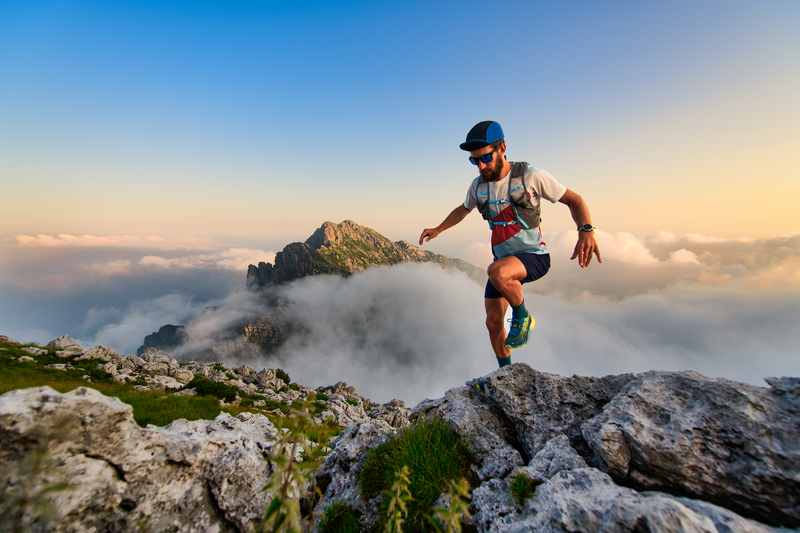
94% of researchers rate our articles as excellent or good
Learn more about the work of our research integrity team to safeguard the quality of each article we publish.
Find out more
ORIGINAL RESEARCH article
Front. Mol. Neurosci. , 06 February 2018
Sec. Neuroplasticity and Development
Volume 11 - 2018 | https://doi.org/10.3389/fnmol.2018.00018
Gamma aminobutyric acid (GABA) subtype A receptors (GABAARs) are integral membrane ion channels composed of five individual proteins or subunits. Up to 19 different GABAAR subunits (α1–6, β1–3, γ1–3, δ, ε, θ, π, and ρ1–3) have been identified, resulting in anatomically, physiologically, and pharmacologically distinct multiple receptor subtypes, and therefore GABA-mediated inhibition, across the central nervous system (CNS). Additionally, GABAAR-modulating drugs are important tools in clinical medicine, although their use is limited by adverse effects. While significant advances have been made in terms of characterizing the GABAAR system within the brain, relatively less is known about the molecular phenotypes within the peripheral nervous system of major organ systems. This represents a potentially missed therapeutic opportunity in terms of utilizing or repurposing clinically available GABAAR drugs, as well as promising research compounds discarded due to their poor CNS penetrance, for the treatment of peripheral disorders. In addition, a broader understanding of the peripheral GABAAR subtype repertoires will contribute to the design of therapies which minimize peripheral side-effects when treating CNS disorders. We have recently provided a high resolution molecular and function characterization of the GABAARs within the enteric nervous system of the mouse colon. In this study, the aim was to determine the constituent GABAAR subunit expression profiles of the mouse bladder, heart, liver, kidney, lung, and stomach, using reverse transcription polymerase chain reaction and western blotting with brain as control. The data indicate that while some subunits are expressed widely across various organs (α3–5), others are restricted to individual organs (γ2, only stomach). Furthermore, we demonstrate complex organ-specific developmental expression plasticity of the transporters which determine the chloride gradient within cells, and therefore whether GABAAR activation has a depolarizing or hyperpolarizing effect. Finally, we demonstrate that prior exposure to early life psychosocial stress induces significant changes in peripheral GABAAR subunit expression and chloride transporters, in an organ- and subunit-specific manner. Collectively, the data demonstrate the molecular diversity of the peripheral GABAAR system and how this changes dynamically in response to life experience. This provides a molecular platform for functional analyses of the GABA–GABAAR system in health, and in diseases affecting various peripheral organs.
The neurotransmitter gamma aminobutyric acid (GABA) is capable of mediating a rich variety of cellular communication patterns, throughout the entire nervous system, by engaging a multitude of molecularly and functionally diverse GABA receptor subtypes (Avoli and Krnjevic, 2016). One such major class of GABA receptors are GABA-A receptors (GABAARs), which are principally engaged in mediating the rapid effects of GABA. GABAARs are composed of individual proteins, called subunits, which assemble in a heteropentameric structure to form an anion-permeable ion channel. Although only five subunits are required to form a functional receptor complex, 19 molecularly distinct subunits have so far been identified; these are classified as α1–6, β1–3, γ1–3, δ, ε, θ, π, and ρ1–3. As a result, GABAARs, composed of various subunit combinations, give rise to numerous receptor subtypes. Characterizing the different GABAAR subtypes, within all the various branches of the nervous system, is essential for determining the contribution of the body’s GABA–GABAAR system, in health and disease.
Within the brain, GABAARs are one of the most comprehensively studied classes of neurotransmitter receptors. Combinatorial evidence over the last ∼30 years has revealed that within the central nervous system (CNS), molecularly distinct GABAAR subunits are diverse according to their cellular and subcellular expression patterns (Fritschy and Panzanelli, 2014), their activation and deactivation kinetics (Farrant and Nusser, 2005), and their gating by different pharmacological ligands (Rudolph and Knoflach, 2011). The overall effect of GABAAR activation on cellular excitability is dependent on the chloride ion gradient across the cell membrane, which is maintained by the potassium-chloride transporter member 5 (KCC2), the Na–K–Cl co-transporter 1 (NKCC1) and Na–K–Cl co-transporter 2 (NKCC2) (Payne et al., 2003; Chamma et al., 2012). Although GABA is the principal inhibitory neurotransmitter within the adult CNS, it initially has an excitatory function in the early postnatal rodent brain (Ben-Ari et al., 1989, 2012). This is due in part to the developmental upregulation in the expression of KCC2 (Rivera et al., 2005). Accordingly, there is a negative shift in the reversal potential for chloride ions with brain maturation. These brain GABAAR profiles are also sensitive to psychosocial stress (Caldji et al., 2004; Corteen et al., 2015), suggesting a class of molecules which change dynamically with life experience. As a result, we have a considerable understanding of the contributions of various GABAAR subtypes to native brain function and various brain disorders. This understanding has contributed to the development of numerous valuable GABAAR-modulating drugs in clinical medicine, primarily for disorders of CNS origin. However, considerable less attention has been paid to the peripheral nervous system (PNS) GABAAR system, despite evidence of its expression within various organ system, thus limiting the possible exploitation of GABAAR-modulating drugs for any associated disorders.
GABA is expressed within various types of peripheral tissue of both rodents and humans, such as pancreatic islet cells, the oviduct, the gastrointestinal tract (GIT) and adrenal chromaffin cells (Tanaka, 1985). GABA has been demonstrated to function as a neurotransmitter within various organ systems such as lung (Yabumoto et al., 2008) and the kidney (Sasaki et al., 2006). Converging evidence points to GABAAR subunit diversity explored across peripheral tissues (Akinci and Schofield, 1999) and in different species such as rat (Akinci and Schofield, 1999; Jin et al., 2008), mouse (Tyagi et al., 2007), and human (Mizuta et al., 2008; Zhang et al., 2013). Inextricably linked to GABAAR function are the ion transporters that maintain the gradients for the chloride ions which permeate such ion channels. Indeed, in contrast to the brain, GABAergic neurotransmission within peripheral branches of the nervous system, such as the enteric nervous system (ENS) of the GIT, have been shown to be excitatory in adulthood (Cherubini and North, 1984; Krantis, 2000). This is due to peripheral excitatory action of GABA within the ENS because of the lack of the KCC2 transporter in adulthood (Gameiro et al., 2005; Xue et al., 2009).
