- Institute of Physiology, University Medical Center of the Johannes Gutenberg University, Mainz, Germany
Throughout early phases of brain development, the two main neural signaling mechanisms—excitation and inhibition—are dynamically sculpted in the neocortex to establish primary functions. Despite its relatively late formation and persistent developmental changes, the GABAergic system promotes the ordered shaping of neuronal circuits at the structural and functional levels. Within this frame, interneurons participate first in spontaneous and later in sensory-evoked activity patterns that precede cortical functions of the mature brain. Upon their subcortical generation, interneurons in the embryonic brain must first orderly migrate to and settle in respective target layers before they can actively engage in cortical network activity. During this process, changes at the molecular and synaptic level of interneurons allow not only their coordinated formation but also the pruning of connections as well as excitatory and inhibitory synapses. At the postsynaptic site, the shift of GABAergic signaling from an excitatory towards an inhibitory response is required to enable synchronization within cortical networks. Concomitantly, the progressive specification of different interneuron subtypes endows the neocortex with distinct local cortical circuits and region-specific modulation of neuronal firing. Finally, the apoptotic process further refines neuronal populations by constantly maintaining a controlled ratio of inhibitory and excitatory neurons. Interestingly, many of these fundamental and complex processes are influenced—if not directly controlled—by electrical activity. Interneurons on the subcellular, cellular, and network level are affected by high frequency patterns, such as spindle burst and gamma oscillations in rodents and delta brushes in humans. Conversely, the maturation of interneuron structure and function on each of these scales feeds back and contributes to the generation of cortical activity patterns that are essential for the proper peri- and postnatal development. Overall, a more precise description of the conducting role of interneurons in terms of how they contribute to specific activity patterns—as well as how specific activity patterns impinge on their maturation as orchestra members—will lead to a better understanding of the physiological and pathophysiological development and function of the nervous system.
Introduction
During early development, mammalian brains can be functionally characterized by the progressive emergence of distinct cortical activity patterns which are essential for the establishment of basic functions of the cerebral cortex (Blankenship and Feller, 2010; Kilb et al., 2011; Kirkby et al., 2013). Underlying this dynamic change in neuronal activity, among other developmental processes, is the structural and functional maturation of the two main signaling principles of neurons: excitation and inhibition (Egorov and Draguhn, 2013; Luhmann et al., 2016; Teppola et al., 2019). While glutamatergic signaling is established already within early, embryonic stages in rodents and humans (Monyer et al., 1994; Bagasrawala et al., 2017), the maturation of the GABAergic system extends into the postnatal period of most mammals. Starting with the formation of the first GABAergic synapse (Khazipov et al., 2001), the maturation of the inhibitory system coincides with the emergence of correlated oscillatory activity patterns, such as spindle burst and gamma oscillations in newborn rodents or delta brushes in prenatal humans (Luhmann and Khazipov, 2018). Here, the thalamus contributes significantly to the generation of these early cortical oscillations (Minlebaev et al., 2011; Yang et al., 2013; Murata and Colonnese, 2016), which conversely also modulate thalamic activity within a cortico-thalamic feedback loop (Yang et al., 2013; Martini et al., 2021). Furthermore, the maturation of the adult inhibitory GABAergic system is still not complete when cortical activity evolves to its final more de-correlated activity state that underlies its later complex functions (Golshani et al., 2009; Rochefort et al., 2009).
Although the contribution of GABA signaling to cortical activities during the perinatal phases is not fully understood, it is often speculated that GABAergic interneurons critically modulate the output of neuronal circuits in the form of spontaneous and sensory-driven activity (Bonifazi et al., 2009; Butt et al., 2017). In general, the importance of distinct cortical activity patterns during cortical development is emphasized by their necessity for and coherent emergence with higher cognitive function (Tort et al., 2009; Bosman et al., 2012). Consistently, in sensory cortical areas during the postnatal period of rodents, stereotypical spontaneous and evoked activity patterns concurrently develop with respective sensory modalities (Rochefort et al., 2009; Yang et al., 2009; Colonnese et al., 2010; Ackman and Crair, 2014; Martini et al., 2021). However, the source of spontaneous activity is still a matter of ongoing research, as well as the root cause and type of evoked activity which varies depending on the region and time point of perinatal development.
Yet, undoubtedly, neuronal activity itself is a key regulator of many—if not all—developmental processes in the cortex. Thus, it comes to no surprise that neuronal activity also strongly impacts the maturation of the GABAergic system, from the cellular to the network level, and fine-tunes excitation/inhibition balance (Turrigiano and Nelson, 2004; Takesian and Hensch, 2013). Besides cortical activity, thalamic inputs also play a role in interneuron maturation on the level of the cortex (Pouchelon et al., 2014; Marques-Smith et al., 2016), while interneurons in turn function as a gate for the thalamus by effecting cortical network activity (Yang et al., 2013; Martini et al., 2021). Therefore, the GABAergic system must permit sufficient excitation to engage in cortical activity and still provide the needed inhibition to prevent over-excitation. In this respect, it is worth mentioning that a certain level of freedom in the excitation/inhibition balance is needed, especially for the establishment of the sensory cortical system during early development (Masquelier et al., 2009; Deidda et al., 2015; Wosniack et al., 2021). Both the GABAergic system and neuronal activity are fulfilling important functions during these critical periods, as discussed in more detail elsewhere (Sale et al., 2010; Reha et al., 2020).
In this article, the focus will be on the interdependency of the maturation of the GABAergic system and cortical network activity throughout the perinatal and postnatal development of the rodent cortex. For this purpose, we will review how neuronal activity impacts the maturation of the GABAergic system on the subcellular (mostly synaptic) level, on the cellular and on the network level and discuss how the maturation on each of these scales feeds back on cortical activity, thus impacting the function of the mature cortex. Finally, we will describe the physiological implications of this interdependency and highlight open questions in this field of research.
The Interplay of Activity and Perinatal Changes of GABAergic System at The Subcellular Level
Before becoming the main inhibitory neurotransmitter in the mature brain, GABA exerts mainly excitatory function in immature neurons (Luhmann and Prince, 1991; Leinekugel et al., 1995; Rheims et al., 2008; Kirmse et al., 2015) and is suggested to regulate spontaneous activity during development (Ben-Ari, 2002; Le Magueresse and Monyer, 2013; Kirmse et al., 2015). In turn, the neuronal activity itself is a key regulator of the subcellular processes that underlie this developmental change in GABA signaling—like the expression of chloride transporters (Fiumelli et al., 2005), GABA-receptor expression, and GABAergic synaptogenesis (Ganguly et al., 2001; Wardle and Poo, 2003; see also Figure 1 for overview).
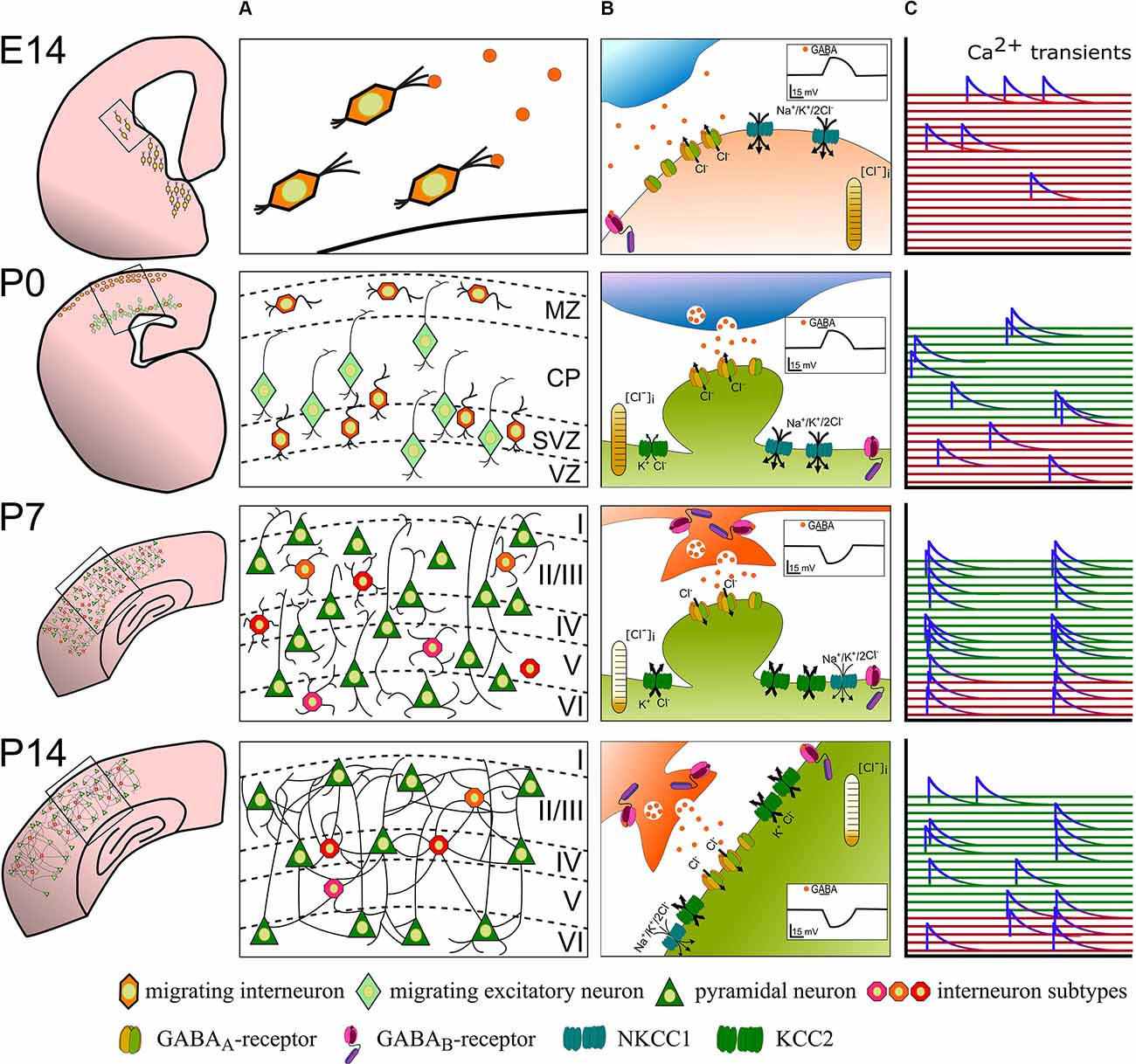
Figure 1. The developmental sequence of perinatal changes in (A) positioning and integration of nascent interneurons, (B) synaptic GABA signaling, and (C) interneuron participation in network activity in the rodent cortex. At E14, GABAergic interneuron precursors tangentially migrate from the ganglionic eminences into the cortex and ambient GABA acts as a chemoattractant. Binding of GABA to GABAA-receptors at this stage depolarizes recipient interneurons and likely contributes to sporadic, uncorrelated Ca2+ transients (red). Around birth (P0), immature GABAergic interneurons are found in the marginal zone and migrate radially concomitant with pyramidal neurons. Here, GABA acts as a Stop and Go signal mainly through GABAA-receptors. At this developmental stage, GABA is still excitatory and contributes to uncorrelated Ca2+ transients in GABAergic interneurons (red traces) and immature pyramidal neurons (green traces). At the end of the 1st postnatal week (P7), layering of the cortex is mostly complete and maturing neurons already begin to form synapses. Vesicular released GABA hyperpolarizes the postsynaptic cell via postsynaptic GABAA- and binds to extra- and pre-synaptic GABAB-receptors. Highly synchronous spontaneous activity in interneurons and pyramidal neurons is a hallmark of this developmental phase. By P14, excess neurons were depleted through developmental apoptosis and stable functional, also perisomatic connections have developed. The cation-chloride transporter profile is fully matured and GABA receptor signaling fulfills its mostly inhibitory function. Spontaneous and sensory-evoked activity shifts to a more decorrelated state.
GABA-Receptor Signaling
At the postsynaptic side, GABA exerts its function via ionotropic GABAA-receptors and metabotropic GABAB-receptors. GABAA-receptors are a heterogenous group of chloride channels with rapid kinetics. Each of them is formed by a heteromeric complex that consists of five of a possible 19 different subunits (α1–6, β1–3, γ1–3, π, θ, δ, ε, Kumada and Fukuda, 2020). Differences in localization of GABAA-receptors lead to two major forms of GABAergic inhibition: phasic inhibition and tonic inhibition. Where the former is mediated by synaptic, low affinity GABAA-receptors, the latter is facilitated by high affinity, extrasynaptic GABAA-receptors (Kumada and Fukuda, 2020). The expression of GABAA-receptor subunits changes with development: some subunits display a consistent increase in expression levels with age (e.g., α1, β1, β2, δ) whereas others instead show a peak, followed by a decline (e.g., α2, α3, α5, β3, γ3, Laurie et al., 1992). Although a large portion of GABAA receptor subunits is only found in postmigratory neurons, others (α2, α3, β1, β3, γ1, γ2) are already detectable in the germinal zone and in migrating neurons in the marginal zone and the cortical plate (Araki et al., 1992; Laurie et al., 1992; Poulter et al., 1992; Van Eden et al., 1995). The early timing of the expression profile supports an effective role of GABAergic signaling before synaptogenesis, i.e., during interneuron migration and maturation of GABAergic synapses.
In contrast to the ionotropic GABAA-receptors, the metabotropic GABAB-receptors consist of two distinct subunits (B1 and B2). Subunit B1 is expressed in two isoforms: namely, B1a and B1b, which require the dimerization with a B2 subunit to form functional heteromeric GABAB-receptors (Terunuma, 2018). Once the receptor is activated by ligand binding on the extracellular domain of the B1 subunit, a G-protein mediated signaling cascade is started which opens K+ channels at the post- and Ca2+ channels at the presynaptic site. In this way, GABAB receptor mediated inhibition leads to hyperpolarization of the postsynaptic neuron and/or to reduced release probability of neurotransmitters in the synaptic cleft. In rodents, GABAB receptors are expressed as early as embryonic day 14 (López-Bendito et al., 2002) and reach their expression level peak in the first postnatal week (Turgeon and Albin, 1994; Behuet et al., 2019). Furthermore, it was shown that the different GABAB subunits have distinct expression levels with GABAB1 playing a more important role during prenatal development of the rat (Li et al., 2004). In addition, GABAB1-receptors are expressed in migrating neurons in the lower intermediate zone, where GABA not only enhances GABAB-receptor expression but also works as a chemo-attractant that promotes motility of migrating neurons (Behar et al., 2001). GABAB receptors are found in dendritic spines and dendritic shafts at extrasynaptic and perisynaptic sites during postnatal development (López-Bendito et al., 2002). Moreover, in the postnatal stage, activity-dependent secretion of brain-derived neurotrophic factor (BDNF) is mainly mediated by activation of GABAB-receptors, which then promote the development of perisomatic GABAergic synapses (Fiorentino et al., 2009).
