- Department of Life Sciences, The National Institute for Biotechnology in the Negev, Ben-Gurion University of the Negev, Beer Sheva, Israel
Cell-non-autonomous signals dictate the functional state of cellular quality control systems, remodeling the ability of cells to cope with stress and maintain protein homeostasis (proteostasis). One highly regulated cell-non-autonomous switch controls proteostatic capacity in Caenorhabditis elegans adulthood. Signals from the reproductive system down-regulate cyto-protective pathways, unless countered by signals reporting on germline proliferation disruption. Here, we utilized dihomo-γ-linolenic acid (DGLA) that depletes the C. elegans germline to ask when cell-non-autonomous signals from the reproductive system determine somatic proteostasis and whether such regulation is reversible. We found that diet supplementation of DGLA resulted in the maintenance of somatic proteostasis after the onset of reproduction. DGLA-dependent proteostasis remodeling was only effective if animals were exposed to DGLA during larval development. A short exposure of 16 h during the second to fourth larval stages was sufficient and required to maintain somatic proteostasis in adulthood but not to extend lifespan. The reproductive system was required for DGLA-dependent remodeling of proteostasis in adulthood, likely via DGLA-dependent disruption of germline stem cells. However, arachidonic acid (AA), a somatic regulator of this pathway that does not require the reproductive system, presented similar regulatory timing. Finally, we showed that DGLA- and AA-supplementation led to activation of the gonadal longevity pathway but presented differential regulatory timing. Proteostasis and stress response regulators, including hsf-1 and daf-16, were only activated if exposed to DGLA and AA during development, while other gonadal longevity factors did not show this regulatory timing. We propose that C. elegans determines its proteostatic fate during development and is committed to either reproduction, and thus present restricted proteostasis, or survival, and thus present robust proteostasis. Given the critical role of proteostatic networks in the onset and progression of many aging-related diseases, such a choice could impact susceptibility to protein misfolding diseases later in life.
Introduction
Cellular quality control networks monitor the cellular proteome by scanning for damaged, misfolded or aggregated proteins and repairing or removing these proteins to maintain protein homeostasis (proteostasis) (Bar-Lavan et al., 2016a; Bett, 2016; Jackson and Hewitt, 2016; McCaffrey and Braakman, 2016; Voos et al., 2016). When proteostasis is breached, cells can activate stress response pathways that induce the expression of various quality control machineries to counter the flux of damaged proteins and restore proteostasis (Morimoto, 2011; Walter and Ron, 2011; Dubnikov et al., 2017; Fiorese and Haynes, 2017). However, recent studies have demonstrated that cell-non-autonomous signals can regulate these responses and inhibit or induce stress response pathways, overriding cellular proteostasis regulation and modulating the onset of protein aggregation and toxicity in models of polyglutamine (polyQ) diseases (Schinzel and Dillin, 2015; Bar-Lavan et al., 2016b; O’Brien and van Oosten-Hawle, 2016; Shemesh and Ben-Zvi, 2016). Understanding how cellular proteostasis is regulated cell-non-autonomously could thus offer novel interventions for the treatment of protein misfolding diseases.
In Caenorhabditis elegans, inefficient stress response activation and reduced protein folding capacity during aging are regulated cell-non-autonomously within a highly specific timeframe, beginning a few hours after the onset of reproduction. Both the basal and induced proteostatic machineries show a sharp functional decline in various somatic cells. This, in turn, results in a reduced ability of cells to protect themselves from acute stresses and chronic challenges associated with aggregation-prone proteins (Vilchez et al., 2012; Shemesh et al., 2013; Yerbury et al., 2016). For stress-induced genes, this collapse is regulated, at least in part, by a remodeling of chromatin accessibility, mediated by signals from the reproductive system (Lapierre et al., 2011; Vilchez et al., 2012; Shemesh et al., 2013; Labbadia and Morimoto, 2015b; Merkwirth et al., 2016).
Signals from the reproductive system are known to regulate organismal metabolism, stress resistance and lifespan (Hsin and Kenyon, 1999; Arantes-Oliveira et al., 2002; Flatt et al., 2008; Mason et al., 2009; Antebi, 2012; Yunger et al., 2017). Specifically, the gonadal longevity pathway mediates hormonal regulation from the somatic gonad in response to the proliferative state of the germline in worms and flies (Antebi, 2012; Amrit and Ghazi, 2017). Proliferation of germline stem cells (GSCs) results in inhibited activity of steroid hormone signaling that causes a switch from the reproductive to the survival mode at the organismal level (Wang et al., 2008; McCormick et al., 2012; Shemesh et al., 2013; Labbadia and Morimoto, 2015a). Moreover, inhibition of GSC proliferation induces transcriptional reprograming, activating a wide range of transcription factors that induce the expression of genes associated with detoxification, the quality control machinery and lipid metabolism (Hsin and Kenyon, 1999; Wang et al., 2008; O’Rourke et al., 2009; Goudeau et al., 2011; Lapierre et al., 2011, 2013; McCormick et al., 2012; Shemesh et al., 2013; Ratnappan et al., 2014; Steinbaugh et al., 2015; Amrit and Ghazi, 2017). This, in turn, negates proteostatic remodeling at the onset of reproduction (Lapierre et al., 2011; Vilchez et al., 2012; Shemesh et al., 2013; Shai et al., 2014; Labbadia and Morimoto, 2015a).
Germline stem cell-dependent lifespan extension is associated with increased production of polyunsaturated fatty acids (PUFAs), such as the ω-6 fatty acids dihomo-γ-linolenic acid (DGLA) and arachidonic acid (AA) (O’Rourke et al., 2013; Folick et al., 2015). Moreover, diet supplementation of DGLA or AA extends C. elegans lifespan and induces autophagy in both C. elegans and tissue culture models (O’Rourke et al., 2013). Given that DGLA and AA are precursors for many signaling molecules (Vrablik and Watts, 2013), these PUFAs could play a role in mediating the transfer of gonadal longevity signals to the soma. Indeed, diet supplementation of AA uncouples somatic proteostasis from the reproductive system, mimicking the proteostatic benefits of GSC arrest without negatively impacting reproduction (Shemesh et al., 2017).
DGLA-derived eicosanoid signals were recently shown to induce sterility by triggering germline depletion during development or adulthood (Watts and Browse, 2006; Webster et al., 2013; Deline et al., 2015). Here, we asked whether DGLA effectively disrupts the germline to activate the gonadal longevity pathway and could be used as a conditional regulator of this pathway and thus, of proteostasis. We found that DGLA diet supplementation resulted in the maintenance of somatic proteostasis past the onset of reproduction in such a manner that was fully dependent on the reproductive system and the gonadal signaling pathway. However, the impact of DGLA on proteostasis and stress response pathways in adulthood was set at a specific time window during development, with only a short exposure (16 h) during the second to fourth larval-stages being required and sufficient for modulating somatic proteostasis in adulthood. This regulation point is shared by the reproductive system and the soma, as a short exposure to AA during the second to fourth larval-stages was also sufficient and required to modulate proteostasis and stress activation of gonad-less animals, independent of the reproductive system. Yet, this timing is not sufficient to activate full transcriptional reprograming of the gonadal longevity pathway and thus, full lifespan extension. We, therefore, suggest that animals are committed to restricted or robust proteostatic states during development and that the choice between reproduction or survival at this point specifically determines proteostatic capacity and hence, susceptibility to protein misfolding diseases later in life.
Results
Dietary Supplementation of DGLA during Development Resulted in Reduced Toxicity in PolyQ-Disease Models
Proteostasis remodeling is thought to aggravate aging-associated protein misfolding diseases. Accordingly, we first asked whether DGLA supplementation could rescue protein toxicity in C. elegans models of polyQ-diseases. For this, embryos expressing 35 glutamine-repeats fused to a fluorescent protein in body wall muscle (Q35m) or 40 glutamine-repeats fused to a fluorescent protein in neurons (Q40n) were transferred to control or DGLA-supplemented plates. Animals were maintained on these plates throughout the experiment and toxicity was examined during adulthood. DGLA supplementation reduced Q35m-associated age-dependent paralysis. By day 6 of adulthood, 63 ± 7% of the animals grown on control plates were paralyzed, as compared to 27 ± 4% of the Q35m animals grown on DGLA-supplemented plates (Figure 1A). However, only a mild effect of DGLA supplementation on the number of Q35m foci was observed (Supplementary Figure S1). Given that toxicity is not always associated with reduced aggregation and that some aggregates are protective (Cohen et al., 2006; Silva et al., 2011), this could be expected. DGLA supplementation also abrogated Q40n-associated age-dependent paralysis. While 49 ± 4% of the Q40n animals grown on control plates were paralyzed by day 6 of adulthood, only 10 ± 1% of the Q40n animals grown on DGLA-supplemented plates were paralyzed (Figure 1B). Thus, DGLA supplementation reduced the toxicity associated with muscular and neuronal models of polyQ-diseases in C. elegans.
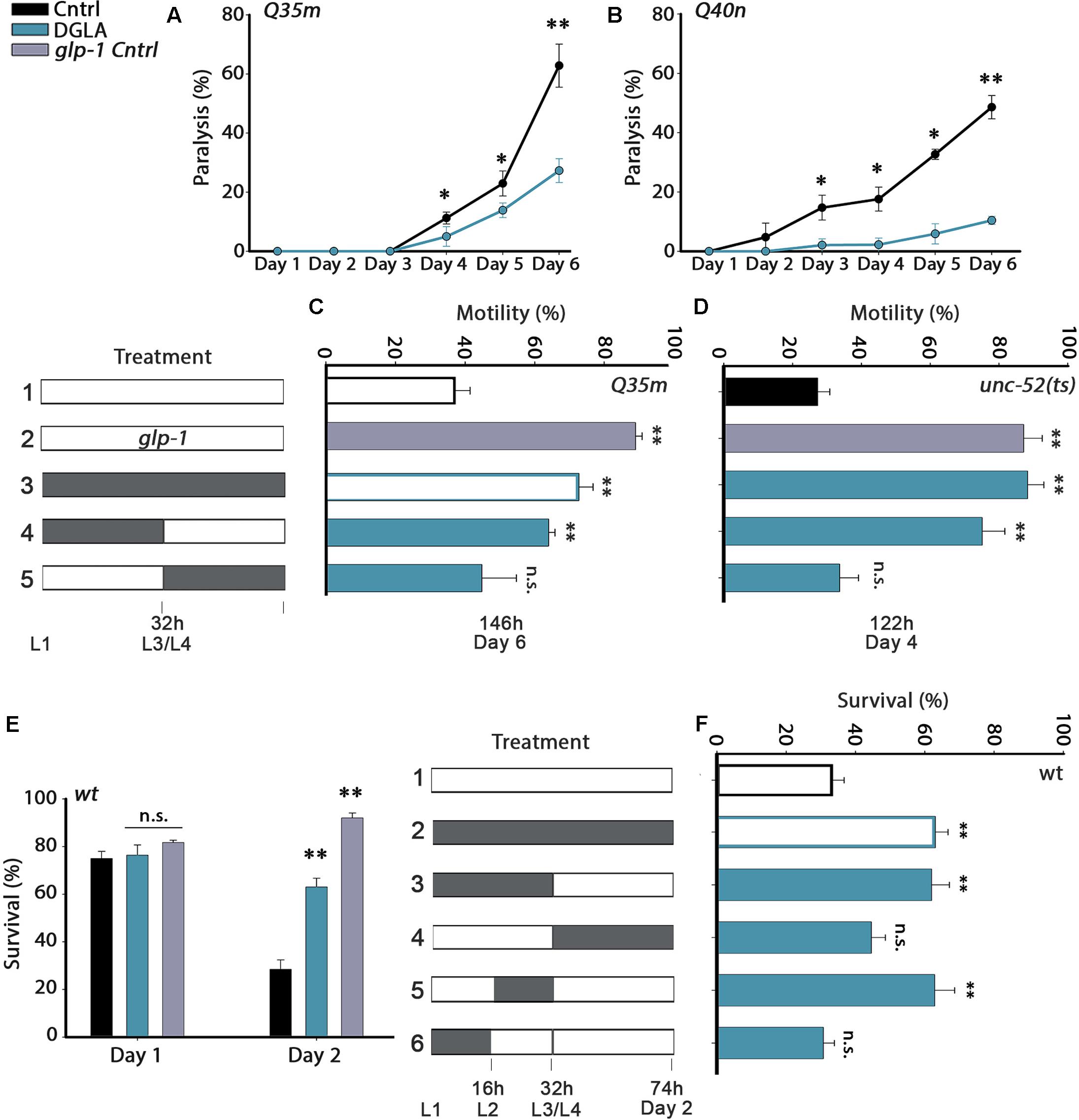
FIGURE 1. Diet supplementation of dihomo-γ-linolenic acid (DGLA) during development modulates proteostasis in adulthood. (A,B) Motility was scored in age-synchronized animals grown on control or DGLA-supplemented plates by determining the percentage of paralyzed (A) Q35m animals (>120 animals per data point, N > 5) or (B) Q40n animals (>45 animals per data point, N = 3) at the indicated times. (C) Motility was scored in age-synchronized Q35m or glp-1;Q35m animals, treated as indicated, by determining the percentage of motile animals (>45 animals per treatment, N > 3). (D) Motility was scored in age-synchronized unc-52(ts) or glp-1;unc-52(ts) animals, treated as indicated, by determining the percentage of motile animals on day 4 of adulthood (>60 animals per treatment, N > 4). (E) Thermo-resistance was examined in age-synchronized wild type or glp-1 animals grown on control or DGLA-supplemented plates. Animals were subjected to heat shock (37°C, 6 h) at the indicated times and survival was assayed (>110 animals per treatment, N > 4). (F) Thermo-resistance was examined in age-synchronized wild type animals, treated as indicated. Animals were subjected to heat shock (37°C, 6 h) on day 2 of adulthood and survival was assayed (>120 animals per treatment, N > 7). Data were compared to age-matched animals grown on control plates. (n.s.) denotes non-significant, ∗P < 0.05, ∗∗P < 0.01.
