- 1State Key Laboratory of Southwestern Chinese Medicine Resources, School of Pharmacy, Chengdu University of Traditional Chinese Medicine, Chengdu, China
- 2School of Medical Information Engineering, Chengdu University of Traditional Chinese Medicine, Chengdu, China
- 3TCM Regulating Metabolic Diseases Key Laboratory of Sichuan Province, Hospital of Chengdu University of Traditional Chinese Medicine, Chengdu, China
Alzheimer's disease (AD) is a common age-related neurodegenerative disease characterized by progressive cognitive decline and irreversible memory impairment. Currently, several studies have failed to fully elucidate AD's cellular and molecular mechanisms. For this purpose, research on related cellular models may propose potential predictive models for the drug development of AD. Therefore, many cells characterized by neuronal properties are widely used to mimic the pathological process of AD, such as PC12, SH-SY5Y, and N2a, especially the PC12 pheochromocytoma cell line. Thus, this review covers the most systematic essay that used PC12 cells to study AD. We depict the cellular source, culture condition, differentiation methods, transfection methods, drugs inducing AD, general approaches (evaluation methods and metrics), and in vitro cellular models used in parallel with PC12 cells.
Introduction
Alzheimer's disease (AD) is one of the most typical forms of dementia and was first proposed by German neuropathologist and psychiatrist Alois Alzheimer in 1906 (Berchtold and Cotman, 1998). AD is a common neurodegenerative disorder that leads to progressive cognitive decline and irreversible memory loss (Association, 2018), eventually causing death from brain failure. It has been reported that 6.2 million people aged 65 years and older in the United States (US) were living with AD in 2021 (2021; Rajan et al., 2021). The number is projected to grow to 82 million in 2030 and 152 million in 2050 (Aminyavari et al., 2019). Additionally, AD deaths have risen markedly according to its death rate, increasing by 145.2% (U.S. Department of Health Human Services, 2020). As a result, health care and long-term care consumption for patients with AD is exceptionally substantial. Alzheimer's and other dementias will cost the nation $355 billion in 2021, while the estimated cost of AD will be more than $1.1 trillion by 2050 in Americans aged 65 and older (2021). Therefore, it has a tremendous impact on the health care system and the quality improvement of the end stage of life.
The main pathological feature of AD is loss of cholinergic neurons, neurofibrillary tangles, senile plaques formed by β-amyloid (Aβ), glial cell activation, and inflammation (Toledo et al., 2015; Weiner et al., 2015; Ossenkoppele et al., 2016; Hampel et al., 2018b; Fu et al., 2019). Thus, the features could be summarized as the cells expressing related proteins expressed by the cells (such as neurons) (Su and Shih, 2015) and pathological properties (such as inflammation, loss of cholinergic neurons, and senile plaques). In particular, the brain's loss of cholinergic neurons and nicotinic acetylcholine receptors (nAChRs) is a significant feature of AD pathology (Yoo et al., 2007). Therefore, the study of the characteristics of cholinergic neurons and cell models could be used to mimic AD pathological damage at the microscopic level. To make effective disease-modifying treatments for AD, sufficient in vivo and in vitro studies must be conducted to comprehend AD's physiological and pathological mechanisms.
PC12 cells are a rat adrenal pheochromocytoma cell line, a monoclonal cell line transplanted from rat adrenal medulloblastoma by Greene and Tischler in 1976 (Greene and Tischler, 1976). As a catecholamine cells, PC12 cells can synthesize, store and release appropriate amounts of catecholamines (mainly dopamine and norepinephrine). PC12 cells are commonly applied to study neuronal cell death and neurotoxic damage (Greene and Tischler, 1976). Thus, PC12 cells are divided into two types: undifferentiated and differentiated. Undifferentiated PCI2 cells synthesize catecholamines. However, PC12 cells differentiate into sympathetic nerve-like cells under the induction of nerve growth factor (NGF), which are close to neurons in terms of morphology, physiological and biochemical functions, such as growing cell protrusions, forming synapse-like structures, and having electrical excitability properties. Furthermore, under the action of NGF, they can synthesize acetylcholine and form neurite structures (Schubert et al., 1977). Additionally, the PC12 cell membrane has IV-methyl-D-aspartic acid (IV-methyl-D-panic acid, NMDA) receptors (NMDARs, as excitatory amino acid receptors in the central nervous system) that regulate synaptic plasticity, memory, and cognitive ability. The weakened nerve conduction function mediated by NMDARs can lead to brain aging, neuroplasticity damage, and cognitive dysfunction. In addition, NMDARs can interact with amyloid β-peptide/amyloid precursor protein and tau protein (Lin et al., 2014). The experimental evidence of NMDA receptor subunits in PC12 cells is shown in Table 1, and experimental evidence of acetylcholine receptor subtypes expressed in PC12 cells is shown in Table 2. Acetylcholine receptors are divided into cholinergic M and N receptors. Ionotropic nicotinic acetylcholine receptors or metabolizing muscarinic acetylcholine receptors could be activated by acetylcholine in the nervous system. Cholinergic M receptors, also named muscarinic receptors (mAChRs), are called G protein-coupled receptors coupled to G proteins and transduce signals. Furthermore, mAChRs can combine with the endogenous neurotransmitter acetylcholine. Five mAChR subtypes (M1-M5) have been identified, as shown in Table 1 (Nathanson, 1987; Caulfield, 1993; Wess, 1996). Nicotinic receptors greatly impact AD pathophysiological research (Jürgensen and Ferreira, 2010; Hernandez and Dineley, 2012). The degeneration of cholinergic neurons and declining activity of choline-acetyltransferase (ChAT), an enzyme that synthesizes ACh, lead to a decrease in cognition (Davies and Maloney, 1976; Bartus et al., 1982; Ballinger et al., 2016; Shimohama and Kawamata, 2018). Choline acetyltransferase (ChAT) is the main enzyme involved in the biosynthesis of the key neurotransmitter acetylcholine (ACh) from choline and acetyl-CoA (ACoA). Acetylcholine (ACh), as a neurotransmitter, could not only be involved in cognitive functions, such as attention (Howe et al., 2017; Urban-Ciecko et al., 2018), but also participate in plasticity and learning; for example, the release of intermittent choline can adjust the plasticity of different types of synapses in the hippocampus and coordinate pre- and post-synaptic activities (Gu and Yakel, 2011; Gu et al., 2012). Moreover, subunits of nicotinic receptors (as a subtype of cholinergic receptors) are expressed on PC12 cells. These subunits are shown in Table 2, especially α3β4 nAChRs (the salient features of α3β4 nAChRs are the lack of sensitivity of the alkaloid nicotine) (Luetje and Patrick, 1991; Figl et al., 1992; Papke and Heinemann, 1994). In addition, the α3 nAChR, α5 nAChR, α7 nAChR, β2 nAChR and β4 nAChR subunits can be expressed by PC12 cells and combined with other subunits, as shown in Table 2. Thus, PC12 cells have the advantages of being easy to obtain and having a high passage number. Therefore, PC12 cells are widely used to study nerve cell function, differentiation methods, apoptosis and neurotransmitter secretion, and determine potential molecular mechanisms (Spicer and Millhorn, 2003). Meanwhile, PC12 cells are generally used as an ideal cellular model to study pathological molecular mechanisms of AD (Parri et al., 2011).
Therefore, based on the above, PC12 cells have neuronal properties. This study aims to provide a systematic review of the standardization of PC12 cells in AD research. We mainly offer a brief overview of PC12 cells in AD research. The study primarily conducted relevant analyses in the following six aspects: cell source and culture condition, neuronal characteristics induced by differentiation of PC12 cells, the transfection method, the general approach to evaluating the AD cellular model, common damaging agents, and in vitro models used in parallel with PC12 cells.
Main text
In this review, we systemically analyzed the literature covering the use of PC12 cells in AD research. The MeSH Database in PubMed searched keywords, subject headings, subheadings and free words, including “Alzheimer's disease”, “AD”, or “Alzheimer”, and “pheochromocytoma”, “PC12”. 1,003 of 1,717 essays from 1988 Jul 8 to 2021 Feb 10 were included, which were original, available and AD-particular. The first screening was performed according to the following criteria: inclusion of articles with AD specificity and use of PC12 as a cell model (rather than only as a tool to express proteins or genes), and excluding articles not specific for AD, methods papers, reviews, articles with “Alzheimer” as an author, documents that mentioned the cell line from previous studies only in the abstract but did not use it, publications in languages other than English, and not accessible articles (all means are shown in Supplementary material 1).
The source and culture condition of PC12 cells
It is estimated that PC12 cells are mainly purchased from the American Type Culture Collection (ATCC), institutes, cell banks, universities and donations. Among the pieces of literature mentioned, 50 % of cell sources were not mentioned, 21 % from ATCC, 8 % from institutes, 6 % from cell banks, and 2 % from universities and donations, respectively.
Due to different sources, the culture media composition required for the growth of PC12 cells is different, according to the ratio that appears in the survey, which is listed in Supplementary material 2. RPMI 1640 medium supplemented with 10 % DHS, 5 % BCS, 2 mM L-glutamine, penicillin at 50 units/ml and streptomycin at 50 mg/ml was proposed by ATCC. DMEM supplemented with 10 % FBS, and an institute obtained 0.3% antibiotics; DMEM supplemented with 10 % FBS, 50 units/ml of penicillin and 100 mg/ml of streptomycin was provided by a cell bank. Among systematic studies involving PC12 cells in AD, the basal media is shown in Supplementary material 2. The basal media is essential for the cultivation of PC12 cells, and DMEM (455 out of 820 articles), RPMI 1,640 (267 out of 820 articles) and DMEM/F12 (19 out of 820 articles) are commonly used. In 82 % of articles, basal media was supplemented with supplement serum in Supplementary material 2.
Among the supplement sera, the primarily supplemented sera were FBS, HS and FCS. The changes in FBS ranged from 0.5 to 15 %; among them, the commonly used concentrations of FBS were (280 out of 816 articles) and 5 % (259 out of 816 articles). The content of HS ranged from 0.5 to 20 %, and the commonly used concentrations of HS were 10 % (276 out of 816 articles) and 5 % (151 out of 816 articles). Moreover, the content of FCS ranged from 2.5 to 20 %, and the commonly used concentrations of FCS are 10 % (72 out of 816 articles) and 5 % (58 out of 816 articles). Statistically, in DMEM, the common supplement sera were 10 % FBS, 5 % FBS and 10 % HS. In RPMI 1640 media, the common supplement sera included 10 % HS, 5 % FBS and 10 % FBS. In DMEM/F12 media, the common supplement sera contained 10% FBS, 7% FBS and 10% HS. Among these, 10% FBS, 5% FBS and 10% HS are frequently applied. In addition, the basal media is supplemented with other types of sera. Different concentrations of serum influence the outcome of the experiment (van der Valk et al., 2010), such as cell differentiation (Medina Benavente et al., 2014) and cell transfection. The supplement serum was used either individually or in combination.
Furthermore, the culture media is also involved in other supplements, as shown in Supplementary material 2. According to relevant statistics, antibiotics/antimycotics and L-glutamine are commonly added to basal media. The widely used antibiotics/antimycotics are mixtures of penicillin and streptomycin. The role of antibiotics in the medium is to avoid the production of other bacteria in the culture fluid, affecting the typical living environment of the cultured cells. L-glutamine is a nonessential amino acid (NEAA). L-glutamine and NEAA can participate in cell signaling, gene expression, and metabolic regulation (Deberardinis et al., 2008). The significant difference is that L-glutamine is relatively essential for cells proliferating at high rates (Wu et al., 2014).
Additionally, G418, sodium pyruvate and NEAAs have a crucial influence on cell growth. All plasmids were subcloned into either a pcDNA3 or pcDNA3.1 vector (Invitrogen) containing antibiotic-resistance genes for selection with G418 (Chi et al., 2010). Excess free oxygen and free radicals are eliminated by sodium pyruvate. In addition, the supplements include Glutamax or other components, such as Na2CO3, NaCl, HEPES, and NaHCO3. The effects of additives on cell culture are shown in Supplementary material 2. The types and compositions of culture media are crucial for cell growth and survival, especially disease research, such as on oxidative stress, cell death (Hwang and Lee, 2008; Jäckel et al., 2011) and the metabolic profile (Dietmair et al., 2012). Therefore, it is necessary to systematically acknowledge various metabolic intermediates, ions, serum components and substrates to affect the growth and differentiation of PC12 cells in future disease-association studies.
The characteristics of PC12 cells after differentiation
PC12 cells are divided into two types: undifferentiated and differentiated. Undifferentiated PC12 cells are small, irregularly shaped, floating cell clusters or scattered lightly attached cells. AD-related studies found that most experiments used undifferentiated PC12 cells because undifferentiated PC12 cells could express nerve growth factor (NGF) receptors and a high transfection capacity (Westerink and Ewing, 2008). Furthermore, PC12 cells contain catecholamine (LDCV) and acetylcholine (LDCV), both of which are found in small transparent follicles (Greene and Tischler, 1976). Moreover, undifferentiated PC12 cells can synthesize acetylcholine and grow neurite structures under the action of NGF (Schubert et al., 1977). In the research using differentiated PC12 cells, differentiated PC12 cells highly express the characteristics of neurons, such as the growth of cell protrusions, the formation of synapse-like structures, and electrical excitability properties. The differentiation condition of PC12 cells for AD research has been described in Table 3 (details are listed in Supplementary material 3). According to statistics, the majority of research used inducers to mediate differentiation. The inducer of differentiation is mainly NGF. NGF plays a vital role in basal forebrain cholinergic neuron differentiation (Thoenen, 1995). NGF is a polypeptide growth factor that has nutritional effects on nerve cells and plays a vital role in nerve cells' growth, differentiation and axon formation (Chao et al., 2006). After adding nerve growth factor (NGF), PC12 cells could be differentiated into sympathetic neurons in the morphology, accompanied by physiological and biochemical changes, and behave like neurons.
Moreover, PC12 cells treated with or without NGF can synthesize, store, uptake and release catecholamines like sympathetic neurons. However, PC12 cells differentiated by NGF will increase their electrical excitability and neurotransmitter sensitivity (Greene and Tischler, 1976; Greene and Rein, 1977). Moreover, an increasing level of nicotinic cholinergic subtypes or mRNA occurs after differentiation (Henderson et al., 1994). Meanwhile, the ACh-mediated channel activity is also increased. The concentration of NGF is between 50 mg/ml and 100 mg/ml. After culturing PC12 cells in serum-free DMEM medium and adding NGF nerve growth factor, it was found that within a certain period of time, nerve growth factor could promote the differentiation of neurites into neuron-like cells, thereby inhibiting the growth of PC12 cells to a certain extent. The latest research has found that NGF can also promote the proliferation of PCI2 cells, but its effect is quickly overshadowed by the significant differentiation effect of the cells themselves (Mouri and Sako, 2013). When PC12 cells were cultured in an NGF medium (Wiatrak et al., 2020) for 3 days, the cells stopped dividing, grew protrusions and gradually differentiated into cells with characteristics of sympathetic neurons. After 5 days, the protrusions gradually increased and extended to form a network structure, and most of the cells progressively transformed into sympathetic cells. The results showed that after 50 ng/L NGF serum-free culture medium induced by PC12 cells to differentiate for 5 days, the cell diameter increased significantly, the protrusions increased, and the cell differentiation rate reached 72.6%. Furthermore, NGF is a neurotrophic factor that can induce neurite outgrowth in neuronal cells (Alipour et al., 2014). Highly differentiated PC12 cells are directly used in the AD experiment. For example, differentiated rat pheochromocytoma PC12 cells have been cultured in RPMI-1640 medium with 10 % (v/v) fetal bovine serum (FBS), 10 U/ml penicillin, and 10 U/ml streptomycins at 37 °C in 95 % humidified air with 5 % CO2 (Ai et al., 2021). Moreover, the highly differentiated PC12 pheochromocytoma cells were cultured in Dulbecco's modified Eagle's medium (DMEM) containing 10 % heat-inactivated fetal bovine serum (FBS), 100 U/ml streptomycin and 100 U/ml penicillin in a humidified 5% CO2 and 95% air incubator (Zhao et al., 2018). Moreover, the neurotrophic factors, NGF and FGF, can activate the MEK-ERK and PI3K-AKT pathways, thereby inducing PC12 cell neurite outgrowth (Lai et al., 2011; Wang et al., 2011). In addition, it has been reported that RA of an appropriate concentration can induce the expression of choline acetyltransferase (ChAT) in PC12 cells, thereby forming pseudo cholinergic neurons, which can be used in some experimental studies of AD. Approximately 7–8 days after induction, the cells could form neurites. Moreover, the studies have shown neuronal characteristics upon differentiation media.
The transfection methods
Differentiation and transfection are two different biological processes of cells. Cell differentiation is a fundamental biological process, and the inducers are essential in addition to the common culture condition. However, distinguished from differentiation, transfection is the transfer of the transfected substance into the cell. Thus, choosing an appropriate cell transfection method is critical to improving the cell transfection rate. The different cell transfection methods used for the AD research are shown in Table 4. According to article statistics, common transfection methods include DNA, RNA, APP and PS. Protein expression was induced by transfection with plasmid DNA as the transfection reagent (Del Toro et al., 2020). Thus, human APP mutation gene-constructed DNA is also applied to transfect PC12 cells. Its main transfection is siRNA transfection in RNA transfection. For cell siRNA transfection, PC12 cells were inoculation in a 6-well plate, and the transfection was performed when the culture was 75–80% confluent. Before transfection, siRNA was incubated with RNAiMAX for 30 min at room temperature. According to the manufacturer's instructions, lipofectamine and reagents (Invitrogen) can transiently transfect PC12 cells containing siRNA (Chen et al., 2019). Furthermore, studies have demonstrated that lipofectamine may be applied as an effective gene carrier for PC12 cells (Lee et al., 2008). Moreover, gene mutations encoded by three essential proteins related to AD may cause familial AD, such as amyloid precursor protein (APP), presenilin 1 (PS1), and presenilin 2 (PS2) (Masters et al., 2015). The APP and PS1 methods are commonly documented by gene transfection. Amyloid (APP) is the amyloid β (Aβ) precursor, a complete membrane protein with a receptor-like structure. In addition, an increase in responsiveness to bFGF stimulation and diminished responsiveness to NGF stimulation could be observed in the transfection of PC12 cells with APPC100 gene construct (Sandhu et al., 1996). Studies have shown that antisense PS2 transfection can prevent neuronal growth factor-induced differentiation or apoptosis of amyloid precursor protein expression in PC12 cells during nutritional deficiency (Wolozin et al., 1996). In addition, some studies have shown that transfection with Bcl-2 gene rescued PC12 cells from apoptotic death and oxidative death caused by H2O2 (Jang and Surh, 2004).
The common damaging agents to mimic AD
To study the process of disease occurrence, the study of pathological processes is an essential part. The mechanisms of AD are described in the text in Figure 1. In the relevant statistical literature, the “cholinergic hypothesis”, “amyloid hypothesis”, “oxidative stress and free radical damage hypothesis”, “inflammation hypothesis”, and “Tau protein phosphorylation hypothesis” are commonly applied in the pathogenesis of AD. The characteristics of the common hypothesis are shown in Figure 1. The “cholinergic hypothesis” is currently widely accepted (Francis et al., 1999; Hampel et al., 2018a). The loss of cholinergic neuron function is directly related to AD cognitive dysfunction. The amyloid hypothesis was proposed by Hardy and Higgins (1992) and is a widely defined hypothesis in the pathogenesis of AD. According to the amyloid cascade hypothesis, the accumulation of amyloid-β initiates a series of downstream molecular events, driving the pathogenesis of AD (Hardy and Selkoe, 2002). Furthermore, Aβ comprises three forms: a monomer, oligomer and fiber. Abundant evidence has revealed (Bjorklund et al., 2012) that oligomers are the factors that cause cognitive dysfunction in AD. The Aβ oligomer combined with an integrator simultaneously induces the excitement of tyrosine kinase dependence on the N-methyl-D-aspartate (NMDA) receptor. The NMDA receptor is expressed by PC12 cells (shown in Table 1).
