- 1Spinal Cord Injury Research Group, Department of Anatomy and Medical Imaging, School of Medical Sciences, University of Auckland, Auckland, New Zealand
- 2Molecular Neurotherapeutics Group, Department of Pharmacology and Clinical Pharmacology, School of Medical Sciences, University of Auckland, Auckland, New Zealand
Different glial cell types are found throughout the central (CNS) and peripheral nervous system (PNS), where they have important functions. These cell types are also involved in nervous system pathology, playing roles in neurodegenerative disease and following trauma in the brain and spinal cord (astrocytes, microglia, oligodendrocytes), nerve degeneration and development of pain in peripheral nerves (Schwann cells, satellite cells), retinal diseases (Müller glia) and gut dysbiosis (enteric glia). These cell type have all been proposed as potential targets for treating these conditions. One approach to target these cell types is the use of gene therapy to modify gene expression. Adeno-associated virus (AAV) vectors have been shown to be safe and effective in targeting cells in the nervous system and have been used in a number of clinical trials. To date, a number of studies have tested the use of different AAV serotypes and cell-specific promoters to increase glial cell tropism and expression. However, true glial-cell specific targeting for a particular glial cell type remains elusive. This review provides an overview of research into developing glial specific gene therapy and discusses some of the issues that still need to be addressed to make glial cell gene therapy a clinical reality.
Introduction
The term glia relates to types of non-neuronal cells in the central nervous system (CNS) and peripheral nervous system (PNS) that maintain homeostasis and are active regulators of numerous physiological functions. The glial cells of the CNS include astrocytes, which support the blood-brain barrier (BBB), provide nutrients to neurons and play a crucial role in maintaining extracellular ion balance and neurotransmitter levels in the CNS. Microglia play roles relating to both the immune response and homeostasis (Kierdorf and Prinz, 2017) and oligodendrocytes primary function is to myelinate axons and provide metabolic support (Bradl and Lassmann, 2010). The retina, which is considered part of the CNS, contains Müller glia, which like astrocytes play a role in regulating blood flow, uptake of neurotransmitters, regulation of ion levels and energy storage (Bringmann et al., 2006).
Several glial cell types play similar roles within the PNS. The gut contains enteric glia, which share many similarities with CNS glia (Grubisic and Gulbransen, 2017) and are crucial for the survival of enteric neurons. Moreover, they play a key role in homeostasis, metabolism and neurotransmission as well as gut epithelial integrity, and regulate gut motility (Ruhl et al., 2004). Schwann cells are the myelinating cells of the PNS and are involved in maintaining ionic balance and providing support to axons (Kidd et al., 2013). Satellite cells are associated with neurons in peripheral ganglia and have similar functions to astrocytes in the CNS (Hanani, 2005, 2010). As well as their role in normal physiological functions of the nervous system, glia are activated under pathological conditions and contribute significantly to disease pathology in many neurodegenerative diseases, neurotrauma, peripheral neuropathies and gut inflammation. Glial cells are therefore a potential cell target for several therapeutic approaches to treat diseases of the nervous system (Ahmed et al., 2017; Spear and Mawe, 2019; Eastlake et al., 2020).
One such approach is the use of gene therapy which employs viral vectors to deliver genetic material with therapeutic potential into a cell. Different viral vector systems have been developed to mediate gene delivery to different organ systems, including the CNS and PNS (Kantor et al., 2014). The use of viral vector gene therapy for the nervous system is appealing as many drugs cannot cross the BBB efficiently and it can overcome the need for repeated delivery of often short-acting drugs into the brain, spinal cord, retina and cochlea by allowing for a single, long-lasting intervention.
One of the most well-characterized vectors for gene therapy is derived from adeno-associated virus (AAV). These are considered the ideal for human gene therapy approaches as they are small and non-replicative, can transduce dividing and non-dividing cells, are non-pathogenic to humans and can provide long-lasting changes in gene expression (Ingusci et al., 2019). AAVs have been used to target a number of different tissue and cell types successfully within the CNS and PNS including neurons, astrocytes, oligodendrocytes, microglia, Müller glia, Schwann cells, and satellite cells (Berns and Giraud, 1996; Rabinowitz and Samulski, 1998; Xiang et al., 2018; Sargiannidou et al., 2020). A large number of clinical trials using AAV have demonstrated the relative safety of AAV gene therapy (Mastakov et al., 2002; Penaud-Budloo et al., 2018). However, to date, these trials have targeted neuronal cell types and retinal pigment epithelium in the retina. An AAV gene therapy approach has real potential for targeting of glial cells and in preclinical studies targeting of different glial cell types has been achieved (Howard et al., 2008; Hammond et al., 2017).
AAV Vectors
Wild type AAVs are small, 4.7 kb, linear, single-stranded DNA (ssDNA) viruses in the Parvovirus family. They are composed of an icosahedral protein capsid of three types of subunit (VP1, VP2, and VP3), totaling 60 copies in a ratio of 1:1:10 (VP1:VP2:VP3). The genome consists of a rep gene, encoding four proteins necessary for viral replication; a cap gene that encodes the three capsid subunits through alternative splicing and translation from different start codons; and a third gene that encodes an assembly activating protein (AAP) which promotes virion assembly. These are flanked by inverted terminal repeats (ITRs) which are needed to direct genome replication and packaging (Samulski and Muzyczka, 2014). For therapeutic use, the rep and cap genes are removed and replaced by an expression cassette containing the therapeutic transgene under the control of a promoter and flanked by the AAV ITRs, forming a recombinant AAV (rAAV) (During et al., 2003). There are hundreds of variants of AAV, including the 11 natural serotypes; AAVs 1–11. The natural serotypes are defined by antigenically distinct viral capsids and although most were first isolated in humans, later serotypes were identified in non-human primate species, including rhesus and cynomolgus macaques (Gao et al., 2004; Mori et al., 2004).
AAV Tropism
In the CNS, while most AAV vectors have a preference for targeting neurons, both naturally-occurring and engineered serotypes have been shown to transduce glia (Figure 1). The tropism of an AAV for a particular cell type is dependent on the interaction of the capsid with cell surface receptors (Lisowski et al., 2015). The vector initially attaches to a cell surface glycan, which acts as a primary receptor. For efficient entry to the cell, the virus must then interact with a co-receptor. Twenty-three different glycan receptors have been identified, although the primary receptor for some serotypes has not yet been determined, whilst a number of co-receptors have also been identified (reviewed in Lisowski et al., 2015; Srivastava, 2016). AAV capsids can be modified, changing their ability to interact with specific receptors and therefore the cell types they will transduce, and this has been used successfully to change AAV tropism for a particular cell or tissue and to improve transduction efficiency.
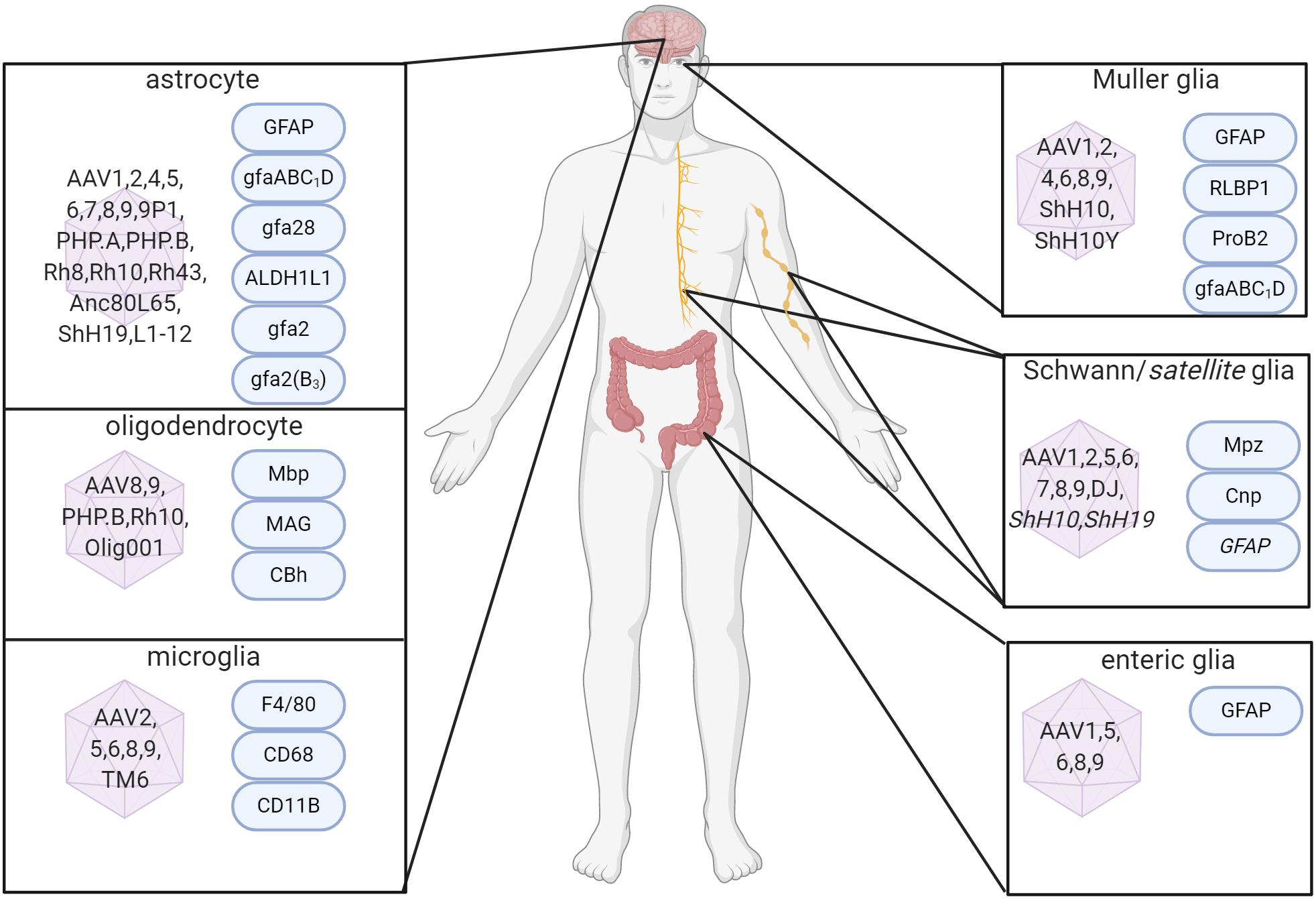
Figure 1. Capsid serotypes and promoters for glial targeting of AAV. Overview depicting naturally-occurring and engineered AAV viral vectors with known glial cell tropism in the CNS and PNS and relevant cell-specific promoters. References used for this figure are detailed and cited in the text. Created with BioRender.com.
Different strategies can be used to alter the tropism of AAV capsids (reviewed in Castle et al., 2016; Deverman et al., 2018). Chemical modification of the virus capsid can lead to improved transduction efficiency and mask native receptors allowing the vector to target alternate receptors (Bartlett et al., 1999; Ponnazhagan et al., 2002; Le et al., 2005; Carlisle et al., 2008; Horowitz et al., 2011), but these have had limited use in vivo. Hybrid capsids that combine the advantageous properties of specific selected AAV serotypes have been developed that lead to improved transgene expression and tropism (Koprich et al., 2010). Short peptides can also be inserted into the capsids, and their presence can allow for interaction with a specific target cell receptor (Chen et al., 2009).
Approaches can involve rational design, which is underpinned by an understanding of the function of capsid protein residues such as key residues involved in receptor binding. Mutation of these residues can lead to unique cellular tropism (Murlidharan et al., 2015), and insertion of specific peptide sequences can change cell tropism and modify the ability of the AAV vector to cross the BBB (Adachi et al., 2014; Albright et al., 2018). Another approach used to develop novel capsids is directed evolution. This involves generating highly diverse capsid libraries and using iterative rounds of selection either in vitro or in vivo to enrich for the most potent AAV variant with the desired tropism. This diversity can be created using capsid-shuffling, which involves the nuclease digestion of different AAV serotype cap genes that are then randomly reassembled to form chimeric genes (Koerber et al., 2009); peptide insertion, where every virus particle is engineered to display a random peptide at the capsid surface (Muller et al., 2003); or error prone PCR, which involves amplifying AAV cap genes in error-prone PCR reaction, with the resulting PCR products cloned to generate a diverse AAV plasmid library (Koerber et al., 2006). A more recent approach called CREATE (Cre-recombination-based AAV targeted evolution) uses Cre/lox technology to generate novel capsids and involves delivering capsid genomes containing loxP sites to animals with Cre expression in a defined cell population and then selective amplification and recovery of Cap sequences that transduced the target population (Deverman et al., 2016). A recent approach called BRAVE (barcoded rational AAV vector evolution), allows for large scale selection of capsids using only a single in vivo round of screening, unlike previous methods that require multiple rounds of enrichment (Davidsson et al., 2019). The directed evolution approach has had the greatest success in shifting AAV tropism toward certain glial cell types, and examples of this are described in the appropriate sections below.
Astrocytes
Astrocytes play a role in several homeostatic functions within the brain and spinal cord, including controlling uptake and release of neurotransmitters, modulating synaptic activity and the supply of metabolites to neurons. Astrocytes are also components of the blood-brain barrier (BBB), where they play a crucial role in BBB integrity and function (Sweeney et al., 2019). As well as these supportive roles in normal CNS function they are well known to respond in a number of CNS disorders including Alzheimer’s disease and other aging-related dementias (Dzamba et al., 2016; Garwood et al., 2017), Parkinson’s disease (Booth et al., 2017), Huntington’s disease (Palpagama et al., 2019), Amyloid Lateral Sclerosis (ALS) (Yamanaka and Komine, 2018) and traumatic conditions such as ischemia (Rossi, 2015), spinal cord injury (Gaudet and Fonken, 2018; Okada et al., 2018), and traumatic brain injury (Burda et al., 2016).
Astrocytes respond to CNS insult by transforming their phenotype via a process called reactive gliosis. Once stimulated by injury or inflammation, several cell pathways are activated that can be either damaging or protective, many of which could be targeted as a treatment. As a result of insult or neurodegeneration, astrocytes produce molecules such as inflammatory cytokines, which activate microglia and infiltration of peripheral immune cells leading to chronic inflammation (Stephenson et al., 2018). Following traumatic injuries of the CNS, activated astrocytes migrate to the lesion where they eventually form a glial scar that produces axonal growth inhibitors, preventing axonal regeneration (Burda et al., 2016; Okada et al., 2018). Astrocyte activation leads to the loss of proper synaptic and plasticity regulation. The control of glutamatergic transmission by astrocytes is adversely affected by oxidative stress and increased production of pro-inflammatory factors (Liu et al., 2006; Santello et al., 2011). Another key role of astrocytes is in the regulation of ion flux, and disruptions to this interfere with neurotransmitter uptake by astrocytes (Djukic et al., 2007; Kucheryavykh et al., 2007). Changes in astrocytic modulation of synaptic function have been demonstrated in a model of ALS (Benkler et al., 2013). Expression of the potassium Kir4.1 channel is lost in the SOD1 mouse (Kaiser et al., 2006) and high levels of endothelin-1, which leads to activation of the AMPA receptor, is produced by activated astrocytes in this model and leads to motor neuron cell death (Ranno et al., 2014). Astrocytes produce a number of growth factors, including nerve growth factor (NGF), brain-derived growth factor (BDNF), and fibroblast growth factor (FGF) which all play an essential role in neuronal function (Miyazaki and Asanuma, 2017). Reduced growth factor levels have been associated with neurodegenerative disease. A decrease in serum BDNF levels is associated with cognitive impairment in dementias and changes in BDNF levels in the hippocampus may be linked with emotional symptoms relating to Alzheimer’s disease (Budni et al., 2015).
As well as the negative effect of reactive gliosis, astrocytes can have protective effects. For example, activating the TGF-β signaling pathway in astrocytes limits the degree of inflammation following stroke (Cekanaviciute et al., 2014). As a reaction to oxidative stress, expression of Toll-like receptor-3 is increased in astrocytes, which upregulates anti-inflammatory cytokines whilst reducing the levels of pro-inflammatory cytokines (Bsibsi et al., 2006). The interferon pathway in astrocytes is also protective. Interferon regulatory factor 3 suppresses astrocyte inflammatory cytokine gene expression following inflammatory insult (Tarassishin et al., 2011). Interferon-1 production by astrocytes is known to regulate immune responses of brain endothelial cells via anti-inflammatory effects (Rothhammer et al., 2016).
