- Department of Radiology, Interventional Radiology Innovation at Stanford (IRIS), Stanford University, Palo Alto, CA, United States
Alzheimer’s disease (AD) is a major cause of age-related dementia and is characterized by progressive brain damage that gradually destroys memory and the ability to learn, which ultimately leads to the decline of a patient’s ability to perform daily activities. Although some of the pharmacological treatments of AD are available for symptomatic relief, they are not able to limit the progression of AD and have several side effects. Mesenchymal stem/stromal cells (MSCs) could be a potential therapeutic option for treating AD due to their immunomodulatory, anti-inflammatory, regenerative, antioxidant, anti-apoptotic, and neuroprotective effects. MSCs not only secret neuroprotective and anti-inflammatory factors to promote the survival of neurons, but they also transfer functional mitochondria and miRNAs to boost their bioenergetic profile as well as improve microglial clearance of accumulated protein aggregates. This review focuses on different clinical and preclinical studies using MSC as a therapy for treating AD, their outcomes, limitations and the strategies to potentiate their clinical translation.
Introduction
Alzheimer’s disease
Alzheimer’s disease (AD) is a neurodegenerative disease and the leading cause of age-associated dementia. It is the eighth leading cause of death in the United States and affects approximately 6.2 million people while accounting for about $305 billion in healthcare costs annually (Wong, 2020). AD is characterized by progressive brain damage that slowly destroys memory and the ability to learn, which eventually hinders patients in performing daily activities. Studies have identified three stages of AD progression: preclinical stage, mild cognitive impairment stage and dementia stage (No author list, 2021). The preclinical stage is of varied duration in which patients demonstrate no observable clinical symptoms but begin to exhibit early pathophysiological signs of AD, such as elevated amyloid-beta (Aβ) peptide and a reduction in glucose metabolism in the brain (No author list, 2021). However, while the brain can compensate for these early AD pathologies for an extent of time, the mild cognitive impairment stage is inevitably expressed as the disease progresses. Approximately one-third of patients with mild cognitive impairment then advance into the dementia stage within 5 years of symptom onset (No author list, 2021).
Alzheimer’s disease can be stratified into two main types: early onset and late-onset AD. Late-onset AD, which is mostly comprised of sporadic AD, is typically diagnosed at age 65 and older, and accounts for about 90% of the total AD cases (Reitz et al., 2020). On the other hand, early onset AD accounts for 5–10% of AD cases and is typically diagnosed before the age of 65 (Reitz et al., 2020). While most AD cases are sporadic, approximately 1% of cases are familial, meaning that the patient inherited AD-inducing genetic mutations from their parents (van der Flier et al., 2011). These familial cases usually manifest as early onset AD and are commonly associated with genetic mutations in amyloid precursor protein (APP), presenilin 1 (PS1), and presenilin 2 (PS2) (Swerdlow, 2007; van der Flier et al., 2011).
Pathophysiology
Although the precise pathophysiology of AD remains inconclusive, the progressive accumulation of Aβ plaques and neurofibrillary tangles (NFTs), which are aggregates of hyperphosphorylated tau protein, have been identified as the primary hallmark of AD. The extracellular deposition of Aβ plaques and the intracellular formation of NFTs leads to synapse loss, dystrophic neurites, microgliosis, and astrogliosis, all of which contribute to AD-associated brain atrophy (Pini et al., 2016). Interestingly, the formation of Aβ plaques is spatially and temporally separated from NFTs (Busche et al., 2019). The aggregation of Aβ plaques is an early event in the AD trajectory and is observed in the preclinical stage, while the accumulation of NFTs occurs at a timepoint closer to the later stages of AD, when neuronal dysfunction and degeneration has begun to induce clinical symptoms (He et al., 2018). Moreover, Aβ plaque accumulation initiates in the neocortex and progresses into deeper brain regions, while NFT accumulation initiates in the medial temporal lobe and spreads outward toward the Aβ-rich neocortex (Busche et al., 2019).
In AD, neuronal loss is found to be focal, predominantly in the cortex and hippocampus regions. Thus, it has been suggested that the coexistence of Aβ plaques and NFTs correlates with AD-driven behavioral symptoms such as memory loss and impaired cognitive abilities. The onset of these symptoms are likely a result of synapse loss, failure to maintain axon and dendrite functions, as well as extensive neuronal damage and death in the cortex and hippocampal regions (Bloom, 2014). Therefore, the transition from the asymptomatic preclinical stage into symptomatic stages of AD may be associated with the propagation of tau pathology into the Aβ plaque-rich cortex, suggesting a collaborative interaction between the two aggregates toward AD progression (Busche et al., 2019).
The aggregation of misfolded proteins, such as Aβ plaques and NFTs, is involved in the pathophysiology of various neurodegenerative diseases. Chaperone proteins, which influence protein folding, can contribute to both AD protection and advancement. For instance, heat shock proteins (HSPs), mainly HSP70 and HSP90, have been described as chaperone proteins that play critical roles in neurodegenerative diseases (Martin-Pena et al., 2018; Gupta et al., 2020). While HSP70 facilitates the clearing and refolding of misfolded proteins in AD, HSP90 stabilizes the misfolded proteins, leading to augmentation of Aβ aggregation and neurodegeneration (Gupta et al., 2020). Based on this hypothesis, HSP70 inducers and HSP90 inhibitors may have therapeutic potential in clearing tau and Aβ aggregates (Gupta et al., 2020).
Genetic mutations account for familial AD cases. Mutations in APP, PS1, and PS2 alter normal APP processing and produce the neurotoxic Aβ oligomer that gives rise to Aβ-induced neuro-toxicity and aggregation of Aβ plagues (Benilova et al., 2012). However, Aβ pathology is not the sole determinant of AD pathology, since disease onset is accompanied by the formation of NFTs in neurons. These intracellular aggregates are composed of hyperphosphorylated tau proteins and are associated with impaired microtubular cytoskeleton formation, synapse loss, and neurodegeneration (Alonso et al., 1996; Guillozet et al., 2003).
The interconnection between Aβ and tau pathology is demonstrated by the synergistic and correlative nature of amyloidosis and tau hyperphosphorylation, which are also interrelated in the induction of neural toxicity (Busche et al., 2019; Griner et al., 2019; Tripathi and Khan, 2020). An example of Aβ and tau interaction occurs in the mitochondria. Studies have shown that APP can be targeted to the mitochondria, and this mitochondria localization of APP correlates with AD adversity and only occurs in pathogenic brain areas of AD patients (Lin and Beal, 2006). The aggregated APP gets processed into the accumulative Aβ peptide by active γ-secretase complex, which leads to Aβ presence in the mitochondria. Aβ presence in the mitochondria then generates hyperphosphorylated tau proteins by interacting with mitochondrial Drp1 protein, which, in turn, disrupts microtubule function and induces neural toxicity (Manczak et al., 2011; Manczak and Reddy, 2012). Mitochondrial Aβ can also interact with Aβ–binding alcohol dehydrogenase (ABAD), leading to mitochondrial dysfunction and the production of reactive oxygen species (ROS) (Lin and Beal, 2006).
The main consequence of mitochondrial dysfunction, ROS accumulation and increased oxidative stress, serve as the major factors involved in AD pathogenesis. Postmortem studies in AD brains have found evidence of oxidative stress in the form of lipid peroxidation, DNA, RNA, and protein oxidation, and decreased antioxidant enzymes (Marcus et al., 1998; Pratico, 2008). This evidence supports the oxidative stress hypothesis as a significant mechanism of AD-induced neurodegeneration and neuron death (Marcus et al., 1998; Pratico, 2008). In fact, both Aβ aggregation and tau hyperphosphorylation can directly increase oxidative stress, since Aβ can act as an oxidant while hyperphosphorylated tau induces neuroinflammation and the consequent microglial ROS production (Nunomura et al., 2006; Alavi Naini and Soussi-Yanicostas, 2015; Cheignon et al., 2018).
Interestingly, postmortem analysis of AD brains from 20 AD patients also identified breakdown of the blood-brain barrier (BBB) (Nelson et al., 2016), likely due to pericyte detachment and degradation that is also observed during AD pathology. The loss of pericytes contribute to reduced BBB and dysregulated BBB transport, which increases the amount of vascular infiltrate entering the brain. Since soluble Aβ peptide is regularly transported across the BBB under normal physiological conditions, the disruption of BBB transport alters the balance between the efflux and influx of Aβ peptides, thereby contributing to reduced Aβ clearance, increased Aβ burden, and the formation of extracellular Aβ plaques in the brain (Deane et al., 2009). Downregulated glucose transport to the brain during AD progression also accelerates BBB breakdown and Aβ pathology, since the loss of glucose contributes to an energy deficiency that induces neuron necrosis and the resulting neuroinflammation (Nelson et al., 2016; Montagne et al., 2017).
Breakdown of the BBB leads to capillary leakage, which fosters neuronal death and injury via the accumulation of vascular-origin neurotoxic products in the brain. These neurotoxic products include RBC-derived hemoglobin, immunoglobulins, fibrinogen, thrombin, and plasminogen. Free Fe2+ from the vasculature can cause further ROS generation, and an increase in oxidative stress. In addition, the entry of albumin from the vasculature to the brain can inhibit blood flow, which contributes to ischemia or hypoxia-induced edema (Zlokovic, 2011). The BBB breakdown enhances neuroinflammation by enabling neutrophils and other immune cells to enter the brain (Zenaro et al., 2015). Enhanced neuroinflammation fosters neurotoxicity by producing ROS from activated immune cells, which causes synapse loss and neuronal damage and ultimately death (Schain and Kreisl, 2017). However, not all neuroinflammation is neurotoxic, and transient neuroinflammation and mobilization of blood-borne myeloid cells to the CNS during early stages of neural damage can have a neuroprotective effect. Alleviation of cognitive symptoms in an AD mouse model has been achieved by blockade of programmed cell death receptor 1 (PD-1/PD-L1), which induces a systemic immune response and enhances recruitment of monocyte-derived macrophages to the brain (Rosenzweig et al., 2019). However, even though immune enhancement achieved by checkpoint blockade has the potential to alleviate both Aβ and tau pathologies in some cases of AD, PD-1 blockade in another study failed to increase the infiltration of monocyte-derived macrophages into the brain and was unable to alter the Aβ burden (Baruch et al., 2016; Latta-Mahieu et al., 2018; Rosenzweig et al., 2019). Thus, additional neuroimmunology mechanisms and treatment options should be considered better to elucidate the role of neuroinflammation in AD pathology.
Current treatment approaches
There are currently two major approaches to AD intervention: symptomatic treatment, and disease-modifying therapy. These interventions are mainly recognized as pharmacological or cellular treatments.
Pharmacological treatments
Pharmacological AD treatment mainly utilizes psychotropic drugs that aim to alleviate behavioral and cognitive AD symptoms such as dementia. For instance, acetylcholinesterase (AChE) inhibitors, responsible for increasing the expression and half-life of the acetylcholine neurotransmitter, have been used to improve AD-induced cognitive function. AChE inhibitors such as rivastigmine, galantamine and donepezil, and NMDA-receptor antagonists like memantine, have been approved by the FDA for AD treatment (Alzheimer’s Association, 2019). However, these drugs are not able to limit the progression of AD and have several side effects. Thus, there is need for development of a molecule that can target multiple factors involved in AD.
Recently, therapies utilizing APP inhibitors, ROS inhibitors, anti-inflammatory, and anti-tau factors have been proposed as potential treatments for AD (Scarpini et al., 2003; Cummings et al., 2019; Xie et al., 2020). However, their efficacy remains uncertain, since some of them are still undergoing clinical trials while others have failed to demonstrate therapeutic efficacy in clinical settings. Recently, a monoclonal antibody targeted against Aβ (aducanumab) was approved for treating AD in the United States; however, the approval was highly controversial due to a lack of evidence that the drug is effective (Rabinovici, 2021).
Cell therapy
In contrast to the symptom-oriented approach of pharmacological therapies, cell therapy aims to target the origin of AD pathologies by replacing lost neurons, clearing toxic aggregates, stimulating neuronal precursors, and enhancing neuroprotection (Si and Wang, 2021). Cell therapy typically utilize stem cells, chosen for their multilineage differentiation and self-renewal capacities. Under the umbrella of the cell therapies are two main therapeutic strategies. The first seeks to directly supply new neurons via engraftment and differentiation of transplanted stem cells (i.e., cell replacement therapy). The second relies on the transplanted cells’ ability to release soluble factors that indirectly stimulate endogenous neural regeneration or promote neuroprotection. Various pre-clinical research and clinical trials have been conducted to determine the therapeutic efficacy of stem cell-based therapies for intractable neurodegenerative diseases like AD.