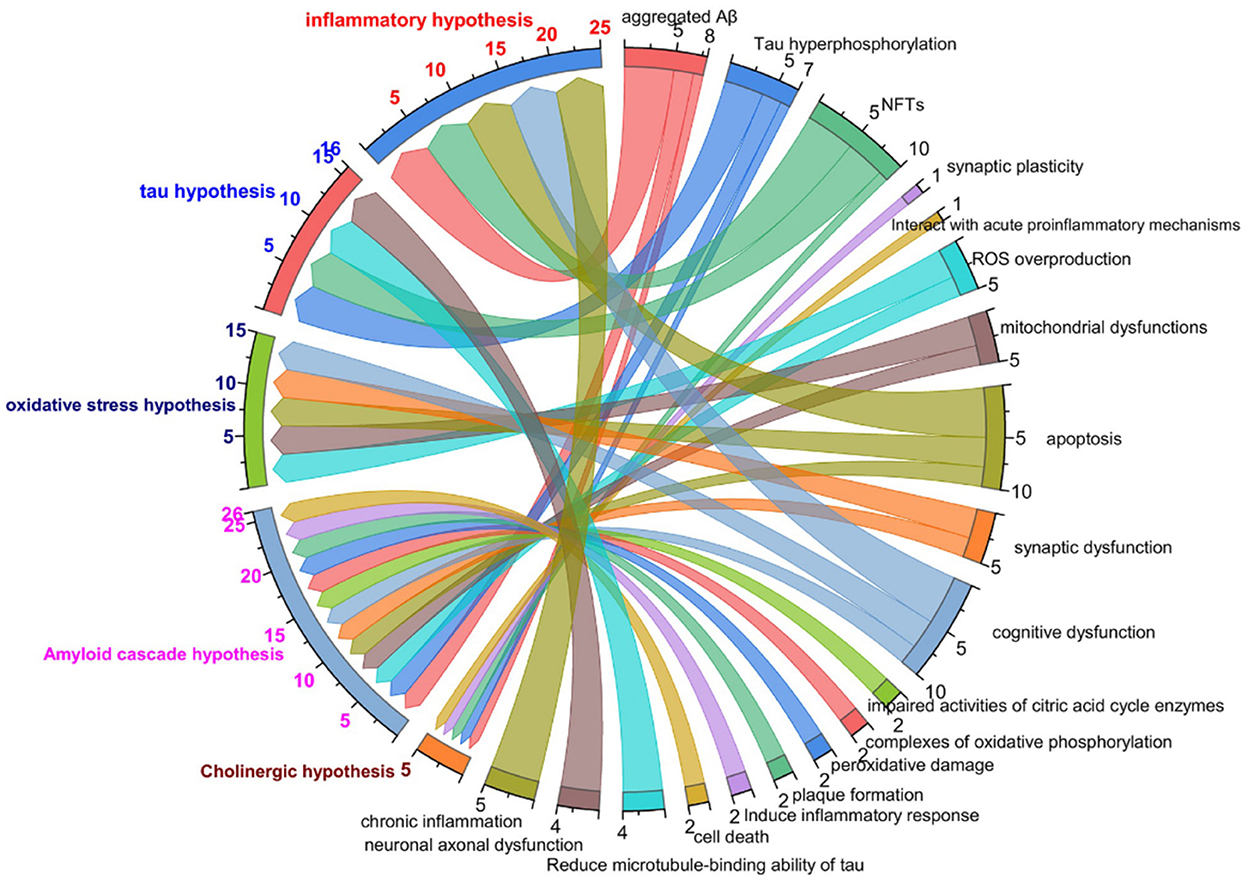
Figure 1. The characteristics of common hypothesis in AD study. Colored text on left side of figure represents cholinergic hypothesis, Aβ hypothesis, Tau protein phosphorylation hypothesis, oxidative stress hypothesis, neuroinflammatory hypothesis, respectively; black text on right side of figure represents relevant pathologic characteristics.
Understanding the pathological mechanism is essential for AD research, and studies often use drugs to establish PC12 cell injury models. PC12 are considered as sympathetic neuron-like cells, and they are sensitive to apoptosis inducers. Statistics on AD injury cellular models show that the main models are oxidative damage and apoptosis, while inflammation models are relatively rare. The occurrence and development of neurodegenerative diseases are closely related to oxidative stress (Thanan et al., 2014). PC12 rat adrenal pheochromocytoma was used as a cell model, and H2O2 (free radical trigger) (Li et al., 2018), Aβ and glutamate could be frequently taken as damage agents in AD research. The evaluation indicators and the use of drugs are shown in Figure 2 (The detailed evaluations are shown in Supplementary material 4). Furthermore, the main damaging agents in AD research are shown in Table 5 (The detailed concentration and relevant time of drugs are shown in Supplementary material 5).
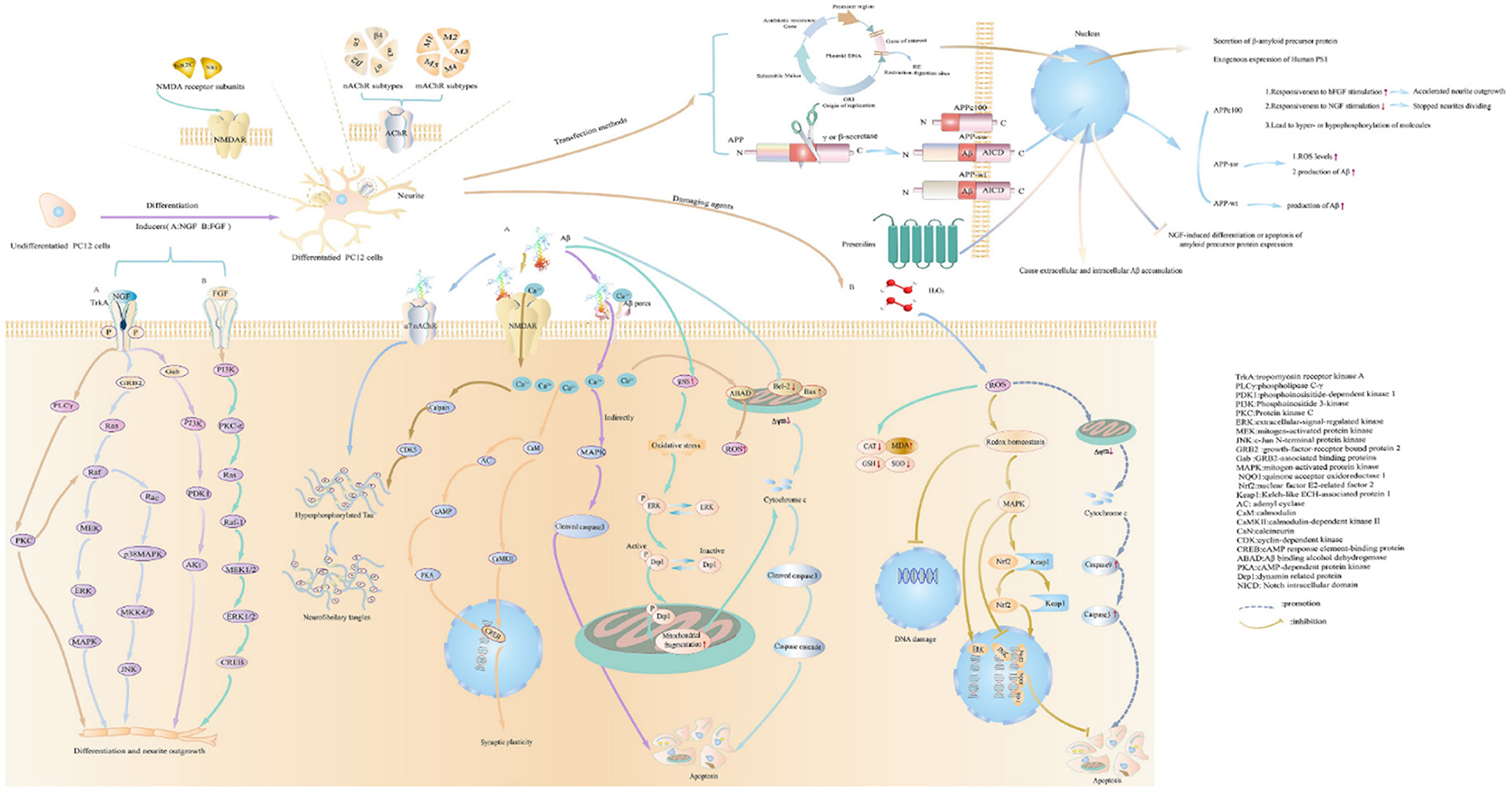
Figure 2. The methods to construct cell models on pathophysiologic processes of AD studies. The figure shows how to cause differentiation, the characteristics of PC12 cells after differentiation, the expression of receptors on PC12 cells, and common damaging agents to mimic AD cell models in AD research.
Aβ induced injury in PC12 cells is shown in Figure 2. The types of Aβ used mainly include Aβ25 − 35, Aβ1 − 42 and Aβ1 − 40. According to relevant statistics, the most used time of Aβ is 24 h and 48h. The concentrations of Aβ25 − 35 is typically 20 μM, 10 μM, 50 μM and 25 μM. Aβ25 − 35 induce oxidative damage in PC12 cells, increase intracellular ROS production and reduce mitochondrial membrane potential (Huang et al., 2019). Cell death was classified as apoptosis and necrosis (Núñez, 2011). Apoptosis, the prevalent form of programmed cell death, is essential for maintaining normal cellular homeostasis (Hengartner, 2000), and could be initiated by an extrinsic death receptor pathway or an intrinsic intracellular pathway, each of which is associated with different molecular pathways (Cazanave and Gores, 2009; Ambjørn et al., 2013). Necrosis is regarded as a degenerative phenomenon that lose their membrane integrity after irreversible injury (Weerasekera et al., 2019). Aβ oligomers exert neurotoxicity via induing caspase-3 mediated apoptosis (Kreutzer et al., 2017). Furthermore, the neurotoxicity of Aβ aggregation involves diverse cellular and molecular mechanisms, such as ROS generation, the increase of intracellular Ca2+ concentrations, and the induction of apoptosis (Arispe et al., 1993; Behl et al., 1994). The inhibition of neuronal apoptosis might be regarded as one of the effective approaches to preventing Aβ-induced neurotoxicity. The finding demonstrated an increase in apoptosis and necrosis of human umbilical vein endothelial cells with 5 μM Aβ25 − 35 treatment for 24 h (Durán-Prado et al., 2014). Additionally, Aβ25 − 35 stimulation caused cell apoptosis, and the apoptotic feature is marked by chromatin condensation (Lee et al., 2017). Statistical results show that the common concentrations of Aβ1 − 42 are 10, 25, and 20 μM and 50 μM, while the concentrations of Aβ1 − 40 are 10, 20, 50, and 25 μM. The studies document that Aβ1 − 42 bind with selectivity to nAChR (Li and Buccafusco, 2003). The monomeric and low oligomeric forms of Aβ1 − 42 increase the expression of acetylcholinesterase as a consequence of the agonist effect of Aβ1 − 42 on the α7 nAChR (Fodero et al., 2004). Meanwhile, human aortic endothelial cells (HAECs) cause toxicity by inducing apoptosis and necrosis after exposure to 10 μM Aβ1-42 for 24 h (Suo et al., 1997). Studies have shown that activating α7 and α4β2 nAChRs reverses the Aβ42-induced hyperexcitability of neurons (Sun et al., 2019). The significant difference is that neurons from Aβ1 − 40 toxicity are protected by M1-acetylcholine-muscarinic-receptor (mAChR) activation (Farías et al., 2004). In addition to the common types of Aβ, Aβ42 are commonly used. The relevant statistics indicated that Aβ42 at 10 μM concentrations commonly are used in experiments. The appropriate time for Aβ42 treatment is typically 24 h. Studies confirmed that Aβ42 is the main component of senile plaques in AD (Iwatsubo et al., 1994). Moreover, the Aβ42 oligomer mediates cell oxidative damage (Zheng et al., 2011; Cecarini et al., 2012) and cell apoptosis (Meng et al., 2016). Studies show that the pathological conformation of the tau protein is changed by the Aβ42 monomer (Manassero et al., 2016).
The peroxide is mainly hydrogen peroxide (H2O2). The use of H2O2 is at a higher concentration. The commonly used concentrations of H2O2 are 150 and 100 μM. The appropriate time for H2O2 is 24 h or 2 h. H2O2 is frequently used as an inducer to induce cell oxidative stress. Thus, ROS oxidative stress induced by H2O2 is regarded as an essential factor for causing oxidative cell damage (Gal et al., 2005). It has a wide range of applications in the establishment of apoptosis models. Studies have shown that H2O2 can induce apoptosis of rat adrenal pheochromocytoma PC12 cells (Lin et al., 2016). Therefore, this study also used H2O2 as an inducer of PC12 cell apoptosis, which is the general approach used in the PC12 cell model of AD. The mainly-used amino acid is mainly glutamate. The common concentration of glutamate is 100 μM and 10 mM. The relevant time for glutamate is 24 and 10 h. The key to glutamate-induced neurotoxicity is to activate NMDAR and increase Ca2+ influx. Furthermore, glutamate could induce the apoptosis model of differentiated PC12 cells (Hu et al., 2016). As a consequence, earlier studies provided evidence that glutamate-induced neurotoxicity is one of the most critical factors leading to the loss of neurons in AD (Bliss and Collingridge, 1993).
Some types are not commonly used. Recently, more evidence showed that a large number of bio-metallics presented in the brains of AD patients (Bush, 2008; Sang et al., 2019; Zhu et al., 2019), such as Cu2+, Zn2+, Al3+ and Fe3+. These metal ions can promote the formation of Aβ plaques and NFTs, catalyse the generation of ROS, and cause oxidative damage. Furthermore, these metal ions are present in senile plaques and aggravate the progression of dementia (Ayton et al., 2013; Li et al., 2017). Among these metal ions, zinc and iron can cause tau hyperphosphorylation, and copper ions may be one of the main cationic elements to form senile plaques (Robert et al., 2015). Copper dysregulation is also associated with tau hyperphosphorylation (the main component of NFT) and aggregation. In addition, ferrous and copper ions participate in the Fenton reaction to generate ROS, which aggravates oxidative stress (Barnham and Bush, 2008). One complimentary strategy to study AD in cells is to interfere directly with one of these processes by administering specific compounds with agonistic or antagonistic activity, such as tert-butyl hydroperoxide (oxidative stress), MGO (a potent inducer of AGEs), bafilomycin (inhibitor of vacuolar H+ATPase, leading to autophagy dysfunction), thapsigargin (inhibitor of the sarco/endoplasmic reticulum Ca2+ATPase, resulting in ER stress and autophagy inhibition), peroxynitrite (a mediator of protein oxidation and nitration, lipid peroxidation, mitochondrial dysfunction, and cell death), and amylin (increased or decreased ERK and Akt phosphorylation in dispersed islets). By simulating the cell model of the disease, the pathological process of the disease can be better understood.
The general approach to evaluating the AD cell model
In addition to studying the pathogenesis of the disease, it also involves various methods in the pathological process of AD. The common mechanism evaluation indexes of PC12 cells are mainly based on hypotheses in AD research, including the cholinergic hypothesis, oxidative stress hypothesis (including secondary apoptosis), inflammation hypothesis, and Tau protein hyperphosphorylation. A series of different approaches could be widely used to analyse cell apoptosis. Flow cytometry (Dimov et al., 2014) and fluorescence microscopy techniques (Jaber et al., 2020) are broadly applied tools for studying biological processes in cell apoptosis. The fluorescein isothiocyanate (FITC) and propidium iodide (PI) coupled with Annexin V (Annexin V-FITC) could be taken as an approach for detecting the process of apoptosis. Additionally, the Hoechst staining analysis showed that the drug's toxicity to PC12 cells was caused by apoptosis. Among the Hoechst, Hoechst 33,342 has been used to distinguish apoptotic cells from healthy or necrotic cells (Zhivotosky and Orrenius, 2001). Hoechst 33258 has been used to quantitatively determine DNA in biological materials (Saleh et al., 2000). It is essential to determine the corresponding proteins, such as caspase and Bcl-2 proteins (fundamental regulators of apoptosis) (Hayakawa et al., 2016). In particular, caspase activation has been recognized as a critical regulator of the apoptotic pathway (Taylor et al., 2008), which could be associated with the maturation of the pro-inflammatory cytokines IL-1β (Creagh et al., 2003). Therefore, western blotting is usually used to measure protein expression levels (Duan et al., 2019). As a kind of oxygen-containing active substance with high reactivity (Steinbrenner and Sies, 2009), reactive oxygen species (ROS) could induce oxidative stress and cause oxidative damage at excessive levels. Moreover, the generation of ROS can be regarded as the result of the neuroinflammatory cycle, and Aβ peptide is taken as a neuroinflammatory factor to promote ROS generation (Holmes et al., 2011). Therefore, ROS could be considered as one of the indicators of oxidative damage (Mittler, 2017), which affects the generation and accumulation of Aβ (Bonda et al., 2010; Jo et al., 2010; Gwon et al., 2012; He et al., 2017). Moreover, measuring the activity of specific antioxidant enzymes could be applied as a means of assessing oxidative stress, such as superoxide dismutase (SOD), glutathione (GSH) and catalase (CAT). Quantitative real-time PCR (qPCR), immunohistochemistry (ICH) and immunofluorescence (IF) could also be used to measure and evaluate indicators of cellular models. Studies have shown that the miRNA signal in AD can be measured by RT-qPCR (Leidinger et al., 2013). In addition, small non-coding RNA profiles are also analyzed by the RT-qPCR method (Leidinger et al., 2013). However, the physiological and pathological changes in the tissue are often described by immunohistochemistry and immunofluorescence. Immunohistochemistry (ICH) is essential for predicting and detecting minimal residual disease (Loghavi et al., 2015; Kurt et al., 2018). However, immunofluorescence (IF) is a technology for visualizing cell types (Im et al., 2019). Consequently, we could judge if a cellular model establishes successfully through the measurement and analysis of the indicator.
In vitro cellular models used in parallel with PC12 cells
The main characteristic of AD is the loss of neurons (Wang and Zhang, 2020). Therefore, primary cultures of neurons may be considered as reliable models to reveal the underlying molecular mechanisms. However, due to the difficulty of maintaining and introducing experimental variability (Al-Ali et al., 2004) (depending on the age of the source animals or the dissection accuracy), the application of primary cultures of neurons is limited. Although PC12 cells are readily available and simple to culture, there might be some limitations. Undifferentiated PC12 cells were poorly adherent and clustered into a mass (Wiatrak et al., 2020). Importantly, undifferentiated PC12 cells have no neurites and are less responsive to the neurotransmitter of the sympathetic nerve compared with cortical neurons (Wang et al., 2015). Furthermore, undifferentiated PC12 cells with a high passage number are insensitive to damage induced by the cytotoxic agent (Kinarivala et al., 2017). Particularly, PC12 cells above the 16th passage, stimulated by NGF, could exhibit morphological alterations correlated with fibroblast-like phenotype, increase resistance to toxics, accelerate cell division, and lose the differentiation capability (Bothwell et al., 1980; Green et al., 1986; Eveleth and Bradshaw, 1992; Mejía et al., 2013). Meanwhile, undifferentiated PC12 cells are unsuitable for research in neural cells due to low dopamine levels (Wang et al., 2015). However, PC12 cells have the potential of gene mutation, thereby contributing to cause the change of phenotype (Chen et al., 2014). Additionally, PC12 cells originated from pheochromocytoma tumors, so there is no guarantee that the same results will be obtained when studying with in vivo models (Grau and Greene, 2012). Owing to the limitations of PC12 cells, so other cell types are also used in many AD studies. In all cited articles, the PC12 cell line was mainly applied in 77% of studies, and 23% of experiments adopted other parallel cell types and PC12 cells. The main alternative cellular models in parallel to PC12 cells in AD research are shown in Table 6 (details are shown in Additional file 6). The parallel cells are primarily sourced from Homo sapiens, Mus musculus, Cricetulus griseus and Cercopithecus aethiops. The Homo sapiens cells mainly comprise SH-SY5Y, HEK293, SK-N-SH and HeLa. The SH-SY5Y (ATCC® CRL-2266™) cells are a subline of the parental line SK-N-SH (ATCC® HTB-11™) (Kovalevich and Langford, 2013). SK-N-SH was subcloned three times: first to SH-SY, then to SH-SY5, and finally to SH-SY5Y. The continuous proliferation, low abundance neuronal markers (Biedler et al., 1978; Påhlman et al., 1984) and the expression of immature neuronal protein are observable characteristics of SH-SY5Y cells. Abundant evidence indicates that SH-SY5Y cells could be differentiated to the cholinergic neuronal phenotype (Kovalevich and Langford, 2013; de Medeiros et al., 2019; Wiedmer et al., 2019). Cholinergic loss is one of the main neuropathological representations of AD (Hampel et al., 2018a). Therefore, the degeneration of cholinergic neurons leads to impaired cognition ability in AD. After differentiation, SH-SY5Y cells could exhibit a phenotype with dopaminergic neurons (Kovalevich and Langford, 2013; Wiedmer et al., 2019) and adrenergic neurons (Kovalevich and Langford, 2013). The loss of dopamine-producing neurons in the substantia nigra pars compacta (Lotharius and Brundin, 2002; Xicoy et al., 2017) is associated with the pathogenesis of PD. During the differentiation process, the increased immunocontent of DJ-1 protein can be found in SH-SY5Y cells. As a neuroprotective protein, DJ-1 could protect dopaminergic neurons from oxidative damage (Björkblom et al., 2013; Choi M. S., et al., 2014; Tanti and Goswami, 2014). Furthermore, the reduction of DJ-1 protein could be extensively related to an early onset of PD (Lopes et al., 2010). Meanwhile, acetylcholine receptors (AChRs) and adrenergic beta2 receptors (B2-ARs) are critical proteins in the neuromuscular junction (NMJ), which is associated with the autophagy and ubiquitin-proteasome system (UPS) in Amyotrophic Lateral Sclerosis (ALS). Thus, SH-SY5Y cells are also broadly applicable to PD and ALS studies (Kovalevich and Langford, 2013; Krishna et al., 2014). Moreover, differentiated SH-SY5Y cells present many neuronal markers at both mRNA and protein levels (Påhlman et al., 1984; Constantinescu et al., 2007). The human embryonic kidney-293(HEK-293) (ATCC® CRL-1573.3) stably expresses rat α3 nAChR and β4 nAChR subunits (Xiao et al., 1998). As a representative cervical carcinoma cell line (Jiang et al., 2010), HeLa cells can express α5 nAChR and β1 nAChR (Calleja-Macias et al., 2009). Furthermore, subcloned M1 mAChR and M2 mAChR genes are permanently expressed in transfected HeLa cells (Pepitoni et al., 1991).
