- 1Athinoula A Martinos Biomedical Imaging Center, Department of Radiology, Massachusetts General Hospital, Harvard Medical School, Charlestown, MA, USA
- 2Ann Romney Center for Neurologic Diseases, Brigham and Women's Hospital, Harvard Medical School, Boston, MA, USA
Non-invasive molecular imaging techniques can enhance diagnosis to achieve successful treatment, as well as reveal underlying pathogenic mechanisms in disorders such as multiple sclerosis (MS). The cooperation of advanced multimodal imaging techniques and increased knowledge of the MS disease mechanism allows both monitoring of neuronal network and therapeutic outcome as well as the tools to discover novel therapeutic targets. Diverse imaging modalities provide reliable diagnostic and prognostic platforms to better achieve precision medicine. Traditionally, magnetic resonance imaging (MRI) has been considered the golden standard in MS research and diagnosis. However, positron emission tomography (PET) imaging can provide functional information of molecular biology in detail even prior to anatomic changes, allowing close follow up of disease progression and treatment response. The recent findings support three major neuroinflammation components in MS: astrogliosis, cytokine elevation, and significant changes in specific proteins, which offer a great variety of specific targets for imaging purposes. Regardless of the fact that imaging of astrocyte function is still a young field and in need for development of suitable imaging ligands, recent studies have shown that inflammation and astrocyte activation are related to progression of MS. MS is a complex disease, which requires understanding of disease mechanisms for successful treatment. PET is a precise non-invasive imaging method for biochemical functions and has potential to enhance early and accurate diagnosis for precision therapy of MS. In this review we focus on modulation of different receptor systems and inflammatory aspect of MS, especially on activation of glial cells, and summarize the recent findings of PET imaging in MS and present the most potent targets for new biomarkers with the main focus on experimental MS research.
Introduction
Multiple sclerosis (MS) is the most common disabling neurologic disease of young people, afflicting approximately a quarter of million Americans (Anderson et al., 1992; Islam et al., 2006; Brody, 2012; Ransohoff et al., 2015). It occurs more in women than in men by a ratio of nearly 2 to 1, and it strikes most often between the ages of 20 and 40 (Compston and Coles, 2008). MS results from the immune-driven demyelination of the central nervous system (CNS), which leads to axonal damage and progressive loss of neurological functions (Sofroniew and Vinters, 2010; Malpass, 2012; Sofroniew, 2015). Based on clinical characteristics, MS pathology can be divided into three different disease courses: relapsing-remitting (RR), secondary progressive (SP), and primary progressive (PP) (Goodin, 2014). Initially, most MS patients exhibit a RR-MS disease course (Morales et al., 2006), experiencing heterogeneous symptoms such as ataxia, visual disturbances, paresthesia, and muscle weakness (Ellwardt and Zipp, 2014). However, eventually the majority of these patients develop SP-MS characterized by the progressive and irreversible accumulation of neurological disability (Lublin and Reingold, 1996). PP-MS patients have continuous disease progression from onset, without relapses or remissions (Morales et al., 2006; Lopez-Diego and Weiner, 2008).
Recent findings of the innate and the adaptive immune system of CNS have shaken up the classical view of MS as being strictly an autoimmune disease of the white matter (Weiner, 2008; Gandhi et al., 2010; Hemmer et al., 2015). The studies have revealed the important role of infiltrating immune cells from the periphery as well as the role of resident activated glial cells leading ultimately to the T cells and macrophages reaction against myelin (see Figure 1) (Frohman et al., 2006; Compston and Coles, 2008). These advances have switched the focus of MS research toward neurodegenerative aspects of the disease, occurring early in the pathological process (Kiferle et al., 2011). Despite the recent progresses in the field of MS therapeutic strategies there is no curative treatment for progressive MS (Lopez-Diego and Weiner, 2008; Derwenskus and Lublin, 2014). Therefore, identifying new specific biomarkers for MS could reveal new potential drug targets and diagnostic markers. Moreover, there is an unmet clinical need for methods to monitor different mechanisms of disease pathogenesis in MS patients, therefore advanced non-invasive molecular imaging technologies are needed to expand our understanding of the controversial aspects of the MS pathology (Kiferle et al., 2011; Jacobs and Tavitian, 2012).
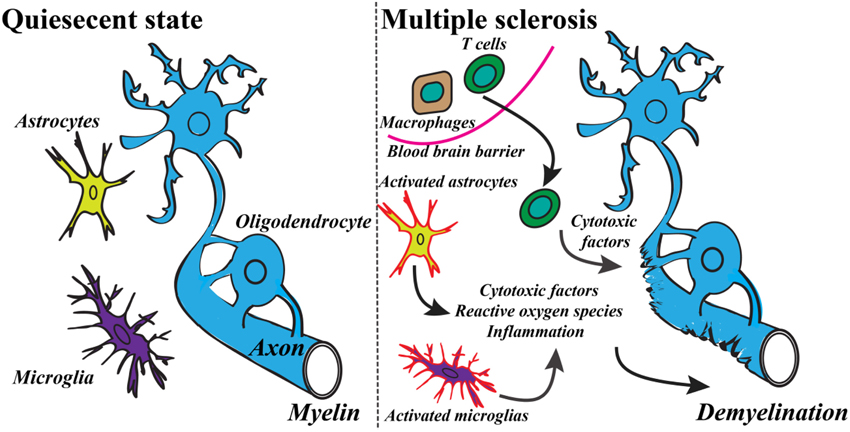
Figure 1. Basic mechanism of the development of MS includes a variety of inflammatory responses and activation of specific cell types. Modified from Criste et al. (2014) and Friese et al. (2014).
Historically, MRI has overruled other imaging technologies in the diagnosis of MS (Traboulsee and Li, 2006; Barkhof and Filippi, 2009). The classical McDonald criterion for MS diagnosis requires objective dissemination of lesions in time and space (Filippi and Rocca, 2011). The literature analysis has shown that the sensitivity of MRI has been between 35 and 100%, and specificity has been between 36 and 92% depending on the research protocol (Schäffler et al., 2011; Tillema and Pirko, 2013). Overall, T2-weighted MRI is effective way to detect MS lesions, but because the signal reflects the water content, it does not provide reliable information about the myelin content (Ge, 2006; Poloni et al., 2011). T1-weighted imaging together with contrast agents such as gadolinium-DTPA has increased the lesion detection sensitivity, however, signal frequency is associated with the opening of the blood brain barrier (BBB; Lund et al., 2013). This leads to the problem that MRI can vary greatly in terms of sensitivity and specificity, especially in MS-related pathological pathways (Barkhof et al., 2009; Lövblad et al., 2010). Early diagnosis and treatment is effective for the therapy and decreases the financial burden of the disease (Noyes et al., 2011; Guo et al., 2014). The annual mean cost is around $47,000 per MS patient, which arises to a national cost of about $13 billion in US per year (Olek, 2012). The disease modifying treatments (DMTs, typically used first-line interferon betas and glatiramer acetate) have been available for the last 25 years and are estimated to account for one third of the total cost. Unfortunately, these treatments suppress the disease only for a few years (Hartung et al., 2015) and the spectrum of treatment options is narrow (Oh and O'Connor, 2015). Conventional MRI gives anatomical information from the progressed lesions in the brain of MS patients but lacks the power to provide target for drug discovery and more specific molecular markers when compared to imaging modalities like positron emission tomography (PET; Filippi et al., 2012; Matthews et al., 2012).
PET research field is emerging and the researchers have been successful in developing novel tracers for multiple different aspects of MS to enhance understanding the pathophysiology of the disease. In this review, we summarize PET imaging in MS research and introduce some of the most potent imaging targets and applications that have been successfully investigated in inflammation and which can be implemented especially to astrocyte activation related pathways, which are presently of high interest in MS research (Maragakis and Rothstein, 2006; Nair et al., 2008; Miljković et al., 2011; Nash et al., 2011; Mayo et al., 2014).
PET Imaging Techniques
PET imaging is based on detection of isotope labeled tracers, which emit beta radiation (see Table 1). These tracers are administered into the subjects to monitor underlying biological processes (Kiferle et al., 2011). The radioisotope undergoes positron emission decay and emits positron, which travels into surrounding tissue until it interacts with an electron and the annihilation process takes part (see Figure 2). The formed two photons travel in approximately opposite directions and can be detected with the imaging device as a coincidence pair. Each detected coincident forms a line of response (LOR) where the point of origin is the location of annihilation event. The combination of LORs can be used for reconstruction of images to provide 3-dimensional (3D) distribution of the radiolabeled tracer (Gambhir, 2002).