Taken together, the results on GABA receptor signaling during brain development illustrate the importance of GABAA-receptor activity for corticogenesis, interneuron migration, and for modulation of synaptic transmission (Cancedda et al., 2007; Patrizi et al., 2008; Fuchs et al., 2013) and indicate a potentially important but largely unresolved role for GABAB-receptors.
Facilitating the Chloride Gradient: The Cotransporters NKKC1 and KCC2
Activation of mature postsynaptic GABAA receptors typically leads to a fast hyperpolarization through anion influx, predominantly by Cl− (Kaila, 1994; Olsen and Sieghart, 2009). However, during brain development, GABA plays a critical role as an excitatory drive relevant for the proper development and establishment of neuronal circuits (Ben-Ari, 2002; Rheims et al., 2008). In fact, in the immature brain GABAA-receptor activation leads to depolarization of neurons due to the high intracellular Cl− concentration (Rivera et al., 1999; Yamada et al., 2004; Rheims et al., 2008; Kirmse et al., 2015). The intracellular concentration is mostly set by two main cation-chloride cotransporters Na+-K+-2Cl−-Cotransporter 1 (NKCC1)—a chloride-importer—and K+-Cl−-cotransporter 2 (KCC2)—a chloride extruder, which play a pivotal role in the polarity of GABAergic action (Rivera et al., 1999; Yamada et al., 2004; Achilles et al., 2007; Rheims et al., 2008; Kirmse et al., 2015). In immature cortical neurons, intracellular chloride is significantly higher than in mature neurons due to the predominant expression of NKCC1 over KCC2. The developmental change in chloride-cotransporter expression, which occurs within the first postnatal week in the rodent cortex (Shimizu-Okabe et al., 2002), is hence effectively reversing GABA action from depolarizing to hyperpolarizing (Rivera et al., 1999; Shimizu-Okabe et al., 2002; Yamada et al., 2004; Rheims et al., 2008). Studies in various animal models have shown that this switch occurs at different time points within different species and have brain region-specific effects (Leinekugel et al., 1995; Reith and Sillar, 1999; Saint-Amant and Drapeau, 2000; Eilers et al., 2001; Gao and Van Den Pol, 2001; Murata and Colonnese, 2020). It also could be demonstrated that the precise time point of the switch is not strictly determined by the genetic program, but might be influenced by neurotrophic factors and neuronal activity (Ganguly et al., 2001; Wardle and Poo, 2003). For example, repetitive fast postsynaptic excitation influences KCC2 expression and therefore affects the chloride reversal potential (Fiumelli et al., 2005). Also, GABA itself can be crucial for the determination of the time point of shift. In the turtle retina, under blockade of GABAA receptors at the developmental time point of the shift, GABA action remains excitatory, through inhibition of KCC2 upregulation (Leitch et al., 2005). On the other hand, experiments in hippocampal slice and dissociated hippocampal cultures do not support a GABA and/or activity dependency of the switch from de- to hyperpolarizing (Ludwig et al., 2003; Titz et al., 2003). Unfortunately, studies aiming to assess the role of activity for the expression of NKCC1 are still difficult to interpret, likely because of broad technical difficulties (Virtanen et al., 2020). A recent study, in which NKCC1 is selectively knocked-out in telencephalic glutamatergic neurons, showed that in the visual cortex NKCC1 is not necessary for the establishment of fully functional networks in adult mice (Graf et al., 2021). Conceptually this is in line with another recent study, in which GABAergic activation did not produce excitation in postsynaptic neurons in the visual cortex of 3 days old mice (Murata and Colonnese, 2020). Supporting brain region specific differences in GABAergic synaptic transmission, glutamatergic hippocampal neurons lacking NKCC1 display significantly lower intracellular chloride concentrations. Despite the alterations in correlated spontaneous activity during development and slightly altered network dynamics in the hippocampus of adult mice, these knock-out mice are perfectly capable of performing hippocampus-dependent behavioral tasks (Graf et al., 2021). However, it remains unclear whether changes in network dynamics are an acute effect of NKCC1 loss, or rather an adaptation to ensure proper functionality in NKCC1 knock-out mouse lines. In support of the latter hypothesis, an earlier study showed that the complete loss of NKCC1 prevents excitation via GABA in hippocampal CA3 neurons, nevertheless, these mice still display typical network activity patterns as seen under physiological conditions (Sipilä et al., 2009). In contrast, another constitutive NKCC1 knock-out mouse line shows impairments in early hippocampal activity patterns and delayed maturation of the network (Pfeffer et al., 2009).
GABAergic Signaling Before and During Synaptogenesis
On a structural level, GABAergic synapses are among the first synapses that are formed in the developing brain (Tyzio et al., 1999; Khazipov et al., 2001; Rymar and Sadikot, 2007). Immature neurons in the hippocampus as well as in the neocortex first receive GABAergic before glutamatergic input (Ben-Ari, 2006; Wang and Kriegstein, 2008). In the neocortex of newborn mice GABAergic vesicle abundance is relatively low and only during the following days the expression of GABAergic synaptic markers increases gradually until it reaches a plateau at the end of the 2nd postnatal week (Minelli et al., 2003). However, not only does the number of GABAergic vesicles increase, but also their overall distribution changes within the developing cortex. While GABAergic vesicles can only be detected in the marginal zone in newborn mice, their distribution gradually extends deeper into the neocortex until finally covering all cortical layers at the end of the second postnatal week (Minelli et al., 2003). Despite the maturation of GABAergic vesicles late in the first postnatal week (Minelli et al., 2003), GABA positive cells can already be found even in the deeper layer of the neocortex at birth (Takayama and Inoue, 2010). These findings support a role of GABAergic signaling before the onset of synaptogenesis, i.e., extrasynaptic transmission. In line with this, GAD67 (the main GABA-producing enzyme isoform) and GABAA receptors can already be detected as early as E17 in the ventricular zone (Ma and Barker, 1995) and throughout the cortical plate (van den Berghe et al., 2013). Paracrine release of GABA was demonstrated to occur in different cell types during development, e.g., in immature neurons, but also in endothelial cells (Taylor and Gordon-Weeks, 1991; Gao and Van Den Pol, 2000; Li et al., 2018). In the latter, partial or complete loss of GABA release during embryogenesis leads to impairment of long-distance migration and positioning of cortical interneurons (Li et al., 2018). In the adult cortex, astrocytes express the GABA transporter GAT1 and thus influence the excitatory and inhibitory transmission through the paracrine spread of GABA (Minelli et al., 1995; Barakat and Bordey, 2002). However, whether or not astrocytes are also a source of GABA during development is yet not clear.
Maturation of GABAergic Synapses
Neuronal activity e.g., via the depolarization of immature neurons, is a key regulator in synaptogenesis. in vitro and in vivo studies show that the excitatory effect of GABA during early development is essential for the normal maturation of dendritic spines (Hensch et al., 1998; Cancedda et al., 2007; Chattopadhyaya et al., 2007; Wang and Kriegstein, 2008; Pfeffer et al., 2009; Oh et al., 2016; Flossmann et al., 2019). In line with an important role for GABAA-receptor-mediated activity during the establishment of neural circuits, the development of synapses between somatostatin-positive (SST) interneurons and pyramidal cells in the hippocampus is NKCC1-dependent (Pfeffer et al., 2009; Flossmann et al., 2019). However, not only GABA-induced activity is required for the proper maturation of GABAergic interneurons, but also, NMDA receptor activity affects the regulation of GABAergic synaptogenesis (Cserép et al., 2012; Gu et al., 2016; Hanson et al., 2019). Tonic NMDA-mediated neuronal activity is important for the maturation and correct integration of parvalbumin-positive (PV) interneurons into the developing cortical network (Hanson et al., 2019). In early development, NMDA-receptors are co-localized with GABAA-receptors at the postsynaptic site (Cserép et al., 2012), where NMDA-receptors act as upstream signaling molecules essential for GABAergic synaptogenesis via Ca2+ transient and calmodulin signaling (Gu et al., 2016). Conversely, GABAA-receptor activation is sufficient to remove the voltage-dependent Mg2+ blockade and thus activate NMDA-receptors (Wang and Kriegstein, 2008). The mutual interplay between GABAA- and NMDA-receptors is thus shown to play an important role in the emergence of spontaneous synchronous activity and the correct balance between excitation and inhibition (E/I) in the neocortex (Wang and Kriegstein, 2008). Of note, also AMPA-receptor expression at the postsynaptic site can be affected by GABAergic action, such that AMPA-receptor levels are downregulated in glutamatergic/GABAergic-mixed synapses (Fattorini et al., 2019). Thereby, a proper E/I balance is ensured and a potential neuroprotective effect is exerted in the developing brain (Fattorini et al., 2019). GABAA-receptors fulfill important functions for GABAergic synapse development not only on the functional but also on the structural level (Chattopadhyaya et al., 2007; Deng et al., 2007; Fuchs et al., 2013; Oh et al., 2016). Along this line, GABA release from SST interneurons leads to the expression of the scaffolding protein gephyrin and dendritic spine formation by recruitment and activation of GABAA receptors in layer 2/3 cortical pyramidal neurons in neonatal mice (Oh et al., 2016). Accordingly, conditionally knocking-out GAD67 in PV basket cells results in less terminal branching, smaller boutons size, and hence, fewer and deficient synaptic contacts (Chattopadhyaya et al., 2007). Depletion of GAD65, the smaller isoform of the GAD protein, leads to impaired formation of cortical networks and over-responsiveness in the visual cortex (Hensch et al., 1998) while overexpression of GAD67 leads to faster perisomatic innervation (Chattopadhyaya et al., 2007). Together, these findings suggest that GABA regulates perisynaptic contact formation during the maturation of neural circuits (Chattopadhyaya et al., 2007) and imply that suppression of electrical activity leads to fewer synaptic contacts via reduced GABA levels (Chattopadhyaya et al., 2004). On the other hand, mice with disturbed GABA homeostasis also display less activity (Fiorentino et al., 2009). This raises the question of whether GABA action on synaptogenesis should be mostly considered as an activity-independent mechanism.
The Interplay of Activity and Perinatal Changes of GABAergic System at The Cellular and Network Level
Not only the maturation of the GABAergic system at the subcellular level is affected by, but also the maturation of the single (inter-)neuron and network level activity shows an activity-dependence. While, on the other hand, network composition in general—and especially the activity of GABAergic subpopulations—significantly influence cortical activity during the postnatal period of rodents (Le Magueresse and Monyer, 2013; Kepecs and Fishell, 2014; Tremblay et al., 2016; for an overview see also Figure 2). Inversely, cortical activity is not only the most relevant cortical output function, but also has an important feedback role as a key regulating factor for many processes at the cellular and network level during early brain development (Luhmann et al., 2016; Okujeni and Egert, 2019). In this way (and as can be seen in Figure 2), cortical activity and especially the activity of interneurons themselves critically control several key steps in the development of GABAergic neurons on the network level, including migration, wiring, and programmed cell death.
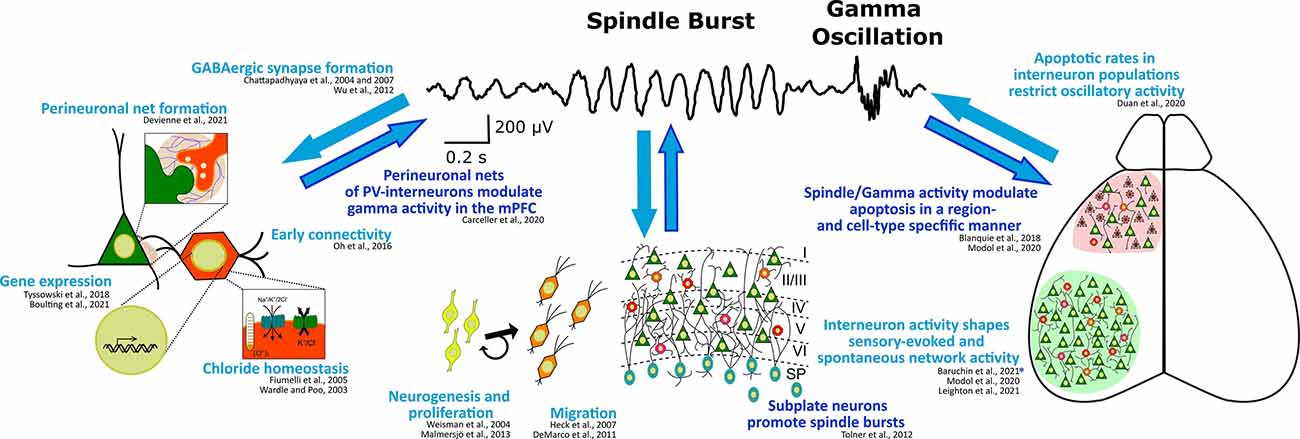
Figure 2. Interdependence of (oscillatory) cortical network activity and development of the GABAergic system at the subcellular, cellular, and network level. Known regulation of or by high frequency spindle burst and gamma activity are highlighted in dark blue. Exemplary references to experimental evidence supporting these processes are listed. *Note, this study suggests that somatostatin interneurons contribute to the early processing of sensory information in a way, that their activity affects spindle burst activity possibly through the plastic arrangement of thalamic innervation to the neocortex.