The Neuro-2a cell line (ATCC® CCL-131™) is derived from mouse brain neuroblastoma. Neuro-2a cells are utilized in neuronal differentiation, axonal growth and signaling pathways studies (Klebe Rj, 1969; Gómez-Villafuertes et al., 2009; Suzuki et al., 2014). Neuro-2a cells could be differentiated into the cholinergic neuronal phenotype (Gomez et al., 1998) and the dopaminergic neuronal phenotype (Tremblay et al., 2010). Generally, to understand the molecular mechanism of muscarinic function, the CHO cells used to be transfected with the human M1, M2, M3, or M4 mAChR genes (Peralta et al., 1987). Glial cells can be primarily divided into three major classes: microglia, astrocytes, and oligodendrocytes (Moalem and Tracey, 2006). Microglial cells are thought to be the resident macrophages of the central nervous system (CNS) (Dexter and Jenner, 2013). Microgliosis (accumulation of activated microglial) is a characterization of PD (Dexter and Jenner, 2013). The α7 nAChR is ubiquitously expressed in microglial cells (Gotti and Clementi, 2004; Shytle et al., 2004) and astrocytes (Sharma and Vijayaraghavan, 2001; Gotti and Clementi, 2004). Additionally, the expressions of α3, α5 and β4 nAChR have been documented in microglia (Rock et al., 2008). BV-2 is a type of microglial cell derived from C57/BL6 murine. α7 nAChR have been suggested to be the predominant component of immune regulation of the cholinergic anti-inflammatory pathway (Wang et al., 2003, 2004). BV-2 can be activated to release pro-inflammatory cytokines by oxidative stress or inflammatory factors. Such stimulus possibly triggers neurodegenerative disorders such as AD; thus, BV-2 is widely used as an alternative model system for neurodegenerative disease models in vitro. In terms of volume and number of cells, astrocytes are the most abundant cells in the CNS (Aldskogius and Kozlova, 1998). C6 cells (ATCC® CCL-10), derived from a rat brain glioma, belong to an astrocyte-like cell line (Shao et al., 2019). At the same time, C6 cells could stimulate acetylcholine synthesis when added to Leibovitz's L-15-CO2 neuronal cultures (Patterson and Chun, 1974). These studies showed that α7 nAChRs could be expressed in C6 cells (Wang and Tang, 2007; Niranjan et al., 2012). COS-7 cells (ATCC® CRL-1651™) are transformed with an origin-defective mutant of Simian Virus 40 (SV40) [As a polyomavirus, SV40 has an intricate structure. The capsid is comprised of the major capsid protein VP1 and the minor capsid proteins VP2 and VP3 (Watanabe et al., 2013)]. The α3 nAChRs, α4 nAChRs and β2 nAChRs have been shown to be expressed on the surface of COS-7 cells (Neff et al., 1995). In addition to these common cells, other cells are also adopted in AD research, such as NT2-N, which is derived from the human teratocarcinoma cell line NT2 (ATCC® CRL-1973TM). NT2 cells can be irreversibly differentiated to NT2-N cells by retinoic acid (RA) treatment (Pleasure et al., 1992; Neelands et al., 1999). NT2-N cells are characterized by a single axon and multiple dendrites (Novak et al., 1999). Meanwhile, the cholinergic properties may be manifested in the NT2-N cells (Zeller and Strauss, 1995; González-Burguera et al., 2016).
In addition to cellular models, molecular genetic studies have proved that the familial form of AD is closely connected with mutations in the amyloid-β precursor protein (APP), presenilin PSEN1 and PSEN2 (Bilkei-Gorzo, 2014). Moreover, mutations in these three genes are correlated with early-onset AD (Stepanichev, 2020). Thus, the pathophysiological or behavioral characterization of AD could be mimicked by the genetic modification of animals. Genetic modification is usually considered as an ideal approach to mimic AD's pathophysiological or behavioral characterization. The transgenic animal models include single transgenic, such as Tg2576 mice, in which the transgene is the human 695 splice-variant of APP, which contains the double mutation K670 M, N671 L driven by a hamster prion protein gene promoter; double transgenic, such as APPswe/PS1dE9 mice, which carries two transgenes, human APP with the Swedish mutation and human PSEN1 lacking exon 9; and triple-transgenic, such as 3xTg-AD mice, which expresses APPSWE, PS1M146V, and tauP301L and demonstrates a clear age-dependent onset of AD neuropathology. Undoubtedly, transgenic animal models could play a pivotal role in insights into the pathophysiological basis of AD.
Discussion
In the past four decades, AD has been the subject of intensive research efforts. In particular, insights into the pathogenesis of AD have been considerably advanced. Patients aged 65 and older waiting for AD-modifying therapy are projected to rose by 34% from 2021 to 2050 (He et al., 2016). One in three seniors dies from Alzheimer's or another dementia (2021). Despite these efforts, no curable or eradicable therapy for AD remains. As of 2020, the drugs for mild to moderate AD or moderate to severe AD treatment that are approved by the FDA mainly include acetylcholinesterase inhibitors, NMDA receptor antagonists, and a fixed-dose combination of an NMDA receptor antagonist and acetylcholinesterase inhibitor, which provide modest and transient cognitive benefits but fail to alter disease process or underlying neurodegeneration (Qaseem et al., 2008; Tan et al., 2014). In the phase I/II/III trial, the continued use of drugs has drawn more attention to passive and active immunotherapy. Compared with the phase II clinical trial (Panza et al., 2019), the drugs numbers in the phase III trial for passive immunotherapy rose by 150%, mainly including aducanumab, solanezumab, gantenerumab and crenezumab. Conversely, the drug numbers in the phase III trial for active immunotherapy fell by 67%. However, aducanumab was the first FDA-approved new drug for the treatment of AD by the “Accelerated Approval pathway” on July 6, 2021. Aducanumab is taken as an anti-amyloid-β antibody, providing potential relief for patients with early AD. As a result, passive immunotherapy offers new insights into future research of new drugs for AD treatment. Therefore, cellular models have served as workhorses in enriching our understanding of the numerous pathophysiological mechanisms associated with disease progression and provide better approaches for mimicking AD in the pre-clinical trial phase of drug development.
The cell culture was markedly affected by the option of media (Weller and Wheeldon, 1982). The cell culture medium plays an essential regulatory role in cell growth and proliferation by providing nutrients and basic materials (Polanco et al., 2020). Meanwhile, the differences in growth indicated that specific cell types require specific media (Bonk et al., 2007). According to the ratio in the survey, DMEM and RPMI 1640 media are frequently used as basal media without the supplemented nutrient (Yuan et al., 2019). The increase in the flattening of the cell and cell-matrix adhesion could be represented after using DMEM culture instead of RPMI 1640 due to the relatively higher concentration of Ca2+ in DMEM (Habauzit et al., 2014). When culturing in RPMI 1640, PC12 cells could retain the sensitivity to NGF protein (Greene and Tischler, 1976) and exert minimal neurite extensions (Chua and Lim, 2021). Several supplements involving serum are added to the basal media to obtain the essential growth nutrients (Brown et al., 2019). The serum contains numerous growth factors, lipoproteins and other crucial nutrients for cell growth (Iscove and Melchers, 1978; Hong et al., 2006). The FBS and HS are common serums in the included pieces of literature, and the commonly used concentrations are 10 and 5%.
FBS, as a medium supplement, provides numerous growth factors and nutrients for cells in culture (Schallmoser et al., 2020). When SH-SY5Y cells were cultured in DMEM containing 0, 1 %, 2.5 %, 5 %, and 10 % (v/v) FBS, the result indicated that the number of viable SH-SY5Y cells was notably enhanced with increasing FBS concentration (Ahmadi, 2020). Furthermore, the study has demonstrated that chick cell proliferation rates and cell density increased as serum concentration raised in the range of 5 and 30 % FBS (Ryan, 1979). When human pterygium fibroblasts (as tumor-like transformed cells) were cultured in a medium supplemented with different FBS concentrations (0, 5, 10, and 15 %), the cell confluence reached higher with the increased concentration of FBS (Lopez-Martinez et al., 2019). Thus, we speculated that the serum might provide more essential nutrients and growth factors that facilitate cell survival with increased FBS concentrations. Yet the less proliferation of human pterygium fibroblasts could be found in 15% FBS cultures on day 2 compared to 5 and 10 % FBS cultures, while optimal cell proliferation can be achieved after using 5% FBS on day 3 compared to 10 and 15 % FBS cultures (Lopez-Martinez et al., 2019). Meanwhile, neuronal cells were maintained in the medium containing 1 % FBS, while the proportion of neurons was reduced in the medium containing 10 % FBS (Hashimoto et al., 2000). Additionally, the high concentrations of FBS (20 and 30 %) increased the cellular reprogramming efficacy (RE) of human adipose-derived stem cells (hADSCs) [hADSCs are differentiated into neuron/motoneuron-like cells (Gao et al., 2019)] to generate iPSCs by approximately twice, while the low concentrations of FBS (5%) reduced the RE of hADSCs (Kwon et al., 2016).
HS, containing more immunoglobulins than FBS, has been regarded as a cost-effective alternative to FBS. When equine bronchial fibroblasts cultured in the medium containing FBS, the alpha-smooth muscle actin expression decreased compared with the medium containing FBS (Franke et al., 2014). Although 10% HS was taken as the most common serum, levels between as low as 5 % (Martin and Grishanin, 2003) and as high as 15% (Iuvone et al., 2004) have been applied. Concentrations up to 20 % or even higher were beneficial for cell attachment but suppressed cell proliferation and differentiation, yet concentrations ranging between 5 and 10 % were beneficial for cell proliferation and differentiation (Fedoroff and Hall, 1979). Therefore, speculation could be proposed that the concentrations ranging between 5 and 10% might be appropriate serum concentrations for cell proliferation and differentiation. Selecting a suitable serum concentration and type might be a crucial determinant of the experiments' success. However, antisera production might occur when cells are cultured in the serum-containing medium (Kerbel and Blakeslee, 1976). Additionally, using serum-free culture medium was more beneficial than using serum-containing culture medium for industrial cell culture (Korke et al., 2002). The cell culture medium and the serum are selected according to the characteristics and types of cells.
As immortalized cell lines, PC12 cells derived from a pheochromocytoma of the rat adrenal medulla have been suggested to be an ideal cell for constructing cell models on pathophysiologic processes of AD in in vitro studies. The methods to build cell models on pathophysiologic processes of AD studies are shown in Figure 2. PC12 cells could synthesize, store, and release norepinephrine and dopamine (Greene and Tischler, 1976). Statistically, PC12 cells could be divided into undifferentiation and differentiation types. Before differentiation, PC12 cells were poorly adherent and clustered into a mass. Furthermore, the morphological changes of the cell were appreciably altered. Under normal culture conditions, PC12 cells possess morphological, biochemical and physiological characterization of adrenal cells (Wiatrak et al., 2020). After differentiation, PC12 cells are completely adherent and include neuronal phenotypes morphologically and biochemically (Greene and Tischler, 1982). The inductive agent has proven to induce the differentiation of cells. Meanwhile, the NGF receptors could be expressed in PC12 cells. The most frequent and commonly used neurotrophic factor is NGF in a statistical representation of the literature. NGF could present the dual biological role of promoting axon growth and nourishing neurons (Xi et al., 2020). After exposure to NGF, PC12 cells halt proliferation, extend neurites and become electrically excitable (Greene and Tischler, 1976). Furthermore, NGF-induced axon growth in PC12 cells could be mediated by activating PI3K and inactivating GSK-3β (Zhou et al., 2004). Between 4 and 14 days, a noticeable increase in the length of neurites was observed (Wiatrak et al., 2020). After 21 days, the length of neurites reached 500–1,000 μm, and PC12 cells eventually reinitiated proliferation within 3 days after the elimination of NGF (Greene and Tischler, 1976). After differentiation, the activity of the NMDAR1 promoter in PC12 cells could be upregulated by NGF (Bai and Kusiak, 1997). Also, PC12 cells could respond to acetylcholine via neuronal-type nicotinic receptors (Casado et al., 1996).
Cell transfection and differentiation are processes that are crucial in developmental biology. Cellular differentiation mainly involves the coordinated regulation of genes by a multitude of cellular pathways, and transfection is the process of artificially introducing nucleic acids (DNA or RNA) into cells. In addition, the main purpose of transfection is to directly investigate the function and products of a gene (Kim and Eberwine, 2010). The general methods are primarily involved in plasmid transfection and gene transfection. Plasmid transfection is frequently accomplished by transfecting DNA or delivering RNA through silencing and overexpressing a wild or mutant gene. DNA transfection provides several advantages compared to RNA transfection, such as targeting specific genomic sites and maintaining transgenes as small episomal plasmids or artificial chromosomes without deleterious consequences (Glover et al., 2005). Plasmid RNA could also be utilized for PC12 cell transfection, in particular for siRNAs, as primers for RNA-dependent RNA polymerase (RdRp), which could synthesize other double-stranded RNA (dsRNA) (Miyagishi and Taira, 2002). The gene transfections in PC12 cells generally include APP and PS. Mutations of PS and APP would result in the autosomal-dominant form in early-onset AD (Lanoiselée et al., 2017; Barthet et al., 2018). In transfected cell lines, mutations in the APP and PS gene could cause extracellular and intracellular Aβ accumulation (Cai et al., 1993; Citron et al., 1997). In particular, the APPsw mutant in PC12 cells could induce oxidative stress and eventually lead to apoptotic cells by activating c-Jun N-terminal kinase and caspase 3 and reducing caspase 9 activity (Marques et al., 2003). In addition, the gene Presenilin mutations in PC12 cells could increase apoptotic cells induced by Aβ or trophic factor withdrawal, especially PS1 (Guo et al., 1997) and PS2 (Wolozin et al., 1996).
Cell differentiation or transfection is also recognized as a fundamental means of establishing a successful cell model. The establishment of cellular models is also critically needed to mimic the pathophysiological hallmark of AD. Among the popularly accepted pathology of AD, the cholinergic system plays an essential role in neuronal function, such as memory, learning, and plasticity. In particular, the loss of acetylcholine in the hippocampus and neocortex is closely associated with AD (Bartus et al., 1982; Babic, 1999). Moreover, cholinergic lesions manifest complex interactions with pathological hallmarks of AD, such as amyloid-β plaques, neurofibrillary tangles and neuroinflammation (Hampel et al., 2018a). Thus, Aβ is regarded as a common damaging agent because it can cause oxidative stress, mitochondrial dysfunction, and apoptosis (Leuner et al., 2012). The Aβ oligomers that precede plaque formation have drawn more attention beyond Aβ fibrils of the insoluble plaques in the research of Aβ toxicity (Bjorklund et al., 2012). Increasing evidence has implicated that Aβ oligomers are viewed as the primary agents for tau hyperphosphorylation (De Felice et al., 2008; Jin et al., 2011), synaptic dysfunction (Benilova et al., 2012) and cellular toxicity (Haass and Selkoe, 2007; Glabe, 2008). Our study provides evidence that Aβ25 − 35, Aβ1 − 42 and Aβ1 − 40 are generally considered as the main damage agents in the amyloid cascade hypothesis. The amino acid sequence and identified methods regarding Aβ peptide are shown in Table 7. According to the literature, the damage action time of 24 h has been generally adopted, while the concentrations of 20, 10, and 25 μM of Aβ25 − 35, Aβ1 − 42 and Aβ1 − 40 have been applied frequently, respectively. As an essential form of ROS (Matsushita et al., 2020), H2O2 is widely used as the inducer for oxidative damage and apoptotic cell death (Maroto and Perez-Polo, 1997). Some intracellular apoptotic pathways, such as the PI3K/Akt signal pathway (Li et al., 2020), could be activated by excessive ROS. The application of H2O2 in PC12 cells could be considered as a proper model system for analyzing antioxidants and the apoptosis prevention mechanism (Hu et al., 2014; Chen et al., 2015), especially regulating apoptosis-related proteins, such as anti-apoptosis/pro-apoptosis proteins, and caspases (Jin et al., 2013). The oxidative damage action time of 24 h and the concentrations of 100 and 150 μM are typically adopted. Besides, H2O2 could increase γ-secretase activity to facilitate Aβ production through c-Jun N-terminal kinase (Shen et al., 2008). In addition, evidence suggests that neuropathological hallmarks of AD include stress-induced hyperphosphorylation of tau (Krstic and Knuesel, 2013). The hyperphosphorylated tau could make a contribution to synaptic dysfunction, mitochondrial dysfunction and cognitive impairment (Di et al., 2016). OKA acts as a potent and highly selective PP2A inhibitor to induce hyperphosphorylated tau (Kamat et al., 2014). The damage action time of 4 h and concentrations of 90 nM are frequently used within the literature. Based on the associated hypothesis of AD, the damaging agent-induced cellular models provide an excellent approach to study the pathological hallmarks of AD.
In an attempt to better reflect the AD-related pathological features, selecting appropriate verifiable methods and indicators for the analysis is equally crucial to establishing successful cellular models. Levels of anti-apoptotic Bcl-2, pro-apoptotic Bax and caspases have been shown to correlate with apoptosis in PC12 cells in vitro. Studies show that PC12 cell apoptosis could be prevented by downregulating the caspase-3 protein and inhibiting the Bax/Bcl-2 ratio (Hao et al., 2015). The ROS, MDA and antioxidant enzyme activities are closely correlated with oxidative stress. DCFH-DA essay is regarded as the dominant method for determining the level of ROS in PC12 cells (Eruslanov and Kusmartsev, 2010), and the increase in ROS leads to damaged mitochondria and activation of the apoptotic cascade (Zeng et al., 2017). Antioxidant enzymes, such as SOD, CAT, and GPx, are suggested to be the oxidative defense system in PC12 cells (Poprac et al., 2017). ACh has a crucial role in the pathophysiology of AD. The level of ACh in the brain is regulated by AChE and butyrylcholinesterase (BChE). The decreased expression of pro-inflammatory cytokines, such as interleukin-1-beta converting enzyme (IL-1β) and tumor necrosis factor-alpha (TNF-α), are taken as common indicators of inflammation (Ma et al., 2019).
However, not all aspects of AD pathogenesis can be covered in PC12 cells. As a result, the in vitro models used in parallel with PC12 cells also play an essential role in AD studies. Of the articles analyzed, the PC12 cells are mainly involved in 77% of the literature. The other 23% of experiments adopt other parallel cell types and PC12 cells, including (mainly cortical) primary neurons, the SH-SY5Y cell line, HEK293, and Neuro-2a cell lines. The expression of nAChR or mAChR could be found on the surface of these cells. The loss of cholinergic neurons is tightly associated with AD pathology. These cells would provide a global overview of the landscape and diversity of cellular models that can be used in AD. These cellular models also presented their own limitations. The primary neuron possessed a limited life span (typically days to weeks) (Li, 2011) and needed to be generated from the embryonic or early postnatal brain (Sahu et al., 2019). The differentiation state of SH-SY5Y cells was undefined and ranged from tumor tissue state (neuroblastoma) to neural progenitor cells or post-mitotic neurons (Feles et al., 2022). Although differentiated SH-SY5Y cells were commonly used to study the growth of neuronal processes (Kovalevich and Langford, 2013; Shipley et al., 2016; Peng et al., 2021), mechanisms involving in neuronal function and excitability cannot be easily compared with the physiological state derived from brain tissue-derived cells. Due to deficient DNA mismatch repair, HEK293 cells were especially vulnerable to genotype drift caused by external disruptions (Panigrahi et al., 2012). However, compared with cerebellar granule neurons, voltage-gated sodium channel expression in Neuro-2a cells was approximately 20-fold lower, and NMDAR expression was also not expressed in Neuro-2a cells (Lepage et al., 2005). Meanwhile, an increase in dopamine neuronal characteristics was manifested only after dbcAMP induced differentiation of Neuro-2a cells (Tremblay et al., 2010). In addition, the cellular metabolism of CHO cells was altered during long-term culture (LTC), including extracellular alanine accumulation and enhanced utilization of glucose and lactate (Bailey et al., 2012). Furthermore, CHO cells was also involved a slower growth rate and low-stress resistance (Fischer et al., 2015). For microglia cells, the limitations probably contributed to the cognitive loss and neuronal damage (Giulian, 1999). BV-2 cells have weaker responses to LPS and interferon-gamma stimuli compared with primary mouse microglia (Stohwasser et al., 2000). Due to the complexity and diversity of AD pathogenesis, these cell models only partly imitate AD pathological microscopic characterizations.
In addition to the above cells, induced pluripotent stem cells (iPSCs), miRNA-induced neurons, neuronal cells, Brain microvascular endothelial cells (BMECs), and genetically engineered cells are broadly applied to construct the AD model. The iPSCs are currently utilized for establishing familial AD cellular models in vitro (Amin et al., 2019). The iPSC from AD patients carried the same AD-causing gene and obtained the same phenotype and function as AD neuronal cells. The iPSC, derived from mouse embryonic fibroblasts, could be generated by transduction of transcription factors with Oct3 /4, Sox2, c-Myc, and Klf4 (Takahashi et al., 2007). Fibroblast of iPSC obtained from AD patients can be used for orthotopic modeling, whose procedure involves seeding tumor cell lines or patient-derived cell xenografts into animal models (Brodaczewska et al., 2016). However, in vitro models of iPSC lack cellular diversity and possess structural complexity in two-dimensional model (Majolo et al., 2019). The microRNA (miRNA)-induced neurons have great potential as neurotoxicity screening. The miRNA plays an essential role in neuronal development, proliferation and differentiation, apoptosis, and homeostasis (Kaur et al., 2012; Denk et al., 2015; Sun and Shi, 2015). The hippocampal neuronal cells and cerebral cortical neural cells are considered as reliable AD cellular models to unravel pathological changes of AD. Hippocampus degeneration is closely associated with learning and memory dysfunction in AD patients (Fujishiro et al., 2006). The cerebral cortex of AD patients appear abundant neurofibrillary lesions and neuritic plaques (Fitzpatrick et al., 2017). Yet the application of neuronal cells was precluded owing to the inaccessibility and lack of proliferation. BMECs are also used to establish the AD cellular model for the following two reasons. BMECs are taken as a crucial component of blood brain barrier (BBB) (Zlokovic, 2008). BBB decomposition is thought to be an initiating factor in the pathogenesis of AD (Yoon et al., 2021). Furthermore, APP and β-secretase enzyme could also be found in BMECs (Bourassa et al., 2019). APP and β-secretase enzyme are co-localized to the site of intracellular Aβ production (Long and Holtzman, 2019). With the development of biomedical and genetic engineering technologies, genetically engineered cells are used to establish the AD cellular model. AD genetically engineered cell lines, particularly PC12, SK-N-SH, and A172, were constructed by the cloned amyloid cDNA that contains a region encoding A4 (beta-polypeptide) amino acids along with recently developed tumor virus vectors derived from simian virus 40 (Marotta et al., 1989). The stable Myh9 in PC12 cells were knocked out by CRISPR/Cas9 nucleases (Wang et al., 2017, 2020), powerful genome engineering tools (Rose et al., 2020). The CRISPR/Cas9-mediated genome editing technology has become a promising approach for the choice of gene targeting (Pelletier et al., 2015), which is broadly applied for gene editing in multiple cell types and organisms (Platt et al., 2014).
