- 1Research Centre, The Seventh Affiliated Hospital of Sun Yat-sen University, Shenzhen, China
- 2School of Life Sciences, University of Technology Sydney, Sydney, NSW, Australia
- 3Chongqing Key Laboratory of Neurobiology, Department of Histology and Embryology, Army Medical University (Third Military Medical University), Chongqing, China
Astrocytes play a crucial role in the maintenance of the normal functions of the Central Nervous System (CNS). During the pathogenesis of neurodegenerative diseases, astrocytes undergo morphological and functional remodeling, a process called reactive astrogliosis, in response to the insults to the CNS. One of the key aspects of the reactive astrocytes is the change in the expression and function of connexins. Connexins are channel proteins that highly expressed in astrocytes, forming gap junction channels and hemichannels, allowing diffusional trafficking of small molecules. Alterations of astrocytic connexin expression and function found in neurodegenerative diseases have been shown to affect the disease progression by changing neuronal function and survival. In this review, we will summarize the role of astroglial connexins in neurodegenerative diseases including Alzheimer’s disease, Huntington’s disease, Parkinson’s disease, and amyotrophic lateral sclerosis. Also, we will discuss why targeting connexins can be a plausible therapeutic strategy to manage these neurodegenerative diseases.
Introduction
Neurodegenerative diseases, presented as the progressive loss of structure or function of neurons, are the main threat to human health, especially for the geriatric population. The most common forms of neurodegenerative diseases include Alzheimer’s disease (AD), Parkinson’s disease (PD), Huntington’s disease (HD), amyotrophic lateral sclerosis (ALS) (Erkkinen et al., 2018). It is believed that different pathophysiological mechanisms causing these diseases are different and thus lead to different neurological outcomes. Some can cause memory and cognitive impairment (e.g., AD and PD), and others can affect people’s ability to move, speak, and breathe (e.g., PD, HD, and ALS) (Abeliovich and Gitler, 2016; Canter et al., 2016; Taylor et al., 2016; Wyss-Coray, 2016). However, treatment strategies which have been developed against the classical mechanisms are in-effective, yet treatments are urgently needed to stop or reverse the neurodegenerative diseases. This suggests that we may have missed some vital aspects in the bigger picture of neurodegenerative diseases.
For a long time, neuron-centered theories dominated the research interest of pathogenesis of neurological disorders, whereas the critical role of astrocytes in this process had been over-looked. In the last two decades, the role of astrocytes in the healthy and diseased brain started to gain some recognition. In the adult brain, astrocytes play several crucial roles in supporting neuronal functions, including forming the blood-brain barrier by interacting with endothelial cells, providing nutrients and metabolites support to neurons, and maintaining extracellular ion balance. These functions highly depend on the coordination of hundreds of astrocytes through the formation of an astrocytic network (Santello et al., 2019), which is crucial for cognition and other CNS function. The impairment of the astrocytic network has been found in neurodegenerative diseases (Cooper et al., 2020), where astrocytes undergo reactive gliosis with morphological and functional remodeling. Such changes have been suggested to contribute to the pathogenesis of neurodegenerative diseases (Pekny and Pekna, 2014).
The communication between astrocytes in the astrocytic network is achieved by sharing cytoplasmic content through specific membrane units called “gap junctions.” Gap junctions allow the transcellular exchange of ions and small molecules, such as Adenosine 5′-diphosphate, glucose, glutamate, glutathione, as well as secondary messengers including cAMP and inositol triphosphate. Connexin (Cx) is a protein family that forms the structural basis of gap junctions. Cx proteins are tetraspanins with two extracellular and one intracellular loop, while the NH2- and COOH-terminal tails are located in the intracellular space (Skerrett and Williams, 2017). Cx monomers are assembled into a hexamer connexon (also called “hemichannel”) on cell membranes, and two adjacently docked connexons in the neighboring cell membranes form gap junction channels (GJCs) (Figure 1). A cluster of GJCs composes the gap junction (Nielsen et al., 2012).
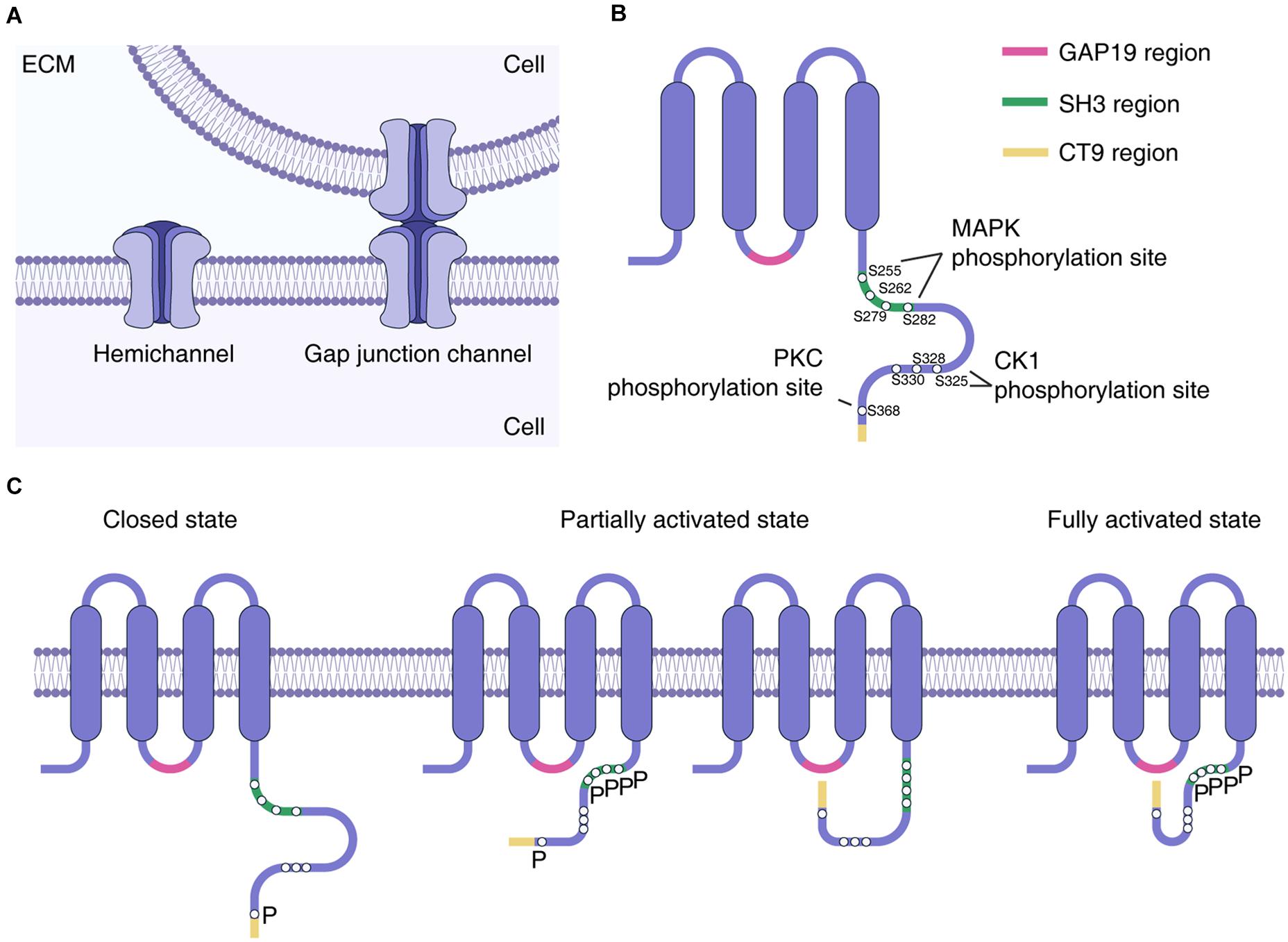
Figure 1. Connexin formation of hemichannel. (A) Connexin hexamer constitutes hemichannel, while hemichannels in the adjacent cells interact to form the gap junction channel. (B) Structure of Cx43 protein. Phosphorylation sites by MAPK, CK1, and PKC in the c-terminal tail are highlighted by white circles. Regions crucial for hemichannel activation regulation was also highlighted. (C) Proposed conformation changes that lead to hemichannel activation. Interaction of either CT9 or SH3-binding region with GAP19 region could achieve partial hemichannel activation, while interaction of both CT9 and SH3-binding region with GAP19 lead to fully activation of hemichannel (Iyyathurai et al., 2018). MAPK phosphorylation at S255, S262, S279, and S282 sites was proposed to facilitate interaction of SH3-binding region to the GAP19 region, enabling hemichannel activation (Freitas-Andrade et al., 2019). PKC phosphorylation at S386 could reduce the permeability of larger molecules such as sucrose (Bao et al., 2007; Hawat and Baroudi, 2008), which might act to interfere with the interaction between CT9 and GAP19 region. CK1 phosphorylation at S325, S328, and S330 has been shown to modulate hemichannel activity (Ek-Vitorín et al., 2018), but the mechanism is yet to be determined. ECM, extracellular matrix; MAPK, mitogen activated protein kinase; PKC, protein kinase C; CK1, casein kinase 1; SH3, SRC Homology 3; CT9, last 9 amino acids of the Cx43 C terminus; P labels phosphorylated amino acid residue.
