- 1Department of Anesthesiology and National Clinical Research Center for Geriatrics, West China Hospital, Sichuan University and The Research Units of West China (2018RU012), Chinese Academy of Medical Sciences, Chengdu, China
- 2Laboratory of Anesthesia and Critical Care Medicine, National-Local Joint Engineering Research Centre of Translational Medicine of Anesthesiology, West China Hospital, Sichuan University, Chengdu, China
- 3Department of Respiratory and Critical Care Medicine, West China Medical School/West China Hospital, Sichuan University, Chengdu, China
- 4Department of Respiratory and Critical Care Medicine, Targeted Tracer Research and Development Laboratory, West China Hospital, Sichuan University, Chengdu, Sichuan, China
Chronic pain (CP) is an unpleasant sensory and emotional experience associated with, or resembling that associated with, actual or potential tissue damage lasting longer than 3 months. CP is the main reason why people seek medical care and exerts an enormous economic burden. Genome-wide expression analysis has revealed that diverse essential genetic elements are altered in CP patients. Although many possible mechanisms of CP have been revealed, we are still unable to meet all the analgesic needs of patients. In recent years, non-coding RNAs (ncRNAs) have been shown to play essential roles in peripheral neuropathy and axon regeneration, which is associated with CP occurrence and development. Multiple key ncRNAs have been identified in animal models of CP, such as microRNA-30c-5p, ciRS-7, and lncRNA MRAK009713. This review highlights different kinds of ncRNAs in the regulation of CP, which provides a more comprehensive understanding of the pathogenesis of the disease. It mainly focuses on the contributions of miRNAs, circRNAs, and lncRNAs to CP, specifically peripheral neuropathic pain (NP), diabetic NP, central NP associated with spinal cord injury, complex regional pain syndrome, inflammatory pain, and cancer-induced pain. In addition, we summarize some potential ncRNAs as novel biomarkers for CP and its complications. With an in-depth understanding of the mechanism of CP, ncRNAs may provide novel insight into CP and could become new therapeutic targets in the future.
Introduction and chronic pain overview
Chronic pain (CP) is an unpleasant sensory and emotional experience associated with, or resembling that associated with, actual or potential tissue damage lasting longer than 3 months (Treede et al., 2015; Raja et al., 2020). Approximately 20% adults suffer pain and another 10% adults are diagnosed with CP worldwide each year (Goldberg and McGee, 2011). Patients with CP are often characterized as having allodynia (innocuous stimulus causing pain), hyperalgesia (noxious stimulus triggering an amplified response), and spontaneous pain (Woolf and Salter, 2000). Several factors are associated with the severity of CP, mainly including age, gender, genetics, ethnicity, cultural background, smoking, alcohol, physical activity, mental health, surgical and medical interventions, and even other attitudes and beliefs about pain, etc. (Mills et al., 2019). Moreover, comorbidities and complications are two difficult problems of CP. Patients with CP often have an increased co-occurrence of depression, cardiovascular disease, clinical insomnia, etc. (Jank et al., 2017; Macfarlane et al., 2017; van Hecke et al., 2017). Several common types of CP are summarized in this review, such as neuropathic pain (NP), complex regional pain syndrome (CRPS), inflammatory pain (IP), and cancer-induced pain (CIP) (Treede et al., 2019). Thereinto, the etiology of chronic NP can be divided into peripheral nerve lesions [such as peripheral nerve injury (PNI)-induced NP, diabetic NP (DNP), etc.] and central nerve lesions [such as spinal cord injury (SCI)] (Treede et al., 2019).
The mechanisms underlying CP have been explored for several decades, mainly including central sensitization, neuroinflammation, oxidative stress, etc. (Ji et al., 2018; Kaushik et al., 2020). However, the pathogenesis of CP has not been completely elucidated. Although several analgesic treatments are available, they are often hampered by side effects or limited efficacy (Sisignano et al., 2019). Elucidating the pathogenic mechanisms of CP may help seek novel specific biomarkers and efficient therapies for controlling the symptoms of CP. Notably, non-coding RNAs (ncRNAs) are altered greatly in both clinical research and pre-clinical models of CP and constitute a regulatory transcriptome network in the pathogenesis of CP (Ciszek et al., 2015; Peng et al., 2017; Du et al., 2022). Multiple ncRNAs are differentially expressed in the animal models of CP (Li et al., 2021b) and are strongly related to various mechanisms, such as pain-related signaling pathways, receptors, cytokines, cell processes, ion channels, and exosomes in cell-to-cell communications (Wang Z. et al., 2018; Xiang et al., 2019; Ma et al., 2021; Felix et al., 2022; Liu et al., 2022; Figure 1).
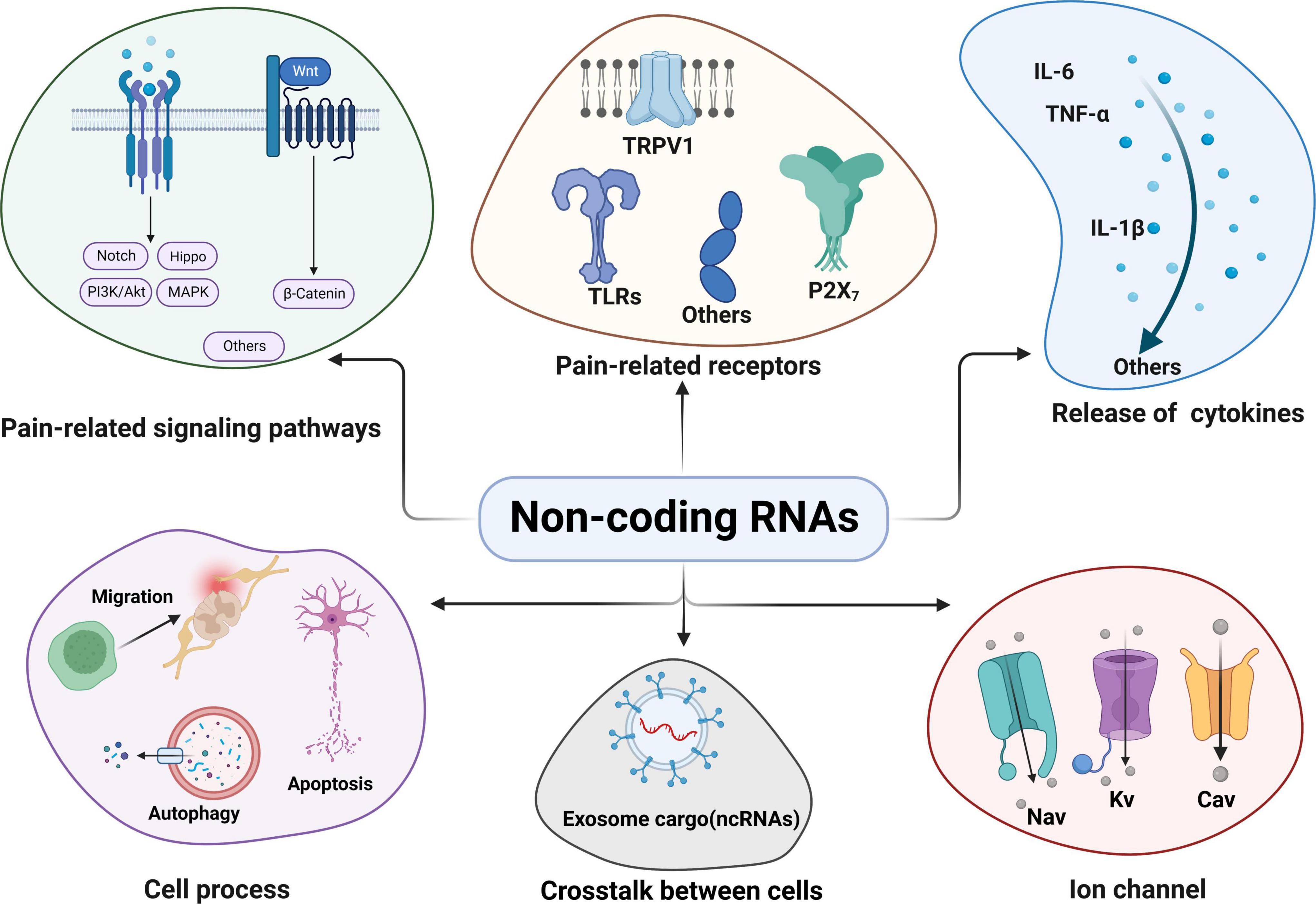
Figure 1. Non-coding RNAs (ncRNAs) and their regulatory mechanism in chronic pain: ncRNAs can participate in pain regulation through various mechanisms. Some common and key mechanisms mainly include pain-related signaling pathways; pain-related receptors and targets; regulation of cytokines release; some cell processes (cell migration, autophagy and apoptosis etc.); exosomal ncRNAs crosstalk between cells and ncRNAs targeting ion channels related to chronic pain. Akt, V-akt murine thymoma viral oncogene homolog; Cav, voltage-gated calcium; IL, interleukin; MAPK, mitogen-activated protein kinases; Kv, voltage-gated potassium channel; Nav, voltage-dependent sodium; ncRNAs, Non-coding RNAs; PI3K, phosphatidylinositol-3-kinase; TLRs, toll-like receptors; TNF, tumor necrosis factor; TRPV1, transient receptor potential vanilloid 1.
This review aims to summarize the recent evidence about the potential role of ncRNAs in CP. Understanding their functions may help to reveal the complex pathogenic mechanisms in CP and identify new potential diagnostic biomarkers and therapeutic targets.
General aspects of non-coding RNAs
Non-coding RNAs are defined as RNAs that are unable to translate into proteins (Beermann et al., 2016). Generally, ncRNAs are divided into two categories according to their sequence length: (I) small ncRNAs (few to 200 nt), including microRNA (miRNA), ribosomal, small nuclear, piwi-interacting, and small interfering RNA; and (II) long ncRNA (lncRNA) (longer than 200 nt) (Beermann et al., 2016; Hombach and Kretz, 2016). We mainly introduce some general aspects of the three common types of ncRNAs: miRNA, circular RNA (circRNA), and lncRNA as follows (Esteller, 2011; Westholm and Lai, 2011; Kristensen et al., 2019; Figure 2).
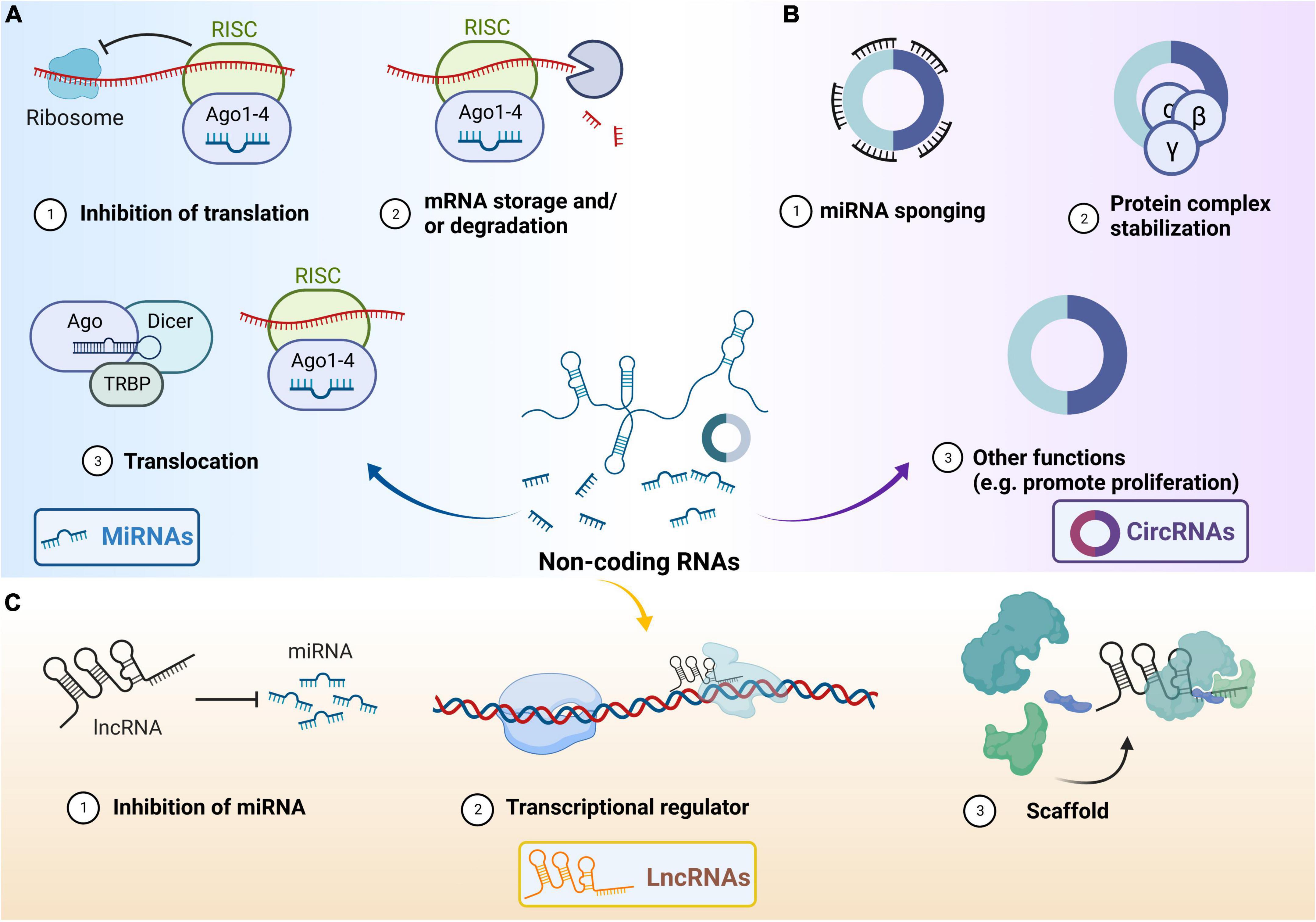
Figure 2. Main functions of three types of non-coding RNAs. (A) General functions of microRNA (miRNA). Unwinding of the miRNA via Argonaute, and transactivation response RNA-binding protein (TRBP)-dependent loading into the RNA-induced silencing complex (RISC). Binding of target mRNAs to miRNAs in RISC can result in translation repression or degradation of the mRNA. MiRNA and RISC can translocate to other cells. The RISC contains RNA-binding proteins including protein kinase RNA activator, TRBP and Dicer. (B) General functions of circular RNA (circRNA). CircRNA can act as miRNA sponge and protein complex stabilization. CircRNAs have other functions (including promoting cell proliferation etc.). (C) General functions of Long non-coding RNA (lncRNA). LncRNA can inhibit miRNA and act as transcriptional regulator. LncRNA participates in scaffolding complexes. The lncRNA possesses different domains that bind distinct effector molecules. The lncRNA can bind its multiple effector partners at the same time, which may have transcriptional activating or repressive activities, together in both time and space. Ago, Argonaute; circRNAs, circular RNAs; lncRNAs, long non-coding RNAs; miRNAs, microRNAs; RISC, RNA-induced silencing complex; TRBP, transactivation response RNA-binding protein.
MicroRNAs, endogenous and single-stranded RNAs ranging between 21 and 25 nucleotides in length, widely regulate gene expression, and more than 60% of genes are targets of miRNAs (Friedman et al., 2009). MiRNA has uridine at its 5′-end and is partially complementary to the 3′-end untranslated regions of the messenger RNA (mRNA). MiRNAs are produced by two RNase III proteins, Drosha and Dicer (Vishnoi and Rani, 2017). Pre-miRNAs are cleaved and processed by Drosha in the nucleus and guided to the cytoplasm (Ma et al., 2008; Westholm and Lai, 2011). Then, the pre-miRNA unwinds and one strand is incorporated into the RNA-induced silencing complex which is composed of Dicer, the double-stranded RNA-binding protein TRBP, and Argonaute2 proteins, ultimately resulting in translation repression or degradation of the mRNA (Gregory et al., 2005; Wu et al., 2006). The critical roles of miRNAs in animal models of CP, such as the severe dysregulation of miRNAs in the dorsal root ganglion (DRG) and the spinal cord regions, have been reported in many studies (Bali et al., 2013; Peng et al., 2017; Sakai et al., 2017).