Additionally, these cell model systems are also used to mimic AD pathophysiology, discover biomarkers or potent therapeutic drugs, or conduct high throughput drug screening. Traditionally, 2D cell culture models do not cover the complex cellular microenvironments in vivo; thus, they are insufficient to predict in vivo efficacy and toxicity. More advanced 3D cell culture models (e.g., organoids, microtissues, spheroids) have been adopted to mimic not only the microenvironment (Lee et al., 2022) but also gene expression and functional characteristics of tissues in vivo (Edmondson et al., 2014). 3D-culture emulate complex cell interactions, multicellular architecture, cell-cell interactions and physical microenvironment of interactions (Ingber, 2018). The 3D cell culture models of human cell lines and primary cells provide a promising approach to improve drug development and screening. In in vitro 3D culture system, neuronal cells differentiated by the iPSCs obtained from familial AD patients accelerate Aβ plaques and neurofibrillary tangles associated (Choi S. H., et al., 2014). As is mentioned above, the 3D cell culture model might provide a promising insight to mimic the complex pathogenesis of AD.
In addition to cellular models, animal models could be widely adapted in the earlier preclinic stage of drug development. Particularly, transgenic animal models contain linear human chromosomes and a similar number of genes as humans (Waterston et al., 2002). In order to dissect the mechanisms underlying AD, transgenic animal models present an efficient pathophysiological process and early typical symptoms in AD, such as memory impairments, Aβ pathology and neuroinflammation (Bilkei-Gorzo, 2014; Saito et al., 2014). At the same time, transgenic models could also be thought as the identification and validation of drug targets for AD (Zahs and Ashe, 2010).
Additionally, metabolomics is widely applied in AD pathogenesis (Arnold et al., 2020). The levels of metabolites were significantly different among the brain tissue and liver of AD wild-type and transgenic mice (Wang et al., 2019). The unusual metabolic pathway of amino acid metabolism, energy metabolism, and gut microbiota could be found in the AD rat model through the metabolomics. Moreover, features of AD could be reproduced by neuronal reprogramming of fibroblasts from familial AD patients into functional neurons (Qiang et al., 2011). Cell-direct reprogramming could convert one fully differentiated cells into another differentiated state (Riva et al., 2022), such as directly reprogramming fibroblasts into neural stem cells (Han et al., 2016), neuronal cells (Treutlein et al., 2016), or mature cardiomyocytes (Herrero and Bernad, 2016). Cell-direct reprogramming technology could supplement iPSC technology and enhance the differentiation of iPSCs via directly reprogramming somatic cells into iPSCs and lineage-restricted stem cells (Ring et al., 2012). Therefore, due to a wide range of sources and a high conversion rate on somatic cells, direct reprogramming technology of somatic cells could outperform traditional iPSC technology. Based on above all, it is speculated that somatic cell reprogramming may have tremendous potential for disease modeling.
Conclusion
Due to the multifactorial and complex pathogenesis of AD, the biomarkers associated with the AD pathogenic mechanism may be crucial for the early detection and prevention of AD (Kapogiannis et al., 2019). Decreased Aβ1 − 42 (as a marker of amyloids) and increased tau (as a marker of neuronal injury) levels in cerebrospinal fluid (CSF) are the most widely used characteristic biomarkers of AD patients (Sunderland et al., 2003; van Maurik et al., 2017), and low CSF levels of Aβ1 − 42 are associated with intracranial amyloid deposition, while high CSF levels of phospho-tau (p-tau) are correlated with tau-associated neurofibrillary tangles (Hampel et al., 2018c).
Research on biomarkers associated with AD in vitro cell models would provide valuable insights into the therapeutic intervention of AD. The cell models can mimic multi-pathophysiological features underlying AD pathogenesis in the micro-changes and processes, which can also be regarded as a promising means for potential therapeutic drug screening. PC12 cells are utilized extensively as a neuronal model in neuroscience research. PC12 cells could synthesize, store, and release norepinephrine and dopamine compared to other cell models. Furthermore, neurotransmitter receptors associated with AD are present on the surface of the PC12 cell membrane (Westerink and Ewing, 2008), especially NMDARs and cholinergic receptors. The attenuation of neurotransmission mediated by NMDAR can lead to neuroplasticity damage and cognitive dysfunction in the aging brain (Lin et al., 2014). NMDAR activation could inhibit Aβ production and release by stimulating nonamyloidogenic APP processes (Marcello et al., 2007; Hoey et al., 2009). The expression of NR1 and NR2 subunits could be manifested in PC12 cells. Meanwhile, the nicotinic receptor-mediated neurotransmitter ACh is closely associated with AD pathology. The degeneration of cholinergic neurons and declining activity of choline-acetyltransferase (ChAT) have contributions to a decrease in cognition (Davies and Maloney, 1976; Bartus et al., 1982; Ballinger et al., 2016; Shimohama and Kawamata, 2018). The α3 nAChR, α5 nAChR, α7 nAChR, β2 nAChR and β4 nAChR subunits can be expressed by PC12 cells. In addition, PC12 cells differentiate into sympathetic nerve-like cells under the induction of NGF and could be sub-cultured indefinitely. Therefore, PC12 cells are not only systematically used to study nerve cell function, differentiation, apoptosis and neurotransmitter secretion (Spicer and Millhorn, 2003), but also usually as an ideal cellular model to extensively explore the pathological molecular mechanisms of AD (Parri et al., 2011).
The development of cellular models reflecting the microscopic pathophysiological features of AD is likely to offer new insights, which also may benefit for exploring the potential therapeutic targets.
Data availability statement
The original contributions presented in the study are included in the article/Supplementary material, further inquiries can be directed to the corresponding authors.
Author contributions
DX, TD, ZZ, YX, and TS contributed to the conception and design of this study, manuscript preparation, and reviewed the final version. DX contributed to the data extraction and analysis. All authors read and approved the final manuscript.
Funding
This study was funded by Sichuan Science and Technology Program (2021YJ0178 and 2020GFW194), Sichuan Provincial Department of Education Project (17ZA0152 and 18ZA0179), and Xinglin Scholar Research Promotion Project of Chengdu University of TCM (ZRQN2020008 and MPRC2021034).
Conflict of interest
The authors declare that the research was conducted in the absence of any commercial or financial relationships that could be construed as a potential conflict of interest.
Publisher's note
All claims expressed in this article are solely those of the authors and do not necessarily represent those of their affiliated organizations, or those of the publisher, the editors and the reviewers. Any product that may be evaluated in this article, or claim that may be made by its manufacturer, is not guaranteed or endorsed by the publisher.
Supplementary material
The Supplementary Material for this article can be found online at: https://www.frontiersin.org/articles/10.3389/fnmol.2022.1016559/full#supplementary-material
References
(2021). 2021 Alzheimer's disease facts and figures. Alzheimers Dement. 17, 327–406. doi: 10.1002/alz.12328
Ahmadi, S. H. (2020). Effect of fetal bovine serum in SH-SY5Y proliferation. Mendeley Data. doi: 10.17632/kjm2fny4z4.1
Ahmed, M., Davis, J., Aucoin, D., Sato, T., Ahuja, S., Aimoto, S., et al. (2010). Structural conversion of neurotoxic amyloid-beta(1-42) oligomers to fibrils. Nat. Struct. Mol. Biol. 17, 561–567. doi: 10.1038/nsmb.1799
Ai, J., Wang, H., Chu, P., Shopit, A., Niu, M., Ahmad, N., et al. (2021). The neuroprotective effects of phosphocreatine on Amyloid Beta 25-35-induced differentiated neuronal cell death through inhibition of AKT /GSK-3β /Tau/APP /CDK5 pathways in vivo and vitro. Free Radic. Biol. Med. 162, 181–190. doi: 10.1016/j.freeradbiomed.2020.10.003
Al-Ali, H., Blackmore, M., Bixby, J. L., and Lemmon, V. P. (2004). “Assay Guidance Manual,” in Markossian, S., Sittampalam, G.S., Grossman, A., Brimacombe, K., Arkin, M., Auld, D., et al. (eds). Bethesda (MD): Eli Lilly and Company and the National Center for Advancing Translational Sciences.
Albillos, A., and Mcintosh, J. M. (2018). Human nicotinic receptors in chromaffin cells, characterization and pharmacology. Pflugers Arch. 470, 21–27. doi: 10.1007/s00424-017-2073-0
Aldskogius, H., and Kozlova, E. N. (1998). Central neuron-glial and glial-glial interactions following axon injury. Prog. Neurobiol. 55, 1–26. doi: 10.1016/s0301-0082(97)00093-2
Alipour, M., Khoobi, M., Moradi, A., Nadri, H., Homayouni Moghadam, F., Emami, S., et al. (2014). Synthesis and anti-cholinesterase activity of new 7-hydroxycoumarin derivatives. Eur. J. Med. Chem. 82, 536–544. doi: 10.1016/j.ejmech.2014.05.056
Ambjørn, M., Ejlerskov, P., Liu, Y., Lees, M., and Issazadeh-Navikas, S. (2013). IFNB1/interferon-β-induced autophagy in MCF-7 breast cancer cells counteracts its proapoptotic function. Autophagy 9, 287–302. doi: 10.4161/auto.22831
Amin, N., Tan, X., Ren, Q., Zhu, N., Botchway, B. O. A., Hu, Z., et al. (2019). Recent advances of induced pluripotent stem cells application in neurodegenerative diseases. Prog. Neuropsychopharmacol. Biol. Psychiatry 95, 109674. doi: 10.1016/j.pnpbp.2019.109674
Aminyavari, S., Zahmatkesh, M., Farahmandfar, M., Khodagholi, F., Dargahi, L., and Zarrindast, M. R. (2019). Protective role of Apelin-13 on amyloid β25-35-induced memory deficit; Involvement of autophagy and apoptosis process. Prog. Neuropsychopharmacol. Biol. Psychiatry 89, 322–334. doi: 10.1016/j.pnpbp.2018.10.005
Anderson, D. H., Talaga, K. C., Rivest, A. J., Barron, E., Hageman, G. S., and Johnson, L. V. (2004). Characterization of beta amyloid assemblies in drusen, the deposits associated with aging and age-related macular degeneration. Exp. Eye Res. 78, 243–256. doi: 10.1016/j.exer.2003.10.011
Arispe, N., Rojas, E., and Pollard, H. B. (1993). Alzheimer disease amyloid beta protein forms calcium channels in bilayer membranes, blockade by tromethamine and aluminum. Proc. Natl. Acad. Sci. USA. 90, 567–571. doi: 10.1073/pnas.90.2.567
Arnold, M., Nho, K., Kueider-Paisley, A., Massaro, T., Huynh, K., Brauner, B., et al. (2020). Sex and APOE ε4 genotype modify the Alzheimer's disease serum metabolome. Nat. Commun. 11, 1148. doi: 10.1038/s41467-020-14959-w
Ashkenazi, A., Ramachandran, J., and Capon, D. J. (1989). Acetylcholine analogue stimulates DNA synthesis in brain-derived cells via specific muscarinic receptor subtypes. Nature. 340, 146–150. doi: 10.1038/340146a0
Association, A. S. (2018). 2018 Alzheimer's disease facts and figures. Alzheimer's Dement. J. Alzheimer's Assoc., 14, 367–429. doi: 10.1002/alz.12068
Ayton, S., Lei, P., and Bush, A. I. (2013). Metallostasis in Alzheimer's disease. Free Radic. Biol. Med. 62, 76–89. doi: 10.1016/j.freeradbiomed.2012.10.558
Babic, T. (1999). The cholinergic hypothesis of Alzheimer's disease, a review of progress. J. Neurol. Neurosurg. Psychiatr. 67, 558. doi: 10.1136/jnnp.67.4.558
Bai, G., and Kusiak, J. W. (1997). Nerve growth factor up-regulates the N-methyl-D-aspartate receptor subunit 1 promoter in PC12 cells. J. Biol. Chem. 272, 5936–5942. doi: 10.1074/jbc.272.9.5936
Bailey, L. A., Hatton, D., Field, R., and Dickson, A. J. (2012). Determination of Chinese hamster ovary cell line stability and recombinant antibody expression during long-term culture. Biotechnol. Bioeng. 109, 2093–2103. doi: 10.1002/bit.24485
Ballinger, E. C., Ananth, M., Talmage, D. A., and Role, L. W. (2016). Basal forebrain cholinergic circuits and signaling in cognition and cognitive decline. Neuron 91, 1199–1218. doi: 10.1016/j.neuron.2016.09.006
Barnham, K. J., and Bush, A. I. (2008). Metals in Alzheimer's and Parkinson's diseases. Curr. Opin. Chem. Biol. 12, 222–228. doi: 10.1016/j.cbpa.2008.02.019
Barthet, G., Jordà-Siquier, T., Rumi-Masante, J., Bernadou, F., Müller, U., and Mulle, C. (2018). Presenilin-mediated cleavage of APP regulates synaptotagmin-7 and presynaptic plasticity. Nat. Commun. 9, 4780. doi: 10.1038/s41467-018-06813-x
Bartolini, M., Bertucci, C., Bolognesi, M. L., Cavalli, A., Melchiorre, C., and Andrisano, V. (2007). Insight into the kinetic of amyloid beta (1-42) peptide self-aggregation, elucidation of inhibitors' mechanism of action. Chembiochem. 8, 2152–2161. doi: 10.1002/cbic.200700427
Bartus, R. T., Dean, R. L. 3rd, Beer, B., and Lippa, A. S. (1982). The cholinergic hypothesis of geriatric memory dysfunction. Science. 217, 408–414. doi: 10.1126/science.7046051
Baumgold, J., and White, T. (1989). Pharmacological differences between muscarinic receptors coupled to phosphoinositide turnover and those coupled to adenylate cyclase inhibition. Biochem. Pharmacol. 38, 1605–1616. doi: 10.1016/0006-2952(89)90308-0
Behl, C., Davis, J. B., Lesley, R., and Schubert, D. (1994). Hydrogen peroxide mediates amyloid beta protein toxicity. Cell. 77, 817–827. doi: 10.1016/0092-8674(94)90131-7
Benilova, I., Karran, E., and De Strooper, B. (2012). The toxic Aβ oligomer and Alzheimer's disease, an emperor in need of clothes. Nat. Neurosci. 15, 349–357. doi: 10.1038/nn.3028
Berchtold, N. C., and Cotman, C. W. (1998). Evolution in the conceptualization of dementia and Alzheimer's disease, Greco-Roman period to the 1960s. Neurobiol. Aging 19, 173–189. doi: 10.1016/s0197-4580(98)00052-9
Berkeley, J. L., and Levey, A. I. (2000). Muscarinic activation of mitogen-activated protein kinase in PC12 cells. J. Neurochem. 75, 487–493. doi: 10.1046/j.1471-4159.2000.0750487.x
Bertini, I., Gonnelli, L., Luchinat, C., Mao, J., and Nesi, A. (2011). A new structural model of Aβ40 fibrils. J. Am. Chem. Soc. 133, 16013–16022. doi: 10.1021/ja2035859
Biedler, J. L., Roffler-Tarlov, S., Schachner, M., and Freedman, L. S. (1978). Multiple neurotransmitter synthesis by human neuroblastoma cell lines and clones. Cancer Res. 38, 3751–3757.
Bilkei-Gorzo, A. (2014). Genetic mouse models of brain ageing and Alzheimer's disease. Pharmacol. Ther. 142, 244–257. doi: 10.1016/j.pharmthera.2013.12.009
Björkblom, B., Adilbayeva, A., Maple-Grødem, J., Piston, D., Ökvist, M., Xu, X M, Brede, C., et al. (2013). Parkinson disease protein DJ-1 binds metals and protects against metal-induced cytotoxicity. J. Biol. Chem. 288, 22809–22820. doi: 10.1074/jbc.M113.482091
Bjorklund, N. L., Reese, L. C., Sadagoparamanujam, V. M., Ghirardi, V., Woltjer, R. L., and Taglialatela, G. (2012). Absence of amyloid β oligomers at the postsynapse and regulated synaptic Zn2+ in cognitively intact aged individuals with Alzheimer's disease neuropathology. Mol. Neurodegener. 7, 23. doi: 10.1186/1750-1326-7-23
Bliss, T. V., and Collingridge, G. L. (1993). A synaptic model of memory, long-term potentiation in the hippocampus. Nature 361, 31–39. doi: 10.1038/361031a0
Boccia, M. M., Blake, M. G., Krawczyk, M. C., and Baratti, C. M. (2010). Hippocampal α7 nicotinic receptors modulate memory reconsolidation of an inhibitory avoidance task in mice. Neuroscience. 171, 531–543. doi: 10.1016/j.neuroscience.2010.08.027
Bonda, D. J., Wang, X., Perry, G., Nunomura, A., Tabaton, M., Zhu, X., et al. (2010). Oxidative stress in Alzheimer disease, a possibility for prevention. Neuropharmacology. 59, 290–294. doi: 10.1016/j.neuropharm.2010.04.005
Bonk, A. J., Cheong, H. T., Li, R., Lai, L., Hao, Y., Liu, Z., et al. (2007). Correlation of developmental differences of nuclear transfer embryos cells to the methylation profiles of nuclear transfer donor cells in Swine. Epigenetics. 2, 179–186. doi: 10.4161/epi.2.3.4844
Boss, V., Talpade, D. J., and Murphy, T. J. (1996). Induction of NFAT-mediated transcription by Gq-coupled receptors in lymphoid and non-lymphoid cells. J. Biol. Chem. 271, 10429–10432. doi: 10.1074/jbc.271.18.10429
Bothwell, M. A., Schechter, A. L., and Vaughn, K. M. (1980). Clonal variants of PC12 pheochromocytoma cells with altered response to nerve growth factor. Cell 21, 857–866. doi: 10.1016/0092-8674(80)90449-3
Boulter, J., Evans, K., Goldman, D., Martin, G., Treco, D., Heinemann, S., et al. (1986). Isolation of a cDNA clone coding for a possible neural nicotinic acetylcholine receptor alpha-subunit. Nature 319, 368–374. doi: 10.1038/319368a0
Boulter, J., O'shea-Greenfield, A., Duvoisin, R. M., Connolly, J. G., Wada, E., Jensen, A., et al. (1990). Alpha 3, alpha 5, and beta 4, three members of the rat neuronal nicotinic acetylcholine receptor-related gene family form a gene cluster. J. Biol. Chem. 265, 4472–4482.
Bourassa, P., Tremblay, C., Schneider, J. A., Bennett, D. A., and Calon, F. (2019). Beta-amyloid pathology in human brain microvessel extracts from the parietal cortex, relation with cerebral amyloid angiopathy and Alzheimer's disease. Acta Neuropathol. 137, 801–823. doi: 10.1007/s00401-019-01967-4
Brodaczewska, K. K., Szczylik, C., Fiedorowicz, M., Porta, C., and Czarnecka, A. M. (2016). Choosing the right cell line for renal cell cancer research. Mol. Cancer. 15, 83. doi: 10.1186/s12943-016-0565-8
Brown, M. J., Bahsoun, S., Morris, M. A., and Akam, A. E. C. (2019). Determining conditions for successful culture of multi-cellular 3D tumour spheroids to investigate the effect of mesenchymal stem cells on breast cancer cell invasiveness. Bioengineering (Basel). 6, 4. doi: 10.3390/bioengineering6040101
Bush, A. I. (2008). Drug development based on the metals hypothesis of Alzheimer's disease. J. Alzheimers. Dis. 15, 223–240. doi: 10.3233/jad-2008-15208
Cai, X. D., Golde, T. E., and Younkin, S. G. (1993). Release of excess amyloid beta protein from a mutant amyloid beta protein precursor. Science. 259, 514–516. doi: 10.1126/science.8424174
Calleja-Macias, I. E., Kalantari, M., and Bernard, H. U. (2009). Cholinergic signaling through nicotinic acetylcholine receptors stimulates the proliferation of cervical cancer cells, an explanation for the molecular role of tobacco smoking in cervical carcinogenesis? Int. J. Cancer 124, 1090–1096. doi: 10.1002/ijc.24053
Casado, M., López-Guajardo, A., Mellström, B., Naranjo, J. R., and Lerma, J. (1996). Functional N-methyl-D-aspartate receptors in clonal rat phaeochromocytoma cells. J Physiol, 490 (Pt 2)(Pt 2), 391–404. doi: 10.1113/jphysiol.1996.sp021153
Caulfield, M. P. (1993). Muscarinic receptors–characterization, coupling and function. Pharmacol. Ther. 58, 319–379. doi: 10.1016/0163-7258(93)90027-b
Cazanave, S. C., and Gores, G. J. (2009). The liver's dance with death, two Bcl-2 guardian proteins from the abyss. Hepatology 50, 1009–1013. doi: 10.1002/hep.23188
Cecarini, V., Bonfili, L., Cuccioloni, M., Mozzicafreddo, M., Rossi, G., Buizza, L., et al. (2012). Crosstalk between the ubiquitin-proteasome system and autophagy in a human cellular model of Alzheimer's disease. Biochim. Biophys. Acta 1822, 1741–1751. doi: 10.1016/j.bbadis.2012.07.015
Chao, M. V., Rajagopal, R., and Lee, F. S. (2006). Neurotrophin signalling in health and disease. Clin. Sci. (Lond). 110, 167–173. doi: 10.1042/cs20050163
Chen, B., Yue, R., Yang, Y., Zeng, H., Chang, W., Gao, N., et al. (2015). Protective effects of (E)-2-(1-hydroxyl-4-oxocyclohexyl) ethyl caffeine against hydrogen peroxide-induced injury in PC12 cells. Neurochem. Res. 40, 531–541. doi: 10.1007/s11064-014-1498-5
Chen, G. F., Xu, T. H., Yan, Y., Zhou, Y. R., Jiang, Y., Melcher, K., et al. (2017). Amyloid beta, structure, biology and structure-based therapeutic development. Acta. Pharmacol. Sin. 38, 1205–1235. doi: 10.1038/aps.2017.28
Chen, J., Venkat, P., Zacharek, A., and Chopp, M. (2014). Neurorestorative therapy for stroke. Front. Hum. Neurosci. 8, 382. doi: 10.3389/fnhum.2014.00382
Chen, L., Liu, Y. C., Tan, H., Zhang, Y., Xu, J., Liu, W. L., et al. (2019). Santacruzamate a ameliorates AD-Like pathology by enhancing er stress tolerance through regulating the functions of KDELR and Mia40-ALR in vivo and in vitro. Front. Cell. Neurosci. 13, 61. doi: 10.3389/fncel.2019.00061
Chi, S. S., Vetiska, S. M., Gill, R. S., Hsiung, M. S., Liu, F., and Van Tol, H. H. (2010). Transactivation of PDGFRbeta by dopamine D4 receptor does not require PDGFRbeta dimerization. Mol. Brain 3, 22. doi: 10.1186/1756-6606-3-22
Choi, M. S., Nakamura, T., Cho, S. J., Han, X., Holland, E. A., Qu, J., et al. (2014). Transnitrosylation from DJ-1 to PTEN attenuates neuronal cell death in parkinson's disease models. J. Neurosci. 34, 15123–15131. doi: 10.1523/jneurosci.4751-13.2014
Choi, S. H., Kim, Y. H., Hebisch, M., Sliwinski, C., Lee, S., D'avanzo, C., et al. (2014). A three-dimensional human neural cell culture model of Alzheimer's disease. Nature. 515, 274–278. doi: 10.1038/nature13800
Chopra, A. (2004). Molecular Imaging and Contrast Agent Database (MICAD). Bethesda (MD): National Center for Biotechnology Information (US).