We have recently provided the first high resolution molecular and function characterization of the GABAARs within the ENS of the mouse colon (Seifi et al., 2014). However, the expression patterns of these receptors within major peripheral organs such as the lung, heart, liver, kidney, and bladder still remain unclear. Furthermore, the developmental expression levels of the chloride transporters that determine the effect of GABAARs on cellular excitability, in other peripheral organs, remains unclear. In this study, we provide the first comprehensive characterization of GABAAR subunits expression within such vital peripheral organs. We also determine the developmental changes in the chloride transporters mRNA expression within various peripheral organs. Finally, we demonstrate that experience of prior early life stress (ELS) induces significant changes in the expression of specific GABAAR subunits and chloride ion transporter in an organ-specific manner, in adulthood.
All procedures involving animal experiments were approved by the Animal Welfare and Ethical Review Body of the University of Portsmouth and were performed by a personal license holder, under a Home Office-issued project license, in accordance with the Animals (Scientific Procedures) Act, 1986 (United Kingdom) and associated procedures.
The first aim of this study was to determine which particular GABAAR subunits are expressed at the mRNA levels within various peripheral organs of the mouse. Therefore, reverse transcription polymerase chain reaction (RT-PCR) was performed on freshly isolated pieces of the mouse lung, heart, liver, stomach, kidney, and bladder with matched brain tissue used as positive control since all the GABAAR subunits investigated have been shown to be expressed within the mouse brain.
Adult male C57BL/6J mice (Charles River Laboratories; N = 3) were killed by cervical dislocation and segments of the lung, heart, liver, stomach, kidney, bladder, and whole brain were removed and snap frozen in liquid nitrogen and stored at -80°C until used. The frozen tissue was then homogenized in appropriate amounts of lysis buffer from which RNA was extracted using an RNeasy mini kit (Qiagen, 74104) according to the manufacturer’s protocol. The quality and quantity of the extracted RNA in each tissue was examined with spectrophotometry (Thermo ScientificTM NanoDropTM).
The reverse transcription and PCR were performed according to our previously published protocols (Seifi et al., 2014). Equal amounts of RNA from each tissue was reverse-transcribed into first-stand cDNA in the following reaction: 2 μl of 10× M-MuLV Reverse Transcriptase Reaction Buffer which included, in the final concentration, 50 mM Tris–HCl, 10 mM DTT, 75 mM KCl and 3 mM MgCl2 (BioLabs), 0.1 mM Oligo(dT)18 Primer (Thermo Fisher Scientific), 1 mM dNTP Mix (Thermo Fisher Scientific), 0.5 μl of M-Mulv reverse transcriptase (Applied Biosystems), and 0.5 μl of riboLock (Thermo Fisher Scientific). Each reaction was then incubated at 37°C for 2 h. Equal amounts of cDNA (1–2 μl) were then used for subsequent PCR using GoTaq Green Master Mix (Promega), PCR grade water and specific primers for 16 different GABAAR subunit. Exon–exon spanning GABAAR subunit specific PCR primers used in the study (Table 1) have been previously published (Glassmeier et al., 1998; Gustincich et al., 1999; Tan K.R. et al., 2011). The RT-PCR transcript products for the GABAAR subunits from all investigated tissues were run on a 2% agarose gel and the DNA was visualized under ultraviolet light using a SYBR green-based DNA stain.
Adult male C57BL/6J mice (Charles River Laboratories; N = 3) were killed by cervical dislocation and segments of the lung, heart, liver, stomach, kidney, bladder, and whole brain were removed and snap frozen in liquid nitrogen and stored at -80°C until used. The tissue was then homogenized and lysed in ice-cold RIPA lysis buffer [25 mM Tris–HCl (pH 7.6), 150 mM NaCl, 1% NP-40, 1% sodium deoxycholate, 0.1% sodium dodecyl sulfate] supplemented with a cocktail of protease and phosphatase inhibitors (Thermo Fisher Scientific). Subsequently, the tissue lysate was clarified by centrifugation of samples at 4°C.
The extracted proteins were then boiled at 95°C for 3 min and separated by 10% sodium dodecyl sulfate polyacrylamide gel electrophoresis. The gels were then placed in a wet transfer tank and the separated proteins were electrotransferred onto an activated polyvinylidene fluoride membrane (Thermo Fisher Scientific). The membranes were then incubated on a shaker for 1 h at room temperature with a blocking solution containing Tris-buffered saline-Tween 0.1% (TBS-Tween; Thermo Fisher Scientific) and 3% non-fat dry milk. After the blocking stage, the membranes were incubated with primary antibodies diluted in the blocking solution over night at 4°C. The next day, membranes were washed four times (5 min each time) with TBS-Tween solution in order to wash the excess primary antibodies off the membranes. Subsequently, membranes were incubated with appropriate horseradish peroxidase (HRP)-conjugated secondary antibody diluted in the blocking solutions for 2 h. The membranes were then washed in TBS-Tween (4 × 5 min) and incubated with an enhanced chemiluminescence development reagent (Luminata Forte; Millipore) for 3 min and visualized with a high sensitivity CCD camera imaging platform (ChemiDoc MP; Bio-Rad, Hemel Hempstead, United Kingdom).
The primary antibodies used were: mouse anti GABAAR α1, 1:500 (NeuroMab clone N95/35); rabbit anti GABAAR α2, 1:1000 (Millipore, catalog number AB5948); rabbit anti GABAAR α3, 1:1000 (Synaptic Systems, catalog number 224 303); mouse anti GABAAR α4, 1:500 (NeuroMab, clone N398A/34); rabbit anti GABAAR α5 (Synaptic Systems, catalog number 224 503), rabbit anti GABAAR γ2, 1:1000 (Synaptic Systems, catalog number 224 003); mouse anti β-actin, 1:1000 (Cell Signaling, catalog number 3700). Secondary antibodies used were donkey anti-rabbit HRP (Promega, Southampton, United Kingdom) and anti-mouse HRP (1:5000) (Promega, Southampton, United Kingdom).
Within the CNS, the expression of the GABAAR system is highly dynamic and changes in response to individual stimuli such as stress (Caldji et al., 2003; Hsu et al., 2003; Verkuyl et al., 2004; Maguire and Mody, 2007; Zheng et al., 2007; Corteen et al., 2015). We therefore investigated whether prior ELS results in altered GABAAR expression within peripheral organs, focusing on the GABAAR α1–5 and γ2 subunits. For these experiments, we exploited a validated animal model of ELS which is based on a fragmented mother–pup interaction during the first week of life (Rice et al., 2008; Gunn et al., 2013).
Briefly, pregnant dams were housed together with male partners and monitored every 12 h for the birth of pups. The day of birth was termed postnatal day 0 (PND 0). Both the control and ELS dams were left undisturbed until PND 2. On PND 2, litters were adjusted to a maximum of eight pups. Only male offspring were used for analyses. Control dams were housed in standard sawdust bedding and provided with sufficient nesting material (1 square; NestletsR, Ancare). In the ELS cages, dams were provided with reduced nesting material (2/3 of a square) placed upon a raised, fine-gauge (5 mm) steel mesh platform. The cage floor was covered with a small amount of sawdust to prevent ammonia build-up. All litters were left undisturbed between PND 2 and 9. On PND 9, both control and ELS pups were returned with the dams to cages with standard bedding and nesting material. Offspring remained with the dams until weaning at PND 22–23. Once the animal reached adulthood (PND 90), the effect of ELS on the expression of various GABAAR subunits in adulthood was assessed within peripheral organs, using quantitative RT-PCR.
