- 1Rudolf-Boehm-Institut für Pharmakologie und Toxikologie, Universität Leipzig, Leipzig, Germany
- 2Acupuncture and Tuina School, Chengdu University of Traditional Chinese Medicine, Chengdu, China
- 3Faculty of Life Sciences, The University of Manchester, Manchester, United Kingdom
- 4Achucarro Centre for Neuroscience, Ikerbasque, Basque Foundation for Science, Bilbao, Spain
The mood disorders, major depression (MD) and bipolar disorder (BD), have a high lifetime prevalence in the human population and accordingly generate huge costs for health care. Efficient, rapidly acting, and side-effect-free pharmaceuticals are hitherto not available, and therefore, the identification of new therapeutic targets is an imperative task for (pre)clinical research. Such a target may be the purinergic P2X7 receptor (P2X7R), which is localized in the central nervous system (CNS) at microglial and neuroglial cells mediating neuroinflammation. MD and BD are due to neuroinflammation caused in the first line by the release of the pro-inflammatory cytokine interleukin-1β (IL-1β) from the microglia. IL-1β in turn induces the secretion of corticotropin-releasing hormone (CRH) and in consequence the secretion of adrenocorticotropic hormone (ACTH) and cortisol, which together with a plethora of further cytokines/chemokines lead to mood disorders. A number of biochemical/molecular biological measurements including the use of P2X7R- or IL-1β-deficient mice confirmed this chain of events. More recent studies showed that a decrease in the astrocytic release of ATP in the prefrontal cortex and hippocampus is a major cause of mood disorders. It is an attractive hypothesis that compensatory increases in P2X7Rs in these areas of the brain are the immediate actuators of MD and BD. Hence, blood-brain barrier-permeable P2X7R antagonists may be promising therapeutic tools to improve depressive disorders in humans.
Introduction
The mood disorder major depression (MD) is characterized by extreme sadness, depressed mood, and loss of interest that persist for at least 2 weeks and interferes with the individual’s social functioning (Harvey et al., 2007; Deussing and Arzt, 2018; Wei et al., 2018; Ribeiro et al., 2019). During bipolar disorder (BD), the mood state cycles between high (mania) and low (depression) episodes. MD and BD arise from complex interactions between genetic, developmental, and environmental factors (Koenig et al., 2011; Sullivan et al., 2012). The lifetime prevalence estimates for MD vary from 11% to 14% with females having an approximately 2-fold higher disease risk than males (Deussing and Arzt, 2018).
In view of the serious limitations these mood disorders impose upon the life quality of patients and because of their relatively frequent occurrence in the human population, it is of eminent importance to find good curative strategies to combat them. Presently, reuptake inhibitors of monoamines [noradrenaline, dopamine, and 5-hydroxytryptamine (5-HT)] are in the forefront of considerations, although significant drawbacks have to be taken into account: (1) the clinical improvement is achieved only after weeks of treatment; (2) there are multiple side effects; and (3) a substantial group of patients is resistant to therapy (Kulkarni and Dhir, 2009; Deussing and Arzt, 2018). Therefore, intensive search for alternative therapeutic targets and tools is a compelling necessity.
Purinergic P2X7 Receptor
Ionotropic P2X7 receptors (P2X7Rs) are members of the P2X purinoceptor family, which were cloned and characterized in 1996 (Surprenant et al., 1996; North, 2002; Burnstock and Knight, 2004). Three properties of the P2X7R are distinguishing characteristics: (1) it is activated by high concentrations of ATP in the millimolar range, clearly surmounting concentrations needed to activate other P2X receptors (P2XRs), which are stimulated by ATP concentrations in the micromolar range; (2) it is a ligand-gated cationic channel, allowing the inward passage of Na+ and Ca2+ and the outward passage of K+ through the cell membrane. However, its repetitive or longer-lasting activation by ATP results in the opening of membrane pores permeable to large organic cations such as the fluorescent dye YO-PRO, which otherwise do not pass the cell membrane; and (3) the P2X7R consists of three subunits (large extracellular loop, two transmembrane regions, and N- and C-terminal ends) forming a receptor, but each subunit has a much longer C-terminus than that of the other P2XRs.
A particularly intensively discussed issue is the transition of the cationic channel to a large membrane pore, because it appears to be essential for cytokine production and secretion (Illes et al., 2019; Martin et al., 2019). Originally, it was suggested based on equilibrium potential (Vrev) measurements with the whole-cell patch-clamp technique that the ion conducting pathway shows progressive dilation (Virginio et al., 1999). However, this suggestion was recently refuted, because the shift in Vrev in a medium in which the counterion of intracellular K+ was NMDG+ instead of Na+, emerged due to time-dependent alterations in the concentration of intracellular ions rather than channel dilation (Li et al., 2015). Moreover, during long-lasting activation of P2X7Rs, the single-channel current amplitude and the permeation characteristics remained constant (Pippel et al., 2017). Although convincing evidence indicates that pore opening is due to the recruitment of an accessory protein, the pannexin-1 channel (Panx-1; Pelegrin and Surprenant, 2006; Gulbransen et al., 2012; Shoji et al., 2014; Chen et al., 2017), the observation that, for example, the P2X7R pore formation is retained in Panx-1−/− cells supports the opposite notion (Hanley et al., 2012).
The P2X7R C-terminal tail constitutes about 40% of the whole protein, and its deletion or massive truncation prevents effects mediated by receptor activation such as dye uptake and membrane blebbing (generation of exosomes) but also alters channel kinetics (Kopp et al., 2019). In addition, the C-terminus was implicated in regulating signaling pathway activation, protein–protein interactions, and posttranslational modification (Costa-Junior et al., 2011).
P2X7Rs are major drivers of inflammation (Di Virgilio et al., 2017; Burnstock and Knight, 2018; Savio et al., 2018). Secretion of several pro-inflammatory cytokines and chemokines depends on the activation of P2X7Rs by large concentrations of ATP outpouring from damaged central nervous system (CNS) cells. The preferential location of P2X7Rs in the CNS is on the microglia, the resident macrophages of the brain (Bhattacharya and Jones, 2018). Microglia are equipped with a battery of pattern recognition receptors that stereotypically detect pathogen-associated molecules (PAMPs) such as lipopolysaccharide (LPS) from bacterial infection or danger-associated molecular patterns (DAMPs), such as ATP (Figure 1; Shao et al., 2015; Young and Górecki, 2018; Illes et al., 2019; Martin et al., 2019). Activation of microglia stimulates the release of interleukin-1β (IL-1β) in a two-step process: the first being the stimulation of toll-like receptor 4 (TLR4) by LPS, leading to accumulation of cytoplasmic pro-IL-1β, and the second being the ATP-dependent stimulation of P2X7Rs, promoting nucleotide-binding, leucine-rich repeat, pyrin domain containing 3 (NLRP3) inflammasome-mediated caspase-1 activation and secretion of IL-1β (Perregaux and Gabel, 1998; Ferrari et al., 2006). Caspase-1 generates IL-1β from pro-IL-1β by enzymatic degradation. It is important to note that the decrease of intracellular K+ is a major stimulus for P2X7R-dependent NLRP3 inflammasome activation (Muñoz-Planillo et al., 2013; Di Virgilio et al., 2017, 2018).
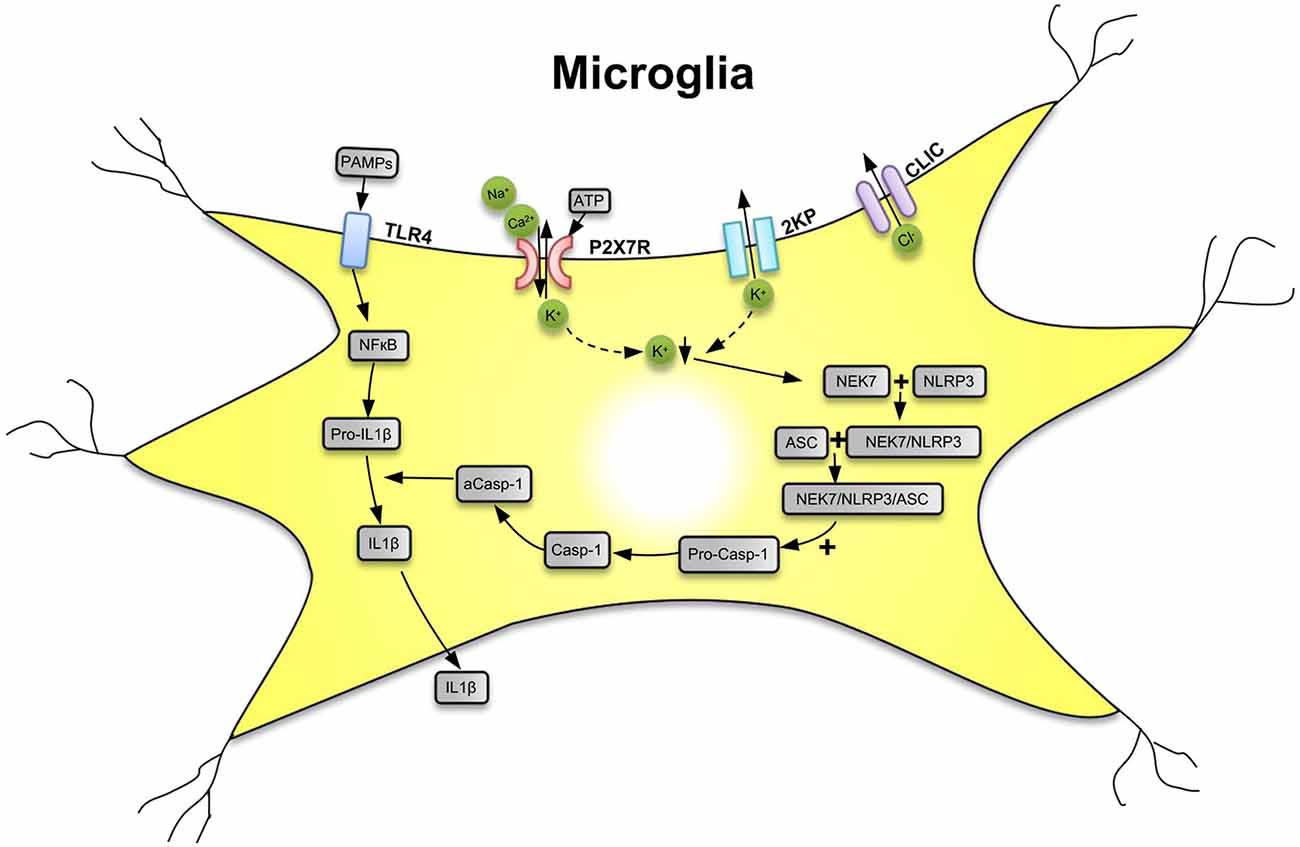
Figure 1. Secretion of interleukin-1β (IL-1β) from microglial cells via involvement of the nucleotide-binding, leucine-rich repeat, pyrin domain containing 3 (NLRP3) inflammasome. Pathogen-associated molecular patterns [PAMPs; e.g., bacterial lipopolysaccharide (LPS)] act on toll-like receptor-4 (TLR4) and cause its phosphorylation. In consequence, in the cell nucleus, NF-κB is activated, which promotes the synthesis of the NLRP3 inflammasome and pro-IL-1β, both accumulating in the cytosol in their inactive forms. The activation of NLRP3 is primarily due to a decrease of the intracellular K+ concentration ([K+]i), initiated by the stimulation of P2X7Rs by high local concentrations of the molecule ATP, which is considered to be a danger-associated molecular pattern (DAMP). P2X7Rs allow the inward flux of Na+/Ca2+ and in exchange the outward flux of K+, leading to a fall in [K+]i. The opening of two-pore domain potassium channels (2KP) may also lead to an impoverishment in cytoplasmic K+. A further stimulus for NLRP3 activation is the outward flux of Cl− through chloride intracellular channels (CLICs). TLR4, P2X7Rs, 2KP channels, and CLIC are all located in the cell membrane of the microglia. A sensor for the fall in [K+]i is the NEC7 serine/threonine kinase. NEC7 is able to form a complex with NLRP3, which is still inactive, but after constitution of a still larger multimeric complex with apoptosis-associated speck-like protein (ASC) recruits pro-caspase-1 (pro-Casp-1). In consequence, pro-Casp-1 in a complex with NLRP3 and ASC is cleaved to Casp-1, which then by its activated form a-Casp-1 degrades pro-IL-1β to IL-1β. Then, IL-1β leaves the cell by a number of mechanisms to the extracellular space and exerts its effects as a neuroinflammatory cytokine. K+ ↓, decrease of the K+ concentration. Artwork by Dr. Hayan Yin.