Chua, P., and Lim, W. K. (2021). Optimisation of a PC12 cell-based in vitro stroke model for screening neuroprotective agents. Sci. Rep. 11, 8096. doi: 10.1038/s41598-021-87431-4
Citron, M., Westaway, D., Xia, W., Carlson, G., Diehl, T., Levesque, G., et al. (1997). Mutant presenilins of Alzheimer's disease increase production of 42-residue amyloid beta-protein in both transfected cells and transgenic mice. Nat. Med. 3, 67–72. doi: 10.1038/nm0197-67
Constantinescu, R., Constantinescu, A. T., Reichmann, H., and Janetzky, B. (2007). Neuronal differentiation and long-term culture of the human neuroblastoma line SH-SY5Y. J. Neural. Transm Suppl. 72, 17–28. doi: 10.1007/978-3-211-73574-9_3
Creagh, E. M., Conroy, H., and Martin, S. J. (2003). Caspase-activation pathways in apoptosis and immunity. Immunol. Rev. 193, 10–21. doi: 10.1034/j.1600-065x.2003.00048.x
Criado, M. (2018). Acetylcholine nicotinic receptor subtypes in chromaffin cells. Pflugers Arch. 470, 13–20. doi: 10.1007/s00424-017-2050-7
Criado, M., Valor, L. M., Mulet, J., Gerber, S., Sala, S., and Sala, F. (2012). Expression and functional properties of α7 acetylcholine nicotinic receptors are modified in the presence of other receptor subunits. J. Neurochem. 123, 504–514. doi: 10.1111/j.1471-4159.2012.07931.x
Davies, P., and Maloney, A. J. (1976). Selective loss of central cholinergic neurons in Alzheimer's disease. Lancet 2, 1403. doi: 10.1016/s0140-6736(76)91936-x
De Felice, F. G., Wu, D., Lambert, M. P., Fernandez, S. J., Velasco, P. T., Lacor, P. N., et al. (2008). Alzheimer's disease-type neuronal tau hyperphosphorylation induced by A beta oligomers. Neurobiol. Aging 29, 1334–1347. doi: 10.1016/j.neurobiolaging.2007.02.029
de Medeiros, L. M., De Bastiani, M. A., Rico, E. P., Schonhofen, P., Pfaffenseller, B., Wollenhaupt-Aguiar, B., et al. (2019). Cholinergic differentiation of human neuroblastoma SH-SY5Y cell line and its potential use as an in vitro model for Alzheimer's Disease studies. Mol. Neurobiol. 56, 7355–7367. doi: 10.1007/s12035-019-1605-3
Deberardinis, R. J., Sayed, N., Ditsworth, D., and Thompson, C. B. (2008). Brick by brick, metabolism and tumor cell growth. Curr. Opin. Genet. Dev. 18, 54–61. doi: 10.1016/j.gde.2008.02.003
Del Toro, D., Carrasquero-Ordaz, M. A., Chu, A., Ruff, T., Shahin, M., Jackson, V. A., et al. (2020). Structural basis of teneurin-latrophilin interaction in repulsive guidance of migrating neurons. Cell, 180, 323–339.e319. doi: 10.1016/j.cell.2019.12.014
Deneris, E. S., Boulter, J., Swanson, L. W., Patrick, J., and Heinemann, S. (1989). Beta 3, a new member of nicotinic acetylcholine receptor gene family is expressed in brain. J. Biol. Chem. 264, 6268–6272.
Denk, J., Boelmans, K., Siegismund, C., Lassner, D., Arlt, S., and Jahn, H. (2015). MicroRNA profiling of CSF reveals potential biomarkers to detect Alzheimer‘s disease. PLoS ONE. 10, e0126423. doi: 10.1371/journal.pone.0126423
Dexter, D. T., and Jenner, P. (2013). Parkinson disease, from pathology to molecular disease mechanisms. Free Radic. Biol. Med. 62, 132–144. doi: 10.1016/j.freeradbiomed.2013.01.018
Di, J., Cohen, L. S., Corbo, C. P., Phillips, G. R., El Idrissi, A., and Alonso, A. D. (2016). Abnormal tau induces cognitive impairment through two different mechanisms, synaptic dysfunction and neuronal loss. Sci. Rep. 6, 20833. doi: 10.1038/srep20833
Dietmair, S., Hodson, M. P., Quek, L. E., Timmins, N. E., Chrysanthopoulos, P., Jacob, S. S., et al. (2012). Metabolite profiling of CHO cells with different growth characteristics. Biotechnol. Bioeng. 109, 1404–1414. doi: 10.1002/bit.24496
Dimov, I. K., Lu, R., Lee, E. P., Seita, J., Sahoo, D., Park, S. M., et al. (2014). Discriminating cellular heterogeneity using microwell-based RNA cytometry. Nat. Commun. 5, 3451. doi: 10.1038/ncomms4451
Drisdel, R. C., and Green, W. N. (2000). Neuronal alpha-bungarotoxin receptors are alpha7 subunit homomers. J. Neurosci. 20, 133–139. doi: 10.1523/jneurosci.20-01-00133.2000
Duan, J., Cui, J., Yang, Z., Guo, C., Cao, J., Xi, M., et al. (2019). Neuroprotective effect of Apelin 13 on ischemic stroke by activating AMPK/GSK-3β/Nrf2 signaling. J. Neuroinflammation 16, 24. doi: 10.1186/s12974-019-1406-7
Durán-Prado, M., Frontiñán, J., Santiago-Mora, R., Peinado, J. R., Parrado-Fernández, C., Gómez-Almagro, M. V., et al. (2014). Coenzyme Q10 protects human endothelial cells from β-amyloid uptake and oxidative stress-induced injury. PLoS ONE. 9, e109223. doi: 10.1371/journal.pone.0109223
Edmondson, R., Broglie, J. J., Adcock, A. F., and Yang, L. (2014). Three-dimensional cell culture systems and their applications in drug discovery and cell-based biosensors. Assay Drug Dev. Technol. 12, 207–218. doi: 10.1089/adt.2014.573
Eruslanov, E., and Kusmartsev, S. (2010). Identification of ROS using oxidized DCFDA and flow-cytometry. Methods Mol. Biol. 594, 57–72. doi: 10.1007/978-1-60761-411-1_4
Eveleth, D. D., and Bradshaw, R. A. (1992). Nerve growth factor nonresponsive pheochromocytoma cells, altered internalization results in signaling dysfunction. J. Cell Biol. 117, 291–299. doi: 10.1083/jcb.117.2.291
Farías, G. G., Godoy, J. A., Hernández, F., Avila, J., Fisher, A., and Inestrosa, N. C. (2004). M1 muscarinic receptor activation protects neurons from beta-amyloid toxicity. A role for Wnt signaling pathway. Neurobiol Dis 17, 337–348. doi: 10.1016/j.nbd.2004.07.016
Fedoroff, S., and Hall, C. (1979). Effect of horse serum on neural cell differentiation in tissue culture. In Vitro. 15, 641–648. doi: 10.1007/bf02623400
Feles, S., Overath, C., Reichardt, S., Diegeler, S., Schmitz, C., Kronenberg, J., et al. (2022). Streamlining culture conditions for the neuroblastoma cell line SH-SY5Y, a prerequisite for functional studies. Methods Protoc 5, 4. doi: 10.3390/mps5040058
Figl, A., Cohen, B. N., Quick, M. W., Davidson, N., and Lester, H. A. (1992). Regions of beta 4.beta 2 subunit chimeras that contribute to the agonist selectivity of neuronal nicotinic receptors. FEBS Lett. 308, 245–248. doi: 10.1016/0014-5793(92)81284-s
Fischer, S., Handrick, R., and Otte, K. (2015). The art of CHO cell engineering: a comprehensive retrospect and future perspectives. Biotechnol. Adv. 33, 1878–1896. https://doi.org/10.1016/j.biotechadv.2015.10.015
Fitzpatrick, A. W. P., Falcon, B., He, S., Murzin, A. G., Murshudov, G., Garringer, H. J., et al. (2017). Cryo-EM structures of tau filaments from Alzheimer's disease. Nature. 547, 185–190. doi: 10.1038/nature23002
Fodero, L. R., Mok, S. S., Losic, D., Martin, L. L., Aguilar, M. I., Barrow, C. J., et al. (2004). Alpha7-nicotinic acetylcholine receptors mediate an Abeta(1-42)-induced increase in the level of acetylcholinesterase in primary cortical neurones. J. Neurochem. 88, 1186–1193. doi: 10.1046/j.1471-4159.2003.02296.x
Foldes, R. L., Rampersad, V., and Kamboj, R. K. (1993). Cloning and sequence analysis of cDNAs encoding human hippocampus N-methyl-D-aspartate receptor subunits, evidence for alternative RNA splicing. Gene. 131, 293–298. doi: 10.1016/0378-1119(93)90309-q
Francis, P. T., Palmer, A. M., Snape, M., and Wilcock, G. K. (1999). The cholinergic hypothesis of Alzheimer's disease, a review of progress. J. Neurol. Neurosurg. Psychiatr. 66, 137–147. doi: 10.1136/jnnp.66.2.137
Franke, J., Abs, V., Zizzadoro, C., and Abraham, G. (2014). Comparative study of the effects of fetal bovine serum versus horse serum on growth and differentiation of primary equine bronchial fibroblasts. BMC Vet. Res. 10, 119. doi: 10.1186/1746-6148-10-119
Fu, W. Y., Wang, X., and Ip, N. Y. (2019). Targeting neuroinflammation as a therapeutic strategy for Alzheimer's Disease: mechanisms, drug candidates, and new opportunities. ACS Chem. Neurosci. 10, 872–879. doi: 10.1021/acschemneuro.8b00402
Fujishiro, H., Umegaki, H., Isojima, D., Akatsu, H., Iguchi, A., and Kosaka, K. (2006). Depletion of cholinergic neurons in the nucleus of the medial septum and the vertical limb of the diagonal band in dementia with Lewy bodies. Acta Neuropathol. 111, 109–114. doi: 10.1007/s00401-005-0004-1
Gal, S., Zheng, H., Fridkin, M., and Youdim, M. B. (2005). Novel multifunctional neuroprotective iron chelator-monoamine oxidase inhibitor drugs for neurodegenerative diseases. In vivo selective brain monoamine oxidase inhibition and prevention of MPTP-induced striatal dopamine depletion. J. Neurochem 95, 79–88. doi: 10.1111/j.1471-4159.2005.03341.x
Gao, S., Guo, X., Zhao, S., Jin, Y., Zhou, F., Yuan, P., et al. (2019). Differentiation of human adipose-derived stem cells into neuron/motoneuron-like cells for cell replacement therapy of spinal cord injury. Cell Death Dis. 10, 597. doi: 10.1038/s41419-019-1772-1
Giulian, D. (1999). Microglia and the immune pathology of Alzheimer disease. Am. J. Hum. Genet. 65, 13–18. doi: 10.1086/302477
Glabe, C. G. (2008). Structural classification of toxic amyloid oligomers. J. Biol. Chem. 283, 29639–29643. doi: 10.1074/jbc.R800016200
Glover, D. J., Lipps, H. J., and Jans, D. A. (2005). Towards safe, non-viral therapeutic gene expression in humans. Nat. Rev. Genet. 6, 299–310. doi: 10.1038/nrg1577
Gomez, J., Boutou, E., Hurel, C., Mamalaki, A., Kentroti, S., Vernadakis, A., et al. (1998). Overexpression of the neuron-specific molecule BM88 in mouse neuroblastoma cells, altered responsiveness to growth factors. J. Neurosci. Res. 51, 119–128. doi: 10.1002/(sici)1097-4547(19980101)51:1<119::Aid-jnr13>3.0.Co;2-6
Gómez-Villafuertes, R., Del Puerto, A., Díaz-Hernández, M., Bustillo, D., Díaz-Hernández, J. I., Huerta, P. G., et al. (2009). Ca2+/calmodulin-dependent kinase II signalling cascade mediates P2X7 receptor-dependent inhibition of neuritogenesis in neuroblastoma cells. FEBS J. 276, 5307–5325. doi: 10.1111/j.1742-4658.2009.07228.x
Gong, Y., Jiang, J. H., and Li, S. T. (2016). Functional expression of human α7 nicotinic acetylcholine receptor in human embryonic kidney 293 cells. Mol. Med. Rep. 14, 2257–2263. doi: 10.3892/mmr.2016.5493
González-Burguera, I., Ricobaraza, A., Aretxabala, X., Barrondo, S., García Del Caño, G., López De Jesús, M., et al. (2016). Highly efficient generation of glutamatergic/cholinergic NT2-derived postmitotic human neurons by short-term treatment with the nucleoside analogue cytosine β-D-arabinofuranoside. Stem Cell Res. 16, 541–551. doi: 10.1016/j.scr.2016.02.038
Gotti, C., and Clementi, F. (2004). Neuronal nicotinic receptors, from structure to pathology. Prog. Neurobiol. 74, 363–396. doi: 10.1016/j.pneurobio.2004.09.006
Grau, C. M., and Greene, L. A. (2012). Use of PC12 cells and rat superior cervical ganglion sympathetic neurons as models for neuroprotective assays relevant to Parkinson's disease. Methods Mol. Biol. 846, 201–211. doi: 10.1007/978-1-61779-536-7_18
Green, S. H., Rydel, R. E., Connolly, J. L., and Greene, L. A. (1986). PC12 cell mutants that possess low- but not high-affinity nerve growth factor receptors neither respond to nor internalize nerve growth factor. J. Cell Biol. 102, 830–843. doi: 10.1083/jcb.102.3.830
Greene, L. A., and Rein, G. (1977). Release, storage and uptake of catecholamines by a clonal cell line of nerve growth factor (NGF) responsive pheo-chromocytoma cells. Brain Res. 129, 247–263. doi: 10.1016/0006-8993(77)90005-1
Greene, L. A., and Tischler, A. S. (1976). Establishment of a noradrenergic clonal line of rat adrenal pheochromocytoma cells which respond to nerve growth factor. Proc. Natl. Acad. Sci. USA. 73, 2424–2428. doi: 10.1073/pnas.73.7.2424
Greene, L. A., and Tischler, A. S. (1982). Advances in Cellular Neurobiology. Fedoroff, S. and Hertz, L. (eds). Amsterdam, Netherlands: Elsevier p. 373–414.
Gu, Z., Lamb, P. W., and Yakel, J. L. (2012). Cholinergic coordination of presynaptic and postsynaptic activity induces timing-dependent hippocampal synaptic plasticity. J. Neurosci. 32, 12337–12348. doi: 10.1523/jneurosci.2129-12.2012
Gu, Z., and Yakel, J. L. (2011). Timing-dependent septal cholinergic induction of dynamic hippocampal synaptic plasticity. Neuron. 71, 155–165. doi: 10.1016/j.neuron.2011.04.026
Guo, Q., Sopher, B. L., Furukawa, K., Pham, D. G., Robinson, N., Martin, G. M., et al. (1997). Alzheimer's presenilin mutation sensitizes neural cells to apoptosis induced by trophic factor withdrawal and amyloid beta-peptide, involvement of calcium and oxyradicals. J. Neurosci. 17, 4212–4222. doi: 10.1523/jneurosci.17-11-04212.1997
Gwon, A. R., Park, J. S., Arumugam, T. V., Kwon, Y. K., Chan, S. L., Kim, S. H., et al. (2012). Oxidative lipid modification of nicastrin enhances amyloidogenic γ-secretase activity in Alzheimer's disease. Aging Cell. 11, 559–568. doi: 10.1111/j.1474-9726.2012.00817.x
Haass, C., and Selkoe, D. J. (2007). Soluble protein oligomers in neurodegeneration, lessons from the Alzheimer's amyloid beta-peptide. Nat. Rev. Mol. Cell Biol. 8, 101–112. doi: 10.1038/nrm2101
Habauzit, D., Ferrière, F., Botherel, N., Flouriot, G., Pakdel, F., and Saligaut, C. (2014). Differentiation of PC12 cells expressing estrogen receptor alpha, a new bioassay for endocrine-disrupting chemicals evaluation. Chemosphere 112, 240–247. doi: 10.1016/j.chemosphere.2014.03.101
Hampel, H., Mesulam, M. M., Cuello, A. C., Farlow, M. R., Giacobini, E., Grossberg, G. T., et al. (2018a). The cholinergic system in the pathophysiology and treatment of Alzheimer's disease. Brain. 141, 1917–1933. doi: 10.1093/brain/awy132
Hampel, H., Toschi, N., Babiloni, C., Baldacci, F., Black, K. L., Bokde, A. L. W., et al. (2018b). Revolution of Alzheimer precision neurology. Passageway of systems biology and neurophysiology. J. Alzheimers Dis. 64, S47–s105. doi: 10.3233/jad-179932
Hampel, H., Toschi, N., Baldacci, F., Zetterberg, H., Blennow, K., Kilimann, I., et al. (2018c). Alzheimer's disease biomarker-guided diagnostic workflow using the added value of six combined cerebrospinal fluid candidates, Aβ(1-42), total-tau, phosphorylated-tau, NFL, neurogranin, and YKL-40. Alzheimers. Dement. 14, 492–501. doi: 10.1016/j.jalz.2017.11.015
Han, Y. C., Lim, Y., Duffieldl, M. D., Li, H., Liu, J., Abdul Manaph, N. P., et al. (2016). Direct Reprogramming of mouse fibroblasts to neural stem cells by small molecules. Stem Cells Int. 2016, 4304916. doi: 10.1155/2016/4304916
Hao, C., Gao, L., Zhang, Y., Wang, W., Yu, G., Guan, H., et al. (2015). Acetylated chitosan oligosaccharides act as antagonists against glutamate-induced PC12 cell death via Bcl-2/Bax signal pathway. Mar. Drugs 13, 1267–1289. doi: 10.3390/md13031267
Hardy, J., and Selkoe, D. J. (2002). The amyloid hypothesis of Alzheimer's disease, progress and problems on the road to therapeutics. Science. 297, 353–356. doi: 10.1126/science.1072994
Hardy, J. A., and Higgins, G. A. (1992). Alzheimer's disease, the amyloid cascade hypothesis. Science. 256, 184–185. doi: 10.1126/science.1566067
Hashimoto, A., Onodera, T., Ikeda, H., and Kitani, H. (2000). Isolation and characterisation of fetal bovine brain cells in primary culture. Res. Vet. Sci. 69, 39–46. doi: 10.1053/rvsc.2000.0382
Hayakawa, K., Formica, A. M., Brill-Dashoff, J., Shinton, S. A., Ichikawa, D., Zhou, Y., et al. (2016). Early generated B1 B cells with restricted BCRs become chronic lymphocytic leukemia with continued c-Myc and low Bmf expression. J. Exp. Med. 213, 3007–3024. doi: 10.1084/jem.20160712
He, H., Zhou, Y., Huang, J., Wu, Z., Liao, Z., Liu, D., et al. (2017). Capsaicin protects cardiomyocytes against anoxia/reoxygenation injury via preventing mitochondrial dysfunction mediated by SIRT1. Oxid. Med. Cell. Longev. 2017, 1035702. doi: 10.1155/2017/1035702
He W. Goodkind D. Kowal P. R. United Bureau of US. (2016). An Aging WORLD 2015. International Population Reports- United States Census Bureau. Boletín-Otras Publicaciones, 1–175. Available online at: http://www.census.gov/library/publications/2016/demo/P95-16-1.html.