Adult male mice from control and ELS groups were killed by cervical dislocation and tissue homogenates of whole brain, lung, heart, liver, stomach, kidney, and bladder prepared. RNA was extracted from the samples and then reverse transcribed into cDNA as described earlier.
Quantitative PCR (qPCR) amplification was performed in 96-well plates in a master mix for probes (Roche, Burgess Hill, United Kingdom) and run on a LightCycler® 96 System (Roche). The qPCR amplifications for the mouse Gabra1 (assay ID: Mm00439046_m1), Gabra2 (assay ID: Mm00433435_m1), Gabra3 (assay ID: Mm01294271_m1), Gabra4 (assay ID: Mm00802631_m1), Gabra5 (assay ID: Mm00621092_m1), Gabrg2 (assay ID: Mm00433489_m1) genes were performed using pre-designed TaqMan primers/probes purchased from Life Technologies (Thermo Fisher scientific). Gapdh (assay ID: Mm99999915_g1) gene expression was used as the housekeeping gene in every reaction. The qPCR cycling conditions entailed 95°C for 10 min and 40 cycles of 95°C for 15 s and 60°C for 60 s (LightCycler® 96 System, Roche). Standard curves were generated for Gabra1–5 and Gabrg2 using serial dilutions of a known amount of mRNA extracted from each organ which were then reverse transcribed into cDNA. Each measurement was performed in duplicate and each Ct value was then converted into ng mRNA using linear regression analysis of the standard curve (Microsoft Excel). Each ng mRNA value was then normalized against the ng housekeeping gene level within the same sample and the mean mRNA levels for every sample was finally calculated and compared across all experimental groups.
In another set of experiments, using normally reared mice, we quantified the developmental mRNA expression levels of the main membrane transporters that determine chloride gradients across cell membranes, namely the KCC2, the NKCC1 and NKCC2, within the different organs, with brain used as a control. Expression levels, using qPCR, were compared between animals aged PND 6 and 60, since developmental changes are known to occur within this time window (Payne et al., 2003; Ben-Ari et al., 2012). The following pre-designed TaqMan primers/probes were used: Slc12a5 (assay ID: Mm00803929_m1), Slc12A2 (assay ID: Mm01265951_m1) and Slc12A1 (assay ID: Mm01275821_m1) genes. Gapdh was used as the housekeeping gene (Thermo Fisher Scientific).
All statistical analyses were performed using GraphPad Prism 7 (GraphPad Inc, La Jolla, CA, United States). Animals were randomly assigned to treatment groups. All results are expressed as mean ± SEM. Statistical comparisons between different animal groups and treatments were assessed using the unpaired Student’s t-test. A P-value less than 0.05 was considered statistically significant.
Previous studies have reported the expression of up to 19 different GABAAR subunits within the rodent CNS (Sieghart, 2006). However, the mammalian gene expression of these receptor subunits within distinct peripheral organs, remains, to a large extent, unclear. GABAAR subunit mRNA expression has only been demonstrated in a few peripheral organs of the rat (Akinci and Schofield, 1999). Here, GABAAR subunit mRNA expression in mouse bladder, heart, kidney, liver, lung, and stomach was investigated, as a surrogate indicator of potential GABAAR subtypes expressed by these organs, with brain tissue used as a positive control. RT-PCR analyses revealed that GABAAR subunits are expressed within all the organs investigated and that the expression of these receptors within peripheral organs is subunit-specific (Figure 1; N = 3 animals). The following GABAAR subunit mRNA expression profiles were detectable in the following organs: (1) Stomach (α1–5, β2 and 3, γ2 and 3, and ε); (2) lung (α1 and 3–5, β1, γ3, and ε); (3) bladder (α1 and 3–5, β1–3, δ, and ε); (4) kidney (α1–5, β1–3, γ1 and 3, δ, and ε); (5) heart (α1–5, β3, and ε); and (6) liver (α1–5, β1 and 3, δ, and ε). Notably, for the ε subunit, the sizes of the amplicons in peripheral organs were noticeably different to that of brain. This is most likely indicative of the expression of different splice variants known to exist and potentially code for truncated protein (Wilke et al., 1997). Therefore, the functional relevance of this subunit in peripheral organ systems is debatable.
FIGURE 1. Diversity of GABAAR subunit expression within various organs of the mouse. Representative gel electrophoresis images of mRNA transcripts for various GABAAR subunits using RT-PCR and homogenates from the whole brain (Br), stomach (St), lung (Lu), bladder (Bl), kidney (Ki), heart (Hr), and liver (Li) obtained from adult male C57BL/6 mice. Corresponding amplicons of the same size bands to those obtained from the brain were consistently detected for various GABAAR subunits within a number of peripheral organs. N = 3 animals.
In summary, the organs which expressed the majority of GABAAR subunits were the stomach (10 different subunits detected) and the kidney (12 different subunits detected). In contrast, the lung (six different subunits) and the heart (five different subunits) expressed significantly fewer numbers of different GABAAR subunits.
The expression of these subunits was then explored at the protein level, using western blotting. This analysis was limited by the availability of antisera which work with this technique, and whose specificity has already been verified in previous studies. As a result, we therefore were unable to investigate all subunits explored at the mRNA level. Therefore, given their known physiological and pharmacological relevance (Rudolph and Knoflach, 2011), we focused only on the α1–5 and γ2 subunits. Representative western blot images are presented in Figure 2. Intense bands for all these subunits were detectable in protein homogenates from brain tissue, at the appropriate molecular weight, indicating the specificity of the antisera used. For the α1 subunit, intense bands were detectable in protein homogenates from bladder and heart tissue, with weaker, though specific bands evident in stomach, lung, kidney, and liver homogenates. For the α2 subunit, intense bands were detectable in heart, with weaker expression in kidney and liver. For the α3 subunit, intense bands were detectable in stomach, kidney, heart, and liver. For the α4 subunit, intense bands were detectable in all peripheral organs apart from the liver. For the α5 subunit, intense bands were detectable in kidney and bladder, with weaker expression evident in stomach, lung and liver. Finally, for the γ2 subunit, strong expression was detectable only in bladder, with weaker expression evident in stomach and lung, which could indicate the antibody recognizing an alternative splice variant of the protein. It is notable that bands from bladder samples were of different sizes to that of the brain. Qualitatively, it is evident that GABAAR subunit protein varies greatly across organs and subunits, when compared against the total amount of protein per organ. This could be due to either a limited expression of a subunit within an organ per se, or strong expression, but within very restricted tissue compartments of an individual organ.
FIGURE 2. Protein expression of the major GABAAR α and γ subunits within various organs of the mouse. Representative western blots for GABAAR α1–5 and γ2 subunits using tissue homogenates from whole brain (Br), stomach (St), lung (Lu), bladder (Bl), kidney (Ki), heart (Hr), and liver (Li) obtained from adult male C57BL/6 mice. Western blots for the different subunits in the peripheral organs corresponded to the sizes of those obtained from the brain. Note that blots for actin were run on separate gels due to the similar molecular weight of this protein compared to GABAAR subunits. N = 3 animals.