During reactive gliosis, the expression and function of these Cx proteins changes in astrocytes (Giaume et al., 2010, 2021), especially the opening of Cx hemichannel. The opening of the hemichannel could be triggered in certain conditions, including lower pH, mechanical stimulation, oxidative stress, as well as inflammation caused by ischemic stroke and other injuries (Retamal et al., 2006, 2007; Sanchez et al., 2014; Turovsky et al., 2020). The opening of Cx hemichannels can release gliotransmitters including ATP, glutamate, and D-serine, to support normal neuronal function in the physiological situation (Meunier et al., 2017). However, overactivation of Cx hemichannels found in reactive astrogliosis during neurodegeneration has been shown to disrupt the microenvironment homeostasis and contribute to disease progression (Vis et al., 1998; Orellana et al., 2011b; Takeuchi et al., 2011; Wang et al., 2013; Almad et al., 2016; Yi et al., 2016; Maatouk et al., 2019).
In addition, the pannexin (Panx) protein family could also perform Cx-hemichannel-like activity (Yeung et al., 2020). Panx usually does not form GJCs (Sosinsky et al., 2011; Sahu et al., 2014) and Panx channels have similar membrane topology and pharmacological properties to Cx hemichannels. However, Panx and Cx exhibit no significant sequence homology (Yeung et al., 2020). Panx1 and Panx2 expression have been found in neurons, however, their expression in astrocytes is still controversial, which may depend on the pathological condition (Vogt et al., 2005; Yeung et al., 2020).
This review will focus on the current understanding of astrocytic Cx in neurodegenerative diseases, including AD, PD, HD, and ALS. We will examine how astroglial Cx, together with Panx, function as hemichannels and contribute toward the development of neurodegenerative diseases. Furthermore, we propose that astroglial hemichannels are potential therapeutic targets for the neurodegenerative diseases.
Connexin Expression and Function in Astrocytes
In astrocytes, the dominant Cx proteins are Cx43 and Cx30, while Cx26 expression is also detectable (Rash et al.,2001a,b). Cx43 and Cx30 normally function as GJCs, as was repeatedly shown by experiments in acute brain slices from knockout mice, including the astrocytic Cx43 conditional knockout mice (hGFAP-cre:Cx43fl/fl), the Cx30 knockout mice (Dere et al., 2003; Theis et al., 2003), and the double KO mice (hGFAP-cre:Cx43fl/fl:Cx30 KO) (Wallraff et al., 2006; Rouach et al., 2008; Pannasch et al., 2011; Roux et al., 2011). The expression levels of these two Cxs in astrocytes varies in different brain regions (Batter et al., 1992; Nadarajah et al., 1996; Nagy et al., 1999), and can be changed in neurodegenerative diseases, such as AD (Mei et al., 2010; Yi et al., 2016; Angeli et al., 2020). Additionally, Cx26 has also been detected in certain astrocytes to a lesser extent (Altevogt and Paul, 2004; Lynn et al., 2011; Nagy et al., 2011). Panx1 was reported to be expressed and also contribute to hemichannel function in reactive astrocytes in disease models (Silverman et al., 2009; Karpuk et al., 2011; Santiago et al., 2011; Orellana et al., 2015; Yi et al., 2016; Maturana et al., 2017).
The CX43- and CX30-formed GJCs organize astrocytic networks with certain selectivity, which is crucial for normal neuronal function (Santello et al., 2019). For example, the astrocytic networks can coordinate the activities of local neuronal networks by transporting glutamate or glutamine (Giaume et al., 2010). In addition, the Cx30 and Cx43 mediated astrocytic networks can nourish distant neurons by mediating the delivery of glucose and lactic acid (Rouach et al., 2008; Clasadonte et al., 2017; Giaume et al., 2021). Cx30 and Cx43 are also present in the astrocyte endfeet which enwrap cerebral microvessels in honeycomb-like large sized puncta that helps to represent the end-feet boundaries. This structure provides a perivascular route to mediate the exchange between neighboring end-feet (Simard et al., 2003; Rouach et al., 2008; De Bock et al., 2017). Additionally, researchers found proliferative parenchymal cells in the hypothalamus in mice were decreased in conditional Cx30 and Cx43 knock out (Recabal et al., 2018), suggesting the potential of promoting neurogenesis by manipulating Cx30 and Cx43 function.
Normally, the permeability of Cx43 hemichannels is low under resting conditions (Contreras et al., 2003). They still act to modulate neuron synaptic function via the release of gliotransmitter, such as D-serine (Meunier et al., 2017). However, during reactive gliosis hemichannel permeability is dysregulated in a series of stress-associated conditions, such as inflammation (Orellana et al., 2009; De Bock et al., 2017), ischemia, oxidative stress (Ramachandran et al., 2007), or increased intracellular free Ca2+ concentration ([Ca2+]i) (De Vuyst et al., 2009). A recent study further revealed that the permeability of Cx43 hemichannels in astrocytes is modulated by cytokines and relies on the permeant species characters (Sáez et al., 2020). Furthermore, the interaction between Cx43 C-terminal tail and its cytoplasmic loop is critical for the hemichannel activity, which, in turn, can affect its GJC function (Iyyathurai et al., 2013). The SH3 binding domain and the last 9 amino acids of the C-terminal tail bind to the L2/GAP19 domain of the cytoplasmic loop, allowing full activation of hemichannels (Iyyathurai et al., 2018; Figure 1). This interaction might be regulated by phosphorylation at serine-residues in the C-terminal tail by kinases including mitogen-activated protein kinase (MAPK), protein kinase C (PKC), and casein kinase 1 (CK1) (Bao et al., 2007; Hawat and Baroudi, 2008; Ek-Vitorín et al., 2018; Freitas-Andrade et al., 2019; Figure 1). The suppression of Cx43 phosphorylation by CK1 delta can promote astrocyte survival and vascular regeneration in proliferative retinopathy (Slavi et al., 2018).
In addition, Panx1 expression has also been found in cultured astrocytes (Huang et al., 2007; Bianco et al., 2009; Iwabuchi and Kawahara, 2011), and the activation of the P2 × 7 receptor by BzATP induced ATP release through Panx1 hemichannels instead of Cx43 hemichannels (Iglesias et al., 2009). Nevertheless, the activation of Cx43 hemichannels but not Panx1 channels in vitro only occurs upon exposure to hypoxia-reoxygenation, pro-inflammatory cytokines, or amyloid-beta (Aβ) treatments (Froger et al., 2010; Orellana et al., 2010; Orellana et al., 2011b). Both Cx43 hemichannels and Panx1 channels were activated in fibroblast growth factor-treated astrocyte from the spinal cord (Garre et al., 2010), and in acute brain slices from a mouse abscess model (Karpuk et al., 2011). The astrocytic Panx1 channels were also found to be activated in the APP/PS1 familial AD mouse model (Yi et al., 2016).
Astroglial Connexins in AD
AD is defined by progressive memory loss, behavioral deficits, and significant personality changes (Soria Lopez et al., 2019). Aβ plaques, neurofibrillary tangles, neuronal death, as well as synapse loss are characteristic features in AD brains. Notably, an invariant feature associated with Aβ plaques is reactive gliosis that includes activated microglia and reactive astrocytes (Nagele et al., 2004).
