- Graduate School of Frontier Biosciences, Osaka University, Suita, Japan
In mammals, the sensory experience can regulate the development of various brain structures, including the cortex, hippocampus, retina, and olfactory bulb (OB). Odor experience-evoked neural activity drives the development of dendrites on excitatory projection neurons in the OB, such as mitral and tufted cells, as well as inhibitory interneurons. OB interneurons are generated continuously in the subventricular zone and differentiate into granule cells (GCs) and periglomerular cells (PGCs). However, it remains unknown what role each type of OB interneuron plays in controlling olfactory behaviors. Recent studies showed that among the various types of OB interneurons, a subtype of GCs expressing oncofetal trophoblast glycoprotein 5T4 is required for simple odor detection and discrimination behaviors. Mouse 5T4 (also known as Tpbg) is a type I membrane glycoprotein whose extracellular domain contains seven leucine-rich repeats (LRRs) sandwiched between characteristic LRR-N and LRR-C regions. Recently, it was found that the developmental expression of 5T4 increases dramatically in the retina just before eye-opening. Single-cell transcriptomics further suggests that 5T4 is involved in the development and maintenance of functional synapses in a subset of retinal interneurons, including rod bipolar cells (RBCs) and amacrine cells (ACs). Collectively, 5T4, expressed in interneurons of the OB and retina, plays a key role in sensory processing in the olfactory and visual systems.
Introduction
Cell adhesion molecules with immunoglobulin, cadherin, and leucine-rich repeat (LRR) domains are involved in target recognition in synaptogenesis (Sanes and Zipursky, 2020). In particular, the membrane proteins containing LRR motifs in the extracellular domain organize excitatory and inhibitory synapses by forming binding interfaces for a broad spectrum of interactions. Several mammalian LRR proteins are implicated in synaptic specificity, although in most cases, it remains unclear whether they promote specificity or synaptogenesis. These include three fibronectin LRR transmembrane proteins (FLRTs), four LRR transmembrane neuronal proteins (LRRTMs), six Slit and neurotrophic receptor tyrosine kinase (NTRK)-like family proteins (Slitrks), five synaptic adhesion-like molecules (SALMs), and three netrin-G ligands (NGLs; Schroeder and de Wit, 2018). Most of these bind heterophilically to other proteins, including FLRTs to latrophilins, LRRTMs to neurexins, and NGLs to netrin G1 and G2 (de Wit and Ghosh, 2014). Here, I show a member of the extracellular-type LRR membrane proteins, which is expressed in interneurons of the olfactory bulb (OB) and retina and plays a key role in sensory processing in the olfactory and visual systems.
LRR-Containing Oncofetal Trophoblast Glycoprotein 5T4
In the neural circuit of the OB, a family of membrane proteins that localize to specific strata was thought to be implicated in the formation of layer-specific dendrodendritic synaptic connections (Figure 1A; Imamura et al., 2006). Mass spectrometry analyses for membrane proteins in the mouse OB identified 5T4 oncofetal trophoblast glycoprotein (termed 5T4 or Tpbg), a member of the LRR membrane protein family, in interneurons at a specific stratum (Figure 1B). Intriguingly, among the extracellular-type LRR membrane proteins, 5T4 is well conserved in mice (King et al., 1999) and humans (Myers et al., 1994), as well as in nonmammalian species, including CG6959 in the fly (Özkan et al., 2013) and Wnt-activated inhibitory factor 1 (WAIF1) in zebrafish (Kagermeier-Schenk et al., 2011).
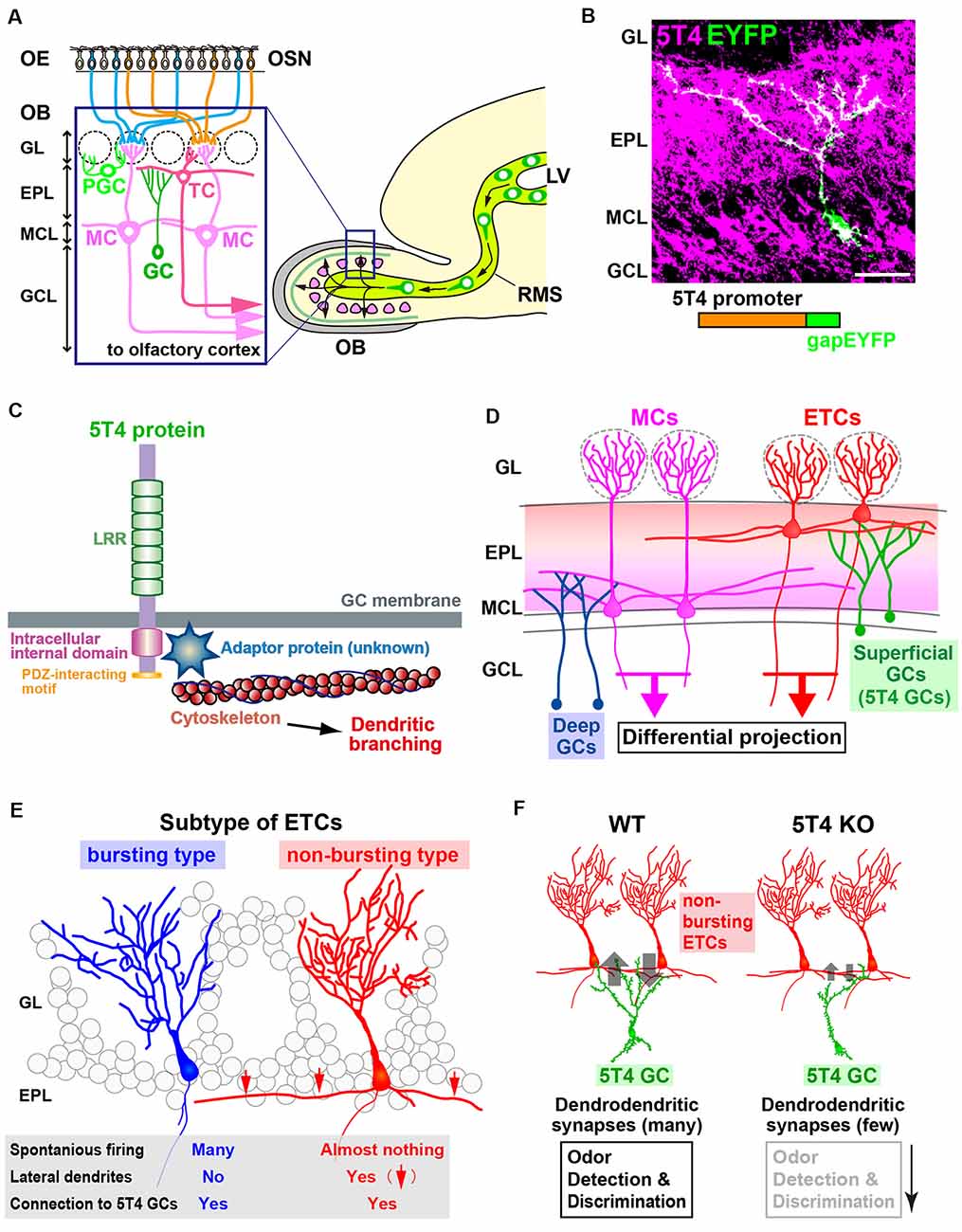
Figure 1. The function of 5T4 in a subtype of granule cells (GCs) in the olfactory bulb (OB). (A) Mammalian OB is composed of a distinct laminar structure. A subset of olfactory sensory neurons (OSNs) in the olfactory epithelium (OE) extend their axons to specific glomeruli in the OB. OSN signals activate a specific neural circuit, promoting the dendritic development of inhibitory interneurons through excitatory projection neurons such as tufted cells (TCs) and mitral cells (MCs). OB interneurons are generated continuously in the subventricular zone within the lateral ventricle (LV), migrate through the rostral migratory stream (RMS), and differentiate into inhibitory interneurons such as GCs and periglomerular cells (PGCs). GL, glomerular layer; EPL, external plexiform layer; MCL, mitral cell layer; GCL, granule cell layer. (B) Dendritic morphology and laminar location of 5T4 GCs. The lentiviral vector carrying 5T4 promoter-driven gapEYFP was injected into the LV of wild-type mice for immunostaining with anti-5T4 and anti-EGFP antibodies. Scale bar, 30 μm. (C) Schematic representations of 5T4 protein and 5T4 signaling pathway. The 5T4-intracellular domain, which lacks the PDZ-interacting motif, is necessary for the dendritic morphology of 5T4 GCs (Yoshihara et al., 2012). Recent studies suggest that a member of Ras-like small GTPase superfamily, Rab11, may