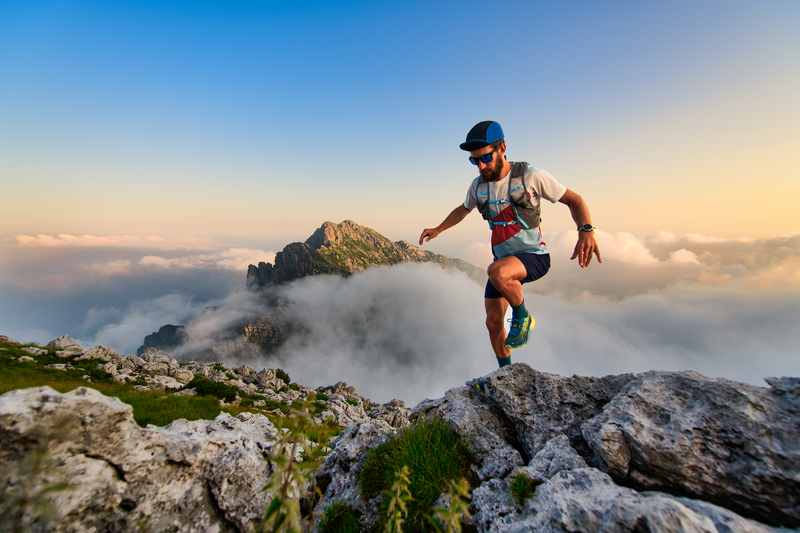
95% of researchers rate our articles as excellent or good
Learn more about the work of our research integrity team to safeguard the quality of each article we publish.
Find out more
ORIGINAL RESEARCH article
Front. Mol. Neurosci. , 26 November 2018
Sec. Neuroplasticity and Development
Volume 11 - 2018 | https://doi.org/10.3389/fnmol.2018.00429
Triclosan, a widely used industrial and household agent, is present as an antiseptic ingredient in numerous products of everyday use, such as toothpaste, cosmetics, kitchenware, and toys. Previous studies have shown that human brain and animal tissues contain triclosan, which has been found also as a contaminant of water and soil. Triclosan disrupts heart and skeletal muscle Ca2+ signaling, damages liver function, alters gut microbiota, causes colonic inflammation, and promotes apoptosis in cultured neocortical neurons and neural stem cells. Information, however, on the possible effects of triclosan on the function of the hippocampus, a key brain region for spatial learning and memory, is lacking. Here, we report that triclosan addition at low concentrations to hippocampal slices from male rats inhibited long-term potentiation but did not affect basal synaptic transmission or paired-pulse facilitation and modified the content or phosphorylation levels of synaptic plasticity-related proteins. Additionally, incubation of primary hippocampal cultures with triclosan prevented both the dendritic spine remodeling induced by brain-derived neurotrophic factor and the emergence of spontaneous oscillatory Ca2+ signals. Furthermore, intra-hippocampal injection of triclosan significantly disrupted rat navigation in the Oasis maze spatial memory task, an indication that triclosan impairs hippocampus-dependent spatial memory performance. Based on these combined results, we conclude that triclosan exerts highly damaging effects on hippocampal neuronal function in vitro and impairs spatial memory processes in vivo.
The production and utilization of chemical agents designed to reduce household and human bacterial load is currently on the rise (Levy, 2001; Aiello and Larson, 2003; Dhillon et al., 2015). Many products for personal care and everyday use contain the antimicrobial agent triclosan [5-chloro-2-(2,4-dichlorophenoxy)phenol], a synthetic broad-spectrum bactericide (Singer et al., 2002). Triclosan (TCS) is a lipophilic molecule extensively used that has an annual production of 1,500 tons worldwide (Dhillon et al., 2015). About 85% of the total triclosan global use stems from its presence in personal care products, compared to 5% in textiles and 10% in plastics and food contact materials. Triclosan was removed in 2010 from the European Union list of additives for use in plastic food contact (European Commission. Directorate General for Health and Consumers, 2010), but it is still present in some soaps, toothpastes, cleaning products, bedding, clothes, fabrics, shoes, carpets, plastics, and medical supplies (Rodricks et al., 2010). In 2016, the United States Food and Drug Administration gave manufacturers of consumer soaps 1 year to remove TCS from their products; yet, this deadline did not apply to other personal products such as toothpaste. Furthermore, TCS remains widely used worldwide in many household and personal care products.
Mounting evidence shows that TCS impairs cardiac and skeletal muscle excitation–contraction coupling, thus disturbing normal Ca2+ signaling in these tissues (Cherednichenko et al., 2012; Fritsch et al., 2013). Additionally, an association between TCS, tumor promotion in liver, and oxidative stress has been reported (Yueh et al., 2014). A recent study in mice reported that TCS causes colonic inflammation and colitis, alters gut microbiota, and worsens colitis-associated colon cancer (Yang et al., 2018). Triclosan induces Fas-dependent apoptosis in neocortical neurons in vitro (Szychowski et al., 2015) and produces toxic effects in neural stem cells through mechanisms involving increased reactive oxygen species (ROS) production and apoptosis (Park et al., 2016), leading to the proposal that TCS acts as a neurotoxic agent (Ruszkiewicz et al., 2017). In consonance with this idea, incubation of primary neocortical neurons with TCS decreases the expression of N-methyl-D-aspartate (NMDA) receptor (NMDAR) subunits and enhances NMDAR-dependent ROS generation and caspase-3-dependent apoptosis (Szychowski et al., 2018).
To our knowledge, however, information is not available regarding the effects of TCS on hippocampal synaptic plasticity and spatial memory processes. The hippocampus, which mediates the formation of long-term memories and is critical for memory consolidation, is a well-established model to study neuronal function (Andersen et al., 2006). Hippocampal synapses exhibit both long-term potentiation (LTP) and long-term depression of synaptic strength; these processes are considered the cellular substrates of memory encoding (Bear and Malenka, 1994; Malenka and Bear, 2004; Whitlock et al., 2006; Citri and Malenka, 2008). In particular, LTP in the CA1 region of the hippocampus may be a primary synaptic mechanism underlying specific types of long-term memory processes (Tsien et al., 1996; Gruart et al., 2006; Whitlock et al., 2006). Additionally, changes in synapse/spine morphology, density, and number have been associated with changes in synaptic efficacy and LTP (Sorra and Harris, 1998; Matsuzaki et al., 2001; Lang et al., 2004).
At present, it is widely accepted that hippocampal LTP requires intracellular Ca2+ signals and evokes dendritic spine remodeling (Lang et al., 2004). Calcium signaling is considered central for normal neuronal function (Berridge, 1998) and plays a pivotal role in neuronal plasticity (Lynch, 2004). Hippocampal structural plasticity entails dendritic spine remodeling (Kitanishi et al., 2009), which associates with memory mechanisms (Segal, 2017), and engages intracellular Ca2+ signals mediated by ryanodine receptor (RyR) Ca2+ release channels present in the endoplasmic reticulum (Adasme et al., 2011; Korkotian and Segal, 2011; Grigoryan et al., 2012; More et al., 2018). Given that TCS disturbs normal Ca2+ signaling in heart and skeletal muscle (Cherednichenko et al., 2012), it is likely to disrupt Ca2+ signaling pathways required for hippocampal neuronal function.
In this work, we report that TCS significantly perturbs hippocampal synaptic plasticity – including LTP and dendritic spine remodeling – and impairs rat performance in a spatial memory task. We conclude, based on these findings, that TCS exerts highly damaging effects on rodent hippocampal neuronal function. If chronic TCS exposure were to produce these damaging effects in the human brain, the presence of TCS in personal care products should be reconsidered.
Minimum essential medium, horse serum, serum-free Neurobasal medium, GIBCOTM B27, Fluo-4-AM, and 2 mM GlutamaxTM were from Thermo Fisher Scientific (Waltham, MA). The pRFP-C-RS vector was from Origene (Rockville, MD), and BDNF was from Chemicon Millipore (Darmstadt, Germany). Triclosan was from Sigma (St. Louis, MO, United States). Enrofloxacin was from Bayer (Pittsburgh, PA, United States) and Ketophen from RhodiaMerieux (Santiago, Chile).
Male Sprague Dawley rats (8 to 10-week-old) were obtained from the animal facility of the Faculty of Medicine, Universidad de Chile. Food and water were provided ad libitum. Animals were maintained in a temperature-controlled room at a 12 h light–dark cycle (lights on at 7 a.m.). All experiments were performed in the light phase. The experimental protocols used in this work complied with the “Guiding Principles for Research Involving Animals and Human Beings” of the American Physiological Society and were approved by the Bioethics Committee on Animal Research, Faculty of Medicine, Universidad de Chile.
