- 1Department of Basic Medical Sciences, Medical School, Universidad de La Laguna, Tenerife, Spain
- 2Instituto de Tecnologias Biomedicas, Universidad de La Laguna, Tenerife, Spain
Within the potassium ion channel family, calcium activated potassium (KCa) channels are unique in their ability to couple intracellular Ca2+ signals to membrane potential variations. KCa channels are diversely distributed throughout the central nervous system and play fundamental roles ranging from regulating neuronal excitability to controlling neurotransmitter release. The physiological versatility of KCa channels is enhanced by alternative splicing and co-assembly with auxiliary subunits, leading to fundamental differences in distribution, subunit composition and pharmacological profiles. Thus, understanding specific KCa channels’ mechanisms in neuronal function is challenging. Based on their single channel conductance, KCa channels are divided into three subtypes: small (SK, 4–14 pS), intermediate (IK, 32–39 pS) and big potassium (BK, 200–300 pS) channels. This review describes the biophysical characteristics of these KCa channels, as well as their physiological roles and pathological implications. In addition, we also discuss the current pharmacological strategies and challenges to target KCa channels for the treatment of various neurological and psychiatric disorders.
Introduction
Ca2+-activated potassium channels (KCa channels) constitute a heterogeneous family of ion channels with variable biophysical and pharmacological properties. These channels share a common functional role by coupling the increase in intracellular Ca2+ concentration to hyperpolarization of the membrane potential. This intrinsic feature allows KCa channels to play key roles in controlling cellular excitability and maintaining K+ homeostasis and cell volume in non-excitable cells. According to their single-channel conductances, KCa channels are divided into three main subfamilies: SK (small conductance; ∼4–14 pS), IK (intermediate conductance; ∼32–39 pS) and BK (big conductance; ∼200–300 pS) channels. KCa channels are expressed in the plasma membrane as tetramers of α subunits. The gene encoding the α subunit (slo; KCNMA1) of BK channels (KCa1.1; slo1) was cloned in the early 1990s from Drosophila (Atkinson et al., 1991; Adelman et al., 1992) and mice (Butler et al., 1993). SKα subunits are products of the KCNN1 (KCa2.1; SK1), KCNN2 (KCa2.2; SK2) and KCNN3 (KCa2.3; SK3) genes (Köhler et al., 1996), whereas IKα (KCa3.1; IK1; SK4) are encoded by the KCNN4 gene (Ishii et al., 1997b; Joiner et al., 1997). Phylogenetic analysis reveals that KCa channels belong to two well defined groups (Wei et al., 2005), a division that is paralleled by biophysical properties regarding voltage dependence or the Ca2+ sensitivity and regulatory mechanisms.
The first group comprises SK and IK channels. As shown in Figure 1A, α subunits of these channels consist on six transmembrane helices (S1–S6; the pore region is formed by helices S5 and S6) and cytosolic N- and C-terminal domains. One of their biophysical characteristics is that their function is independent of membrane voltage (Hirschberg et al., 1999). These channels are activated by low intracellular Ca2+ concentrations (≈ 0.5 μM) through a mechanism consisting on the existence of a calmodulin (CaM) binding-domain (CaMBD) within the channel’s protein (Fanger et al., 1999; Adelman, 2016). The C-lobe region of CaM is associated to the CaMBD domains following a 1:1 stoichiometry, in a Ca2+-independent manner (Lee and MacKinnon, 2018) (Figure 1B). Binding of Ca2+ to the N-lobes of CaM forces the CaM-CaMBD monomers to arrange structurally into a “dimer of dimers” conformation, pulling the bundle-crossed helices of the pore to open the channel (Schumacher et al., 2001; Lee and MacKinnon, 2018).
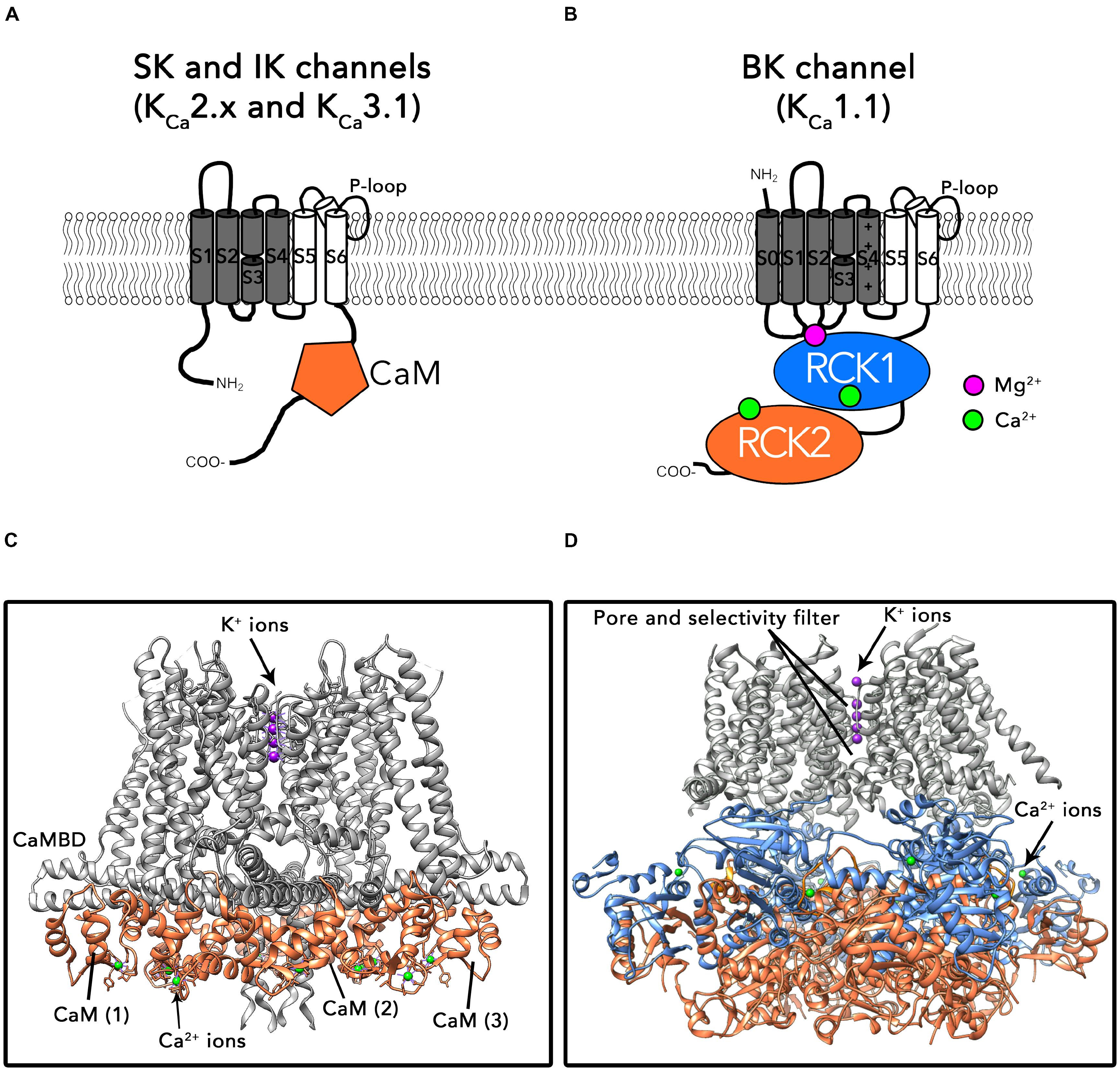
FIGURE 1. Topology and structures of SK/IK and BK channels. (A) Schematic protein topology of one SKα-subunit including the CaM bound to the CaMBD (represented as an orange hexagon). (B) Full-length structure of the Ca2+-CaM bound IK channel (PDB: 6CNN). The CaM N-lobe binds Ca2+ ions after association of CaM to the SK channel C-lobe. (C) Schematic topology of one BKα-subunit. Binding sites for divalent cations are located in the cytosolic C-terminal region of the channel. Each α subunit contains two high affinity Ca2+ binding sites (represented as green circles) and one low affinity Ca2+ and Mg2+ binding site, formed by residues from RCK1, S0–S1 and S2–S3 intracellular loops (purple circle). (D) Ca2+-bound BKα homotetramer full-length structure from Aplysia californica (PDB: 5TJ6).
BK channels belong to the second group in the KCa family (for a recent review, see Latorre et al., 2017). The topology of BK α subunits differs from the SK/IK group in the existence of an additional transmembrane helix S0 which drives the N-terminus to the extracellular side of the plasma membrane (Figure 1C). The large cytosolic C-terminal region of these channels is composed of two non-identical “regulator for conductance of potassium” domains (RCK1 and RCK2), each one containing a high affinity Ca2+ binding site (Kd = 0.8–11 μM; (Schreiber et al., 1999; Xia et al., 2002; Sweet and Cox, 2009). In the tetramer, four RCK1-RCK2 tandems form a structure known as the “gating ring” (Wu et al., 2010; Yuan et al., 2011; Hite et al., 2017; Tao et al., 2017) (Figure 1D). An additional regulatory site located at the interface between the gating ring and transmembrane region exhibits millimolar affinity for divalent cations such as Mg2+ (Figure 1D) (Shi et al., 2002; Xia et al., 2002; Yang et al., 2008). In contrast to SK and IK channels, BK are allosterically activated by both changes in the membrane voltage and intracellular Ca2+ (Horrigan and Aldrich, 2002). Similarly to other voltage-gated ion channels, the membrane voltage is sensed by charged amino acids within segments S2, S3, and S4 in the transmembrane region (Diaz et al., 1998; Ma et al., 2006; Lee and Cui, 2010; Tao et al., 2017). In contrast to SK and IK channels, Ca2+ directly activates BK channels by binding to the specific Ca2+ binding sites in the gating ring region, without involving CaM. Binding of Ca2+ results on structural rearrangements of the gating ring region, ultimately leading to pore opening (Yusifov et al., 2008, 2010; Lee and Cui, 2010; Javaherian et al., 2011; Yuan et al., 2011; Savalli et al., 2012; Hoshi et al., 2013). It has been proposed that the interaction of the gating ring with the voltage-sensing region of the channel plays an important role in this process (Hite et al., 2017; but see also Zhou et al., 2017) Importantly, the biophysical properties of KCa channels such as the voltage and Ca2+ sensitivities as well as their pharmacological characteristics are greatly influenced by the co-assembly with auxiliary subunits (e.g., β1-4, γ1-4; see below).
Members of the two groups comprising the KCa channels family also differ in their structural features. Available crystal structures of the SK CaMBD region bound to CaM have contributed to advance our knowledge about the regulation of these channels by various effectors (Schumacher et al., 2001, 2004; Zhang et al., 2012a,b, 2013; Zhang M. et al., 2014; Nam et al., 2017). Until very recently, structural information about BK channels was the most extensive, including X-ray crystal structures of the isolated C-terminal region known as the “gating ring” (Wu et al., 2010; Yuan et al., 2011) as well as cryo-EM structures of the full-length channel obtained in the presence and absence of Ca2+ (Hite et al., 2017; Tao et al., 2017; see also Giraldez and Rothberg, 2017; Zhou et al., 2017) (Figure 1D). Exciting new results from the MacKinnon laboratory bring now to light the cryo-electron microscopy (cryo-EM) structures of a human IK-CaM complex in closed and activated states, unveiling unprecedented insights about their gating mechanism (Lee and MacKinnon, 2018) (Figure 1B).
The SK, IK, and BK subfamilies show distinct tissue distribution and pharmacology. SK channels are predominantly expressed in the nervous system and are typically inhibited by the bee venom apamin (Park, 1994; Stocker and Pedarzani, 2000). IK channels are mainly distributed in blood, epithelial cells and in some peripheral neurons and are sensitive to the antifungal drug clotrimazole (Gardos, 1958; Jensen et al., 1998). BK channels are the most diverse group of KCa channels. They are ubiquitously expressed and sensitive to iberiotoxin, charybdotoxin, and paxilline (Latorre et al., 2017). The diversity of KCa physiological roles is widened by the existence of numerous splicing variants (specially for BK and SK channels) in different tissues (Navaratnam et al., 1997; Shipston, 2001; Chen et al., 2005; Scholl et al., 2014), as well as their association with auxiliary subunits. In vertebrates, BK channels co-assemble with modulatory β (β1-4) and γ (γ1-4) subunits to modify the channel functional properties and pharmacology (Latorre et al., 2017). In addition, KCa channels in neurons are closely associated to Ca2+ sources such as voltage-gated Ca2+ channels (VGCC) (Marrion and Tavalin, 1998; Tonini et al., 2013; Rudolph and Thanawala, 2015; Vivas et al., 2017), transient receptor potential vanilloid (TRPV) channels (Wu et al., 2013; Feetham et al., 2015) and N-methyl-D-aspartate receptors (NMDAR) (Isaacson and Murphy, 2001; Ngo-Anh et al., 2005) allowing the precise regulation of membrane excitability and spike firing patterns.
Mutation of genes encoding KCa channels and subsequent loss- or gain-of-function of these channels has been directly linked to a wide range of human diseases (Table 1). Over the last two decades, numerous studies have contributed to decipher the potential role of KCa channels in these diseases at the molecular level, paving the way for novel therapies. However, despite our current understanding about tissue specific distribution, functional diversity and clinical impact of KCa channels, they still remain underexploited as targets for drug discovery. In this review, we summarize the most recent knowledge about KCa channels, focusing on their potential as therapeutic targets in the nervous system and analyzing the factors that could contribute to the difficulties to develop novel KCa channel modulators. Some excellent reviews in the last few years relate to individual members of the KCa family, or are centered on particular aspects of KCa physiopathology (Faber, 2009; Lam et al., 2013; Gueguinou et al., 2014; Contet et al., 2016; Sugunan et al., 2016). Here we initially describe the functional roles of KCa channels in the nervous system, including their protective roles. Subsequently, the pathologies associated to malfunction of these channels are detailed. Known activators and inhibitors of KCa channels are presented in the third section of the review, as they may constitute the molecular basis of potential therapeutic strategies to treat a wide range of neurological disorders. Finally, we discuss the possible reasons why little progress has been made toward development of such strategies despite intense research efforts in academia and industry.
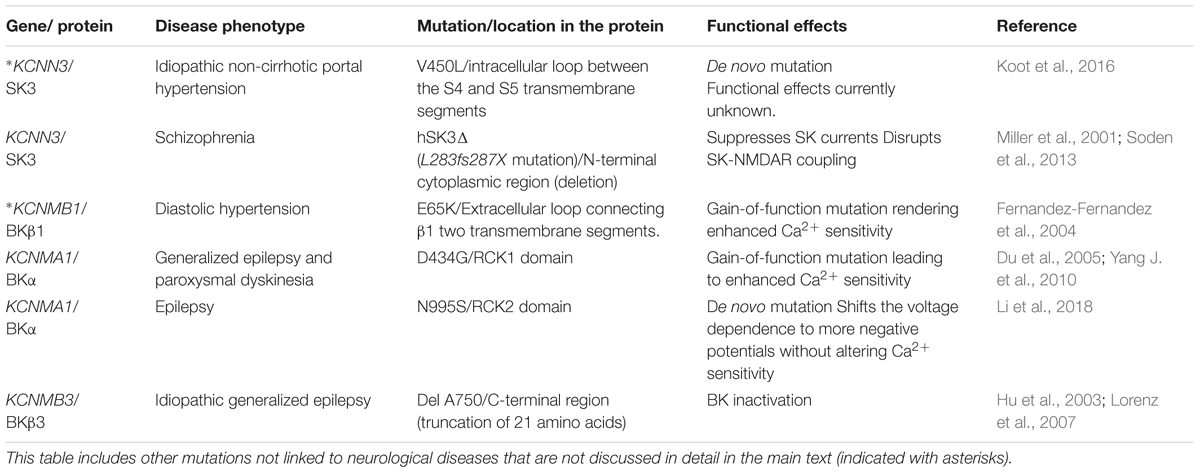
TABLE 1. Mutations in KCa channels that are associated in human diseases, their location in the channel protein, functional implications and related references.