Mutations in key astrocyte genes have been associated with neurodegenerative disease. Mutations of Fyn tyrosine kinase are associated with increased inflammatory responses in Alzheimer’s disease (Lee et al., 2016, 2017). Mutations in the gene encoding TGF-β have been associated with AD risk (Caraci et al., 2012, 2018). Mutations in the astrocyte protein apolipoprotein E4 can impair amyloid-beta (Aβ) clearance (Liu et al., 2013) and may be linked to oxidative stress and inflammation (Liu et al., 2015). PARK7, a regulator of astrocyte metabolism has been found to be mutated in cases of familial Parkinson’s disease (Bandopadhyay et al., 2004), is important for astrocyte mitochondrial function and its loss leads to oxidative stress (Kumaran et al., 2007; Larsen et al., 2011). Therefore, astrocytes are a potential cell target for AAV-mediated gene therapy strategies that modulate either the inflammatory or protective effects of reactive gliosis or through potentially expressing normal copies of mutated genes expressed in astrocytes.
AAV-Based Approaches for Targeting Transgene Expression to Astrocytes
A number of studies have looked at the tropism of AAV vectors for astrocytes. Most AAV serotypes demonstrate broad tropism without absolute specificity, but some differ in their absolute levels of transgene delivery to specific tissues. This depends on the experimental model as cell receptors for AAVs are likely expressed differently in vitro and in vivo (Royo et al., 2008). This is reflected in the variation of AAV tropism observed in each study as shown in Table 1.
In primary cultures of rat CNS cells, AAV5 appears to demonstrate the strongest glial tropism under the control of a constitutively active CAG or CMV promoter (Harding et al., 2006; Howard et al., 2008). Further, AAVs 1, 2, 6, 7, 8, and especially 9 can transduce both neurons and astrocytes (Howard et al., 2008; Royo et al., 2008; Schober et al., 2016). Newer, more novel serotypes have expanded the repertoire and potential for glial transduction, but many of these have yet to be compared with the naturally-occurring serotypes (Cearley et al., 2008).
In vivo animal studies have demonstrated some astroglial transduction with AAVs 1, 2, 5, 6, and 8 (Davidson et al., 2000; Wang et al., 2003; Harding et al., 2006; Klein et al., 2008; Hutson et al., 2012; Schober et al., 2016; Hammond et al., 2017), but AAVrh43 has been shown to have more specific astrocyte targeting when compared to AAV8 (Lawlor et al., 2009). In a separate study, AAV4 has demonstrated strong transduction of astrocytes when injected into the brain parenchyma (Liu et al., 2005). However, this has not been replicated in side-by-side comparisons with other serotypes. AAVs rh8 and rh10, in addition to rh43 and 9 can penetrate the BBB and transduce both neurons and glial cells (Foust et al., 2009; Gray et al., 2011; Yang et al., 2014). Glial transduction was more robust in adult animals, while transduction in neonatal animals was primarily neuronal (Foust et al., 2009; Gray et al., 2011; Yang et al., 2014). This may be an example of differential receptor expression causing altered tropism, in this case between neonatal and mature adult mice. Despite the existence of certain trends, many of the studies only compare a limited number of AAV serotypes and other variables are not controlled for between studies. Even AAV purification methods have led to differences in tropism (Klein et al., 2008). This indicates that for new experiments, it is worth comparing as many serotypes as possible to ensure the best choice for a specific set of experimental conditions.
Several synthetic AAVs that have been developed to improve transduction of the CNS have demonstrated improved ability to target astrocytes. AAV9P1 is a synthetic AAV9 variant that produces selective and robust astrocyte transduction in vitro (Kunze et al., 2018). This vector was identified from a screen of 30 artificial AAV variants, generated by introducing specific peptides into the AAV capsid sequence of AAV1, 2, 6, 8, and 9. While this variant was shown to have relatively good astrocyte specificity in vitro (the transduction rate for primary human neurons was around 10%), to date no data is available on whether this specificity is still seen in vivo. The CREATE approach led to the discovery of a variant AAV-PHP.B, which can transduce the CNS much more efficiently than AAV9, and is able to transduce the majority of astrocytes (> 75%) in multiple CNS regions of the mouse brain (Deverman et al., 2016). However, this does not have selectivity for astrocytes as it can effectively transduce neurons and oligodendrocytes. Another variant AAV-PHP.A improved the selective targeting to more than 80% of transduced cells being ALDH1L1 + ve. However, when AAV-PHP.A and AAV-PHP.B were used to transduce human iPSC-derived cortical spheroids, only around 15% of AAV-PHP.A transduced cells were glial fibrillary acidic protein (GFAP)-positive, compared with 40% for AAV-PHP.B. There did not appear to be any difference in selectivity for astrocytes between the two variants (Deverman et al., 2016). The AAV capsid Anc80L65, developed using in silico reconstruction of the viral evolutionary lineage transduces astrocytes with around four times the efficacy of AAV9 (Hudry et al., 2018). A study that utilized molecular evolution to engineer novel AAV variants using directed evolution and a panel of 4 distinct AAV libraries found variants that had increased astrocyte transduction. Two AAV mutants, ShH19 and L1–12, transduced astrocytes 5.5- and 3.3-fold, respectively, compared to the parent AAV2. However, the percentage of astrocytes showing expression from these vectors was very low; 15% for ShH19 and 9% for L1–12 (Koerber et al., 2009). While these vectors described above are not astrocyte-specific, the use of these with astrocyte-specific promoters could have potential.
Most of the work to date developing astrocyte-specific promoters for gene therapy has focused on the use of the promoter for GFAP. GFAP is an intermediate filament protein that is expressed almost exclusively by astrocytes (Yang and Wang, 2015). This fact has led to its promoter being used to direct transgene activity to astrocytes, and there is a large amount of literature that shows this can be achieved. Many studies have tested the incorporation of GFAP promoters to drive astrocytic-specific expression using viral vectors. Transgene expression under the transcriptional control of the 2.2 kb human GFAP promoter, gfa2, has been shown to be expressed in astrocytes throughout the brain (Lee et al., 2008). However, as with many cell-type-specific promoters, the large size of this promoter has severe limitations when used with AAV vectors, due to it occupying a considerable amount of the vector genome. Different strategies have been employed to shorten the GFAP promoter to make it more suitable for use in AAV vectors. An AAV vector containing a truncated 448 base-pair gfa28 promoter (Lee et al., 2006, 2008), was able to drive gene expression much more strongly than the full-length promoter when tested in vitro. However, when this promoter was used in vivo in mice, the level of transgene expression driven by this promoter was comparable to the full-length gfa2 promoter, expression was restricted to certain CNS regions, and neuronal expression was observed as well as in astrocytes. Based on this finding Lee et al. (2008) created transgenic mice with promoters containing different enhancer fragments to determine which were required to silence neuronal signaling, and to restrict expression to specific brain regions. This work led to the discovery of a 681 bp GFAP promoter, gfaABC1D, which exhibited mostly the same expression pattern in the brain as the full-length 2,210 bp gfa2 promoter but had a twofold greater expression that was largely restricted to astrocytes (Lee et al., 2008). Similarly a 681 bp gfaABC1(mC1.1)D variant had expression limited to astrocytes in the dorsal and caudal cortex, hippocampus and caudal vermis of the cerebellum. This study demonstrates that it may be possible to further limit gene expression to specific glial populations by modifying cell-specific promoters (Lee et al., 2008). de Leeuw et al. inserted additional copies of the GFAP enhancer regions to determine if these would increase its transcriptional activity. Injection of an adenoviral construct containing the gfa2 promoter engineered to contain three copies of the B enhancer region [gfa2(B3)] resulted in greater gene expression in astrocyte cell cultures and expression that was limited to GFAP-positive cells when injected into the basal ganglia of mice (de Leeuw et al., 2006). However, again due to its size, there are issues in using this in the context of AAV vectors.
A number of studies have used AAV vectors containing the 681 bp gfaABC1D with the goal of obtaining astrocyte specificity (Xie et al., 2010; Theofilas et al., 2011; Dirren et al., 2014; Dvorzhak et al., 2016; Vagner et al., 2016; Taschenberger et al., 2017; Griffin et al., 2019, 2020; Testen et al., 2020). While several studies show good evidence of astrocyte specificity (Xie et al., 2010; Theofilas et al., 2011), other studies report transduction of other cell types (Taschenberger et al., 2017; Griffin et al., 2019). For instance, we observed transgene expression in lower motor neurons but not in neurons of the dorsal horn following vector infusion in the adult rat spinal cord (Griffin et al., 2019). Very high levels of transgene expression in lower motor neurons was also reported with the full-length GFAP promoter (Peel and Klein, 2000). One approach that has been used to overcome the issue of lack of astrocyte specificity is the incorporation of cell-specific microRNAs (miRNAs) to suppress off-target transgene expression in particular cell types (Brown et al., 2006). Endogenous expression of miR124, which is specific to neurons is able to repress gene expression in neuronal cells (Colin et al., 2009) and addition of miRNA recognition sequences to viral constructs can suppress leaky gene expression from AAV vectors (Shimizu et al., 2014). When target sequences for miR124 were included in the 3′ UTR of an AAV expression plasmid containing a transgene under the control of the gfaABC1D promoter, neuronal transgene expression in the rat striatum was completely absent compared to around 10% neuronal expression with the gfaABC1D promoter alone (Taschenberger et al., 2017). However, the presence of the miR sequence strongly reduces the number of astrocytes expressing the transgene to around 10% of that seen with the gfaABC1D promoter alone, calling into question the usefulness of this approach for improving astrocyte specificity.
One issue with the use of a GFAP promoter is that the levels of GFAP expression can be variable in different parts of the CNS and relatively low in some brain regions (Hajos and Kalman, 1989; Kalman and Hajos, 1989). Therefore using this promoter may not always be appropriate. Aldehyde dehydrogenase family 1, member L1 (ALDH1L1) has been characterized as a pan-astrocytic marker that is found more homogeneously throughout the brain than GFAP (Cahoy et al., 2008). Mudannayake et al. (2016) tested several different AAV serotypes under the control of a putative rat Aldh1l1 promoter for astrocyte selectivity in the rat substantia nigra pars compacta (SNpc) brain region and found transgene expression was exclusively expressed in neurons and independent of AAV serotype used. Neuronal-specific transgene expression was also found following intrahippocampal vector infusion, but expression was found in both neurons and astrocytes in the striatum following intrastriatal vector infusion. In a later study by Koh et al. (2017) using a human ALDH1L1 promoter, an AAV-hALDH1L1-Cre vector was injected into several brain regions of the Ail4 (RCL-tdTomato) mouse, found tdTomato gene expression was also seen to be predominantly neuronal in most brain regions analyzed. Interestingly, in the thalamus, this expression pattern was reversed, with the majority of tdTomato expression found in astrocytes (92%) with minimal neuronal expression (2%). Therefore, the use of the ALDH1L1 promoter may have the potential for targeting astrocyte expression in the thalamus, especially as GFAP expression in this region appears to be very low (Kalman and Hajos, 1989).
Other potential astrocyte-specific gene promoters have also been suggested (Kery et al., 2020) including Slc1a3, which codes for the glutamate transporter SLC1A3 (also known as GLAST or EAAT1) (Sery et al., 2015). A 636 bp region 5′ upstream of the gene can drive strong gene expression, and so this relatively small promoter might have potential use in AAV vectors (Hagiwara et al., 1996). Another potential promoter is Gjb6, which codes for the gap junction protein Connexin30. Connexin30 is only expressed in gray matter astrocytes and so this promoter could be used to specifically target these populations (Nagy et al., 1999; Sohl et al., 2004).
Oligodendrocytes
Oligodendrocytes are the myelin-producing cells of the CNS. This myelin forms an insulating membrane that wraps tightly around axons that allows for rapid signal conduction and is crucial for normal CNS function (Kuhn et al., 2019). Oligodendrocytes and the myelin sheath also provide trophic support for axons, such as the production of neurotrophic factors (Bradl and Lassmann, 2010) and lactate that is passed to axons to partake in the metabolic pathways involved in producing ATP (Bercury and Macklin, 2015). Oligodendrocytes are particularly sensitive to excitotoxic and cytotoxic factors and damage of the CNS. The high metabolic rate required for myelination and the presence of high levels of iron, which is required as a co-factor for this process, can lead to high levels of reactive oxygen species, free radical formation and lipid peroxidation. This combined with the presence of low levels of the antioxidant enzyme glutathione in oligodendrocytes makes this cell type particularly sensitive (Bradl and Lassmann, 2010). Oligodendrocyte pathology is, therefore, present in a range of CNS disorders (Fern et al., 2014). This includes leukodystrophies, which are a group of inherited disorders that lead to white matter degeneration (Vanderver et al., 2015), multiple sclerosis (Procaccini et al., 2015), Alzheimer’s disease (Nasrabady et al., 2018), Parkinson’s disease (Bohnen and Albin, 2011), Fragile X syndrome (Filley, 2016), ischemic stroke (Wang et al., 2016), spinal cord, and traumatic brain injury (Fern et al., 2014; Hassannejad et al., 2019; Pukos et al., 2019), as well as in conditions such as schizophrenia and depression (Wang et al., 2014; Najjar and Pearlman, 2015). Gene therapy approaches have the potential to protect against toxicity or to promote remyelination.
AAV-Based Approaches for Targeting Transgene Expression in Oligodendrocytes
No natural AAV capsid that exhibits primary oligodendrocyte tropism has been described. While a small number of serotypes can transduce this cell type when combined with pan-cellular markers, the overall transduction efficiency is low (Lawlor et al., 2009). These include AAV8 and 9 when paired with a cytomegalovirus (CMV) promoter (Hutson et al., 2012; Bucher et al., 2014) and AAV8 with a chicken β-actin (CBA) promoter (Gray et al., 2010). In a preclinical study of metachromatic leukodystrophy, AAVrh10 has been found to transduce oligodendrocytes when driven by a cytomegalovirus/β-actin hybrid (CAG/cu) promoter (Piguet et al., 2012). AAV-PHP.B’s widespread transduction of the mouse CNS includes oligodendrocytes when using a CAG promoter (Deverman et al., 2016). DNA shuffling and directed evolution approaches have also produced a chimeric capsid that transduced both neurons and oligodendrocytes (Gray et al., 2010), with a novel AAV capsid shown to have excellent oligodendrocyte preference (Powell et al., 2016). The Olig001 vector, which contains a chimeric mixture of AAVs 1, 2, 6, 8, and 9 had a > 95% specificity for oligodendrocytes (as assessed by GFP expression) following striatal vector infusion into rats even though transgene expression was under the control of the CBA promoter. The other 5% of cells transduced were neurons, and no astrocyte or microglial expression was seen.
In order to specifically target transgene expression to oligodendrocytes, a number of cell-specific promoters have been trialed. Initial studies used the promoter from the gene for myelin basic protein (MBP), which is a major constituent of the myelin sheath of both oligodendrocytes and Schwann cells. A 1.9 kb Mbp promoter was able to drive GFP expression in the MOCH-1 transformed oligodendrocyte cell line and primary rat oligodendrocyte cultures (Chen et al., 1998). In primary cultures, GFP expression was almost exclusively in oligodendrocytes, although some expression was observed in astrocyte-like cells. When AAV vector was injected into the cerebral hemisphere of mice, GFP expression appeared to be only in oligodendrocytes and was not seen in astrocytes, microglia or neuronal filaments. Building on this work, the authors then investigated cell and tissue specificity and the duration of transgene expression following injection of the vector into different regions of the mouse brain (Chen et al., 1999). High-levels of GFP expression were almost exclusively seen in white matter areas of the brain with very limited expression in areas of gray matter. When cell-specificity was determined based on morphology, anatomic location, and cell-type specific immunohistochemistry, GFP expression was found to be almost exclusively in oligodendrocytes with no expression seen in neurons, astrocytes or microglia.
While this data suggests that cell-specific promoters such as those for MBP can be used to target oligodendrocytes specifically, it is also important to determine how the stage of development would impact this. This is essential to understand when using somatic gene transfer approaches for glia in the developing brain to treat genetic conditions such as leukodystrophies. von Jonquieres et al. (2013) tested this by injecting a chimeric AAV 1/2 vector expressing GFP under the control of the Mbp promoter into the striatum of mice at postnatal day 0 (P0) (neonates), P10 and P90 (adults). While in the P10 and P90 animals, the majority of GFP staining was localized to oligodendrocytes, for the P0 animals only around 25% of GFP + ve cells were oligodendrocytes, with the majority (56%) being astrocytes and the number of oligodendrocytes transduced was very low at only 3%. While for the P10 and P90 animals the majority of transduced cells were oligodendrocytes, there was a different pattern in the degree of transduction of other cell types. In the P90 animals, around 20% of transduced cells were neurons, and no GFP was detected in astrocytes. However, in the P10 animals, no GFP was detected in neurons, but it was seen in astrocytes, although in small numbers (3.6% of GFP + ve cells). Perhaps most interestingly at P10, transduction was almost exclusively in oligodendrocytes (96%), compared with around 75% in the adult mice. The authors suggested that this vector could allow for treatment of developmental gliopathies as brain development in the P10 mouse corresponds to that seen at the beginning of the last trimester in human pregnancy (Clancy et al., 2001). When the vector was injected into the brains of P10 homozygous ASPAlacZ/lacZ mice, which are a model of the early onset leukodystrophy Canavan disease, a similar pattern of oligodendrocyte specificity was seen as in WT mice (von Jonquieres et al., 2013). While this is promising for the potential to treat developmental gliopathies such as leukodystrophies, due to the marked difference in cell expression patterns seen between P0 and P10 animals, much more work would be required to understand changes in expression during human development before it could be used for such a purpose. In a subsequent paper these authors looked at transduction of non-chimeric AAV variants rh20, rh39, and cy5 and their ability to drive expression under the control of the Mbp promoter, in the striatum of adult mice. They showed that oligodendrocyte specificity was greater for rh39 (91%) and cy5 (87%) when compared to AAV 1/2 (78%) (von Jonquieres et al., 2016).