Different types of stem cells have been utilized for cell therapy in AD. Cell replacement therapies rely on transplanted cells engrafting and differentiating into neuronal fates, and thus must use competent cells to give rise to neurons. These include induced pluripotent stem cells (iPS), embryonic stem cells (ES), and neural stem cells (NSCs). However, ES and iPS cells have inherent risks of teratoma formation and immune rejection. Additionally, transplanted cells would have to demonstrate robust engraftment and site-appropriate neuronal differentiation and synaptic integration, which has not been achieved. Due to these difficulties, some have given up on direct engraftment of stem cells and have turned instead to the ability of adult stem cells to stimulate endogenous neural regeneration via paracrine effects. Mesenchymal stem/stromal cells (MSCs) have been a popular platform for cell therapy in general. While they are not competent to give rise to neural cell types by themselves, they have been described to have anti-inflammatory and immunomodulatory properties. Preclinical studies have reported the therapeutic efficacy of MSC-based therapies, and several clinical trials have explored the potential of clinically translating these therapies.
Characteristics of mesenchymal stem/stromal cells
When they were first discovered, MSCs were recognized as a subcomponent of the bone marrow (BM) cell population. They were defined by the International Society of Cellular Therapy (ISCT) as adherent fibroblastic cells that can differentiate into osteocytes, chondrocytes, and adipocytes (Dominici et al., 2006). In addition, MSCs express cell surface markers such as CD105, CD73, and CD90, and do not express CD45, CD19, CD11b, and HLA-DR surface molecules (Dominici et al., 2006; Maleki et al., 2014). MSCs cannot be characterized by the expression of a single, specific marker, and they behave differently under different conditions like hypoxia and inflammation. The metabolic profile, secretomes, and proteome of MSCs may also vary based on their origin, culture conditions and microenvironment. These environment and origin-based variations make it challenging to identify and characterize MSCs, and the criteria provided by ISCT might not be enough for the proper characterization of MSCs (Maleki et al., 2014). Indeed, it is generally agreed that MSCs are not a coherent cell type, but rather a mix of various stem, progenitor, and mature cell types. Despite these issues, MSCs have remained popular for cellular therapy research, as they are easy to obtain and expand in vitro.
Applications of mesenchymal stem/stromal cells and mesenchymal stem/stromal cell-derived therapeutics
Mesenchymal stem/stromal cells have been established as an important platform for cell therapy for treating various injuries and illnesses. Several clinical trials have been conducted to assess the therapeutic efficacy of MSC-derived therapies for a variety of diseases, but only 9 hMSC-based products have acquired legal approval for clinical application. In South Korea, Cellgram AMI, Cartistem, Cupistem, and Neuronata-R have been approved for the treatment of myocardial infarction, cartilage defects, Crohn’s diseases and amyotrophic lateral sclerosis, respectively. Similarly, Prochymal, an intravenous formulation of mesenchymal stem cells, has been approved in Canada, New Zealand, and Australia while TEMCELLS has been approved in Japan for the treatment of acute graft versus host disease (GVHD). Recently, STEMIRAC and Stempeucel have been approved for the treatment of spinal cord injury and Buerger’s disease-induced critical limb ischemia, respectively (Lopez-Beas et al., 2020). Some of these commercial MSC-based therapeutics have shown beneficial effects whereas the results of a few other products have not been published yet.
Therapeutic potential of mesenchymal stem/stromal cells for AD treatment
Neurodegenerative diseases, like AD, are characterized by abnormal protein aggregation leading to neuroinflammation and neuronal cell damage. The neuronal loss leads to synaptic dysfunction that fosters clinical symptoms such as memory, cognitive, and behavioral impediments (No author list, 2021). MSC-based therapies have been investigated as a cell therapy for AD due the paracrine ability of MSCs to secrete growth factors, anti-inflammatory proteins, membrane receptors, and microRNAs (miRNA) that aid in the reduction of neuronal loss by blocking apoptosis and increasing neurogenesis, synaptogenesis, and angiogenesis (Nooshabadi et al., 2018).
Mesenchymal stem/stromal cells are commonly derived from bone marrow and adipose tissues, and are capable of secreting factors that promote recruitment, proliferation and differentiation of other neural stem cells. Their role as an antioxidant coupled with their anti-apoptotic effects foster the inhibition of neuronal cell death, and secrets growth factors, such as brain-derived neurotrophic factor (BDNF) and glial cell line-derived neurotrophic factor (GDNF), to promote neurogenesis via the stimulation of neural progenitor cells (Harris et al., 2012; Van Velthoven et al., 2012; Reza-Zaldivar et al., 2018). In addition, MSCs exert immunomodulatory effects by inhibiting the activation of inflammatory microglia (M1) and promoting the activation of the anti-inflammatory microglia (M2) to prevent further tissue damage induced by chronic neuroinflammation. MSCs also accelerate the accumulation of microglia around Aβ deposits to promote Aβ clearance, and studies have revealed that MSCs can enhance activation of autophagy, which might be responsible for lysosomal clearance of Aβ plaques (Shin et al., 2014; Yokokawa et al., 2019; Figure 1).
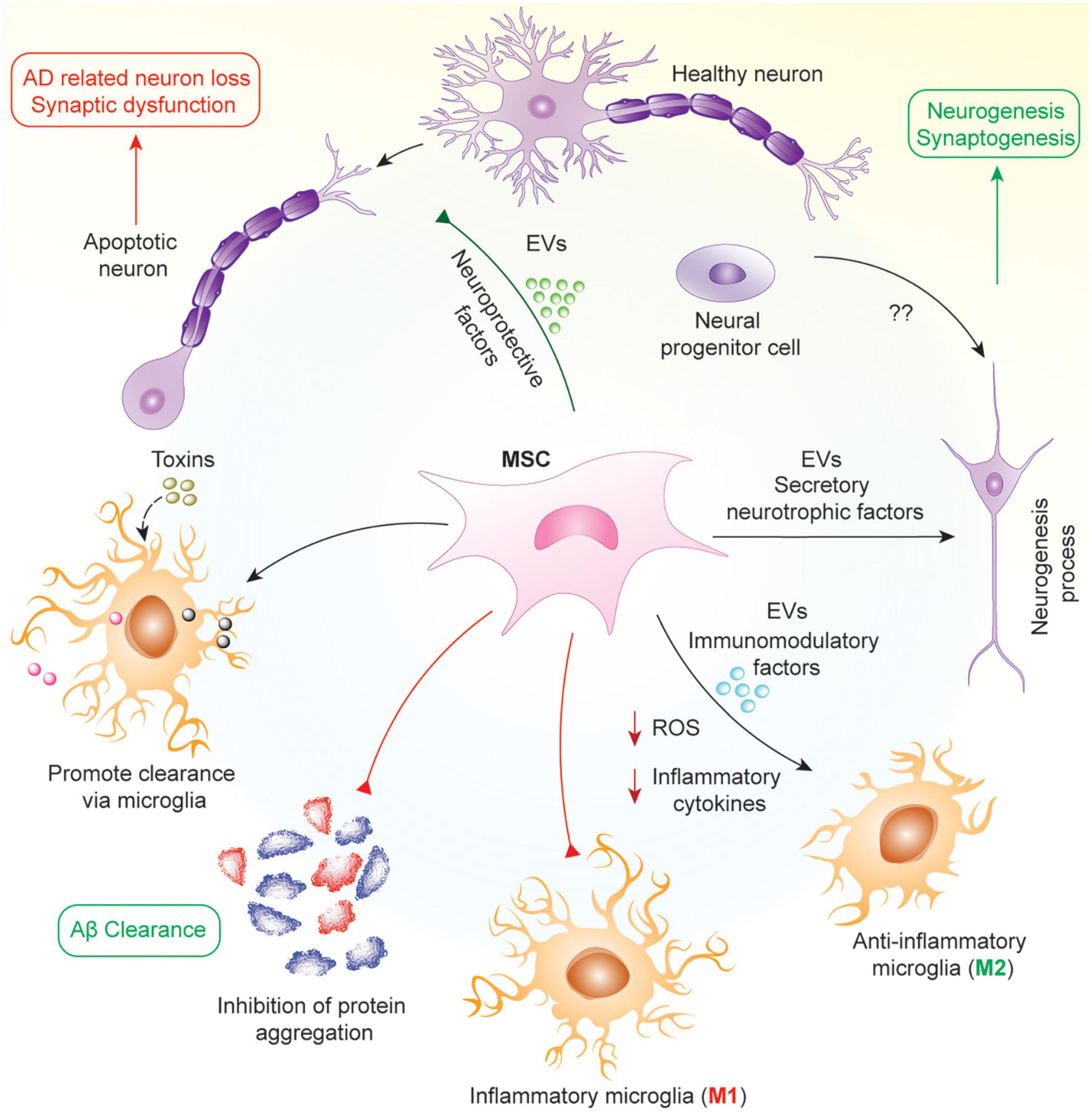
Figure 1. Mechanism of protective effects of MSCs in AD. In AD, neuronal loss and synaptic dysfunction occur due to the apoptosis of neurons by accumulating different proteins such as Aβ and hyperphosphorylated tau. MSCs work in pleiotropic mechanisms in this pathology to attenuate AD via secretion of various neuroprotective and neurotrophic factors such as VEGF, GDNF, BDNF, and several miRNAs in soluble or insoluble form as EVs. As a result, MSCs help in the clearance of aggregated proteins by increasing microglial phagocytosis, reprogramming microglia into anti-inflammatory phenotype, attenuating oxidative stress and neuronal apoptosis, and promoting neurogenesis from neural progenitor cells.
In early stage AD, there is aggregation of Aβ and inefficient clearance of these aggregates resulting in the formation of amyloid plaques. In mid-stage AD, there is the formation of neuro-fibrillary tangles and neuronal cell death due to these aggregates. Finally, in late-stage AD, there is inflammation and oxidative stress mediated by reactive microglia. Hence, the mechanism by which MSCs work will be dependent on the stage of the disease in which they are being used. In early and mid-stages, MSCs will work to inhibit Aβ generation and promote its effective clearance, alter APP processing, decrease tau phosphorylation and increase proteasomal activity resulting in reduced accumulation of ubiquitin-conjugated proteins (Lee et al., 2017; Mendonça et al., 2019; Neves et al., 2021). In later stages, their effects will be more geared toward microglial reprograming, reducing the number of reactive microglia in the brain and promoting anti-inflammatory/anti-oxidant strategies (Cui et al., 2017; Zhao et al., 2018; Peruzzaro et al., 2019). Furthermore, MSCs have also been shown to promote microglia and autophagy mediated clearance of protein aggregates including Aβ (Kim et al., 2012; Shin et al., 2014; Yokokawa et al., 2019). Throughout all stages of AD, MSCs are able to protect neurons from cell death via secretion of different neuroprotective factors, growth factors and, mitochondrial transfer (Cui et al., 2017; Wang et al., 2018; Zhang et al., 2020). Hence, MSCs have multiple effects based on the disease condition, with their activity determined by the surrounding microenvironment in which they finally reside following their administration.
As stated earlier, the anti-inflammatory, immunomodulatory, and neuro-regenerative aspects of MSCs highlight them as potential therapeutics for AD. The therapeutic potential of MSCs has been suggested by various studies, in which intravenous injection of placenta-derived MSCs in Aβ-infused mice significantly inhibited neuroinflammation while improving cognitive function (Yun et al., 2013). The intravenous delivery of human umbilical cord mesenchymal stem cells (UC-MSCs) in transgenic AD mice (Tg2576) inhibited oxidative stress while fostering neuro-repair and neurogenesis (Cui et al., 2017). The therapeutic effect of UC-MSC engraftment was enhanced when UC-MSCs were treated with resveratrol, which is an activator that has been suggested to rejuvenate and improve the survival and differentiation of resident stem cells (Gorbunov et al., 2012; Wang et al., 2018). In addition, Aβ accumulation was significantly decreased by UC-MSC infusion (Lykhmus et al., 2019). Aβ deposition in the hippocampus and cortex regions of APP/PS1 mice was reduced after intra-carotid arterial injection of UC-MSCs, which is also associated with improved cognitive function in vivo (Boutajangout et al., 2017).
In addition to UC-MSCs, those from other sites of origin have also demonstrated therapeutic efficacy for AD. For instance, stereotactic injection of amniotic MSCs into bilateral hippocampi of APP/PS1 mice significantly stimulated microglial activation and phagocytic activity, resulting in Aβ clearance, neurogenesis and cognitive improvement (Zheng et al., 2017). Intracerebral injection of menstrual blood-derived MSCs correlates with reduced BACE1 (β-APP cleaving enzyme 1) and β-CTF in the cortex and hippocampus of APP/PS1 mice, suggesting an inhibition of β-secretase activity that diminished the formation of Aβ plagues (Zhao et al., 2018). Preclinical studies on the effectiveness of MSC-derived treatments in AD are listed in Table 1.
