical consequences. Rapid protocols for effective modeling of these conditions, which are increasingly being reported, are pivotal to tackling the underlying mechanisms and prompt patient care. On the other hand, increasing experimental evidence from the field of comparative neurobiology shows that the teleost brain, considered “simple” for long time, exhibits high cognitive functions, some of the underlying core cell types, development strategies and circuits which are closely related to those build within mammalian pallium derivatives. However, analysis of the extent of similarity and shared ancestry between the pallium ontogeny, morphology, and function in teleosts and amniotes (and gyrencephalic) mammals just began. From a technical point of view, compared to classical and innovative mammalian models, in both the context of brain EvoDevo and disease, teleosts offer a distinctive readiness for generating affordable models with a broad and fast interrogation capacity of nano-scale dynamics within precursor cells and up to neuronal circuits logics of entire brain territories at the whole-organism scale. Furthermore, quantifiable behavioral readouts can be performed simultaneously, creating an ideal setting for scalable preclinical drug testing. Specifically, for disease modeling, limitations exist, however, when researching biological processes which could be altered in MCD using zebrafish and other teleosts, given the differences discussed so far and the clear lack of a bona fide cortex in this species. Not with understanding these differences, fish have indeed already demonstrated their usefulness in speeding up functional validations of new MCD-causing variants and disease genes. In this context, they can often model basal defects in brain/forebrain development, at the level of proliferation, migration and connectivity, which recapitulate those found in patients (Table 2). It is without a doubt that zebrafish work has already benefited our understanding of rare neurodevelopmental diseases. However, the speed by which teleosts are being used, calls for a careful interpretation of the data obtained in these models, which should be assessed against complementary systems more closely resembling human cortical features. Mammalian 2D and 3D neuronal cultures -especially if patient-derived- begin to provide interesting possibilities. We are at the beginning of a new era for brain disorder modeling. Understanding the extent to which small vertebrate fish and 3D organoid cultures can be used as high-fidelity/informative systems recapitulating human brain development is compulsory. As shown, examples of successful in vivo fish models for MCD exist, which expand the possibilities offered by COs and validate the use of teleost fish in the translational research of MCD. Complementary studies combining “fish and dish”-based brain models are accumulating, which demonstrate how they may be extremely compelling to dissect different aspects of human rare disorders (Ayala-Nunez et al., 2019; Pini et al., 2020) and to counterbalance possible pitfalls of the single models (Hengel et al., 2020). A robust integration of patients’ genomic sequencing and functional investigations in zebrafish, iPSCs and organoids exhibiting diverse and unbeatable advantages promises to be a simplified yet efficient multimodal paradigm of contemporary translational research on MCD.
AL conceived the manuscript and generated the illustration. AL, GF, and CC wrote the manuscript. MT and BD revised the final manuscript. GF and AL conceived and generated the tables. All authors contributed to the article and approved the submitted version.
This work was funded by grants from European Commission (individual fellowship Marie Skłodowska-Curie, 844636 – Innervate, Ricerca Corrente RC2020, RC2021, AL), Italian Ministry of Health (Ricerca 2019 5 × 1000, CCR-2017-23669081 and RCR-2020-23670068_001, MT, Ricerca Finalizzata Giovani Ricercatori GR-2019-12368907, CC and AL), Fondazione Bambino Gesù (Vite Coraggiose, MT), and Ministero dell’Università e della Ricerca (Sviluppo di protocolli innovativi e applicazione di nuovi strumenti-omici nei pazienti orfani di diagnosi, MT).
The authors apologize to colleagues whose work was not cited due to limited space and gratefully acknowledge all the researchers who have contributed to the original works cited here.
The authors declare that the research was conducted in the absence of any commercial or financial relationships that could be construed as a potential conflict of interest.
All claims expressed in this article are solely those of the authors and do not necessarily represent those of their affiliated organizations, or those of the publisher, the editors and the reviewers. Any product that may be evaluated in this article, or claim that may be made by its manufacturer, is not guaranteed or endorsed by the publisher.
Abdel-Salam, G. M., Halász, A. A., and Czeizel, A. E. (2000). Association of epilepsy with different groups of microcephaly. Dev. Med. Child Neurol. 42, 760–767. doi: 10.1017/s0012162200001419
Abdollahi, M. R., Morrison, E., Sirey, T., Molnar, Z., Hayward, B. E., Carr, I. M., et al. (2009). Mutation of the variant alpha-tubulin TUBA8 results in polymicrogyria with optic nerve hypoplasia. Am J Hum Genet 85, 737–744. doi: 10.1016/j.ajhg.2009.10.007
Ablain, J., Durand, E. M., Yang, S., Zhou, Y., and Zon, L. I. (2015). A CRISPR/Cas9 vector system for tissue-specific gene disruption in zebrafish. Dev. Cell 32, 756–764. doi: 10.1016/j.devcel.2015.01.032
Ackerman, S. D., Garcia, C., Piao, X., Gutmann, D. H., and Monk, K. R. (2015). The adhesion GPCR Gpr56 regulates oligodendrocyte development via interactions with Gα12/13 and RhoA. Nat Commun 6, 6122. doi: 10.1038/ncomms7122
Adams, M., Simms, R. J., Abdelhamed, Z., Dawe, H. R., Szymanska, K., Logan, C. V., et al. (2012). A meckelin–filamin A interaction mediates ciliogenesis. Hum Mol Genet 21, 1272–1286. doi: 10.1093/hmg/ddr557
Ahrens, M. B., Orger, M. B., Robson, D. N., Li, J. M., and Keller, P. J. (2013). Whole-brain functional imaging at cellular resolution using light-sheet microscopy. Nat. Methods 10, 413–420. doi: 10.1038/nmeth.2434
Akerboom, J., Chen, T.-W., Wardill, T. J., Tian, L., Marvin, J. S., Mutlu, S., et al. (2012). Optimization of a GCaMP calcium indicator for neural activity imaging. J. Neurosci. 32, 13819–13840. doi: 10.1523/JNEUROSCI.2601-12.2012
Alcantara, D., Timms, A. E., Gripp, K., Baker, L., Park, K., Collins, S., et al. (2017). Mutations of AKT3 are associated with a wide spectrum of developmental disorders including extreme megalencephaly. Brain 140, 2610–2622. doi: 10.1093/brain/awx203
Aleström, P., D’Angelo, L., Midtlyng, P. J., Schorderet, D. F., Schulte-Merker, S., Sohm, F., et al. (2020). Zebrafish: housing and husbandry recommendations. Lab. Anim. 54, 213–224. doi: 10.1177/0023677219869037
Ali, S., Champagne, D., and Richardson, M. (2012). Behavioral profiling of zebrafish embryos exposed to a panel of 60 water-soluble compounds. Behav. Brain Res. 228, 272–283. doi: 10.1016/j.bbr.2011.11.020
Alié, A., Devos, L., Torres-Paz, J., Prunier, L., Boulet, F., Blin, M., et al. (2018). Developmental evolution of the forebrain in cavefish, from natural variations in neuropeptides to behavior. eLife 7:e32808. doi: 10.7554/eLife.32808
Alvarez-Buylla, A., Kohwi, M., Nguyen, T. M., and Merkle, F. T. (2008). The heterogeneity of adult neural stem cells and the emerging complexity of their niche. Cold Spring Harb. Symp. Quant. Biol. 73, 357–365. doi: 10.1101/sqb.2008.73.019
Amsterdam, A., Burgess, S., Golling, G., Chen, W., Sun, Z., Townsend, K., et al. (1999). A large-scale insertional mutagenesis screen in zebrafish. Genes Dev. 13, 2713–2724.
André, V. M., Cepeda, C., Vinters, H. V., Huynh, M., Mathern, G. W., and Levine, M. S. (2010). Interneurons, GABAA currents, and subunit composition of the GABAA receptor in type I and type II cortical dysplasia. Epilepsia 51, (Suppl. 3) 166–170. doi: 10.1111/j.1528-1167.2010.02634.x
Antinucci, P., and Hindges, R. (2016). A crystal-clear zebrafish for in vivo imaging. Sci. Rep. 6:29490. doi: 10.1038/srep29490
Asakawa, K., Handa, H., and Kawakami, K. (2020). Optogenetic modulation of TDP-43 oligomerization accelerates ALS-related pathologies in the spinal motor neurons. Nat. Commun. 11:1004. doi: 10.1038/s41467-020-14815-x
Ayala-Nunez, N. V., Follain, G., Delalande, F., Hirschler, A., Partiot, E., Hale, G. L., et al. (2019). Zika virus enhances monocyte adhesion and transmigration favoring viral dissemination to neural cells. Nat. Commun. 10:4430. doi: 10.1038/s41467-019-12408-x
Bachy, I., Berthon, J., and Rétaux, S. (2002). Defining pallial and subpallial divisions in the developing Xenopus forebrain. Mech. Dev. 117, 163–172. doi: 10.1016/S0925-4773(02)00199-5
Bamba, Y., Shofuda, T., Kato, M., Pooh, R. K., Tateishi, Y., Takanashi, J., et al. (2016). In vitro characterization of neurite extension using induced pluripotent stem cells derived from lissencephaly patients with TUBA1A missense mutations. Molecular Brain 9, 70. doi: 10.1186/s13041-016-0246-y
Banne, E., Atawneh, O., Henneke, M., Brockmann, K., Gärtner, J., Elpeleg, O., et al. (2013). West syndrome, microcephaly, grey matter heterotopia and hypoplasia of corpus callosum due to a novel ARFGEF2 mutation. J. Med. Genet. 50, 772–775. doi: 10.1136/jmedgenet-2013-101752
Bao, W., Wang, X., Luo, L., and Ni, R. (2021). The Lysosomal Storage Disorder Due to fig4a Mutation Causes Robust Liver Vacuolation in Zebrafish. Zebrafish 18, 175–183. doi: 10.1089/zeb.2020.1911
Baraban, S. C., Dinday, M. T., and Hortopan, G. A. (2013). Drug screening in Scn1a zebrafish mutant identifies clemizole as a potential Dravet syndrome treatment. Nat. Commun. 4:2410. doi: 10.1038/ncomms3410