Proteostasis remodeling occurs between days 1 and 2 of adulthood, at the onset of reproduction (Shemesh et al., 2013). We, therefore, asked when diet supplementation of DGLA is required, i.e., during development or adulthood, to rescue polyQ toxicity. As such, we shifted animals from DGLA-supplemented plates to control plates or from control to DGLA-supplemented plates at the transition to the fourth larval stage (L3/L4) and examined proteostatic functions later in adulthood. Q35m animals were grown on plates supplemented with DGLA during development (0–32 h) and then shifted to control plates for the reminder of the experiment (until day 6 of adulthood), when motility was examined. Similar to animals grown continuously on DGLA, most animals exposed to DGLA only during development were motile on day 6 of adulthood (73 ± 4% and 64 ± 2%, respectively; Figure 1C). DGLA-dependent rescue of motility was comparable to germline-less glp-1(e2141) mutant animals crossed to Q35m (Q35m; glp-1; 89 ± 2%). In contrast, when animals were exposed to DGLA starting from the L3/L4 transition (>32 h), motility did not improve (even after 6 days on DGLA). Only 45 ± 10% of the animals was motile, similar to animals grown continuously on control plates (37 ± 4%; Figure 1C). Thus, DGLA supplementation only during development was sufficient and required to reduce polyQ-associated toxicity in C. elegans.
Dietary Supplementation of DGLA during Development Led to Proteostasis Rescue in Adulthood But Had No Effect on Lifespan
To extend our analysis to basal proteostatic capacity, we next asked whether DGLA supplementation during development could rescue the function of unc-52(ts), an established folding reporter that shows an age-dependent decline in motility (stiff body paralysis) under permissive conditions (Ben-Zvi et al., 2009). We monitored unc-52(ts)-dependent paralysis on day 4 of adulthood, by which point only 27 ± 4% of the animals grown on control plates were motile. DGLA diet supplementation rescued unc-52(ts)-dependent paralysis (93 ± 2.5% motile animals), similar to unc-52(ts) animals crossed to glp-1(e2141) (unc-52(ts);glp-1; 88 ± 5% motile animals, Figure 1D). Moreover, motility was improved to a similar extent when unc-52(ts) animals were grown on DGLA-supplemented plates only during development (0–32 h; 79 ± 7%). Motility of unc-52(ts) animals shifted to DGLA-supplemented plates after the L3/L4 transition showed no improvement and was similar to animals grown continuously on control plates (34 ± 6%; Figure 1D). Thus, exposure to DGLA only during development was also sufficient and required to modulate basal proteostasis in adulthood.
We next examined whether dietary supplementation of DGLA could abrogate the decline in heat shock survival rates at the transition to reproductive adulthood. While survival rates of wild type young adults grown on control plates declined sharply between days 1 and 2 of adulthood (75 ± 3% and 28 ± 4%, respectively), survival rates of days 1 and 2 adults grown on DGLA-supplemented plates did not decline (76 ± 4% and 69 ± 2%, respectively; Figure 1E), similar to glp-1(e2141) animals (82 ± 1% and 92 ± 3%, Figure 1E). We then asked whether heat shock survival rates of animals grown on DGLA only during development or adulthood are comparable to the survival of animals grown on DGLA-supplemented plates over the entire duration of the experiment. Exposure to DGLA only during development (0–32 h) was as efficient as constant exposure to DGLA for rescuing heat shock survival rates of adult animals on day 2 of adulthood (63 ± 4%; Figure 1F). In contrast, transfer to DGLA-supplemented plates at the L3/L4 stage did not significantly rescue heat shock survival rates (44 ± 4%; Figure 1F). We then further restricted the time on DGLA-supplemented plates to either the first 16 h period (L1–L2) or second 16 h period (L2–L4) of the 32 h window. A short exposure to DGLA during L2–L4 but not during the L1–L2 stages was sufficient to maintain high heat shock survival rates on day 2 of adulthood (63 ± 6% and 31 ± 3%, respectively; Figure 1F). Thus, a short exposure to DGLA during larval development was sufficient and required to modulate heat shock survival in adulthood.
Finally, we asked whether a short exposure to DGLA during development (0–32 h) would also be sufficient to extend the lifespan of wild type animals, similar to glp-1(e2141) animals. Wild type animals were either exposed to DGLA during development (0–32 h) or during adulthood (transferred to DGLA after 32 h on control plates) and lifespan was compared to wild type animals maintained continuously on control or DGLA-supplemented plates at 25°C. While continuous exposure to DGLA resulted in ∼15% lifespan extension, a short exposure during development (0–32 h) or exposure to DGLA only during adulthood (starting from the L4 transition stage) resulted in a more moderate effect on lifespan (∼5–10%; Supplementary Figure S2 and Table S1). Thus, exposure to DGLA during development was not sufficient or required for DGLA to impact lifespan, supporting the hypothesis that proteostasis regulation is not necessarily associated with lifespan extension (Cohen et al., 2010; El-Ami et al., 2014).
DGLA-Dependent Remodeling of Proteostasis Requires the Gonad
Dietary supplementation of DGLA during development resulted in proteostasis remodeling (Figure 1). Because GSC arrest is associated with increased production of DGLA and DGLA-derived eicosanoids induce GSC depletion, we subsequently asked whether DGLA required the reproductive system to elicit this effect. For this, we considered whether dietary supplementation of DGLA affected the proteostasis of gon-2(9388) (gon-2) mutant animals lacking the entire reproductive system. We first crossed gon-2 animals to Q40n (gon-2;Q40n) or unc-52(ts) (gon-2;unc-52) animals and compared their motility after continuous growth on control or DGLA-supplemented plates (Q35m animals could not be tested as the array was integrated close to the gon-2 gene). While DGLA supplementation resulted in improved motility of Q40n animals (Figure 1B), the percent of motile gon-2;Q40n animals was not significantly different between animals grown on control or DGLA-supplemented plates (Figure 2A). Similarly, the percent of motile gon-2;unc-52 animals on control or DGLA-supplemented plates was not significantly increased (17 ± 4% and 25 ± 4%, respectively; Figure 2B). Finally, DGLA supplementation improved wild type heat shock survival rates (Figure 1E) but could not improve survival rates of gon-2 mutant animals when grown on control or DGLA-supplemented plates (37 ± 7% and 30 ± 6%, respectively; Figure 2C). As expected, supplementation of DGLA only during development (0–32 h) was as ineffective as continuous supplementation in rescuing heat shock survival rates of gon-2(ts) (36 ± 12% and 37 ± 4%, respectively, Supplementary Figure S3). Thus, DGLA-dependent proteostasis remodeling requires the somatic gonad.
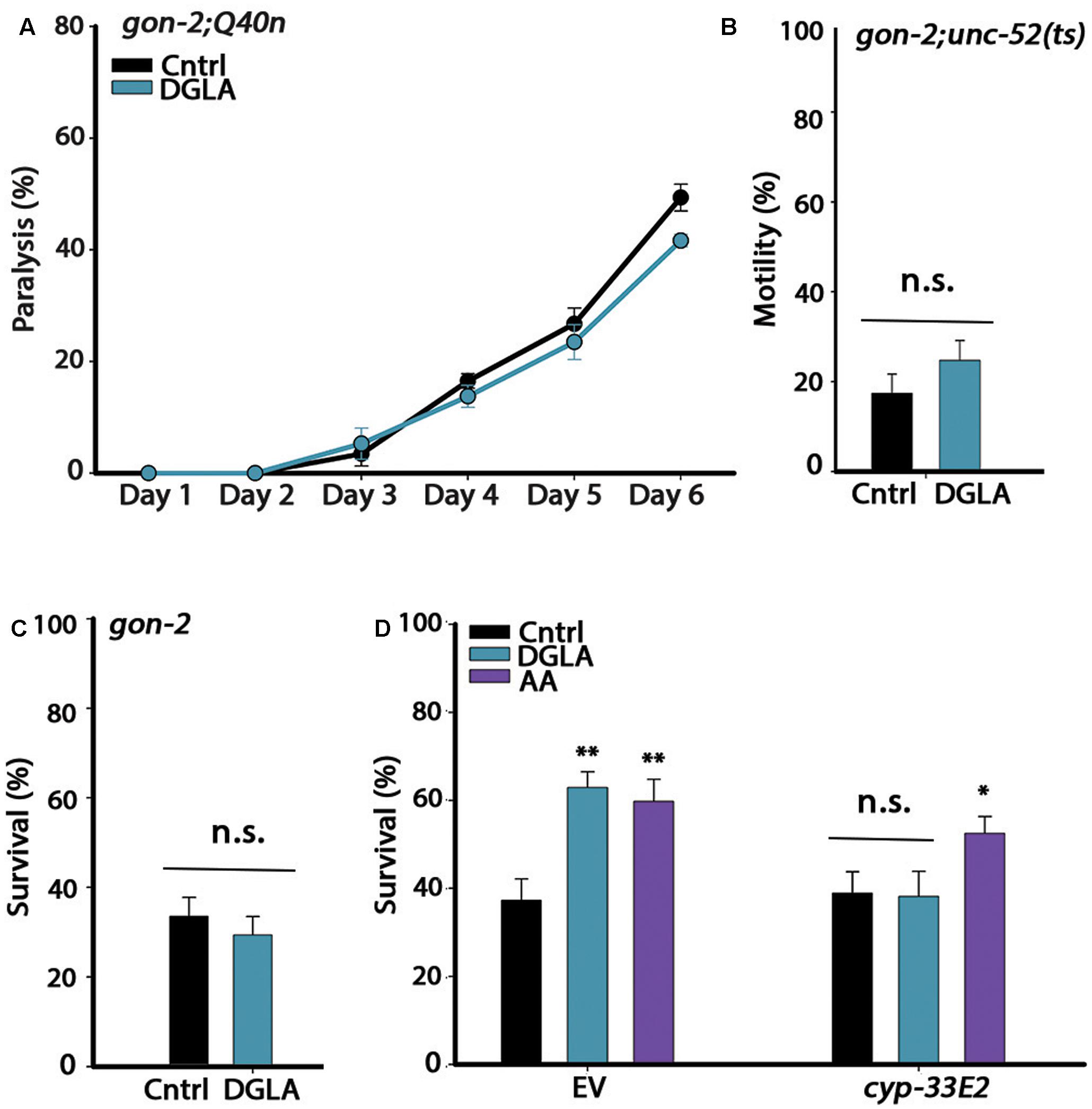
FIGURE 2. The gonad is required for DGLA-dependent proteostasis remodeling in adulthood. (A) Motility was scored in age-synchronized gon-2;Q40n animals grown on control or DGLA-supplemented plates by determining the percentages of paralyzed animals at the indicated times (>45 animals per data point, N > 3). (B) Motility was scored in age-synchronized gon-2;unc-52(ts) animals grown on control or DGLA-supplemented plates by scoring for stiff-body paralysis on day 4 of adulthood (>65 animals per data point, N > 5). (C) Thermo-resistance was examined in age-synchronized gon-2(q388ts) animals grown on control or DGLA-supplemented plates. Animals were subjected to a prolonged heat shock treatment (37°C, 6 h) on day 2 of adulthood and survival was assayed (>65 animals per data point, N > 5). (D) Thermo-resistance was examined in age-synchronized wild type animals grown on control, or DGLA- or AA-supplemented RNAi plates. Animals treated with empty vector (EV) or cyp-32E2 RNAi, were subjected to heat shock (37°C, 6 h) on day 3 of adulthood and survival was assayed (>80 animals per data point, N > 5). Data were compared to age-matched animals grown on control plates. (n.s.) denotes non-significant, ∗P < 0.05, ∗∗P < 0.01.
