- The Picower Institute for Learning and Memory, Department of Biology, Department of Brain and Cognitive Sciences, Massachusetts Institute of Technology, Cambridge, MA, United States
Voltage-gated Ca2+ channels (VGCCs) mediate Ca2+ influx to trigger neurotransmitter release at specialized presynaptic sites termed active zones (AZs). The abundance of VGCCs at AZs regulates neurotransmitter release probability (Pr), a key presynaptic determinant of synaptic strength. Given this functional significance, defining the processes that cooperate to establish AZ VGCC abundance is critical for understanding how these mechanisms set synaptic strength and how they might be regulated to control presynaptic plasticity. VGCC abundance at AZs involves multiple steps, including channel biosynthesis (transcription, translation, and trafficking through the endomembrane system), forward axonal trafficking and delivery to synaptic terminals, incorporation and retention at presynaptic sites, and protein recycling. Here we discuss mechanisms that control VGCC abundance at synapses, highlighting findings from invertebrate and vertebrate models.
Introduction
Electrical signaling within the nervous system provides a fast and robust mechanism for transmitting action potentials to synaptic terminals. Voltage-gated calcium channels (VGCCs) are essential for translating electrical propagation of action potentials into intracellular chemical signals. When the membrane voltage passes a critical threshold, VGCCs open and allow influx of Ca2+ ions into the cell. Baseline Ca2+ concentrations in the cytosol are kept extremely low through extensive buffering and fast extrusion via pumps, allowing Ca2+ to act as a potent intracellular signal to regulate a diversity of processes, such as vesicle fusion, phosphorylation, or transcriptional changes (Berridge et al., 2003). At chemical synapses, presynaptic VGCCs trigger neurotransmitter release from synaptic vesicles (SVs) by mediating Ca2+ influx, which activates the SV protein Synaptotagmin 1 (Syt1) to drive fusion of the SV and plasma membranes (Littleton et al., 1993, 1994; DiAntonio and Schwarz, 1994; Sauvola and Littleton, 2021).
Presynaptic neurotransmitter release lags behind intracellular Ca2+ influx by less than a millisecond (Katz and Miledi, 1965; Borst and Sakmann, 1996; Sabatini and Regehr, 1996; Neher, 1998). This incredible speed reflects the tight spatial organization of fusion-primed SVs near VGCCs. This spatial coordination occurs at active zones (AZs), specialized domains within the presynaptic membrane where a macromolecular complex of conserved scaffold proteins recruits SVs to clusters of VGCCs for efficient Ca2+ use (Kawasaki et al., 2004; Bucurenciu et al., 2008; Catterall and Few, 2008; Wang et al., 2008; Fouquet et al., 2009; Eggermann et al., 2011; Chen et al., 2015; Nakamura et al., 2015). Although the opening of a single VGCC can trigger SV release at some synapses, VGCCs are typically clustered at AZs to produce a larger transient domain of intracellular Ca2+ (Jarsky et al., 2010; Bartoletti et al., 2011; Nakamura et al., 2015). AZs in different neurons and species differ in their ultrastructure. For example, AZs at the Drosophila neuromuscular junction (NMJ) show an electron dense “T-bar” structure composed primarily of the scaffold protein Bruchpilot (BRP) when viewed by EM (Figure 1A; Fouquet et al., 2009). In contrast, sensory synapses within photoreceptors and hair cells contain a long synaptic ribbon that is predicted to facilitate robust SV release at these terminals (Figure 1B). Mammalian CNS synapses lack such a striking dense projection but still have an increased electron density that corresponds to the dense network of AZ scaffold proteins (Figure 1C). Despite these ultrastructural differences, scaffolding proteins present at synapses are generally conserved. The major scaffolds that cluster VGCCs at AZs are Rab3-interacting molecules (RIMs), RIM-binding proteins (RBPs), ELKS/CAST, and Bassoon/Piccolo/Fife (Kittel et al., 2006; Müller et al., 2010; Han et al., 2011; Kaeser et al., 2011; Liu et al., 2011; Graf et al., 2012; Kiyonaka et al., 2012; Davydova et al., 2014; Bruckner et al., 2017; Xuan et al., 2017). These proteins form multiple binding interactions with VGCCs to provide functional redundancy in VGCC clustering at AZs.
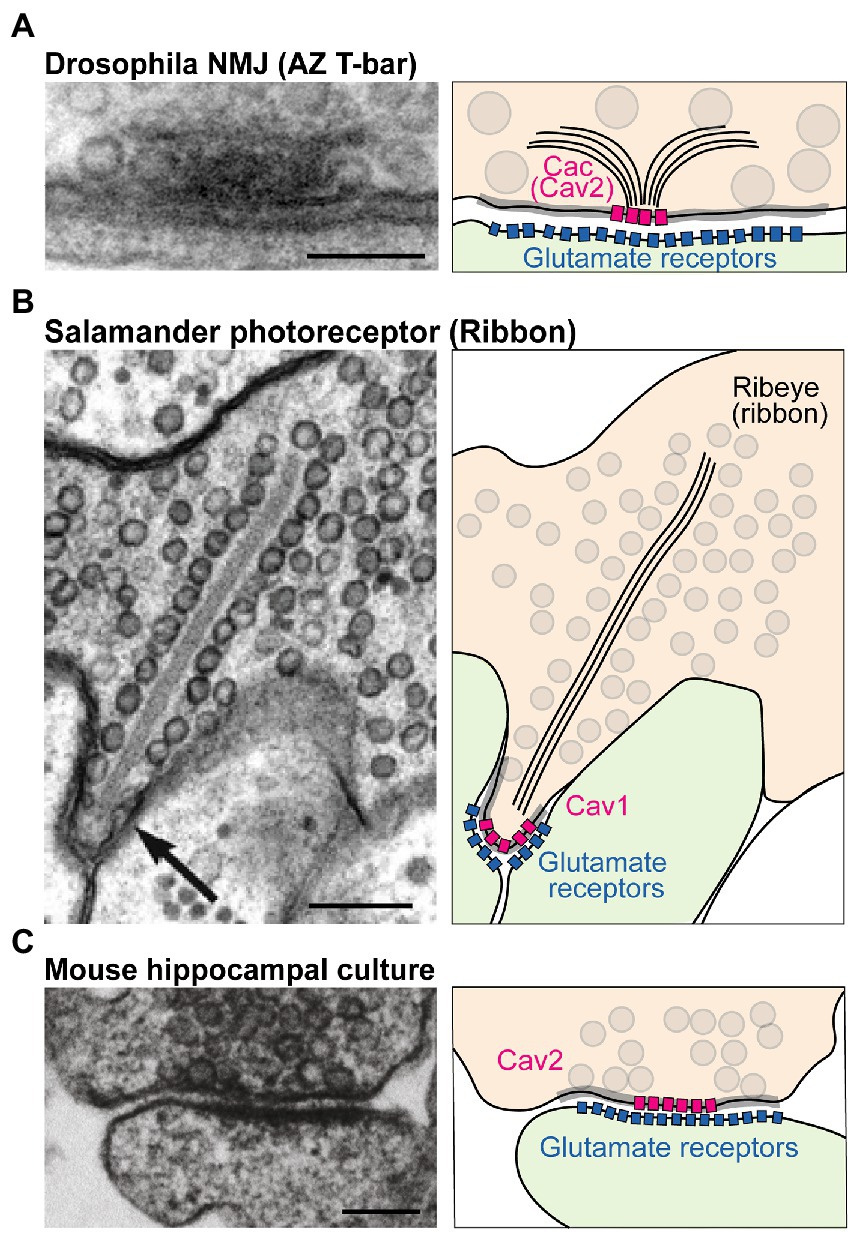
Figure 1. Structure and molecular composition of AZs. (A) Left: EM of an AZ at the Drosophila NMJ (modified from Fouquet et al., 2009; scale bar: 100 nm). Right: molecular depiction of the AZ. The presynaptic terminal is colored in orange, and the postsynaptic cell green. Gray circles represent SVs. The gray highlighted zone along the presynaptic membrane marks the AZ area. Bruchpilot (BRP; black lines) forms the “T-bar” structure together with other scaffolds (not shown). The presynaptic Cav2 channel Cacophony (Cac) clusters at the base of the T-bar, while Glutamate Receptors cluster postsynaptically. (B) EM (modified from Thoreson et al., 2004; scale bar: 200 nm) and depiction of a salamander photoreceptor ribbon synapse. The ribbon is an electron dense projection (formed by the protein Ribeye) and is lined with SVs. Cav1 family channels mediate fusion at this synapse. (C) EM (modified from Kaeser et al., 2011; scale bar: 100 nm) and model of a mouse hippocampal cultured synapse. SV fusion at mammalian CNS neurons is primarily supported by Cav2.1 and Cav2.2 channels (pink).
Although AZs are specialized for SV fusion, not every AZ releases a SV following an action potential. Instead, neurons display a wide range of synaptic efficacies. Synaptic strength is a composite of both pre- and post-synaptic factors, and its regulation increases diversity for supporting circuit function and plasticity (Atwood and Karunanithi, 2002). A key presynaptic determinant of synaptic strength is neurotransmitter release probability (Pr), the likelihood of SV fusion after an action potential. Evoked Pr is partially regulated at the AZ-level and can vary dramatically across AZs formed by a single neuron (Figure 2A; Peled and Isacoff, 2011; Melom et al., 2013; Akbergenova et al., 2018; Sauvola et al., 2021; Newman et al., 2022). The amount of presynaptic Ca2+ influx at AZs, regulated in large part by the number of VGCCs clustered at the AZ, is a major determinant of Pr (Augustine et al., 1985; Borst and Sakmann, 1996; Wang et al., 2008; Bartoletti et al., 2011; Ariel et al., 2012; Sheng et al., 2012; Akbergenova et al., 2018; Newman et al., 2022). As such, VGCC function, regulation, and localization is central to how neurons control presynaptic output.
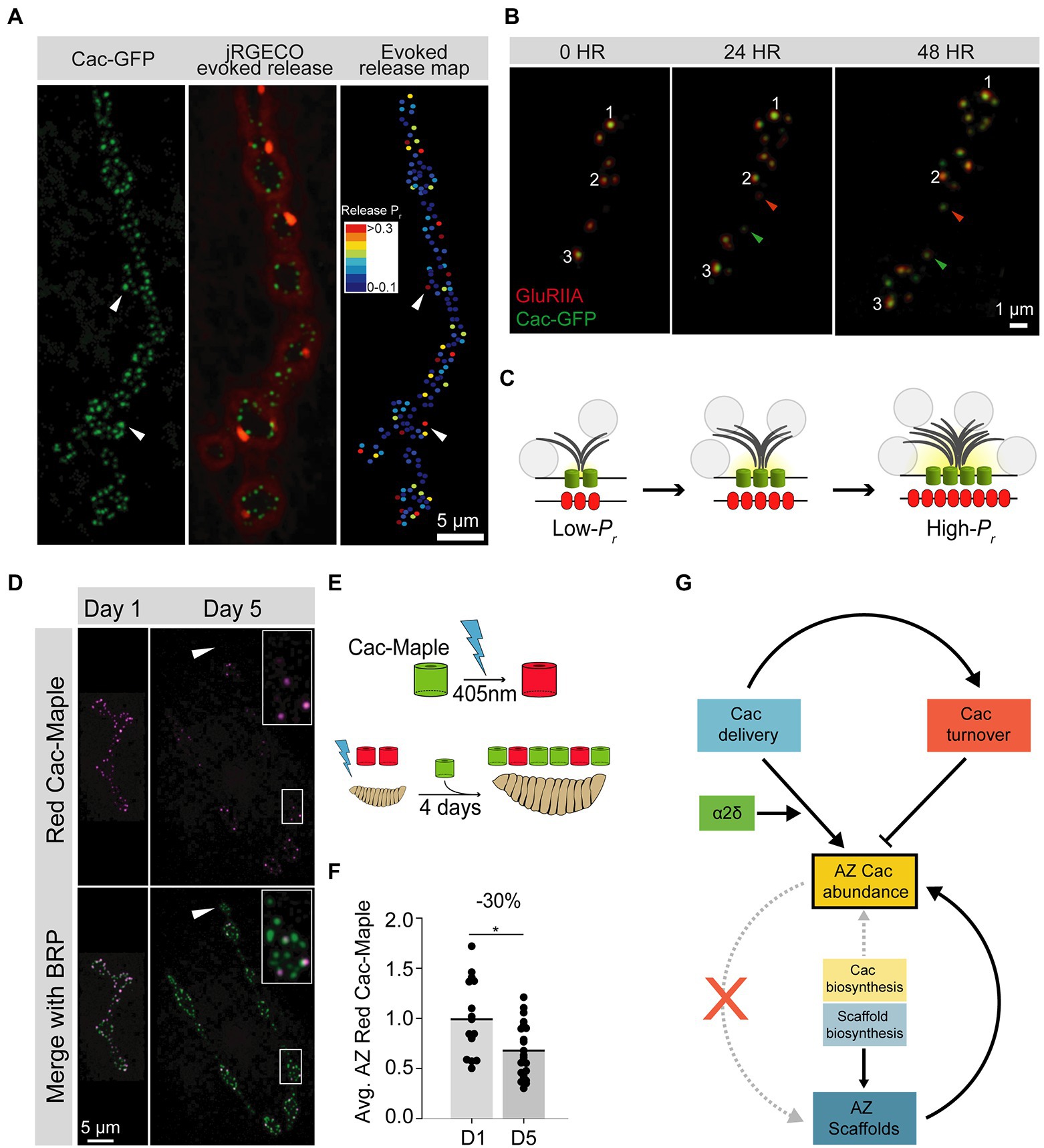
Figure 2. Cac regulation at the Drosophila third instar larval NMJ. (A) Representative image of a Drosophila larval NMJ. AZs are marked by clusters of Cac-GFP (green), and the jRGECO calcium sensor (red) is tethered to the postsynaptic membrane and indicates evoked release events at individual AZs. An evoked release heat map (right); generated from videos of jRGECO responses following a series of individual APs indicates Pr for each AZ, with high-Pr AZs in red and low-Pr sites in blue. (B) Sequential intravital imaging of a growing NMJ in an intact, anesthetized animal. Glutamate Receptor subunit GluRIIA-RFP marks postsynaptic densities and Cac-GFP marks presynaptic AZs. Arrows mark several new AZs formed after the 0-h timepoint, and white numbers track individual AZs through the image series. (C) Schematic of an AZ as it structurally and functionally matures from a young (low-Pr) site to an old (high-Pr) site through the multi-day acquisition of key components including BRP (gray), and Cac (green). Postsynaptic Glutamate Receptor abundance (red) also increases throughout maturation. SVs are marked as gray circles. (D) Representative images of Cac-Maple (magenta) and the AZ scaffold BRP (green) at AZs of the Drosophila NMJ, 1 day or 5 days after complete photoconversion. White arrows mark a bouton that formed after photoconversion, and is completely devoid of red Cac-Maple channels. The bouton outlined in white is enlarged in the upper right corner of each Day 5 image. (E) Schematic of Cac-Maple photoconversion (top panel) and experimental approach to measuring Cac turnover rate at AZs (bottom panel). Cac-Maple is green, and photoconverts permanently to red upon illumination with a 405 nm light (blue lightning bolt). Photoconversion of an entire first instar larva, followed by 4 days of growth (during which time new green Cac-Maple channels are added to growing NMJs) results in a mixed pool of AZs with some AZs having only green channels (those that were added to the NMJ after the photoconversion event) and some AZs having red signal as well (representing channels present at the initial photoconversion timepoint). (F) Quantification of average red Cac-Maple abundance per AZ 1 day and 5 days post-photoconversion. A 30% reduction in red Cac-Maple levels occurs over this 4-day window. (G) Model of VGCC (Cac) regulation at the Drosophila NMJ. Cac delivery and turnover rates can be measured in vivo at this synapse. Both delivery (blue) and turnover (orange) cooperate to establish AZ Cac abundance. The α2δ subunit (green) positively regulates Cac delivery. AZ Cac abundance is only weakly regulated by Cac biosynthesis levels, as AZ Cac levels are highly buffered against changes in biosynthesis (yellow). In contrast, AZ scaffold biosynthesis plays a larger role in regulating AZ levels of the scaffold protein BRP (blue). While the presence of the AZ scaffold BRP is required for proper AZ Cac abundance (upward curved solid arrow), AZ Cac is not required for scaffold formation (red X). Figure panels (A,B) were adapted from Akbergenova et al. (2018). Figure panels (D,G) were adapted from Cunningham et al. (2022).
This review explores current models for how VGCC abundance is regulated at presynaptic AZs, with an emphasis on Cav2 family channels, which are the primary mediators of neurotransmission at most synapses (Dolphin and Lee, 2020). We focus exclusively on processes that mediate channel localization and abundance, as the structure and function of the channel has been extensively reviewed elsewhere (Catterall et al., 2020). We examine the regulation of VGCC localization at all stages of the channel’s life, beginning with biosynthesis and progression through the ER and Golgi that requires auxiliary subunits. After axonal trafficking to synaptic terminals, channels are stabilized at AZs through multiple binding interactions with conserved AZ scaffold proteins including ELKS/CAST, RIM and RBP. We review evidence for the “slot” model of VGCC AZ abundance that suggests excess VGCCs compete for limited AZ localization through rate-limiting binding interactions downstream of channel biosynthesis (Cao et al., 2004; Cao and Tsien, 2010). Next, we focus on the channel’s mobility once incorporated into AZs. Despite binding to multiple scaffolds, single-molecule tracking studies indicate VGCCs are highly mobile within the AZ (Mercer et al., 2011, 2012; Thoreson et al., 2013; Schneider et al., 2015; Voigt et al., 2016; Ghelani et al., 2022). In addition to VGCC mobility, we review molecular pathways facilitating endocytosis, although limited in situ information is available to contextualize these molecular pathways in intact circuits. Finally, we discuss how these processes are regulated during synaptic plasticity. Each of these steps—biosynthesis, trafficking, AZ scaffold-binding, mobility, and turnover, provide points of VGCC regulation that can be modulated to control presynaptic Pr.
The VGCC is a multisubunit complex
High resolution structures are available of the VGCC and its auxiliary subunits (Wu et al., 2015, 2016). Like other ion channels, VGCCs have a pore-forming α1 subunit that selectively fluxes Ca2+, as well as several auxiliary subunits that regulate channel trafficking, stabilization, and function. The α1 subunit of the VGCC is closely related to voltage-gated sodium (Nav) channels, and only several amino acid changes in the pore region are required to convert a Nav channel into one capable of fluxing Ca2+ (Tang et al., 2014). The VGCC α1 subunit contains four domains, each with six transmembrane spanning segments. Transmembrane segments I–IV comprise the voltage-sensing module of the channel, while segments V and VI form the Ca2+ selective pore (Figure 3A; Catterall et al., 2020). Despite conservation of α1 structure with other voltage-gated ion channels, the set of auxiliary subunits that regulates VGCCs are unique in the voltage-gated ion channel superfamily (Witcher et al., 1993; Yu et al., 2005). An extracellular α2δ subunit facilitates forward trafficking of the VGCC and modulates its gating and conductance properties (Dolphin, 2016). In addition, a cytosolic ß subunit acts as a chaperone during biosynthesis and is required for VGCC membrane expression (Figure 3A, Pragnell et al., 1994; Gregg et al., 1996; Cantí et al., 2001; Van Petegem et al., 2004; Weissgerber et al., 2006; Altier et al., 2011; Waithe et al., 2011; Dolphin, 2016). These subunits also support interactions between the channel and its signaling or scaffolding partners (Müller et al., 2010).