Circular RNAs are generally produced through back-splicing or exon skipping of pre-mRNAs, while a single genomic location can generate multiple types of circRNAs. CircRNAs are notable for their continuous closed-loop structure, named a “back-splicing” structure, which is primarily formed through the junction of a downstream 3′ splice site with an upstream 5′ splice site (Zhang et al., 2016). Due to these structures, circRNAs are abnormally stable for exonucleases and are widely involved in various life processes such as cell proliferation, differentiation, and apoptosis (Haque and Harries, 2017). CircRNAs play key roles in mRNA stability and function through their activities as miRNA sponges, translation modulators, protein complex stabilization, and biomarkers (Hansen et al., 2013). CircRNAs are stable in the human bloodstream under physiological or pathological conditions and may be a potential biomarker easily obtained for clinical CP patients in the future (Vea et al., 2018).
Long non-coding RNAs have more than 200 nucleotides and can interact with proteins, DNAs, and multiple RNAs (Ulitsky and Bartel, 2013). LncRNAs lack protein-coding potential and often harbor a poly-A tail and can be spliced, which has been shown to play vital roles in a variety of biological processes such as translational inhibition, transcriptional silencing, promoter-specific gene regulation, X-chromosome inactivation, imprinting, maintenance of nuclear architecture and modulation of multiprotein complexes (Lee, 2009; Ponting et al., 2009; Statello et al., 2021). An estimated abundance of 5,400 to more than 10,000 lncRNA transcripts has been reported in humans (Jia et al., 2010; Djebali et al., 2012; Lorenzi et al., 2021) and several studies have shown that lncRNAs contribute to the occurrence of CP in some pre-clinical models by targeting pain-associated genes or other downstream molecules and increasing neuronal excitability in DRG primary sensory neurons (Zhao et al., 2013; Du et al., 2022).
Several factors are associated with expression of ncRNA. Current evidence has demonstrated that common traits, including age, sex, smoking, body mass, and physical activity, can influence circulating small ncRNA expression levels (Rounge et al., 2018), among which aging is a strong factor. For example, a previous study has analyzed miRNAs from the whole blood of 5,221 individuals and found that 127 of 150 miRNAs are affected by age (Huan et al., 2018). In addition, different exercise training programs greatly alter ncRNAs. Researchers have identified 204 differentially expressed lncRNAs (DELs) from untrained young individuals after 12 weeks of high-intensity interval training, whereas 43 DELs are identified after resistance training (Bonilauri and Dallagiovanna, 2020).
In this review, we provide an overview of the recent research progress and specific roles of ncRNAs in CP and focus on the alterations of ncRNAs reported mainly in the blood samples, DRG, spinal cord dorsal horn (SDH), and injured peripheral nerves of animal models and patients suffering CP.
Non-coding RNAs: Insights into the molecular mechanism of chronic pain
Peripheral neuropathic pain-associated with peripheral nerve injury
Neuropathic pain affects 8% of adults worldwide and is commonly caused by somatosensory system lesions or diseases, such as PNI (Colloca et al., 2017; Finnerup et al., 2021). PNI is a major onset cause of NP in adults, characterized by allodynia and pain hypersensitivity, and accompanied by ncRNAs alterations in the DRG or peripheral blood in patients (Arthur-Farraj et al., 2017; Hu et al., 2021). An increasing number of researchers have focused on the potential of ncRNAs as biomarkers in PNI-induced NP (Table 1).
MicroRNAs
Peripheral nerve injury leading to NP is usually accompanied by miRNA alterations in DRG or other tissues, which has been proven to be associated with the severity of pain hypersensitivity (Adilakshmi et al., 2012; Chang et al., 2017; Wang Y. et al., 2021). MiRNAs bind to the 3′-untranslated region of target mRNAs, causing mRNA splicing and destabilization (Bartel, 2009). This is a crucial mechanism by which miRNAs are involved in NP. MiR-124-3p is downregulated in the DRG and SDH in a spared sciatic nerve injury (SNI)-induced NP rat model, leading to upregulation of Egr1 (Jiang et al., 2021). Analogously, miR-206-3p is decreased in the DRG of chronic constriction injury (CCI) rats and histone deacetylase 4 (HDAC4) is identified as a potential target of miR-206-3p. Intrathecal injection a lentivirus encoding miR-206-3p (LV-miR-206-3p mimic) upregulates the level of miR-206-3p in DRG of rat and alleviates NP induced by PNI (Wen et al., 2019). In addition, accumulative studies have shown that miR-223, miR-144, miR-140-3p, miR-122-5p, miR-30b-5p, and miR-340-5p, are highly decreased in the CCI model and verify their roles in the development of NP (Gao et al., 2019; Zhang X. et al., 2020; Cheng et al., 2021; Wan et al., 2021; Zhu et al., 2021; Liao et al., 2022). However, some miRNAs contributed to NP are contrary to the conventional trend. Upregulation of miR-30c-5p is observed in the DRG, spinal cord, plasma, and cerebrospinal fluid (CSF) of SNI rats, and is robustly consistent with the severity of allodynia (Tramullas et al., 2018).
Furthermore, the regulation of voltage-gated channels in DRGs by miRNAs has been considered another critical mechanism in CP in recent years. Following PNI-induced NP in rats, for instance, voltage-dependent sodium (Nav) 1.7 encoded by the SCN9A gene in the afferent fiber is proven to be involved in the development of NP (Xue et al., 2021). Interestingly, intrathecal administration of miR-30b agomir, chemically modified double-strand miR-30b mimic, shows an obvious decrease in Nav1.7 in DRG neurons and alleviates pain behaviors remarkably (Shao et al., 2016). The above evidence has indicated the regulatory effect of miRNAs on voltage-gated sodium channels in DRGs. In addition to miR-30b, upregulation of miR-137 suppresses the expression of voltage-gated potassium channel (Kv) Kv1.2 and increases mechanical allodynia and thermal hyperalgesia in CCI rats (Zhang J. et al., 2021). Downregulation of miR-137 restores the abnormal Kv currents and excitability in DRG neurons and thus attenuating NP. Moreover, voltage-gated calcium channels related to CP are also regulated by miRNA. MiR-34c-5p could bidirectionally regulate voltage-gated calcium (Cav) 1.2 channels, which proves that they can interact with each other to regulate hyperalgesia (Favereaux et al., 2011).
In addition to acting on voltage-gated channels, miRNAs also interact with numerous receptors in PNI-induced NP. Taking toll-like receptors (TLRs) as a common example, the expression of TLR8 is highly increased in IB4+ DRG neurons in a spinal nerve ligation (SNL) model, and inhibition of TLR8 alleviates SNL-induced pain (Zhang et al., 2018). Moreover, miRNAs interact with TLR8 highly associated with NP. Mechanistically, the miR-21-TLR8 signaling axis is identified as an important target in the SNL model (Zhang et al., 2018). TLR5 is also observed to be upregulated in bilateral CCI rats regulated by miR-217 (Jiang et al., 2019). Downregulation of the level of miR-217 exerts an inhibition effect of TLR5 through suppressing neuroinflammation and alleviates NP in vivo ultimately. Additionally, the C-X-C motif chemokine receptor (CXCR) is reported to be involved in the regulation of NP. Dong et al. (2021) have proved that the C-X-C motif chemokine ligand-12 (CXCL12)-CXCR4 pathway is regulated by miR-130a-5p in the development of NP. MiR-130a-5p mimics can attenuate CCI-induced NP in vivo by inhibiting the activation and inflammatory response of astrocytes, as well as inactivating CXCR4 and its downstream targets, such as Rac1 and nuclear factor-κB (NF-κB), etc. (Dong et al., 2021). Further study might be performed to explore more novel receptors contributing to the pathogenesis of PNI related to NP, providing for more strategies to treat NP.
The crosstalk between immune system and nervous system may be a novel pathogenic mechanism (Pinho-Ribeiro et al., 2017). It has been reported that exosomes and its cargos, including miRNAs, mediate the crucial crosstalk between different cells, organs, or systems, and are involved in the regulation of NP (Simeoli et al., 2017; D’Agnelli et al., 2020). Simeoli et al. (2017) have verified that exosomal miR-21-5p, as one of the cargos of sensory neuron-derived exosomes, is released after capsaicin activation of transient receptor potential vanilloid 1 (TRPV1) receptor in cultured DRG. In vivo, intrathecal injection of miR-21-5p antagomir, the inhibitor of miR-21-5p, attenuates neuropathic hypersensitivity and reduces the extent of inflammatory macrophage recruitment in the DRG. In brief, exosomal cargos play critical roles in sensory neuron–macrophage communication after damage to the peripheral nerve, and similar remote communication in the NP deserves further investigation.
Circular RNAs
Accumulative studies have also shown that circRNAs are significantly altered in the SDH in rats with NP. Bioinformatics analysis has shown that the expression levels of three circRNAs, circRNA_013779, circRNA_008008, and circRNA_003724, are increased more than 10 fold in the SDH of the CCI model compared with the control group (Cao et al., 2017), indicating that these three circRNAs are involved in the pathogenesis of NP. In addition, circRNA_008008 and circRNA_013779, as the two largest nodes in the circRNA-miRNA interaction network, can interact with eight miRNAs (Cao et al., 2017).
Mechanistically, current studies have found that circRNAs regulate pain by acting as miRNA sponges and through the circRNA-miRNA-downstream molecular axis. CIRCZNF609 acts as miRNA sponge to inhibit the expression of miR-22-3p, which alleviates mechanical allodynia and thermal hyperalgesia levels through regulation of the expression of Tumor Necrosis Factor-alpha (TNF-α), interleukin-1 (IL-1), and IL-6 in L4-L6 spinal cord tissue of CCI rats (Li et al., 2020). CircRNA.2837 attenuates nerve damage by regulating autophagy by targeting the miR-34 family (including miR-34a, miR-34b, and miR-34c) (Zhou et al., 2018). Besides, some other circRNAs are also involved in PNI through a similar mechanism, such as circ_0005075 as miR-151a-3p sponge in CCI rats (Zhang J. Y. et al., 2021), circ-Ankib1 as miR-423-5p, miR-485-5p, and miR-666-3p sponges in PNI (Mao et al., 2019) and ciRS-7 as the miR-135a-5p sponge in CCI rats (Cai et al., 2020).
In addition, circRNAs play roles in regulation of various pain-related signaling pathways. Several studies have performed Kyoto Encyclopedia of Genes and Genomes (KEGG) analysis, showing that the Hippo signaling pathway, MAPK signaling pathway, and endocytosis are the most key pathways related to NP (Li N. et al., 2013; Chen and Lu, 2017; Huang et al., 2017). CircRNAs alleviate or aggravate pain by controlling signaling pathways, ultimately affecting the associated inflammatory levels. For example, circ_0005075 affects the expression level of Notch2 by inhibiting the level of miR-151a-3p, eventually leading to changes in cyclooxygenase-2 (COX-2), IL-6, TNF-α, and other cytokines in the CCI model (Zhang J. Y. et al., 2021). Previous studies have demonstrated that Notch signaling is activated, mediating mechanical hyperalgesia induction and maintenance in a rat model of NP (Xie et al., 2015). Additionally, circRNA ZRANB1 mediates the Wnt5a/β-catenin signaling pathway to aggravate NP by acting on the miR-24-3p/LPAR3 axis (Wei et al., 2020). However, regulation of circRNAs in the complex signaling pathway network of NP is still very limited, and further studies are needed to elucidate the downstream pathways participating in the regulation of pain.
Long non-coding RNAs
Similar to miRNAs and circRNAs, lncRNAs also play roles in regulating the process of PNI. Since there is abundant evidence that includes lncRNAs in PNI, we mainly focus on the common downstream targets related to PNI regulated by lncRNAs in the latest findings. However, current research on lncRNA with its targets related to NP is not in-depth. Most current research mainly focuses on the mechanism of the lncRNA/miRNA/target axis. LncRNAs can directly bind to several targets, such as many receptors, ligands or enzyme, and participate in the pathogenesis of PNI-induced NP. Although receptors are of various types, they can be roughly divided into ion channel receptors and non-ion channel receptors. The typical ionic receptors are the P2X receptor and EPHB1-NMDA. The other targets mainly include CXCL, Janus kinase (JAK), brain-derived neurotrophic factor (BDNF), high mobility group box-1 (HMGB1), cyclin-dependent kinases (CDKs) and programmed cell death factor-4 (PDCD4), etc.
P2X receptors, members of an ATP-gated ion channels family, are widely involved in CP regulated by ncRNAs (Xiong et al., 2019). LncRNA MRAK009713 is markedly increased and interacts with P2X3 to enhance mechanical and thermal hyperalgesia in CCI rats (Li et al., 2017). LncRNA LOC100911498 plays a key role in the pathophysiological process of NP and LOC100911498 siRNA can block P2X4Rs-mediated p38MAPK activation and BDNF release, attenuating NP in rats (Tang et al., 2021). Additional evidence of ion channel receptors involved in PNI is that overexpression of lncRNA uc.153 in SC increases NP by targeting miR-182-5p and subsequent modulation of EphB1-NMDA receptors (Zhang C. et al., 2020).
On the other hand, some targets related to inflammation and the cell cycle are identified to be related to PNI-induced NP regulated by various lncRNAs. HMGB1, as a critical target in NP, is expressed in multiple cells (Andersson et al., 2002), and can promote neuroinflammation and NP in animal models (Wen et al., 2021). Diverse lncRNAs, such as lncRNA FIRRE, lncRNA NEAT1, and lncRNA MALAT1, can regulate HMGB1 and are involved in PNI-induced NP (Xia et al., 2018; Ma et al., 2020; Wen et al., 2021). Inhibition of lncRNA MALAT1 reverses the abnormal increasing expression level of HMGB1 caused by CCI surgery in rats, which attenuates the development of NP and neuroinflammation (Ma et al., 2020). The LncRNA MALAT1/miR-129-5p/HMGB1 axis is confirmed to play a critical role. Another study has reported that downregulation of lncRNA FIRRE suppresses the secretion of microglial cell-derived proinflammatory cytokines and reduces NP by also suppressing HMGB1 expression (Wen et al., 2021). Additionally, the lncRNA NEAT1/miR-381/HMGB1 axis highly contributes to PNI-induced NP (Xia et al., 2018).
Cyclin-dependent kinases, cell cycle-related proteins, have also been shown to be engaged in pain-related behavior (Yokoyama et al., 2020). The lncRNA PKIA-AS1 directly regulates the expression and function of CDK6 in SNL-induced NP, exerting an effect on maintaining neuroinflammation and NP (Hu et al., 2019). Inhibition of lncRNA PKIA-AS1 decreases the expression of CDK6 and alleviates SNL-induced NP (Hu et al., 2019). The lncRNA SNHG1 induces NP by directly regulating CDK4 levels (Zhang J. Y. et al., 2020). WNT5A, a member of the WNT family, is reported to be related to pain behaviors, and inflammatory responses and could sensibilize spinal nerves in rat models of NP (Simonetti and Kuner, 2020). The lncRNA CRNDE/miR-146a-5p/WNT5A axis plays a central role in rats with CCI-induced NP (Zhang Q. et al., 2021). CXCL has the normal physiological function of inducing directed chemotaxis of nearby reactive cells and is also believed to play a role in peripheral NP (Braga et al., 2020). Knockdown of LncRNA SNHG5 in the DRG of SNL mice alleviates NP and inhibits the activation of astrocytes and microglia by targeting the miR-154-5p/CXCL13 axis (Chen et al., 2020). The JAK-STAT pathway is an original signal transduction pathway shared by multiple cytokines (Dominguez et al., 2008; Wang et al., 2016a) and has been reported to be regulated by lncRNAs in PNI-induced NP. Downregulation of lncRNA DILC in the spinal cord attenuates NP through SOCS3-induced suppression of the JAK2/STAT3 pathway while increasing the viability of primary microglia, suppressing apoptosis and inhibiting the production of interleukin (IL)-6 and IL-1β in microglia (Liu Y. et al., 2020). STAT3 is also regulated by lncRNA XIST. Increasing the lncRNA XIST in the spinal cord can contribute to CCI-induced NP in rats by downregulating miR-544 and upregulating STAT3 (Jin et al., 2018). The lncRNA Linc00052/miR-448/JAK1 axis also plays a key role in SNL-induced NP (Wang L. et al., 2020). PDCD4, a translation factor that binds to eIF4A, is regulated by various lncRNAs under different pathological conditions. For instance, miR-155 regulate the inflammatory response through SOCS1–STAT3–PDCD4 in atherogenesis (Ye et al., 2016) and is recently found its concrete role in NP. Overexpression of lncRNA DGCR5 in the spinal cord attenuates NP by sponging miR-330-3p and targeting PDCD4 in CCI rats (Peng et al., 2019). Zeste homolog 2 (EZH2), a contributor to microglial activation and NP, can bind to lncRNAs. The LncRNA Lncenc1 contribute to NP by targeting EZH2 and downregulating the brain angiogenesis inhibitor 1 (BAI1) gene in mouse microglia (Zhang Z. et al., 2021). Furthermore, upregulation of lncRNA GAS5 in the spinal cord decreases pain behaviors by targeting miR-452-5p/CELF2 (Tian et al., 2020).
