- 1Department of Medical Imaging, The First Affiliated Hospital of Anhui Medical University, Hefei, China
- 2Department of Orthopedics (Spinal Surgery), The First Affiliated Hospital of Anhui Medical University, Hefei, China
- 3Department of Clinical Laboratory, No. 2 People’s Hospital of Fuyang, Fuyang, China
- 4Department of Orthopedics, No. 2 People’s Hospital of Fuyang, Fuyang, China
Spinal cord injury (SCI) often causes neuronal and axonal damage, resulting in permanent neurological impairments. Mesenchymal stem cells (MSCs) and extracellular vesicles (EVs) are promising treatments for SCI. However, the underlying mechanisms remain unclear. Herein, we demonstrated that EVs from bone marrow-derived MSCs promoted the differentiation of neural stem cells (NSCs) into the neurons and outgrowth of neurites that are extending into astrocytic scars in SCI rats. Further study found that let-7a-5p exerted a similar biological effect as MSC-EVs in regulating the differentiation of NSCs and leading to neurological improvement in SCI rats. Moreover, these MSC-EV-induced effects were attenuated by let-7a-5p inhibitors/antagomirs. When investigating the mechanism, bioinformatics predictions combined with western blot and RT-PCR analyses showed that both MSC-EVs and let-7a-5p were able to downregulate the expression of SMAD2 by inhibiting HMGA2. In conclusion, MSC-EV-secreted let-7a-5p promoted the regrowth of neurons and improved neurological recovery in SCI rats by targeting the HMGA2/SMAD2 axis.
Introduction
Spinal cord injury (SCI) causes necrosis, demyelination of axons, and neuronal apoptosis, resulting in the disruption of the motor and sensory tracts of the spinal cord, which leads to severe neurological impairment (Assinck et al., 2017; O’Shea et al., 2017). The discovery of endogenous neural stem cells (NSCs) in the spinal cord has been a promising strategy for the treatment of SCI (Stenudd et al., 2015). However, further studies have shown that although these endogenous NSCs could be activated and then migrate into injured lesions after SCI, most of them differentiated into astrocytes forming glial scars due to the severe microenvironment following SCI. The over-formation of astrocytic scars haven been proved to be one of the reasons for the failure of the reorganization and regeneration of neural circuits. Therefore, it is critical to promote the differentiation of endogenous NSCs into neurons and oligodendrocytes.
Mesenchymal stem cells (MSCs) were first found in the bone marrow due to their self-renewing, multipotent abilities; they are obtained from various sources, and have been widely used for the treatment of SCI (Prockop et al., 2000; Cofano et al., 2019; Yamazaki et al., 2020). Initially, MSCs were transplanted to injured lesions in an attempt to differentiate them into oligodendrocytes and neurons, replacing the damaged nerve cells (Brazelton et al., 2000; Mezey et al., 2000). However, emerging studies have found that the beneficial biological effects of transplanted MSCs are mainly associated with their paracrine activity (Nakajima et al., 2012). Based on this evidence, conditioned medium (CM), which is composed of various factors secreted by MSCs, is widely used in the treatment of neurological disorders (Gnecchi and Melo, 2009; Cantinieaux et al., 2013). In addition, studies have reported that CM from MSCs can promote the regeneration of neruons and remyelination of axons by regulating the differentiation of endogenous NSCs, as well as protect these NSCs from apoptosis by generating a favorable environment against secondary injury following primary SCI (Fang et al., 2018; Niu et al., 2019; Song et al., 2020).
With further understanding of paracrine signaling from cells, extracellular vesicles (EVs) in MSC-CM were discovered (Chen et al., 2010; Keshtkar et al., 2018). EVs are membrane particles secreted by nearly all cell types and carry various bioactive cargos, including microRNAs (miRNAs) (Phinney et al., 2015). miRNAs are short RNA molecules, 20–22 nucleotides in length, that mainly work as negative regulators by degrading or repressing the targeted mRNAs in a variety of cellular processes (Vishnoi and Rani, 2017). Further studies have shown that miRNAs are critical regulators of stem cell biology, including proliferation and differentiation (Mens and Ghanbari, 2018; Vieira et al., 2018). Many miRNAs have been reported to be present in MSC-EVs (including the let-7 family, miRNA-21, miRNA-22, and miRNA-133) (Phinney and Pittenger, 2017). Moreover, some of these miRNAs secreted by MSCs play a crucial role in mediating axonal sparing, glial formation, and neural plasticity (Ghibaudi et al., 2017; Fang et al., 2021). However, whether these miRNAs could regulate the differentiation of endogenous NSCs, which are activated following SCI, and their molecular and cellular mechanisms remain unclear.
In this study, we focused on let-7a-5p, a member of the let-7 family, which contributes to axonal sparing, myelination, and neural regeneration (Kawahara et al., 2012). We demonstrated that both MSC-EVs and let-7a-5p are able to promote the differentiation of NSCs into neurons and that these MSC-EV-induced biological effects could be attenuated by the knockdown of let-7a-5p, indicating that MSC-EV-mediated differentiation of NSCs partly depends on let-7a-5p. Moreover, by detecting the expression of high-mobility group A 2 (HMGA2) and SMAD2, we found that let-7a-5p downregulated the TGF-beta/Smad signaling pathway by targeting HMGA2. In vivo, treatment with let-7a-5p and MSC-EVs promoted neurons and neurite outgrowth around the cavity in SCI rats. Moreover, the MSC-EVS-induced effects on neurite outgrowth and regulation of SMAD2 expression in injured lesions were suppressed by inhibiting let-7a-5p in SCI rats.
Materials and Methods
Mesenchymal Stem Cells Culture and the Collection of Mesenchymal Stem Cell-Extracellular Vesicles
The details of MSC culture have been described in our previous studies (Fang et al., 2018). MSCs were obtained from the bone marrow and cultured in low-glucose DMEM (Hyclone, United States) with 10% fetal bovine serum (FBS, Gibco, United States) in the presence of a 1% antibiotic solution. The cells were passaged when 90% confluence was reached. Passage 3 BMSCs were reseed and used to collect EVs. The supernatant was harvested when the BMSCs reached 90% confluence. EV collection was performed as described in our previous study (Han et al., 2021). Briefly, the harvested supernatant was centrifuged at 500 × g for 10 min, followed by centrifugation at 2,000 × g for 20 min and 10,000 × g for 45 min at 4°C to remove debris. Subsequently, the collected medium was re-centrifuged at 100,000 × g for 70 min at 4°C to obtain BMSC-EVs. Transmission electron microscopy, dynamic light scattering, and western blot analysis (CD 63, CD9, and TSG 101) were used to identify EVs (Supplementary Figure 1; Han et al., 2021). The EVSs were then dissolved in 100 μL of PBS and stored at −80°C.
Neural Stem Cells Culture, Differentiation, and Transfection
Neural stem cells were cultured as described in our previous study (Fang et al., 2018). The cells were isolated from the subventricular zone and cultured as suspended neurospheres for 7 days in DMEM/F12 (Gibco, United States) containing 2% B27 (Gibco, United States), 20 ng/mL epidermal growth factor (EGF) (Gibco, United States), and 10 ng/mL basic fibroblast growth factor (bFGF) (Gibco, United States).
The overexpression or knockdown of let-7a-5p in NSCs was performed using 50 nM let-7a-5p mimics or inhibitors (Nanjing Kaien, China), non-target control miRNA mimics (NC mimics), or a scrambled control sequence (anti-NC) for NCs or anti-NCs. The HiPerfect transfection reagent (Hilden, Germany) was used following the manufacturer’s instructions. The sequences were as follows: let-7a-5p mimic, 5′-UGAGGUAGUAGGUUGUAUA GUU-3′; let-7a-5p inhibitor, 5′-AACUAUACAACCUACUAC CUCA-3′; NC mimic: 5′-UUCUCCGAACGUGUCACGUTT-3′; anti-NC: 5′-CAGUACUUUUGUGUAGUACAA-3′.
Passage 2 neurospheres or transfected neurospheres were dissociated by trypsin and cultured in 10% FBS-DMEM/F12 for 24 h. The medium was changed to DMEFM/F12 with or without BMSC-EVs. NSCs were cultured for 7 days and then used for immunofluorescence.
RNA Extraction and Quantitative PCR
Total RNA of NSCs and 3 cm length of spinal cord tissues (center of the epicenter of the injured lesion) were extracted by using TRIzol (Gibco) following the manufacturer’s instructions, and cDNA was synthesized using the Superscript III RT Reaction Mix (Invitrogen). Quantitative PCR was performed using the SYBR Green master mix (Applied Biosystems) and a RealPlex2 Mastercycler (Eppendorf). mRNA expression was normalized to that of GAPDH and U6 was used to normalize miRNA expression. Sequences of transcript-specific primers were as followings: HMGA2 5′-GAGACCATTGGAGAAAAACGGC-3′, 5′-AATCTTCCTCTGCGGACTCTTGCG-3′; SMAD2 5′-CAGG AAGAGAAGTGGTGTGA-3′, 5′-ATACTGGAGGCAGAACTG GT-3′; GAPDH 5′-ACAGTCCATGCCATCACTGCC-3′, 5′-AC AGTCCATGCCATCACTGCC-3′; let-7a-5p 5′-CGGTGAGGTA GTAGGTTG-3′, 5′-GCAGGGTCCGAGGTATTC-3′; U6 5′-CT CGCTTCGGCAGCACA-3′, AACGCTTCACGAATTTGCGT.