interact with 5T4 to regulate dendritic branching of 5T4 GCs in the OB (Harris et al., 2018; Siri et al., 2020). (D) Schematic drawing of the OB neural circuit. Superficial GCs, including 5T4 GCs, connect preferentially to the lateral dendrites of external TCs (ETCs) at the upper EPL, whereas deep GCs connect mainly to MCs at the deeper EPL. Parallel ETC and MC pathways send distinct odor information via their specific routes to different areas in the olfactory cortex. (E) ETCs are divided into two different subtypes. Bursting ETCs without lateral dendrites frequently fire the spontaneously, whereas non-bursting ETCs with lateral dendrites do not. 5T4 GCs connect to both bursting and non-bursting ETCs via dendrodendritic synapses (Takahashi et al., 2016). (F) 5T4 GCs connect to non-bursting ETCs. The dendritic branching of 5T4 GCs is more reduced in 5T4-knockout (KO) mice (Yoshihara et al., 2012). Notably, GABAergic inputs into non-bursting ETCs are significantly reduced in 5T4-KO mice, while those into bursting ETCs are unaffected. This gives rise to alterations of olfactory behaviors such as odor detection and discrimination in 5T4-KO mice.
5T4 is a type I transmembrane glycoprotein with an N-terminal extracellular domain comprising seven LRRs (24 amino acids each), flanked by characteristic LRR-N and LRR-C regions, and interspersed by seven N-linked glycosylation sites (Figure 1C; King et al., 1999; Imamura et al., 2006; Zhao et al., 2014). The intracellular domain of 5T4 is capped by a class 1 PDZ-interacting motif (Figure 1C; Imamura et al., 2006; Zhao et al., 2014) and contains two serine residues, which are likely phosphorylated by protein kinase Cα (PKCα; Wakeham et al., 2019, 2020).
5T4 was originally identified while searching for molecules with invasive properties that are likely shared by placental trophoblasts and cancer cells (Hole and Stern, 1990). 5T4 is normally expressed at high levels in the brain and ovaries (King et al., 1999; Barrow et al., 2005) and at low levels in other tissues, but is highly expressed in a variety of carcinomas (Southall et al., 1990). Overexpression of 5T4 in murine epithelial cells downregulates E-cadherin, disrupts of cell-to-cell contacts, alters their morphology, and increases their motility (Carsberg et al., 1996). However, 5T4 upregulation is also associated with the differentiation of embryonic stem cells and is essential for epithelial-to-mesenchymal transition (Eastham et al., 2007; Spencer et al., 2007). In embryonic cell lines, 5T4 affects the cytoskeletal organization and cell motility by modulating Wnt/β-catenin signaling (Kagermeier-Schenk et al., 2011). A recent study also reported the expression of 5T4 in epithelial progenitors, such as taste stem and progenitor cells, and taste bud cell precursors, suggesting that it contributes to the maintenance of taste papillae throughout life (Takahashi et al., 2019).
The Function of 5T4 in a Granule Cell Subtype Within the Olfactory Bulb
Odorants activate specific olfactory sensory neurons (OSNs) expressing the corresponding odorant receptors (Mori and Sakano, 2011). OSN axons project to specific glomeruli in the OB to comprise a specific neural circuit involving glutamatergic excitatory projections of external tufted cells (ETCs) and mitral cells (MCs) that also promote the dendritic development of inhibitory interneurons (Figure 1A; Mori and Sakano, 2011; Lepousez et al., 2013). OB interneurons, such as granule cells (GCs) and periglomerular cells (PGCs), are generated continuously in the subventricular zone within the lateral ventricle (LV) and migrate through the rostral migratory stream (RMS) to the OB, where they differentiate into γ-aminobutyric acid (GABA)-releasing inhibitory interneurons (Figure 1A; Imayoshi et al., 2008; Lledo et al., 2008; Whitman and Greer, 2009; Adam and Mizrahi, 2010; Kaneko et al., 2010; Sakamoto et al., 2011). Odor-rich environment and odor deprivation promote and suppress, respectively, dendritic morphogenesis and spinogenesis in newborn OB interneurons (Saghatelyan et al., 2005; Livneh et al., 2014), which are essential for odor detection and discrimination, olfactory learning and memory, and innate olfactory responses including avoidance and sexual behaviors (Breton-Provencher et al., 2009; Sakamoto et al., 2011, 2014; Alonso et al., 2012; Nunes and Kuner, 2015). Cell morphology and lineage analyses revealed that GCs are the largest population of OB interneurons and are subdivided into several subtypes (Orona et al., 1983; Shepherd et al., 2007; Merkle et al., 2014) according to their expression of calretinin, Ca2+/calmodulin-dependent protein kinase II α subunit (CaMKIIα), 5T4, metabotropic glutamate receptor 2 (mGluR2), and neurogranin (Imamura et al., 2006; Batista-Brito et al., 2008; Gribaudo et al., 2009; Murata et al., 2011; Merkle et al., 2014; Malvaut et al., 2017). However, the functional specificity that distinguishes each of these GC subtypes in the OB remains unknown, in part because of the paucity of genetically altered mouse lines.