While the Mbp promoter seems to have potential for oligodendrocyte targeting, its relatively large size and the poor oligodendrocyte specificity following early neonatal vector delivery (von Jonquieres et al., 2013) would limit its usefulness. However, MBP-driven transgene expression from an AAV vector has been shown to be effective in a model of oligodendrocyte disease. The Cx32/Cx47 double-knockout mouse is a well-characterized model of hypomyelinating leukodystrophy-2. An AAV vector containing the Gjc2/Cx47 gene under the Mbp promoter was delivered to the internal capsule of P10 animals. This resulted in greater survival and significant motor improvement, improved myelination and reduced oligodendrocyte apoptosis, inflammation and astrogliosis (Georgiou et al., 2017).
The myelin-associated glycoprotein (MAG) has been tested for its ability to drive oligodendrocyte-specific expression using AAV (von Jonquieres et al., 2016). MAG is a protein responsible for recognition of axons and maintenance of myelin. Based on an in silico analysis of the MAG promoter, the authors generated AAV plasmids expressing GFP under the control of either a 2.2 kb MAG promoter or truncated 1.5 and 0.3 kb fragments. All three AAV constructs were packaged into cy5 vectors and injected into the striatum of adult mice. All three constructs showed very good oligodendrocyte specificity, with GFP expression almost exclusively confined to oligodendrocytes, with between 98.4% for the 2.2 kb to 90.7% for the 0.3 kb promoter. The percentage of oligodendrocytes transduced was 65, 82, and 57% for the 2.2, 1.5, and 0.3 kb promoters, respectively. When the vector containing the 2.2 kb promoter was injected into the brains of P0 pups, the specificity for oligodendrocytes was seen to be around 80% in comparison to the Mbp promoter where only around 25% specificity for oligodendrocytes was seen, suggesting use of the MAG promoter may be better suited to treatment of developmental gliopathies. However, the authors did not test the smaller promoters. The specificity and percentage of oligodendrocytes transduced appeared to be less for the smaller promoters. It would have been interesting to look at their profile in P0 pups, especially as the 0.3 kb promoter is likely to be the most useful for use with AAV vectors due to its small size.
A recent report by Powell et al. (2020) demonstrated that modification of constitutive promoters can shift gene expression from neurons to oligodendrocytes. Infusion of an AAV9 vector with a full-length CBA promoter into the rat striatum led to predominantly neuronal (88.4%) transgene expression. However, the use of a truncated CBA promoter (CBh) showed only 46% of neurons but 38% of oligodendrocytes were labeled. When an AAV2 vector was used, expression was predominantly neuronal for both promoters. This suggests that certain AAV capsids can influence promoter activity between different cell types. When six glutamate residues were inserted into the VP2 region of the AAV9 capsid, this shifted the transgene expression profile from the full-length promoter from neurons to oligodendrocytes (80%). However, when these amino acids were changed in AAV2, no change in expression was seen. This ability for capsid sequence to influence gene expression could be used to target particular cell types.
Microglia
Studies have tested various AAV serotypes for their ability to transduce microglia, and the level of transduction is generally low (Maes et al., 2019). In early studies, Bartlett et al. (1998) found that while AAV2 was able to transduce microglia, as determined using Cy3-labeled virions, no transgene expression was observed. Similarly, no transgene expression was observed in primary microglia cultures following application of AAV1–9 or rh10 vectors (Rosario et al., 2016) although others showed 80% of the cells expressed GFP when AAV2-CMV-eGFP was applied to primary neonatal and adult microglia (Su et al., 2016). When different serotypes (AAV2, AAV5, AAV6, AAV8, and AAV9) were applied to cultured neonatal microglia, AAV6-CMV-eGFP produced an 80-fold increase in transgene expression compared to AAV2-CMV-eGFP while AAV8 resulted in a 25-fold increase in transgene expression. However, when the expression of microglial M1 and M2 activation markers was assessed to determine the effect of viral vector-mediated transduction and/or transgene expression, AAV6 and AAV9 elevated expression of the scavenger receptor MARCO, while the M2 phenotype marker YM1 was down-regulated in cells treated with AAV5 and AAV8. AAV2 did not produce any significant change in any activation markers assessed or influence the phagocytic activity of the microglia (Su et al., 2016). Therefore, development of an AAV to target microglia in vivo will require an understanding of the effect a serotype will have on the activation state of microglia, to prevent any unwanted, potentially detrimental inflammatory side-effects of the treatment.
Several macrophage-specific promoter sequences, human CD11B and CD68, and murine F4/80, have been assessed for their ability to provide specific microglial targeting both in vitro and in vivo. When AAV2 and AAV5 vectors expressing transgenes under control of these promoters were applied to rat primary microglia cultures, transduction efficiencies of around 25% for F4/80, 10% for CD68, and only one cell per field-of-view for CD11B were reported for both vectors. While these transduction efficiencies are low, transgene expression did appear to be microglia-specific as no expression was detected following transduction of rat primary neuronal cultures (Cucchiarini et al., 2003). When AAV5 vector containing the F4/80 promoter was injected in to brains of adult rats, transgene expression appeared largely localized to F4/80-labeled cells.
Modified AAV capsids have also shown some success in improving transduction of microglia. Rosario et al. modified the AAV6 capsid (AAV6) through site-directed mutagenesis of two tyrosine residues to phenylalanine and a threonine to valine (Y731F/Y705F/T492V), which has been shown to increase transduction efficiency in monocyte-derived dendritic cells (Pandya et al., 2014). The combination of this modified AAV capsid and either the F4/80 or CD68 promoter resulted in > 95% transduction of microglia and no neuronal or astrocytic expression in mixed glial or primary microglial cultures. When tested in vivo, microglial specificity was shown to be around 75% for F4/80 and 20% for CD68 following injection into P0 rat pups and the adult rat hippocampus, although the total numbers of microglia showing expression appeared to be low (Rosario et al., 2016).
An important consideration is the impact that the activation state of microglia will have on expression from specific vectors used. For example, levels of CD68 are greater in pro-inflammatory microglia, which could explain the low level of expression seen in a healthy brain. This expression pattern could potentially be of use when targeting activated microglia, where it is likely that gene expression would be highest in this population of cells.
Müller Cells
Müller glial cells are a major cellular component of the retina and engage in numerous roles vital to retinal function, such as structural, nutritional, homeostatic, osmotic, metabolic, and growth factor support to retinal neurons (Bringmann et al., 2006; Reichenbach and Bringmann, 2013). These glial cells also interact with blood vessels and are involved in the function of the blood–retina barrier and regulating retinal blood flow (Newman, 2015). In response to retinal injury, stress, or degeneration, Müller glial cells undergo active gliosis (Graca et al., 2018). A number of diseases including diabetes, macular edema, and ischemia lead to hypertrophy and hyperplasia of the Müller glia that contributes to a chronic inflammatory retinal environment and ultimately cell death (Coughlin et al., 2017). It has been demonstrated that Müller cells have a neuroprotective phenotype. For example, they respond to disease or injury-mediated photoreceptor stress by upregulating secretion of neurotrophic factors such as basic fibroblast growth factor (bFGF) and ciliary neurotrophic factor (CNTF) (Greenberg et al., 2007). Mutations in several genes expressed in Müller glial cells have been linked to retinal dystrophies (Maw et al., 1997; Zhao et al., 2015). Therefore Müller glial cells have been proposed as targets for therapeutic approaches to retinal diseases such as gene replacement therapy or boosting neurotrophin secretion to enhance their neuroprotective properties (Dorrell et al., 2009; Pellissier et al., 2015). As these cells transverse the entire thickness of the retina, they would have the ability to facilitate expression of factors throughout all layers of the retina (Reichenbach and Bringmann, 2013).
A number of different AAVs have been shown to have tropism for Müller cells. When AAV 1, 2, and 5 were delivered to the retinas of E13 mice via subretinal injection, only AAV1 and 2 transduced Müller cells (Surace et al., 2003). Intravitreal injection of AAV 1, 5, 8, and 9 showed occasional Müller cell transduction in 2 month-old C57BL/6 mice (Lebherz et al., 2008). Subretinal injection of AAV8 and 9 also shows Müller cell transduction in 4 week-old mice, but transduction was not seen with AAV5 (Allocca et al., 2007). When retinal transduction by AAV2 was compared following intravitreal injection into P0, P14 and adult mice, transduction was seen in Müller cells at all ages with highest levels of transduction observed in adults, where around 10% of AAV transgene expression was observed (Harvey et al., 2002). AAV4 and 6 also have tropism for Müller cells (Hellstrom et al., 2009). In all these cases the number of Müller cells transduced was low, compared with other retinal cell populations. However, Pellissier et al. (2014) reported that use of AAV9 vectors led to transduction of around 30% of Müller cells along with photoreceptor cells and retinal pigment epithelia. These authors used AAV9 under the control of a CMV promoter to deliver the Crumbs-homolog-2 (CRB2) protein, which is expressed in Müller cells and photoreceptors, to the retinas of Crb2 and Crb1Crb2F/+conditional knock-out mice, which are both models of severe, progressive retinal degeneration (Pellissier et al., 2015). This led to improved retinal function and morphology compared to untreated animals, demonstrating the potential for AAV to target and modify Müller cell-related gene mutations.
As Müller cells express GFAP, this has been used to target transgene expression to these cells. AAV-mediated delivery of neurotrophic factors, under control of the GFAP promoter has been shown to be protective in animal models of retinal disease. A mouse model that has a defective gene for the VLDL receptor (VLDLR) shows excessive retinal neo-vascularization (NV), which is associated with common causes of vision loss (Klein et al., 2004). The retinal phenotype of these animals is similar to that seen in human patients with certain retinal NV diseases, including glial abnormalities as evidenced by increased Müller cell activation associated with the area of NV. In order to target activated Müller cells as a potential therapy, Dorrell et al. (2009) tested an AAV2 vector containing GFP under the control of a minimal 0.35 kb human GFAP promoter consisting of the A/B and D sequences (Besnard et al., 1991). When the vector was delivered by intravitreal injection to wild-type mice, limited GFP expression was noted in Müller cells while in Vldlr–/– mice, a greater number of Müller cells expressed GFP, and this was also associated with transgene expression in processes adjacent to areas of NV. GFP expression appeared to be Müller cell-specific, unlike non-specific expression mainly localized to ganglion cells that was found using a non-selective CAG promoter. The authors then used this system to deliver neurotrophin-4 (NT-4), which is known to protect neurons in models of retinal degeneration. Using an AAV-GFAP-NT4 vector, a similar expression pattern of NT-4 as found for GFP was observed, and this was associated with protection of the retina from neuronal degeneration.
A number of AAV variants have shown enhanced targeting for Müller cells. Based on work which determined novel AAV capsids that more efficiently transduced both primary human astrocytes in vitro and rat astrocytes in vivo (Koerber et al., 2009) and the shared properties between astrocytes and Müller cells, Klimczak et al. (2009) explored the effectiveness of a number of AAV variants for their potential for intravitreal transduction of Müller cells. Compared to very low transduction from the parent AAV6, one novel variant named ShH10 demonstrated a striking increase in both transduction efficiency and specificity for Müller cells. ShH10 was able to produce diffuse expression throughout the retina with approximately 94% of transduced cells being Müller cells, with limited transduction in interneurons (2%), and retinal ganglion cells (4%) compared with approximately 76% of transduced cells being Müller cells, 3% interneurons, and 21% retinal ganglion cells for the related AAV2 virus. ShH10 was also more efficient at transducing Müller cells, transducing 22 vs. 14% of total Müller cells compared with AAV2. These authors then extended to testing the ability of the ShH10 vector to deliver GDNF to the retina via Müller cells, and its ability to modulate retinal degeneration in the S334-4ter rat model of retinitis pigmentosa (Dalkara et al., 2011). To optimize GDNF production by improving the transduction capability of the vector, they modified ShH10 via a tyrosine to phenylalanine amino acid change to create ShH10.Y445F. Mutations in conserved tyrosine residues in AAV capsids have been shown to enhance vector transduction including in the retina (Zhong et al., 2008; Petrs-Silva et al., 2009). This vector led to transduction of approximately 50% of Müller cells in the retina of TgS334 rats. This was over 100% greater transduction than that seen in WT rats, demonstrating that this mutation allows for a greater transduction efficiency, although the authors also acknowledge that the degenerating retina may be a more permissive environment for AAV transduction. When this vector was used to deliver a GDNF transgene in the diseased retina, they were able to demonstrate long-term expression of therapeutic and safe levels of GDNF for up to 5 months, and this was accompanied by a slowdown in retinal degeneration, as assessed by electroretinogram and histology. Interestingly, when the ShH10Y vector was used to specifically target Müller cells in the Crb2 and Crb1Crb2F/+ models described above, no improvement in retinal function was seen (Pellissier et al., 2015), as opposed to AAV9, which targeted Müller cells and photoreceptor cells and led to functional improvement. This demonstrates that depending in the disease, specific targeting of just one cell type with an AAV may not be appropriate. These vectors combined with the use of cell-specific promoters have been used in an attempt to further improve Müller cell specificity (Pellissier et al., 2014). Pellissier et al. (2014) looked at the transduction profile of AAV6 and the two AAV6-derived variants ShH10 and ShH10Y via injection into the vitreous of Crb1–/– mice, a model of retinal dystrophy. The ShH10Y variant had an enhanced ability to transduce Müller cells, with 2–3 times the transduction efficiency of the other vectors. This supports the idea that the increase in transduction seen by Dalkara et al. (2011) is due to the vector modification and not the diseased environment. The authors tested ShH10Y vectors with GFP expression under the control of a full-length (1.8 kb) or minimal (0.4 kb) Cd44 promoter or the 2.8 kb RLBP1 promoter (Pellissier et al., 2014). However, the Cd44 promoters showed only low levels of expression, that was not restricted to Müller cells. Conversely, the RLBP1 promoter caused high levels of expression that was restricted to Müller cells. However, due to its size, a shortened promoter would likely be required for it to be of practical use for expression of most genes. More recently Cao et al. (2020) found using an AAV2/6 mutant rAAV2/6-S663L led to increased tropism for Müller cells when injected intravitreally into 5 week-old mice and when gene expression as driven by the GFAP promoter that gene expression was specifically seen in Müller cells.
Due to the fact that natural “cell-specific” promoters often target expression to more than one cell type, attempts have been made to screen a library of promoters driving transgene expression in regions of interest. Jüttner et al. developed a library of 230 AAVs, each with a different synthetic promoter and tested their transduction in the retina of mice, non-human primates and humans (Juttner et al., 2019). This screen found a number of synthetic promoters that targeted Müller cells with 100% specificity and one in particular (labeled ProB2) transduced 45% of Müller cells.
Attempts have been made to create vectors that will restrict gene expression to an injured retina. Hypoxia in the eye is known to be a causative factor in a number of retinal diseases, such as diabetic retinopathy and age-related macular degeneration (Campochiaro, 2015). When an AAV2 vector containing a hybrid promoter consisting of the GfaABC1D promoter and hypoxia-responsive and aerobically silenced elements (HRSE) (Wenger, 2002) was injected into a mouse model of oxygen-induced retinopathy, high levels of gene expression was seen in Müller cells of damaged eyes but was completely absent in mice exposed to normoxia (Prentice et al., 2011). This approach would allow for gene expression only in regions of hypoxia, which could be beneficial in reducing off-target effects of gene expression in healthy tissue.