Animal Protocols
Details of the SCI procedure have been outlined in our previous study (Song et al., 2020). Briefly, laminectomy was performed at the T10 level in female Wistar rats. Rats were divided randomly into sham, SCI (control, treated with PBS), BMSC-EV-treated, let-7a-5p agomir-treated, and BMSC-EVs + let-7a-5p-antagomir-treated groups. A direct weight-drop injury to the spinal cord was induced via an infinite-horizon spinal cord impactor (IH-0400). A polyethylene catheter (PE-10, o.d. 0.61 mm) was placed at the injured level for intrathecal injection. The injured rats received a continuous injection of 25 μl PBS, 25 μl ml BMSC-EV-injection, 50 nmol let-7a-5p agomir (Ribobio, China), and 25 μl BMSC-EVs + 50 nmol let-7a-5p-antagomir (Ribobio, China) for 3 days. The Basso, Beattie, and Bresnahan (BBB) open-field test was used to investigate the motor function of the lower extremities of SCI rats blindly by two independent individuals at different time points (days 1, 4, 7, 14, 17, 21, 24, and 28) post-injury. The inclined plane test was also used to evaluate the neurological outcomes. It was performed on a testing apparatus and the maximum angle was recorded as the rat retained its position for more than 5 s without falling. Animal procedures were approved by the Ethics Committee of Anhui Medical University (No. 20191064) in accordance with the guidelines of the Declaration of Helsinki revised in Edinburgh in 2000.
Tissue Processing and Immunofluorescence Staining
Spinal cord tissues were obtained from the rats 4-weeks post-injury and fixed in 4% paraformaldehyde. A 4-μm-thick longitudinal slice was obtained using a Leica RM2135 electric slicer. The following primary antibodies were used in the overnight incubation at 4°C: rabbit anti-Map-2 for neurons (1:500; Abcam, United Kingdom), mouse anti-glial fibrillary acidic protein (GFAP) for astroglia (1:1,000; Abcam, United Kingdom), and myelin basic protein (MBP) for oligodendrocytes (1:1,000; Abcam, United Kingdom) followed by incubation with primary antibodies for 1 h at room temperature: Alexa Fluor 488 (green, 1:50; Elabscience, China) and Cy3 (red, 1:50; Elabscience, China). The sections were observed and photographed using a DM-6B fluorescence microscope (Leica, Germany) connected to a computer screen. The percentage of positive areas was calculated using Image J.
Dual-Luciferase Reporter Analysis
The HMGA2 3-UTR sequence containing the target gene of let-7a-5p or a mutant reporter of HMGA2 was cloned into a luciferase vector to construct the HMGA2-wt or HMGA2-mut (GenScript, China). NSCs were co-transfected with 50 nM of let-7a-5p mimics or NC mimics and HMGA2-wt or HMGA2-mut. After transfection for 48 h, luciferase activity was determined using the Dual-luciferase Reporter Assay System.
Statistical Analysis
Data are presented as mean ± standard deviation, and statistical analyses were performed using SPSS software (version 16.0; Chicago, IL, United States). The data between two groups were analyzed using Student’s t-test to test the statistical significance. Statistical significance was set at a p-value of <0.05. For cell counting, 5–20 random fields containing a total of 500–1,000 cells were randomly selected. The number of positive cells was blindly quantified by two different individuals.
Results
BMSC-Extracellular Vesicles and let-7a-5p Promoted the Differentiation of Neural Stem Cells Into Neurons
As previously reported (Kim et al., 2017; Harrell et al., 2019), miRNAs are one of the components of EVs and play a key role in exerting their biological effects. To determine whether let-7a-5p was present in the BMSC-EVs, we first obtained EVs from bone marrow-derived MSCs and detected let-7a-5p expression by RT-PCR (Figure 1A). The results showed that let-7a-5p was present in all the samples from five individual rats. Next, to investigate the effects of BMSC-EVs and let-7a-5p on the differentiation of NSCs, NSCs were co-cultured with BMSC-EVs or let-7a-5p mimics for 7 days and the percentage of neurons and astrocytes was calculated by immunofluorescence. In the control group, approximately 70% of the cells were GFAP-positive astrocytes and 15% were microtubule-associated protein 2 (Map-2)-positive neurons (Figure 1B). In contrast, the addition of BMSC-EVs to NSCs increased the percentage of Map-2 positive neurons to 40%, and the proportion of GFAP-positive astrocytes decreased to 44% (Figure 1B). Similar to the biological effects of BMSC-EVs, the transfection of let-7a-5p mimics to NSCs increased the percentage of neurons to 27% with a reduction in the proportion of astrocytes compared with the control groups (Figure 1B). All these results indicated that in mediating the differentiation of NSCs, BMSC-EVs and let-7a-5p had similar biological functions.
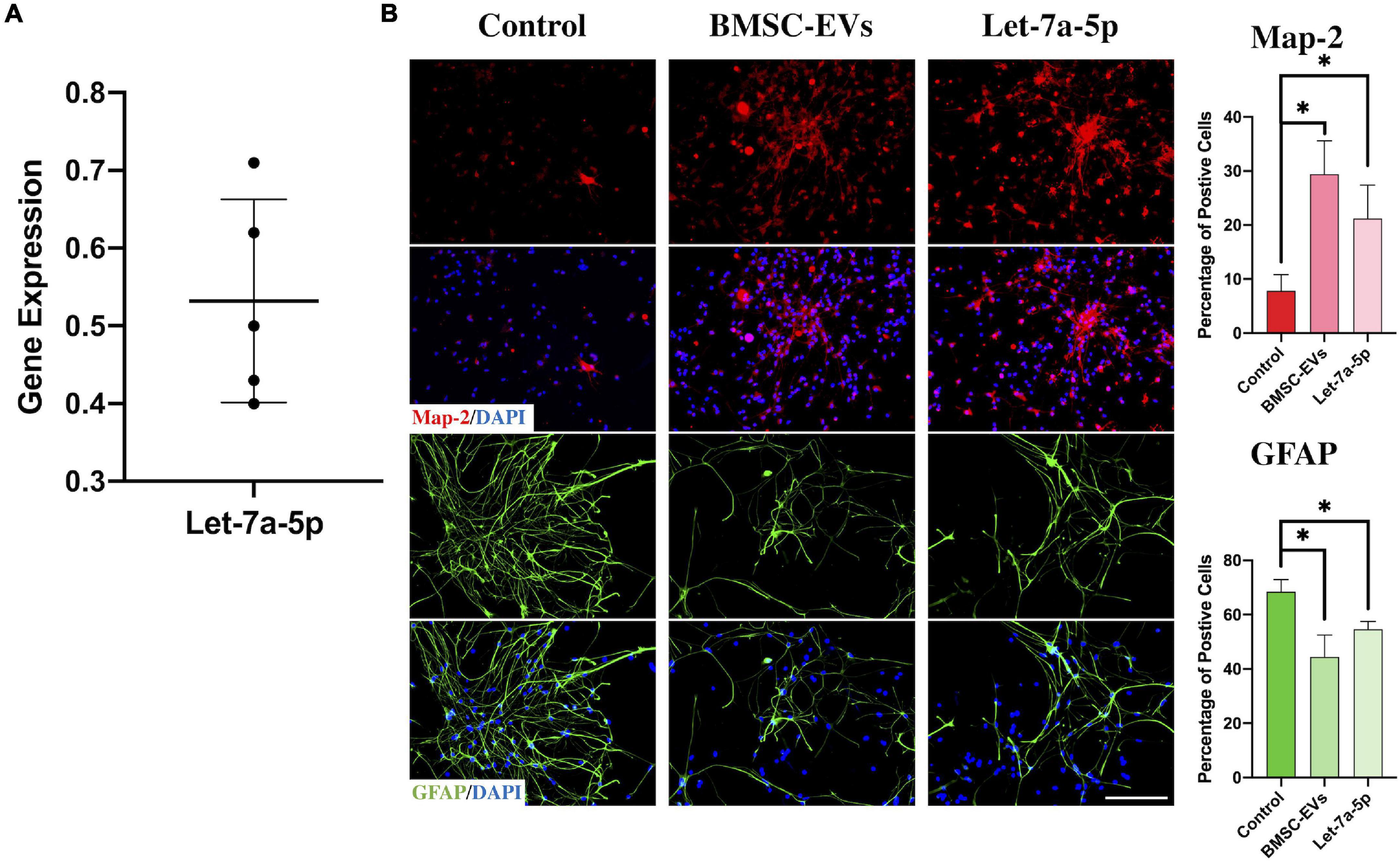
Figure 1. BMSC-EVs and let-7a-5p promoted the differentiation of NSCs into neurons. (A) PCR confirmed the presence of let-7a-5p in BMSC-EVs (n = 5). (B) The BMSC-EVs or let-7a-5p mimics were added to the NSCs and co-cultured for 7 days. The results showed that the percentage of Map-2 positive neurons was increased along with a reduction of GFAP positive astrocytes compared with the control groups by the addition of BMSC-EVs or let-7a-5p (n = 5; data are mean ± S.D; Student’s t-test was used for comparisons. *p < 0.05; scale bars, 100 μm).
In addition, we detected the histology of injured lesions and neurological function in rats injected with BMSC-EVs or let-7a-5p agomirs. The histological immunofluorescence results showed that in SCI rats, the glial scar boundary consisting of GFAP-positive astrocytes was formed around the cavity within few neurons at 4 weeks post-injury (Figures 2A,B). In contrast, in the glial scar boundary, the BMSC-EVs or let-7a-5p-agomir-treated rats had more Map-2-postive neurons in gray matters and MBP-positive myelin sheaths in white matter (Figures 2A,B). Moreover, neurite outgrowth of neurons and the axons extended into the scar and toward the cavity (Figures 2A,B). Consistent with the histology of the injured lesions, the BMSC-EVs or let-7a-5p-agomir-treated rats had better neurological outcomes than SCI rats (Figures 2C,D). All these results indicated that both BMSC-EVs and let-7a-5p were able to promote the regeneration of neurons and their neurite outgrowth, as well as inhibit the over-formation of astrocyte scars, resulting in a better neurological recovery following SCI.
