- Laboratory of Neuroepigenetics, Brain Mind Institute, School of Life Sciences, École Polytechnique Fédérale de Lausanne (EPFL), Lausanne, Switzerland
Whether the attenuation of traumatic memories is mediated through the suppression of the original memory trace of fear by a new memory trace of safety, or through an updating of the original fear trace towards safety has been a long-standing question at the interface of neuroscience and psychology. This matter is of particular importance for remote fear memories as they lie at the core of stress- and anxiety-related disorders. Recently, we have found that in the dentate gyrus, the effective attenuation of remote fear memories is accompanied by a reactivation of memory recall-induced neurons and that the continued activity of these neurons is critical for fear reduction. However, whether this also applies to other brain areas implicated in the storage of remote fear memories remains to be determined. Here, we show—by cellular compartment analysis of temporal activity using fluorescence in situ hybridization—that such reactivation also occurs in the basolateral amygdala and the infralimbic cortex, two brain areas known to be involved in fear memory attenuation. These results provide further experimental support for effective traumatic memory attenuation likely being mediated by an updating of the original fear trace towards safety.
Introduction
Post-traumatic stress and other anxiety disorders range among the most enduring forms of memories. Remembrances of traumata months later in rodents (Debiec et al., 2002; Frankland et al., 2006) and years after the original insult in humans are commonplace (Ringburg et al., 2011; Haagsma et al., 2012). The lifetime prevalence of post-traumatic stress disorder (PTSD) in the general population is estimated at 7% (Kessler et al., 2005), and this number at least quadruples among individuals having suffered severe traumata such as war or sexual assault (Davidson et al., 2004; Javidi and Yadollahie, 2012). Because of the persistent nature of traumatic memories, early interventions are considered of prime importance (Davidson et al., 2004; Kearns et al., 2012). Yet, such interventions are oftentimes not readily available, which places a strong emphasis on better understanding treatment approaches for remote traumata (McCleery and Harvey, 2004; Centonze et al., 2005).
Among the most effective treatments for traumatic memories are exposure-based therapies (Foa and Kozak, 1986; Foa, 2000). In these therapies, patients are repeatedly confronted with the trauma-eliciting stimulus in a safe environment, with the premise that the fear associated with this stimulus will eventually subside. On the one hand, such repetitive re-exposure is thought to induce the formation of a new memory trace of safety, one that suppresses the original memory trace of fear, and thus leads to the extinction of the original fear memory (Bouton, 2004; Myers and Davis, 2007; Quirk and Mueller, 2008; Pape and Pare, 2010). Indeed, several studies have shown that during memory extinction of an aversive tone, a different set of neuronal subpopulations in the basolateral nucleus of the amygdala (BLA) is recruited than for its initial memory formation (Herry et al., 2008; Ehrlich et al., 2009; Trouche et al., 2013). Such suppression is likely to be mediated by inhibitory circuits projecting from the infralimbic (IL) region of the prefrontal cortex, whereas the expression of the original fear memory was shown to depend on excitatory projections from the prelimbic (PL) area of the prefrontal cortex (Herry et al., 2008; Ehrlich et al., 2009; Maren et al., 2013).
On the other hand, the cellular mechanisms of exposure-based therapies may also be mediated by a process referred to as reconsolidation-updating (Tronson and Taylor, 2007; Monfils et al., 2009; Nader and Hardt, 2009; Schiller et al., 2010; Clem and Schiller, 2016). Each time a memory is being recalled, it enters a period of lability (Misanin et al., 1968; Nader et al., 2000), the so-called reconsolidation window. This time-limited window is thought to allow the reactivated memory to incorporate new information pertinent to the present environmental contingencies that might no longer be the same as at the time of encoding (Dudai, 2006; Hupbach et al., 2007; Tronson and Taylor, 2007; Lee, 2008; Nader and Hardt, 2009; McKenzie and Eichenbaum, 2011). Thereby, memory reconsolidation helps the memory to be either maintained—when similar situations are encountered at learning and recall, strengthened—when a higher valence is encountered at recall, or weakened—when a lower valence is encountered at recall (Sandrini et al., 2015; Clem and Schiller, 2016). This third scenario is ideally suited to incorporate safe or non fear-eliciting information into a fearful memory trace so that its fear component is updated towards one of safety and no longer persists in its original form (Parsons and Ressler, 2013; Sandrini et al., 2015).
Surprisingly, the vast majority of studies aimed at deciphering extinction from reconsolidation-updating processes have been conducted for 1 day-old fear memories, leaving it unclear which of these mechanisms takes place when remote fear memories are being attenuated. Recently, we showed that reconsolidation-updating mechanisms are critically involved in the attenuation of remote traumatic memories (Khalaf et al., 2018). Focusing on the hippocampus because of its documented re-engagement upon remote memory recall (Debiec et al., 2002; Goshen et al., 2011; Gräff et al., 2014), we demonstrated that the reactivation of recall-induced neurons in the dentate gyrus (DG) not only accompanied behavioral attenuation of a 4-week-old fear memory, but that the continued activity of recall-induced neurons is necessary for memory attenuation (Khalaf et al., 2018).
Notwithstanding, whether similar processes also occur in other brain areas remains unexplored. Given the more distributed nature of remote contextual fear memory storage, which involves areas of the prefrontal cortex as well as of the amygdala (Frankland and Bontempi, 2005; Wheeler et al., 2013; Kitamura et al., 2017; Albo and Gräff, 2018; Silva et al., 2019; Zhou et al., 2018), this question is of considerable interest.
