- 1Department of Neuromuscular Diseases, UCL Queen Square Institute of Neurology, University College London, London, United Kingdom
- 2UK Dementia Research Institute, University College London, London, United Kingdom
Virus-mediated gene therapy has the potential to deliver exogenous genetic material into specific cell types to promote survival and counteract disease. This is particularly enticing for neuronal conditions, as the nervous system is renowned for its intransigence to therapeutic targeting. Administration of gene therapy viruses into skeletal muscle, where distal terminals of motor and sensory neurons reside, has been shown to result in extensive transduction of cells within the spinal cord, brainstem, and sensory ganglia. This route is minimally invasive and therefore clinically relevant for gene therapy targeting to peripheral nerve soma. For successful transgene expression, viruses administered into muscle must undergo a series of processes, including host cell interaction and internalization, intracellular sorting, long-range retrograde axonal transport, endosomal liberation, and nuclear import. In this review article, we outline key characteristics of major gene therapy viruses—adenovirus, adeno-associated virus (AAV), and lentivirus—and summarize the mechanisms regulating important steps in the virus journey from binding at peripheral nerve terminals to nuclear delivery. Additionally, we describe how neuropathology can negatively influence these pathways, and conclude by discussing opportunities to optimize the intramuscular administration route to maximize gene delivery and thus therapeutic potential.
Introduction
With thousands of clinical trials to date, gene therapy is a flourishing strategy with great promise for the treatment of diseases impacting the nervous system. Indeed, virus-mediated gene therapies have now been approved by the FDA in the US for RPE65-associated retinal dystrophy (voretigene neparvovec marketed as Luxturna) and SMN1-linked spinal muscular atrophy (SMA; onasemnogene abeparvovec marketed as Zolgenmsa), as well as non-neuronal conditions (High and Roncarolo, 2019). Gene therapy viruses are non-replicating, but still hijack host cell machinery to express transgenes of interest in the nucleus. Crucially, some viral vectors (i.e., viruses specifically used to deliver genetic material into cells) have the potential to circumvent the blood-brain- (BBB) and blood-spinal cord barriers (BSCB) when intravenously injected. Similarly, direct injection of viruses into the cerebrospinal fluid (e.g., via lumbar puncture in humans) also permits targeting of the peripheral (PNS) and central nervous systems (CNS). These two administration routes for neuronal delivery have been extensively covered in recent reviews (Hocquemiller et al., 2016; Deverman et al., 2018; Hudry and Vandenberghe, 2019). A complementary, and perhaps sometimes superior (Benkhelifa-Ziyyat et al., 2013), method to introduce genetic material into select neuronal populations is by virus administration into muscle, which is the focus of this review. Muscles contain the synaptic connection between lower motor neurons and muscle fibers, i.e., the neuromuscular junction (NMJ), as well as specialized sensory nerve endings (e.g., muscle spindles). Viruses can be internalized into peripheral nerve terminals and subsequently retrogradely transported along axons to deliver viral payloads into corresponding motor and sensory neurons, with scope for widespread transfer to additional cells throughout the spinal cord and brain (Benkhelifa-Ziyyat et al., 2013; Chen et al., 2020).
The NMJ is a tripartite synapse comprised of a pre-synaptic motor nerve terminal, a post-synaptic muscle fiber, and several terminal Schwann cells (Li et al., 2018a). Moreover, the synaptic cleft consists of a complex and dynamic extracellular matrix (ECM) that contributes to receptor translocation and internalization of a variety of molecules (Heikkinen et al., 2020). Targeting muscles with viruses can transduce all three cellular constituents of the NMJ (Mazarakis et al., 2001; Homs et al., 2011)—by “transduction,” we mean the introduction of genetic material into target cells. Furthermore, uptake at sensory nerve terminals can lead to transgene expression in dorsal root ganglia (DRG), trigeminal ganglia, and dorsal horn nerve fibers (Watson et al., 2016; Chen et al., 2020). When injected into a muscle, viruses are close to nerve endings for longer periods and at higher concentrations than when systemically injected. Moreover, limiting widespread virus distribution is likely to decrease safety risks due to immunogenicity or toxicity, while possible negative effects caused by central injections will be avoided. Hence, targeting muscle may prove to be a useful method to introduce viral vectors to certain central and peripheral neurons and/or glia.
For this strategy to be exploited, viruses must undergo several major processes, including host cell binding, internalization, intracellular sorting, and retrograde axonal trafficking to neuronal soma before nuclear entry. In this review article, we outline these mechanisms for major gene therapy viruses—adenovirus (AdV), adeno-associated virus (AAV) and lentivirus (LV; Table 1)—with a focus on peripheral neurons. We also comment on the impact of neuropathology on using intramuscular virus injection as an administration route. To conclude, we discuss opportunities to optimize gene therapy delivery to muscle for nervous system targeting.
Gene Therapy Viruses
Adenovirus
First isolated in the 1950s, AdVs are non-enveloped, double-stranded DNA viruses with an icosahedral-shaped capsid comprised mainly of hexon and penton capsomeres (Greber and Flatt, 2019). Adenoviridae encompasses more than 300 different vertebrate-infecting types, including seven human AdV (HAdV) species (A to G) currently comprised of ≈80 types classified by serology or sequencing. HAdVs primarily cause ocular, gastrointestinal, or respiratory infections (Ghebremedhin, 2014). It is estimated that more than 80% of the human population has been exposed to HAdV and develop type-specific humoral and cross-reactive cellular immunity (Ahi et al., 2011), hence, for utilization as a gene therapy vector, strategies to circumvent the host immune response have been examined (Duffy et al., 2012). In the 1990s, AdV became the first gene therapy virus to be tested in human clinical trials and currently remains the most investigated (Lee et al., 2017). The more common human serotypes 2 and 5 belonging to species C have been the focus for gene therapy development. E1/E3-deleted AdVs have a relatively large packaging capacity of ≈8 kb, can transduce many different cell types, and form episomes rather than integrating into the host genome. Moreover, AdVs can be efficiently produced in large, concentrated quantities. In some hosts and some organs, transgene expression using AdV can be transient, likely due to host-specific responses, while in other cases, transgene expression remains robust for months (Li et al., 2016). In this regard, the transient expression can be advantageous for scenarios requiring short-term upregulation of therapeutic genes and for limiting deleterious consequences that may arise from long-term expression (discussed in Tosolini and Morris, 2016b). However, the transgene capacity of AdV can be increased up to ≈36 kb by removing essential elements and exogenously providing them for in vitro packaging, and with this approach, they lack the elements that usually activate host immunity, which can thereby facilitate prolonged-expression (Ricobaraza et al., 2020). Permitting much broader options for transgene incorporation, this expansive packaging capacity is one major advantage of AdV over other viral vectors.
AdVs display broad cell and tissue tropisms mediated by the interaction between their capsid and specific cellular receptors (Arnberg, 2012). Capsid modification, for instance by altering the virus genome or adding ligands, can widen or narrow tissue specificity depending on the required strategy (Worgall and Crystal, 2014). Direct intracranial injection of HAdV has been shown to result in the transduction of several different neuronal and non-neuronal cell types in the rodent CNS (Akli et al., 1993; Davidson et al., 1993; Le Gal La Salle et al., 1993). Furthermore, intramuscular administration of AdVs can result in their uptake at rodent NMJs and sensory terminals before retrograde transport to cell bodies (Finiels et al., 1995; Ghadge et al., 1995; Tosolini and Morris, 2016a), which is a viable strategy to counteract neuromuscular disease (Haase et al., 1998; Acsadi et al., 2002) and peripheral nerve injury (Giménez y Ribotta et al., 1997; Baumgartner and Shine, 1998). Of note, the canine adenovirus serotype 2 (CAV-2; also known as CAdV-2), which can cause mild respiratory infections in Canidae, has become the AdV of choice for neuronal transduction (Del Rio et al., 2019). Due to possessing greater specificity in host cell receptor binding than HAdVs, CAV-2 preferentially targets neurons (Soudais et al., 2001). Furthermore, it is efficiently retrogradely transported along axons (Salinas et al., 2009), while a helper-dependent CAV-2 has been shown to drive transgene expression in the rodent CNS for over a year (Soudais et al., 2004). CAV-2 injection into craniofacial muscles of rhesus monkeys caused robust motor neuron transduction (Bohlen et al., 2019), while intramuscular administration in rats results in superior motor neuron uptake and transport compared to AdV serotype 5 (Soudais et al., 2001), which together highlight the potential of CAV-2 for motor neuron targeting via skeletal muscle.
Adeno-Associated Virus
Belonging to the Dependoparvovirus genus and thus needing factors from helper viruses (e.g., AdV) to replicate, AAVs are non-enveloped, single-stranded DNA viruses discovered as AdV preparation contaminants (Zinn and Vandenberghe, 2014). More than 100 natural AAV variants, including 13 serotypes from primates, have been identified, each with differing tissue tropisms, transduction efficiencies, and antigenicities, all resulting from their distinct protein capsids (Zincarelli et al., 2008; Srivastava, 2016). Additional synthetic AAV subtypes have been derived/engineered in the laboratory to optimize these features for gene transfer (Kotterman and Schaffer, 2014). Impinging considerably upon its tractability, the packaging capacity of AAV is limited to ≈4.7 kb, which is halved in the more rapidly expressing self-complementary AAV (for simplicity, we refer to single-stranded and self-complementary AAV as one), although DNA delivery across separate AAV particles is possible (Patel et al., 2019). In most cases, AAV vectors induce limited immunogenicity in naïve hosts (Ronzitti et al., 2020), and have a good safety record, although there may be toxicity issues when administered at high doses (Hinderer et al., 2018). However, the AAV vector effect on brain homeostasis has not been completely addressed and is an important consideration (He et al., 2019). Forming stable, non-replicating episomes for sustained transgene expression, AAV is largely non-integrating (Schnepp et al., 2005), although insertional mutagenesis has been reported (Chandler et al., 2017). These combined features have led to AAV becoming the premier clinical gene therapy vector and its recent regulatory approval for the treatment of several conditions (High and Roncarolo, 2019). However, AAV gene therapy is not entirely infallible, as wild type AAV infections have been linked with human disease (Nault et al., 2016); however, potential solutions to overcome these and other concerns to drive human AAV gene therapy are continuing (Colella et al., 2018). Nonetheless, many more clinical trials of AAV-mediated gene therapy are ongoing or planned, including several involving intramuscular administration (although not necessarily for neuronal transduction).
AAVs have been used for many years in the laboratory to drive transgene expression in the nervous system (Hudry and Vandenberghe, 2019). Due to its ability to cross the BBB, AAV serotype 9 (AAV9) has become the principal serotype for CNS-targeting upon systemic administration (Foust et al., 2009; Bevan et al., 2011; Samaranch et al., 2012), although superior serotypes, such as AAVrh10, have also emerged (Tanguy et al., 2015). However, cell binding and transduction can change with age (Chakrabarty et al., 2013), thus engineered serotypes with greater neuronal tropism, at least in mice, are being developed (Choudhury et al., 2016; Deverman et al., 2016). Nervous system delivery has also been achieved by AAV injection into muscle; intramuscular administration of several AAV serotypes (e.g., AAV2, AAV9) results in AAV uptake into the motor and sensory neurons in rodents (Hollis Ii et al., 2008; Zheng et al., 2010; Benkhelifa-Ziyyat et al., 2013; Jan et al., 2019; Chen et al., 2020) and motor neurons in non-human primates (Towne et al., 2010). Consequently, this method of gene delivery has proven beneficial in mouse models of motor neuron diseases amyotrophic lateral sclerosis (ALS), and SMA (Tosolini and Sleigh, 2017). Increasing the possible clinical applicability of AAV, single intramuscular injections of rAAV2-retro, a newly evolved variant with robust retrograde transport capacity (Tervo et al., 2016), were recently shown to result in broad transgene expression across ipsilateral and contralateral motor neurons along the length of the spinal cord, as well as brainstem motor nuclei, DRG, trigeminal ganglia and dorsal horn nerve fibers (Chen et al., 2020). Importantly, AAV targeting of peripheral neurons is therefore not limited to those cells innervating the injected muscle.
Lentivirus
Belonging to the Retroviridae family, LV possesses a single-stranded RNA genome and can infect both dividing and non-dividing cells (Parr-Brownlie et al., 2015). LV is an enveloped virus with a packaging capacity of ≈8 kb and it relies on reverse transcription of its single-stranded RNA genome to generate corresponding double-stranded DNA for integration into the host genome (Mátrai et al., 2010). This provides benefits of long-term transgene expression and inheritance of genetic material in dividing cells; however, integration also has the major disadvantage that it can disrupt host gene function through insertional mutagenesis, which poses a safety risk. Incorporation into the host genome is not random, as there are preferential sites and conditions for integration (e.g., highly expressed and intron-rich genes), but it is unpredictable (Lesbats et al., 2016). Nonetheless, this has not prevented several LV-mediated gene therapies being approved for human use, albeit being utilized for ex vivo modification of autologous immune cells (High and Roncarolo, 2019). For gene delivery, essential viral coding regions (e.g., gag, pol, and env) are removed from the LV genome, and instead provided by separate expression plasmids for in vitro packaging (Milone and O’Doherty, 2018). This removal of viral genes ensures that the immunogenicity of LV is relatively low, although not absent (Annoni et al., 2019).