Twenty years ago, Nagy and colleagues have firstly demonstrated that astrocyte Cx43 protein levels are increased in the brain tissue of AD patients, especially around the Aβ plaques (Nagy et al., 1995), which has been repeatedly confirmed (Kajiwara et al., 2018), and is also found in the APP/PS1 mouse model (Mei et al., 2010; Yi et al., 2016). However, a recent study showed that the mRNA level of Cx43 is decreased in the cortex and thalamus area of another mouse model of AD, 5xFAD mice, albeit the increased protein levels (Angeli et al., 2020). Treatment of Aβ25–35 on primary astrocytes also results in a similar negative correlation between Cx43 mRNA and protein levels (Maulik et al., 2020). These pieces of evidence imply a possible unknown mechanism of Cx43 protein expression or turnover in AD pathology. Additionally, results from primary astrocyte culture suggested that Aβ25–35 does not alter de novo synthesized Cx43 membrane forward trafficking, but increases the internalization of Cx43, which may be responsible for the decreased GJC coupling and the increased hemichannel activity (Maulik et al., 2020).
The role of astrocytic Cxs functional alteration in AD has only been identified recently, revealing that the increased Cx HC opening in AD might contribute to neuronal dysfunction. Aβ aggregates and dense core Aβ plaques can induce reactive astrogliosis in AD patients and murine AD models (Nagele et al., 2004; Verkhratsky et al., 2010). The treatment of Aβ peptide in cultured astrocytes as well as in acute hippocampal slices has been shown to induce hemichannel opening, which releases glutamate and ATP, resulting in neuronal death (Orellana et al., 2011a). Similarly, in APP/PS1 mice, there is not only increased Cx43 and Cx30 expression in reactive astrocytes surrounding Aβ plaques, but also increased Cx43 hemichannel activity as shown in acute hippocampal slices; however, the GJC function was unaltered (Yi et al., 2016). Furthermore, conditional knockout of astrocytic Cx43 in APP/PS1 mice can block hemichannel activation and lead to reduced neuronal damage in the hippocampus (Yi et al., 2016). A more recent study has also shown that specific deletion of Cx43 in astrocytes ameliorates cognitive dysfunction in APP/PS1 mice (Ren et al., 2018). These studies confirmed a critical role of astrocytic Cx43 in causing neuronal damage in the AD model, suggesting that astrocytic Cx hemichannels function could be a possible therapeutic target of AD (Figure 2).
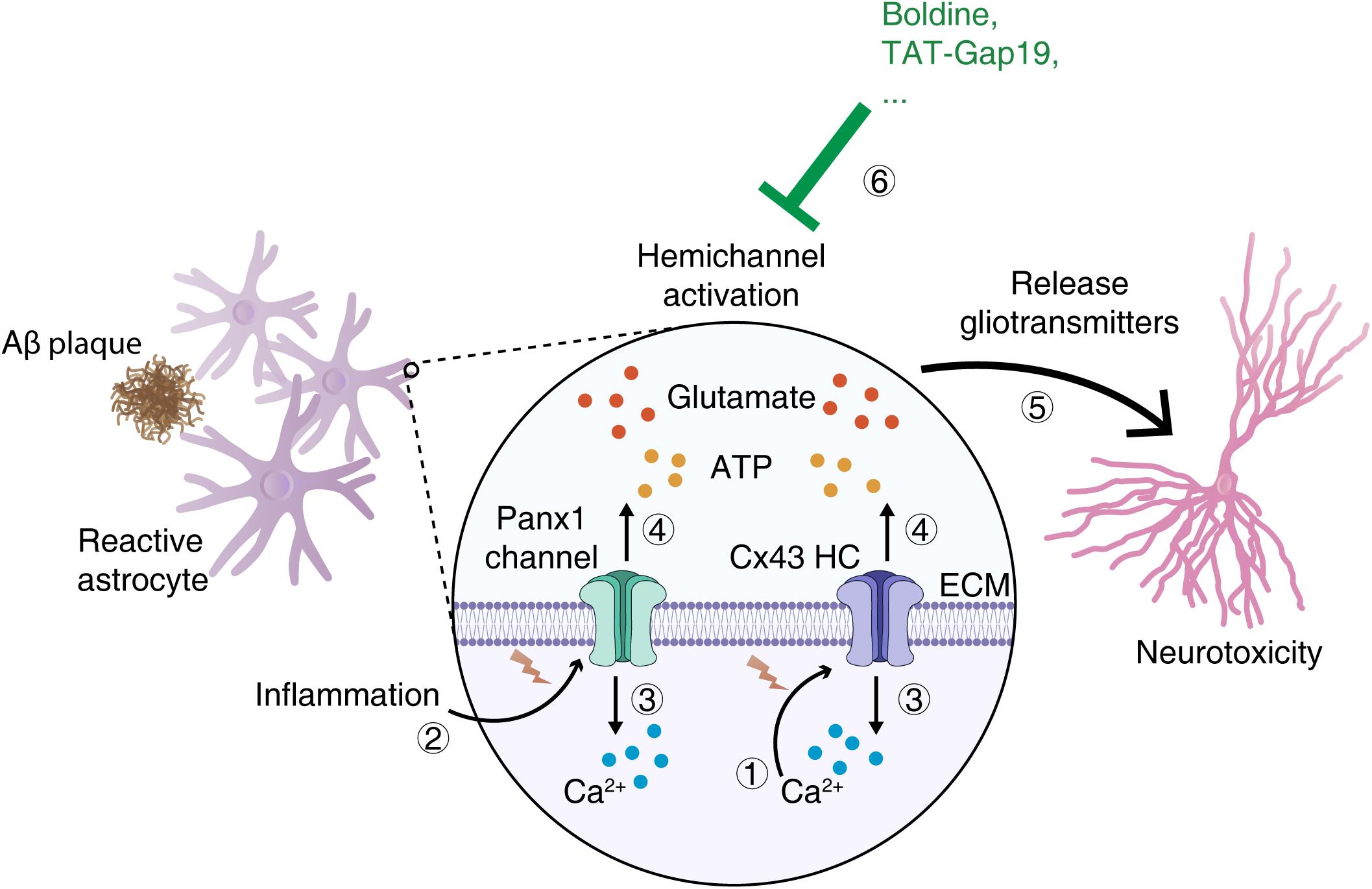
Figure 2. Schematic illustration of the role of astroglial hemichannels in neurodegeneration in an AD mouse model (APP/PS1). In the hippocampus, Cx43 HCs are activated in astrocytes contacting Aβ plaques which are triggered by high [Ca2+]i ①, while Panx1 hemichannels are only activated as a minor contributor triggered by proinflammatory cytokines ② (Yi et al., 2016). HC opening results in the influx of Ca2+ from extracellular to cytoplasm, allowing the high [Ca2+]i maintenance ③ (Yi et al., 2016). HCs activation in astrocytes can lead to gliotransmitter release including glutamate and ATP ④, which then stimulate the intracellular neurotoxic cascades and resulting in neurodegeneration ⑤ (Yi et al., 2016). The astroglial connexin hemichannel blockers [such as Boldine (Yi et al., 2017) and TAT-Gap19 (Abudara et al., 2014)] may become new pharmaceutical tools that can alleviate the neuronal damage in AD ⑥. AD, Alzheimer’s disease; HC, hemichannel; ECM, extracellular matrix.
Efforts have been made to screen or design compounds targeting astrocytic Cx proteins, in particular their hemichannel function, to ameliorate AD progression. It was reported that an alkaloid from the boldo tree called boldine could block the activation of hemichannels in astrocytes and microglia without affecting GJC both in cell culture and in acute hippocampal slices (Yi et al., 2017). In the AD murine model (APP/PS1), long-term oral administration of boldine could inhibit hemichannel activation in astrocytes, accompanied by reduced intracellular Ca2+ in astrocytes, decreased gliotransmitter release, and alleviated neuronal damage in the hippocampus (Yi et al., 2017). It was also found that endogenous and synthetic cannabinoid administration can reduce astrocyte Cx43 hemichannels activity and thereafter alleviate the neuronal damage in hippocampal slices exposed to Aβ (Gajardo-Gomez et al., 2017). However, more studies are required to confirm if pharmacological Cx hemichannel blockers could rescue cognitive function in AD, in order to pave the way for clinical applications.
Astroglial Connexins in PD
PD, as the second most common neurodegenerative disease, is characterized by progressive dopaminergic neuronal loss in the striatum and substantia nigra (Beitz, 2014). The most characteristic hallmark of PD is Lewy bodies, which are cytoplasmic protein-based aggregations of α-synuclein. The clinical manifestations of PD include several motor dysfunction such as postural and movement disability, and non-motor symptoms including depression, psychosis, and dementia (Fernandez, 2012). Notably, astrogliosis in the substantia nigra plays a crucial role in PD pathogenesis (Cabezas et al., 2014).
