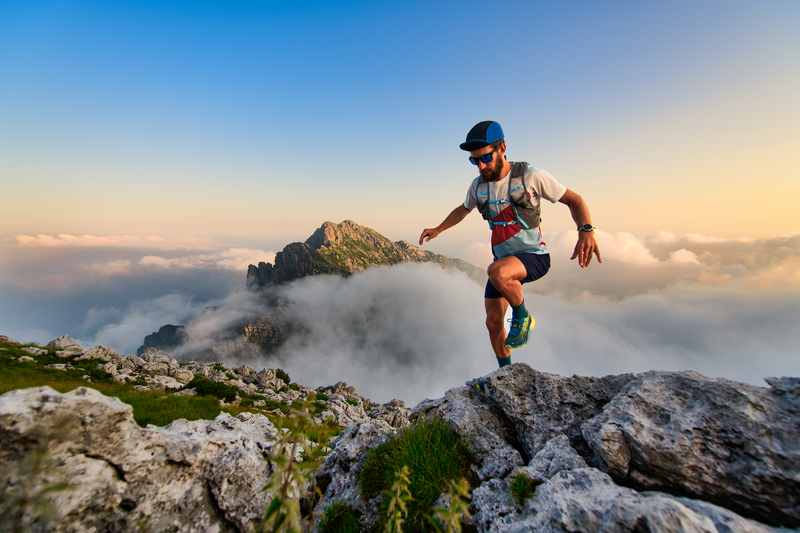
95% of researchers rate our articles as excellent or good
Learn more about the work of our research integrity team to safeguard the quality of each article we publish.
Find out more
REVIEW article
Front. Mol. Neurosci. , 12 October 2022
Sec. Methods and Model Organisms
Volume 15 - 2022 | https://doi.org/10.3389/fnmol.2022.1028125
This article is part of the Research Topic Gene Therapy for Hearing Loss: From Mechanism to Clinic View all 12 articles
The prevalence of hearing loss-related diseases caused by different factors is increasing worldwide year by year. Currently, however, the patient’s hearing loss has not been effectively improved. Therefore, there is an urgent need to adopt new treatment measures and treatment techniques to help improve the therapeutic effect of hearing loss. G protein-coupled receptors (GPCRs), as crucial cell surface receptors, can widely participate in different physiological and pathological processes, particularly play an essential role in many disease occurrences and be served as promising therapeutic targets. However, no specific drugs on the market have been found to target the GPCRs of the cochlea. Interestingly, many recent studies have demonstrated that GPCRs can participate in various pathogenic process related to hearing loss in the cochlea including heredity, noise, ototoxic drugs, cochlear structure, and so on. In this review, we comprehensively summarize the functions of 53 GPCRs known in the cochlea and their relationships with hearing loss, and highlight the recent advances of new techniques used in cochlear study including cryo-EM, AI, GPCR drug screening, gene therapy vectors, and CRISPR editing technology, as well as discuss in depth the future direction of novel GPCR-based drug development and gene therapy for cochlear hearing loss. Collectively, this review is to facilitate basic and (pre-) clinical research in this area, and provide beneficial help for emerging GPCR-based cochlear therapies.
Hearing disease currently affects nearly 1.5 billion people worldwide.1 Most hearing loss is mainly occurred in the cochlea of the inner ear. The cochlea can be divided into five parts: Organ of Corti (OC), Stria Vascularis (SV), Reissner’s Membrane (RM), Mesenchymal Cell (MC), Bony Labyrinth (BL), and Spiral Ganglion Neurons (SGNs) (Figure 1A; van der Valk et al., 2021; Kelley, 2022). Among them, the OC is the main organ of sound perception in the cochlea, particularly the hair cells (HCs) and various supporting cells (SCs) of the OC are essential for hearing (Wagner and Shin, 2019; Driver and Kelley, 2020), but cell abnormalities in other areas of the cochlea can also cause hearing damage (Jang et al., 2022). Hearing damage can be caused by a variety of factors, including heredity, noise, ototoxic drugs, damage to the cochlear environment and structure. However, current treatment options (cochlear implants and hearing aids) mainly depend on the capacity of residual HCs and SGNs to improve the patient’s hearing level to a certain extent (Wolf et al., 2022).
Figure 1. Structure of the cochlea and the GPCR related to human hearing disease. (A) Schematic of the mammalian mature cochlea (cross-section). The cochlea can be divided into five parts, including Organ of Corti (OC), Stria Vascularis (SV), Reissner’s Membrane (RM), Spiral Ganglion Neurons (SGNs), Bony Labyrinth (BL) (not shown), and Mesenchymal Cell (MC) (not shown). Among them, two kinds of hair cell (HC) and various kinds of supporting cell (SC) can be subdivided in OC. (B) The summary of five key GPCRs (V2R, EDNRB, S1PR, VLGR1, and mGluR7) in cochlea related to human hearing disease (USH2C, WS-IV, NIHL, ARHL, and MD).
G protein-coupled receptors (GPCRs) with seven transmembrane domains are the largest superfamily of mammalian cell surface receptors, which have more than 800 members (Foord et al., 2005; Wingler and Lefkowitz, 2020). GPCRs govern a wide range of physiological processes, such as hormone release, neurotransmitter transmission, and immune responses, mainly through the recognition and activation of heterotrimeric G proteins (Gα, Gβ, and Gγ) by binding to a variety of ligands (proteins, peptides, and lipids, etc.), as well as GPCRs phosphorylated by G protein-coupled receptor kinases (GRKs) to recruit β-arrestins and internalize and inactivate GPCRs (Wang et al., 2018; Insel et al., 2019). Based on structural similarity, GPCRs in humans are divided into five major families: Rhodopsin receptors (Class A), Secretin receptors (Class B1), Adhesion receptors (Class B2), Glutamate receptors (Class C), and Frizz/Taste 2 (Class F) (Kochman, 2014; Hauser et al., 2017). Currently, more than 100 GPCRs could be regarded as therapeutic targets, especially more than 30% of marketed drugs have been designed for GPCRs (Hauser et al., 2017).
Compared with extensive studies on GPCRs in mental illness (Pasquini et al., 2022) and cancer (Chaudhary and Kim, 2021), the function studies of GPCRs in the cochlea are very limited, scattered, and unsystematic. In particular, there are still no specific drugs on the market targeting the GPCR of the cochlea. With the in-depth study of GPCRs in the cochlea, we believe that more GPCR functions in the cochlea will be revealed, and more drugs and treatment programs targeting cochlear GPCRs will be discovered. In this review, we therefore characterized the distribution and function of 53 GPCRs expressed in the cochlea, as well as their relationships with hearing loss. Notably, five GPCRs (V2R, EDNRB, S1PR, VLGR1, and mGluR7) of them in the cochlea have been reported to be directly associated with human hearing disorders (Figure 1B). We also summarize the new advances in cochlear research techniques, and suggest the future direction of novel GPCR-based drug development and gene therapy for cochlear hearing loss.
Vasopressin type 2 receptor (V2R) is primarily expressed in the kidney and participates in controlling water homeostasis (Kim et al., 2021; Zhou et al., 2021). V2R is activated by arginine vasopressin (AVP), which in turn induces the buildup of downstream cAMP (Wang et al., 2021). Numerous V2R-related human diseases have been identified, including nephrotic syndrome of inappropriate diuresis (NSIAD), X-linked congenital nephrogenic diabetes insipidus (NDI), and hyponatremia (Makita et al., 2020). V2R-related antagonists have also been extensively studied, such as Tolvaptan (de la Nuez Veulens et al., 2022).
In the cochlea, V2R is mainly expressed in HCs, SGNs, and SVs (Takumida et al., 2012). V2R is thought to play a role in endolymphatic hydrops (EH). EH is caused by an imbalance in the volume of endolymph and is thought to be associated with the pathology of Menière’s disease (MD) that is a kind of hearing loss’s inner ear disease (Zou et al., 2019; Wang et al., 2022). EH can be also inhibited via reducing the expression of V2R. The degree of cochlear hydrops can be alleviated by applying the V2R antagonist (OPC-41061/Tolvaptan) (Egami et al., 2016). Additionally, the expression level of V2R in the cochlea can be significantly inhibited by vincamine, thereby reducing EH and regulating hearing (Li et al., 2018). However, studies found that EH is significantly attenuated by electroacupuncture (EA), but V2R is up-regulated (Jiang L. et al., 2019; Jiang L. Y. et al., 2019). Therefore, it is still needed to validate the function of V2R in the regulation of EH.
Endothelin receptor B (EDNRB) is activated by endothelins (ETs), and three ETs (ET-1, ET-2, and ET-3) have equal affinity for EDNRB (Shihoya et al., 2016; Izume et al., 2020). EDNRB is widely expressed in circulatory organs including vascular endothelium, brain, and intestine, especially endothelin receptor antagonists have been used to treat circulatory system diseases (Bondurand et al., 2018; Shihoya et al., 2018). Heterozygous and homozygous mutations in EDNRB and ET-3 are found in patients of Waardenburg-Shah syndrome (WS-IV) that is one kind of syndromes with genetic hearing loss (GHL) (Huang et al., 2021). Homozygous mutations of the EDNRB gene can be also identified in Moroccan deaf patients (AitRaise et al., 2022).
EDNRB is expressed in SV’s melanocytes and the SGN of the cochlea, which is important for postnatal hearing development (Ida-Eto et al., 2011; Renauld et al., 2021). In mouse models, both EDNRB spontaneously mutated and EDNRB homozygous knockout (EDNRB–/–) mice developed severe congenital deafness (Gariepy et al., 1996; Matsushima et al., 2002). In the case of EDNRB–/–, melanocytes in the SV of the cochlea are defective and the SGN undergoes postnatal degeneration (Ida-Eto et al., 2011). The introduction of human DBH-EDNRB transgene can restore the SGN of EDNRB–/– mice to a certain extent and improve the hearing levels, but the defect of melanocytes does not change (Ida-Eto et al., 2011). Therefore, targeted modulation of EDNRB expression in SGN might be a new strategy to treat congenital hearing loss patients with WS-IV.
Thyrotropin receptor (TSHR) can be activated by thyrotropin (TSH), which then stimulates thyroid hormone production through the Gs and Gq signaling pathways (Tuncel, 2017; Vieira et al., 2022). Abnormalities in TSHR can lead to autoimmune diseases such as hypothyroidism and hyperthyroidism. Recent studies have elucidated the structures of the activated and inactivated states of TSHR with different types of antibodies, which provides a structural basis for subsequent antibody drug and small molecule drug discovery (Duan et al., 2022; Faust et al., 2022).
In a mouse model, TSHRhyt/hyt mutant mice express the gene encoding the TSHR with a point mutation in the highly conserved transmembrane structure, rendering TSHR unable to bind with TSH (Gu et al., 1995). The autosomal recessive TSHRhyt/hyt mutant mice develop severe hearing loss, and outer hair cells (OHCs) of the cochlea exhibit developmental defects and loss of functional integrity (O’Malley et al., 1995; Li et al., 1999; Song et al., 2006). Of note, the exact role of TSHR in the cochlea remains currently in a stagnant state.
Sphingosine-1-phosphate receptors (S1PR1 to S1PR5) can be involved in the regulation of immune and vascular systems after activation by sphingosine-1-phosphate (S1P) (Cartier and Hla, 2019). Three inherited missense mutations (R108P, R108Q, and Y140C) in S1PR2 are found in patients with deaf (Santos-Cortez et al., 2016; Hofrichter et al., 2018). A recent protein structure study revealed that three mutation sites associated with human autosomal recessive hearing loss (ARHL) lead to changes of the protein structure of S1PR2 (Chen et al., 2022). These structural changes affect the binding of S1PR2 to the ligand S1P, to G13, suggesting that the S1PR2-G13 signaling complex plays a role in maintaining normal hearing function of the cochlea.
S1PR1-3 was also found to be expressed in the mouse cochlea (Nakayama et al., 2014). Among them, S1PR2 can be specifically expressed in HCs, SCs, SGNs, and SVs (Ingham et al., 2016). In mouse models, both mutation (S1PR2stdf/stdf) and knockout of S1PR2 (S1PR2–/–) result in progressive hearing loss, essentially starting at 2–4 weeks postnatally with varying degrees of hearing impairment to complete deafness, which is characterized by the defect of SV at the onset and followed by decreased intracochlear potential (EP) and subsequent loss of HCs and SGNs (Herr et al., 2007, 2016; Kono et al., 2007; Ingham et al., 2016). Remarkably, mice lacking the S1PR3 did not develop a hearing impairment phenotype (Kono et al., 2007). S1PR2 is also a potential target to protect hearing loss by preventing the ototoxic drugs induced apoptosis of HC and SGN. Administration of an S1PR2 antagonist (JTE013) resulted in the increase of gentamicin ototoxicity (Nakayama et al., 2014), whereas administration of an S1PR2 agonist (CYM-5478) reduced the cisplatin ototoxicity by reducing ROS accumulation (Wang et al., 2020). Of note, antagonists of S1PR1 and S1PR3 failed to increase gentamicin ototoxicity (Nakayama et al., 2014). Overall, S1PR2 plays a key role in maintaining hearing function and inhibiting damage caused by ototoxicity, particularly it is worthy of in-depth study as an ear protection therapeutic drug target.
P2Y receptor (P2YR), as a GPCR subfamily of eight subunits (P2YR1, 2, 4, 6, 11–14) known, can respond to extracellular nucleotides (Cabou and Martinez, 2022). Among them, P2YR1, 11–13 are activated by ATP/ADP, P2YR4, 6, 14 are activated by UTP/UDP, and P2YR2 receptors are activated by ATP/UTP (Abbracchio et al., 2006; Koles et al., 2019). The expression of six P2YRs (P2YR1, 2, 4, 6, 12, 14) could be detected in the cochlea (Parker et al., 2003; Huang et al., 2010; O’Keeffe et al., 2010; Koles et al., 2019). Before hearing maturation (<P15), five P2YRs except P2YR1 could be detected in both sensory and non-sensory cells in the cochlea. After hearing maturation (>P15), only P2YR12 and P2YR14 could not be detected in HCs. The specific expressions of six P2YRs in the cochlea are shown in Table 1.
P2YR1, 2, 4 have been functionally studied to some extent in the cochlea. Among them, P2YR1 plays an important role in the burst firing before hearing onset. The maturation of emerging neural circuits is facilitated by spontaneous bursts of electrical activity in the developing nervous system (Blankenship and Feller, 2010). K+ release can be triggered when P2RY1 is activated in SCs, thereby activating inner hair cells (IHCs) and SGNs (Babola et al., 2020, 2021). Both pharmacological (MRS2500) inhibition of P2RY1 or P2RY1 deletion significantly reduced burst firing in SGNs (Babola et al., 2020, 2021). Moreover, P2YR4 mediated the inhibition of Na+ uptake in cochlear RMs, possibly in response to noise exposure (Kim et al., 2010). In particular, P2YR2 and P2YR4 in the cochlea can also induce the propagation of Ca2+ waves (Piazza et al., 2007).
G protein-coupled dopamine receptors execute almost all the physiological functions of catecholaminergic neurotransmitter dopamine. This dopamine receptor family includes five GPCR subtypes, and can be divided into two categories: DRD1 (dopamine receptor D1) and DRD5 binding to G proteins and activating adenylyl cyclase; DRD2, DRD3, and DRD4 binding to G proteins and inhibiting adenylyl cyclase (Beaulieu and Gainetdinov, 2011). Transcriptome sequencing of whole cochlear samples from adult mice revealed the presence of DRD1, DRD2, DRD4, and DRD5 transcripts, but not DRD3 mRNA. DRD1, DRD5, and DRD2 receptors were expressed in OHCs and SGNs, but DRD4 receptors were expressed only in SGNs (Maison et al., 2012).
There is no consensus on which receptors can mediate the hearing (Meredith and Rennie, 2021), but it is widely accepted that activated dopamine receptors can decrease the excitotoxicity of IHC synapses through the effect of dopamine on afferents (Oestreicher et al., 1997). Current biochemical and pharmacological evidence suggests that dopamine release from lateral cochlear efferent neurons can inhibit the cochlear nerve fiber activity (Gaborjan et al., 1999; Ruel et al., 2001). Studies have also shown that the administration of dopamine and dopaminergic agonists may reduce the action potentials’ firing rate in frog semicircular canal afferents (Andrianov et al., 2009). In guinea pigs and rats, dopamine reduced the rate of action potential firing from cochlear auditory afferents (Oestreicher et al., 1997; Wu et al., 2020). Exposure to sound also raise dopamine in mouse efferent neurons, revealing that dopamine has very vital neuroprotective effect (Maison et al., 2012; Wu et al., 2020). When the DRD1, DRD2, DRD4, and DRD5 dopamine receptor knockout mice were respectively exposed to noise, all four mutants demonstrated increased vulnerability (Maison et al., 2012). These studies not also support the role of dopaminergic signaling in the HC system of different species, but reveal its potential application value in hearing protection.