LVs are typically derived from primate or non-primate immunodeficiency viruses [e.g., human immunodeficiency virus type 1 (HIV-1) or equine infectious anemia virus (EIAV)]. LV tropism is mediated by the viral envelope, which is engineered to include glycoproteins from other enveloped viruses in a process called pseudotyping (Cronin et al., 2005). The most common virus used to pseudotype LV is the vesicular stomatitis virus (VSV), but heterologous envelope proteins from many other viruses have been used to target LV to particular cells and tissues, e.g., measles virus, murine leukemia virus and influenza viruses (Joglekar and Sandoval, 2017). The VSV glycoprotein (VSV-G) binds to a widely expressed receptor, leading to broad tropism when integrated into the LV envelope. In contrast, LVs pseudotyped with rabies virus (RV) display greater neuronal selectivity and have been shown to aid efficient transduction of neurons both in vitro and in vivo. Compared to VSV, LV pseudotyped with RV glycoprotein (LV-RV) shows superior neuronal transduction and transport when injected into the rat striatum and spinal cord (Mazarakis et al., 2001). A similar high efficiency has been reported when injected into the primate brain (Kato et al., 2007), while distal uptake and efficient retrograde trafficking occurs in rodent primary motor neurons (Hislop et al., 2014). Moreover, LV-RV administration into gastrocnemius muscle results in effective transgene expression in spinal cord motor neurons, while LV-VSV remains restricted to the muscle injection site (Mazarakis et al., 2001), which was confirmed with additional RV strains (Wong et al., 2004; Mentis et al., 2006). Pseudotyping with several different hybrid glycoproteins has since shown improved targeting of motor neurons when delivered to muscle, which can be further enhanced by the coupling of antibodies against NMJ receptors to the virus surface (Hirano et al., 2013; Eleftheriadou et al., 2014). As a consequence, numerous different LV-mediated therapeutic strategies that target motor neurons via muscle have proven successful in mouse models of ALS and SMA (Azzouz et al., 2004a,b; Ralph et al., 2005; Raoul et al., 2005; Benkler et al., 2016; Eleftheriadou et al., 2016).
From Virus Binding to Nuclear Entry
For viruses injected into a muscle to express transgenes in neurons, they must undergo a series of events: host cell binding and internalization, intracellular sorting, retrograde axonal transport, liberation from the transporting structure/organelle and nuclear entry (Figure 1). AdV, AAV, and LV rely on the same or similar mechanisms for several parts of this journey which are also shared by botulinum and tetanus neurotoxins (Surana et al., 2018). For instance, they all hijack retrograde axonal transport (Merino-Gracia et al., 2011), which is dependent on active, processive movement along microtubules by the motor protein complex cytoplasmic dynein-dynactin (Schiavo et al., 2013). By trafficking towards the stable minus ends of the microtubule, which are located at the cell body end of an axon, cytoplasmic dynein enables long-range retrograde delivery of cargoes, such as autophagosomes and neurotrophin-containing signaling endosomes. Additionally, the Rab (Ras-related proteins in the brain) GTPase protein family is specifically required for signaling endosome trafficking (Villarroel-Campos et al., 2018). Target tissue-derived (e.g., muscle) neurotrophins transition from early Rab5-positive endosomes into retrogradely transported Rab7-positive signaling endosomes (Deinhardt et al., 2006). Unlike in the canonical endolysosomal pathway, retrograde Rab7-endosomes within axons display a tightly regulated neutral pH value that is maintained during transport (Bohnert and Schiavo, 2005). All three gene therapy viruses have been shown to localize to these axonal Rab7-endosomes, indicating that they share a common compartment when voyaging to the nucleus. Retrograde trafficking is a rapid and constitutive process that delivers large quantities of endosomes to the motor and sensory soma; it is thus unlikely to be a rate-limiting step in virus transgene expression. Rather, idiosyncratic aspects of the journey of each virus, e.g., binding to specific receptors or endosomal liberation at the cell body, probably have a greater impact on overall transduction efficiency.
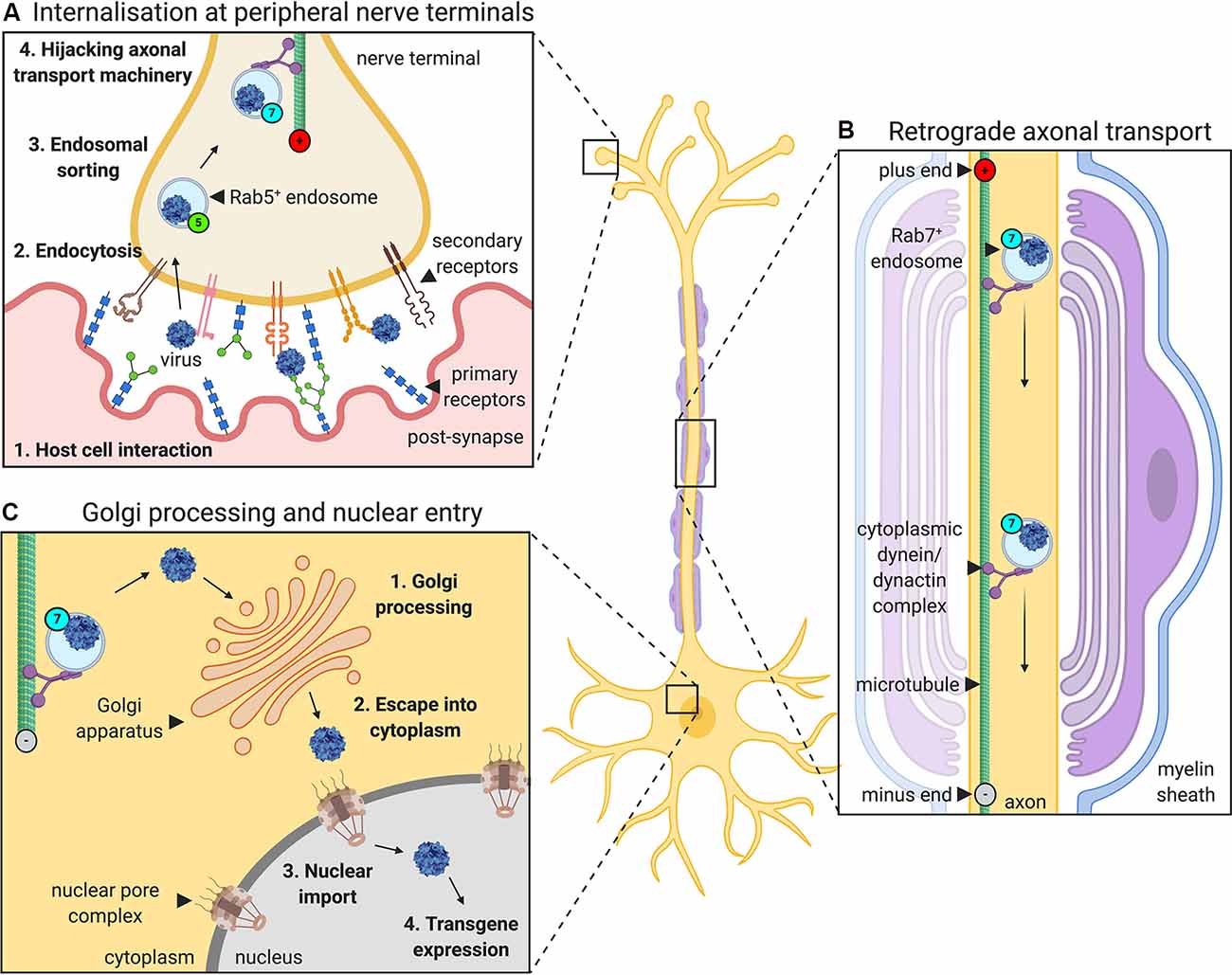
Figure 1. The journey of gene therapy viruses from peripheral nerve terminals to the nucleus. Viruses used to deliver gene therapy must access cell nuclei to express their packaged genetic material. When administered into muscles for targeting of peripheral nerve somas, viruses such as adenovirus, adeno-associated virus (AAV) and lentivirus, undergo a series of processes that aid their transfer from the periphery to CNS (depicted here using AAV as an example). (A) First, the virus interacts with specific host cell surfaces. This entails primary receptor binding (e.g., glycans) followed by internalization, which is often mediated, at least in part, by a secondary receptor (e.g., AAV receptor, AAVR or fibroblast growth factor receptor, FGFR). Internalization at nerve terminals is regulated by a variety of endocytic pathways. Post-internalisation, viruses hijack the Rab GTPase-mediated endosomal sorting system, transitioning through Rab5-positive early endosomes to non-acidic Rab7-positive late endosomes. (B) Virus-containing Rab7-positive signaling endosomes are actively transported along microtubules by cytoplasmic dynein-dynactin complexes towards nerve cell bodies (i.e., retrogradely). (C) At the neuronal soma, viruses escape endosomes and are processed, sometimes through the Golgi apparatus, before entry into the nucleus (e.g., via the nuclear pore complex), where the virus can begin to drive transgene expression.
Highlighting similarities and differences, we now describe the individual journeys that each virus must take to migrate from muscle to peripheral nerve soma for transgene expression.
Adenovirus
Similar to most viruses, AdV is typically internalized in a two-step, receptor-mediated fashion that is dependent on the viral capsid, although non-specific, large-scale internalization has also been reported (Meier et al., 2002). Primary receptors that mediate AdV attachment to cells include, heparan sulfate proteoglycans, CD46, and sialic acid, which selectively interact with different serotypes (Arnberg, 2012); however, the appears to be the major initial binding partner for AdVs (Bergelson et al., 1997; Arnberg, 2012). Coxsackie and adenovirus receptor (CAR) is a widely expressed cell adhesion protein critical for heart development (Dorner et al., 2005), and is involved in neurogenesis through its synaptic expression throughout the mature brain (Zussy et al., 2016). CAR serves as the primary receptor for several different HAdV species (i.e., A, C-F) and serotypes, including 2 and 5, as well as CAV-2 (Arnberg, 2012; Loustalot et al., 2016). The second step of AdV internalization (i.e., entry) is facilitated by penton capsomere binding to members of the integrin receptor family, e.g., αVβ3 and αVβ5 (Wickham et al., 1993). Facilitating cell-to-cell and cell-to-ECM interactions, integrins are expressed in a tissue-specific fashion and can in some instances mediate AdV attachment in the absence of CAR (Huang et al., 1996).
Despite extensive knowledge on AdV receptors, relatively little is known about the specific entry of AdV at the NMJ or sensory nerve terminals. Intramuscular injections of AdV result in the targeting of both muscle fibers and innervating motor neurons in juvenile and adult mice (Tosolini and Morris, 2016a), which is consistent with the reported expression of CAR in muscle fibers (Nalbantoglu et al., 1999) and at both mouse and human NMJs (Shaw et al., 2004; Sinnreich et al., 2005). However, one of the major issues with AdV-mediated gene therapy is the relatively poor transduction of neurons in adults compared to young mice, including upon intramuscular injection (Acsadi et al., 1994; Huard et al., 1995; Tosolini and Morris, 2016a). This is somewhat unsurprising as CAR is downregulated post-natally in several neuronal subtypes (Hotta et al., 2003) and muscle (Nalbantoglu et al., 1999). Indeed, CAR is highly expressed in immature skeletal muscle fibers but is drastically downregulated after birth (Nalbantoglu et al., 1999) becoming restricted to the NMJ (Shaw et al., 2004; Sinnreich et al., 2005). Nevertheless, to better understand the limited uptake of AdV into adult motor neurons, further investigation is required to provide a thorough longitudinal assessment of CAR levels at post-natal neuromuscular synapses. Upon muscle damage caused by Duchenne muscular dystrophy or polymyositis, CAR expression increases within muscle fibers and co-localizes with markers of regeneration (Sinnreich et al., 2005); given the parallels between mechanisms of muscle development and regeneration, this suggests that CAR may indeed be developmentally regulated at the NMJ and serve in the synaptic response to regeneration (Sinnreich et al., 2005).
After binding to CAR, AdVs are internalized and processed in a cell type-dependent manner. Experiments in immortalized non-neuronal cells describe AdV internalization into endosomes via clathrin-coated pits (Meier et al., 2002) and subsequent endosomal liberation via acidification (Leopold et al., 1998). The intracellular domain of CAR plays a critical role in this by recruiting the endocytic machinery and influencing subsequent intracellular AdV trafficking (Loustalot et al., 2015). AdVs are then transported towards the nucleus by cytoplasmic dynein-mediated trafficking along with the microtubule network (Kelkar et al., 2004), impairments in which drastically disrupt this nuclear targeting (Suomalainen et al., 1999; Leopold et al., 2000). The AdV capsid directly interacts with cytoplasmic dynein via hexon capsomeres (Bremner et al., 2009), suggesting that in non-neuronal cells AdVs are transported as “naked particles” rather than in membrane-bound organelles (e.g., endosomes; Scherer et al., 2020). Moreover, this interaction appears to be dependent on exposure to low pH, suggesting that AdV binding to the motor protein is primed by transition through the early endosomal system (Bremner et al., 2009). AdV serotype 5 has also been shown to interact with the Kif5B subunit of kinesin-1, a motor protein that drives transport in the opposite direction to cytoplasmic dynein (i.e., towards dynamic plus ends), possibly as an evolutionary strategy for increased cellular exploration (Zhou et al., 2018).
In primary neurons, AdVs are also internalized in a CAR-dependent manner (Loustalot et al., 2016), facilitated by CAR enrichment in actin-domains of neuronal growth cones as well as lipid rafts (Huang et al., 2007). Internalization occurs through a lipid microdomain-, actin- and dynamin-dependent manner before the receptors are eventually targeted for lysosomal degradation (Salinas et al., 2014). The major difference between neuronal and non-neuronal AdV trafficking is that in neurons, CAR does not undergo lysis during intracellular sorting, and is instead transported to the neuronal soma as part of non-acidic, Rab7-positive endosomes, thus preventing pH-induced conformational changes to the AdV capsid and restricting endosomal liberation (Salinas et al., 2009). CAR-positive organelles favor the retrograde direction but can also be anterogradely transported by kinesin motor proteins (Salinas et al., 2009). Again confirming the essential nature of transport to AdV migration, in vivo pharmacological blockade of microtubule dynamics inhibits the delivery of AdV to the neuron (Boulis et al., 2003). Once in the soma, AdV accesses the nucleus at the nuclear pore complex via histone H1 (Trotman et al., 2001) or the nucleoporin receptors (Trotman et al., 2001; Cassany et al., 2015), with the route also appearing to be cell type-dependent (Kremer and Nemerow, 2015).