Neuronal Expression and Functional Roles of KCa Channels
KCa channels are expressed in neurons and other cell types in the central nervous system (CNS), where they are involved in a large variety of physiological functions. Due to the vast distribution of KCa channels and their pleiotropic roles in the CNS, functional studies have been performed in different neuronal types, developmental stages or animal species. It is important to take into account the diversity of these approaches when interpreting the results described in the present review.
Activation of SK channels regulates firing in pacemaker neurons of midbrain and cerebellum, which is critical for muscle coordination and movement (Wolfart and Roeper, 2002; Womack and Khodakhah, 2003). In many neurons, action potentials are followed by a medium-duration after-hyperpolarization (mAHP) that affects their intrinsic excitability (Adelman et al., 2012). In numerous neuron types, the mAHP underlying current (ImAHP), which can last up to hundreds of milliseconds, is Ca2+-dependent but voltage-insensitive and is blocked by apamin but not by BK channel blockers, suggesting the involvement of SK channels (Sah, 1996; Stocker et al., 1999; but see Gu et al., 2005). The three SK channel α subunits, SK1, SK2 and SK3 display characteristics similar to ImAHP in heterologous expression systems but fail to fully reproduce the pharmacological and biophysical properties observed in vivo (Stocker et al., 1999). This conundrum may be explained by the existence of SK heteromultimeric channels, which have been also described in heterologous expression systems (Ishii et al., 1997a). The idea that SK channels can assemble either as homo- or heteromultimeric channels in vivo is supported by their expression pattern in brain, which is overlapping in many regions (Stocker and Pedarzani, 2000). Interestingly, recent evidence shows that SK channels preferentially form species-specific heteromultimers when expressed in HEK cells, by interaction of their C-terminal regions (Tuteja et al., 2010; Church et al., 2015). These authors also show the physiological relevance of SK heteromultimers in CA1 pyramidal neurons (Church et al., 2015) and cardiomyocytes (Tuteja et al., 2010), highlighting the need of further studies to address the implications of heteromeric SK channels in brain and heart.
A role of SK channels in dendritic spines of pyramidal neurons in hippocampus and amygdala has been postulated, where they may regulate the amplitude of excitatory postsynaptic potentials (EPSPs) (Faber et al., 2005; Ngo-Anh et al., 2005; Bloodgood and Sabatini, 2007). During EPSPs, activation of SK channels limits Ca2+ influx into the spines and dendrites, thus creating a negative feedback loop controlling EPSPs amplitude. In addition, this activation of SK channels may influence NMDAR receptor activation (via voltage-dependent Mg2+ block), consequently affecting synaptic plasticity (Ngo-Anh et al., 2005). In agreement with this, blockade of SK channels in pyramidal neurons from hippocampus (Stackman et al., 2002) and amygdala (Faber et al., 2005; Ngo-Anh et al., 2005) enhanced long-term potentiation (LTP), suggesting that dendritic SK channels may play an important role in memory and learning processes (Stackman et al., 2002).
IK channels are widely expressed throughout the body, excluding some excitable tissues such as cardiac muscle or skeletal muscle. IK channels regulate the membrane potential and Ca2+ signaling and are responsible of activation and proliferation of vascular smooth cells and lymphocytes (Toyama et al., 2008; Di et al., 2010; Yu et al., 2013). Immunohistochemical staining experiments indicate that human enteric, sensory and sympathetic neurons also express IK channels (Furness et al., 2004; Bahia et al., 2005; Mongan et al., 2005). In the CNS, IK channels are mainly localized in microglia and endothelial cells (Pedarzani and Stocker, 2008). Microglial IK channels participate in controlling several functions including respiratory burst, proliferation, migration as well as lipopolysaccharide mediated nitric oxide production (Kaushal et al., 2007; Nguyen et al., 2017). Accumulating evidence indicates that IK channels are involved in the process of astrogliosis, which occurs in most forms of CNS insults (Bouhy et al., 2011; Chen et al., 2011; Yu et al., 2014). It has been proposed that, in addition to BK (see below), IK channels also contribute to neurovascular coupling in astrocytes (Longden et al., 2011).
In many neuron types including hippocampal CA1 and CA3 pyramidal cells, repetitive firing stimulus elicits a slow, long lasting hyperpolarization, which is referred to as slow AHP (sAHP) (Adelman et al., 2012). The development of sAHP prevents the generation of new action potentials, limiting hyperexcitation of neurons in response to a long lasting or repetitive stimulus. Several studies have suggested that both SK (Lima and Marrion, 2007) and Kv7 channels (Tzingounis and Nicoll, 2008; Tzingounis et al., 2010) may contribute to sAHP. However, this assertion is contradicted by other observations showing that blockers of those channels produce only a minor reduction of the sAHP current (IsAHP), suggesting that other channels are involved (Womble and Moises, 1993; Power and Sah, 2008). Furthermore, genetic ablation of SK1, SK2 or SK3 does not affect sAHP in CA1 pyramidal neurons (Bond et al., 2004). Although IK channels have been suggested to underlay sAHP (King et al., 2015), a recent study ruled out this hypothesis based on the pharmacological properties of IsAHP, which were unaltered in IK knockout mice (Wang K. et al., 2016). The molecular identity of the sAHP channels remains unknown.
BK channels are localized throughout the dendrites, axon, soma and synaptic terminals of neurons in different brain regions, including cortex and hippocampus (Knaus et al., 1996; Grunnet and Kaufmann, 2004; Wang et al., 2014), where they play key roles in action potential duration, firing frequency and neurotransmitter release (for a recent review, see Contet et al., 2016). It should be noted that depending on the subcellular location where they are expressed, BK channel isoforms may exhibit different biophysical and trafficking properties, determined by the subunit composition (assembly with auxiliary subunits) and alternative splicing (Contet et al., 2016). At the synaptic terminals, it has been proposed that tight spatial coupling between BK channels and VGCCs (Marrion and Tavalin, 1998) constitutes a molecular brake for neurotransmitter release, by the BK-mediated termination of action potentials (Bielefeldt and Jackson, 1993; Hu et al., 2001; Muller et al., 2007; Contet et al., 2016). In dendrites, this association is also relevant, contributing to regulation of the magnitude and duration of dendritic Ca2+ spikes to influence action potential output (Engbers et al., 2013; Indriati et al., 2013). BK channels have the ability to shape the action potential waveforms and influence the frequency of firing in different ways. Pharmacological or genetic deletion of BK channels can increase or decrease the evoked or spontaneous firing frequency (Bielefeldt and Jackson, 1993; Nelson et al., 2003; Gu et al., 2007). Many studies have shown that blockade of BK channels using TEA, charybdotoxin or paxilline slows action potential repolarization and reduces the magnitude of the fast-duration AHP (Adams et al., 1982; Storm, 1987; Sah and McLachlan, 1992; Zhang and McBain, 1995; Faber and Sah, 2002). In the suprachiasmatic nucleus, BK channels contribute to spontaneous firing rate related to normal circadian behavioral rhythm (Meredith et al., 2006; Whitt et al., 2016; Whitt et al., 2018). Genetic ablation of the BKβ4 subunit in neurons from the dentate gyrus (DG) augmented the fast AHP amplitude, sharpened the action potential and increased spike frequency (Brenner et al., 2005). These findings support the idea that BK channels are not strictly excitatory or inhibitory but can be dynamically regulated to control neuronal excitability (Contet et al., 2016). Regulatory mechanisms include coupling to accessory subunits (Brenner et al., 2005; Whitt et al., 2016) and different sources of intracellular Ca2+ (Wang B. et al., 2016; Whitt et al., 2018).
Expression of BK has been also reported in astrocytes (Seidel et al., 2011; Seifert et al., 2018), where they have been related to astrocytic function connecting neuronal signaling to CNS vasculature (Howarth, 2014). Many studies have highlighted the role of astrocytic processes (endfeet) in modulating the vascular response by dynamically controlling intracellular Ca2+ (Filosa et al., 2006; Takano et al., 2006; Girouard et al., 2010). It has been proposed that activation of BK channels by elevated Ca2+ in astrocytic endfeet leads to vasodilation by the release of K+ into the perivascular space (Price et al., 2002; Filosa et al., 2006). Interestingly, another study demonstrated that this mechanism can also mediate vasoconstriction, leading the authors to propose that BK channels may produce dual vascular responses depending on the Ca2+ levels at the astrocytic endfoot (Girouard et al., 2010).
Neuroprotective Roles of KCa Channels
Dopamine (DA) neurons in the midbrain retrorubral field (RRF), substantia nigra pars compacta (SNc) and ventral tegmental area (VTA) influence the neurons in target areas due to their extensive axonal projections (Matsuda et al., 2009). Thus, DA neurons are involved in regulating essential processes such as fine motor control (Bernheimer et al., 1973) learning and memory (Matsumoto and Takada, 2013), cognitive processes (Nieoullon, 2002), response to stress (Ungless et al., 2010) and noxious stimuli (Brischoux et al., 2009). SK channels control the pattern of single spike firing of DA neurons both in vivo and in vitro, as demonstrated by the reduction in firing regularity observed after treating the cells with apamin (Waroux et al., 2005; Ji and Shepard, 2006). Some studies have postulated that SK channels may exert neuroprotective effects against progressive DA neuronal death (Dolga et al., 2014), by a proposed mechanism in which activation of SK channels in SNc neurons counteracts excitotoxicity associated to NMDAR overactivation (Dolga et al., 2014; Duda et al., 2016). Additionally, SK channels could directly regulate NAPDH oxidase function, thus hampering neuronal damage by preventing production of reactive oxygen species (ROS) in mitochondria (Fay et al., 2006; Dolga et al., 2014). In mouse CA1 pyramidal neurons, a protective role of SK2 channels has been described, based on the observation that direct activation of SK channels by 1-ethyl-2- benzimidazolinone (1-EBIO) significantly reduced neuronal death in a model of cerebral ischemia induced by cardiac arrest/cardiopulmonary resuscitation. This finding points to activation of SK channels as a potential neuroprotective target in cerebral ischemia (Allen et al., 2011).
Accumulating evidence supports multifaceted functions of IK channels (Kaushal et al., 2007; Dolga and Culmsee, 2012). Specifically, IK channels have been related to microglia activation by modulating Ca2+ signaling, oxidative burst, proinflammatory cytokines production and microglia-mediated neuronal cell death (Kaushal et al., 2007). According to these proposed physiological roles, a recent study demonstrated that genetic ablation or in vivo pharmacological blockade of IK channels in the middle cerebral artery occlusion (MCAO) mouse model reduced the infarct area as well as improved neurological deficit by reducing microglia-associated neuronal death (Chen Y.J. et al., 2016).
A protective role of BK channels in the context of acute focal cerebral ischemia has been tested in vivo by performing MCAO in BK knockout mouse models, which showed higher post-ischemic mortality than their WT littermates (Liao et al., 2010). In this context, it was suggested that BK plays a neuroprotective role by counteracting NMDA-induced neurotoxicity, since genetic silencing or blockade of BK channels in organotypic hippocampal slice cultures (Liao et al., 2010) or cortico-striatal brain slice cultures (Katsuki et al., 2005) showed higher levels of NMDA-induced neuronal death when they were exposed to ischemic-like conditions.
KCa channels are also expressed at inner mitochondrial membranes (mitoKCa), where they have been suggested to be involved in neuroprotection by different mechanisms, some of which are still unknown (Bednarczyk, 2009). The activation of mitoSK channels in conditions of glutamate toxicity enhanced the mitochondrial resilience and reduced cell death in mouse hippocampal cell lines (Dolga et al., 2013; Honrath et al., 2017). Additionally, mitoBK channels were found to preserve neuronal cell viability by attenuating the overall ROSs production (Kulawiak et al., 2008; Skalska et al., 2009). In addition to these reports, several others have reviewed the importance of mitoKCa channels as potential targets for neuroprotection (Szewczyk et al., 2010; Balderas et al., 2015; Krabbendam et al., 2018).
Role of KCa Channels in Neurological and Psychiatric Diseases
Concomitant with their physiological roles, alterations in KCa function lead to excitability disorders that are characterized by abnormal firing of neuronal networks. The advancement of genetic linkage analysis over the past decades significantly facilitated the identification of specific loci associated to disease, many of which include genes encoding KCa channels subunits (Cooper and Jan, 1999; see Table 1). The role of these channels in disease has been also addressed using pharmacological tools. In this section we review the proposed contribution of BK and SK channels to neurological diseases. It is worth noting that, to our knowledge, no human diseases have been described in the literature involving KCa3.1 (IK) channel mutations. Their potential as drug targets in neurodegenerative diseases and ischemic stroke is reviewed in latter sections referring to KCa modulators.
Role of SK Channels
Malfunction of SK channels has been related to epilepsy. In pilocarpine-treated epileptic rats, the expression and function of SK channels was significantly reduced (Oliveira et al., 2010). Using thalamic slices treated with bicuculline methiodide as an experimental model of absence epilepsy, Kleiman-Weiner et al. (2009) found that blockade of SK channels modulated epileptiform oscillations by reducing intrinsic excitability of reticular neurons with no effect on relay neurons, pointing to SK channels as potential therapeutical targets for this disease. The involvement of SK in epilepsy has been further studied by using different modulators (see following section in this review).
Schizophrenia is a progressive neurodegenerative disorder that is characterized by disintegration of processes involved in thinking and emotional responsiveness (Postmes et al., 2014), that has been linked to DA imbalance (Brisch et al., 2014). A spontaneous mutation of the SK3 channel gene (L283fs287X) resulting in the deletion of the protein N-terminal region was identified in human schizophrenia patients (Bowen et al., 2001) and the resulting altered channels (hSK3Δ) were found to dominantly suppress SK channel currents. (Miller et al., 2001). Selective expression of this SK3 mutant in mice DA neurons additionally reduced the coupling between the SK channels and NMDAR, leading to increased burst firing as well as amplification of DA release, ultimately impacting animal behavior (Soden et al., 2013). The results by Soden et al. (2013) additionally showed that functional deficits of SK channels can alter the balance between phasic and tonic DA signals, which are normally associated to the pathogenesis of schizophrenia (Grace, 1991).
Pathogenesis of Parkinson’s disease (PD), which is associated to death of DA neurons in the SNc (Surmeier et al., 2010) has been also related to K+ channels dysfunction, including SK channels (Wang et al., 2008; Chen et al., 2018). This hypothesis is consistent with the observed roles of SK channels as regulators of dopaminergic neurotransmission in the SNc, protecting DA neurons from excitotoxic death (as reviewed in the previous section; see also Dolga et al., 2014). However, the role of SK channels in the etiology of PD remains elusive due to contradictory evidence. Some reports showed that activation of SK channels by NS309 in human DA neurons inhibited spontaneous firing, enhancing mAHP (Ji and Shepard, 2006), and reducing neurotoxicity (Dolga et al., 2014). These results suggest that increasing SK channel activity could boost or at least preserve levels of DA synthesis, which would in turn mitigate the long-term motor symptoms of PD. On the other hand, several studies have provided evidence that blockade of SK channels by apamin improved the symptoms of PD in vitro and in vivo (Doo et al., 2010; Kim et al., 2011; Alvarez-Fischer et al., 2013). This idea was further strengthened by the fact that blocking SK channels restored minimal DA activity in the striatum, alleviating the non-motor symptoms induced by partial striatal DA lesions (Chen et al., 2014). A possible interpretation of these apparently contradictory results is that SK channel positive or negative modulators could be beneficial depending on the stage (early or late) of the disease.