Barber, M., and Pierani, A. (2016). Tangential migration of glutamatergic neurons and cortical patterning during development: lessons from Cajal-Retzius cells. Dev. Neurobiol. 76, 847–881. doi: 10.1002/dneu.22363
Barbosa, J. S., Sanchez-Gonzalez, R., Di Giaimo, R., Baumgart, E. V., Theis, F. J., Götz, M., et al. (2015). Neurodevelopment. Live imaging of adult neural stem cell behavior in the intact and injured zebrafish brain. Science 348, 789–793. doi: 10.1126/science.aaa2729
Barkovich, A. J., Dobyns, W. B., and Guerrini, R. (2015). Malformations of cortical development and epilepsy. Cold Spring Harb. Perspect. Med. 5:a022392. doi: 10.1101/cshperspect.a022392
Baumgart, E. V., Barbosa, J. S., Bally-Cuif, L., Götz, M., and Ninkovic, J. (2012). Stab wound injury of the zebrafish telencephalon: a model for comparative analysis of reactive gliosis. Glia 60, 343–357. doi: 10.1002/glia.22269
Becerra-Solano, L. E., Mateos-Sánchez, L., and López-Muñoz, E. (2021). Microcephaly, an etiopathogenic vision. Pediatr. Neonatol. 62, 354–360. doi: 10.1016/j.pedneo.2021.05.008
Bellion, A., Baudoin, J.-P., Alvarez, C., Bornens, M., and Métin, C. (2005). Nucleokinesis in tangentially migrating neurons comprises two alternating phases: forward migration of the Golgi/centrosome associated with centrosome splitting and myosin contraction at the rear. J. Neurosci. 25, 5691–5699. doi: 10.1523/JNEUROSCI.1030-05.2005
Bem, D., Yoshimura, S.-I., Nunes-Bastos, R., Bond, F. F., Kurian, M. A., Rahman, F., et al. (2011). Loss-of-Function Mutations in RAB18 Cause Warburg Micro Syndrome. Am J Hum Genet 88, 499–507. doi: 10.1016/j.ajhg.2011.03.012
Ben Jehuda, R., Shemer, Y., and Binah, O. (2018). Genome editing in induced pluripotent stem cells using CRISPR/Cas9. Stem Cell Rev. Rep. 14, 323–336. doi: 10.1007/s12015-018-9811-3
Bercier, V., Rosello, M., Del Bene, F., and Revenu, C. (2019). Zebrafish as a model for the study of live in vivo processive transport in neurons. Front. Cell Dev. Biol. 7:17. doi: 10.3389/fcell.2019.00017
Bernardos, R. L., and Raymond, P. A. (2006). GFAP transgenic zebrafish. Gene Expr. Patterns 6, 1007–1013. doi: 10.1016/j.modgep.2006.04.006
Bershteyn, M., Nowakowski, T. J., Pollen, A. A., Di Lullo, E., Nene, A., Wynshaw-Boris, A., et al. (2017). Human iPSC-derived cerebral organoids model cellular features of lissencephaly and reveal prolonged mitosis of outer radial Glia. Cell Stem Cell 20, 435.e–449.e. doi: 10.1016/j.stem.2016.12.007
Betizeau, M., Cortay, V., Patti, D., Pfister, S., Gautier, E., Bellemin-Ménard, A., et al. (2013). Precursor diversity and complexity of lineage relationships in the outer subventricular zone of the primate. Neuron 80, 442–457. doi: 10.1016/j.neuron.2013.09.032
Birey, F., Li, M.-Y., Gordon, A., Thete, M. V., Valencia, A. M., Revah, O., et al. (2022). Dissecting the molecular basis of human interneuron migration in forebrain assembloids from Timothy syndrome. Cell Stem Cell 29, 248.e–264.e. doi: 10.1016/j.stem.2021.11.011
Blair, J. D., Hockemeyer, D., and Bateup, H. S. (2018). Genetically engineered human cortical spheroid models of tuberous sclerosis. Nat. Med. 24, 1568–1578. doi: 10.1038/s41591-018-0139-y
Bobone, S., Pannone, L., Biondi, B., Solman, M., Flex, E., Canale, V. C., et al. (2021). Targeting oncogenic src homology 2 domain-containing phosphatase 2 (SHP2) by inhibiting its protein-protein interactions. J. Med. Chem. 64, 15973–15990. doi: 10.1021/acs.jmedchem.1c01371
Bonduelle, T., Hartlieb, T., Baldassari, S., Sim, N. S., Kim, S. H., Kang, H.-C., et al. (2021). Frequent SLC35A2 brain mosaicism in mild malformation of cortical development with oligodendroglial hyperplasia in epilepsy (MOGHE). Acta Neuropathol Commun 9, 3. doi: 10.1186/s40478-020-01085-3
Bouwmeester, T., Kim, S., Sasai, Y., Lu, B., and De Robertis, E. M. (1996). Cerberus is a head-inducing secreted factor expressed in the anterior endoderm of Spemann’s organizer. Nature 382, 595–601. doi: 10.1038/382595a0
Buchsbaum, I. Y., and Cappello, S. (2019). Neuronal migration in the CNS during development and disease: insights from in vivo and in vitro models. Development 146:dev163766. doi: 10.1242/dev.163766
Buchsbaum, I. Y., Kielkowski, P., Giorgio, G., O’Neill, A. C., Di Giaimo, R., Kyrousi, C., et al. (2020). ECE2 regulates neurogenesis and neuronal migration during human cortical development. EMBO Rep. 21:e48204. doi: 10.15252/embr.201948204
Buysse, K., Riemersma, M., Powell, G., van Reeuwijk, J., Chitayat, D., Roscioli, T., et al. (2013). Missense mutations in β-1,3-N-acetylglucosaminyltransferase 1 (B3GNT1) cause Walker-Warburg syndrome. Hum Mol Genet 22, 1746–1754. doi: 10.1093/hmg/ddt021
Byrd, C. A., and Brunjes, P. C. (2001). Neurogenesis in the olfactory bulb of adult zebrafish. Neuroscience 105, 793–801. doi: 10.1016/s0306-4522(01)00215-9
Cakir, B., Xiang, Y., Tanaka, Y., Kural, M. H., Parent, M., Kang, Y.-J., et al. (2019). Engineering of human brain organoids with a functional vascular-like system. Nat. Methods 16, 1169–1175. doi: 10.1038/s41592-019-0586-5
Calcagnotto, M. E., Paredes, M. F., Tihan, T., Barbaro, N. M., and Baraban, S. C. (2005). Dysfunction of synaptic inhibition in epilepsy associated with focal cortical dysplasia. J. Neurosci. 25, 9649–9657. doi: 10.1523/JNEUROSCI.2687-05.2005
Carabalona, A., Beguin, S., Pallesi-Pocachard, E., Buhler, E., Pellegrino, C., Arnaud, K., et al. (2012). A glial origin for periventricular nodular heterotopia caused by impaired expression of Filamin-A. Hum. Mol. Genet. 21, 1004–1017. doi: 10.1093/hmg/ddr531
Cárdenas, A., and Borrell, V. (2020). Molecular and cellular evolution of corticogenesis in amniotes. Cell Mol. Life Sci. 77, 1435–1460. doi: 10.1007/s00018-019-03315-x
Carpentieri, J. A., Di Cicco, A., Lampic, M., Andreau, D., Del Maestro, L., El Marjou, F., et al. (2022). Endosomal trafficking defects alter neural progenitor proliferation and cause microcephaly. Nat. Commun. 13:16. doi: 10.1038/s41467-021-27705-7
Carvajal-Gonzalez, J. M., Balmer, S., Mendoza, M., Dussert, A., Collu, G., Roman, A.-C., et al. (2015). The clathrin adaptor AP-1 complex and Arf1 regulate planar cell polarity in vivo. Nat Commun 6, 6751. doi: 10.1038/ncomms7751
Cavallin, M., Mine, M., Philbert, M., Boddaert, N., Lepage, J. M., Coste, T., et al. (2018). Further refinement of COL4A1 and COL4A2 related cortical malformations. Eur. J. Med. Genet 61, 765–772. doi: 10.1016/j.ejmg.2018.10.004
Cavallin, M., Rujano, M. A., Bednarek, N., Medina-Cano, D., Bernabe Gelot, A., Drunat, S., et al. (2017). WDR81 mutations cause extreme microcephaly and impair mitotic progression in human fibroblasts and Drosophila neural stem cells. Brain 140, 2597–2609. doi: 10.1093/brain/awx218
Cavodeassi, F., and Houart, C. (2012). Brain regionalization: of signaling centers and boundaries. Dev. Neurobiol. 72, 218–233. doi: 10.1002/dneu.20938
Cederquist, G. Y., Asciolla, J. J., Tchieu, J., Walsh, R. M., Cornacchia, D., Resh, M. D., et al. (2019). Specification of positional identity in forebrain organoids. Nat. Biotechnol. 37, 436–444. doi: 10.1038/s41587-019-0085-3
Cepeda, C., André, V. M., Flores-Hernández, J., Nguyen, O. K., Wu, N., Klapstein, G. J., et al. (2005). Pediatric cortical dysplasia: correlations between neuroimaging, electrophysiology and location of cytomegalic neurons and balloon cells and glutamate/GABA synaptic circuits. Dev. Neurosci. 27, 59–76. doi: 10.1159/000084533
Chakraborti, S., Natarajan, K., Curiel, J., Janke, C., and Liu, J. (2016). The emerging role of the tubulin code: from the tubulin molecule to neuronal function and disease. Cytoskeleton (Hoboken) 73, 521–550. doi: 10.1002/cm.21290
Chiaradia, I., and Lancaster, M. A. (2020). Brain organoids for the study of human neurobiology at the interface of in vitro and in vivo. Nat. Neurosci. 23, 1496–1508. doi: 10.1038/s41593-020-00730-3
Cong, L., Wang, Z., Chai, Y., Hang, W., Shang, C., Yang, W., et al. (2017). Rapid whole brain imaging of neural activity in freely behaving larval zebrafish (Danio rerio). elife 6:e28158. doi: 10.7554/eLife.28158
Coolen, M., Labusch, M., Mannioui, A., and Bally-Cuif, L. (2020). Mosaic heterochrony in neural progenitors sustains accelerated brain growth and neurogenesis in the Juvenile Killifish N. furzeri. Curr. Biol. 30, 736.e–745.e. doi: 10.1016/j.cub.2019.12.046
Costamagna, G., Comi, G. P., and Corti, S. (2021). Advancing drug discovery for neurological disorders using iPSC-derived neural organoids. Int. J. Mol. Sci. 22:2659. doi: 10.3390/ijms22052659
Coutinho, P., Pavlou, S., Bhatia, S., Chalmers, K. J., Kleinjan, D. A., and van Heyningen, V. (2011). Discovery and assessment of conserved Pax6 target genes and enhancers. Genome Res. 21, 1349–1359. doi: 10.1101/gr.124115.111
D’Arcangelo, G., Miao, G. G., Chen, S. C., Soares, H. D., Morgan, J. I., and Curran, T. (1995). A protein related to extracellular matrix proteins deleted in the mouse mutant reeler. Nature 374, 719–723. doi: 10.1038/374719a0
Darvish, H., Esmaeeli-Nieh, S., Monajemi, G. B., Mohseni, M., Ghasemi-Firouzabadi, S., Abedini, S. S., et al. (2010). A clinical and molecular genetic study of 112 Iranian families with primary microcephaly. J. Med. Genet. 47, 823–828. doi: 10.1136/jmg.2009.076398
De Robertis, E. M. (2009). Spemann’s organizer and the self-regulation of embryonic fields. Mech. Dev. 126, 925–941. doi: 10.1016/j.mod.2009.08.004
Deán-Ben, X. L., Sela, G., Lauri, A., Kneipp, M., Ntziachristos, V., Westmeyer, G. G., et al. (2016). Functional optoacoustic neuro-tomography for scalable whole-brain monitoring of calcium indicators. Light Sci. Appl. 5:e16201. doi: 10.1038/lsa.2016.201
Dehay, C., Kennedy, H., and Kosik, K. S. (2015). The outer subventricular zone and primate-specific cortical complexification. Neuron 85, 683–694. doi: 10.1016/j.neuron.2014.12.060
Devisme, L., Bouchet, C., Gonzalès, M., Alanio, E., Bazin, A., Bessières, B., et al. (2012). Cobblestone lissencephaly: neuropathological subtypes and correlations with genes of dystroglycanopathies. Brain 135, 469–482. doi: 10.1093/brain/awr357