Materials and Methods
Animals
Wild-type C57BL/6 male mice were used. All mice had food and water ad libitum. Mice were at least 10–12 week old at the start of the experiments. All animal experimentations were done and approved under the cantonal veterinary authority in Switzerland (VD2808 and VD2808.1).
Behavioral Paradigms
Contextual Fear Conditioning (CFC)
Animals were acclimatized for 2 days to handling several times a day. Contextual fear conditioning (CFC) training consisted of a 3-min habituation of the mice to the conditioning chamber (TSE systems) followed by three 2 s foot shocks (0.8 mA) with an intertrial interval of 28 s. After the shocks, the animals remained in the chamber for an additional 15 s. Three weeks later (spent in the home cage, during which animals were monitored for their overall health), the massed fear extinction paradigm was carried out. The home cage control group consisted of mice being exposed to the CFC, but without a recall nor the massed extinction session.
Massed Extinction
Animals were re-exposed to the conditioning chamber for 3 min without receiving the foot shock (to recall the memory), and returned to their home cage for 45 min, after which they were once again exposed to the training chamber for a total of 18 min. Extinction memory (EM) was tested by a 3-min context exposure 24 h after the last extinction trial.
Cellular Compartment Analysis of Temporal Activity by Fluorescence in situ Hybridization (catFISH)
C57Bl6/J mice were contextually fear conditioned and tested for the memory 21 days later. Forty-five minutes following the recall session, the animals underwent the massed extinction paradigm, after which they were sacrificed at specific timepoints by cervical dislocation, and their brains were extracted and snap frozen directly using isopentane and dry-ice. For the home cage control group, animals were taken out of their home cage and sacrificed straightaway. All brains were cut with a cryostat (CM3050S, Leica Biosystems), and the coronal slices (20 μm) were attached on charged super frost plus slides (Thermo Fisher). The in situ hybridization was carried out at the EPFL histology core facility following the manufacturer’s protocol of the RNA probes (RNAscope, ACDBio). Two RNA probes were used against the immediate early gene (IEG) markers Homer1a (H1a), and cFos. The H1a probe was conjugated with Alexa Flour488 fluorophore, whereas the cFos probe was conjugated with Atto550. For each slide used for catFISH, an internal control was performed to detect the housekeeping gene Ppib and the bacterial gene Dapb, which served as positive and negative control, respectively.
Image Acquisition and Quantification
Images were acquired using a Zeiss LSM700 laser scanning confocal microscope. Four different brain slices from different animals [six for the conditioned stimulus (CS)-unconditioned stimulus (US), and four for the home cage control, respectively] were quantified. The images were acquired with a frame size of 1,024 × 1,024 pixels using tiling mode and a 40× oil-immersion objective to achieve the highest resolution. The cells were counted with the cell counter plugin of Fiji. H1a and cFos positive cells were quantified in their corresponding separate channels, and then both channels were overlapped with their markers to identify the double positive population. The rates were calculated according to the formulas below.
Statistics
Statistical analysis was done using Prism 6.0 (Graph Pad) as described in the figure legends. All t-tests were two-tailed unless otherwise indicated, and the level of significance (alpha) was set at p < 0.05.
Results
In order to investigate the cellular processes of remote fear memory attenuation in brain areas other than the hippocampus, we used a previously described massed extinction paradigm in mice (Khalaf et al., 2018; Figure 1A), which effectively reduces remote fear memories (Figure 1B, Supplementary Figure S1). This paradigm consists of the repeated exposure (6 × 3 min) of the animals to the context, which was paired with the foot shock by CFC 3 weeks earlier (Figure 1A). In parallel, we employed catFISH to harvest the intracellular spatiotemporal characteristics of different IEG mRNA species (Guzowski et al., 1999; Nonaka et al., 2014). Five minutes after the last extinction session, we identified neuronal populations activated at remote fear memory recall by the presence of cytoplasmic Homer1a mRNA transcripts (which appear 75 min after bouts of neuronal activity), while the neuronal populations activated by extinction were visualized with nuclear cFos mRNA transcripts (which remain nucleus-bound for 5 min after neuronal activity; Figure 1A). With this tool, we assessed extra-hippocampal brain areas implicated in remote memory storage, namely the amygdala and the prefrontal cortex (Kitamura et al., 2017; Figure 1C), more precisely the BLA and central amygdala (CeA; Figure 1D), as well as the anterior cingulate cortex (ACC), the PL and the IL (Figure 1E).
