- 1Section of Physiology and Biochemistry, Department of Experimental Medicine, University of Perugia School of Medicine, Perugia, Italy
- 2Department of Physiology and Biochemistry, Faculty of Medicine and Surgery, University of Malta, Msida, Malta
- 3Department of Chemistry, Biology and Biotechnology, University of Perugia, Perugia, Italy
- 4Department of Medical-Surgical Sciences and Biotechnologies, University of Rome “Sapienza”, Polo Pontino, Latina, Italy
- 5Section of Pharmacology, Department of Medicine, University of Perugia School of Medicine, Perugia, Italy
Glioblastoma multiforme (GBM) is the most common and malignant of the glial tumors. The world-wide estimates of new cases and deaths annually are remarkable, making GBM a crucial public health issue. Despite the combination of radical surgery, radio and chemotherapy prognosis is extremely poor (median survival is approximately 1 year). Thus, current therapeutic interventions are highly unsatisfactory. For many years, GBM-induced brain oedema and inflammation have been widely treated with dexamethasone (DEX), a synthetic glucocorticoid (GC). A number of studies have reported that DEX also inhibits GBM cell proliferation and migration. Nevertheless, recent controversial results provided by different laboratories have challenged the widely accepted dogma concerning DEX therapy for GBM. Here, we have reviewed the main clinical features and genetic and epigenetic abnormalities underlying GBM. Finally, we analyzed current notions and concerns related to DEX effects on cerebral oedema, cancer cell proliferation and migration and clinical outcome.
Introduction
Glioblastoma multiforme (GBM) is the most aggressive form of brain tumor, accounting for 54% of all gliomas (Dolecek et al., 2012). The estimated number of annual new cases of GBM are: Japan 2,200, UK 2,531, France 3,000, Germany 3,500, USA 18,000. Globally, over 100,000 patients die each year as a result of this type of brain cancer. It is highly invasive and not amenable to current treatment such as surgical management, chemo- and radio-therapy (Furnari et al., 2007) and affected individuals have very poor life expectancy (Miller and Perry, 2007). The highly aggressive course and poor response to treatments likely result from uncontrolled cellular proliferation, migration, presence of a cancer stem-like cell population, neo-angiogenesis and severe brain oedema.
GBM-induced cerebral oedema is currently treated with corticosteroids due to their ability to decrease the permeability of the blood brain barrier (BBB; Salvador et al., 2014). Dexamethasone (DEX) represents the drug of choice among the synthetic glucocorticoids (GCs) by virtue of its minimal mineralocorticoid activity, long half-life and high potency (Kostaras et al., 2014). By evaluating the typical features of GBM aggressiveness, several studies have shown positive effects of DEX on GBM cells both in vitro and cancer volume, in vivo (Guerin et al., 1992; Wolff et al., 1997; Kaup et al., 2001; Villeneuve et al., 2008; Piette et al., 2009; Fan et al., 2014) although, the mechanisms accounting for these actions remain unclear. Strikingly, contradictory evidence has been published generating controversies that are still debated. As a resource for those interested in this issue, we have reviewed relevant critical articles from various research groups describing DEX actions in GBM and discussed proposed mechanisms and controversies.
Glioblastoma Multiforme: Clinical and Molecular Features
GBM is known as grade IV astrocytoma according to the World Health Organization (WHO) classification. “Primary GBM” accounts for some 90% of cases, arises de novo and affects older patients. “Secondary GBM” develops from preceding low-grade astrocytomas and is more common in patients that are ≤45 years old (Crespo et al., 2015; Louis et al., 2016). Neurological signs depend on the location of the tumor within the brain and can be either focal or generalized. Frequently, symptoms include headache, seizures, cognitive dysfunction, ataxia, vomiting, vision disturbance. Unusual symptoms such as syncope, vertigo, hypoesthesia or psychiatric manifestations could result in misdiagnosis and mistreatment. In some instances, GBM infiltrate the brain as finger-like tentacles making surgical resection of the tumor very difficult, especially when it develops near eloquent regions of the brain. GBM could be considered a non-metastatic cancer, as it rarely spreads from the primary site to other remote tissues of the body thanks to the confining properties of the BBB (Beauchesne, 2011). Another typical feature is the presence of hypoxic and necrotic regions within the cancer mass (Lara-Velazquez et al., 2017). Microscopic analyses showed a central large necrotic core that takes up to 80% of tumor mass and multiple thrombotic foci surrounded by pseudopalisading cells migrating towards more oxygenated areas (Rong et al., 2006; Amberger-Murphy, 2009; Sforna et al., 2017). Hypoxia induces important changes on tumor cell genome and proteome, which increase its aggressiveness (Amberger-Murphy, 2009). In particular, hypoxia promotes invasiveness, radio- and chemo-resistance, glioma stem cell (GSC) development and angiogenesis (Jensen, 2009; Yang et al., 2012; Sforna et al., 2015). The vascular endothelial growth factor (VEGF) is over-expressed by cancer cells under hypoxia and promotes neo-angiogenesis (Soda et al., 2013; Dubois et al., 2014). Indeed, GBM is a highly vascularized tumor and the degree of vascularization influences prognosis, remarkably (Takano et al., 2010; Dubois et al., 2014).
Histological evaluations have shown extensive variability of cell morphology that is characterized by the coexistence of small cells and multinucleated giant cells (Meyer-Puttlitz et al., 1997; Kleihues, 1998). A growing body of evidence supports the notion that GBM cell heterogeneity results also from the presence of GSCs, a small subpopulation of tumor cells that show features of self-renewal, resistance to radiotherapy (RT) and chemotherapy, ability to differentiate in vitro and promote tumor recurrence. These properties are shared in part with neuronal stem cells (NSCs; Reya et al., 2001; Bao et al., 2006; Vescovi et al., 2006; Park and Rich, 2009; Rosen and Jordan, 2009; Frank et al., 2010; Heddleston et al., 2010). Of note is that, GSCs are also responsible for the angiogenic potential of the tumor by expressing high levels of VEGF (Bao et al., 2006; Li et al., 2009). Initially, the term multiforme referred to a broad spectrum in cellular heterogeneity. However, in addition to the histological features, GBM is characterized by several genetic and epigenetic alterations. Thus, multiforme currently refers to the latter multifaceted features of the cancer (DeAngelis and Mellinghoff, 2011; Stoyanov et al., 2018). Indeed, genomic investigations resulted in the identification of a number of genetic abnormalities underlying the molecular transcriptional subtypes of GBM. Verhaak et al. (2010) proposed the classification of GBM into four distinct subtypes: classical, mesenchymal, proneural and neural.
The classical subtype is characterized by amplification or mutation in the epidermal growth factor receptor (EGFR) and deletion mutations in the cyclin-dependent kinase inhibitor 2A (CDKN2A) gene, coding for the p16INK4A and p14arf protein tumor suppressors. Genomic amplification of EGFR is observed in 97% of the classical subtype, which leads to the four-fold increase in EGFR expression. The most common point mutation results in the deletion variant EGFRvIII, lacking exons 2–7, which encode the extracellular domain. The EGFRvIII alteration prevents EGF binding and confers ligand-independent signaling, thereby activating pathways other than those controlled by the wild-type EGFR.
The mesenchymal subtype is featured by deletions of a region at 17q11.2 containing the gene NF1 (neurofibromin). Moreover, genes in the tumor necrosis factor (TNF) super family pathway are highly expressed in this subtype, potentially as a consequence of higher overall necrosis (Verhaak et al., 2010; DeAngelis and Mellinghoff, 2011; Lara-Velazquez et al., 2017).
The proneural subtype is characterized by high levels of platelet-derived growth factor receptor A (PDGFRA) expression and point mutations of both isocitrate dehydrogenase 1 (IDH1) and p53. Although, focal amplifications of the locus at 4q12 harboring the PDGFRA gene were observed in all GBM subtypes, the proneural samples possess a much higher degree. Multiple PDGFRA point mutations have been identified in the Ig-domain, potentially disrupting ligand interaction. Moreover, a rare in frame deletion of the Ig-domain of PDGFRA has been described (Kumabe et al., 1992; Rand et al., 2005). The IDH family includes three enzymes with different locations: IDH1 found in cytosol and peroxisome and IDH2 and IDH3 located in mitochondria. These are involved in the biosynthesis of central metabolites in the tricarboxylic acid (TCA) cycle and catalyze the oxidative decarboxylation of isocitrate to α-ketoglutarate (α-KG), producing NADPH (Leonardi et al., 2012; Miller and Perry, 2007). The proneural subtype is also characterized by point mutations in the IDH1 gene. These mutations produce d-2-hydroxyglutarate (d-2HG), a competitive inhibitor of alpha-ketoglutarate-dependent dioxygenase which induces epigenetic changes, including hypermethylation (Waitkus et al., 2016; Czapski et al., 2018; Lee et al., 2018).
The neural subtype is characterized by expression of neural markers such as neurofilament light (NEFL), gamma-aminobutyric acid type A receptor alpha-1 subunit (GABRA1) and the SLC12A5 (K +/Cl− co-transporter 2).
According to recent WHO guidelines, GBMs could be subdivided into IDH wild-type and IDH-mutant (Louis et al., 2016). GBM/IDH wild-type are more common (~90%), tend to be more aggressive, and have worse prognosis than GBM/IDH mutant. Mutations in the IDH-encoding gene have been found in ~10% of GBM (Parsons et al., 2008) and are associated with altered cell metabolism. IDH mutations were mainly found in secondary GBM, which develops from low-grade gliomas (Han and Batchelor, 2017).
IDH1 mutations occur in 50%–80% of grade II and III astrocytoma, oligodendroglioma and secondary GBM. IDH2 mutations occur in the same tumor with less frequency (Hartmann et al., 2009). The most common mutation in IDH1 results in arginine 132 to histidine substitution (R132H). This mutation converts α-KG to the R(−)-2-hydroxyglutarate (2-HG), considered as a potential “oncometabolite.” It has been shown that accumulation of 2HG affects histone and DNA demethylases resulting in a hypermethylation phenotype with chromatin modifications and gene expression dysregulation (Staedtke et al., 2016; Han and Batchelor, 2017).
The Epigenetic Origin of GBM and Therapeutic Potential of Epigenetic Modifiers Synergized by DEX
Epigenetic modifications refer to changes in gene expression and cellular phenotype without alterations in the DNA sequence. A number of studies over the past years have provided greater knowledge and insight concerning the epigenetic origin of GBM. Hypermethylation represents an epigenetic mechanism frequently observed in GBM that occurs at genes involved in cell cycle regulation, DNA repair, apoptosis, angiogenesis and invasion (Alaminos et al., 2005; Tews et al., 2007; Martinez and Esteller, 2010). DNA hypermethylation of promoter regions can silence tumor suppressor genes or pro-apoptotic genes, or even favor the response to chemotherapy and RT in tumor cells (Kanazawa et al., 2019). Remarkably, 90% of high-grade gliomas contained methylated gene promoters. Affected individuals were found to have large amounts of DNA in the plasma and the same methylated promoters present in the tumor were also found in the plasma in 60% of the cases. This represents the first step towards the development of quantitative plasma biomarkers that could be used to monitor glioma status (Weaver et al., 2006). Methylation of the O6-methylguanine DNA methyltransferase (MGMT) promoter is frequently observed in secondary GBM, associated with p53 mutations (Nakamura et al., 2001; Martinez and Esteller, 2010). MGMT is involved in DNA repair and its activity is down-regulated by methylation.