IL-1β is co-produced/secreted with other pro-inflammatory cytokines such as IL-6 and IL-18 as well as tumor necrosis factor-α (TNF-α). A convincing argument for the idea that P2X7R activation provides the signal that leads to maturation and release of IL-1β and initiation of the cytokine cascade stemmed from experiments showing that P2X7R−/− cells or animals primed with LPS failed to produce IL-1β on the application/injection of ATP (Solle et al., 2001).
The majority of the fully sequenced mammalian genomes include representatives of all vertebrate P2X genes, including P2X4, which in humans is located on chromosome 12 in close proximity to P2X7 (Suurväli et al., 2017). The overlapping expression of P2X4 and P2X7Rs has been documented in macrophages and microglia (Dubyak, 2007; Suurväli et al., 2017). The reason for the co-expression may be the involvement of both receptors in inflammatory processes (de Rivero Vaccari et al., 2012; Hung et al., 2013; Sakaki et al., 2013). Originally, it has been assumed that subunits of P2X4 and P2X7Rs form the heteromeric complex P2X4/P2X7 (Guo et al., 2007), although more recent data lend support to the existence of independent receptors tightly interacting with each other (Nicke, 2008; Antonio et al., 2011). The agonist binding affinities largely differ between P2X7 and P2X4 receptors (P2X4Rs); while the former one is activated by millimolar ATP concentrations, the latter one responds to ATP in the micromolar range (Kaczmarek-Hájek et al., 2012). Hence, non-cell-lytic micromolar ATP release cannot directly stimulate P2X7Rs but easily activates its more sensitive partner, the P2X4R, thereby modifying the function of the P2X4–P2X7R multiprotein complex.
Association of P2X7 Gene Polymorphism and Mood Disorders
Linkage studies suggested that variations of the chromosome 12q24,31 containing candidate genes for the P2X7R and calmodulin-dependent protein kinase b (CaMKKb) may be associated with MD and BD. It has been repeatedly reported that the nonsynonymous single-nucleotide polymorphism (NS-SNP) rs2230912 coding for Gln460Arg-P2X7R is associated with MD (McQuillin et al., 2009; Soronen et al., 2011; Sperlágh and Illes, 2014). However, in the meantime this association has been questioned. Although further studies have supported the possible role of this NS-SNP in mood disorders (Halmai et al., 2013; Vereczkei et al., 2019), other authors failed to detect any association (Green et al., 2009; Grigoroiu-Serbanescu et al., 2009). Two recent meta-analyses also yielded divergent results, one of them confirming (Czamara et al., 2018) and the other one refuting (Feng et al., 2014) the hypothesis on the causal role of the NS-SNP rs2230912 in MD and BD. Eventually, this led the Psychiatric Genomics Consortium to deny the P2RX7 gene as a genetic risk factor for mood disorders in large-scale genome-wide association studies (Mühleisen et al., 2014; Wray et al., 2018).
When various P2RX7 single-nucleotide polymorphism were investigated by electrophysiology/dye uptake studies either in native cells or in HEK293 cells transfected with the respective plasmids, several gain-of-function or loss-of-function allelic mutations were identified (Gu et al., 2001; Roger et al., 2010; Sun et al., 2010). Surprisingly, the ATP-induced inward current was the same through the wild-type (WT) receptor and the Gln460Arg polymorphic receptor (Roger et al., 2010), leading to the suggestion that a haplotype block may explain the lack of the expected decrease of ATP effects (Sluyter et al., 2010). This riddle was dissolved by Aprile-Garcia et al. (2016) who reported that although the variant per se is not compromised in its function, co-expression of WT P2X7R with the Gln460Arg-P2X7R results in inhibition of calcium influx, channel current, and intracellular signaling. Moreover, co-immunoprecipitation and FRET studies demonstrated that the Gln460Arg-P2X7R variant physically interacts with the WT P2X7R. The same group of authors found that humanized mice co-expressing both P2X7R variants showed alterations in their sleep quality resembling signs of a prodromal MD state (Metzger et al., 2017a).
In conclusion, the evidence for an association of the SNP rs2230912 as an etiologic factor for hereditary mood disorders is far from being equivocal, although its role in P2X7R involvement cannot be excluded either (see above controversial results of epidemiological studies).
The P2X7R Triggers Neuroinflammation and Subsequent Mood Disorders
Activation of the inflammasome, which precipitates the release of pro-inflammatory cytokines, and activation and migration of microglia and reactive astrogliosis are key regulators of the neuroinflammatory response (Beamer et al., 2016; Liu and Quan, 2018). IL-1β is a master regulator of inflammatory reactions, capable of activating innate immunity by inducing the expression of inflammatory cytokines and chemokines, eliciting leukocyte infiltration into the inflammatory loci, increasing the phagocytic and bactericidal activity of immune cells, enhancing the activity of the complement system, and facilitating the activation of the adaptive immune responses (Dinarello, 2009; Liu and Quan, 2018).
Stress exposure is considered to be the main environmental factor instigating mood disorders in humans, and all animal models of MD are based on exposure to inescapable stress (Ribeiro et al., 2019). Psychological and metabolic stress could induce adrenocorticotropic hormone (ACTH) and glucocorticoid secretion in mice, which were reduced in IL-1 knockouts (KOs) or transgenic animals overexpressing brain IL-1ra, a naturally occurring IL-1 antagonist (Goshen et al., 2003; Liu and Quan, 2018). Intracerebral administration of IL-1 induces corticotropin-releasing hormone (CRH) release in rats (Barbanel et al., 1990), and psychological stress causes brain IL-1 expression (Gadek-Michalska and Bugajski, 2010). Thus, brain IL-1 could mediate physiological responses to stress by stimulating the production of the immunosuppressive glucocorticoid hormone cortisol from the adrenal medulla (Liu and Quan, 2018). In perfect correlation with this idea, IL-1ra suppresses stress-induced depression in animal models (Koo and Duman, 2009; Maes et al., 2012). Consequently, disturbances of the main neuroendocrine stress response system, the hypothalamic–pituitary–adrenal axis including the main initiator CRH and effector glucocorticoids, have been suggested to cause depression (de Kloet et al., 2005; Deussing and Arzt, 2018).
As outlined previously, the primary function of P2X7Rs in the CNS is to initiate (neuro)inflammation. Therefore, it was deduced that the receptor might cause MD and BD, which are reportedly accompanied by neuroimmunological alterations (Bhattacharya and Jones, 2018). The chain of events may be the following: stress causes a massive outflow of ATP in the brain stimulating P2X7Rs, which on their behalf trigger the release of IL-1β. Then, IL-1β induces the secretion of CRH and the consecutive production of ACTH/glucocorticoids, resulting in mood disorders. In fact, acute restraint stress rapidly increases extracellular ATP, the inflammatory cytokine IL-1β, and the active form of the NLRP3 inflammasome in the hippocampus of rodents (Iwata et al., 2016).
Acute and chronic stress may induce in rodent models depressive-like behavior, which can be used to investigate antidepressive pharmaceuticals (Figure 2). In contrast to the acute stress models shown in this figure, unpredictable chronic mild stress (UCMS) is delivered for prolonged periods of 8–12 weeks and includes once daily, for example, immobilization, food deprivation, light/dark phase reversal, hot environment, and cage shaking. This procedure leads to depressive-like behavior as measured by reduced sucrose consumption and prolonged immobility in the tail suspension test (TST) and forced swim test (FST) in rodents (Zhang et al., 2015; Su et al., 2017; Wang et al., 2018; Feng et al., 2019). UCMS also resulted in higher protein levels of NLRP3, caspase-1, and IL-1β in the hippocampus of stress-exposed mice (Zhang et al., 2015) and rats (Wang et al., 2018; Feng et al., 2019). Pharmacological blockade of NLRP3 (Zhang et al., 2015) or its genetic deletion (Su et al., 2017) decreased the level of inflammatory mediators and counteracted the symptoms of depressive-like behavior. Microglia has been shown to be essential for these effects, because chronic minocycline treatment known to block the activation of microglia inhibited the following engagement of the NLRP3 inflammasome and the ensuing increased release of inflammatory mediators (Wang et al., 2018). Further, the inflammasome inhibitor Ac-Tyr-Val-Ala-Asp-chloromethyl ketone blocked the behavioral alterations and the production of inflammatory mediators caused by systemic injection of LPS to mice (Zhu et al., 2017). Chronic treatment with the standard antidepressant drug fluoxetine suppressed all symptoms induced by UCMS in rodents (Pan et al., 2014; Du et al., 2016). There is a multitude of review articles available which give further insight to the causal relationship between inflammasome activation and MD (Alcocer-Gómez et al., 2016; Kaufmann et al., 2017; Franklin et al., 2018; Herman and Pasinetti, 2018).