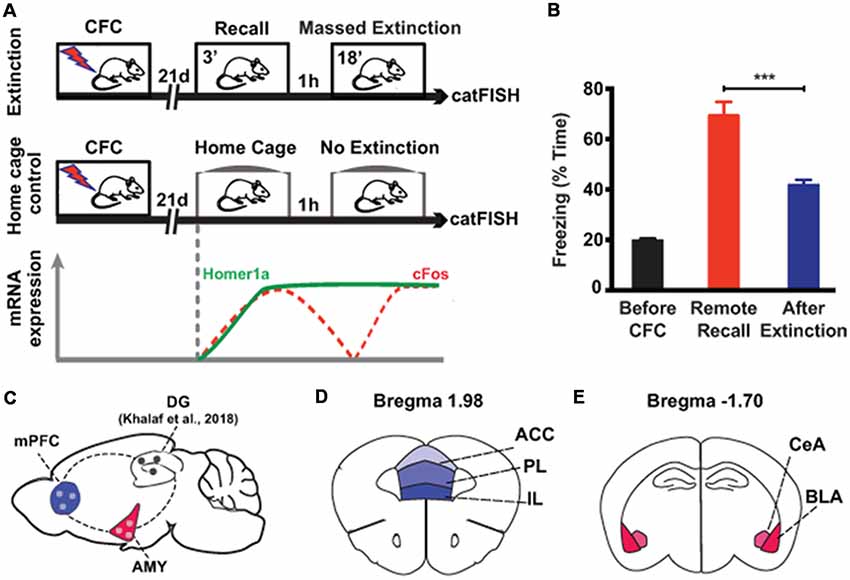
Figure 1. Experimental setup to study brain areas involved in remote memory attenuation by catFISH. (A) Schematic representation of the two experimental groups used in the study. The lower part indicates the time course of the intracellular dynamics of the RNA transcripts Homer1A and cFos, which were employed in the catFISH experiment to study brain areas engaged in remote memory recall and after the massed extinction paradigm. (B) Behavioral results showing the efficiency of the massed extinction paradigm to attenuate remote fear memories (n = 8/group, p < 0.001). (C) Schematic representation of the brain structures selected for catFISH analysis as compared to the results obtained in the dentate gyrus (DG; Khalaf et al., 2018). (D,E) Schematic representation of the cortical and amygdalar substructures analyzed by catFISH, respectively. CFC, contextual fear conditioning; ACC, anterior cingulate cortex; BLA, basolateral amygdala; CeA, central amygdala; IL, infralimbic cortex; PL, prelimbic cortex. ***p < 0.001, two-tailed t-test.
First, we investigated the amygdala, a key limbic structure for the encoding, recall and attenuation of recent fear memories (Trouche et al., 2013; Silva et al., 2016; Tovote et al., 2016), and for the storage of remote fear memories (Maren et al., 1996; Kitamura et al., 2017; Supplementary Figure S2). For the BLA, we found a significant engagement at both memory recall (i.e., the amount of cytoplasmic Homer1a+ cells normalized to the total amount of cells) and after the last extinction trial (i.e., the amount of nuclear cFos+ cells normalized to the total amount of cells) when compared to a home cage control group (Figures 1A, 2A–C). These results reflect that the BLA is activated at both time points. What is more, we found a significantly elevated reactivation rate (calculated as the amount of double positive cytoplasmic Homer1a+/nuclear cFos+ cells normalized to the total amount of cytoplasmic Homer1a+ cells) in the group that underwent massed extinction compared to the home cage control group (Figure 2D), indicating that upon remote fear attenuation a substantial proportion of the original memory trace active when behavioral expression of fear was high becomes reactivated when fear expression is low.
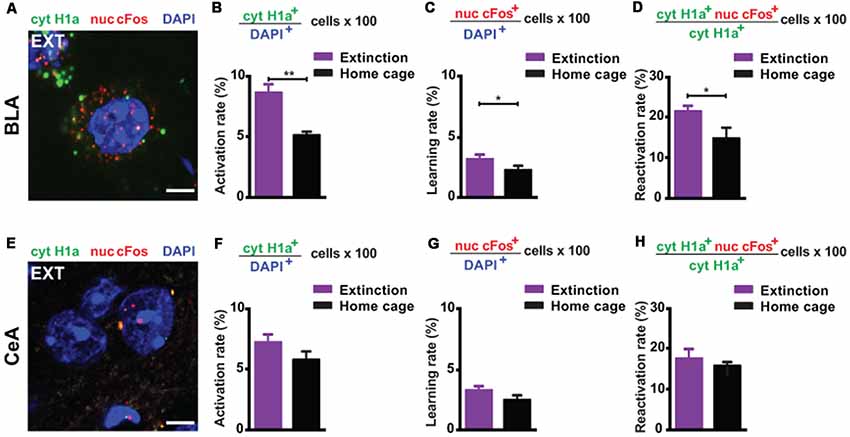
Figure 2. catFISH-deduced engagement of amygdala subregions upon remote fear memory recall and attenuation. (A) Representative image of the mRNA transcripts Homer1a and cFos in the BLA upon remote fear memory attenuation. Scale bar = 5 μm. (B) Homer1a-deduced activation rate in the BLA (n = 8–10 mice/group, p < 0.01). (C) cFos-deduced extinction learning rate in the BLA (n = 8–13 mice/group, p < 0.05). (D) Reactivation rate in the BLA (n = 8–13 mice/group, p < 0.05). (E) Representative image of the mRNA transcripts Homer1a and cFos in the CeA upon remote fear attenuation. Scale bar = 5 μm. (F) Homer1a-deduced activation rate in the CeA (n = 8–13 mice/group, n.s.). (G) cFos-deduced extinction learning rate in the CeA (n = 8–13 mice/group, n.s.). (H) Reactivation rate in the CeA (n = 8–13 mice/group, n.s.). BLA, basolateral amygdala, CeA, central amygdala. **p < 0.01, two-tailed t-test; *p < 0.05, two-tailed t-test.
In the CeA (Figure 2E), neither the recall-induced activation rate (Figure 2F), nor the extinction-induced learning rate (Figure 2G), nor the reactivation rate (Figure 2H) was different between the extinction and the home cage control group. These results suggest that the CeA is not engaged upon remote memory recall, does not become activated by massed extinction and that the memory trace active at recall is not re-engaged by the extinction procedure.