Peripheral Nerve
Schwann Cells
Schwann cells are the major glia of the PNS. Two types of Schwann cells are found; myelinating, which form a myelin sheath around peripheral axons and non-myelinating, which are involved in maintaining ionic balance and providing support to axons (Kidd et al., 2013). Many neuropathies are related to mutations or inflammation of immune cells (Martini and Willison, 2016). Charcot-Marie-Tooth (CMT) disease, or non-syndromic inherited peripheral neuropathy, is one of the most common neurogenic disorders. Clinical characteristics include distal weakness, sensory loss and deformities of the feet. It is a genetically heterogeneous set of disorders with over 100 different genes implicated in disease causation. Mutations can occur in myelinating Schwann cells, and these cause demyelinating forms of neuropathy (Laura et al., 2019; Sargiannidou et al., 2020). A number of gene therapy approaches using lentiviral vectors for gene replacement strategies in models of CMT have been trialed and demonstrated promise (Eggers et al., 2013; Allodi et al., 2014; Sargiannidou et al., 2015; Kagiava et al., 2016). To date, only a few studies have looked at the feasibility of using AAV vectors to target Schwann cells in CMT.
Several AAV vectors demonstrate tropism for Schwann cells. Hoyng et al. (2015) tested AAV 1–9 for their ability to transduce cultured primary rat and human Schwann cells and rat and human nerve segments. This study showed a few differences in tropism between the species and between cells and nerve segments. AAV1 was the most efficient at transducing rat Schwann cells, with twice the number of transgene-expressing cells compared to any other AAV tested. In human Schwann cells, AAV2 and AAV6 were seen to perform equally well. However, a different transduction pattern was seen in nerve segments. AAV 1, 5, 7, and 9 were all equally successful in transducing rat nerve segments, whereas AAV2 was superior in human nerve segments. More recently, AAV1, AAV2, AAV6, and AAV-DJ were found to efficiently transduce primary human Schwann cells, with levels of transgene expression for AAV6 and AAV-DJ being 2–3 times that seen in AAV1 or 2 (Bai et al., 2019). A study by Homs et al. (2011) to determine whether AAV vectors could specifically target Schwann cells found that sciatic nerve injection of AAV8 led to specific Schwann cell expression with limited (< 1%) neuronal gene expression, unlike the CNS where significant neuronal tropism is seen. This could be explained by differences in the expression of receptors between the CNS and PNS (Homs et al., 2011).
Due to the presence of common transcription binding elements, a number of oligodendrocyte promoters are also able to drive gene expression in Schwann cells. However, the size of these promoters precludes their use in AAV vectors. A full-length Mbp promoter is capable of driving expression in Schwann cells but the shorter 1.3 or 1.9 kb fragments shown to drive expression in oligodendrocytes do not contain the enhancer elements required for Schwann cell expression (Mathis et al., 2000; Forghani et al., 2001). The 2,3-cyclic nucleotide (Cnp) and proteolipid protein (Plp) promoters have been shown to be expressed in Schwann cells, and a Cnp promoter has been used to drive expression in oligodendrocytes using lentivirus, but are again too big to use with AAV (Kagiava et al., 2014; Schiza et al., 2015). The 2.2, 1.5, and 0.3 kb fragments of the MAG promoter should drive expression in Schwann cells, but this has not been tested. These promoter fragments contain an RNF10 site which has been suggested would allow transgene expression in Schwann cells (von Jonquieres et al., 2016). The myelin-specific myelin protein zero (Mpz) promoter has been shown to have high selectivity for Schwann cells. This along with its relatively short length (1.1 kb) (Messing et al., 1992) and its successful use in targeting Schwann cells using lentivirus (Sargiannidou et al., 2020), suggests it is likely to be a good candidate for use with AAV vectors.
Satellite Cells
Another glial cell type found in peripheral nerves are satellite cells. These are associated with neurons in the sensory, sympathetic and parasympathetic ganglia and are thought to play similar functions to astrocytes in the CNS (Hanani, 2005, 2010). Following nerve injury, satellite cells become activated, leading to chemokine/cytokine release (Ohara et al., 2009; Souza et al., 2013). This activation is an important component of pain signaling, and dysregulation can lead to chronic pain (Gosselin et al., 2010; Ji et al., 2013). Modulation of satellite cells can alter the pain responses after nerve injury, and genetic manipulation of satellite cells has been proposed as a potential treatment for pain control (Jasmin et al., 2010). A recent study tested different AAV vectors for their ability to transduce satellite cells. AAV6 as well as AAV shH10, and AAV shH19, which have been shown to have a preference for transduction of Müller glia in the retina were used and injected into the dorsal root ganglion (DRG) of adult rats. Strong expression that was restricted to neurons was observed when transgene expression was under the control of a CMV promoter. Conversely, when expression was driven by a GFAP promoter, expression was almost exclusively in satellite cells (Xiang et al., 2018). Interestingly the AAV shH10 vector, the novel capsid variant of AAV6 that demonstrates almost exclusive glial tropism in the retina, was almost exclusively neuronal on the DRG. This suggests that cellular receptors for different AAVs can vary between different glial populations in the CNS and PNS.
Enteric Glia
The gut contains its own nervous system, termed the enteric nervous system (ENS) which regulates gastrointestinal functions such as motility, local blood flow, transport of molecules across the mucosa and modulates endocrine and immune functions (Costa et al., 2000). It consists of two interconnected ganglionated plexuses that surround the digestive tract. As well as enteric neurons, the ENS contains enteric glia, which are present in numbers up to 6 times higher than the number of neurons. These glia express astrocyte markers such as GFAP and S100 and are typically thought of as astrocytes of the gut. The role of enteric glia in gut function is not completely understood, but they are involved in regulating motility via interactions with enteric neurons (McClain et al., 2014). Furthermore, they also play a role in the maintenance of gut epithelial integrity and loss of enteric glia has been shown to lead to intestinal inflammation (Aube et al., 2006). It has also been suggested that enteric glia may influence barrier integrity through interaction with immune cells (Ibiza et al., 2016). The ability of enteric glia to mediate immune responses could be a possible underlying mechanism for Crohn’s disease (Pochard et al., 2018) and they are known to contribute to the inflammation that occurs with conditions of the gut including irritable bowel disease, enterocolitis, and gut infections (von Boyen et al., 2004; Linan-Rico et al., 2016; Lilli et al., 2018). An age-related decrease in the number of enteric glia may play a role in chronic, low-grade inflammation that is associated with age-related gut motility disorders (Franceschi et al., 2007). Treatments for Crohn’s disease are limited and can have serious side-effects (Seyedian et al., 2019). Therefore, gene therapy targeting of enteric glia with neuroprotective strategies in the early stage of such diseases, may be a useful approach to help treat these disorders.
Several studies have looked at the delivery of AAV to the gastrointestinal tract. Oral or enema delivery of AAV serotypes 1–10 have shown transduction of GI tissue including the lamina propria and endothelial cells, but transduction of the ENS was not determined (Shao et al., 2006). When AAV vectors were injected directly into the descending colon, neuronal and enteric glia transduction was observed in the myenteric and submucosal plexuses (Benskey et al., 2015). Of the serotypes tested, AAV serotypes 1, 5, 6, 8, and AAV8-double Y-F + T-V showed both neuronal and glial transduction. However, the authors note that glial transduction was rare for most serotypes except for AAV6, where the level of transduction was roughly equal between neurons and glia. Systemic delivery of AAV serotypes has demonstrated tropism for enteric glia, although few studies have attempted to target glia directly. Both intravenous and intrathecal delivery of AAV9 to juvenile mice leads to transduction of enteric ganglia, but no glial transduction was reported (Schuster et al., 2014). Gombash et al. (2014) showed that intravenous injection of scAAV8 and scAAV9 (which have known tropism for astrocytes) containing a GFP reporter into neonatal and juvenile mice led to GFP expression that was found exclusively in myenteric neurons. GFP expression was occasionally detected in S100 positive glia in neonatal animals for scAAV8. To determine if tropism could be directed toward glia, the authors engineered an AAV9 vector with GFP expression under the control of the GFAP promoter, as GFAP expression is present in enteric glia, similar to astrocytes in the CNS. Following intravenous injection of an AAV9-GFAP-GFP vector into neonates, GFP expression was found principally in enteric glia of the myenteric ganglia. However, it should be noted that the number of glial cells transduced was less than 5%. This study also trialed AAV 6, which has been shown to cross the BBB (Zhang et al., 2011) but no transgene expression was detected. This is likely due to differences in vasculature between the ENS and CNS that impact on the virus’s ability to cross the endothelial barrier. In another study (Buckinx et al., 2016) using AAV8 and AAV9 to transduce myenteric and submucosal neurons, about 25–30% of neurons were found to be expressing eGFP. All subtypes of neurons expressed GFP, but no expression was seen in glia (assessed using S100 and GFAP). While it appears that AAV-mediated transgene expression can be somewhat tailored toward enteric glia, further work is required to make this a viable approach to target these cells. One issue is the use of a GFAP promoter and systemic delivery of AAV9, as this would make specific targeting of enteric glia, without potential off-target effects on other GFAP-expressing cells, impossible. Currently, an enteric-glia-specific promoter has not been identified. However, transcriptional profiling of enteric glia suggests that they are developmentally closer to oligodendrocytes and Schwann cells than astrocytes and can adopt some Schwann cell markers (Rao and Gershon, 2018). However, the gut microenvironment shifts these cells toward an astrocyte-like phenotype as shown by expression of GFAP, which is not seen in Schwann cells (Gulbransen and Christofi, 2018). Therefore, enteric glia are considered a novel type of glia, and that an enteric glia-specific promoter may need to be developed. This different phenotype may also explain the low transduction efficiency of enteric glia compared with other glial types. Further testing of novel AAVs would likely be needed to make this a viable approach to therapy.
Conclusion
Through the use of specific serotypes and cell-specific promoters, glial cell targeting is possible and has shown some promise in experimental models, particularly in the retina. However, there are still several hurdles that need to be overcome for truly glial-specific AAV tropism to be achieved for use in human gene therapy. While several AAV serotypes have increased tropism toward glia, they are not glia-specific, and so any gene therapy transgene will be present in other cell types. Furthermore, the percentage of glial cells that are transduced is also often low, raising the question of whether transgene expression levels would be enough to have a therapeutic effect. The development of promoters that are highly specific for a particular glial cell type would be beneficial to avoid the possibility of off-target effects which might be likely with systemic delivery of an AAV vector expressing transgenes under the control of the GFAP promoter for example, due to it being expressed in a number of glial cell types throughout the body. An issue with the use of cell-specific promoters is that they are often large, which can preclude their use in an AAV vector context. However, a number of studies have demonstrated the use of shortened promoter sequences for driving glial cell-specific transgene expression. The development of new methods for synthetic promoter design holds real promise (Juttner et al., 2019). This study used a number of approaches to design synthetic promoters based on conserved upstream sequences of highly cell-specific genes and cis-regulatory regions active in cell types of interest. This approach led to the discovery of promoters that provide 100% specific gene expression in different retinal cell types, including the 500 bp ProB2 promoter, which shows 100% specificity for Müller cells. Applying this approach to other cell types could lead to the discovery of highly cell-type-specific promoters of appropriate size for use in AAV vectors.
The AAV literature also reports differences in transduction results depending on the model used and the method of delivery. Observations from in vitro studies often do not translate to the in vivo situation, and differences can be seen depending on the animal species or strain used or the method of delivery. For example, the ability to systemically deliver an AAV vector with a particular cellular tropism or cellular promoter would be beneficial. The variants AAV9-PHP.B and PHP.eB have been reported to allow for significant transduction of the CNS following intravenous infusion but depending on the animal model or method of delivery used, different results are seen (Hordeaux et al., 2018; Simpson et al., 2019). While enhanced CNS tropism has been shown in a number of mouse strains including C57BL/6J mouse where it was first demonstrated, no such increase in transduction efficiency is seen in the BALB/cJ mouse strain (Hordeaux et al., 2018; Matsuzaki et al., 2019). These results are explained by lower levels of the receptor for AAV9-PHP.B, LY6A, in BALB/cJ mice (Huang et al., 2019; Batista et al., 2020). When this virus was delivered to cats, sheep and non-human primates the efficiency of transduction of the CNS was low (Hordeaux et al., 2018; Matsuzaki et al., 2018; Liguore et al., 2019; Batista et al., 2020; Liu et al., 2020) and these species, as well as humans, have no known ortholog for Ly6a (Batista et al., 2020). This demonstrates that the use of non-human species to select novel AAV capsid variants could inadvertently limit the usefulness of candidate capsids that are isolated. These differences can also be more subtle. Using the cell-type-specific markers GFAP and Olig-2, He et al. (2019) assessed the cell-specific tropism of AAV serotypes 2, 5, 7, 8, and 9 in C57 and FVB mice. In the case of oligodendrocyte transduction, AAV8 resulted in 23% of oligodendrocytes being EGFP positive in C57 mice, significantly more than was seen measured in FVB mice at only 4.4%. In FVB mice, AAV7 vectors transduced a significantly larger number of oligodendrocytes than AAV8 vectors. Therefore, the choice of model can have a significant impact on the results that are obtained, and it will be important to understand the species and cell-type specific localization of AAV receptors to ensure accurate targeting of AAVs.
In a review by Maes et al. (2019) on the use of gene therapy to target microglia, the authors proposed guidelines for the reporting of viral transduction of this cell type. A similar set of guidelines for design and reporting of glial-targeting gene therapy studies could be of benefit for moving these approaches to clinically useful interventions. In order to confirm the results observed in animals, experiments using human model systems are crucial. While a number of non-human primate studies of AAV tropism have been carried out, these are not practical or financially viable for many research groups to undertake. Studies could be carried out in primary cells derived from the human brain; however, these may not express the same AAV receptors and lack the differentiation of the in vivo environment. In order to better understand the tropism and glial cell-specific transduction of AAV vectors, studies using primary human cells as close to their in vivo context are needed (Lisowski et al., 2015). One example that has been proposed is the use of humanized mouse models containing chimeric tissues, such as the FRG mouse model that allows animals to be generated with chimeric mouse-human livers (Azuma et al., 2007). While such an approach does not apply to the nervous system, the use of induced pluripotent stem cells (iPSCs) and human brain organoids have potential to understand tropism and expression of transgenes in human cells. Recent studies have used this approach to study AAV transduction and expression in the retina (Mookherjee et al., 2018; Quinn et al., 2019; Tornabene et al., 2019; Garita-Hernandez et al., 2020; Lane et al., 2020). The study by Quinn et al. (2019) specifically looked at the expression in Müller cells within the organoids. Using AAV and ShH10Y445F vectors with either the CMV or RLBP1 promoter showed good Müller cell transduction, with the authors reporting a ShH10Y445F-RLBP1-GFP vector being specific for transduction in Müller cells. A similar tropism and expression potency was seen in cultured adult human retinal explants. This is an important observation, as it demonstrates findings seen in organoids may recapitulate what would be seen in adult human tissue. Studies have also used human iPSC-derived cerebral organoids to study AAV transduction (Latour et al., 2019; Depla et al., 2020). However, these studies only looked at neuronal transgene expression achieved from the AAV vectors used. While organoids are likely useful for testing AAV for treating developmental disorders, using optimal differentiation conditions enables the creation of cerebral organoids containing mature neurons and astrocytes (Yakoub, 2019) that could be used to study AAV glial tropism. Models of human intestinal organoids that contain an ENS (including glia) could potentially be used for this purpose (Schlieve et al., 2017).
Author Contributions
SO’C, WC, and DY wrote the manuscript. All authors contributed to the article and approved the submitted version.
Funding
This work was supported by the funding from the Catwalk Trust Spinal Cord Injury Research Trust (SO’C), the Neurological Foundation of New Zealand (DY; 2009 PRG), and the Auckland Medical Research Foundation (DY; 1120003).
Conflict of Interest
The authors declare that the research was conducted in the absence of any commercial or financial relationships that could be construed as a potential conflict of interest.
