- Centre for Neuroscience, Indian Institute of Science, Bengaluru, India
Continued mRNA translation and protein production are critical for various neuronal functions. In addition to the precise sorting of proteins from cell soma to distant locations, protein synthesis allows a dynamic remodeling of the local proteome in a spatially variable manner. This spatial heterogeneity of protein synthesis is shaped by several factors such as injury, guidance cues, developmental cues, neuromodulators, and synaptic activity. In matured neurons, thousands of synapses are non-uniformly distributed throughout the dendritic arbor. At any given moment, the activity of individual synapses varies over a wide range, giving rise to the variability in protein synthesis. While past studies have primarily focused on the translation factors or the identity of translated mRNAs to explain the source of this variation, the role of ribosomes in this regard continues to remain unclear. Here, we discuss how several stochastic mechanisms modulate ribosomal functions, contributing to the variability in neuronal protein expression. Also, we point out several underexplored factors such as local ion concentration, availability of tRNA or ATP during translation, and molecular composition and organization of a compartment that can influence protein synthesis and its variability in neurons.
Introduction
Careful decoding of mRNA messages is a fundamental yet complex challenge for any cell. Highly arborized morphology of neurons demands precise spatial and temporal control of mRNA translation in response to synaptic or network activation (Kapur et al., 2017; Rangaraju et al., 2017; Holt et al., 2019). The last few decades of research have pointed out that mRNA translation is regulated locally in neuronal dendrites, axons, dendritic spines, presynaptic boutons, or axonal growth cones (Biever et al., 2019; Fernandopulle et al., 2021). In matured neurons, the cumulative extent of dendritic translation exceeds that of cell soma, allowing spatial variability required for network integrity and homeostasis in the brain (Job and Eberwine, 2001; Hanus and Schuman, 2013; Spillane et al., 2013). Other contemporary studies have confirmed that variability in local protein synthesis is critical for neuronal development, maintenance, synaptic signaling, and plasticity (Holt et al., 2019; Fernandopulle et al., 2021). Studies also suggest that disruptions of activity-induced local translation have drastic consequences leading to cognitive deficits observed in several neurodevelopmental disorders and neurodegenerative diseases (Bear et al., 2004; Oddo, 2012; Deshpande et al., 2020; Ma, 2020).
Ribosomes are the central components of the translation machinery (Baßler and Hurt, 2019). For a long time, all cytosolic ribosomes were believed to act similarly, following a sequence of well-defined steps (Ramakrishnan, 2002; Wilson and Cate, 2012). However, in the past 5 years, an emerging body of evidence indicates the presence of significant heterogeneity in cellular ribosomes (Genuth and Barna, 2018; Gay et al., 2022). In addition, translation factors often shape ribosomal performance by controlling numerous aspects such as subunit loading on mRNAs, translational fidelity, ribosomal processivity, speed of translocation, probability of reloading, and more (Jackson et al., 2010; Kapur et al., 2017; Neelagandan et al., 2020). Within neurons, ribosomes are produced in the nucleolus and transported to the cytosol (Stoykova et al., 1985; Baßler and Hurt, 2019). A fraction of this population is then sorted to distant locations from the cell soma to participate in local translation (Tiedge and Brosius, 1996; Scarnati et al., 2018; Hafner et al., 2019; Koltun et al., 2020). Further, remodeling of existing ribosomes can occur in remote locations in a biogenesis-independent fashion (Sotelo-Silveira et al., 2006; Shigeoka et al., 2019; Mofatteh, 2020; Fernandopulle et al., 2021). Such remodeling events together with other parameters such as local ion concentrations, mRNA and tRNA availability, steady-state ATP content, and signaling pathways govern ribosomal activity and translation in neuronal compartments (Holt and Schuman, 2013; Rangaraju et al., 2017; Biever et al., 2019, 2020; Holt et al., 2019).
Here, we review the current understanding of ribosome biogenesis and heterogeneity within a neuron. We highlight the mechanisms and local parameters that influence stochasticity in ribosomal performance. Also, we discuss the importance of studying ribosomal dynamics to explain this translational variability required for neuronal functions.
Regulation of Ribosome Biogenesis Within Neurons
“Ribosome Biogenesis” results in the production of functionally matured ribosomes that determines the protein synthetic ability of a living cell (Stoykova et al., 1985; Chau et al., 2018; Hetman and Slomnicki, 2019; Figure 1). The biogenesis process happens primarily in the nucleolus and is guided at least by 200 associating factors (AFs) and 80 different types of small nucleolar RNAs (snoRNA) (Baßler and Hurt, 2019).
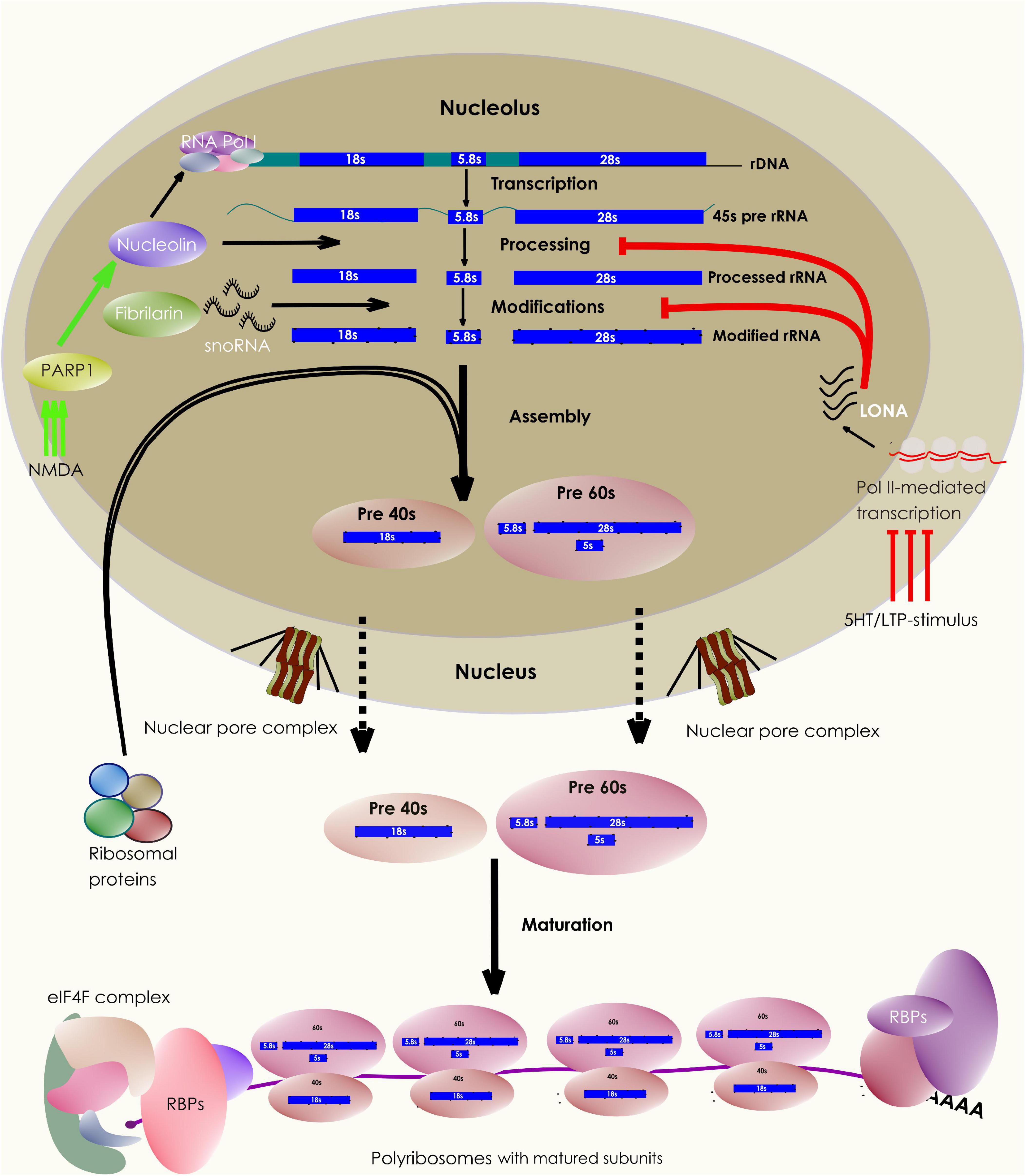
Figure 1. Neuronal control of ribosome biogenesis. The schematic depicts various stages of ribosome biogenesis within the nucleolar compartment. The rRNA synthesis, modification, and maturation takes place in the nucleolus following which, they associate with ribosomal proteins produced in the cytosol. The assembled subunits are then exported out of the nucleus to undergo the final steps of cytoplasmic maturation. A pool of these matured subunits engages in translating mRNAs, a process facilitated by multiple other protein factors. Interestingly, the nucleolar biogenesis process can be influenced by synaptic stimulation through a variety of synapse to nucleus (retrograde) signaling pathways.
Within the brain, three of the four rRNA species are synthesized together as a single polycistronic ∼45s rRNA precursor (pre rRNA) (Thomson et al., 2013; Baßler and Hurt, 2019). In general, the ribosomal DNA (rDNA) genes are present in multiple copies within the genome grouped into 7 different clusters, known as variants. Of these variants, 5 are expressed in the brain (Tseng et al., 2008; Allen et al., 2018). However, the rRNA content in individual brain cells can vary in a cell-type-specific manner. For example, studies have revealed that rat neurons possess fourfold higher pre rRNA content than oligodendrocytes due to a reduced turnover rate (Stoykova et al., 1985). In addition, ribosome biogenesis dynamically alters with stages of brain development. For example, the nucleolar number in rat and chicken cerebellar Purkinje neurons increases from the embryonic stage to the post-natal/hatching period (Lafarga et al., 1995). In mice, the synthesis of a few ribosomal proteins (RP), ribosome biogenesis factors, and translation factors are repressed in the neuronal progenitor cells following neural tube closure. The resulting dip in protein synthesis capacity is required for a timed reduction in the rate of proliferation of these cells which, otherwise causes macrocephaly (Chau et al., 2018). However, later during forebrain development, a surge in ribosome biosynthesis promotes dendritic development and arborisation (Slomnicki et al., 2016; Chau et al., 2018). Studies in mouse hippocampal neurons have shown that a moderate depletion of ribosomal proteins S6, S14, or L4, required for subunit export, perturbs dendritic growth and development. This is due to the reduced ribosomal recruitment and translation of BDNF target mRNAs despite having signaling pathways from the TrkB receptors intact (Slomnicki et al., 2016). In addition, the ribosome content is depleted within axons during synaptogenesis (Costa et al., 2019). Together these results highlight that ribosome biogenesis and assembly are regulated in a dynamic and site-specific manner during brain and neuronal development.
Also, neuronal stimulation affects rDNA transcription. For example, 1-h stimulation of auditory nerves results in a significant rise in the rRNA content of chicken cochlear neurons (Hyson and Rubel, 1995). In Aplysia neurons, 5-HT or LTF-inducing stimulus leads to an elevation in pol I-mediated rRNA synthesis. Translocation of the chromatin remodeling protein PARP1, following the activation of the PKA-ERK pathway, mediates this rapid synapse to nucleus signaling (Hernandez et al., 2009; Figure 1). Another study has observed that on NMDA stimulation, AIDA1, a synaptic PSD-interacting protein, translocates from the synapse to the nucleus to regulate nucleolar numbers (Jordan et al., 2007). These observations explain the mechanistic basis of the dynamic communication between the nucleolus and synapse that shape ribosome biogenesis in response to synaptic signaling.