Adeno-Associated Virus
AAV also gains cellular access via a two-step process involving primary cell surface receptors with a secondary receptor mediating entry. Negatively charged glycans or glycoconjugates serve as primary attractants with which AAVs initially interact allowing extracellular viral accumulation and co-receptor access. These include heparan sulfate proteoglycans for AAV2, AAV3, AAV6 and AAV13, N-terminal galactose for AAV9, and specific N- and O-linked sialic acid moieties for AAV1, AAV4, AAV5 and AAV6 (Huang et al., 2014). The wide expression of surface glycans, including in neuronal extracellular matrices (Broadie et al., 2011; Singhal and Martin, 2011), explains the broad infectivity of AAV, while glycan diversity and relative density likely dictates selectivity of AAV serotype tropism.
Several serotype-specific co-receptors have also been identified that after glycan binding, facilitate AAV uptake. These co-receptors include fibroblast growth factor receptor (FGFR) and hepatocyte growth factor receptor (HGFR) for both AAV2 and AAV3, platelet-derived growth factor receptor (PDGF) for AAV5, and epidermal growth factor receptor (EGFR) for AAV6 (Madigan and Asokan, 2016). Signaling through each of these receptors has been linked to NMJ formation/function (Zhao et al., 1999; Li et al., 2012; Taetzsch et al., 2018), consistent with their synaptic availability. Additional receptors have been identified for engineered serotypes contributing to distinct tropisms (Hordeaux et al., 2019; Huang et al., 2019). However, a common receptor required for endocytosis of most natural primate AAV serotypes was recently identified (Pillay et al., 2016). Originally called KIAA0319L and linked with dyslexia and functions of neuronal migration and axon guidance (Poon et al., 2011), the AAV receptor (AAVR) possesses an N-terminal MANSC domain, several immunoglobulin-like PKD domains, a C6 domain, and a transmembrane region before a short C-terminal tail (Poon et al., 2011). As expected given the broad cellular and tissue infectivity of AAV, AAVR is expressed across many human tissues, including muscle and nerve, and can be found as several spliced variants and post-translationally modified isoforms (Poon et al., 2011; Gostic et al., 2019). AAVR knockout rendered mammalian HeLa cells highly resistant to infection with AAV serotypes 1, 2, 3b, 5, 6, 8, and 9, with a similar finding in AAV9-injected AAVR knockout mice in vivo (Pillay et al., 2016). The removal of AAVR resulted in no obvious phenotype, suggesting that AAVR is non-essential or there is genetic compensation. In subsequent work from the same group and others, AAV serotypes have been shown to differentially interact with AAVR PKD domains (Pillay et al., 2017; Zhang et al., 2019), while AAV4 gains full cellular access in absence of the receptor, suggesting that some serotypes can utilize non-AAVR internalization pathways (Dudek et al., 2018). In immortalized cells, AAVR localizes to the cytoplasm and perinuclear region where it associates with the Golgi network (Poon et al., 2011; Pillay et al., 2016). Several hypotheses as to where exactly AAV interacts with AAVR have been put forward, including on the cell surface, in the endolysosomal system and at the Golgi apparatus; however, this requires further clarification (Summerford et al., 2016; Pillay and Carette, 2017).
Data are supporting several distinct AAV internalization mechanisms, including clathrin-dependent endocytosis (Uhrig et al., 2012), caveolar endocytosis (Sanlioglu et al., 2000), and the clathrin-independent carriers and GPI-enriched endocytic compartments (CLIC/GEEC) pathway (Nonnenmacher and Weber, 2011). However, not all routes result in an efficient delivery to the nucleus, rather they traffic AAV through unproductive paths leading to a viral cul-de-sac (Nonnenmacher and Weber, 2012; Pillay and Carette, 2017); only ≈30% of internalized AAV is estimated to enter the nucleus (Zhong et al., 2008; Xiao et al., 2012). Nonetheless, there are distinctions in AAV uptake depending on cell type and serotype (Weinberg et al., 2014), thus future work identifying neuronal-specific internalization mechanisms is required.
Upon cellular entry, AAVs have been reported to be retrogradely transported from the cell surface to Golgi in a syntaxin 5-dependent mechanism (Nonnenmacher et al., 2015), before escaping into the cytoplasm and entering into the nucleus via the nuclear pore complex (Nicolson and Samulski, 2014). However, before reaching the Golgi, AAV must transit through various acidic endosomal compartments to drive pH- and cathepsin-mediated conformational changes in the capsid (Akache et al., 2007; Salganik et al., 2012). Indeed, the passage of AAV through the endosome to Golgi system appears to be necessary for transgene expression, as AAV directly injected into cytosol do not migrate to the nucleus (Sonntag et al., 2006). AAV has been reported to localize to Rab5-, Rab7-, and Rab11-positive (recycling) endosomes (Berry and Asokan, 2016), and, as expected, requires a functioning microtubule network for transport (Xiao and Samulski, 2012). Nevertheless, its exact route through the cell requires further elucidation, especially its transit through long and highly polarized peripheral nerves, as little data have been generated in neurons.
That being said, there is ample indirect evidence that AAVs are transported in axons in vivo both in peripheral (Hollis Ii et al., 2008; Towne et al., 2010; Zheng et al., 2010; Benkhelifa-Ziyyat et al., 2013; Jan et al., 2019) and CNS (Salegio et al., 2013; Castle et al., 2014a,b) neurons, suggesting the availability of AAV receptors and uptake mechanisms; however, observations of AAV being actively trafficked are limited. Nevertheless, peripherally administered AAV likely hijacks Rab-positive endosomes in peripheral nerves to reach the CNS, like that of AdV. Indeed, in primary cortical neurons grown in microfluidic chambers to separate axons and soma, AAV9 was shown to localize in a time-dependent fashion to several different endosomes/vesicles (e.g., Rab5-, Rab7-, Rab11-positive; Castle et al., 2014b). AAV9 internalized at axon tips was retrogradely transported in cytoplasmic dynein-dynactin-driven Rab7-positive endosomes and was subsequently capable of inducing transgene expression post-transition through the Golgi (Castle et al., 2014b). Moreover, in a companion study it was shown that AAV1, AAV8, and AAV9 share the same intra-axonal compartment when being transported in primary cortical neurons, indicating that once they have gained access to the endosomal sorting system, AAV serotypes harness common axonal transport mechanisms (Castle et al., 2014a). However, direct evidence from the motor and sensory neurons remains unavailable.
Lentivirus
LV tropism is dictated by the envelope glycoproteins with which it has been pseudotyped (Cronin et al., 2005). VSV-G interacts with the low-density lipoprotein receptor (LDLR; Finkelshtein et al., 2013). LDLR mediates uptake of cholesterol-rich LDL and is broadly expressed, thus LV-VSV is pan-tropic. A measure of cell-type selectivity can be achieved with cell/tissue-specific promoters, which is a strategy used with all three gene therapy viruses. For example, LV-VSV combined with an hGFAP promoter induces astrocytic expression, whereas LV-VSV with an rNSE promoter selectively expresses in neurons (Jakobsson et al., 2003). Alternatively, envelope modification coupled with surface antibody-mediated targeting can confer tissue specificity and improve virus uptake (Yang et al., 2006; Eleftheriadou et al., 2014). In contrast, LV-RV interacts with receptors that are predominantly expressed by neurons, including the pan-neurotrophin receptor p75NTR (Tuffereau et al., 1998), neuronal cell adhesion molecule (NCAM; Thoulouze et al., 1998) and nicotinic acetylcholine receptor (nAChR; Hanham et al., 1993). p75NTR non-selectively binds to all neurotrophins (i.e., BDNF, NGF, NT-3, and NT-4/5) and, depending on the active co-receptor, can activate both pro-survival or pro-death signaling (Gentry et al., 2004). NCAM is an immunoglobulin-like glycoprotein that mediates cell-to-cell contact and functions in adhesion, guidance, and differentiation during neuronal growth (Weledji and Assob, 2014). nAChRs bind to the excitatory neurotransmitter acetylcholine secreted into the synaptic cleft to facilitate depolarization of the postsynaptic cell. All three LV-RV receptors are integral constituents of the NMJ (although nAChRs are post-synaptic), explaining the efficient in vivo uptake into motor neurons of these RV pseudotyped viruses when injected into a muscle (Mazarakis et al., 2001; Azzouz et al., 2004a,b; Wong et al., 2004).
After receptor-mediated internalization, most likely in clathrin-coated pits as dictated by their neuronal receptors (i.e., p75NTR; Bronfman et al., 2003), RV-LVs migrate through the endolysosomal system transitioning from Rab5-positive early endosomes to the non-acidic Rab7-positive compartment (Hislop et al., 2014). In non-neuronal cells, endosome acidification causes a conformational change in LV glycoproteins, which initiates membrane fusion between the viral envelope and endosome membrane to permit the escape of the virus into the cytoplasm (Gaudin et al., 1993; Gaudin, 2000). However, in neurons, LVs are retrogradely transported within neutral Rab7-positive signaling endosomes towards peripheral nerve cell bodies through the same motor protein-driven process as AdV and AAV. In rat primary motor neuron cultures, LV-RV was shown to co-localize in axons with all three receptors (i.e., p75NTR, NCAM, and nAChR) with co-migration confirmed for p75NTR (Hislop et al., 2014). However, despite transport being rapid and effective, neuronal transduction was comparatively inefficient, suggesting that post-trafficking processes are suboptimal in neurons (Hislop et al., 2014). Upon arrival at the cell body, LV must undergo a process known as uncoating, in which several viral proteins (e.g., Gag structural proteins) are removed to permit reverse transcription of the viral RNA (Matreyek and Engelman, 2013). The resulting double-stranded DNA then complexes with virus proteins for entry into the nucleus via the nuclear pore complex, before integration into the DNA of the host neuron. Improving understanding of these processes in motor and sensory neurons will be key to optimizing the effectiveness of intramuscular virus delivery.
Influence of Pathology
Neuropathology will impact most, if not all, major steps in the journey of viruses from the nerve terminal to the nucleus (Figure 2). Neurodegeneration of peripheral nerves results in the loss of axon terminals within muscles (Figure 2A). Motor neuron retraction from the NMJ, i.e., denervation, is an early feature of motor neuron diseases [e.g., ALS, SMA and Charcot-Marie-Tooth disease (CMT; Goulet et al., 2013; Moloney et al., 2014; Sleigh et al., 2014; Spaulding et al., 2016)], and will limit neuron-virus interactions within muscles. Sensory degeneration observed in conditions like CMT (Sleigh et al., 2017), will have a similar restrictive effect. Nonetheless, motor neurons branch frequently within muscles resulting in multiple contacts across the entire muscle; thus, if one or several NMJs become denervated, there is likely to be a window of time in which at least some neuromuscular contacts of a pathological neuron remain viable. In ALS mice, for instance, rather than all neuromuscular contacts of a single motor neuron denervating simultaneously, healthy synapses close to degenerating NMJs are more likely to denervate than those located further away, suggestive of localized pathological transfer (Martineau et al., 2018). It is therefore conceivable that functional synapses may facilitate virus uptake and nuclear delivery to preserve the integrity of NMJs that remain. Moreover, once delivered, viral vectors encoding secretable proteins (e.g., neurotrophins) can influence central networks through both autocrine and paracrine mechanisms (Baumgartner and Shine, 1997; Benkhelifa-Ziyyat et al., 2013). NMJs resident in different muscles, and even within a single muscle, can show large differences in both pre- and post-synaptic structures (Mech et al., 2020) as well as levels of key synaptic proteins (Allodi et al., 2016), thus virus binding and uptake are likely to differ across motor nerve terminals.
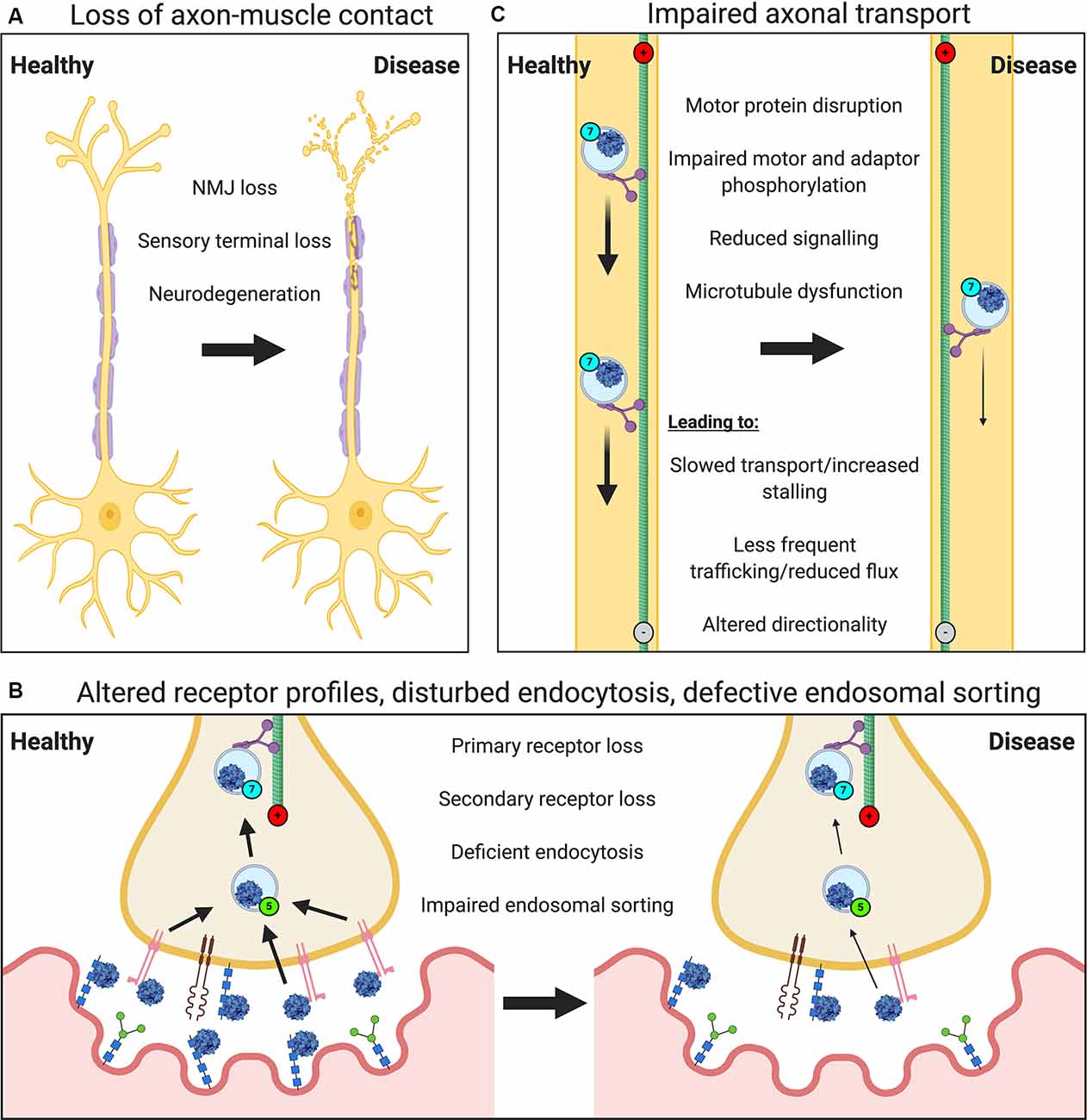
Figure 2. Neuropathological events impair the viral transduction of peripheral neurons. Several general and virus-specific pathological events caused by neurological disease diminish the effectiveness of gene therapy delivery to the nervous system via muscle. (A) Loss of motor and sensory nerve endings due to neurodegeneration will restrict nerve-muscle connections and the frequency of virus-nerve interaction. (B) Alterations in the expression or availability of certain primary or secondary receptors will affect virus attraction and binding. Deficits in endocytosis, as seen in spinal muscular atrophy (SMA), or impaired endosomal sorting, as identified in amyotrophic lateral sclerosis (ALS) and some forms of Charcot-Marie Tooth disease (CMT), could reduce virus uptake into peripheral nerve terminals. Defects in Golgi processing and nuclear import may also decrease viral transduction (not depicted). (C) A variety of impairments affecting axonal transport machinery (e.g., microtubule dysfunction) are known to cause defects in cargo trafficking (e.g., slowed transport or reduced quantity/flux), which will limit viral delivery.