Role of BK Channels in Neurological Diseases
BK malfunction has been related to seizures and epilepsy, although the role of these channels in this pathological condition seems to be complex. KCNMA1 knockout mice did not show spontaneous seizures, suggesting that CNS excitability is unaffected and discarding a prominent role of BK hypoactivity in epileptogenesis (Sausbier et al., 2004). However, growing evidence associates BKα gain-of-function or loss-of-function to neuronal pro-excitatory or pro-inhibitory effects, which may depend on the cellular context (for recent reviews, see (N’Gouemo, 2014; Contet et al., 2016). A BKα gain-of-function mutation (D434G) showing larger macroscopic currents, increased intrinsic gating and Ca2+ sensitivity was associated with a human syndrome of generalized epilepsy and paroxysmal dyskinesia (Du et al., 2005). Studies addressed to characterizing the mechanism underlying the strong functional impact of this mutation have greatly contributed to a better understanding of the biophysics of BK channels. Since the D434 site is located in the vicinity of the RCK1 Ca2+ binding site of the BK channel, its alteration was found to affect the allosteric coupling between the Ca2+ binding and the opening of the channel (Yang J. et al., 2010). Enhanced neuronal BK channel activity as a result of the D434G mutation would increase the fAHP, resulting in faster recovery of sodium channels from inactivation, enabling neuronal firing at higher frequency and ultimately leading to increased neuronal excitability and seizures (Du et al., 2005; Diez-Sampedro et al., 2006; Wang et al., 2009; Yang J. et al., 2010). In a recent study, Li et al. (2018) identified a de novo mutation in the BKα gene (N995S) associated with epilepsy but not with paroxysmal dyskinesia. In contrast to the D434G mutant, the N995S gain-of-function variant shows alterations of the channel’s sensitivity to voltage, but not to Ca2+ (Li et al., 2018). This notion that hyperactivity of BK channels is related to hyperexcitability and seizures is further supported by their implication in pathogenesis of alcohol withdrawal seizures (Ghezzi et al., 2014). Furthermore, blockade of BK has been shown to reduce neuronal hyperexcitability in animal models of epilepsy and seizures, but does not present anticonvulsant effects in animals with no previous seizure episodes (Jin et al., 2000; Sheehan et al., 2009). BK pro-excitatory or pro-inhibitory effects can be additionally determined and modulated by association to regulatory subunits. A recent study by Whitmire et al. (2017) showed that β4 expression in hippocampal DG granule neurons is downregulated following pilocarpine-induced seizures, leading to gain-of-function BK and increased excitability. This result is consistent with previously observed effects in β4 knockout mice (Wang et al., 2009; Whitmire et al., 2017). Alteration of the BKβ3 regulatory subunit as a consequence of a single base pair deletion (delA750) in the KCNMB3 gene has also been associated to idiopathic generalized epilepsy in humans (Lorenz et al., 2007). This variant confers loss-of-function characteristics to BKα in heterologous expression systems by enhancing inactivation (Hu et al., 2003).
Two independent genome-wide association studies reported that single nucleotide polymorphisms in the KCNMA1 gene encoding the BKα subunit (Beecham et al., 2009) and in the KCNMAB2 gene (regulatory subunit BKβ2) (Beecham et al., 2014) are strongly linked to the pathophysiology of Alzheimer’s disease (AD). Intracellular application of amyloid β peptide (β) in neocortical pyramidal neurons from rats and mice increased neuronal excitability by reducing function of BK channels (Yamamoto et al., 2011). In AD and depression mice models, transcranial magnetic stimulation (TMS) reduced the depression-like behavior by a proposed mechanism in which the activation of BK channels by TMS would enhance hippocampal LTP and inhibit cortical excitability (Sun et al., 2011; Wang F. et al., 2015). Interestingly, TMS was found to improve cognitive functions in human AD patients (Cotelli et al., 2006; Ahmed et al., 2012).
BK malfunction has been also related to Fragile X syndrome (FXS), a monogenic form of intellectual disability and autism, associated to transcriptional silencing of the Fmr1 gene encoding Fragile X mental retardation protein (FMRP) (Verkerk et al., 1991). Recent evidence has shown that FMRP regulates neurotransmitter release in CA3 neurons by directly interacting with BKβ4 subunits (Deng et al., 2013). Consistent with this hypothesis, Hebert et al. (2014) restored the FXS behavioral deficits in Fmr1 knockout mice by treating the animals with selective BK channel openers (see below). These results support the idea that BK channels may be potential therapeutic targets for FXS (Brager and Johnston, 2014; Contractor et al., 2015).
Habituation is a form of learning in which the behavioral response to a stimulus decreases following repeated exposure to the stimulus (Rankin et al., 2009). Habituation to the acoustic startle response is impaired in many psychiatric disorders including schizophrenia and autism (Braff et al., 1992; Ornitz et al., 1993). BK channels have been implicated in habituation by controlling synaptic transmission (Typlt et al., 2013). Recently, it has been shown that activation of BK channels and their subsequent phosphorylation are essential for synaptic depression underlying habituation (Zaman et al., 2017).
Regulation of KCa Channels and Implications for Therapeutic Approaches
As described in the previous sections, alterations in KCa channels are associated to several genetically linked and acquired diseases. In this section we review the use of various KCa modulators oriented toward disease treatment (summarized in Table 2), including some pharmacological considerations. The current state-of-the-art knowledge on the potential of KCa channels as therapeutic targets is summarized in Figure 2 by representing as a weighing scale the beneficial effects resulting from either activation (left side) or blockade (right side) of each KCa subtype. Interestingly, SK and BK channels’ balance is tipped toward the activation side, which seems to be more beneficial than blockade. Conversely, for IK channels blockade is more favorable than activation.
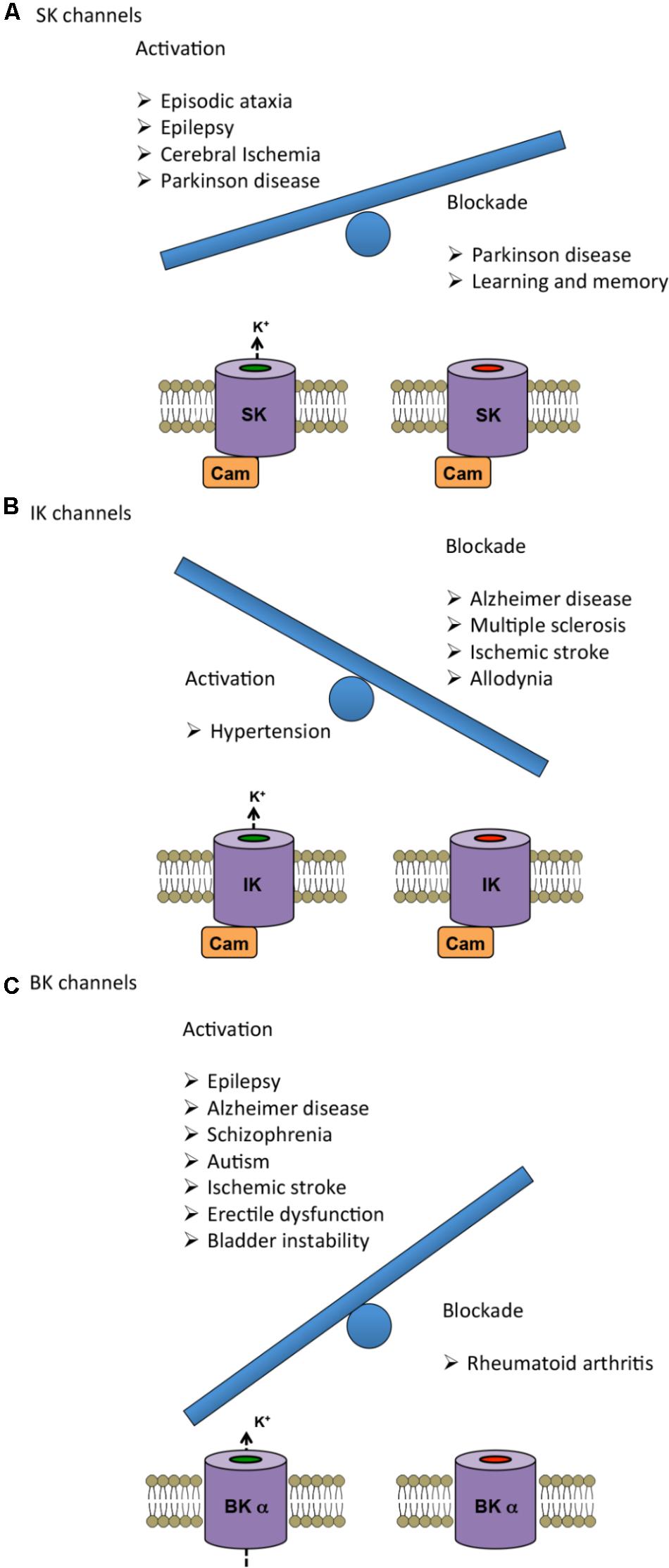
FIGURE 2. Therapeutic benefits of either activation (left) or blockade (right) of KCa channels represented as a weighing scale. The heavier balance indicates the modulation (activation or blockade) that has been reported advantageous in a larger number of diseases. (A,C) In the case of SK and BK channels, activation shows more beneficial effects than inhibition. (B) For IK channels, pharmacological blockade has proven to be more advantageous than activation. For further information about the specific effects, see Tables and main text.
Modulators of SK Channels
Several modulators of SK function have been identified as the basis for potential treatment of neurological diseases. Chlorzoxazone (CZX) is a SK channel activator, which has been approved by the U.S. Food and Drug Administration (FDA) as a muscle relaxant to treat spasticity (Losin and McKean, 1966). In addition, it has been proposed to be a viable pharmacotherapeutic approach for episodic ataxia type-2 (EA2) treatment. Oral administration of CZX in an EA2 mouse model improved motor coordination and reduced the severity, frequency and duration of dyskinesia episodes with no side effects, by a proposed mechanism of restoring SK channels function (Alvina and Khodakhah, 2010).
Riluzole is the first FDA-approved treatment for amyotrophic lateral sclerosis (ALS) (Miller et al., 2012). Although the molecular target of riluzole remains elusive, many studies have proposed that it works by modulating SK channels (Dimitriadi et al., 2013; Lam et al., 2013). In spinal muscular atrophy animal models, treatment with riluzole ameliorated disease-related loss-of-function defects by acting on SK channels (Dimitriadi et al., 2013). A recent study demonstrated that riluzole inhibits supraspinally organized pain behavior in a rat model of arthritic pain by activating SK channels (Thompson et al., 2015). Beneficial effects of riluzole have also been reported for the treatment of spinocerebellar ataxia type 2 (SCA2), a disease involving irregular firing and eventual neuronal death of Purkinje cells (PC) (Kasumu and Bezprozvanny, 2012). Administration of riluzole in patients improved ataxia-related symptoms (Ristori et al., 2010). This result was paralleled in a SCA2 transgenic mouse model, in which treatment with the SK selective activator NS13001 alleviated the symptoms of the disease (Kasumu et al., 2012). The mechanism underlying the effect of riluzole seems to reside in the activation of SK2 channels predominant in PC (Durr, 2010), which has been implicated in regulation of tonic firing (Ito, 2002; Walter et al., 2006). Consistently with this hypothesis, Walter et al. (2006) observed an improvement in motor coordination as well as restored PC firing regularity after treatment of an ataxia mouse model with SK channel modulators.
Selective activation of SK channels could be an effective therapy in some epileptic conditions. Potentiation of SK channels using ethylbenzimidazolinone (EBIO) was shown to inhibit epileptiform bursting in rat hippocampal CA3 neurons (Lappin et al., 2005). Additionally, this activator also reduced seizure incidence following maximal electroshock and increased the threshold to pentylenetetrazole-induced seizures in mice (Anderson et al., 2006).
In vivo infusion of SK activator 5,6-Dichloro-1-ethyl-1,3-dihydro-2H-benzimidazol-2-one (DCEBIO) in rats reduced recall of extinction memory, leading the authors to propose SK as a therapeutic target in anxiety disorders (Criado-Marrero et al., 2014). However, this result also suggests that the effect of SK activators on learning and memory should be taken into account in potential therapies.
The use of SK channel blockers has been also explored for disease treatment. Initial studies showed that SK blockers are not neuroprotective and might detrimentally accelerate neurodegeneration (Mourre et al., 1997). However, administration of apamin in rats and mice accelerated spatial learning (Deschaux et al., 1997; Stackman et al., 2002), promoted object recognition memory (Mpari et al., 2005) and facilitated the recall of extinction memory (Criado-Marrero et al., 2014). Additionally, apamin also mitigated the memory deficits associated to scopolamine- or electroconvulsive shock-induced amnesia (Inan et al., 2000). In summary, SK activators and blockers seem to be involved in distinct beneficial effects that would need to be balanced in order to design specific therapies.
Modulators of IK Channels
Consistent with the physiological roles of IK channels described in previous sections of this review, the selective inhibitor TRAM-34 (1-[(2- chlorophenyl)diphenylmethyl]-1H-pyrazole) decreased astrogliosis and microglial activation and attenuated memory loss in an AD mouse model (Yi et al., 2016). Furthermore, reduced microglia activation after blocking IK channels in an ischemic stroke mouse model has also been documented, which resulted in reduced infarction size and improved neurological deficit (Chen Y.J. et al., 2016). Additionally, application of TRAM-34 alleviated the symptoms of experimental autoimmune encephalomyelitis, a murine model of multiple sclerosis (Reich et al., 2005). Together, these data suggest that pharmacological blockade or genetic deletion of IK channels may represent a therapeutic target for various neurodegenerative disorders and ischemic stroke. Nonetheless, it is important to point out that although pharmacological blockade of IK channels appeared to be safe and well tolerated in animal models and humans, the genetic deletion induced an increase in blood pressure (Si et al., 2006). On the other hand, no study has yet reported increased blood pressure with IK channel blockers including treatment of mice with TRAM-34 (Toyama et al., 2008) or human volunteers taking Senicapoc (Ataga et al., 2008). Finally, recent evidence suggests that IK inhibitors should be used with caution since they may also increase tonic pain in certain clinical conditions. A recent study demonstrated that pharmacological inhibition of IK channels using TRAM-34 in mice increased formalin-induced nociceptive behavior (Lu et al., 2017). Conversely, another study showed that blocking IK channels with Senicapoc reversed tactile allodynia in rats with peripheral nerve injury (Staal et al., 2017).
Modulators of BK Channels
Zonisamide is an antiepileptic drug used clinically as adjunctive therapy in refractory partial-onset seizures that has also shown beneficial effects in a wide range of epilepsies and neuropsychiatric disorders (Biton, 2007). In addition to inhibiting neuronal Na+ and Ca2+ ion channels, Huang et al. showed that zonisamide activated BK channels in a hippocampal neuron-derived cell line, suggesting that the effects of zonisamide may be mediated, at least partly, by modulation of these channels (Huang et al., 2007).
A recent study suggests that BK channel activators could have therapeutic potential for cognitive defects in AD patients. Chronic application of the BK channel activator isoprimaric acid (ISO) enhanced non-spatial memory in AD mice, restoring the basic synaptic transmission and LTP at the hippocampal CA1 synapses, which were elevated and suppressed respectively in the disease model (Wang L. et al., 2015). Interestingly, ISO treatment not only enhanced BK channel activity but also reduced the Aβ fraction suggesting that a two-way relationship exists between BK channels and the Aβ plaque (Wang L. et al., 2015).
Treatment of FMR1 knockout mice, an animal model of FXS, with the selective BK channel opener BMS-204352 reversed the abnormal dendritic spine phenotype in vitro. Additionally, direct injection of the activator restored hippocampal glutamate homeostasis and corrected disturbances in social recognition and interaction, non-social anxiety and spatial memory (Hebert et al., 2014). In another study, BMS-204352 efficiently reversed the cortical hyperexcitability and increased acoustic startle seen in FMR1 knockout mice (Carreno-Munoz et al., 2018), suggesting that this treatment could alleviate sensory hypersensitivity symptoms in FXS patients.
Finally, BK channel openers have been tested to restore impaired habituation associated to some psychiatric disorders. Systemic administration of BMS-204352 greatly attenuated synaptic depression in the sensorimotor neurons of the caudal pontine reticular nucleus in the brain stem, which was associated with enhanced short-term habituation (Zaman et al., 2017).