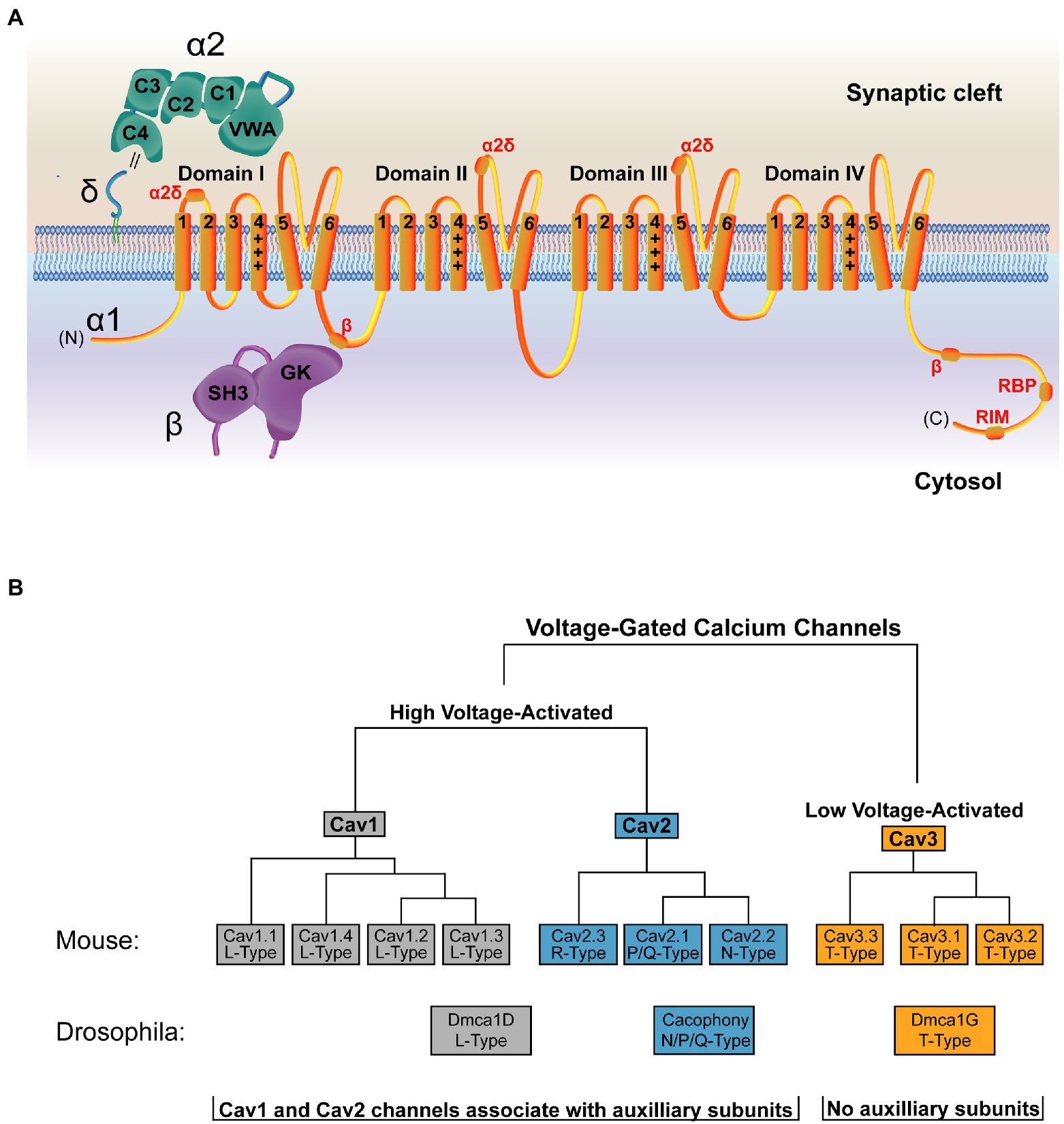
Figure 3. Structure and organization of the VGCC family. (A) Structure of a VGCC complex with the α1 pore-forming subunit in orange. VGCCs have four domains with six transmembrane segments each. Transmembrane segments I-IV comprise the voltage-sensing module, with transmembrane segment IV (marked +++) as the voltage sensing segment. Transmembrane segments V and VI form the ion-conducting pore. The cytoplasmic C-terminal tail interacts with multiple binding partners, including a secondary Cavß interaction site (Walker et al., 1998) and binding regions for RBP and RIM (Lübbert et al., 2017). The α2δ subunit (green) is extracellular and comprised of α2 and δ peptides linked via a disulfide bond (double black line) and anchored to the outer membrane leaflet via a lipid anchor (Davies et al., 2010; Wu et al., 2015). α2δ contains five domains, with the Von Willebrand Factor-A (VWA) and the first two Cache domains (C1 and C2) interacting with α1 (Wu et al., 2016). Sites of α2δ-interaction on the α1 subunit are marked in red. The Cavß subunit (purple) is cytosolic and comprised of an SH3 domain and a Guanylate Kinase (GK) domain. The primary α1 interaction site is mediated through an intracellular loop in domain I of the channel and the Cavß GK domain (Pragnell et al., 1994; Cantí et al., 2001; Chen et al., 2004; Opatowsky et al., 2004; Van Petegem et al., 2004; Wu et al., 2016). (B) The mammalian VGCC family is comprised of 7 high voltage-activated VGCCs (Cav1 and Cav2 family channels) and 3 low voltage-activated VGCCs (Cav3 family channels). Drosophila (shown below the mammalian tree) encodes one VGCC per class, with Dmca1D encoding the sole L-Type Cav1 channel (Eberl et al., 1998; Hara et al., 2015), Cacophony (Cac) encoding the sole N/Q/P type Cav2 channel that mediates synaptic transmission (Smith et al., 1996; Kawasaki et al., 2000, 2004), and Dmca1G encoding the sole T-Type Cav3 channel (Jeong et al., 2015). Cav1 and Cav2 channels associate with auxiliary subunits, while Cav3 channels do not.
Mammals encode three VGCC families (Cav1–Cav3) defined by their pore-forming α1 subunit (Figure 3B; Dolphin, 2016). Of these, the four Cav1 channels (also called L-type based on initial current characterization) and the three Cav2 channels (P/Q-, N-, and R-type) are high-voltage activated and are the dominant contributors to synaptic transmission at presynaptic AZs (Luebke et al., 1993; Takahashi and Momiyama, 1993; Wheeler et al., 1994; Reuter, 1995; Reid et al., 1997; Wu et al., 1999; Dolphin and Lee, 2020). Cav2 channels mediate the majority of neurotransmission in the CNS, while Cav1 channels are important in sensory neurons like inner hair cells and photoreceptors. The three Cav3 channels (T-type) are low-voltage activated, do not play a central role in mediating evoked synaptic transmission, and do not require the canonical auxiliary subunits (Dolphin and Lee, 2020). Invertebrate VGCCs also mediate synaptic transmission but have less redundancy. Drosophila encodes one family member from each of the three VGCC families, and the single Cav2 family VGCC (Cacophony; Cac) complexes with a single α2δ family member (Straightjacket) to mediate neurotransmission at synapses (Kawasaki et al., 2004; Ly et al., 2008; Ryglewski et al., 2012; Heinrich and Ryglewski, 2020; Figure 3B; Table 1).
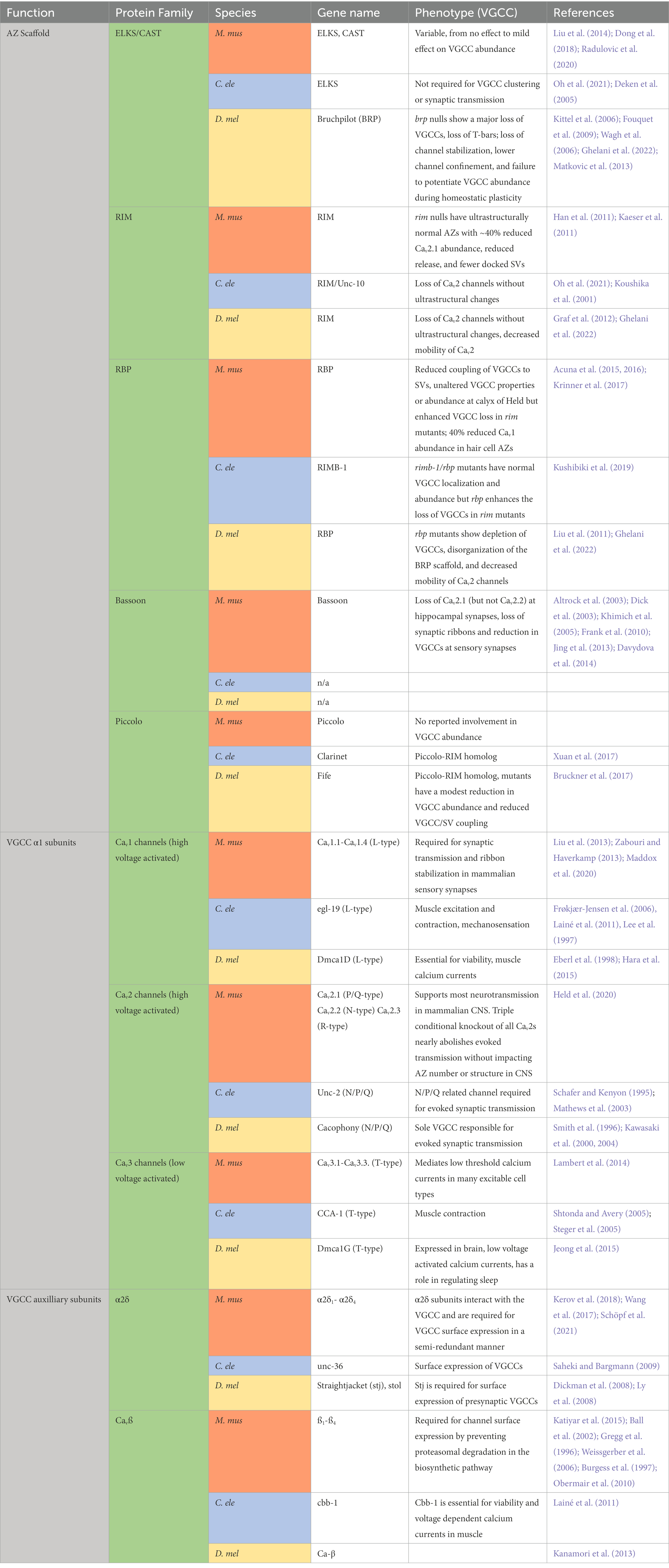
Table 1. Summary of AZ structure and VGCC localization/abundance phenotypes in Mus musculus (M. mus), Caenorhabditis elegans (C. ele), and Drosophila melanogaster (D. mel) AZ and VGCC mutants.
VGCC auxiliary subunits promote cell surface expression
During biosynthesis, VGCCs are translated into the ER and move to the Golgi where they are extensively modified before delivery to the cell surface (Figure 4; step 2). The pore-forming α1 subunit requires co-expression of its auxiliary α2δ and Cavß subunits to reach the plasma membrane (Brice et al., 1997; Ball et al., 2002; Cassidy et al., 2014). Mammals encode 4 Cavß genes (ß1-ß4) that are essential for channel function and result in lethality or severe phenotypes when disrupted (Gregg et al., 1996; Burgess et al., 1997; Weissgerber et al., 2006). Four α2δ subunits (α2δ1–α2δ4) that are important for survival and display some functional redundancy are also expressed in mammals (Schöpf et al., 2021). While Cavß is essential for surface expression, α2δ plays a secondary trafficking role that rate-limits presynaptic expression of functional channels. In rodent cultured neurons, overexpression of either α2δ or Cavß alone dramatically increases presynaptic VGCC abundance, but only α2δ overexpression increases SV fusion (Hoppa et al., 2012). In addition to their requirements in promoting surface expression, these auxiliary subunits play extensive roles in modulating channel properties, including activation, inactivation, and gating, as well as mediating modulation by other regulatory pathways. In addition, α2δ subunits control synapse morphology independent of their role as channel subunits, and can localize to synapses without VGCCs (Kurshan et al., 2009; Dolphin, 2018; Held et al., 2020; Schöpf et al., 2021). These non-localizing or VGCC-independent roles of α2δ and Cavß subunits have been reviewed elsewhere (Buraei and Yang, 2010; Dolphin, 2018).
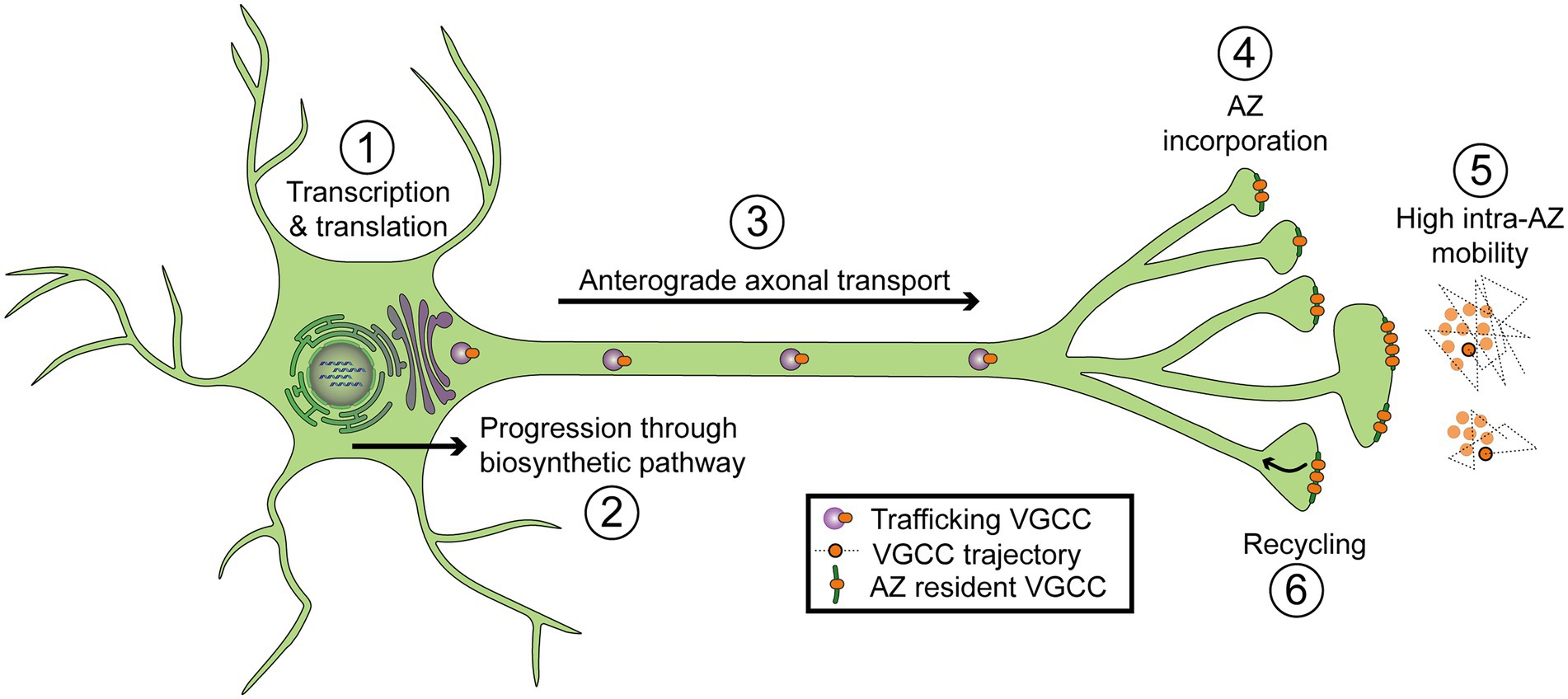
Figure 4. Diagram of regulatory steps involved in setting VGCC abundance at synaptic AZs. (1) Transcription and translation. (2) Progression through the biosynthetic pathway, including the endoplasmic reticulum and Golgi. (3) Forward axonal trafficking. (4) Incorporation into AZs through local interactions with scaffolding proteins. (5) High mobility within individual AZs (intra-AZ mobility) and low inter-AZ mobility. Cartoon on the right depicts a top-down view of VGCCs residing in two nearby AZs. Dotted lines represent short-term trajectories of VGCCs outlined in black. (6) Recycling of VGCCs.
Cavß is a conserved intracellular subunit that controls channel progression through the biosynthetic pathway, determining whether nascent channels are destined for degradation or surface expression. Cavß contains SH3 and guanylate kinase (GK) domains through which it associates with an intracellular loop between domains I and II of the α1 subunit of Cav1 and Cav2 family VGCCs (Figure 3A; Pragnell et al., 1994; Cantí et al., 2001; Chen et al., 2004; Opatowsky et al., 2004; Van Petegem et al., 2004). Cavß binding at this site is predicted to promote proper folding of the channel (Opatowsky et al., 2004). In heterologous expression systems, α1 expression alone is insufficient for channel surface expression, but co-expression with Cavß promotes high levels of surface-expressed α1 (Brice et al., 1997; He et al., 2007; Cassidy et al., 2014). Likewise, in vivo knockdown of Cavß or disruption of the Cavß binding site on α1 inhibits VGCC surface expression (Berrow et al., 1995; Obermair et al., 2010). Cav1.2 α1 subunits are ubiquitinated and subsequently degraded without Cavß, and preventing this degradation by pharmacologically blocking the proteasome restores channel surface expression. This data suggests Cavß is not required for surface expression beyond its role in promoting protein stability in the ER (Altier et al., 2011; Waithe et al., 2011). A secondary Cavß-binding site is present in the VGCC C-terminal tail (Figure 3A). However structure–function studies at the calyx of Held demonstrated effective rescue of Cav2.1 function by Cav2.1 C-terminal truncation constructs lacking this Cavß-interaction domain (Walker et al., 1998; Lübbert et al., 2017). Overall these studies indicate Cavß functions as a chaperone by promoting α1 folding in the ER to prevent degradation.
The conserved VGCC subunit α2δ is also required for VGCC surface expression, though its mechanism of action is less clear. It is entirely extracellular, heavily glycosylated, and anchored to the external leaflet of the presynaptic membrane via a glycosyl-phosphatidyl inositol (GPI) anchor (Figure 3A; Davies et al., 2010; Wu et al., 2015). During processing, the α2δ precursor polypeptide is cleaved into α2 and δ subunits that are then linked together via disulfide bonds (De Jongh et al., 1990; Jay et al., 1991). Five domains have been identified in α2δ: a Von Willebrand Factor-A (VWA) domain and four Cache domains, with high-resolution structures suggesting multiple interactions between α2δ and the external face of the α1 channel (Figure 3A; Whittaker and Hynes, 2002; Wu et al., 2015, 2016; Cantí et al., 2001). The α2δ subunit can remain associated with the α1 subunit at synapses and modulate channel function, though it is unclear if continued α2δ- α1 interactions are absolutely essential for VGCC activity. Indeed, unlike Cavß that associates tightly to the α1 subunit with 1:1 stoichiometry, studies have reported a range of interaction strengths between α2δ and α1 ranging from stable to transient association modes (Pragnell et al., 1994; Müller et al., 2010; Cassidy et al., 2014; Voigt et al., 2016). Like Cavß, α2δ is required for proper surface expression of the channel. While the exact mechanism is unknown, this role of α2δ is likely performed by promoting forward trafficking rather than preventing channel endocytosis (Cantí et al., 2001; Dickman et al., 2008; Ly et al., 2008; Saheki and Bargmann, 2009; Hoppa et al., 2012; Cassidy et al., 2014; Cunningham et al., 2022). Understanding the structure and function of α2δ is of special importance due to its pharmacological targeting by the widely prescribed drugs gabapentin and pregabalin (Gee et al., 1996; Taylor et al., 2007; Eroglu et al., 2009; Dolphin, 2016).