Next, we investigated the prefrontal cortex, a crucial structure for remote memory storage (Frankland and Bontempi, 2005; Kitamura et al., 2017; Supplementary Figure S3). For the IL, we observed a strong engagement at remote memory recall (Figures 3A,B). Upon remote memory extinction, the activity of the IL was also elevated in the extinction group compared to the home cage control group (Figure 3C). Furthermore, we found a significant reactivation of recall-induced neurons by the extinction procedure (Figure 3D), indicating that a part of the original fear memory trace in the IL is still active after fear attenuation.
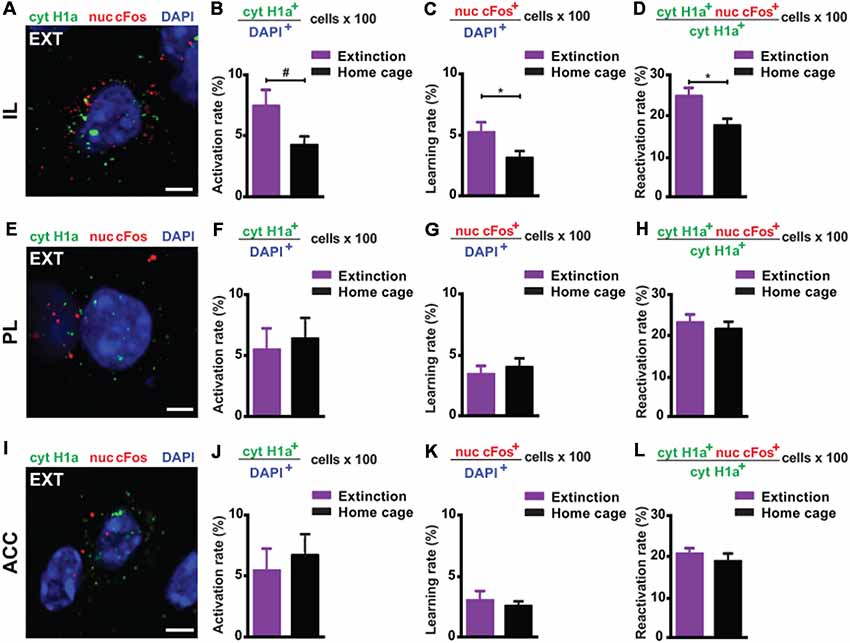
Figure 3. catFISH-deduced engagement of cortical subregions upon remote fear memory recall and attenuation. (A) Representative image of the mRNA transcripts Homer1a and cFos in the IL upon remote fear attenuation. Scale bar = 5 μm. (B) Homer1a-deduced activation rate in the IL (n = 5–9 mice/group, p = 0.0595). (C) cFos-deduced extinction learning rate in the IL (n = 5–6 mice/group, p < 0.05). (D) Reactivation rate in the IL (n = 5–6 mice/group, p < 0.05). (E) Representative image of the mRNA transcripts Homer1a and cFos in the PL upon remote fear attenuation. Scale bar = 5 μm. (F) Homer1a-deduced activation rate in the PL (n = 8–9 mice/group, n.s.). (G) cFos-deduced extinction learning rate in the PL (n = 8–9 mice/group, n.s.). (H) Reactivation rate in the PL (n = 8–9 mice/group, n.s.). (I) Representative image of the mRNA species Homer1a and cFos in the ACC upon remote fear attenuation. Scale bar = 5 μm. (J) Homer1a-deduced activation rate in the ACC (n = 8–9 mice/group, n.s.). (K) cFos-deduced extinction learning rate in the ACC (n = 4–8 mice/group, n.s.). (L) Reactivation rate in the ACC. (n = 4–8 mice/group, n.s.). ACC, anterior cingulate cortex; IL, infralimbic cortex; PL, prelimbic cortex. #p < 0.06; *p < 0.05, two-tailed t-test.
Conversely, in the ACC and PL, we did not find any activation at remote recall (Figures 3E,F,I,J) and after behavioral extinction (Figures 3G,K). Likewise, no reactivation was observed in either structure (Figures 3H,L). These results suggest—based on the methodology employed here—that neither the ACC nor the PL is engaged by remote fear memory recall and its attenuation.
Discussion
Here, using catFISH of the IEGs Homer1a and cFos, we found that the BLA and IL were not only activated by remote fear memory recall and upon remote fear memory attenuation but also that a significant proportion of recall-induced neurons in these structures was reactivated when behavioral expression of fear was low. In contrast, none of these changes were observed for the CeA, the PL and the ACC (Silva et al., 2019).
This study is only the second of its kind to simultaneously study the involvement of different brain areas in remote fear memory attenuation (Silva et al., 2019), which spotlights the paucity of research conducted in this domain. Interestingly, although these studies used different IEG visualization tools (i.e., mRNA vs. protein level), different IEGs (Homer1a and cFos vs. cFos alone) and different paradigms of remote fear memory attenuation (massed vs. spaced extinction), both found the BLA and the IL to be engaged upon remote fear memory recall and its attenuation. These findings are also in line with a persistent implication of the BLA in fear memory storage over time (Maren et al., 1996; Goshen et al., 2011; Do-Monte et al., 2015; Kitamura et al., 2017), and thus expand the well-established role of this structure in recent fear memory attenuation (Phelps et al., 2004; Herry et al., 2010) to remote fear memories. Likewise, the results presented here further translate the importance of the IL for the attenuation of recent fear memories (Santini et al., 2008; Rosas-Vidal et al., 2014; Awad et al., 2015) to remote ones (Silva et al., 2019). In contrast, no engagement of the CeA and the ACC was detected for either remote fear recall or its attenuation, suggesting these subregions to be of minimal importance for remote fear memory attenuation. In the case of the ACC, this finding is discordant with earlier studies that had implicated the ACC in the recall of remote fear memories (Bontempi et al., 1999; Frankland et al., 2004; Goshen et al., 2011; Silva et al., 2019). Lastly, by protein-based IEG cFos studies the PL has recently been shown to be involved in both remote memory storage (Wheeler et al., 2013; Kitamura et al., 2017; Silva et al., 2019) and attenuation (Silva et al., 2019), while the current study did not reflect such a role. These discrepancies are likely due to the subtle differences in memory age, the different IEG methodologies or conditioning paradigms employed, and await functional investigations to be resolved.