Chemokines regulate cell migration and proliferation, as well as immune and inflammatory responses. Twenty chemokine receptors have been identified, including four subfamilies (Sanchez et al., 2019). Chemokine receptors and chemokines can participate in various physiological and pathological processes, including cancer cell growth (Smith et al., 2004) and metastasis (Zlotnik et al., 2011), angiogenesis (Keeley et al., 2010; Lin et al., 2015), and immune responses of patient prognosis (He et al., 2022). Chemokine receptors reported in the cochlea include CXCR4, CX3CR1, CCR2, and CCR7.
CXCR4 and its ligand CXC chemokine ligand 12 (CXCL12, also called as stromal cell-derived factor-1) involve in regulating neural stem cell migration, differentiation and maturation (Zhang et al., 2015), vertebrate embryogenesis (Peyvandi et al., 2018b). In the cochlea, CXCR4 protein is mainly expressed in SGNs. CXCR4/CXCL12 participates in cochlear development in neonatal mice and rats (Zhang et al., 2015, 2016), and stem cell homing in noise-induced injury areas in adult rats (Zhang et al., 2014; Peyvandi et al., 2018a). CX3CR1, as a receptor for the chemokine Fractalkine, is found to express in NK cells, macrophages, monocytes, microglia, and partly T cells (Jung et al., 2000). CX3CR1 is also expressed in macrophages and monocytes of the mouse cochlea (Claussen et al., 2022). In response to the transformation of monocytes and migration of macrophages in hearing damage caused by noise stimulation (Shin et al., 2022a,b), CX3CR1 regulates the inflammatory response caused by cochlear injury (Zhang et al., 2021). CX3CR1-deficient cochlear macrophages can also aggravate the ototoxicity of kanamycin (Sato et al., 2010). Remarkably, CCR2 and CCR7 may be also involved in regulating inflammatory response in hearing impairment induced by noise and drugs (Sautter et al., 2006; Maeda et al., 2018; Hirose and Li, 2019). Therefore, chemokine receptors and chemokines play important roles in cochlear development, stem cell homing and immune response after hearing damage, suggesting them with a potential to repair hearing damage and protect nerves.
Cannabinoid 2 receptors (CB2Rs), one type of cannabinoid receptor, are found in peripheral tissues of immunological origin (Munro et al., 1993; Brown et al., 2002) and are distributed in different brain regions (Ishiguro et al., 2022). CB2Rs are post-synaptically expressed and up-regulated in response to injury and inflammation (Ishiguro et al., 2022). CB2Rs are mainly distributed in OC, spiral ligament and SGN cells in the cochlea of rats and mice (Kim et al., 2014; Kaur et al., 2016; Ghosh et al., 2018). CB2Rs can protect the cochlea and reduce ototoxicity, inflammation and oxidative stress with cisplatin treatment in rats (Vlajkovic et al., 2006; Dhukhwa et al., 2021). The effect of CB2Rs on preventing cisplatin induced hearing loss was blocked by injection of the antagonist AM630, but HC loss was reduced by injection of JWH105 (one agonist of CB2R). Of note, after knock-down of CB2Rs by siRNA, the cochlea is more sensitive to cisplatin induced hearing loss (Ghosh et al., 2018). Therefore, CB2Rs may be an important therapeutic target against ototoxicity.
Apelin receptor (APJ) and its ligand Apelin are key participators involved in the regulation of oxidative stress. Among various subtypes of Apelin, Apelin-13 has the strongest biological activity (Niknazar et al., 2019). APJ/Apelin is widely expressed in the heart, brain, kidney, stomach and intestines (Fournel et al., 2017; Lv et al., 2017), and has antioxidant and apoptotic effects in distinct cell types (Bircan et al., 2016; Aminyavari et al., 2019). Apelin attenuates DNA damage caused by ROS accumulation in cisplatin-induced myocardial toxicity (Zhang P. et al., 2017). Apelin-13 protects cardiomyocytes by reducing oxidative damage in a rat model of myocardial infarction (Azizi et al., 2013).
Both APJ and Apelin are expressed in mouse cochlear HCs and HEI-OC1 cells, and the expression of APJ in OHCs is significantly higher than that in IHCs (Yin et al., 2020). Cisplatin can down-regulate the expression of Apelin in HCs and HEI-OC1 cells, and treatment with Apelin in advance can improve the survival rate of HEI-OC1 cells under cischloride ototoxicity and alleviate the damage of cochlear mitochondrial membrane potential by ROS (Yin et al., 2020). In addition, noise-induced oxidative stress and DPOAE response were significantly altered and inhibited by Apelin-13 pretreatment (Khoshsirat et al., 2021).
This adenosine receptor family includes four GPCRs, designated as A1, A2A, A2B, and A3. Adenosine A1 receptor (AA1R) and adenosine A3 receptor (AA3R) coupled with Gi/o proteins to inhibit adenylate cyclase activity, whereas adenosine A2A receptor (AA2AR) and adenosine A2B receptor (AA2BR) coupled with Gs proteins to activate adenylate cyclase (Vlajkovic et al., 2007). Together, the adenosine receptor family and its signaling molecules regulate cellular activity in peripheral organs.
The distribution of four adenosine receptors in the cochlea is diverse (Vlajkovic et al., 2009; Manalo et al., 2020). AA1R is distributed in SGNs, and in SCs and IHCs of the OC, but AA2AR and AA2BR localize to SGNs, OC, and cochlear vessels. AA3R is mainly expressed in SCs and inner HCs of the OC. The balance between AA1R and AA2AR determines the cochlear response to oxidative stress. AA1R can protect the cochlea of mice from noise injury, cisplatin-induced ototoxicity and age-related hearing loss (Vlajkovic et al., 2011, 2017; Sheth et al., 2019). Similar results have been reported in rat, chinchilla and guinea pig (Ramkumar et al., 1994, 2004; Tabuchi et al., 2012). Administration of AA1R probiotics R-PIA and ADAC was more significant in inhibiting cisplatin-induced ototoxicity (Vlajkovic et al., 2010; Kaur et al., 2016), and the protective effect of R-PIA was inhibited by combined use of AA1R antagonist DPCPX (Whitworth et al., 2004). In contrast, AA2AR and AA2BR play a negative regulatory role in hearing loss, with cochlear protection achieved by the use of the inhibitor (istradefylline) (Han et al., 2019; Manalo et al., 2020; Shin et al., 2021).
GPR26, one class of orphan receptors for Class A, is mainly expressed in the brain and attracted attention due to its role in central nervous system diseases (Alavi et al., 2018; Watkins and Orlandi, 2020). GPR26 is deleted along with two other genes (CPMX2 and CHST15) in recessive mouse mutant mice (hb/hb) that exhibit severe hearing impairment (Buniello et al., 2013). Symptoms of anxiety and depression were presented in GPR26 knockout mice, but no hearing function was reported (Zhang et al., 2011). In the mouse cochlea, GPR26 expression was detected in spiral ligament fibrocytes (SLF) and SGNs (Buniello et al., 2013), but hb/hb mutant mice in the cochlea without GPR26 expression, indicating that it is worth using the GPR26–/– mice to examine the role of GPR26 in the cochlea.
Another class of orphan receptors for Class A is one member of the leucine-rich repeat-containing G-protein-coupled receptors (LGRs) family. LGR4, LGR5, and LGR6 could be expressed in the cochlea (Zak et al., 2016; Zhang Y. et al., 2018; Smith-Cortinez et al., 2021). LGR5 is considered as a marker of cochlear stem cells and participates in the development of auditory HCs (Smith-Cortinez et al., 2021). LGR5 regulates cochlear development by enhancing the Wnt/β-catenin signaling pathway (Cheng et al., 2017; McLean et al., 2017), especially LGR5-positive SCs have the potential to transdifferentiate into HCs, suggesting that it may be acted as a therapeutic target for hearing loss (Cox et al., 2014; Zhang S. et al., 2017; Smith-Cortinez et al., 2021; Ma et al., 2022). LGR5-deficient mice produce additional HCs, and LGR4-deficient mice show similar results (Zak et al., 2016). LGR6+ cells, a subtype of LGR5+ progenitor cells, also regulate progenitor cell proliferation and HC production (Zhang Y. et al., 2018).
Vasoactive intestinal peptide (VIP) and pituitary adenylate cyclase-activating peptide (PACAP) receptors include VPAC1R, VPAC2R, and PAC1R, which are activated by VIP and PACAP (Hollenstein et al., 2014; Langer et al., 2022). Among them, unlike VPAC1R and VPAC2R, PAC1R can specifically binds to PACAP but has a lower affinity toward VIP (Vaudry et al., 2009). These two neuropeptides are widely distributed and involved in development, anti-apoptosis, and neuroprotection together with their receptors (Langer et al., 2022). In the cochlea, VIP and VIP receptors are mainly expressed in SGNs (Kitanishi et al., 1998). In addition, the levels of VIP and VPAC1R were down-regulated in the cochlea of chronic alcoholic rats, implying that they might act as neurotransmitters (Feng and Liu, 2015). However, there are still few studies on VIP and VIP receptors, and their specific mechanisms of action in the cochlea need to be further studied.
PACAP and PAC1R are expressed in HCs, SCs, SGNs, afferent, and efferent nerve fibers, and stria vascularis of the cochlea (Abu-Hamdan et al., 2006; Drescher et al., 2006; Ruel et al., 2021). Endogenous PACAP plays important roles in protection against noise-induced hearing loss (NIHL) (Ruel et al., 2021), maintenance of hearing during aging in mice (Fulop et al., 2019) and against oxidative stress-induced apoptosis (Racz et al., 2010). Current functional studies focused on the role of PACAP in the cochlea as well as the PAC1R in the protection of NIHL (Ruel et al., 2021). After noise injury, the PAC1R–/– knockout mice exhibited a significant increase in hearing threshold, but the humanized mice expressing human PAC1R (TgHPAC1R) showed a relatively small increase in hearing threshold. Taken together, with the establishment of a mouse model corresponding to the PAC1R, other roles of the PAC1R in the cochlea will be gradually uncovered.
Corticotropin-releasing factor receptors (CRFRs) in mammals only express CRFR1 and CRFR2, which are activated by corticotropin-releasing factor (CRF). As the main regulator of stress response, they participate in neuroendocrine, metabolism and response to stress (Vetter, 2015; Dedic et al., 2018). Among them, CRFR1 has a higher affinity for CRF than CRFR2 does.
CRFR1 is mainly localized in inner sulcus cells (ISCs), Hensen cells (HSCs), Deiters’ cells (DCs), and border cells (BCs) in the cochlea (Graham and Vetter, 2011), but no expression in HCs and SGNs. CRFR1 plays an important role in maintaining normal auditory function, IHC and HC innervation development. In CRFR1–/– mice, both ABR thresholds and DP thresholds were elevated, suggesting that elimination of CRFR1 might result in decreased cochlear sensitivity and impaired OHC motility (Graham and Vetter, 2011), as well as defects in IHC, afferent and efferent innervation (Graham and Vetter, 2011).
Likewise, the effects of CRFR2 on the cochlea are diverse. CRFR2 is mainly expressed in ISCs, DCs, inner border cells (IBCs), SGNs, Claudius cells (CCs), and Boettcher cells (BoCs) (Graham et al., 2010), but not in HCs. CRFR2 constitutively modulates hearing sensitivity under normal conditions and performs an important protective function in noise-induced hearing loss. Mice lacking CRFR2 exhibited significantly lower hearing thresholds under normal conditions, but more severe hearing impairment when exposed to noise (Graham et al., 2010). Interestingly, there was no loss of IHCs or OHCs in the cochlea of CRFR2–/– mice exposed to moderate ambient noise (Graham et al., 2010). CRFR2 affects cochlear hearing function by acting on glutamatergic transmission, purinergic signaling and activation of Akt/PKB signaling in the cochlea (Graham et al., 2010). Currently CRFR1 and CRFR2 have been considered as promising targets for the treatment of asthma and alcoholism drug therapy (Tantisira et al., 2004; Lowery and Thiele, 2010).
Calcitonin Gene-Related Peptide Receptor (CGRPR) is a heterodimeric membrane protein complex composed of receptor activity-modifying protein 1 (RAMP1) and calcitonin receptor-like receptor (CLR) with the ability to bind to CGRP (Liang et al., 2018). As an important sensory neuropeptide, CGRP is widely expressed in the nervous system and exists in two forms, i.e., α-CGRP and β-CGRP (Lv et al., 2022). CGRP plays important roles in migraine pathophysiology, inflammatory response, and blood pressure (Mehkri et al., 2022). At present, good progress has been made in the drug research of GCRP and CGRPR, and four related monoclonal antibodies have been developed (Deganutti et al., 2021). In addition, GCRP plays an important role in the cochlea. GCRP is expressed in the lateral olivocochlear (LOC), medial olivocochlear (MOC) efferent neurons and type II SGNs (SGN IIs) and up-regulates excitatory of auditory nerve (AN) activity (Schrott-Fischer et al., 2007; Vyas et al., 2019; Le Prell et al., 2021). In αCGRP knockout mice, ABR thresholds were reduced and hearing impairment was presented (Maison et al., 2003). Additionally, the CGRPR complex in the cochlea exhibits maturation during the first 3 months, which corresponds to an increase in cochlear nerve activity (Dickerson et al., 2016). However, research on CGRPR in the cochlea is relatively lagging, particularly the location and specific function of CGRPR in the cochlea are currently unknown.
Cadherin EGF LAG Seven-pass G-type Receptor 1 (CELSR1), also named as Adhesion G-protein Receptor C1 (ADGRC1), is mainly distributed in the nervous system. In humans and mice, mutations in CELSR1 strongly affect neural tube development (Ravni et al., 2009; Allache et al., 2012).
CELSR1 is expressed in both inner ear HCs and SCs, and is considered as a key Planar cell polarity (PCP) protein in the cochlea to be involved in cellular communication and coordination between HCs and SCs (Shima et al., 2002; Curtin et al., 2003; Davies et al., 2005). In two CELSR1 mutant mice (Scy and Crsh), the OHCs were massively misoriented, most severely at the apex of the cochlea (Curtin et al., 2003). Interestingly, no significant auditory HC dislocation and hearing impairment were observed in CELSR1 knockout mice but not in mutant strains, which may be due to compensatory effects from other CELSR genes (e.g., CELSR2, 3) (Tissir and Goffinet, 2006; Duncan et al., 2017). In addition, there are some PCP proteins in cochlear HCs, but whether and how CELSR1 cooperates with other PCP proteins to effect on the plane polarity of HCs, which is largely unknown and needs to further study.
Very large G protein-coupled receptor 1 (VLGR1), known as MASS1, Adhesion G-protein Receptor V1 (ADGRV1), Neurepin and G protein-coupled receptor 98 (GPR98), is to date the largest known protein in GPCR super-families including about 6,300 amino acid residues (Sun et al., 2013). Several VLGR1 mutations have been reported to cause Usher syndrome type IIC (USH2C) in humans, a genetically heterogeneous autosomal recessive disorder characterized by hearing impairment and epileptic seizures (Kimberling et al., 1995; Ebermann et al., 2007; Bonnet and El-Amraoui, 2012).
In the inner ear, VLGR1 is expressed in the ankle region of HCs stereocilia, which can form the ankle-link complex with Usherin, Vezatin, and Whirlin (Michalski et al., 2007). VLGR1 was identified to form a complex with Clarin-1, CDH23, and PCDH15 at the ribbon synapses of HCs (Zallocchi et al., 2012b), and can also interact with various proteins including Harmony (Verpy et al., 2000), PDZ7 (Colcombet-Cazenave et al., 2022), MyosinVIIa (Michalski et al., 2007), and SNAP25 (Zallocchi et al., 2012a). In various VLGR1 mutant or knockout mouse models, the stereociliary development of auditory HCs is impaired, the ankle-links are absent, and hearing impairment of varying degrees occurs (Skradski et al., 2001; McMillan and White, 2004; Yagi et al., 2005). Collectively, VLGR1 can carry out the stereociliary development and hearing function.
G protein-coupled receptor 125 (GPR125) is also named as adhesion G protein-coupled receptor A3 (ADGRA3), and involve in regulating planar cell polarity signaling (Li et al., 2013). GPR125 is widely expressed in the cochlea, especially in OHCs, SGNs, and interdental cells (ICs) (Sun et al., 2021). However, in GP125-deficient mice, various types of cells developed normally, and hearing function was not impaired (Sun et al., 2021), implying that GPR125 may not regulate the planar cell polarity in the cochlea.
The metabotropic glutamate receptor (mGluR) family includes eight known subtypes (mGluR1∼8) that are subdivided into three groups (group I-III) (Niswender and Conn, 2010; Reiner and Levitz, 2018; Ge and Wang, 2022). In general, group I (mGluR1 and mGluR5) mainly positively regulate the activity of glutamatergic synapses. In contrast, both group II (mGluR2 and mGluR3) and III mGluR (mGluR4, mGluR6∼8) function in limiting the release of neurotransmitters. In addition, most mGluRs can be alternatively spliced at the intracellular C-terminus to generate isoforms such as mGluR7a and mGluR7b, and then form homo- and heterodimers for dynamic regulation (Seebahn et al., 2011; Habrian et al., 2019).