Loss, reduced numbers or disruption of GSCs and sterility were observed in animals supplemented with DGLA either intermittently (during development or adulthood) or continuously (Watts and Browse, 2006) (Supplementary Figure S4). Given that even a short disruption to GSC proliferation during development or adulthood extends lifespan and induces GSC-dependent transcription (Arantes-Oliveira et al., 2002; Wang et al., 2008), we considered that the impact of DGLA on proteostasis could be mediated by GSC disruption. Specifically, the cytochrome P450 monooxygenase CYP-32E2 that is expressed in the pharynx was shown to produce various DGLA-derived eicosanoids that act in the gonad to induce germline defects and sterility (Deline et al., 2015). As such, we next examined whether RNAi knockdown of cyp-33E2, known to inhibit DGLA-dependent sterility (Deline et al., 2015), could affect DGLA-mediated rescue of heat shock survival rates. While animals grown on empty vector (EV)-containing control bacteria and DGLA-supplemented plates were able to survive heat shock treatment on day 3 of adulthood, the survival of animals grown on cyp-33E2(RNAi) bacteria supplemented with DGLA was similar to that of animals grown on control plates (38 ± 6% and 39 ± 6%, respectively; Figure 2D). The gonadal longevity pathway also elevates AA levels (O’Rourke et al., 2013; Folick et al., 2015). AA modulates proteostasis of gonad-less animals but does not affect GSC proliferation or reproduction (Shemesh et al., 2017). In agreement, survival of animals grown on cyp-33E2(RNAi) bacteria supplemented with AA was similar to their survival on EV control plates (53 ± 4% and 60 ± 5%, respectively; Figure 2D). cyp-33E2-mediated sterility is, therefore, specifically required for DGLA-mediated protective effects in the soma. This was supported by DGLA supplementation to germline-less glp-1(e2141) or glp-4(bn2) mutant animals that did not further improve (and even slightly reduced) heat shock survival rates on day 2 of adulthood, as compared to the survival rates of wild type animals supplemented with DGLA (Supplementary Figure S5). Thus, local DGLA-derived eicosanoids that act specifically in the gonad and disrupt GSC proliferation (Deline et al., 2015) could potentially activate the gonadal-longevity signaling pathway.
AA Supplementation during Development Led to Remodeling of Proteostasis in Gonad-Less Animals
The timing of DGLA requirement could simply depend on the ability of DGLA to eliminate GSCs. Although, under our conditions, DGLA supplementation only induced partial sterility and reduced brood size (Supplementary Figure S4), addition of DGLA at the L2–L4 larval stages is most effective to eliminate GSCs (Watts and Browse, 2006). As noted above, AA also modulates proteostasis in adulthood, in a manner independent of the reproductive system (Shemesh et al., 2017). To examine whether the L2–L4 window is associated with GSC disruption, we next examined the timing of AA requirement for proteostasis remodeling in wild type or gonad-less animals. AA diet supplementation rescued gon-2;Q40n-dependent paralysis (89 ± 1.5% motile animals by day 6 of adulthood) and motility was improved to a similar extent when gon-2;Q40n animals were grown on AA-supplemented plates only during development (0–32 h; 94 ± 2%; Figure 3A). In contrast, motility of gon-2;Q40n animals shifted to AA-supplemented plates after the L3/L4 transition was similar to animals grown continuously on control plates (61 ± 3% and 53 ± 3%, respectively; Figure 3A). AA supplementation to gon-2 animals only during development (0–32 h), specifically in the 16–32 h window, was also sufficient to modulate heat shock survival rates in adulthood (55 ± 5% and 55 ± 4%, respectively; Figure 3B), similar to the wild type (50 ± 5% and 49 ± 5%, respectively; Supplementary Figure S6). In contrast, AA supplementation starting from L3/L4 transition of either wild type or gon-2 animals had no effect on survival (35 ± 2% and 35 ± 6%, respectively; Figure 3B and Supplementary Figure S6). Thus, our data demonstrate that the L2–L4 period is critical for determining proteostatic capacity during adulthood even in gonad-less animals, suggesting that this period is detrimental to proteostasis in adulthood of both the soma and the reproductive system. However, this timing was not required for lifespan extension, similar to DGLA (Supplementary Table S1).
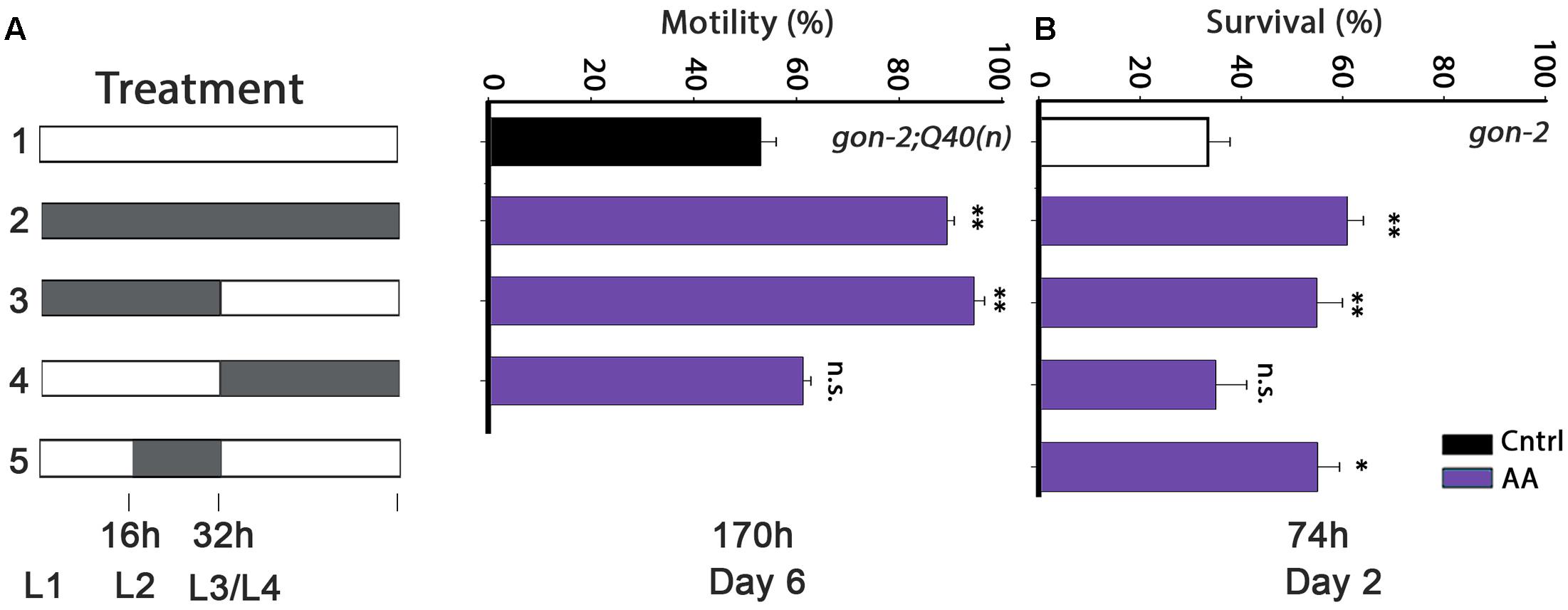
FIGURE 3. Diet supplementation of arachidonic acid (AA) during development modulates proteostasis of gonad-less animals in adulthood. (A) Motility was scored in age-synchronized gon-2:Q40n animals, treated as indicated, by determining the percentage of motile animals (>60 animals per treatment, N > 4). (B) Thermo-resistance was examined in age-synchronized gon-2(q388ts) animals, treated as indicated. Animals were subjected to a prolonged heat shock treatment (37°C, 6 h) on day 2 of adulthood and survival was assayed (>65 animals per data point, N > 5). Data were compared to age-matched animals grown on control plates. (n.s.) denotes non-significant, ∗P < 0.05, ∗∗P < 0.01.
DGLA- and AA-Supplementation Led to Remodeling of the Heat Shock Response Switch at the Onset of Reproduction
Having established that the L2–L4 period is critical for proteostasis remodeling but not for lifespan extension, we next asked whether DGLA and AA supplementation resulted in the activation of the different stress and metabolic transcription factors associated with the gonadal longevity pathway and if so, when. We first examined whether DGLA and AA supplementation remodeled the heat shock response switch regulated by the gonadal longevity pathway at the onset of reproduction. Exposing animals to stress even 6 h after the onset of reproduction results in limited induction of cyto-protective heat shock genes required to confer resistance to the stress. The decline in response activation is associated with chromatin remodeling and is reflected in reduced levels of heat shock gene induction (Shemesh et al., 2013; Labbadia and Morimoto, 2015b). To examine whether DGLA and AA supplementation led to modulation of this switch, as in the gonadal longevity pathway, the induction of heat shock genes was examined. For this, hsp-16.2-regulated green fluorescent protein (GFP) that served as a reporter expressed in different somatic tissues upon heat shock was monitored. GFP-regulated expression was not significantly different between days 1 and 3 of adulthood in animals grown on DGLA-supplemented plates (68 ± 9% and 79 ± 7%, respectively) or AA-supplemented plates (71 ± 6% and 59 ± 4%, respectively) but declined sharply in control-treated animals (68 ± 4% and 36 ± 7%, respectively; Figures 4A,B). Likewise, hsp-70 and F44E5.4 induction, examined using qPCR, was two–four fold lower on day 2, as compared to day 1 adults grown on control plates, yet remained high when animals were grown on DGLA-supplemented plates (Supplementary Figure S7). Thus, dietary supplementation of DGLA or AA can negate the decline in heat shock activation at the onset of reproduction.