In addition to the receptors above, amniotic aquaporins (AQP), special channel proteins with roles in PNI-induced NP, has been reported recently. Knockdown of AQP9 promotes the development of NP, and further investigation have revealed that the lncRNA MALA T1/miR-154-5p/AQP9 axis acts as a novel target for NP (Wu J. et al., 2020). In summary, multiple lncRNAs can bind to the same receptor, while a single lncRNA can target numerous receptors in the development of PNI-induced NP. More research is needed to elucidate the huge network between lncRNAs and their receptors.
Peripheral neuropathic pain-associated with diabetic neuropathic pain
Diabetic neuropathic pain is a general complication of diabetes mellitus (DM), and patients with DNP suffer various degrees of pain (Feldman et al., 2017). Oral treatment is the most frequent topical treatment for DNP, but its use is often limited by systemic side effects (Yang et al., 2019). Since a large population suffers from DM worldwide with a high risk of DNP (Sloan et al., 2018), we must seek better treatment to alleviate DNP. Much evidence has shown that ncRNAs are dysregulated in DNP (Table 1) and have the potential to be a novel strategy (Figure 3).
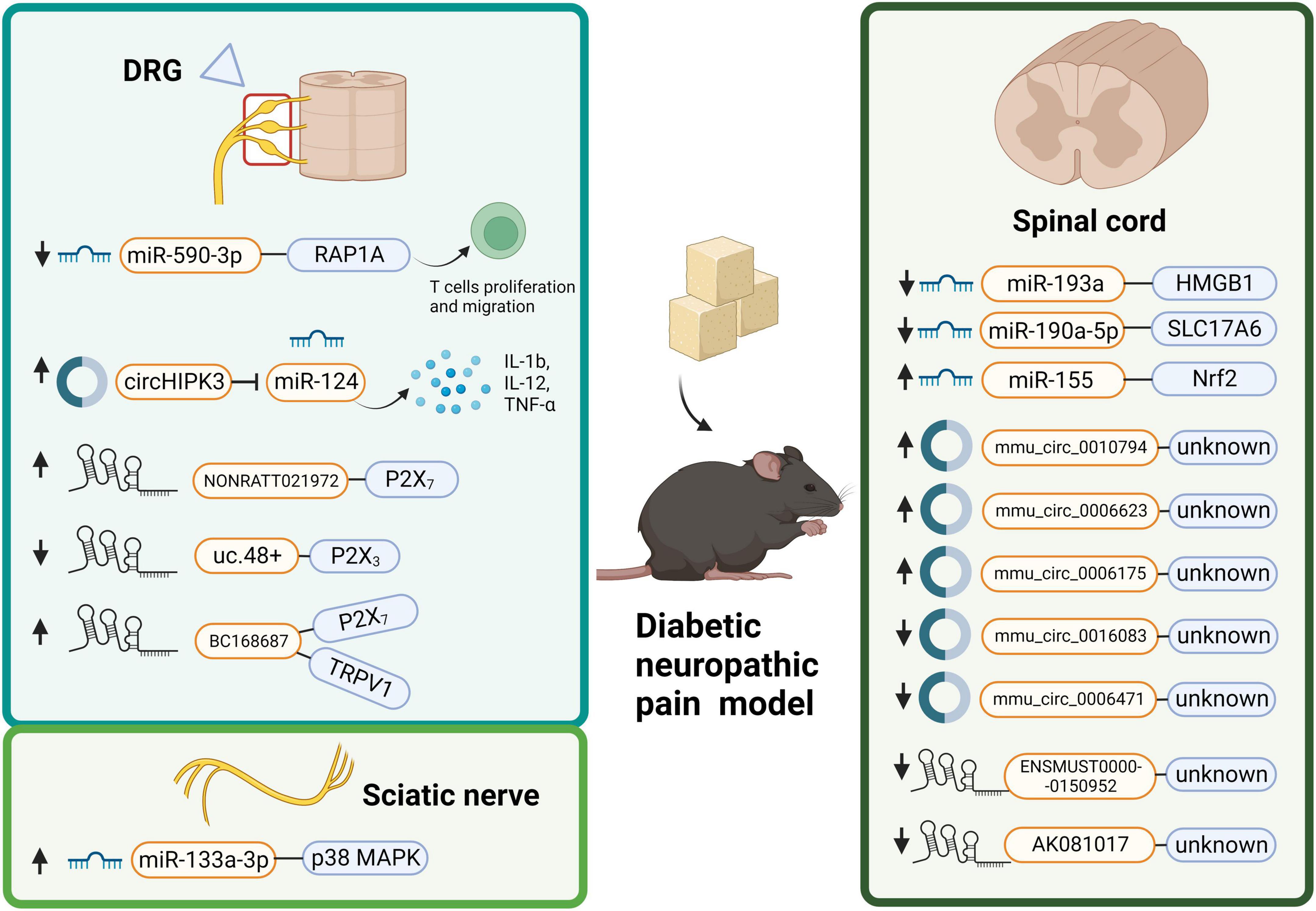
Figure 3. Distribution of dysregulated non-coding RNAs (ncRNAs) with the respective associated targets in diabetic neuropathic pain (DNP): ncRNAs (including microRNA, circular RNA, and long non-coding RNA) can participate in the development of DNP through various targets and mechanisms. NcRNAs displays different expressions in DRG, spinal cord and sciatic nerve of DNP models in DNP. DRG, dorsal root ganglion; HMGB1, high mobility group box-1; IL, interleukin; MAPK, mitogen-activated protein kinases; Nrf2, nuclear factor erythroid 2-related factor 2; RAP1A, Ras-associated protein-1 A; TNF, tumor necrosis factor; TRPV1, transient receptor potential vanilloid 1.
MicroRNAs
A recent sequencing analysis has found that miRNAs are altered greatly in the spinal cord of a mouse model with streptozotocin (STZ)-induced DNP, while a total of 791 miRNAs are detected (He et al., 2021). Therein, 148 miRNAs, including 68 upregulated and 80 downregulated miRNAs, exhibit remarkably dysregulated expression 42 days after STZ injection in mice compared with those in the control group (He et al., 2021). KEGG signaling pathway analysis indicates that the “Rap1 signaling pathway,” “human T-lymphotropic virus-I infection,” and the “MAPK signaling pathway” are the top three pathways related to the differentially expressed miRNAs. Meanwhile, GO analysis in the category of the biological process shows that “multicellular organism development,” “developmental process,” and “regulation of cellular metabolic process” are the top three enriched processes among the differentially expressed miRNA target genes. Based on the research above, miRNAs exert potential functions in the development of DNP.
MicroRNAs affect diverse progress related to DNP, including inflammation-related cytokines, signaling pathways and interaction with various cells. For example, Yang et al. (2017) have revealed that the expression of miR-190a-5p is decreased and SLC17A6 is increased in the spinal tissue of the DNP model. In addition, reversing the expression of miR-190a-5p and SLC17A6 alleviates the nociceptive response and decreases IL-1β and IL-6 levels in DNP (Yang et al., 2017). Another study has demonstrated that miR-155 suppresses Nrf2 expression level (Chen et al., 2019). Silencing miR-155 alleviates SNI by promoting cell proliferation, inhibiting apoptosis, and decreasing inflammation in DNP (Chen et al., 2019). Also, miR-193a is decreased in the lumbar SDH of STZ-induced diabetic mice (Wu et al., 2019). Overexpression of miR-193a inhibits HMGB1 expression in the lumbar SDH accompanied by suppressing peripheral neuroinflammation to alleviate DNP (Wu et al., 2019).
In addition, miRNAs inhibiting or interfering with cell processes, such as migration, apoptosis, and infiltration may become one of the pathogenic mechanisms of DNP (Gumy et al., 2008; Wang et al., 2014; Feng et al., 2018). Regulation of these miRNAs to improve cell state will be a prospective idea in the treatments of DNP. T cells can migrate into the spinal cord in several pain models, exerting a pro-inflammatory effect, and immune cell infiltration is involved in DNP (Wu Y. et al., 2020). MiR-590-3p inhibit T cell infiltration to alleviate DNP (Wu Y. et al., 2020). Chang et al. (2020) have found that miR-133a-3p is upregulated in the sciatic nerves of DM rats. MiR-133a-3p mimics are transfected into RSC96 Schwann cells, increasing p-p38 MAPK levels in the sciatic nerve and contributing to the development of NP (Chang et al., 2020). In summary, miRNAs could be a new direction for the treatment and diagnosis of DNP and further explorations will be needed to reveal their application and extra functions in DNP.
Circular RNAs
He et al. (2021) have identified 2,118 distinct circRNAs in the spinal cord of STZ-induced DNP mice. Among them, 1,552 circRNAs are <1,000 nt, and the median length is 620 nt. Further analysis has indicated that the majority of circRNAs are derived from exons. Thereinto, mmu_circ_0010794, mmu_circ_0006623, and mmu_circ_0006175 are more significantly upregulated circRNAs, while mmu_circ_0016083 and mmu_circ_0006471 show the opposite trends (He et al., 2021). Specifically, Wang Z. et al. (2018) have explored the role of circHIPK3 in DNP. CircHIPK3 is abundant in serum of diabetes patients who suffer DNP and in DRG from STZ-induced DNP rats (Wang L. et al., 2018). Knockdown of circHIPK3 alleviates DNP by inhibiting IL-1b, IL-6, IL-12, and TNF-α protein expression in the DRG of diabetic rats. Furthermore, they have verified that circHIPK3 interacts with miR-124, decreasing its expression and that overexpression of miR-124 greatly decreases mechanical allodynia and thermal hyperalgesia in vivo. This research provides us with a novel pathway to treat DNP by interfering with the circHIPK3/miR-124 axis. Evidence above shows a strong connection between circRNAs and DNP. However, research on circRNAs and DNP is very limited, and further research is urgently needed.
Long non-coding RNAs
Long non-coding RNAs are involved in the processes of DNP. Clinical evidence that have enrolled 154 patients with DM type 2 reveals an upregulation of lncRNA NONRATT021972 associated with NP scores of DM type 2 (Yu et al., 2017). Animal experiments have demonstrated that lncRNA NONRATT021972 siRNA decreases inflammation by inhibiting TNF-α and attenuates NP in rats of STZ-induced DNP (Liu et al., 2016). Indeed, the expression of lncRNA NONRATT021972 is significantly higher in the DRG of the DM group than in the control group. Wang et al. (2016b) have found that lncRNA uc.48+ is downregulated in DM and further explored their roles in DNP. Upregulation of lncRNA uc.48+ can attenuate DNP by inhibiting the phosphorylation and activation of ERK1/2 and targeting the P2X3 receptor in DRG. In addition to the P2X3 receptor, P2X receptors have been widely identified in DNP (Wang et al., 2016b). P2X7 receptors, as ligand-gated ion channels, are verified in occurrence of CP in both pre-clinical and clinical research (Sorge et al., 2012; Hu et al., 2020) and recently find its role in regulation of DNP hypersensitivity (Liu et al., 2016; Wang A. et al., 2021). Knock-out of the P2X7 receptor decreases mechanical and thermal hypersensitivities in mice and activation of the P2X7 receptor shows an adverse effect (Zhao et al., 2016). Interestingly, both lncRNA BC168687 siRNA and lncRNA NONRATT021972 siRNA are identified to regulate DNP by targeting the P2X7 receptor in DRG (Liu et al., 2016, 2017). LncRNA BC168687 siRNA is also involved in TRPV1-mediated DNP in rats (Liu et al., 2018).
Genome-wide expression patterns of lncRNAs in the SDH of mice with DNP have shown that 1,481 lncRNAs are differentially expressed and 289 neighboring and 57 overlapping lncRNA-mRNA 15 pairs are identified (Du et al., 2019). Researchers have verified that lncRNA-mRNA, such as ENSMUST00000150952-Mbp and AK081017-Usp15, may play a critical role in DNP pathogenesis (Du et al., 2019). In summary, lncRNAs are widely altered in the occurrence of DNP, and more lncRNAs need to be elucidated as potential treatment targets for DNP patients in the future.
Central neuropathic pain associated with spinal cord injury
Spinal cord injury is a kind of general central nervous system trauma accompanied by a common complication: CNP as well as an abnormality of autonomic function (Eldahan and Rabchevsky, 2018; Viswanath et al., 2020). SCI patients with NP find it to be unbearable, and the long-term pain affects their sleep, work, and life (Siddall and Middleton, 2015). NcRNAs are involved in the development of CNP after SCI.
MicroRNAs
Multiple studies have focused on miRNA microarrays or genome-wide miRNA expression profiling screens to confirm their alterations after SCI-induced NP in clinical patients (Zhao et al., 2021), trying to find a new mechanism for this type of pain. A total of 2,367 distinct miRNAs in adults with pain after SCI are identified, and 71 miRNAs are differentially expressed in chronic NP (Ye et al., 2021). Among these, hsa-miR-19a-3p and hsa-miR-19b-3p are remarkably higher in chronic SCI with NP, and their potential clinical value for discriminating between patients with and without pain is verified (Ye et al., 2021). Moreover, miRNA-targeted therapy and its roles in SCI-induced CNP are increasingly studied. Yao et al. (2021) have elucidated that miR-130a-3p is upregulated in the spinal cord lesions of SCI rats. The inhibition of miR-130a-3p represses inflammatory cytokines, such as IL-1β, IL-6, and TNF-α, and upregulates the IGF-1/IGF-1R signaling pathway to alleviate NP caused by SCI (Yao et al., 2021). Upregulation of miR-139-5p improves locomotor functional recovery and attenuates both mechanical and thermal hypersensitivities, as well as promotes neuronal survival in the SCI model (Wang P. et al., 2021). These studies suggest that it would be beneficial to explore the mechanism of miRNAs as a strategy to treat pain following SCI.
Circular RNAs
There are very few studies of circRNAs directly involved in CNP in SCI models. However, circRNAs are proven to be associated with nerve cell inflammation, microglial activation, neuronal death, etc., after SCI (He et al., 2020; Tong et al., 2021). These pathophysiological processes are highly related to CNP, and circRNAs are verified for their direct role in CNP associated with SCI in further exploration. He et al. (2020) have observed that quietness circ 0000962 expression is downregulated in SCI model rats and overexpression of quietness circ 0000962 exerts an effect of inhibiting nerve cell inflammation in an in vitro model of SCI through activation of PI3K/Akt and suppression of NF-κB. Another study has found that circ-Usp10, as a competing endogenous RNA, could promote microglial activation and induce neuronal death by targeting miR-152-5p/CD84 in SCI (Tong et al., 2021). Additionally, circ-HIPK3 sponges miR-558 to inhibit neuronal cell apoptosis after SCI, exerting its neuroprotective effect (Wang L. et al., 2018). Although some of the diverse roles of circRNAs on neurons or microglia after SCI have been elucidated, whether their direct neuroprotective effects and possible mechanisms on sensory neurons in SCI deserve more original research.