Despite the effectiveness of MSC-based therapies in preclinical studies, they have largely failed to be clinically translated. Although MSC therapies are feasible, safe, and well tolerated when delivered into the brain via stereotactic injection, a recent clinical trial found minimal therapeutic improvements in a 24-month follow-up period (Kim H. J. et al., 2015). Nevertheless, this phase 1 study paved way for further evaluation of MSC therapies in larger cohorts with long-term follow-up, and many clinical trials are currently being conducted in AD patients. In another clinical trial, a single dose I.V. injection of MSCs showed improvement in inflammation and neurocognitive function compared to a placebo (Oliva et al., 2021; Brody et al., 2022; Table 2). The dose of MSCs via stereotactic injection into the hippocampus was 3-to-33 times less than the clinical trial with I.V. injection. Both of these clinical trials support the potential of MSCs in treating AD. However, the variation in doses, MSCs source, disease onset time, route of delivery, and small study population hinder any definitive conclusions. Nevertheless, local injection of MSCs might be an effective strategy in decreasing the dose of MSCs being used for therapy due to minimal loss in the systemic circulation.
Impact of mesenchymal stem/stromal cells on neurons and neural progenitor cells
Mesenchymal stem/stromal cells are involved in neuroprotection via the secretion of anti-inflammatory cytokines and anti-apoptotic, angiogenic, and neurotrophic factors. Direct contact between primary neurons and MSCs is believed to enhance the long-term survival of neurons and may play a vital role in neuronal maturation and differentiation (Scuteri et al., 2006). Furthermore, MSCs have been shown to prevent oxidative stress in neurons as well as Aβ/tau-induced toxicity by clearing Aβ or tau via autophagy activation, increasing proteasome activity and promoting microglial phagocytosis (Yan et al., 2014b; Lee et al., 2017; Zhao et al., 2018; Neves et al., 2021; Santamaria et al., 2021). It had been postulated that MSCs could transdifferentiate into neuron-like cells, which would serve as an asset in AD therapies (Yang et al., 2013). The differentiation of MSCs into a neuronal lineage is still controversial, even though some research shows the ability of MSCs to differentiate into neural-like cells (Scuteri et al., 2011a; Divya et al., 2012; Jeong et al., 2013; Feng et al., 2014; Haragopal et al., 2015; Joe et al., 2015; Hernández et al., 2020; Zhou et al., 2020). Interestingly, a study by Lu et al. showed morphological and immunocytochemical changes in MSCs after their exposure to a medium for neuronal induction and differentiation, which they attributed to not be the consequence of true neuronal differentiation but rather a biological response to chemical stress (Lu et al., 2004). In another study, it was shown that newly generated neuronal cells regained their MSC morphology as soon as the neuronal induction therapy stopped, indicating that this change is reversible in many instances (Lukomska et al., 2019). Other studies have also shown a beneficial interaction between MSCs and NSCs in promoting neurogenesis (i.e., differentiation, proliferation and survival of NSCs), neuroprotection as well as facilitating the differentiation of MSCs to neural cells (Alexanian, 2005; Oh et al., 2011; Haragopal et al., 2015; Ju et al., 2015; Kim D. H. et al., 2015). Hence, the co-transplantation of NSCs and MSCs. or transplantation of NSCs-primed MSCs. could be an effective strategy for neuroregeneration and neuroprotection.
Neuroprotective effect of mesenchymal stem/stromal cells on co-cultured neurons
Co-culturing MSCs with primary rat cortical neurons has been shown to protect neurons from apoptosis and enhance their survival (Scuteri et al., 2011b; Scheibe et al., 2012). MSC-co-cultured neurons demonstrated no signs of degeneration and survived 60 days or more, while primary neuronal cultures without MSC exposure experienced cellular death in a few days (Scuteri et al., 2011b). This MSC-induced neuroprotection and promotion of long-term neuron survival was suggested to be the result of downregulated matrix metalloproteinase (MMP) activity and the fostering of neuronal maturation (Scuteri et al., 2006, 2011b).
Co-culture of MSCs with hippocampal neurons in a transwell system protected neurons from Aβ-induced oxidative stress and prevented the synapse loss that is typically associated with Aβ exposure (de Godoy et al., 2018). This protective effect was speculated to be a consequence of MSC-mediated Aβ clearance (de Godoy et al., 2018). The study further reported that MSCs are capable of internalizing fibrillary Aβ and neurotoxic Aβ oligomers without compromising their own viability, proliferation and ROS levels, and that the internalized Aβ oligomers and fibrils then undergo endosomal and lysosomal clearance (de Godoy et al., 2018). This MSC-induced autophagy and lysosomal clearance correlates with neuroprotection in vivo and in vitro and insinuates a connection between MSCs and microglial activation (Shin et al., 2014). For instance, when LPS-stimulated microglia and dopaminergic neurons were co-cultured with MSCs, the anti-inflammatory and clearance-inducing effects of MSC led to a decrease in LPS-induced damage and suppression of dopaminergic neuronal loss (Kim et al., 2009).
Role of mesenchymal stem/stromal cell-secreted neuroprotective factors
Mesenchymal stem/stromal cell-induced neuron survival and neuroprotection have been suggested to be a result of various MSC-secreted factors. For instance, thrombospondin-1 secreted by UC-MSCs has been shown to rescue synaptic dysfunction induced by Aβ deposition in hippocampal neurons via the upregulation of neuroligin-1 (NLGN1) and the voltage-activated Ca2+ channel subunit α2δ-1, both of which are involved in glutamatergic synapses and mediation of long-term potentiation (Kim et al., 2018b). In addition, MSCs have been shown to secrete BDNF growth factor to upregulate AKT phosphorylation while downregulating p38 phosphorylation in order to protect neurons against trophic factor withdrawal and ROS exposure (Wilkins et al., 2009). BDNF-overexpressing hMSCs have been shown to have an augmented neuroprotective effect (Scheper et al., 2019).
Other MSC-secreted neuroprotective factors include the antioxidant enzyme superoxide dismutase 3 (SOD3) and agouti-related peptide (AgRP) (Kemp et al., 2010). Inflammatory cytokines, specifically tumor necrosis factor alpha (TNF-α) and interferon-gamma (IFN-γ), can induce MSCs to secrete SOD3, thereby reducing the build-up of excess superoxide and enhancing neuronal and axonal survival in vitro (Kemp et al., 2010). Pretreating MSCs with the antioxidant tanshinone has been reported to reduce neuroinflammation and suppress ROS in rats with Aβ-induced neuroinflammation (Huang et al., 2019). In another study, when SH-SY5Y neuroblastoma cells were co-cultured with MSCs, the ubiquitin proteasomal system was significantly upregulated in a dose-dependent manner based on the concentration of the MSC-secreted cytokine AgRP (Lee et al., 2017). This upregulation in proteasome activity fosters the formation of autolysosomes, which propels the clearance of abnormal protein aggregates, enhances neuron survival and reduces cognitive impairment in AD (Lee et al., 2017).
Neuroprotective effects of mesenchymal stem/stromal cell-secreted extracellular vesicles
The neuroprotective effect of MSCs is also exerted by secreted extracellular vesicles (EVs), which deliver antioxidant catalase and other MSC-derived factors (de Godoy et al., 2018). miRNA-21 derived from MSC-secreted exosomes has been shown to inhibit neuron apoptosis by downregulating the expression of phosphatase and tensin homolog (PTEN) and programmed cell death protein 4 (PDCD4) in rats with spinal cord injury (Kang et al., 2019). Interestingly, the secretome of MSCs varies based on the time that the conditioned media (CM) is collected, exerting different effects on neurons and glial cells. The CM collected during earlier time points (24 h) enhanced neuron viability while CM collected at later time points (96 h) contributed to higher glial viability (Ribeiro et al., 2011), though the factors responsible for this difference were not determined. Exosomal miR-21 from MSCs have been shown to not only inhibit neuronal apoptosis but also improve the cognition and memory in transgenic APP/PS1 mice, thereby reducing Aβ deposition and downregulating pro-inflammatory cytokines. This effect was further improved with exosomes from hypoxia pre-conditioned MSCs (Cui et al., 2018). miR-146a in BM-MSC derived exosomes decreased NF-kB in astrocytes thereby restoring their function, which, in turn, promoted synaptogenesis and improved cognitive function (Nakano et al., 2020). Recently, BM-MSC derived EVs exhibited a protective effect on hippocampal neurons by decreasing amyloid beta deposition and reducing inflammatory cytokines in an amyloid beta-induced rat model of AD; these effects were deeded to be mediated by miR-29c-3p which was shown to activate the Wnt/β-catenin pathway (Sha et al., 2021). In another study, miR-29b overexpressed exosomes from MSCs reduced neuronal cell death and the pathology of Aβ in a rat model of AD while also showing improvement in spatial learning and memory (Jahangard et al., 2020). Similarly, miR-455-3p from BM-MSCs also ameliorated neuronal injury in the hippocampus (Gan and Ouyang, 2022).
Role of mesenchymal stem/stromal cells on microglia
Microglia are the resident phagocytic immune cells of the central nervous system (CNS). They are involved in immune surveillance, pathogen defense, and maintenance of homeostasis in the brain (Li and Barres, 2018). Microglia are also crucial for the development of the brain and play a critical role in neurogenesis, myelin turnover, and the modeling and pruning of synaptic architecture and network (Li and Barres, 2018).
Under normal brain physiology, microglia are responsible for the clearance and degradation of extracellular Aβ, which they recognize through microglial integrin receptors and recruitment of several enzymes such as the pro-inflammatory matrix metalloproteinases (MMPs) to restrict the formation of Aβ plaques (Konnecke and Bechmann, 2013; Doens and Fernandez, 2014; Hansen et al., 2018). However, in AD pathology, the accumulation of Aβ aggregates and other chemokines released from injured neurons prolongs the activation of microglia, which can lead to chronic neuroinflammation and subsequent accumulation of ROS (Leng and Edison, 2021). Activated microglia are characterized by elevated expressions of CD86, CD40 and Iba-1, and are also responsible for secretion of inflammatory cytokines and chemokines to recruit peripheral immune cells into the brain (Leng and Edison, 2021). However, while transient neuroinflammation is beneficial for neurogenesis and aggregate clearance, excessive or chronic neuroinflammation that fails to resolve itself can worsen AD symptoms due to ROS generation, necrosis, and collateral tissue damage in the brain (DiSabato et al., 2016; Schain and Kreisl, 2017; Yong et al., 2019). Thus, microglial activation plays a critical role in AD pathology and has been proposed as a target for AD therapy (Solito and Sastre, 2012; Siskova and Tremblay, 2013).
Mesenchymal stem/stromal cells have been proposed as mediators of AD therapy by targeting microglia due to their immunomodulatory effects. As a result, interactions between MSCs and microglia have been studied extensively in the context of neurological diseases. Intraparenchymal MSC transplantation in rats with traumatic brain injury has been shown to improve fine motor function, an effect the authors attribute to MSCs shifting microglia from a classical inflammation (CD86) to an alternative inflammation state (CD163) (Peruzzaro et al., 2019). MSCs transplanted in AD mice have been reported to reduce microglial production of pro-inflammatory factors TNF-α, IL-1β, iNOS, and COX-2, and upregulate the expression of Aβ-degrading enzymes such as insulin-degrading enzyme (IDE) and neprilysin (NEP) (Zhao et al., 2018). This led to an improved clearance of abnormal protein aggregates, including Aβ plaques and hyperphosphorylated tau, without causing chronic neuroinflammation (Zhao et al., 2018).
Interestingly, studies suggest that MSCs have the ability to reprogram microglia into an “M2-like” phenotype that is characterized by an increase in phagocytic activity and a reduction in neuroinflammation. In fact, MSCs induce a mixed microglial phenotype that is CD206-high, Arg1-high, CD86-high, IL-10-high, MCP-1/CCL2-high, PGE2-high, IL-1β-moderate, TNF-α-low, and NALP-3-low (Hegyi et al., 2014). Thus, it has been speculated that the therapeutic effect of MSCs in AD pathology is related to their ability to alter microglia cells from the inflammatory, neurotoxic phenotype to a neuroprotective, anti-inflammatory phenotype that fosters neuro-regeneration and repair (Hegyi et al., 2014).