Molecular Strategies and Current Challenges to Target KCa Channels in Drug Discovery
Ion channels are expressed at distinct physiological niches in the human body where they mediate diverse functions. Although numerous drugs are available to modulate K+ channels, currently no KCa modulators are clinically used. One potential reason for this comparative failure in the drug discovery process relates to the fact that the study of channel complexes in physiological conditions is challenging, due to their intricate functional mechanisms. For instance, the diversity of physiological roles fulfilled by BKα channels in neurons is greatly enhanced by coexpression with tissue specific auxiliary β subunits, which confer tissue- and subcellular-specific characteristics to the BK currents determining the action potential shape, firing rate and pharmacology (Jaffe et al., 2011; Wang et al., 2014; Whitt et al., 2016). This otherwise beautiful example of evolutionary complexity imposes an arduous task on the drug designing process, which must specifically target the various subunit compositions. Although some encouraging results may have been obtained in heterologous expression systems, quite often the potential compounds modulating KCa channels are less potent in vivo, leading to undesirable side effects. This section reviews some considerations about the existing technical approaches to test the efficiency and efficacy of potential modulators, as well as the use of other structural and genetic approaches to improve targeting of specific KCa channel subunits to particular tissues.
Electrophysiological testing of ion channel function on heterologous expression systems still remains the backbone for functional validation of potential modulators. However, the major downside of both conventional and automated electrophysiology is that throughput efficiency is limited and operational costs are high, so there is a significant need for the development of novel robust, affordable and effective high-throughput screening methods (Picones et al., 2016). Membrane potential-based drug assays constitute a robust method for assessing the K+ channel response to numerous drug candidates, although they seem to be subject to a large number of false positives (Solly et al., 2008). Ion flux assays are useful tools for screening compounds in drug discovery processes (Gill et al., 2003) including BK channel modulators (Jorgensen et al., 2008, 2013). This approach utilizes thallium or rubidium radioisotopes as K+ ion substitutes providing sensitive, precise and reproducible results, yet with some limitations related to the radioactivity and toxicity of these ions. A novel and imaginative cell free assay is the liposome flux assay (LFA), where purified K+ channels are reconstituted into lipid vesicles from which K+ flux is recorded and optically monitored with a high signal-to-noise ratio. Su et al. (2016) demonstrated the robustness of this technique by screening a library of 300,000 compounds targetting K+ ion channels, including BK.
Structural data is an essential tool supporting and complementing the discovery of ion channel-modulating drugs by providing information about drug–protein binding interactions. This knowledge is in turn necessary to improve the potency, efficacy and selectivity of the candidate drug compounds. Until very recently, available SK crystal structures were restricted to the Ca2+-gating domains (CaM and CaMBD) (Schumacher et al., 2001, 2004; Li et al., 2009; Zhang et al., 2012a,b, 2013; Zhang M. et al., 2014; Nam et al., 2017), lacking information about the transmembrane domains, therefore making the structure-based drug discovery a complicated task. Based on available structural information, some positive modulators of SK channels including PHU, 1-EBIO and NS309 were proposed to bind to the CaM/CaMBD interface (Strobaek et al., 2004; Zhang et al., 2012b, 2013; Morales et al., 2013; Brown et al., 2017; Nam et al., 2017). However, these conclusions are now challenged by the cryo-EM full-length IK-CaM structures only recently unveiled by the MacKinnon laboratory, which redefine the native interface between the CaM N-lobe and the C-terminal end of S6, showing a predominant role of the S4-S5 linker in Ca-CaM mediated channel gating (Lee and MacKinnon, 2018). Previous considerations should be revisited in light of these novel findings, which undoubtedly set an unprecedented ground for SK channels drug discovery. These new IK structures appear barely 1 year after the cryo-EM structures of the full-length BK channel in the open and closed states was obtained (Hite et al., 2017; Tao et al., 2017), revealing new and interesting insights in the channel structure–function relationships (Giraldez and Rothberg, 2017; Zhou et al., 2017; Kshatri et al., 2018). In this context, other techniques such as patch-clamp fluorometry (Zheng and Zagotta, 2003; Wulf and Pless, 2018) have proven to be useful tools to study conformational changes between subunits at the level of the gating ring during activation of functional BK channels (Giraldez et al., 2005; Miranda et al., 2013, 2016), and could also be extended to study other members of the KCa subfamily. Altogether, these approaches are revolutionizing our knowledge about how these ion channels can be selectively drug-targeted.
Developing specific rodent disease models based on characterized mutations has become a standard procedure to understanding their physiological relevance. In most cases, these models recapitulate the disease phenotypes and are useful as probing tools to characterize the channel’s cellular and neuronal functions. Furthermore, they might constitute preclinical tools to attain a better understanding of the disease-causing mechanisms, consequently helping to build therapeutic strategies targeting the disease. The development of the CRISPR genome editing technique has facilitated generation of disease models by introducing loss- or gain-of-function mutations, allowing to study the effects of underlying genetic aberrations (Chen S. et al., 2016).
In addition, taking advantage of novel molecular techniques, new strategies have been developed to target the mutated genes in vivo. One example consists on the administration of antisense oligonucleotides to reduce the levels of mutant proteins that could be responsible for the neurodegenerative diseases. In an animal model of ALS caused by a mutation in the SOD1 protein, direct delivery of antisense oligonucleotides significantly slowed disease progression (Smith et al., 2006). In this context, the CRISPR technique undoubtedly constitutes a promising molecular approach to reverse the alterations in proteins and restore their physiological activity. Cheng et al. (2013) demonstrated that injection of CRISPR components into mouse zygotes efficiently activated several endogenous genes. The application of these techniques to target KCa channels and associated pathologies needs further exploration.
A strategy that has progressed toward clinical trials is gene-based therapy. The first and only phase I trial using this approach was performed on human patients with erectile dysfunction (ED). Plasmids encoding BK channels were injected directly into the penis corpus cavernosum of patients with ED, rendering positive encouraging results, suggesting that gene therapy represents a valuable treatment for ED (Melman et al., 2006, 2007) and could be considered for other KCa-related pathologies.
Finally, combination therapies that integrate tissue delivery of genes and drugs has been shown to be a viable approach enhancing drug specificity (Chen J. et al., 2016). This is especially relevant in the case of BK channels, which are expressed ubiquitously throughout the body, so systemic drug administration often results in off-target effects (Lappin et al., 2005).
Conclusion and Future Perspectives
KCa channels are relevant determinants of neuronal excitability and their dysfunction is implicated in many inherited and acquired neurological and psychiatric diseases, thus constituting appealing targets for pharmacological intervention. Over the last few years, the drug discovery field has significantly advanced to identify lead compounds for KCa channel modulators. Available therapeutic strategies to target these channels are challenging due to their diverse physiological roles and tissue distribution, so the design of modulators that recognize tissue-specific subunit combinations is of special relevance. Structural biology has dramatically advanced over the last decade to reveal many ion channel structures with atomic resolution, including the long-awaited structure of the full-length BK channel and of the human IK-CaM complex. Knowledge of genetic- and drug-induced regulation of KCa channels coupled to investigation of their structure–function relationships will facilitate the development of disease-specific treatment strategies.
Author Contributions
AK, AG-H, and TG designed and wrote the manuscript.
Funding
The authors’ work is supported by grants from the Spanish Ministry of Economy and Competitiveness (BFU2015-66490 and RYC-2012-11349 to TG; FPU15/02528 to AG-H) and the European Research Council (ERC) under the European Union’s Horizon 2020 Research and Innovation Program (Grant Agreement 648936 to TG).
Conflict of Interest Statement
The authors declare that the research was conducted in the absence of any commercial or financial relationships that could be construed as a potential conflict of interest.
Acknowledgments
We thank Ricardo Gomez-Garcia, David Bartolome-Martin, Diego Alvarez de la Rosa, and Tomas Gonzalez-Hernandez for useful comments on the manuscript.
References
Adams, P. R., Constanti, A., Brown, D. A., and Clark, R. B. (1982). Intracellular Ca2+ activates a fast voltage-sensitive K+ current in vertebrate sympathetic neurones. Nature 296, 746–749. doi: 10.1038/296746a0
Adelman, J. P. (2016). SK channels and calmodulin. Channels (Austin) 10, 1–6. doi: 10.1080/19336950.2015.1029688
Adelman, J. P., Maylie, J., and Sah, P. (2012). Small-conductance Ca2+-activated K+ channels: form and function. Annu. Rev. Physiol. 74, 245–269. doi: 10.1146/annurev-physiol-020911-153336
Adelman, J. P., Shen, K. Z., Kavanaugh, M. P., Warren, R. A., Wu, Y. N., Lagrutta, A., et al. (1992). Calcium-activated potassium channels expressed from cloned complementary DNAs. Neuron 9, 209–216. doi: 10.1016/0896-6273(92)90160-F
Ahmed, M. A., Darwish, E. S., Khedr, E. M., El Serogy, Y. M., and Ali, A. M. (2012). Effects of low versus high frequencies of repetitive transcranial magnetic stimulation on cognitive function and cortical excitability in Alzheimer’s dementia. J. Neurol. 259, 83–92. doi: 10.1007/s00415-011-6128-4
Allen, D., Nakayama, S., Kuroiwa, M., Nakano, T., Palmateer, J., Kosaka, Y., et al. (2011). SK2 channels are neuroprotective for ischemia-induced neuronal cell death. J. Cereb. Blood Flow Metab. 31, 2302–2312. doi: 10.1038/jcbfm.2011.90
Alvarez-Fischer, D., Noelker, C., Vulinovic, F., Grunewald, A., Chevarin, C., Klein, C., et al. (2013). Bee venom and its component apamin as neuroprotective agents in a Parkinson disease mouse model. PLoS One 8:e61700. doi: 10.1371/journal.pone.0061700
Alvina, K., and Khodakhah, K. (2010). KCa channels as therapeutic targets in episodic ataxia type-2. J. Neurosci. 30, 7249–7257. doi: 10.1523/JNEUROSCI.6341-09.2010
Anderson, N.J., Slough, S., and Watson, W.P. (2006). In vivo characterisation of the small-conductance KCa (SK) channel activator 1-ethyl-2-benzimidazolinone (1-EBIO) as a potential anticonvulsant. Eur. J. Pharmacol. 546, 48–53. doi: 10.1016/j.ejphar.2006.07.007
Ataga, K. I., Smith, W. R., De Castro, L. M., Swerdlow, P., Saunthararajah, Y., Castro, O., et al. (2008). Efficacy and safety of the gardos channel blocker, senicapoc (ICA-17043), in patients with sickle cell anemia. Blood 111, 3991–3997. doi: 10.1182/blood-2007-08-110098
Atkinson, N. S., Robertson, G. A., and Ganetzky, B. (1991). A component of calcium-activated potassium channels encoded by the Drosophila slo locus. Science 253, 551–555. doi: 10.1126/science.1857984
Bahia, P. K., Suzuki, R., Benton, D. C., Jowett, A. J., Chen, M. X., Trezise, D. J., et al. (2005). A functional role for small-conductance calcium-activated potassium channels in sensory pathways including nociceptive processes. J. Neurosci. 25, 3489–3498. doi: 10.1523/JNEUROSCI.0597-05.2005
Balderas, E., Zhang, J., Stefani, E., and Toro, L. (2015). Mitochondrial BKCa channel. Front. Physiol. 6:104. doi: 10.3389/fphys.2015.00104
Beecham, G. W., Hamilton, K., Naj, A. C., Martin, E. R., Huentelman, M., Myers, A. J., et al. (2014). Genome-wide association meta-analysis of neuropathologic features of Alzheimer’s disease and related dementias. PLoS Genet. 10:e1004606. doi: 10.1371/journal.pgen.1004606
Beecham, G. W., Martin, E. R., Li, Y. J., Slifer, M. A., Gilbert, J. R., Haines, J. L., et al. (2009). Genome-wide association study implicates a chromosome 12 risk locus for late-onset Alzheimer disease. Am. J. Hum. Genet. 84, 35–43. doi: 10.1016/j.ajhg.2008.12.008
Bernheimer, H., Birkmayer, W., Hornykiewicz, O., Jellinger, K., and Seitelberger, F. (1973). Brain dopamine and the syndromes of Parkinson and Huntington. Clinical, morphological and neurochemical correlations. J. Neurol. Sci. 20, 415–455. doi: 10.1016/0022-510X(73)90175-5
Bielefeldt, K., and Jackson, M. B. (1993). A calcium-activated potassium channel causes frequency-dependent action-potential failures in a mammalian nerve terminal. J. Neurophysiol. 70, 284–298. doi: 10.1152/jn.1993.70.1.284
Biton, V. (2007). Clinical pharmacology and mechanism of action of zonisamide. Clin. Neuropharmacol. 30, 230–240.