Henderson, L. P., Gdovin, M. J., Liu, C., Gardner, P. D., and Maue, R. A. (1994). Nerve growth factor increases nicotinic ACh receptor gene expression and current density in wild-type and protein kinase A-deficient PC12 cells. J. Neurosci. 14, 1153–1163. doi: 10.1523/jneurosci.14-03-01153.1994
Hengartner, M. O. (2000). The biochemistry of apoptosis. Nature. 407, 770–776. doi: 10.1038/35037710
Hernandez, C. M., and Dineley, K. T. (2012). α7 nicotinic acetylcholine receptors in Alzheimer's disease, neuroprotective, neurotrophic or both? Curr. Drug Target.s 13, 613–622. doi: 10.2174/138945012800398973
Herrero, D., and Bernad, A. (2016). Bmi1-mediated epigenetic signature acts as a critical barrier for direct reprogramming to mature cardiomyocytes. Stem Cell Investig. 3, 28. doi: 10.21037/sci.2016.07.05
Hoey, S. E., Williams, R. J., and Perkinton, M. S. (2009). Synaptic NMDA receptor activation stimulates alpha-secretase amyloid precursor protein processing and inhibits amyloid-beta production. J. Neurosci. 29, 4442–4460. doi: 10.1523/jneurosci.6017-08.2009
Holmes, C., Cunningham, C., Zotova, E., Culliford, D., and Perry, V. H. (2011). Proinflammatory cytokines, sickness behavior, and Alzheimer disease. Neurology 77, 212–218. doi: 10.1212/WNL.0b013e318225ae07
Hong, S., Ergezen, E., Lec, R., and Barbee, K. A. (2006). Real-time analysis of cell-surface adhesive interactions using thickness shear mode resonator. Biomaterials. 27, 5813–5820. doi: 10.1016/j.biomaterials.2006.07.031
Howe, W. M., Gritton, H. J., Lusk, N. A., Roberts, E. A., Hetrick, V. L., Berke, J. D., et al. (2017). Acetylcholine release in prefrontal cortex promotes gamma oscillations and theta-gamma coupling during cue detection. J. Neurosci. 37, 3215–3230. doi: 10.1523/jneurosci.2737-16.2017
Hu, S., Wang, D., Zhang, J., Du, M., Cheng, Y., Liu, Y., et al. (2016). Mitochondria related pathway is essential for polysaccharides purified from sparassis crispa mediated neuro-protection against glutamate-induced toxicity in differentiated PC12 cells. Int. J. Mol. Sci. 17, 33. doi: 10.3390/ijms17020133
Hu, W., Wang, G., Li, P., Wang, Y., Si, C. L., He, J., et al. (2014). Neuroprotective effects of macranthoin G from Eucommia ulmoides against hydrogen peroxide-induced apoptosis in PC12 cells via inhibiting NF-κB activation. Chem. Biol. Interact. 224, 108–116. doi: 10.1016/j.cbi.2014.10.011
Huang, X., Zhen, J., Dong, S., Zhang, H., Van Halm-Lutterodt, N., and Yuan, L. (2019). DHA and vitamin E antagonized the Aβ(25-35)-mediated neuron oxidative damage through activation of Nrf2 signaling pathways and regulation of CD36, SRB1 and FABP5 expression in PC12 cells. Food Funct. 10, 1049–1061. doi: 10.1039/c8fo01713a
Hwang, S. O., and Lee, G. M. (2008). Nutrient deprivation induces autophagy as well as apoptosis in Chinese hamster ovary cell culture. Biotechnol. Bioeng. 99, 678–685. doi: 10.1002/bit.21589
Hynd, M. R., Scott, H. L., and Dodd, P. R. (2004a). Differential expression of N-methyl-D-aspartate receptor NR2 isoforms in Alzheimer's disease. J. Neurochem. 90, 913–919. doi: 10.1111/j.1471-4159.2004.02548.x
Hynd, M. R., Scott, H. L., and Dodd, P. R. (2004b). Selective loss of NMDA receptor NR1 subunit isoforms in Alzheimer's disease. J. Neurochem. 89, 240–247. doi: 10.1111/j.1471-4159.2003.02330.x
Im, K., Mareninov, S., Diaz, M. F. P., and Yong, W. H. (2019). An introduction to performing immunofluorescence staining. Methods Mol. Biol. 1897, 299–311. doi: 10.1007/978-1-4939-8935-5_26
Ingber, D. E. (2018). Developmentally inspired human 'organs on chips'. Development. 145, 16. doi: 10.1242/dev.156125
Inouye, H., and Kirschner, D. A. (2005). Alzheimer's beta-amyloid, insights into fibril formation and structure from Congo red binding. Subcell. Biochem. 38, 203–224. doi: 10.1007/0-387-23226-5_10
Iscove, N. N., and Melchers, F. (1978). Complete replacement of serum by albumin, transferrin, and soybean lipid in cultures of lipopolysaccharide-reactive B lymphocytes. J. Exp. Med. 147, 923–933. doi: 10.1084/jem.147.3.923
Iuvone, T., Esposito, G., Esposito, R., Santamaria, R., Di Rosa, M., and Izzo, A. A. (2004). Neuroprotective effect of cannabidiol, a non-psychoactive component from Cannabis sativa, on beta-amyloid-induced toxicity in PC12 cells. J. Neurochem. 89, 134–141. doi: 10.1111/j.1471-4159.2003.02327.x
Iwatsubo, T., Odaka, A., Suzuki, N., Mizusawa, H., Nukina, N., and Ihara, Y. (1994). Visualization of A beta 42(43) and A beta 40 in senile plaques with end-specific A beta monoclonals, evidence that an initially deposited species is A beta 42(43). Neuron. 13, 45–53. doi: 10.1016/0896-6273(94)90458-8
Jaber, Q. Z., Bibi, M., Ksiezopolska, E., Gabaldon, T., Berman, J., and Fridman, M. (2020). Elevated vacuolar uptake of fluorescently labeled antifungal drug caspofungin predicts echinocandin resistance in pathogenic yeast. ACS Cent. Sci. 6, 1698–1712. doi: 10.1021/acscentsci.0c00813
Jäckel, T., Knels, L., Valtink, M., Funk, R. H., and Engelmann, K. (2011). Serum-free corneal organ culture medium (SFM) but not conventional minimal essential organ culture medium (MEM) protects human corneal endothelial cells from apoptotic and necrotic cell death. Br. J. Ophthalmol. 95, 123–130. doi: 10.1136/bjo.2010.183418
Jang, J. H., and Surh, Y. J. (2004). Bcl-2 attenuation of oxidative cell death is associated with up-regulation of gamma-glutamylcysteine ligase via constitutive NF-kappaB activation. J. Biol. Chem. 279, 38779–38786. doi: 10.1074/jbc.M406371200
Jiang, L., Zeng, X., Wang, Z., Ji, N., Zhou, Y., Liu, X., et al. (2010). Oral cancer overexpressed 1 (ORAOV1) regulates cell cycle and apoptosis in cervical cancer HeLa cells. Mol. Cancer 9, 20. doi: 10.1186/1476-4598-9-20
Jin, M., Shepardson, N., Yang, T., Chen, G., Walsh, D., and Selkoe, D. J. (2011). Soluble amyloid beta-protein dimers isolated from Alzheimer cortex directly induce Tau hyperphosphorylation and neuritic degeneration. Proc. Natl. Acad. Sci. USA. 108, 5819–5824. doi: 10.1073/pnas.1017033108
Jin, M. M., Zhang, L., Yu, H. X., Meng, J., Sun, Z., and Lu, R. R. (2013). Protective effect of whey protein hydrolysates on H2O2-induced PC12 cells oxidative stress via a mitochondria-mediated pathway. Food Chem. 141, 847–852. doi: 10.1016/j.foodchem.2013.03.076
Jo, D. G., Arumugam, T. V., Woo, H. N., Park, J. S., Tang, S. C., Mughal, M., et al. (2010). Evidence that gamma-secretase mediates oxidative stress-induced beta-secretase expression in Alzheimer's disease. Neurobiol. Aging. 31, 917–925. doi: 10.1016/j.neurobiolaging.2008.07.003
Jürgensen, S., and Ferreira, S. T. (2010). Nicotinic receptors, amyloid-beta, and synaptic failure in Alzheimer's disease. J. Mol. Neurosci. 40, 221–229. doi: 10.1007/s12031-009-9237-0
Kamat, P. K., Rai, S., Swarnkar, S., Shukla, R., and Nath, C. (2014). Molecular and cellular mechanism of okadaic acid (OKA)-induced neurotoxicity, a novel tool for Alzheimer's disease therapeutic application. Mol. Neurobiol. 50, 852–865. doi: 10.1007/s12035-014-8699-4
Kapogiannis, D., Mustapic, M., Shardell, M. D., Berkowitz, S. T., Diehl, T. C., Spangler, R. D., et al. (2019). Association of extracellular vesicle biomarkers with alzheimer disease in the baltimore longitudinal study of aging. JAMA Neurol. 76, 1340–1351. doi: 10.1001/jamaneurol.2019.2462
Kaur, P., Armugam, A., and Jeyaseelan, K. (2012). MicroRNAs in neurotoxicity. J. Toxicol. 2012, 870150. doi: 10.1155/2012/870150
Kerbel, R. S., and Blakeslee, D. (1976). Rapid adsorption of a foetal calf serum component by mammalian cells in culture. A potential source of artifacts in studies of antisera to cell-specific antigens. Immunology. 31, 881–891.
Kim, T. K., and Eberwine, J. H. (2010). Mammalian cell transfection, the present and the future. Anal. Bioanal. Chem. 397, 3173–3178. doi: 10.1007/s00216-010-3821-6
Kinarivala, N., Shah, K., Abbruscato, T. J., and Trippier, P. C. (2017). Passage variation of PC12 cells results in inconsistent susceptibility to externally induced apoptosis. ACS Chem. Neurosci. 8, 82–88. doi: 10.1021/acschemneuro.6b00208
King, J. R., Nordman, J. C., Bridges, S. P., Lin, M. K., and Kabbani, N. (2015). Identification and characterization of a G protein-binding cluster in α7 nicotinic acetylcholine receptors. J. Biol. Chem. 290, 20060–20070. doi: 10.1074/jbc.M115.647040
King, J. R., Ullah, A., Bak, E., Jafri, M. S., and Kabbani, N. (2018). Ionotropic and metabotropic mechanisms of allosteric modulation of α7 nicotinic receptor intracellular calcium. Mol. Pharmacol. 93, 601–611. doi: 10.1124/mol.117.111401
Klebe Rj, R. F. (1969). Neuroblastoma, cell culture analysis of a differentiating stem cell system. J. Cell Biol. 43:69a.
Kobayashi, S., Yokoyama, S., Maruta, T., Negami, M., Muroyama, A., Mitsumoto, Y., et al. (2013). Autoantibody-induced internalization of nicotinic acetylcholine receptor α3 subunit exogenously expressed in human embryonic kidney cells. J. Neuroimmunol. 257, 102–106. doi: 10.1016/j.jneuroim.2012.12.010
Korke, R., Rink, A., Seow, T. K., Chung, M. C., Beattie, C. W., and Hu, W. S. (2002). Genomic and proteomic perspectives in cell culture engineering. J. Biotechnol. 94, 73–92. doi: 10.1016/s0168-1656(01)00420-5
Kovalevich, J., and Langford, D. (2013). Considerations for the use of SH-SY5Y neuroblastoma cells in neurobiology. Methods Mol. Biol. 1078, 9–21. doi: 10.1007/978-1-62703-640-5_2
Kreutzer, A. G., Yoo, S., Spencer, R. K., and Nowick, J. S. (2017). Stabilization, assembly, and toxicity of trimers derived from Aβ. J. Am. Chem. Soc. 139, 966–975. doi: 10.1021/jacs.6b11748
Krishna, A., Biryukov, M., Trefois, C., Antony, P. M., Hussong, R., Lin, J., et al. (2014). Systems genomics evaluation of the SH-SY5Y neuroblastoma cell line as a model for Parkinson's disease. BMC Genomics. 15, 1154. doi: 10.1186/1471-2164-15-1154
Krstic, D., and Knuesel, I. (2013). Deciphering the mechanism underlying late-onset Alzheimer disease. Nat. Rev. Neurol. 9, 25–34. doi: 10.1038/nrneurol.2012.236
Kubo, T., Nishimura, S., Kumagae, Y., and Kaneko, I. (2002). In vivo conversion of racemized beta-amyloid ([D-Ser 26]A beta 1-40) to truncated and toxic fragments ([D-Ser 26]A beta 25-35/40) and fragment presence in the brains of Alzheimer's patients. J. Neurosci. Res. 70, 474–483. doi: 10.1002/jnr.10391
Kurt, H., Bueso-Ramos, C. E., Khoury, J. D., Routbort, M. J., Kanagal-Shamanna, R., Patel, U. V., et al. (2018). Characterization of IDH1 p.R132H mutant clones using mutation-specific antibody in myeloid neoplasms. Am. J. Surg. Pathol. 42, 569–577. doi: 10.1097/pas.0000000000000970
Kuryatov, A., Onksen, J., and Lindstrom, J. (2008). Roles of accessory subunits in alpha4beta2(*) nicotinic receptors. Mol. Pharmacol. 74, 132–143. doi: 10.1124/mol.108.046789
Kwon, D., Kim, J. S., Cha, B. H., Park, K. S., Han, I., Park, K. S., et al. (2016). The effect of fetal bovine serum (FBS) on efficacy of cellular reprogramming for induced pluripotent stem cell (iPSC) generation. Cell Transplant. 25, 1025–1042. doi: 10.3727/096368915x689703
Lai, H. C., Wu, M. J., Chen, P. Y., Sheu, T. T., Chiu, S. P., Lin, M. H., et al. (2011). Neurotrophic effect of citrus 5-hydroxy-3,6,7,8,3',4'-hexamethoxyflavone, promotion of neurite outgrowth via cAMP/PKA/CREB pathway in PC12 cells. PLoS ONE. 6, e28280. doi: 10.1371/journal.pone.0028280
Lanoiselée, H. M., Nicolas, G., Wallon, D., Rovelet-Lecrux, A., Lacour, M., Rousseau, S., et al. (2017). APP, PSEN1, and PSEN2 mutations in early-onset Alzheimer disease: a genetic screening study of familial and sporadic cases. PLoS Med. 14, e1002270. doi: 10.1371/journal.pmed.1002270
Laube, B., Kuhse, J., and Betz, H. (1998). Evidence for a tetrameric structure of recombinant NMDA receptors. J. Neurosci. 18, 2954–2961. doi: 10.1523/jneurosci.18-08-02954.1998
Lee, J. H., Ahn, H. H., Kim, K. S., Lee, J. Y., Kim, M. S., Lee, B., et al. (2008). Polyethyleneimine-mediated gene delivery into rat pheochromocytoma PC-12 cells. J. Tissue Eng. Regen. Med. 2, 288–295. doi: 10.1002/term.94
Lee, J. W., Park, B. C., Jang, N. Y., Lee, S., Cho, Y. K., Sharma, P., et al. (2022). Inducing ectopic T cell clusters using stromal vascular fraction spheroid-based immunotherapy to enhance anti-tumor immunity. Adv Sci (Weinh). 9, e2203842. doi: 10.1002/advs.202203842
Lee, S., Youn, K., Jeong, W. S., Ho, C. T., and Jun, M. (2017). Protective effects of red ginseng oil against Aβ(25-35)-induced neuronal apoptosis and inflammation in PC12 cells. Int. J. Mol. Sci. 18, 2218. doi: 10.3390/ijms18102218
Leidinger, P., Backes, C., Deutscher, S., Schmitt, K., Mueller, S. C., Frese, K., et al. (2013). A blood based 12-miRNA signature of Alzheimer disease patients. Genome Biol. 14, R78. doi: 10.1186/gb-2013-14-7-r78
Lepage, K. T., Dickey, R. W., Gerwick, W. H., Jester, E. L., and Murray, T. F. (2005). On the use of neuro-2a neuroblastoma cells versus intact neurons in primary culture for neurotoxicity studies. Crit. Rev. Neurobiol. 17, 27–50. doi: 10.1615/critrevneurobiol.v17.i1.20
Leuner, K., Müller, W. E., and Reichert, A. S. (2012). From mitochondrial dysfunction to amyloid beta formation: novel insights into the pathogenesis of Alzheimer's disease. Mol. Neurobiol. 46, 186–193. doi: 10.1007/s12035-012-8307-4
Levey, A. I., Edmunds, S. M., Koliatsos, V., Wiley, R. G., and Heilman, C. J. (1995). Expression of m1-m4 muscarinic acetylcholine receptor proteins in rat hippocampus and regulation by cholinergic innervation. J. Neurosci. 15, 4077–4092. doi: 10.1523/jneurosci.15-05-04077.1995
Levin, E. D., Bradley, A., Addy, N., and Sigurani, N. (2002). Hippocampal alpha 7 and alpha 4 beta 2 nicotinic receptors and working memory. Neuroscience. 109, 757–765. doi: 10.1016/s0306-4522(01)00538-3
Li, M., Xu, T., Zhou, F., Wang, M., Song, H., Xiao, X., et al. (2018). Neuroprotective effects of four phenylethanoid glycosides on H2O2-induced apoptosis on PC12 cells via the Nrf2/ARE pathway. Int. J. Mol. Sci. 19, 4. doi: 10.3390/ijms19041135
Li, R. L., Zhang, Q., Liu, J., Sun, J. Y., He, L. Y., Duan, H. X., et al. (2020). Hydroxy-α-sanshool possesses protective potentials on H(2)O(2)-stimulated PC12 cells by suppression of oxidative stress-induced apoptosis through regulation of PI3K/AKT signal pathway. Oxid. Med. Cell. Longev. 2020, 3481758. doi: 10.1155/2020/3481758
Li, X. D., and Buccafusco, J. J. (2003). Effect of beta-amyloid peptide 1-42 on the cytoprotective action mediated by alpha7 nicotinic acetylcholine receptors in growth factor-deprived differentiated PC-12 cells. J. Pharmacol. Exp. Ther. 307, 670–675. doi: 10.1124/jpet.103.053785
Li, Y., Jiao, Q., Xu, H., Du, X., Shi, L., Jia, F., et al. (2017). Biometal dyshomeostasis and toxic metal accumulations in the development of Alzheimer's disease. Front. Mol. Neurosci. 10, 339. doi: 10.3389/fnmol.2017.00339
Li, Z. (2011). Comprehensive Biotechnology (Second Edition). Moo-Young, M. (ed). Burlington: Academic Press. p. 551–563
Lin, C. H., Huang, Y. J., Lin, C. J., Lane, H. Y., and Tsai, G. E. (2014). NMDA neurotransmission dysfunction in mild cognitive impairment and Alzheimer's disease. Curr. Pharm. Des. 20, 5169–5179. doi: 10.2174/1381612819666140110115603
Lin, X., Wu, S., Wang, Q., Shi, Y., Liu, G., Zhi, J., et al. (2016). Saikosaponin-D reduces H(2)O(2)-induced PC12 cell apoptosis by removing ros and blocking mapk-dependent oxidative damage. Cell. Mol. Neurobiol. 36, 1365–1375. doi: 10.1007/s10571-016-0336-5
Liu, Q., Huang, Y., Shen, J., Steffensen, S., and Wu, J. (2012). Functional α7β2 nicotinic acetylcholine receptors expressed in hippocampal interneurons exhibit high sensitivity to pathological level of amyloid β peptides. BMC Neurosci. 13, 155. doi: 10.1186/1471-2202-13-155
Loghavi, S., Al-Ibraheemi, A., Zuo, Z., Garcia-Manero, G., Yabe, M., Wang, S. A., et al. (2015). TP53 overexpression is an independent adverse prognostic factor in de novo myelodysplastic syndromes with fibrosis. Br. J. Haematol. 171, 91–99. doi: 10.1111/bjh.13529
Long, J. M., and Holtzman, D. M. (2019). Alzheimer disease, an update on pathobiology and treatment strategies. Cell 179, 312–339. doi: 10.1016/j.cell.2019.09.001
Lopes, F. M., Schröder, R., Da Frota, M. L. Jr., Zanotto-Filho, A., Müller, C. B., Pires, A. S., et al. (2010). Comparison between proliferative and neuron-like SH-SY5Y cells as an in vitro model for Parkinson disease studies. Brain Res. 1337, 85–94. doi: 10.1016/j.brainres.2010.03.102
Lopez-Martinez, M., Gomez-Elizondo, D., Guerrero-Ramirez, G., Morales-Aseff, D., Zavala, J., and Valdez-García, J. E. (2019). Effect of different FBS concentrations on the proliferation of primary subculture of human pterygium fibroblasts. Invest. Ophthalmol. Vis. Sci. 60, 6267–6267.