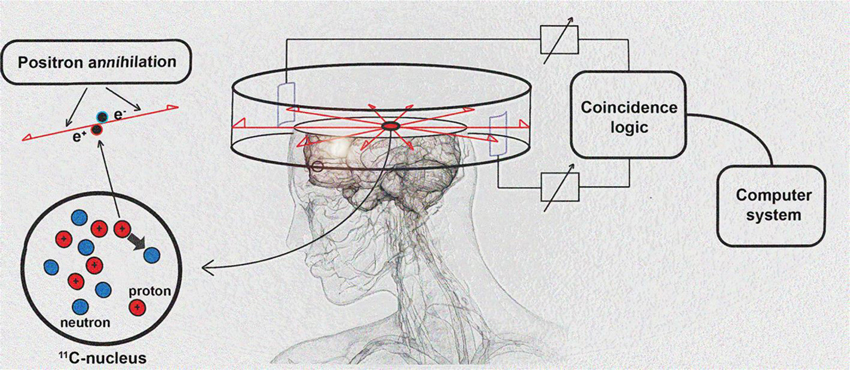
Figure 2. Schematic diagram of positron detection. Modified from Brownell (2008).
The clinical history of positron emission techniques started in 1952 when Gordon Brownell was able to localize brain tumors from patients (Brownell and Sweet, 1953). Further technical progression led to 3D tomographical positron imaging by 1971 (Pizer et al., 1971). However, even today PET suffers from high cost because the production of radiopharmaceutical agents increase the imaging cost compared to CT or MRI, both which became available later. After the development of [18F]fluorodeoxy glucose ([18F]FDG) PET imaging received more significant clinical role especially in oncological diagnosis (Portnow et al., 2013). In MS however, MRI has been regarded as the golden standard in assessing patients (Filippi et al., 2012; Miller et al., 2012). Combined PET/MRI imaging with high specificity to MS lesions, would have a potential to become a practical tool in clinics to follow up the treatment of MS patients and increase cost-effectiveness. This approach could reveal an optimized treatment regimen; increase the treatment effectiveness and safety of patients, especially in early stage and patients with aggressive disease (Catana et al., 2013). When comparing these two imaging techniques, PET imaging has at least four major advantages over conventional MR imaging: (Massoud, 2003; Kiferle et al., 2011; Poloni et al., 2011; Jacobs and Tavitian, 2012; Miller et al., 2012; Torigian et al., 2013; Faria Dde et al., 2014; Jadvar and Colletti, 2014; Bodini et al., 2015) (1) Specific information of disease mechanism and molecular contributors, (2) Enhance development of new medicines and therapeutic targets, (3) Efficient allocation of new costly therapeutics and personalized medicine, and (4) Improved prognostic method for the MS patients.
Although the first clinical positron emission imaging studies were done over 60 years ago, the spectrum of applications of PET imaging is still limited due to the high cost and lack of validated traces and state-of-the-art facilities including availability of cyclotrons and automated radiopharmaceutical production laboratories (Jones et al., 2012). Complete knowledge about pharmacokinetic and pharmacodynamic properties of injected tracers can assure the correct interpretation of the images from preclinical and clinical studies. Overall, PET is an extremely powerful technology and the in vivo receptor occupancy can help answer many vital questions in the MS research (Matthews et al., 2012; Bodini et al., 2015). Furthermore, PET offers an opportunity for the detection of enzyme reactions, ligand-receptor interactions, cellular metabolism, cell proliferation, protein-protein interactions, as well as gene and cell therapy (Herschman, 2003; Ono, 2009; Thorek et al., 2013). The development of new PET tracers is challenging because the binding affinity and selectivity of the tracer have to be high and the dissociation must be fast enough to obtain the binding equilibrium in time frame of scan (1–2 h) (Hicks, 2006; Sharma et al., 2010). The tracer should penetrate the BBB, but too lipophilic compound might have strong non-specific binding (Liu et al., 2008). The optimal radiotracer should have minimum amount of unwanted metabolism and fast synthetic method (usually in single half-life of the radioisotope).
PET imaging systems have been developed also for small animals enhancing significantly basic research. Modern micro-PET instrumentation (resolution < 1 mm) is rapidly expanding the use of non-invasive PET imaging techniques in basic research. These advances have been progressively translated to human studies (Herschman, 2003; Liang et al., 2007; Lancelot and Zimmer, 2010). PET imaging offers tools to evaluate a great variety of molecular aspects of MS and neurodegeneration in animal models as well as in clinics (see Figures 3, 4).
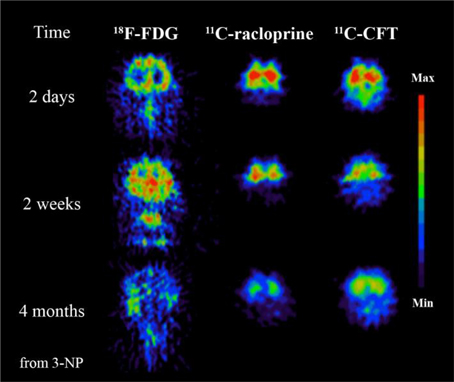
Figure 3. 3-Nitropropionic acid (3-NP, a naturally occurring plant toxin and mycotoxin) could be involved to the development of MS. This study demonstrates the advantages of PET imaging where specific tracers can be used to reveal different time dependent neurochemical processes. In this case significant decrease of glucose metabolism imaged by 18F-FDG, decrease of dopamine D2 receptor function imaged by 11C-raclopride and decrease of dopamine transporter function imaged with 11C-CFT follow after 3-NP administration. Modified from Brownell et al. (2004).
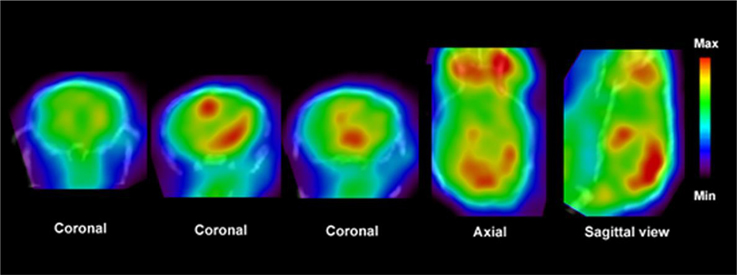
Figure 4. PET images show distribution of [11C]PBR28 in the brain of an EAE mouse model. Fused CT images show the boundaries of the skull. Enhanced accumulation of [11C]PBR28 in the hind brain and cerebellum is an indication of regionally activated microglia. Modified from Radu et al. (2007) and Arsenault et al. (2014).
Preclinical Models for MS
Several animal models are used to study mechanisms of disease pathogenesis relevant for MS (Furlan et al., 2009; Denic et al., 2011; Ransohoff, 2012). Experimental autoimmune encephalomyelitis (EAE) is the most vividly used animal model especially to study the inflammation aspects of MS. In this model, rodents are immunized with myelin antigens to activate peripheral antigen specific T-cells, which travel to CNS and induce formation of demyelinating lesion (Baxter, 2007; Constantinescu et al., 2011). Based on the hypothesis that viral infections may cause MS, virus-induced demyelination animal models are also used to study the disease (Gilden, 2005; Owens et al., 2011; Tselis, 2012). A disadvantage of this model is that the experimental disease manifests months after the initial infection (Olson et al., 2001; Fatima et al., 2010). Demyelination and spontaneous remyelination processes relevant to MS are predominantly studied using toxin-induced models (Blakemore and Franklin, 2008). The induction with copper chelating agent, Cuprizone [oxalic acid bis(cyclohexylidene hydrazide)], is one of the frequently used methods, since it is highly reproducible, relatively simple, induces fast demyelination, and the model has spontaneous remyelination after halting the toxin exposure (Torkildsen et al., 2008; Kipp et al., 2009). The focal demyelination lesions are commonly induced with ethidium bromide and lysolecithin (Woodruff and Franklin, 1999). The small size of the disease model increases the technical aspects for imaging technology. In the following chapters we will discuss the current tracers designed to detect the main pathological features of MS.