References
Adachi, K., Enoki, T., Kawano, Y., Veraz, M., and Nakai, H. (2014). Drawing a high-resolution functional map of adeno-associated virus capsid by massively parallel sequencing. Nat. Commun. 5:3075. doi: 10.1038/ncomms4075
Ahmed, S., Gull, A., Khuroo, T., Aqil, M., and Sultana, Y. (2017). Glial cell: a potential target for cellular and drug based therapy in various CNS diseases. Curr. Pharm. Des. 23, 2389–2399. doi: 10.2174/1381612823666170316124500
Albright, B. H., Storey, C. M., Murlidharan, G., Castellanos Rivera, R. M., Berry, G. E., Madigan, V. J., et al. (2018). Mapping the structural determinants required for AAVrh.10 transport across the blood-brain barrier. Mol. Ther. 26, 510–523. doi: 10.1016/j.ymthe.2017.10.017
Allocca, M., Mussolino, C., Garcia-Hoyos, M., Sanges, D., Iodice, C., Petrillo, M., et al. (2007). Novel adeno-associated virus serotypes efficiently transduce murine photoreceptors. J. Virol. 81, 11372–11380. doi: 10.1128/JVI.01327-07
Allodi, I., Mecollari, V., Gonzalez-Perez, F., Eggers, R., Hoyng, S., Verhaagen, J., et al. (2014). Schwann cells transduced with a lentiviral vector encoding Fgf-2 promote motor neuron regeneration following sciatic nerve injury. Glia 62, 1736–1746. doi: 10.1002/glia.22712
Aube, A. C., Cabarrocas, J., Bauer, J., Philippe, D., Aubert, P., Doulay, F., et al. (2006). Changes in enteric neurone phenotype and intestinal functions in a transgenic mouse model of enteric glia disruption. Gut 55, 630–637. doi: 10.1136/gut.2005.067595
Azuma, H., Paulk, N., Ranade, A., Dorrell, C., Al-Dhalimy, M., Ellis, E., et al. (2007). Robust expansion of human hepatocytes in Fah-/-/Rag2-/-/Il2rg-/- mice. Nat. Biotechnol. 25, 903–910. doi: 10.1038/nbt1326
Bai, R. Y., Esposito, D., Tam, A. J., McCormick, F., Riggins, G. J., Wade Clapp, D., et al. (2019). Feasibility of using NF1-GRD and AAV for gene replacement therapy in NF1-associated tumors. Gene Ther. 26, 277–286. doi: 10.1038/s41434-019-0080-9
Bandopadhyay, R., Kingsbury, A. E., Cookson, M. R., Reid, A. R., Evans, I. M., Hope, A. D., et al. (2004). The expression of DJ-1 (PARK7) in normal human CNS and idiopathic Parkinson’s disease. Brain 127, 420–430. doi: 10.1093/brain/awh054
Bartlett, J. S., Kleinschmidt, J., Boucher, R. C., and Samulski, R. J. (1999). Targeted adeno-associated virus vector transduction of nonpermissive cells mediated by a bispecific F(ab’gamma)2 antibody. Nat. Biotechnol. 17, 181–186. doi: 10.1038/6185
Bartlett, J. S., Samulski, R. J., and McCown, T. J. (1998). Selective and rapid uptake of adeno-associated virus type 2 in brain. Hum. Gene Ther. 9, 1181–1186. doi: 10.1089/hum.1998.9.8-1181
Batista, A. R., King, O. D., Reardon, C. P., Davis, C., Shankaracharya, Philip, V., et al. (2020). Ly6a differential expression in blood-brain barrier is responsible for strain specific central nervous system transduction profile of AAV-PHP.B. Hum. Gene Ther. 31, 90–102. doi: 10.1089/hum.2019.186
Benkler, C., Ben-Zur, T., Barhum, Y., and Offen, D. (2013). Altered astrocytic response to activation in SOD1(G93A) mice and its implications on amyotrophic lateral sclerosis pathogenesis. Glia 61, 312–326. doi: 10.1002/glia.22428
Benskey, M. J., Kuhn, N. C., Galligan, J. J., Garcia, J., Boye, S. E., Hauswirth, W. W., et al. (2015). Targeted gene delivery to the enteric nervous system using AAV: a comparison across serotypes and capsid mutants. Mol. Ther. 23, 488–500. doi: 10.1038/mt.2015.7
Bercury, K. K., and Macklin, W. B. (2015). Dynamics and mechanisms of CNS myelination. Dev. Cell 32, 447–458. doi: 10.1016/j.devcel.2015.01.016
Berns, K. I., and Giraud, C. (1996). Biology of adeno-associated virus. Curr. Top. Microbiol. Immunol. 218, 1–23.
Besnard, F., Brenner, M., Nakatani, Y., Chao, R., Purohit, H. J., and Freese, E. (1991). Multiple interacting sites regulate astrocyte-specific transcription of the human gene for glial fibrillary acidic protein. J. Biol. Chem. 266, 18877–18883.
Bohnen, N. I., and Albin, R. L. (2011). White matter lesions in Parkinson disease. Nat. Rev. Neurol. 7, 229–236. doi: 10.1038/nrneurol.2011.21
Booth, H. D. E., Hirst, W. D., and Wade-Martins, R. (2017). The role of astrocyte dysfunction in Parkinson’s disease pathogenesis. Trends Neurosci. 40, 358–370. doi: 10.1016/j.tins.2017.04.001
Bradl, M., and Lassmann, H. (2010). Oligodendrocytes: biology and pathology. Acta Neuropathol. 119, 37–53. doi: 10.1007/s00401-009-0601-5
Bringmann, A., Pannicke, T., Grosche, J., Francke, M., Wiedemann, P., Skatchkov, S. N., et al. (2006). Muller cells in the healthy and diseased retina. Prog. Retin Eye Res. 25, 397–424. doi: 10.1016/j.preteyeres.2006.05.003
Brown, B. D., Venneri, M. A., Zingale, A., Sergi Sergi, L., and Naldini, L. (2006). Endogenous microRNA regulation suppresses transgene expression in hematopoietic lineages and enables stable gene transfer. Nat. Med. 12, 585–591. doi: 10.1038/nm1398
Bsibsi, M., Persoon-Deen, C., Verwer, R. W., Meeuwsen, S., Ravid, R., and Van Noort, J. M. (2006). Toll-like receptor 3 on adult human astrocytes triggers production of neuroprotective mediators. Glia 53, 688–695. doi: 10.1002/glia.20328
Bucher, T., Dubreil, L., Colle, M. A., Maquigneau, M., Deniaud, J., Ledevin, M., et al. (2014). Intracisternal delivery of AAV9 results in oligodendrocyte and motor neuron transduction in the whole central nervous system of cats. Gene Ther. 21, 522–528. doi: 10.1038/gt.2014.16
Buckinx, R., Van Remoortel, S., Gijsbers, R., Waddington, S. N., and Timmermans, J. P. (2016). Proof-of-concept: neonatal intravenous injection of adeno-associated virus vectors results in successful transduction of myenteric and submucosal neurons in the mouse small and large intestine. Neurogastroenterol. Motil. 28, 299–305. doi: 10.1111/nmo.12724
Budni, J., Bellettini-Santos, T., Mina, F., Garcez, M. L., and Zugno, A. I. (2015). The involvement of BDNF, NGF and GDNF in aging and Alzheimer’s disease. Aging Dis. 6, 331–341. doi: 10.14336/AD.2015.0825
Burda, J. E., Bernstein, A. M., and Sofroniew, M. V. (2016). Astrocyte roles in traumatic brain injury. Exp. Neurol. 275(Pt 3), 305–315. doi: 10.1016/j.expneurol.2015.03.020
Cahoy, J. D., Emery, B., Kaushal, A., Foo, L. C., Zamanian, J. L., Christopherson, K. S., et al. (2008). A transcriptome database for astrocytes, neurons, and oligodendrocytes: a new resource for understanding brain development and function. J. Neurosci. 28, 264–278. doi: 10.1523/JNEUROSCI.4178-07.2008
Campochiaro, P. A. (2015). Molecular pathogenesis of retinal and choroidal vascular diseases. Prog. Retin Eye Res. 49, 67–81. doi: 10.1016/j.preteyeres.2015.06.002
Cao, J., Liu, X., Yuan, Y., Wang, F., Kong, W., Shi, G., et al. (2020). A rAAV2/6 mutant with enhanced targeting for mouse retinal muller cells. Curr. Eye Res. 45, 64–71. doi: 10.1080/02713683.2019.1639768
Caraci, F., Bosco, P., Signorelli, M., Spada, R. S., Cosentino, F. I., Toscano, G., et al. (2012). The CC genotype of transforming growth factor-beta1 increases the risk of late-onset Alzheimer’s disease and is associated with AD-related depression. Eur. Neuropsychopharmacol. 22, 281–289. doi: 10.1016/j.euroneuro.2011.08.006
Caraci, F., Spampinato, S. F., Morgese, M. G., Tascedda, F., Salluzzo, M. G., Giambirtone, M. C., et al. (2018). Neurobiological links between depression and AD: the role of TGF-beta1 signaling as a new pharmacological target. Pharmacol. Res. 130, 374–384. doi: 10.1016/j.phrs.2018.02.007
Carlisle, R. C., Benjamin, R., Briggs, S. S., Sumner-Jones, S., McIntosh, J., Gill, D., et al. (2008). Coating of adeno-associated virus with reactive polymers can ablate virus tropism, enable retargeting and provide resistance to neutralising antisera. J. Gene Med. 10, 400–411. doi: 10.1002/jgm.1161
Castle, M. J., Turunen, H. T., Vandenberghe, L. H., and Wolfe, J. H. (2016). Controlling AAV tropism in the nervous system with natural and engineered capsids. Methods Mol. Biol. 1382, 133–149. doi: 10.1007/978-1-4939-3271-9_10
Cearley, C. N., Vandenberghe, L. H., Parente, M. K., Carnish, E. R., Wilson, J. M., and Wolfe, J. H. (2008). Expanded repertoire of AAV vector serotypes mediate unique patterns of transduction in mouse brain. Mol. Ther. 16, 1710–1718. doi: 10.1038/mt.2008.166
Cekanaviciute, E., Fathali, N., Doyle, K. P., Williams, A. M., Han, J., and Buckwalter, M. S. (2014). Astrocytic transforming growth factor-beta signaling reduces subacute neuroinflammation after stroke in mice. Glia 62, 1227–1240. doi: 10.1002/glia.22675
Chen, H., McCarty, D. M., Bruce, A. T., and Suzuki, K. (1999). Oligodendrocyte-specific gene expression in mouse brain: use of a myelin-forming cell type-specific promoter in an adeno-associated virus. J. Neurosci. Res. 55, 504–513. doi: 10.1002/(SICI)1097-4547(19990215)55:4<504::AID-JNR10<3.0.CO;2-0
Chen, H., McCarty, D. M., Bruce, A. T., Suzuki, K., and Suzuki, K. (1998). Gene transfer and expression in oligodendrocytes under the control of myelin basic protein transcriptional control region mediated by adeno-associated virus. Gene Ther. 5, 50–58. doi: 10.1038/sj.gt.3300547
Chen, Y. H., Chang, M., and Davidson, B. L. (2009). Molecular signatures of disease brain endothelia provide new sites for CNS-directed enzyme therapy. Nat. Med. 15, 1215–1218. doi: 10.1038/nm.2025
Clancy, B., Darlington, R. B., and Finlay, B. L. (2001). Translating developmental time across mammalian species. Neuroscience 105, 7–17. doi: 10.1016/s0306-4522(01)00171-3
Colin, A., Faideau, M., Dufour, N., Auregan, G., Hassig, R., Andrieu, T., et al. (2009). Engineered lentiviral vector targeting astrocytes in vivo. Glia 57, 667–679. doi: 10.1002/glia.20795
Costa, M., Brookes, S. J., and Hennig, G. W. (2000). Anatomy and physiology of the enteric nervous system. Gut 47(Suppl. 4), iv15–iv19. doi: 10.1136/gut.47.suppl_4.iv15
Coughlin, B. A., Feenstra, D. J., and Mohr, S. (2017). Muller cells and diabetic retinopathy. Vision Res. 139, 93–100. doi: 10.1016/j.visres.2017.03.013
Cucchiarini, M., Ren, X. L., Perides, G., and Terwilliger, E. F. (2003). Selective gene expression in brain microglia mediated via adeno-associated virus type 2 and type 5 vectors. Gene Ther. 10, 657–667. doi: 10.1038/sj.gt.3301925
Dalkara, D., Kolstad, K. D., Guerin, K. I., Hoffmann, N. V., Visel, M., Klimczak, R. R., et al. (2011). AAV mediated GDNF secretion from retinal glia slows down retinal degeneration in a rat model of retinitis pigmentosa. Mol. Ther. 19, 1602–1608. doi: 10.1038/mt.2011.62
Davidson, B. L., Stein, C. S., Heth, J. A., Martins, I., Kotin, R. M., Derksen, T. A., et al. (2000). Recombinant adeno-associated virus type 2, 4, and 5 vectors: transduction of variant cell types and regions in the mammalian central nervous system. Proc. Natl. Acad. Sci. U.S.A. 97, 3428–3432. doi: 10.1073/pnas.97.7.3428
Davidsson, M., Wang, G., Aldrin-Kirk, P., Cardoso, T., Nolbrant, S., Hartnor, M., et al. (2019). A systematic capsid evolution approach performed in vivo for the design of AAV vectors with tailored properties and tropism. Proc. Natl. Acad. Sci. U.S.A. 116, 27053–27062. doi: 10.1073/pnas.1910061116
de Leeuw, B., Su, M., ter Horst, M., Iwata, S., Rodijk, M., Hoeben, R. C., et al. (2006). Increased glia-specific transgene expression with glial fibrillary acidic protein promoters containing multiple enhancer elements. J. Neurosci. Res. 83, 744–753. doi: 10.1002/jnr.20776
Depla, J. A., Sogorb-Gonzalez, M., Mulder, L. A., Heine, V. M., Konstantinova, P., van Deventer, S. J., et al. (2020). Cerebral organoids: a human model for AAV capsid selection and therapeutic transgene efficacy in the brain. Mol. Ther. Methods Clin. Dev. 18, 167–175. doi: 10.1016/j.omtm.2020.05.028
Deverman, B. E., Pravdo, P. L., Simpson, B. P., Kumar, S. R., Chan, K. Y., Banerjee, A., et al. (2016). Cre-dependent selection yields AAV variants for widespread gene transfer to the adult brain. Nat. Biotechnol. 34, 204–209. doi: 10.1038/nbt.3440
Deverman, B. E., Ravina, B. M., Bankiewicz, K. S., Paul, S. M., and Sah, D. W. Y. (2018). Gene therapy for neurological disorders: progress and prospects. Nat. Rev. Drug Discov. 17:767. doi: 10.1038/nrd.2018.158
Dirren, E., Towne, C. L., Setola, V., Redmond, D. E. Jr., Schneider, B. L., and Aebischer, P. (2014). Intracerebroventricular injection of adeno-associated virus 6 and 9 vectors for cell type-specific transgene expression in the spinal cord. Hum. Gene Ther. 25, 109–120. doi: 10.1089/hum.2013.021
Djukic, B., Casper, K. B., Philpot, B. D., Chin, L. S., and McCarthy, K. D. (2007). Conditional knock-out of Kir4.1 leads to glial membrane depolarization, inhibition of potassium and glutamate uptake, and enhanced short-term synaptic potentiation. J. Neurosci. 27, 11354–11365. doi: 10.1523/JNEUROSCI.0723-07.2007
Dorrell, M. I., Aguilar, E., Jacobson, R., Yanes, O., Gariano, R., Heckenlively, J., et al. (2009). Antioxidant or neurotrophic factor treatment preserves function in a mouse model of neovascularization-associated oxidative stress. J. Clin. Invest. 119, 611–623. doi: 10.1172/JCI35977
During, M. J., Young, D., Baer, K., Lawlor, P., and Klugmann, M. (2003). Development and optimization of adeno-associated virus vector transfer in the central nervous system. Methods Mol. Med. 76, 221–236.
Dvorzhak, A., Vagner, T., Kirmse, K., and Grantyn, R. (2016). Functional indicators of glutamate transport in single striatal astrocytes and the influence of Kir4.1 in normal and huntington mice. J. Neurosci. 36, 4959–4975. doi: 10.1523/JNEUROSCI.0316-16.2016
Dzamba, D., Harantova, L., Butenko, O., and Anderova, M. (2016). Glial cells–the key elements of Alzheimer’s disease. Curr. Alzheimer Res. 13, 894–911. doi: 10.2174/1567205013666160129095924
Eastlake, K., Luis, J., and Limb, G. A. (2020). Potential of muller glia for retina neuroprotection. Curr. Eye Res. 45, 339–348. doi: 10.1080/02713683.2019.1648831
Eggers, R., de Winter, F., Hoyng, S. A., Roet, K. C., Ehlert, E. M., Malessy, M. J., et al. (2013). Lentiviral vector-mediated gradients of GDNF in the injured peripheral nerve: effects on nerve coil formation, Schwann cell maturation and myelination. PLoS One 8:e71076. doi: 10.1371/journal.pone.0071076
Fern, R. F., Matute, C., and Stys, P. K. (2014). White matter injury: ischemic and nonischemic. Glia 62, 1780–1789. doi: 10.1002/glia.22722
Filley, C. M. (2016). Fragile X tremor ataxia syndrome and white matter dementia. Clin. Neuropsychol. 30, 901–912. doi: 10.1080/13854046.2016.1165805
Forghani, R., Garofalo, L., Foran, D. R., Farhadi, H. F., Lepage, P., Hudson, T. J., et al. (2001). A distal upstream enhancer from the myelin basic protein gene regulates expression in myelin-forming schwann cells. J. Neurosci. 21, 3780–3787.