Following the synthesis of 45s pre-rRNA transcript, several molecules take part in rRNA maturation (Thomson et al., 2013; Sen Gupta et al., 2018). While a key enzyme Nucleolin is involved in transcriptional regulation and cleavage of the 45s rRNA, other critical proteins like Fibrillarin catalyze the modifications of hundreds of bases and backbone residues of the processed rRNA (Boisvert et al., 2007; Sen Gupta et al., 2018). These modifications, such as 2′ O-methylations, can influence rRNA secondary structures, the subunit RP compositions, ribosomal association with various RNA-binding proteins (RBPs), and thus allowing ribosomes with specific rRNA modifications to translate a distinct set of mRNA targets (Kondrashov et al., 2011; Polikanov et al., 2015; Simsek et al., 2017; Sloan et al., 2017; D’Souza et al., 2018; Merkurjev et al., 2018; Figure 1). In addition, activity-dependent changes in rRNA modifications can alter global mRNA translation. For example, the induction of experience-dependent plasticity leads to an increased rRNA production and translation in neurons. Neurons express a long nucleolus-specific lncRNA (LONA) that precludes both Nucleolin and Fibrillarin activity, reducing the pro-translational 2′ O-methyl marks on rRNA. However, synaptic activation causes degradation of LONA, relieving the inhibition of global translation and upscaling the rRNA production (Li et al., 2018; Figure 1). However, the evidence for other types of rRNA modifications impacting local ribosomal performance in neurons is yet to come. Further experiments can reveal whether individual ribosomes carrying unique combinations of rRNA modifications (an epitranscriptomic code) can influence their functions distinctly (Li and Wang, 2020). Nevertheless, rRNA modifications represent an additional layer of ribosomal regulation in neurons (Figure 2).
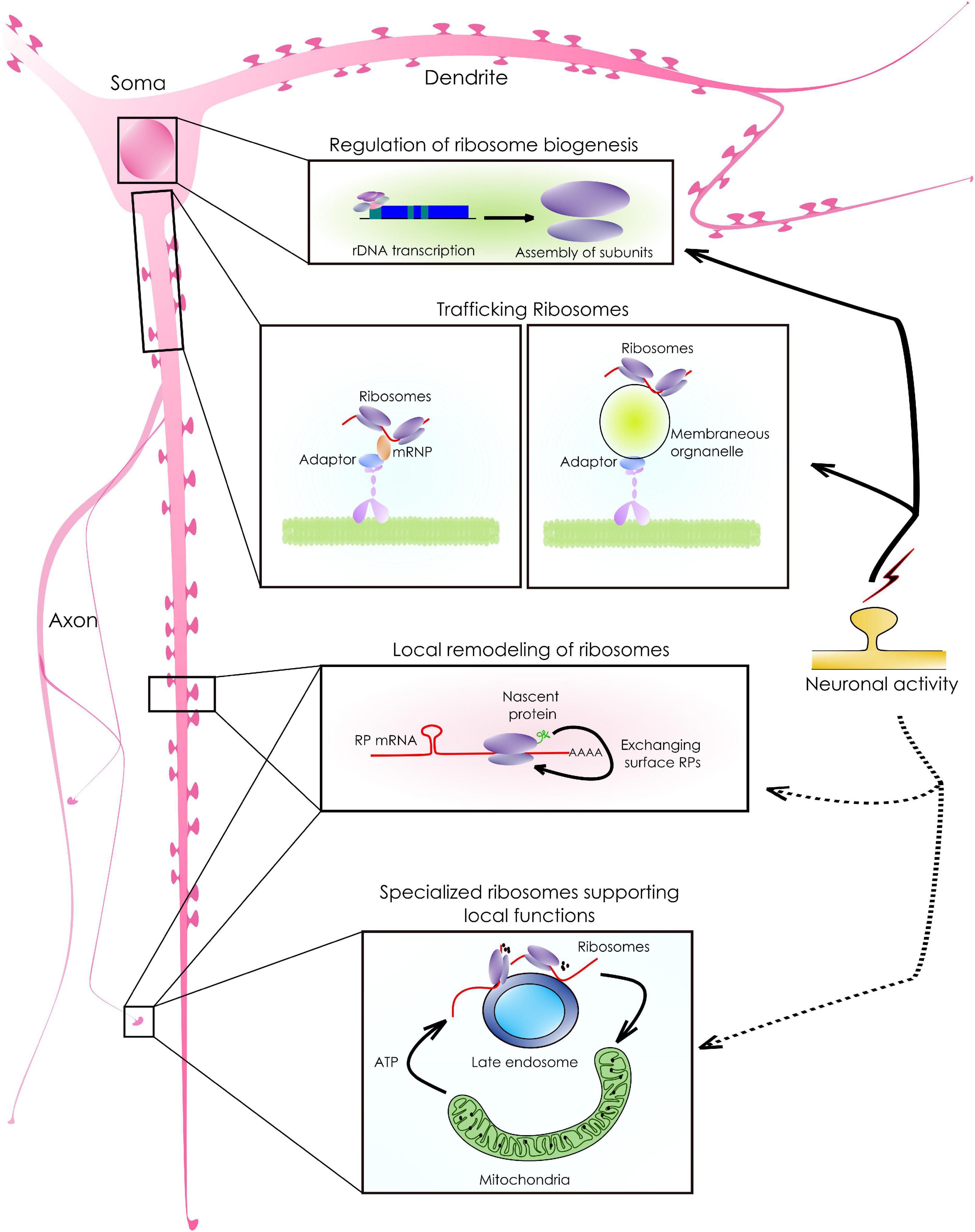
Figure 2. Ribosomal synthesis, sorting, and remodeling to support local functions. As shown in the schematic, neurons have an arborized morphology. mRNA abundant. As a result, the availability of ribosomes and translation machinery in these remote locations is crucial for the abundant local mRNA translation that supports neuronal functions. Following biosynthesis, ribosomal sorting in neurons happens either via diffusion or by active transport. The active sorting, is achieved either by associating with mRNPs or by tethering to membrane-bound organelles targeted to distant locations. In addition, existing ribosomes can exchange locally synthesized surface proteins (RPs) giving rise to ribosomes with specialized functions. For example, RP mRNAs with a specific sequence motif are translated into the axons which, can then associate with the surface components of existing ribosomes. Such modifications allow ribosomes to translate a distinct set of transcripts that support local functions.
Ribosomal Sorting and Distribution
Localization of ribosomes to subcellular compartments allows neurons to mount a rapid translation response upon stimulation (Figure 2). It is currently believed that while the subunits diffuse freely anywhere in the cell, most assembled ribosomes reach their target location by associating with various RBPs, membrane-less granules, or membrane-bound organelles (Rolls et al., 2002; Fernandopulle et al., 2021). Such association can also lead to the functional compartmentalization of ribosomes. For example, a recent study has reported that the presynaptic ribosomes, tethered to Rab7a-containing endosomal vesicles, drive the translation of mRNAs relevant to mitochondrial functions (Cioni et al., 2019; Figure 2). In axonal growth cones, the DCC receptor and its ligand netrin regulate the translation of their target mRNAs by immobilizing the ribosomes locally (Martin, 2010; Tcherkezian et al., 2010; Kim and Martin, 2015; Koppers et al., 2019). These results highlight the importance of spatial confinement of ribosomes for compartmentalizing translation (Reid and Nicchitta, 2015; Cioni et al., 2019).
A large fraction of the cytosolic ribosome functions as polyribosomes. They often reach their target locations by piggybacking partner mRNPs or transporting granules (Fernandopulle et al., 2021). For example, TDP43 and FMRP/FUS containing transport granules are shown to carry polyribosomes to neuronal axons and dendrites, respectively (Miyashiro et al., 2003; Simsek et al., 2017; Thelen and Kye, 2020; Nagano et al., 2020). Both motor proteins such as Kinesin I, II, Myosin II, and V and adaptor proteins like RACK1 help in the precise targeting of polysomes (Hirokawa et al., 2010; Ceci et al., 2012; Spillane et al., 2013). However, given the heterogeneous properties of ribosome-associated mRNPs and the contradictory nature of evidence, it is not clear whether moving polysomes can translate actively (Wu et al., 2015; Katz et al., 2016; Mateju et al., 2020). This necessitates the study of the translatability of moving polysomes in greater depth.
While ample evidence supports the polyribosomal translation of mRNAs at somatic and dendritic compartments, evidence for axonal ribosomes has been hard to come by for a long period (Ostroff et al., 2002, 2018, 2017; Biever et al., 2019). Eventually, improved paradigms have allowed the detection of mRNAs, rRNAs, actively translating polysomes, ER-Golgi-mediated protein synthesis, and their membrane targeting in axons (Giuditta et al., 1968, 1980, 1986; Tennyson, 1970; Bassell et al., 1998; Willis, 2005; Merianda et al., 2009). Recent investigations have discovered additional mechanisms that aid in axonal ribosomal homeostasis. For example, studies on injured sciatic nerves have shown that polyribosomes are transferred to the axons from the neighboring Schwann cells through tunneling nanotubes or exosomes (Court et al., 2008). In addition, a few other current studies suggest that in the axons, monosome-mediated translation is abundant compared to polysomes (Biever et al., 2019, 2020; Koltun et al., 2020). These findings imply that neurons utilize a range of mechanisms to supply ribosomes to axons, where the translation program is modified uniquely to meet the local protein demands. The fact that several stimulations uniquely impact axonal translation corroborates this idea further (Hafner et al., 2019; Koltun et al., 2020).
Local Remodeling of Ribosomes
Ribosomal subunits are composed of ∼80 ribosomal proteins and 4 different rRNA species. Conventionally it is believed that the protein or rRNA composition of the ribosomal population within a cell is consistent over time (Ferretti and Karbstein, 2019). However, multiple recent reports suggest otherwise. Studies in various non-neuronal systems have detected differential transcription and splicing of RP mRNAs and change in the stoichiometry of ribosomal core proteins across a range of physiological states (Bortoluzzi et al., 2001; Sharov et al., 2003; Richards, 2004; Adjaye et al., 2005; Kondrashov et al., 2011; Slavov et al., 2015). Some of these studies also show that ribosomes with distinct RP compositions can preferentially translate a subpool of mRNAs. For example, ribosomes containing RPL10A/uL1 protein selectively translate the internal ribosome entry site (IRES)-containing mRNAs (Shi et al., 2017). Interestingly, neurons also contain a large proportion of RPL10A containing ribosomes at the dendrites (Koltun et al., 2020; Sun et al., 2021). However, it is not known whether they translate a selected set of mRNAs in these compartments. Other than the RP composition, their posttranslational modifications are necessary for ribosomal function and can be a source of significant functional variability (Belin et al., 2010; Loenarz et al., 2014; Simsek and Barna, 2017). For example, in RPL12/uS23, hydroxylation of a proline residue is required for polysome formation, without which, human patients develop microcephaly and hearing loss (Loenarz et al., 2014). In addition, phosphorylated forms of various ribosomal proteins are studied widely in the context of synaptic signaling and are implicated in Parkinson’s disease (Simsek and Barna, 2017; Martin et al., 2011). Besides, a combination of methylated, acetylated, and ubiquitylated ribosomal proteins can define some form of post-translational modifications code (PTM code) that can direct a ribosome to function uniquely (Nesterchuk et al., 2011; Simsek and Barna, 2017). Such functional specifications corroborate the “ribosome filter hypothesis” that considers ribosomes as active components of the gene regulatory framework (Mauro and Edelman, 2002).
In neurons, a consistent yet intriguing finding has been an abundance of RP mRNAs in the neuronal branches away from the cell body (Poon et al., 2006; Cajigas et al., 2012; Rangaraju et al., 2017). The targeting of these mRNAs to the distal processes cannot be explained by the Brownian diffusion and requires a reassessment of their physiological roles in these compartments. Also, there is evidence to support the ubiquitous synthesis of ribosomal proteins at distant locations, where they can physically associate with the pre-existing ribosomes. For example, RP mRNAs with CUIC-sequence motifs are translated locally and are incorporated into the axonal ribosomes required to translate critical mRNAs for axonal maintenance and branching (Shigeoka et al., 2019). The translation of RP mRNAs in axons requires the survival of the motor neuron (SMN) protein in the absence of which, axonal ribosomal content dips by 27% (Fallini et al., 2012, 2016). In dendrites, 17 ribosomal proteins are synthesized locally while 12 of them are incorporated into the existing ribosomes rapidly (Fusco et al., 2021). These proteins are short-lived and are associated with the solvent-accessible surfaces of ribosomes (Shigeoka et al., 2019; Fusco et al., 2021). Such dynamic exchanges of subunit proteins are necessary for the maintenance, repair, or modification of their functions in the local compartments (Figure 2). Interestingly, ribosomes are often located at both dendritic and axonal branch points. Here, they colocalize with mitochondria and other RBPs to translate mRNAs critical for stabilizing branches (Cui-Wang et al., 2012; Spillane et al., 2013).