PET Imaging of Axonal Degeneration
The complex network of conditions leading to neuroaxonal degeneration and neuronal loss contribute the permanent disability related to MS pathology (Friese et al., 2014). Even though during the earlier days of MS research, axonal loss was considered to be late-occurring, it is now discovered to happen also in the early stages of MS (Trapp and Nave, 2008; Trapp and Stys, 2009). In the earlier phases of the disease axonal damage can occur acutely in the new inflammatory lesions. Whereas later in the disease progression axonal damage is usually related to chronic and demyelinated regions and there is only little if any active inflammation present (Criste et al., 2014). In addition, there is growing amount of evidence from both MRI and histological studies proposing that the axonal degeneration contributes to the development of clinical disability (Edgar and Nave, 2009; Nave, 2010). These interesting facts further highlight the need of specific markers for imaging of disease stage.
Currently, the most promising marker for neuronal integrity is benzodiazepine site on the GABAA receptor (Sigel and Buhr, 1997). Flumazenil, antagonist for benzodiazepine site, has been already labeled with 18F and 11C (Suzuki et al., 1985; The MICAD Research Team, 2004). Interestingly, in MS patients the axonal reduction has been demonstrated with the use of [11C]flumazenil (Barkhof et al., 2009). Furthermore, it has been shown that focal brain inflammation causes reduced GABAA mediated inhibition in neurons (Rossi et al., 2012). In addition, the inhibition is also induced in gray matter in the acute relapsing phases of MS (Rossi et al., 2012). Rossi et al. suggest that neurodegeneration in white and gray matter lesions are accompanied by a loss of GABAA receptors. PET could visualize this with radiolabeled flumazenil. However, this strategy remains yet to be tested.
Another cell type, which suffers from axonal loss during MS, is cholinergic neurons (D'Intino et al., 2005). Degradation of these neurons can, at least party, contribute to the cognitive impairment of the MS patients (Kooi et al., 2011). Interestingly, when assessing the acetylcolinesterase (AChE) activity by [11C]MP4A (11C-methyl-4-piper-idinylpropionate), an inverse correlation with the activity of AChE and cognitive impairment was observed in MS patients (Virta et al., 2011). This result is contradicting the demonstrated positive response seen in MS patients with AChE inhibitors (Krupp et al., 2004; Tsao and Heilman, 2005). However, it has been hypothesized that the controversial results with increased AChE expression might be due to induction by inflammatory response in glial cells (Virta et al., 2011).
In addition, the reduction of glucose metabolism in the degenerated regions has shown correlation between disease activity, hypo-metabolism and specific cognitive functions during the MS progression (Bakshi et al., 1998). [18F]FDG has some valuable characteristics for monitoring cognitive and mental dysfunctions associated with MS (Paulesu et al., 1996; Zarei, 2003; Buck et al., 2012; Colasanti et al., 2014).
PET Imaging of Demyelination
Demyelination, the pathological removal of myelin sheaths surrounding the axons, has been thought to be an integral part of axonal degeneration, as chronic CNS demyelination has been demonstrated to lead to axonal pathology and degeneration (Wilkins et al., 2010). However, these two events can happen independently from one another as axonal degeneration has been demonstrated to occur without myelin loss (Nave, 2010) and recently it has been demonstrated that the loss of myelin does not necessarily lead to axonal degeneration (Smith et al., 2013).
Classically in MS, demyelination is thought to cause the axonal dysfunction and disease-related pathogen conditions (Lucchinetti et al., 2000). On the other hand, spontaneous remyelination, executed by oligodendrocytes that mature from oligodendrocyte precursor cells, may occur following demyelination, presumably allowing a partial, if not complete, recovery from disability (Brück, 2005; Compston and Coles, 2008). Both adaptive and innate immune systems control the fine balance between demyelination and remyelination during MS and determine the outcome of the disease (Zhang et al., 2013). However, recently researchers have demonstrated early loss of both neurons and oligodendrocytes, leading to the question whether inflammatory demyelination is primary or secondary in the disease process of MS (Trapp and Nave, 2008). Remyelination is usually seen to occur in the early phases of the disease, whereas in the later phases it fails to recover the demyelinated areas leading to chronic demyelinated lesions (Chang et al., 2002; Kuhlmann et al., 2008).
Several tracers have been developed to target the β-sheet structures of intact myelin (Wu et al., 2006; Mallik et al., 2014). The first tracer, [11C]BMB (1,4-bis(p-aminostyryl)-[11C]2-methoxy benzene), had significant off-target affinity toward white and gray matter (Stankoff et al., 2006). Some of these downsides have been overcome with Congo red derivatives (e.q. [11C]CIC, Case Imaging Compound) and thioflavine-T derivatives (e.q. [11C]PIB, N-methyl-[11C]2-(4′-methylaminophenyl)-6-hydroxybenzothyazole). Moreover, these tracers have more reliable production and BBB penetration (Wang et al., 2009; Stankoff et al., 2011). Recent comparisons between these series and new [11C]MeDAS, (N-[11C]methyl-4,4′-diaminostilbene) tracers prefer the latter compound as the most promising ligand so far to detect MS-like lesions and spinal cord imaging (de Paula Faria et al., 2014b). [11C]MeDAS has been successfully used to image acute focal neuroinflammation in the brain, lyso-phosphatidyl choline induced focal demyelination in the spinal cord and EAE rodent models of MS (Wu et al., 2013). Furthermore, [11C]MeDAS was also able to highlight both demyelination and remyelination processes in cuprizone mouse model (de Paula Faria et al., 2014b). Interestingly, the uptake of [11C]MeDAS was not interfered by inflammation (Wu et al., 2013). The current literature suggest that [11C]MeDAS is the most preferred PET agent so far to highlight the lesions as well as the myelin content in the spinal cord in motor disability related MS (de Paula Faria et al., 2014a). To this point the only PET tracer used to image myelin in MS patients is [11C]PIB, a tracer widely utilized to visualize β-amyloid plaques in Alzheimer's disease (Stankoff et al., 2011; Zhang et al., 2014).
Altogether, PET imaging of myelin integrity shows great potential in animal models of MS. It is interesting to validate these methods in patients, especially now that new remyelination therapies are introduced in clinical trials (Brugarolas and Popko, 2014).
PET Imaging of Microglial Activation
Neuroinflammation is a common characteristic of numerous neurodegenerative disorders, including MS (Glass et al., 2010). Reactive states of astrocytes (astrogliosis), and microglia (microgliosis), as well as the infiltration of the lymphocytes are the hallmarks of neuroinflammation (Carson et al., 2006). Although factors inducing inflammation vary between CNS related diseases, there is evidence that convergence mechanisms are accountable for the sensing, transduction, and amplification of inflammatory processes that eventually lead to the production of neurotoxic mediators (Glass et al., 2010). In fact, neuroinflammation is a highly dynamic and complex process combining local and systemic reactions of multiple cell types, chemical signals, and signaling pathways to adaptive response for restoring tissue homeostasis (Medzhitov, 2008; Aguzzi et al., 2013; Naegele and Martin, 2014). In the following, we will discuss the PET tracers used to visualize microglial and astrocytic activation.
Microglia are of mesenchymal origin and constantly monitor the extracellular environment as well as interact closely with astrocytes and neurons (Yamasaki et al., 2014; Michell-Robinson et al., 2015). As macrophages in the periphery, microglia are the first line of defense against infections or insults in the CNS (Olson et al., 2001; Hanisch and Kettenmann, 2007; Nau et al., 2014). Upon activation, microglia acquire an amoeboid appearance and secrete pro-inflammatory molecules such as interleukin 1β, interferon γ, and tumor necrosis factor-α (TNFα) (Boche et al., 2013): a classically activated M1 state (Mills et al., 2000; Martinez and Gordon, 2014). The aim of the pro-inflammatory reaction is to clear the hazardous material and correct the inflicted damage (Gordon, 2003; Martinez et al., 2009). Usually, the pro-inflammatory reaction is down-regulated by the anti-inflammatory molecules (Tambuyzer et al., 2009; Scheller et al., 2011). In addition to pro-inflammatory molecules, microglia can release trophic and anti-inflammatory factors such as interleukins 4 and 10 as well as insulin-like growth factor 1 (Cherry et al., 2014). These factors are aimed to contribute to the repair and limitation of the inflammation (Mantovani et al., 2004; Hanisch and Kettenmann, 2007; Michelucci et al., 2009). Astrocytes and inflammatory T-cell subsets surrounding microglia influence the state of microglia, and determine whether they are releasing pro- or anti-inflammatory factors (Shih, 2006; Goverman, 2009; Mayo et al., 2012, 2014; Quintana et al., 2014).