Bloodgood, B. L., and Sabatini, B. L. (2007). Nonlinear regulation of unitary synaptic signals by CaV(2.3) voltage-sensitive calcium channels located in dendritic spines. Neuron 53, 249–260. doi: 10.1016/j.neuron.2006.12.017
Bond, C. T., Herson, P. S., Strassmaier, T., Hammond, R., Stackman, R., Maylie, J., et al. (2004). Small conductance Ca2+-activated K+ channel knock-out mice reveal the identity of calcium-dependent afterhyperpolarization currents. J. Neurosci. 24, 5301–5306. doi: 10.1523/JNEUROSCI.0182-04.2004
Bouhy, D., Ghasemlou, N., Lively, S., Redensek, A., Rathore, K. I., Schlichter, L. C., et al. (2011). Inhibition of the Ca(2)(+)-dependent K(+) channel, KCNN4/KCa3.1, improves tissue protection and locomotor recovery after spinal cord injury. J. Neurosci. 31, 16298–16308. doi: 10.1523/JNEUROSCI.0047-11.2011
Bowen, T., Williams, N., Norton, N., Spurlock, G., Wittekindt, O. H., Morris-Rosendahl, D. J., et al. (2001). Mutation screening of the KCNN3 gene reveals a rare frameshift mutation. Mol. Psychiatry 6, 259–260. doi: 10.1038/sj.mp.4000128
Braff, D. L., Grillon, C., and Geyer, M. A. (1992). Gating and habituation of the startle reflex in schizophrenic patients. Arch. Gen. Psychiatry 49, 206–215. doi: 10.1001/archpsyc.1992.01820030038005
Brager, D. H., and Johnston, D. (2014). Channelopathies and dendritic dysfunction in fragile X syndrome. Brain Res. Bull. 103, 11–17. doi: 10.1016/j.brainresbull.2014.01.002
Brenner, R., Chen, Q. H., Vilaythong, A., Toney, G. M., Noebels, J. L., and Aldrich, R. W. (2005). BK channel beta4 subunit reduces dentate gyrus excitability and protects against temporal lobe seizures. Nat. Neurosci. 8, 1752–1759. doi: 10.1038/nn1573
Brisch, R., Saniotis, A., Wolf, R., Bielau, H., Bernstein, H. G., Steiner, J., et al. (2014). The role of dopamine in schizophrenia from a neurobiological and evolutionary perspective: old fashioned, but still in vogue. Front. Psychiatry 5:47. doi: 10.3389/fpsyt.2014.00047
Brischoux, F., Chakraborty, S., Brierley, D. I., and Ungless, M. A. (2009). Phasic excitation of dopamine neurons in ventral VTA by noxious stimuli. Proc. Natl. Acad. Sci. U.S.A. 106, 4894–4899. doi: 10.1073/pnas.0811507106
Brown, B. M., Shim, H., Zhang, M., Yarov-Yarovoy, V., and Wulff, H. (2017). Structural Determinants for the Selectivity of the Positive KCa3.1 Gating Modulator 5-Methylnaphtho[2,1-d]oxazol-2-amine (SKA-121). Mol. Pharmacol. 92, 469–480. doi: 10.1124/mol.117.109421
Brown, D. A., and Passmore, G. M. (2009). Neural KCNQ (Kv7) channels. Br. J. Pharmacol. 156, 1185–1195. doi: 10.1111/j.1476-5381.2009.00111.x
Butler, A., Tsunoda, S., Mccobb, D. P., Wei, A., and Salkoff, L. (1993). mSlo, a complex mouse gene encoding “maxi” calcium-activated potassium channels. Science 261, 221–224. doi: 10.1126/science.7687074
Carreno-Munoz, M. I., Martins, F., Medrano, M. C., Aloisi, E., Pietropaolo, S., Dechaud, C., et al. (2018). Potential involvement of impaired BKCa channel function in sensory defensiveness and some behavioral disturbances induced by unfamiliar environment in a mouse model of Fragile × Syndrome. Neuropsychopharmacology 43, 492–502. doi: 10.1038/npp.2017.149
Chen, J., Guo, Z., Tian, H., and Chen, X. (2016). Production and clinical development of nanoparticles for gene delivery. Mol. Ther. Methods Clin. Dev. 3, 16023. doi: 10.1038/mtm.2016.23
Chen, L., Deltheil, T., Turle-Lorenzo, N., Liberge, M., Rosier, C., Watabe, I., et al. (2014). SK channel blockade reverses cognitive and motor deficits induced by nigrostriatal dopamine lesions in rats. Int. J. Neuropsychopharmacol. 17, 1295–1306. doi: 10.1017/S1461145714000236
Chen, L., Tian, L., Macdonald, S. H., Mcclafferty, H., Hammond, M. S., Huibant, J. M., et al. (2005). Functionally diverse complement of large conductance calcium- and voltage-activated potassium channel (BK) alpha-subunits generated from a single site of splicing. J. Biol. Chem. 280, 33599–33609. doi: 10.1074/jbc.M505383200
Chen, S., Sun, H., Miao, K., and Deng, C. X. (2016). CRISPR-Cas9: from genome editing to cancer research. Int. J. Biol. Sci. 12, 1427–1436. doi: 10.7150/ijbs.17421
Chen, X., Xue, B., Wang, J., Liu, H., Shi, L., and Xie, J. (2018). Potassium Channels: a potential therapeutic target for parkinson’s disease. Neurosci. Bull. 34, 341–348. doi: 10.1007/s12264-017-0177-3
Chen, Y. J., Nguyen, H. M., Maezawa, I., Grossinger, E. M., Garing, A. L., Kohler, R., et al. (2016). The potassium channel KCa3.1 constitutes a pharmacological target for neuroinflammation associated with ischemia/reperfusion stroke. J. Cereb. Blood Flow Metab. 36, 2146–2161. doi: 10.1177/0271678X15611434
Chen, Y. J., Raman, G., Bodendiek, S., O’donnell, M. E., and Wulff, H. (2011). The KCa3.1 blocker TRAM-34 reduces infarction and neurological deficit in a rat model of ischemia/reperfusion stroke. J. Cereb. Blood Flow Metab. 31, 2363–2374. doi: 10.1038/jcbfm.2011.101
Cheng, A. W., Wang, H., Yang, H., Shi, L., Katz, Y., Theunissen, T. W., et al. (2013). Multiplexed activation of endogenous genes by CRISPR-on, an RNA-guided transcriptional activator system. Cell Res. 23, 1163–1171. doi: 10.1038/cr.2013.122
Church, T. W., Weatherall, K. L., Correa, S. A., Prole, D. L., Brown, J. T., and Marrion, N. V. (2015). Preferential assembly of heteromeric small conductance calcium-activated potassium channels. Eur. J. Neurosci. 41, 305–315. doi: 10.1111/ejn.12789
Contet, C., Goulding, S. P., Kuljis, D. A., and Barth, A. L. (2016). BK channels in the central nervous system. Int. Rev. Neurobiol. 128, 281–342. doi: 10.1016/bs.irn.2016.04.001
Contractor, A., Klyachko, V. A., and Portera-Cailliau, C. (2015). Altered neuronal and circuit excitability in Fragile × Syndrome. Neuron 87, 699–715. doi: 10.1016/j.neuron.2015.06.017
Cooper, E. C., and Jan, L. Y. (1999). Ion channel genes and human neurological disease: recent progress, prospects, and challenges. Proc. Natl. Acad. Sci. U.S.A. 96, 4759–4766. doi: 10.1073/pnas.96.9.4759
Cotelli, M., Manenti, R., Cappa, S. F., Geroldi, C., Zanetti, O., Rossini, P. M., et al. (2006). Effect of transcranial magnetic stimulation on action naming in patients with Alzheimer disease. Arch. Neurol. 63, 1602–1604. doi: 10.1001/archneur.63.11.1602
Criado-Marrero, M., Santini, E., and Porter, J. T. (2014). Modulating fear extinction memory by manipulating SK potassium channels in the infralimbic cortex. Front. Behav. Neurosci. 8:96. doi: 10.3389/fnbeh.2014.00096
Deng, P. Y., Rotman, Z., Blundon, J. A., Cho, Y., Cui, J., Cavalli, V., et al. (2013). FMRP regulates neurotransmitter release and synaptic information transmission by modulating action potential duration via BK channels. Neuron 77, 696–711. doi: 10.1016/j.neuron.2012.12.018
Deschaux, O., Bizot, J. C., and Goyffon, M. (1997). Apamin improves learning in an object recognition task in rats. Neurosci. Lett. 222, 159–162. doi: 10.1016/S0304-3940(97)13367-5
Di, L., Srivastava, S., Zhdanova, O., Ding, Y., Li, Z., Wulff, H., et al. (2010). Inhibition of the K+ channel KCa3.1 ameliorates T cell-mediated colitis. Proc. Natl. Acad. Sci. U.S.A. 107, 1541–1546. doi: 10.1073/pnas.0910133107
Diaz, L., Meera, P., Amigo, J., Stefani, E., Alvarez, O., Toro, L., et al. (1998). Role of the S4 segment in a voltage-dependent calcium-sensitive potassium (hSlo) channel. J. Biol. Chem. 273, 32430–32436. doi: 10.1074/jbc.273.49.32430
Diez-Sampedro, A., Silverman, W. R., Bautista, J. F., and Richerson, G. B. (2006). Mechanism of increased open probability by a mutation of the BK channel. J. Neurophysiol. 96, 1507–1516. doi: 10.1152/jn.00461.2006
Dimitriadi, M., Kye, M. J., Kalloo, G., Yersak, J. M., Sahin, M., and Hart, A. C. (2013). The neuroprotective drug riluzole acts via small conductance Ca2+-activated K+ channels to ameliorate defects in spinal muscular atrophy models. J. Neurosci. 33, 6557–6562. doi: 10.1523/JNEUROSCI.1536-12.2013
Dolga, A. M., and Culmsee, C. (2012). Protective roles for potassium SK/K(Ca)2 channels in microglia and Neurons. Front. Pharmacol. 3:196. doi: 10.3389/fphar.2012.00196
Dolga, A. M., De Andrade, A., Meissner, L., Knaus, H. G., Hollerhage, M., Christophersen, P., et al. (2014). Subcellular expression and neuroprotective effects of SK channels in human dopaminergic neurons. Cell Death Dis. 5:e999. doi: 10.1038/cddis.2013.530
Dolga, A. M., Netter, M. F., Perocchi, F., Doti, N., Meissner, L., Tobaben, S., et al. (2013). Mitochondrial small conductance SK2 channels prevent glutamate-induced oxytosis and mitochondrial dysfunction. J. Biol. Chem. 288, 10792–10804. doi: 10.1074/jbc.M113.453522
Doo, A. R., Kim, S. T., Kim, S. N., Moon, W., Yin, C. S., Chae, Y., et al. (2010). Neuroprotective effects of bee venom pharmaceutical acupuncture in acute 1-methyl-4-phenyl-1,2,3,6-tetrahydropyridine-induced mouse model of Parkinson’s disease. Neurol. Res. 32 (Suppl 1), 88–91. doi: 10.1179/016164109X12537002794282
Du, W., Bautista, J. F., Yang, H., Diez-Sampedro, A., You, S. A., Wang, L., et al. (2005). Calcium-sensitive potassium channelopathy in human epilepsy and paroxysmal movement disorder. Nat. Genet. 37, 733–738. doi: 10.1038/ng1585
Duda, J., Potschke, C., and Liss, B. (2016). Converging roles of ion channels, calcium, metabolic stress, and activity pattern of Substantia nigra dopaminergic neurons in health and Parkinson’s disease. J. Neurochem. 139(Suppl 1), 156–178. doi: 10.1111/jnc.13572
Durr, A. (2010). Autosomal dominant cerebellar ataxias: polyglutamine expansions and beyond. Lancet Neurol. 9, 885–894. doi: 10.1016/S1474-4422(10)70183-6
Engbers, J. D., Anderson, D., Zamponi, G. W., and Turner, R. W. (2013). Signal processing by T-type calcium channel interactions in the cerebellum. Front. Cell Neurosci. 7:230. doi: 10.3389/fncel.2013.00230
Faber, E. S. (2009). Functions and modulation of neuronal SK channels. Cell Biochem. Biophys. 55, 127–139. doi: 10.1007/s12013-009-9062-7
Faber, E. S., and Sah, P. (2002). Physiological role of calcium-activated potassium currents in the rat lateral amygdala. J. Neurosci. 22, 1618–1628. doi: 10.1523/JNEUROSCI.22-05-01618.2002
Faber, E. S., Delaney, A. J., and Sah, P. (2005). SK channels regulate excitatory synaptic transmission and plasticity in the lateral amygdala. Nat. Neurosci. 8, 635–641. doi: 10.1038/nn1450
Fanger, C. M., Ghanshani, S., Logsdon, N. J., Rauer, H., Kalman, K., Zhou, J., et al. (1999). Calmodulin mediates calcium-dependent activation of the intermediate conductance KCa Channel, IKCa1. J. Biol. Chem. 274, 5746–5754. doi: 10.1074/jbc.274.9.5746
Fay, A. J., Qian, X., Jan, Y. N., and Jan, L. Y. (2006). SK channels mediate NADPH oxidase-independent reactive oxygen species production and apoptosis in granulocytes. Proc. Natl. Acad. Sci. U.S.A. 103, 17548–17553. doi: 10.1073/pnas.0607914103
Feetham, C. H., Nunn, N., Lewis, R., Dart, C., and Barrett-Jolley, R. (2015). TRPV4 and K(Ca) ion channels functionally couple as osmosensors in the paraventricular nucleus. Br. J. Pharmacol. 172, 1753–1768. doi: 10.1111/bph.13023
Fernandez-Fernandez, J. M., Tomas, M., Vazquez, E., Orio, P., Latorre, R., Senti, M., et al. (2004). Gain-of-function mutation in the KCNMB1 potassium channel subunit is associated with low prevalence of diastolic hypertension. J. Clin. Invest. 113, 1032–1039. doi: 10.1172/JCI200420347
Filosa, J. A., Bonev, A. D., Straub, S. V., Meredith, A. L., Wilkerson, M. K., Aldrich, R. W., et al. (2006). Local potassium signaling couples neuronal activity to vasodilation in the brain. Nat. Neurosci. 9, 1397–1403. doi: 10.1038/nn1779
Furness, J. B., Kearney, K., Robbins, H. L., Hunne, B., Selmer, I. S., Neylon, C. B., et al. (2004). Intermediate conductance potassium (IK) channels occur in human enteric neurons. Auton. Neurosci. 112, 93–97. doi: 10.1016/j.autneu.2004.02.003
Gardos, G. (1958). The function of calcium in the potassium permeability of human erythrocytes. Biochim. Biophys. Acta 30, 653–654. doi: 10.1016/0006-3002(58)90124-0
Ghezzi, A., Krishnan, H. R., and Atkinson, N. S. (2014). Susceptibility to ethanol withdrawal seizures is produced by BK channel gene expression. Addict. Biol. 19, 332–337. doi: 10.1111/j.1369-1600.2012.00465.x
Gill, S., Gill, R., Lee, S. S., Hesketh, J. C., Fedida, D., Rezazadeh, S., et al. (2003). Flux assays in high throughput screening of ion channels in drug discovery. Assay Drug Dev. Technol. 1, 709–717. doi: 10.1089/154065803770381066
Giraldez, T., and Rothberg, B. S. (2017). Understanding the conformational motions of RCK gating rings. J. Gen. Physiol. 149, 431–441. doi: 10.1085/jgp.201611726
Giraldez, T., Hughes, T. E., and Sigworth, F. J. (2005). Generation of functional fluorescent BK channels by random insertion of GFP variants. J. Gen. Physiol. 126, 429–438. doi: 10.1085/jgp.200509368
Girouard, H., Bonev, A. D., Hannah, R. M., Meredith, A., Aldrich, R. W., and Nelson, M. T. (2010). Astrocytic endfoot Ca2+ and BK channels determine both arteriolar dilation and constriction. Proc. Natl. Acad. Sci. U.S.A. 107, 3811–3816. doi: 10.1073/pnas.0914722107
Grace, A. A. (1991). Phasic versus tonic dopamine release and the modulation of dopamine system responsivity: a hypothesis for the etiology of schizophrenia. Neuroscience 41, 1–24. doi: 10.1016/0306-4522(91)90196-U
Grunnet, M., and Kaufmann, W. A. (2004). Coassembly of big conductance Ca2+-activated K+ channels and L-type voltage-gated Ca2+ channels in rat brain. J. Biol. Chem. 279, 36445–36453. doi: 10.1074/jbc.M402254200
Gu, N., Vervaeke, K., and Storm, J. F. (2007). BK potassium channels facilitate high-frequency firing and cause early spike frequency adaptation in rat CA1 hippocampal pyramidal cells. J. Physiol. 580, 859–882. doi: 10.1113/jphysiol.2006.126367
Gu, N., Vervaeke, K., Hu, H., and Storm, J. F. (2005). Kv7/KCNQ/M and HCN/h, but not KCa2/SK channels, contribute to the somatic medium after-hyperpolarization and excitability control in CA1 hippocampal pyramidal cells. J. Physiol. 566, 689–715. doi: 10.1113/jphysiol.2005.086835
Gueguinou, M., Chantome, A., Fromont, G., Bougnoux, P., Vandier, C., and Potier-Cartereau, M. (2014). KCa and Ca(2+) channels: the complex thought. Biochim. Biophys. Acta 1843, 2322–2333. doi: 10.1016/j.bbamcr.2014.02.019