Methylation, demethylation and acetylation are among the main epigenetic modifications of histone. Histone methylation and demethylation are involved in reprogramming GBM cell metabolism and occur by action of histone methyltransferases (HMTs) and demethylases (HDMs), respectively (Dong and Cui, 2018). Histone acetyltransferases (HATs) and deacetylases (HDACs) control the acetylation state of histones, modulating chromatin structure and function and promote the transcriptional activation or repression (Bezecny, 2014). HDACs also change non-histone proteins which regulate important cellular functions such as cell-cycle progression, differentiation and apoptosis (Lee et al., 2015). The HDAC family includes four classes: Zn2+-dependent (classes I, II and IV), Zn2+-independent (class III) and nicotinamide-adenine dinucleotide-dependent enzymes (Lee et al., 2017). In GBM, HDACs are considered the main effectors of epigenetic alterations and are implicated in tumorigenesis. Indeed, mutations and alterations of HDAC expression have been identified and associated to GBM pathogenesis and progression (Was et al., 2019). In particular, mutations in both HDAC2 and HDAC9 genes have been found through sequencing of GBM biopsies (Parsons et al., 2008). It has been reported that GBM displays decreased mRNA expression in class II and class IV HDACs. On the other hand, recent studies have shown increased levels of HDAC1, HDAC3, and HDAC6 (Staberg et al., 2017), as well as overexpression of HDAC9 (Yang, 2015).
The reversibility of epigenetic modifications opens new therapeutic perspectives for GBM. As such, the search for new drugs targeting epigenetic modifications has been expanded over the past years. DNA methyltransferase inhibitors (DNMTi) and histone deacetylase inhibitors (HDACi) have been tested in multiple cancers. However, only HDACi have been approved in clinical trials (Romani et al., 2018). HDACi are divided into seven categories: short chain fatty acids, benzamides, cyclic peptides, electrophilic ketones, hydro-xamines, sirtuin inhibitors and other miscellaneous forms (Lee et al., 2015). Their anti-cancer effects include the induction of cell-cycle arrest, differentiation, apoptosis, mitotic cell death and autophagic cell death (Bezecny, 2014). Vorinostat, Romidepsin and Valproic Acid are among the HDCAi that managed to find their way in clinical trials. The efficacy of Vorinostat has been tested in primary GBM explants, as well as in murine GBM cell lines, in vitro and in vivo. The drug induced accumulation of cells in the G2-M phase, increased the expression of p21WAF1, p27KIP1, DR5 and TNFα, and decreased the levels of the pro-growth genes CDK2, CDK4, cyclin D1 and cyclin D2. In addition, it reduced the invasiveness in a number of GBM cell lines with different PTEN and p53 mutations (An et al., 2010; Xu et al., 2011). Romidepsin is a class I HDACi and exerts its functions by down-regulating Bcl-xL and up-regulating p21 expression (Lee et al., 2015).
The Enhancer of Zeste Homolog 2 (EZH2) is a histone-lysine N-methyltransferase enzyme that participates in histone methylation. EZH2 is the functional enzymatic component of the Polycomb Repressive Complex 2 (PRC2) involved in a wide range of glioma processes, including cell cycle, invasion, GSC maintenance, drug and RT resistance (Yin et al., 2016). A recent study showed the effects of the SAM-competitive EZH2 inhibitor UNC1999 in different stem cell-like glioma cells, named brain tumor-initiating cells (BTICs). By evaluating cell growth, the combination of UNC1999 with DEX exerted a synergistic effect in two different BTICs type and suppressed tumor growth, in vivo (Grinshtein et al., 2016). Therefore, therapeutic anti-cancer benefits may result from the combination of drugs which enhance therapeutic efficacy compared to the mono-therapy approach derived through targeting key pathways in a characteristically synergistic or additive manner. However, a common obstacle shared by all these therapeutic approaches is the low permeability of the BBB. It is hoped that the use of easily penetrating drugs, such as DEX, together with newly selected epigenetic compounds that are able to cross the BBB could contribute to finding a better therapy for GBM.
GBM-Induced Cerebral Oedema
Cerebral oedema is a hallmark of malignant brain tumors that influences the clinical course and the prognosis of the disease (Stummer, 2007; Lin et al., 2016). It represents a major cause of morbidity and mortality in GBM due to the high risk of brain herniation in up to 60% of patients (Silbergeld et al., 1991). Indeed, the accumulation of fluids inside the cerebral parenchyma induces a rapid increase in brain volume leading to a sharp increase in intracranial pressure (ICP), which may cause ischemia, herniation and ultimately death (Papadopoulos et al., 2004). The main processes involved in cerebral oedema are vasogenic and cytotoxic oedema. Vasogenic oedema results from accumulation of fluid in the cerebral parenchyma caused by disruption and increased permeability of the BBB. Cytotoxic oedema results from failure in cell metabolism leading to impairment of the Na+/K+ pump (Michinaga and Koyama, 2015). In gray and white matter, astrocytes may swell as a result of cytoplasmic retention of Na+ ions and water. Typically, GBM-associated cerebral oedema is vasogenic in nature. It is characterized by breakdown of the BBB, resulting in extracellular accumulation of fluid with disruption of homeostasis around the microenvironment of the compromised parenchyma.
The BBB represents a structure that separates the brain parenchyma from the circulatory system, allowing maintenance of central nervous system (CNS) fluid homeostasis and passage of substances to brain cells by mechanisms that rely on simple diffusion or active transport (Campos-Bedolla et al., 2014). It is formed by brain capillary endothelial cells (BCECs) that are supported by neighboring glial cells (microglia and astrocytes; Kaur and Ling, 2008), neurons and perivascular pericytes (Wolburg and Lippoldt, 2002; Zlokovic, 2008; Zozulya et al., 2008). The BCECs are joined together by means of tight junctions (TJs), composed of several proteins, among which are claudins and occludins (Furuse et al., 1993, 1996, 1998; Kubota et al., 1999). These transmembrane proteins bind intracellular proteins such as zonula occludens-1 and -2 (ZO1; ZO2) allowing the coupling of TJs to the cytoskeleton elements of endothelial cells (Stummer, 2007). The TJ formation is promoted by growth factors secreted by astrocytes which therefore play an important role in controlling the unique tightness of the BBB (Wolburg et al., 2012). TJ proteins with altered expression or function affect the tightness of epithelial surfaces, causing BBB hyper-permeability and consequently vasogenic oedema (Figure 1). It has been shown that occludin is downregulated and subsequently phosphorylated in human high-grade gliomas, thus causing increased permeability of the TJs as a result of the altered interaction between phosphorylated occludin and ZO1, ZO2 and ZO3 (Rubin and Staddon, 1999; Papadopoulos et al., 2001; Kale et al., 2003). Liebner et al. (2000) have shown that the expression of the TJ protein claudin 1 is lost in the majority of tumor micro vessels and claudin 5 down-regulated in hyperplastic vessels, leading to increased endothelial permeability. GBM cells secrete several factors including VEGF, stromal cell-derived factor-1 (SDF-1α) and Angiopoietin 1 (Ang-1) that increase the proliferation of endothelial cells and promote development of new vessels. To further aggravate the condition, the tumor’s vascularization is immature and morphologically abnormal. Indeed, the new vessels are disorganized, deformed, tortuous, partially occluded, excessively leaky and dysfunctional (Hardee and Zagzag, 2012; Dubois et al., 2014; Salmaggi et al., 2004). The main factor that promotes neo-angiogenesis is VEGF, which is up-regulated in brain tumors associated with oedema (Lin and Wang, 2016; Lin et al., 2016). VEGF affects the vascular endothelium, stimulating the proliferation and migration of endothelial cells, decreasing the expression of TJ proteins, whilst increasing the hydraulic permeability of vessels (Hardee and Zagzag, 2012; Stokum et al., 2016). Overall, altered TJs in endothelial cells and abnormal vascularization result in fluid buildup in cerebral parenchyma, leading to increased brain volume and ICP (Papadopoulos et al., 2004).
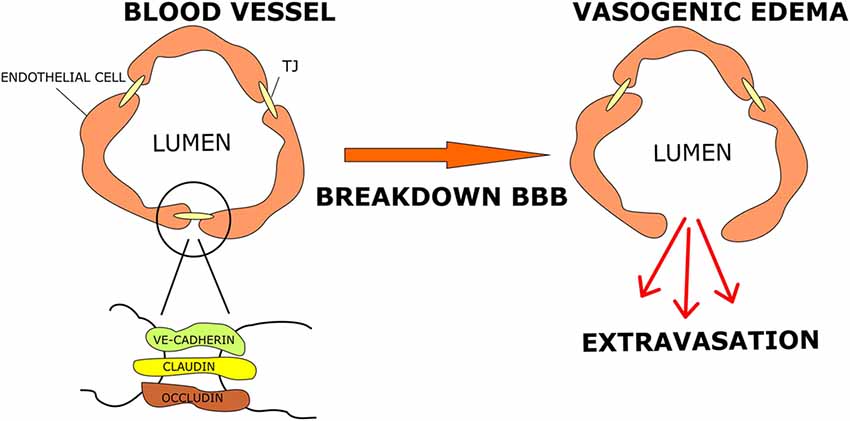
Figure 1. Tumor-related oedema. Brain capillary endothelial cells (BCECs) are connected via tight junction (TJ) protein complexes that fuse endothelial cells together. When a brain tumor develops, particularly high-grade gliomas, TJs become permeable due to release of angiogenic factors and changes in protein component. These events cause blood brain barrier (BBB) breakdown and vasogenic oedema.
Management of Brain Oedema With DEX
GCs are steroid hormones produced by the adrenal cortex under the control of the hypothalamic–pituitary–adrenal axis (HPA) that regulate carbohydrate metabolism, inflammatory and immune responses. Their effects are mediated by binding to GC receptor (GR) on a time scale from hours to days (genomic effects). The best known and well characterized isoform of GR is GR-α. The complex GC-GR binds to DNA at particular glucocorticoid responsive elements (GRE) and regulates the transcription of a number of genes (Piette et al., 2006).
GCs reduce oedema by affecting BBB functionality, particularly through modulation of gene expression and function of claudins, occludins, and vascular endothelial (VE)-cadherin that regulate endothelial permeability (Hue et al., 2015). In rodent brain tumors, DEX decreases BBB permeability by up-regulating the expression of occludin (Gu et al., 2009a,b).
The stability of the BBB is regulated by Ang-1 and Ang-2, as well as VEGF (Kaal and Vecht, 2004; Nag et al., 2005). Ang-1 binds to the receptor tyrosine kinase Tie-2 expressed on endothelial cells, whereas, Ang-2 participates in BBB breakdown through vessel destabilization (Maisonpierre et al., 1997). Up-regulation of Ang-1 and down-regulation of VEGF by DEX treatment have been reported in human brain astrocytes and pericytes (Kim et al., 2008). DEX ameliorates oedema not only by influencing the factors that regulate the permeability of the capillary bed, but also by inhibiting their production (Figure 2). Indeed in C6 cells, DEX down-regulates VEGF mRNA and protein expression in a dose-dependent manner both in normoxic and hypoxic conditions. This effect was found to be mediated by GR (Finkenzeller et al., 1995; Nauck et al., 1998; Machein et al., 1999).