Among all mGluRs, mGluR1 is present both in the SGNs and HCs (Ye et al., 2017). mGluR4, mGluR7a, mGluR7b, and mGluR8b were found at the pre-synaptic ribbons of IHCs, while mGluR2 is localized at post-synaptic type I SGNs (SGN Is) and efferent lateral olivocochlear GABAergic fibers (Doleviczenyi et al., 2005; Klotz et al., 2019; Klotz and Enz, 2021). Moreover, mGluR7 and mGluR8 can be detected at the OHCs (Friedman et al., 2009; Girotto et al., 2014). Especially, mGluR1 can enhance efferent inhibition of developing IHCs and promote excitatory neurotransmission in SGN Is (Peng et al., 2004; Ye et al., 2017). In contrast, mGluR2 can protect cochlea from damage by inhibiting efferent dopamine release onto IHCs (Doleviczenyi et al., 2005). Interestingly, mGluR7 is also associated with ARHL and NIHL in humans (Friedman et al., 2009; Newman et al., 2012; Chang et al., 2018; Yu et al., 2018; Matyas et al., 2019), and mGluR7 knockout mice exhibited hearing deficits (Fisher et al., 2020). These studies suggest that mGluRs, especially group II and III mGluRs, can play a key role in preventing excitotoxicity induced by excessive glutamate release from IHCs. However, mGluR4 and mGluR8b, which co-localize with the mGluR7, warrant further investigation of their specific functions in IHCs. In addition, whether mGluR4, mGluR7a, mGluR7b, and mGluR8b can form different homologous and/or heterodimeric receptors to execute diverse roles in IHCs deserves further investigation.
The γ-aminobutyric acid receptor type B (GABA B receptor), as a metabotropic receptor, can be activated by γ-aminobutyric acid (GABA) and mediate long-term, slow signaling responses mainly in the form of heterodimers (Mao et al., 2020; Fritzius et al., 2022; Vlachou, 2022). GABAB receptors are composed of two distinct subunits, GB1 and GB2. Due to the alternative splicing of GB1 subunit mRNA, 14 different GB1 isoforms can be generated, of which GB1a and GB1b are most widely studied (Bowery and Enna, 2000; Shaye et al., 2021; Shen et al., 2021). What’s more, GABA B receptors were found to be localized at all mature or nascent cochlear SGNs, including SGN I and SGN II, but not in HCs (Lin et al., 2000; Reijntjes and Pyott, 2016). GABA B receptors affect OHC function in the cochlea. In mice knocked out of GABA B1, hearing thresholds increased by about 10 dB (Maison et al., 2009). In addition, GABA B(1a,2) on the SGN modulates the strength of the SGN-HC synapse by inhibiting the release of acetylcholine (Ach) following GABA activation (Wedemeyer et al., 2013). In contrast to the few studies that work in the cochlea, research on GABA B receptors in the auditory domain is currently focused on the cochlear nuclear complex (CNC) of the brain (Kou et al., 2013; Qu et al., 2015). Whether there is a potential correlation between the GABA B receptors of the cochlea and CNC is worthy of follow-up study.
The calcium-sensing receptor (CaSR) works as a key regulator by sensing extracellular Ca2+ fluctuations to affect downstream intracellular signaling pathways (Tuffour et al., 2021). Thus, CaSR plays a key role in maintaining cellular Ca2+ homeostasis. In cochlea, Ca2+ homeostasis is also essential for acoustic transduction and proper development of cochlea, including synaptic transmission, mechanoelectrical transduction and the network of SCs (Ceriani and Mammano, 2012; Sirko et al., 2019). CaSR expression is detected in fibrocytes of the spiral ligament and spiral limbus, smooth muscle cells (SMCs) of the spiral modiolar arteries and epithelia of the osseous spiral lamina (Wonneberger et al., 2000; Minakata et al., 2019). Only one work has reported the role of CaSR in cochlear fibrocytes, where CaSR can regulate Ca2+ concentration (Minakata et al., 2019). When the CaSR inhibitor (NPS2143 and Calhex231) was used, the hearing threshold increased by 20–30 dB, indicating that the Ca2+ signal mediated by CaSR is required for hearing. Therefore, the study of the complete regulatory pathway of CaSR to maintain cochlear Ca2+ homeostasis will help to treat hearing loss.
GPR156, as an orphan GPCR of class C, has a significant sequence homology with GABA B receptor (Calver et al., 2003), and has a high Gi/o constitutive activity (Watkins and Orlandi, 2021). GPR156 is currently only reported as a key regulator of orientation in sensory HCs (Kindt et al., 2021). GPR156 is expressed in all HCs of the cochlea, and knockout of GPR156 causes hearing loss but not HC death (Kindt et al., 2021). GPR156 distribution can be polarized by the transcription factor EMX2, which is then signaled by Gαi to trigger a 180° reversal of HC orientation (Kindt et al., 2021). The EMX2 > GPR156 > Gαi signaling cascade is therefore required for HC orientation (especially OHC1 and OHC2) and hearing function. In this signaling cascade, how EMX2 affects GPR156 and whether there are agonists combined with GPR156 to participate in the reversal of HCs worth further research.
Class F of GPCR or frizzled GPCR family includes ten Frizzleds (FZD1-10) and Smoothened (SMO), all of them have this cysteine-rich domain (CRD) in their extracellular region (Schulte and Wright, 2018; Zhang X. et al., 2018; Kozielewicz et al., 2020; Schulte and Kozielewicz, 2020). These receptors play key roles in embryonic development, cellular polarity, proliferation, differentiation, and maintenance of stem cells.
The 10 FZDs coordinate the Wnt signaling in two ways: through disheveled (DVL1-3)–dependent pathway (Clevers and Nusse, 2012; Grainger and Willert, 2018) and through heterotrimeric G-protein-mediated pathway (Dijksterhuis et al., 2014; Kilander et al., 2014). In addition to FZD5 and FZD8, the other eight FZDs have been reported to couple to various types of G proteins (Schulte and Wright, 2018). Most of these FZDs were detected by RT-PCR in the rat cochlea (Daudet et al., 2002) and RNA in situ hybridization in the chicken cochlea (Sienknecht and Fekete, 2008). In the mammalian cochlea, FZD1 and FZD2 were both expressed at lower levels in sensory HCs, but at higher levels in SCs (Yu et al., 2010). And the expression of FZD4 can be detected in auditory and vestibular HCs (Wang et al., 2001). Furthermore, both FZD3 and FZD6 are expressed in cochlear SCs (Ghimire and Deans, 2019) and the medial side of HCs (Montcouquiol et al., 2006; Chang et al., 2016), but FZD3 is expressed in SGNs (Duncan et al., 2019; Stoner et al., 2021). While the expression of FZD9 can be detected in early cochlear inner phalangeal cells (IPhCs), IBCs, and the third-row DCs (Zhang et al., 2019).
Among the 10 FZDs, FZD 2, 3, 4, 6, and 9 play different important roles in the cochlea. Knockout of FZD2 in the mouse cochlea results in defects in OHC number and orientation, which are most severe in the apical region. In addition, a recent study identified FZD2 as a signature gene in one of three distinct SGN I populations by using single-cell transcriptome (Grandi et al., 2020). In the mouse cochlea, planar polarity is guided by the PCP proteins FZD3 and FZD6. However, FZD3 and FZD6 are functionally redundant, and the orientation of HCs is severely affected only in FZD3/6 double knockout mice (Wang et al., 2006). Moreover, FZD3 and FZD6 play a key role in guiding SGN II peripheral axons turning (Ghimire and Deans, 2019). A recent study uncovered SPAG6 as a regulator of FZD6, because FZD6 lost its normal polarized distribution in SPAG6–/– mice (Li et al., 2021). Furthermore, a novel non-canonical Wnt pathway was identified in cochlear HCs that signals through PI3K, Rac1 and Gsk3β to regulate the PCP pathway by promoting the junctional localization of core PCP proteins such as FZD6 (Landin Malt et al., 2021). Compared with the above-mentioned FZDs, FZD4 does not affect HC survival but only affects HC function, so only the late onset hearing loss can be found in FZD4–/– mice (Wang et al., 2001). The main role of FZD9 in cochlea is HC regeneration, and FZD9+ cells have strong ability of proliferation, differentiation and HC generation. It still has HC-generating capacity at 6 days after treatment in vivo lineage tracing, especially it can act as an effective marker for HC progenitors (Zhang et al., 2019). Therefore, FZD9 has clinical translational value for the regeneration of HCs.
SMO mediates the Hedgehog (Hh) signaling pathway, activated SMO lead Gli to translocate into the nucleus to activate target genes, in which SMO can couple to Gi/o and G12/13 (Gorojankina, 2016; Qi et al., 2019; Okashah et al., 2020). In the auditory field, SMO is found to participate in cochlear development, HC differentiation and hearing function. In mouse embryos carried inner ear conditional knockout of Smoothened (SMOecko), the cochlea exhibited hypoplasia, in which the cochlear duct and saccule were completely absent in SMOecko embryos (Brown and Epstein, 2011; Muthu et al., 2019). What’s more, in SMOcko mouse (similar to SMOecko mouse) early cochlea, apex HCs preferentially accelerate differentiation (Tateya et al., 2013). Although SMOcko mice survive after birth, HCs in the apical region appear disorganized and reduced in number, causing hearing loss which predominantly at low frequencies. And SMOecko has also been used to identify key genes that are activated and repressed by Shh signaling in the cochlea during the initial stages of growth (Muthu et al., 2019). In addition, SMO may be associated with otosclerosis (Brown and Epstein, 2011) and cochlear neural stem cell (NSC) transplantation (Huang et al., 2018). The use of taurine during transplantation can up-regulate Hh pathway proteins such as SMO, thereby stimulating the cell proliferation and differentiation of NSCs into SGNs. These results suggest that SMO has potential applications in the treatment of hearing impairment and cochlear NSC transplantation.
G protein-coupled receptors are keeping great advantages as drug targets, thanks to the rapid development of single-particle cryo-electron microscopy (cryo-EM) technology and artificial intelligence (AI) technology in recent years. Since the first use of cryo-EM to resolve the complex structure of GPCR and G protein in 2017, the number of GPCR structures resolved every year has grown exponentially (Liang et al., 2017; Kooistra et al., 2021). Compared with previous X-ray crystallography studies required higher thermal stability, cryo-EM can obtain different conformations of stable GPCRs and structures of complexes with G proteins that are closer to the native state, which greatly improves the efficiency of ligand screening or drug design (Renaud et al., 2018). So far, the structures of 30 GPCRs functioning in the cochlea have been successfully resolved (Figures 2, 3; Supplementary Table 1). In recent years, the application of AI in structural biology has also greatly promoted the elucidation of GPCRs and the corresponding drug design. For example, AlphaFold2 and RosettaFold are the most typical applications at present (Baek et al., 2021; Jumper et al., 2021) with high accuracy, high speed, and convenience for GPCR structure-oriented drug design. In addition, according to statistics, GPCRs remain one of the most important drug targets (Hauser et al., 2017).
Figure 2. The summary of reported 30 Class A GPCRs in cochlea. The blue circle indicates that GPCR are functional in the cochlea. Pink circle indicates GPCR associated with hearing loss. Red circle indicates GPCR associated with human hearing disorders. Green circle indicates GPCR involved in hearing protection. The orange circle indicates that the GPCR has resolved the protein structure. Open circles indicate conjectures or confirmed conclusions.
Figure 3. The summary of reported Class B1/B2/C/F GPCRs in cochlea. (A) The summary of reported 5 Class B1 GPCRs in cochlea. (B) The summary of reported 3 Class B2 GPCRs in cochlea. (C) The summary of reported 8 Class C GPCRs in cochlea. (D) The summary of reported 7 Class F GPCRs in cochlea.
There are many types of GPCRs targeting drugs in clinical trials, including peptides, monoclonal antibodies, recombinant proteins, small molecules, and nanobodies (Saikia et al., 2019). According to the mode of action, it can be divided into agonists, antagonists, positive allosteric modulators (PAM), negative allosteric modulators (NAM) and so on (Hauser et al., 2017; Odoemelam et al., 2020). When a ligand binds to a GPCR, the receptor undergoes a conformational change in which agonists can activate and antagonists can inhibit signal transduction pathways. In contrast to orthosteric ligands such as agonists and antagonists, allosteric modulators (PAM or NAM) as promising therapeutic agents can infiltrate into a pocket that is different in space than the orthotopic site, and modulate signaling only in the presence of the natural ligand to prevent adverse side effects (Massink et al., 2020; Yang et al., 2022).
In cochlear research and treatment, GPCR-targeted drugs have also been used to some extent. For example, S1PR2 agonist (CYM-5478) (Wang et al., 2020), CaSR inhibitors (NPS2143 and Calhex231) (Minakata et al., 2019), V2R antagonist (OPC-41061/Tolvaptan) (Egami et al., 2016), CB2R agonist (JWH105) (Vlajkovic et al., 2006; Dhukhwa et al., 2021), AA1R agonists (R-PIA and ADAC) (Vlajkovic et al., 2010; Kaur et al., 2016), AA2AR antagonist (istradefylline) (Han et al., 2019; Manalo et al., 2020; Shin et al., 2021) all have a therapeutic and protective effect on the cochlea. Among these GPCR-targeted drugs, V2R antagonist (OPC-41061/Tolvaptan) and AA2AR antagonist (istradefylline) have been approved by the US Food and Drug Administration (FDA) (NDA022075, NDA022275). V2R antagonist (OPC-41061/Tolvaptan) is approved for the treatment of autosomal dominant polycystic kidney disease, fibrosis, hyponatremia, heart failure, and the syndrome of dysregulated antidiuretic hormone secretion in humans (Cao et al., 2022; Martin-Grace et al., 2022). AA2AR antagonist (istradefylline) is widely used to treat Parkinson’s disease in humans (Merighi et al., 2022). However, many GPCRs extensively developed in the cochlea, such as CRFR1 (Graham and Vetter, 2011), CRFR2 (Graham et al., 2010), and CGRPR (Maison et al., 2003) mentioned above, also have great potential in the future of cochlear therapy to treat or prevent of noise- and pharmaceutical-induced auditory toxicity (Lowery and Thiele, 2010; Hauser et al., 2017; Deganutti et al., 2021). Therefore, besides existing drugs targeting GPCRs that can be further tried to be applied to the treatment of the cochlea, more structures of potential GPCRs targets are helpful to design drugs in cochlear therapy.
The use of drugs generally only works when the target protein exists and expresses. Considering that one out of every 1,000 births in the world is hereditary deafness (Ajay et al., 2022), and mutations or deletions of 124 genes have been found to cause hearing loss,2 so these hereditary hearing impairments are difficult to treat with drugs. Therefore, gene therapy provides a therapeutic direction for hearing loss caused by gene mutation or deletion (Lee et al., 2020; Maguire and Corey, 2020). Briefly, the introducing a normal gene into the target cell through a delivery vector to replace or enhance the defective gene, can restore a normal level to avoid loss of function. In the field of cochlear therapy, gene therapy was first used to rescue hearing loss in the VGLUT3 knockout mouse, a model of congenital deafness, as early as 2012 (Akil et al., 2012). Subsequently, many deafness caused by gene mutation or deletion were studied by similar gene therapy approaches, including TMC1 (Nist-Lund et al., 2019), GJB2 (Iizuka et al., 2015), USH1C (Pan et al., 2017), and so on.
In recent years, the CRISPR (clustered regularly interspaced short palindromic repeats) based gene editing methods have opened up new avenues for gene therapy in the field of hearing (Zuris et al., 2015). It can target gene disruption or repair mutations to restore gene function, no need to consider how to produce adequate levels of exogenous transgene expression. At present, the exploration of gene editing methods for cochlear gene therapy is mainly based on CRISPR-Cas9 and CRISPR-Cas13 (Geleoc and El-Amraoui, 2020; Botto et al., 2021). The CRISPR-Cas9 system edits DNA, and the cure may be permanent after the correction of disease-causing mutation in vivo. It has been studied in a series of hearing treatments, for example, the dominant mutation of the TMC1 gene in a Beethoven mouse model of hearing loss has been successfully corrected. Hearing was significantly restored in the treated mice, and this effect was stable for up to a year (Gao et al., 2018; Gyorgy et al., 2019). Unlike the CRISPR-Cas9 system targeting DNA, CRISPR-Cas13 system edits disease-associated RNA transcripts, which is transient and potentially reversible, thus also offering improved safety. Two recent studies have revealed the potential of the CRISPR-Cas13 system in gene therapy for the repair of hearing loss caused by mutations, including CRISPR-Cas13X (Xiao et al., 2022) and CRISPR-CasRx (Guo et al., 2022).