The role of microglial activation in MS progression has remained enigmatic (Correale, 2014). However, several theories have been offered. The first theory suggests that inflammatory processes similar to those observed in RR-MS cause the brain damage (Kutzelnigg and Lassmann, 2014). However, during the progressive disease stages, a microenvironment is created within the brain favoring the homing and retention of inflammatory cells, finally resulting in the failure of disease-modifying therapies (Frischer et al., 2009). According to the second theory, MS starts out as an inflammatory disease and after several years, neurodegeneration, a process autonomous of inflammatory response, becomes the mechanism responsible for progression of the disease (Meuth et al., 2008; Kutzelnigg and Lassmann, 2014). Finally, MS could be seen primarily as a neurodegenerative disease, where inflammation occurs as a secondary response, augmenting and modifying progressive stages (Kassmann et al., 2007; Fitzner and Simons, 2010). Needless to say, these theories are not mutually exclusive. Furthermore, it has been postulated that the lack of understanding the exact microglial function during course of MS, has led to the absence of therapies for SP-MS (Correale, 2014). Altogether, this clearly demonstrates the need for a consensus and better understanding of microglial activation, which can only be achieved by using appropriate methodology.
Inflammation related PET studies in MS are traditionally focused on monitoring changes in glucose metabolism and the presence of activated microglia/macrophages in sclerotic lesions (Schiepers et al., 1997; Kiferle et al., 2011). [18F]FDG was recently used to evaluate the inflammation in the spinal cord in the EAE rat model (Buck et al., 2012). However, the basal uptake of glucose is elevated in the brain reducing the usability of [18F]FDG as a marker for brain lesions. Results of different stage patients indicate that [18F]FDG could be used to classify white matter lesions as either acute (hyper metabolism) or chronic (hypo metabolism) based on the glucose consumption (Paulesu et al., 1996; Dimitrakopoulou-Strauss et al., 2004; Buck et al., 2012). It is obvious that more specific markers are required to image the inflammation related metabolism in MS.
The majority of current PET tracers used to detect microglial activation utilize the expression of the peripheral benzodiazepine receptor (PBR), also known as the translocator protein TSPO (18 kDa) (Ryu et al., 2005; Ching et al., 2012). Translocator protein is expressed in the outer mitochondrial membrane. It was assumed to contribute through the cholesterol transportation into mitochondria regulating the rate of the synthesis of neurosteroids. However, these views have recently been challenged (Rupprecht et al., 2010; Selvaraj and Stocco, 2015). Gene-expression studies in the brain of rodents, primates, and humans have shown that TSPO expression is nearly absent in microglia patrolling the intact CNS parenchyma but rapidly increases in inflammation (Venneti et al., 2008; Ching et al., 2012). TSPO is highly expressed in activated microglia, in the choroid plexus and in reactive astrocytes, but its expression is globally low in the normal brain (Chauveau et al., 2008; Banati et al., 2014; Liu et al., 2014). These findings indicate that TSPO is a biomarker and an attractive target for the imaging microglial activation and reactive gliosis in cerebral inflammation (Rupprecht et al., 2010; Ching et al., 2012).
The isoquinoline carboxamide derivate PK11195 (N-butan-2-yl-1-(2-chlorophenyl)-N-methylisoquinoline-3-carboxamide), a nonbenzodiazepine ligand specifically binding to TSPO, has been widely used for its functional characterization and for the identification of its cellular origin in brain tissue (Banati et al., 1997, 2000; Chauveau et al., 2008). The issues regarding sensitivity and specificity of traditional PK11195 has been discussed (Venneti et al., 2008; Dickens et al., 2014; Boutin et al., 2015). Fortunately, recently developed radioligands such as DPA-714 (James et al., 2008; Chauveau et al., 2009), PBR28 (Imaizumi et al., 2007), PBR111 (Van Camp et al., 2010), SSR18075 (Chauveau et al., 2011), CLINME (Arlicot et al., 2008; Van Camp et al., 2010), and GE-180 (Dickens et al., 2014) have demonstrated better binding potency and bioavailability compared to the classical PK11195 and could overcome the problems of the classical tracers in MS and its models. A number of other TSPO targeting tracers have been developed to study the inflammation including but not limiting to DAA1106, FE-DAA1106, DPA-713, and vinpocetine, and reviewed by (Chauveau et al., 2008; James et al., 2008; Winkeler et al., 2010; Ciarmiello, 2011; Kiferle et al., 2011). Microglial activation was demonstrated in clinical MS studies with [11C]PK11195, unfortunately only in a limited number of patients (Banati et al., 2000; Debruyne et al., 2003; Versijpt et al., 2005; Vas et al., 2008). Radiotracer binding was increased in areas of acute and relapse-associated inflammation detected by classical Gd-DTPA enhanced T1-weighted MRI imaging (Rissanen et al., 2014). Interestingly, a significant increase in [11C]PK11195 binding was observed in activated microglia outside the histopathologically or MRI defined borders of MS plaques in both cerebral central gray-matter areas, which are not normally reported as sites of pathology in MS, as well as in normal appearing white matter (Banati et al., 2000; Debruyne et al., 2003). Unfortunately, 2nd generation TSPO targeting agents suffer from unexpected low binding status in over 30% of the population, which limits their use in clinics and demands genetic testing of the TSPO polymorphism. However, clinical studies have shown increased uptake with 18F-PBR111 and 11C-PBR28 in white matter lesions but not with all 2nd generation compounds like 18F-FEDAA1106. Additional studies are required to further investigate the specificity of these radiotracers for activated microglia over other activated glial cells. Overall, imaging of microglial activation in MS patients may serve as a complementary biomarker for disease progress (Abourbeh et al., 2012; Airas et al., 2015).
The type 2 cannabinoid receptor (CB2R) is part of the human endocannabinoid system and is involved in both central and peripheral inflammatory processes (Ehrhart et al., 2005; Pacher, 2006; Chiurchiù et al., 2014). CB2R can be found in immune cells, such as macrophages, perivascular T lymphocytes, astrocytes and reactive microglia, and it is thought to mediate anti-inflammatory as well as immunomodulatory effects (Docagne et al., 2008; Rodgers et al., 2013). 2-oxoquinoline and oxadiazolyle derivatives have been synthesized and radiolabeled with 11C and 18F, representing promising candidates for brain imaging in mice (Evens et al., 2009; Teodoro et al., 2013; Slavik et al., 2015). CB2R is almost undetectable in a healthy brain, whereas it is expressed in the activated glial cells (Stella, 2004; Cabral et al., 2008; Atwood and Mackie, 2010). This demonstrates that the effective PET ligands for CB receptors have the potential to act as biomarkers in the studies of pathophysiology of MS (Sanchez-Pernaute et al., 2008; Evens et al., 2009; Horti et al., 2010; Turkman et al., 2011; Vandeputte et al., 2011). In addition, new microglial targets, like P2X purinoceptor 7 (P2X7; Yiangou et al., 2006; Monif et al., 2009) and matrix metalloproteinases (Wagner et al., 2007; Iwama et al., 2011), have been explored for imaging of MS.
Overall, the benefits of PET contribute to the understanding of personalized status of MS patients, disease profiling, prognosis, and response, which are all combined in precision medicine. Specific biomarkers are the backbone for capturing the different aspects of MS heterogeneity, which could be useful for diagnosis, treatment stratification, and personalization of the therapeutic approach. Simplified, the precision medicine aims to provide the right drug with the right dose for the right indication in the right patient at the right time. Such as the case with the current 2nd generation TSPO markers, precision medicine relies on variability of genes, environment and life style of each person rather than on the data from large clinical trials. The customization of the treatment is based on the characterization of the genotype and phenotype induced effects on imaging in the individual patient. Biomedical imaging offers a great tool for mapping data from biomarkers, genomics, and physiology. There is a great interest for the monitoring of microglial activation in MS. However, the recent results with TSPO ligands suggest that the reactive astrocytes might increase the signal levels in MS (Lavisse et al., 2012). Since the role of reactive astrocytes in MS is recently of great interest, more specific markers are needed for reliable imaging of neuroinflammation (Rostami and Ciric, 2014; Zeis et al., 2015).
Imaging Astrocyte Activation
Astrocytes are one of the most abundant cell types in the CNS. They have complex function ranging from supporting the surrounding neurons to the regulation of synaptic activity and BBB integrity (Sofroniew and Vinters, 2010). Although astrocytes are not immune cells per-se they can in specific conditions, such as in CNS inflammation, exert both pro- and anti-inflammatory effects on microglia (Min et al., 2006; Farina et al., 2007; Sofroniew, 2015).