Impact of mesenchymal stem/stromal cell-secreted factors on microglia
The therapeutic benefits and tissue repair observed in MSC transplantation has been associated with their ability to modulate the functional behavior of cells in the brain via paracrine mechanisms associated with MSC-secreted cytokines, growth factors, chemokines, and EVs (Gnecchi et al., 2016). For instance, human UC-MSCs secrete soluble intercellular adhesion molecule-1 (sICAM-1) after co-culturing with BV2 microglia which, in turn, diminish accumulation of Aβ plaques (Kim et al., 2012). Release of sICAM-1 increased after Aβ induction in the BV2 microglia, and an up-regulation of NEP enzyme was observed in co-cultured microglia as compared to those that were not exposed to MSCs (Kim et al., 2012). sICAM-1 also interrupts CD40/CD40L activity, thereby reducing pro-inflammatory signaling and enhancing microglial phagocytosis for Aβ and tau deposits (Kim et al., 2012). In addition, MSCs also up-regulate the expression of CD14, an important receptor for Aβ uptake, in microglia to facilitate microglial internalization and clearance of Aβ deposits, both in vitro and in vivo (Yokokawa et al., 2019). Growth differentiation factor-15 (GDF-15) secreted by UC-MSCs has also been associated with enhanced BV2 microglial Aβ clearance, in vitro and in vivo, through the upregulation of IDE (Kim et al., 2018a).
The ability of MSCs to regulate microglia activation also relies on its secreted paracrine factors. For instance, transwell co-culture of MSCs and LPS-stimulated microglia attenuated the activation of microglia with increased IL-6, IL-10, and TGF-β expressions, and reduced NO and TNF-α production (Kim et al., 2009). MSC-secreted CX3CL1 also exerts a regulatory effect on microglia by inhibiting expression of TNF-α, inducible nitric oxide synthase (iNOS), and ROS (Giunti et al., 2012). This enables an alternate microglial activation that enhances microglial phagocytic capacity without the onset of neurotoxic, chronic neuroinflammation, thereby reducing cellular damage and apoptosis (Giunti et al., 2012). Thus, it has been suggested that the ability of MSCs to improve microglial phagocytic activity under the anti-inflammatory, neuroprotective phenotype is dependent on the secretion of CX3CL1 (Giunti et al., 2012). While MSCs induce functional changes to microglia, they do not appear to promote microglial proliferation (Giunti et al., 2012). In fact, studies suggest that MSCs actually exhibit anti-proliferative effect toward BV2 microglia by reducing TNF-α expression, increasing the percentage of BV2 microglia that are under G0/G1 cell cycle arrest even in the face of LPS stimulation (Jose et al., 2014). Table 3 summarize different studies showing the effect of MSCs in modulating microglia for decreasing neuroinflammation and neuroprotection.
Limitations of mesenchymal stem/stromal cells for the treatment of Alzheimer’s disease
The therapeutic efficacy of MSC-based therapies depends on a variety of factors, including a homogenous cell population, the source of MSCs, the optimal dose of transplanted cells, time of transplantation, and suitable route for cell delivery. However, an optimal protocol for the isolation, characterization, and expansion of MSCs remains poorly characterized, and there is an unmet need to determine the appropriate dose, route and time for MSC transplantation. The occurrence of immune responses, especially from allogeneic transplantations of cells, also complicates the application of MSC therapies. While some clinical trials reported evidence of therapeutic efficacy, many studies failed to observe clinical improvement after MSC therapy. In addition to the above-mentioned variables, the lack of homing of MSCs to brain is another hurdle in the treatment of neurodegenerative diseases as blood-brain barrier (BBB) also increases the difficulty of MSC delivery to the brain.
Strategies to overcome limitations
Facilitating mesenchymal stem/stromal cell delivery to the brain
Although MSCs possess some homing capacity to sites of injury, very few intravenously injected cells successfully migrate to the target site, and the majority end up entrapped in the lung microvasculature instead of the brain (Ullah et al., 2019). The BBB is another major limiting factor that compromises the delivery of therapeutics, including MSCs, into the brain for treatment of neurodegenerative diseases (Hour et al., 2020). As a result, several studies have attempted to bypass the BBB by delivering MSCs via intraparenchymal or intracerebroventricular routes (Ma et al., 2013; Zheng et al., 2017; Zhao et al., 2018; Reza-Zaldivar et al., 2019). Delivering MSCs via the intrathecal route has also been identified as a less invasive delivery route, since it does not require brain surgery (Kim et al., 2020).
Focused ultrasound is another technology that has been leveraged to improve MSC homing (Liu et al., 2020). Application of focused ultrasound to the brain transiently ruptures the capillary lining of the BBB and supports delivery of therapeutic molecules into the brain through increased capillary permeability. Focused ultrasound application has also been shown to upregulate intercellular adhesion molecules (ICAMs), stromal cell-derived factor 1α (SDF-1α), monocyte chemotactic protein 1 (MCP-1), matrix metalloproteinase 9 (MMP9), and immune cell trophic factors that contribute to transient BBB opening and tropism of MSCs to the brain (Kovacs et al., 2017; Yang et al., 2019). Preclinical studies in rats have demonstrated that focused ultrasound to the hippocampus increases local expression of vascular cell adhesion molecule 1 (VCAM-1) and ICAM-1, and results in a more than 2-fold increase in the number of MSCs found in the sonicated region following intravenous infusion (Lee et al., 2020). Focused ultrasound has also been combined with contrast agents such as microbubbles to enhance BBB permeabilization. In response to ultrasound, microbubbles cavitate and locally exert various physical forces, a technique known as ultrasound-mediated microbubble destruction. In a rat model of brain ischemia, ultrasound-mediated microbubble destruction has been shown to increase the number of intravenously infused MSCs found in the brain parenchyma by 2.3-fold (Cui et al., 2020). The increased MSC homing appeared to correlate with greater recovery of neurological function, though the ultrasound-only control, which would be necessary to make this conclusion, was not included.
Magnetic targeting has also been investigated for improving MSC delivery to the brain. By labeling MSCs with superparamagnetic nanoparticles, they can be guided to the brain using external magnets. One such study found that magnetic targeting of intravenously infused MSCs improved their migration into the brains of AD rats, to levels comparable to those of intracerebroventricularly injected MSCs (Hour et al., 2020). Magnetic targeting also appeared to improve certain measures of cognitive function as well as expression of cholinergic signaling molecules.
Genetic modification of mesenchymal stem/stromal cells
Studies have explored various genetic modifications of MSCs to enhancing their function or survival. For instance, MSCs overexpressing CX3CL1 have been shown to attenuate synaptic loss and the levels of pro-inflammatory cytokines in APP/PS1 transgenic AD mice (Li et al., 2020). While CX3CL1 overexpression alone did not lead to cognitive improvement, transplantation of MSCs overexpressing both CX3CL1 and Wnt3a successfully fostered hippocampal neurogenesis, improved cognitive function, and reduced microglia neurotoxicity (Li et al., 2020). In the same mouse model, MSCs overexpressing VEGF have been shown to improve hippocampal neovascularization, diminish senile plaques, inhibit inflammation, and rescue behavioral and cognitive functions as compared to control MSCs (Garcia et al., 2014).
Studies have revealed that microRNA-modified MSCs may play a beneficial role in treatment of neurodegenerative diseases like AD (Liu et al., 2015; Han et al., 2018). For instance, MSCs overexpressing BDNF have been shown to promote neuron survival when they are co-cultured with primary neurons isolated from APP/PS1 mice (Song et al., 2015). It was revealed that MSCs express low levels of Brn-4 protein despite the high expression of the transcription factor Brn-4 mRNA due to the presence of miR-937, which inhibits the translation of Brn-4 mRNA (Liu et al., 2015). However, bone marrow MSCs induced to express the antisense of miR-937 (as-miR-937) successfully suppressed miR-937 to increase expression of transcription factor Brn-4, which in turn increased BDNF protein levels and significantly enhanced the therapeutic effect of MSCs in the APP/PS1 mice (Liu et al., 2015). Additionally, MSCs overexpressing as-miR-937 had reduced Aβ accumulation, and enhanced cognitive function (Liu et al., 2015).
It has been observed that MSCs exposed to Aβ express early apoptosis and increased levels of the apoptosis mediating protease caspase 3, along with a decrease in levels of the microRNA let-7f-5p (Han et al., 2018). Upregulation of let-7f-5p countered the Aβ-induced apoptosis in MSCs by decreasing the levels of caspase-3, thereby prolonging MSC retention in the brain (Han et al., 2018). Elevation of let-7f-5p also reduced Aβ-induced cytotoxicity and enhanced survival of engrafted MSCs by targeting caspase-3 (Han et al., 2018).
Glucagon-like peptide-1 (GLP-1) has also been suggested to protect neurons from Aβ-mediated toxicity by preventing neuronal apoptosis, oxidative injury, and the generation and accumulation of Aβ deposits from APP (Perry and Greig, 2002). One study has shown that intracerebroventricular transplantation of GLP-1 overexpressing MSCs into AD mice reduced Aβ deposition and a downregulated microglial and astrocytic immunoreactivity in the brain (Klinge et al., 2011), though the reductions were moderate.
Priming of mesenchymal stem/stromal cells
Priming MSCs with cytokines, hypoxia, nutrition deficiency, and various small molecules has been conducted to enhance the therapeutic efficacy and long-term survival of MSCs. For instance, pre-treating gingiva-derived MSCs (G-MSCs) with cannabidiol (CBD) modified the transcriptional profile of these MSCs to downregulate expression of proteins that are potentially involved in tau phosphorylation and Aβ production while upregulating genes involved in Aβ clearance and degradation (Libro et al., 2016). CBD-treated G-MSCs exhibited a downregulation of β- and γ-secretases which are usually responsible for Aβ production, and an upregulation of α-secretases which are responsible for the normal cleavage of APP (Libro et al., 2016). CBD treatment also upregulated the expressions of HSPs (HSP70s and HSP90s) and ubiquitin-conjugating enzymes, which enhances the clearance of aberrant proteins associated with AD pathology, such as Aβ plaques and neurofibrillary tangles. CBD was also found to bind to the vanilloid receptor 1 (TRPV1) to promote PI3K/Akt signaling and inhibit GSK3β, the latter of which is believed to be responsible for tau hyperphosphorylation. In another study, pre-treating MSCs with melatonin improved survival of transplanted adipose tissue-derived MSCs (ADMSCs) and better preserved the cognitive, learning, and memory functions in Aβ-treated AD rats (Nasiri et al., 2019). The number of activated microglia was also significantly decreased in melatonin-pre-treated ADMSCs group, and Aβ clearance was increased relative to untreated ADMSCs (Nasiri et al., 2019).
Extracellular vesicles as cell-free therapy
The primary mechanism of action for MSC-based therapies is believed to be their paracrine activities via release of various secretory factors to facilitate tissue repair and immunomodulation. Due to this, many groups are investigating cell-free therapies based on MSC-derived EVs which have been shown to be sufficient for inhibiting apoptosis and reducing neuroinflammation (de Godoy et al., 2018; Kang et al., 2019). Conditioned media (CM) collected from different types of MSCs have been shown to reduce cognitive impairment in AD mice (Mita et al., 2015).
The use of MSC-derived EVs confers several advantages, including low immunogenicity, higher safety profile, ease of injection, and enhanced ability to trespass biological barriers. These attributes circumvent complications such as tumor formation, immune rejection, and undesired entrapment in the lung microvasculature. Moreover, MSC-derived EVs express the same set of membrane receptors and surface markers as MSCs, which may allow them to retain some of the homing capabilities possessed by their parent MSCs (Grange et al., 2014).
Mesenchymal stem/stromal cell-secreted EVs have demonstrated therapeutic efficacy in several AD studies. For instance, treating neural stem cells derived from the Tg2576 AD mouse model with ADMSC-derived EVs successfully reduced Aβ levels and neuronal apoptosis while fostering neuronal growth in vitro (Lee et al., 2018). It has been demonstrated that AD-MSC-derived EVs carry enzymatically active NEP and successfully degrade secreted and intracellular Aβ levels in Aβ-overexpressing neuroblastoma cells (Katsuda et al., 2013). Interestingly, the Aβ inhibition of these AD-MSC-derived EVs exceeded that of BM-MSCs (Katsuda et al., 2013). UC-MSC-derived EVs have also been shown to ameliorate cognitive dysfunction and neuroinflammation by modulating microglia activation in a mouse AD model (Ding et al., 2018).
Extracellular vesicles secreted from 3D-cultured MSCs seem to demonstrate higher therapeutic effect in several studies relative to those derived from 2D-cultured MSCs. 3D-cultured MSCs produced higher yield of EVs, and these EVs exhibited improved efficiency in delivering their contents to neurons (Haraszti et al., 2018). EVs secreted from UC-MSCs cultured in 3D graphene scaffold have been shown to differentially express hundreds of miRNAs compared to those collected from 2D-cultured UC-MSCs, and were enriched for several genes relevant to AD therapy such as HSP90, NEP, and IDE (Yang et al., 2020). These differences highlight the large variation in EV functional properties depending on the culture conditions, which will be a crucial factor to consider for successful clinical translation.