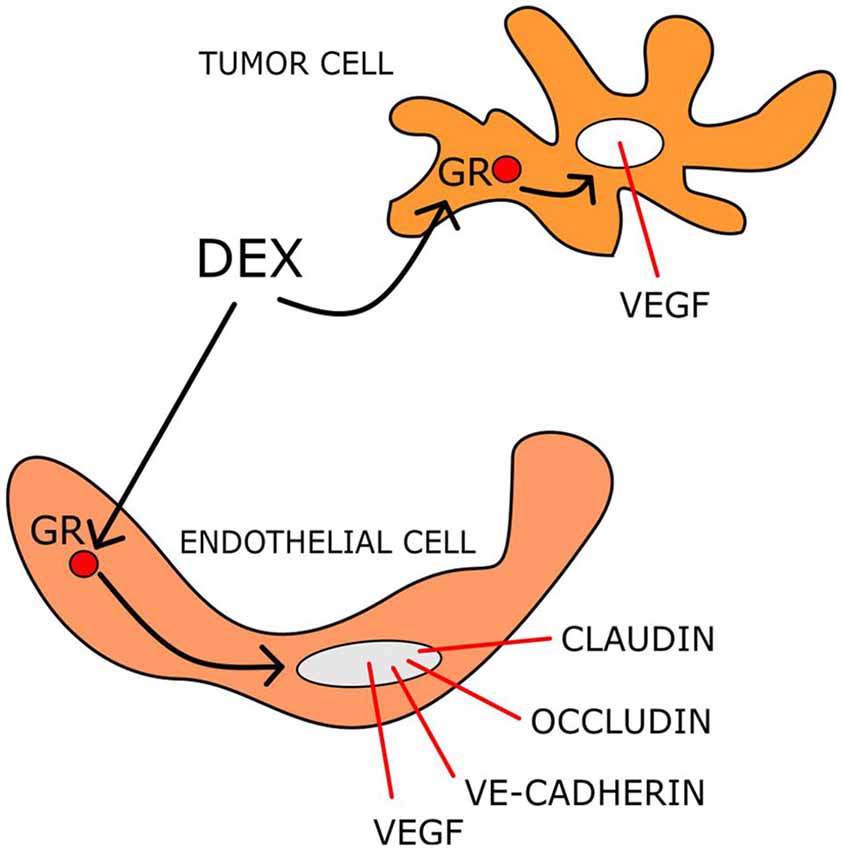
Figure 2. DEX mechanisms reducing tumor-induced brain oedema. DEX acting on both endothelial and tumor cells modulates the transcription of several gene products that control the BBB permeability.
The ability of DEX to modulate the expression of several potassium channel types in the BBB of glioma has also been demonstrated (Zhang et al., 2007). In particular, DEX treatment increased the expression of calcium-activated K+ channels in the BBB of rat brain glioma (Gu et al., 2007; Gu et al., 2009b). In the same glioma model, up-regulation of ATP sensitive-K+ channel (KATP) expression by DEX has also been reported (Gu et al., 2007). Through up-regulation of these K+ channel types, DEX could regulate transcellular pathways of brain tumor microvessels controlling BBB permeability. Although DEX seems to reduce oedema formation by modifying the permeability of capillaries within the tumor and enhancing the clearance of extracellular water (Swaroops et al., 2001; Sinha et al., 2004), the mechanisms of its actions are as to date not fully understood.
GBM-Induced Abnormal Cell-Proliferation
Cell proliferation is a physiological process that involves the activation of many signaling pathways. GBM and several genetic abnormalities associated with this cancer alter various signaling pathways that control cell proliferation.
EGFR plays an important role in cell proliferation principally through receptor-mediated activation of PI3K/AKT/mTOR and GRB2/MEK/ERK/MAPK signaling pathways (Chistiakov et al., 2017). Amplification or mutations of EGFR are frequently found in GBM (Maire and Ligon, 2014). Indeed, 30% of GBM cases express the truncated mutant EGFRvIII receptor that is deprived from its extracellular ligand binding site and as a consequence is activated, constitutively (Frederick et al., 2000; Paff et al., 2014). By stimulating the downstream signal transduction pathways promotes tumorigenicity (Wang et al., 2009; Chistiakov et al., 2017). Importantly, the genetic analysis of EGFR in GBM is widely used as a diagnostic biomarker (Maire and Ligon, 2014).
Phosphatase and tensin homolog (PTEN) is a tumor suppressor that dephosphorylates the second messenger phosphatidylinositol 3, 4, 5-triphosphate, inhibits the PI3K/AKT pathway and reduces cyclin D1 accumulation, inducing cell cycle arrest at the G0/G1 phase. Mutations in PTEN are found in 20%–40% of cases that are mainly affected by primary GBM. Loss of function mutations in PTEN activate signaling molecules including phosphatidylinositol-dependent kinases (PDKs), serine/threonine kinases, AKT/protein kinase B, S6 kinase, and mTOR, as well as the small GTPases Rac1 and Cdc42 (Alexiou and Voulgaris, 2010). The overall effects resulting from activation of these signaling pathways are increased cell proliferation and tumor growth. In addition to cell proliferation, the PI3K/Akt/mTOR pathway regulates apoptosis and angiogenesis. Notably, this pathway is up-regulated in GBM and mediates uncontrolled cell proliferation, tumor growth and multidrug resistance (Zhao et al., 2017). As such, intense investigations are aimed to identify therapeutic strategies to inhibit the PI3K/AKT/mTOR pathway (Ströbele et al., 2015; Li et al., 2016).
Recent studies, have highlighted the important role of ion channels in GBM cell proliferation and aggressiveness (Sforna et al., 2017; Wong et al., 2018; Yang et al., 2017, 2018; D’Alessandro et al., 2018). In particular, it has been shown that the swelling-activated Cl− current is over-expressed in GBM cell lines (Catacuzzeno et al., 2014; Sforna et al., 2017), and contributes substantially to cell proliferation through activation of JAK2/STAT3 and PI3K/AKT signaling pathways (Wong et al., 2018). Indeed, the inhibition of this Cl− current reduced cell viability, proliferation and migration. However, cell volume changes are detected by the Volume Regulated Anion Channel (VRAC) that is composed of members of the leucine-rich repeat-containing protein 8 (LRRC8) gene family, LRRC8A–E (Qiu et al., 2014; Voss et al., 2014). Downregulation of LRRC8A expression reduces GBM cell proliferation and increases sensitivity to the clinically used Temozolomide (TMZ) and Carmustine (Rubino et al., 2018).
The Anti- and Pro-Proliferative Effects of DEX
The effects of DEX on GBM cell proliferation depend on cell type, drug concentration and experimental conditions (Piette et al., 2006). The anti-proliferative effect of DEX has been shown in several cell lines (e.g., T98G, A172, 86HG39, F98, GL261, U87; Kaup et al., 2001; Fan et al., 2014). By contrast, Fan et al. (2014) found that DEX did not compromise the vitality of T98G and U251 cells. However, they showed that DEX significantly decreased the percentage of F98 cells in the S-phase favoring the G0/G1 phase of cell cycle, thus highlighting its cytostatic effect (Fan et al., 2014). Piette et al. (2009) reported that DEX attenuated cell proliferation, through inhibition of the ERK1/2 MAPK pathway. It has also been shown that DEX interrupted cell cycle progression through down-regulation of cyclin D1 and inhibition of ERK1/2 phosphorylation, without altering the overall expression level of ERK1/2 (Liu et al., 2009). Necrotic death of C6 cells can be induced by serum deprivation and DEX enhanced this cytotoxic effect through GR activation (Morita et al., 1999). In the same cell line, the anti-proliferative effect of DEX was shown to be dose and time dependent and to involve Aquaporin 1 (AQP1; Guan et al., 2018). Indeed, DEX increased the mRNA and protein expression of AQP1. Moreover, the effect of DEX on cell proliferation was abolished upon AQP1 gene silencing (Guan et al., 2018). Surprisingly, by using U373 cells, Gündisch et al. (2012) showed a pro-proliferative effect of DEX (Table 1).
In murine xenograph models, in vivo, DEX reduced tumor mass (Villeneuve et al., 2008). GL261 cells were implanted in the brains of male C57BL/6 mice and DEX treatment (1 mg/kg) was administered twice daily. After 20 days of treatment, this resulted in a 33% reduction in glioma volume (Villeneuve et al., 2008). Although, DEX did not influence the in vitro proliferation of GL261 cells, the decrease in tumor mass could be accounted for its effects on Ang-2 expression (Villeneuve et al., 2008).
DEX increases the chemotherapeutic action of several drugs. Indeed, in a human glioma U87 xenograph model, DEX significantly increased the therapeutic effects of carboplatin/gemcitabine combined therapy (Wang et al., 2004). However, the opposite effect of DEX has also been observed, in vitro. In particular, the strong apoptotic effect of TMZ in T98G and U87 cells was antagonized by DEX pre-treatment (Das et al., 2004, 2008; Sur et al., 2005). Notably, DEX administration pre-RT reduced the survival of glioma-bearing mice (Pitter et al., 2016).
GBM-Induced Cell Migration
The ability of GBM cells to migrate and invade healthy brain tissue is remarkable, and makes GBM cells excellent experimental models for studying migratory processes. Cell invasion comprises four basic steps: detachment of invasive cells from the primary tumor mass, adhesion to the extracellular matrix (ECM), matrix degradation and cell migration (Onishi et al., 2011). Matrix metalloproteinases (MMPs) have been implicated in the degradation of ECM in various physiological and pathological conditions. They are believed to be important factors in tumor invasion through their effects on components of the ECM (Nakada et al., 2003). A decisive role in glioma invasion is played by the gelatinases MMP-2 and MMP-9 and increased expression levels of MMP-2 is associated with glioma invasiveness (Rao, 2003).
Full surgical resection of GBM is impossible as cancer cells migrate actively along brain structures, including white matter tracks, interstitial space of the brain parenchyma, blood vessels and the subarachnoid space (Bellail et al., 2004; Cuddapah et al., 2014). Migration also requires physical contacts that the GBM cells establish with molecules of the vascular walls. It has been shown that bradykinin induces Ca2+ release from intracellular stores of GBM cells, promoting cell migration (Montana and Sontheimer, 2011). In addition, GBM cells release large amounts of glutamate in the brain parenchyma that kill neurons effectively and acts as an autocrine and paracrine signal to promote migration of cancer cells by triggering cytoplasmic Ca2+ oscillations (Ye and Sontheimer, 1999;Lyons et al., 2007).
To assume their characteristic migratory shape, GBM cells undergo cell volume changes and cytoskeletal remodeling. These processes are strictly dependent on the activity of several ion channel types. Indeed, Cl− and K+ channels are over-expressed in GBM cells and facilitate their migratory features. The Cl− and K+ ion flux through chloride channels and Ca2+-activated K+ channels (KCa1.1 and KCa3.1) allows cell volume changes that are essential events for effective GBM cell migration (Turner and Sontheimer, 2014; Catacuzzeno et al., 2015; Rosa et al., 2017). ClC-3 expression is up-regulated in glioma and correlates with WHO histological grade (Wang et al., 2017). Previous studies have shown the important role of ClC-3 in human glioma cells invasion through the application of pharmacological inhibitors or ClC-3 siRNA transfection (Lui et al., 2010; Cuddapah and Sontheimer, 2010). Recently, Wang et al. (2017) reported that knock-down of ClC-3, by using recombinant adenovirus expressing short hairpin RNA targeting human ClC-3 gene, inhibited the migration of U87MG cells significantly and reduced volume-regulated chloride currents in U87MG and SNB19 cells.
Previous studies have shown that hypoxia promotes GBM cell migration and increases tumor aggressiveness (Jensen, 2009; Yang et al., 2012). Extensive hypoxic areas are found within GBM mass that distinguish this tumor from those of low-grade malignancy. A distinctive histological pattern observed within the GBM is the palisade characterized by the presence of occluded vessels, around an extensive necrotic area and migrating cancerous cells (Rong et al., 2006; Amberger-Murphy, 2009). Sforna et al. (2017) reported that the swelling-activated Cl− current is up-regulated during hypoxia, an effect that further facilitates GBM cell migration and brain infiltration (Wong et al., 2018).
