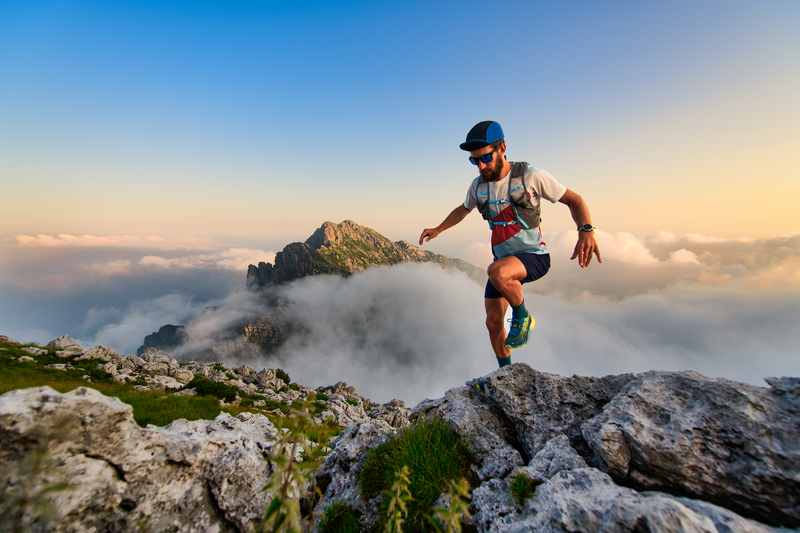
94% of researchers rate our articles as excellent or good
Learn more about the work of our research integrity team to safeguard the quality of each article we publish.
Find out more
REVIEW article
Front. Mol. Neurosci. , 05 May 2021
Sec. Molecular Signalling and Pathways
Volume 14 - 2021 | https://doi.org/10.3389/fnmol.2021.665693
The development and function of the central nervous system rely on the microtubule (MT) and actin cytoskeletons and their respective effectors. Although the structural role of the cytoskeleton has long been acknowledged in neuronal morphology and activity, it was recently recognized to play the role of a signaling platform. Following this recognition, research into Microtubule Associated Proteins (MAPs) diversified. Indeed, historically, structural MAPs—including MAP1B, MAP2, Tau, and MAP6 (also known as STOP);—were identified and described as MT-binding and -stabilizing proteins. Extensive data obtained over the last 20 years indicated that these structural MAPs could also contribute to a variety of other molecular roles. Among multi-role MAPs, MAP6 provides a striking example illustrating the diverse molecular and cellular properties of MAPs and showing how their functional versatility contributes to the central nervous system. In this review, in addition to MAP6’s effect on microtubules, we describe its impact on the actin cytoskeleton, on neuroreceptor homeostasis, and its involvement in signaling pathways governing neuron development and maturation. We also discuss its roles in synaptic plasticity, brain connectivity, and cognitive abilities, as well as the potential relationships between the integrated brain functions of MAP6 and its molecular activities. In parallel, the Collapsin Response Mediator Proteins (CRMPs) are presented as examples of how other proteins, not initially identified as MAPs, fall into the broader MAP family. These proteins bind MTs as well as exhibiting molecular and cellular properties very similar to MAP6. Finally, we briefly summarize the multiple similarities between other classical structural MAPs and MAP6 or CRMPs.In summary, this review revisits the molecular properties and the cellular and neuronal roles of the classical MAPs, broadening our definition of what constitutes a MAP.
Microtubule-Associated Proteins (MAPs) were discovered in the context of the study of microtubule (MT) stability in neurons during the 1970s (Weingarten et al., 1975; Sloboda et al., 1976). Indeed, in neurons, MTs composed of α- and β-tubulin heterodimers forming 25 nm wide hollow tubes can either exhibit dynamic properties with phases of polymerization/depolymerization (Mitchison and Kirschner, 1984), or be particularly stable with a slow tubulin turnover (Okabe and Hirokawa, 1990; Li and Black, 1996). In testimony to their stability, neuronal MTs can resist conditions usually causing MT depolymerization, such as exposure to tubulin poison (nocodazole) or to cold temperatures (Webb and Wilson, 1980; Brady et al., 1984; Baas and Black, 1990). The search for the neuronal effectors leading to this stability has long been a focus of research. Various factors were shown to modulate neuronal MT stability, including: (1) the nature of tubulin isotypes, (2) post-translational tubulin modifications, and (3) binding of MAPs (Baas et al., 2016).
The nature of tubulin isotypes was clearly demonstrated in mice, where α and β tubulin are each coded by eight genes (Findeisen et al., 2014; Hausrat et al., 2020). The different isoforms combine to produce MTs with distinct dynamic parameters. For example, the βIII/βII tubulin MT isoform displays antagonist effects on dynamicity/stability (Panda et al., 1994; Pamula et al., 2016; Vemu et al., 2016; Ti et al., 2018).
Post-translational modifications of tubulin—including tyrosination, polyamination, polyglutamylation, and acetylation—were shown to modulate MT stability by altering their dynamic properties (Moutin et al., 2020), resistance to cold exposure (Song et al., 2013), sensitivity to severing enzymes (Lacroix et al., 2010; Valenstein and Roll-Mecak, 2016), and flexibility (Portran et al., 2017; Xu et al., 2017). Finally, the so-called structural MAPs which bind throughout the MT lattice also influence MT stabilization. As indicated above, these structural MAPs were first discovered in the 1970s, when they were found to be associated with purified brain tubulin preparations. The group includes MAP1, MAP2, MAP4, Tau, and MAP6 (also known as STOP; Cleveland et al., 1977; Herzog and Weber, 1978; Huber and Matus, 1984; Margolis et al., 1986; Job et al., 1987; Chapin and Bulinski, 1994). Other members were identified more recently: DCX, MAP7, and MAP9 (Gleeson et al., 1999; Yadav et al., 2014; Monroy et al., 2020).
Since the initial discovery of these MAPs based on their ability to bind and stabilize MTs, studies have increasingly pointed toward a wide array of other cellular functions (Dehmelt and Halpain, 2005; Morris et al., 2011; Dent and Baas, 2014; Bodakuntla et al., 2019). Thus, structural MAPs have been shown to: regulate actin cytoskeleton dynamics; be amenable to post-translational modifications which target them to membrane compartments; interact with a huge number of partners which are then involved in neuroreceptor homeostasis and signaling cascades. These additional abilities stem from molecular features including actin-binding domains, stretches of cysteine residues for palmitoylation-driven membrane association, and Proline-Rich-Domain (PRDs) to mediate binding to SH3-containing signaling proteins.
In this review, we will present results obtained over almost 40 years of research on MAP6 proteins to illustrate the wide range of MT-dependent and -independent molecular properties that MAPs can exhibit. Using the Collapsin Response Mediator Proteins (CRMPs) as an example, we will show how other proteins not initially identified as MAPs also fulfill molecular and cellular functions initially attributed to the classical structural MAPs. We will also discuss the multiple cellular and physiological roles of MAPs in neurons and in the central nervous system. The review will, in particular, illustrate the crucial implication of MAPs in neuronal plasticity and cognition in accordance with their dysfunctions in neuropsychiatric diseases. Overall, we aim at demonstrating that the initial definition of classical MAPs (i.e., MT binding and stabilization) should now encompass the MT-dependent and -independent functions of these proteins.
Like the other structural MAPs, MAP6 protein was initially identified thanks to its ability to interact with tubulin/MTs. Subsequent studies identified a large number of MAP6 partners related to various cellular functions including neuroreceptor homeostasis, endocytosis, nuclear function, and signaling pathways. The different partners are summarized in Figure 1, and further details are provided in Supplementary Table 1. In the following sections, the contribution of each MAP6 sub-domain to its molecular, cellular, and physiological functions are detailed. A summary of MAP6 domains and their roles is presented in Figure 2.
Figure 1. MAP6 protein and its interactors. All the known MAP6 interactors (see Supplementary Table 1) are presented and grouped based on their functions.
Figure 2. MAP6 domains and functions. Schematic representation of MAP6, color-coded arrows indicate the different domains identified so far: the N-terminal domain shared with MAP6d1 protein (violet box); the three palmitoylation sites (red bars); the proline-rich domain involved in transduction of Semaphorin 3A signals (black box); the three microtubule-stabilizing Mn modules (orange boxes); the tandem repeats corresponding to microtubule-stabilizing Mc modules (dark gray boxes); the C-terminal repeats (light gray boxes). Below the representation, the corresponding roles of these domains are indicated, covering molecular and cellular roles, and tissues and integrated functions.
The exceptional stability of brain-extracted MTs when exposed to cold was first described almost half a century ago (Brinkley and Cartwright, 1975; Lee et al., 1975; Webb and Wilson, 1980). Subsequent work led to the identification of several polypeptides that co-purify with cold-stable MTs (Job et al., 1981, 1982; Margolis and Rauch, 1981; Pabion et al., 1984) and are retained on calmodulin-affinity columns (Job et al., 1982; Pirollet et al., 1983). The unique ability of these polypeptides to confer cold stability on MTs led Job and collaborators to call them STOP proteins, for Stable-Tubule-Only Polypeptides (they were later renamed MAP6 proteins). The MT stabilization properties of MAP6 are Ca2+-calmodulin sensitive (Job et al., 1981; Pirollet et al., 1983, 1992a,b) and the 145-kDa STOP isoform from the rat brain was shown to confer a super-stable state on MTs, in a dose-dependent manner (Job et al., 1987).
Bosc et al. (1996) first cloned MAP6 cDNA by immuno-screening of a DNA expression library with MAP6 monoclonal antibodies (Pirollet et al., 1989). These experiments provided clear identification of MAP6 and made further studies to gather specific information on the protein possible. MAP6 isoforms are restricted to vertebrates, where they are expressed in several tissues (Pirollet et al., 1989; Bosc et al., 2001). In mice, a single four-exon gene, Map6 (formerly Mtap6), produces several isoforms of MAP6 proteins as a result of RNA splicing and the use of alternative promoters (Bosc et al., 1996; Denarier et al., 1998a; Aguezzoul et al., 2003). Murine neurons express MAP6-E (MAP6-Early, formerly E-STOP, apparent MW 79 kDa) from early developmental stages to adulthood at constant expression levels, whereas expression of the longest isoform MAP6-N (MAP6-Neuronal, formerly N-STOP, apparent MW 120 kDa) increases between birth and adulthood (up to 10-fold higher expression than MAP6-E in adults) (Guillaud et al., 1998; Galiano et al., 2004; Tortosa et al., 2017). These two variants can be found associated with MTs in neurons and stabilize neuronal MTs when exposed to cold or nocodazole (Pirollet et al., 1989; Margolis et al., 1990; Bosc et al., 1996; Guillaud et al., 1998; Andrieux et al., 2002). Glial cells also express some MAP6 isoforms, with MAP6-O (apparent MW 89 kDa) found in oligodendrocytes, where they provide resistance to both cold and nocodazole. In contrast, astrocytes express MAP6-A (apparent MW 66 kDa), which only provides cold resistance (Galiano et al., 2004). Like MAP6-A, the ubiquitous MAP6-F isoform (apparent MW 42 kDa) provides only cold resistance (Denarier et al., 1998b).
The identification of 12 calmodulin-binding domains on MAP6-N revealed the existence of three domains that partially overlap some of the calmodulin-binding motifs. These three domains are known as Mn modules (Mn1–3, Figure 2) and stabilize MTs against both cold and nocodazole-induced depolymerization (Bosc et al., 2001). Mn1 and Mn2 modules were shown to reproduce the function of full-length MAP6-N with regard to MT stabilization, both in vitro and in cellulo (Bosc et al., 2001; Lefevre et al., 2013). Thus, these modules may stabilize MTs exposed to cold and nocodazole by forming bridges with adjacent tubulin heterodimers either between protofilaments, or longitudinally within the same protofilament (Lefevre et al., 2013).
In addition to its Mn modules, MAP6-N contains central repeats, or Mc modules, each of which encompass a calmodulin-binding domain (Figure 2). As indicated above, MAP6 proteins are found only in vertebrates, but Mc modules are further restricted. Thus, these domains have only been identified in mammals and are absent from MAP6 homologs expressed in fish, frogs, lizards or birds (Bosc et al., 2001, 2003). Among mammals, the number of Mc modules varies depending on the species and/or individual (4–6 in non-inbred rats, 3–6 in mice depending on the strain, and only 1 in higher primates) (Bosc et al., 2003). Mc modules have been shown to be responsible for conferring cold-stability of MTs in cells, even though they display no MT-binding capacity at physiological temperatures (Denarier et al., 1998b; Delphin et al., 2012). In vitro studies demonstrated that cold temperatures induce conformational changes in the Mc modules which allow them to interact with MTs (Delphin et al., 2012). Thus, Mc modules behave like cold sensors, stabilizing MTs when temperatures drop, for example during hibernation or torpor.
The high abundance of calmodulin-binding domains overlapping the Mn and Mc modules in MAP6 hints that MAP6 binding to MTs is likely to be tightly regulated in cells. Indeed, it was shown in vitro that Ca2+-Calmodulin (CaM) binding to MAP6 occurs in an unusual manner (Bouvier et al., 2003) and prevents MAP6 binding to MTs (Lefevre et al., 2013). MAP6/MTs interaction is also prevented by phosphorylation of MAP6 by CAMKII and favors MAP6/F-actin interaction in neurons (Baratier et al., 2006). Based on the available experimental data and as proposed by Ramkumar and collaborators (Ramkumar et al., 2018), the regulation of MAP6 by Ca2+-CaM in dendritic spines might follow this sequence: upon synaptic activity, the Ca2+-CaM complex forms, detaches MAP6 from adjacent MTs and activates CAMKII (Fink and Meyer, 2002). When Ca2+ level decreases, CaM is released from MAP6 allowing its phosphorylation by CAMKII. Then phosphorylated MAP6 is unable to re-associate with MTs but rather binds and stabilizes the synaptic F-actin (Baratier et al., 2006; Peris et al., 2018).
Recently, in cell-free systems, recombinant MAP6-N was shown to exert some stabilizing effects on MT dynamics by promoting rescue (Cuveillier et al., 2020), although the precise contribution of the various MAP6-N MT-binding domains (Mn and/or Mc modules) remains to be clarified. Indeed, the specific role of MAP6-related MT stabilization in developing neurons remains unclear. For example, MT-dependent parameters of neuronal differentiation and morphology (e.g., neurite elongation and branching, axonal polarization) are not dramatically altered in neurons expressing reduced MAP6 levels. Indeed, MAP6 deficient neurons exhibited only moderate morphological defects with a slight increase of the axonal length and a decreased spine density (Andrieux et al., 2002, 2006; Peris et al., 2018; Boulan et al., 2020), as well as a reduced growth cone size and an increased dendrite branching (Schwenk et al., 2014; Qiang et al., 2018). Those defects are not striking possibly due to compensatory mechanisms by other MAPs. Indeed, double-KO neurons for Tau/MAP1B or MAP2/MAP1B display stronger alterations of neuronal differentiation (Takei et al., 2000; Teng et al., 2001) than single KO neurons (Harada et al., 1994; Takei et al., 1997; Gonzalez-Billault et al., 2001). In this respect, an increased expression of Tau has been observed when MAP6 expression is knocked-down even though Tau and MAP6 role in MT stabilization are not similar in neurons (Qiang et al., 2018). In developing neurons, MAP6 was found enriched in the proximal part of the future axon (Tortosa et al., 2017) and a recent proteomic analysis pointed out MAP6 as a specific component of the AIS (Hamdan et al., 2020). MAP6-N localization in the proximal part of axons has been shown to be protective toward the formation of axonal varicosities induced by mechanical stress (Gu et al., 2017). Still, the exact relationships between MAP6-dependent MT stabilization, axonal polarization and AIS functions remains elusive.
Although most structural MAPs exhibit repeated MT-binding motifs, the repeated motifs in MAP6—its Mn and Mc modules—are unique, and share no homology with MT-binding domains found in Tau or MAP1B (Bosc et al., 1996). However, bioinformatic analysis of the MAP6 sequence has revealed three proteins that share homology in MAP6 functional domains.
The first one is MAP6d1, for MAP6-domain-containing protein 1 (formerly SL21 for 21-kDa STOP-like protein). MAP6d1 is expressed at high levels in the brain and shares two major similarities with MAP6: 80% sequence homology in its N-terminal domain, and 72% sequence homology with the Mn3 module of MAP6 (Bosc et al., 2001; Gory-Fauré et al., 2006). Since the N-terminal domain of both proteins contains the main calmodulin-binding site (Bosc et al., 2001), its functional role might be important.
The original MAP6 family was enlarged following the discovery of homologs of SAXO proteins (SAXO1 and SAXO2 in mammals, formerly named FAM154A and FAM154B, respectively). These proteins were originally identified in the protozoan Trypanosoma brucei and in ciliated/flagellated cells (Dacheux et al., 2012). Like neuronal MTs, cilia and flagella are characterized by a high level of MT stability displaying resistance to cold- and nocodazole-induced depolymerization. The human isoform hSAXO1 is ubiquitously expressed and specifically associates with centrioles, basal bodies and cilia (Dacheux et al., 2015). Little is known about SAXO2 beyond the fact that its expression appears to be enriched in ciliated cells.
SAXO proteins share homologies with the Mn modules of MAP6/MAP6d1, and their N-terminal sequence, although distinct, is also rich in cysteines (Dacheux et al., 2012). Interestingly, 80% of hSAXO1 consists of 12 tandem Mn modules that, like the equivalent modules in MAP6 and MAP6d1, are involved in MT binding and cold stabilization. The high number of Mn modules is important since hSAXO1 overexpression results in an increased primary cilia length in RPE1 cells, through a mechanism requiring the Mn modules (Dacheux et al., 2015).
Cryo-EM experiments revealed neuronal MTs to contain visible intraluminal densities (Burton, 1984; Garvalov et al., 2006; Atherton et al., 2018). These densities correspond to Microtubule Inner Proteins (MIPs), the molecular identity of which was totally unknown until MAP6 was identified as a MIP (Cuveillier et al., 2020). The capacity of MAP6 to enter the lumen of MTs was demonstrated using cell-free systems in which MTs were copolymerized with MAP6, yielding MTs with regularly spaced intraluminal densities. When using MTs derived from murine MAP6 KO neurons, a net reduction in the numbers of intraluminal densities was observed. The presence of MAP6 inside MTs would explain, in part, the extremely slow turnover of MAP6 on neuronal microtubules described by Tortosa et al. (2017). Strikingly, intraluminal MAP6 induced MT coiling in vitro, leading to the formation of helical MTs with a highly conserved pitch and width associated with a specific tubulin compaction state in the MT lattice. This coiling pattern requires the Mn and Mc modules, as well as the first 35 N-terminal residues.
In neurons, the functions of such stable helical MAP6-containing MTs remains to be determined. By occupying a greater width, helical MTs could influence the spatial organization of the MT network, help determine neurite or axonal width, or confer resistance to compressive loads, such as those encountered during development. The unhabitual tubulin compaction state of helical MTs could also be a mean to specifically recruit a set of proteins such as molecular motors.