Despite our knowledge of dendritic and axonal translation, we know relatively little about the synthesis of ribosomal proteins at the synapse. Ribosome profiling from cortical synaptoneurosomes has revealed that a few RP mRNAs are synthesized locally at the synapse upon NMDAR stimulation (Kuzniewska et al., 2020). Whether they can modulate existing ribosomal function requires verification. Moreover, since solitary monosomes translate the bulk of the synaptic mRNAs, especially with shorter ORFs (Biever et al., 2020; Koltun et al., 2020), conceptually it is feasible to selectively alter the translation of such mRNAs by modifying single ribosomes. Whether such mechanisms operate at the synapse requires validation through experimentation.
Also, these studies have opened up a flurry of other questions that remain unanswered. For instance, given the distinct translation states of various neuronal compartments, whether ribosomes with unique RP compositions or rRNA modifications populate these compartments differentially remains to be seen. In addition, it is not clear whether the type of synaptic activity (i.e., excitatory vs. inhibitory) has distinct impacts on the ribosomal properties. Besides, within a synapse, there can be multiple functional zones with heterogeneous molecular compositions (Nanguneri et al., 2019; Venkatesan et al., 2020). Whether ribosomes proximal to these zones function uniquely is not understood. Also, specific biochemical mechanisms that control ribosome-RBP association needs to be identified. Intriguingly, a large part of the variability in protein expression is contributed by the stochasticity of translation elongation (Datta and Seed, 2018; Koltun et al., 2020; Sun et al., 2021). Whether rRNA modifications and subunit protein compositions contribute to the stochastic aspects of protein synthesis are not well characterized. Also, it needs to be investigated whether specialized ribosomes necessarily need to function as monosomes. In the case of a polysome, do all ribosomes harbor similar subunit compositions? Whether sequence and structural features of mRNAs dictate their affinity toward ribosomes? How does the presence or absence of an RBP affect ribosome-mRNA recognition? Some of these questions need to be addressed thoroughly for a better understanding of translation regulation in neurons. Besides, mRNA nucleotides often undergo chemical modifications posttranscriptionally, known as the epitranscriptomic changes, which represent an additional layer of translation regulation. A large number of synaptic mRNAs harbor critical modifications such as N6-methyladenosine, m5-cytosine, etc., because of the actions of a group of depositing (writer), binding (reader), and removing (eraser) enzymes (Flamand and Meyer, 2019). For example, almost 3,000 synaptic mRNAs are m6A methylated (Merkurjev et al., 2018). These modifications either facilitate or occlude the binding of modified mRNAs to RBPs and translation machinery, thus, tuning translation kinetics (Merkurjev et al., 2018; Li et al., 2019). A study has shown in the past that mRNA modification such as N6-methyladenosine (m6A) alters the recognition time and binding probability of a cognate tRNA to an mRNA codon and consequently alters elongation dynamics (Choi et al., 2016). Also, it is established that the absence of these RNA modifying enzymes affects synaptic translation and plasticity (Li et al., 2019). Further insight would clarify whether the deficiency of such enzymes impacts the ribosome-mRNA recognition, binding, and translatability of these mRNAs. Finally, how these processes are altered in pathological conditions or with aging should be monitored. Nonetheless, on-site remodeling of ribosomes through various mechanisms represents an exciting ramification of our understanding of spatiotemporal gene expression regulation in neurons.
Local Factors That Affect Translation Kinetics
For the holistic understanding of neuronal translation, numerous other factors need considerations. Since distant branches with smaller diameters present significant diffusion barriers, local factors are bound to influence ribosomal function. However, such studies are limited in the neuronal context. We discuss a few components from the local microenvironment that could be critical in our opinion to shape ribosomal function.
Concentration of Cations
K+
K+ is a major intracellular monovalent cation in neurons. Recent studies have revealed the role of K+ ions as integral components of the ribosomal structure (Khatter et al., 2015; Rozov et al., 2019). In eukaryotic ribosomes, K+ ions strengthen the intersubunit interactions and mediate the interactions between tRNAs and ribosomal components (Wilson and Cate, 2012; Khatter et al., 2015). This is unlike the prokaryotic ribosomes, where the role of Mg2+ ions is more prominent (Ramakrishnan, 2002; Nierhaus, 2014). Intriguingly, recent studies from prokaryotic ribosomes also have suggested diverse roles for K+ ions (Rozov et al., 2019). However, our current understanding of the influence of K+ ions upon ribosomal performance in the cellular context is limited. In the case of hippocampal neurons, while the steady-state concentration of K+ [(K+)i] is ∼140 mM, it can reduce rapidly up to almost ∼43 percent following glutamate-mediated depolarization (Ballanyi et al., 1984; Müller and Somjen, 2000; Somjen and Müller, 2000; Nierhaus, 2014; Shen et al., 2019). Similar observations were made for carbachol or GABA treatment [∼20% and 8.5% (K+)i respectively (Ballanyi et al., 1984)]. Such changes would be pronounced in the small volume compartments like dendritic spines or axonal growth cones and are likely to impact ribosomal subunit interactions and subsequent functions. Considering a large body of evidence pointing to the fact that neuronal depolarization can affect protein synthesis (Sutton et al., 2004; Hsu et al., 2015; Kos et al., 2016; Brigidi et al., 2019; Dastidar et al., 2020), it is worth investigating whether such effects are mediated by the changes in [K+]i. In addition, multiple reports suggest altered neuronal firing and membrane properties upon treatment with global protein synthesis inhibitors (Kleim et al., 2003; Sharma et al., 2012; Scavuzzo et al., 2019). Whether this effect is due to the release of a large amount of ribosome-bound K+ ions following the inhibitor actions needs verification.
Mg2+
Magnesium is an abundant divalent cation within mammalian cells. In neurons, the intracellular Mg2+ concentration ranges between 17–20 mM (Romani, 2011). Interestingly, the concentration difference of Mg2+ ions across the plasma membrane is only twofold (compared to 20,000-fold of calcium). There are various mechanisms by which Mg2+ ions are stored in a cell, of which ribosome-bound Mg2+ pool represents a considerable fraction. In humans, each ribosome is bound to 239 Mg2+ ions (Khatter et al., 2015). Studies of diverse backgrounds have revealed that Mg2+ is a small electron-dense ion that helps in stabilizing the negative charges of the rRNA backbone residues (counterions) (Nierhaus, 2014). Given a neuron can have as many as 106–107 ribosomes, the amount of ribosome-bound Mg2+ is substantial. However, due to the shallow concentration gradient of Mg2+ across the membrane and the continuous exchange between their bound and the free form in the cytosol, changes in extracellular Mg2+ availability can severely impact the intracellular Mg2+ concentration and ribosomal functions. Also, the Mg2+ deficiency within neurons can be sensed by mTOR signaling that can alter translation in several ways (Shindo et al., 2020).
Ca2+ and Mn2+
Apart from being a cation, Ca2+ acts as an important intracellular second messenger in neurons. However, its role in the context of neuronal ribosomes is less studied. Earlier observations on purified E. coli ribosomes have established that the presence of Ca2+ in the reaction buffer improves ribosomal performance (Gordon and Lipmann, 1967). However, later studies have found that another divalent cation Mn2+ can substitute Mg2+ in both subunits while Ca2+ could do so only in the small subunit (Weiss et al., 1973). In sync with these observations, Mg2+ depletion in pituitary GH3 cells leads to a complete abolishment of polysomes. However, replenishing Ca2+ quickly recovers the polysomal functions, highlighting a positive influence of calcium over eukaryotic polysomes (Chin et al., 1987). In addition, Ca2+ promotes the association between purified human ribosomes and the Ca2+-sensitive protein Calmodulin (Behnen et al., 2012). Interestingly, at the neuronal synapse, Ca2+ activates several EF-hand-containing proteins such as Calmodulin, Caldendrin, Calbindin, Calreticulin, Calneuron, and others (Chard et al., 1995; Seidenbecher et al., 1998; Pangršič et al., 2015; Mundhenk et al., 2019). Some of them, such as Calneuron 1 and 2, have the highest affinity toward Ca2+. Functionally, they are implicated in Golgi to plasma membrane protein trafficking (Mundhenk et al., 2019). However, not much is known whether they can influence protein synthesis. Since neuronal activation alters intracellular Ca2+ levels, an exciting direction would be to investigate how these various Ca2+-binding proteins act together to regulate ribosomal functions following neuronal stimulation.
ATP Level
Intracellular ATP concentration is a major rate-limiting factor for almost all anabolic pathways. ATP level is particularly important for protein synthesis due to multiple ATP-consuming steps in mRNA translation (Schwanhäusser et al., 2011). Our previous study has demonstrated that neuronal activity-induced protein synthesis is responsible for a significant ATP expenditure (Dastidar et al., 2020). Others have observed that a deficiency in energy biosynthesis can attenuate activity-induced synaptic translation (Rangaraju et al., 2019). Moreover, perturbing mitochondrial function that colocalizes with the translational hotspots at the neurite branch points impairs brach-point protein synthesis and branch stabilization (Hill et al., 2012; Spillane et al., 2013; Li et al., 2004). Conversely, new proteins can support mitochondrial function locally. For example, local translation of lamin B2 at the retinal ganglionic axons of Xenopus maintains mitochondrial morphology and function (Yoon et al., 2012). In this context, an intriguing observation has been that an excess of intracellular ATP can negatively impact translation by binding additional Mg2+ ions, thus limiting Mg2+-dependent ribosomal assembly (Pontes et al., 2015). Therefore, a coordinated regulation between protein synthesis and energy biosynthesis is necessary for neuronal functions and can be achieved by the actions of intracellular metabolic sensors like phosphofructokinase 1 (PFK1) or AMP-activated protein kinase (AMPK) (Jang et al., 2016; Marinangeli et al., 2018; Dastidar et al., 2020). In general, the interplay between metabolic and translation regulatory pathways remains incompletely understood and would be an active area of future research.
tRNA Availability
Transfer RNAs (tRNA) canonically function as adapter molecules during mRNA translation. The availability of tRNAs, therefore, is one of the rate-limiting parameters of the ribosomal function. The redundancy in the genetic code allows most amino acids to be encoded by multiple codons. Also, these codons are distributed non-randomly along the length of an mRNA message (Komar, 2016) and are related to the decoding times for each codon (Gardin et al., 2014; Quax et al., 2015). Indeed eukaryotic ribosomes can accommodate frequent codons more rapidly at the A site than the rare codons (Gardin et al., 2014). The codon usage bias has been estimated to account for 30% of the variation in mRNA-protein correlation in human cells (Schwanhäusser et al., 2011). Also, the difference in decoding time determines the speed of polypeptide emergence and co-translational protein folding (Waudby et al., 2019). In other words, any change in the mRNA codons influences their average decoding time, the rate of ribosomal translocation, the rate of polypeptide emergence, and hence the folding probability of a protein toward its native state. Since tRNA availability influences the average decoding time, the rapid activity-induced translation response would require various tRNA species to be readily available within local compartments. Toward this end, the presence of tRNAs was detected within neuronal dendrites back in 1996 (Tiedge and Brosius, 1996). In addition, a more recent study has determined their kinetics inside neurons (Koltun et al., 2020). In this study, the exogenously labeled tRNAs introduced in the cortical neurons showed punctate structures. In general, the tRNA puncta were bidirectionally transported in the dendrites and a fraction of them (generally larger in size) could be destabilized with puromycin, a translation inhibitor that disengages elongating ribosomes, indicating they were part of actively translating complexes. However, chemical LTP induction led to a further increase in these large tRNA aggregation and potentially mRNA translation (Koltun et al., 2020).