Hannigan, K. I., Griffin, C. S., Large, R. J., Sergeant, G. P., Hollywood, M. A., Mchale, N. G., et al. (2017). The role of Ca(2+)-activated Cl(–) current in tone generation in the rabbit corpus cavernosum. Am. J. Physiol. Cell Physiol. 313, C475-C486. doi: 10.1152/ajpcell.00025.2017
Hebert, B., Pietropaolo, S., Meme, S., Laudier, B., Laugeray, A., Doisne, N., et al. (2014). Rescue of fragile X syndrome phenotypes in Fmr1 KO mice by a BKCa channel opener molecule. Orphanet. J. Rare Dis. 9, 124. doi: 10.1186/s13023-014-0124-6
Hirschberg, B., Maylie, J., Adelman, J. P., and Marrion, N. V. (1999). Gating Properties of single SK channels in hippocampal CA1 pyramidal neurons. Biophys. J. 77, 1905–1913. doi: 10.1016/S0006-3495(99)77032-3
Hite, R. K., Tao, X., and Mackinnon, R. (2017). Structural basis for gating the high-conductance Ca2+-activated K+ channel. Nature 541, 52–57. doi: 10.1038/nature20775
Honrath, B., Matschke, L., Meyer, T., Magerhans, L., Perocchi, F., Ganjam, G. K., et al. (2017). SK2 channels regulate mitochondrial respiration and mitochondrial Ca(2+) uptake. Cell Death Differ. 24, 761–773. doi: 10.1038/cdd.2017.2
Horrigan, F. T., and Aldrich, R. W. (2002). Coupling between voltage sensor activation, Ca2+ Binding and channel opening in large conductance (BK) potassium channels. J. Gen. Physiol. 120, 267–305. doi: 10.1085/jgp.20028605
Hoshi, T., Pantazis, A., and Olcese, R. (2013). Transduction of voltage and Ca2+ signals by Slo1 BK channels. Physiology (Bethesda) 28, 172–189. doi: 10.1152/physiol.00055.2012
Howarth, C. (2014). The contribution of astrocytes to the regulation of cerebral blood flow. Front. Neurosci. 8:103. doi: 10.3389/fnins.2014.00103
Hu, H., Shao, L. R., Chavoshy, S., Gu, N., Trieb, M., Behrens, R., et al. (2001). Presynaptic Ca2+-activated K+ channels in glutamatergic hippocampal terminals and their role in spike repolarization and regulation of transmitter release. J. Neurosci. 21, 9585–9597. doi: 10.1523/JNEUROSCI.21-24-09585.2001
Hu, S., Labuda, M. Z., Pandolfo, M., Goss, G. G., Mcdermid, H. E., and Ali, D. W. (2003). Variants of the KCNMB3 regulatory subunit of maxi BK channels affect channel inactivation. Physiol. Genom. 15, 191–198. doi: 10.1152/physiolgenomics.00110.2003
Huang, C. W., Huang, C. C., and Wu, S. N. (2007). Activation by zonisamide, a newer antiepileptic drug, of large-conductance calcium-activated potassium channel in differentiated hippocampal neuron-derived H19-7 cells. J. Pharmacol. Exp. Ther. 321, 98–106. doi: 10.1124/jpet.106.116954
Inan, S. Y., Aksu, F., and Baysal, F. (2000). The effects of some K(+) channel blockers on scopolamine- or electroconvulsive shock-induced amnesia in mice. Eur. J. Pharmacol. 407, 159–164. doi: 10.1016/S0014-2999(00)00736-6
Indriati, D. W., Kamasawa, N., Matsui, K., Meredith, A. L., Watanabe, M., and Shigemoto, R. (2013). Quantitative localization of Cav2.1 (P/Q-type) voltage-dependent calcium channels in Purkinje cells: somatodendritic gradient and distinct somatic coclustering with calcium-activated potassium channels. J. Neurosci. 33, 3668–3678. doi: 10.1523/JNEUROSCI.2921-12.2013
Isaacson, J. S., and Murphy, G. J. (2001). Glutamate-mediated extrasynaptic inhibition: direct coupling of NMDA receptors to Ca(2+)-activated K+ channels. Neuron 31, 1027–1034. doi: 10.1016/S0896-6273(01)00428-7
Ishii, T. M., Maylie, J., and Adelman, J. P. (1997a). Determinants of apamin and d-tubocurarine block in SK potassium channels. J. Biol. Chem. 272, 23195–23200. doi: 10.1074/jbc.272.37.23195
Ishii, T. M., Silvia, C., Hirschberg, B., Bond, C. T., Adelman, J. P., and Maylie, J. (1997b). A human intermediate conductance calcium-activated potassium channel. Proc. Natl. Acad. Sci. U.S.A. 94, 11651–11656. doi: 10.1073/pnas.94.21.11651
Ito, M. (2002). Historical review of the significance of the cerebellum and the role of Purkinje cells in motor learning. Ann. N. Y. Acad. Sci. 978, 273–288. doi: 10.1111/j.1749-6632.2002.tb07574.x
Jaffe, D. B., Wang, B., and Brenner, R. (2011). Shaping of action potentials by type I and type II large-conductance Ca(2)+-activated K+ channels. Neuroscience 192, 205–218. doi: 10.1016/j.neuroscience.2011.06.028
Javaherian, A. D., Yusifov, T., Pantazis, A., Franklin, S., Gandhi, C. S., and Olcese, R. (2011). Metal-driven operation of the human large-conductance voltage- and Ca2+-dependent potassium channel (BK) gating ring apparatus. J. Biol. Chem. 286, 20701–20709. doi: 10.1074/jbc.M111.235234
Jensen, B. S. (2002). BMS-204352: a potassium channel opener developed for the treatment of stroke. CNS Drug Rev. 8, 353–360. doi: 10.1111/j.1527-3458.2002.tb00233.x
Jensen, B. S., Strobaek, D., Christophersen, P., Jorgensen, T. D., Hansen, C., Silahtaroglu, A., et al. (1998). Characterization of the cloned human intermediate-conductance Ca2+-activated K+ channel. Am. J. Physiol. 275, C848–C856. doi: 10.1152/ajpcell.1998.275.3.C848
Ji, H., and Shepard, P. D. (2006). SK Ca2+-activated K+ channel ligands alter the firing pattern of dopamine-containing neurons in vivo. Neuroscience 140, 623–633. doi: 10.1016/j.neuroscience.2006.02.020
Jin, W., Sugaya, A., Tsuda, T., Ohguchi, H., and Sugaya, E. (2000). Relationship between large conductance calcium-activated potassium channel and bursting activity. Brain Res. 860, 21–28. doi: 10.1016/S0006-8993(00)01943-0
Joiner, W. J., Wang, L. Y., Tang, M. D., and Kaczmarek, L. K. (1997). hSK4, a member of a novel subfamily of calcium-activated potassium channels. Proc. Natl. Acad. Sci. U.S.A. 94, 11013–11018. doi: 10.1073/pnas.94.20.11013
Jorgensen, S., Dyhring, T., Brown, D. T., Strobaek, D., Christophersen, P., and Demnitz, J. (2013). A high-throughput screening campaign for detection of ca(2+)-activated k(+) channel activators and inhibitors using a fluorometric imaging plate reader-based tl(+)-influx assay. Assay Drug Dev. Technol. 11, 163–172. doi: 10.1089/adt.2012.479
Jorgensen, S., Johansen, T. H., and Dyhring, T. (2008). Fluorescence-based Tl(+)-influx assays as a novel approach for characterization of small-conductance Ca(2+)-activated K (+) channel modulators. Methods Mol. Biol. 491, 257–266. doi: 10.1007/978-1-59745-526-8_20
Kasumu, A. W., Hougaard, C., Rode, F., Jacobsen, T. A., Sabatier, J. M., Eriksen, B. L., et al. (2012). Selective positive modulator of calcium-activated potassium channels exerts beneficial effects in a mouse model of spinocerebellar ataxia type 2. Chem. Biol. 19, 1340–1353. doi: 10.1016/j.chembiol.2012.07.013
Kasumu, A., and Bezprozvanny, I. (2012). Deranged calcium signaling in Purkinje cells and pathogenesis in spinocerebellar ataxia 2 (SCA2) and other ataxias. Cerebellum 11, 630–639. doi: 10.1007/s12311-010-0182-9
Katsuki, H., Shinohara, A., Fujimoto, S., Kume, T., and Akaike, A. (2005). Tetraethylammonium exacerbates ischemic neuronal injury in rat cerebrocortical slice cultures. Eur. J. Pharmacol. 508, 85–91. doi: 10.1016/j.ejphar.2004.11.058
Kaushal, V., Koeberle, P. D., Wang, Y., and Schlichter, L. C. (2007). The Ca2+-activated K+ channel KCNN4/KCa3.1 contributes to microglia activation and nitric oxide-dependent neurodegeneration. J. Neurosci. 27, 234–244. doi: 10.1523/JNEUROSCI.3593-06.2007
Kim, J. I., Yang, E. J., Lee, M. S., Kim, Y. S., Huh, Y., Cho, I. H., et al. (2011). Bee venom reduces neuroinflammation in the MPTP-induced model of Parkinson’s disease. Int. J. Neurosci. 121, 209–217. doi: 10.3109/00207454.2010.548613
King, B., Rizwan, A. P., Asmara, H., Heath, N. C., Engbers, J. D., Dykstra, S., et al. (2015). IKCa channels are a critical determinant of the slow AHP in CA1 pyramidal neurons. Cell Rep. 11, 175–182. doi: 10.1016/j.celrep.2015.03.026
Kleiman-Weiner, M., Beenhakker, M. P., Segal, W. A., and Huguenard, J. R. (2009). Synergistic roles of GABAA receptors and SK channels in regulating thalamocortical oscillations. J. Neurophysiol. 102, 203–213. doi: 10.1152/jn.91158.2008
Knaus, H. G., Schwarzer, C., Koch, R. O., Eberhart, A., Kaczorowski, G. J., Glossmann, H., et al. (1996). Distribution of high-conductance Ca(2+)-activated K+ channels in rat brain: targeting to axons and nerve terminals. J. Neurosci. 16, 955–963. doi: 10.1523/JNEUROSCI.16-03-00955.1996
Köhler, M., Hirschberg, B., Bond, C. T., Kinzie, J. M., Marrion, N. V., Maylie, J., et al. (1996). Small-conductance, calcium-activated potassium channels from mammalian brain. Science 273, 1709–1714. doi: 10.1126/science.273.5282.1709
Koot, B. G., Alders, M., Verheij, J., Beuers, U., and Cobben, J. M. (2016). A de novo mutation in KCNN3 associated with autosomal dominant idiopathic non-cirrhotic portal hypertension. J. Hepatol. 64, 974–977. doi: 10.1016/j.jhep.2015.11.027
Krabbendam, I. E., Honrath, B., Culmsee, C., and Dolga, A. M. (2018). Mitochondrial Ca(2+)-activated K(+) channels and their role in cell life and death pathways. Cell Calcium 69, 101–111. doi: 10.1016/j.ceca.2017.07.005
Kshatri, A. S., Gonzalez-Hernandez, A. J., and Giraldez, T. (2018). Functional validation of Ca(2+)-binding residues from the crystal structure of the BK ion channel. Biochim. Biophys. Acta 1860, 943–952. doi: 10.1016/j.bbamem.2017.09.023
Kulawiak, B., Kudin, A. P., Szewczyk, A., and Kunz, W. S. (2008). BK channel openers inhibit ROS production of isolated rat brain mitochondria. Exp. Neurol. 212, 543–547. doi: 10.1016/j.expneurol.2008.05.004
Lam, J., Coleman, N., Garing, A. L., and Wulff, H. (2013). The therapeutic potential of small-conductance KCa2 channels in neurodegenerative and psychiatric diseases. Expert Opin. Ther. Targets 17, 1203–1220. doi: 10.1517/14728222.2013.823161
Lappin, S. C., Dale, T. J., Brown, J. T., Trezise, D. J., and Davies, C. H. (2005). Activation of SK channels inhibits epileptiform bursting in hippocampal CA3 neurons. Brain Res. 1065, 37–46. doi: 10.1016/j.brainres.2005.10.024
Large, R. J., Kshatri, A., Webb, T. I., Roy, S., Akande, A., Bradley, E., et al. (2015). Effects of the novel BK (KCa 1.1) channel opener GoSlo-SR-5-130 are dependent on the presence of BKbeta subunits. Br. J. Pharmacol. 172, 2544–2556. doi: 10.1111/bph.13085
Latorre, R., Castillo, K., Carrasquel-Ursulaez, W., Sepulveda, R. V., Gonzalez-Nilo, F., Gonzalez, C., et al. (2017). Molecular determinants of BK channel functional diversity and functioning. Physiol. Rev. 97, 39–87. doi: 10.1152/physrev.00001.2016
Lee, C., and MacKinnon, R. (2018). Activation mechanism of a human SK-calmodulin channel complex elucidated by cryo-EM structures. Science 360, 508–513. doi: 10.1126/science.aas9466
Lee, U. S., and Cui, J. (2010). BK channel activation: structural and functional insights. Trends Neurosci. 33, 415–423. doi: 10.1016/j.tins.2010.06.004
Li, W., Halling, D. B., Hall, A. W., and Aldrich, R. W. (2009). EF hands at the N-lobe of calmodulin are required for both SK channel gating and stable SK-calmodulin interaction. J. Gen. Physiol. 134, 281–293. doi: 10.1085/jgp.200910295
Li, X., Poschmann, S., Chen, Q., Fazeli, W., Oundjian, N. J., Snoeijen-Schouwenaars, F. M., et al. (2018). De novo BK channel variant causes epilepsy by affecting voltage gating but not Ca(2+) sensitivity. Eur. J. Hum. Genet. 26, 220–229. doi: 10.1038/s41431-017-0073-3
Liao, Y., Kristiansen, A. M., Oksvold, C. P., Tuvnes, F. A., Gu, N., Runden-Pran, E., et al. (2010). Neuronal Ca2+-activated K+ channels limit brain infarction and promote survival. PLoS One 5:e15601. doi: 10.1371/journal.pone.0015601
Lima, P. A., and Marrion, N. V. (2007). Mechanisms underlying activation of the slow AHP in rat hippocampal neurons. Brain Res. 1150, 74–82. doi: 10.1016/j.brainres.2007.02.067
Longden, T. A., Dunn, K. M., Draheim, H. J., Nelson, M. T., Weston, A. H., and Edwards, G. (2011). Intermediate-conductance calcium-activated potassium channels participate in neurovascular coupling. Br. J. Pharmacol. 164, 922–933. doi: 10.1111/j.1476-5381.2011.01447.x
Lorenz, S., Heils, A., Kasper, J. M., and Sander, T. (2007). Allelic association of a truncation mutation of the KCNMB3 gene with idiopathic generalized epilepsy. Am. J. Med. Genet. B Neuropsychiatr. Genet. 144B, 10–13. doi: 10.1002/ajmg.b.30369
Losin, S., and McKean, C. M. (1966). Chlorzoxazone (paraflex) in the treatment of severe spasticity. Dev. Med. Child Neurol. 8, 768–769. doi: 10.1111/j.1469-8749.1966.tb01839.x
Lu, R., Flauaus, C., Kennel, L., Petersen, J., Drees, O., Kallenborn-Gerhardt, W., et al. (2017). KCa3.1 channels modulate the processing of noxious chemical stimuli in mice. Neuropharmacology 125, 386–395. doi: 10.1016/j.neuropharm.2017.08.021
Ma, Z., Lou, X. J., and Horrigan, F. T. (2006). Role of charged residues in the S1-S4 voltage sensor of BK channels. J. Gen. Physiol. 127, 309–328. doi: 10.1085/jgp.200509421
Malerba, M.,Amato, D. M., Radaeli, A., Giacovelli, G., Rovati, L., Arshad, S. H., et al. (2015). Efficacy of andolast in mild to moderate asthma: a randomized, controlled, double-blind multicenter study (the andast trial). Curr. Pharm. Des. 21, 3835–3843. doi: 10.2174/1381612821666150407101614
Marrion, N. V., and Tavalin, S. J. (1998). Selective activation of Ca2+-activated K+ channels by co-localized Ca2+ channels in hippocampal neurons. Nature 395, 900–905. doi: 10.1038/27674
Matsuda, W., Furuta, T., Nakamura, K. C., Hioki, H., Fujiyama, F., Arai, R., et al. (2009). Single nigrostriatal dopaminergic neurons form widely spread and highly dense axonal arborizations in the neostriatum. J. Neurosci. 29, 444–453. doi: 10.1523/JNEUROSCI.4029-08.2009
Matsumoto, M., and Takada, M. (2013). Distinct representations of cognitive and motivational signals in midbrain dopamine neurons. Neuron 79, 1011–1024. doi: 10.1016/j.neuron.2013.07.002
Melman, A., Bar-Chama, N., Mccullough, A., Davies, K., and Christ, G. (2006). hMaxi-K gene transfer in males with erectile dysfunction: results of the first human trial. Hum. Gene Ther. 17, 1165–1176. doi: 10.1089/hum.2006.17.1165
Melman, A., Bar-Chama, N., Mccullough, A., Davies, K., and Christ, G. (2007). Plasmid-based gene transfer for treatment of erectile dysfunction and overactive bladder: results of a phase I trial. ISR Med. Assoc. J. 9, 143–146.