Dhaliwal, N., Choi, W. W. Y., Muffat, J., and Li, Y. (2021). Modeling PTEN overexpression-induced microcephaly in human brain organoids. Mol. Brain 14:131. doi: 10.1186/s13041-021-00841-3
Di Donato, N., Chiari, S., Mirzaa, G. M., Aldinger, K., Parrini, E., Olds, C., et al. (2017). Lissencephaly: expanded imaging and clinical classification. Am. J. Med. Genet. A 173, 1473–1488. doi: 10.1002/ajmg.a.38245
Dinday, M. T., and Baraban, S. C. (2015). Large-scale phenotype-based antiepileptic drug screening in a zebrafish model of dravet syndrome. eNeuro 2, ENEURO.68–ENEURO.15. doi: 10.1523/ENEURO.0068-15.2015
Doetsch, F., García-Verdugo, J. M., and Alvarez-Buylla, A. (1997). Cellular composition and three-dimensional organization of the subventricular germinal zone in the adult mammalian brain. J. Neurosci. 17, 5046–5061. doi: 10.1523/JNEUROSCI.17-13-05046.1997
Dou, C. L., Li, S., and Lai, E. (1999). Dual role of brain factor-1 in regulating growth and patterning of the cerebral hemispheres. Cereb. Cortex 9, 543–550. doi: 10.1093/cercor/9.6.543
Duerinckx, S., Jacquemin, V., Drunat, S., Vial, Y., Passemard, S., Perazzolo, C., et al. (2020). Digenic inheritance of human primary microcephaly delineates centrosomal and non-centrosomal pathways. Hum Mutat 41, 512–524. doi: 10.1002/humu.23948
Dunlap, K. D., Corbo, J. H., Vergara, M. M., Beston, S. M., and Walsh, M. R. (2019). Predation drives the evolution of brain cell proliferation and brain allometry in male Trinidadian killifish, Rivulus hartii. Proc. R. Soc. B Biol. Sci. 286:20191485. doi: 10.1098/rspb.2019.1485
Espuny-Camacho, I., Michelsen, K. A., Gall, D., Linaro, D., Hasche, A., Bonnefont, J., et al. (2013). Pyramidal neurons derived from human pluripotent stem cells integrate efficiently into mouse brain circuits in vivo. Neuron 77, 440–456. doi: 10.1016/j.neuron.2012.12.011
Eura, N., Matsui, T. K., Luginbühl, J., Matsubayashi, M., Nanaura, H., Shiota, T., et al. (2020). Brainstem organoids from human pluripotent stem cells. Front. Neurosci. 14:538. doi: 10.3389/fnins.2020.00538
Eze, U. C., Bhaduri, A., Haeussler, M., Nowakowski, T. J., and Kriegstein, A. R. (2021). Single-cell atlas of early human brain development highlights heterogeneity of human neuroepithelial cells and early radial glia. Nat. Neurosci. 24, 584–594. doi: 10.1038/s41593-020-00794-1
Fasano, G., Muto, V., Radio, F. C., Venditti, M., Ziegler, A., Chillemi, G., et al. (2021). Dominantly acting variants in ARF3 have disruptive consequences on Golgi integrity and cause microcephaly recapitulated in zebrafish. Res. Square [Preprint]. doi: 10.21203/rs.3.rs-678090/v1
Faux, C., Rakic, S., Andrews, W., and Britto, J. M. (2012). Neurons on the move: migration and lamination of cortical interneurons. Neurosignals 20, 168–189. doi: 10.1159/000334489
Fernandez, A. S., Pieau, C., Repérant, J., Boncinelli, E., and Wassef, M. (1998). Expression of the Emx-1 and Dlx-1 homeobox genes define three molecularly distinct domains in the telencephalon of mouse, chick, turtle and frog embryos: implications for the evolution of telencephalic subdivisions in amniotes. Development 125, 2099–2111. doi: 10.1242/dev.125.11.2099
Fernández, V., Llinares-Benadero, C., and Borrell, V. (2016). Cerebral cortex expansion and folding: what have we learned? EMBO J. 35, 1021–1044. doi: 10.15252/embj.201593701
Fietz, S. A., Kelava, I., Vogt, J., Wilsch-Bräuninger, M., Stenzel, D., Fish, J. L., et al. (2010). OSVZ progenitors of human and ferret neocortex are epithelial-like and expand by integrin signaling. Nat. Neurosci. 13, 690–699. doi: 10.1038/nn.2553
Fietz, S. A., Lachmann, R., Brandl, H., Kircher, M., Samusik, N., Schröder, R., et al. (2012). Transcriptomes of germinal zones of human and mouse fetal neocortex suggest a role of extracellular matrix in progenitor self-renewal. Proc. Natl. Acad. Sci. 109, 11836–11841. doi: 10.1073/pnas.1209647109
Filges, I., Nosova, E., Bruder, E., Tercanli, S., Townsend, K., Gibson, W. T., et al. (2014). Exome sequencing identifies mutations in KIF14 as a novel cause of an autosomal recessive lethal fetal ciliopathy phenotype. Clin. Genet. 86, 220–228. doi: 10.1111/cge.12301
Fitzgerald, J. A., Kirla, K. T., Zinner, C. P., and Vom Berg, C. M. (2019). Emergence of consistent intra-individual locomotor patterns during zebrafish development. Sci. Rep. 9:13647. doi: 10.1038/s41598-019-49614-y
Flex, E., Niceta, M., Cecchetti, S., Thiffault, I., Au, M. G., Capuano, A., et al. (2016). Biallelic mutations in TBCD, encoding the tubulin folding cofactor D, perturb microtubule dynamics and cause early-onset encephalopathy. Am. J. Hum. Genet. 99, 962–973. doi: 10.1016/j.ajhg.2016.08.003
Florio, M., Albert, M., Taverna, E., Namba, T., Brandl, H., Lewitus, E., et al. (2015). Human-specific gene ARHGAP11B promotes basal progenitor amplification and neocortex expansion. Science 347, 1465–1470. doi: 10.1126/science.aaa1975
Florio, M., and Huttner, W. B. (2014). Neural progenitors, neurogenesis and the evolution of the neocortex. Development 141, 2182–2194. doi: 10.1242/dev.090571
Florio, M., Namba, T., Pääbo, S., Hiller, M., and Huttner, W. B. (2016). A single splice site mutation in human-specific ARHGAP11B causes basal progenitor amplification. Sci. Adv. 2:e1601941. doi: 10.1126/sciadv.1601941
Folgueira, M., Bayley, P., Navratilova, P., Becker, T. S., Wilson, S. W., and Clarke, J. D. (2012). Morphogenesis underlying the development of the everted teleost telencephalon. Neural Dev. 7:212. doi: 10.1186/1749-8104-7-32
Fotowat, H., Lee, C., Jun, J. J., and Maler, L. (2019). Neural activity in a hippocampus-like region of the teleost pallium is associated with active sensing and navigation. eLife 8:e44119. doi: 10.7554/eLife.44119
Furlan, G., Cuccioli, V., Vuillemin, N., Dirian, L., Muntasell, A. J., Coolen, M., et al. (2017). Life-long neurogenic activity of individual neural stem cells and continuous growth establish an outside-in architecture in the teleost pallium. Curr. Biol. 27, 3288.e–3301.e. doi: 10.1016/j.cub.2017.09.052
Gabriel, E., Wason, A., Ramani, A., Gooi, L. M., Keller, P., Pozniakovsky, A., et al. (2016). CPAP promotes timely cilium disassembly to maintain neural progenitor pool. EMBO J 35, 803–819. doi: 10.15252/embj.201593679
Ganz, J., Kaslin, J., Freudenreich, D., Machate, A., Geffarth, M., and Brand, M. (2012). Subdivisions of the adult zebrafish subpallium by molecular marker analysis. J. Comp. Neurol. 520, 633–655. doi: 10.1002/cne.22757
García-Verdugo, J. M., Ferrón, S., Flames, N., Collado, L., Desfilis, E., and Font, E. (2002). The proliferative ventricular zone in adult vertebrates: a comparative study using reptiles, birds, and mammals. Brain Res. Bull. 57, 765–775. doi: 10.1016/s0361-9230(01)00769-9
Garreta, E., Kamm, R. D., Chuva de Sousa Lopes, S. M., Lancaster, M. A., Weiss, R., Trepat, X., et al. (2021). Rethinking organoid technology through bioengineering. Nat Mater 20, 145–155. doi: 10.1038/s41563-020-00804-4
Gilardi, C., and Kalebic, N. (2021). The ferret as a model system for neocortex development and evolution. Front. Cell Dev. Biol. 9:1004. doi: 10.3389/fcell.2021.661759
Godfrey, C., Clement, E., Mein, R., Brockington, M., Smith, J., Talim, B., et al. (2007). Refining genotype–phenotype correlations in muscular dystrophies with defective glycosylation of dystroglycan. Brain 130, 2725–2735. doi: 10.1093/brain/awm212
Götz, M., and Huttner, W. B. (2005). The cell biology of neurogenesis. Nat. Rev. Mol. Cell Biol. 6, 777–788. doi: 10.1038/nrm1739
Grandel, H., Kaslin, J., Ganz, J., Wenzel, I., and Brand, M. (2006). Neural stem cells and neurogenesis in the adult zebrafish brain: origin, proliferation dynamics, migration and cell fate. Dev. Biol. 295, 263–277. doi: 10.1016/j.ydbio.2006.03.040
Griffin, A., Carpenter, C., Liu, J., Paterno, R., Grone, B., Hamling, K., et al. (2021). Phenotypic analysis of catastrophic childhood epilepsy genes. Epilepsy Zebrafish Project 4. doi: 10.1101/2021.02.11.430844
Griffin, A., Hamling, K. R., Knupp, K., Hong, S., Lee, L. P., and Baraban, S. C. (2017). Clemizole and modulators of serotonin signalling suppress seizures in Dravet syndrome. Brain 140, 669–683. doi: 10.1093/brain/aww342
Grosenick, L., Clement, T. S., and Fernald, R. D. (2007). Fish can infer social rank by observation alone. Nature 445, 429–432. doi: 10.1038/nature05511
Guarnieri, F. C., de Chevigny, A., Falace, A., and Cardoso, C. (2018). Disorders of neurogenesis and cortical development. Dialogues Clin. Neurosci. 20, 255–266. doi: 10.31887/DCNS.2018.20.4/ccardoso
Guemez-Gamboa, A., Nguyen, L. N., Yang, H., Zaki, M. S., Kara, M., Ben-Omran, T., et al. (2015). Inactivating mutations in MFSD2A, required for omega-3 fatty acid transport in brain, cause a lethal microcephaly syndrome. Nat Genet 47, 809–813. doi: 10.1038/ng.3311
Guerrini, R., and Dobyns, W. B. (2014). Malformations of cortical development: clinical features and genetic causes. Lancet Neurol. 13, 710–726. doi: 10.1016/S1474-4422(14)70040-7
Guerrini, R., and Parrini, E. (2010). Neuronal migration disorders. Neurobiol. Dis. 38, 154–166. doi: 10.1016/j.nbd.2009.02.008
Gupta, T., Marquart, G. D., Horstick, E. J., Tabor, K. M., Pajevic, S., and Burgess, H. A. (2018). Morphometric analysis and neuroanatomical mapping of the zebrafish brain. Methods 150, 49–62. doi: 10.1016/j.ymeth.2018.06.008
Gupta, V. A., Kawahara, G., Myers, J. A., Chen, A. T., Hall, T. E., Manzini, M. C., et al. (2012). A Splice Site Mutation in Laminin-α2 Results in a Severe Muscular Dystrophy and Growth Abnormalities in Zebrafish. PLoS One 7:e43794. doi: 10.1371/journal.pone.0043794
Haines, D. E., and Mihailoff, G. A. (2018). Fundamental Neuroscience for Basic and Clinical Applications, 5th Edn. Available online at: https://evolve.elsevier.com/cs/product/9780323396325?role=student [Accessed April 11, 2022].