Despite the advances, appreciating various dimensions of tRNA function in neuronal mRNA translation would require more information. In particular, how the concentration of individual tRNA species affects ribosomal processivity is not established clearly. Also, considering the differences in decoding time for various codons (Gardin et al., 2014), it would be compelling to probe whether mRNAs in a compartment show distinct codon usage patterns and whether the tRNAs for those codons are enriched in those locations. Finally, given the rising evidence of on-site ribosomal remodeling, one would be intrigued to know if the remodeled ribosomes prefer to bind specific tRNA species and translate mRNAs with distinct codon-usage patterns.
In cellulo Dynamics of Neuronal Ribosomes
Decades of work in biochemistry and insight from high-resolution structures have elucidated the founding principles of mRNA translation and ribosomal function. But, these approaches provide very little dynamic information in the cellular realm. More recent studies based on single-molecule tracking have been instrumental in describing the dynamic properties of ribosomes and mRNAs within cells (Volkov and Johansson, 2019). Both prokaryotic and eukaryotic ribosomes have been visualized by tagging RPs with fluorescent proteins (Katz et al., 2016; Bayas et al., 2018). These experiments have been critical in understanding the population behavior of ribosomes arising from thousands of single-molecule detection events. Especially, live tracking of ribosomal subunits allows correlating their dynamics to mRNA translation within a cell (Prabhakar et al., 2019; Volkov and Johansson, 2019). For example, single-molecule diffusion studies on E. coli ribosomes have found that while the subunits themselves can diffuse freely across the entire cell, elongating ribosomes are excluded from the nucleoid (Sanamrad et al., 2014). Besides, simultaneous tracking of mRNPs and ribosomal particles on mouse fibroblast lines has revealed that polysome-associated mRNPs move slower than the mRNPs alone. Ribosomes in these cells show two prominent diffusional states (Katz et al., 2016). While the majority display relatively less mobility, a smaller freely diffusing fraction moves more rapidly (Katz et al., 2016; Donlin-Asp et al., 2021). Interestingly, treatment with puromycin increases the mobility of both ribosomes and mRNPs in these cells. Another study noted a similar effect of puromycin treatment on the mRNP dynamics in neurons (Donlin-Asp et al., 2021). These results suggest that polyribosomes impede the movement of mRNP complexes by making them heavier while destabilizing them with puromycin increases their mobility (Katz et al., 2016). However, one problem with single-molecule experiments is that the fluorescent proteins coexpressed with the proteins of interest often show moderate brightness and photostability (Chudakov et al., 2010; Volkov and Johansson, 2019). In addition, the presence of a large number of subdiffraction ribosomal particles can complicate the detection and analysis of single-molecule trajectories (Volkov and Johansson, 2019). To this end, a combination of photoactivable or photoswitchable fluorescent proteins/fluorophores and imaging techniques that can overcome the diffraction barrier such as STED, RESOLFT, and PALM/STORM have enabled molecular tracking with relatively lesser complications (Manley et al., 2008; Nair et al., 2013; Shcherbakova et al., 2014; Kedia et al., 2021). For example, observations made through sptPALM trajectories in migrating mouse fibroblast cells describe that ribosomes near focal adhesions of the leading edge show much-confined movement compared to elsewhere in the cytosol. Since ribosomes tend to dwell more at the site of translation, such interchanges between diffusion states are envisioned to drive compartmentalization of protein synthesis (Katz et al., 2016).
Despite considerable progress, much of our understanding of the dynamics of individual ribosomes in neurons remain elusive. Considering the large variability in the mRNP composition, kinetics, and biochemical properties (Formicola et al., 2019; Tauber et al., 2020), it is necessary to probe how mRNP properties affect ribosomal movement and function. In the same context, it would be useful to generate a neuron-wide ribosomal mobility map with nanometer precision. In addition, compartments like dendritic spines or growth cones show non-homogeneous molecular distribution and organization (Frost et al., 2010; Igarashi, 2019). While functional zones like post-synaptic density (PSD) are protein-dense regions, other areas of a spine are more dynamic with varied molecular compositions (Kaizuka and Takumi, 2018; Venkatesan et al., 2020; Helm et al., 2021). Interestingly, similar to focal adhesions, PSD is also reported to associate with polyribosomes, RBPs and act as a platform of translation (Ostroff et al., 2017; Yoon et al., 2016; Zhang et al., 2012). However, ribosomal diffusion kinetics in and around PSD has not been examined yet. Using single-molecule localization microscopy (SMLM), it is now possible to track the mean square displacement of ribosomes and calculate their diffusion parameters from HMM-bayesian modeling. Given the strong correlation between ribosomal confinement and active translation, the analysis can reveal the spatial distribution of translation “hotspots” within spines in live neurons (Monnier et al., 2015; Katz et al., 2016; Volkov and Johansson, 2019; Figure 3). In addition, we are yet to know how neuronal stimulation may impact ribosomal localization and their dynamics within spines (Ostroff et al., 2017). As discussed before, synaptic molecules often organize into functional zones by forming nanoclusters. Signaling through these nanodomains is crucial for synaptic plasticity and protein synthesis (Nair et al., 2013; Goncalves et al., 2020; Sun et al., 2021). For example, GluA1 or GluA2 and PSD 95 nanodomains at the dendritic spines are the hubs of intracellular signaling. The efficacy of signaling depends on nanodomain sizes and the localization of receptors in these clusters (Nair et al., 2013; Tang et al., 2016; Nanguneri et al., 2019; Venkatesan et al., 2020). In addition, it is observed that within translationally active spines, PSD microdomains often associate with newly synthesized proteins which, in turn, are closely apposed to ribosomes (Sun et al., 2021). However, little is known whether the molecular organization of the PSD can sequester or trap ribosomes to initiate localized translation. Also, the precise nanometer level localization maps of receptors, PSD, ribosome distribution, and protein synthesis hotspots together are not available yet. These finer insights into spatio-temporal correlation and regulation of ribosomal functions would be valuable to understand the stochastic constraints that control local rates of translation. In parallel, direct visualization of single ribosomes and their kinetics can verify whether their sequestration at the PSD is necessary for localized translation. Perturbing nanodomain properties using pharmacochemical or optogenetic approaches followed by ribo-tracking can indeed delineate the relationship between the molecular organization, and protein synthesis at the individual spine level. Altogether, these approaches provide us with the opportunity to answer the previously underexplored questions of mRNA translation. They also allow us to appreciate the tremendous variability in ribosomal functions within neurons with unprecedented details.
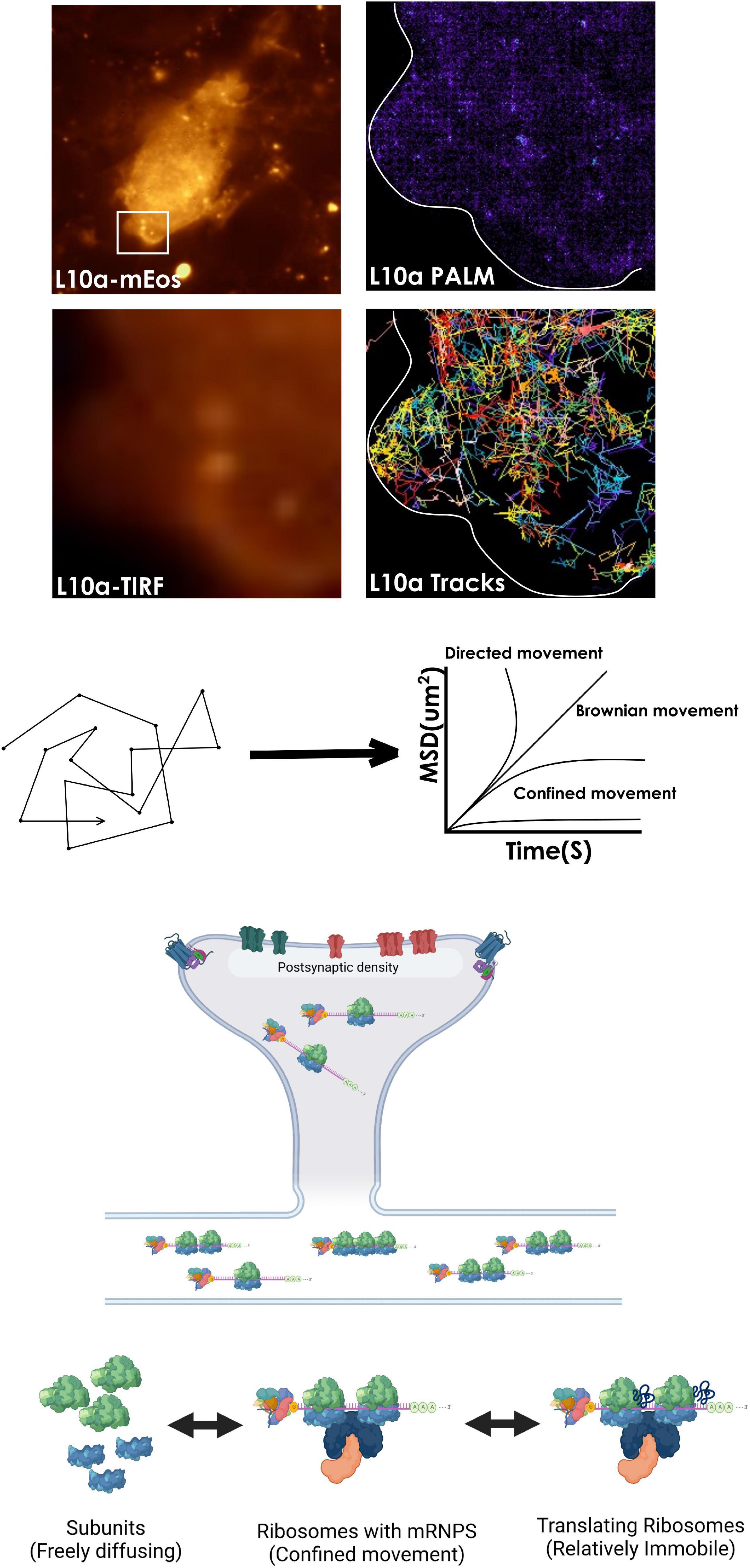
Figure 3. Tracking ribosomal mobility for locating “hotspots” of active translation. Visualizing and tracking ribosomal subunits at the single-particle level is possible by tagging a subunit protein such as L10a with photoactivable fluorescent proteins such as mEos within a cell. Analysis of their mean square displacement (MSD) trajectories of single protein molecules can then be used to calculate the diffusion coefficients of various ribosomal populations, that switch between diffusional states while functioning within cells. In addition, perturbations with pharmacological agents that destabilize ribosomes can confirm their engagement in active translation. Also, an HMM-based analysis of the trajectories can be used to reveal the mechanisms underpinning the conversion between diffusional states of ribosomes (not shown).
Concluding Remarks
Proteome remodeling is a critical component of the neuronal response to several incoming stimuli. The complex morphology of neurons requires the remodeling to be done locally in a compartmentalized manner. Together with various other mechanisms, ribosomal modulation (through biogenesis, sorting, local remodeling, and dynamic properties) provides a way to create variability in protein expression. However, the spatiotemporal kinetics of such changes and their relation with synaptic signaling remains to be determined. During memory formation, information is stored from shorter to longer time scales at the synapse. Yet the mechanistic connections between the various phases of memory formation remain to be uncovered. Considering signaling events and instantaneous molecular organizations encode information for a short period while protein synthesis dictates its long-term storage, a rigorous connection between the events of these two timescales can explain how memories are stored permanently from their shorter labile versions. With the emergence of suitable technologies with high spatial and temporal precisions, we are finally in a position to address some of these pressing questions.
Author Contributions
SD conceptualized and designed the manuscript. Both authors wrote and edited the manuscript and approved the submitted version.
Funding
This work was supported by Department of Biotechnology, Govt. of India grant (Department of Biotechnology Genomics Engineering Taskforce to DN), DBT-IISc Partnership program to DN, IISC-STAR program grant to DN, and Institute of Eminence grant from IISc to DN. Department of Biotechnology research associateship grant to SD.
