- 1Neuroscience Graduate Program, University of California, Riverside, Riverside, CA, United States
- 2Division of Biomedical Sciences, School of Medicine, University of California, Riverside, Riverside, CA, United States
- 3Psychology Graduate Program, Department of Psychology, University of California, Riverside, Riverside, CA, United States
Perineuronal nets (PNN) are extracellular matrix (ECM) assemblies that preferentially ensheath parvalbumin (PV) expressing interneurons. Converging evidence indicates that PV cells and PNN are impaired in a variety of neurological disorders. PNN development and maintenance is necessary for a number of processes within the CNS, including regulation of GABAergic cell function, protection of neurons from oxidative stress, and closure of developmental critical period plasticity windows. Understanding PNN functions may be essential for characterizing the mechanisms of altered cortical excitability observed in neurodegenerative and neurodevelopmental disorders. Indeed, PNN abnormalities have been observed in post-mortem brain tissues of patients with schizophrenia and Alzheimer’s disease. There is impaired development of PNNs and enhanced activity of its key regulator matrix metalloproteinase-9 (MMP-9) in Fragile X Syndrome, a common genetic cause of autism. MMP-9, a protease that cleaves ECM, is differentially regulated in a number of these disorders. Despite this, few studies have addressed the interactions between PNN expression, MMP-9 activity and neuronal excitability. In this review, we highlight the current evidence for PNN abnormalities in CNS disorders associated with altered network function and MMP-9 levels, emphasizing the need for future work targeting PNNs in pathophysiology and therapeutic treatment of neurological disorders.
Introduction
First described by Golgi and Cajal (Golgi, 1893; Ramón and Cajal, 1897), PNNs are assemblies of ECM proteins, which form net-like structures around neurons (Brauer et al., 1982, 1984). While PNNs were first identified in the late 1800s, much of our current knowledge about PNN structure and function can be attributed to a renewed interest in PNNs almost a century later (Brückner et al., 1993; Celio and Blümcke, 1994; Celio et al., 1998). Since then, PNNs have been implicated in a wide range of functions including neuroprotection, regulation of neural activity and experience-dependent plasticity. Excellent reviews have been written about the role of PNNs in brain development (Berardi et al., 2004; Hensch, 2005; Karetko and Skangiel-Kramska, 2009; Wang and Fawcett, 2012). Recently, increasing evidence suggests that PNNs are altered in various neurodevelopmental and neurodegenerative disorders associated with changes in brain activity (De Luca and Papa, 2016; Sorg et al., 2016; Härtig et al., 2016). In parallel, a number of these CNS diseases exhibit dysregulated expression of enzymes necessary for PNN cleavage and reorganization (Backstrom et al., 1996; Lorenzl et al., 2003; Rybakowski et al., 2009; Kim et al., 2009; Zhang et al., 2011; Yamamori et al., 2013; Liu et al., 2014; Rankin-Gee et al., 2015; Lepeta et al., 2017). However, the role of PNNs in neural and network excitability is only beginning to be understood (Balmer, 2016; Murase et al., 2017; Wen et al., 2017). Based on evidence from recent studies in the mouse model of FXS, we propose a novel working hypothesis in which MMP-9 mediated cleavage and/or reorganization of PNN structures around PV-expressing cells contributes to abnormal PV cell development and cortical hyperexcitability (Figure 1). Using this hypothesis as a scaffold, we bring together evidence from studies of schizophrenia, AD, and epilepsy to suggest that systematic studies of the interplay between MMP-9, PNN and PV cells may be key to understanding mechanisms of multiple CNS disorders at the cellular and circuit levels.
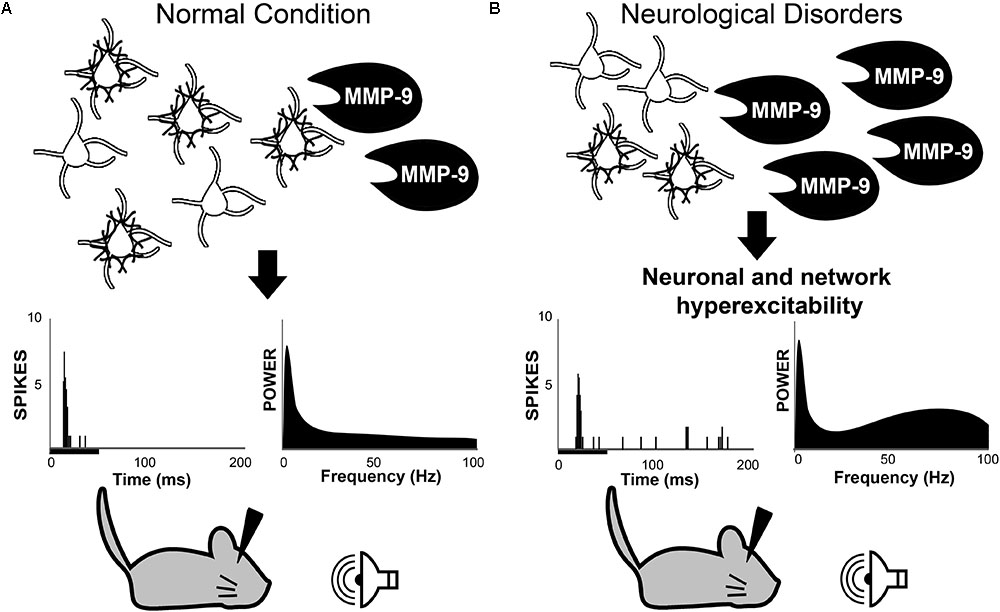
FIGURE 1. Working hypothesis in which MMP-9 is necessary for normal PV cell function and consequently normal neuronal and network excitability (A). Top row: schematic of PV/PNN cells and cleavage of PNN by MMP-9. Middle row: single unit responses (each vertical line is an action potential in the post-stimulus time histogram) to sound stimulus (black bar under the abscissa) and spectral power of baseline EEGs. (B) Increased MMP-9 levels in disorders such as Fragile X Syndrome cause altered PNN formation/composition around PV cells. This may contribute to increased response to sensory stimuli and enhanced gamma power in baseline EEGs. The review argues that multiple neurological and neurodevelopmental disorders may include altered PV/PNN/MMP-9 interactions leading to circuit dysfunction. PV, parvalbumin; PNN, perineuronal nets; MMP-9, matrix metalloproteinase-9.
PNN Structure and Developmental Regulation of its Components
Perineuronal nets are specialized assemblies of ECM proteins which ensheath neurons and proximal dendrites (Celio et al., 1998). PNNs are composed of CSPGs, tenascin, and link proteins, which facilitate binding to a hyaluronan backbone (Yamaguchi, 1999) (Figure 2). Developmental regulation of ECM proteins, which make up PNNs, may underlie PNN formation, composition, and function (Köppe et al., 1997). The developmental changes in major PNN proteins are discussed in the following sections:
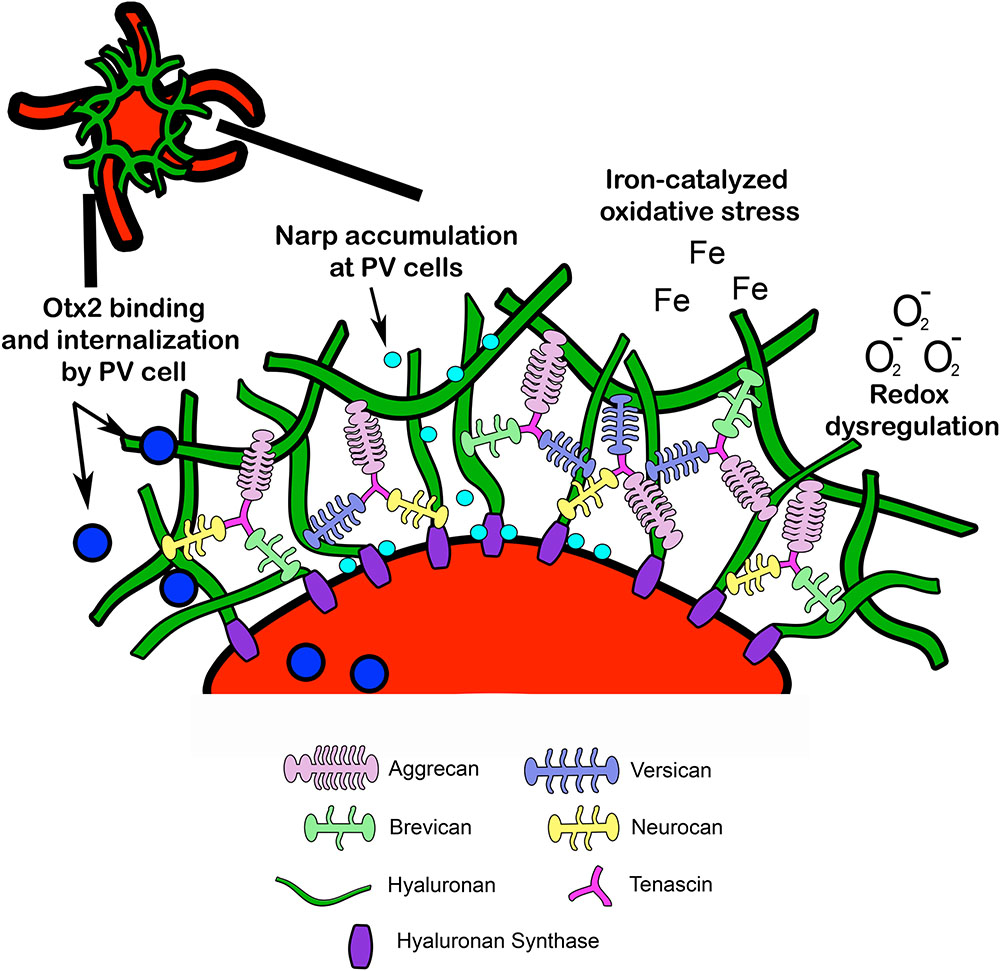
FIGURE 2. Structural composition of PNN. Lecticans are attached to a hyaluronan backbone via link proteins such as cartilage link protein and hyaluronan link proteins (not pictured). Hyaluronan synthase contributes to hyaluronan synthesis and anchors hyaluronan to the cell membrane. Tenascin promotes crosslinking of lecticans and helps maintain PNN structural integrity. PNN around PV cells may provide protection from redox dysregulation and oxidative stressors such as superoxide free radicals (O2-) and metal ions (Fe). In addition, PNNs may facilitate PV maturation through internalization of Otx2 homeoproteins and regulate PV cell excitability through accumulation of the immediate early gene and synaptic scaling molecule Narp (see Fragile X Syndrome). Narp, neuronal activity-regulated pentraxin; Otx2, orthodenticle homeoprotein 2.
Chondroitin Sulfate Proteoglycans
Chondroitin sulfate proteoglycans consist of a core protein and varying amounts of chondroitin sulfate chains covalently bound to a central region of the protein. These chondroitin sulfate side chains are a type of GAG polysaccharide consisting of disaccharide blocks of N-acetylgalactosamine and glucuronic acid (Sherman and Back, 2007). Various CSPGs exist in the ECM throughout the body, but the lectican family of CSPGs is most prominent in PNNs of the CNS. These include aggrecan, neurocan, versican, and brevican, which vary in core protein size and the number of GAG side chains (Yamaguchi, 1999).
Although lecticans are developmentally regulated, their function in CNS development remains unclear. A developmental upregulation of aggrecan and brevican, which peak in adulthood, was reported in rat brain (Milev et al., 1998; Brückner et al., 2000; Popp et al., 2003). Versican consists of two different alpha and beta isoforms, which are differentially expressed during development. The expression of alpha isoform was low during embryonic development, first and second postnatal weeks, and then increased steadily until around 3.5 months of age (Bignami et al., 1993b). In contrast, the expression of beta isoform increased 2–3 fold from embryonic day (E) 14 until birth and then decreased in adulthood. Neurocan expression also increased between E14 and postnatal day (P) 3 and then decreased during early-late adolescence and into adulthood (Meyer-Puttlitz et al., 1996; Miller et al., 1996; Milev et al., 1998; Brückner et al., 2000). Studies of postmortem human tissues also suggest developmental changes in lectican levels in human CNS. For example, high levels of brevican mRNA expression were detected in postmortem human cortex during early development until 8 years of age, and the expression levels were lower in the adult human brain (Gary et al., 2000). These developmental changes in lectican expression levels suggest that the PNNs themselves may also be developmentally regulated, underlying maturation of circuit function (Köppe et al., 1997). However, the functional impact of developmental changes in PNN lectican composition is unknown.
Tenascins and Hyaluronan
Tenascins and hyaluronan are also part of PNN structure. Tenascins are fibrous, matricellular proteins, which bind to the C-terminal domain of lecticans (Aspberg et al., 1997; Day et al., 2004). Hyaluronan is an unsulfated GAG that is synthesized at the cell surface by the membrane-bound enzymes called HAS and protrudes through the plasma membrane into the extracellular space (Fraser et al., 1997). Hyaluronan binds to other ECM molecules via N-terminal hyaluronan binding domains, including lecticans and link proteins such as cartilage link protein, Ctrl1, and brain-derived link proteins Bral1 and Bral2 (Frischknecht and Seidenbecher, 2008). This complex of hyaluronan with ECM proteins forms the basis for PNN structure. Similar to lecticans, tenascin and hyaluronan are also developmentally regulated. In the mouse cortex, tenascin immunolabeling is first detected around E16 (Sheppard et al., 1991) and tenascin levels increase after birth until the third postnatal week (Brückner et al., 2000). By week 4, tenascin expression is reduced to adult levels (Brückner et al., 2000). Hyaluronan expression precedes the detection of both tenascin and lecticans, and is first observed near the notochord in E11-12 rat embryos (Delpech and Delpech, 1984; Bignami et al., 1993a). Hyaluronan is expressed during postnatal development and adulthood but peaks during embryonic development (Bignami et al., 1993a).
While lecticans, tenascins, and hyaluronan are expressed during embryonic development, the classic net-like structure of PNNs is not typically seen until later during postnatal development. It is unclear how these molecules are assembled to form PNN around neurons, but some studies suggest that both neurons and glial cells are involved in the process (Miyata et al., 2007; Pyka et al., 2011). Astrocytes extend their processes into PNNs localized near the synapse (Brückner et al., 1993; Blümcke et al., 1995), suggesting a tetrapartite synaptic organization involving ECM, pre/post synaptic sites and astrocytes (Dityatev and Rusakov, 2011; Smith et al., 2015) whose synergistic functions remain unexplored.