At embryonic day, 18 pregnant rats were sacrificed by decapitation under isoflurane anesthesia, and primary cultures were prepared from the hippocampus dissected from the embryos (Adasme et al., 2011). Cells were plated in minimum essential medium plus 10% horse serum for 40 min and were maintained for 14 days at 37°C under 5% CO2/95% O2 in serum-free Neurobasal medium supplemented with GIBCOTM B27 and 2 mM GlutamaxTM. Primary hippocampal cultures were used at 14 days in vitro (DIV).
Experiments were performed as described earlier (Adasme et al., 2011). Briefly, primary hippocampal cultures were plated over polylysine-coated 35 mm plates (∼500,000 cells/cover). Cultures were transiently transfected at 13 DIV with the pRFP-C-RS vector to visualize cellular structures through red fluorescence detection; 24 h after transfection (14 DIV), cultures were tested for changes in spine density elicited by incubation for 6 h with BDNF (50 ng/ml), as described (Adasme et al., 2011). To evaluate the effects of triclosan on BDNF-induced spine density changes, cultures were pre-incubated for 1 h with 1 μM triclosan prior to addition of BDNF. As control, we added the vehicle DMSO (final concentration <0.01%). Images of dendrites were acquired by confocal microscope (Carl Zeiss, Axiovert 200, LSM 5 Pascal, Jena, Germany) of living cells maintained during the determination in Tyrode medium, under the following conditions: 63× oil objective, 4× digital zoom, NA 1.4, excitation/emission 543/530–600 nm. For spine density determinations, randomly selected dendrites present in 30–50 μm proximity to the soma were analyzed independently; 2–3 dendrites from each one of eight different cultures were subject to blind analysis in each case. The number of spines was quantified in 36.6 μm × 36.6 μm fields, in which dendrite segments were placed in the diagonal (corresponding to 51.7 μm). Dendritic spine density was analyzed by measuring the number of spines present in a dendritic length of 50 μm. The IMAGE J image program (National Institutes of Health, United States) was used for image deconvolution and to construct z-project images from 9–15 stacks (0.4 μm each).
After 14 days in culture, neurons were transferred to modified Tyrode solution (in mM: 129 NaCl, 5 KCl, 2 CaCl2, 1 MgCl2, 30 glucose, 25 Hepes, pH 7.3), preloaded for 30 min at 37°C with 2 μM Fluo-4-AM and washed three times with Tyrode solution to allow complete dye de-esterification. These conditions were chosen to avoid deleterious dye effects (Smith et al., 2018). Fluorescence images of intracellular Ca2+ signals in primary hippocampal neurons were acquired at 1 s intervals in an inverted epifluorescence microscope (Carl Zeiss, Axiovert A1, Colibri system, Jena, Germany), utilizing the 63× objective and excitation at 470 nm with a LED module. Images from cell bodies were collected and analyzed. Ca2+ signals are expressed as ΔF/F0, where F and F0 correspond to the experimental and the basal fluorescence levels, respectively. All experiments were performed at room temperature (∼24°C). Calcium signals were analyzed as described elsewhere (Uhlen, 2004; Maggio and Vlachos, 2014).
We evaluated the effect of triclosan on membrane capacity and membrane resistance of primary hippocampal neurons under the cell-attach configuration, as described (Bournaud et al., 1998).
Male rats (3–4 weeks) under isofluorane anesthesia were euthanized by decapitation and their brains were quickly removed. The hippocampus was dissected in cold dissection buffer containing (in mM: 212.7 sucrose, 5 KCl, 1 MgCl2, 2 CaCl2, 10 glucose, 1.25 NaH2PO4, 26 NaHCO3, pH 7.4) and was cut into 400 μm transversal slices using a vibratome (Vibratome 1000 plus, Ted Pella Inc., CA, United States), as detailed elsewhere (Munoz et al., 2011). Hippocampal slices were transferred to an immersion storage chamber kept at room temperature in artificial cerebrospinal fluid (ACSF) containing (in mM: 124 NaCl, 5 KCl, 1.25 NaH2PO4, 1 MgCl2, 2 CaCl2, 10 glucose, 26 NaHCO3, pH 7.4), in 95% O2/5% CO2. Slices were kept in this solution for at least 1 h before recording at 30 ± 2°C. Stock triclosan solutions (100 mM) were prepared in dimethylsulfoxide (DMSO). Hippocampal slices were incubated with ACSF solutions containing 1, 5, or 10 μM triclosan or vehicle (up to 0.01%, DMSO) as control.
Electrophysiological experiments were performed in an immersion-recording chamber. To evaluate field excitatory postsynaptic potentials (fEPSPs), hippocampal slices were superfused with ACSF (in 95% O2/5% CO2) at a rate of 2 ml/min at 30 ± 2°C. fEPSP, evoked by square current pulses (0.2 ms) delivered with a concentric bipolar stimulating electrode (FHC Inc., Bowdoinham, ME, United States) located in the Schaeffer collateral–commissural fibers, were recorded using glass microelectrodes (2–3 MΩ) filled with ACSF placed into the stratum radiatum of the CA1 region. To evaluate basal excitatory synaptic transmission, pulses of 25, 50, 75, 100, 150, and 200 μA were applied to construct an input/output curve. Results are presented as stimulus intensity versus fiber volley (FV) amplitude, or as stimulus intensity versus fEPSP slope. To evaluate pre-synaptic components of the responses, we used the following paired-pulse stimulation protocol: two pulses were applied every 15 s, with inter-stimulus intervals starting with 20 ms and ending with 640 ms, doubling the interval after each trial. The results are presented as the ratio between the initial fEPSP slopes evoked by the second over the first stimulus. After monitoring both basal synaptic transmission and pre-synaptic responses, we evaluated LTP adjusting the stimulus intensity to generate fEPSPs to half of the maximal evoked response, with pulses applied every 15 s until a stable baseline was attained for at least 15 min. To induce LTP, we applied the TBS protocol, consisting of 4 trains of 10 bursts at 5 Hz each (1 burst = 4 pulses at 100 Hz). In all experiments, fEPSP recordings were continued for 60 min after applying the TBS protocol. Recordings were filtered at 10 kHz and were digitized at 5 kHz, using Igor Pro (WaveMetrics Inc., Lake Oswego, OR, United States). Synaptic responses, quantified as the initial slope of the evoked fEPSPs, were plotted as percentage of basal change relative to the slope of the baseline record, considered as 100%. Triclosan stocks (100 mM) were prepared fresh in dry DMSO and were diluted in aqueous ACSF solution to the indicated concentration.
Male Sprague-Dawley rats (2.5 months average age) weighing 230–250 g were used in these experiments. Animals were maintained with a light/dark cycle of 12 h, at an average temperature of 22°C with food and water ad libitum, and were handled daily for 2 weeks prior to surgery. Rats were anesthetized prior to surgery with isoflurane in oxygen (2.5% for induction, 1.5% for maintenance; 1 L/min oxygen flow). Sedation depth was monitored by the absence of the toe pinch withdrawal reflex. Cannulas were placed in all rats used in this work (control and TCS-injected). The animal head was restrained with a stereotaxic frame; an incision on the skin and a small craniotomy was conducted to implant two bilateral stainless-steel cannula guides (21-gauge, plastics one). To target the dorsal CA3 region of the hippocampus, we used the following stereotaxic coordinates according to the rat brain atlas (Paxinos and Watson, 2007): anteroposterior: 2.5 mm; laterality ±3.5 mm and 2.7 mm in depth. Cannulas were fixed to the skull using as anchors jewelry stainless steel screws set with dental acrylic. Antibiotic (Enrofloxacin 5%, 19 mg/kg i.p.) and anti-inflammatory (Ketophen 0.2 mg/kg i.p.) drugs were administered at the end of surgery and during three consecutive days.
