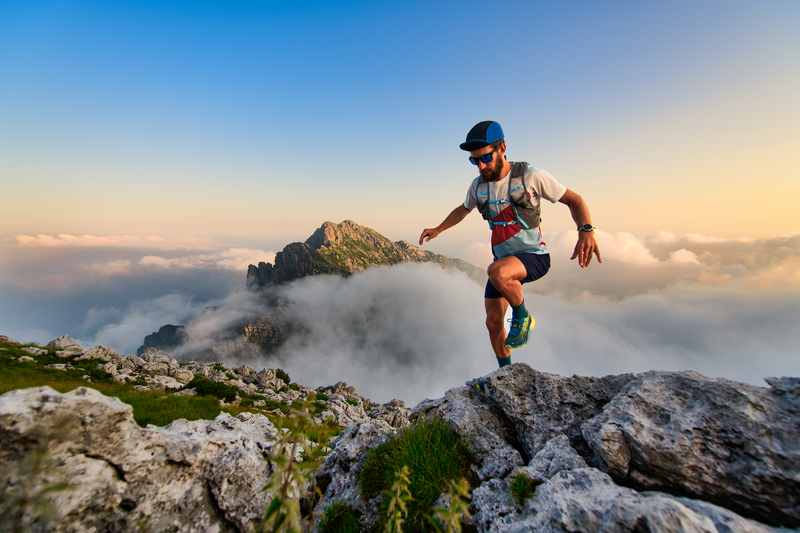
94% of researchers rate our articles as excellent or good
Learn more about the work of our research integrity team to safeguard the quality of each article we publish.
Find out more
REVIEW article
Front. Mol. Neurosci. , 05 June 2019
Sec. Brain Disease Mechanisms
Volume 12 - 2019 | https://doi.org/10.3389/fnmol.2019.00136
Mood disorders have multiple phenotypes and complex underlying biological mechanisms and, as such, there are no effective therapeutic strategies. A review of recent work on the role of astrocytes in mood disorders is thus warranted, which we embark on here. We argue that there is tremendous potential for novel strategies for therapeutic interventions based on the role of astrocytes. Astrocytes are traditionally considered to have supporting roles within the brain, yet emerging evidence has shown that astrocytes have more direct roles in influencing brain function. Notably, evidence from postmortem human brain tissues has highlighted changes in glial cell morphology, density and astrocyte-related biomarkers and genes following mood disorders, indicating astrocyte involvement in mood disorders. Findings from animal models strongly imply that astrocytes not only change astrocyte morphology and physiological characteristics but also influence neural circuits via synapse structure and formation. This review pays particular attention to interactions between astrocytes and neurons and argues that astrocyte dysfunction affects the monoaminergic system, excitatory–inhibitory balance and neurotrophic states of local networks. Together, these studies provide a foundation of knowledge about the exact role of astrocytes in mood disorders. Importantly, we then change the focus from neurons to glial cells and the interactions between the two, so that we can understand newly proposed mechanisms underlying mood disorders, and to identify more diagnostic indicators or effective targets for treatment of these diseases.
Mood disorders are a group of illnesses that describe a serious disturbance in a person’s mood (Sadock and Sadock, 2011), such as major depression disorder (MDD) and bipolar disorder (American Psychiatric Pub Association, 2013), and are a worldwide problem in modern society. According to the World Health Organization (WHO), more than 300 million people are living with depression disorder and this disorder has been ranked as the largest contributor to non-fatal health loss (World Health Organization [WHO], 2017).
Mood disorders lead to substantial personal and social burdens, yet efficacious therapeutic targets for these disorders are currently lacking (Nemeroff and Owens, 2002; Colpo et al., 2018; Boas et al., 2019), due to our relatively poor mechanistic understanding of the neurobiology involved. Determining the cellular mechanisms and neural circuits involved and how they operate is, therefore, an important step to find better therapeutic intervention targets.
Astrocytes, star-shaped non-neuronal cells found in the central nervous system, are traditionally thought to be supporting cells that provide homeostatic control and trophic support within the brain (Lindsay, 1979; Walz and Hertz, 1983; Schousboe et al., 1997; Parpura and Verkhratsky, 2012). Given that a single astrocyte may interact with as many as 100,000 synapses in mice and possibly up to 2,000,000 synapses in humans (Bushong et al., 2002; Oberheim et al., 2009), astrocytes are likely more than simple support cells. We also know that the dysfunction of astrocytes influences synaptic activity; evidence has shown that astrocytes can modulate neuronal circuits and influence behavior (Volterra and Meldolesi, 2005). Astrocytes also exert significant control over synapse formation, adult neurogenesis, and vascular tone (Song et al., 2002; Filosa and Iddings, 2013; Chung et al., 2015). As more and more astrocyte-derived active substances are found, such as glutamate and D-serine, the concept of the “tripartite synapse” has been established (Araque et al., 1999; Perea et al., 2009; Perez-Alvarez and Araque, 2013), which represents the ability of astrocytes to participate in synaptic activity. Based on numerous observations of reduced glial cell numbers from postmortem histopathologic studies of depressed patients, it has been posited that abnormal astrocyte function may contribute to the pathophysiology of mood disorders (Cotter D. R. et al., 2001; Rajkowska and Miguel-Hidalgo, 2007; Hercher et al., 2009).
We review current evidence, mainly on the mechanisms involving mood disorders through which astrocytes are thought to function, with a particular emphasis on depression. We also add evidence from anxiety disorders due to the comorbidity between depression and anxiety disorder (Mineka et al., 1998; Watson, 2005). We first discuss some human studies that have helped clarify astrocyte function in depression before examining some hypotheses that have been proposed to explain the roles that astrocytes play in depression and anxiety disorders. Notably, these multiple hypotheses attempt to explain the same question, and it may be possible to reconcile them rather than discard any. We underscore the utility of a global view when approaching mechanistic questions about depression due to complex diagnostic indicators and widespread biological effects of the disorder. Finally, we discuss some issues arising from astrocyte heterogeneity, such as interspecies differences, subtypes of astrocytes and different interactions between astrocytes and neurons (Oberheim et al., 2009; Haim and Rowitch, 2017; Lin et al., 2017). This heterogeneity should be taken into consideration when studying the relationship between astrocytes and mood disorders. The aim of this review is to promote the idea that astrocytes influence mood disorders and to give a brief view about the current understanding of the possible mechanisms through which astrocytes can be a target for mood disorder interventions.
Negative emotions, like anxiety and fear, can aid survival by increasing awareness of possible imminent harm. However, mood disorders may occur when negative emotions become persistent, disruptive or inappropriate to the perceived threat. According to the WHO, the number of people suffering from depression increased by more than 18% between 2005 and 2015 (World Health Organization [WHO], 2017). Although there is a lack of effective therapies to treat these mood disorders, there is human evidence showing that abnormalities in glial cells may alter normal brain function and likely contribute to mood disorder development (Rajkowska and Stockmeier, 2013). As such, these human data should not only guide animal model-based research programs but also be used to identify diagnostic indicators.
The first type of evidence connecting astrocytes to mood disorders is through cell counting studies and cell morphology in patients who had mood disorders. In subjects diagnosed with MDD, cell counting studies report that glial cell number and density were decreased in many brain regions, including the anterior cingulate cortex (Cotter D. et al., 2001; Gittins and Harrison, 2011), the dorsolateral prefrontal cortex (PFC) (Cotter et al., 2002), and the amygdala (Bowley et al., 2002), compared to non-psychiatric control subjects. In addition, a decrease of glial cell density has also been observed in subjects diagnosed with bipolar disorder (Grazyna Rajkowska et al., 2001). However, some postmortem work found no change in glia density in the orbitofrontal cortex (Khundakar A. et al., 2011), anterior cingulate cortex (Khundakar A. A. et al., 2011) or hippocampus (Cobb et al., 2013) in subjects that had MMD in life. In addition to changes in glial cell density, an increased glial cell nuclei size has been observed in the dorsolateral PFC in MMD patients (Rajkowska et al., 1999). Hypertrophy of astrocyte cell bodies and processes have also been observed in the anterior cingulate cortex (Torres-Platas et al., 2011). Together, this provides associational evidence of a relationship between mood disorders and abnormal glia pathology.
The second line of evidence from humans relates to altered levels of potentially astrocyte-specific biomarkers in postmortem brain specimens of individuals that had suffered from mood disorders. Low levels of a traditional astrocyte marker, glial fibrillary acidic protein (GFAP), have been found in the hippocampus, PFC, anterior cingulate, and amygdala (Müller et al., 2001; Webster et al., 2001; Altshuler et al., 2010; Gittins and Harrison, 2011).
Interestingly, a consistent finding is that young and mixed age groups of MDD patients have lower GFAP- immunoreactive (IR) astrocyte density in cortical areas than control patients (Öngür et al., 1998; Gittins and Harrison, 2011). However, studies performed on late-onset depression patients (commonly defined as occurring after age 50 or 60) have reported an increase in the density of GFAP-IR astrocytes than younger MDD patients (Khundakar and Thomas, 2009; Paradise et al., 2012). This indicates the astrocyte pathology in cortical areas is different in younger and older patients with depression (Miguel-Hidalgo et al., 2000; Khundakar and Thomas, 2009). Moreover, it is not known whether GFAP simply reflects the astrocytic function and/or whether it is directly associated with the symptoms of mood disorders. Another astrocyte marker, S100B, is a calcium-binding protein predominantly expressed in the cytoplasm that can be secreted to extracellular space and thus be detected in the serum (Gerlach et al., 2006; Andreazza et al., 2007). Mood disorder patients have increased S100B levels (Schroeter et al., 2002), and serum concentration of S100B may be a possible predictor of antidepressant response in patients (Arts et al., 2006; Ambree et al., 2015). Damaged astrocytes release an excess S100B into the serum (Rothermundt et al., 2003), so this should be taken into consideration when increased S100 levels are detected in serum.