The commonly used animal model of PD is 1-methyl-4-phenyl-1,2,3,6-tet- rahydropyridine (MPTP)-lesioned striatum which leads to neurodegeneration of dopaminergic neurons. In this PD model, the expression of Cx43 and Cx30 in the striatum is increased (Rufer et al., 1996; Fujita et al., 2018). A recent study showed that astrocytic Cx43 hemichannel permeability was also increased in the MPTP model, accompanied by elevated intracellular Ca2+ levels in the astrocytes of acute midbrain slices (Maatouk et al., 2019). The administration of a hemichannel inhibitor TAT-Gap19 peptide (Abudara et al., 2014), is able to rescue dopaminergic neuronal loss and inhibit microglial activation (Maatouk et al., 2019). These data suggest that astrocytic Cx hemichannel opening is detrimental to the neurons in the MPTP model. However, it appears that other aspects of astrocytic Cx function might be required for neuronal survival, as Cx30 KO enhanced the loss of dopaminergic neurons in MPTP treatment (Fujita et al., 2018). In Cx30 knockout mice receiving MPTP, reactive gliosis was suppressed and the expression of neuroprotective astrocytic genes was reduced, which may contribute to the exaggerated neuronal damage (Fujita et al., 2018). However, the exact function of Cx30 in the development of PD remained unknown. Rotenone, a mitochondrial complex I inhibitor, is another neurotoxic substance commonly used to generate rodent models of PD. Rotenone administration in vivo or in vitro can increase Cx43 protein level and its phosphorylation, and GJC function in astrocytes (Kawasaki et al., 2009).
Researchers also examined how α-synuclein affects astrocytic hemichannel function. It has been shown that α-synuclein also enhances the opening of Cx43 and Panx1 hemichannels in mouse cortical astrocytes, which results in the alterations in the intracellular Ca2+ dynamics, nitric oxide production, gliotransmitter release, mitochondrial morphology, and astrocyte survival (Díaz et al., 2019). This suggests that Cx43 and Panx 1 hemichannels may be involved in the pathogenesis of PD.
Astroglial Connexins in HD and ALS
HD is characterized as a progressively autosomal-dominant neurodegenerative disorder, The features of HD include chorea, dystonia, cognition deficits, as well as behavioral impairments (Walker, 2007). In both healthy and diseased human brains, the distribution of Cx43 in the globus pallidus is homogeneously in the neuropil. However, in the caudate nucleus, the density of Cx43 is increased, which is formed in patches in HD. The immunoreactivity of the staining for glial fibrillary acidic protein (GFAP) in the astrocytes is also significantly higher in the caudate nucleus in HD brains compared to in healthy brains, and there is also increased reactive astrogliosis with elevated Cx43 expression associated with degenerating neurons (Vis et al., 1998). However, the contributions of Cx hemichannels in HD have been rarely reported in recent years and thus remain to be elucidated.
ALS is characterized by progressively weakened voluntary skeletal muscles, as well as those controlling swallowing, speech, and respiration (Oskarsson et al., 2018). It is a progressive and fatal neurodegenerative disease that occurs in the younger population compared with AD and PD. Cx43 expression was found to be upregulated in the motor cortex and spinal cord of patients with ALS and in a murine model of ALS (SOD1G93A) (Díaz-Amarilla et al., 2011; Almad et al., 2016). This upregulated Cx43 expression was accompanied by an increased hemichannel activity and gap junction coupling, and subsequently elevated concentration of intracellular Ca2+, which led to motor neuron damage. In addition, the administration of pan Cx43 blocker and Cx43 hemichannel inhibitors in the ALS mouse model can alleviate the neuronal toxicity (Takeuchi et al., 2011; Almad et al., 2016), suggesting that targeting Cx43 hemichannel function is a potential ALS treatment strategy. The upregulation of Panx1 expression is also found in the spinal cord of SOD1G93A mice when the symptoms become apparent (Cunha et al., 2018). However, the role of Panx1 in ALS development has not been comprehensively studied, therefore its role is still unknown.
Perspectives
The astrocytic GJCs and hemichannels formed by Cx proteins play important roles in neuroglial interactions. GJCs maintain neuronal homeostasis via astroglial and panglial networks for the trafficking of metabolic substances and elimination of potassium and glutamate. Under pathological conditions, the maintenance of GJC function may be beneficial as it is required for astrocytes to resist oxidative stress (Le et al., 2014). In contrast, while proper astroglial hemichannels opening is required for neuronal function under physiological conditions, hemichannel overactivation plays a detrimental role in several neurodegenerative disorders, such as AD, PD, and ALS.
Although it has been shown that Cx proteins could directly cause neuronal damage via hemichannel function in neurodegenerative diseases, they might also implicate in the disease pathogenesis by alternative mechanisms. Cx43 and Cx30 protein expression is enriched at the astrocyte endfeet at the gliovascular interface, and the absence of these astrocytic Cx proteins weakens the blood-brain barrier function (Ezan et al., 2012; Boulay et al., 2015), indicating a critical role of Cx proteins in the maintenance of the blood-brain barrier. Blood-brain barrier disruption has been found in neurodegenerative diseases including AD, PD, HD, and ALS (Sweeney et al., 2018; Huang et al., 2020). However, whether astrocytic Cx proteins contribute to these disease processes remains to be studied. In addition, astrocytic Cx proteins might also regulate the glymphatic pathway, which is constituted by the perivascular space wrapped by astrocytic endfeet and involved in protein waste clearance from the CNS (Rasmussen et al., 2018). Disruption of the glymphatic system has been identified in AD, which might hinder the export of Aβ protein (Nedergaard and Goldman, 2020). Considering the enrichment of Cx proteins at the astrocytic endfeet, they might also regulate glymphatic system function in neurodegenerative diseases.
Given their role in several neurodegenerative diseases, Cx and Panx hemichannels can be considered as promising alternative therapeutic targets. Hemichannels appear to be more associated with neurotoxicity compared to GJCs (Froger et al., 2010; Orellana et al., 2011a; Yi et al., 2016) and their cellular localizations enable pharmacological interventions. Indeed, several strategies using genetic or pharmacological tools to block hemichannel activity have been developed in recent years (Huang et al., 2012; O’Carroll et al., 2013; Bravo et al., 2014; Chen et al., 2014). Most of them inhibit the expression and/or function of Cx43, which is regarded as the major hemichannel component in astrocytes (Nagy et al., 2004). However, they also seem to impact astroglial GJC function, which results in an inaccurate interpretation of the findings. Therefore, a tool that can specifically block hemichannel function in glial cells may delineate the future direction that reduces potential off-target effects.
In neurodegenerative diseases, the development of a potential treatment must consider the needs of long-term treatment and also the use of molecules with the ability to cross the blood-brain-barrier. As such, boldine, an alkaloid compound as mentioned in earlier session, can block Cx43 hemichannels in astrocytes and microglia without affecting GJCs in vitro and in acute hippocampal slices from APP/PS1 mice at the age of 9 months (Yi et al., 2017). Three-month oral administration of boldine in APP/PS1 mice blocked the activation of astroglial hemichannels and ameliorated hippocampal neuritic dystrophies around the Aβ plaques (Yi et al., 2017). These results suggest that boldine seems to be a promising small molecule drug, which opens the revenue to design novel protective molecules that can alleviate neuronal toxicity under neurodegenerative conditions, especially the amyloid pathology. However, it needs to be noted that boldine has other functions, such as antioxidant and anti-inflammatory effects (Schulz et al., 2015), which can also participate in the protection of neurodegeneration in AD. Furthermore, several TAT-conjugated Cx43 peptidomimetics have been shown to block Cx43 hemichannel activity (Evans et al., 2012). For example, TAT-Gap19, a nonapeptide targeting on Cx43 extracellular loop, has been reported to exclusively block astroglial Cx43 hemichannel in a dose-dependent manner, without affecting GJCs (Abudara et al., 2014). Furthermore, in a mouse model of PD, TAT-Gap19 can protect against dopaminergic neuron degeneration and microglial activation (Maatouk et al., 2019). However, TAT peptides are susceptible to proteolytic cleavage in the blood (with a half-life less than 10 min, as determined by MALDI-TOF MS Analysis) (Grunwald et al., 2009), which limits its application in chronic diseases. Structural modification is needed to increase their half-life or slow down their release in the blood. More research is also needed to identify other inhibitors with high specificity to hemichannels and long half-life to enable later clinical translation.