Hall, Z. J., and Tropepe, V. (2020). Using teleost fish to discern developmental signatures of evolutionary adaptation from phenotypic plasticity in brain structure. Front. Neuroanat. 14:10. doi: 10.3389/fnana.2020.00010
Hashiguchi, M., and Mullins, M. C. (2013). Anteroposterior and dorsoventral patterning are coordinated by an identical patterning clock. Development 140, 1970–1980. doi: 10.1242/dev.088104
Haubensak, W., Attardo, A., Denk, W., and Huttner, W. B. (2004). Neurons arise in the basal neuroepithelium of the early mammalian telencephalon: a major site of neurogenesis. Proc. Natl. Acad. Sci. U.S.A. 101, 3196–3201. doi: 10.1073/pnas.0308600100
Heide, M., Haffner, C., Murayama, A., Kurotaki, Y., Shinohara, H., Okano, H., et al. (2020). Human-specific ARHGAP11B increases size and folding of primate neocortex in the fetal marmoset. Science 369, 546–550. doi: 10.1126/science.abb2401
Heinzen, E. L., O’Neill, A. C., Zhu, X., Allen, A. S., Bahlo, M., Chelly, J., et al. (2018). De novo and inherited private variants in MAP1B in periventricular nodular heterotopia. PLoS Genet. 14:e1007281. doi: 10.1371/journal.pgen.1007281
Heisenberg, C. P., Brand, M., Jiang, Y. J., Warga, R. M., Beuchle, D., van Eeden, F. J., et al. (1996). Genes involved in forebrain development in the zebrafish, Danio rerio. Development 123, 191–203. doi: 10.1242/dev.123.1.191
Hengel, H., Bosso-Lefèvre, C., Grady, G., Szenker-Ravi, E., Li, H., Pierce, S., et al. (2020). Loss-of-function mutations in UDP-Glucose 6-Dehydrogenase cause recessive developmental epileptic encephalopathy. Nat. Commun. 11:595. doi: 10.1038/s41467-020-14360-7
Hevner, R. F. (2015). Brain overgrowth in disorders of RTK-PI3K-AKT signaling: a mosaic of malformations. Semin. Perinatol. 39, 36–43. doi: 10.1053/j.semperi.2014.10.006
Hirota, Y., and Nakajima, K. (2017). Control of neuronal migration and aggregation by reelin signaling in the developing cerebral cortex. Front Cell Dev. Biol. 5:40. doi: 10.3389/fcell.2017.00040
Hong, S. E., Shugart, Y. Y., Huang, D. T., Shahwan, S. A., Grant, P. E., Hourihane, J. O., et al. (2000). Autosomal recessive lissencephaly with cerebellar hypoplasia is associated with human RELN mutations. Nat. Genet. 26, 93–96. doi: 10.1038/79246
Hontani, Y., Akbari, N., Kolkman, K. E., Wu, C., Xia, F., Choe, K., et al. (2022). Deep-tissue three-photon fluorescence microscopy in intact mouse and zebrafish brain. J. Vis. Exp. e63213. doi: 10.3791/63213
Hoshijima, K., Jurynec, M. J., Klatt Shaw, D., Jacobi, A. M., Behlke, M. A., and Grunwald, D. J. (2019). Highly efficient CRISPR-Cas9-based methods for generating deletion mutations and F0 embryos that lack gene function in zebrafish. Dev. Cell 51, 645.e–657.e. doi: 10.1016/j.devcel.2019.10.004
Houart, C., Caneparo, L., Heisenberg, C., Barth, K., Take-Uchi, M., and Wilson, S. (2002). Establishment of the telencephalon during gastrulation by local antagonism of Wnt signaling. Neuron 35, 255–265. doi: 10.1016/s0896-6273(02)00751-1
Houart, C., Westerfield, M., and Wilson, S. W. (1998). A small population of anterior cells patterns the forebrain during zebrafish gastrulation. Nature 391, 788–792. doi: 10.1038/35853
Howe, K., Clark, M. D., Torroja, C. F., Torrance, J., Berthelot, C., Muffato, M., et al. (2013). The zebrafish reference genome sequence and its relationship to the human genome. Nature 496, 498–503. doi: 10.1038/nature12111
Huang, C.-Y., Liu, C.-L., Ting, C.-Y., Chiu, Y.-T., Cheng, Y.-C., Nicholson, M. W., et al. (2019). Human iPSC banking: barriers and opportunities. J. Biomed. Sci. 26:87. doi: 10.1186/s12929-019-0578-x
Hutsler, J. J., Lee, D.-G., and Porter, K. K. (2005). Comparative analysis of cortical layering and supragranular layer enlargement in rodent carnivore and primate species. Brain Res. 1052, 71–81. doi: 10.1016/j.brainres.2005.06.015
Hwang, W. Y., Fu, Y., Reyon, D., Maeder, M. L., Tsai, S. Q., Sander, J. D., et al. (2013). Efficient genome editing in zebrafish using a CRISPR-Cas system. Nat. Biotechnol. 31, 227–229. doi: 10.1038/nbt.2501
Iefremova, V., Manikakis, G., Krefft, O., Jabali, A., Weynans, K., Wilkens, R., et al. (2017). An organoid-based model of cortical development identifies non-cell-autonomous defects in wnt signaling contributing to miller-dieker syndrome. Cell Rep. 19, 50–59. doi: 10.1016/j.celrep.2017.03.047
Indrieri, A., Conte, I., Chesi, G., Romano, A., Quartararo, J., Tatè, R., et al. (2013). The impairment of HCCS leads to MLS syndrome by activating a non-canonical cell death pathway in the brain and eyes. EMBO Mol. Med. 5, 280–293. doi: 10.1002/emmm.201201739
Insinna, C., Baye, L. M., Amsterdam, A., Besharse, J. C., and Link, B. A. (2010). Analysis of a zebrafish dync1h1 mutant reveals multiple functions for cytoplasmic dynein 1 during retinal photoreceptor development. Neural Dev 5, 12. doi: 10.1186/1749-8104-5-12
Ito, H., and Yamamoto, N. (2009). Non-laminar cerebral cortex in teleost fishes? Biol. Lett. 5, 117–121. doi: 10.1098/rsbl.2008.0397
Ito, H., Ishikawa, Y., Yoshimoto, M., and Yamamoto, N. (2007). Diversity of brain morphology in teleosts: brain and ecological niche. Brain Behav. Evol. 69, 76–86. doi: 10.1159/000095196
Jansen, L. A., Mirzaa, G. M., Ishak, G. E., O’Roak, B. J., Hiatt, J. B., Roden, W. H., et al. (2015). PI3K/AKT pathway mutations cause a spectrum of brain malformations from megalencephaly to focal cortical dysplasia. Brain 138, 1613–1628. doi: 10.1093/brain/awv045
Johnson, M. B., Sun, X., Kodani, A., Borges-Monroy, R., Girskis, K. M., Ryu, S. C., et al. (2018). Aspm knockout ferret reveals an evolutionary mechanism governing cerebral cortical size. Nature 556, 370–375. doi: 10.1038/s41586-018-0035-0
Kalebic, N., Gilardi, C., Albert, M., Namba, T., Long, K. R., Kostic, M., et al. (2018). Human-specific ARHGAP11B induces hallmarks of neocortical expansion in developing ferret neocortex. elife 7:e41241. doi: 10.7554/eLife.41241
Kalebic, N., Gilardi, C., Stepien, B., Wilsch-Bräuninger, M., Long, K. R., Namba, T., et al. (2019). Neocortical expansion due to increased proliferation of basal progenitors is linked to changes in their morphology. Cell Stem Cell 24, 535.e–550.e. doi: 10.1016/j.stem.2019.02.017
Kanton, S., Boyle, M. J., He, Z., Santel, M., Weigert, A., Sanchís-Calleja, F., et al. (2019). Organoid single-cell genomic atlas uncovers human-specific features of brain development. Nature 574, 418–422. doi: 10.1038/s41586-019-1654-9
Kawakami, K. (2005). Transposon tools and methods in zebrafish. Dev. Dyn. 234, 244–254. doi: 10.1002/dvdy.20516
Kawano, Y., and Kypta, R. (2003). Secreted antagonists of the Wnt signalling pathway. J. Cell Sci. 116, 2627–2634. doi: 10.1242/jcs.00623
Kedra, M., Banasiak, K., Kisielewska, K., Wolinska-Niziol, L., Jaworski, J., and Zmorzynska, J. (2020). TrkB hyperactivity contributes to brain dysconnectivity, epileptogenesis, and anxiety in zebrafish model of tuberous sclerosis complex. Proc. Natl. Acad. Sci. 117, 2170–2179. doi: 10.1073/pnas.1910834117
Kelava, I., Lewitus, E., and Huttner, W. B. (2013). The secondary loss of gyrencephaly as an example of evolutionary phenotypical reversal. Front. Neuroanat. 7:16. doi: 10.3389/fnana.2013.00016
Kernohan, K. D., Tétreault, M., Liwak-Muir, U., Geraghty, M. T., Qin, W., Venkateswaran, S., et al. (2015). Homozygous mutation in the eukaryotic translation initiation factor 2alpha phosphatase gene, PPP1R15B, is associated with severe microcephaly, short stature and intellectual disability. Hum. Mol. Genet. 24, 6293–6300. doi: 10.1093/hmg/ddv337
Khan, T. N., Khan, K., Sadeghpour, A., Reynolds, H., Perilla, Y., McDonald, M. T., et al. (2019). Mutations in NCAPG2 Cause a Severe Neurodevelopmental Syndrome that Expands the Phenotypic Spectrum of Condensinopathies. Am J Hum Genet 104, 94–111. doi: 10.1016/j.ajhg.2018.11.017
Kim, H.-T., Lee, M.-S., Choi, J.-H., Jung, J.-Y., Ahn, D.-G., Yeo, S.-Y., et al. (2011a). The microcephaly gene aspm is involved in brain development in zebrafish. Biochem Biophys. Res. Commun. 409, 640–644. doi: 10.1016/j.bbrc.2011.05.056
Kim, S.-H., Speirs, C. K., Solnica-Krezel, L., and Ess, K. C. (2011b). Zebrafish model of tuberous sclerosis complex reveals cell-autonomous and non-cell-autonomous functions of mutant tuberin. Dis. Model Mech. 4, 255–267. doi: 10.1242/dmm.005587
Kim, O.-H., Cho, H.-J., Han, E., Hong, T. I., Ariyasiri, K., Choi, J.-H., et al. (2017). Zebrafish knockout of Down syndrome gene, DYRK1A, shows social impairments relevant to autism. Molecular Autism 8, 50. doi: 10.1186/s13229-017-0168-2
Kirchmaier, S., Naruse, K., Wittbrodt, J., and Loosli, F. (2015). The genomic and genetic toolbox of the teleost medaka (Oryzias latipes). Genetics 199, 905–918. doi: 10.1534/genetics.114.173849
Kitagawa, D., Watanabe, T., Saito, K., Asaka, S., Sasado, T., Morinaga, C., et al. (2004). Genetic dissection of the formation of the forebrain in Medaka, Oryzias latipes. Mech. Dev. 121, 673–685. doi: 10.1016/j.mod.2004.03.010
Klaus, J., Kanton, S., Kyrousi, C., Ayo-Martin, A. C., Di Giaimo, R., Riesenberg, S., et al. (2019). Altered neuronal migratory trajectories in human cerebral organoids derived from individuals with neuronal heterotopia. Nat. Med. 25, 561–568. doi: 10.1038/s41591-019-0371-0
Koenig, M., Dobyns, W. B., and Donato, N. D. (2021). Lissencephaly: update on diagnostics and clinical management. Eur. J. Paediatr. Neurol. 35, 147–152. doi: 10.1016/j.ejpn.2021.09.013
Krefft, O., Koch, P., and Ladewig, J. (2021). Cerebral organoids to unravel the mechanisms underlying malformations of human cortical development. Semin. Cell Dev. Biol. 111, 15–22. doi: 10.1016/j.semcdb.2020.06.001
Kriegstein, A. R., and Götz, M. (2003). Radial glia diversity: a matter of cell fate. Glia 43, 37–43. doi: 10.1002/glia.10250
Kriegstein, A., and Alvarez-Buylla, A. (2009). The glial nature of embryonic and adult neural stem cells. Annu. Rev. Neurosci. 32, 149–184. doi: 10.1146/annurev.neuro.051508.135600
Kroll, F., Powell, G. T., Ghosh, M., Gestri, G., Antinucci, P., Hearn, T. J., et al. (2021). A simple and effective F0 knockout method for rapid screening of behaviour and other complex phenotypes. elife 10:e59683. doi: 10.7554/eLife.59683
Kunst, M., Laurell, E., Mokayes, N., Kramer, A., Kubo, F., Fernandes, A. M., et al. (2019). A cellular-resolution atlas of the larval zebrafish brain. Neuron 103, 21.e–38.e. doi: 10.1016/j.neuron.2019.04.034
Kuo, G., Arnaud, L., Kronstad-O’Brien, P., and Cooper, J. A. (2005). Absence of fyn and src causes a reeler-like phenotype. J. Neurosci. 25, 8578–8586. doi: 10.1523/JNEUROSCI.1656-05.2005
Kurahashi, H., Taniguchi, M., Meno, C., Taniguchi, Y., Takeda, S., Horie, M., et al. (2005). Basement membrane fragility underlies embryonic lethality in fukutin-null mice. Neurobiol. Dis. 19, 208–217. doi: 10.1016/j.nbd.2004.12.018
Kyrousi, C., O’Neill, A. C., Brazovskaja, A., He, Z., Kielkowski, P., Coquand, L., et al. (2021). Extracellular LGALS3BP regulates neural progenitor position and relates to human cortical complexity. Nat. Commun. 12:6298. doi: 10.1038/s41467-021-26447-w
Laclef, C., and Métin, C. (2018). Conserved rules in embryonic development of cortical interneurons. Semin. Cell Dev. Biol. 76, 86–100. doi: 10.1016/j.semcdb.2017.09.017
LaFave, M. C., Varshney, G. K., Vemulapalli, M., Mullikin, J. C., and Burgess, S. M. (2014). A defined zebrafish line for high-throughput genetics and genomics: NHGRI-1. Genetics 198, 167–170. doi: 10.1534/genetics.114.166769
Lal, P., Tanabe, H., Suster, M. L., Ailani, D., Kotani, Y., Muto, A., et al. (2018). Identification of a neuronal population in the telencephalon essential for fear conditioning in zebrafish. BMC Biol. 16:45. doi: 10.1186/s12915-018-0502-y
Lancaster, M. A., and Knoblich, J. A. (2014). Generation of cerebral organoids from human pluripotent stem cells. Nat. Protoc. 9, 2329–2340. doi: 10.1038/nprot.2014.158
Lancaster, M. A., Renner, M., Martin, C.-A., Wenzel, D., Bicknell, L. S., Hurles, M. E., et al. (2013). Cerebral organoids model human brain development and microcephaly. Nature 501, 373–379. doi: 10.1038/nature12517
Lange, C., Rost, F., Machate, A., Reinhardt, S., Lesche, M., Weber, A., et al. (2020). Single cell sequencing of radial glia progeny reveals the diversity of newborn neurons in the adult zebrafish brain. Development 147:dev185595. doi: 10.1242/dev.185595
Lauri, A., Fasano, G., Venditti, M., Dallapiccola, B., and Tartaglia, M. (2021). In vivo functional genomics for undiagnosed patients: the impact of small GTPases signaling dysregulation at pan-embryo developmental scale. Front. Cell Dev. Biol. 9:642235. doi: 10.3389/fcell.2021.642235
Lavdas, A. A., Grigoriou, M., Pachnis, V., and Parnavelas, J. G. (1999). The medial ganglionic eminence gives rise to a population of early neurons in the developing cerebral cortex. J. Neurosci. 19, 7881–7888. doi: 10.1523/JNEUROSCI.19-18-07881.1999
Levin, E. D., and Cerutti, D. T. (2009). “Behavioral neuroscience of zebrafish,” in Methods of Behavior Analysis in Neuroscience Frontiers in Neuroscience, ed. J. J. Buccafusco (Boca Raton, FL: CRC Press/Taylor & Francis).
