- 1Department of Applied Life Sciences, Graduate School, Konkuk University, Chungju, South Korea
- 2Department of Integrated Bioscience and Biotechnology, College of Biomedical and Health Sciences, Research Institute of Inflammatory Diseases (RID), Konkuk University, Chungju, South Korea
- 3Nanotechnology Research Center, Konkuk University, Chungju, South Korea
Glutamate receptors play a crucial role in the central nervous system and are implicated in different brain disorders. They play a significant role in the pathogenesis of neurodegenerative diseases (NDDs) such as Alzheimer’s disease, Parkinson’s disease, and amyotrophic lateral sclerosis. Although many studies on NDDs have been conducted, their exact pathophysiological characteristics are still not fully understood. In in vivo and in vitro models of neurotoxic-induced NDDs, neurotoxic agents are used to induce several neuronal injuries for the purpose of correlating them with the pathological characteristics of NDDs. Moreover, therapeutic drugs might be discovered based on the studies employing these models. In NDD models, different neurotoxic agents, namely, kainic acid, domoic acid, glutamate, β-N-Methylamino-L-alanine, amyloid beta, 1-methyl-4-phenyl-1,2,3,6-tetrahydropyridine, 1-methyl-4-phenylpyridinium, rotenone, 3-Nitropropionic acid and methamphetamine can potently impair both ionotropic and metabotropic glutamate receptors, leading to the progression of toxicity. Many other neurotoxic agents mainly affect the functions of ionotropic glutamate receptors. We discuss particular neurotoxic agents that can act upon glutamate receptors so as to effectively mimic NDDs. The correlation of neurotoxic agent-induced disease characteristics with glutamate receptors would aid the discovery and development of therapeutic drugs for NDDs.
Background
Glutamate receptors are the most abundant type of excitatory neurotransmitter receptors, and they are involved in mediating common excitatory synaptic transmissions in the brain and spinal cord (Jensen, 2009; Byrne et al., 2014). These receptors are very complex in nature and more than 20 different glutamate receptors have been recognized in the mammalian central nervous system (Altevogt et al., 2011). Glutamate receptors are categorized into two groups: ionotropic and metabotropic. iGluRs are voltage-sensitive, whereas mGluRs are ligand-sensitive (Altevogt et al., 2011). The subtypes of iGluRs are named for the chemical agonists that selectively bind to these subfamily members: NMDARs, AMPARs, and KARs (Conn and Pin, 1997). These subtypes have different subunits: the NMDAR subunits are GluN1, GluN2A, GluN2B, GluN2C, GluN2D, GluN3A, and GluN3B; the AMPAR subunits are GluA1, GluA2, GluA3, and GluA4; and the KAR subunits are GluK1, GluK2, GluK3, GluK4, and GluK5 (Tang et al., 2004). According to their pharmacological and signal transduction properties, mGluRs are divided into three broad groups that comprise eight subtypes (Jia et al., 2014). Group I and II mGluRs have two subunits: mGluR1 and mGluR5, and mGluR2 and mGluR3, respectively. Group III mGluRs have four subunits: mGluR4, mGluR6, mGluR7, and mGluR8 (Wong et al., 2005).
Glutamate receptors are best known for their mediation of glutamate in learning and memory through plasticity, that is the modification of channel properties; enhanced glutamate neurotransmission; and gene expression (Barco et al., 2006). They are involved in the pathogenesis of a variety of neurological disorders, including anxiety, schizophrenia, and epilepsy. (Rubio et al., 2012; Barker-Haliski and White, 2015; De Filippis et al., 2015). In addition to these disorders, various studies have reported that iGluRs and mGluRs play crucial roles in the pathogenesis of NDDs, such as AD, PD, Huntington’s disease (HD), amyotrophic lateral sclerosis (ALS), multiple sclerosis, and spinocerebellar ataxia (Geurts et al., 2003; Jin and Smith, 2007; O’Neill and Witkin, 2007; Fernandes and Raymond, 2009; André et al., 2010; Fallarino et al., 2010; Kwak et al., 2010; Ahmed et al., 2011; Spalloni et al., 2013; Titulaer et al., 2014; van Beugen et al., 2014; Iizuka et al., 2015; Guntupalli et al., 2016; Ishibashi et al., 2016; Zhang Y. et al., 2016; Ribeiro et al., 2017).
Based on the complexity of the mechanistic progression of NDDs, elucidating the proper disease pathophysiology and therapeutics of NDDs remains a major challenge. Recently, many neurotoxic agents have been employed in experiments in order to explore cellular functions and dysfunctions (Kumar and Kumar, 2010; van der Star et al., 2012; Abbasi et al., 2013; Jiang et al., 2015; Parekh, 2015; Ahmad et al., 2017; Kim et al., 2017). Their correlation with pathological characteristics of diseases via the utilization of neurotoxic agent-induced models is a helpful way to screen and discover potential therapeutic drugs for NDDs. Prior studies have also documented the actions of common neurotoxic agents that cause injury in NDD models (Schober, 2004; Bové et al., 2005; Hisahara and Shimohama, 2011; More et al., 2016). With respect to diverse neuronal functions, we focus on glutamate receptors as a target in neurotoxic agent-induced injury that may aid in the discovery of therapeutic drugs specifically for NDDs.
Glutamate Receptors as Potential Targets in Neurotoxic Agent-Induced NDD Models
Agents That Act on iGluRs and mGluRs
Glutamate, Kainic Acid, and Domoic Acid
Glutamate, a major excitatory neurotransmitter in the central nervous system, plays a vital role in neuronal cell differentiation, migration, and survival in the developing brain, basically via facilitating the entry of Ca2+ (Llorente-Folch et al., 2016). Kainic acid (KA), an agonist for the iGluR subtype which is known as a non-degradable analog of the excitotoxin, glutamate, offers a well-characterized model for the study of NDDs (Wang et al., 2005; Zhang et al., 2010; Zheng et al., 2010). DomA, a naturally occurring marine neurotoxin produced by members of the diatom genus, Pseudo-nitzschia, is a structural relative of KA (Lu et al., 2013).
Glutamate is capable of binding to and activating both iGluRs and mGluRs. In the brain, intracellular glutamate concentrations are in the millimolar range, and extracellular glutamate concentrations remain in the low micromolar range. These concentrations are achieved through the action of excitatory amino acid transporters that import glutamate and aspartate into astrocytes and neurons. Excess extracellular glutamate may lead to excitotoxicity in vitro and in vivo through the overactivation of iGluRs (Lewerenz and Maher, 2015). In general, glutamate triggers neuroinflammation while glutamate-induced excitotoxicity may contribute to neuronal cell death in NDDs (Lee et al., 2017). Glutamate-induced excitotoxicity causes cell death, apoptosis, and autophagy in both hippocampal cells (HT22) and primary cultured hippocampal neuron cells with neurotoxicity in differentiated Y-79 cells, BV-2 cells, and PC12 cells. Mitochondrial dysfunction, oxidative damage, and neuroinflammation are also key toxic effects in the glutamate-induced neurotoxicity model (Bak et al., 2016; Shinoda et al., 2016; Wang K. et al., 2016; Xu et al., 2016; Chen Z.W. et al., 2017). Numerous studies have described glutamate-induced neurotoxicity through the action of glutamate receptors. In human embryonic stem cell-derived neurons, glutamate produces NMDAR-dependent excitotoxicity. On the other hand, an NMDAR antagonist reduces glutamate-induced Ca2+ influx, which leads to the reduction of excitotoxicity (Gupta et al., 2013). In addition, berberine-induced mitochondria and NMDAR-dependent toxicity sensitize neurons to glutamate injury. Memantine (an NMDARs antagonist) and dizocilpine (MK-801) (a non-competitive NMDARs antagonist) completely block berberine-induced neurotoxicity (Kysenius et al., 2014). Another study found that MK-801 and γ-D-glutamylaminomethyl sulfonic acid (a KARs/AMPARs antagonist) wholly prevents glutamate-induced impairment in hippocampal cells. An p38 MAPK inhibitor, SB203580, also prevents glutamate-induced cell damage, but an MEK1 inhibitor, PD98059, does not alter glutamate-induced cell death in the intracellular signaling pathways (Molz et al., 2008). According to the most recent research on glutamate-induced toxicity in differentiated PC12 cells, the glutamate-induced dysfunction of Ca2+ homeostasis is protected by FAM3A upregulation. This activity is accomplished through the inhibition of mGluR1/5-dependent Ca2+ release by the endoplasmic reticulum (ER) and attenuation of the stromal interaction molecule-1 (STIM1)-Orai1 channel interactions that modulate store-operated Ca2+ entry (Song et al., 2017). Further, mGluR5 is expressed on astrocytes and through its activation, aquaporin 4-mediated glutamate-induced neurotoxicity causes partial mediation of astrocyte swelling. An mGluR5 agonist, (S)-3,5-dihydroxyphenylglycine (DHPG), which activates mGluR5 in cultured astrocytes, mimics the effect of glutamate. Incubation of DHPG with fenobam (an mGluR5 antagonist) negates this, and DL-threo-β-benzyloxyaspartic acid (DL-TBOA), a glutamate transporter inhibitor, does not abolish this agonistic effect (Shi et al., 2017).
The KA-induced neurotoxicity model is suitable for both in vivo and in vitro studies using rodents and several cell lines, such as BV-2 microglia, PC12 cells, and SH-SY5Y cells (Zhang et al., 2010; Hsieh et al., 2011; Xie et al., 2011; Luo et al., 2013; Nampoothiri et al., 2014; Nabeka et al., 2015). By acting on KARs, KA causes neuroexcitotoxic and epileptogenic properties. KA induces behavioral changes in rodents and causes a variety of cellular events to take place, including the influx of cellular Ca2+, neuroinflammation, production of reactive oxygen species (ROS) and mitochondrial dysfunction. It eventually leads to neuronal apoptosis and necrosis in many regions of the brain, particularly in the hippocampal subregions, cornu ammonis 1 (CA1), cornu ammonis 3 (CA3), and hilus of dentate gyrus (Wang et al., 2005; Zhang et al., 2010; Xie et al., 2011; Nabeka et al., 2015). Moreover, in cellular models, KA produces effects similar to those seen in rodent models (Hsieh et al., 2011; Nampoothiri et al., 2014). According to a recent study involving KA-induced excitotoxic hippocampal neuronal damage in rats, 2-Methyl-6-(phenylethynyl)-pyridine (a negative allosteric modulator of mGluR5) and LY354740 (an agonist of mGluR2) treatments ameliorate KA-induced neuronal cell death. Based on these results, both KARs and mGluRs may be involved in the KA-induced neuronal toxicity (Pershina et al., 2017).
As a KAR agonist, DomA is considered a potent neurotoxin and is used in experimental models to cause neurotoxicity. DomA-induced neurotoxicity causes neuroinflammation, mitochondrial dysfunction, oxidative stress, apoptosis, cognitive impairment, and neuronal cell death (Ananth et al., 2003; Chandrasekaran et al., 2004; Giordano et al., 2009; Lu et al., 2013; Wang D. et al., 2016). It is also employed in order to induce the symptoms of epilepsy in animal models (Buckmaster et al., 2014). The modulation of iGluRs may play a part in DomA-induced excitotoxicity (Qiu et al., 2005). In a neonatal rat model, a very low dose of DomA was shown to elicit a conditioned odor preference, and this was partly attributed to NMDARs involvement (Tasker et al., 2005). According to another investigation, AMPARs/KARs primarily regulate the neurotoxic effects of DomA. NBQX (a AMPARs/KARs antagonist) completely prevents DomA-induced toxic effects, whereas the NMDARs antagonist, (2R)-amino-5-phosphonopentanoate (APV), only partially blocks these effects (Hogberg and Bal-Price, 2011). The glutamate-, DomA-, and KA-induced progressions of major neurotoxicity via glutamate receptors are depicted in Figure 1.
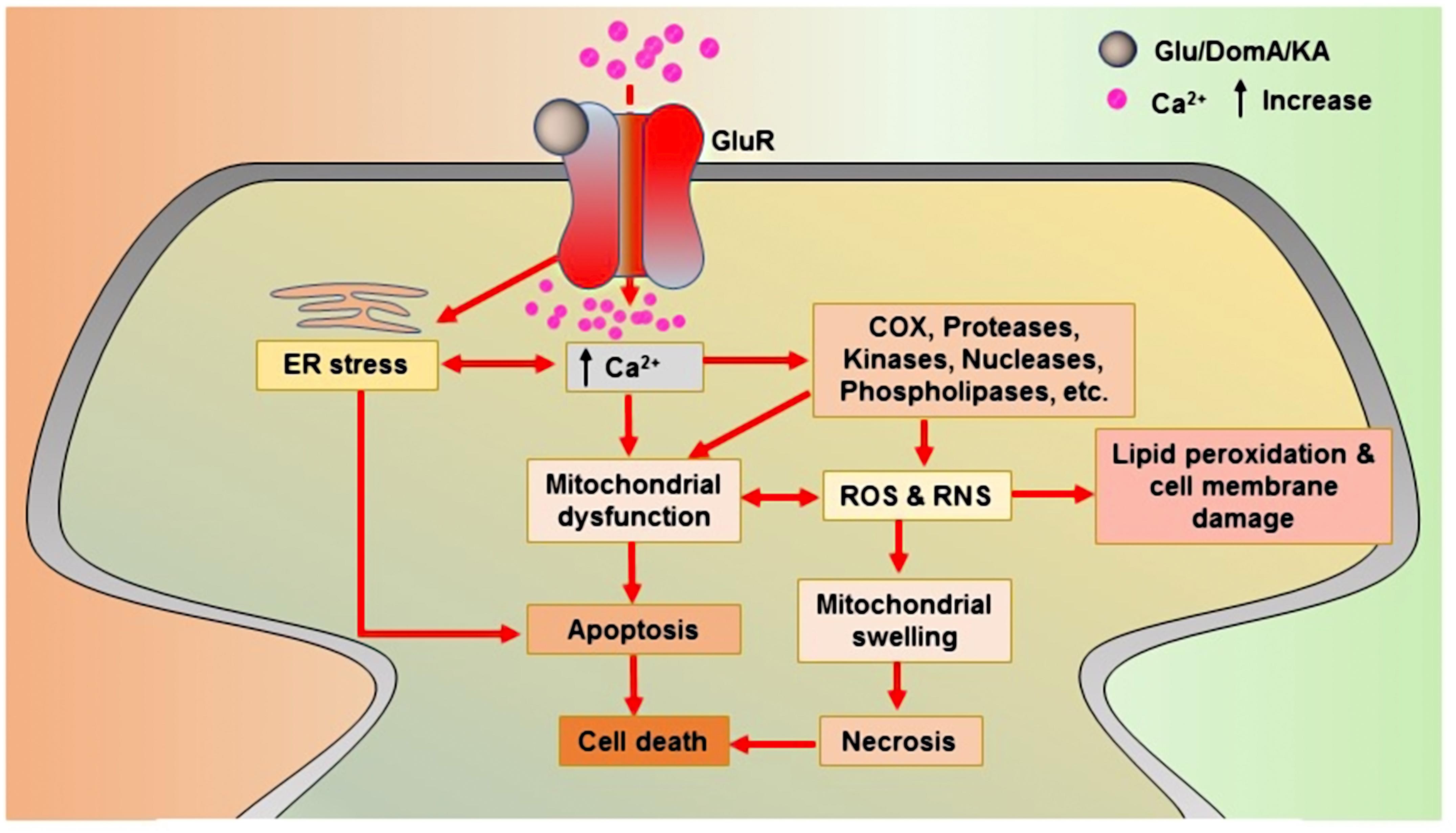
FIGURE 1. Glutamate receptor-mediated neurotoxic actions of glutamate, domoic acid, and kainic acid. Upon binding to glutamate receptors, all toxins produce an agonistic reaction that lead to cell death. Glu: Glutamate; KA: Kainic acid; DomA: Domoic acid; COX: Cyclooxygenase; ROS: Reactive oxygen species; and RNS: Reactive nitrogen species.
1-Methyl-4-phenyl-1,2,3,6-tetrahydropyridine and 1-Methyl-4-phenylpyridinium
1-methyl-4-phenyl-1,2,3,6-tetrahydropyridine (MPTP) is a common neurotoxic agent used to induce PD in animal models. MPTP yields large variations in nigral cell loss, striatal dopamine (DA) loss, and behavioral deficits (Meredith and Rademacher, 2011). 1-methyl-4-phenylpyridinium (MPP+), a metabolite of MPTP by monoamine oxidase-B, is also considered to be a neurotoxic agent and is also commonly used in both in vitro and in vivo PD models (Gasparini et al., 2013). MPTP activates the NMDARs and increases glutamate release in the striatum, which causes a large influx of Ca2+-induced neuronal excitotoxicity (Wang et al., 2010). The modulation of glutamate receptors by MPTP may be responsible for producing the associated neurotoxic effects. In addition, certain iGluRs antagonists have shown antiparkinsonian and anti-dyskinetic activities (Gasparini et al., 2013). Moreover, MPTP upregulates mGluR5 in monkey model. Chronic treatment with an mGluR5 antagonist, (3-[(2-methyl-1,3-thiazol-4-yl) ethynyl] pyridine) (MTEP), significantly protects dopaminergic and noradrenergic neurons from MPTP-induced toxicity (Samadi et al., 2008; Masilamoni et al., 2011). MPP+ interacts with NMDARs as a partial agonist and may dysregulate receptor functioning. Concerning the available research, NMDAR antagonists inhibit the actions of MPP+, specifically by decreasing MPP+-induced mitochondrial dysfunction (Camins et al., 1997). In a study, an mGluR8 agonist, (S)-3,4-Dicarboxyphenylglycine, demonstrates neuroprotective activity against MPP+-induced cell death in SH-SY5Y cells (Jantas et al., 2014).
Rotenone
Rotenone is a commonly used pesticide and fish toxin that impedes mitochondrial respiratory chain complex I (Zhang et al., 2006). It a valuable tool for PD research and exposure to rotenone causes the induction of parkinsonism in rodents. Nowadays, rotenone is an extensively used toxin to induce neurotoxicity in both in vitro and in vivo models of PD. It causes aging-related SN dopaminergic neurodegeneration in rats (Wang X. et al., 2015). Rotenone potently augmented NMDA-evoked currents in rat DA neurons through a tyrosine kinase-dependent mechanism. Further study showed that the potentiation of NMDA currents by a tyrosine kinase-dependent process attenuates the voltage-dependent Mg2+ block of NMDA-gated channels (Wu and Johnson, 2009). In addition, rotenone modulates mGluRs as it damages DNA through mGluR5. The selective mGluR5 antagonist protects rotenone-induced neurotoxicity by mitigating the oxidative stress-related damage to DNA associated with 8-hydroxy-2′-deoxyguanosine production, and also decreases the phosphorylation of extracellular-signal-regulated kinase activity and thioredoxin-2 expression (Xia et al., 2015).
