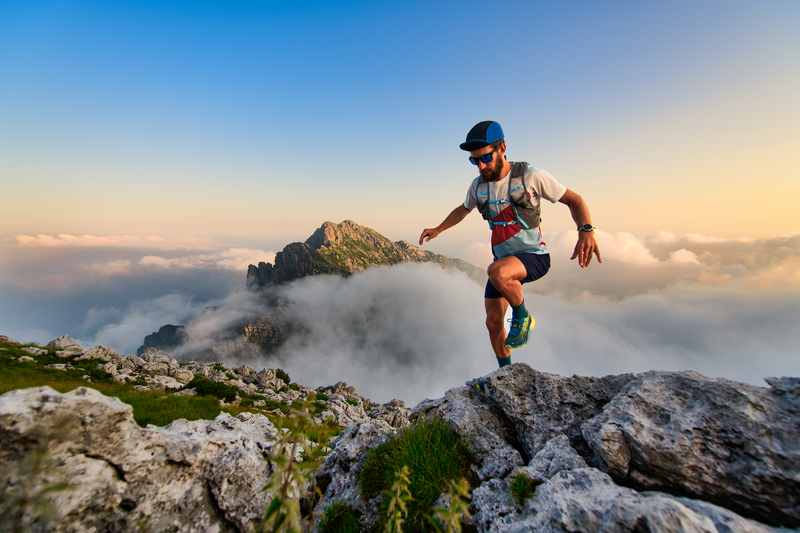
95% of researchers rate our articles as excellent or good
Learn more about the work of our research integrity team to safeguard the quality of each article we publish.
Find out more
REVIEW article
Front. Mol. Neurosci. , 11 October 2022
Sec. Methods and Model Organisms
Volume 15 - 2022 | https://doi.org/10.3389/fnmol.2022.1031989
This article is part of the Research Topic Gene Therapy for Hearing Loss: From Mechanism to Clinic View all 12 articles
The Kölliker’s organ is a transient cellular cluster structure in the development of the mammalian cochlea. It gradually degenerates from embryonic columnar cells to cuboidal cells in the internal sulcus at postnatal day 12 (P12)–P14, with the cochlea maturing when the degeneration of supporting cells in the Kölliker’s organ is complete, which is distinct from humans because it disappears at birth already. The supporting cells in the Kölliker’s organ play a key role during this critical period of auditory development. Spontaneous release of ATP induces an increase in intracellular Ca2+ levels in inner hair cells in a paracrine form via intercellular gap junction protein hemichannels. The Ca2+ further induces the release of the neurotransmitter glutamate from the synaptic vesicles of the inner hair cells, which subsequently excite afferent nerve fibers. In this way, the supporting cells in the Kölliker’s organ transmit temporal and spatial information relevant to cochlear development to the hair cells, promoting fine-tuned connections at the synapses in the auditory pathway, thus facilitating cochlear maturation and auditory acquisition. The Kölliker’s organ plays a crucial role in such a scenario. In this article, we review the morphological changes, biological functions, degeneration, possible trans-differentiation of cochlear hair cells, and potential molecular mechanisms of supporting cells in the Kölliker’s organ during the auditory development in mammals, as well as future research perspectives.
Mammals are not born with a sense of hearing, which gradually matures during the 12th to 14th day of life (Geal-Dor et al., 1993). During the development of hearing, the Kölliker’s organ supporting cells play a key regulatory role (Tritsch et al., 2007; Dayaratne et al., 2014), changing cell morphology and numbers during the embryonic and postnatal periods, eventually degenerating and disappearing after the external auditory canal opens, the endocochlear potential is established, and the cochlea can receive external acoustic stimuli, and then eventually diffuse into internal sulcus cells, at which point the cochlear hearing becomes mature (Lim and Anniko, 1985). Delayed degeneration or dysfunction of Kölliker’s organ-supporting cells can lead to abnormal cochlear hearing development (Lim, 1986). In addition, Kölliker’s organ-supporting cells can be induced to differentiate into new hair cells as inner ear progenitors for hair cell regeneration, which in turn can repair damaged hearing (Chai et al., 2011, 2012; Wang et al., 2015; Cheng et al., 2017; Zhang et al., 2017, 2018; You et al., 2018). Although the Kölliker’s organ was described more than a century ago, the molecular mechanisms regulating cochlear auditory development are still far beyond understanding. In this article, we review the morphological changes, biological functions, degeneration, possible trans-differentiation into hair cells, and potential molecular mechanisms of Kölliker’s organ-supporting cells during development, as well as future research perspectives to gain insight and understanding of the role of Kölliker’s organ-supporting cells in cochlear auditory development.
The organ of Corti consists of a chimera of hair cells and supporting cells connected in a highly ordered manner, and is an important component of the inner ear auditory receptors (Lim, 1986). At the base of the inner spiral sulcus is a single layer of cuboidal epithelium, which is derived from the degenerated Kölliker’s organ-supporting cells (Lim and Anniko, 1985).
The Kölliker’s organ is the earliest visible epithelial structure, present in the late embryonic and early postnatal periods (P0–P14), which is a marker of cochlear immaturity (Dayaratne et al., 2014). The differentiated Kölliker’s organ consists mainly of closely spaced columnar cells, and due to the dense distribution, the nucleus can appear in different areas of the cell (mostly at the base of the Kölliker’s organ), thus appearing stratified in cross-section (Hinojosa, 1977). Kölliker’s organ-supporting cells have elongated cytosomes, usually separated by an extracellular space of about 200 Å, with some extracellular gaps down to 30 Å, with bipolar or unipolar protrusions at the top of the cell and microvilli visible at the end, which were shown to be about 3–4 μm long in mice and appeared within 10 days after birth (Hinojosa, 1977). Some of these cells can be mitotically active in late embryonic and early postnatal stages and have the potential to trans-differentiate into hair cells (Wu and Kelley, 2012; Dayaratne et al., 2014).
The morphology and structure of Kölliker’s organ-supporting cells undergo programmed changes and alterations during the embryonic period and after birth: the cytoplasm moves away from the cell membrane, the cells develop folds, and the cell gaps increase in size. After this critical period, the columnar cells gradually degenerate and their number decreases dramatically to about 12% of the original number of cells in the adult cochlea, and are eventually replaced by cuboidal-like cells that form a mature internal sulcus before degenerating and disappearing (Figure 1), at which point the cochlea matures in hearing (Tritsch et al., 2007). The origin of the cuboidal-like epithelial cells that replace the columnar supporting cells of the Kölliker’s organ during cochlear developmental maturation and remodeling of the Kölliker’s organ is unclear.
Figure 1. The morphology and structure of Kölliker’s organ-supporting cells from immature to mature stages. From P1 to P14, the cytoplasm moves away from the cell membrane, the cells develop folds, and the cell gaps increase in size. Also, during this critical period, the columnar cells gradually degenerate and their number decreases dramatically, and are eventually replaced by cuboidal-like cells which form a mature internal sulcus, at which point the cochlea matures in hearing. KO, Kölliker’s organ; GER, Greater epithelial ridge; LER, Lesser epithelial ridge; OHC, Outer hair cells; IHC, inner hair cells.
The role of ATP in initiating spontaneous morphological changes in Kölliker’s organ-supporting cells was first described by Tritsch et al. (2007). After ATP is released into the extracellular space through gap-linked hemichannels on the surface of Kölliker’s organ-supporting cells, it in turn, acts on the purine P2X and P2Y receptors on the surface of the cells themselves receptors on the cell surface, causing intracellular Ca2+ elevation and inward current generation (Tritsch et al., 2007; Liang et al., 2009). Using real-time imaging, the study found that these rhythmic structural changes first start in a small group of cells within the Kölliker’s organ and then radially spread to neighboring cells through gap junctions (Tritsch and Bergles, 2010). Much like inward currents, the frequency of these morphological events was significantly increased in border cells adjacent to the hair cells within the Kölliker’s organ region, suggesting that border cells around the hair cells first initiate this spontaneous activity and play a key regulatory role (Nishani Dayaratne et al., 2015).
This spontaneous morphological change in the rodent Kölliker’s organ does not occur at birth, and studies suggest that cellular morphological changes need to be initiated when the cochlea develops to a certain extent. The low level of purinergic receptor expression on the surface of Kölliker’s organ-supporting cells in the early postnatal period results in ATP-induced inward currents and calcium waves at low levels, which are insufficient to initiate this process (Tritsch and Bergles, 2010). It was found that neither strong current stimulation nor elevated extracellular K+ ion concentrations induced spontaneous morphological changes; whereas when the increased intracellular Ca2+ concentrations, cells underwent depolarization, activated Kölliker’s organ-supporting cells, and further promoted changes in cell diameter in neighboring cells (Tritsch et al., 2007; Tritsch and Bergles, 2010). These results suggest that the intracellular Ca2+ concentration is a key factor in triggering rhythmic morphological changes in cells that diffuse into adjacent cells within the Kölliker’s organ (Tritsch et al., 2007; Peng et al., 2012).
It is now believed that one of the key factors initiating this morphological change is the Ca2+-activated Cl− channel, leading to Cl− efflux, which decreases the cell membrane threshold potential level and further causes an efflux of intracellular fluid due to the osmotic gradient, resulting in sawtooth morphological changes in Kölliker’s organ-supporting cells (Tritsch et al., 2010). Furthermore, this outflow of intracellular fluid may be influenced by the distribution of water channel proteins (especially AQP4) within the Kölliker’s organ-supporting cells (Nishani Dayaratne et al., 2015), and additionally, the activation of intracellular contractile proteins may also be responsible for the altered morphology of the supporting cells (Tritsch et al., 2010; Liu Y. et al., 2019).
Studies have shown that afferent nerve fibers are not in a silent state before the onset of hearing and that the hair cells continuously release low levels of glutamate, triggering spontaneous electrical activity that plays an important role in promoting the survival and maturation of auditory neurons, the development of synapses, and the refinement of audio localization (Lelli et al., 2009; Blankenship and Feller, 2010; Wang and Bergles, 2015). Rodents are not auditory at birth and do not respond to external sounds until 2 weeks after birth, and Kölliker’s organ-supporting cells play a key role in this auditory maturation process (Forsythe, 2007; He and Yang, 2011).
The inner and outer hair cells generate spontaneous calcium action potentials in the first week of life. Spontaneous calcium action potentials begin to guide cochlear development before the appearance of hearing (Beurg et al., 2008; Marcotti, 2012; Sendin et al., 2014). While this spontaneous calcium action potential of immature inner and outer hair cells is certainly important in cochlear development, the developmental maturation process of hearing also relies on the cellular communication network formed by Kölliker’s organ-supporting cells through gap junctions. In the second week of life, Kölliker’s organ-supporting cells begin to release adenosine triphosphate (ATP) spontaneously and release ATP to the endolymphatic surface via gap junction hemichannels composed of Cx26 and Cx30 between Kölliker’s organ-supporting cells and act as paracrine receptors on the surface of neighboring hair cells to produce phospholipase C (PLC)-dependent diacylglycerol and inositol triphosphate (IP3; Rodriguez et al., 2012; Giorgi et al., 2018). The intracellular diffusion of IP3 and subsequent binding to IP3 receptors in hair cells triggers the release of Ca2+ from the endoplasmic reticulum (ER) calcium pool and an increase in cytoplasmic Ca2+ concentration, which further induces the release of the neurotransmitter glutamate from synaptic vesicles in inner hair cells, excites hair cells and their afferent nerve fibers, activates type I spiral neurons (SGNs) to generate action potentials and in this way transmits cochlear development to the upstream auditory pathway. This transmits temporal and spatial information about cochlear development to the upstream auditory pathway and refines synaptic connections in the auditory pathway, thus promoting cochlear maturation and auditory acquisition (Tritsch and Bergles, 2010; Rodriguez et al., 2012; Johnson et al., 2017). It has been suggested that ATP released by Kölliker’s organ-supporting cells primarily coordinates spontaneous electrical activity with its neighboring inner hair cells, with little association with the more distant inner hair cells, and it is hypothesized that this mechanism may be critical for the establishment of audio localization in the cochlear nucleus before the emergence of hearing (Tritsch et al., 2007).