Parvalbumin Expressing Interneurons, PNNs and MMP-9
In the forebrain, PNNs are often found on PV expressing GABAergic neurons (Table 1). Other cell types, including putative excitatory neurons also contain PNNs but they are less numerous compared to GABAergic-PNN expressing cells (Wegner et al., 2003). Most studies detecting PNNs in the CNS use fluorescently coupled lectins, such as WFA and vicia villosa agglutinin, which bind N-acetylgalactosamine on chondroitin sulfate GAG side-chains of CSPGs (Nakagawa et al., 1986; Kosaka and Heizmann, 1989; Härtig et al., 1992). Immunolabeling using antibodies against specific PNN proteins, including aggrecan, tenascin and hyaluronan can also be used to visualize PNNs (Matthews et al., 2002; Dityatev et al., 2007; Giamanco et al., 2010; Yamada and Jinno, 2017). Studies comparing PNNs detected with lectins or specific antibodies indicate substantial levels of molecular heterogeneity (Matthews et al., 2002; Irvine and Kwok, 2018), highlighting the need for better approaches which address PNN heterogeneity (Irvine and Kwok, 2018). In the following discussion, most studies use lectin based detection of PNNs unless noted otherwise.
Parvalbumin cells are a major class of GABAergic, fast-spiking inhibitory interneurons in the neocortex. Cortical PV cells contribute to synchronous oscillatory activity (Cardin et al., 2009; Sohal et al., 2009; Uhlhaas et al., 2009), particularly in the gamma range (∼30–100 Hz). Like PNNs, PV expression is developmentally regulated and experience-dependent (Hensch, 2005). In fact, PV and PNN colocalization is correlated with critical period closure and reduced synaptic plasticity, particularly in sensory cortices (Nowicka et al., 2009; Beurdeley et al., 2012). A large proportion of PNN-expressing PV cells is found within layer 4 of neocortex (Table 1). Interestingly, developing auditory cortex appears to exhibit fewer PNN and PV expressing cells than visual or somatosensory cortex, but in adult sensory cortices, a majority of PV cells express PNNs as seen with WFA staining. PNNs may regulate PV cell functions (Hensch, 2005; Cabungcal et al., 2013; Bernard and Prochiantz, 2016), as PNN reorganization is shown to result in abnormal CNS development and altered neural excitability (Pizzorusso et al., 2002; Dityatev et al., 2007; Liu et al., 2013; Balmer, 2016; Lensjø et al., 2017).
MMP-9 is a zinc-dependent endopeptidase expressed in various cells within the CNS which is capable of cleaving ECM proteins, including collagen and laminin (reviewed in Reinhard et al., 2015). Peak MMP-9 expression is observed during postnatal development and is reduced during adulthood (Bednarek et al., 2009; Reinhard et al., 2015; Wen et al., 2017). High levels of MMP-9 contribute to proteolytic cleavage of ECM, creating an extracellular environment that is permissive for synaptic plasticity. As aggrecan has been previously identified as an MMP-9 target (Mercuri et al., 2000; Madsen et al., 2010; Rankin-Gee et al., 2015), aggrecan-rich PNNs may be unstable in the presence of MMP-9, leading to abnormal development and neural excitability (Murase et al., 2017; Wen et al., 2017). A recent study by Murase and colleagues demonstrated that PV, PNN, and MMP-9 interactions are important for driving adult plasticity (Murase et al., 2017). In this study, monocular deprivation followed by light exposure in adult mice was associated with reduced WFA-expressing PNNs, lower aggrecan levels, and increased MMP-9 activity, as well as reduced PV cell output, increased firing of regular-spiking cells, and reduced gamma power (Murase et al., 2017). These findings suggest a link between PNNs, MMP-9 activity and PV activity that shapes normal brain development. Conversely, abnormal interactions may lead to neurological disorders.
Fragile X Syndrome
Recently, we proposed a hypothesis for increased sensory hyperexcitability in Fragile X syndrome (FXS) through increased MMP-9 levels and abnormal PNN development (Figure 1). FXS is a leading genetic cause of autism, occurring in 1 in 4000 males and 1 in 8000 females (Murray et al., 1997). The most common cause of FXS is an expansion of CGG trinucleotide repeats in 5′ region of the Fragile X mental retardation 1 (Fmr1) gene that results in its hypermethylation and inactivation. This leads to loss of Fragile X Mental Retardation protein (FMRP), which is necessary for regulation of protein synthesis (Verkerk et al., 1991). Individuals with this neurodevelopmental disorder suffer from social and communication deficits, sensory hypersensitivity, and anxiety disorders (Abbeduto and Hagerman, 1997; Rogers et al., 2001). Auditory hypersensitivity is common in humans with FXS as measured by increased sound evoked responses, reduced habituation and expansion of brain regions activated by auditory stimuli (Rojas et al., 2001; Castrén et al., 2003; Van der Molen et al., 2012; Ethridge et al., 2016). These auditory processing deficits may drive social, cognitive and language delays and deficits in FXS (Rotschafer and Razak, 2014; Sinclair et al., 2017a,b).
Auditory hypersensitivity is also evident in Fmr1 knockout (KO) mice, a mouse model of FXS, which exhibit increased susceptibility to audiogenic seizures (Musumeci et al., 2000) and abnormal auditory startle (Frankland et al., 2004). In vivo electrophysiological studies demonstrate that neurons in adult Fmr1 KO mouse auditory cortex (1) are hyperexcitable, exhibiting increased responses per stimulus, (2) show more variable response latencies, and (3) display broader frequency tuning curves (Rotschafer and Razak, 2013). Recent work using EEG/ERP recordings shows that mature Fmr1 KO mouse auditory cortex exhibits reduced habituation to repeated sound presentations (Lovelace et al., 2016). When resting EEG is analyzed for spectral power distribution, Fmr1 KO mouse cortex shows increased gamma power. There is also enhanced N1 amplitude and reduced gamma phase locking in auditory ERPs (Lovelace et al., 2018). These phenotypes in the mouse model are remarkably similar to those seen in humans with FXS (Castrén et al., 2003; Ethridge et al., 2016; Wang et al., 2017). These results indicate that there is altered E/I balance in auditory cortex, which may underlie auditory hypersensitivity phenotypes. As PNNs are thought to be critical for maintaining E/I balance by modulating PV neuron activity, we tested the hypothesis that disruption of PNN development may underlie auditory hypersensitivity in FXS.
We found delayed PNN development and increased MMP-9 activity levels in the developing auditory cortex of Fmr1 KO mice (Wen et al., 2017), which may lead to impaired PV cell development and reduced inhibition. These phenotypes were mostly observed between P14 and P21 suggesting a window of abnormal development that can be targeted for therapeutic applications. At P14, the responses of single neurons were similar across genotypes, while at P21, Fmr1 KO neurons show hyper-responsiveness. Genetic reduction of MMP-9 levels in the Fmr1 KO mice normalized PV/PNN development and also reversed the hyperresponsiveness of auditory cortical neurons. These findings suggest that MMP-9-mediated cleavage of PNNs during development may underlie sensory hypersensitivity in FXS. Reduction of MMP-9 levels with minocycline in humans with FXS (Dziembowska et al., 2013) contributes to reversal of several FXS phenotypes, including behavioral deficits and enhanced auditory electrocortical activity in adults and/or adolescents with FXS (Paribello et al., 2010; Leigh et al., 2013; Schneider et al., 2013). Minocycline administration in Fmr1 KO mice prevents abnormal dendritic spine development in the hippocampus, improves anxiety-like behaviors (Bilousova et al., 2009; Dansie et al., 2013) and corrects ultrasonic vocalizations (Rotschafer et al., 2012). Genetic reduction of MMP-9 in Fmr1 KO mice normalizes PNN formation and prevents the development of neuronal hyperexcitability (Lovelace et al., 2016; Wen et al., 2017), emphasizing the therapeutic potential of targeting MMP-9 and PNN in treatment of FXS.
In addition to regulating cortical development, MMP-9 cleavage of PNNs may drive changes in PV expression and/or PV cell functions seen in FXS. The somatosensory cortex of adult FXS mice exhibit delayed PV cell maturation (Nomura et al., 2017). In developing mice, PV interneurons receive reduced excitatory drive from neighboring pyramidal cells in layer 4 of somatosensory cortex (Gibson et al., 2008). PV cell maturation is characterized by gradual increases in signaling, including faster firing rates and IPSC kinetics (Lazarus and Huang, 2011). An orthodenticle homeoprotein, Otx2, may play a role in PV cell maturation and refinement of cortical inhibition. Otx2 expression coincides with both PV and PNN expression in the cortex during critical period plasticity (Sugiyama et al., 2008). Studies of rodent visual cortex development indicate that Otx2 is localized to GABAergic cells, and is more likely found in PV cells that are enwrapped with PNNs, which contain a specific Otx2 binding site (Beurdeley et al., 2012). PNNs are necessary for the uptake of Otx2 by PV cells, which promotes PV cell maturation (Beurdeley et al., 2012). RNA sequencing analysis of PV cells containing Otx2 indicates that Otx2 may be necessary for mitochondrial function, cellular respiration, and redox regulation (Sakai et al., 2017). These data suggest that PNNs may drive PV cell maturation by directing transcription or translation of specific genes necessary for PV cell function. In FXS, delayed PNN expression around PV cells during development may contribute to abnormal PV cell maturation.
Network excitability in FXS may also result from impaired synaptic scaling at PV cells due to PNN dysfunction. There is evidence for a role of PNNs in mediating synaptic scaling via neuronal activity-regulated pentraxin, also known as Narp. Narp can interact with the N-terminal domain of AMPA receptors localized extracellularly and facilitate AMPA receptor clustering at both the pre- and post-synaptic cell in response to stimulation (O’Brien et al., 1999). Studies by Chang et al. (2011) in developing mouse hippocampus demonstrated that (1) Narp was highly expressed at excitatory synapses on PV interneurons, (2) PNN expression facilitated Narp expression (and conversely PNN degradation contributed to reduced Narp levels), and (3) Narp expression at PV interneurons was necessary for increased AMPA receptor-mediated mEPSC amplitude in response to stimulation both in vitro and in vivo. This suggests that during development, sensory stimuli can activate Narp expression which promotes PV interneuron excitation, leading to feed-forward inhibition (O’Brien et al., 1999; Gu et al., 2013). In FXS, delayed development of PNNs may contribute to abnormal Narp expression, impairing the ability of PV cells to scale cortical networks, resulting in cortical hyperexcitability.
Schizophrenia
One mechanism that has been proposed to explain some symptoms of schizophrenia is a reduction in GABAergic signaling in specific cortical regions. This is evident from a number of studies characterizing GABAergic cells using antibodies which target GABA-synthesizing enzymes glutamate decarboxylase 65 and 67 (GAD65 and GAD67) in brain tissues from schizophrenia patients. In situ hybridization and RT-PCR studies indicate that post-mortem brain tissues from schizophrenia patients exhibit reduced PV and/or GAD67 mRNA, particularly in prefrontal cortex (Akbarian et al., 1995; Volk et al., 2000; Hashimoto et al., 2003, 2008a,b; Mellios et al., 2009). Reduced PV and GAD67 protein levels are also seen in both prefrontal and temporal cortex of post-mortem brain tissues (Beasley and Reynolds, 1997; Curley et al., 2011; Glausier et al., 2014). Cortical gamma band oscillations have been attributed to GABAergic cell activity, in particular PV interneurons (Sohal et al., 2009). Increased gamma-band power was observed in baseline EEG recordings from humans with schizophrenia (Gandal et al., 2012). In contrast, gamma-band activity that is driven by rapid presentation of auditory stimuli and synchrony or phase-locking of electrocortical activity to these stimuli are reduced in schizophrenic patients compared to healthy controls (Kwon et al., 1999; Light et al., 2006). These data suggest altered PV cell function in schizophrenia, but why these cells are susceptible is unclear.
Redox dysregulation has been observed in schizophrenic patients, who have defective antioxidant systems and exhibit increased lipid peroxidation (Do et al., 2000; Yao et al., 2006; Flatow et al., 2013). Genetic analysis of single nucleotide polymorphisms in schizophrenia patients demonstrates mutations in the gene encoding GCL, an enzyme that is important for the production of the cellular antioxidant GSH (Tosic et al., 2006). PV cells are particularly susceptible to oxidative stress given their fast cellular metabolism and increased mitochondrial function (Hasenstaub et al., 2010; Kann et al., 2014; Inan et al., 2016). Recent studies show that PNNs may protect PV cells from oxidative stress (Cabungcal et al., 2013; Morishita et al., 2015), and thus serve as a ‘safety net’ to support the rapid firing properties of PV cells. Cabungcal and colleagues analyzed the levels of oxidative stress in PV cells with and without PNNs in GCL KO mice, a mouse model of schizophrenia, by measuring the levels of 8-hydroxy 2 deoxyguanosine (8-oxo-dG). During development, GCL KO mice had fewer PNN-expressing PV cells in anterior cingulate cortex, and the injection of a dopamine reuptake inhibitor to promote more oxidative stress via buildup of reactive oxygen species contributed to increased 8-oxo-dG signal and PV cell loss. Further analysis indicated that while PV neurons with PNNs were less susceptible to oxidative stress, chABC-mediated degradation of PNNs made PV cells more vulnerable (Cabungcal et al., 2013). As chABC removes GAG side chains from CSPGs (Bradbury et al., 2002), these results suggest that the neuroprotective effects of PNNs may be attributed to the CS-side chains of CSPGs. Specifically, in vitro studies conducted in human neuroblastoma SH-SY5Y cells indicate that chondroitin sulfate application prior to exposure to oxidative-stress inducing agents, such as hydrogen peroxide, can prevent cell death and attenuate the production of reactive oxygen species. Analysis of molecular mechanisms indicates that chondroitin sulfate promotes activation of protein kinase pathways including PKC and PI3K/Akt, and subsequent production of a neuronal antioxidant, heme oxygenase-1 (Cañas et al., 2007).
Perineuronal net dysfunction in schizophrenia may also drive changes in GABAergic cell function leading to network dysfunction, as normal PNN expression regulates excitability of PV cells (Liu et al., 2013; Balmer, 2016). Härtig et al. (1999) speculated that the large, negatively charged sugar chains, which are enriched in PNNs, might allow for buffering of sodium and potassium ions at synapses. While this mechanism has not been confirmed, chABC-mediated removal of chondroitin sulfate side chains from PNNs reduced spiking of PV cells in adult rats (Balmer, 2016; Lensjø et al., 2017) and increased spiking variability (Lensjø et al., 2017). In addition, brevican conditional knockdown in PV-expressing cells demonstrated that changes in the expression of specific lecticans alter synaptic input and firing frequency in PV cells (Favuzzi et al., 2017). These findings lend support to the idea that PNN composition plays an important role in regulating PV cell function.