Methamphetamine
As a sympathomimetic amine, METH belongs to the phenethylamine and amphetamine classes of psychoactive drugs. It is abused extensively for its euphoric, stimulant, empathogenic, and hallucinogenic properties (Yu et al., 2015). As a neurotoxic agent, METH can be used to induce neurotoxicity in a study model, which may be helpful in the study of NDDs, particularly in study of PD. METH-induced neurotoxicity is characterized by a long-lasting depletion of striatal DA and serotonin as well as by damage to striatal dopaminergic and serotonergic nerve terminals (Kita et al., 2003). The principal neurotoxic effects caused by METH are oxidative stress, neuroinflammation, and apoptosis leading to neuronal cell death (Choi et al., 2002; Raineri et al., 2012; Shin et al., 2012; Jayanthi et al., 2014; Jumnongprakhon et al., 2014). Studies have reported that METH downregulates the glutamate receptors. In the hippocampus, METH induction decreases the permeability and/or functionality of NMDARs and AMPARs, impairing spatial working memory (Simoes et al., 2007). In the rat striatum and frontal cortex, METH changes the NMDAR and AMPAR subunit levels (Simões et al., 2008). Besides, the cortical iGluR antagonism protects against METH-induced striatal neurotoxicity (Gross et al., 2011). A few studies have correlated METH-induced neurotoxicity with mGluRs. Antagonism of mGluR1 by its selective antagonist JNJ16259685 [(3,4-dihydro-2H-pyrano-[2,3-b]quinolin-7-yl)-(cis-4-methoxycyclohexyl) metha-none] attenuates cocaine- and METH- treated behavioral effects in squirrel monkeys (Achat-Mendes et al., 2012). In addition to mGluR1, mGluR5 receptors play an important role in METH reinforcement and METH-seeking behavior. An mGluR5 antagonist, 2-methyl-6-(phenylethynyl)-pyridine, dose-dependently reduces the reinforcing effects of METH under a fixed-ratio 1 and a progressive ratio schedule of reinforcement without altering overall responding for food. It also dose-dependently prevents the cue and drug-induced reinstatement of METH-seeking behavior (Gass et al., 2009). Another study reported that mGluR5 plays a role in the maintenance of place preference memory and that its negative allosteric modulators could be potentially used in METH addiction therapy (Herrold et al., 2013).
Amyloid Beta
Amyloid beta (Aβ) acts as a neurotoxic agent by initiating biochemical cascades that ultimately lead to synaptotoxicity and neurodegeneration (Walsh and Selkoe, 2004). It can interrupt excitatory synaptic transmission and plasticity in the brain via the dysregulation of AMPARs and NMDARs (Guntupalli et al., 2016). Recent research employing Aβ-induced neurotoxicity models have shown that the altered activity of NMDARs plays a major role in disease pathogenesis. Aβ enhances the activation of extrasynaptic NMDARs by decreasing neuronal glutamate uptake and inducing glutamate spillover (Wang Z.-C. et al., 2013). It binds to hippocampal neuron NMDAR subunits GluN1 and GluN2B (Lacor et al., 2004; Lacor et al., 2007). The GluN2B subunit is involved in regulating the action of Aβ oligomers by increasing intracellular Ca2+ in dendritic spines, resulting in the reduction of dendritic spine and synaptic density, which leads to early synaptic dysfunction (Shankar et al., 2007). In an Aβ-induced model, synaptic alterations were mitigated by blocking glutamate from binding to NMDARs though the use of an NMDARs antagonist (Birnbaum et al., 2015). Correspondingly, the stimulation of GluN2B by Aβ oligomer triggers the activation of MAPK and the subsequent downregulation of cAMP response element-binding protein (CREB) (Li et al., 2011). Indeed, Aβ reduces BDNF signaling by impairing axonal transport, which leads to synaptic dysfunction (Poon et al., 2011). A recent report showed that inhibition of NMDARs prevents the Aβ-induced loss of BDNF function (Tanqueiro et al., 2018). In addition, through in an α7-nicotinic acetylcholine receptor (α7-nAChR)-dependent manner, Aβ oligomers induce the endocytosis of NMDARs (Snyder et al., 2005). Likewise, α7-nAChRs are linked to the deregulation of NMDA signaling pathways (Roselli et al., 2005; Shankar et al., 2007). Interestingly, relatively low doses of NMDA-antagonists have been shown to reverse Aβ-induced synaptic disruption (Li et al., 2011; Rönicke et al., 2011). Aβ downregulates the caspase-mediated loss of two synaptic proteins, PSD-95 and synaptophysin. It suppresses NR2A function and activates NR2B following the induction of caspase-8 and caspase-3 activities. On the other hand, MK-801 and ifenprodil (an NR2B antagonist) prevent Aβ-induced toxicity (Liu et al., 2010). In hippocampal neurons, Aβ oligomers disrupt axonal transport initiated by NMDAR-dependent mechanisms, and this is modulated by the enzyme glycogen synthase kinase-3β (Decker et al., 2010). In another study, the activation of Aβ-induced striatal-enriched protein tyrosine phosphatase was shown to lead to the dephosphorylation of tyrosine residues on NMDARs. Dephosphorylation of the GluN2B subunit correlates with increased NMDARs endocytosis and suppression of its synaptic function. On the other hand, reelin activates Src family tyrosine kinases and enhances tyrosine phosphorylation of the GluN2A and GluN2B subunits. Reelin signaling may prevent Aβ-induced NMDARs endocytosis and Src family tyrosine kinases activation (Durakoglugil et al., 2009). A proposed mechanism of Aβ-induced impairment in major types of signaling through NMDARs that leads to synaptic dysfunction is presented in Figure 2.
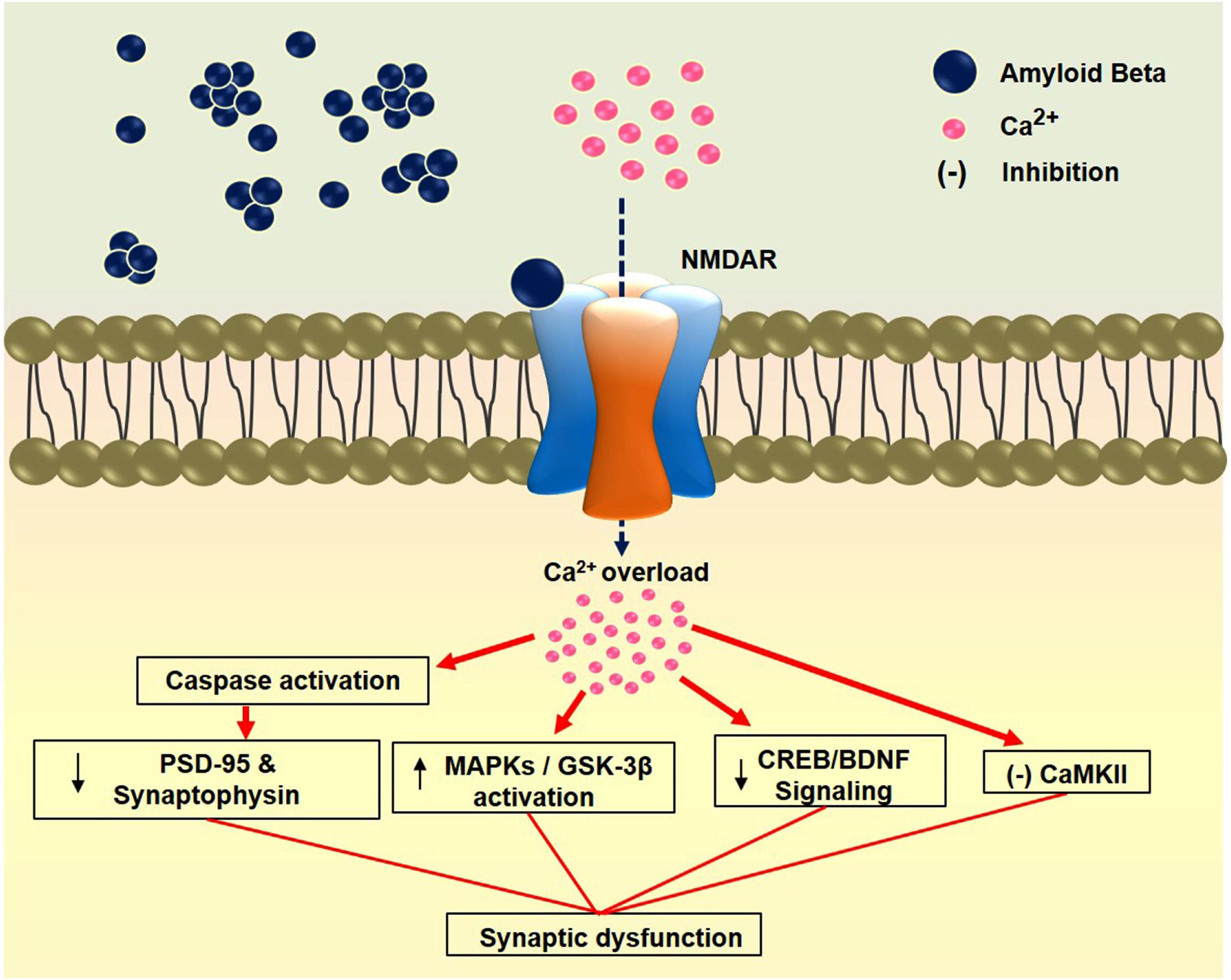
FIGURE 2. Amyloid beta-induced synaptic dysfunction through NMDAR. Binding of Aβ to the NMDAR causes calcium overload in the synapse leading to impairment in various signaling pathways. Finally, these impairments cause synaptic dysfunction. PSD-95: Postsynaptic density protein 95; MAPKs: Mitogen-activated protein kinases; GSK-3β: Glycogen synthase kinase-3 beta; CREB: cAMP-response element binding protein; BDNF: Brain-derived neurotrophic factor; CaMKII: Calcium/calmodulin-dependent protein kinase II
Aβ-induced synaptic dysfunction has been attributed to the synaptic removal of AMPARs. Aβ-induced change in the subcellular distribution of Ca2+/calmodulin-dependent protein kinase II may drive the removal of AMPARs from the synaptic membrane by Aβ (Gu et al., 2009). Aβ initiates synaptic and memory deficits by removing GluA3-containing AMPARs from synapses (Reinders et al., 2016). It disrupts mitochondrial trafficking, which may contribute to AMPAR removal as well as trafficking defects that cause synaptic inhibition (Rui et al., 2010). Furthermore, Aβ-induced dendritic spine loss and reductions in pre- and post-synaptic protein levels in hippocampal slice cultures can impair hippocampal LTP (Zhang et al., 2009; Wang Z.-C. et al., 2013; Birnbaum et al., 2015).
Aβ-induced ectopic NMDA and mGluR-mediated signaling coupled with caspase-3 activation may inhibit LTP and also facilitate long-term depression (LTD) (Hu et al., 2012). Within the synaptic space, membrane-bound Aβ oligomers accumulate and via a lateral diffusion process, gradually aggregate in order to form large non-mobile clusters. Aβ pathological clusters form complexes with mGluR5 receptors, which decreases the mobility of mGluR5 and causes its anomalous accumulation at the postsynaptic membrane. This is followed by calcium deregulation, synaptic disruption, and loss of NMDARs (Renner et al., 2010). In a transgenic model study, Aβ oligomer-cellular prion protein complexes activated mGluR5 at the neuronal surface, which led to the disruption of neuronal function (Um et al., 2013). The downregulation of mGluR and desensitization of the frontal cortex in AD patients correlated with AD-related neuropathological variations. Furthermore, the chronic activation of mGluR5 increased NMDA-dependent Aβ neurotoxicity, whereas mGluR5 antagonism exhibited neuroprotective effects against Aβ excitotoxic processes and prevented impairments in learning, memory and synaptic density (Rammes et al., 2011; Um et al., 2013).
Homocysteine
Homocysteine (Hcy), a sulfur-containing amino acid derived from the metabolism of methionine, is an independent risk factor for AD (Ataie et al., 2010c; Li et al., 2016). As a known neurotoxic agent, Hcy is used to induce neurotoxicity. In several animal model studies, Hcy brought about synaptic dysfunction, oxidative stress, neurochemical imbalance, and apoptosis, resulting in cognitive impairment and neuronal cell death. Thus, an Hcy-induced neurotoxicity model might be suitable for the study of AD (Ataie et al., 2010a,b,c; Wei et al., 2014; Kamat et al., 2016; Li T. et al., 2017). Hcy has been shown to modulate glutamate receptors, which leads to various neurotoxic effects. The activation of Hcy-NMDAR-mediated extracellular signal-regulated kinase causes neuronal cell death. Further, it modulates hippocampal glutamate and the NMDAR/AMPAR ratio in a rat model of chronic unpredictable mild stress-induced depression (Poddar and Paul, 2009; Liu et al., 2013; Moustafa et al., 2014; Poddar et al., 2017). Moreover, Hcy modulates mGluRs. An mGluR1 antagonist produces neuroprotective effect in the Hcy-induced neurodegenerative model (Yeganeh et al., 2013).
β-N-methylamino-L-alanine
The non-proteinogenic amino acid β-N-methylamino-L-alanine (BMAA), was first identified in the seeds of Cycas micronesica in 1967 (McCarron et al., 2014), though a wide range of cyanobacteria are now known to produce BMAA. Recently, the most common group of algae (diatoms) was also found to produce it (Delzor et al., 2014). BMAA is considered one of the first environmental factors that contributes to the etiologies of AD, PD, and ALS (Zhou et al., 2010). It is a common neurotoxin utilized in the study of neurodegeneration in cellular and animal models, specifically those for the study of ALS/Parkinsonism-dementia complex. BMAA causes neuroinflammation, oxidative stress, apoptosis and cognitive impairment (Brownson et al., 2002; Lobner, 2009; Santucci et al., 2009; Zhou et al., 2010; Muñoz-Saez et al., 2013; Al-Sammak et al., 2015; Takser et al., 2016; Laugeray et al., 2017; Petrozziello et al., 2017). It elicits neurotoxicity by acting as an agonist for glutamate receptors such as AMPARs/KARs, NMDARs, and mGluR5 (Lobner, 2009; Delzor et al., 2014). BMAA causes a significant increase in Ca2+ influx and enhanced ROS production, while also disrupting mitochondrial activity in rat olfactory ensheathing cells (Chiu et al., 2013). In addition, it interferes with neurotransmission in human neuroblastoma cells. BMAA alters alanine, aspartate, and glutamate metabolism and also modifies numerous neurotransmitters/neuromodulators, such as GABA and taurine (Engskog et al., 2017). However, the mechanism of BMAA-induced neurotoxicity is not yet fully understood, and its role as a glutamate receptor agonist may in fact lead to excitotoxicity that hampers glutamate transport systems (Zimmerman et al., 2016).
3-Nitropropionic Acid
3-Nitropropionic acid (3-NP) is a common neurotoxic agent used to study HD and is an irreversible inhibitor of mitochondrial complex-II. In the experimental animal model, it caused mitochondrial dysfunction, oxidative stress, biochemical imbalance, neuroinflammation, apoptosis, and autophagy, leading to neuronal cell death (Binawade and Jagtap, 2013; Solesio et al., 2013; Shetty et al., 2015; Jamwal and Kumar, 2016; Thangarajan et al., 2016). 3-NP was shown to produce neurotoxicity via the modulation of glutamate receptors. One study has suggested that 3-NP produces a neurotoxic effect through GluN2B-containing NMDARs (Centonze et al., 2006). In addition, the glutamate receptor antagonist, (2R)-amino-5-phosphonovaleric acid (AP5), negates the 3-NP-induced NMDAR-mediated second peak in ROS, mitochondrial fission, and cell death (Liot et al., 2009). The cannabinoid agonist WIN55,212-2 has been shown to produce a neuroprotective effect against 3-NP-induced striatal neurotoxicity via the induction of NMDARs hypofunction (Maya-López et al., 2017). Moreover, mGluR5 may be involved in 3-NP-induced neurotoxicity. In a study, MTEP produced neuroprotective activity in a 3-NP-induced neuronal injury model (Souza et al., 2014).
Cuprizone
Cuprizone is a common neurotoxic agent indicated to induce neurotoxicity to study NDDs. It is particularly used to induce multiple sclerosis-like syndromes. In the experimental animal model, it has shown to cause demyelination, oxidative stress, neuroinflammation, and apoptosis leading to neuronal cell death (Gudi et al., 2014; Zimmermann et al., 2014; Slowik et al., 2015; Sághy et al., 2016; Ragerdi Kashani et al., 2017; Sanadgol et al., 2017). In the cuprizone model of demyelination, the NMDARs specific antagonist MK-801 delays remyelination. NMDARs plays a critical role in the regulation of oligodendrocyte precursor cell differentiation in vitro and remyelination in cuprizone model, which may provide a potential target for the treatment of demyelination disease (Li et al., 2013). Another study reported that cuprizone treatment affected glutamate-receptors and -transporters differently in the gray and white matter areas of the brain, specifically, showed that it regulates the glutamate-aspartate transporter (Slc1a3) and neutral amino acid transporter A (Slc1a4) genes compared with other genes. Among the different NMDAR subunits, GluN2A was upregulated in the demyelinated corpus callosum (CC) and mGluR2 was downregulated in the demyelinated gray matter (Tameh et al., 2013).
Doxorubicin
Doxorubicin (DOX) is a known neurotoxic agent suitable for both in vivo and in vitro studies. In experimental models, DOX induction of neurotoxicity causes mitochondrial dysfunction, oxidative stress, neuroinflammation, apoptosis, cognitive impairments, and neuronal cell death (Shokoohinia et al., 2014; Siswanto et al., 2016; Cheruku et al., 2017). Several molecular studies have shown that DOX impairs glutamate receptors, leading to neuronal toxicity. In a DOX-induced neurotoxicity study, memantine was shown to counter neuronal cell death by blocking NMDARs (Jantas and Lason, 2009). In addition, mGluR II and III activators produced neuroprotective effects on DOX-induced cellular injury (Jantas et al., 2015). Furthermore, LTP and AMPARs impairment has been shown in DOX-treated animals. Compared to controls, the expression of the AMPAR subunit, GluA1, was significantly decreased, whereas the expression of the GluA2 subunit was significantly elevated (Alhowail, 2014). Another study showed that NMDARs and AMPAR might be involved in DOX-induced damage to DNA in neurons (Manchon et al., 2016).
Glucocorticoid
The hormone, GC, rises in concentration response to stress, which can cause neuronal loss (Kim et al., 2010). GC-induced neurotoxicity might be an appropriate model for the study of NDDs. It causes oxidative stress, memory impairment, neuronal cell death, and apoptosis (De Quervain et al., 2003; Lu et al., 2003; Roozendaal et al., 2003; You et al., 2009; Xu Y. et al., 2013) the latter through iGluRs and mGluRs (Lu et al., 2003). The stress-induced elevation of GC increases microglia proliferation through the activation of NMDARs. MK-801 has been shown to prevent the increase of microglia following the administration of exogenous GC corticosterone (Nair and Bonneau, 2006).
Harmaline
Harmaline-induced neurotoxicity in animals also could be a robust model for the study of NDDs (Iseri et al., 2011; Dahmardeh et al., 2017). Harmaline modulates both iGluRs and mGluRs (Kolasiewicz et al., 2009; Iseri et al., 2011). As an inverse agonist of NMDARs, it produces tremors (Du et al., 1997). The synchronous activation of the olivocerebellar pathway and release of glutamate in the cerebellum (which acts on NMDARs and AMPARs) is proposed to be main cause of harmaline-induced tremors (Shourmasti et al., 2014). In addition, the agonistic action of harmaline on mGluR1 produces motor disturbances (Kolasiewicz et al., 2009). On the other hand, memantine produces neuroprotective and anti-tremorgenic activities against harmaline-induced tremors and neurodegeneration (Iseri et al., 2011).