Meredith, A. L., Wiler, S. W., Miller, B. H., Takahashi, J. S., Fodor, A. A., Ruby, N. F., et al. (2006). BK calcium-activated potassium channels regulate circadian behavioral rhythms and pacemaker output. Nat. Neurosci. 9, 1041–1049. doi: 10.1038/nn1740
Miller, M. J., Rauer, H., Tomita, H., Rauer, H., Gargus, J. J., Gutman, G. A., et al. (2001). Nuclear localization and dominant-negative suppression by a mutant SKCa3 N-terminal channel fragment identified in a patient with schizophrenia. J. Biol. Chem. 276, 27753–27756. doi: 10.1074/jbc.C100221200
Miller, R. G., Mitchell, J. D., and Moore, D. H. (2012). Riluzole for amyotrophic lateral sclerosis (ALS)/motor neuron disease (MND). Cochrane Database Syst. Rev. 2:CD001447. doi: 10.1002/14651858.CD001447.pub3
Miranda, P., Contreras, J. E., Plested, A. J., Sigworth, F. J., Holmgren, M., and Giraldez, T. (2013). State-dependent FRET reports calcium- and voltage-dependent gating-ring motions in BK channels. Proc. Natl. Acad. Sci. U.S.A. 110, 5217–5222. doi: 10.1073/pnas.1219611110
Miranda, P., Giraldez, T., and Holmgren, M. (2016). Interactions of divalent cations with calcium binding sites of BK channels reveal independent motions within the gating ring. Proc. Natl. Acad. Sci. U.S.A. 113, 14055–14060. doi: 10.1073/pnas.1611415113
Mongan, L. C., Hill, M. J., Chen, M. X., Tate, S. N., Collins, S. D., Buckby, L., et al. (2005). The distribution of small and intermediate conductance calcium-activated potassium channels in the rat sensory nervous system. Neuroscience 131, 161–175. doi: 10.1016/j.neuroscience.2004.09.062
Morales, P., Garneau, L., Klein, H., Lavoie, M. F., Parent, L., and Sauve, R. (2013). Contribution of the KCa3.1 channel-calmodulin interactions to the regulation of the KCa3.1 gating process. J. Gen. Physiol. 142, 37–60. doi: 10.1085/jgp.201210933
Mourre, C., Fournier, C., and Soumireu-Mourat, B. (1997). Apamin, a blocker of the calcium-activated potassium channel, induces neurodegeneration of Purkinje cells exclusively. Brain Res. 778, 405–408. doi: 10.1016/S0006-8993(97)01165-7
Mpari, B., Regaya, I., Escoffier, G., and Mourre, C. (2005). Differential effects of two blockers of small conductance Ca2+-activated K+ channels, apamin and lei-Dab7, on learning and memory in rats. J. Integr. Neurosci. 4, 381–396. doi: 10.1142/S0219635205000884
Muller, A., Kukley, M., Uebachs, M., Beck, H., and Dietrich, D. (2007). Nanodomains of single Ca2+ channels contribute to action potential repolarization in cortical neurons. J. Neurosci. 27, 483–495. doi: 10.1523/JNEUROSCI.3816-06.2007
Nam, Y. W., Orfali, R., Liu, T., Yu, K., Cui, M., Wulff, H., et al. (2017). Structural insights into the potency of SK channel positive modulators. Sci. Rep. 7:7178. doi: 10.1038/s41598-017-16607-8
Navaratnam, D. S., Bell, T. J., Tu, T. D., Cohen, E. L., and Oberholtzer, J. C. (1997). Differential distribution of Ca2+-activated K+ channel splice variants among hair cells along the tonotopic axis of the chick cochlea. Neuron 19, 1077–1085. doi: 10.1016/S0896-6273(00)80398-0
Nelson, A. B., Krispel, C. M., Sekirnjak, C., and Du Lac, S. (2003). Long-lasting increases in intrinsic excitability triggered by inhibition. Neuron 40, 609–620. doi: 10.1016/S0896-6273(03)00641-X
Ngo-Anh, T. J., Bloodgood, B. L., Lin, M., Sabatini, B. L., Maylie, J., and Adelman, J. P. (2005). SK channels and NMDA receptors form a Ca2+-mediated feedback loop in dendritic spines. Nat. Neurosci. 8, 642–649. doi: 10.1038/nn1449
N’Gouemo, P. (2014). BKCa channel dysfunction in neurological diseases. Front. Physiol. 5:373. doi: 10.3389/fphys.2014.00373
Nguyen, H. M., Grossinger, E. M., Horiuchi, M., Davis, K. W., Jin, L. W., Maezawa, I., et al. (2017). Differential Kv1.3, KCa3.1, and Kir2.1 expression in “classically” and “alternatively” activated microglia. Glia 65, 106–121. doi: 10.1002/glia.23078
Nieoullon, A. (2002). Dopamine and the regulation of cognition and attention. Prog. Neurobiol. 67, 53–83. doi: 10.1016/S0301-0082(02)00011-4
Oliveira, M. S., Skinner, F., Arshadmansab, M. F., Garcia, I., Mello, C. F., Knaus, H. G., et al. (2010). Altered expression and function of small-conductance (SK) Ca(2+)-activated K+ channels in pilocarpine-treated epileptic rats. Brain Res. 1348, 187–199. doi: 10.1016/j.brainres.2010.05.095
Ornitz, E. M., Lane, S. J., Sugiyama, T., and De Traversay, J. (1993). Startle modulation studies in autism. J. Autism. Dev. Disord. 23, 619–637. doi: 10.1007/BF01046105
Park, Y. B. (1994). Ion selectivity and gating of small conductance Ca(2+)-activated K+ channels in cultured rat adrenal chromaffin cells. J. Physiol. 481 (Pt 3), 555–570. doi: 10.1113/jphysiol.1994.sp020463
Pedarzani, P., and Stocker, M. (2008). Molecular and cellular basis of small–and intermediate-conductance, calcium-activated potassium channel function in the brain. Cell Mol. Life Sci. 65, 3196–3217. doi: 10.1007/s00018-008-8216-x
Picones, A., Loza-Huerta, A., Segura-Chama, P., and Lara-Figueroa, C. O. (2016). Contribution of automated technologies to ion channel drug discovery. Adv. Protein Chem. Struct. Biol. 104, 357–378. doi: 10.1016/bs.apcsb.2016.01.002
Postmes, L., Sno, H. N., Goedhart, S., Van Der Stel, J., Heering, H. D., and De Haan, L. (2014). Schizophrenia as a self-disorder due to perceptual incoherence. Schizophr. Res. 152, 41–50. doi: 10.1016/j.schres.2013.07.027
Power, J. M., and Sah, P. (2008). Competition between calcium-activated K+ channels determines cholinergic action on firing properties of basolateral amygdala projection neurons. J. Neurosci. 28, 3209–3220. doi: 10.1523/JNEUROSCI.4310-07.2008
Price, D. L., Ludwig, J. W., Mi, H., Schwarz, T. L., and Ellisman, M. H. (2002). Distribution of rSlo Ca2+-activated K+ channels in rat astrocyte perivascular endfeet. Brain Res. 956, 183–193. doi: 10.1016/S0006-8993(02)03266-3
Rankin, C. H., Abrams, T., Barry, R. J., Bhatnagar, S., Clayton, D. F., Colombo, J., et al. (2009). Habituation revisited: an updated and revised description of the behavioral characteristics of habituation. Neurobiol. Learn. Mem. 92, 135–138. doi: 10.1016/j.nlm.2008.09.012
Reich, E. P., Cui, L., Yang, L., Pugliese-Sivo, C., Golovko, A., Petro, M., et al. (2005). Blocking ion channel KCNN4 alleviates the symptoms of experimental autoimmune encephalomyelitis in mice. Eur. J. Immunol. 35, 1027–1036. doi: 10.1002/eji.200425954
Ristori, G., Romano, S., Visconti, A., Cannoni, S., Spadaro, M., Frontali, M., et al. (2010). Riluzole in cerebellar ataxia: a randomized, double-blind, placebo-controlled pilot trial. Neurology 74, 839–845. doi: 10.1212/WNL.0b013e3181d31e23
Rudolph, S., and Thanawala, M. S. (2015). Location matters: somatic and dendritic SK channels answer to distinct calcium signals. J. Neurophysiol. 114, 1–5. doi: 10.1152/jn.00181.2014
Sah, P. (1996). Ca(2+)-activated K+ currents in neurones: types, physiological roles and modulation. Trends Neurosci. 19, 150–154. doi: 10.1016/S0166-2236(96)80026-9
Sah, P., and McLachlan, E. M. (1992). Potassium currents contributing to action potential repolarization and the afterhyperpolarization in rat vagal motoneurons. J. Neurophysiol. 68, 1834–1841. doi: 10.1152/jn.1992.68.5.1834
Sausbier, M., Hu, H., Arntz, C., Feil, S., Kamm, S., Adelsberger, H., et al. (2004). Cerebellar ataxia and Purkinje cell dysfunction caused by Ca2+-activated K+ channel deficiency. Proc. Natl. Acad. Sci. U.S.A. 101, 9474–9478. doi: 10.1073/pnas.0401702101
Savalli, N., Pantazis, A., Yusifov, T., Sigg, D., and Olcese, R. (2012). The contribution of RCK domains to human BK channel allosteric activation. J. Biol. Chem. 287, 21741–21750. doi: 10.1074/jbc.M112.346171
Scholl, E. S., Pirone, A., Cox, D. H., Duncan, R. K., and Jacob, M. H. (2014). Alternative splice isoforms of small conductance calcium-activated SK2 channels differ in molecular interactions and surface levels. Channels (Austin) 8, 62–75. doi: 10.4161/chan.27470
Schreiber, M., Yuan, A., and Salkoff, L. (1999). Transplantable sites confer calcium sensitivity to BK channels. Nat. Neurosci. 2, 416–421. doi: 10.1038/8077
Schumacher, M. A., Crum, M., and Miller, M. C. (2004). Crystal structures of apocalmodulin and an apocalmodulin/SK potassium channel gating domain complex. Structure 12, 849–860. doi: 10.1016/j.str.2004.03.017
Schumacher, M. A., Rivard, A. F., Bächinger, H. P., and Adelman, J. P. (2001). Structure of the gating domain of a Ca2+-activated K+ channel complexed with Ca2+/calmodulin. Nature 410, 1120–1124. doi: 10.1038/35074145
Seidel, K. N., Derst, C., Salzmann, M., Holtje, M., Priller, J., Markgraf, R., et al. (2011). Expression of the voltage- and Ca2+-dependent BK potassium channel subunits BKbeta1 and BKbeta4 in rodent astrocytes. Glia 59, 893–902. doi: 10.1002/glia.21160
Seifert, G., Henneberger, C., and Steinhauser, C. (2018). Diversity of astrocyte potassium channels: an update. Brain Res. Bull. 136, 26–36. doi: 10.1016/j.brainresbull.2016.12.002
Sheehan, J. J., Benedetti, B. L., and Barth, A. L. (2009). Anticonvulsant effects of the BK-channel antagonist paxilline. Epilepsia 50, 711–720. doi: 10.1111/j.1528-1167.2008.01888.x
Shi, J., Krishnamoorthy, G., Yang, Y., Hu, L., Chaturvedi, N., Harilal, D., et al. (2002). Mechanism of magnesium activation of calcium-activated potassium channels. Nature 418, 876–880. doi: 10.1038/nature00941
Shipston, M. J. (2001). Alternative splicing of potassium channels: a dynamic switch of cellular excitability. Trends Cell Biol. 11, 353–358. doi: 10.1016/S0962-8924(01)02068-2
Si, H., Heyken, W. T., Wolfle, S. E., Tysiac, M., Schubert, R., Grgic, I., et al. (2006). Impaired endothelium-derived hyperpolarizing factor-mediated dilations and increased blood pressure in mice deficient of the intermediate-conductance Ca2+-activated K+ channel. Circ. Res. 99, 537–544. doi: 10.1161/01.RES.0000238377.08219.0c
Skalska, J., Bednarczyk, P., Piwonska, M., Kulawiak, B., Wilczynski, G., Dolowy, K., et al. (2009). Calcium ions regulate K(+) uptake into brain mitochondria: the evidence for a novel potassium channel. Int. J. Mol. Sci. 10, 1104–1120. doi: 10.3390/ijms10031104
Smith, R. A., Miller, T. M., Yamanaka, K., Monia, B. P., Condon, T. P., Hung, G., et al. (2006). Antisense oligonucleotide therapy for neurodegenerative disease. J. Clin. Invest. 116, 2290–2296. doi: 10.1172/JCI25424
Soden, M. E., Jones, G. L., Sanford, C. A., Chung, A. S., Guler, A. D., Chavkin, C., et al. (2013). Disruption of dopamine neuron activity pattern regulation through selective expression of a human KCNN3 mutation. Neuron 80, 997–1009. doi: 10.1016/j.neuron.2013.07.044
Solly, K., Cassaday, J., Felix, J. P., Garcia, M. L., Ferrer, M., Strulovici, B., et al. (2008). Miniaturization and HTS of a FRET-based membrane potential assay for K(ir) channel inhibitors. Assay Drug Dev. Technol. 6, 225–234. doi: 10.1089/adt.2008.123
Staal, R. G. W., Khayrullina, T., Zhang, H., Davis, S., Fallon, S. M., Cajina, M., et al. (2017). Inhibition of the potassium channel KCa3.1 by senicapoc reverses tactile allodynia in rats with peripheral nerve injury. Eur. J. Pharmacol. 795, 1–7. doi: 10.1016/j.ejphar.2016.11.031
Stackman, R. W., Hammond, R. S., Linardatos, E., Gerlach, A., Maylie, J., Adelman, J. P., et al. (2002). Small conductance Ca2+-activated K+ channels modulate synaptic plasticity and memory encoding. J. Neurosci. 22, 10163–10171. doi: 10.1523/JNEUROSCI.22-23-10163.2002
Stocker, M., and Pedarzani, P. (2000). Differential distribution of three Ca(2+)-activated K(+) channel subunits, SK1, SK2, and SK3, in the adult rat central nervous system. Mol. Cell. Neurosci. 15, 476–493. doi: 10.1006/mcne.2000.0842
Stocker, M., Krause, M., and Pedarzani, P. (1999). An apamin-sensitive Ca2+-activated K+ current in hippocampal pyramidal neurons. Proc. Natl. Acad. Sci. U.S.A. 96, 4662–4667. doi: 10.1073/pnas.96.8.4662
Storm, J. F. (1987). Action potential repolarization and a fast after-hyperpolarization in rat hippocampal pyramidal cells. J. Physiol. 385, 733–759. doi: 10.1113/jphysiol.1987.sp016517
Strobaek, D., Teuber, L., Jorgensen, T. D., Ahring, P. K., Kjaer, K., Hansen, R. S., et al. (2004). Activation of human IK and SK Ca2+ -activated K+ channels by NS309 (6,7-dichloro-1H-indole-2,3-dione 3-oxime). Biochim. Biophys. Acta 1665, 1–5. doi: 10.1016/j.bbamem.2004.07.006
Su, Z., Brown, E. C., Wang, W., and Mackinnon, R. (2016). Novel cell-free high-throughput screening method for pharmacological tools targeting K+ channels. Proc. Natl. Acad. Sci. U.S.A. 113, 5748–5753. doi: 10.1073/pnas.1602815113
Sugunan, S., Nampoothiri, S. S., Garg, T., and Krishnamurthy, R. G. (2016). Role of KCa3.1 Channels in CNS diseases: a concise review. CNS Neurol. Disord. Drug Targets 15, 1299–1305. doi: 10.2174/1871527315666160822111913
Sun, P., Wang, F., Wang, L., Zhang, Y., Yamamoto, R., Sugai, T., et al. (2011). Increase in cortical pyramidal cell excitability accompanies depression-like behavior in mice: a transcranial magnetic stimulation study. J. Neurosci. 31, 16464–16472. doi: 10.1523/JNEUROSCI.1542-11.2011
Surmeier, D. J., Guzman, J. N., Sanchez-Padilla, J., and Goldberg, J. A. (2010). What causes the death of dopaminergic neurons in Parkinson’s disease? Prog. Brain Res. 183, 59–77. doi: 10.1016/S0079-6123(10)83004-3
Sweet, T. B., and Cox, D. H. (2009). Measuring the influence of the BKCa {beta}1 subunit on Ca2+ binding to the BKCa channel. J. Gen. Physiol. 133, 139–150. doi: 10.1085/jgp.200810129