Axonal trafficking and the “prefabricated synapse” hypothesis
After progression through the biosynthetic pathway, presynaptic VGCCs are trafficked down the axon to synaptic terminals (Figure 4; step 3). VGCC axonal trafficking is largely mysterious, but the trafficking of other presynaptic components such as AZ scaffolds and SV proteins has been extensively studied. Plus-end directed microtubule-based transport mediated by motor proteins of the Kinesin family is the dominant mode of trafficking to terminals (Vale et al., 1996; Goldstein et al., 2008; Hirokawa et al., 2010). Specifically, the anterograde Kinesin-3 family motor Unc-104/KIF1A plays a conserved role in the transport of many synaptic cargoes (Hall and Hedgecock, 1991; Okada et al., 1995; Yonekawa et al., 1998; Pack-Chung et al., 2007; Rivière et al., 2011; Maeder et al., 2014; Zhang et al., 2016, 2017). Kinesin-1 family members have also been implicated in synaptic transport (Gho et al., 1992; Hurd and Saxton, 1996; Gindhart et al., 1998). Since Kinesin diversity alone is insufficient to support the wide need of unique cellular trafficking processes, adaptor proteins that associate with cargo and Kinesins provide additional levels of regulation (Goldstein et al., 2008; Tempes et al., 2020). A conserved adaptor role for the GTPase Arl-8 in supporting Unc-104 mediated synaptic transport was first described in C. elegans where SV and AZ proteins are co-transported in an Unc-104/KIF1A dependent pathway (Hall and Hedgecock, 1991; Wu et al., 2013). In a forward genetic screen for genes involved in synapse formation, Arl-8 disruptions were found to deplete SV components from synapses and promote the ectopic accumulation of AZ and SV proteins along axons (Klassen et al., 2010). Likewise, Arl-8 co-traffics with several synaptic proteins, and its loss severely disrupts their synaptic localization as well as synapse growth in Drosophila (Vukoja et al., 2018). These studies demonstrate an important and conserved role for Unc-104 and its Arl-8 adaptor in axonal trafficking of synaptic proteins.
Do presynaptic proteins co-transport or arrive independently at developing synapses? Through studies of transport packets containing AZ and SV proteins, several synapse-specific transport organelles have been identified (Goldstein et al., 2008; Vukoja et al., 2018). In mammals, Golgi-derived ~80 nm dense core vesicles called Piccolo-Bassoon transport vesicles (PTVs) traffic many AZ proteins including Piccolo, Bassoon, RIM, CAST, N-Cadherin, Rab3, and Munc18, but lack the SV proteins Syt1 and VAMP (Zhai et al., 2001; Ohtsuka et al., 2002; Shapira et al., 2003; Dresbach et al., 2006; Cai et al., 2007; Maas et al., 2012). The α1 and Cavß subunits of the Cav2.2 channel were biochemically suggested to reside on these compartments, but confirmation of their presence through microscopy was inaccessible due to technical limitations (Shapira et al., 2003). The other major transport organelle that has been identified is the Synaptic Vesicle Precursor (SVP), which carries SV markers including Synaptotagmin, Synaptophysin, and Rab3, and has not been shown to contain VGCCs (Okada et al., 1995; Maeder et al., 2014; Guedes-Dias et al., 2019; De Pace et al., 2020). SVP trafficking is primarily Kinesin-3 mediated (Okada et al., 1995). In addition to PTVs and SVPs, other transport organelles also likely exist. For example, mobile Neurexin puncta in axons do not colocalize with Bassoon but partially colocalize with other AZ proteins including CASK, RIM, and Mint, as well as the Cav2.2 channel, suggesting the identity of a third AZ transport packet that has so far been minimally studied (Fairless et al., 2008).
One hypothesis for the bulk transport of AZ proteins (on PTVs) and SV proteins (on SVPs) is that this packaging allows robust and efficient maturation of new AZs into functional release sites. It has even been suggested that AZ and SV transport packets may be coordinated and comprehensive enough to be considered “pre-fabricated synapses” (Ahmari et al., 2000; Shapira et al., 2003; Tao-Cheng, 2007; Bury and Sabo, 2011; Vukoja et al., 2018). Indeed, comparisons of Piccolo and Bassoon abundance at synaptic and non-synaptic puncta suggest that only several PTVs are required to populate an AZ with its full complement of these proteins (Shapira et al., 2003; Tao-Cheng, 2007). Additionally, live imaging of movement and pausing suggests that although PTVs and SVPs represent separate organelles, they partially co-transport and share defined pause sites along axons (Bury and Sabo, 2011). Evidence from EM also points to bundled transport organelles, as Bassoon/Piccolo-positive aggregates of proteins, dense core vesicles, and smaller clear vesicles carrying SV markers can be seen in axons (Ahmari et al., 2000; Tao-Cheng, 2007). Light microscopy suggests VGCC subunits may colocalize with these organelle aggregates, but the lack of spatial resolution precludes determination of whether channels are present on PTVs, SVPs or a separate (and perhaps co-bundled) compartment (Ahmari et al., 2000).
Invertebrates were long thought to lack Piccolo and Bassoon (although Drosophila and C. elegans encode Piccolo-RIM homologs called Fife and Clarinet, respectively), calling into question whether they use PTV-like organelles to transport AZ proteins (Bruckner et al., 2017; Xuan et al., 2017). Like mammalian neurons, imaging in Drosophila axons revealed coordinated transport of some presynaptic proteins. The core AZ scaffold BRP partially co-transports with the SV protein Syt1. Interestingly, these transport packets colocalize with markers of non-degradative lysosomes and have thus been termed presynaptic lysosome-related vesicles (PLVs; Vukoja et al., 2018). Consistent with the role of Arl-8 in mediating synaptic protein and lysosome transport, arl-8 mutations block trafficking of these PLV packets, resulting in their buildup in the cell soma. EM visualization of these stalled packets indicate they are ~60–70 nm in diameter and have variable electron densities resembling a mix of dense-core and clear transport vesicles as seen in mammals (Khatter et al., 2015; Vukoja et al., 2018). In mammalian neurons, the SV marker VGlut1 and the AZ marker Bassoon also co-transport with the lysosome marker Lamp1, and reductions in Arl-8 caused a buildup of Bassoon in the soma, suggesting this lysosomal transport mechanism also occurs in mammals (Vukoja et al., 2018). In contrast to the “pre-fabricated synapse” hypothesis, sequential steps of AZ assembly are clearly temporally and genetically separable in Drosophila, as some proteins populate AZs ahead of others and rab3 mutations produce a sub-population of AZs that have only early AZ scaffolds but not late scaffolds or VGCCs (Fouquet et al., 2009; Graf et al., 2009; Owald et al., 2010; Böhme et al., 2016; Ghelani and Sigrist, 2018). Though a picture is emerging for the trafficking of core AZ scaffolds and SV components, how VGCCs traffic to synapses is still a major unknown. Determining if VGCCs travel in association with PTV/SVP aggregates or other post-Golgi vesicles, and which motor proteins mediate their transport, will require developing new tools to visualize VGCC trafficking in live neurons.
The AZ clusters VGCCs
Once VGCCs arrive at the synaptic terminal, they are incorporated into an AZ (Figure 4 step 4). The AZ is a defined region of presynaptic membrane featuring a dense protein matrix that functions as a scaffold to cluster SVs near VGCCs for efficient Ca2+-mediated fusion (Südhof, 2012). The structure of the AZ scaffold differs between species and neuron types, but it is comprised of several conserved proteins (including RIM, RIM-binding protein, and ELKS/CAST) that help cluster VGCCs (Table 1; Figure 1; Zhai and Bellen, 2004). The interactome of Cav2 channels has been analyzed in rodent brains using multi-epitope affinity purification and mass spectrometry, revealing a large cohort of ~200 proteins that interact with the channel (Müller et al., 2010). Multiple protein classes were identified, including known AZ scaffolds (CASK, RIM, RBP, Piccolo, etc) and other proteins that may regulate or function downstream of the channel. Given the multitude of proteins that bind VGCCs and contribute to their abundance and localization, a key question arises: which of these interactions is rate-limiting for VGCC accumulation? Identifying proteins that regulate VGCC abundance in a dosage-sensitive manner is critical, as these rate-limiting interactions may represent candidates for modulation during synaptic plasticity. The molecular constituents of the AZ and their roles in promoting VGCC clustering have been reviewed in depth (Dolphin and Lee, 2020; Gandini and Zamponi, 2022). Here we review the interactions of AZ proteins with VGCCs with a focus on distinguishing requirement versus rate-limiting roles.
RIM and RBP
RIM-interacting molecule and RBP are central scaffolds that semi-redundantly regulate VGCC abundance at AZs (Han et al., 2011; Kaeser et al., 2011; Liu et al., 2011; Graf et al., 2012; Jung et al., 2015; Oh et al., 2021). RIM was identified through its interaction with the SV protein Rab3 (Wang et al., 1997), but it also interacts with multiple AZ-resident proteins including Cav2 family VGCCs (via RIM’s PDZ domain), ELKS/CAST, RBP, Munc-13, and Liprin-α (Wang et al., 2000; Betz et al., 2001; Coppola et al., 2001; Ohtsuka et al., 2002; Schoch et al., 2002; Kiyonaka et al., 2007; Müller et al., 2010; Kaeser et al., 2011). Conditional knock-out of all PDZ-domain-containing rim genes from mammalian neurons results in ultrastructurally normal AZs with ~40% reduced Cav2.1 channel abundance, similar to the partial loss of Cav2 channels in Drosophila and C. elegans rim mutants (Koushika et al., 2001; Kaeser et al., 2011; Graf et al., 2012; Oh et al., 2021). Mouse rim mutants show a dramatic reduction in evoked release that is secondary to a decrease in presynaptic Ca2+ influx and fewer docked SVs (Han et al., 2011; Kaeser et al., 2011). RIM’s PDZ domain is required to rescue Cav2.1 AZ abundance, while its RBP-binding sequences are required for normal [Ca2+]-dependence of release, indicating that both RIM’s direct interaction with Cav2.1 and indirect interactions through RBP contribute to Cav2.1 channel localization (Hibino et al., 2002; Kaeser et al., 2011).
RBPs-interacting molecule-binding proteins were identified through their binding interaction with RIMs, but they also directly bind VGCCs and Liprin-α (Wang et al., 2000; Hibino et al., 2002; Müller et al., 2010; Liu et al., 2011). RBPs role in setting VGCC abundance varies between neuron types and is semi-redundant with RIM. In C. elegans, rbp deletion alone did not change VGCC abundance at AZs, but rim/rbp double nulls had more severe VGCC depletion than either individual mutation (Kushibiki et al., 2019). Similarly, conditional deletion of both neuronally-expressed rbp genes in mammalian neurons did not alter VGCC abundance, but removing both RIM and RBP families at the calyx of Held resulted in a more severe (~75%) disruption in presynaptic Ca2+ influx (and in AZ ultrastructure) than deletions of RIMs alone, suggesting partially redundant roles for RIM and RBP in localizing VGCCs (Acuna et al., 2015, 2016). Though Drosophila rpb mutations do independently disrupt VGCC clustering (possibly downstream of a disorganized BRP scaffold), rim/rbp double heterozygotes have severely reduced release despite normal function in each individual heterozygote, further suggesting functional redundancy (Kittel et al., 2006; Fouquet et al., 2009; Liu et al., 2011; Müller et al., 2015; Bruckner et al., 2017; Ortega et al., 2018). Though RBP plays a secondary role to RIM in many systems, RBP does independently regulate VGCCs in hair cells, where rbp mutants display ~40% reduction in presynaptic Ca2+ influx and a similarly reduced level of synaptic Cav1.3 immunofluorescence (Krinner et al., 2017).
These studies suggest that while RIM and RBP both bind to VGCCs, RIM is the dominant regulator of VGCC abundance at many synapses with partially redundant functions to RBP. This picture is complicated by in vivo structure–function studies at the calyx of Held, where Cav2.1 C-terminal truncation constructs lacking the known RIM and RBP binding domains rescue presynaptic currents in cav2.1 conditional knockouts, suggesting these binding domains are dispensable for Cav2.1 localization (Lübbert et al., 2017). This finding likely reflects redundancy in binding interactions that localize the channel to presynaptic membranes and may indicate that the known binding interactions of RIM to Cavß, or perhaps direct or indirect binding to another unknown site on the α1 subunit, provides an additional mechanism of interaction (Kiyonaka et al., 2007). Functional redundancy in localizing VGCCs presents a challenge in deciphering whether RIMs or RBPs play a dosage-sensitive role in fine-tuning VGCC AZ abundance. Future experiments testing whether RIM or RBP levels can be bidirectionally modulated to fine-tune VGCC abundance at AZs would provide insights into whether the abundance of these components rate-limits VGCC clustering.
CAST/ELKS
In addition to RIM and RBP, the two semi-redundant CAST/ELKS family proteins (CAST and ELKS) are conserved core AZ scaffolds, initially discovered through biochemical analysis (Ohtsuka et al., 2002; Wang et al., 2002). In mammals, CAST/ELKS interacts directly with VGCCs, and other core AZ proteins including RIM, Munc13, and Liprin-α (Ohtsuka et al., 2002; Ko et al., 2003; Wang et al., 2009; Chen et al., 2011; Billings et al., 2012; Kiyonaka et al., 2012). Similarly, the Drosophila CAST/ELKS homolog BRP diverges from mammalian ELKS in its C-terminal domain but interacts with presynaptic Cav2 channels through its ELKS-homologous N-terminal domain (Wagh et al., 2006; Fouquet et al., 2009). Despite their presence at synapses and direct interactions with VGCCs, the role of CAST/ELKS proteins in regulating VGCC abundance varies across systems. In mouse hippocampal synapses, conditional knockout of both elks genes after synapse formation resulted in a 30% decrease in presynaptic Ca2+ influx without any change in presynaptic VGCC abundance or synaptic ultrastructure (Liu et al., 2014). However, this manipulation was made after Cav2 channels had already populated synapses, so whether this timeframe is long enough to see an ELKS-dependent effect on Cav2 levels depends both on the AZ half-life of Cav2 channels and the role of the ELKS-VGCC binding interactions. At mature (Cav2.1-exclusive) and immature (Cav2-mixed) mouse calyx of Held synapses, conditional knockout of elks in the cast null line caused mildly decreased Cav1.3 abundance (Dong et al., 2018; Radulovic et al., 2020). In C. elegans, ELKS does not play a major role in clustering VGCCs at AZs (Deken et al., 2005; Oh et al., 2021). In contrast, the Drosophila CASK/ELKS homolog BRP plays a central role in forming the core of the AZ “T-bar” scaffold, promoting VGCC clustering and recruiting SVs to AZs (Kittel et al., 2006; Wagh et al., 2006; Fouquet et al., 2009). Brp mutants lack consolidated Cav2 clusters and have a large decrease in evoked synaptic transmission (Kittel et al., 2006; Fouquet et al., 2009). Unlike mammalian CAST/ELKS which is not always required for AZ morphology and structure (Dong et al., 2018), brp null mutants lack the AZ “T-bar” (Kittel et al., 2006; Fouquet et al., 2009). Despite this requirement for BRP in clustering VGCCs at the AZ, BRP is not a rate-limiting regulator of VGCC abundance, as ~35% reductions in AZ BRP have no impact on VGCC abundance and do not change single AP-evoked SV release (Müller et al., 2015; Cunningham et al., 2022).
Bassoon
The remaining core AZ proteins that are well characterized are Piccolo and Bassoon, Liprin-α, Syd-1, and Munc-13 (Cases-Langhoff et al., 1996; Tom Dieck et al., 1998; Fenster et al., 2000; Ackermann et al., 2015; Gundelfinger et al., 2015). Of these, Bassoon plays the most prominent role in VGCC localization, although Liprin-α is required for channel localization in C. elegans (Oh et al., 2021). Bassoon is a large multi-domain scaffolding protein that co-immunoprecipitates with Cavß and promotes VGCC clustering in some systems (Frank et al., 2010; Chen et al., 2011; Davydova et al., 2014). Binding between Bassoon and the VGCC-interaction partner RBP is important for recruiting Cav2.1 (but not Cav2.2) channels to hippocampal synapses (Davydova et al., 2014). At ribbon synapses in mammalian sensory neurons, the major AZ phenotype in bassoon mutants is loss of the ribbons (Dick et al., 2003; Khimich et al., 2005;Frank et al., 2010; Jing et al., 2013). At inner hair cell synapses, Cav1.3 abundance is reduced even though some AZs have intact ribbons, indicating the bassoon VGCC-reduction phenotype is not completely downstream of ribbon loss (Frank et al., 2010; Jing et al., 2013). The Bassoon homolog Piccolo does not have an established role in promoting VGCC abundance, although it has been suggested to bind to VGCCs (Müller et al., 2010) and interacts with L-type VGCCs in pancreatic cells (Shibasaki et al., 2004). In contrast to the requirement of Bassoon for proper VGCC abundance and ribbon attachment at mammalian sensory synapses, Bassoon plays more minor roles in synaptic ultrastructure at mammalian central synapses (Altrock et al., 2003; Mukherjee et al., 2010). Although invertebrates were thought to lack Piccolo/Bassoon homologs, the Piccolo/Rim related proteins (Fife and Clarinet) were recently identified in Drosophila and C. elegans, respectively (Bruckner et al., 2017; Xuan et al., 2017). Fife mutants display a modest reduction in VGCC abundance at AZs (Bruckner et al., 2017). In summary, redundant interactions between the core AZ scaffolds (RIMs, RBPs, ELKS/CAST, and Bassoon) with each other and VGCCs provide a robust mechanism to ensure AZs are populated with VGCCs required to support synaptic transmission.
The slot model for VGCC accumulation at AZs
A popular slot model of VGCC AZ abundance was originally proposed to explain several observations of competition among VGCCs for AZ localization in cultured hippocampal neurons with mixed Cav2.1 and Cav2.2 synapses (Cao et al., 2004). Overexpressing Cav2.1 did not increase Cav2.1-mediated release at synaptic terminals, suggesting the number of Cav2.1 channels that localize to AZs is limited downstream of Cav2.1 biosynthesis. Overexpression of mutant Ca2+-impermeant Cav2.1 channels reduced the contribution of Cav2.1 to total release, further indicating that mutant Cav2.1 channels compete with their wildtype counterparts for AZ localization “slots” (Cao et al., 2004). Because whole cell (somatodendritic) Cav2.1 currents were normal despite mutant channel overexpression, and were increased 5-fold by WT Cav2.1 overexpression, the rate-limiting factor in AZ localization is proposed to be downstream of channel biosynthesis and surface expression (Cao et al., 2004). Additionally, Cav2.2 influx was unaltered by Cav2.1 overexpression, suggesting the existence of “Cav2.2 specific slots” that cannot be occupied by Cav2.1 (Cao et al., 2004). In a similar series of experiments, overexpressing Ca2+-impermeant Cav2.2 reduced synaptic release, further indicating competition for saturated VGCC “slots” (Cao and Tsien, 2010). While Cav2.2 overexpression failed to increase total presynaptic release, Cav2.2 channels could displace Cav2.1 channels, suggesting “Cav2.1-preferring slots” can accommodate Cav2.2 under conditions of Cav2.2 excess (Cao and Tsien, 2010).