Using real time qPCR, we compared the relative mRNA expression of KCC2, NKCC1, and NKCC2 transporters within various organ systems of mice aged PND 6 and 60. Within the mouse brain, as expected, we observed a steep developmental upregulation in the mRNA expression of KCC2 and downregulation of NKCC1 and NKCC2 (Figure 3A). A similar trend was evident in tissue from lung (Figure 3B) and heart (Figure 3C). This suggests that similar to the brain, activation of GABAARs within these peripheral organs will have a depolarizing effect during early postnatal development and a hyperpolarizing effect during adulthood.
FIGURE 3. Developmental changes in the mRNA expression level of the KCC2, NKCC1, and NKCC2 chloride ion transporters within various peripheral organs. Quantification of the mRNA expression levels of the potassium-chloride transporter member 5 (KCC2), Na–K–Cl cotransporter 1 (NKCC1), and Na–K–Cl cotransporter 2 (NKCC2) in tissue from mice aged postnatal day 6 (PND 6) and adulthood (PND 60), using qPCR. Within the (A) brain, (B) lung, and (C) heart, a significant increase in KCC2 was detected and a decrease in the NKCCs mRNA levels as the mice age. However, within the (D) stomach, the contrary is true. In addition, there is an increase in both KCC2 and NKCCs mRNA expression levels from PND 6 to 60 within the mouse bladder (E) and liver (F). Within the mouse (G) kidney, KCC2 mRNA is expressed at very low levels in compare to the NKCCs and aging induces a decrease in the expression of NKCCs. Data represent mean ± SEM; N = 5 animals. NS, not significant; ND, not detected. ∗P < 0.05, Student’s unpaired t-test.
In stark contrast, within the mouse stomach, aging resulted in a significant decrease in the expression of the KCC2 and an increase in the mRNA expression of NKCC1 (Figure 3D). This finding is in agreement with the current dogma that GABA has a depolarizing and thus excitatory effect within the GI tract and the ENS. There was a detectable increase in both KCC2 and NKCC1 mRNA expression levels within the mouse bladder (Figure 3E) and liver (Figure 3F).
Within the mouse kidney, NKCC1 and NKCC2 are the predominant Cl ion transporters (Figure 3G). This suggests an excitatory effect for GABA within the mouse kidney. Collectively, the data suggest that, in adulthood, cells from different organs may produce contrasting chloride gradients across their membranes. When associated with GABAARs, the implication is that the activation of a specific GABAAR subtype may opposite effects on cellular excitation, that is, either hyperpolarization or depolarization, in different organs.
Psychosocial stress, experienced either in adulthood or during development, alters the expression and function of rodent GABAARs within the CNS, in a receptor subtype and brain region–specific manner (Caldji et al., 2003; Lamy and Beck, 2010; Martisova et al., 2012; Crawford et al., 2013; Liu et al., 2014; Corteen et al., 2015). However, the effect of stress on peripheral GABAARs is poorly understood. Using a mouse model of ELS that robustly imparts an enduring hyper-stress phenotype throughout adulthood (Gunn et al., 2013), we quantified the corresponding changes in the mRNA expression levels of the major α and γ GABAAR subunits within our target organs, using qPCR.
RT-PCR evidence for the expression of the GABAAR α1 subunit was detected within mouse stomach, kidney, and liver (see Figure 1). However, when using qPCR and assessed relative to the housekeeping gene Gapdh, the GABAAR α1 subunit expression was detectable only within the mouse stomach (Figure 4A). Within this organ, there were no significant differences in GABAAR α1 subunit mRNA expression between control and ELS subjects (P = 0.44, unpaired Student’s t-test; N = 5 control and ELS animals).
FIGURE 4. Early life stress (ELS)-induced changes in the level of GABAAR subunit mRNA expression within various peripheral organs. Quantification of the ELS-induced changes in the expression levels of the (A) α1-GABAAR subunit mRNA, (B) α2-GABAAR subunit mRNA, (C) α3-GABAAR subunit mRNA, (D) α4-GABAAR subunit mRNA, (E) α5-GABAAR subunit mRNA, and (F) γ2-GABAAR subunit mRNA, within mouse stomach (St), lung (Lu), bladder (Bl), kidney (Ki), heart (Hr), and liver (Li) tissue, relative to the housekeeping gene GAPDH, using qPCR. Note that the mRNA expression of some subunits, relative to GAPDH, was not always detectable (ND) using qPCR. Data represent mean ± SEM; N = 5 animals. NS, not significant. Student’s unpaired t-test. ∗P < 0.05.
Using qPCR the levels of GABAAR α2 subunit expression in comparison to the housekeeping gene Gapdh was negligible in all organs investigated (Figure 4B). Within the brain, stress has been shown to significantly increase the expression of the GABAAR α3 subunit (Corteen et al., 2014). Here, ELS induced a significant decrease in the mRNA expression of the GABAAR α3 subunit within the mouse bladder (P = 0.04, unpaired Student’s t-test; N = 5 control and ELS animals) and liver (P < 0.0001, unpaired Student’s t-test; N = 5 control and ELS animals) (Figure 4C). In contrast, within the heart, ELS induced a significant increase in the expression of this GABAAR subunit (P = 0.03, unpaired Student’s t-test; N = 5 control and ELS animals) (Figure 4C). There were no significant changes in GABAAR α3 subunit expression within the stomach, lung, and kidney.
Stress-induced GABAAR α4 subunit expression plasticity has yet to be reported within the CNS. Here, ELS induced a significant increase in the expression of the α4 subunit within mouse lung (P = 0.0024, unpaired Student’s t-test; N = 5 control and ELS animals) (Figure 4D). In stark contrast, ELS induced a significant decrease in the expression of the GABAAR α4 within the mouse bladder (P = 0.01, unpaired Student’s t-test; N = 5 control and ELS animals). However, the expression of the α4 subunit within the mouse stomach and kidney was not significantly altered as a result of ELS.
Using qPCR, GABAAR α5 subunit was detectable within the mouse kidney but not in the lung, bladder, stomach, heart, or liver (Figure 4E). Within the kidney, ELS resulted in a significant decrease in the expression of the GABAAR α5 subunit (P = 0.0024, unpaired Student’s t-test; N = 5 control and ELS animals).
Using qPCR, the GABAAR γ2 subunit mRNA expression was only detectable within the mouse stomach and not the lung, bladder, kidney, heart, or liver (Figure 4F). Within the stomach, ELS induced a significant decrease in the expression of the GABAAR γ2 subunit (P = 0.01, unpaired Student’s t-test; N = 5 control and ELS animals). All these data are summarized in Table 1.
Collectively, these data demonstrate that early life environment has an enduring impact on the expression levels of GABAAR subunits, within various organs, in adulthood. Furthermore, the trajectory of ELS-induced GABAAR subunit expression plasticity is organ-specific.
Given the importance of KCC2, NKCC1, and NKCC2 expression to GABAAR function, and the impact that ELS had on GABAAR expression in adulthood, we investigated whether ELS also altered the adulthood phenotype of these transporters in peripheral organs. ELS induced a significant decrease in the mRNA expression of KCC2 in the brain. No significant differences were detected for NKCC1 and NKCC2 (Figure 5A). Within the stomach, ELS significantly decreased the expression of NKCC1 (Figure 5B). ELS significantly decreased the expression of KCC2, and increased the expression of NKCC1 in lung (Figure 5C). ELS had a profound effect on the expression of these transporters in the bladder by dramatically decreasing the expression of both KCC2 and NKCC1 (Figure 5D). There were no significant effects of ELS on the expression of these transporters in kidney, heart, and liver (Figures 5E–G, respectively). Thus, early life environment not only engages peripheral GABAAR but also the transporters that determine the gradients of ions which permeate these ion channels.