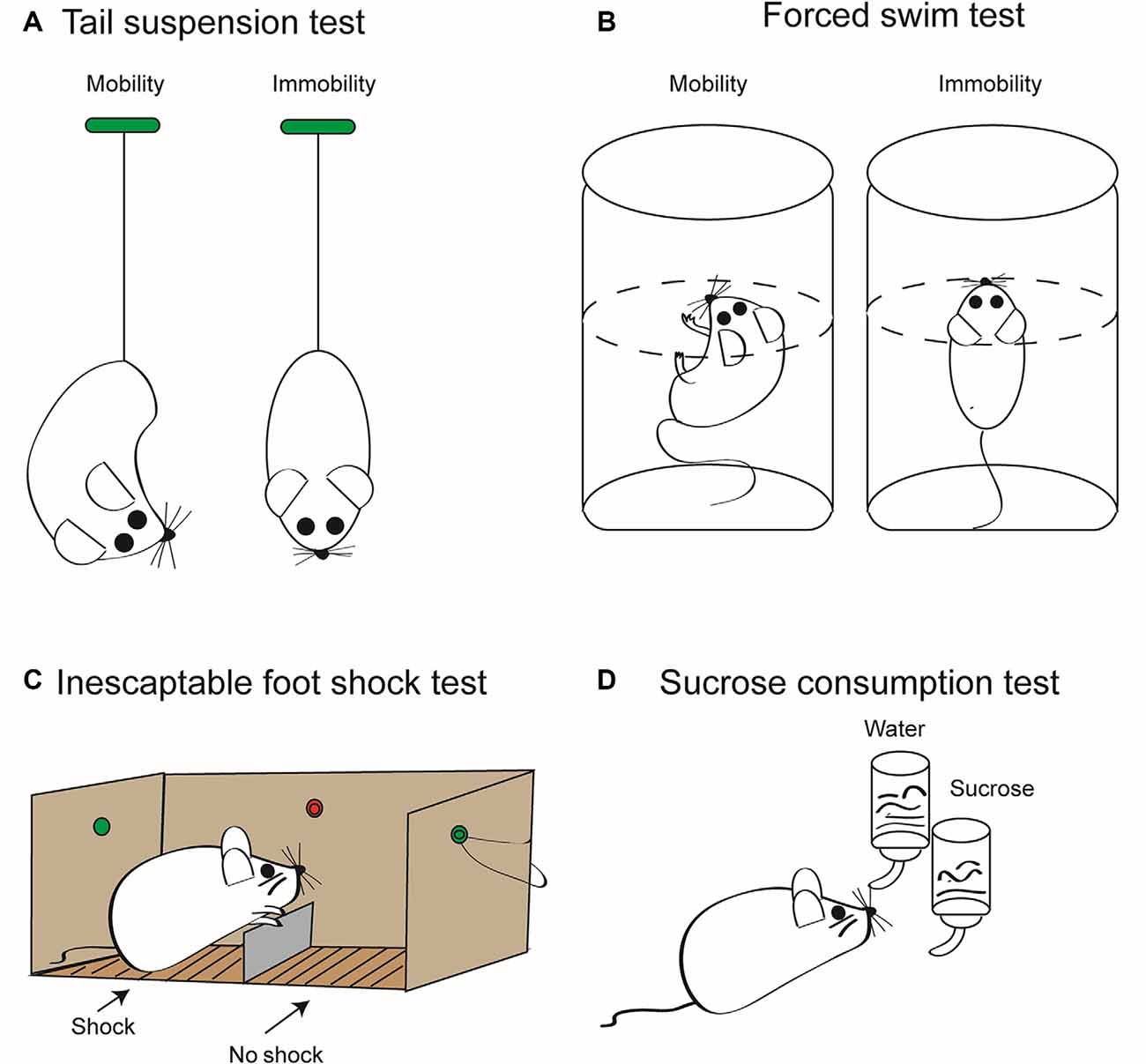
Figure 2. Some relevant tests to measure depressive-like behavior in rodents induced by stressors. These tests are employed to quantify the extent of “learned helplessness” of rats/mice, and thereby, with the necessary precaution, they are supposed to model major depression (MD) in humans. In consequence, they are routinely used to determine the effectiveness of antidepressant pharmacological agents. (A) Tail suspension test (TST). Mice are suspended by their tails with tape, in such a position that they cannot escape or hold on to nearby surfaces. Then, the sum of the time periods is measured during which they stop escape reactions; that is, they become immobile. This time period is considered to be a measure of the depressive-like behavior. The duration of the test is maximized usually at 6 min, in order to avoid unnecessary suffering of the animals. Because the weight of rats is much larger than that of mice, rats are not considered to be an appropriate rodent species for this test method. (B) Forced swim test (FST). Mice or rats are put into a tank containing water whose temperature is kept at about 23°C. The dimensions of the tank and the depth of water are such that the animals are forced to swim as an escape reaction. Swimming is stopped when the animal notices that it cannot escape and starts to float on the surface of the water. The length of the immobile periods is measured during a maximum of 6 min and is considered to be a measure of the degree of depressive-like behavior. (C) Inescapable foot shock test (IFST). The electric foot shock paradigm includes acute or chronic exposures of shocks of varying intensity and duration on an electrified grid floor in a foot shock apparatus. In contrast to the scheme shown, the mice or rat is not able to escape from the chamber where it is subjected to electric shocks to the other chamber where there is no comparable painful stimulation. Animals generally do not habituate to foot shocks in comparison to other stressors, including loud noise, bright light, and hot and cold temperatures. (D) Sucrose consumption test (SCT). The two-bottle choice procedure for assessing sucrose preference is a useful test to investigate anhedonia (i.e., inability to feel pleasure) in laboratory rodents. It allows for a comparison between the preference for sucrose solution in drinking water and that for water only. This preference is measured by volume and/or weight of liquid consumed daily, which is then converted to a percent sucrose solution consumed compared to a water only baseline period. As a result of the anhedonia induced by inescapable foot shock, the preference for choosing a sucrose solution decreases in mice or rats. It is important to verify the results of all these tests with separate behavioral tests that measure overall activity such as the open-field test. Moreover, TST, FST, and IFST/SCT should be used in combination to minimize false positivity, and it should be kept in mind that depressive-like behavior in laboratory rodents is not identical with the clinical state of MD in human beings (see above). Artwork by Ms. Lumei Huang.
The role of P2X7Rs as essential activators of NLRP3 was also convincingly demonstrated by showing that UCMS elevates hippocampal P2X7R levels (Tan et al., 2017). The selective P2X7R antagonist Brilliant Blue G (BBG) attenuated the increase of immobility time in TST and FST in mice after activation of the inflammasome by LPS (Ma et al., 2014). Similarly, BBG reversed the behavioral deterioration induced by UCMS in mice (Farooq et al., 2018), and clemastine, a nonselective antagonist of P2X7Rs, counteracted the prolonged duration of immobility in TST (Su et al., 2018).
Another piece of evidence for the participation of P2X7Rs in depressive-like behavior was supplied by the use of KO animals. P2RX7−/− mice exhibited an antidepressant-like profile in TST and FST; this effect was not accompanied by changes in spontaneous locomotor activity (Basso et al., 2009). In these animals, decreased behavioral despair in FST, reduced immobility in TST, and attenuated amphetamine-induced hyperactivity were detected, indicating an antidepressant phenotype (Sperlágh et al., 2012; Csölle et al., 2013a,b). In addition, several potential mechanisms were identified for these mice such as elevated basal production of brain-derived neurotrophic factor (BDNF), enhanced neurogenesis, and increased 5-HT bioavailability in the hippocampus (Csölle et al., 2013b). In contrast to these findings, equivalent levels of immobility were observed in P2RX7−/− and WT mice on the first exposure to forced swim, but much greater immobility was seen in the WT animals on second and third exposures (Boucher et al., 2011). An explanation for this discrepancy may be that the FST was recently questioned to be an adequate model of despair or helplessness (Molendijk and de Kloet, 2019).
Another factor of insecurity inherent to the P2X7R-deficient mice is that with the two types used routinely for experimentation, some splice variants of the P2RX7 gene escape inactivation (Bartlett et al., 2014; Sperlágh and Illes, 2014). Experiments with a recently generated conditional humanized P2X7R-deficient mouse, supposed to be devoid of active splice variants of the receptor, could be helpful in this respect (Metzger et al., 2017b).
A further argument for the involvement of P2X7Rs in the etiology of MD is supplied by studies which show that inhibition or genetic abrasion of the P2X7R–Panx-1 pore complex suppresses spreading depolarization and neuroinflammation in mice (Chen et al., 2017). This is in perfect agreement with findings that Cx43- and Panx-1-based channels participate in the induction of neuroinflammation and cerebral neuropathies (Sarrouilhe et al., 2017; for further considerations on the role of connexins/pannexins in MD, see the section “Inhibited Astrocytic ATP Release in the Pre-frontal Cortex”).
Microglial and Astroglial Functions; Cell Death and Proliferation by P2X7 Receptors
Microglia are the resident immunocytes of the CNS; unlike other tissue macrophages, they persist for the life of the organism with negligible turnover rates at steady state (Tay et al., 2016; Anderson and Vetter, 2019). Microglia are instrumental in the maintenance of biochemical homeostasis, neuronal circuit maturation during development, and experience-dependent remodeling of neuronal circuits in the adult brain (Szepesi et al., 2018; Anderson and Vetter, 2019; Illes et al., 2019). The cellular processes of quiescent or “resting” microglia are highly mobile (extension and withdrawal) by scanning the environment for disruptions of brain homeostasis (Davalos et al., 2005). When microglia detect danger signals, they rapidly become activated by shortening their processes, eventually being transformed to amoeboid microglia, which produces a number of cytokines, chemokines, and growth factors, as well as developing phagocytotic activity (Kettenmann et al., 2011).
Microglia establish close contact with both neurons (Eyo and Wu, 2013) and astrocytes (Jha et al., 2019), supplementing the “tripartite synapse” (see below) with a microglial component (“quadripartite synapse”; Schafer et al., 2013; Illes et al., 2019). An important regulator of this interaction is ATP/ADP, which is released from neurons and astrocytes/microglia by exocytotic and non-exocytotic mechanisms (Calovi et al., 2019). Microglia possess a range of P2Y receptors (P2YRs). P2Y1 receptors (P2Y1Rs) steer microglial migration (De Simone et al., 2010), P2Y6 receptors (P2Y6Rs) regulate microglial phagocytosis (Koizumi et al., 2007), and P2Y12 receptors (P2Y12Rs) are responsible for chemoattraction of microglial branches to the site of ATP accumulation (Ohsawa et al., 2010).
P2X7, the archetypical macrophage/microglial receptor, mediates two diametrically opposite functions of microglia such as, firstly, proliferation, most likely via calcium signaling (Monif et al., 2010, 2016), and, secondly, necrosis/apoptosis via the generation of transmembrane pores and activation of the caspase enzymatic cascade (Bartlett et al., 2014; He et al., 2017). Whereas microglial phagocytosis of bacteria and cellular debris is under the regulation of ATP/ADP, P2X7 has been shown to be a scavenger receptor for apoptotic cells even in the absence of its ligand ATP (Gu et al., 2011).
Astrocytes to a large extent define synaptic connectivity. Indirect effects are exerted by changes in astrocytic functions due to modifications in K+ uptake and redistribution; Cl− and water fluxes; Na+/K+, Na+/Ca2+, or Na+/HCO3− exchange; neurotransmitter uptake; etc (Verkhratsky et al., 2017; Mederos et al., 2018; Illes et al., 2019). Astrocytes also directly modify synaptic transmission, because they contact and partially ensheathe synapses with their perisynaptic processes (Allen and Eroglu, 2017). Astrocytes may release “gliotransmitters” [e.g., glutamate, γ-aminobutyric acid (GABA), and ATP] by an exocytotic mechanism modulating neuronal functions; the structural basis for this effect is the “tripartite synapse,” which consists of the presynaptic elements, the postsynaptic/dendritic structures, and the astrocytic processes terminating at the synapse (Araque et al., 1999; Halassa and Haydon, 2010; Illes et al., 2019). More recently, the tripartite synapse hypothesis has evolved into the idea of an “astroglial cradle” summarizing all aspects of the synapse function not only those mediated by neurotransmitters (Verkhratsky and Nedergaard, 2018). In addition, astrocytes may also deliver ATP/ADP into the extracellular space by non-exocytotic mechanisms via, for example, connexin hemichannels, pannexin channels, maxi-anion channels, and volume-regulated anion channels, contributing to the exocytotic release (Cheung et al., 2014; Dahl, 2015).