From an evolutionary point of view, it will be interesting to discover when did the ability of mammalian neuronal MAP6 to behave as a MIP emerge? In other words, does the MAP6 ancestor SAXO in Trypanosoma (Dacheux et al., 2012) behave as a MIP only, as a MAP only, or does it exhibit both properties?
The first indication that MAP6 can bind to actin was obtained when CaMKII-phosphorylated MAP6 was found to be unable to bind MTs, but that it binds actin in vitro and in the growth cones of neurons (Baratier et al., 2006). More recently, the central Mc modules of MAP6 were shown to bind actin and to induce specific rearrangements—leading to straightening and bundling of actin filaments (Peris et al., 2018). Moreover, MAP6-mediated stabilization of synaptic actin following synaptic activation was shown to be crucial for maintaining mature dendritic spines, the postsynaptic compartments of synapses (Peris et al., 2018). Other effects of MAP6 on the dynamic parameters of actin, such as nucleation, remain to be investigated, as does the possibility that MAP6 mediates cross-linking between actin and MT networks in neurons. MAP6 may also indirectly influence the actin cytoskeleton through an effect on signaling cascades as it has been shown to interact with the small GTPase protein Rac2 (Figure 1; Capala et al., 2015). Several MAP6 partners have also been shown to interact with actin (Supplementary Table 1), such as spinophilin (Grossman et al., 2004) or α-synuclein (Oliveira da Silva and Liz, 2020). These interactions support specific actin-related cellular functions for MAP6 and its partners.
The N-terminal domains of MAP6 and MAP6d1 contain a stretch of cysteines with C5, C10, and C11 residues (Figure 2). These positions have been shown to be palmitoylated by a subset of palmitoylating enzymes containing a DHHC motif. The proteins are then targeted to the Golgi apparatus or the plasma membrane (Gory-Fauré et al., 2006, 2014). In addition, MAP6 interacts directly with ankyrin repeats present in the palmitoylating enzymes zDHHC13 and zDHHC17 (Lemonidis et al., 2015). During neuronal development, palmitoylated forms of MAP6 were identified on the Golgi and on secretory vesicles, and depalmitoylation by α/β hydrolase-domain-containing 17 proteins (ABHD17 proteins) induced MAP6 relocalization to MTs in the proximal part of the axon (Tortosa et al., 2017). In neurons, non-centrosomal MT nucleation is crucial, and through its interactions with MTs as well as with the Golgi apparatus or Golgi outposts which are nucleation sites for non-centrosomal MTs (Sanders and Kaverina, 2015; Valenzuela et al., 2020; Yang and Wildonger, 2020), MAP6 might contribute to such events.
MAP6 and MAP6d1 proteins were also shown to localize to mitochondria. This localization involved their N-terminal domain, but not palmitoylation (Gory-Fauré et al., 2014).
Finally, indirect evidence links MAP6 to lysosomes: KIF5B (Kinesin 1 heavy-chain) preferentially binds to MAP6-positive MTs (Tortosa et al., 2017) and lysosomal transport—which is dependent on KIF5B and on TMEM106B, a MAP6 partner (Figure 1)—is perturbed when MAP6 expression is impaired (Schwenk et al., 2014).
Overall, these results show that MAP6’s association with membranous compartments plays important roles in establishing and maintaining neuronal morphology. The several endocytosis-related proteins such as Varp, dynamin 1 or endophilin 1 (Craft et al., 2008; Vikhreva et al., 2009) which have been found to interact with MAP6 (Supplementary Table 1) suggests that the roles played by MAP6 in intracellular organelle trafficking still holds many secrets.
Proteomic analysis of the nano-environment of calcium channels (Cav2.1, Cav2.2, Cav2.3, CavBeta3, CavBeta4) revealed the presence of MAP6 (Müller et al., 2010; Figure 1). More recently, MAP6, through its Mn3 module, was found to associate with Tctex1 (Brocard et al., 2017), a dynein light chain interacting with Cav2.2/N-type calcium channels (Lai et al., 2005). In conjunction with Tctex1, MAP6 is involved in sorting and trafficking Cav2.2 channels, as shown by impaired calcium signaling in MAP6 KO neurons (Brocard et al., 2017).
Even if MTs are only transiently present in both pre- and post-synaptic compartments of synapses (axonal boutons and dendritic spines), MAP6 was consistently identified in synaptic proteomes, suggesting MT-independent roles (Peng et al., 2004; Baratier et al., 2006; Cheng et al., 2006; Collins et al., 2006; Munton et al., 2007; Weingarten et al., 2014). In a study related to subicular neurons from the hippocampus, MAP6 was associated with the receptors Neuropilin1, Plexin D1, and VEGFR2—which together make up the tripartite Semaphorin 3E receptor (Deloulme et al., 2015; Figure 1).
In addition to its associations with subcellular compartments and receptors, MAP6 protein contains proline-rich domains (PRD), which are involved in the binding of SH3-domain-containing proteins (Figure 1 and Supplementary Table 1). Binding of Intersectin 1, cSrc, PLC-γ, or PI3K have all been described (Morderer et al., 2012; Deloulme et al., 2015). One of the PRD domains in MAP6 is essential to the Semaphorin 3E signaling cascade (Deloulme et al., 2015), driving the formation of the fornix, an axonal tract which requires Semaphorin 3E signaling.
Although some interactions with kinases and phosphatases have been reported (Figure 1), almost nothing is known about how MAP6’s functions are regulated by phosphorylation enzymes, with the exception of CaMKII. MAP6-N protein contains four CaMKII phosphorylation sites located within its calmodulin/MT-binding domains. These domains can be phosphorylated in vitro and in vivo. Phosphorylation induces MAP6 to detach from MTs and delocalize to actin within growth cones or dendritic spines (Baratier et al., 2006; Peris et al., 2018).
Finally, MAP6 was shown to associate with the highly brain-enriched BCH (Cdc42GAP Homology)-domain-containing protein Bmcc1/Prune2 (Figure 1) which negatively regulates the actin cytoskeleton regulator RhoA (Soh and Low, 2008). This association promotes the emergence of membrane protrusions (Arama et al., 2012). However, the relationship between a direct or indirect effect on the cytoskeleton is not clear.
As neuronal MAPs, especially MAP6, were thought to be strong MT stabilizers, its deletion in mice was expected to be lethal due to major MT-breakdown in neurons. But in fact, MAP6 KO mice (also known as STOP KO mice) are viable, with an apparently normal brain organization (Andrieux et al., 2002). However, these mice display a wide range of biological and behavioral impairments reminiscent of symptoms displayed by patients suffering from psychiatric disorders, especially schizophrenia, as detailed below.
MAP6 KO mice show hyperactivity, fragmentation of normal activity, anxiety-like behavior, social withdrawal, and impaired maternal behavior leading to the death of pups (Andrieux et al., 2002). These defects are associated with altered synapse-functioning, particularly during synaptic plasticity events when the synapses need to adapt their reactivity. These alterations lead, for example, to strong deficits in potentiation or depression of the synaptic responses. These biological and behavioral defects were shown to respond to long-term treatment with antipsychotics, the gold standard in schizophrenia treatment, thus positioning MAP6 KO mice as a useful model for the study of the physiopathology of this disease (Andrieux et al., 2002).
Schizophrenia is a chronic debilitating neurodevelopmental disorder that affects approximately 1% of the population worldwide; the first symptoms emerge during adolescence and early adulthood. It is characterized by a combination of positive (auditory and visual hallucination), negative (social withdrawal, anxiety), and cognitive symptoms (impaired memory, decision-making difficulties; Joseph et al., 2015). Following our seminal 2002 article (Andrieux et al., 2002), numerous studies were performed and their findings reinforced the validity of the MAP6 KO model for the study of schizophrenia. Indeed, MAP6 KO mice fulfill the three—construct/face/predictive—criteria for the validity of an animal model (Belzung and Lemoine, 2011; Jones et al., 2011) with similar underlying molecular defects/phenotypes/pharmacological responses, respectively (Delotterie et al., 2010; Volle et al., 2013; Deloulme et al., 2015; Bouet et al., 2021).
In further support of the validity of this model, subsequent studies revealed defects in MAP6 expression in humans presenting developmental delay, corpus callosum dysgenesis, autistic or schizophrenic symptoms (Shimizu et al., 2006; Choi et al., 2009; Martins-de-Souza et al., 2009; Xiao et al., 2015; Coumans et al., 2016; Wei et al., 2016a, b; Chen et al., 2021).
In tight correlation with the hyper-dopaminergia observed in schizophrenic patients (Carlsson et al., 2000; Kapur, 2003), MAP6 KO mice present increased dopamine overflow in the mesolimbic pathway. This system plays a significant role in mediating pleasure and rewarding experiences (Brun et al., 2005; Bouvrais-Veret et al., 2007, 2008). Schizophrenia also presents positive symptoms, and MAP6 KO mice exhibited increased locomotor activity (Andrieux et al., 2002; Brun et al., 2005; Fradley et al., 2005; Bouvrais-Veret et al., 2007) associated with hypersensitivity to novelty or to the psychostimulant amphetamine (Brun et al., 2005; Bégou et al., 2007; Bouvrais-Veret et al., 2008), along with extensive disruption of sleep patterns (Profitt et al., 2016). All these processes crucially depend on dopaminergic neurotransmission.
Negative symptoms in schizophrenia are mediated by alterations in both the glutamatergic and serotoninergic (5-HT) neurotransmission systems (Aghajanian and Marek, 2000; Carlsson et al., 2000). MAP6 KO mice exhibited abnormal glutamatergic neurotransmission with altered synaptic plasticity in the hippocampus, leading to totally defective Long-Term Potentiation (LTP) and Long-Term Depression (LTD), as measured by electrophysiology techniques (Andrieux et al., 2002). These defects are related to a smaller presynaptic vesicle pool (Andrieux et al., 2002), a decreased level of glutamate and the glutamate precursor glutamine in the forebrain (Brenner et al., 2007), as well as decreased mRNA levels of the glutamate transporter-1, Vglut1 (Eastwood et al., 2007). MAP6 KO mice are thus characterized by an overall hypo-glutamatergia.
If we now focus on serotoninergic neurotransmission, serotonin biosynthesis and expression of the serotonin (5-HT) receptors are highly perturbed in MAP6 KO mice, with a 70% increase in 5HT-1A expression in the raphe nuclei for example (Fournet et al., 2010), as well as half reduction of serotonin (5-HT) in the substantia nigra, the ventral tegmental area and the hippocampus (Fournet et al., 2012b). In addition, the levels of serotonin transporters (SERT) recapturing the serotonin (5-HT) released into the synaptic cleft is very severely affected in MAP6 KO mice with a decreased expression ranging from 30% to 90% in some brain areas whereas the expression increase up to 120% in other brain regions (Fournet et al., 2010). MAP6 KO mice thus display an extreme imbalance in serotoninergic transmission.
Finally, sensory-motor gating is also altered in MAP6 KO mice (Fradley et al., 2005; Volle et al., 2013). This effect might be linked to perturbed dopamine-, glutamate-, and serotonin-mediated neurotransmission, but could also be related to activation of opioid mu receptors (Quednow et al., 2008) which is altered in MAP6 KO mice (Charlet et al., 2010).
In summary, basal neurotransmission for all the major neurotransmitters is strongly perturbed in MAP6 KO mice. Strikingly, serotoninergic neurons—the longest and most extensively branched neurons in the brain—display very severe morphological defects, suggesting complementary roles of MAP6 in these neurons. These roles may include MT stabilization, modulation of serotonin neuroreceptors/transporters, and involvement in signaling cascades.
In terms of cognitive symptoms, MAP6 KO mice exhibit severe defects in both short-and long-term hippocampal synaptic plasticity. Indeed, glutamatergic hippocampal neurons in the CA1 region display severely defective Post-Tetanus Potentiation (PTP), and, as indicated above, cannot support LTP and LTD (Andrieux et al., 2002). Synaptic deficits exist in the presynaptic compartment, the axonal bouton, where a two-fold decrease in synaptic vesicles is reported (Andrieux et al., 2002) with a possible glutamate-release defect due to the absence of interaction between MAP6 and the SNARE protein SNAP25 (Figure 1). One can speculate on the formation of a transient complex between MAP6 and SNAP25 at the plasma membrane, in the presynaptic release zone, as the membrane targeting of both proteins is induced by similar palmitoylating enzymes (Greaves and Chamberlain, 2011; Gory-Fauré et al., 2014). In addition, the absence of MAP6 induces a general decrease in spine density which is related to altered actin dynamics in dendritic spines (Peris et al., 2018). MAP6 could also contribute to the entry of MTs into the synaptic compartments, and their residence time, thus influencing synaptic strength. Overall, these synaptic defects are most probably a consequence of MAP6’s involvement in signaling cascades, receptor homeostasis, and cytoskeleton regulation.
Although the first rough anatomical study of MAP6 KO mice revealed no obvious defects in brain anatomy (Andrieux et al., 2002), subsequent detailed studies showed that MAP6 KO mice have a reduced brain volume associated with an increased ventricular volume and a reduced cerebellum and thalamus size (Powell et al., 2007; Deloulme et al., 2015). Through the use of brain imaging techniques, MAP6 KO mice were found to exhibit a decrease in myelinated tract volumes (e.g., in the internal capsule and cerebellar peduncle) as well as a strong decrease in cortico-spinal tract fasciculation (Deloulme et al., 2015; Gimenez et al., 2017). A very intriguing structural defect is the absence of an important component of the limbic system in MAP6 KO mice—the post-commissural part of the fornix (Deloulme et al., 2015). The fornix is a tract that emerges from the subiculum, the most inferior component of the hippocampal formation of both hemispheres and extends to the mammillary bodies, within the hypothalamus. As part of the Papez circuit, the lack of the fornix leads to a dis-connectivity between the hippocampus and the hypothalamus and certainly contributes to the behavioral defects observed in MAP6 KO mice. During the formation of the fornix, Semaphorin 3E is an attractive guidance molecule for subicular neurons (Chauvet et al., 2007) as it binds to its tripartite receptor (Neuropilin 1/PlexinD1/VEGFR2) to induce activation of downstream signaling cascades involving SH3-containing proteins. Interestingly, the impaired fornix formation observed in MAP6 KO mice is not dependent on MAP6’s MT-binding domains; rather it is driven by the interaction of MAP6 PRD domains with key SH3-domain-containing proteins, including Intersectin 1, PI3K, and Src (Figure 1; Deloulme et al., 2015). The axonal tract defects identified in MAP6 KO mice correlate to a striking extent with anomalies identified on brain images from patients with schizophrenia (McCarley et al., 1999; De Peri et al., 2012; Shepherd et al., 2012; Bopp et al., 2017), including alteration of the fornix or of the cortico-spinal tract (Douaud et al., 2007; Kendi et al., 2008; Fitzsimmons et al., 2009; Luck et al., 2010; Davidson et al., 2012; Lee et al., 2013).
MAP6 is highly expressed in the olfactory bulb and the hippocampus (Couégnas et al., 2007; Richard et al., 2009), two regions where adult neurogenesis is known to occur. Several studies investigated adult neurogenesis in MAP6 KO mice. Benardais et al. (2010) reported an increased number of proliferating cells in the olfactory epithelium with increased apoptosis, whereas Fournet et al. (2010) discovered decreased cell proliferation in the hippocampus. At the molecular level, how MAP6 regulates neurogenesis remains a completely open question, although it is possible that MAP6 through its binding to the retinoic receptors RAR-beta and RAR-gamma (Figure 1) can modulate their functions known to be active during neurogenesis (Jacobs et al., 2006; Maden, 2007; Mishra et al., 2018).
As the defects observed in MAP6 KO mice are reminiscent of schizophrenia symptoms, the gold standard treatments for psychiatric diseases were the most obvious approach to try in an attempt to alleviate MAP6 KO deficiencies. Alternatively, new pharmacological approaches targeting the neuro-cytoskeleton represent promising avenues of investigation.
Classical treatments of psychiatric diseases. In accordance with their defects in almost all neuro-transmission systems, the defects observed in MAP6 KO mice are sensitive to neuroactive molecules. Thus, long-term treatments with both typical antipsychotics, such as haloperidol (Haldol) (dopamine antagonist), and atypical antipsychotics, such as risperidone (Risperdal) or clozapine (dopamine and serotonin antagonists), alleviate behavioral defects in MAP6 KO mice (Andrieux et al., 2002; Bégou et al., 2008; Delotterie et al., 2010). Moreover, clozapine improves alterations to synaptic plasticity (Delotterie et al., 2010) via its known positive effect on glutamatergic synapses (Fukuyama et al., 2019). Similarly, treatment of MAP6 KO mice with anti-depressants such as fluoxetine/Prozac (a Selective Serotonin Reuptake Inhibitor, gold standard treatment for depression) alleviated anxiety-like behavior and cognitive defects (Fournet et al., 2012a). The depressive-like behavior of MAP6 KO mice and their impaired hippocampal neurogenesis were alleviated by the use of electroconvulsive stimulation (ECS) (Jonckheere et al., 2018). ECS is the animal equivalent of Electroconvulsive Therapy (ECT), which remains a very powerful and useful treatment for major depression.
Cytoskeleton-related drugs represent a new pharmacological approach to psychiatric disorders. MAP6 KO mice were the first animal model to establish a link between cytoskeleton defects and the cognitive impairment characteristics of psychiatric disorders, in particular schizophrenia (Andrieux et al., 2002). Subsequently, other cytoskeletal components including actin and tubulin themselves, as well as CRMP1, CRMP2, MAP2, MAP1B, and LIM kinases were also linked to psychiatric disorders (see below and for review: Benitez-King et al., 2007; Gardiner et al., 2011; Zhao et al., 2015; Marchisella et al., 2016; Lasser et al., 2018). In addition, proteins that were initially identified as risk factors for mental illnesses, such as DISC1 (Disrupted In Schizophrenia) or dysbindin, were later shown to interact with MTs, MAPs, and actin (Morris et al., 2003; Hayashi et al., 2005; Talbot et al., 2006; Taya et al., 2007; Shimizu et al., 2008; Marley and von Zastrow, 2010; Bader et al., 2012).