In addition to being activated by remote fear memory recall and its attenuation, both the BLA and the IL showed a significantly elevated reactivation rate. At cellular resolution, these findings extend a previous report documenting a similar reactivation in another brain area, namely the DG (Khalaf et al., 2018). Given the reduced expression of fear after the massed extinction procedure, this could stipulate that the continued engagement of the original memory trace of fear in the BLA and IL is needed for fear reduction to occur, in analogy to the findings in the DG. While this interpretation remains speculative at this point and the functional experiments to address it beyond the scope of the present manuscript, several lines of evidence nevertheless point in its favor. First, by engram-specific gain and loss-of-function experiments of recall-induced neurons in the DG, a brain region that had previously been implicated in behavioral extinction (Bernier et al., 2017), such reactivation was shown to be essential for the occurrence of remote fear memory attenuation (Khalaf et al., 2018). Second, such reactivation of the fear memory engram might represent a physiological basis for learning inside the original memory trace. This renewed learning period may then serve the purpose to re-learn (or disassociate) the association formed between environmental happenstances present at encoding and the ensuing fearful response, akin to reconsolidation-updating (Morris et al., 2006). In line, empirical evidence from psychology and psychotherapy emphasizes that the recall of a trauma ought to be as complete as possible for exposure-based therapies to be effective (Foa and Kozak, 1986; Foa, 2000; Nemeroff et al., 2006), which cannot be solely explained by fear memory inhibition through extinction. Nevertheless, the participation of extinction-specific inhibitory processes cannot be ruled out with the present results, and indeed is likely given the low percentage of reactivated cells. Thus, a parsimonious explanation for the cellular processes underlying remote fear memory attenuation combines both extinction-specific, inhibitory processes mediated by a newly learned memory trace of safety that is different from the original trace of fear, together with a reconsolidation-updating process that is mediated by a re-learning of the original memory trace of fear towards safety, which occurs in recall-induced neurons.
Future studies should thus be aimed at further disentangling extinction from reconsolidation-updating processes, for example by addressing the causal implication of recall-induced neurons in memory attenuation, or by addressing the behavioral consequence of functionally manipulating remote extinction-induced neurons. Anatomically, this is of special interest for the two key brain areas emanating from the present study, the BLA and the IL, as the IL is known to send monosynaptic inhibitory projections to the BLA that are important for the attenuation of recent fear memories (Herry et al., 2008, 2010; Awad et al., 2015) and to store memories of past extinction trials (Milad and Quirk, 2002). In the BLA, it would further be interesting to decipher whether the reactivated neurons are those responsible for creating negative or positive associations (Namburi et al., 2015), and how they dynamically develop over the course of extinction (Grewe et al., 2017). This is of particular relevance for long-lasting memories since the vast majority of findings concerning extinction and reconsolidation-updating have been obtained by studying day-old fear memories, despite the fact that traumatic memories are extremely persistent and can impinge on one’s emotional well-being for a long time after the trauma. As remote fear memories are stored differently than recent ones (Frankland and Bontempi, 2005; Frankland et al., 2006; Khalaf and Gräff, 2016; Kitamura et al., 2017; Albo and Gräff, 2018; Tonegawa et al., 2018) and appear to more difficult to attenuate (Milekic and Alberini, 2002; Costanzi et al., 2011; Gräff et al., 2014; Tsai and Gräff, 2014), this represents a fundamental gap in memory research.
In sum, this study shows that recall-induced neurons in both the BLA and IL become reactivated upon remote fear memory attenuation, which extends previous findings from the DG (Khalaf et al., 2018). Together, these results indicate that an active participation of the original fear trace towards fear memory attenuation may be a conserved mechanism across brain areas that are engaged by remote fear memory recall.
Author Contributions
OK and JG designed the experiments and wrote the article. OK carried out the experiments and analyzed the data.
Funding
This research was supported by the Swiss National Science Foundation (Schweizerischer Nationalfonds zur Förderung der Wissenschaftlichen Forschung; 31003A_155898), the National Competence Center for Research “Synapsy” (51NF40-185897), and the European Research Council (ERC-2015-StG 678832). JG is an MQ fellow and a National Alliance for Research on Schizophrenia and Depression (NARSAD) Independent Investigator.
Conflict of Interest Statement
The authors declare that the research was conducted in the absence of any commercial or financial relationships that could be construed as a potential conflict of interest.