Neurogenesis and Proliferation of Interneuron Precursors
In chronological order, the first step to consider is the embryonic generation of GABAergic interneurons, i.e., the neurogenesis and proliferation of interneuron precursor cells in the ventral telencephalon—in particular the medial and caudal ganglionic eminences (MGE and CGE, respectively), with a minor contribution of the preoptic area and the lateral ganglionic eminence (POA and LGE; Gelman and Marín, 2010; Sultan et al., 2013). Expression of homeobox transcription factors of the Dlx family is of essential importance for GABAergic precursors proliferation, as well as for the differentiation of interneurons (Petryniak et al., 2007). Additionally, both processes are activity-dependent. The proliferation of neuronal progenitors, in general, has been shown to be influenced by spontaneous calcium activity (Weissman et al., 2004; Malmersjö et al., 2013). Meanwhile, spontaneous calcium activity in parallel also critically impacts the further specification of neuronal phenotypes (Ciccolini et al., 2003; Borodinsky et al., 2004), which is no surprise considering the tight link between neuronal gene expression and neuronal activity (Flavell and Greenberg, 2008). In this regard, it should be highlighted that the increasing complexity of activity patterns in developing neurons that follows the occurrence of simple calcium transients in progenitor cells also offers a higher order complexity on the level of gene regulation (Tyssowski et al., 2018). Activity-dependent regulation of the proliferation and differentiation of neural stem cells and oligodendrocyte precursors has also been shown in the postnatal brain (Káradóttir and Kuo, 2018). Thus, neuronal activity is not only an import modulator determining the extent and type of interneurons during development but also remains important in adult neuro- and gliogenesis. Moreover, via the direct action of synaptically released GABA (Andäng et al., 2008) as well as through cortical activity that is in turn significantly influenced by GABAergic neuronal population sizes (Modol et al., 2020), cross-talk of interneuron proliferation and cortical activity should be carefully considered as a regulatory mechanism that shapes neuronal circuitry.
Migration of Interneurons
Upon their generation, interneurons need to migrate from their places of origin in the subpallium along the subventricular and marginal zone to reach their final place of destination in the postnatal cortex. This location is spatially characterized by a distinct radial position within a certain cortical region and also a distinct laminar location within a certain cortical layer (Faux et al., 2012). Early experiments in vitro have already shown that migration of immature neurons is generally dependent on spontaneous calcium activity (Komuro and Rakic, 1996; Komuro and Kumada, 2005). These experiments were later confirmed in vivo, where the pharmacological or genetic reduction of activity also altered the migration of excitatory and inhibitory neurons (Heck et al., 2007; De Marco García et al., 2011). In addition to this effect of neuronal activity on migration, one has to note that various neurotransmitters—including GABA—act as chemoattractants for targeting migratory streams and thus, can directly modulate neuronal migration (Behar et al., 2000; Inada et al., 2011). Since GABA release itself is regulated in an activity-dependent manner, this implies a further level of regulation on account of this (Luhmann et al., 2015). Further, more recent studies show that silencing neuronal activity e.g., by the overexpression of the Kir2.1 channel, results in mispositioning of specific interneuron subpopulations by affecting the expression of Dlx genes (De Marco García et al., 2011). This suggests that genetic programs initiated at the progenitor stage are modulated during development by activity (Bando et al., 2016; Hurni et al., 2017). Nevertheless, the experimental disentangling of direct causal links between activity and differentiation, migration, and/or integration of interneurons remains challenging and requires the careful analysis of subtype-specific differences in these relationships (Bugeon et al., 2021). Moreover, the simultaneous maturation of the inhibitory GABAergic response from its immature excitatory function (Ben-Ari, 2002) with the migration of interneurons adds another layer to the complex and multidimensional regulation needed for the correct laminar positioning of interneurons. The importance of which is also experimentally supported by the halt of neuronal motility induced by upregulation of KCC2 or pharmacological interference with GABAA receptor function (Heck et al., 2007; Bortone and Polleux, 2009).
Thus, activity on the single interneuron level but also on the network level critically regulates the migration of immature neurons. However, the extent to which spatial and temporal changes in the migration of interneurons impacts cortical activity and function needs further investigations, especially since interneuron migration is tightly linked to the specification of interneurons (Lim et al., 2018b).
Specification of Interneuron Subtypes
In the neocortex, the vast majority of GABAergic cells are represented by local circuit interneurons, which are traditionally classified as aspiny neurons (Lodato and Arlotta, 2015). All GABAergic interneurons produce GABA, the hyperpolarizing action of which in the mature brain accounts for their definition as inhibitory neurons. GABAergic neurons form a heterogeneous population, of which classification is an ongoing effort that encompasses several morphological, electrophysiological, molecular, connectivity, and transcriptomic properties (Kepecs and Fishell, 2014). The broadest and most widely adopted classification relies on molecular markers, two of which (namely: parvalbumin and somatostatin) label around 70% of cortical interneurons. The remaining 30% are instead identified by a handful of markers, among which the most prevalent one is the serotonin receptor 5hT3aR. Other markers—such as the vasointestinal peptide (VIP), reelin (RELN), cholecystokinin (CCK), and calretinin (CR)—label smaller subclasses.
The maturation of subtype-specific properties of inhibitory interneurons mainly occurs during the first weeks of postnatal development in rodents and the different types of interneurons become only observable after the migration is complete at the end of the 1st postnatal week (Lim et al., 2018b). Whether the lineage specification is already predetermined during the embryonic stage or is (partially) acquired during the postnatal period through a microenvironment-mediated influence is still a matter of debate (Wamsley and Fishell, 2017; Lim et al., 2018a). However, it is becoming evident that activity-dependent mechanisms impinge on cellular properties of interneurons, such as morphology, synapse specificity, and connectivity (De Marco García et al., 2011; Dehorter et al., 2015). Indeed, many supporting findings are coming out from studies that manipulate or abolish the activity of certain cell-type precursors (MGE or CGE derived) (Chattopadhyaya et al., 2004, 2007; De Marco García et al., 2011). Some of these findings suggest an activity-dependent regulation of molecular and electrophysiological properties of different interneuron subtypes (Miller et al., 2011; Dehorter et al., 2015) and thereby contribute to subtype-specific differences in gene expression (Batista-Brito et al., 2008; Paul et al., 2017), which have now been resolved with increasing depth (Joglekar et al., 2021; Scala et al., 2021). Furthermore, the onset and duration of these activity modulations have differential effects on the different interneurons subtypes, reflecting the timeline with which they differentiate from the respective ganglionic eminences (Wamsley and Fishell, 2017). Mostly early maturational aspects of MGE- and CGE-derived interneuron specification are hereby discussed, since general morphological and electrophysiological characteristics that distinguish the different interneuron subpopulations are extensively described elsewhere (Gelman and Marín, 2010; Rudy et al., 2011; Pfeffer et al., 2013; Lim et al., 2018a; Fishell and Kepecs, 2020).
Early Maturation of MGE-Derived Interneurons
In the rodent cortex, the earliest developing interneurons are SST and PV interneurons originating from the MGE. As mentioned before, the first GABAergic neurons start to populate the cortical plate very early in development, already between E9.5 and E12.5 in deep cortical laminas (Miyoshi et al., 2007). At first, SST interneurons are generated from the dorsal division of MGE. Around E17.5, they are found in the deep layer of the cortical plate (Miyoshi and Fishell, 2011), whereas at the end of the 1st postnatal week, they are visible across all cortical layers (Liguz-Lecznar et al., 2016). During early postnatal days, SST interneurons play transient and instrumental functions in shaping neural circuits in the cortex. At P4–6 in the mouse somatosensory cortex, SST interneurons receive dense innervation from the thalamus, and in turn, give inputs to pyramidal cells, spiny stellate cells, and recently migrated prospective PV neurons (Marques-Smith et al., 2016; Tuncdemir et al., 2016). Remarkably, these connections are fundamental for the proper formation of thalamo-cortical feedforward circuits (Tuncdemir et al., 2016), the coordinated activation of PV cells (Modol et al., 2020), and functional topography (Duan et al., 2020), since conditional ablation or silencing of SST neurons drastically impairs these processes. Conversely, it has been shown that the functional maturation of SST interneurons is delayed if afferent excitatory inputs from pyramidal neurons are decreased at early (P1) rather than later (P8) postnatal stages (Pan et al., 2019). Furthermore, besides direct synaptic inputs, it was recently shown that SST interneurons also exert a paracrine role through the release of synaptogenic extracellular matrix proteins such as collagen XIX (Su et al., 2020). During the 2nd postnatal week, SST interneurons are involved in the control of sensory-evoked activity, such as spontaneous retinally-driven activity in the visual cortex (Leighton et al., 2021), or multi-whisking activity in the barrel cortex (Kastli et al., 2020). In line with these findings, conditional silencing of SST interneurons leads to a decrease in spontaneous spindle-burst activity and abolished facilitation in sensory adaptation (Baruchin et al., 2021).
Later on, PV neurons originate from the ventral division of MGE (Bandler et al., 2017) and start to radially migrate between E18 and P2, not reaching their final position until P6 (Bartholome et al., 2020). Between the 2nd and the 4th week, cortical PV neurons start expressing PV and defining ion channel composition that characterizes their peculiar electrophysiological properties (Bartholome et al., 2020). Upon arrival into the cortical layer, a particular type of extracellular matrix dubbed the perineuronal net (PNN), plays a critical role in the correct settling of PV interneurons by influencing their connectivity. The PNN can control synaptic plasticity by preventing spine formation (Vo et al., 2013). In support of this, degradation of PNNs leads to reduced gamma activity in juvenile mice (Carceller et al., 2020), which is in line with recent discovery linking PV to gamma activity (Bitzenhofer et al., 2020) and the finding that altered PNNs lead to abnormal activity (Wingert and Sorg, 2021). In addition to the role of PNNs, other molecular mechanisms can influence PV development, such as tonic activation of NMDA receptor (Hanson et al., 2019), BDNF (Lau et al., 2021), or retinoic acid (Larsen et al., 2019), whose receptor expression is also dependent on activity.
Early Maturation of CGE-Derived Interneuron
In the rodent brain, CGE-derived interneurons are produced at first at E12.5, reaching a peak around E16.5 (Miyoshi et al., 2010). Unlike MGE-derived cortical interneurons, they do not populate the cortex in an inside-out manner, but the vast majority are located in superficial cortical layers, and only acquire their final position around P4 (Miyoshi et al., 2010). Remarkably, the integration into the neocortex of CGE-derived interneurons depends on serotonin signaling (Murthy et al., 2014): impairment of which leads to their mispositioning (Frazer et al., 2015). Although it has been shown that a common feature of most, if not all, CGE-derived interneurons is the expression of the serotonin ionotropic receptor 5HT3aR (Lee et al., 2010) most of our understanding nonetheless remains built upon traditional molecular markers that identify specific subclasses (Tremblay et al., 2016). Of these, the best characterized is probably the VIP interneuron subclass, which accounts for around 40% of all CGE-derived interneurons, and the reelin subclass which labels around 60% of them (Wamsley and Fishell, 2017). However, our knowledge on the early developmental phases of CGE-derived interneurons is still limited and, only recently, CGE-specific transcriptional factors and activity-dependent mechanisms began to be explored (De Marco García et al., 2011; Miyoshi et al., 2015; Wei et al., 2019). Of note, it was shown that Prox1 is fundamental for the acquisition of CGE-derived interneuron properties both in the embryonic and postnatal stage (Miyoshi et al., 2015), with its conditional knock-out during early postnatal days leading to impairment of excitatory inputs onto the VIP multipolar subtype (Stachniak et al., 2021). Remarkably, it has been shown that network activity critically affects the proper morphological development of CR-positive VIP bipolar cells and RELN interneurons, but not that of CCK-positive VIP multipolar interneurons (De Marco García et al., 2011, 2015). Thus, activity and genetic program might act in a subtype-specific manner onto CGE-derived interneuron developmental steps. Finally, with the introduction of subtype-specific driver Cre-lines early functions and regulatory mechanisms have also begun to be studied in more depth (Taniguchi et al., 2011). In the barrel cortex, for example, VIP interneurons show a transient preferential response to multi-whisking that is lost during the 3rd postnatal week (Kastli et al., 2020), and their conditional silencing influence the onset of active whisking (Baruchin et al., 2021).
Connectivity Within GABAergic Populations and Across Transient Neuronal Populations
Interneurons are not only integrated into nascent and mature cortical networks via chemical synapses—of which many previously discussed pre- and postsynaptic GABAergic elements critically impact the emergence of cortical activity but also via gap junctions which are ubiquitous in the cortex. Gap junctions form connections mainly amongst GABAergic interneurons of the same functional class, but also across functionally distinct classes in the mature and immature cortex (Peinado et al., 1993; Hatch et al., 2017). Interestingly, gap junctions are generally described to be essential for oscillatory activity (Tchumatchenko and Clopath, 2014; Pernelle et al., 2018) and bidirectional activity-dependent plasticity is shown (Haas et al., 2016). Yet, the concise contribution of electrical coupling to distinct activity patterns during peri- and postnatal development remains unknown. Integration of GABAergic interneurons into developing cortical circuits via chemical synapses can be measured as spontaneous and evoked GABAergic inputs onto cortical plate neurons in the rodent cortex as early as E19 and P3, respectively (Owens et al., 1999; Daw et al., 2007). Instead, functional synaptic connections between GABAergic interneurons have only been shown after P4 in the visual cortex (Pangratz-Fuehrer and Hestrin, 2011). Prior to this, transient cortical populations already show GABAergic inputs (Kilb and Luhmann, 2001; Soda et al., 2003). However, the contribution of interneurons towards GABAergic signaling to transient cell populations like Cajal Retzius neurons, or subplate neurons that precede the integration of GABAergic cells into immature but persistent cortical circuits—is the subject of ongoing research (Molnár et al., 2020). The prerequisite for the functional integration of GABAergic interneurons is the maturation of their electrophysiological as well as their morphological features at the presynapse, but also the maturation of GABAergic synapses on the postsynaptic side of the recipient cells. This includes the aforementioned expression of GABAergic receptors and the setting of chloride and bicarbonate gradients. As discussed above, this structural and functional maturation of the GABAergic synapse occurs in an largely activity-independent manner (le Magueresse et al., 2011). Not only does neuronal activity influence the initial formation of perisomatic synapses by interneurons (Chattopadhyaya et al., 2004), but it also remains a key influencer of plastic changes on the structure and function of GABAergic synapses in the adult brain (Flores and Méndez, 2014). On the other side of the coin, many important key cortical functions depend on the proper integration of GABAergic interneurons into the cortical network, like selectivity of sensory modalities, gain control, range modulation and plasticity of cortical circuits, regulation of firing rates and bursting activity with high temporal precision, generation and synchronization of cortical rhythms, as well as the maintenance of the excitatory and inhibitory balance (Tremblay et al., 2016; Fishell and Kepecs, 2020).