Li, R., Sun, L., Fang, A., Li, P., Wu, Q., and Wang, X. (2017a). Recapitulating cortical development with organoid culture in vitro and modeling abnormal spindle-like (ASPM related primary) microcephaly disease. Protein Cell 8, 823–833. doi: 10.1007/s13238-017-0479-2
Li, Y., Muffat, J., Omer, A., Bosch, I., Lancaster, M. A., Sur, M., et al. (2017b). Induction of expansion and folding in human cerebral organoids. Cell Stem Cell 20, 385.e–396.e. doi: 10.1016/j.stem.2016.11.017
Lin, Y.-Y., White, R. J., Torelli, S., Cirak, S., Muntoni, F., and Stemple, D. L. (2011). Zebrafish Fukutin family proteins link the unfolded protein response with dystroglycanopathies. Hum. Mol. Genet. 20, 1763–1775. doi: 10.1093/hmg/ddr059
Liu, Y., Yu, M., Shang, X., Nguyen, M. H. H., Balakrishnan, S., Sager, R., et al. (2020). Eyes shut homolog (EYS) interacts with matriglycan of O-mannosyl glycans whose deficiency results in EYS mislocalization and degeneration of photoreceptors. Sci Rep 10, 7795. doi: 10.1038/s41598-020-64752-4
Lodato, S., and Arlotta, P. (2015). Generating neuronal diversity in the mammalian cerebral cortex. Annu. Rev. Cell Dev. Biol. 31, 699–720. doi: 10.1146/annurev-cellbio-100814-125353
Long, K. R., Newland, B., Florio, M., Kalebic, N., Langen, B., Kolterer, A., et al. (2018). Extracellular matrix components HAPLN1, lumican, and collagen I cause hyaluronic acid-dependent folding of the developing human neocortex. Neuron 99, 702.e–719.e. doi: 10.1016/j.neuron.2018.07.013
Loosli, F., Köster, R. W., Carl, M., Kühnlein, R., Henrich, T., Mücke, M., et al. (2000). A genetic screen for mutations affecting embryonic development in medaka fish (Oryzias latipes). Mech. Dev. 97, 133–139. doi: 10.1016/s0925-4773(00)00406-8
Lu, X., Yang, J., and Xiang, Y. (2022). Modeling human neurodevelopmental diseases with brain organoids. Cell Regen. 11:1. doi: 10.1186/s13619-021-00103-6
Lui, J. H., Hansen, D. V., and Kriegstein, A. R. (2011). Development and evolution of the human neocortex. Cell 146, 18–36. doi: 10.1016/j.cell.2011.06.030
Ma, H., Blake, T., Chitnis, A., Liu, P., and Balla, T. (2009). Crucial role of phosphatidylinositol 4-kinase IIIα in development of zebrafish pectoral fin is linked to phosphoinositide 3-kinase and FGF signaling. J Cell Sci 122, 4303–4310. doi: 10.1242/jcs.057646
Mack, A. F., DeOliveira-Mello, L., Mattheus, U., and Neckel, P. H. (2021). Organization of radial glia reveals growth pattern in the telencephalon of a percomorph fish Astatotilapia burtoni. J. Comp. Neurol. 529, 2813–2823. doi: 10.1002/cne.25126
Marino, S., Pavone, P., Marino, L., Rapisarda, F. A. S., and Falsaperla, R. (2020). Congenital genetic microcephaly: clinical diagnostic approach. J. Pediatr. Neurol. 18, 131–134. doi: 10.1055/s-0039-1692970
Marinowic, D. R., Majolo, F., Sebben, A. D., da Silva, V. D., Lopes, T. G., Paglioli, E., et al. (2017). Induced pluripotent stem cells from patients with focal cortical dysplasia and refractory epilepsy. Mol. Med. Rep. 15, 2049–2056. doi: 10.3892/mmr.2017.6264
Marin-Valencia, I., Novarino, G., Johansen, A., Rosti, B., Issa, M. Y., Musaev, D., et al. (2018). A homozygous founder mutation in TRAPPC6B associates with a neurodevelopmental disorder characterised by microcephaly, epilepsy and autistic features. J Med Genet 55, 48–54. doi: 10.1136/jmedgenet-2017-104627
Martínez-Cerdeño, V., Cunningham, C. L., Camacho, J., Antczak, J. L., Prakash, A. N., Cziep, M. E., et al. (2012). Comparative analysis of the subventricular zone in rat, ferret and macaque: evidence for an outer subventricular zone in rodents. PLoS One 7:e30178. doi: 10.1371/journal.pone.0030178
März, M., Schmidt, R., Rastegar, S., and Strähle, U. (2011). Regenerative response following stab injury in the adult zebrafish telencephalon. Dev. Dyn. 240, 2221–2231. doi: 10.1002/dvdy.22710
Masselink, W. (2021). Crispants take the spotlight. Lab. Anim. 50, 95–96. doi: 10.1038/s41684-021-00739-6
Mastrodonato, V., Beznoussenko, G., Mironov, A., Ferrari, L., Deflorian, G., and Vaccari, T. (2019). A genetic model of CEDNIK syndrome in zebrafish highlights the role of the SNARE protein Snap29 in neuromotor and epidermal development. Sci Rep 9, 1211. doi: 10.1038/s41598-018-37780-4
Masuda, K., Toda, T., Shinmyo, Y., Ebisu, H., Hoshiba, Y., Wakimoto, M., et al. (2015). Pathophysiological analyses of cortical malformation using gyrencephalic mammals. Sci. Rep. 5:15370. doi: 10.1038/srep15370
Matsui, T., and Shinozawa, T. (2021). Human organoids for predictive toxicology research and drug development. Front. Genet. 12:767621. doi: 10.3389/fgene.2021.767621
Medina, L., and Abellán, A. (2009). Development and evolution of the pallium. Semin. Cell Dev. Biol. 20, 698–711. doi: 10.1016/j.semcdb.2009.04.008
Medina, L., Brox, A., Legaz, I., García-López, M., and Puelles, L. (2005). Expression patterns of developmental regulatory genes show comparable divisions in the telencephalon of Xenopus and mouse: insights into the evolution of the forebrain. Brain Res. Bull. 66, 297–302. doi: 10.1016/j.brainresbull.2005.02.003
Menuet, A., Alunni, A., Joly, J.-S., Jeffery, W. R., and Rétaux, S. (2007). Expanded expression of Sonic Hedgehog in Astyanax cavefish: multiple consequences on forebrain development and evolution. Development 134, 845–855. doi: 10.1242/dev.02780
Messina, A., Potrich, D., Schiona, I., Sovrano, V. A., Fraser, S. E., Brennan, C. H., et al. (2022). Neurons in the dorso-central division of zebrafish pallium respond to change in visual numerosity. Cereb. Cortex 32, 418–428. doi: 10.1093/cercor/bhab218
Mione, M., Baldessari, D., Deflorian, G., Nappo, G., and Santoriello, C. (2008). How neuronal migration contributes to the morphogenesis of the CNS: insights from the zebrafish. Dev. Neurosci. 30, 65–81. doi: 10.1159/000109853
Mishra-Gorur, K., Çağlayan, A. O., Schaffer, A. E., Chabu, C., Henegariu, O., Vonhoff, F., et al. (2014). Mutations in KATNB1 Cause Complex Cerebral Malformations by Disrupting Asymmetrically Dividing Neural Progenitors. Neuron 84, 1226–1239. doi: 10.1016/j.neuron.2014.12.014
Miyoshi, G., Hjerling-Leffler, J., Karayannis, T., Sousa, V. H., Butt, S. J. B., Battiste, J., et al. (2010). Genetic fate mapping reveals that the caudal ganglionic eminence produces a large and diverse population of superficial cortical interneurons. J. Neurosci. 30, 1582–1594. doi: 10.1523/JNEUROSCI.4515-09.2010
Montgomery, S. H., Mundy, N. I., and Barton, R. A. (2016). Brain evolution and development: adaptation, allometry and constraint. Proc. R. Soc. B Biol. Sci. 283:20160433. doi: 10.1098/rspb.2016.0433
Mora-Bermúdez, F., Badsha, F., Kanton, S., Camp, J. G., Vernot, B., Köhler, K., et al. (2016). Differences and similarities between human and chimpanzee neural progenitors during cerebral cortex development. elife 5:e18683. doi: 10.7554/eLife.18683
Moreno-Layseca, P., and Streuli, C. H. (2014). Signalling pathways linking integrins with cell cycle progression. Matrix Biol. 34, 144–153. doi: 10.1016/j.matbio.2013.10.011
Mueller, T., Dong, Z., Berberoglu, M. A., and Guo, S. (2011). The dorsal pallium in zebrafish, Danio rerio (Cyprinidae, Teleostei). Brain Res. 1381, 95–105. doi: 10.1016/j.brainres.2010.12.089
Mukhopadhyay, M., Shtrom, S., Rodriguez-Esteban, C., Chen, L., Tsukui, T., Gomer, L., et al. (2001). Dickkopf1 is required for embryonic head induction and limb morphogenesis in the mouse. Dev. Cell 1, 423–434. doi: 10.1016/s1534-5807(01)00041-7
Murakami, Y., Uchida, K., Rijli, F. M., and Kuratani, S. (2005). Evolution of the brain developmental plan: insights from agnathans. Dev. Biol. 280, 249–259. doi: 10.1016/j.ydbio.2005.02.008
Murata, K., Kinoshita, M., Naruse, K., Tanaka, M., and Kamei, Y. (2019). Medaka: Biology, Management, and Experimental Protocols. Chichester, UK: John Wiley & Sons, Ltd. doi: 10.1002/9781119575399
Nadarajah, B., Brunstrom, J. E., Grutzendler, J., Wong, R. O., and Pearlman, A. L. (2001). Two modes of radial migration in early development of the cerebral cortex. Nat. Neurosci. 4, 143–150. doi: 10.1038/83967
Nageshappa, S., Carromeu, C., Trujillo, C. A., Mesci, P., Espuny-Camacho, I., Pasciuto, E., et al. (2016). Altered neuronal network and rescue in a human MECP2 duplication model. Mol. Psychiatry 21, 178–188. doi: 10.1038/mp.2015.128
Najm, I. M., Sarnat, H. B., and Blümcke, I. (2018). Review: The international consensus classification of Focal Cortical Dysplasia - a critical update 2018. Neuropathol. Appl. Neurobiol. 44, 18–31. doi: 10.1111/nan.12462
Nakagawa, J. M., Donkels, C., Fauser, S., Schulze-Bonhage, A., Prinz, M., Zentner, J., et al. (2017). Characterization of focal cortical dysplasia with balloon cells by layer-specific markers: evidence for differential vulnerability of interneurons. Epilepsia 58, 635–645. doi: 10.1111/epi.13690
Nakanishi, E., Uemura, N., Akiyama, H., Kinoshita, M., Masanori, S., Taruno, Y., et al. (2021). Impact of Gba2 on neuronopathic Gaucher’s disease and α-synuclein accumulation in medaka (Oryzias latipes). Mol. Brain 14:80. doi: 10.1186/s13041-021-00790-x
Namba, T., Vaid, S., and Huttner, W. B. (2019). Primate neocortex development and evolution: conserved versus evolved folding. J. Comp. Neurol. 527, 1621–1632. doi: 10.1002/cne.24606
Nieuwenhuys, R. (2009). The forebrain of actinopterygians revisited. Brain Behav. Evol. 73, 229–252. doi: 10.1159/000225622
Nimura, T., Itoh, T., Hagio, H., Hayashi, T., Di Donato, V., Takeuchi, M., et al. (2019). Role of Reelin in cell positioning in the cerebellum and the cerebellum-like structure in zebrafish. Developmental Biology 455, 393–408. doi: 10.1016/j.ydbio.2019.07.010
Noctor, S. C., Flint, A. C., Weissman, T. A., Dammerman, R. S., and Kriegstein, A. R. (2001). Neurons derived from radial glial cells establish radial units in neocortex. Nature 409, 714–720. doi: 10.1038/35055553