Astrocytes were regarded to be non-participating bystanders in MS, responding secondarily to insults by undergoing astrogliosis and producing a glial scar (Brosnan and Raine, 2013). However, since the T-cell mediated immunity has been strongly associated with MS there are several plausible means by which astrocytes could contribute to autoimmunity. Astrocytes may facilitate immune cell extravasation into the CNS by releasing chemokines. They can modulate the activity of innate immune cells, such as microglia and inflammatory monocytes recruited to the CNS, by boosting their ability to promote neurodegeneration. Finally, astrocytes also have direct neurodegenerative functions mediated by the production of TNFα and nitric oxide (NO). However, these actions can also represent potential mechanisms by which astrocytes could reduce inflammation to promote remyelination (Claycomb et al., 2013).
Activation of glial cells is a common feature of MS as discussed earlier. Acetate is reported to accumulate into astrocytes and the [11C]acetate accumulation is increased in MS lesions (Takata et al., 2014). However, brain uptake of [11C]acetate is insufficient for obtaining a quantitative image of astrocytes' oxidative metabolism (Okada et al., 2013). To overcome this drawback benzyl [11C]acetate has been synthesized (Okada et al., 2013). Although the quantitative measurement remains under development, acetate is specific for astrocyte lipid metabolism (Brekke et al., 2015) and could serve as a marker for activated astrocyte metabolism in MS (Takata et al., 2014). In addition, 18F labeled derivative of acetate could increase the signal to noise ratio compared to 11C analog. It is expected that this tracer will be used in MS (Ponde et al., 2007).
One critical function of astrocytes is acting as sentinels and monitoring the BBB, a complex barrier composed of endothelial cells, astrocytes, pericytes, and myeloid cells such as perivascular macrophages and mast cells (Abbott et al., 2006). BBB functions as an anatomical mechanism for the highly selective passage of water, ions, nutrients, and cells from peripheral circulation into and out of the brain parenchyma (Abbott et al., 2006; Daneman and Rescigno, 2009; Larochelle et al., 2011). Under inflammatory conditions the BBB opens and it enables higher leukocyte passage into the CNS (Claycomb et al., 2013). Astrocytes play a critical role in shielding and protecting the CNS under inflammatory conditions (Voskuhl et al., 2009). Furthermore, astrocyte ablation has been shown to cause enhanced monocyte, but not T-cell, migration into the CNS (Toft-Hansen et al., 2011). To date, there is no clinically relevant PET tracer for BBB integrity, although several candidates have been proposed [13N]glutamate, [82Rb]Cl, or 68Gallium-ethylene-diamine-tetra-acetic acid (EDTA) (Saha et al., 1994; Wunder et al., 2012).
Imaging of astrocyte function is still a young field and it needs development of suitable imaging ligands. Astrocytes are involved in several neurological diseases and the main obstacle using imaging techniques has been the lack of proper tracers.
Targets for PET Imaging in MS
The recent increased availability of PET tracers to assess activated glial cells, disease pathology, and signaling pathways give PET a promising role in MS research. Since the underlying mechanisms of neurodegeneration and regeneration are still poorly understood the non-invasive techniques will enhance understanding these processes to develop better drug candidates, early diagnosis, and reliable monitoring of the treatment response. Several possible targets for PET imaging in MS are discussed in this section. These candidates may serve as more specific targets and may reveal some of the missing links in MS treatment and pathology, especially in the glial cell mediated actions.
Neuroinflammation is a dynamic and complex adaptive response process, which involves multiple cell types and various signaling routes, pathways, and receptors (Singhal et al., 2014). As discussed earlier, neuroinflammation can be imaged in MS. However, new tracers are needed to gain practical importance in clinics. The greatest potential may lay in the imaging of the dynamic interplay between neuroinflammation and the molecular mechanisms that contributes to the disease progression. The recent findings support three major neuroinflammation components in MS: astrogliosis, cytokine elevation, and significant changes in specific proteins, which offer a great variety of specific targets for imaging purposes.
TNF-α is associated with self-propagation of neuroinflammation and the expression of TNF-α is elevated in MS patients (Rossi et al., 2014). Microglia, inflammatory monocytes recruited to the CNS and astrocytes are major sources of TNF-α in CNS, interestingly proposing TNF-α expression as a marker in MS (Welser-Alves and Milner, 2013). PET tracers, like [64Cu]DOTA-etanercept and [64Cu]pegylated dimeric c(RGDyK), have been developed to target TNF-α in both acute and chronic inflammation in mice (Cao et al., 2007). TNF-α may be a target for MS imaging in the future. Overall, cytokines are highly related to oxidative stress in the brain (Di Penta et al., 2013). The expression of inducible nitric oxide synthase (iNOS) is increased in MS lesions, increasing generation of NO as well as reactive nitrogen species like peroxynitrite (Kröncke et al., 1998; Ortiz et al., 2013). The accumulation of these molecules induces lipid peroxidation, resulting in damage to DNA and neuronal degeneration (Haider et al., 2011).
In the healthy CNS tissues, the expression levels of iNOS are low but become highly expressed in astrocytes and neurons during inflammation (Saha and Pahan, 2006). In chronic pathology the reactive nitrogen species produced by iNOS are not efficiently eliminated, which leads to cellular dysfunctions (Fulda et al., 2010). The number of tracers for iNOS is minimal and the current [18F]NOS (6-(1/2)(2-[18F]fluoropropyl)-4-methylpyridin-2-amine) needs further modification and improvement. Importantly, the feasibility of iNOS PET imaging has been demonstrated in human inflammation (Herrero et al., 2012; Huang et al., 2015). In addition, active iNOS enzyme has been demonstrated in astrocytes in both acute and chronic active MS lesions and might therefore be an interesting target for imaging purposes (Liu et al., 2001).
The expression of another proinflammatory cytokine mediator, cyclooxygenase-2 (COX-2), is extensively increased in MS lesions and it has been tightly linked to increased iNOS expression (Rose et al., 2004). Furthermore, COX-2 expression was found in the cells expressing microglial marker, highlighting the importance of immune-derived cells. COX-2 has also been suggested to act as a link between neuroinflammation and glutamate mediated neuronal excitotoxicity (Kelley et al., 1999). These facts clearly indicate a need for methods to detect COX-2 expression. PET tracers for COX-2 have been developed, but the in vivo imaging properties have not been very effective (de Vries et al., 2003, 2008; Takashima-Hirano et al., 2011; Ji et al., 2013). The most promising COX-2 tracer so far is [11C]Rofecoxib (4-(4-methylsulfonylphenyl)-3-phenyl-5H-furan-2-one), demonstrating in vitro usability, but lacking necessary affinity for in vivo studies (Ji et al., 2013). Nevertheless, cyclooxygenases is presently an important target for PET tracer development.
Besides stimulating production of reactive oxygen species, cytokines are known to modulate the lipid metabolism and increase the production of neurodegeneration promoters such as eicosanoids and ceramides (Adibhatla and Hatcher, 2007). As previously mentioned, acetate is converted into fatty acid by acetyl-CoA synthase and [11C]acetate PET has proven useful for imaging in several diseases (Grassi et al., 2012). In addition, acetate is preferentially absorbed into astrocytes by the monocarboxylate transporter, which is overexpressed in MS (Nijland et al., 2014). Moreover, bioactive lipids exert significant effects on inflammation during autoimmunity targets or regulators of the immune response (Rinaldi et al., 2009). In addition, the appearance of cytosolic lipid synthesis is one the corner stones of macrophage foam cell formation (Matthäus et al., 2012). The intracellular concentrations of different individual lipids or the receptors involved the synthesis of particular bioactive lipids could reveal novel aspects of the disease progression (Mayo et al., 2014). Recently β-1,4-galactosyltransferase 6 (B4GALT6) was found to promote astrocyte activation and neuroinflammation during chronic EAE. The lactosylceramide (LacCer) synthesized by B4GALT6 in astrocytes controls the production of chemokines and cytokines, such as CCL2 and GM-CSF, which regulates the recruitment and activation of inflammatory monocytes and microglia and clearly highlights the importance of a specific lipid profile for disease progression (Mayo et al., 2014).
In summary, comprehensive profiling of lipid metabolism and the BBB function are likely to reveal new targets for therapeutic intervention in MS as well as for other neurological disorders where astrocyte activation contributes to disease pathology (Neu and Woelk, 1982; Pannu et al., 2005; Adibhatla and Hatcher, 2007; Wheeler et al., 2008; Kooij et al., 2012; Prüss et al., 2013). The imaging of specific bioactive lipids, receptors or enzymes that are involved in their synthesis may be novel targets for PET imaging.