Szewczyk, A., Kajma, A., Malinska, D., Wrzosek, A., Bednarczyk, P., Zablocka, B., et al. (2010). Pharmacology of mitochondrial potassium channels: dark side of the field. FEBS Lett. 584, 2063–2069. doi: 10.1016/j.febslet.2010.02.048
Takano, T., Tian, G. F., Peng, W., Lou, N., Libionka, W., Han, X., et al. (2006). Astrocyte-mediated control of cerebral blood flow. Nat. Neurosci. 9, 260–267. doi: 10.1038/nn1623
Tanner, M. R., Pennington, M. W., Chamberlain, B. H., Huq, R., Gehrmann, E. J., Laragione, T., et al. (2018). Targeting KCa1.1 channels with a scorpion venom peptide for the therapy of rat models of rheumatoid arthritis. J. Pharmacol. Exp. Ther. 365, 227–236. doi: 10.1124/jpet.117.245118
Tao, X., Hite, R. K., and Mackinnon, R. (2017). Cryo-EM structure of the open high-conductance Ca2+-activated K+ channel. Nature 541, 46–51. doi: 10.1038/nature20608
Thompson, J. M., Ji, G., and Neugebauer, V. (2015). Small-conductance calcium-activated potassium (SK) channels in the amygdala mediate pain-inhibiting effects of clinically available riluzole in a rat model of arthritis pain. Mol. Pain 11, 51. doi: 10.1186/s12990-015-0055-9
Tonini, R., Ferraro, T., Sampedro-Castaneda, M., Cavaccini, A., Stocker, M., Richards, C. D., et al. (2013). Small-conductance Ca2+-activated K+ channels modulate action potential-induced Ca2+ transients in hippocampal neurons. J. Neurophysiol. 109, 1514–1524. doi: 10.1152/jn.00346.2012
Toyama, K., Wulff, H., Chandy, K. G., Azam, P., Raman, G., Saito, T., et al. (2008). The intermediate-conductance calcium-activated potassium channel KCa3.1 contributes to atherogenesis in mice and humans. J. Clin. Invest. 118, 3025–3037. doi: 10.1172/JCI30836
Tuteja, D., Rafizadeh, S., Timofeyev, V., Wang, S., Zhang, Z., Li, N., et al. (2010). Cardiac small conductance Ca2+-activated K+ channel subunits form heteromultimers via the coiled-coil domains in the C termini of the channels. Circ. Res. 107, 851–859. doi: 10.1161/CIRCRESAHA.109.215269
Typlt, M., Mirkowski, M., Azzopardi, E., Ruth, P., Pilz, P. K., and Schmid, S. (2013). Habituation of reflexive and motivated behavior in mice with deficient BK channel function. Front. Integr. Neurosci. 7:79. doi: 10.3389/fnint.2013.00079
Tzingounis, A. V., and Nicoll, R. A. (2008). Contribution of KCNQ2 and KCNQ3 to the medium and slow afterhyperpolarization currents. Proc. Natl. Acad. Sci. U.S.A. 105, 19974–19979. doi: 10.1073/pnas.0810535105
Tzingounis, A. V., Heidenreich, M., Kharkovets, T., Spitzmaul, G., Jensen, H. S., Nicoll, R. A., et al. (2010). The KCNQ5 potassium channel mediates a component of the afterhyperpolarization current in mouse hippocampus. Proc. Natl. Acad. Sci. U.S.A. 107, 10232–10237. doi: 10.1073/pnas.1004644107
Ungless, M. A., Argilli, E., and Bonci, A. (2010). Effects of stress and aversion on dopamine neurons: implications for addiction. Neurosci. Biobehav. Rev. 35, 151–156. doi: 10.1016/j.neubiorev.2010.04.006
Verkerk, A. J., Pieretti, M., Sutcliffe, J. S., Fu, Y. H., Kuhl, D. P., Pizzuti, A., et al. (1991). Identification of a gene (FMR-1) containing a CGG repeat coincident with a breakpoint cluster region exhibiting length variation in fragile X syndrome. Cell 65, 905–914. doi: 10.1016/0092-8674(91)90397-H
Vivas, O., Moreno, C. M., Santana, L. F., and Hille, B. (2017). Proximal clustering between BK and CaV1.3 channels promotes functional coupling and BK channel activation at low voltage. Elife 6:e28029. doi: 10.7554/eLife.28029
Walter, J. T., Alvina, K., Womack, M. D., Chevez, C., and Khodakhah, K. (2006). Decreases in the precision of Purkinje cell pacemaking cause cerebellar dysfunction and ataxia. Nat. Neurosci. 9, 389–397. doi: 10.1038/nn1648
Wang, B., Bugay, V., Ling, L., Chuang, H. H., Jaffe, D. B., and Brenner, R. (2016). Knockout of the BK beta4-subunit promotes a functional coupling of BK channels and ryanodine receptors that mediate a fAHP-induced increase in excitability. J. Neurophysiol. 116, 456–465. doi: 10.1152/jn.00857.2015
Wang, B., Jaffe, D. B., and Brenner, R. (2014). Current understanding of iberiotoxin-resistant BK channels in the nervous system. Front. Physiol. 5:382. doi: 10.3389/fphys.2014.00382
Wang, B., Rothberg, B. S., and Brenner, R. (2009). Mechanism of increased BK channel activation from a channel mutation that causes epilepsy. J. Gen. Physiol. 133, 283–294. doi: 10.1085/jgp.200810141
Wang, F., Zhang, Y., Wang, L., Sun, P., Luo, X., Ishigaki, Y., et al. (2015). Improvement of spatial learning by facilitating large-conductance calcium-activated potassium channel with transcranial magnetic stimulation in Alzheimer’s disease model mice. Neuropharmacology 97, 210–219. doi: 10.1016/j.neuropharm.2015.05.027
Wang, K., Mateos-Aparicio, P., Honigsperger, C., Raghuram, V., Wu, W. W., Ridder, M. C., et al. (2016). IK1 channels do not contribute to the slow afterhyperpolarization in pyramidal neurons. Elife 5:e11206. doi: 10.7554/eLife.11206
Wang, L., Kang, H., Li, Y., Shui, Y., Yamamoto, R., Sugai, T., et al. (2015). Cognitive recovery by chronic activation of the large-conductance calcium-activated potassium channel in a mouse model of Alzheimer’s disease. Neuropharmacology 92, 8–15. doi: 10.1016/j.neuropharm.2014.12.033
Wang, Y., Yang, P. L., Tang, J. F., Lin, J. F., Cai, X. H., Wang, X. T., et al. (2008). Potassium channels: possible new therapeutic targets in Parkinson’s disease. Med. Hypotheses 71, 546–550. doi: 10.1016/j.mehy.2008.05.021
Waroux, O., Massotte, L., Alleva, L., Graulich, A., Thomas, E., Liegeois, J. F., et al. (2005). SK channels control the firing pattern of midbrain dopaminergic neurons in vivo. Eur. J. Neurosci. 22, 3111–3121. doi: 10.1111/j.1460-9568.2005.04484.x
Wei, A. D., Gutman, G. A., Aldrich, R., Chandy, K. G., Grissmer, S., and Wulff, H. (2005). International Union of Pharmacology. LII. Nomenclature and molecular relationships of calcium-activated potassium channels. Pharmacol. Rev. 57, 463–472. doi: 10.1124/pr.57.4.9
Whitmire, L. E., Ling, L., Bugay, V., Carver, C. M., Timilsina, S., Chuang, H. H., et al. (2017). Downregulation of KCNMB4 expression and changes in BK channel subtype in hippocampal granule neurons following seizure activity. PLoS One 12:e0188064. doi: 10.1371/journal.pone.0188064
Whitt, J. P., Mcnally, B. A., and Meredith, A. L. (2018). Differential contribution of Ca(2+) sources to day and night BK current activation in the circadian clock. J. Gen. Physiol. 150, 259–275. doi: 10.1085/jgp.201711945
Whitt, J. P., Montgomery, J. R., and Meredith, A. L. (2016). BK channel inactivation gates daytime excitability in the circadian clock. Nat. Commun. 7:10837. doi: 10.1038/ncomms10837
Wolfart, J., and Roeper, J. (2002). Selective coupling of T-type calcium channels to SK potassium channels prevents intrinsic bursting in dopaminergic midbrain neurons. J. Neurosci. 22, 3404–3413. doi: 10.1523/JNEUROSCI.22-09-03404.2002
Womack, M. D., and Khodakhah, K. (2003). Somatic and dendritic small-conductance calcium-activated potassium channels regulate the output of cerebellar Purkinje neurons. J. Neurosci. 23, 2600–2607. doi: 10.1523/JNEUROSCI.23-07-02600.2003
Womble, M. D., and Moises, H. C. (1993). Muscarinic modulation of conductances underlying the afterhyperpolarization in neurons of the rat basolateral amygdala. Brain Res. 621, 87–96. doi: 10.1016/0006-8993(93)90301-3
Wu, Y., Liu, Y., Hou, P., Yan, Z., Kong, W., Liu, B., et al. (2013). TRPV1 channels are functionally coupled with BK(mSlo1) channels in rat dorsal root ganglion (DRG) neurons. PLoS One 8:e78203. doi: 10.1371/journal.pone.0078203
Wu, Y., Yang, Y., Ye, S., and Jiang, Y. (2010). Structure of the gating ring from the human large-conductance Ca(2+)-gated K(+) channel. Nature 466, 393–397. doi: 10.1038/nature09252
Wulf, M., and Pless, S. A. (2018). High-sensitivity fluorometry to resolve ion channel conformational dynamics. Cell Rep. 22, 1615–1626. doi: 10.1016/j.celrep.2018.01.029
Wulff, H., and Castle, N. A. (2010). Therapeutic potential of KCa3.1 blockers: recent advances and promising trends. Exp. Rev. Clin. Pharmacol. 3, 385–396. doi: 10.1586/ecp.10.11
Xia, X. M., Zeng, X., and Lingle, C. J. (2002). Multiple regulatory sites in large-conductance calcium-activated potassium channels. Nature 418, 880–884. doi: 10.1038/nature00956
Yamamoto, K., Ueta, Y., Wang, L., Yamamoto, R., Inoue, N., Inokuchi, K., et al. (2011). Suppression of a neocortical potassium channel activity by intracellular amyloid-beta and its rescue with Homer1a. J. Neurosci. 31, 11100–11109. doi: 10.1523/JNEUROSCI.6752-10.2011
Yang, E. J., Jiang, J. H., Lee, S. M., Yang, S. C., Hwang, H. S., Lee, M. S., et al. (2010). Bee venom attenuates neuroinflammatory events and extends survival in amyotrophic lateral sclerosis models. J. Neuroinflammat. 7:69. doi: 10.1186/1742-2094-7-69
Yang, H., Shi, J., Zhang, G., Yang, J., Delaloye, K., and Cui, J. (2008). Activation of Slo1 BK channels by Mg2+ coordinated between the voltage sensor and RCK1 domains. Nat. Struct. Mol. Biol. 15, 1152–1159. doi: 10.1038/nsmb.1507
Yang, J., Krishnamoorthy, G., Saxena, A., Zhang, G., Shi, J., Yang, H., et al. (2010). An epilepsy/dyskinesia-associated mutation enhances BK channel activation by potentiating Ca2+ sensing. Neuron 66, 871–883. doi: 10.1016/j.neuron.2010.05.009
Yi, M., Yu, P., Lu, Q., Geller, H. M., Yu, Z., and Chen, H. (2016). KCa3.1 constitutes a pharmacological target for astrogliosis associated with Alzheimer’s disease. Mol. Cell. Neurosci. 76, 21–32. doi: 10.1016/j.mcn.2016.08.008
Yu, Z. H., Wang, Y. X., Song, Y., Lu, H. Z., Hou, L. N., Cui, Y. Y., et al. (2013). Up-regulation of KCa3.1 promotes human airway smooth muscle cell phenotypic modulation. Pharmacol. Res. 77, 30–38. doi: 10.1016/j.phrs.2013.09.002
Yu, Z., Yu, P., Chen, H., and Geller, H. M. (2014). Targeted inhibition of KCa3.1 attenuates TGF-beta-induced reactive astrogliosis through the Smad2/3 signaling pathway. J. Neurochem. 130, 41–49. doi: 10.1111/jnc.12710
Yuan, P., Leonetti, M. D., Hsiung, Y., and Mackinnon, R. (2011). Open structure of the Ca2+ gating ring in the high-conductance Ca2+-activated K+ channel. Nature 481, 94–97. doi: 10.1038/nature10670
Yusifov, T., Javaherian, A. D., Pantazis, A., Gandhi, C. S., and Olcese, R. (2010). The RCK1 domain of the human BKCa channel transduces Ca2+ binding into structural rearrangements. J. Gen. Physiol. 136, 189–202. doi: 10.1085/jgp.200910374
Yusifov, T., Savalli, N., Gandhi, C. S., Ottolia, M., and Olcese, R. (2008). The RCK2 domain of the human BKCa channel is a calcium sensor. Proc. Natl. Acad. Sci. U.S.A. 105, 376–381. doi: 10.1073/pnas.0705261105
Zaman, T., De Oliveira, C., Smoka, M., Narla, C., Poulter, M. O., and Schmid, S. (2017). BK channels mediate synaptic plasticity underlying habituation in rats. J. Neurosci. 37, 4540–4551. doi: 10.1523/JNEUROSCI.3699-16.2017
Zhang, L., and McBain, C. J. (1995). Potassium conductances underlying repolarization and after-hyperpolarization in rat CA1 hippocampal interneurones. J. Physiol. 488(Pt 3), 661–672. doi: 10.1113/jphysiol.1995.sp020998
Zhang, M., Abrams, C., Wang, L., Gizzi, A., He, L., Lin, R., et al. (2012a). Structural basis for calmodulin as a dynamic calcium sensor. Structure 20, 911–923. doi: 10.1016/j.str.2012.03.019
Zhang, M., Pascal, J. M., Schumann, M., Armen, R. S., and Zhang, J. F. (2012b). Identification of the functional binding pocket for compounds targeting small-conductance Ca(2)(+)-activated potassium channels. Nat. Commun. 3:1021. doi: 10.1038/ncomms2017
Zhang, M., Meng, X. Y., Cui, M., Pascal, J. M., Logothetis, D. E., and Zhang, J. F. (2014). Selective phosphorylation modulates the PIP2 sensitivity of the CaM-SK channel complex. Nat. Chem. Biol. 10, 753–759. doi: 10.1038/nchembio.1592
Zhang, M., Pascal, J. M., and Zhang, J. (2013). Unstructured to structured transition of an intrisically disordered protein peptide in coupling Ca2+-sensing and SK channel activation. Proc. Natl. Acad. Sci. U.S.A. 110, 4828–4833. doi: 10.1073/pnas.1220253110
Zheng, J., and Zagotta, W. N. (2003). Patch-clamp fluorometry recording of conformational rearrangements of ion channels. Sci. STKE 2003:PL7. doi: 10.1126/stke.2003.176.pl7
Keywords: SK channels, IK channels, BK channels, modulators, drug discovery, nervous system, neurological disease
Citation: Kshatri AS, Gonzalez-Hernandez A and Giraldez T (2018) Physiological Roles and Therapeutic Potential of Ca2+ Activated Potassium Channels in the Nervous System. Front. Mol. Neurosci. 11:258. doi: 10.3389/fnmol.2018.00258
Received: 04 May 2018; Accepted: 06 July 2018;
Published: 30 July 2018.
Edited by:
Florian Lesage, Centre National de la Recherche Scientifique (CNRS), FranceReviewed by:
Ramon Latorre, Universidad de Valparaíso, ChileJames S. Trimmer, University of California, Davis, United States
Copyright © 2018 Kshatri, Gonzalez-Hernandez and Giraldez. This is an open-access article distributed under the terms of the Creative Commons Attribution License (CC BY). The use, distribution or reproduction in other forums is permitted, provided the original author(s) and the copyright owner(s) are credited and that the original publication in this journal is cited, in accordance with accepted academic practice. No use, distribution or reproduction is permitted which does not comply with these terms.
*Correspondence: Teresa Giraldez, Z2lyYWxkZXpAdWxsLmVkdS5lcw==