Noctor, S. C., Martínez-Cerdeño, V., and Kriegstein, A. R. (2008). Distinct behaviors of neural stem and progenitor cells underlie cortical neurogenesis. J. Comp. Neurol. 508, 28–44. doi: 10.1002/cne.21669
Noctor, S. C., Martínez-Cerdeño, V., Ivic, L., and Kriegstein, A. R. (2004). Cortical neurons arise in symmetric and asymmetric division zones and migrate through specific phases. Nat Neurosci 7, 136–144. doi: 10.1038/nn1172
Nonaka-Kinoshita, M., Reillo, I., Artegiani, B., Martínez-Martínez, M. Á, Nelson, M., Borrell, V., et al. (2013). Regulation of cerebral cortex size and folding by expansion of basal progenitors. EMBO J. 32, 1817–1828. doi: 10.1038/emboj.2013.96
Noreikiene, K., Herczeg, G., Gonda, A., Balázs, G., Husby, A., and Merilä, J. (2015). Quantitative genetic analysis of brain size variation in sticklebacks: support for the mosaic model of brain evolution. Proc. Biol. Sci. 282:20151008. doi: 10.1098/rspb.2015.1008
Northcutt, R. G. (1995). The forebrain of gnathostomes: in search of a morphotype. Brain Behav. Evol. 46, 275–318. doi: 10.1159/000113279
Novorol, C., Burkhardt, J., Wood, K. J., Iqbal, A., Roque, C., Coutts, N., et al. (2013). Microcephaly models in the developing zebrafish retinal neuroepithelium point to an underlying defect in metaphase progression. Open Biol. 3:130065. doi: 10.1098/rsob.130065
Ohtsuka, T., and Kageyama, R. (2019). Regulation of temporal properties of neural stem cells and transition timing of neurogenesis and gliogenesis during mammalian neocortical development. Semin. Cell Dev. Biol. 95, 4–11. doi: 10.1016/j.semcdb.2019.01.007
Orger, M. B., Gahtan, E., Muto, A., Page-McCaw, P., Smear, M. C., and Baier, H. (2004). Behavioral screening assays in zebrafish. Methods Cell Biol. 77, 53–68. doi: 10.1016/S0091-679X(04)77003-X
Ossola, C., and Kalebic, N. (2021). Roots of the malformations of cortical development in the cell biology of neural progenitor cells. Front. Neurosci. 15:817218. doi: 10.3389/fnins.2021.817218
Otani, T., Marchetto, M. C., Gage, F. H., Simons, B. D., and Livesey, F. J. (2016). 2D and 3D stem cell models of primate cortical development identify species-specific differences in progenitor behavior contributing to brain size. Cell Stem Cell 18, 467–480. doi: 10.1016/j.stem.2016.03.003
Palechor-Ceron, N., Krawczyk, E., Dakic, A., Simic, V., Yuan, H., Blancato, J., et al. (2019). Conditional reprogramming for patient-derived cancer models and next-generation living biobanks. Cells 8:1327. doi: 10.3390/cells8111327
Pancoast, M., Dobyns, W., and Golden, J. A. (2005). Interneuron deficits in patients with the miller-dieker syndrome. Acta Neuropathol. 109, 400–404. doi: 10.1007/s00401-004-0979-z
Parnavelas, J. G., Anderson, S. A., Lavdas, A. A., Grigoriou, M., Pachnis, V., and Rubenstein, J. L. (2000). The contribution of the ganglionic eminence to the neuronal cell types of the cerebral cortex. Novartis Found. Symp. 228, 129–139. doi: 10.1002/0470846631.ch10
Parrini, E., Ramazzotti, A., Dobyns, W. B., Mei, D., Moro, F., Veggiotti, P., et al. (2006). Periventricular heterotopia: phenotypic heterogeneity and correlation with filamin a mutations. Brain 129, 1892–1906. doi: 10.1093/brain/awl125
Partoens, M., De Meulemeester, A.-S., Giong, H.-K., Pham, D.-H., Lee, J.-S., de Witte, P. A., et al. (2021). Modeling Neurodevelopmental Disorders and Epilepsy Caused by Loss of Function of kif2a in Zebrafish. eNeuro 8, ENEURO.55–ENEURO.21. doi: 10.1523/ENEURO.0055-21.2021
Paşca, A. M., Sloan, S. A., Clarke, L. E., Tian, Y., Makinson, C. D., Huber, N., et al. (2015). Functional cortical neurons and astrocytes from human pluripotent stem cells in 3D culture. Nat. Methods 12, 671–678. doi: 10.1038/nmeth.3415
Passemard, S., Perez, F., Colin-Lemesre, E., Rasika, S., Gressens, P., and El Ghouzzi, V. (2017). Golgi trafficking defects in postnatal microcephaly: the evidence for “Golgipathies.”. Prog. Neurobiol. 153, 46–63. doi: 10.1016/j.pneurobio.2017.03.007
Paulsen, B., Velasco, S., Kedaigle, A. J., Pigoni, M., Quadrato, G., Deo, A. J., et al. (2022). Autism genes converge on asynchronous development of shared neuron classes. Nature 602, 268–273. doi: 10.1038/s41586-021-04358-6
Pei, D.-S., and Strauss, P. R. (2013). Zebrafish as a model system to study DNA damage and repair. Mutat. Res. 743–744, 151–159. doi: 10.1016/j.mrfmmm.2012.10.003
Pellegrini, L., Bonfio, C., Chadwick, J., Begum, F., Skehel, M., and Lancaster, M. A. (2020). Human CNS barrier-forming organoids with cerebrospinal fluid production. Science 369, eaaz5626. doi: 10.1126/science.aaz5626
Peluso, I., Conte, I., Testa, F., Dharmalingam, G., Pizzo, M., Collin, R. W., et al. (2013). The ADAMTS18 gene is responsible for autosomal recessive early onset severe retinal dystrophy. Orphanet J. Rare Dis. 8:16. doi: 10.1186/1750-1172-8-16
Perez, Y., Bar-Yaacov, R., Kadir, R., Wormser, O., Shelef, I., Birk, O. S., et al. (2019). Mutations in the microtubule-associated protein MAP11 (C7orf43) cause microcephaly in humans and zebrafish. Brain 142, 574–585. doi: 10.1093/brain/awz004
Piccolo, S., Agius, E., Leyns, L., Bhattacharyya, S., Grunz, H., Bouwmeester, T., et al. (1999). The head inducer cerberus is a multifunctional antagonist of Nodal, BMP and Wnt signals. Nature 397, 707–710. doi: 10.1038/17820
Pini, J., Kueper, J., Hu, Y. D., Kawasaki, K., Yeung, P., Tsimbal, C., et al. (2020). ALX1-related frontonasal dysplasia results from defective neural crest cell development and migration. EMBO Mol. Med. 12:e12013. doi: 10.15252/emmm.202012013
Pinson, A., Namba, T., and Huttner, W. B. (2019). Malformations of human neocortex in development – their progenitor cell basis and experimental model systems. Front. Cell. Neurosci. 13:305. doi: 10.3389/fncel.2019.00305
Pode-Shakked, B., Barash, H., Ziv, L., Gripp, K. W., Flex, E., Barel, O., et al. (2017). Microcephaly, intractable seizures and developmental delay caused by biallelic variants in TBCD: further delineation of a new chaperone-mediated tubulinopathy. Clin Genet 91, 725–738. doi: 10.1111/cge.12914
Pollen, A. A., Bhaduri, A., Andrews, M. G., Nowakowski, T. J., Meyerson, O. S., Mostajo-Radji, M. A., et al. (2019). Establishing cerebral organoids as models of human-specific brain evolution. Cell 176, 743.e–756.e. doi: 10.1016/j.cell.2019.01.017
Popova, G., Soliman, S. S., Kim, C. N., Keefe, M. G., Hennick, K. M., Jain, S., et al. (2021). Human microglia states are conserved across experimental models and regulate neural stem cell responses in chimeric organoids. Cell Stem Cell 28, 2153.e–2166.e. doi: 10.1016/j.stem.2021.08.015
Portavella, M., Vargas, J. P., Torres, B., and Salas, C. (2002). The effects of telencephalic pallial lesions on spatial, temporal, and emotional learning in goldfish. Brain Res. Bull. 57, 397–399. doi: 10.1016/s0361-9230(01)00699-2
Porter, B. A., and Mueller, T. (2020). The zebrafish amygdaloid complex – functional ground plan, molecular delineation, and everted topology. Front. Neurosci. 14:608. doi: 10.3389/fnins.2020.00608
Puelles, L. (1995). A segmental morphological paradigm for understanding vertebrate forebrains. Brain Behav. Evol. 46, 319–337. doi: 10.1159/000113282
Puelles, L., and Rubenstein, J. L. R. (2003). Forebrain gene expression domains and the evolving prosomeric model. Trends Neurosci. 26, 469–476. doi: 10.1016/S0166-2236(03)00234-0
Puelles, L., Kuwana, E., Puelles, E., Bulfone, A., Shimamura, K., Keleher, J., et al. (2000). Pallial and subpallial derivatives in the embryonic chick and mouse telencephalon, traced by the expression of the genes Dlx-2, Emx-1, Nkx-2.1, Pax-6, and Tbr-1. J. Comp. Neurol. 424, 409–438. doi: 10.1002/1096-9861(20000828)424:3<409::aid-cne3<3.0.co;2-7
Pulvers, J. N., Bryk, J., Fish, J. L., Wilsch-Bräuninger, M., Arai, Y., Schreier, D., et al. (2010). Mutations in mouse Aspm (abnormal spindle-like microcephaly associated) cause not only microcephaly but also major defects in the germline. Proc. Natl. Acad. Sci. U.S.A. 107, 16595–16600. doi: 10.1073/pnas.1010494107
Qian, X., Nguyen, H. N., Song, M. M., Hadiono, C., Ogden, S. C., Hammack, C., et al. (2016). Brain-region-specific organoids using mini-bioreactors for modeling ZIKV exposure. Cell 165, 1238–1254. doi: 10.1016/j.cell.2016.04.032
Quadrato, G., Brown, J., and Arlotta, P. (2016). The promises and challenges of human brain organoids as models of neuropsychiatric disease. Nat. Med. 22, 1220–1228. doi: 10.1038/nm.4214
Quadrato, G., Nguyen, T., Macosko, E. Z., Sherwood, J. L., Min Yang, S., Berger, D. R., et al. (2017). Cell diversity and network dynamics in photosensitive human brain organoids. Nature 545, 48–53. doi: 10.1038/nature22047
Quiñonez-Silvero, C., Hübner, K., and Herzog, W. (2020). Development of the brain vasculature and the blood-brain barrier in zebrafish. Dev. Biol. 457, 181–190. doi: 10.1016/j.ydbio.2019.03.005
Rakic, P. (1974). Neurons in rhesus monkey visual cortex: systematic relation between time of origin and eventual disposition. Science 183, 425–427. doi: 10.1126/science.183.4123.425
Rakic, P. (2009a). Evolution of the neocortex: a perspective from developmental biology. Nat. Rev. Neurosci. 10, 724–735. doi: 10.1038/nrn2719
Rakic, P., Ayoub, A. E., Breunig, J. J., and Dominguez, M. H. (2009b). Decision by division: making cortical maps. Trends Neurosci. 32, 291–301. doi: 10.1016/j.tins.2009.01.007
Rallu, M., Corbin, J. G., and Fishell, G. (2002). Parsing the prosencephalon. Nat. Rev. Neurosci. 3, 943–951. doi: 10.1038/nrn989
Randlett, O., Wee, C. L., Naumann, E. A., Nnaemeka, O., Schoppik, D., Fitzgerald, J. E., et al. (2015). Whole-brain activity mapping onto a zebrafish brain atlas. Nat. Methods 12, 1039–1046. doi: 10.1038/nmeth.3581