Effects of DEX on Cell Migration
Scant and inconsistent information is available on the effects of DEX on cell migration and this varies with the cell type under investigation. Inhibitory effects of DEX in migration/invasion of several glioma cell lines (e.g., C6, U251, U373, and A172), have been previously reported (Bauman et al., 1999). DEX inhibited the migration of U87 cells by reducing MMP-2 secretion (Lin et al., 2008). On the other hand, Piette et al. (2009) have shown that DEX treatment significantly reduced the migration and invasion of U373 cells through GR-dependent ERK1/2 MAPK pathway. It should be recalled that in GBM the ERK1/2 MAPK pathway is activated remarkably through EGFR and is linked to cell invasion and migration, as well as proliferation (Huang et al., 2009). Moreover, the isoforms p44 (ERK1) and p42 (ERK2) are stimulated by a wide variety of growth factors and mitogens (Johnson and Lapadat, 2002).
In stark contrast with previous studies, a recent report has shown that DEX facilitated C6 cells migration through up-regulation of AQP1 expression (Guan et al., 2018).
DEX Therapy in GBM
Patients with primary brain tumors are commonly treated with DEX before and after biopsy or resection and during RT. Unfortunately, DEX causes many side effects including myopathy, abnormal glucose metabolism, gastrointestinal complications, irritability, anxiety, insomnia, and is linked to high risk of pneumonia infection (Kostaras et al., 2014). Although, myopathy is reversible upon DEX discontinuation, almost 50% of patients develop disturbed glucose metabolism which persists even after dose reduction (Wen et al., 2006). Most psychiatric complications occur within the 1st week of therapy. The severity of side effects increases with the dose regimen and length of treatment. Thus, it is recommended that DEX therapy be reduced once symptoms commence to improve (Kostaras et al., 2014).
Upon DEX treatment apoptosis of T cell is observed, accounting for its immunosuppressant effects (Dietrich et al., 2011). The potent immunosuppressive actions of GCs could raise the question as to whether these drugs could weaken the body immune response against cancer cells and contribute to the short survival of GBM-affected individuals. However, due to its immunosuppressant activity, DEX interfered with conventional chemotherapies or electric field-based therapy delivered by the NovoTTF-100A (Hughes et al., 2005; Grossman et al., 2011; Wong et al., 2015). This is a new medical device used for chronic treatment of patients with recurrent or progressive GBM that exploit alternating electric fields (termed TTFields).
A number of studies have demonstrated DEX effectiveness in management of patients with brain peri-tumor oedema by ameliorating the symptoms associated with vasogenic oedema and intracranial high pressure within 48 h of treatment (Galicich et al., 1961; Hossmann et al., 1983; Ostergaard et al., 1999; Sinha et al., 2004; Dietrich et al., 2011; Kostaras et al., 2014). An excellent technique for monitoring tumor-related oedema in patients is magnetic resonance imaging (MRI) that allows the evaluation of various water diffusion parameters (Yamasaki et al., 2012; Bode et al., 2006; Kural et al., 2018). Using diffusion tensor imaging, Sinha et al. (2004) examined individuals with intracranial tumors and observed a significant reduction in the mean diffusivity of brain oedema after 48–72 h post-DEX treatment. However, a recent study conducted in 28 patients with different degrees of glioma, brain metastases and neurological deficits, reported that DEX had no significant effect on the volume of peritumoral oedema in 19 patients, while some improvements were observed in the remaining (Kural et al., 2018). Given the very frequent use of DEX in GBM therapy and the controversies concerning its effects there is now a renewed interest to evaluate the correlation between the use of this drug and the overall survival (OS) rate of treated patients. A recent study showed that administration of DEX during RT in patients treated with TMZ, was a poor prognostic indicator of both OS and progression-free survival (PFS). Therefore, DEX treatment before or concomitantly with TMZ was not recommended. However, the OS and PFS were not affected by DEX in patients who received a combination therapy with both TMZ and Bevacizumab (BEV), during RT (Shields et al., 2015). Important observations about the use of DEX in GBM therapy have been provided by Wong et al. (2015). Indeed, they showed that patients treated with higher DEX doses (>4.1 mg daily) had significantly shorter OS than those treated with lower doses (<4.1 mg daily). DEX-treatment at high doses (6–16 mg/day) resulted in the disappearance of the tumor mass in some patients. Remarkably, the tumor reappeared after 1–4 weeks and was much more aggressive (Buxton et al., 1997; Zaki et al., 2004; Goh et al., 2009). It has also been observed that DEX therapy reduced the OS of a particular subgroup of GBM patients (Dubinski et al., 2018). In this study, 35 patients received preoperative DEX and compared to 78 control patients. The OS value was not different between these two main groups. However, within the subgroup of patients who received DEX, 22 individuals showed DEX-induced leukocytosis (DIL) and had shorter OS that those without DIL. As such, patients with DIL were considered at a high risk of poor outcome (Dubinski et al., 2018).
In patients with cancer, inter-individual differences in drug response influences both the outcome to therapy, as well as the prevalence of adverse effects. Along with the progress in diagnosis, based on tumor molecular characteristics, genomic and proteomic approaches continue to be developed to ameliorate individualized treatment (Shai et al., 2008). Changes in protein expression due to altered gene regulation in drug-target genes can affect the efficacy or toxicity of the drug. This concept applies especially to GCs, as well as to other drugs used in the management of GBM. Indeed, GCs are known to regulate the expression of hundreds of genes, whose dysregulation could favor a poor prognosis for GBM patients. In a study that analysed the type of genes affected by DEX treatment from patient-derived GSC lines several genes associated with cell proliferation and migration were either up-regulated or down-regulated by the drug (Luedi et al., 2017). Importantly, DEX-responsive genes were found to be prognostic of poor outcome in two independent GBM cohorts (Luedi et al., 2017). Both, in vitro and in vivo evaluation of DEX effects along with the analysis of clinical information from The Cancer Genome Atlas (TCGA) cohort, important correlations have been derived between DEX treatment and alterations in gene expression profiles. The comparison between patients with mesenchymal and proneural GBM showed that the former subgroup had significant up-regulation of DEX-controlled gene network as well as pathways closely related to proliferation, invasion, and angiogenesis. The effects of DEX on invasion, proliferation and angiogenesis in GBM patient–derived GSCs with IDH1 wild-type (GSC3) and with IDH1 mutant (GSC6) were very recently evaluated. DEX significantly increased the invasive properties of the GSC3 cell line, both in vitro and in vivo. Moreover, using both GSC3 and GSC6 lines, the drug promoted proliferation and angiogenesis, in vivo (Luedi et al., 2018). The results of this interesting study, have questioned the use of DEX in GBM therapy, highlighting its ability to increase the aggressiveness of the tumor.
Concluding Remarks
Since 1960, DEX represented the gold standard for treatment to reduce oedema caused by brain tumors, although current literature fails to clearly delineate the mechanism of action. In this review, we have discussed several critical factors regarding the influence of DEX in GBM. Overall, contradictory effects of DEX opposing or favoring GBM aggressiveness have emerged from a number of in vitro, in vivo and clinical studies. It has been argued that this conflicting data was a result of different experimental conditions (Mealey et al., 1971; Grasso et al., 1977; Pinski et al., 1993; Langeveld et al., 1992; Morita et al., 1999; Piette et al., 2006). Considering this variability and current controversial issues, investigators are focusing on genetic biomarkers and transcriptional signature that are influenced by DEX. Knowledge derived through these studies is highly needed to provide prediction in patient OS. The urge to evaluate other subtypes of GBM that are distinguished by different genetic and epigenetic alterations, the distinct susceptibility to DEX and their changes in the degree of malignancy upon drug exposure is inevitable in future studies. Indeed, establishing the GBM type that can or cannot be treated with DEX along with early diagnosis shall increase patients’ life expectancy, considerably.
Pharmacogenomics promise the advent of precision medicine. Thus, to optimize DEX therapy with respect to patients’ genotype and ensure maximum efficiency with minimal adverse effects, genome-wide association investigations incorporating genomics and epigenetic approaches, should be considered. The recognition of the various desirable and undesirable pathways that are activated in GBM by GCs could pave the way towards a combinational therapy, consisting in the co-administration of a classical GC with drugs that inhibit the unwanted pathways activated by that specific GC. Furthermore, a road map guiding progress towards development of selective GR agonists and modulators with a more restricted GR activity, which retain efficacy without eliciting unwanted adverse effects should be established (Sundahl et al., 2016). Overall, these strategies could settle the “DEX issue in GBM” and allow the prescription of this drug to distinct subsets of patients or through individual therapy depending on one’s genetic makeup.
Author Contributions
MC, MV, SB, MD and MP contributed to writing the manuscript. LS, PR and SR contributed to revising the manuscript. MP coordinated the writing, revised and submitted the manuscript.
Funding
This work was supported by the University of Malta—Research, Innovation and Development Trust (RIDT) and Internal Research Grant. MV was supported by the Alfred Mizzi Foundation, Malta through the RIDT.
Conflict of Interest Statement
The authors declare that the research was conducted in the absence of any commercial or financial relationships that could be construed as a potential conflict of interest.
Acknowledgments
We thank Eleanor Harold-Barry for reading and commenting our manuscript.