To evaluate hippocampal-dependent spatial memory, we used the Oasis maze task, a modified dry-land version of the Morris water maze (Kesner et al., 1991; Clark et al., 2005; Martinez et al., 2016; More et al., 2018). In brief, the task consisted of a circular open field arena of 1.4 m in diameter containing 21 evenly spaced wells (4.5 cm diameter, 2 cm height), placed 50 cm above the floor, and limited by a wall of 20 cm in height. All experiments were conducted in a dedicated room with distal visual cues. Rats were first exposed for three consecutive days to pre-training sessions, during which rats – water-deprived for 23 h – were trained to seek water-containing wells. To this aim, the animals were allowed to explore the arena for 10 min, in conditions in which all wells contained one drop of water (∼50 μL). The following day, the animals were surgically implanted with injection cannulas placed in the CA3 hippocampal region (Supplementary Figure S2, left panel). After a recuperation period of 7 days, the pre-training sessions were repeated for two consecutive days. The following day, rats were tested in the spatial memory task, which entailed one daily session over six consecutive days; each session comprised 15 trials of up to 1 min duration. Before each session, rats were enclosed within a black cylinder (22 cm diameter, 27 cm height). The trial started after placing the rat in the arena and removing the cylinder, and it ended when the rat reached the baited well or at the end of the 1-min exploration time. An inter-trial interval of 20–30 s was used. During this inter-trial period, the animal was enclosed with the cylinder and was gently moved to a different starting position randomly assigned to prevent stereotyped trajectory or procedural learning of the animal to solve the maze. The reward was maintained every day in the same location.
All animals were tested for three consecutive days. Two hours after completing the training session on the third day, one group of animals (n = 6) was injected with TCS (0.5 μl of 10 μM, equivalent to 5 pmol) or with 0.5 μl of saline (Supplementary Figure S2, left panel). Triclosan or saline injection was repeated later on the same day and on the following day. Considering that the rat hippocampus has a volume of ∼0.8 ml (Le Duigou et al., 2005) and assuming homogenous TCS distribution into this hippocampal volume, each TCS injection would yield a final TCS concentration of ∼6 nM. Rats were tested in three consecutive daily sessions following TCS injections. Animal behavior was recorded with a video camera placed in the zenithal position. Off-line analysis of the videos was conducted by using a custom-made MATLAB routine to reconstruct the trajectory of the animal (More et al., 2018). Three behavioral parameters of learning were quantified: hit ratio (number of correct hits in each 15 trial session); latency, defined as the time taken to find the baited well, and distance ratio, defined as the ratio between the observed and the straight path lengths from the starting point to the reward.
Five hippocampal slices (400 μm each) from each animal were combined, and extracts were prepared as described (Arias-Cavieres et al., 2017). Proteins were resolved by SDS-PAGE using 3.5–8% Tris-acetate gels for RyR2 determination or 10% gels for assaying Synapsin I and CaMKII protein content and phosphorylation status. Following SDS-PAGE, proteins were transferred to polyvinylidenedifluoride (PVDF) membranes and were probed with one of the following antibodies: anti-RyR2 mouse monoclonal antibody (1:1,000; Thermo Scientific, Rockford, IL, United States), anti-CamKII (pan) (D11A10) rabbit monoclonal antibody (#4436, 1:3,000; Cell Signaling Technology, Danvers, MA, United States). PhosphoCaMKII (phospho T286) rabbit polyclonal antibody (ab32678, 1:5,000), anti-Synapsin I rabbit polyclonal antibody (ab64581, 1:2,500), and anti-Synapsin I (phospho-S603) rabbit polyclonal antibody (ab13879, 1:1,500) were from Abcam (Cambridge, MA, United States). Image acquisition and densitometric analysis of band density were performed, respectively, by means of the ChemidocTM MP System (Bio-Rad laboratories, Hercules, CA, United States), and the Image Lab software.
The type of analysis used in each experimental determination is described in detail in each figure legend. Statistical significance was considered at p < 0.05. The statistical Power was calculated with alpha = 0.05, using the Sigma Plot program.
We evaluated the effects of TCS (1, 5, and 10 μM) on hippocampal basal transmission in the CA3-CA1 hippocampal circuit. As illustrated by the representative records of field excitatory postsynaptic potentials (fEPSP) shown in Figure 1A, addition of 1 μM TCS to acute hippocampal slices did not modify fEPSP slopes and basal synaptic transmission (Figure 1B). In contrast, addition of 5 or 10 μM TCS significantly decreased both the slope of the fEPSP records (Figure 1A) and the basal response (Figure 1B). The average values of fEPSP slopes – collected 30 min after TCS addition – are summarized in Figure 1C. Based on these results, we conclude that TCS concentrations ≥5 μM exert significant inhibitory effects on basal synaptic transmission.
FIGURE 1. Concentration-dependent effects of triclosan on basal synaptic transmission (CA3-CA1). (A) Representative fEPSP traces recorded in hippocampal slices treated with different TCS concentrations, as indicated in the figure. The numbers indicated in the traces correspond to the times of record collection, as indicated in (B). (B) Time course records showing the effects of TCS addition (arrow) on basal transmission; numbers in parenthesis represent the number of animals followed by the number of slices used in each determination. (C) Bar graph showing average values of fEPSP slopes, collected at the end of the respective records. All values represent Mean ± SE; control (n = 7), 1 μM TCS (n = 6), 5 μm TCS (n = 6), and 10 μm TCS (n = 5). Statistical analysis was performed by two-way ANOVA followed by Bonferroni’s post hoc test; ∗∗∗p < 0.001; statistical power: 1.0.
To further analyze whether 1 μM TCS impairs other synaptic properties, we measured fiber volley amplitude (FV), a parameter that reflects the number of fibers activated as a function of stimulus intensity (Bodhinathan et al., 2010; Ardiles et al., 2012). Representative traces recorded in control slices or in slices incubated with 1 μM TCS for 15 min are presented in Supplementary Figure S1A. Quantification of FV amplitude versus stimulus intensity showed that slices treated with 1 μM TCS displayed FV amplitude values undistinguishable from controls (Supplementary Figure S1B), an indication that TCS does not interfere with pre-synaptic fiber recruitment. Next, we evaluated the effects of TCS on fEPSP slopes determined at increasing stimulus intensities and assessed whether TCS affected paired-pulse facilitation responses (Schulz et al., 1994). When stimulated at <150 μA slices treated with 1 μM TCS displayed similar fEPSP slopes as controls, but exhibited reduced responses when stimulated at 150 or 200 μA (Supplementary Figure S1C). Slices treated with 1 μM TCS for 15 min displayed paired-pulse facilitation responses undistinguishable from controls (Supplementary Figure S1D), an indication of unaffected presynaptic transmitter release.
To characterize the effects of TCS on neuronal synaptic plasticity, we tested the effects of 1 μM TCS, a concentration that did not affect fEPSP slopes, basal synaptic transmission, fiber volley amplitude, or paired-pulse facilitation responses, on LTP induced by the theta-burst stimulation (TBS) protocol (Larson et al., 1986; Raymond, 2007). Representative fEPSP traces recorded before (1) and ∼60 min after applying the TBS protocol (2) show significant reduction in the responses recorded at 60 min in TCS-treated slices (Figure 2A). Addition of 1 μM TCS to slices 15 min before TBS caused significant reduction in the LTP response relative to controls (Figure 2B). Average values of the responses collected 60 min after LTP induction (Figure 2C) showed that slices treated with 1 μM TCS displayed (in %) significantly lower values (122.6 ± 10.6) relative to controls (182.0 ± 10.2). These results show that 1 μM TCS impairs LTP presumably by interfering with postsynaptic pathways.
FIGURE 2. Triclosan impairs LTP (CA3-CA1). (A) Representative fEPSP traces recorded in control or slices treated with 1 μM TCS (added 5 min after starting the record). Representative fEPSP traces were collected 10 min before applying the TBS protocol (number 1 in graph B) and 60 min after TBS (number 2 in graph B). (B) The LTP-inducing TBS protocol (four trains) was delivered at the time indicated by the arrows. Open circles: Control; black circles: 1 μM TCS. Values presented in each trace represent Mean ± SE; control (n = 6); TCS-treated (n = 7). (C) Average values of the fEPSP slopes recorded during the last 10 min of the records; open bar: control; solid bar: treated with 1 μM TCS. Statistical data analysis was performed with the Mann–Whitney U-test; ∗∗∗p < 0.001.
Triclosan is a highly hydrophobic molecule (DeSalva et al., 1989) likely to partition into the lipid component of cellular membranes. We found that incubation of primary hippocampal cultures for 20 min with 5 μM TCS, a concentration of TCS that significantly decreased both the slope of the fEPSP records and basal synaptic transmission (Figure 1), did not have significant effects on neuronal membrane capacity (pF, C: 35.8 ± 8.4, n = 12; TCS: 26.0 ± 4.4) or membrane resistance (MΩ, C: 98.8 ± 13.9, n = 12; TCS: 103.0 ± 13.2, n = 11).