Significantly, intramuscular injections of gene therapy viruses can result in efficient and extensive transgene expression within the neonatal and adult mouse spinal cord, brainstem, and sensory ganglia, likely via the cerebrospinal fluid (Benkhelifa-Ziyyat et al., 2013; Chen et al., 2020). This finding is particularly important, as it suggests that injecting one muscle can result in viral transduction of an array of central neurons (Chen et al., 2020), meaning that not all muscles require injection for potential widespread motor and sensory neuron transduction; although injecting more muscles can cause greater therapeutic benefit (Benkhelifa-Ziyyat et al., 2013). Furthermore, muscle transduction can be used to promote synaptogenesis and/or reinnervation after neuromuscular pathology (Darabid et al., 2014). In this regard, collateral sprouting and dynamic remodeling of the NMJ, as is observed in ALS mice (Martineau et al., 2018), may also be therapeutically targeted.
In addition to the loss of peripheral nerve endings in muscle, deficiencies in endocytosis (e.g., in SMA; Dimitriadi et al., 2016), endolysosomal sorting (observed in many conditions; Neefjes and van der Kant, 2014), Golgi processing (e.g., in ALS; van Dis et al., 2014), and nuclear import (e.g., in ALS; Dormann and Haass, 2011) would all likely reduce the efficiency of viral transgene expression (Figure 2B). As would pathology-associated restrictions in axonal transport (Figure 2C), which have been reported in many neurodevelopmental and neurodegenerative conditions (Sleigh et al., 2019), such as the signaling endosome transport deficits observed in ALS mice (Bilsland et al., 2010; Sleigh et al., 2020a). Nevertheless, Rab7-positive endosomes containing AAV have been shown in primary cortical neurons in vitro to increase retrograde transport speeds compared to non-AAV containing Rab7 organelles (Castle et al., 2014b), which could perhaps counteract transport dysfunction.
Only a few studies are have investigated the impact of disease on virus transduction after intramuscular delivery. Despite downregulation during development, CAR expression is upregulated in regenerating adult skeletal muscle in response to disease (Nalbantoglu et al., 1999; Shaw et al., 2004; Sinnreich et al., 2005), which will likely positively impact AdV uptake. Increased levels of sialic acid, a known AAV9 inhibitor, in the CNS of a mouse model of lysosomal storage disorder have been shown to severely limit the effectiveness of AAV9-mediated gene therapy (Chen et al., 2012). Nonetheless, the opposite may be true for particular AdV and other AAV serotypes, which use sialic acid as a primary attachment factor. Involved in pro-apoptotic signaling during development, but downregulated in the mature nervous system, the p75NTR receptor is also re-expressed in neurons after disease or trauma (Dechant and Barde, 2002), possibly impacting LV efficacy. For example, p75NTR expression is increased in SOD1G93A mice motor neurons and human ALS tissue (Lowry et al., 2001), and plays a key role in organizing and maintaining NMJ connectivity (Pérez et al., 2019). Moreover, NCAM expression is a major regulator of synaptic remodeling in pre-synaptic NMJ terminals (Chipman et al., 2014) and levels are dysregulated in ALS (Jensen et al., 2016), which could also affect LV binding. Also, the background of the experimental animal can influence the transduction efficiency of some vectors and must be carefully considered (He et al., 2019). Overall, these studies warn against the assumption of similar virus binding and uptake profiles between healthy and disease states and indicate that further studies in disease models at symptomatic stages are required.
Despite these hurdles, intramuscular injections of gene therapies have proved successful at symptomatic stages in ALS mice (Tosolini and Sleigh, 2017), hence the above-discussed effects of pathology do not abolish virus transduction. Furthermore, symptomatic SMA patients treated with onasemnogene abeparvovec to augment SMN protein levels respond positively to treatment (Mendell et al., 2017), albeit with AAV administered intravenously. Nevertheless, while it remains unclear precisely how and to what extent specific diseases and associated pathologies will impact the transduction of peripheral neurons, the described viral vectors have the undisputed potential for the treatment of neuromuscular disorders when delivered to skeletal muscle.
Optimizing Intramuscular Gene Therapy
One of the biggest challenges facing gene therapy is achieving sufficient delivery to target cells/tissues to combat disease. This is particularly difficult for peripheral nerve disorders in which pathological cells are located deep within the spinal cord and behind the BBB and BSCB. Several investigator-independent factors such as nervous system maturity (Foust et al., 2009; Tosolini and Morris, 2016a) and pathology influence viral transduction and transgene expression, but these cannot be modified in a clinical setting. However, varied investigator-driven factors also impact the effectiveness and should be carefully considered when designing gene therapy for intramuscular administration. Differences in tropism, infectivity, and transport between viruses and their serotypes will impact the success of this delivery method; for example, in a side-by-side comparison, muscle injection of rAAV2-retro was shown to have superior capacity to transduce peripheral neurons compared to AAV serotypes 1, 2, and 5–9 (Chen et al., 2020). Similarly, superior LV pseudotypes based on hybrid glycoproteins have also been identified (Hirano et al., 2013; Eleftheriadou et al., 2016). Moreover, vector purity and concentration will impact transduction levels (Hollis Ii et al., 2008; Klein et al., 2008), as will the efficiency and specificity of the promoter (von Jonquieres et al., 2013; Borel et al., 2016), the choice of which can also reduce off-target expression, and hence further enhance therapeutic potential (Parr-Brownlie et al., 2015).
Several different methods have been pioneered that can enhance peripheral neuron transduction upon intramuscular virus administration. As may be expected, these techniques focus on enhancing virus uptake rather than other processes essential to transduction. For instance, a complementary viral strategy can be used to boost the expression of the virus receptor(s) at peripheral nerve terminals that can then be therapeutically targeted with a different virus, as has been demonstrated with AAV-mediated CAR expression for increased AdV binding and uptake (Larochelle et al., 2010; Li et al., 2018b). Receptor expression may also be selectively increased by genetic overexpression (Nalbantoglu et al., 2001) or administration of drugs that enhance transcription, albeit non-specifically (e.g., histone deacetylase inhibitors; Larochelle et al., 2010). Similarly, genetic screens are beginning to identify a variety of viral restriction factors (i.e., proteins that constrain uptake and transduction), which could also be genetically or chemically manipulated, perhaps in a tissue-specific fashion, to aid uptake (Mano et al., 2015; Madigan et al., 2019). Alternatively, approaches are being developed in which recombinant viral receptor proteins are conjugated to biomaterials and pre-loaded with gene therapy viruses before injection. Indeed, intramuscular administration of recombinant cysteine-tagged AAVR chemically linked to polyester microspheres and pre-incubated with AAV resulted in local and prolonged gene delivery with reduced spread compared to AAV alone (Kim et al., 2019). However, it remains to be seen whether this system can be adapted to increase uptake into peripheral nerve terminals, which would require the release of AAV from the receptor microspheres. Similarly, viral capsids can be chemically modified with a variety of different substances that may aid peripheral nerve binding (e.g., conjugation with neuron-specific homing peptides; Terashima et al., 2009), or antibodies against key neuronal receptor proteins (e.g., p75NTR and CAR; Hedley et al., 2006; Eleftheriadou et al., 2014). Furthermore, motor neuron transduction efficiency upon intramuscular administration of AdV was shown to be enhanced by pre-treatment with flaccid paralysis-causing botulinum toxin type A (BoNT/A; Millecamps et al., 2002). Likely mediated by enhanced motor terminal sprouting, this enhancement was even greater in the SOD1G93A ALS mouse (Millecamps et al., 2001, 2002).
Unfortunately, many of these strategies are not currently a clinical possibility, for obvious reasons. Nonetheless, their implementation in the laboratory to deliver genes within the therapeutic range, along with the development of novel and improved tools to assess virus transduction and treatment efficacy (Han et al., 2019; Chen et al., 2020; Sleigh et al., 2020b; Surana et al., 2020; Ueda et al., 2020), will undoubtedly lead to improved understanding of disease mechanisms and assessment of potential gene therapy strategies.
Conclusion
Gene therapy injected into the skeletal muscle for delivery to neurons holds therapeutic promise for peripheral nerve disorders. Motor and sensory nerve terminals located within muscles can act as therapeutic conduits not only for the innervating neurons (Figure 1) but also neighboring nerve and glial cells via paracrine mechanisms. Moreover, some viruses can escape from the initially transduced neurons, resulting in widespread gene delivery throughout the spinal cord, brain stem, and sensory ganglia. Importantly, this indicates that not all muscles need to be injected to obtain broad cellular dosing. Unfortunately, neuropathology is likely to hinder the effectiveness of intramuscular gene therapy delivery (Figure 2); but innovative pre-clinical methods are being developed that will enhance peripheral neuron transduction via this method. Also, the intramuscular administration could be combined with, for example, intrathecal delivery to further enhance CNS uptake. However, due to the immune response, repeated successful dosing is unlikely, and hence such treatments need to be given within a short time frame to circumvent this impediment. Nevertheless, by factoring in a detailed understanding of the dynamics of viruses and host cell receptors, especially in the context of peripheral nerve biology and neuromuscular pathology, perhaps this minimally invasive delivery method can contribute to successful gene therapy in the future.
Author Contributions
AT and JS wrote the manuscript and have approved the submission of this work.
Funding
This work was funded by a postdoctoral position (AT) supported by a Wellcome Trust Senior Investigator Award (107116/Z/15/Z) to Prof. Giampietro Schiavo (Institute of Neurology, University College London) and by the Medical Research Council Career Development Award (MR/S006990/1; JS).
Conflict of Interest
The authors declare that the research was conducted in the absence of any commercial or financial relationships that could be construed as a potential conflict of interest.
Acknowledgments
We would like to thank Prof. Giampietro Schiavo (University College London) for his enduring support and mentorship. The figures were created with BioRender (https://biorender.com).