Conflict of Interest
The authors declare that the research was conducted in the absence of any commercial or financial relationships that could be construed as a potential conflict of interest.
Publisher’s Note
All claims expressed in this article are solely those of the authors and do not necessarily represent those of their affiliated organizations, or those of the publisher, the editors and the reviewers. Any product that may be evaluated in this article, or claim that may be made by its manufacturer, is not guaranteed or endorsed by the publisher.
References
Adjaye, J., John, H., Ralf, H., BenKahla, A., Brink, T. C., Wierling, C., et al. (2005). “Primary Differentiation in the Human Blastocyst: Comparative Molecular Portraits of Inner Cell Mass and Trophectoderm Cells.”. Stem Cells 23, 1514–1525. doi: 10.1634/stemcells.2005-0113
Allen, K. D., Regier, M. J., Hsieh, C., Tsokas, P., Barnard, M., Phatarpekar, S., et al. (2018). “Learning-Induced Ribosomal RNA Is Required for Memory Consolidation in Mice—Evidence of Differentially Expressed rRNA Variants in Learning and Memory.” Edited by Michal Hetman. PLoS One 13:e0203374. doi: 10.1371/journal.pone.0203374
Ballanyi, K., Bruggencate, T., Grafe, P., and Reddy, M. M. (1984). “Different types of potassium transport linked to carbachol and y-aminobutyric acid actions in rat sympathetic neurons.”. Neuroscience 12, 917–927. doi: 10.1016/0306-4522(84)90179-9
Bassell, G. J., Zhang, H., Byrd, A. L., Femino, A. M., Singer, R. H., Taneja, K. L., et al. (1998). “Sorting of beta-actin mRNA and protein to neurites and growth cones in culture,”. J. Neurosci. 18, 251–265. doi: 10.1523/JNEUROSCI.18-01-00251.1998
Baßler, J., and Hurt, E. (2019). “Eukaryotic Ribosome Assembly.”. Annu. Rev. Biochem. 88, 281–306. doi: 10.1146/annurev-biochem-013118-110817
Bayas, C. A., Wang, J., Lee, M. K., Schrader, J. M., Shapiro, L., and Moerner, W. E. (2018). “Spatial Organization and Dynamics of RNase E and Ribosomes in Caulobacter Crescentus.”. Proc. Natl. Acad. Sci. 115, E3712–E3721. doi: 10.1073/pnas.1721648115
Bear, M. F., Huber, K. M., and Warren, S. T. (2004). “The mGluR Theory of Fragile X Mental Retardation.”. Trends Neurosci. 27, 370–377. doi: 10.1016/j.tins.2004.04.009
Behnen, P., Davis, E., Delaney, E., Frohm, B., Bauer, M., Cedervall, T., et al. (2012). “Calcium-Dependent Interaction of Calmodulin with Human 80S Ribosomes and Polyribosomes.”. Biochemistry 51, 6718–6727. doi: 10.1021/bi3005939
Belin, S., Hacot, S., Daudignon, L., Therizols, G., Pourpe, S., Mertani, H. C., et al. (2010). “Purification of Ribosomes from Human Cell Lines.”. Curr. Protoc. Cell Biol. 49:cb0340s49. doi: 10.1002/0471143030.cb0340s49
Biever, A., Donlin-Asp, P. G., and Schuman, E. M. (2019). “Local Translation in Neuronal Processes.”. Curr. Opin. Neurobiol. 57, 141–148. doi: 10.1016/j.conb.2019.02.008
Biever, A., Glock, C., Tushev, G., Ciirdaeva, E., Dalmay, T., Langer, J. D., et al. (2020). “Monosomes Actively Translate Synaptic mRNAs in Neuronal Processes.”. Science 367:eaay4991. doi: 10.1126/science.aay4991
Boisvert, F., Koningsbruggen, S. V., Navascués, J., and Lamond, A. I. (2007). “The Multifunctional Nucleolus.”. Nat. Rev. Mol. Cell Biol. 8, 574–585. doi: 10.1038/nrm2184
Bortoluzzi, S., D’Alessi, F., Romualdi, C., and Danieli, G. A. (2001). “Differential Expression of Genes Coding for Ribosomal Proteins in Different Human Tissues.”. Bioinformatics 17, 1152–1157. doi: 10.1093/bioinformatics/17.12.1152
Brigidi, G. S., Hayes, M. G. B., Santos, N. P. D., Hartzell, A. L., Texari, L., Lin, P., et al. (2019). “Genomic Decoding of Neuronal Depolarization by Stimulus-Specific NPAS4 Heterodimers.”. Cell 179, 373.e–391.e. doi: 10.1016/j.cell.2019.09.004
Cajigas, I. J., Tushev, G., Will, T. J., Dieck, S. T., Fuerst, N., and Schuman, E. M. (2012). “The Local Transcriptome in the Synaptic Neuropil Revealed by Deep Sequencing and High-Resolution Imaging.”. Neuron 74, 453–466. doi: 10.1016/j.neuron.2012.02.036
Ceci, M., Welshhans, K., Ciotti, M. T., Brandi, R., Parisi, C., Paoletti, F., et al. (2012). “RACK1 Is a Ribosome Scaffold Protein for β-Actin mRNA/ZBP1 Complex.”. PLoS One 7:e35034. doi: 10.1371/journal.pone.0035034
Chard, P. S., Jordan, J., Marcuccilli, C. J., Miller, R. J., Leiden, J. M., Roos, R. P., et al. (1995). “Regulation of Excitatory Transmission at Hippocampal Synapses by Calbindin D28k.”. Proc. Natl. Acad. Sci. 92, 5144–5148. doi: 10.1073/pnas.92.11.5144
Chau, K. F., Shannon, M. L., Fame, R. M., Fonseca, E., Mullan, H., Johnson, M. B., et al. (2018). “Downregulation of Ribosome Biogenesis during Early Forebrain Development.”. ELife 7:e36998. doi: 10.7554/eLife.36998
Chin, K. V., Cade, C., Brostrom, C. O., Galuska, E. M., and Brostrom, M. A. (1987). “Calcium-Dependent Regulation of Protein Synthesis at Translational Initiation in Eukaryotic Cells.”. J. Biol. Chem. 262, 16509–16514. doi: 10.1016/S0021-9258(18)49285-X
Choi, J., Ieong, K., Demirci, H., Chen, J., Petrov, A., Prabhakar, A., et al. (2016). “N6-Methyladenosine in mRNA Disrupts tRNA Selection and Translation-Elongation Dynamics.”. Nat. Struct. Mol. Biol. 23, 110–115. doi: 10.1038/nsmb.3148
Chudakov, D. M., Matz, M. V., Lukyanov, S., and Lukyanov, K. A. (2010). “Fluorescent Proteins and Their Applications in Imaging Living Cells and Tissues.”. Physiol. Rev. 90, 1103–1163. doi: 10.1152/physrev.00038.2009
Cioni, J., Lin, J. Q., Holtermann, A. V., Koppers, M., Jakobs, M. A. H., Azizi, A., et al. (2019). Late Endosomes Act as mRNA Translation Platforms and Sustain Mitochondria in Axons. Cell 176, 56.e–72.e. doi: 10.1016/j.cell.2018.11.030
Costa, R. O., Martins, H., Martins, L. F., Cwetsch, A. W., Mele, M., Pedro, J. R., et al. (2019). Synaptogenesis Stimulates a Proteasome-Mediated Ribosome Reduction in Axons. Cell Rep. 28, 864.e–876.e. doi: 10.1016/j.celrep.2019.06.080
Court, F. A., Hendriks, W. T. J., MacGillavry, H. D., Alvarez, J., and Minnen, J. V. (2008). “Schwann Cell to Axon Transfer of Ribosomes: Toward a Novel Understanding of the Role of Glia in the Nervous System.”. J. Neurosci. 28, 11024–11029. doi: 10.1523/Jneurosci.2429-08.2008
Cui-Wang, T., Hanus, C., Cui, T., Helton, T., Bourne, J., Watson, D., et al. (2012). “Local Zones of Endoplasmic Reticulum Complexity Confine Cargo in Neuronal Dendrites.”. Cell 148, 309–321. doi: 10.1016/j.cell.2011.11.056
D’Souza, M. N., Gowda, N. K. C., Tiwari, V., Babu, R. O., Anand, P., Dastidar, S. G., et al. (2018). “FMRP Interacts with C/D Box snoRNA in the Nucleus and Regulates Ribosomal RNA Methylation.”. IScience 9, 399–411. doi: 10.1016/j.isci.2018.11.007
Dastidar, S. G., Sharma, S. D., Chakraborty, S., Chattarji, S., Bhattacharya, A., and Muddashetty, R. S. (2020). Distinct Regulation of Bioenergetics and Translation by Group I mGluR and NMDAR. EMBO Rep. 2020:201948037. doi: 10.15252/embr.201948037
Datta, S., and Seed, B. (2018). “Influence of Multiplicative Stochastic Variation on Translational Elongation Rates.”. PLoS One 13:e0191152. doi: 10.1371/journal.pone.0191152
Deshpande, P., Flinkman, D., Hong, Y., Goltseva, E., Siino, V., Sun, L., et al. (2020). “Protein Synthesis Is Suppressed in Sporadic and Familial Parkinson’s Disease by LRRK2.”. FASEB J. 34, 14217–14233. doi: 10.1096/fj.202001046R
Donlin-Asp, P. G., Polisseni, C., Klimek, R., Heckel, A., and Schuman, E. M. (2021). “Differential Regulation of Local mRNA Dynamics and Translation Following Long-Term Potentiation and Depression.”. Proc. Natl. Acad. Sci. 118:e2017578118. doi: 10.1073/pnas.2017578118
Fallini, C., Bassell, G. J., and Rossoll, W. (2012). “Spinal Muscular Atrophy: The Role of SMN in Axonal mRNA Regulation.”. Brain Res. 1462, 81–92. doi: 10.1016/j.brainres.2012.01.044
Fallini, C., Donlin-Asp, P. G., Rouanet, J. P., Bassell, G. J., and Rossoll, W. (2016). Deficiency of the Survival of Motor Neuron Protein Impairs mRNA Localization and Local Translation in the Growth Cone of Motor Neurons. J. Neurosci. 36, 3811–3820. doi: 10.1523/JNEUROSCI.2396-15.2016
Fernandopulle, M. S., Lippincott-Schwartz, J., and Ward, M. E. (2021). “RNA Transport and Local Translation in Neurodevelopmental and Neurodegenerative Disease.”. Nat. Neurosci. 24, 622–632. doi: 10.1038/s41593-020-00785-2
Ferretti, M. B., and Karbstein, K. (2019). “Does Functional Specialization of Ribosomes Really Exist?”. RNA 25, 521–538. doi: 10.1261/rna.069823.118
Flamand, M. N., and Meyer, K. D. (2019). “The Epitranscriptome and Synaptic Plasticity.”. Curr. Opin. Neurobiol. 59, 41–48. doi: 10.1016/j.conb.2019.04.007
Formicola, N., Vijayakumar, J., and Besse, F. (2019). Neuronal Ribonucleoprotein Granules: Dynamic Sensors of Localized Signals. Traffic 2019:12672. doi: 10.1111/tra.12672
Frost, N. A., Shroff, H., Kong, H., Betzig, E., and Blanpied, T. A. (2010). “Single-Molecule Discrimination of Discrete Perisynaptic and Distributed Sites of Actin Filament Assembly within Dendritic Spines.”. Neuron 67, 86–99. doi: 10.1016/j.neuron.2010.05.026
Fusco, C. M., Desch, K., Dörrbaum, A. R., Wang, M., Staab, A., Chan, I. C. W., et al. (2021). “Neuronal Ribosomes Exhibit Dynamic and Context-Dependent Exchange of Ribosomal Proteins.”. Nat. Commun. 12:6127. doi: 10.1038/s41467-021-26365-x
Gardin, J., Yeasmin, R., Yurovsky, A., Cai, Y., Skiena, S., and Futcher, et al.(2014). “Measurement of Average Decoding Rates of the 61 Sense Codons in Vivo.”. ELife 3:e03735. doi: 10.7554/eLife.03735
Gay, D. M., Lund, A. H., and Jansson, M. D. (2022). “Translational Control through Ribosome Heterogeneity and Functional Specialization.”. Trends Biochem. Sci. 47, 66–81. doi: 10.1016/j.tibs.2021.07.001
Genuth, N. R., and Barna, M. (2018). “The Discovery of Ribosome Heterogeneity and Its Implications for Gene Regulation and Organismal Life.”. Mol. Cell 71, 364–374. doi: 10.1016/j.molcel.2018.07.018
Giuditta, A., Cupellot, A., and Lazzarini, G. (1980). “Ribosomal RNA in the Axoplasm of the Squid Giant Axon.”. J. Neurochem. 34, 1757–1760. doi: 10.1111/j.1471-4159.1980.tb11271.x
Giuditta, A., Dettbarn, W. D., and Brzin, M. (1968). “Protein Synthesis in the Isolated Giant Axon of the Squid.”. Proc. Natl. Acad. Sci. 59, 1284–1287. doi: 10.1073/pnas.59.4.1284
Giuditta, A., Tim, H., and Luigia, S. (1986). “Rapid Important Paper.”. Neurochem. Int. 8, 435–442. doi: 10.1016/0197-0186(86)90019-7
Goncalves, J., Bartol, T. M., Camus, C., Levet, F., Menegolla, A. P., Sejnowski, T. J., et al. (2020). “Nanoscale Co-Organization and Coactivation of AMPAR, NMDAR, and mGluR at Excitatory Synapses.”. Proc. Natl. Acad. Sci. 117, 14503–14511. doi: 10.1073/pnas.1922563117
Gordon, J., and Lipmann, F. (1967). “Role of Divalent Ions in Poly U-Directed Phenylalanine Polymerization.”. J. Mol. Biol. 23, 23–33. doi: 10.1016/S0022-2836(67)80064-0
Hafner, A., Donlin-Asp, P. G., Leitch, B., Herzog, E., and Schuman, E. M. (2019). Local Protein Synthesis Is a Ubiquitous Feature of Neuronal Pre- and Postsynaptic Compartments. Science 364:eaau3644. doi: 10.1126/science.aau3644
Hanus, C., and Schuman, E. M. (2013). “Proteostasis in Complex Dendrites.”. Nat. Rev. Neurosci. 14, 638–648. doi: 10.1038/nrn3546
Helm, M. S., Dankovich, T. M., Mandad, S., Rammner, B., Jähne, S., Salimi, V., et al. (2021). “A Large-Scale Nanoscopy and Biochemistry Analysis of Postsynaptic Dendritic Spines.”. Nat. Neurosci. 24, 1151–1162. doi: 10.1038/s41593-021-00874-w
Hernandez, A. I., Wolk, J., Hu, J.-Y., Liu, J., Kurosu, T., Schwartz, J. H., et al. (2009). Poly-(ADP-Ribose) Polymerase-1 Is Necessary for Long-Term Facilitation in Aplysia. J. Neurosci. 29, 9553–9562.