References
Alaminos, M., Dávalos, V., Ropero, S., Setién, F., Paz, M. F., Herranz, M., et al. (2005). EMP3, a myelin-related gene located in the critical 19q13.3 region, is epigenetically silenced and exhibits features of a candidate tumor suppressor in glioma and neuroblastoma. Cancer Res. 65, 2565–2571. doi: 10.1158/0008-5472.can-04-4283
Alexiou, G. A., and Voulgaris, S. (2010). The role of the PTEN gene in malignant gliomas. Neurol. Neurochir. Pol. 44, 80–86. doi: 10.1016/s0028-3843(14)60408-4
Amberger-Murphy, V. (2009). Hypoxia helps glioma to fight therapy. Curr. Cancer Drug Targets 9, 381–390. doi: 10.2174/156800909788166637
An, Z., Gluck, C. B., Choy, M. L., and Kaufman, L. I. (2010). Suberoylanilide hydroxamic acid limits migration and invasion of glioma cells in two and three dimensional culture. Cancer Lett. 292, 215–227. doi: 10.1016/j.canlet.2009.12.006
Bao, S., Wu, Q., McLendon, R. E., Hao, Y., Shi, Q., Hjelmeland, A. B., et al. (2006). Glioma stem cells promote radioresistance by preferential activation of the DNA damage response. Nature 444, 756–760. doi: 10.1038/nature05236
Bauman, G. S., MacDonald, W., Moore, E., Ramsey, D. A., Fisher, B. J., Amberger, V. R., et al. (1999). Effects of radiation on a model of malignant glioma invasion. J. Neurooncol. 44, 223–231. doi: 10.1023/A:1006319417077
Beauchesne, P. (2011). Extra-neural metastases of malignant gliomas: myth or reality? Cancers 3, 461–477. doi: 10.3390/cancers3010461
Bellail, A. C., Hunter, S. B., Brat, D. J., Tan, C., and Van Meir, E. G. (2004). Microregional extracellular matrix heterogeneity in brain modulates glioma cell invasion. Int. J. Biochem. Cell Biol. 36, 1046–1069. doi: 10.1016/j.biocel.2004.01.013
Bezecny, P. (2014). Histone deacetylase inhibitors in glioblastoma: pre-clinical and clinical experience. Med. Oncol. 31:985. doi: 10.1007/s12032-014-0985-5
Bode, M. K., Ruohonen, J., Nieminen, M. T., and Pyhtinen, J. (2006). Potential of diffusion imaging in brain tumors: a review. Acta Radiol. 47, 585–594. doi: 10.1080/02841850600580598
Buxton, N., Phillips, N., and Robertson, I. (1997). The case of the disappearing glioma. J. Neurol. Neurosurg. Psychiatry 63, 520–521. doi: 10.1136/jnnp.63.4.520
Campos-Bedolla, P., Walter, F. R., Veszelka, S., and Deli, M. A. (2014). Role of the blood-brain barrier in the nutrition of the central nervous system. Arch. Med. Res. 45, 610–638. doi: 10.1016/j.arcmed.2014.11.018
Catacuzzeno, L., Caramia, M., Sforna, L., Belia, S., Guglielmi, L., D’Adamo, M. C., et al. (2015). Reconciling the discrepancies on the involvement of large-conductance Ca2+-activated K channels in glioblastoma cell migration. Front. Cell. Neurosci. 9:152. doi: 10.3389/fncel.2015.00152
Catacuzzeno, L., Michelucci, A., Sforna, L., Aiello, F., Sciaccaluga, M., Fioretti, B., et al. (2014). Identification of key signaling molecules involved in the activation of the swelling-activated chloride current in human glioblastoma cells. J. Membr. Biol. 247, 45–55. doi: 10.1007/s00232-013-9609-9
Chistiakov, D. A., Chekhonin, I. V., and Chekhonin, V. P. (2017). The EGFR variant III mutant as a target for immunotherapy of glioblastoma multiforme. Eur. J. Pharmacol. 810, 70–82. doi: 10.1016/j.ejphar.2017.05.064
Crespo, I., Vital, A. L., Gonzalez-Tablas, M., Patino Mdel, C., Otero, A., Lopes, M. C., et al. (2015). Molecular and genomic alterations in glioblastoma multiforme. Am. J. Pathol. 185, 1820–1833. doi: 10.1016/j.ajpath.2015.02.023
Cuddapah, V. A., Robel, S., Watkins, S., and Sontheimer, H. (2014). A neurocentric perspective on glioma invasion. Nat. Rev. Neurosci. 15, 455–465. doi: 10.1038/nrn3765
Cuddapah, V. A., and Sontheimer, H. (2010). Molecular interaction and functional regulation of ClC-3 by Ca2+/calmodulin-dependent protein kinase II (CaMKII) in human malignant glioma. J. Biol. Chem. 285, 11188–11196. doi: 10.1074/jbc.M109.097675
Czapski, B., Baluszek, S., Herold-Mende, C., and Kaminska, B. (2018). Clinical and immunological correlates of long term survival in glioblastoma. Contemp. Oncol. 22, 81–85. doi: 10.5114/wo.2018.73893
D’Alessandro, G., Limatola, C., and Catalano, M. (2018). Functional roles of the Ca2+-activated K+ channel, KCa3.1, in brain tumors. Curr. Neuropharmacol. 16, 636–643. doi: 10.2174/0929867324666170713103621
Das, A., Banik, N. L., Patel, S. J., and Ray, S. K. (2004). Dexamethasone protected human glioblastoma U87MG cells from temozolomide induced apoptosis by maintaining Bax:Bcl-2 ratio and preventing proteolytic activities. Mol. Cancer 3:36. doi: 10.1186/1476-4598-3-36
Das, A., Banik, N. L., and Ray, S. K. (2008). Modulatory effects of acetazolomide and dexamethasone on temozolomide-mediated apoptosis in human glioblastoma T98G and U87MG cells. Cancer Invest. 26, 352–358. doi: 10.1080/07357900701788080
DeAngelis, L. M., and Mellinghoff, I. K. (2011). Virchow 2011 or how to ID(H) human glioblastoma. J. Clin. Oncol. 29, 4473–4474. doi: 10.1200/JCO.2011.37.5873
Dietrich, J., Rao, K., Pastorino, S., and Kesari, S. (2011). Corticosteroids in brain cancer patients: benefits and pitfalls. Expert Rev. Clin. Pharmacol. 4, 233–242. doi: 10.1586/ecp.11.1
Dolecek, T. A., Propp, J. M., Stroup, N. E., and Kruchko, C. (2012). CBTRUS statistical report: primary brain and central nervous system tumors diagnosed in the United States in 2005–2009. Neuro Oncol. 14, v1–v49. doi: 10.1093/neuonc/nos218
Dong, Z., and Cui, H. (2018). Epigenetic modulation of metabolism in glioblastoma. Semin. Cancer Biol. doi: 10.1016/j.semcancer.2018.09.002 [Epub ahead of print].
Dubinski, D., Won, S. Y., Gessler, F., Quick-Weller, J., Behmanesh, B., Bernatz, S., et al. (2018). Dexamethasone-induced leukocytosis is associated with poor survival in newly diagnosed glioblastoma. J. Neurooncol. 137, 503–510. doi: 10.1007/s11060-018-2761-4
Dubois, L. G., Campanati, L., Righy, C., D’Andrea-Meira, I., Spohr, T. C., Porto-Carreiro, I., et al. (2014). Gliomas and the vascular fragility of the blood brain barrier. Front. Cell. Neurosci. 8:418. doi: 10.3389/fncel.2014.00418
Fan, Z., Sehm, T., Rauh, M., Buchfelder, M., Eyupoglu, I. Y., and Savaskan, N. E. (2014). Dexamethasone alleviates tumor-associated brain damage and angiogenesis. PLoS One 9:e93264. doi: 10.1371/journal.pone.0093264
Finkenzeller, G., Technau, A., and Marmé, D. (1995). Hypoxia-induced transcription of the vascular endothelial growth factor gene is independent of functional AP-1 transcription factor. Biochem. Biophys. Res. Commun. 208, 432–439. doi: 10.1006/bbrc.1995.1356
Frank, N. Y., Schatton, T., and Frank, M. H. (2010). The therapeutic promise of the cancer stem cell concept. J. Clin. Invest. 120, 41–50. doi: 10.1172/JCI41004
Frederick, L., Wang, X. Y., Eley, G., and James, C. D. (2000). Diversity and frequency of epidermal growth factor receptor mutations in human glioblastomas. Cancer Res. 60, 1383–1387.
Furnari, F. B., Fenton, T., Bachoo, R. M., Mukasa, A., Stommel, J. M., Stegh, A., et al. (2007). Malignant astrocytic glioma: genetics, biology and paths to treatment. Genes Dev. 21, 2683–2710. doi: 10.1101/gad.1596707
Furuse, M., Fujimoto, K., Sato, N., Hirase, T., Tsukita, S., and Tsukita, S. (1996). Overexpression of occludin, a tight junction-associated integral membrane protein, induces the formation of intracellular multilamellar bodies bearing tight junction-like structures. J. Cell Sci. 109, 429–435.
Furuse, M., Hirase, T., Itoh, M., Nagafuchi, A., Yonemura, S., Tsukita, S., et al. (1993). Occludin: a novel integral membrane protein localizing at tight junctions. J. Cell Biol. 123, 1777–1788. doi: 10.1083/jcb.123.6.1777
Furuse, M., Sasaki, H., Fujimoto, K., and Tsukita, S. (1998). A single gene product, claudin-1 or -2, reconstitutes tight junction strands and recruits occludin in fibroblasts. J. Cell Biol. 143, 391–401. doi: 10.1083/jcb.143.2.391
Galicich, J. H., French, L. A., and Melby, J. C. (1961). Use of dexamethasone in treatment of cerebral oedema associated with brain tumors. J. Lancet 81, 46–53.
Goh, J. J., See, S. J., Ang, E., and Ng, W. H. (2009). Vanishing glioblastoma after corticosteroid therapy. J. Clin. Neurosci. 16, 1226–1228. doi: 10.1016/j.jocn.2008.10.029
Grasso, R. J., Johnson, C. E., Boler, R. K., and Moore, N. A. (1977). Combined growth-inhibitory responses and ultrastructural alterations produced by 1,3-bis(2-chloroethyl)-1-nitrosourea and dexamethasone in rat glioma cell cultures. Cancer Res. 37, 585–594.
Grinshtein, N., Rioseco, C. C., Marcellus, R., Uehling, D., Aman, A., Lun, X., et al. (2016). Small molecule epigenetic screen identifies novel EZH2 and HDAC inhibitors that target glioblastoma brain tumor-initiating cells. Oncotarget 7, 59360–59376. doi: 10.18632/oncotarget.10661
Grossman, S. A., Ye, X., Lesser, G., Sloan, A., Carraway, H., Desideri, S., et al. (2011). Immunosuppression in patients with high-grade gliomas treated with radiation and temozolomide. Clin. Cancer Res. 17, 5473–5480. doi: 10.1158/1078-0432.CCR-11-0774
Gu, Y. T., Qin, L. J., Qin, X., and Xu, F. (2009a). The molecular mechanism of dexamethasone-mediated effect on the blood-brain tumor barrier permeability in a rat brain tumor model. Neurosci. Lett. 452, 114–118. doi: 10.1016/j.neulet.2008.12.047
Gu, Y. T., Xue, Y. X., Wang, P., Zhang, H., Qin, L. J., and Liu, L. B. (2009b). Dexamethasone enhances calcium-activated potassium channel expression in blood-brain tumor barrier in a rat brain tumor model. Brain Res. 1259, 1–6. doi: 10.1016/j.brainres.2008.12.080
Gu, Y. T., Zhang, H., and Xue, Y. X. (2007). Dexamethasone enhances adenosine 5′-triphosphate-sensitive potassium channel expression in the blood-brain tumor barrier in a rat brain tumor model. Brain Res. 1162, 1–8. doi: 10.1016/j.brainres.2007.05.053
Guan, Y., Chen, J., Zhan, Y., and Lu, H. (2018). Effects of dexamethasone on C6 cell proliferation, migration and invasion through the upregulation of AQP1. Oncol. Lett. 15, 7595–7602. doi: 10.3892/ol.2018.8269
Guerin, C., Wolff, J. E., Laterra, J., Drewes, L. R., Brem, H., and Goldstein, G. W. (1992). Vascular differentiation and glucose transporter expression in rat gliomas: effects of steroids. Ann. Neurol. 31, 481–487. doi: 10.1002/ana.410310504
Gündisch, S., Boeckeler, E., Behrends, U., Amtmann, E., Ehrhardt, H., and Jeremias, I. (2012). Glucocorticoids augment survival and proliferation of tumor cells. Anticancer Res. 32, 4251–4261.