As PNNs regulate GABAergic cell function and oxidative stress levels, cortical abnormalities observed in schizophrenia may be attributed to PNN dysfunction (reviewed in Berretta, 2012; Berretta et al., 2015). Indeed, several studies indicate that schizophrenia is associated with the loss of PNNs. Post-mortem brains from patients with schizophrenia exhibit reduced density of WFA-labeled cells (Mauney et al., 2013) and reduced levels of CSPGs and PV expression in prefrontal cortex (Enwright et al., 2016). A mouse model of schizophrenia also exhibits reduced PNN levels in prefrontal cortex (Cabungcal et al., 2014). In this model, schizophrenia-like abnormalities are reported in the adult mouse prefrontal cortex as a result of neonatal lesion of ventral hippocampus. The effects of dysregulated dopamine signaling on prefrontal cortex oscillatory activity, which are suggested to drive schizophrenia phenotypes, can be also regulated by PNNs. For example, network oscillatory activity that was increased by drug-induced changes in dopaminergic signaling in mouse prefrontal cortex was further enhanced following chABC-mediated degradation of PNNs (Steullet et al., 2014).
Recent studies have hinted at the potential role of MMP-9 in the manifestation of schizophrenia phenotypes. A functional polymorphism of the MMP-9 gene was discovered in human schizophrenia patients (Rybakowski et al., 2009; Lepeta et al., 2017). Additionally, Yamamori et al. (2013) showed elevated MMP-9 levels in the plasma of schizophrenic patients. Various clinical trials have been conducted using minocycline alone or as an adjunct to risperidone in patients with schizophrenia and have demonstrated overall improvement in negative symptoms and executive functioning (Miyaoka et al., 2008; Levkovitz et al., 2010; Khodaie-Ardakani et al., 2014). Beside its antibiotic activity, minocycline can reduce plasma levels of MMP-9 (Dziembowska et al., 2013) and inhibit MMP-9 activity (Lee et al., 2006), suggesting a possible role of MMP-9 inhibition in the beneficial effects of minocycline in schizophrenia. Clearly additional studies are needed to test whether elevated MMP-9 levels do indeed drive PNN changes observed in prefrontal cortex of schizophrenia mouse models and how it relates to the human condition. Future studies can help to determine whether ECM abnormalities underlie the changes in GABAergic signaling and redox dysregulation associated with schizophrenia.
Seizures and Epilepsy
Epilepsy occurs unpredictably and affects people of all ages, although onset commonly occurs in childhood (Hauser, 1992). It is idiopathic and can include generalized seizures, which involve epileptiform activity emanating from the entire cortex, as well as focal or partial seizures, which result from epileptiform activity from a single part of the cortex (Hauser, 1992). The molecular mechanisms underlying seizure onset include impaired PV cell functions. Analysis of post-mortem human brain tissues demonstrated reduced PV cell density and immunoreactivity at epileptic foci in the hippocampus and neocortex (Sloviter et al., 1991; Ferrer et al., 1994; Andrioli et al., 2007). Genomic sequencing data indicate that a number of mutations in the Scn1a gene contribute to a spectrum of different epileptic disorders affecting children, including de novo (Claes et al., 2001; Fujiwara et al., 2003) as well as missense, frame-shift, and nonsense mutations (Fujiwara et al., 2003). Scn1a is a gene that encodes Nav1.1, a sodium channel necessary for PV cell firing (Ogiwara et al., 2007; Hedrich et al., 2014). Recent studies demonstrate that reduced PV cell spike output may contribute to seizures in mice with genetic knock-in of mutated human Scn1a, which results in subtle changes in Nav1.1 functionality (Ogiwara et al., 2007; Hedrich et al., 2014). Several rodent models of epilepsy, which use drugs to induce seizures, also shed some light on the role of PV cells. For example, PV KO mice are more likely to experience status epilepticus compared to controls following increased doses of pentylenetetrazol (PTZ), a GABAA receptor blocker (Schwaller et al., 2004).
As PNNs regulate PV cell activity (Balmer, 2016; Lensjø et al., 2017) and protect PV cells from oxidative stress (Cabungcal et al., 2013), PNNs can be potentially targeted to control seizures. Recent work has reported changes in PNN integrity in epilepsy. Drug-induced seizures led to increased expression of aggrecan cleavage products around PV cells and reduced expression of PNN components in rodent hippocampus, including Ctrl1 and hyaluronan synthase 3, both of which help to stabilize PNNs (McRae and Porter, 2012; Rankin-Gee et al., 2015). Drug-induced seizures increased unbound CSPG levels and decreased the levels of CSPGs associated with PNNs around PV cells, which were detected with WFA in cerebral cortex (Yutsudo and Kitagawa, 2015). In rodent piriform cortex, kindling-induced seizures caused PNN degradation and increased GABAergic synapses, contributing to the rewiring of cortical networks (Pollock et al., 2014). Moreover, PNN breakdown induced by seizures was at least partially facilitated by increased enzymatic activity of MMPs (Kim et al., 2009; Rankin-Gee et al., 2015), and seizure susceptibility was reduced in MMP-9 KO mice (Wilczynski et al., 2008). This suggests that PNN breakdown via MMP-9 following seizure induction contributes to network rewiring, leading to pathological hyperexcitability. Future studies should explore this PNN loss-hyperexcitability connection further and determine whether there would be therapeutic benefit to MMP inhibition and PNN restoration in epilepsy.
CNS Injury and Stroke
In CNS injury and stroke, re-establishing neuronal plasticity appears to be key for re-innervation and recovery. A full summary of this literature is outside the scope this review, so only a few exemplars are mentioned to make the point that understanding the contributions of PNN and other ECM molecules may be critical in developing treatments, because the CSPGs present in PNNs appear to inhibit axonal growth (Kwok et al., 2011). In rat spinal cord injury models, increased concentrations of CSPGs and PNN expression were found at injury sites and surrounding secondary dorsal column relay neurons (Massey et al., 2008). When sensory axons were transplanted into adult rats with dorsal column lesions, neurons were able to sprout and extend their processes to additional denervated areas, but failed to penetrate the original lesion sites, which lead to denervation of upstream pathways and inactivation of parts of somatosensory cortex (Davies et al., 1999; Kaas et al., 2008). CSPG degradation via application of chABC promoted regeneration of dorsal column axons after injury. This not only resulted in an increase in terminals from initially injured afferents, but also contributed to expanded receptive fields of uninjured primary afferents (Bradbury et al., 2002; Massey et al., 2006).
Studies in stroke models indicate that injury onset leads to two processes. On the one hand, focal cortical stroke in rats contributes to upregulation of a number of CSPG-encoding genes particularly near the infarct and glial scar. RT-PCR analysis of the affected tissue indicated upregulation of neurocan, which peaked on the third day following middle cerebral artery occlusion, as well as versican and brevican, both of which peaked by day 28 (Carmichael et al., 2005). In contrast, in cortical areas surrounding the infarct and outside of the glial scar, WFA-expressing PNNs are completely degraded by day 7, and CSPG immunoreactivity is down regulated although they remain detectable up to 14 days post-injury (Hobohm et al., 2005). Similar degradation of WFA-expressing PNNs in the neocortex has also been observed following thromboembolic middle cerebral artery occlusion in rats, permanent middle artery occlusion in mice, and focal cerebral ischemia in sheep (Härtig et al., 2017). Interestingly, reduced PV-immunoreactivity and PV cell function have also been observed following stroke and this decrease in inhibitory cell function may underlie epilepsy-like activity following stroke (Hobohm et al., 2005; Xie et al., 2014). As CSPGs inhibit growth, these findings indicate that spinal cord injury, stroke and/or traumatic brain injury may contribute to ECM reorganization, allowing neuronal growth and plasticity in areas free from damage and inhibiting growth where injury-induced cellular damage is more severe (Hobohm et al., 2005; Harris et al., 2010). MMP-9 may be responsible for this ECM reorganization as a number of studies have demonstrated increased MMP-9 levels following CNS injury and stroke in humans as well as animal models (Zhang et al., 2011; Chaturvedi and Kaczmarek, 2014; Liu et al., 2014).
Alzheimer’S Disease
Alzheimer’s disease is associated with memory impairment, which may be attributed to intracellular buildup of neurofibrillary tangles and the accumulation of extracellular beta-amyloid plaques in hippocampus and cortex. This may lead to hippocampal and cortical hyperexcitability as well as increased seizure susceptibility in both AD humans and rodent models (Palop et al., 2007; Roberson et al., 2011). Post-mortem brains of AD patients exhibit reduced expression of PNNs, particularly in the hippocampus (Baig et al., 2005; Lendvai et al., 2013). Neurons that exhibit buildup of tau protein and neurofibrillary tangles are more likely to lack PNNs (Brückner et al., 1999; Morawski et al., 2010). Direct support for the neuroprotective effects of PNNs from AD-related pathology was demonstrated in rodent cortical neurons in vitro. These experiments showed that PNN-ensheathed cells were more resistant to cell death when neurons were incubated with exogenous monomeric Aβ1-42 peptide for 48 h. More cells died when pretreated with chABC (Miyata et al., 2007). The neuroprotective abilities of PNNs may stem from their ability to act as a barrier for oxidative stress-inducing molecules, such as metal ions. The molecules can react with free radicals generated by cellular metabolism to generate even more free radicals, resulting in lipid peroxidation, oxidative stress, and cell death (Stohs and Bagchi, 1995). Indeed, cortical cells expressing PNNs exhibited accumulation of iron in post-fixed brain tissues obtained from both AD and normally aged controls (Reinart et al., 2003). Accumulation of lipofuscin, a byproduct of iron-catalyzed oxidative stress, was also observed in cells without PNN (Morawski et al., 2004). These results indicate that cells without PNNs are more susceptible to cell death (Morawski et al., 2004). Similar results have been obtained in AD rodent models, which rely on bilateral or unilateral microinjection of iron chloride into mouse cortex to mimic AD-related oxidative stress. While iron-induced oxidative stress resulted in apoptotic cell death in cells without PNNs, neurons with PNNs were more likely to survive (Suttkus et al., 2012, 2014). Genetic reduction of aggrecan, which has the highest number of CS-side chains and presumably the largest negative charge, resulted in reduced neuroprotective effects (Suttkus et al., 2014). These results lend further support to the idea that the CS-side chains of PNNs mediate its neuroprotective effects.
Perineuronal net loss in AD may contribute to altered E/I balance (Palop et al., 2007; Sun et al., 2009), synaptic loss (Scheibel and Tomiyasu, 1978; Ruiter and Uyling, 1987; Hoover et al., 2010) and increased susceptibility to oxidative stress (Deibel et al., 1996; Varadarajan et al., 2000; Manczak et al., 2006; De Felice et al., 2007). Interestingly, some studies suggest that MMP-9 may be a key molecular target in treating AD, as MMP-9 is capable of cleaving Aβ in vitro (Backstrom et al., 1996), and plasma levels of MMP-9 are elevated in AD patients (Backstrom et al., 1996; Lorenzl et al., 2003). As MMP-9 is also involved in PNN maintenance, elevated MMP-9 levels may contribute to both PNN degradation and altered levels of Aβ in AD.
Hearing Loss and Central Auditory Processing
Recent studies suggest that loss of PNNs around PV cells occurs following noise-induced and age-related hearing loss. Overexposure to loud sounds can cause hair cell damage (Puel et al., 1998), hearing loss and reduced afferent input in the auditory system (Syka, 2002). Noise-induced hearing loss leads to increased excitability (gain) in the central auditory system, but the cellular mechanisms are not clear. PNNs are impaired following noise induced hearing loss, even within 1 day after noise exposure. Specifically, PNN intensity is reduced in layers 1–4 of auditory cortex of adult CBA mice 1, 10, and 30 days post-noise exposure. In layers 5–6, PNN intensity decreases 10 days post-noise exposure (Nguyen et al., 2017). Reduced PNN intensity may be a result of increased cleavage or degradation of various PNN components, such as CSPGs, hyaluronan, or link proteins. In brainstem auditory nuclei of mice, genetic loss of brevican contributes to loss of brevican-expressing PNNs as well as reduced sound-evoked firing rates and increased neuronal response thresholds (Blosa et al., 2015). chABC-mediated degradation of PNNs around PV cells in brainstem and central auditory neurons has similar effects, resulting in reduced PV cell excitability and gain (Balmer, 2016). These results indicate that PNNs may be necessary for neuronal excitability throughout the auditory pathway. Understanding the auditory system’s ability to control excitability as a result of exposure to traumatic sounds and the potential role of PNNs in regulating this process may help to characterize molecular targets in treatment of noise induced hearing loss and tinnitus.
Age-related hearing loss, also known as presbycusis, is associated with difficulty interpreting speech and slower auditory processing (Gates and Mills, 2005) which may lead to social isolation and cognitive decline. Hearing aids amplify sounds, but may not improve speech processing, suggesting central auditory system changes. Neuronal response properties susceptible to presbycusis include frequency selectivity, spontaneous activity levels and spectrotemporal selectivity (Trujillo and Razak, 2013). GABAergic signaling is involved in shaping these properties, with fast-spiking PV cells shaping both spectral and temporal selectivity (Moore and Wehr, 2012). Indeed, several studies have demonstrated reduced GABAergic signaling in the aged auditory system of rodents and humans (Caspary et al., 1990; Ouda et al., 2008; Martin del Campo et al., 2012; Gao et al., 2015).
Perineuronal nets may play a major role in PV neuron susceptibility and altered cortical processing in presbycusis. The number of PNN expressing cells is reduced in aging mouse auditory cortex with a particular impact on PV/PNN co-localized cells (Brewton et al., 2016). As PNNs protect PV cells from oxidative stress, these data indicate that PNN downregulation with age may make PV cells more susceptible to cell death. Altered PNN formation may contribute to abnormal GABAergic signaling and impaired temporal processing with age, leading to deficits in speech comprehension. Additional studies are required to identify the specific components of PNNs that are altered in noise- and age-related hearing loss.