Combinatory screening with DNA microarray and in situ hybridization for unilaterally naris-occluded (i.e., odor-deprived) mice revealed that 5T4 expression in OB interneurons is dependent on odor-evoked neural activity (Yoshihara et al., 2012). Imamura et al. (2006) and Yoshihara et al. (2012) observed 5T4 expression in subpopulations of GCs and PGCs, suggesting its role in sensory processing. GCs of the 5T4-genetic lineage (hereinafter termed 5T4 GCs) in the OB have unique morphological features: their cell bodies are located mostly in the MC layer and some in the inner plexiform layer (IPL) and superficial GC layer; their dendrites ramify in the superficial external plexiform layer (EPL; Figure 1B; Imamura et al., 2006; Yoshihara et al., 2012). 5T4 loss- and gain-of-function experiments via knockout (KO) mice and lentiviral vector expression, respectively, demonstrated that 5T4 is necessary and sufficient for dendritic branching of 5T4 GCs in response to odor stimuli (Yoshihara et al., 2012). Takahashi et al. (2016) also used 5T4-KO and wild-type mice to show that 5T4 GCs play an important role in processing odor information in the OB neural circuit, as described below.
Inhibitory GCs synapse with MCs and ETCs, excitatory projection neurons of the OB (Figure 1D; Mori et al., 1983; Orona et al., 1984). ETCs are further divided into two distinct types: bursting ETCs without lateral dendrites that frequently fire spontaneously and non-bursting ETCs with lateral dendrites that do not fire spontaneously (Figure 1E; Ma and Lowe, 2010). To identify which type synapses with 5T4 GCs, GABAA-mediated postsynaptic currents were recorded for individual ETCs after Channelrhodopsin-2-expressing 5T4 GCs were stimulated by light (Madisen et al., 2012; Takahashi et al., 2016). These experiments revealed that the apical dendrites of 5T4 GCs form GABAergic synapses with both non-bursting ETCs and bursting ETCs (Figures 1D,E), as well as with MCs. Also, studies on OB slices from 5T4-KO mice showed that electrode stimulation evoked GABAA-mediated postsynaptic currents in bursting ETCs, whereas the currents in non-bursting ETCs were significantly reduced (Figure 1F; Takahashi et al., 2016). As GCs in the OB form reciprocal dendrodendritic synapses with projection neurons (Shepherd et al., 2004), excitatory inputs from ETCs to 5T4 GCs were also examined in wild-type and 5T4-KO mice. Importantly, the excitatory inputs from ETCs to 5T4 GCs were significantly fewer in 5T4-KO mice than in the wild type (Figure 1F), consistent with the reduced dendritic branching of 5T4-deficient GCs (Yoshihara et al., 2012). Taken together, these results demonstrate that 5T4 GCs regulate neural activity in non-bursting ETCs (Takahashi et al., 2016).
The reduced inhibition of non-bursting ETCs combined with the reduced excitation of 5T4-deficient GCs may affect olfactory behaviors in 5T4-KO mice (Figure 1F). To assess the physiological role of 5T4 GCs in odor information processing in the OB neural circuit, odor-detection thresholds in wild-type and 5T4-KO mice were examined by using a habituation-dishabituation test (Takahashi et al., 2016). The sensitivity of odor detection in 5T4-KO mice was approximately 100-fold lower than in the wild type. Moreover, 5T4-KO mice were unable to discriminate between two odorants presented simultaneously, although they showed no deficit when the odorants were presented separately in an odor discrimination learning task (Takahashi et al., 2016). Impaired odor discrimination in 5T4-KO mice was also demonstrated by the prolonged time they spent searching for a buried food pellet when a nonfood-related odorant was presented. Notably, they showed no deficit when searching for the buried food pellet in the absence of the distracting odor. Thus, 5T4 GCs in the OB play a crucial role in both odor detection and discrimination behaviors (Figure 1F; Takahashi et al., 2016, 2018). However, I cannot exclude the possibility that the behavioral changes were due to the elimination of 5T4 PGCs.
Recently, it was reported that zebrafish MCs receive direct interhemispheric projections from their contralateral counterparts, whereas interneurons receive interhemispheric top-down inputs from the contralateral zebrafish homolog of the olfactory cortex (Kermen et al., 2020). Mouse MCs/TCs receive indirect interhemispheric projections from their contralateral counterparts via the anterior olfactory nucleus pars externa (Grobman et al., 2018), whereas interneurons receive top-down inputs mostly from the ipsilateral olfactory cortex (Niedworok et al., 2012). The interhemispheric connections of ETCs, whose neural activity is regulated by 5T4 GCs, may enable modulation of odor responses and contribute to the detection of specific odors in a noisy odor background.
Expression of 5T4 in Rod Bipolar Cells and a Subtype of Amacrine Cells Within the Retina
Like the OB, the mammalian retina also has a distinct laminar structure (Sanes and Yamagata, 2009). The unique output element is the retinal ganglion cell (RGC) in the ganglion cell layer (Figure 2A). RGC dendrites extend into the IPL and receive inputs from bipolar cells (BCs) and amacrine cells (ACs), the retinal interneurons (Figure 2A; Sterling and Demb, 2004). In the rod pathways of the retina, rod BCs (RBCs) are the first excitatory interneurons in the rod circuit to receive light-dependent synaptic input from rod photoreceptors in the outer plexiform layer (OPL), and give rise to retinal output via AII ACs in the IPL (Figure 2A). Although RBCs are primarily responsible for dark-adapted low-light vision, they contribute to retinal output under a diverse range of lighting conditions (Euler et al., 2014); however, the molecular mechanisms involved in RBC adaptation to changing luminance conditions remain unknown (Rampino and Nawy, 2011; Wakeham et al., 2019).