Conclusion
There is still a need for more in-depth investigations of astroglial Cx proteins, especially Cx43, in the pathology of neurodegenerative diseases not only in AD and PD but also in HD and ALS. Targeting astroglial Cx has become a potential strategy for the intervention or treatment of neurodegenerative diseases. Recent advances in the hemichannel opening mechanism have identified several regulatory regions in Cx43, which could facilitate the drug development targeting Cx hemichannel.
Author Contributions
XH wrote the first draft of the manuscript. YS and HL revised the manuscript. NW, ZL, and GY edited the manuscript. HC, JN, and CY revised, edited, and supervised the manuscript. All authors contributed to the article and approved the submitted version.
Funding
This work was supported by grants from the National Nature Science Foundation of China (NSFC 81971309), the Guangdong Basic and Applied Basic Research Foundation (2019A1515011333), the Fundamental Research Funds for the Central Universities (19ykzd04), and Shenzhen Fundamental Research Program (JCYJ20190809161405495 and RCYX20200714114644167).
Conflict of Interest
The authors declare that the research was conducted in the absence of any commercial or financial relationships that could be construed as a potential conflict of interest.
References
Abeliovich, A., and Gitler, A. D. (2016). Defects in trafficking bridge Parkinson’s disease pathology and genetics. Nature 539, 207–216. doi: 10.1038/nature20414
Abudara, V., Bechberger, J., Freitas-Andrade, M., De Bock, M., Wang, N., Bultynck, G., et al. (2014). The connexin43 mimetic peptide Gap19 inhibits hemichannels without altering gap junctional communication in astrocytes. Front. Cell. Neurosci. 8:306. doi: 10.3389/fncel.2014.00306
Almad, A. A., Doreswamy, A., Gross, S. K., Richard, J. P., Huo, Y., Haughey, N., et al. (2016). Connexin 43 in astrocytes contributes to motor neuron toxicity in amyotrophic lateral sclerosis. Glia 64, 1154–1169. doi: 10.1002/glia.22989
Altevogt, B. M., and Paul, D. L. (2004). Four classes of intercellular channels between glial cells in the CNS. J. Neurosci. 24, 4313–4323. doi: 10.1523/JNEUROSCI.3303-03.2004
Angeli, S., Kousiappa, I., Stavrou, M., Sargiannidou, I., Georgiou, E., Papacostas, S. S., et al. (2020). Altered expression of glial gap junction proteins Cx43, Cx30, and Cx47 in the 5XFAD model of Alzheimer’s Disease. Front. Neurosci. 14:582934. doi: 10.3389/fnins.2020.582934
Bao, X., Lee, S. C., Reuss, L., and Altenberg, G. A. (2007). Change in permeant size selectivity by phosphorylation of connexin 43 gap-junctional hemichannels by PKC. Proc. Natl. Acad. Sci. U S A 104, 4919–4924. doi: 10.1073/pnas.0603154104
Batter, D. K., Corpina, R. A., Roy, C., Spray, D. C., Hertzberg, E. L., and Kessler, J. A. (1992). Heterogeneity in gap junction expression in astrocytes cultured from different brain regions. Glia 6, 213–221. doi: 10.1002/glia.440060309
Bianco, F., Colombo, A., Saglietti, L., Lecca, D., Abbracchio, M. P., Matteoli, M., et al. (2009). Different properties of P2X(7) receptor in hippocampal and cortical astrocytes. Purinergic. Signal. 5, 233–240. doi: 10.1007/s11302-009-9137-3
Boulay, A. C., Mazeraud, A., Cisternino, S., Saubaméa, B., Mailly, P., Jourdren, L., et al. (2015). Immune quiescence of the brain is set by astroglial connexin 43. J. Neurosci. 35, 4427–4439. doi: 10.1523/jneurosci.2575-14.2015
Bravo, D., Ibarra, P., Retamal, J., Pelissier, T., Laurido, C., Hernandez, A., et al. (2014). Pannexin 1: A novel participant in neuropathic pain signaling in the rat spinal cord. Pain 155, 2108–2115. doi: 10.1016/j.pain.2014.07.024
Cabezas, R., Avila, M., Gonzalez, J., El-Bacha, R. S., Baez, E., Garcia-Segura, L. M., et al. (2014). Astrocytic modulation of blood brain barrier: perspectives on Parkinson’s disease. Front. Cell. Neurosci. 8:211. doi: 10.3389/fncel.2014.00211
Canter, R. G., Penney, J., and Tsai, L. H. (2016). The road to restoring neural circuits for the treatment of Alzheimer’s disease. Nature 539, 187–196. doi: 10.1038/nature20412
Chen, G., Park, C. K., Xie, R. G., Berta, T., Nedergaard, M., and Ji, R. R. (2014). Connexin-43 induces chemokine release from spinal cord astrocytes to maintain late-phase neuropathic pain in mice. Brain 137, 2193–2209. doi: 10.1093/brain/awu140
Clasadonte, J., Scemes, E., Wang, Z., Boison, D., and Haydon, P. G. (2017). Connexin 43-mediated astroglial metabolic networks contribute to the regulation of the sleep-wake cycle. Neuron 95, 1365–1380. doi: 10.1016/j.neuron.2017.08.022
Contreras, J. E., Sáez, J. C., Bukauskas, F. F., and Bennett, M. V. (2003). Gating and regulation of connexin 43 (Cx43) hemichannels. Proc. Natl. Acad. Sci. U S A 100, 11388–11393. doi: 10.1073/pnas.1434298100
Cooper, M. L., Pasini, S., Lambert, W. S., D’Alessandro, K. B., Yao, V., Risner, M. L., et al. (2020). Redistribution of metabolic resources through astrocyte networks mitigates neurodegenerative stress. Proc. Natl. Acad. Sci. U S A 117, 18810–18821. doi: 10.1073/pnas.2009425117
Cunha, C., Santos, C., Gomes, C., Fernandes, A., Correia, A. M., Sebastião, A. M., et al. (2018). Downregulated Glia Interplay and increased miRNA-155 as promising markers to track ALS at an early stage. Mol. Neurobiol. 55, 4207–4224. doi: 10.1007/s12035-017-0631-2
De Bock, M., Leybaert, L., and Giaume, C. (2017). Connexin channels at the Glio-Vascular interface: Gatekeepers of the brain. Neurochem. Res. 42, 2519–2536. doi: 10.1007/s11064-017-2313-x
De Vuyst, E., Wang, N., Decrock, E., De Bock, M., Vinken, M., Van Moorhem, M., et al. (2009). Ca(2+) regulation of connexin 43 hemichannels in C6 glioma and glial cells. Cell. Calcium 46, 176–187. doi: 10.1016/j.ceca.2009.07.002
Dere, E., De Souza-Silva, M. A., Frisch, C., Teubner, B., Sohl, G., Willecke, K., et al. (2003). Connexin30-deficient mice show increased emotionality and decreased rearing activity in the open-field along with neurochemical changes. Eur. J. Neurosci. 18, 629–638. doi: 10.1046/j.1460-9568.2003.02784.x
Díaz, E. F., Labra, V. C., Alvear, T. F., Mellado, L. A., Inostroza, C. A., Oyarzún, J. E., et al. (2019). Connexin 43 hemichannels and pannexin-1 channels contribute to the α-synuclein-induced dysfunction and death of astrocytes. Glia 67, 1598–1619. doi: 10.1002/glia.23631
Díaz-Amarilla, P., Olivera-Bravo, S., Trias, E., Cragnolini, A., Martínez-Palma, L., Cassina, P., et al. (2011). Phenotypically aberrant astrocytes that promote motoneuron damage in a model of inherited amyotrophic lateral sclerosis. Proc. Natl. Acad. Sci. U S A 108, 18126–18131. doi: 10.1073/pnas.1110689108
Ek-Vitorín, J. F., Pontifex, T. K., and Burt, J. M. (2018). Cx43 channel gating and permeation: multiple phosphorylation-dependent roles of the Carboxyl Terminus. Int. J. Mol. Sci. 19:1659. doi: 10.3390/ijms19061659