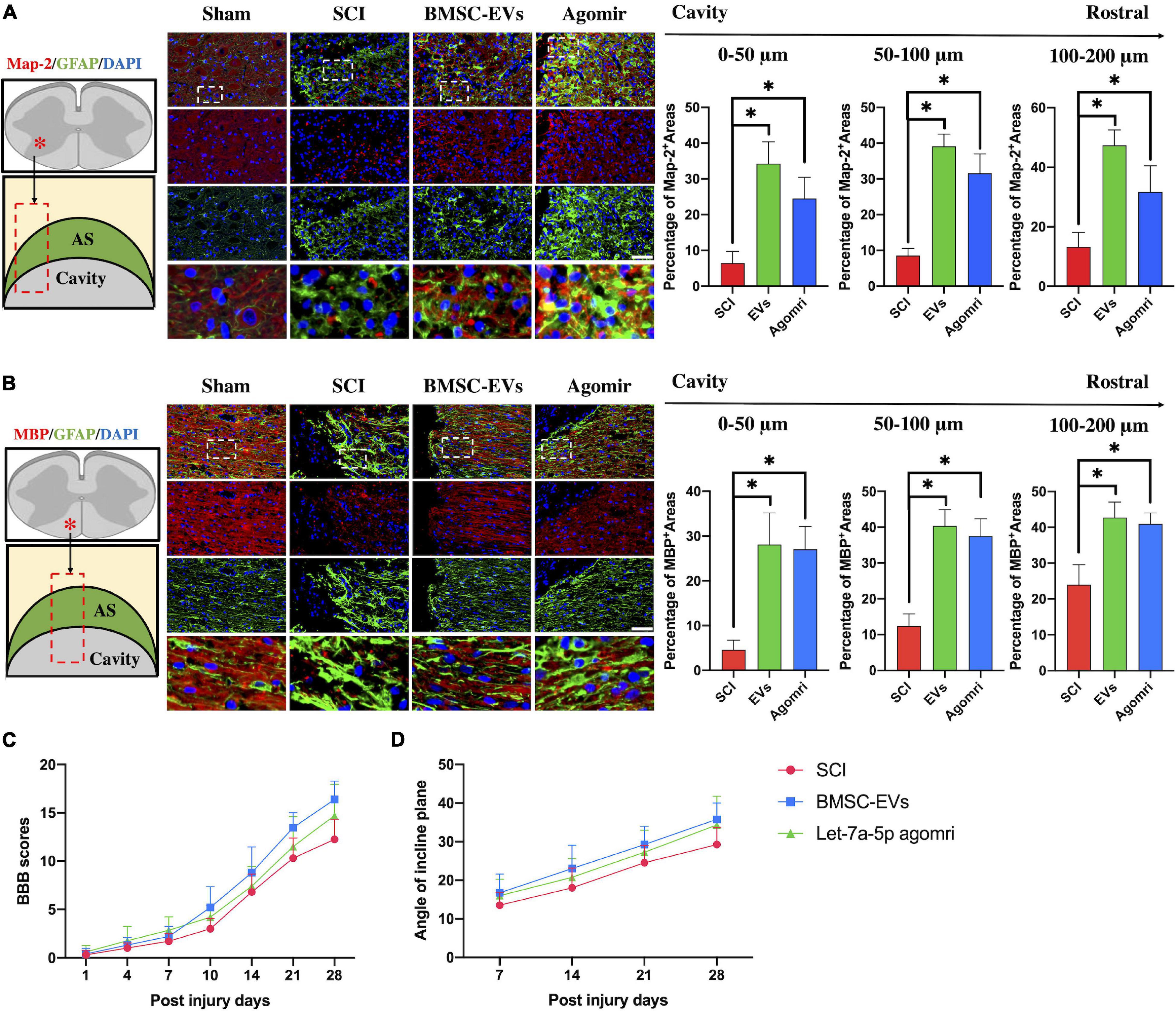
Figure 2. BMSC-EVs and let-7a-5p agomirs promoted the regeneration of neurons and their outgrowth in the glial scar surrounding the cavity, resulting in a better neurological outcome compared with the SCI rats. (A,B) In SCI rats, an astrocytic scar boundary was formed around the cavity, and Map-2 and MBP positive neurons were seldom present within the astrocytic scar. In contrast, the rats that received either BMSC-EVS or let-7a-5p agomir treatment had a significantly increased amounts of Map-2 and MBP positive neurons in the glial scars around the cavity (n = 5; data are mean ± S.D; Student’s t-test was used for comparisons. *p < 0.05; scar bar, 50 μm). (C,D) BBB scores and incline plane test showed that the neurological outcomes were improved in rats that received either BMSC-EVs or let-7a-5p agomirs compared with SCI rats (n = 10; data shown as mean ± S.D). (The term BMSC-EVs is abbreviated as EVs and let-7a-5p agomir is abbreviated as agomir).
The Inhibition of let-7a-5p Attenuated the BMSC Extracellular Vesicle-Induced Effects in vitro and in vivo
To determine whether qrKindly confirm whether the term [BMSC] is fine in line XXX.let-7a-5p secreted from BMSCs participated in mediating the differentiation of NSCs, let-7a-5p inhibitors were transfected into NSCs and co-cultured with BMSC-EVs for 7 days. The results of immunofluorescence revealed that compared with the BMSC-EV-treated NSCs, the let-7a-5p inhibitor-transfected NSCs had a lower percentage of Map-2-postive neurons and a higher proportion of GFAP-positive astrocytes following 7 days of co-culture with BMSC-EVs (Figure 3A), indicating that the BMSC-EV-induced biological effects on the differentiation of NSCs were partly repressed by the transfection of let-7a-5p inhibitors.
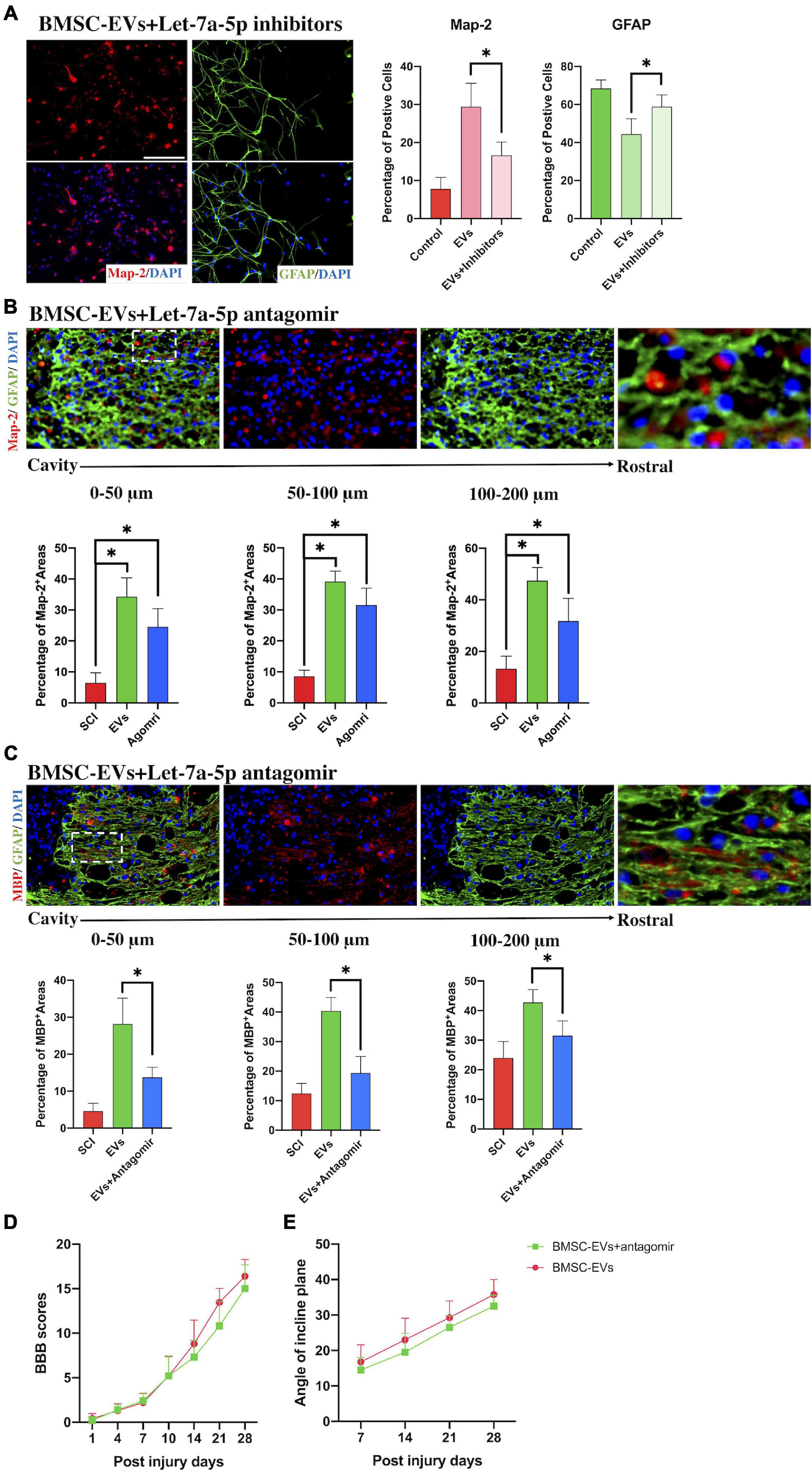
Figure 3. The inhibition of let-7a-5p attenuated the BMSC-EV-induced effects in vitro and in vivo. (A) The transfection of let-7a-5p inhibitors to NSCs in the presence of BMSC-EVs reduced the percentage of neurons and increased the proportion of astrocytes compared with the NSCs that only received the BMSC-EV treatment (n = 5; data are mean ± S.D; Student’s t-test was used for comparisons. *p < 0.05; scale bar, 100 μm). (B,C) The addition of let-7a-5p antagomirs reduced the Map-2 and MBP positive areas in glial scars in BMSC-EV-treated SCI rats (n = 5, data are mean ± S.D; Student’s t-test was used for comparisons. *p < 0.05; scar bar, 50 μm) and resulted in a worse BBB score (D) and lower max angle of incline plane tests (E) compared with the rats that only received BMSC-EV treatment (n = 10; data shown as mean ± S.D). The term BMSC-EVs is abbreviated as EVs, let-7a-5p agomir is abbreviated as agomir, and let-7a-5p inhibitors are abbreviated as inhibitors.