Foust, K. D., Nurre, E., Montgomery, C. L., Hernandez, A., Chan, C. M., and Kaspar, B. K. (2009). Intravascular AAV9 preferentially targets neonatal neurons and adult astrocytes. Nat. Biotechnol. 27, 59–65. doi: 10.1038/nbt.1515
Franceschi, C., Capri, M., Monti, D., Giunta, S., Olivieri, F., Sevini, F., et al. (2007). Inflammaging and anti-inflammaging: a systemic perspective on aging and longevity emerged from studies in humans. Mech. Ageing Dev. 128, 92–105. doi: 10.1016/j.mad.2006.11.016
Gao, G., Vandenberghe, L. H., Alvira, M. R., Lu, Y., Calcedo, R., Zhou, X., et al. (2004). Clades of adeno-associated viruses are widely disseminated in human tissues. J. Virol. 78, 6381–6388. doi: 10.1128/JVI.78.12.6381
Garita-Hernandez, M., Routet, F., Guibbal, L., Khabou, H., Toualbi, L., Riancho, L., et al. (2020). AAV-mediated gene delivery to 3D retinal organoids derived from human induced pluripotent stem cells. Int. J. Mol. Sci. 21:994. doi: 10.3390/ijms21030994
Garwood, C. J., Ratcliffe, L. E., Simpson, J. E., Heath, P. R., Ince, P. G., and Wharton, S. B. (2017). Review: astrocytes in Alzheimer’s disease and other age-associated dementias: a supporting player with a central role. Neuropathol. Appl. Neurobiol. 43, 281–298. doi: 10.1111/nan.12338
Gaudet, A. D., and Fonken, L. K. (2018). Glial cells shape pathology and repair after spinal cord injury. Neurotherapeutics 15, 554–577. doi: 10.1007/s13311-018-0630-7
Georgiou, E., Sidiropoulou, K., Richter, J., Papaneophytou, C., Sargiannidou, I., Kagiava, A., et al. (2017). Gene therapy targeting oligodendrocytes provides therapeutic benefit in a leukodystrophy model. Brain 140, 599–616. doi: 10.1093/brain/aww351
Gombash, S. E., Cowley, C. J., Fitzgerald, J. A., Hall, J. C., Mueller, C., Christofi, F. L., et al. (2014). Intravenous AAV9 efficiently transduces myenteric neurons in neonate and juvenile mice. Front. Mol. Neurosci. 7:81. doi: 10.3389/fnmol.2014.00081
Gosselin, R. D., Suter, M. R., Ji, R. R., and Decosterd, I. (2010). Glial cells and chronic pain. Neuroscientist 16, 519–531. doi: 10.1177/1073858409360822
Graca, A. B., Hippert, C., and Pearson, R. A. (2018). Muller glia reactivity and development of gliosis in response to pathological conditions. Adv. Exp. Med. Biol. 1074, 303–308. doi: 10.1007/978-3-319-75402-4_37
Gray, S. J., Blake, B. L., Criswell, H. E., Nicolson, S. C., Samulski, R. J., and McCown, T. J. (2010). Directed evolution of a novel adeno-associated virus (AAV) vector that crosses the seizure-compromised blood-brain barrier (BBB). Mol. Ther. 18, 570–578. doi: 10.1038/mt.2009.292
Gray, S. J., Matagne, V., Bachaboina, L., Yadav, S., Ojeda, S. R., and Samulski, R. J. (2011). Preclinical differences of intravascular AAV9 delivery to neurons and glia: a comparative study of adult mice and nonhuman primates. Mol. Ther. J. Am. Soc. Gene Ther. 19, 1058–1069. doi: 10.1038/mt.2011.72
Greenberg, K. P., Geller, S. F., Schaffer, D. V., and Flannery, J. G. (2007). Targeted transgene expression in muller glia of normal and diseased retinas using lentiviral vectors. Invest. Ophthalmol. Vis. Sci. 48, 1844–1852. doi: 10.1167/iovs.05-1570
Griffin, J. M., Fackelmeier, B., Clemett, C. A., Fong, D. M., Mouravlev, A., Young, D., et al. (2020). Astrocyte-selective AAV-ADAMTS4 gene therapy combined with hindlimb rehabilitation promotes functional recovery after spinal cord injury. Exp. Neurol. 327:113232. doi: 10.1016/j.expneurol.2020.113232
Griffin, J. M., Fackelmeier, B., Fong, D. M., Mouravlev, A., Young, D., and O’Carroll, S. J. (2019). Astrocyte-selective AAV gene therapy through the endogenous GFAP promoter results in robust transduction in the rat spinal cord following injury. Gene Ther. 26, 198–210. doi: 10.1038/s41434-019-0075-6
Grubisic, V., and Gulbransen, B. D. (2017). Enteric glia: the most alimentary of all glia. J. Physiol. 595, 557–570. doi: 10.1113/JP271021
Gulbransen, B. D., and Christofi, F. L. (2018). Are we close to targeting enteric glia in gastrointestinal diseases and motility disorders? Gastroenterology 155, 245–251. doi: 10.1053/j.gastro.2018.06.050
Hagiwara, T., Tanaka, K., Takai, S., Maeno-Hikichi, Y., Mukainaka, Y., and Wada, K. (1996). Genomic organization, promoter analysis, and chromosomal localization of the gene for the mouse glial high-affinity glutamate transporter Slc1a3. Genomics 33, 508–515. doi: 10.1006/geno.1996.0226
Hajos, F., and Kalman, M. (1989). Distribution of glial fibrillary acidic protein (GFAP)-immunoreactive astrocytes in the rat brain. II. Mesencephalon, rhombencephalon and spinal cord. Exp. Brain Res. 78, 164–173. doi: 10.1007/BF00230695
Hammond, S. L., Leek, A. N., Richman, E. H., and Tjalkens, R. B. (2017). Cellular selectivity of AAV serotypes for gene delivery in neurons and astrocytes by neonatal intracerebroventricular injection. PLoS One 12:e0188830. doi: 10.1371/journal.pone.0188830
Hanani, M. (2005). Satellite glial cells in sensory ganglia: from form to function. Brain Res. Brain Res. Rev. 48, 457–476. doi: 10.1016/j.brainresrev.2004.09.001
Hanani, M. (2010). Satellite glial cells in sympathetic and parasympathetic ganglia: in search of function. Brain Res. Rev. 64, 304–327. doi: 10.1016/j.brainresrev.2010.04.009
Harding, T. C., Dickinson, P. J., Roberts, B. N., Yendluri, S., Gonzalez-Edick, M., LeCouteur, R. A., et al. (2006). Enhanced gene transfer efficiency in the murine striatum and an orthotopic glioblastoma tumor model, using AAV-7- and AAV-8-pseudotyped vectors. Hum. Gene Ther. 17, 807–820. doi: 10.1089/hum.2006.17.807
Harvey, A. R., Kamphuis, W., Eggers, R., Symons, N. A., Blits, B., Niclou, S., et al. (2002). Intravitreal injection of adeno-associated viral vectors results in the transduction of different types of retinal neurons in neonatal and adult rats: a comparison with lentiviral vectors. Mol. Cell Neurosci. 21, 141–157. doi: 10.1006/mcne.2002.1168
Hassannejad, Z., Shakouri-Motlagh, A., Mokhatab, M., Zadegan, S. A., Sharif-Alhoseini, M., Shokraneh, F., et al. (2019). Oligodendrogliogenesis and axon remyelination after traumatic spinal cord injuries in animal studies: a systematic review. Neuroscience 402, 37–50. doi: 10.1016/j.neuroscience.2019.01.019
He, T., Itano, M. S., Earley, L. F., Hall, N. E., Riddick, N., Samulski, R. J., et al. (2019). The influence of murine genetic background in adeno-associated virus transduction of the mouse brain. Hum. Gene Ther. Clin. Dev. 30, 169–181. doi: 10.1089/humc.2019.030
Hellstrom, M., Ruitenberg, M. J., Pollett, M. A., Ehlert, E. M., Twisk, J., Verhaagen, J., et al. (2009). Cellular tropism and transduction properties of seven adeno-associated viral vector serotypes in adult retina after intravitreal injection. Gene Ther. 16, 521–532. doi: 10.1038/gt.2008.178
Homs, J., Ariza, L., Pages, G., Udina, E., Navarro, X., Chillon, M., et al. (2011). Schwann cell targeting via intrasciatic injection of AAV8 as gene therapy strategy for peripheral nerve regeneration. Gene Ther. 18, 622–630. doi: 10.1038/gt.2011.7
Hordeaux, J., Wang, Q., Katz, N., Buza, E. L., Bell, P., and Wilson, J. M. (2018). The neurotropic properties of AAV-PHP.B are limited to C57BL/6J mice. Mol. Ther. 26, 664–668. doi: 10.1016/j.ymthe.2018.01.018
Horowitz, E. D., Weinberg, M. S., and Asokan, A. (2011). Glycated AAV vectors: chemical redirection of viral tissue tropism. Bioconjug. Chem. 22, 529–532. doi: 10.1021/bc100477g
Howard, D. B., Powers, K., Wang, Y., and Harvey, B. K. (2008). Tropism and toxicity of adeno-associated viral vector serotypes 1, 2, 5, 6, 7, 8, and 9 in rat neurons and glia in vitro. Virology 372, 24–34. doi: 10.1016/j.virol.2007.10.007
Hoyng, S. A., De Winter, F., Gnavi, S., van Egmond, L., Attwell, C. L., Tannemaat, M. R., et al. (2015). Gene delivery to rat and human Schwann cells and nerve segments: a comparison of AAV 1-9 and lentiviral vectors. Gene Ther. 22, 767–780. doi: 10.1038/gt.2015.47
Huang, Q., Chan, K. Y., Tobey, I. G., Chan, Y. A., Poterba, T., Boutros, C. L., et al. (2019). Delivering genes across the blood-brain barrier: LY6A, a novel cellular receptor for AAV-PHP.B capsids. PLoS One 14:e0225206. doi: 10.1371/journal.pone.0225206
Hudry, E., Andres-Mateos, E., Lerner, E. P., Volak, A., Cohen, O., Hyman, B. T., et al. (2018). Efficient gene transfer to the central nervous system by single-stranded Anc80L65. Mol. Ther. Methods Clin. Dev. 10, 197–209. doi: 10.1016/j.omtm.2018.07.006
Hutson, T. H., Verhaagen, J., Yáñez-Muñoz, R. J., and Moon, L. D. F. (2012). Corticospinal tract transduction: a comparison of seven adeno-associated viral vector serotypes and a non-integrating lentiviral vector. Gene Ther. 19, 49–60. doi: 10.1038/gt.2011.71
Ibiza, S., Garcia-Cassani, B., Ribeiro, H., Carvalho, T., Almeida, L., Marques, R., et al. (2016). Glial-cell-derived neuroregulators control type 3 innate lymphoid cells and gut defence. Nature 535, 440–443. doi: 10.1038/nature18644
Ingusci, S., Verlengia, G., Soukupova, M., Zucchini, S., and Simonato, M. (2019). Gene therapy tools for brain diseases. Front. Pharmacol. 10:724. doi: 10.3389/fphar.2019.00724
Jasmin, L., Vit, J. P., Bhargava, A., and Ohara, P. T. (2010). Can satellite glial cells be therapeutic targets for pain control? Neuron Glia Biol. 6, 63–71. doi: 10.1017/S1740925X10000098
Ji, R. R., Berta, T., and Nedergaard, M. (2013). Glia and pain: is chronic pain a gliopathy? Pain 154(Suppl. 1), S10–S28. doi: 10.1016/j.pain.2013.06.022
Juttner, J., Szabo, A., Gross-Scherf, B., Morikawa, R. K., Rompani, S. B., Hantz, P., et al. (2019). Targeting neuronal and glial cell types with synthetic promoter AAVs in mice, non-human primates and humans. Nat. Neurosci. 22, 1345–1356. doi: 10.1038/s41593-019-0431-2
Kagiava, A., Sargiannidou, I., Bashiardes, S., Richter, J., Schiza, N., Christodoulou, C., et al. (2014). Gene delivery targeted to oligodendrocytes using a lentiviral vector. J. Gene Med. 16, 364–373. doi: 10.1002/jgm.2813
Kagiava, A., Sargiannidou, I., Theophilidis, G., Karaiskos, C., Richter, J., Bashiardes, S., et al. (2016). Intrathecal gene therapy rescues a model of demyelinating peripheral neuropathy. Proc. Natl. Acad. Sci. U.S.A. 113, E2421–E2429. doi: 10.1073/pnas.1522202113
Kaiser, M., Maletzki, I., Hulsmann, S., Holtmann, B., Schulz-Schaeffer, W., Kirchhoff, F., et al. (2006). Progressive loss of a glial potassium channel (KCNJ10) in the spinal cord of the SOD1 (G93A) transgenic mouse model of amyotrophic lateral sclerosis. J. Neurochem. 99, 900–912. doi: 10.1111/j.1471-4159.2006.04131.x
Kalman, M., and Hajos, F. (1989). Distribution of glial fibrillary acidic protein (GFAP)-immunoreactive astrocytes in the rat brain. I. Forebrain. Exp. Brain Res. 78, 147–163. doi: 10.1007/BF00230694
Kantor, B., Bailey, R. M., Wimberly, K., Kalburgi, S. N., and Gray, S. J. (2014). Methods for gene transfer to the central nervous system. Adv. Genet. 87, 125–197. doi: 10.1016/B978-0-12-800149-3.00003-2
Kery, R., Chen, A. P. F., and Kirschen, G. W. (2020). Genetic targeting of astrocytes to combat neurodegenerative disease. Neural Regen. Res. 15, 199–211. doi: 10.4103/1673-5374.265541
Kidd, G. J., Ohno, N., and Trapp, B. D. (2013). Biology of Schwann cells. Handb. Clin. Neurol. 115, 55–79. doi: 10.1016/B978-0-444-52902-2.00005-9
Kierdorf, K., and Prinz, M. (2017). Microglia in steady state. J. Clin. Invest. 127, 3201–3209. doi: 10.1172/JCI90602
Klein, R., Peto, T., Bird, A., and Vannewkirk, M. R. (2004). The epidemiology of age-related macular degeneration. Am. J. Ophthalmol. 137, 486–495. doi: 10.1016/j.ajo.2003.11.069
Klein, R. L., Dayton, R. D., Tatom, J. B., Henderson, K. M., and Henning, P. P. (2008). AAV8, 9, Rh10, Rh43 vector gene transfer in the rat brain: effects of serotype, promoter and purification method. Mol. Ther. 16, 89–96. doi: 10.1038/sj.mt.6300331
Klimczak, R. R., Koerber, J. T., Dalkara, D., Flannery, J. G., and Schaffer, D. V. (2009). A novel adeno-associated viral variant for efficient and selective intravitreal transduction of rat Muller cells. PLoS One 4:e7467. doi: 10.1371/journal.pone.0007467
Koerber, J. T., Klimczak, R., Jang, J. H., Dalkara, D., Flannery, J. G., and Schaffer, D. V. (2009). Molecular evolution of adeno-associated virus for enhanced glial gene delivery. Mol. Ther. 17, 2088–2095. doi: 10.1038/mt.2009.184
Koerber, J. T., Maheshri, N., Kaspar, B. K., and Schaffer, D. V. (2006). Construction of diverse adeno-associated viral libraries for directed evolution of enhanced gene delivery vehicles. Nat. Protoc. 1, 701–706. doi: 10.1038/nprot.2006.93
Koh, W., Park, Y. M., Lee, S. E., and Lee, C. J. (2017). AAV-mediated astrocyte-specific gene expression under human ALDH1L1 promoter in mouse thalamus. Exp. Neurobiol. 26, 350–361. doi: 10.5607/en.2017.26.6.350
Koprich, J. B., Johnston, T. H., Reyes, M. G., Sun, X., and Brotchie, J. M. (2010). Expression of human A53T alpha-synuclein in the rat substantia nigra using a novel AAV1/2 vector produces a rapidly evolving pathology with protein aggregation, dystrophic neurite architecture and nigrostriatal degeneration with potential to model the pat. Mol. Neurodegen. 5, 1–13. doi: 10.1186/1750-1326-5-43
Kucheryavykh, Y. V., Kucheryavykh, L. Y., Nichols, C. G., Maldonado, H. M., Baksi, K., Reichenbach, A., et al. (2007). Downregulation of Kir4.1 inward rectifying potassium channel subunits by RNAi impairs potassium transfer and glutamate uptake by cultured cortical astrocytes. Glia 55, 274–281. doi: 10.1002/glia.20455
Kuhn, S., Gritti, L., Crooks, D., and Dombrowski, Y. (2019). Oligodendrocytes in development, myelin generation and beyond. Cells 8:1424. doi: 10.3390/cells8111424