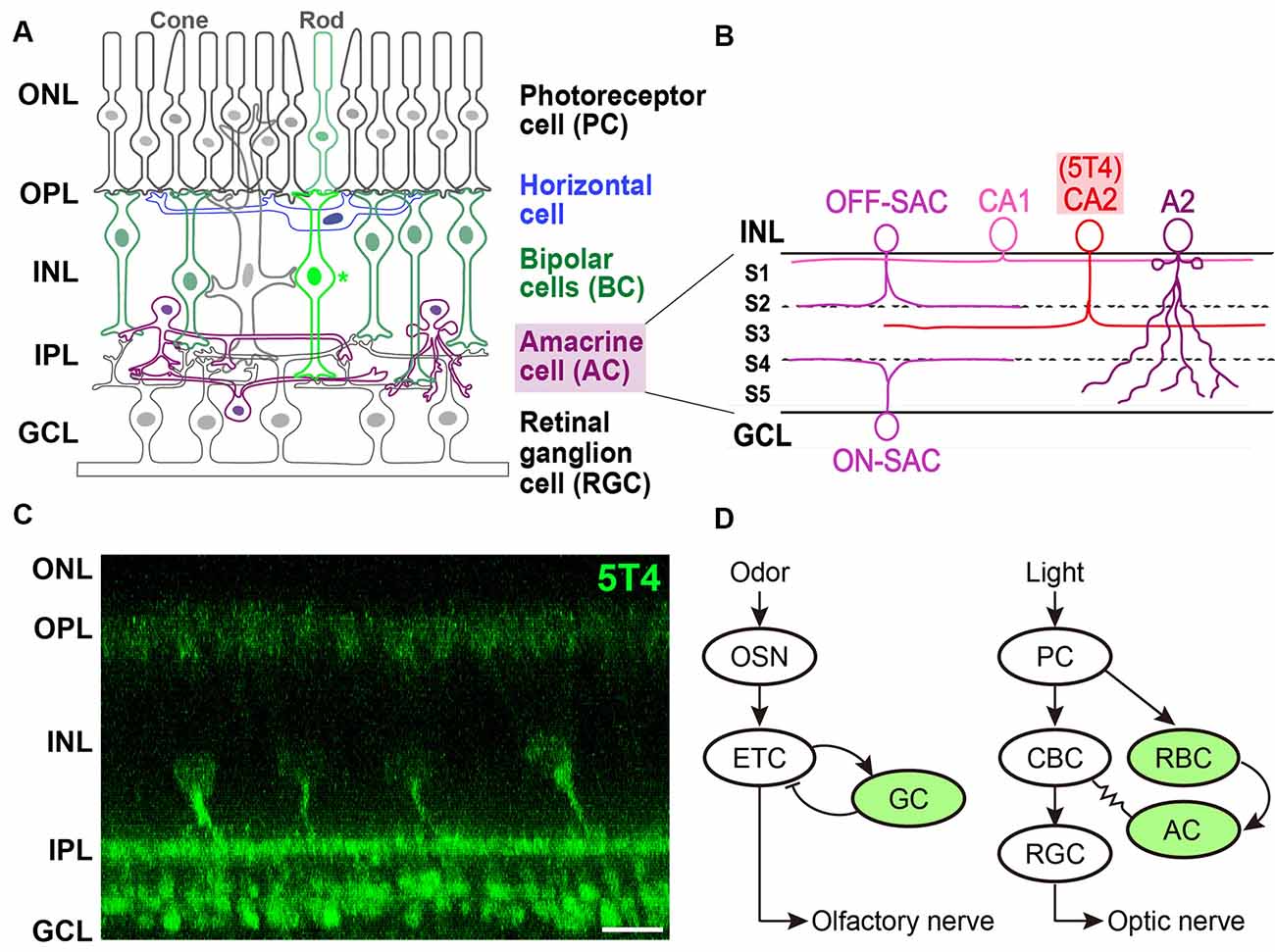
Figure 2. Expression of 5T4 in rod bipolar cells (RBCs) and a subtype of amacrine cells (ACs) in the retina. (A) Mammalian retina is composed of a distinct laminar structure. Photoreceptor (rod and cone) cells, interneurons such as horizontal cells, BCs, and ACs; and retinal ganglion cells (RGCs) are drawn schematically. Photoreceptor cells (PCs) form synapses with horizontal cells and BCs at the outer plexiform layer (OPL); BCs and ACs form synapses with each other as well as with RGCs at the inner plexiform layer (IPL). Remarkably, recent single-cell transcriptomics revealed that 5T4 belongs to one of 15 mouse BC clusters (Shekhar et al., 2016) that contains rod BCs (shown as an asterisk), whose processes extend to RGCs in the lower half of the IPL near the ganglion cell layer (GCL; shown in panel C), consistent with the previous observations (Imamura et al., 2006; Wakeham et al., 2019, 2020). Axons of RGCs extend through the optic nerve to the brain. ONL, outer nuclear layer; INL, inner nuclear layer. (B) Single-cell transcriptomics separated mouse ACs into 63 clusters. Based on the expression of established type-specific markers, several clusters are assigned to known AC types such as starburst ACs (SACs; C17 cluster) and AII (also A2; C3 cluster) ACs (Yan et al., 2020a). Importantly, 5T4 belongs to AC clusters, C25 and C31: glutamate decarboxylase 1 (Gad1) is highly expressed in C25 and C31; and tyrosine hydroxylase (Th) is highly expressed in C25. These results suggest that 5T4+ ACs are GABAergic and catecholaminergic (CAII or CA2) cells, whose dendrites branch at the sublamina (S3) between ON and OFF sublayers in the IPL (C), consistent with the previous observations (Imamura et al., 2006; Wakeham et al., 2019, 2020). Note that the IPL is divided into five sublaminae, S1–S5, with processes of each neuronal type confined to one or a few of them. Panels (A,B) are modified from Figure 1 in the article by Yan et al. (2020a), with permission of the journal for use. (C) Immunohistochemistry of the adult mouse retina with anti-5T4 antibody (kindly provided by Dr. Keisuke Yonehara at DANDRITE). Scale bar, 10 μm. (D) Schematic drawings of neuronal circuits in OB (left) and retina (right). Green color depicts 5T4-positive cells. (Left) Odor stimulation depolarizes OSNs, and connections from OSNs to ETCs produce excitatory outputs. Glutamatergic ETCs are reciprocally connected to GABAergic GCs and receive inhibitory feedback. ETCs extend their axons, forming the olfactory nerve, to the olfactory cortex. (Right) Light stimulation hyperpolarizes PCs, which connect to BCs, leading to the production of both ON-type and OFF-type signals. ON-type RGCs receive indirect excitation from rod BCs (RBC), via ACs and ON-type cone BCs (CBCs), and direct excitation from the terminals of ON-type CBCs via chemical synapses. RGC axons form the optic nerve, which projects to the visual cortex.
Imamura et al. (2006) identified 5T4-positive interneurons in the adult mouse retina. 5T4 is expressed mainly by RBCs whose processes are dispersed in the lower half of the IPL near the ganglion cell layer, as well as by a subpopulation of ACs whose dendrites branch at a single sublamina between ON and OFF sublayers in the IPL (Figures 2A,C). Recently, Wakeham et al. (2019) performed mass spectroscopy with multiplexed tandem mass tags to reveal that 5T4 acts as a PKCα-dependent phosphoprotein in RBCs. These authors then showed that 5T4 protein localizes to the somas, dendrites, and axon terminals of RBCs, as well as the somas and dendrites of an uncharacterized group of ACs (Figures 2B,C; Wakeham et al., 2020). Interestingly, 5T4 protein is undetectable in the neuron at birth but appears in the putative ACs by postnatal day 6 followed by an expression in RBCs by postnatal day 11. 5T4 expression in RBCs increases remarkably between postnatal days 11 and 12, just before eye-opening, suggesting that it plays a role in the development and maintenance of RBCs and ACs. Possibly, 5T4 expression in RBCs may be induced in response to glutamate-driven spontaneous retinal activity beginning a few days before eye-opening or in response to light-mediated activity, since BCs become light-responsive around postnatal day 10, probably via light entering the retina through closed eyelids (Tian and Copenhagen, 2003).