Long non-coding RNAs
Spinal cord injury with CNP is a multifactorial pathological process, and lncRNAs have recently been regarded as promising biomarkers for CNP-associated SCI. Zhao et al. (2021) have detected the expression of lncRNAs and mRNAs in peripheral blood samples of patients who suffer from SCI with NP and without NP. Screening results have demonstrated that seven genes and two lncRNAs are directly involved in the pain pathway: E2F1, MAX, MITF, CTNNA1, ADORA2B, GRIK3, OXTR, LINC01119, and LINC02447 (Zhao et al., 2021). LINC01119 and LINC02447 have a positive correlation with these genes (Zhao et al., 2021). The expression of lncRNA NEAT1 is distinctly higher in rats with SCI with CNP (Xian et al., 2021). Mechanistically, overexpression of lncRNA NEAT1 mediates the expression of IL-6, IL-1β, and TNFα and further exploration have indicated that lncRNA NEAT1 is involved in SCI-induced NP progression through the lncRNA NEAT1/miR-128-3p/AQP4 axis (Xian et al., 2021). Another study has found that LncRNA PVT1 depletion significantly alleviates SCI with NP by inhibiting astrocytic activation and reducing the expression of neuroinflammatory factors (Zhang P. et al., 2021). The lncRNAPVT1/miR-186-5p/CXCL13/CXCR5 axis is regarded as a new therapeutic target for NP (Zhang P. et al., 2021). Therefore, it is critical to explore the interaction network between multiple lncRNAs and its targets.
Chronic regional pain syndrome
Complex regional pain syndrome is characterized by megalgia and inflammation, often affecting a single limb and following an injury (Kessler et al., 2020). It is difficult to meet a satisfactory analgesic treatment for CRPS. However, the pathogenesis of CRPS is still unclear. Here we focus on the role of ncRNAs in the development of CRPS, and almost all research concentrated on microRNAs in CRPS.
Circulating miRNAs, most encapsulated in small extracellular vehicles (sEVs) or exosomes, are stable in intercellular communication and may become an original biomarker or risk factor for CRPS (Ramanathan et al., 2019a; Wickman et al., 2021). Wickman et al. (2021) have purified and identified both cytokine and miRNA levels in serum sEVs in a tibia fracture model (TFM) induced-CRPS. The results have shown an unchanged level of cytokines but significantly differential expression of miRNAs. Thereinto, thirty sEV miRNAs are identified and correlated with CRPS clinical patients, which suggests a prospective prediction and diagnosis biomarker for CRPS. Another similar study has shown that miR-939 is robustly upregulation in sEVs from serum of CRPS patients (Ramanathan et al., 2019b). Levels of miR-939 in CRPS patients varies greatly in different immune cell-derived sEVs, especially higher in sEVs derived from B cells, T cells, and NK cells relative to monocytes in controls (Ramanathan et al., 2019b). We speculate that accurate detection of miRNAs in some related cell-derived sEVs is of great clinical significance and requires further research. In addition, Pande et al. (2021) have revealed 22-fold downregulation of the miRNA hsa-miR-605 in the blood of CRPS patients with poor analgesic effects of ketamine. Moreover, the level of serum hsa-miR-605 is highly correlated with CRPS, which regulates the proinflammatory chemokine CXCL5 and plays a critical role in leukocyte recruitment and activation (Pande et al., 2021).
Complex regional pain syndrome is more common in women than men (van Velzen et al., 2019). Interestingly, a recent study has confirmed that miRNAs and their roles can also lead to different gender incidences of CRPS. MiR-34a targets X inactivation-specific transcript (XIST) through the proinflammatory transcription factor Yin–Yang one under inflammation directly (Shenoda et al., 2018). XIST mediating X-chromosome inactivation causes a different incidence of CRPS in sex (Patrat et al., 2020). XIST is critical for dosage compensation in females, and thus downregulating XIST completely or directly could be an infeasible intervention strategy for female patients with CRPS (Shenoda et al., 2018). The findings above suggest that miRNA-mediated downregulation of XIST may be a potential method for pain relief in female patients. Although several studies have explored multiple mechanisms of miRNAs in CRPS, whether some miRNAs can be used as post-treatment efficacy evaluation of CRPS is still worthy of further study and exerts its broad prospects for diagnosis and therapeutic effect prediction.
Inflammatory pain
Inflammatory pain is a very common type of pain, especially in bone and joint diseases, such as osteoarthritis (OA), rheumatoid arthritis (RA), and multiple sclerosis (MS) (Muley et al., 2016; Conaghan et al., 2019; Mailhot et al., 2020). Clinically, we have very limited treatments for this severe type of pain. Recent evidence has shown that ncRNAs play an essential role in IP in both patients and rat models (Cheng et al., 2018; Pan et al., 2019).
MicroRNAs
MicroRNAs have proven to be promising targets for refractory IP (Descalzi et al., 2015). Exosomal miRNAs can mediate pain through communication between sensory neurons and immune cells (Simeoli et al., 2017). MiR-21 deletion in sensory neurons inhibits macrophage infiltration and attenuates pain in mice (Simeoli et al., 2017). This evidence indirectly indicates that miRNAs have a potential effect on pain-related immune response.
MiR-544-3p is markedly reduced in the DRG of RA mouse models and targets neuronal FcgRI to mediate acute joint pain hypersensitivity induced by IgG immune complexes (Liu et al., 2021). Similarly, miR-146a and the miR-183 cluster are also robustly downregulated in the DRG (L4/L5) and spinal cord after experiencing knee joint OA pain in rats (Li X. et al., 2013). Interestingly, miR-146a and the miR-183 cluster could mediate hyperalgesia by regulating diverse factors associated with inflammation in glial cells, such as IL-6, IL-1β, TNFα, NF-κB, TLR-2, and TLR-4. The miR-183 cluster can even affect pain-related ion channel genes (i.e., Nav1.3 and TRPV1) in microglial cells (Li X. et al., 2013). Besides, miR-485-5p (Xu et al., 2020), miR-16 (Chen et al., 2016), miR-211 (Sun et al., 2019), miR-451 (Sun and Zhang, 2018), and miR-34a (Liang et al., 2019) are decreased in IP animal models while miRNA-107 (Zhang L. et al., 2021) is proven to be upregulated in IP.
MicroRNAs can also regulate IP by participating in various signaling pathways. For instance, microRNA-211-5p enhances the analgesic effect of dexmedetomidine on TNBS-induced chronic inflammatory visceral pain in rats through the MEK/ERK/CREB pathway (Sun et al., 2019). Another study has observed that miR-216a-5p is downregulated and HMGB1 is upregulated in a complete Freund’s adjuvant-induced IP model (Zhenzhen et al., 2021). Furthermore, it is proven that miR-216a-5p targets the HMGB1-TLR4-NF-κB pathway and finally alters microglia-mediated neuroinflammation to alleviate inflammatory behavioral hypersensitivity (Zhenzhen et al., 2021). In summary, miRNAs can mediate IP or neuroinflammation through various mechanisms. We mainly review its role in interacting with downstream gene expression and molecules or targeting diverse signaling pathways.
Circular RNAs
Circular RNAs also play an essential role in IP. Pan et al. (2019) have found that circRNA-filip1L is notably increased in the ipsilateral SDH of mice with IP and that miRNA-1224 knockdown or Ago2 overexpression induces nociceptive behaviors in naive mice. Functional evidence has suggested that circRNA-Filip1l regulates IP by targeting Ubr5 in an Ago2-dependent manner (Pan et al., 2019). This study shows a novel epigenetic mechanism of interaction between miRNA and circRNA in chronic IP.
Circular RNAs can be used as biomarkers for many joint diseases. With similar mechanism as above, the role of the circRNF121/miR-665/MYD88 axis becomes a potential target for OA patients through the NF-κB pathway (Wang T. et al., 2020). Other evidence has shown that the levels of hsa_circ_0000175 and hsa_circ_0008410 in peripheral blood monocytes improve the diagnostic accuracy of rheumatoid joints and are associated with the activation, severity, and the amount of joint tenderness of RA (Luo et al., 2020b). Similarly, the expression level of hsa_circ_0079787 is related to the severity of axial spondylarthritis (Luo et al., 2020a). The evidence above has shown that circRNAs may be related to the severity of joint diseases or pain biomarkers. However, whether hsa_circ_0008410 are involved in RA-induced pain or not needs to be further studied.
Additionally, several studies have focused on the circRNAs involved in the pathogenesis of low back pain and intervertebral disc degeneration (Li et al., 2021a). Downregulation of circRNAs such as circRNA-CIDN, circSEMA4B, and circRNA GRB10 promotes low back pain by regulating apoptosis, signaling pathways, and sponging different miRNAs (Guo et al., 2018; Wang X. et al., 2018; Xiang et al., 2020). Circ-FAM169A may act as a competitive endogenous RNA to sponge miR-583 and regulate intervertebral disc degeneration pathological process (Li et al., 2021a). These studies have described circRNAs as novel diagnostic biomarkers of diseases and have showed effective strategies to alleviate the severity of disease and pain.
Long non-coding RNAs
Accumulated studies have indicated the essential role of lncRNAs in IP, such as OA and RA (Budd et al., 2018; Lü et al., 2020). In an experiment on the treatment of OA, Yang et al. (2021) have tried to determine the underlying mechanism of lncRNA H19 secreted by umbilical cord blood mesenchymal stem cells (UCBMSCs) in the treatment of advanced OA-induced pain. LncRNA H19 is enriched in the exosomes of UCBMSCs and lncRNA H19 affects pain and central sensitization of advanced OA through the microRNA-29a-3p/FOS axis (Yang et al., 2021). Another study has shown that the expression of lncRNA SNHG14 and IL-1β mRNA are both upregulated in OA. Downregulation of lncRNA SNHG14 enhances the proliferation of IL-1β-treated chondrocytes and inhibits cell apoptosis (Wang B. et al., 2021). Moreover, knocking down lncRNA SNHG14 decreases the progression of OA in rats by inhibiting inflammatory responses. Downregulation of lncRNA SNHG14 inhibits FSTL-1-mediated activation of NLRP3 and TLR4/NF-κB signaling pathway activation through miR-124-3p to alleviate inflammatory reactions in OA (Wang B. et al., 2021). However, whether lncRNA SNHG14 and its target has an effect on OA-induced pain needs further confirmation.
In addition to OA, researchers have established a rat model of neuroinflammatory pain by human immunodeficiency virus envelope glycoprotein 120 (gp120) treatment (Peng et al., 2021). Downregulation of lncRNA uc.48+ significantly alleviates hyperalgesia in gp120-treated rats (Peng et al., 2021). P2Y12 receptors are finally found to be a downstream molecular target for lncRNA uc.48+ in neurons and heterologous cells (Peng et al., 2021). LncRNA-mediated gene regulation occurs in primary sensory neurons of the DRG during RA-like joint inflammation or RA-induced pain (Bai et al., 2020). A lncRNA-mRNA microarray has shown that 69 lncRNAs (42 up and 27 down) are significantly altered in a rat model of joint inflammation (Bai et al., 2020). Thereinto, the remarkable upregulation lncRNA MRAK163594 and lncRNA uc.247+ are co-expressed with the pain-related gene Scn2a, which contributes to pain conditions, such as inflammatory joint pain (Bai et al., 2020). The evidence above provides us with numerous potential targets for the diagnosis or therapy of diverse IP.
Cancer-induced pain
Cancer-induced pain is caused by primary cancer itself or metastases with moderate to severe pain among 90% of individuals with advanced cancer, which is an increasingly prominent public health problem (Treede et al., 2019). The current therapeutic regimen for CIP could not meet all needs for analgesia. Clinically, bone cancer pain (BCP) is a common type of CIP. We mainly focus on the role of ncRNAs in BCP as well as other types of CIP.
MicroRNAs
Overexpression of miR-300 suppresses expression of its target HMGB1 in rat model of cancer-induced bone pain, which significantly attenuates BCP (Liu C. et al., 2020). In addition to BCP, Zhu et al. (2020) have found that miR-330 is highly expressed in the SDH of nude mice with pancreatic cancer pain and microinjection of miR-330 mimic induces abdominal mechanical hypersensitivity in normal nude mice. MiR-330 robustly suppresses the expression of GABABR2 in the SDH of nude mice with pancreatic cancer pain (Zhu et al., 2020).
Alterations in gene expression, such as miRNAs, play a crucial role and affect the degree of pain. Bali et al. (2013) have found that 57 miRNAs are dysregulated in sensory neurons corresponding to tumor-related areas with genome-wide miRNA screening. Among these, miR-1a-3p could target Clcn3, an important gene encoding the chloride channel in CIP (Bali et al., 2013). Knocking down Clcn3 expression with Clcn3-siRNA enhances tumor-induced mechanical allodynia. Similarly, the effect of miRNA on Cav also plays an important role in the pathogenesis of CIP. For instance, knocking down the expression of Cav2.3 specifically in DRG neurons leads to hypersensitivity in mice (Gandla et al., 2017). Besides, miR-34c-5p and Cav2.3 are co-expressed both in cultured sensory neurons and DRG in mice with CIP. Researchers have concluded that miR-34c-5p target Cav2.3, exerting a pro-nociceptive effect (Gandla et al., 2017). Above all, miR-34c-5p might be a promising strategy to reach a satisfactory effect in further clinical therapy. Moreover, miRNAs can also affect synapses related to algesia conduction. MiR-124 is a specific inhibitor of synaptopodin (Synpo), a key protein for synaptic transmission (Elramah et al., 2017). MiR-124 is decreased and Synpo is increased in the spinal cord under CIP conditions. Elramah et al. (2017) have found that Synpo acts as a crucial component in nociceptive pathways. Intrathecal administration of miR-124 mimics in mice with BCP decreases Synpo expression and completely alleviates CIP in the early phase of cancer (Elramah et al., 2017). In conclusion, the evidence above provides us with broad insight into seeking better analgesia therapy plans for patients who suffer CIP.
Circular RNAs
Limited studies have shown that circRNAs have a potential effect on the pathogenesis of CIP. Chen et al. (2021) have validated eight differentially expressed circRNAs in the rat spinal cord by agarose gel electrophoresis and Sanger sequencing. CircSlc7a11 is selected for further functional study. Overexpression of circSlc7a11 greatly promotes cell proliferation and represses apoptosis of LLC-WRC 256 and UMR-106 cells (Chen et al., 2021). Besides, several signaling pathways, such as the chemokine signaling pathway, are verified to be linked to changes in circSlc7a11. Several potential targets of circSlc7a11, such as Pax8, Isg15, and CxCL10, and a complex circRNA/miRNA/mRNA network are involved in BCP (Chen et al., 2021). The effect of circSlc7a11 on the pathogenesis of BCP could be found through multiple pathways such as tumor cell invasion and proliferation, expression of multiple miRNAs and functional genes, etc. Screening differentially expressed circRNAs in BCP shows that 850 are obviously upregulated whereas 644 are significantly downregulated in the BCP group (Zhang Y. et al., 2020). Specifically focused on the role of circStrn3 in BCP, knockdown of circStrn3 can promote bone cancer cell apoptosis and proliferation (Zhang Y. et al., 2020). CircStrn3 regulates rno-miR-9a-5p to target Nfkb1, and Nfkb1 is found to be positively expressed in BCP rats and associated with the NF-κB pathway, indicating that circStrn3 might be a potential biomarker for BCP (Zhang Y. et al., 2020).
Long non-coding RNAs
Many lncRNAs have been verified to play a crucial role in cancer or cancer metastasis. However, we have ignored the functions of lncRNAs in CIP before and an increasing number of studies have focused on lncRNAs related to the occurrence of CIP. Microarray analysis has identified the differentially expressed lncRNAs and mRNAs in the DRG 14-days post-operation in the BCP rat model (Wei et al., 2021). A total of 73 lncRNAs and 187 mRNAs are dysregulated. The top 30 lncRNA-predicted mRNAs are mainly related to peroxisome, double-stranded RNA-binding, DNA-dependent DNA replication, tuberculosis, and purine metabolism (Wei et al., 2021). These findings suggest a strong correlation between lncRNAs and CIP.
Interestingly, upregulation of lncRNA-NONRATT021203.2 in the DRG contributes to cancer-induced hyperalgesia (Sun et al., 2020). In the underlying mechanism, CXCL9, mainly expressed in CGRP-positive and IB4-positive DRG neurons, is found to act as a target of lncRNA-NONRATT021203.2 by colocalizing with lncRNA-NONRATT021203.2 in DRG neurons (Sun et al., 2020). Moreover, lncRNA-NONRATT009773.2 is also identified to be involved in BCP. Depletion of lncRNA NONRATT009773.2 alleviates BCP and further lncRNA NONRATT009773.2/miR-708-5p/CXCL13 axis is confirmed to be engaged in the initiation and maintenance of neuroinflammation and hyperalgesia of BCP (Chen et al., 2022). The findings above indicate that the lncRNA/miRNA axis will be a novel strategy for the treatment of CIP.
