- UCLA MS Program, Department of Neurology, David Geffen School of Medicine, University of California, Los Angeles, Los Angeles, CA, United States
Animal models of multiple sclerosis (MS), specifically experimental autoimmune encephalomyelitis (EAE), have been used extensively to develop anti-inflammatory treatments. However, the similarity between MS and one particular EAE model does not end at inflammation. MS and chronic EAE induced in C57BL/6 mice using myelin oligodendrocyte glycoprotein (MOG) peptide 35–55 share many neuropathologies. Beyond both having white matter lesions in spinal cord, both also have widespread neuropathology in the cerebral cortex, hippocampus, thalamus, striatum, cerebellum, and retina/optic nerve. In this review, we compare neuropathologies in each of these structures in MS with chronic EAE in C57BL/6 mice, and find evidence that this EAE model is well suited to study neuroaxonal degeneration in MS.
Introduction
Multiple sclerosis (MS) is an autoimmune and neurodegenerative disease of the central nervous system (CNS). Axonal degeneration, synaptic and neuronal loss, and demyelination in the context of inflammation are characteristic of the disease (Trapp et al., 1998, 1999; Peterson et al., 2001; Stadelmann et al., 2011; Friese et al., 2014; Mock et al., 2021). Over time these processes result in atrophy that can be visualized by magnetic resonance imaging (MRI) (Miller et al., 2002; Zivadinov and Bakshi, 2004; Hulst and Geurts, 2011). Brain parenchymal fraction atrophy (Rudick et al., 1999; Fisher et al., 2002) and whole brain gray matter (GM) atrophy (Fisher et al., 2008) have been documented in both relapsing-remitting MS (RRMS) (Chard et al., 2002) and secondary progressive MS (SPMS) (Larochelle et al., 2016). Further, GM atrophy of neuroanatomical structures has been associated with specific disabilities (Bakshi et al., 2001; Calabrese et al., 2009, 2010; MacKenzie-Graham et al., 2016) and cumulative disease progression (Simon, 2001). Thus, GM substructure atrophy and neurodegenerative processes therein are highly relevant to disability progression in MS (Bermel and Bakshi, 2006).
Inflammation plays a critical role in MS; however, it is not clear how inflammation causes neurodegeneration. Correlations between early T2-lesion loads and subsequent atrophy (Chard et al., 2003), as well as gadolinium-enhancing lesions and brain atrophy have been shown (Lin and Blumhardt, 2001) and there is clearly a neuropathologic relationship between inflammation and axonal transection in RRMS (Trapp et al., 1998). There is also a relationship between inflammation and both demyelination and neurodegeneration in progressive MS (Frischer et al., 2009), albeit not as strongly as in RRMS. Anti-inflammatory disease-modifying treatments (DMTs) reduce relapses and have robust immunomodulatory effects, but there is still an unmet need for direct neuroprotective treatments that target cells within the CNS. Understanding mechanisms that drive neurodegeneration are crucial to identifying targets for therapeutic interventions to halt and repair disabilities.
There is no perfect model for MS. The most appropriate model should be chosen based on the question asked. Experimental autoimmune encephalomyelitis (EAE) has been widely used as an animal model for immune studies in MS (Steinman and Zamvil, 2005; Ransohoff, 2012) and almost all DMTs have been tested in the EAE model (Denic et al., 2011; Ransohoff, 2012). MS has multiple presentations, from relapsing to secondary progressive to primary progressive MS. There are multiple presentations of EAE as well, from monophasic disease in the B10.PL mouse immunized with myelin basic protein (MBP) peptide Ac1-9 (Ando et al., 1989; Pastor et al., 2009) to relapsing-remitting disease in the SJL mouse immunized with proteolipid protein (PLP) peptide 139–151 (Brocke et al., 1996; Voskuhl, 1996) to chronic disease in the C57BL6 mouse immunized with myelin oligodendrocyte glycoprotein (MOG) peptide 35–55 (Hjelmstrom et al., 1998; Meyer et al., 2020; Figure 1). As we will review, chronic EAE induced using MOG 35–55 peptide in the C57BL/6J mouse strain (MOG 35–55 EAE) shares many neuropathologies with MS.
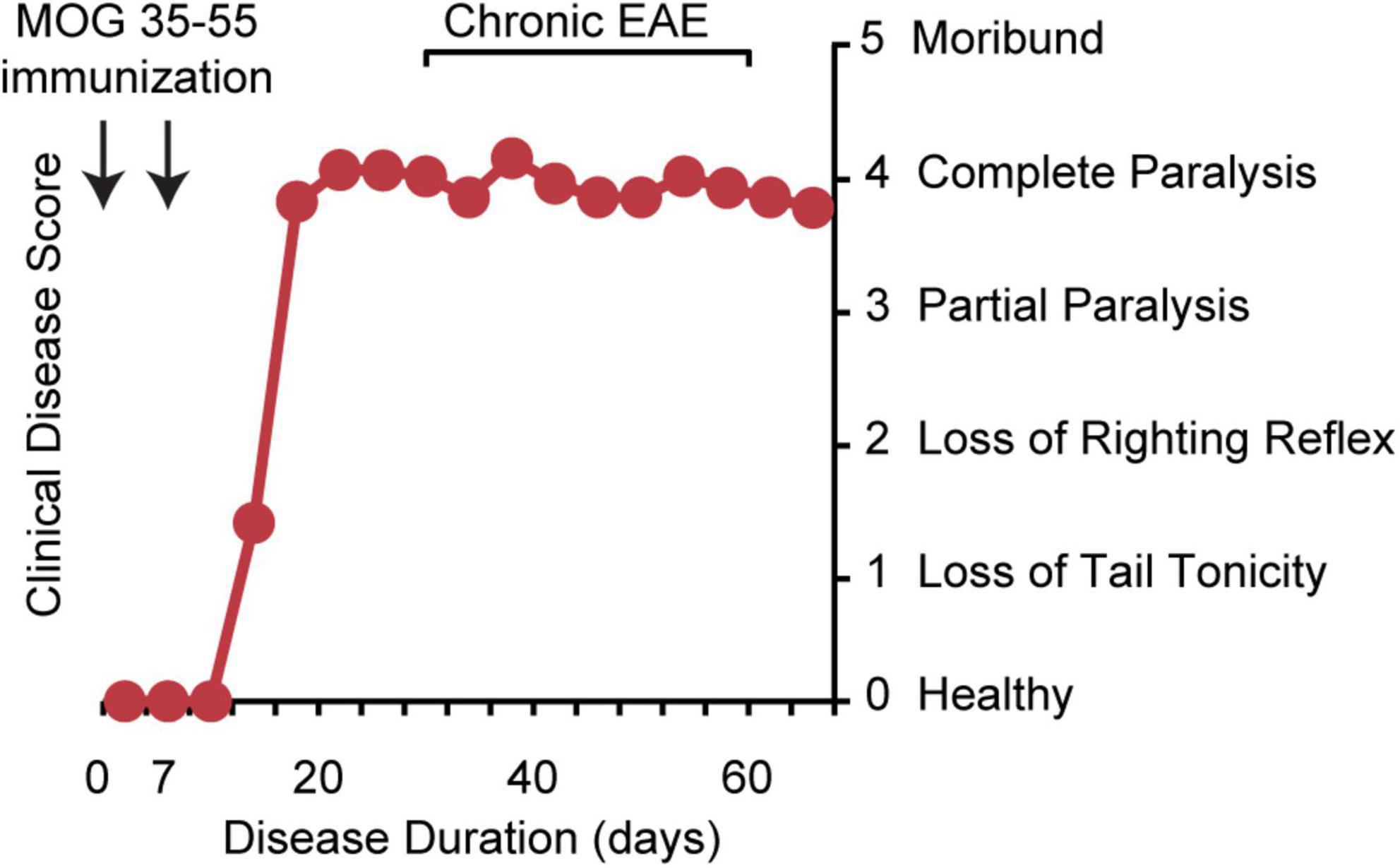
Figure 1. Chronic EAE disease induction and clinical disease course. Chronic EAE is induced by subcutaneous immunization with MOG peptide 35–55 and mycobacterium tuberculosis in complete Freund’s adjuvant at day 0 and again at day 7. Pertussis toxin is injected intraperitoneally on day 0 and day 2. Clinical disease is scored from 0 to 5 with 0 being healthy, 1 complete loss of tail tonicity, 2 loss of righting reflex, 3 partial paralysis of a limb, 4 complete paralysis of one or both hind limbs, and 5 moribund. Clinical signs of disease are usually first observed between days 10 and 15, followed by the chronic phase of disease, characterized by severe walking disability and progressive gray matter atrophy.
It is believed that MS is caused by the autoimmune activation of peripheral T cells that then migrate to the CNS and initiate disease (Baecher-Allan et al., 2018). In EAE, disease is induced by immunization with CNS antigens to produce autoimmune activation (Voskuhl, 1996; Hjelmstrom et al., 1998) or by the adoptive transfer of T-cell clones specific to myelin proteins that infiltrate the CNS (Zamvil S. et al., 1985; Zamvil S. S. et al., 1985). Once in the CNS, immune cells lead to classic inflammatory WM lesions, consisting of T-cells and macrophages with activation of resident glia (astrocytes and microglia), in both MS and EAE (Lassmann, 1998; Lassmann and Ransohoff, 2004; Wu et al., 2008; MacKenzie-Graham et al., 2009). Axonal damage characterized by axonal ovoids and end bulbs has been shown in both chronic EAE in C57BL/6 mice (Spence et al., 2014; Meyer et al., 2020) and in MS (Trapp et al., 1998, 1999). Further, chronic EAE also exhibits WM hyperintensities on T2-weighted MRI (MacKenzie-Graham et al., 2006) and axonal damage has been demonstrated using diffusion tensor imaging (DTI) (Budde et al., 2008, 2009), again as in MS (Klawiter, 2013). These observations suggest that EAE is a good model to study WM lesions and axonal loss in MS.
Beyond these WM lesions, there are also changes in normal-appearing white matter (NAWM). DTI and magnetization transfer ratio (MTR) abnormalities are common in MS (Moll et al., 2011; Elliott et al., 2021). DTI abnormalities in NAWM (Ahrens et al., 1998; Sun et al., 2007; MacKenzie-Graham et al., 2012a; Aharoni et al., 2013) and decreased MTR in NAWM have also been reported in EAE (Aharoni et al., 2013). NAWM in MS is associated with axonal pathology, activated astrocytes and microglia (Moll et al., 2011; Lassmann et al., 2012), all elements that are observed in NAWM in the corpus callosum and spinal cord in EAE (Wu et al., 2008; Crawford et al., 2010; Mangiardi et al., 2011).