Biomarkers for the Early Phases of MS
In the search of better treatments for MS, cerebrospinal fluid (CSF) biomarkers have been used to identify high risk MS patients as well as patients with other neuronal disorders. Recently, high levels of astrocyte derived chitinase 3-like protein 1 (CHI3L1) were associated with the strong prediction of MS. This finding further demonstrates the increased importance of astrocyte activation and the specific role of astrocyte as a source for biomarkers in MS, already at the early disease phase.
The activated lipid metabolism in astrocytes demands increased acetate and lipid transportation (Lev, 2012). ATP and glutamate stimulation can significantly enhance the dynamin-independent endocytosis and their receptors control the microglial physiology and pathology (Jiang and Chen, 2009). For example ATP related purinergic receptors control microglial cytokine release among several other functions (Sperlágh and Illes, 2007). Moreover, purinergic pathways regulate neuroinflammation (Burnstock, 2008). The increasing evidence suggests that the P2X7 receptor is an interesting neuroinflammation associated molecular target (Lister et al., 2007; Monif et al., 2009; Gandelman et al., 2010). PET tracers have been developed to image P2X7 receptor, [11C]A-740003 (N-[1-[[(Cyanoamino)(5-quinolinylamino)methylene]amino]-2,2-dimethylpropyl]-3,4-dimethoxybenzeneacetamide) and [11C]GSK1482160 ((S)-N-(2-chloro-3-(trifluoromethyl)benzyl)-1-[11C]methyl-5-oxopyrro-lidine-2-carboxamide; Janssen et al., 2014; Gao et al., 2015). Purinergic system might serve as a sensitive target for MS imaging.
Furthermore, the adenosine receptors, whose expression is modulated by microglial activation, moderate immune function (Haskó et al., 2008; Orr et al., 2009; Domercq et al., 2013; Luongo et al., 2014). Especially A2A receptors are up-regulated during inflammation (Rissanen et al., 2013). It is clear that adenosine signaling play a significant role in MS as a neuromodulator and the clinical studies with [11C]TMSX (7-methyl-[11C]-(E)-8-(3,4,5-Trimethoxystyryl)-1,3,7-trimethylxanthine) PET will likely open new perspective to develop new tracers to this target in the future (Rissanen et al., 2015).
In addition, the cholinergic system shows decreased function in MS patients (Kooi et al., 2011). PET imaging studies of cholinergic activity may define which patient will respond to the treatment which will further increase the knowledge of MS. A similar approach has been already used in Alzheimer's disease using radiolabeled choline derivatives and these techniques could be easily transferred to MS clinical research (Volkow et al., 2001; Rinne, 2003; Kooi et al., 2011).
The cannabinoid receptors CB2 are expressed in very low levels in a healthy brain, but the expression increases during microglial activation (Benito et al., 2007). CB2 is an interesting target for PET imaging in MS models especially with [11C]A836339 (2,2,3,3-Tetramethylcyclopropanecarboxylic acid [3-(2-[11C]methoxyethyl)-4,5-dimethyl-3H-thiazol-(2Z)-ylidene]amide) and [11C]NE40. Recently there has been great progress in developing new tracers for this target (Docagne et al., 2008; Horti et al., 2010; Evens et al., 2011, 2012; Slavik et al., 2015; Yrjölä et al., 2015).
During inflammation microglia will release glutamate in response to the production of reactive oxygen species (ROS; Bal-Price and Brown, 2001; Brown and Neher, 2010; Takaki et al., 2012). The cysteine-glutamate exchange modulates the release of ROS and cytokines which impairs the function of glutamate transporters and leads to increased extracellular glutamate levels as well as excitotoxicity (Rao et al., 2003; Matute et al., 2006). Metabotropic glutamate receptors (mGluRs) are transmembrane proteins that are expressed in glial cells and play a pivotal role in cell function and glial-neuronal co-operation (Kritis et al., 2015). Immunohistochemical analyses have revealed that mGluR5 is expressed in reactive astrocytes surrounding the MS lesion site and the expression is higher than in non-activated astrocytes (Geurts et al., 2003). In addition, the activation of mGluR5 reduced the microglial activation in an inflammation model (Byrnes et al., 2009; Loane et al., 2009). During the last 15 years the subtype selective allosteric modulators have been identified for different mGluRs (see Figure 5; Zhang and Brownell, 2012). Many PET tracers have been synthesized by radiolabeling the derivatives of MPEP and MTEP and to date over 15 mGluR5 targeting PET ligands have been reported (Mu et al., 2010; Zhang and Brownell, 2012). Tracers like [18F]FPEB ((3-[18F]Fluoro-5-(2-pyridinylethynyl)benzonitrile) have been already developed for automated synthesis and evaluated in humans (Lim et al., 2014).
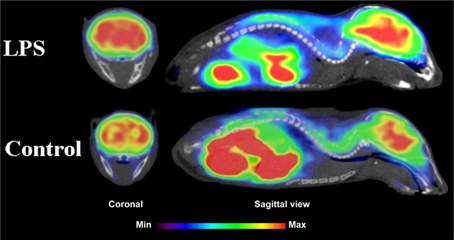
Figure 5. Coronal and sagittal sections of fused PET and CT images in 10 days old pups of mice. PET studies using [18F]FPEB show enhanced mGluR5 expression in the brain of the pups, whose mothers were injected with LPS compared to saline injection (control). Coronal slices show highest accumulation in the hippocampal area of the mouse, whose mother had LPS administration. Sagittal images show spine based on CT images and high accumulation of [18F]FPEB in the brain and gut. Modified from Arsenault et al. (2014).
Monoamine oxidase type B located in the outer membrane of mitochondria and is expressed in astrocytes, where its activity is increased in neurodegenerative diseases (Mallajosyula et al., 2008; Veitinger et al., 2014). It catalyzes the deamination reaction thus modulating neurotransmitter concentrations and has been a major target for drug development, especially in movement related diseases (Talati et al., 2009; Deftereos et al., 2012). The 11C-L-deprenyl indicates increased monoamine oxidase type B content in reactive and proliferating astrocytes in AD (Gulyás et al., 2011). Results from the MS patients studies are likely to be published soon (Hurley, 2015).
The above exploration shows that the combined PET imaging of activated microglia and astrocytes is presently of special interest in MS research.
PET as a Tool for Precision Medicine in MS
More specific features of MS lesions have been described in parallel with the identification of body fluid markers such as CHI3L1 and B4GALT6. Recent progress with biomarkers and imaging tracers suggests that precision medicine is becoming a reality in MS. The prevalence of MS is increasing and there is relatively little data available to personalize the treatments and increase the cost effectiveness. Sophisticated tools are needed to handle the complex data to obtain more detailed insight of the clinical status of the patient's condition. The combined information from various biomarkers and imaging studies can be used to predict the disease evolution individually. PET imaging can provide precise data for the cross-roads of multiple fields, like biomedical imaging, pharmacology, neurology, genomics etc. Achieving precision medicine in MS requires high quality data, large samples, and consistent interdisciplinary approach.
Conclusion
Inflammation and glial activation play an important role in numerous neurodegenerative diseases, such as Alzheimer's disease, Parkinson disease, amyotrophic lateral sclerosis and MS. Although factors inducing inflammation vary between diseases, there is evidence of greatly converging mechanisms for the sensing, transduction, and amplification of inflammatory processes that eventually lead to the production of neurotoxic mediators. PET imaging provides a powerful method for dynamic imaging of these events. The full potential of PET is not yet recognized, mainly due to the lack of validated tracers; the complicated and costly process of validating new tracers needs partnerships, human resources, expertise, funding, and access to patients, but it is something that needs to be focused on to obtain the essential information of the biological processes in disease pathology. This will ultimately produce more reliable diagnosis, better treatments and effective prevention methods for MS. The role of PET imaging will increase in clinics, when onsite cyclotrons, the development of new tracers, and imaging equipment become available.
[18F]-FDG is still the most extensively used PET imaging tracer for inflammation even though it tends to produce controversial results. New imaging tracers for TSPO ([11C]PK11195, [11C]PBR28, etc.) have gained a great interest for detection of inflammation and evaluation of therapy. Using these new tracers, PET imaging has greatly improved our understanding of the mechanism of inflammation and increased the diagnostic specificity and accuracy of inflammation. As summarized in Table 2, various radiopharmaceutical approaches have been developed for PET imaging to detect inflammation, including biomarkers targeting to specific receptors and lipid metabolism.