Synaptic plasticity encompasses structural plasticity; a process characterized by dendritic spine remodeling that entails generation and growth of dendritic spines (Kitanishi et al., 2009). We tested whether incubation with 1 μM TCS, which impaired the LTP response, also affected structural plasticity. To this aim, we determined if incubation with 1 μM TCS affected the spine remodeling induced by incubation of primary hippocampal cultures for 6 h with brain-derived neurotrophic factor (BDNF), a neurotrophin known to promote dendritic spine remodeling (Tyler and Pozzo-Miller, 2001). As illustrated by the representative images (Figure 3A) and the average results from four independent experiments (Figure 3B), pre-incubation for 30 min with 1 μM TCS, which was maintained during the subsequent 6-h incubation period with BDNF or vehicle, abolished BDNF-induced spine remodeling but did not modify basal spine density.
FIGURE 3. Triclosan prevents BDNF-induced spine remodeling in hippocampal neurons and disrupts spontaneous Ca2+ oscillations. (A) Representative images of neuronal projections acquired from control cultures (plus or minus TCS) or from cultures treated with BDNF (plus or minus TCS). Cultures were pre-incubated with TCS (1 μM) for 30 min before subsequent incubation with BDNF (50 ng/mL) or vehicle for 6 h. Scale bar, 5 μm. (B) Quantification of spine density (Mean ± SE, n = 8). Statistical analysis was performed with One-way ANOVA; ∗∗∗p < 0.001; Power = 0.99. (C) Representative recordings of the spontaneous Ca2+ oscillations in neurons loaded with the Ca2+ probe Fluo-4. Triclosan (1 μM) was added at the arrow. (D) Quantification of the frequency of oscillations measured before and after TCS addition (Mean ± SE, n = 5). The statistical analysis of spine remodeling data was performed by one-way ANOVA followed by Bonferroni’s post hoc test. Calcium oscillation data were compared using Student’s paired t-test. ∗∗p < 0.01; statistical power = 0.84.
Previous work showed that TCS disrupts voltage-dependent Ca2+ signals in skeletal and cardiac muscle (Cherednichenko et al., 2012). Addition of 1 μM TCS (arrow) to primary hippocampal cultures (Figure 3C) caused a significant inhibition of the spontaneous Ca2+ oscillations displayed by hippocampal neurons before TCS addition (Figure 3D). The implications of these results are addressed in the Discussion section.
The LTP response is currently considered a substrate of memory processes (Morris et al., 1986). The LTP inhibition produced by TCS led us to test whether TCS affects spatial memory formation in rats. To this aim, we injected TCS into the CA3 region of rat hippocampus (Supplementary Figure S2) and evaluated spatial performance in the Oasis maze task (see section “Materials and Methods”). The representative experiment shown in Figure 4A illustrates the remarkable effects of TCS on rat performance in the Oasis maze task. The TCS-injected rat displayed noticeably longer navigation trajectories than the control rat in three separate trials recorded at 5, 10, and 15 min during session 4 and exhibited many periods in which the animals stayed in the same place undergoing rotating movements (black arrows); control animals rarely displayed this behavior along their trajectories. In addition, TCS injections significantly impaired hit rates, latency times, and the distance traveled to the reward relative to the controls (Figures 4B,C), typical parameters used to assess navigation in the Oasis maze task (Clark et al., 2005; More et al., 2018). Rats injected with TCS displayed similar exploration velocities as vehicle injected rats (Supplementary Figure S2, right panel), an indication that TCS did not affect rat motor ability. Of note, TCS injection deteriorated the spatial performance of rats in the Oasis maze despite the fact that before injection the same rats successfully navigated and effectively learned to find the baited well (Figure 4C).
FIGURE 4. Triclosan impairs hippocampus-dependent spatial memory. Triclosan (0.5 μl, 10 μM) was injected three times into the CA3 region of rat hippocampus (Supplementary Figure S2, left panel), after which animals were trained in a spatial memory task (for details, see text). (A) Representative experiment showing how the animals explored the maze during the Oasis maze task. The animal location was tracked by video recordings and was analyzed using a MATLAB routine. Walked (solid lines) and expected/shortest (dotted lines) distance records were acquired in session 5 (see Supplementary Figure S2, left panel). The black stars indicate the bait location. The thicker regions of the tracking solid line correspond to periods where the animal remained rotating in the same place (black arrows). (B) The arrows over each graph indicate the times of TCS injections. Left panel: Hit rate, defined as the relative ratio between the successful hits and the 15 trials performed in each of six daily sessions. Center panel: Latency, defined as the time it takes the animal to find the reward. Right panel: Ratio between the distance covered by the animal and the shortest linear distance to the baited well. (C) Average values of hit rates, latencies, and distance ratios evaluated in sessions 1–3, and sessions 4–6 in TCS-injected or vehicle-injected rats. Statistical analysis in (B) was performed using two-way ANOVA followed by Holm-Sidak post hoc test. Statistical analysis in (C) was performed using paired Student’s t-test. Values represent Mean ± SE (n = 6); ∗p < 0.05; ∗∗∗p < 0.001; statistical power = 1.0.
Based on these combined results, we strongly suggest that TCS exerts severe negative effects on activity-dependent hippocampal neuronal function and on spatial memory performance.
A previous study reported that prolonged incubation (≥3 h) of primary neocortical neurons with 10 μM TCS reduced the protein expression of several NMDAR sub-units (Szychowski et al., 2018). Hence, we tested whether TCS modified the protein contents of two NMDAR downstream targets, the CaMKII enzyme (Bayer et al., 2001) and RyR channels (Riquelme et al., 2011). To this aim, we used 5 μM TCS, a concentration that caused ∼20% inhibition of fEPSP slopes (Figure 1). Acute hippocampal slices incubated for 30 min with 5 μM TCS did not display changes in the protein content of CaMKII-α or CaMKII-β, but caused a modest but significant reduction of CaMKII-α phosphorylation levels while CaMKII-β phosphorylation levels did not change (Figure 5A). In addition, incubation with TCS caused a significant reduction of RyR2 protein content (Figure 5B), the predominant RyR isoform expressed in rat hippocampus that has a central role in synaptic plasticity and memory processes (More et al., 2018). In contrast, the protein content and the phosphorylation levels of the presynaptic protein Synapsin I did not change following incubation of acute hippocampal slices with 5 μM TCS for 30 min (Figure 5C).
FIGURE 5. Phosphorylation levels of CaMKII and Synapsin I and RyR2 protein content in control and TCS-injected rat hippocampus. (A) Representative Western blots and quantification of phosphorylation levels of CaMKII-α and CaMKII-β assessed in control and TCS-incubated (30 min, 5 mM) hippocampal slices. (B) Representative Western blots and quantification of RyR2 protein levels in control and TCS-incubated (30 min, 5 mM) hippocampal slices. (C) Representative Western blots and quantification of phosphorylation levels of Synapsin I assessed in control and TCS-incubated (30 min, 5 μM) hippocampal slices. Statistical analysis was performed with unpaired Student’s t-test. Values represent Mean ± SE (n = 4); ∗p < 0.05. CaMKII: statistical power = 0.35; RyR2: statistical power = 0.68.
In this work, we report that low concentrations of TCS (1–5 μM) affected hippocampal neuronal function at various levels. In particular, TCS disrupted hippocampal synaptic plasticity, evidenced by impaired TBS-prompted LTP induction and defective BDNF-induced dendritic spine remodeling, and also reduced CaMKII-α phosphorylation levels and RyR2 protein content in acute hippocampal slices. In addition, primary hippocampal neurons exposed to TCS displayed a significant decrease in the frequency of spontaneous Ca2+ signals. Moreover, TCS injected intra-hippocampus (CA3) caused marked defects in spatial memory performance. Altogether, these results provide novel information on the deleterious effects of TCS on rodent neuronal Ca2+ signaling, synaptic plasticity, and spatial memory processes.
Synaptic plasticity denotes changes in the efficacy of synaptic transmission in response to neuronal activity (Bliss et al., 2014); it also promotes related structural plasticity responses exemplified by dendritic spine remodeling (Bailey et al., 2004). Here, we report that acute exposure to a low TCS concentration (1 μM) markedly inhibited LTP induction in hippocampal slices and significantly decreased BDNF-induced dendritic spine remodeling in primary hippocampal neurons. Rodent models of depression present a reduction in dendrite complexity and spine density in the hippocampus (Duman and Aghajanian, 2012). Accordingly, future studies should address if chronic TCS exposure impairs activity-dependent dendritic spine remodeling in human hippocampal neurons, since this reduction might be a contributing factor to human depression.
Present evidence supports LTP and structural plasticity as the biological substrates for associative learning and long-term memory (Bliss and Collingridge, 1993; Martin et al., 2000; Leuner et al., 2003; Bailey et al., 2004, 2013; Lynch, 2004; Gruart et al., 2006; Whitlock et al., 2006; Fedulov et al., 2007; Holtmaat and Svoboda, 2009; Kasai et al., 2010; Giachero et al., 2013; Baudry et al., 2015; Lynch et al., 2015). In agreement with the LTP and structural plasticity impairments caused by TCS, we found that after three intra-hippocampal TCS injections, which presumably yielded a final hippocampal TCS concentration of up to ∼18 nM (see Materials and Methods section), rats displayed significant defects in the performance of a spatial memory task.