Conclusion and Future Studies
Accumulating evidence suggests that PNN loss is associated with a number of neurological disorders. PNNs are also implicated in experience-dependent plasticity during development. In fact, activity-dependent synaptogenesis and the development of stable neuronal networks may be a result of normal PNN interactions with inhibitory interneurons, in particular fast spiking PV cells. Despite the evidence for developmental and pathological changes in PNNs, their precise role in network function and development is not known. Additional studies are needed that compare intrinsic and synaptic properties of the same cell types with and without PNNs. While the use of chABC has been useful in addressing PNN function, this enzyme likely has broader effects. Additional methods such as genetic removal of specific PNN components are needed.
Considering the prominent role of PNNs in many processes within the CNS, more focus should be placed on characterizing the mechanisms of PNN regulation in the healthy and diseased brain. Some of the work reviewed here indicates that PNNs are necessary for PV cell function, yet in several brain areas not all PV cells are ensheathed by PNNs as labeled with WFA (Table 1). PNNs are heterogeneous structures (Ueno et al., 2017b; Yamada and Jinno, 2017; Irvine and Kwok, 2018), and PNN composition may be brain region- and developmental age-specific. For instance, in the rodent hippocampus, aggrecan-expressing PNNs overlap with WFA labeling in stratum oriens, but stratum pyramidale exhibits aggrecan-rich PNNs, which are not WFA-positive (Yamada and Jinno, 2017). PNNs containing aggrecan and brevican do not always overlap in mouse somatosensory cortex (Galtrey et al., 2008; Ueno et al., 2017b). Tenascin-R expressing PNNs are not observed in rodent PFC, but are prominent in other cortical areas (Ueno et al., 2017a). These findings suggest that future work characterizing other PNN components such as hyaluronan (Oohashi et al., 2015) will be helpful in understanding PNN development and re-organization in health and disease. New models that allow for the examination of PNN formation and restructuring in the living brain will contribute to a better understanding of its role in circuit function. Genetic approaches to visualize and disassemble PNNs will also be very important moving forward.
Mechanisms of PNN loss and restructuring in disease are still not clear. One of the ECM modifying enzymes, MMP-9, has been hypothesized to play a role in pathophysiology of schizophrenia, AD and FXS. As MMP-9 is capable of cleaving ECM, elevated MMP-9 levels can drive PNN loss and re-organization. As there is evidence for abnormal MMP-9 regulation in FXS, schizophrenia and AD as well as altered PNNs in presbycusis and acoustic trauma models, studies of how MMP-9 is involved in PNN regulation are crucial for the development and validation of future therapeutics. Recent studies indicate that minocycline administration may reverse both schizophrenia and FXS phenotypes, perhaps due to its ability to lower MMP-9 levels or activity (Dziembowska et al., 2013). As MMP-9 cleaves PNN components, perhaps the use of more specific MMP-9 inhibitors may yield higher efficacy in treatment of these and other neurological disorders.
While we have focused on the potential role of MMP-9 in regulating PNNs and neural excitability in disease pathology, the ADAMTs family of aggrecanases may also cause PNN cleavage and reorganization. Indeed, several ADAMTs are upregulated following spinal cord injury, stroke, and epilepsy (Lemarchant et al., 2013; Kim et al., 2016). In addition, a number of molecules can regulate MMP-9 activity itself, including tissue inhibitors of MMPs, known as TIMPs, which form 1:1 complexes with MMPs in order to inhibit MMP activity (Gomis-Rüth et al., 1997; Brew and Nagase, 2010). TIMP levels are increased in postmortem brains of AD patients (Peress et al., 1995), and may be indicators for risk of stroke mortality (Hansson et al., 2011). Schizophrenia is associated with higher MMP-9/TIMP1 ratios compared to healthy controls (Rahimi et al., 2017). Future work characterizing the expression of other MMPs in disease pathology may also be useful as a number of MMPs can directly or indirectly activate MMP-9, including MMP-7, MMP-3, and MMP-14. Quantitative PCR analysis indicates that transcripts from MMP-9, 3, 7, and 14 are upregulated in rodent models of spinal cord injury (Zhang et al., 2011). Understanding how other MMP-9 and PNN modifying enzymes are regulated may contribute to the development of new targets in treating neurological disorders.
As PNNs may play a major role in disease pathology, the development of therapeutic interventions, which target these structures, may be key in restoring neuronal excitability and cortical function. For instance, recent findings in rodent stroke models indicate that rats housed in enriched environments following ischemic damage exhibited faster neurological recovery compared to rats placed in standard housing (Madinier et al., 2014; Quattromani et al., 2018). Quatrromani and colleagues suggest that this phenomenon may be attributed to sensorimotor stimulation which results in increased protease activity and reduced expression of PNNs. As the absence of PNNs is associated with increased plasticity (Pizzorusso et al., 2002; Nowicka et al., 2009), this may allow for axonal outgrowth and synaptogenesis, particularly in surviving cortical areas, which exhibit reduced CSPG and PNN expression and are therefore growth permissive (2014). These findings suggest that comparable human treatments, which target PNNs specifically, might involve non-invasive brain stimulation or combination brain stimulation/protease inhibitor treatments. While brain stimulation techniques are already currently utilized to treat neurological disorders (Cirillo et al., 2017), understanding how PNNs are modified following stimulation in rodents would lend support for use of this treatment paradigm, particularly in disorders involving abnormal PNNs.
While we do not emphasize inflammatory response pathways in the present review, these may be crucial in understanding MMP-9 dysregulation in CNS disorders as there is evidence for neuro-inflammation in injury models, AD, epilepsy, and schizophrenia (Papa et al., 2014). Cirillo and colleagues suggest that following insult, reactive gliosis triggers activation of MMPs and maladaptive plasticity, perhaps due to increased degradation of neurotrophins such as NGF (De Luca et al., 2016; Cirillo et al., 2016). Neurotrophins are necessary for neuronal growth and plasticity, and increased MMP-9 activity has been shown to degrade mature NGF (Bruno and Cuello, 2006). Moreover, recent work in peripheral nerve injury models indicates that co-treatment with MMP-9 inhibitors and NGF peptide following insult can restore synaptic homeostasis, i.e., normalize glutamate/GABA ratio and expression of glial amino acid transporters (De Luca et al., 2016; Cirillo et al., 2016). Unlike FXS, where MMP-9 upregulation can likely be attributed to loss of the Fmr1 gene (Janusz et al., 2013), these findings provide important mechanistic insight for how MMP-9 dysregulation might arise in other CNS diseases. Whether NGF downregulation alters PNN expression remains to be studied. However, if NGF does play a role, this has important implications for the development of new PNN-targeting therapies.
Author Contributions
TW reviewed the literature and wrote the manuscript. IE, DB, and KR were involved in writing and editing the manuscript.
Funding
Research on PV/PNN in FXS was funded by NIH grant U54 HD082008 to IE, KR, and DB.
Conflict of Interest Statement
The authors declare that the research was conducted in the absence of any commercial or financial relationships that could be construed as a potential conflict of interest.
The reviewer GC and handling Editor declared their shared affiliation.
Acknowledgments
We thank members of IE, KR, and DB labs for valuable inputs on ideas presented in this review.
Abbreviations
AD, Alzheimer’s disease; ADAMTs, a disintegrin and metalloproteinase with thrombospondin motif; chABC, chondroitinase ABC; CNS, central nervous system; CSPG, chondroitin sulfate proteoglycan; E, embryonic; E/I, excitatory/inhibitory; ECM, extracellular matrix; EEG, electroencephalography; FXS, Fragile X Syndrome; GAD, glutamate decarboxylase; GAG, glycosaminoglycan; GCL, glutamate cysteine ligase; GSH, glutathione; HAS, hyaluronan synthase; MMP-9, matrix metalloproteinase-9; NGF, nerve growth factor; Otx2, orthodenticle homeoprotein 2; P, postnatal; PNN, perineuronal net; PV, parvalbumin; TIMPs, tissue inhibitor of MMPs; WFA, wisteria floribunda agglutinin.
References
Abbeduto, L., and Hagerman, R. (1997). Language and communication in fragile X syndrome. Dev. Disabil. Res. Rev. 3, 313–322. doi: 10.1002/(SICI)1098-2779(1997)3:4<313::AID-MRDD6>3.0.CO;2-O
Akbarian, S., Kim, J. J., Potkin, S. G., Hagman, J. O., Tafazzoli, A., Bunney, W. E. Jr., et al. (1995). Gene expression for glutamic acid decarboxylase is reduced without loss of neurons in prefrontal cortex of schizophrenics. Arch. Gen. Psychiatry 52, 258–266. doi: 10.1001/archpsyc.1995.03950160008002
Andrioli, A., Alonso-Nanclares, L., Arellano, J. I., and DeFelipe, J. (2007). Quantitative analysis of parvalbumin-immunoreactive cells in the human epileptic hippocampus. Neuroscience. 149, 131–143. doi: 10.1016/j.neuroscience.2007.07.029
Aspberg, A., Miura, R., Bourdoulous, S., Shimonaka, M., Heinegård, D., Schachner, M., et al. (1997). The C-type lectin domains of lecticans, a family of aggregating chondroitin sulfate proteoglycans, bind tenascin-R by protein– protein interactions independent of carbohydrate moiety. Proc. Natl. Acad. Sci. U.S.A. 94, 10116–10121. doi: 10.1073/pnas.94.19.10116
Backstrom, J. R., Lim, G. P., Cullen, M. J., and Tökés, Z. A. (1996). Matrix metalloproteinase-9 (MMP-9) is synthesized in neurons of the human hippocampus and is capable of degrading the amyloid-β peptide (1–40). J. Neurosci. 16, 7910–7919. doi: 10.1523/JNEUROSCI.16-24-07910.1996
Baig, S., Wilcock, G. K., and Love, S. (2005). Loss of perineuronal net N-acetylgalactosamine in Alzheimer’s Disease. Acta Neuropathol. 110, 393–401. doi: 10.1007/s00401-005-1060-2
Balmer, T.S. (2016). Perineuronal nets enhance the excitability of fast-spiking neurons. eNeuro 3, ENEURO.0112-16.2016. doi: 10.1523/ENEURO.0112-16.2016
Beasley, C. L., and Reynolds, G. P. (1997). Parvalbumin-immunoreactive neurons are reduced in the prefrontal cortex of schizophrenics. Schizophr. Res. 24, 349–355. doi: 10.1016/S0920-9964(96)00122-3
Bednarek, N., Clément, Y., Lelièvre, V., Olivier, P., Loron, G., Garnotel, R., et al. (2009). Ontogeny of MMPs and TIMPs in the murine neocortex. Pediatr. Res. 65, 296–300. doi: 10.1203/PDR.0b013e3181973aee
Berardi, N., Pizzorusso, T., and Maffei, L. (2004). Extracellular matrix and visual cortical plasticity: freeing the synapse. Neuron 44, 905–908. doi: 10.1016/j.neuron.2004.12.008
Bernard, C., and Prochiantz, A. (2016). Otx2-PNN interaction to regulate cortical plasticity. Neural Plast. 2016:7931693. doi: 10.1155/2016/7931693
Berretta, S. (2012). Extracellular matrix abnormalities in schizophrenia. Neuropharmacology 62, 1584–1597. doi: 10.1016/j.neuropharm.2011.08.010
Berretta, S., Pantazopoulos, H., Markota, M., Brown, C., and Batzianouli, E. T. (2015). Losing the sugar coating: potential impact of perineuronal net abnormalities on interneurons in schizophrenia. Schizophr. Res. 167, 18–27. doi: 10.1016/j.schres.2014.12.040
Beurdeley, M., Spatazza, J., Lee, H. H., Sugiyama, S., Bernard, C., Di Nardo, A. A., et al. (2012). Otx2 binding to perineuronal nets persistently regulates plasticity in the mature visual cortex. J. Neurosci. 32, 9429–9437. doi: 10.1523/JNEUROSCI.0394-12.2012
Bignami, A., Hosley, M., and Dahl, D. (1993a). Hyaluronic acid and hyaluronic acid-binding proteins in brain extracellular matrix. Anat. Embryol. 188, 419–433.
Bignami, A., Perides, G., and Rahemtulla, F. (1993b). Versican, a hyaluronate-binding proteoglycan of embryonal precartilaginous mesenchyma, is mainly expressed postnatally in rat brain. J. Neurosci. Res. 34, 97–106.
Bilousova, T. V., Dansie, L., Ngo, M., Aye, J., Charles, J. R., Ethell, D. W., et al. (2009). Minocycline promotes dendritic spine maturation and improves behavioural performance in the fragile X mouse model. J. Med. Genet. 46, 94–102. doi: 10.1136/jmg.2008.061796
Blosa, M., Sonntag, M., Jäger, C., Weigel, S., Seeger, J., Frischknecht, R., et al. (2015). The extracellular matrix molecule brevican is an integral component of the machinery mediating fast synaptic transmission at the calyx of Held. J. Physiol. 593, 4341–4360. doi: 10.1113/JP270849
Blümcke, I., Eggli, P., and Celio, M. R. (1995). Relationship between astrocytic processes and “perineuronal nets” in rat neocortex. Glia 15, 131–140. doi: 10.1002/glia.440150205
Bradbury, E. J., Moon, L. D., Popat, R. J., King, V. R., Bennett, G. S., Patel, P. N., et al. (2002). Chondroitinase ABC promotes functional recovery after spinal cord injury. Nature 416, 636–640. doi: 10.1038/416636a
Brauer, K., Brückner, G., Leibnitz, L., and Werner, L. (1984). Structural and cytochemical features of perineuronal glial nets in the rat brain. Acta Histochem. 74, 53–60. doi: 10.1016/S0065-1281(84)80026-4
Brauer, K., Werner, L., and Leibnitz, L. (1982). Perineuronal nets of glia. J. Brain Res. 23, 701–708.