So far, the clinical PET studies in MS are limited to evaluation of two biological processes: glucose metabolism and inflammation. It is clear that the use of combined PET/MR imaging is increasing also in MS research. One of the main interests is to develop combined imaging markers and methods for MS pathology to stage, cell type and record activity related changes in lesions. However, presently PET imaging is relatively expensive and it also requires sophisticated quantification, which demands special software and skilled operators. MS is a complex disease, which remains difficult to treat before more specific disease mechanisms are revealed. PET research community is looking for the first ligands to be recommended for routine clinical practice in MS diagnosis and follow up of therapy. PET has already shown to be one of the most sophisticated, sensitive, reliable, effective, and safest tools for the monitoring of several cancers in clinics and there is no reason why it could not be same in the neurodegenerative disorders as well. Clinical imaging and research modalities should be combined to expand the knowledge of clinical findings, genetics, phenotyping, pharmacology, and drug targeting. Advanced imaging technologies, including PET, could be used to reveal the causes of MS rather than concentrating on correlations. MS is a complex and heterogeneous disease, which could benefit from precision medicine in the future. The genomic approach can be used to individualize the imaging data as presently done with 2nd generation TSPO markers ([11C]PBR28 etc.). Astrocyte activation and their ability to modulate the complex neuronal network and inflammation related pathways have a great potential to reveal disease stage specific markers for personalized medicine. Despite the astrocyte related research in MS is still in early stages, and the recent promising results suggest new techniques to diagnose, monitor and treat this cruel disease. The combined pathogenic characteristics of MS are still unknown and the key to prevent and cure this devastating disease is still waiting for discovery.
Author Contributions
PP did literature search, prepared tables and participated writing. MJ participated literature search, writing and composing the manuscript. FQ did critical evaluation of the manuscript. AB participated writing, preparation of figures, and final evaluation of the manuscript.
Conflict of Interest Statement
The authors declare that the research was conducted in the absence of any commercial or financial relationships that could be construed as a potential conflict of interest.
Acknowledgments
AB was supported by the grant NIBIB-R01EB012864. PP was supported by the Orion Farmos Research Foundation and Kuopio University Foundation, Finland. MJ was supported by the Sigrid Juselius Foundation, Finnish MS-Society, Orion Farmos Research Foundation and Saastamoinen Foundation, Finland.
References
Abbott, N. J., Rönnbäck, L., and Hansson, E. (2006). Astrocyte-endothelial interactions at the blood-brain barrier. Nat. Rev. Neurosci. 7, 41–53. doi: 10.1038/nrn1824
Abourbeh, G., Thézé, B., Maroy, R., Dubois, A., Brulon, V., Fontyn, Y., et al. (2012). Imaging microglial/macrophage activation in spinal cords of experimental autoimmune encephalomyelitis rats by positron emission tomography using the mitochondrial 18 kDa translocator protein radioligand [18F]DPA-714. J. Neurosci. 32, 5728–5736. doi: 10.1523/JNEUROSCI.2900-11.2012
Adibhatla, R. M., and Hatcher, J. F. (2007). Role of lipids in brain injury and diseases. Future Lipidol. 2, 403–422. doi: 10.2217/17460875.2.4.403
Aguzzi, A., Barres, B. A., and Bennett, M. L. (2013). Microglia: scapegoat, saboteur, or something else? Science 339, 156–161. doi: 10.1126/science.1227901
Airas, L., Dickens, A. M., Elo, P., Marjamaki, P., Johansson, J., Eskola, O., et al. (2015). In vivo PET imaging demonstrates diminished microglial activation after fingolimod treatment in an animal model of multiple sclerosis. J. Nucl. Med. 56, 305–310. doi: 10.2967/jnumed.114.149955
Ametamey, S. M., Kessler, L. J., Honer, M., Wyss, M. T., Buck, A., Hintermann, S., et al. (2006). Radiosynthesis and preclinical evaluation of 11C-ABP688 as a probe for imaging the metabotropic glutamate receptor subtype 5. J. Nucl. Med. 47, 698–705.
Anderson, D. W., Ellenberg, J. H., Leventhal, C. M., Reingold, S. C., Rodriguez, M., and Silberberg, D. H. (1992). Revised estimate of the prevalence of multiple sclerosis in the United States. Ann. Neurol. 31, 333–336. doi: 10.1002/ana.410310317
Arlicot, N., Katsifis, A., Garreau, L., Mattner, F., Vergote, J., Duval, S., et al. (2008). Evaluation of CLINDE as potent translocator protein (18 kDa) SPECT radiotracer reflecting the degree of neuroinflammation in a rat model of microglial activation. Eur. J. Nucl. Med. Mol. Imaging 35, 2203–2211. doi: 10.1007/s00259-008-0834-x
Arsenault, D., Zhu, A., Gong, C., Kil, K.-E., Kura, S., Choi, J.-K., et al. (2014). Hypo-anxious phenotype of adolescent offspring prenatally exposed to LPS is associated with reduced mGluR5 expression in hippocampus. Open J. Med. Psychol. 3, 202–211. doi: 10.4236/ojmp.2014.33022
Atwood, B. K., and Mackie, K. (2010). CB2: a cannabinoid receptor with an identity crisis. Br. J. Pharmacol. 160, 467–479. doi: 10.1111/j.1476-5381.2010.00729.x
Bakshi, R., Miletich, R. S., Kinkel, P. R., Emmet, M. L., and Kinkel, W. R. (1998). High-resolution fluorodeoxyglucose positron emission tomography shows both global and regional cerebral hypometabolism in multiple sclerosis. J. Neuroimaging 8, 228–234. doi: 10.1111/jon199884228
Bal-Price, A., and Brown, G. C. (2001). Inflammatory neurodegeneration mediated by nitric oxide from activated glia-inhibiting neuronal respiration, causing glutamate release and excitotoxicity. J. Neurosci. 21, 6480–6491.
Banati, R. B., Middleton, R. J., Chan, R., Hatty, C. R., Wai-Ying Kam, W., Quin, C., et al. (2014). Positron emission tomography and functional characterization of a complete PBR/TSPO knockout. Nat. Commun. 5:5452. doi: 10.1038/ncomms6452
Banati, R. B., Myers, R., and Kreutzberg, G. W. (1997). PK (‘peripheral benzodiazepine’)–binding sites in the CNS indicate early and discrete brain lesions: microautoradiographic detection of [3H]PK11195 binding to activated microglia. J. Neurocytol. 26, 77–82. doi: 10.1023/A:1018567510105
Banati, R. B., Newcombe, J., Gunn, R. N., Cagnin, A., Turkheimer, F., Heppner, F., et al. (2000). The peripheral benzodiazepine binding site in the brain in multiple sclerosis: quantitative in vivo imaging of microglia as a measure of disease activity. Brain J. Neurol. 123 (Pt 11), 2321–2337. doi: 10.1093/brain/123.11.2321
Barkhof, F., Calabresi, P. A., Miller, D. H., and Reingold, S. C. (2009). Imaging outcomes for neuroprotection and repair in multiple sclerosis trials. Nat. Rev. Neurol. 5, 256–266. doi: 10.1038/nrneurol.2009.41
Barkhof, F., and Filippi, M. (2009). Multiple sclerosis: MRI—the perfect surrogate marker for multiple sclerosis? Nat. Rev. Neurol. 5, 182–183. doi: 10.1038/nrneurol.2009.31
Baxter, A. G. (2007). The origin and application of experimental autoimmune encephalomyelitis. Nat. Rev. Immunol. 7, 904–912. doi: 10.1038/nri2190
Benito, C., Romero, J. P., Tolón, R. M., Clemente, D., Docagne, F., Hillard, C. J., et al. (2007). Cannabinoid CB1 and CB2 receptors and fatty acid amide hydrolase are specific markers of plaque cell subtypes in human multiple sclerosis. J. Neurosci. 27, 2396–2402. doi: 10.1523/JNEUROSCI.4814-06.2007
Blakemore, W. F., and Franklin, R. J. M. (2008). Remyelination in experimental models of toxin-induced demyelination. Curr. Top. Microbiol. Immunol. 318, 193–212. doi: 10.1007/978-3-540-73677-6_8
Boche, D., Perry, V. H., and Nicoll, J. A. (2013). Review: activation patterns of microglia and their identification in the human brain. Neuropathol. Appl. Neurobiol. 39, 3–18. doi: 10.1111/nan.12011
Bodini, B., Louapre, C., and Stankoff, B. (2015). Advanced imaging tools to investigate multiple sclerosis pathology. Presse Medicale. 44, e159–e167. doi: 10.1016/j.lpm.2015.02.011
Boutin, H., Chauveau, F., Thominiaux, C., Grégoire, M.-C., James, M. L., Trebossen, R., et al. (2007a). 11C-DPA-713: a novel peripheral benzodiazepine receptor PET ligand for in vivo imaging of neuroinflammation. J. Nucl. Med. 48, 573–581. doi: 10.2967/jnumed.106.036764
Boutin, H., Chauveau, F., Thominiaux, C., Kuhnast, B., Grégoire, M.-C., Jan, S., et al. (2007b). In vivo imaging of brain lesions with [(11)C]CLINME, a new PET radioligand of peripheral benzodiazepine receptors. Glia 55, 1459–1468. doi: 10.1002/glia.20562
Boutin, H., Murray, K., Pradillo, J., Maroy, R., Smigova, A., Gerhard, A., et al. (2015). 18F-GE-180: a novel TSPO radiotracer compared to 11C-R-PK11195 in a preclinical model of stroke. Eur. J. Nucl. Med. Mol. Imaging 42, 503–511. doi: 10.1007/s00259-014-2939-8
Brekke, E., Morken, T. S., and Sonnewald, U. (2015). Glucose metabolism and astrocyte-neuron interactions in the neonatal brain. Neurochem. Int. 82, 33–41. doi: 10.1016/j.neuint.2015.02.002
Brody, A. L., Okita, K., Shieh, J., Liang, L., Hubert, R., Mamoun, M., et al. (2014). Radiation dosimetry and biodistribution of the translocator protein radiotracer [(11)C]DAA1106 determined with PET/CT in healthy human volunteers. Nucl. Med. Biol. 41, 871–875. doi: 10.1016/j.nucmedbio.2014.07.004
Brosnan, C. F., and Raine, C. S. (2013). The astrocyte in multiple sclerosis revisited. Glia 61, 453–465. doi: 10.1002/glia.22443
Brown, G. C., and Neher, J. J. (2010). Inflammatory neurodegeneration and mechanisms of microglial killing of neurons. Mol. Neurobiol. 41, 242–247. doi: 10.1007/s12035-010-8105-9
Brownell, A.-L. (2008). “Positron emission tomography (PET),” in Encyclopedia of Biomaterials and Biomedical Engineering, 2nd Edn., eds G. E. Wnek and G. L. Bowlin (Boca Raton, FL: CRC Press), 2314–2325.