Here we will focus on GM pathologies, comparing chronic EAE in C57BL/6 mice to that which occurs in MS. These pathologies include astrocyte and microglia activation, demyelination, axonal loss, synaptic loss, and neuronal loss in the cerebral cortex, hippocampus, thalamus, striatum, cerebellum, retina/optic nerve, and corpus callosum. Based on this review, we conclude that chronic EAE is an excellent model to study GM atrophy and neuroaxonal degeneration in MS.
Cerebral cortex
Atrophy of cerebral cortex was recognized almost two decades ago (De Stefano et al., 2003; Calabrese et al., 2007). Interestingly, cerebral cortex atrophy and clinical disability are consistently associated (Amato et al., 2004; Charil et al., 2007; Rocca et al., 2021). It has been recently demonstrated that there is a direct correlation between regional cerebral cortex atrophy and specific disabilities (MacKenzie-Graham et al., 2016). Cerebral cortex atrophy in MOG 35–55 induced chronic EAE in C57BL/6 mice was first demonstrated in 2012 (MacKenzie-Graham et al., 2012b) and has been validated multiple times since then (Itoh et al., 2017; Hamilton et al., 2019; Meyer et al., 2020). Landmark MS studies demonstrated axonal damage and transection in lesions in subcortical WM (Ferguson et al., 1997; Trapp et al., 1998) and subsequent analyses demonstrated transected neurites and apoptotic neurons in the cerebral cortex (Kidd et al., 1999; Peterson et al., 2001), cementing the importance of cortical pathology in MS. Axonal loss (using B3-tubulin and NF200 staining) in the cerebral cortex has been demonstrated in chronic EAE (MacKenzie-Graham et al., 2012b). Decreased neuronal density has been shown in the cerebral cortices of MS patients (Vercellino et al., 2005, 2009; Bevan et al., 2018) and pyramidal neuronal loss (annexin V, encephalopsin, NeuN, and Thy1-YFP) has also been shown multiple times in chronic EAE (Mangiardi et al., 2011; MacKenzie-Graham et al., 2012b; Burns et al., 2014; Stanojlovic et al., 2016), highlighting that cortical pathology is important in this MS model as well. A systematic study of cortical demyelination performed by Bo et al. (2003) observed extensive myelin loss and these findings have been validated (Lucchinetti et al., 2011; Kutzelnigg and Lassmann, 2014; Bevan et al., 2018). Small areas of cortical demyelination have been reported many times in chronic EAE (Errede et al., 2012; MacKenzie-Graham et al., 2012b; Burns et al., 2014). Furthermore, microglial and astrocyte activation have been shown in the cerebral cortices of MS patients (Vercellino et al., 2009), as has synaptic loss (Mock et al., 2021). Diffuse astrocyte and microglia activation in cerebral cortex also occurs in chronic EAE (Errede et al., 2012; Burns et al., 2014; Meyer et al., 2020), as well as synaptic loss (Burns et al., 2014; Itoh et al., 2017; Meyer et al., 2020). We summarize cerebral cortex pathologies in chronic EAE in Table 1.
Hippocampus
Hippocampal involvement in depression and in learning and memory dysfunction has been well documented in MS (Rocca et al., 2018). Hippocampal atrophy has been demonstrated in patients with MS, with worse atrophy in SPMS patients than in RRMS patients (Sicotte et al., 2008). Interneuron loss (parvalbumin) in the CA1 region and hippocampal atrophy was shown in chronic EAE (Ziehn et al., 2010; Hamilton et al., 2019). Interestingly, mice with chronic MOG 35–55 EAE exhibited a deficit in hippocampal-dependent spatial learning and memory in the Barnes maze (Ziehn et al., 2010), as well as altered excitatory synaptic transmission and paired-pulse facilitation (Ziehn et al., 2012a). Neuropathology revealed demyelination in the hippocampus in MS (Vercellino et al., 2005; Geurts et al., 2007; Dutta et al., 2011, 2013), a pathology also observed in chronic EAE (Ziehn et al., 2012b; Bellizzi et al., 2016). Hippocampal demyelination is often associated with synaptic reduction (Dutta et al., 2011; Michailidou et al., 2015) and changes in synaptic composition and transmission in MS (Dutta et al., 2011, 2013). Hippocampal demyelination, synaptic loss and changes in synaptic transmission have also been demonstrated in chronic EAE (Ziehn et al., 2012a,b). Microglial activation has been shown in hippocampal tissues in both humans with MS (Geurts et al., 2007; Dutta et al., 2011) and mice with chronic EAE (Aharoni et al., 2005; Ziehn et al., 2012b; Hammond et al., 2020). Table 2 summarizes hippocampal pathologies in MOG 35–55 EAE.
Thalamus
The thalamus has extensive cortical and sub-cortical connections. The involvement of the thalamus in MS is well documented, with early reports demonstrating thalamic atrophy in MS patients (Cifelli et al., 2002; Wylezinska et al., 2003) and later work demonstrating that thalamic atrophy is detectable early in disease (Brex et al., 2000; Calabrese et al., 2011; Zivadinov et al., 2013; Voskuhl et al., 2020). In fact, thalamic atrophy is a strong predictor for cognitive decline in MS patients (Houtchens et al., 2007; Batista et al., 2012; Schoonheim et al., 2012; Benedict et al., 2013) and is correlated with the accumulation of disability in patients with MS (Rocca et al., 2010; Magon et al., 2020). Early thalamic atrophy has also been reported in chronic EAE (Meyer et al., 2020). Demyelination was reported in postmortem thalami of MS patients (Vercellino et al., 2005), with a later study validating that finding and reporting microglial activation (Vercellino et al., 2009). In parallel, demyelination, astrocyte and microglial activation have been shown in the thalamus in chronic EAE (Aharoni et al., 2005, 2013; Wagenknecht et al., 2016; Werneburg et al., 2020). Neuronal loss in the thalamus in MS has been shown repeatedly (Cifelli et al., 2002; Vercellino et al., 2005, 2009) and neuronal loss (NeuN) in the ventral posterolateral nucleus was observed in chronic EAE (Wagenknecht et al., 2016). Interestingly, synaptic loss in the thalamus in both MS and EAE was shown in the same study (Werneburg et al., 2020). Despite a very large literature on thalamic involvement in MS, there are relatively fewer reports on the thalamus compared to other substructures in EAE. That said, those that do documented thalamic atrophy, demyelination, microglial activation, synaptic and neuronal loss in chronic EAE, as shown in Table 3.
Striatum
The striatum comprises the caudate, putamen, and nucleus accumbens, structures associated with both sensory and motor function, as well as cognition and emotion processing. Early studies demonstrated caudate atrophy in MS patients compared to healthy controls (Bermel et al., 2002, 2003), a finding that was replicated in chronic EAE (Hamilton et al., 2019; Meyer et al., 2020). In a comprehensive study of deep GM pathology in postmortem MS patients, demyelination, microglial activation, and neuronal loss were observed in the striatum (Vercellino et al., 2009) and another study by the same group also observed synaptic loss (Vercellino et al., 2007). Astrocyte and microglia activation and synaptic loss have all been shown in chronic EAE (Aharoni et al., 2005; Centonze et al., 2009; Ruffini et al., 2013). Furthermore, alternations in synaptic transmission in the striatum of mice with EAE has been demonstrated many times (Centonze et al., 2007; Gentile et al., 2015; Kammel et al., 2018). Striatal pathologies in MOG 35–55 EAE are listed in Table 4.
Cerebellum
The cerebellum plays a critical role in coordination and balance. Cerebellar deficits have been shown in MS patients (Weinshenker et al., 1996; Alusi et al., 2001) and cerebellar dysfunction was correlated with cerebellar atrophy (Edwards et al., 1999; Liu et al., 1999). Cerebellar atrophy has been shown multiple times in chronic EAE (MacKenzie-Graham et al., 2006, 2009; Hamilton et al., 2019). Interestingly, cerebellar atrophy has been shown to be very strongly correlated with cumulative disease score in chronic EAE (MacKenzie-Graham et al., 2012a), indicating an intimate relationship between cerebellar volume and walking disability. Demyelination in both the cerebellar cortex and cerebellar WM, combined with Purkinje cell loss, was described in postmortem MS patients (Kutzelnigg et al., 2007; Redondo et al., 2015). Similarly, cerebellar cortex and cerebellar WM demyelination, as well as Purkinje cell loss (calbindin and parvalbumin), has been reported in chronic EAE (MacKenzie-Graham et al., 2009, 2012a; Stanojlovic et al., 2016). Other studies have validated cerebellar demyelination and observed extensive microglial activation in postmortem MS tissue (Kemp et al., 2016), as well as astrocyte activation (Albert et al., 2017). Astrocyte and microglia activation have also been shown in chronic EAE (MacKenzie-Graham et al., 2012a; Mandolesi et al., 2013, 2017). Synaptic loss (Albert et al., 2017), as well as axonal damage and transection, have been reported in cerebellum of MS patients (Redondo et al., 2015), and similarly axonal damage (NF200) and synaptic loss have been shown in chronic EAE (MacKenzie-Graham et al., 2009). We have summarized these findings in the cerebellum in chronic EAE in Table 5.
Retina/optic nerve
Optic neuritis is a common first presentation of MS (Ransohoff et al., 2015) and MS patients can have a persistent reduction in vision after optic neuritis (Cole et al., 2000). Atrophy of the optic nerve has been observed in MS patients with optic neuritis (Hickman et al., 2001; Harrigan et al., 2017) and also in mice with chronic EAE (Hamilton et al., 2019). Demyelination of the optic nerve has been well documented for many years in both MS (Toussaint et al., 1983; Mogensen, 1990) and EAE (Sun et al., 2007; Horstmann et al., 2013; Tassoni et al., 2019). A comprehensive analysis of retinal and optic nerve head neuropathology in MS patients by Green et al. (2010) demonstrated astrocyte and microglia activation, axonal damage and loss, and the loss of retinal ganglion cells. Similarly, astrocyte and microglia activation, axonal damage and loss (APP, NF200, SMI-31, and SMI-312), and the loss of retinal ganglion cells (Brn3a) have also been seen in chronic EAE (Larabee et al., 2016; Jin et al., 2019; Tassoni et al., 2019). Interestingly, retinal nerve fiber layer (RNFL) thinning (Tassoni et al., 2019) and ganglion cell complex (GCC) thinning (Nishioka et al., 2019) has been observed using optical coherence tomograph (OCT), and very strong correlations between GCC thickness and DTI measures of fractional anisotropy and radial diffusivity have also been shown in chronic EAE (Nishioka et al., 2019). Visual acuity was also found to be significantly decreased in mice with chronic EAE (Lin et al., 2014a). Extensive work done in the retina and optic nerve (anterior visual pathway) in chronic EAE is summarized in Table 6.