A third line of studies from postmortem brain tissue show astrocyte dysfunction of gene transcription and protein expression in patients diagnosed with mood disorders. For example, gene and protein expression of some astrocyte function-related proteins including glutamine synthetase, glutamate transporters, and even gap junction proteins are down regulated in patients with depression (Sequeira et al., 2009; Bernard et al., 2011). Astrocytes convert glutamate into glutamine by glutamine synthetase, so glutamine synthetase and glutamate transporters associated with astrocytes reflect astrocytic function in glutamate transmission (Norenberg and Martinez-Hernandez, 1979; Sonnewald et al., 1997; Anderson and Swanson, 2000). Expression of glutamine synthetase by mRNA is down-regulated in the dorsolateral PFC, premotor cortex and the amygdala of depressed patients (Sequeira et al., 2009) and microarray analysis of specific areas of MDD patient-cerebral cortex show down-regulation of SLC1A2 and SLC1A3, two glial high-affinity glutamate transporters (Choudary et al., 2005). Connexin 30 and connexin 43 are gap junction-forming membrane proteins located on astrocyte endfeet, and dysfunction of the two proteins may alter calcium wave propagation and communication between astrocytes (Blomstrand et al., 1999; Giaume and Theis, 2010). Interestingly, the decreased expression of connexin 30 and connexin 43 has been observed in the dorsolateral PFC of suicide completers with MDD (Blomstrand et al., 1999). It has been reported recently that aquaporin-4 (AQP4), a protein located predominantly in astrocytic endfeet, has lower expression levels in MDD patients compared to non-psychiatric control subjects (Rajkowska et al., 2013). The reduction of AQP4 may influence many astrocytic functions, such as maintenance of the blood brain barrier’s integrity (Nico et al., 2001), glutamate turnover (Zeng et al., 2007), and synaptic plasticity (Li et al., 2012).
Here, we need to bear in mind that studies based on humans, especially postmortem studies, have many limitations, including individual differences and multiple causes of death. For example, the age at which mood disorder onset occurs may influence the number of GFAP-IR astrocytes (Öngür et al., 1998; Khundakar and Thomas, 2009) and postmortem interval may influence the quality of RNA obtained from postmortem brains (Lipska et al., 2006). These limitations make it difficult to generalize results and so, at this stage, appropriate animal models and experimental studies are of equal importance.
A wide body of evidence has shown that depression reshapes brain structures, leading to changes at the level of both synapse and behavior (Christoffel et al., 2011b). Changes in dendritic spines can be roughly divided into three categories: changes in density, in morphology, and in function. Studies from a stress model of depression have reported that spine density is increased or decreased in a region dependent manner; for instance, decreased spine density has been observed in hippocampus CA1 and CA3 cells (Magariños et al., 1997; Qiao et al., 2014), whereas increased spine density has been found in amygdala (Vyas et al., 2006) and NAc (Christoffel et al., 2011a). Spines can be categorized into three subtypes: mushroom, thin and stubby spines, according to their length, the diameter of spine head and the diameter of spine neck (Nimchinsky et al., 2002). Different spine subtypes have different functions and the ratio of these spines can influence neuronal excitability and function (Qiao et al., 2016). Decreased spine volume and surface area have been observed in the brains of stressed rodent models (Radley et al., 2008). Along with changes in spine density and morphology, synapse function also becomes abnormal (Liu and Aghajanian, 2008; Christoffel et al., 2011a). The shift in spine density and morphology may result in a decrease in the number of functional mature spines on neurons and may reflect the dysfunction of synaptic efficacy (Duman and Duman, 2015).
Interestingly, changes in dendritic spines in stress models is not only related to stress stimulation but is also affected by other physiological variables, such as gender and age. Dendritic morphology and spine density of pyramidal neurons in layers II–III of the prelimbic cortex vary with rat gender during recovery from chronic restraint stress (Moench and Wellman, 2017). In addition, stress stimulation leads to dendritic spine loss and changes of spine morphology in prefrontal cortical neurons in young rats but not in middle-aged and aged rats (Bloss et al., 2011). Not all neurons are affected by stress to the same degree; for example, it has been found in rats that a subpopulation of infralimbic neurons in the mPFC that project to the basolateral amygdala are resilient against the effects of stress (Shansky et al., 2009).
Synaptic changes in neurons is a hallmark of depression and astrocytes, as integral components of tripartite synapses, very likely participate in the pathology of depression and anxiety disorder (Bender et al., 2016). Although astrocytes cannot produce action potentials, evidence has shown that astrocytes change morphology and alter the expression of some proteins in response to stimuli (Allen and Barres, 2005). In fact, astrocyte morphology is now known to be very dynamic, with filopodia-like processes moving or growing in the space of only a few minutes (Bernardinelli et al., 2014). Multiple lines of evidence indicate that astrocytes can influence the synaptic plasticity of neurons, not only during synaptogenesis (Christopherson et al., 2005), but also in mature synapses (Jones et al., 2011). There is accumulating evidence that astrocytes remodel synapses, coming from mice strains that are knocked-out or knocked-down for astrocyte-secreted synapse modifying factors [for example, hevin (Singh et al., 2016), SPARC (Jones et al., 2011), and TNF-α (Stellwagen and Malenka, 2006)].
Depression can be divided into subtypes according to psychiatric symptoms and is thought to share some biological mechanisms with anxiety disorder. In some cases, depression can arise in people with anxiety disorder and symptoms of depression and abnormal anxiety levels are often observed together. In humans, exposure to stress is a predominant risk factor for depression (Kessler, 1997) and may trigger some susceptible genes that are associated with depression (Caspi et al., 2003; Kaufman et al., 2006). As such, stress paradigms are often adopted in studies investigating depression because of their co-incidence. In this section, the focus is on possible mechanisms of depression and anxiety disorder generally; most studies are from rodent models and we do not distinguish among subtypes unless stated specifically.
Numerous studies have suggested that astrocytes can respond to external signals and release transmitters like glutamate, ATP, D-serine, and lactate, as well as gamma-aminobutyric acid (GABA) (Sahlender et al., 2014; Martín et al., 2015; Machler et al., 2016; Papouin et al., 2017; Tan et al., 2017). Interactions between neurons and astrocytes through gliotransmitters is a possible mechanism in the development and maintaining of mood disorders. Although the mechanism through which astrocytes release these transmitters remains controversial, two hypotheses have been proposed, including vesicular exocytotic release and non-exocytotic release mechanisms.
The vesicular exocytotic release theory holds that astrocytes release gliotransmitters through exocytosis. The existence of synaptic-like vesicles in astrocytes in different brain areas has been observed using electron microscopy (Stranna et al., 2011; Dickens et al., 2017). The SNARE (soluble N-ethylmaleimide-sensitive factor attachment protein receptor) complex, which is Ca2+dependent, is widely accepted as the main biochemical driver of exocytosis. SNARE complexes in astrocytes are comprised of different subunits to those in neuronal SNARE complexes. The subunit synaptosomal-associated protein 23 (SNAP23) undertakes an analogous role to neuronal SNAP25 (Hepp et al., 1999), and the vesicle-associated membrane protein 3 (VAMP3) subunit has an analogous role to neuronal VAMP2 (Bezzi et al., 2004; Schubert et al., 2011). Two genetic mouse models [the dominant negative (dn) SNARE mouse (Pascual et al., 2005) and the iBot mouse (Slezak et al., 2012)] based on the variant forms of the astrocyte SNARE complex have been used to demonstrate roles that astrocytes play in several physiological and pathological processes (Hines and Haydon, 2013; Nadjar et al., 2013; Turner et al., 2013).
Alternatively, non-exocytotic release mechanisms are also possible. Firstly, there are some astrocyte channels that can mediate gliotransmitter release, such as Bestrophin-1 (BEST1) (Lee et al., 2010; Woo et al., 2012; Oh and Lee, 2017) and astrocyte gap junction hemichannels (Contreras et al., 2002; Stout et al., 2002; Ye et al., 2003). There is evidence that BEST1 is permeable to GABA and glutamate (Lee et al., 2010; Woo et al., 2012; Oh and Lee, 2017), however, studies that focus on structure show that this channel excludes larger molecules such as amino acids (Dickson et al., 2014; Vaisey et al., 2016). The mechanisms for anion selectivity of BEST1 still need to be explored. Secondly, the function of some astrocytic channels and transporters, for example, P2X purinoceptor 7 (P2X7) receptor channels (Virginio et al., 1999; Duan et al., 2003) and astrocytic glutamate re-uptake transporter (Rossi et al., 2000), may change under certain conditions and thereby release gliotransmitters to neurons. Third, exocytotic release itself brings vesicular membranes to the plasma membrane, which may contain channels or transporters that can release gliotransmitters to the surface of the astrocytes, thus leading to non-exocytotic release (Bowser and Khakh, 2007; Parpura et al., 2010).
Although mechanisms that underlie gliotransmission remain controversial, the pathways that astrocytes accept signals from, prior to releasing gliotransmitters, has been widely accepted to be dependent on intracellular Ca2+. Infusion of Ca2+ buffer solutions in astrocytes can influence synaptic activity (Di Castro et al., 2011; Panatier et al., 2011). What’s more, chelating astrocytic Ca2+ via patch clamp through chelators can block the effect that astrocytes have on neurons (Chen et al., 2016; Tan et al., 2017). In addition, fluorescent calcium sensors, Fura-2 and GCaMP6s, have been used to detect changes in astrocyte cytosolic Ca2+ and exocytosis-related elevation of Ca2+ concentration has been demonstrated (Parpura et al., 1994; Perea et al., 2014; Ma et al., 2016; Tan et al., 2017).
Although the physiological mechanisms whereby astrocytes modulate neurons is not completely determined, many studies have begun to reveal the mechanisms by which astrocytes may contribute to mood disorders.