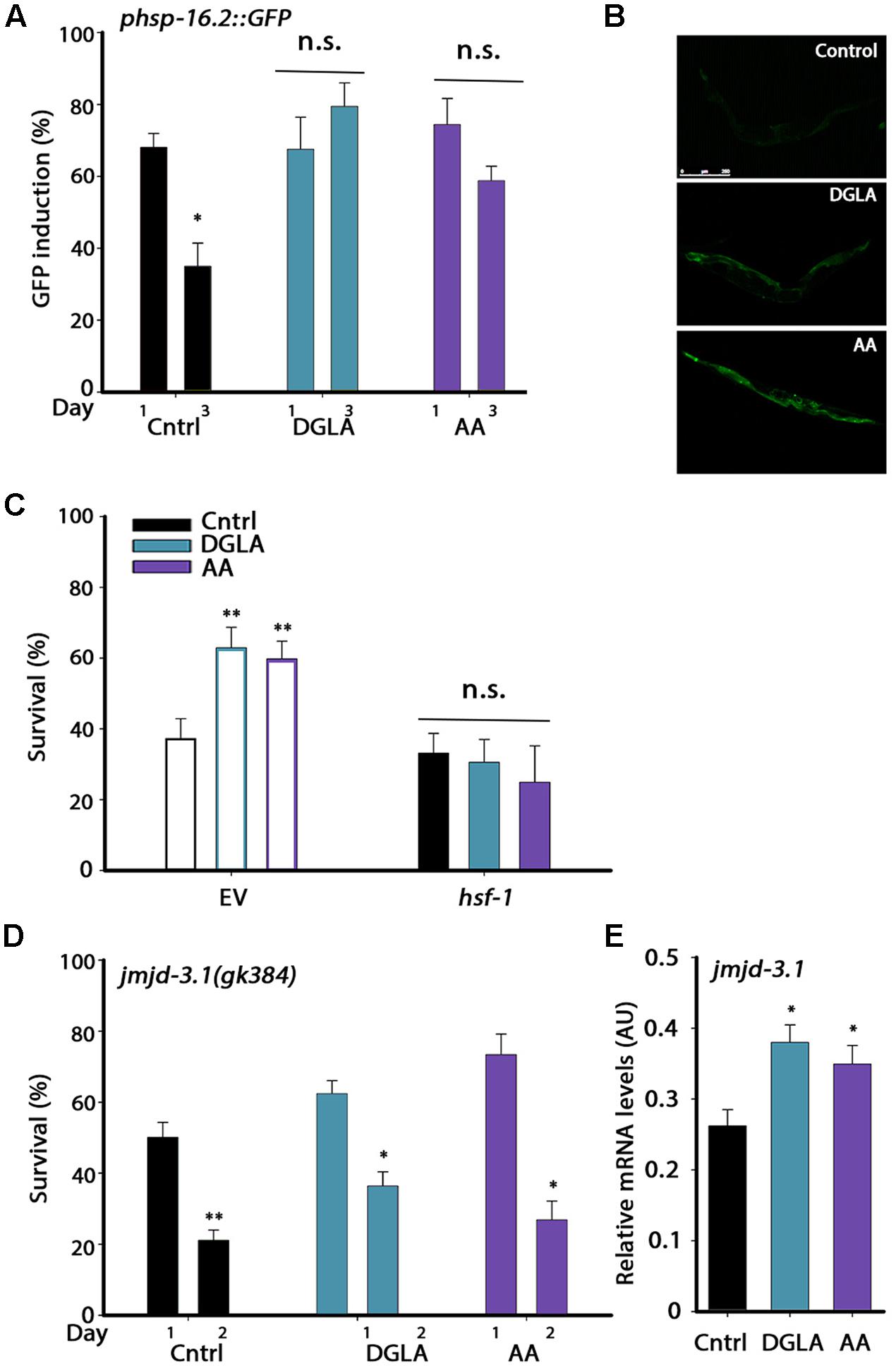
FIGURE 4. DGLA and AA supplementation results in remodeling of the heat shock response switch in adulthood. (A) Heat shock gene induction was examined in age-synchronized animals expressing phsp-16.2::GFP grown on control, DGLA- or AA-supplemented plates. Animals were subjected to heat shock (37°C, 90 min) at the indicated times and fluorescence was monitored after a 24 h recovery period. Animals expressing GFP in somatic tissues (other than the pharynx) were scored (>60 animals per treatment, N > 3). (B) Representative images of day 3 adult animals expressing phsp-16.2::GFP grown on control, or DGLA- or AA-supplemented plates as in A. (C) Thermo-resistance was examined in age-synchronized wild type animals grown on control, or DGLA- or AA-supplemented RNAi plates. Animals treated with EV or hsf-1 RNAi were subjected to heat shock (37°C, 6 h) on day 3 of adulthood and survival was examined (>70 animals per treatment, N > 4). (D) Thermo-resistance was examined in age-synchronized jmjd-3.1(gk384) animals grown on control, or DGLA- or AA-supplemented plates. Animals were subjected to heat shock treatment (37°C, 6 h) at the indicted times and survival was examined (>130 animals per treatment, N > 5). (E) Quantification of jmjd-3.1 mRNA levels from age-synchronized animals grown on control, or DGLA- or AA-supplemented plates (N > 5). For (A,D) data were compared to similarly treated animals on day 1 of adulthood. For (C,E), data were compared to age-matched animals grown on control plates. (n.s.) denotes non-significant, ∗P < 0.05, ∗∗P < 0.01.
The methyltransferase JMJD-3.1 is required for HSF-1-dependent heat shock response activation and expression of heat shock genes in the soma after reproduction onset. jmjd-3.1 expression is reduced at the transition to reproductive adulthood and its increased expression is associated with GSC-dependent rescue of proteostasis remodeling (Labbadia and Morimoto, 2015b). We, therefore, examined whether HSF-1 and JMJD-3.1 are required for DGLA- and AA-mediated rescue of heat shock survival. Animals were grown on EV- or hsf-1 RNAi-expressing bacteria with or without dietary supplementation of DGLA or AA and heat shock survival was examined on day 3 of adulthood. While ∼60% of day 3 adults grown on EV bacteria supplemented with DGLA or AA survived the stress, growth on hsf-1(RNAi) abolished DGLA- and AA-associated beneficial effects (31 ± 6%, 25 ± 10%, respectively), reducing survival to control levels (33 ± 5%; Figure 4C). HSF-1 is thus required for DGLA- and AA-dependent rescue of heat shock survival. We next examined heat shock survival rates in a jmjd-3.1(gk384) mutant. Mutant jmjd-3.1(gk384) animals grown on plates supplemented with DGLA or AA showed a ∼2-fold decline in survival rates between days 1 and 2 of adulthood (62 ± 4% to 36 ± 4% and 73 ± 6% to 27 ± 5%, respectively), similar to animals grown on control plates (50 ± 4% and 23 ± 3.5%, respectively; Figure 4D). In agreement, jmjd-3.1 levels, examined using qPCR, were significantly higher (∼1.4-fold) in animals grown on DGLA or AA than in animals grown on control plates (Figure 4E). These data suggest that dietary supplementation of DGLA or AA abrogates the decline in heat shock activation in adulthood by remodeling the JMJD-3.1- and HSF-1-regulated switch and thus, the ability to induce heat shock gene expression, similar to the gonadal longevity pathway.
DGLA- and AA-Supplementation Resulted in the Induction of Transcriptional Reprograming Associated with the Gonadal-Longevity Pathway
DGLA and AA levels are elevated by overexpression of lipl-4 that is regulated by the gonadal-longevity signaling pathway and specifically, DAF-16 (O’Rourke et al., 2013; Folick et al., 2015). We thus asked whether the gonadal longevity pathway could, in turn, be activated in DGLA- or AA-supplemented animals. Activation of the gonadal longevity pathway triggers the nuclear localization of DAF-16 in the intestine (Berman and Kenyon, 2006). To examine whether DAF-16 is activated by DGLA or AA supplementation, we monitored the localization of DAF-16 tagged with GFP (DAF-16::GFP). Animals expressing DAF-16::GFP were grown on control, DGLA- or AA-supplemented plates and DAF-16 localization was monitored. While DAF-16::GFP was equally localized in the cytoplasm and nuclei of animals grown on control plates, DGLA or AA supplementation enhanced DAF-16::GFP nuclear localization in the intestine of animals grown on DGLA- or AA-supplemented plates (6+4%, 60 ± 10%, and 31 ± 9%, respectively; Figures 5A,B). DGLA- and AA-dependent changes in DAF-16::GFP localization were associated with increased expression of genes regulated by DAF-16. dod-8 and sod-3 mRNA levels increased ∼2-fold in animals supplemented with DGLA or AA (Figure 5C). Moreover, diet supplementation of DGLA or AA to animals harboring a mutation in the gene encoding DAF-16/FOXO did not increase their heat shock survival (34 ± 8% or 38 ± 8, respectively; Figure 5D). These data suggest that DGLA and AA levels are not only regulated by the gonadal longevity pathway (O’Rourke et al., 2013; Folick et al., 2015) but can also induce DAF-16 nuclear localization and expression associated with activation of this pathway.
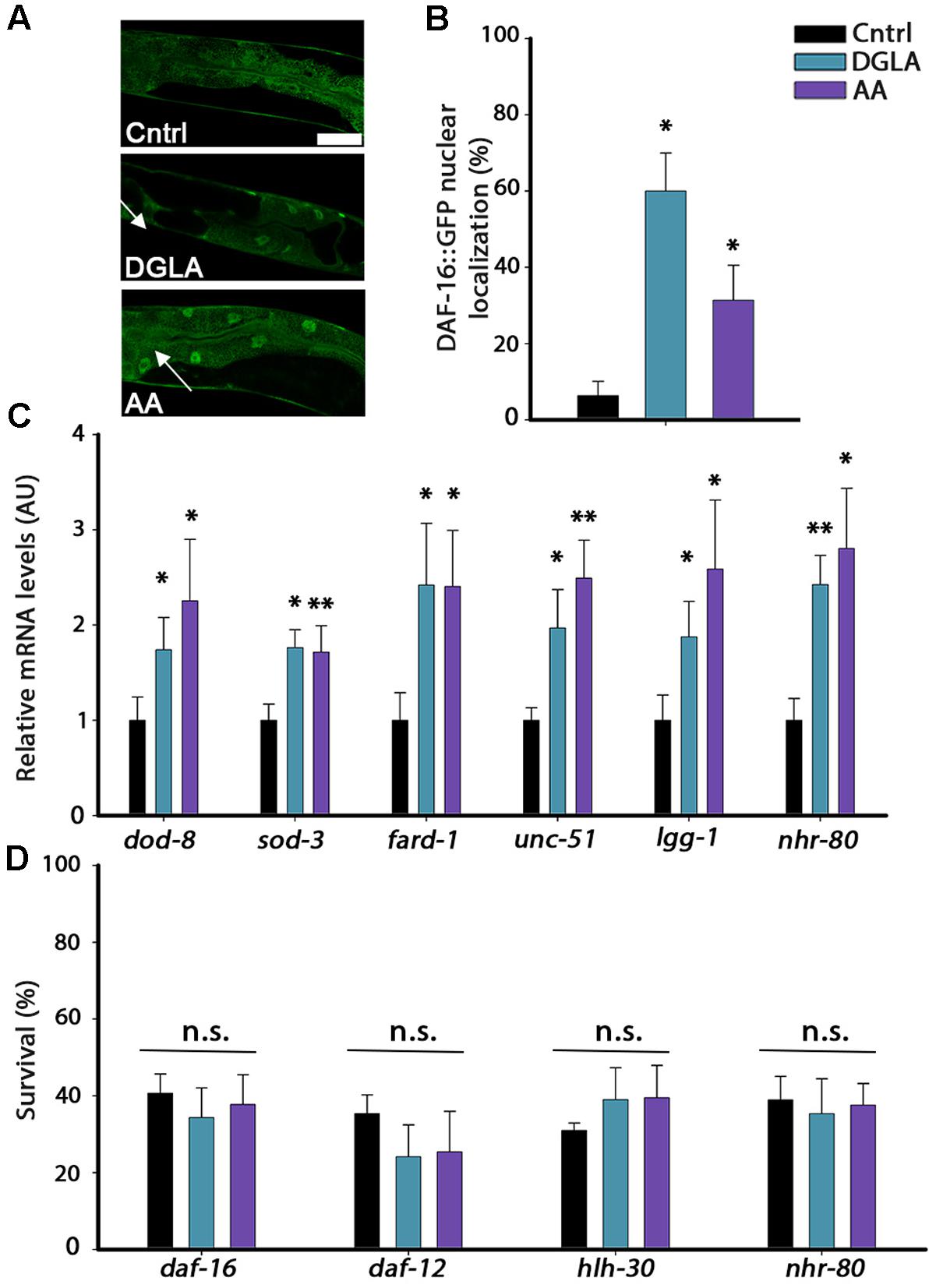
FIGURE 5. DGLA and AA supplementation results in the activation of the gonadal longevity pathway. (A) Representative images of age-synchronized animals expressing DAF-16::GFP grown on control, or DGLA- or AA-supplemented plates. Animals were fixed and imaged on day 2 of adulthood. (B) Age-synchronized DAF-16::GFP animals were grown on control, or DGLA- or AA-supplemented plates, as indicated. Animals were fixed on day 2 of adulthood and the percentage of animals showing nuclear localization of DAF-16::GFP (arrows) was scored (>400 cells per treatment, N > 3). (C) Quantification of mRNA levels of dod-8, sod-1, fard-1, unc-51, lgg-1, or nhr-80 from age-synchronized animals grown on control, or DGLA- or AA-supplemented plates. Data were normalized to those obtained with controls (N > 4). (D) Thermo-resistance was examined in age-synchronized mutant, daf-16, daf-12, hlh-30, or nhr-80 animals, grown on control, or DGLA- or AA-supplemented plates. Animals were subjected to heat shock (37°C, 6 h) on day 2 of adulthood and survival was examined (>65 animals per treatment, N > 3). Data were compared to age-matched animals grown on control plates. (n.s.) denotes non-significant, ∗P < 0.05, ∗∗P < 0.01.