Corpus callosum
Early MS studies using MRI showed not only corpus callosum atrophy, but that callosal atrophy was associated with brain atrophy and the duration and severity of clinical symptoms (Dietemann et al., 1988), findings that have been reproduced many times (Pelletier et al., 2001; Granberg et al., 2015). Callosal atrophy has also been shown recently in chronic EAE (Hamilton et al., 2019). Callosal demyelination has been shown by histological staining (Barnard and Triggs, 1974) and diffusion MRI (Ozturk et al., 2010) in MS. Similarly, studies using chronic EAE have also shown demyelination in the corpus callosum (Aharoni et al., 2013; Moore et al., 2013). Postmortem studies in MS demonstrated substantial regional axonal loss in the corpus callosum that correlated with regional lesion load and callosal atrophy (Evangelou et al., 2000a,b). Axonal damage and loss (APP, NF200, and SMI-32) and decreased synaptic transmission have also been shown in the corpus callosum in chronic EAE (Mangiardi et al., 2011).
Discussion
Progressive GM atrophy is strongly associated with clinical disability, making it an important marker for disease progression in MS (Chard et al., 2002; Pirko et al., 2007; MacKenzie-Graham et al., 2016). Understanding neurodegenerative mechanisms that lead to GM atrophy are thus critical. Inflammation, demyelination, and neurodegeneration are intimately connected in MS. In order to understand neurodegeneration in MS, we need models that exhibit neurodegeneration and atrophy in the GM in the context of inflammation and demyelination in the WM. That model is chronic EAE induced with MOG 35–55 peptide in C57BL/6 mice.
Multiple sclerosis and chronic EAE exhibit many of the same pathologies associated with neurodegeneration such as axonal ovoids and end bulbs, synaptic loss, and even neuronal loss in multiple neuroanatomical structures. Atrophy in the cerebral cortex, hippocampus, thalamus, striatum, and cerebellum occur in both MS and chronic EAE. The presence of these key elements of neurodegeneration in chronic EAE suggest that it is a good model to study neurodegeneration in MS.
Outside of relapses, clinical disability worsening in MS is progressive and does not improve significantly. Clinical disabilities are heterogeneous in MS, affecting walking, vision, cognition, and coordination. Standard MS clinical scales such as the Expanded Disability Status Scale (EDSS) are more sensitive to walking disability than other disabilities. At high scores the EDSS scale becomes insensitive to progression, even in walking disability. Progression of GM atrophy continues even during the time when high EDSS scores have plateaued. Other more sensitive outcome measures are used to evaluate progression of disability in vision and cognition. Clinical disability in the chronic EAE model as measured by standard EAE walking scores also reaches a plateau, without improvement. Progression of GM atrophy on MRI continues in chronic EAE over time during the plateau (MacKenzie-Graham et al., 2012b). Beyond walking, disability in vision and cognition have also been shown in chronic EAE using other more sensitive outcome measures (Ziehn et al., 2010; Lin et al., 2014a; Itoh et al., 2018; Tassoni et al., 2019).
Cuprizone toxicity has been suggested as a model to study demyelination in MS. Short-term cuprizone treatment in the diet induces demyelination without axonal damage and complete remyelination after return to normal diet. Chronic cuprizone treatment causes some axonal damage with only partial remyelination (Crawford et al., 2009; Voskuhl et al., 2019), and is thereby more similar to MS. However, the cuprizone model does not entail systemic autoimmunity nor immune cell infiltration into the CNS. Thus, while the cuprizone model is useful to study demyelination and remyelination, it lacks a pathology crucial to MS, namely autoimmunity and infiltrating immune cells. Clearly, chronic demyelination is an important pathology and remyelination is a goal in MS, but inflammation critically affects these processes. It has been shown that the inflammatory microenvironment in MS lesions inhibits the remyelinating capacity of oligodendrocytes in MS and chronic EAE (Kim et al., 2018; Starost et al., 2020). Thus, the presence of inflammation is an essential element in a model that seeks to assess treatments aiming to induce remyelination in MS.
Lysolecithin injections into the CNS induce localized demyelination. The injection is often followed by immune infiltration at the site that appears to be beneficial for remyelination (Kotter et al., 2001; Bieber et al., 2003; Miron et al., 2013). However, there are two important aspects related to neuroaxonal degeneration that warrant consideration in the lysolecithin model. First, axonal damage is not a feature of the lysolecithin model, while axonal damage is known to be an important characteristic of MS (Trapp et al., 1998, 1999). This is key since axonal integrity affects remyelination. Second, MS lesions do not occur in an otherwise healthy CNS. Instead, MS lesions occur in CNS tissues characterized by multifocal pre-existing WM lesions as well as GM pathology (Trapp et al., 1998, 1999; Peterson et al., 2001; Stadelmann et al., 2011; Friese et al., 2014; Mock et al., 2021). Wallerian degeneration from remote lesions could negatively impact remyelination of a new lesion. Even in clinically isolated syndrome (CIS), MRI often demonstrates multiple lesions and early GM atrophy (Brex et al., 2000; Dalton et al., 2004). Thus, identification of remyelination strategies in the lysolecithin model would be most appropriate for determining how to remyelinate a single demyelinating lesion in an otherwise normal CNS, but less aligned with modeling remyelination in a multifocal disease with ongoing pathologies over time.
No animal model recapitulates all aspects of human disease. So too, chronic EAE is not MS and it also has limitations. Ultimately, the MS model chosen depends on the question asked. B-cell involvement in MS is well documented (Meinl et al., 2006; von Budingen et al., 2011) and MOG 35–55 EAE does not require B-cells to induce disease (Hjelmström et al., 1998). Further, MOG 35–55 peptide does not efficiently activate B cells nor promote MOG-specific antibody production (Weber et al., 2010), both hallmarks of MS. That said, EAE induction with recombinant human MOG induces a B cell–dependent disease (Oliver et al., 2003; Marta et al., 2005) suggesting it as a model to study the role of B-cells in MS.
Cortical lesions are characteristic in MS (Geurts et al., 2005; Filippi et al., 2010; Lucchinetti et al., 2011). Although small demyelinating lesions have been reported in MOG 35–55 induced chronic EAE (Mangiardi et al., 2011; MacKenzie-Graham et al., 2012b), there are not large demyelinating cortical lesions. Recent work has produced large demyelinating lesions using a modified MOG 35–55 EAE induction protocol which entailed additional intrathecal injections of TNF-alpha and IFN-gamma into the primary somatosensory cortex (Orefice et al., 2020). This more complex induction paradigm also exhibits neuronal loss, making it appealing for studying large demyelinating cortical lesions in MS.
Experimental autoimmune encephalomyelitis induced by immunization of non-obese diabetic (NOD) mice with MOG 35–55 peptide has been described as having relapses with worsening disability (Ignatius Arokia Doss et al., 2015). The NOD EAE model exhibits gadolinium-enhancing lesions as well as WM damage as detected by DTI (Levy et al., 2010; Levy Barazany et al., 2014). Neuropathology includes WM inflammation, demyelination, and glial activation. Notably however, GM atrophy and GM neuropathologies are important features of disability progression in MS, and these have not been described in the NOD model like they have been in chronic EAE in C57BL/6 mice (Tables 1–6). Also, the vast array of genetically modified (transgenic and gene-targeted knock-out) mice that exist on the C57BL/6 background serve as tools which are more readily amenable for use in addressing mechanistic questions in the C57BL/6 EAE model than in the NOD EAE model.
In summary, we have reviewed neuropathologies in MS and chronic EAE in C57BL/6 mice focusing on various neuroanatomical structures. Although originally developed as a WM inflammatory model of MS, chronic EAE also exhibits neurodegeneration in several key GM structures, indeed those affected in MS. The combination of inflammation, demyelination, and neuroaxonal degeneration that characterizes chronic EAE in C57BL/6 mice makes it an excellent model for the development of new therapies targeting CNS cells to halt and repair disability progression in MS.
Author contributions
Both authors wrote, read, and approved the final manuscript.
Funding
This work was generously supported by NINDS/NIH R01NS109670 (RV), NINDS/NIH R01NS096748 (RV), NINDS/NIH R01NS086981 (AMG), NINDS/NIH R01NS121761 (AMG), NINDS/NIH R21NS121806 (AMG), the Conrad N. Hilton Foundation 201918394 (RV), Nancy Davis Race to Erase MS (RV), the Tom Sherak MS Hope Foundation, the Rhoda Goetz Foundation for MS, and the Dunk MS Foundation. We are grateful for the generous support from the Brain Mapping Medical Research Organization, Brain Mapping Support Foundation, Pierson-Lovelace Foundation, The Ahmanson Foundation, Capital Group Companies Charitable Foundation, William M. and Linda R. Dietel Philanthropic Fund, and Northstar Fund. Research reported in this publication was also partially supported by the National Center for Research Resources and by the Office of the Director of the National Institutes of Health under award numbers C06RR012169, C06RR015431, and S10OD011939.
Conflict of interest
The authors declare that the research was conducted in the absence of any commercial or financial relationships that could be construed as a potential conflict of interest.
Publisher’s note
All claims expressed in this article are solely those of the authors and do not necessarily represent those of their affiliated organizations, or those of the publisher, the editors and the reviewers. Any product that may be evaluated in this article, or claim that may be made by its manufacturer, is not guaranteed or endorsed by the publisher.
Author disclaimer
The content is solely the responsibility of the authors and does not necessarily represent the official views of the National Institutes of Health.
References
Aharoni, R., Arnon, R., and Eilam, R. (2005). Neurogenesis and neuroprotection induced by peripheral immunomodulatory treatment of experimental autoimmune encephalomyelitis. J. Neurosci. 25, 8217–8228. doi: 10.1523/JNEUROSCI.1859-05.2005
Aharoni, R., Sasson, E., Blumenfeld-Katzir, T., Eilam, R., Sela, M., Assaf, Y., et al. (2013). Magnetic resonance imaging characterization of different experimental autoimmune encephalomyelitis models and the therapeutic effect of glatiramer acetate. Exp. Neurol. 240, 130–144. doi: 10.1016/j.expneurol.2012.11.004
Ahrens, E. T., Laidlaw, D. H., Readhead, C., Brosnan, C. F., Fraser, S. E., and Jacobs, R. E. (1998). MR microscopy of transgenic mice that spontaneously acquire experimental allergic encephalomyelitis. Magn. Reson. Med. 40, 119–132. doi: 10.1002/mrm.1910400117
Albert, M., Barrantes-Freer, A., Lohrberg, M., Antel, J. P., Prineas, J. W., Palkovits, M., et al. (2017). Synaptic pathology in the cerebellar dentate nucleus in chronic multiple sclerosis. Brain Pathol. 27, 737–747. doi: 10.1111/bpa.12450
Alusi, S. H., Worthington, J., Glickman, S., and Bain, P. G. (2001). A study of tremor in multiple sclerosis. Brain 124(Pt 4), 720–730.