Three key elements define the slot model for limiting VGCC accumulation at AZs. First, Cav2.1/Cav2.2 mixed synapses are proposed to have “Cav2.1-preferring slots” and “Cav2.2 specific slots” that limit the number of VGCCs at the AZ. Second, “slots” are typically saturated, supported by the observation that channel overexpression does not increase AZ channel levels (Cao et al., 2004). Third, “slots” may not represent a limited number of rigid locations at the AZ where VGCCs are physically tethered, but may instead include competition for binding partners at any stage of VGCC localization all the way from axonal trafficking to channel incorporation or stabilization at AZs. Since Cav2.1 and Cav2.2 overexpression increased cell body Ca2+ currents, the competition for rate-limiting binding partners (“slots”) are proposed to be downstream of ER exit and cell surface expression (Cao and Tsien, 2010). The slot model predicts Cav2.2 channels should compensate for loss of Cav2.1 expression, whereas Cav2.1 channels should be unable to occupy Cav2.2-specific slots in Cav2.2 mutants. Indeed, Cav2.2 channels partially compensate in cav2.1 knockout mice at the mature calyx of Held, but Cav2.1 does not increase in cav2.2 mutant hippocampal neurons (Kim et al., 2001; Inchauspe et al., 2004; Ishikawa et al., 2005; Jeon et al., 2007). Additionally, Cav2 α1 subunit overexpression in dissociated rat neurons and Drosophila NMJs does not increase Cav2 levels at synapses, providing further support for a competition model (Hoppa et al., 2012; Cunningham et al., 2022).
Despite evidence supporting the slot model, its predictions partially fail at the calyx of Held. At immature (Cav2.1/Cav2.2 mixed) and mature (Cav2.1 exclusive) calyx neurons, Cav2.1 overexpression increased Cav2.1 number at AZs, indicating that if Cav2.1 slots exist, they are not saturated at this synapse (Lübbert et al., 2019). However, some evidence of competition was still observed, as Cav2.1 overexpression outcompeted Cav2.2 channels in the immature calyx. These data support an alternative model where Cav2.1 channels are not saturated at AZs, and Cav2.2 slots are Cav2.2-preferred rather than Cav2.2-specific (Lübbert et al., 2019). Contrasting findings in hippocampal vs. calyx of Held neurons could be due to several factors. VGCC regulation could differ between cultured neurons vs. in vivo neurons embedded in native circuitry. In addition, rules for mixed synapses may differ and change during development. Finally, previous studies used human Cav2.1 and Cav2.2 overexpression in mouse neurons. Even though these constructs rescued their respective knockouts, human and mouse VGCCs could differ in their regulation (Cao et al., 2004; Cao and Tsien, 2010). Further experiments are needed to define which aspects of the slot model represent general principles versus synapse-specific regulation that reflect neuronal diversity.
Several important questions still need to be addressed in the classical slot model for AZ VGCC abundance. If slots exist, what do they physically represent? Is the slot mechanism implemented locally at AZs (by limiting incorporation or retention of channels) or upstream of AZ localization, perhaps through limited binding to axonal trafficking partners? The key criterion for identifying a protein that regulates competition is that the level of that protein should affect VGCC AZ abundance in a dosage sensitive manner. The conserved AZ scaffold proteins are attractive candidates for locally mediating slots at the AZ. Drosophila BRP is well situated to be a slot protein, as it binds directly to the Cav2 channel and is required for channel accumulation and stabilization at AZs (Fouquet et al., 2009; Ghelani et al., 2022). However, reductions in AZ BRP abundance have no effect on AZ VGCC levels, indicating this protein is likely not a rate-limiting regulator of VGCCs at AZs (Cunningham et al., 2022). RIMs and RBPs initially were compelling candidates for a slot protein because they both bind to VGCCs and are required (albeit redundantly at different synapses) for VGCC localization (Han et al., 2011; Kaeser et al., 2011; Liu et al., 2011; Graf et al., 2012; Jung et al., 2015; Oh et al., 2021). Additionally, RIM interacts stoichiometrically with Cav2 (Kaeser et al., 2011; Oh et al., 2021). However, in mammalian central synapses and Drosophila NMJs, loss of RIM and RBP binding to the C-terminal of VGCCs did not reduce channel AZ localization (Schneider et al., 2015; Lübbert et al., 2017; Ghelani et al., 2022). Indeed, several studies reported the lack of RIM and RBP interactions actually promotes channel stability at AZs, opposite to what would be expected for a protein functioning as a VGCC slot interactor (Schneider et al., 2015; Ghelani et al., 2022). Bassoon has also been proposed to contribute to defining Cav2.1 slots, as the Bassoon-RBP interaction recruits Cav2.1 (but not Cav2.2) channels to hippocampal synapses (Davydova et al., 2014). However, Bassoon does not appear to regulate VGCC abundance in all neurons (Altrock et al., 2003; Mukherjee et al., 2010). The α2δ subunit is another possible “slot” protein, as it plays a dosage-sensitive role in promoting AZ VGCC abundance. Overexpression of α2δ leads to increased VGCC levels at synapses, while heterozygous mutations in this subunit moderately decrease Cav2 levels at AZs (Hoppa et al., 2012; Cunningham et al., 2022). A role for α2δ as the slot factor would likely be in its capacity as a VGCC trafficking regulator, as α2δ mutants actually show increased Cav2 retention at synapses, arguing against AZ-localized α2δ as the regulator of slot number (Cunningham et al., 2022). Another possibility is the slot interaction is lipid-mediated, as cholesterol has been shown to restrict VGCC domain size at AZs in photoreceptors (Mercer et al., 2011). Deciphering which molecules and binding interactions are rate limiting for channel localization to AZs is an important goal but is complicated by functional redundancy and other potential compensatory mechanisms.
VGCCs are mobile within the AZ
Characterizing mobility of VGCCs within the AZ is a topic of interest, given positional coupling of VGCCs and docked SVs is a major determinant of Pr (Bucurenciu et al., 2008; Eggermann et al., 2011; Chen et al., 2015). VGCC mobility within the AZ could represent a fast method of Pr regulation by altering the channel’s coupling distance to docked SVs. The idea that VGCCs occupy defined spots at the AZ arose from studies at the frog NMJ, where freeze fracture EM showed an array-like organization of particles, generating questions of whether these particles represent statically arranged VGCCs (Heuser et al., 1974; Pumplin et al., 1981; Cohen et al., 1991; Harlow et al., 2001). However modeling and experimental estimation of VGCC number at the frog NMJ suggests not all of these intramembrane particles can be channels, and Cav-immunogold EM reveal a less orderly, but non-randomly clustered, array of VGCCs (Luo et al., 2011; Holderith et al., 2012; Althof et al., 2015; Miki et al., 2017; Lübbert et al., 2019; Eguchi et al., 2022). The model of an orderly array of VGCCs is also at odds with more recent evidence from in vivo tracking of single VGCCs at synapses, showing that a fraction of AZ-resident VGCCs are mobile within a defined region of membrane, with low exit of channels from the AZ area (Mercer et al., 2011, 2012; Thoreson et al., 2013; Schneider et al., 2015; Figure 4; step 5).
At photoreceptor ribbon synapses of the salamander retina (populated with L-type VGCCs), quantum dots tagged to the extracellular α2δ-4 subunit of the channel revealed mobility within a defined ~0.18 μm2 region of presynaptic membrane under the ribbon (Mercer et al., 2011, 2012). In addition to baseline VGCC movements, SV fusion briefly displaced VGCCs toward the outer rim of the membrane region (Mercer et al., 2011). In both photoreceptors and bipolar cells, actin restricts the size of the VGCC-mobile area, consistent with studies showing actin disruption promotes VGCC internalization (Cristofanilli et al., 2007; Mercer et al., 2011; Thoreson et al., 2013). Cholesterol levels also regulate VGCC mobility within photoreceptor synapses, as cholesterol depletion widened the VGCC-mobile area and reduced Pr without altering Ca2+ influx, suggesting mobility may be regulated to tune VGCC-SV coupling distances (Mercer et al., 2011, 2012). Additionally, movement of an open VGCC could spread Ca2+ over a larger area, reducing the effective peak Ca2+ concentration compared to influx from stabilized VGCCs. This “smearing” factor may be especially relevant at highly sensitive synapses in photoreceptors or bipolar cells where the opening of only one or a few VGCCs is sufficient to trigger SV fusion (Jarsky et al., 2010; Bartoletti et al., 2011; Kim et al., 2013). Though modeling suggests the expanded VGCC-domain size is sufficient to account for decreased release, the effect of cholesterol depletion on other proteins involved in SV fusion cannot be ruled out (Mercer et al., 2012). These tracking experiments provide insights into the mobility of VGCCs, but it is unclear if α2δ-4-QDot tagging is a robust proxy for VGCC α1 subunit localization and mobility, as α2δ subunits regulate synapse development independent of their canonical position as VGCC subunits and can localize to synaptic terminals independent of the VGCC (Kurshan et al., 2009; Dolphin, 2018; Held et al., 2020). Furthermore, studies in hippocampal cultured neurons suggest association of VGCC α1 and α2δ is dynamic, with α2δ showing more mobility than the VGCC α1 subunit (Voigt et al., 2016).
Direct single-particle tracking of VGCC α1 subunits in mammalian cultured neurons have circumvented this caveat. SptPALM imaging of cytoplasmic mEOS2-tagged Cav2.1 and Cav2.2 channels in hippocampal neurons revealed the population of VGCCs within clusters is comprised of a mobile fraction (~60% of channels) and a smaller immobile fraction (Schneider et al., 2015). Channel mobility was largely confined within individual synapses, exhibited transient (~80 ms) confinement within synaptic nanodomains, and was similar for both Cav2.1 and Cav2.2, in contrast to mEOS2-tagged Syntaxin-1A (Schneider et al., 2015; Heck et al., 2019). Interestingly, reducing intracellular Ca2+ using BAPTA increased the fraction of immobile VGCCs, hinting at a possible mechanism to modulate VGCC mobility during plasticity (Schneider et al., 2015). In addition to regulation by Ca2+ buffering, VGCC mobility is activity-dependent, as blocking action potentials or postsynaptic glutamate receptors results in channel stabilization (Heck et al., 2019). Scaffold-channel interactions also regulate mobility; though surprisingly, the Cav2.1 splice variant lacking a C-terminal exon that encodes both RIM and RBP binding domains displays decreased mobility and supports more efficient SV release (Heck et al., 2019). These α1 tracking experiments in cell culture represent exciting steps forward in understanding channel mobility, as they reveal direct localization of the channel without relying on α2δ as a localization proxy. However, whether the lack of in vivo connections and a native synaptic environment abnormally influences channel mobility is currently unclear. Similar single-VGCC tracking experiments are currently being performed in vivo at Drosophila NMJs, where VGCCs appear to undergo high intra-AZ mobility as well (Ghelani et al., 2022). In addition, insights into longer-term VGCC mobility at Drosophila NMJs using photoconvertible Cac channels reveal they do not appear to laterally diffuse between neighboring AZs over multiple days, suggesting low inter-AZ movement despite high intra-AZ mobility (Cunningham et al., 2022).
VGCC internalization from AZs
The lifetime of surface expression for transmembrane proteins varies widely and is regulated in part by re-internalization through endocytosis (Figure 4; step 6). Most endocytosis is through a relatively slow Clathrin-mediated process, with adaptor proteins concentrating cargo and recruiting Clathrin, which assembles to deform the membrane into a pit. Subsequently, a burst of Actin to budding endocytic vesicles and membrane pinching by the GTPase Dynamin completes the endocytic process (Kaksonen and Roux, 2018). Faster Clathrin-independent modes of endocytosis have also been described at synapses. Bulk and ultrafast endocytosis are thought to quickly retrieve synaptic membrane after SV fusion (Watanabe and Boucrot, 2017). In addition, fast Endophilin mediated endocytosis (FEME) can be initiated to internalize specific membrane proteins, including some G Protein-Coupled Receptors (GPCRs; Moo et al., 2021). GPCRs are inhibited by their own agonist-stimulated endocytosis, a process which is canonically initiated by the binding of endocytic adaptor proteins of the Arrestin family, but that can also proceed through non-canonical pathways (Moo et al., 2021; von Zastrow and Sorkin, 2021). Receptor Tyrosine Kinases (RTKs) are also endocytosed after ligand binding, with internalization initiated either though RTK ubiquitination or binding to Clathrin-adapter proteins (von Zastrow and Sorkin, 2021). AMPA Receptors are glutamate-gated cation channels that mediate most excitatory neurotransmission in the mammalian CNS and their internalization regulates synaptic strength during several forms of synaptic plasticity (Citri and Malenka, 2008; Hastings and Man, 2018). AMPA Receptors can dissociate from scaffolds within the postsynaptic density and move into endocytic zones where they associate with Clathrin adaptor proteins and become internalized. In contrast to GPCRs, RTKs, and AMPA receptors, little is known about the role of internalization in VGCC regulation at AZs. How big of a role does VGCC internalization play in regulating synaptic strength? What regulates VGCC internalization and what molecular pathways facilitate this process? Does channel endocytosis occur within AZs or elsewhere on the presynaptic membrane?
Some evidence for GPCR-regulated VGCC internalization has come from studies of Cav2.2 channels in cultured neurons and DRG neurons involved in pain signaling (Bourinet et al., 2014). In this circuit, the GPCR opioid receptor (ORL1) forms a complex with Cav2.2 channels and its activation via the agonist nociceptin results in ORL1/Cav2.2 complex internalization (Beedle et al., 2004; Altier et al., 2006). This internalization can be directly visualized using GFP-tagged Cav2.2 α1 subunits and red-tagged ORL receptors. Upon ORL activation, Cav2.2 and ORL exclusively colocalize in intracellular puncta that label with a lysosomal marker (Altier et al., 2006). In acutely dissociated DRG neurons, Cav2.2 channels are internalized following nociceptin exposure, leading to a decrease in Cav2.2-mediated Ca2+ influx (Altier et al., 2006). Though lysosome marker colocalization suggests internalized channels may be degraded, the fate of these channels is unknown. Agonist washout results in loss of intracellular VGCCs after several hours, but whether channels were returned to the surface or targeted for degradation is unclear (Altier et al., 2006). Dopamine (DA) receptors are another family of GPCRs that regulate VGCCs in the mammalian CNS, and DA receptors can promote internalization of Cav2.2 through direct protein–protein interactions (Kisilevsky et al., 2008; Kisilevsky and Zamponi, 2008). Along with GPCR regulation, the Actin cytoskeleton plays a role in regulating presynaptic VGCC internalization in some systems (Furukawa et al., 1997; Cristofanilli et al., 2007; Mizuno et al., 2010; Tseng et al., 2017).
Studies of molecular mechanisms of VGCC internalization in cell culture is facilitated by molecular and imaging access, but in vivo experiments are required to understand the timescales and patterns of VGCC internalization at native synapses. Do AZ-localized VGCCs become internalized through similar pathways? How long do VGCCs remain at the presynaptic membrane and how is their internalization regulated? Animal-wide isotopic labeling has been employed as a high-throughput strategy for measuring protein half-lives in vivo (Price et al., 2010; Fornasiero et al., 2018; Heo et al., 2018). This approach can measure degradation rates of newly synthesized proteins across the entire proteome, but has limited spatial resolution to measure turnover in specific compartments or individual neuronal populations. Given degradation of VGCCs can occur in the biosynthetic pathway before channels reach the synapse, whole-brain turnover measurements may not accurately reflect rates of AZ-localized VGCCs dynamics. Despite these drawbacks, it is worth noting that VGCCs display a half-life of around 8 days when assayed by isotopic labeling (Fornasiero et al., 2018).
Studies of AZ-resident VGCC half-life and turnover have also been performed at the Drosophila NMJ, a synapse with hundreds of AZs that are individually resolvable by conventional light microscopy in intact animals, allowing multi-day experiments using intravital imaging (Figures 2B,C). Red-to-green photoconversion of endogenously Maple-tagged Cac (the sole VGCC mediating synaptic transmission in flies) allowed measurements of Cac removal from AZs over a multi-day period. On average, 30% of photoconverted Cac signal intensity was lost from AZs over 4 days, indicating turnover plays an important role in regulating the abundance of the channel at AZs (Figures 2D–G; Cunningham et al., 2022). This 30% loss over 4 days predicts a half-life of about 8 days, consistent with isotopic labeling measurements of VGCC stability (Fornasiero et al., 2018; Cunningham et al., 2022). Measurements of new Cac delivery at individual AZs indicates turnover contributes to a leveling-off of Cac abundance at mature AZs. Furthermore, Cac loss from AZs is predicted to occur primarily through re-internalization of the channel, as lateral transfer of Cac channels was not observed (Cunningham et al., 2022). In mutants with either reduced levels of α2δ or reduced levels of the α1 subunit, turnover was reduced, indicating new channel delivery regulates channel turnover at this synapse rather than a fixed VGCC lifespan (Cunningham et al., 2022).
Presynaptic VGCCs: Beyond evoked neurotransmission
Aside from the canonical role of presynaptic VGCCs as mediators of evoked neurotransmission, non-AZ resident VGCCs can regulate other Ca2+-dependent aspects of presynaptic function, including SV endocytosis and presynaptic plasticity. At presynaptic terminals, Ca2+-dependent endocytosis immediately follows SV fusion (Hosoi et al., 2009; Wu et al., 2009, 2014). Temporal coordination between exo- and endocytosis ensures prompt recycling of SVs after fusion, and maintains presynaptic membrane homeostasis (for a detailed review of presynaptic exo-endocytic coupling, see Wu et al., 2014; Maritzen and Haucke, 2018). Ca2+ influx through VGCCs has been proposed to mediate this coupling (Wu et al., 2009, 2014; Xue et al., 2012; Krick et al., 2021). In addition to SV fusion and endocytosis, Ca2+ signaling through VGCCs can contribute to short term plasticity without altering baseline Pr, reflecting functional separation between VGCC subtypes within the presynaptic membrane (Jensen and Mody, 2001; Dietrich et al., 2003; Krick et al., 2021).
Given multiple processes—including neurotransmission, endocytosis, and plasticity—are controlled by VGCC-dependent Ca2+ signaling within a relatively small area, how are these Ca2+ signals separated to avoid crosstalk? Precise positioning of different VGCC subtypes within subdomains of the presynaptic membrane is one mechanism by which synapses can reduce crosstalk. This spatial separation of distinct VGCC populations in the presynaptic terminal is illustrated at the Drosophila NMJ, where the sole Cav2 channel (Cac) localizes to AZs and mediates neurotransmission, while the Cav1 channel (Dmca1D) localizes to non-AZ domains within the presynaptic membrane and regulates Ca2+-dependent endocytosis and short-term plasticity (Krick et al., 2021). In addition to the distinct localizations of these channel types, cytosolic Ca2+ buffers and active extrusion of Ca2+ by the PMCA pump further reduces crosstalk between Cav1 and Cav2 signaling (Krick et al., 2021). Similar to AZ-resident VGCCs, the mechanisms that regulate the abundance and subcellular localization of other VGCC populations within the presynaptic membrane are unknown.