Erkkinen, M. G., Kim, M. O., and Geschwind, M. D. (2018). Clinical neurology and epidemiology of the major neurodegenerative diseases. Cold Spring Harb. Perspect. Biol. 10:a033118. doi: 10.1101/cshperspect.a033118
Evans, W. H., Bultynck, G., and Leybaert, L. (2012). Manipulating connexin communication channels: use of peptidomimetics and the translational outputs. J. Membr. Biol. 245, 437–449. doi: 10.1007/s00232-012-9488-5
Ezan, P., Andre, P., Cisternino, S., Saubamea, B., Boulay, A. C., Doutremer, S., et al. (2012). Deletion of astroglial connexins weakens the blood-brain barrier. J. Cereb. Blood. Flow Metab. 32, 1457–1467. doi: 10.1038/jcbfm.2012.45
Fernandez, H. H. (2012). Updates in the medical management of Parkinson disease. Cleve Clin. J. Med. 79, 28–35. doi: 10.3949/ccjm.78gr.11005
Freitas-Andrade, M., Wang, N., Bechberger, J. F., De Bock, M., Lampe, P. D., Leybaert, L., et al. (2019). Targeting MAPK phosphorylation of Connexin43 provides neuroprotection in stroke. J. Exp. Med. 216, 916–935. doi: 10.1084/jem.20171452
Froger, N., Orellana, J. A., Calvo, C. F., Amigou, E., Kozoriz, M. G., Naus, C. C., et al. (2010). Inhibition of cytokine-induced connexin43 hemichannel activity in astrocytes is neuroprotective. Mol. Cell. Neurosci. 45, 37–46. doi: 10.1016/j.mcn.2010.05.007
Fujita, A., Yamaguchi, H., Yamasaki, R., Cui, Y., Matsuoka, Y., Yamada, K. I., et al. (2018). Connexin 30 deficiency attenuates A2 astrocyte responses and induces severe neurodegeneration in a 1-methyl-4-phenyl-1,2,3,6-tetrahydropyridine hydrochloride Parkinson’s disease animal model. J. Neuroinflam. 15:227. doi: 10.1186/s12974-018-1251-0
Gajardo-Gomez, R., Labra, V. C., Maturana, C. J., Shoji, K. F., Santibanez, C. A., Saez, J. C., et al. (2017). Cannabinoids prevent the amyloid beta-induced activation of astroglial hemichannels: A neuroprotective mechanism. Glia 65, 122–137. doi: 10.1002/glia.23080
Garre, J. M., Retamal, M. A., Cassina, P., Barbeito, L., Bukauskas, F. F., Saez, J. C., et al. (2010). FGF-1 induces ATP release from spinal astrocytes in culture and opens pannexin and connexin hemichannels. Proc. Natl. Acad. Sci. U S A 107, 22659–22664. doi: 10.1073/pnas.1013793107
Giaume, C., Koulakoff, A., Roux, L., Holcman, D., and Rouach, N. (2010). Astroglial networks: a step further in neuroglial and gliovascular interactions. Nat. Rev. Neurosci. 11, 87–99. doi: 10.1038/nrn2757
Giaume, C., Naus, C. C., Saez, J. C., and Leybaert, L. (2021). Glial connexins and pannexins in the healthy and diseased brain. Physiol. Rev. 101, 93–145. doi: 10.1152/physrev.00043.2018
Grunwald, J., Rejtar, T., Sawant, R., Wang, Z., and Torchilin, V. P. (2009). TAT peptide and its conjugates: proteolytic stability. Bioconjug. Chem. 20, 1531–1537. doi: 10.1021/bc900081e
Hawat, G., and Baroudi, G. (2008). Differential modulation of unapposed connexin 43 hemichannel electrical conductance by protein kinase C isoforms. Pflugers Arch. 456, 519–527. doi: 10.1007/s00424-007-0426-9
Huang, C., Han, X., Li, X., Lam, E., Peng, W., Lou, N., et al. (2012). Critical role of connexin 43 in secondary expansion of traumatic spinal cord injury. J. Neurosci. 32, 3333–3338. doi: 10.1523/JNEUROSCI.1216-11.2012
Huang, Y., Grinspan, J. B., Abrams, C. K., and Scherer, S. S. (2007). Pannexin1 is expressed by neurons and glia but does not form functional gap junctions. Glia 55, 46–56. doi: 10.1002/glia.20435
Huang, Z., Wong, L. W., Su, Y., Huang, X., Wang, N., Chen, H., et al. (2020). Blood-brain barrier integrity in the pathogenesis of Alzheimer’s disease. Front. Neuroendocrinol. 59:100857. doi: 10.1016/j.yfrne.2020.100857
Iglesias, R., Dahl, G., Qiu, F., Spray, D. C., and Scemes, E. (2009). Pannexin 1: the molecular substrate of astrocyte “hemichannels”. J. Neurosci. 29, 7092–7097. doi: 10.1523/JNEUROSCI.6062-08.2009
Iwabuchi, S., and Kawahara, K. (2011). Functional significance of the negative-feedback regulation of ATP release via pannexin-1 hemichannels under ischemic stress in astrocytes. Neurochem. Int. 58, 376–384. doi: 10.1016/j.neuint.2010.12.013
Iyyathurai, J., D’Hondt, C., Wang, N., De Bock, M., Himpens, B., Retamal, M. A., et al. (2013). Peptides and peptide-derived molecules targeting the intracellular domains of Cx43: gap junctions versus hemichannels. Neuropharmacology 75, 491–505. doi: 10.1016/j.neuropharm.2013.04.050
Iyyathurai, J., Wang, N., D’Hondt, C., Jiang, J. X., Leybaert, L., and Bultynck, G. (2018). The SH3-binding domain of Cx43 participates in loop/tail interactions critical for Cx43-hemichannel activity. Cell Mol. Life Sci. 75, 2059–2073. doi: 10.1007/s00018-017-2722-7
Kajiwara, Y., Wang, E., Wang, M., Sin, W. C., Brennand, K. J., Schadt, E., et al. (2018). GJA1 (connexin43) is a key regulator of Alzheimer’s disease pathogenesis. Acta Neuropathol. Commun. 6:144. doi: 10.1186/s40478-018-0642-x
Karpuk, N., Burkovetskaya, M., Fritz, T., Angle, A., and Kielian, T. (2011). Neuroinflammation leads to region-dependent alterations in astrocyte gap junction communication and hemichannel activity. J. Neurosci. 31, 414–425. doi: 10.1523/JNEUROSCI.5247-10.2011
Kawasaki, A., Hayashi, T., Nakachi, K., Trosko, J. E., Sugihara, K., Kotake, Y., et al. (2009). Modulation of connexin 43 in rotenone-induced model of Parkinson’s disease. Neuroscience 160, 61–68. doi: 10.1016/j.neuroscience.2009.01.080
Le, H. T., Sin, W. C., Lozinsky, S., Bechberger, J., Vega, J. L., Guo, X. Q., et al. (2014). Gap junction intercellular communication mediated by connexin43 in astrocytes is essential for their resistance to oxidative stress. J. Biol. Chem. 289, 1345–1354. doi: 10.1074/jbc.M113.508390
Lynn, B. D., Tress, O., May, D., Willecke, K., and Nagy, J. I. (2011). Ablation of connexin30 in transgenic mice alters expression patterns of connexin26 and connexin32 in glial cells and leptomeninges. Eur. J. Neurosci. 34, 1783–1793. doi: 10.1111/j.1460-9568.2011.07900.x
Maatouk, L., Yi, C., Carrillo-de Sauvage, M. A., Compagnion, A. C., Hunot, S., Ezan, P., et al. (2019). Glucocorticoid receptor in astrocytes regulates midbrain dopamine neurodegeneration through connexin hemichannel activity. Cell Death Differ. 26, 580–596. doi: 10.1038/s41418-018-0150-3
Maturana, C. J., Aguirre, A., and Saez, J. C. (2017). High glucocorticoid levels during gestation activate the inflammasome in hippocampal oligodendrocytes of the offspring. Dev. Neurobiol. 77, 625–642. doi: 10.1002/dneu.22409
Maulik, M., Vasan, L., Bose, A., Dutta Chowdhury, S., Sengupta, N., and Das Sarma, J. (2020). Amyloid-β regulates gap junction protein connexin 43 trafficking in cultured primary astrocytes. J. Biol. Chem. 295, 15097–15111. doi: 10.1074/jbc.RA120.013705
Mei, X., Ezan, P., Giaume, C., and Koulakoff, A. (2010). Astroglial connexin immunoreactivity is specifically altered at β-amyloid plaques in β-amyloid precursor protein/presenilin1 mice. Neuroscience 171, 92–105. doi: 10.1016/j.neuroscience.2010.08.001