Hetman, M., and Slomnicki, L. P. (2019). “Ribosomal Biogenesis as an Emerging Target of Neurodevelopmental Pathologies.”. J. Neurochem. 148, 325–347. doi: 10.1111/jnc.14576
Hill, S. E., Parmar, M., Gheres, K. W., Guignet, M. A., Huang, Y., Jackson, F., et al. (2012). “Development of Dendrite Polarity in Drosophila Neurons.”. Neural Dev. 7:34. doi: 10.1186/1749-8104-7-34
Hirokawa, N., Niwa, S., and Tanaka, Y. (2010). “Molecular Motors in Neurons: Transport Mechanisms and Roles in Brain Function, Development, and Disease.”. Neuron 68, 610–638. doi: 10.1016/j.neuron.2010.09.039
Holt, C. E., and Schuman, E. M. (2013). “The Central Dogma Decentralized: New Perspectives on RNA Function and Local Translation in Neurons.”. Neuron 80, 648–657. doi: 10.1016/j.neuron.2013.10.036
Holt, C. E., Martin, K. C., and Schuman, E. M. (2019). “Local Translation in Neurons: Visualization and Function.”. Nat. Struct. Mol. Biol. 26, 557–566. doi: 10.1038/s41594-019-0263-5
Hsu, W., Chung, H., Wu, C., Wu, H., Lee, Y., Chen, E., et al. (2015). “Glutamate Stimulates Local Protein Synthesis in the Axons of Rat Cortical Neurons by Activating α-Amino-3-Hydroxy-5-Methyl-4-Isoxazolepropionic Acid (AMPA) Receptors and Metabotropic Glutamate Receptors.”. J. Biol. Chem. 290, 20748–20760. doi: 10.1074/jbc.M115.638023
Hyson, R. L., and Rubel, E. W. (1995). “Activity-Dependent Regulation of a Ribosomal RNA Epitope in the Chick Cochlear Nucleus.”. Brain Res. 672, 196–204. doi: 10.1016/0006-8993(94)01390-4
Igarashi, M. (2019). “Molecular Basis of the Functions of the Mammalian Neuronal Growth Cone Revealed Using New Methods.”. Proc. Jap. Acad. Ser. B 95, 358–377. doi: 10.2183/pjab.95.026
Jackson, R. J., Hellen, C. U. T., and Pestova, T. V. (2010). “The Mechanism of Eukaryotic Translation Initiation and Principles of Its Regulation.”. Nat. Rev. Mol. Cell Biol. 11, 113–127. doi: 10.1038/nrm2838
Jang, S., Nelson, J. C., Bend, E. G., Rodríguez-Laureano, L., Tueros, F. G., Cartagenova, L., et al. (2016). “Glycolytic Enzymes Localize to Synapses under Energy Stress to Support Synaptic Function.”. Neuron 90, 278–291. doi: 10.1016/j.neuron.2016.03.011
Job, C., and Eberwine, J. (2001). “Identification of Sites for Exponential Translation in Living Dendrites.”. Proc. Natl. Acad. Sci. 98, 13037–13042. doi: 10.1073/pnas.231485698
Jordan, B. A., Fernholz, B. D., Khatri, L., and Ziff, E. B. (2007). “Activity-Dependent AIDA-1 Nuclear Signaling Regulates Nucleolar Numbers and Protein Synthesis in Neurons.”. Nat. Neurosci. 10, 427–435. doi: 10.1038/nn1867
Kaizuka, T., and Takumi, T. (2018). “Postsynaptic Density Proteins and Their Involvement in Neurodevelopmental Disorders.”. J. Biochem. 163, 447–455. doi: 10.1093/jb/mvy022
Kapur, M., Monaghan, C. E., and Ackerman, S. L. (2017). “Regulation of mRNA Translation in Neurons—A Matter of Life and Death.”. Neuron 96, 616–637. doi: 10.1016/j.neuron.2017.09.057
Katz, Z. B., English, B. P., Lionnet, T., Yoon, Y. J., Monnier, N., Ovryn, B., et al. (2016). “Mapping Translation ‘hot-Spots’ in Live Cells by Tracking Single Molecules of mRNA and Ribosomes.”. ELife 5:e10415. doi: 10.7554/eLife.10415
Kedia, S., Ramakrishna, P., Netrakanti, P. R., Singh, N., Sisodia, S. S., Jose, M., et al. (2021). “Alteration in Synaptic Nanoscale Organization Dictates Amyloidogenic Processing in Alzheimer’s Disease.”. IScience 24:101924. doi: 10.1016/j.isci.2020.101924
Khatter, H., Myasnikov, A. G., Natchiar, S. K., and Klaholz, B. P. (2015). “Structure of the Human 80S Ribosome.”. Nature 520, 640–645. doi: 10.1038/nature14427
Kim, S., and Martin, K. C. (2015). “Neuron-Wide RNA Transport Combines with Netrin-Mediated Local Translation to Spatially Regulate the Synaptic Proteome.”. ELife 4:e04158. doi: 10.7554/eLife.04158
Kleim, J. A., Bruneau, R., Calder, K., Pocock, D., Vandenberg, P. M., MacDonald, E., et al. (2003). “Functional Organization of Adult Motor Cortex Is Dependent upon Continued Protein Synthesis.”. Neuron 40, 167–176. doi: 10.1016/S0896-6273(03)00592-0
Koltun, B., Ironi, S., Gershoni-Emek, N., Barrera, I., Hleihil, M., Nanguneri, S., et al. (2020). “Measuring mRNA Translation in Neuronal Processes and Somata by tRNA-FRET.”. Nucleic Acids Res. 48, e32–e32. doi: 10.1093/nar/gkaa042
Komar, A. A. (2016). The Yin and Yang of Codon Usage. Huma. Mol. Genet. 25, R77–R85. doi: 10.1093/hmg/ddw207
Kondrashov, N., Pusic, A., Stumpf, C. R., Shimizu, K., Hsieh, A. C., Xue, S., et al. (2011). “Ribosome-Mediated Specificity in Hox mRNA Translation and Vertebrate Tissue Patterning.”. Cell 145, 383–397. doi: 10.1016/j.cell.2011.03.028
Koppers, M., Cagnetta, S., Shigeoka, T., Wunderlich, L. C. S., Vallejo-Ramirez, P., Lin, J. Q., et al. (2019). “Receptor-Specific Interactome as a Hub for Rapid Cue-Induced Selective Translation in Axons.”. ELife 8:e48718. doi: 10.7554/eLife.48718
Kos, A., Wanke, K. A., Gioio, A., Martens, G. J., Kaplan, B. B., and Aschrafi, A. (2016). “Monitoring mRNA Translation in Neuronal Processes Using Fluorescent Non-Canonical Amino Acid Tagging.”. J. Histochem. Cytochem. 64, 323–333. doi: 10.1369/0022155416641604
Kuzniewska, B., Cysewski, D., Wasilewski, M., Sakowska, P., Milek, J., Kulinski, T. M., et al. (2020). Mitochondrial Protein Biogenesis in the Synapse Is Supported by Local Translation. EMBO Rep. 2020:201948882. doi: 10.15252/embr.201948882
Lafarga, M., Andres, M. A., Fernandez-Viadero, C., Villegas, J., and Berciano, M. T. (1995). “Number of Nucleoli and Coiled Bodies and Distribution of Fibrillar Centres in Differentiating Purkinje Neurons of Chick and Rat Cerebellum.”. Anat. Embryol. 191, 359–367. doi: 10.1007/BF00534689
Li, D., and Wang, J. (2020). Ribosome heterogeneity in stem cells and development. J. Cell Biol. 219:e202001108. doi: 10.1083/jcb.202001108
Li, D., Zhang, J., Wang, M., Li, X., Gong, H., Tang, H., et al. (2018). “Activity-Dependent LoNA Regulates Translation by Coordinating rRNA Transcription and Methylation.”. Nat. Commun. 9:1726. doi: 10.1038/s41467-018-04072-4
Li, J., Yang, X., Qi, Z., Sang, Y., Liu, Y., Xu, B., et al. (2019). “The Role of mRNA M6A Methylation in the Nervous System.”. Cell Biosci. 9:66. doi: 10.1186/s13578-019-0330-y
Li, Z., Okamoto, K., Hayashi, Y., and Sheng, M. (2004). “The Importance of Dendritic Mitochondria in the Morphogenesis and Plasticity of Spines and Synapses.”. Cell 119, 873–887. doi: 10.1016/j.cell.2004.11.003
Loenarz, C., Sekirnik, R., Thalhammer, A., Ge, W., Spivakovsky, E., Mackeen, M. M., et al. (2014). “Hydroxylation of the Eukaryotic Ribosomal Decoding Center Affects Translational Accuracy.”. Proc. Natl. Acad. Sci. 111, 4019–4024. doi: 10.1073/pnas.1311750111
Ma, T. (2020). “Dysregulation of Neuronal Protein Synthesis in Alzheimer’s Disease,” in The Oxford Handbook of Neuronal Protein Synthesis, by Tao Ma, ed. W. Sossin (Oxford: Oxford University Press), doi: 10.1093/oxfordhb/9780190686307.013.18
Manley, S., Gillette, J. M., Patterson, G. H., Shroff, H., Hess, H. F., Betzig, E., et al. (2008). High-Density Mapping of Single-Molecule Trajectories with Photoactivated Localization Microscopy. Nat. Methods 5, 155–157. doi: 10.1038/nmeth.1176
Marinangeli, C., Didier, S., Ahmed, T., Caillerez, R., Domise, M., Laloux, C., et al. (2018). “AMP-Activated Protein Kinase Is Essential for the Maintenance of Energy Levels during Synaptic Activation.”. IScience 9, 1–13. doi: 10.1016/j.isci.2018.10.006
Martin, I., Dawson, V. L., and Dawson, T. M. (2011). “Recent Advances in the Genetics of Parkinson’s Disease.”. Annu. Rev. Genomics Hum. Genet. 12, 301–325. doi: 10.1146/annurev-genom-082410-101440
Martin, K. C. (2010). “Anchoring Local Translation in Neurons.”. Cell 141, 566–568. doi: 10.1016/j.cell.2010.04.031
Mateju, D., Eichenberger, B., Voigt, F., Eglinger, J., Roth, G., and Chao, J. A. (2020). Single-Molecule Imaging Reveals Translation of mRNAs Localized to Stress Granules. Cell 183, 1801.e–1812.e. doi: 10.1016/j.cell.2020.11.010
Mauro, V. P., and Edelman, G. M. (2002). “The Ribosome Filter Hypothesis.”. Proc. Natl. Acad. Sci. 99, 12031–12036. doi: 10.1073/pnas.192442499
Merianda, T. T., Lin, A. C., Lam, J. S. Y., Vuppalanchi, D., Willis, D. E., Karin, N., et al. (2009). “A Functional Equivalent of Endoplasmic Reticulum and Golgi in Axons for Secretion of Locally Synthesized Proteins.”. Mol. Cell. Neurosci. 40, 128–142. doi: 10.1016/j.mcn.2008.09.008