Han, C. H., and Batchelor, T. T. (2017). Isocitrate dehydrogenase mutation as a therapeutic target in gliomas. Chin. Clin. Oncol. 6:33. doi: 10.21037/cco.2017.06.11
Hardee, M. E., and Zagzag, D. (2012). Mechanisms of glioma-associated neovascularization. Am. J. Pathol. 181, 1126–1141. doi: 10.1016/j.ajpath.2012.06.030
Hartmann, C., Meyer, J., Balss, J., Capper, D., Mueller, W., Christians, A., et al. (2009). Type and frequency of IDH1 and IDH2 mutations are related to astrocytic and oligodendroglial differentiation and age:a study of 1,010 diffuse gliomas. Acta Neuropathol. 118, 469–474. doi: 10.1007/s00401-009-0561-9
Heddleston, J. M., Li, Z., Lathia, J. D., Bao, S., Hjelmeland, A. B., and Rich, J. N. (2010). Hypoxia inducible factors in cancer stem cells. Br. J. Cancer 102, 789–795. doi: 10.1038/sj.bjc.6605551
Hossmann, K. A., Hürter, T., and Oschlies, U. (1983). The effect of dexamethasone on serum protein extravasation and oedema development in experimental brain tumors of cat. Acta Neuropathol. 60, 223–231. doi: 10.1007/bf00691870
Huang, P. H., Xu, A. M., and White, F. M. (2009). Oncogenic EGFR signaling networks in glioma. Sci. Signal 2:re6. doi: 10.1126/scisignal.287re6
Hue, C. D., Cho, F. S., Cao, S., Dale Bass, C. R., Meaney, D. F., and Morrison, B. (2015). Dexamethasone potentiates in vitro blood-brain barrier recovery after primary blast injury by glucocorticoid receptor-mediated upregulation of ZO-1 tight junction protein. J. Cereb. Blood Flow Metab. 35, 1191–1198. doi: 10.1038/jcbfm.2015.38
Hughes, M. A., Parisi, M., Grossman, S., and Kleinberg, L. (2005). Primary brain tumors treated with steroids and radiotherapy: low CD4 counts and risk of infection. Int. J. Radiat. Oncol. Biol. Phys. 62, 1423–1426. doi: 10.1016/j.ijrobp.2004.12.085
Jensen, R. L. (2009). Brain tumor hypoxia: tumorigenesis, angiogenesis, imaging, pseudoprogression and as a therapeutic target. J. Neurooncol. 92, 317–335. doi: 10.1007/s11060-009-9827-2
Johnson, G. L., and Lapadat, R. (2002). Mitogen-activated protein kinase pathways mediated by ERK, JNK, and p38 protein kinases. Science 298, 1911–1912. doi: 10.1126/science.1072682
Kaal, E. C., and Vecht, C. J. (2004). The management of brain oedema in brain tumors. Curr. Opin. Oncol. 16, 593–600. doi: 10.1097/01.cco.0000142076.52721.b3
Kale, G., Naren, A. P., Sheth, P., and Rao, R. K. (2003). Tyrosine phosphorylation of occludin attenuates its interactions with ZO-1, ZO-2, and ZO-3. Biochem. Biophys. Res. Commun. 302, 324–329. doi: 10.1016/S0006-291X(03)00167-0
Kanazawa, T., Minami, Y., Jinzaki, M., Toda, M., Yoshida, K., and Sasaki, H. (2019). Predictive markers for MGMT promoter methylation in glioblastomas. Neurosurg. Rev. doi: 10.1007/s10143-018-01061-5 [Epub ahead of print].
Kaup, B., Schindler, I., Knüpfer, H., Schlenzka, A., Preiss, R., and Knüpfer, M. M. (2001). Time-dependent inhibition of glioblastoma cell proliferation by dexamethasone. J. Neurooncol. 51, 105–110. doi: 10.1023/A:1010684921099
Kaur, C., and Ling, E. A. (2008). Blood brain barrier in hypoxic-ischemic conditions. Curr. Neurovasc. Res. 5, 71–81. doi: 10.2174/156720208783565645
Kim, H., Lee, J. M., Park, J. S., Jo, S. A., Kim, Y. O., Kim, C. W., et al. (2008). Dexamethasone coordinately regulates angiopoietin-1 and VEGF: a mechanism of glucocorticoid-induced stabilization of blood-brain barrier. Biochem. Biophys. Res. Commun. 372, 243–248. doi: 10.1016/j.bbrc.2008.05.025
Kleihues, P. (1998). Subsets of glioblastoma: clinical and histological vs. Brain Pathol. 8, 667–668. doi: 10.1111/j.1750-3639.1998.tb00192.x
Kostaras, X., Cusano, F., Kline, G. A., Roa, W., and Easaw, J. (2014). Use of dexamethasone in patients with high-grade glioma: a clinical practice guideline. Curr. Oncol. 21, e493–e503. doi: 10.3747/co.21.1769
Kubota, K., Furuse, M., Sasaki, H., Sonoda, N., Fujita, K., Nagafuchi, A., et al. (1999). Ca2+-independent cell-adhesion activity of claudins, a family of integral membrane proteins localized at tight junctions. Curr. Biol. 9, 1035–1038. doi: 10.1016/s0960-9822(99)80452-7
Kumabe, T., Sohma, Y., Kayama, T., Yoshimoto, T., and Yamamoto, T. (1992). Overexpression and amplification of α-PDGF receptor gene lacking exons coding for a portion of the extracellular region in a malignant glioma. Tohoku J. Exp. Med. 168, 265–269. doi: 10.1620/tjem.168.265
Kural, C., Atac, G. K., Tehli, O., Solmaz, I., Temiz, C., Hodaj, I., et al. (2018). The evaluation of the effects of steroid treatment on the tumor and peritumoral oedema by DWI and MR spectroscopy in brain tumors. Neurol. Neurochir. Pol. 52, 495–504. doi: 10.1016/j.pjnns.2018.03.002
Langeveld, C. H., van Waas, M. P., Stoof, J. C., Sutanto, W., de Kloet, E. R., Wolbers, J. G., et al. (1992). Implication of glucocorticoid receptors in the stimulation of human glioma cell proliferation by dexamethasone. J. Neurosci. Res. 31, 524–531. doi: 10.1002/jnr.490310316
Lara-Velazquez, M., Al-Kharboosh, R., Jeanneret, S., Vazquez-Ramos, C., Mahato, D., Tavanaiepour, D., et al. (2017). Advances in brain tumor surgery for glioblastoma in adults. Brain Sci. 7:E166. doi: 10.3390/brainsci7120166
Lee, P., Murphy, B., Miller, R., Menon, V., Banik, N. L., Giglio, P., et al. (2015). Mechanisms and clinical significance of histone deacetylase inhibitors: epigenetic glioblastoma therapy. Anticancer Res. 35, 615–625.
Lee, D. H., Ryu, H. W., Won, H. R., and Kwon, S. H. (2017). Advances in epigenetic glioblastoma therapy. Oncotarget 8, 18577–18589. doi: 10.18632/oncotarget.14612
Lee, E., Yong, R. L., Paddison, P., and Zhu, J. (2018). Comparison of glioblastoma (GBM) molecular classification methods. Semin. Cancer Biol. 53, 201–211. doi: 10.1016/j.semcancer.2018.07.006
Leonardi, R., Subramanian, C., Jackowski, S., and Rock, C. O. (2012). Cancer associated isocitrate dehydrogenase mutations inactivate NADPH-dependent reductive carboxylation. J. Biol. Chem. 287, 14615–14620. doi: 10.1074/jbc.C112.353946
Li, X., Wu, C., Chen, N., Gu, H., Yen, A., Cao, L., et al. (2016). PI3K/Akt/mTOR signaling pathway and targeted therapy for glioblastoma. Oncotarget 7, 33440–33450. doi: 10.18632/oncotarget.7961
Li, Z., Bao, S., Wu, Q., Wang, H., Eyler, C., Sathornsumetee, S., et al. (2009). Hypoxia-inducible factors regulate tumorigenic capacity of glioma stem cells. Cancer Cell 15, 501–513. doi: 10.1016/j.ccr.2009.03.018
Liebner, S., Fischmann, A., Rascher, G., Duffner, F., Grote, E. H., Kalbacher, H., et al. (2000). Claudin-1 and claudin-5 expression and tight junction morphology are altered in blood vessels of human glioblastoma multiforme. Acta Neuropathol. 100, 323–331. doi: 10.1007/s004010000180
Liu, H., Huang, X., Wang, H., Shen, A., and Cheng, C. (2009). Dexamethasone inhibits proliferation and stimulates SSeCKS expression in C6 rat glioma cell line. Brain Res. 1265, 1–12. doi: 10.1016/j.brainres.2009.01.050
Lin, Y. M., Jan, H. J., Lee, C. C., Tao, H. Y., Shih, Y. L., Wei, H. W., et al. (2008). Dexamethasone reduced invasiveness of human malignant glioblastoma cells through a MAPK phosphatase-1(MKP-1) dependent mechanism. Eur. J. Pharmacol. 593, 1–9. doi: 10.1016/j.ejphar.2008.06.111
Lin, K. T., and Wang, L. H. (2016). New dimension of glucocorticoids in cancer treatment. Steroids 111, 84–88. doi: 10.1016/j.steroids.2016.02.019
Lin, L., Xue, Y., Duan, Q., Sun, B., Lin, H., Huang, X., et al. (2016). The role of cerebral blood flow gradient in peritumoral oedema for differentiation of glioblastomas from solitary metastatic lesions. Oncotarget 7, 69051–69059. doi: 10.18632/oncotarget.12053
Louis, D. N., Perry, A., Reifenberger, G., von Deimling, A., Figarella-Branger, D., Cavenee, W. K., et al. (2016). The 2016 world health organization classification of tumors of the central nervous system: a summary. Acta Neuropathol. 131, 803–820. doi: 10.1007/s00401-016-1545-1
Luedi, M. M., Singh, S. K., Mosley, J. C., Hassan, I. S. A., Hatami, M., Gumin, J., et al. (2018). Dexamethasone-mediated oncogenicity in vitro and in an animal model of glioblastoma. J. Neurosurg. 129, 1446–1455. doi: 10.3171/2017.7.jns17668
Luedi, M. M., Singh, S. K., Mosley, J. C., Hatami, M., Gumin, J., Sulman, E. P., et al. (2017). A dexamethasone-regulated gene signature is prognostic for poor survival in glioblastoma patients. J. Neurosurg. Anesthesiol. 29, 46–58. doi: 10.1097/ANA.0000000000000368
Lui, V. C., Lung, S. S., Pu, J. K., Hung, K. N., and Leung, G. K. (2010). Invasion of human glioma cells is regulated by multiple chloride channels including ClC-3. Anticancer Res. 30, 4515–4524.
Lyons, S. A., Chung, W. J., Weaver, A. K., Ogunrinu, T., and Sontheimer, H. (2007). Autocrine glutamate signaling promotes glioma cell invasion. Cancer Res. 67, 9463–9471. doi: 10.1158/0008-5472.can-07-2034
Machein, M. R., Kullmer, J., Rönicke, V., Machein, U., Krieg, M., Damert, A., et al. (1999). Differential downregulation of vascular endothelial growth factor by dexamethasone in normoxic and hypoxic rat glioma cells. Neuropathol. Appl. Neurobiol. 25, 104–112. doi: 10.1046/j.1365-2990.1999.00166.x
Maire, C. L., and Ligon, K. L. (2014). Molecular pathologic diagnosis of epidermal growth factor receptor. Neuro Oncol. 16, viii1–viii6. doi: 10.1093/neuonc/nou294
Maisonpierre, P. C., Suri, C., Jones, P. F., Bartunkova, S., Wiegand, S. J., Radziejewski, C., et al. (1997). Angiopoietin-2, a natural antagonist for Tie2 that disrupts in vivo angiogenesis. Science 277, 55–60.
Martinez, R., and Esteller, M. (2010). The DNA methylome of glioblastoma multiforme. Neurobiol. Dis. 39, 40–46. doi: 10.1016/j.nbd.2009.12.030
Mealey, J. Jr., Chen, T. T., and Schanz, G. P. (1971). Effects of dexamethasone and methylprednisolone on cell cultures of human glioblastomas. J. Neurosurg. 34, 324–334. doi: 10.3171/jns.1971.34.3.0324
Meyer-Puttlitz, B., Hayashi, Y., Waha, A., Rollbrocker, B., Boström, J., Wiestler, O. D., et al. (1997). Molecular genetic analysis of giant cell glioblastomas. Am. J. Pathol. 151, 853–857.