Conclusion and future perspectives
In summary, CP is a major health problem affecting the quality of life because of the lack of understanding of the underlying pathophysiology and effective and safe treatments. CP is a multifactorial disease and multiple mechanisms are involved in its pathogenesis (Cohen et al., 2021). For example, low socioeconomic status, poor working conditions, unstable home life, low education levels, and living in deprived environments are associated with increased pain (van Hecke et al., 2013). NcRNAs are one of the factors that can affect CP and have been studied extensively and are considered to be novel biomarkers and pharmacological applications in CP (Lötsch et al., 2013; Bali and Kuner, 2014; Lopez-Gonzalez et al., 2017). However, there are some limitations in the field of ncRNAs and CP. Most existing studies are confined to the ncRNA/receptor axis or RNA sequencing in DRG or spinal cord tissue in rodent animal models of CP. MiRNAs are the most well studied among the family of ncRNAs, their presence at the forefront of ncRNA-based CP therapeutics is not yet satisfied, and it is difficult to definitively categorize the majority of miRNAs as neither suppressors nor promoters of CP. Additionally, it is still unclear how ncRNAs directly bind to the target and the connection of diverse complex ncRNAs in different CP conditions, which deserves further investigation. Recent technological advancements might not allow a comprehensive assessment of their functional roles in multiple types of CP.
Although ncRNAs are promising for clinical applications in CP, there are still many challenges we face. Concrete issues must be considered, such as the quality, stability, safety of ncRNAs, and even biological activity until reaching the target location. Regarding the quality and stability of ncRNAs, degradation by ubiquitous RNase is an unneglectable problem if ncRNAs are considered to be pharmaceuticals for CP patients. More technological advances are needed to synthesize and manufacture multiple standard ncRNA mimics and inhibitors for clinical use in the future. Secondly, whether exogenous administration of ncRNAs for clinical use will produce unpredictable immune responses is a tricky problem we need to evaluate in advance. In addition, off-target effects are the main reason for side effects and poor healing efficacy in miRNA-based therapy (Jackson and Linsley, 2010). The properties of ncRNAs, including high molecular weight and hydrophilia determine the difficulties of traversing biological membranes if their targets are intracellular or intranuclear. Therefore, ncRNA-based therapeutics require a reliable delivery system across the membrane obstacle and protection from degradation and clearance, such as exosomes, liposomes, lentivirus, etc., as a delivery system (Vickers et al., 2011; El Andaloussi et al., 2013). Additionally, existing studies are limited to cell or animal models, and more pre-clinical studies and clinical trials are needed to verify the potential therapeutic effects of ncRNAs in CP. It is still a challenge to apply ncRNA-based strategies for diagnosis and therapeutics to the human body.
Author contributions
CZ and RG mainly contributed to designing and writing the manuscript. RZ, HC, and CL retrieved literature. TZ provided administrative support. CC critically revised the manuscript. All authors wrote the article and read and approved the final manuscript.
Funding
This study was supported by the National Natural Science Foundation of China (grant nos. 81870858, 82171185, and 81500937 to CC), the National Key R&D Program of China (grant no. 2018YFC2001800 to TZ), the National Natural Science Foundation of China (grant no. 81671062 to TZ), the Natural Science Foundation of Sichuan Province (grant no. 2022NSFSC1322 to RG), Sichuan Province Science and Technology Support Program (grant no. 2020YJ0051 to CC), China Post-doctoral Science Foundation (grant no. 2020 M673234 to RG), Post-doctoral Research Project, West China Hospital, Sichuan University (grant no. 2020HXBH022 to RG), and China Post-doctoral Science Foundation (grant no. 2017 M610603 to CC).
Conflict of interest
The authors declare that the research was conducted in the absence of any commercial or financial relationships that could be construed as a potential conflict of interest.
Publisher’s note
All claims expressed in this article are solely those of the authors and do not necessarily represent those of their affiliated organizations, or those of the publisher, the editors and the reviewers. Any product that may be evaluated in this article, or claim that may be made by its manufacturer, is not guaranteed or endorsed by the publisher.
Abbreviations
AQP, amniotic aquaporins; BAI1, brain angiogenesis inhibitor 1; BCP, bone cancer pain; BDNF, brain-derived neurotrophic factor; Cav, voltage-gated calcium; CCI, chronic constriction injury; CDKs, cyclin-dependent kinases; CELF2, CUGBP Elav-like family member 2; CIP, cancer-induced pain; circRNAs, circular RNAs; CNP, central neuropathic pain; CP, chronic pain; CRPS, complex regional pain syndrome; CSF, cerebrospinal fluid; CXCR, C-X-C motif chemokine receptor; COX, cyclooxygenase; DM, diabetes mellitus; DNP, diabetic neuropathic pain; DRG, dorsal root ganglion; gp120, glycoprotein 120; ELAVL1, ELAV-like RNA-binding protein 1; ENO1, Alpha-enolase; EphB1, Eph receptor B1; EZH, Zeste homolog; HDAC, histone deacetylase; HMGB-1, high mobility group box-1; IL, interleukin; IP, inflammatory pain; IRAK1, interleukin-1 receptor-associated kinases 1; JAG1, Jagged1; JAK, Janus kinase; KEGG, Kyoto Encyclopedia of Genes and Genomes; Kv, voltage-gated potassium; LPAR3, lysophosphatidic acid receptor 3; lncRNAs, long non-coding RNAs; MAPK, mitogen-activated protein kinases; miRNAs, microRNAs; mRNA, messenger RNA; MS, multiple sclerosis; Nav, voltage-dependent sodium; ncRNAs, non-coding RNAs; NF-κB, nuclear factor-κB; NLRP3, NOD-like receptor family pyrin domain containing 3; NMDA, N-methyl-d-aspartate; NP, neuropathic pain; Nrf2, nuclear factor erythroid 2-related factor 2; OA, osteoarthritis; PDCD4, programmed cell death factor-4; PDK4, pyruvate dehydrogenase kinase 4; PNI, peripheral nerve injury; P2XR, P2X receptor; RA, rheumatoid arthritis; RAP1A, Ras-associated protein-1 A; RISC, RNA-induced silencing complex; SC, spinal cord; SCI, spinal cord injury; SDH, spinal cord dorsal horn; sEVs, small extracellular vesicles; SN, sciatic nerve; SNI, spared sciatic nerve injury; SNL, spinal nerve ligation; STAT3, signal transducer and activator of transcription 3; STZ, streptozotocin; Synpo, synaptopodin; TGF-β, transforming growth factor-β; TLRs, toll-like receptors; TNF, tumor necrosis factor; TRAF, TNF receptor associated factor; TRBP, transactivation response RNA-binding protein; TRPV1, transient receptor potential vanilloid 1; UCBMSCs, umbilical cord blood mesenchymal stem cells; XIST, X inactivation-specific transcript; ZEB1, Zinc finger E-box binding homeobox 1.
References
Adilakshmi, T., Sudol, I., and Tapinos, N. (2012). Combinatorial action of miRNAs regulates transcriptional and post-transcriptional gene silencing following in vivo PNS injury. PLoS One 7:e39674. doi: 10.1371/journal.pone.0039674
Andersson, U., Erlandsson-Harris, H., Yang, H., and Tracey, K. J. (2002). HMGB1 as a DNA-binding cytokine. J. Leukoc. Biol. 72, 1084–1091.
Arthur-Farraj, P. J., Morgan, C. C., Adamowicz, M., Gomez-Sanchez, J. A., Fazal, S. V., Beucher, A., et al. (2017). Changes in the coding and non-coding transcriptome and DNA methylome that define the schwann cell repair phenotype after nerve injury. Cell Rep. 20, 2719–2734. doi: 10.1016/j.celrep.2017.08.064
Bai, Q., Cao, J., Dong, T., and Tao, F. (2020). Transcriptome Analysis of Dorsal Root Ganglion in Rats with Knee Joint Inflammation. J. Pain Res. 13, 2709–2720. doi: 10.2147/JPR.S278474
Bali, K. K., and Kuner, R. (2014). Noncoding RNAs: Key molecules in understanding and treating pain. Trends Mol. Med. 20, 437–448. doi: 10.1016/j.molmed.2014.05.006
Bali, K. K., Selvaraj, D., Satagopam, V. P., Lu, J., Schneider, R., and Kuner, R. (2013). Genome-wide identification and functional analyses of microRNA signatures associated with cancer pain. EMBO Mol. Med. 5, 1740–1758. doi: 10.1002/emmm.201302797
Bartel, D. P. (2009). MicroRNAs: Target recognition and regulatory functions. Cell 136, 215–233. doi: 10.1016/j.cell.2009.01.002
Beermann, J., Piccoli, M. T., Viereck, J., and Thum, T. (2016). Non-coding RNAs in development and disease: Background, mechanisms, and therapeutic approaches. Physiol. Rev. 96, 1297–1325. doi: 10.1152/physrev.00041.2015
Bonilauri, B., and Dallagiovanna, B. (2020). Long non-coding RNAs Are differentially expressed after different exercise training programs. Front. Physiol. 11:567614. doi: 10.3389/fphys.2020.567614
Braga, A. V., Costa, S., Rodrigues, F. F., Melo, I. S. F., Morais, M. I., Coelho, M. M., et al. (2020). Thiamine, riboflavin, and nicotinamide inhibit paclitaxel-induced allodynia by reducing TNF-α and CXCL-1 in dorsal root ganglia and thalamus and activating ATP-sensitive potassium channels. Inflammopharmacology 28, 201–213. doi: 10.1007/s10787-019-00625-1
Budd, E., Nalesso, G., and Mobasheri, A. (2018). Extracellular genomic biomarkers of osteoarthritis. Expert Rev. Mol. Diagn. 18, 55–74. doi: 10.1080/14737159.2018.1415757
Cai, W., Zhang, Y., and Su, Z. (2020). ciRS-7 targeting miR-135a-5p promotes neuropathic pain in CCI rats via inflammation and autophagy. Gene 736:144386. doi: 10.1016/j.gene.2020.144386
Cao, S., Deng, W., Li, Y., Qin, B., Zhang, L., Yu, S., et al. (2017). Chronic constriction injury of sciatic nerve changes circular RNA expression in rat spinal dorsal horn. J. Pain Res. 10, 1687–1696. doi: 10.2147/jpr.S139592
Chang, H. L., Wang, H. C., Chunag, Y. T., Chou, C. W., Lin, I. L., Lai, C. S., et al. (2017). miRNA expression change in dorsal root ganglia after peripheral nerve injury. J. Mol. Neurosci. 61, 169–177. doi: 10.1007/s12031-016-0876-7
Chang, L. L., Wang, H. C., Tseng, K. Y., Su, M. P., Wang, J. Y., Chuang, Y. T., et al. (2020). Upregulation of miR-133a-3p in the sciatic nerve contributes to neuropathic pain development. Mol. Neurobiol. 57, 3931–3942. doi: 10.1007/s12035-020-01999-y
Chen, H. W., Zhang, X. X., Peng, Z. D., Xing, Z. M., Zhang, Y. W., and Li, Y. L. (2021). The circular RNA circSlc7a11 promotes bone cancer pain pathogenesis in rats by modulating LLC-WRC 256 cell proliferation and apoptosis. Mol. Cell. Biochem. 476, 1751–1763. doi: 10.1007/s11010-020-04020-1
Chen, J., Li, C., Liu, W., Yan, B., Hu, X., and Yang, F. (2019). miRNA-155 silencing reduces sciatic nerve injury in diabetic peripheral neuropathy. J. Mol. Endocrinol. 63, 227–238. doi: 10.1530/jme-19-0067
Chen, J., Lu, C., Wang, X., Wang, L., Chen, J., and Ji, F. (2022). LncRNA NONRATT009773.2 promotes bone cancer pain progression through the miR-708-5p/CXCL13 axis. Eur. J. Neurosci. 55, 661–674. doi: 10.1111/ejn.15607
Chen, M., Yang, Y., Zhang, W., Li, X., Wu, J., Zou, X., et al. (2020). Long noncoding RNA SNHG5 knockdown alleviates neuropathic pain by targeting the miR-154-5p/CXCL13 Axis. Neurochem. Res. 45, 1566–1575. doi: 10.1007/s11064-020-03021-2
Chen, W., Guo, S., and Wang, S. (2016). MicroRNA-16 Alleviates Inflammatory Pain by Targeting Ras-Related Protein 23 (RAB23) and Inhibiting p38 MAPK Activation. Med. Sci. Monit. 22, 3894–3901. doi: 10.12659/msm.897580
Chen, W., and Lu, Z. (2017). Upregulated TLR3 promotes neuropathic pain by regulating autophagy in rat With L5 spinal nerve ligation model. Neurochem. Res. 42, 634–643. doi: 10.1007/s11064-016-2119-2
Cheng, F., Qin, W., Yang, A. X., Yan, F. F., Chen, Y., and Ma, J. X. (2021). Propofol alleviates neuropathic pain in chronic constriction injury rat models via the microRNA-140-3p/Jagged-1 peptide/Notch signaling pathway. Synapse 75:e22219. doi: 10.1002/syn.22219
Cheng, X., Zhang, L., Zhang, K., Zhang, G., Hu, Y., Sun, X., et al. (2018). Circular RNA VMA21 protects against intervertebral disc degeneration through targeting miR-200c and X linked inhibitor-of-apoptosis protein. Ann. Rheum. Dis. 77, 770–779. doi: 10.1136/annrheumdis-2017-212056
Ciszek, B. P., Khan, A. A., Dang, H., Slade, G. D., Smith, S., Bair, E., et al. (2015). MicroRNA expression profiles differentiate chronic pain condition subtypes. Transl. Res. 166, 706–720.e711. doi: 10.1016/j.trsl.2015.06.008
Cohen, S. P., Vase, L., and Hooten, W. M. (2021). Chronic pain: An update on burden, best practices, and new advances. Lancet 397, 2082–2097. doi: 10.1016/s0140-6736(21)00393-7
Colloca, L., Ludman, T., Bouhassira, D., Baron, R., Dickenson, A. H., Yarnitsky, D., et al. (2017). Neuropathic pain. Nat. Rev. Dis. Primers 3:17002. doi: 10.1038/nrdp.2017.2