Modulation of VGCC abundance during plasticity: Insights from Drosophila
Voltage-gated Ca2+ channels are key regulators of presynaptic Pr, placing them in a prime position to be targeted by plasticity pathways that modulate synaptic strength (Augustine et al., 1985; Borst and Sakmann, 1996; Wang et al., 2008; Bartoletti et al., 2011; Ariel et al., 2012; Sheng et al., 2012; Newman et al., 2022). Indeed, acute modulation of VGCC activation, inactivation, and conductance have all been shown to contribute to various presynaptic plasticity pathways (Nanou and Catterall, 2018). More recently, studies at the Drosophila NMJ indicate plastic regulation of channel abundance and mobility at the presynaptic membrane can also occur. Due to robust genetic, imaging, and electrophysiological approaches that enable studies of individual AZs in vivo, this model has emerged as a key system for characterizing how presynaptic plasticity impinges on the abundance and mobility of AZ components. Indeed, changes in the abundance and organization of VGCCs and the AZ scaffold have been reported at the NMJ during expression of acute and chronic forms of plasticity.
When postsynaptic Glutamate Receptors are blocked acutely with a toxin or chronically via genetic mutations at Drosophila NMJs, the decrease in postsynaptic responsiveness to neurotransmitter (quantal size) triggers a compensatory upregulation of presynaptic Pr. Increased SV fusion precisely offsets the reduction in quantal size, homeostatically preserving overall synaptic strength. This homeostatic synaptic potentiation (HSP) can happen strikingly fast, occurring on the scale of minutes after application of a Glutamate Receptor toxin (Frank et al., 2006, 2009; Müller and Davis, 2012; Frank, 2014). The Cac channel is mechanistically implicated in HSP plasticity. Ca2+ imaging directly demonstrates an increase in presynaptic Ca2+ influx during HSP, and hypomorphic mutations in cac block potentiation of Ca2+ influx and homeostatic potentiation (Frank et al., 2006; Müller and Davis, 2012). Additionally, the extracellular Cac subunit α2δ is required for HSP, independent of its effect on baseline Ca2+ influx (Wang et al., 2016). An opposing form of presynaptic homeostatic plasticity is homeostatic synaptic depression (HSD). When quantal size is chronically increased through overexpression of the SV glutamate transporter VGlut, HSD compensates by reducing presynaptic Pr. Imaging of Ca2+ influx and AZ Cac-GFP abundance demonstrates this form of plasticity also targets the Cac channel by decreasing its abundance at AZs (Gaviño et al., 2015). Together these findings suggest Ca2+ influx through VGCCs is modulated bidirectionally to influence Pr during multiple forms of presynaptic plasticity.
While initial evidence for Cac involvement in HSP did not resolve whether channel properties or abundance were altered to trigger increased Ca2+ influx, several studies suggest AZ Cac abundance may increase during this form of potentiation (Gratz et al., 2019; Ghelani et al., 2022). Similarly, BRP puncta observed in AZ rings increased in number during HSP (Hong et al., 2020). Although these studies suggest elevated levels of BRP and Cac, studies employing STORM imaging indicate the increased fluorescent intensity is secondary to compaction of AZ material that occurs during plasticity rather than increases in protein content across AZs (Mrestani et al., 2021). Work in the Drosophila CNS indicates Cac transcription may also be a target for certain forms of presynaptic potentiation. In the Drosophila CNS, Kenyon Cell neurons form boutons along compartmentalized regions of the mushroom body to drive associative learning. Monitoring of presynaptic Ca2+ during behavior reveals compartment-specific modulation of Ca2+ influx along Kenyon Cell axons during learning that is mediated by neuromodulatory neuron dopamine release and presumed GPCR-mediated silencing of VGCC function (Bilz et al., 2020). Although reducing VGCC biosynthesis by modest levels does not alter baseline transmission at these synapses, the same manipulation impairs presynaptic potentiation, indicating Cac biosynthesis becomes rate-limiting during certain forms of presynaptic plasticity (Stahl et al., 2022). Together, these studies suggest VGCC abundance, location, and mobility at AZs may represent important targets for fine-tuning of presynaptic output.
Conclusion and future directions
Pathways regulating the surface abundance of presynaptic VGCCs, including progression through the biosynthetic pathway, transport to the synapse, stabilization and mobility at AZs, and turnover by endocytosis, have emerged as important mechanisms to set baseline synaptic strength and as potential targets to change output during plasticity. Despite the importance of VGCC dynamics and regulation, many questions remain unsolved. In particular, identifying which VGCC-regulatory components are rate-limiting in setting channel abundance at AZs will provide insights into the fine-tuning and regulation of channel surface expression. Additionally, the precise mechanisms and timescales of channel delivery and turnover at individual presynaptic AZs are still unclear, precluding a clear understanding of how delivery and recycling modulate synaptic development and presynaptic strength in growing circuits.
Author contributions
All authors listed have made a substantial, direct, and intellectual contribution to the work and approved it for publication.
Funding
The authors’ work has been funded by NIH grants NS40296, NS117588, and MH104536 and the JPB Foundation.
Conflict of interest
The authors declare that the research was conducted in the absence of any commercial or financial relationships that could be construed as a potential conflict of interest.
Publisher’s note
All claims expressed in this article are solely those of the authors and do not necessarily represent those of their affiliated organizations, or those of the publisher, the editors and the reviewers. Any product that may be evaluated in this article, or claim that may be made by its manufacturer, is not guaranteed or endorsed by the publisher.
References
Ackermann, F., Waites, C. L., and Garner, C. C. (2015). Presynaptic active zones in invertebrates and vertebrates. EMBO Rep. 16, 923–938. doi: 10.15252/embr.201540434
Acuna, C., Liu, X., Gonzalez, A., and Südhof, T. C. (2015). RIM-BPs mediate tight coupling of action potentials to Ca(2+)-triggered neurotransmitter release. Neuron 87, 1234–1247. doi: 10.1016/j.neuron.2015.08.027
Acuna, C., Liu, X., and Südhof, T. C. (2016). How to make an active zone: unexpected universal functional redundancy between RIMs and RIM-BPs. Neuron 91, 792–807. doi: 10.1016/j.neuron.2016.07.042
Ahmari, S. E., Buchanan, J., and Smith, S. J. (2000). Assembly of presynaptic active zones from cytoplasmic transport packets. Nat. Neurosci. 3, 445–451. doi: 10.1038/74814
Akbergenova, Y., Cunningham, K. L., Zhang, Y. V., Weiss, S., and Littleton, J. T. (2018). Characterization of developmental and molecular factors underlying release heterogeneity at drosophila synapses. elife 7. doi: 10.7554/eLife.38268
Althof, D., Baehrens, D., Watanabe, M., Suzuki, N., Fakler, B., and Kulik, Á. (2015). Inhibitory and excitatory axon terminals share a common nano-architecture of their Cav2.1 (P/Q-type) Ca(2+) channels. Front. Cell. Neurosci. 9:315. doi: 10.3389/fncel.2015.00315
Altier, C., Garcia-Caballero, A., Simms, B., You, H., Chen, L., Walcher, J., et al. (2011). The Cavβ subunit prevents RFP2-mediated ubiquitination and proteasomal degradation of L-type channels. Nat. Neurosci. 14, 173–180. doi: 10.1038/nn.2712
Altier, C., Khosravani, H., Evans, R. M., Hameed, S., Peloquin, J. B., Vartian, B. A., et al. (2006). ORL1 receptor-mediated internalization of N-type calcium channels. Nat. Neurosci. 9, 31–40. doi: 10.1038/nn1605
Altrock, W. D., tom Dieck, S., Sokolov, M., Meyer, A. C., Sigler, A., Brakebusch, C., et al. (2003). Functional inactivation of a fraction of excitatory synapses in mice deficient for the active zone protein bassoon. Neuron 37, 787–800. doi: 10.1016/s0896-6273(03)00088-6
Ariel, P., Hoppa, M. B., and Ryan, T. A. (2012). Intrinsic variability in Pv, RRP size, Ca(2+) channel repertoire, and presynaptic potentiation in individual synaptic boutons. Front. Synap. Neurosci. 4:9. doi: 10.3389/fnsyn.2012.00009
Atwood, H. L., and Karunanithi, S. (2002). Diversification of synaptic strength: presynaptic elements. Nat. Rev. Neurosci. 3, 497–516. doi: 10.1038/nrn876
Augustine, G. J., Charlton, M. P., and Smith, S. J. (1985). Calcium entry and transmitter release at voltage-clamped nerve terminals of squid. J. Physiol. Lond. 367, 163–181. doi: 10.1113/jphysiol.1985.sp015819
Ball, S. L., Powers, P. A., Shin, H. S., Morgans, C. W., Peachey, N. S., and Gregg, R. G. (2002). Role of the β2 subunit of voltage-dependent calcium channels in the retinal outer plexiform layer. Invest. Ophthalmol. Vis. Sci. 43, 1595–1603.
Bartoletti, T. M., Jackman, S. L., Babai, N., Mercer, A. J., Kramer, R. H., and Thoreson, W. B. (2011). Release from the cone ribbon synapse under bright light conditions can be controlled by the opening of only a few Ca(2+) channels. J. Neurophysiol. 106, 2922–2935. doi: 10.1152/jn.00634.2011
Beedle, A. M., McRory, J. E., Poirot, O., Doering, C. J., Altier, C., Barrere, C., et al. (2004). Agonist-independent modulation of N-type calcium channels by ORL1 receptors. Nat. Neurosci. 7, 118–125. doi: 10.1038/nn1180
Berridge, M. J., Bootman, M. D., and Roderick, H. L. (2003). Calcium signalling: dynamics, homeostasis and remodelling. Nat. Rev. Mol. Cell Biol. 4, 517–529. doi: 10.1038/nrm1155
Berrow, N. S., Campbell, V., Fitzgerald, E. M., Brickley, K., and Dolphin, A. C. (1995). Antisense depletion of beta-subunits modulates the biophysical and pharmacological properties of neuronal calcium channels. J. Physiol. Lond. 482, 481–491. doi: 10.1113/jphysiol.1995.sp020534
Betz, A., Thakur, P., Junge, H. J., Ashery, U., Rhee, J. S., Scheuss, V., et al. (2001). Functional interaction of the active zone proteins Munc13-1 and RIM1 in synaptic vesicle priming. Neuron 30, 183–196. doi: 10.1016/s0896-6273(01)00272-0
Billings, S. E., Clarke, G. L., and Nishimune, H. (2012). ELKS1 and Ca(2+) channel subunit β4 interact and colocalize at cerebellar synapses. Neuroreport 23, 49–54. doi: 10.1097/WNR.0b013e32834e7deb
Bilz, F., Geurten, B. R. H., Hancock, C. E., Widmann, A., and Fiala, A. (2020). Visualization of a distributed synaptic memory code in the drosophila brain. Neuron 106, 963–976.e4. doi: 10.1016/j.neuron.2020.03.010
Böhme, M. A., Beis, C., Reddy-Alla, S., Reynolds, E., Mampell, M. M., Grasskamp, A. T., et al. (2016). Active zone scaffolds differentially accumulate Unc13 isoforms to tune Ca(2+) channel-vesicle coupling. Nat. Neurosci. 19, 1311–1320. doi: 10.1038/nn.4364
Borst, J. G., and Sakmann, B. (1996). Calcium influx and transmitter release in a fast CNS synapse. Nature 383, 431–434. doi: 10.1038/383431a0
Bourinet, E., Altier, C., Hildebrand, M. E., Trang, T., Salter, M. W., and Zamponi, G. W. (2014). Calcium-permeable ion channels in pain signaling. Physiol. Rev. 94, 81–140. doi: 10.1152/physrev.00023.2013
Brice, N. L., Berrow, N. S., Campbell, V., Page, K. M., Brickley, K., Tedder, I., et al. (1997). Importance of the different beta subunits in the membrane expression of the alpha1A and alpha2 calcium channel subunits: studies using a depolarization-sensitive alpha1A antibody. Eur. J. Neurosci. 9, 749–759. doi: 10.1111/j.1460-9568.1997.tb01423.x
Bruckner, J. J., Zhan, H., Gratz, S. J., Rao, M., Ukken, F., Zilberg, G., et al. (2017). Fife organizes synaptic vesicles and calcium channels for high-probability neurotransmitter release. J. Cell Biol. 216, 231–246. doi: 10.1083/jcb.201601098
Bucurenciu, I., Kulik, A., Schwaller, B., Frotscher, M., and Jonas, P. (2008). Nanodomain coupling between Ca2+ channels and Ca2+ sensors promotes fast and efficient transmitter release at a cortical GABAergic synapse. Neuron 57, 536–545. doi: 10.1016/j.neuron.2007.12.026
Buraei, Z., and Yang, J. (2010). The ß subunit of voltage-gated Ca2+ channels. Physiol. Rev. 90, 1461–1506. doi: 10.1152/physrev.00057.2009
Burgess, D. L., Jones, J. M., Meisler, M. H., and Noebels, J. L. (1997). Mutation of the Ca2+ channel beta subunit gene Cchb4 is associated with ataxia and seizures in the lethargic (lh) mouse. Cells 88, 385–392. doi: 10.1016/s0092-8674(00)81877-2
Bury, L. A. D., and Sabo, S. L. (2011). Coordinated trafficking of synaptic vesicle and active zone proteins prior to synapse formation. Neural Dev. 6:24. doi: 10.1186/1749-8104-6-24
Cai, Q., Pan, P.-Y., and Sheng, Z.-H. (2007). Syntabulin-kinesin-1 family member 5B-mediated axonal transport contributes to activity-dependent presynaptic assembly. J. Neurosci. 27, 7284–7296. doi: 10.1523/JNEUROSCI.0731-07.2007
Cantí, C., Davies, A., Berrow, N. S., Butcher, A. J., Page, K. M., and Dolphin, A. C. (2001). Evidence for two concentration-dependent processes for beta-subunit effects on alpha1B calcium channels. Biophys. J. 81, 1439–1451. doi: 10.1016/S0006-3495(01)75799-2
Cao, Y.-Q., Piedras-Rentería, E. S., Smith, G. B., Chen, G., Harata, N. C., and Tsien, R. W. (2004). Presynaptic Ca2+ channels compete for channel type-preferring slots in altered neurotransmission arising from Ca2+ channelopathy. Neuron 43, 387–400. doi: 10.1016/j.neuron.2004.07.014
Cao, Y.-Q., and Tsien, R. W. (2010). Different relationship of N- and P/Q-type Ca2+ channels to channel-interacting slots in controlling neurotransmission at cultured hippocampal synapses. J. Neurosci. 30, 4536–4546. doi: 10.1523/JNEUROSCI.5161-09.2010
Cases-Langhoff, C., Voss, B., Garner, A. M., Appeltauer, U., Takei, K., Kindler, S., et al. (1996). Piccolo, a novel 420 kDa protein associated with the presynaptic cytomatrix. Eur. J. Cell Biol. 69, 214–223.
Cassidy, J. S., Ferron, L., Kadurin, I., Pratt, W. S., and Dolphin, A. C. (2014). Functional exofacially tagged N-type calcium channels elucidate the interaction with auxiliary α2δ-1 subunits. Proc. Natl. Acad. Sci. U. S. A. 111, 8979–8984. doi: 10.1073/pnas.1403731111
Catterall, W. A., and Few, A. P. (2008). Calcium channel regulation and presynaptic plasticity. Neuron 59, 882–901. doi: 10.1016/j.neuron.2008.09.005
Catterall, W. A., Lenaeus, M. J., and Gamal El-Din, T. M. (2020). Structure and pharmacology of voltage-gated sodium and calcium channels. Annu. Rev. Pharmacol. Toxicol. 60, 133–154. doi: 10.1146/annurev-pharmtox-010818-021757
Chen, J., Billings, S. E., and Nishimune, H. (2011). Calcium channels link the muscle-derived synapse organizer laminin β2 to bassoon and CAST/Erc2 to organize presynaptic active zones. J. Neurosci. 31, 512–525. doi: 10.1523/JNEUROSCI.3771-10.2011
Chen, Z., Das, B., Nakamura, Y., DiGregorio, D. A., and Young, S. M. (2015). Ca2+ channel to synaptic vesicle distance accounts for the readily releasable pool kinetics at a functionally mature auditory synapse. J. Neurosci. 35, 2083–2100. doi: 10.1523/JNEUROSCI.2753-14.2015
Chen, Y.-H., Li, M.-H., Zhang, Y., He, L.-L., Yamada, Y., Fitzmaurice, A., et al. (2004). Structural basis of the alpha1-beta subunit interaction of voltage-gated Ca2+ channels. Nature 429, 675–680. doi: 10.1038/nature02641
Citri, A., and Malenka, R. C. (2008). Synaptic plasticity: multiple forms, functions, and mechanisms. Neuropsychopharmacology 33, 18–41. doi: 10.1038/sj.npp.1301559
Cohen, M. W., Jones, O. T., and Angelides, K. J. (1991). Distribution of Ca2+ channels on frog motor nerve terminals revealed by fluorescent omega-conotoxin. J. Neurosci. 11, 1032–1039. doi: 10.1523/JNEUROSCI.11-04-01032.1991
Coppola, T., Magnin-Luthi, S., Perret-Menoud, V., Gattesco, S., Schiavo, G., and Regazzi, R. (2001). Direct interaction of the Rab3 effector RIM with Ca2+ channels, SNAP-25, and synaptotagmin. J. Biol. Chem. 276, 32756–32762. doi: 10.1074/jbc.M100929200
Cristofanilli, M., Mizuno, F., and Akopian, A. (2007). Disruption of actin cytoskeleton causes internalization of Cav1.3 (alpha 1D) L-type calcium channels in salamander retinal neurons. Mol. Vis. 13, 1496–1507.
Cunningham, K. L., Sauvola, C. W., Tavana, S., and Littleton, J. T. (2022). Regulation of presynaptic Ca2+ channel abundance at active zones through a balance of delivery and turnover. elife 11:e78648. doi: 10.7554/eLife.78648
Davies, A., Kadurin, I., Alvarez-Laviada, A., Douglas, L., Nieto-Rostro, M., Bauer, C. S., et al. (2010). The α2δ subunits of voltage-gated calcium channels form GPI-anchored proteins, a posttranslational modification essential for function. Proc. Natl. Acad. Sci. 107, 1654–1659. doi: 10.1073/pnas.0908735107
Davydova, D., Marini, C., King, C., Klueva, J., Bischof, F., Romorini, S., et al. (2014). Bassoon specifically controls presynaptic P/Q-type Ca(2+) channels via RIM-binding protein. Neuron 82, 181–194. doi: 10.1016/j.neuron.2014.02.012
De Jongh, K. S., Warner, C., and Catterall, W. A. (1990). Subunits of purified calcium channels. Alpha 2 and delta are encoded by the same gene. J. Biol. Chem. 265, 14738–14741. doi: 10.1016/S0021-9258(18)77174-3
De Pace, R., Britt, D. J., Mercurio, J., Foster, A. M., Djavaherian, L., Hoffmann, V., et al. (2020). Synaptic vesicle precursors and lysosomes are transported by different mechanisms in the axon of mammalian neurons. Cell reports. 31:107775.