Ca2+ is the primary messenger for intercellular messaging in the cochlea and is involved in the transduction of a large number of signaling pathways (Tritsch et al., 2010). ATP in the cochlea triggers changes in intracellular Ca2+ concentration in Kölliker’s organ-supporting cells and intercellular calcium wave transmission, which in turn transmits important biological information to the cochlear sensory epithelium. Organ-on-chip model studies have shown that developing cochlear Kölliker’s organ-supporting cells release ATP to drive spontaneous Ca2+ signals via gap junction protein hemichannels (Mazzarda et al., 2020). Before the appearance of hearing, the spontaneous release of ATP from the Kölliker’s organ further mediates the release of Ca2+ within Kölliker’s organ-supporting cells after release into the extracellular compartment via hemichannels, initially inducing the release of Ca2+ from only a small group of Kölliker’s organ-supporting cells, which subsequently propagate rapidly through the intercellular gap junction channels, further initiating the release of Ca2+ from the calcium pool in neighboring Kölliker’s organ-supporting cells. The release of large amounts of Ca2+ promotes the synchronization of neighboring cells, which further causes the release of ATP (Zhao et al., 2005). In this way, the P2 purinoceptor/phospholipase C/IP3/Ca2+ signaling cascade is maintained in an orderly and positive feedback manner, causing sustained spontaneous electrical activity, and promoting cochlear development.
Kölliker’s organ-supporting cells form a functional syncytium through extensive intercellular gap junctions (Goodenough and Paul, 2009), through which intercellular Ca2+ propagation and ATP release occur, providing a Kölliker’s organ cell-to-cell signaling metabolic basis, i.e., facilitating intercellular signaling when the gap junctions are open and closing them under some specific physiological conditions (Goodenough and Paul, 2009; Mammano, 2013). GJB2 mutations are mainly characterized by degeneration of outer hair cells and hypoplasia of the vascular stripe and are the main cause of deafness in 50% of pre-speech deafness (Hochman et al., 2010). In addition, GJB6 mutation in rodents was found to reduce Ca2+ in Kölliker’s organ-supporting cells, leading to an increased hearing threshold (Rodriguez et al., 2012; Chen et al., 2021a); while Cx26 cKD mice also showed reduced ATP release, downregulation of ATP-dependent calcium signaling, the disappearance of calcium waves and increased hearing threshold in Kölliker’s organ-supporting cells (Sun et al., 2022).
Studies have shown that glycoproteins secreted by Kölliker’s organ-supporting cells, such as otocollagen and capsid protein, are involved in the formation of the tectorial membrane (Hinojosa, 1977; Lim and Anniko, 1985; Zine and Romand, 1996). During early developmental stages, the tectorial membrane is attached to the Kölliker’s organ-supporting cells by a network of thin filaments, which gradually separate as the cochlea matures, and about 2 weeks after birth, the tectorial membrane is completely separated from the Kölliker’s organ and eventually extends to the out hair cells. Thyroid hormone is a key factor in regulating this process, and thyroid hormone deficiency prolongs the survival of Kölliker’s organ-supporting cells and causes tectorial membrane malformations (Uziel et al., 1981).
PR-domain-containing 16 (PRDM16) is a key transcriptional regulator in craniofacial, adipose, and neural tissue development (Fumasoni et al., 2007; Shull et al., 2020). It was found that PRDM16 is highly expressed in the nuclei of Kölliker’s organ-supporting cells in the bottom and middle cochlear apex by embryonic day 13.5 and decreases rapidly at birth. Its expression in the Kölliker’s organ continued throughout development until postnatal day 7, and PRDM16 was thus suggested as a possible specific marker for Kölliker’s organ-supporting cells (Ebeid et al., 2022). Further, by studying the model with Prdm16cGT double allele deletion mice, it was found that the cochlear Kölliker’s organ of Prdm16 gene-deficient mice at day P0 had poor development, shortened cochlear ducts, reduced proliferation of Kölliker’s organ-supporting cells, increased density of hair cells and supporting cells in the parietal ring, and loss of anchoring ability of the lid membrane to the Kölliker’s organ, indicating that Prdm16 is a regulatory gene necessary for Kölliker’s organ-supporting cells’ proliferation (Ebeid et al., 2022). Cochlear duct development requires the proliferation of these cells for convergent extension and to achieve normal hair cells and supporting cell densities within the organ of Corti (Chen et al., 2002; Driver et al., 2017). In addition, gene expression analysis revealed that α-tectorin (TECTA) expression was downregulated and β-tectorin (TECTB) appeared upregulated in the cochlea of Prdm16cGT-deficient mice. α-TECTA and TECTB are essential proteins in the tectorial membrane (Richardson et al., 2008), and mutant mice with α-tectorin exhibit abnormal tectorial membrane and hearing loss (Legan et al., 2014; Kim et al., 2019), and the study hypothesized that deletion of the Prdm16cGT gene leads to dysregulation of tectorin subunit expression, resulting in abnormal tectorial membrane morphology.
Single-cell RNA transcriptome sequencing-based studies have revealed that Kölliker’s organ-supporting cells also have different cellular subtypes (Chen et al., 2021b). The study, based on clustering analysis of cells with similar gene expression patterns, revealed the existence of four different cell subtypes in Kölliker’s organ-supporting cells (Kolla et al., 2020; Chen et al., 2021b; Kubota et al., 2021). The Kubota et al. (2021) study of organoid development classified Kölliker’s organ-supporting cells into three different cell subtypes. Gene expression localization showed that specific overexpressed genes were displayed in different regions of the Kölliker’s organ and gradually degenerated and disappeared as the cochlea matured (Kubota et al., 2021). Chen et al. (2021b) identified four cell subsets from P1 to P14, of which the number of cells decreased gradually, while the other four cell subsets proliferated at P1 to P7 and completely disappeared at P14. These eight cell subpopulations were found to be closely linked on the t-SEN cell space map and had very similar gene expression patterns and biological properties (Kolla et al., 2020; Chen et al., 2021b; Kubota et al., 2021). Kamiya et al. (2001) also found that apoptosis and mitosis co-exist in Kölliker’s organ-supporting cells at P7 postnatally and that the cells not only degenerate but also regenerate before disappearing around P12, demonstrating that mitotic proliferation of Kölliker’s organ-supporting cells occurs not only in embryonic development but also in the development of the normal auditory system after birth.
Impaired or delayed degeneration of the Kölliker’s organ can lead to structural deformities of the organ of Corti, especially the organ of Corti lid (Uziel, 1986). However, the molecular mechanisms of Kölliker’s organ-supporting cell degeneration are not clear.
He and Yang (2015b) found that the morphology of Kölliker’s organ-supporting cells in the neonatal P1, P3, P5, P10, P12, and P14 rat cochlea gradually changed from short columnar to tall columnar cell morphology from the basal turn to the apical turn and the number of cells also gradually decreased in addition to the change in cell morphology, presuming that apoptosis of Kölliker’s organ-supporting cells exists during the development of the neonatal rat cochlea (He and Yang, 2015b). He and Yang (2015a,b) showed that Kölliker’s organ-supporting cells exhibited a programmed apoptotic process from basal turn to the apical turn, but in vitro tests showed proliferation, suggesting that the initiating factor of apoptosis may come from outside the Kölliker’s organ-supporting cells rather than from intrinsic cellular factors. On this basis, Hou et al. (2019, 2020) further found that the expression levels of caspase-3, caspase-8, caspase-9, and BCL-gene mRNA and protein in the cochlear basement membrane of rats at different days of age after birth were significantly time-dependent, and the study concluded that in vivo Kölliker’s organ-supporting cells not only existed in apoptosis but also in proliferation. The number of proliferating cells is much less than the number of apoptotic cells, which eventually manifests in the tissue structure as the disappearance of Kölliker’s organ-supporting cells (Hou et al., 2019, 2020).
Liu et al. (2017) investigated the morphological changes of Kölliker’s organ-supporting cells and the expression of apoptosis-related factors during early postnatal development. It was found that on day P5, the Kölliker’s organ in the basal turn of the cochlea became significantly smaller, while the Kölliker’s organ in the middle and apical turn became smaller. On day P12 the cochlea showed the disappearance of the Kölliker’s organ in the basal turn and middle turn, and at this stage, the Kölliker’s organ in the apical turn was still present, but the number of cells was significantly reduced and its morphology changed from a high columnar to a short columnar shape. The results of immunohistochemistry and TUNEL staining showed the presence of necrotic cytomorphological changes in some TUNEL-positive Kölliker’s organ-supporting cells at day P7, showing chromatin condensation and vacuole-like changes (Liu et al., 2017).
It was concluded that exogenous and endogenous apoptotic pathways exist during the apoptosis of supporting cells as the rat cochlear Kölliker’s organ develops in vivo. After birth, the Kölliker’s organ in the cochlea degenerates in a time-dependent pattern in which cysteine proteases are involved in apoptotic cell death during postnatal development, suggesting the involvement of endogenous factors (Adrain and Martin, 2001).
Apoptosis and proliferation co-exist during the degeneration of the Kölliker’s organ, and the degenerative disappearance of the Kölliker’s organ is thought to be a disturbance of the balance between apoptosis and mitosis (Kamiya et al., 2001). It is still unclear why such cell proliferation exists in Kölliker’s organ-supporting cells at a later stage of their degenerative disappearance.
The thyroid hormone is a key factor in guiding the orderly degeneration of the Kölliker’s organ during cochlear development (Mustapha et al., 2009; Peeters et al., 2015; Sundaresan et al., 2016). Hypothyroidism and mutations in the thyroid hormone receptor beta gene cause delayed degeneration of the Kölliker’s organ, while ectopic treatment with T3 (3,5-triiodo-L-thyroxine) on days P0 and P1 leads to earlier degeneration of the Kölliker’s organ and hearing loss (Rusch et al., 2001; Peeters et al., 2015; Borse et al., 2021). Peeters et al. (2015) investigated the time course of apoptosis support by the Kölliker’s organ and the effect of ectopic T3 on apoptosis. Furthermore, in methimazole-treated hypothyroid rat cochlea studies showed that Kölliker’s organ-supporting cells lacked TUNEL-stained positive apoptotic cells at day P7. It has been reported that apoptosis in Kölliker’s organ-supporting cells may be regulated by some unknown mechanism regulating the expression of the thyroid hormone receptor β gene in Kölliker’s supporting cells and thus regulating this programmed apoptosis (Ng et al., 2013).