The momoaminergic hypothesis postulates that depressive symptoms are due to a deficit or imbalance in the central monoaminergic system, which includes serotonergic, dopaminergic, and/or noradrenergic neurotransmission (Tissot, 1975; Syvälahti, 1987). We know that serotonin and noradrenaline play a role in depression and anxiety disorder because of two antidepressant drugs, iproniazid and imipramine. Iproniazid, a monoamine oxidase inhibitor, was first used to treat tuberculosis (Bloch et al., 1954; Ogilvie, 1955), whereas imipramine, a tricyclic antidepressant, was originally developed as an antipsychotic compound to treat schizophrenia (Campbell et al., 1971). Both drugs have been found to improve symptoms of depression, possibly by increasing the efficiency of synaptic monoaminergic neurotransmitters (Smith et al., 1963; Molina et al., 1990; Siris et al., 1994). Because of the existence of the drug-resistant depression (Thase et al., 1992) and drug side effects (Crane, 1956; Saraf et al., 1974), these two drugs are no longer commonly used. Second generation medications, such as serotonin selective reuptake inhibitors (SSRIs) and norepinephrine selective reuptake inhibitors have since been developed and are still widely used today (Nestler et al., 2002).
In the adult rodent brain, many lines of evidence indicate that astrocytes cannot only detect serotonin (5-HT) and noradrenaline (Porter and Mccarthy, 1997) but can also uptake these transmitters. To detect these neurotransmitters, astrocytes express 5-HT receptors, such as 5-HT1A and 5-HT7 (Whitaker-Azmitia et al., 1993; Shimizu et al., 1996), and also adrenergic receptors (targets for noradrenaline), such as α1, α2, β1, and β2 (Junker et al., 2002). Moving various neurotransmitters, including monoamines, from the synaptic cleft is one of multiple astrocytic duties and, in order to meet this duty, astrocytes contain many transporters, for instance, glial serotonin transporter (SERT) (Sur et al., 1996) and noradrenaline transporter (NET) (Inazu et al., 2003). SSRIs and tricyclic antidepressants can downregulate the expression of SERT (Inazu et al., 2001), leading to an increase of 5-HT and it is known that the tricyclic antidepressant imipramine decreases the density of astrocytic β1 adrenergic receptors in the rat forebrain (Sapena et al., 1996). This suggests that astrocytes may contribute to depression and anxiety disorder through a deficit in the central monoaminergic system.
Excitement and inhibition are two opposing processes that control the activity of neural populations. An imbalance between excitatory and inhibitory neurotransmissions may lead to aberrant functional connectivity patterns within brain circuits. Many clinical imaging studies, such as magnetic resonance spectroscopy (MRS) work (Salvadore et al., 2012; Milak et al., 2016), has shown changes in glutamate and GABA concentrations and activity in patients with depression, suggesting that an imbalance in excitatory and inhibitory neurotransmission may play a role in depression.
One important astrocyte task is to take up, metabolize, and recycle glutamate that is released into the synapses (Magistretti, 2006). When glutamatergic neurons are excited, glutamate is released into the synaptic cleft and bonds to the receptors in the post-synaptic membrane, thus transmitting the signal downstream (Traynelis et al., 2010). The remaining glutamate in the cleft then needs to be removed so that the signal can be stopped. Glutamate removal and recycling is mediated by surrounding astrocytes (Schousboe et al., 2014). The first aspect of astrocyte participation in the excitatory–inhibitory imbalance hypothesis of depression is, therefore, related to deficient glial re-uptake of glutamate. Some proteins, such as glutamine synthetase, are astrocyte-specific and are necessary in the recycling of glutamate (Norenberg, 1979; Rothstein et al., 1994). Reduced expression and content of glial-specific glutamine synthetase and glutamate transporter 1 (GLT1) has been observed in postmortem studies of patients with depression (Choudary et al., 2005), suggesting that glutamate clearance and metabolism are likely impaired in some brain regions. If glutamate re-uptake is blocked, excessive glutamate may stimulate extrasynaptic NMDA receptors, thus promoting cell death (Hardingham and Bading, 2010). Some NMDA receptor antagonists, like ketamine, can produce a rapid antidepressant effect (Newport et al., 2015). Moreover, excessive extrasynaptic glutamate is also taken up by presynaptic metabotropic glutamate receptors and this leads to a reduction in synaptic glutamate transmission (Figure 1) (McEwen et al., 2016). Although we don’t fully understand the mechanism behind astrocyte dysfunction in glutamate re-uptake, inflammation may be a key factor in this (McNally et al., 2008). In patients with depression and anxiety disorders, increased inflammation [as judged by excess levels of inflammatory mediators such as high-sensitivity C-reactive protein level (Danese et al., 2009)] has been detected (Felger, 2018) and inflammation causes impaired astrocytic glutamate uptake (Haroon et al., 2016; Felger, 2018). Astrocytes respond to infection by synthesizing pro-inflammatory and anti-inflammatory cytokines, such as interleukin-1β and tumor necrosis factor-α (TNF-α) (Dantzer et al., 2008). Cytokines such as these stimulate a cascade of inflammatory changes that include activation of proteins such as mitogen-activated protein kinases (MAPK) (Ji et al., 2002; Gorina et al., 2011).
Figure 1. (A) Astrocytes can take up, metabolize, and recycle glutamate that is released into the synapses. (B) In depression and anxiety disorder conditions, astrocyte dysfunction may lead to excitatory–inhibitory imbalance within neural networks.
Besides deficient glial re-uptake of glutamate, depression and anxiety are also associated with glutamate receptors, including NMDA and AMPA receptors. Some NMDA receptor antagonists produce antidepressive effects in animal models of depression (Skolnick et al., 1996; Li et al., 2010). A second aspect of astrocyte participation in the excitatory–inhibitory imbalance hypothesis of depression and anxiety disorder is, therefore, related to altered glutamate receptor function. Astrocytes can secrete proteins such as glypicans, and by doing so, recruit additional AMPA receptors to synapses, which will amplify neuronal transmission (Liddelow and Barres, 2015).
In rats, astroglial degeneration in the PFC is a useful depression model (Domin et al., 2014). In addition, 3-((2-Methyl-4-thiazolyl)ethynyl)pyridine (MTEP), a mGluR5 antagonist, may alleviate depression symptoms of the astroglial degeneration model through inhibition of glutamatergic transmission (Domin et al., 2014). Blocking astrocyte-specific GLT1 receptors using pharmacological inhibitors induces depressive-like phenotypes in rats (Bechtholt-Gompf et al., 2010). In summary, astrocyte dysfunction may lead to excitatory–inhibitory imbalance within neural networks, which eventually results in depression and anxiety disorder.
Lower serum levels of neurotrophic factors, such as brain-derived neurotrophic factor (BDNF) are often observed in patients with depression (Pisoni et al., 2018) and increased expression of neurotrophic factors, such as BDNF or glial cell-derived neurotrophic factor (GDNF), have been reported in multiple studies as a response to antidepressant treatment. As a result, the neurotrophic hypothesis of depression was proposed. In addition, evidence that reduced neurotrophic factor levels are tightly linked with neuronal atrophy in certain brain areas in individuals with MDD, such as the PFC and the hippocampus (Duman and Monteggia, 2006), led to the neurotrophic hypothesis of depression. Interestingly, in MDD patients with suicidal ideation, serum BDNF levels are significantly lower than MDD patients with no suicidal ideation (Khan et al., 2019), which suggests that serum BDNF levels may have a complex relationship with MDD symptoms.
Neurotrophic factors can promote neurogenesis, gliogenesis, and synaptic structure remodeling (Koyama, 2015). Astrocytes are a source of these neurotrophic factors (Chen et al., 2006) and so a decrease in such factors may be a mechanism through which astrocytes influence mood disorders, in particular considering that in hippocampus, BDNF overexpression in astrocytes leads to anxiolytic-/antidepressant-like activity in mice (Quesseveur et al., 2013).
Whilst there are different opinions on the mechanisms underlying mood disorders, it is likely that there are multiple factors involved. When searching for the mechanisms by which astrocytes influence mood disorders, a combination of current hypotheses is needed. For example, excessive glutamate may stimulate extrasynaptic NMDA receptors as mentioned above, and the activated extrasynaptic NMDA receptors inhibit the BDNF expression pathway (Hardingham et al., 2002). Additionally, monoamine dopamine induces BDNF upregulation in astrocytes, mainly through β adrenoreceptors (Koppel et al., 2018).
There is also evidence suggesting that astrocytes influence mood disorder through other mechanisms, for example, through gap junctions and hormones. Inhibition of CX43, a main component of astrocytic gap junctions, can lead to depressive-like behavior in rodents (Sun et al., 2012). In addition, the knockout of insulin receptors in astrocytes results in depression-like behavior in mice (Cai et al., 2018). On account of the complexity of depression and anxiety disorders, more detailed mechanisms and effective drug targets should arise following enhancement of understanding of the neurobiology underlying these mood disorders.
Mood disorders are measured by a strong psychological component in humans; they are difficult to quantify and studying their physiopathology remains challenging. However, there are some physical and behavioral symptoms that we can identify, including loss of appetite and abnormal anxiety levels. These are emotional-related behaviors that are thought to be largely preserved during evolution and can be identified across species (Darwin, 1998; Anderson and Adolphs, 2014; Janak and Tye, 2015). Thus, with appropriate methods, we can use animal models to study astrocytic mechanisms involved in mood disorders. We should also take differences across species into consideration because astrocyte number and size increases reflecting brain size and cognitive capabilities (Allen, 2014; Stogsdill and Eroglu, 2017). Rodents are one of the most widely used animal models and there are many differences in rodent astrocytes compared to human astrocytes. Astrocytes in human brains have larger populations, signal faster, are bigger, and are more structurally complex than those of rodents (Oberheim et al., 2009). Gene expression studies have also identified novel human-specific astrocytes (Zhang et al., 2016). Interestingly, transplanting human glial progenitors into the adult mouse brain enhances synaptic plasticity and behavioral learning (Han et al., 2013).
With more and more studies focusing on astrocytes, our understanding is developing. Yet, more problems arise when studying and interpreting astrocyte function. One is related to different astrocyte populations in different brain regions or even within the same region. A recent study identified five distinct astrocyte subpopulations, and these populations differentially support synaptogenesis between neurons (Lin et al., 2017). In glial scar area, reactive astrocytes have also shown heterogeneity, findings that have contributed to debate about whether or not glial scar aids CNS regeneration (Adams and Gallo, 2018). Even within one pathological or physiological condition, astrocytes may be able to play different roles in different brain regions or show heterogenetic influences on the same neurons (Haim and Rowitch, 2017; Martin-Fernandez et al., 2017). Deciphering the diversity of astrocytes and elucidating their functions in vivo is an important next step. These heterogenetic astrocytes may add more complexity to studies but should also help understand the complex mechanisms behind mood disorders and confirm the view that astrocytes are more than just “glue.”