Pentylenetetrazol
The tetrazole derivative, PTZ, causes convulsions in mice, rats, cats, and primates, likely by interfering with GABA-mediated inhibition. PTZ is best known for its use in the screening of antiepileptic drugs (Zhao, 2006). It causes neuroinflammation and oxidative stress, which affects cognition. In addition, PTZ has been observed to cause amnesia in animal models (Oscós-Alvarado and de Muñoz, 1981; Pourmotabbed et al., 2011; dos Santos Branco et al., 2013). It is also known to modulate glutamate receptors. In a PTZ-induced kindling model of epilepsy, NMDARs are upregulated in both the hippocampus and cortex. KARs/AMPARs antagonists act as anticonvulsants against the tonic hind limb component of PTZ-induced seizures in developing rats (Velíšek et al., 1995; Ekonomou and Angelatou, 1999; Rogawski, 2011). The group III mGluR agonist CPPG [(RS)-α-cyclopropyl-4-phosphonophenylglycine] has been shown to attenuate PTZ-induced seizures. Moreover, it increases glutamate concentrations in the hippocampus of non-kindled control rats (Maciejak et al., 2009). The effects of several neurotoxic agents on both iGluRs and mGluRs are listed in Table 1.
Agents That Act on iGluRs
Ethanol
Chronic exposure to ethanol has complex and long-lasting effects on the function and expression of innumerable neuroreceptors as well as their modulators (Lovinger, 1997). Ethanol-induced neurotoxicity models have previously been used to study NDDs (Naseer et al., 2014; Saito et al., 2016). In vivo and in vitro studies have shown that chronic ethanol exposure elevates GluN1 and GluN2B gene expression and their polypeptide levels. In animal models, ethanol-induced neurotoxicity has been shown to cause oxidative stress, apoptosis, and neuroinflammation, ultimately causing neurodegeneration (Ullah et al., 2012, 2013; Naseer et al., 2014; Tajuddin et al., 2014; Ahmad et al., 2016; Wang P. et al., 2017). Ethanol has been shown to produce neurotoxicity in cellular studies on BV-2 microglia, PC12 cells, and HT22 cells (Casañas-Sánchez et al., 2016; Zhang J. et al., 2016; Huang et al., 2017). As a potent inhibitor of NMDARs, it acts on glutamate receptors and impairs the functionality of NMDARs and AMPARs. Moreover, ethanol inhibits glutamate receptor-mediated synaptic plasticity such as NMDA-dependent LTP (Wirkner et al., 1999; Möykkynen et al., 2003, 2009; Hicklin et al., 2011; Möykkynen and Korpi, 2012; He et al., 2013). The effect of alcohol and molecular changes within the regulatory process that modulates NMDARs functions, including factors that alter transcription, translation, post-translational modifications, and protein expression along with those that influence their interactions with different regulatory proteins (downstream effectors) constantly increases at the cellular level. Epigenetic dimension (i.e., histone modification-induced chromatin remodeling and DNA methylation that occurs in the process of alcohol-related neuroadaptation) is a key molecular mechanism in alcohol-mediated NMDARs alteration (Chandrasekar, 2013).
Ammonia
An ammonia-induced neurotoxicity model uncovered potential therapeutic strategies (Hadjihambi et al., 2014). Elevated ammonia levels damage motor neurons through numerous means, including endoplasmic reticulum (ER) stress, cyclin-dependent kinase 5 activation, macroautophagy-endolysosomal pathway impairment, oxidative and nitrosative stress, neuronal hyperexcitability, and neuroinflammation (Parekh, 2015). In order to produce neurotoxicity, ammonia modulates glutamate receptors and activates NMDARs which in turn leads to the following: (i) adenosine triphosphate (ATP) is depleted in the brain, causing glutamate release; (ii) calcineurin and dephosphorylation are activated and Na+/K+-ATPase is activated in the brain, increasing ATP consumption; (iii) mitochondrial function and calcium homeostasis are impaired at different levels, thus reducing ATP synthesis; (iv) calpain activation degrades microtubule-associated protein-2, thus altering the microtubular network; and (v) nitric oxide (NO) formation rises, reducing the activity of glutamine synthetase, thus decreasing the elimination of ammonia in the brain (Monfort et al., 2002; Kosenko et al., 2004). In addition, through an excitatory amino acid transporter, ammonia mediates METH-induced increases in extracellular glutamate in order to cause excitotoxicity (Halpin et al., 2014). Interestingly, NMDARs and AMPARs antagonists prevent ammonia-induced toxicity in experimental models (Monfort et al., 2002; Halpin and Yamamoto, 2012).
Hydrogen Peroxide
Hydrogen peroxide (H2O2) is a common neurotoxic agent that induces neurotoxicity in cellular models. Cell lines, including BV-2 microglia, PC12 cells, and SH-SY5Y cells, are ideal for studying H2O2-induced neurotoxicity. In experimental models, H2O2-induced neurotoxicity causes mitochondrial dysfunction, oxidative stress, cytotoxicity, apoptosis, and neuronal cell death (Han et al., 2014; Morelli et al., 2014; Ismail et al., 2015; Mesbah-Ardakani et al., 2016; Zhong et al., 2016; Masilamani et al., 2017; Oh et al., 2017) while it also modulates glutamate receptors. Exposure to H2O2 can activate normally silent NMDARs, potentially via the inhibition of redox-sensitive glutamate uptake. H2O2 affects synaptic transmission and oxidative stress through the activation of NMDARs (Avshalumov and Rice, 2002). In striatal medium spiny neurons, the generation of AMPAR-dependent H2O2 is one source of retrograde signals that can block the release of DA (Avshalumov et al., 2008).
Cisplatin
Cisplatin (cis-diaminodichloroplatinum) was developed in the 1970s as the first platinum-based antineoplastic agent. Neurotoxicity, a common side effect of cisplatin leads to chemotherapy-induced peripheral neuropathy. An accumulation of cis-platinum in dorsal root ganglion neurons in the form of platinum-DNA adducts is thought to be a key mechanism driving such neurotoxicity (Zhu et al., 2016). In addition, as a known neurotoxic agent, cisplatin is suitable for use in NDD models, because it is known to cause memory and learning impairment through oxidative stress and neuroinflammation (Moneim, 2014; Oz et al., 2015; Zhou et al., 2016; Chen C. et al., 2017). Cisplatin modulates glutamate receptors and induces neural activation through the central upregulation of AMPARs and NMDARs (Holland et al., 2014). NMDARs antagonist protects against cisplatin-induced depression (Lehmann and Kärrberg, 1996).
Lead
Lead (Pb) is a well-known neurotoxic agent that brings about cognitive deficits in animal models. As a neurotoxic agent in different study models, Pb caused oxidative stress, synaptic and cholinergic dysfunction, neuroinflammation, autophagy, apoptosis, cognitive deficits, and neuronal cell death (Phyu and Tangpong, 2014; Ye et al., 2015; Chibowska et al., 2016; Meng et al., 2016; Tang et al., 2017; Xue et al., 2017). Pb-induced neurotoxicity may be based on the modulation of glutamate receptors, especially iGluRs. During synaptogenesis, it inhibits the functionality of NMDARs. Moreover, Pb exposure causes a decrease in the expression of AMPAR subunits (GluA1, GluA2, GluA3, and GluA4) with the observed decrease in GluA2 expression being particularly remarkable. Pb-induced neuronal cell death was rescued by three glutamate receptor antagonists: 6-cyano-7-nitroquinoxaline-2,3-dione (CNQX), MK-801, and 1-naphthyl acetyl spermine (a specific Ca2+-permeable AMPARs blocker) (Mußhoff et al., 1995; Neal et al., 2011; Ishida et al., 2017).
Manganese
Manganese (Mn) can be employed in order to induce neurotoxicity in studies on NDDs, especially PD. Mn-induced neurotoxicity brings about mitochondrial dysfunction, oxidative and nitrosative stress, neuroinflammation, and apoptosis, leading to cell death in different animal and cellular models (Chtourou et al., 2011; Moreno et al., 2011; da Silva Santos et al., 2014; Chen et al., 2016; Li S.J. et al., 2017; Shibata et al., 2017). It alters the functionality of glutamate receptors and it has been reported that Mn inhibits the functionality of NMDAR channels with an implication for psychiatric and cognitive impairment (Guilarte and Chen, 2007). Furthermore, by increasing extracellular glutamate levels and modifying the expression of NMDAR subunits, Mn induces nerve cell damage in mRNAs and proteins in rat striatum (Xu B. et al., 2010). In addition to NMDARs, it may also modulate AMPARs. Co-administration of the excitatory agonist, AMPA, with Mn enhances Mn-enhanced magnetic resonance imaging signals. However, this was attenuated by the co-administration of either the Na+ channel blocker tetrodotoxin (TTX), or the broad-spectrum Ca2+ channel blocker Ni2+ (Wang L. et al., 2015).
Mercury
Mercury (Hg) is a naturally occurring heavy metal (Moneim, 2015), and a well-known neurotoxic agent for inducing neurotoxicity. A Hg-induced neurotoxicity model is a powerful tool in the study of neurodegeneration. Hg-induced neurotoxicity in animals causes mitochondrial dysfunction, oxidative stress, neuroinflammation, and apoptosis, overall resulting in cognitive deficits and neuronal cell death (Kempuraj et al., 2010; Ayyathan et al., 2015; Adedara et al., 2016). Hg-induced neuronal death is dependent on glutamate-mediated excitotoxicity. Hg has been found to affect NMDARs by increasing their expression and enhancing their responsiveness. The overactivation of NMDA-type glutamate receptors increases Ca2+ influx into neurons, which leads to the activation of important pathways involved in neuronal cell death. Furthermore, Ca2+ stimulates ROS generation through the mitochondrial pathway as a result of Hg-induced overactivation of NMDARs that take part in neuronal cell death (Farina et al., 2011; Xu et al., 2012; Moneim, 2015; Engin et al., 2017).
Melamine
Another powerful tool in the study of NDDs is melamine-induced neurotoxicity. In studies involving animal models, it has been shown to cause synaptic dysfunction and oxidative stress, leading to cognitive impairment (An et al., 2011, 2012; An and Zhang, 2014). In recent studies, it presynaptically altered the glutamatergic transmission of the hippocampal CA3-CA1 synapses in vitro. This alteration is likely linked to a modification to autophagy. Acute melamine exposure impaired spatial memory consolidation by disrupting hippocampal NMDAR-dependent LTD (Zhang H. et al., 2016; An and Sun, 2017).
Sodium Azide
NaN3 is a neurotoxic agent commonly used for neurotoxicity in both in vivo and in vitro models. It causes mitochondrial dysfunction, oxidative stress, neuroinflammation, apoptosis, and autophagy, ultimately leading to neuronal cell death (Selvatici et al., 2009; Zhang et al., 2011; Olajide et al., 2016; Shan et al., 2017). Upon the glutamate receptors modulation by NaN3 produces neurotoxicity. Studies have found that MK-801 blocks NaN3-induced cell death, signifying that NMDARs contributes to mediated cell death (Grammatopoulos et al., 2002; Selvatici et al., 2009).
Isoflurane
In experimental animal models, isoflurane brings about mitochondrial dysfunction, neuroinflammation, and apoptosis, leading to cognitive impairments and neuronal cell death (Li et al., 2014; Hu X. et al., 2017; Liang et al., 2017; Su et al., 2017; Wu et al., 2017; Xu et al., 2017). Furthermore, it has been observed to modulate glutamate receptors. One study has reported that NMDAR-mediated excitatory synaptic transmission is more sensitive to isoflurane than non-NMDAR-mediated excitatory synaptic transmission (Xu et al., 2017). In addition, at minimum alveolar concentrations, isoflurane causes GABAA receptor antagonism and increases NMDARs inhibition (Nishikawa and MacIver, 2000).
3-Acetylpyridine
As a potent neurotoxic agent, 3-acetylpyridine (3-AP) readily induces neurodegeneration. 3-AP-induced neurotoxicity causes degeneration in the nigrostriatal dopaminergic system within experimental animal models. Furthermore, 3-AP-induced neurotoxicity can be used for both PD and spinocerebellar ataxia models (Deutch et al., 1989; Weller et al., 1992; Janahmadi et al., 2009; Abbasi et al., 2013; Medina et al., 2015). 3-AP modulates NMDARs and also yields neurotoxicity. Pre-exposure to subtoxic concentrations of NMDA enhances 3-AP toxicity, but the NMDARs antagonists, MK-801 or APV, as well as deprenyl, mazindol, and tetrahydrofolic acid, have no effect on 3-AP toxicity (Weller et al., 1992). Another study has suggested that thyrotropin-releasing hormone receptor agonists ameliorate 3-AP-induced ataxia in rats via NMDARs (Kinoshita et al., 1998).
6-Hydroxydopamine
6-Hydroxydopamine (6-OHDA) is employed to induce PD and study the mechanism of dopaminergic neuron cell death in animal models. It is a selective agent for the nigrostriatal pathway. As 6-OHDA shares certain structural similarities with DA, it can enter dopaminergic neurons via DA transporters, and consequently cause toxicity (Shi et al., 2011). It produces diverse toxic effects, such as mitochondrial dysfunction and oxidative stress, apoptosis, neuroinflammation, and dopaminergic cell death (Chan et al., 2009; Liang et al., 2011; Shi et al., 2011; Thornton and Vink, 2012; Guo et al., 2013; Pyo et al., 2013; Wang J. Y. et al., 2013; Elyasi et al., 2014; Magalingam et al., 2014; Mu et al., 2014; De Jesús-Cortés et al., 2015; Liu et al., 2015; Yan et al., 2015; Zhang et al., 2015; Mirzaie et al., 2016). Disturbance of the dopaminergic system may also cause glutamatergic NMDARs imbalances in the brain (Hallett et al., 2006). In addition, 6-OHDA dysregulates NMDAR functions. NMDARs antagonists increase dopaminergic neuronal survival and prevent a levodopa-induced abnormal motor response (Bibbiani et al., 2005; Yan et al., 2014).
Ketamine
Ketamine is considered to be a general anesthetic and is used extensively in pediatric surgery. It is an NMDARs antagonist and is increasingly employed in pre-clinical studies in order to induce psychosis in experimental models (Malhotra et al., 1997; Powers et al., 2015; Wang Q. et al., 2017). As a neurotoxic agent, it is utilized in animal models to induce cognitive impairment. This produces diverse toxic effects such as oxidative stress and apoptosis, leading to cognitive impairment. Ketamine-induced cognitive impairment models have long been employed to study NDDs (Malhotra et al., 1997; Duan et al., 2014; Gazal et al., 2014; Wang et al., 2014; Palsa et al., 2016; Wang Q. et al., 2017). Ketamine-induced neurotoxicity causes a use-dependent blockade of NMDARs. This excitatory synaptic blockade activity possibly causes the loss of responsiveness linked to ketamine anesthesia (Sleigh et al., 2014). Furthermore, in a rat model of ketamine-induced neurotoxicity, extended ketamine exposure produces an increase in the expression of NMDAR (GluN1) (compensatory upregulation). This permits a higher toxic influx of Ca2+ into neurons once ketamine is removed from the system, increasing the generation of ROS and neuronal cell death (Liu et al., 2012). In addition, ketamine modulates the AMPARs. However, ketamine-induced inhibition of glycogen synthase kinase-3 contributes to the increase in AMPAR signaling that leads to ketamine antidepressant activity (Beurel et al., 2016). Recently, ketamine-induced impairment of the AMPARs potentiator, PF-04958242, has been shown to affect the verbal learning and memories of healthy volunteers (Ranganathan et al., 2017). In addition, an updated study was carried out with primary cultured cortical neurons and PC12 cells using a ketamine-induced neurotoxicity model (Liu et al., 2011).
Colchicine
Colchicine is a plant alkaloid that is regarded as a potent inhibitor of physiological processes. It specifically binds to a receptor site on tubulin and blocks mitosis (Karami et al., 2013). It is one of the major neurotoxins used in the study of AD (Mohamed et al., 2015; Sil et al., 2016b). Colchicine-induced neurotoxicity might be caused by glutamate receptor modulation. Colchicine-induced neuroinflammation leading to neurodegeneration in rats might be linked to NMDARs and therapy with memantine mitigates that toxicity (Sil et al., 2016a).
Bisphenol-A
As a neurotoxic agent, BPA mainly affects hippocampal neurogenesis and causes cognitive impairment in animal models (Castro et al., 2013; Tiwari et al., 2016; El Tabaa et al., 2017). Various studies have reported that BPA affects glutamate receptors (NMDARs and AMPARs) and produces neurotoxicity (Xu X. et al., 2010; Xu X.H. et al., 2010; Xu X. et al., 2013). According to a recent study, GluN2A and GluA1 (LTP-related glutamate receptors) were significantly downregulated in BPA-exposed rats (Hu F. et al., 2017). iGluRs that modulate the actions of miscellaneous neurotoxic agents are summarized in Table 2.
Concluding Remarks
Apart from the transgenic model of NDDs, animal and cellular models that make use of neurotoxic agent-induced neurotoxicity are very popular in the study of disease progression and potential therapeutic drugs. However, numerous pharmaceutical research projects have so far failed to discover therapeutic drugs for NDDs using these toxic agent-induced models.
Currently, the use of receptors for targeting drug discovery is an efficient approach. Diversity in the class and structural features of glutamate receptors plays a large role in the pathogenesis of NDDs and serves as a target for drugs for treating neurological disorders. We discussed here numerous neurotoxic agents that are capable of producing neuronal toxicity by altering the functionality of glutamate receptors. Several covered neurotoxic agents produced neuronal toxicity mainly by activating receptors where both ionotropic and metabotropic receptors are important for inducing neurotoxicity. As iGluRs are non-selective cation channels, upon binding to an agonist, they permit the passage of Na+ and K+, and in certain cases, limited quantities of Ca2+. Hence, excitotoxicity that induces neuronal damage might be one of the principal mechanisms of neurotoxic agents. On the other hand, upon an agonist binding to mGluRs, a post-synaptic membrane-bound G-protein is activated. This triggers a second messenger system that opens an ion channel for the mediation of signals. G-protein activation also triggers functional changes within the cytoplasm, culminating in gene expression and protein synthesis and specifically, the activation of diverse signaling systems. The mechanic progression of neuronal damage exerted by neurotoxic agents might be complex because the agents act on metabotropic receptors.
Many studies that use neurotoxic agent-induced models reveal the receptors modulating effects, but do not correlate with associated molecular signaling pathways. Despite knowing the mechanism involving glutamate receptors and neurotoxic agents, future studies focusing on glutamate receptors should still be designed. First, considering the pathological actions of currently available neurotoxic agents, the development of new natural, semi-synthetic, and synthetic neurotoxic agents is recommended. Second, more studies using the potent neurotoxic agents discussed here should be conducted. Third, molecular docking should be performed in order to identify receptor-neurotoxic agent interaction at the molecular level based on updated structural features. Finally, laboratory-based in vitro studies in which neuronal cell lines are used should be designed. In addition, in vivo studies on model organisms to identify molecular signals that impair by neurotoxic agents should be designed and conducted. By understanding the neurotoxic agent-induced neurotoxicity model, screening of agonist, antagonist and allosteric modulators might be of value for NDD therapy. In summary, by targeting glutamate receptors and their associated signals, a neurotoxic agent-induced neurotoxicity model has the potential to correlate a neurotoxic agent with disease pathophysiology. By considering that pathophysiology, the design and discovery of new and modified therapeutic molecules may be a feasible treatment strategy for NDD therapy.
Author Contributions
MJ and D-KC conceived and designed the study. MJ performed the literature review, wrote the manuscript, produced the figures, and compiled the table. S-YP performed the literature review and MH edited the data. GK contributed by drawing the figures. I-SK and PG arranged the data. D-KC also supervised and handled correspondence. All authors read and approved the final manuscript.