References
Acsadi, G., Anguelov, R. A., Yang, H., Toth, G., Thomas, R., Jani, A., et al. (2002). Increased survival and function of SOD1 mice after glial cell-derived neurotrophic factor gene therapy. Hum. Gene Ther. 13, 1047–1059. doi: 10.1089/104303402753812458
Acsadi, G., Jani, A., Massie, B., Simoneau, M., Holland, P., Blaschuk, K., et al. (1994). A differential efficiency of adenovirus-mediated in vivo gene transfer into skeletal muscle cells of different maturity. Hum. Mol. Genet. 3, 579–584. doi: 10.1093/hmg/3.4.579
Ahi, Y. S., Bangari, D. S., and Mittal, S. K. (2011). Adenoviral vector immunity: its implications and circumvention strategies. Curr. Gene Ther. 11, 307–320. doi: 10.2174/156652311796150372
Akache, B., Grimm, D., Shen, X., Fuess, S., Yant, S. R., Glazer, D. S., et al. (2007). A two-hybrid screen identifies cathepsins B and L as uncoating factors for adeno-associated virus 2 and 8. Mol. Ther. 15, 330–339. doi: 10.1038/sj.mt.6300053
Akli, S., Caillaud, C., Vigne, E., Stratford-Perricaudet, L. D., Poenaru, L., Perricaudet, M., et al. (1993). Transfer of a foreign gene into the brain using adenovirus vectors. Nat. Genet. 3, 224–228. doi: 10.1038/ng0393-224
Allodi, I., Comley, L., Nichterwitz, S., Nizzardo, M., Simone, C., Benitez, J. A., et al. (2016). Differential neuronal vulnerability identifies IGF-2 as a protective factor in ALS. Sci. Rep. 6:25960. doi: 10.1038/srep25960
Annoni, A., Gregori, S., Naldini, L., and Cantore, A. (2019). Modulation of immune responses in lentiviral vector-mediated gene transfer. Cell. Immunol. 342:103802. doi: 10.1016/j.cellimm.2018.04.012
Arnberg, N. (2012). Adenovirus receptors: implications for targeting of viral vectors. Trends Pharmacol. Sci. 33, 442–448. doi: 10.1016/j.tips.2012.04.005
Azzouz, M., Le, T., Ralph, G. S., Walmsley, L., Monani, U. R., Lee, D. C. P., et al. (2004a). Lentivector-mediated SMN replacement in a mouse model of spinal muscular atrophy. J. Clin. Invest. 114, 1726–1731. doi: 10.1172/JCI22922
Azzouz, M., Ralph, G. S., Storkebaum, E., Walmsley, L. E., Mitrophanous, K. A., Kingsman, S. M., et al. (2004b). VEGF delivery with retrogradely transported lentivector prolongs survival in a mouse ALS model. Nature 429, 413–417. doi: 10.1038/nature02544
Baumgartner, B. J., and Shine, H. D. (1997). Targeted transduction of CNS neurons with adenoviral vectors carrying neurotrophic factor genes confers neuroprotection that exceeds the transduced population. J. Neurosci. 17, 6504–6511. doi: 10.1523/JNEUROSCI.17-17-06504.1997
Baumgartner, B. J., and Shine, H. D. (1998). Neuroprotection of spinal motoneurons following targeted transduction with an adenoviral vector carrying the gene for glial cell line-derived neurotrophic factor. Exp. Neurol. 153, 102–112. doi: 10.1006/exnr.1998.6878
Benkhelifa-Ziyyat, S., Besse, A., Roda, M., Duque, S., Astord, S., Carcenac, R., et al. (2013). Intramuscular scAAV9-SMN injection mediates widespread gene delivery to the spinal cord and decreases disease severity in SMA mice. Mol. Ther. 21, 282–290. doi: 10.1038/mt.2012.261
Benkler, C., Barhum, Y., Ben-Zur, T., and Offen, D. (2016). Multifactorial gene therapy enhancing the glutamate uptake system and reducing oxidative stress delays symptom onset and prolongs survival in the SOD1–G93A ALS mouse model. J. Mol. Neurosci. 58, 46–58. doi: 10.1007/s12031-015-0695-2
Bergelson, J. M., Cunningham, J. A., Droguett, G., Kurt-Jones, E. A., Krithivas, A., Hong, J. S., et al. (1997). Isolation of a common receptor for Coxsackie B viruses and adenoviruses 2 and 5. Science 275, 1320–1323. doi: 10.1126/science.275.5304.1320
Berry, G. E., and Asokan, A. (2016). Cellular transduction mechanisms of adeno-associated viral vectors. Curr. Opin. Virol. 21, 54–60. doi: 10.1016/j.coviro.2016.08.001
Bevan, A. K., Duque, S., Foust, K. D., Morales, P. R., Braun, L., Schmelzer, L., et al. (2011). Systemic gene delivery in large species for targeting spinal cord, brain and peripheral tissues for pediatric disorders. Mol. Ther. 19, 1971–1980. doi: 10.1038/mt.2011.157
Bilsland, L. G., Sahai, E., Kelly, G., Golding, M., Greensmith, L., and Schiavo, G. (2010). Deficits in axonal transport precede ALS symptoms in vivo. Proc. Natl. Acad. Sci. U S A 107, 20523–20528. doi: 10.1073/pnas.1006869107
Bohlen, M. O., El-Nahal, H. G., and Sommer, M. A. (2019). Transduction of craniofacial motoneurons following intramuscular injections of canine adenovirus type-2 (CAV-2) in rhesus macaques. Front. Neuroanat. 13:84. doi: 10.3389/fnana.2019.00084
Bohnert, S., and Schiavo, G. (2005). Tetanus toxin is transported in a novel neuronal compartment characterized by a specialized pH regulation. J. Biol. Chem. 280, 42336–42344. doi: 10.1074/jbc.m506750200
Borel, F., Gernoux, G., Cardozo, B., Metterville, J. P., Toro Cabrera, G. C., Song, L., et al. (2016). Therapeutic rAAVrh10 mediated SOD1 silencing in adult SOD1G93A mice and nonhuman primates. Hum. Gene Ther. 27, 19–31. doi: 10.1089/hum.2015.122
Boulis, N. M., Willmarth, N. E., Song, D. K., Feldman, E. L., and Imperiale, M. J. (2003). Intraneural colchicine inhibition of adenoviral and adeno-associated viral vector remote spinal cord gene delivery. Neurosurgery 52, 381–387. doi: 10.1227/01.neu.0000044459.24519.3e
Bremner, K. H., Scherer, J., Yi, J., Vershinin, M., Gross, S. P., and Vallee, R. B. (2009). Adenovirus transport via direct interaction of cytoplasmic dynein with the viral capsid hexon subunit. Cell Host Microbe. 6, 523–535. doi: 10.1016/j.chom.2009.11.006
Broadie, K., Baumgartner, S., and Prokop, A. (2011). Extracellular matrix and its receptors in Drosophila neural development. Dev. Neurobiol. 71, 1102–1130. doi: 10.1002/dneu.20935
Bronfman, F. C., Tcherpakov, M., Jovin, T. M., and Fainzilber, M. (2003). Ligand-induced internalization of the p75 neurotrophin receptor: a slow route to the signaling endosome. J. Neurosci. 23, 3209–3220. doi: 10.1523/JNEUROSCI.23-08-03209.2003
Cassany, A., Ragues, J., Guan, T., Bégu, D., Wodrich, H., Kann, M., et al. (2015). Nuclear import of adenovirus DNA involves direct interaction of hexon with an N-terminal domain of the nucleoporin Nup214. J. Virol. 89, 1719–1730. doi: 10.1128/jvi.02639-14
Castle, M. J., Gershenson, Z. T., Giles, A. R., Holzbaur, E. L. F., and Wolfe, J. H. (2014a). Adeno-associated virus serotypes 1, 8, and 9 share conserved mechanisms for anterograde and retrograde axonal transport. Hum. Gene Ther. 25, 705–720. doi: 10.1089/hum.2013.189
Castle, M. J., Perlson, E., Holzbaur, E. L., and Wolfe, J. H. (2014b). Long-distance axonal transport of AAV9 is driven by dynein and kinesin-2 and is trafficked in a highly motile Rab7-positive compartment. Mol. Ther. 22, 554–566. doi: 10.1038/mt.2013.237
Chakrabarty, P., Rosario, A., Cruz, P., Siemienski, Z., Ceballos-Diaz, C., Crosby, K., et al. (2013). Capsid serotype and timing of injection determines AAV transduction in the neonatal mice brain. PLoS One 8:e67680. doi: 10.1371/journal.pone.0067680
Chandler, R. J., Sands, M. S., and Venditti, C. P. (2017). Recombinant adeno-associated viral integration and genotoxicity: insights from animal models. Hum. Gene Ther. 28, 314–322. doi: 10.1089/hum.2017.009
Chen, Y. H., Claflin, K., Geoghegan, J. C., and Davidson, B. L. (2012). Sialic acid deposition impairs the utility of AAV9, but not peptide-modified AAVs for brain gene therapy in a mouse model of lysosomal storage disease. Mol. Ther. 20, 1393–1399. doi: 10.1038/mt.2012.100
Chen, Z., Fan, G., Li, A., Yuan, J., and Xu, T. (2020). rAAV2-retro enables extensive and high-efficient transduction of lower motor neurons following intramuscular injection. Mol. Ther. Methods Clin. Dev. 17, 21–33. doi: 10.1016/j.omtm.2019.11.006
Chipman, P. H., Schachner, M., and Rafuse, V. F. (2014). Presynaptic NCAM is required for motor neurons to functionally expand their peripheral field of innervation in partially denervated muscles. J. Neurosci. 34, 10497–10510. doi: 10.1523/JNEUROSCI.0697-14.2014
Choudhury, S. R., Fitzpatrick, Z., Harris, A. F., Maitland, S. A., Ferreira, J. S., Zhang, Y., et al. (2016). In vivo selection yields AAV-B1 capsid for central nervous system and muscle gene therapy. Mol. Ther. 24, 1247–1257. doi: 10.1038/mt.2016.84
Colella, P., Ronzitti, G., and Mingozzi, F. (2018). Emerging issues in AAV-mediated in vivo gene therapy. Mol. Ther. Methods Clin. Dev. 8, 87–104. doi: 10.1016/j.omtm.2017.11.007
Cronin, J., Zhang, X.-Y., and Reiser, J. (2005). Altering the tropism of lentiviral vectors through pseudotyping. Curr. Gene Ther. 5, 387–398. doi: 10.2174/1566523054546224
Darabid, H., Perez-Gonzalez, A. P., and Robitaille, R. (2014). Neuromuscular synaptogenesis: coordinating partners with multiple functions. Nat. Rev. Neurosci. 15, 703–718. doi: 10.1038/nrn3821
Davidson, B. L., Allen, E. D., Kozarsky, K. F., Wilson, J. M., and Roessler, B. J. (1993). A model system for in vivo gene transfer into the central nervous system using an adenoviral vector. Nat. Genet. 3, 219–223. doi: 10.1038/ng0393-219
Dechant, G., and Barde, Y.-A. (2002). The neurotrophin receptor p75NTR: novel functions and implications for diseases of the nervous system. Nat. Neurosci. 5, 1131–1136. doi: 10.1038/nn1102-1131
Deinhardt, K., Salinas, S., Verastegui, C., Watson, R., Worth, D., Hanrahan, S., et al. (2006). Rab5 and Rab7 control endocytic sorting along the axonal retrograde transport pathway. Neuron 52, 293–305. doi: 10.1016/j.neuron.2006.08.018
Del Rio, D., Beucher, B., Lavigne, M., Wehbi, A., Gonzalez Dopeso-Reyes, I., Saggio, I., et al. (2019). CAV-2 vector development and gene transfer in the central and peripheral nervous systems. Front. Mol. Neurosci. 12:71. doi: 10.3389/fnmol.2019.00071
Deverman, B. E., Pravdo, P. L., Simpson, B. P., Kumar, S. R., Chan, K. Y., Banerjee, A., et al. (2016). Cre-dependent selection yields AAV variants for widespread gene transfer to the adult brain. Nat. Biotechnol. 34, 204–209. doi: 10.1038/nbt.3440
Deverman, B. E., Ravina, B. M., Bankiewicz, K. S., Paul, S. M., and Sah, D. W. Y. (2018). Gene therapy for neurological disorders: progress and prospects. Nat. Rev. Drug Discov. 17, 641–659. doi: 10.1038/nrd.2018.110
Dimitriadi, M., Derdowski, A., Kalloo, G., Maginnis, M. S., O’Hern, P., Bliska, B., et al. (2016). Decreased function of survival motor neuron protein impairs endocytic pathways. Proc. Natl. Acad. Sci. U S A 113, E4377–E4386. doi: 10.1073/pnas.1600015113
Dormann, D., and Haass, C. (2011). TDP-43 and FUS: a nuclear affair. Trends Neurosci. 34, 339–348. doi: 10.1016/j.tins.2011.05.002
Dorner, A. A., Wegmann, F., Butz, S., Wolburg-Buchholz, K., Wolburg, H., Mack, A., et al. (2005). Coxsackievirus-adenovirus receptor (CAR) is essential for early embryonic cardiac development. J. Cell Sci. 118, 3509–3521. doi: 10.1242/jcs.02476
Dudek, A. M., Pillay, S., Puschnik, A. S., Nagamine, C. M., Cheng, F., Qiu, J., et al. (2018). An alternate route for adeno-associated virus (AAV) entry independent of AAV receptor. J. Virol. 92:e02213-17. doi: 10.1128/jvi.02213-17
Duffy, M. R., Parker, A. L., Bradshaw, A. C., and Baker, A. H. (2012). Manipulation of adenovirus interactions with host factors for gene therapy applications. Nanomedicine 7, 271–288. doi: 10.2217/nnm.11.186
Eleftheriadou, I., Manolaras, I., Irvine, E. E., Dieringer, M., Trabalza, A., and Mazarakis, N. D. (2016). αCAR IGF-1 vector targeting of motor neurons ameliorates disease progression in ALS mice. Ann. Clin. Transl. Neurol. 3, 752–768. doi: 10.1002/acn3.335
Eleftheriadou, I., Trabalza, A., Ellison, S. M., Gharun, K., and Mazarakis, N. D. (2014). Specific retrograde transduction of spinal motor neurons using lentiviral vectors targeted to presynaptic NMJ receptors. Mol. Ther. 22, 1285–1298. doi: 10.1038/mt.2014.49
Finiels, F., Gimenez y Ribotta, M., Barkats, M., Samolyk, M. L., Robert, J. J., Privat, A., et al. (1995). Specific and efficient gene transfer strategy offers new potentialities for the treatment of motor neurone diseases. Neuroreport 7, 373–378. doi: 10.1097/00001756-199512290-00088
Finkelshtein, D., Werman, A., Novick, D., Barak, S., and Rubinstein, M. (2013). LDL receptor and its family members serve as the cellular receptors for vesicular stomatitis virus. Proc. Natl. Acad. Sci. U S A 110, 7306–7311. doi: 10.1073/pnas.1214441110
Foust, K. D., Nurre, E., Montgomery, C. L., Hernandez, A., Chan, C. M., and Kaspar, B. K. (2009). Intravascular AAV9 preferentially targets neonatal neurons and adult astrocytes. Nat. Biotechnol. 27, 59–65. doi: 10.1038/nbt.1515
Gaudin, Y. (2000). Rabies virus-induced membrane fusion pathway. J. Cell Biol. 150, 601–612. doi: 10.1083/jcb.150.3.601
Gaudin, Y., Ruigrok, R. W., Knossow, M., and Flamand, A. (1993). Low-pH conformational changes of rabies virus glycoprotein and their role in membrane fusion. J. Virol. 67, 1365–1372. doi: 10.1128/jvi.67.3.1365-1372.1993
Gentry, J. J., Barker, P. A., and Carter, B. D. (2004). The p75 neurotrophin receptor: multiple interactors and numerous functions. Prog. Brain Res. 146, 25–39. doi: 10.1016/S0079-6123(03)46002-0
Ghadge, G. D., Roos, R. P., Kang, U. J., Wollmann, R., Fishman, P. S., Kalynych, A. M., et al. (1995). CNS gene delivery by retrograde transport of recombinant replication-defective adenoviruses. Gene Ther. 2, 132–137.