The gonadal longevity pathway is also associated with the activation of other transcription factors, including the steroid nuclear receptor DAF-12 and the lipid metabolism, lipolysis and autophagy transcription regulators PHA-4, HLH-30, and NHR-80 (Amrit and Ghazi, 2017). The mRNA levels of fard-1 regulated by DAF-12, of unc-51 and lgg-1 regulated by PHA-4 and HLH-30 and of nhr-80 regulated by NHR-80 (Goudeau et al., 2011; Lapierre et al., 2011, 2013; McCormick et al., 2012) were, therefore, examined using qPCR. The mRNA levels of these genes were elevated by two–three fold in animals grown on DGLA- or AA-supplemented plates, compared to controls (Figure 5C). In agreement, diet supplementation of DGLA or AA to animals harboring mutations in daf-12, hlh-30, or nhr-80 did not increase their heat shock survival (Figure 5D). Thus, various transcription factors that are regulated by the gonadal longevity pathway are activated by exposure to DGLA or AA and are required for DGLA- or AA-dependent effects on proteostasis remodeling in adulthood. These data suggest the continuous exposure to DGLA and AA activate the gonadal longevity pathway.
DGLA- and AA-Supplementation during Development Is Sufficient to Activate Proteostasis-Associated Pathways
We next asked whether DGLA or AA supplementation during development was sufficient to activate the different factors associated with the gonadal longevity pathway or whether it was specific to only some of factors in this pathway. Accordingly, animals were either grown on DGLA- or AA-supplemented plates during development (until L3/L4 transition) and then shifted to control plates, or first grown on control plates (until L3/L4 transition) and then shifted to DGLA- or AA-supplemented plates. In either growth protocol, gene regulation was examined. First, DAF-16 nuclear localization was examined. DGLA- or AA-supplementation before L3/L4 transition was sufficient to induce DAF-16::GFP nuclear localization in adulthood (34 ± 4% and 55 ± 9%, respectively), while supplementation of DGLA or AA starting at L3/L4 was not (12 ± 12% and 5 ± 5%, respectively; Figure 6A). In agreement, the expression of dod-8 and sod-3 was induced two–three fold by DGLA or AA supplementation before L3/L4 transition but not after (Figure 6B and Supplementary Figure S8). Thus, proteostasis regulation pathways linked to the gonadal longevity pathway were specifically activated early in a development.
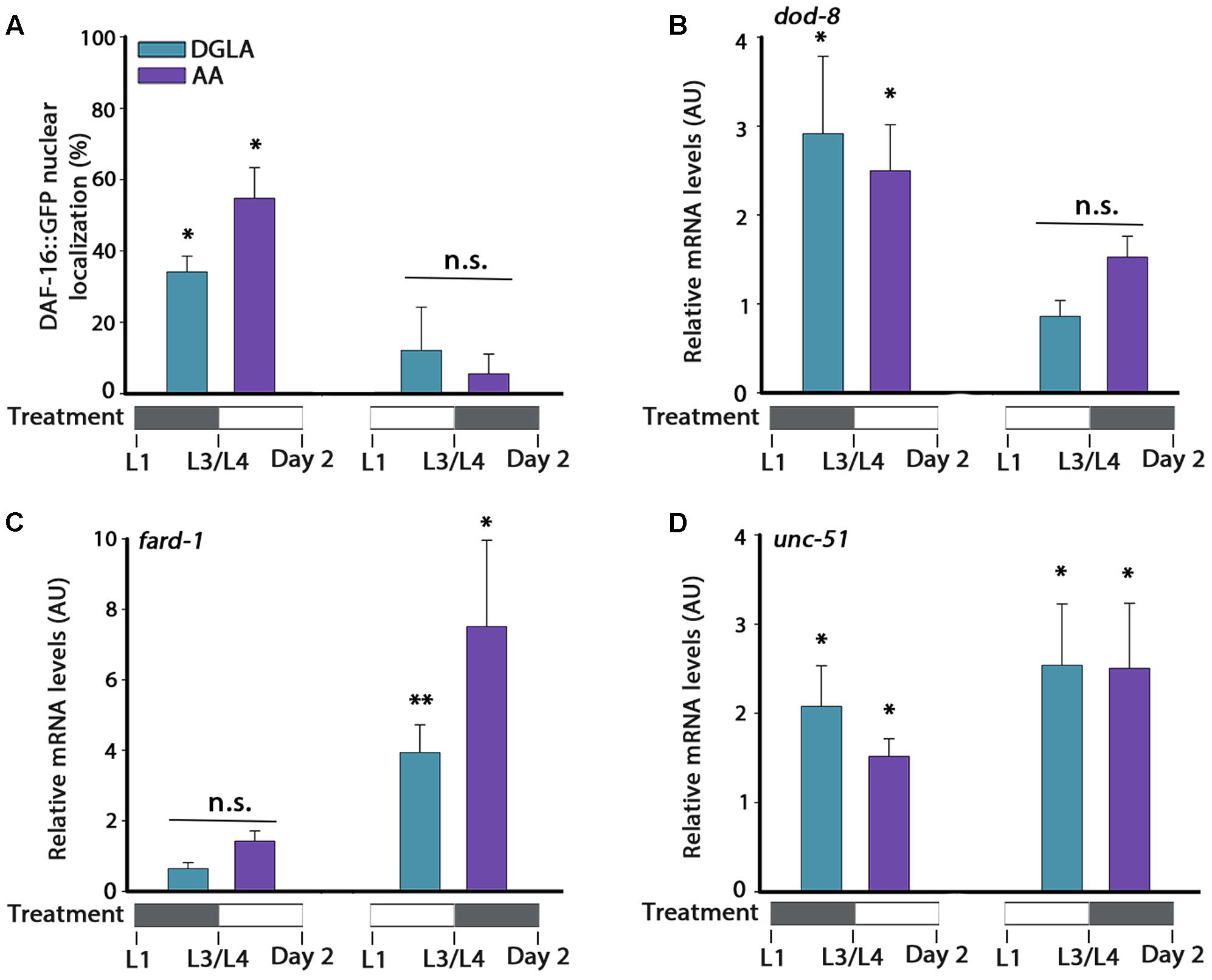
FIGURE 6. DGLA and AA supplementation during development results in partial activation of the gonadal longevity pathway. (A) Age-synchronized DAF-16::GFP animals, treated as indicated, were fixed on day 2 of adulthood and the percentage of animals showing nuclear localization of DAF-16::GFP was scored (>400 cells per treatment, N > 3). (B–D) Quantification of dod-8 (B), fard-1 (C), or unc-51 (D) mRNA levels from age-synchronized animals, treated as indicated. Data were normalized to those obtained with controls (N > 4). Data were compared to age-matched animals grown on control plates. (n.s.) denotes non-significant, ∗P < 0.05, ∗∗P < 0.01.
The expression of genes regulated by other gonadal longevity pathways, such as daf-12, hlh-30, and nhr-80, showed different regulation behavior in time (Figures 6C,D and Supplementary Figure S8). Whereas expression levels of fard-1 were not significantly induced by supplementation of DGLA or AA during development, they were induced by DGLA or AA supplementation after L3/L4 transition. On the other hand, expression levels of unc-51 were induced regardless of the timing of DGLA or AA supplementation (Figures 6C,D). These data suggest that early signals can determine somatic proteostatic capacity in adulthood, although the timing of DGLA or AA supplementation differs for other factors in the gonadal longevity pathway. We, therefore, propose that the L2–L4 period is specific for proteostasis remodeling in adulthood but that other pathways, and thus lifespan, could be differentially regulated.
Discussion
Proteostasis remodeling at the onset of C. elegans reproduction is a highly regulated switch, activated by signals from the reproductive system that result in the inability of somatic tissues to maintain proteostasis (Figure 7A). GSC arrest mitigates this response, activating the gonadal longevity pathway and maintaining juvenile proteostasis (Figure 7B). To understand when the fate of proteostasis is set and whether it is reversible, we employed DGLA, known to disrupt GSCs (Watts and Browse, 2006; Deline et al., 2015) and associated with extended lifespan (O’Rourke et al., 2013; Folick et al., 2015), as a conditional agent to remodel proteostasis. We found that diet supplementation of DGLA rescued the maintenance of proteostasis past the onset of reproduction only if supplemented during development. This rescue required the gonad and different effectors in the gonadal longevity pathway. Specifically, for the heat shock response, this rescue required DAF-16, HSF-1, and JMJD-3.1, suggesting that DGLA is a modulator of the proteostatic switch early in adulthood (Figure 7C). Although AA rescued proteostatic function in the soma, like DGLA, it was only effective when applied during development so as to rescue proteostasis in adulthood (Figure 7D). We, therefore, propose that proteostasis in adulthood is set during development. This regulation timing, however, was specific for proteostasis and stress response pathways. Other transcription factors that are regulated by the gonadal longevity signaling, such as metabolic and lipolysis regulators, were not specifically activated during development.