Amato, M. P., Bartolozzi, M. L., Zipoli, V., Portaccio, E., Mortilla, M., Guidi, L., et al. (2004). Neocortical volume decrease in relapsing-remitting MS patients with mild cognitive impairment. Neurology 63, 89–93.
Ando, D. G., Clayton, J., Kono, D., Urban, J. L., and Sercarz, E. E. (1989). Encephalitogenic T cells in the B10.PL model of experimental allergic encephalomyelitis (EAE) are of the Th-1 lymphokine subtype. Cell. Immunol. 124, 132–143. doi: 10.1016/0008-8749(89)90117-2
Baecher-Allan, C., Kaskow, B. J., and Weiner, H. L. (2018). Multiple sclerosis: Mechanisms and immunotherapy. Neuron 97, 742–768. doi: 10.1016/j.neuron.2018.01.021
Bakshi, R., Benedict, R. H., Bermel, R. A., and Jacobs, L. (2001). Regional brain atrophy is associated with physical disability in multiple sclerosis: Semiquantitative magnetic resonance imaging and relationship to clinical findings. J. Neuroimaging 11, 129–136. doi: 10.1111/j.1552-6569.2001.tb00022.x
Barnard, R. O., and Triggs, M. (1974). Corpus callosum in multiple sclerosis. J. Neurol. Neurosurg. Psychiatry 37, 1259–1264. doi: 10.1136/jnnp.37.11.1259
Batista, S., Zivadinov, R., Hoogs, M., Bergsland, N., Heininen-Brown, M., Dwyer, M. G., et al. (2012). Basal ganglia, thalamus and neocortical atrophy predicting slowed cognitive processing in multiple sclerosis. J. Neurol. 259, 139–146. doi: 10.1007/s00415-011-6147-1
Bellizzi, M. J., Geathers, J. S., Allan, K. C., and Gelbard, H. A. (2016). Platelet-activating factor receptors mediate excitatory postsynaptic hippocampal injury in experimental autoimmune encephalomyelitis. J. Neurosci. 36, 1336–1346. doi: 10.1523/JNEUROSCI.1171-15.2016
Benedict, R. H., Hulst, H. E., Bergsland, N., Schoonheim, M. M., Dwyer, M. G., Weinstock-Guttman, B., et al. (2013). Clinical significance of atrophy and white matter mean diffusivity within the thalamus of multiple sclerosis patients. Mult. Scler. 19, 1478–1484. doi: 10.1177/1352458513478675
Bermel, R. A., and Bakshi, R. (2006). The measurement and clinical relevance of brain atrophy in multiple sclerosis. Lancet Neurol. 5, 158–170. doi: 10.1016/S1474-4422(06)70349-0
Bermel, R. A., Bakshi, R., Tjoa, C., Puli, S. R., and Jacobs, L. (2002). Bicaudate ratio as a magnetic resonance imaging marker of brain atrophy in multiple sclerosis. Arch. Neurol. 59, 275–280. doi: 10.1001/archneur.59.2.275
Bermel, R. A., Innus, M. D., Tjoa, C. W., and Bakshi, R. (2003). Selective caudate atrophy in multiple sclerosis: A 3D MRI parcellation study. Neuroreport 14, 335–339. doi: 10.1097/01.wnr.0000059773.23122.ce
Bevan, R. J., Evans, R., Griffiths, L., Watkins, L. M., Rees, M. I., Magliozzi, R., et al. (2018). Meningeal inflammation and cortical demyelination in acute multiple sclerosis. Ann. Neurol. 84, 829–842. doi: 10.1002/ana.25365
Bieber, A. J., Kerr, S., and Rodriguez, M. (2003). Efficient central nervous system remyelination requires T cells. Ann. Neurol. 53, 680–684. doi: 10.1002/ana.10578
Bo, L., Vedeler, C. A., Nyland, H. I., Trapp, B. D., and Mork, S. J. (2003). Subpial demyelination in the cerebral cortex of multiple sclerosis patients. J. Neuropathol. Exp. Neurol. 62, 723–732.
Bourel, J., Planche, V., Dubourdieu, N., Oliveira, A., Sere, A., Ducourneau, E. G., et al. (2021). Complement C3 mediates early hippocampal neurodegeneration and memory impairment in experimental multiple sclerosis. Neurobiol. Dis. 160:105533. doi: 10.1016/j.nbd.2021.105533
Brex, P. A., Jenkins, R., Fox, N. C., Crum, W. R., O’Riordan, J. I., Plant, G. T., et al. (2000). Detection of ventricular enlargement in patients at the earliest clinical stage of MS. Neurology 54, 1689–1691. doi: 10.1212/wnl.54.8.1689
Brocke, S., Quigley, L., McFarland, H. F., and Steinman, L. (1996). Isolation and characterization of autoreactive T cells in experimental autoimmune encephalomyelitis of the mouse. Methods 9, 458–462. doi: 10.1006/meth.1996.0053
Budde, M. D., Kim, J. H., Liang, H. F., Russell, J. H., Cross, A. H., and Song, S. K. (2008). Axonal injury detected by in vivo diffusion tensor imaging correlates with neurological disability in a mouse model of multiple sclerosis. NMR Biomed. 21, 589–597. doi: 10.1002/nbm.1229
Budde, M. D., Xie, M., Cross, A. H., and Song, S. K. (2009). Axial diffusivity is the primary correlate of axonal injury in the experimental autoimmune encephalomyelitis spinal cord: A quantitative pixelwise analysis. J. Neurosci. 29, 2805–2813. doi: 10.1523/JNEUROSCI.4605-08.2009
Burns, T., Miers, L., Xu, J., Man, A., Moreno, M., Pleasure, D., et al. (2014). Neuronopathy in the motor neocortex in a chronic model of multiple sclerosis. J. Neuropathol. Exp. Neurol. 73, 335–344. doi: 10.1097/NEN.0000000000000058
Calabrese, M., Agosta, F., Rinaldi, F., Mattisi, I., Grossi, P., Favaretto, A., et al. (2009). Cortical lesions and atrophy associated with cognitive impairment in relapsing-remitting multiple sclerosis. Arch. Neurol. 66, 1144–1150. doi: 10.1001/archneurol.2009.174
Calabrese, M., Atzori, M., Bernardi, V., Morra, A., Romualdi, C., Rinaldi, L., et al. (2007). Cortical atrophy is relevant in multiple sclerosis at clinical onset. J. Neurol. 254, 1212–1220. doi: 10.1007/s00415-006-0503-6
Calabrese, M., Mattisi, I., Rinaldi, F., Favaretto, A., Atzori, M., Bernardi, V., et al. (2010). Magnetic resonance evidence of cerebellar cortical pathology in multiple sclerosis. J. Neurol. Neurosurg. Psychiatry 81, 401–404. doi: 10.1136/jnnp.2009.177733
Calabrese, M., Rinaldi, F., Mattisi, I., Bernardi, V., Favaretto, A., Perini, P., et al. (2011). The predictive value of gray matter atrophy in clinically isolated syndromes. Neurology 77, 257–263. doi: 10.1212/WNL.0b013e318220abd4
Centonze, D., Bari, M., Rossi, S., Prosperetti, C., Furlan, R., Fezza, F., et al. (2007). The endocannabinoid system is dysregulated in multiple sclerosis and in experimental autoimmune encephalomyelitis. Brain 130(Pt 10), 2543–2553. doi: 10.1093/brain/awm160
Centonze, D., Muzio, L., Rossi, S., Cavasinni, F., De Chiara, V., Bergami, A., et al. (2009). Inflammation triggers synaptic alteration and degeneration in experimental autoimmune encephalomyelitis. J. Neurosci. 29, 3442–3452. doi: 10.1523/JNEUROSCI.5804-08.2009
Chard, D. T., Brex, P. A., Ciccarelli, O., Griffin, C. M., Parker, G. J., Dalton, C., et al. (2003). The longitudinal relation between brain lesion load and atrophy in multiple sclerosis: A 14 year follow up study. J. Neurol. Neurosurg. Psychiatry 74, 1551–1554. doi: 10.1136/jnnp.74.11.1551
Chard, D. T., Griffin, C. M., Parker, G. J., Kapoor, R., Thompson, A. J., and Miller, D. H. (2002). Brain atrophy in clinically early relapsing-remitting multiple sclerosis. Brain 125(Pt 2), 327–337.
Charil, A., Dagher, A., Lerch, J. P., Zijdenbos, A. P., Worsley, K. J., and Evans, A. C. (2007). Focal cortical atrophy in multiple sclerosis: Relation to lesion load and disability. Neuroimage 34, 509–517. doi: 10.1016/j.neuroimage.2006.10.006
Chaudhary, P., Marracci, G., Yu, X., Galipeau, D., Morris, B., and Bourdette, D. (2011). Lipoic acid decreases inflammation and confers neuroprotection in experimental autoimmune optic neuritis. J. Neuroimmunol. 233, 90–96. doi: 10.1016/j.jneuroim.2010.12.002
Cifelli, A., Arridge, M., Jezzard, P., Esiri, M. M., Palace, J., and Matthews, P. M. (2002). Thalamic neurodegeneration in multiple sclerosis. Ann. Neurol. 52, 650–653. doi: 10.1002/ana.10326
Cole, S. R., Beck, R. W., Moke, P. S., Gal, R. L., and Long, D. T. (2000). The national eye institute visual function questionnaire: Experience of the ONTT. Optic neuritis treatment trial. Invest. Ophthalmol. Vis. Sci. 41, 1017–1021.