Here, we report that exposure of primary hippocampal neurons to TCS caused a significant decrease in the frequency of spontaneous Ca2+ signals. Addition of up to 10 μM TCS to RyR channels from brain cortex incorporated in planar lipid bilayers does not affect RyR single channel properties (Bull et al., manuscript in preparation). Based on these combined results, we suggest that TCS disturbs Ca2+ entry pathways required for neuronal calcium-dependent responses, including CamKII-α phosphorylation, LTP, dendritic spine remodeling, and hippocampus-dependent spatial memory. Studies showing that TCS disrupts Ca2+ signaling in both cardiac and skeletal muscle by inhibiting Ca2+ currents mediated by voltage-dependent Ca2+ channels (Cherednichenko et al., 2012), support this proposal. Of note, TCS did not modify two pre-synaptic responses, paired-pulse facilitation, and Synapsin I phosphorylation. Therefore, we propose that TCS primarily affects postsynaptic Ca2+ signaling pathways.
These novel findings complement and expand a recent report showing that neocortical neurons in primary culture exhibit decreased protein levels of several NMDAR subunits after 3, 6, or 24 h post-treatment with 10 μM TCS (Szychowski et al., 2018). In particular, the significant RyR2 protein decrease induced by TCS reported here, which occurred within minutes after TCS addition, may contribute to the defective spatial memory displayed by TCS-injected rats since a decrease in RyR2 expression markedly impairs this process (More et al., 2018). Therefore, we propose that TCS disrupts Ca2+ signaling pathways required for synaptic plasticity and memory processes (Berridge et al., 2003; Maggio and Vlachos, 2014).
Disruption of neuronal Ca2+ signaling has deleterious effects on experience-dependent dendritic plasticity during rat development (Lohmann and Wong, 2005; Yang et al., 2009; More et al., 2018) and perturbs synaptic plasticity responses (Zundorf and Reiser, 2011; Pchitskaya et al., 2017). There is evidence linking anomalous intracellular Ca2+ signaling with autism spectrum disorder (Gargus, 2009; Vallipuram et al., 2010; Wayman et al., 2012; Shen et al., 2015), Alzheimer’s disease (Mattson and Chan, 2001; Berridge et al., 2003; Paula-Lima et al., 2011; Popugaeva et al., 2018), and other neurodegenerative diseases (Mattson and Chan, 2001; Bezprozvanny, 2010). Through disruption of normal neuronal Ca2+ signaling, chronic TCS exposure may contribute to cause the memory defects associated with these pathological conditions.
Several reports indicate that TCS accumulates in experimental animals (Pannu et al., 2012), as well as in liver and kidney samples from birds (Tanoue et al., 2014), and distributes into the whole body, including the brain of experimental animals exposed to commercial forms of TCS (Fang et al., 2016). Earlier works reporting that TCS did not accumulate in human tissues (Bagley and Lin, 2000; Lin, 2000), suggested that TCS is metabolized and eliminated from the body. Subsequent studies refuted these earlier reports by showing the presence of TCS in human adipose tissue (Wang et al., 2015), breast milk (Allmyr et al., 2006), and brain tissue (Geens et al., 2012). Moreover, a study performed on 181 pregnant women showed that TCS was present in all urine samples, as well as in 51% of cord blood samples (Pycke et al., 2014); these findings raise the possibility that intrauterine TCS exposure may affect brain development and function at early embryonic stages. Likewise, a recent United States study carried out in 12,793 individuals reported that ∼98% of them had TCS concentrations in the 30 nM – 2 μM range (mean = 0.6 μM) in their urine (Pycke et al., 2014). Other studies reported that in the Chinese population TCS was present in 80% of 209 tested subjects (Yin et al., 2016), while in Australia TCS was detected in all urine samples of 2,400 tested subjects (Heffernan et al., 2015). These results show that TCS presence in human urine is a worldwide and common occurrence, which reflects significant exposure of the human population to TCS. The highly lipophilic properties of TCS render it readily absorbable by mucous membranes (Lin, 2000), skin (Moss et al., 2000; Chedgzoy et al., 2002), and following oral intake (Bagley and Lin, 2000; Lin, 2000; Sandborgh-Englund et al., 2006; Weatherly and Gosse, 2017). After oral administration, TCS plasma levels increase rapidly reaching the maximum concentration in ∼1–3 h, with a terminal plasma half-life of ∼21 h; the accumulated urinary excretion ranges from 24 to 83% of the oral dose within the first 4 days after TCS administration (Sandborgh-Englund et al., 2006). In humans, TCS percutaneous absorption is estimated to be around 6% of the exposure dose, and a main proportion of the absorbed TCS is excreted in the urine within the first day after the exposure (Queckenberg et al., 2010).
Triclosan is present in several commercial products at a concentration of ∼15 mM. Therefore, nanomolar TCS concentrations may be present in the brain after daily and multiple exposures to products that contain this chemical, such as toothpastes and soaps. Consequently, and considering the widespread exposure to TCS-containing personal care products – plus the fact that this chemical has been found in dust from private houses and workplaces (Canosa et al., 2007; Geens et al., 2009) – we posit that the presence of TCS in household products may have deleterious effects on human neurological health. Nevertheless, although TCS has been found with low frequency in human post-mortem brain tissue (Geens et al., 2012), to our knowledge direct determinations of TCS concentrations in human cerebrospinal fluid (CSF) have not been reported. Consequently, it is not possible to discern how TCS concentrations detected in human urine samples correlate with TCS brain levels. We propose that in order to ascertain whether TCS levels in CSF correlate with clinical neurological performance and neurological diseases, the actual TCS concentrations in human CSF samples should be evaluated. These determinations would provide sound information to evaluate the safety of TCS presence in daily use products.
AA-C performed the electrophysiology experiments in slices, analyzed the data, and contributed to manuscript writing. JM and JV performed the behavioral experiments and analyzed the data. TA determined the dendritic spine changes and analyzed the data. JH performed the single-cell electrophysiological experiments and analyzed the data. JV performed the behavioral experiments and analyzed the data. AH contributed to manuscript writing. IV-U performed the electrophysiology experiments in slices and analyzed the data. GS performed the Western blot experiments and analyzed the data. CH designed the experiments, analyzed the results, and wrote the final version of the manuscript. GB designed the experiments, performed the neuronal Ca2+ determinations and electrophysiology experiments in slices, analyzed the data, and wrote the final version of the manuscript.
This work was supported by Fondo Nacional de Desarrollo Científico y Tecnológico (FONDECYT) Grants Nos. 1170053 and 11140580 and by Biomedical Neuroscience Institute (Grant No. BNI P09-015-F).
The authors declare that the research was conducted in the absence of any commercial or financial relationships that could be construed as a potential conflict of interest.
We thank the expert technical assistance provided by Nicole Henriquez and Luis Montecinos.
The Supplementary Material for this article can be found online at: https://www.frontiersin.org/articles/10.3389/fnmol.2018.00429/full#supplementary-material
Adasme, T., Haeger, P., Paula-Lima, A. C., Espinoza, I., Casas-Alarcon, M. M., Carrasco, M. A., et al. (2011). Involvement of ryanodine receptors in neurotrophin-induced hippocampal synaptic plasticity and spatial memory formation. Proc. Natl. Acad. Sci. U.S.A. 108, 3029–3034. doi: 10.1073/pnas.1013580108
Aiello, A. E., and Larson, E. (2003). Antibacterial cleaning and hygiene products as an emerging risk factor for antibiotic resistance in the community. Lancet Infect. Dis. 3, 501–506. doi: 10.1016/S1473-3099(03)00723-0
Allmyr, M., Adolfsson-Erici, M., McLachlan, M. S., and Sandborgh-Englund, G. (2006). Triclosan in plasma and milk from Swedish nursing mothers and their exposure via personal care products. Sci. Total Environ. 372, 87–93. doi: 10.1016/j.scitotenv.2006.08.007
Andersen, P., Morris, R., Amaral, D., Bliss, T., O’Keefe, J., Oxford University, et al. (2006). The Hippocampus Book. New York, NY: Oxford University Press. doi: 10.1093/acprof:oso/9780195100273.001.0001
Ardiles, A. O., Tapia-Rojas, C. C., Mandal, M., Alexandre, F., Kirkwood, A., Inestrosa, N. C., et al. (2012). Postsynaptic dysfunction is associated with spatial and object recognition memory loss in a natural model of Alzheimer’s disease. Proc. Natl. Acad. Sci. U.S.A. 109, 13835–13840. doi: 10.1073/pnas.1201209109
Arias-Cavieres, A., Adasme, T., Sanchez, G., Munoz, P., and Hidalgo, C. (2017). Aging Impairs hippocampal- dependent recognition memory and LTP and prevents the associated RyR up-regulation. Front. Aging Neurosci. 9:111. doi: 10.3389/fnagi.2017.00111
Bagley, D. M., and Lin, Y. J. (2000). Clinical evidence for the lack of triclosan accumulation from daily use in dentifrices. Am. J. Dent. 13, 148–152.