Developmental Apoptosis
Besides genetic programs, trophic support, and pro- and anti-apoptotic factors, neuronal activity also has a major impact on cell death and survival rates in the developing cortical network (Blanquie et al., 2017a; Wong and Marín, 2019). Here, increases in neuronal activity are associated with elevated survival rates in principal neurons and interneurons, whereas blockade or attenuation of activity is generally associated with higher apoptotic rates (Ruijter et al., 1991; Ikonomidou et al., 1999; Heck et al., 2008; Southwell et al., 2012). However, cell-type-specific peculiarities exist, for example in the transient cell population of Cajal Retzius neurons, where activity even fulfills an antithetic pro-apoptotic function (Del Río et al., 1995; Blanquie et al., 2017b). Whether this effect of activity for the survival of developing neurons is controlled by a cell-autonomous process or by network-dependent mechanisms is the subject of current investigations (Southwell et al., 2012; Blanquie et al., 2017c; Wong et al., 2018). Most recent evidence suggests that not only the level of neuronal activity but also the temporal pattern of activity affects neuronal survival rates in vivo (Blanquie et al., 2017c) and in vitro (Wong Fong Sang et al., 2021). This also applies to interneurons, as different evidence supports that positive or negative alterations in network activity result in a respective change of survival rates in GABAergic interneurons (Wong et al., 2018; Duan et al., 2020; Bitzenhofer et al., 2021). Notably, the most potent neuroprotective patterns highlighted within these studies are of a high-frequency oscillatory nature and resemble activity which typically occurs at the end of the 1st postnatal week in vivo (Yang et al., 2009; Luhmann and Khazipov, 2018) or is reflected in vitro by reminiscent patterns such as recurrent bursts (Wagenaar et al., 2006; Sun et al., 2010). Interestingly, GABAergic neurons themselves are essential for the modulation of these cortical activity patterns (Bonifazi et al., 2009; Isaacson and Scanziani, 2011; Modol et al., 2020). Thus, as far as the understanding of the mutual dependency of activity and apoptosis in interneurons goes until now, cortical activity acts as a master regulator of apoptotic rates in both interneurons and pyramidal neurons (Wong et al., 2018), even in a region-specific manner (Blanquie et al., 2017c). Herewith, activity-dependent regulation of developmental cell death can be seen as a bona fide homeostatic system (Blanquie et al., 2017a; Causeret et al., 2018) with the GABAergic interneurons in the perfect position to orchestrate this cortical activity set point (Duan et al., 2020).
How Do Dynamic Changes in the GABAergic Neuron Fraction During Perinatal Development Affect Network Activity in the Developing Cortex?
The sequential generation, migration, and apoptotic removal of interneurons during early brain development eventually influence GABAergic population sizes in the mature cortex, but also cause a dynamic variation in the absolute GABAergic neuron population size in the cortex during the developmental phase. Yet, the relative GABAergic neuron fraction is maintained throughout the embryonic and postnatal development and into adulthood (Sahara et al., 2012). Experimental manipulations of excitation/inhibition ratio are effectively compensated for, either through adjustments in the number of connections (Sukenik et al., 2021) or changes in synaptic strength (Southwell et al., 2012). Similar adaptive mechanisms also stabilize cortical inhibition on the network level under physiological (Southwell et al., 2012; Field et al., 2020; Romagnoni et al., 2020) and pathophysiological conditions (Hunt et al., 2013). Thus, in line with the dispensability of NKCC1-mediated depolarizing GABA responses for the establishment of cortical activity patterns (Graf et al., 2021), cortical networks adapt surprisingly well to alterations in the relative GABAergic fraction (Liu, 2004; Sukenik et al., 2021) and thereby keep the network activity level and patterning mostly stable. Both phenomena—specifically the stable expression of network activity despite the physiological changes in absolute GABAergic population during development, but also the tight homeostatic regulation of activity upon pathological or experimental perturbations of the GABAergic system—emphasize the importance of network activity as the most relevant output function. At the same time, these findings do not exclude that the developmental changes in interneuron function and network composition cause per se physiologically relevant difference in this output, i.e., merging network activity patterns throughout development and differences in activity patterns across models (Luhmann et al., 2016). Deciphering the multi-layered developmental processes in GABA signaling discussed above is necessary for the future assessment of the exact contribution of these processes to cortical activity patterns seen during development and in adult cortical networks. Certain partly-transient network structures, such as clustered GABAergic assemblies (Tuncdemir et al., 2016; Modol et al., 2020), subplate neurons (Kanold and Shatz, 2006; Molnár et al., 2020), and subcortical thalamic regions (Minlebaev et al., 2011; Yang et al., 2013; Murata and Colonnese, 2016), are surely essential and thus not dispensable for the establishment of cortical network activity and function during early brain development (Tolner et al., 2012).
Conclusion and Outlook
Immature cortical networks have a unique capacity to stabilize their network activity, even if strong changes in GABA signaling are introduced e.g., by alterations in the absolute number of GABAergic interneurons in neocortical cultures (Sukenik et al., 2021; Xing et al., 2021), genetic changes of total GABA content in the brain (Tamamaki et al., 2003), or modulations of chloride homeostasis (Pfeffer et al., 2009; Graf et al., 2021). This stability underlines the great source of plasticity of the neuronal system in general, but is especially remarkable given the suggested key function of GABAergic interneurons for the balancing of excitation and inhibition, and thus coordinating network activity during development (Bonifazi et al., 2009; Le Magueresse and Monyer, 2013; Modol et al., 2020; Baruchin et al., 2021). By and large, GABAergic interneurons keep this crucial role in mature networks with some critical modifications (Markram et al., 2004; Bartos et al., 2007; Tremblay et al., 2016). While it is well accepted that GABAergic neuron-mediated inhibition is essential for the regulation of synchronized oscillations in adult cortical networks (Klausberger and Somogyi, 2008; Gonzalez-Burgos et al., 2010), the functional role of interneurons during development is still less clear. It remains to be seen, if the activity of distinct interneuron subclasses during development is crucial per se for brain development, as suggested by recent studies (Modol et al., 2020; Baruchin et al., 2021; Leighton et al., 2021), or if only certain network activity patterns must be played in distinct cortical compartments or temporal windows for proper brain development-regardless of the GABAergic contribution. Interestingly, a prolonged developmental timeline for GABAergic interneurons is an amplified trait in higher order gyrencephalic mammals, which suggests that a protracted development of interneurons through neurogenesis, neuronal migration, and network integration is a mechanism for increased complexity and cognitive flexibility in cortex function (Kim and Paredes, 2021).
In view of the above, the association of pathophysiological changes in interneuron function or excitation/inhibition balance with neurological and psychological conditions in humans are to be expected and have been well described (Marín, 2012; Nelson and Valakh, 2015). With pharmacological GABAergic modulators such as benzodiazepines as first-line treatment options in acute epileptic emergencies in children and adults (Glauser et al., 2016), the direct intervention with GABAA receptor signaling is already common practice in the clinic and will likely profit from future advances in this field of research. Additionally, the absence of certain activity patterns during critical developmental periods, to which GABAergic interneurons significantly contribute, is associated with unfavorable outcomes in humans and animal models (Ranasinghe et al., 2015; Whitehead et al., 2016). Thus, scientific progress will likely also provide important insights to the clinically relevant questions: (I) how pre- and early postnatal pathophysiological insults (e.g., in utero inflammation/infection, perinatal hypoxia-ischemia); or (II) certain drugs that impact GABAergic signaling (e.g., medications or drug abuse during pregnancy) change spontaneous activity; (III) how these activity changes ultimately affect clinical outcomes; and (IV) which clinical interventions could be advisable (ter Horst et al., 2004; Iyer et al., 2014).
Besides the manifold developmental changes in both interneuron function and cortical activity which are described in this review, in addition to the pathophysiological changes in this mutual interaction (described in more detail elsewhere; Marín, 2012), makes it more and more evident that physiological conditions—as well as anatomical and even subcellular compartment location—critically impact the contribution of GABA signaling to neuronal activity, and vice versa (Raimondo et al., 2017; Düsterwald et al., 2018). While current research in this field has already begun to understand these subcellular effects of ionic plasticity (Blaesse et al., 2009) and coincidence membrane depolarization (Doyon et al., 2011; Raimondo et al., 2012) on network activity in the adult brain (Jedlička and Backus, 2006; Raimondo et al., 2017), the relevance of subcellular as well as regional or state-dependent differences in GABA signaling and their impact on cortical network activity during development, remains largely unexploited. Hence, the final portrait of interneurons as replaceable or unique orchestra members and/or designated conductors of cortical activity within the orchestra line-up of the immature cortex remains a vibrant field of research with many open questions. We are only beginning to understand: (I) how interneuron subpopulations and subcellular processes contribute to spontaneous and evoked activity patterns on the network level; (II) how the GABAergic contribution differs across functionally distinct cortical regions and converging periods of development; and (III) how cortical network activity eventually feeds back on nascent interneuron function. However, it is becoming more evident that cortical network activity should be considered as the most significant output in development or, in the figurative sense, as the most sonorous symphony that the heterogenous orchestra of the developing neocortex has to play.
Author Contributions
DW and JS contributed equally to this manuscript. All authors contributed to the article and approved the submitted version.
Funding
This study was supported by funding from the Deutsche Forschungsgemeinschaft (DFG) to AS (CRC 1080, A01), a FTN stipend to DW, and intramural funding to AS (Stufe1).
Conflict of Interest
The authors declare that the research was conducted in the absence of any commercial or financial relationships that could be construed as a potential conflict of interest.
Publisher’s Note
All claims expressed in this article are solely those of the authors and do not necessarily represent those of their affiliated organizations, or those of the publisher, the editors and the reviewers. Any product that may be evaluated in this article, or claim that may be made by its manufacturer, is not guaranteed or endorsed by the publisher.
Acknowledgments
We thank Heiko J. Luhmann and all members of the Institute of Physiology Mainz for their continuous support as well as Davide Bassetti, Elena Nigi, and Celine Gallagher for helpful comments on the manuscript.
References
Achilles, K., Okabe, A., Ikeda, M., Shimizu-Okabe, C., Yamada, J., Fukuda, A., et al. (2007). Kinetic properties of Cl− uptake mediated by Na+-dependent K+-2Cl− cotransport in immature rat neocortical neurons. J. Neurosci. 27, 8616–8627. doi: 10.1523/JNEUROSCI.5041-06.2007
Ackman, J. B., and Crair, M. C. (2014). Role of emergent neural activity in visual map development. Curr. Opin. Neurobiol. 24, 166–175. doi: 10.1016/j.conb.2013.11.011
Andäng, M., Hjerling-Leffler, J., Moliner, A., Lundgren, T. K., Castelo-Branco, G., Nanou, E., et al. (2008). Histone H2AX-dependent GABAA receptor regulation of stem cell proliferation. Nature 451, 460–464. doi: 10.1038/nature06488
Araki, T., Kiyama, H., and Tohyama, M. (1992). GABAA Receptor subunit messenger RNAs show differential expression during cortical development in the rat brain. Neuroscience 51, 583–591. doi: 10.1016/0306-4522(92)90298-g
Bagasrawala, I., Memi, F., Radonjić, N. V., and Zecevic, N. (2017). N-methyl d-aspartate receptor expression patterns in the human fetal cerebral cortex. Cereb. Cortex 27, 5041–5053. doi: 10.1093/cercor/bhw289
Bandler, R. C., Mayer, C., and Fishell, G. (2017). Cortical interneuron specification: the juncture of genes, time and geometry. Curr. Opin. Neurobiol. 42, 17–24. doi: 10.1016/j.conb.2016.10.003
Bando, Y., Irie, K., Shimomura, T., Umeshima, H., Kushida, Y., Kengaku, M., et al. (2016). Control of spontaneous Ca2+ transients is critical for neuronal maturation in the developing neocortex. Cereb. Cortex 26, 106–117. doi: 10.1093/cercor/bhu180
Barakat, L., and Bordey, A. (2002). GAT-1 and reversible GABA transport in Bergmann glia in slices. J. Neurophysiol. 88, 1407–1419. doi: 10.1152/jn.2002.88.3.1407
Bartholome, O., de la Brassinne Bonardeaux, O., Neirinckx, V., and Rogister, B. (2020). A composite sketch of fast-spiking parvalbumin-positive neurons. Cereb. Cortex Commun. 1, 1–15. doi: 10.1093/texcom/tgaa026
Bartos, M., Vida, I., and Jonas, P. (2007). Synaptic mechanisms of synchronized gamma oscillations in inhibitory interneuron networks. Nat. Rev. Neurosci. 8, 45–56. doi: 10.1038/nrn2044
Baruchin, L. J., Ghezzi, F., Kohl, M. M., and Butt, S. J. B. (2021). Contribution of interneuron subtype-specific gabaergic signaling to emergent sensory processing in mouse somatosensory whisker barrel cortex. Cereb. Cortex 2021:bhab363. doi: 10.1093/cercor/bhab363
Batista-Brito, R., MacHold, R., Klein, C., and Fishell, G. (2008). Gene expression in cortical interneuron precursors is prescient of their mature function. Cereb. Cortex 18, 2306–2317. doi: 10.1093/cercor/bhm258
Behar, T. N., Schaffner, A. E., Scott, C. A., Greene, C. L., and Barker, J. L. (2000). GABA receptor antagonists modulate postmitotic cell migration in slice cultures of embryonic rat cortex. Cereb. Cortex 10, 899–909. doi: 10.1093/cercor/10.9.899
Behar, T. N., Smith, S. V., Kennedy, R. T., Mckenzi, J. M., Maric, I., and Barker, J. L. (2001). GABAB receptors mediate motility signals for migrating embryonic cortical cells. Cereb. Cortex 11, 744–753. doi: 10.1093/cercor/11.8.744
Behuet, S., Cremer, J. N., Cremer, M., Palomero-Gallagher, N., Zilles, K., and Amunts, K. (2019). Developmental changes of glutamate and GABA receptor densities in wistar rats. Front. Neuroanat. 13:100. doi: 10.3389/fnana.2019.00100
Ben-Ari, Y. (2002). Excitatory actions of GABA during development: the nature of the nurture. Nat. Rev. Neurosci. 3, 728–739. doi: 10.1038/nrn920
Ben-Ari, Y. (2006). Basic developmental rules and their implications for epilepsy in the immature brain. Epileptic Disord. 8, 91–102.