Funding
This research was supported by Basic Science Research Program through the National Research Foundation of Korea (NRF) funded by the Ministry of Science and ICT (Grant No. 2017R1A2A2A07001035).
Conflict of Interest Statement
The authors declare that the research was conducted in the absence of any commercial or financial relationships that could be construed as a potential conflict of interest.
Abbreviations
α-Syn, alpha-synuclein; 6-OHDA, 6-Hydroxydopamine; AD, Alzheimer’s disease; AMPARs, α-amino-3-hydroxyl-5-methyl-4-isoxazole-propionate receptors; Aβ, amyloid beta; Al, aluminum; BMAA, β-N-methylamino-L-alanine; BPA, bisphenol A; DomA, domoic acid; DZP, diazepam; DOX, doxorubicin; DSP-4, [N-(2-chloroethyl)-N-ethyl-2-bromobenzylamine]; GC, glucocorticoid; Hg, mercury; Hcy, homocysteine; iGluRs, ionotropic glutamate receptors; KARs, kainate receptors; LTP, long term potentiation; mGluRs, metabotropic glutamate receptors; MPTP, 1-methyl-4-phenyl-1,2,3,6-tetrahydropyridine; MTEP, 3-[(2-methyl-1,3-thiazol-4-yl) ethynyl]pyridine; Mn, manganese; METH, methamphetamine; NDDs, neurodegenerative diseases; NMDARs, N-methyl-D-aspartate receptors; NaN3, sodium azide; PD, Parkinson’s disease; PTZ, pentylenetetrazol
References
Abbasi, S., Edrisi, M., Mahnam, A., and Janahmadi, M. (2013). Computational insights into the neuroprotective action of riluzole on 3-acetylpyridine-induced ataxia in rats. Cell J. 15, 98–107.
Achat-Mendes, C., Platt, D. M., and Spealman, R. D. (2012). Antagonism of metabotropic glutamate 1 receptors attenuates behavioral effects of cocaine and methamphetamine in squirrel monkeys. J. Pharmacol. Exp. Ther. 343, 214–224. doi: 10.1124/jpet.112.196295
Adedara, I. A., Rosemberg, D. B., Souza, D. O., Farombi, E. O., Aschner, M., and Rocha, J. B. (2016). Neuroprotection of luteolin against methylmercury-induced toxicity in lobster cockroach Nauphoeta cinerea. Environ. Toxicol. Pharmacol. 42, 243–251. doi: 10.1016/j.etap.2016.02.001
Ahmad, A., Ali, T., Park, H. Y., Badshah, H., Rehman, S. U., and Kim, M. O. (2017). Neuroprotective effect of fisetin against amyloid-beta-induced cognitive/synaptic dysfunction, neuroinflammation, and neurodegeneration in adult mice. Mol. Neurobiol. 54, 2269–2285. doi: 10.1007/s12035-016-9795-4
Ahmad, A., Shah, S. A., Badshah, H., Kim, M. J., Ali, T., Yoon, G. H., et al. (2016). Neuroprotection by vitamin C against ethanol-induced neuroinflammation associated neurodegeneration in developing rat brain. CNS Neurol. Disord. Drug Targets 15, 360–370. doi: 10.2174/1871527315666151110130139
Ahmed, I., Bose, S. K., Pavese, N., Ramlackhansingh, A., Turkheimer, F., Hotton, G., et al. (2011). Glutamate NMDA receptor dysregulation in Parkinson’s disease with dyskinesias. Brain 134, 979–986. doi: 10.1093/brain/awr028
Alhowail, A. (2014). Elucidating the Cognitive Deficits following Doxorubicin Treatment. Master thesis, Auburn University, Auburn, AL.
Al-Sammak, M. A., Rogers, D. G., and Hoagland, K. D. (2015). Acute β-N-methylamino-L-alanine toxicity in a mouse model. J. Toxicol. 2015:739746. doi: 10.1155/2015/739746
Altevogt, B. M., Davis, M., and Pankevich, D. E. (2011). Glutamate-Related Biomarkers in Drug Development for Disorders of the Nervous System: Workshop Summary. Washington, DC: National Academies Press.
An, L., Li, Z., Yang, Z., and Zhang, T. (2011). Cognitive deficits induced by melamine in rats. Toxicol. Lett. 206, 276–280. doi: 10.1016/j.toxlet.2011.08.009
An, L., Li, Z., Yang, Z., and Zhang, T. (2012). Melamine induced cognitive impairment associated with oxidative damage in rat’s hippocampus. Pharmacol. Biochem. Behav. 102, 196–202. doi: 10.1016/j.pbb.2012.04.009
An, L., and Sun, W. (2017). Acute melamine affects spatial memory consolidation via inhibiting hippocampal NMDAR-dependent LTD in rats. Toxicol. Sci. 163, 385–396. doi: 10.1093/toxsci/kfx039
An, L., and Zhang, T. (2014). Vitamins C and E reverse melamine-induced deficits in spatial cognition and hippocampal synaptic plasticity in rats. Neurotoxicology 44, 132–139. doi: 10.1016/j.neuro.2014.06.009
Ananth, C., Gopalakrishnakone, P., and Kaur, C. (2003). Protective role of melatonin in domoic acid-induced neuronal damage in the hippocampus of adult rats. Hippocampus 13, 375–387. doi: 10.1002/hipo.10090
André, V. M., Cepeda, C., and Levine, M. S. (2010). Dopamine and glutamate in Huntington’s disease: a balancing act. CNS Neurosci. Ther. 16, 163–178. doi: 10.1111/j.1755-5949.2010.00134.x
Ataie, A., Sabetkasaei, M., Haghparast, A., Moghaddam, A. H., Ataie, R., and Moghaddam, S. N. (2010a). An investigation of the neuroprotective effects of Curcumin in a model of Homocysteine-induced oxidative stress in the rat’s brain. Daru 18:128.
Ataie, A., Sabetkasaei, M., Haghparast, A., Moghaddam, A. H., Ataee, R., and Moghaddam, S. N. (2010b). Curcumin exerts neuroprotective effects against homocysteine intracerebroventricular injection-induced cognitive impairment and oxidative stress in rat brain. J. Med. Food 13, 821–826. doi: 10.1089/jmf.2009.1278
Ataie, A., Sabetkasaei, M., Haghparast, A., Moghaddam, A. H., and Kazeminejad, B. (2010c). Neuroprotective effects of the polyphenolic antioxidant agent, Curcumin, against homocysteine-induced cognitive impairment and oxidative stress in the rat. Pharmacol. Biochem. Behav. 96, 378–385. doi: 10.1016/j.pbb.2010.06.009
Avshalumov, M. V., Patel, J. C., and Rice, M. E. (2008). AMPA receptor-dependent H 2 O 2 generation in striatal medium spiny neurons but not dopamine axons: one source of a retrograde signal that can inhibit dopamine release. J. Neurophysiol. 100, 1590–1601. doi: 10.1021/cn300130b
Avshalumov, M. V., and Rice, M. E. (2002). NMDA receptor activation mediates hydrogen peroxide–induced pathophysiology in rat hippocampal slices. J. Neurophysiol. 87, 2896–2903. doi: 10.1152/jn.2002.87.6.2896
Ayyathan, D. M., Chandrasekaran, R., and Thiagarajan, K. (2015). Neuroprotective effect of Brahmi, an ayurvedic drug against oxidative stress induced by methyl mercury toxicity in rat brain mitochondrial-enriched fractions. Nat. Prod. Res. 29, 1046–1051. doi: 10.1080/14786419.2014.968153
Bak, D.-H., Kim, H. D., Kim, Y. O., Park, C. G., Han, S.-Y., and Kim, J.-J. (2016). Neuroprotective effects of 20 (S)-protopanaxadiol against glutamate-induced mitochondrial dysfunction in PC12 cells. Int. J. Mol. Med. 37, 378–386. doi: 10.3892/ijmm.2015.2440
Barco, A., Bailey, C. H., and Kandel, E. R. (2006). Common molecular mechanisms in explicit and implicit memory. J. Neurochem. 97, 1520–1533. doi: 10.1111/j.1471-4159.2006.03870.x
Barker-Haliski, M., and White, H. S. (2015). Glutamatergic mechanisms associated with seizures and epilepsy. Cold Spring Harb. Perspect. Med. 5:a022863. doi: 10.1101/cshperspect.a022863
Beurel, E., Grieco, S. F., Amadei, C., Downey, K., and Jope, R. S. (2016). Ketamine-induced inhibition of glycogen synthase kinase-3 contributes to the augmentation of α-amino-3-hydroxy-5-methylisoxazole-4-propionic acid (AMPA) receptor signaling. Bipolar Disord. 18, 473–480. doi: 10.1111/bdi.12436
Bibbiani, F., Oh, J., Kielaite, A., Collins, M., Smith, C., and Chase, T. (2005). Combined blockade of AMPA and NMDA glutamate receptors reduces levodopa-induced motor complications in animal models of PD. Exp. Neurol. 196, 422–429. doi: 10.1016/j.expneurol.2005.08.017
Binawade, Y., and Jagtap, A. (2013). Neuroprotective effect of lutein against 3-nitropropionic acid–induced Huntington’s disease–like symptoms: possible behavioral, biochemical, and cellular alterations. J. Med. Food 16, 934–943. doi: 10.1089/jmf.2012.2698
Birnbaum, J., Bali, J., Rajendran, L., Nitsch, R., and Tackenberg, C. (2015). Calcium flux-independent NMDA receptor activity is required for Aβ oligomer-induced synaptic loss. Cell Death Dis. 6:e1791. doi: 10.1038/cddis.2015.160
Bové, J., Prou, D., Perier, C., and Przedborski, S. (2005). Toxin-induced models of Parkinson’s disease. NeuroRx 2, 484–494. doi: 10.1602/neurorx.2.3.484
Brownson, D. M., Mabry, T. J., and Leslie, S. W. (2002). The cycad neurotoxic amino acid, ß-N-methylamino-l-alanine (BMAA), elevates intracellular calcium levels in dissociated rat brain cells. J. Ethnopharmacol. 82, 159–167. doi: 10.1016/S0378-8741(02)00170-8
Buckmaster, P. S., Wen, X., Toyoda, I., Gulland, F., and Van Bonn, W. (2014). Hippocampal neuropathology of domoic acid–induced epilepsy in California sea lions (Zalophus californianus). J. Comp. Neurol. 522, 1691–1706. doi: 10.1002/cne.23509
Byrne, J. H., Heidelberger, R., and Waxham, M. N. (2014). From Molecules to Networks: An Introduction to Cellular and Molecular Neuroscience. Cambridge, MA: Academic Press.
Camins, A., Sureda, F., Gabriel, C., Pallas, M., Escubedo, E., and Camarasa, J. (1997). Effect of 1-methyl-4-phenylpyridinium (MPP+) on mitochondrial membrane potential in cerebellar neurons: interaction with the NMDA receptor. J. Neural Transm. 104, 569–577. doi: 10.1007/BF01291876
Casañas-Sánchez, V., Pérez, J. A., Quinto-Alemany, D., and Díaz, M. (2016). Sub-toxic ethanol exposure modulates gene expression and enzyme activity of antioxidant systems to provide neuroprotection in hippocampal HT22 cells. Front. Physiol. 7:312. doi: 10.3389/fphys.2016.00312
Castro, B., Sánchez, P., Torres, J. M., and Ortega, E. (2013). Effects of adult exposure to bisphenol A on genes involved in the physiopathology of rat prefrontal cortex. PLoS One 8:e73584. doi: 10.1371/journal.pone.0073584
Centonze, D., Prosperetti, C., Barone, I., Rossi, S., Picconi, B., Tscherter, A., et al. (2006). NR2B-containing NMDA receptors promote the neurotoxic effects of 3-nitropropionic acid but not of rotenone in the striatum. Exp. Neurol. 202, 470–479. doi: 10.1016/j.expneurol.2006.07.009
Chan, W.-S., Durairajan, S. S. K., Lu, J.-H., Wang, Y., Xie, L.-X., Kum, W.-F., et al. (2009). Neuroprotective effects of Astragaloside IV in 6-hydroxydopamine-treated primary nigral cell culture. Neurochem. Int. 55, 414–422. doi: 10.1016/j.neuint.2009.04.012
Chandrasekar, R. (2013). Alcohol and NMDA receptor: current research and future direction. Front. Mol. Neurosci. 6:14. doi: 10.3389/fnmol.2013.00014
Chandrasekaran, A., Ponnambalam, G., and Kaur, C. (2004). Domoic acid-induced neurotoxicity in the hippocampus of adult rats. Neurotox. Res. 6, 105–117. doi: 10.1007/BF03033213
Chen, C., Cao, J., Ma, X., Wang, X., Chen, Q., Yan, S., et al. (2016). Neuroprotection by polynitrogen manganese complexes: regulation of reactive oxygen species-related pathways. Sci. Rep. 6:20853. doi: 10.1038/srep20853
Chen, C., Zhang, H., Xu, H., Zheng, Y., Wu, T., and Lian, Y. (2017). Ginsenoside Rb1 ameliorates cisplatin-induced learning and memory impairments. J. Ginseng Res.
Chen, Z.-W., Liu, A., Liu, Q., Chen, J., Li, W.-M., Chao, X.-J., et al. (2017). MEF2D mediates the neuroprotective effect of methylene blue against glutamate-induced oxidative damage in HT22 hippocampal cells. Mol. Neurobiol. 54, 2209–2222. doi: 10.1007/s12035-016-9818-1
Cheruku, S. P., Ramalingayya, G. V., Chamallamudi, M. R., Biswas, S., Nandakumar, K., Nampoothiri, M., et al. (2017). Catechin ameliorates doxorubicin-induced neuronal cytotoxicity in in vitro and episodic memory deficit in in vivo in Wistar rats. Cytotechnology 70, 245–259. doi: 10.1007/s10616-017-0138-8
Chibowska, K., Baranowska-Bosiacka, I., Falkowska, A., Gutowska, I., Goschorska, M., and Chlubek, D. (2016). Effect of lead (Pb) on inflammatory processes in the brain. Int. J. Mol. Sci. 17:2140. doi: 10.3390/ijms17122140
Chiu, A. S., Gehringer, M. M., Braidy, N., Guillemin, G. J., Welch, J. H., and Neilan, B. A. (2013). Gliotoxicity of the cyanotoxin, β-methyl-amino-L-alanine (BMAA). Sci. Rep. 3:1482. doi: 10.1038/srep01482
Choi, H. J., Yoo, T. M., Chung, S. Y., Yang, J. S., Kim, J.-I., Ha, E. S., et al. (2002). Methamphetamine-induced apoptosis in a CNS-derived catecholaminergic cell line. Mol. Cells 13, 221–227.
Chtourou, Y., Trabelsi, K., Fetoui, H., Mkannez, G., Kallel, H., and Zeghal, N. (2011). Manganese induces oxidative stress, redox state unbalance and disrupts membrane bound ATPases on murine neuroblastoma cells in vitro: protective role of silymarin. Neurochem. Res. 36, 1546–1557. doi: 10.1007/s11064-011-0483-5
Conn, P. J., and Pin, J.-P. (1997). Pharmacology and functions of metabotropic glutamate receptors. Annu. Rev. Pharmacol. Toxicol. 37, 205–237. doi: 10.1146/annurev.pharmtox.37.1.205
da Silva Santos, V., Bisen-Hersh, E., Yu, Y., Cabral, I. S., Nardini, V., Culbreth, M., et al. (2014). Anthocyanin-rich acai (Euterpe oleracea Mart.) extract attenuates manganese-induced oxidative stress in rat primary astrocyte cultures. J. Toxicol. Environ. Health A 77, 390–404. doi: 10.1080/15287394.2014.880392
Dahmardeh, N., Asadi-Shekaari, M., Arjmand, S., Kalantaripour, T., Basiri, M., and Shabani, M. (2017). Modulation of sphingosine-1-phosphate receptor ameliorates harmaline-induced essential tremor in rat. Neurosci. Lett. 653, 376–381. doi: 10.1016/j.neulet.2017.06.015
De Filippis, B., Lyon, L., Taylor, A., Lane, T., Burnet, P. W., Harrison, P. J., et al. (2015). The role of group II metabotropic glutamate receptors in cognition and anxiety: comparative studies in GRM2-/-, GRM3-/- and GRM2/3-/- knockout mice. Neuropharmacology 89, 19–32. doi: 10.1016/j.neuropharm.2014.08.010
De Jesús-Cortés, H., Miller, A. D., Britt, J. K., DeMarco, A. J., De Jesús-Cortés, M., Stuebing, E., et al. (2015). Protective efficacy of P7C3-S243 in the 6-hydroxydopamine model of Parkinson’s disease. NPJ Parkinsons Dis. 1:15010. doi: 10.1038/npjparkd.2015.10
De Quervain, D. J. F., Henke, K., Aerni, A., Treyer, V., McGaugh, J. L., Berthold, T., et al. (2003). Glucocorticoid-induced impairment of declarative memory retrieval is associated with reduced blood flow in the medial temporal lobe. Eur. J. Neurosci. 17, 1296–1302. doi: 10.1046/j.1460-9568.2003.02542.x
Decker, H., Lo, K. Y., Unger, S. M., Ferreira, S. T., and Silverman, M. A. (2010). Amyloid-β peptide oligomers disrupt axonal transport through an NMDA receptor-dependent mechanism that is mediated by glycogen synthase kinase 3β in primary cultured hippocampal neurons. J. Neurosci. 30, 9166–9171. doi: 10.1523/JNEUROSCI.1074-10.2010
Delzor, A., Couratier, P., Boumédiène, F., Nicol, M., Druet-Cabanac, M., Paraf, F., et al. (2014). Searching for a link between the L-BMAA neurotoxin and amyotrophic lateral sclerosis: a study protocol of the French BMAALS programme. BMJ Open 4:e005528. doi: 10.1136/bmjopen-2014-005528
Deutch, A. Y., Rosin, D. L., Goldstein, M., and Roth, R. H. (1989). 3-Acetylpyridine-induced degeneration of the nigrostriatal dopamine system: an animal model of olivopontocerebellar atrophy-associated parkinsonism. Exp. Neurol. 105, 1–9. doi: 10.1016/0014-4886(89)90166-0
dos Santos Branco, C., Scola, G., Rodrigues, A. D., Cesio, V., Laprovitera, M., Heinzen, H., et al. (2013). Anticonvulsant, neuroprotective and behavioral effects of organic and conventional yerba mate (Ilex paraguariensis St. Hil.) on pentylenetetrazol-induced seizures in Wistar rats. Brain Res. Bull. 92, 60–68. doi: 10.1016/j.brainresbull.2012.11.008
Du, W., Aloyo, V. J., and Harvey, J. A. (1997). Harmaline competitively inhibits [3H] MK-801 binding to the NMDA receptor in rabbit brain. Brain Res. 770, 26–29. doi: 10.1016/S0006-8993(97)00606-9
Duan, X., Li, Y., Zhou, C., Huang, L., and Dong, Z. (2014). Dexmedetomidine provides neuroprotection: impact on ketamine-induced neuroapoptosis in the developing rat brain. Acta Anaesthesiol. Scand. 58, 1121–1126. doi: 10.1111/aas.12356
Durakoglugil, M. S., Chen, Y., White, C. L., Kavalali, E. T., and Herz, J. (2009). Reelin signaling antagonizes β-amyloid at the synapse. Proc. Natl. Acad. Sci. U.S.A. 106, 15938–15943. doi: 10.1073/pnas.0908176106
Ekonomou, A., and Angelatou, F. (1999). Upregulation of NMDA receptors in hippocampus and cortex in the pentylenetetrazol-induced “kindling” model of epilepsy. Neurochem. Res. 24, 1515–1522. doi: 10.1023/A:1021143813935
El Tabaa, M. M., Sokkar, S. S., Ramadan, E. S., Abd El Salam, I. Z., and Zaid, A. (2017). Neuroprotective role of Ginkgo biloba against cognitive deficits associated with Bisphenol A exposure: an animal model study. Neurochem. Int. 108, 199–212. doi: 10.1016/j.neuint.2017.03.019
Elyasi, L., Eftekhar-Vaghefi, S. H., and Esmaeili-Mahani, S. (2014). Morphine protects SH-SY5Y human neuroblastoma cells against 6-hydroxydopamine–induced cell damage: involvement of anti-oxidant, calcium blocking, and anti-apoptotic properties. Rejuvenation Res. 17, 255–263. doi: 10.1089/rej.2013.1473
Engin, A. B., Engin, E. D., Golokhvast, K., Spandidos, D. A., and Tsatsakis, A. M. (2017). Glutamate-mediated effects of caffeine and interferon-γ on mercury-induced toxicity. Int. J. Mol. Med. 39, 1215–1223. doi: 10.3892/ijmm.2017.2937
Engskog, M. K., Ersson, L., Haglöf, J., Arvidsson, T., Pettersson, C., and Brittebo, E. (2017). β-N-Methylamino-l-alanine (BMAA) perturbs alanine, aspartate and glutamate metabolism pathways in human neuroblastoma cells as determined by metabolic profiling. Amino Acids 49, 905–919. doi: 10.1007/s00726-017-2391-8
Fallarino, F., Volpi, C., Fazio, F., Notartomaso, S., Vacca, C., Busceti, C., et al. (2010). Metabotropic glutamate receptor-4 modulates adaptive immunity and restrains neuroinflammation. Nat. Med. 16, 897–902. doi: 10.1038/nm.2183
Farina, M., Rocha, J. B., and Aschner, M. (2011). Mechanisms of methylmercury-induced neurotoxicity: evidence from experimental studies. Life Sci. 89,555–563. doi: 10.1016/j.lfs.2011.05.019
Fernandes, H. B., and Raymond, L. A. (2009). “NMDA receptors and Huntington’s disease,” in Biology of the NMDA Receptor, ed. A. M. Van Dongen (Boca Raton, FL: CRC Press), 17–40.