Studies on single-cell transcriptomics have been recently performed to classify neuronal types and reveal the recognition molecules they express (Sanes and Zipursky, 2020). Shekhar et al. (2016) utilized massively parallel single-cell RNA-Seq and optimized computations to reveal 15 clusters of retinal BCs in mice, one of which could be distinguished by its expression of PKCα (Puthussery et al., 2010). This rod BC cluster contains more than 100 enriched genes (Figure 2C; Shekhar et al., 2016), including all previously reported RBC markers such as 5T4 (Imamura et al., 2006). Intriguingly, single-cell RNAseq analysis revealed that 5T4 is expressed in another retinal cell type, a subtype of GABAergic ACs (Macosko et al., 2015; Shekhar et al., 2016). Indeed, high-throughput single-cell RNA-Seq analyses (Yan et al., 2020a) identified 63 distinct AC clusters, including those expressing 5T4 (clusters 25 and 31; Figure 2C). Clusters 25 and 31 also contained Gad1 encoding glutamate decarboxylase 1, suggesting that these ACs are GABAergic, along with Tac1, encoding tachykinin precursor 1. Furthermore, the Th gene for tyrosine hydroxylase was expressed by ACs in cluster 25, suggesting that 5T4+ ACs are also catecholaminergic (CAII or CA2; Figure 2B). These results strongly suggested that 5T4 is expressed not only by excitatory RBCs but also by inhibitory ACs (Figure 2C; Imamura et al., 2006; Wakeham et al., 2019). However, the relative amount of TPBG (the 5T4 homolog) mRNA seems to be low in macaque (Peng et al., 2019) or human (Yan et al., 2020b) retina. TPBG is expressed in a subtype of RGCs (18 types in macaque or 12 in human), termed ON midget RGCs (Peng et al., 2019; Yan et al., 2020b). Notably, the contrast-response functions of ON midget RGCs have lower thresholds, higher gain, and are more linear than those of OFF midget RGCs in humans (Soto et al., 2020).
Perspectives of 5T4 Function in the Retinal Neural Circuit
The functional organization of neuronal circuits for signal processing in the OB may be more similar to that in the retina than previously thought (Gollisch and Meister, 2010; Gire et al., 2013). In the OB circuit, odor stimuli depolarize OSNs, which connect to glutamatergic ETCs to produce excitatory outputs. ETCs also receive inhibitory feedback from reciprocal connections with GCs, and project to the olfactory cortex through the olfactory nerve. In the retinal circuit, light stimuli hyperpolarize photoreceptor cells (PCs), which connect to BCs to produce both ON-type and OFF-type bipolar signals. ON-type RGCs receive indirect excitation from rod BCs (RBCs) via AII ACs and ON-type cone BCs, and direct excitation from the terminals of ON-type cone BCs via chemical synapses (Figure 2D). RGCs, which represent the output layer of the retina, project their axons via the optic nerve to the lateral geniculate nucleus and the superior colliculus.
Intriguingly, these observations suggest a similarity between the OB and retinal circuits. In the OB, 5T4 expression is required for odor stimulation-dependent dendritic branching of GCs (Yoshihara et al., 2012) and crucial for odor detection and discrimination behaviors (Figure 1D; Takahashi et al., 2016, 2018). I hypothesize that 5T4 expression by excitatory RBCs and inhibitory ACs in the retina may regulate dendritic branching in response to light stimuli and contribute to dim-light detection and visual pattern discrimination.
In other systems, 5T4 changes Wnt signaling to modulate cytoskeletal rearrangement and cell morphology during embryonic development and cancer progression (Kagermeier-Schenk et al., 2011; Stern et al., 2014). In the OB, Wnt5a is expressed in interneurons, and a disruption of this reduces the extension of dendrites from GCs (Pino et al., 2011). Thus, Wnt5a production may regulate the Wnt-signaling pathway to promote the dendritic branching of GCs in the OB (van Amerongen and Nusse, 2009). In the developing mouse retina, Wnt signaling between rods and RBCs is involved in functional synaptic targeting and OPL lamination. In Wnt5a-KO mice, RBCs are mistargeted and give rise to the formation of an ectopic OPL (Sarin et al., 2018). This suggests that a Wnt-dependent mechanism is activated during the development of RBC dendrites and axons. Furthermore, 5T4 expression increases concomitantly with the development processes of RBCs that depend on the Wnt-signaling pathway (Wakeham et al., 2019), and regulates Wnt signaling in other embryonic and cancer tissues (Kagermeier-Schenk et al., 2011; Stern et al., 2014). These results further suggest that 5T4 may modulate similar signaling pathways in the retina and OB, and thus play crucial roles in the development and maintenance of neurites of retinal and OB interneurons. Future electrophysiological analyses of 5T4-expressing RBCs and ACs and behavioral analyses of 5T4-KO mice will help to elucidate its functional role in visual processing in the retinal neural circuit.
Yoshihara et al. (2012) revealed that the intracellular domain of 5T4 is necessary and sufficient for dendritic branching of 5T4 GCs, based on results of domain deletion and swapping experiments. Further, the 5T4 intracellular domain that lacks the PDZ-interacting motif is crucial (Figure 1C). They attempted to identify the molecules that interact with this domain by a yeast two-hybrid screen but failed. Harris et al. (2018) used a proteomic screen to identify ARF6, Rab18, and Rab11 as interacting proteins that control the expression and distribution of 5T4 in breast cancer cells. Interestingly, loss of Rab11, encoding a member of the Ras superfamily of small GTPases prevents the endocytosis of 5T4, resulting in its accumulation in the plasma membrane. Hence, evolutionarily conserved Rab11 may be a critical regulator for the sorting and trafficking of 5T4-containing vesicles to the cytoplasmic membrane. Indeed, Rab11-recycling endosomes are essential for growth cones, synapse architecture regulation, and neuronal migration (Welz et al., 2014). Siri et al. (2020) recently showed that the localization of these endosomes correlates with the developmental stage of hippocampal neurons, and that suppression of Rab11 expression increases dendritic branching (but not total dendritic length) and results in a misdistribution of dendritic proteins in vitro and in vivo. The interaction between Rab11-recycling endosomes and 5T4 may be required for proper dendritic branching of 5T4 GCs, thus controlling key aspects of synaptic plasticity (Figure 1C). Future studies on the molecular targets that interact with extracellular and intracellular domains of 5T4 will shed light on its physiological roles in the neural circuitry driving odor- and vision-associated behaviors.
Author Contributions
AT wrote the article.
Funding
This work was supported by Grants-in-Aid for Scientific Research on Basic Research-B (19H03340) and for Challenging Exploratory Research (19K22687), from the Ministry of Education, Culture, Sports, Science and Technology (MEXT), Japan, and by a grant for the SEEDS-A Program (A106) in Project of Translational and Clinical Research Core Center in Osaka University from Agency for Medical Research and Development (AMED), Japan. AT was supported by grants from the Smoking Science Research Foundation and the Takeda Science Foundation for Collaborative Research Projects, Japan.
Conflict of Interest
The author declares that the research was conducted in the absence of any commercial or financial relationships that could be construed as a potential conflict of interest.
Acknowledgments
I thank Dr. Keisuke Yonehara for kindly providing a figure and helpful discussion, Ms. Hisae Tsuboi for schematic drawing, and Drs. Hiroo Takahashi and Nobuhiko Yamamoto for their supports.
Abbreviations
AC, amacrine cell; BC, bipolar cell; CaMKIIα, Ca2+/calmodulin-dependent protein kinase II α subunit; EPL, external plexiform layer; ETC, external tufted cell; 5T4 (Tpbg), 5T4 oncofetal trophoblast glycoprotein in rodents; GABA, γ-aminobutyric acid; GC, granule cell; IPL, inner plexiform layer; KO, knockout; LRR, leucine-rich repeat; LV, lateral ventricle; MC, mitral cell; mGluR2, metabotropic glutamate receptor 2; OB, olfactory bulb; OPL, outer plexiform layer; OSN, olfactory sensory neurons; PKCα, protein kinase Cα; RBC, rod bipolar cell; RGC, retinal ganglion cell.