FIGURE 5. Early life stress (ELS)-induced changes in the level of the potassium-chloride transporter member 5 (KCC2), Na–K–Cl cotransporter 1 (NKCC1), and Na–K–Cl cotransporter 2 (NKCC2) within various peripheral organs. Quantification of the mRNA expression levels of the KCC2, NKCC1, and NKCC2 in (A) brain, (B) stomach, (C) lung, (D) bladder, (E) kidney, (F) heart, and (G) liver tissue from adult mice (PND 60) exposed to ELS. Data represent mean ± SEM; N = 5 animals. NS, not significant. Student’s unpaired t-test. Note that the ELS induced significant changes in the mRNA expression of ion transporters specifically within the brain, stomach, lung, and bladder of the mouse. ∗P < 0.05.
The current study advances our understanding of the rich diversity of GABAAR subunit expression patterns beyond the CNS, by demonstrating potentially unique receptor subtype profiles within various organ systems. Furthermore, the ontogenic expression profiles of the chloride transporters that determine whether the activation of such GABAARs leads to cellular activation or inhibition, are also organ specific. Finally, we provide evidence that early life psychological stress directly engages the transcriptional machinery of the peripheral GABAAR system, in an organ- and subunit-specific manner.
For most of this study, mRNA was used as a surrogate measure of the active expression of specific subunits by individual organs. The obvious caveat is that mRNA expression on its own does not unequivocally prove the expression of the functional protein product. However, initial GABAAR mRNA screens within the brain (Wisden et al., 1992) have proved to be indispensable in the design of future analyses within the CNS, and thus the overall advancement of this field within this organ. Nevertheless, to provide a translational insight to these expression profiles, we undertook a protein analysis of the major GABAAR subunits in these peripheral organs. Unfortunately, our analyses were limited by the availability of GABAAR subunit antisera which have been confirmed to exhibit subunit specificity, as well as perform in western blots as opposed to other protein assays such as immunohistochemistry. There was general agreement between the datasets with subunit protein being detected only if mRNA expression was evident within a particular organ. The only outlier was the γ2 subunit in the bladder. We did not detect any mRNA for this subunit, but did obtain a band within this organ. However, the size of the band observed in the bladder was different to that of the brain. There were of course some organs that expressed subunit mRNA and not protein, suggesting that for these subunits, their expression may be limited to the transcriptional level.
A technical confound was the discrepancies in subunit mRNA expression patterns obtained in peripheral organs when using the RT-PCR and qPCR methods. Essentially, the qPCR method failed to detect some subunits which were revealed using RT-PCR, in specific peripheral organs. A possible explanation is the differences in primer sequences or their amplification efficiencies. We therefore performed RT-PCR using our qPCR primers and reliably detected amplicons for these subunits in these organs (Supplementary Figure 1). Therefore, the most likely explanation relates to the relative expression of the subunit that is reported with qPCR, when normalized against a housekeeping gene. If the subunit is expressed in discrete cells of the entire organ, or in limited amounts compared to the housekeeping gene, then it is likely that such signal will be difficult to detect, when normalized to that of an abundant housekeeping gene found throughout the organ. Therefore, the suggested future, high resolution molecular and histological analyses in individual organs (see below) will be required to place the role of individual subunits within the wider context of organ function. Nevertheless, the data presented here provide a platform for guiding further analyses on the candidate subunits which are most likely to be relevant in terms of GABAAR-mediated regulation of these major organs.
Since whole tissue homogenates were used for RNA extraction in this study, we were unable to identify which particular cell types, within a specific organ, express distinct GABAAR subunits. Such information is particularly important for predicting the potential functional consequences of GABAAR activation on overall organ function. However, technical difficulties relating to the separation of various cell types within the tissue and extracting mRNA from such cell types individually, precluded such analyses. Therefore, future immunohistochemical studies aimed at investigating the cellular and subcellular expression pattern of GABAAR subunits within peripheral organs are essential, informed by these data.
Our data largely correspond to previous reports on the repertoire of GABAAR subunits expressed in the peripheral organs of mouse (Tyagi et al., 2007), and the lungs of rat (Jin et al., 2008) and human (Mizuta et al., 2008; Zhang et al., 2013). The major discrepancy between this report and a related study in mouse is that they (Tyagi et al., 2007) did not detect α1, β1, β3, and γ1 subunit expression in any peripheral organs even though these were detected in rat (Akinci and Schofield, 1999). Given the time-frame between these studies, a parsimonious explanation could be differences in reagents and technical protocols.
The current data reveal that, unlike the CNS which expresses all known GABAAR subunits, mouse peripheral organs express only subsets of subunit combinations. Although co-expression does not necessarily imply co-assembly, these data do invite cautious extrapolations of potential receptor subtypes, within individual organ systems, compared to the brain. Firstly, within the brain, the α1 and 2 subunits are considered to be the most widely expressed of all α subunits. However, the current data indicate that the expression of these two subunits is rather limited across all the organs surveyed. Instead, the α3–5 subunits are more widespread, being detectable in all organs investigated. Once again, this is in contrast to the brain where these particular α subunits are enriched within specific regions (Hortnagl et al., 2013).
Another striking difference between the brain and peripheral GABAAR subunit expression profiles is the restricted distribution of the γ2 subunit in the organs surveyed; indeed, transcripts for this subunit were detected only in stomach. In contrast, the ε subunit was expressed throughout all organs. An important caveat is that amplicons for the ε subunit in peripheral organs were notably of a different size compared to brain. Since a number of ε splice variants, which putatively encode for truncated proteins, are thought to be expressed particularly within peripheral organs (Wilke et al., 1997), the role for subunits auxiliary to α/β counterparts may be occupied by γ/δ subunits rather than ε. No specific parcellation of individual β subunits, within specific organs was noticeable. In this understandably limited set of organs, the major peripheral receptor subtypes are most likely to be combinations of: (α) α3–5 > α1 > α2; (β) β3 > β1 > β2; (γ/δ/ε) ε > δ–γ3 > γ1–2. If the conventional CNS GABAAR subunit stoichiometry of 2α/2β/1γ–δ–ε holds true for the PNS as well, then these data suggest that the major peripheral GABAAR subtypes are likely to be composed of α3–5/β3/ε subunits.
These potential GABAAR subtypes have implications for drug design if off-target effects are to be avoided. This is important given the growing evidence of different GABAAR subtypes having marked contributions to the functioning of various peripheral organs. Many clinically available GABAAR-targeting drugs for CNS disorders require the presence of the γ2 subunit. The current study highlights the predominance of the ε subunit over the γ2 within the periphery. This could be exploited in future drug design strategies in order to minimize unwanted effects when targeting either CNS or PNS disorders with GABAAR-based therapies.
Emerging evidence indicates the widespread effects of GABAARs on the native functioning and pathologies of various organs. For example, GABAARs have been shown to have a pronounced effect on various facets of lung function, ranging from inflammation (Yocum et al., 2017), asthma (Forkuo et al., 2017), lung cancer (Liu et al., 2016) to airway smooth muscle contractility (Gallos et al., 2015). However, within other peripheral organs such as kidney, relatively less is known about the role of GABA–GABAAR system. Importantly, many of the actions arise from a direct effect on the organs, rather than through centrally mediated mechanisms. This could therefore represent opportunities for the repurposing and use in peripheral disorders, of discarded GABAAR subtype-specific ligands because of their inability to cross the blood–brain barrier.