Deken, S. L., Vincent, R., Hadwiger, G., Liu, Q., Wang, Z.-W., and Nonet, M. L. (2005). Redundant localization mechanisms of RIM and ELKS in Caenorhabditis elegans. J. Neurosci. 25, 5975–5983. doi: 10.1523/JNEUROSCI.0804-05.2005
DiAntonio, A., and Schwarz, T. L. (1994). The effect on synaptic physiology of synaptotagmin mutations in drosophila. Neuron 12, 909–920. doi: 10.1016/0896-6273(94)90342-5
Dick, O., tom Dieck, S., Altrock, W. D., Ammermüller, J., Weiler, R., Garner, C. C., et al. (2003). The presynaptic active zone protein bassoon is essential for photoreceptor ribbon synapse formation in the retina. Neuron 37, 775–786. doi: 10.1016/s0896-6273(03)00086-2
Dickman, D. K., Kurshan, P. T., and Schwarz, T. L. (2008). Mutations in a drosophila alpha2delta voltage-gated calcium channel subunit reveal a crucial synaptic function. J. Neurosci. 28, 31–38. doi: 10.1523/JNEUROSCI.4498-07.2008
Dietrich, D., Kirschstein, T., Kukley, M., Pereverzev, A., von der Brelie, C., Schneider, T., et al. (2003). Functional specialization of presynaptic Cav2. 3 Ca2+ channels. Neuron 39, 483–496. doi: 10.1016/S0896-6273(03)00430-6
Dolphin, A. C. (2016). Voltage-gated calcium channels and their auxiliary subunits: physiology and pathophysiology and pharmacology. J. Physiol. Lond. 594, 5369–5390. doi: 10.1113/JP272262
Dolphin, A. C. (2018). Voltage-gated calcium channel α 2δ subunits: an assessment of proposed novel roles. [version 1; peer review: 2 approved]. F1000Res 7:1830. doi: 10.12688/f1000research.16104.1
Dolphin, A. C., and Lee, A. (2020). Presynaptic calcium channels: specialized control of synaptic neurotransmitter release. Nat. Rev. Neurosci. 21, 213–229. doi: 10.1038/s41583-020-0278-2
Dong, W., Radulovic, T., Goral, R. O., Thomas, C., Suarez Montesinos, M., Guerrero-Given, D., et al. (2018). CAST/ELKS proteins control voltage-gated Ca2+ channel density and synaptic release probability at a mammalian central synapse. Cell Rep. 24, 284–293.e6. doi: 10.1016/j.celrep.2018.06.024
Dresbach, T., Torres, V., Wittenmayer, N., Altrock, W. D., Zamorano, P., Zuschratter, W., et al. (2006). Assembly of active zone precursor vesicles: obligatory trafficking of presynaptic cytomatrix proteins bassoon and piccolo via a trans-Golgi compartment. J. Biol. Chem. 281, 6038–6047. doi: 10.1074/jbc.M508784200
Eberl, D. F., Ren, D., Feng, G., Lorenz, L. J., Van Vactor, D., and Hall, L. M. (1998). Genetic and developmental characterization of Dmca1D, a calcium channel alpha1 subunit gene in Drosophila melanogaster. Genetics 148, 1159–1169. doi: 10.1093/genetics/148.3.1159
Eggermann, E., Bucurenciu, I., Goswami, S. P., and Jonas, P. (2011). Nanodomain coupling between Ca2+ channels and sensors of exocytosis at fast mammalian synapses. Nat. Rev. Neurosci. 13, 7–21. doi: 10.1038/nrn3125
Eguchi, K., Montanaro, J., Le Monnier, E., and Shigemoto, R. (2022). The number and distinct clustering patterns of voltage-gated calcium channels in nerve terminals. Front. Neuroanat. 16:846615. doi: 10.3389/fnana.2022.846615
Eroglu, C., Allen, N. J., Susman, M. W., O’Rourke, N. A., Park, C. Y., Ozkan, E., et al. (2009). Gabapentin receptor alpha2delta-1 is a neuronal thrombospondin receptor responsible for excitatory CNS synaptogenesis. Cells 139, 380–392. doi: 10.1016/j.cell.2009.09.025
Fairless, R., Masius, H., Rohlmann, A., Heupel, K., Ahmad, M., Reissner, C., et al. (2008). Polarized targeting of neurexins to synapses is regulated by their C-terminal sequences. J. Neurosci. 28, 12969–12981. doi: 10.1523/JNEUROSCI.5294-07.2008
Fenster, S. D., Chung, W. J., Zhai, R., Cases-Langhoff, C., Voss, B., Garner, A. M., et al. (2000). Piccolo, a presynaptic zinc finger protein structurally related to bassoon. Neuron 25, 203–214. doi: 10.1016/s0896-6273(00)80883-1
Fornasiero, E. F., Mandad, S., Wildhagen, H., Alevra, M., Rammner, B., Keihani, S., et al. (2018). Precisely measured protein lifetimes in the mouse brain reveal differences across tissues and subcellular fractions. Nat. Commun. 9:4230. doi: 10.1038/s41467-018-06519-0
Fouquet, W., Owald, D., Wichmann, C., Mertel, S., Depner, H., Dyba, M., et al. (2009). Maturation of active zone assembly by drosophila Bruchpilot. J. Cell Biol. 186, 129–145. doi: 10.1083/jcb.200812150
Frank, C. A. (2014). How voltage-gated calcium channels gate forms of homeostatic synaptic plasticity. Front. Cell. Neurosci. 8:40. doi: 10.3389/fncel.2014.00040
Frank, C. A., Kennedy, M. J., Goold, C. P., Marek, K. W., and Davis, G. W. (2006). Mechanisms underlying the rapid induction and sustained expression of synaptic homeostasis. Neuron 52, 663–677. doi: 10.1016/j.neuron.2006.09.029
Frank, C. A., Pielage, J., and Davis, G. W. (2009). A presynaptic homeostatic signaling system composed of the Eph receptor, ephexin, Cdc42, and CaV2.1 calcium channels. Neuron 61, 556–569. doi: 10.1016/j.neuron.2008.12.028
Frank, T., Rutherford, M. A., Strenzke, N., Neef, A., Pangršič, T., Khimich, D., et al. (2010). Bassoon and the synaptic ribbon organize Ca2+ channels and vesicles to add release sites and promote refilling. Neuron 68, 724–738. doi: 10.1016/j.neuron.2010.10.027
Frøkjær-Jensen, C., Kindt, K. S., Kerr, R. A., Suzuki, H., Melnik-Martinez, K., Gerstbreih, B., et al. (2006). Effects of voltage-gated calcium channel subunit genes on calcium influx in cultured C. elegans mechanosensory neurons. J. Neurobiol. 66, 1125–1139. doi: 10.1002/neu.20261
Furukawa, K., Fu, W., Li, Y., Witke, W., Kwiatkowski, D. J., and Mattson, M. P. (1997). The actin-severing protein gelsolin modulates calcium channel and NMDA receptor activities and vulnerability to excitotoxicity in hippocampal neurons. J. Neurosci. 17, 8178–8186. doi: 10.1523/JNEUROSCI.17-21-08178.1997
Gandini, M. A., and Zamponi, G. W. (2022). Voltage-gated calcium channel nanodomains: molecular composition and function. FEBS J. 289, 614–633. doi: 10.1111/febs.15759
Gaviño, M. A., Ford, K. J., Archila, S., and Davis, G. W. (2015). Homeostatic synaptic depression is achieved through a regulated decrease in presynaptic calcium channel abundance. elife 4:e05473. doi: 10.7554/eLife.05473
Gee, N. S., Brown, J. P., Dissanayake, V. U., Offord, J., Thurlow, R., and Woodruff, G. N. (1996). The novel anticonvulsant drug, gabapentin (Neurontin), binds to the alpha2delta subunit of a calcium channel. J. Biol. Chem. 271, 5768–5776. doi: 10.1074/jbc.271.10.5768
Ghelani, T., Escher, M., Thomas, U., Esch, K., Lützkendorf, J., Depner, H., et al. (2022). An active zone state switch concentrates and immobilizes voltage-gated Ca2+ channels to promote long-term plasticity. Research Square [Preprint]. doi: 10.21203/rs.3.rs-1292687/v1
Ghelani, T., and Sigrist, S. J. (2018). Coupling the structural and functional assembly of synaptic release sites. Front. Neuroanat. 12:81. doi: 10.3389/fnana.2018.00081
Gho, M., McDonald, K., Ganetzky, B., and Saxton, W. M. (1992). Effects of kinesin mutations on neuronal functions. Science 258, 313–316. doi: 10.1126/science.1384131
Gindhart, J. G., Desai, C. J., Beushausen, S., Zinn, K., and Goldstein, L. S. (1998). Kinesin light chains are essential for axonal transport in drosophila. J. Cell Biol. 141, 443–454. doi: 10.1083/jcb.141.2.443
Goldstein, A. Y. N., Wang, X., and Schwarz, T. L. (2008). Axonal transport and the delivery of pre-synaptic components. Curr. Opin. Neurobiol. 18, 495–503. doi: 10.1016/j.conb.2008.10.003
Graf, E. R., Daniels, R. W., Burgess, R. W., Schwarz, T. L., and DiAntonio, A. (2009). Rab3 dynamically controls protein composition at active zones. Neuron 64, 663–677. doi: 10.1016/j.neuron.2009.11.002
Graf, E. R., Valakh, V., Wright, C. M., Wu, C., Liu, Z., Zhang, Y. Q., et al. (2012). RIM promotes calcium channel accumulation at active zones of the drosophila neuromuscular junction. J. Neurosci. 32, 16586–16596. doi: 10.1523/JNEUROSCI.0965-12.2012
Gratz, S. J., Goel, P., Bruckner, J. J., Hernandez, R. X., Khateeb, K., Macleod, G. T., et al. (2019). Endogenous tagging reveals differential regulation of Ca2+ channels at single active zones during presynaptic homeostatic potentiation and depression. J. Neurosci. 39, 2416–2429. doi: 10.1523/JNEUROSCI.3068-18.2019
Gregg, R. G., Messing, A., Strube, C., Beurg, M., Moss, R., Behan, M., et al. (1996). Absence of the beta subunit (cchb1) of the skeletal muscle dihydropyridine receptor alters expression of the alpha 1 subunit and eliminates excitation-contraction coupling. Proc. Natl. Acad. Sci. U. S. A. 93, 13961–13966. doi: 10.1073/pnas.93.24.13961
Guedes-Dias, P., Nirschl, J. J., Abreu, N., Tokito, M. K., Janke, C., Magiera, M. M., et al. (2019). Kinesin-3 responds to local microtubule dynamics to target synaptic cargo delivery to the Presynapse. Curr. Biol. 29, 268–282.e8. doi: 10.1016/j.cub.2018.11.065
Gundelfinger, E. D., Reissner, C., and Garner, C. C. (2015). Role of bassoon and piccolo in assembly and molecular Organization of the Active Zone. Front. Synap. Neurosci. 7:19. doi: 10.3389/fnsyn.2015.00019
Hall, D. H., and Hedgecock, E. M. (1991). Kinesin-related gene unc-104 is required for axonal transport of synaptic vesicles in C. elegans. Cells 65, 837–847. doi: 10.1016/0092-8674(91)90391-b
Han, Y., Kaeser, P. S., Südhof, T. C., and Schneggenburger, R. (2011). RIM determines Ca2+ channel density and vesicle docking at the presynaptic active zone. Neuron 69, 304–316. doi: 10.1016/j.neuron.2010.12.014
Hara, Y., Koganezawa, M., and Yamamoto, D. (2015). The Dmca1D channel mediates Ca(2+) inward currents in drosophila embryonic muscles. J. Neurogenet. 29, 117–123. doi: 10.3109/01677063.2015.1054991
Harlow, M. L., Ress, D., Stoschek, A., Marshall, R. M., and McMahan, U. J. (2001). The architecture of active zone material at the frog’s neuromuscular junction. Nature 409, 479–484. doi: 10.1038/35054000
Hastings, M. H., and Man, H.-Y. (2018). Synaptic capture of laterally diffusing AMPA receptors - an idea that stuck. Trends Neurosci. 41, 330–332. doi: 10.1016/j.tins.2018.03.016
He, L.-L., Zhang, Y., Chen, Y.-H., Yamada, Y., and Yang, J. (2007). Functional modularity of the beta-subunit of voltage-gated Ca2+ channels. Biophys. J. 93, 834–845. doi: 10.1529/biophysj.106.101691
Heck, J., Parutto, P., Ciuraszkiewicz, A., Bikbaev, A., Freund, R., Mitlöhner, J., et al. (2019). Transient confinement of CaV2.1 Ca2+-channel splice variants shapes synaptic short-term plasticity. Neuron 103, 66–79.e12. doi: 10.1016/j.neuron.2019.04.030
Heinrich, L., and Ryglewski, S. (2020). Different functions of two putative drosophila α2δ subunits in the same identified motoneurons. Sci. Rep. 10:13670. doi: 10.1038/s41598-020-69748-8
Held, R. G., Liu, C., Ma, K., Ramsey, A. M., Tarr, T. B., De Nola, G., et al. (2020). Synapse and active zone assembly in the absence of presynaptic ca2+ channels and ca2+ entry. Neuron 107, 667–683.e9. doi: 10.1016/j.neuron.2020.05.032
Heo, S., Diering, G. H., Na, C. H., Nirujogi, R. S., Bachman, J. L., Pandey, A., et al. (2018). Identification of long-lived synaptic proteins by proteomic analysis of synaptosome protein turnover. Proc. Natl. Acad. Sci. U. S. A. 115, E3827–E3836. doi: 10.1073/pnas.1720956115
Heuser, J. E., Reese, T. S., and Landis, D. M. (1974). Functional changes in frog neuromuscular junctions studied with freeze-fracture. J. Neurocytol. 3, 109–131. doi: 10.1007/BF01111936
Hibino, H., Pironkova, R., Onwumere, O., Vologodskaia, M., Hudspeth, A. J., and Lesage, F. (2002). RIM binding proteins (RBPs) couple Rab3-interacting molecules (RIMs) to voltage-gated Ca(2+) channels. Neuron 34, 411–423. doi: 10.1016/s0896-6273(02)00667-0
Hirokawa, N., Niwa, S., and Tanaka, Y. (2010). Molecular motors in neurons: transport mechanisms and roles in brain function, development, and disease. Neuron 68, 610–638. doi: 10.1016/j.neuron.2010.09.039
Holderith, N., Lorincz, A., Katona, G., Rózsa, B., Kulik, A., Watanabe, M., et al. (2012). Release probability of hippocampal glutamatergic terminals scales with the size of the active zone. Nat. Neurosci. 15, 988–997. doi: 10.1038/nn.3137
Hong, H., Zhao, K., Shiyan, H., Sheng, H., Yao, A., Jiang, Y., et al. (2020). Structural remodeling of active zones is associated with synaptic homeostasis. J. Neurosci. 40, 2817–2827. doi: 10.1523/JNEUROSCI.2002-19.2020
Hoppa, M. B., Lana, B., Margas, W., Dolphin, A. C., and Ryan, T. A. (2012). α2δ expression sets presynaptic calcium channel abundance and release probability. Nature 486, 122–125. doi: 10.1038/nature11033
Hosoi, N., Holt, M., and Sakaba, T. (2009). Calcium dependence of exo-and endocytotic coupling at a glutamatergic synapse. Neuron 63, 216–229. doi: 10.1016/j.neuron.2009.06.010
Hurd, D. D., and Saxton, W. M. (1996). Kinesin mutations cause motor neuron disease phenotypes by disrupting fast axonal transport in drosophila. Genetics 144, 1075–1085. doi: 10.1093/genetics/144.3.1075
Inchauspe, C. G., Martini, F. J., Forsythe, I. D., and Uchitel, O. D. (2004). Functional compensation of P/Q by N-type channels blocks short-term plasticity at the calyx of Held presynaptic terminal. J. Neurosci. 24, 10379–10383. doi: 10.1523/JNEUROSCI.2104-04.2004
Ishikawa, T., Kaneko, M., Shin, H.-S., and Takahashi, T. (2005). Presynaptic N-type and P/Q-type Ca2+ channels mediating synaptic transmission at the calyx of Held of mice. J. Physiol. Lond. 568, 199–209. doi: 10.1113/jphysiol.2005.089912
Jarsky, T., Tian, M., and Singer, J. H. (2010). Nanodomain control of exocytosis is responsible for the signaling capability of a retinal ribbon synapse. J. Neurosci. 30, 11885–11895. doi: 10.1523/JNEUROSCI.1415-10.2010
Jay, S. D., Sharp, A. H., Kahl, S., Vedvick, T. S., Harpold, M. M., and Campbell, K. P. (1991). Structural characterization of the dihydropyridine-sensitive calcium channel alpha 2-subunit and the associated delta peptides. J. Biol. Chem. 266, 3287–3293. doi: 10.1016/S0021-9258(18)49986-3
Jensen, K., and Mody, I. (2001). L-type Ca2+ channel-mediated short-term plasticity of GABAergic synapses. Nat. Neurosci. 4, 975–976. doi: 10.1038/nn722
Jeon, D., Kim, C., Yang, Y. M., Rhim, H., Yim, E., Oh, U., et al. (2007). Impaired long-term memory and long-term potentiation in N-type Ca2+ channel-deficient mice. Genes Brain Behav. 6, 375–388. doi: 10.1111/j.1601-183X.2006.00267.x
Jeong, K., Lee, S., Seo, H., Oh, Y., Jang, D., Choe, J., et al. (2015). Ca-α1T, a fly T-type Ca2+ channel, negatively modulates sleep. Sci. Rep. 5:17893. doi: 10.1038/srep17893
Jing, Z., Rutherford, M. A., Takago, H., Frank, T., Fejtova, A., Khimich, D., et al. (2013). Disruption of the presynaptic cytomatrix protein bassoon degrades ribbon anchorage, multiquantal release, and sound encoding at the hair cell afferent synapse. J. Neurosci. 33, 4456–4467. doi: 10.1523/JNEUROSCI.3491-12.2013
Jung, S., Oshima-Takago, T., Chakrabarti, R., Wong, A. B., Jing, Z., Yamanbaeva, G., et al. (2015). Rab3-interacting molecules 2α and 2β promote the abundance of voltage-gated CaV1.3 Ca2+ channels at hair cell active zones. Proc. Natl. Acad. Sci. U. S. A. 112, E3141–E3149. doi: 10.1073/pnas.1417207112
Kaeser, P. S., Deng, L., Wang, Y., Dulubova, I., Liu, X., Rizo, J., et al. (2011). RIM proteins tether Ca2+ channels to presynaptic active zones via a direct PDZ-domain interaction. Cells 144, 282–295. doi: 10.1016/j.cell.2010.12.029
Kaksonen, M., and Roux, A. (2018). Mechanisms of clathrin-mediated endocytosis. Nat. Rev. Mol. Cell Biol. 19, 313–326. doi: 10.1038/nrm.2017.132
Kanamori, T., Kanai, M. I., Dairyo, Y., Yasunaga, K. I., Morikawa, R. K., and Emoto, K. (2013). Compartmentalized calcium transients trigger dendrite pruning in drosophila sensory neurons. Science 340, 1475–1478. doi: 10.1126/science.1234879
Katiyar, R., Weissgerber, P., Roth, E., Dörr, J., Sothilingam, V., Garrido, M. G., et al. (2015). Influence of the β2-subunit of L-type voltage-gated Cav channels on the structural and functional development of photoreceptor ribbon synapses. Invest. Ophthalmol. Vis. Sci. 56, 2312–2324. doi: 10.1167/iovs.15-16654