For a couple of years, it was doubted whether astrocytes possess the prototypic microglial P2X7R (see, e.g., Jabs et al., 2007); however, more recently convincing functional evidence corroborated this notion (Duan et al., 2003; Oliveira et al., 2011; Illes et al., 2012, 2017). Immunohistochemical investigations in the nucleus accumbens of rats showed that after stab wound injury, P2X7R immunoreactivity was observed in glial fibrillary acidic protein (GFAP)-positive astrocytes (Franke et al., 2001). Similar findings were reported for the cerebral cortex of spontaneously hypertensive rats, where the occlusion of the medial cerebral artery led to the upregulation of P2X7Rs in the penumbra surrounding the necrotic region (Franke et al., 2004). Thus, it was concluded that P2X7Rs induce proliferation of astrocytes upon their stimulation by ATP possibly released from the nearby, massively damaged CNS tissue (Franke et al., 2012; Franke and Illes, 2014; Martin et al., 2019).
Inhibited Astrocytic ATP Release in the Prefrontal Cortex and Hippocampus Is a Possible Link to Mood Disorders
After having discussed the role of P2X7Rs in MD and BD, we turn our attention to a possible role of astrocytes in the pathogenesis of mood disorders by their impeded release of ATP. Numerous lines of evidence support the contention that modification of astrocytic functions or decreased density of astrocytes in the frontolimbic and hippocampal regions is associated with depression (Rajkowska and Stockmeier, 2013; Peng et al., 2015; Rial et al., 2016). Astrocytes are integrated into networks where individual cells communicate with each other via gap junctions. Connexins, mainly represented by Cx43, provide the molecular basis for gap junction channels, connecting the cytoplasm of adjacent glial cells (Theis and Giaume, 2012; Verkhratsky and Nedergaard, 2018; Illes et al., 2019). These channels allow direct exchange of a variety of small molecules of less than about 1 kDa, including ions (most importantly Ca2+), energy metabolites, neurotransmitters, and signaling molecules coordinating metabolic and functional activities of connected cells (Pannasch and Rouach, 2013; Cheung et al., 2014). In addition, unopposed connexin hemichannels and Panx-1 channels are conduits for ATP release from astrocytes (Huang et al., 2012; Beckel et al., 2014).
Rats exposed to chronic unpredictable stress exhibited deficits in the sucrose preference test, which signals anhedonic behavior, a core symptom of depression. In the prefrontal cortex of these animals, the diffusion of gap junction channel-permeable dyes as well as the expression of Cx43 is decreased (Sun et al., 2010; Xia et al., 2018). The infusion into the prefrontal cortex of both the gap junction blocker carbenoxolone and the Cx43 mimetic, antagonistic peptide Gap27 caused anhedonia. Similarly, exposure to chronic unpredictable stress of rats also caused a decrease in the expression of prefrontal cortical connexins, while long-lasting treatment with antidepressants with unrelated structure and mode of action invariably increased the expression of connexins (Ren et al., 2018).
In the case of the blockade of connexins, it is unclear whether the gap junction property or the outflow of various neuroactive substances, for example, ATP through (hemi)channels, has been inhibited in the above experiments. However, Panx-1 works only as a channel, and therefore, its blockade in the medial prefrontal cortex by carbenoxolone, 40Panx, and mefloquine appeared to be due to impaired release of an astrocytic signaling molecule (Ni et al., 2018). This molecule may be ATP, because the mefloquine-induced depressive-like behavior was prevented by preconditioning with ATP.
However, opposite results have also been published. Dye uptake experiments in hippocampal slices demonstrated that acute restraint stress, known to instigate depressive-like behavior, induced opening of both Cx43 and Panx-1 channels (Orellana et al., 2015). Moreover, incubation of cultured astrocytes with seven antidepressants inhibited Cx43 channels with different efficacies depending on their therapeutic potencies (Jeanson et al., 2015). An explanation for these divergent results may be that conclusions were drawn based on investigations carried out on different organizational structures (cell culture/brain slice vs. whole animal) and different areas of the brain (hippocampus vs. prefrontal cortex).
When mice susceptible or non-susceptible to social defeat were compared to each other, the brains of the susceptible mice contained lower ATP levels than those of the non-susceptible ones (Cao et al., 2013). Further, FST also caused ATP deficiency in the brain and decreased the ATP content in the microdialysates of their prefrontal cortices. The infusion of ATP into the lateral ventricle of the mouse brain decreased the duration of immobility in the FST. Inositol 1,4,5-trisphosphate (IP3) triggers the release of Ca2+ from the endoplasmic reticulum which is a prerequisite for the exocytotic release of ATP. In consequence, IP3 receptor type 2 KO mice exhibited lower ATP release from astrocytes compared with their WT counterparts, as well as a depressive-like phenotype. Comparably, the astrocytic, vesicular release of ATP was blocked, when in mice, a dominant negative domain of vesicular soluble N-ethylmaleimide-sensitive fusion protein attachment protein receptor (SNARE) was selectively overexpressed in astrocytes. These transgenic animals also exhibited depressive-like behaviors (Halassa and Haydon, 2010).
Conventional KO and conditioned astrocytic KO of the calcium homeostasis modulator 2 channel (Calhm2) initiated depression-like behaviors in mice (TST and FST), indicating that this channel is the exit pathway for the release of ATP (Jun et al., 2018). In partial disagreement with these findings, the effect of the antidepressant drug fluoxetine has been shown to increase ATP exocytosis (Kinoshita et al., 2018). In consequence, the authors of this latter study concluded that the astrocytic release of ATP involved in depression operates by vesicular exocytosis rather than by Calhm2 opening.
Thus, ample evidence supports the notion that an impaired ATP release from prefronto-cortical astrocytes is the primary reason for depressive-like behavior and probably also MD. However, there is disagreement on whether this damage may be confined to connexin/pannexin hemichannels, the Ca2+-dependent exocytotic machinery, or Calhm2 channels as exit pathways for ATP. In view of the already discussed idea that hyperreactivity of microglial/astrocytic P2X7Rs is causally involved in the pathogenesis of MD/BD, it is quite attractive to hypothesize that the decreased ATP concentration in the prefrontal cortex (and hippocampus) leads to upregulation of P2X7Rs in this area of the brain.
P2X7R Antagonists as Possible Therapeutic Agents to Treat Mood Disorders
Because P2X7Rs mediate peripheral and central inflammation, a number of pharmaceutical companies developed ligands for this target, and some of them advanced P2X7R antagonistic compounds even to clinical trials. A major advantage of a P2X7R antagonistic drug would be that the receptor is stimulated only by pathologically high extracellular concentrations of ATP; thus, its blockade will not interfere with effects due to the more physiological release of smaller quantities of ATP (Illes et al., 2019). Although, against expectations, P2X7R antagonists did not produce a beneficial effect on rheumatoid arthritis (Keystone et al., 2012; Stock et al., 2012), they improved symptoms in patients with moderate-to-severe Crohn’s disease (Eser et al., 2015). Nonetheless, the development of such compounds for both therapeutic indications was terminated by Pfizer and Astra-Zeneca (Rech et al., 2016; Young and Górecki, 2018), in the case of Crohn’s disease probably also because of insufficient safety margins (Bhattacharya and Biber, 2016).
It can be derived from the available literature as discussed in our review that P2X7Rs may be promising targets to treat MD and BD (Bhattacharya, 2018; Wei et al., 2018). However, the following three difficulties are major obstacles in developing new P2X7R antagonists for the treatment of mood disorders: (1) a number of P2X7R antagonists act in rodent receptor orthologs but not in human receptor orthologs when investigated under in vitro conditions (Bhattacharya and Biber, 2016); (2) the disease can be modeled by depressive-like states induced by applying acute or chronic inescapable stress to rodents; however, it is most likely that there is no perfect analogy with the human disease (Ribeiro et al., 2019); and (3) P2X7R antagonists have to pass the blood-brain barrier in order to exert effects in the CNS.
The majority of compounds disclosed in the last decade are human-specific P2X7R antagonists with no or weak rodent activity but suffer from lack of robust CNS permeability. However, numerous blood-brain barrier-permeable P2X7R antagonists have been developed by Abbott, Astra-Zeneca, GlaxoSmithKline, and especially Janssen more recently (Bhattacharya, 2018; Wei et al., 2018). The Janssen compounds JNJ-47965567 (Bhattacharya et al., 2013) and JNJ-42253432 (Lord et al., 2014) demonstrated activity in rodent and human P2X7Rs, had good rat pharmacokinetic profiles, and had excellent brain penetration, when dosed subcutaneously.
Conclusions and Perspectives
A tight causal relationship of P2X7Rs with mood disorders is imperatively suggested by their involvement in neuroinflammation and the subsequent modulation/damage of neuronal circuits in mood-relevant areas of the brain. Functional changes in long-term synaptic potentiation (LTP) in the lateral habenula have been observed in rats exposed to inescapable stressors leading to learned helplessness (Li et al., 2011; Park et al., 2017). Similarly, in models of learned helplessness, the expression of synapse-related genes decreased, indicating the loss of synaptic structures. The morphological alterations were manifest as a decrease in spine synapse density in the CA1, CA3, and dentate gyrus of the hippocampus (Hajszan et al., 2009) and were absent in P2X7R-deficient mice (Otrokócsi et al., 2017).
In short, neuroinflammation triggered by inescapable stressors activates microglial cells outpouring cytokines/chemokines, proteases, reactive oxygen, and nitrogen species which damage neurons in the prefrontal cortex and hippocampus (Box 1). Microglia also acquires phagocytotic properties, thereby shaping adult neuronal circuits by phagocytosis and synaptic stripping. The classical DAMP ATP initiates the transformation of ramified microglia to microglia with a rounded surface. P2X7Rs have a key role in microglial activation causing via multiple signal transduction pathways functional/morphological changes leading to depressive-like reactions in animals and possible also in humans.
Box 1. Microglial cellular effectors modulating neuronal functions
• Resting (ramified) microglia constantly scan their environment for exogenous and endogenous signals indicating a threat to the neuronal homeostasis. They detect PAMPs such as LPS from bacterial infection or DAMPs, such as ATP. DAMPs initiate the transformation of ramified microglia after withdrawal of their cellular processes to microglia with a rounded surface.
• In activated microglia, the assembly/activation of the inflammasome converts pro-caspase-1 to caspase-1, which in turn cleaves the biologically inactive pro-IL-1β to IL-1β. Caspase-1 also activates the apoptotic caspase enzyme cascade to induce programmed cell death (apoptosis). After LPS priming, P2X7Rs largely boost the inflammatory cytokine response executed in the first line by IL-1β, but also by IL-6 and TNF-α.
• Microglial P2X7Rs are termed “suicide receptors” because their activation causes necrosis/cell death.
• Activated microglia release proteases as well as reactive oxygen and nitrogen species into their cellular environment. In addition, they secrete diacylglycerol lipase responsible for endocannabinoid production. These microglia also release ATP and probably also glutamate by vesicular exocytotic mechanisms.