To achieve this, it is essential for dedicated studies on individual organ systems to identify the roles of individual receptor subtypes in the functioning of specific organs. The availability of GABAAR transgenic mouse models, already generated for CNS studies (Crestani and Rudolph, 2015), will be indispensable for this purpose. However, the data also suggest the need for additional models targeting other subunits, such as epsilon, which, in terms of CNS GABAAR studies may have not been warranted given its restricted expression in the brain. Indeed, given the ubiquity of the epsilon subunit in the periphery demonstrated by these data, it is reasonable to predict a significant functional role, thus placing it as a prime target for future such studies.
The results of this study suggest that the function of the peripheral GABAAR subtypes, and associated transporters, are likely to change dynamically, at least with age, as well as with early life experience. The effect of the GABA–GABAAR system on cellular excitability, namely hyperpolarization or depolarization, is determined by the directional flow of chloride ions, down a concentration gradient upon agonist binding. The expression levels of chloride transporters are thus integral to GABAAR-mediated cellular excitability as this determines the relative chloride ion concentrations across the plasma membranes of the cell system of interest. This is elegantly illustrated in the developing CNS where an initial excitatory role for GABAARs during development is transformed to that of neuronal inhibition in adulthood, due to the increased expression of the KCC2 with age. Such ontogenic expression profiles of associated chloride transporters are poorly understood in peripheral organs and the PNS. The current study indicates that the developmental expression profiles of the major chloride transporters that determine cellular chloride gradients, namely the NKCC1, NKCC2, and KCC2, change dynamically during development, in an organ-specific manner. The implication is that an individual GABAAR subtype could have contrasting effects on cellular excitability depending on the individual organ.
A striking finding of this study was that prior experience of ELS had a robust effect on expression of GABAAR subunit and ion transporter levels in adulthood, although in an organ-specific manner. Convergent lines of evidence indicate that various forms of stress robustly engage brain GABA–GABAAR systems (Maguire, 2014). Importantly, early life adversity is known to have a profound effect on multiple organ systems, not only the nervous system. Indeed, ELS confers an enduring vulnerability to developing a range of illnesses later on in life, such as mental illnesses, cardiovascular and metabolic disorders (Shonkoff et al., 2009; Miller et al., 2011). With an ever aging population, there is a growing urgency to identify the underlying biological mechanisms through which experiences in childhood engage such a wide variety of body systems, resulting in a multitude of pathologies in later life. A considerable body of evidence indicates that brain disorders in adulthood due to prior ELS arise from epigenetic, morphological, and physiological changes in various CNS pathways (McEwen et al., 2015). However, the role of the PNS in mediating the negative effects of ELS in various peripheral organs is less well understood. To the best of our knowledge, we provide the first demonstration that ELS engages different branches of the PNS, in an interaction that involves specific organs and GABAAR subunits. A tantalizing proposition is that such ELS-induced GABAAR expression phenotypes contribute to changes in organ function later on in life. Functional verification of this proposition could provide unique molecular targets for addressing such medical conditions.
Conceptually, the question arises as to how one’s environment, or experience thereof, could alter the activity of different GABAAR genes, divergently in various organs. Since ELS has been shown to alter gene activity epigenetically within the CNS and such epigenetic alterations result directly in changes at transcriptional levels (Anacker et al., 2014), the questions emerges as to whether the ELS-induced changes in the mRNA expression of GABAAR subunits within peripheral organs are due to epigenetic modifications. Hence, the data in this study could provide a platform for further epigenetic studies aimed at identifying drug targets which may counteract the negative impacts of ELS on major peripheral organs via modification of the peripheral GABA–GABAAR system. Furthermore, intracellular chloride concentration has been shown to influence the subunit composition of GABAAR subtypes, in particular α3-GABAAR (Succol et al., 2012). Coincidently, we have previously demonstrated repeated stress in adulthood increases the expression of brain α3-GABAARs (Corteen et al., 2015). The current data indicate that ELS alters the expression of membrane transporters integral to regulating intracellular chloride concentration. Therefore, some of the ELS induced changes in the expression of GABAAR subunits demonstrated in this study, or in previous reports (Corteen et al., 2015), could be due to alterations in chloride gradients arising from the associated changes in the expression of the relevant membrane transporters. If so, and given the ubiquity of other cellular processes underpinned by such ionic gradients, such ELS-induced changes have the potential to impart significant changes on a variety of cellular processes, which could underlie the associated pathologies.
In summary, the study provides an outline of the diversity of GABAAR subunits, expressed at the mRNA level, in major peripheral organs. These data provide a platform for future functional analyses of the contribution of the GABA–GABAAR system to the health, and associated diseases of these particular organ systems.
JS and MS designed the research. EE, AG, JS, and MS performed the experiments. EE, AG, and MS analyzed the data. JS and MS wrote the manuscript.
The authors declare that the research was conducted in the absence of any commercial or financial relationships that could be construed as a potential conflict of interest.
The reviewer TJ and the handling editor declared their shared affiliation.
The Supplementary Material for this article can be found online at: https://www.frontiersin.org/articles/10.3389/fnmol.2018.00018/full#supplementary-material
FIGURE S1 | Performance of qPCR primers using the RT-PCR methods. Representative gel electrophoresis image of mRNA transcripts for various GABAAR subunits, demonstrating the performance of qPCR primers using the RT-PCR method, on homogenates from the whole brain (Br), stomach (St), lung (Lu), bladder (Bl), kidney (Ki), heart (Hr), and liver (Li) obtained from adult male C57BL/6 mice.
CNS, central nervous system; ELS, early life stress; ENS, enteric nervous system; GABA, gamma aminobutyric acid; KCC2, potassium-chloride transporter member 5; NKCC1, Na–K–Cl cotransporter 1; NKCC2, Na–K–Cl cotransporter 2; PND, postnatal day; PNS, peripheral nervous system.
Akinci, M. K., and Schofield, P. R. (1999). Widespread expression of GABA(A) receptor subunits in peripheral tissues. Neurosci. Res. 35, 145–153. doi: 10.1016/S0168-0102(99)00078-4
Anacker, C., O’donnell, K. J., and Meaney, M. J. (2014). Early life adversity and the epigenetic programming of hypothalamic-pituitary-adrenal function. Dialogues Clin. Neurosci. 16, 321–333.