The good news is that the first gene therapy for a disease caused by a specific genetic mutation has been approved by the FDA at 2017 (Maguire et al., 2021), supporting the huge clinical potential of gene therapy. In the field of GPCR-related gene therapy, considerable progress has been made in the study of rhodopsin (RHO) in the retina (Athanasiou et al., 2018). Multiple works rescue retinal degeneration in RHO mutant mice for up to 6–9 months by supplementing exogenous RHO (O’Reilly et al., 2007; Chadderton et al., 2009; Mao et al., 2011). In addition, there is some work to correct RHO mutations by CRISPR/Cas9 gene editing for the treatment of inherited retinal degeneration (Bakondi et al., 2016; Burnight et al., 2017). Among the nine genes with mutations or deletions of GPCR-encoding genes that cause hearing loss, including GABA B1 (Maison et al., 2009), GPR156 (Kindt et al., 2021), mGluR7 (Friedman et al., 2009), VLGR1 (Kimberling et al., 1995), PAC1R (Ruel et al., 2021), EDNRB (Huang et al., 2021), S1PR2 (Santos-Cortez et al., 2016), TSHR (Li et al., 1999), and CRFR1 (Graham and Vetter, 2011), five of them mGluR7 (Friedman et al., 2009), VLGR1 (Kimberling et al., 1995), EDNRB (Huang et al., 2021), V2R (Zou et al., 2019; Wang et al., 2022), and S1PR2 (Santos-Cortez et al., 2016) are directly related to human deafness, which are especially worth developing for gene therapy.
In this review, we mainly summarize the expression of five subfamilies of GPCRs in the cochlea, their functions, their relationship with hearing loss, and their potential therapeutic directions (Tables 1–5). A total of 53 GPCRs have been reported to be expressed in the cochlea, of which 38 have been shown to function in the cochlea (Figures 2, 3; Supplementary Table 1). Most importantly, 27 GPCRs were found to be associated with hearing loss, 5 of which were directly associated with human hearing disorders (VLGR1, mGluR7, V2R, EDNRB, and S1PR2). In addition, 13 GPCRs (CXCR4, CX3CR1, CCR2, CCR7, CB2R, APJ, AA1R, AA2AR, AA2BR, PAC1R, CRFR2, mGluR7, and S1PR2) were confirmed to play a hearing protective role in noise and ototoxicity. We also prospect the GPCR-targeted drug development and gene therapies in the future. In conclusion, GPCRs have great potential in the treatment of hearing loss, so more GPCR functions in the cochlea, more GPCRs related to hearing loss, and more GPCR-based treatment regimens remain to be further explored.
YY, JF, and RC conceived this review. XM and JG wrote the manuscript. YF, CS, PJ, YZ, LZ, YY, JF, and RC revised the manuscript. All authors have read and approved the final manuscript.
This work was supported by grants from National Key R&D Program of China (No. 2017YFA0103903), Strategic Priority Research Program of the Chinese Academy of Sciences (XDA16010303), National Natural Science Foundation of China (No. 81970882 and 81970892), Natural Science Foundation from Jiangsu Province (BE2019711 and BK20190062), Shenzhen Fundamental Research Program (JCYJ20190814093401920), Open Research Fund of State Key Laboratory of Genetic Engineering, Fudan University (No. SKLGE1809), and Postdoctoral Innovative Talent Support Program (BX20220122).
The authors declare that the research was conducted in the absence of any commercial or financial relationships that could be construed as a potential conflict of interest.
The reviewers FC and YC declared a past co-authorship with the authors XM, YZ, and RC to the handling editor.
All claims expressed in this article are solely those of the authors and do not necessarily represent those of their affiliated organizations, or those of the publisher, the editors and the reviewers. Any product that may be evaluated in this article, or claim that may be made by its manufacturer, is not guaranteed or endorsed by the publisher.
The Supplementary Material for this article can be found online at: https://www.frontiersin.org/articles/10.3389/fnmol.2022.1028125/full#supplementary-material
Abbracchio, M. P., Burnstock, G., Boeynaems, J. M., Barnard, E. A., Boyer, J. L., Kennedy, C., et al. (2006). International Union of Pharmacology LVIII: Update on the P2Y G protein-coupled nucleotide receptors: From molecular mechanisms and pathophysiology to therapy. Pharmacol. Rev. 58, 281–341. doi: 10.1124/pr.58.3.3
Abu-Hamdan, M. D., Drescher, M. J., Ramakrishnan, N. A., Khan, K. M., Toma, V. S., Hatfield, J. S., et al. (2006). Pituitary adenylyl cyclase-activating polypeptide (PACAP) and its receptor (PAC1-R) in the cochlea: Evidence for specific transcript expression of PAC1-R splice variants in rat microdissected cochlear subfractions. Neuroscience 140, 147–161. doi: 10.1016/j.neuroscience.2006.01.019
AitRaise, I., Amalou, G., Bousfiha, A., Charoute, H., Rouba, H., Abdelghaffar, H., et al. (2022). Genetic heterogeneity in GJB2, COL4A3, ATP6V1B1 and EDNRB variants detected among hearing impaired families in Morocco. Mol. Biol. Rep. 49, 3949–3954. doi: 10.1007/s11033-022-07245-z
Ajay, E., Gunewardene, N., and Richardson, R. (2022). Emerging therapies for human hearing loss. Expert Opin. Biol. Ther. 22, 689–705. doi: 10.1080/14712598.2022.2072208
Akil, O., Seal, R. P., Burke, K., Wang, C., Alemi, A., During, M., et al. (2012). Restoration of hearing in the VGLUT3 knockout mouse using virally mediated gene therapy. Neuron 75, 283–293. doi: 10.1016/j.neuron.2012.05.019
Alavi, M. S., Shamsizadeh, A., Azhdari-Zarmehri, H., and Roohbakhsh, A. (2018). Orphan G protein-coupled receptors: The role in CNS disorders. Biomed. Pharmacother. 98, 222–232. doi: 10.1016/j.biopha.2017.12.056
Allache, R., De Marco, P., Merello, E., Capra, V., and Kibar, Z. (2012). Role of the planar cell polarity gene CELSR1 in neural tube defects and caudal agenesis. Birth Defects Res. A Clin. Mol. Teratol. 94, 176–181. doi: 10.1002/bdra.23002
Aminyavari, S., Zahmatkesh, M., Farahmandfar, M., Khodagholi, F., Dargahi, L., and Zarrindast, M. R. (2019). Protective role of Apelin-13 on amyloid beta25-35-induced memory deficit; involvement of autophagy and apoptosis process. Prog. Neuropsychopharmacol. Biol. Psychiatry 89, 322–334. doi: 10.1016/j.pnpbp.2018.10.005
Andrianov, G. N., Ryzhova, I. V., and Tobias, T. V. (2009). Dopaminergic modulation of afferent synaptic transmission in the semicircular canals of frogs. Neurosignals 17, 222–228. doi: 10.1159/000224632
Athanasiou, D., Aguila, M., Bellingham, J., Li, W., McCulley, C., Reeves, P. J., et al. (2018). The molecular and cellular basis of rhodopsin retinitis pigmentosa reveals potential strategies for therapy. Prog. Retin. Eye Res. 62, 1–23. doi: 10.1016/j.preteyeres.2017.10.002
Azizi, Y., Faghihi, M., Imani, A., Roghani, M., and Nazari, A. (2013). Post-infarct treatment with [Pyr1]-apelin-13 reduces myocardial damage through reduction of oxidative injury and nitric oxide enhancement in the rat model of myocardial infarction. Peptides 46, 76–82. doi: 10.1016/j.peptides.2013.05.006
Babola, T. A., Kersbergen, C. J., Wang, H. C., and Bergles, D. E. (2020). Purinergic signaling in cochlear supporting cells reduces hair cell excitability by increasing the extracellular space. Elife 9:e52160. doi: 10.7554/eLife.52160
Babola, T. A., Li, S., Wang, Z., Kersbergen, C. J., Elgoyhen, A. B., Coate, T. M., et al. (2021). Purinergic signaling controls spontaneous activity in the auditory system throughout early development. J. Neurosci. 41, 594–612. doi: 10.1523/JNEUROSCI.2178-20.2020
Baek, M., DiMaio, F., Anishchenko, I., Dauparas, J., Ovchinnikov, S., Lee, G. R., et al. (2021). Accurate prediction of protein structures and interactions using a three-track neural network. Science 373, 871–876. doi: 10.1126/science.abj8754
Bakondi, B., Lv, W., Lu, B., Jones, M. K., Tsai, Y., Kim, K. J., et al. (2016). In vivo CRISPR/Cas9 gene editing corrects retinal dystrophy in the S334ter-3 rat model of autosomal dominant retinitis pigmentosa. Mol. Ther. 24, 556–563. doi: 10.1038/mt.2015.220
Beaulieu, J. M., and Gainetdinov, R. R. (2011). The physiology, signaling, and pharmacology of dopamine receptors. Pharmacol. Rev. 63, 182–217. doi: 10.1124/pr.110.002642
Bircan, B., Cakir, M., Kirbag, S., and Gul, H. F. (2016). Effect of apelin hormone on renal ischemia/reperfusion induced oxidative damage in rats. Ren. Fail. 38, 1122–1128. doi: 10.1080/0886022X.2016.1184957
Blankenship, A. G., and Feller, M. B. (2010). Mechanisms underlying spontaneous patterned activity in developing neural circuits. Nat. Rev. Neurosci. 11, 18–29. doi: 10.1038/nrn2759
Bondurand, N., Dufour, S., and Pingault, V. (2018). News from the endothelin-3/EDNRB signaling pathway: Role during enteric nervous system development and involvement in neural crest-associated disorders. Dev. Biol. 4, S156–S169. doi: 10.1016/j.ydbio.2018.08.014
Bonnet, C., and El-Amraoui, A. (2012). Usher syndrome (sensorineural deafness and retinitis pigmentosa): Pathogenesis, molecular diagnosis and therapeutic approaches. Curr. Opin. Neurol. 25, 42–49. doi: 10.1097/WCO.0b013e32834ef8b2
Botto, C., Dalkara, D., and El-Amraoui, A. (2021). Progress in gene editing tools and their potential for correcting mutations underlying hearing and vision loss. Front. Genome Ed. 3:737632. doi: 10.3389/fgeed.2021.737632
Bowery, N. G., and Enna, S. J. (2000). gamma-aminobutyric acid(B) receptors: First of the functional metabotropic heterodimers. J. Pharmacol. Exp. Ther. 292, 2–7.
Brown, A. S., and Epstein, D. J. (2011). Otic ablation of smoothened reveals direct and indirect requirements for Hedgehog signaling in inner ear development. Development 138, 3967–3976. doi: 10.1242/dev.066126
Brown, S. M., Wager-Miller, J., and Mackie, K. (2002). Cloning and molecular characterization of the rat CB2 cannabinoid receptor. Biochim. Biophys. Acta 1576, 255–264. doi: 10.1016/s0167-4781(02)00341-x
Buniello, A., Hardisty-Hughes, R. E., Pass, J. C., Bober, E., Smith, R. J., and Steel, K. P. (2013). Headbobber: A combined morphogenetic and cochleosaccular mouse model to study 10qter deletions in human deafness. PLoS One 8:e56274. doi: 10.1371/journal.pone.0056274
Burnight, E. R., Gupta, M., Wiley, L. A., Anfinson, K. R., Tran, A., Triboulet, R., et al. (2017). Using CRISPR-Cas9 to generate gene-corrected autologous iPSCs for the treatment of inherited retinal degeneration. Mol. Ther. 25, 1999–2013. doi: 10.1016/j.ymthe.2017.05.015
Cabou, C., and Martinez, L. O. (2022). The interplay of endothelial P2Y receptors in cardiovascular health: From vascular physiology to pathology. Int. J. Mol. Sci. 23:5883. doi: 10.3390/ijms23115883
Calver, A. R., Michalovich, D., Testa, T. T., Robbins, M. J., Jaillard, C., Hill, J., et al. (2003). Molecular cloning and characterisation of a novel GABAB-related G-protein coupled receptor. Brain Res. Mol. Brain Res. 110, 305–317. doi: 10.1016/s0169-328x(02)00662-9
Cao, X., Wang, P., Yuan, H., Zhang, H., He, Y., Fu, K., et al. (2022). Benzodiazepine derivatives as potent vasopressin V2 receptor antagonists for the treatment of autosomal dominant kidney disease. J. Med. Chem. 65, 9295–9311. doi: 10.1021/acs.jmedchem.2c00567
Cartier, A., and Hla, T. (2019). Sphingosine 1-phosphate: Lipid signaling in pathology and therapy. Science 366:eaar5551. doi: 10.1126/science.aar5551
Ceriani, F., and Mammano, F. (2012). Calcium signaling in the cochlea–molecular mechanisms and physiopathological implications. Cell Commun. Signal. 10:20. doi: 10.1186/1478-811X-10-20
Chadderton, N., Millington-Ward, S., Palfi, A., O’Reilly, M., Tuohy, G., Humphries, M. M., et al. (2009). Improved retinal function in a mouse model of dominant retinitis pigmentosa following AAV-delivered gene therapy. Mol. Ther. 17, 593–599. doi: 10.1038/mt.2008.301
Chang, H., Smallwood, P. M., Williams, J., and Nathans, J. (2016). The spatio-temporal domains of Frizzled6 action in planar polarity control of hair follicle orientation. Dev. Biol. 409, 181–193. doi: 10.1016/j.ydbio.2015.10.027
Chang, N. C., Dai, C. Y., Lin, W. Y., Yang, H. L., Wang, H. M., Chien, C. Y., et al. (2018). The association of GRM7 single nucleotide polymorphisms with age-related hearing impairment in a Taiwanese population. J. Int. Adv. Otol. 14, 170–175. doi: 10.5152/iao.2018.5109
Chaudhary, P. K., and Kim, S. (2021). An insight into GPCR and G-proteins as cancer drivers. Cells 10:3288. doi: 10.3390/cells10123288
Chen, H., Chen, K., Huang, W., Staudt, L. M., Cyster, J. G., and Li, X. (2022). Structure of S1PR2-heterotrimeric G13 signaling complex. Sci. Adv. 8:eabn0067. doi: 10.1126/sciadv.abn0067
Cheng, C., Guo, L., Lu, L., Xu, X., Zhang, S., Gao, J., et al. (2017). Characterization of the transcriptomes of Lgr5+ hair cell progenitors and Lgr5- supporting cells in the mouse cochlea. Front. Mol. Neurosci. 10:122. doi: 10.3389/fnmol.2017.00122
Claussen, A. D., Quevedo, R. V., Kirk, J. R., Higgins, T., Mostaert, B., Rahman, M. T., et al. (2022). Chronic cochlear implantation with and without electric stimulation in a mouse model induces robust cochlear influx of CX3CR1+/GFP macrophages. Hear. Res. 108510. doi: 10.1016/j.heares.2022.108510
Clevers, H., and Nusse, R. (2012). Wnt/beta-catenin signaling and disease. Cell 149, 1192–1205. doi: 10.1016/j.cell.2012.05.012
Colcombet-Cazenave, B., Cordier, F., Zhu, Y., Bouvier, G., Litsardaki, E., Laserre, L., et al. (2022). Deciphering the molecular interaction between the adhesion G protein-coupled receptor ADGRV1 and its PDZ-containing regulator PDZD7. Front. Mol. Biosci. 9:923740. doi: 10.3389/fmolb.2022.923740
Cox, B. C., Chai, R., Lenoir, A., Liu, Z., Zhang, L., Nguyen, D. H., et al. (2014). Spontaneous hair cell regeneration in the neonatal mouse cochlea in vivo. Development 141, 816–829. doi: 10.1242/dev.103036
Curtin, J. A., Quint, E., Tsipouri, V., Arkell, R. M., Cattanach, B., Copp, A. J., et al. (2003). Mutation of Celsr1 disrupts planar polarity of inner ear hair cells and causes severe neural tube defects in the mouse. Curr. Biol. 13, 1129–1133. doi: 10.1016/s0960-9822(03)00374-9
Daudet, N., Ripoll, C., Moles, J. P., and Rebillard, G. (2002). Expression of members of Wnt and Frizzled gene families in the postnatal rat cochlea. Brain Res. Mol. Brain Res. 105, 98–107. doi: 10.1016/s0169-328x(02)00397-2
Davies, A., Formstone, C., Mason, I., and Lewis, J. (2005). Planar polarity of hair cells in the chick inner ear is correlated with polarized distribution of c-flamingo-1 protein. Dev. Dyn. 233, 998–1005. doi: 10.1002/dvdy.20376
de la Nuez Veulens, A., Ginarte, Y. M. A., Fernandez, R. E. R., Leclerc, F., and Cabrera, L. A. M. (2022). Prediction of molecular interactions and physicochemical properties relevant for vasopressin V2 receptor antagonism. J. Mol. Model. 28:31. doi: 10.1007/s00894-021-05022-6
Dedic, N., Chen, A., and Deussing, J. M. (2018). The CRF family of neuropeptides and their receptors–mediators of the central stress response. Curr. Mol. Pharmacol. 11, 4–31. doi: 10.2174/1874467210666170302104053
Deganutti, G., Atanasio, S., Rujan, R. M., Sexton, P. M., Wootten, D., and Reynolds, C. A. (2021). Exploring ligand binding to calcitonin gene-related peptide receptors. Front. Mol. Biosci. 8:720561. doi: 10.3389/fmolb.2021.720561
Dhukhwa, A., Al Aameri, R. F. H., Sheth, S., Mukherjea, D., Rybak, L., and Ramkumar, V. (2021). Regulator of G protein signaling 17 represents a novel target for treating cisplatin induced hearing loss. Sci. Rep. 11:8116. doi: 10.1038/s41598-021-87387-5
Dickerson, I. M., Bussey-Gaborski, R., Holt, J. C., Jordan, P. M., and Luebke, A. E. (2016). Maturation of suprathreshold auditory nerve activity involves cochlear CGRP-receptor complex formation. Physiol. Rep. 4:e12869. doi: 10.14814/phy2.12869
Dijksterhuis, J. P., Petersen, J., and Schulte, G. (2014). WNT/Frizzled signalling: Receptor-ligand selectivity with focus on FZD-G protein signalling and its physiological relevance: IUPHAR Review 3. Br. J. Pharmacol. 171, 1195–1209. doi: 10.1111/bph.12364
Doleviczenyi, Z., Halmos, G., Repassy, G., Vizi, E. S., Zelles, T., and Lendvai, B. (2005). Cochlear dopamine release is modulated by group II metabotropic glutamate receptors via GABAergic neurotransmission. Neurosci. Lett. 385, 93–98. doi: 10.1016/j.neulet.2005.05.017
Drescher, M. J., Drescher, D. G., Khan, K. M., Hatfield, J. S., Ramakrishnan, N. A., Abu-Hamdan, M. D., et al. (2006). Pituitary adenylyl cyclase-activating polypeptide (PACAP) and its receptor (PAC1-R) are positioned to modulate afferent signaling in the cochlea. Neuroscience 142, 139–164. doi: 10.1016/j.neuroscience.2006.05.065
Driver, E. C., and Kelley, M. W. (2020). Development of the cochlea. Development 147:dev162263. doi: 10.1242/dev.162263
Duan, J., Xu, P., Luan, X., Ji, Y., He, X., Song, N., et al. (2022). Hormone- and antibody-mediated activation of the thyrotropin receptor. Nature 609, 854–859. doi: 10.1038/s41586-022-05173-3
Duncan, J. S., Fritzsch, B., Houston, D. W., Ketchum, E. M., Kersigo, J., Deans, M. R., et al. (2019). Topologically correct central projections of tetrapod inner ear afferents require Fzd3. Sci. Rep. 9:10298. doi: 10.1038/s41598-019-46553-6
Duncan, J. S., Stoller, M. L., Francl, A. F., Tissir, F., Devenport, D., and Deans, M. R. (2017). Celsr1 coordinates the planar polarity of vestibular hair cells during inner ear development. Dev. Biol. 423, 126–137. doi: 10.1016/j.ydbio.2017.01.020
Ebermann, I., Scholl, H. P., Charbel Issa, P., Becirovic, E., Lamprecht, J., Jurklies, B., et al. (2007). A novel gene for Usher syndrome type 2: Mutations in the long isoform of whirlin are associated with retinitis pigmentosa and sensorineural hearing loss. Hum. Genet. 121, 203–211. doi: 10.1007/s00439-006-0304-0
Egami, N., Kakigi, A., Takeda, T., and Yamasoba, T. (2016). Dehydration effects of a V2 antagonist on endolymphatic hydrops in guinea pigs. Hear. Res. 332, 151–159. doi: 10.1016/j.heares.2015.12.017
Faust, B., Billesbolle, C. B., Suomivuori, C. M., Singh, I., Zhang, K., Hoppe, N., et al. (2022). Autoantibody mimicry of hormone action at the thyrotropin receptor. Nature 609, 846–853. doi: 10.1038/s41586-022-05159-1
Feng, J., and Liu, H. (2015). [The expression and significance of VIP and its receptor in the cochlea of different degrees of chronic alcoholism rats]. Lin Chung Er Bi Yan Hou Tou Jing Wai Ke Za Zhi 29, 1295–1298.