To further study whether the BMSC-EV-induced neurological improvement in SCI rats was associated with let-7a-5p, SCI rats were treated with the let-7a-5p antagomirs in the presence of BMSC-EVs. Compared with the BMSC-EV-treated SCI rats, the SCI rats that received both BMSC-EVs and antagomirs had fewer neurons and axons in the astrocytic scar around the cavity (Figures 3B,C), resulting in a decrease in BBB scores and the max angle of incline plane tests (Figures 3D,E). This indicated that the BMSC-EV-induced improvement was partly associated with let-7a-5p within EVs.
By Targeting HMGA2, let-7a-5p Inhibited SMAD2 Expression
To further study the mechanism by which let-7a-5p mediates the differentiation of NSCs, the TargetScan database was used to predict the downstream molecular genes. It showed that a binding site of let-7a-5p was found at position 21–28 of the high mobility group protein A2 (HMGA2) 3′UTR. HMGA2 has been reported to play important roles in cell proliferation, differentiation, and migration by activating transforming growth factor beta (TGF-β)/Smad signaling (Dutta et al., 2017; Zhou et al., 2020). The TGF-beta/Smad signaling pathway could be significantly inhibited by the silencing of HMGA2, resulting in the alteration of apoptosis, migration, and invasion of cancer (Shi et al., 2016a,b). In addition, TGF-β has also been shown to be upregulated in injured lesions following SCI and is closely associated with the formation of glial scars (Buss et al., 2008; Zhang, 2009; Song et al., 2019). Therefore, we hypothesized that let-7a-5p mediates the differentiation of NSCs by targeting HMGA2, which represses TGF-beta/Smad signaling.
To test this hypothesis, we first used dual-luciferase reporter assays to identify the correlation between HMGA2 and let-7a-5p (Figure 4A). Co-transfection of HMGA 3UTR-wt and let-7a-5p mimics resulted in reduction in luciferase activity (Figure 4B), indicating that let-7a-5p mimics targeted the 3′UTR binding site of HMGA2. Next, to investigate whether let-7a-5p was able to affect the expression of HMGA2 in NSCs, let-7a-5p mimics/inhibitors were transfected into NSCs. PCR analysis revealed that the expression of let-7a-5p in NSCs increased/decreased according to transfection of let-7a-5p mimics/inhibitors compared with the NC group (Figure 4C). Subsequently, the expression of HMGA2 and SMAD2 in NSCs was detected after transfection with let-7a-5p mimics or inhibitors. As expect, the transfection of let-7a-5p markedly reduced the expression of HMGA2 and SMAD2 in NSCs (Figure 4D). In contrast, HMGA2 and SMAD2 expression was significantly increased by transfection with the let-7a-5p inhibitor. In addition, we used western blotting to detect the expression of SMAD 2 and p-SMAD2, revealing that the transfection of let-7a-5p mimics to NSCs significantly reduced both SMAD2 and p-SMAD2 expression, whereas let-7a-5p inhibitor transfection increased the expression of SMAD2 and p-SMAD2 (Figure 4E). All these results indicated that let-7a-5p was able to downregulate the expression of SMAD2 by targeting HMGA2.
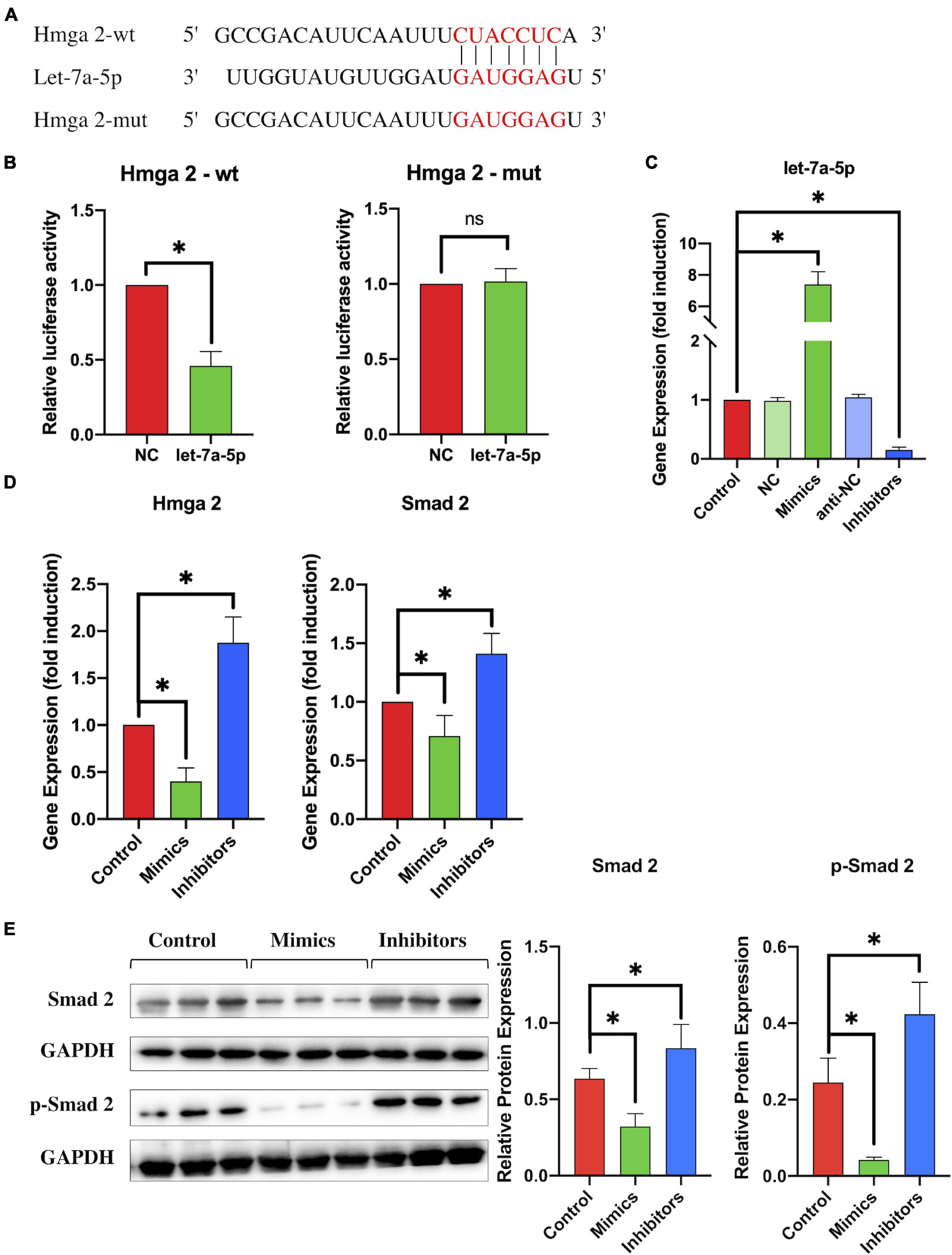
Figure 4. By targeting HMGA2, let-7a-5p downregulated the expression of SMAD2. (A) The target sequence for let-7a-5p in the 3′-UTR of HMGA2 and the mutated target sequence. (B) HMGA2-wt or HMGA2-mut was transfected into NSCs together with let-7a-5p mimics or NC mimics. Dual luciferase reporter analysis confirmed the direct recognition of the HMGA2 3′-UTR by let-7f-5p (n = 3; data are mean ± S.D; Student’s t-test was used for comparisons; *p < 0.05, n.s. p > 0.05). (C) NSCs were transfected with NC mimics, let-7a-5p mimics, anti-NCs, or let-7a-5p inhibitors respectively; RT-PCR analysis was used to detect the expression of let-7a-5p in NSCs. The analysis showed that transfection of let-7a-5p mimics was able to increase the expression of let-7a-5p. In contrast, the expression of let-7a-5p was reduced by the transfection of let-7a-5p inhibitors after 3 days of NSC culturing (n = 3). (D) RT-PCR detected the expression of HMGA2 and SMAD2 in NSCs with the addition of let-7a-5p mimics or inhibitors (n = 3). (E) Western-blot analysis showed that the SMAD2 and p-SMAD2 expression was repressed by the transfection of let-7a-5p mimics. In contrast, the transfection of let-7a-5p increased SMAD2 and p-SMAD2 expression after 3 days of NSC culture (n = 3; data are mean ± S.D; Student’s t-test was used for comparisons., gene expressions were normalized to the GAPDH (for mRNA) and U6 snRNA (for miRNA), *p < 0.05, ns p > 0.05. The term let-7a-5p mimics is abbreviated as mimics and let-7a-5p inhibitors is abbreviated as inhibitors).
In vivo, HMGA2 and SMAD2 expression was analyzed in the injured lesion sites from SCI, BMSC-EV-treated, and let-7a-5p agomir-treated rats, respectively. In SCI rats, the expression of let-7a-5p was significantly reduced at days 3 and 7 post-injury (Figure 5A) compared with the sham rats. In contrast, let-7a-5p expression was markedly increased after treatment with BMSC-EVs (Figure 5A). In accordance with the alternation of let-7a-5p expression, the expressions of HMGA2 and SMAD2 was upregulated in the injured lesion sites following SCI (Figures 5B,C). Moreover, the injection of BMSC-EVS or let-7a-5p markedly reduced the expression of HMGA2 and SMAD2 (Figures 5B,C). This indicated that BMSC-EVS was able to upregulate the expression of let-7a-5p, which, in turn, repressed HMGA2 and SMAD2 expression in the injured lesion sites following SCI.