Conclusion and future implications
Mesenchymal stem/stromal cells have been extensively investigated as a therapeutic strategy for treating neurodegenerative diseases such as AD. However, while preclinical studies have demonstrated some therapeutic potential of MSC-based therapies, several limitations mentioned above have hindered their effectiveness in clinical trials. Since intravenously administration of MSCs results in majority being trapped in the microvasculature of the lungs, finding a path of delivery that enables efficient MSC delivery and homing to the brain remains a challenge. In order to foster MSC migration to the brain and improve clinical therapeutic efficacy, studies have explored applications such as focused ultrasound, genetic modification, MSC conditioning, and local delivery of MSC into the brain. In addition to MSC-based cell therapy, cell free therapy based on MSC-derived EVs has also demonstrated potential in the treatment of AD.
As we have seen in this review, much of the preclinical literatures investigating MSCs for AD therapy have utilized widely different AD animal models, MSC sources, culture conditions and administration routes; while the reported therapeutic effects often attain statistical significance but are yet to be established in clinics. Nevertheless, the mechanistic understanding of how MSCs might ameliorate AD pathology still remains to be proven. The potential future of MSC-based therapy for AD hinges on a thorough scientific inquiry and mechanistic insight into the interaction between MSCs and neural cells, followed by rigorous preclinical studies that evaluate clinically meaningful endpoints. Majority of clinical trials have not lived up to their potential despite having good pre-clinical data due to limited consideration in pathological variability corresponding to different stages of the disease and its resulting impact on therapeutic effect of MSC-based therapies. Hence, well defined pre-clinical study protocols will be essential for effective clinical translation of novel therapeutics. Indeed, this can include testing specific animal models with therapeutic interventions mapped to specific stages of the disease. Furthermore, the actual therapeutic candidate should be controlled and evaluated for at the pre-clinical stage, in terms of its source, dose and route of administration. These specific parameters should then not be changed to ensure that the clinical translation is optimal less likely to fail. Finally, given that each patient will have their own unique physiology and microenvironment, modulation of the brain tissue at target sites may in fact help to ensure consistent and reproducible regenerative and protective responses. Without this groundwork, it is highly possible that clinical trials will continue to generate disappointing results.
Author contributions
SR and AST contributed to conceptualization. SR, MS, and DDL contributed to writing the review. SR, MS, DDL, AG, RP, SC, RY, and AST contributed to editing and review. BDK contributed to preparing the figures. AST contributed to supervision. All authors contributed to the article and approved the submitted version.
Funding
This study was supported by the Radiology Research Fund for Alzheimer’s disease at Stanford University.
Conflict of interest
The authors declare that the research was conducted in the absence of any commercial or financial relationships that could be construed as a potential conflict of interest.
Publisher’s note
All claims expressed in this article are solely those of the authors and do not necessarily represent those of their affiliated organizations, or those of the publisher, the editors and the reviewers. Any product that may be evaluated in this article, or claim that may be made by its manufacturer, is not guaranteed or endorsed by the publisher.
References
Alavi Naini, S. M., and Soussi-Yanicostas, N. (2015). Tau Hyperphosphorylation and oxidative stress, a critical vicious circle in neurodegenerative tauopathies? Oxid. Med. Cell Longev. 2015:151979. doi: 10.1155/2015/151979
Alexanian, A. R. (2005). Neural stem cells induce bone-marrow-derived mesenchymal stem cells to generate neural stem-like cells via juxtacrine and paracrine interactions. Exp. Cell Res. 310, 383–391. doi: 10.1016/j.yexcr.2005.08.015
Alonso, A. C., Grundke-Iqbal, I., and Iqbal, K. (1996). Alzheimer’s disease hyperphosphorylated tau sequesters normal tau into tangles of filaments and disassembles microtubules. Nat. Med. 2, 783–787. doi: 10.1038/nm0796-783
Alzheimer’s Association (2019). 2019 Alzheimer’s disease facts and figures. Alzheimer Dement 15, 321–387. doi: 10.1016/j.jalz.2019.01.010
Baruch, K., Deczkowska, A., Rosenzweig, N., Tsitsou-Kampeli, A., Sharif, A. M., Matcovitch-Natan, O., et al. (2016). PD-1 immune checkpoint blockade reduces pathology and improves memory in mouse models of Alzheimer’s disease. Nat. Med. 22:135. doi: 10.1038/nm.4022
Benilova, I., Karran, E., and De Strooper, B. (2012). The toxic Abeta oligomer and Alzheimer’s disease: An emperor in need of clothes. Nat. Neurosci. 15, 349–357. doi: 10.1038/nn.3028
Bloom, G. S. (2014). Amyloid-beta and tau: The trigger and bullet in Alzheimer disease pathogenesis. JAMA Neurol. 71, 505–508. doi: 10.1001/jamaneurol.2013.5847
Boutajangout, A., Noorwali, A., Atta, H., and Wisniewski, T. (2017). Human umbilical cord stem cell xenografts improve cognitive decline and reduce the amyloid burden in a mouse model of Alzheimer’s Disease. Curr. Alzheimer Res. 14, 104–111. doi: 10.2174/1567205013666161004151416
Brody, M., Agronin, M., Herskowitz, B. J., Bookheimer, S. Y., Small, G. W., Hitchinson, B., et al. (2022). Results and insights from a phase I clinical trial of Lomecel-B for Alzheimer’s disease. Alzheimers Dement. 2022, 1–13. doi: 10.1002/alz.12651
Busche, M. A., Wegmann, S., Dujardin, S., Commins, C., Schiantarelli, J., Klickstein, N., et al. (2019). Tau impairs neural circuits, dominating amyloid-beta effects, in Alzheimer models in vivo. Nat. Neurosci. 22, 57–64. doi: 10.1038/s41593-018-0289-8
Cheignon, C., Tomas, M., Bonnefont-Rousselot, D., Faller, P., Hureau, C., and Collin, F. (2018). Oxidative stress and the amyloid beta peptide in Alzheimer’s disease. Redox Biol. 14, 450–464. doi: 10.1016/j.redox.2017.10.014
Cui, G. H., Guo, H. D., Li, H., Zhai, Y., Gong, Z. B., Wu, J., et al. (2019). RVG-modified exosomes derived from mesenchymal stem cells rescue memory deficits by regulating inflammatory responses in a mouse model of Alzheimer’s disease. Immun. Ageing 16:10. doi: 10.1186/s12979-019-0150-2
Cui, G. H., Wu, J., Mou, F. F., Xie, W. H., Wang, F. B., Wang, Q. L., et al. (2018). Exosomes derived from hypoxia-preconditioned mesenchymal stromal cells ameliorate cognitive decline by rescuing synaptic dysfunction and regulating inflammatory responses in APP/PS1 mice. FASEB J. 32, 654–668. doi: 10.1096/fj.201700600R
Cui, H., Zhu, Q., Xie, Q., Liu, Z., Gao, Y., He, Y., et al. (2020). Low intensity ultrasound targeted microbubble destruction assists MSCs delivery and improves neural function in brain ischaemic rats. J. Drug Target 28, 320–329. doi: 10.1080/1061186X.2019.1656724
Cui, Y., Ma, S., Zhang, C., Cao, W., Liu, M., Li, D., et al. (2017). Human umbilical cord mesenchymal stem cells transplantation improves cognitive function in Alzheimer’s disease mice by decreasing oxidative stress and promoting hippocampal neurogenesis. Behav. Brain Res. 320, 291–301. doi: 10.1016/j.bbr.2016.12.021
Cummings, J., Lee, G., Ritter, A., Sabbagh, M., and Zhong, K. (2019). Alzheimer’s disease drug development pipeline: 2019. Alzheimers Dement (N Y) 5, 272–293. doi: 10.1016/j.trci.2019.05.008
de Godoy, M. A., Saraiva, L. M., De Carvalho, L. R. P., Vasconcelos-Dos-Santos, A., Beiral, H. J. V., Ramos, A. B., et al. (2018). Mesenchymal stem cells and cell-derived extracellular vesicles protect hippocampal neurons from oxidative stress and synapse damage induced by amyloid-beta oligomers. J. Biol. Chem. 293, 1957–1975. doi: 10.1074/jbc.M117.807180
Deane, R., Bell, R. D., Sagare, A., and Zlokovic, B. V. (2009). Clearance of amyloid-beta peptide across the blood-brain barrier: Implication for therapies in Alzheimer’s disease. CNS Neurol. Disord Drug Targets 8, 16–30. doi: 10.2174/187152709787601867
Ding, M., Shen, Y., Wang, P., Xie, Z., Xu, S., Zhu, Z., et al. (2018). Exosomes isolated from human umbilical cord mesenchymal stem cells alleviate neuroinflammation and reduce amyloid-beta deposition by modulating microglial activation in Alzheimer’s Disease. Neurochem. Res. 43, 2165–2177. doi: 10.1007/s11064-018-2641-5
DiSabato, D. J., Quan, N., and Godbout, J. P. (2016). Neuroinflammation: The devil is in the details. J. Neurochem. 139 Suppl 2, 136–153. doi: 10.1111/jnc.13607
Divya, M. S., Roshin, G. E., Divya, T. S., Rasheed, V. A., Santhoshkumar, T. R., Elizabeth, K. E., et al. (2012). Umbilical cord blood-derived mesenchymal stem cells consist of a unique population of progenitors co-expressing mesenchymal stem cell and neuronal markers capable of instantaneous neuronal differentiation. Stem Cell Res. Ther. 3:57. doi: 10.1186/scrt148
Doens, D., and Fernandez, P. L. (2014). Microglia receptors and their implications in the response to amyloid beta for Alzheimer’s disease pathogenesis. J. Neuroinflammation 11:48. doi: 10.1186/1742-2094-11-48
Dominici, M., Le Blanc, K., Mueller, I., Slaper-Cortenbach, I., Marini, F., Krause, D., et al. (2006). Minimal criteria for defining multipotent mesenchymal stromal cells. The International society for cellular therapy position statement. Cytotherapy 8, 315–317. doi: 10.1080/14653240600855905
Feng, N., Han, Q., Li, J., Wang, S., Li, H., Yao, X., et al. (2014). Generation of highly purified neural stem cells from human adipose-derived mesenchymal stem cells by Sox1 activation. Stem Cells Dev. 23, 515–529. doi: 10.1089/scd.2013.0263
Gan, C., and Ouyang, F. (2022). Exosomes released from bone-marrow stem cells ameliorate hippocampal neuronal injury through transferring miR-455-3p. J. Stroke Cerebrovasc. Dis. 31:106142. doi: 10.1016/j.jstrokecerebrovasdis.2021.106142
Garcia, K. O., Ornellas, F. L., Martin, P. K., Patti, C. L., Mello, L. E., Frussa-Filho, R., et al. (2014). Therapeutic effects of the transplantation of VEGF overexpressing bone marrow mesenchymal stem cells in the hippocampus of murine model of Alzheimer’s disease. Front. Aging Neurosci. 6:30. doi: 10.3389/fnagi.2014.00030
Giunti, D., Parodi, B., Usai, C., Vergani, L., Casazza, S., Bruzzone, S., et al. (2012). Mesenchymal stem cells shape microglia effector functions through the release of CX3CL1. Stem Cells 30, 2044–2053. doi: 10.1002/stem.1174