XZ, QX, and JT conceived of and wrote this manuscript. LX, FY, and LW provided suggestions.
This work was funded by the National Natural Science Foundation of China (31671116 JT, 31761163005 JT, 81471164 FY, 31800881 LW, and 91132306 LW), the International Big Science Program Cultivating Project of CAS (172644KYS820170004 LW), the National Basic Research Program of China (973 program: 2015CB856402 JT), the External Cooperation Program of the Chinese Academy of Sciences (172644KYSB20160057 JT), the Key Research Program of Frontier Science of CAS (QYZDB-SSW-SMC056 FY), the Shenzhen Government Basic Research Grants (JCYJ20160429190927063 JT, JCYJ20170413165807008, and JCYJ20170413164535041 LW), the Shenzhen Discipline Construction Project for Neurobiology DRCSM [2016]1379 (LW), and the Guangdong Provincial Key S&T Program (2018B030336001).
The authors declare that the research was conducted in the absence of any commercial or financial relationships that could be construed as a potential conflict of interest.
Adams, K. L., and Gallo, V. (2018). The diversity and disparity of the glial scar. Nat. Neurosci. 21, 9–15. doi: 10.1038/s41593-017-0033-9
Allen, N. J. (2014). Astrocyte regulation of synaptic behavior. Annu. Rev. Cell Dev. Biol. 30, 439–463. doi: 10.1146/annurev-cellbio-100913-013053
Allen, N. J., and Barres, B. A. (2005). Signaling between glia and neurons: focus on synaptic plasticity. Curr. Opin. Neurobiol. 15, 542–548. doi: 10.1016/j.conb.2005.08.006
Altshuler, L. L., Abulseoud, O. A., Foland-Ross, L., Bartzokis, G., Chang, S., Mintz, J., et al. (2010). Amygdala astrocyte reduction in subjects with major depressive disorder but not bipolar disorder. Bipolar Disord. 12, 541–549. doi: 10.1111/j.1399-5618.2010.00838.x
Ambree, O., Bergink, V., Grosse, L., Alferink, J., Drexhage, H. A., Rothermundt, M., et al. (2015). S100B serum levels predict treatment response in patients with melancholic depression. Int. J. Neuropsychopharmacol. 19:yv103. doi: 10.1093/ijnp/pyv103
American Psychiatric Pub Association (2013). Diagnostic and Statistical Manual of Mental Disorders (DSM-5®). Washington, DC: American Psychiatric Pub.
Anderson, C. M., and Swanson, R. A. (2000). Astrocyte glutamate transport: review of properties, regulation, and physiological functions. Glia 32, 1–14. doi: 10.1002/1098-1136(200010)32:1<1::aid-glia10>3.3.co;2-n
Anderson, D. J., and Adolphs, R. (2014). A framework for studying emotions across species. Cell 157, 187–200. doi: 10.1016/j.cell.2014.03.003
Andreazza, A. C., Cassini, C., Rosa, A. R., Leite, M. C., de Almeida, L. M., Cunha, A. B., et al. (2007). Serum S100B and antioxidant enzymes in bipolar patients. J. Psychiatr. Res. 41, 523–529. doi: 10.1016/j.jpsychires.2006.07.013
Araque, A., Parpura, V., Sanzgiri, R. P., and Haydon, P. G. (1999). Tripartite synapses: glia, the unacknowledged partner. Trends Neurosci. 22, 208–215. doi: 10.1016/s0166-2236(98)01349-6
Arts, B., Peters, M., Ponds, R., Honig, A., Menheere, P., and van Os, J. (2006). S100 and impact of ECT on depression and cognition. J. ECT 22, 206–212. doi: 10.1097/01.yct.0000235925.37494.2c
Bechtholt-Gompf, A. J., Walther, H. V., Adams, M. A., Carlezon, W. A. Jr., Öngür, D., and Cohen, B. M. (2010). Blockade of astrocytic glutamate uptake in rats induces signs of anhedonia and impaired spatial memory. Neuropsychopharmacology 35:2049. doi: 10.1038/npp.2010.74
Bender, C. L., Calfa, G. D., and Molina, V. A. (2016). Astrocyte plasticity induced by emotional stress: a new partner in psychiatric physiopathology? Prog. Neuropsychopharmacol. Biol. Psychiatry 65, 68–77. doi: 10.1016/j.pnpbp.2015.08.005
Bernard, R., Kerman, I. A., Thompson, R. C., Jones, E. G., Bunney, W. E., Barchas, J. D., et al. (2011). Altered expression of glutamate signaling, growth factor, and glia genes in the locus coeruleus of patients with major depression. Mol. Psychiatry 16, 634–646. doi: 10.1038/mp.2010.44
Bernardinelli, Y., Muller, D., and Nikonenko, I. (2014). Astrocyte-synapse structural plasticity. Neural Plast. 2014:13.
Bezzi, P., Gundersen, V., Galbete, J. L., Seifert, G., Steinhäuser, C., Pilati, E., et al. (2004). Astrocytes contain a vesicular compartment that is competent for regulated exocytosis of glutamate. Nat. Neurosci. 7, 613–620. doi: 10.1038/nn1246
Bloch, R. G., Dooneief, A. S., Buchberg, A. S., and Spellman, S. (1954). The clinical effect of isoniazid and iproniazid in the treatment of pulmonary tuberculosis. Ann. Intern. Med. 40, 881–900.
Blomstrand, F., Åberg, N., Eriksson, P., Hansson, E., and Rönnbäck, L. (1999). Extent of intercellular calcium wave propagation is related to gap junction permeability and level of connexin-43 expression in astrocytes in primary cultures from four brain regions. Neuroscience 92, 255–265. doi: 10.1016/s0306-4522(98)00738-6
Bloss, E. B., Janssen, W. G., Ohm, D. T., Yuk, F. J., Wadsworth, S., Saardi, K. M., et al. (2011). Evidence for reduced experience-dependent dendritic spine plasticity in the aging prefrontal cortex. J. Neurosci. 31, 7831–7839. doi: 10.1523/JNEUROSCI.0839-11.2011
Boas, G. R. V., de Lacerda, R. B., Paes, M. M., Gubert, P., da Cruz Almeida, W. L., Rescia, V. C., et al. (2019). Molecular aspects of depression: a review from neurobiology to treatment. Eur. J. Pharmacol. 851, 99–121. doi: 10.1016/j.ejphar.2019.02.024
Bowley, M. P., Drevets, W. C., Ongur, D., and Price, J. L. (2002). Low glial numbers in the amygdala in major depressive disorder. Biol. Psychiatry 52, 404–412. doi: 10.1016/s0006-3223(02)01404-x
Bowser, D. N., and Khakh, B. S. (2007). Two forms of single-vesicle astrocyte exocytosis imaged with total internal reflection fluorescence microscopy. Proc. Natl. Acad. Sci. U.S.A. 104, 4212–4217. doi: 10.1073/pnas.0607625104
Bushong, E. A., Martone, M. E., Jones, Y. Z., and Ellisman, M. H. (2002). Protoplasmic astrocytes in CA1 stratum radiatum occupy separate anatomical domains. J. Neurosci. 22, 183–192. doi: 10.1523/jneurosci.22-01-00183.2002
Cai, W., Xue, C., Sakaguchi, M., Konishi, M., Shirazian, A., Ferris, H. A., et al. (2018). Insulin regulates astrocyte gliotransmission and modulates behavior. J. Clin. Investig. 128, 2914–2926. doi: 10.1172/JCI99366
Campbell, M., Fish, B., Shapiro, T., and Floyd, A. (1971). Imipramine in preschool autistic and schizophrenic children. J. Autism Childhood Schizophr. 1, 267–282. doi: 10.1007/bf01557348
Caspi, A., Sugden, K., Moffitt, T. E., Taylor, A., Craig, I. W., Harrington, H., et al. (2003). Influence of life stress on depression: moderation by a polymorphism in the 5-HTT gene. Science 301, 386–389. doi: 10.1126/science.1083968
Chen, N., Sugihara, H., Kim, J., Fu, Z., Barak, B., Sur, M., et al. (2016). Direct modulation of GFAP-expressing glia in the arcuate nucleus bi-directionally regulates feeding. Elife 5:e18716. doi: 10.7554/eLife.18716
Chen, P. S., Peng, G., Li, G., Yang, S., Wu, X., Wang, C., et al. (2006). Valproate protects dopaminergic neurons in midbrain neuron/glia cultures by stimulating the release of neurotrophic factors from astrocytes. Mol. Psychiatry 11:1116. doi: 10.1038/sj.mp.4001893
Choudary, P. V., Molnar, M., Evans, S. J., Tomita, H., Li, J. Z., Vawter, M. P., et al. (2005). Altered cortical glutamatergic and GABAergic signal transmission with glial involvement in depression. Proc. Natl. Acad. Sci. U.S.A. 102, 15653–15658. doi: 10.1073/pnas.0507901102
Christoffel, D. J., Golden, S. A., Dumitriu, D., Robison, A. J., Janssen, W. G., Ahn, H. F., et al. (2011a). IκB kinase regulates social defeat stress-induced synaptic and behavioral plasticity. J. Neurosci. 31, 314–321. doi: 10.1523/JNEUROSCI.4763-10.2011
Christoffel, D. J., Golden, S. A., and Russo, S. J. (2011b). Structural and synaptic plasticity in stress-related disorders. Rev. Neurosci. 22, 535–549. doi: 10.1515/RNS.2011.044
Christopherson, K. S., Ullian, E. M., Stokes, C. C., Mullowney, C. E., Hell, J. W., Agah, A., et al. (2005). Thrombospondins are astrocyte-secreted proteins that promote CNS synaptogenesis. Cell 120, 421–433. doi: 10.1016/j.cell.2004.12.020
Chung, W.-S., Allen, N. J., and Eroglu, C. (2015). Astrocytes control synapse formation, function, and elimination. Cold Spring Harb. Perspect. Biol. 7:a020370. doi: 10.1101/cshperspect.a020370
Cobb, J. A., Simpson, J., Mahajan, G. J., Overholser, J. C., Jurjus, G. J., Dieter, L., et al. (2013). Hippocampal volume and total cell numbers in major depressive disorder. J. Psychiatr. Res. 47, 299–306. doi: 10.1016/j.jpsychires.2012.10.020
Colpo, G. D., Leboyer, M., Dantzer, R., Trivedi, M. H., and Teixeira, A. L. (2018). Immune-based strategies for mood disorders: facts and challenges. Exp. Rev. Neurotherapeut. 18, 139–152. doi: 10.1080/14737175.2018.1407242
Contreras, J. E., Sánchez, H. A., Eugenín, E. A., Speidel, D., Theis, M., Willecke, K., et al. (2002). Metabolic inhibition induces opening of unapposed connexin 43 gap junction hemichannels and reduces gap junctional communication in cortical astrocytes in culture. Proc. Natl. Acad. Sci. U.S.A. 99, 495–500. doi: 10.1073/pnas.012589799
Cotter, D., Mackay, D., Chana, G., Beasley, C., Landau, S., and Everall, I. P. (2002). Reduced neuronal size and glial cell density in area 9 of the dorsolateral prefrontal cortex in subjects with major depressive disorder. Cereb. Cortex 12, 386–394. doi: 10.1093/cercor/12.4.386
Cotter, D., Mackay, D., Landau, S., Kerwin, R., and Everall, I. (2001). Reduced glial cell density and neuronal size in the anterior cingulate cortex in major depressive disorder. Arch. Gen. Psychiatry 58, 545–553.