Brew, K., and Nagase, H. (2010). The tissue inhibitors of metalloproteinases (TIMPs): an ancient family with structural and functional diversity. Biochim. Biophys. Acta 1803, 55–71. doi: 10.1016/j.bbamcr.2010.01.003
Brewton, D. H., Kokash, J., Jimenez, O., Pena, E. R., and Razak, K. A. (2016). Age-related deterioration of perineuronal nets in the primary auditory cortex of mice. Front. Aging Neurosci. 8:270. doi: 10.3389/fnagi.2016.00270
Brückner, G., Brauer, K., Härtig, W., Wolff, J. R., Rickmann, M. J., Derouiche, A., et al. (1993). Perineuronal nets provide a polyanionic, glia-associated form of microenviornment around certain neurons in many parts of the rat brain. Glia 8, 183–200. doi: 10.1002/glia.440080306
Brückner, G., Grosche, J., Schmidt, S., Härtig, W., Margolis, R. U., Delpech, B., et al. (2000). Postnatal development of perineuronal nets in wild type mice and in a mutant deficient in tenascin R. J. Comp. Neurol. 428, 616–629. doi: 10.1002/1096-9861(20001225)428:4<616::AID-CNE3>3.0.CO;2-K
Brückner, G., Hausen, D., Härtig, W., Drlicek, M., Arendt, T., and Brauer, K. (1999). Cortical areas abundant in extracellular matrix chondroitin sulphate proteoglycans are less affected by cytoskeletal changes in Alzheimer’s disease. Neuroscience 92, 719–805. doi: 10.1016/S0306-4522(99)00071-8
Bruno, M. A., and Cuello, A. C. (2006). Activity-dependent release of precursor nerve growth factor, conversion to mature nerve growth factor, and its degradation by a protease cascade. Proc. Natl. Acad. Sci. U.S.A. 103, 6735–6740. doi: 10.1073/pnas.0510645103
Cabungcal, J. H., Counotte, D. S., Lewis, E., Tejeda, H. A., Piantadosi, P., Pollock, C., et al. (2014). Juvenile antioxidant treatment prevents adult deficits in a developmental model of schizophrenia. Neuron 83, 1073–1084. doi: 10.1016/j.neuron.2014.07.028
Cabungcal, J. H., Steullet, P., Morishita, H., Kraftsik, R., Cuenod, M., Hensch, T. K., et al. (2013). Perineuronal nets protect fast-spiking interneurons against oxidative stress. Proc. Natl. Acad. Sci. U.S.A. 110, 9130–9135. doi: 10.1073/pnas.1300454110
Cañas, N., Valero, T., Villarroya, M., Montell, E., Vergés, J., García, A. G., et al. (2007). Chondroitin sulfate protects SH-SY5Y cells from oxidative stress by inducing heme oxygenase-1 via phosphatidylinositol 3-kinase/AKT. J. Pharmacol. Exp. Ther. 323, 946–953. doi: 10.1124/jpet.107.123505
Cardin, J. A., Carlén, M., Meletis, K., Knoblich, U., Zhang, F., Deisseroth, K., et al. (2009). Driving fast-spiking cells induces gamma rhythm and controls sensory responses. Nature 459, 663–667. doi: 10.1038/nature08002
Carmichael, S. T., Archibeque, I., Luke, L., Nolan, T., Momiy, J., and Li, S. (2005). Growth-associated gene expression after stroke: evidence for a growth-promoting region in peri-infarct cortex. Exp. Neurol. 193, 291–311. doi: 10.1016/j.expneurol.2005.01.004
Caspary, D. M., Raza, A., Lawhorn Armour, B. A., Pippin, J., and Arneriæ, S. P. (1990). Immunocytochemical and neurochemical evidence for age-related loss of GABA in the inferior colliculus: implications for neural presbycusis. J. Neurosci. 10, 2363–2372. doi: 10.1523/JNEUROSCI.10-07-02363.1990
Castrén, M., Pääkkönen, A., Tarkka, I. M., Ryynänen, M., and Partanen, J. (2003). Augmentation of auditory N1 in children with fragile X syndrome. Brain Topogr. 15, 165–171. doi: 10.1023/A:1022606200636
Celio, M. R., and Blümcke, I. (1994). Perineuronal nets—a specialized form of extracellular matrix in the adult nervous system. Brain Res. Brain Res. Rev. 19, 128–145. doi: 10.1016/0165-0173(94)90006-X
Celio, M. R., Spreafico, R., De Biasi, S., and Vitellaro-Zuccarello, L. (1998). Perineuronal nets: past and present. Trends Neurosci. 21, 510–515. doi: 10.1016/S0166-2236(98)01298-3
Chang, M. C., Park, J. M., Pelkey, K. A., Grabenstatter, H. L., Xu, D., Linden, D. J., et al. (2011). Narp regulates homeostatic scaling of excitatory synapses on parvalbumin-expressing interneurons. Nature 13, 1090–1097. doi: 10.1038/nn.2621
Chaturvedi, M., and Kaczmarek, L. (2014). MMP-9 inhibition: a therapeutic strategy in ischemic stroke. Mol. Neurobiol. 49, 563–573. doi: 10.1007/s12035-013-8538-z
Cirillo, G., Colangelo, A. M., De Luca, C., Savarese, L., Barillari, M. R., Alberghina, L., et al. (2016). Modulation of matrix metalloproteinases activity in the ventral horn of the spinal cord re-stores neuroglial synaptic homeostasis and neurotrophic support following peripheral nerve injury. PLoS One 11:e0152750. doi: 10.1371/journal.pone.0152750
Cirillo, G., Di Pino, G., Capone, F., Ranieri, F., Florio, L., Todisco, V., et al. (2017). Neurobiological after-effects of non-invasive brain stimulation. Brain Stimul. 10, 1–18. doi: 10.1016/j.brs.2016.11.009
Claes, L., Del-Favero, J., Ceulemans, B., Lagae, L., Van Broeckhoven, C., and De Jonghe, P. (2001). De novo mutations in the sodium-channel gene SCN1A cause severe myoclonic epilepsy of infancy. Am. J. Hum. Genet. 68, 1327–1332. doi: 10.1086/320609
Curley, A. A., Arion, D., Volk, D. W., Asafu-Adjei, J. K., Sampson, A. R., Fish, K. N., et al. (2011). Cortical deficits of glutamic acid decarboxylase 67 expression in schizophrenia: clinical, protein, and cell type-specific features. Am. J. Psychiatry 168, 921–929. doi: 10.1176/appi.ajp.2011.11010052
Dansie, L. E., Phommahaxay, K., Okusanya, A. G., Uwadia, J., Huang, M., Rotschafer, S. E., et al. (2013). Long-lasting effects of minocycline on behavior in young but not adult Fragile X mice. Neuroscience 246, 186–198. doi: 10.1016/j.neuroscience.2013.04.058
Davies, S. J., Goucher, D. R., Doller, C., and Silver, J. (1999). Robust regeneration of adult sensory axons in degenerating white matter of the adult rat spinal cord. J. Neurosci. 19, 5810–5822. doi: 10.1523/JNEUROSCI.19-14-05810.1999
Day, J. M., Olin, A. I., Murdoch, A. D., Canfield, A., Sasaki, T., Timpl, R., et al. (2004). Alternative splicing in the aggrecan G3 domain influences binding interactions with tenascin-C and other extracellular matrix proteins. J. Biol. Chem. 279, 12511–12518. doi: 10.1074/jbc.M400242200
De Felice, F., Velasco, P. T., Lambert, M. P., Viola, K., Fernandez, S. J., Ferreira, S. T., et al. (2007). Aβ oligomers induce neuronal oxidative stress through an N-methyl-D-aspartate receptor-dependent mechanism that is blocked by the Alzheimer drug memantine. J. Biol. Chem. 282, 11590–11601. doi: 10.1074/jbc.M607483200
De Luca, C., and Papa, M. (2016). Looking inside the matrix: perineuronal nets in plasticity, maladaptive plasticity and neurological disorders. Neurochem. Res. 41, 1507–1515. doi: 10.1007/s11064-016-1876-2
De Luca, C., Savarese, L., Colangelo, A. M., Bianco, M. R., Cirillo, G., Alberghina, L., et al. (2016). Astrocytes and microglia-mediated immune response in maladaptive plasticity is differently modulated by NGF in the ventral horn of the spinal cord following peripheral nerve injury. Cell. Mol. Neurobiol. 36, 37–46. doi: 10.1007/s10571-015-0218-2
Deibel, M. A., Ehmann, W. D., and Markesbery, W. R. (1996). Copper, iron, and zinc imbalances in severely degenerated brain regions in Alzheimer’s disease: possible relation to oxidative stress. J. Neurol. Sci. 143, 137–142. doi: 10.1016/S0022-510X(96)00203-1
Delpech, A., and Delpech, B. (1984). Expression of hyaluronic acid-binding glycoprotein, hyaluronectin, in the developing rat embryo. Dev. Biol. 101, 391–400. doi: 10.1016/0012-1606(84)90153-2
Dityatev, A., Brückner, G., Dityateva, G., Grosche, J., Kleene, R., and Schachner, M. (2007). Activity-dependent formation and functions of chondroitin sulfate rich extracellular matrix of perineuronal nets. Dev. Biol. 67, 570–588. doi: 10.1002/dneu.20361
Dityatev, A., and Rusakov, D. (2011). Molecular signals of plasticity at the tetrapartite synapse. Curr. Opin. Neurobiol. 21, 353–359. doi: 10.1016/j.conb.2010.12.006
Do, K. Q., Trabesinger, A. H., Kirsten-Krüger, M., Lauer, C. J., Dydak, U., Hell, D., et al. (2000). Schizophrenia: glutathione deficit in cerebrospinal fluid and prefrontal cortex in vivo. Eur. J. Neurosci. 12, 3721–3728. doi: 10.1046/j.1460-9568.2000.00229.x
Dziembowska, M., Pretto, D. I., Janusz, A., Kaczmarek, L., Leigh, M. J., Gabriel, N., et al. (2013). High MMP-9 activity levels in fragile X syndrome are lowered by minocycline. Am. J. Med. Genet. A 161, 1897–1903. doi: 10.1002/ajmg.a.36023
Enwright, J. F., Sanapala, S., Foglio, A., Berry, R., Fish, K. N., and Lewis, D. A. (2016). Reduced labeling of parvalbumin neurons and perineuronal nets in the dorsolateral prefrontal cortex of subjects with schizophrenia. Neuropyschopharmacology 41, 2206–2214. doi: 10.1038/npp.2016.24
Ethridge, L. E., White, S. P., Mosconi, M. W., Wang, J., Byerly, M. J., and Sweeney, J. A. (2016). Reduced habituation of auditory evoked potentials indicate cortical hyper-excitability in Fragile X Syndrome. Transl. Psychiatry 6:e787. doi: 10.1038/tp.2016.48
Favuzzi, E., Marques-Smith, A., Deogracias, R., Winterflood, C. M., Sánchez-Aguilera, A., Mantoan, L., et al. (2017). Activity-dependent gating of parvalbumin interneuron function by the perineuronal net protein brevican. Neuron 95, 639–655. doi: 10.1016/j.neuron.2017.06.028
Ferrer, I., Oliver, B., Russi, A., Casas, R., and Rivera, R. (1994). Parvalbumin and calbindin-D28k immunocytochemistry in human neocortical epileptic foci. J. Neurol. Sci. 123, 18–25. doi: 10.1016/0022-510X(94)90198-8
Flatow, J., Buckley, P., and Miller, B. J. (2013). Meta-analysis of oxidative stress in schizophrenia. Biol. Psychiatry 74, 400–409. doi: 10.1016/j.biopsych.2013.03.018
Frankland, P. W., Wang, Y., Rosner, B., Shimizu, T., Balleine, B. W., Dykens, E. M., et al. (2004). Sensorimotor gating abnormalities in young males with fragile X syndrome and Fmr1-knockout mice. Mol. Psychiatry 9, 417–425. doi: 10.1038/sj.mp.4001432
Fraser, J. R., Laurent, T. C., and Laurent, U. B. (1997). Hyaluronan: its nature, distribution, functions and turnover. J. Intern. Med. 242, 27–33. doi: 10.1046/j.1365-2796.1997.00170.x
Frischknecht, R., and Seidenbecher, C. (2008). The crosstalk of hyaluronan-based extracellular matrix and synapses. Neuron Glia Biol. 4, 249–257. doi: 10.1017/S1740925X09990226
Fujiwara, T., Sugawara, T., Mazaki-Miyazaki, E., Takahashi, Y., Fukushima, K., Watanabe, M., et al. (2003). Mutations of sodium channel α subunit type 1 (SCN1A) in intractable childhood epilepsies with frequent generalized tonic–clonic seizures. Brain 126, 531–546. doi: 10.1093/brain/awg053
Galtrey, C. M., Kwok, J. C., Carulli, D., Rhodes, K. E., and Fawcett, J. W. (2008). Distribution and synthesis of extracellular matrix proteoglycans, hyaluronan, link proteins and tenascin-R in the rat spinal cord. Eur. J. Neurosci. 27, 1373–1390. doi: 10.1111/j.1460-9568.2008.06108.x
Gandal, M. J., Edgar, J. C., Klook, K., and Siegel, S. J. (2012). Gamma synchrony: towards a translational biomarker for the treatment-resistant symptoms of schizophrenia. Neuropharmacology 62, 1504–1518. doi: 10.1016/j.neuropharm.2011.02.007
Gao, F., Wang, G., Ma, W., Ren, F., Li, M., Dong, Y., et al. (2015). Decreased auditory GABA + concentrations in presbycusis demonstrated by edited magnetic resonance. Neuroimage 106, 311–316. doi: 10.1016/j.neuroimage.2014.11.023
Gary, S. C., Zerillo, C. A., Chiang, V. L., Gaw, J. U., Gray, G., and Hockfield, S. (2000). cDNA cloning, chromosomal localization, and expression analysis of human BEHAB/brevican, a brain specific proteoglycan regulated during cortical development and in glioma. Gene 256, 139–147. doi: 10.1016/S0378-1119(00)00362-0
Gates, G., and Mills, J. (2005). Presbycusis. Lancet 366, 1111–1120. doi: 10.1016/S0140-6736(05)67423-5
Giamanco, K. A., Morawski, M., and Matthews, R. T. (2010). Perineuronal net formation and structure in aggrecan knockout mice. Neuroscience 170, 1314–1327. doi: 10.1016/j.neuroscience.2010.08.032
Gibson, J. R., Bartley, A. F., Hays, S. A., and Huber, K. M. (2008). Imbalance of neocortical excitation and inhibition and altered UP states reflect network hyperexcitability in the mouse model of fragile X syndrome. J. Neurophysiol. 100, 2615–2626. doi: 10.1152/jn.90752.2008
Glausier, J. R., Fish, K. N., and Lewis, D. A. (2014). Altered parvalbumin basket cell inputs in the dorsolateral prefrontal cortex of schizophrenia subjects. Mol. Psychiatry 19, 30–36. doi: 10.1038/mp.2013.152
Golgi, C. (1893). Intorno all’origine del quarto nervo cerebrale e una questione istofisiologica che a questo argomento si collega. Rend. R Accademia Lincei 2, 378–389.