• ATP through activation of microglial P2X7Rs releases extracellular vesicles from the plasma membrane (microvesicles and exosomes), inducing a robust inflammatory reaction in glial cells.
• Activated microglia also acquires phagocytotic properties, thereby eliminating not only cellular debris or pathogenic bacteria but also surplus neurons during development and thereby shaping adult neuronal circuits by phagocytosis or synaptic stripping.
In view of good blood-brain barrier-permeable P2X7R antagonists at our disposal and the intensive research activities carried out in academic and pharmaceutical institutions, there is strong hope that newly synthesized and clinically tested drugs of this family will be soon available as potent and side-effect-free antidepressants.
Author Contributions
PI drafted the manuscript. All authors contributed to manuscript revision and read and approved the submitted version.
Funding
Our work was made possible by a generous grant (“The Project First-Class Disciplines Development”; CZYHW1901) of the Chengdu University of Traditional Chinese Medicine to PI, AV, and YT in order to build up “The International Collaborative Center for Purinergic Signaling”, and grants of the Sichuan Provincial Administration of Foreign Affairs to support the stay of PI and AV in Chengdu (SZD201731 and SZD201846).
Conflict of Interest
The authors declare that the research was conducted in the absence of any commercial or financial relationships that could be construed as a potential conflict of interest.
Acknowledgments
We are grateful to Dr. Hayan Yin and Ms. Lumei Huang for the expert drawing of Figures 1, 2, respectively.
References
Alcocer-Gómez, E., Ulecia-Morón, C., Marín-Aguilar, F., Rybkina, T., Casas-Barquero, N., Ruiz-Cabello, J., et al. (2016). Stress-induced depressive behaviors require a functional NLRP3 inflammasome. Mol. Neurobiol. 53, 4874–4882. doi: 10.1007/s12035-015-9408-7
Allen, N. J., and Eroglu, C. (2017). Cell biology of astrocyte-synapse interactions. Neuron 96, 697–708. doi: 10.1016/j.neuron.2017.09.056
Anderson, S. R., and Vetter, M. L. (2019). Developmental roles of microglia: a window into mechanisms of disease. Dev. Dyn. 248, 98–117. doi: 10.1002/dvdy.1
Antonio, L. S., Stewart, A. P., Xu, X. J., Varanda, W. A., Murrell-Lagnado, R. D., and Edwardson, J. M. (2011). P2X4 receptors interact with both P2X2 and P2X7 receptors in the form of homotrimers. Br. J. Pharmacol. 163, 1069–1077. doi: 10.1111/j.1476-5381.2011.01303.x
Aprile-Garcia, F., Metzger, M. W., Paez-Pereda, M., Stadler, H., Acuña, M., Liberman, A. C., et al. (2016). Co-expression of wild-type P2X7R with Gln460Arg variant alters receptor function. PLoS One 11:e0151862. doi: 10.1371/journal.pone.0151862
Araque, A., Parpura, V., Sanzgiri, R. P., and Haydon, P. G. (1999). Tripartite synapses: glia, the unacknowledged partner. Trends Neurosci. 22, 208–215. doi: 10.1016/s0166-2236(98)01349-6
Barbanel, G., Ixart, G., Szafarczyk, A., Malaval, F., and Assenmacher, I. (1990). Intrahypothalamic infusion of interleukin-1β increases the release of corticotropin-releasing hormone (CRH 41) and adrenocorticotropic hormone (ACTH) in free-moving rats bearing a push-pull cannula in the median eminence. Brain Res. 516, 31–36. doi: 10.1016/0006-8993(90)90893-g
Bartlett, R., Stokes, L., and Sluyter, R. (2014). The P2X7 receptor channel: recent developments and the use of P2X7 antagonists in models of disease. Pharmacol. Rev. 66, 638–675. doi: 10.1124/pr.113.008003
Basso, A. M., Bratcher, N. A., Harris, R. R., Jarvis, M. F., Decker, M. W., and Rueter, L. E. (2009). Behavioral profile of P2X7 receptor knockout mice in animal models of depression and anxiety: relevance for neuropsychiatric disorders. Behav. Brain Res. 198, 83–90. doi: 10.1016/j.bbr.2008.10.018
Beamer, E., Gölöncsér, F., Horváth, G., Bekö, K., Otrokocsi, L., Koványi, B., et al. (2016). Purinergic mechanisms in neuroinflammation: an update from molecules to behavior. Neuropharmacology 104, 94–104. doi: 10.1016/j.neuropharm.2015.09.019
Beckel, J. M., Argall, A. J., Lim, J. C., Xia, J., Lu, W., Coffey, E. E., et al. (2014). Mechanosensitive release of adenosine 5’-triphosphate through pannexin channels and mechanosensitive upregulation of pannexin channels in optic nerve head astrocytes: a mechanism for purinergic involvement in chronic strain. Glia 62, 1486–1501. doi: 10.1002/glia.22695
Bhattacharya, A. (2018). Recent advances in CNS P2X7 physiology and pharmacology: focus on neuropsychiatric disorders. Front. Pharmacol. 9:30. doi: 10.3389/fphar.2018.00030
Bhattacharya, A., and Biber, K. (2016). The microglial ATP-gated ion channel P2X7 as a CNS drug target. Glia 64, 1772–1787. doi: 10.1002/glia.23001
Bhattacharya, A., and Jones, D. N. C. (2018). Emerging role of the P2X7-NLRP3-IL1β pathway in mood disorders. Psychoneuroendocrinology 98, 95–100. doi: 10.1016/j.psyneuen.2018.08.015
Bhattacharya, A., Wang, Q., Ao, H., Shoblock, J. R., Lord, B., Aluisio, L., et al. (2013). Pharmacological characterization of a novel centrally permeable P2X7 receptor antagonist: JNJ-47965567. Br. J. Pharmacol. 170, 624–640. doi: 10.1111/bph.12314
Boucher, A. A., Arnold, J. C., Hunt, G. E., Spiro, A., Spencer, J., Brown, C., et al. (2011). Resilience and reduced c-Fos expression in P2X7 receptor knockout mice exposed to repeated forced swim test. Neuroscience 189, 170–177. doi: 10.1016/j.neuroscience.2011.05.049
Burnstock, G., and Knight, G. E. (2004). Cellular distribution and functions of P2 receptor subtypes in different systems. Int. Rev. Cytol. 240, 31–304. doi: 10.1016/s0074-7696(04)40002-3
Burnstock, G., and Knight, G. E. (2018). The potential of P2X7 receptors as a therapeutic target, including inflammation and tumour progression. Purinergic Signal. 14, 1–18. doi: 10.1007/s11302-017-9593-0
Calovi, S., Mut-Arbona, P., and Sperlagh, B. (2019). Microglia and the purinergic signaling system. Neuroscience 405, 137–147. doi: 10.1016/j.neuroscience.2018.12.021
Cao, X., Li, L. P., Wang, Q., Wu, Q., Hu, H. H., Zhang, M., et al. (2013). Astrocyte-derived ATP modulates depressive-like behaviors. Nat. Med. 19, 773–777. doi: 10.1038/nm.3162
Chen, S. P., Qin, T., Seidel, J. L., Zheng, Y., Eikermann, M., Ferrari, M. D., et al. (2017). Inhibition of the P2X7-PANX1 complex suppresses spreading depolarization and neuroinflammation. Brain 140, 1643–1656. doi: 10.1093/brain/awx085
Cheung, G., Chever, O., and Rouach, N. (2014). Connexons and pannexons: newcomers in neurophysiology. Front. Cell. Neurosci. 8:348. doi: 10.3389/fncel.2014.00348
Costa-Junior, H. M., Sarmento, V. F., and Coutinho-Silva, R. (2011). C terminus of the P2X7 receptor: treasure hunting. Purinergic Signal. 7, 7–19. doi: 10.1007/s11302-011-9215-1
Csölle, C., Andó, R. D., Kittel, Á., Gölöncsér, F., Baranyi, M., Soproni, K., et al. (2013a). The absence of P2X7 receptors (P2rx7) on non-haematopoietic cells leads to selective alteration in mood-related behaviour with dysregulated gene expression and stress reactivity in mice. Int. J. Neuropsychopharmacol. 16, 213–233. doi: 10.1017/s1461145711001933
Csölle, C., Baranyi, M., Zsilla, G., Kittel, A., Gölöncsér, F., Illes, P., et al. (2013b). Neurochemical changes in the mouse hippocampus underlying the antidepressant effect of genetic deletion of P2X7 receptors. PLoS One 8:e66547. doi: 10.1371/journal.pone.0066547
Czamara, D., Müller-Myhsok, B., and Lucae, S. (2018). The P2RX7 polymorphism rs2230912 is associated with depression: a meta-analysis. Prog. Neuropsychopharmacol. Biol. Psychiatry 82, 272–277. doi: 10.1016/j.pnpbp.2017.11.003
Dahl, G. (2015). ATP release through pannexon channels. Philos. Trans. R. Soc. Lond. B Biol. Sci. 370:20140191. doi: 10.1098/rstb.2014.0191
Davalos, D., Grutzendler, J., Yang, G., Kim, J. V., Zuo, Y., Jung, S., et al. (2005). ATP mediates rapid microglial response to local brain injury in vivo. Nat. Neurosci. 8, 752–758. doi: 10.1038/nn1472
de Kloet, E. R., Joëls, M., and Holsboer, F. (2005). Stress and the brain: from adaptation to disease. Nat. Rev. Neurosci. 6, 463–475. doi: 10.1038/nrn1683
de Rivero Vaccari, J. P., Bastien, D., Yurcisin, G., Pineau, I., Dietrich, W. D., De Koninck, Y., et al. (2012). P2X4 receptors influence inflammasome activation after spinal cord injury. J. Neurosci. 32, 3058–3066. doi: 10.1523/JNEUROSCI.4930-11.2012
De Simone, R., Niturad, C. E., De Nuccio, C., Ajmone-Cat, M. A., Visentin, S., and Minghetti, L. (2010). TGF-β and LPS modulate ADP-induced migration of microglial cells through P2Y1 and P2Y12 receptor expression. J. Neurochem. 115, 450–459. doi: 10.1111/j.1471-4159.2010.06937.x
Deussing, J. M., and Arzt, E. (2018). P2X7 receptor: a potential therapeutic target for depression? Trends Mol. Med. 24, 736–747. doi: 10.1016/j.molmed.2018.07.005
Di Virgilio, F., Dal Ben, D., Sarti, A. C., Giuliani, A. L., and Falzoni, S. (2017). The P2X7 receptor in infection and inflammation. Immunity 47, 15–31. doi: 10.1016/j.immuni.2017.06.020
Di Virgilio, F., Schmalzing, G., and Markwardt, F. (2018). The elusive P2X7 macropore. Trends Cell Biol. 28, 392–404. doi: 10.1016/j.tcb.2018.01.005
Dinarello, C. A. (2009). Immunological and inflammatory functions of the interleukin-1 family. Annu. Rev. Immunol. 27, 519–550. doi: 10.1146/annurev.immunol.021908.132612
Du, R. H., Tan, J., Sun, X. Y., Lu, M., Ding, J. H., and Hu, G. (2016). Fluoxetine inhibits NLRP3 inflammasome activation: implication in depression. Int. J. Neuropsychopharmacol. 19:pyw037. doi: 10.1093/ijnp/pyw037
Duan, S., Anderson, C. M., Keung, E. C., Chen, Y., Chen, Y., and Swanson, R. A. (2003). P2X7 receptor-mediated release of excitatory amino acids from astrocytes. J. Neurosci. 23, 1320–1328. doi: 10.1523/JNEUROSCI.23-04-01320.2003
Dubyak, G. R. (2007). Go it alone no more—P2X7 joins the society of heteromeric ATP-gated receptor channels. Mol. Pharmacol. 72, 1402–1405. doi: 10.1124/mol.107.042077