Rash, B. G., Duque, A., Morozov, Y. M., Arellano, J. I., Micali, N., and Rakic, P. (2019). Gliogenesis in the outer subventricular zone promotes enlargement and gyrification of the primate cerebrum. PNAS 116, 7089–7094. doi: 10.1073/pnas.1822169116
Rashidi-Nezhad, A., Talebi, S., Saebnouri, H., Akrami, S. M., and Reymond, A. (2014). The effect of homozygous deletion of the BBOX1 and Fibin genes on carnitine level and acyl carnitine profile. BMC Medical Genetics 15:75. doi: 10.1186/1471-2350-15-75
Reijnders, M. R. F., Kousi, M., van Woerden, G. M., Klein, M., Bralten, J., Mancini, G. M. S., et al. (2017). Variation in a range of mTOR-related genes associates with intracranial volume and intellectual disability. Nat Commun 8, 1052. doi: 10.1038/s41467-017-00933-6
Reilly, M. L., Stokman, M. F., Magry, V., Jeanpierre, C., Alves, M., Paydar, M., et al. (2019). Loss-of-function mutations in KIF14 cause severe microcephaly and kidney development defects in humans and zebrafish. Hum Mol Genet 28, 778–795. doi: 10.1093/hmg/ddy381
Reinert, S., Hübener, M., Bonhoeffer, T., and Goltstein, P. M. (2021). Mouse prefrontal cortex represents learned rules for categorization. Nature 593, 411–417. doi: 10.1038/s41586-021-03452-z
Represa, A. (2019). Why malformations of cortical development cause epilepsy. Front. Neurosci. 13:250. doi: 10.3389/fnins.2019.00250
Rétaux, S., Bourrat, F., Joly, J.-S., and Hinaux, H. (2013). “Perspectives in evo-devo of the vertebrate brain,” in Advances in Evolutionary Developmental Biology, ed. J. T. Streelman (Hoboken, NJ: Wiley), 151–172. doi: 10.1002/9781118707449.ch8
Rodríguez, F., Quintero, B., Amores, L., Madrid, D., Salas-Peña, C., and Salas, C. (2021). Spatial cognition in teleost fish: strategies and mechanisms. Animals (Basel) 11:2271. doi: 10.3390/ani11082271
Romero, D. M., Bahi-Buisson, N., and Francis, F. (2018). Genetics and mechanisms leading to human cortical malformations. Semin. Cell Dev. Biol. 76, 33–75. doi: 10.1016/j.semcdb.2017.09.031
Rosch, R. E., Hunter, P. R., Baldeweg, T., Friston, K. J., and Meyer, M. P. (2018). Calcium imaging and dynamic causal modelling reveal brain-wide changes in effective connectivity and synaptic dynamics during epileptic seizures. PLoS Comput. Biol. 14:e1006375. doi: 10.1371/journal.pcbi.1006375
Roscioli, T., Kamsteeg, E.-J., Buysse, K., Maystadt, I., van Reeuwijk, J., van den Elzen, C., et al. (2012). Mutations in ISPD cause Walker-Warburg syndrome and defective glycosylation of α-dystroglycan. Nat Genet 44, 581–585. doi: 10.1038/ng.2253
Rosello, M., Vougny, J., Czarny, F., Mione, M. C., Concordet, J.-P., Albadri, S., et al. (2021). Precise base editing for the in vivo study of developmental signaling and human pathologies in zebrafish. eLife 10:e65552. doi: 10.7554/eLife.65552
Rubenstein, J. L., and Beachy, P. A. (1998). Patterning of the embryonic forebrain. Curr. Opin. Neurobiol. 8, 18–26. doi: 10.1016/s0959-4388(98)80004-4
Rubenstein, J. L., Martinez, S., Shimamura, K., and Puelles, L. (1994). The embryonic vertebrate forebrain: the prosomeric model. Science 266, 578–580. doi: 10.1126/science.7939711
Saberi, A., Aldenkamp, A. P., Kurniawan, N. A., and Bouten, C. V. C. (2022). In-vitro engineered human cerebral tissues mimic pathological circuit disturbances in 3D. Commun. Biol. 5:254. doi: 10.1038/s42003-022-03203-4
Sakaguchi, H., Kadoshima, T., Soen, M., Narii, N., Ishida, Y., Ohgushi, M., et al. (2015). Generation of functional hippocampal neurons from self-organizing human embryonic stem cell-derived dorsomedial telencephalic tissue. Nat. Commun. 6:8896. doi: 10.1038/ncomms9896
Sakai, C., Ijaz, S., and Hoffman, E. J. (2018). Zebrafish models of neurodevelopmental disorders: past, present, and future. Front. Mol. Neurosci. 11:294. doi: 10.3389/fnmol.2018.00294
Salas, C., Broglio, C., and Rodríguez, F. (2003). Evolution of forebrain and spatial cognition in vertebrates: conservation across diversity. Brain Behav. Evol. 62, 72–82. doi: 10.1159/000072438
Salomoni, P., and Calegari, F. (2010). Cell cycle control of mammalian neural stem cells: putting a speed limit on G1. Trends Cell Biol. 20, 233–243. doi: 10.1016/j.tcb.2010.01.006
Scheldeman, C., Mills, J. D., Siekierska, A., Serra, I., Copmans, D., Iyer, A. M., et al. (2017). mTOR-related neuropathology in mutant tsc2 zebrafish: phenotypic, transcriptomic and pharmacological analysis. Neurobiol. Dis. 108, 225–237. doi: 10.1016/j.nbd.2017.09.004
Schier, A. F., Neuhauss, S. C., Harvey, M., Malicki, J., Solnica-Krezel, L., Stainier, D. Y., et al. (1996). Mutations affecting the development of the embryonic zebrafish brain. Development 123, 165–178. doi: 10.1242/dev.123.1.165
Schmidt, R., Strähle, U., and Scholpp, S. (2013). Neurogenesis in zebrafish – from embryo to adult. Neural Dev. 8:3. doi: 10.1186/1749-8104-8-3
Severino, M., Geraldo, A. F., Utz, N., Tortora, D., Pogledic, I., Klonowski, W., et al. (2020). Definitions and classification of malformations of cortical development: practical guidelines. Brain 143, 2874–2894. doi: 10.1093/brain/awaa174
Shahsavani, M., Pronk, R. J., Falk, R., Lam, M., Moslem, M., Linker, S. B., et al. (2018). An in vitro model of lissencephaly: expanding the role of DCX during neurogenesis. Mol Psychiatry 23, 1674–1684. doi: 10.1038/mp.2017.175
Shanmugalingam, S., Houart, C., Picker, A., Reifers, F., Macdonald, R., Barth, A., et al. (2000). Ace/Fgf8 is required for forebrain commissure formation and patterning of the telencephalon. Development 127, 2549–2561. doi: 10.1242/dev.127.12.2549
Shao, D. D., Achkar, C. M., Lai, A., Srivastava, S., Doan, R. N., Rodan, L. H., et al. (2020). Polymicrogyria is Associated With Pathogenic Variants in PTEN. Ann. Neurol. 88, 1153–1164. doi: 10.1002/ana.25904
Shcheglovitov, A., and Peterson, R. T. (2021). Screening platforms for genetic epilepsies—zebrafish, iPSC-derived neurons, and organoids. Neurotherapeutics 18, 1478–1489. doi: 10.1007/s13311-021-01115-5
Sheen, V. L., Ferland, R. J., Harney, M., Hill, R. S., Neal, J., Banham, A. H., et al. (2006). Impaired proliferation and migration in human miller-dieker neural precursors. Ann. Neurol. 60, 137–144. doi: 10.1002/ana.20843
Sheen, V. L., Ganesh, V. S., Topcu, M., Sebire, G., Bodell, A., Hill, R. S., et al. (2004). Mutations in ARFGEF2 implicate vesicle trafficking in neural progenitor proliferation and migration in the human cerebral cortex. Nat. Genet. 36, 69–76. doi: 10.1038/ng1276
Sheppard, A. M., and Pearlman, A. L. (1997). Abnormal reorganization of preplate neurons and their associated extracellular matrix: an early manifestation of altered neocortical development in the reeler mutant mouse. J. Comp. Neurol. 378, 173–179. doi: 10.1002/(sici)1096-9861(19970210)378:2<173::aid-cne2<3.0.co;2-0
Shi, Y., Kirwan, P., and Livesey, F. J. (2012). Directed differentiation of human pluripotent stem cells to cerebral cortex neurons and neural networks. Nat. Protoc. 7, 1836–1846. doi: 10.1038/nprot.2012.116
Shinya, M., Koshida, S., Sawada, A., Kuroiwa, A., and Takeda, H. (2001). Fgf signalling through MAPK cascade is required for development of the subpallial telencephalon in zebrafish embryos. Development 128, 4153–4164. doi: 10.1242/dev.128.21.4153
Siekierska, A., Stamberger, H., Deconinck, T., Oprescu, S. N., Partoens, M., Zhang, Y., et al. (2019). Biallelic VARS variants cause developmental encephalopathy with microcephaly that is recapitulated in vars knockout zebrafish. Nat. Commun. 10:708. doi: 10.1038/s41467-018-07953-w
Silva, C. G., Peyre, E., Adhikari, M. H., Tielens, S., Tanco, S., Van Damme, P., et al. (2018). Cell-Intrinsic control of interneuron migration drives cortical Morphogenesis. Cell 172, 1063.e–1078.e. doi: 10.1016/j.cell.2018.01.031
Simmich, J., Staykov, E., and Scott, E. (2012). Zebrafish as an appealing model for optogenetic studies. Prog. Brain Res. 196, 145–162. doi: 10.1016/B978-0-444-59426-6.00008-2
Smits, D. J., Schot, R., Wilke, M., van Slegtenhorst, M., de Wit, M. C. Y., Dremmen, M. H. G., et al. (2021). Biallelic DAB1 Variants Are Associated With Mild Lissencephaly and Cerebellar Hypoplasia. Neurol Genet 7, e558. doi: 10.1212/NXG.0000000000000558
Sneddon, L. U., Halsey, L. G., and Bury, N. R. (2017). Considering aspects of the 3Rs principles within experimental animal biology. J. Exp. Biol. 220, 3007–3016. doi: 10.1242/jeb.147058
Stepien, B. K., Naumann, R., Holtz, A., Helppi, J., Huttner, W. B., and Vaid, S. (2020). Lengthening neurogenic period during neocortical development causes a hallmark of neocortex expansion. Curr. Biol. 30, 4227.e–4237.e. doi: 10.1016/j.cub.2020.08.046
Stepien, B. K., Vaid, S., and Huttner, W. B. (2021). Length of the neurogenic period—a key determinant for the generation of upper-layer neurons during neocortex development and evolution. Front. Cell Dev. Biol. 9:676911. doi: 10.3389/fcell.2021.676911
Stern, C. D. (2001). Initial patterning of the central nervous system: how many organizers? Nat. Rev. Neurosci. 2, 92–98. doi: 10.1038/35053563
Stevens, E., Carss, K. J., Cirak, S., Foley, A. R., Torelli, S., Willer, T., et al. (2013). Mutations in B3GALNT2 cause congenital muscular dystrophy and hypoglycosylation of α-dystroglycan. Am. J. Hum. Genet. 92, 354–365. doi: 10.1016/j.ajhg.2013.01.016
Strobl-Mazzulla, P. H., Nuñez, A., Pellegrini, E., Gueguen, M.-M., Kah, O., and Somoza, G. M. (2010). Progenitor radial cells and neurogenesis in pejerrey fish forebrain. Brain Behav. Evol. 76, 20–31. doi: 10.1159/000316022
Stutterd, C. A., Dobyns, W. B., Jansen, A., Mirzaa, G., and Leventer, R. J. (1993). “Polymicrogyria Overview,” in GeneReviews, eds M. P. Adam, H. H. Ardinger, R. A. Pagon, S. E. Wallace, L. J. Bean, K. W. Gripp, et al. (Seattle WA: University of Washington, Seattle).