Gomis-Rüth, F. X., Maskos, K., Betz, M., Bergner, A., Huber, R., Suzuki, K., et al. (1997). Mechanism of inhibition of the human matrix metalloproteinase stromelysin-1 by TIMP-1. Nature 389, 77–81. doi: 10.1038/37995
Gu, Y., Huang, S., Chang, M. C., Worley, P., Kirkwood, A., and Quinlan, E. M. (2013). Obligatory role for the immediate early gene NARP in critical period plasticity. Neuron 79, 335–346. doi: 10.1016/j.neuron.2013.05.016
Hansson, J., Vasan, R. S., Ärnlöv, J., Ingelsson, E., Lind, L., Larsson, A., et al. (2011). Biomarkers of extracellular matrix metabolism (MMP-9 and TIMP-1) and risk of stroke, myocardial infarction, and cause-specific mortality: cohort study. PLoS One 6:e16185. doi: 10.1371/journal.pone.0016185
Harris, N. G., Mironova, Y. A., Hovda, D. A., and Sutton, R. L. (2010). Pericontusion axon sprouting is spatially and temporally consistent with a growth-permissive environment after traumatic brain injury. J. Neuropathol. Exp. Neurol. 69, 139–154. doi: 10.1097/NEN.0b013e3181cb5bee
Härtig, W., Appel, S., Suttkus, A., Grosche, J., and Michalski, D. (2016). Abolished perineuronal nets and altered parvalbumin-immunoreactivity in the nucleus reticularis thalami of wildtype and 3xTg mice after experimental stroke. Neuroscience 337, 66–87. doi: 10.1016/j.neuroscience.2016.09.004
Härtig, W., Brauer, K., and Brückner, G. (1992). Wisteria floribunda agglutinin-labelled nets surround parvalbumin-containing neurons. Neuroreport 3, 869–872. doi: 10.1097/00001756-199210000-00012
Härtig, W., Derouiche, A., Welt, K., Brauer, K., Grosche, J., Mäder, M., et al. (1999). Cortical neurons immunoreactive for the potassium channel Kv3.1b subunit are predominantly surrounded by perineuronal nets presumed as a buffering system for cations. Brain Res. 842, 15–29. doi: 10.1016/S0006-8993(99)01784-9
Härtig, W., Mages, B., Aleithe, S., Nitzsche, B., Altmann, S., Barthel, H., et al. (2017). Damaged neocortical perineuronal nets due to experimental focal cerebral ischemia in mice. rats and sheep. Front. Intergr. Neurosci. 11:15. doi: 10.3389/fnint.2017.00015
Hasenstaub, A., Otte, S., Callaway, E., and Sejnowski, T. J. (2010). Metabolic cost as a unifying principle governing neuronal biophysics. Proc. Natl. Acad. Sci. U.S.A. 107, 12329–12334. doi: 10.1073/pnas.0914886107
Hashimoto, T., Arion, D., Unger, T., Maldonado-Avilés, J. G., Morris, H. M., Volk, D. W., et al. (2008a). Alterations in GABA-related transcriptome in the dorsolateral prefrontal cortex of subjects with schizophrenia. Mol. Psychiatry 13, 147–161.
Hashimoto, T., Bazmi, H. H., Mirnics, K., Wu, Q., Sampson, A. R., and Lewis, D. A. (2008b). Conserved regional patterns of GABA-related transcript expression in the neocortex of subjects with schizophrenia. Am. J. Psychiatry 165, 479–489. doi: 10.1176/appi.ajp.2007.07081223
Hashimoto, T., Volk, D. W., Eggan, S. M., Mirnics, K., Pierri, J. N., Sun, Z., et al. (2003). Gene expression deficits in a subclass of GABA neurons in the prefrontal cortex of subjects with schizophrenia. J. Neurosci. 23, 6315–6326. doi: 10.1523/JNEUROSCI.23-15-06315.2003
Hauser, W. A. (1992). The natural history of drug resistant epilepsy: epidemiologic considerations. Epilepsy Res. Suppl. 5, 25–28.
Hedrich, U. B., Liautard, C., Kirschenbaum, D., Pofahl, M., Lavigne, J., Liu, Y., et al. (2014). Impaired action potential initiation in GABAergic interneurons causes hyperexcitable networks in an epileptic mouse model carrying a human NaV1.1 mutation. J. Neurosci. 34, 14874–14889. doi: 10.1523/JNEUROSCI.0721-14.2014
Hensch, T. (2005). Critical period plasticity in local cortical circuits. Nat. Rev. Neurosci. 6, 877–888. doi: 10.1038/nrn1787
Hobohm, C., Günther, A., Grosche, J., Rossner, S., Schneider, D., and Brückner, G. (2005). Decomposition and long-lasting downregulation of extracellular matrix in perineuronal nets induced by focal cerebral ischemia in rats. J. Neurosci. Res. 80, 539–548. doi: 10.1002/jnr.20459
Hoover, B. R., Reed, M. N., Su, J., Penrod, R. D., Kotilinek, L. A., Grant, M. K., et al. (2010). Tau mislocalization to dendritic spines mediates synaptic dysfunction independently of neurodegeneration. Neuron 68, 1067–1081. doi: 10.1016/j.neuron.2010.11.030
Inan, M., Zhao, M., Manuszak, M., Karakaya, C., Rajadhyaksha, A. M., Pickel, V. M., et al. (2016). Energy deficit in parvalbumin neurons leads to circuit dysfunction, impaired sensory gating and social disability. Neurobiol. Dis. 93, 35–46. doi: 10.1016/j.nbd.2016.04.004
Irvine, S. F., and Kwok, J. C. (2018). Perineuronal nets in spinal motoneurones: chondroitin sulphate proteoglycan around alpha motoneurones. Int. J. Mol. Sci. 19:1172. doi: 10.3390/ijms19041172
Janusz, A., Milek, J., Perycz, M., Pacini, L., Bagni, C., Kaczmarek, L., et al. (2013). The Fragile X mental retardation protein regulates matrix metalloproteinase 9 mRNA at synapses. J. Neurosci. 33, 18234–18241. doi: 10.1523/JNEUROSCI.2207-13.2013
Kaas, J. H., Qi, H. X., Burish, M. J., Gharbawie, O. A., Onifer, S. M., and Massey, J. M. (2008). Cortical and subcortical plasticity in the brains of humans, primates, and rats after damage to sensory afferents in the dorsal columns of the spinal cord. Exp. Neurol. 209, 407–416. doi: 10.1016/j.expneurol.2007.06.014
Kann, O., Papageorgiou, I. E., and Draguhn, A. (2014). Highly energized inhibitory interneurons are a central element for information processing in cortical networks. J. Cereb. Blood Flow Metab. 34, 1270–1282. doi: 10.1038/jcbfm.2014.104
Karetko, M., and Skangiel-Kramska, J. (2009). Diverse functions of perineuronal nets. Acta Neurobiol. Exp. 69, 564–577.
Karetko-Sysa, M., Skangiel-Kramska, J., and Nowicka, D. (2014). Aging somatosensory cortex displays increased density of WFA-binding perineuronal nets associated with GAD-negative neurons. Neuroscience 277, 734–746. doi: 10.1016/j.neuroscience.2014.07.049
Khodaie-Ardakani, M. R., Mirshafiee, O., Farokhnia, M., Tajdini, M., Hosseini, S. M., Modabbernia, A., et al. (2014). Minocycline add-on to risperidone for treatment of negative symptoms in patients with stable schizophrenia: randomized double-blind placebo-controlled study. Psychiatry Res. 215, 540–546. doi: 10.1016/j.psychres.2013.12.051
Kim, G. W., Kim, H. J., Cho, K. J., Kim, H. W., Cho, Y. J., and Lee, B. I. (2009). The role of MMP-9 in integrin-mediated hippocampal cell death after pilocarpine-induced status epilepticus. Neurobiol. Dis. 36, 169–180. doi: 10.1016/j.nbd.2009.07.008
Kim, S. Y., Porter, B. E., Friedman, A., and Kaufer, D. (2016). A potential role for glia-derived extracellular matrix remodeling in postinjury epilepsy. J. Neurosci. Res. 94, 794–803. doi: 10.1002/jnr.23758
Köppe, G., Brückner, G., Brauer, K., Härtig, W., and Bigl, V. (1997). Developmental patterns of proteoglycan-containing extracellular matrix in perineuronal nets and neuropil of the postnatal rat brain. Cell Tissue Res. 288, 33–41. doi: 10.1007/s004410050790
Kosaka, T., and Heizmann, C. W. (1989). Selective staining of a population of parvalbumin-containing GABAergic neurons in the rat cerebral cortex by lectins with specific affinity for terminal N-acetylgalactosamine. Brain Res. 483, 158–163. doi: 10.1016/0006-8993(89)90048-6
Kwok, J. C., Dick, G., Wang, D., and Fawcett, J. W. (2011). Extracellular matrix and perineuronal nets in CNS repair. Dev. Neurobiol. 71, 1073–1089. doi: 10.1002/dneu.20974
Kwon, J. S., O’Donnell, B. F., Wallenstein, G. V., Greene, R. W., Hirayasu, Y., Nestor, P. G., et al. (1999). Gamma frequency–range abnormalities to auditory stimulation in schizophrenia. Arch. Gen. Psychiatry 56, 1001–1005. doi: 10.1001/archpsyc.56.11.1001
Lazarus, M., and Huang, Z. (2011). Distinct maturation profiles of perisomatic and dendritic targeting GABAergic interneurons in the mouse primary visual cortex during the critical period of ocular dominance plasticity. J. Neurophysiol. 106, 775–787. doi: 10.1152/jn.00729.2010
Lee, C., Yao, J. S., Huang, Y., Zhai, W., Liu, W., Guglielmo, B. J., et al. (2006). Dose–response effect of tetracyclines on cerebral matrix metalloproteinase-9 after vascular endothelial growth factor hyperstimulation. J. Cereb. Blood Flow Metab. 26, 1157–1164. doi: 10.1038/sj.jcbfm.9600268
Leigh, M. J., Nguyen, D. V., Mu, Y., Winarni, T. I., Schneider, A., Chechi, T., et al. (2013). A randomized double-blind, placebo-controlled trial of minocycline in children and adolescents with Fragile X Syndrome. J. Dev. Behav. Pediatr. 34, 147–155. doi: 10.1097/DBP.0b013e318287cd17
Lemarchant, S., Pruvost, M., Montaner, J., Emery, E., Vivien, D., Kanninen, K., et al. (2013). ADAMTS proteoglycanases in the physiological and pathological central nervous system. J. Neuroinflammation 10:133. doi: 10.1186/1742-2094-10-133
Lendvai, D., Morawski, M., Négyessy, L., Gáti, G., Jäger, C., Baksa, G., et al. (2013). Neurochemical mapping of the human hippocampus reveals perisynaptic matrix around functional synapses in Alzheimer’s disease. Acta Neuropathol. 125, 215–229. doi: 10.1007/s00401-012-1042-0
Lensjø, K. K., Lepperød, M. E., Dick, G., Hafting, T., and Fyhn, M. (2017). Removal of perineuronal nets unlocks juvenile plasticity through network mechanisms of decreased inhibition and increased gamma activity. J. Neurosci. 37, 1269–1283. doi: 10.1523/JNEUROSCI.2504-16.2016
Lepeta, K., Purzycka, K. J., Pachulska-Wieczorek, K., Mitjans, M., Begemann, M., Vafadari, B., et al. (2017). A normal genetic variation modulates synaptic MMP-9 protein levels and the severity of schizophrenia symptoms. EMBO Mol. Med. 9, 1100–1116. doi: 10.15252/emmm.201707723
Levkovitz, Y., Mendlovich, S., Riwkes, S., Braw, Y., Levkovitch-Verbin, H., Gal, G., et al. (2010). A double-blind, randomized study of minocycline for the treatment of negative and cognitive symptoms in early-phase schizophrenia. J. Clin. Psychiatry 71, 138–149. doi: 10.4088/JCP.08m04666yel
Light, G. A., Hsu, J. L., Hsieh, M. H., Meyer-Gomes, K., Sprock, J., Swerdlow, N. R., et al. (2006). Gamma band oscillations reveal neural network cortical coherence dysfunction in schizophrenia patients. Biol. Psychiatry 60, 1231–1240. doi: 10.1016/j.biopsych.2006.03.055
Liu, H., Gao, P. F., Xu, H. W., Liu, M. M., Yu, T., Yao, J. P., et al. (2013). Perineuronal nets increase inhibitory GABAergic currents during the critical period in rats. Int. J. Opthalmol. 6, 120–125. doi: 10.3980/j.issn.2222-3959.2013.02.02
Liu, J., Wang, Y., Akamatsu, Y., Lee, C. C., Stetler, R. A., Lawton, M. T., et al. (2014). Vascular remodeling after ischemic stroke: mechanisms and therapeutic potentials. Prog. Neurobiol. 115, 138–156. doi: 10.1016/j.pneurobio.2013.11.004
Lorenzl, S., Albers, D. S., Relkin, N., Ngyuen, T., Hilgenberg, S. L., Chirichigno, J., et al. (2003). Increased plasma levels of matrix metalloproteinase-9 in patients with Alzheimer’s disease. Neurochem. Int. 43, 191–196. doi: 10.1016/S0197-0186(03)00004-4
Lovelace, J. W., Ethell, I. M., Binder, D. K., and Razak, K. A. (2018). Translation-relevant EEG phenotypes in a mouse model of Fragile X Syndrome. Neurobiol. Dis. 115, 39–48. doi: 10.1016/j.nbd.2018.03.012
Lovelace, J. W., Wen, T. H., Reinhard, S., Hsu, M. S., Sidhu, H., Ethell, I. M., et al. (2016). Matrix metalloproteinase-9 deletion rescues auditory evoked potential habituation deficit in a mouse model of fragile X syndrome. Neurobiol. Dis. 89, 126–135. doi: 10.1016/j.nbd.2016.02.002