Crawford, D. K., Mangiardi, M., Song, B., Patel, R., Du, S., Sofroniew, M. V., et al. (2010). Oestrogen receptor beta ligand: A novel treatment to enhance endogenous functional remyelination. Brain 133, 2999–3016. doi: 10.1093/brain/awq237
Crawford, D. K., Mangiardi, M., Xia, X., Lopez-Valdes, H. E., and Tiwari-Woodruff, S. K. (2009). Functional recovery of callosal axons following demyelination: A critical window. Neuroscience 164, 1407–1421. doi: 10.1016/j.neuroscience.2009.09.069
Dalton, C. M., Chard, D. T., Davies, G. R., Miszkiel, K. A., Altmann, D. R., Fernando, K., et al. (2004). Early development of multiple sclerosis is associated with progressive grey matter atrophy in patients presenting with clinically isolated syndromes. Brain 127(Pt 5), 1101–1107. doi: 10.1093/brain/awh126
De Stefano, N., Matthews, P. M., Filippi, M., Agosta, F., De Luca, M., Bartolozzi, M. L., et al. (2003). Evidence of early cortical atrophy in MS: Relevance to white matter changes and disability. Neurology 60, 1157–1162. doi: 10.1212/01.wnl.0000055926.69643.03
Denic, A., Johnson, A. J., Bieber, A. J., Warrington, A. E., Rodriguez, M., and Pirko, I. (2011). The relevance of animal models in multiple sclerosis research. Pathophysiology 18, 21–29. doi: 10.1016/j.pathophys.2010.04.004
Di Filippo, M., Chiasserini, D., Gardoni, F., Viviani, B., Tozzi, A., Giampa, C., et al. (2013). Effects of central and peripheral inflammation on hippocampal synaptic plasticity. Neurobiol. Dis. 52, 229–236. doi: 10.1016/j.nbd.2012.12.009
Di Filippo, M., de Iure, A., Giampa, C., Chiasserini, D., Tozzi, A., Orvietani, P. L., et al. (2016). Persistent activation of microglia and NADPH oxidase [corrected] drive hippocampal dysfunction in experimental multiple sclerosis. Sci. Rep. 6:20926. doi: 10.1038/srep20926
Di Filippo, M., Mancini, A., Bellingacci, L., Gaetani, L., Mazzocchetti, P., Zelante, T., et al. (2021). Interleukin-17 affects synaptic plasticity and cognition in an experimental model of multiple sclerosis. Cell Rep. 37:110094. doi: 10.1016/j.celrep.2021.110094
Dietemann, J. L., Beigelman, C., Rumbach, L., Vouge, M., Tajahmady, T., Faubert, C., et al. (1988). Multiple sclerosis and corpus callosum atrophy: Relationship of MRI findings to clinical data. Neuroradiology 30, 478–480. doi: 10.1007/BF00339686
Dutta, R., Chang, A., Doud, M. K., Kidd, G. J., Ribaudo, M. V., Young, E. A., et al. (2011). Demyelination causes synaptic alterations in hippocampi from multiple sclerosis patients. Ann. Neurol. 69, 445–454. doi: 10.1002/ana.22337
Dutta, R., Chomyk, A. M., Chang, A., Ribaudo, M. V., Deckard, S. A., Doud, M. K., et al. (2013). Hippocampal demyelination and memory dysfunction are associated with increased levels of the neuronal microRNA miR-124 and reduced AMPA receptors. Ann. Neurol. 73, 637–645. doi: 10.1002/ana.23860
Edwards, S. G., Gong, Q. Y., Liu, C., Zvartau, M. E., Jaspan, T., Roberts, N., et al. (1999). Infratentorial atrophy on magnetic resonance imaging and disability in multiple sclerosis. Brain 122(Pt 2), 291–301.
Elliott, C., Momayyezsiahkal, P., Arnold, D. L., Liu, D., Ke, J., Zhu, L., et al. (2021). Abnormalities in normal-appearing white matter from which multiple sclerosis lesions arise. Brain Commun. 3:fcab176. doi: 10.1093/braincomms/fcab176
Errede, M., Girolamo, F., Ferrara, G., Strippoli, M., Morando, S., Boldrin, V., et al. (2012). Blood-brain barrier alterations in the cerebral cortex in experimental autoimmune encephalomyelitis. J. Neuropathol. Exp. Neurol. 71, 840–854. doi: 10.1097/NEN.0b013e31826ac110
Evangelou, N., Esiri, M. M., Smith, S., Palace, J., and Matthews, P. M. (2000a). Quantitative pathological evidence for axonal loss in normal appearing white matter in multiple sclerosis. Ann. Neurol. 47, 391–395.
Evangelou, N., Konz, D., Esiri, M. M., Smith, S., Palace, J., and Matthews, P. M. (2000b). Regional axonal loss in the corpus callosum correlates with cerebral white matter lesion volume and distribution in multiple sclerosis. Brain 123(Pt 9), 1845–1849.
Ferguson, B., Matyszak, M. K., Esiri, M. M., and Perry, V. H. (1997). Axonal damage in acute multiple sclerosis lesions. Brain 120(Pt 3), 393–399.
Filippi, M., Rocca, M. A., Calabrese, M., Sormani, M. P., Rinaldi, F., Perini, P., et al. (2010). Intracortical lesions: Relevance for new MRI diagnostic criteria for multiple sclerosis. Neurology 75, 1988–1994. doi: 10.1212/WNL.0b013e3181ff96f6
Fisher, E., Lee, J. C., Nakamura, K., and Rudick, R. A. (2008). Gray matter atrophy in multiple sclerosis: A longitudinal study. Ann. Neurol. 64, 255–265. doi: 10.1002/ana.21436
Fisher, E., Rudick, R. A., Simon, J. H., Cutter, G., Baier, M., Lee, J. C., et al. (2002). Eight-year follow-up study of brain atrophy in patients with MS. Neurology 59, 1412–1420.
Friese, M. A., Schattling, B., and Fugger, L. (2014). Mechanisms of neurodegeneration and axonal dysfunction in multiple sclerosis. Nat. Rev. Neurol. 10, 225–238. doi: 10.1038/nrneurol.2014.37
Frischer, J. M., Bramow, S., Dal-Bianco, A., Lucchinetti, C. F., Rauschka, H., Schmidbauer, M., et al. (2009). The relation between inflammation and neurodegeneration in multiple sclerosis brains. Brain 132(Pt 5), 1175–1189. doi: 10.1093/brain/awp070
Gentile, A., Fresegna, D., Federici, M., Musella, A., Rizzo, F. R., Sepman, H., et al. (2015). Dopaminergic dysfunction is associated with IL-1beta-dependent mood alterations in experimental autoimmune encephalomyelitis. Neurobiol. Dis. 74, 347–358. doi: 10.1016/j.nbd.2014.11.022
Gentile, A., Musella, A., Bullitta, S., Fresegna, D., De Vito, F., Fantozzi, R., et al. (2016). Siponimod (BAF312) prevents synaptic neurodegeneration in experimental multiple sclerosis. J. Neuroinflammation 13:207. doi: 10.1186/s12974-016-0686-4
Geurts, J. J., Bo, L., Pouwels, P. J., Castelijns, J. A., Polman, C. H., and Barkhof, F. (2005). Cortical lesions in multiple sclerosis: Combined postmortem MR imaging and histopathology. AJNR Am. J. Neuroradiol. 26, 572–577.
Geurts, J. J., Bo, L., Roosendaal, S. D., Hazes, T., Daniels, R., Barkhof, F., et al. (2007). Extensive hippocampal demyelination in multiple sclerosis. J. Neuropathol. Exp. Neurol. 66, 819–827. doi: 10.1097/nen.0b013e3181461f54
Girolamo, F., Ferrara, G., Strippoli, M., Rizzi, M., Errede, M., Trojano, M., et al. (2011). Cerebral cortex demyelination and oligodendrocyte precursor response to experimental autoimmune encephalomyelitis. Neurobiol. Dis. 43, 678–689. doi: 10.1016/j.nbd.2011.05.021
Granberg, T., Martola, J., Bergendal, G., Shams, S., Damangir, S., Aspelin, P., et al. (2015). Corpus callosum atrophy is strongly associated with cognitive impairment in multiple sclerosis: Results of a 17-year longitudinal study. Mult. Scler. 21, 1151–1158. doi: 10.1177/1352458514560928
Grasselli, G., Rossi, S., Musella, A., Gentile, A., Loizzo, S., Muzio, L., et al. (2013). Abnormal NMDA receptor function exacerbates experimental autoimmune encephalomyelitis. Br. J. Pharmacol. 168, 502–517. doi: 10.1111/j.1476-5381.2012.02178.x
Green, A. J., McQuaid, S., Hauser, S. L., Allen, I. V., and Lyness, R. (2010). Ocular pathology in multiple sclerosis: Retinal atrophy and inflammation irrespective of disease duration. Brain 133(Pt 6), 1591–1601. doi: 10.1093/brain/awq080
Guo, J., Wang, J., Guo, R., Shao, H., and Guo, L. (2022). Pterostilbene protects the optic nerves and retina in a murine model of experimental autoimmune encephalomyelitis via activation of SIRT1 signaling. Neuroscience 487, 35–46. doi: 10.1016/j.neuroscience.2022.01.016
Hamilton, A. M., Forkert, N. D., Yang, R., Wu, Y., Rogers, J. A., Yong, V. W., et al. (2019). Central nervous system targeted autoimmunity causes regional atrophy: A 9.4T MRI study of the EAE mouse model of multiple sclerosis. Sci. Rep. 9:8488. doi: 10.1038/s41598-019-44682-6
Hammond, J. W., Bellizzi, M. J., Ware, C., Qiu, W. Q., Saminathan, P., Li, H., et al. (2020). Complement-dependent synapse loss and microgliosis in a mouse model of multiple sclerosis. Brain Behav. Immun. 87, 739–750. doi: 10.1016/j.bbi.2020.03.004
Harrigan, R. L., Smith, A. K., Lyttle, B., Box, B., Landman, B. A., Bagnato, F., et al. (2017). Quantitative characterization of optic nerve atrophy in patients with multiple sclerosis. Mult. Scler. J. Exp. Transl. Clin. 3:2055217317730097. doi: 10.1177/2055217317730097
Hickman, S. J., Brex, P. A., Brierley, C. M., Silver, N. C., Barker, G. J., Scolding, N. J., et al. (2001). Detection of optic nerve atrophy following a single episode of unilateral optic neuritis by MRI using a fat-saturated short-echo fast FLAIR sequence. Neuroradiology 43, 123–128. doi: 10.1007/s002340000450
Hjelmstrom, P., Juedes, A. E., and Ruddle, N. H. (1998). Cytokines and antibodies in myelin oligodendrocyte glycoprotein-induced experimental allergic encephalomyelitis. Res. Immunol. 149, 794–804; discussion 847–898, 855–860.
Hjelmström, P., Juedes, A. E., Fjell, J., and Ruddle, N. H. (1998). B-cell-deficient mice develop experimental allergic encephalomyelitis with demyelination after myelin oligodendrocyte glycoprotein sensitization. J. Immunol. 161, 4480–4483.