Kumaran, R., Kingsbury, A., Coulter, I., Lashley, T., Williams, D., de Silva, R., et al. (2007). DJ-1 (PARK7) is associated with 3R and 4R tau neuronal and glial inclusions in neurodegenerative disorders. Neurobiol. Dis. 28, 122–132. doi: 10.1016/j.nbd.2007.07.012
Kunze, C., Borner, K., Kienle, E., Orschmann, T., Rusha, E., Schneider, M., et al. (2018). Synthetic AAV/CRISPR vectors for blocking HIV-1 expression in persistently infected astrocytes. Glia 66, 413–427. doi: 10.1002/glia.23254
Lane, A., Jovanovic, K., Shortall, C., Ottaviani, D., Panes, A. B., Schwarz, N., et al. (2020). Modeling and rescue of RP2 retinitis pigmentosa using iPSC-derived retinal organoids. Stem Cell Reports 15, 67–79. doi: 10.1016/j.stemcr.2020.05.007
Larsen, N. J., Ambrosi, G., Mullett, S. J., Berman, S. B., and Hinkle, D. A. (2011). DJ-1 knock-down impairs astrocyte mitochondrial function. Neuroscience 196, 251–264. doi: 10.1016/j.neuroscience.2011.08.016
Latour, Y. L., Yoon, R., Thomas, S. E., Grant, C., Li, C., Sena-Esteves, M., et al. (2019). Human GLB1 knockout cerebral organoids: a model system for testing AAV9-mediated GLB1 gene therapy for reducing GM1 ganglioside storage in GM1 gangliosidosis. Mol. Genet. Metab. Rep. 21:100513. doi: 10.1016/j.ymgmr.2019.100513
Laura, M., Pipis, M., Rossor, A. M., and Reilly, M. M. (2019). Charcot-marie-tooth disease and related disorders: an evolving landscape. Curr. Opin. Neurol. 32, 641–650. doi: 10.1097/WCO.0000000000000735
Lawlor, P. A., Bland, R. J., Mouravlev, A., Young, D., and During, M. J. (2009). Efficient gene delivery and selective transduction of glial cells in the mammalian brain by AAV serotypes isolated from nonhuman primates. Mol. Ther. 17, 1692–1702. doi: 10.1038/mt.2009.170
Le, H. T., Yu, Q. C., Wilson, J. M., and Croyle, M. A. (2005). Utility of PEGylated recombinant adeno-associated viruses for gene transfer. J. Control Release 108, 161–177. doi: 10.1016/j.jconrel.2005.07.019
Lebherz, C., Maguire, A., Tang, W., Bennett, J., and Wilson, J. M. (2008). Novel AAV serotypes for improved ocular gene transfer. J. Gene Med. 10, 375–382. doi: 10.1002/jgm.1126
Lee, C., Low, C. Y., Francis, P. T., Attems, J., Wong, P. T., Lai, M. K., et al. (2016). An isoform-specific role of FynT tyrosine kinase in Alzheimer’s disease. J. Neurochem. 136, 637–650. doi: 10.1111/jnc.13429
Lee, C., Low, C. Y., Wong, S. Y., Lai, M. K., and Tan, M. G. (2017). Selective induction of alternatively spliced FynT isoform by TNF facilitates persistent inflammatory responses in astrocytes. Sci. Rep. 7:43651. doi: 10.1038/srep43651
Lee, Y., Messing, A., Su, M., and Brenner, M. (2008). GFAP promoter elements required for region-specific and astrocyte-specific expression. Glia 56, 481–493. doi: 10.1002/glia.20622
Lee, Y., Su, M., Messing, A., and Brenner, M. (2006). Astrocyte heterogeneity revealed by expression of a GFAP-LacZ transgene. Glia 53, 677–687. doi: 10.1002/glia.20320
Liguore, W. A., Domire, J. S., Button, D., Wang, Y., Dufour, B. D., Srinivasan, S., et al. (2019). AAV-PHP.B administration results in a differential pattern of CNS biodistribution in non-human primates compared with mice. Mol. Ther. 27, 2018–2037. doi: 10.1016/j.ymthe.2019.07.017
Lilli, N. L., Queneherve, L., Haddara, S., Brochard, C., Aubert, P., Rolli-Derkinderen, M., et al. (2018). Glioplasticity in irritable bowel syndrome. Neurogastroenterol. Motil. 30:e13232. doi: 10.1111/nmo.13232
Linan-Rico, A., Turco, F., Ochoa-Cortes, F., Harzman, A., Needleman, B. J., Arsenescu, R., et al. (2016). Molecular signaling and dysfunction of the human reactive enteric glial cell phenotype: implications for GI infection, IBD, POI, neurological, motility, and GI disorders. Inflamm. Bowel Dis. 22, 1812–1834. doi: 10.1097/MIB.0000000000000854
Lisowski, L., Tay, S. S., and Alexander, I. E. (2015). Adeno-associated virus serotypes for gene therapeutics. Curr. Opin. Pharmacol. 24, 59–67. doi: 10.1016/j.coph.2015.07.006
Liu, C. C., Liu, C. C., Kanekiyo, T., Xu, H., and Bu, G. (2013). Apolipoprotein E and Alzheimer disease: risk, mechanisms and therapy. Nat. Rev. Neurol. 9, 106–118. doi: 10.1038/nrneurol.2012.263
Liu, D., Zhu, M., Zhang, Y., and Diao, Y. (2020). Crossing the blood-brain barrier with AAV vectors. Metab. Brain Dis. 36, 45–52. doi: 10.1007/s11011-020-00630-2
Liu, G., Martins, I. H., Chiorini, J. A., and Davidson, B. L. (2005). Adeno-associated virus type 4 (AAV4) targets ependyma and astrocytes in the subventricular zone and RMS. Gene Ther. 12, 1503–1508. doi: 10.1038/sj.gt.3302554
Liu, H. T., Tashmukhamedov, B. A., Inoue, H., Okada, Y., and Sabirov, R. Z. (2006). Roles of two types of anion channels in glutamate release from mouse astrocytes under ischemic or osmotic stress. Glia 54, 343–357. doi: 10.1002/glia.20400
Liu, L., Zhang, K., Sandoval, H., Yamamoto, S., Jaiswal, M., Sanz, E., et al. (2015). Glial lipid droplets and ROS induced by mitochondrial defects promote neurodegeneration. Cell 160, 177–190. doi: 10.1016/j.cell.2014.12.019
Maes, M. E., Colombo, G., Schulz, R., and Siegert, S. (2019). Targeting microglia with lentivirus and AAV: recent advances and remaining challenges. Neurosci. Lett. 707, 134310. doi: 10.1016/j.neulet.2019.134310
Martini, R., and Willison, H. (2016). Neuroinflammation in the peripheral nerve: cause, modulator, or bystander in peripheral neuropathies? Glia 64, 475–486. doi: 10.1002/glia.22899
Mastakov, M. Y., Baer, K., Symes, C. W., Leichtlein, C. B., Kotin, R. M., and During, M. J. (2002). Immunological aspects of recombinant adeno-associated virus delivery to the mammalian brain. J. Virol. 76, 8446–8454. doi: 10.1128/jvi.76.16.8446-8454.2002
Mathis, C., Hindelang, C., LeMeur, M., and Borrelli, E. (2000). A transgenic mouse model for inducible and reversible dysmyelination. J. Neurosci. 20, 7698–7705.
Matsuzaki, Y., Konno, A., Mochizuki, R., Shinohara, Y., Nitta, K., Okada, Y., et al. (2018). Intravenous administration of the adeno-associated virus-PHP.B capsid fails to upregulate transduction efficiency in the marmoset brain. Neurosci. Lett. 665, 182–188. doi: 10.1016/j.neulet.2017.11.049
Matsuzaki, Y., Tanaka, M., Hakoda, S., Masuda, T., Miyata, R., Konno, A., et al. (2019). Neurotropic properties of AAV-PHP.B are shared among diverse inbred strains of mice. Mol. Ther. 27, 700–704. doi: 10.1016/j.ymthe.2019.02.016
Maw, M. A., Kennedy, B., Knight, A., Bridges, R., Roth, K. E., Mani, E. J., et al. (1997). Mutation of the gene encoding cellular retinaldehyde-binding protein in autosomal recessive retinitis pigmentosa. Nat. Genet. 17, 198–200. doi: 10.1038/ng1097-198
McClain, J., Grubisic, V., Fried, D., Gomez-Suarez, R. A., Leinninger, G. M., Sevigny, J., et al. (2014). Ca2+ responses in enteric glia are mediated by connexin-43 hemichannels and modulate colonic transit in mice. Gastroenterology 146, 497–507.e491. doi: 10.1053/j.gastro.2013.10.061
Messing, A., Behringer, R. R., Hammang, J. P., Palmiter, R. D., Brinster, R. L., and Lemke, G. (1992). P0 promoter directs expression of reporter and toxin genes to Schwann cells of transgenic mice. Neuron 8, 507–520. doi: 10.1016/0896-6273(92)90279-m
Miyazaki, I., and Asanuma, M. (2017). Therapeutic strategy of targeting astrocytes for neuroprotection in Parkinson’s disease. Curr. Pharm. Des. 23, 4936–4947. doi: 10.2174/1381612823666170710163731
Mookherjee, S., Chen, H. Y., Isgrig, K., Yu, W., Hiriyanna, S., Levron, R., et al. (2018). A CEP290 C-terminal domain complements the mutant CEP290 of Rd16 mice in trans and rescues retinal degeneration. Cell Rep. 25, 611–623.e616. doi: 10.1016/j.celrep.2018.09.043
Mori, S., Wang, L., Takeuchi, T., and Kanda, T. (2004). Two novel adeno-associated viruses from cynomolgus monkey: pseudotyping characterization of capsid protein. Virology 330, 375–383. doi: 10.1016/j.virol.2004.10.012
Mudannayake, J. M., Mouravlev, A., Fong, D. M., and Young, D. (2016). Transcriptional activity of novel ALDH1L1 promoters in the rat brain following AAV vector-mediated gene transfer. Mol. Ther. Methods Clin. Dev. 3:16075. doi: 10.1038/mtm.2016.75
Muller, O. J., Kaul, F., Weitzman, M. D., Pasqualini, R., Arap, W., Kleinschmidt, J. A., et al. (2003). Random peptide libraries displayed on adeno-associated virus to select for targeted gene therapy vectors. Nat. Biotechnol. 21, 1040–1046. doi: 10.1038/nbt856
Murlidharan, G., Corriher, T., Ghashghaei, H. T., and Asokan, A. (2015). Unique glycan signatures regulate adeno-associated virus tropism in the developing brain. J. Virol. 89, 3976–3987. doi: 10.1128/JVI.02951-14
Nagy, J. I., Patel, D., Ochalski, P. A., and Stelmack, G. L. (1999). Connexin30 in rodent, cat and human brain: selective expression in gray matter astrocytes, co-localization with connexin43 at gap junctions and late developmental appearance. Neuroscience 88, 447–468. doi: 10.1016/s0306-4522(98)00191-2
Najjar, S., and Pearlman, D. M. (2015). Neuroinflammation and white matter pathology in schizophrenia: systematic review. Schizophr. Res. 161, 102–112. doi: 10.1016/j.schres.2014.04.041
Nasrabady, S. E., Rizvi, B., Goldman, J. E., and Brickman, A. M. (2018). White matter changes in Alzheimer’s disease: a focus on myelin and oligodendrocytes. Acta Neuropathol. Commun. 6:22. doi: 10.1186/s40478-018-0515-3
Newman, E. A. (2015). Glial cell regulation of neuronal activity and blood flow in the retina by release of gliotransmitters. Philos. Trans. R. Soc. Lond. B Biol. Sci. 370:20140195. doi: 10.1098/rstb.2014.0195
Ohara, P. T., Vit, J. P., Bhargava, A., Romero, M., Sundberg, C., Charles, A. C., et al. (2009). Gliopathic pain: when satellite glial cells go bad. Neuroscientist 15, 450–463. doi: 10.1177/1073858409336094
Okada, S., Hara, M., Kobayakawa, K., Matsumoto, Y., and Nakashima, Y. (2018). Astrocyte reactivity and astrogliosis after spinal cord injury. Neurosci. Res. 126, 39–43. doi: 10.1016/j.neures.2017.10.004
Palpagama, T. H., Waldvogel, H. J., Faull, R. L. M., and Kwakowsky, A. (2019). The role of microglia and astrocytes in Huntington’s disease. Front. Mol. Neurosci. 12:258. doi: 10.3389/fnmol.2019.00258
Pandya, J., Ortiz, L., Ling, C., Rivers, A. E., and Aslanidi, G. (2014). Rationally designed capsid and transgene cassette of AAV6 vectors for dendritic cell-based cancer immunotherapy. Immunol. Cell Biol. 92, 116–123. doi: 10.1038/icb.2013.74
Peel, A. L., and Klein, R. L. (2000). Adeno-associated virus vectors: activity and applications in the CNS. J. Neurosci. Methods 98, 95–104. doi: 10.1016/s0165-0270(00)00183-7
Pellissier, L. P., Hoek, R. M., Vos, R. M., Aartsen, W. M., Klimczak, R. R., Hoyng, S. A., et al. (2014). Specific tools for targeting and expression in Muller glial cells. Mol. Ther. Methods Clin. Dev. 1:14009. doi: 10.1038/mtm.2014.9
Pellissier, L. P., Quinn, P. M., Alves, C. H., Vos, R. M., Klooster, J., Flannery, J. G., et al. (2015). Gene therapy into photoreceptors and Muller glial cells restores retinal structure and function in CRB1 retinitis pigmentosa mouse models. Hum. Mol. Genet. 24, 3104–3118. doi: 10.1093/hmg/ddv062
Penaud-Budloo, M., François, A., Clément, N., and Ayuso, E. (2018). Pharmacology of recombinant adeno-associated virus production. Mol. Ther. Methods Clin. Dev. 8, 166–180. doi: 10.1016/j.omtm.2018.01.002
Petrs-Silva, H., Dinculescu, A., Li, Q., Min, S. H., Chiodo, V., Pang, J. J., et al. (2009). High-efficiency transduction of the mouse retina by tyrosine-mutant AAV serotype vectors. Mol. Ther. 17, 463–471. doi: 10.1038/mt.2008.269
Piguet, F., Sondhi, D., Piraud, M., Fouquet, F., Hackett, N. R., Ahouansou, O., et al. (2012). Correction of brain oligodendrocytes by AAVrh.10 intracerebral gene therapy in metachromatic leukodystrophy mice. Hum. Gene Ther. 23, 903–914. doi: 10.1089/hum.2012.015
Pochard, C., Coquenlorge, S., Freyssinet, M., Naveilhan, P., Bourreille, A., Neunlist, M., et al. (2018). The multiple faces of inflammatory enteric glial cells: is Crohn’s disease a gliopathy? Am. J. Physiol. Gastrointest. Liver Physiol. 315, G1–G11. doi: 10.1152/ajpgi.00016.2018
Ponnazhagan, S., Mahendra, G., Kumar, S., Thompson, J. A., and Castillas, M. Jr. (2002). Conjugate-based targeting of recombinant adeno-associated virus type 2 vectors by using avidin-linked ligands. J. Virol. 76, 12900–12907. doi: 10.1128/jvi.76.24.12900-12907.2002
Powell, S. K., Khan, N., Parker, C. L., Samulski, R. J., Matsushima, G., Gray, S. J., et al. (2016). Characterization of a novel adeno-associated viral vector with preferential oligodendrocyte tropism. Gene Ther. 23, 807–814. doi: 10.1038/gt.2016.62
Powell, S. K., Samulski, R. J., and McCown, T. J. (2020). AAV capsid-promoter interactions determine CNS cell-selective gene expression in vivo. Mol. Ther. 28, 1373–1380. doi: 10.1016/j.ymthe.2020.03.007
Prentice, H. M., Biswal, M. R., Dorey, C. K., and Blanks, J. C. (2011). Hypoxia-regulated retinal glial cell-specific promoter for potential gene therapy in disease. Invest. Ophthalmol. Vis. Sci. 52, 8562–8570. doi: 10.1167/iovs.10-6835
Procaccini, C., De Rosa, V., Pucino, V., Formisano, L., and Matarese, G. (2015). Animal models of multiple sclerosis. Eur. J. Pharmacol. 759, 182–191. doi: 10.1016/j.ejphar.2015.03.042
Pukos, N., Goodus, M. T., Sahinkaya, F. R., and McTigue, D. M. (2019). Myelin status and oligodendrocyte lineage cells over time after spinal cord injury: what do we know and what still needs to be unwrapped? Glia 67, 2178–2202. doi: 10.1002/glia.23702
Quinn, P. M., Buck, T. M., Mulder, A. A., Ohonin, C., Alves, C. H., Vos, R. M., et al. (2019). Human iPSC-derived retinas recapitulate the fetal CRB1 CRB2 complex formation and demonstrate that photoreceptors and muller glia are targets of AAV5. Stem Cell Reports 12, 906–919. doi: 10.1016/j.stemcr.2019.03.002