Studies have reported that immunoreactivity for the neurotrophin receptor p75NTR, a mediator of neuronal survival or differentiation, is detected in the Kölliker’s organ of P5 rats and that this immunoreactivity changes in response to thyroxine (T4; Knipper et al., 1999). It is unclear whether p75NTR contributes to Kölliker’s organ-supporting cell apoptosis (Sato et al., 2006). T3-induced Kölliker’s organ-supporting cells’ apoptosis is a unique cell- and time-specific form of apoptosis in the late mitotic phase of tissue maturation and may involve a series of pre-apoptotic events, including cellular reorganization and cellular translocation before the cell death phase, in addition to how this process is coordinated with other unknown signals together with other unknown signals, still need to be investigated in depth (Coen et al., 2007). Although it is not clear how premature remodeling leads to deafness, these studies suggest that cochlear development is a precisely ordered regulatory process and that T3 provides an important molecular signaling role in the orderly regulation of these remodeling events.
Several studies have reported the relationship between autophagy and hearing loss, and activation of autophagy can reduce noisiness (Guo et al., 2021), drug resistance (He et al., 2017; Liu et al., 2021; Zhang et al., 2022), and the degree of inner ear damage and hearing loss in the elderly (He et al., 2020, 2021). It was found that autophagy is closely related to the occurrence and development of sensorineural deafness (Zhou et al., 2020) and that autophagy activators can effectively reduce the level of oxidative stress in hair cells and decrease hair cell death, thus attenuating high-intensity noise-induced damage to hair cells (Li et al., 2018). In addition, autophagy also plays an important regulatory role during inner ear development and is essential for maintaining the morphology and function of auditory hair cells (Zhou et al., 2020).
The existence of autophagy during Kölliker’s organ-supporting cell degeneration was investigated and a large number of autophagic vacuoles containing organelles was first detected by Hinojosa (Hinojosa, 1977). Autophagy markers, including LC3-II, SQSTM1/p62, and Beclin1, were detected in Kölliker’s organ-supporting cells by immunohistochemical staining. In addition, it showed that during early postnatal cochlear development, the expression of LC3-II gradually decreased and the expression of p62 increased, compared with that between P7 and P10. Compared to apoptotic markers that peak at P1, autophagic flux peaks earlier at P1, and activated autophagic flux gradually decreases with the degeneration of Kölliker’s organ-supporting cells from P1 until P14 (Hou et al., 2019).
Apoptosis and autophagy in Kölliker’s organ-supporting cells show different time-dependent and sophisticated expression patterns, with the peak of autophagic activity during postnatal maturation of cochlear hearing development located on postnatal day 1 (P1) or earlier, while the expression of apoptotic markers (Bcl-2, caspase-3, caspase-8, and caspase-9) follows a bell-shaped curve, with peak expression at P3 (Qu et al., 2007). Organelles are digested by autophagy before apoptosis, and autophagy can provide a source of energy for the removal of aggregated proteins and damaged organelles, both of which play different roles at different stages of cochlear development (Aburto et al., 2012; Liu et al., 2017). These results suggest that autophagy plays an important early role in the pre-apoptotic transformation and degeneration of the Kölliker’s organ during early postnatal development and that any disturbance in autophagy or apoptosis of Kölliker’s organ-supporting cells during development will lead to impaired cochlear development and eventually cause hearing loss (He et al., 2017). Therefore, the dynamic balance between autophagy and apoptosis regulates the normal differentiation and orderly organization of developing cochlear supporting cells, but the regulatory mechanism of the dynamic balance between autophagy and apoptosis is currently unknown (He et al., 2017; Zhou et al., 2020). Abnormal intracellular calcium signaling mediated by Gjb2 may be a key regulator of abnormal alterations in the autophagy-apoptosis signaling pathway and still requires in-depth study (Inoshita et al., 2014; Sun et al., 2022).
Studies have confirmed that some important genes and signaling pathways play an important role in cochlear hearing development, such as Sox2 (Cheng et al., 2019), Pou4f3, and Atoh1 genes (Chen et al., 2021c); FGF (Tang et al., 2016), Notch (Ni et al., 2016; Waqas et al., 2016), FoxG1 (He et al., 2020, 2021), Strip1 (Zhang et al., 2021), mTOR (Fu et al., 2018, 2022) and Wnt signaling pathway (Chai et al., 2012; Liu et al., 2016). However, the molecular mechanisms of signaling pathways associated with the degenerative disappearance of Kölliker’s organ-supporting cells early in postnatal cochlear hearing development are not clear. It has been proposed that Hedgehog signaling inhibits the sensory differentiation ability of Kölliker’s organ-supporting cells, regulates the fate of Kölliker’s organ-supporting cells, and induces cochlear developmental maturation (Driver et al., 2008).
Chen et al. (2021b) performed KEGG signaling pathway analysis in different subtypes of Kölliker’s organ-supporting cells by scRNA-seq and found that genes in different subtypes of Kölliker’s organ-supporting cells were significantly enriched in the Ribosome signaling pathway; however, in the Cluster 3, a large number of genes were enriched in the Ribosome signaling pathway and Protein digestion and absorption signaling pathway. The Ribosome signaling pathway is an important signaling pathway that regulates cell proliferation and development (Pelletier et al., 2018). The results of scRNA-seq showed that from P1 to P7, there was a significant proliferation of cell numbers, but cell numbers showed a decrease throughout Kölliker’s organ-supporting cells development, presumably mainly associated with the PI3K-Akt signaling pathway, a negative regulatory pathway of gene expression in the Cluster 3. The PI3K-Akt signaling pathway is an important signaling pathway that regulates cell proliferation, differentiation, apoptosis, and migration (Jia et al., 2019; Bu et al., 2022). It has also been shown to regulate cochlear hair cell regeneration (Mullen et al., 2012; Xia W. et al., 2019). A large number of Col family genes on Cluster 3 are enriched in the PI3K-Akt signaling pathway, including Col4a1, Col4a2, Col6a1, Col6a2, and Col1a1, which are presumed to regulate the dynamic balance of proliferation and apoptosis in Kölliker’s organ-supporting cells. The Col family genes Col3a1, Col5a1, Col5a2, Col6a1, and Col1a1 in the Cluster 0 regulate the autophagy of these apoptotic proteins and cellular debris and provide the energy required to promote the apoptotic process through autophagic catabolism (Komatsu et al., 2005), which in turn directs the Kölliker’s organ-supporting cells under the sequential degeneration or trans-differentiation of Kölliker’s organ-supporting cells. The role of this signaling pathway and related genes in regulating Kölliker’s organ-supporting cell degeneration and auditory development needs to be further investigated. The proposed signal pathway diagram of the molecular mechanism of degeneration and trans-differentiation of Kölliker’s organ-supporting cells has been shown in Figure 2.
Figure 2. The proposed signal pathway diagram of the molecular mechanism of Kölliker’s organ-supporting cells degeneration and trans-differentiation. It is shown that Col family genes on KO 3 and the PI3K-Akt signaling pathway are presumed to regulate the dynamic balance of proliferation and apoptosis in Kölliker’s organ-supporting cells, and also the Col family genes in the KO 0 regulate the autophagy of these apoptotic proteins and cellular debris and provide the energy required to promote the apoptotic process. In addition, it has been proposed that the PI3K-Akt-mTOR signaling pathway may be one of the underlying mechanisms to induce the differentiation process of Kölliker’s organ-supporting cells to hair cells. IHC, inner hair cells; KO 0, Cluster 0 of Kölliker’s organ-supporting cells; KO 3, Cluster 3 of Kolliker’s organ-supporting cells.
Adult mammalian cochlear hair cells have no regenerative capacity, but studies have shown that newborn rodent cochlear basement membrane cells have a limited but transient regenerative potential, which is mainly derived from non-sensory cells in the cochlea (Chai et al., 2018). Under normal conditions, mitotic cells in the neonatal mouse cochlea are relatively quiescent, and early damage caused by various factors will indirectly activate these non-sensory cells to exert their limited regenerative potential to restore the damaged hair cell population and thus repair hearing (Gao et al., 2019; Liu W. et al., 2019; Chai et al., 2022). Kölliker’s organ-supporting cells normally exist in the late mid-embryonic and early postnatal stages as a transient neonatal cell population, and it was found that some cell populations located in the Kölliker’s organ region retain the properties of precursor cells, which can regenerate and transform and are the precursor cell pool for hair cell regeneration (Chai et al., 2011, 2012; Bramhall et al., 2014).
Math1 is a homolog of the Drosophila gene, the deletion of which leads to failure of inner ear hair cell differentiation (Chen et al., 2002). It was found that induction of Math1 overexpression in Kölliker’s organ-supporting cells led to the generation of a large number of ectopic hair cells, and these newborn ectopic hair cells were positive for myosin VIIa (a hair cell-specific marker) and could form keratin plates and stereocilia bundles (Zheng and Gao, 2000; Zhang et al., 2007).
Overexpression of Math1 resulted in the interdental cells of the organ of Corti and its adjacent organ of Corti, the internal sulcus, and the Hensen’s cells regions, and axonal extension from the auditory nerve bundle to some of the new hair cells regions, suggesting that the new hair cells attract the guided regeneration of auditory spiral neurons (Gubbels et al., 2008).
The Kölliker’s organ does not express the Math1 gene after birth and is normally expressed only in the embryonic sensory epithelium starting at E12.5, suggesting that the reason why Kölliker’s organ-supporting cells do not continue to differentiate into hair cells after birth but gradually transform into internal sulci, most likely because of the absence of Math1 expression (Bermingham et al., 1999). Math1 is expressed in differentiated hair cells in the early embryonic stage but is downregulated in supporting cells in the late embryonic stage and is absent in cells outside the sensory epithelium (Chen et al., 2002; Woods et al., 2004). In addition, it was found that when cochlear organ cultures overexpressing Math1 caused the production of ectopic hair cells within the large and small epithelial crest regions, while knockdown of Hes1 also resulted in the production of ectopic hair cells and these hair cells showed elevated levels of Math1 expression (Zheng and Gao, 2000). The Hes1 gene is normally expressed only in the Kölliker’s organ of P0–P3, suggesting that Hes1 may repress Math1 expression to some extent early in life, consistent with the idea that Hes5 is primarily involved in the precise regulation of ectopic hair cells (Zine et al., 2001; McGovern et al., 2018). Math1 is an essential gene for hair cell differentiation and plays a positive regulatory role, while Hes1 and Hes5 are negative regulators of hair cell differentiation (Zine et al., 2001; Su et al., 2015). Future attempts might be made to continue the differentiation of postnatal Kölliker’s organ-supporting cells to hair cells by upregulating the expression levels of Math1 and or downregulating Hes1 in Kölliker’s organ-supporting cells.