Madinier, A., Quattromani, M. J., Sjölund, C., Ruscher, K., and Wieloch, T. (2014). Enriched housing enhances recovery of limb placement ability and reduces aggrecan-containing perineuronal nets in the rat somatosensory cortex after experimental stroke. PLoS One 9:e93121. doi: 10.1371/journal.pone.0093121
Madsen, S. H., Sumer, E. U., Bay-Jensen, A. C., Sondergaard, B. C., Qvist, P., and Karsdal, M. A. (2010). Aggrecanase-and matrix metalloproteinase-mediated aggrecan degradation is associated with different molecular characteristics of aggrecan and separated in time ex vivo. Biomarkers 15, 266–276. doi: 10.3109/13547500903521810
Manczak, M., Anekonda, T. S., Henson, E., Park, B. S., Quinn, J., Reddy, P. H., et al. (2006). Mitochondria are a direct site of Aβ accumulation in Alzheimer’s disease neurons: implications for free radical generation and oxidative damage in disease progression. Hum. Mol. Genet. 15, 1437–1449. doi: 10.1093/hmg/ddl066
Martin del Campo, H. N., Measor, K. R., and Razak, K. A. (2012). Parvalbumin immunoreactivity in the auditory cortex of a mouse model of presbycusis. Hear. Res. 294, 31–39. doi: 10.1016/j.heares.2012.08.017
Massey, J. M., Amps, J., Viapiano, M. S., Matthews, R. T., Wagoner, M. R., Whitaker, C. M., et al. (2008). Increased chondroitin sulfate proteoglycan expression in denervated brainstem targets following spinal cord injury creates a barrier to axonal regeneration overcome by chondroitinase ABC and neurotrophin-3. Exp. Neurol. 209, 426–445. doi: 10.1016/j.expneurol.2007.03.029
Massey, J. M., Hubscher, C. H., Wagoner, M. R., Decker, J. A., Amps, J., Silver, J., et al. (2006). Chondroitinase ABC digestion of the perineuronal net promotes functional collateral sprouting in the cuneate nucleus after cervical spinal cord injury. J. Neurosci. 26, 4406–4414. doi: 10.1523/JNEUROSCI.5467-05.2006
Matthews, R. T., Kelly, G. M., Zerillo, C. A., Gray, G., Tiemeyer, M., and Hockfield, S. (2002). Aggrecan glycoforms contribute to the molecular heterogeneity of perineuronal nets. J. Neurosci. 22, 7536–7547. doi: 10.1523/JNEUROSCI.22-17-07536.2002
Mauney, S. A., Athanas, K. M., Pantazopoulos, H., Shaskan, N., Passeri, E., Berretta, S., et al. (2013). Developmental pattern of perineuronal nets in the human prefrontal cortex and their deficit in schizophrenia. Biol. Psychiatry 74, 427–435. doi: 10.1016/j.biopsych.2013.05.007
McRae, P., and Porter, B. (2012). The perineuronal net component of the extracellular matrix in plasticity and epilepsy. Neurochem. Int. 61, 963–972. doi: 10.1016/j.neuint.2012.08.007
Mellios, N., Huang, H. S., Baker, S. P., Galdzicka, M., Ginns, E., and Akbarian, S. (2009). Molecular determinants of dysregulated GABAergic gene expression in the prefrontal cortex of subjects with schizophrenia. Biol. Psychiatry 65, 1006–1014. doi: 10.1016/j.biopsych.2008.11.019
Mercuri, F. A., Maciewicz, R. A., Tart, J., Last, K., and Fosang, A. J. (2000). Mutations in the interglobular domain of aggrecan alter matrix metalloproteinase and aggrecanase cleavage patterns. Evidence that matrix metalloproteinase cleavage interferes with aggrecanase activity. J. Biol. Chem. 275, 33038–33045. doi: 10.1074/jbc.275.42.33038
Meyer-Puttlitz, B., Junker, E., Margolis, R. U., and Margolis, R. K. (1996). Chondroitin sulfate proteoglycans in the developing central nervous system. II. Immunocytochemical localization of neurocan and phosphacan. J. Comp. Neurol. 366, 44–54. doi: 10.1002/(SICI)1096-9861(19960226)366:1<44::AID-CNE4>3.0.CO;2-K
Milev, P., Maurel, P., Chiba, A., Mevissen, M., Popp, S., Yamaguchi, Y., et al. (1998). Differential regulation of expression of hyaluronan-binding proteoglycans in developing brain: aggrecan, versican, neurocan, and brevican. Biochem. Biophys. Res. Commun. 247, 207–212. doi: 10.1006/bbrc.1998.8759
Miller, B., Sheppard, A. M., Bicknese, A. R., and Pearlman, A. L. (1996). Chondroitin sulphate proteoglycans in the developing cerebral cortex: The distribution of neurocan distinguishes forming afferent and efferent axonal pathways. J. Comp. Neurol. 355, 615–628. doi: 10.1002/cne.903550410
Miyaoka, T., Yasukawa, R., Yasuda, H., Hayashida, M., Inagaki, T., and Horiguchi, J. (2008). Minocycline as adjunctive therapy for schizophrenia: an open-label study. Clin. Neuropharmacol. 31, 287–292. doi: 10.1097/WNF.0b013e3181593d45
Miyata, S., Nishimura, Y., and Nakashima, T. (2007). Perineuronal nets protect against amyloid β-protein neurotoxicity in cultured cortical neurons. Brain Res. 1150, 200–206. doi: 10.1016/j.brainres.2007.02.066
Moore, A., and Wehr, M. (2012). Parvalbumin-expressing inhibitory interneurons in auditory cortex are well-tuned for frequency. J. Neurosci. 33, 13713–13723. doi: 10.1523/JNEUROSCI.0663-13.2013
Morawski, M., Brückner, G., Jäger, C., Seeger, G., and Arendt, T. (2010). Neurons associated with aggrecan-based perineuronal nets are protected against tau pathology in subcortical regions in Alzheimer’s disease. J. Neurosci. 169, 1347–1363. doi: 10.1016/j.neuroscience.2010.05.022
Morawski, M., Brückner, M. K., Riederer, P., Brückner, G., and Arendt, T. (2004). Perineuronal nets potentially protect against oxidative stress. Exp. Neurol. 188, 309–315. doi: 10.1016/j.expneurol.2004.04.017
Morishita, H., Cabungcal, J. H., Chen, Y., Do, K. Q., and Hensch, T. K. (2015). Prolonged period of cortical plasticity upon redox dysregulation in fast-spiking interneurons. Biol. Psychiatry 78, 396–402. doi: 10.1016/j.biopsych.2014.12.026
Murase, S., Lantz, C. L., and Quinlan, E. M. (2017). Light reintroduction after dark exposure reactivates plasticity in adults via perisynaptic activation of MMP-9. eLife 6:e27345. doi: 10.7554/eLife.27345
Murray, J., Cuckle, H., Taylor, G., and Hewison, J. (1997). Screening for fragile X syndrome. Health Technol. Assess. 1, i–iv, 1–71.
Musumeci, S. A., Bosco, P., Calabrese, G., Bakker, C., De Sarro, G. B., Elia, M., et al. (2000). Audiogenic seizures susceptibility in transgenic mice with fragile X syndrome. Epilepsia 41, 19–23. doi: 10.1111/j.1528-1157.2000.tb01499.x
Nakagawa, F., Schulte, B. A., Wu, J. Y., and Spicer, S. S. (1986). GABAergic neurons of rodent brain correspond partially with those staining for glycoconjugate with terminal N-acetylgalactosamine. J. Neurocytol. 15, 389–396. doi: 10.1007/BF01611440
Nguyen, A., Khaleel, H. M., and Razak, K. A. (2017). Effects of noise-induced hearing loss on parvalbumin and perineuronal net expression in the mouse primary auditory cortex. Hear. Res. 350, 82–90. doi: 10.1016/j.heares.2017.04.015
Nomura, T., Musial, T. F., Marshall, J. J., Zhu, Y., Remmers, C. L., Xu, J., et al. (2017). Delayed maturation of fast-spiking interneurons is rectified by activation of the TrkB receptor in the mouse model of fragile X syndrome. J. Neurosci. 37, 11298–11310. doi: 10.1523/JNEUROSCI.2893-16.2017
Nowicka, D., Soulsby, S., Skangiel-Kramska, J., and Glazewski, S. (2009). Parvalbumin-containing neurons, perineuronal nets and experience-dependent plasticity in murine barrel cortex. Eur. J. Neurosci. 30, 2053–2063. doi: 10.1111/j.1460-9568.2009.06996.x
O’Brien, R. J., Xu, D., Petralia, R. S., Steward, O., Huganir, R. L., and Worley, P. (1999). Synaptic clustering of AMPA receptors by the extracellular immediate-early gene product narp. Neuron 23, 309–323. doi: 10.1016/S0896-6273(00)80782-5
Ogiwara, I., Miyamoto, H., Morita, N., Atapour, N., Mazaki, E., Inoue, I., et al. (2007). Nav1.1 localizes to axons of parvalbumin-positive inhibitory interneurons: a circuit basis for epileptic seizures in mice carrying an Scn1a gene mutation. J. Neurosci. 27, 5903–5914. doi: 10.1523/JNEUROSCI.5270-06.2007
Oohashi, T., Edamatsu, M., Bekku, Y., and Carulli, D. (2015). The hyaluronan and proteoglycan link proteins: organizers of the brain extracellular matrix and key molecules for neuronal function and plasticity. Exp. Neurol. 274, 134–144. doi: 10.1016/j.expneurol.2015.09.010
Ouda, L., Druga, R., and Syka, J. (2008). Changes in parvalbumin immunoreactivity with aging in the central auditory system of the rat. Exp. Gerontol. 43, 782–789. doi: 10.1016/j.exger.2008.04.001
Palop, J. J., Chin, J., Roberson, E. D., Wang, J., Thwin, M. T., Bien-Ly, N., et al. (2007). Aberrant excitatory neuronal activity and compensatory remodeling of inhibitory hippocampal circuits in mouse models of alzheimer’s disease. Neuron 55, 697–711. doi: 10.1016/j.neuron.2007.07.025
Papa, M., De Luca, C., Petta, F., Alberghina, L., and Cirillo, G. (2014). Astrocyte–neuron interplay in maladaptive plasticity. Neurosci. Biobehav. Rev. 42, 35–54. doi: 10.1016/j.neubiorev.2014.01.010
Paribello, C., Tao, L., Folino, A., Berry-Kravis, E., Tranfaglia, M., Ethell, I. M., et al. (2010). Open-label add-on treatment trial of minocycline in fragile X syndrome. BMC Neurol. 10:91. doi: 10.1186/1471-2377-10-91
Peress, N., Perillo, E., and Zucker, S. (1995). Localization of tissue inhibitor of matrix metalloproteinases in Alzheimer’s disease and normal brain. J. Neuropathol. Exp. Neurol. 54, 16–22. doi: 10.1097/00005072-199501000-00002
Pizzo, R., Gurgone, A., Castroflorio, E., Amendola, E., Gross, C., Sassoè-Pognetto, M., et al. (2016). Lack of Cdkl5 disrupts the organization of excitatory and inhibitory synapses and parvalbumin interneurons in the primary visual cortex. Front. Cell. Neurosci. 10:261. doi: 10.3389/fncel.2016.00261
Pizzorusso, T., Medini, P., Berardi, N., Chierzi, S., Fawcett, J. W., and Maffei, L. (2002). Reactivation of ocular dominance plasticity in the adult visual cortex. Science 298, 1248–1251. doi: 10.1126/science.1072699
Pollock, E., Everest, M., Brown, A., and Poulter, M. O. (2014). Metalloproteinase inhibition prevents inhibitory synapse reorganization and seizure genesis. Neurobiol. Dis. 70, 21–31. doi: 10.1016/j.nbd.2014.06.003
Popp, S., Andersen, J. S., Maurel, P., and Margolis, R. U. (2003). Localization of aggrecan and versican in the developing rat central nervous system. Dev. Dyn. 227, 143–149. doi: 10.1002/dvdy.10282
Puel, J. L., Ruel, J., Gervais d’Aldin, C., and Pujol, R. (1998). Excitotoxicity and repair of cochlear synapses after noise-trauma induced hearing loss. Neuroreport 9, 2109–2114. doi: 10.1097/00001756-199806220-00037
Pyka, M., Wetzel, C., Aguado, A., Geissler, M., Hatt, H., and Faissner, A. (2011). Chondroitin sulfate proteoglycans regulate astrocyte-dependent synaptogenesis and modulate synaptic activity in primary embryonic hippocampal neurons. Eur. J. Neurosci. 33, 2187–2202. doi: 10.1111/j.1460-9568.2011.07690.x
Quattromani, M. J., Pruvost, M., Guerreiro, C., Backlund, F., Englund, E., Aspberg, A., et al. (2018). Extracellular matrix modulation is driven by experience-dependent plasticity during stroke recovery. Mol. Neurobiol. 55, 2196–2213. doi: 10.1007/s12035-017-0461-2
Rahimi, S., Sayad, A., Moslemi, E., Ghafouri-Fard, S., and Taheri, M. (2017). Blood assessment of the expression levels of matrix metalloproteinase 9 (MMP9) and its natural inhibitor, TIMP1 genes in Iranian schizophrenic patients. Metab. Brain Dis. 32, 1537–1542. doi: 10.1007/s11011-017-0043-z
Ramón, Y., and Cajal, S. (1897). Las células de cilindro-eje corto de la capa molecular del cerebro. Rev. Trim Micrográf. 2, 105–127.