Lotharius, J., and Brundin, P. (2002). Pathogenesis of Parkinson's disease, dopamine, vesicles and alpha-synuclein. Nat. Rev. Neurosci. 3, 932–942. doi: 10.1038/nrn983
Luetje, C. W., and Patrick, J. (1991). Both alpha- and beta-subunits contribute to the agonist sensitivity of neuronal nicotinic acetylcholine receptors. J. Neurosci. 11, 837–845. doi: 10.1523/jneurosci.11-03-00837.1991
Lukas, R. J., Norman, S. A., and Lucero, L. (1993). Characterization of nicotinic acetylcholine receptors expressed by cells of the sh-sy5y human neuroblastoma clonal line. Mol. Cell. Neurosci. 4, 1–12. doi: 10.1006/mcne.1993.1001
Ma, P., Li, Y., Zhang, W., Fang, F., Sun, J., Liu, M., et al. (2019). Long non-coding RNA MALAT1 inhibits neuron apoptosis and neuroinflammation while stimulates neurite outgrowth and its correlation with MiR-125b mediates PTGS2, CDK5 and FOXQ1 in Alzheimer's Disease. Curr. Alzheimer Res. 16, 596–612. doi: 10.2174/1567205016666190725130134
Majolo, F., Marinowic, D. R., Machado, D. C., and Da Costa, J. C. (2019). Important advances in Alzheimer's disease from the use of induced pluripotent stem cells. J. Biomed. Sci. 26, 15. doi: 10.1186/s12929-019-0501-5
Manassero, G., Guglielmotto, M., Zamfir, R., Borghi, R., Colombo, L., Salmona, M., et al. (2016). Beta-amyloid 1-42 monomers, but not oligomers, produce PHF-like conformation of Tau protein. Aging Cell 15, 914–923. doi: 10.1111/acel.12500
Mao, L. M., Wang, H. H., and Wang, J. Q. (2017). Antagonism of muscarinic acetylcholine receptors alters synaptic ERK phosphorylation in the rat forebrain. Neurochem. Res. 42, 1202–1210. doi: 10.1007/s11064-016-2157-9
Marcello, E., Gardoni, F., Mauceri, D., Romorini, S., Jeromin, A., Epis, R., et al. (2007). Synapse-associated protein-97 mediates alpha-secretase ADAM10 trafficking and promotes its activity. J. Neurosci. 27, 1682–1691. doi: 10.1523/jneurosci.3439-06.2007
Maroto, R., and Perez-Polo, J. R. (1997). BCL-2-related protein expression in apoptosis, oxidative stress versus serum deprivation in PC12 cells. J. Neurochem. 69, 514–523. doi: 10.1046/j.1471-4159.1997.69020514.x
Marotta, C. A., Chou, W. G., Majocha, R. E., Watkins, R., Labonne, C., and Zain, S. B. (1989). Overexpression of amyloid precursor protein A4 (beta-amyloid) immunoreactivity in genetically transformed cells, implications for a cellular model of Alzheimer amyloidosis. Proc. Natl. Acad. Sci. USA. 86, 337–341. doi: 10.1073/pnas.86.1.337
Marques, C. A., Keil, U., Bonert, A., Steiner, B., Haass, C., Muller, W. E., et al. (2003). Neurotoxic mechanisms caused by the Alzheimer's disease-linked Swedish amyloid precursor protein mutation: oxidative stress, caspases, and the JNK pathway. J. Biol. Chem. 278, 28294–28302. doi: 10.1074/jbc.M212265200
Martin, T. F., and Grishanin, R. N. (2003). PC12 cells as a model for studies of regulated secretion in neuronal and endocrine cells. Methods Cell Biol. 71, 267–286. doi: 10.1016/s0091-679x(03)01012-4
Masters, C. L., Bateman, R., Blennow, K., Rowe, C. C., Sperling, R. A., and Cummings, J. L. (2015). Alzheimer's disease. Nat Rev Dis Primers 1, 15056. doi: 10.1038/nrdp.2015.56
Matsushita, M., Nakamura, T., Moriizumi, H., Miki, H., and Takekawa, M. (2020). Stress-responsive MTK1 SAPKKK serves as a redox sensor that mediates delayed and sustained activation of SAPKs by oxidative stress. Sci. Adv. 6, eaay9778. doi: 10.1126/sciadv.aay9778
Mcbain, C. J., and Mayer, M. L. (1994). N-methyl-D-aspartic acid receptor structure and function. Physiol. Rev. 74, 723–760. doi: 10.1152/physrev.1994.74.3.723
Medina Benavente, J. J., Mogami, H., Sakurai, T., and Sawada, K. (2014). Evaluation of silicon nitride as a substrate for culture of PC12 cells, an interfacial model for functional studies in neurons. PLoS ONE. 9, e90189. doi: 10.1371/journal.pone.0090189
Meinhardt, J., Sachse, C., Hortschansky, P., Grigorieff, N., and Fändrich, M. (2009). Abeta(1-40) fibril polymorphism implies diverse interaction patterns in amyloid fibrils. J. Mol. Biol. 386, 869–877. doi: 10.1016/j.jmb.2008.11.005
Mejía, M., Salgado-Bustamante, M., Castillo, C. G., and Jiménez-Capdeville, M. E. (2013). Passage determines toxicity and neuronal markers expression in PC12 cells with altered phenotype. Toxicol. Res. 2, 388–396. doi: 10.1039/c3tx50010a
Meng, X., Luo, Y., Liang, T., Wang, M., Zhao, J., Sun, G., et al. (2016). Gypenoside XVII enhances lysosome biogenesis and autophagy flux and accelerates autophagic clearance of amyloid-β through TFEB activation. J. Alzheimers. Dis. 52, 1135–1150. doi: 10.3233/jad-160096
Middleton, D. A. (2007). Solid-state NMR spectroscopy as a tool for drug design, from membrane-embedded targets to amyloid fibrils. Biochem. Soc. Trans. 35, 985–990. doi: 10.1042/bst0350985
Miyagishi, M., and Taira, K. (2002). U6 promoter-driven siRNAs with four uridine 3' overhangs efficiently suppress targeted gene expression in mammalian cells. Nat. Biotechnol. 20, 497–500. doi: 10.1038/nbt0502-497
Moalem, G., and Tracey, D. J. (2006). Immune and inflammatory mechanisms in neuropathic pain. Brain Res. Rev. 51, 240–264. doi: 10.1016/j.brainresrev.2005.11.004
Moretti, M., Zoli, M., George, A. A., Lukas, R. J., Pistillo, F., Maskos, U., et al. (2014). The novel α7β2-nicotinic acetylcholine receptor subtype is expressed in mouse and human basal forebrain, biochemical and pharmacological characterization. Mol. Pharmacol. 86, 306–317. doi: 10.1124/mol.114.093377
Mouri, K., and Sako, Y. (2013). Optimality conditions for cell-fate heterogeneity that maximize the effects of growth factors in PC12 cells. PLoS Comput. Biol. 9, e1003320. doi: 10.1371/journal.pcbi.1003320
Mullaney, I., Dodd, M. W., Buckley, N., and Milligan, G. (1993). Agonist activation of transfected human M1 muscarinic acetylcholine receptors in CHO cells results in down-regulation of both the receptor and the alpha subunit of the G-protein Gq. Biochem J. 289, 125–131. doi: 10.1042/bj2890125
Murray, T. A., Bertrand, D., Papke, R. L., George, A. A., Pantoja, R., Srinivasan, R., et al. (2012). α7β2 nicotinic acetylcholine receptors assemble, function, and are activated primarily via their α7-α7 interfaces. Mol. Pharmacol. 81, 175–188. doi: 10.1124/mol.111.074088
Naiki, H., and Gejyo, F. (1999). Kinetic analysis of amyloid fibril formation. Meth. Enzymol. 309, 305–318. doi: 10.1016/s0076-6879(99)09022-9
Naldi, M., Fiori, J., Pistolozzi, M., Drake, A. F., Bertucci, C., Wu, R., et al. (2012). Amyloid β-peptide 25-35 self-assembly and its inhibition, a model undecapeptide system to gain atomistic and secondary structure details of the Alzheimer's disease process and treatment. ACS Chem. Neurosci. 3, 952–962. doi: 10.1021/cn3000982
Nathanson, N. M. (1987). Molecular properties of the muscarinic acetylcholine receptor. Annu. Rev. Neurosci. 10, 195–236. doi: 10.1146/annurev.ne.10.030187.001211
Neelands, T. R., Zhang, J., and Macdonald, R. L. (1999). GABA(A) receptors expressed in undifferentiated human teratocarcinoma NT2 cells differ from those expressed by differentiated NT2-N cells. J. Neurosci. 19, 7057–7065. doi: 10.1523/jneurosci.19-16-07057.1999
Neff, S., Dineley-Miller, K., Char, D., Quik, M., and Patrick, J. (1995). Production of polyclonal antisera that recognize and distinguish between the extracellular domains of neuronal nicotinic acetylcholine receptor subunits. J. Neurochem. 64, 332–339. doi: 10.1046/j.1471-4159.1995.64010332.x
Nery, A. A., Resende, R. R., Martins, A. H., Trujillo, C. A., Eterovic, V. A., and Ulrich, H. (2010). Alpha 7 nicotinic acetylcholine receptor expression and activity during neuronal differentiation of PC12 pheochromocytoma cells. J. Mol. Neurosci. 41, 329–339. doi: 10.1007/s12031-010-9369-2
Niranjan, R., Nath, C., and Shukla, R. (2012). Melatonin attenuated mediators of neuroinflammation and alpha-7 nicotinic acetylcholine receptor mRNA expression in lipopolysaccharide (LPS) stimulated rat astrocytoma cells, C6. Free Radic. Res. 46, 1167–1177. doi: 10.3109/10715762.2012.697626
Novak, J. E., Turner, R. S., Agranoff, B. W., and Fisher, S. K. (1999). Differentiated human NT2-N neurons possess a high intracellular content of myo-inositol. J. Neurochem. 72, 1431–1440. doi: 10.1046/j.1471-4159.1999.721431.x
Núñez, G. (2011). Intracellular sensors of microbes and danger. Immunol. Rev. 243, 5–8. doi: 10.1111/j.1600-065X.2011.01058.x
Ossenkoppele, R., Schonhaut, D. R., Schöll, M., Lockhart, S. N., Ayakta, N., Baker, S. L., et al. (2016). Tau PET patterns mirror clinical and neuroanatomical variability in Alzheimer's disease. Brain, 139(Pt 5), 1551–1567. doi: 10.1093/brain/aww027
Påhlman, S., Ruusala, A. I., Abrahamsson, L., Mattsson, M. E., and Esscher, T. (1984). Retinoic acid-induced differentiation of cultured human neuroblastoma cells, a comparison with phorbolester-induced differentiation. Cell Differ. 14, 135–144. doi: 10.1016/0045-6039(84)90038-1
Pals-Rylaarsdam, R., Gurevich, V. V., Lee, K. B., Ptasienski, J. A., Benovic, J. L., and Hosey, M. M. (1997). Internalization of the m2 muscarinic acetylcholine receptor. Arrestin-independent and -dependent pathways. J. Biol. Chem. 272, 23682–23689. doi: 10.1074/jbc.272.38.23682
Panigrahi, G. B., Slean, M. M., Simard, J. P., and Pearson, C. E. (2012). Human mismatch repair protein hMutLα is required to repair short slipped-DNAs of trinucleotide repeats. J. Biol. Chem. 287, 41844–41850. doi: 10.1074/jbc.M112.420398
Panza, F., Lozupone, M., Logroscino, G., and Imbimbo, B. P. (2019). A critical appraisal of amyloid-β-targeting therapies for Alzheimer disease. Nat. Rev. Neurol. 15, 73–88. doi: 10.1038/s41582-018-0116-6
Papke, R. L., and Heinemann, S. F. (1994). Partial agonist properties of cytisine on neuronal nicotinic receptors containing the beta 2 subunit. Mol. Pharmacol. 45, 142–149.
Parri, H. R., Hernandez, C. M., and Dineley, K. T. (2011). Research update, Alpha7 nicotinic acetylcholine receptor mechanisms in Alzheimer's disease. Biochem. Pharmacol. 82, 931–942. doi: 10.1016/j.bcp.2011.06.039
Patterson, P. H., and Chun, L. L. (1974). The influence of non-neuronal cells on catecholamine and acetylcholine synthesis and accumulation in cultures of dissociated sympathetic neurons. Proc. Natl. Acad. Sci. USA. 71, 3607–3610. doi: 10.1073/pnas.71.9.3607
Peddie, C. J., Davies, H. A., Colyer, F. M., Stewart, M. G., and Rodríguez, J. J. (2008). Colocalisation of serotonin2A receptors with the glutamate receptor subunits NR1 and GluR2 in the dentate gyrus, an ultrastructural study of a modulatory role. Exp. Neurol. 211, 561–573. doi: 10.1016/j.expneurol.2008.03.003
Pelletier, S., Gingras, S., and Green, D. R. (2015). Mouse genome engineering via CRISPR-Cas9 for study of immune function. Immunity. 42, 18–27. doi: 10.1016/j.immuni.2015.01.004
Peng, X., Katz, M., Gerzanich, V., Anand, R., and Lindstrom, J. (1994). Human alpha 7 acetylcholine receptor, cloning of the alpha 7 subunit from the SH-SY5Y cell line and determination of pharmacological properties of native receptors and functional alpha 7 homomers expressed in Xenopus oocytes. Mol. Pharmacol. 45, 546–554.
Peng, Y., Chu, S., Yang, Y., Zhang, Z., Pang, Z., and Chen, N. (2021). Neuroinflammatory In vitro cell culture models and the potential applications for neurological disorders. Front. Pharmacol. 12, 671734. doi: 10.3389/fphar.2021.671734
Pepitoni, S., Mallon, R. G., Pai, J. K., Borkowski, J. A., Buck, M. A., and Mcquade, R. D. (1991). Phospholipase D activity and phosphatidylethanol formation in stimulated HeLa cells expressing the human m1 muscarinic acetylcholine receptor gene. Biochem. Biophys. Res. Commun. 176, 453–458. doi: 10.1016/0006-291x(91)90945-4
Peralta, E. G., Ashkenazi, A., Winslow, J. W., Smith, D. H., Ramachandran, J., and Capon, D. J. (1987). Distinct primary structures, ligand-binding properties and tissue-specific expression of four human muscarinic acetylcholine receptors. EMBO J. 6, 3923–3929.
Platt, R. J., Chen, S., Zhou, Y., Yim, M. J., Swiech, L., Kempton, H. R., et al. (2014). CRISPR-Cas9 knockin mice for genome editing and cancer modeling. Cell. 159, 440–455. doi: 10.1016/j.cell.2014.09.014
Pleasure, S. J., Page, C., and Lee, V. M. (1992). Pure, postmitotic, polarized human neurons derived from NTera 2 cells provide a system for expressing exogenous proteins in terminally differentiated neurons. J. Neurosci. 12, 1802–1815. doi: 10.1523/jneurosci.12-05-01802.1992
Polanco, A., Kuang, B., and Yoon, S. (2020). Bioprocess technologies that preserve the quality of iPSCs. Trends Biotechnol. 38, 1128–1140. doi: 10.1016/j.tibtech.2020.03.006
Poprac, P., Jomova, K., Simunkova, M., Kollar, V., Rhodes, C. J., and Valko, M. (2017). Targeting free radicals in oxidative stress-related human diseases. Trends Pharmacol. Sci. 38, 592–607. doi: 10.1016/j.tips.2017.04.005
Poulin, B., Butcher, A., Mcwilliams, P., Bourgognon, J. M., Pawlak, R., Kong, K. C., et al. (2010). The M3-muscarinic receptor regulates learning and memory in a receptor phosphorylation/arrestin-dependent manner. Proc. Natl. Acad. Sci. USA. 107, 9440–9445. doi: 10.1073/pnas.0914801107
Qaseem, A., Snow, V., Cross, J. T. Jr., Forciea, M. A., Hopkins, R., Shekelle, P., et al. (2008). Current pharmacologic treatment of dementia, a clinical practice guideline from the American College of Physicians and the American Academy of Family Physicians. Ann. Intern. Med. 148, 370–378. doi: 10.7326/0003-4819-148-5-200803040-00008
Qiang, L., Fujita, R., Yamashita, T., Angulo, S., Rhinn, H., Rhee, D., et al. (2011). Directed conversion of Alzheimer's disease patient skin fibroblasts into functional neurons. Cell. 146, 359–371. doi: 10.1016/j.cell.2011.07.007
Rajan, K. B., Weuve, J., Barnes, L. L., Mcaninch, E. A., Wilson, R. S., and Evans, D. A. (2021). Population estimate of people with clinical Alzheimer's disease and mild cognitive impairment in the United States (2020-2060). Alzheimers. Dement. 17, 1966–1975. doi: 10.1002/alz.12362
Ring, K. L., Tong, L. M., Balestra, M. E., Javier, R., Andrews-Zwilling, Y., Li, G., et al. (2012). Direct reprogramming of mouse and human fibroblasts into multipotent neural stem cells with a single factor. Cell Stem Cell. 11, 100–109. doi: 10.1016/j.stem.2012.05.018
Riva, C., Hajduskova, M., Gally, C., Suman, S. K., Ahier, A., and Jarriault, S. (2022). A natural transdifferentiation event involving mitosis is empowered by integrating signaling inputs with conserved plasticity factors. Cell Rep. 40, 111365. doi: 10.1016/j.celrep.2022.111365
Robert, A., Liu, Y., Nguyen, M., and Meunier, B. (2015). Regulation of copper and iron homeostasis by metal chelators, a possible chemotherapy for Alzheimer's disease. Acc. Chem. Res. 48, 1332–1339. doi: 10.1021/acs.accounts.5b00119
Rock, R. B., Gekker, G., Aravalli, R. N., Hu, S., Sheng, W. S., and Peterson, P. K. (2008). Potentiation of HIV-1 expression in microglial cells by nicotine: involvement of transforming growth factor-beta 1. J. Neuroimmune Pharmacol. 3, 143–149. doi: 10.1007/s11481-007-9098-7
Rogers, S. W., Mandelzys, A., Deneris, E. S., Cooper, E., and Heinemann, S. (1992). The expression of nicotinic acetylcholine receptors by PC12 cells treated with NGF. J. Neurosci. 12, 4611–4623. doi: 10.1523/jneurosci.12-12-04611.1992
Rose, J. C., Popp, N. A., Richardson, C. D., Stephany, J. J., Mathieu, J., Wei, C. T., et al. (2020). Suppression of unwanted CRISPR-Cas9 editing by co-administration of catalytically inactivating truncated guide RNAs. Nat. Commun. 11, 2697. doi: 10.1038/s41467-020-16542-9
Ryan, J. M. (1979). Effect of different fetal bovine serum concentrations on the replicative life span of cultured chick cells. In Vitro. 15, 895–899. doi: 10.1007/bf02618046
Sachse, C., Xu, C., Wieligmann, K., Diekmann, S., Grigorieff, N., and Fändrich, M. (2006). Quaternary structure of a mature amyloid fibril from Alzheimer's Abeta(1-40) peptide. J. Mol. Biol. 362, 347–354. doi: 10.1016/j.jmb.2006.07.011
Sahu, M. P., Lågas, S., Kolehmainen, S., and Castrén, E. (2019). Culturing primary neurons from rat hippocampus and cortex. Neuronal Signal. 3, Ns20180207. doi: 10.1042/ns20180207
Saito, T., Matsuba, Y., Mihira, N., Takano, J., Nilsson, P., Itohara, S., et al. (2014). Single App knock-in mouse models of Alzheimer's disease. Nat. Neurosci. 17, 661–663. doi: 10.1038/nn.3697
Saleh, H., Schlatter, E., Lang, D., Pauels, H. G., and Heidenreich, S. (2000). Regulation of mesangial cell apoptosis and proliferation by intracellular Ca(2+) signals. Kidney Int. 58, 1876–1884. doi: 10.1111/j.1523-1755.2000.00359.x
Sandhu, F. A., Kim, Y., Lapan, K. A., Salim, M., Aliuddin, V., and Zain, S. B. (1996). Expression of the C terminus of the amyloid precursor protein alters growth factor responsiveness in stably transfected PC12 cells. Proc. Natl. Acad. Sci. USA. 93, 2180–2185. doi: 10.1073/pnas.93.5.2180
Sang, Z., Wang, K., Shi, J., Liu, W., and Tan, Z. (2019). Design, synthesis, in-silico and biological evaluation of novel chalcone-O-carbamate derivatives as multifunctional agents for the treatment of Alzheimer's disease. Eur. J. Med. Chem. 178, 726–739. doi: 10.1016/j.ejmech.2019.06.026
Sato, K., Wakamiya, A., Maeda, T., Noguchi, K., Takashima, A., and Imahori, K. (1995). Correlation among secondary structure, amyloid precursor protein accumulation, and neurotoxicity of amyloid beta(25-35) peptide as analyzed by single alanine substitution. J. Biochem. 118, 1108–1111. doi: 10.1093/oxfordjournals.jbchem.a124994
Schallmoser, K., Henschler, R., Gabriel, C., Koh, M. B. C., and Burnouf, T. (2020). Production and Quality Requirements of Human Platelet Lysate, A Position Statement from the Working Party on Cellular Therapies of the International Society of Blood Transfusion. Trends Biotechnol. 38, 13–23. doi: 10.1016/j.tibtech.2019.06.002
Schubert, D., Heinemann, S., and Kidokoro, Y. (1977). Cholinergic metabolism and synapse formation by a rat nerve cell line. Proc. Natl. Acad. Sci. USA. 74, 2579–2583. doi: 10.1073/pnas.74.6.2579
Schwartz, E. J., Rothman, J. S., Diana, M., Rousseau, C., Silver, R. A., et al. (2012). NMDA receptors with incomplete Mg2+ block enable low-frequency transmission through the cerebellar cortex. J. Neurosci. 32, 6878–6893. doi: 10.1523/jneurosci.5736-11.2012
Shao, Y., Tan, B., Shi, J., and Zhou, Q. (2019). Methotrexate induces astrocyte apoptosis by disrupting folate metabolism in the mouse juvenile central nervous system. Toxicol. Lett. 301, 146–156. doi: 10.1016/j.toxlet.2018.11.016
Sharma, G., and Vijayaraghavan, S. (2001). Nicotinic cholinergic signaling in hippocampal astrocytes involves calcium-induced calcium release from intracellular stores. Proc. Natl. Acad. Sci. USA. 98, 4148–4153. doi: 10.1073/pnas.071540198
Shen, C., Chen, Y., Liu, H., Zhang, K., Zhang, T., Lin, A., et al. (2008). Hydrogen peroxide promotes Abeta production through JNK-dependent activation of gamma-secretase. J. Biol. Chem. 283, 17721–17730. doi: 10.1074/jbc.M800013200
Shimohama, S., and Kawamata, J. (2018). Nicotinic Acetylcholine Receptor Signaling in Neuroprotection. Akaike, A., Shimohama, S., and Misu, Y. (eds). Singapore: Springer Copyright 2018. p. 137–158.