Ghebremedhin, B. (2014). Human adenovirus: viral pathogen with increasing importance. Eur. J. Microbiol. Immunol. 4, 26–33. doi: 10.1556/EuJMI.4.2014.1.2
Giménez y Ribotta, M., Revah, F., Pradier, L., Loquet, I., Mallet, J., and Privat, A. (1997). Prevention of motoneuron death by adenovirus-mediated neurotrophic factors. J. Neurosci. Res. 48, 281–285. doi: 10.1002/(sici)1097-4547(19970501)48:3<281::aid-jnr11>3.3.co;2-i
Gostic, M., Martinelli, A., Tucker, C., Yang, Z., Gasparoli, F., Ewart, J.-Y., et al. (2019). The dyslexia susceptibility KIAA0319 gene shows a specific expression pattern during zebrafish development supporting a role beyond neuronal migration. J. Comp. Neurol. 527, 2634–2643. doi: 10.1002/cne.24696
Goulet, B. B., Kothary, R., and Parks, R. J. (2013). At the “junction” of spinal muscular atrophy pathogenesis: the role of neuromuscular junction dysfunction in SMA disease progression. Curr. Mol. Med. 13, 1160–1174. doi: 10.2174/15665240113139990044
Greber, U. F., and Flatt, J. W. (2019). Adenovirus entry: from infection to immunity. Annu. Rev. Virol. 6, 177–197. doi: 10.1146/annurev-virology-092818-015550
Haase, G., Pettmann, B., Vigne, E., Castelnau-Ptakhine, L., Schmalbruch, H., and Kahn, A. (1998). Adenovirus-mediated transfer of the neurotrophin-3 gene into skeletal muscle of pmn mice: therapeutic effects and mechanisms of action. J. Neurol. Sci. 160, S97–S105. doi: 10.1016/s0022-510x(98)00207-x
Han, S., Li, D., Kou, Y., Fu, Z., and Yin, X. (2019). Multiple retrograde tracing methods compatible with 3DISCO clearing. Artif. Cells Nanomed. Biotechnol. 47, 4240–4247. doi: 10.1080/21691401.2019.1687493
Hanham, C. A., Zhao, F., and Tignor, G. H. (1993). Evidence from the anti-idiotypic network that the acetylcholine receptor is a rabies virus receptor. J. Virol. 67, 530–542. doi: 10.1128/jvi.67.1.530-542.1993
He, T., Itano, M. S., Earley, L. F., Hall, N. E., Riddick, N., Samulski, R. J., et al. (2019). The influence of murine genetic background in adeno-associated virus transduction of the mouse brain. Hum. Gene Ther. Clin. Dev. 30, 169–181. doi: 10.1089/humc.2019.030
Hedley, S. J., Auf der Maur, A., Hohn, S., Escher, D., Barberis, A., Glasgow, J. N., et al. (2006). An adenovirus vector with a chimeric fiber incorporating stabilized single chain antibody achieves targeted gene delivery. Gene Ther. 13, 88–94. doi: 10.1038/sj.gt.3302603
Heikkinen, A., Härönen, H., Norman, O., and Pihlajaniemi, T. (2020). Collagen XIII and other ECM components in the assembly and disease of the neuromuscular junction. Anat. Rec. 303, 1653–1663. doi: 10.1002/ar.24092
High, K. A., and Roncarolo, M. G. (2019). Gene therapy. N. Engl. J. Med. 381, 455–464. doi: 10.1056/NEJMra1706910
Hinderer, C., Katz, N., Buza, E. L., Dyer, C., Goode, T., Bell, P., et al. (2018). Severe toxicity in nonhuman primates and piglets following high-dose intravenous administration of an adeno-associated virus vector expressing human SMN. Hum. Gene Ther. 29, 285–298. doi: 10.1089/hum.2018.015
Hirano, M., Kato, S., Kobayashi, K., Okada, T., Yaginuma, H., and Kobayashi, K. (2013). Highly efficient retrograde gene transfer into motor neurons by a lentiviral vector pseudotyped with fusion glycoprotein. PLoS One 8:e75896. doi: 10.1371/journal.pone.0075896
Hislop, J. N., Islam, T. A., Eleftheriadou, I., Carpentier, D. C. J., Trabalza, A., Parkinson, M., et al. (2014). Rabies virus envelope glycoprotein targets lentiviral vectors to the axonal retrograde pathway in motor neurons. J. Biol. Chem. 289, 16148–16163. doi: 10.1074/jbc.M114.549980
Hocquemiller, M., Giersch, L., Audrain, M., Parker, S., and Cartier, N. (2016). Adeno-associated virus-based gene therapy for CNS diseases. Hum. Gene Ther. 27, 478–496. doi: 10.1089/hum.2016.087
Hollis Ii, E. R., Kadoya, K., Hirsch, M., Samulski, R. J., and Tuszynski, M. H. (2008). Efficient retrograde neuronal transduction utilizing self-complementary AAV1. Mol. Ther. 16, 296–301. doi: 10.1038/sj.mt.6300367
Homs, J., Ariza, L., Pagès, G., Udina, E., Navarro, X., Chillón, M., et al. (2011). Schwann cell targeting via intrasciatic injection of AAV8 as gene therapy strategy for peripheral nerve regeneration. Gene Ther. 18, 622–630. doi: 10.1038/gt.2011.7
Hordeaux, J., Yuan, Y., Clark, P. M., Wang, Q., Martino, R. A., Sims, J. J., et al. (2019). The GPI-linked protein LY6A drives AAV-PHP.B transport across the blood-brain barrier. Mol. Ther. 27, 912–921. doi: 10.1016/j.ymthe.2019.02.013
Hotta, Y., Honda, T., Naito, M., and Kuwano, R. (2003). Developmental distribution of coxsackie virus and adenovirus receptor localized in the nervous system. Brain Res. Dev. Brain Res. 143, 1–13. doi: 10.1016/s0165-3806(03)00035-x
Huang, Q., Chan, K. Y., Tobey, I. G., Chan, Y. A., Poterba, T., Boutros, C. L., et al. (2019). Delivering genes across the blood-brain barrier: LY6A, a novel cellular receptor for AAV-PHP.B capsids. PLoS One 14:e0225206. doi: 10.1371/journal.pone.0225206
Huang, L.-Y., Halder, S., and Agbandje-McKenna, M. (2014). Parvovirus glycan interactions. Curr. Opin. Virol. 7, 108–118. doi: 10.1016/j.coviro.2014.05.007
Huang, S., Kamata, T., Takada, Y., Ruggeri, Z. M., and Nemerow, G. R. (1996). Adenovirus interaction with distinct integrins mediates separate events in cell entry and gene delivery to hematopoietic cells. J. Virol. 70, 4502–4508. doi: 10.1128/jvi.70.7.4502-4508.1996
Huang, K.-C., Yasruel, Z., Guérin, C., Holland, P. C., and Nalbantoglu, J. (2007). Interaction of the Coxsackie and adenovirus receptor (CAR) with the cytoskeleton: binding to actin. FEBS Lett. 581, 2702–2708. doi: 10.1016/j.febslet.2007.05.019
Huard, J., Lochmüller, H., Acsadi, G., Jani, A., Holland, P., Guérin, C., et al. (1995). Differential short-term transduction efficiency of adult versus newborn mouse tissues by adenoviral recombinants. Exp. Mol. Pathol. 62, 131–143. doi: 10.1006/exmp.1995.1015
Hudry, E., and Vandenberghe, L. H. (2019). Therapeutic AAV gene transfer to the nervous system: a clinical reality. Neuron 102:263. doi: 10.1016/j.neuron.2019.03.020
Jakobsson, J., Ericson, C., Jansson, M., Björk, E., and Lundberg, C. (2003). Targeted transgene expression in rat brain using lentiviral vectors. J. Neurosci. Res. 73, 876–885. doi: 10.1002/jnr.10719
Jan, A., Richner, M., Vægter, C. B., Nyengaard, J. R., and Jensen, P. H. (2019). Gene transfer in rodent nervous tissue following hindlimb intramuscular delivery of recombinant adeno-associated virus serotypes AAV2/6, AAV2/8 and AAV2/9. Neurosci. Insights 14:1179069519889022. doi: 10.1177/1179069519889022
Jensen, L., Jørgensen, L. H., Bech, R. D., Frandsen, U., and Schrøder, H. D. (2016). Skeletal muscle remodelling as a function of disease progression in amyotrophic lateral sclerosis. Biomed. Res. Int. 2016:5930621. doi: 10.1155/2016/5930621
Joglekar, A. V., and Sandoval, S. (2017). Pseudotyped lentiviral vectors: one vector, many guises. Hum. Gene Ther. Methods 28, 291–301. doi: 10.1089/hgtb.2017.084
Kariyawasam, D., Alexander, I. E., Kurian, M., and Farrar, M. A. (2020). Great expectations: virus-mediated gene therapy in neurological disorders. J. Neurol. Neurosurg. Psychiatry doi: 10.1136/jnnp-2019-322327 [Epub ahead of print].
Kato, S., Inoue, K., Kobayashi, K., Yasoshima, Y., Miyachi, S., Inoue, S., et al. (2007). Efficient gene transfer via retrograde transport in rodent and primate brains using a human immunodeficiency virus type 1-based vector pseudotyped with rabies virus glycoprotein. Hum. Gene Ther. 18, 1141–1151. doi: 10.1089/hum.2007.082
Kelkar, S. A., Pfister, K. K., Crystal, R. G., and Leopold, P. L. (2004). Cytoplasmic dynein mediates adenovirus binding to microtubules. J. Virol. 78, 10122–10132. doi: 10.1128/JVI.78.18.10122-10132.2004
Kim, S.-H., Lee, S., Lee, H., Cho, M., Schaffer, D. V., and Jang, J.-H. (2019). AAVR-displaying interfaces: serotype-independent adeno-associated virus capture and local delivery systems. Mol. Ther. Nucleic Acids 18, 432–443. doi: 10.1016/j.omtn.2019.09.015
Klein, R. L., Dayton, R. D., Tatom, J. B., Henderson, K. M., and Henning, P. P. (2008). AAV8, 9, Rh10, Rh43 vector gene transfer in the rat brain: effects of serotype, promoter and purification method. Mol. Ther. 16, 89–96. doi: 10.1038/sj.mt.6300331
Kotterman, M. A., and Schaffer, D. V. (2014). Engineering adeno-associated viruses for clinical gene therapy. Nat. Rev. Genet. 15, 445–451. doi: 10.1038/nrg3742
Kremer, E. J., and Nemerow, G. R. (2015). Adenovirus tales: from the cell surface to the nuclear pore complex. PLoS Pathog. 11:e1004821. doi: 10.1371/journal.ppat.1004821
Larochelle, N., Teng, Q., Gilbert, R., Deol, J. R., Karpati, G., Holland, P. C., et al. (2010). Modulation of coxsackie and adenovirus receptor expression for gene transfer to normal and dystrophic skeletal muscle. J. Gene Med. 12, 266–275. doi: 10.1002/jgm.1433
Le Gal La Salle, G., Robert, J. J., Berrard, S., Ridoux, V., Stratford-Perricaudet, L. D., Perricaudet, M., et al. (1993). An adenovirus vector for gene transfer into neurons and glia in the brain. Science 259, 988–990. doi: 10.1126/science.8382374
Lee, C. S., Bishop, E. S., Zhang, R., Yu, X., Farina, E. M., Yan, S., et al. (2017). Adenovirus-mediated gene delivery: potential applications for gene and cell-based therapies in the new era of personalized medicine. Genes Dis. 4, 43–63. doi: 10.1016/j.gendis.2017.04.001
Leopold, P. L., Ferris, B., Grinberg, I., Worgall, S., Hackett, N. R., and Crystal, R. G. (1998). Fluorescent virions: dynamic tracking of the pathway of adenoviral gene transfer vectors in living cells. Hum. Gene Ther. 9, 367–378. doi: 10.1089/hum.1998.9.3-367
Leopold, P. L., Kreitzer, G., Miyazawa, N., Rempel, S., Pfister, K. K., Rodriguez-Boulan, E., et al. (2000). Dynein- and microtubule-mediated translocation of adenovirus serotype 5 occurs after endosomal lysis. Hum. Gene Ther. 11, 151–165. doi: 10.1089/10430340050016238
Lesbats, P., Engelman, A. N., and Cherepanov, P. (2016). Retroviral DNA integration. Chem. Rev. 116, 12730–12757. doi: 10.1021/acs.chemrev.6b00125
Li, Y., Hickey, L., Perrins, R., Werlen, E., Patel, A. A., Hirschberg, S., et al. (2016). Retrograde optogenetic characterization of the pontospinal module of the locus coeruleus with a canine adenoviral vector. Brain Res. 1641, 274–290. doi: 10.1016/j.brainres.2016.02.023
Li, P. P., Madhavan, R., and Peng, H. B. (2012). Differential regulation of axonal growth and neuromuscular junction assembly by HGF/c-Met signaling. Dev. Dyn. 241, 1562–1574. doi: 10.1002/dvdy.23845
Li, S.-L., Vaughan, A., Sturgill, J. F., and Kepecs, A. (2018b). A viral receptor complementation strategy to overcome CAV-2 tropism for efficient retrograde targeting of neurons. Neuron 98, 905.e5–917.e5. doi: 10.1016/j.neuron.2018.05.028
Li, L., Xiong, W.-C., and Mei, L. (2018a). Neuromuscular junction formation, aging and disorders. Annu. Rev. Physiol. 80, 159–188. doi: 10.1146/annurev-physiol-022516-034255
Loustalot, F., Kremer, E. J., and Salinas, S. (2015). The intracellular domain of the coxsackievirus and adenovirus receptor differentially influences adenovirus entry. J. Virol. 89, 9417–9426. doi: 10.1128/jvi.01488-15
Loustalot, F., Kremer, E. J., and Salinas, S. (2016). Membrane dynamics and signaling of the coxsackievirus and adenovirus receptor. Int. Rev. Cell Mol. Biol. 322, 331–362. doi: 10.1016/bs.ircmb.2015.10.006
Lowry, K. S., Murray, S. S., McLean, C. A., Talman, P., Mathers, S., Lopes, E. C., et al. (2001). A potential role for the p75 low-affinity neurotrophin receptor in spinal motor neuron degeneration in murine and human amyotrophic lateral sclerosis. Amyotroph. Lateral Scler. Other Motor Neuron Disord. 2, 127–134. doi: 10.1080/146608201753275463
Madigan, V. J., and Asokan, A. (2016). Engineering AAV receptor footprints for gene therapy. Curr. Opin. Virol. 18, 89–96. doi: 10.1016/j.coviro.2016.05.001
Madigan, V. J., Tyson, T. O., Yuziuk, J. A., Pillai, M., Moller-Tank, S., and Asokan, A. (2019). A CRISPR screen identifies the cell polarity determinant crumbs 3 as an adeno-associated virus restriction factor in hepatocytes. J. Virol. 93:e00943-19. doi: 10.1128/jvi.00943-19
Mano, M., Ippodrino, R., Zentilin, L., Zacchigna, S., and Giacca, M. (2015). Genome-wide RNAi screening identifies host restriction factors critical for in vivo AAV transduction. Proc. Natl. Acad. Sci. U S A 112, 11276–11281. doi: 10.1073/pnas.1503607112
Martineau, É., Di Polo, A., Vande Velde, C., and Robitaille, R. (2018). Dynamic neuromuscular remodeling precedes motor-unit loss in a mouse model of ALS. eLife 7:e41973. doi: 10.7554/eLife.41973
Mátrai, J., Chuah, M. K. L., and VandenDriessche, T. (2010). Recent advances in lentiviral vector development and applications. Mol. Ther. 18, 477–490. doi: 10.1038/mt.2009.319
Matreyek, K. A., and Engelman, A. (2013). Viral and cellular requirements for the nuclear entry of retroviral preintegration nucleoprotein complexes. Viruses 5, 2483–2511. doi: 10.3390/v5102483
Mazarakis, N. D., Azzouz, M., Rohll, J. B., Ellard, F. M., Wilkes, F. J., Olsen, A. L., et al. (2001). Rabies virus glycoprotein pseudotyping of lentiviral vectors enables retrograde axonal transport and access to the nervous system after peripheral delivery. Hum. Mol. Genet. 10, 2109–2121. doi: 10.1093/hmg/10.19.2109
Mech, A. M., Brown, A. L., Schiavo, G., and Sleigh, J. N. (2020). Morphological variability is greater at developing than mature mouse neuromuscular junctions. J. Anat. doi: 10.1111/joa.13228 [Epub ahead of print].