Horstmann, L., Kuehn, S., Pedreiturria, X., Haak, K., Pfarrer, C., Dick, H. B., et al. (2016). Microglia response in retina and optic nerve in chronic experimental autoimmune encephalomyelitis. J. Neuroimmunol. 298, 32–41. doi: 10.1016/j.jneuroim.2016.06.008
Horstmann, L., Schmid, H., Heinen, A. P., Kurschus, F. C., Dick, H. B., and Joachim, S. C. (2013). Inflammatory demyelination induces glia alterations and ganglion cell loss in the retina of an experimental autoimmune encephalomyelitis model. J. Neuroinflammation 10:120. doi: 10.1186/1742-2094-10-120
Houtchens, M. K., Benedict, R. H., Killiany, R., Sharma, J., Jaisani, Z., Singh, B., et al. (2007). Thalamic atrophy and cognition in multiple sclerosis. Neurology 69, 1213–1223. doi: 10.1212/01.wnl.0000276992.17011.b5
Hulst, H. E., and Geurts, J. J. (2011). Gray matter imaging in multiple sclerosis: What have we learned? BMC Neurol. 11:153. doi: 10.1186/1471-2377-11-153
Ignatius Arokia Doss, P. M., Roy, A. P., Wang, A., Anderson, A. C., and Rangachari, M. (2015). The non-obese diabetic mouse strain as a model to study CD8(+) T cell function in relapsing and progressive multiple sclerosis. Front. Immunol. 6:541. doi: 10.3389/fimmu.2015.00541
Itoh, N., Itoh, Y., Tassoni, A., Ren, E., Kaito, M., Ohno, A., et al. (2018). Cell-specific and region-specific transcriptomics in the multiple sclerosis model: Focus on astrocytes. Proc. Natl. Acad. Sci. U.S.A. 115, E302–E309. doi: 10.1073/pnas.1716032115
Itoh, N., Kim, R., Peng, M., DiFilippo, E., Johnsonbaugh, H., MacKenzie-Graham, A., et al. (2017). Bedside to bench to bedside research: Estrogen receptor beta ligand as a candidate neuroprotective treatment for multiple sclerosis. J. Neuroimmunol. 304, 63–71. doi: 10.1016/j.jneuroim.2016.09.017
Jin, J., Smith, M. D., Kersbergen, C. J., Kam, T. I., Viswanathan, M., Martin, K., et al. (2019). Glial pathology and retinal neurotoxicity in the anterior visual pathway in experimental autoimmune encephalomyelitis. Acta Neuropathol. Commun. 7:125. doi: 10.1186/s40478-019-0767-6
Kammel, L. G., Wei, W., Jami, S. A., Voskuhl, R. R., and O’Dell, T. J. (2018). Enhanced GABAergic tonic inhibition reduces intrinsic excitability of hippocampal CA1 pyramidal cells in experimental autoimmune encephalomyelitis. Neuroscience 395, 89–100. doi: 10.1016/j.neuroscience.2018.11.003
Kemp, K., Redondo, J., Hares, K., Rice, C., Scolding, N., and Wilkins, A. (2016). Oxidative injury in multiple sclerosis cerebellar grey matter. Brain Res. 1642, 452–460. doi: 10.1016/j.brainres.2016.04.027
Kidd, D., Barkhof, F., McConnell, R., Algra, P. R., Allen, I. V., and Revesz, T. (1999). Cortical lesions in multiple sclerosis. Brain 122(Pt 1), 17–26.
Kim, R. Y., Mangu, D., Hoffman, A. S., Kavosh, R., Jung, E., Itoh, N., et al. (2018). Oestrogen receptor beta ligand acts on CD11c+ cells to mediate protection in experimental autoimmune encephalomyelitis. Brain 141, 132–147. doi: 10.1093/brain/awx315
Klawiter, E. C. (2013). Current and new directions in MRI in multiple sclerosis. Continuum (Minneap. Minn) 19(4 Multiple Sclerosis), 1058–1073. doi: 10.1212/01.CON.0000433283.00221.37
Kotter, M. R., Setzu, A., Sim, F. J., Van Rooijen, N., and Franklin, R. J. (2001). Macrophage depletion impairs oligodendrocyte remyelination following lysolecithin-induced demyelination. Glia 35, 204–212. doi: 10.1002/glia.1085
Kutzelnigg, A., and Lassmann, H. (2014). Pathology of multiple sclerosis and related inflammatory demyelinating diseases. Handb. Clin. Neurol. 122, 15–58. doi: 10.1016/B978-0-444-52001-2.00002-9
Kutzelnigg, A., Faber-Rod, J. C., Bauer, J., Lucchinetti, C. F., Sorensen, P. S., Laursen, H., et al. (2007). Widespread demyelination in the cerebellar cortex in multiple sclerosis. Brain Pathol. 17, 38–44.
Larabee, C. M., Desai, S., Agasing, A., Georgescu, C., Wren, J. D., Axtell, R. C., et al. (2016). Loss of Nrf2 exacerbates the visual deficits and optic neuritis elicited by experimental autoimmune encephalomyelitis. Mol. Vis. 22, 1503–1513.
Larochelle, C., Uphaus, T., Prat, A., and Zipp, F. (2016). Secondary progression in multiple sclerosis: Neuronal exhaustion or distinct pathology? Trends Neurosci. 39, 325–339. doi: 10.1016/j.tins.2016.02.001
Lassmann, H. (1998). Neuropathology in multiple sclerosis: New concepts. Mult. Scler. 4, 93–98. doi: 10.1177/135245859800400301
Lassmann, H., and Ransohoff, R. M. (2004). The CD4-Th1 model for multiple sclerosis: A critical [correction of crucial] re-appraisal. Trends Immunol. 25, 132–137. doi: 10.1016/j.it.2004.01.007
Lassmann, H., van Horssen, J., and Mahad, D. (2012). Progressive multiple sclerosis: Pathology and pathogenesis. Nat. Rev. Neurol. 8, 647–656. doi: 10.1038/nrneurol.2012.168
Levy Barazany, H., Barazany, D., Puckett, L., Blanga-Kanfi, S., Borenstein-Auerbach, N., Yang, K., et al. (2014). Brain MRI of nasal MOG therapeutic effect in relapsing-progressive EAE. Exp. Neurol. 255, 63–70. doi: 10.1016/j.expneurol.2014.02.010
Levy, H., Assaf, Y., and Frenkel, D. (2010). Characterization of brain lesions in a mouse model of progressive multiple sclerosis. Exp. Neurol. 226, 148–158. doi: 10.1016/j.expneurol.2010.08.017
Lin, T. H., Kim, J. H., Perez-Torres, C., Chiang, C. W., Trinkaus, K., Cross, A. H., et al. (2014a). Axonal transport rate decreased at the onset of optic neuritis in EAE mice. Neuroimage 100, 244–253. doi: 10.1016/j.neuroimage.2014.06.009
Lin, T. H., Spees, W. M., Chiang, C. W., Trinkaus, K., Cross, A. H., and Song, S. K. (2014b). Diffusion fMRI detects white-matter dysfunction in mice with acute optic neuritis. Neurobiol. Dis. 67, 1–8. doi: 10.1016/j.nbd.2014.02.007
Lin, X., and Blumhardt, L. D. (2001). Inflammation and atrophy in multiple sclerosis: MRI associations with disease course. J. Neurol. Sci. 189, 99–104. doi: 10.1016/s0022-510x(01)00576-7
Liu, C., Edwards, S., Gong, Q., Roberts, N., and Blumhardt, L. D. (1999). Three dimensional MRI estimates of brain and spinal cord atrophy in multiple sclerosis. J. Neurol. Neurosurg. Psychiatry 66, 323–330.
Lucchinetti, C. F., Popescu, B. F., Bunyan, R. F., Moll, N. M., Roemer, S. F., Lassmann, H., et al. (2011). Inflammatory cortical demyelination in early multiple sclerosis. N. Engl. J. Med. 365, 2188–2197. doi: 10.1056/NEJMoa1100648
MacKenzie-Graham, A. J., Rinek, G. A., Avedisian, A., Morales, L. B., Umeda, E., Boulat, B., et al. (2012a). Estrogen treatment prevents gray matter atrophy in experimental autoimmune encephalomyelitis. J. Neurosci. Res. 90, 1310–1323. doi: 10.1002/jnr.23019
MacKenzie-Graham, A., Rinek, G. A., Avedisian, A., Gold, S. M., Frew, A. J., Aguilar, C., et al. (2012b). Cortical atrophy in experimental autoimmune encephalomyelitis: In vivo imaging. Neuroimage 60, 95–104. doi: 10.1016/j.neuroimage.2011.11.099
MacKenzie-Graham, A., Kurth, F., Itoh, Y., Wang, H. J., Montag, M. J., Elashoff, R., et al. (2016). Disability-specific atlases of gray matter loss in relapsing-remitting multiple sclerosis. JAMA Neurol. 73, 944–953. doi: 10.1001/jamaneurol.2016.0966
MacKenzie-Graham, A., Tinsley, M. R., Shah, K. P., Aguilar, C., Strickland, L. V., Boline, J., et al. (2006). Cerebellar cortical atrophy in experimental autoimmune encephalomyelitis. Neuroimage 32, 1016–1023.