Meunier, C., Wang, N., Yi, C., Dallerac, G., Ezan, P., Koulakoff, A., et al. (2017). Contribution of astroglial Cx43 Hemichannels to the modulation of glutamatergic currents by D-Serine in the mouse prefrontal cortex. J. Neurosci. 37, 9064–9075. doi: 10.1523/jneurosci.2204-16.2017
Nadarajah, B., Thomaidou, D., Evans, W. H., and Parnavelas, J. G. (1996). Gap junctions in the adult cerebral cortex: regional differences in their distribution and cellular expression of connexins. J. Comp. Neurol. 376, 326–342. doi: 10.1002/(sici)1096-9861(19961209)376:2<326::Aid-cne13<3.0.Co;2-j
Nagele, R. G., Wegiel, J., Venkataraman, V., Imaki, H., Wang, K. C., and Wegiel, J. (2004). Contribution of glial cells to the development of amyloid plaques in Alzheimer’s disease. Neurobiol. Aging 25, 663–674. doi: 10.1016/j.neurobiolaging.2004.01.007
Nagy, J. I., Dudek, F. E., and Rash, J. E. (2004). Update on connexins and gap junctions in neurons and glia in the mammalian nervous system. Brain Res. Brain Res. Rev. 47, 191–215. doi: 10.1016/j.brainresrev.2004.05.005
Nagy, J. I., Li, W., Hertzberg, E. L., and Marotta, C. A. (1995). Elevated connexin43 immunoreactivity at sites of amyloid plaques in Alzheimer’s disease. Brain Res. 717, 173–178. doi: 10.1016/0006-8993(95)01526-4
Nagy, J. I., Lynn, B. D., Tress, O., Willecke, K., and Rash, J. E. (2011). Connexin26 expression in brain parenchymal cells demonstrated by targeted connexin ablation in transgenic mice. Eur. J. Neurosci. 34, 263–271. doi: 10.1111/j.1460-9568.2011.07741.x
Nagy, J. I., Patel, D., Ochalski, P. A., and Stelmack, G. L. (1999). Connexin30 in rodent, cat and human brain: selective expression in gray matter astrocytes, co-localization with connexin43 at gap junctions and late developmental appearance. Neuroscience 88, 447–468. doi: 10.1016/s0306-4522(98)00191-2
Nedergaard, M., and Goldman, S. A. (2020). Glymphatic failure as a final common pathway to dementia. Science 370, 50–56. doi: 10.1126/science.abb8739
Nielsen, M. S., Axelsen, L. N., Sorgen, P. L., Verma, V., Delmar, M., and Holstein-Rathlou, N. H. (2012). Gap junctions. Compr. Physiol. 2, 1981–2035. doi: 10.1002/cphy.c110051
O’Carroll, S. J., Becker, D. L., Davidson, J. O., Gunn, A. J., Nicholson, L. F., and Green, C. R. (2013). The use of connexin-based therapeutic approaches to target inflammatory diseases. Methods Mol. Biol. 1037, 519–546. doi: 10.1007/978-1-62703-505-7_31
Orellana, J. A., Froger, N., Ezan, P., Jiang, J. X., Bennett, M. V. L., Naus, C. C., et al. (2011a). ATP and glutamate released via astroglial connexin 43 hemichannels mediate neuronal death through activation of pannexin 1 hemichannels. J. Neurochem. 118, 826–840. doi: 10.1111/j.1471-4159.2011.07210.x
Orellana, J. A., Shoji, K. F., Abudara, V., Ezan, P., Amigou, E., Saez, P. J., et al. (2011b). Amyloid beta-induced death in neurons involves glial and neuronal hemichannels. J. Neurosci. 31, 4962–4977. doi: 10.1523/jneurosci.6417-10.2011
Orellana, J. A., Hernandez, D. E., Ezan, P., Velarde, V., Bennett, M. V., Giaume, C., et al. (2010). Hypoxia in high glucose followed by reoxygenation in normal glucose reduces the viability of cortical astrocytes through increased permeability of connexin 43 hemichannels. Glia 58, 329–343. doi: 10.1002/glia.20926
Orellana, J. A., Moraga-Amaro, R., Diaz-Galarce, R., Rojas, S., Maturana, C. J., Stehberg, J., et al. (2015). Restraint stress increases hemichannel activity in hippocampal glial cells and neurons. Front. Cell. Neurosci. 9:102. doi: 10.3389/fncel.2015.00102
Orellana, J. A., Saez, P. J., Shoji, K. F., Schalper, K. A., Palacios-Prado, N., Velarde, V., et al. (2009). Modulation of brain hemichannels and gap junction channels by pro-inflammatory agents and their possible role in neurodegeneration. Antioxid. Redox. Signal. 11, 369–399. doi: 10.1089/ars.2008.2130
Oskarsson, B., Gendron, T. F., and Staff, N. P. (2018). Amyotrophic lateral sclerosis: an update for 2018. Mayo. Clin. Proc. 93, 1617–1628. doi: 10.1016/j.mayocp.2018.04.007
Pannasch, U., Vargova, L., Reingruber, J., Ezan, P., Holcman, D., Giaume, C., et al. (2011). Astroglial networks scale synaptic activity and plasticity. Proc. Natl. Acad. Sci. U S A 108, 8467–8472. doi: 10.1073/pnas.1016650108
Pekny, M., and Pekna, M. (2014). Astrocyte reactivity and reactive astrogliosis: costs and benefits. Physiol. Rev. 94, 1077–1098. doi: 10.1152/physrev.00041.2013
Ramachandran, S., Xie, L. H., John, S. A., Subramaniam, S., and Lal, R. (2007). A novel role for connexin hemichannel in oxidative stress and smoking-induced cell injury. PLoS One 2:e712. doi: 10.1371/journal.pone.0000712
Rash, J. E., Yasumura, T., Dudek, F. E., and Nagy, J. I (2001a). Cell-specific expression of connexins and evidence of restricted gap junctional coupling between glial cells and between neurons. J. Neurosci. 21, 1983–2000. doi: 10.1523/JNEUROSCI.21-06-01983.2001
Rash, J. E., Yasumura, T., Davidson, K. G., Furman, C. S., Dudek, F. E., and Nagy, J. I. (2001b). Identification of cells expressing Cx43, Cx30, Cx26, Cx32 and Cx36 in gap junctions of rat brain and spinal cord. Cell Commun. Adhes 8, 315–320. doi: 10.3109/15419060109080745
Rasmussen, M. K., Mestre, H., and Nedergaard, M. (2018). The glymphatic pathway in neurological disorders. Lancet Neurol. 17, 1016–1024. doi: 10.1016/S1474-4422(18)30318-1
Recabal, A., Elizondo-Vega, R., Philippot, C., Salgado, M., López, S., Palma, A., et al. (2018). Connexin-43 gap junctions are responsible for the hypothalamic tanycyte-coupled network. Front. Cell. Neurosci. 12:406. doi: 10.3389/fncel.2018.00406
Ren, R., Zhang, L., and Wang, M. (2018). Specific deletion connexin43 in astrocyte ameliorates cognitive dysfunction in APP/PS1 mice. Life Sci. 208, 175–191. doi: 10.1016/j.lfs.2018.07.033
Retamal, M. A., Cortés, C. J., Reuss, L., Bennett, M. V., and Sáez, J. C. (2006). S-nitrosylation and permeation through connexin 43 hemichannels in astrocytes: induction by oxidant stress and reversal by reducing agents. Proc. Natl. Acad. Sci. U S A 103, 4475–4480. doi: 10.1073/pnas.0511118103
Retamal, M. A., Froger, N., Palacios-Prado, N., Ezan, P., Sáez, P. J., Sáez, J. C., et al. (2007). Cx43 hemichannels and gap junction channels in astrocytes are regulated oppositely by proinflammatory cytokines released from activated microglia. J. Neurosci. 27, 13781–13792. doi: 10.1523/jneurosci.2042-07.2007
Rouach, N., Koulakoff, A., Abudara, V., Willecke, K., and Giaume, C. (2008). Astroglial metabolic networks sustain hippocampal synaptic transmission. Science 322, 1551–1555. doi: 10.1126/science.1164022
Roux, L., Benchenane, K., Rothstein, J. D., Bonvento, G., and Giaume, C. (2011). Plasticity of astroglial networks in olfactory glomeruli. Proc. Natl. Acad. Sci. U S A 108, 18442–18446. doi: 10.1073/pnas.1107386108