Gasparini, F., Di Paolo, T., and Gomez-Mancilla, B. (2013). Metabotropic glutamate receptors for Parkinson’s disease therapy. Parkinsons Dis. 2013:196028. doi: 10.1155/2013/196028
Gass, J. T., Osborne, M. P., Watson, N. L., Brown, J. L., and Olive, M. F. (2009). mGluR5 antagonism attenuates methamphetamine reinforcement and prevents reinstatement of methamphetamine-seeking behavior in rats. Neuropsychopharmacology 34, 820–833. doi: 10.1038/npp.2008.140
Gazal, M., Valente, M. R., Acosta, B. A., Kaufmann, F. N., Braganhol, E., Lencina, C. L., et al. (2014). Neuroprotective and antioxidant effects of curcumin in a ketamine-induced model of mania in rats. Eur. J. Pharmacol. 724, 132–139. doi: 10.1016/j.ejphar.2013.12.028
Geurts, J., Wolswijk, G., Bö, L., Van Der Valk, P., Polman, C., Troost, D., et al. (2003). Altered expression patterns of group I and II metabotropic glutamate receptors in multiple sclerosis. Brain 126, 1755–1766. doi: 10.1093/brain/awg179
Giordano, G., Li, L., White, C. C., Farin, F. M., Wilkerson, H. W., Kavanagh, T. J., et al. (2009). Muscarinic receptors prevent oxidative stress-mediated apoptosis induced by domoic acid in mouse cerebellar granule cells. J. Neurochem. 109, 525–538. doi: 10.1111/j.1471-4159.2009.05969.x
Grammatopoulos, T., Morris, K., Ferguson, P., and Weyhenmeyer, J. (2002). Angiotensin protects cortical neurons from hypoxic-induced apoptosis via the angiotensin type 2 receptor. Mol. Brain Res. 99, 114–124. doi: 10.1016/S0169-328X(02)00101-8
Gross, N. B., Duncker, P. C., and Marshall, J. F. (2011). Cortical ionotropic glutamate receptor antagonism protects against methamphetamine-induced striatal neurotoxicity. Neuroscience 199, 272–283. doi: 10.1016/j.neuroscience.2011.09.014
Gu, Z., Liu, W., and Yan, Z. (2009). β-Amyloid impairs AMPA receptor trafficking and function by reducing Ca2+/calmodulin-dependent protein kinase II synaptic distribution. J. Biol. Chem. 284, 10639–10649. doi: 10.1074/jbc.M806508200
Gudi, V., Gingele, S., Skripuletz, T., and Stangel, M. (2014). Glial response during cuprizone-induced de-and remyelination in the CNS: lessons learned. Front. Cell. Neurosci. 8:73. doi: 10.3389/fncel.2014.00073
Guilarte, T. R., and Chen, M. K. (2007). Manganese inhibits NMDA receptor channel function: implications to psychiatric and cognitive effects. Neurotoxicology 28, 1147–1152. doi: 10.1016/j.neuro.2007.06.005
Guntupalli, S., Widagdo, J., and Anggono, V. (2016). Amyloid-β-induced dysregulation of AMPA receptor trafficking. Neural Plast. 2016:3204519. doi: 10.1155/2016/3204519
Guo, D.-J., Li, F., Yu, P. H.-F., and Chan, S.-W. (2013). Neuroprotective effects of luteolin against apoptosis induced by 6-hydroxydopamine on rat pheochromocytoma PC12 cells. Pharm. Biol. 51, 190–196. doi: 10.3109/13880209.2012.716852
Gupta, K., Hardingham, G. E., and Chandran, S. (2013). NMDA receptor-dependent glutamate excitotoxicity in human embryonic stem cell-derived neurons. Neurosci. Lett. 543, 95–100. doi: 10.1016/j.neulet.2013.03.010
Hadjihambi, A., Rose, C. F., and Jalan, R. (2014). Novel insights into ammonia-mediated neurotoxicity pointing to potential new therapeutic strategies. Hepatology 60, 1101–1103. doi: 10.1002/hep.27282
Hallett, P. J., Spoelgen, R., Hyman, B. T., Standaert, D. G., and Dunah, A. W. (2006). Dopamine D1 activation potentiates striatal NMDA receptors by tyrosine phosphorylation-dependent subunit trafficking. J. Neurosci. 26, 4690–4700. doi: 10.1523/JNEUROSCI.0792-06.2006
Halpin, L. E., Northrop, N. A., and Yamamoto, B. K. (2014). Ammonia mediates methamphetamine-induced increases in glutamate and excitotoxicity. Neuropsychopharmacology 39:1031. doi: 10.1038/npp.2013.306
Halpin, L. E., and Yamamoto, B. K. (2012). Peripheral ammonia as a mediator of methamphetamine neurotoxicity. J. Neurosci. 32, 13155–13163. doi: 10.1523/JNEUROSCI.2530-12.2012
Han, S. M., Kim, J. M., Park, K. K., Chang, Y. C., and Pak, S. C. (2014). Neuroprotective effects of melittin on hydrogen peroxide-induced apoptotic cell death in neuroblastoma SH-SY5Y cells. BMC Complement. Altern. Med. 14:286. doi: 10.1186/1472-6882-14-286
He, Q., Titley, H., Grasselli, G., Piochon, C., and Hansel, C. (2013). Ethanol affects NMDA receptor signaling at climbing fiber-Purkinje cell synapses in mice and impairs cerebellar LTD. J. Neurophysiol. 109, 1333–1342. doi: 10.1152/jn.00350.2012
Herrold, A., Voigt, R., and Napier, T. (2013). mGluR5 is necessary for maintenance of methamphetamine-induced associative learning. Eur. Neuropsychopharmacol. 23, 691–696. doi: 10.1016/j.euroneuro.2012.05.014
Hicklin, T. R., Wu, P. H., Radcliffe, R. A., Freund, R. K., Goebel-Goody, S. M., Correa, P. R., et al. (2011). Alcohol inhibition of the NMDA receptor function, long-term potentiation, and fear learning requires striatal-enriched protein tyrosine phosphatase. Proc. Natl. Acad. Sci. U.S.A. 108, 6650–6655. doi: 10.1073/pnas.1017856108
Hisahara, S., and Shimohama, S. (2011). Toxin-induced and genetic animal models of Parkinson’s disease. Parkinsons Dis. 2011:951709. doi: 10.4061/2011/951709
Hogberg, H. T., and Bal-Price, A. K. (2011). Domoic acid-induced neurotoxicity is mainly mediated by the AMPA/KA receptor: comparison between immature and mature primary cultures of neurons and glial cells from rat cerebellum. J. Toxicol. 2011:543512. doi: 10.1155/2011/543512
Holland, R. A., Leonard, J. J., Kensey, N. A., Hannikainen, P. A., and De Jonghe, B. C. (2014). Cisplatin induces neuronal activation and increases central AMPA and NMDA receptor subunit gene expression in mice. Physiol. Behav. 136, 79–85. doi: 10.1016/j.physbeh.2014.02.038
Hsieh, P. F., Hou, C.-W., Yao, P.-W., Wu, S.-P., Peng, Y.-F., Shen, M.-L., et al. (2011). Sesamin ameliorates oxidative stress and mortality in kainic acid-induced status epilepticus by inhibition of MAPK and COX-2 activation. J. Neuroinflammation 8:57. doi: 10.1186/1742-2094-8-57
Hu, F., Li, T., Gong, H., Chen, Z., Jin, Y., Xu, G., et al. (2017). Bisphenol A impairs synaptic plasticity by both pre-and postsynaptic mechanisms. Adv. Sci. 4:1600493. doi: 10.1002/advs.201600493
Hu, N.-W., Ondrejcak, T., and Rowan, M. J. (2012). Glutamate receptors in preclinical research on Alzheimer’s disease: update on recent advances. Pharmacol. Biochem. Behav. 100, 855–862. doi: 10.1016/j.pbb.2011.04.013
Hu, X., Luan, L., Guan, W., Zhang, S., Li, B., Ji, W., et al. (2017). Hydrogen sulfide attenuates isoflurane-induced neuroapoptosis and cognitive impairment in the developing rat brain. BMC Anesthesiol. 17:123. doi: 10.1186/s12871-017-0419-y
Huang, J., Xiao, L., Wei, J. X., Shu, Y. H., Fang, S. Q., Wang, Y. T., et al. (2017). Protective effect of arctigenin on ethanol-induced neurotoxicity in PC12 cells. Mol. Med. Rep. 15, 2235–2240. doi: 10.3892/mmr.2017.6222
Iizuka, A., Nakamura, K., and Hirai, H. (2015). Long-term oral administration of the NMDA receptor antagonist memantine extends life span in spinocerebellar ataxia type 1 knock-in mice. Neurosci. Lett. 592, 37–41. doi: 10.1016/j.neulet.2015.02.055
Iseri, P. K., Karson, A., Gullu, K. M., Akman, O., Kokturk, S., Yardýmoglu, M., et al. (2011). The effect of memantine in harmaline-induced tremor and neurodegeneration. Neuropharmacology 61, 715–723. doi: 10.1016/j.neuropharm.2011.05.015
Ishibashi, K., Miura, Y., Ishikawa, K., Zhang, M.-R., Toyohara, J., Ishiwata, K., et al. (2016). Relationship between type 1 metabotropic glutamate receptors and cerebellar ataxia. J. Neurol. 263, 2179–2187. doi: 10.1007/s00415-016-8248-3
Ishida, K., Kotake, Y., Sanoh, S., and Ohta, S. (2017). Lead-Induced ERK Activation Is Mediated by GluR2 Non-containing AMPA Receptor in Cortical Neurons. Biol. Pharm. Bull. 40, 303–309. doi: 10.1248/bpb.b16-00784
Ismail, N., Ismail, M., Azmi, N. H., Abu Bakar, M. F., Basri, H., and Abdullah, M. A. (2015). Modulation of hydrogen peroxide-induced oxidative stress in human neuronal cells by Thymoquinone-rich fraction and Thymoquinone via transcriptomic regulation of antioxidant and apoptotic signaling genes. Oxid. Med. Cell. Longev. 2016:2528935. doi: 10.1155/2016/2528935
Jamwal, S., and Kumar, P. (2016). Spermidine ameliorates 3-nitropropionic acid (3-NP)-induced striatal toxicity: possible role of oxidative stress, neuroinflammation, and neurotransmitters. Physiol. Behav. 155, 180–187. doi: 10.1016/j.physbeh.2015.12.015
Janahmadi, M., Goudarzi, I., Kaffashian, M. R., Behzadi, G., Fathollahi, Y., and Hajizadeh, S. (2009). Co-treatment with riluzole, a neuroprotective drug, ameliorates the 3-acetylpyridine-induced neurotoxicity in cerebellar Purkinje neurones of rats: behavioural and electrophysiological evidence. Neurotoxicology 30, 393–402. doi: 10.1016/j.neuro.2009.02.014
Jantas, D., Greda, A., Golda, S., Korostynski, M., Grygier, B., Roman, A., et al. (2014). Neuroprotective effects of metabotropic glutamate receptor group II and III activators against MPP (+)-induced cell death in human neuroblastoma SH-SY5Y cells: the impact of cell differentiation state. Neuropharmacology 83, 36–53. doi: 10.1016/j.neuropharm.2014.03.019
Jantas, D., Greda, A., Leskiewicz, M., Grygier, B., Pilc, A., and Lason, W. (2015). Neuroprotective effects of mGluR II and III activators against staurosporine-and doxorubicin-induced cellular injury in SH-SY5Y cells: new evidence for a mechanism involving inhibition of AIF translocation. Neurochem. Int. 88, 124–137. doi: 10.1016/j.neuint.2014.12.011
Jantas, D., and Lason, W. (2009). Protective effect of memantine against Doxorubicin toxicity in primary neuronal cell cultures: influence a development stage. Neurotox. Res. 15, 24–37. doi: 10.1007/s12640-009-9002-8
Jayanthi, S., McCoy, M. T., Chen, B., Britt, J. P., Kourrich, S., Yau, H.-J., et al. (2014). Methamphetamine downregulates striatal glutamate receptors via diverse epigenetic mechanisms. Biol. Psychiatry 76, 47–56. doi: 10.1016/j.biopsych.2013.09.034
Jensen, F. E. (2009). “GLUTAMATE | glutamate-mediated excitation and mechanisms of epileptogenesis in the immature brain A 2,” in Encyclopedia of Basic Epilepsy Research, ed. P. A. Schwartzkroin (Oxford: Academic Press.), 429–436.
Jia, W., Zhang, R., Wu, B., Dai, Z.-X., Zhu, Y.-S., Li, P.-P., et al. (2014). Metabotropic glutamate receptor 3 is associated with heroin dependence but not depression or schizophrenia in a Chinese population. PLoS One 9:e87247. doi: 10.1371/journal.pone.0087247
Jiang, Y.-Y., Cao, B.-B., Wang, X.-Q., Peng, Y.-P., and Qiu, Y.-H. (2015). Cerebellar ataxia induced by 3-AP affects immunological function. Neuroendocrinol. Lett. 36, 246–256.