Conaghan, P. G., Cook, A. D., Hamilton, J. A., and Tak, P. P. (2019). Therapeutic options for targeting inflammatory osteoarthritis pain. Nat. Rev. Rheumatol. 15, 355–363. doi: 10.1038/s41584-019-0221-y
D’Agnelli, S., Gerra, M. C., Bignami, E., and Arendt-Nielsen, L. (2020). Exosomes as a new pain biomarker opportunity. Mol. Pain 16:1744806920957800. doi: 10.1177/1744806920957800
Descalzi, G., Ikegami, D., Ushijima, T., Nestler, E. J., Zachariou, V., and Narita, M. (2015). Epigenetic mechanisms of chronic pain. Trends Neurosci. 38, 237–246. doi: 10.1016/j.tins.2015.02.001
Djebali, S., Davis, C. A., Merkel, A., Dobin, A., Lassmann, T., Mortazavi, A., et al. (2012). Landscape of transcription in human cells. Nature 489, 101–108. doi: 10.1038/nature11233
Dominguez, E., Rivat, C., Pommier, B., Mauborgne, A., and Pohl, M. (2008). JAK/STAT3 pathway is activated in spinal cord microglia after peripheral nerve injury and contributes to neuropathic pain development in rat. J. Neurochem. 107, 50–60. doi: 10.1111/j.1471-4159.2008.05566.x
Dong, J., Xia, R., Zhang, Z., and Xu, C. (2021). lncRNA MEG3 aggravated neuropathic pain and astrocyte overaction through mediating miR-130a-5p/CXCL12/CXCR4 axis. Aging 13, 23004–23019. doi: 10.18632/aging.203592
Du, H., Liu, Z., Tan, X., Ma, Y., and Gong, Q. (2019). Identification of the genome-wide expression patterns of long non-coding RNAs and mRNAs in Mice with Streptozotocin-induced Diabetic Neuropathic Pain. Neuroscience 402, 90–103. doi: 10.1016/j.neuroscience.2018.12.040
Du, S., Wu, S., Feng, X., Wang, B., Xia, S., Liang, L., et al. (2022). A nerve injury-specific long noncoding RNA promotes neuropathic pain by increasing Ccl2 expression. J. Clin. Invest. 132:e153563. doi: 10.1172/jci153563
El Andaloussi, S., Lakhal, S., Mäger, I., and Wood, M. J. (2013). Exosomes for targeted siRNA delivery across biological barriers. Adv. Drug Deliv. Rev. 65, 391–397. doi: 10.1016/j.addr.2012.08.008
Eldahan, K. C., and Rabchevsky, A. G. (2018). Autonomic dysreflexia after spinal cord injury: Systemic pathophysiology and methods of management. Auton. Neurosci. 209, 59–70. doi: 10.1016/j.autneu.2017.05.002
Elramah, S., López-González, M. J., Bastide, M., Dixmérias, F., Roca-Lapirot, O., Wielanek-Bachelet, A. C., et al. (2017). Spinal miRNA-124 regulates synaptopodin and nociception in an animal model of bone cancer pain. Sci. Rep. 7:10949. doi: 10.1038/s41598-017-10224-1
Esteller, M. (2011). Non-coding RNAs in human disease. Nat. Rev. Genet. 12, 861–874. doi: 10.1038/nrg3074
Favereaux, A., Thoumine, O., Bouali-Benazzouz, R., Roques, V., Papon, M. A., Salam, S. A., et al. (2011). Bidirectional integrative regulation of Cav1.2 calcium channel by microRNA miR-103: Role in pain. EMBO J. 30, 3830–3841. doi: 10.1038/emboj.2011.249
Feldman, E. L., Nave, K. A., Jensen, T. S., and Bennett, D. L. H. (2017). New horizons in diabetic neuropathy: Mechanisms, bioenergetics, and pain. Neuron 93, 1296–1313. doi: 10.1016/j.neuron.2017.02.005
Felix, R., Muñoz-Herrera, D., Corzo-López, A., Fernández-Gallardo, M., Leyva-Leyva, M., González-Ramírez, R., et al. (2022). Ion channel long non-coding RNAs in neuropathic pain. Pflugers Arch. 474, 457–468. doi: 10.1007/s00424-022-02675-x
Feng, Y., Chen, L., Luo, Q., Wu, M., Chen, Y., and Shi, X. (2018). Involvement of microRNA-146a in diabetic peripheral neuropathy through the regulation of inflammation. Drug Des. Devel. Ther. 12, 171–177. doi: 10.2147/dddt.S157109
Finnerup, N. B., Kuner, R., and Jensen, T. S. (2021). Neuropathic pain: From mechanisms to treatment. Physiol. Rev. 101, 259–301. doi: 10.1152/physrev.00045.2019
Friedman, R. C., Farh, K. K., Burge, C. B., and Bartel, D. P. (2009). Most mammalian mRNAs are conserved targets of microRNAs. Genome Res. 19, 92–105. doi: 10.1101/gr.082701.108
Gandla, J., Lomada, S. K., Lu, J., Kuner, R., and Bali, K. K. (2017). miR-34c-5p functions as pronociceptive microRNA in cancer pain by targeting Cav2.3 containing calcium channels. Pain 158, 1765–1779. doi: 10.1097/j.pain.0000000000000971
Gao, L., Pu, X., Huang, Y., and Huang, J. (2019). MicroRNA-340-5p relieved chronic constriction injury-induced neuropathic pain by targeting Rap1A in rat model. Genes Genomics 41, 713–721. doi: 10.1007/s13258-019-00802-0
Goldberg, D. S., and McGee, S. J. (2011). Pain as a global public health priority. BMC Public Health 11:770. doi: 10.1186/1471-2458-11-770
Gregory, R. I., Chendrimada, T. P., Cooch, N., and Shiekhattar, R. (2005). Human RISC couples microRNA biogenesis and posttranscriptional gene silencing. Cell 123, 631–640. doi: 10.1016/j.cell.2005.10.022
Gumy, L. F., Bampton, E. T., and Tolkovsky, A. M. (2008). Hyperglycaemia inhibits Schwann cell proliferation and migration and restricts regeneration of axons and Schwann cells from adult murine DRG. Mol. Cell. Neurosci. 37, 298–311. doi: 10.1016/j.mcn.2007.10.004
Guo, W., Zhang, B., Mu, K., Feng, S. Q., Dong, Z. Y., Ning, G. Z., et al. (2018). Circular RNA GRB10 as a competitive endogenous RNA regulating nucleus pulposus cells death in degenerative intervertebral disk. Cell Death Dis. 9:319. doi: 10.1038/s41419-017-0232-z
Hansen, T. B., Jensen, T. I., Clausen, B. H., Bramsen, J. B., Finsen, B., Damgaard, C. K., et al. (2013). Natural RNA circles function as efficient microRNA sponges. Nature 495, 384–388. doi: 10.1038/nature11993
Haque, S., and Harries, L. W. (2017). Circular RNAs (circRNAs) in Health and Disease. Genes 8:353. doi: 10.3390/genes8120353
He, J., Wang, H. B., Huang, J. J., Zhang, L., Li, D. L., He, W. Y., et al. (2021). Diabetic neuropathic pain induced by streptozotocin alters the expression profile of non-coding RNAs in the spinal cord of mice as determined by sequencing analysis. Exp. Ther. Med. 22:775. doi: 10.3892/etm.2021.10207
He, R., Tang, G. L., Niu, L., Ge, C., Zhang, X. Q., Ji, X. F., et al. (2020). Quietness Circ 0000962 promoted nerve cell inflammation through PIK3CA/Akt/NF-κB signaling by miR-302b-3p in spinal cord injury. Ann. Palliat. Med. 9, 190–198. doi: 10.21037/apm.2020.02.13
Hombach, S., and Kretz, M. (2016). Non-coding RNAs: Classification, biology and functioning. Adv. Exp. Med. Biol. 937, 3–17. doi: 10.1007/978-3-319-42059-2_1
Hu, C., He, M., Xu, Q., and Tian, W. (2021). Advances With Non-coding RNAs in neuropathic pain. Front. Neurosci. 15:760936. doi: 10.3389/fnins.2021.760936
Hu, J. Z., Rong, Z. J., Li, M., Li, P., Jiang, L. Y., Luo, Z. X., et al. (2019). Silencing of lncRNA PKIA-AS1 attenuates spinal nerve ligation-induced neuropathic pain through epigenetic downregulation of CDK6 Expression. Front. Cell. Neurosci. 13:50. doi: 10.3389/fncel.2019.00050
Hu, X., Liu, Y., Wu, J., Liu, Y., Liu, W., Chen, J., et al. (2020). Inhibition of P2X7R in the amygdala ameliorates symptoms of neuropathic pain after spared nerve injury in rats. Brain Behav. Immun. 88, 507–514. doi: 10.1016/j.bbi.2020.04.030
Huan, T., Chen, G., Liu, C., Bhattacharya, A., Rong, J., Chen, B. H., et al. (2018). Age-associated microRNA expression in human peripheral blood is associated with all-cause mortality and age-related traits. Aging Cell 17, doi: 10.1111/acel.12687
Huang, Q., Mao, X. F., Wu, H. Y., Liu, H., Sun, M. L., Wang, X., et al. (2017). Cynandione A attenuates neuropathic pain through p38β MAPK-mediated spinal microglial expression of β-endorphin. Brain Behav. Immun. 62, 64–77. doi: 10.1016/j.bbi.2017.02.005
Jackson, A. L., and Linsley, P. S. (2010). Recognizing and avoiding siRNA off-target effects for target identification and therapeutic application. Nat. Rev. Drug Discov. 9, 57–67. doi: 10.1038/nrd3010
Jank, R., Gallee, A., Boeckle, M., Fiegl, S., and Pieh, C. (2017). Chronic pain and sleep disorders in primary care. Pain Res. Treat. 2017:9081802. doi: 10.1155/2017/9081802
Ji, R. R., Nackley, A., Huh, Y., Terrando, N., and Maixner, W. (2018). Neuroinflammation and central sensitization in chronic and widespread pain. Anesthesiology 129, 343–366. doi: 10.1097/aln.0000000000002130
Jia, H., Osak, M., Bogu, G. K., Stanton, L. W., Johnson, R., and Lipovich, L. (2010). Genome-wide computational identification and manual annotation of human long noncoding RNA genes. RNA 16, 1478–1487. doi: 10.1261/rna.1951310
Jiang, M., Zhang, X., Wang, X., Xu, F., Zhang, J., Li, L., et al. (2021). MicroRNA-124-3p attenuates the development of nerve injury-induced neuropathic pain by targeting early growth response 1 in the dorsal root ganglia and spinal dorsal horn. J. Neurochem. 158, 928–942. doi: 10.1111/jnc.15433
Jiang, W., Wang, Q., Yu, X., Lu, T., and Zhang, P. (2019). MicroRNA-217 relieved neuropathic pain through targeting toll-like receptor 5 expression. J. Cell. Biochem. 120, 3009–3017. doi: 10.1002/jcb.27269
Jin, H., Du, X. J., Zhao, Y., and Xia, D. L. (2018). XIST/miR-544 axis induces neuropathic pain by activating STAT3 in a rat model. J. Cell. Physiol. 233, 5847–5855. doi: 10.1002/jcp.26376
Kaushik, A. S., Strath, L. J., and Sorge, R. E. (2020). Dietary interventions for treatment of chronic pain: Oxidative stress and inflammation. Pain Ther. 9, 487–498. doi: 10.1007/s40122-020-00200-5
Kessler, A., Yoo, M., and Calisoff, R. (2020). Complex regional pain syndrome: An updated comprehensive review. NeuroRehabilitation 47, 253–264. doi: 10.3233/nre-208001
Kristensen, L. S., Andersen, M. S., Stagsted, L. V. W., Ebbesen, K. K., Hansen, T. B., and Kjems, J. (2019). The biogenesis, biology and characterization of circular RNAs. Nat. Rev. Genet. 20, 675–691. doi: 10.1038/s41576-019-0158-7
Lee, J. T. (2009). Lessons from X-chromosome inactivation: Long ncRNA as guides and tethers to the epigenome. Genes Dev. 23, 1831–1842. doi: 10.1101/gad.1811209
Li, G., Jiang, H., Zheng, C., Zhu, G., Xu, Y., Sheng, X., et al. (2017). Long noncoding RNA MRAK009713 is a novel regulator of neuropathic pain in rats. Pain 158, 2042–2052. doi: 10.1097/j.pain.0000000000001013
Li, L., Luo, Y., Zhang, Y., Wei, M., Zhang, M., Liu, H., et al. (2020). CircZNF609 aggravates neuropathic pain via miR-22-3p/ENO1 axis in CCI rat models. Gene 763:145069. doi: 10.1016/j.gene.2020.145069
Li, N., Lim, G., Chen, L., McCabe, M. F., Kim, H., Zhang, S., et al. (2013). Spinal expression of Hippo signaling components YAP and TAZ following peripheral nerve injury in rats. Brain Res. 1535, 137–147. doi: 10.1016/j.brainres.2013.08.049
Li, X., Kroin, J. S., Kc, R., Gibson, G., Chen, D., Corbett, G. T., et al. (2013). Altered spinal microRNA-146a and the microRNA-183 cluster contribute to osteoarthritic pain in knee joints. J. Bone Miner. Res. 28, 2512–2522. doi: 10.1002/jbmr.2002
Li, Y., Yin, C., Liu, B., Nie, H., Wang, J., Zeng, D., et al. (2021b). Transcriptome profiling of long noncoding RNAs and mRNAs in spinal cord of a rat model of paclitaxel-induced peripheral neuropathy identifies potential mechanisms mediating neuroinflammation and pain. J. Neuroinflammation 18:48. doi: 10.1186/s12974-021-02098-y
Li, Y., Pan, D., Liu, S., Xing, X., Zhou, H., Zhang, B., et al. (2021a). Identification of circ-FAM169A sponges miR-583 involved in the regulation of intervertebral disc degeneration. J. Orthop. Transl. 26, 121–131. doi: 10.1016/j.jot.2020.07.007
Liang, M., Shao, A., Tang, X., Feng, M., Wang, J., and Qiu, Y. (2019). MiR-34a affects dexmedetomidine-inhibited chronic inflammatory visceral pain by targeting to HDAC2. BMC Anesthesiol. 19:131. doi: 10.1186/s12871-019-0801-z
Liao, J., Liu, J., Long, G., and Lv, X. (2022). MiR-30b-5p attenuates neuropathic pain by the CYP24A1-Wnt/beta-catenin signaling in CCI rats. Exp. Brain Res. 240, 263–277. doi: 10.1007/s00221-021-06253-y
Liu, C., Li, C., Deng, Z., Du, E., and Xu, C. (2018). Long Non-coding RNA BC168687 is involved in TRPV1-mediated Diabetic Neuropathic Pain in Rats. Neuroscience 374, 214–222. doi: 10.1016/j.neuroscience.2018.01.049
Liu, C., Tao, J., Wu, H., Yang, Y., Chen, Q., Deng, Z., et al. (2017). Effects of LncRNA BC168687 siRNA on diabetic neuropathic pain mediated by P2X7 receptor on SGCs in DRG of Rats. Biomed Res. Int. 2017:7831251. doi: 10.1155/2017/7831251
Liu, Y., Feng, L., Ren, S., Zhang, Y., and Xue, J. (2020). Inhibition of lncRNA DILC attenuates neuropathic pain via the SOCS3/JAK2/STAT3 pathway. Biosci. Rep. 40:BSR20194486. doi: 10.1042/BSR20194486
Liu, C., Yang, J., Liu, H., Xia, T., and Zhang, F. (2020). miR-300 mitigates cancer-induced bone pain through targeting HMGB1 in rat models. Genes Genomics 42, 309–316. doi: 10.1007/s13258-019-00904-9
Liu, S., Zou, L., Xie, J., Xie, W., Wen, S., Xie, Q., et al. (2016). LncRNA NONRATT021972 siRNA regulates neuropathic pain behaviors in type 2 diabetic rats through the P2X7 receptor in dorsal root ganglia. Mol. Brain 9:44. doi: 10.1186/s13041-016-0226-2
Liu, Y., Jeon, S. M., Caterina, M. J., and Qu, L. (2021). miR-544-3p mediates arthritis pain through regulation of FcgammaRI. Pain 163, 1497–1510.