Katz, B., and Miledi, R. (1965). The measurement of synaptic delay, and the time course of acetylcholine release at the neuromuscular junction. Proc. R. Soc. Lond. B Biol. Sci. 161, 483–495. doi: 10.1098/rspb.1965.0016
Kawasaki, F., Felling, R., and Ordway, R. W. (2000). A temperature-sensitive paralytic mutant defines a primary synaptic calcium channel in drosophila. J. Neurosci. 20, 4885–4889. doi: 10.1523/JNEUROSCI.20-13-04885.2000
Kawasaki, F., Zou, B., Xu, X., and Ordway, R. W. (2004). Active zone localization of presynaptic calcium channels encoded by the cacophony locus of drosophila. J. Neurosci. 24, 282–285. doi: 10.1523/JNEUROSCI.3553-03.2004
Kerov, V., Laird, J. G., Joiner, M.-L., Knecht, S., Soh, D., Hagen, J., et al. (2018). α2δ-4 is required for the molecular and structural Organization of rod and Cone Photoreceptor Synapses. J. Neurosci. 38, 6145–6160. doi: 10.1523/JNEUROSCI.3818-16.2018
Khatter, D., Sindhwani, A., and Sharma, M. (2015). Arf-like GTPase Arl8: moving from the periphery to the center of lysosomal biology. Cell Logist. 5:e1086501. doi: 10.1080/21592799.2015.1086501
Khimich, D., Nouvian, R., Pujol, R., Tom Dieck, S., Egner, A., Gundelfinger, E. D., et al. (2005). Hair cell synaptic ribbons are essential for synchronous auditory signalling. Nature 434, 889–894. doi: 10.1038/nature03418
Kim, C., Jun, K., Lee, T., Kim, S. S., McEnery, M. W., Chin, H., et al. (2001). Altered nociceptive response in mice deficient in the α1B subunit of the voltage-dependent calcium channel. Mol. Cell. Neurosci. 18, 235–245. doi: 10.1006/mcne.2001.1013
Kim, M.-H., Li, G.-L., and von Gersdorff, H. (2013). Single Ca2+ channels and exocytosis at sensory synapses. J. Physiol. Lond. 591, 3167–3178. doi: 10.1113/jphysiol.2012.249482
Kisilevsky, A. E., Mulligan, S. J., Altier, C., Iftinca, M. C., Varela, D., Tai, C., et al. (2008). D1 receptors physically interact with N-type calcium channels to regulate channel distribution and dendritic calcium entry. Neuron 58, 557–570. doi: 10.1016/j.neuron.2008.03.002
Kisilevsky, A. E., and Zamponi, G. W. (2008). D2 dopamine receptors interact directly with N-type calcium channels and regulate channel surface expression levels. Channels 2, 269–277. doi: 10.4161/chan.2.4.6402
Kittel, R. J., Wichmann, C., Rasse, T. M., Fouquet, W., Schmidt, M., Schmid, A., et al. (2006). Bruchpilot promotes active zone assembly, Ca2+ channel clustering, and vesicle release. Science 312, 1051–1054. doi: 10.1126/science.1126308
Kiyonaka, S., Nakajima, H., Takada, Y., Hida, Y., Yoshioka, T., Hagiwara, A., et al. (2012). Physical and functional interaction of the active zone protein CAST/ERC2 and the β-subunit of the voltage-dependent Ca(2+) channel. J. Biochem. 152, 149–159. doi: 10.1093/jb/mvs054
Kiyonaka, S., Wakamori, M., Miki, T., Uriu, Y., Nonaka, M., Bito, H., et al. (2007). RIM1 confers sustained activity and neurotransmitter vesicle anchoring to presynaptic Ca2+ channels. Nat. Neurosci. 10, 691–701. doi: 10.1038/nn1904
Klassen, M. P., Wu, Y. E., Maeder, C. I., Nakae, I., Cueva, J. G., Lehrman, E. K., et al. (2010). An Arf-like small G protein, ARL-8, promotes the axonal transport of presynaptic cargoes by suppressing vesicle aggregation. Neuron 66, 710–723. doi: 10.1016/j.neuron.2010.04.033
Ko, J., Na, M., Kim, S., Lee, J.-R., and Kim, E. (2003). Interaction of the ERC family of RIM-binding proteins with the liprin-alpha family of multidomain proteins. J. Biol. Chem. 278, 42377–42385. doi: 10.1074/jbc.M307561200
Koushika, S. P., Richmond, J. E., Hadwiger, G., Weimer, R. M., Jorgensen, E. M., and Nonet, M. L. (2001). A post-docking role for active zone protein rim. Nat. Neurosci. 4, 997–1005. doi: 10.1038/nn732
Krick, N., Ryglewski, S., Pichler, A., Bikbaev, A., Götz, T., Kobler, O., et al. (2021). Separation of presynaptic Cav2 and Cav1 channel function in synaptic vesicle exo-and endocytosis by the membrane anchored Ca2+ pump PMCA. Proc. Natl. Acad. Sci. 118:e2106621118. doi: 10.1073/pnas.2106621118
Krinner, S., Butola, T., Jung, S., Wichmann, C., and Moser, T. (2017). RIM-binding protein 2 promotes a large number of CaV1.3 Ca2+-channels and contributes to fast synaptic vesicle replenishment at hair cell active zones. Front. Cell. Neurosci. 11:334. doi: 10.3389/fncel.2017.00334
Kurshan, P. T., Oztan, A., and Schwarz, T. L. (2009). Presynaptic alpha2delta-3 is required for synaptic morphogenesis independent of its Ca2+−channel functions. Nat. Neurosci. 12, 1415–1423. doi: 10.1038/nn.2417
Kushibiki, Y., Suzuki, T., Jin, Y., and Taru, H. (2019). RIMB-1/RIM-binding protein and UNC-10/RIM redundantly regulate presynaptic localization of the voltage-gated Calcium Channel in Caenorhabditis elegans. J. Neurosci. 39, 8617–8631. doi: 10.1523/JNEUROSCI.0506-19.2019
Lainé, V., Frøkjær-Jensen, C., Couchoux, H., and Jospin, M. (2011). The alpha1 subunit EGL-19, the alpha2/delta subunit UNC-36, and the beta subunit CCB-1 underlie voltage-dependent calcium currents in Caenorhabditis elegans striated muscle. J. Biol. Chem. 286, 36180–36187. doi: 10.1074/jbc.M111.256149
Lambert, R. C., Bessaïh, T., Crunelli, V., and Leresche, N. (2014). The many faces of T-type calcium channels. Pflugers Arch. 466, 415–423. doi: 10.1007/s00424-013-1353-6
Lee, R. Y., Lobel, L., Hengartner, M., Horvitz, H. R., and Avery, L. (1997). Mutations in the alpha1 subunit of an L-type voltage-activated Ca2+ channel cause myotonia in Caenorhabditis elegans. EMBO J. 16, 6066–6076. doi: 10.1093/emboj/16.20.6066
Littleton, J. T., Stern, M., Perin, M., and Bellen, H. J. (1994). Calcium dependence of neurotransmitter release and rate of spontaneous vesicle fusions are altered in drosophila synaptotagmin mutants. Proc. Natl. Acad. Sci. U. S. A. 91, 10888–10892. doi: 10.1073/pnas.91.23.10888
Littleton, J. T., Stern, M., Schulze, K., Perin, M., and Bellen, H. J. (1993). Mutational analysis of drosophila synaptotagmin demonstrates its essential role in Ca(2+)-activated neurotransmitter release. Cells 74, 1125–1134. doi: 10.1016/0092-8674(93)90733-7
Liu, C., Bickford, L. S., Held, R. G., Nyitrai, H., Südhof, T. C., and Kaeser, P. S. (2014). The active zone protein family ELKS supports Ca2+ influx at nerve terminals of inhibitory hippocampal neurons. J. Neurosci. 34, 12289–12303. doi: 10.1523/JNEUROSCI.0999-14.2014
Liu, X., Kerov, V., Haeseleer, F., Majumder, A., Artemyev, N., Baker, S. A., et al. (2013). Dysregulation of Ca(v)1.4 channels disrupts the maturation of photoreceptor synaptic ribbons in congenital stationary night blindness type 2. Channels 7, 514–523. doi: 10.4161/chan.26376
Liu, K. S. Y., Siebert, M., Mertel, S., Knoche, E., Wegener, S., Wichmann, C., et al. (2011). RIM-binding protein, a central part of the active zone, is essential for neurotransmitter release. Science 334, 1565–1569. doi: 10.1126/science.1212991
Lübbert, M., Goral, R. O., Keine, C., Thomas, C., Guerrero-Given, D., Putzke, T., et al. (2019). Cav2.1 α1 subunit expression regulates presynaptic cav2.1 abundance and synaptic strength at a central synapse. Neuron 101, 260–273.e6. doi: 10.1016/j.neuron.2018.11.028
Lübbert, M., Goral, R. O., Satterfield, R., Putzke, T., van den Maagdenberg, A. M., Kamasawa, N., et al. (2017). A novel region in the CaV2.1 α1 subunit C-terminus regulates fast synaptic vesicle fusion and vesicle docking at the mammalian presynaptic active zone. elife 6. doi: 10.7554/eLife.28412
Luebke, J. I., Dunlap, K., and Turner, T. J. (1993). Multiple calcium channel types control glutamatergic synaptic transmission in the hippocampus. Neuron 11, 895–902. doi: 10.1016/0896-6273(93)90119-c
Luo, F., Dittrich, M., Stiles, J. R., and Meriney, S. D. (2011). Single-pixel optical fluctuation analysis of calcium channel function in active zones of motor nerve terminals. J. Neurosci. 31, 11268–11281. doi: 10.1523/JNEUROSCI.1394-11.2011
Ly, C. V., Yao, C.-K., Verstreken, P., Ohyama, T., and Bellen, H. J. (2008). Straightjacket is required for the synaptic stabilization of cacophony, a voltage-gated calcium channel alpha1 subunit. J. Cell Biol. 181, 157–170. doi: 10.1083/jcb.200712152
Maas, C., Torres, V. I., Altrock, W. D., Leal-Ortiz, S., Wagh, D., Terry-Lorenzo, R. T., et al. (2012). Formation of Golgi-derived active zone precursor vesicles. J. Neurosci. 32, 11095–11108. doi: 10.1523/JNEUROSCI.0195-12.2012
Maddox, J. W., Randall, K. L., Yadav, R. P., Williams, B., Hagen, J., Derr, P. J., et al. (2020). A dual role for Cav1.4 Ca2+ channels in the molecular and structural organization of the rod photoreceptor synapse. elife 9. doi: 10.7554/eLife.62184
Maeder, C. I., San-Miguel, A., Wu, E. Y., Lu, H., and Shen, K. (2014). In vivo neuron-wide analysis of synaptic vesicle precursor trafficking. Traffic 15, 273–291. doi: 10.1111/tra.12142
Maritzen, T., and Haucke, V. (2018). Coupling of exocytosis and endocytosis at the presynaptic active zone. Neurosci. Res. 127, 45–52. doi: 10.1016/j.neures.2017.09.013
Mathews, E. A., García, E., Santi, C. M., Mullen, G. P., Thacker, C., Moerman, D. G., et al. (2003). Critical residues of the Caenorhabditis elegans unc-2 voltage-gated calcium channel that affect behavioral and physiological properties. J. Neurosci. 23, 6537–6545. doi: 10.1523/JNEUROSCI.23-16-06537.2003
Matkovic, T., Siebert, M., Knoche, E., Depner, H., Mertel, S., Owald, D., et al. (2013). The Bruchpilot cytomatrix determines the size of the readily releasable pool of synaptic vesicles. J. Cell Biol. 202, 667–683. doi: 10.1083/jcb.201301072
Melom, J. E., Akbergenova, Y., Gavornik, J. P., and Littleton, J. T. (2013). Spontaneous and evoked release are independently regulated at individual active zones. J. Neurosci. 33, 17253–17263. doi: 10.1523/JNEUROSCI.3334-13.2013
Mercer, A. J., Chen, M., and Thoreson, W. B. (2011). Lateral mobility of presynaptic L-type calcium channels at photoreceptor ribbon synapses. J. Neurosci. 31, 4397–4406. doi: 10.1523/JNEUROSCI.5921-10.2011
Mercer, A. J., Szalewski, R. J., Jackman, S. L., Van Hook, M. J., and Thoreson, W. B. (2012). Regulation of presynaptic strength by controlling Ca2+ channel mobility: effects of cholesterol depletion on release at the cone ribbon synapse. J. Neurophysiol. 107, 3468–3478. doi: 10.1152/jn.00779.2011
Miki, T., Kaufmann, W. A., Malagon, G., Gomez, L., Tabuchi, K., Watanabe, M., et al. (2017). Numbers of presynaptic Ca2+ channel clusters match those of functionally defined vesicular docking sites in single central synapses. Proc. Natl. Acad. Sci. U. S. A. 114, E5246–E5255. doi: 10.1073/pnas.1704470114
Mizuno, F., Barabas, P., Krizaj, D., and Akopian, A. (2010). Glutamate-induced internalization of Ca(v)1.3 L-type Ca(2+) channels protects retinal neurons against excitotoxicity. J. Physiol. Lond. 588, 953–966. doi: 10.1113/jphysiol.2009.181305
Moo, E. V., van Senten, J. R., Bräuner-Osborne, H., and Møller, T. C. (2021). Arrestin-dependent and -independent internalization of G protein-coupled receptors: methods, mechanisms, and implications on cell signaling. Mol. Pharmacol. 99, 242–255. doi: 10.1124/molpharm.120.000192
Mrestani, A., Pauli, M., Kollmannsberger, P., Repp, F., Kittel, R. J., Eilers, J., et al. (2021). Active zone compaction correlates with presynaptic homeostatic potentiation. Cell Rep. 37:109770. doi: 10.1016/j.celrep.2021.109770
Mukherjee, K., Yang, X., Gerber, S. H., Kwon, H.-B., Ho, A., Castillo, P. E., et al. (2010). Piccolo and bassoon maintain synaptic vesicle clustering without directly participating in vesicle exocytosis. Proc. Natl. Acad. Sci. U. S. A. 107, 6504–6509. doi: 10.1073/pnas.1002307107
Müller, M., and Davis, G. W. (2012). Transsynaptic control of presynaptic Ca2+ influx achieves homeostatic potentiation of neurotransmitter release. Curr. Biol. 22, 1102–1108. doi: 10.1016/j.cub.2012.04.018
Müller, M., Genç, Ö., and Davis, G. W. (2015). RIM-binding protein links synaptic homeostasis to the stabilization and replenishment of high release probability vesicles. Neuron 85, 1056–1069. doi: 10.1016/j.neuron.2015.01.024
Müller, C. S., Haupt, A., Bildl, W., Schindler, J., Knaus, H.-G., Meissner, M., et al. (2010). Quantitative proteomics of the Cav2 channel nano-environments in the mammalian brain. Proc. Natl. Acad. Sci. U. S. A. 107, 14950–14957. doi: 10.1073/pnas.1005940107
Nakamura, Y., Harada, H., Kamasawa, N., Matsui, K., Rothman, J. S., Shigemoto, R., et al. (2015). Nanoscale distribution of presynaptic Ca(2+) channels and its impact on vesicular release during development. Neuron 85, 145–158. doi: 10.1016/j.neuron.2014.11.019
Nanou, E., and Catterall, W. A. (2018). Calcium channels, synaptic plasticity, and neuropsychiatric disease. Neuron 98, 466–481. doi: 10.1016/j.neuron.2018.03.017
Neher, E. (1998). Vesicle pools and Ca2+ microdomains: new tools for understanding their roles in neurotransmitter release. Neuron 20, 389–399. doi: 10.1016/s0896-6273(00)80983-6
Newman, Z. L., Bakshinskaya, D., Schultz, R., Kenny, S. J., Moon, S., Aghi, K., et al. (2022). Determinants of synapse diversity revealed by super-resolution quantal transmission and active zone imaging. Nat. Commun. 13:229. doi: 10.1038/s41467-021-27815-2
Obermair, G. J., Schlick, B., Di Biase, V., Subramanyam, P., Gebhart, M., Baumgartner, S., et al. (2010). Reciprocal interactions regulate targeting of calcium channel beta subunits and membrane expression of alpha1 subunits in cultured hippocampal neurons. J. Biol. Chem. 285, 5776–5791. doi: 10.1074/jbc.M109.044271
Oh, K. H., Krout, M. D., Richmond, J. E., and Kim, H. (2021). UNC-2 CaV2 channel localization at presynaptic active zones depends on UNC-10/RIM and SYD-2/Liprin-α in Caenorhabditis elegans. J. Neurosci. 41, 4782–4794. doi: 10.1523/JNEUROSCI.0076-21.2021
Ohtsuka, T., Takao-Rikitsu, E., Inoue, E., Inoue, M., Takeuchi, M., Matsubara, K., et al. (2002). Cast: a novel protein of the cytomatrix at the active zone of synapses that forms a ternary complex with RIM1 and munc13-1. J. Cell Biol. 158, 577–590. doi: 10.1083/jcb.200202083
Okada, Y., Yamazaki, H., Sekine-Aizawa, Y., and Hirokawa, N. (1995). The neuron-specific kinesin superfamily protein KIF1A is a uniqye monomeric motor for anterograde axonal transport of synaptic vesicle precursors. Cells 81, 769–780. doi: 10.1016/0092-8674(95)90538-3
Opatowsky, Y., Chen, C.-C., Campbell, K. P., and Hirsch, J. A. (2004). Structural analysis of the voltage-dependent calcium channel beta subunit functional core and its complex with the alpha 1 interaction domain. Neuron 42, 387–399. doi: 10.1016/s0896-6273(04)00250-8
Ortega, J. M., Genç, Ö., and Davis, G. W. (2018). Molecular mechanisms that stabilize short term synaptic plasticity during presynaptic homeostatic plasticity. elife 7. doi: 10.7554/eLife.40385
Owald, D., Fouquet, W., Schmidt, M., Wichmann, C., Mertel, S., Depner, H., et al. (2010). A Syd-1 homologue regulates pre- and postsynaptic maturation in drosophila. J. Cell Biol. 188, 565–579. doi: 10.1083/jcb.200908055
Pack-Chung, E., Kurshan, P. T., Dickman, D. K., and Schwarz, T. L. (2007). A drosophila kinesin required for synaptic Bouton formation and synaptic vesicle transport. Nat. Neurosci. 10, 980–989. doi: 10.1038/nn1936
Peled, E. S., and Isacoff, E. Y. (2011). Optical quantal analysis of synaptic transmission in wild-type and rab3-mutant drosophila motor axons. Nat. Neurosci. 14, 519–526. doi: 10.1038/nn.2767
Pragnell, M., De Waard, M., Mori, Y., Tanabe, T., Snutch, T. P., and Campbell, K. P. (1994). Calcium channel beta-subunit binds to a conserved motif in the I-II cytoplasmic linker of the alpha 1-subunit. Nature 368, 67–70. doi: 10.1038/368067a0
Price, J. C., Guan, S., Burlingame, A., Prusiner, S. B., and Ghaemmaghami, S. (2010). Analysis of proteome dynamics in the mouse brain. Proc. Natl. Acad. Sci. U. S. A. 107, 14508–14513. doi: 10.1073/pnas.1006551107