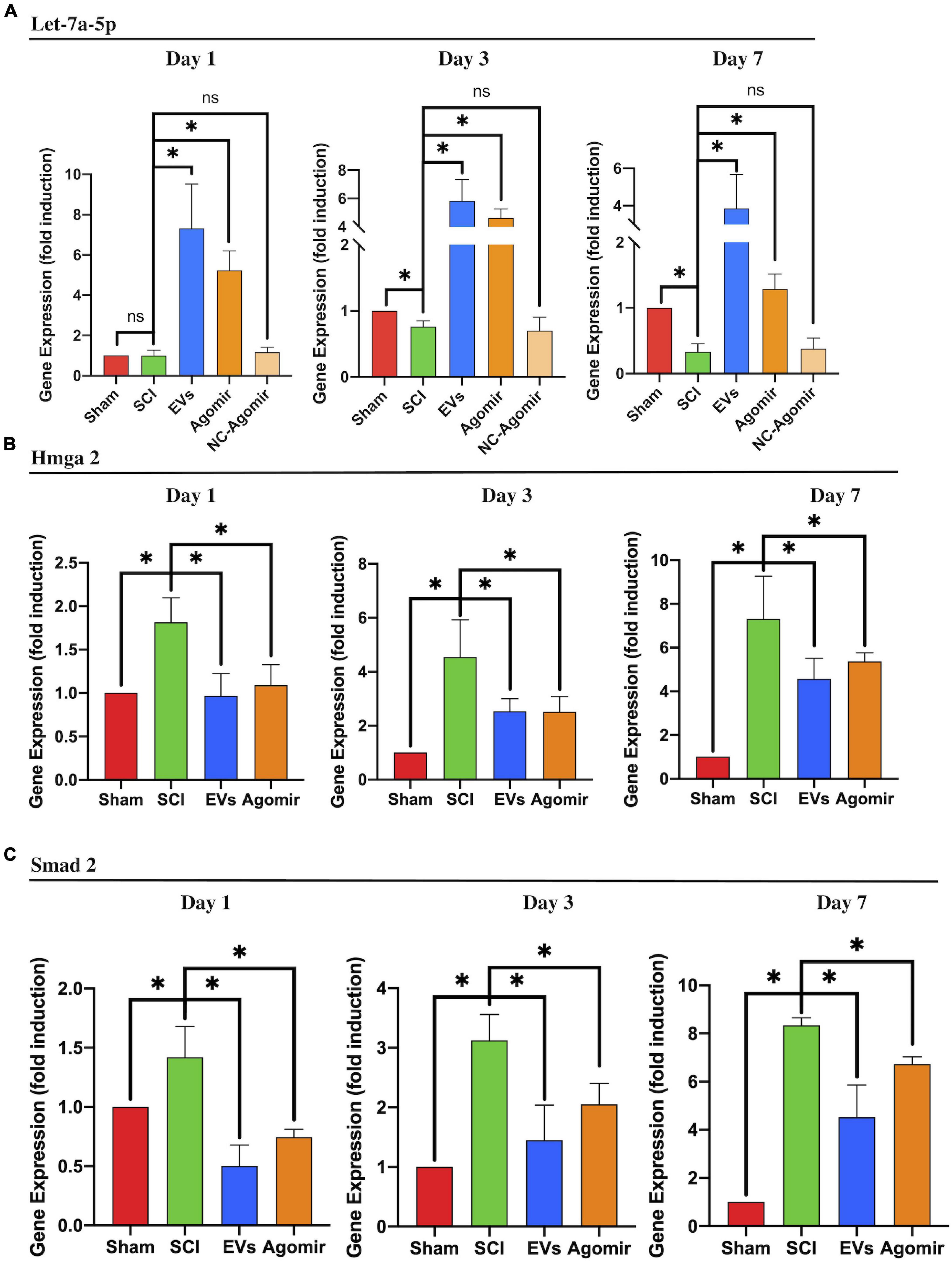
Figure 5. BMSC-EVs and let-7a-5p agomirs inhibited the expression of SMAD2 by targeting HMGA2. (A) The injection of BMSC-EVs increased let-7a-5p expression in injured lesion at different time points following SCI. Similarly, the let-7a-5p agomirs increased let-7a-5p expression in the early time points following SCI (n = 3). (B,C) In SCI rats, the expression of HMGA2 and SMAD2 in the injured lesion was markedly upregulated compared with the sham rats. Both BMSC-EVs or let-7a-5p agomirs were able to reduce HMGA2 and SMAD2 expression in SCI rats (n = 3; data are mean ± S.D; Student’s t-test was used for comparisons; gene expressions were normalized to GAPDH (for mRNA) and U6 snRNA (for miRNA). *p < 0.05. The term BMSC-EVs is abbreviated as EVs and let-7a-5p agomir is abbreviated as agomir.
The Transfection of let-7a-5p Inhibitors or Antagomirs Abolished the BMSC-Extracellular Vesicle-Induced Regulation of the HMGA2/SMAD2 Axis
To further identify that the BMSC-EV-regulated effects on the HMGA2/SMAD2 axis were associated with let-7a-5p within the EVs, we transfected the let-7a-5p inhibitors into NSCs in the presence of BMSC-EVs and detected the expression of HMGA2 and SMAD2 after 3 days of culture. Compared with the control groups, the addition of BMSC-EVs markedly reduced the expression of HMGA2 and SMAD2 in NSCs (Figure 6A). Moreover, the BMSC-EV-induced downregulation of HMGA2 and SMAD2 expression was partly abolished by transfection with let-7a-5p inhibitors (Figure 6A). These results were consistent with the western blot analysis, showing that the transfection of let-7a-5p inhibitors increased p-SMAD2 expression in the presence of BMSC-EVs (Figure 6B). In addition, we detected HMGA2 and SMAD2 in SCI rats that received BMSC-EV injection with or without let-7a-5p antagomirs. This revealed that the BMSC-EV-induced downregulation of HMGA2 and SMAD2 expression was partly attenuated by the addition of let-7a-5p antagomirs (Figures 6C,D,E). These data suggest that the BMSC-EV-related regulation of HMGA2 and SMAD2 expression was partly associated with let-7a-5p.
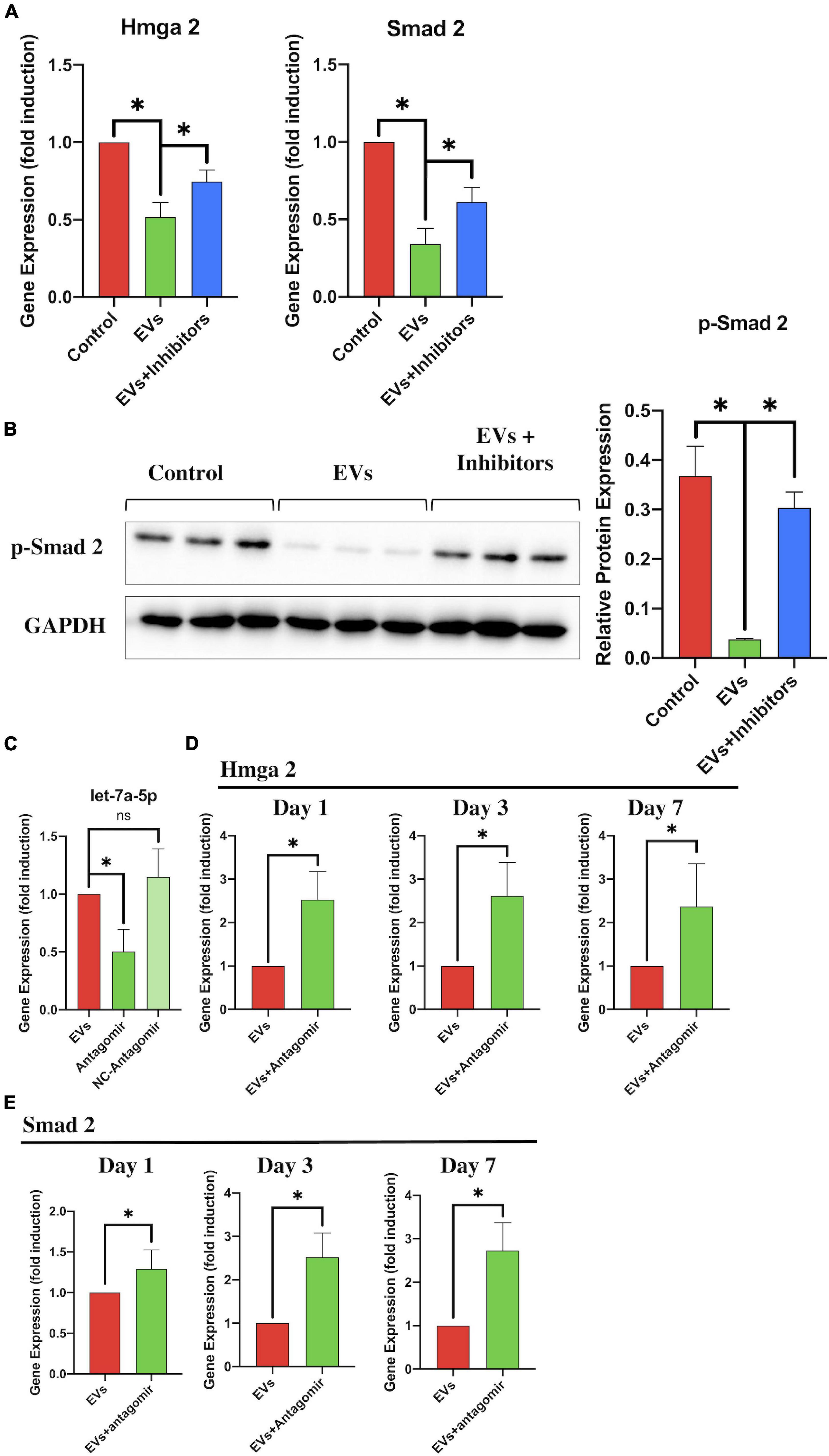
Figure 6. let-7a-5p inhibitors repressed the BMSC-EV-induced effects on the HMGA2/SMAD2 axis. (A) RT-PCR analysis revealed that the expression of HMGA2 and SMAD2 in NSCs was reduced by the addition of let-7a-5p inhibitors in the presence of BMSC-EVs (n = 3). (B) Western blot analysis also revealed that BMSC-EVs were able to reduce the expression of p-SMAD2 and that this BMSC-EV-induced regulation was partly attenuated by the addition of let-7a-5p antagomirs after 3 days of NSC culturing. (C) PCR analysis confirmed that the let-7a-5p antagomirs could reduce let-7a-5p expression (n = 3). (D,E) Compared with the BMSC-EV-treated rats, the let-7a-5p antagomir injection repressed the BMSC-EV-associated downregulation of HMGA2 and SMAD2 expression in the early phase of SCI, leading to an increase in HMGA2 and SMAD2 expression in the injured lesion (n = 3; data are shown as mean ± S.D; Student’s t-test was used for comparisons). Gene expressions were normalized to the GAPDH (for mRNA) and U6 snRNA (for miRNA). *p < 0.05. The term BMSC-EVs is abbreviated as EVs and let-7a-5p agomir is abbreviated as agomir.