Merkurjev, D., Hong, W., Iida, K., Oomoto, I., Goldie, B. J., Yamaguti, H., et al. (2018). “Synaptic N6-Methyladenosine (M6A) Epitranscriptome Reveals Functional Partitioning of Localized Transcripts.”. Nat. Neurosci. 21, 1004–1014. doi: 10.1038/s41593-018-0173-6
Miyashiro, K. Y., Beckel-Mitchener, A., Park, T. P., Becker, K. G., Barret, T., Liu, L., et al. (2003). “RNA Cargoes Associating with FMRP Reveal Deficits in Cellular Functioning in Fmr1 Null Mice.”. Neuron 37, 417–431. doi: 10.1016/S0896-6273(03)00034-5
Mofatteh, M. (2020). “mRNA Localization and Local Translation in Neurons.”. AIMS Neurosci. 7, 299–310. doi: 10.3934/Neuroscience.2020016
Monnier, N., Barry, Z., Park, H. Y., Su, K., Katz, Z., English, B. P., et al. (2015). “Inferring Transient Particle Transport Dynamics in Live Cells.”. Nat. Methods 12, 838–840. doi: 10.1038/nmeth.3483
Müller, M., and Somjen, G. G. (2000). “Na + and K + Concentrations, Extra- and Intracellular Voltages, and the Effect of TTX in Hypoxic Rat Hippocampal Slices.”. J. Neurophysiol. 83, 735–745. doi: 10.1152/jn.2000.83.2.735
Mundhenk, J., Fusi, C., and Kreutz, M. R. (2019). “Caldendrin and Calneurons—EF-Hand CaM-Like Calcium Sensors With Unique Features and Specialized Neuronal Functions.”. Front. Mol. Neurosci. 12:16. doi: 10.3389/fnmol.2019.00016
Nagano, S., Jinno, J., Abdelhamid, R. F., Jin, Y., Shibata, M., Watanabe, S., et al. (2020). “TDP-43 Transports Ribosomal Protein mRNA to Regulate Axonal Local Translation in Neuronal Axons.”. Acta Neuropathol. 140, 695–713. doi: 10.1007/s00401-020-02205-y
Nair, D., Hosy, E., Petersen, J. D., Constals, A., Giannone, G., Choquet, D., et al. (2013). Super-Resolution Imaging Reveals That AMPA Receptors Inside Synapses Are Dynamically Organized in Nanodomains Regulated by PSD95. J. Neurosci. 33, 13204–13224. doi: 10.1523/JNEUROSCI.2381-12.2013
Nanguneri, S., Pramod, R. T., Efimova, N., Das, D., Jose, M., Svitkina, T., et al. (2019). Characterization of Nanoscale Organization of F-Actin in Morphologically Distinct Dendritic Spines In Vitro Using Supervised Learning. Eneuro 6, ENEURO.425–ENEURO.418. doi: 10.1523/ENEURO.0425-18.2019
Neelagandan, N., Lamberti, I., Carvalho, H. J. F., Gobet, C., and Naef, F. (2020). “What Determines Eukaryotic Translation Elongation: Recent Molecular and Quantitative Analyses of Protein Synthesis.”. Open Biol. 10:200292. doi: 10.1098/rsob.200292
Nesterchuk, M. V., Sergiev, P. V., and Dontsova, O. A. (2011). “Posttranslational Modifications of Ribosomal Proteins in Escherichia Coli.”. Acta Naturae 3, 22–33. doi: 10.32607/20758251-2011-3-2-22-33
Nierhaus, K. H. (2014). “Mg2+, K+, and the Ribosome.”. J. Bacteriol. 196, 3817–3819. doi: 10.1128/JB.02297-14
Oddo, S. (2012). “The Role of mTOR Signaling in Alzheimer Disease.”. Front. Biosci. 4:941–952. doi: 10.2741/s310
Ostroff, L. E., Botsford, B., Gindina, S., Cowansage, K. K., LeDoux, J. E., Klann, E., et al. (2017). Accumulation of Polyribosomes in Dendritic Spine Heads, But Not Bases and Necks, during Memory Consolidation Depends on Cap-Dependent Translation Initiation. J. Neurosci. 37, 1862–1872. doi: 10.1523/JNEUROSCI.3301-16.2017
Ostroff, L. E., Fiala, J. C., Allwardt, B., and Harris, K. M. (2002). “Polyribosomes Redistribute from Dendritic Shafts into Spines with Enlarged Synapses during LTP in Developing Rat Hippocampal Slices.”. Neuron 35, 535–545. doi: 10.1016/S0896-6273(02)00785-7
Ostroff, L. E., Watson, D. J., Cao, G., Parker, P. H., Smith, H., and Harris, K. M. (2018). “Shifting Patterns of Polyribosome Accumulation at Synapses over the Course of Hippocampal Long-Term Potentiation.”. Hippocampus 28, 416–430. doi: 10.1002/hipo.22841
Pangršič, T., Gabrielaitis, M., Michanski, S., Schwaller, B., Wolf, F., Strenzke, N., et al. (2015). “EF-Hand Protein Ca 2+ Buffers Regulate Ca 2+ Influx and Exocytosis in Sensory Hair Cells.”. Proc. Natl. Acad. Sci. 112, E1028–E1037. doi: 10.1073/pnas.1416424112
Polikanov, Y. S., Melnikov, S. V., Söll, D., and Steitz, T. A. (2015). “Structural Insights into the Role of RNA Modifications in Protein Synthesis and Ribosome Assembly.”. Nat. Struct. Mol. Biol. 22, 342–344. doi: 10.1038/nsmb.2992
Pontes, M. H., Sevostyanova, A., and Groisman, E. A. (2015). “When Too Much ATP Is Bad for Protein Synthesis.”. J. Mol. Biol. 427, 2586–2594. doi: 10.1016/j.jmb.2015.06.021
Poon, M. M., Choi, S., Jamieson, C. A. M., Geschwind, D. H., and Martin, K. C. (2006). Identification of Process-Localized mRNAs from Cultured Rodent Hippocampal Neurons. J. Neurosci. 26, 13390–13399. doi: 10.1523/JNEUROSCI.3432-06.2006
Prabhakar, A., Puglisi, E. V., and Puglisi, J. D. (2019). “Single-Molecule Fluorescence Applied to Translation.”. Cold Spring Harb. Perspect. Biol. 11:a032714. doi: 10.1101/cshperspect.a032714
Quax, T. E. F., Claassens, N. J., Söll, D., and Oost, J. V. D. (2015). “Codon Bias as a Means to Fine-Tune Gene Expression.”. Mol. Cell 59, 149–161. doi: 10.1016/j.molcel.2015.05.035
Ramakrishnan, V. (2002). “Ribosome Structure and the Mechanism of Translation.”. Cell 108, 557–572. doi: 10.1016/S0092-8674(02)00619-0
Rangaraju, V., Dieck, S. T., and Schuman, E. M. (2017). “Local Translation in Neuronal Compartments: How Local Is Local?”. EMBO Rep. 18, 693–711. doi: 10.15252/embr.201744045
Rangaraju, V., Lauterbach, M., and Schuman, E. M. (2019). Spatially Stable Mitochondrial Compartments Fuel Local Translation during Plasticity. Cell 176, 73.e–84.e. doi: 10.1016/j.cell.2018.12.013
Reid, D. W., and Nicchitta, C. V. (2015). “Diversity and Selectivity in mRNA Translation on the Endoplasmic Reticulum.”. Nat. Rev. Mol. Cell Biol. 16, 221–231. doi: 10.1038/nrm3958
Richards, M. (2004). “The Transcriptome Profile of Human Embryonic Stem Cells as Defined by SAGE.”. Stem Cells 22, 51–64. doi: 10.1634/stemcells.22-1-51
Rolls, M. M., Hall, D. H., Victor, M., Stelzer, E. H. K., Tom, A., and Rapoport. (2002). “Targeting of Rough Endoplasmic Reticulum Membrane Proteins and Ribosomes in Invertebrate Neurons.”. Mol. Biol. Cell 13, 1778–1791. doi: 10.1091/mbc.01-10-0514
Romani, A. M. P. (2011). “Cellular Magnesium Homeostasis.”. Arch. Biochem. Biophys. 512, 1–23. doi: 10.1016/j.abb.2011.05.010
Rozov, A., Khusainov, I., Omari, K. E., Duman, R., Mykhaylyk, V., Yusupov, M., et al. (2019). “Importance of Potassium Ions for Ribosome Structure and Function Revealed by Long-Wavelength X-Ray Diffraction.”. Nat. Commun. 10:2519. doi: 10.1038/s41467-019-10409-4
Sanamrad, A., Persson, F., Lundius, E. G., Fange, D., Gynna, A. H., and Elf, J. (2014). “Single-Particle Tracking Reveals That Free Ribosomal Subunits Are Not Excluded from the Escherichia Coli Nucleoid.”. Proc. Natl. Acad. Sci. 111, 11413–11418. doi: 10.1073/pnas.1411558111
Scarnati, M. S., Kataria, R., Biswas, M., and Paradiso, K. G. (2018). “Active Presynaptic Ribosomes in the Mammalian Brain, and Altered Transmitter Release after Protein Synthesis Inhibition.”. ELife 7:e36697. doi: 10.7554/eLife.36697
Scavuzzo, C. J., LeBlancq, M. J., Nargang, F., Lemieux, H., Hamilton, T. J., and Dickson, C. T. (2019). “The Amnestic Agent Anisomycin Disrupts Intrinsic Membrane Properties of Hippocampal Neurons via a Loss of Cellular Energetics.”. J. Neurophysiol. 122, 1123–1135. doi: 10.1152/jn.00370.2019
Schwanhäusser, B., Busse, D., Li, N., Dittmar, G., Schuchhardt, J., Wolf, J., et al. (2011). “Global Quantification of Mammalian Gene Expression Control.”. Nature 473, 337–342. doi: 10.1038/nature10098
Seidenbecher, C. I., Langnaese, K., Sanmartıì-Vila, L., Boeckers, T. M., Smalla, K., Sabel, B. A., et al. (1998). Caldendrin, a Novel Neuronal Calcium-Binding Protein Confined to the Somato-Dendritic Compartment. J. Biol. Chem. 273, 21324–21331. doi: 10.1074/jbc.273.33.21324
Sen Gupta, A., Joshi, G., Pawar, S., and Sengupta, K. (2018). “Nucleolin Modulates Compartmentalization and Dynamics of Histone 2B-ECFP in the Nucleolus.”. Nucleus 9, 350–367. doi: 10.1080/19491034.2018.1471936
Sharma, A. V., Nargang, F. E., and Dickson, C. T. (2012). Neurosilence: Profound Suppression of Neural Activity Following Intracerebral Administration of the Protein Synthesis Inhibitor Anisomycin. J. Neurosci. 32, 2377–2387. doi: 10.1523/JNEUROSCI.3543-11.2012
Sharov, A. A., Piao, Y., Matoba, R., Dudekula, D. D., Qian, Y., VanBuren, C., et al. (2003). “Transcriptome Analysis of Mouse Stem Cells and Early Embryos.”. PLoS Biol. 1:e74. doi: 10.1371/journal.pbio.0000074