Cotter, D. R., Pariante, C. M., and Everall, I. P. (2001). Glial cell abnormalities in major psychiatric disorders: the evidence and implications. Brain Res. Bull. 55, 585–595. doi: 10.1016/s0361-9230(01)00527-5
Crane, G. E. (1956). The psychiatric side-effects of iproniazid. Am. J. Psychiatry 112, 494–501. doi: 10.1176/ajp.112.7.494
Danese, A., Moffitt, T. E., Harrington, H., Milne, B. J., Polanczyk, G., Pariante, C. M., et al. (2009). Adverse childhood experiences and adult risk factors for age-related disease: depression, inflammation, and clustering of metabolic risk markers. Arch. Pediatr. Adolesc. Med. 163, 1135–1143. doi: 10.1001/archpediatrics.2009.214
Dantzer, R., O’Connor, J. C., Freund, G. G., Johnson, R. W., and Kelley, K. W. (2008). From inflammation to sickness and depression: when the immune system subjugates the brain. Nat. Rev. Neurosci. 9:46. doi: 10.1038/nrn2297
Darwin, C. (1998). The Expression of the Emotions in Man and Animals. Oxford, MS: Oxford University Press.
Di Castro, M. A., Chuquet, J., Liaudet, N., Bhaukaurally, K., Santello, M., Bouvier, D., et al. (2011). Local Ca2+ detection and modulation of synaptic release by astrocytes. Nat. Neurosci. 14, 1276–1284. doi: 10.1038/nn.2929
Dickens, A. M., Tovar, Y. R. L. B., Yoo, S. W., Trout, A. L., Bae, M., Kanmogne, M., et al. (2017). Astrocyte-shed extracellular vesicles regulate the peripheral leukocyte response to inflammatory brain lesions. Sci. Signal. 10:eaai7696. doi: 10.1126/scisignal.aai7696
Dickson, V. K., Pedi, L., and Long, S. B. (2014). Structure and insights into the function of a Ca 2+-activated Cl- channel. Nature 516:213. doi: 10.1038/nature13913
Domin, H., Szewczyk, B., Wozniak, M., Wawrzak-Wlecial, A., and Smialowska, M. (2014). Antidepressant-like effect of the mGluR5 antagonist MTEP in an astroglial degeneration model of depression. Behav. Brain Res. 273, 23–33. doi: 10.1016/j.bbr.2014.07.019
Duan, S., Anderson, C. M., Keung, E. C., Chen, Y., Chen, Y., and Swanson, R. A. (2003). P2X7 receptor-mediated release of excitatory amino acids from astrocytes. J. Neurosci. 23, 1320–1328. doi: 10.1523/jneurosci.23-04-01320.2003
Duman, C. H., and Duman, R. S. (2015). Spine synapse remodeling in the pathophysiology and treatment of depression. Neurosci. Lett. 601, 20–29. doi: 10.1016/j.neulet.2015.01.022
Duman, R. S., and Monteggia, L. M. (2006). A neurotrophic model for stress-related mood disorders. Biol. Psychiatry 59, 1116–1127. doi: 10.1016/j.biopsych.2006.02.013
Felger, J. C. (2018). Imaging the role of inflammation in mood and anxiety-related disorders. Curr. Neuropharmacol. 16, 533–558. doi: 10.2174/1570159X15666171123201142
Filosa, J. A., and Iddings, J. A. (2013). Astrocyte regulation of cerebral vascular tone. Am. J. Physiol. Heart Circ. Physiol. 305, H609–H619. doi: 10.1152/ajpheart.00359.2013
Gerlach, R., Demel, G., König, H.-G., Gross, U., Prehn, J., Raabe, A., et al. (2006). Active secretion of S100B from astrocytes during metabolic stress. Neuroscience 141, 1697–1701. doi: 10.1016/j.neuroscience.2006.05.008
Giaume, C., and Theis, M. (2010). Pharmacological and genetic approaches to study connexin-mediated channels in glial cells of the central nervous system. Brain Res. Rev. 63, 160–176. doi: 10.1016/j.brainresrev.2009.11.005
Gittins, R. A., and Harrison, P. J. (2011). A morphometric study of glia and neurons in the anterior cingulate cortex in mood disorder. J. Affect. Disord. 133, 328–332. doi: 10.1016/j.jad.2011.03.042
Gorina, R., Font-Nieves, M., Márquez-Kisinousky, L., Santalucia, T., and Planas, A. M. (2011). Astrocyte TLR4 activation induces a proinflammatory environment through the interplay between MyD88-dependent NFκB signaling, MAPK, and Jak1/Stat1 pathways. Glia 59, 242–255. doi: 10.1002/glia.21094
Grazyna Rajkowska, A. H., and Selemon, L. D. (2001). Reductions in neuronal and glial density characterize the dorsolateral prefrontal cortex in bipolar disorder. Biol. Psychiatry 49, 741–752. doi: 10.1016/s0006-3223(01)01080-0
Haim, L. B., and Rowitch, D. H. (2017). Functional diversity of astrocytes in neural circuit regulation. Nat. Rev. Neurosci. 18:31. doi: 10.1038/nrn.2016.159
Han, X., Chen, M., Wang, F., Windrem, M., Wang, S., Shanz, S., et al. (2013). Forebrain engraftment by human glial progenitor cells enhances synaptic plasticity and learning in adult mice. Cell Stem Cell 12, 342–353. doi: 10.1016/j.stem.2012.12.015
Hardingham, G. E., and Bading, H. (2010). Synaptic versus extrasynaptic NMDA receptor signalling: implications for neurodegenerative disorders. Nat. Rev. Neurosci. 11, 682–696. doi: 10.1038/nrn2911
Hardingham, G. E., Fukunaga, Y., and Bading, H. (2002). Extrasynaptic NMDARs oppose synaptic NMDARs by triggering CREB shut-off and cell death pathways. Nat. Neurosci. 5, 405–414. doi: 10.1038/nn835
Haroon, E., Miller, A. H., and Sanacora, G. (2016). Inflammation, glutamate and glia: a trio of trouble in mood disorders. Neuropsychopharmacology 42, 193–215. doi: 10.1038/npp.2016.199
Hepp, R., Perraut, M., Chasserot-Golaz, S., Galli, T., Aunis, D., Langley, K., et al. (1999). Cultured glial cells express the SNAP-25 analogue SNAP-23. Glia 27, 181–187. doi: 10.1002/(sici)1098-1136(199908)27:2<181::aid-glia8>3.0.co;2-9
Hercher, C., Turecki, G., and Mechawar, N. (2009). Through the looking glass: examining neuroanatomical evidence for cellular alterations in major depression. J. Psychiatr. Res. 43, 947–961. doi: 10.1016/j.jpsychires.2009.01.006
Hines, D. J., and Haydon, P. G. (2013). Inhibition of a SNARE-sensitive pathway in astrocytes attenuates damage following stroke. J. Neurosci. 33, 4234–4240. doi: 10.1523/JNEUROSCI.5495-12.2013
Inazu, M., Takeda, H., Ikoshi, H., Sugisawa, M., Uchida, Y., and Matsumiya, T. (2001). Pharmacological characterization and visualization of the glial serotonin transporter. Neurochem. Int. 39, 39–49. doi: 10.1016/s0197-0186(01)00010-9
Inazu, M., Takeda, H., and Matsumiya, T. (2003). Functional expression of the norepinephrine transporter in cultured rat astrocytes. J. Neurochem. 84, 136–144. doi: 10.1046/j.1471-4159.2003.01514.x
Janak, P. H., and Tye, K. M. (2015). From circuits to behaviour in the amygdala. Nature 517:284. doi: 10.1038/nature14188
Ji, R.-R., Samad, T. A., Jin, S.-X., Schmoll, R., and Woolf, C. J. (2002). p38 MAPK activation by NGF in primary sensory neurons after inflammation increases TRPV1 levels and maintains heat hyperalgesia. Neuron 36, 57–68. doi: 10.1016/s0896-6273(02)00908-x
Jones, E. V., Bernardinelli, Y., Tse, Y. C., Chierzi, S., Wong, T. P., and Murai, K. K. (2011). Astrocytes control glutamate receptor levels at developing synapses through SPARC-β-integrin interactions. J. Neurosci. 31, 4154–4165. doi: 10.1523/JNEUROSCI.4757-10.2011
Junker, V., Becker, A., Hühne, R., Zembatov, M., Ravati, A., Culmsee, C., et al. (2002). Stimulation of β-adrenoceptors activates astrocytes and provides neuroprotection. Eur. J. Pharmacol. 446, 25–36. doi: 10.1016/s0014-2999(02)01814-9
Kaufman, J., Yang, B.-Z., Douglas-Palumberi, H., Grasso, D., Lipschitz, D., Houshyar, S., et al. (2006). Brain-derived neurotrophic factor–5-HTTLPR gene interactions and environmental modifiers of depression in children. Biol. Psychiatry 59, 673–680. doi: 10.1016/j.biopsych.2005.10.026
Kessler, R. C. (1997). The effects of stressful life events on depression. Annu. Rev. Psychol. 48, 191–214. doi: 10.1146/annurev.psych.48.1.191