MacKenzie-Graham, A., Tiwari-Woodruff, S. K., Sharma, G., Aguilar, C., Vo, K. T., Strickland, L. V., et al. (2009). Purkinje cell loss in experimental autoimmune encephalomyelitis. Neuroimage 48, 637–651. doi: 10.1016/j.neuroimage.2009.06.073
Magon, S., Tsagkas, C., Gaetano, L., Patel, R., Naegelin, Y., Amann, M., et al. (2020). Volume loss in the deep gray matter and thalamic subnuclei: A longitudinal study on disability progression in multiple sclerosis. J. Neurol. 267, 1536–1546. doi: 10.1007/s00415-020-09740-4
Mandolesi, G., De Vito, F., Musella, A., Gentile, A., Bullitta, S., Fresegna, D., et al. (2017). miR-142-3p is a key regulator of il-1beta-dependent synaptopathy in neuroinflammation. J. Neurosci. 37, 546–561. doi: 10.1523/JNEUROSCI.0851-16.2016
Mandolesi, G., Musella, A., Gentile, A., Grasselli, G., Haji, N., Sepman, H., et al. (2013). Interleukin-1beta alters glutamate transmission at purkinje cell synapses in a mouse model of multiple sclerosis. J. Neurosci. 33, 12105–12121. doi: 10.1523/JNEUROSCI.5369-12.2013
Mangiardi, M., Crawford, D. K., Xia, X., Du, S., Simon-Freeman, R., Voskuhl, R. R., et al. (2011). An animal model of cortical and callosal pathology in multiple sclerosis. Brain Pathol. 21, 263–278. doi: 10.1111/j.1750-3639.2010.00444.x
Manogaran, P., Samardzija, M., Schad, A. N., Wicki, C. A., Walker-Egger, C., Rudin, M., et al. (2019). Retinal pathology in experimental optic neuritis is characterized by retrograde degeneration and gliosis. Acta Neuropathol. Commun. 7:116. doi: 10.1186/s40478-019-0768-5
Marenna, S., Huang, S. C., Castoldi, V., d’Isa, R., Costa, G. D., Comi, G., et al. (2020). Functional evolution of visual involvement in experimental autoimmune encephalomyelitis. Mult. Scler. J. Exp. Transl. Clin. 6:2055217320963474. doi: 10.1177/2055217320963474
Marta, C. B., Oliver, A. R., Sweet, R. A., Pfeiffer, S. E., and Ruddle, N. H. (2005). Pathogenic myelin oligodendrocyte glycoprotein antibodies recognize glycosylated epitopes and perturb oligodendrocyte physiology. Proc. Natl. Acad. Sci. U.S.A. 102, 13992–13997. doi: 10.1073/pnas.0504979102
Meinl, E., Krumbholz, M., and Hohlfeld, R. (2006). B lineage cells in the inflammatory central nervous system environment: Migration, maintenance, local antibody production, and therapeutic modulation. Ann. Neurol. 59, 880–892. doi: 10.1002/ana.20890
Meyer, C. E., Gao, J. L., Cheng, J. Y., Oberoi, M. R., Johnsonbaugh, H., Lepore, S., et al. (2020). Axonal damage in spinal cord is associated with gray matter atrophy in sensorimotor cortex in experimental autoimmune encephalomyelitis. Mult. Scler. 26, 294–303. doi: 10.1177/1352458519830614
Michailidou, I., Willems, J. G., Kooi, E. J., van Eden, C., Gold, S. M., Geurts, J. J., et al. (2015). Complement C1q-C3-associated synaptic changes in multiple sclerosis hippocampus. Ann. Neurol. 77, 1007–1026. doi: 10.1002/ana.24398
Miller, D. H., Barkhof, F., Frank, J. A., Parker, G. J., and Thompson, A. J. (2002). Measurement of atrophy in multiple sclerosis: Pathological basis, methodological aspects and clinical relevance. Brain 125(Pt 8), 1676–1695. doi: 10.1093/brain/awf177
Miron, V. E., Boyd, A., Zhao, J. W., Yuen, T. J., Ruckh, J. M., Shadrach, J. L., et al. (2013). M2 microglia and macrophages drive oligodendrocyte differentiation during CNS remyelination. Nat. Neurosci. 16, 1211–1218. doi: 10.1038/nn.3469
Mock, E. E. A., Honkonen, E., and Airas, L. (2021). Synaptic loss in multiple sclerosis: A systematic review of human post-mortem studies. Front. Neurol. 12:782599. doi: 10.3389/fneur.2021.782599
Mogensen, P. H. (1990). Histopathology of anterior parts of the optic pathway in patients with multiple sclerose. Acta Ophthalmol. (Copenh) 68, 218–220. doi: 10.1111/j.1755-3768.1990.tb01908.x
Moll, N. M., Rietsch, A. M., Thomas, S., Ransohoff, A. J., Lee, J. C., Fox, R., et al. (2011). Multiple sclerosis normal-appearing white matter: Pathology-imaging correlations. Ann. Neurol. 70, 764–773. doi: 10.1002/ana.22521
Moore, S., Khalaj, A. J., Yoon, J., Patel, R., Hannsun, G., Yoo, T., et al. (2013). Therapeutic laquinimod treatment decreases inflammation, initiates axon remyelination, and improves motor deficit in a mouse model of multiple sclerosis. Brain Behav. 3, 664–682. doi: 10.1002/brb3.174
Nishioka, C., Liang, H. F., Barsamian, B., and Sun, S. W. (2019). Sequential phases of RGC axonal and somatic injury in EAE mice examined using DTI and OCT. Mult. Scler. Relat. Disord. 27, 315–323. doi: 10.1016/j.msard.2018.11.010
Nistico, R., Mango, D., Mandolesi, G., Piccinin, S., Berretta, N., Pignatelli, M., et al. (2013). Inflammation subverts hippocampal synaptic plasticity in experimental multiple sclerosis. PLoS One 8:e54666. doi: 10.1371/journal.pone.0054666
Novkovic, T., Shchyglo, O., Gold, R., and Manahan-Vaughan, D. (2015). Hippocampal function is compromised in an animal model of multiple sclerosis. Neuroscience 309, 100–112. doi: 10.1016/j.neuroscience.2015.03.008
Oliver, A. R., Lyon, G. M., and Ruddle, N. H. (2003). Rat and human myelin oligodendrocyte glycoproteins induce experimental autoimmune encephalomyelitis by different mechanisms in C57BL/6 mice. J. Immunol. 171, 462–468. doi: 10.4049/jimmunol.171.1.462
Orefice, N. S., Guillemot-Legris, O., Capasso, R., Bottemanne, P., Hantraye, P., Caraglia, M., et al. (2020). miRNA profile is altered in a modified EAE mouse model of multiple sclerosis featuring cortical lesions. Elife 9:e56916. doi: 10.7554/eLife.56916
Ozturk, A., Smith, S. A., Gordon-Lipkin, E. M., Harrison, D. M., Shiee, N., Pham, D. L., et al. (2010). MRI of the corpus callosum in multiple sclerosis: Association with disability. Mult. Scler. 16, 166–177. doi: 10.1177/1352458509353649
Parodi, B., Rossi, S., Morando, S., Cordano, C., Bragoni, A., Motta, C., et al. (2015). Fumarates modulate microglia activation through a novel HCAR2 signaling pathway and rescue synaptic dysregulation in inflamed CNS. Acta Neuropathol. 130, 279–295. doi: 10.1007/s00401-015-1422-3
Pastor, S., Minguela, A., Mi, W., and Ward, E. S. (2009). Autoantigen immunization at different sites reveals a role for anti-inflammatory effects of IFN-gamma in regulating susceptibility to experimental autoimmune encephalomyelitis. J. Immunol. 182, 5268–5275. doi: 10.4049/jimmunol.0800681
Pelletier, J., Suchet, L., Witjas, T., Habib, M., Guttmann, C. R., Salamon, G., et al. (2001). A longitudinal study of callosal atrophy and interhemispheric dysfunction in relapsing-remitting multiple sclerosis. Arch. Neurol. 58, 105–111. doi: 10.1001/archneur.58.1.105
Peterson, J. W., Bo, L., Mork, S., Chang, A., and Trapp, B. D. (2001). Transected neurites, apoptotic neurons, and reduced inflammation in cortical multiple sclerosis lesions. Ann. Neurol. 50, 389–400. doi: 10.1002/ana.1123
Pirko, I., Lucchinetti, C. F., Sriram, S., and Bakshi, R. (2007). Gray matter involvement in multiple sclerosis. Neurology 68, 634–642. doi: 10.1212/01.wnl.0000250267.85698.7a
Planche, V., Panatier, A., Hiba, B., Ducourneau, E. G., Raffard, G., Dubourdieu, N., et al. (2017). Selective dentate gyrus disruption causes memory impairment at the early stage of experimental multiple sclerosis. Brain Behav. Immun. 60, 240–254. doi: 10.1016/j.bbi.2016.11.010
Potter, L. E., Paylor, J. W., Suh, J. S., Tenorio, G., Caliaperumal, J., Colbourne, F., et al. (2016). Altered excitatory-inhibitory balance within somatosensory cortex is associated with enhanced plasticity and pain sensitivity in a mouse model of multiple sclerosis. J. Neuroinflammation 13:142. doi: 10.1186/s12974-016-0609-4
Ransohoff, R. M. (2012). Animal models of multiple sclerosis: The good, the bad and the bottom line. Nat. Neurosci. 15, 1074–1077. doi: 10.1038/nn.3168
Ransohoff, R. M., Hafler, D. A., and Lucchinetti, C. F. (2015). Multiple sclerosis-a quiet revolution. Nat. Rev. Neurol. 11, 134–142. doi: 10.1038/nrneurol.2015.14
Ravelli, K. G., Santos, G. D., Dos Santos, N. B., Munhoz, C. D., Azzi-Nogueira, D., Campos, A. C., et al. (2019). Nox2-dependent neuroinflammation in an EAE model of multiple sclerosis. Transl. Neurosci. 10, 1–9. doi: 10.1515/tnsci-2019-0001
Redondo, J., Kemp, K., Hares, K., Rice, C., Scolding, N., and Wilkins, A. (2015). Purkinje cell pathology and loss in multiple sclerosis cerebellum. Brain Pathol. 25, 692–700. doi: 10.1111/bpa.12230
Rocca, M. A., Barkhof, F., De Luca, J., Frisen, J., Geurts, J. J. G., Hulst, H. E., et al. (2018). The hippocampus in multiple sclerosis. Lancet Neurol. 17, 918–926. doi: 10.1016/S1474-4422(18)30309-0
Rocca, M. A., Mesaros, S., Pagani, E., Sormani, M. P., Comi, G., and Filippi, M. (2010). Thalamic damage and long-term progression of disability in multiple sclerosis. Radiology 257, 463–469. doi: 10.1148/radiol.10100326
Rocca, M. A., Valsasina, P., Meani, A., Gobbi, C., Zecca, C., Rovira, A., et al. (2021). Association of gray matter atrophy patterns with clinical phenotype and progression in multiple sclerosis. Neurology 96, e1561–e1573. doi: 10.1212/WNL.0000000000011494
Rossi, S., Muzio, L., De Chiara, V., Grasselli, G., Musella, A., Musumeci, G., et al. (2011). Impaired striatal GABA transmission in experimental autoimmune encephalomyelitis. Brain Behav. Immun. 25, 947–956. doi: 10.1016/j.bbi.2010.10.004
Rudick, R. A., Fisher, E., Lee, J. C., Simon, J., and Jacobs, L. (1999). Use of the brain parenchymal fraction to measure whole brain atrophy in relapsing-remitting MS. Multiple sclerosis collaborative research group. Neurology 53, 1698–1704. doi: 10.1212/wnl.53.8.1698
Ruffini, F., Rossi, S., Bergamaschi, A., Brambilla, E., Finardi, A., Motta, C., et al. (2013). Laquinimod prevents inflammation-induced synaptic alterations occurring in experimental autoimmune encephalomyelitis. Mult. Scler. 19, 1084–1094. doi: 10.1177/1352458512469698
Schoonheim, M. M., Popescu, V., Rueda Lopes, F. C., Wiebenga, O. T., Vrenken, H., Douw, L., et al. (2012). Subcortical atrophy and cognition: Sex effects in multiple sclerosis. Neurology 79, 1754–1761. doi: 10.1212/WNL.0b013e3182703f46
Sicotte, N. L., Kern, K. C., Giesser, B. S., Arshanapalli, A., Schultz, A., Montag, M., et al. (2008). Regional hippocampal atrophy in multiple sclerosis. Brain 131(Pt 4), 1134–1141. doi: 10.1093/brain/awn030
Simon, J. H. (2001). Brain and spinal cord atrophy in multiple sclerosis: Role as a surrogate measure of disease progression. CNS Drugs 15, 427–436. doi: 10.2165/00023210-200115060-00001
Spence, R. D., Kurth, F., Itoh, N., Mongerson, C. R., Wailes, S. H., Peng, M. S., et al. (2014). Bringing CLARITY to gray matter atrophy. Neuroimage 101, 625–632. doi: 10.1016/j.neuroimage.2014.07.017
Stadelmann, C., Wegner, C., and Bruck, W. (2011). Inflammation, demyelination, and degeneration - recent insights from MS pathology. Biochim. Biophys. Acta 1812, 275–282. doi: 10.1016/j.bbadis.2010.07.007
Stanojlovic, M., Pang, X., Lin, Y., Stone, S., Cvetanovic, M., and Lin, W. (2016). Inhibition of vascular endothelial growth factor receptor 2 exacerbates loss of lower motor neurons and axons during experimental autoimmune encephalomyelitis. PLoS One 11:e0160158. doi: 10.1371/journal.pone.0160158
Starost, L., Lindner, M., Herold, M., Xu, Y. K. T., Drexler, H. C. A., Hess, K., et al. (2020). Extrinsic immune cell-derived, but not intrinsic oligodendroglial factors contribute to oligodendroglial differentiation block in multiple sclerosis. Acta Neuropathol. 140, 715–736. doi: 10.1007/s00401-020-02217-8
Steinman, L., and Zamvil, S. S. (2005). Virtues and pitfalls of EAE for the development of therapies for multiple sclerosis. Trends Immunol. 26, 565–571. doi: 10.1016/j.it.2005.08.014
Sun, J. J., Ren, Q. G., Xu, L., and Zhang, Z. J. (2015). LINGO-1 antibody ameliorates myelin impairment and spatial memory deficits in experimental autoimmune encephalomyelitis mice. Sci. Rep. 5:14235. doi: 10.1038/srep14235
Sun, S. W., Liang, H. F., Schmidt, R. E., Cross, A. H., and Song, S. K. (2007). Selective vulnerability of cerebral white matter in a murine model of multiple sclerosis detected using diffusion tensor imaging. Neurobiol. Dis. 28, 30–38. doi: 10.1016/j.nbd.2007.06.011
Tassoni, A., Farkhondeh, V., Itoh, Y., Itoh, N., Sofroniew, M. V., and Voskuhl, R. R. (2019). The astrocyte transcriptome in EAE optic neuritis shows complement activation and reveals a sex difference in astrocytic C3 expression. Sci. Rep. 9:10010. doi: 10.1038/s41598-019-46232-6
Toussaint, D., Perier, O., Verstappen, A., and Bervoets, S. (1983). Clinicopathological study of the visual pathways, eyes, and cerebral hemispheres in 32 cases of disseminated sclerosis. J. Clin. Neuroophthalmol. 3, 211–220.