Fisher, N. M., Gould, R. W., Gogliotti, R. G., McDonald, A. J., Badivuku, H., Chennareddy, S., et al. (2020). Phenotypic profiling of mGlu7 knockout mice reveals new implications for neurodevelopmental disorders. Genes Brain Behav. 19:e12654. doi: 10.1111/gbb.12654
Foord, S. M., Bonner, T. I., Neubig, R. R., Rosser, E. M., Pin, J. P., Davenport, A. P., et al. (2005). International Union of Pharmacology. XLVI. G protein-coupled receptor list. Pharmacol. Rev. 57, 279–288. doi: 10.1124/pr.57.2.5
Fournel, A., Drougard, A., Duparc, T., Marlin, A., Brierley, S. M., Castro, J., et al. (2017). Apelin targets gut contraction to control glucose metabolism via the brain. Gut 66, 258–269. doi: 10.1136/gutjnl-2015-310230
Friedman, R. A., Van Laer, L., Huentelman, M. J., Sheth, S. S., Van Eyken, E., Corneveaux, J. J., et al. (2009). GRM7 variants confer susceptibility to age-related hearing impairment. Hum. Mol. Genet. 18, 785–796. doi: 10.1093/hmg/ddn402
Fritzius, T., Stawarski, M., Isogai, S., and Bettler, B. (2022). Structural basis of GABAB receptor regulation and signaling. Curr. Top. Behav. Neurosci. 52, 19–37. doi: 10.1007/7854_2020_147
Fulop, D. B., Humli, V., Szepesy, J., Ott, V., Reglodi, D., Gaszner, B., et al. (2019). Hearing impairment and associated morphological changes in pituitary adenylate cyclase activating polypeptide (PACAP)-deficient mice. Sci. Rep. 9:14598. doi: 10.1038/s41598-019-50775-z
Gaborjan, A., Lendvai, B., and Vizi, E. S. (1999). Neurochemical evidence of dopamine release by lateral olivocochlear efferents and its presynaptic modulation in guinea-pig cochlea. Neuroscience 90, 131–138. doi: 10.1016/s0306-4522(98)00461-8
Gao, X., Tao, Y., Lamas, V., Huang, M., Yeh, W. H., Pan, B., et al. (2018). Treatment of autosomal dominant hearing loss by in vivo delivery of genome editing agents. Nature 553, 217–221. doi: 10.1038/nature25164
Gariepy, C. E., Cass, D. T., and Yanagisawa, M. (1996). Null mutation of endothelin receptor type B gene in spotting lethal rats causes aganglionic megacolon and white coat color. Proc. Natl. Acad. Sci. U.S.A. 93, 867–872. doi: 10.1073/pnas.93.2.867
Ge, Y., and Wang, Y. T. (2022). Postsynaptic signaling at glutamatergic synapses as therapeutic targets. Curr. Opin. Neurobiol. 75:102585. doi: 10.1016/j.conb.2022.102585
Geleoc, G. G. S., and El-Amraoui, A. (2020). Disease mechanisms and gene therapy for Usher syndrome. Hear. Res. 394:107932. doi: 10.1016/j.heares.2020.107932
Ghimire, S. R., and Deans, M. R. (2019). Frizzled3 and Frizzled6 cooperate with Vangl2 to direct cochlear innervation by type II spiral ganglion neurons. J. Neurosci. 39, 8013–8023. doi: 10.1523/JNEUROSCI.1740-19.2019
Ghosh, S., Sheth, S., Sheehan, K., Mukherjea, D., Dhukhwa, A., Borse, V., et al. (2018). The endocannabinoid/cannabinoid receptor 2 system protects against cisplatin-induced hearing loss. Front. Cell. Neurosci. 12:271. doi: 10.3389/fncel.2018.00271
Girotto, G., Vuckovic, D., Buniello, A., Lorente-Canovas, B., Lewis, M., Gasparini, P., et al. (2014). Expression and replication studies to identify new candidate genes involved in normal hearing function. PLoS One 9:e85352. doi: 10.1371/journal.pone.0085352
Gorojankina, T. (2016). Hedgehog signaling pathway: A novel model and molecular mechanisms of signal transduction. Cell. Mol. Life Sci. 73, 1317–1332. doi: 10.1007/s00018-015-2127-4
Graham, C. E., Basappa, J., and Vetter, D. E. (2010). A corticotropin-releasing factor system expressed in the cochlea modulates hearing sensitivity and protects against noise-induced hearing loss. Neurobiol. Dis. 38, 246–258. doi: 10.1016/j.nbd.2010.01.014
Graham, C. E., and Vetter, D. E. (2011). The mouse cochlea expresses a local hypothalamic-pituitary-adrenal equivalent signaling system and requires corticotropin-releasing factor receptor 1 to establish normal hair cell innervation and cochlear sensitivity. J. Neurosci. 31, 1267–1278. doi: 10.1523/JNEUROSCI.4545-10.2011
Grainger, S., and Willert, K. (2018). Mechanisms of Wnt signaling and control. Wiley Interdiscip. Rev. Syst. Biol. Med. 10:e1422. doi: 10.1002/wsbm.1422
Grandi, F. C., De Tomasi, L., and Mustapha, M. (2020). Single-cell RNA analysis of type I spiral ganglion neurons reveals a Lmx1a population in the cochlea. Front. Mol. Neurosci. 13:83. doi: 10.3389/fnmol.2020.00083
Gu, W. X., Du, G. G., Kopp, P., Rentoumis, A., Albanese, C., Kohn, L. D., et al. (1995). The thyrotropin (TSH) receptor transmembrane domain mutation (Pro556-Leu) in the hypothyroid hyt/hyt mouse results in plasma membrane targeting but defective TSH binding. Endocrinology 136, 3146–3153. doi: 10.1210/endo.136.7.7789342
Guo, Y., Han, L., Han, S., Tang, H., Wang, S., Cui, C., et al. (2022). Specific knockdown of Htra2 by CRISPR-CasRx prevents acquired sensorineural hearing loss in mice. Mol. Ther. Nucleic Acids 28, 643–655. doi: 10.1016/j.omtn.2022.04.014
Gyorgy, B., Nist-Lund, C., Pan, B., Asai, Y., Karavitaki, K. D., Kleinstiver, B. P., et al. (2019). Allele-specific gene editing prevents deafness in a model of dominant progressive hearing loss. Nat. Med. 25, 1123–1130. doi: 10.1038/s41591-019-0500-9
Habrian, C. H., Levitz, J., Vyklicky, V., Fu, Z., Hoagland, A., McCort-Tranchepain, I., et al. (2019). Conformational pathway provides unique sensitivity to a synaptic mGluR. Nat. Commun. 10:5572. doi: 10.1038/s41467-019-13407-8
Han, B. R., Lin, S. C., Espinosa, K., Thorne, P. R., and Vlajkovic, S. M. (2019). Inhibition of the adenosine A2A receptor mitigates excitotoxic injury in organotypic tissue cultures of the rat cochlea. Cells 8:877. doi: 10.3390/cells8080877
Hauser, A. S., Attwood, M. M., Rask-Andersen, M., Schioth, H. B., and Gloriam, D. E. (2017). Trends in GPCR drug discovery: New agents, targets and indications. Nat. Rev. Drug Discov. 16, 829–842. doi: 10.1038/nrd.2017.178
He, C., He, L., Lu, Q., Xiao, J., and Dong, W. (2022). The functions and prognostic values of chemokine and chemokine receptors in gastric cancer. Am. J. Cancer Res. 12, 3034–3050.
Herr, D. R., Grillet, N., Schwander, M., Rivera, R., Muller, U., and Chun, J. (2007). Sphingosine 1-phosphate (S1P) signaling is required for maintenance of hair cells mainly via activation of S1P2. J. Neurosci. 27, 1474–1478. doi: 10.1523/JNEUROSCI.4245-06.2007
Herr, D. R., Reolo, M. J., Peh, Y. X., Wang, W., Lee, C. W., Rivera, R., et al. (2016). Sphingosine 1-phosphate receptor 2 (S1P2) attenuates reactive oxygen species formation and inhibits cell death: Implications for otoprotective therapy. Sci. Rep. 6:24541. doi: 10.1038/srep24541
Hirose, K., and Li, S. Z. (2019). The role of monocytes and macrophages in the dynamic permeability of the blood-perilymph barrier. Hear. Res. 374, 49–57. doi: 10.1016/j.heares.2019.01.006
Hofrichter, M. A. H., Mojarad, M., Doll, J., Grimm, C., Eslahi, A., Hosseini, N. S., et al. (2018). The conserved p.Arg108 residue in S1PR2 (DFNB68) is fundamental for proper hearing: Evidence from a consanguineous Iranian family. BMC Med. Genet. 19:81. doi: 10.1186/s12881-018-0598-5
Hollenstein, K., de Graaf, C., Bortolato, A., Wang, M. W., Marshall, F. H., and Stevens, R. C. (2014). Insights into the structure of class B GPCRs. Trends Pharmacol. Sci. 35, 12–22. doi: 10.1016/j.tips.2013.11.001
Huang, L. C., Thorne, P. R., Vlajkovic, S. M., and Housley, G. D. (2010). Differential expression of P2Y receptors in the rat cochlea during development. Purinergic Signal. 6, 231–248. doi: 10.1007/s11302-010-9191-x
Huang, S., Song, J., He, C., Cai, X., Yuan, K., Mei, L., et al. (2021). Genetic insights, disease mechanisms, and biological therapeutics for Waardenburg syndrome. Gene Ther. 29, 479–497. doi: 10.1038/s41434-021-00240-2
Huang, X., Wu, W., Hu, P., and Wang, Q. (2018). Taurine enhances mouse cochlear neural stem cells proliferation and differentiation to sprial gangli through activating sonic hedgehog signaling pathway. Organogenesis 14, 147–157. doi: 10.1080/15476278.2018.1477462
Ida-Eto, M., Ohgami, N., Iida, M., Yajima, I., Kumasaka, M. Y., Takaiwa, K., et al. (2011). Partial requirement of endothelin receptor B in spiral ganglion neurons for postnatal development of hearing. J. Biol. Chem. 286, 29621–29626. doi: 10.1074/jbc.M111.236802
Iizuka, T., Kamiya, K., Gotoh, S., Sugitani, Y., Suzuki, M., Noda, T., et al. (2015). Perinatal Gjb2 gene transfer rescues hearing in a mouse model of hereditary deafness. Hum. Mol. Genet. 24, 3651–3661. doi: 10.1093/hmg/ddv109
Ingham, N. J., Carlisle, F., Pearson, S., Lewis, M. A., Buniello, A., Chen, J., et al. (2016). S1PR2 variants associated with auditory function in humans and endocochlear potential decline in mouse. Sci. Rep. 6:28964. doi: 10.1038/srep28964
Insel, P. A., Sriram, K., Gorr, M. W., Wiley, S. Z., Michkov, A., Salmeron, C., et al. (2019). GPCRomics: An approach to discover GPCR drug targets. Trends Pharmacol. Sci. 40, 378–387. doi: 10.1016/j.tips.2019.04.001
Ishiguro, H., Kibret, B. G., Horiuchi, Y., and Onaivi, E. S. (2022). Potential role of cannabinoid type 2 receptors in neuropsychiatric and neurodegenerative disorders. Front. Psychiatry 13:828895. doi: 10.3389/fpsyt.2022.828895
Izume, T., Miyauchi, H., Shihoya, W., and Nureki, O. (2020). Crystal structure of human endothelin ETB receptor in complex with sarafotoxin S6b. Biochem. Biophys. Res. Commun. 528, 383–388. doi: 10.1016/j.bbrc.2019.12.091
Jang, M. W., Lim, J., Park, M. G., Lee, J. H., and Lee, C. J. (2022). Active role of glia-like supporting cells in the organ of Corti: Membrane proteins and their roles in hearing. Glia 70, 1799–1825. doi: 10.1002/glia.24229
Jiang, L., He, J., Chen, X., and Chen, H. (2019). Effect of electroacupuncture on arginine vasopressin-induced endolymphatic hydrops. J. Tradit. Chin. Med. 39, 221–228.