Liu, Y., Jeon, S. M., Caterina, M. J., and Qu, L. (2022). miR-544-3p mediates arthritis pain through regulation of FcγRI. Pain 163, 1497–1510. doi: 10.1097/j.pain.0000000000002531
Lopez-Gonzalez, M. J., Landry, M., and Favereaux, A. (2017). MicroRNA and chronic pain: From mechanisms to therapeutic potential. Pharmacol. Ther. 180, 1–15. doi: 10.1016/j.pharmthera.2017.06.001
Lorenzi, L., Chiu, H. S., Avila Cobos, F., Gross, S., Volders, P. J., Cannoodt, R., et al. (2021). The RNA Atlas expands the catalog of human non-coding RNAs. Nat. Biotechnol. 39, 1453–1465. doi: 10.1038/s41587-021-00936-1
Lötsch, J., Doehring, A., Mogil, J. S., Arndt, T., Geisslinger, G., and Ultsch, A. (2013). Functional genomics of pain in analgesic drug development and therapy. Pharmacol. Ther. 139, 60–70. doi: 10.1016/j.pharmthera.2013.04.004
Lü, S., Liu, Y., Cui, J., Yang, B., Li, G., Guo, Y., et al. (2020). Mechanism of Caulophyllum robustum Maxim against rheumatoid arthritis using LncRNA-mRNA chip analysis. Gene 722:144105. doi: 10.1016/j.gene.2019.144105
Luo, Q., Zeng, L., Zeng, L., Rao, J., Zhang, L., Guo, Y., et al. (2020b). Expression and clinical significance of circular RNAs hsa_circ_0000175 and hsa_circ_0008410 in peripheral blood mononuclear cells from patients with rheumatoid arthritis. Int. J. Mol. Med. 45, 1203–1212. doi: 10.3892/ijmm.2020.4498
Luo, Q., Fu, B., Zhang, L., Guo, Y., Huang, Z., and Li, J. (2020a). Expression and clinical significance of circular RNA hsa_circ_0079787 in the peripheral blood of patients with axial spondyloarthritis. Mol. Med. Rep. 22, 4197–4206. doi: 10.3892/mmr.2020.11520
Ma, E., MacRae, I. J., Kirsch, J. F., and Doudna, J. A. (2008). Autoinhibition of human dicer by its internal helicase domain. J. Mol. Biol. 380, 237–243. doi: 10.1016/j.jmb.2008.05.005
Ma, X., Wang, H., Song, T., Wang, W., and Zhang, Z. (2020). lncRNA MALAT1 contributes to neuropathic pain development through regulating miR-129-5p/HMGB1 axis in a rat model of chronic constriction injury. Int. J. Neurosci. 130, 1215–1224. doi: 10.1080/00207454.2020.1731508
Ma, Y., Deng, Q., Li, S., Chen, M., Jin, B., and Wang, M. (2021). TRPV1, Targeted by miR-338-3p, Induces Neuropathic Pain by Interacting with NECAB2. J. Mol. Neurosci. 71, 55–65. doi: 10.1007/s12031-020-01626-4
Macfarlane, G. J., Barnish, M. S., and Jones, G. T. (2017). Persons with chronic widespread pain experience excess mortality: Longitudinal results from UK Biobank and meta-analysis. Ann. Rheum. Dis. 76, 1815–1822. doi: 10.1136/annrheumdis-2017-211476
Mailhot, B., Christin, M., Tessandier, N., Sotoudeh, C., Bretheau, F., Turmel, R., et al. (2020). Neuronal interleukin-1 receptors mediate pain in chronic inflammatory diseases. J. Exp. Med. 217:e20191430. doi: 10.1084/jem.20191430
Mao, S., Zhang, S., Zhou, S., Huang, T., Feng, W., Gu, X., et al. (2019). A Schwann cell-enriched circular RNA circ-Ankib1 regulates Schwann cell proliferation following peripheral nerve injury. FASEB J. 33, 12409–12424. doi: 10.1096/fj.201900965R
Mills, S. E. E., Nicolson, K. P., and Smith, B. H. (2019). Chronic pain: A review of its epidemiology and associated factors in population-based studies. Br. J. Anaesth. 123, e273–e283. doi: 10.1016/j.bja.2019.03.023
Muley, M. M., Krustev, E., and McDougall, J. J. (2016). Preclinical assessment of inflammatory pain. CNS Neurosci. Ther. 22, 88–101. doi: 10.1111/cns.12486
Pan, Z., Li, G. F., Sun, M. L., Xie, L., Liu, D., Zhang, Q., et al. (2019). MicroRNA-1224 splicing circularRNA-Filip1l in an Ago2-dependent manner regulates chronic inflammatory pain via targeting Ubr5. J. Neurosci. 39, 2125–2143. doi: 10.1523/jneurosci.1631-18.2018
Pande, R., Parikh, A., Shenoda, B., Ramanathan, S., Alexander, G. M., Schwartzman, R. J., et al. (2021). Hsa-miR-605 regulates the proinflammatory chemokine CXCL5 in complex regional pain syndrome. Biomed. Pharmacother. 140:111788. doi: 10.1016/j.biopha.2021.111788
Patrat, C., Ouimette, J. F., and Rougeulle, C. (2020). X chromosome inactivation in human development. Development 147:dev183095. doi: 10.1242/dev.183095
Peng, C., Li, L., Zhang, M. D., Bengtsson Gonzales, C., Parisien, M., Belfer, I., et al. (2017). miR-183 cluster scales mechanical pain sensitivity by regulating basal and neuropathic pain genes. Science 356, 1168–1171. doi: 10.1126/science.aam7671
Peng, C., Zhang, C., Su, Z., and Lin, D. (2019). DGCR5 attenuates neuropathic pain through sponging miR-330-3p and regulating PDCD4 in CCI rat models. J. Cell. Physiol. 234, 7292–7300. doi: 10.1002/jcp.27487
Peng, L., Wu, B., Shi, L., Zou, L., Li, L., Yang, R., et al. (2021). Long Non-coding RNA Uc.48+ small interfering RNA alleviates neuroinflammatory hyperalgesia in Gp120-Treated Rats via the P2Y12 Receptor. Front. Neurosci. 15:663962. doi: 10.3389/fnins.2021.663962
Pinho-Ribeiro, F. A., Verri, W. A. Jr., and Chiu, I. M. (2017). Nociceptor Sensory Neuron-Immune Interactions in Pain and Inflammation. Trends Immunol. 38, 5–19. doi: 10.1016/j.it.2016.10.001
Ponting, C. P., Oliver, P. L., and Reik, W. (2009). Evolution and functions of long noncoding RNAs. Cell 136, 629–641. doi: 10.1016/j.cell.2009.02.006
Raja, S. N., Carr, D. B., Cohen, M., Finnerup, N. B., Flor, H., Gibson, S., et al. (2020). The revised International Association for the Study of Pain definition of pain: Concepts, challenges, and compromises. Pain 161, 1976–1982. doi: 10.1097/j.pain.0000000000001939
Ramanathan, S., Douglas, S. R., Alexander, G. M., Shenoda, B. B., Barrett, J. E., Aradillas, E., et al. (2019a). Exosome microRNA signatures in patients with complex regional pain syndrome undergoing plasma exchange. J. Transl. Med. 17:81. doi: 10.1186/s12967-019-1833-3
Ramanathan, S., Shenoda, B. B., Lin, Z., Alexander, G. M., Huppert, A., Sacan, A., et al. (2019b). Inflammation potentiates miR-939 expression and packaging into small extracellular vesicles. J. Extracell. Vesicles 8:1650595. doi: 10.1080/20013078.2019.1650595
Rounge, T. B., Umu, S. U., Keller, A., Meese, E., Ursin, G., Tretli, S., et al. (2018). Circulating small non-coding RNAs associated with age, sex, smoking, body mass and physical activity. Sci. Rep. 8:17650. doi: 10.1038/s41598-018-35974-4
Sakai, A., Saitow, F., Maruyama, M., Miyake, N., Miyake, K., Shimada, T., et al. (2017). MicroRNA cluster miR-17-92 regulates multiple functionally related voltage-gated potassium channels in chronic neuropathic pain. Nat. Commun. 8:16079. doi: 10.1038/ncomms16079
Shao, J., Cao, J., Wang, J., Ren, X., Su, S., Li, M., et al. (2016). MicroRNA-30b regulates expression of the sodium channel Nav1.7 in nerve injury-induced neuropathic pain in the rat. Mol. Pain 12:1744806916671523. doi: 10.1177/1744806916671523
Shenoda, B. B., Tian, Y., Alexander, G. M., Aradillas-Lopez, E., Schwartzman, R. J., and Ajit, S. K. (2018). miR-34a-mediated regulation of XIST in female cells under inflammation. J. Pain Res. 11, 935–945. doi: 10.2147/jpr.S159458
Siddall, P. J., and Middleton, J. W. (2015). Spinal cord injury-induced pain: Mechanisms and treatments. Pain Manag. 5, 493–507. doi: 10.2217/pmt.15.47
Simeoli, R., Montague, K., Jones, H. R., Castaldi, L., Chambers, D., Kelleher, J. H., et al. (2017). Exosomal cargo including microRNA regulates sensory neuron to macrophage communication after nerve trauma. Nat. Commun. 8:1778. doi: 10.1038/s41467-017-01841-5
Simonetti, M., and Kuner, R. (2020). Spinal Wnt5a plays a key role in spinal dendritic spine remodeling in neuropathic and inflammatory pain models and in the proalgesic effects of peripheral Wnt3a. J. Neurosci. 40, 6664–6677. doi: 10.1523/jneurosci.2942-19.2020
Sisignano, M., Parnham, M. J., and Geisslinger, G. (2019). Novel approaches to persistent pain therapy. Trends Pharmacol. Sci. 40, 367–377. doi: 10.1016/j.tips.2019.04.003
Sloan, G., Shillo, P., Selvarajah, D., Wu, J., Wilkinson, I. D., Tracey, I., et al. (2018). A new look at painful diabetic neuropathy. Diabetes Res. Clin. Pract. 144, 177–191. doi: 10.1016/j.diabres.2018.08.020
Sorge, R. E., Trang, T., Dorfman, R., Smith, S. B., Beggs, S., Ritchie, J., et al. (2012). Genetically determined P2X7 receptor pore formation regulates variability in chronic pain sensitivity. Nat. Med. 18, 595–599. doi: 10.1038/nm.2710
Statello, L., Guo, C. J., Chen, L. L., and Huarte, M. (2021). Gene regulation by long non-coding RNAs and its biological functions. Nat. Rev. Mol. Cell Biol. 22, 96–118. doi: 10.1038/s41580-020-00315-9
Sun, L., Zhou, J., and Sun, C. (2019). MicroRNA-211-5p enhances analgesic effect of dexmedetomidine on inflammatory visceral pain in rats by suppressing ERK signaling. J. Mol. Neurosci. 68, 19–28. doi: 10.1007/s12031-019-01278-z
Sun, R. M., Wei, J., Wang, S. S., Xu, G. Y., and Jiang, G. Q. (2020). Upregulation of lncRNA-NONRATT021203.2 in the dorsal root ganglion contributes to cancer-induced pain via CXCL9 in rats. Biochem. Biophys. Res. Commun. 524, 983–989. doi: 10.1016/j.bbrc.2020.01.163
Sun, X., and Zhang, H. (2018). miR-451 elevation relieves inflammatory pain by suppressing microglial activation-evoked inflammatory response via targeting TLR4. Cell Tissue Res. 374, 487–495. doi: 10.1007/s00441-018-2898-7
Tang, W., Zhang, L., and Li, Z. (2021). Long noncoding RNA LOC100911498 is a novel regulator of neuropathic pain in rats. Brain Behav. 11:e01966. doi: 10.1002/brb3.1966
Tian, Y., Sun, L., and Qi, T. (2020). Long noncoding RNA GAS5 ameliorates chronic constriction injury induced neuropathic pain in rats by modulation of the miR-452-5p/CELF2 axis. Can. J. Physiol. Pharmacol. 98, 870–877. doi: 10.1139/cjpp-2020-0036
Tong, D., Zhao, Y., Tang, Y., Ma, J., Wang, Z., and Li, C. (2021). Circ-Usp10 promotes microglial activation and induces neuronal death by targeting miRNA-152-5p/CD84. Bioengineered 12, 10812–10822. doi: 10.1080/21655979.2021.2004362
Tramullas, M., Francés, R., de la Fuente, R., Velategui, S., Carcelén, M., García, R., et al. (2018). MicroRNA-30c-5p modulates neuropathic pain in rodents. Sci. Transl. Med. 10:eaao6299. doi: 10.1126/scitranslmed.aao6299
Treede, R. D., Rief, W., Barke, A., Aziz, Q., Bennett, M. I., Benoliel, R., et al. (2015). A classification of chronic pain for ICD-11. Pain 156, 1003–1007. doi: 10.1097/j.pain.0000000000000160
Treede, R. D., Rief, W., Barke, A., Aziz, Q., Bennett, M. I., Benoliel, R., et al. (2019). Chronic pain as a symptom or a disease: The IASP Classification of Chronic Pain for the International Classification of Diseases (ICD-11). Pain 160, 19–27. doi: 10.1097/j.pain.0000000000001384
Ulitsky, I., and Bartel, D. P. (2013). lincRNAs: Genomics, evolution, and mechanisms. Cell 154, 26–46. doi: 10.1016/j.cell.2013.06.020
van Hecke, O., Hocking, L. J., Torrance, N., Campbell, A., Padmanabhan, S., Porteous, D. J., et al. (2017). Chronic pain, depression and cardiovascular disease linked through a shared genetic predisposition: Analysis of a family-based cohort and twin study. PLoS One 12:e0170653. doi: 10.1371/journal.pone.0170653
van Hecke, O., Torrance, N., and Smith, B. H. (2013). Chronic pain epidemiology - where do lifestyle factors fit in? Br. J. Pain 7, 209–217. doi: 10.1177/2049463713493264
van Velzen, G. A. J., Huygen, F., van Kleef, M., van Eijs, F. V., Marinus, J., and van Hilten, J. J. (2019). Sex matters in complex regional pain syndrome. Eur. J. Pain 23, 1108–1116. doi: 10.1002/ejp.1375
Vea, A., Llorente-Cortes, V., and de Gonzalo-Calvo, D. (2018). Circular RNAs in blood. Adv. Exp. Med. Biol. 1087, 119–130. doi: 10.1007/978-981-13-1426-1_10
Vickers, K. C., Palmisano, B. T., Shoucri, B. M., Shamburek, R. D., and Remaley, A. T. (2011). MicroRNAs are transported in plasma and delivered to recipient cells by high-density lipoproteins. Nat. Cell Biol. 13, 423–433. doi: 10.1038/ncb2210
Vishnoi, A., and Rani, S. (2017). MiRNA biogenesis and regulation of diseases: An overview. Methods Mol. Biol. 1509, 1–10. doi: 10.1007/978-1-4939-6524-3_1
Viswanath, O., Urits, I., Burns, J., Charipova, K., Gress, K., McNally, A., et al. (2020). Central neuropathic mechanisms in pain signaling pathways: Current evidence and recommendations. Adv. Ther. 37, 1946–1959. doi: 10.1007/s12325-020-01334-w
Wan, L., Su, Z., Li, F., Gao, P., and Zhang, X. (2021). MiR-122-5p suppresses neuropathic pain development by targeting PDK4. Neurochem. Res. 46, 957–963. doi: 10.1007/s11064-020-03213-w
Wang, Y., Wang, S., and He, J. H. (2021). Transcriptomic analysis reveals essential microRNAs after peripheral nerve injury. Neural Regen. Res. 16, 1865–1870. doi: 10.4103/1673-5374.306092
Wang, A., Shi, X., Yu, R., Qiao, B., Yang, R., and Xu, C. (2021). The P2X(7) receptor is involved in diabetic neuropathic pain hypersensitivity mediated by TRPV1 in the rat dorsal root ganglion. Front. Mol. Neurosci. 14:663649. doi: 10.3389/fnmol.2021.663649
Wang, P., Zhang, Y., Xia, Y., Xu, D., Wang, H., Liu, D., et al. (2021). MicroRNA-139-5p promotes functional recovery and reduces pain hypersensitivity in mice with spinal cord injury by targeting Mammalian Sterile 20-like Kinase 1. Neurochem. Res. 46, 349–357. doi: 10.1007/s11064-020-03170-4
Wang, B., Li, J., and Tian, F. (2021). Downregulation of lncRNA SNHG14 attenuates osteoarthritis by inhibiting FSTL-1 mediated NLRP3 and TLR4/NF-kappaB pathway through miR-124-3p. Life Sci. 270:119143. doi: 10.1016/j.lfs.2021.119143