Bailey, C. H., Kandel, E. R., and Si, K. (2004). The persistence of long-term memory: a molecular approach to self-sustaining changes in learning-induced synaptic growth. Neuron 44, 49–57. doi: 10.1016/j.neuron.2004.09.017
Bailey, D. J., Ma, C., Soma, K. K., and Saldanha, C. J. (2013). Inhibition of hippocampal aromatization impairs spatial memory performance in a male songbird. Endocrinology 154, 4707–4714. doi: 10.1210/en.2013-1684
Baudry, M., Zhu, G., Liu, Y., Wang, Y., Briz, V., and Bi, X. (2015). Multiple cellular cascades participate in long-term potentiation and in hippocampus-dependent learning. Brain Res. 1621, 73–81. doi: 10.1016/j.brainres.2014.11.033
Bayer, K. U., De Koninck, P., Leonard, A. S., Hell, J. W., and Schulman, H. (2001). Interaction with the NMDA receptor locks CaMKII in an active conformation. Nature 411, 801–805. doi: 10.1038/35081080
Bear, M. F., and Malenka, R. C. (1994). Synaptic plasticity: LTP and LTD. Curr. Opin. Neurobiol. 4, 389–399. doi: 10.1016/0959-4388(94)90101-5
Berridge, M. J. (1998). Neuronal calcium signaling. Neuron 21, 13–26. doi: 10.1016/S0896-6273(00)80510-3
Berridge, M. J., Bootman, M. D., and Roderick, H. L. (2003). Calcium signalling: dynamics, homeostasis and remodelling. Nat. Rev. Mol. Cell Biol. 4, 517–529. doi: 10.1038/nrm1155
Bliss, T. V., and Collingridge, G. L. (1993). A synaptic model of memory: long-term potentiation in the hippocampus. Nature 361, 31–39. doi: 10.1038/361031a0
Bliss, T. V., Collingridge, G. L., and Morris, R. G. (2014). Synaptic plasticity in health and disease: introduction and overview. Philos. Trans. R. Soc. Lond. B Biol. Sci. 369, 20130129. doi: 10.1098/rstb.2013.0129
Bodhinathan, K., Kumar, A., and Foster, T. C. (2010). Intracellular redox state alters NMDA receptor response during aging through Ca2+/calmodulin-dependent protein kinase II. J. Neurosci. 30, 1914–1924. doi: 10.1523/JNEUROSCI.5485-09.2010
Bournaud, R., Hidalgo, J., Melliti, K., and Shimahara, T. (1998). The action of diltiazem and gallopamil (D600) on calcium channel current and charge movement in mouse Purkinje neurons. Neurosci. Lett. 241, 163–166. doi: 10.1016/S0304-3940(97)00970-1
Canosa, P., Rodriguez, I., Rubi, E., and Cela, R. (2007). Determination of parabens and triclosan in indoor dust using matrix solid-phase dispersion and gas chromatography with tandem mass spectrometry. Anal. Chem. 79, 1675–1681. doi: 10.1021/ac061896e
Chedgzoy, P., Winckle, G., and Heard, C. M. (2002). Triclosan: release from transdermal adhesive formulations and in vitro permeation across human epidermal membranes. Int. J. Pharm. 235, 229–236. doi: 10.1016/S0378-5173(01)00992-9
Cherednichenko, G., Zhang, R., Bannister, R. A., Timofeyev, V., Li, N., Fritsch, E. B., et al. (2012). Triclosan impairs excitation-contraction coupling and Ca2+ dynamics in striated muscle. Proc. Natl. Acad. Sci. U.S.A. 109, 14158–14163. doi: 10.1073/pnas.1211314109
Citri, A., and Malenka, R. C. (2008). Synaptic plasticity: multiple forms, functions, and mechanisms. Neuropsychopharmacology 33, 18–41. doi: 10.1038/sj.npp.1301559
Clark, R. E., Broadbent, N. J., and Squire, L. R. (2005). Hippocampus and remote spatial memory in rats. Hippocampus 15, 260–272. doi: 10.1002/hipo.20056
DeSalva, S. J., Kong, B. M., and Lin, Y. J. (1989). Triclosan: a safety profile. Am. J. Dent. 2, 185–196.
Dhillon, G. S., Kaur, S., Pulicharla, R., Brar, S. K., Cledon, M., Verma, M., et al. (2015). Triclosan: current status, occurrence, environmental risks and bioaccumulation potential. Int. J. Environ. Res. Public Health 12, 5657–5684. doi: 10.3390/ijerph120505657
Duman, R. S., and Aghajanian, G. K. (2012). Synaptic dysfunction in depression: potential therapeutic targets. Science 338, 68–72. doi: 10.1126/science.1222939
European Commission. Directorate General for Health and Consumers (2010). Opinion on Triclosan Colipa n°P32. Brussels: European Commission, Directorate General for Health & Consumers.
Fang, J. L., Vanlandingham, M., da Costa, G. G., and Beland, F. A. (2016). Absorption and metabolism of triclosan after application to the skin of B6C3F1 mice. Environ. Toxicol. 31, 609–623. doi: 10.1002/tox.22074
Fedulov, V., Rex, C. S., Simmons, D. A., Palmer, L., Gall, C. M., and Lynch, G. (2007). Evidence that long-term potentiation occurs within individual hippocampal synapses during learning. J. Neurosci. 27, 8031–8039. doi: 10.1523/JNEUROSCI.2003-07.2007
Fritsch, E. B., Connon, R. E., Werner, I., Davies, R. E., Beggel, S., Feng, W., et al. (2013). Triclosan impairs swimming behavior and alters expression of excitation-contraction coupling proteins in fathead minnow (Pimephales promelas). Environ. Sci. Technol. 47, 2008–2017. doi: 10.1021/es303790b
Gargus, J. J. (2009). Genetic calcium signaling abnormalities in the central nervous system: seizures, migraine, and autism. Ann. N. Y. Acad. Sci. 1151, 133–156. doi: 10.1111/j.1749-6632.2008.03572.x
Geens, T., Neels, H., and Covaci, A. (2012). Distribution of bisphenol-A, triclosan and n-nonylphenol in human adipose tissue, liver and brain. Chemosphere 87, 796–802. doi: 10.1016/j.chemosphere.2012.01.002
Geens, T., Roosens, L., Neels, H., and Covaci, A. (2009). Assessment of human exposure to Bisphenol-A, triclosan and tetrabromobisphenol-A through indoor dust intake in Belgium. Chemosphere 76, 755–760. doi: 10.1016/j.chemosphere.2009.05.024
Giachero, M., Calfa, G. D., and Molina, V. A. (2013). Hippocampal structural plasticity accompanies the resulting contextual fear memory following stress and fear conditioning. Learn. Mem. 20, 611–616. doi: 10.1101/lm.031724.113
Grigoryan, G., Korkotian, E., and Segal, M. (2012). Selective facilitation of LTP in the ventral hippocampus by calcium stores. Hippocampus 22, 1635–1644. doi: 10.1002/hipo.22000
Gruart, A., Munoz, M. D., and Delgado-Garcia, J. M. (2006). Involvement of the CA3-CA1 synapse in the acquisition of associative learning in behaving mice. J. Neurosci. 26, 1077–1087. doi: 10.1523/JNEUROSCI.2834-05.2006
Heffernan, A. L., Baduel, C., Toms, L. M., Calafat, A. M., Ye, X., Hobson, P., et al. (2015). Use of pooled samples to assess human exposure to parabens, benzophenone-3 and triclosan in Queensland, Australia. Environ. Int. 85, 77–83. doi: 10.1016/j.envint.2015.09.001
Holtmaat, A., and Svoboda, K. (2009). Experience-dependent structural synaptic plasticity in the mammalian brain. Nat. Rev. Neurosci. 10, 647–658. doi: 10.1038/nrn2699
Kasai, H., Fukuda, M., Watanabe, S., Hayashi-Takagi, A., and Noguchi, J. (2010). Structural dynamics of dendritic spines in memory and cognition. Trends Neurosci. 33, 121–129. doi: 10.1016/j.tins.2010.01.001
Kesner, R. P., Farnsworth, G., and Kametani, H. (1991). Role of parietal cortex and hippocampus in representing spatial information. Cereb. Cortex 1, 367–373. doi: 10.1093/cercor/1.5.367
Kitanishi, T., Ikegaya, Y., Matsuki, N., and Yamada, M. K. (2009). Experience-dependent, rapid structural changes in hippocampal pyramidal cell spines. Cereb. Cortex 19, 2572–2578. doi: 10.1093/cercor/bhp012
Korkotian, E., and Segal, M. (2011). Synaptopodin regulates release of calcium from stores in dendritic spines of cultured hippocampal neurons. J. Physiol. 589(Pt 24), 5987–5995. doi: 10.1113/jphysiol.2011.217315
Lang, C., Barco, A., Zablow, L., Kandel, E. R., Siegelbaum, S. A., and Zakharenko, S. S. (2004). Transient expansion of synaptically connected dendritic spines upon induction of hippocampal long-term potentiation. Proc. Natl. Acad. Sci. U.S.A. 101, 16665–16670. doi: 10.1073/pnas.0407581101
Larson, J., Wong, D., and Lynch, G. (1986). Patterned stimulation at the theta frequency is optimal for the induction of hippocampal long-term potentiation. Brain Res. 368, 347–350. doi: 10.1016/0006-8993(86)90579-2
Le Duigou, C., Wittner, L., Danglot, L., and Miles, R. (2005). Effects of focal injection of kainic acid into the mouse hippocampus in vitro and ex vivo. J. Physiol. 569(Pt 3), 833–847. doi: 10.1113/jphysiol.2005.094599
Leuner, B., Falduto, J., and Shors, T. J. (2003). Associative memory formation increases the observation of dendritic spines in the hippocampus. J. Neurosci. 23, 659–665. doi: 10.1523/JNEUROSCI.23-02-00659.2003
Levy, S. B. (2001). Antibacterial household products: cause for concern. Emerg. Infect. Dis. 7(Suppl. 3), 512–515. doi: 10.3201/eid0707.010705
Lin, Y. J. (2000). Buccal absorption of triclosan following topical mouthrinse application. Am. J. Dent. 13, 215–217.