Meier, O., Boucke, K., Hammer, S. V., Keller, S., Stidwill, R. P., Hemmi, S., et al. (2002). Adenovirus triggers macropinocytosis and endosomal leakage together with its clathrin-mediated uptake. J. Cell Biol. 158, 1119–1131. doi: 10.1083/jcb.200112067
Mendell, J. R., Al-Zaidy, S., Shell, R., Arnold, W. D., Rodino-Klapac, L. R., Prior, T. W., et al. (2017). Single-dose gene-replacement therapy for spinal muscular atrophy. N. Engl. J. Med. 377, 1713–1722. doi: 10.1056/NEJMoa1706198
Mentis, G. Z., Gravell, M., Hamilton, R., Shneider, N. A., O’Donovan, M. J., and Schubert, M. (2006). Transduction of motor neurons and muscle fibers by intramuscular injection of HIV-1-based vectors pseudotyped with select rabies virus glycoproteins. J. Neurosci. Methods 157, 208–217. doi: 10.1016/j.jneumeth.2006.04.011
Merino-Gracia, J., García-Mayoral, M. F., and Rodríguez-Crespo, I. (2011). The association of viral proteins with host cell dynein components during virus infection. FEBS J. 278, 2997–3011. doi: 10.1111/j.1742-4658.2011.08252.x
Millecamps, S., Mallet, J., and Barkats, M. (2002). Adenoviral retrograde gene transfer in motoneurons is greatly enhanced by prior intramuscular inoculation with botulinum toxin. Hum. Gene Ther. 13, 225–232. doi: 10.1089/10430340252769752
Millecamps, S., Nicolle, D., Ceballos-Picot, I., Mallet, J., and Barkats, M. (2001). Synaptic sprouting increases the uptake capacities of motoneurons in amyotrophic lateral sclerosis mice. Proc. Natl. Acad. Sci. U S A 98, 7582–7587. doi: 10.1073/pnas.131031098
Milone, M. C., and O’Doherty, U. (2018). Clinical use of lentiviral vectors. Leukemia 32, 1529–1541. doi: 10.1038/s41375-018-0106-0
Moloney, E. B., de Winter, F., and Verhaagen, J. (2014). ALS as a distal axonopathy: molecular mechanisms affecting neuromuscular junction stability in the presymptomatic stages of the disease. Front. Neurosci. 8:252. doi: 10.3389/fnins.2014.00252
Nalbantoglu, J., Larochelle, N., Wolf, E., Karpati, G., Lochmuller, H., and Holland, P. C. (2001). Muscle-specific overexpression of the adenovirus primary receptor CAR overcomes low efficiency of gene transfer to mature skeletal muscle. J. Virol. 75, 4276–4282. doi: 10.1128/jvi.75.9.4276-4282.2001
Nalbantoglu, J., Pari, G., Karpati, G., and Holland, P. C. (1999). Expression of the primary coxsackie and adenovirus receptor is downregulated during skeletal muscle maturation and limits the efficacy of adenovirus-mediated gene delivery to muscle cells. Hum. Gene Ther. 10, 1009–1019. doi: 10.1089/10430349950018409
Nault, J.-C., Mami, I., La Bella, T., Datta, S., Imbeaud, S., Franconi, A., et al. (2016). Wild-type AAV insertions in hepatocellular carcinoma do not inform dbate over genotoxicity risk of vectorized AAV. Mol. Ther. 24, 660–661. doi: 10.1038/mt.2016.47
Neefjes, J., and van der Kant, R. (2014). Stuck in traffic: an emerging theme in diseases of the nervous system. Trends Neurosci. 37, 66–76. doi: 10.1016/j.tins.2013.11.006
Nicolson, S. C., and Samulski, R. J. (2014). Recombinant adeno-associated virus utilizes host cell nuclear import machinery to enter the nucleus. J. Virol. 88, 4132–4144. doi: 10.1128/JVI.02660-13
Nonnenmacher, M. E., Cintrat, J.-C., Gillet, D., and Weber, T. (2015). Syntaxin 5-dependent retrograde transport to the trans-Golgi network is required for adeno-associated virus transduction. J. Virol. 89, 1673–1687. doi: 10.1128/JVI.02520-14
Nonnenmacher, M., and Weber, T. (2011). Adeno-associated virus 2 infection requires endocytosis through the CLIC/GEEC pathway. Cell Host Microbe. 10, 563–576. doi: 10.1016/j.chom.2011.10.014
Nonnenmacher, M., and Weber, T. (2012). Intracellular transport of recombinant adeno-associated virus vectors. Gene Ther. 19, 649–658. doi: 10.1038/gt.2012.6
Parr-Brownlie, L. C., Bosch-Bouju, C., Schoderboeck, L., Sizemore, R. J., Abraham, W. C., and Hughes, S. M. (2015). Lentiviral vectors as tools to understand central nervous system biology in mammalian model organisms. Front. Mol. Neurosci. 8:14. doi: 10.3389/fnmol.2015.00014
Patel, A., Zhao, J., Duan, D., and Lai, Y. (2019). Design of AAV vectors for delivery of large or multiple transgenes. Methods Mol. Biol. 1950, 19–33. doi: 10.1007/978-1-4939-9139-6_2
Pérez, V., Bermedo-Garcia, F., Zelada, D., Court, F. A., Pérez, M. Á., Fuenzalida, M., et al. (2019). The p75NTR neurotrophin receptor is required to organize the mature neuromuscular synapse by regulating synaptic vesicle availability. Acta Neuropathol. Commun. 7:147. doi: 10.1186/s40478-019-0802-7
Pillay, S., and Carette, J. E. (2017). Host determinants of adeno-associated viral vector entry. Curr. Opin. Virol. 24, 124–131. doi: 10.1016/j.coviro.2017.06.003
Pillay, S., Meyer, N. L., Puschnik, A. S., Davulcu, O., Diep, J., Ishikawa, Y., et al. (2016). An essential receptor for adeno-associated virus infection. Nature 530, 108–112. doi: 10.1038/nature16465
Pillay, S., Zou, W., Cheng, F., Puschnik, A. S., Meyer, N. L., Ganaie, S. S., et al. (2017). Adeno-associated virus (AAV) serotypes have distinctive interactions with domains of the cellular AAV receptor. J. Virol. 91:e00391-17. doi: 10.1128/jvi.00391-17
Poon, M. W., Tsang, W. H., Chan, S. O., Li, H. M., Ng, H. K., and Waye, M. M. (2011). The dyslexia candidate gene KIAA0319L encodes N-glycosylated isoforms that form homodimers. Cell Mol. Neurobiol. 31, 27–35. doi: 10.1007/s10571-010-9549-1
Ralph, G. S., Radcliffe, P. A., Day, D. M., Carthy, J. M., Leroux, M. A., Lee, D. C. P., et al. (2005). Silencing mutant SOD1 using RNAi protects against neurodegeneration and extends survival in an ALS model. Nat. Med. 11, 429–433. doi: 10.1038/nm1205
Raoul, C., Abbas-Terki, T., Bensadoun, J.-C., Guillot, S., Haase, G., Szulc, J., et al. (2005). Lentiviral-mediated silencing of SOD1 through RNA interference retards disease onset and progression in a mouse model of ALS. Nat. Med. 11, 423–428. doi: 10.1038/nm1207
Ricobaraza, A., Gonzalez-Aparicio, M., Mora-Jimenez, L., Lumbreras, S., and Hernandez-Alcoceba, R. (2020). High-capacity adenoviral vectors: expanding the scope of gene therapy. Int. J. Mol. Sci. 21:3643. doi: 10.3390/ijms21103643
Ronzitti, G., Gross, D.-A., and Mingozzi, F. (2020). human immune responses to adeno-associated virus (AAV) vectors. Front. Immunol. 11:670. doi: 10.3389/fimmu.2020.00670
Salegio, E. A., Samaranch, L., Kells, A. P., Mittermeyer, G., San Sebastian, W., Zhou, S., et al. (2013). Axonal transport of adeno-associated viral vectors is serotype-dependent. Gene Ther. 20, 348–352. doi: 10.1038/gt.2012.27
Salganik, M., Venkatakrishnan, B., Bennett, A., Lins, B., Yarbrough, J., Muzyczka, N., et al. (2012). Evidence for pH-dependent protease activity in the adeno-associated virus capsid. J. Virol. 86, 11877–11885. doi: 10.1128/jvi.01717-12
Salinas, S., Bilsland, L. G., Henaff, D., Weston, A. E., Keriel, A., Schiavo, G., et al. (2009). CAR-associated vesicular transport of an adenovirus in motor neuron axons. PLoS Pathog. 5:e1000442. doi: 10.1371/journal.ppat.1000442
Salinas, S., Zussy, C., Loustalot, F., Henaff, D., Menendez, G., Morton, P. E., et al. (2014). Disruption of the coxsackievirus and adenovirus receptor-homodimeric interaction triggers lipid microdomain- and dynamin-dependent endocytosis and lysosomal targeting. J. Biol. Chem. 289, 680–695. doi: 10.1074/jbc.m113.518365
Samaranch, L., Salegio, E. A., San Sebastian, W., Kells, A. P., Foust, K. D., Bringas, J. R., et al. (2012). Adeno-associated virus serotype 9 transduction in the central nervous system of nonhuman primates. Hum. Gene Ther. 23, 382–389. doi: 10.1089/hum.2011.200
Sanlioglu, S., Benson, P. K., Yang, J., Atkinson, E. M., Reynolds, T., and Engelhardt, J. F. (2000). Endocytosis and nuclear trafficking of adeno-associated virus type 2 are controlled by rac1 and phosphatidylinositol-3 kinase activation. J. Virol. 74, 9184–9196. doi: 10.1128/jvi.74.19.9184-9196.2000
Scherer, J., Yi, J., and Vallee, R. B. (2020). Role of cytoplasmic dynein and kinesins in adenovirus transport. FEBS Lett. 594, 1838–1847. doi: 10.1002/1873-3468.13777
Schiavo, G., Greensmith, L., Hafezparast, M., and Fisher, E. M. C. (2013). Cytoplasmic dynein heavy chain: the servant of many masters. Trends Neurosci. 36, 641–651. doi: 10.1016/j.tins.2013.08.001
Schnepp, B. C., Jensen, R. L., Chen, C.-L., Johnson, P. R., and Clark, K. R. (2005). Characterization of adeno-associated virus genomes isolated from human tissues. J. Virol. 79, 14793–14803. doi: 10.1128/jvi.79.23.14793-14803.2005
Shaw, C. A., Holland, P. C., Sinnreich, M., Allen, C., Sollerbrant, K., Karpati, G., et al. (2004). Isoform-specific expression of the Coxsackie and adenovirus receptor (CAR) in neuromuscular junction and cardiac intercalated discs. BMC Cell Biol. 5:42. doi: 10.1186/1471-2121-5-42
Singhal, N., and Martin, P. T. (2011). Role of extracellular matrix proteins and their receptors in the development of the vertebrate neuromuscular junction. Dev. Neurobiol. 71, 982–1005. doi: 10.1002/dneu.20953
Sinnreich, M., Shaw, C. A., Pari, G., Nalbantoglu, J., Holland, P. C., and Karpati, G. (2005). Localization of coxsackie virus and adenovirus receptor (CAR) in normal and regenerating human muscle. Neuromuscul. Disord. 15, 541–548. doi: 10.1016/j.nmd.2005.05.007
Sleigh, J. N., Dawes, J. M., West, S. J., Wei, N., Spaulding, E. L., Gómez-Martín, A., et al. (2017). Trk receptor signaling and sensory neuron fate are perturbed in human neuropathy caused by Gars mutations. Proc. Natl. Acad. Sci. U S A 114, E3324–E3333. doi: 10.1073/pnas.1614557114
Sleigh, J. N., Grice, S. J., Burgess, R. W., Talbot, K., and Cader, M. Z. (2014). Neuromuscular junction maturation defects precede impaired lower motor neuron connectivity in Charcot-Marie-Tooth type 2D mice. Hum. Mol. Genet. 23, 2639–2650. doi: 10.1093/hmg/ddt659
Sleigh, J. N., Rossor, A. M., Fellows, A. D., Tosolini, A. P., and Schiavo, G. (2019). Axonal transport and neurological disease. Nat. Rev. Neurol. 15, 691–703. doi: 10.1038/s41582-019-0257-2
Sleigh, J. N., Tosolini, A. P., Gordon, D., Devoy, A., Fratta, P., Fisher, E. M. C., et al. (2020a). Mice carrying ALS mutant TDP-43, but not mutant FUS, display in vivo defects in axonal transport of signaling endosomes. Cell Rep. 30, 3655.e2–3662.e2. doi: 10.1016/j.celrep.2020.02.078
Sleigh, J. N., Tosolini, A. P., and Schiavo, G. (2020b). in vivo imaging of anterograde and retrograde axonal transport in rodent peripheral nerves. Methods Mol. Biol. 2143, 271–292. doi: 10.1007/978-1-0716-0585-1_20