Khan, M. S., Wu, G. W. Y., Reus, V. I., Hough, C. M., Lindqvist, D., Westrin, A., et al. (2019). Low serum brain-derived neurotrophic factor is associated with suicidal ideation in major depressive disorder. Psychiatry Res. 273, 108–113. doi: 10.1016/j.psychres.2019.01.013
Khundakar, A., Morris, C., Oakley, A., and Thomas, A. J. (2011). A morphometric examination of neuronal and glial cell pathology in the orbitofrontal cortex in late-life depression. Int. Psychogeriatr. 23, 132–140. doi: 10.1017/S1041610210000700
Khundakar, A. A., Morris, C. M., Oakley, A. E., and Thomas, A. J. (2011). Cellular pathology within the anterior cingulate cortex of patients with late-life depression: a morphometric study. Psychiatry Res. Neuroimag. 194, 184–189. doi: 10.1016/j.pscychresns.2011.04.008
Khundakar, A. A., and Thomas, A. J. (2009). Morphometric changes in early-and late-life major depressive disorder: evidence from postmortem studies. Int. Psychogeriatr. 21, 844–854. doi: 10.1017/S104161020999007X
Koppel, I., Jaanson, K., Klasche, A., Tuvikene, J., Tiirik, T., Parn, A., et al. (2018). Dopamine cross-reacts with adrenoreceptors in cortical astrocytes to induce BDNF expression, CREB signaling and morphological transformation. Glia 66, 206–216. doi: 10.1002/glia.23238
Koyama, Y. (2015). Functional alterations of astrocytes in mental disorders: pharmacological significance as a drug target. Front. Cell. Neurosci. 9:261. doi: 10.3389/fncel.2015.00261
Lee, S., Yoon, B.-E., Berglund, K., Oh, S.-J., Park, H., Shin, H.-S., et al. (2010). Channel-mediated tonic GABA release from glia. Science 330, 790–796. doi: 10.1126/science.1184334
Li, N., Lee, B., Liu, R.-J., Banasr, M., Dwyer, J. M., Iwata, M., et al. (2010). mTOR-dependent synapse formation underlies the rapid antidepressant effects of NMDA antagonists. Science 329, 959–964. doi: 10.1126/science.1190287
Li, Y.-K., Wang, F., Wang, W., Luo, Y., Wu, P.-F., Xiao, J.-L., et al. (2012). Aquaporin-4 deficiency impairs synaptic plasticity and associative fear memory in the lateral amygdala: involvement of downregulation of glutamate transporter-1 expression. Neuropsychopharmacology 37:1867. doi: 10.1038/npp.2012.34
Liddelow, S., and Barres, B. (2015). SnapShot: astrocytes in health and disease. Cell 162, 1170.e1–1170.e1. doi: 10.1016/j.cell.2015.08.029
Lin, C.-C. J., Yu, K., Hatcher, A., Huang, T.-W., Lee, H. K., Carlson, J., et al. (2017). Identification of diverse astrocyte populations and their malignant analogs. Nat. Neurosci. 20:396. doi: 10.1038/nn.4493
Lindsay, R. M. (1979). Adult rat brain astrocytes support survival of both NGF-dependent and NGF-insensitive neurones. Nature 282, 80–82. doi: 10.1038/282080a0
Lipska, B. K., Deep-Soboslay, A., Weickert, C. S., Hyde, T. M., Martin, C. E., Herman, M. M., et al. (2006). Critical factors in gene expression in postmortem human brain: focus on studies in schizophrenia. Biol. Psychiatry 60, 650–658. doi: 10.1016/j.biopsych.2006.06.019
Liu, R.-J., and Aghajanian, G. K. (2008). Stress blunts serotonin-and hypocretin-evoked EPSCs in prefrontal cortex: role of corticosterone-mediated apical dendritic atrophy. Proc. Natl. Acad. Sci. U.S.A. 105, 359–364. doi: 10.1073/pnas.0706679105
Ma, Z., Stork, T., Bergles, D. E., and Freeman, M. R. (2016). Neuromodulators signal through astrocytes to alter neural circuit activity and behaviour. Nature 539:428. doi: 10.1038/nature20145
Machler, P., Wyss, M. T., Elsayed, M., Stobart, J., Gutierrez, R., von Faber-Castell, A., et al. (2016). In vivo evidence for a lactate gradient from astrocytes to neurons. Cell Metab. 23, 94–102. doi: 10.1016/j.cmet.2015.10.010
Magariños, A. M., Verdugo, J. M. G., and McEwen, B. S. (1997). Chronic stress alters synaptic terminal structure in hippocampus. Proc. Natl. Acad. Sci. U.S.A. 94, 14002–14008. doi: 10.1073/pnas.94.25.14002
Magistretti, P. J. (2006). Neuron-glia metabolic coupling and plasticity. J. Exp. Biol. 209, 2304–2311. doi: 10.1242/jeb.02208
Martín, R., Bajo-Graneras, R., Moratalla, R., Perea, G., and Araque, A. (2015). Circuit-specific signaling in astrocyte-neuron networks in basal ganglia pathways. Science 349, 730–734. doi: 10.1126/science.aaa7945
Martin-Fernandez, M., Jamison, S., Robin, L. M., Zhao, Z., Martin, E. D., Aguilar, J., et al. (2017). Synapse-specific astrocyte gating of amygdala-related behavior. Nat. Neurosci. 20:1540. doi: 10.1038/nn.4649
McEwen, B. S., Nasca, C., and Gray, J. D. (2016). Stress effects on neuronal structure: hippocampus, amygdala, and prefrontal cortex. Neuropsychopharmacology 41, 3–23. doi: 10.1038/npp.2015.171
McNally, L., Bhagwagar, Z., and Hannestad, J. (2008). Inflammation, glutamate, and glia in depression: a literature review. CNS Spectr. 13, 501–510. doi: 10.1017/s1092852900016734
Miguel-Hidalgo, J. J., Baucom, C., Dilley, G., Overholser, J. C., Meltzer, H. Y., Stockmeier, C. A., et al. (2000). Glial fibrillary acidic protein immunoreactivity in the prefrontal cortex distinguishes younger from older adults in major depressive disorder. Biol. Psychiatry 48, 861–873. doi: 10.1016/s0006-3223(00)00999-9
Milak, M. S., Proper, C. J., Mulhern, S. T., Parter, A. L., Kegeles, L. S., Ogden, R. T., et al. (2016). A pilot in vivo proton magnetic resonance spectroscopy study of amino acid neurotransmitter response to ketamine treatment of major depressive disorder. Mol. Psychiatry 21, 320–327. doi: 10.1038/mp.2015.83
Mineka, S., Watson, D., and Clark, L. A. (1998). Comorbidity of anxiety and unipolar mood disorders. Annu. Rev. Psychol. 49, 377–412. doi: 10.1146/annurev.psych.49.1.377
Moench, K. M., and Wellman, C. L. (2017). Differential dendritic remodeling in prelimbic cortex of male and female rats during recovery from chronic stress. Neuroscience 357, 145–159. doi: 10.1016/j.neuroscience.2017.05.049
Molina, V., Volosin, M., Cancela, L., Keller, E., Murua, V., and Basso, A. (1990). Effect of chronic variable stress on monoamine receptors: influence of imipramine administration. Pharmacol. Biochem. Behav. 35, 335–340. doi: 10.1016/0091-3057(90)90165-e
Müller, M. B., Lucassen, P. J., Yassouridis, A., Hoogendijk, W. J., Holsboer, F., and Swaab, D. F. (2001). Neither major depression nor glucocorticoid treatment affects the cellular integrity of the human hippocampus. Eur. J. Neurosci. 14, 1603–1612. doi: 10.1046/j.0953-816x.2001.01784.x
Nadjar, A., Blutstein, T., Aubert, A., Laye, S., and Haydon, P. G. (2013). Astrocyte-derived adenosine modulates increased sleep pressure during inflammatory response. Glia 61, 724–731. doi: 10.1002/glia.22465
Nestler, E. J., Barrot, M., DiLeone, R. J., Eisch, A. J., Gold, S. J., and Monteggia, L. M. (2002). Neurobiology of depression. Neuron 34, 13–25.
Newport, D. J., Carpenter, L. L., McDonald, W. M., Potash, J. B., Tohen, M., Nemeroff, C. B., et al. (2015). Ketamine and other NMDA antagonists: early clinical trials and possible mechanisms in depression. Am. J. Psychiatry 172, 950–966. doi: 10.1176/appi.ajp.2015.15040465
Nico, B., Frigeri, A., Nicchia, G. P., Quondamatteo, F., Herken, R., Errede, M., et al. (2001). Role of aquaporin-4 water channel in the development and integrity of the blood-brain barrier. J. Cell Sci. 114, 1297–1307.
Nimchinsky, E. A., Sabatini, B. L., and Svoboda, K. (2002). Structure and function of dendritic spines. Annu. Rev. Physiol. 64, 313–353.