Subramanian, L., Calcagnotto, M. E., and Paredes, M. F. (2020). Cortical malformations: lessons in human brain development. Front. Cell. Neurosci. 13:576. doi: 10.3389/fncel.2019.00576
Susaimanickam, P. J., Kiral, F. R., and Park, I.-H. (2022). Region specific brain organoids to study neurodevelopmental disorders. Int. J. Stem Cells 15, 26–40. doi: 10.15283/ijsc22006
Sylvester, J. B., Rich, C. A., Yi, C., Peres, J. N., Houart, C., and Streelman, J. T. (2013). Competing signals drive telencephalon diversity. Nat. Commun. 4:1745. doi: 10.1038/ncomms2753
Symvoulidis, P., Lauri, A., Stefanoiu, A., Cappetta, M., Schneider, S., Jia, H., et al. (2017). NeuBtracker-imaging neurobehavioral dynamics in freely behaving fish. Nat. Methods 14, 1079–1082. doi: 10.1038/nmeth.4459
Tao, W., and Lai, E. (1992). Telencephalon-restricted expression of BF-1, a new member of the HNF-3/fork head gene family, in the developing rat brain. Neuron 8, 957–966. doi: 10.1016/0896-6273(92)90210-5
Tassi, L., Colombo, N., Garbelli, R., Francione, S., Lo Russo, G., Mai, R., et al. (2002). Focal cortical dysplasia: neuropathological subtypes, EEG, neuroimaging and surgical outcome. Brain 125, 1719–1732. doi: 10.1093/brain/awf175
Taverna, E., Götz, M., and Huttner, W. B. (2014). The cell biology of neurogenesis: toward an understanding of the development and evolution of the neocortex. Annu. Rev. Cell Dev. Biol. 30, 465–502. doi: 10.1146/annurev-cellbio-101011-155801
Terrone, G., Voisin, N., Abdullah Alfaiz, A., Cappuccio, G., Vitiello, G., Guex, N., et al. (2016). De novo PIK3R2 variant causes polymicrogyria, corpus callosum hyperplasia and focal cortical dysplasia. Eur J Hum Genet 24, 1359–1362. doi: 10.1038/ejhg.2016.7
Toi, A., Chitayat, D., and Blaser, S. (2009). Abnormalities of the foetal cerebral cortex. Prenat. Diagn. 29, 355–371. doi: 10.1002/pd.2211
Tomer, R., Denes, A. S., Tessmar-Raible, K., and Arendt, D. (2010). Profiling by image registration reveals common origin of annelid mushroom bodies and vertebrate pallium. Cell 142, 800–809. doi: 10.1016/j.cell.2010.07.043
Trommsdorff, M., Gotthardt, M., Hiesberger, T., Shelton, J., Stockinger, W., Nimpf, J., et al. (1999). Reeler/Disabled-like disruption of neuronal migration in knockout mice lacking the VLDL receptor and ApoE receptor 2. Cell 97, 689–701. doi: 10.1016/s0092-8674(00)80782-5
Turrini, L., Fornetto, C., Marchetto, G., Müllenbroich, M. C., Tiso, N., Vettori, A., et al. (2017). Optical mapping of neuronal activity during seizures in zebrafish. Sci. Rep. 7:3025. doi: 10.1038/s41598-017-03087-z
Uzquiano, A., Gladwyn-Ng, I., Nguyen, L., Reiner, O., Götz, M., Matsuzaki, F., et al. (2018). Cortical progenitor biology: key features mediating proliferation versus differentiation. J. Neurochem. 146, 500–525. doi: 10.1111/jnc.14338
Vacaru, A. M., Unlu, G., Spitzner, M., Mione, M., Knapik, E. W., and Sadler, K. C. (2014). In vivo cell biology in zebrafish – providing insights into vertebrate development and disease. J. Cell Sci. 127, 485–495. doi: 10.1242/jcs.140194
Van Essen, D. C., Donahue, C. J., and Glasser, M. F. (2018). Development and evolution of cerebral and cerebellar cortex. BBE 91, 158–169. doi: 10.1159/000489943
Varshney, G. K., Pei, W., LaFave, M. C., Idol, J., Xu, L., Gallardo, V., et al. (2015). High-throughput gene targeting and phenotyping in zebrafish using CRISPR/Cas9. Genome Res. 25, 1030–1042. doi: 10.1101/gr.186379.114
Velasco, S., Kedaigle, A. J., Simmons, S. K., Nash, A., Rocha, M., Quadrato, G., et al. (2019). Individual brain organoids reproducibly form cell diversity of the human cerebral cortex. Nature 570, 523–527. doi: 10.1038/s41586-019-1289-x
von Trotha, J. W., Vernier, P., and Bally-Cuif, L. (2014). Emotions and motivated behavior converge on an amygdala-like structure in the zebrafish. Eur. J. Neurosci. 40, 3302–3315. doi: 10.1111/ejn.12692
Wang, L., Li, Z., Sievert, D., Smith, D. E. C., Mendes, M. I., Chen, D. Y., et al. (2020). Loss of NARS1 impairs progenitor proliferation in cortical brain organoids and leads to microcephaly. Nat Commun 11, 4038. doi: 10.1038/s41467-020-17454-4
Wang, X., Tsai, J.-W., LaMonica, B., and Kriegstein, A. R. (2011). A new subtype of progenitor cell in the mouse embryonic neocortex. Nat. Neurosci. 14, 555–561. doi: 10.1038/nn.2807
Waters, A. M., Asfahani, R., Carroll, P., Bicknell, L., Lescai, F., Bright, A., et al. (2015). The kinetochore protein, CENPF, is mutated in human ciliopathy and microcephaly phenotypes. J Med Genet 52, 147–156. doi: 10.1136/jmedgenet-2014-102691
Watrin, F., Manent, J.-B., Cardoso, C., and Represa, A. (2015). Causes and consequences of gray matter heterotopia. CNS Neurosci. Ther. 21, 112–122. doi: 10.1111/cns.12322
Weiss, P., and Taylor, A. C. (1960). Reconstitution of complete organs from single-cell suspensions of chick embryos in advanced stages of differentiation. Proc. Natl. Acad. Sci. U.S.A. 46, 1177–1185. doi: 10.1073/pnas.46.9.1177
White, R. M., Sessa, A., Burke, C., Bowman, T., LeBlanc, J., Ceol, C., et al. (2008). Transparent adult zebrafish as a tool for in vivo transplantation analysis. Cell Stem Cell 2, 183–189. doi: 10.1016/j.stem.2007.11.002
Williams, S. N., Locke, C. J., Braden, A. L., Caldwell, K. A., and Caldwell, G. A. (2004). Epileptic-like convulsions associated with LIS-1 in the cytoskeletal control of neurotransmitter signaling in Caenorhabditis elegans. Hum. Mol. Genet. 13, 2043–2059. doi: 10.1093/hmg/ddh209
Wilson, S. W., and Houart, C. (2004). Early steps in the development of the forebrain. Dev. Cell 6, 167–181. doi: 10.1016/S1534-5807(04)00027-9
Wilson, S. W., and Rubenstein, J. L. (2000). Induction and dorsoventral patterning of the telencephalon. Neuron 28, 641–651. doi: 10.1016/s0896-6273(00)00171-9
Wong, K.-L., Akiyama, R., Bessho, Y., and Matsui, T. (2019). ERK activity dynamics during zebrafish embryonic development. Int. J. Mol. Sci. 20:109. doi: 10.3390/ijms20010109
Wong, M., and Roper, S. N. (2016). Genetic animal models of malformations of cortical development and epilepsy. J. Neurosci. Methods 260, 73–82. doi: 10.1016/j.jneumeth.2015.04.007
Woods, C. G. (2004). Human microcephaly. Curr. Opin. Neurobiol. 14, 112–117. doi: 10.1016/j.conb.2004.01.003
Wullimann, M. F., and Mueller, T. (2004). Teleostean and mammalian forebrains contrasted: evidence from genes to behavior. J. Comp. Neurol. 475, 143–162. doi: 10.1002/cne.20183
Wullimann, M. F., Rupp, B., and Reichert, H. (1996). “Functional anatomy of the zebrafish brain: a comparative evaluation,” in Neuroanatomy of the Zebrafish Brain: A Topological Atlas, eds M. F. Wullimann, B. Rupp, and H. Reichert (Basel: Birkhäuser), 89–101. doi: 10.1007/978-3-0348-8979-7_6
Xu, Y., Xi, J., Wang, G., Guo, Z., Sun, Q., Lu, C., et al. (2021). PAUPAR and PAX6 sequentially regulate human embryonic stem cell cortical differentiation. Nucleic Acids Res. 49, 1935–1950. doi: 10.1093/nar/gkab030
Yaksi, E., Jamali, A., Diaz Verdugo, C., and Jurisch-Yaksi, N. (2021). Past, present and future of zebrafish in epilepsy research. FEBS J. 288, 7243–7255. doi: 10.1111/febs.15694
Yamaguchi, T. P. (2001). Heads or tails: Wnts and anterior-posterior patterning. Curr. Biol. 11, R713–R724. doi: 10.1016/s0960-9822(01)00417-1
Yamamoto, N. (2009). Studies on the teleost brain morphology in search of the origin of cognition. Jap. Psychol. Res. 51, 154–167. doi: 10.1111/j.1468-5884.2009.00397.x
Zhang, W., Yang, S. L., Yang, M., Herrlinger, S., Shao, Q., Collar, J. L., et al. (2019). Modeling microcephaly with cerebral organoids reveals a WDR62–CEP170–KIF2A pathway promoting cilium disassembly in neural progenitors. Nature Communications 10, 2612. doi: 10.1038/s41467-019-10497-2
Zhang, X., Ling, J., Barcia, G., Jing, L., Wu, J., Barry, B. J., et al. (2014). Mutations in QARS, encoding glutaminyl-tRNA synthetase, cause progressive microcephaly, cerebral-cerebellar atrophy, and intractable seizures. Am J Hum Genet 94, 547–558. doi: 10.1016/j.ajhg.2014.03.003
Zhao, S., Li, Z., Zhang, M., Zhang, L., Zheng, H., Ning, J., et al. (2019). A brain somatic RHEB doublet mutation causes focal cortical dysplasia type II. Exp Mol Med 51, 1–11. doi: 10.1038/s12276-019-0277-4
Ziffra, R. S., Kim, C. N., Ross, J. M., Wilfert, A., Turner, T. N., Haeussler, M., et al. (2021). Single-cell epigenomics reveals mechanisms of human cortical development. Nature 598, 205–213. doi: 10.1038/s41586-021-03209-8
Zilles, K., Palomero-Gallagher, N., and Amunts, K. (2013). Development of cortical folding during evolution and ontogeny. Trends Neurosci. 36, 275–284. doi: 10.1016/j.tins.2013.01.006
Zilova, L., Weinhardt, V., Tavhelidse, T., Schlagheck, C., Thumberger, T., and Wittbrodt, J. (2021). Fish primary embryonic pluripotent cells assemble into retinal tissue mirroring in vivo early eye development. eLife 10:e66998. doi: 10.7554/eLife.66998
Zimmer, T. S., Broekaart, D. W. M., Gruber, V.-E., van Vliet, E. A., Mühlebner, A., and Aronica, E. (2020). Tuberous Sclerosis Complex as Disease Model for Investigating mTOR-Related Gliopathy During Epileptogenesis. Frontiers in Neurology 11:1028. doi: 10.3389/fneur.2020.01028
Keywords: forebrain, cortical malformations, rare neurodevelopmental diseases, teleosts, organoids
Citation: Fasano G, Compagnucci C, Dallapiccola B, Tartaglia M and Lauri A (2022) Teleost Fish and Organoids: Alternative Windows Into the Development of Healthy and Diseased Brains. Front. Mol. Neurosci. 15:855786. doi: 10.3389/fnmol.2022.855786
Received: 19 January 2022; Accepted: 16 May 2022;
Published: 11 August 2022.
Edited by:
Robert Gerlai, University of Toronto, CanadaReviewed by:
Eve Seuntjens, KU Leuven, BelgiumCopyright © 2022 Fasano, Compagnucci, Dallapiccola, Tartaglia and Lauri. This is an open-access article distributed under the terms of the Creative Commons Attribution License (CC BY). The use, distribution or reproduction in other forums is permitted, provided the original author(s) and the copyright owner(s) are credited and that the original publication in this journal is cited, in accordance with accepted academic practice. No use, distribution or reproduction is permitted which does not comply with these terms.
*Correspondence: Antonella Lauri, YW50b25lbGxhLmxhdXJpQG9wYmcubmV0
Disclaimer: All claims expressed in this article are solely those of the authors and do not necessarily represent those of their affiliated organizations, or those of the publisher, the editors and the reviewers. Any product that may be evaluated in this article or claim that may be made by its manufacturer is not guaranteed or endorsed by the publisher.
Research integrity at Frontiers
Learn more about the work of our research integrity team to safeguard the quality of each article we publish.