Eser, A., Colombel, J. F., Rutgeerts, P., Vermeire, S., Vogelsang, H., Braddock, M., et al. (2015). Safety and efficacy of an oral inhibitor of the purinergic receptor P2X7 in adult patients with moderately to severely active crohn’s disease: a randomized placebo-controlled, double-blind, phase IIa study. Inflamm. Bowel Dis. 21, 2247–2253. doi: 10.1097/mib.0000000000000514
Eyo, U. B., and Wu, L. J. (2013). Bidirectional microglia-neuron communication in the healthy brain. Neural Plast. 2013:456857. doi: 10.1155/2013/456857
Farooq, R. K., Tanti, A., Ainouche, S., Roger, S., Belzung, C., and Camus, V. (2018). A P2X7 receptor antagonist reverses behavioural alterations, microglial activation and neuroendocrine dysregulation in an unpredictable chronic mild stress (UCMS) model of depression in mice. Psychoneuroendocrinology 97, 120–130. doi: 10.1016/j.psyneuen.2018.07.016
Feng, W. P., Zhang, B., Li, W., and Liu, J. (2014). Lack of association of P2RX7 gene rs2230912 polymorphism with mood disorders: a meta-analysis. PLoS One 9:e88575. doi: 10.1371/journal.pone.0088575
Feng, X., Zhao, Y., Yang, T., Song, M., Wang, C., Yao, Y., et al. (2019). Glucocorticoid-driven NLRP3 inflammasome activation in hippocampal microglia mediates chronic stress-induced depressive-like behaviors. Front. Mol. Neurosci. 12:210. doi: 10.3389/fnmol.2019.00210
Ferrari, D., Pizzirani, C., Adinolfi, E., Lemoli, R. M., Curti, A., Idzko, M., et al. (2006). The P2X7 receptor: a key player in IL-1 processing and release. J. Immunol. 176, 3877–3883. doi: 10.4049/jimmunol.176.7.3877
Franke, H., and Illes, P. (2014). Nucleotide signaling in astrogliosis. Neurosci. Lett. 565, 14–22. doi: 10.1016/j.neulet.2013.09.056
Franke, H., Grosche, J., Schädlich, H., Krügel, U., Allgaier, C., and Illes, P. (2001). P2X receptor expression on astrocytes in the nucleus accumbens of rats. Neuroscience 108, 421–429. doi: 10.1016/s0306-4522(01)00416-x
Franke, H., Günther, A., Grosche, J., Schmidt, R., Rossner, S., Reinhardt, R., et al. (2004). P2X7 receptor expression after ischemia in the cerebral cortex of rats. J. Neuropathol. Exp. Neurol. 63, 686–699. doi: 10.1093/jnen/63.7.686
Franke, H., Verkhratsky, A., Burnstock, G., and Illes, P. (2012). Pathophysiology of astroglial purinergic signalling. Purinergic Signal. 8, 629–657. doi: 10.1007/s11302-012-9300-0
Franklin, T. C., Xu, C., and Duman, R. S. (2018). Depression and sterile inflammation: essential role of danger associated molecular patterns. Brain Behav. Immun. 72, 2–13. doi: 10.1016/j.bbi.2017.10.025
Gadek-Michalska, A., and Bugajski, J. (2010). Interleukin-1 (IL-1) in stress-induced activation of limbic-hypothalamic-pituitary adrenal axis. Pharmacol. Rep. 62, 969–982. doi: 10.1016/s1734-1140(10)70359-5
Goshen, I., Yirmiya, R., Iverfeldt, K., and Weidenfeld, J. (2003). The role of endogenous interleukin-1 in stress-induced adrenal activation and adrenalectomy-induced adrenocorticotropic hormone hypersecretion. Endocrinology 144, 4453–4458. doi: 10.1210/en.2003-0338
Green, E. K., Grozeva, D., Raybould, R., Elvidge, G., Macgregor, S., Craig, I., et al. (2009). P2RX7: a bipolar and unipolar disorder candidate susceptibility gene? Am. J. Med. Genet. B Neuropsychiatr. Genet. 150B, 1063–1069. doi: 10.1002/ajmg.b.30931
Grigoroiu-Serbanescu, M., Herms, S., Mühleisen, T. W., Georgi, A., Diaconu, C. C., Strohmaier, J., et al. (2009). Variation in P2RX7 candidate gene (rs2230912) is not associated with bipolar I disorder and unipolar major depression in four European samples. Am. J. Med. Genet. B Neuropsychiatr. Genet. 150B, 1017–1021. doi: 10.1002/ajmg.b.30952
Gu, B. J., Saunders, B. M., Petrou, S., and Wiley, J. S. (2011). P2X7 is a scavenger receptor for apoptotic cells in the absence of its ligand, extracellular ATP. J. Immunol. 187, 2365–2375. doi: 10.4049/jimmunol.1101178
Gu, B. J., Zhang, W., Worthington, R. A., Sluyter, R., Dao-Ung, P., Petrou, S., et al. (2001). A Glu-496 to Ala polymorphism leads to loss of function of the human P2X7 receptor. J. Biol. Chem. 276, 11135–11142. doi: 10.1074/jbc.m010353200
Gulbransen, B. D., Bashashati, M., Hirota, S. A., Gui, X., Roberts, J. A., MacDonald, J. A., et al. (2012). Activation of neuronal P2X7 receptor-pannexin-1 mediates death of enteric neurons during colitis. Nat. Med. 18, 600–604. doi: 10.1038/nm.2679
Guo, C., Masin, M., Qureshi, O. S., and Murrell-Lagnado, R. D. (2007). Evidence for functional P2X4/P2X7 heteromeric receptors. Mol. Pharmacol. 72, 1447–1456. doi: 10.1124/mol.107.035980
Hajszan, T., Dow, A., Warner-Schmidt, J. L., Szigeti-Buck, K., Sallam, N. L., Parducz, A., et al. (2009). Remodeling of hippocampal spine synapses in the rat learned helplessness model of depression. Biol. Psychiatry 65, 392–400. doi: 10.1016/j.biopsych.2008.09.031
Halassa, M. M., and Haydon, P. G. (2010). Integrated brain circuits: astrocytic networks modulate neuronal activity and behavior. Annu. Rev. Physiol. 72, 335–355. doi: 10.1146/annurev-physiol-021909-135843
Halmai, Z., Dome, P., Vereczkei, A., Abdul-Rahman, O., Szekely, A., Gonda, X., et al. (2013). Associations between depression severity and purinergic receptor P2RX7 gene polymorphisms. J. Affect. Disord. 150, 104–109. doi: 10.1016/j.jad.2013.02.033
Hanley, P. J., Kronlage, M., Kirschning, C., del Rey, A., Di Virgilio, F., Leipziger, J., et al. (2012). Transient P2X7 receptor activation triggers macrophage death independent of Toll-like receptors 2 and 4, caspase-1, and pannexin-1 proteins. J. Biol. Chem. 287, 10650–10663. doi: 10.1074/jbc.m111.332676
Harvey, M., Belleau, P., and Barden, N. (2007). Gene interactions in depression: pathways out of darkness. Trends Genet. 23, 547–556. doi: 10.1016/j.tig.2007.08.011
He, Y., Taylor, N., Fourgeaud, L., and Bhattacharya, A. (2017). The role of microglial P2X7: modulation of cell death and cytokine release. J. Neuroinflammation 14:135. doi: 10.1186/s12974-017-0904-8
Herman, F. J., and Pasinetti, G. M. (2018). Principles of inflammasome priming and inhibition: implications for psychiatric disorders. Brain Behav. Immun. 73, 66–84. doi: 10.1016/j.bbi.2018.06.010
Huang, C., Han, X., Li, X., Lam, E., Peng, W., Lou, N., et al. (2012). Critical role of connexin 43 in secondary expansion of traumatic spinal cord injury. J. Neurosci. 32, 3333–3338. doi: 10.1523/JNEUROSCI.1216-11.2012
Hung, S. C., Choi, C. H., Said-Sadier, N., Johnson, L., Atanasova, K. R., Sellami, H., et al. (2013). P2X4 assembles with P2X7 and pannexin-1 in gingival epithelial cells and modulates ATP-induced reactive oxygen species production and inflammasome activation. PLoS One 8:e70210. doi: 10.1371/journal.pone.0070210
Illes, P., Khan, T. M., and Rubini, P. (2017). Neuronal P2X7 receptors revisited: do they really exist? J. Neurosci. 37, 7049–7062. doi: 10.1523/JNEUROSCI.3103-16.2017
Illes, P., Rubini, P., Huang, L., and Tang, Y. (2019). The P2X7 receptor: a new therapeutic target in Alzheimer’s disease. Expert Opin. Ther. Targets 23, 165–176. doi: 10.1080/14728222.2019.1575811
Illes, P., Verkhratsky, A., Burnstock, G., and Franke, H. (2012). P2X receptors and their roles in astroglia in the central and peripheral nervous system. Neuroscientist 18, 422–438. doi: 10.1177/1073858411418524
Iwata, M., Ota, K. T., Li, X. Y., Sakaue, F., Li, N., Dutheil, S., et al. (2016). Psychological stress activates the inflammasome via release of adenosine triphosphate and stimulation of the purinergic type 2X7 receptor. Biol. Psychiatry 80, 12–22. doi: 10.1016/j.biopsych.2015.11.026
Jabs, R., Matthias, K., Grote, A., Grauer, M., Seifert, G., and Steinhäuser, C. (2007). Lack of P2X receptor mediated currents in astrocytes and GluR type glial cells of the hippocampal CA1 region. Glia 55, 1648–1655. doi: 10.1002/glia.20580
Jeanson, T., Pondaven, A., Ezan, P., Mouthon, F., Charvériat, M., and Giaume, C. (2015). Antidepressants impact connexin 43 channel functions in astrocytes. Front. Cell. Neurosci. 9:495. doi: 10.3389/fncel.2015.00495
Jha, M. K., Jo, M., Kim, J. H., and Suk, K. (2019). Microglia-astrocyte crosstalk: an intimate molecular conversation. Neuroscientist 25, 227–240. doi: 10.1177/1073858418783959
Jun, M., Xiaolong, Q., Chaojuan, Y., Ruiyuan, P., Shukun, W., Junbing, W., et al. (2018). Calhm2 governs astrocytic ATP releasing in the development of depression-like behaviors. Mol. Psychiatry 23:1091. doi: 10.1038/mp.2017.254
Kaczmarek-Hájek, K., Lörinczi, E., Hausmann, R., and Nicke, A. (2012). Molecular and functional properties of P2X receptors—recent progress and persisting challenges. Purinergic Signal. 8, 375–417. doi: 10.1007/s11302-012-9314-7
Kaufmann, F. N., Costa, A. P., Ghisleni, G., Diaz, A. P., Rodrigues, A. L. S., Peluffo, H., et al. (2017). NLRP3 inflammasome-driven pathways in depression: clinical and preclinical findings. Brain Behav. Immun. 64, 367–383. doi: 10.1016/j.bbi.2017.03.002
Kettenmann, H., Hanisch, U. K., Noda, M., and Verkhratsky, A. (2011). Physiology of microglia. Physiol Rev. 91, 461–553. doi: 10.1152/physrev.00011.2010
Keystone, E. C., Wang, M. M., Layton, M., Hollis, S., and McInnes, I. B. (2012). Clinical evaluation of the efficacy of the P2X7 purinergic receptor antagonist AZD9056 on the signs and symptoms of rheumatoid arthritis in patients with active disease despite treatment with methotrexate or sulphasalazine. Ann. Rheum. Dis. 71, 1630–1635. doi: 10.1136/annrheumdis-2011-143578
Kinoshita, M., Hirayama, Y., Fujishita, K., Shibata, K., Shinozaki, Y., Shigetomi, E., et al. (2018). Anti-depressant fluoxetine reveals its therapeutic effect via astrocytes. EBioMedicine 32, 72–83. doi: 10.1016/j.ebiom.2018.05.036
Koenig, J. I., Walker, C. D., Romeo, R. D., and Lupien, S. J. (2011). Effects of stress across the lifespan. Stress 14, 475–480. doi: 10.3109/10253890.2011.604879
Koizumi, S., Shigemoto-Mogami, Y., Nasu-Tada, K., Shinozaki, Y., Ohsawa, K., Tsuda, M., et al. (2007). UDP acting at P2Y6 receptors is a mediator of microglial phagocytosis. Nature 446, 1091–1095. doi: 10.1038/nature05704
Koo, J. W., and Duman, R. S. (2009). Evidence for IL-1 receptor blockade as a therapeutic strategy for the treatment of depression. Curr. Opin. Investig. Drugs 10, 664–671.