Norenberg, M. D. (1979). Distribution of glutamine synthetase in the rat central nervous system. J. Histochem. Cytochem. 27, 756–762. doi: 10.1177/27.3.39099
Norenberg, M. D., and Martinez-Hernandez, A. (1979). Fine structural localization of glutamine synthetase in astrocytes of rat brain. Brain Res. 161, 303–310. doi: 10.1016/0006-8993(79)90071-4
Oberheim, N. A., Takano, T., Han, X., He, W., Lin, J. H., Wang, F., et al. (2009). Uniquely hominid features of adult human astrocytes. J. Neurosci. 29, 3276–3287. doi: 10.1523/JNEUROSCI.4707-08.2009
Ogilvie, C. (1955). The treatment of pulmonary tuberculosis with iproniazid (1-isonicotinyl-2-isopropyl hydrazine) and isoniazid (isonicotinyl hydrazine). Quart. J. Med. 24, 175–189.
Oh, S.-J., and Lee, C. J. (2017). Distribution and function of the bestrophin-1 (Best1) channel in the brain. Exp. Neurobiol. 26, 113–121. doi: 10.5607/en.2017.26.3.113
Öngür, D., Drevets, W. C., and Price, J. L. (1998). Glial reduction in the subgenual prefrontal cortex in mood disorders. Proc. Natl. Acad. Sci. U.S.A. 95, 13290–13295. doi: 10.1073/pnas.95.22.13290
Panatier, A., Vallée, J., Haber, M., Murai, K. K., Lacaille, J.-C., and Robitaille, R. (2011). Astrocytes are endogenous regulators of basal transmission at central synapses. Cell 146, 785–798. doi: 10.1016/j.cell.2011.07.022
Papouin, T., Dunphy, J. M., Tolman, M., Dineley, K. T., and Haydon, P. G. (2017). Septal cholinergic neuromodulation tunes the astrocyte-dependent gating of hippocampal NMDA receptors to wakefulness. Neuron 94, 840.e7–854.e7. doi: 10.1016/j.neuron.2017.04.021
Paradise, M. B., Naismith, S. L., Norrie, L. M., Graeber, M. B., and Hickie, I. B. (2012). The role of glia in late-life depression. Int. Psychogeriatr. 24, 1878–1890. doi: 10.1017/S1041610212000828
Parpura, V., Baker, B. J., Jeras, M., and Zorec, R. (2010). Regulated exocytosis in astrocytic signal integration. Neurochem. Int. 57, 451–459. doi: 10.1016/j.neuint.2010.02.007
Parpura, V., Basarsky, T. A., Liu, F., Jeftinija, K., Jeftinija, S., and Haydon, P. G. (1994). Glutamate-mediated astrocyte-neuron signalling. Nature 369, 744–747. doi: 10.1038/369744a0
Parpura, V., and Verkhratsky, A. (2012). Homeostatic function of astrocytes: Ca(2+) and Na(+) signalling. Transl. Neurosci. 3, 334–344.
Pascual, O., Casper, K. B., Kubera, C., Zhang, J., Revilla-Sanchez, R., Sul, J.-Y., et al. (2005). Astrocytic purinergic signaling coordinates synaptic networks. Science 310, 113–116. doi: 10.1126/science.1116916
Perea, G., Navarrete, M., and Araque, A. (2009). Tripartite synapses: astrocytes process and control synaptic information. Trends Neurosci. 32, 421–431. doi: 10.1016/j.tins.2009.05.001
Perea, G., Yang, A., Boyden, E. S., and Sur, M. (2014). Optogenetic astrocyte activation modulates response selectivity of visual cortex neurons in vivo. Nat. Commun. 5:3262. doi: 10.1038/ncomms4262
Perez-Alvarez, A., and Araque, A. (2013). Astrocyte-neuron interaction at tripartite synapses. Curr. Drug Targets 14, 1220–1224. doi: 10.2174/13894501113149990203
Pisoni, A., Strawbridge, R., Hodsoll, J., Powell, T. R., Breen, G., Hatch, S., et al. (2018). Growth factor proteins and treatment-resistant depression: a place on the path to precision. Front. Psychiatry 9:386. doi: 10.3389/fpsyt.2018.00386
Porter, J. T., and Mccarthy, K. D. (1997). Astrocytic neurotransmitter receptors in situ and in vivo. Progr. Neurobiol. 51, 439–455. doi: 10.1016/s0301-0082(96)00068-8
Qiao, H., An, S.-C., Ren, W., and Ma, X.-M. (2014). Progressive alterations of hippocampal CA3-CA1 synapses in an animal model of depression. Behav. Brain Res. 275, 191–200. doi: 10.1016/j.bbr.2014.08.040
Qiao, H., Li, M. X., Xu, C., Chen, H. B., An, S. C., and Ma, X. M. (2016). Dendritic spines in depression: what we learned from animal models. Neural Plast. 2016:8056370. doi: 10.1155/2016/8056370
Quesseveur, G., David, D. J., Gaillard, M. C., Pla, P., Wu, M. V., Nguyen, H. T., et al. (2013). BDNF overexpression in mouse hippocampal astrocytes promotes local neurogenesis and elicits anxiolytic-like activities. Transl. Psychiatry 3:e253. doi: 10.1038/tp.2013.30
Radley, J. J., Rocher, A. B., Rodriguez, A., Ehlenberger, D. B., Dammann, M., McEwen, B. S., et al. (2008). Repeated stress alters dendritic spine morphology in the rat medial prefrontal cortex. J. Compar. Neurol. 507, 1141–1150. doi: 10.1002/cne.21588
Rajkowska, G., Hughes, J., Stockmeier, C. A., Miguel-Hidalgo, J. J., and Maciag, D. (2013). Coverage of blood vessels by astrocytic endfeet is reduced in major depressive disorder. Biol. Psychiatry 73, 613–621. doi: 10.1016/j.biopsych.2012.09.024
Rajkowska, G., and Miguel-Hidalgo, J. J. (2007). Gliogenesis and glial pathology in depression. CNS Neurol. Disord. Drug Targets 6, 219–233. doi: 10.2174/187152707780619326
Rajkowska, G., Miguel-Hidalgo, J. J., Wei, J., Dilley, G., Pittman, S. D., Meltzer, H. Y., et al. (1999). Morphometric evidence for neuronal and glial prefrontal cell pathology in major depression. Biol. Psychiatry 45, 1085–1098. doi: 10.1016/s0006-3223(99)00041-4
Rajkowska, G., and Stockmeier, C. A. (2013). Astrocyte pathology in major depressive disorder: insights from human postmortem brain tissue. Curr. Drug Targets 14, 1225–1236. doi: 10.2174/13894501113149990156
Rossi, D. J., Oshima, T., and Attwell, D. (2000). Glutamate release in severe brain ischaemia is mainly by reversed uptake. Nature 403, 316–321. doi: 10.1038/35002090
Rothermundt, M., Peters, M., Prehn, J. H., and Arolt, V. (2003). S100B in brain damage and neurodegeneration. Microsc. Res. Tech. 60, 614–632. doi: 10.1002/jemt.10303
Rothstein, J. D., Martin, L., Levey, A. I., Dykes-Hoberg, M., Jin, L., Wu, D., et al. (1994). Localization of neuronal and glial glutamate transporters. Neuron 13, 713–725. doi: 10.1016/0896-6273(94)90038-8
Sadock, B. J., and Sadock, V. A. (2011). Kaplan and Sadock’s Synopsis of Psychiatry: Behavioral Sciences/Clinical Psychiatry. Philadelphia, PA: Lippincott Williams & Wilkins.
Sahlender, D. A., Savtchouk, I., and Volterra, A. (2014). What do we know about gliotransmitter release from astrocytes? Philos. Trans. R. Soc. Lond. B Biol. Sci. 369:20130592. doi: 10.1098/rstb.2013.0592
Salvadore, G., van der Veen, J. W., Zhang, Y., Marenco, S., Machado-Vieira, R., Baumann, J., et al. (2012). An investigation of amino-acid neurotransmitters as potential predictors of clinical improvement to ketamine in depression. Int. J. Neuropsychopharmacol. 15, 1063–1072. doi: 10.1017/S1461145711001593
Sapena, R., Morin, D., Zini, R., Morin, C., and Tillement, J.-P. (1996). Desipramine treatment differently down-regulates β-adrenoceptors of freshly isolated neurons and astrocytes. Eur. J. Pharmacol. 300, 159–162. doi: 10.1016/0014-2999(96)00060-x
Saraf, K. R., Klein, D. F., Gittelman-Klein, R., and Groff, S. (1974). Imipramine side effects in children. Psychopharmacologia 37, 265–274. doi: 10.1007/bf00421540
Schousboe, A., Scafidi, S., Bak, L. K., Waagepetersen, H. S., and McKenna, M. C. (2014). “Glutamate metabolism in the brain focusing on astrocytes,” in Glutamate and ATP at the Interface of Metabolism and Signaling in the Brain, eds V. Parpura, A. Schousboe, and A. Verkhratsky (Berlin: Springer), 13–30. doi: 10.1007/978-3-319-08894-5_2
Schousboe, A., Sonnewald, U., Civenni, G., and Gegelashvili, G. (1997). Role of astrocytes in glutamate homeostasis. Implications for excitotoxicity. Adv. Exp. Med. Biol. 429, 195–206. doi: 10.1007/978-1-4757-9551-6_14
Schroeter, M. L., Abdul-Khaliq, H., Diefenbacher, A., and Blasig, I. E. (2002). S100B is increased in mood disorders and may be reduced by antidepressive treatment. Neuroreport 13, 1675–1678. doi: 10.1097/00001756-200209160-00021
Schubert, V., Bouvier, D., and Volterra, A. (2011). SNARE protein expression in synaptic terminals and astrocytes in the adult hippocampus: a comparative analysis. Glia 59, 1472–1488. doi: 10.1002/glia.21190
Sequeira, A., Mamdani, F., Ernst, C., Vawter, M. P., Bunney, W. E., Lebel, V., et al. (2009). Global brain gene expression analysis links glutamatergic and GABAergic alterations to suicide and major depression. PLoS One 4:e6585. doi: 10.1371/journal.pone.0006585
Shansky, R. M., Hamo, C., Hof, P. R., McEwen, B. S., and Morrison, J. H. (2009). Stress-induced dendritic remodeling in the prefrontal cortex is circuit specific. Cereb. Cortex 19, 2479–2484. doi: 10.1093/cercor/bhp003
Shimizu, M., Nishida, A., Zensho, H., and Yamawaki, S. (1996). Chronic antidepressant exposure enhances 5-hydroxytryptamine7 receptor-mediated cyclic adenosine monophosphate accumulation in rat frontocortical astrocytes. J. Pharmacol. Exp. Therapeut. 279, 1551–1558.