Supplementary Material
The Supplementary Material for this article can be found online at: https://www.frontiersin.org/articles/10.3389/fnmol.2019.00070/full#supplementary-material
References
Albo, Z., and Gräff, J. (2018). The mysteries of remote memory. Philos. Trans. R. Soc. B. Biol. Sci. 373:20170029. doi: 10.1098/rstb.2017.0029
Awad, W., Ferreira, G., and Maroun, M. (2015). Dissociation of the role of infralimbic cortex in learning and consolidation of extinction of recent and remote aversion memory. Neuropsychopharmacology 40, 2566–2575. doi: 10.1038/npp.2015.103
Bernier, B. E., Lacagnina, A. F., Ayoub, A., Shue, F., Zemelman, B. V., Krasne, F. B., et al. (2017). Dentate gyrus contributes to retrieval as well as encoding: evidence from context fear conditioning, recall and extinction. J. Neurosci. 37, 6359–6371. doi: 10.1523/JNEUROSCI.3029-16.2017
Bontempi, B., Laurent-Demir, C., Destrade, C., and Jaffard, R. (1999). Time-dependent reorganization of brain circuitry underlying long-term memory storage. Nature 400, 671–675. doi: 10.1038/23270
Bouton, M. E. (2004). Context and behavioral processes in extinction. Learn. Mem. 11, 485–494. doi: 10.1101/lm.78804
Centonze, D., Siracusano, A., Calabresi, P., and Bernardi, G. (2005). Removing pathogenic memories: a neurobiology of psychotherapy. Mol. Neurobiol. 32, 123–132. doi: 10.1385/mn:32:2:123
Clem, R. L., and Schiller, D. (2016). New learning and unlearning: strangers or accomplices in threat memory attenuation? Trends Neurosci. 39, 340–351. doi: 10.1016/j.tins.2016.03.003
Costanzi, M., Cannas, S., Saraulli, D., Rossi-Arnaud, C., and Cestari, V. (2011). Extinction after retrieval: effects on the associative and nonassociative components of remote contextual fear memory. Learn. Mem. 18, 508–518. doi: 10.1101/lm.2175811
Davidson, J. R., Stein, D. J., Shalev, A. Y., and Yehuda, R. (2004). Posttraumatic stress disorder: acquisition, recognition, course and treatment. J. Neuropsychiatry Clin. Neurosci. 16, 135–147. doi: 10.1176/jnp.16.2.135
Debiec, J., LeDoux, J. E., and Nader, K. (2002). Cellular and systems reconsolidation in the hippocampus. Neuron 36, 527–538. doi: 10.1016/s0896-6273(02)01001-2
Do-Monte, F. H., Quiñones-Laracuente, K., and Quirk, G. J. (2015). A temporal shift in the circuits mediating retrieval of fear memory. Nature 519, 460–463. doi: 10.1038/nature14030
Dudai, Y. (2006). Reconsolidation: the advantage of being refocused. Curr. Opin. Neurobiol. 16, 174–178. doi: 10.1016/j.conb.2006.03.010
Ehrlich, I., Humeau, Y., Grenier, F., Ciocchi, S., Herry, C., and Lüthi, A. (2009). Amygdala inhibitory circuits and the control of fear memory. Neuron 62, 757–771. doi: 10.1016/j.neuron.2009.05.026
Foa, E. B. (2000). Psychosocial treatment of posttraumatic stress disorder. The J. Clin. Psychiatry 61, 43–48; discussion 49–51. doi: 10.1093/med:psych/9780195304145.003.0018
Foa, E. B., and Kozak, M. J. (1986). Emotional processing of fear: exposure to corrective information. Psychol. Bull. 99, 20–35. doi: 10.1037/0033-2909.99.1.20
Frankland, P. W., and Bontempi, B. (2005). The organization of recent and remote memories. Nat. Rev. Neurosci. 6, 119–130. doi: 10.1038/nrn1607
Frankland, P. W., Bontempi, B., Talton, L. E., Kaczmarek, L., and Silva, A. J. (2004). The involvement of the anterior cingulate cortex in remote contextual fear memory. Science 304, 881–883. doi: 10.1126/science.1094804
Frankland, P. W., Ding, H. K., Takahashi, E., Suzuki, A., Kida, S., and Silva, A. J. (2006). Stability of recent and remote contextual fear memory. Learn. Mem. 13, 451–457. doi: 10.1101/lm.183406
Goshen, I., Brodsky, M., Prakash, R., Wallace, J., Gradinaru, V., Ramakrishnan, C., et al. (2011). Dynamics of retrieval strategies for remote memories. Cell 147, 678–689. doi: 10.1016/j.cell.2011.09.033
Gräff, J., Joseph, N. F., Horn, M. E., Samiei, A., Meng, J., Seo, J., et al. (2014). Epigenetic priming of memory updating during reconsolidation to attenuate remote fear memories. Cell 156, 261–276. doi: 10.1016/j.cell.2013.12.020
Grewe, B. F., Gründemann, J., Kitch, L. J., Lecoq, J. A., Parker, J. G., Marshall, J. D., et al. (2017). Neural ensemble dynamics underlying a long-term associative memory. Nature 543, 670–675. doi: 10.1038/nature21682
Guzowski, J. F., McNaughton, B. L., Barnes, C. A., and Worley, P. F. (1999). Environment-specific expression of the immediate-early gene Arc in hippocampal neuronal ensembles. Nat. Neurosci. 2, 1120–1124. doi: 10.1038/16046
Haagsma, J. A., Ringburg, A. N., van Lieshout, E. M., van Beeck, E. F., Patka, P., Schipper, I. B., et al. (2012). Prevalence rate, predictors and long-term course of probable posttraumatic stress disorder after major trauma: a prospective cohort study. BMC Psychiatry 12:236. doi: 10.1186/1471-244x-12-236
Herry, C., Ciocchi, S., Senn, V., Demmou, L., Müller, C., and Lüthi, A. (2008). Switching on and off fear by distinct neuronal circuits. Nature 454, 600–606. doi: 10.1038/nature07166
Herry, C., Ferraguti, F., Singewald, N., Letzkus, J. J., Ehrlich, I., and Lüthi, A. (2010). Neuronal circuits of fear extinction. Eur. J. Neurosci. 31, 599–612. doi: 10.1111/j.1460-9568.2010.07101.x
Hupbach, A., Gomez, R., Hardt, O., and Nadel, L. (2007). Reconsolidation of episodic memories: a subtle reminder triggers integration of new information. Learn. Mem. 14, 47–53. doi: 10.1101/lm.365707
Javidi, H., and Yadollahie, M. (2012). Post-traumatic stress disorder. Int. J. Occup. Environ. Med. 3, 2–9.