Kopp, R., Krautloher, A., Ramírez-Fernéndez, A., and Nicke, A. (2019). P2X7 interactions and signaling—making head or tail of it. Front. Mol. Neurosci. 12:183. doi: 10.3389/fnmol.2019.00183
Kulkarni, S. K., and Dhir, A. (2009). Current investigational drugs for major depression. Expert Opin. Investig. Drugs 18, 767–788. doi: 10.1517/13543780902880850
Li, B., Piriz, J., Mirrione, M., Chung, C., Proulx, C. D., Schulz, D., et al. (2011). Synaptic potentiation onto habenula neurons in the learned helplessness model of depression. Nature 470, 535–539. doi: 10.1038/nature09742
Li, M., Toombes, G. E., Silberberg, S. D., and Swartz, K. J. (2015). Physical basis of apparent pore dilation of ATP-activated P2X receptor channels. Nat. Neurosci. 18, 1577–1583. doi: 10.1038/nn.4120
Liu, X., and Quan, N. (2018). Microglia and CNS interleukin-1: beyond immunological concepts. Front. Neurol. 9:8. doi: 10.3389/fneur.2018.00008
Lord, B., Aluisio, L., Shoblock, J. R., Neff, R. A., Varlinskaya, E. I., Ceusters, M., et al. (2014). Pharmacology of a novel central nervous system-penetrant P2X7 antagonist JNJ-42253432. J. Pharmacol. Exp. Ther. 351, 628–641. doi: 10.1124/jpet.114.218487
Ma, M., Ren, Q., Zhang, J. C., and Hashimoto, K. (2014). Effects of brilliant blue G on serum tumor necrosis factor-α levels and depression-like behavior in mice after lipopolysaccharide administration. Clin. Psychopharmacol. Neurosci. 12, 31–36. doi: 10.9758/cpn.2014.12.1.31
Martin, E., Amar, M., Dalle, C., Youssef, I., Boucher, C., Le Duigou, C., et al. (2019). New role of P2X7 receptor in an Alzheimer’s disease mouse model. Mol. Psychiatry 24, 108–125. doi: 10.1038/s41380-018-0108-3
Maes, M., Song, C., and Yirmiya, R. (2012). Targeting IL-1 in depressions. Expert Opin. Ther. Targets 16, 1097–1112. doi: 10.1517/14728222.2012.718331
McQuillin, A., Bass, N. J., Choudhury, K., Puri, V., Kosmin, M., Lawrence, J., et al. (2009). Case-control studies show that a non-conservative amino-acid change from a glutamine to arginine in the P2RX7 purinergic receptor protein is associated with both bipolar- and unipolar-affective disorders. Mol. Psychiatry 14, 614–620. doi: 10.1038/mp.2008.6
Mederos, S., González-Arias, C., and Perea, G. (2018). Astrocyte-neuron networks: a multilane highway of signaling for homeostatic brain function. Front. Synaptic Neurosci. 10:45. doi: 10.3389/fnsyn.2018.00045
Metzger, M. W., Walser, S. M., Aprile-Garcia, F., Dedic, N., Chen, A., Holsboer, F., et al. (2017a). Genetically dissecting P2rx7 expression within the central nervous system using conditional humanized mice. Purinergic Signal. 13, 153–170. doi: 10.1007/s11302-016-9546-z
Metzger, M. W., Walser, S. M., Dedic, N., Aprile-Garcia, F., Jakubcakova, V., Adamczyk, M., et al. (2017b). Heterozygosity for the mood disorder-associated variant Gln460Arg alters P2X7 receptor function and sleep quality. J. Neurosci. 37, 11688–11700. doi: 10.1523/JNEUROSCI.3487-16.2017
Molendijk, M. L., and de Kloet, E. R. (2019). Coping with the forced swim stressor: current state-of-the-art. Behav. Brain Res. 364, 1–10. doi: 10.1016/j.bbr.2019.02.005
Monif, M., Burnstock, G., and Williams, D. A. (2010). Microglia: proliferation and activation driven by the P2X7 receptor. Int. J. Biochem. Cell Biol. 42, 1753–1756. doi: 10.1016/j.biocel.2010.06.021
Monif, M., Reid, C. A., Powell, K. L., Drummond, K. J., O’Brien, T. J., and Williams, D. A. (2016). Interleukin-1β has trophic effects in microglia and its release is mediated by P2X7R pore. J. Neuroinflammation 13:173. doi: 10.1186/s12974-016-0621-8
Mühleisen, T. W., Leber, M., Schulze, T. G., Strohmaier, J., Degenhardt, F., Treutlein, J., et al. (2014). Genome-wide association study reveals two new risk loci for bipolar disorder. Nat. Commun. 5:3339. doi: 10.1038/ncomms4339
Muñoz-Planillo, R., Kuffa, P., Martínez-Colón, G., Smith, B. L., Rajendiran, T. M., and Núñez, G. (2013). K+ efflux is the common trigger of NLRP3 inflammasome activation by bacterial toxins and particulate matter. Immunity 38, 1142–1153. doi: 10.1016/j.immuni.2013.05.016
Ni, M., He, J. G., Zhou, H. Y., Lu, X. J., Hu, Y. L., Mao, L., et al. (2018). Pannexin-1 channel dysfunction in the medial prefrontal cortex mediates depressive-like behaviors induced by chronic social defeat stress and administration of mefloquine in mice. Neuropharmacology 137, 256–267. doi: 10.1016/j.neuropharm.2017.12.004
Nicke, A. (2008). Homotrimeric complexes are the dominant assembly state of native P2X7 subunits. Biochem. Biophys. Res. Commun. 377, 803–808. doi: 10.1016/j.bbrc.2008.10.042
North, R. A. (2002). Molecular physiology of P2X receptors. Physiol. Rev. 82, 1013–1067. doi: 10.1152/physrev.00015.2002
Ohsawa, K., Irino, Y., Sanagi, T., Nakamura, Y., Suzuki, E., Inoue, K., et al. (2010). P2Y12 receptor-mediated integrin-β1 activation regulates microglial process extension induced by ATP. Glia 58, 790–801. doi: 10.1002/glia.20963
Oliveira, J. F., Riedel, T., Leichsenring, A., Heine, C., Franke, H., Krugel, U., et al. (2011). Rodent cortical astroglia express in situ functional P2X7 receptors sensing pathologically high ATP concentrations. Cereb. Cortex 21, 806–820. doi: 10.1093/cercor/bhq154
Orellana, J. A., Moraga-Amaro, R., Díaz-Galarce, R., Rojas, S., Maturana, C. J., Stehberg, J., et al. (2015). Restraint stress increases hemichannel activity in hippocampal glial cells and neurons. Front. Cell. Neurosci. 9:102. doi: 10.3389/fncel.2015.00102
Otrokócsi, L., Kittel, A., and Sperlágh, B. (2017). P2X7 receptors drive spine synapse plasticity in the learned helplessness model of depression. Int. J. Neuropsychopharmacol. 20, 813–822. doi: 10.1093/ijnp/pyx046
Pan, Y., Chen, X. Y., Zhang, Q. Y., and Kong, L. D. (2014). Microglial NLRP3 inflammasome activation mediates IL-1β-related inflammation in prefrontal cortex of depressive rats. Brain Behav. Immun. 41, 90–100. doi: 10.1016/j.bbi.2014.04.007
Pannasch, U., and Rouach, N. (2013). Emerging role for astroglial networks in information processing: from synapse to behavior. Trends Neurosci. 36, 405–417. doi: 10.1016/j.tins.2013.04.004
Park, H., Rhee, J., Park, K., Han, J. S., Malinow, R., and Chung, C. (2017). Exposure to stressors facilitates long-term synaptic potentiation in the lateral habenula. J. Neurosci. 37, 6021–6030. doi: 10.1523/JNEUROSCI.2281-16.2017
Pelegrin, P., and Surprenant, A. (2006). Pannexin-1 mediates large pore formation and interleukin-1β release by the ATP-gated P2X7 receptor. EMBO J. 25, 5071–5082. doi: 10.1038/sj.emboj.7601378
Peng, L., Verkhratsky, A., Gu, L., and Li, B. (2015). Targeting astrocytes in major depression. Expert Rev. Neurother. 15, 1299–1306. doi: 10.1586/14737175.2015.1095094
Perregaux, D. G., and Gabel, C. A. (1998). Post-translational processing of murine IL-1: evidence that ATP-induced release of IL-1α and IL-1β occurs via a similar mechanism. J. Immunol. 160, 2469–2477.