Shcherbakova, D. M., Sengupta, P., Lippincott-Schwartz, J., and Verkhusha, V. V. (2014). “Photocontrollable Fluorescent Proteins for Superresolution Imaging.”. Annu. Rev. Biophys. 43, 303–329. doi: 10.1146/annurev-biophys-051013-022836
Shen, Y., Wu, S., Rancic, C., Aggarwal, A., Qian, Y., Miyashita, S., et al. (2019). “Genetically Encoded Fluorescent Indicators for Imaging Intracellular Potassium Ion Concentration.”. Commun. Biol. 2:18. doi: 10.1038/s42003-018-0269-2
Shi, Z., Fujii, K., Kovary, K. M., Genuth, N. R., Röst, H. L., Teruel, M. N., et al. (2017). Heterogeneous Ribosomes Preferentially Translate Distinct Subpools of mRNAs Genome-Wide. Mol. Cell 67, 71.e–83.e. doi: 10.1016/j.molcel.2017.05.021
Shigeoka, T., Koppers, M., Wong, H. H., Lin, J. Q., Cagnetta, R., Dwivedy, A., et al. (2019). On-Site Ribosome Remodeling by Locally Synthesized Ribosomal Proteins in Axons. Cell Rep. 29, 3605.e–3619.e. doi: 10.1016/j.celrep.2019.11.025
Shindo, Y., Yamanaka, R., Hotta, K., and Oka, K. (2020). “Inhibition of Mg2+ Extrusion Attenuates Glutamate Excitotoxicity in Cultured Rat Hippocampal Neurons.”. Nutrients 12:2768. doi: 10.3390/nu12092768
Simsek, D., and Barna, M. (2017). “An Emerging Role for the Ribosome as a Nexus for Post-Translational Modifications.”. Curr. Opin. Cell Biol. 45, 92–101. doi: 10.1016/j.ceb.2017.02.010
Simsek, D., Tiu, G. C., Flynn, R. A., Byeon, G. W., Leppek, K., Xu, A. F., et al. (2017). The Mammalian Ribo-Interactome Reveals Ribosome Functional Diversity and Heterogeneity. Cell 169, 1051.e–1065.e. doi: 10.1016/j.cell.2017.05.022
Slavov, N., Semrau, S., Airoldi, E., Budnik, B., and Oudenaarden, A. V. (2015). “Differential Stoichiometry among Core Ribosomal Proteins.”. Cell Rep. 13, 865–873. doi: 10.1016/j.celrep.2015.09.056
Sloan, K. E., Warda, A. S., Sharma, S., Entian, K., Lafontaine, D., and Bohnsack, M. T. (2017). Tuning the Ribosome: The Influence of rRNA Modification on Eukaryotic Ribosome Biogenesis and Function. RNA Biol. 14, 1138–1152. doi: 10.1080/15476286.2016.1259781
Slomnicki, L. P., Pietrzak, M., Vashishta, A., Jones, J., Lynch, N., Elliot, S., et al. (2016). “Requirement of Neuronal Ribosome Synthesis for Growth and Maintenance of the Dendritic Tree.”. J. Biol. Chem. 291, 5721–5739. doi: 10.1074/jbc.M115.682161
Somjen, G. G., and Müller, M. (2000). “Potassium-Induced Enhancement of Persistent Inward Current in Hippocampal Neurons in Isolation and in Tissue Slices.”. Brain Res. 885, 102–110. doi: 10.1016/S0006-8993(00)02948-6
Sotelo-Silveira, J. R., Calliari, A., Kun, A., Koenig, E., and Sotelo, J. R. (2006). “RNA Trafficking in Axons: RNA in Axons.”. Traffic 7, 508–515. doi: 10.1111/j.1600-0854.2006.00405.x
Spillane, M., Ketschek, A., Merianda, T. T., Twiss, J. L., and Gallo, G. (2013). “Mitochondria Coordinate Sites of Axon Branching through Localized Intra-Axonal Protein Synthesis.”. Cell Rep. 5, 1564–1575. doi: 10.1016/j.celrep.2013.11.022
Stoykova, A. S., Dabeva, M. D., Dimova, R. N., and Hadjiolov, A. A. (1985). “Ribosome Biogenesis and Nucleolar Ultrastructure in Neuronal and Oligodendroglial Rat Brain Cells.”. J. Neurochem. 45, 1667–1676. doi: 10.1111/j.1471-4159.1985.tb10521.x
Sun, C., Nold, A., Fusco, C. M., Rangaraju, V., Tchumatchenko, T., Heilemann, M., et al. (2021). “The Prevalence and Specificity of Local Protein Synthesis during Neuronal Synaptic Plasticity.”. Sci. Adv. 7:eabj0790. doi: 10.1126/sciadv.abj0790
Sutton, M. A., Wall, N. R., Akalu, G. L., and Schuman, E. M. (2004). “Regulation of Dendritic Protein Synthesis by Miniature Synaptic Events.”. Science 304, 1979–1983. doi: 10.1126/science.1096202
Tang, A., Chen, H., Li, T. P., Metzbower, S. R., MacGillavry, H. D., and Blanpied, T. A. (2016). “A trans-synaptic Nanocolumn Aligns Neurotransmitter Release to Receptors.”. Nature 536, 210–214. doi: 10.1038/nature19058
Tauber, D., Tauber, G., and Parker, R. (2020). “Mechanisms and Regulation of RNA Condensation in RNP Granule Formation.”. Trends Biochem. Sci. 45, 764–778. doi: 10.1016/j.tibs.2020.05.002
Tcherkezian, J., Brittis, P. A., Thomas, F., Roux, P. P., and Flanagan, J. G. (2010). “Transmembrane Receptor DCC Associates with Protein Synthesis Machinery and Regulates Translation.”. Cell 141, 632–644. doi: 10.1016/j.cell.2010.04.008
Tennyson, V. M. (1970). “The Fine Structure of The Axon and Growth Cone of the Dorsal Root Neuroblast of the Rabbit Embryo.”. J. Cell Biol. 44, 62–79. doi: 10.1083/jcb.44.1.62
Thelen, M. P., and Kye, M. J. (2020). “The Role of RNA Binding Proteins for Local mRNA Translation: Implications in Neurological Disorders.”. Front. Mol. Biosci. 6:161. doi: 10.3389/fmolb.2019.00161
Thomson, E., Ferreira-Cerca, S., and Hurt, E. (2013). “Eukaryotic Ribosome Biogenesis at a Glance.”. J. Cell Sci. 126, 4815–4821. doi: 10.1242/jcs.111948
Tiedge, H., and Brosius, J. (1996). Translational Machinery in Dendrites of Hippocampal Neurons in Culture. J. Neurosci. 16, 7171–7181. doi: 10.1523/JNEUROSCI.16-22-07171.1996
Tseng, H., Chou, W., Wang, J., Zhang, X., Zhang, S., and Schultz, R. M. (2008). “Mouse Ribosomal RNA Genes Contain Multiple Differentially Regulated Variants.”. PLoS One 3:e1843. doi: 10.1371/journal.pone.0001843
Venkatesan, S., Subramaniam, S., Rajeev, P., Chopra, Y., Jose, M., and Nair, D. (2020). Differential Scaling of Synaptic Molecules within Functional Zones of an Excitatory Synapse during Homeostatic Plasticity. Eneuro 7, ENEURO.407–ENEURO.419. doi: 10.1523/ENEURO.0407-19.2020
Volkov, I. L., and Johansson, M. (2019). “Single-Molecule Tracking Approaches to Protein Synthesis Kinetics in Living Cells.”. Biochemistry 58, 7–14. doi: 10.1021/acs.biochem.8b00917
Waudby, C. A., Dobson, C. M., and Christodoulou, J. (2019). “Nature and Regulation of Protein Folding on the Ribosome.”. Trends Biochem. Sci. 44, 914–926. doi: 10.1016/j.tibs.2019.06.008
Weiss, R. L., Kimes, B. W., and Morris, D. R. (1973). Cations and Ribosome Structure. 111. Effects on the 30s and 50s Subunits of Replacing Bound Mg2+; by Inorganic Cations. Biochemistry 12, 450–456. doi: 10.1021/bi00727a014
Willis, D. (2005). Differential Transport and Local Translation of Cytoskeletal, Injury-Response, and Neurodegeneration Protein mRNAs in Axons. J. Neurosci. 25, 778–791. doi: 10.1523/JNEUROSCI.4235-04.2005
Wilson, D. N., and Cate, J. H. D. (2012). “The Structure and Function of the Eukaryotic Ribosome.”. Cold Spring Harb. Perspect. Biol. 4, a011536–a011536. doi: 10.1101/cshperspect.a011536
Wu, B., Buxbaum, A. R., Katz, Z. B., Yoon, Y. J., and Singer, R. H. (2015). “Quantifying Protein-mRNA Interactions in Single Live Cells.”. Cell 162, 211–220. doi: 10.1016/j.cell.2015.05.054
Yoon, B. C., Jung, H., Dwivedy, A., O’Hare, C. M., Zivraj, K. H., and Holt, C. E. (2012). “Local Translation of Extranuclear Lamin B Promotes Axon Maintenance.”. Cell 148, 752–764. doi: 10.1016/j.cell.2011.11.064
Yoon, Y. J., Wu, B., Buxbaum, A. R., Das, S., Tsai, A., English, B. P., et al. (2016). “Glutamate-Induced RNA Localization and Translation in Neurons.”. Proc. Natl. Acad. Sci. 113, E6877–E6886. doi: 10.1073/pnas.1614267113
Keywords: neurons, synapse, local translation, ribosome remodeling, in vivo dynamics
Citation: Dastidar SG and Nair D (2022) A Ribosomal Perspective on Neuronal Local Protein Synthesis. Front. Mol. Neurosci. 15:823135. doi: 10.3389/fnmol.2022.823135
Received: 26 November 2021; Accepted: 17 January 2022;
Published: 23 February 2022.
Edited by:
James P. Clement, Jawaharlal Nehru Center for Advanced Scientific Research, IndiaReviewed by:
Rosalina Fonseca, New University of Lisbon, PortugalZach T. Campbell, The University of Texas at Dallas, United States
Dan Ohtan Wang, Kyoto University, Japan
Copyright © 2022 Dastidar and Nair. This is an open-access article distributed under the terms of the Creative Commons Attribution License (CC BY). The use, distribution or reproduction in other forums is permitted, provided the original author(s) and the copyright owner(s) are credited and that the original publication in this journal is cited, in accordance with accepted academic practice. No use, distribution or reproduction is permitted which does not comply with these terms.
*Correspondence: Sudhriti Ghosh Dastidar, c3VkaHJpdGlnQGlpc2MuYWMuaW4=; Deepak Nair, ZGVlcGFrQGlpc2MuYWMuaW4=