In this context, cytoskeleton-related drugs have been investigated to determine their ability to influence biological and behavioral defects in MAP6 KO mice. Firstly, chronic exposure of MAP6 KO mice to Epothilone D (EpoD), a modulator of MT dynamics known to stabilize MTs in vitro (Chou et al., 1998; Kolman, 2004), leads to behavioral changes (improved maternal behavior, and concomitant pup survival), and improves short-term memory, associated with restoration of synaptic plasticity (LTP) in the hippocampus (Andrieux et al., 2006; Fournet et al., 2012a), as well as restoring neuronal transport deficits (Daoust et al., 2014). Second, a small peptide motif called NAP—present in Activity-Dependent Neuroprotective Protein (ADNP), which is dysregulated in schizophrenia and in autism (Gozes, 2011; Hacohen-Kleiman et al., 2018; Van Dijck et al., 2019)—partially alleviates cognitive impairments in MAP6 heterozygous mice (Merenlender-Wagner et al., 2010; Volle et al., 2013). NAP directly interacts with tubulin (Divinski et al., 2004) and also promotes MT growth and stability by its interaction with MT plus-end binding proteins of EB family (+TIPs proteins) (Gozes and Divinski, 2007; Oz et al., 2014). In addition, NAP has been shown to bind to Tau (Ivashko-Pachima et al., 2019) and to enhance its binding to MTs in cells (Ivashko-Pachima et al., 2017; Ivashko-Pachima and Gozes, 2019). Thus, NAP protective activity involves MT dynamics modulation via direct binding to tubulin and interaction with MT-associated proteins (Oz et al., 2014; Ivashko-Pachima et al., 2017). However, NAP’s effects in MAP6 KO mice might not be exclusively related to its MT-related properties as chronic NAP treatment restores normal levels of Beclin1 mRNA in MAP6-deficient mice (Merenlender-Wagner et al., 2014). Beclin1 is a key regulator of autophagy and its expression is strongly decreased in brains from patients with schizophrenia (Merenlender-Wagner et al., 2015).
Overall, these studies with EpoD and NAP highlight MAP6-mediated MT stabilization as an important feature for synaptic plasticity and behavior. The results presented also suggest that the cytoskeleton might be a relevant target for drug development to treat psychiatric disorders including schizophrenia.
MAP6 was the first neuronal MIP to be identified (Cuveillier et al., 2020). MIPs were originally described in the MT doublet of motile cilia and flagella axonemes (Kirima and Oiwa, 2018; Owa et al., 2019), where MTs needed to be highly stable to support the strong deformations required to produce a beating motion. The question of whether MAP6 also localizes to non-motile (primary) cilia or to motile cilia within the brain remains open. Future studies assessing these possibilities may provide additional molecular explanations for some of the cognitive impairments observed in MAP6 KO mice. Indeed, ciliopathies can lead to brain malformation and/or mental retardation (Reiter and Leroux, 2017). More precisely, primary cilia which act as signaling platforms participating in Sonic hedgehog or Wnt signaling pathways for example (Lee and Gleeson, 2010) have been shown to modulate neurotransmission through dopamine receptors expressed on their surface (Domire et al., 2011; Iwanaga et al., 2011; Leaf and Von Zastrow, 2015). Interestingly, Inversin and APT1—both MAP6 partners—have been shown to be part of the Wnt pathway (Supplementary Table 1). More directly, a reduced number of primary cilia was observed in the olfactory neuroepithelium in patients with schizophrenia (Muñoz-Estrada et al., 2018) and DISC1 was shown to be necessary for the formation of neuronal cilia (Marley and von Zastrow, 2010; Wang and Brandon, 2011). It would be interesting to investigate a possible role for MAP6 acting as a MIP in controlling the number and integrity of neuronal primary cilia.
Research on the structural MAPs revealed over time that their functions are not restricted to MT regulation. Simultaneously, other proteins that were first identified in signaling cascades, for example, were subsequently found to belong to the family of structural MAPs. Collapsin Response Mediator Proteins (CRMPs) are a perfect example of such proteins. Indeed, in addition to their well-known roles in signal transduction and neuronal physiology, we will summarize how, since the 1990s, CRMPs have been documented to play roles in regulating the cytoskeleton and especially in MT dynamics.
Originally discovered in C. elegans and named ULIP (UNC-33 like phosphoprotein), members of the CRMP family are involved in neuronal connectivity for sensory and motor neurons (Brenner, 1974; Hedgecock et al., 1985). These proteins originated from various genes and were subsequently renamed Collapsin Response Mediator Proteins (CRMPs) due to their involvement in Semaphorin 3A guidance molecule signaling (Collapsin was the original name of Semaphorin 3A; Goshima et al., 1995).
CRMPs are substrates for a large number of kinases, and high levels of phosphorylation have been reported, mostly in the C-terminal domain (Cole et al., 2004, 2006; Zheng et al., 2018); CRMP phosphorylation has been extensively studied in the context of the axonal guidance signaling pathway induced by Semaphorin 3A. Several kinases involved in this signaling pathway phosphorylate CRMPs. First, Cdk5 acts as the priming kinase, phosphorylating CRMP1, 2, 4, and 5 in response to a Semaphorin 3A-signal in vitro and in vivo (Brown et al., 2004; Uchida et al., 2005). This phosphorylation is required for subsequent phosphorylation of CRMPs by GSK3β, shown to be a key factor in modulating CRMPs’ interaction with the cytoskeleton (Uchida et al., 2005; Yoshimura et al., 2005). Thus, the Semaphorin 3A-induced phosphorylation of CRMP2 by Cdk5 and GSK3β blocks its capacity to bind tubulin and MTs (Uchida et al., 2005; Yoshimura et al., 2005). This lack of interaction leads to cytoskeleton breakdown and the resulting collapse of the growth cone, as the functional consequences of Semaphorin 3A signaling.
Semaphorin 3A-stimulation of the growth of dendritic spines also involves CRMP1 phosphorylation by Cdk5, blocking its interaction with actin (Yamashita et al., 2011; Yao et al., 2016).
Phosphorylation events on CRMPs, thus regulate the proteins’ capacity to interact with the cytoskeleton while also modulating their interaction with other partners, such as RhoA (Alabed et al., 2007), the guidance cue co-receptor PlexinA1 (Deo et al., 2004), or the ion channels Cav2.2 (Brittain et al., 2009), and Nav1.7 (Dustrude et al., 2016).
At the same time, the C-terminal part of CRMP2 shares similarities with Tau PRD domains (Hensley and Kursula, 2016), opening up the possibility that CRMPs can bind to SH3-domain-containing proteins.
From the time of their discovery in 1985, CRMPs were linked to MTs since mutations induced an over-abundance of MTs in axonal shafts (Hedgecock et al., 1985). It was therefore proposed that CRMP could modulate axonal outgrowth by stabilizing the cytoskeleton (Hedgecock et al., 1985). More recent work has indicated that all CRMPs bind tubulin both in vitro and in vivo (Fukata et al., 2002a; Lin et al., 2011; Khazaei et al., 2014), although the interaction between CRMP2 and MTs has been the focus of this particular study. Indeed, for CRMP2, its interaction with MTs was recently found to involve two distinct domains: the N-terminal domain, which is essential for binding to soluble tubulin, thus promoting MT polymerization; and the C-terminal region, which interacts with and stabilizes polymerized microtubules (Niwa et al., 2017). Although the ability to bind MTs is shared by all CRMPs, the MT-stabilizing capacity reported for CRMP2 is only shared by CRMP1 and CRMP4 (Lin et al., 2011). In contrast, CRMP5 does not influence MT dynamics (Brot et al., 2010), and CRMP3 was shown to inhibit MT polymerization (Aylsworth et al., 2009). The role of CRMP2 in MT assembly was found to be crucial for neurite formation and axon development (Fukata et al., 2002b) whereas CRMP4-dependent MT organizations contribute to growth-cone dynamics (progression, pausing, and retraction) as well as to axon elongation and regeneration (Khazaei et al., 2014).
Of all the isoforms, only CRMP3 presents weaker or no interaction with actin (Tan et al., 2015). All other CRMPs have an actin-binding site located at their C-terminal end, adjoining their MT-binding site. This actin-binding site was initially characterized in CRMP4 and promotes the formation of F-actin both in vitro and in vivo (Rosslenbroich et al., 2005; Khazaei et al., 2014; Cha et al., 2016). In neurons, through their action on the actin cytoskeleton, CRMP4 and CRMP5 contribute to neurite outgrowth and growth-cone remodeling (Khazaei et al., 2014; Gong et al., 2016), and CRMP4’s actin-binding capacity also contributes to dendrite maturation in hippocampal neurons (Cha et al., 2016). The effects of CRMP1 and CRMP2 on actin dynamics are more indirect, as interactions with VASP family proteins or the Sra-1 / WAVE1 complex are required for these proteins to influence axon formation and growth (Kawano et al., 2005; Yu-Kemp and Brieher, 2016; Yu-Kemp et al., 2017).
The role of CRMPs in vesicular trafficking and plasma membrane targeting of several transmembrane proteins is well-documented. Thus, CRMP2 is known to bind to the vesicle-associated proteins Slp1 and Rab27 allowing anterograde transport of BDNF receptor TrkB (Arimura et al., 2009). It also binds to the endocytic adaptor Eps15, the ubiquitin ligase Nedd4.2, and to α-adaptin which regulates clathrin-dependent endocytosis of the cellular adhesion molecule L1 and the sodium channel Nav1.7 (Nishimura et al., 2003; Kawano et al., 2005; Dustrude et al., 2016). CRMP1 modulates Na+ currents by interacting with Nav1.7 (Yamane et al., 2017) whereas, like MAP6, CRMP2, and CRMP3 respectively interact with voltage-gated Cav2.2/N-type and L-type channels (Brittain et al., 2009; Chi et al., 2009; Quach et al., 2013). CRMP4 is involved in vesicular trafficking through mechanisms involving binding to the SH3 domains of the scaffolding protein Intersectin 1 (Quinn et al., 2003)—which is also a MAP6 partner (Figure 1).
Despite the early discovery of the involvement of CRMPs in the Semaphorin 3A signaling pathway (Goshima et al., 1995), little is known about direct interactions between CRMPs and the numerous semaphorin receptors represented by the Neuropilin and Plexin families. CRMP1, 2, 3, and 4 complexes with Plexin-A1 have been reported following over-expression of the different isoforms in the COS7 cell line (Deo et al., 2004), and associations with the mono-oxygenases MICALs have also been described (Schmidt et al., 2008). In addition, CRMP2 was shown to interact with Plexin-A2 and A3 in the Nogo and Semaphorin 3A signaling pathways, respectively (Sekine et al., 2019; Jiang et al., 2020).
As part of their regulation of neuronal development and plasticity, CRMPs are involved in many neurodevelopmental processes including neural progenitor proliferation (Charrier et al., 2006), neuronal migration (Yamashita et al., 2006), and neuronal morphogenesis with both axonal and dendritic maturation influences. The study of CRMP1, CRMP2, and CRMP4 KO neurons revealed morphological defects, especially in dendritic development and branching, but also in migration and positioning (Yamashita et al., 2006, 2007; Niisato et al., 2012). These phenotypes are more severe in double KO neurons, whether the combination is CRMP1 and CRMP4, or CRMP2 and CRMP4 (Tan et al., 2015; Yamazaki et al., 2020). In vivo, KO of any CRMP (CRMP1, CRMP2, CRMP3, or CRMP4) leads to robust alteration of dendritic morphogenesis in several brain regions including the hippocampus and the cortex (Yamashita et al., 2007; Quach et al., 2008; Niisato et al., 2012, 2013; Yamashita and Goshima, 2012; Tsutiya et al., 2015, 2016). CRMP2 is also strongly associated with axonal specification (Nishimura et al., 2003; Kawano et al., 2005; Yoshimura et al., 2005; Morita and Sobue, 2009). The extensive effects of CRMPs on neuronal differentiation are stronger than those observed for the deletion of classical MAPs. This enhanced effect might be due to the crucial roles of CRMPs at the interface between elements of the cytoskeleton and signaling proteins. Impairment of CRMPs functions also leads to synaptic-plasticity defects with abnormal LTP (Su et al., 2007; Quach et al., 2008, 2011; Yamashita et al., 2011), linked to impaired learning and memory (Su et al., 2007; Yamashita et al., 2013).
In humans, CRMPs are associated with several psychiatric disorders, with striking numbers of publications providing evidence of links between CRMPs and schizophrenia (Nakata et al., 2003; Nakata and Ujike, 2004; Ujike et al., 2006; Koide et al., 2010; Hensley et al., 2011; Bader et al., 2012; Lee et al., 2015; Quach et al., 2015; Toyoshima et al., 2019). For example, polymorphisms in the genes encoding CRMP1 and CRMP2, as well as altered hippocampal expression of CRMP2 and CRMP4 have been reported in patients with schizophrenia (Edgar et al., 2000; Beasley et al., 2006; Föcking et al., 2011; Bader et al., 2012). In addition, the abnormal sensory-motor gating abilities reported in psychiatric patients and described for MAP6 KO mice (Fradley et al., 2005), were also replicated in CRMP1 and CRMP3 KO mice (Quach et al., 2008; Yamashita et al., 2013). Importantly, these defects could be alleviated through the use of the antipsychotic chlorpromazine (Yamashita et al., 2013), and CRMP2 and CRMP4 phosphorylation states were shown to be downregulated by the antipsychotics Clozapine and Risperidone (Kedracka-Krok et al., 2015).
In summary, the ability of CRMPs to bind MTs, and thus promote their polymerization and stabilization, are major arguments to consider them as members of the wider MAP family. Moreover, and as indicated above, like other MAPs, CRMPs can also bind actin and are involved in signaling cascades. As for conventional MAPs, deletion of CRMPs in mice does not lead to severe cytoskeleton alterations, but rather to subtler neurodevelopmental defects and cognitive dysfunctions similar to those encountered in psychiatric diseases. The history of protein identification draws attention toward specific research. In the case of CRMPs, although data regarding their interactions with MTs have been produced they have not been extensively investigated in the field of cytoskeleton research. In particular, they have never been used in in vitro cell-free systems. Several basic questions thus remain open, such as: Do CRMPs modulate specific parameters of microtubule dynamics? Do they induce MT bundling or simply MT polymerization?
Our demonstration that CRMPs can be considered as MAPs could be extended to other proteins like for example, the Huntingtin protein or α-synuclein. These two proteins have been actively studied in the context of neurodegenerative diseases. Indeed, HTT binds to MTs and actin, is involved in signaling cascades, is palmitoylated by the same DHCC as MAP6 (Lemonidis et al., 2015), and interacts with neuroreceptors (for review see Saudou and Humbert, 2016). Similarly, α-synuclein, a MAP6 partner (Figure 1), interacts with and nucleates MTs (Cartelli et al., 2016). α-synuclein also interacts with actin and reduces F-actin polymerization speed (Sousa et al., 2009; Cartelli et al., 2016; Oliveira da Silva and Liz, 2020). It also participates in signaling cascades and neuroreceptor functions (for review see Emamzadeh, 2016; Bernal-Conde et al., 2019). In addition to these examples, proteins related to schizophrenia susceptibility could be mentioned, such as DISC1 (Disrupted In Schizophrenia) or dysbindin, both of which have been shown to interact with MTs, MAPs, and actin (Morris et al., 2003; Hayashi et al., 2005; Talbot et al., 2006; Taya et al., 2007; Shimizu et al., 2008; Marley and von Zastrow, 2010; Bader et al., 2012).
In this section, we will briefly summarize the properties of the classical neuronal structural MAPs (Tau, MAP1, and MAP2) and compare them to those described for MAP6 and CRMPs. We will focus particularly on properties that are just coming to light.
All the classical MAPs bind to MTs and induce various levels of stability (Baas et al., 1994; Bulinski and Bossler, 1994; Vandecandelaere et al., 1996; Faller et al., 2009; Kadavath et al., 2015; Qiang et al., 2018). Recent advances in cryo-EM have made it possible to visualize how Tau binds along protofilaments at the interface between tubulin dimers (Kellogg et al., 2018). Elucidating the near-atomic structure of complexes between the other MAPs and MTs is essential if we wish to reveal the common and specific mechanisms behind MT stabilization for each MAP. Interestingly, several classical MAPs such as MAP1B, MAP2, and Tau, are also able to indirectly modulate MT dynamics via their interaction with EB proteins (Kapitein et al., 2011; Tortosa et al., 2013; Sayas et al., 2015; Ramirez-Rios et al., 2016). Such a possibility regarding MAP6 and CRMPs has not yet been investigated and could be of interest.
With regard to the ability of MAP6 to behave as a MIP, future studies will reveal whether this property is shared by other structural MAPs. Although some previous works suggested that Tau binds to the luminal side of MTs (Kar et al., 2003; Inaba et al., 2019), the answer to this question remains elusive. Interestingly, actin was very recently discovered inside the MT lumen (Paul et al., 2020), opening the possibility that MAPs/MIPs might be involved in the crosstalk between the two cytoskeletons in the MT lumen like they are in the cytoplasm.
Like MAP6, the other structural MAPs—Tau, MAP1, and MAP2—were shown to bind actin (Griffith and Pollard, 1982; Pedrotti et al., 1994; Ozer and Halpain, 2000; Roger et al., 2004; Ding et al., 2006) and to regulate synaptic plasticity through actin-dependent mechanisms (Davidkova and Carroll, 2007; Tortosa et al., 2011; Benoist et al., 2013; Takei et al., 2015). Interestingly, Tau was directly shown to co-organize actin and MT networks in vitro and in the neuronal growth cone (Elie et al., 2015; Biswas and Kalil, 2018), this ability may be shared by the other MAPs (Mohan and John, 2015).
In relation to neuroreceptors, like MAP6 (Figure 1), MAP1 and MAP2 proteins interact with the Cav2.2/N-type calcium channel or with BKCa potassium channels (Davare et al., 1999; Park et al., 2004; Leenders et al., 2008). In terms of membrane-association, Tau and MAP2 interact with the membranes of the Golgi and the endoplasmic reticulum (Farah et al., 2005, 2006), whereas MAP1S was shown to link mitochondria and autophagosomes to MTs (Xie et al., 2011).
Several other MAPs in addition to MAP6 contain PRD domains and thus interact with SH3-containing proteins. For example, Tau and MAP2 bind to various SH3-containing proteins including the non-receptor tyrosine kinase Src (MAP6 partner, Figure 1; Lim and Halpain, 2000). Interestingly, in link with Alzheimer’s disease, a general inhibition of Tau’s interactions with SH3-domain-containing proteins was shown to reduce Amyloid β-induced membrane trafficking abnormalities and neurite degeneration (Rush et al., 2020).
Finally, as with MAP6 deletion, invalidation of other MAPs in mice did not result in major MT-breakdown or in lethal neurodevelopmental defects but rather produced viable mice (Harada et al., 1994; Takei et al., 1997, 2015; Teng et al., 2001). However, all KO mice display cognitive dysfunctions similar to those associated with psychiatric diseases. Thus, Tau KO mice exhibit impaired neurogenesis (Dioli et al., 2017), hippocampal synaptic plasticity, and cognitive defects (Ikegami et al., 2000; Lei et al., 2012; Ahmed et al., 2014; Ma et al., 2014; Regan et al., 2015), as well as age-dependent brain atrophy associated with loss of neurons and synapses (Lei et al., 2012). MAP1B-deficient mice also display abnormal synapse maturation (Tortosa et al., 2011; Bodaleo et al., 2016), along with synaptic transmission defects due to deregulation of AMPA receptor trafficking (Benoist et al., 2013; Palenzuela et al., 2017), and impaired synaptic plasticity with abnormal LTP (Zervas et al., 2005). Importantly, MAP1B has been linked to the protein KIRREL3 which is associated with autism/intellectual disability (Liu et al., 2015). This protein is associated with the altered working memory observed in young people with attention-deficit/hyperactivity disorder (Salatino-Oliveira et al., 2016). Mutated forms of MAP1B have been linked to intellectual disability and extensive white-matter deficits in humans (Walters et al., 2018).