Bitzenhofer, S. H., Pöpplau, J. A., Chini, M., Marquardt, A., and Hanganu-Opatz, I. L. (2021). A transient developmental increase in prefrontal activity alters network maturation and causes cognitive dysfunction in adult mice. Neuron 109, 1350–1364.e6. doi: 10.1016/j.neuron.2021.02.011
Bitzenhofer, S. H., Pöpplau, J. A., and Hanganu-Opatz, I. L. (2020). Gamma activity accelerates during prefrontal development. eLife 9:e56795. doi: 10.7554/eLife.56795
Blaesse, P., Airaksinen, M. S., Rivera, C., and Kaila, K. (2009). Cation-chloride cotransporters and neuronal function. Neuron 61, 820–838. doi: 10.1016/j.neuron.2009.03.003
Blankenship, A. G., and Feller, M. B. (2010). Mechanisms underlying spontaneous patterned activity in developing neural circuits. Nat. Rev. Neurosci. 11, 18–29. doi: 10.1038/nrn2759
Blanquie, O., Kilb, W., Sinning, A., and Luhmann, H. J. (2017a). Homeostatic interplay between electrical activity and neuronal apoptosis in the developing neocortex. Neuroscience 358, 190–200. doi: 10.1016/j.neuroscience.2017.06.030
Blanquie, O., Liebmann, L., Hübner, C. A., Luhmann, H. J., and Sinning, A. (2017b). NKCC1-mediated GABAergic signaling promotes postnatal cell death in neocortical cajal-retzius cells. Cereb. Cortex 27, 1644–1659. doi: 10.1093/cercor/bhw004
Blanquie, O., Yang, J. W., Kilb, W., Sharopov, S., Sinning, A., and Luhmann, H. J. (2017c). Electrical activity controls area-specific expression of neuronal apoptosis in the mouse developing cerebral cortex. eLife 6:e27696. doi: 10.7554/eLife.27696
Bonifazi, P., Goldin, M., Picardo, M. A., Jorquera, I., Cattani, A., Bianconi, G., et al. (2009). GABAergic hub neurons orchestrate synchrony in developing hippocampal networks. Science 326, 1419–1424. doi: 10.1126/science.1175509
Borodinsky, L. N., Root, C. M., Cronin, J. A., Sann, S. B., Gu, X., and Spitzer, N. C. (2004). Activity-dependent homeostatic specification of transmitter expression in embryonic neurons. Nature 429, 523–530. doi: 10.1038/nature02518
Bortone, D., and Polleux, F. (2009). KCC2 expression promotes the termination of cortical interneuron migration in a voltage-sensitive calcium-dependent manner. Neuron 62, 53–71. doi: 10.1016/j.neuron.2009.01.034
Bosman, C. A., Schoffelen, J. M., Brunet, N., Oostenveld, R., Bastos, A. M., Womelsdorf, T., et al. (2012). Attentional stimulus selection through selective synchronization between monkey visual areas. Neuron 75, 875–888. doi: 10.1016/j.neuron.2012.06.037
Bugeon, S., Haubold, C., Ryzynski, A., Cremer, H., and Platel, J. C. (2021). Intrinsic neuronal activity during migration controls the recruitment of specific interneuron subtypes in the postnatal mouse olfactory bulb. J. Neurosci. 41, 2630–2644. doi: 10.1523/JNEUROSCI.1960-20.2021
Butt, S. J., Stacey, J. A., Teramoto, Y., and Vagnoni, C. (2017). A role for GABAergic interneuron diversity in circuit development and plasticity of the neonatal cerebral cortex. Curr. Opin. Neurobiol. 43, 149–155. doi: 10.1016/j.conb.2017.03.011
Cancedda, L., Fiumelli, H., Chen, K., and Poo, M. M. (2007). Excitatory GABA action is essential for morphological maturation of cortical neurons in vivo. J. Neurosci. 27, 5224–5235. doi: 10.1523/JNEUROSCI.5169-06.2007
Carceller, H., Guirado, R., Ripolles-Campos, E., Teruel-Marti, V., and Nacher, J. (2020). Perineuronal nets regulate the inhibitory perisomatic input onto parvalbumin interneurons and γ activity in the prefrontal cortex. J. Neurosci. 40, 5008–5018. doi: 10.1523/JNEUROSCI.0291-20.2020
Causeret, F., Coppola, E., and Pierani, A. (2018). Cortical developmental death: selected to survive or fated to die. Curr. Opin. Neurobiol. 53, 35–42. doi: 10.1016/j.conb.2018.04.022
Chattopadhyaya, B., Di Cristo, G., Higashiyama, H., Knott, G. W., Kuhlman, S. J., Welker, E., et al. (2004). Experience and activity-dependent maturation of perisomatic GABAergic innervation in primary visual cortex during a postnatal critical period. J. Neurosci. 24, 9598–9611. doi: 10.1523/JNEUROSCI.1851-04.2004
Chattopadhyaya, B., Di Cristo, G., Wu, C. Z., Knott, G., Kuhlman, S., Fu, Y., et al. (2007). GAD67-mediated GABA synthesis and signaling regulate inhibitory synaptic innervation in the visual cortex. Neuron 54, 889–903. doi: 10.1016/j.neuron.2007.05.015
Ciccolini, F., Collins, T. J., Sudhoelter, J., Lipp, P., Berridge, M. J., and Bootman, M. D. (2003). Local and global spontaneous calcium events regulate neurite outgrowth and onset of GABAergic phenotype during neural precursor differentiation. J. Neurosci. 23, 103–111. doi: 10.1523/JNEUROSCI.23-01-00103.2003
Colonnese, M. T., Kaminska, A., Minlebaev, M., Milh, M., Bloem, B., Lescure, S., et al. (2010). A conserved switch in sensory processing prepares developing neocortex for vision. Neuron 67, 480–498. doi: 10.1016/j.neuron.2010.07.015
Cserép, C., Szabadits, E., Szonyi, A., Watanabe, M., Freund, T. F., and Nyiri, G. (2012). NMDA receptors in GABAergic synapses during postnatal development. PLoS One 7:e37753. doi: 10.1371/journal.pone.0037753
Daw, M. I., Ashby, M. C., and Isaac, J. T. R. (2007). Coordinated developmental recruitment of latent fast spiking interneurons in layer IV barrel cortex. Nat. Neurosci. 10, 453–461. doi: 10.1038/nn1866
De Marco García, N. V., Karayannis, T., and Fishell, G. (2011). Neuronal activity is required for the development of specific cortical interneuron subtypes. Nature 472, 351–355. doi: 10.1038/nature09865
De Marco García, N. V., Priya, R., Tuncdemir, S. N., Fishell, G., and Karayannis, T. (2015). Sensory inputs control the integration of neurogliaform interneurons into cortical circuits. Nat. Neurosci. 18, 393–403. doi: 10.1038/nn.3946
Dehorter, N., Ciceri, G., Bartolini, G., Lim, L., Del Pino, I., and Marín, O. (2015). Tuning of fast-spiking interneuron properties by an activity-dependent transcriptional switch. Science 349, 1216–1220. doi: 10.1126/science.aab3415
Deidda, G., Allegra, M., Cerri, C., Naskar, S., Bony, G., Zunino, G., et al. (2015). Early depolarizing GABA controls critical-period plasticity in the rat visual cortex. Nat. Neurosci. 18, 87–96. doi: 10.1038/nn.3890
Del Río, J. A., Martinez, A., Fonseca, M., Auladell, C., and Soriano, E. (1995). Glutamate-like immunoreactivity and fate of cajal-retzius cells in the murine cortex as identified with calretinin antibody. Cereb. Cortex 5, 13–21. doi: 10.1093/cercor/5.1.13
Deng, L., Yao, J., Fang, C., Dong, N., Luscher, B., and Chen, G. (2007). Sequential postsynaptic maturation governs the temporal order of gabaergic and glutamatergic synaptogenesis in rat embryonic cultures. J. Neurosci. 27, 10860–10869. doi: 10.1523/JNEUROSCI.2744-07.2007
Doyon, N., Prescott, S. A., Castonguay, A., Godin, A. G., Kröger, H., and de Koninck, Y. (2011). Efficacy of synaptic inhibition depends on multiple, dynamically interacting mechanisms implicated in chloride homeostasis. PLoS Comput. Biol. 7:e1002149. doi: 10.1371/journal.pcbi.1002149
Duan, Z. R. S., Che, A., Chu, P., Modol, L., Bollmann, Y., Babij, R., et al. (2020). GABAergic restriction of network dynamics regulates interneuron survival in the developing cortex. Neuron 105, 75–92.e5. doi: 10.1016/j.neuron.2019.10.008
Düsterwald, K. M., Currin, C. B., Burman, R. J., Akerman, C. J., Kay, A. R., and Raimondo, J. V. (2018). Biophysical models reveal the relative importance of transporter proteins and impermeant anions in chloride homeostasis. eLife 7:e39575. doi: 10.7554/eLife.39575
Egorov, A. V., and Draguhn, A. (2013). Development of coherent neuronal activity patterns in mammalian cortical networks: common principles and local hetereogeneity. Mech. Dev. 130, 412–423. doi: 10.1016/j.mod.2012.09.006
Eilers, J., Plant, T. D., Marandi, N., and Konnerth, A. (2001). GABA-mediated Ca2+ signalling in developing rat cerebellar Purkinje neurones. J. Physiol. 536, 429–437. doi: 10.1111/j.1469-7793.2001.0429c.xd
Fattorini, G., Ripoli, C., Cocco, S., Spinelli, M., Mattera, A., Grassi, C., et al. (2019). Glutamate/GABA co-release selectively influences postsynaptic glutamate receptors in mouse cortical neurons. Neuropharmacology 161:107737. doi: 10.1016/j.neuropharm.2019.107737
Faux, C., Rakic, S., Andrews, W., and Britto, J. M. (2012). Neurons on the move: migration and lamination of cortical interneurons. NeuroSignals 20, 168–189. doi: 10.1159/000334489
Field, R. E., D’amour, J. A., Tremblay, R., Miehl, C., Rudy, B., Gjorgjieva, J., et al. (2020). Heterosynaptic plasticity determines the set point for cortical excitatory-inhibitory balance. Neuron 106, 842–854.e4. doi: 10.1016/j.neuron.2020.03.002
Fiorentino, H., Kuczewski, N., Diabira, D., Ferrand, N., Pangalos, M. N., Porcher, C., et al. (2009). GABAB receptor activation triggers BDNF release and promotes the maturation of GABAergic synapses. J. Neurosci. 29, 11650–11661. doi: 10.1523/JNEUROSCI.3587-09.2009
Fishell, G., and Kepecs, A. (2020). Interneuron types as attractors and controllers. Annu. Rev. Neurosci. 43, 1–30. doi: 10.1146/annurev-neuro-070918-050421
Fiumelli, H., Cancedda, L., and Poo, M. M. (2005). Modulation of GABAergic transmission by activity via postsynaptic Ca2+-dependent regulation of KCC2 function. Neuron 48, 773–786. doi: 10.1016/j.neuron.2005.10.025
Flavell, S. W., and Greenberg, M. E. (2008). Signaling mechanisms linking neuronal activity to gene expression and plasticity of the nervous system. Annu. Rev. Neurosci. 31, 563–590. doi: 10.1146/annurev.neuro.31.060407.125631
Flores, C. E., and Méndez, P. (2014). Shaping inhibition: activity dependent structural plasticity of GABAergic synapses. Front. Cell. Neurosci. 8:327. doi: 10.3389/fncel.2014.00327
Flossmann, T., Kaas, T., Rahmati, V., Kiebel, S. J., Witte, O. W., Holthoff, K., et al. (2019). Somatostatin interneurons promote neuronal synchrony in the neonatal hippocampus. Cell Rep. 26, 3173–3182.e5. doi: 10.1016/j.celrep.2019.02.061
Frazer, S., Otomo, K., and Dayer, A. (2015). Early-life serotonin dysregulation affects the migration and positioning of cortical interneuron subtypes. Transl. Psychiatry 5:e644. doi: 10.1038/tp.2015.147
Fuchs, C., Abitbol, K., Burden, J. J., Mercer, A., Brown, L., Iball, J., et al. (2013). GABAA receptors can initiate the formation of functional inhibitory GABAergic synapses. Eur. J. Neurosci. 38, 3146–3158. doi: 10.1111/ejn.12331
Ganguly, K., Schinder, A. F., Wong, S. T., and Poo, M. M. (2001). GABA itself promotes the developmental switch of neuronal GABAergic responses from excitation to inhibition. Cell 105, 521–532. doi: 10.1016/s0092-8674(01)00341-5
Gao, X. B., and Van Den Pol, A. N. (2000). GABA release from mouse axonal growth cones. J. Physiol. 523, 629–637. doi: 10.1111/j.1469-7793.2000.t01-1-00629.x
Gao, X. B., and Van Den Pol, A. N. (2001). GABA, not glutamate, a primary transmitter driving action potentials in developing hypothalamic neurons. J. Neurophysiol. 85, 425–434. doi: 10.1152/jn.2001.85.1.425
Gelman, D. M., and Marín, O. (2010). Generation of interneuron diversity in the mouse cerebral cortex. Eur. J. Neurosci. 31, 2136–2141. doi: 10.1111/j.1460-9568.2010.07267.x
Glauser, T., Shinnar, S., Gloss, D., Alldredge, B., Arya, R., Bainbridge, J., et al. (2016). Evidence-based guideline: treatment of convulsive status epilepticus in children and adults: report of the guideline committee of the american epilepsy society. Epilepsy Curr. 16, 48–61. doi: 10.5698/1535-7597-16.1.48
Golshani, P., Gonçalves, J. T., Khoshkhoo, S., Mostany, R., Smirnakis, S., and Portera-Cailliau, C. (2009). Internally mediated developmental desynchronization of neocortical network activity. J. Neurosci. 29, 10890–10899. doi: 10.1523/JNEUROSCI.2012-09.2009
Gonzalez-Burgos, G., Hashimoto, T., and Lewis, D. A. (2010). Alterations of cortical GABA neurons and network oscillations in schizophrenia. Curr. Psychiatry Rep. 12, 335–344. doi: 10.1007/s11920-010-0124-8