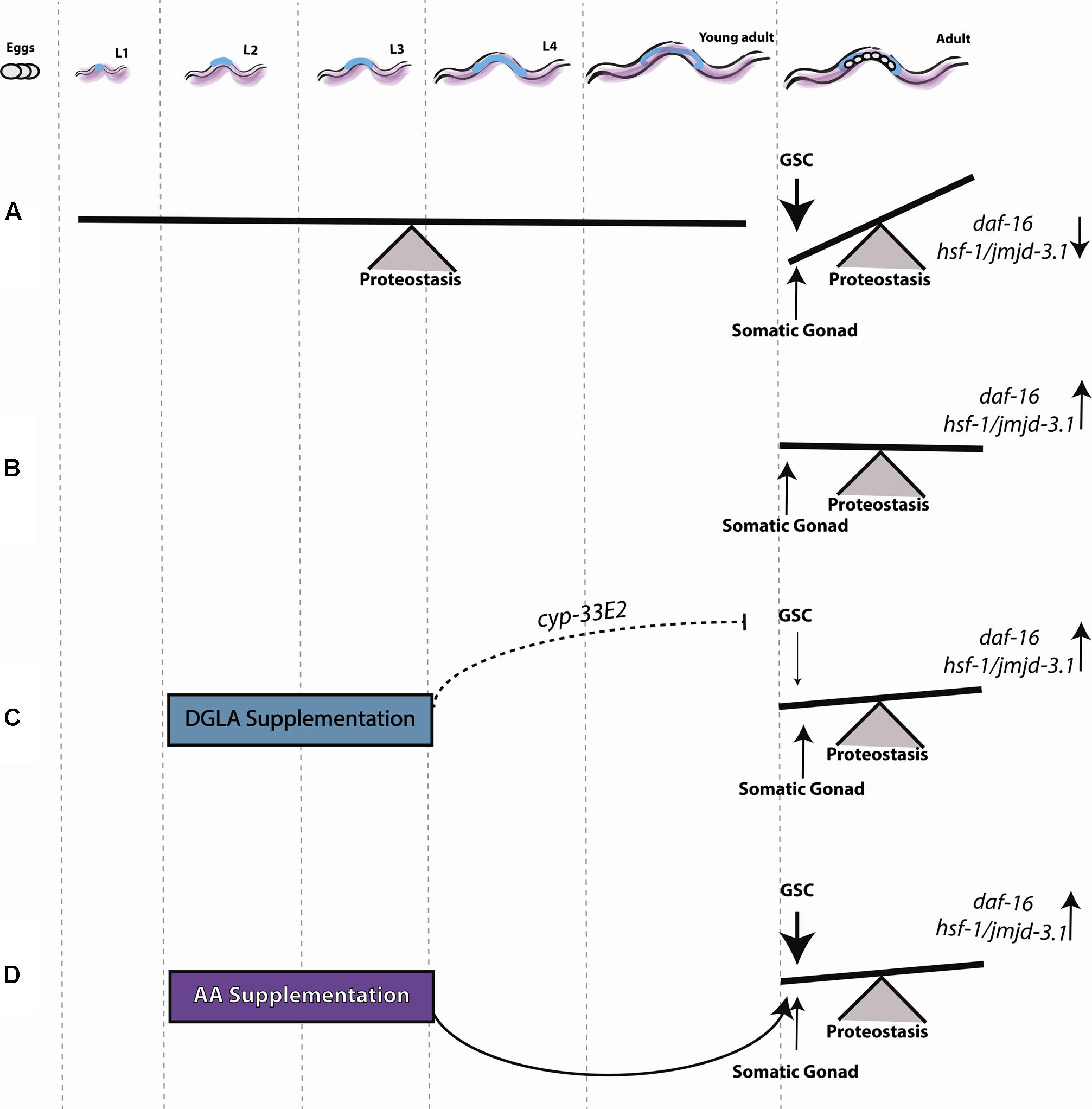
FIGURE 7. Early signals from the reproductive system determine somatic maintenance. (A) Proteostasis of young adult animals is robust, showing low levels of protein damage, effective activation of stress responses and high rates of stress survival. Following the onset of reproduction, signals from GSCs activate a regulatory switch that changes the proteostatic capacity of the soma. (B) Inhibiting GSC proliferation induces sterility and allows the organism to maintain robust proteostasis by somatic gonad signals, as seen in young animals. (C) DGLA supplementation during the L2–L4 stage has a beneficial effect on proteostatic collapse, depending on somatic gonad function, and a negative effect on fecundity. (D) AA supplementation during the L2–L4 stage has a beneficial effect on proteostasis during adulthood.
Temporal Resolution of the Gonadal Longevity Pathway
The gonadal longevity pathway responds to GSC status by regulating a number transcription factors required for detoxification, quality control and lipid metabolism (Hsin and Kenyon, 1999; Wang et al., 2008; O’Rourke et al., 2009; Lapierre et al., 2011, 2013; Goudeau et al., 2011; McCormick et al., 2012; Shemesh et al., 2013; Ratnappan et al., 2014; Steinbaugh et al., 2015; Amrit and Ghazi, 2017). Our data demonstrate that continuous supplementation of DGLA or AA required key transcription factors that are an integral part of this pathway, such as HSF-1/JMJD-3.1, DAF-16, DAF-12, NHR-80, and HLH-30, and induced their transcriptional activities. However, our data also indicate that the timing of activation of these transcription factors differs. While supplementation of DGLA or AA during the L2–L4 stages led to remodeling of the heat shock response and the activation of HSF-1 and DAF-16, it was not required for the transcriptional activation of other transcription factors. For example, unc-51 expression that is regulated by PHA-4 and HLH-30 was also induced when DGLA and AA were supplemented only after L4 (O’Rourke et al., 2009) and was not restricted to development (Figure 6D). Differential transcriptional activation is likely to be a feature of GSC signaling as Nile Red staining, indicative of lysosome-related organelles, is regulated by GSC arrest in development and adulthood (Wang et al., 2008; O’Rourke et al., 2013). Temporal differences in transcriptional activation are also evident in lifespan regulation. Arantes-Oliveira et al. (2002) specifically examined the timing of GSC-dependent regulation of lifespan and showed that GSC arrest starting from L4 stage could modulate lifespan in adulthood but that this timing resulted in a milder effect than starting during larval development. In agreement, DGLA and AA supplementation starting from L4 can extend lifespan (O’Rourke et al., 2013) but has a milder effect than continuous exposure during both development and adulthood (Supplementary Figure S2 and Table S1). We, therefore, propose that protesotasis regulation during larval development can extend lifespan but other transcription factors, such as DAF-12, PHA-4, and HLH-30 that regulate lipid metabolism and lipolysis (Lapierre et al., 2011, 2013; O’Rourke and Ruvkun, 2013), can be activated during adulthood to promote longevity.
One implication of this work is that cell-non-autonomous signals from the reproductive system cannot be employed to reverse proteostatic collapse in adult animals unless triggered during development, at least in C. elegans. Restoring proteostasis by modulating the gonadal longevity pathway as a possible intervention in protein misfolding diseases is, therefore, not likely. However, other cell-non-autonomous signaling pathways, including other gonadal longevity pathway-regulated transcription factors, could serve such a function (Dillin et al., 2002a; Cohen et al., 2010).
DGLA-Mediated Actions Require the Reproductive System
The combination of signals from GSCs and the gonad were suggested to report on reproductive potential to the soma and thus modulate somatic survival and proteostatic capacity, accordingly (Shemesh et al., 2013). Our data suggest that DGLA supplementation causes GSC disruption, since: (1) DGLA-mediated rescue of somatic proteostasis required the gonad and could not further improve rescue in GSC-arrested animals (Figure 2 and Supplementary Figures S3, S5); (2) DGLA impact required the gonadal longevity pathway. DGLA supplementation resulted in DAF-16 nuclear localization in the soma, transcriptional activation of the gonadal signaling pathway and mutant animals that were unable to activate the gonadal signaling pathway were unaffected by DGLA supplementation (Figures 5, 6 and Supplementary Figure 8); (3) DGLA-dependent rescue of proteostasis required CYP-33E2 (Figure 2D), shown to locally produce DGLA-derived eicosanoids that harm GSCs (Deline et al., 2015); and (4) the L2-L4 window that was sufficient for full somatic rescue of proteostasis (Figure 1) was also sufficient to disrupt reproduction (Watts and Browse, 2006) (Supplementary Figure S4). When these observations are taken together, they lead us to propose that DGLA likely acts within the reproduction system by disrupting GSC, placing it upstream to the GSCs. However, a short supplementation of AA during development was also sufficient to improve proteostasis in adulthood (Figure 3 and Supplementary Figure S6), even though AA acts in the soma (Shemesh et al., 2017). Given that AA is also required in the gonadal longevity pathway to modulate proteostatic capacity during adulthood, it would appear that both DGLA and AA are associated with mediating signals received from the reproductive system.
A Developmental Regulatory Checkpoint Sets Proteostatic Collapse
The second and third larval stages are critical periods in the development of the reproduction system and GSCs (Killian and Hubbard, 2005). During this window, GSCs proliferate in the gonad arm (Killian and Hubbard, 2005) and their proliferation depends on interactions with the somatic gonad (McCarter et al., 1997). Moreover, when GSC proliferation is inhibited, the somatic gonad activates the steroid nuclear receptor DAF-12 (Hsin and Kenyon, 1999; Yamawaki et al., 2010; Shen et al., 2012). Of note, DAF-12 functions in the heterochronic pathway, which controls larval development timing. Specifically, DAF-12 is involved in L2–L3 transition and this role is associated with GSC-dependent regulation of lifespan (Antebi, 2012; Shen et al., 2012). The fact that DAF-12 function coincides with the timing of DGLA and AA commitment suggests that DAF-12 function during this phase could be critical for somatic maintenance in adulthood. However, the expression of fard-1, regulated by DAF-12, was not induced upon DGLA or AA supplementation when animals were treated only during development (Figure 6C).
There are other checkpoints that determine whether or not conditions are suitable for reproduction, depending on environmental conditions and food availability (Dillin et al., 2002b; Volovik et al., 2012; Levi-Ferber et al., 2014; Schindler et al., 2014; Thondamal et al., 2014; Wang et al., 2017). One checkpoint that impacts the reproduction system is the L3 stage, when food deprivation can result in arrested development of the reproductive system. This signal is associated with daf-16 and daf-9 (Schindler et al., 2014) that also function in the gonadal longevity pathway (Hsin and Kenyon, 1999; Gerisch et al., 2007; Antebi, 2012). Moreover, the L3 stage is also a metabolic checkpoint, impacted by cell-non-autonomous signaling from neurons responding to mitochondrial function (Durieux et al., 2011; Merkwirth et al., 2016) or GSCs (Wei and Kenyon, 2016). In turn, disruption of mitochondrial function could also cause larval arrest at L3 (Tsang et al., 2001; Tsang and Lemire, 2003). We, therefore, propose that a balance of environmental conditions, metabolic state and reproduction potential are weighed in the L2–L3 window to determine the fate of the organism in terms of its ability to reproduce or to maintain proteostasis in the soma.
Materials and Methods
Nematodes and Growth Conditions
Nematodes were grown on NGM plates seeded with the Escherichia coli OP50-1 strain. Diet-supplemented fatty acids were prepared as previously described (Deline et al., 2013). Plates contained the detergent Tergitol (0.1%, NP-40; Sigma) were used as control or were supplemented with DGLA (90 μM; Santa Cruz Biotechnology, dissolved in Tergitol) or AA (60 μM; TCI Chemical, dissolved in Tergitol). Unless otherwise stated, 30–80 embryos, laid at 15°C, were transferred to the indicated plates (control, DGLA, or AA) and grown at 25°C for the duration of an experiment. The first day of adulthood (day 1) was set before the onset of egg-laying (50 h after temperature shift). To avoid progeny contamination, animals were moved to new plates during the reproductive period.
Statistical Analysis
Experiments were repeated at least three times and >15 animals per experimental condition were scored. Data are presented as means ± SEM. P-values were calculated using the Wilcoxon Mann–Whitney rank sum test to compare two independent populations. (n.s.) denotes non-significant (∗) denotes P < 0.05, (∗∗) denotes P < 0.01. OASIS2, an online application for survival analysis (Han et al., 2016) was used to analyze lifespan assays (data included in Supplementary Figure S2 and Table S1). Mean life spans were calculated using Kaplan–Meier survival curves and P-values were determined using log-rank (Mantel-Cox) statistics.
Aggregates Quantification
Age-synchronized animals (n > 50) that express punc-54::Q35::YFP (Q35m) were imaged using a Leica M165 FC fluorescent stereoscope with a YFP filter and the number of aggregates was counted. Aggregates were defined as discrete structures that are brighter and clearly distinguished from the surrounding fluorescence (bright foci) (Morley et al., 2002).
Paralysis Assay
Age-synchronized animals (n > 30) were moved every day, and animals were scored by monitoring their movement 5 min after transfer to a new plate. Animals that did not move were scored as paralyzed (Karady et al., 2013).
Stiff Body Paralysis Assay
Age-synchronized (n > 30) unc-52(ts) mutant animals were grown at 25°C until day 1 of adulthood. Animals were then shifted to 15°C and paralysis was scored on day 4 of adulthood.