A review of the literature relating to MAPs, in particular, Tau (which is by far the most extensively studied MAP), reveals that some MAP features have not yet been reported for MAP6 and CRMPs. Firstly, it is quite clear that Tau is a bona fide nuclear protein (for review see Bukar Maina et al., 2016) mainly contributing to defending the genome against cellular stress. As shown in Supplementary Table 1, MAP6 has been shown to interact with various nuclear receptors and effectors, but nothing is known about its possible roles in the nucleus. Secondly, Tau is an unstructured protein that has been shown to promote phase-separation events in cells, both as part of physiological processes such as axonal transport and in pathological conditions such as during Tau aggregation (Hernández-Vega et al., 2017; Wegmann et al., 2018; Siahaan et al., 2019). How the capacity of Tau to promote phase separation affects its non-cytoskeleton-related functions still remains to be determined. As most MAPs including MAP6 are unstructured proteins, it appears logical that the ability to contribute to phase separation events will be shared by many MAPs.
Structural MAPs, presented here through the examples of MAP6 and CRMPs, are highly versatile proteins with multiple partners and functions, playing major roles in several brain functions.
The original classification of MAPs was based on their ability to bind MTs. This binding may contribute to MT stability, but it might also be crucial to ensure MAPs presence all over the cell in order to be available to promote signal propagation and/or to form multi-protein complexes (post-synaptic densities) or regulate the protein composition of membrane compartments. In other words, the MT-binding ability of MAPs is probably required for all their other functions as it is essential to ensure specific spatial and temporal localization. These dual abilities of MAPs to stabilize MTs and to use them as a means to gain access to all regions of the cell for other functions makes it experimentally impossible to distinguish between their MT-related and -unrelated functions.
CC, BB, CR, ED, J-CD, SG-F, CD, CB, IA and AA wrote the manuscript. All authors contributed to the article and approved the submitted version.
This work was supported by funding from INSERM, CEA, CNRS, University Grenoble Alpes and in part by awards from the French Agence Nationale de la Recherche to AA and IA (2017-CE11-0026, MAMAs).
The authors declare that the research was conducted in the absence of any commercial or financial relationships that could be construed as a potential conflict of interest.
The Supplementary Material for this article can be found online at: https://www.frontiersin.org/articles/10.3389/fnmol.2021.665693/full#supplementary-material.
Aghajanian, G. K., and Marek, G. J. (2000). Serotonin model of schizophrenia: emerging role of glutamate mechanisms. Brain Res. Brain Res. Rev. 31, 302–312. doi: 10.1016/s0165-0173(99)00046-6
Aguezzoul, M., Andrieux, A., and Denarier, E. (2003). Overlap of promoter and coding sequences in the mouse STOP gene (Mtap6). Genomics 81, 623–627. doi: 10.1016/s0888-7543(03)00053-3
Ahmed, T., Van der Jeugd, A., Blum, D., Galas, M. C., D’Hooge, R., Buee, L., et al. (2014). Cognition and hippocampal synaptic plasticity in mice with a homozygous tau deletion. Neurobiol. Aging 35, 2474–2478. doi: 10.1016/j.neurobiolaging.2014.05.005
Alabed, Y. Z., Pool, M., Tone, S. O., and Fournier, A. E. (2007). Identification of CRMP4 as a convergent regulator of axon outgrowth inhibition. J. Neurosci. 27, 1702–1711. doi: 10.1523/JNEUROSCI.5055-06.2007
Andrieux, A., Salin, P., Schweitzer, A., Begou, M., Pachoud, B., Brun, P., et al. (2006). Microtubule stabilizer ameliorates synaptic function and behavior in a mouse model for schizophrenia. Biol. Psychiatry 60, 1224–1230. doi: 10.1016/j.biopsych.2006.03.048
Andrieux, A., Salin, P. A., Vernet, M., Kujala, P., Baratier, J., Gory-Faure, S., et al. (2002). The suppression of brain cold-stable microtubules in mice induces synaptic defects associated with neuroleptic-sensitive behavioral disorders. Genes Dev. 16, 2350–2364. doi: 10.1101/gad.223302
Arama, J., Boulay, A. C., Bosc, C., Delphin, C., Loew, D., Rostaing, P., et al. (2012). Bmcc1s, a novel brain-isoform of Bmcc1, affects cell morphology by regulating MAP6/STOP functions. PLoS One 7:e35488. doi: 10.1371/journal.pone.0035488
Arimura, N., Kimura, T., Nakamuta, S., Taya, S., Funahashi, Y., Hattori, A., et al. (2009). Anterograde transport of TrkB in axons is mediated by direct interaction with Slp1 and Rab27. Dev. Cell 16, 675–686. doi: 10.1016/j.devcel.2009.03.005
Atherton, J., Stouffer, M., Francis, F., and Moores, C. A. (2018). Microtubule architecture in vitro and in cells revealed by cryo-electron tomography. Acta Crystallogr. D Struct. Biol. 74, 572–584. doi: 10.1107/S2059798318001948
Aylsworth, A., Jiang, S. X., Desbois, A., and Hou, S. T. (2009). Characterization of the role of full-length CRMP3 and its calpain-cleaved product in inhibiting microtubule polymerization and neurite outgrowth. Exp. Cell Res. 315, 2856–2868. doi: 10.1016/j.yexcr.2009.06.014
Baas, P. W., and Black, M. M. (1990). Individual microtubules in the axon consist of domains that differ in both composition and stability. J. Cell Biol. 111, 495–509. doi: 10.1083/jcb.111.2.495
Baas, P. W., Pienkowski, T. P., Cimbalnik, K. A., Toyama, K., Bakalis, S., Ahmad, F. J., et al. (1994). Tau confers drug stability but not cold stability to microtubules in living cells. J. Cell Sci. 107, 135–143.
Baas, P. W., Rao, A. N, Matamoros, A. J. and Leo, L. (2016). Stability properties of neuronal microtubules. Cytoskeleton (Hoboken) 73, 442–460. doi: 10.1002/cm.21286
Bader, V., Tomppo, L., Trossbach, S. V., Bradshaw, N. J., Prikulis, I., Leliveld, S. R., et al. (2012). Proteomic, genomic and translational approaches identify CRMP1 for a role in schizophrenia and its underlying traits. Hum. Mol. Genet. 21, 4406–4418. doi: 10.1093/hmg/dds273
Baratier, J., Peris, L., Brocard, J., Gory-Faure, S., Dufour, F., Bosc, C., et al. (2006). Phosphorylation of microtubule-associated protein STOP by calmodulin kinase II. J. Biol. Chem. 281, 19561–19569. doi: 10.1074/jbc.M509602200
Beasley, C. L., Pennington, K., Behan, A., Wait, R., Dunn, M. J., and Cotter, D. (2006). Proteomic analysis of the anterior cingulate cortex in the major psychiatric disorders: evidence for disease-associated changes. Proteomics 6, 3414–3425. doi: 10.1002/pmic.200500069
Bégou, M., Brun, P., Bertrand, J. B., Job, D., Schweitzer, A., D’Amato, T., et al. (2007). Post-pubertal emergence of alterations in locomotor activity in stop null mice. Synapse 61, 689–697. doi: 10.1002/syn.20409
Bégou, M., Volle, J.-B., Bertrand, J. B., Brun, P., Job, D., Schweitzer, A., et al. (2008). The stop null mice model for schizophrenia displays [corrected] cognitive and social deficits partly alleviated by neuroleptics. Neuroscience 157, 29–39. doi: 10.1016/j.neuroscience.2008.07.080
Belzung, C., and Lemoine, M. (2011). Criteria of validity for animal models of psychiatric disorders: focus on anxiety disorders and depression. Biol. Mood Anxiety Disord. 1:9. doi: 10.1186/2045-5380-1-9
Benardais, K., Kasem, B., Couegnas, A., Samama, B., Fernandez, S., Schaeffer, C., et al. (2010). Loss of STOP protein impairs peripheral olfactory neurogenesis. PLoS One 5:e12753. doi: 10.1371/journal.pone.0012753
Benitez-King, G., Ramirez-Rodriguez, G., and Ortiz-López, L. (2007). Altered microtubule associated proteins in schizophrenia. Neuroquantology 5:1. doi: 10.14704/nq.2007.5.1.117
Benoist, M., Palenzuela, R., Rozas, C., Rojas, P., Tortosa, E., Morales, B., et al. (2013). MAP1B-dependent Rac activation is required for AMPA receptor endocytosis during long-term depression. EMBO J. 32, 2287–2299. doi: 10.1038/emboj.2013.166
Bernal-Conde, L. D., Ramos-Acevedo, R., Reyes-Hernandez, M. A., Balbuena-Olvera, A. J., Morales-Moreno, I. D., Arguero-Sanchez, R., et al. (2019). α-synuclein physiology and pathology: a perspective on cellular structures and organelles. Front. Neurosci. 13:1399. doi: 10.3389/fnins.2019.01399
Biswas, S., and Kalil, K. (2018). The microtubule-associated protein tau mediates the organization of microtubules and their dynamic exploration of actin-rich lamellipodia and filopodia of cortical growth cones. J. Neurosci. 38, 291–307. doi: 10.1523/JNEUROSCI.2281-17.2017
Bodakuntla, S., Jijumon, A. S., Villablanca, C., Gonzalez-Billault, C., and Janke, C. (2019). Microtubule-associated proteins: structuring the cytoskeleton. Trends Cell Biol. 29, 804–819. doi: 10.1016/j.tcb.2019.07.004
Bodaleo, F. J., Montenegro-Venegas, C., Henriquez, D. R., Court, F. A., and Gonzalez-Billault, C. (2016). Microtubule-associated protein 1B (MAP1B)-deficient neurons show structural presynaptic deficiencies in vitro and altered presynaptic physiology. Sci. Rep. 6:30069. doi: 10.1038/srep30069
Bopp, M. H. A., Zöllner, R., Jansen, A., Dietsche, B., Krug, A., and Kircher, T. T. J. (2017). White matter integrity and symptom dimensions of schizophrenia: a diffusion tensor imaging study. Schizophr. Res. 184, 59–68. doi: 10.1016/j.schres.2016.11.045
Bosc, C., Andrieux, A., and Job, D. (2003). STOP proteins. Biochemistry 42, 12125–12132. doi: 10.1021/bi0352163
Bosc, C., Cronk, J. D., Pirollet, F., Watterson, D. M., Haiech, J., Job, D., et al. (1996). Cloning, expression and properties of the microtubule-stabilizing protein STOP. Proc. Natl. Acad. Sci. U S A 93, 2125–2130. doi: 10.1073/pnas.93.5.2125
Bosc, C., Frank, R., Denarier, E., Ronjat, M., Schweitzer, A., Wehland, J., et al. (2001). Identification of novel bifunctional calmodulin-binding and microtubule-stabilizing motifs in STOP proteins. J. Biol. Chem. 276, 30904–30913. doi: 10.1074/jbc.M011614200
Bouet, V., Percelay, S., Leroux, E., Diarra, B., Leger, M., Delcroix, N., et al. (2021). A new 3-hit mouse model of schizophrenia built on genetic, early and late factors. Schizophr. Res. 228, 519–528. doi: 10.1016/j.schres.2020.11.043
Boulan, B., Beghin, A., Ravanello, C., Deloulme, J. C., Gory-Faure, S., Andrieux, A., et al. (2020). AutoNeuriteJ: an ImageJ plugin for measurement and classification of neuritic extensions. PLoS One 15:e0234529. doi: 10.1371/journal.pone.0234529
Bouvier, D., Vanhaverbeke, C., Simorre, J. P., Arlaud, G. J., Bally, I., Forge, V., et al. (2003). Unusual Ca2+-calmodulin binding interactions of the microtubule-associated protein F-STOP. Biochemistry 42, 11484–11493. doi: 10.1021/bi034746w
Bouvrais-Veret, C., Weiss, S., Andrieux, A., Schweitzer, A., McIntosh, J. M., Job, D., et al. (2007). Sustained increase of α7 nicotinic receptors and choline-induced improvement of learning deficit in STOP knock-out mice. Neuropharmacology 52, 1691–1700. doi: 10.1016/j.neuropharm.2007.03.015
Bouvrais-Veret, C., Weiss, S., Hanoun, N., Andrieux, A., Schweitzer, A., Job, D., et al. (2008). Microtubule-associated STOP protein deletion triggers restricted changes in dopaminergic neurotransmission. J. Neurochem. 104, 745–756. doi: 10.1111/j.1471-4159.2007.05025.x
Brady, S. T., Tytell, M., and Lasek, R. J. (1984). Axonal tubulin and axonal microtubules: biochemical evidence for cold stability. J. Cell Biol. 99, 1716–1724. doi: 10.1083/jcb.99.5.1716
Brenner, S. (1974). The genetics of Caenorhabditis elegans. Genetics 77, 71–94. doi: 10.1093/genetics/77.1.71
Brenner, E., Sonnewald, U., Schweitzer, A., Andrieux, A., and Nehlig, A. (2007). Hypoglutamatergic activity in the STOP knockout mouse: a potential model for chronic untreated schizophrenia. J. Neurosci. Res. 85, 3487–3493. doi: 10.1002/jnr.21200
Brinkley, B. R., and Cartwright, J. Jr. (1975). Cold-labile and cold-stable microtubules in the mitotic spindle of mammalian cells. Ann. N Y Acad. Sci. 253, 428–439. doi: 10.1111/j.1749-6632.1975.tb19218.x
Brittain, J. M., Piekarz, A. D., Wang, Y., Kondo, T., Cummins, T. R., and Khanna, R. (2009). An atypical role for collapsin response mediator protein 2 (CRMP-2) in neurotransmitter release via interaction with presynaptic voltage-gated calcium channels. J. Biol. Chem. 284, 31375–31390. doi: 10.1074/jbc.M109.009951
Brocard, J., Dufour, F., Gory-Fauré, S., Arnoult, C., Bosc, C., Denarier, E., et al. (2017). MAP6 interacts with Tctex1 and Cav2.2/N-type calcium channels to regulate calcium signalling in neurons. Eur. J. Neurosci. 46, 2754–2767. doi: 10.1111/ejn.13766
Brot, S., Rogemond, V., Perrot, V., Chounlamountri, N., Auger, C., Honnorat, J., et al. (2010). CRMP5 interacts with tubulin to inhibit neurite outgrowth, thereby modulating the function of CRMP2. J. Neurosci. 30, 10639–10654. doi: 10.1523/JNEUROSCI.0059-10.2010
Brown, M., Jacobs, T., Eickholt, B., Ferrari, G., Teo, M., Monfries, C., et al. (2004). α2-chimaerin, cyclin-dependent kinase 5/p35 and its target collapsin response mediator protein-2 are essential components in semaphorin 3A-induced growth-cone collapse. J. Neurosci. 24, 8994–9004. doi: 10.1523/JNEUROSCI.3184-04.2004
Brun, P., Begou, M., Andrieux, A., Mouly-Badina, L., Clerget, M., Schweitzer, A., et al. (2005). Dopaminergic transmission in STOP null mice. J. Neurochem. 94, 63–73. doi: 10.1111/j.1471-4159.2005.03166.x
Bukar Maina, M., Al-Hilaly, Y. K., and Serpell, L. C. (2016). Nuclear tau and its potential role in Alzheimer’s disease. Biomolecules 6:9. doi: 10.3390/biom6010009
Bulinski, J. C., and Bossler, A. (1994). Purification and characterization of ensconsin, a novel microtubule stabilizing protein. J. Cell Sci. 107, 2839–2849.
Burton, P. R. (1984). Luminal material in microtubules of frog olfactory axons: structure and distribution. J. Cell Biol. 99, 520–528. doi: 10.1083/jcb.99.2.520
Capala, M. E., Maat, H., Bonardi, F., van den Boom, V., Kuipers, J., Vellenga, E., et al. (2015). Mitochondrial dysfunction in human leukemic stem/progenitor cells upon loss of RAC2. PLoS One 10:e0128585. doi: 10.1371/journal.pone.0128585
Carlsson, A., Waters, N., Waters, S., and Carlsson, M. L. (2000). Network interactions in schizophrenia—therapeutic implications. Brain Res. Brain Res. Rev. 31, 342–349. doi: 10.1016/s0165-0173(99)00050-8
Cartelli, D., Aliverti, A., Barbiroli, A., Santambrogio, C., Ragg, E. M., Casagrande, F. V., et al. (2016). α-synuclein is a novel microtubule dynamase. Sci. Rep. 6:33289. doi: 10.1038/srep33289
Cha, C., Zhang, J., Ji, Z., Tan, M., Li, S., Wu, F., et al. (2016). CRMP4 regulates dendritic growth and maturation via the interaction with actin cytoskeleton in cultured hippocampal neurons. Brain Res. Bull. 124, 286–294. doi: 10.1016/j.brainresbull.2016.06.008
Chapin, S. J., and Bulinski, J. C. (1994). Cellular microtubules heterogeneous in their content of microtubule-associated protein 4 (MAP4). Cell Motil. Cytoskeleton. 27, 133–149. doi: 10.1002/cm.970270205
Charlet, A., Muller, A. H., Laux, A., Kemmel, V., Schweitzer, A., Deloulme, J. C., et al. (2010). Abnormal nociception and opiate sensitivity of STOP null mice exhibiting elevated levels of the endogenous alkaloid morphine. Mol. Pain 6:96. doi: 10.1186/1744-8069-6-96
Charrier, C., Coronas, V., Fombonne, J., Roger, M., Jean, A., Krantic, S., et al. (2006). Characterization of neural stem cells in the dorsal vagal complex of adult rat by in vivo proliferation labeling and in vitro neurosphere assay. Neuroscience 138, 5–16. doi: 10.1016/j.neuroscience.2005.10.046
Chauvet, S., Cohen, S., Yoshida, Y., Fekrane, L., Livet, J., Gayet, O., et al. (2007). Gating of Sema3E/PlexinD1 signaling by neuropilin-1 switches axonal repulsion to attraction during brain development. Neuron 56, 807–822. doi: 10.1016/j.neuron.2007.10.019
Chen, C. P., Lin, S. P., Chern, S. R., Wu, P. S., Chen, S. W., Wu, F. T., et al. (2021). Tetrasomy of 11q13.4–q14.3 due to an intrachromosomal triplication associated with paternal uniparental isodisomy for 11q14.3-qter, intrauterine growth restriction, developmental delay, corpus callosum dysgenesis, microcephaly, congenital heart defects and facial dysmorphism. Taiwan J. Obstet. Gynecol. 60, 169–172. doi: 10.1016/j.tjog.2020.11.027
Cheng, D., Hoogenraad, C. C., Rush, J., Ramm, E., Schlager, M. A., Duong, D. M., et al. (2006). Relative and absolute quantification of postsynaptic density proteome isolated from rat forebrain and cerebellum. Mol. Cell. Proteomics 5, 1158–1170. doi: 10.1074/mcp.D500009-MCP200
Chi, X. X., Schmutzler, B. S., Brittain, J. M., Wang, Y., Hingtgen, C. M., Nicol, G. D., et al. (2009). Regulation of N-type voltage-gated calcium channels (Cav2.2) and transmitter release by collapsin response mediator protein-2 (CRMP-2) in sensory neurons. J. Cell Sci. 122, 4351–4362. doi: 10.1242/jcs.053280
Choi, K. H., Zepp, M. E., Higgs, B. W., Weickert, C. S., and Webster, M. J. (2009). Expression profiles of schizophrenia susceptibility genes during human prefrontal cortical development. J. Psychiatry Neurosci. 34, 450–458.