It was found that after induction of Atoh1 overexpression, ectopic hair cell regeneration occurred in different subtypes of Corti organelle supporting cells (Liu et al., 2012; Richardson and Atkinson, 2015; Walters et al., 2017; Yamashita et al., 2018). Kelly et al. (2012) identified a large number of isolated ectopic hair cells in the non-sensory Kölliker’s organ region by constructing a triple allele TGATOH1 mouse model. These ectopic hair cells displayed a stereocilia structure similar to that of mature hair cells, but exhibited different maturation and were scattered within the Kölliker’s organ (Kelly et al., 2012). Ectopic hair cells regeneration occurs most vigorously in the Kölliker’s organ region, but the efficiency of induced transformed hair cells remains low, compared to ectopic expression of Atoh1 alone, and it was shown that co-activation of Atoh1 with other transcription factors, such as Pou4f3, Gfi1, Gata3, and Nymc, induces more stem cells to trans-differentiate into hair cell-like cells, and the newly generated cells are more mature in both neonatal and mature mouse cochlea (Liu et al., 2012; Walters et al., 2017; Chen et al., 2021c). Xu et al. (2021) induced Atoh1 in Sox2-overexpressing positive progenitor cells in Kölliker’s organ and found that ectopic expression of Atoh1 promoted the trans-differentiation of progenitor cells to hair cells regeneration while demonstrating the importance of the Isl1/Tub/Znf532 pathway in promoting Atoh1-mediated hair cells regeneration. All these studies suggest that the supporting cells in the lateral of the Kölliker’s organ can serve as a source of progenitor cells for hair cell regeneration in the early postnatal period.
In the neonatal mouse cochlea, Wnt-responsive Lgr5-expressing cells are progenitors of regenerative hair cells, and upregulation of Wnt signaling stimulates the proliferation of Lgr5-positive progenitors (Chai et al., 2011, 2012). Lgr5 expression decreases gradually during cochlear development and maturation, mainly in IPs, and IPCs. In the adult cochlea, Lgr5 is only expressed in D3 s (Chai et al., 2012). Several studies have shown that Lgr5-labeled cochlear progenitor cells, following hair cell injury, can regenerate by mitosis and/or directly differentiate to generate new cochlear hair cells in the early postnatal period (Shi et al., 2012, 2013; Cox et al., 2014).
Recent studies have shown that multiple signaling pathways play an important role in hair cell regeneration by inducing proliferation and differentiation of Lg5 progenitor cells (Chen et al., 2021d). Activation of Wnt/β-linked protein signaling and inhibition of Notch signaling can induce Lgr5 progenitor cells to regenerate Myo7a-positive hair cells (Chai et al., 2012; Korrapati et al., 2013; Mizutari et al., 2013). Lgr5 progenitor cells can be regulated by many other factors and signaling pathways such as Shh, Foxg1, and Hippo (Gregorieff et al., 2015; Chen et al., 2017; Cheng et al., 2017; Zhang et al., 2020). The efficiency of regenerating hair cells from inner ear Lgr5 progenitor cells remains very limited, suggesting that hair cells regeneration involves other factors or signaling pathways (Wu et al., 2016; Lu et al., 2017; Fang et al., 2019; Xia L. et al., 2019). A previous study of hair cell regeneration and differentiation in the presence of SEC inhibitor flavopiridol showed that flavopiridol decreased the proliferative capacity of Lgr5 progenitor cells, but with no effect on the differentiation ability (Chen et al., 2021d). These results suggest that SEC plays an important role in regulating inner ear progenitor cells and thus influencing hair cells regeneration, and further in vivo studies are needed to elucidate the role of SEC in the inner ear, which will lay the experimental foundation for using cochlear progenitor cells to regenerate functional cochlea for the treatment of patients with sensorineural deafness.
Zhang et al. (2020) studied using Sox2CreER/Foxg1loxp/loxp mice and Lgr5EGFPCreER/Foxg1loxp/loxp mice to conditionally and specifically knock down Foxg1 in Sox2supporting cells and Lgr5 progenitor cells of neonatal mice, respectively and found that conditional knockdown of Foxg1 in both Sox2 supporting cells and Lgr5 progenitor cells at postnatal day 1 (P; cKD) resulted in the appearance of a large number of additional hair cells that In particular, more additional internal hair cells were generated at day P7, and these nascent hair cells with normal cilia bundles and synapses survived until at least P30. In addition, sphere formation assays showed that Foxg1 cKD in Lgr5 progenitor cells did not significantly alter their sphere formation capacity, and the findings suggest that Foxg1 cKD may directly trans-differentiate supporting cells and progenitor cells by inducing This study provides new evidence for the role of Foxg1 in regulating hair cell regeneration from supporting cells and progenitor cells in the neonatal mouse cochlea (Zhang et al., 2020).
It was shown that Bmi1 regulates redox homeostasis and reactive oxygen species (ROS) levels and is expressed in hair cells and supporting cells in addition to spiral ligament and spiral ganglion cells, thus playing an important role in the survival of auditory hair cells (Chen et al., 2015). It was found that Bmi1 knockdown significantly reduced the sphere formation ability of neonatal mouse Corti organ-supporting cells and Lgr5-positive progenitor cells, suggesting that Bmi1 is required to initiate the proliferation of neonatal mouse supporting cells and progenitor cells (Lu et al., 2017). It was also found that in Bmi1 knockout neonatal mice, DKK1 expression was significantly upregulated, while β-linked protein and Lgr5 expression were significantly downregulated, suggesting that Bmi1 indirectly exercises its role as an activator of the Wnt signaling pathway by inhibiting the DKK family of Wnt inhibitors (Cho et al., 2013). Bmi1 plays an important role in regulating the proliferation of neonatal mouse cochlear supporting cells and Lgr5-positive progenitor cells through the Wnt signaling pathway, suggesting that Bmi1 may be a new therapeutic target for hair cell regeneration.
Ephrins and their Eph receptors are the large Eph family genes that control tissue morphogenesis and are divided into two subclasses, EphB and EphA, based on their affinity for transmembrane EphB ligands or the glycosylphosphatidylinositol-anchored ephrin-A (Gale et al., 1996). EphB and EphA play an important role in the boundary formation between adjacent cell types by interacting with specific EphB or EphA4 receptors (Dahmann et al., 2011). The previous study found that EphA4 receptors interacting with Ephrin-B2 (EphBs at the end of EphA4), is the only receptor to be exclusively expressed in the inner and outer hair cells (Bianchi and Liu, 1999; Miko et al., 2008). While Ephrin-B2 specifically restricted expression in the Kölliker’s organ-supporting cells located medially to the inner hair cell layer from embryonic day (E) 16 and formed a gene-specific expression boundary. Such expression profile can distinguish adjacent supporting cells from hair cells and persists in the post-hearing onset (Defourny et al., 2015). Ephrin-B2 and EphA4 show complementary expression patterns in the developing organ of Corti, participate in sorting at the hair cell/support cell layer and play a key regulatory role in inducing the formation of different cell types in the organ of Corti. It has been hypothesized that cells migrating across non-lineage boundaries transfer their phenotype to fit the identity of their local neighbors, and thus manipulating these boundaries may be an unexpected strategy for generating additional hair cells (Defourny et al., 2011).
Defourny et al. (2015) showed that hair cells could arise directly from Kölliker’s organ-supporting cells located at the medial border with inner hair cell layers by inhibiting Ephrin-B2 signaling. Through using soluble inhibitors, short hairpin RNA (shRNA)-mediated gene silencing, and Ephrin-B2 conditional knockout mice, it was found that at late embryonic stages (the normal hair cell generation phase), downregulation of Ephrin-B2 signaling resulted in translocation of supporting cells to the hair cells layers and subsequent switch in cell identity from supporting cell to hair cell fate. This study demonstrates that manipulation of Ephrin-B2 gene expression in the Kölliker’s organ-supporting cells located at the medial border of the inner hair cell layer at the early stage can serve as a novel hair cell regeneration strategy to directly convert support cells to trans-differentiation hair cells.
Nevertheless, the exact mechanism of this cell fate change precisely occurs remains unclear. One possible explanation is that specific expression of the Sox2 transcription factor in supporting cells induces the differentiation process of supporting cells to hair cells (Millimaki et al., 2010). Furthermore, throughout cochlear development, ephrin-B2 and Notch have similar expression patterns in supporting cell types (Lanford et al., 1999), similar to the vascular regeneration model (Swift and Weinstein, 2009), and Ephrin-B2 may be a downstream target of Notch signaling involved in Notch signaling to couple cell separation and differentiation (Cheng et al., 2004; Tossell et al., 2011). Thus, the downregulation of Ephrin-B2 expression resulting in the absence of Notch signaling pathway components may weaken the fate of supporting cells and make them more susceptible to transition to the hair cell phenotype (Defourny et al., 2015). Further studies are still needed to reveal the underlying mechanisms of this trans-differentiation process.
Kolla et al. (2020) based on scRNA sequencing technology in E14 and E16 day mouse cochlea found two types of precursor cells expressing Cdkn1b and Sox2 marker genes, which these two groups of cells are located in the medial and lateral regions of the Kölliker’ organ structure, respectively (Kolla et al., 2020). Furthermore, Kubota et al. (2021) used P2 day mice and similarly found that Kölliker’s organ-supporting cell subtypes located in the lateral and medial regions can regenerate into hair cells and supporting cells.
Chen et al. (2021b) also showed that four cell subtypes gradually developed into two different trajectory directions, with one part towards the outer hair cells subtype, and the other part eventually shifting toward the fate of the inner hair cells. The analysis of cell developmental trajectories suggests that these four cell subtypes have the potential to trans-differentiate into inner and outer hair cells, two of which may have the potential to trans-differentiate into outer hair cells, and the other two subtypes have the potential to trans-differentiate into inner hair cells (Chen et al., 2021b).
Differentiation of Kölliker’s organ-supporting cells to the hair cell phenotype can be induced by overexpression of Math1 and Atoh1 genes, such cells with differentiation potential are fewer and the cell transformation rate is lower (Zheng and Gao, 2000; Shou et al., 2003; Kelly et al., 2012). Therefore, future research should be devoted to improving the efficiency of Kölliker’s organ-supporting cell trans-differentiation into hair cells, and establishing an efficient and safe induction system. Meanwhile, how to induce the transformed neonatal hair cells to form functional mature cilia bundles and establish normal and durable synaptic connections with spiral ganglia (Chai et al., 2017; Li et al., 2019; Xia et al., 2020), to truly achieve functional recovery of neonatal hair cells, needs further study, and immortalized cell lines of hair cell progenitors need to be established.
In the future, there is still a need to optimize the conditions of mouse inner ear stem cell isolated culture and proliferation, to establish a safe and efficient technology system for differentiation of inner ear stem cells into mature hair cells (Wei et al., 2021; Guo et al., 2022; Hu et al., 2022; Tao et al., 2022), to screen mouse stem cell lines that can be used for stem cell therapy (Liu et al., 2018; Tang et al., 2019); discovering new molecular markers specific for inner ear hair cells and their precursor cells (Qi et al., 2019), thus providing conditions for the establishment of rapid and non-destructive cell identification and sorting techniques, and thus laying a solid theoretical foundation and providing technical support for stem cell therapy for sensorineural deafness technical support.
JC and DG drafted the manuscript. JY and LS revised the manuscript. All authors contributed to the article and approved the submitted version.
This study was funded by the National Natural Science Foundation of China (81873689, 82271179, and 82230035).