Thermo-Resistance Assay
Animals were moved to a 24-well plate containing heat shock buffer (100 mM Tris-HCl, pH 7.4, 17 mM NaCl and 1% cholesterol supplemented with bacteria) at the indicated ages and subjected to a 37°C heat shock for 6 h. Animal survival was scored by monitoring uptake of SYTOX orange (Invitrogen) dye supplemented to the buffer following heat shock, using a Leica M165 FC fluorescent stereoscope with a TXR filter. Fluorescent animals were scored as dead (Karady et al., 2013). Heat shock-treated animals were discarded after scoring.
Lifespan Analysis
Synchronized embryos [N2 or glp-1(e2141)], laid at 15°C, were transferred to the indicated plates (control, DGLA or AA) and maintained at 25°C for the duration of an experiment. At L4 (after 32 h), animals were shifted to new plates (15–30 animals per plate), as indicated (control, DGLA or AA). For each treatment condition, ∼50–200 L4 animals were monitored. During the reproductive period, animals were transferred to freshly seeded plates every day. Animals were considered dead when they no longer responded to gentle prodding with a platinum wire. Scoring was performed every day. Animals that crawled off the plate, were contaminated or displayed a bagging phenotype (matricide due to internal hatching of embryos) were censored.
DAPI Staining
Gonads were dissected as described (Colaiacovo et al., 2003), fixed and stained as previously described (Karady et al., 2013) and subsequently mounted and imaged using an Olympus Fluoview FV1000 confocal microscope through a 60× 1.0 numerical aperture objective with a 405 nm line for excitation.
Sterility
Age-synchronized animals (n > 30) were grown on control or DGLA- or AA-supplemented plates at 25°C were imaged using a Leica M165 FC fluorescent stereoscope and examined for the presence of oocytes and embryo in the uterus.
Progeny Quantification
Age-synchronized animals of all tested condition (in parallel) were allowed to lay eggs on fresh plates at 25°C. Individual animals were set of separate plates and moved every 24 h during the first 5 days of adulthood (i.e., past the reproduction span). The number of offspring was scored 48–72 h later and the progeny of >15 animals per condition were scored.
RNA Interference (RNAi)
Embryos (n > 15) were placed on E. coli strain HT115(DE3) transformed with the indicated RNAi vectors (obtained from the Ahringer or Vidal RNAi libraries) or the empty vector (pL4440), as previously described (Shemesh et al., 2013).
DAF-16 Nuclear Localization Assay
Age-synchronized DAF-16::GFP animals (n > 20) were grown on control or DGLA- or AA-supplemented plates at 25°C. Animals were fixed on day 2 of adulthood and mounted as previously described. DAF-16 nuclear localization in intestinal cells was imaged using a Leica DM5500 confocal microscope through a 40× 1.0 numerical aperture objective with a 488 nm line for excitation. Animals were scored as having nuclear-localized DAF-16 when the majority of their intestinal cells showed GFP accumulation in the nucleus (Berman and Kenyon, 2006). Animals were scored blind.
Heat Shock Treatment
Age-synchronized animals (n > 30) were placed in a 37°C bath for 90 min in sealed plates. Following treatment, the animals were frozen or fixed immediately.
RNA Levels
Forty to sixty animals were collected per condition. RNA was extracted using the TRIzol reagent (Invitrogen). mRNA was reverse-transcribed using the iScript cDNA Synthesis Kit (Bio-Rad) for cDNA synthesis. Quantitative PCR was performed on a C1000 Thermal Cycler (Bio-Rad) with KAPA SYBER FAST (KAPA BIOSYSTEMS) (Shemesh et al., 2013).
Author Contributions
NeS and AB-Z designed the experiments. NeS, LM, and NuS performed the experiments, analyzed the data and interpreted the results. NeS and AB-Z wrote the manuscript.
Funding
This research was supported by a grant from the Legacy Heritage Biomedical Science Partnership Program of the Israel Science Foundation (grant No. 804/13; https://www.isf.org.il/#/). NeS was supported by Kreitman Negev scholarship and a Kreitman short-term post-doctoral scholarship.
Conflict of Interest Statement
The authors declare that the research was conducted in the absence of any commercial or financial relationships that could be construed as a potential conflict of interest.
Acknowledgments
Some nematode strains used in this work were provided by the Caenorhabditis Genetics Center, which is funded by the NIH National Center for Research Resources (NCRR). We thank all the members of the AB-Z laboratory and specifically to Shiran Dror and Mehtap Abu-Qarn for technical support with the revisions. Finally, we thank Yarden Cohen-Shemesh for coming early into this world and turning our world upside down.
Supplementary Material
The Supplementary Material for this article can be found online at: http://journal.frontiersin.org/article/10.3389/fnmol.2017.00254/full#supplementary-material
References
Amrit, F. R., and Ghazi, A. (2017). “Influences of germline cells on organismal lifespan and healthspan,” in Ageing: Lessons from C. elegans, eds M. S. Gill and A. Olsen (Cham: Springer International Publishing),109–135.
Antebi, A. (2012). Regulation of longevity by the reproductive system. Exp. Gerontol. 48, 596–602. doi: 10.1016/j.exger.2012.09.009
Arantes-Oliveira, N., Apfeld, J., Dillin, A., and Kenyon, C. (2002). Regulation of life-span by germ-line stem cells in Caenorhabditis elegans. Science 295, 502–505. doi: 10.1126/science.1065768
Bar-Lavan, Y., Shemesh, N., and Ben-Zvi, A. (2016a). Chaperone families and interactions in metazoa. Essays Biochem. 60, 237–253.
Bar-Lavan, Y., Shemesh, N., Dror, S., Ofir, R., Yeger-Lotem, E., and Ben-Zvi, A. (2016b). A differentiation transcription factor establishes muscle-specific proteostasis in Caenorhabditis elegans. PLoS Genet. 12:e1006531. doi: 10.1371/journal.pgen.1006531
Ben-Zvi, A., Miller, E. A., and Morimoto, R. I. (2009). Collapse of proteostasis represents an early molecular event in Caenorhabditis elegans aging. Proc. Natl. Acad. Sci. U.S.A. 106, 14914–14919. doi: 10.1073/pnas.0902882106
Berman, J. R., and Kenyon, C. (2006). Germ-cell loss extends C. elegans life span through regulation of DAF-16 by kri-1 and lipophilic-hormone signaling. Cell 124, 1055–1068. doi: 10.1016/j.cell.2006.01.039
Bett, J. S. (2016). Proteostasis regulation by the ubiquitin system. Essays Biochem. 60, 143–151. doi: 10.1042/EBC20160001
Cohen, E., Bieschke, J., Perciavalle, R. M., Kelly, J. W., and Dillin, A. (2006). Opposing activities protect against age-onset proteotoxicity. Science 313, 1604–1610. doi: 10.1126/science.1124646
Cohen, E., Du, D., Joyce, D., Kapernick, E. A., Volovik, Y., Kelly, J. W., et al. (2010). Temporal requirements of insulin/IGF-1 signaling for proteotoxicity protection. Aging Cell 9, 126–134. doi: 10.1111/j.1474-9726.2009.00541.x
Colaiacovo, M. P., MacQueen, A. J., Martinez-Perez, E., McDonald, K., Adamo, A., La Volpe, A., et al. (2003). Synaptonemal complex assembly in C. elegans is dispensable for loading strand-exchange proteins but critical for proper completion of recombination. Dev. Cell 5, 463–474. doi: 10.1016/S1534-5807(03)00232-6
Deline, M., Keller, J., Rothe, M., Schunck, W. H., Menzel, R., and Watts, J. L. (2015). Epoxides derived from dietary dihomo-gamma-linolenic acid induce germ cell death in C. elegans. Sci. Rep. 5:15417. doi: 10.1038/srep15417
Deline, M. L., Vrablik, T. L., and Watts, J. L. (2013). Dietary supplementation of polyunsaturated fatty acids in Caenorhabditis elegans. J. Vis. Exp. 81:e50879.
Dillin, A., Crawford, D. K., and Kenyon, C. (2002a). Timing requirements for insulin/IGF-1 signaling in C. elegans. Science 298, 830–834.
Dillin, A., Hsu, A. L., Arantes-Oliveira, N., Lehrer-Graiwer, J., Hsin, H., Fraser, A. G., et al. (2002b). Rates of behavior and aging specified by mitochondrial function during development. Science 298, 2398–2401.
Dubnikov, T., Ben-Gedalya, T., and Cohen, E. (2017). Protein quality control in health and disease. Cold Spring Harb. Perspect. Biol. 9:a023523. doi: 10.1101/cshperspect.a023523
Durieux, J., Wolff, S., and Dillin, A. (2011). The cell-non-autonomous nature of electron transport chain-mediated longevity. Cell 144, 79–91. doi: 10.1016/j.cell.2010.12.016
El-Ami, T., Moll, L., Carvalhal Marques, F., Volovik, Y., Reuveni, H., and Cohen, E. (2014). A novel inhibitor of the insulin/IGF signaling pathway protects from age-onset, neurodegeneration-linked proteotoxicity. Aging Cell 13, 165–174. doi: 10.1111/acel.12171
Fiorese, C. J., and Haynes, C. M. (2017). Integrating the UPRmt into the mitochondrial maintenance network. Crit. Rev. Biochem. Mol. Biol. 52, 304–313. doi: 10.1080/10409238.2017.1291577
Flatt, T., Min, K. J., D’Alterio, C., Villa-Cuesta, E., Cumbers, J., Lehmann, R., et al. (2008). Drosophila germ-line modulation of insulin signaling and lifespan. Proc. Natl. Acad. Sci. U.S.A. 105, 6368–6373. doi: 10.1073/pnas.0709128105
Folick, A., Oakley, H. D., Yu, Y., Armstrong, E. H., Kumari, M., Sanor, L., et al. (2015). Aging. Lysosomal signaling molecules regulate longevity in Caenorhabditis elegans. Science 347, 83–86. doi: 10.1126/science.1258857
Gerisch, B., Rottiers, V., Li, D., Motola, D. L., Cummins, C. L., Lehrach, H., et al. (2007). A bile acid-like steroid modulates Caenorhabditis elegans lifespan through nuclear receptor signaling. Proc. Natl. Acad. Sci. U.S.A. 104, 5014–5019. doi: 10.1073/pnas.0700847104
Goudeau, J., Bellemin, S., Toselli-Mollereau, E., Shamalnasab, M., Chen, Y., and Aguilaniu, H. (2011). Fatty acid desaturation links germ cell loss to longevity through NHR-80/HNF4 in C. elegans. PLoS Biol. 9:e1000599. doi: 10.1371/journal.pbio.1000599
Han, S. K., Lee, D., Lee, H., Kim, D., Son, H. G., Yang, J. S., et al. (2016). OASIS 2: online application for survival analysis 2 with features for the analysis of maximal lifespan and healthspan in aging research. Oncotarget 7, 56147–56152. doi: 10.18632/oncotarget.11269
Hsin, H., and Kenyon, C. (1999). Signals from the reproductive system regulate the lifespan of C. elegans. Nature 399, 362–366. doi: 10.1038/20694
Jackson, M. P., and Hewitt, E. W. (2016). Cellular proteostasis: degradation of misfolded proteins by lysosomes. Essays Biochem. 60, 173–180. doi: 10.1042/EBC20160005
Karady, I., Frumkin, A., Dror, S., Shemesh, N., Shai, N., and Ben-Zvi, A. (2013). Using Caenorhabditis elegans as a model system to study protein homeostasis in a multicellular organism. J. Vis. Exp. 82:e50840. doi: 10.3791/50840