Lohmann, C., and Wong, R. O. (2005). Regulation of dendritic growth and plasticity by local and global calcium dynamics. Cell Calcium 37, 403–409. doi: 10.1016/j.ceca.2005.01.008
Lynch, G., Kramar, E. A., and Gall, C. M. (2015). Protein synthesis and consolidation of memory-related synaptic changes. Brain Res. 1621, 62–72. doi: 10.1016/j.brainres.2014.11.060
Lynch, M. A. (2004). Long-term potentiation and memory. Physiol. Rev. 84, 87–136. doi: 10.1152/physrev.00014.2003
Maggio, N., and Vlachos, A. (2014). Synaptic plasticity at the interface of health and disease: new insights on the role of endoplasmic reticulum intracellular calcium stores. Neuroscience 281, 135–146. doi: 10.1016/j.neuroscience.2014.09.041
Malenka, R. C., and Bear, M. F. (2004). LTP and LTD: an embarrassment of riches. Neuron 44, 5–21. doi: 10.1016/j.neuron.2004.09.012
Martin, S. J., Grimwood, P. D., and Morris, R. G. (2000). Synaptic plasticity and memory: an evaluation of the hypothesis. Annu. Rev. Neurosci. 23, 649–711. doi: 10.1146/annurev.neuro.23.1.649
Martinez, G., Vidal, R. L., Mardones, P., Serrano, F. G., Ardiles, A. O., Wirth, C., et al. (2016). Regulation of memory formation by the transcription factor XBP1. Cell Rep. 14, 1382–1394. doi: 10.1016/j.celrep.2016.01.028
Matsuzaki, M., Ellis-Davies, G. C., Nemoto, T., Miyashita, Y., Iino, M., and Kasai, H. (2001). Dendritic spine geometry is critical for AMPA receptor expression in hippocampal CA1 pyramidal neurons. Nat. Neurosci. 4, 1086–1092. doi: 10.1038/nn736
Mattson, M. P., and Chan, S. L. (2001). Dysregulation of cellular calcium homeostasis in Alzheimer’s disease: bad genes and bad habits. J. Mol. Neurosci. 17, 205–224. doi: 10.1385/JMN:17:2:205
More, J. Y., Bruna, B. A., Lobos, P. E., Galaz, J. L., Figueroa, P. L., Namias, S., et al. (2018). Calcium release mediated by redox-sensitive RyR2 channels has a central role in hippocampal structural plasticity and spatial memory. Antioxid. Redox Signal. 29, 1125–1146. doi: 10.1089/ars.2017.7277
Morris, R. G., Anderson, E., Lynch, G. S., and Baudry, M. (1986). Selective impairment of learning and blockade of long-term potentiation by an N-methyl-D-aspartate receptor antagonist, AP5. Nature 319, 774–776. doi: 10.1038/319774a0
Moss, T., Howes, D., and Williams, F. M. (2000). Percutaneous penetration and dermal metabolism of triclosan (2,4, 4’-trichloro-2’-hydroxydiphenyl ether). Food Chem. Toxicol. 38, 361–370. doi: 10.1016/S0278-6915(99)00164-7
Munoz, P., Humeres, A., Elgueta, C., Kirkwood, A., Hidalgo, C., and Nunez, M. T. (2011). Iron mediates N-methyl-D-aspartate receptor-dependent stimulation of calcium-induced pathways and hippocampal synaptic plasticity. J. Biol. Chem. 286, 13382–13392. doi: 10.1074/jbc.M110.213785
Pannu, M. W., O’Connor, G. A., and Toor, G. S. (2012). Toxicity and bioaccumulation of biosolids-borne triclosan in terrestrial organisms. Environ. Toxicol. Chem. 31, 646–653. doi: 10.1002/etc.1721
Park, B. K., Gonzales, E. L., Yang, S. M., Bang, M., Choi, C. S., and Shin, C. Y. (2016). Effects of triclosan on neural stem cell viability and survival. Biomol. Ther. 24, 99–107. doi: 10.4062/biomolther.2015.164
Paula-Lima, A. C., Adasme, T., SanMartin, C., Sebollela, A., Hetz, C., Carrasco, M. A., et al. (2011). Amyloid beta-peptide oligomers stimulate RyR-mediated Ca2+ release inducing mitochondrial fragmentation in hippocampal neurons and prevent RyR-mediated dendritic spine remodeling produced by BDNF. Antioxid. Redox. Signal. 14, 1209–1223. doi: 10.1089/ars.2010.3287
Pchitskaya, E., Popugaeva, E., and Bezprozvanny, I. (2017). Calcium signaling and molecular mechanisms underlying neurodegenerative diseases. Cell Calcium 70, 87–94. doi: 10.1016/j.ceca.2017.06.008
Popugaeva, E., Pchitskaya, E., and Bezprozvanny, I. (2018). Dysregulation of intracellular calcium signaling in Alzheimer’s disease. Antioxid. Redox Signal. 29, 1176–1188. doi: 10.1089/ars.2018.7506
Pycke, B. F., Geer, L. A., Dalloul, M., Abulafia, O., Jenck, A. M., and Halden, R. U. (2014). Human fetal exposure to triclosan and triclocarban in an urban population from Brooklyn, New York. Environ. Sci. Technol. 48, 8831–8838. doi: 10.1021/es501100w
Queckenberg, C., Meins, J., Wachall, B., Doroshyenko, O., Tomalik-Scharte, D., Bastian, B., et al. (2010). Absorption, pharmacokinetics, and safety of triclosan after dermal administration. Antimicrob. Agents Chemother. 54, 570–572. doi: 10.1128/AAC.00615-09
Raymond, C. R. (2007). LTP forms 1, 2 and 3: different mechanisms for the “long” in long-term potentiation. Trends Neurosci. 30, 167–175. doi: 10.1016/j.tins.2007.01.007
Riquelme, D., Alvarez, A., Leal, N., Adasme, T., Espinoza, I., Valdes, J. A., et al. (2011). High-frequency field stimulation of primary neurons enhances ryanodine receptor-mediated Ca2+ release and generates hydrogen peroxide, which jointly stimulate NF-kappaB activity. Antioxid. Redox Signal. 14, 1245–1259. doi: 10.1089/ars.2010.3238
Rodricks, J. V., Swenberg, J. A., Borzelleca, J. F., Maronpot, R. R., and Shipp, A. M. (2010). Triclosan: a critical review of the experimental data and development of margins of safety for consumer products. Crit. Rev. Toxicol. 40, 422–484. doi: 10.3109/10408441003667514
Ruszkiewicz, J. A., Li, S., Rodriguez, M. B., and Aschner, M. (2017). Is Triclosan a neurotoxic agent? J. Toxicol. Environ. Health B Crit. Rev. 20, 104–117. doi: 10.1080/10937404.2017.1281181
Sandborgh-Englund, G., Adolfsson-Erici, M., Odham, G., and Ekstrand, J. (2006). Pharmacokinetics of triclosan following oral ingestion in humans. J. Toxicol. Environ. Health A 69, 1861–1873. doi: 10.1080/15287390600631706
Schulz, P. E., Cook, E. P., and Johnston, D. (1994). Changes in paired-pulse facilitation suggest presynaptic involvement in long-term potentiation. J. Neurosci. 14, 5325–5337. doi: 10.1523/JNEUROSCI.14-09-05325.1994
Segal, M. (2017). Dendritic spines: morphological building blocks of memory. Neurobiol. Learn. Mem. 138, 3–9. doi: 10.1016/j.nlm.2016.06.007
Shen, C., Huo, L. R., Zhao, X. L., Wang, P. R., and Zhong, N. (2015). Novel interactive partners of neuroligin 3: new aspects for pathogenesis of autism. J. Mol. Neurosci. 56, 89–101. doi: 10.1007/s12031-014-0470-9