Kearns, M. C., Ressler, K. J., Zatzick, D., and Rothbauml, B. O. (2012). Early interventions for PTSD: a review. Depress. Anxiety 29, 833–842. doi: 10.1002/da.21997
Kessler, R. C., Berglund, P., Demler, O., Jin, R., Merikangas, K. R., and Walters, E. E. (2005). Lifetime prevalence and age-of-onset distributions of DSM-IV disorders in the national comorbidity survey replication. Arch. Gen. Psychiatry 62, 593–602. doi: 10.1001/archpsyc.62.6.593
Khalaf, O., and Gräff, J. (2016). Structural, synaptic, and epigenetic dynamics of enduring memories. Neural Plast. 2016:3425908. doi: 10.1155/2016/3425908
Khalaf, O., Resch, S., Dixsaut, L., Gorden, V., Glauser, L., and Gräff, J. (2018). Reactivation of recall-induced neurons contributes to remote fear memory attenuation. Science 360, 1239–1242. doi: 10.1126/science.aas9875
Kitamura, T., Ogawa, S. K., Roy, D. S., Okuyama, T., Morrissey, M. D., Smith, L. M., et al. (2017). Engrams and circuits crucial for systems consolidation of a memory. Science 356, 73–78. doi: 10.1126/science.aam6808
Lee, J. L. (2008). Memory reconsolidation mediates the strengthening of memories by additional learning. Nat. Neurosci. 11, 1264–1266. doi: 10.1038/nn.2205
Maren, S., Aharonov, G., and Fanselow, M. S. (1996). Retrograde abolition of conditional fear after excitotoxic lesions in the basolateral amygdala of rats: absence of a temporal gradient. Behav. Neurosci. 110, 718–726. doi: 10.1037/0735-7044.110.4.718
Maren, S., Phan, K. L., and Liberzon, I. (2013). The contextual brain: implications for fear conditioning, extinction and psychopathology. Nat. Rev. Neurosci. 14, 417–428. doi: 10.1038/nrn3492
McCleery, J. M., and Harvey, A. G. (2004). Integration of psychological and biological approaches to trauma memory: implications for pharmacological prevention of PTSD. J. Trauma. Stress 17, 485–496. doi: 10.1007/s10960-004-5797-5
McKenzie, S., and Eichenbaum, H. (2011). Consolidation and reconsolidation: two lives of memories? Neuron 71, 224–233. doi: 10.1016/j.neuron.2011.06.037
Milad, M. R., and Quirk, G. J. (2002). Neurons in medial prefrontal cortex signal memory for fear extinction. Nature 420, 70–74. doi: 10.1038/nature01138
Milekic, M. H., and Alberini, C. M. (2002). Temporally graded requirement for protein synthesis following memory reactivation. Neuron 36, 521–525. doi: 10.1016/S0896-6273(02)00976-5
Misanin, J. R., Miller, R. R., and Lewis, D. J. (1968). Retrograde amnesia produced by electroconvulsive shock after reactivation of a consolidated memory trace. Science 160, 554–555. doi: 10.1126/science.160.3827.554
Monfils, M. H., Cowansage, K. K., Klann, E., and LeDoux, J. E. (2009). Extinction-reconsolidation boundaries: key to persistent attenuation of fear memories. Science 324, 951–955. doi: 10.1126/science.1167975
Morris, R. G., Inglis, J., Ainge, J. A., Olverman, H. J., Tulloch, J., Dudai, Y., et al. (2006). Memory reconsolidation: sensitivity of spatial memory to inhibition of protein synthesis in dorsal hippocampus during encoding and retrieval. Neuron 50, 479–489. doi: 10.1016/j.neuron.2006.04.012
Myers, K. M., and Davis, M. (2007). Mechanisms of fear extinction. Mol. Psychiatry 12, 120–150. doi: 10.1038/sj.mp.4001939
Nader, K., and Hardt, O. (2009). A single standard for memory: the case for reconsolidation. Nat. Rev. Neurosci. 10, 224–234. doi: 10.1038/nrn2590
Nader, K., Schafe, G. E., and Le Doux, J. E. (2000). Fear memories require protein synthesis in the amygdala for reconsolidation after retrieval. Nature 406, 722–726. doi: 10.1038/35021052
Namburi, P., Beyeler, A., Yorozu, S., Calhoon, G. G., Halbert, S. A., Wichmann, R., et al. (2015). A circuit mechanism for differentiating positive and negative associations. Nature 520, 675–678. doi: 10.1038/nature14366
Nemeroff, C. B., Bremner, J. D., Foa, E. B., Mayberg, H. S., North, C. S., and Stein, M. B. (2006). Posttraumatic stress disorder: a state-of-the-science review. J. Psychiatr. Res. 40, 1–21. doi: 10.1016/j.jpsychires.2005.07.005
Nonaka, A., Toyoda, T., Miura, Y., Hitora-Imamura, N., Naka, M., Eguchi, M., et al. (2014). Synaptic plasticity associated with a memory engram in the basolateral amygdala. J. Neurosci. 34, 9305–9309. doi: 10.1523/JNEUROSCI.4233-13.2014
Pape, H. C., and Pare, D. (2010). Plastic synaptic networks of the amygdala for the acquisition, expression and extinction of conditioned fear. Physiol. Rev. 90, 419–463. doi: 10.1152/physrev.00037.2009