Discussion
Mesenchymal stem cells are one of the most widely used cell types in cell-based clinical trials for neurological impairments such as spinal cord injury, stroke, Alzheimer’s disease, and neurodegenerative diseases (Karussis et al., 2008; Assinck et al., 2017; Dabrowska et al., 2019; Staff et al., 2019). They exert beneficial biological effects largely via the following: mediating the inflammatory process by limiting the pro-inflammatory cytokines and increasing the anti-inflammatory cytokines; protecting the surviving nerve cells from apoptosis; providing various neurotrophies to promote the regrowth of neurons, accelerating the remyelination of axons, and preventing the over-formation of glial scars. Moreover, a large number of studies have reported that most of these biological effects of MSCs contribute to MSC-secreted EVs, which enrich various proteins, lipids, and miRNAs (Phinney et al., 2015; Keshtkar et al., 2018; Harrell et al., 2019). Therefore, MSC-EV-based therapies have been used in many different animal models and have achieved promising outcomes. In spinal cord injury, a recent study by Li et al. (2020) revealed that mobilization of MSC-EVs in an adhesive hydrogel markedly improved neurological recovery by promoting neuronal regrowth and limiting secondary damage. Consistent with the present study, treatment with MSC-EVs significantly increased the regrowth of neurons and their outgrowth in the astrocytic scar boundary around the cavity.
Although the biological effects of MSC-EVs have been confirmed to be beneficial, the miRNAs and their mechanisms responsible for MSC-EV-induced effects have not been identified. Among all the miRNAs secreted from BMSCs, let-7 is the first known human miRNA, identified in Caenorhabditis elegans (Pasquinelli et al., 2000; Reinhart et al., 2000). It is abundantly expressed in the central nervous system (CNS) and has been reported to contribute to the development of the CNS and neurogliogenic decisions (Pena et al., 2009; Rehfeld et al., 2015; Xia and Ahmad, 2016). For example, in a neural development model, Balzer et al. (2010) found that during neurogenesis, Lin 28 was able to influence cell fate by regulating let-7. Further study by Patterson revealed that let-7 itself was able to switch the differentiation of neural progenitors from glial lineages directly toward neuronal lineages (Patterson et al., 2014). In the present study, it was revealed that in vitro, the addition of let-7a-5p mimics to NSCS increased the percentage of neurons. In vivo, the treatment of let-7a-5p could promote the outgrow of neurite into the astrocytic scar. These results, consistent with previous studies, indicated that the let-7a-5p was able to promote the differentiation of NSCs into neurons.
TGF-β plays a critical role in the regulation of a wide array of biological processes in various systems, including the CNS (Luo, 2017; Miyazawa and Miyazono, 2017; Stewart et al., 2018). The activation of TGF-β phosphorylates its downstream protein SMAD2, which in turn forms a complex with the co-Smad and translocases to the nucleus, where the complex exerts its function via the activation or repression of gene expression (Han et al., 2021). TGF-β has been reported to have a closely relationship with the balance between astrocytes and oligodendrocytes, the upregulation of TGF-beta signaling pathway could promote the differentiation of oligodendrocyte progenitors toward astrocytes (Zhang et al., 2010). Moreover, recent studies have shown that the activation of TGF-β also contributes to the upregulation of chondroitin sulfate proteoglycans (CSPGs) (Susarla et al., 2011; Jahan and Hannila, 2015), which are known as axon inhibitors. The overexpression of CSPGs in spinal cord lesions, especially in astrocytic scars, could act as barriers to stop the neurite outgrow or axons growth through the astrocytic scars, resulting in the neuroplasticity failure following SCI (Tran et al., 2018). Therefore, the inhibition of the TGF-β/Smad signaling pathway is considered a promising therapy to promote neurite outgrowth of neurons and avoid the over-formation of astrocytic scars.
In addition, HMGA2 has been reported to be an enhanced regulator that promotes TGF-beta/Smad signaling. A study by Xia and Ahmad (2016) revealed that let-7a could affect the generation of neurospheres and their differentiation likely by inhibiting HMGA2 expression. They also revealed that the let-7a-induced effects were abolished in the presence of ΔHMGA2. Similarly, in the present study, we found that treatment with let-7a-5p could inhibit the expression of HMGA2, inducing downregulation of SMAD2 expression. Taken together, let-7a-5p was able to promote the differentiation of NSCs into neurons and the regrowth of neurons and their outgrowth of neurites in SCI rats by targeting HMGA2.
Nevertheless, TGF-β has also been shown to be an anti-inflammatory cytokine. It could directly repress the upregulation of pro-inflammatory cytokines (Rustenhoven et al., 2016) or attenuate inflammation through inhibition of the NF-κB pathway (Miyazawa and Miyazono, 2017). Studies by Noh et al. (2016) and Xu et al. (2020) have pointed out that MSCs can regulate the properties of microglia and suppress neuroinflammation via TGF-β secretion. In a recent study (Han et al., 2021), we found that blocking TGF-β signaling in different phases of SCI exerts a distinct outcome. In the early phase of SCI, blocking TGF-β signaling might attenuate the anti-inflammatory process causing the spread of destructive inflammation, resulting in increased apoptosis or death of the adjacent nerve cells in the lesion sites. Therefore, in the acute phase of SCI, TGF-β is considered to be a beneficial anti-inflammatory cytokine that limits inflammation following SCI. In the present study, although the addition of let-7a-5p reduced SMAD2 expression in the early stage of SCI post-injury, the spread of the destructive inflammation was not noted. This could be because let-7a-5p only partially repressed the activation of SMAD2 and did not hinder the TGF-β’s downstream signaling pathways or the crosstalk with other signaling pathways (Luo, 2017). Moreover, it has been reported that let-7a itself had anti-inflammatory effects, which might compensated for the loss of TGF-β-induced anti-inflammatory effects (Li et al., 2019; Sui et al., 2019; Zhang et al., 2019). However, in the present study, we did not have direct evidence to prove whether let-7a-5p could increase the nerve cells survival by mediating the inflammation following SCI.
In addition, evidence from the present study indicated that the BMSC-EV-induced effects on the mediation of NSC differentiation were partly dependent on the secretion of let-7a-5p. For example, BMSC-EVs and let-7a-5p had similar effects on mediating the differentiation of NSCs, both of which were able to promote the differentiation of NSCs into neurons and increase the amount of neurons and neuron outgrowths in SCI rats. Likewise, the mechanisms of BMSC-EVs and let-7a-5p are similar, both reducing SMAD2 expression by targeting HMGA2; therefore, inhibiting let-7a-5p function blocked the effects of BMSC-EVs. In vitro, the BMSC-EV-induced effects on NSC differentiation were partly abolished by the addition of let-7a-5p inhibitors, resulting in a reduction in the percentage of neurons. In vivo, co-treatment with BMSC-EVs and let-7a-5p antagomirs inhibited neurite outgrowth in astrocytic scars compared with rats that received only BMSC-EVs. When attempting to study the mechanisms involved, HMGA2 and SMAD2 expression was notably decreased by treatment with BMSC-EVs in vitro and in vivo. This BMSC-EV-associated downregulation of HMGA2 and SMAD2 was abolished by the addition of let-7a-5p inhibitors/antagomirs. These results indicated that the effects of BMSC-EVs were associated with the secretion of let-7a-5p.
Another notable result is that although the BMSC-EV-induced regrowth of neurons in SCI rats was markedly repressed by the addition of let-7-5p antagomir, no significant difference of the neurological outcome was noted in these two groups. It could be explained by two reasons: firstly, let-7a-5p was one of the miRNAs secreted by BMSCs, some other miRNAs or proteins were also reported to be include in the BMSC-EVs and to be related with the mediation of the differentiation of NSCs (Song et al., 2020; Han et al., 2021; Zhang et al., 2021). Therefore, although the effects of let-7a-5p was abolished, the other miRNAs or proteins within BMSC-EVs might exert their effect to partly compensate for the let-7a-5p-induced effects. Secondly, the ability of the reorganization of the neural circuit was also closely associated with the neurological recovery following SCI (Freund et al., 2019). The appropriate reorganization could promote the recovery of motor or sensation, but inappropriate reorganization might lead to the neuropathic pain or spasm, which even worsens the neurological outcomes (Murray and Goldberger, 1974; Tan and Waxman, 2012; Tan et al., 2012). It explained that in some SCI rats, although the histology of injured lesions was similar, the neurological outcome might be distinct.
In conclusion, BMSC-EV-secreted let-7a-5p mediates the differentiation of NSCs and promotes the regeneration of neurons and their neurite outgrowth in glial scars by targeting HMGA2.
Data Availability Statement
The raw data supporting the conclusions of this article will be made available by the authors, without undue reservation.
Ethics Statement
The animal study was reviewed and approved by the Ethics Committee of Anhui Medical University.
Author Contributions
CS and YW contributed to the research design. YW contributed to the manuscript writing. CS contributed to the manuscript editing. TH and PS contributed to the injury testing on the rats, tissue processing, and immunohistochemistry. ZW and RG contributed to the cell culturing, Western-blot, and ELISA arrays. YL and JA contributed to the RT-PCR analysis. All authors read and approved the final manuscript.
Funding
This work was supported by grants from the National Natural Science Foundation of China (No. 81472088) and the Natural Science Foundation of Anhui Province (No. 1608085MH205).