References
Adam, Y., and Mizrahi, A. (2010). Circuit formation and maintenance—perspectives from the mammalian olfactory bulb. Curr. Opin. Neurobiol. 20, 134–140. doi: 10.1016/j.conb.2009.11.001
Alonso, M., Lepousez, G., Wagner, S., Bardy, C., Gabellec, M. M., Torquet, N., et al. (2012). Activation of adult-born neurons facilitates learning and memory. Nat. Neurosci. 15, 897–904. doi: 10.1038/nn.3108
Barrow, K. M., Ward, C. M., Rutter, J., Ali, S., and Stern, P. L. (2005). Embryonic expression of murine 5T4 oncofoetal antigen is associated with morphogenetic events at implantation and in developing epithelia. Dev. Dyn. 233, 1535–1545. doi: 10.1002/dvdy.20482
Batista-Brito, R., Close, J., Machold, R., and Fishell, G. (2008). The distinct temporal origins of olfactory bulb interneuron subtypes. J. Neurosci. 28, 3966–3975. doi: 10.1523/JNEUROSCI.5625-07.2008
Breton-Provencher, V., Lemasson, M., Peralta, M. R. III., and Saghatelyan, A. (2009). Interneurons produced in adulthood are required for the normal functioning of the olfactory bulb network and for the execution of selected olfactory behaviors. J. Neurosci. 29, 15245–15257. doi: 10.1523/JNEUROSCI.3606-09.2009
Carsberg, C. J., Myers, K. A., and Stern, P. L. (1996). Metastasis-associated 5T4 antigen disrupts cell-cell contacts and induces cellular motility in epithelial cells. Int. J. Cancer 68, 84–92. doi: 10.1002/(SICI)1097-0215(19960927)68:1<84::AID-IJC15>3.0.CO;2-6
de Wit, J., and Ghosh, A. (2014). Control of neural circuit formation by leucine-rich repeat proteins. Trends Neurosci. 37, 539–550. doi: 10.1016/j.tins.2014.07.004
Eastham, A. M., Spencer, H., Soncin, F., Ritson, S., Merry, C. L., Stern, P. L., et al. (2007). Epithelial-mesenchymal transition events during human embryonic stem cell differentiation. Cancer Res. 67, 11254–11262. doi: 10.1158/0008-5472.CAN-07-2253
Euler, T., Haverkamp, S., Schubert, T., and Baden, T. (2014). Retinal bipolar cells: elementary building blocks of vision. Nat. Rev. Neurosci. 15, 507–519. doi: 10.1038/nrn3783
Gire, D. H., Restrepo, D., Sejnowski, T. J., Greer, C., De Carlos, J. A., and Lopez-Mascaraque, L. (2013). Temporal processing in the olfactory system: can we see a smell? Neuron 78, 416–432. doi: 10.1016/j.neuron.2013.04.033
Gollisch, T., and Meister, M. (2010). Eye smarter than scientists believed: neural computations in circuits of the retina. Neuron 65, 150–164. doi: 10.1016/j.neuron.2009.12.009
Gribaudo, S., Bovetti, S., Garzotto, D., Fasolo, A., and De Marchis, S. (2009). Expression and localization of the calmodulin-binding protein neurogranin in the adult mouse olfactory bulb. J. Comp. Neurol. 517, 683–694. doi: 10.1002/cne.22177
Grobman, M., Dalal, T., Lavian, H., Shmuel, R., Belelovsky, K., Xu, F., et al. (2018). A mirror-symmetric excitatory link coordinates odor maps across olfactory bulbs and enables odor perceptual unity. Neuron 99, 800.e6–813.e6. doi: 10.1016/j.neuron.2018.07.012
Harris, J. L., Dave, K., Gorman, J., and Khanna, K. K. (2018). The breast cancer antigen 5T4 interacts with Rab11 and is a target and regulator of Rab11 mediated trafficking. Int. J. Biochem. Cell. Biol. 99, 28–37. doi: 10.1016/j.biocel.2018.03.002
Hole, N., and Stern, P. L. (1990). Isolation and characterization of 5T4, a tumour-associated antigen. Int. J. Cancer 45, 179–184. doi: 10.1002/ijc.2910450132
Imamura, F., Nagao, H., Naritsuka, H., Murata, Y., Taniguchi, H., and Mori, K. (2006). A leucine-rich repeat membrane protein, 5T4, is expressed by a subtype of granule cells with dendritic arbors in specific strata of the mouse olfactory bulb. J. Comp. Neurol. 495, 754–768. doi: 10.1002/cne.20896
Imayoshi, I., Sakamoto, M., Ohtsuka, T., Takao, K., Miyakawa, T., Yamaguchi, M., et al. (2008). Roles of continuous neurogenesis in the structural and functional integrity of the adult forebrain. Nat. Neurosci. 11, 1153–1161. doi: 10.1038/nn.2185
Kagermeier-Schenk, B., Wehner, D., Ozhan-Kizil, G., Yamamoto, H., Li, J., Kirchner, K., et al. (2011). Waif1/5T4 inhibits Wnt/β-catenin signaling and activates noncanonical Wnt pathways by modifying LRP6 subcellular localization. Dev. Cell 21, 1129–1143. doi: 10.1016/j.devcel.2011.10.015
Kaneko, N., Marin, O., Koike, M., Hirota, Y., Uchiyama, Y., Wu, J. Y., et al. (2010). New neurons clear the path of astrocytic processes for their rapid migration in the adult brain. Neuron 67, 213–223. doi: 10.1016/j.neuron.2010.06.018
Kermen, F., Lal, P., Faturos, N. G., and Yaksi, E. (2020). Interhemispheric connections between olfactory bulbs improve odor detection. PLoS Biol. 18:e3000701. doi: 10.1371/journal.pbio.3000701
King, K. W., Sheppard, F. C., Westwater, C., Stern, P. L., and Myers, K. A. (1999). Organisation of the mouse and human 5T4 oncofoetal leucine-rich glycoprotein genes and expression in foetal and adult murine tissues. Biochim. Biophys. Acta. 1445, 257–270. doi: 10.1016/s0167-4781(99)00055-x
Lepousez, G., Valley, M. T., and Lledo, P. M. (2013). The impact of adult neurogenesis on olfactory bulb circuits and computations. Annu. Rev. Physiol. 75, 339–363. doi: 10.1146/annurev-physiol-030212-183731
Livneh, Y., Adam, Y., and Mizrahi, A. (2014). Odor processing by adult-born neurons. Neuron 81, 1097–1110. doi: 10.1016/j.neuron.2014.01.007
Lledo, P. M., Merkle, F. T., and Alvarez-Buylla, A. (2008). Origin and function of olfactory bulb interneuron diversity. Trends. Neurosci. 31, 392–400. doi: 10.1016/j.tins.2008.05.006
Ma, J., and Lowe, G. (2010). Correlated firing in tufted cells of mouse olfactory bulb. Neuroscience 169, 1715–1738. doi: 10.1016/j.neuroscience.2010.06.033
Macosko, E. Z., Basu, A., Satija, R., Nemesh, J., Shekhar, K., Goldman, M., et al. (2015). Highly parallel genome-wide expression profiling of individual cells using nanoliter droplets. Cell 161, 1202–1214. doi: 10.1016/j.cell.2015.05.002