Chou, T. C., Zhang, X. G., Balog, A., Su, D. S., Meng, D., Savin, K., et al. (1998). Desoxyepothilone B: an efficacious microtubule-targeted antitumor agent with a promising in vivo profile relative to epothilone B. Proc. Natl. Acad. Sci. U S A 95, 9642–9647. doi: 10.1073/pnas.95.16.9642
Cleveland, D. W., Hwo, S. Y., and Kirschner, M. W. (1977). Purification of tau, a microtubule-associated protein that induces assembly of microtubules from purified tubulin. J. Mol. Biol. 116, 207–225. doi: 10.1016/0022-2836(77)90213-3
Cole, A. R., Causeret, F., Yadirgi, G., Hastie, C. J., McLauchlan, H., McManus, E. J., et al. (2006). Distinct priming kinases contribute to differential regulation of collapsin response mediator proteins by glycogen synthase kinase-3 in vivo*. J. Biol. Chem. 281, 16591–16598. doi: 10.1074/jbc.M513344200
Cole, A. R., Knebel, A., Morrice, N. A., Robertson, L. A., Irving, A. J., Connolly, C. N., et al. (2004). GSK-3 phosphorylation of the Alzheimer epitope within collapsin response mediator proteins regulates axon elongation in primary neurons. J. Biol. Chem. 279, 50176–50180. doi: 10.1074/jbc.C400412200
Collins, M. O., Husi, H., Yu, L., Brandon, J. M., Anderson, C. N., Blackstock, W. P., et al. (2006). Molecular characterization and comparison of the components and multiprotein complexes in the postsynaptic proteome. J. Neurochem. 97, 16–23. doi: 10.1111/j.1471-4159.2005.03507.x
Couégnas, A., Schweitzer, A., Andrieux, A., Ghandour, M. S., and Boehm, N. (2007). Expression pattern of STOP lacZ reporter gene in adult and developing mouse brain. J. Neurosci. Res. 85, 1515–1527. doi: 10.1002/jnr.21278
Coumans, J. V., Palanisamy, S. K., McFarlane, J., and Moens, P. D. (2016). Proteomic and microscopic strategies towards the analysis of the cytoskeletal networks in major neuropsychiatric disorders. Int. J. Mol. Sci. 17:581. doi: 10.3390/ijms17040581
Craft, G. E., Graham, M. E., Bache, N., Larsen, M. R., and Robinson, P. J. (2008). The in vivo phosphorylation sites in multiple isoforms of amphiphysin I from rat brain nerve terminals. Mol. Cell Proteomics 7, 1146–1161. doi: 10.1074/mcp.M700351-MCP200
Cuveillier, C., Delaroche, J., Seggio, M., Gory-Faure, S., Bosc, C., Denarier, E., et al. (2020). MAP6 is an intraluminal protein that induces neuronal microtubules to coil. Sci. Adv. 6:eaaz4344. doi: 10.1126/sciadv.aaz4344
Dacheux, D., Landrein, N., Thonnus, M., Gilbert, G., Sahin, A., Wodrich, H., et al. (2012). A MAP6-related protein is present in protozoa and is involved in flagellum motility. PLoS One 7:e31344. doi: 10.1371/journal.pone.0031344
Dacheux, D., Roger, B., Bosc, C., Landrein, N., Roche, E., Chansel, L., et al. (2015). Human FAM154A (SAXO1) is a microtubule-stabilizing protein specific to cilia and related structures. J. Cell Sci. 128, 1294–1307. doi: 10.1242/jcs.155143
Daoust, A., Bohic, S., Saoudi, Y., Debacker, C., Gory-Faure, S., Andrieux, A., et al. (2014). Neuronal transport defects of the MAP6 KO mouse—a model of schizophrenia—and alleviation by Epothilone D treatment, as observed using MEMRI. NeuroImage 96, 133–142. doi: 10.1016/j.neuroimage.2014.03.071
Davare, M. A., Dong, F., Rubin, C. S., and Hell, J. W. (1999). The A-kinase anchor protein MAP2B and cAMP-dependent protein kinase are associated with class C L-type calcium channels in neurons. J. Biol. Chem. 274, 30280–30287. doi: 10.1074/jbc.274.42.30280
Davidkova, G., and Carroll, R. C. (2007). Characterization of the role of microtubule-associated protein 1B in metabotropic glutamate receptor-mediated endocytosis of AMPA receptors in hippocampus. J. Neurosci. 27, 13273–13278. doi: 10.1523/JNEUROSCI.3334-07.2007
Davidson, C. A., Kuroki, N., Alvarado, J. L., Niznikiewicz, M. A., McCarley, R. W., and Levitt, J. J. (2012). An MRI study of septi pellucidi in relation to hippocampus volume and fornix integrity in schizophrenia. Schizophr. Res. 134, 165–170. doi: 10.1016/j.schres.2011.11.012
De Peri, L., Crescini, A., Deste, G., Fusar-Poli, P., Sacchetti, E., and Vita, A. (2012). Brain structural abnormalities at the onset of schizophrenia and bipolar disorder: a meta-analysis of controlled magnetic resonance imaging studies. Curr. Pharm. Des. 18, 486–494. doi: 10.2174/138161212799316253
Dehmelt, L., and Halpain, S. (2005). The MAP2/Tau family of microtubule-associated proteins. Genome Biol. 6:204. doi: 10.1186/gb-2004-6-1-204
Delotterie, D., Ruiz, G., Brocard, J., Schweitzer, A., Roucard, C., Roche, Y., et al. (2010). Chronic administration of atypical antipsychotics improves behavioral and synaptic defects of STOP null mice. Psychopharmacology 208, 131–141. doi: 10.1007/s00213-009-1712-3
Deloulme, J.-C., Gory-Fauré, S., Mauconduit, F., Chauvet, S., Jonckheere, J., Boulan, B., et al. (2015). Microtubule-associated protein 6 mediates neuronal connectivity through Semaphorin 3E-dependent signalling for axonal growth. Nat. Commun. 6:7246. doi: 10.1038/ncomms8246
Delphin, C., Bouvier, D., Seggio, M., Couriol, E., Saoudi, Y., Denarier, E., et al. (2012). MAP6-F is a temperature sensor that directly binds to and protects microtubules from cold-induced depolymerization. J. Biol. Chem. 287, 35127–35138. doi: 10.1074/jbc.M112.398339
Denarier, E., Aguezzoul, M., Jolly, C., Vourc’h, C., Roure, A., Andrieux, A., et al. (1998a). Genomic structure and chromosomal mapping of the mouse STOP gene (Mtap6). Biochem. Biophys. Res. Commun. 243, 791–796. doi: 10.1006/bbrc.1998.8179
Denarier, E., Fourest-Lieuvin, A., Bosc, C., Pirollet, F., Chapel, A., Margolis, R. L., et al. (1998b). Nonneuronal isoforms of STOP protein are responsible for microtubule cold stability in mammalian fibroblasts. Proc. Natl. Acad. Sci. U S A 95, 6055–6060. doi: 10.1073/pnas.95.11.6055
Dent, E. W., and Baas, P. W. (2014). Microtubules in neurons as information carriers. J. Neurochem. 129, 235–239. doi: 10.1111/jnc.12621
Deo, R. C., Schmidt, E. F., Elhabazi, A., Togashi, H., Burley, S. K., and Strittmatter, S. M. (2004). Structural bases for CRMP function in plexin-dependent semaphorin3A signaling. EMBO J. 23, 9–22. doi: 10.1038/sj.emboj.7600021
Ding, J., Valle, A., Allen, E., Wang, W., Nardine, T., Zhang, Y., et al. (2006). Microtubule-associated protein 8 contains two microtubule binding sites. Biochem. Biophys. Res. Commun. 339, 172–179. doi: 10.1016/j.bbrc.2005.10.199
Dioli, C., Patrício, P., Trindade, R., Pinto, L. G., Silva, J. M., Morais, M., et al. (2017). Tau-dependent suppression of adult neurogenesis in the stressed hippocampus. Mol. Psychiatry 22, 1110–1118. doi: 10.1038/mp.2017.103
Divinski, I., Mittelman, L., and Gozes, I. (2004). A femtomolar acting octapeptide interacts with tubulin and protects astrocytes against zinc intoxication. J. Biol. Chem. 279, 28531–28538. doi: 10.1074/jbc.M403197200
Domire, J. S., Green, J. A., Lee, K. G., Johnson, A. D., Askwith, C. C., and Mykytyn, K. (2011). Dopamine receptor 1 localizes to neuronal cilia in a dynamic process that requires the Bardet-Biedl syndrome proteins. Cell. Mol. Life Sci. 68, 2951–2960. doi: 10.1007/s00018-010-0603-4
Douaud, G., Smith, S., Jenkinson, M., Behrens, T., Johansen-Berg, H., Vickers, J., et al. (2007). Anatomically related grey and white matter abnormalities in adolescent-onset schizophrenia. Brain 130, 2375–2386. doi: 10.1093/brain/awm184
Dustrude, E. T., Moutal, A., Yang, X., Wang, Y., Khanna, M., and Khanna, R. (2016). Hierarchical CRMP2 posttranslational modifications control NaV1.7 function. Proc. Natl. Acad. Sci. U S A 113, E8443–E8452. doi: 10.1073/pnas.1610531113
Eastwood, S. L., Lyon, L., George, L., Andrieux, A., Job, D., and Harrison, P. J. (2007). Altered expression of synaptic protein mRNAs in STOP (MAP6) mutant mice. J. Psychopharmacol. 21, 635–644. doi: 10.1177/0269881106068825
Edgar, P. F., Douglas, J. E., Cooper, G. J., Dean, B., Kydd, R., and Faull, R. L. (2000). Comparative proteome analysis of the hippocampus implicates chromosome 6q in schizophrenia. Mol. Psychiatry 5, 85–90. doi: 10.1038/sj.mp.4000580
Elie, A., Prezel, E., Guerin, C., Denarier, E., Ramirez-Rios, S., Serre, L., et al. (2015). Tau co-organizes dynamic microtubule and actin networks. Sci. Rep. 5:9964. doi: 10.1038/srep09964
Emamzadeh, F. N. (2016). α-synuclein structure, functions, and interactions. J. Res. Med. Sci. 21:29. doi: 10.4103/1735-1995.181989
Faller, E. M., Villeneuve, T. S., and Brown, D. L. (2009). MAP1a associated light chain 3 increases microtubule stability by suppressing microtubule dynamics. Mol. Cell. Neurosci. 41, 85–93. doi: 10.1016/j.mcn.2009.02.001
Farah, C. A., Liazoghli, D., Perreault, S., Desjardins, M., Guimont, A., Anton, A., et al. (2005). Interaction of microtubule-associated protein-2 and p63: a new link between microtubules and rough endoplasmic reticulum membranes in neurons. J. Biol. Chem. 280, 9439–9449. doi: 10.1074/jbc.M412304200
Farah, C. A., Perreault, S., Liazoghli, D., Desjardins, M., Anton, A., Lauzon, M., et al. (2006). Tau interacts with Golgi membranes and mediates their association with microtubules. Cell Motil. Cytoskeleton 63, 710–724. doi: 10.1002/cm.20157
Findeisen, P., Muhlhausen, S., Dempewolf, S., Hertzog, J., Zietlow, A., Carlomagno, T., et al. (2014). Six subgroups and extensive recent duplications characterize the evolution of the eukaryotic tubulin protein family. Genome Biol. Evol. 6, 2274–2288. doi: 10.1093/gbe/evu187
Fink, C. C., and Meyer, T. (2002). Molecular mechanisms of CaMKII activation in neuronal plasticity. Curr. Opin. Neurobiol. 12, 293–299. doi: 10.1016/s0959-4388(02)00327-6
Fitzsimmons, J., Kubicki, M., Smith, K., Bushell, G., Estepar, R. S., Westin, C. F., et al. (2009). Diffusion tractography of the fornix in schizophrenia. Schizophr. Res. 107, 39–46. doi: 10.1016/j.schres.2008.10.022
Föcking, M., Dicker, P., English, J. A., Schubert, K. O., Dunn, M. J., and Cotter, D. R. (2011). Common proteomic changes in the hippocampus in schizophrenia and bipolar disorder and particular evidence for involvement of cornu ammonis regions 2 and 3. Arch. Gen. Psychiatry 68, 477–488. doi: 10.1001/archgenpsychiatry.2011.43
Fournet, V., de Lavilleon, G., Schweitzer, A., Giros, B., Andrieux, A., and Martres, M. P. (2012a). Both chronic treatments by epothilone D and fluoxetine increase the short-term memory and differentially alter the mood status of STOP/MAP6 KO mice. J. Neurochem. 123, 982–996. doi: 10.1111/jnc.12027
Fournet, V., Jany, M., Fabre, V., Chali, F., Orsal, D., Schweitzer, A., et al. (2010). The deletion of the microtubule-associated STOP protein affects the serotonergic mouse brain network. J. Neurochem. 115, 1579–1594. doi: 10.1111/j.1471-4159.2010.07064.x
Fournet, V., Schweitzer, A., Chevarin, C., Deloulme, J. C., Hamon, M., Giros, B., et al. (2012b). The deletion of STOP/MAP6 protein in mice triggers highly altered mood and impaired cognitive performances. J. Neurochem. 121, 99–114. doi: 10.1111/j.1471-4159.2011.07615.x
Fradley, R. L., O’Meara, G. F., Newman, R. J., Andrieux, A., Job, D., and Reynolds, D. S. (2005). STOP knockout and NMDA NR1 hypomorphic mice exhibit deficits in sensorimotor gating. Behav. Brain Res. 163, 257–264. doi: 10.1016/j.bbr.2005.05.012
Fukata, Y., Itoh, T. J., Kimura, T., Menager, C., Nishimura, T., Shiromizu, T., et al. (2002a). CRMP-2 binds to tubulin heterodimers to promote microtubule assembly. Nat. Cell Biol. 4, 583–591. doi: 10.1038/ncb825
Fukata, Y., Kimura, T., and Kaibuchi, K. (2002b). Axon specification in hippocampal neurons. Neurosci. Res. 43, 305–315. doi: 10.1016/s0168-0102(02)00062-7
Fukuyama, K., Kato, R., Murata, M., Shiroyama, T., and Okada, M. (2019). Clozapine normalizes a glutamatergic transmission abnormality induced by an impaired NMDA receptor in the thalamocortical pathway via the activation of a group III metabotropic glutamate receptor. Biomolecules 9:234. doi: 10.3390/biom9060234
Galiano, M. R., Bosc, C., Schweitzer, A., Andrieux, A., Job, D., and Hallak, M. E. (2004). Astrocytes and oligodendrocytes express different STOP protein isoforms. J. Neurosci. Res. 78, 329–337. doi: 10.1002/jnr.20260
Gardiner, J., Overall, R., and Marc, J. (2011). The microtubule cytoskeleton acts as a key downstream effector of neurotransmitter signaling. Synapse 65, 249–256. doi: 10.1002/syn.20841
Garvalov, B. K., Zuber, B., Bouchet-Marquis, C., Kudryashev, M., Gruska, M., Beck, M., et al. (2006). Luminal particles within cellular microtubules. J. Cell Biol. 174, 759–765. doi: 10.1083/jcb.200606074
Gimenez, U., Boulan, B., Mauconduit, F., Taurel, F., Leclercq, M., Denarier, E., et al. (2017). 3D imaging of the brain morphology and connectivity defects in a model of psychiatric disorders: MAP6-KO mice. Sci. Rep. 7:10308. doi: 10.1038/s41598-017-10544-2
Gleeson, J. G., Lin, P. T., Flanagan, L. A., and Walsh, C. A. (1999). Doublecortin is a microtubule-associated protein and is expressed widely by migrating neurons. Neuron 23, 257–271. doi: 10.1016/s0896-6273(00)80778-3
Gong, X., Tan, M., Gao, Y., Chen, K., and Guo, G. (2016). CRMP-5 interacts with actin to regulate neurite outgrowth. Mol. Med. Rep. 13, 1179–1185. doi: 10.3892/mmr.2015.4662
Gonzalez-Billault, C., Avila, J., and Cáceres, A. (2001). Evidence for the role of MAP1B in axon formation. Mol. Biol. Cell 12, 2087–2098. doi: 10.1091/mbc.12.7.2087
Gory-Fauré, S., Windscheid, V., Bosc, C., Peris, L., Proietto, D., Franck, R., et al. (2006). STOP-like protein 21 is a novel member of the STOP family, revealing a Golgi localization of STOP proteins. J. Biol. Chem. 281, 28387–28396. doi: 10.1074/jbc.M603380200
Gory-Fauré, S., Windscheid, V., Brocard, J., Montessuit, S., Tsutsumi, R., Denarier, E., et al. (2014). Non-microtubular localizations of microtubule-associated protein 6 (MAP6). PLoS One 9:e114905. doi: 10.1371/journal.pone.0114905
Goshima, Y., Nakamura, F., Strittmatter, P., and Strittmatter, S. M. (1995). Collapsin-induced growth cone collapse mediated by an intracellular protein related to UNC-33. Nature 376, 509–514. doi: 10.1038/376509a0
Gozes, I. (2011). Microtubules, schizophrenia and cognitive behavior: preclinical development of davunetide (NAP) as a peptide-drug candidate. Peptides 32, 428–431. doi: 10.1016/j.peptides.2010.10.030
Gozes, I., and Divinski, I. (2007). NAP, a neuroprotective drug candidate in clinical trials, stimulates microtubule assembly in the living cell. Curr. Alzheimer Res. 4, 507–509. doi: 10.2174/156720507783018208
Greaves, J., and Chamberlain, L. H. (2011). DHHC palmitoyl transferases: substrate interactions and (patho)physiology. Trends Biochem. Sci. 36, 245–253. doi: 10.1016/j.tibs.2011.01.003
Griffith, L. M., and Pollard, T. D. (1982). Cross-linking of actin filament networks by self-association and actin-binding macromolecules. J. Biol. Chem. 257, 9135–9142. doi: 10.1016/s0021-9258(18)34253-4
Grossman, S. D., Futter, M., Snyder, G. L., Allen, P. B., Nairn, A. C., Greengard, P., et al. (2004). Spinophilin is phosphorylated by Ca2+/calmodulin-dependent protein kinase II resulting in regulation of its binding to F-actin. J. Neurochem. 90, 317–324. doi: 10.1111/j.1471-4159.2004.02491.x
Gu, Y., Jukkola, P., Wang, Q., Esparza, T., Zhao, Y., Brody, D., et al. (2017). Polarity of varicosity initiation in central neuron mechanosensation. J. Cell Biol. 216, 2179–2199. doi: 10.1083/jcb.201606065
Guillaud, L., Bosc, C., Fourest-Lieuvin, A., Denarier, E., Pirollet, F., Lafanechere, L., et al. (1998). STOP proteins are responsible for the high degree of microtubule stabilization observed in neuronal cells. J. Cell Biol. 142, 167–179. doi: 10.1083/jcb.142.1.167
Hacohen-Kleiman, G., Sragovich, S., Karmon, G., Gao, A. Y. L., Grigg, I., Pasmanik-Chor, M., et al. (2018). Activity-dependent neuroprotective protein deficiency models synaptic and developmental phenotypes of autism-like syndrome. J. Clin. Invest. 128, 4956–4969. doi: 10.1172/JCI98199
Hamdan, H., Lim, B. C., Torii, T., Joshi, A., Konning, M., Smith, C., et al. (2020). Mapping axon initial segment structure and function by multiplexed proximity biotinylation. Nat. Commun. 11:100. doi: 10.1038/s41467-019-13658-5
Harada, A., Oguchi, K., Okabe, S., Kuno, J., Terada, S., Ohshima, T., et al. (1994). Altered microtubule organization in small-calibre axons of mice lacking tau protein. Nature 369, 488–491. doi: 10.1038/369488a0
Hausrat, T. J., Radwitz, J., Lombino, F. L., Breiden, P., and Kneussel, M. (2020). α- and β-tubulin isotypes are differentially expressed during brain development. Dev. Neurobiol. [Epub ahead of print]. doi: 10.1002/dneu.22745
Hayashi, M. A., Portaro, F. C., Bastos, M. F., Guerreiro, J. R., Oliveira, V., Gorrao, S. S., et al. (2005). Inhibition of NUDEL (nuclear distribution element-like)-oligopeptidase activity by disrupted-in-schizophrenia 1. Proc. Natl. Acad. Sci. U S A 102, 3828–3833. doi: 10.1073/pnas.0500330102