Avoli, M., and Krnjevic, K. (2016). The long and winding road to gamma-amino-butyric acid as neurotransmitter. Can. J. Neurol. Sci. 43, 219–226. doi: 10.1017/cjn.2015.333
Ben-Ari, Y., Cherubini, E., Corradetti, R., and Gaiarsa, J. L. (1989). Giant synaptic potentials in immature rat CA3 hippocampal neurones. J. Physiol. 416, 303–325. doi: 10.1113/jphysiol.1989.sp017762
Ben-Ari, Y., Khalilov, I., Kahle, K. T., and Cherubini, E. (2012). The GABA excitatory/inhibitory shift in brain maturation and neurological disorders. Neuroscientist 18, 467–486. doi: 10.1177/1073858412438697
Caldji, C., Diorio, J., Anisman, H., and Meaney, M. J. (2004). Maternal behavior regulates benzodiazepine/GABAA receptor subunit expression in brain regions associated with fear in BALB/c and C57BL/6 mice. Neuropsychopharmacology 29, 1344–1352. doi: 10.1038/sj.npp.1300436
Caldji, C., Diorio, J., and Meaney, M. J. (2003). Variations in maternal care alter GABA(A) receptor subunit expression in brain regions associated with fear. Neuropsychopharmacology 28, 1950–1959. doi: 10.1038/sj.npp.1300237
Chamma, I., Chevy, Q., Poncer, J. C., and Levi, S. (2012). Role of the neuronal K-Cl co-transporter KCC2 in inhibitory and excitatory neurotransmission. Front. Cell. Neurosci. 6:5. doi: 10.3389/fncel.2012.00005
Cherubini, E., and North, R. A. (1984). Actions of gamma-aminobutyric acid on neurones of guinea-pig myenteric plexus. Br. J. Pharmacol. 82, 93–100. doi: 10.1111/j.1476-5381.1984.tb16445.x
Corteen, N. L., Carter, J. A., Rudolph, U., Belelli, D., Lambert, J. J., and Swinny, J. D. (2014). Localisation and stress-induced plasticity of GABA receptor subunits within the cellular networks of the mouse dorsal raphe nucleus. Brain Struct. Funct. 220, 2739–2763. doi: 10.1007/s00429-014-0824-7
Corteen, N. L., Carter, J. A., Rudolph, U., Belelli, D., Lambert, J. J., and Swinny, J. D. (2015). Localisation and stress-induced plasticity of GABAA receptor subunits within the cellular networks of the mouse dorsal raphe nucleus. Brain Struct. Funct. 220, 2739–2763. doi: 10.1007/s00429-014-0824-7
Crawford, L. K., Rahman, S. F., and Beck, S. G. (2013). Social stress alters inhibitory synaptic input to distinct subpopulations of raphe serotonin neurons. ACS Chem. Neurosci. 4, 200–209. doi: 10.1021/cn300238j
Crestani, F., and Rudolph, U. (2015). Behavioral functions of GABAA receptor subtypes–the Zurich experience. Adv. Pharmacol. 72, 37–51. doi: 10.1016/bs.apha.2014.10.001
Farrant, M., and Nusser, Z. (2005). Variations on an inhibitory theme: phasic and tonic activation of GABA(A) receptors. Nat. Rev. Neurosci. 6, 215–229. doi: 10.1038/nrn1625
Forkuo, G. S., Nieman, A. N., Yuan, N. Y., Kodali, R., Yu, O. B., Zahn, N. M., et al. (2017). Alleviation of multiple asthmatic pathologic features with orally available and subtype selective GABAA receptor modulators. Mol. Pharm. 14, 2088–2098. doi: 10.1021/acs.molpharmaceut.7b00183
Fritschy, J. M., and Panzanelli, P. (2014). GABAA receptors and plasticity of inhibitory neurotransmission in the central nervous system. Eur. J. Neurosci. 39, 1845–1865. doi: 10.1111/ejn.12534
Gallos, G., Yocum, G. T., Siviski, M. E., Yim, P. D., Fu, X. W., Poe, M. M., et al. (2015). Selective targeting of the alpha5-subunit of GABAA receptors relaxes airway smooth muscle and inhibits cellular calcium handling. Am. J. Physiol. Lung Cell. Mol. Physiol. 308, L931–L942. doi: 10.1152/ajplung.00107.2014
Gameiro, A., Reimann, F., Habib, A. M., O’malley, D., Williams, L., Simpson, A. K., et al. (2005). The neurotransmitters glycine and GABA stimulate glucagon-like peptide-1 release from the GLUTag cell line. J. Physiol. 569, 761–772. doi: 10.1113/jphysiol.2005.098962
Glassmeier, G., Herzig, K. H., Opfner, M. H., Lemmer, K., Jansen, A., and Scherubl, H. (1998). Expression of functional GABAA receptors in cholecystokinin-secreting gut neuroendocrine murine STC-1 cells. J. Physiol. 510, 805–814. doi: 10.1111/j.1469-7793.1998.805bj.x
Gunn, B. G., Cunningham, L., Cooper, M. A., Corteen, N. L., Seifi, M., Swinny, J. D., et al. (2013). Dysfunctional astrocytic and synaptic regulation of hypothalamic glutamatergic transmission in a mouse model of early-life adversity: relevance to neurosteroids and programming of the stress response. J. Neurosci. 33, 19534–19554. doi: 10.1523/JNEUROSCI.1337-13.2013
Gustincich, S., Feigenspan, A., Sieghart, W., and Raviola, E. (1999). Composition of the GABA(A) receptors of retinal dopaminergic neurons. J. Neurosci. 19, 7812–7822.
Hortnagl, H., Tasan, R. O., Wieselthaler, A., Kirchmair, E., Sieghart, W., and Sperk, G. (2013). Patterns of mRNA and protein expression for 12 GABAA receptor subunits in the mouse brain. Neuroscience 236, 345–372. doi: 10.1016/j.neuroscience.2013.01.008
Hsu, F. C., Zhang, G. J., Raol, Y. S., Valentino, R. J., Coulter, D. A., and Brooks-Kayal, A. R. (2003). Repeated neonatal handling with maternal separation permanently alters hippocampal GABAA receptors and behavioral stress responses. Proc. Natl. Acad. Sci. U.S.A. 100, 12213–12218. doi: 10.1073/pnas.2131679100
Jin, N., Guo, Y., Sun, P., Bell, A., Chintagari, N. R., Bhaskaran, M., et al. (2008). Ionotropic GABA receptor expression in the lung during development. Gene Expr. Patterns 8, 397–403. doi: 10.1016/j.gep.2008.04.008
Krantis, A. (2000). GABA in the mammalian enteric nervous system. News Physiol. Sci. 15, 284–290. doi: 10.1152/physiologyonline.2000.15.6.284
Lamy, C. M., and Beck, S. G. (2010). Swim stress differentially blocks CRF receptor mediated responses in dorsal raphe nucleus. Psychoneuroendocrinology 35, 1321–1332. doi: 10.1016/j.psyneuen.2010.03.003
Liu, L., Yang, C., Shen, J., Huang, L., Lin, W., Tang, H., et al. (2016). GABRA3 promotes lymphatic metastasis in lung adenocarcinoma by mediating upregulation of matrix metalloproteinases. Oncotarget 7, 32341–32350. doi: 10.18632/oncotarget.8700
Liu, Z. P., Song, C., Wang, M., He, Y., Xu, X. B., Pan, H. Q., et al. (2014). Chronic stress impairs GABAergic control of amygdala through suppressing the tonic GABAA receptor currents. Mol. Brain 7:32. doi: 10.1186/1756-6606-7-32
Maguire, J. (2014). Stress-induced plasticity of GABAergic inhibition. Front. Cell Neurosci. 8:157. doi: 10.3389/fncel.2014.00157
Maguire, J., and Mody, I. (2007). Neurosteroid synthesis-mediated regulation of GABA(A) receptors: relevance to the ovarian cycle and stress. J. Neurosci. 27, 2155–2162. doi: 10.1523/JNEUROSCI.4945-06.2007
Martisova, E., Solas, M., Horrillo, I., Ortega, J. E., Meana, J. J., Tordera, R. M., et al. (2012). Long lasting effects of early-life stress on glutamatergic/GABAergic circuitry in the rat hippocampus. Neuropharmacology 62, 1944–1953. doi: 10.1016/j.neuropharm.2011.12.019