Singh, S. K., Stogsdill, J. A., Pulimood, N. S., Dingsdale, H., Kim, Y. H., Pilaz, L.-J., et al. (2016). Astrocytes assemble thalamocortical synapses by bridging NRX1α and NL1 via hevin. Cell 164, 183–196. doi: 10.1016/j.cell.2015.11.034
Siris, S. G., Bermanzohn, P. C., Mason, S. E., and Shuwall, M. A. (1994). Maintenance imipramine therapy for secondary depression in schizophrenia: a controlled trial. Arch. Gen. Psychiatry 51, 109–115.
Skolnick, P., Layer, R., Popik, P., Nowak, G., Paul, I., and Trullas, R. (1996). Adaptation of N-methyl-D-aspartate NMDA receptors following antidepressant treatment: implications for the pharmacotherapy of depression. Pharmacopsychiatry 29, 23–26. doi: 10.1055/s-2007-979537
Slezak, M., Grosche, A., Niemiec, A., Tanimoto, N., Pannicke, T., Münch, T. A., et al. (2012). Relevance of exocytotic glutamate release from retinal glia. Neuron 74, 504–516. doi: 10.1016/j.neuron.2012.03.027
Smith, T. E., Weissbach, H., and Udenfriend, S. (1963). Studies on monoamine oxidase: the mechanism of inhibition of monoamine oxidase by iproniazid. Biochemistry 2, 746–751. doi: 10.1021/bi00904a021
Song, H., Stevens, C. F., and Gage, F. H. (2002). Astroglia induce neurogenesis from adult neural stem cells. Nature 417:39. doi: 10.1038/417039a
Sonnewald, U., Westergaard, N., and Schousboe, A. (1997). Glutamate transport and metabolism in astrocytes. Glia 21, 56–63. doi: 10.1002/(sici)1098-1136(199709)21:1<56::aid-glia6>3.0.co;2-#
Stellwagen, D., and Malenka, R. C. (2006). Synaptic scaling mediated by glial TNF-α. Nature 440:1054. doi: 10.1038/nature04671
Stogsdill, J. A., and Eroglu, C. (2017). The interplay between neurons and glia in synapse development and plasticity. Curr. Opin. Neurobiol. 42, 1–8. doi: 10.1016/j.conb.2016.09.016
Stout, C. E., Costantin, J. L., Naus, C. C., and Charles, A. C. (2002). Intercellular calcium signaling in astrocytes via ATP release through connexin hemichannels. J. Biol. Chem. 277, 10482–10488. doi: 10.1074/jbc.m109902200
Stranna, A., Røe,ÅT., Morland, C., Rinholm, J. E., Wold, J. F. H., Ormel, L., et al. (2011). Immunogold detection of L-glutamate and D-serine in small synaptic-like microvesicles in adult hippocampal astrocytes. Cereb. Cortex 22, 1690–1697. doi: 10.1093/cercor/bhr254
Sun, J. D., Liu, Y., Yuan, Y. H., Li, J., and Chen, N. H. (2012). Gap junction dysfunction in the prefrontal cortex induces depressive-like behaviors in rats. Neuropsychopharmacology 37, 1305–1320. doi: 10.1038/npp.2011.319
Sur, C., Betz, H., and Schloss, P. (1996). Immunocytochemical detection of the serotonin transporter in rat brain. Neuroscience 73, 217–231. doi: 10.1016/0306-4522(96)00030-9
Tan, Z., Liu, Y., Xi, W., Lou, H.-F., Zhu, L., Guo, Z., et al. (2017). Glia-derived ATP inversely regulates excitability of pyramidal and CCK-positive neurons. Nat. Commun. 8:13772. doi: 10.1038/ncomms13772
Thase, M. E., Frank, E., Mallinger, A. G., Hamer, T., and Kupfer, D. (1992). Treatment of imipramine-resistant recurrent depression: III. Efficacy of monoamine oxidase inhibitors. J. Clin. Psychiatry 53, 5–11.
Tissot, R. (1975). The common pathophysiology of monoaminergic psychoses: a new hypothesis. Neuropsychobiology 1, 243–260. doi: 10.1159/000117498
Torres-Platas, S. G., Hercher, C., Davoli, M. A., Maussion, G., Labonté, B., Turecki, G., et al. (2011). Astrocytic hypertrophy in anterior cingulate white matter of depressed suicides. Neuropsychopharmacology 36:2650. doi: 10.1038/npp.2011.154
Traynelis, S. F., Wollmuth, L. P., McBain, C. J., Menniti, F. S., Vance, K. M., Ogden, K. K., et al. (2010). Glutamate receptor ion channels: structure, regulation, and function. Pharmacol. Rev. 62, 405–496. doi: 10.1124/pr.109.002451
Turner, J. R., Ecke, L. E., Briand, L. A., Haydon, P. G., and Blendy, J. A. (2013). Cocaine-related behaviors in mice with deficient gliotransmission. Psychopharmacology 226, 167–176. doi: 10.1007/s00213-012-2897-4
Vaisey, G., Miller, A. N., and Long, S. B. (2016). Distinct regions that control ion selectivity and calcium-dependent activation in the bestrophin ion channel. Proc. Natl. Acad. Sci. U.S.A. 113, E7399–E7408.
Virginio, C., MacKenzie, A., Rassendren, F. A., North, R. A., and Surprenant, A. (1999). Pore dilation of neuronal P2X receptor channels. Nat. Neurosci. 2:315. doi: 10.1038/7225
Volterra, A., and Meldolesi, J. (2005). Astrocytes, from brain glue to communication elements: the revolution continues. Nat. Rev. Neurosci. 6, 626–640. doi: 10.1038/nrn1722
Vyas, A., Jadhav, S., and Chattarji, S. (2006). Prolonged behavioral stress enhances synaptic connectivity in the basolateral amygdala. Neuroscience 143, 387–393. doi: 10.1016/j.neuroscience.2006.08.003
Walz, W., and Hertz, L. (1983). Functional interactions between neurons and astrocytes. II. Potassium homeostasis at the cellular level. Progr. Neurobiol. 20, 133–183. doi: 10.1016/0301-0082(83)90013-8
Watson, D. (2005). Rethinking the mood and anxiety disorders: a quantitative hierarchical model for DSM-V. J. Abnorm. Psychol. 114:522. doi: 10.1037/0021-843x.114.4.522
Webster, M., Knable, M., Johnston-Wilson, N., Nagata, K., Inagaki, M., and Yolken, R. (2001). Immunohistochemical localization of phosphorylated glial fibrillary acidic protein in the prefrontal cortex and hippocampus from patients with schizophrenia, bipolar disorder, and depression. Brain Behav. Immun. 15, 388–400. doi: 10.1006/brbi.2001.0646
Whitaker-Azmitia, P. M., Clarke, C., and Azmitia, E. C. (1993). Localization of 5-HT1A receptors to astroglial cells in adult rats: implications for neuronal-glial interactions and psychoactive drug mechanism of action. Synapse 14, 201–205. doi: 10.1002/syn.890140303
Woo, D. H., Han, K.-S., Shim, J. W., Yoon, B.-E., Kim, E., Bae, J. Y., et al. (2012). TREK-1 and Best1 channels mediate fast and slow glutamate release in astrocytes upon GPCR activation. Cell 151, 25–40. doi: 10.1016/j.cell.2012.09.005
World Health Organization [WHO] (2017). Depression and Other Common Mental Disorders: Global Health Estimates. Geneva: World Health Organization.
Ye, Z.-C., Wyeth, M. S., Baltan-Tekkok, S., and Ransom, B. R. (2003). Functional hemichannels in astrocytes: a novel mechanism of glutamate release. J. Neurosci. 23, 3588–3596. doi: 10.1523/jneurosci.23-09-03588.2003
Zeng, X.-N., Sun, X.-L., Gao, L., Fan, Y., Ding, J.-H., and Hu, G. (2007). Aquaporin-4 deficiency down-regulates glutamate uptake and GLT-1 expression in astrocytes. Mol. Cell. Neurosci. 34, 34–39. doi: 10.1016/j.mcn.2006.09.008
Keywords: depression, anxiety disorder, neuron, gliotransmitters, astrocytes
Citation: Zhou X, Xiao Q, Xie L, Yang F, Wang L and Tu J (2019) Astrocyte, a Promising Target for Mood Disorder Interventions. Front. Mol. Neurosci. 12:136. doi: 10.3389/fnmol.2019.00136
Received: 11 March 2019; Accepted: 09 May 2019;
Published: 05 June 2019.
Edited by:
Julie A. Chowen, Hospital Infantil Universitario Niño Jesús, SpainReviewed by:
Gertrudis Perea, Cajal Institute (CSIC), SpainCopyright © 2019 Zhou, Xiao, Xie, Yang, Wang and Tu. This is an open-access article distributed under the terms of the Creative Commons Attribution License (CC BY). The use, distribution or reproduction in other forums is permitted, provided the original author(s) and the copyright owner(s) are credited and that the original publication in this journal is cited, in accordance with accepted academic practice. No use, distribution or reproduction is permitted which does not comply with these terms.
*Correspondence: Jie Tu, amllLnR1QHNpYXQuYWMuY24=
†These authors have contributed equally to this work
Disclaimer: All claims expressed in this article are solely those of the authors and do not necessarily represent those of their affiliated organizations, or those of the publisher, the editors and the reviewers. Any product that may be evaluated in this article or claim that may be made by its manufacturer is not guaranteed or endorsed by the publisher.
Research integrity at Frontiers
Learn more about the work of our research integrity team to safeguard the quality of each article we publish.