Gnecchi, M., Danieli, P., Malpasso, G., and Ciuffreda, M. C. (2016). Paracrine mechanisms of mesenchymal stem cells in tissue repair. Methods Mol. Biol. 1416, 123–146. doi: 10.1007/978-1-4939-3584-0_7
Gorbunov, N., Petrovski, G., Gurusamy, N., Ray, D., Kim, D. H., and Das, D. K. (2012). Regeneration of infarcted myocardium with resveratrol-modified cardiac stem cells. J. Cell Mol. Med. 16:174–184. doi: 10.1111/j.1582-4934.2011.01281.x
Grange, C., Tapparo, M., Bruno, S., Chatterjee, D., Quesenberry, P. J., Tetta, C., et al. (2014). Biodistribution of mesenchymal stem cell-derived extracellular vesicles in a model of acute kidney injury monitored by optical imaging. Int. J. Mol. Med. 33, 1055–1063. doi: 10.3892/ijmm.2014.1663
Griner, S. L., Seidler, P., Bowler, J., Murray, K. A., Yang, T. P., Sahay, S., et al. (2019). Structure-based inhibitors of amyloid beta core suggest a common interface with tau. Elife 8:e46924. doi: 10.7554/eLife.46924
Guillozet, A. L., Weintraub, S., Mash, D. C., and Mesulam, M. M. (2003). Neurofibrillary tangles, amyloid, and memory in aging and mild cognitive impairment. Arch. Neurol. 60, 729–736. doi: 10.1001/archneur.60.5.729
Gupta, A., Bansal, A., and Hashimoto-Torii, K. (2020). HSP70 and HSP90 in neurodegenerative diseases. Neurosci. Lett. 716:134678. doi: 10.1016/j.neulet.2019.134678
Han, L., Zhou, Y., Zhang, R., Wu, K., Lu, Y., Li, Y., et al. (2018). MicroRNA Let-7f-5p Promotes bone marrow mesenchymal stem cells survival by targeting Caspase-3 in Alzheimer Disease Model. Front. Neurosci. 12:333. doi: 10.3389/fnins.2018.00333
Hansen, D. V., Hanson, J. E., and Sheng, M. (2018). Microglia in Alzheimer’s disease. J. Cell Biol. 217, 459–472. doi: 10.1083/jcb.201709069
Haragopal, H., Yu, D., Zeng, X., Kim, S. W., Han, I. B., Ropper, A. E., et al. (2015). Stemness enhancement of human neural stem cells following bone marrow MSC coculture. Cell Transplant. 24, 645–659. doi: 10.3727/096368915X687561
Haraszti, R. A., Miller, R., Stoppato, M., Sere, Y. Y., Coles, A., Didiot, M.-C., et al. (2018). Exosomes produced from 3D Cultures of MSCs by tangential flow filtration show higher yield and improved activity. Mol. Ther. 26, 2838–2847. doi: 10.1016/j.ymthe.2018.09.015
Harris, V. K., Faroqui, R., Vyshkina, T., and Sadiq, S. A. (2012). Characterization of autologous mesenchymal stem cell-derived neural progenitors as a feasible source of stem cells for central nervous system applications in multiple sclerosis. Stem Cells Transl. Med. 1, 536–547. doi: 10.5966/sctm.2012-0015
He, Z., Guo, J. L., Mcbride, J. D., Narasimhan, S., Kim, H., Changolkar, L., et al. (2018). Amyloid-beta plaques enhance Alzheimer’s brain tau-seeded pathologies by facilitating neuritic plaque tau aggregation. Nat. Med. 24, 29–38. doi: 10.1038/nm.4443
Hegyi, B., Környei, Z., Ferenczi, S., Fekete, R., Kudlik, G., Kovács, K. J., et al. (2014). Regulation of mouse microglia activation and effector functions by bone marrow-derived mesenchymal stem cells. Stem Cells Dev. 23, 2600–2612. doi: 10.1089/scd.2014.0088
Hernández, R., Jiménez-Luna, C., Perales-Adán, J., Perazzoli, G., Melguizo, C., and Prados, J. (2020). Differentiation of human mesenchymal stem cells towards neuronal lineage: Clinical trials in nervous system disorders. Biomol. Ther (Seoul) 28, 34–44. doi: 10.4062/biomolther.2019.065
Hour, F. Q., Moghadam, A. J., Shakeri-Zadeh, A., Bakhtiyari, M., Shabani, R., and Mehdizadeh, M. (2020). Magnetic targeted delivery of the SPIONs-labeled mesenchymal stem cells derived from human Wharton’s jelly in Alzheimer’s rat models. J. Control Release 321, 430–441. doi: 10.1016/j.jconrel.2020.02.035
Huang, N., Li, Y., Zhou, Y., Zhou, Y., Feng, F., Shi, S., et al. (2019). Neuroprotective effect of tanshinone IIA-incubated mesenchymal stem cells on Aβ25-35-induced neuroinflammation. Behav. Brain Res. 365, 48–55. doi: 10.1016/j.bbr.2019.03.001
Jahangard, Y., Monfared, H., Moradi, A., Zare, M., Mirnajafi-Zadeh, J., and Mowla, S. J. (2020). Therapeutic effects of transplanted exosomes containing miR-29b to a rat model of Alzheimer’s Disease. Front. Neurosci. 14:564. doi: 10.3389/fnins.2020.00564
Jeong, S. G., Ohn, T., Kim, S. H., and Cho, G. W. (2013). Valproic acid promotes neuronal differentiation by induction of neuroprogenitors in human bone-marrow mesenchymal stromal cells. Neurosci. Lett. 554, 22–27. doi: 10.1016/j.neulet.2013.08.059
Joe, I. S., Jeong, S. G., and Cho, G. W. (2015). Resveratrol-induced SIRT1 activation promotes neuronal differentiation of human bone marrow mesenchymal stem cells. Neurosci. Lett. 584, 97–102. doi: 10.1016/j.neulet.2014.10.024
Jose, S., Tan, S. W., Ooi, Y. Y., Ramasamy, R., and Vidyadaran, S. (2014). Mesenchymal stem cells exert anti-proliferative effect on lipopolysaccharide-stimulated BV2 microglia by reducing tumour necrosis factor-α levels. J. Neuroinflammation 11:149. doi: 10.1186/s12974-014-0149-8
Ju, R., Zeng, W., Wu, R., and Feng, Z. (2015). Interaction between neural stem cells and bone marrow derived-mesenchymal stem cells during differentiation. Biomed. Rep. 3, 242–246. doi: 10.3892/br.2014.405
Kang, J., Li, Z., Zhi, Z., Wang, S., and Xu, G. (2019). MiR-21 derived from the exosomes of MSCs regulates the death and differentiation of neurons in patients with spinal cord injury. Gene Ther. 26, 491–503. doi: 10.1038/s41434-019-0101-8
Katsuda, T., Tsuchiya, R., Kosaka, N., Yoshioka, Y., Takagaki, K., Oki, K., et al. (2013). Human adipose tissue-derived mesenchymal stem cells secrete functional neprilysin-bound exosomes. Sci. Rep. 3:1197. doi: 10.1038/srep01197
Kemp, K., Gray, E., Mallam, E., Scolding, N., and Wilkins, A. (2010). Inflammatory cytokine induced regulation of superoxide dismutase 3 expression by human mesenchymal stem cells. Stem Cell Rev. Rep. 6, 548–559. doi: 10.1007/s12015-010-9178-6
Kim, D. H., Lee, D., Chang, E. H., Kim, J. H., Hwang, J. W., Kim, J.-Y., et al. (2015). GDF-15 secreted from human umbilical cord blood mesenchymal stem cells delivered through the cerebrospinal fluid promotes hippocampal neurogenesis and synaptic activity in an Alzheimer’s disease model. Stem Cells Dev. 24, 2378–2390. doi: 10.1089/scd.2014.0487
Kim, D. H., Lee, D., Lim, H., Choi, S. J., Oh, W., Yang, Y. S., et al. (2018a). Effect of growth differentiation factor-15 secreted by human umbilical cord blood-derived mesenchymal stem cells on amyloid beta levels in in vitro and in vivo models of Alzheimer’s disease. Biochem. Biophys. Res. Commun. 504, 933–940. doi: 10.1016/j.bbrc.2018.09.012
Kim, D. H., Lim, H., Lee, D., Choi, S. J., Oh, W., Yang, Y. S., et al. (2018b). Thrombospondin-1 secreted by human umbilical cord blood-derived mesenchymal stem cells rescues neurons from synaptic dysfunction in Alzheimer’s disease model. Sci. Rep. 8:354. doi: 10.1038/s41598-017-18542-0
Kim, H. J., Seo, S. W., Chang, J. W., Lee, J. I., Kim, C. H., Chin, J., et al. (2015). Stereotactic brain injection of human umbilical cord blood mesenchymal stem cells in patients with Alzheimer’s disease dementia: A phase 1 clinical trial. Alzheimers Dement (N Y) 1, 95–102. doi: 10.1016/j.trci.2015.06.007
Kim, H., Na, D. L., Lee, N. K., Kim, A. R., Lee, S., and Jang, H. (2020). Intrathecal injection in a rat model: A potential route to deliver human wharton’s jelly-derived mesenchymal stem cells into the brain. Int. J. Mol. Med. Sci. 21:1272. doi: 10.3390/ijms21041272
Kim, J. Y., Kim, D. H., Kim, J. H., Lee, D., Jeon, H. B., Kwon, S. J., et al. (2012). Soluble intracellular adhesion molecule-1 secreted by human umbilical cord blood-derived mesenchymal stem cell reduces amyloid-β plaques. Cell Death Differ. 19, 680–691. doi: 10.1038/cdd.2011.140
Kim, Y. J., Park, H. J., Lee, G., Bang, O. Y., Ahn, Y. H., Joe, E., et al. (2009). Neuroprotective effects of human mesenchymal stem cells on dopaminergic neurons through anti-inflammatory action. Glia 57, 13–23. doi: 10.1002/glia.20731
Klinge, P. M., Harmening, K., Miller, M. C., Heile, A., Wallrapp, C., Geigle, P., et al. (2011). Encapsulated native and glucagon-like peptide-1 transfected human mesenchymal stem cells in a transgenic mouse model of Alzheimer’s disease. Neurosci. Lett. 497, 6–10. doi: 10.1016/j.neulet.2011.03.092
Konnecke, H., and Bechmann, I. (2013). The role of microglia and matrix metalloproteinases involvement in neuroinflammation and gliomas. Clin. Dev. Immunol. 2013:914104. doi: 10.1155/2013/914104
Kovacs, Z. I., Kim, S., Jikaria, N., Qureshi, F., Milo, B., Lewis, B. K., et al. (2017). Disrupting the blood-brain barrier by focused ultrasound induces sterile inflammation. Proc. Natl. Acad. Sci. U.S.A. 114, E75–E84. doi: 10.1073/pnas.1614777114
Latta-Mahieu, M., Elmer, B., Bretteville, A., Wang, Y., Lopez-Grancha, M., Goniot, P., et al. (2018). Systemic immune-checkpoint blockade with anti-PD1 antibodies does not alter cerebral amyloid−β burden in several amyloid transgenic mouse models. Glia 66, 492–504. doi: 10.1002/glia.23260
Lee, J. K., Schuchman, E. H., Jin, H. K., and Bae, J.-S. (2012). Soluble CCL5 derived from bone marrow-derived mesenchymal stem cells and activated by Amyloid β Ameliorates Alzheimer’s Disease in mice by recruiting bone marrow-induced microglia immune responses. Stem Cells 30, 1544–1555. doi: 10.1002/stem.1125
Lee, J., Chang, W. S., Shin, J., Seo, Y., Kong, C., Song, B.-W., et al. (2020). Non-invasively enhanced intracranial transplantation of mesenchymal stem cells using focused ultrasound mediated by overexpression of cell-adhesion molecules. Stem Cell Res. 43:101726. doi: 10.1016/j.scr.2020.101726
Lee, M., Ban, J.-J., Yang, S., Im, W., and Kim, M. (2018). The exosome of adipose-derived stem cells reduces β-amyloid pathology and apoptosis of neuronal cells derived from the transgenic mouse model of Alzheimer’s disease. Brain Res. 1691, 87–93. doi: 10.1016/j.brainres.2018.03.034
Lee, N. K., Park, S. E., Kwon, S. J., Shim, S., Byeon, Y., Kim, J. H., et al. (2017). Agouti related peptide secreted via human mesenchymal stem cells upregulates proteasome activity in an Alzheimer’s Disease Model. Sci. Rep. 7:39340. doi: 10.1038/srep39340
Leng, F., and Edison, P. (2021). Neuroinflammation and microglial activation in Alzheimer disease: Where do we go from here? Nat. Rev. Neurol. 17, 157–172. doi: 10.1038/s41582-020-00435-y
Li, A., Zhao, J., Fan, C., Zhu, L., Huang, C., Li, Q., et al. (2020). Delivery of exogenous proteins by mesenchymal stem cells attenuates early memory deficits in a murine model of Alzheimer’s disease. Neurobiol. Aging 86, 81–91. doi: 10.1016/j.neurobiolaging.2019.10.012
Li, Q., and Barres, B. A. (2018). Microglia and macrophages in brain homeostasis and disease. Nat. Rev. Immunol. 18, 225–242. doi: 10.1038/nri.2017.125