Jiang, L. Y., He, J. J., Chen, X. X., Sun, X. J., Wang, X. Z., Zhong, S., et al. (2019). Arginine vasopressin-aquaporin-2 pathway-mediated dehydration effects of electroacupuncture in guinea pig model of AVP-induced eendolymphatic hydrops. Chin. J. Integr. Med. 25, 763–769. doi: 10.1007/s11655-017-2411-2
Jumper, J., Evans, R., Pritzel, A., Green, T., Figurnov, M., Ronneberger, O., et al. (2021). Highly accurate protein structure prediction with AlphaFold. Nature 596, 583–589. doi: 10.1038/s41586-021-03819-2
Jung, S., Aliberti, J., Graemmel, P., Sunshine, M. J., Kreutzberg, G. W., Sher, A., et al. (2000). Analysis of fractalkine receptor CX(3)CR1 function by targeted deletion and green fluorescent protein reporter gene insertion. Mol. Cell. Biol. 20, 4106–4114. doi: 10.1128/MCB.20.11.4106-4114.2000
Kaur, T., Borse, V., Sheth, S., Sheehan, K., Ghosh, S., Tupal, S., et al. (2016). Adenosine A1 receptor protects against cisplatin ototoxicity by suppressing the NOX3/STAT1 inflammatory pathway in the cochlea. J. Neurosci. 36, 3962–3977. doi: 10.1523/JNEUROSCI.3111-15.2016
Keeley, E. C., Mehrad, B., and Strieter, R. M. (2010). CXC chemokines in cancer angiogenesis and metastases. Adv. Cancer Res. 106, 91–111. doi: 10.1016/S0065-230X(10)06003-3
Kelley, M. W. (2022). Cochlear development; new tools and approaches. Front. Cell Dev. Biol. 10:884240. doi: 10.3389/fcell.2022.884240
Khoshsirat, S., Abbaszadeh, H. A., Peyvandi, A. A., Heidari, F., Peyvandi, M., Simani, L., et al. (2021). Apelin-13 prevents apoptosis in the cochlear tissue of noise-exposed rat via Sirt-1 regulation. J. Chem. Neuroanat. 114:101956. doi: 10.1016/j.jchemneu.2021.101956
Kilander, M. B., Petersen, J., Andressen, K. W., Ganji, R. S., Levy, F. O., Schuster, J., et al. (2014). Disheveled regulates precoupling of heterotrimeric G proteins to Frizzled 6. FASEB J. 28, 2293–2305. doi: 10.1096/fj.13-246363
Kim, C. H., Kim, H. Y., Lee, H. S., Chang, S. O., Oh, S. H., and Lee, J. H. (2010). P2Y4-mediated regulation of Na+ absorption in the Reissner’s membrane of the cochlea. J. Neurosci. 30, 3762–3769. doi: 10.1523/JNEUROSCI.3300-09.2010
Kim, H. J., Ryu, J., Woo, H. M., Cho, S. S., Sung, M. K., Kim, S. C., et al. (2014). Patterns of gene expression associated with Pten deficiency in the developing inner ear. PLoS One 9:e97544. doi: 10.1371/journal.pone.0097544
Kim, S., Jo, C. H., and Kim, G. H. (2021). The role of vasopressin V2 receptor in drug-induced hyponatremia. Front. Physiol. 12:797039. doi: 10.3389/fphys.2021.797039
Kimberling, W. J., Weston, M. D., Moller, C., van Aarem, A., Cremers, C. W., Sumegi, J., et al. (1995). Gene mapping of Usher syndrome type IIa: Localization of the gene to a 2.1-cM segment on chromosome 1q41. Am. J. Hum. Genet. 56, 216–223.
Kindt, K. S., Akturk, A., Jarysta, A., Day, M., Beirl, A., Flonard, M., et al. (2021). EMX2-GPR156-Galphai reverses hair cell orientation in mechanosensory epithelia. Nat. Commun. 12:2861. doi: 10.1038/s41467-021-22997-1
Kitanishi, T., Suzuki, M., Kitano, H., Yazawa, Y., Yamada, H., and Kitajima, K. (1998). Immunohistochemical detection of vasoactive intestinal polypeptide (VIP) and the VIP receptor in the rat inner ear. Acta Otolaryngol. Suppl. 539, 52–56.
Klotz, L., and Enz, R. (2021). MGluR7 is a presynaptic metabotropic glutamate receptor at ribbon synapses of inner hair cells. FASEB J. 35:e21855. doi: 10.1096/fj.202100672R
Klotz, L., Wendler, O., Frischknecht, R., Shigemoto, R., Schulze, H., and Enz, R. (2019). Localization of group II and III metabotropic glutamate receptors at pre- and postsynaptic sites of inner hair cell ribbon synapses. FASEB J. 33, 13734–13746. doi: 10.1096/fj.201901543R
Kochman, K. (2014). Superfamily of G-protein coupled receptors (GPCRs)–extraordinary and outstanding success of evolution. Postepy Hig. Med. Dosw. 68, 1225–1237. doi: 10.5604/17322693.1127326
Koles, L., Szepesy, J., Berekmeri, E., and Zelles, T. (2019). Purinergic signaling and cochlear injury-targeting the immune system? Int. J. Mol. Sci. 20:2979. doi: 10.3390/ijms20122979
Kono, M., Belyantseva, I. A., Skoura, A., Frolenkov, G. I., Starost, M. F., Dreier, J. L., et al. (2007). Deafness and stria vascularis defects in S1P2 receptor-null mice. J. Biol. Chem. 282, 10690–10696. doi: 10.1074/jbc.M700370200
Kooistra, A. J., Mordalski, S., Pandy-Szekeres, G., Esguerra, M., Mamyrbekov, A., Munk, C., et al. (2021). GPCRdb in 2021: Integrating GPCR sequence, structure and function. Nucleic Acids Res. 49, D335–D343. doi: 10.1093/nar/gkaa1080
Kou, Z. Z., Qu, J., Zhang, D. L., Li, H., and Li, Y. Q. (2013). Noise-induced hearing loss is correlated with alterations in the expression of GABAB receptors and PKC gamma in the murine cochlear nucleus complex. Front. Neuroanat. 7:25. doi: 10.3389/fnana.2013.00025
Kozielewicz, P., Turku, A., and Schulte, G. (2020). Molecular pharmacology of class F receptor activation. Mol. Pharmacol. 97, 62–71. doi: 10.1124/mol.119.117986
Landin Malt, A., Clancy, S., Hwang, D., Liu, A., Smith, C., Smith, M., et al. (2021). Non-canonical Wnt signaling regulates cochlear outgrowth and planar cell polarity via Gsk3beta inhibition. Front. Cell Dev. Biol. 9:649830. doi: 10.3389/fcell.2021.649830
Langer, I., Jeandriens, J., Couvineau, A., Sanmukh, S., and Latek, D. (2022). Signal transduction by VIP and PACAP receptors. Biomedicines 10:406. doi: 10.3390/biomedicines10020406
Le Prell, C. G., Hughes, L. F., Dolan, D. F., and Bledsoe, S. C. Jr. (2021). Effects of calcitonin-gene-related-peptide on auditory nerve activity. Front. Cell Dev. Biol. 9:752963. doi: 10.3389/fcell.2021.752963
Lee, S., Dondzillo, A., Gubbels, S. P., and Raphael, Y. (2020). Practical aspects of inner ear gene delivery for research and clinical applications. Hear. Res. 394:107934. doi: 10.1016/j.heares.2020.107934
Li, D., Henley, C. M., and O’Malley, B. W. Jr. (1999). Distortion product otoacoustic emissions and outer hair cell defects in the hyt/hyt mutant mouse. Hear. Res. 138, 65–72. doi: 10.1016/s0378-5955(99)00150-1
Li, X., Roszko, I., Sepich, D. S., Ni, M., Hamm, H. E., Marlow, F. L., et al. (2013). Gpr125 modulates Dishevelled distribution and planar cell polarity signaling. Development 140, 3028–3039. doi: 10.1242/dev.094839
Li, X., Zhang, D., Xu, L., Han, Y., Liu, W., Li, W., et al. (2021). Planar cell polarity defects and hearing loss in sperm-associated antigen 6 (Spag6)-deficient mice. Am. J. Physiol. Cell Physiol. 320, C132–C141. doi: 10.1152/ajpcell.00166.2020
Li, Y., Zou, Q., and Zhang, J. (2018). Vincamine exerts protective effect on spiral ganglion neurons in endolymphatic hydrops guinea pig models. Am. J. Transl. Res. 10, 3650–3663.
Liang, Y. L., Khoshouei, M., Deganutti, G., Glukhova, A., Koole, C., Peat, T. S., et al. (2018). Cryo-EM structure of the active, Gs-protein complexed, human CGRP receptor. Nature 561, 492–497. doi: 10.1038/s41586-018-0535-y
Liang, Y. L., Khoshouei, M., Radjainia, M., Zhang, Y., Glukhova, A., Tarrasch, J., et al. (2017). Phase-plate cryo-EM structure of a class B GPCR-G-protein complex. Nature 546, 118–123. doi: 10.1038/nature22327
Lin, L., Chen, Y. S., Yao, Y. D., Chen, J. Q., Chen, J. N., Huang, S. Y., et al. (2015). CCL18 from tumor-associated macrophages promotes angiogenesis in breast cancer. Oncotarget 6, 34758–34773. doi: 10.18632/oncotarget.5325
Lin, X., Chen, S., and Chen, P. (2000). Activation of metabotropic GABAB receptors inhibited glutamate responses in spiral ganglion neurons of mice. Neuroreport 11, 957–961. doi: 10.1097/00001756-200004070-00012
Lowery, E. G., and Thiele, T. E. (2010). Pre-clinical evidence that corticotropin-releasing factor (CRF) receptor antagonists are promising targets for pharmacological treatment of alcoholism. CNS Neurol. Disord. Drug Targets 9, 77–86. doi: 10.2174/187152710790966605
Lv, X., Chen, Q., Zhang, S., Gao, F., and Liu, Q. (2022). CGRP: A new endogenous cell stemness maintenance molecule. Oxid. Med. Cell. Longev. 2022:4107433. doi: 10.1155/2022/4107433
Lv, X., Kong, J., Chen, W. D., and Wang, Y. D. (2017). The role of the Apelin/APJ system in the regulation of liver disease. Front. Pharmacol. 8:221. doi: 10.3389/fphar.2017.00221
Ma, X., Zhang, S., Qin, S., Guo, J., Yuan, J., Qiang, R., et al. (2022). Transcriptomic and epigenomic analyses explore the potential role of H3K4me3 in neomycin-induced cochlear Lgr5+ progenitor cell regeneration of hair cells. Hum. Cell 35, 1030–1044. doi: 10.1007/s13577-022-00727-z
Maeda, Y., Kariya, S., Omichi, R., Noda, Y., Sugaya, A., Fujimoto, S., et al. (2018). Targeted PCR array analysis of genes in innate immunity and glucocorticoid signaling pathways in mice cochleae following acoustic trauma. Otol. Neurotol. 39, e593–e600. doi: 10.1097/MAO.0000000000001874
Maguire, A. M., Bennett, J., Aleman, E. M., Leroy, B. P., and Aleman, T. S. (2021). Clinical perspective: Treating RPE65-associated retinal dystrophy. Mol. Ther. 29, 442–463. doi: 10.1016/j.ymthe.2020.11.029
Maguire, C. A., and Corey, D. P. (2020). Viral vectors for gene delivery to the inner ear. Hear. Res. 394:107927. doi: 10.1016/j.heares.2020.107927
Maison, S. F., Casanova, E., Holstein, G. R., Bettler, B., and Liberman, M. C. (2009). Loss of GABAB receptors in cochlear neurons: Threshold elevation suggests modulation of outer hair cell function by type II afferent fibers. J. Assoc. Res. Otolaryngol. 10, 50–63. doi: 10.1007/s10162-008-0138-7
Maison, S. F., Emeson, R. B., Adams, J. C., Luebke, A. E., and Liberman, M. C. (2003). Loss of alpha CGRP reduces sound-evoked activity in the cochlear nerve. J. Neurophysiol. 90, 2941–2949. doi: 10.1152/jn.00596.2003
Maison, S. F., Liu, X. P., Eatock, R. A., Sibley, D. R., Grandy, D. K., and Liberman, M. C. (2012). Dopaminergic signaling in the cochlea: Receptor expression patterns and deletion phenotypes. J. Neurosci. 32, 344–355. doi: 10.1523/JNEUROSCI.4720-11.2012
Makita, N., Manaka, K., Sato, J., and Iiri, T. (2020). V2 vasopressin receptor mutations. Vitam. Horm. 113, 79–99. doi: 10.1016/bs.vh.2019.08.012
Manalo, J. M., Liu, H., Ding, D., Hicks, J., Sun, H., Salvi, R., et al. (2020). Adenosine A2B receptor: A pathogenic factor and a therapeutic target for sensorineural hearing loss. FASEB J. 34, 15771–15787. doi: 10.1096/fj.202000939R
Mao, C., Shen, C., Li, C., Shen, D. D., Xu, C., Zhang, S., et al. (2020). Cryo-EM structures of inactive and active GABAB receptor. Cell Res. 30, 564–573. doi: 10.1038/s41422-020-0350-5
Mao, H., James, T. Jr., Schwein, A., Shabashvili, A. E., Hauswirth, W. W., Gorbatyuk, M. S., et al. (2011). AAV delivery of wild-type rhodopsin preserves retinal function in a mouse model of autosomal dominant retinitis pigmentosa. Hum. Gene Ther. 22, 567–575. doi: 10.1089/hum.2010.140
Martin-Grace, J., Tomkins, M., O’Reilly, M. W., Thompson, C. J., and Sherlock, M. (2022). Approach to the patient: Hyponatremia and the syndrome of inappropriate antidiuresis (SIAD). J. Clin. Endocrinol. Metab. 107, 2362–2376. doi: 10.1210/clinem/dgac245
Massink, A., Amelia, T., Karamychev, A., and Ap, I. J. (2020). Allosteric modulation of G protein-coupled receptors by amiloride and its derivatives. Perspectives for drug discovery? Med. Res. Rev. 40, 683–708. doi: 10.1002/med.21633
Matsushima, Y., Shinkai, Y., Kobayashi, Y., Sakamoto, M., Kunieda, T., and Tachibana, M. (2002). A mouse model of Waardenburg syndrome type 4 with a new spontaneous mutation of the endothelin-B receptor gene. Mamm. Genome 13, 30–35. doi: 10.1007/s00335-001-3038-2
Matyas, P., Postyeni, E., Komlosi, K., Szalai, R., Bene, J., Magyari, L., et al. (2019). Age-related hearing impairment associated NAT2, GRM7, GRHL2 susceptibility gene polymorphisms and haplotypes in roma and Hungarian populations. Pathol. Oncol. Res. 25, 1349–1355. doi: 10.1007/s12253-018-0388-6
McLean, W. J., Yin, X., Lu, L., Lenz, D. R., McLean, D., Langer, R., et al. (2017). Clonal expansion of Lgr5-positive cells from mammalian cochlea and high-purity generation of sensory hair cells. Cell Rep. 18, 1917–1929. doi: 10.1016/j.celrep.2017.01.066
McMillan, D. R., and White, P. C. (2004). Loss of the transmembrane and cytoplasmic domains of the very large G-protein-coupled receptor-1 (VLGR1 or Mass1) causes audiogenic seizures in mice. Mol. Cell. Neurosci. 26, 322–329. doi: 10.1016/j.mcn.2004.02.005
Mehkri, Y., Hanna, C., Sriram, S., Lucke-Wold, B., Johnson, R. D., and Busl, K. (2022). Calcitonin gene-related peptide and neurologic injury: An emerging target for headache management. Clin. Neurol. Neurosurg. 220:107355. doi: 10.1016/j.clineuro.2022.107355
Meredith, F. L., and Rennie, K. J. (2021). Dopaminergic inhibition of Na+ currents in vestibular inner ear afferents. Front. Neurosci. 15:710321. doi: 10.3389/fnins.2021.710321
Merighi, S., Borea, P. A., Varani, K., Vincenzi, F., Travagli, A., Nigro, M., et al. (2022). Pathophysiological role and medicinal chemistry of A2A adenosine receptor antagonists in Alzheimer’s disease. Molecules 27:2680. doi: 10.3390/molecules27092680
Michalski, N., Michel, V., Bahloul, A., Lefevre, G., Barral, J., Yagi, H., et al. (2007). Molecular characterization of the ankle-link complex in cochlear hair cells and its role in the hair bundle functioning. J. Neurosci. 27, 6478–6488. doi: 10.1523/JNEUROSCI.0342-07.2007
Minakata, T., Inagaki, A., Yamamura, A., Yamamura, H., Sekiya, S., and Murakami, S. (2019). Calcium-sensing receptor is functionally expressed in the cochlear perilymphatic compartment and essential for hearing. Front. Mol. Neurosci. 12:175. doi: 10.3389/fnmol.2019.00175
Montcouquiol, M., Sans, N., Huss, D., Kach, J., Dickman, J. D., Forge, A., et al. (2006). Asymmetric localization of Vangl2 and Fz3 indicate novel mechanisms for planar cell polarity in mammals. J. Neurosci. 26, 5265–5275. doi: 10.1523/JNEUROSCI.4680-05.2006
Munro, S., Thomas, K. L., and Abu-Shaar, M. (1993). Molecular characterization of a peripheral receptor for cannabinoids. Nature 365, 61–65. doi: 10.1038/365061a0
Muthu, V., Rohacek, A. M., Yao, Y., Rakowiecki, S. M., Brown, A. S., Zhao, Y. T., et al. (2019). Genomic architecture of Shh-dependent cochlear morphogenesis. Development 146:dev181339. doi: 10.1242/dev.181339
Nakayama, M., Tabuchi, K., Hoshino, T., Nakamagoe, M., Nishimura, B., and Hara, A. (2014). The influence of sphingosine-1-phosphate receptor antagonists on gentamicin-induced hair cell loss of the rat cochlea. Neurosci. Lett. 561, 91–95. doi: 10.1016/j.neulet.2013.12.063
Newman, D. L., Fisher, L. M., Ohmen, J., Parody, R., Fong, C. T., Frisina, S. T., et al. (2012). GRM7 variants associated with age-related hearing loss based on auditory perception. Hear. Res. 294, 125–132. doi: 10.1016/j.heares.2012.08.016
Niknazar, S., Abbaszadeh, H. A., Peyvandi, H., Rezaei, O., Forooghirad, H., Khoshsirat, S., et al. (2019). Protective effect of [Pyr1]-apelin-13 on oxidative stress-induced apoptosis in hair cell-like cells derived from bone marrow mesenchymal stem cells. Eur. J. Pharmacol. 853, 25–32. doi: 10.1016/j.ejphar.2019.03.012
Nist-Lund, C. A., Pan, B., Patterson, A., Asai, Y., Chen, T., Zhou, W., et al. (2019). Improved TMC1 gene therapy restores hearing and balance in mice with genetic inner ear disorders. Nat. Commun. 10:236. doi: 10.1038/s41467-018-08264-w
Niswender, C. M., and Conn, P. J. (2010). Metabotropic glutamate receptors: Physiology, pharmacology, and disease. Annu. Rev. Pharmacol. Toxicol. 50, 295–322. doi: 10.1146/annurev.pharmtox.011008.145533
Odoemelam, C. S., Percival, B., Wallis, H., Chang, M. W., Ahmad, Z., Scholey, D., et al. (2020). G-Protein coupled receptors: Structure and function in drug discovery. RSC Adv. 10, 36337–36348. doi: 10.1039/d0ra08003a
Oestreicher, E., Arnold, W., Ehrenberger, K., and Felix, D. (1997). Dopamine regulates the glutamatergic inner hair cell activity in guinea pigs. Hear. Res. 107, 46–52. doi: 10.1016/s0378-5955(97)00023-3
Okashah, N., Wright, S. C., Kawakami, K., Mathiasen, S., Zhou, J., Lu, S., et al. (2020). Agonist-induced formation of unproductive receptor-G12 complexes. Proc. Natl. Acad. Sci. U.S.A. 117, 21723–21730. doi: 10.1073/pnas.2003787117
O’Keeffe, M. G., Thorne, P. R., Housley, G. D., Robson, S. C., and Vlajkovic, S. M. (2010). Developmentally regulated expression of ectonucleotidases NTPDase5 and NTPDase6 and UDP-responsive P2Y receptors in the rat cochlea. Histochem. Cell Biol. 133, 425–436. doi: 10.1007/s00418-010-0682-1
O’Malley, B. W. Jr., Li, D., and Turner, D. S. (1995). Hearing loss and cochlear abnormalities in the congenital hypothyroid (hyt/hyt) mouse. Hear. Res. 88, 181–189. doi: 10.1016/0378-5955(95)00111-g
O’Reilly, M., Palfi, A., Chadderton, N., Millington-Ward, S., Ader, M., Cronin, T., et al. (2007). RNA interference-mediated suppression and replacement of human rhodopsin in vivo. Am. J. Hum. Genet. 81, 127–135. doi: 10.1086/519025
Pan, B., Askew, C., Galvin, A., Heman-Ackah, S., Asai, Y., Indzhykulian, A. A., et al. (2017). Gene therapy restores auditory and vestibular function in a mouse model of Usher syndrome type 1c. Nat. Biotechnol. 35, 264–272. doi: 10.1038/nbt.3801
Parker, M. S., Onyenekwu, N. N., and Bobbin, R. P. (2003). Localization of the P2Y4 receptor in the guinea pig organ of Corti. J. Am. Acad. Audiol. 14, 286–295.