Graf, J., Zhang, C., Marguet, S. L., Herrmann, T., Flossmann, T., Hinsch, R., et al. (2021). A limited role of NKCC1 in telencephalic glutamatergic neurons for developing hippocampal network dynamics and behavior. Proc. Natl. Acad. Sci. U S A 118:e2014784118. doi: 10.1073/pnas.2014784118
Gu, X., Zhou, L., and Lu, W. (2016). An NMDA receptor-dependent mechanism underlies inhibitory synapse development. Cell Rep. 14, 471–478. doi: 10.1016/j.celrep.2015.12.061
Haas, J. S., Greenwald, C. M., and Pereda, A. E. (2016). Activity-dependent plasticity of electrical synapses: increasing evidence for its presence and functional roles in the mammalian brain. BMC Cell Biol. 17:14. doi: 10.1186/s12860-016-0090-z
Hanson, E., Armbruster, M., Lau, L. A., Sommer, M. E., Klaft, Z. J., Swanger, S. A., et al. (2019). Tonic activation of GluN2C/GluN2D-containing NMDA receptors by ambient glutamate facilitates cortical interneuron maturation. J. Neurosci. 39, 3611–3626. doi: 10.1523/JNEUROSCI.1392-18.2019
Hatch, R. J., Mendis, G. D. C., Kaila, K., Reid, C. A., and Petrou, S. (2017). Gap junctions link regular-spiking and fast-spiking interneurons in layer 5 somatosensory cortex. Front. Cell. Neurosci. 11:204. doi: 10.3389/fncel.2017.00204
Heck, N., Golbs, A., Riedemann, T., Sun, J.-J., Lessmann, V., and Luhmann, H. J. (2008). Activity-dependent regulation of neuronal apoptosis in neonatal mouse cerebral cortex. Cereb. Cortex 18, 1335–1349. doi: 10.1093/cercor/bhm165
Heck, N., Kilb, W., Reiprich, P., Kubota, H., Furukawa, T., Fukuda, A., et al. (2007). GABA-A receptors regulate neocortical neuronal migration in vitro and in vivo. Cereb. Cortex 17, 138–148. doi: 10.1093/cercor/bhj135
Hensch, T. K., Fagiolini, M., Mataga, N., Stryker, M. P., Baekkeskov, S., and Kash, S. F. (1998). Local GABA circuit control of experience-dependent plasticity in developing visual cortex. Science 282, 1504–1508. doi: 10.1126/science.282.5393.1504
Hunt, R. F., Girskis, K. M., Rubenstein, J. L., Alvarez-Buylla, A., and Baraban, S. C. (2013). GABA progenitors grafted into the adult epileptic brain control seizures and abnormal behavior. Nat. Neurosci. 16, 692–697. doi: 10.1038/nn.3392
Hurni, N., Kolodziejczak, M., Tomasello, U., Badia, J., Jacobshagen, M., Prados, J., et al. (2017). Transient cell-intrinsic activity regulates the migration and laminar positioning of cortical projection neurons. Cereb. Cortex 27, 3052–3063. doi: 10.1093/cercor/bhx059
Ikonomidou, C., Bosch, F., Miksa, M., Bittigau, P., Vöckler, J., Dikranian, K., et al. (1999). Blockade of NMDA receptors and apoptotic neurodegeneration in the developing brain. Science 283, 70–74. doi: 10.1126/science.283.5398.70
Inada, H., Watanabe, M., Uchida, T., Ishibashi, H., Wake, H., Nemoto, T., et al. (2011). GABA regulates the multidirectional tangential migration of GABAergic interneurons in living neonatal mice. PLoS One 6:e27048. doi: 10.1371/journal.pone.0027048
Isaacson, J. S., and Scanziani, M. (2011). How inhibition shapes cortical activity. Neuron 72, 231–243. doi: 10.1016/j.neuron.2011.09.027
Iyer, K. K., Roberts, J. A., Metsäranta, M., Finnigan, S., Breakspear, M., and Vanhatalo, S. (2014). Novel features of early burst suppression predict outcome after birth asphyxia. Ann. Clin. Transl. Neurol. 1, 209–214. doi: 10.1002/acn3.32
Jedlička, P., and Backus, K. H. (2006). Inhibitory transmission, activity-dependent ionic changes and neuronal network oscillations. Physiol. Res. 55, 139–149.
Joglekar, A., Prjibelski, A., Mahfouz, A., Collier, P., Lin, S., Schlusche, A. K., et al. (2021). A spatially resolved brain region- and cell type-specific isoform atlas of the postnatal mouse brain. Nat. Commun. 12:463. doi: 10.1038/s41467-020-20343-5
Kaila, K. (1994). Ionic basis of GABAA receptor channel function in the nervous system. Prog. Neurobiol. 42, 489–537. doi: 10.1016/0301-0082(94)90049-3
Kanold, P. O., and Shatz, C. J. (2006). Subplate neurons regulate maturation of cortical inhibition and outcome of ocular dominance plasticity. Neuron 51, 627–638. doi: 10.1016/j.neuron.2006.07.008
Káradóttir, R. T., and Kuo, C. T. (2018). Neuronal activity-dependent control of postnatal neurogenesis and gliogenesis. Annu. Rev. Neurosci. 41, 139–161. doi: 10.1146/annurev-neuro-072116-031054
Kastli, R., Vighagen, R., van der Bourg, A., Argunsah, A. Ö., Iqbal, A., Voigt, F. F., et al. (2020). Developmental divergence of sensory stimulus representation in cortical interneurons. Nat. Commun. 11:5729. doi: 10.1038/s41467-020-19427-z
Kepecs, A., and Fishell, G. (2014). Interneuron cell types are fit to function. Nature 505, 318–326. doi: 10.1038/nature12983
Khazipov, R., Esclapez, M., Caillard, O., Bernard, C., Khalilov, I., Tyzio, R., et al. (2001). Early development of neuronal activity in the primate hippocampus in utero. J. Neurosci. 21, 9770–9781. doi: 10.1523/JNEUROSCI.21-24-09770.2001
Kilb, W., and Luhmann, H. J. (2001). Spontaneous GABAergic postsynaptic currents in Cajal-Retzius cells in neonatal rat cerebral cortex. Eur. J. Neurosci. 13, 1387–1390. doi: 10.1046/j.0953-816x.2001.01514.x
Kilb, W., Kirischuk, S., and Luhmann, H. J. (2011). Electrical activity patterns and the functional maturation of the neocortex. Eur. J. Neurosci. 34, 1677–1686. doi: 10.1111/j.1460-9568.2011.07878.x
Kim, J. Y., and Paredes, M. F. (2021). Implications of extended inhibitory neuron development. Int. J. Mol. Sci. 22:5113. doi: 10.3390/ijms22105113
Kirkby, L. A., Sack, G. S., Firl, A., and Feller, M. B. (2013). A role for correlated spontaneous activity in the assembly of neural circuits. Neuron 80, 1129–1144. doi: 10.1016/j.neuron.2013.10.030
Kirmse, K., Kummer, M., Kovalchuk, Y., Witte, O. W., Garaschuk, O., and Holthoff, K. (2015). GABA depolarizes immature neurons and inhibits network activity in the neonatal neocortex in vivo. Nat. Commun. 6:7750. doi: 10.1038/ncomms8750
Klausberger, T., and Somogyi, P. (2008). Neuronal diversity and temporal dynamics: the unity of hippocampal circuit operations. Science 321, 53–57. doi: 10.1126/science.1149381
Komuro, H., and Kumada, T. (2005). Ca2+ transients control CNS neuronal migration. Cell Calcium 37, 387–393. doi: 10.1016/j.ceca.2005.01.006
Komuro, H., and Rakic, P. (1996). Intracellular Ca2+ fluctuations modulate the rate of neuronal migration. Neuron 17, 275–285. doi: 10.1016/s0896-6273(00)80159-2
Kumada, T., and Fukuda, A. (2020). “Multimodal GABAA receptor functions in the development of the central nervous system,” in Synapse Development and Maturation, (Cambridge, MA: Elsevier), 323–343. doi: 10.1016/b978-0-12-823672-7.00014-4
Larsen, R., Proue, A., Scott, E. P., Christiansen, M., and Nakagawa, Y. (2019). The thalamus regulates retinoic acid signaling and development of parvalbumin interneurons in postnatal mouse prefrontal cortex. eNeuro 6:30868103. doi: 10.1523/ENEURO.0018-19.2019
Lau, C. G., Zhang, H., and Murthy, V. N. (2021). Deletion of TrkB in parvalbumin interneurons alters cortical neural dynamics. J. Cell. Physiol. [Online ahead of print]. doi: 10.1002/jcp.30571
Laurie, D. J., Wisden, W., and Seeburg, P. H. (1992). The distribution of thirteen GABA(A) receptor subunit mRNAs in the rat brain. III. embryonic and postnatal development. J. Neurosci. 12, 4151–4172. doi: 10.1523/JNEUROSCI.12-11-04151.1992
le Magueresse, C., Alfonso, J., Khodosevich, K., Martín, Á. A. A., Bark, C., and Monyer, H. (2011). “Small axonless neurons”: postnatally generated neocortical interneurons with delayed functional maturation. J. Neurosci. 31, 16731–16747. doi: 10.1523/JNEUROSCI.4273-11.2011
Le Magueresse, C., and Monyer, H. (2013). GABAergic interneurons shape the functional maturation of the cortex. Neuron 77, 388–405. doi: 10.1016/j.neuron.2013.01.011
Leighton, A. H., Cheyne, J. E., Houwen, G. J., Maldonado, P. P., De Winter, F., Levelt, C. N., et al. (2021). Somatostatin interneurons restrict cell recruitment to retinally driven spontaneous activity in the developing cortex. Cell Rep. 36:109316. doi: 10.1016/j.celrep.2021.109316
Leinekugel, X., Tseeb, V., Ben-Ari, Y., and Bregestovski, P. (1995). Synaptic GABAA activation induces Ca2+ rise in pyramidal cells and interneurons from rat neonatal hippocampal slices. J. Physiol. 487, 319–329. doi: 10.1113/jphysiol.1995.sp020882
Leitch, E., Coaker, J., Young, C., Mehta, V., and Sernagor, E. (2005). GABA type-A activity controls its own developmental polarity switch in the maturing retina. J. Neurosci. 25, 4801–4805. doi: 10.1523/JNEUROSCI.0172-05.2005
Lee, S., Hjerling-Leffler, J., Zagha, E., Fishell, G., and Rudy, B. (2010). The largest group of superficial neocortical GABAergic interneurons expresses ionotropic serotonin receptors. J. Neurosci. 30, 16796–16808. doi: 10.1523/JNEUROSCI.1869-10.2010
Li, S., Kumar, P., Joshee, S., Kirschstein, T., Subburaju, S., Khalili, J. S., et al. (2018). Endothelial cell-derived GABA signaling modulates neuronal migration and postnatal behavior. Cell Res. 28, 221–248. doi: 10.1038/cr.2017.135
Li, S., Park, M. S., and Kim, M. O. (2004). Prenatal alteration and distribution of the GABAB1 and GABAB2 receptor subunit mRNAs during rat central nervous system development. Brain Res. Dev. Brain Res. 150, 141–150. doi: 10.1016/j.devbrainres.2004.03.009
Liguz-Lecznar, M., Urban-Ciecko, J., and Kossut, M. (2016). Somatostatin and somatostatin-containing neurons in shaping neuronal activity and plasticity. Front. Neural Circuits 10:48. doi: 10.3389/fncir.2016.00048
Lim, L., Mi, D., Llorca, A., and Marín, O. (2018a). Development and functional diversification of cortical interneurons. Neuron 100, 294–313. doi: 10.1016/j.neuron.2018.10.009
Lim, L., Pakan, J. M. P., Selten, M. M., Marques-Smith, A., Llorca, A., Bae, S. E., et al. (2018b). Optimization of interneuron function by direct coupling of cell migration and axonal targeting. Nat. Neurosci. 21, 920–931. doi: 10.1038/s41593-018-0162-9
Liu, G. (2004). Local structural balance and functional interaction of excitatory and inhibitory synapses in hippocampal dendrites. Nat. Neurosci. 7, 373–379. doi: 10.1038/nn1206
Lodato, S., and Arlotta, P. (2015). Generating neuronal diversity in the mammalian cerebral cortex. Annu. Rev. Cell Dev. Biol. 31, 699–720. doi: 10.1146/annurev-cellbio-100814-125353
López-Bendito, G., Shigemoto, R., Kulik, A., Paulsen, O., Fairén, A., and Luján, R. (2002). Expression and distribution of metabotropic GABA receptor subtypes GABABR1 and GABABR2 during rat neocortical development. Eur. J. Neurosci. 15, 1766–1778. doi: 10.1046/j.1460-9568.2002.02032.x
Ludwig, A., Li, H., Saarma, M., Kaila, K., and Rivera, C. (2003). Developmental up-regulation of KCC2 in the absence of GABAergic and glutamatergic transmission. Eur. J. Neurosci. 18, 3199–3206. doi: 10.1111/j.1460-9568.2003.03069.x
Luhmann, H. J., Fukuda, A., and Kilb, W. (2015). Control of cortical neuronal migration by glutamate and GABA. Front. Cell. Neurosci. 9:4. doi: 10.3389/fncel.2015.00004
Luhmann, H. J., and Khazipov, R. (2018). Neuronal activity patterns in the developing barrel cortex. Neuroscience 368, 256–267. doi: 10.1016/j.neuroscience.2017.05.025
Luhmann, H. J., and Prince, D. A. (1991). Postnatal maturation of the GABAergic system in rat neocortex. J. Neurophysiol. 65, 247–263. doi: 10.1152/jn.1991.65.2.247
Luhmann, H. J., Sinning, A., Yang, J. W., Reyes-Puerta, V., Stüttgen, M. C., Kirischuk, S., et al. (2016). Spontaneous neuronal activity in developing neocortical networks: from single cells to large-scale interactions. Front. Neural Circuits 10:40. doi: 10.3389/fncir.2016.00040