Rufer, M., Wirth, S. B., Hofer, A., Dermietzel, R., Pastor, A., Kettenmann, H., et al. (1996). Regulation of connexin-43, GFAP, and FGF-2 is not accompanied by changes in astroglial coupling in MPTP-lesioned, FGF-2-treated parkinsonian mice. J. Neurosci. Res. 46, 606–617. doi: 10.1002/(SICI)1097-4547(19961201)46:5<606::AID-JNR9<3.0.CO;2-N
Sáez, J. C., Vargas, A. A., Hernández, D. E., Ortiz, F. C., Giaume, C., and Orellana, J. A. (2020). Permeation of molecules through astroglial connexin 43 hemichannels is modulated by cytokines with parameters depending on the permeant species. Int. J. Mol. Sci. 21:3970. doi: 10.3390/ijms21113970
Sahu, G., Sukumaran, S., and Bera, A. K. (2014). Pannexins form gap junctions with electrophysiological and pharmacological properties distinct from connexins. Sci. Rep. 4:4955. doi: 10.1038/srep04955
Sanchez, H. A., Bienkowski, R., Slavi, N., Srinivas, M., and Verselis, V. K. (2014). Altered inhibition of Cx26 hemichannels by pH and Zn2+ in the A40V mutation associated with keratitis-ichthyosis-deafness syndrome. J. Biol. Chem. 289, 21519–21532. doi: 10.1074/jbc.M114.578757
Santello, M., Toni, N., and Volterra, A. (2019). Astrocyte function from information processing to cognition and cognitive impairment. Nat. Neurosci. 22, 154–166. doi: 10.1038/s41593-018-0325-8
Santiago, M. F., Veliskova, J., Patel, N. K., Lutz, S. E., Caille, D., Charollais, A., et al. (2011). Targeting pannexin1 improves seizure outcome. PLoS One 6:e25178. doi: 10.1371/journal.pone.0025178
Schulz, R., Gorge, P. M., Gorbe, A., Ferdinandy, P., Lampe, P. D., and Leybaert, L. (2015). Connexin 43 is an emerging therapeutic target in ischemia/reperfusion injury, cardioprotection and neuroprotection. Pharmacol. Ther. 153, 90–106. doi: 10.1016/j.pharmthera.2015.06.005
Silverman, W. R., de Rivero, Vaccari JP, Locovei, S., Qiu, F., Carlsson, S. K., Scemes, E., et al. (2009). The pannexin 1 channel activates the inflammasome in neurons and astrocytes. J. Biol. Chem. 284, 18143–18151. doi: 10.1074/jbc.M109.004804
Simard, M., Arcuino, G., Takano, T., Liu, Q. S., and Nedergaard, M. (2003). Signaling at the gliovascular interface. J. Neurosci. 23, 9254–9262. doi: 10.1523/JNEUROSCI.23-27-09254.2003
Skerrett, I. M., and Williams, J. B. (2017). A structural and functional comparison of gap junction channels composed of connexins and innexins. Dev. Neurobiol. 77, 522–547. doi: 10.1002/dneu.22447
Slavi, N., Toychiev, A. H., Kosmidis, S., Ackert, J., Bloomfield, S. A., Wulff, H., et al. (2018). Suppression of connexin 43 phosphorylation promotes astrocyte survival and vascular regeneration in proliferative retinopathy. Proc. Natl. Acad. Sci. 115, E5934–E5943. doi: 10.1073/pnas.1803907115
Soria Lopez, J. A., Gonzalez, H. M., and Leger, G. C. (2019). Alzheimer’s disease. Handb. Clin. Neurol. 167, 231–255. doi: 10.1016/B978-0-12-804766-8.00013-3
Sosinsky, G. E., Boassa, D., Dermietzel, R., Duffy, H. S., Laird, D. W., MacVicar, B., et al. (2011). Pannexin channels are not gap junction hemichannels. Channels (Austin) 5, 193–197. doi: 10.4161/chan.5.3.15765
Sweeney, M. D., Sagare, A. P., and Zlokovic, B. V. (2018). Blood-brain barrier breakdown in Alzheimer disease and other neurodegenerative disorders. Nat. Rev. Neurol. 14, 133–150. doi: 10.1038/nrneurol.2017.188
Takeuchi, H., Mizoguchi, H., Doi, Y., Jin, S., Noda, M., Liang, J., et al. (2011). Blockade of gap junction hemichannel suppresses disease progression in mouse models of amyotrophic lateral sclerosis and Alzheimer’s disease. PLoS One 6:e21108. doi: 10.1371/journal.pone.0021108
Taylor, J. P., Brown, R. H. Jr., and Cleveland, D. W. (2016). Decoding ALS: from genes to mechanism. Nature 539, 197–206. doi: 10.1038/nature20413
Theis, M., Jauch, R., Zhuo, L., Speidel, D., Wallraff, A., Döring, B., et al. (2003). Accelerated hippocampal spreading depression and enhanced locomotory activity in mice with astrocyte-directed inactivation of connexin43. J. Neurosci. 23, 766–776. doi: 10.1523/JNEUROSCI.23-03-00766.2003
Turovsky, E. A., Braga, A., Yu, Y., Esteras, N., Korsak, A., Theparambil, S. M., et al. (2020). Mechanosensory signaling in astrocytes. J. Neurosci. 40, 9364–9371. doi: 10.1523/jneurosci.1249-20.2020
Verkhratsky, A., Olabarria, M., Noristani, H. N., Yeh, C. Y., and Rodriguez, J. J. (2010). Astrocytes in Alzheimer’s disease. Neurotherapeutics 7, 399–412. doi: 10.1016/j.nurt.2010.05.017
Vis, J. C., Nicholson, L. F., Faull, R. L., Evans, W. H., Severs, N. J., and Green, C. R. (1998). Connexin expression in Huntington’s diseased human brain. Cell. Biol. Int. 22, 837–847. doi: 10.1006/cbir.1998.0388
Vogt, A., Hormuzdi, S. G., and Monyer, H. (2005). Pannexin1 and Pannexin2 expression in the developing and mature rat brain. Mol. Brain Res. 141, 113–120. doi: 10.1016/j.molbrainres.2005.08.002
Wallraff, A., Kohling, R., Heinemann, U., Theis, M., Willecke, K., and Steinhauser, C. (2006). The impact of astrocytic gap junctional coupling on potassium buffering in the hippocampus. J. Neurosci. 26, 5438–5447. doi: 10.1523/JNEUROSCI.0037-06.2006
Wang, Y., Wu, Z., Liu, X., and Fu, Q. (2013). Gastrodin ameliorates Parkinson’s disease by downregulating connexin 43. Mol. Med. Rep. 8, 585–590. doi: 10.3892/mmr.2013.1535
Wyss-Coray, T. (2016). Ageing, neurodegeneration and brain rejuvenation. Nature 539, 180–186. doi: 10.1038/nature20411
Yeung, A. K., Patil, C. S., and Jackson, M. F. (2020). Pannexin-1 in the CNS: Emerging concepts in health and disease. J. Neurochem. 154, 468–485. doi: 10.1111/jnc.15004
Yi, C., Ezan, P., Fernandez, P., Schmitt, J., Saez, J. C., Giaume, C., et al. (2017). Inhibition of glial hemichannels by boldine treatment reduces neuronal suffering in a murine model of Alzheimer’s disease. Glia 65, 1607–1625. doi: 10.1002/glia.23182
Keywords: astrocyte, connexin, hemichannel, gap junction, neurodegenerative disease, Alzheimer’s disease
Citation: Huang X, Su Y, Wang N, Li H, Li Z, Yin G, Chen H, Niu J and Yi C (2021) Astroglial Connexins in Neurodegenerative Diseases. Front. Mol. Neurosci. 14:657514. doi: 10.3389/fnmol.2021.657514
Received: 23 January 2021; Accepted: 05 May 2021;
Published: 28 May 2021.
Edited by:
Michele Papa, University of Campania Luigi Vanvitelli, ItalyReviewed by:
Ciro De Luca, Second University of Naples, ItalyJuan Andrés Orellana, Pontificia Universidad Católica de Chile, Chile
Copyright © 2021 Huang, Su, Wang, Li, Li, Yin, Chen, Niu and Yi. This is an open-access article distributed under the terms of the Creative Commons Attribution License (CC BY). The use, distribution or reproduction in other forums is permitted, provided the original author(s) and the copyright owner(s) are credited and that the original publication in this journal is cited, in accordance with accepted academic practice. No use, distribution or reproduction is permitted which does not comply with these terms.
*Correspondence: Jianqin Niu, amlhbnFpbm5pdUAxNjMuY29t; Chenju Yi, eWljaGpAbWFpbC5zeXN1LmVkdS5jbg==
†These authors have contributed equally to this work