Parsons, R. G., and Ressler, K. J. (2013). Implications of memory modulation for post-traumatic stress and fear disorders. Nat. Neurosci. 16, 146–153. doi: 10.1038/nn.3296
Phelps, E. A., Delgado, M. R., Nearing, K. I., and LeDoux, J. E. (2004). Extinction learning in humans: role of the amygdala and vmPFC. Neuron 43, 897–905. doi: 10.1016/j.neuron.2004.08.042
Quirk, G. J., and Mueller, D. (2008). Neural mechanisms of extinction learning and retrieval. Neuropsychopharmacology 33, 56–72. doi: 10.1038/sj.npp.1301555
Ringburg, A. N., Polinder, S., van Ierland, M. C., Steyerberg, E. W., van Lieshout, E. M., Patka, P., et al. (2011). Prevalence and prognostic factors of disability after major trauma. J. Trauma. 70, 916–922. doi: 10.1097/ta.0b013e3181f6bce8
Rosas-Vidal, L. E., DoMonte, F. H., Sotres-Bayon, F., and Quirk, G. J. (2014). Hippocampal’prefrontal BDNF and memory for fear extinction. Neuropsychopharmacology 39, 2161–2169. doi: 10.1038/npp.2014.64
Sandrini, M., Cohen, L. G., and Censor, N. (2015). Modulating reconsolidation: a link to causal systems-level dynamics of human memories. Trends Cogn. Sci. 19, 475–482. doi: 10.1016/j.tics.2015.06.002
Santini, E., Quirk, G. J., and Porter, J. T. (2008). Fear conditioning and extinction differentially modify the intrinsic excitability of infralimbic neurons. J. Neurosci. 28, 4028–4036. doi: 10.1523/jneurosci.2623-07.2008
Schiller, D., Monfils, M. H., Raio, C. M., Johnson, D. C., Ledoux, J. E., and Phelps, E. A. (2010). Preventing the return of fear in humans using reconsolidation update mechanisms. Nature 463, 49–53. doi: 10.1038/nature08637
Silva, B. A., Burns, A. M., and Gräff, J. (2019). A cFos activation map of remote fear memory attenuation. Psychopharmacology 236, 369–381. doi: 10.1007/s00213-018-5000-y
Silva, B. A., Gross, C. T., and Gräff, J. (2016). The neural circuits of innate fear: detection, integration, action and memorization. Learn. Mem. 23, 544–555. doi: 10.1101/lm.042812.116
Tonegawa, S., Morrissey, M. D., and Kitamura, T. (2018). The role of engram cells in the systems consolidation of memory. Nat. Rev. Neurosci. 19, 485–498. doi: 10.1038/s41583-018-0031-2
Tovote, P., Esposito, M. S., Botta, P., Chaudun, F., Fadok, J. P., Markovic, M., et al. (2016). Midbrain circuits for defensive behaviour. Nature 534, 206–212. doi: 10.1038/nature17996
Tronson, N. C., and Taylor, J. R. (2007). Molecular mechanisms of memory reconsolidation. Nat. Rev. Neurosci. 8, 262–275. doi: 10.1038/nrn2090
Trouche, S., Sasaki, J. M., Tu, T., and Reijmers, L. G. (2013). Fear extinction causes target-specific remodeling of perisomatic inhibitory synapses. Neuron 80, 1054–1065. doi: 10.1016/j.neuron.2013.07.047
Tsai, L. H., and Gräff, J. (2014). On the resilience of remote traumatic memories against exposure therapy-mediated attenuation. EMBO Rep. 15, 853–861. doi: 10.15252/embr.201438913
Wheeler, A. J., Teixeira, C. M., Wang, A. H., Xiong, X., Kovacevic, N., Lerch, J. P., et al. (2013). Identification of a functional connectome for long-term fear memory in mice. PLoS Comput. Biol. 9:e1002853. doi: 10.1371/journal.pcbi.1002853
Keywords: engram, basolateral amydala, infralimbic cortex, fear extinction, reconsolidation, remote memory, updating, memory trace
Citation: Khalaf O and Gräff J (2019) Reactivation of Recall-Induced Neurons in the Infralimbic Cortex and the Basolateral Amygdala After Remote Fear Memory Attenuation. Front. Mol. Neurosci. 12:70. doi: 10.3389/fnmol.2019.00070
Received: 10 January 2019; Accepted: 06 March 2019;
Published: 17 April 2019.
Edited by:
Jason D. Shepherd, The University of Utah, United StatesReviewed by:
Sungmo Park, Hospital for Sick Children, CanadaMiou Zhou, Western University of Health Sciences, United States
Copyright © 2019 Khalaf and Gräff. This is an open-access article distributed under the terms of the Creative Commons Attribution License (CC BY). The use, distribution or reproduction in other forums is permitted, provided the original author(s) and the copyright owner(s) are credited and that the original publication in this journal is cited, in accordance with accepted academic practice. No use, distribution or reproduction is permitted which does not comply with these terms.
*Correspondence: Johannes Gräff, am9oYW5uZXMuZ3JhZWZmQGVwZmwuY2g=