Pasquini, S., Contri, C., Merighi, S., Gessi, S., Borea, P. A., Varani, K., et al. (2022). Adenosine receptors in neuropsychiatric disorders: Fine regulators of neurotransmission and potential therapeutic targets. Int. J. Mol. Sci. 23:1219. doi: 10.3390/ijms23031219
Peng, B. G., Li, Q. X., Ren, T. Y., Ahmad, S., Chen, S. P., Chen, P., et al. (2004). Group I metabotropic glutamate receptors in spiral ganglion neurons contribute to excitatory neurotransmissions in the cochlea. Neuroscience 123, 221–230. doi: 10.1016/j.neuroscience.2003.09.010
Peyvandi, A. A., Roozbahany, N. A., Peyvandi, H., Abbaszadeh, H. A., Majdinasab, N., Faridan, M., et al. (2018b). Critical role of SDF-1/CXCR4 signaling pathway in stem cell homing in the deafened rat cochlea after acoustic trauma. Neural Regen. Res. 13, 154–160. doi: 10.4103/1673-5374.224382
Peyvandi, A. A., Abbaszadeh, H. A., Roozbahany, N. A., Pourbakht, A., Khoshsirat, S., Niri, H. H., et al. (2018a). Deferoxamine promotes mesenchymal stem cell homing in noise-induced injured cochlea through PI3K/AKT pathway. Cell Prolif. 51:e12434. doi: 10.1111/cpr.12434
Piazza, V., Ciubotaru, C. D., Gale, J. E., and Mammano, F. (2007). Purinergic signalling and intercellular Ca2+ wave propagation in the organ of Corti. Cell Calcium 41, 77–86. doi: 10.1016/j.ceca.2006.05.005
Qi, X., Liu, H., Thompson, B., McDonald, J., Zhang, C., and Li, X. (2019). Cryo-EM structure of oxysterol-bound human smoothened coupled to a heterotrimeric Gi. Nature 571, 279–283. doi: 10.1038/s41586-019-1286-0
Qu, J., Liao, Y. H., Kou, Z. Z., Wei, Y. Y., Huang, J., Chen, J., et al. (2015). Puerarin alleviates noise-induced hearing loss via affecting PKCgamma and GABAB receptor expression. J. Neurol. Sci. 349, 110–116. doi: 10.1016/j.jns.2014.12.038
Racz, B., Horvath, G., Reglodi, D., Gasz, B., Kiss, P., and Gallyas, F. Jr., et al. (2010). PACAP ameliorates oxidative stress in the chicken inner ear: An in vitro study. Regul. Pept. 160, 91–98. doi: 10.1016/j.regpep.2009.12.003
Ramkumar, V., Ravi, R., Wilson, M. C., Gettys, T. W., Whitworth, C., and Rybak, L. P. (1994). Identification of A1 adenosine receptors in rat cochlea coupled to inhibition of adenylyl cyclase. Am. J. Physiol. 267(3 Pt 1), C731–C737. doi: 10.1152/ajpcell.1994.267.3.C731
Ramkumar, V., Whitworth, C. A., Pingle, S. C., Hughes, L. F., and Rybak, L. P. (2004). Noise induces A1 adenosine receptor expression in the chinchilla cochlea. Hear. Res. 188, 47–56. doi: 10.1016/S0378-5955(03)00344-7
Ravni, A., Qu, Y., Goffinet, A. M., and Tissir, F. (2009). Planar cell polarity cadherin Celsr1 regulates skin hair patterning in the mouse. J. Invest. Dermatol. 129, 2507–2509. doi: 10.1038/jid.2009.84
Reijntjes, D. O. J., and Pyott, S. J. (2016). The afferent signaling complex: Regulation of type I spiral ganglion neuron responses in the auditory periphery. Hear. Res. 336, 1–16. doi: 10.1016/j.heares.2016.03.011
Reiner, A., and Levitz, J. (2018). Glutamatergic signaling in the central nervous system: Ionotropic and metabotropic receptors in concert. Neuron 98, 1080–1098. doi: 10.1016/j.neuron.2018.05.018
Renaud, J. P., Chari, A., Ciferri, C., Liu, W. T., Remigy, H. W., Stark, H., et al. (2018). Cryo-EM in drug discovery: Achievements, limitations and prospects. Nat. Rev. Drug Discov. 17, 471–492. doi: 10.1038/nrd.2018.77
Renauld, J. M., Davis, W., Cai, T., Cabrera, C., and Basch, M. L. (2021). Transcriptomic analysis and ednrb expression in cochlear intermediate cells reveal developmental differences between inner ear and skin melanocytes. Pigment Cell Melanoma Res. 34, 585–597. doi: 10.1111/pcmr.12961
Ruel, J., Guitton, M. J., Gratias, P., Lenoir, M., Shen, S., Puel, J. L., et al. (2021). Endogenous pituitary adenylate cyclase-activating polypeptide (PACAP) plays a protective effect against noise-induced hearing loss. Front. Cell Neurosci. 15:658990. doi: 10.3389/fncel.2021.658990
Ruel, J., Nouvian, R., Gervais d’Aldin, C., Pujol, R., Eybalin, M., and Puel, J. L. (2001). Dopamine inhibition of auditory nerve activity in the adult mammalian cochlea. Eur. J. Neurosci. 14, 977–986. doi: 10.1046/j.0953-816x.2001.01721.x
Saikia, S., Bordoloi, M., and Sarmah, R. (2019). Established and in-trial GPCR families in clinical trials: A review for target selection. Curr. Drug Targets 20, 522–539. doi: 10.2174/1389450120666181105152439
Sanchez, J., Lane, J. R., Canals, M., and Stone, M. J. (2019). Influence of chemokine N-terminal modification on biased agonism at the chemokine receptor CCR1. Int. J. Mol. Sci. 20:2417. doi: 10.3390/ijms20102417
Santos-Cortez, R. L., Faridi, R., Rehman, A. U., Lee, K., Ansar, M., Wang, X., et al. (2016). Autosomal-recessive hearing impairment due to rare missense variants within S1PR2. Am. J. Hum. Genet. 98, 331–338. doi: 10.1016/j.ajhg.2015.12.004
Sato, E., Shick, H. E., Ransohoff, R. M., and Hirose, K. (2010). Expression of fractalkine receptor CX3CR1 on cochlear macrophages influences survival of hair cells following ototoxic injury. J. Assoc. Res. Otolaryngol. 11, 223–234. doi: 10.1007/s10162-009-0198-3
Sautter, N. B., Shick, E. H., Ransohoff, R. M., Charo, I. F., and Hirose, K. (2006). CC chemokine receptor 2 is protective against noise-induced hair cell death: Studies in CX3CR1+/GFP mice. J. Assoc. Res. Otolaryngol. 7, 361–372. doi: 10.1007/s10162-006-0051-x
Schrott-Fischer, A., Kammen-Jolly, K., Scholtz, A., Rask-Andersen, H., Glueckert, R., and Eybalin, M. (2007). Efferent neurotransmitters in the human cochlea and vestibule. Acta Otolaryngol. 127, 13–19. doi: 10.1080/00016480600652123
Schulte, G., and Kozielewicz, P. (2020). Structural insight into Class F receptors–what have we learnt regarding agonist-induced activation? Basic Clin. Pharmacol. Toxicol. 126(Suppl. 6), 17–24. doi: 10.1111/bcpt.13235
Schulte, G., and Wright, S. C. (2018). Frizzleds as GPCRs–more conventional than we thought! Trends Pharmacol. Sci. 39, 828–842. doi: 10.1016/j.tips.2018.07.001
Seebahn, A., Dinkel, H., Mohrluder, J., Hartmann, R., Vogel, N., Becker, C. M., et al. (2011). Structural characterization of intracellular C-terminal domains of group III metabotropic glutamate receptors. FEBS Lett. 585, 511–516. doi: 10.1016/j.febslet.2010.12.042
Shaye, H., Stauch, B., Gati, C., and Cherezov, V. (2021). Molecular mechanisms of metabotropic GABAB receptor function. Sci. Adv. 7:eabg3362. doi: 10.1126/sciadv.abg3362
Shen, C., Mao, C., Xu, C., Jin, N., Zhang, H., Shen, D. D., et al. (2021). Structural basis of GABAB receptor-Gi protein coupling. Nature 594, 594–598. doi: 10.1038/s41586-021-03507-1
Sheth, S., Sheehan, K., Dhukhwa, A., Al Aameri, R. F. H., Mamillapalli, C., Mukherjea, D., et al. (2019). Oral administration of caffeine exacerbates cisplatin-induced hearing loss. Sci. Rep. 9:9571. doi: 10.1038/s41598-019-45964-9
Shihoya, W., Izume, T., Inoue, A., Yamashita, K., Kadji, F. M. N., Hirata, K., et al. (2018). Crystal structures of human ETB receptor provide mechanistic insight into receptor activation and partial activation. Nat. Commun. 9:4711. doi: 10.1038/s41467-018-07094-0
Shihoya, W., Nishizawa, T., Okuta, A., Tani, K., Dohmae, N., Fujiyoshi, Y., et al. (2016). Activation mechanism of endothelin ETB receptor by endothelin-1. Nature 537, 363–368. doi: 10.1038/nature19319
Shima, Y., Copeland, N. G., Gilbert, D. J., Jenkins, N. A., Chisaka, O., Takeichi, M., et al. (2002). Differential expression of the seven-pass transmembrane cadherin genes Celsr1-3 and distribution of the Celsr2 protein during mouse development. Dev. Dyn. 223, 321–332. doi: 10.1002/dvdy.10054
Shin, M., Pandya, M., Espinosa, K., Telang, R., Boix, J., Thorne, P. R., et al. (2021). Istradefylline mitigates age-related hearing loss in C57BL/6J mice. Int. J. Mol. Sci. 22:8000. doi: 10.3390/ijms22158000
Shin, S. H., Jung, J., Park, H. R., Sim, N. S., Choi, J. Y., and Bae, S. H. (2022a). The Time course of monocytes infiltration after acoustic overstimulation. Front. Cell. Neurosci. 16:844480. doi: 10.3389/fncel.2022.844480
Shin, S. H., Yoo, J. E., Jung, J., Choi, J. Y., and Bae, S. H. (2022b). Inflammatory monocytes infiltrate the spiral ligament and migrate to the basilar membrane after noise exposure. Clin. Exp. Otorhinolaryngol. 15, 153–159. doi: 10.21053/ceo.2021.00857
Sienknecht, U. J., and Fekete, D. M. (2008). Comprehensive Wnt-related gene expression during cochlear duct development in chicken. J. Comp. Neurol. 510, 378–395. doi: 10.1002/cne.21791
Sirko, P., Gale, J. E., and Ashmore, J. F. (2019). Intercellular Ca2+ signalling in the adult mouse cochlea. J. Physiol. 597, 303–317. doi: 10.1113/JP276400
Skradski, S. L., Clark, A. M., Jiang, H., White, H. S., Fu, Y. H., and Ptacek, L. J. (2001). A novel gene causing a mendelian audiogenic mouse epilepsy. Neuron 31, 537–544. doi: 10.1016/s0896-6273(01)00397-x
Smith, M. C., Luker, K. E., Garbow, J. R., Prior, J. L., Jackson, E., Piwnica-Worms, D., et al. (2004). CXCR4 regulates growth of both primary and metastatic breast cancer. Cancer Res. 64, 8604–8612. doi: 10.1158/0008-5472.CAN-04-1844
Smith-Cortinez, N., Yadak, R., Hendriksen, F. G. J., Sanders, E., Ramekers, D., Stokroos, R. J., et al. (2021). LGR5-positive supporting cells survive ototoxic trauma in the adult mouse cochlea. Front. Mol. Neurosci. 14:729625. doi: 10.3389/fnmol.2021.729625
Song, L., McGee, J. A., and Walsh, E. J. (2006). Consequences of combined maternal, fetal and persistent postnatal hypothyroidism on the development of auditory function in Tshrhyt mutant mice. Brain Res. 1101, 59–72. doi: 10.1016/j.brainres.2006.05.027
Stoner, Z. A., Ketchum, E. M., Sheltz-Kempf, S., Blinkiewicz, P. V., Elliott, K. L., and Duncan, J. S. (2021). Fzd3 expression within inner ear afferent neurons is necessary for central pathfinding. Front. Neurosci. 15:779871. doi: 10.3389/fnins.2021.779871
Sun, H., Wang, T., Atkinson, P. J., Billings, S. E., Dong, W., and Cheng, A. G. (2021). Gpr125 marks distinct cochlear cell types and is dispensable for cochlear development and hearing. Front. Cell Dev. Biol. 9:690955. doi: 10.3389/fcell.2021.690955
Sun, J. P., Li, R., Ren, H. Z., Xu, A. T., Yu, X., and Xu, Z. G. (2013). The very large G protein coupled receptor (Vlgr1) in hair cells. J. Mol. Neurosci. 50, 204–214. doi: 10.1007/s12031-012-9911-5
Tabuchi, K., Sakai, S., Nakayama, M., Nishimura, B., Hayashi, K., Hirose, Y., et al. (2012). The effects of A1 and A2A adenosine receptor agonists on kainic acid excitotoxicity in the guinea pig cochlea. Neurosci. Lett. 518, 60–63. doi: 10.1016/j.neulet.2012.04.057
Takumida, M., Kakigi, A., Egami, N., Nishioka, R., and Anniko, M. (2012). Localization of aquaporins 1, 2, and 3 and vasopressin type 2 receptor in the mouse inner ear. Acta Otolaryngol. 132, 807–813. doi: 10.3109/00016489.2012.662718
Tantisira, K. G., Lake, S., Silverman, E. S., Palmer, L. J., Lazarus, R., Silverman, E. K., et al. (2004). Corticosteroid pharmacogenetics: Association of sequence variants in CRHR1 with improved lung function in asthmatics treated with inhaled corticosteroids. Hum. Mol. Genet. 13, 1353–1359. doi: 10.1093/hmg/ddh149
Tateya, T., Imayoshi, I., Tateya, I., Hamaguchi, K., Torii, H., Ito, J., et al. (2013). Hedgehog signaling regulates prosensory cell properties during the basal-to-apical wave of hair cell differentiation in the mammalian cochlea. Development 140, 3848–3857. doi: 10.1242/dev.095398
Tissir, F., and Goffinet, A. M. (2006). Expression of planar cell polarity genes during development of the mouse CNS. Eur. J. Neurosci. 23, 597–607. doi: 10.1111/j.1460-9568.2006.04596.x
Tuffour, A., Kosiba, A. A., Zhang, Y., Peprah, F. A., Gu, J., and Shi, H. (2021). Role of the calcium-sensing receptor (CaSR) in cancer metastasis to bone: Identifying a potential therapeutic target. Biochim. Biophys. Acta Rev. Cancer 1875:188528. doi: 10.1016/j.bbcan.2021.188528
Tuncel, M. (2017). Thyroid stimulating hormone receptor. Mol. Imaging Radionucl. Ther. 26(Suppl. 1), 87–91. doi: 10.4274/2017.26.suppl.10
van der Valk, W. H., Steinhart, M. R., Zhang, J., and Koehler, K. R. (2021). Building inner ears: Recent advances and future challenges for in vitro organoid systems. Cell Death Differ. 28, 24–34. doi: 10.1038/s41418-020-00678-8
Vaudry, D., Falluel-Morel, A., Bourgault, S., Basille, M., Burel, D., Wurtz, O., et al. (2009). Pituitary adenylate cyclase-activating polypeptide and its receptors: 20 years after the discovery. Pharmacol. Rev. 61, 283–357. doi: 10.1124/pr.109.001370
Verpy, E., Leibovici, M., Zwaenepoel, I., Liu, X. Z., Gal, A., Salem, N., et al. (2000). A defect in harmonin, a PDZ domain-containing protein expressed in the inner ear sensory hair cells, underlies Usher syndrome type 1C. Nat. Genet. 26, 51–55. doi: 10.1038/79171