Hedgecock, E. M., Culotti, J. G., Thomson, J. N., and Perkins, L. A. (1985). Axonal guidance mutants of Caenorhabditis elegans identified by filling sensory neurons with fluorescein dyes. Dev. Biol. 111, 158–170. doi: 10.1016/0012-1606(85)90443-9
Hensley, K., and Kursula, P. (2016). Collapsin response mediator protein-2 (CRMP2) is a plausible etiological factor and potential therapeutic target in Alzheimer’s disease: comparison and contrast with microtubule-associated protein tau. J. Alzheimers Dis. 53, 1–14. doi: 10.3233/JAD-160076
Hensley, K., Venkova, K., Christov, A., Gunning, W., and Park, J. (2011). Collapsin response mediator protein-2: an emerging pathologic feature and therapeutic target for neurodisease indications. Mol. Neurobiol. 43, 180–191. doi: 10.1007/s12035-011-8166-4
Hernández-Vega, A., Braun, M., Scharrel, L., Jahnel, M., Wegmann, S., Hyman, B. T., et al. (2017). Local nucleation of microtubule bundles through tubulin concentration into a condensed tau phase. Cell Rep. 20, 2304–2312. doi: 10.1016/j.celrep.2017.08.042
Herzog, W., and Weber, K. (1978). Fractionation of brain microtubule-associated proteins. Isolation of two different proteins which stimulate tubulin polymerization in vitro. Eur. J. Biochem. 92, 1–8. doi: 10.1111/j.1432-1033.1978.tb12716.x
Huber, G., and Matus, A. (1984). Differences in the cellular distributions of two microtubule-associated proteins, MAP1 and MAP2, in rat brain. J. Neurosci. 4, 151–160. doi: 10.1523/JNEUROSCI.04-01-00151.1984
Ikegami, S., Harada, A., and Hirokawa, N. (2000). Muscle weakness, hyperactivity and impairment in fear conditioning in tau-deficient mice. Neurosci. Lett. 279, 129–132. doi: 10.1016/s0304-3940(99)00964-7
Inaba, H., Yamamoto, T., Iwasaki, T., Kabir, A. M. R., Kakugo, A., Sada, K., et al. (2019). Stabilization of microtubules by encapsulation of the GFP using a Tau-derived peptide. Chem. Commun. 55, 9072–9075. doi: 10.1039/c9cc04345d
Ivashko-Pachima, Y., and Gozes, I. (2019). A novel microtubule-tau association enhancer and neuroprotective drug candidate: Ac-SKIP. Front. Cell. Neurosci. 13:435. doi: 10.3389/fncel.2019.00435
Ivashko-Pachima, Y., Maor-Nof, M., and Gozes, I. (2019). NAP (davunetide) preferential interaction with dynamic 3-repeat Tau explains differential protection in selected tauopathies. PLoS One 14:e0213666. doi: 10.1371/journal.pone.0213666
Ivashko-Pachima, Y., Sayas, C. L., Malishkevich, A., and Gozes, I. (2017). ADNP/NAP dramatically increase microtubule end-binding protein-Tau interaction: a novel avenue for protection against tauopathy. Mol. Psychiatry 22, 1335–1344. doi: 10.1038/mp.2016.255
Iwanaga, T., Hozumi, Y., and Takahashi-Iwanaga, H. (2011). Immunohistochemical demonstration of dopamine receptor D2R in the primary cilia of the mouse pituitary gland. Biomed. Res. 32, 225–235. doi: 10.2220/biomedres.32.225
Jacobs, S., Lie, D. C., DeCicco, K. L., Shi, Y., DeLuca, L. M., Gage, F. H., et al. (2006). Retinoic acid is required early during adult neurogenesis in the dentate gyrus. Proc. Natl. Acad. Sci. U S A 103, 3902–3907. doi: 10.1073/pnas.0511294103
Jiang, T., Zhang, G., Liang, Y., Cai, Z., Liang, Z., Lin, H., et al. (2020). PlexinA3 interacts with CRMP2 to mediate sema3A signalling during dendritic growth in cultured cerebellar granule neurons. Neuroscience 434, 83–92. doi: 10.1016/j.neuroscience.2020.02.008
Job, D., Fischer, E. H., and Margolis, R. L. (1981). Rapid disassembly of cold-stable microtubules by calmodulin. Proc. Natl. Acad. Sci. U S A 78, 4679–4682. doi: 10.1073/pnas.78.8.4679
Job, D., Rauch, C. T., Fischer, E. H., and Margolis, R. L. (1982). Recycling of cold-stable microtubules: evidence that cold stability is due to substoichiometric polymer blocks. Biochemistry 21, 509–515. doi: 10.1021/bi00532a015
Job, D., Rauch, C. T., and Margolis, R. L. (1987). High concentrations of STOP protein induce a microtubule super-stable state. Biochem. Biophys. Res. Commun. 148, 429–434. doi: 10.1016/0006-291x(87)91129-6
Jonckheere, J., Deloulme, J.-C., Dall’Igna, G., Chauliac, N., Pelluet, A., Nguon, A.-S., et al. (2018). Short- and long-term efficacy of electroconvulsive stimulation in animal models of depression: the essential role of neuronal survival. Brain Stimul. 11, 1336–1347. doi: 10.1016/j.brs.2018.08.001
Jones, C., Watson, D., and Fone, K. (2011). Animal models of schizophrenia: animal models of schizophrenia. Br. J. Pharmacol. 164, 1162–1194. doi: 10.1111/j.1476-5381.2011.01386.x
Joseph, B., Narayanaswamy, J. C., and Venkatasubramanian, G. (2015). Insight in schizophrenia: relationship to positive, negative and neurocognitive dimensions. Indian J. Psychol. Med. 37, 5–11. doi: 10.4103/0253-7176.150797
Kadavath, H., Hofele, R. V., Biernat, J., Kumar, S., Tepper, K., Urlaub, H., et al. (2015). Tau stabilizes microtubules by binding at the interface between tubulin heterodimers. Proc. Natl. Acad. Sci. U S A 112, 7501–7506. doi: 10.1073/pnas.1504081112
Kapitein, L. C., Yau, K. W., Gouveia, S. M., van der Zwan, W. A., Wulf, P. S., Keijzer, N., et al. (2011). NMDA receptor activation suppresses microtubule growth and spine entry. J. Neurosci. 31, 8194–8209. doi: 10.1523/JNEUROSCI.6215-10.2011
Kapur, S. (2003). Psychosis as a state of aberrant salience: a framework linking biology, phenomenology, and pharmacology in schizophrenia. Am. J. Psychiatry 160, 13–23. doi: 10.1176/appi.ajp.160.1.13
Kar, S., Fan, J., Smith, M. J., Goedert, M., and Amos, L. A. (2003). Repeat motifs of tau bind to the insides of microtubules in the absence of taxol. EMBO J. 22, 70–77. doi: 10.1093/emboj/cdg001
Kawano, Y., Yoshimura, T., Tsuboi, D., Kawabata, S., Kaneko-Kawano, T., Shirataki, H., et al. (2005). CRMP-2 is involved in kinesin-1-dependent transport of the Sra-1/WAVE1 complex and axon formation. Mol. Cell. Biol. 25, 9920–9935. doi: 10.1128/MCB.25.22.9920-9935.2005
Kedracka-Krok, S., Swiderska, B., Jankowska, U., Skupien-Rabian, B., Solich, J., Buczak, K., et al. (2015). Clozapine influences cytoskeleton structure and calcium homeostasis in rat cerebral cortex and has a different proteomic profile than risperidone. J. Neurochem. 132, 657–676. doi: 10.1111/jnc.13007
Kellogg, E. H., Hejab, N. M. A., Poepsel, S., Downing, K. H., DiMaio, F., and Nogales, E. (2018). Near-atomic model of microtubule-tau interactions. Science 360, 1242–1246. doi: 10.1126/science.aat1780
Kendi, M., Kendi, A. T. K., Lehericy, S., Ducros, M., Lim, K. O., Ugurbil, K., et al. (2008). Structural and diffusion tensor imaging of the fornix in childhood- and adolescent-onset schizophrenia. J. Am. Acad. Child Adolesc. Psychiatry 47, 826–832. doi: 10.1097/CHI.Ob013e318172ef36
Khazaei, M. R., Girouard, M. P., Alchini, R., Ong Tone, S., Shimada, T., Bechstedt, S., et al. (2014). Collapsin response mediator protein 4 regulates growth cone dynamics through the actin and microtubule cytoskeleton. J. Biol. Chem. 289, 30133–30143. doi: 10.1074/jbc.M114.570440
Kirima, J., and Oiwa, K. (2018). Flagellar-associated Protein FAP85 is a microtubule inner protein that stabilizes microtubules. Cell Struct. Funct. 43, 1–14. doi: 10.1247/csf.17023
Koide, T., Aleksic, B., Ito, Y., Usui, H., Yoshimi, A., Inada, T., et al. (2010). A two-stage case-control association study of the dihydropyrimidinase-like 2 gene (DPYSL2) with schizophrenia in Japanese subjects. J. Hum. Genet. 55, 469–472. doi: 10.1038/jhg.2010.38
Lacroix, B., van Dijk, J., Gold, N. D., Guizetti, J., Aldrian-Herrada, G., Rogowski, K., et al. (2010). Tubulin polyglutamylation stimulates spastin-mediated microtubule severing. J. Cell Biol. 189, 945–954. doi: 10.1083/jcb.201001024
Lai, M., Wang, F., Rohan, J. G., Maeno-Hikichi, Y., Chen, Y., Zhou, Y., et al. (2005). A tctex1-Ca2+ channel complex for selective surface expression of Ca2+ channels in neurons. Nat. Neurosci. 8, 435–442. doi: 10.1038/nn1418
Lasser, M., Tiber, J., and Lowery, L. A. (2018). The role of the microtubule cytoskeleton in neurodevelopmental disorders. Front. Cell. Neurosci. 12:165. doi: 10.3389/fncel.2018.00165
Leaf, A., and Von Zastrow, M. (2015). Dopamine receptors reveal an essential role of IFT-B, KIF17 and Rab23 in delivering specific receptors to primary cilia. Elife 4:e06996. doi: 10.7554/eLife.06996
Lee, J. C., Frigon, R. P., and Timasheff, S. N. (1975). Structural stability of calf brain microtubule protein. Ann. N Y Acad. Sci. 253, 284–291. doi: 10.1111/j.1749-6632.1975.tb19207.x
Lee, J. H., and Gleeson, J. G. (2010). The role of primary cilia in neuronal function. Neurobiol. Dis. 38, 167–172. doi: 10.1016/j.nbd.2009.12.022
Lee, H., Joo, J., Nah, S. S., Kim, J. W., Kim, H. K., Kwon, J. T., et al. (2015). Changes in Dpysl2 expression are associated with prenatally stressed rat offspring and susceptibility to schizophrenia in humans. Int. J. Mol. Med. 35, 1574–1586. doi: 10.3892/ijmm.2015.2161
Lee, S.-H., Kubicki, M., Asami, T., Seidman, L. J., Goldstein, J. M., Mesholam-Gately, R. I., et al. (2013). Extensive white matter abnormalities in patients with first-episode schizophrenia: a diffusion tensor iimaging (DTI) study. Schizophr. Res. 143, 231–238. doi: 10.1016/j.schres.2012.11.029
Leenders, A. G., Lin, L., Huang, L.-D., Gerwin, C., Lu, P. H., and Sheng, Z. H. (2008). The role of MAP1A light chain 2 in synaptic surface retention of Cav2.2 channels in hippocampal neurons. J. Neurosci. 28, 11333–11346. doi: 10.1523/JNEUROSCI.3078-08.2008
Lefevre, J., Savarin, P., Gans, P., Hamon, L., Clement, M. J., David, M. O., et al. (2013). Structural basis for the association of MAP6 protein with microtubules and its regulation by calmodulin. J. Biol. Chem. 288, 24910–24922. doi: 10.1074/jbc.M113.457267
Lei, P., Ayton, S., Finkelstein, D. I., Spoerri, L., Ciccotosto, G. D., Wright, D. K., et al. (2012). Tau deficiency induces parkinsonism with dementia by impairing APP-mediated iron export. Nat. Med. 18, 291–295. doi: 10.1038/nm.2613
Lemonidis, K., Sanchez-Perez, M. C., and Chamberlain, L. H. (2015). Identification of a novel sequence motif recognized by the ankyrin repeat domain of zDHHC17/13 S-acyltransferases. J. Biol. Chem. 290, 21939–21950. doi: 10.1074/jbc.M115.657668
Li, Y., and Black, M. (1996). Microtubule assembly and turnover in growing axons. J. Neurosci. 16, 531–544. doi: 10.1523/JNEUROSCI.16-02-00531.1996
Lim, R. W., and Halpain, S. (2000). Regulated association of microtubule-associated protein 2 (MAP2) with Src and Grb2: evidence for MAP2 as a scaffolding protein. J. Biol. Chem. 275, 20578–20587. doi: 10.1074/jbc.M001887200
Lin, P.-C., Chan, P. M., Hall, C., and Manser, E. (2011). Collapsin response mediator proteins (CRMPs) are a new class of microtubule-associated protein (MAP) that selectively interacts with assembled microtubules via a taxol-sensitive binding interaction. J. Biol. Chem. 286, 41466–41478. doi: 10.1074/jbc.M111.283580
Liu, Y. F., Sowell, S. M., Luo, Y., Chaubey, A., Cameron, R. S., Kim, H. G., et al. (2015). Autism and intellectual disability-associated KIRREL3 interacts with neuronal proteins MAP1B and MYO16 with potential roles in neurodevelopment. PLoS One 10:e0123106. doi: 10.1371/journal.pone.0123106
Luck, D., Malla, A. K., Joober, R., and Lepage, M. (2010). Disrupted integrity of the fornix in first-episode schizophrenia. Schizophr. Res. 119, 61–64. doi: 10.1016/j.schres.2010.03.027
Ma, Q.-L., Zuo, X., Yang, F., Ubeda, O. J., Gant, D. J., Alaverdyan, M., et al. (2014). Loss of MAP function leads to hippocampal synapse loss and deficits in the Morris Water Maze with aging. J. Neurosci. 34, 7124–7136. doi: 10.1523/JNEUROSCI.3439-13.2014
Maden, M. (2007). Retinoic acid in the development, regeneration and maintenance of the nervous system. Nat. Rev. Neurosci. 8, 755–765. doi: 10.1038/nrn2212
Marchisella, F., Coffey, E. T., and Hollos, P. (2016). Microtubule and microtubule associated protein anomalies in psychiatric disease. Cytoskeleton 73, 596–611. doi: 10.1002/cm.21300
Margolis, R. L., and Rauch, C. T. (1981). Characterization of rat brain crude extract microtubule assembly: correlation of cold stability with the phosphorylation state of a microtubule-associated 64K protein. Biochemistry 20, 4451–4458. doi: 10.1021/bi00518a033
Margolis, R. L., Rauch, C. T., and Job, D. (1986). Purification and assay of cold-stable microtubules and STOP protein. Methods Enzymol. 134, 160–170. doi: 10.1016/0076-6879(86)34085-0
Margolis, R. L., Rauch, C. T., Pirollet, F., and Job, D. (1990). Specific association of STOP protein with microtubules in vitro and with stable microtubules in mitotic spindles of cultured cells. EMBO J. 9, 4095–4102. doi: 10.1002/j.1460-2075.1990.tb07631.x
Marley, A., and von Zastrow, M. (2010). DISC1 regulates primary cilia that display specific dopamine receptors. PLoS One 5:e10902. doi: 10.1371/journal.pone.0010902
Martins-de-Souza, D., Gattaz, W. F., Schmitt, A., Rewerts, C., Maccarrone, G., Dias-Neto, E., et al. (2009). Prefrontal cortex shotgun proteome analysis reveals altered calcium homeostasis and immune system imbalance in schizophrenia. Eur. Arch. Psychiatry Clin. Neurosci. 259, 151–163. doi: 10.1007/s00406-008-0847-2
McCarley, R. W., Wible, C. G., Frumin, M., Hirayasu, Y., Levitt, J. J., Fischer, I. A., et al. (1999). MRI anatomy of schizophrenia. Biol. Psychiatry 45, 1099–1119. doi: 10.1016/s0006-3223(99)00018-9
Merenlender-Wagner, A., Malishkevich, A., Shemer, Z., Udawela, M., Gibbons, A., Scarr, E., et al. (2015). Autophagy has a key role in the pathophysiology of schizophrenia. Mol. Psychiatry 20, 126–132. doi: 10.1038/mp.2013.174
Merenlender-Wagner, A., Pikman, R., Giladi, E., Andrieux, A., and Gozes, I. (2010). NAP (davunetide) enhances cognitive behavior in the STOP heterozygous mouse—a microtubule-deficient model of schizophrenia. Peptides 31, 1368–1373. doi: 10.1016/j.peptides.2010.04.011
Merenlender-Wagner, A., Shemer, Z., Touloumi, O., Lagoudaki, R., Giladi, E., Andrieux, A., et al. (2014). New horizons in schizophrenia treatment: autophagy protection is coupled with behavioral improvements in a mouse model of schizophrenia. Autophagy 10, 2324–2332. doi: 10.4161/15548627.2014.984274
Mishra, S., Kelly, K. K., Rumian, N. L., and Siegenthaler, J. A. (2018). Retinoic acid is required for neural stem and progenitor cell proliferation in the adult hippocampus. Stem Cell Reports 10, 1705–1720. doi: 10.1016/j.stemcr.2018.04.024
Mitchison, T., and Kirschner, M. (1984). Dynamic instability of microtubule growth. Nature 312, 237–242. doi: 10.1038/312237a0
Mohan, R., and John, A. (2015). Microtubule-associated proteins as direct crosslinkers of actin filaments and microtubules. IUBMB Life 67, 395–403. doi: 10.1002/iub.1384
Monroy, B. Y., Tan, T. C., Oclaman, J. M., Han, J. S., Simo, S., Niwa, S., et al. (2020). A combinatorial MAP code dictates polarized microtubule transport. Dev. Cell 53, 60.e4–72.e4. doi: 10.1016/j.devcel.2020.01.029
Morderer, D., Nikolaienko, O., Skrypkina, I., Cherkas, V., Tsyba, L., Belan, P., et al. (2012). Endocytic adaptor protein intersectin 1 forms a complex with microtubule stabilizer STOP in neurons. Gene 505, 360–364. doi: 10.1016/j.gene.2012.06.061
Morita, T., and Sobue, K. (2009). Specification of neuronal polarity regulated by local translation of CRMP2 and Tau via the mTOR-p70S6K pathway. J. Biol. Chem. 284, 27734–27745. doi: 10.1074/jbc.M109.008177
Morris, J. A., Kandpal, G., Ma, L., and Austin, C. P. (2003). DISC1 (Disrupted-In-Schizophrenia 1) is a centrosome-associated protein that interacts with MAP1A, MIPT3, ATF4/5 and NUDEL: regulation and loss of interaction with mutation. Hum. Mol. Genet. 12, 1591–1608. doi: 10.1093/hmg/ddg162
Morris, M., Maeda, S., Vossel, K., and Mucke, L. (2011). The many faces of tau. Neuron 70, 410–426. doi: 10.1016/j.neuron.2011.04.009
Moutin, M.-J., Bosc, C., Peris, L., and Andrieux, A. (2020). Tubulin post-translational modifications control neuronal development and functions. Dev. Neurobiol. [Epub ahead of print]. doi: 10.1002/dneu.22774
Müller, C. S., Haupt, A., Bildl, W., Schindler, J., Knaus, H. G., Meissner, M., et al. (2010). Quantitative proteomics of the Cav2 channel nano-environments in the mammalian brain. Proc. Natl. Acad. Sci. U S A 107, 14950–14957. doi: 10.1073/pnas.1005940107
Muñoz-Estrada, J., Lora-Castellanos, A., Meza, I., Alarcón Elizalde, S., and Benítez-King, G. (2018). Primary cilia formation is diminished in schizophrenia and bipolar disorder: a possible marker for these psychiatric diseases. Schizophr. Res. 195, 412–420. doi: 10.1016/j.schres.2017.08.055
Munton, R. P., Tweedie-Cullen, R., Livingstone-Zatchej, M., Weinandy, F., Waidelich, M., Longo, D., et al. (2007). Qualitative and quantitative analyses of protein phosphorylation in naive and stimulated mouse synaptosomal preparations. Mol. Cell. Proteomics 6, 283–293. doi: 10.1074/mcp.M600046-MCP200
Nakata, K., and Ujike, H. (2004). [The human dihydropyrimidinase-related protein 2 (DRP-2) gene on chromosome 8p21 is associated with paranoid-type schizophrenia]. Nihon Shinkei Seishin Yakurigaku Zasshi 24, 33–37.