Pippel, A., Stolz, M., Woltersdorf, R., Kless, A., Schmalzing, G., and Markwardt, F. (2017). Localization of the gate and selectivity filter of the full-length P2X7 receptor. Proc. Natl. Acad. Sci. U S A 114, E2156–E2165. doi: 10.1073/pnas.1610414114
Rajkowska, G., and Stockmeier, C. A. (2013). Astrocyte pathology in major depressive disorder: insights from human postmortem brain tissue. Curr. Drug Targets 14, 1225–1236. doi: 10.2174/13894501113149990156
Rech, J. C., Bhattacharya, A., Letavic, M. A., and Savall, B. M. (2016). The evolution of P2X7 antagonists with a focus on CNS indications. Bioorg. Med. Chem. Lett. 26, 3838–3845. doi: 10.1016/j.bmcl.2016.06.048
Ren, Q., Wang, Z. Z., Chu, S. F., Xia, C. Y., and Chen, N. H. (2018). Gap junction channels as potential targets for the treatment of major depressive disorder. Psychopharmacology 235, 1–12. doi: 10.1007/s00213-017-4782-7
Rial, D., Lemos, C., Pinheiro, H., Duarte, J. M., Gonçalves, F. Q., Real, J. I., et al. (2016). Depression as a glial-based synaptic dysfunction. Front. Cell. Neurosci. 9:521. doi: 10.3389/fncel.2015.00521
Ribeiro, D. E., Roncalho, A. L., Glaser, T., Ulrich, H., Wegener, G., and Joca, S. (2019). P2X7 receptor signaling in stress and depression. Int. J. Mol. Sci. 20:E2778. doi: 10.3390/ijms20112778
Roger, S., Mei, Z. Z., Baldwin, J. M., Dong, L., Bradley, H., Baldwin, S. A., et al. (2010). Single nucleotide polymorphisms that were identified in affective mood disorders affect ATP-activated P2X7 receptor functions. J. Psychiatr. Res. 44, 347–355. doi: 10.1016/j.jpsychires.2009.10.005
Sakaki, H., Fujiwaki, T., Tsukimoto, M., Kawano, A., Harada, H., and Kojima, S. (2013). P2X4 receptor regulates P2X7 receptor-dependent IL-1β and IL-18 release in mouse bone marrow-derived dendritic cells. Biochem. Biophys. Res. Commun. 432, 406–411. doi: 10.1016/j.bbrc.2013.01.135
Sarrouilhe, D., Dejean, C., and Mesnil, M. (2017). Connexin43- and pannexin-based channels in neuroinflammation and cerebral neuropathies. Front. Mol. Neurosci. 10:320. doi: 10.3389/fnmol.2017.00320
Savio, L. E. B., de Andrade, M. P., da Silva, C. G., and Coutinho-Silva, R. (2018). The P2X7 receptor in inflammatory diseases: angel or demon? Front. Pharmacol. 9:52. doi: 10.3389/fphar.2018.00052
Schafer, D. P., Lehrman, E. K., and Stevens, B. (2013). The “quad-partite” synapse: microglia-synapse interactions in the developing and mature CNS. Glia 61, 24–36. doi: 10.1002/glia.22389
Shao, B. Z., Xu, Z. Q., Han, B. Z., Su, D. F., and Liu, C. (2015). NLRP3 inflammasome and its inhibitors: a review. Front. Pharmacol. 6:262. doi: 10.3389/fphar.2015.00262
Shoji, K. F., Sáez, P. J., Harcha, P. A., Aguila, H. L., and Sáez, J. C. (2014). Pannexin1 channels act downstream of P2X7 receptors in ATP-induced murine T-cell death. Channels 8, 142–156. doi: 10.4161/chan.28122
Sluyter, R., Stokes, L., Fuller, S. J., Skarratt, K. K., Gu, B. J., and Wiley, J. S. (2010). Functional significance of P2RX7 polymorphisms associated with affective mood disorders. J. Psychiatr. Res. 44, 1116–1117. doi: 10.1016/j.jpsychires.2010.04.013
Solle, M., Labasi, J., Perregaux, D. G., Stam, E., Petrushova, N., Koller, B. H., et al. (2001). Altered cytokine production in mice lacking P2X7 receptors. J. Biol. Chem. 276, 125–132. doi: 10.1074/jbc.M006781200
Soronen, P., Mantere, O., Melartin, T., Suominen, K., Vuorilehto, M., Rytsala, H., et al. (2011). P2RX7 gene is associated consistently with mood disorders and predicts clinical outcome in three clinical cohorts. Am. J. Med. Genet. B Neuropsychiatr. Genet. 156B, 435–447. doi: 10.1002/ajmg.b.31179
Sperlágh, B., and Illes, P. (2014). P2X7 receptor: an emerging target in central nervous system diseases. Trends Pharmacol. Sci. 35, 537–547. doi: 10.1016/j.tips.2014.08.002
Sperlágh, B., Csölle, C., Andó, R. D., Gölöncser, F., Kittel, A., and Baranyi, M. (2012). The role of purinergic signaling in depressive disorders. Neuropsychopharmacol. Hung. 14, 231–238.
Stock, T. C., Bloom, B. J., Wei, N., Ishaq, S., Park, W., Wang, X., et al. (2012). Efficacy and safety of CE-224,535, an antagonist of P2X7 receptor, in treatment of patients with rheumatoid arthritis inadequately controlled by methotrexate. J. Rheumatol. 39, 720–727. doi: 10.3899/jrheum.110874
Su, W. J., Zhang, T., Jiang, C. L., and Wang, W. (2018). Clemastine alleviates depressive-like behavior through reversing the imbalance of microglia-related pro-inflammatory state in mouse hippocampus. Front. Cell. Neurosci. 12:412. doi: 10.3389/fncel.2018.00412
Su, W. J., Zhang, Y., Chen, Y., Gong, H., Lian, Y. J., Peng, W., et al. (2017). NLRP3 gene knockout blocks NF-κB and MAPK signaling pathway in CUMS-induced depression mouse model. Behav. Brain Res. 322, 1–8. doi: 10.1016/j.bbr.2017.01.018
Sullivan, P. F., Daly, M. J., and O’Donovan, M. (2012). Genetic architectures of psychiatric disorders: the emerging picture and its implications. Nat. Rev. Genet. 13, 537–551. doi: 10.1038/nrg3240
Sun, C., Chu, J., Singh, S., and Salter, R. D. (2010). Identification and characterization of a novel variant of the human P2X7 receptor resulting in gain of function. Purinergic Signal. 6, 31–45. doi: 10.1007/s11302-009-9168-9
Surprenant, A., Rassendren, F., Kawashima, E., North, R. A., and Buell, G. (1996). The cytolytic P2Z receptor for extracellular ATP identified as a P2X receptor (P2X7). Science 272, 735–738. doi: 10.1126/science.272.5262.735
Suurväli, J., Boudinot, P., Kanellopoulos, J., and Rüütel Boudinot, S. (2017). P2X4: a fast and sensitive purinergic receptor. Biomed. J. 40, 245–256. doi: 10.1016/j.bj.2017.06.010
Szepesi, Z., Manouchehrian, O., Bachiller, S., and Deierborg, T. (2018). Bidirectional microglia-neuron communication in health and disease. Front. Cell. Neurosci. 12:323. doi: 10.3389/fncel.2018.00323
Tan, S., Wang, Y., Chen, K., Long, Z., and Zou, J. (2017). Ketamine alleviates depressive-like behaviors via down-regulating inflammatory cytokines induced by chronic restraint stress in mice. Biol. Pharm. Bull. 40, 1260–1267. doi: 10.1248/bpb.b17-00131
Tay, T. L., Hagemeyer, N., and Prinz, M. (2016). The force awakens: insights into the origin and formation of microglia. Curr. Opin. Neurobiol. 39, 30–37. doi: 10.1016/j.conb.2016.04.003
Theis, M., and Giaume, C. (2012). Connexin-based intercellular communication and astrocyte heterogeneity. Brain Res. 1487, 88–98. doi: 10.1016/j.brainres.2012.06.045
Vereczkei, A., Abdul-Rahman, O., Halmai, Z., Nagy, G., Szekely, A., Somogyi, A., et al. (2019). Association of purinergic receptor P2RX7 gene polymorphisms with depression symptoms. Prog. Neuropsychopharmacol. Biol. Psychiatry 92, 207–216. doi: 10.1016/j.pnpbp.2019.01.006
Verkhratsky, A., and Nedergaard, M. (2018). Physiology of astroglia. Physiol. Rev. 98, 239–389. doi: 10.1152/physrev.00042.2016
Verkhratsky, A., Zorec, R., and Parpura, V. (2017). Stratification of astrocytes in healthy and diseased brain. Brain Pathol. 27, 629–644. doi: 10.1111/bpa.12537
Virginio, C., MacKenzie, A., Rassendren, F. A., North, R. A., and Surprenant, A. (1999). Pore dilation of neuronal P2X receptor channels. Nat. Neurosci. 2, 315–321. doi: 10.1038/7225
Wang, Y. L., Han, Q. Q., Gong, W. Q., Pan, D. H., Wang, L. Z., Hu, W., et al. (2018). Microglial activation mediates chronic mild stress-induced depressive- and anxiety-like behavior in adult rats. J. Neuroinflammation 15:21. doi: 10.1186/s12974-018-1054-3
Wei, L., Syed Mortadza, S. A., Yan, J., Zhang, L., Wang, L., Yin, Y., et al. (2018). ATP-activated P2X7 receptor in the pathophysiology of mood disorders and as an emerging target for the development of novel antidepressant therapeutics. Neurosci. Biobehav. Rev. 87, 192–205. doi: 10.1016/j.neubiorev.2018.02.005
Wray, N. R., Ripke, S., Mattheisen, M., Trzaskowski, M., Byrne, E. M., Abdellaoui, A., et al. (2018). Genome-wide association analyses identify 44 risk variants and refine the genetic architecture of major depression. Nat. Genet. 50, 668–681. doi: 10.1038/s41588-018-0090-3
Xia, C. Y., Wang, Z. Z., Zhang, Z., Chen, J., Wang, Y. Y., Lou, Y. X., et al. (2018). Corticosterone impairs gap junctions in the prefrontal cortical and hippocampal astrocytes via different mechanisms. Neuropharmacology 131, 20–30. doi: 10.1016/j.neuropharm.2017.12.003
Young, C. N. J., and Górecki, D. C. (2018). P2RX7 purinoceptor as a therapeutic target—the second coming? Front. Chem. 6:248. doi: 10.3389/fchem.2018.00248
Zhang, Y., Liu, L., Liu, Y. Z., Shen, X. L., Wu, T. Y., Zhang, T., et al. (2015). NLRP3 inflammasome mediates chronic mild stress-induced depression in mice via neuroinflammation. Int. J. Neuropsychopharmacol. 18:pyv006. doi: 10.1093/ijnp/pyv006
Keywords: P2X7 receptor, mood disorders, hippocampus, microglia, astroglia
Citation: Illes P, Verkhratsky A and Tang Y (2020) Pathological ATPergic Signaling in Major Depression and Bipolar Disorder. Front. Mol. Neurosci. 12:331. doi: 10.3389/fnmol.2019.00331
Received: 03 November 2019; Accepted: 26 December 2019;
Published: 31 January 2020.
Edited by:
Tobias Engel, Royal College of Surgeons in Ireland, IrelandReviewed by:
Chiara Parisi, Institute of Cell Biology (CNR), ItalyValery I. Shestopalov, University of Miami Health System, United States
Copyright © 2020 Illes, Verkhratsky and Tang. This is an open-access article distributed under the terms of the Creative Commons Attribution License (CC BY). The use, distribution or reproduction in other forums is permitted, provided the original author(s) and the copyright owner(s) are credited and that the original publication in this journal is cited, in accordance with accepted academic practice. No use, distribution or reproduction is permitted which does not comply with these terms.
*Correspondence: Peter Illes, cGV0ZXIuaWxsZXNAbWVkaXppbi51bmktbGVpcHppZy5kZQ==