The authors declare that the research was conducted in the absence of any commercial or financial relationships that could be construed as a potential conflict of interest.
We are grateful to the National Natural Science Foundation for supporting this study, and to LC Sciences for their support and guidance.
Aburto, M. R., Sánchez-Calderón, H., Hurlé, J. M., Varela-Nieto, I., and Magariños, M. (2012). Early otic development depends on autophagy for apoptotic cell clearance and neural differentiation. Cell Death Dis. 3:e394. doi: 10.1038/cddis.2012.132
Adrain, C., and Martin, S. J. (2001). The mitochondrial apoptosome: a killer unleashed by the cytochrome seas. Trends Biochem. Sci. 26, 390–397. doi: 10.1016/s0968-0004(01)01844-8
Bermingham, N. A., Hassan, B. A., Price, S. D., Vollrath, M. A., Ben-Arie, N., Eatock, R. A., et al. (1999). Math1: an essential gene for the generation of inner ear hair cells. Science 284, 1837–1841. doi: 10.1126/science.284.5421.1837
Beurg, M., Safieddine, S., Roux, I., Bouleau, Y., Petit, C., Dulon, D., et al. (2008). Calcium- and otoferlin-dependent exocytosis by immature outer hair cells. J. Neurosci. 28, 1798–1803. doi: 10.1523/JNEUROSCI.4653-07.2008
Bianchi, L. M., and Liu, H. (1999). Comparison of ephrin-A ligand EphA receptor distribution in the developing inner ear. Anat. Rec. 254, 127–134. doi: 10.1002/(SICI)1097-0185(19990101)254:1<127::AID-AR16>3.0.CO;2-Q
Blankenship, A. G., and Feller, M. B. (2010). Mechanisms underlying spontaneous patterned activity in developing neural circuits. Nat. Rev. Neurosci. 11, 18–29. doi: 10.1038/nrn2759
Borse, V., Kaur, T., Hinton, A., Ohlemiller, K., and Warchol, M. E. (2021). Programmed cell death recruits macrophages into the developing mouse cochlea. Front. Cell. Dev. Biol. 9:777836. doi: 10.3389/fcell.2021.777836
Bramhall, N. F., Shi, F., Arnold, K., Hochedlinger, K., and Edge, A. S. (2014). Lgr5-positive supporting cells generate new hair cells in the postnatal cochlea. Stem Cell Rep. 2, 311–322. doi: 10.1016/j.stemcr.2014.01.008
Bu, C., Xu, L., Han, Y., Wang, M., Wang, X., Liu, W., et al. (2022). c-Myb protects cochlear hair cells from cisplatin-induced damage via the PI3K/Akt signaling pathway. Cell Death Discov. 8:78. doi: 10.1038/s41420-022-00879-9
Chai, R., Kuo, B., Wang, T., Liaw, E. J., Xia, A., Jan, T. A., et al. (2012). Wnt signaling induces proliferation of sensory precursors in the postnatal mouse cochlea. Proc. Natl. Acad. Sci. U S A 109, 8167–8172. doi: 10.1073/pnas.1202774109
Chai, R., Li, G. L., Wang, J., and Huang, H. (2018). Hearing loss: reestablish the neural plasticity in regenerated spiral ganglion neurons and sensory hair cells 2018. Neural Plast. 2018:4759135. doi: 10.1155/2018/4759135
Chai, R., Li, G. L., Wang, J., and Zou, J. (2017). Hearing loss: reestablish the neural plasticity in regenerated spiral ganglion neurons and sensory hair cells. Neural Plast. 2017:1807581. doi: 10.1155/2017/1807581
Chai, R., Li, H., Yang, T., Sun, S., and Yuan, Y. (2022). Editorial: hearing loss: mechanisms and prevention. Front. Cell. Dev. Biol. 10:838271. doi: 10.3389/fcell.2022.838271
Chai, R., Xia, A., Wang, T., Jan, T. A., Hayashi, T., Bermingham-McDonogh, O., et al. (2011). Dynamic expression of Lgr5, a Wnt target gene, in the developing and mature mouse cochlea. J. Assoc. Res. Otolaryngol. 12, 455–469. doi: 10.1007/s10162-011-0267-2
Chen, J., Chen, P., He, B., Gong, T., Li, Y., Zhang, J., et al. (2021a). Connexin30-deficiency causes mild hearing loss with the reduction of endocochlear potential and ATP release. Front. Cell. Neurosci. 15:819194. doi: 10.3389/fncel.2021.819194
Chen, J., Gao, D., Chen, J., Hou, S., He, B., Li, Y., et al. (2021b). Single-Cell RNA sequencing analysis reveals greater epithelial ridge cells degeneration during postnatal development of cochlea in rats. Front. Cell. Dev. Biol. 9:719491. doi: 10.3389/fcell.2021.719491
Chen, P., Johnson, J. E., Zoghbi, H. Y., and Segil, N. (2002). The role of Math1 in inner ear development: uncoupling the establishment of the sensory primordium from hair cell fate determination. Development 129, 2495–2505. doi: 10.1242/dev.129.10.2495
Chen, Y., Li, L., Ni, W., Zhang, Y., Sun, S., Miao, D., et al. (2015). Bmi1 regulates auditory hair cell survival by maintaining redox balance. Cell Death Dis. 6:e1605. doi: 10.1038/cddis.2014.549
Chen, Y., Gu, Y., Li, Y., Li, G. L., Chai, R., Li, W., et al. (2021c). Generation of mature and functional hair cells by co-expression of Gfi1, Pou4f3 and Atoh1 in the postnatal mouse cochlea. Cell Rep. 35:109016. doi: 10.1016/j.celrep.2021.109016
Chen, Y., Qiang, R., Zhang, Y., Cao, W., Wu, L., Jiang, P., et al. (2021d). The expression and roles of the super elongation complex in mouse cochlear Lgr5+ progenitor cells. Front. Cell. Neurosci. 15:735723. doi: 10.3389/fncel.2021.735723
Chen, Y., Lu, X., Guo, L., Ni, W., Zhang, Y., Zhao, L., et al. (2017). Hedgehog signaling promotes the proliferation and subsequent hair cell formation of progenitor cells in the neonatal mouse cochlea. Front. Mol. Neurosci. 10:426. doi: 10.3389/fnmol.2017.00426
Cheng, C., Guo, L., Lu, L., Xu, X., Zhang, S., Gao, J., et al. (2017). Characterization of the transcriptomes of Lgr5+ hair cell progenitors and Lgr5- supporting cells in the mouse cochlea. Front. Mol. Neurosci. 10:122. doi: 10.3389/fnmol.2017.00122
Cheng, C., Wang, Y., Guo, L., Lu, X., Zhu, W., Muhammad, W., et al. (2019). Age-related transcriptome changes in Sox2+ supporting cells in the mouse cochlea. Stem Cell Res. Ther. 10:365. doi: 10.1186/s13287-019-1437-0
Cheng, Y. C., Amoyel, M., Qiu, X., Jiang, Y. J., Xu, Q., Wilkinson, D. G., et al. (2004). Notch activation regulates the segregation and differentiation of rhombomere boundary cells in the zebrafish hindbrain. Dev. Cell 6, 539–550. doi: 10.1016/s1534-5807(04)00097-8
Cho, J. H., Dimri, M., and Dimri, G. P. (2013). A positive feedback loop regulates the expression of polycomb group protein BMI1 via WNT signaling pathway. J. Biol. Chem. 288, 3406–3418. doi: 10.1074/jbc.M112.422931
Coen, L., Le Blay, K., Rowe, I., and Demeneix, B. A. (2007). Caspase-9 regulates apoptosis/proliferation balance during metamorphic brain remodeling in Xenopus. Proc. Natl. Acad. Sci. U S A 104, 8502–8507. doi: 10.1073/pnas.0608877104
Cox, B. C., Chai, R., Lenoir, A., Liu, Z., Zhang, L., Nguyen, D. H., et al. (2014). Spontaneous hair cell regeneration in the neonatal mouse cochlea in vivo. Development 141, 816–829. doi: 10.1242/dev.103036
Dahmann, C., Oates, A. C., and Brand, M. (2011). Boundary formation and maintenance in tissue development. Nat. Rev. Genet. 12, 43–55. doi: 10.1038/nrg2902
Dayaratne, M. W., Vlajkovic, S. M., Lipski, J., and Thorne, P. R. (2014). Kölliker’s organ and the development of spontaneous activity in the auditory system: implications for hearing dysfunction. Biomed. Res. Int. 2014:367939. doi: 10.1155/2014/367939
Defourny, J., Lallemend, F., and Malgrange, B. (2011). Structure and development of cochlear afferent innervation in mammals. Am. J. Physiol. Cell Physiol. 301, C750–C761. doi: 10.1152/ajpcell.00516.2010
Defourny, J., Mateo Sánchez, S., Schoonaert, L., Robberecht, W., Davy, A., Nguyen, L., et al. (2015). Cochlear supporting cell transdifferentiation and integration into hair cell layers by inhibition of ephrin-B2 signalling. Nat. Commun. 6:7017. doi: 10.1038/ncomms8017
Driver, E. C., Northrop, A., and Kelley, M. W. (2017). Cell migration, intercalation and growth regulate mammalian cochlear extension. Development 144, 3766–3776. doi: 10.1242/dev.151761
Driver, E. C., Pryor, S. P., Hill, P., Turner, J., Rüther, U., Biesecker, L. G., et al. (2008). Hedgehog signaling regulates sensory cell formation and auditory function in mice and humans. J. Neurosci. 28, 7350–7358. doi: 10.1523/JNEUROSCI.0312-08.2008
Ebeid, M., Barnas, K., Zhang, H., Yaghmour, A., Noreikaite, G., and Bjork, B. C. (2022). PRDM16 expression and function in mammalian cochlear development. Dev. Dyn. doi: 10.1002/dvdy.480
PubMed Abstract | CrossRef Full Text | Google Scholar[Online ahead of print].