Libro, R., Diomede, F., Scionti, D., Piattelli, A., Grassi, G., Pollastro, F., et al. (2016). Cannabidiol modulates the expression of Alzheimer’s Disease-related genes in mesenchymal stem cells. Int. J. Mol. Sci. 18:26. doi: 10.3390/ijms18010026
Lim, H., Lee, D., Choi, W. K., Choi, S. J., Oh, W., and Kim, D. H. (2020). Galectin-3 secreted by human umbilical cord blood-derived mesenchymal stem cells reduces aberrant tau phosphorylation in an Alzheimer Disease Model. Stem Cells Int. 2020:8878412. doi: 10.1155/2020/8878412
Lin, M. T., and Beal, M. F. (2006). Alzheimer’s APP mangles mitochondria. Nat. Med. 12, 1241–1243. doi: 10.1038/nm1106-1241
Liu, D. D., Ullah, M., Concepcion, W., Dahl, J. J., and Thakor, A. S. (2020). The role of ultrasound in enhancing mesenchymal stromal cell-based therapies. Stem Cells Transl. Med. 9, 850–866. doi: 10.1002/sctm.19-0391
Liu, Y., Zhang, R., Yan, K., Chen, F., Huang, W., Lv, B., et al. (2014). Mesenchymal stem cells inhibit lipopolysaccharide-induced inflammatory responses of BV2 microglial cells through TSG-6. J. Neuroinflammation 11:135. doi: 10.1186/1742-2094-11-135
Liu, Z., Wang, C., Wang, X., and Xu, S. (2015). Therapeutic effects of transplantation of As-MiR-937-Expressing mesenchymal stem cells in murine model of Alzheimer’s Disease. Cell Physiol. Biochem. 37, 321–330. doi: 10.1159/000430356
Lopez-Beas, J., Guadix, J. A., Clares, B., Soriano-Ruiz, J. L., Zugaza, J. L., and Gálvez-Martín, P. (2020). An overview of international regulatory frameworks for mesenchymal stromal cell-based medicinal products: From laboratory to patient. Med. Res. Rev. 40, 1315–1334. doi: 10.1002/med.21659
Losurdo, M., Pedrazzoli, M., D’agostino, C., Elia, C. A., Massenzio, F., Lonati, E., et al. (2020). Intranasal delivery of mesenchymal stem cell-derived extracellular vesicles exerts immunomodulatory and neuroprotective effects in a 3xTg model of Alzheimer’s disease. Stem Cells Transl. Med. 9, 1068–1084. doi: 10.1002/sctm.19-0327
Lu, P., Blesch, A., and Tuszynski, M. H. (2004). Induction of bone marrow stromal cells to neurons: Differentiation, transdifferentiation, or artifact? J. Neurosci. Res. 77, 174–191. doi: 10.1002/jnr.20148
Lukomska, B., Stanaszek, L., Zuba-Surma, E., Legosz, P., Sarzynska, S., and Drela, K. (2019). Challenges and controversies in human mesenchymal stem cell therapy. Stem Cells Int. 2019:9628536. doi: 10.1155/2019/9628536
Lykhmus, O., Koval, L., Voytenko, L., Uspenska, K., Komisarenko, S., Deryabina, O., et al. (2019). Intravenously injected mesenchymal stem cells penetrate the brain and treat inflammation-induced brain damage and memory impairment in mice. Front. Pharmacol. 10:355. doi: 10.3389/fphar.2019.00355
Ma, T., Gong, K., Ao, Q., Yan, Y., Song, B., Huang, H., et al. (2013). Intracerebral transplantation of adipose-derived mesenchymal stem cells alternatively activates microglia and ameliorates neuropathological deficits in Alzheimer’s Disease Mice. Cell Transplant. 22, 113–126. doi: 10.3727/096368913X672181
Maleki, M., Ghanbarvand, F., Reza Behvarz, M., Ejtemaei, M., and Ghadirkhomi, E. (2014). Comparison of mesenchymal stem cell markers in multiple human adult stem cells. Int. J. Stem Cells 7, 118–126. doi: 10.15283/ijsc.2014.7.2.118
Manczak, M., and Reddy, P. H. (2012). Abnormal interaction between the mitochondrial fission protein Drp1 and hyperphosphorylated tau in Alzheimer’s disease neurons: Implications for mitochondrial dysfunction and neuronal damage. Hum. Mol. Genet. 21, 2538–2547. doi: 10.1093/hmg/dds072
Manczak, M., Calkins, M. J., and Reddy, P. H. (2011). Impaired mitochondrial dynamics and abnormal interaction of amyloid beta with mitochondrial protein Drp1 in neurons from patients with Alzheimer’s disease: Implications for neuronal damage. Hum. Mol. Genet. 20, 2495–2509. doi: 10.1093/hmg/ddr139
Marcus, D. L., Thomas, C., Rodriguez, C., Simberkoff, K., Tsai, J. S., Strafaci, J. A., et al. (1998). Increased peroxidation and reduced antioxidant enzyme activity in Alzheimer’s Disease. Exp. Neurol. 150, 40–44. doi: 10.1006/exnr.1997.6750
Martin-Pena, A., Rincon-Limas, D. E., and Fernandez-Funez, P. (2018). Engineered Hsp70 chaperones prevent Abeta42-induced memory impairments in a Drosophila model of Alzheimer’s disease. Sci. Rep. 8:9915. doi: 10.1038/s41598-018-28341-w
Mehrabadi, S., Motevaseli, E., Sadr, S. S., and Moradbeygi, K. (2020). Hypoxic-conditioned medium from adipose tissue mesenchymal stem cells improved neuroinflammation through alternation of toll like receptor (TLR) 2 and TLR4 expression in model of Alzheimer’s disease rats. Behav. Brain Res. 379:112362. doi: 10.1016/j.bbr.2019.112362
Mendonça, C. F., Kuras, M., Nogueira, F. C. S., Plá, I., Hortobágyi, T., Csiba, L., et al. (2019). Proteomic signatures of brain regions affected by tau pathology in early and late stages of Alzheimer’s disease. Neurobiol. Dis. 130:104509. doi: 10.1016/j.nbd.2019.104509
Mita, T., Furukawa-Hibi, Y., Takeuchi, H., Hattori, H., Yamada, K., Hibi, H., et al. (2015). Conditioned medium from the stem cells of human dental pulp improves cognitive function in a mouse model of Alzheimer’s disease. Behav. Brain Res. 293, 189–197. doi: 10.1016/j.bbr.2015.07.043
Montagne, A., Zhao, Z., and Zlokovic, B. V. (2017). Alzheimer’s disease: A matter of blood-brain barrier dysfunction? J. Exp. Med. 214, 3151–3169. doi: 10.1084/jem.20171406
Naaldijk, Y., Jäger, C., Fabian, C., Leovsky, C., Blüher, A., Rudolph, L., et al. (2017). Effect of systemic transplantation of bone marrow-derived mesenchymal stem cells on neuropathology markers in APP/PS1 Alzheimer mice. Neuropathol. Appl. Neurobiol. 43, 299–314. doi: 10.1111/nan.12319
Nakano, M., Kubota, K., Kobayashi, E., Chikenji, T. S., Saito, Y., Konari, N., et al. (2020). Bone marrow-derived mesenchymal stem cells improve cognitive impairment in an Alzheimer’s disease model by increasing the expression of microRNA-146a in hippocampus. Sci. Rep. 10:10772. doi: 10.1038/s41598-020-67460-1
Nasiri, E., Alizadeh, A., Roushandeh, A. M., Gazor, R., Hashemi-Firouzi, N., and Golipoor, Z. (2019). Melatonin-pretreated adipose-derived mesenchymal stem cells efficeintly improved learning, memory, and cognition in an animal model of Alzheimer’s disease. Metab. Brain Dis. 34, 1131–1143. doi: 10.1007/s11011-019-00421-4
Nelson, A. R., Sweeney, M. D., Sagare, A. P., and Zlokovic, B. V. (2016). Neurovascular dysfunction and neurodegeneration in dementia and Alzheimer’s disease. Biochim. Biophys. Acta 1862, 887–900. doi: 10.1016/j.bbadis.2015.12.016
Neves, A. F., Camargo, C., Premer, C., Hare, J. M., Baumel, B. S., and Pinto, M. (2021). Intravenous administration of mesenchymal stem cells reduces Tau phosphorylation and inflammation in the 3xTg-AD mouse model of Alzheimer’s disease. Exp. Neurol. 341:113706. doi: 10.1016/j.expneurol.2021.113706
No author list. (2021). 2021 Alzheimer’s disease facts and figures. Alzheimer’s Dement 17, 327–406. doi: 10.1002/alz.12328
Noh, M. Y., Lim, S. M., Oh, K.-W., Cho, K.-A., Park, J., Kim, K.-S., et al. (2016). Mesenchymal stem cells modulate the functional properties of microglia via TGF−β secretion. Stem Cells Transl. Med. 5, 1538–1549. doi: 10.5966/sctm.2015-0217
Nooshabadi, V. T., Mardpour, S., Yousefi-Ahmadipour, A., Allahverdi, A., Izadpanah, M., Daneshimehr, F., et al. (2018). The extracellular vesicles-derived from mesenchymal stromal cells: A new therapeutic option in regenerative medicine. J. Cell Biochem. 119, 8048–8073. doi: 10.1002/jcb.26726
Nunomura, A., Castellani, R. J., Zhu, X., Moreira, P. I., Perry, G., and Smith, M. A. (2006). Involvement of Oxidative Stress in Alzheimer Disease. J. Neuropathol. Exp. Neurol. 65, 631–641. doi: 10.1097/01.jnen.0000228136.58062.bf
Oh, J. S., Kim, K. N., An, S. S., Pennant, W. A., Kim, H. J., Gwak, S. J., et al. (2011). Cotransplantation of mouse neural stem cells (mNSCs) with adipose tissue-derived mesenchymal stem cells improves mNSC survival in a rat spinal cord injury model. Cell Transplant. 20, 837–849. doi: 10.3727/096368910X539083
Oliva, A. A. Jr., Brody, M., Agronin, M., Herskowitz, B., Bookheimer, S. Y., Hitchinson, B., et al. (2021). Safety and efficacy of Lomecel-B in patients with mild Alzheimer’s disease: Results of a double-blinded, randomized, placebo-controlled phase 1 clinical trial. Alzheimers Dement 17, e057581. doi: 10.1002/alz.057581
Park, B. N., Lim, T. S., Yoon, J. K., and An, Y. S. (2018). In vivo tracking of intravenously injected mesenchymal stem cells in an Alzheimer’s animal model. Cell Transplant. 27, 1203–1209. doi: 10.1177/0963689718788067
Park, S. E., Kim, H. S., Kwon, S. J., Kim, M. J., Choi, S. J., Oh, S. Y., et al. (2021). Exposure of mesenchymal stem cells to an Alzheimer’s Disease environment enhances therapeutic effects. Stem Cells Int. 2021:6660186. doi: 10.1155/2021/6660186
Perry, T., and Greig, N. H. (2002). The glucagon-like peptides: A new genre in therapeutic targets for intervention in Alzheimer’s disease. J. Alzheimers Dis. 4, 487–496. doi: 10.3233/JAD-2002-4605
Peruzzaro, S. T., Andrews, M. M. M., Al-Gharaibeh, A., Pupiec, O., Resk, M., Story, D., et al. (2019). Transplantation of mesenchymal stem cells genetically engineered to overexpress interleukin-10 promotes alternative inflammatory response in rat model of traumatic brain injury. J. Neuroinflammation 16:2. doi: 10.1186/s12974-018-1383-2
Pini, L., Pievani, M., Bocchetta, M., Altomare, D., Bosco, P., Cavedo, E., et al. (2016). Brain atrophy in Alzheimer’s Disease and aging. Ageing Res. Rev. 30, 25–48. doi: 10.1016/j.arr.2016.01.002
Pratico, D. (2008). Oxidative stress hypothesis in Alzheimer’s disease: A reappraisal. Trends Pharmacol. Sci. 29, 609–615. doi: 10.1016/j.tips.2008.09.001
Rabinovici, G. D. (2021). Controversy and Progress in Alzheimer’s Disease — FDA Approval of Aducanumab. N. Engl. J. Med. 385, 771–774. doi: 10.1056/NEJMp2111320
Reitz, C., Rogaeva, E., and Beecham, G. W. (2020). Late-onset vs nonmendelian early-onset Alzheimer disease: A distinction without a difference? Neurol. Genet. 6:e512. doi: 10.1212/NXG.0000000000000512
Reza-Zaldivar, E. E., Hernández-Sapiéns, M. A., Gutiérrez-Mercado, Y. K., Sandoval-Ávila, S., Gomez-Pinedo, U., Márquez-Aguirre, A. L., et al. (2019). Mesenchymal stem cell-derived exosomes promote neurogenesis and cognitive function recovery in a mouse model of Alzheimer’s disease. Neural. Regen. Res. 14, 1626–1634. doi: 10.4103/1673-5374.255978
Reza-Zaldivar, E. E., Hernández-Sapiéns, M. A., Minjarez, B., Gutiérrez-Mercado, Y. K., Márquez-Aguirre, A. L., and Canales-Aguirre, A. A. (2018). Potential effects of MSC-derived exosomes in neuroplasticity in Alzheimer’s disease. Front. Cell Neurosci. 12:317. doi: 10.3389/fncel.2018.00317