Trapp, B. D., Peterson, J., Ransohoff, R. M., Rudick, R., Mork, S., and Bo, L. (1998). Axonal transection in the lesions of multiple sclerosis. N. Engl. J. Med. 338, 278–285.
Trapp, B. D., Ransohoff, R., and Rudick, R. (1999). Axonal pathology in multiple sclerosis: Relationship to neurologic disability. Curr. Opin. Neurol. 12, 295–302.
Vercellino, M., Masera, S., Lorenzatti, M., Condello, C., Merola, A., Mattioda, A., et al. (2009). Demyelination, inflammation, and neurodegeneration in multiple sclerosis deep gray matter. J. Neuropathol. Exp. Neurol. 68, 489–502. doi: 10.1097/NEN.0b013e3181a19a5a
Vercellino, M., Merola, A., Piacentino, C., Votta, B., Capello, E., Mancardi, G. L., et al. (2007). Altered glutamate reuptake in relapsing-remitting and secondary progressive multiple sclerosis cortex: Correlation with microglia infiltration, demyelination, and neuronal and synaptic damage. J. Neuropathol. Exp. Neurol. 66, 732–739. doi: 10.1097/nen.0b013e31812571b0
Vercellino, M., Plano, F., Votta, B., Mutani, R., Giordana, M. T., and Cavalla, P. (2005). Grey matter pathology in multiple sclerosis. J. Neuropathol. Exp. Neurol. 64, 1101–1107.
von Budingen, H. C., Bar-Or, A., and Zamvil, S. S. (2011). B cells in multiple sclerosis: Connecting the dots. Curr. Opin. Immunol. 23, 713–720. doi: 10.1016/j.coi.2011.09.003
Voskuhl, R. R. (1996). Chronic relapsing experimental allergic encephalomyelitis in the SJL mouse: Relevant techniques. Methods 10, 435–439.
Voskuhl, R. R., Itoh, N., Tassoni, A., Matsukawa, M. A., Ren, E., Tse, V., et al. (2019). Gene expression in oligodendrocytes during remyelination reveals cholesterol homeostasis as a therapeutic target in multiple sclerosis. Proc. Natl. Acad. Sci. U.S.A. 116, 10130–10139. doi: 10.1073/pnas.1821306116
Voskuhl, R. R., Patel, K., Paul, F., Gold, S. M., Scheel, M., Kuchling, J., et al. (2020). Sex differences in brain atrophy in multiple sclerosis. Biol. Sex Differ. 11:49. doi: 10.1186/s13293-020-00326-3
Wagenknecht, N., Becker, B., Scheld, M., Beyer, C., Clarner, T., Hochstrasser, T., et al. (2016). Thalamus degeneration and inflammation in two distinct multiple sclerosis animal models. J. Mol. Neurosci. 60, 102–114. doi: 10.1007/s12031-016-0790-z
Weber, M. S., Prod’homme, T., Patarroyo, J. C., Molnarfi, N., Karnezis, T., Lehmann-Horn, K., et al. (2010). B-cell activation influences T-cell polarization and outcome of anti-CD20 B-cell depletion in central nervous system autoimmunity. Ann. Neurol. 68, 369–383. doi: 10.1002/ana.22081
Weinshenker, B. G., Issa, M., and Baskerville, J. (1996). Long-term and short-term outcome of multiple sclerosis: A 3-year follow-up study. Arch. Neurol. 53, 353–358. doi: 10.1001/archneur.1996.00550040093018
Werneburg, S., Jung, J., Kunjamma, R. B., Ha, S. K., Luciano, N. J., Willis, C. M., et al. (2020). Targeted complement inhibition at synapses prevents microglial synaptic engulfment and synapse loss in demyelinating disease. Immunity 52, 167–182.e7. doi: 10.1016/j.immuni.2019.12.004
Wu, J., Ohlsson, M., Warner, E. A., Loo, K. K., Hoang, T. X., Voskuhl, R. R., et al. (2008). Glial reactions and degeneration of myelinated processes in spinal cord gray matter in chronic experimental autoimmune encephalomyelitis. Neuroscience 156, 586–596. doi: 10.1016/j.neuroscience.2008.07.037
Wu, Q., Miao, X., Zhang, J., Xiang, L., Li, X., Bao, X., et al. (2021). Astrocytic YAP protects the optic nerve and retina in an experimental autoimmune encephalomyelitis model through TGF-beta signaling. Theranostics 11, 8480–8499. doi: 10.7150/thno.60031
Wylezinska, M., Cifelli, A., Jezzard, P., Palace, J., Alecci, M., and Matthews, P. M. (2003). Thalamic neurodegeneration in relapsing-remitting multiple sclerosis. Neurology 60, 1949–1954.
Yang, R., Lin, T. H., Zhan, J., Lai, S., Song, C., Sun, P., et al. (2021). Diffusion basis spectrum imaging measures anti-inflammatory and neuroprotective effects of fingolimod on murine optic neuritis. Neuroimage Clin. 31:102732. doi: 10.1016/j.nicl.2021.102732
Zamvil, S., Nelson, P., Trotter, J., Mitchell, D., Knobler, R., Fritz, R., et al. (1985). T-cell clones specific for myelin basic protein induce chronic relapsing paralysis and demyelination. Nature 317, 355–358. doi: 10.1038/317355a0
Zamvil, S. S., Nelson, P. A., Mitchell, D. J., Knobler, R. L., Fritz, R. B., and Steinman, L. (1985). Encephalitogenic T cell clones specific for myelin basic protein. An unusual bias in antigen recognition. J. Exp. Med. 162, 2107–2124. doi: 10.1084/jem.162.6.2107
Ziehn, M. O., Avedisian, A. A., Dervin, S. M., O’Dell, T. J., and Voskuhl, R. R. (2012a). Estriol preserves synaptic transmission in the hippocampus during autoimmune demyelinating disease. Lab. Invest. 92, 1234–1245. doi: 10.1038/labinvest.2012.76
Ziehn, M. O., Avedisian, A. A., Dervin, S. M., Umeda, E. A., O’Dell, T. J., and Voskuhl, R. R. (2012b). Therapeutic testosterone administration preserves excitatory synaptic transmission in the hippocampus during autoimmune demyelinating disease. J. Neurosci. 32, 12312–12324. doi: 10.1523/JNEUROSCI.2796-12.2012
Ziehn, M. O., Avedisian, A. A., Tiwari-Woodruff, S., and Voskuhl, R. R. (2010). Hippocampal CA1 atrophy and synaptic loss during experimental autoimmune encephalomyelitis, EAE. Lab. Invest. 90, 774–786. doi: 10.1038/labinvest.2010.6
Zivadinov, R., and Bakshi, R. (2004). Role of MRI in multiple sclerosis II: Brain and spinal cord atrophy. Front. Biosci. 9:647–664. doi: 10.2741/1262
Keywords: atrophy, cerebral cortex, demyelination, inflammation, neurodegeneration, multiple sclerosis, experimental autoimmune encephalomyelitis
Citation: Voskuhl RR and MacKenzie-Graham A (2022) Chronic experimental autoimmune encephalomyelitis is an excellent model to study neuroaxonal degeneration in multiple sclerosis. Front. Mol. Neurosci. 15:1024058. doi: 10.3389/fnmol.2022.1024058
Received: 20 August 2022; Accepted: 30 September 2022;
Published: 19 October 2022.
Edited by:
Olaf Stuve, University of Texas Southwestern Medical Center, United StatesReviewed by:
Li-Jin Chew, Brown University, United StatesMatthew N. Rasband, Baylor College of Medicine, United States
Copyright © 2022 Voskuhl and MacKenzie-Graham. This is an open-access article distributed under the terms of the Creative Commons Attribution License (CC BY). The use, distribution or reproduction in other forums is permitted, provided the original author(s) and the copyright owner(s) are credited and that the original publication in this journal is cited, in accordance with accepted academic practice. No use, distribution or reproduction is permitted which does not comply with these terms.
*Correspondence: Rhonda R. Voskuhl, cnZvc2t1aGxAbWVkbmV0LnVjbGEuZWR1