Jin, X.-T., and Smith, Y. (2007). Activation of presynaptic kainate receptors suppresses GABAergic synaptic transmission in the rat globus pallidus. Neuroscience 149, 338–349. doi: 10.1016/j.neuroscience.2007.07.017
Jumnongprakhon, P., Govitrapong, P., Tocharus, C., Tungkum, W., and Tocharus, J. (2014). Protective effect of melatonin on methamphetamine-induced apoptosis in glioma cell line. Neurotox. Res. 25, 286–294. doi: 10.1007/s12640-013-9419-y
Kamat, P. K., Kyles, P., Kalani, A., and Tyagi, N. (2016). Hydrogen sulfide ameliorates homocysteine-induced Alzheimer’s disease-like pathology, blood–brain barrier disruption, and synaptic disorder. Mol. Neurobiol. 53, 2451–2467. doi: 10.1007/s12035-015-9212-4
Karami, M., Riahi, N., and Nadoushan, M. R. J. (2013). Injection of colchicine intra-hippocampal cortical area 1 enhances novelty seeking behavior. Indian J. Pharmacol. 45, 274–277. doi: 10.4103/0253-7613.111945
Kempuraj, D., Asadi, S., Zhang, B., Manola, A., Hogan, J., Peterson, E., et al. (2010). Mercury induces inflammatory mediator release from human mast cells. J. Neuroinflammation 7:20. doi: 10.1186/1742-2094-7-20
Kim, B.-W., Koppula, S., Kumar, H., Park, J.-Y., Kim, I.-W., More, S. V., et al. (2017). Corrigendum to “α-Asarone attenuates microglia-mediated neuroinflammation by inhibiting NF kappa B activation and mitigates MPTP-induced behavioral deficits in a mouse model of Parkinson’s disease” [Neuropharm 97 (2015) 46-57]. Neuropharmacology 116, 444–445. doi: 10.1016/j.neuropharm.2017.02.023
Kim, S.-O., You, J.-M., Yun, S.-J., Son, M.-S., Nam, K. N., Hong, J.-W., et al. (2010). Ginsenoside Rb1 and Rg3 attenuate glucocorticoid-induced neurotoxicity. Cell Mol. Neurobiol. 30, 857–862. doi: 10.1007/s10571-010-9513-0
Kinoshita, K., Watanabe, Y., Yamamura, M., and Matsuoka, Y. (1998). TRH receptor agonists ameliorate 3-acetylpyridine-induced ataxia through NMDA receptors in rats. Eur. J. Pharmacol. 343, 129–133. doi: 10.1016/S0014-2999(97)01539-2
Kita, T., Wagner, G. C., and Nakashima, T. (2003). Current research on methamphetamine-induced neurotoxicity: animal models of monoamine disruption. J. Pharmacol. Sci. 92, 178–195. doi: 10.1254/jphs.92.178
Kolasiewicz, W., Kuter, K., Wardas, J., and Ossowska, K. (2009). Role of the metabotropic glutamate receptor subtype 1 in the harmaline-induced tremor in rats. J. Neural Transm. 116, 1059–1063. doi: 10.1007/s00702-009-0254-5
Kosenko, E., Montoliu, C., Giordano, G., Kaminsky, Y., Venediktova, N., Buryanov, Y., et al. (2004). Acute ammonia intoxication induces an NMDA receptor-mediated increase in poly (ADP-ribose) polymerase level and NAD+ metabolism in nuclei of rat brain cells. J. Neurochem. 89, 1101–1110. doi: 10.1111/j.1471-4159.2004.02426.x
Kumar, P., and Kumar, A. (2010). Protective effect of hesperidin and naringin against 3-nitropropionic acid induced Huntington’s like symptoms in rats: possible role of nitric oxide. Behav. Brain Res. 206, 38–46. doi: 10.1016/j.bbr.2009.08.028
Kwak, S., Hideyama, T., Yamashita, T., and Aizawa, H. (2010). AMPA receptor-mediated neuronal death in sporadic ALS. Neuropathology 30, 182–188. doi: 10.1111/j.1440-1789.2009.01090.x
Kysenius, K., Brunello, C. A., and Huttunen, H. J. (2014). Mitochondria and NMDA receptor-dependent toxicity of berberine sensitizes neurons to glutamate and rotenone injury. PLoS One 9:e107129. doi: 10.1371/journal.pone.0107129
Lacor, P. N., Buniel, M. C., Chang, L., Fernandez, S. J., Gong, Y., Viola, K. L., et al. (2004). Synaptic targeting by Alzheimer’s-related amyloid β oligomers. J. Neurosci. 24, 10191–10200. doi: 10.1523/JNEUROSCI.3432-04.2004
Lacor, P. N., Buniel, M. C., Furlow, P. W., Clemente, A. S., Velasco, P. T., Wood, M., et al. (2007). Aβ oligomer-induced aberrations in synapse composition, shape, and density provide a molecular basis for loss of connectivity in Alzheimer’s disease. J. Neurosci. 27, 796–807. doi: 10.1523/JNEUROSCI.3501-06.2007
Laugeray, A., Oummadi, A., Jourdain, C., Feat, J., Meyer-Dilhet, G., Menuet, A., et al. (2017). Perinatal exposure to the cyanotoxin β-N-méthylamino-l-alanine (BMAA) results in long-lasting behavioral changes in offspring—potential involvement of DNA damage and oxidative stress. Neurotox. Res. 33, 87–112. doi: 10.1007/s12640-017-9802-1
Lee, H. Y., Weon, J. B., Ryu, G., Yang, W. S., Kim, N. Y., Kim, M. K., et al. (2017). Neuroprotective effect of Aronia melanocarpa extract against glutamate-induced oxidative stress in HT22 cells. BMC Complement. Altern. Med. 17:207. doi: 10.1186/s12906-017-1716-1
Lehmann, A., and Kärrberg, L. (1996). Effects of N-methyl-D-aspartate receptor antagonists on cisplatin-induced emesis in the ferret. Neuropharmacology 35, 475–481. doi: 10.1016/0028-3908(96)00008-1
Lewerenz, J., and Maher, P. (2015). Chronic glutamate toxicity in neurodegenerative diseases—what is the evidence? Front. Neurosci. 9:469. doi: 10.3389/fnins.2015.00469
Li, C., Xiao, L., Liu, X., Yang, W., Shen, W., Hu, C., et al. (2013). A functional role of NMDA receptor in regulating the differentiation of oligodendrocyte precursor cells and remyelination. Glia 61, 732–749. doi: 10.1002/glia.22469
Li, M., Zhang, P., Wei, H.-J., Li, M.-H., Zou, W., Li, X., et al. (2016). Hydrogen sulfide ameliorates homocysteine-induced cognitive dysfunction by inhibition of reactive aldehydes involving upregulation of ALDH2. Int. J. Neuropsychopharmacol. 20, 305–315. doi: 10.1093/ijnp/pyw103
Li, S., Jin, M., Koeglsperger, T., Shepardson, N. E., Shankar, G. M., and Selkoe, D. J. (2011). Soluble Aβ oligomers inhibit long-term potentiation through a mechanism involving excessive activation of extrasynaptic NR2B-containing NMDA receptors. J. Neurosci. 31, 6627–6638. doi: 10.1523/JNEUROSCI.0203-11.2011
Li, S. J., Qin, W. X., Peng, D. J., Yuan, Z. X., He, S. N., Luo, Y. N., et al. (2017). Sodium P-aminosalicylic acid inhibits sub-chronic manganese-induced neuroinflammation in rats by modulating MAPK and COX-2. Neurotoxicology 64, 219–229. doi: 10.1016/j.neuro.2017.06.012
Li, T., Wang, L., Hu, Q., Liu, S., Bai, X., Xie, Y., et al. (2017). Neuroprotective roles of l-cysteine in attenuating early brain injury and improving synaptic density via the CBS/H2S pathway following subarachnoid hemorrhage in rats. Front. Neurol. 8:176. doi: 10.3389/fneur.2017.00176
Li, Y., Zeng, M., Chen, W., Liu, C., Wang, F., Han, X., et al. (2014). Dexmedetomidine reduces isoflurane-induced neuroapoptosis partly by preserving PI3K/Akt pathway in the hippocampus of neonatal rats. PLoS One 9:e93639. doi: 10.1371/journal.pone.0093639
Liang, L., Ma, Z., Dong, M., Ma, J., Jiang, A., and Sun, X. (2017). Protective effects of salidroside against isoflurane-induced cognitive impairment in rats. Hum. Exp. Toxicol. 36, 1295–1302. doi: 10.1177/0960327116688068
Liang, Z., Shi, F., Wang, Y., Lu, L., Zhang, Z., Wang, X., et al. (2011). Neuroprotective effects of tenuigenin in a SH-SY5Y cell model with 6-OHDA-induced injury. Neurosci. Lett. 497, 104–109. doi: 10.1016/j.neulet.2011.04.041
Liot, G., Bossy, B., Lubitz, S., Kushnareva, Y., Sejbuk, N., and Bossy-Wetzel, E. (2009). Complex II inhibition by 3-NP causes mitochondrial fragmentation and neuronal cell death via an NMDA-and ROS-dependent pathway. Cell Death Differ. 16, 899–909. doi: 10.1038/cdd.2009.22
Liu, F., Patterson, T. A., Sadovova, N., Zhang, X., Liu, S., Zou, X., et al. (2012). Ketamine-induced neuronal damage and altered N-methyl-D-aspartate receptor function in rat primary forebrain culture. Toxicol. Sci. 131, 548–557. doi: 10.1093/toxsci/kfs296
Liu, F., Paule, M. G., Ali, S., and Wang, C. (2011). Ketamine-induced neurotoxicity and changes in gene expression in the developing rat brain. Curr. Neuropharmacol. 9, 256–261. doi: 10.2174/157015911795017155
Liu, H., Mao, P., Wang, J., Wang, T., and Xie, C.-H. (2015). Allicin protects PC12 cells against 6-OHDA-induced oxidative stress and mitochondrial dysfunction via regulating mitochondrial dynamics. Cell Physiol. Biochem. 36, 966–979. doi: 10.1159/000430271
Liu, H., Wen, L., Qiao, H., and An, S. (2013). Modulation of hippocampal glutamate and NMDA/AMPA receptor by homocysteine in chronic unpredictable mild stress-induced rat depression. Sheng Li Xue Bao 65, 61–71.
Liu, J., Chang, L., Roselli, F., Almeida, O. F., Gao, X., Wang, X., et al. (2010). Amyloid-β induces caspase-dependent loss of PSD-95 and synaptophysin through NMDA receptors. J. Alzheimers Dis. 22, 541–556. doi: 10.3233/JAD-2010-100948
Llorente-Folch, I., Rueda, C. B., Pérez-Liébana, I., Satrústegui, J., and Pardo, B. (2016). L-lactate-mediated neuroprotection against glutamate-induced excitotoxicity requires ARALAR/AGC1. J. Neurosci. 36, 4443–4456. doi: 10.1523/JNEUROSCI.3691-15.2016
Lobner, D. (2009). Mechanisms of β-N-methylamino-L-alanine induced neurotoxicity. Amyotroph. Lateral Scler. 10, 56–60. doi: 10.3109/17482960903269062
Lovinger, D. M. (1997). Alcohols and neurotransmitter gated ion channels: past, present and future. Naunyn Schmiedebergs Arch. Pharmacol. 356, 267–282.
Lu, J., Goula, D., Sousa, N., and Almeida, O. (2003). Ionotropic and metabotropic glutamate receptor mediation of glucocorticoid-induced apoptosis in hippocampal cells and the neuroprotective role of synaptic N-methyl-D-aspartate receptors. Neuroscience 121, 123–131. doi: 10.1016/S0306-4522(03)00421-4
Lu, J., Wu D-m, Zheng Y-l, Hu, B., Cheng, W., Zhang, Z.-F., et al. (2013). Troxerutin counteracts domoic acid–induced memory deficits in mice by inhibiting CCAAT/enhancer binding protein β–mediated inflammatory response and oxidative stress. J. Immunol. 190, 3466–3479. doi: 10.4049/jimmunol.1202862
Luo, L., Jin, Y., Kim, I.-D., and Lee, J.-K. (2013). Glycyrrhizin attenuates kainic Acid-induced neuronal cell death in the mouse hippocampus. Exp. Neurobiol. 22, 107–115. doi: 10.5607/en.2013.22.2.107
Maciejak, P., Szyndler, J., Turzyńska, D., Sobolewska, A., Taracha, E., Skórzewska, A., et al. (2009). The effects of group III mGluR ligands on pentylenetetrazol-induced kindling of seizures and hippocampal amino acids concentration. Brain Res. 1282, 20–27. doi: 10.1016/j.brainres.2009.05.049
Magalingam, K. B., Radhakrishnan, A., and Haleagrahara, N. (2014). Protective effects of flavonol isoquercitrin, against 6-hydroxy dopamine (6-OHDA)-induced toxicity in PC12 cells. BMC Res. Notes 7:49. doi: 10.1186/1756-0500-7-49
Malhotra, A. K., Pinals, D. A., Adler, C. M., Elman, I., Clifton, A., Pickar, D., et al. (1997). Ketamine-induced exacerbation of psychotic symptoms and cognitive impairment in neuroleptic-free schizophrenics. Neuropsychopharmacology 17, 141–150. doi: 10.1016/S0893-133X(97)00036-5
Manchon, J. F. M., Dabaghian, Y., Uzor, N.-E., Kesler, S. R., Wefel, J. S., and Tsvetkov, A. S. (2016). Levetiracetam mitigates doxorubicin-induced DNA and synaptic damage in neurons. Sci. Rep. 6:25705. doi: 10.1038/srep25705
Masilamani, T., Subramaniam, T., Nordin, N., and Rosli, R. (2017). Neuroprotective effects of Peltophorum pterocarpum leaf extract against hydrogen peroxide induced oxidative stress and cytotoxicity. Clin. Phytosci. 3:16. doi: 10.1186/s40816-017-0054-7
Masilamoni, G. J., Bogenpohl, J. W., Alagille, D., Delevich, K., Tamagnan, G., Votaw, J. R., et al. (2011). Metabotropic glutamate receptor 5 antagonist protects dopaminergic and noradrenergic neurons from degeneration in MPTP-treated monkeys. Brain 134, 2057–2073. doi: 10.1093/brain/awr137
Maya-López, M., Colín-González, A. L., Aguilera, G., de Lima, M. E., Colpo-Ceolin, A., Rangel-López, E., et al. (2017). Neuroprotective effect of WIN 55, 212-2 against 3-nitropropionic acid-induced toxicity in the rat brain: involvement of CB1 and NMDA receptors. Am. J. Transl. Res. 9, 261–274.
McCarron, P., Logan, A. C., Giddings, S. D., and Quilliam, M. A. (2014). Analysis of β-N-methylamino-L-alanine (BMAA) in spirulina-containing supplements by liquid chromatography-tandem mass spectrometry. Aquat. Biosyst. 10:5. doi: 10.1186/2046-9063-10-5
Medina, B. N., Santos de Abreu, I., Cavalcante, L. A., Silva, W. A., da Fonseca, R. N., Allodi, S., et al. (2015). 3-acetylpyridine-induced degeneration in the adult ascidian neural complex: reactive and regenerative changes in glia and blood cells. Dev. Neurobiol. 75, 877–893. doi: 10.1002/dneu.22255
Meng, H., Wang, L., He, J., and Wang, Z. (2016). The protective effect of gangliosides on lead (Pb)-induced neurotoxicity is mediated by autophagic pathways. Int. J. Environ. Res. Public Health 13:365. doi: 10.3390/ijerph13040365 Epub 2016/03/31.,
Meredith, G. E., and Rademacher, D. J. (2011). MPTP mouse models of Parkinson’s disease: an update. J. Parkinsons Dis. 1, 19–33. doi: 10.3233/JPD-2011-11023
Mesbah-Ardakani, M., Homayouni-Moghadam, F., and Izadi, M. (2016). Effects of hydrogen peroxide-induced oxidative stress on the pattern of pro-apoptotic and anti-apoptotic genes expression during PC12 cells differentiation. J. Basic Clin. Pathophysiol. 4, 27–34.
Mirzaie, M., Khalili, M., Kiasalari, Z., and Roghani, M. (2016). Neuroprotective and antiapoptotic potential of trigonelline in a striatal 6-hydroxydopamine rat model of Parkinson’s disease. Neurophysiology 48, 176–183. doi: 10.1007/s11062-016-9586-6
Mohamed, A. R., Soliman, G. Y., Ismail, C. A., and Mannaa, H. F. (2015). Neuroprotective role of vitamin D3 in colchicine-induced Alzheimer’s disease in rats. Alex. J. Med. 51, 127–136. doi: 10.1016/j.ajme.2014.05.005
Molz, S., Decker, H., Dal-Cim, T., Cremonez, C., Cordova, F. M., Leal, R. B., et al. (2008). Glutamate-induced toxicity in hippocampal slices involves apoptotic features and p38MAPK signaling. Neurochem. Res. 33, 27–36. doi: 10.1007/s11064-007-9402-1
Moneim, A. E. A. (2014). Azadirachta indica attenuates cisplatin-induced neurotoxicity in rats. Indian J. Pharmacol. 46, 316–321. doi: 10.4103/0253-7613.132182
Moneim, A. E. A. (2015). Mercury-induced neurotoxicity and neuroprotective effects of berberine. Neural Regen. Res. 10, 881–882. doi: 10.4103/1673-5374.158336
Monfort, P., Kosenko, E., Erceg, S., Canales, J.-J., and Felipo, V. (2002). Molecular mechanism of acute ammonia toxicity: role of NMDA receptors. Neurochem. Int. 41, 95–102. doi: 10.1016/S0197-0186(02)00029-3
More, S. V., Kumar, H., Cho, D.-Y., Yun, Y.-S., and Choi, D.-K. (2016). Toxin-induced experimental models of learning and memory impairment. Int. J. Mol. Sci. 17:E1447. doi: 10.3390/ijms17091447
Morelli, S., Piscioneri, A., Salerno, S., Al-Fageeh, M. B., Drioli, E., and De Bartolo, L. (2014). Neuroprotective effect of didymin on hydrogen peroxide-induced injury in the neuronal membrane system. Cells Tissues Organs 199, 184–200. doi: 10.1159/000365072
Moreno, J. A., Streifel, K. M., Sullivan, K. A., Hanneman, W. H., and Tjalkens, R. B. (2011). Manganese-induced NF-kappaB activation and nitrosative stress is decreased by estrogen in juvenile mice. Toxicol. Sci. 122, 121–133. doi: 10.1093/toxsci/kfr091
Moustafa, A. A., Hewedi, D. H., Eissa, A. M., Frydecka, D., and Misiak, B. (2014). Homocysteine levels in schizophrenia and affective disorders—focus on cognition. Front. Behav. Neurosci. 8:343. doi: 10.3389/fnbeh.2014.00343
Möykkynen, T., and Korpi, E. R. (2012). Acute effects of ethanol on glutamate receptors. Basic Clin. Pharmacol. Toxicol. 111, 4–13. doi: 10.1111/j.1742-7843.2012.00879.x
Möykkynen, T., Korpi, E. R., and Lovinger, D. M. (2003). Ethanol inhibits α-amino-3-hydyroxy-5-methyl-4-isoxazolepropionic acid (AMPA) receptor function in central nervous system neurons by stabilizing desensitization. J. Pharmacol. Exp. Ther. 306, 546–555. doi: 10.1124/jpet.103.050666
Möykkynen, T. P., Coleman, S. K., Keinänen, K., Lovinger, D. M., and Korpi, E. R. (2009). Ethanol increases desensitization of recombinant GluR-D AMPA receptor and TARP combinations. Alcohol 43, 277–284. doi: 10.1016/j.alcohol.2009.04.005
Mu, X., Lu, Y., and Du, G. (2014). Baicalein exerts neuroprotective effects in 6-hydroxydopamine induced experimental Parkisonism (LB625). FASEB J. 28:LB625.
Muñoz-Saez, E., de Munck, E., Arahuetes, R. M., Solas, M. T., Martínez, A. M., and Miguel, B. G. (2013). β-N-methylamino-L-alanine induces changes in both GSK3 and TDP-43 in human neuroblastoma. J. Toxicol. Sci. 38, 425–430. doi: 10.2131/jts.38.425
Mußhoff, U., Madeja, M., Binding, N., Witting, U., and Speckmann, E.-J. (1995). Lead-induced blockade of kainate-sensitive receptor channels. Naunyn Schmiedebergs Arch. Pharmacol. 353, 42–45.
Nabeka, H., Shimokawa, T., Doihara, T., Saito, S., Wakisaka, H., Hamada, F., et al. (2015). A prosaposin-derived peptide alleviates kainic acid-induced brain injury. PLoS One 10:e0126856. doi: 10.1371/journal.pone.0126856
Nair, A., and Bonneau, R. H. (2006). Stress-induced elevation of glucocorticoids increases microglia proliferation through NMDA receptor activation. J. Neuroimmunol. 171, 72–85. doi: 10.1016/j.jneuroim.2005.09.012
Nampoothiri, M., Reddy, N. D., John, J., Kumar, N., Kutty Nampurath, G., and Rao Chamallamudi, M. (2014). Insulin blocks glutamate-induced neurotoxicity in differentiated SH-SY5Y neuronal cells. Behav. Neurol. 2014:674164. doi: 10.1155/2014/674164
Naseer, M., Ullah, I., Narasimhan, M., Lee, H., Bressan, R., Yoon, G., et al. (2014). Neuroprotective effect of osmotin against ethanol-induced apoptotic neurodegeneration in the developing rat brain. Cell Death Dis. 5:e1150. doi: 10.1038/cddis.2014.53
Neal, A. P., Worley, P. F., and Guilarte, T. R. (2011). Lead exposure during synaptogenesis alters NMDA receptor targeting via NMDA receptor inhibition. Neurotoxicology 32, 281–289. doi: 10.1016/j.neuro.2010.12.013
Nishikawa, K.-I., and MacIver, M. B. (2000). Excitatory synaptic transmission mediated by NMDA receptors is more sensitive to isoflurane than are non-NMDA receptor-mediated responses. Anesthesiology 92, 228–228. doi: 10.1097/00000542-200001000-00035
Oh, S.-H., Ryu, B., Ngo, D.-H., Kim, W.-S., Kim, D. G., and Kim, S.-K. (2017). 4-hydroxybenzaldehyde-chitooligomers suppresses H 2 O 2-induced oxidative damage in microglia BV-2 cells. Carbohydr. Res. 440, 32–37. doi: 10.1016/j.carres.2017.01.007
Olajide, O. J., Enaibe, B. U., Bankole, O. O., Akinola, O. B., Laoye, B. J., and Ogundele, O. M. (2016). Kolaviron was protective against sodium azide (NaN3) induced oxidative stress in the prefrontal cortex. Metab. Brain Dis. 31, 25–35. doi: 10.1007/s11011-015-9674-0
O’Neill, M. J., and Witkin, J. M. (2007). AMPA receptor potentiators: application for depression and Parkinson’s disease. Curr. Drug Targets 8, 603–620. doi: 10.2174/138945007780618517
Oscós-Alvarado, A., and de Muñoz, D. M. (1981). Effects of 4-hydroxy, 4-phenyl, caproamide on pentylenetetrazol-induced amnesia. Psychopharmacology 74, 93–96. doi: 10.1007/BF00431764
Oz, M., Atalik, K. E. N., Yerlikaya, F. H., and Demir, E. A. (2015). Curcumin alleviates cisplatin-induced learning and memory impairments. Neurobiol. Learn. Mem. 123, 43–49. doi: 10.1016/j.nlm.2015.05.001
Palsa, D., Begum, N., Kommidi, J., Singh, K., Yellu, M., and Bakshi, V. (2016). Neuroprotective effect of pterostilbene on ketamine induced schizophrenia in mice. Int. J. Appl. Pharm. Sci. Res. 1, 96–103.