McEwen, B. S., Bowles, N. P., Gray, J. D., Hill, M. N., Hunter, R. G., Karatsoreos, I. N., et al. (2015). Mechanisms of stress in the brain. Nat. Neurosci. 18, 1353–1363. doi: 10.1038/nn.4086
Miller, G. E., Chen, E., and Parker, K. J. (2011). Psychological stress in childhood and susceptibility to the chronic diseases of aging: moving toward a model of behavioral and biological mechanisms. Psychol. Bull. 137, 959–997. doi: 10.1037/a0024768
Mizuta, K., Xu, D., Pan, Y., Comas, G., Sonett, J. R., Zhang, Y., et al. (2008). GABAA receptors are expressed and facilitate relaxation in airway smooth muscle. Am. J. Physiol. Lung Cell. Mol. Physiol. 294, L1206–L1216. doi: 10.1152/ajplung.00287.2007
Payne, J. A., Rivera, C., Voipio, J., and Kaila, K. (2003). Cation-chloride co-transporters in neuronal communication, development and trauma. Trends Neurosci. 26, 199–206. doi: 10.1016/S0166-2236(03)00068-7
Rice, C. J., Sandman, C. A., Lenjavi, M. R., and Baram, T. Z. (2008). A novel mouse model for acute and long-lasting consequences of early life stress. Endocrinology 149, 4892–4900. doi: 10.1210/en.2008-0633
Rivera, C., Voipio, J., and Kaila, K. (2005). Two developmental switches in GABAergic signalling: the K+-Cl- cotransporter KCC2 and carbonic anhydrase CAVII. J. Physiol. 562, 27–36. doi: 10.1113/jphysiol.2004.077495
Rudolph, U., and Knoflach, F. (2011). Beyond classical benzodiazepines: novel therapeutic potential of GABAA receptor subtypes. Nat. Rev. Drug Discov. 10, 685–697. doi: 10.1038/nrd3502
Sasaki, S., Yokozawa, T., Cho, E. J., Oowada, S., and Kim, M. (2006). Protective role of gamma-aminobutyric acid against chronic renal failure in rats. J. Pharm. Pharmacol. 58, 1515–1525. doi: 10.1211/jpp.58.11.0013
Seifi, M., Brown, J. F., Mills, J., Bhandari, P., Belelli, D., Lambert, J. J., et al. (2014). Molecular and functional diversity of GABA-A receptors in the enteric nervous system of the mouse colon. J. Neurosci. 34, 10361–10378. doi: 10.1523/JNEUROSCI.0441-14.2014
Shonkoff, J. P., Boyce, W. T., and Mcewen, B. S. (2009). Neuroscience, molecular biology, and the childhood roots of health disparities: building a new framework for health promotion and disease prevention. JAMA 301, 2252–2259. doi: 10.1001/jama.2009.754
Sieghart, W. (2006). Structure, pharmacology, and function of GABAA receptor subtypes. Adv. Pharmacol. 54, 231–263. doi: 10.1016/S1054-3589(06)54010-4
Succol, F., Fiumelli, H., Benfenati, F., Cancedda, L., and Barberis, A. (2012). Intracellular chloride concentration influences the GABAA receptor subunit composition. Nat. Commun. 3:738. doi: 10.1038/ncomms1744
Tan, K. R., Rudolph, U., and Luscher, C. (2011). Hooked on benzodiazepines: GABAA receptor subtypes and addiction. Trends Neurosci. 34, 188–197. doi: 10.1016/j.tins.2011.01.004
Tan, S., Rudd, J. A., and Yew, D. T. (2011). Gene expression changes in gabaa receptors and cognition following chronic ketamine administration in mice. PLOS ONE 6:e21328. doi: 10.1371/journal.pone.0021328
Tanaka, C. (1985). gamma-Aminobutyric acid in peripheral tissues. Life Sci. 37, 2221–2235. doi: 10.1016/0024-3205(85)90013-X
Tyagi, N., Lominadze, D., Gillespie, W., Moshal, K. S., Sen, U., Rosenberger, D. S., et al. (2007). Differential expression of gamma-aminobutyric acid receptor A (GABA(A)) and effects of homocysteine. Clin. Chem. Lab. Med. 45, 1777–1784. doi: 10.1515/CCLM.2007.342
Verkuyl, J. M., Hemby, S. E., and Joels, M. (2004). Chronic stress attenuates GABAergic inhibition and alters gene expression of parvocellular neurons in rat hypothalamus. Eur. J. Neurosci. 20, 1665–1673. doi: 10.1111/j.1460-9568.2004.03568.x
Wilke, K., Gaul, R., Klauck, S. M., and Poustka, A. (1997). A gene in human chromosome band Xq28 (GABRE) defines a putative new subunit class of the GABAA neurotransmitter receptor. Genomics 45, 1–10. doi: 10.1006/geno.1997.4885
Wisden, W., Laurie, D. J., Monyer, H., and Seeburg, P. H. (1992). The distribution of 13 GABAA receptor subunit mRNAs in the rat brain. I. Telencephalon, diencephalon, mesencephalon. J. Neurosci. 12, 1040–1062.
Xue, H., Liu, S., Ji, T., Ren, W., Zhang, X. H., Zheng, L. F., et al. (2009). Expression of NKCC2 in the rat gastrointestinal tract. Neurogastroenterol. Motil. 21, e1068–e1089. doi: 10.1111/j.1365-2982.2009.01334.x
Yabumoto, Y., Watanabe, M., Ito, Y., Maemura, K., Otsuki, Y., Nakamura, Y., et al. (2008). Expression of GABAergic system in pulmonary neuroendocrine cells and airway epithelial cells in GAD67-GFP knock-in mice. Med. Mol. Morphol. 41, 20–27. doi: 10.1007/s00795-007-0391-6
Yocum, G. T., Turner, D. L., Danielsson, J., Barajas, M. B., Zhang, Y., Xu, D., et al. (2017). GABAA receptor alpha4 subunit knockout enhances lung inflammation and airway reactivity in a murine asthma model. Am. J. Physiol. Lung Cell. Mol. Physiol. 313, L406–L415. doi: 10.1152/ajplung.00107.2017
Zhang, X., Zhang, R., Zheng, Y., Shen, J., Xiao, D., Li, J., et al. (2013). Expression of gamma-aminobutyric acid receptors on neoplastic growth and prediction of prognosis in non-small cell lung cancer. J. Transl. Med. 11:102. doi: 10.1186/1479-5876-11-102
Keywords: chloride transporters, GABA, KCC2, peripheral nervous system, psychosocial stress, NKCC1/2
Citation: Everington EA, Gibbard AG, Swinny JD and Seifi M (2018) Molecular Characterization of GABA-A Receptor Subunit Diversity within Major Peripheral Organs and Their Plasticity in Response to Early Life Psychosocial Stress. Front. Mol. Neurosci. 11:18. doi: 10.3389/fnmol.2018.00018
Received: 24 August 2017; Accepted: 12 January 2018;
Published: 06 February 2018.
Edited by:
Gregg E. Homanics, University of Pittsburgh, United StatesReviewed by:
Hartmut Lüddens, Johannes Gutenberg-Universität Mainz, GermanyCopyright © 2018 Everington, Gibbard, Swinny and Seifi. This is an open-access article distributed under the terms of the Creative Commons Attribution License (CC BY). The use, distribution or reproduction in other forums is permitted, provided the original author(s) and the copyright owner are credited and that the original publication in this journal is cited, in accordance with accepted academic practice. No use, distribution or reproduction is permitted which does not comply with these terms.
*Correspondence: Jerome D. Swinny, amVyb21lLnN3aW5ueUBwb3J0LmFjLnVr Mohsen Seifi, bW9oc2VuLnNlaWZpQHBvcnQuYWMudWs=
†These authors have contributed equally to this work.
Disclaimer: All claims expressed in this article are solely those of the authors and do not necessarily represent those of their affiliated organizations, or those of the publisher, the editors and the reviewers. Any product that may be evaluated in this article or claim that may be made by its manufacturer is not guaranteed or endorsed by the publisher.
Research integrity at Frontiers
Learn more about the work of our research integrity team to safeguard the quality of each article we publish.