Pumplin, D. W., Reese, T. S., and Llinás, R. (1981). Are the presynaptic membrane particles the calcium channels? Proc. Natl. Acad. Sci. U. S. A. 78, 7210–7213. doi: 10.1073/pnas.78.11.7210
Radulovic, T., Dong, W., Goral, R. O., Thomas, C. I., Veeraraghavan, P., Montesinos, M. S., et al. (2020). Presynaptic development is controlled by the core active zone proteins CAST/ELKS. J. Physiol. Lond. 598, 2431–2452. doi: 10.1113/JP279736
Reid, C. A., Clements, J. D., and Bekkers, J. M. (1997). Nonuniform distribution of Ca2+ channel subtypes on presynaptic terminals of excitatory synapses in hippocampal cultures. J. Neurosci. 17, 2738–2745. doi: 10.1523/JNEUROSCI.17-08-02738.1997
Reuter, H. (1995). Measurements of exocytosis from single presynaptic nerve terminals reveal heterogeneous inhibition by Ca(2+)-channel blockers. Neuron 14, 773–779. doi: 10.1016/0896-6273(95)90221-x
Rivière, J.-B., Ramalingam, S., Lavastre, V., Shekarabi, M., Holbert, S., Lafontaine, J., et al. (2011). KIF1A, an axonal transporter of synaptic vesicles, is mutated in hereditary sensory and autonomic neuropathy type 2. Am. J. Hum. Genet. 89, 219–230. doi: 10.1016/j.ajhg.2011.06.013
Ryglewski, S., Lance, K., Levine, R. B., and Duch, C. (2012). Ca(v)2 channels mediate low and high voltage-activated calcium currents in drosophila motoneurons. J. Physiol. Lond. 590, 809–825. doi: 10.1113/jphysiol.2011.222836
Sabatini, B. L., and Regehr, W. G. (1996). Timing of neurotransmission at fast synapses in the mammalian brain. Nature 384, 170–172. doi: 10.1038/384170a0
Saheki, Y., and Bargmann, C. I. (2009). Presynaptic CaV2 calcium channel traffic requires CALF-1 and the alpha(2)delta subunit UNC-36. Nat. Neurosci. 12, 1257–1265. doi: 10.1038/nn.2383
Sauvola, C. W., Akbergenova, Y., Cunningham, K. L., Aponte-Santiago, N. A., and Littleton, J. T. (2021). The decoy SNARE Tomosyn sets tonic versus phasic release properties and is required for homeostatic synaptic plasticity. elife 10:e72841. doi: 10.7554/eLife.72841
Sauvola, C. W., and Littleton, J. T. (2021). SNARE regulatory proteins in synaptic vesicle fusion and recycling. Front. Mol. Neurosci. 14:733138. doi: 10.3389/fnmol.2021.733138
Schafer, W. R., and Kenyon, C. J. (1995). A calcium-channel homologue required for adaptation to dopamine and serotonin in Caenorhabditis elegans. Nature 375, 73–78. doi: 10.1038/375073a0
Schneider, R., Hosy, E., Kohl, J., Klueva, J., Choquet, D., Thomas, U., et al. (2015). Mobility of calcium channels in the presynaptic membrane. Neuron 86, 672–679. doi: 10.1016/j.neuron.2015.03.050
Schoch, S., Castillo, P. E., Jo, T., Mukherjee, K., Geppert, M., Wang, Y., et al. (2002). RIM1alpha forms a protein scaffold for regulating neurotransmitter release at the active zone. Nature 415, 321–326. doi: 10.1038/415321a
Schöpf, C. L., Ablinger, C., Geisler, S. M., Stanika, R. I., Campiglio, M., Kaufmann, W. A., et al. (2021). Presynaptic α2δ subunits are key organizers of glutamatergic synapses. Proc. Natl. Acad. Sci. U. S. A. 118. doi: 10.1073/pnas.1920827118
Shapira, M., Zhai, R. G., Dresbach, T., Bresler, T., Torres, V. I., Gundelfinger, E. D., et al. (2003). Unitary assembly of presynaptic active zones from piccolo-bassoon transport vesicles. Neuron 38, 237–252. doi: 10.1016/s0896-6273(03)00207-1
Sheng, J., He, L., Zheng, H., Xue, L., Luo, F., Shin, W., et al. (2012). Calcium-channel number critically influences synaptic strength and plasticity at the active zone. Nat. Neurosci. 15, 998–1006. doi: 10.1038/nn.3129
Shibasaki, T., Sunaga, Y., Fujimoto, K., Kashima, Y., and Seino, S. (2004). Interaction of ATP sensor, cAMP sensor, Ca2+ sensor, and voltage-dependent Ca2+ channel in insulin granule exocytosis. J. Biol. Chem. 279, 7956–7961. doi: 10.1074/jbc.M309068200
Shtonda, B., and Avery, L. (2005). CCA-1, EGL-19 and EXP-2 currents shape action potentials in the Caenorhabditis elegans pharynx. J. Exp. Biol. 208, 2177–2190. doi: 10.1242/jeb.01615
Smith, L. A., Wang, X., Peixoto, A. A., Neumann, E. K., Hall, L. M., and Hall, J. C. (1996). A drosophila calcium channel alpha1 subunit gene maps to a genetic locus associated with behavioral and visual defects. J. Neurosci. 16, 7868–7879. doi: 10.1523/JNEUROSCI.16-24-07868.1996
Stahl, A., Noyes, N. C., Boto, T., Botero, V., Broyles, C. N., Jing, M., et al. (2022). Associative learning drives longitudinally graded presynaptic plasticity of neurotransmitter release along axonal compartments. elife 11:11. doi: 10.7554/eLife.76712
Steger, K. A., Shtonda, B. B., Thacker, C., Snutch, T. P., and Avery, L. (2005). The C. elegans T-type calcium channel CCA-1 boosts neuromuscular transmission. J. Exp. Biol. 208, 2191–2203. doi: 10.1242/jeb.01616
Südhof, T. C. (2012). The presynaptic active zone. Neuron 75, 11–25. doi: 10.1016/j.neuron.2012.06.012
Takahashi, T., and Momiyama, A. (1993). Different types of calcium channels mediate central synaptic transmission. Nature 366, 156–158. doi: 10.1038/366156a0
Tang, L., Gamal El-Din, T. M., Payandeh, J., Martinez, G. Q., Heard, T. M., Scheuer, T., et al. (2014). Structural basis for Ca2+ selectivity of a voltage-gated calcium channel. Nature 505, 56–61. doi: 10.1038/nature12775
Tao-Cheng, J. H. (2007). Ultrastructural localization of active zone and synaptic vesicle proteins in a preassembled multi-vesicle transport aggregate. Neuroscience 150, 575–584. doi: 10.1016/j.neuroscience.2007.09.031
Taylor, C. P., Angelotti, T., and Fauman, E. (2007). Pharmacology and mechanism of action of pregabalin: the calcium channel alpha2-delta (alpha2-delta) subunit as a target for antiepileptic drug discovery. Epilepsy Res. 73, 137–150. doi: 10.1016/j.eplepsyres.2006.09.008
Tempes, A., Weslawski, J., Brzozowska, A., and Jaworski, J. (2020). Role of dynein-dynactin complex, kinesins, motor adaptors, and their phosphorylation in dendritogenesis. J. Neurochem. 155, 10–28. doi: 10.1111/jnc.15010
Thoreson, W. B., Mercer, A. J., Cork, K. M., and Szalewski, R. J. (2013). Lateral mobility of L-type calcium channels in synaptic terminals of retinal bipolar cells. Mol. Vis. 19, 16–24.
Thoreson, W. B., Rabl, K., Townes-Anderson, E., and Heidelberger, R. (2004). A highly Ca2+−sensitive pool of vesicles contributes to linearity at the rod photoreceptor ribbon synapse. Neuron 42, 595–605. doi: 10.1016/s0896-6273(04)00254-5
Tom Dieck, S., Sanmartí-Vila, L., Langnaese, K., Richter, K., Kindler, S., Soyke, A., et al. (1998). Bassoon, a novel zinc-finger CAG/glutamine-repeat protein selectively localized at the active zone of presynaptic nerve terminals. J. Cell Biol. 142, 499–509. doi: 10.1083/jcb.142.2.499
Tseng, P.-Y., Henderson, P. B., Hergarden, A. C., Patriarchi, T., Coleman, A. M., Lillya, M. W., et al. (2017). α-Actinin promotes surface localization and current density of the Ca2+ channel CaV1.2 by binding to the IQ region of the α1 subunit. Biochemistry 56, 3669–3681. doi: 10.1021/acs.biochem.7b00359
Vale, R. D., Funatsu, T., Pierce, D. W., Romberg, L., Harada, Y., and Yanagida, T. (1996). Direct observation of single kinesin molecules moving along microtubules. Nature 380, 451–453. doi: 10.1038/380451a0
Van Petegem, F., Clark, K. A., Chatelain, F. C., and Minor, D. L. (2004). Structure of a complex between a voltage-gated calcium channel beta-subunit and an alpha-subunit domain. Nature 429, 671–675. doi: 10.1038/nature02588
Voigt, A., Freund, R., Heck, J., Missler, M., Obermair, G. J., Thomas, U., et al. (2016). Dynamic association of calcium channel subunits at the cellular membrane. Neurophotonics 3:041809. doi: 10.1117/1.NPh.3.4.041809
von Zastrow, M., and Sorkin, A. (2021). Mechanisms for regulating and organizing receptor signaling by endocytosis. Annu. Rev. Biochem. 90, 709–737. doi: 10.1146/annurev-biochem-081820-092427
Vukoja, A., Rey, U., Petzoldt, A. G., Ott, C., Vollweiter, D., Quentin, C., et al. (2018). Presynaptic biogenesis requires axonal transport of lysosome-related vesicles. Neuron 99, 1216–1232.e7. doi: 10.1016/j.neuron.2018.08.004
Wagh, D. A., Rasse, T. M., Asan, E., Hofbauer, A., Schwenkert, I., Dürrbeck, H., et al. (2006). Bruchpilot, a protein with homology to ELKS/CAST, is required for structural integrity and function of synaptic active zones in drosophila. Neuron 49, 833–844. doi: 10.1016/j.neuron.2006.02.008
Waithe, D., Ferron, L., Page, K. M., Chaggar, K., and Dolphin, A. C. (2011). Beta-subunits promote the expression of Ca(V)2.2 channels by reducing their proteasomal degradation. J. Biol. Chem. 286, 9598–9611. doi: 10.1074/jbc.M110.195909
Walker, D., Bichet, D., Campbell, K. P., and De Waard, M. (1998). A beta 4 isoform-specific interaction site in the carboxyl-terminal region of the voltage-dependent Ca2+ channel alpha 1A subunit. J. Biol. Chem. 273, 2361–2367. doi: 10.1074/jbc.273.4.2361
Wang, Y., Fehlhaber, K. E., Sarria, I., Cao, Y., Ingram, N. T., Guerrero-Given, D., et al. (2017). The auxiliary Calcium Channel subunit α2δ4 is required for axonal elaboration, synaptic transmission, and wiring of rod photoreceptors. Neuron 93, 1359–1374.e6. doi: 10.1016/j.neuron.2017.02.021
Wang, X., Hu, B., Zieba, A., Neumann, N. G., Kasper-Sonnenberg, M., Honsbein, A., et al. (2009). A protein interaction node at the neurotransmitter release site: domains of Aczonin/piccolo, bassoon, CAST, and rim converge on the N-terminal domain of Munc13-1. J. Neurosci. 29, 12584–12596. doi: 10.1523/JNEUROSCI.1255-09.2009
Wang, T., Jones, R. T., Whippen, J. M., and Davis, G. W. (2016). α2δ-3 is required for rapid Transsynaptic homeostatic signaling. Cell Rep. 16, 2875–2888. doi: 10.1016/j.celrep.2016.08.030
Wang, Y., Liu, X., Biederer, T., and Südhof, T. C. (2002). A family of RIM-binding proteins regulated by alternative splicing: implications for the genesis of synaptic active zones. Proc. Natl. Acad. Sci. U. S. A. 99, 14464–14469. doi: 10.1073/pnas.182532999
Wang, L.-Y., Neher, E., and Taschenberger, H. (2008). Synaptic vesicles in mature calyx of Held synapses sense higher nanodomain calcium concentrations during action potential-evoked glutamate release. J. Neurosci. 28, 14450–14458. doi: 10.1523/JNEUROSCI.4245-08.2008
Wang, Y., Okamoto, M., Schmitz, F., Hofmann, K., and Südhof, T. C. (1997). Rim is a putative Rab3 effector in regulating synaptic-vesicle fusion. Nature 388, 593–598. doi: 10.1038/41580
Wang, Y., Sugita, S., and Sudhof, T. C. (2000). The RIM/NIM family of neuronal C2 domain proteins. Interactions with Rab3 and a new class of Src homology 3 domain proteins. J. Biol. Chem. 275, 20033–20044. doi: 10.1074/jbc.M909008199
Watanabe, S., and Boucrot, E. (2017). Fast and ultrafast endocytosis. Curr. Opin. Cell Biol. 47, 64–71. doi: 10.1016/j.ceb.2017.02.013
Weissgerber, P., Held, B., Bloch, W., Kaestner, L., Chien, K. R., Fleischmann, B. K., et al. (2006). Reduced cardiac L-type Ca2+ current in Ca(V)beta2−/− embryos impairs cardiac development and contraction with secondary defects in vascular maturation. Circ. Res. 99, 749–757. doi: 10.1161/01.RES.0000243978.15182.c1
Wheeler, D. B., Randall, A., and Tsien, R. W. (1994). Roles of N-type and Q-type Ca2+ channels in supporting hippocampal synaptic transmission. Science 264, 107–111. doi: 10.1126/science.7832825
Whittaker, C. A., and Hynes, R. O. (2002). Distribution and evolution of von Willebrand/integrin a domains: widely dispersed domains with roles in cell adhesion and elsewhere. Mol. Biol. Cell 13, 3369–3387. doi: 10.1091/mbc.E02-05-0259
Witcher, D. R., De Waard, M., Sakamoto, J., Franzini-Armstrong, C., Pragnell, M., Kahl, S. D., et al. (1993). Subunit identification and reconstitution of the N-type Ca2+ channel complex purified from brain. Science 261, 486–489. doi: 10.1126/science.8392754
Wu, L. G., Hamid, E., Shin, W., and Chiang, H. C. (2014). Exocytosis and endocytosis: modes, functions, and coupling mechanisms. Annu. Rev. Physiol. 76, 301–331. doi: 10.1146/annurev-physiol-021113-170305
Wu, Y. E., Huo, L., Maeder, C. I., Feng, W., and Shen, K. (2013). The balance between capture and dissociation of presynaptic proteins controls the spatial distribution of synapses. Neuron 78, 994–1011. doi: 10.1016/j.neuron.2013.04.035
Wu, X. S., McNeil, B. D., Xu, J., Fan, J., Xue, L., Melicoff, E., et al. (2009). Ca2+ and calmodulin initiate all forms of endocytosis during depolarization at a nerve terminal. Nat. Neurosci. 12, 1003–1010. doi: 10.1038/nn.2355
Wu, L. G., Westenbroek, R. E., Borst, J. G., Catterall, W. A., and Sakmann, B. (1999). Calcium channel types with distinct presynaptic localization couple differentially to transmitter release in single calyx-type synapses. J. Neurosci. 19, 726–736. doi: 10.1523/JNEUROSCI.19-02-00726.1999
Wu, J., Yan, Z., Li, Z., Qian, X., Lu, S., Dong, M., et al. (2016). Structure of the voltage-gated calcium channel Ca(v)1.1 at 3.6 Å resolution. Nature 537, 191–196. doi: 10.1038/nature19321
Wu, J., Yan, Z., Li, Z., Yan, C., Lu, S., Dong, M., et al. (2015). Structure of the voltage-gated calcium channel Cav1.1 complex. Science 350:aad2395. doi: 10.1126/science.aad2395
Xuan, Z., Manning, L., Nelson, J., Richmond, J. E., Colón-Ramos, D. A., Shen, K., et al. (2017). Clarinet (CLA-1), a novel active zone protein required for synaptic vesicle clustering and release. elife 6. doi: 10.7554/eLife.29276
Xue, L., Zhang, Z., McNeil, B. D., Luo, F., Wu, X. S., Sheng, J., et al. (2012). Voltage-dependent calcium channels at the plasma membrane, but not vesicular channels, couple exocytosis to endocytosis. Cell Rep. 1, 632–638. doi: 10.1016/j.celrep.2012.04.011
Yonekawa, Y., Harada, A., Okada, Y., Funakoshi, T., Kanai, Y., Takei, Y., et al. (1998). Defect in synaptic vesicle precursor transport and neuronal cell death in KIF1A motor protein-deficient mice. J. Cell Biol. 141, 431–441. doi: 10.1083/jcb.141.2.431
Yu, F. H., Yarov-Yarovoy, V., Gutman, G. A., and Catterall, W. A. (2005). Overview of molecular relationships in the voltage-gated ion channel superfamily. Pharmacol. Rev. 57, 387–395. doi: 10.1124/pr.57.4.13
Zabouri, N., and Haverkamp, S. (2013). Calcium channel-dependent molecular maturation of photoreceptor synapses. PLoS One 8:e63853. doi: 10.1371/journal.pone.0063853
Zhai, R. G., and Bellen, H. J. (2004). The architecture of the active zone in the presynaptic nerve terminal. Physiology (Bethesda) 19, 262–270. doi: 10.1152/physiol.00014.2004
Zhai, R. G., Vardinon-Friedman, H., Cases-Langhoff, C., Becker, B., Gundelfinger, E. D., Ziv, N. E., et al. (2001). Assembling the presynaptic active zone: a characterization of an active one precursor vesicle. Neuron 29, 131–143. doi: 10.1016/s0896-6273(01)00185-4
Zhang, Y. V., Hannan, S. B., Kern, J. V., Stanchev, D. T., Koç, B., Jahn, T. R., et al. (2017). The KIF1A homolog Unc-104 is important for spontaneous release, postsynaptic density maturation and perisynaptic scaffold organization. Sci. Rep. 7:38172. doi: 10.1038/srep38172
Keywords: voltage-gated calcium channel, synapse, active zone, neurotransmitter release, protein trafficking
Citation: Cunningham KL and Littleton JT (2023) Mechanisms controlling the trafficking, localization, and abundance of presynaptic Ca2+ channels. Front. Mol. Neurosci. 15:1116729. doi: 10.3389/fnmol.2022.1116729
Edited by:
Chen Gu, The Ohio State University, United StatesReviewed by:
Hee Jung Chung, University of Illinois at Urbana-Champaign, United StatesMartin Heine, Johannes Gutenberg University Mainz, Germany
Copyright © 2023 Cunningham and Littleton. This is an open-access article distributed under the terms of the Creative Commons Attribution License (CC BY). The use, distribution or reproduction in other forums is permitted, provided the original author(s) and the copyright owner(s) are credited and that the original publication in this journal is cited, in accordance with accepted academic practice. No use, distribution or reproduction is permitted which does not comply with these terms.
*Correspondence: Karen L. Cunningham, ✉ a2FyZW41MjFAbWl0LmVkdQ==