Nakata, K., Ujike, H., Sakai, A., Takaki, M., Imamura, T., Tanaka, Y., et al. (2003). The human dihydropyrimidinase-related protein 2 gene on chromosome 8p21 is associated with paranoid-type schizophrenia. Biol. Psychiatry 53, 571–576. doi: 10.1016/s0006-3223(02)01729-8
Niisato, E., Nagai, J., Yamashita, N., Abe, T., Kiyonari, H., Goshima, Y., et al. (2012). CRMP4 suppresses apical dendrite bifurcation of CA1 pyramidal neurons in the mouse hippocampus. Dev. Neurobiol. 72, 1447–1457. doi: 10.1002/dneu.22007
Niisato, E., Nagai, J., Yamashita, N., Nakamura, F., Goshima, Y., and Ohshima, T. (2013). Phosphorylation of CRMP2 is involved in proper bifurcation of the apical dendrite of hippocampal CA1 pyramidal neurons. Dev. Neurobiol. 73, 142–151. doi: 10.1002/dneu.22048
Nishimura, T., Fukata, Y., Kato, K., Yamaguchi, T., Matsuura, Y., Kamiguchi, H., et al. (2003). CRMP-2 regulates polarized Numb-mediated endocytosis for axon growth. Nat. Cell Biol. 5, 819–826. doi: 10.1038/ncb1039
Niwa, S., Nakamura, F., Tomabechi, Y., Aoki, M., Shigematsu, H., Matsumoto, T., et al. (2017). Structural basis for CRMP2-induced axonal microtubule formation. Sci. Rep. 7:10681. doi: 10.1038/s41598-017-11031-4
Okabe, S., and Hirokawa, N. (1990). Turnover of fluorescently labelled tubulin and actin in the axon. Nature 343, 479–482. doi: 10.1038/343479a0
Oliveira da Silva, M. I., and Liz, M. A. (2020). Linking α-synuclein to the actin cytoskeleton: consequences to neuronal function. Front. Cell Dev. Biol. 8:787. doi: 10.3389/fcell.2020.00787
Owa, M., Uchihashi, T., Yanagisawa, H. A., Yamano, T., Iguchi, H., Fukuzawa, H., et al. (2019). Inner lumen proteins stabilize doublet microtubules in cilia and flagella. Nat. Commun. 10:1143. doi: 10.1038/s41467-019-09051-x
Oz, S., Kapitansky, O., Ivashco-Pachima, Y., Malishkevich, A., Giladi, E., Skalka, N., et al. (2014). The NAP motif of activity-dependent neuroprotective protein (ADNP) regulates dendritic spines through microtubule end binding proteins. Mol. Psychiatry 19, 1115–1124. doi: 10.1038/mp.2014.97
Ozer, R. S., and Halpain, S. (2000). Phosphorylation-dependent localization of microtubule-associated protein MAP2c to the actin cytoskeleton. Mol. Biol. Cell 11, 3573–3587. doi: 10.1091/mbc.11.10.3573
Pabion, M., Job, D., and Margolis, R. L. (1984). Sliding of STOP proteins on microtubules. Biochemistry 23, 6642–6648. doi: 10.1021/bi00321a055
Palenzuela, R., Gutiérrez, Y., Draffin, J. E., Lario, A., Benoist, M., and Esteban, J. A. (2017). MAP1B light chain modulates synaptic transmission via AMPA receptor intracellular trapping. J. Neurosci. 37, 9945–9963. doi: 10.1523/JNEUROSCI.0505-17.2017
Pamula, M. C., Ti, S.-C., and Kapoor, T. M. (2016). The structured core of human β tubulin confers isotype-specific polymerization properties. J. Cell Biol. 213, 425–433. doi: 10.1083/jcb.201603050
Panda, D., Miller, H. P., Banerjee, A., Ludueña, R. F., and Wilson, L. (1994). Microtubule dynamics in vitro are regulated by the tubulin isotype composition. Proc. Natl. Acad. Sci. U S A 91, 11358–11362. doi: 10.1073/pnas.91.24.11358
Park, S. M., Liu, G., Kubal, A., Fury, M., Cao, L., and Marx, S. O. (2004). Direct interaction between BKCa potassium channel and microtubule-associated protein 1A. FEBS Lett. 570, 143–148. doi: 10.1016/j.febslet.2004.06.037
Paul, D. M., Mantell, J., Borucu, U., Coombs, J., Surridge, K. J., Squire, J. M., et al. (2020). in situ cryo-electron tomography reveals filamentous actin within the microtubule lumen. J. Cell Biol. 219:e201911154. doi: 10.1083/jcb.201911154
Pedrotti, B., Colombo, R., and Islam, K. (1994). Interactions of microtubule-associated protein MAP2 with unpolymerized and polymerized tubulin and actin using a 96-well microtiter plate solid-phase immunoassay. Biochemistry 33, 8798–8806. doi: 10.1021/bi00195a023
Peng, J., Kim, M. J., Cheng, D., Duong, D. M., Gygi, S. P., and Sheng, M. (2004). Semiquantitative proteomic analysis of rat forebrain postsynaptic density fractions by mass spectrometry. J. Biol. Chem. 279, 21003–21011. doi: 10.1074/jbc.M400103200
Peris, L., Bisbal, M., Martinez-Hernandez, J., Saoudi, Y., Jonckheere, J., Rolland, M., et al. (2018). A key function for microtubule-associated-protein 6 in activity-dependent stabilisation of actin filaments in dendritic spines. Nat. Commun. 9:3775. doi: 10.1038/s41467-018-05869-z
Pirollet, F., Derancourt, J., Haiech, J., Job, D., and Margolis, R. L. (1992a). Ca2+-calmodulin regulated effectors of microtubule stability in bovine brain. Biochemistry 31, 8849–8855. doi: 10.1021/bi00152a022
Pirollet, F., Job, D., Fischer, E. H., and Margolis, R. L. (1983). Purification and characterization of sheep brain cold-stable microtubules. Proc. Natl. Acad. Sci. U S A 80, 1560–1564. doi: 10.1073/pnas.80.6.1560
Pirollet, F., Margolis, R. L., and Job, D. (1992b). Ca2+-calmodulin regulated effectors of microtubule stability in neuronal tissues. Biochim. Biophys. Acta 1160, 113–119. doi: 10.1016/0167-4838(92)90044-e
Pirollet, F., Rauch, C. T., Job, D., and Margolis, R. L. (1989). Monoclonal antibody to microtubule-associated STOP protein: affinity purification of neuronal STOP activity and comparison of antigen with activity in neuronal and nonneuronal cell extracts. Biochemistry 28, 835–842. doi: 10.1021/bi00428a064
Portran, D., Schaedel, L., Xu, Z., Thery, M., and Nachury, M. V. (2017). Tubulin acetylation protects long-lived microtubules against mechanical ageing. Nat. Cell Biol. 19, 391–398. doi: 10.1038/ncb3481
Powell, K. J., Hori, S. E., Leslie, R., Andrieux, A., Schellinck, H., Thorne, M., et al. (2007). Cognitive impairments in the STOP null mouse model of schizophrenia. Behav. Neurosci. 121, 826–835. doi: 10.1037/0735-7044.121.5.826
Profitt, M. F., Deurveilher, S., Robertson, G. S., Rusak, B., and Semba, K. (2016). Disruptions of sleep/wake patterns in the stable tubule only polypeptide (STOP) null mouse model of schizophrenia. Schizophr. Bull. 42, 1207–1215. doi: 10.1093/schbul/sbw017
Qiang, L., Sun, X., Austin, T. O., Muralidharan, H., Jean, D. C., Liu, M., et al. (2018). Tau does not stabilize axonal microtubules but rather enables them to have long labile domains. Curr. Biol. 28, 2181.e4–2189.e4. doi: 10.1016/j.cub.2018.05.045
Quach, T. T., Honnorat, J., Kolattukudy, P. E., Khanna, R., and Duchemin, A. M. (2015). CRMPs: critical molecules for neurite morphogenesis and neuropsychiatric diseases. Mol. Psychiatry 20, 1037–1045. doi: 10.1038/mp.2015.77
Quach, T. T., Massicotte, G., Belin, M. F., Honnorat, J., Glasper, E. R., Devries, A. C., et al. (2008). CRMP3 is required for hippocampal CA1 dendritic organization and plasticity. FASEB J. 22, 401–409. doi: 10.1096/fj.07-9012com
Quach, T. T., Wang, Y., Khanna, R., Chounlamountri, N., Auvergnon, N., Honnorat, J., et al. (2011). Effect of CRMP3 expression on dystrophic dendrites of hippocampal neurons. Mol. Psychiatry 16, 689–691. doi: 10.1038/mp.2011.6
Quach, T. T., Wilson, S. M., Rogemond, V., Chounlamountri, N., Kolattukudy, P. E., Martinez, S., et al. (2013). Mapping CRMP3 domains involved in dendrite morphogenesis and voltage-gated calcium channel regulation. J. Cell Sci. 126, 4262–4273. doi: 10.1242/jcs.131409
Quednow, B. B., Csomor, P. A., Chmiel, J., Beck, T., and Vollenweider, F. X. (2008). Sensorimotor gating and attentional set-shifting are improved by the mu-opioid receptor agonist morphine in healthy human volunteers. Int. J. Neuropsychopharmacol. 11, 655–669. doi: 10.1017/S1461145707008322
Quinn, C. C., Chen, E., Kinjo, T. G., Kelly, G., Bell, A. W., Elliott, R. C., et al. (2003). TUC-4b, a novel TUC family variant, regulates neurite outgrowth and associates with vesicles in the growth cone. J. Neurosci. 23, 2815–2823. doi: 10.1523/JNEUROSCI.23-07-02815.2003
Ramirez-Rios, S., Denarier, E., Prezel, E., Vinit, A., Stoppin-Mellet, V., Devred, F., et al. (2016). Tau antagonizes end-binding protein tracking at microtubule ends through a phosphorylation-dependent mechanism. Mol. Biol. Cell 27, 2924–2934. doi: 10.1091/mbc.E16-01-0029
Ramkumar, A., Jong, B. Y., and Ori-McKenney, K. M. (2018). ReMAPping the microtubule landscape: how phosphorylation dictates the activities of microtubule-associated proteins: phosphorylation of microtubule-associated proteins. Dev. Dyn. 247, 138–155. doi: 10.1002/dvdy.24599
Regan, P., Piers, T., Yi, J.-H., Kim, D.-H., Huh, S., Park, S. J., et al. (2015). Tau phosphorylation at serine 396 residue is required for hippocampal LTD. J. Neurosci. 35, 4804–4812. doi: 10.1523/JNEUROSCI.2842-14.2015
Reiter, J. F., and Leroux, M. R. (2017). Genes and molecular pathways underpinning ciliopathies. Nat. Rev. Mol. Cell Biol. 18, 533–547. doi: 10.1038/nrm.2017.60
Richard, M., Sacquet, J., Jany, M., Schweitzer, A., Jourdan, F., Andrieux, A., et al. (2009). STOP proteins contribute to the maturation of the olfactory system. Mol. Cell. Neurosci. 41, 120–134. doi: 10.1016/j.mcn.2009.02.004
Roger, B., Al-Bassam, J., Dehmelt, L., Milligan, R. A., and Halpain, S. (2004). MAP2c, but not tau, binds and bundles F-actin via its microtubule binding domain. Curr. Biol. 14, 363–371. doi: 10.1016/j.cub.2004.01.058
Rosslenbroich, V., Dai, L., Baader, S. L., Noegel, A. A., Gieselmann, V., and Kappler, J. (2005). Collapsin response mediator protein-4 regulates F-actin bundling. Exp. Cell Res. 310, 434–444. doi: 10.1016/j.yexcr.2005.08.005
Rush, T., Roth, J. R., Thompson, S. J., Aldaher, A. R., Cochran, J. N., and Roberson, E. D. (2020). A peptide inhibitor of Tau-SH3 interactions ameliorates amyloid-β toxicity. Neurobiol. Dis. 134:104668. doi: 10.1016/j.nbd.2019.104668
Salatino-Oliveira, A., Wagner, F., Akutagava-Martins, G. C., Bruxel, E. M., Genro, J. P., Zeni, C., et al. (2016). MAP1B and NOS1 genes are associated with working memory in youths with attention-deficit/hyperactivity disorder. Eur. Arch. Psychiatry Clin. Neurosci. 266, 359–366. doi: 10.1007/s00406-015-0626-9
Sanders, A. A. W. M., and Kaverina, I. (2015). Nucleation and dynamics of golgi-derived microtubules. Front. Neurosci. 9:431. doi: 10.3389/fnins.2015.00431
Saudou, F., and Humbert, S. (2016). The biology of huntingtin. Neuron 89, 910–926. doi: 10.1016/j.neuron.2016.02.003
Sayas, C. L., Tortosa, E., Bollati, F., Ramirez-Rios, S., Arnal, I., and Avila, J. (2015). Tau regulates the localization and function of End Binding proteins in neuronal cells. Springerplus 4:L16. doi: 10.1186/2193-1801-4-S1-L16
Schmidt, E. F., Shim, S.-O., and Strittmatter, S. M. (2008). Release of MICAL autoinhibition by semaphorin-plexin signaling promotes interaction with collapsin response mediator protein. J. Neurosci. 28, 2287–2297. doi: 10.1523/JNEUROSCI.5646-07.2008
Schwenk, B. M., Lang, C. M., Hogl, S., Tahirovic, S., Orozco, D., Rentzsch, K., et al. (2014). The FTLD risk factor TMEM106B and MAP6 control dendritic trafficking of lysosomes. EMBO J. 33, 450–467. doi: 10.1002/embj.201385857
Sekine, X. Y., Algarate, P. T., Cafferty, X. W. B. J., and Strittmatter, X. S. M. (2019). Plexina2 and CRMP2 signaling complex is activated by nogo-a-liganded Ngr1 to restrict corticospinal axon sprouting after trauma. J. Neurosci. 39, 3204–3216. doi: 10.1523/JNEUROSCI.2996-18.2019
Shepherd, A. M., Laurens, K. R., Matheson, S. L., Carr, V. J., and Green, M. J. (2012). Systematic meta-review and quality assessment of the structural brain alterations in schizophrenia. Neurosci. Biobehav. Rev. 36, 1342–1356. doi: 10.1016/j.neubiorev.2011.12.015
Shimizu, H., Iwayama, Y., Yamada, K., Toyota, T., Minabe, Y., Nakamura, K., et al. (2006). Genetic and expression analyses of the STOP (MAP6) gene in schizophrenia. Schizophr. Res. 84, 244–252. doi: 10.1016/j.schres.2006.03.017
Shimizu, S., Matsuzaki, S., Hattori, T., Kumamoto, N., Miyoshi, K., Katayama, T., et al. (2008). DISC1-kendrin interaction is involved in centrosomal microtubule network formation. Biochem. Biophys. Res. Commun. 377, 1051–1056. doi: 10.1016/j.bbrc.2008.10.100
Siahaan, V., Krattenmacher, J., Hyman, A. A., Diez, S., Hernandez-Vega, A., Lansky, Z., et al. (2019). Kinetically distinct phases of tau on microtubules regulate kinesin motors and severing enzymes. Nat. Cell Biol. 21, 1086–1092. doi: 10.1038/s41556-019-0374-6
Sloboda, R. D., Dentler, W. L., and Rosenbaum, J. L. (1976). Microtubule-associated proteins and the stimulation of tubulin assembly in vitro. Biochemistry 15, 4497–4505. doi: 10.1021/bi00665a026
Soh, U. J. K., and Low, B. C. (2008). BNIP2 extra long inhibits RhoA and cellular transformation by Lbc RhoGEF via its BCH domain. J. Cell Sci. 121, 1739–1749. doi: 10.1242/jcs.021774
Song, Y., Kirkpatrick, L. L., Schilling, A. B., Helseth, D. L., Chabot, N., Keillor, J. W., et al. (2013). Transglutaminase and polyamination of tubulin: posttranslational modification for stabilizing axonal microtubules. Neuron 78, 109–123. doi: 10.1016/j.neuron.2013.01.036
Sousa, V. L., Bellani, S., Giannandrea, M., Yousuf, M., Valtorta, F., Meldolesi, J., et al. (2009). α-synuclein and its A30P mutant affect actin cytoskeletal structure and dynamics. Mol. Biol. Cell 20, 3725–3739. doi: 10.1091/mbc.e08-03-0302
Su, K.-Y., Chien, W.-L., Fu, W.-M., Yu, I.-S., Huang, H.-P., Huang, P.-H., et al. (2007). Mice deficient in collapsin response mediator protein-1 exhibit impaired long-term potentiation and impaired spatial learning and memory. J. Neurosci. 27, 2513–2524. doi: 10.1523/JNEUROSCI.4497-06.2007
Takei, Y., Kikkawa, Y. S., Atapour, N., Hensch, T. K., and Hirokawa, N. (2015). Defects in synaptic plasticity, reduced NMDA-receptor transport, and instability of postsynaptic density proteins in mice lacking microtubule-associated protein 1A. J. Neurosci. 35, 15539–15554. doi: 10.1523/JNEUROSCI.2671-15.2015