Michinaga, S., and Koyama, Y. (2015). Pathogenesis of brain oedema and investigation into anti-oedema drugs. Int. J. Mol. Sci. 16, 9949–9975. doi: 10.3390/ijms16059949
Miller, C. R., and Perry, A. (2007). Glioblastoma. Arch. Pathol. Lab. Med. 131, 397–406. doi: 10.1043/1543-2165(2007)131[397:G]2.0.CO;2
Montana, V., and Sontheimer, H. (2011). Bradykinin promotes the chemotactic invasion of primary brain tumors. J. Neurosci. 31, 4858–4867. doi: 10.1523/JNEUROSCI.3825-10.2011
Morita, K., Ishimura, K., Tsuruo, Y., and Wong, D. L. (1999). Dexamethasone enhances serum deprivation-induced necrotic death of rat C6 glioma cells through activation of glucocorticoid receptors. Brain Res. 816, 309–316. doi: 10.1016/s0006-8993(98)01093-2
Nag, S., Papneja, T., Venugopalan, R., and Stewart, D. J. (2005). Increased angiopoietin2 expression is associated with endothelial apoptosis and blood-brain barrier breakdown. Lab. Invest. 85, 1189–1198. doi: 10.1038/labinvest.3700325
Nakada, M., Okada, Y., and Yamashita, J. (2003). The role of matrix metalloproteinases in glioma invasion. Front. Biosci. 8, e261–e269. doi: 10.2741/1016
Nakamura, M., Watanabe, T., Yonekawa, Y., Kleihues, P., and Ohgaki, H. (2001). Promoter methylation of the DNA repair gene MGMT in astrocytomas is frequently associated with G:C –>A:T mutations of the TP53 tumor suppressor gene. Carcinogenesis 22, 1715–1719. doi: 10.1093/carcin/22.10.1715
Nauck, M., Karakiulakis, G., Perruchoud, A. P., Papakonstantinou, E., and Roth, M. (1998). Corticosteroids inhibit the expression of the vascular endothelial growth factor gene in human vascular smooth muscle cells. Eur. J. Pharmacol. 341, 309–315. doi: 10.1016/s0014-2999(97)01464-7
Onishi, M., Ichikawa, T., Kurozumi, K., and Date, I. (2011). Angiogenesis and invasion in glioma. Brain Tumor Pathol. 28, 13–24. doi: 10.1007/s10014-010-0007-z
Ostergaard, L., Hochberg, F. H., Rabinov, J. D., Sorensen, A. G., Lev, M., Kim, L., et al. (1999). Early changes measured by magnetic resonance imaging in cerebral blood flow, blood volume and blood-brain barrier permeability following dexamethasone treatment in patients with brain tumors. J. Neurosurg. 90, 300–305. doi: 10.3171/jns.1999.90.2.0300
Paff, M., Alexandru-Abrams, D., Hsu, F. P., and Bota, D. A. (2014). The evolution of the EGFRvIII (rindopepimut) immunotherapy for glioblastoma multiforme patients. Hum. Vaccin. Immunother. 10, 3322–3331. doi: 10.4161/21645515.2014.983002
Papadopoulos, M. C., Saadoun, S., Binder, D. K., Manley, G. T., Krishna, S., and Verkman, A. S. (2004). Molecular mechanisms of brain tumor oedema. Neuroscience 129, 1011–1020. doi: 10.1016/j.neuroscience.2004.05.044
Papadopoulos, M. C., Saadoun, S., Woodrow, C. J., Davies, D. C., Costa-Martins, P., Moss, R. F., et al. (2001). Occludin expression in microvessels of neoplastic and non-neoplastic human brain. Neuropathol. Appl. Neurobiol. 27, 384–395. doi: 10.1046/j.0305-1846.2001.00341.x
Park, D. M., and Rich, J. N. (2009). Biology of glioma cancer stem cells. Mol. Cells 28, 7–12. doi: 10.1007/s10059-009-0111-2
Parsons, D. W., Jones, S., Zhang, X., Lin, J. C., Leary, R. J., Angenendt, P., et al. (2008). An integrated genomic analysis of human glioblastoma multiforme. Science 321, 1807–1812. doi: 10.1126/science.1164382
Piette, C., Deprez, M., Roger, T., Noël, A., Foidart, J. M., and Munaut, C. (2009). The dexamethasone-induced inhibition of proliferation, migration, and invasion in glioma cell lines is antagonized by macrophage migration inhibitory factor (MIF) and can be enhanced by specific MIF inhibitors. J. Biol. Chem. 284, 32483–32492. doi: 10.1074/jbc.m109.014589
Piette, C., Munaut, C., Foidart, J. M., and Deprez, M. (2006). Treating gliomas with glucocorticoids: from bedside to bench. Acta Neuropathol. 112, 651–664. doi: 10.1007/s00401-006-0100-x
Pinski, J., Halmos, G., Shirahige, Y., Wittliff, J. L., and Schally, A. V. (1993). Inhibition of growth of the human malignant glioma cell line (U87MG) by the steroid hormone antagonist RU486. J. Clin. Endocrinol. Metab. 77, 1388–1392. doi: 10.1210/jcem.77.5.8077338
Pitter, K. L., Tamagno, I., Alikhanyan, K., Hosni-Ahmed, A., Pattwell, S. S., Donnola, S., et al. (2016). Corticosteroids compromise survival in glioblastoma. Brain 139, 1458–1471. doi: 10.1093/brain/aww046
Qiu, Z., Dubin, A. E., Mathur, J., Tu, B., Reddy, K., Miraglia, L. J., et al. (2014). SWELL1, a plasma membrane protein, is an essential component of volume-regulated anion channel. Cell 157, 447–458. doi: 10.1016/j.cell.2014.03.024
Rand, V., Huang, J., Stockwell, T., Ferriera, S., Buzko, O., Levy, S., et al. (2005). Sequence survey of receptor tyrosine kinases reveals mutations in glioblastomas. Proc. Natl. Acad. Sci. U S A 102, 14344–14349. doi: 10.1073/pnas.0507200102
Rao, J. S. (2003). Molecular mechanisms of glioma invasiveness: the role of proteases. Nat. Rev. Cancer 3, 489–501. doi: 10.1038/nrc1121
Reya, T., Morrison, S. J., Clarke, M. F., and Weissman, I. L. (2001). Stem cells, cancer and cancer stem cells. Nature 414, 105–111. doi: 10.1038/35102167
Romani, M., Pistillo, M. P., and Banelli, B. (2018). Epigenetic targeting of glioblastoma. Front. Oncol. 8:448. doi: 10.3389/fonc.2018.00448
Rong, Y., Durden, D. L., Van Meir, E. G., and Brat, D. J. (2006). ‘Pseudopalisading’ necrosis in glioblastoma: a familiar morphologic feature that links vascular pathology, hypoxia, and angiogenesis. J. Neuropathol. Exp. Neurol. 65, 529–539. doi: 10.1097/00005072-200606000-00001
Rosa, P., Sforna, L., Carlomagno, S., Mangino, G., Miscusi, M., Pessia, M., et al. (2017). Overexpression of large-conductance calcium-activated potassium channels in human glioblastoma stem-like cells and their role in cell migration. J. Cell. Physiol. 232, 2478–2488. doi: 10.1002/jcp.25592
Rosen, J. M., and Jordan, C. T. (2009). The increasing complexity of the cancer stem cell paradigm. Science 324, 1670–1673. doi: 10.1126/science.1171837
Rubin, L. L., and Staddon, J. M. (1999). The cell biology of the blood-brain barrier. Annu. Rev. Neurosci. 22, 11–28. doi: 10.1146/annurev.neuro.22.1.11
Rubino, S., Bach, M. D., Schober, A. L., Lambert, I. H., and Mongin, A. A. (2018). Downregulation of leucine-rich repeat-containing 8A limits proliferation and increases sensitivity of glioblastoma to temozolomide and carmustine. Front. Oncol. 8:142. doi: 10.3389/fonc.2018.00142
Salmaggi, A., Gelati, M., Pollo, B., Frigerio, S., Eoli, M., Silvani, A., et al. (2004). CXCL12 in malignant glial tumors: a possible role in angiogenesis and cross-talk between endothelial and tumoral cells. J. Neurooncol. 67, 305–317. doi: 10.1023/b:neon.0000024241.05346.24
Salvador, E., Shityakov, S., and Förster, C. (2014). Glucocorticoids and endothelial cell barrier function. Cell Tissue Res. 355, 597–605. doi: 10.1007/s00441-013-1762-z
Sforna, L., Cenciarini, M., Belia, S., D’Adamo, M. C., Pessia, M., Franciolini, F., et al. (2015). The role of ion channels in the hypoxia-induced aggressiveness of glioblastoma. Front. Cell. Neurosci. 8:467. doi: 10.3389/fncel.2014.00467
Sforna, L., Cenciarini, M., Belia, S., Michelucci, A., Pessia, M., Franciolini, F., et al. (2017). Hypoxia modulates the swelling-activated Cl current in human glioblastoma cells: role in volume regulation and cell survival. J. Cell. Physiol. 232, 91–100. doi: 10.1002/jcp.25393
Shai, R. M., Reichardt, J. K., and Chen, T. C. (2008). Pharmacogenomics of brain cancer and personalized medicine in malignant gliomas. Future Oncol. 4, 525–534. doi: 10.2217/14796694.4.4.525
Shields, L. B., Shelton, B. J., Shearer, A. J., Chen, L., Sun, D. A., Parsons, S., et al. (2015). Dexamethasone administration during definitive radiation and temozolomide renders a poor prognosis in a retrospective analysis of newly diagnosed glioblastoma patients. Radiat. Oncol. 10:222. doi: 10.1186/s13014-015-0527-0
Silbergeld, D. L., Rostomily, R. C., and Alvord, E. C. Jr. (1991). The cause of death in patients with glioblastoma is multifactorial: clinical factors and autopsy findings in 117 cases of supratentorial glioblastoma in adults. J. Neurooncol. 10, 179–185. doi: 10.1007/bf00146880
Sinha, S., Bastin, M. E., Wardlaw, J. M., Armitage, P. A., and Whittle, I. R. (2004). Effects of dexamethasone on peritumoral oedematous brain: a DT-MRI study. J. Neurol. Neurosurg. Psychiatry 75, 1632–1635. doi: 10.1136/jnnp.2003.028647
Soda, Y., Myskiw, C., Rommel, A., and Verma, I. M. (2013). Mechanisms of neovascularization and resistance to anti-angiogenic therapies in glioblastoma multiforme. J. Mol. Med. 91, 439–448. doi: 10.1007/s00109-013-1019-z
Staberg, M., Michaelsen, S. R., Rasmussen, R. D., Villingshøj, M., Poulsen, H. S., and Hamerlik, P. (2017). Inhibition of histone deacetylases sensitizes glioblastoma cells to lomustine. Cell. Oncol. 40, 21–32. doi: 10.1007/s13402-016-0301-9
Staedtke, V., Bai, R. Y., and Laterra, J. (2016). Investigational new drugs for brain cancer. Expert Opin. Investig. Drugs 25, 937–956. doi: 10.1080/13543784.2016.1182497
Stokum, J. A., Gerzanich, V., and Simard, J. M. (2016). Molecular pathophysiology of cerebral oedema. J. Cereb. Blood Flow Metab. 36, 513–538. doi: 10.1177/0271678X15617172