Fang, Q., Zhang, Y., Chen, X., Li, H., Cheng, L., Zhu, W., et al. (2019). Three-dimensional graphene enhances neural stem cell proliferation through metabolic regulation. Front. Bioeng. Biotechnol. 7:436. doi: 10.3389/fbioe.2019.00436
Forsythe, I. D. (2007). Hearing: a fantasia on Kölliker’s organ. Nature 450, 43–44. doi: 10.1038/450043a
Fu, X., Li, P., Zhang, L., Song, Y., An, Y., Zhang, A., et al. (2022). Activation of Rictor/mTORC2 signaling acts as a pivotal strategy to protect against sensorineural hearing loss. Proc. Natl. Acad. Sci. U S A 119:e2107357119. doi: 10.1073/pnas.2107357119
Fu, X., Sun, X., Zhang, L., Jin, Y., Chai, R., Yang, L., et al. (2018). Tuberous sclerosis complex-mediated mTORC1 overactivation promotes age-related hearing loss. J. Clin. Invest. 128, 4938–4955. doi: 10.1172/JCI98058
Fumasoni, I., Meani, N., Rambaldi, D., Scafetta, G., Alcalay, M., Ciccarelli, F. D., et al. (2007). Family expansion and gene rearrangements contributed to the functional specialization of PRDM genes in vertebrates. BMC Evol. Biol. 7:187. doi: 10.1186/1471-2148-7-187
Gale, N. W., Holland, S. J., Valenzuela, D. M., Flenniken, A., Pan, L., Ryan, T. E., et al. (1996). Eph receptors and ligands comprise two major specificity subclasses and are reciprocally compartmentalized during embryogenesis. Neuron 17, 9–19. doi: 10.1016/s0896-6273(00)80276-7
Gao, S., Cheng, C., Wang, M., Jiang, P., Zhang, L., Wang, Y., et al. (2019). Blebbistatin inhibits neomycin-induced apoptosis in hair cell-like HEI-OC-1 cells and in cochlear hair cells. Front. Cell. Neurosci. 13:590. doi: 10.3389/fncel.2019.00590
Geal-Dor, M., Freeman, S., Li, G., and Sohmer, H. (1993). Development of hearing in neonatal rats: air and bone conducted ABR thresholds. Hear. Res. 69, :236–242. doi: 10.1016/0378-5955(93)90113-f
Giorgi, C., Danese, A., Missiroli, S., Patergnani, S., and Pinton, P. (2018). Calcium dynamics as a machine for decoding signals. Trends Cell Biol. 28, 258–273. doi: 10.1016/j.tcb.2018.01.002
Goodenough, D. A., and Paul, D. L. (2009). Gap junctions. Cold. Spring. Harb. Perspect. Biol. 1:a002576. doi: 10.1101/cshperspect.a002576
Gregorieff, A., Liu, Y., Inanlou, M. R., Khomchuk, Y., and Wrana, J. L. (2015). Yap-dependent reprogramming of Lgr5+ stem cells drives intestinal regeneration and cancer. Nature 526, 715–718. doi: 10.1038/nature15382
Gubbels, S. P., Woessner, D. W., Mitchell, J. C., Ricci, A. J., and Brigande, J. V. (2008). Functional auditory hair cells produced in the mammalian cochlea by in utero gene transfer. Nature 455, 537–541. doi: 10.1038/nature07265
Guo, L., Cao, W., Niu, Y., He, S., Chai, R., Yang, J., et al. (2021). Autophagy regulates the survival of hair cells and spiral ganglion neurons in cases of noise, ototoxic drug and age-induced sensorineural hearing loss. Front. Cell. Neurosci. 15:760422. doi: 10.3389/fncel.2021.760422
Guo, R., Xiao, M., Zhao, W., Zhou, S., Hu, Y., Liao, M., et al. (2022). 2D Ti(3)C(2)T(x)MXene couples electrical stimulation to promote proliferation and neural differentiation of neural stem cells. Acta Biomater. 139, 105–117. doi: 10.1016/j.actbio.2020.12.035
He, S., and Yang, J. (2011). Maturation of neurotransmission in the developing rat cochlea: immunohistochemical evidence from differential expression of synaptophysin and synaptobrevin 2. Eur. J. Histochem. 55:e2. doi: 10.4081/ejh.2011.e2
He, Y., and Yang, J. (2015a). ATP release mechanism from the supporting cells in the Kölliker organ in vitro in the cochlea of newborn rat. Zhonghua Er Bi Yan Hou Tou Jing Wai Ke Za Zhi 50, 43–49. doi: 10.3760/cma.j.issn.1673-0860.2015.01.010
He, Y., and Yang, J. (2015b). The study on the proliferation and the apoptosis factors in vitro of Kölliker organ supporting cells in the cochlea of newborn rat. Lin Chung Er Bi Yan Hou Tou Jing Wai Ke Za Zhi 29, 152–159. doi: 10.13201/j.issn.1001-1781.2015.02.015
He, Z., Guo, L., Shu, Y., Fang, Q., Zhou, H., Liu, Y., et al. (2017). Autophagy protects auditory hair cells against neomycin-induced damage. Autophagy 13, 1884–1904. doi: 10.1080/15548627.2017.1359449
He, Z. H., Li, M., Fang, Q. J., Liao, F. L., Zou, S. Y., Wu, X., et al. (2021). FOXG1 promotes aging inner ear hair cell survival through activation of the autophagy pathway. Autophagy 17, 4341–4362. doi: 10.1080/15548627.2021.1916194
He, Z. H., Zou, S. Y., Li, M., Liao, F. L., Wu, X., Sun, H. Y., et al. (2020). The nuclear transcription factor FoxG1 affects the sensitivity of mimetic aging hair cells to inflammation by regulating autophagy pathways. Redox. Biol. 28:101364. doi: 10.1016/j.redox.2019.101364
Hinojosa, R. (1977). A note on development of Corti’s organ. Acta Otolaryngol. 84, 238–251. doi: 10.3109/00016487709123963
Hochman, J. B., Stockley, T. L., Shipp, D., Lin, V. Y., Chen, J. M., Nedzelski, J. M., et al. (2010). Prevalence of Connexin 26 (GJB2) and Pendred (SLC26A4) mutations in a population of adult cochlear implant candidates. Otol. Neurotol. 31, 919–922. doi: 10.1097/MAO.0b013e3181e3d324
Hou, S., Chen, P., Chen, J., Chen, J., Sun, L., Chen, J., et al. (2020). Distinct expression patterns of apoptosis and autophagy-associated proteins and genes during postnatal development of spiral ganglion neurons in rat. Neural Plast. 2020:9387560. doi: 10.1155/2020/9387560
Hou, S., Chen, J., and Yang, J. (2019). Autophagy precedes apoptosis during degeneration of the Kölliker’s organ in the development of rat cochlea. Eur. J. Histochem. 63:3025. doi: 10.4081/ejh.2019.3025
Hu, Y., Chen, Z., Wang, H., Guo, J., Cai, J., Chen, X., et al. (2022). Conductive nerve guidance conduits based on morpho butterfly wings for peripheral nerve repair. ACS Nano 16, 1868–1879. doi: 10.1021/acsnano.1c11627
Inoshita, A., Karasawa, K., Funakubo, M., Miwa, A., Ikeda, K., and Kamiya, K. (2014). Dominant negative connexin26 mutation R75W causing severe hearing loss influences normal programmed cell death in postnatal organ of Corti. BMC Genet. 15:1. doi: 10.1186/1471-2156-15-1
Jia, X., Wen, Z., Sun, Q., Zhao, X., Yang, H., Shi, X., et al. (2019). Apatinib suppresses the proliferation and apoptosis of gastric cancer cells via the PI3K/Akt signaling pathway. J. BUON 24, 1985–1991.
Johnson, S. L., Ceriani, F., Houston, O., Polishchuk, R., Polishchuk, E., Crispino, G., et al. (2017). Connexin-mediated signaling in nonsensory cells is crucial for the development of sensory inner hair cells in the mouse cochlea. J. Neurosci. 37, 258–268. doi: 10.1523/JNEUROSCI.2251-16.2016
Kamiya, K., Takahashi, K., Kitamura, K., Momoi, T., and Yoshikawa, Y. (2001). Mitosis and apoptosis in postnatal auditory system of the C3H/He strain. Brain Res. 901, 296–302. doi: 10.1016/s0006-8993(01)02300-9
Kelly, M. C., Chang, Q., Pan, A., Lin, X., and Chen, P. (2012). Atoh1 directs the formation of sensory mosaics and induces cell proliferation in the postnatal mammalian cochlea in vivo. J. Neurosci. 32, 6699–6710. doi: 10.1523/JNEUROSCI.5420-11.2012
Kim, D. K., Kim, J. A., Park, J., Niazi, A., Almishaal, A., and Park, S. (2019). The release of surface-anchored α-tectorin, an apical extracellular matrix protein, mediates tectorial membrane organization. Sci. Adv. 5:eaay6300. doi: 10.1126/sciadv.aay6300
Knipper, M., Gestwa, L., Ten Cate, W. J., Lautermann, J., Brugger, H., Maier, H., et al. (1999). Distinct thyroid hormone-dependent expression of TrKB and p75NGFR in nonneuronal cells during the critical TH-dependent period of the cochlea. J. Neurobiol. 38, 338–356.
Kolla, L., Kelly, M. C., Mann, Z. F., Anaya-Rocha, A., Ellis, K., Lemons, A., et al. (2020). Characterization of the development of the mouse cochlear epithelium at the single cell level. Nat. Commun. 11:2389. doi: 10.1038/s41467-020-16113-y
Komatsu, M., Waguri, S., Ueno, T., Iwata, J., Murata, S., Tanida, I., et al. (2005). Impairment of starvation-induced and constitutive autophagy in Atg7-deficient mice. J. Cell. Biol. 169, 425–434. doi: 10.1083/jcb.200412022
Korrapati, S., Roux, I., Glowatzki, E., and Doetzlhofer, A. (2013). Notch signaling limits supporting cell plasticity in the hair cell-damaged early postnatal murine cochlea. PLoS One 8:e73276. doi: 10.1371/journal.pone.0073276
Kubota, M., Scheibinger, M., Jan, T. A., and Heller, S. (2021). Greater epithelial ridge cells are the principal organoid-forming progenitors of the mouse cochlea. Cell Rep. 34:108646. doi: 10.1016/j.celrep.2020.108646
Lanford, P. J., Lan, Y., Jiang, R., Lindsell, C., Weinmaster, G., Gridley, T., et al. (1999). Notch signalling pathway mediates hair cell development in mammalian cochlea. Nat. Genet. 21, 289–292. doi: 10.1038/6804
Legan, P. K., Goodyear, R. J., Morín, M., Mencia, A., Pollard, H., Olavarrieta, L., et al. (2014). Three deaf mice: mouse models for TECTA-based human hereditary deafness reveal domain-specific structural phenotypes in the tectorial membrane. Hum. Mol. Genet. 23, 2551–2568. doi: 10.1093/hmg/ddt646
Lelli, A., Asai, Y., Forge, A., Holt, J. R., and Géléoc, G. S. (2009). Tonotopic gradient in the developmental acquisition of sensory transduction in outer hair cells of the mouse cochlea. J. Neurophysiol. 101, 2961–2973. doi: 10.1152/jn.00136.2009
Li, G., Chen, K., You, D., Xia, M., Li, W., Fan, S., et al. (2019). Laminin-coated electrospun regenerated silk fibroin mats promote neural progenitor cell proliferation, differentiation and survival in vitro. Front. Bioeng. Biotechnol. 7:190. doi: 10.3389/fbioe.2019.00190
Li, H., Song, Y., He, Z., Chen, X., Wu, X., Li, X., et al. (2018). Meclofenamic acid reduces reactive oxygen species accumulation and apoptosis, inhibits excessive autophagy and protects hair cell-like HEI-OC1 cells from cisplatin-induced damage. Front. Cell. Neurosci. 12:139. doi: 10.3389/fncel.2018.00139
Liang, Y., Huang, L., and Yang, J. (2009). Differential expression of ryanodine receptor in the developing rat cochlea. Eur. J. Histochem. 53:e30. doi: 10.4081/ejh.2009.e30
Lim, D. J. (1986). Functional structure of the organ of Corti: a review. Hear. Res. 22, 117–146. doi: 10.1016/0378-5955(86)90089-4
Lim, D. J., and Anniko, M. (1985). Developmental morphology of the mouse inner ear. A scanning electron microscopic observation. Acta Otolaryngol. Suppl. 422, 1–69.