Madisen, L., Mao, T., Koch, H., Zhuo, J. M., Berenyi, A., Fujisawa, S., et al. (2012). A toolbox of Cre-dependent optogenetic transgenic mice for light-induced activation and silencing. Nat. Neurosci. 15, 793–802. doi: 10.1038/nn.3078
Malvaut, S., Gribaudo, S., Hardy, D., David, L. S., Daroles, L., Labrecque, S., et al. (2017). CaMKIIα expression defines two functionally distinct populations of granule cells involved in different types of odor behavior. Curr. Biol. 27, 3315.e6–3329.e6. doi: 10.1016/j.cub.2017.09.058
Merkle, F. T., Fuentealba, L. C., Sanders, T. A., Magno, L., Kessaris, N., and Alvarez-Buylla, A. (2014). Adult neural stem cells in distinct microdomains generate previously unknown interneuron types. Nat. Neurosci. 17, 207–214. doi: 10.1038/nn.3610
Mori, K., and Sakano, H. (2011). How is the olfactory map formed and interpreted in the mammalian brain? Annu. Rev. Neurosci. 34, 467–499. doi: 10.1146/annurev-neuro-112210-112917
Mori, K., Kishi, K., and Ojima, H. (1983). Distribution of dendrites of mitral, displaced mitral, tufted and granule cells in the rabbit olfactory bulb. J. Comp. Neurol. 219, 339–355. doi: 10.1002/cne.902190308
Murata, K., Imai, M., Nakanishi, S., Watanabe, D., Pastan, I., Kobayashi, K., et al. (2011). Compensation of depleted neuronal subsets by new neurons in a local area of the adult olfactory bulb. J. Neurosci. 31, 10540–10557. doi: 10.1523/JNEUROSCI.1285-11.2011
Myers, K. A., Rahi-Saund, V., Davison, M. D., Young, J. A., Cheater, A. J., and Stern, P. L. (1994). Isolation of a cDNA encoding 5T4 oncofetal trophoblast glycoprotein. An antigen associated with metastasis contains leucine-rich repeats. J. Biol. Chem. 269, 9319–9324.
Niedworok, C. J., Schwarz, I., Ledderose, J., Giese, G., Conzelmann, K. K., and Schwarz, M. K. (2012). Charting monosynaptic connectivity maps by two-color light-sheet fluorescence microscopy. Cell Rep. 2, 1375–1386. doi: 10.1016/j.celrep.2012.10.008
Nunes, D., and Kuner, T. (2015). Disinhibition of olfactory bulb granule cells accelerates odour discrimination in mice. Nat. Commun. 6:8950. doi: 10.1038/ncomms9950
Orona, E., Rainer, E. C., and Scott, J. W. (1984). Dendritic and axonal organization of mitral and tufted cells in the rat olfactory bulb. J. Comp. Neurol. 226, 346–356. doi: 10.1002/cne.902260305
Orona, E., Scott, J. W., and Rainer, E. C. (1983). Different granule cell populations innervate superficial and deep regions of the external plexiform layer in rat olfactory bulb. J. Comp. Neurol. 217, 227–237. doi: 10.1002/cne.902170209
Özkan, E., Carrillo, R. A., Eastman, C. L., Weiszmann, R., Waghray, D., Johnson, K. G., et al. (2013). An extracellular interactome of immunoglobulin and LRR proteins reveals receptor-ligand networks. Cell 154, 228–239. doi: 10.1016/j.cell.2013.06.006
Peng, Y. R., Shekhar, K., Yan, W., Herrmann, D., Sappington, A., Bryman, G. S., et al. (2019). Molecular classification and comparative taxonomics of foveal and peripheral cells in primate retina. Cell 176, 1222.e22–1237.e22. doi: 10.1016/j.cell.2019.01.004
Pino, D., Choe, Y., and Pleasure, S. J. (2011). Wnt5a controls neurite development in olfactory bulb interneurons. ASN Neuro 3:e00059. doi: 10.1042/AN20100038
Puthussery, T., Gayet-Primo, J., and Taylor, W. R. (2010). Localization of the calcium-binding protein secretagogin in cone bipolar cells of the mammalian retina. J. Comp. Neurol. 518, 513–525. doi: 10.1002/cne.22234
Rampino, M. A., and Nawy, S. A. (2011). Relief of Mg2+-dependent inhibition of TRPM1 by PKCα at the rod bipolar cell synapse. J. Neurosci. 31, 13596–13603. doi: 10.1523/JNEUROSCI.2655-11.2011
Saghatelyan, A., Roux, P., Migliore, M., Rochefort, C., Desmaisons, D., Charneau, P., et al. (2005). Activity-dependent adjustments of the inhibitory network in the olfactory bulb following early postnatal deprivation. Neuron 46, 103–116. doi: 10.1016/j.neuron.2005.02.016
Sakamoto, M., Ieki, N., Miyoshi, G., Mochimaru, D., Miyachi, H., Imura, T., et al. (2014). Continuous postnatal neurogenesis contributes to formation of the olfactory bulb neural circuits and flexible olfactory associative learning. J. Neurosci. 34, 5788–5799. doi: 10.1523/JNEUROSCI.0674-14.2014
Sakamoto, M., Imayoshi, I., Ohtsuka, T., Yamaguchi, M., Mori, K., and Kageyama, R. (2011). Continuous neurogenesis in the adult forebrain is required for innate olfactory responses. Proc. Natl. Acad. Sci. U S A 108, 8479–8484. doi: 10.1073/pnas.1018782108
Sanes, J. R., and Yamagata, M. (2009). Many paths to synaptic specificity. Annu. Rev. Cell. Dev. Biol. 25, 161–195. doi: 10.1146/annurev.cellbio.24.110707.175402
Sanes, J. R., and Zipursky, S. L. (2020). Synaptic specificity, recognition molecules, and assembly of neural circuits. Cell 181, 536–556. doi: 10.1016/j.cell.2020.04.008
Sarin, S., Zuniga-Sanchez, E., Kurmangaliyev, Y. Z., Cousins, H., Patel, M., Hernandez, J., et al. (2018). Role for Wnt signaling in retinal neuropil development: analysis via RNA-Seq and in vivo somatic CRISPR mutagenesis. Neuron 98, 109.e8–126.e8. doi: 10.1016/j.neuron.2018.03.004
Schroeder, A., and de Wit, J. (2018). Leucine-rich repeat-containing synaptic adhesion molecules as organizers of synaptic specificity and diversity. Exp. Mol. Med. 50:10. doi: 10.1038/s12276-017-0023-8
Shekhar, K., Lapan, S. W., Whitney, I. E., Tran, N. M., Macosko, E. Z., Kowalczy, K. M., et al. (2016). Comprehensive classification of retinal bipolar neurons by single-cell transcriptomics. Cell 166, 1308.e30–1323.e30. doi: 10.1016/j.cell.2016.07.054
Shepherd, G. M., Chen, W. R., and Greer, C. A. (2004). “Olfactory bulb,” in The synaptic Organization of the Brain, ed. G. M. Shepherd (Oxford: Oxford University Press), 165–216.