Rabinowitz, J. E., and Samulski, J. (1998). Adeno-associated virus expression systems for gene transfer. Curr. Opin. Biotechnol. 9, 470–475. doi: 10.1016/S0958-1669(98)80031-1
Ranno, E., D’Antoni, S., Spatuzza, M., Berretta, A., Laureanti, F., Bonaccorso, C. M., et al. (2014). Endothelin-1 is over-expressed in amyotrophic lateral sclerosis and induces motor neuron cell death. Neurobiol. Dis. 65, 160–171. doi: 10.1016/j.nbd.2014.01.002
Rao, M., and Gershon, M. D. (2018). Enteric nervous system development: what could possibly go wrong? Nat. Rev. Neurosci. 19, 552–565. doi: 10.1038/s41583-018-0041-0
Reichenbach, A., and Bringmann, A. (2013). New functions of Muller cells. Glia 61, 651–678. doi: 10.1002/glia.22477
Rosario, A. M., Cruz, P. E., Ceballos-Diaz, C., Strickland, M. R., Siemienski, Z., Pardo, M., et al. (2016). Microglia-specific targeting by novel capsid-modified AAV6 vectors. Mol. Ther. Methods Clin. Dev. 3:16026. doi: 10.1038/mtm.2016.26
Rossi, D. (2015). Astrocyte physiopathology: at the crossroads of intercellular networking, inflammation and cell death. Prog. Neurobiol. 130, 86–120. doi: 10.1016/j.pneurobio.2015.04.003
Rothhammer, V., Mascanfroni, I. D., Bunse, L., Takenaka, M. C., Kenison, J. E., Mayo, L., et al. (2016). Type I interferons and microbial metabolites of tryptophan modulate astrocyte activity and central nervous system inflammation via the aryl hydrocarbon receptor. Nat. Med. 22, 586–597. doi: 10.1038/nm.4106
Royo, N. C., Vandenberghe, L. H., Ma, J. Y., Hauspurg, A., Yu, L. Y., Maronski, M., et al. (2008). Specific AAV serotypes stably transduce primary hippocampal and cortical cultures with high efficiency and low toxicity. Brain Res. 1190, 15–22. doi: 10.1016/j.brainres.2007.11.015
Ruhl, A., Nasser, Y., and Sharkey, K. A. (2004). Enteric glia. Neurogastroenterol. Motil. 16(Suppl. 1), 44–49. doi: 10.1111/j.1743-3150.2004.00474.x
Samulski, R. J., and Muzyczka, N. (2014). AAV-mediated gene therapy for research and therapeutic purposes. Annu. Rev. Virol. 1, 427–451. doi: 10.1146/annurev-virology-031413-085355
Santello, M., Bezzi, P., and Volterra, A. (2011). TNFalpha controls glutamatergic gliotransmission in the hippocampal dentate gyrus. Neuron 69, 988–1001. doi: 10.1016/j.neuron.2011.02.003
Sargiannidou, I., Kagiava, A., Bashiardes, S., Richter, J., Christodoulou, C., Scherer, S. S., et al. (2015). Intraneural GJB1 gene delivery improves nerve pathology in a model of X-linked charcot-marie-tooth disease. Ann. Neurol. 78, 303–316. doi: 10.1002/ana.24441
Sargiannidou, I., Kagiava, A., and Kleopa, K. A. (2020). Gene therapy approaches targeting Schwann cells for demyelinating neuropathies. Brain Res. 1728:146572. doi: 10.1016/j.brainres.2019.146572
Schiza, N., Sargiannidou, I., Kagiava, A., Karaiskos, C., Nearchou, M., and Kleopa, K. A. (2015). Transgenic replacement of Cx32 in gap junction-deficient oligodendrocytes rescues the phenotype of a hypomyelinating leukodystrophy model. Hum. Mol. Genet. 24, 2049–2064. doi: 10.1093/hmg/ddu725
Schlieve, C. R., Fowler, K. L., Thornton, M., Huang, S., Hajjali, I., Hou, X., et al. (2017). Neural crest cell implantation restores enteric nervous system function and alters the gastrointestinal transcriptome in human tissue-engineered small intestine. Stem Cell Reports 9, 883–896. doi: 10.1016/j.stemcr.2017.07.017
Schober, A. L., Gagarkin, D. A., Chen, Y., Gao, G., Jacobson, L., and Mongin, A. A. (2016). Recombinant adeno-associated virus serotype 6 (rAAV6) potently and preferentially transduces rat astrocytes in vitro and in vivo. Front. Cell. Neurosci. 10:262. doi: 10.3389/fncel.2016.00262
Schuster, D. J., Dykstra, J. A., Riedl, M. S., Kitto, K. F., Belur, L. R., McIvor, R. S., et al. (2014). Biodistribution of adeno-associated virus serotype 9 (AAV9) vector after intrathecal and intravenous delivery in mouse. Front. Neuroanat. 8:42. doi: 10.3389/fnana.2014.00042
Sery, O., Sultana, N., Kashem, M. A., Pow, D. V., and Balcar, V. J. (2015). GLAST but not least–distribution, function, genetics and epigenetics of L-glutamate transport in brain–focus on GLAST/EAAT1. Neurochem. Res. 40, 2461–2472. doi: 10.1007/s11064-015-1605-2
Seyedian, S. S., Nokhostin, F., and Malamir, M. D. (2019). A review of the diagnosis, prevention, and treatment methods of inflammatory bowel disease. J. Med. Life 12, 113–122. doi: 10.25122/jml-2018-0075
Shao, G., Greathouse, K., Huang, Q., Wang, C. M., and Sferra, T. J. (2006). Gene transfer to the gastrointestinal tract after peroral administration of recombinant adeno-associated virus type 2 vectors. J. Pediatr. Gastroenterol. Nutr. 43, 168–179. doi: 10.1097/01.mpg.0000228118.59853.ba
Shimizu, K., Sakurai, F., Tomita, K., Nagamoto, Y., Nakamura, S., Katayama, K., et al. (2014). Suppression of leaky expression of adenovirus genes by insertion of microRNA-targeted sequences in the replication-incompetent adenovirus vector genome. Mol. Ther. Methods Clin. Dev. 1:14035. doi: 10.1038/mtm.2014.35
Simpson, C. P., Bolch, S. N., Zhu, P., Weidert, F., Dinculescu, A., and Lobanova, E. S. (2019). Systemic delivery of genes to retina using adeno-associated viruses. Adv. Exp. Med. Biol. 1185, 109–112. doi: 10.1007/978-3-030-27378-1_18
Sohl, G., Odermatt, B., Maxeiner, S., Degen, J., and Willecke, K. (2004). New insights into the expression and function of neural connexins with transgenic mouse mutants. Brain Res. Brain Res. Rev. 47, 245–259. doi: 10.1016/j.brainresrev.2004.05.006
Souza, G. R., Talbot, J., Lotufo, C. M., Cunha, F. Q., Cunha, T. M., and Ferreira, S. H. (2013). Fractalkine mediates inflammatory pain through activation of satellite glial cells. Proc. Natl. Acad. Sci. U.S.A. 110, 11193–11198. doi: 10.1073/pnas.1307445110
Spear, E. T., and Mawe, G. M. (2019). Enteric neuroplasticity and dysmotility in inflammatory disease: key players and possible therapeutic targets. Am. J. Physiol. Gastrointest. Liver Physiol. 317, G853–G861. doi: 10.1152/ajpgi.00206.2019
Srivastava, A. (2016). In vivo tissue-tropism of adeno-associated viral vectors. Curr. Opin. Virol. 21, 75–80. doi: 10.1016/j.coviro.2016.08.003
Stephenson, J., Nutma, E., van der Valk, P., and Amor, S. (2018). Inflammation in CNS neurodegenerative diseases. Immunology 154, 204–219. doi: 10.1111/imm.12922
Su, W., Kang, J., Sopher, B., Gillespie, J., Aloi, M. S., Odom, G. L., et al. (2016). Recombinant adeno-associated viral (rAAV) vectors mediate efficient gene transduction in cultured neonatal and adult microglia. J. Neurochem. 136(Suppl. 1), 49–62. doi: 10.1111/jnc.13081
Surace, E. M., Auricchio, A., Reich, S. J., Rex, T., Glover, E., Pineles, S., et al. (2003). Delivery of adeno-associated virus vectors to the fetal retina: impact of viral capsid proteins on retinal neuronal progenitor transduction. J. Virol. 77, 7957–7963. doi: 10.1128/jvi.77.14.7957-7963.2003
Sweeney, M. D., Zhao, Z., Montagne, A., Nelson, A. R., and Zlokovic, B. V. (2019). Blood-brain barrier: from physiology to disease and back. Physiol. Rev. 99, 21–78. doi: 10.1152/physrev.00050.2017
Tarassishin, L., Loudig, O., Bauman, A., Shafit-Zagardo, B., Suh, H. S., and Lee, S. C. (2011). Interferon regulatory factor 3 inhibits astrocyte inflammatory gene expression through suppression of the proinflammatory miR-155 and miR-155∗. Glia 59, 1911–1922. doi: 10.1002/glia.21233
Taschenberger, G., Tereshchenko, J., and Kugler, S. (2017). A MicroRNA124 target sequence restores astrocyte specificity of gfaABC1D-driven transgene expression in AAV-mediated gene transfer. Mol. Ther. Nucleic Acids 8, 13–25. doi: 10.1016/j.omtn.2017.03.009
Testen, A., Kim, R., and Reissner, K. J. (2020). High-resolution three-dimensional imaging of individual astrocytes using confocal microscopy. Curr. Protoc. Neurosci. 91:e92. doi: 10.1002/cpns.92
Theofilas, P., Brar, S., Stewart, K. A., Shen, H. Y., Sandau, U. S., Poulsen, D., et al. (2011). Adenosine kinase as a target for therapeutic antisense strategies in epilepsy. Epilepsia 52, 589–601. doi: 10.1111/j.1528-1167.2010.02947.x
Tornabene, P., Trapani, I., Minopoli, R., Centrulo, M., Lupo, M., de Simone, S., et al. (2019). Intein-mediated protein trans-splicing expands adeno-associated virus transfer capacity in the retina. Sci. Transl. Med. 11:eaav4523. doi: 10.1126/scitranslmed.aav4523
Vagner, T., Dvorzhak, A., Wojtowicz, A. M., Harms, C., and Grantyn, R. (2016). Systemic application of AAV vectors targeting GFAP-expressing astrocytes in Z-Q175-KI Huntington’s disease mice. Mol. Cell Neurosci. 77, 76–86. doi: 10.1016/j.mcn.2016.10.007
Vanderver, A., Prust, M., Tonduti, D., Mochel, F., Hussey, H. M., Helman, G., et al. (2015). Case definition and classification of leukodystrophies and leukoencephalopathies. Mol. Genet. Metab. 114, 494–500. doi: 10.1016/j.ymgme.2015.01.006
von Boyen, G. B., Steinkamp, M., Reinshagen, M., Schafer, K. H., Adler, G., and Kirsch, J. (2004). Proinflammatory cytokines increase glial fibrillary acidic protein expression in enteric glia. Gut 53, 222–228. doi: 10.1136/gut.2003.012625
von Jonquieres, G., Frohlich, D., Klugmann, C. B., Wen, X., Harasta, A. E., Ramkumar, R., et al. (2016). Recombinant human myelin-associated glycoprotein promoter drives selective AAV-mediated transgene expression in oligodendrocytes. Front. Mol. Neurosci. 9:13. doi: 10.3389/fnmol.2016.00013
von Jonquieres, G., Mersmann, N., Klugmann, C. B., Harasta, A. E., Lutz, B., Teahan, O., et al. (2013). Glial promoter selectivity following AAV-delivery to the immature brain. PLoS One 8:e65646. doi: 10.1371/journal.pone.0065646
Wang, C., Wang, C. M., Clark, K. R., and Sferra, T. J. (2003). Recombinant AAV serotype 1 transduction efficiency and tropism in the murine brain. Gene Ther. 10, 1528–1534.
Wang, L., Leonards, C. O., Sterzer, P., and Ebinger, M. (2014). White matter lesions and depression: a systematic review and meta-analysis. J. Psychiatr. Res. 56, 56–64. doi: 10.1016/j.jpsychires.2014.05.005
Wang, Y., Liu, G., Hong, D., Chen, F., Ji, X., and Cao, G. (2016). White matter injury in ischemic stroke. Prog. Neurobiol. 141, 45–60. doi: 10.1016/j.pneurobio.2016.04.005
Wenger, R. H. (2002). Cellular adaptation to hypoxia: O2-sensing protein hydroxylases, hypoxia-inducible transcription factors, and O2-regulated gene expression. FASEB J. 16, 1151–1162. doi: 10.1096/fj.01-0944rev
Xiang, H., Xu, H., Fan, F., Shin, S. M., Hogan, Q. H., and Yu, H. (2018). Glial fibrillary acidic protein promoter determines transgene expression in satellite glial cells following intraganglionic adeno-associated virus delivery in adult rats. J. Neurosci. Res. 96, 436–448. doi: 10.1002/jnr.24183
Xie, Y., Wang, T., Sun, G. Y., and Ding, S. (2010). Specific disruption of astrocytic Ca2+ signaling pathway in vivo by adeno-associated viral transduction. Neuroscience 170, 992–1003. doi: 10.1016/j.neuroscience.2010.08.034
Yakoub, A. M. (2019). Cerebral organoids exhibit mature neurons and astrocytes and recapitulate electrophysiological activity of the human brain. Neural Regen. Res. 14, 757–761. doi: 10.4103/1673-5374.249283
Yamanaka, K., and Komine, O. (2018). The multi-dimensional roles of astrocytes in ALS. Neurosci. Res. 126, 31–38. doi: 10.1016/j.neures.2017.09.011
Yang, B., Li, S., Wang, H., Guo, Y., Gessler, D. J., Cao, C., et al. (2014). Global CNS transduction of adult mice by intravenously delivered rAAVrh.8 and rAAVrh.10 and nonhuman primates by rAAVrh.10. Mol. Ther. 22, 1299–1309. doi: 10.1038/mt.2014.68
Yang, Z., and Wang, K. K. (2015). Glial fibrillary acidic protein: from intermediate filament assembly and gliosis to neurobiomarker. Trends Neurosci. 38, 364–374. doi: 10.1016/j.tins.2015.04.003
Zhang, H., Yang, B., Mu, X., Ahmed, S. S., Su, Q., He, R., et al. (2011). Several rAAV vectors efficiently cross the blood-brain barrier and transduce neurons and astrocytes in the neonatal mouse central nervous system. Mol. Ther. 19, 1440–1448. doi: 10.1038/mt.2011.98
Zhao, M., Andrieu-Soler, C., Kowalczuk, L., Paz Cortes, M., Berdugo, M., Dernigoghossian, M., et al. (2015). A new CRB1 rat mutation links Muller glial cells to retinal telangiectasia. J. Neurosci. 35, 6093–6106. doi: 10.1523/JNEUROSCI.3412-14.2015
Zhong, L., Li, B., Mah, C. S., Govindasamy, L., Agbandje-McKenna, M., Cooper, M., et al. (2008). Next generation of adeno-associated virus 2 vectors: point mutations in tyrosines lead to high-efficiency transduction at lower doses. Proc. Natl. Acad. Sci. U.S.A. 105, 7827–7832. doi: 10.1073/pnas.0802866105
Keywords: gene therapy, AAV, glia, astrocyte, oligodendrocyte, microglia, peripheral nerve, Müller glia cell
Citation: O’Carroll SJ, Cook WH and Young D (2021) AAV Targeting of Glial Cell Types in the Central and Peripheral Nervous System and Relevance to Human Gene Therapy. Front. Mol. Neurosci. 13:618020. doi: 10.3389/fnmol.2020.618020
Received: 16 October 2020; Accepted: 11 December 2020;
Published: 11 January 2021.
Edited by:
Andreas Toft Sørensen, University of Copenhagen, DenmarkReviewed by:
Jan Wijnholds, Leiden University Medical Center, NetherlandsJonathan T. Ting, Allen Institute for Brain Science, United States
Copyright © 2021 O’Carroll, Cook and Young. This is an open-access article distributed under the terms of the Creative Commons Attribution License (CC BY). The use, distribution or reproduction in other forums is permitted, provided the original author(s) and the copyright owner(s) are credited and that the original publication in this journal is cited, in accordance with accepted academic practice. No use, distribution or reproduction is permitted which does not comply with these terms.
*Correspondence: Simon J. O’Carroll, s.ocarroll@auckland.ac.nz