Liu, J., Cai, L., He, Y., and Yang, J. (2017). Apoptosis pattern and alterations of expression of apoptosis-related factors of supporting cells in Kölliker’s organ in vivo in early stage after birth in rats. Eur. J. Histochem. 61:2706. doi: 10.4081/ejh.2017.2706
Liu, L., Chen, Y., Qi, J., Zhang, Y., He, Y., Ni, W., et al. (2016). Wnt activation protects against neomycin-induced hair cell damage in the mouse cochlea. Cell Death Dis. 7:e2136. doi: 10.1038/cddis.2016.35
Liu, Z., Dearman, J. A., Cox, B. C., Walters, B. J., Zhang, L., Ayrault, O., et al. (2012). Age-dependent in vivo conversion of mouse cochlear pillar and Deiters’ cells to immature hair cells by Atoh1 ectopic expression. J. Neurosci. 32, 6600–6610. doi: 10.1523/JNEUROSCI.0818-12.2012
Liu, Y., Qi, J., Chen, X., Tang, M., Chu, C., Zhu, W., et al. (2019). Critical role of spectrin in hearing development and deafness. Sci. Adv. 5:eaav7803. doi: 10.1126/sciadv.aav7803
Liu, Z., Tang, M., Zhao, J., Chai, R., and Kang, J. (2018). Looking into the future: toward advanced 3D biomaterials for stem-cell-based regenerative medicine. Adv. Mater. 30:e1705388. doi: 10.1002/adma.201705388
Liu, W., Xu, X., Fan, Z., Sun, G., Han, Y., Zhang, D., et al. (2019). Wnt signaling activates TP53-induced glycolysis and apoptosis regulator and protects against cisplatin-induced spiral ganglion neuron damage in the mouse cochlea. Antioxid. Redox Signal. 30, 1389–1410. doi: 10.1089/ars.2017.7288
Liu, W., Xu, L., Wang, X., Zhang, D., Sun, G., Wang, M., et al. (2021). PRDX1 activates autophagy via the PTEN-AKT signaling pathway to protect against cisplatin-induced spiral ganglion neuron damage. Autophagy 17, 4159–4181. doi: 10.1080/15548627.2021.1905466
Lu, X., Sun, S., Qi, J., Li, W., Liu, L., Zhang, Y., et al. (2017). Bmi1 regulates the proliferation of cochlear supporting cells via the canonical Wnt signaling pathway. Mol. Neurobiol. 54, 1326–1339. doi: 10.1007/s12035-016-9686-8
Mammano, F. (2013). ATP-dependent intercellular Ca2+ signaling in the developing cochlea: facts, fantasies and perspectives. Semin. Cell Dev. Biol. 24, 31–39. doi: 10.1016/j.semcdb.2012.09.004
Marcotti, W. (2012). Functional assembly of mammalian cochlear hair cells. Exp. Physiol. 97, 438–451. doi: 10.1113/expphysiol.2011.059303
Mazzarda, F., D’Elia, A., Massari, R., De Ninno, A., Bertani, F. R., Businaro, L., et al. (2020). Organ-on-chip model shows that ATP release through connexin hemichannels drives spontaneous Ca2+ signaling in non-sensory cells of the greater epithelial ridge in the developing cochlea. Lab Chip 20, 3011–3023. doi: 10.1039/d0lc00427h
McGovern, M. M., Zhou, L., Randle, M. R., and Cox, B. C. (2018). Spontaneous hair cell regeneration is prevented by increased notch signaling in supporting cells. Front. Cell Neurosci. 12:120. doi: 10.3389/fncel.2018.00120
Miko, I. J., Henkemeyer, M., and Cramer, K. S. (2008). Auditory brainstem responses are impaired in EphA4 and ephrin-B2 deficient mice. Hear. Res. 235, 39–46. doi: 10.1016/j.heares.2007.09.003
Millimaki, B. B., Sweet, E. M., and Riley, B. B. (2010). Sox2 is required for maintenance and regeneration, but not initial development, of hair cells in the zebrafish inner ear. Dev. Biol. 338, 262–269. doi: 10.1016/j.ydbio.2009.12.011
Mizutari, K., Fujioka, M., Hosoya, M., Bramhall, N., Okano, H. J., Okano, H., et al. (2013). Notch inhibition induces cochlear hair cell regeneration and recovery of hearing after acoustic trauma. Neuron 77, 58–69. doi: 10.1016/j.neuron.2012.10.032
Mullen, L. M., Pak, K. K., Chavez, E., Kondo, K., Brand, Y., and Ryan, A. F. (2012). Ras/p38 and PI3K/Akt but not Mek/Erk signaling mediate BDNF-induced neurite formation on neonatal cochlear spiral ganglion explants. Brain Res. 1430, 25–34. doi: 10.1016/j.brainres.2011.10.054
Mustapha, M., Fang, Q., Gong, T. W., Dolan, D. F., Raphael, Y., Camper, S. A., et al. (2009). Deafness and permanently reduced potassium channel gene expression and function in hypothyroid Pit1dw mutants. J. Neurosci. 29, 1212–1223. doi: 10.1523/JNEUROSCI.4957-08.2009
Ng, L., Kelley, M. W., and Forrest, D. (2013). Making sense with thyroid hormone–the role of T3 in auditory development. Nat. Rev. Endocrinol. 9, 296–307. doi: 10.1038/nrendo.2013.58
Ni, W., Zeng, S., Li, W., Chen, Y., Zhang, S., Tang, M., et al. (2016). Wnt activation followed by Notch inhibition promotes mitotic hair cell regeneration in the postnatal mouse cochlea. Oncotarget 7, 66754–66768. doi: 10.18632/oncotarget.11479
Nishani Dayaratne, M. W., Vlajkovic, S. M., Lipski, J., and Thorne, P. R. (2015). Putative role of border cells in generating spontaneous morphological activity within Kölliker’s organ. Hear. Res. 330, 90–97. doi: 10.1016/j.heares.2015.06.017
Peeters, R. P., Ng, L., Ma, M., and Forrest, D. (2015). The timecourse of apoptotic cell death during postnatal remodeling of the mouse cochlea and its premature onset by triiodothyronine (T3). Mol. Cell. Endocrinol. 407, 1–8. doi: 10.1016/j.mce.2015.02.025
Pelletier, J., Thomas, G., and Volarević, S. (2018). Ribosome biogenesis in cancer: new players and therapeutic avenues. Nat. Rev. Cancer 18, 51–63. doi: 10.1038/nrc.2017.104
Peng, Y., Chen, J., He, S., Yang, J., and Wu, H. (2012). Release of ATP from marginal cells in the cochlea of neonatal rats can be induced by changes in extracellular and intracellular ion concentrations. PLoS One 7:e47124. doi: 10.1371/journal.pone.0047124
Qi, J., Liu, Y., Chu, C., Chen, X., Zhu, W., Shu, Y., et al. (2019). A cytoskeleton structure revealed by super-resolution fluorescence imaging in inner ear hair cells. Cell Discov. 5:12. doi: 10.1038/s41421-018-0076-4
Qu, X., Zou, Z., Sun, Q., Luby-Phelps, K., Cheng, P., Hogan, R. N., et al. (2007). Autophagy gene-dependent clearance of apoptotic cells during embryonic development. Cell 128, 931–946. doi: 10.1016/j.cell.2006.12.044
Richardson, R. T., and Atkinson, P. J. (2015). Atoh1 gene therapy in the cochlea for hair cell regeneration. Expert Opin. Biol. Ther. 15, 417–430. doi: 10.1517/14712598.2015.1009889
Richardson, G. P., Lukashkin, A. N., and Russell, I. J. (2008). The tectorial membrane: one slice of a complex cochlear sandwich. Curr. Opin. Otolaryngol. Head Neck Surg. 16, 458–464. doi: 10.1097/MOO.0b013e32830e20c4
Rodriguez, L., Simeonato, E., Scimemi, P., Anselmi, F., Calì, B., Crispino, G., et al. (2012). Reduced phosphatidylinositol 4,5-bisphosphate synthesis impairs inner ear Ca2+ signaling and high-frequency hearing acquisition. Proc. Natl. Acad. Sci. U S A 109, 14013–14018. doi: 10.1073/pnas.1211869109
Rusch, A., Ng, L., Goodyear, R., Oliver, D., Lisoukov, I., Vennstrom, B., et al. (2001). Retardation of cochlear maturation and impaired hair cell function caused by deletion of all known thyroid hormone receptors. J. Neurosci. 21, 9792–9800. doi: 10.1523/JNEUROSCI.21-24-09792.2001
Sato, T., Doi, K., Taniguchi, M., Yamashita, T., Kubo, T., and Tohyama, M. (2006). Progressive hearing loss in mice carrying a mutation in the p75 gene. Brain Res. 1091, 224–234. doi: 10.1016/j.brainres.2005.12.104
Sendin, G., Bourien, J., Rassendren, F., Puel, J. L., and Nouvian, R. (2014). Spatiotemporal pattern of action potential firing in developing inner hair cells of the mouse cochlea. Proc. Natl. Acad. Sci. U S A 111, 1999–2004. doi: 10.1073/pnas.1319615111
Shi, F., Hu, L., and Edge, A. S. (2013). Generation of hair cells in neonatal mice by β-catenin overexpression in Lgr5-positive cochlear progenitors. Proc. Natl. Acad. Sci. U S A 110, 13851–13856. doi: 10.1073/pnas.1219952110
Shi, F., Kempfle, J. S., and Edge, A. S. (2012). Wnt-responsive Lgr5-expressing stem cells are hair cell progenitors in the cochlea. J. Neurosci. 32, 9639–9648. doi: 10.1523/JNEUROSCI.1064-12.2012
Shou, J., Zheng, J. L., and Gao, W. Q. (2003). Robust generation of new hair cells in the mature mammalian inner ear by adenoviral expression of Hath1. Mol. Cell. Neurosci. 23, 169–179. doi: 10.1016/s1044-7431(03)00066-6
Shull, L. C., Sen, R., Menzel, J., Goyama, S., Kurokawa, M., and Artinger, K. B. (2020). The conserved and divergent roles of Prdm3 and Prdm16 in zebrafish and mouse craniofacial development. Dev. Biol. 461, 132–144. doi: 10.1016/j.ydbio.2020.02.006
Su, Y. X., Hou, C. C., and Yang, W. X. (2015). Control of hair cell development by molecular pathways involving Atoh1, Hes1 and Hes5. Gene 558, 6–24. doi: 10.1016/j.gene.2014.12.054
Sun, L., Gao, D., Chen, J., Hou, S., Li, Y., Huang, Y., et al. (2022). Failure Of hearing acquisition in mice with reduced expression of connexin 26 correlates with the abnormal phasing of apoptosis relative to autophagy and defective ATP-dependent Ca2+ signaling in Kölliker’s Organ. Front. Cell. Neurosci. 16:816079. doi: 10.3389/fncel.2022.816079
Sundaresan, S., Kong, J. H., Fang, Q., Salles, F. T., Wangsawihardja, F., Ricci, A. J., et al. (2016). Thyroid hormone is required for pruning, functioning and long-term maintenance of afferent inner hair cell synapses. Eur. J. Neurosci. 43, 148–161. doi: 10.1111/ejn.13081
Swift, M. R., and Weinstein, B. M. (2009). Arterial-venous specification during development. Circ. Res. 104, 576–588. doi: 10.1161/CIRCRESAHA.108.188805