Shepherd, G. M., Chen, W. R., Willhite, D., Migliore, M., and Greer, C. A. (2007). The olfactory granule cell: from classical enigma to central role in olfactory processing. Brain Res. Rev. 55, 373–382. doi: 10.1016/j.brainresrev.2007.03.005
Siri, S. O., Rozés-Salvador, V., de la Villarmois, E. A., Ghersi, M. S., Quassollo, G., Pérez, M. F., et al. (2020). Decrease of Rab11 prevents the correct dendritic arborization, synaptic plasticity and spatial memory formation. Biochim. Biophys. Acta Mol. Cell. Res 1867:118735. doi: 10.1016/j.bbamcr.2020.118735
Soto, F., Hsiang, J. C., Rajagopal, R., Piggott, K., Harocopos, G. J., Couch, S. M., et al. (2020). Efficient coding by midget and parasol ganglion cells in the human retina. Neuron 107, 656.e5–666.e5. doi: 10.1016/j.neuron.2020.05.030
Southall, P. J., Boxer, G. M., Bagshawe, K. D., Hole, N., Bromley, M., and Stern, P. L. (1990). Immunohistological distribution of 5T4 antigen in normal and malignant tissues. Br. J. Cancer 61, 89–95. doi: 10.1038/bjc.1990.20
Spencer, H. L., Eastham, A. M., Merry, C. L., Southgate, T. D., Perez-Campo, F., Soncin, F., et al. (2007). E-cadherin inhibits cell surface localization of the pro-migratory 5T4 oncofetal antigen in mouse embryonic stem cells. Mol. Biol. Cell 18, 2838–2851. doi: 10.1091/mbc.e06-09-0875
Sterling, P., and Demb, J. B. (2004). “Retina,” in The Synaptic Organization of the Brain, ed. G. M. Shepherd (New York, NY: Oxford UP), 217–269.
Stern, P. L., Brazzatti, J., Sawan, S., and McGinn, O. J. (2014). Understanding and exploiting 5T4 oncofoetal glycoprotein expression. Semin. Cancer Biol. 29, 13–20. doi: 10.1016/j.semcancer.2014.07.004
Takahashi, H., Ogawa, Y., Yoshihara, S., Asahina, R., Kinoshita, M., Kitano, T., et al. (2016). A subtype of olfactory bulb interneurons is required for odor detection and discrimination behaviors. J. Neurosci. 36, 8210–8227. doi: 10.1523/JNEUROSCI.2783-15.2016
Takahashi, Y., Takahashi, H., Stern, P. L., Kirita, T., and Tsuboi, A. (2019). Expression of oncofetal antigen 5T4 in murine taste papillae. Front. Cell. Neurosci. 13:343. doi: 10.3389/fncel.2019.00343
Takahashi, H., Yoshihara, S., and Tsuboi, A. (2018). The functional role of olfactory bulb granule cell subtypes derived from embryonic and postnatal neurogenesis. Front. Mol. Neurosci. 11:229. doi: 10.3389/fnmol.2018.00229
Tian, N., and Copenhagen, D. R. (2003). Visual stimulation is required for refinement of ON and OFF pathways in postnatal retina. Neuron 39, 85–96. doi: 10.1016/s0896-6273(03)00389-1
van Amerongen, R., and Nusse, R. (2009). Towards an integrated view of Wnt signaling in development. Development 136, 3205–3214. doi: 10.1242/dev.033910
Wakeham, C. M., Ren, G., and Morgans, C. W. (2020). Expression and distribution of trophoblast glycoprotein in the mouse retina. J. Comp. Neurol. 528, 1660–1671. doi: 10.1002/cne.24850
Wakeham, C. M., Wilmarth, P. A., Cunliffe, J. M., Klimek, J. E., Ren, G., David, L. L., et al. (2019). Identification of PKCα-dependent phosphoproteins in mouse retina. J. Proteomics 206:103423. doi: 10.1016/j.jprot.2019.103423
Welz, T., Wellbourne-Wood, J., and Kerkhoff, E. (2014). Orchestration of cell surface proteins by Rab11. Trends Cell. Biol. 24, 407–415. doi: 10.1016/j.tcb.2014.02.004
Whitman, M. C., and Greer, C. A. (2009). Adult neurogenesis and the olfactory system. Prog. Neurobiol. 89, 162–175. doi: 10.1016/j.pneurobio.2009.07.003
Yan, W., Laboulaye, M. A., Tran, N. M., Whitney, I. E., Benhar, I., and Sanes, J. R. (2020a). Mouse retinal cell atlas: molecular identification of over sixty amacrine cell types. J. Neurosci. 40, 5177–5195. doi: 10.1523/JNEUROSCI.0471-20.2020
Yan, W., Peng, Y. R., van Zyl, T., Regev, A., Shekhar, K., Juric, D., et al. (2020b). Cell atlas of the human fovea and peripheral retina. Sci. Rep. 10:9802. doi: 10.1038/s41598-020-66092-9
Yoshihara, S., Takahashi, H., Nishimura, N., Naritsuka, H., Shirao, T., Hirai, H., et al. (2012). 5T4 glycoprotein regulates the sensory input-dependent development of a specific subtype of newborn interneurons in the mouse olfactory bulb. J. Neurosci. 32, 2217–2226. doi: 10.1523/JNEUROSCI.5907-11.2012
Keywords: LRR-containing membrane protein, 5T4 oncofetal trophoblast glycoprotein, olfactory bulb interneuron, retinal interneuron, odor detection and discrimination
Citation: Tsuboi A (2020) LRR-Containing Oncofetal Trophoblast Glycoprotein 5T4 Shapes Neural Circuits in Olfactory and Visual Systems. Front. Mol. Neurosci. 13:581018. doi: 10.3389/fnmol.2020.581018
Received: 07 July 2020; Accepted: 22 September 2020;
Published: 28 October 2020.
Edited by:
Masahito Yamagata, Harvard University, United StatesReviewed by:
Catherine Morgans, Oregon Health and Science University, United StatesFumiaki Imamura, Penn State Milton S. Hershey Medical Center, United States
Takeshi Imai, Kyushu University, Japan
Copyright © 2020 Tsuboi. This is an open-access article distributed under the terms of the Creative Commons Attribution License (CC BY). The use, distribution or reproduction in other forums is permitted, provided the original author(s) and the copyright owner(s) are credited and that the original publication in this journal is cited, in accordance with accepted academic practice. No use, distribution or reproduction is permitted which does not comply with these terms.
*Correspondence: Akio Tsuboi, YWtpb0BmYnMub3Nha2EtdS5hYy5qcA==; dC5ha2lvcmV4QGdtYWlsLmNvbQ==