Wang, L., Chopp, M., Szalad, A., Zhang, Y., Wang, X., Zhang, R. L., et al. (2014). The role of miR-146a in dorsal root ganglia neurons of experimental diabetic peripheral neuropathy. Neuroscience 259, 155–163. doi: 10.1016/j.neuroscience.2013.11.057
Wang, Z., Liu, F., Wei, M., Qiu, Y., Ma, C., Shen, L., et al. (2018). Chronic constriction injury-induced microRNA-146a-5p alleviates neuropathic pain through suppression of IRAK1/TRAF6 signaling pathway. J. Neuroinflammation 15:179. doi: 10.1186/s12974-018-1215-4
Wang, L., Luo, T., Bao, Z., Li, Y., and Bu, W. (2018). Intrathecal circHIPK3 shRNA alleviates neuropathic pain in diabetic rats. Biochem. Biophys. Res. Commun. 505, 644–650. doi: 10.1016/j.bbrc.2018.09.158
Wang, X., Wang, B., Zou, M., Li, J., Lü, G., Zhang, Q., et al. (2018). CircSEMA4B targets miR-431 modulating IL-1β-induced degradative changes in nucleus pulposus cells in intervertebral disc degeneration via Wnt pathway. Biochim. Biophys. Acta Mol. Basis Dis. 1864, 3754–3768. doi: 10.1016/j.bbadis.2018.08.033
Wang, L., Zhu, K., Yang, B., and Cai, Y. (2020). Knockdown of Linc00052 alleviated spinal nerve ligation-triggered neuropathic pain through regulating miR-448 and JAK1. J. Cell. Physiol. 235, 6528–6535. doi: 10.1002/jcp.29465
Wang, T., Hao, Z., Liu, C., Yuan, L., Li, L., Yin, M., et al. (2020). LEF1 mediates osteoarthritis progression through circRNF121/miR-665/MYD88 axis via NF-κB signaling pathway. Cell Death Dis. 11:598. doi: 10.1038/s41419-020-02769-3
Wang, S., Li, A., and Guo, S. (2016a). Ligustrazine attenuates neuropathic pain by inhibition of JAK/STAT3 pathway in a rat model of chronic constriction injury. Pharmazie 71, 408–412. doi: 10.1691/ph.2016.6546
Wang, S., Xu, H., Zou, L., Xie, J., Wu, H., Wu, B., et al. (2016b). LncRNA uc.48+ is involved in diabetic neuropathic pain mediated by the P2X3 receptor in the dorsal root ganglia. Purinergic Signal. 12, 139–148. doi: 10.1007/s11302-015-9488-x
Wei, J., Dou, Q., Ba, F., Xu, G. Y., and Jiang, G. Q. (2021). Identification of lncRNA and mRNA expression profiles in dorsal root ganglion in rats with cancer-induced bone pain. Biochem. Biophys. Res. Commun. 572, 98–104. doi: 10.1016/j.bbrc.2021.07.063
Wei, M., Li, L., Zhang, Y., Zhang, M., and Su, Z. (2020). Downregulated circular RNA zRANB1 mediates Wnt5a/β-Catenin signaling to promote neuropathic pain via miR-24-3p/LPAR3 axis in CCI rat models. Gene 761:145038. doi: 10.1016/j.gene.2020.145038
Wen, J., He, T., Qi, F., and Chen, H. (2019). MiR-206-3p alleviates chronic constriction injury-induced neuropathic pain through targeting HDAC4. Exp. Anim. 68, 213–220. doi: 10.1538/expanim.18-0091
Wen, Y., Fan, X., Bu, H., Ma, L., Kong, C., Huang, C., et al. (2021). Downregulation of lncRNA FIRRE relieved the neuropathic pain of female mice by suppressing HMGB1 expression. Mol. Cell. Biochem. 476, 841–852. doi: 10.1007/s11010-020-03949-7
Westholm, J. O., and Lai, E. C. (2011). Mirtrons: microRNA biogenesis via splicing. Biochimie 93, 1897–1904. doi: 10.1016/j.biochi.2011.06.017
Wickman, J. R., Luo, X., Li, W., Jean-Toussaint, R., Sahbaie, P., Sacan, A., et al. (2021). Circulating microRNAs from the mouse tibia fracture model reflect the signature from patients with complex regional pain syndrome. Pain Rep. 6:e950. doi: 10.1097/PR9.0000000000000950
Woolf, C. J., and Salter, M. W. (2000). Neuronal plasticity: Increasing the gain in pain. Science 288, 1765–1769. doi: 10.1126/science.288.5472.1765
Wu, B., Guo, Y., Chen, Q., Xiong, Q., and Min, S. (2019). MicroRNA-193a Downregulates HMGB1 to Alleviate Diabetic Neuropathic Pain in a Mouse Model. Neuroimmunomodulation 26, 250–257. doi: 10.1159/000503325
Wu, J., Wang, C., and Ding, H. (2020). LncRNA MALAT1 promotes neuropathic pain progression through the miR1545p/AQP9 axis in CCI rat models. Mol. Med. Rep. 21, 291–303. doi: 10.3892/mmr.2019.10829
Wu, Y., Gu, Y., and Shi, B. (2020). miR-590-3p Alleviates diabetic peripheral neuropathic pain by targeting RAP1A and suppressing infiltration by the T cells. Acta Biochim. Pol. 67, 587–593. doi: 10.18388/abp.2020_5451
Wu, L., Fan, J., and Belasco, J. G. (2006). MicroRNAs direct rapid deadenylation of mRNA. Proc. Natl. Acad. Sci. U.S.A. 103, 4034–4039. doi: 10.1073/pnas.0510928103
Xia, L. X., Ke, C., and Lu, J. M. (2018). NEAT1 contributes to neuropathic pain development through targeting miR-381/HMGB1 axis in CCI rat models. J. Cell. Physiol. 233, 7103–7111. doi: 10.1002/jcp.26526
Xian, S., Ding, R., Li, M., and Chen, F. (2021). LncRNA NEAT1/miR-128-3p/AQP4 axis regulating spinal cord injury-induced neuropathic pain progression. J. Neuroimmunol. 351:577457. doi: 10.1016/j.jneuroim.2020.577457
Xiang, H. C., Lin, L. X., Hu, X. F., Zhu, H., Li, H. P., Zhang, R. Y., et al. (2019). AMPK activation attenuates inflammatory pain through inhibiting NF-κB activation and IL-1β expression. J. Neuroinflammation 16:34. doi: 10.1186/s12974-019-1411-x
Xiang, Q., Kang, L., Wang, J., Liao, Z., Song, Y., Zhao, K., et al. (2020). CircRNA-CIDN mitigated compression loading-induced damage in human nucleus pulposus cells via miR-34a-5p/SIRT1 axis. EBioMedicine 53:102679. doi: 10.1016/j.ebiom.2020.102679
Xie, K., Jia, Y., Hu, Y., Sun, Y., Hou, L., and Wang, G. (2015). Activation of notch signaling mediates the induction and maintenance of mechanical allodynia in a rat model of neuropathic pain. Mol. Med. Rep. 12, 639–644. doi: 10.3892/mmr.2015.3379
Xiong, W., Tan, M., Tong, Z., Yin, C., He, L., Liu, L., et al. (2019). Effects of long non-coding RNA uc.48+ on pain transmission in trigeminal neuralgia. Brain Res. Bull. 147, 92–100. doi: 10.1016/j.brainresbull.2019.02.009
Xu, M., Wu, R., Zhang, L., Zhu, H. Y., Xu, G. Y., Qian, W., et al. (2020). Decreased MiR-485-5p contributes to inflammatory pain through post-transcriptional upregulation of ASIC1 in rat dorsal root ganglion. J. Pain Res. 13, 3013–3022. doi: 10.2147/jpr.S279902
Xue, Y., Chidiac, C., Herault, Y., and Gaveriaux-Ruff, C. (2021). Pain behavior in SCN9A (Nav1.7) and SCN10A (Nav1.8) mutant rodent models. Neurosci. Lett. 753:135844. doi: 10.1016/j.neulet.2021.135844
Yang, D., Yang, Q., Wei, X., Liu, Y., Ma, D., Li, J., et al. (2017). The role of miR-190a-5p contributes to diabetic neuropathic pain via targeting SLC17A6. J. Pain Res. 10, 2395–2403. doi: 10.2147/jpr.S133755
Yang, Q., Yao, Y., Zhao, D., Zou, H., Lai, C., Xiang, G., et al. (2021). LncRNA H19 secreted by umbilical cord blood mesenchymal stem cells through microRNA-29a-3p/FOS axis for central sensitization of pain in advanced osteoarthritis. Am. J. Transl. Res. 13, 1245–1256.
Yang, X. D., Fang, P. F., Xiang, D. X., and Yang, Y. Y. (2019). Topical treatments for diabetic neuropathic pain. Exp. Ther. Med. 17, 1963–1976. doi: 10.3892/etm.2019.7173
Yao, L., Guo, Y., Wang, L., Li, G., Qian, X., Zhang, J., et al. (2021). Knockdown of miR-130a-3p alleviates spinal cord injury induced neuropathic pain by activating IGF-1/IGF-1R pathway. J. Neuroimmunol. 351:577458. doi: 10.1016/j.jneuroim.2020.577458
Ye, J., Guo, R., Shi, Y., Qi, F., Guo, C., and Yang, L. (2016). miR-155 regulated inflammation response by the SOCS1-STAT3-PDCD4 Axis in Atherogenesis. Mediators Inflamm. 2016:8060182. doi: 10.1155/2016/8060182
Ye, L., Morse, L. R., Falci, S. P., Olson, J. K., Shrivastava, M., Nguyen, N., et al. (2021). hsa-MiR-19a-3p and hsa-MiR-19b-3p are associated with spinal cord injury-induced neuropathic pain: Findings from a genome-wide MicroRNA Expression Profiling Screen. Neurotrauma Rep. 2, 424–439. doi: 10.1089/neur.2021.0011
Yokoyama, H., Hirai, T., Nagata, T., Enomoto, M., Kaburagi, H., Leiyo, L., et al. (2020). DNA microarray analysis of differential gene expression in the dorsal root ganglia of four different neuropathic pain mouse models. J. Pain Res. 13, 3031–3043. doi: 10.2147/jpr.S272952
Yu, W., Zhao, G. Q., Cao, R. J., Zhu, Z. H., and Li, K. (2017). LncRNA NONRATT021972 was associated with neuropathic pain scoring in patients with type 2 Diabetes. Behav. Neurol. 2017:2941297. doi: 10.1155/2017/2941297
Zhang, X., Guo, H., Xie, A., Liao, O., Ju, F., and Zhou, Y. (2020). MicroRNA-144 relieves chronic constriction injury-induced neuropathic pain via targeting RASA1. Biotechnol Appl Biochem 67, 294–302. doi: 10.1002/bab.1854
Zhang, C., Peng, Y., Wang, Y., Xu, H., and Zhou, X. (2020). Transcribed ultraconserved noncoding RNA uc.153 is a new player in neuropathic pain. Pain 161, 1744–1754. doi: 10.1097/j.pain.0000000000001868
Zhang, J. Y., Lv, D. B., Su, Y. N., Wang, X. L., Sheng, W. C., Yang, G., et al. (2020). LncRNA SNHG1 attenuates neuropathic pain following spinal cord injury by regulating CDK4 level. Eur. Rev. Med. Pharmacol. Sci. 24, 12034–12040. doi: 10.26355/eurrev_202012_23992
Zhang, Y., Zhang, X., Xing, Z., Tang, S., Chen, H., Zhang, Z., et al. (2020). circStrn3 is involved in bone cancer pain regulation in a rat model. Acta Biochim. Biophys. Sin. 52, 495–505. doi: 10.1093/abbs/gmaa018
Zhang, J., Rong, L., Shao, J., Zhang, Y., Liu, Y., Zhao, S., et al. (2021). Epigenetic restoration of voltage-gated potassium channel Kv1.2 alleviates nerve injury-induced neuropathic pain. J. Neurochem. 156, 367–378. doi: 10.1111/jnc.15117
Zhang, Y., Gao, T., Li, X., Wen, C. C., Yan, X. T., Peng, C., et al. (2021). Circ_0005075 targeting miR-151a-3p promotes neuropathic pain in CCI rats via inducing NOTCH2 expression. Gene 767:145079. doi: 10.1016/j.gene.2020.145079
Zhang, Q., Zhu, D., and Li, Q. (2021). LncRNA CRNDE exacerbates neuropathic pain in chronic constriction injury-induced(CCI) rats through regulating miR-146a-5p/WNT5A pathway. Bioengineered 12, 7348–7359. doi: 10.1080/21655979.2021.1972901
Zhang, Z., Sun, X., Zhao, G., Ma, Y., and Zeng, G. (2021). LncRNA embryonic stem cells expressed 1 (Lncenc1) is identified as a novel regulator in neuropathic pain by interacting with EZH2 and downregulating the expression of Bai1 in mouse microglia. Exp. Cell Res. 399:112435. doi: 10.1016/j.yexcr.2020.112435
Zhang, P., Sun, H., and Ji, Z. (2021). Downregulating lncRNA PVT1 relieves astrocyte overactivation induced neuropathic pain through targeting miR-186-5p/CXCL13/CXCR5 Axis. Neurochem. Res. 46, 1457–1469. doi: 10.1007/s11064-021-03287-0
Zhang, L., Wu, R., Xu, M. J., Sha, J., Xu, G. Y., Wu, J., et al. (2021). MiRNA-107 contributes to inflammatory pain by down-regulating GLT-1 expression in rat spinal dorsal horn. Eur. J. Pain 25, 1254–1263. doi: 10.1002/ejp.1745
Zhang, X. O., Dong, R., Zhang, Y., Zhang, J. L., Luo, Z., Zhang, J., et al. (2016). Diverse alternative back-splicing and alternative splicing landscape of circular RNAs. Genome Res. 26, 1277–1287. doi: 10.1101/gr.202895.115
Zhang, Z. J., Guo, J. S., Li, S. S., Wu, X. B., Cao, D. L., Jiang, B. C., et al. (2018). TLR8 and its endogenous ligand miR-21 contribute to neuropathic pain in murine DRG. J. Exp. Med. 215, 3019–3037. doi: 10.1084/jem.20180800
Zhao, J., Yang, L., Huang, L., and Li, Z. (2021). Screening of disease-related biomarkers related to neuropathic pain (NP) after spinal cord injury (SCI). Hum. Genomics 15:5. doi: 10.1186/s40246-021-00303-w
Zhao, X., Liu, H. Z., and Zhang, Y. Q. (2016). [Effect of P2X7 receptor knock-out on bone cancer pain in mice]. Sheng Li Xue Bao 68, 224–232.
Zhao, X., Tang, Z., Zhang, H., Atianjoh, F. E., Zhao, J. Y., Liang, L., et al. (2013). A long noncoding RNA contributes to neuropathic pain by silencing Kcna2 in primary afferent neurons. Nat. Neurosci. 16, 1024–1031. doi: 10.1038/nn.3438
Zhenzhen, Z., Fenghao, L., Meina, M., Rui, L., Wenbo, S., and Qi, W. (2021). Targeting HMGB1-TLR4 signaling by miR-216a-5p elevation alleviates the inflammatory behavioral hypersensitivity. Neurosci. Lett. 759:136043. doi: 10.1016/j.neulet.2021.136043
Zhou, Z. B., Niu, Y. L., Huang, G. X., Lu, J. J., Chen, A., and Zhu, L. (2018). Silencing of circRNA.2837 plays a protective role in sciatic nerve injury by sponging the miR-34 family via regulating neuronal autophagy. Mol. Ther. Nucleic Acids 12, 718–729. doi: 10.1016/j.omtn.2018.07.011
Zhu, J., Yang, J., and Xu, J. (2021). miR-223 inhibits the polarization and recruitment of macrophages via NLRP3/IL-1beta pathway to meliorate neuropathic pain. Pain Res. Manag. 2021, 6674028. doi: 10.1155/2021/6674028
Keywords: non-coding RNA, microRNA, long non-coding RNA, circular RNA, chronic pain, biomarker
Citation: Zhang C, Gao R, Zhou R, Chen H, Liu C, Zhu T and Chen C (2022) The emerging power and promise of non-coding RNAs in chronic pain. Front. Mol. Neurosci. 15:1037929. doi: 10.3389/fnmol.2022.1037929
Received: 06 September 2022; Accepted: 07 October 2022;
Published: 03 November 2022.
Edited by:
Dong Huang, Central South University, ChinaReviewed by:
John Wood, University College London, United KingdomJeffrey C. Petruska, University of Louisville, United States
Copyright © 2022 Zhang, Gao, Zhou, Chen, Liu, Zhu and Chen. This is an open-access article distributed under the terms of the Creative Commons Attribution License (CC BY). The use, distribution or reproduction in other forums is permitted, provided the original author(s) and the copyright owner(s) are credited and that the original publication in this journal is cited, in accordance with accepted academic practice. No use, distribution or reproduction is permitted which does not comply with these terms.
*Correspondence: Chan Chen, Y2hlbmNoYW5Ac2N1LmVkdS5jbg==, eHljaGVuY2hhbkBnbWFpbC5jb20=; Rui Gao, c29waGllZ2FvcnVpQGZveG1haWwuY29t
†These authors have contributed equally to this work