Takei, Y., Kondo, S., Harada, A., Inomata, S., Noda, T., and Hirokawa, N. (1997). Delayed development of nervous system in mice homozygous for disrupted microtubule-associated protein 1B (MAP1B) gene. J. Cell Biol. 137, 1615–1626. doi: 10.1083/jcb.137.7.1615
Takei, Y., Teng, J., Harada, A., and Hirokawa, N. (2000). Defects in axonal elongation and neuronal migration in mice with disrupted tau and map1b genes. J. Cell Biol. 150, 989–1000. doi: 10.1083/jcb.150.5.989
Talbot, K., Cho, D.-S., Ong, W.-Y., Benson, M. A., Han, L.-Y., Kazi, H. A., et al. (2006). Dysbindin-1 is a synaptic and microtubular protein that binds brain snapin. Hum. Mol. Genet. 15, 3041–3054. doi: 10.1093/hmg/ddl246
Tan, M., Cha, C., Ye, Y., Zhang, J., Li, S., Wu, F., et al. (2015). CRMP4 and CRMP2 interact to coordinate cytoskeleton dynamics, regulating growth cone development and axon elongation. Neural Plast. 2015:947423. doi: 10.1155/2015/947423
Taya, S., Shinoda, T., Tsuboi, D., Asaki, J., Nagai, K., Hikita, T., et al. (2007). DISC1 regulates the transport of the NUDEL/LIS1/14–3-3epsilon complex through kinesin-1. J. Neurosci. 27, 15–26. doi: 10.1523/JNEUROSCI.3826-06.2006
Teng, J., Takei, Y., Harada, A., Nakata, T., Chen, J., and Hirokawa, N. (2001). Synergistic effects of MAP2 and MAP1B knockout in neuronal migration, dendritic outgrowth, and microtubule organization. J. Cell Biol. 155, 65–76. doi: 10.1083/jcb.200106025
Ti, S.-C., Alushin, G. M., and Kapoor, T. M. (2018). Human β-tubulin isotypes can regulate microtubule protofilament number and stability. Dev. Cell 47, 175.e5–190.e5. doi: 10.1016/j.devcel.2018.08.014
Tortosa, E., Adolfs, Y., Fukata, M., Pasterkamp, R. J., Kapitein, L. C., and Hoogenraad, C. C. (2017). Dynamic palmitoylation targets MAP6 to the axon to promote microtubule stabilization during neuronal polarization. Neuron 94, 809.e7–825.e7. doi: 10.1016/j.neuron.2017.04.042
Tortosa, E., Galjart, N., Avila, J., and Sayas, C. L. (2013). MAP1B regulates microtubule dynamics by sequestering EB1/3 in the cytosol of developing neuronal cells. EMBO J. 32, 1293–1306. doi: 10.1038/emboj.2013.76
Tortosa, E., Montenegro-Venegas, C., Benoist, M., Härtel, S., González-Billault, C., Esteban, J. A., et al. (2011). Microtubule-associated protein 1B (MAP1B) is required for dendritic spine development and synaptic maturation. J. Biol. Chem. 286, 40638–40648. doi: 10.1074/jbc.M111.271320
Toyoshima, M., Jiang, X., Ogawa, T., Ohnishi, T., Yoshihara, S., Balan, S., et al. (2019). Enhanced carbonyl stress induces irreversible multimerization of CRMP2 in schizophrenia pathogenesis. Life Sci. Alliance 2:e201900478. doi: 10.26508/lsa.201900478
Tsutiya, A., Nishihara, M., Goshima, Y., and Ohtani-Kaneko, R. (2015). Mouse pups lacking collapsin response mediator protein 4 manifest impaired olfactory function and hyperactivity in the olfactory bulb. Eur. J. Neurosci. 42, 2335–2345. doi: 10.1111/ejn.12999
Tsutiya, A., Watanabe, H., Nakano, Y., Nishihara, M., Goshima, Y., and Ohtani-Kaneko, R. (2016). Deletion of collapsin response mediator protein 4 results in abnormal layer thickness and elongation of mitral cell apical dendrites in the neonatal olfactory bulb. J. Anat. 228, 792–804. doi: 10.1111/joa.12434
Uchida, Y., Ohshima, T., Sasaki, Y., Suzuki, H., Yanai, S., Yamashita, N., et al. (2005). Semaphorin3A signalling is mediated via sequential Cdk5 and GSK3β phosphorylation of CRMP2: implication of common phosphorylating mechanism underlying axon guidance and Alzheimer’s disease. Genes Cells 10, 165–179. doi: 10.1111/j.1365-2443.2005.00827.x
Ujike, H., Sakai, A., Nakata, K., Tanaka, Y., Kodaka, T., Okahisa, Y., et al. (2006). Association study of the dihydropyrimidinase-related protein 2 gene and methamphetamine psychosis. Ann. N Y Acad. Sci. 1074, 90–96. doi: 10.1196/annals.1369.008
Valenstein, M. L., and Roll-Mecak, A. (2016). Graded control of microtubule severing by tubulin glutamylation. Cell 164, 911–921. doi: 10.1016/j.cell.2016.01.019
Valenzuela, A., Meservey, L., Nguyen, H., and Fu, M. M. (2020). Golgi outposts nucleate microtubules in cells with specialized shapes. Trends Cell Biol. 30, 792–804. doi: 10.1016/j.tcb.2020.07.004
Van Dijck, A., Vulto-van Silfhout, A. T., Cappuyns, E., van der Werf, I. M., Mancini, G. M., Tzschach, A., et al. (2019). Clinical presentation of a complex neurodevelopmental disorder caused by mutations in ADNP. Biol. Psychiatry 85, 287–297. doi: 10.1016/j.biopsych.2018.02.1173
Vandecandelaere, A., Pedrotti, B., Utton, M. A., Calvert, R. A., and Bayley, P. M. (1996). Differences in the regulation of microtubule dynamics by microtubule-associated proteins MAP1B and MAP2. Cell Motil. Cytoskeleton. 35, 134–146. doi: 10.1002/(SICI)1097-0169(1996)35:2<134::AID-CM6>3.0.CO;2-A
Vemu, A., Atherton, J., Spector, J. O., Szyk, A., Moores, C. A., and Roll-Mecak, A. (2016). Structure and dynamics of single-isoform recombinant neuronal human tubulin. J. Biol. Chem. 291, 12907–12915. doi: 10.1074/jbc.C116.731133
Vikhreva, P. N., Korobko, E. V., and Korobko, I. V. (2009). Identification of novel proteins, possible interaction partners for guanine nucleotide exchange factor Varp. Dokl. Biochem. Biophys. 429, 323–325. doi: 10.1134/s160767290906009x
Volle, J., Brocard, J., Saoud, M., Gory-Faure, S., Brunelin, J., Andrieux, A., et al. (2013). Reduced expression of STOP/MAP6 in mice leads to cognitive deficits. Schizophr. Bull. 39, 969–978. doi: 10.1093/schbul/sbs113
Walters, G. B., Gustafsson, O., Sveinbjornsson, G., Eiriksdottir, V. K., Agustsdottir, A. B., Jonsdottir, G. A., et al. (2018). MAP1B mutations cause intellectual disability and extensive white matter deficit. Nat. Commun. 9:3456. doi: 10.1038/s41467-018-05595-6
Wang, Q., and Brandon, N. J. (2011). Regulation of the cytoskeleton by Disrupted-in-schizophrenia 1 (DISC1). Mol. Cell. Neurosci. 48, 359–364. doi: 10.1016/j.mcn.2011.06.004
Webb, B. C., and Wilson, L. (1980). Cold-stable microtubules from brain. Biochemistry 19, 1993–2001. doi: 10.1021/bi00550a041
Wegmann, S., Eftekharzadeh, B., Tepper, K., Zoltowska, K. M., Bennett, R. E., Dujardin, S., et al. (2018). Tau protein liquid-liquid phase separation can initiate tau aggregation. EMBO J. 37:e98049. doi: 10.15252/embj.201798049
Wei, H., Ma, Y., Liu, J., Ding, C., Hu, F., and Yu, L. (2016a). Proteomic analysis of cortical brain tissue from the BTBR mouse model of autism: evidence for changes in STOP and myelin-related proteins. Neuroscience 312, 26–34. doi: 10.1016/j.neuroscience.2015.11.003
Wei, H., Sun, S., Li, Y., and Yu, S. (2016b). Reduced plasma levels of microtubule-associated STOP/MAP6 protein in autistic patients. Psychiatry Res. 245, 116–118. doi: 10.1016/j.psychres.2016.08.024
Weingarten, J., Lassek, M., Mueller, B. F., Rohmer, M., Lunger, I., Baeumlisberger, D., et al. (2014). The proteome of the presynaptic active zone from mouse brain. Mol. Cell. Neurosci. 59, 106–118. doi: 10.1016/j.mcn.2014.02.003
Weingarten, M. D., Lockwood, A. H., Hwo, S. Y., and Kirschner, M. W. (1975). A protein factor essential for microtubule assembly. Proc. Natl. Acad. Sci. U S A 72, 1858–1862. doi: 10.1073/pnas.72.5.1858
Xiao, B., Xu, H., Ye, H., Hu, Q., Chen, Y., and Qiu, W. (2015). De novo 11q13.4q14.3 tetrasomy with uniparental isodisomy for 11q14.3qter. Am. J. Med. Genet. A 167A, 2327–2333. doi: 10.1002/ajmg.a.37179
Xie, R., Nguyen, S., McKeehan, K., Wang, F., McKeehan, W. L., and Liu, L. (2011). Microtubule-associated protein 1S (MAP1S) bridges autophagic components with microtubules and mitochondria to affect autophagosomal biogenesis and degradation. J. Biol. Chem. 286, 10367–10377. doi: 10.1074/jbc.M110.206532
Xu, Z., Schaedel, L., Portran, D., Aguilar, A., Gaillard, J., Marinkovich, M. P., et al. (2017). Microtubules acquire resistance from mechanical breakage through intralumenal acetylation. Science 356, 328–332. doi: 10.1126/science.aai8764
Yadav, S., Verma, P. J., and Panda, D. (2014). C-terminal region of MAP7 domain containing protein 3 (MAP7D3) promotes microtubule polymerization by binding at the C-terminal tail of tubulin. PLoS One 9:e99539. doi: 10.1371/journal.pone.0099539
Yamane, M., Yamashita, N., Hida, T., Kamiya, Y., Nakamura, F., Kolattukudy, P., et al. (2017). A functional coupling between CRMP1 and Nav1.7 for retrograde propagation of Semaphorin3A signaling. J. Cell Sci. 130, 1393–1403. doi: 10.1242/jcs.199737
Yamashita, N., and Goshima, Y. (2012). Collapsin response mediator proteins regulate neuronal development and plasticity by switching their phosphorylation status. Mol. Neurobiol. 45, 234–246. doi: 10.1007/s12035-012-8242-4
Yamashita, N., Morita, A., Uchida, Y., Nakamura, F., Usui, H., Ohshima, T., et al. (2007). Regulation of spine development by semaphorin3A through cyclin-dependent kinase 5 phosphorylation of collapsin response mediator protein 1. J. Neurosci. 27, 12546–12554. doi: 10.1523/JNEUROSCI.3463-07.2007
Yamashita, N., Mosinger, B., Roy, A., Miyazaki, M., Ugajin, K., Nakamura, F., et al. (2011). CRMP5 (collapsin response mediator protein 5) regulates dendritic development and synaptic plasticity in the cerebellar Purkinje cells. J. Neurosci. 31, 1773–1779. doi: 10.1523/JNEUROSCI.5337-10.2011
Yamashita, N., Takahashi, A., Takao, K., Yamamoto, T., Kolattukudy, P., Miyakawa, T., et al. (2013). Mice lacking collapsin response mediator protein 1 manifest hyperactivity, impaired learning and memory, and impaired prepulse inhibition. Front. Behav. Neurosci. 7:216. doi: 10.3389/fnbeh.2013.00216
Yamashita, N., Uchida, Y., Ohshima, T., Hirai, S. I., Nakamura, F., Taniguchi, M., et al. (2006). Collapsin response mediator protein 1 mediates reelin signaling in cortical neuronal migration. J. Neurosci. 26, 13357–13362. doi: 10.1523/JNEUROSCI.4276-06.2006
Yamazaki, Y., Nagai, J., Akinaga, S., Koga, Y., Hasegawa, M., Takahashi, M., et al. (2020). Phosphorylation of CRMP2 is required for migration and positioning of Purkinje cells: redundant roles of CRMP1 and CRMP4. Brain Res. 1736:146762. doi: 10.1016/j.brainres.2020.146762
Yang, S. Z., and Wildonger, J. (2020). Golgi outposts locally regulate microtubule orientation in neurons but are not required for the overall polarity of the dendritic cytoskeleton. Genetics 215, 435–447. doi: 10.1534/genetics.119.302979
Yao, L., Liu, Y.-H., Li, X., Ji, Y.-H., Yang, X.-J., Hang, X.-T., et al. (2016). CRMP1 interacted with Spy1 during the collapse of growth cones induced by Sema3A and acted on regeneration after sciatic nerve crush. Mol. Neurobiol. 53, 879–893. doi: 10.1007/s12035-014-9049-2
Yoshimura, T., Kawano, Y., Arimura, N., Kawabata, S., Kikuchi, A., and Kaibuchi, K. (2005). GSK-3β regulates phosphorylation of CRMP-2 and neuronal polarity. Cell 120, 137–149. doi: 10.1016/j.cell.2004.11.012
Yu-Kemp, H.-C., and Brieher, W. M. (2016). Collapsin response mediator protein-1 regulates Arp2/3-dependent actin assembly. J. Biol. Chem. 291, 658–664. doi: 10.1074/jbc.C115.689265
Yu-Kemp, H.-C., Kemp, J. P. Jr., and Brieher, W. M. (2017). CRMP-1 enhances EVL-mediated actin elongation to build lamellipodia and the actin cortex. J. Cell Biol. 216, 2463–2479. doi: 10.1083/jcb.201606084
Zervas, M., Opitz, T., Edelmann, W., Wainer, B., Kucherlapati, R., and Stanton, P. K. (2005). Impaired hippocampal long-term potentiation in microtubule-associated protein 1B-deficient mice. J. Neurosci. Res. 82, 83–92. doi: 10.1002/jnr.20624
Zhao, Z., Xu, J., Chen, J., Kim, S., Reimers, M., Bacanu, S. A., et al. (2015). Transcriptome sequencing and genome-wide association analyses reveal lysosomal function and actin cytoskeleton remodeling in schizophrenia and bipolar disorder. Mol. Psychiatry 20, 563–572. doi: 10.1038/mp.2014.82
Keywords: microtubule, microtubule-associated-proteins, actin, neuron, synapse, psychiatric disease, cognition
Citation: Cuveillier C, Boulan B, Ravanello C, Denarier E, Deloulme J-C, Gory-Fauré S, Delphin C, Bosc C, Arnal I and Andrieux A (2021) Beyond Neuronal Microtubule Stabilization: MAP6 and CRMPs, Two Converging Stories. Front. Mol. Neurosci. 14:665693. doi: 10.3389/fnmol.2021.665693
Received: 08 February 2021; Accepted: 09 April 2021;
Published: 05 May 2021.
Edited by:
David Blum, INSERM U1172 Centre de Recherche Jean Pierre Aubert, FranceReviewed by:
Christian Gonzalez-Billault, University of Chile, ChileCopyright © 2021 Cuveillier, Boulan, Ravanello, Denarier, Deloulme, Gory-Fauré, Delphin, Bosc, Arnal and Andrieux. This is an open-access article distributed under the terms of the Creative Commons Attribution License (CC BY). The use, distribution or reproduction in other forums is permitted, provided the original author(s) and the copyright owner(s) are credited and that the original publication in this journal is cited, in accordance with accepted academic practice. No use, distribution or reproduction is permitted which does not comply with these terms.
*Correspondence: Annie Andrieux, YW5uaWUuYW5kcmlldXhAdW5pdi1ncmVub2JsZS1hbHBlcy5mcg==; Isabelle Arnal, aXNhYmVsbGUuYXJuYWxAdW5pdi1ncmVub2JsZS1hbHBlcy5mcg==
Disclaimer: All claims expressed in this article are solely those of the authors and do not necessarily represent those of their affiliated organizations, or those of the publisher, the editors and the reviewers. Any product that may be evaluated in this article or claim that may be made by its manufacturer is not guaranteed or endorsed by the publisher.
Research integrity at Frontiers
Learn more about the work of our research integrity team to safeguard the quality of each article we publish.