Tang, M., Li, J., He, L., Guo, R., Yan, X., Li, D., et al. (2019). Transcriptomic profiling of neural stem cell differentiation on graphene substrates. Colloids Surf. B Biointerfaces 182:110324. doi: 10.1016/j.colsurfb.2019.06.054
Tang, D., Lin, Q., He, Y., Chai, R., and Li, H. (2016). Inhibition of H3K9me2 reduces hair cell regeneration after hair cell loss in the zebrafish lateral line by down-regulating the Wnt and Fgf signaling pathways. Front. Mol. Neurosci. 9:39. doi: 10.3389/fnmol.2016.00039
Tao, Y., Liu, X., Yang, L., Chu, C., Tan, F., Yu, Z., et al. (2022). AAV-ie-K558R mediated cochlear gene therapy and hair cell regeneration. Signal Transduct. Target. Ther. 7:109. doi: 10.1038/s41392-022-00938-8
Tossell, K., Kiecker, C., Wizenmann, A., Lang, E., and Irving, C. (2011). Notch signalling stabilises boundary formation at the midbrain-hindbrain organiser. Development 138, 3745–3757. doi: 10.1242/dev.070318
Tritsch, N. X., and Bergles, D. E. (2010). Developmental regulation of spontaneous activity in the Mammalian cochlea. J. Neurosci. 30, 1539–1550. doi: 10.1523/JNEUROSCI.3875-09.2010
Tritsch, N. X., Yi, E., Gale, J. E., Glowatzki, E., and Bergles, D. E. (2007). The origin of spontaneous activity in the developing auditory system. Nature 450, 50–55. doi: 10.1038/nature06233
Tritsch, N. X., Zhang, Y. X., Ellis-Davies, G., and Bergles, D. E. (2010). ATP-induced morphological changes in supporting cells of the developing cochlea. Purinergic Signal. 6, 155–166. doi: 10.1007/s11302-010-9189-4
Uziel, A. (1986). Periods of sensitivity to thyroid hormone during the development of the organ of Corti. Acta Otolaryngol. Suppl. 429, 23–27. doi: 10.3109/00016488609122726
Uziel, A., Gabrion, J., Ohresser, M., and Legrand, C. (1981). Effects of hypothyroidism on the structural development of the organ of Corti in the rat. Acta Otolaryngol. 92, 469–480. doi: 10.3109/00016488109133286
Walters, B. J., Coak, E., Dearman, J., Bailey, G., Yamashita, T., Kuo, B., et al. (2017). In vivo interplay between p27Kip1, GATA3, ATOH1 and POU4F3 converts non-sensory cells to hair cells in adult mice. Cell Rep. 19, 307–320. doi: 10.1016/j.celrep.2017.03.044
Wang, H. C., and Bergles, D. E. (2015). Spontaneous activity in the developing auditory system. Cell Tissue Res. 361, 65–75. doi: 10.1007/s00441-014-2007-5
Wang, T., Chai, R., Kim, G. S., Pham, N., Jansson, L., Nguyen, D. H., et al. (2015). Lgr5+ cells regenerate hair cells via proliferation and direct transdifferentiation in damaged neonatal mouse utricle. Nat. Commun. 6:6613. doi: 10.1038/ncomms7613
Waqas, M., Zhang, S., He, Z., Tang, M., and Chai, R. (2016). Role of Wnt and Notch signaling in regulating hair cell regeneration in the cochlea. Front. Med. 10, 237–249. doi: 10.1007/s11684-016-0464-9
Wei, H., Chen, Z., Hu, Y., Cao, W., Ma, X., Zhang, C., et al. (2021). Topographically conductive butterfly wing substrates for directed spiral ganglion neuron growth. Small 17:e2102062. doi: 10.1002/smll.202102062
Woods, C., Montcouquiol, M., and Kelley, M. W. (2004). Math1 regulates development of the sensory epithelium in the mammalian cochlea. Nat. Neurosci. 7, 1310–1318. doi: 10.1038/nn1349
Wu, D. K., and Kelley, M. W. (2012). Molecular mechanisms of inner ear development. Cold Spring Harb. Perspect. Biol. 4:a008409. doi: 10.1101/cshperspect.a008409
Wu, J., Li, W., Lin, C., Chen, Y., Cheng, C., Sun, S., et al. (2016). Co-regulation of the Notch and Wnt signaling pathways promotes supporting cell proliferation and hair cell regeneration in mouse utricles. Sci. Rep. 6:29418. doi: 10.1038/srep29418
Xia, W., Hu, J., Ma, J., Huang, J., Jing, T., Deng, L., et al. (2019). Mutations in TOP2B cause autosomal-dominant hereditary hearing loss via inhibition of the PI3K-Akt signalling pathway. FEBS Lett. 593, 2008–2018. doi: 10.1002/1873-3468.13482
Xia, L., Shang, Y., Chen, X., Li, H., Xu, X., Liu, W., et al. (2020). Oriented neural spheroid formation and differentiation of neural stem cells guided by anisotropic inverse opals. Front. Bioeng. Biotechnol. 8:848. doi: 10.3389/fbioe.2020.00848
Xia, L., Zhu, W., Wang, Y., He, S., and Chai, R. (2019). Regulation of neural stem cell proliferation and differentiation by graphene-based biomaterials. Neural Plast. 2019:3608386. doi: 10.1155/2019/3608386
Xu, Z., Rai, V., and Zuo, J. (2021). TUB and ZNF532 promote the Atoh1-mediated hair cell regeneration in mouse cochleae. Front. Cell. Neurosci. 15:759223. doi: 10.3389/fncel.2021.759223
Yamashita, T., Zheng, F., Finkelstein, D., Kellard, Z., Carter, R., Rosencrance, C. D., et al. (2018). High-resolution transcriptional dissection of in vivo Atoh1-mediated hair cell conversion in mature cochleae identifies Isl1 as a co-reprogramming factor. PLoS Genet. 14:e1007552. doi: 10.1371/journal.pgen.1007552
You, D., Guo, L., Li, W., Sun, S., Chen, Y., Chai, R., et al. (2018). Characterization of Wnt and Notch-responsive Lgr5+ hair cell progenitors in the striolar region of the neonatal mouse utricle. Front. Mol. Neurosci. 11:137. doi: 10.3389/fnmol.2018.00137
Zhang, S., Dong, Y., Qiang, R., Zhang, Y., Zhang, X., Chen, Y., et al. (2021). Characterization of Strip1 expression in mouse cochlear hair cells. Front. Genet. 12:625867. doi: 10.3389/fgene.2021.625867
Zhang, Y., Fang, Q., Wang, H., Qi, J., Sun, S., Liao, M., et al. (2022). Increased mitophagy protects cochlear hair cells from aminoglycoside-induced damage. Autophagy 1–17. . [Online ahead of print]. doi: 10.1080/15548627.2022.2062872
Zhang, Y., Guo, L., Lu, X., Cheng, C., Sun, S., Li, W., et al. (2018). Characterization of Lgr6+ cells as an enriched population of hair cell progenitors compared to Lgr5+ cells for hair cell generation in the neonatal mouse cochlea. Front. Mol. Neurosci. 11:147. doi: 10.3389/fnmol.2018.00147
Zhang, Y., Zhai, S. Q., Shou, J., Song, W., Sun, J. H., Guo, W., et al. (2007). Isolation, growth and differentiation of hair cell progenitors from the newborn rat cochlear greater epithelial ridge. J. Neurosci. Methods 164, 271–279. doi: 10.1016/j.jneumeth.2007.05.009
Zhang, S., Zhang, Y., Dong, Y., Guo, L., Zhang, Z., Shao, B., et al. (2020). Knockdown of Foxg1 in supporting cells increases the trans-differentiation of supporting cells into hair cells in the neonatal mouse cochlea. Cell. Mol. Life Sci. 77, 1401–1419. doi: 10.1007/s00018-019-03291-2
Zhang, S., Zhang, Y., Yu, P., Hu, Y., Zhou, H., Guo, L., et al. (2017). Characterization of Lgr5+ progenitor cell transcriptomes after neomycin injury in the neonatal mouse cochlea. Front. Mol. Neurosci. 10:213. doi: 10.3389/fnmol.2017.00213
Zhao, H. B., Yu, N., and Fleming, C. R. (2005). Gap junctional hemichannel-mediated ATP release and hearing controls in the inner ear. Proc. Natl. Acad. Sci. U S A 102, 18724–18729. doi: 10.1073/pnas.0506481102
Zheng, J. L., and Gao, W. Q. (2000). Overexpression of Math1 induces robust production of extra hair cells in postnatal rat inner ears. Nat. Neurosci. 3, 580–586. doi: 10.1038/75753
Zhou, H., Qian, X., Xu, N., Zhang, S., Zhu, G., Zhang, Y., et al. (2020). Disruption of Atg7-dependent autophagy causes electromotility disturbances, outer hair cell loss and deafness in mice. Cell Death Dis. 11:913. doi: 10.1038/s41419-020-03110-8
Zine, A., Aubert, A., Qiu, J., Therianos, S., Guillemot, F., Kageyama, R., et al. (2001). Hes1 and Hes5 activities are required for the normal development of the hair cells in the mammalian inner ear. J. Neurosci. 21, 4712–4720. doi: 10.1523/JNEUROSCI.21-13-04712.2001
Keywords: Kölliker’s organ supporting cells, cochlear auditory development, degeneration, trans-differentiation, hair cells
Citation: Chen J, Gao D, Sun L and Yang J (2022) Kölliker’s organ-supporting cells and cochlear auditory development. Front. Mol. Neurosci. 15:1031989. doi: 10.3389/fnmol.2022.1031989
Received: 30 August 2022; Accepted: 23 September 2022;
Published: 11 October 2022.
Edited by:
Zuhong He, Department of Otolaryngology, Zhongnan Hospital, Wuhan University, ChinaReviewed by:
Zheng-De Du, Department of Otolaryngology, Affiliated Beijing Friendship Hospital, Capital Medical University, ChinaCopyright © 2022 Chen, Gao, Sun and Yang. This is an open-access article distributed under the terms of the Creative Commons Attribution License (CC BY). The use, distribution or reproduction in other forums is permitted, provided the original author(s) and the copyright owner(s) are credited and that the original publication in this journal is cited, in accordance with accepted academic practice. No use, distribution or reproduction is permitted which does not comply with these terms.
*Correspondence: Lianhua Sun, c3VubGlhbmh1YUB4aW5odWFtZWQuY29tLmNu; Jun Yang, eWFuZ2p1bkB4aW5odWFtZWQuY29tLmNu
† These authors have contributed equally to this work
Disclaimer: All claims expressed in this article are solely those of the authors and do not necessarily represent those of their affiliated organizations, or those of the publisher, the editors and the reviewers. Any product that may be evaluated in this article or claim that may be made by its manufacturer is not guaranteed or endorsed by the publisher.
Research integrity at Frontiers
Learn more about the work of our research integrity team to safeguard the quality of each article we publish.