Conflict of Interest
The authors declare that the research was conducted in the absence of any commercial or financial relationships that could be construed as a potential conflict of interest.
Publisher’s Note
All claims expressed in this article are solely those of the authors and do not necessarily represent those of their affiliated organizations, or those of the publisher, the editors and the reviewers. Any product that may be evaluated in this article, or claim that may be made by its manufacturer, is not guaranteed or endorsed by the publisher.
Acknowledgments
We would like to thank the Center for Scientific Research of Anhui Medical University for valuable help in our experiment.
Supplementary Material
The Supplementary Material for this article can be found online at: https://www.frontiersin.org/articles/10.3389/fnmol.2022.850364/full#supplementary-material
References
Assinck, P., Duncan, G. J., Hilton, B. J., Plemel, J. R., and Tetzlaff, W. (2017). Cell transplantation therapy for spinal cord injury. Nat. Neurosci. 20, 637–647. doi: 10.1038/nn.4541
Balzer, E., Heine, C., Jiang, Q., Lee, V. M., and Moss, E. G. (2010). LIN28 alters cell fate succession and acts independently of the let-7 microRNA during neurogliogenesis in vitro. Development 137, 891–900. doi: 10.1242/dev.042895
Brazelton, T. R., Rossi, F. M., Keshet, G. I., and Blau, H. M. (2000). From marrow to brain: expression of neuronal phenotypes in adult mice. Science 290, 1775–1779. doi: 10.1126/science.290.5497.1775
Buss, A., Pech, K., Kakulas, B. A., Martin, D., Schoenen, J., Noth, J., et al. (2008). TGF-beta1 and TGF-beta2 expression after traumatic human spinal cord injury. Spinal Cord 46, 364–371. doi: 10.1038/sj.sc.3102148
Cantinieaux, D., Quertainmont, R., Blacher, S., Rossi, L., Wanet, T., Noël, A., et al. (2013). Conditioned medium from bone marrow-derived mesenchymal stem cells improves recovery after spinal cord injury in rats: an original strategy to avoid cell transplantation. PLoS One 8:e69515. doi: 10.1371/journal.pone.0069515
Chen, T. S., Lai, R. C., Lee, M. M., Choo, A. B., Lee, C. N., and Lim, S. K. (2010). Mesenchymal stem cell secretes microparticles enriched in pre-microRNAs. Nucleic Acids Res. 38, 215–224. doi: 10.1093/nar/gkp857
Cofano, F., Boido, M., Monticelli, M., Zenga, F., Ducati, A., Vercelli, A., et al. (2019). Mesenchymal Stem Cells for Spinal Cord Injury: current Options, Limitations, and Future of Cell Therapy. Int. J. Mol. Sci. 20:2698. doi: 10.3390/ijms20112698
Dabrowska, S., Andrzejewska, A., Lukomska, B., and Janowski, M. (2019). Neuroinflammation as a target for treatment of stroke using mesenchymal stem cells and extracellular vesicles. J. Neuroinflamm. 16:178. doi: 10.1186/s12974-019-1571-8
Dutta, A., Hutchison, R. E., and Mohi, G. (2017). Hmga2 promotes the development of myelofibrosis in Jak2(V617F) knockin mice by enhancing TGF-β1 and Cxcl12 pathways. Blood 130, 920–932. doi: 10.1182/blood-2016-12-757344
Fang, H., Song, P., Shen, Y., Shen, C., and Liu, X. (2018). Bone mesenchymal stem cell-conditioned medium decreases the generation of astrocytes during the process of neural stem cells differentiation. J. Spinal Cord Med. 41, 10–16. doi: 10.1080/10790268.2017.1314880
Fang, H., Yang, M., Pan, Q., Jin, H. L., Li, H. F., Wang, R. R., et al. (2021). MicroRNA-22-3p alleviates spinal cord ischemia/reperfusion injury by modulating M2 macrophage polarization via IRF5. J. Neurochem. 156, 106–120. doi: 10.1111/jnc.15042
Freund, P., Seif, M., Weiskopf, N., Friston, K., Fehlings, M. G., Thompson, A. J., et al. (2019). MRI in traumatic spinal cord injury: from clinical assessment to neuroimaging biomarkers. Lancet Neurol. 18, 1123–1135. doi: 10.1016/s1474-4422(19)30138-3
Ghibaudi, M., Boido, M., and Vercelli, A. (2017). Functional integration of complex miRNA networks in central and peripheral lesion and axonal regeneration. Prog. Neurobiol. 158, 69–93. doi: 10.1016/j.pneurobio.2017.07.005
Gnecchi, M., and Melo, L. G. (2009). Bone marrow-derived mesenchymal stem cells: isolation, expansion, characterization, viral transduction, and production of conditioned medium. Meth. Mol. Biol. 482, 281–294. doi: 10.1007/978-1-59745-060-7_18
Han, T., Song, P., Wu, Z., Xiang, X., Liu, Y., Wang, Y., et al. (2021). MSC secreted extracellular vesicles carrying TGF-beta upregulate Smad 6 expression and promote the regrowth of neurons in spinal cord injured rats. Stem Cell Rev. Rep. [Epub online ahead of print] doi: 10.1007/s12015-021-10219-6
Harrell, C. R., Jovicic, N., Djonov, V., Arsenijevic, N., and Volarevic, V. (2019). Mesenchymal Stem Cell-Derived Exosomes and Other Extracellular Vesicles as New Remedies in the Therapy of Inflammatory Diseases. Cells 8:1605. doi: 10.3390/cells8121605
Jahan, N., and Hannila, S. S. (2015). Transforming growth factor β-induced expression of chondroitin sulfate proteoglycans is mediated through non-Smad signaling pathways. Exp. Neurol. 263, 372–384. doi: 10.1016/j.expneurol.2014.10.023
Karussis, D., Kassis, I., Kurkalli, B. G., and Slavin, S. (2008). Immunomodulation and neuroprotection with mesenchymal bone marrow stem cells (MSCs): a proposed treatment for multiple sclerosis and other neuroimmunological/neurodegenerative diseases. J. Neurol. Sci. 265, 131–135. doi: 10.1016/j.jns.2007.05.005
Kawahara, H., Imai, T., and Okano, H. (2012). MicroRNAs in Neural Stem Cells and Neurogenesis. Front. Neurosci. 6:30. doi: 10.3389/fnins.2012.00030
Keshtkar, S., Azarpira, N., and Ghahremani, M. H. (2018). Mesenchymal stem cell-derived extracellular vesicles: novel frontiers in regenerative medicine. Stem Cell Res. Ther. 9:63. doi: 10.1186/s13287-018-0791-7
Kim, K. M., Abdelmohsen, K., Mustapic, M., Kapogiannis, D., and Gorospe, M. (2017). RNA in extracellular vesicles. Wiley Interdiscip. Rev. RNA 8:10.1002/wrna.1413. doi: 10.1002/wrna.1413
Li, L., Zhang, Y., Mu, J., Chen, J., Zhang, C., Cao, H., et al. (2020). Transplantation of Human Mesenchymal Stem-Cell-Derived Exosomes Immobilized in an Adhesive Hydrogel for Effective Treatment of Spinal Cord Injury. Nano Lett. 20, 4298–4305. doi: 10.1021/acs.nanolett.0c00929
Li, Z. H., Wang, Y. F., He, D. D., Zhang, X. M., Zhou, Y. L., Yue, H., et al. (2019). Let-7f-5p suppresses Th17 differentiation via targeting STAT3 in multiple sclerosis. Aging 11, 4463–4477. doi: 10.18632/aging.102093
Luo, K. (2017). Signaling Cross Talk between TGF-β/Smad and Other Signaling Pathways. Cold Spring Harb. Perspect. Biol. 9:a022137. doi: 10.1101/cshperspect.a022137
Mens, M. M. J., and Ghanbari, M. (2018). Cell Cycle Regulation of Stem Cells by MicroRNAs. Stem Cell Rev. Rep. 14, 309–322. doi: 10.1007/s12015-018-9808-y
Mezey, E., Chandross, K. J., Harta, G., Maki, R. A., and McKercher, S. R. (2000). Turning blood into brain: cells bearing neuronal antigens generated in vivo from bone marrow. Science 290, 1779–1782. doi: 10.1126/science.290.5497.1779
Miyazawa, K., and Miyazono, K. (2017). Regulation of TGF-β Family Signaling by Inhibitory Smads. Cold Spring Harb. Perspect. Biol. 9:a022095. doi: 10.1101/cshperspect.a022095
Murray, M., and Goldberger, M. E. (1974). Restitution of function and collateral sprouting in the cat spinal cord: the partially hemisected animal. J. Comp. Neurol. 158, 19–36. doi: 10.1002/cne.901580103
Nakajima, H., Uchida, K., Guerrero, A. R., Watanabe, S., Sugita, D., Takeura, N., et al. (2012). Transplantation of mesenchymal stem cells promotes an alternative pathway of macrophage activation and functional recovery after spinal cord injury. J. Neurotrauma. 29, 1614–1625. doi: 10.1089/neu.2011.2109
Niu, Y., Xia, X., Song, P., Fang, H., Dong, F., Tao, H., et al. (2019). Bone mesenchymal stem cell-conditioned medium attenuates the effect of oxidative stress injury on NSCs by inhibiting the Notch1 signaling pathway. Cell Biol. Int. 43, 1267–1275. doi: 10.1002/cbin.11126
Noh, M. Y., Lim, S. M., Oh, K. W., Cho, K. A., Park, J., Kim, K. S., et al. (2016). Mesenchymal Stem Cells Modulate the Functional Properties of Microglia via TGF-β Secretion. Stem Cells Transl. Med. 5, 1538–1549. doi: 10.5966/sctm.2015-0217