Parekh, B. (2015). A (a) LS: ammonia-induced amyotrophic lateral sclerosis. F1000Res. 4:119. doi: 10.12688/f1000research.6364.1
Pershina, E., Kapralova, M., and Arkhipov, V. (2017). Effect of pharmacological modulation of activity of metabotropic glutamate receptors on their gene expression after excitotoxic damage in hippocampal neurons. Bull. Exp. Biol. Med. 162, 784–787. doi: 10.1007/s10517-017-3713-2
Petrozziello, T., Secondo, A., Tedeschi, V., Esposito, A., Sisalli, M., Scorziello, A., et al. (2017). ApoSOD1 lacking dismutase activity neuroprotects motor neurons exposed to beta-methylamino-L-alanine through the Ca2+/Akt/ERK1/2 prosurvival pathway. Cell Death Differ. 24, 511. doi: 10.1038/cdd.2016.154
Phyu, M. P., and Tangpong, J. (2014). Neuroprotective effects of xanthone derivative of Garcinia mangostana against lead-induced acetylcholinesterase dysfunction and cognitive impairment. Food Chem. Toxicol. 70, 151–156. doi: 10.1016/j.fct.2014.04.035
Poddar, R., Chen, A., Winter, L., Rajagopal, S., and Paul, S. (2017). Role of AMPA receptors in homocysteine-NMDAR induced crosstalk between ERK and p38 MAP kinase. J. Neurochem. 142, 560–573. doi: 10.1111/jnc.14078
Poddar, R., and Paul, S. (2009). Homocysteine–NMDA receptor-mediated activation of extracellular signal-regulated kinase leads to neuronal cell death. J. Neurochem. 110, 1095–1106. doi: 10.1111/j.1471-4159.2009.06207.x
Poon, W. W., Blurton-Jones, M., Tu, C. H., Feinberg, L. M., Chabrier, M. A., Harris, J. W., et al. (2011). β-Amyloid impairs axonal BDNF retrograde trafficking. Neurobiol. Aging 32, 821–833. doi: 10.1016/j.neurobiolaging.2009.05.012
Pourmotabbed, A., Nedaei, S., Cheraghi, M., Moradian, S., Touhidi, A., Aeinfar, M., et al. (2011). Effect of prenatal pentylenetetrazol-induced kindling on learning and memory of male offspring. Neuroscience 172, 205–211. doi: 10.1016/j.neuroscience.2010.11.001
Powers, A. R. I. I. I., Gancsos, M. G., Finn, E. S., Morgan, P. T., and Corlett, P. R. (2015). Ketamine-induced hallucinations. Psychopathology 48, 376–385. doi: 10.1159/000438675
Pyo, J.-H., Jeong, Y.-K., Yeo, S., Lee, J.-H., Jeong, M.-Y., Kim, S.-H., et al. (2013). Neuroprotective effect of trans-cinnamaldehyde on the 6-hydroxydopamine-induced dopaminergic injury. Biol. Pharm. Bull. 36, 1928–1935. doi: 10.1248/bpb.b13-00537
Qiu, S., Pak, C. W., and Currás-Collazo, M. C. (2005). Sequential involvement of distinct glutamate receptors in domoic acid-induced neurotoxicity in rat mixed cortical cultures: effect of multiple dose/duration paradigms, chronological age, and repeated exposure. Toxicol. Sci. 89, 243–256. doi: 10.1093/toxsci/kfj008
Ragerdi Kashani, I., Chavoshi, H., Pasbakhsh, P., Hassani, M., Omidi, A., Mahmoudi, R., et al. (2017). Protective effects of erythropoietin against cuprizone-induced oxidative stress and demyelination in the mouse corpus callosum. Iran. J. Basic Med. Sci. 20, 886–893. doi: 10.22038/IJBMS.2017.9110
Raineri, M., Gonzalez, B., Goitia, B., Garcia-Rill, E., Krasnova, I. N., Cadet, J. L., et al. (2012). Modafinil abrogates methamphetamine-induced neuroinflammation and apoptotic effects in the mouse striatum. PLoS One 7:e46599. doi: 10.1371/journal.pone.0046599
Rammes, G., Hasenjäger, A., Sroka-Saidi, K., Deussing, J. M., and Parsons, C. G. (2011). Therapeutic significance of NR2B-containing NMDA receptors and mGluR5 metabotropic glutamate receptors in mediating the synaptotoxic effects of β-amyloid oligomers on long-term potentiation (LTP) in murine hippocampal slices. Neuropharmacology 60, 982–990. doi: 10.1016/j.neuropharm.2011.01.051
Ranganathan, M., DeMartinis, N., Huguenel, B., Gaudreault, F., Bednar, M., Shaffer, C., et al. (2017). Attenuation of ketamine-induced impairment in verbal learning and memory in healthy volunteers by the AMPA receptor potentiator PF-04958242. Mol. Psychiatry 22, 1633–1640. doi: 10.1038/mp.2017.6
Reinders, N. R., Pao, Y., Renner, M. C., da Silva-Matos, C. M., Lodder, T. R., Malinow, R., et al. (2016). Amyloid-β effects on synapses and memory require AMPA receptor subunit GluA3. Proc. Natl. Acad. Sci. U.S.A. 113, E6526–E6534. doi: 10.1073/pnas.1614249113
Renner, M., Lacor, P. N., Velasco, P. T., Xu, J., Contractor, A., Klein, W. L., et al. (2010). Deleterious effects of amyloid β oligomers acting as an extracellular scaffold for mGluR5. Neuron 66, 739–754. doi: 10.1016/j.neuron.2010.04.029
Ribeiro, F. M., Vieira, L. B., Pires, R. G., Olmo, R. P., and Ferguson, S. S. (2017). Metabotropic glutamate receptors and neurodegenerative diseases. Pharmacol. Res. 115, 179–191. doi: 10.1016/j.phrs.2016.11.013
Rogawski, M. A. (2011). Revisiting AMPA receptors as an antiepileptic drug target. Epilepsy Curr. 11, 56–63. doi: 10.5698/1535-7511-11.2.56
Rönicke, R., Mikhaylova, M., Rönicke, S., Meinhardt, J., Schröder, U. H., Fändrich, M., et al. (2011). Early neuronal dysfunction by amyloid β oligomers depends on activation of NR2B-containing NMDA receptors. Neurobiol. Aging 32, 2219–2228.
Roozendaal, B., Griffith, Q. K., Buranday, J., Dominique, J.-F., and McGaugh, J. L. (2003). The hippocampus mediates glucocorticoid-induced impairment of spatial memory retrieval: dependence on the basolateral amygdala. Proc. Natl. Acad. Sci. U.S.A. 100, 1328–1333. doi: 10.1073/pnas.0337480100
Roselli, F., Tirard, M., Lu, J., Hutzler, P., Lamberti, P., Livrea, P., et al. (2005). Soluble β-amyloid1-40 induces NMDA-dependent degradation of postsynaptic density-95 at glutamatergic synapses. J. Neurosci. 25, 11061–11070. doi: 10.1523/JNEUROSCI.3034-05.2005
Rubio, M. D., Drummond, J. B., and Meador-Woodruff, J. H. (2012). Glutamate receptor abnormalities in schizophrenia: implications for innovative treatments. Biomol. Ther. 20, 1–18. doi: 10.4062/biomolther.2012.20.1.001
Rui, Y., Gu, J., Yu, K., Hartzell, H. C., and Zheng, J. Q. (2010). Inhibition of AMPA receptor trafficking at hippocampal synapses by β-amyloid oligomers: the mitochondrial contribution. Mol. Brain 3:10. doi: 10.1186/1756-6606-3-10
Sághy, É, Sipos, É, Ács, P., Bölcskei, K., Pohóczky, K., Kemény, Á, et al. (2016). TRPA1 deficiency is protective in cuprizone-induced demyelination—A new target against oligodendrocyte apoptosis. Glia 64, 2166–2180. doi: 10.1002/glia.23051
Saito, M., Chakraborty, G., Hui, M., Masiello, K., and Saito, M. (2016). Ethanol-induced neurodegeneration and glial activation in the developing brain. Brain Sci. 6:E31. doi: 10.3390/brainsci6030031
Samadi, P., Grégoire, L., Morissette, M., Calon, F., Tahar, A. H., Dridi, M., et al. (2008). mGluR5 metabotropic glutamate receptors and dyskinesias in MPTP monkeys. Neurobiol. Aging 29, 1040–1051. doi: 10.1016/j.neurobiolaging.2007.02.005
Sanadgol, N., Golab, F., Tashakkor, Z., Taki, N., Moradi Kouchi, S., Mostafaie, A., et al. (2017). Neuroprotective effects of ellagic acid on cuprizone-induced acute demyelination through limitation of microgliosis, adjustment of CXCL12/IL-17/IL-11 axis and restriction of mature oligodendrocytes apoptosis. Pharm. Biol. 55, 1679–1687. doi: 10.1080/13880209.2017.1319867
Santucci, S., Zsürger, N., and Chabry, J. (2009). β-N-methylamino-l-alanine induced in vivo retinal cell death. J. Neurochem. 109, 819–825. doi: 10.1111/j.1471-4159.2009.06022.x
Schober, A. (2004). Classic toxin-induced animal models of Parkinson’s disease: 6-OHDA and MPTP. Cell Tissue Res. 318, 215–224. doi: 10.1007/s00441-004-0938-y
Selvatici, R., Previati, M., Marino, S., Marani, L., Falzarano, S., Lanzoni, I., et al. (2009). Sodium azide induced neuronal damage in vitro: evidence for non-apoptotic cell death. Neurochem. Res. 34, 909–916. doi: 10.1007/s11064-008-9852-0
Shan, H., Chu, Y., Chang, P., Yang, L., Wang, Y., Zhu, S., et al. (2017). Neuroprotective effects of hydrogen sulfide on sodium azide-induced autophagic cell death in PC12 cells. Mol. Med. Rep. 16, 5938–5946. doi: 10.3892/mmr.2017.7363
Shankar, G. M., Bloodgood, B. L., Townsend, M., Walsh, D. M., Selkoe, D. J., and Sabatini, B. L. (2007). Natural oligomers of the Alzheimer amyloid-β protein induce reversible synapse loss by modulating an NMDA-type glutamate receptor-dependent signaling pathway. J. Neurosci. 27, 2866–2875. doi: 10.1523/JNEUROSCI.4970-06.2007
Shetty, S., Hariharan, A., Shirole, T., and Jagtap, A. G. (2015). Neuroprotective potential of escitalopram against behavioral, mitochondrial and oxidative dysfunction induced by 3-nitropropionic acid. Ann. Neurosci. 22, 11–18. doi: 10.5214/ans.0972.7531.220104
Shi, X. R., Hong, Z. Y., Liu, H. R., Zhang, Y. C., and Zhu, Y. Z. (2011). Neuroprotective effects of SCM198 on 6-hydroxydopamine-induced behavioral deficit in rats and cytotoxicity in neuronal SH-SY5Y cells. Neurochem. Int. 58, 851–860. doi: 10.1016/j.neuint.2010.11.007
Shi, Z., Zhang, W., Lu, Y., Lu, Y., Xu, L., Fang, Q., et al. (2017). Aquaporin 4-mediated glutamate-induced astrocyte swelling is partially mediated through metabotropic glutamate receptor 5 activation. Front. Cell. Neurosci. 11:116. doi: 10.3389/fncel.2017.00116
Shibata, S., Furuta, K., Oh-Hashi, K., Ueda, H., Kiuchi, K., and Hirata, Y. (2017). Prevention of oxytosis-induced c-Raf down-regulation by (arylthio)cyclopentenone prostaglandins is neuroprotective. Toxicology 390, 83–87. doi: 10.1016/j.tox.2017.09.006
Shin, E.-J., Duong, C. X., Nguyen, X.-K. T., Li, Z., Bing, G., Bach, J.-H., et al. (2012). Role of oxidative stress in methamphetamine-induced dopaminergic toxicity mediated by protein kinase Cδ. Behav. Brain Res. 232, 98–113. doi: 10.1016/j.bbr.2012.04.001
Shinoda, Y., Nakajima, Y., Iguchi, H., Tatsumi, S., Kitaoka, M., Nakajima, M., et al. (2016). Galacto-N-biose is neuroprotective against glutamate-induced excitotoxicity in vitro. Eur. J. Pharmacol. 791, 711–717. doi: 10.1016/j.ejphar.2016.10.010
Shokoohinia, Y., Hosseinzadeh, L., Moieni-Arya, M., Mostafaie, A., and Mohammadi-Motlagh, H.-R. (2014). Osthole attenuates doxorubicin-induced apoptosis in PC12 cells through inhibition of mitochondrial dysfunction and ROS production. Biomed. Res. Int. 2014:156848. doi: 10.1155/2014/156848
Shourmasti, F. R., Goudarzi, I., Abrari, K., Salmani, M. E., and Laskarbolouki, T. (2014). Riluzole ameliorates harmaline-induced tremor in rat. Basic Clin. Neurosci. 5, 138–143.
Sil, S., Ghosh, T., and Ghosh, R. (2016a). NMDA receptor is involved in neuroinflammation in intracerebroventricular colchicine-injected rats. J. Immunotoxicol. 13, 474–489. doi: 10.3109/1547691X.2015.1130760
Sil, S., Ghosh, T., Gupta, P., Ghosh, R., Kabir, S. N., and Roy, A. (2016b). Dual role of vitamin C on the neuroinflammation mediated neurodegeneration and memory impairments in colchicine induced rat model of Alzheimer disease. J. Mol. Neurosci. 60, 421–435.