Sonntag, F., Bleker, S., Leuchs, B., Fischer, R., and Kleinschmidt, J. A. (2006). Adeno-associated virus type 2 capsids with externalized VP1/VP2 trafficking domains are generated prior to passage through the cytoplasm and are maintained until uncoating occurs in the nucleus. J. Virol. 80, 11040–11054. doi: 10.1128/jvi.01056-06
Soudais, C., Laplace-Builhe, C., Kissa, K., and Kremer, E. J. (2001). Preferential transduction of neurons by canine adenovirus vectors and their efficient retrograde transport in vivo. FASEB J. 15, 2283–2285. doi: 10.1096/fj.01-0321fje
Soudais, C., Skander, N., and Kremer, E. J. (2004). Long-term in vivo transduction of neurons throughout the rat CNS using novel helper-dependent CAV-2 vectors. FASEB J. 18, 391–393. doi: 10.1096/fj.03-0438fje
Spaulding, E. L., Sleigh, J. N., Morelli, K. H., Pinter, M. J., Burgess, R. W., and Seburn, K. L. (2016). Synaptic deficits at neuromuscular junctions in two mouse models of Charcot-Marie-Tooth type 2d. J. Neurosci. 36, 3254–3267. doi: 10.1523/JNEUROSCI.1762-15.2016
Srivastava, A. (2016). in vivo tissue-tropism of adeno-associated viral vectors. Curr. Opin. Virol. 21, 75–80. doi: 10.1016/j.coviro.2016.08.003
Summerford, C., Johnson, J. S., and Samulski, R. J. (2016). AAVR: a multi-serotype receptor for AAV. Mol. Ther. 24, 663–666. doi: 10.1038/mt.2016.49
Suomalainen, M., Nakano, M. Y., Keller, S., Boucke, K., Stidwill, R. P., and Greber, U. F. (1999). Microtubule-dependent plus- and minus end-directed motilities are competing processes for nuclear targeting of adenovirus. J. Cell Biol. 144, 657–672. doi: 10.1083/jcb.144.4.657
Surana, S., Tosolini, A. P., Meyer, I. F. G., Fellows, A. D., Novoselov, S. S., and Schiavo, G. (2018). The travel diaries of tetanus and botulinum neurotoxins. Toxicon 147, 58–67. doi: 10.1016/j.toxicon.2017.10.008
Surana, S., Villarroel-Campos, D., Lazo, O. M., Moretto, E., Tosolini, A. P., Rhymes, E. R., et al. (2020). The evolution of the axonal transport toolkit. Traffic 21, 13–33. doi: 10.1111/tra.12710
Taetzsch, T., Brayman, V. L., and Valdez, G. (2018). FGF binding proteins (FGFBPs): modulators of FGF signaling in the developing, adult and stressed nervous system. Biochim. Biophys. Acta Mol. Basis Dis. 1864, 2983–2991. doi: 10.1016/j.bbadis.2018.06.009
Tanguy, Y., Biferi, M. G., Besse, A., Astord, S., Cohen-Tannoudji, M., Marais, T., et al. (2015). Systemic AAVrh10 provides higher transgene expression than AAV9 in the brain and the spinal cord of neonatal mice. Front. Mol. Neurosci. 8:36. doi: 10.3389/fnmol.2015.00036
Terashima, T., Oka, K., Kritz, A. B., Kojima, H., Baker, A. H., and Chan, L. (2009). DRG-targeted helper-dependent adenoviruses mediate selective gene delivery for therapeutic rescue of sensory neuronopathies in mice. J. Clin. Invest. 119, 2100–2112. doi: 10.1172/jci39038
Tervo, D. G. R., Hwang, B.-Y., Viswanathan, S., Gaj, T., Lavzin, M., Ritola, K. D., et al. (2016). A designer AAV variant permits efficient retrograde access to projection neurons. Neuron 92, 372–382. doi: 10.1016/j.neuron.2016.09.021
Thoulouze, M. I., Lafage, M., Schachner, M., Hartmann, U., Cremer, H., and Lafon, M. (1998). The neural cell adhesion molecule is a receptor for rabies virus. J. Virol. 72, 7181–7190. doi: 10.1128/jvi.72.9.7181-7190.1998
Tosolini, A. P., and Morris, R. (2016a). Targeting motor end plates for delivery of adenoviruses: an approach to maximize uptake and transduction of spinal cord motor neurons. Sci. Rep. 6:33058. doi: 10.1038/srep33058
Tosolini, A. P., and Morris, R. (2016b). Viral-mediated gene therapy for spinal cord injury (SCI) from a translational neuroanatomical perspective. Neural Regen. Res. 11, 743–744. doi: 10.4103/1673-5374.182698
Tosolini, A. P., and Sleigh, J. N. (2017). Motor neuron gene therapy: lessons from spinal muscular atrophy for amyotrophic lateral sclerosis. Front. Mol. Neurosci. 10:405. doi: 10.3389/fnmol.2017.00405
Towne, C., Schneider, B. L., Kieran, D., Redmond, D. E., and Aebischer, P. (2010). Efficient transduction of non-human primate motor neurons after intramuscular delivery of recombinant AAV serotype 6. Gene Ther. 17, 141–146. doi: 10.1038/gt.2009.119
Trotman, L. C., Mosberger, N., Fornerod, M., Stidwill, R. P., and Greber, U. F. (2001). Import of adenovirus DNA involves the nuclear pore complex receptor CAN/Nup214 and histone H1. Nat. Cell Biol. 3, 1092–1100. doi: 10.1038/ncb1201-1092
Tuffereau, C., Bénéjean, J., Blondel, D., Kieffer, B., and Flamand, A. (1998). Low-affinity nerve-growth factor receptor (P75NTR) can serve as a receptor for rabies virus. EMBO J. 17, 7250–7259. doi: 10.1093/emboj/17.24.7250
Ueda, H. R., Ertürk, A., Chung, K., Gradinaru, V., Chédotal, A., Tomancak, P., et al. (2020). Tissue clearing and its applications in neuroscience. Nat. Rev. Neurosci. 21, 61–79. doi: 10.1038/s41583-019-0250-1
Uhrig, S., Coutelle, O., Wiehe, T., Perabo, L., Hallek, M., and Büning, H. (2012). Successful target cell transduction of capsid-engineered rAAV vectors requires clathrin-dependent endocytosis. Gene Ther. 19, 210–218. doi: 10.1038/gt.2011.78
van Dis, V., Kuijpers, M., Haasdijk, E. D., Teuling, E., Oakes, S. A., Hoogenraad, C. C., et al. (2014). Golgi fragmentation precedes neuromuscular denervation and is associated with endosome abnormalities in SOD1-ALS mouse motor neurons. Acta Neuropathol. Commun. 2:38. doi: 10.1186/2051-5960-2-38
Villarroel-Campos, D., Schiavo, G., and Lazo, O. M. (2018). The many disguises of the signalling endosome. FEBS Lett. 592, 3615–3632. doi: 10.1002/1873-3468.13235
von Jonquieres, G., Mersmann, N., Klugmann, C. B., Harasta, A. E., Lutz, B., Teahan, O., et al. (2013). Glial promoter selectivity following AAV-delivery to the immature brain. PLoS One 8:e65646. doi: 10.1371/journal.pone.0065646
Watson, Z. L., Ertel, M. K., Lewin, A. S., Tuli, S. S., Schultz, G. S., Neumann, D. M., et al. (2016). Adeno-associated virus vectors efficiently transduce mouse and rabbit sensory neurons coinfected with herpes simplex virus 1 following peripheral inoculation. J. Virol. 90, 7894–7901. doi: 10.1128/jvi.01028-16
Weinberg, M. S., Nicolson, S., Bhatt, A. P., McLendon, M., Li, C., and Samulski, R. J. (2014). Recombinant adeno-associated virus utilizes cell-specific infectious entry mechanisms. J. Virol. 88, 12472–12484. doi: 10.1128/jvi.01971-14
Weledji, E. P., and Assob, J. C. (2014). The ubiquitous neural cell adhesion molecule (N-CAM). Ann. Med. Surg. 3, 77–81. doi: 10.1016/j.amsu.2014.06.014
Wickham, T. J., Mathias, P., Cheresh, D. A., and Nemerow, G. R. (1993). Integrins α v β 3 and α v β 5 promote adenovirus internalization but not virus attachment. Cell 73, 309–319. doi: 10.1016/0092-8674(93)90231-e
Wong, L.-F., Azzouz, M., Walmsley, L. E., Askham, Z., Wilkes, F. J., Mitrophanous, K. A., et al. (2004). Transduction patterns of pseudotyped lentiviral vectors in the nervous system. Mol. Ther. 9, 101–111. doi: 10.1016/j.ymthe.2003.09.017
Worgall, S., and Crystal, R. G. (2014). “Gene therapy,” in Principles of Tissue Engineering, 4th edn. eds R. Lanza, R. Langer and J. P. Vacanti (Academic Press), 657–686.
Xiao, P.-J., Li, C., Neumann, A., and Samulski, R. J. (2012). Quantitative 3D tracing of gene-delivery viral vectors in human cells and animal tissues. Mol. Ther. 20, 317–328. doi: 10.1038/mt.2011.250
Xiao, P.-J., and Samulski, R. J. (2012). Cytoplasmic trafficking, endosomal escape and perinuclear accumulation of adeno-associated virus type 2 particles are facilitated by microtubule network. J. Virol. 86, 10462–10473. doi: 10.1128/jvi.00935-12
Yang, L., Bailey, L., Baltimore, D., and Wang, P. (2006). Targeting lentiviral vectors to specific cell types in vivo. Proc. Natl. Acad. Sci. U S A 103, 11479–11484. doi: 10.1073/pnas.0604993103
Zhang, R., Xu, G., Cao, L., Sun, Z., He, Y., Cui, M., et al. (2019). Divergent engagements between adeno-associated viruses with their cellular receptor AAVR. Nat. Commun. 10:3760. doi: 10.1038/s41467-019-11668-x
Zhao, Y., Haginoya, K., and Iinuma, K. (1999). Strong immunoreactivity of platelet-derived growth factor and its receptor at human and mouse neuromuscular junctions. Tohoku J. Exp. Med. 189, 239–244. doi: 10.1620/tjem.189.239
Zheng, H., Qiao, C., Wang, C.-H., Li, J., Li, J., Yuan, Z., et al. (2010). Efficient retrograde transport of adeno-associated virus type 8 to spinal cord and dorsal root ganglion after vector delivery in muscle. Hum. Gene Ther. 21, 87–97. doi: 10.1089/hum.2009.131
Zhong, L., Li, B., Jayandharan, G., Mah, C. S., Govindasamy, L., Agbandje-McKenna, M., et al. (2008). Tyrosine-phosphorylation of AAV2 vectors and its consequences on viral intracellular trafficking and transgene expression. Virology 381, 194–202. doi: 10.1016/j.virol.2008.08.027
Zhou, J., Scherer, J., Yi, J., and Vallee, R. B. (2018). Role of kinesins in directed adenovirus transport and cytoplasmic exploration. PLoS Pathog. 14:e1007055. doi: 10.1371/journal.ppat.1007055
Zincarelli, C., Soltys, S., Rengo, G., and Rabinowitz, J. E. (2008). Analysis of AAV serotypes 1-9 mediated gene expression and tropism in mice after systemic injection. Mol. Ther. 16, 1073–1080. doi: 10.1038/mt.2008.76
Zinn, E., and Vandenberghe, L. H. (2014). Adeno-associated virus: fit to serve. Curr. Opin. Virol. 8, 90–97. doi: 10.1016/j.coviro.2014.07.008
Keywords: adenovirus (AdV), adeno-associated virus (AAV), axonal transport, lentivirus, motor neuron, neuromuscular junction (NMJ), peripheral nerve, sensory neuron
Citation: Tosolini AP and Sleigh JN (2020) Intramuscular Delivery of Gene Therapy for Targeting the Nervous System. Front. Mol. Neurosci. 13:129. doi: 10.3389/fnmol.2020.00129
Received: 12 May 2020; Accepted: 26 June 2020;
Published: 17 July 2020.
Edited by:
Marco Ledri, Lund University, SwedenReviewed by:
Eric J. Kremer, UMR5535 Institut de Génétique Moléculaire de Montpellier (IGMM), FranceKenta Kobayashi, National Institute for Physiological Sciences (NIPS), Japan
Copyright © 2020 Tosolini and Sleigh. This is an open-access article distributed under the terms of the Creative Commons Attribution License (CC BY). The use, distribution or reproduction in other forums is permitted, provided the original author(s) and the copyright owner(s) are credited and that the original publication in this journal is cited, in accordance with accepted academic practice. No use, distribution or reproduction is permitted which does not comply with these terms.
*Correspondence: Andrew P. Tosolini, YS50b3NvbGluaUB1Y2wuYWMudWs=; James N. Sleigh, ai5zbGVpZ2hAdWNsLmFjLnVr
† ORCID: Andrew P. Tosolini orcid.org/0000-0001-7651-7442
James N. Sleigh orcid.org/0000-0002-3782-9045