Singer, H., Muller, S., Tixier, C., and Pillonel, L. (2002). Triclosan: occurrence and fate of a widely used biocide in the aquatic environment: field measurements in wastewater treatment plants, surface waters, and lake sediments. Environ. Sci. Technol. 36, 4998–5004. doi: 10.1021/es025750i
Smith, N. A., Kress, B. T., Lu, Y., Chandler-Militello, D., Benraiss, A., and Nedergaard, M. (2018). Fluorescent Ca(2+) indicators directly inhibit the Na,K-ATPase and disrupt cellular functions. Sci. Signal. 11:515. doi: 10.1126/scisignal.aal2039
Sorra, K. E., and Harris, K. M. (1998). Stability in synapse number and size at 2 hr after long-term potentiation in hippocampal area CA1. J. Neurosci. 18, 658–671. doi: 10.1523/JNEUROSCI.18-02-00658.1998
Szychowski, K. A., Sitarz, A. M., and Wojtowicz, A. K. (2015). Triclosan induces Fas receptor-dependent apoptosis in mouse neocortical neurons in vitro. Neuroscience 284, 192–201. doi: 10.1016/j.neuroscience.2014.10.001
Szychowski, K. A., Wnuk, A., Rzemieniec, J., Kajta, M., Leszczynska, T., and Wojtowicz, A. K. (2018). Triclosan-evoked neurotoxicity involves NMDAR subunits with the specific role of GluN2A in caspase-3-dependent apoptosis. Mol Neurobiol. doi: 10.1007/s12035-018-1083-z [Epub ahead of print].
Tanoue, R., Nomiyama, K., Nakamura, H., Hayashi, T., Kim, J. W., Isobe, T., et al. (2014). Simultaneous determination of polar pharmaceuticals and personal care products in biological organs and tissues. J. Chromatogr. A 1355, 193–205. doi: 10.1016/j.chroma.2014.06.016
Tsien, J. Z., Huerta, P. T., and Tonegawa, S. (1996). The essential role of hippocampal CA1 NMDA receptor-dependent synaptic plasticity in spatial memory. Cell 87, 1327–1338. doi: 10.1016/S0092-8674(00)81827-9
Tyler, W. J., and Pozzo-Miller, L. D. (2001). BDNF enhances quantal neurotransmitter release and increases the number of docked vesicles at the active zones of hippocampal excitatory synapses. J. Neurosci. 21, 4249–4258. doi: 10.1523/JNEUROSCI.21-12-04249.2001
Uhlen, P. (2004). Spectral analysis of calcium oscillations. Science’s STKE 2004:l15. doi: 10.1126/stke.2582004pl15
Vallipuram, J., Grenville, J., and Crawford, D. A. (2010). The E646D-ATP13A4 mutation associated with autism reveals a defect in calcium regulation. Cell. Mol. Neurobiol. 30, 233–246. doi: 10.1007/s10571-009-9445-8
Wang, L., Asimakopoulos, A. G., and Kannan, K. (2015). Accumulation of 19 environmental phenolic and xenobiotic heterocyclic aromatic compounds in human adipose tissue. Environ. Int. 78, 45–50. doi: 10.1016/j.envint.2015.02.015
Wayman, G. A., Bose, D. D., Yang, D., Lesiak, A., Bruun, D., Impey, S., et al. (2012). PCB-95 modulates the calcium-dependent signaling pathway responsible for activity-dependent dendritic growth. Environ. Health Perspect. 120, 1003–1009. doi: 10.1289/ehp.1104833
Weatherly, L. M., and Gosse, J. A. (2017). Triclosan exposure, transformation, and human health effects. J. Toxicol. Environ. Health B. Crit. Rev. 20, 447–469. doi: 10.1080/10937404.2017.1399306
Whitlock, J. R., Heynen, A. J., Shuler, M. G., and Bear, M. F. (2006). Learning induces long-term potentiation in the hippocampus. Science 313, 1093–1097. doi: 10.1126/science.1128134
Yang, D., Kim, K. H., Phimister, A., Bachstetter, A. D., Ward, T. R., Stackman, R. W., et al. (2009). Developmental exposure to polychlorinated biphenyls interferes with experience-dependent dendritic plasticity and ryanodine receptor expression in weanling rats. Environ. Health Perspect. 117, 426–435. doi: 10.1289/ehp.11771
Yang, H., Wang, W., Romano, K. A., Gu, M., Sanidad, K. Z., Kim, D., et al. (2018). A common antimicrobial additive increases colonic inflammation and colitis-associated colon tumorigenesis in mice. Sci. Transl. Med. 10:443. doi: 10.1126/scitranslmed.aan4116
Yin, J., Wei, L., Shi, Y., Zhang, J., Wu, Q., and Shao, B. (2016). Chinese population exposure to triclosan and triclocarban as measured via human urine and nails. Environ. Geochem. Health 38, 1125–1135. doi: 10.1007/s10653-015-9777-x
Yueh, M. F., Taniguchi, K., Chen, S., Evans, R. M., Hammock, B. D., Karin, M., et al. (2014). The commonly used antimicrobial additive triclosan is a liver tumor promoter. Proc. Natl. Acad. Sci. U.S.A. 111, 17200–17205. doi: 10.1073/pnas.1419119111
Keywords: hippocampus, structural plasticity, Ca2+ signals, synaptic transmission, antimicrobial agents
Citation: Arias-Cavieres A, More J, Vicente JM, Adasme T, Hidalgo J, Valdés JL, Humeres A, Valdés-Undurraga I, Sánchez G, Hidalgo C and Barrientos G (2018) Triclosan Impairs Hippocampal Synaptic Plasticity and Spatial Memory in Male Rats. Front. Mol. Neurosci. 11:429. doi: 10.3389/fnmol.2018.00429
Received: 18 July 2018; Accepted: 05 November 2018;
Published: 26 November 2018.
Edited by:
Victor Ramírez-Amaya, Instituto de Investigación Médica Mercedes y Martín Ferreyra (INIMEC), ArgentinaReviewed by:
Almira Vazdarjanova, Augusta University, United StatesCopyright © 2018 Arias-Cavieres, More, Vicente, Adasme, Hidalgo, Valdés, Humeres, Valdés-Undurraga, Sánchez, Hidalgo and Barrientos. This is an open-access article distributed under the terms of the Creative Commons Attribution License (CC BY). The use, distribution or reproduction in other forums is permitted, provided the original author(s) and the copyright owner(s) are credited and that the original publication in this journal is cited, in accordance with accepted academic practice. No use, distribution or reproduction is permitted which does not comply with these terms.
*Correspondence: Genaro Barrientos, Z2JhcnJpZW50b3NAbWVkLnVjaGlsZS5jbA==
Disclaimer: All claims expressed in this article are solely those of the authors and do not necessarily represent those of their affiliated organizations, or those of the publisher, the editors and the reviewers. Any product that may be evaluated in this article or claim that may be made by its manufacturer is not guaranteed or endorsed by the publisher.
Research integrity at Frontiers
Learn more about the work of our research integrity team to safeguard the quality of each article we publish.