Vetter, D. E. (2015). Cellular signaling protective against noise-induced hearing loss–a role for novel intrinsic cochlear signaling involving corticotropin-releasing factor? Biochem. Pharmacol. 97, 1–15. doi: 10.1016/j.bcp.2015.06.011
Vieira, I. H., Rodrigues, D., and Paiva, I. (2022). The mysterious universe of the TSH receptor. Front. Endocrinol. 13:944715. doi: 10.3389/fendo.2022.944715
Vlachou, S. (2022). A brief history and the significance of the GABAB receptor. Curr. Top. Behav. Neurosci. 52, 1–17. doi: 10.1007/7854_2021_264
Vlajkovic, S. M., Abi, S., Wang, C. J., Housley, G. D., and Thorne, P. R. (2007). Differential distribution of adenosine receptors in rat cochlea. Cell Tissue Res. 328, 461–471. doi: 10.1007/s00441-006-0374-2
Vlajkovic, S. M., Ambepitiya, K., Barclay, M., Boison, D., Housley, G. D., and Thorne, P. R. (2017). Adenosine receptors regulate susceptibility to noise-induced neural injury in the mouse cochlea and hearing loss. Hear. Res. 345, 43–51. doi: 10.1016/j.heares.2016.12.015
Vlajkovic, S. M., Guo, C. X., Telang, R., Wong, A. C., Paramananthasivam, V., Boison, D., et al. (2011). Adenosine kinase inhibition in the cochlea delays the onset of age-related hearing loss. Exp. Gerontol. 46, 905–914. doi: 10.1016/j.exger.2011.08.001
Vlajkovic, S. M., Housley, G. D., and Thorne, P. R. (2009). Adenosine and the auditory system. Curr. Neuropharmacol. 7, 246–256. doi: 10.2174/157015909789152155
Vlajkovic, S. M., Lee, K. H., Wong, A. C., Guo, C. X., Gupta, R., Housley, G. D., et al. (2010). Adenosine amine congener mitigates noise-induced cochlear injury. Purinergic Signal. 6, 273–281. doi: 10.1007/s11302-010-9188-5
Vlajkovic, S. M., Vinayagamoorthy, A., Thorne, P. R., Robson, S. C., Wang, C. J., and Housley, G. D. (2006). Noise-induced up-regulation of NTPDase3 expression in the rat cochlea: Implications for auditory transmission and cochlear protection. Brain Res. 1104, 55–63. doi: 10.1016/j.brainres.2006.05.094
Vyas, P., Wu, J. S., Jimenez, A., Glowatzki, E., and Fuchs, P. A. (2019). Characterization of transgenic mouse lines for labeling type I and type II afferent neurons in the cochlea. Sci. Rep. 9:5549. doi: 10.1038/s41598-019-41770-5
Wagner, E. L., and Shin, J. B. (2019). Mechanisms of hair cell damage and repair. Trends Neurosci. 42, 414–424. doi: 10.1016/j.tins.2019.03.006
Wang, L., Xu, J., Cao, S., Sun, D., Liu, H., Lu, Q., et al. (2021). Cryo-EM structure of the AVP-vasopressin receptor 2-Gs signaling complex. Cell Res. 31, 932–934. doi: 10.1038/s41422-021-00483-z
Wang, S. Q., Li, C. L., Xu, J. Q., Chen, L. L., Xie, Y. Z., Dai, P. D., et al. (2022). The effect of endolymphatic hydrops and mannitol dehydration treatment on guinea pigs. Front. Cell. Neurosci. 16:836093. doi: 10.3389/fncel.2022.836093
Wang, W., Qiao, Y., and Li, Z. (2018). New insights into modes of GPCR activation. Trends Pharmacol. Sci. 39, 367–386. doi: 10.1016/j.tips.2018.01.001
Wang, W., Shanmugam, M. K., Xiang, P., Yam, T. Y. A., Kumar, V., Chew, W. S., et al. (2020). Sphingosine 1-phosphate receptor 2 induces otoprotective responses to cisplatin treatment. Cancers 12:211. doi: 10.3390/cancers12010211
Wang, Y., Guo, N., and Nathans, J. (2006). The role of Frizzled3 and Frizzled6 in neural tube closure and in the planar polarity of inner-ear sensory hair cells. J. Neurosci. 26, 2147–2156. doi: 10.1523/JNEUROSCI.4698-05.2005
Wang, Y., Huso, D., Cahill, H., Ryugo, D., and Nathans, J. (2001). Progressive cerebellar, auditory, and esophageal dysfunction caused by targeted disruption of the frizzled-4 gene. J. Neurosci. 21, 4761–4771. doi: 10.1523/JNEUROSCI.21-13-04761.2001
Watkins, L. R., and Orlandi, C. (2020). Orphan G protein coupled receptors in affective disorders. Genes 11:694. doi: 10.3390/genes11060694
Watkins, L. R., and Orlandi, C. (2021). In vitro profiling of orphan G protein coupled receptor (GPCR) constitutive activity. Br. J. Pharmacol. 178, 2963–2975. doi: 10.1111/bph.15468
Wedemeyer, C., Zorrilla de San Martin, J., Ballestero, J., Gomez-Casati, M. E., Torbidoni, A. V., Fuchs, P. A., et al. (2013). Activation of presynaptic GABA(B(1a,2)) receptors inhibits synaptic transmission at mammalian inhibitory cholinergic olivocochlear-hair cell synapses. J. Neurosci. 33, 15477–15487. doi: 10.1523/JNEUROSCI.2554-13.2013
Whitworth, C. A., Ramkumar, V., Jones, B., Tsukasaki, N., and Rybak, L. P. (2004). Protection against cisplatin ototoxicity by adenosine agonists. Biochem. Pharmacol. 67, 1801–1807. doi: 10.1016/j.bcp.2004.01.010
Wingler, L. M., and Lefkowitz, R. J. (2020). Conformational basis of G protein-coupled receptor signaling versatility. Trends Cell Biol. 30, 736–747. doi: 10.1016/j.tcb.2020.06.002
Wolf, B. J., Kusch, K., Hunniford, V., Vona, B., Kuhler, R., Keppeler, D., et al. (2022). Is there an unmet medical need for improved hearing restoration? EMBO Mol. Med. 14, e15798. doi: 10.15252/emmm.202215798
Wonneberger, K., Scofield, M. A., and Wangemann, P. (2000). Evidence for a calcium-sensing receptor in the vascular smooth muscle cells of the spiral modiolar artery. J. Membr. Biol. 175, 203–212. doi: 10.1007/s00232001068
Wu, J. S., Yi, E., Manca, M., Javaid, H., Lauer, A. M., and Glowatzki, E. (2020). Sound exposure dynamically induces dopamine synthesis in cholinergic LOC efferents for feedback to auditory nerve fibers. Elife 9:e52419. doi: 10.7554/eLife.52419
Xiao, Q., Xu, Z., Xue, Y., Xu, C., Han, L., Liu, Y., et al. (2022). Rescue of autosomal dominant hearing loss by in vivo delivery of mini dCas13X-derived RNA base editor. Sci. Transl. Med. 14:eabn0449. doi: 10.1126/scitranslmed.abn0449
Yagi, H., Takamura, Y., Yoneda, T., Konno, D., Akagi, Y., Yoshida, K., et al. (2005). Vlgr1 knockout mice show audiogenic seizure susceptibility. J. Neurochem. 92, 191–202. doi: 10.1111/j.1471-4159.2004.02875.x
Yang, X., Wang, X., Xu, Z., Wu, C., Zhou, Y., Wang, Y., et al. (2022). Molecular mechanism of allosteric modulation for the cannabinoid receptor CB1. Nat. Chem. Biol. 18, 831–840. doi: 10.1038/s41589-022-01038-y
Ye, Z., Goutman, J. D., Pyott, S. J., and Glowatzki, E. (2017). mGluR1 enhances efferent inhibition of inner hair cells in the developing rat cochlea. J. Physiol. 595, 3483–3495. doi: 10.1113/JP272604
Yin, H., Zhang, H., Kong, Y., Wang, C., Guo, Y., Gao, Y., et al. (2020). Apelin protects auditory cells from cisplatin-induced toxicity in vitro by inhibiting ROS and apoptosis. Neurosci. Lett. 728:134948. doi: 10.1016/j.neulet.2020.134948
Yu, H., Smallwood, P. M., Wang, Y., Vidaltamayo, R., Reed, R., and Nathans, J. (2010). Frizzled 1 and Frizzled 2 genes function in palate, ventricular septum and neural tube closure: General implications for tissue fusion processes. Development 137, 3707–3717. doi: 10.1242/dev.052001
Yu, P., Jiao, J., Chen, G., Zhou, W., Zhang, H., Wu, H., et al. (2018). Effect of GRM7 polymorphisms on the development of noise-induced hearing loss in Chinese Han workers: A nested case-control study. BMC Med. Genet. 19:4. doi: 10.1186/s12881-017-0515-3
Zak, M., van Oort, T., Hendriksen, F. G., Garcia, M. I., Vassart, G., and Grolman, W. (2016). LGR4 and LGR5 regulate hair cell differentiation in the sensory epithelium of the developing mouse cochlea. Front. Cell. Neurosci. 10:186. doi: 10.3389/fncel.2016.00186
Zallocchi, M., Meehan, D. T., Delimont, D., Rutledge, J., Gratton, M. A., Flannery, J., et al. (2012b). Role for a novel Usher protein complex in hair cell synaptic maturation. PLoS One 7:e30573. doi: 10.1371/journal.pone.0030573
Zallocchi, M., Delimont, D., Meehan, D. T., and Cosgrove, D. (2012a). Regulated vesicular trafficking of specific PCDH15 and VLGR1 variants in auditory hair cells. J. Neurosci. 32, 13841–13859. doi: 10.1523/JNEUROSCI.1242-12.2012
Zhang, C., Frye, M. D., Riordan, J., Sharma, A., Manohar, S., Salvi, R., et al. (2021). Loss of CX3CR1 augments neutrophil infiltration into cochlear tissues after acoustic overstimulation. J. Neurosci. Res. 99, 2999–3020. doi: 10.1002/jnr.24925
Zhang, L. L., Wang, J. J., Liu, Y., Lu, X. B., Kuang, Y., Wan, Y. H., et al. (2011). GPR26-deficient mice display increased anxiety- and depression-like behaviors accompanied by reduced phosphorylated cyclic AMP responsive element-binding protein level in central amygdala. Neuroscience 196, 203–214. doi: 10.1016/j.neuroscience.2011.08.069
Zhang, P., Yi, L. H., Meng, G. Y., Zhang, H. Y., Sun, H. H., and Cui, L. Q. (2017). Apelin-13 attenuates cisplatin-induced cardiotoxicity through inhibition of ROS-mediated DNA damage and regulation of MAPKs and AKT pathways. Free Radic. Res. 51, 449–459. doi: 10.1080/10715762.2017.1313414
Zhang, P. Z., Cao, X. S., Jiang, X. W., Wang, J., Liang, P. F., Wang, S. J., et al. (2014). Acoustical stimulus changes the expression of stromal cell-derived factor-1 in the spiral ganglion neurons of the rat cochlea. Neurosci. Lett. 561, 140–145. doi: 10.1016/j.neulet.2013.12.061
Zhang, S., Liu, D., Dong, Y., Zhang, Z., Zhang, Y., Zhou, H., et al. (2019). Frizzled-9+ supporting cells are progenitors for the generation of hair cells in the postnatal mouse cochlea. Front. Mol. Neurosci. 12:184. doi: 10.3389/fnmol.2019.00184
Zhang, S., Zhang, Y., Yu, P., Hu, Y., Zhou, H., Guo, L., et al. (2017). Characterization of Lgr5+ progenitor cell transcriptomes after neomycin injury in the neonatal mouse cochlea. Front. Mol. Neurosci. 10:213. doi: 10.3389/fnmol.2017.00213
Zhang, W., Sun, J. Z., Han, Y., Chen, J., Liu, H., Wang, Y., et al. (2016). CXCL12/CXCR4 signaling pathway regulates cochlear development in neonatal mice. Mol. Med. Rep. 13, 4357–4364. doi: 10.3892/mmr.2016.5085
Zhang, W., Zhang, F., Han, Y., Liu, H., Wang, Y., Yue, B., et al. (2015). Auditory stimulation modulates CXCL12/CXCR4 expression in postnatal development of the newborn rat cochlea. Neuroreport 26, 681–687. doi: 10.1097/WNR.0000000000000408
Zhang, X., Dong, S., and Xu, F. (2018). Structural and druggability landscape of Frizzled G protein-coupled receptors. Trends Biochem. Sci. 43, 1033–1046. doi: 10.1016/j.tibs.2018.09.002
Zhang, Y., Guo, L., Lu, X., Cheng, C., Sun, S., Li, W., et al. (2018). Characterization of Lgr6+ cells as an enriched population of hair cell progenitors compared to Lgr5+ cells for hair cell generation in the neonatal mouse cochlea. Front. Mol. Neurosci. 11:147. doi: 10.3389/fnmol.2018.00147
Zhou, F., Ye, C., Ma, X., Yin, W., Croll, T. I., Zhou, Q., et al. (2021). Molecular basis of ligand recognition and activation of human V2 vasopressin receptor. Cell Res. 31, 929–931. doi: 10.1038/s41422-021-00480-2
Zlotnik, A., Burkhardt, A. M., and Homey, B. (2011). Homeostatic chemokine receptors and organ-specific metastasis. Nat. Rev. Immunol. 11, 597–606. doi: 10.1038/nri3049
Zou, J., Wang, Z., Chen, Y., Zhang, G., Chen, L., and Lu, J. (2019). MRI detection of endolymphatic hydrops in Meniere’s disease in 8 minutes using MIIRMR and a 20-channel coil after targeted gadolinium delivery. World J. Otorhinolaryngol. Head Neck Surg. 5, 180–187. doi: 10.1016/j.wjorl.2019.04.001
Keywords: hearing loss, G protein-coupled receptors, cochlea, drug therapy, gene therapy
Citation: Ma X, Guo J, Fu Y, Shen C, Jiang P, Zhang Y, Zhang L, Yu Y, Fan J and Chai R (2022) G protein-coupled receptors in cochlea: Potential therapeutic targets for hearing loss. Front. Mol. Neurosci. 15:1028125. doi: 10.3389/fnmol.2022.1028125
Received: 25 August 2022; Accepted: 21 September 2022;
Published: 12 October 2022.
Edited by:
Qingyin Zheng, Case Western Reserve University, United StatesReviewed by:
Yan Chen, Fudan University, ChinaCopyright © 2022 Ma, Guo, Fu, Shen, Jiang, Zhang, Zhang, Yu, Fan and Chai. This is an open-access article distributed under the terms of the Creative Commons Attribution License (CC BY). The use, distribution or reproduction in other forums is permitted, provided the original author(s) and the copyright owner(s) are credited and that the original publication in this journal is cited, in accordance with accepted academic practice. No use, distribution or reproduction is permitted which does not comply with these terms.
*Correspondence: Yafeng Yu, eWZ5dTEwMjRAMTYzLmNvbQ==; Jiangang Fan, ZW50c2NmamdAMTYzLmNvbQ==; Renjie Chai, cmVuamllY0BzZXUuZWR1LmNu
†These authors have contributed equally to this work and share first authorship
Disclaimer: All claims expressed in this article are solely those of the authors and do not necessarily represent those of their affiliated organizations, or those of the publisher, the editors and the reviewers. Any product that may be evaluated in this article or claim that may be made by its manufacturer is not guaranteed or endorsed by the publisher.
Research integrity at Frontiers
Learn more about the work of our research integrity team to safeguard the quality of each article we publish.