Killian, D. J., and Hubbard, E. J. (2005). Caenorhabditis elegans germline patterning requires coordinated development of the somatic gonadal sheath and the germ line. Dev. Biol. 279, 322–335. doi: 10.1016/j.ydbio.2004.12.021
Labbadia, J., and Morimoto, R. I. (2015a). The biology of proteostasis in aging and disease. Annu. Rev. Biochem. 84, 435–464. doi: 10.1146/annurev-biochem-060614-033955
Labbadia, J., and Morimoto, R. I. (2015b). Repression of the heat shock response is a programmed event at the onset of reproduction. Mol. Cell 59, 639–650. doi: 10.1016/j.molcel.2015.06.027
Lapierre, L. R., De Magalhaes Filho, C. D., McQuary, P. R., Chu, C. C., Visvikis, O., Chang, J. T., et al. (2013). The TFEB orthologue HLH-30 regulates autophagy and modulates longevity in Caenorhabditis elegans. Nat. Commun. 4:2267. doi: 10.1038/ncomms3267
Lapierre, L. R., Gelino, S., Melendez, A., and Hansen, M. (2011). Autophagy and lipid metabolism coordinately modulate life span in germline-less C. elegans. Curr. Biol. 21, 1507–1514. doi: 10.1016/j.cub.2011.07.042
Levi-Ferber, M., Salzberg, Y., Safra, M., Haviv-Chesner, A., Bulow, H. E., and Henis-Korenblit, S. (2014). It’s all in your mind: determining germ cell fate by neuronal IRE-1 in C. elegans. PLoS Genet. 10:e1004747. doi: 10.1371/journal.pgen.1004747
Mason, J. B., Cargill, S. L., Anderson, G. B., and Carey, J. R. (2009). Transplantation of young ovaries to old mice increased life span in transplant recipients. J. Gerontol. A Biol. Sci. Med. Sci. 64, 1207–1211. doi: 10.1093/gerona/glp134
McCaffrey, K., and Braakman, I. (2016). Protein quality control at the endoplasmic reticulum. Essays Biochem. 60, 227–235. doi: 10.1042/EBC20160003
McCarter, J., Bartlett, B., Dang, T., and Schedl, T. (1997). Soma-germ cell interactions in Caenorhabditis elegans: multiple events of hermaphrodite germline development require the somatic sheath and spermathecal lineages. Dev. Biol. 181, 121–143. doi: 10.1006/dbio.1996.8429
McCormick, M., Chen, K., Ramaswamy, P., and Kenyon, C. (2012). New genes that extend Caenorhabditis elegans’ lifespan in response to reproductive signals. Aging Cell 11, 192–202. doi: 10.1111/j.1474-9726.2011.00768.x
Merkwirth, C., Jovaisaite, V., Durieux, J., Matilainen, O., Jordan, S. D., Quiros, P. M., et al. (2016). Two conserved histone demethylases regulate mitochondrial stress-induced longevity. Cell 165, 1209–1223. doi: 10.1016/j.cell.2016.04.012
Morimoto, R. I. (2011). The heat shock response: systems biology of proteotoxic stress in aging and disease. Cold Spring Harb. Symp. Quant. Biol. 76, 91–99. doi: 10.1101/sqb.2012.76.010637
Morley, J. F., Brignull, H. R., Weyers, J. J., and Morimoto, R. I. (2002). The threshold for polyglutamine-expansion protein aggregation and cellular toxicity is dynamic and influenced by aging in Caenorhabditis elegans. Proc. Natl. Acad. Sci. U.S.A. 99, 10417–10422. doi: 10.1073/pnas.152161099
O’Brien, D., and van Oosten-Hawle, P. (2016). Regulation of cell-non-autonomous proteostasis in metazoans. Essays Biochem. 60, 133–142. doi: 10.1042/EBC20160006
O’Rourke, E. J., Kuballa, P., Xavier, R., and Ruvkun, G. (2013). omega-6 Polyunsaturated fatty acids extend life span through the activation of autophagy. Genes Dev. 27, 429–440. doi: 10.1101/gad.205294.112
O’Rourke, E. J., and Ruvkun, G. (2013). MXL-3 and HLH-30 transcriptionally link lipolysis and autophagy to nutrient availability. Nat. Cell Biol. 15, 668–676. doi: 10.1038/ncb2741
O’Rourke, E. J., Soukas, A. A., Carr, C. E., and Ruvkun, G. (2009). C. elegans major fats are stored in vesicles distinct from lysosome-related organelles. Cell Metab. 10, 430–435. doi: 10.1016/j.cmet.2009.10.002
Ratnappan, R., Amrit, F. R., Chen, S. W., Gill, H., Holden, K., Ward, J., et al. (2014). Germline signals deploy NHR-49 to modulate fatty-acid beta-oxidation and desaturation in somatic tissues of C. elegans. PLoS Genet. 10:e1004829. doi: 10.1371/journal.pgen.1004829
Schindler, A. J., Baugh, L. R., and Sherwood, D. R. (2014). Identification of late larval stage developmental checkpoints in Caenorhabditis elegans regulated by insulin/IGF and steroid hormone signaling pathways. PLoS Genet. 10:e1004426. doi: 10.1371/journal.pgen.1004426
Schinzel, R., and Dillin, A. (2015). Endocrine aspects of organelle stress-cell non-autonomous signaling of mitochondria and the ER. Curr. Opin. Cell Biol. 33, 102–110. doi: 10.1016/j.ceb.2015.01.006
Shai, N., Shemesh, N., and Ben-Zvi, A. (2014). Remodeling of proteostasis upon transition to adulthood is linked to reproduction onset. Curr. Genomics 15, 122–129. doi: 10.2174/1389202915666140221005023
Shemesh, N., and Ben-Zvi, A. (2016). “HSF1 regulation in aging and its role in longevity,” in Heat Shock Factor, ed. A. Nakai (Osaka: Springer), 93–114.
Shemesh, N., Shai, N., and Ben-Zvi, A. (2013). Germline stem cell arrest inhibits the collapse of somatic proteostasis early in Caenorhabditis elegans adulthood. Aging Cell 12, 814–822. doi: 10.1111/acel.12110
Shemesh, N., Shai, N., Meshnik, L., Katalan, R., and Ben-Zvi, A. (2017). Uncoupling the trade-off between somatic proteostasis and reproduction in Caenorhabditis elegans models of polyglutamine diseases. Front. Mol. Neurosci. 10:101. doi: 10.3389/fnmol.2017.00101
Shen, Y., Wollam, J., Magner, D., Karalay, O., and Antebi, A. (2012). A steroid receptor-microRNA switch regulates life span in response to signals from the gonad. Science 338, 1472–1476. doi: 10.1126/science.1228967
Silva, M. C., Fox, S., Beam, M., Thakkar, H., Amaral, M. D., and Morimoto, R. I. (2011). A genetic screening strategy identifies novel regulators of the proteostasis network. PLoS Genet. 7:e1002438. doi: 10.1371/journal.pgen.1002438
Steinbaugh, M. J., Narasimhan, S. D., Robida-Stubbs, S., Moronetti Mazzeo, L. E., Dreyfuss, J. M., Hourihan, J. M., et al. (2015). Lipid-mediated regulation of SKN-1/Nrf in response to germ cell absence. eLife 4:e07836. doi: 10.7554/eLife.07836
Thondamal, M., Witting, M., Schmitt-Kopplin, P., and Aguilaniu, H. (2014). Steroid hormone signalling links reproduction to lifespan in dietary-restricted Caenorhabditis elegans. Nat. Commun. 5:4879. doi: 10.1038/ncomms5879
Tsang, W. Y., and Lemire, B. D. (2003). Mitochondrial ATP synthase controls larval development cell nonautonomously in Caenorhabditis elegans. Dev. Dyn. 226, 719–726. doi: 10.1002/dvdy.10272
Tsang, W. Y., Sayles, L. C., Grad, L. I., Pilgrim, D. B., and Lemire, B. D. (2001). Mitochondrial respiratory chain deficiency in Caenorhabditis elegans results in developmental arrest and increased life span. J. Biol. Chem. 276, 32240–32246. doi: 10.1074/jbc.M103999200
Vilchez, D., Morantte, I., Liu, Z., Douglas, P. M., Merkwirth, C., Rodrigues, A. P., et al. (2012). RPN-6 determines C. elegans longevity under proteotoxic stress conditions. Nature 489, 263–268. doi: 10.1038/nature11315
Volovik, Y., Maman, M., Dubnikov, T., Bejerano-Sagie, M., Joyce, D., Kapernick, E. A., et al. (2012). Temporal requirements of heat shock factor-1 for longevity assurance. Aging Cell 11, 491–499. doi: 10.1111/j.1474-9726.2012.00811.x
Voos, W., Jaworek, W., Wilkening, A., and Bruderek, M. (2016). Protein quality control at the mitochondrion. Essays Biochem. 60, 213–225. doi: 10.1042/EBC20160009
Vrablik, T. L., and Watts, J. L. (2013). Polyunsaturated fatty acid derived signaling in reproduction and development: insights from Caenorhabditis elegans and Drosophila melanogaster. Mol. Reprod. Dev. 80, 244–259. doi: 10.1002/mrd.22167
Walter, P., and Ron, D. (2011). The unfolded protein response: from stress pathway to homeostatic regulation. Science 334, 1081–1086. doi: 10.1126/science.1209038
Wang, D., Hou, L., Nakamura, S., Su, M., Li, F., Chen, W., et al. (2017). LIN-28 balances longevity and germline stem cell number in Caenorhabditis elegans through let-7/AKT/DAF-16 axis. Aging Cell 16, 113–124. doi: 10.1111/acel.12539
Wang, M. C., O’Rourke, E. J., and Ruvkun, G. (2008). Fat metabolism links germline stem cells and longevity in C. elegans. Science 322, 957–960. doi: 10.1126/science.1162011
Watts, J. L., and Browse, J. (2006). Dietary manipulation implicates lipid signaling in the regulation of germ cell maintenance in C. elegans. Dev. Biol. 292, 381–392. doi: 10.1016/j.ydbio.2006.01.013
Webster, C. M., Deline, M. L., and Watts, J. L. (2013). Stress response pathways protect germ cells from omega-6 polyunsaturated fatty acid-mediated toxicity in Caenorhabditis elegans. Dev. Biol. 373, 14–25. doi: 10.1016/j.ydbio.2012.10.002
Wei, Y., and Kenyon, C. (2016). Roles for ROS and hydrogen sulfide in the longevity response to germline loss in Caenorhabditis elegans. Proc. Natl. Acad. Sci. U.S.A. 113, E2832–E2841. doi: 10.1073/pnas.1524727113
Yamawaki, T. M., Berman, J. R., Suchanek-Kavipurapu, M., McCormick, M., Gaglia, M. M., Lee, S. J., et al. (2010). The somatic reproductive tissues of C. elegans promote longevity through steroid hormone signaling. PLoS Biol. 8:e1000468. doi: 10.1371/journal.pbio.1000468
Yerbury, J. J., Ooi, L., Dillin, A., Saunders, D. N., Hatters, D. M., Beart, P. M., et al. (2016). Walking the tightrope: proteostasis and neurodegenerative disease. J. Neurochem. 137, 489–505. doi: 10.1111/jnc.13575
Keywords: aging, arachidonic acid (AA), Caenorhabditis elegans, dihomo-γ-linolenic acid (DGLA), germline stem cells (GSCs), neurodegenerative diseases, proteostasis, reproduction
Citation: Shemesh N, Meshnik L, Shpigel N and Ben-Zvi A (2017) Dietary-Induced Signals That Activate the Gonadal Longevity Pathway during Development Regulate a Proteostasis Switch in Caenorhabditis elegans Adulthood. Front. Mol. Neurosci. 10:254. doi: 10.3389/fnmol.2017.00254
Received: 06 April 2017; Accepted: 27 July 2017;
Published: 09 August 2017.
Edited by:
Serena Carra, University of Modena and Reggio Emilia, ItalyReviewed by:
Sivan Henis-Korenblit, Bar-Ilan University, IsraelPatricija Van Oosten-Hawle, University of Leeds, United Kingdom
Copyright © 2017 Shemesh, Meshnik, Shpigel and Ben-Zvi. This is an open-access article distributed under the terms of the Creative Commons Attribution License (CC BY). The use, distribution or reproduction in other forums is permitted, provided the original author(s) or licensor are credited and that the original publication in this journal is cited, in accordance with accepted academic practice. No use, distribution or reproduction is permitted which does not comply with these terms.
*Correspondence: Anat Ben-Zvi, YW5hdGJ6QGJndS5hYy5pbA==
†These authors have contributed equally to this work.