O’Shea, T. M., Burda, J. E., and Sofroniew, M. V. (2017). Cell biology of spinal cord injury and repair. J. Clin. Invest. 127, 3259–3270. doi: 10.1172/JCI90608
Pasquinelli, A. E., Reinhart, B. J., Slack, F., Martindale, M. Q., Kuroda, M. I., Maller, B., et al. (2000). Conservation of the sequence and temporal expression of let-7 heterochronic regulatory RNA. Nature 408, 86–89. doi: 10.1038/35040556
Patterson, M., Gaeta, X., Loo, K., Edwards, M., Smale, S., Cinkornpumin, J., et al. (2014). let-7 miRNAs can act through notch to regulate human gliogenesis. Stem Cell Rep. 3, 758–773. doi: 10.1016/j.stemcr.2014.08.015
Pena, J. T., Sohn-Lee, C., Rouhanifard, S. H., Ludwig, J., Hafner, M., Mihailovic, A., et al. (2009). miRNA in situ hybridization in formaldehyde and EDC-fixed tissues. Nat. Meth. 6, 139–141. doi: 10.1038/nmeth.1294
Phinney, D. G., Di Giuseppe, M., Njah, J., Sala, E., Shiva, S., St Croix, C. M., et al. (2015). Mesenchymal stem cells use extracellular vesicles to outsource mitophagy and shuttle microRNAs. Nat. Commun. 6:8472. doi: 10.1038/ncomms9472
Phinney, D. G., and Pittenger, M. F. (2017). Concise Review: MSC-Derived Exosomes for Cell-Free Therapy. Stem Cells 35, 851–858. doi: 10.1002/stem.2575
Prockop, D. J., Azizi, S. A., Phinney, D. G., Kopen, G. C., and Schwarz, E. J. (2000). Potential use of marrow stromal cells as therapeutic vectors for diseases of the central nervous system. Prog. Brain Res. 128, 293–297. doi: 10.1016/s0079-6123(00)28026-6
Rehfeld, F., Rohde, A. M., Nguyen, D. T., and Wulczyn, F. G. (2015). Lin28 and let-7: ancient milestones on the road from pluripotency to neurogenesis. Cell Tissue Res. 359, 145–160. doi: 10.1007/s00441-014-1872-2
Reinhart, B. J., Slack, F. J., Basson, M., Pasquinelli, A. E., Bettinger, J. C., Rougvie, A. E., et al. (2000). The 21-nucleotide let-7 RNA regulates developmental timing in Caenorhabditis elegans. Nature 403, 901–906. doi: 10.1038/35002607
Rustenhoven, J., Aalderink, M., Scotter, E. L., Oldfield, R. L., Bergin, P. S., Mee, E. W., et al. (2016). TGF-beta1 regulates human brain pericyte inflammatory processes involved in neurovasculature function. J. Neuroinflamm. 13:37. doi: 10.1186/s12974-016-0503-0
Shi, Z., Li, X., Wu, D., Tang, R., Chen, R., Xue, S., et al. (2016a). Silencing of HMGA2 suppresses cellular proliferation, migration, invasion, and epithelial-mesenchymal transition in bladder cancer. Tumour Biol. 37, 7515–7523. doi: 10.1007/s13277-015-4625-2
Shi, Z., Wu, D., Tang, R., Li, X., Chen, R., Xue, S., et al. (2016b). Silencing of HMGA2 promotes apoptosis and inhibits migration and invasion of prostate cancer cells. J. Biosci. 41, 229–236. doi: 10.1007/s12038-016-9603-3
Song, G., Yang, R., Zhang, Q., Chen, L., Huang, D., Zeng, J., et al. (2019). TGF-β Secretion by M2 Macrophages Induces Glial Scar Formation by Activating Astrocytes In Vitro. J. Mol. Neurosci. 69, 324–332. doi: 10.1007/s12031-019-01361-5
Song, P., Han, T., Xiang, X., Wang, Y., Fang, H., Niu, Y., et al. (2020). The role of hepatocyte growth factor in mesenchymal stem cell-induced recovery in spinal cord injured rats. Stem Cell Res. Ther. 11:178. doi: 10.1186/s13287-020-01691-x
Staff, N. P., Jones, D. T., and Singer, W. (2019). Mesenchymal Stromal Cell Therapies for Neurodegenerative Diseases. Mayo Clin. Proc. 94, 892–905. doi: 10.1016/j.mayocp.2019.01.001
Stenudd, M., Sabelstrom, H., and Frisen, J. (2015). Role of endogenous neural stem cells in spinal cord injury and repair. JAMA Neurol. 72, 235–237. doi: 10.1001/jamaneurol.2014.2927
Stewart, A. G., Thomas, B., and Koff, J. (2018). TGF-β: master regulator of inflammation and fibrosis. Respirology 23, 1096–1097. doi: 10.1111/resp.13415
Sui, C., Zhang, L., and Hu, Y. (2019). MicroRNA-let-7a inhibition inhibits LPS-induced inflammatory injury of chondrocytes by targeting IL6R. Mol. Med. Rep. 20, 2633–2640. doi: 10.3892/mmr.2019.10493
Susarla, B. T., Laing, E. D., Yu, P., Katagiri, Y., Geller, H. M., and Symes, A. J. (2011). Smad proteins differentially regulate transforming growth factor-β-mediated induction of chondroitin sulfate proteoglycans. J. Neurochem. 119, 868–878. doi: 10.1111/j.1471-4159.2011.07470.x
Tan, A. M., Chakrabarty, S., Kimura, H., and Martin, J. H. (2012). Selective corticospinal tract injury in the rat induces primary afferent fiber sprouting in the spinal cord and hyperreflexia. J. Neurosci. 32, 12896–12908. doi: 10.1523/jneurosci.6451-11.2012
Tan, A. M., and Waxman, S. G. (2012). Spinal cord injury, dendritic spine remodeling, and spinal memory mechanisms. Exp. Neurol. 235, 142–151. doi: 10.1016/j.expneurol.2011.08.026
Tran, A. P., Warren, P. M., and Silver, J. (2018). The Biology of Regeneration Failure and Success After Spinal Cord Injury. Physiol. Rev. 98, 881–917. doi: 10.1152/physrev.00017.2017
Vieira, M. S., Santos, A. K., Vasconcellos, R., Goulart, V. A. M., Parreira, R. C., Kihara, A. H., et al. (2018). Neural stem cell differentiation into mature neurons: mechanisms of regulation and biotechnological applications. Biotechnol. Adv. 36, 1946–1970. doi: 10.1016/j.biotechadv.2018.08.002
Vishnoi, A., and Rani, S. (2017). MiRNA Biogenesis and Regulation of Diseases: an Overview. Meth. Mol. Biol. 1509, 1–10. doi: 10.1007/978-1-4939-6524-3_1
Xia, X., and Ahmad, I. (2016). let-7 microRNA regulates neurogliogenesis in the mammalian retina through Hmga2. Dev. Biol. 410, 70–85. doi: 10.1016/j.ydbio.2015.12.010
Xu, C., Diao, Y. F., Wang, J., Liang, J., Xu, H. H., Zhao, M. L., et al. (2020). Intravenously Infusing the Secretome of Adipose-Derived Mesenchymal Stem Cells Ameliorates Neuroinflammation and Neurological Functioning After Traumatic Brain Injury. Stem Cells Dev. 29, 222–234. doi: 10.1089/scd.2019.0173
Yamazaki, K., Kawabori, M., Seki, T., and Houkin, K. (2020). Clinical Trials of Stem Cell Treatment for Spinal Cord Injury. Int. J. Mol. Sci. 21:3994. doi: 10.3390/ijms21113994
Zhang, J., Dongwei, Z., Zhang, Z., Xinhui, Q., Kunwang, B., Guohui, L., et al. (2019). miR-let-7a suppresses α-Synuclein-induced microglia inflammation through targeting STAT3 in Parkinson’s disease. Biochem. Biophys. Res. Commun. 519, 740–746. doi: 10.1016/j.bbrc.2019.08.140
Zhang, Y., Liu, J., Su, M., Wang, X., and Xie, C. (2021). Exosomal microRNA-22-3p alleviates cerebral ischemic injury by modulating KDM6B/BMP2/BMF axis. Stem Cell Res. Ther. 12:111. doi: 10.1186/s13287-020-02091-x
Zhang, Y., Zhang, J., Navrazhina, K., Argaw, A. T., Zameer, A., Gurfein, B. T., et al. (2010). TGFbeta1 induces Jagged1 expression in astrocytes via ALK5 and Smad3 and regulates the balance between oligodendrocyte progenitor proliferation and differentiation. Glia 58, 964–974. doi: 10.1002/glia.20978
Zhang, Y. E. (2009). Non-Smad pathways in TGF-beta signaling. Cell Res. 19, 128–139. doi: 10.1038/cr.2008.328
Keywords: spinal cord injury (SCI), mesenchymal stem cells (MeSH ID D059630), extracellular vesicles (EVs), neural stem cells, SMAD 2
Citation: Wang Y, Han T, Guo R, Song P, Liu Y, Wu Z, Ai J and Shen C (2022) Micro-RNA let-7a-5p Derived From Mesenchymal Stem Cell-Derived Extracellular Vesicles Promotes the Regrowth of Neurons in Spinal-Cord-Injured Rats by Targeting the HMGA2/SMAD2 Axis. Front. Mol. Neurosci. 15:850364. doi: 10.3389/fnmol.2022.850364
Received: 07 January 2022; Accepted: 01 March 2022;
Published: 25 March 2022.
Edited by:
Benoit Laurent, Université de Sherbrooke, CanadaReviewed by:
Jinghui Huang, Fourth Military Medical University, ChinaBing Hu, University of Science and Technology of China, China
Copyright © 2022 Wang, Han, Guo, Song, Liu, Wu, Ai and Shen. This is an open-access article distributed under the terms of the Creative Commons Attribution License (CC BY). The use, distribution or reproduction in other forums is permitted, provided the original author(s) and the copyright owner(s) are credited and that the original publication in this journal is cited, in accordance with accepted academic practice. No use, distribution or reproduction is permitted which does not comply with these terms.
*Correspondence: Cailiang Shen, c2hlbmNsc3BpbmVAMTYzLmNvbQ==
†These authors have contributed equally to this work and share first authorship