Ribeiro, C., Salgado, A., Fraga, J., Silva, N., Reis, R., and Sousa, N. (2011). The secretome of bone marrow mesenchymal stem cells-conditioned media varies with time and drives a distinct effect on mature neurons and glial cells (primary cultures). J. Regen. Med. Tissue Eng. 5, 668–672. doi: 10.1002/term.365
Rosenzweig, N., Dvir-Szternfeld, R., Tsitsou-Kampeli, A., Keren-Shaul, H., Ben-Yehuda, H., Weill-Raynal, P., et al. (2019). PD-1/PD-L1 checkpoint blockade harnesses monocyte-derived macrophages to combat cognitive impairment in a tauopathy mouse model. Nat. Commun. 10, 1–15. doi: 10.1038/s41467-019-08352-5
Santamaria, G., Brandi, E., Vitola, P., Grandi, F., Ferrara, G., Pischiutta, F., et al. (2021). Intranasal delivery of mesenchymal stem cell secretome repairs the brain of Alzheimer’s mice. Cell Death Differ. 28, 203–218. doi: 10.1038/s41418-020-0592-2
Scarpini, E., Schelterns, P., and Feldman, H. (2003). Treatment of Alzheimer’s disease; current status and new perspectives. Lancet Neurol. 2, 539–547. doi: 10.1016/S1474-4422(03)00502-7
Schain, M., and Kreisl, W. C. (2017). Neuroinflammation in neurodegenerative disorders-a review. Curr. Neurol. Neurosci. Rep. 17:25. doi: 10.1007/s11910-017-0733-2
Scheibe, F., Klein, O., Klose, J., and Priller, J. (2012). Mesenchymal stromal cells rescue cortical neurons from apoptotic cell death in an in vitro model of cerebral ischemia. Cell Mol. Neurobiol. 32, 567–576. doi: 10.1007/s10571-012-9798-2
Scheper, V., Schwieger, J., Hamm, A., Lenarz, T., and Hoffmann, A. (2019). BDNF-overexpressing human mesenchymal stem cells mediate increased neuronal protection in vitro. J. Neurosci. Res. 97, 1414–1429. doi: 10.1002/jnr.24488
Scuteri, A., Cassetti, A., and Tredici, G. (2006). Adult mesenchymal stem cells rescue dorsal root ganglia neurons from dying. Brain Res. 1116, 75–81. doi: 10.1016/j.brainres.2006.07.127
Scuteri, A., Miloso, M., Foudah, D., Orciani, M., Cavaletti, G., and Tredici, G. (2011a). Mesenchymal stem cells neuronal differentiation ability: A real perspective for nervous system repair? Curr. Stem Cell Res. Ther. 6, 82–92. doi: 10.2174/157488811795495486
Scuteri, A., Ravasi, M., Pasini, S., Bossi, M., and Tredici, G. (2011b). Mesenchymal stem cells support dorsal root ganglion neurons survival by inhibiting the metalloproteinase pathway. Neuroscience 172, 12–19. doi: 10.1016/j.neuroscience.2010.10.065
Sha, S., Shen, X., Cao, Y., and Qu, L. (2021). Mesenchymal stem cells-derived extracellular vesicles ameliorate Alzheimer’s disease in rat models via the microRNA-29c-3p/BACE1 axis and the Wnt/β-catenin pathway. Aging (Albany NY) 13, 15285–15306. doi: 10.18632/aging.203088
Shin, J. Y., Park, H. J., Kim, H. N., Oh, S. H., Bae, J.-S., Ha, H.-J., et al. (2014). Mesenchymal stem cells enhance autophagy and increase β-amyloid clearance in Alzheimer disease models. Autophagy 10, 32–44. doi: 10.4161/auto.26508
Si, Z., and Wang, X. (2021). Stem Cell Therapies in Alzheimer’s Disease: Applications for disease modeling. J. Pharmacol. Exp. Ther. 377, 207–217. doi: 10.1124/jpet.120.000324
Siskova, Z., and Tremblay, M. -È (2013). Microglia and Synapse: Interactions in health and neurodegeneration. Neural plasticity 2013, 425845. doi: 10.1155/2013/425845
Solito, E., and Sastre, M. (2012). Microglia Function in Alzheimer’s Disease. Front. Pharmacol. 3:14. doi: 10.3389/fphar.2012.00014
Song, M. S., Learman, C. R., Ahn, K. C., Baker, G. B., Kippe, J., Field, E. M., et al. (2015). In vitro validation of effects of BDNF-expressing mesenchymal stem cells on neurodegeneration in primary cultured neurons of APP/PS1 mice. Neuroscience 307, 37–50. doi: 10.1016/j.neuroscience.2015.08.011
Tripathi, T., and Khan, H. (2020). Direct Interaction between the beta-Amyloid Core and Tau Facilitates Cross-Seeding: A Novel Target for Therapeutic Intervention. Biochemistry 59, 341–342. doi: 10.1021/acs.biochem.9b01087
Ullah, M., Liu, D. D., and Thakor, A. S. (2019). Mesenchymal stromal cell homing: Mechanisms and strategies for improvement. iScience 15, 421–438. doi: 10.1016/j.isci.2019.05.004
van der Flier, W. M., Pijnenburg, Y. A., Fox, N. C., and Scheltens, P. (2011). Early-onset versus late-onset Alzheimer’s disease: The case of the missing APOE varepsilon4 allele. Lancet Neurol. 10, 280–288. doi: 10.1016/S1474-4422(10)70306-9
Van Velthoven, C. T., Kavelaars, A., and Heijnen, C. J. (2012). Mesenchymal stem cells as a treatment for neonatal ischemic brain damage. Pediatr. Res. 71, 474–481. doi: 10.1038/pr.2011.64
Villar, M. F. Z., Hanotte, J. L., Pardo, J., Morel, G. R., Mazzolini, G., García, M. G., et al. (2020). Mesenchymal stem cells therapy improved the streptozotocin-induced behavioral and hippocampal impairment in rats. Mol. Neurobiol. 57, 600–615. doi: 10.1007/s12035-019-01729-z
Wang, X., Ma, S., Yang, B., Huang, T., Meng, N., Xu, L., et al. (2018). Resveratrol promotes hUC-MSCs engraftment and neural repair in a mouse model of Alzheimer’s disease. Behav. Brain Res. 339, 297–304. doi: 10.1016/j.bbr.2017.10.032
Wei, Y., Xie, Z., Bi, J., and Zhu, Z. (2018). Anti-inflammatory effects of bone marrow mesenchymal stem cells on mice with Alzheimer’s disease. Exp. Ther. Med. 16, 5015–5020. doi: 10.3892/etm.2018.6857
Wilkins, A., Kemp, K., Ginty, M., Hares, K., Mallam, E., and Scolding, N. (2009). Human bone marrow-derived mesenchymal stem cells secrete brain-derived neurotrophic factor which promotes neuronal survival in vitro. Stem Cell Res. 3, 63–70. doi: 10.1016/j.scr.2009.02.006
Wong, W. (2020). Economic burden of Alzheimer disease and managed care considerations. Am. J. Manag. Care 26, S177–S183. doi: 10.37765/ajmc.2020.88482
Xie, J., Liang, R., Wang, Y., Huang, J., Cao, X., and Niu, B. (2020). Progress in Target Drug Molecules for Alzheimer’s Disease. Curr. Top Med. Chem. 20, 4–36. doi: 10.2174/1568026619666191203113745
Xie, Z. H., Liu, Z., Zhang, X. R., Yang, H., Wei, L. F., Wang, Y., et al. (2016). Wharton’s Jelly-derived mesenchymal stem cells alleviate memory deficits and reduce amyloid-beta deposition in an APP/PS1 transgenic mouse model. Clin. Exp. Med. 16, 89–98. doi: 10.1007/s10238-015-0375-0
Yan, K., Zhang, R., Chen, L., Chen, F., Liu, Y., Peng, L., et al. (2014a). Nitric oxide-mediated immunosuppressive effect of human amniotic membrane-derived mesenchymal stem cells on the viability and migration of microglia. Brain Res. 1590, 1–9. doi: 10.1016/j.brainres.2014.05.041
Yan, Y., Ma, T., Gong, K., Ao, Q., Zhang, X., and Gong, Y. (2014b). Adipose-derived mesenchymal stem cell transplantation promotes adult neurogenesis in the brains of Alzheimer’s disease mice. Neural. Regen. Res. 9, 798–805. doi: 10.4103/1673-5374.131596
Yang, C., Li, Y., Du, M., and Chen, Z. (2019). Recent advances in ultrasound-triggered therapy. J. Drug Target 27, 33–50. doi: 10.1080/1061186X.2018.1464012
Yang, H., Xie, Z. H., Wei, L. F., Yang, H. N., Yang, S. N., Zhu, Z. Y., et al. (2013). Human umbilical cord mesenchymal stem cell-derived neuron-like cells rescue memory deficits and reduce amyloid-beta deposition in an AβPP/PS1 transgenic mouse model. Stem Cell Res. Ther. 4:76. doi: 10.1186/scrt227
Yang, L., Zhai, Y., Hao, Y., Zhu, Z., and Cheng, G. (2020). The regulatory functionality of exosomes derived from hUMSCs in 3D Culture for Alzheimer’s Disease Therapy. Small 16:1906273. doi: 10.1002/smll.201906273
Yokokawa, K., Iwahara, N., Hisahara, S., Emoto, M. C., Saito, T., Suzuki, H., et al. (2019). Transplantation of mesenchymal stem cells improves Amyloid-β pathology by modifying microglial function and suppressing oxidative stress. J. Alzheimers Dis. 72, 867–884.
Yong, H. Y. F., Rawji, K. S., Ghorbani, S., Xue, M., and Yong, V. W. (2019). The benefits of neuroinflammation for the repair of the injured central nervous system. Cell Mol. Immunol. 16, 540–546. doi: 10.1038/s41423-019-0223-3
Yun, H., Kim, H., Park, K., Shin, J., Kang, A., Il Lee, K., et al. (2013). Placenta-derived mesenchymal stem cells improve memory dysfunction in an A β 1–42-infused mouse model of Alzheimer’s disease. Cell Death Dis. 4, e958–e958. doi: 10.1038/cddis.2013.490
Zenaro, E., Pietronigro, E., Della Bianca, V., Piacentino, G., Marongiu, L., Budui, S., et al. (2015). Neutrophils promote Alzheimer’s disease-like pathology and cognitive decline via LFA-1 integrin. Nat. Med. 21, 880–886. doi: 10.1038/nm.3913
Zhang, Z., Sheng, H., Liao, L., Xu, C., Zhang, A., Yang, Y., et al. (2020). Mesenchymal stem cell-conditioned medium improves mitochondrial dysfunction and suppresses apoptosis in okadaic acid-Treated SH-SY5Y Cells by extracellular vesicle mitochondrial transfer. J. Alzheimers Dis. 78, 1161–1176. doi: 10.3233/JAD-200686
Zhao, Y., Chen, X., Wu, Y., Wang, Y., Li, Y., and Xiang, C. (2018). Transplantation of human menstrual blood-derived mesenchymal stem cells alleviates Alzheimer’s Disease-Like Pathology in APP/PS1 Transgenic Mice. Front. Mol. Neurosci. 11:140. doi: 10.3389/fnmol.2018.00140
Zheng, X.-Y., Wan, Q.-Q., Zheng, C.-Y., Zhou, H.-L., Dong, X.-Y., Deng, Q.-S., et al. (2017). Amniotic mesenchymal stem cells decrease Aβ deposition and improve memory in APP/PS1 transgenic mice. Neurochem. Res. 42, 2191–2207. doi: 10.1007/s11064-017-2226-8
Zhou, L. N., Wang, J. C., Zilundu, P. L. M., Wang, Y. Q., Guo, W. P., Zhang, S. X., et al. (2020). A comparison of the use of adipose-derived and bone marrow-derived stem cells for peripheral nerve regeneration in vitro and in vivo. Stem Cell Res. Ther. 11:153. doi: 10.1186/s13287-020-01661-3
Keywords: mesenchymal stromal cells, mesenchymal stem cells, Alzheimer’s disease, microglia, neurons, neuroprotection
Citation: Regmi S, Liu DD, Shen M, Kevadiya BD, Ganguly A, Primavera R, Chetty S, Yarani R and Thakor AS (2022) Mesenchymal stromal cells for the treatment of Alzheimer’s disease: Strategies and limitations. Front. Mol. Neurosci. 15:1011225. doi: 10.3389/fnmol.2022.1011225
Received: 04 August 2022; Accepted: 20 September 2022;
Published: 06 October 2022.
Edited by:
Jiehui Jiang, Shanghai University, ChinaReviewed by:
Francesca Massenzio, Università di Bologna, ItalySun Young Park, Pusan National University, South Korea
Copyright © 2022 Regmi, Liu, Shen, Kevadiya, Ganguly, Primavera, Chetty, Yarani and Thakor. This is an open-access article distributed under the terms of the Creative Commons Attribution License (CC BY). The use, distribution or reproduction in other forums is permitted, provided the original author(s) and the copyright owner(s) are credited and that the original publication in this journal is cited, in accordance with accepted academic practice. No use, distribution or reproduction is permitted which does not comply with these terms.
*Correspondence: Avnesh S. Thakor, YXN0aGFrb3JAc3RhbmZvcmQuZWR1