Ma, W., and Barker, J. L. (1995). Complementary expressions of transcripts encoding GAD67 and GABA(A) receptor α4, β1 and γ1 subunits in the proliferative zone of the embryonic rat central nervous system. J. Neurosci. 15, 2547–2560. doi: 10.1523/JNEUROSCI.15-03-02547.1995
Malmersjö, S., Rebellato, P., Smedler, E., Planert, H., Kanatani, S., Liste, I., et al. (2013). Neural progenitors organize in small-world networks to promote cell proliferation. Proc. Natl. Acad. Sci. U S A 110, E1524–E1532. doi: 10.1073/pnas.1220179110
Marín, O. (2012). Interneuron dysfunction in psychiatric disorders. Nat. Rev. Neurosci. 13, 107–120. doi: 10.1038/nrn3155
Markram, H., Toledo-Rodriguez, M., Wang, Y., Gupta, A., Silberberg, G., and Wu, C. (2004). Interneurons of the neocortical inhibitory system. Nat. Rev. Neurosci. 5, 793–807. doi: 10.1038/nrn1519
Marques-Smith, A., Lyngholm, D., Kaufmann, A. K., Stacey, J. A., Hoerder-Suabedissen, A., Becker, E. B. E., et al. (2016). A transient translaminar GABAergic interneuron circuit connects thalamocortical recipient layers in neonatal somatosensory cortex. Neuron 89, 536–549. doi: 10.1016/j.neuron.2016.01.015
Martini, F. J., Guillamón-Vivancos, T., Moreno-Juan, V., Valdeolmillos, M., and López-Bendito, G. (2021). Spontaneous activity in developing thalamic and cortical sensory networks. Neuron 109, 2519–2534. doi: 10.1016/j.neuron.2021.06.026
Masquelier, T., Hugues, E., Deco, G., and Thorpe, S. J. (2009). Oscillations, phase-of-firing coding and spike timing-dependent plasticity: an efficient learning scheme. J. Neurosci. 29, 13484–13493. doi: 10.1523/JNEUROSCI.2207-09.2009
Miller, M. N., Okaty, B. W., Kato, S., and Nelson, S. B. (2011). Activity-dependent changes in the firing properties of neocortical fast-spiking interneurons in the absence of large changes in gene expression. Dev. Neurobiol. 71, 62–70. doi: 10.1002/dneu.20811
Minelli, A., Alonso-Nanclares, L., Edwards, R. H., Defelipe, J., and Conti, F. (2003). Postnatal development of the vesicular GABA transporter in rat cerebral cortex. Neuroscience 117, 337–346. doi: 10.1016/s0306-4522(02)00864-3
Minelli, A., Brecha, N. C., Karschin, C., DeBiasi, S., and Conti, F. (1995). GAT-1, a high-affinity GABA plasma membrane transporter, is localized to neurons and astroglia in the cerebral cortex. J. Neurosci. 15, 7734–7746. doi: 10.1523/JNEUROSCI.15-11-07734.1995
Minlebaev, M., Colonnese, M., Tsintsadze, T., Sirota, A., and Khazipov, R. (2011). Early gamma oscillations synchronize developing thalamus and cortex. Science 334, 226–229. doi: 10.1126/science.1210574
Miyoshi, G., Butt, S. J. B., Takebayashi, H., and Fishell, G. (2007). Physiologically distinct temporal cohorts of cortical interneurons arise from telencephalic Olig2-expressing precursors. J. Neurosci. 27, 7786–7798. doi: 10.1523/JNEUROSCI.1807-07.2007
Miyoshi, G., and Fishell, G. (2011). GABAergic interneuron lineages selectively sort into specific cortical layers during early postnatal development. Cereb. Cortex 21, 845–852. doi: 10.1093/cercor/bhq155
Miyoshi, G., Hjerling-Leffler, J., Karayannis, T., Sousa, V. H., Butt, S. J. B., Battiste, J., et al. (2010). Genetic fate mapping reveals that the caudal ganglionic eminence produces a large and diverse population of superficial cortical interneurons. J. Neurosci. 30, 1582–1594. doi: 10.1523/JNEUROSCI.4515-09.2010
Miyoshi, G., Young, A., Petros, T., Karayannis, T., Chang, M. M. K., Lavado, A., et al. (2015). Prox1 regulates the subtype-specific development of caudal ganglionic eminence-derived GABAergic cortical interneurons. J. Neurosci. 35, 12869–12889. doi: 10.1523/JNEUROSCI.1164-15.2015
Modol, L., Bollmann, Y., Tressard, T., Baude, A., Che, A., Duan, Z. R. S., et al. (2020). Assemblies of perisomatic GABAergic neurons in the developing barrel cortex. Neuron 105, 93–105.e4. doi: 10.1016/j.neuron.2019.10.007
Molnár, Z., Luhmann, H. J., and Kanold, P. O. (2020). Transient cortical circuits match spontaneous and sensory-driven activity during development. Science 370:eabb2153. doi: 10.1126/science.abb2153
Monyer, H., Burnashev, N., Laurie, D. J., Sakmann, B., and Seeburg, P. H. (1994). Developmental and regional expression in the rat brain and functional properties of four NMDA receptors. Neuron 12, 529–540. doi: 10.1016/0896-6273(94)90210-0
Murata, Y., and Colonnese, M. T. (2016). An excitatory cortical feedback loop gates retinal wave transmission in rodent thalamus. eLife 5:e18816. doi: 10.7554/eLife.18816
Murata, Y., and Colonnese, M. T. (2020). GABAergic interneurons excite neonatal hippocampus in vivo. Sci. Adv. 6:eaba1430. doi: 10.1126/sciadv.aba1430
Murthy, S., Niquille, M., Hurni, N., Limoni, G., Frazer, S., Chameau, P., et al. (2014). Serotonin receptor 3A controls interneuron migration into the neocortex. Nat. Commun. 5:5524. doi: 10.1038/ncomms6524
Nelson, S. B., and Valakh, V. (2015). Excitatory/inhibitory balance and circuit homeostasis in autism spectrum disorders. Neuron 87, 684–698. doi: 10.1016/j.neuron.2015.07.033
Oh, W. C., Lutzu, S., Castillo, P. E., and Kwon, H. B. (2016). De novo synaptogenesis induced by GABA in the developing mouse cortex. Science 353, 1037–1040. doi: 10.1126/science.aaf5206
Okujeni, S., and Egert, U. (2019). Self-organization of modular network architecture by activity-dependent neuronal migration and outgrowth. eLife 8:e47996. doi: 10.7554/eLife.47996
Olsen, R. W., and Sieghart, W. (2009). GABAA receptors: subtypes provide diversity of function and pharmacology. Neuropharmacology 56, 141–148. doi: 10.1016/j.neuropharm.2008.07.045
Owens, D. F., Liu, X., and Kriegstein, A. R. (1999). Changing properties of GABA(A) receptor-mediated signaling during early neocortical development. J. Neurophysiol. 82, 570–583. doi: 10.1152/jn.1999.82.2.570
Pan, N. C., Fang, A., Shen, C., Sun, L., Wu, Q., and Wang, X. (2019). Early excitatory activity-dependent maturation of somatostatin interneurons in cortical layer 2/3 of mice. Cereb. Cortex 29, 4107–4118. doi: 10.1093/cercor/bhy293
Pangratz-Fuehrer, S., and Hestrin, S. (2011). Synaptogenesis of electrical and GABAergic synapses of fast-spiking inhibitory neurons in the neocortex. J. Neurosci. 31, 10767–10775. doi: 10.1523/JNEUROSCI.6655-10.2011
Patrizi, A., Scelfo, B., Viltono, L., Briatore, F., Fukaya, M., Watanabe, M., et al. (2008). Synapse formation and clustering of neuroligin-2 in the absence of GABAA receptors. Proc. Natl. Acad. Sci. U S A 105, 13151–13156. doi: 10.1073/pnas.0802390105
Paul, A., Crow, M., Raudales, R., He, M., Gillis, J., and Huang, Z. J. (2017). Transcriptional architecture of synaptic communication delineates GABAergic neuron identity. Cell 171, 522–539.e20. doi: 10.1016/j.cell.2017.08.032
Peinado, A., Yuste, R., and Katz, L. C. (1993). Extensive dye coupling between rat neocortical neurons during the period of circuit formation. Neuron 10, 103–114. doi: 10.1016/0896-6273(93)90246-n
Pernelle, G., Nicola, W., and Clopath, C. (2018). Gap junction plasticity as a mechanism to regulate network-wide oscillations. PLoS Comput. Biol. 14:e1006025. doi: 10.1371/journal.pcbi.1006025
Petryniak, M. A., Potter, G. B., Rowitch, D. H., and Rubenstein, J. L. R. (2007). Dlx1 and Dlx2 control neuronal versus oligodendroglial cell fate acquisition in the developing forebrain. Neuron 55, 417–433. doi: 10.1016/j.neuron.2007.06.036
Pfeffer, C. K., Stein, V., Keating, D. J., Maier, H., Rinke, I., Rudhard, Y., et al. (2009). NKCC1-dependent GABAergic excitation drives synaptic network maturation during early hippocampal development. J. Neurosci. 29, 3419–3430. doi: 10.1523/JNEUROSCI.1377-08.2009
Pfeffer, C. K., Xue, M., He, M., Huang, Z. J., and Scanziani, M. (2013). Inhibition of inhibition in visual cortex: the logic of connections between molecularly distinct interneurons. Nat. Neurosci. 16, 1068–1076. doi: 10.1038/nn.3446
Pouchelon, G., Gambino, F., Bellone, C., Telley, L., Vitali, I., Lüscher, C., et al. (2014). Modality-specific thalamocortical inputs instruct the identity of postsynaptic L4 neurons. Nature 511, 471–474. doi: 10.1038/nature13390
Poulter, M. O., Barker, J. L., O’Carroll, A. M., Lolait, S. J., and Mahan, L. C. (1992). Differential and transient expression of GABA(A) receptor α-subunit mRNAs in the developing rat CNS. J. Neurosci. 12, 2888–2900. doi: 10.1523/JNEUROSCI.12-08-02888.1992
Raimondo, J. V., Markram, H., and Akerman, C. J. (2012). Short-term ionic plasticity at GABAergic synapses. Front. Synaptic Neurosci. 4:5. doi: 10.3389/fnsyn.2012.00005
Raimondo, J. V., Richards, B. A., and Woodin, M. A. (2017). Neuronal chloride and excitability - the big impact of small changes. Curr. Opin. Neurobiol. 43, 35–42. doi: 10.1016/j.conb.2016.11.012
Ranasinghe, S., Or, G., Wang, E. Y., Ievins, A., McLean, M. A., Niell, C. M., et al. (2015). Reduced cortical activity impairs development and plasticity after neonatal hypoxia ischemia. J. Neurosci. 35, 11946–11959. doi: 10.1523/JNEUROSCI.2682-14.2015
Reha, R. K., Dias, B. G., Nelson, C. A., Kaufer, D., Werker, J. F., Kolbh, B., et al. (2020). Critical period regulation across multiple timescales. Proc. Natl. Acad. Sci. U S A 117, 23242–23251. doi: 10.1073/pnas.1820836117
Reith, C. A., and Sillar, K. T. (1999). Development and role of GABAA receptor-mediated synaptic potentials during swimming in postembryonic Xenopus laevis tadpoles. J. Neurophysiol. 82, 3175–3187. doi: 10.1152/jn.1999.82.6.3175
Rheims, S., Minlebaev, M., Ivanov, A., Represa, A., Khazipov, R., Holmes, G. L., et al. (2008). Excitatory GABA in rodent developing neocortex in vitro. J. Neurophysiol. 100, 609–619. doi: 10.1152/jn.90402.2008
Rivera, C., Voipio, J., Payne, J. A., Ruusuvuori, E., Lahtinen, H., Lamsa, K., et al. (1999). The K+/Cl− co-transporter KCC2 renders GABA hyperpolarizing during neuronal maturation. Nature 397, 251–255. doi: 10.1038/16697
Rochefort, N. L., Garaschuk, O., Milos, R. I., Narushima, M., Marandi, N., Pichler, B., et al. (2009). Sparsification of neuronal activity in the visual cortex at eye-opening. Proc. Natl. Acad. Sci. U S A 106, 15049–15054. doi: 10.1073/pnas.0907660106
Romagnoni, A., Colonnese, M. T., Touboul, J. D., and Gutkin, B. S. (2020). Progressive alignment of inhibitory and excitatory delay may drive a rapid developmental switch in cortical network dynamics. J. Neurophysiol. 123, 1583–1599. doi: 10.1152/jn.00402.2019
Rudy, B., Fishell, G., Lee, S. H., and Hjerling-Leffler, J. (2011). Three groups of interneurons account for nearly 100% of neocortical GABAergic neurons. Dev. Neurobiol. 71, 45–61. doi: 10.1002/dneu.20853
Ruijter, J. M., Baker, R. E., De Jong, B. M., and Romijn, H. J. (1991). Chronic blockade of bioelectric activity in neonatal rat cortex grown in vitro: morphological effects. Int. J. Dev. Neurosci. 9, 331–333. doi: 10.1016/0736-5748(91)90054-p
Rymar, V. V., and Sadikot, A. F. (2007). Laminar fate of cortical GABAergic interneurons is dependent on both birthdate and phenotype. J. Comp. Neurol. 501, 369–380. doi: 10.1002/cne.21250
Sahara, S., Yanagawa, Y., O’Leary, D. D. M., and Stevens, C. F. (2012). The fraction of cortical GABAergic neurons is constant from near the start of cortical neurogenesis to adulthood. J. Neurosci. 32, 4755–4761. doi: 10.1523/JNEUROSCI.6412-11.2012
Saint-Amant, L., and Drapeau, P. (2000). Motoneuron activity patterns related to the earliest behavior of the zebrafish embryo. J. Neurosci. 20, 3964–3972. doi: 10.1523/JNEUROSCI.20-11-03964.2000
Sale, A., Berardi, N., Spolidoro, M., Baroncelli, L., and Maffei, L. (2010). GABAergic inhibition in visual cortical plasticity. Front. Cell. Neurosci. 4:10. doi: 10.3389/fncel.2010.00010
Scala, F., Kobak, D., Bernabucci, M., Bernaerts, Y., Cadwell, C. R., Castro, J. R., et al. (2021). Phenotypic variation of transcriptomic cell types in mouse motor cortex. Nature 598, 144–150. doi: 10.1038/s41586-020-2907-3