Shipley, M. M., Mangold, C. A., and Szpara, M. L. (2016). Differentiation of the SH-SY5Y human neuroblastoma cell line. J. Vis. Exp. 108, 53193. doi: 10.3791/53193
Shytle, R. D., Mori, T., Townsend, K., Vendrame, M., Sun, N., Zeng, J., et al. (2004). Cholinergic modulation of microglial activation by alpha 7 nicotinic receptors. J. Neurochem. 89, 337–343. doi: 10.1046/j.1471-4159.2004.02347.x
Soll, L. G., Grady, S. R., Salminen, O., Marks, M. J., and Tapper, A. R. (2013). A role for α4(non-α6)* nicotinic acetylcholine receptors in motor behavior. Neuropharmacology. 73, 19–30. doi: 10.1016/j.neuropharm.2013.05.001
Spicer, Z., and Millhorn, D. E. (2003). Oxygen sensing in neuroendocrine cells and other cell types, pheochromocytoma (PC12) cells as an experimental model. Endocr. Pathol. 14, 277–291. doi: 10.1385/ep:14:4:277
Srinivasan, R., Richards, C. I., Xiao, C., Rhee, D., Pantoja, R., Dougherty, D. A., et al. (2012). Pharmacological chaperoning of nicotinic acetylcholine receptors reduces the endoplasmic reticulum stress response. Mol. Pharmacol. 81, 759–769. doi: 10.1124/mol.112.077792
Steinbrenner, H., and Sies, H. (2009). Protection against reactive oxygen species by selenoproteins. Biochim. Biophys. Acta 1790, 1478–1485. doi: 10.1016/j.bbagen.2009.02.014
Stepanichev, M. (2020). Gene editing and alzheimer's disease, is there light at the end of the tunnel? Front. Genome Editing. 2, 4. doi: 10.3389/fgeed.2020.00004
Stohwasser, R., Giesebrecht, J., Kraft, R., Müller, E. C., Häusler, K. G., Kettenmann, H., et al. (2000). Biochemical analysis of proteasomes from mouse microglia, induction of immunoproteasomes by interferon-gamma and lipopolysaccharide. Glia. 29, 355–365.
Su, W. T., and Shih, Y. A. (2015). Nanofiber containing carbon nanotubes enhanced PC12 cell proliferation and neuritogenesis by electrical stimulation. Biomed. Mater. Eng. 26, S189–195. doi: 10.3233/bme-151305
Sun, E., and Shi, Y. (2015). MicroRNAs: small molecules with big roles in neurodevelopment and diseases. Exp. Neurol. 268, 46–53. doi: 10.1016/j.expneurol.2014.08.005
Sun, J. L., Stokoe, S. A., Roberts, J. P., Sathler, M. F., Nip, K. A., Shou, J., et al. (2019). Co-activation of selective nicotinic acetylcholine receptors is required to reverse beta amyloid-induced Ca(2+) hyperexcitation. Neurobiol. Aging. 84, 166–177. doi: 10.1016/j.neurobiolaging.2019.09.005
Sunderland, T., Linker, G., Mirza, N., Putnam, K. T., Friedman, D. L., Kimmel, L. H., et al. (2003). Decreased beta-amyloid1-42 and increased tau levels in cerebrospinal fluid of patients with Alzheimer disease. JAMA. 289, 2094–2103. doi: 10.1001/jama.289.16.2094
Suo, Z., Fang, C., Crawford, F., and Mullan, M. (1997). Superoxide free radical and intracellular calcium mediate A beta(1-42) induced endothelial toxicity. Brain Res. 762, 144–152. doi: 10.1016/s0006-8993(97)00383-1
Suzuki, N., Numakawa, T., Chou, J., De Vega, S., Mizuniwa, C., Sekimoto, K., et al. (2014). Teneurin-4 promotes cellular protrusion formation and neurite outgrowth through focal adhesion kinase signaling. FASEB J. 28, 1386–1397. doi: 10.1096/fj.13-241034
Takahashi, K., Tanabe, K., Ohnuki, M., Narita, M., Ichisaka, T., Tomoda, K., et al. (2007). Induction of pluripotent stem cells from adult human fibroblasts by defined factors. Cell. 131, 861–872. doi: 10.1016/j.cell.2007.11.019
Takahashi, T., Yamashita, H., Nakamura, S., Ishiguro, H., Nagatsu, T., and Kawakami, H. (1999). Effects of nerve growth factor and nicotine on the expression of nicotinic acetylcholine receptor subunits in PC12 cells. Neurosci. Res. 35, 175–181. doi: 10.1016/s0168-0102(99)00064-4
Tan, C. C., Yu, J. T., Wang, H. F., Tan, M. S., Meng, X. F., Wang, C., et al. (2014). Efficacy and safety of donepezil, galantamine, rivastigmine, and memantine for the treatment of Alzheimer's disease, a systematic review and meta-analysis. J. Alzheimers. Dis. 41, 615–631. doi: 10.3233/jad-132690
Tanti, G. K., and Goswami, S. K. (2014). SG2NA recruits DJ-1 and Akt into the mitochondria and membrane to protect cells from oxidative damage. Free Radic. Biol. Med. 75, 1–13. doi: 10.1016/j.freeradbiomed.2014.07.009
Taylor, R. C., Cullen, S. P., and Martin, S. J. (2008). Apoptosis, controlled demolition at the cellular level. Nat. Rev. Mol. Cell Biol. 9, 231–241. doi: 10.1038/nrm2312
Terpinskaya, T. I., Osipov, A. V., Kryukova, E. V., Kudryavtsev, D. S., Kopylova, N. V., Yanchanka, T. L., et al. (2021). α-Conotoxins and α-cobratoxin promote, while lipoxygenase and cyclooxygenase inhibitors suppress the proliferation of glioma C6 cells. Mar. Drugs. 19, 2. doi: 10.3390/md19020118
Terzi, E., Hölzemann, G., and Seelig, J. (1994). Reversible random coil-beta-sheet transition of the Alzheimer beta-amyloid fragment (25-35). Biochemistry 33, 1345–1350. doi: 10.1021/bi00172a009
Thanan, R., Oikawa, S., Hiraku, Y., Ohnishi, S., Ma, N., Pinlaor, S., et al. (2014). Oxidative stress and its significant roles in neurodegenerative diseases and cancer. Int. J. Mol. Sci. 16, 193–217. doi: 10.3390/ijms16010193
Thoenen, H. (1995). Neurotrophins and neuronal plasticity. Science. 270, 593–598. doi: 10.1126/science.270.5236.593
Toledo, J. B., Zetterberg, H., Van Harten, A. C., Glodzik, L., Martinez-Lage, P., Bocchio-Chiavetto, L., et al. (2015). Alzheimer's disease cerebrospinal fluid biomarker in cognitively normal subjects. Brain. 138, 2701–2715. doi: 10.1093/brain/awv199
Tremblay, R. G., Sikorska, M., Sandhu, J. K., Lanthier, P., Ribecco-Lutkiewicz, M., and Bani-Yaghoub, M. (2010). Differentiation of mouse Neuro 2A cells into dopamine neurons. J. Neurosci. Methods. 186, 60–67. doi: 10.1016/j.jneumeth.2009.11.004
Treutlein, B., Lee, Q. Y., Camp, J. G., Mall, M., Koh, W., Shariati, S. A., et al. (2016). Dissecting direct reprogramming from fibroblast to neuron using single-cell RNA-seq. Nature. 534, 391–395. doi: 10.1038/nature18323
U.S. Department of Health Human Services Centers for Disease Control Prevention National Center for Health Statistics. (2020). CDC WONDER online database: About Underlying Cause of Death, 1999-2019. Available online at: http://wonder.cdc.gov/ucd-icd10.html
Uhász, G. J., Barkóczi, B., Vass, G., Datki, Z., Hunya, A., Fülöp, L., et al. (2010). Fibrillar Abeta (1-42) enhances NMDA receptor sensitivity via the integrin signaling pathway. J. Alzheimers. Dis. 19, 1055–1067. doi: 10.3233/jad-2009-1301
Urbanc, B., Betnel, M., Cruz, L., Bitan, G., and Teplow, D. B. (2010). Elucidation of amyloid beta-protein oligomerization mechanisms, discrete molecular dynamics study. J. Am. Chem. Soc. 132, 4266–4280. doi: 10.1021/ja9096303
Urbanc, B., Cruz, L., Yun, S., Buldyrev, S. V., Bitan, G., Teplow, D. B., et al. (2004). In silico study of amyloid beta-protein folding and oligomerization. Proc. Natl. Acad. Sci. USA. 101, 17345–17350. doi: 10.1073/pnas.0408153101
Urban-Ciecko, J., Jouhanneau, J. S., Myal, S. E., Poulet, J. F. A., and Barth, A. L. (2018). Precisely timed nicotinic activation drives SST inhibition in neocortical circuits. Neuron. 97, 611-625.e615. doi: 10.1016/j.neuron.2018.01.037
van der Valk, J., Brunner, D., De Smet, K., Fex Svenningsen, A., Honegger, P., Knudsen, L. E., et al. (2010). Optimization of chemically defined cell culture media–replacing fetal bovine serum in mammalian in vitro methods. Toxicol. In Vitro. 24, 1053–1063. doi: 10.1016/j.tiv.2010.03.016
van Maurik, I. S., Zwan, M. D., Tijms, B. M., Bouwman, F. H., Teunissen, C. E., Scheltens, P., et al. (2017). Interpreting biomarker results in individual patients with mild cognitive impairment in the Alzheimer's biomarkers in daily practice (ABIDE) project. JAMA Neurol. 74, 1481–1491. doi: 10.1001/jamaneurol.2017.2712
Vazhappilly, R., Wee, K. S., Sucher, N. J., and Low, C. M. (2010). A non-muscle myosin II motor links NR1 to retrograde trafficking and proteasomal degradation in PC12 cells. Neurochem. Int. 56, 569–576. doi: 10.1016/j.neuint.2009.12.020
Vögler, O., Bogatkewitsch, G. S., Wriske, C., Krummenerl, P., Jakobs, K. H., and Van Koppen, C. J. (1998). Receptor subtype-specific regulation of muscarinic acetylcholine receptor sequestration by dynamin. Distinct sequestration of m2 receptors. J. Biol. Chem. 273, 12155–12160. doi: 10.1074/jbc.273.20.12155
Volpato, D., and Holzgrabe, U. (2018). Designing hybrids targeting the cholinergic system by modulating the muscarinic and nicotinic receptors, a concept to treat Alzheimer's disease. Molecules 23, 3230. doi: 10.3390/molecules23123230
Wang, G., Wang, T., Hu, Y., Wang, J., Wang, Y., Zhang, Y., et al. (2020). NMMHC IIA triggers neuronal autophagic cell death by promoting F-actin-dependent ATG9A trafficking in cerebral ischemia/reperfusion. Cell Death Dis. 11, 428. doi: 10.1038/s41419-020-2639-1
Wang, H., Liao, H., Ochani, M., Justiniani, M., Lin, X., Yang, L., et al. (2004). Cholinergic agonists inhibit HMGB1 release and improve survival in experimental sepsis. Nat. Med. 10, 1216–1221. doi: 10.1038/nm1124
Wang, H., Yu, M., Ochani, M., Amella, C. A., Tanovic, M., Susarla, S., et al. (2003). Nicotinic acetylcholine receptor alpha7 subunit is an essential regulator of inflammation. Nature. 421, 384–388. doi: 10.1038/nature01339
Wang, L., and Zhang, L. (2020). Circulating exosomal miRNA as diagnostic biomarkers of neurodegenerative diseases. Front. Mol. Neurosci. 13, 53. doi: 10.3389/fnmol.2020.00053
Wang, T. C., Chiu, H., Chang, Y. J., Hsu, T. Y., Chiu, I. M., and Chen, L. (2011). The adaptor protein SH2B3 (Lnk) negatively regulates neurite outgrowth of PC12 cells and cortical neurons. PLoS ONE. 6, e26433. doi: 10.1371/journal.pone.0026433
Wang, W.-L., Dai, R., Yan, H., Han, C.-N., Liu, L.-S., and Duan, X. (2015). Current situation of PC12 cell use in neuronal injury study. Int. J. Biotechnol. Wellness Ind. 4, 61–66.
Wang, X., Han, W., Yang, J., Westaway, D., and Li, L. (2019). Development of chemical isotope labeling LC-MS for tissue metabolomics and its application for brain and liver metabolome profiling in Alzheimer's disease mouse model. Anal. Chim. Acta. 1050, 95–104. doi: 10.1016/j.aca.2018.10.060
Wang, Y., Xu, Y., Liu, Q., Zhang, Y., Gao, Z., Yin, M., et al. (2017). Myosin IIA-related actomyosin contractility mediates oxidative stress-induced neuronal apoptosis. Front. Mol. Neurosci. 10, 75. doi: 10.3389/fnmol.2017.00075
Wang, Z. F., and Tang, X. C. (2007). Huperzine A protects C6 rat glioma cells against oxygen-glucose deprivation-induced injury. FEBS Lett. 581, 596–602. doi: 10.1016/j.febslet.2007.01.016
Watanabe, M., Phamduong, E., Huang, C. H., Itoh, N., Bernal, J., Nakanishi, A., et al. (2013). Formation of covalently modified folding intermediates of simian virus 40 Vp1 in large T antigen-expressing cells. J. Virol. 87, 5053–5064. doi: 10.1128/jvi.00955-12
Waterston, R. H., Lindblad-Toh, K., Birney, E., Rogers, J., Abril, J. F., Agarwal, P., et al. (2002). Initial sequencing and comparative analysis of the mouse genome. Nature 420, 520–562. doi: 10.1038/nature01262
Weerasekera, D., Hahn, J., Herrmann, M., and Burkovski, A. (2019). Induction of necrosis in human macrophage cell lines by corynebacterium diphtheriae and corynebacterium ulcerans strains isolated from fatal cases of systemic infections. Int. J. Mol. Sci. 20, 4109. doi: 10.3390/ijms20174109
Weiner, M. W., Veitch, D. P., Aisen, P. S., Beckett, L. A., Cairns, N. J., Cedarbaum, J., et al. (2015). 2014 Update of the Alzheimer's disease neuroimaging initiative: a review of papers published since its inception. Alzheimers. Dement. 11, e1–120. doi: 10.1016/j.jalz.2014.11.001
Weller, T. H., and Wheeldon, S. K. (1982). The cultivation in vitro of cells derived from adult Schistosoma mansoni. I. methodology; criteria for evaluation of cultures; and development of media. Am. J. Trop. Med. Hyg. 31, 335–348. doi: 10.4269/ajtmh.1982.31.335
Wess, J. (1996). Molecular biology of muscarinic acetylcholine receptors. Crit. Rev. Neurobiol. 10, 69–99. doi: 10.1615/critrevneurobiol.v10.i1.40
Westerink, R. H., and Ewing, A. G. (2008). The PC12 cell as model for neurosecretion. Acta Physiol (Oxf). 192, 273–285. doi: 10.1111/j.1748-1716.2007.01805.x
Wiatrak, B., Kubis-Kubiak, A., Piwowar, A., and Barg, E. (2020). PC12 cell line, cell types, coating of culture vessels, differentiation and other culture conditions. Cells 9, 958. doi: 10.3390/cells9040958
Wiedmer, L., Ducray, A. D., Frenz, M., Stoffel, M. H., Widmer, H. R., and Mevissen, M. (2019). Silica nanoparticle-exposure during neuronal differentiation modulates dopaminergic and cholinergic phenotypes in SH-SY5Y cells. J. Nanobiotechnology 17, 46. doi: 10.1186/s12951-019-0482-2
Williams, A. D., Sega, M., Chen, M., Kheterpal, I., Geva, M., Berthelier, V., et al. (2005). Structural properties of Abeta protofibrils stabilized by a small molecule. Proc. Natl. Acad. Sci. USA. 102, 7115–7120. doi: 10.1073/pnas.0408582102
Wolozin, B., Iwasaki, K., Vito, P., Ganjei, J. K., Sunderland, T., et al. (1996). Participation of presenilin 2 in apoptosis, enhanced basal activity conferred by an Alzheimer mutation. Science. 274, 1710–1713. doi: 10.1126/science.274.5293.1710
Wu, E. H., and Wong, Y. H. (2006). Activation of muscarinic M4 receptor augments NGF-induced pro-survival Akt signaling in PC12 cells. Cell. Signal. 18, 285–293. doi: 10.1016/j.cellsig.2005.04.009
Wu, G., Bazer, F. W., Dai, Z., Li, D., Wang, J., and Wu, Z. (2014). Amino acid nutrition in animals, protein synthesis and beyond. Annu. Rev. Anim. Biosci. 2, 387–417. doi: 10.1146/annurev-animal-022513-114113
Xi, K., Gu, Y., Tang, J., Chen, H., Xu, Y., Wu, L., et al. (2020). Microenvironment-responsive immunoregulatory electrospun fibers for promoting nerve function recovery. Nat. Commun. 11, 4504. doi: 10.1038/s41467-020-18265-3
Xiao, C., Srinivasan, R., Drenan, R. M., Mackey, E. D., Mcintosh, J. M., and Lester, H. A. (2011). Characterizing functional α6β2 nicotinic acetylcholine receptors in vitro, mutant β2 subunits improve membrane expression, and fluorescent proteins reveal responsive cells. Biochem. Pharmacol. 82, 852–861. doi: 10.1016/j.bcp.2011.05.005
Xiao, Y., Meyer, E. L., Thompson, J. M., Surin, A., Wroblewski, J., and Kellar, K. J. (1998). Rat alpha3/beta4 subtype of neuronal nicotinic acetylcholine receptor stably expressed in a transfected cell line, pharmacology of ligand binding and function. Mol. Pharmacol. 54, 322–333. doi: 10.1124/mol.54.2.322
Xicoy, H., Wieringa, B., and Martens, G. J. (2017). The SH-SY5Y cell line in Parkinson's disease research, a systematic review. Mol. Neurodegener. 12, 10. doi: 10.1186/s13024-017-0149-0
Yankner, B. A., Duffy, L. K., and Kirschner, D. A. (1990). Neurotrophic and neurotoxic effects of amyloid beta protein, reversal by tachykinin neuropeptides. Science. 250, 279–282. doi: 10.1126/science.2218531
Yoo, J. H., Valdovinos, M. G., and Williams, D. C. (2007). Relevance of donepezil in enhancing learning and memory in special populations, a review of the literature. J. Autism Dev. Disord. 37, 1883–1901. doi: 10.1007/s10803-006-0322-8
Yoon, J. K., Kim, J., Shah, Z., Awasthi, A., Mahajan, A., and Kim, Y. (2021). Advanced human BBB-on-a-Chip: a new platform for alzheimer's disease studies. Adv. Healthc. Mater. 10, e2002285. doi: 10.1002/adhm.202002285
Yuan, M., Kremer, D. M., Huang, H., Breitkopf, S. B., Ben-Sahra, I., Manning, B. D., et al. (2019). Ex vivo and in vivo stable isotope labelling of central carbon metabolism and related pathways with analysis by LC-MS/MS. Nat. Protoc. 14, 313–330. doi: 10.1038/s41596-018-0102-x
Zahs, K. R., and Ashe, K. H. (2010). 'Too much good news' - are Alzheimer mouse models trying to tell us how to prevent, not cure, Alzheimer's disease? Trends Neurosci. 33, 381–389. doi: 10.1016/j.tins.2010.05.004
Zeller, M., and Strauss, W. L. (1995). Retinoic acid induces cholinergic differentiation of NTera 2 human embryonal carcinoma cells. Int. J. Dev. Neurosci. 13, 437–445. doi: 10.1016/0736-5748(95)00025-c
Zeng, Z., Xu, J., and Zheng, W. (2017). Artemisinin protects PC12 cells against β-amyloid-induced apoptosis through activation of the ERK1/2 signaling pathway. Redox Biol. 12, 625–633. doi: 10.1016/j.redox.2017.04.003
Zhao, L., Zhu, L., and Guo, X. (2018). Valproic acid attenuates Aβ(25-35)-induced neurotoxicity in PC12 cells through suppression of mitochondria-mediated apoptotic pathway. Biomed. Pharmacother. 106, 77–82. doi: 10.1016/j.biopha.2018.06.080
Zheng, L., Terman, A., Hallbeck, M., Dehvari, N., Cowburn, R. F., Benedikz, E., et al. (2011). Macroautophagy-generated increase of lysosomal amyloid β-protein mediates oxidant-induced apoptosis of cultured neuroblastoma cells. Autophagy 7, 1528–1545. doi: 10.4161/auto.7.12.18051
Zhivotosky, B., and Orrenius, S. (2001). Assessment of apoptosis and necrosis by DNA fragmentation and morphological criteria. Curr. Protoc. Cell Biol. 18, 18.13.11–18.13.23. doi: 10.1002/0471143030.cb1803s12
Zhou, F. Q., Zhou, J., Dedhar, S., Wu, Y. H., and Snider, W. D. (2004). NGF-induced axon growth is mediated by localized inactivation of GSK-3beta and functions of the microtubule plus end binding protein APC. Neuron. 42, 897–912. doi: 10.1016/j.neuron.2004.05.011
Zhu, Z., Yang, T., Zhang, L., Liu, L., Yin, E., Zhang, C., et al. (2019). Inhibiting Aβ toxicity in Alzheimer's disease by a pyridine amine derivative. Eur. J. Med. Chem. 168, 330–339. doi: 10.1016/j.ejmech.2019.02.052
Keywords: Alzheimer's disease, PC12 cells, culture condition, differentiation methods, transfection methods, drugs inducing AD, in vitro cellular models used in parallel with PC12 cells
Citation: Xie D, Deng T, Zhai Z, Sun T and Xu Y (2023) The cellular model for Alzheimer's disease research: PC12 cells. Front. Mol. Neurosci. 15:1016559. doi: 10.3389/fnmol.2022.1016559
Received: 11 August 2022; Accepted: 08 December 2022;
Published: 04 January 2023.
Edited by:
Mukesh K. Pandey, Mayo Clinic, United StatesReviewed by:
Nicolas Sergeant, Institut National de la Santé et de la Recherche Médicale (INSERM), FranceGuang Hu, Chongqing University of Technology, China
Peng Li, University of Macau, China
Copyright © 2023 Xie, Deng, Zhai, Sun and Xu. This is an open-access article distributed under the terms of the Creative Commons Attribution License (CC BY). The use, distribution or reproduction in other forums is permitted, provided the original author(s) and the copyright owner(s) are credited and that the original publication in this journal is cited, in accordance with accepted academic practice. No use, distribution or reproduction is permitted which does not comply with these terms.
*Correspondence: Tao Sun, c3VudGFvNTEzJiN4MDAwNDA7Z21haWwuY29t; Ying Xu,
eHV5aW5nLmRvY3RvciYjeDAwMDQwO2dtYWlsLmNvbQ==
†These authors have contributed equally to this work