Simoes, P., Silva, A., Pereira, F., Marques, E., Grade, S., Milhazes, N., et al. (2007). Methamphetamine induces alterations on hippocampal NMDA and AMPA receptor subunit levels and impairs spatial working memory. Neuroscience 150, 433–441. doi: 10.1016/j.neuroscience.2007.09.044
Simões, P. F., Silva, A. P., Pereira, F. C., Marques, E., Milhazes, N., Borges, F., et al. (2008). Methamphetamine changes NMDA and AMPA glutamate receptor subunit levels in the rat striatum and frontal cortex. Ann. N. Y. Acad. Sci. 1139, 232–241. doi: 10.1196/annals.1432.028
Siswanto, S., Arozal, W., Juniantito, V., Grace, A., and Agustini, F. D. (2016). The effect of mangiferin against brain damage caused by oxidative stress and inflammation induced by doxorubicin. HAYATI J. Biosci. 23, 51–55. doi: 10.1016/j.hjb.2016.02.001
Sleigh, J., Harvey, M., Voss, L., and Denny, B. (2014). Ketamine–More mechanisms of action than just NMDA blockade. Trends Anaesth. Crit. Care 4, 76–81. doi: 10.1016/j.tacc.2014.03.002
Slowik, A., Schmidt, T., Beyer, C., Amor, S., Clarner, T., and Kipp, M. (2015). The sphingosine 1-phosphate receptor agonist FTY720 is neuroprotective after cuprizone-induced CNS demyelination. Br. J. Pharmacol. 172, 80–92. doi: 10.1111/bph.12938
Snyder, E. M., Nong, Y., Almeida, C. G., Paul, S., Moran, T., Choi, E. Y., et al. (2005). Regulation of NMDA receptor trafficking by amyloid-beta. Nat. Neurosci. 8, 1051–1058. doi: 10.1038/nn1503
Solesio, M. E., Saez-Atienzar, S., Jordan, J., and Galindo, M. F. (2013). 3-Nitropropionic acid induces autophagy by forming mitochondrial permeability transition pores rather than activatiing the mitochondrial fission pathway. Br. J. Pharmacol. 168, 63–75. doi: 10.1111/j.1476-5381.2012.01994.x
Song, Q., Gou, W.-L., and Zou, Y.-L. (2017). FAM3A protects against glutamate-induced toxicity by preserving calcium homeostasis in differentiated PC12 cells. Cell. Physiol. Biochem. 44, 2029–2041. doi: 10.1159/000485943
Souza, L. C., Wilhelm, E. A., Bortolatto, C. F., Nogueira, C. W., Boeira, S. P., and Jesse, C. R. (2014). Involvement of mGlu5 receptor in 3-nitropropionic acid-induced oxidative stress in rat striatum. Neurol. Res. 36, 833–840. doi: 10.1179/1743132814Y.0000000334
Spalloni, A., Nutini, M., and Longone, P. (2013). Role of the N-methyl-d-aspartate receptors complex in amyotrophic lateral sclerosis. Biochim. Biophys. Acta 1832, 312–322. doi: 10.1016/j.bbadis.2012.11.013
Su, Z.-Y., Ye, Q., Liu, X.-B., Chen, Y.-Z., Zhan, H., and Xu, S.-Y. (2017). Dexmedetomidine mitigates isoflurane-induced neurodegeneration in fetal rats during the second trimester of pregnancy. Neural Regen. Res. 12, 1329–1337. doi: 10.4103/1673-5374.213554
Tajuddin, N., Moon, K.-H., Marshall, S. A., Nixon, K., Neafsey, E. J., Kim, H.-Y., et al. (2014). Neuroinflammation and neurodegeneration in adult rat brain from binge ethanol exposure: abrogation by docosahexaenoic acid. PLoS One 9:e101223. doi: 10.1371/journal.pone.0101223
Takser, L., Benachour, N., Husk, B., Cabana, H., and Gris, D. (2016). Cyanotoxins at low doses induce apoptosis and inflammatory effects in murine brain cells: potential implications for neurodegenerative diseases. Toxicol. Rep. 3, 180–189. doi: 10.1016/j.toxrep.2015.12.008
Tameh, A. A., Clarner, T., Beyer, C., Atlasi, M. A., Hassanzadeh, G., and Naderian, H. (2013). Regional regulation of glutamate signaling during cuprizone-induced demyelination in the brain. Ann. Anat. 195, 415–423. doi: 10.1016/j.aanat.2013.03.004
Tang, L. M., Wang, L. X., Wang, Z. Y., Sun, L. F., Pan, X. D., and Pan, G. Q. (2017). Tanshinone IIA ameliorates lead (Pb)-induced cognitive deficits and oxidative stress in a rat pup model. Bratisl. Lek. Listy 118, 196–201. doi: 10.4149/BLL_2017_039
Tang, W., Wesley, M., Freeman, W. M., Liang, B., and Hemby, S. E. (2004). Alterations in ionotropic glutamate receptor subunits during binge cocaine self-administration and withdrawal in rats. J. Neurochem. 89, 1021–1033. doi: 10.1111/j.1471-4159.2004.02392.x
Tanqueiro, S. R., Ramalho, R. M., Sebastião, A. M., and Diógenes, M. J. (2018). Inhibition of NMDA receptors prevents the loss of BDNF function induced by amyloid β. Front. Pharmacol. 9:237. doi: 10.3389/fphar.2018.00237
Tasker, R., Perry, M., Doucette, T. A., and Ryan, C. L. (2005). NMDA receptor involvement in the effects of low dose domoic acid in neonatal rats. Amino Acids 28, 193–196. doi: 10.1007/s00726-005-0167-z
Thangarajan, S., Ramachandran, S., and Krishnamurthy, P. (2016). Chrysin exerts neuroprotective effects against 3-Nitropropionic acid induced behavioral despair—mitochondrial dysfunction and striatal apoptosis via upregulating Bcl-2 gene and downregulating Bax—Bad genes in male wistar rats. Biomed. Pharmacother. 84, 514–525. doi: 10.1016/j.biopha.2016.09.070
Thornton, E., and Vink, R. (2012). Treatment with a substance P receptor antagonist is neuroprotective in the intrastriatal 6-hydroxydopamine model of early Parkinson’s disease. PLoS One 7:e34138. doi: 10.1371/journal.pone.0034138
Titulaer, M. J., Höftberger, R., Iizuka, T., Leypoldt, F., McCracken, L., Cellucci, T., et al. (2014). Overlapping demyelinating syndromes and anti–N-methyl-D-aspartate receptor encephalitis. Ann. Neurol. 75, 411–428. doi: 10.1002/ana.24117
Tiwari, S. K., Agarwal, S., Tripathi, A., and Chaturvedi, R. K. (2016). Bisphenol-A mediated inhibition of hippocampal neurogenesis attenuated by curcumin via canonical Wnt pathway. Mol. Neurobiol. 53, 3010–3029. doi: 10.1007/s12035-015-9197-z
Ullah, I., Ullah, N., Naseer, M. I., Lee, H. Y., and Kim, M. O. (2012). Neuroprotection with metformin and thymoquinone against ethanol-induced apoptotic neurodegeneration in prenatal rat cortical neurons. BMC Neurosci. 13:11. doi: 10.1186/1471-2202-13-11
Ullah, N., Naseer, M. I., Ullah, I., Kim, T. H., Lee, H. Y., and Kim, M. O. (2013). Neuroprotective profile of pyruvate against ethanol-induced neurodegeneration in developing mice brain. Neurol. Sci. 34, 2137–2143. doi: 10.1007/s10072-013-1350-8
Um, J. W., Kaufman, A. C., Kostylev, M., Heiss, J. K., Stagi, M., Takahashi, H., et al. (2013). Metabotropic glutamate receptor 5 is a coreceptor for Alzheimer aβ oligomer bound to cellular prion protein. Neuron 79, 887–902. doi: 10.1016/j.neuron.2013.06.036
van Beugen, B. J., Qiao, X., Simmons, D. H., De Zeeuw, C. I., and Hansel, C. (2014). Enhanced AMPA receptor function promotes cerebellar long-term depression rather than potentiation. Learn. Mem. 21, 662–667. doi: 10.1101/lm.035220.114
van der Star, B. J., Ys Vogel, D., Kipp, M., Puentes, F., Baker, D., and Amor, S. (2012). In vitro and in vivo models of multiple sclerosis. CNS Neurol. Disord. Drug Targets 11, 570–588. doi: 10.2174/187152712801661284
Velíšek, L., Kubová, H., Mareš, P., and Vachová, D. (1995). Kainate/AMPA receptor antagonists are anticonvulsant against the tonic hindlimb component of pentylenetetrazol-induced seizures in developing rats. Pharmacol. Biochem. Behav. 51, 153–158. doi: 10.1016/0091-3057(94)00329-H
Walsh, D. M., and Selkoe, D. J. (2004). Deciphering the molecular basis of memory failure in Alzheimer’s disease. Neuron 44, 181–193. doi: 10.1016/j.neuron.2004.09.010
Wang, A.-L., Liou, Y.-M., Pawlak, C. R., and Ho, Y.-J. (2010). Involvement of NMDA receptors in both MPTP-induced neuroinflammation and deficits in episodic-like memory in Wistar rats. Behav. Brain Res. 208, 38–46. doi: 10.1016/j.bbr.2009.11.006
Wang, D., Zhao, J., Li, S., Shen, G., and Hu, S. (2016). Quercetin attenuates domoic acid-induced cognitive deficits in mice. Nutr. Neurosci. 21, 123–131. doi: 10.1080/1028415X.2016.1231438
Wang, J., Zhou, M., Wang, X., Yang, X., Wang, M., Zhang, C., et al. (2014). Impact of ketamine on learning and memory function, neuronal apoptosis and its potential association with miR-214 and PTEN in adolescent rats. PLoS One 9:e99855. doi: 10.1371/journal.pone.0099855
Wang, J. Y., Yang, J. Y., Wang, F., Fu, S. Y., Hou, Y., Jiang, B., et al. (2013). Neuroprotective effect of pseudoginsenoside-f11 on a rat model of Parkinson’s disease induced by 6-hydroxydopamine. Evid. Based Complement. Alternat. Med. 2013:152798. doi: 10.1155/2013/152798
Wang, K., Zhu, X., Zhang, K., Wu, Z., Sun, S., Zhou, F., et al. (2016). Neuroprotective effect of puerarin on glutamate-induced cytotoxicity in differentiated Y-79 cells via inhibition of ROS generation and Ca2+ influx. Int. J. Mol. Sci. 17:1109. doi: 10.3390/ijms17071109
Wang, L., Lu, H., Brown, P. L., Rea, W., Vaupel, B., Yang, Y., et al. (2015). Manganese-enhanced MRI reflects both activity-independent and activity-dependent uptake within the rat habenulomesencephalic pathway. PLoS One 10:e0127773. doi: 10.1371/journal.pone.0127773
Wang, P., Luo, Q., Qiao, H., Ding, H., Cao, Y., Yu, J., et al. (2017). The neuroprotective effects of carvacrol on ethanol-induced hippocampal neurons impairment via the antioxidative and antiapoptotic pathways. Oxid. Med. Cell. Longev. 2017:4079425. doi: 10.1155/2017/4079425
Wang, Q., Shen, F.-Y., Zou, R., Zheng, J.-J., Yu, X., and Wang, Y.-W. (2017). Ketamine-induced apoptosis in the mouse cerebral cortex follows similar characteristic of physiological apoptosis and can be regulated by neuronal activity. Mol. Brain 10:24. doi: 10.1186/s13041-017-0302-2
Wang, Q., Yu, S., Simonyi, A., Sun, G. Y., and Sun, A. Y. (2005). Kainic acid-mediated excitotoxicity as a model for neurodegeneration. Mol. Neurobiol. 31, 3–16. doi: 10.1385/MN:31:1-3:003
Wang, X., Guan, Q., Wang, M., Yang, L., Bai, J., Yan, Z., et al. (2015). Aging-related rotenone-induced neurochemical and behavioral deficits: role of SIRT2 and redox imbalance, and neuroprotection by AK-7. Drug Des. Dev. Ther. 9, 2553–2563. doi: 10.2147/DDDT.S81539
Wang, Z.-C., Zhao, J., and Li, S. (2013). Dysregulation of synaptic and extrasynaptic N-methyl-D-aspartate receptors induced by amyloid-β. Neurosci. Bull. 29, 752–760. doi: 10.1007/s12264-013-1383-2
Wei, H.-J., Xu, J.-H., Li, M.-H., Tang, J.-P., Zou, W., Zhang, P., et al. (2014). Hydrogen sulfide inhibits homocysteine-induced endoplasmic reticulum stress and neuronal apoptosis in rat hippocampus via upregulation of the BDNF-TrkB pathway. Acta Pharmacol. Sin. 35, 707–715. doi: 10.1038/aps.2013.197
Weller, M., Marini, A. M., and Paul, S. M. (1992). Niacinamide blocks 3-acetylpyridine toxicity of cerebellar granule cells in vitro. Brain Res. 594, 160–164. doi: 10.1016/0006-8993(92)91043-E
Wirkner, K., Poelchen, W., Köles, L., Mühlberg, K., Scheibler, P., Allgaier, C., et al. (1999). Ethanol-induced inhibition of NMDA receptor channels. Neurochem. Int. 35, 153–162. doi: 10.1016/S0197-0186(99)00057-1
Wong, R. K., Bianchi, R., Chuang, S. C., and Merlin, L. R. (2005). Group I mGluR-induced epileptogenesis: distinct and overlapping roles of mGluR1 and mGluR5 and implications for antiepileptic drug design. Epilepsy Curr. 5, 63–68. doi: 10.1111/j.1535-7597.2005.05207.x
Wu, J., Hao, S., Sun, X.-R., Zhang, H., Li, H., Zhao, H., et al. (2017). Elamipretide (SS-31) ameliorates isoflurane-induced long-term impairments of mitochondrial morphogenesis and cognition in developing rats. Front. Cell. Neurosci. 11:119. doi: 10.3389/fncel.2017.00119
Wu, Y.-N., and Johnson, S. W. (2009). Rotenone reduces Mg2+-dependent block of NMDA currents in substantia nigra dopamine neurons. Neurotoxicology 30, 320–325. doi: 10.1016/j.neuro.2009.01.002
Xia, N., Zhang, Q., Wang, S. T., Gu, L., Yang, H. M., Liu, L., et al. (2015). Blockade of metabotropic glutamate receptor 5 protects against DNA damage in a rotenone-induced Parkinson’s disease model. Free Radic. Biol. Med. 89, 567–580. doi: 10.1016/j.freeradbiomed.2015.09.017
Xie, C., Sun, J., Qiao, W., Lu, D., Wei, L., Na, M., et al. (2011). Administration of simvastatin after kainic acid-induced status epilepticus restrains chronic temporal lobe epilepsy. PLoS One 6:e24966. doi: 10.1371/journal.pone.0024966
Xu, B., Xu, Z. F., and Deng, Y. (2010). Manganese exposure alters the expression of N-methyl-D-aspartate receptor subunit mRNAs and proteins in rat striatum. J. Biochem. Mol. Toxicol. 24, 1–9. doi: 10.1002/jbt.20306
Xu, D., Chen, H., Mak, S., Hu, S., Tsim, K. W., Hu, Y., et al. (2016). Neuroprotection against glutamate-induced excitotoxicity and induction of neurite outgrowth by T-006, a novel multifunctional derivative of tetramethylpyrazine in neuronal cell models. Neurochem. Int. 99, 194–205. doi: 10.1016/j.neuint.2016.07.006
Xu, F., Farkas, S., Kortbeek, S., Zhang, F.-X., Chen, L., Zamponi, G. W., et al. (2012). Mercury-induced toxicity of rat cortical neurons is mediated through N-methyl-D-Aspartate receptors. Mol. Brain 5:30. doi: 10.1186/1756-6606-5-30
Xu, G., Huang, Y. L., Li, P. L., Guo, H. M., and Han, X. P. (2017). Neuroprotective effects of artemisinin against isoflurane-induced cognitive impairments and neuronal cell death involve JNK/ERK1/2 signalling and improved hippocampal histone acetylation in neonatal rats. J. Pharm. Pharmacol. 69, 684–697. doi: 10.1111/jphp.12704
Xu, X., Liu, X., Zhang, Q., Zhang, G., Lu, Y., Ruan, Q., et al. (2013). Sex-specific effects of bisphenol-A on memory and synaptic structural modification in hippocampus of adult mice. Horm. Behav. 63, 766–775. doi: 10.1016/j.yhbeh.2013.03.004
Xu, X., Ye, Y., Li, T., Chen, L., Tian, D., Luo, Q., et al. (2010). Bisphenol-A rapidly promotes dynamic changes in hippocampal dendritic morphology through estrogen receptor-mediated pathway by concomitant phosphorylation of NMDA receptor subunit NR2B. Toxicol. Appl. Pharmacol. 249, 188–196. Epub 2010/09/23., doi: 10.1016/j.taap.2010.09.007
Xu, X. H., Wang, Y. M., Zhang, J., Luo, Q. Q., Ye, Y. P., and Ruan, Q. (2010). Perinatal exposure to bisphenol-A changes N-methyl-D-aspartate receptor expression in the hippocampus of male rat offspring. Environ. Toxicol. Chem. 29, 176–181. doi: 10.1002/etc.18
Xu, Y., Pan, J., Chen, L., Zhang, C., Sun, J., Li, J., et al. (2013). Phosphodiesterase-2 inhibitor reverses corticosterone-induced neurotoxicity and related behavioural changes via cGMP/PKG dependent pathway. Int. J. Neuropsychopharmacol. 16, 835–847. doi: 10.1017/S146114571200065X
Xue, W.-Z., Yang, Q.-Q., Chen, Y., Zou, R.-X., Xing, D., Xu, Y., et al. (2017). Kiwifruit alleviates learning and memory deficits induced by Pb through antioxidation and inhibition of microglia activation in vitro and in vivo. Oxid. Med. Cell. Longev. 2017:5645324. doi: 10.1155/2017/5645324
Yan, J.-Q., Ma, Y.-J., Sun, J.-C., Bai, S.-F., and Huang, L.-N. (2014). Neuroprotective effect of lovastatin by inhibiting NMDA receptor1 in 6-hydroxydopamine treated PC12 cells. Int. J. Clin. Exp. Med. 7, 3313–3319.
Yan, J.-Q., Sun, J.-C., Zhai, M.-M., Cheng, L.-N., Bai, X.-L., and Feng, C.-L. (2015). Lovastatin induces neuroprotection by inhibiting inflammatory cytokines in 6-hydroxydopamine treated microglia cells. Int. J. Clin. Exp. Med. 8,9030–9037.
Ye, F., Li, X., Li, L., Yuan, J., and Chen, J. (2015). t-BHQ provides protection against lead neurotoxicity via Nrf2/HO-1 pathway. Oxid. Med. Cell. Longev. 2016:2075915. doi: 10.1155/2016/2075915
Yeganeh, F., Nikbakht, F., Bahmanpour, S., Rastegar, K., and Namavar, R. (2013). Neuroprotective effects of NMDA and group I metabotropic glutamate receptor antagonists against neurodegeneration induced by homocysteine in rat hippocampus: in vivo study. J. Mol. Neurosci. 50, 551–557. doi: 10.1007/s12031-013-9996-5
You, J.-M., Yun, S.-J., Nam, K. N., Kang, C., Won, R., and Lee, E. H. (2009). Mechanism of glucocorticoid-induced oxidative stress in rat hippocampal slice cultures. Can. J. Physiol. Pharmacol. 87, 440–447. doi: 10.1139/y09-027
Yu, S., Zhu, L., Shen, Q., Bai, X., and Di, X. (2015). Recent advances in methamphetamine neurotoxicity mechanisms and its molecular pathophysiology. Behav. Neurol. 2015:103969. doi: 10.1155/2015/103969
Zhang, H., Wang, H., Xiao, X., and Zhang, T. (2016). Melamine alters glutamatergic synaptic transmission of CA3–CA1 synapses presynaptically through autophagy activation in the rat hippocampus. Neurotox. Res. 29, 135–142. doi: 10.1007/s12640-015-9570-8
Zhang, J., Fan, W., Wang, H., Bao, L., Li, G., Li, T., et al. (2015). Resveratrol protects PC12 cell against 6-OHDA damage via CXCR4 signaling pathway. Evid. Based Complement. Alternat. Med. 2015:730121. doi: 10.1155/2015/730121
Zhang, J., Hou, L., Gao, X., Guo, F., Jing, W., Qi, J., et al. (2009). Amyloid β-protein differentially affects NMDA receptor-and GABA A receptor-mediated currents in rat hippocampal CA1 neurons. Prog. Nat. Sci. 19, 963–972. doi: 10.1016/j.pnsc.2008.11.006
Zhang, J., Wu, J., Zeng, W., Yao, K., Zu, H., and Zhao, Y. (2016). Function of thymosin Beta-4 in ethanol-induced microglial activation. Cell. Physiol. Biochem. 38, 2230–2238. doi: 10.1159/000445578
Zhang, L., Cheng, X.-R., Juan-Juan, H., Lan, S., and Guan-Hua, D. (2011). Neuroprotective effects of hyperoside on sodium azide-induced apoptosis in PC12 cells. Chin. J. Nat. Med. 9, 450–455.
Zhang, X., Jones, D., and Gonzalez-Lima, F. (2006). Neurodegeneration produced by rotenone in the mouse retina: a potential model to investigate environmental pesticide contributions to neurodegenerative diseases. J. Toxicol. Environ. Health A 69, 1681–1697. doi: 10.1080/15287390600630203
Zhang, X.-M., Jin, T., Quezada, H. C., Mix, E., Winblad, B., and Zhu, J. (2010). Kainic acid-induced microglial activation is attenuated in aged interleukin-18 deficient mice. J. Neuroinflammation 7:26. doi: 10.1186/1742-2094-7-26
Zhang, Y., Li, P., Feng, J., and Wu, M. (2016). Dysfunction of NMDA receptors in Alzheimer’s disease. Neurol. Sci. 37, 1039–1047. doi: 10.1007/s10072-016-2546-5
Zhao, Q. (2006). “Repetitive seizures in the immature brain,” in Models of Seizures and Epilepsy, eds A. Pitkänen, P. Buckmaster, A. S. Galanopoulou, and S. L. Moshé (San Diego, CA: Academic Press), 341–350. doi: 10.1016/B978-012088554-1/50029-3
Zheng, X.-Y., Zhang, H.-L., Luo, Q., and Zhu, J. (2010). Kainic acid-induced neurodegenerative model: potentials and limitations. Biomed. Res. Int. 2011:457079. doi: 10.1155/2011/457079
Zhong, L., Zhou, J., Chen, X., Lou, Y., Liu, D., Zou, X., et al. (2016). Quantitative proteomics study of the neuroprotective effects of B12 on hydrogen peroxide-induced apoptosis in SH-SY5Y cells. Sci. Rep. 6:22635. doi: 10.1038/srep22635
Zhou, W., Kavelaars, A., and Heijnen, C. J. (2016). Metformin prevents cisplatin-induced cognitive impairment and brain damage in mice. PLoS One 11:e0151890. doi: 10.1371/journal.pone.0151890
Zhou, X., Escala, W., Papapetropoulos, S., and Zhai, R. G. (2010). β-N-methylamino-L-alanine induces neurological deficits and shortened life span in Drosophila. Toxins 2, 2663–2679. doi: 10.3390/toxins2112663
Zhu, J., Carozzi, V. A., Reed, N., Mi, R., Marmiroli, P., Cavaletti, G., et al. (2016). Ethoxyquin provides neuroprotection against cisplatin-induced neurotoxicity. Sci. Rep. 6:28861. doi: 10.1038/srep28861
Zimmerman, D., Goto, J. J., and Krishnan, V. V. (2016). Equilibrium dynamics of β-n-methylamino-l-alanine (bmaa) and its carbamate adducts at physiological conditions. PLoS One 11:e0160491. doi: 10.1371/journal.pone.0160491
Keywords: glutamate receptors, neurodegenerative diseases, neurotoxic agents, nerve tissue, neuronal toxicity
Citation: Jakaria M, Park S-Y, Haque ME, Karthivashan G, Kim I-S, Ganesan P and Choi D-K (2018) Neurotoxic Agent-Induced Injury in Neurodegenerative Disease Model: Focus on Involvement of Glutamate Receptors. Front. Mol. Neurosci. 11:307. doi: 10.3389/fnmol.2018.00307
Received: 04 May 2018; Accepted: 13 August 2018;
Published: 29 August 2018.
Edited by:
Ildikó Rácz, Universitätsklinikum Bonn, GermanyReviewed by:
Patrizia Longone, Fondazione Santa Lucia (IRCCS), ItalyWladyslaw Lason, Polish Academy of Sciences, Poland
Copyright © 2018 Jakaria, Park, Haque, Karthivashan, Kim, Ganesan and Choi. This is an open-access article distributed under the terms of the Creative Commons Attribution License (CC BY). The use, distribution or reproduction in other forums is permitted, provided the original author(s) and the copyright owner(s) are credited and that the original publication in this journal is cited, in accordance with accepted academic practice. No use, distribution or reproduction is permitted which does not comply with these terms.
*Correspondence: Dong-Kug Choi, Y2hvaWRrQGtrdS5hYy5rcg==