Shimizu-Okabe, C., Yokokura, M., Okabe, A., Ikeda, M., Sato, K., Kilb, W., et al. (2002). Layer-specific expression of Cl− transporters and differential [Cl−]i in newborn rat cortex. Neuroreport 13, 2433–2437. doi: 10.1097/00001756-200212200-00012
Sipilä, S. T., Huttu, K., Yamada, J., Afzalov, R., Voipio, J., Blaesse, P., et al. (2009). Compensatory enhancement of intrinsic spiking upon NKCC1 disruption in neonatal hippocampus. J. Neurosci. 29, 6982–6988. doi: 10.1038/s41467-021-22339-1
Soda, T., Nakashima, R., Watanabe, D., Nakajima, K., Pastan, I., and Nakanishi, S. (2003). Segregation and coactivation of developing neocortical layer 1 neurons. J. Neurosci. 23, 6272–6279. doi: 10.1523/JNEUROSCI.23-15-06272.2003
Southwell, D. G., Paredes, M. F., Galvao, R. P., Jones, D. L., Froemke, R. C., Sebe, J. Y., et al. (2012). Intrinsically determined cell death of developing cortical interneurons. Nature 491, 109–113. doi: 10.1038/nature11523
Stachniak, T. J., Kastli, R., Hanley, O., Argunsah, A. Ö., van der Valk, E. G. T., Kanatouris, G., et al. (2021). Postmitotic Prox1 expression controls the final specification of cortical VIP interneuron subtypes. J. Neurosci. 41, 8150–8162. doi: 10.1523/JNEUROSCI.1021-21.2021
Su, J., Basso, D., Iyer, S., Su, K., Wei, J., and Fox, M. A. (2020). Paracrine role for somatostatin interneurons in the assembly of perisomatic inhibitory synapses. J. Neurosci. 40, 7421–7435. doi: 10.1523/JNEUROSCI.0613-20.2020
Sukenik, N., Vinogradov, O., Weinreb, E., Segal, M., Levina, A., and Moses, E. (2021). Neuronal circuits overcome imbalance in excitation and inhibition by adjusting connection numbers. Proc. Natl. Acad. Sci. U S A 118:e2018459118. doi: 10.1073/pnas.2018459118
Sultan, K. T., Brown, K. N., and Shi, S. H. (2013). Production and organization of neocortical interneurons. Front. Cell. Neurosci. 7:221. doi: 10.1073/pnas.2018459118
Sun, J. J., Kilb, W., and Luhmann, H. J. (2010). Self-organization of repetitive spike patterns in developing neuronal networks in vitro. Eur. J. Neurosci. 32, 1289–1299. doi: 10.1111/j.1460-9568.2010.07383.x
Takayama, C., and Inoue, Y. (2010). Developmental localization of potassium chloride co-transporter 2 (KCC2), GABA and vesicular GABA transporter (VGAT) in the postnatal mouse somatosensory cortex. Neurosci. Res. 67, 137–148. doi: 10.1016/j.neures.2010.02.010
Takesian, A. E., and Hensch, T. K. (2013). Balancing plasticity/stability across brain development. Prog Brain Res. 207, 3–34. doi: 10.1016/B978-0-444-63327-9.00001-1
Tamamaki, N., Yanagawa, Y., Tomioka, R., Miyazaki, J. I., Obata, K., and Kaneko, T. (2003). Green fluorescent protein expression and colocalization with calretinin, parvalbumin and somatostatin in the GAD67-GFP knock-in mouse. J. Comp. Neurol. 467, 60–79. doi: 10.1002/cne.10905
Taniguchi, H., He, M., Wu, P., Kim, S., Paik, R., Sugino, K., et al. (2011). A resource of cre driver lines for genetic targeting of GABAergic neurons in cerebral cortex. Neuron 71, 995–1013. doi: 10.1016/j.neuron.2011.07.026
Taylor, J., and Gordon-Weeks, P. R. (1991). Calcium-independent γ-aminobutyric acid release from growth cones: role of γ-aminobutyric acid transport. J. Neurochem. 56, 273–280. doi: 10.1111/j.1471-4159.1991.tb02592.x
Tchumatchenko, T., and Clopath, C. (2014). Oscillations emerging from noise-driven steady state in networks with electrical synapses and subthreshold resonance. Nat. Commun. 5:5512. doi: 10.1038/ncomms6512
Teppola, H., Aćimović, J., and Linne, M. L. (2019). Unique features of network bursts emerge from the complex interplay of excitatory and inhibitory receptors in rat neocortical networks. Front. Cell. Neurosci. 13:377. doi: 10.3389/fncel.2019.00377
Terunuma, M. (2018). Diversity of structure and function of GABAB receptors: a complexity of GABAB-mediated signaling. Proc. Jpn. Acad. Ser. B Phys. Biol. Sci. 94, 390–411. doi: 10.2183/pjab.94.026
ter Horst, H. J., Sommer, C., Bergman, K. A., Fock, J. M., van Weerden, T. W., and Bos, A. F. (2004). Prognostic significance of amplitude-integrated EEG during the first 72 hours after birth in severely asphyxiated neonates. Pediatr. Res. 55, 1026–1033. doi: 10.1203/01.pdr.0000127019.52562.8c
Titz, S., Hans, M., Kelsch, W., Lewen, A., Swandulla, D., and Misgeld, U. (2003). Hyperpolarizing inhibition develops without trophic support by GABA in cultured rat midbrain neurons. J. Physiol. 550, 719–730. doi: 10.1113/jphysiol.2003.041863
Tolner, E. A., Sheikh, A., Yukin, A. Y., Kaila, K., and Kanold, P. O. (2012). Subplate neurons promote spindle bursts and thalamocortical patterning in the neonatal rat somatosensory cortex. J. Neurosci. 32, 692–702. doi: 10.1523/JNEUROSCI.1538-11.2012
Tort, A. B. L., Komorowski, R. W., Manns, J. R., Kopell, N. J., and Eichenbaum, H. (2009). Theta-gamma coupling increases during the learning of item-context associations. Proc. Natl. Acad. Sci. U S A 106, 20942–20947. doi: 10.1073/pnas.0911331106
Tremblay, R., Lee, S., and Rudy, B. (2016). GABAergic interneurons in the neocortex: from cellular properties to circuits. Neuron 91, 260–292. doi: 10.1016/j.neuron.2016.06.033
Tuncdemir, S. N., Wamsley, B., Stam, F. J., Osakada, F., Goulding, M., Callaway, E. M., et al. (2016). Early somatostatin interneuron connectivity mediates the maturation of deep layer cortical circuits. Neuron 89, 521–535. doi: 10.1016/j.neuron.2015.11.020
Turgeon, S. M., and Albin, R. L. (1994). Postnatal ontogeny of GABAB binding in rat brain. Neuroscience 62, 601–613. doi: 10.1016/0306-4522(94)90392-1
Turrigiano, G. G., and Nelson, S. B. (2004). Homeostatic plasticity in the developing nervous system. Nat. Rev. Neurosci. 5, 97–107. doi: 10.1038/nrn1327
Tyssowski, K. M., DeStefino, N. R., Cho, J. H., Dunn, C. J., Poston, R. G., Carty, C. E., et al. (2018). Different neuronal activity patterns induce different gene expression programs. Neuron 98, 530–546.e11. doi: 10.1016/j.neuron.2018.04.001
Tyzio, R., Represa, A., Jorquera, I., Ben-Ari, Y., Gozlan, H., and Aniksztejn, L. (1999). The establishment of GABAergic and glutamatergic synapses on CA1 pyramidal neurons is sequential and correlates with the development of the apical dendrite. J. Neurosci. 19, 10372–10382. doi: 10.1523/JNEUROSCI.19-23-10372.1999
van den Berghe, V., Stappers, E., Vandesande, B., Dimidschstein, J., Kroes, R., Francis, A., et al. (2013). Directed migration of cortical interneurons depends on the cell-autonomous action of sip1. Neuron 77, 70–82. doi: 10.1016/j.neuron.2012.11.009
Van Eden, C. G., Parmar, R., Lichtensteiger, W., and Schlumpf, M. (1995). Laminar distribution of GABAA receptor α1, β2 and γ2 subunit mRNAs in the granular and agranular frontal cortex of the rat during pre- and postnatal development. Cereb. Cortex 5, 234–246. doi: 10.1093/cercor/5.3.234
Virtanen, M. A., Uvarov, P., Hübner, C. A., and Kaila, K. (2020). NKCC1, an elusive molecular target in brain development: making sense of the existing data. Cells 9:2607. doi: 10.3390/cells9122607
Vo, T., Carulli, D., Ehlert, E. M. E., Kwok, J. C. F., Dick, G., Mecollari, V., et al. (2013). The chemorepulsive axon guidance protein semaphorin3A is a constituent of perineuronal nets in the adult rodent brain. Mol. Cell. Neurosci. 56, 186–200. doi: 10.1016/j.mcn.2013.04.009
Wagenaar, D. A., Pine, J., and Potter, S. M. (2006). An extremely rich repertoire of bursting patterns during the development of cortical cultures. BMC Neurosci. 7:11. doi: 10.1186/1471-2202-7-11
Wamsley, B., and Fishell, G. (2017). Genetic and activity-dependent mechanisms underlying interneuron diversity. Nat. Rev. Neurosci. 18, 299–309. doi: 10.1038/nrn.2017.30
Wang, D. D., and Kriegstein, A. R. (2008). GABA regulates excitatory synapse formation in the neocortex via NMDA receptor activation. J. Neurosci. 28, 5547–5558. doi: 10.1523/JNEUROSCI.5599-07.2008
Wardle, R. A., and Poo, M. M. (2003). Brain-derived neurotrophic factor modulation of GABAergic synapses by postsynaptic regulation of chloride transport. J. Neurosci. 23, 8722–8732. doi: 10.1523/JNEUROSCI.23-25-08722.2003
Wei, S., Du, H., Li, Z., Tao, G., Xu, Z., Song, X., et al. (2019). Transcription factors Sp8 and Sp9 regulate the development of caudal ganglionic eminence-derived cortical interneurons. J. Comp. Neurol. 527, 2860–2874. doi: 10.1002/cne.24712
Weissman, T. A., Riquelme, P. A., Ivic, L., Flint, A. C., and Kriegstein, A. R. (2004). Calcium waves propagate through radial glial cells and modulate proliferation in the developing neocortex. Neuron 43, 647–661. doi: 10.1016/j.neuron.2004.08.015
Whitehead, K., Pressler, R., and Fabrizi, L. (2016). Characteristics and clinical significance of delta brushes in the EEG of premature infants. Clin. Neurophysiol. Pract. 2, 12–18. doi: 10.1016/j.cnp.2016.11.002
Wingert, J. C., and Sorg, B. A. (2021). Impact of perineuronal nets on electrophysiology of parvalbumin interneurons, principal neurons and brain oscillations: a review. Front. Synaptic Neurosci. 13:673210. doi: 10.3389/fnsyn.2021.673210
Wong Fong Sang, I. E., Schroer, J., Halbhuber, L., Warm, D., Yang, J. W., Luhmann, H. J., et al. (2021). Optogenetically controlled activity pattern determines survival rate of developing neocortical neurons. Int. J. Mol. Sci. 22:6575. doi: 10.3390/ijms22126575
Wong, F. K., Bercsenyi, K., Sreenivasan, V., Portalés, A., Fernández-Otero, M., and Marín, O. (2018). Pyramidal cell regulation of interneuron survival sculpts cortical networks. Nature 557, 668–673. doi: 10.1038/s41586-018-0139-6
Wong, F. K., and Marín, O. (2019). Developmental cell death in the cerebral cortex. Annu. Rev. Cell Dev. Biol. 35, 523–542. doi: 10.1146/annurev-cellbio-100818-125204
Wosniack, M. E., Kirchner, J. H., Chao, L. Y., Zabouri, N., Lohmann, C., and Gjorgjieva, J. (2021). Adaptation of spontaneous activity in the developing visual cortex. eLife 10:e61619. doi: 10.7554/eLife.61619
Xing, W., de Lima, A. D., and Voigt, T. (2021). The structural E/I balance constrains the early development of cortical network activity. Front. Cell. Neurosci. 15:687306. doi: 10.3389/fncel.2021.687306
Yamada, J., Okabe, A., Toyoda, H., Kilb, W., Luhmann, H. J., and Fukuda, A. (2004). Cl- uptake promoting depolarizing GABA actions in immature rat neocortical neurones is mediated by NKCC1. J. Physiol. 557, 829–841. doi: 10.1113/jphysiol.2004.062471
Yang, J. W., An, S., Sun, J. J., Reyes-Puerta, V., Kindler, J., Berger, T., et al. (2013). Thalamic network oscillations synchronize ontogenetic columns in the newborn rat barrel cortex. Cereb. Cortex 23, 1299–1316. doi: 10.1093/cercor/bhs103
Keywords: cortex, development, activity patterns, interneuron, GABA shift, apoptosis, migration, synaptogenesis
Citation: Warm D, Schroer J and Sinning A (2022) GABAergic Interneurons in Early Brain Development: Conducting and Orchestrated by Cortical Network Activity. Front. Mol. Neurosci. 14:807969. doi: 10.3389/fnmol.2021.807969
Received: 02 November 2021; Accepted: 06 December 2021;
Published: 03 January 2022.
Edited by:
Atsuo Fukuda, Hamamatsu University School of Medicine, JapanReviewed by:
Matthew T. Colonnese, George Washington University, United StatesMiao He, Fudan University, China
Copyright © 2022 Warm, Schroer and Sinning. This is an open-access article distributed under the terms of the Creative Commons Attribution License (CC BY). The use, distribution or reproduction in other forums is permitted, provided the original author(s) and the copyright owner(s) are credited and that the original publication in this journal is cited, in accordance with accepted academic practice. No use, distribution or reproduction is permitted which does not comply with these terms.
*Correspondence: Anne Sinning, YXNpbm5pbmdAdW5pLW1haW56LmRl
† These authors have contributed equally to this work