Rankin-Gee, E. K., McRae, P. A., Baranov, E., Rogers, S., Wandrey, L., and Porter, B. E. (2015). Perineuronal net degradation in epilepsy. Epilepsia 56, 1124–1133. doi: 10.1111/epi.13026
Reinart, T., Morawskib, T., Arendtb, T., and Butz, T. (2003). Quantitative microanalysis of perineuronal nets in brain tissue. Nucl. Instrum. Methods Phys. Res. B 210, 395–400. doi: 10.1016/S0168-583X(03)01041-3
Reinhard, S. M., Razak, K., and Ethell, I. M. (2015). A delicate balance: role of MMP-9 in brain development and pathophysiology of neurodevelopmental disorders. Front. Cell. Neurosci. 9:280. doi: 10.3389/fncel.2015.00280
Roberson, E., Halabisky, B., Yoo, J. W., Yao, J., Chin, J., Yan, F., et al. (2011). Amyloid-β/fyn–induced synaptic, network, and cognitive impairments depend on tau levels in multiple mouse models of Alzheimer’s Disease. J. Neurosci. 31, 700–711. doi: 10.1523/JNEUROSCI.4152-10.2011
Rogers, S. J., Wehner, D. E., and Hagerman, R. (2001). The behavioral phenotype in fragile X: symptoms of autism in very young children with fragile X syndrome, idiopathic autism, and other developmental disorders. J. Dev. Behav. Pediatr. 22, 409–417. doi: 10.1097/00004703-200112000-00008
Rojas, D. C., Benkers, T. L., Rogers, S. J., Teale, P. D., Reite, M. L., and Hagerman, R. J. (2001). Auditory evoked magnetic fields in adults with fragile X syndrome. Neuroreport 12, 2573–2576. doi: 10.1097/00001756-200108080-00056
Rotschafer, S., and Razak, K. (2013). Altered auditory processing in a mouse model of fragile X syndrome. Brain Res. 1506, 12–24. doi: 10.1016/j.brainres.2013.02.038
Rotschafer, S., and Razak, K. (2014). Auditory processing in fragile X syndrome. Front. Cell. Neurosci. 8:19. doi: 10.3389/fncel.2014.00019
Rotschafer, S. E., Trujillo, M. S., Dansie, L. E., Ethell, I. M., and Razak, K. A. (2012). Minocycline treatment reverses ultrasonic vocalization production deficit in a mouse model of Fragile X Syndrome. Brain Res. 1439, 7–14. doi: 10.1016/j.brainres.2011.12.041
Ruiter, J., and Uyling, H. (1987). Morphometric and dendritic analysis of fascia dentata granule cells in human again and senile dementia. Brain Res. 402, 217–229. doi: 10.1016/0006-8993(87)90028-X
Rybakowski, J. K., Skibinska, M., Kapelski, P., Kaczmarek, L., and Hauser, J. (2009). Functional polymorphism of the matrix metalloproteinase-9 (MMP-9) gene in schizophrenia. Schizohr. Res. 109, 90–93. doi: 10.1016/j.schres.2009.02.005
Sakai, A., Nakato, R., Ling, Y., Hou, X., Hara, N., Iijima, T., et al. (2017). Genome-wide target analyses of Otx2 homeoprotein in postnatal cortex. Front. Neurosci. 11:307. doi: 10.3389/fnins.2017.00307
Scheibel, A., and Tomiyasu, U. (1978). Dendritic sprouting in alzheimer’s presenile dementia. Exp. Neurol. 60, 1–8. doi: 10.1016/0014-4886(78)90164-4
Schneider, A., Leigh, M. J., Adams, P., Nanakul, R., Chechi, T., Olichney, J., et al. (2013). Electrocortical changes associated with minocycline treatment in fragile X syndrome. J. Psychopharmacol. 27, 956–963. doi: 10.1177/0269881113494105
Schwaller, B., Tetko, I. V., Tandon, P., Silveira, D. C., Vreugdenhil, M., Henzi, T., et al. (2004). Parvalbumin deficiency affects network properties resulting in increased susceptibility to epileptic seizures. Mol. Cell. Neurosci. 25, 650–663. doi: 10.1016/j.mcn.2003.12.006
Sheppard, A. M., Hamilton, S. K., and Pearlman, A. L. (1991). Changes in the distribution of extracellular matrix components accompany early morphogenetic events of mammalian cortical development. J. Neurosci. 11, 3928–3942. doi: 10.1523/JNEUROSCI.11-12-03928.1991
Sherman, L., and Back, S. (2007). A ‘GAG’ reflex prevents repair of the damage CNS. Trends Neurosci. 31, 44–52. doi: 10.1016/j.tins.2007.11.001
Sinclair, D., Featherstone, R., Naschek, M., Nam, J., Du, A., Wright, S., et al. (2017a). GABA-B agonist baclofen normalizes auditory-evoked neural oscillations and behavioral deficits in the Fmr1 knockout mouse model of Fragile X Syndrome. eNeuro 4, ENEURO.0380-16.2017. doi: 10.1523/ENEURO.0380-16.2017
Sinclair, D., Oranje, B., Razak, K. A., Siegel, S. J., and Schmid, S. (2017b). Sensory processing in autism spectrum disorders and Fragile X syndrome—From the clinic to animal models. Neurosci. Biobehav. Rev. 76, 235–253. doi: 10.1016/j.neubiorev.2016.05.029
Sloviter, R. S., Sollas, A. L., Barbaro, N. M., and Laxer, K. D. (1991). Calcium-binding protein (calbindin-D28K) and parvalbumin immunocytochemistry in the normal and epileptic human hippocampus. J. Comp. Neurol. 308, 381–396. doi: 10.1002/cne.903080306
Smith, A. C., Scofield, M. D., and Kalivas, P. W. (2015). The tetrapartite synapse: extracellular matrix remodeling contributes to corticoaccumbens plasticity underlying drug addiction. Brain Res. 1628, 29–39. doi: 10.1016/j.brainres.2015.03.027
Sohal, V. S., Zhang, F., Yizhar, O., and Deisseroth, K. (2009). Parvalbumin neurons and gamma rhythms enhance cortical circuit performance. Nature 459, 698–702. doi: 10.1038/nature07991
Sorg, B. A., Berretta, S., Blacktop, J. M., Fawcett, J. W., Kitagawa, H., Kwok, J. C., et al. (2016). Casting a wide net: role of perineuronal nets in neural plasticity. J. Neurosci. 36, 11459–11468. doi: 10.1523/JNEUROSCI.2351-16.2016
Steullet, P., Cabungcal, J. H., Cuénod, M., and Do, K. Q. (2014). Fast oscillatory activity in the anterior cingulate cortex: dopaminergic modulation and effect of perineuronal net loss. Front. Cell. Neurosci. 8:244. doi: 10.3389/fncel.2014.00244
Stohs, W., and Bagchi, D. (1995). Oxidative mechanisms in the toxicity of metal ions. Free Radic. Biol. Med. 18, 321–336. doi: 10.1016/0891-5849(94)00159-H
Sugiyama, S., Di Nardo, A. A., Aizawa, S., Matsuo, I., Volovitch, M., Prochiantz, A., et al. (2008). Experience-dependent transfer of Otx2 homeoprotein into the visual cortex activates postnatal plasticity. Cell 134, 508–520. doi: 10.1016/j.cell.2008.05.054
Sun, B., Halabisky, B., Zhou, Y., Palop, J. J., Yu, G., Mucke, L., et al. (2009). Imbalance between GABAergic and glutamatergic transmission impairs adult neurogenesis in an animal model of Alzheimer’s Disease. Cell Stem Cell 5, 624–633. doi: 10.1016/j.stem.2009.10.003
Suttkus, A., Rohn, S., Jäger, C., Arendt, T., and Morawski, M. (2012). Neuroprotection against iron-induced cell death by perineuronal nets - an in vivo analysis of oxidative stress. Am. J. Neurodegener. Dis. 1, 122–129.
Suttkus, A., Rohn, S., Weigel, S., Glöckner, P., Arendt, T., and Morawski, M. (2014). Aggrecan, link protein and tenascin-R are essential components of the perineuronal net to protect neurons against iron-induced oxidative stress. Cell Death Dis. 5:e1119. doi: 10.1038/cddis.2014.25
Syka, J. (2002). Plastic changes in the central auditory system after hearing loss, restoration of function, and during learning. Physiol. Rev. 82, 601–636. doi: 10.1152/physrev.00002.2002
Tosic, M., Ott, J., Barral, S., Bovet, P., Deppen, P., Gheorghita, F., et al. (2006). Schizophrenia and oxidative stress: glutamate cysteine ligase modifier as a susceptibility gene. Am. J. Hum. Genet. 79, 586–592. doi: 10.1086/507566
Trujillo, M., and Razak, K. (2013). Altered cortical spectrotemporal processing with age-related hearing loss. J. Neurophysiol. 110, 2873–2886. doi: 10.1152/jn.00423.2013
Ueno, H., Suemitsu, S., Okamoto, M., Matsumoto, Y., and Ishihara, T. (2017a). Parvalbumin neurons and perineuronal nets in the mouse prefrontal cortex. Neuroscience 343, 115–127. doi: 10.1016/j.neuroscience.2016.11.035
Ueno, H., Suemitsu, S., Okamoto, M., Matsumoto, Y., and Ishihara, T. (2017b). Sensory experience-dependent formation of perineuronal nets and expression of Cat-315 immunoreactive components in the mouse somatosensory cortex. Neuroscience 355, 161–174. doi: 10.1016/j.neuroscience.2017.04.041
Ueno, H., Takao, K., Suemitsu, S., Murakami, S., Kitamura, N., Wani, K., et al. (2017c). Age-dependent and region-specific alteration of parvalbumin neurons and perineuronal nets in the mouse cerebral cortex. Neurochem. Int. 112, 59–70. doi: 10.1016/j.neuint.2017.11.001
Uhlhaas, P. J., Roux, F., Singer, W., Haenschel, C., Sireteanu, R., and Rodriguez, E. (2009). The development of neural synchrony reflects late maturation and restructuring of functional networks in humans. Proc. Natl. Acad. Sci. U.S.A. 106, 9866–9871. doi: 10.1073/pnas.0900390106
Van der Molen, M. J., Van der Molen, M. W., Ridderinkhof, K. R., Hamel, B. C., Curfs, L. M., and Ramakers, G. J. (2012). Auditory and visual cortical activity during selective attention in fragile X syndrome: a cascade of processing deficiencies. Clin. Neurophysiol. 123, 720–729. doi: 10.1016/j.clinph.2011.08.023
Varadarajan, S., Yatin, S., Aksenova, M., and Butterfield, D. A. (2000). Alzheimer’s amyloid β-peptide-associated free radical oxidative stress and neurotoxicity. J. Struct. Biol. 130, 184–208. doi: 10.1006/jsbi.2000.4274
Verkerk, A. J., Pieretti, M., Sutcliffe, J. S., Fu, Y. H., Kuhl, D. P., Pizzuti, A., et al. (1991). Identification of a gene (FMR-1) containing a CGG repeat coincident with a breakpoint cluster region exhibiting length variation in fragile X syndrome. Cell 65, 905–914. doi: 10.1016/0092-8674(91)90397-H
Volk, D. W., Austin, M. C., Pierri, J. N., Sampson, A. R., and Lewis, D. A. (2000). Decreased GAD67 mRNA expression in a subset of prefrontal cortical GABA neurons in subjects with schizophrenia. Arch. Gen. Psychiatry 57, 237–245. doi: 10.1001/archpsyc.57.3.237
Wang, D., and Fawcett, J. (2012). The perineuronal net and the control of CNS plasticity. Cell Tissue Res. 349, 147–160. doi: 10.1007/s00441-012-1375-y
Wang, J., Ethridge, L. E., Mosconi, M. W., White, S. P., Binder, D. K., Pedapati, E. V., et al. (2017). A resting EEG study of neocortical hyperexcitability and altered functional connectivity in fragile X syndrome. J. Neurodev. Disord. 9:11. doi: 10.1186/s11689-017-9191-z
Wegner, F., Härtig, W., Bringmann, A., Grosche, J., Wohlfarth, K., et al. (2003). Diffuse perineuronal nets and modified pyramidal cells immunoreactive for glutamate and the GABA A receptor α1 subunit form a unique entity in rat cerebral cortex. Exp. Neurol. 184, 705–714. doi: 10.1016/S0014-4886(03)00313-3
Wen, T. H., Afroz, S., Reinhard, S. M., Palacios, A. R., Tapia, K., Binder, D. K., et al. (2017). Genetic reduction of matrix metalloproteinase-9 promotes formation of perineuronal nets around parvalbumin-expressing interneurons and normalizes auditory cortex responses in developing Fmr1 knock-out mice. Cereb. Cortex 13, 1–14. doi: 10.1093/cercor/bhx258
Wilczynski, G. M., Konopacki, F. A., Wilczek, E., Lasiecka, Z., Gorlewicz, A., Michaluk, P., et al. (2008). Important role of matrix metalloproteinase 9 in epileptogenesis. J. Cell Biol. 180, 1021–1035. doi: 10.1083/jcb.200708213
Xie, Y., Chen, S., Wu, Y., and Murphy, T. H. (2014). Prolonged deficits in parvalbumin neuron stimulation-evoked network activity despite recovery of dendritic structure and excitability in the somatosensory cortex following global ischemia in mice. J. Neurosci. 34, 14890–14900. doi: 10.1523/JNEUROSCI.1775-14.2014
Yamada, J., and Jinno, S. (2017). Molecular heterogeneity of aggrecan-based perineuronal nets around five subclasses of parvalbumin-expressing neurons in the mouse hippocampus. J. Comp. Neurol. 525, 1234–1249. doi: 10.1002/cne.24132
Yamaguchi, Y. (1999). Lecticans: organizers of the brain extracellular matrix. Cell. Mol. Life Sci. 57, 276–289. doi: 10.1007/PL00000690
Yamamori, H., Hashimoto, R., Ishima, T., Kishi, F., Yasuda, Y., Ohi, K., et al. (2013). Plasma levels of mature brain-derived neurotrophic factor (BDNF) and matrix metalloproteinase-9 (MMP-9) in treatment-resistant schizophrenia treated with clozapine. Neurosci. Lett. 556, 37–41. doi: 10.1016/j.neulet.2013.09.059
Yao, J., Leonard, S., and Reddy, R. (2006). Altered glutathione redox state in schizophrenia. Dis. Markers 22, 83–93. doi: 10.1155/2006/248387
Yutsudo, N., and Kitagawa, H. (2015). Involvement of chondroitin 6-sulfation in temporal lobe epilepsy. Exp. Neurol. 274, 126–133. doi: 10.1016/j.expneurol.2015.07.009
Keywords: perineuronal nets, extracellular matrix, interneurons, excitation/inhibition balance, sensory cortex, Fragile X Syndrome, autism
Citation: Wen TH, Binder DK, Ethell IM and Razak KA (2018) The Perineuronal ‘Safety’ Net? Perineuronal Net Abnormalities in Neurological Disorders. Front. Mol. Neurosci. 11:270. doi: 10.3389/fnmol.2018.00270
Received: 03 April 2018; Accepted: 17 July 2018;
Published: 03 August 2018.
Edited by:
Michele Papa, Università degli Studi della Campania Luigi Vanvitelli” Caserta, ItalyReviewed by:
Wolfgang Härtig, Leipzig University, GermanyGiovanni Cirillo, Università degli Studi della Campania “Luigi Vanvitelli” Naples, Italy
Michael Fox, Virginia Tech Carilion Research Institute, United States
Copyright © 2018 Wen, Binder, Ethell and Razak. This is an open-access article distributed under the terms of the Creative Commons Attribution License (CC BY). The use, distribution or reproduction in other forums is permitted, provided the original author(s) and the copyright owner(s) are credited and that the original publication in this journal is cited, in accordance with accepted academic practice. No use, distribution or reproduction is permitted which does not comply with these terms.
*Correspondence: Iryna M. Ethell, aXJ5bmEuZXRoZWxsQG1lZHNjaC51Y3IuZWR1 Khaleel A. Razak, a2hhbGVlbEB1Y3IuZWR1