Stoyanov, G. S., Dzhenkov, D., Ghenev, P., Iliev, B., Enchev, Y., and Tonchev, A. B. (2018). Cell biology of glioblastoma multiforme: from basic science to diagnosis and treatment. Med. Oncol. 35:27. doi: 10.1007/s12032-018-1083-x
Ströbele, S., Schneider, M., Schneele, L., Siegelin, M. D., Nonnenmacher, L., Zhou, S., et al. (2015). A potential role for the inhibition of PI3K signaling in glioblastoma therapy. PLoS One 10:e0131670. doi: 10.1371/journal.pone.0131670
Sundahl, N., Clarisse, D., Bracke, M., Offner, F., Berghe, W. V., and Beck, I. M. (2016). Selective glucocorticoid receptor-activating adjuvant therapy in cancer treatments. Oncoscience 3, 188–202. doi: 10.18632/oncoscience.315
Sur, P., Sribnick, E. A., Patel, S. J., Ray, S. K., and Banik, N. L. (2005). Dexamethasone decreases temozolomide-induced apoptosis in human gliobastoma T98G cells. Glia 50, 160–167. doi: 10.1002/glia.20168
Swaroops, G. R., Kelly, P. A., Holmes, M. C., Shinoda, J., and Whittle, I. R. (2001). The effects of dexamethasone therapy on permeability, blood flow and iNOS expression in experimental glioma. J. Clin. Neurosci. 8, 35–39. doi: 10.1054/jocn.2000.0817
Takano, S., Yamashita, T., and Ohneda, O. (2010). Molecular therapeutic targets for glioma angiogenesis. J. Oncol. 2010:351908. doi: 10.1155/2010/351908
Tews, B., Roerig, P., Hartmann, C., Hahn, M., Felsberg, J., Blaschke, B., et al. (2007). Hypermethylation and transcriptional downregulation of the CITED4 gene at 1p34.2 in oligodendroglial tumors with allelic losses on 1p and 19q. Oncogene 26, 5010–5016. doi: 10.1038/sj.onc.1210297
Turner, K. L., and Sontheimer, H. (2014). Cl− and K+ channels and their role in primary brain tumor biology. Philos. Trans. R. Soc. Lond. B Biol. Sci. 369:20130095. doi: 10.1098/rstb.2013.0095
Verhaak, R. G., Hoadley, K. A., Purdom, E., Wang, V., Qi, Y., Wilkerson, M. D., et al. (2010). Integrated genomic analysis identifies clinically relevant subtypes of glioblastoma characterized by abnormalities in PDGFRA, IDH1, EGFR, and NF1. Cancer Cell. 17, 98–110. doi: 10.1016/j.ccr.2009.12.020
Vescovi, A. L., Galli, R., and Reynolds, B. A. (2006). Brain tumor stem cells. Nat. Rev. Cancer 6, 425–436. doi: 10.1038/nrc1889
Villeneuve, J., Galarneau, H., Beaudet, M. J., Tremblay, P., Chernomoretz, A., and Vallières, L. (2008). Reduced glioma growth following dexamethasone or anti-angiopoietin 2 treatment. Brain Pathol. 18, 401–414. doi: 10.1111/j.1750-3639.2008.00139.x
Voss, F. K. F., Ullrich, J., Münch, K., Lazarow, D., Lutter, N., Mah, M. A., et al. (2014). Identification of LRRC8 heteromers as an essential component of the volume-regulated anion channel VRAC. Science 344, 634–638. doi: 10.1126/science.1252826
Waitkus, M. S., Diplas, B. H., and Yan, H. (2016). Isocitrate dehydrogenase mutations in gliomas. Neuro Oncol. 18, 16–26. doi: 10.1093/neuonc/nov136
Wang, H., Jiang, H., Zhou, M., Xu, Z., Liu, S., Shi, B., et al. (2009). Epidermal growth factor receptor vIII enhances tumorigenicity and resistance to 5-fluorouracil in human hepatocellular carcinoma. Cancer Lett. 279, 30–38. doi: 10.1016/j.canlet.2009.01.019
Wang, H., Li, M., Rinehart, J. J., and Zhang, R. (2004). Pretreatment with dexamethasone increases antitumor activity of carboplatin and gemcitabine in mice bearing human cancer xenografts: in vivo activity, pharmacokinetics, and clinical implications for cancer chemotherapy. Clin. Cancer Res. 10, 1633–1644. doi: 10.1158/1078-0432.ccr-0829-3
Wang, B., Xie, J., He, H. Y., Huang, E. W., Cao, Q. H., Luo, L., et al. (2017). Suppression of CLC-3 chloride channel reduces the aggressiveness of glioma through inhibiting nuclear factor-κB pathway. Oncotarget 8, 63788–63798. doi: 10.18632/oncotarget.19093
Was, H., Krol, S. K., Rotili, D., Mai, A., Wojtas, B., Kaminska, B., et al. (2019). Histone deacetylase inhibitors exert anti-tumor effects on human adherent and stem-like glioma cells. Clin. Epigenetics 11:11. doi: 10.1186/s13148-018-0598-5
Weaver, K. D., Grossman, S. A., and Herman, J. G. (2006). Methylated tumor-specific DNA as a plasma biomarker in patients with glioma. Cancer Invest. 24, 35–40. doi: 10.1080/07357900500449546
Wen, P. Y., Schiff, D., Kesari, S., Drappatz, J., Gigas, D. C., and Doherty, L. (2006). Medical management of patients with brain tumors. J. Neurooncol. 80, 313–332. doi: 10.1212/01.con.0000464172.50638.21
Wolburg, H., and Lippoldt, A. (2002). Tight junctions of the blood-brain barrier: development, composition and regulation. Vascul Pharmacol. 38, 323–337. doi: 10.1016/S1537-1891(02)00200-8
Wolff, J. E., Mölenkamp, G., Hotfilder, M., and Laterra, J. (1997). Dexamethasone inhibits glioma-induced formation of capillary like structures in vitro and angiogenesis in vivo. Klin. Padiatr. 209, 275–277. doi: 10.1055/s-2008-1043962
Wong, R., Chen, W., Zhong, X., Rutka, J. T., Feng, Z. P., and Sun, H. S. (2018). Swelling-induced chloride current in glioblastoma proliferation, migration, and invasion. J. Cell. Physiol. 233, 363–370. doi: 10.1002/jcp.25891
Wolburg, H., Noell, S., Fallier-Becker, P., Mack, A. F., and Wolburg-Buchholz, K. (2012). The disturbed blood-brain barrier in human glioblastoma. Mol. Aspects. Med. 33, 579–589. doi: 10.1016/j.mam.2012.02.003
Wong, E. T., Lok, E., Gautam, S., and Swanson, K. D. (2015). Dexamethasone exerts profound immunologic interference on treatment efficacy for recurrent glioblastoma. Br. J. Cancer 113:1642. doi: 10.1038/bjc.2015.404
Xu, J., Sampath, D., Lang, F. F., Prabhu, S., Rao, G., Fuller, G. N., et al. (2011). Vorinostat modulates cell cycle regulatory proteins in glioma cells and human glioma slice cultures. J. Neurooncol. 105, 241–251. doi: 10.1007/s11060-011-0604-7
Yamasaki, F., Kurisu, K., Aoki, T., Yamanaka, M., Kajiwara, Y., Watanabe, Y., et al. (2012). Advantages of high b-value diffusion-weighted imaging to diagnose pseudo-responses in patients with recurrent glioma after bevacizumab treatment. Eur. J. Radiol. 81, 2805–2810. doi: 10.1016/j.ejrad.2011.10.018
Yang, Y. (2015). Cancer immunotherapy: harnessing the immune system to battle cancer. J. Clin. Invest. 125, 3335–3337. doi: 10.1172/JCI83871
Yang, L., Lin, C., Wang, L., Guo, H., and Wang, X. (2012). Hypoxia and hypoxia-inducible factors in glioblastoma multiforme progression and therapeutic implications. Exp. Cell Res. 318, 2417–2426. doi: 10.1016/j.yexcr.2012.07.017
Yang, W. Y., Tan, Z. F., Dong, D. W., Ding, Y., Meng, H., Zhao, Y., et al. (2018). Association of aquaporin—1 with tumor migration, invasion and vasculogenic mimicry in glioblastoma multiforme. Mol. Med. Rep. 17, 3206–3211. doi: 10.3892/mmr.2017.8265
Yang, J., Zhang, J. N., Chen, W. L., Wang, G. S., Mao, Q., Li, S. Q., et al. (2017). Effects of AQP5 gene silencing on proliferation, migration and apoptosis of human glioma cells through regulating EGFR/ERK/ p38 MAPK signalling pathway. Oncotarget 8, 38444–38455. doi: 10.18632/oncotarget.16461
Ye, Z. C., and Sontheimer, H. (1999). Glioma cells release excitotoxic concentrations of glutamate. Cancer Res. 59, 4383–4391.
Yin, Y., Qiu, S., and Peng, Y. (2016). Functional roles of enhancer of zeste homolog 2 in gliomas. Gene 576, 189–194. doi: 10.1016/j.gene.2015.09.080
Zaki, H. S., Jenkinson, M. D., Du Plessis, D. G., Smith, T., and Rainov, N. G. (2004). Vanishing contrastenhancement in malignant glioma after corticosteroid treatment. Acta Neurochir. 146, 841–845. doi: 10.1007/s00701-004-0282-8
Zhang, H., Gu, Y. T., and Xue, Y. X. (2007). Bradykinin-induced blood-brain tumor barrier permeability increase is mediated by adenosine 5’-triphosphate-sensitive potassium channel. Brain Res. 1144, 33–41. doi: 10.1016/j.brainres.2007.01.133
Zhao, H. F., Wang, J., Shao, W., Wu, C. P., Chen, Z. P., To, S. T., et al. (2017). Recent advances in the use of PI3K inhibitors for glioblastoma multiforme: current preclinical and clinical development. Mol. Cancer 16:100. doi: 10.1186/s12943-017-0670-3
Zlokovic, B. V. (2008). The blood-brain barrier in health and chronic neurodegenerative disorders. Neuron 57, 178–201. doi: 10.1016/j.neuron.2008.01.003
Keywords: GBM, dexamethasone, cerebral oedema, pharmacogenomics, glioblastoma multiforme therapy
Citation: Cenciarini M, Valentino M, Belia S, Sforna L, Rosa P, Ronchetti S, D’Adamo MC and Pessia M (2019) Dexamethasone in Glioblastoma Multiforme Therapy: Mechanisms and Controversies. Front. Mol. Neurosci. 12:65. doi: 10.3389/fnmol.2019.00065
Received: 16 January 2019; Accepted: 26 February 2019;
Published: 29 March 2019.
Edited by:
Vittorio Maglione, Mediterranean Neurological Institute (IRCCS), ItalyReviewed by:
Giuseppe Giannini, Sapienza University of Rome, ItalyStefania Crispi, Institute of Bioscience and Bioresources, National Research Council, Italy
Copyright © 2019 Cenciarini, Valentino, Belia, Sforna, Rosa, Ronchetti, D’Adamo and Pessia. This is an open-access article distributed under the terms of the Creative Commons Attribution License (CC BY). The use, distribution or reproduction in other forums is permitted, provided the original author(s) and the copyright owner(s) are credited and that the original publication in this journal is cited, in accordance with accepted academic practice. No use, distribution or reproduction is permitted which does not comply with these terms.
*Correspondence: Mauro Pessia, bWF1cm8ucGVzc2lhQHVtLmVkdS5tdA==; bWF1cm8ucGVzc2lhQHVuaXBnLml0