Brownell, A.-L., Chen, Y. I., Yu, M., Wang, X., Dedeoglu, A., Cicchetti, C., et al. (2004). 3- Nitropropionic acid induced neurotoxicity – assessed by ultra high resolution PET with comparison to MRS. J. Neurochem. 89, 1206–1214. doi: 10.1111/j.1471-4159.2004.02408.x
Brownell, G. L, and Sweet, W. H. (1953). Localization of brain tumors with positron emitters. Nucleonics 11, 40–45.
Brück, W. (2005). Clinical implications of neuropathological findings in multiple sclerosis. J. Neurol. 252(Suppl. 3), iii10–iii14. doi: 10.1007/s00415-005-2011-5
Brugarolas, P., and Popko, B. (2014). Remyelination therapy goes to trial for multiple sclerosis. Neurol. Neuroimmunol. Neuroinflammation 1:e26. doi: 10.1212/nxi.0000000000000026
Buck, D., Forschler, A., Lapa, C., Schuster, T., Vollmar, P., Korn, T., et al. (2012). 18F-FDG PET detects inflammatory infiltrates in spinal cord experimental autoimmune encephalomyelitis lesions. J. Nucl. Med. 53, 1269–1276. doi: 10.2967/jnumed.111.102608
Burnstock, G. (2008). Purinergic signalling and disorders of the central nervous system. Nat. Rev. Drug Discov. 7, 575–590. doi: 10.1038/nrd2605
Byrnes, K. R., Stoica, B., Loane, D. J., Riccio, A., Davis, M. I., and Faden, A. I. (2009). Metabotropic glutamate receptor 5 activation inhibits microglial associated inflammation and neurotoxicity. Glia 57, 550–560. doi: 10.1002/glia.20783
Cabral, G. A., Raborn, E. S., Griffin, L., Dennis, J., and Marciano-Cabral, F. (2008). CB 2 receptors in the brain: role in central immune function. Br. J. Pharmacol. 153, 240–251. doi: 10.1038/sj.bjp.0707584
Cao, Q., Cai, W., Li, Z.-B., Chen, K., He, L., Li, H.-C., et al. (2007). PET imaging of acute and chronic inflammation in living mice. Eur. J. Nucl. Med. Mol. Imaging 34, 1832–1842. doi: 10.1007/s00259-007-0451-0
Carson, M. J., Cameron Thrash, J., and Walter, B. (2006). The cellular response in neuroinflammation: the role of leukocytes, microglia and astrocytes in neuronal death and survival. Clin. Neurosci. Res. 6, 237–245. doi: 10.1016/j.cnr.2006.09.004
Catana, C., Guimaraes, A. R., and Rosen, B. R. (2013). PET and MR imaging: the odd couple or a match made in heaven? J. Nucl. Med. 54, 815–824. doi: 10.2967/jnumed.112.112771
Chang, A., Tourtellotte, W. W., Rudick, R., and Trapp, B. D. (2002). Premyelinating oligodendrocytes in chronic lesions of multiple sclerosis. N. Engl. J. Med. 346, 165–173. doi: 10.1056/NEJMoa010994
Chauveau, F., Boutin, H., Van Camp, N., Dollé, F., and Tavitian, B. (2008). Nuclear imaging of neuroinflammation: a comprehensive review of [11C]PK11195 challengers. Eur. J. Nucl. Med. Mol. Imaging 35, 2304–2319. doi: 10.1007/s00259-008-0908-9
Chauveau, F., Boutin, H., Van Camp, N., Thominiaux, C., Hantraye, P., Rivron, L., et al. (2011). In vivo imaging of neuroinflammation in the rodent brain with [11C]SSR180575, a novel indoleacetamide radioligand of the translocator protein (18 kDa). Eur. J. Nucl. Med. Mol. Imaging 38, 509–514. doi: 10.1007/s00259-010-1628-5
Chauveau, F., Van Camp, N., Dollé, F., Kuhnast, B., Hinnen, F., Damont, A., et al. (2009). Comparative evaluation of the translocator protein radioligands 11C-DPA-713, 18F-DPA-714, and 11C-PK11195 in a rat model of acute neuroinflammation. J. Nucl. Med. 50, 468–476. doi: 10.2967/jnumed.108.058669
Cherry, J. D., Olschowka, J. A., and O'Banion, M. (2014). Neuroinflammation and M2 microglia: the good, the bad, and the inflamed. J. Neuroinflammation 11:98. doi: 10.1186/1742-2094-11-98
Ching, A. S. C., Kuhnast, B., Damont, A., Roeda, D., Tavitian, B., and Dollé, F. (2012). Current paradigm of the 18-kDa translocator protein (TSPO) as a molecular target for PET imaging in neuroinflammation and neurodegenerative diseases. Insights Imaging 3, 111–119. doi: 10.1007/s13244-011-0128-x
Chiurchiù, V., Lanuti, M., Catanzaro, G., Fezza, F., Rapino, C., and Maccarrone, M. (2014). Detailed characterization of the endocannabinoid system in human macrophages and foam cells, and anti-inflammatory role of type-2 cannabinoid receptor. Atherosclerosis 233, 55–63. doi: 10.1016/j.atherosclerosis.2013.12.042
Ciarmiello, A. (2011). Imaging of neuroinflammation. Eur. J. Nucl. Med. Mol. Imaging 38, 2198–2201. doi: 10.1007/s00259-011-1959-x
Claycomb, K. I., Johnson, K. M., Winokur, P. N., Sacino, A. V., and Crocker, S. J. (2013). Astrocyte regulation of CNS inflammation and remyelination. Brain Sci. 3, 1109–1127. doi: 10.3390/brainsci3031109
Colasanti, A., Guo, Q., Muhlert, N., Giannetti, P., Onega, M., Newbould, R. D., et al. (2014). In vivo assessment of brain white matter inflammation in multiple sclerosis with 18F-PBR111 PET. J. Nucl. Med. 55, 1112–1118. doi: 10.2967/jnumed.113.135129
Compston, A., and Coles, A. (2008). Multiple sclerosis. Lancet 372, 1502–1517. doi: 10.1016/S0140-6736(08)61620-7
Constantinescu, C. S., Farooqi, N., O'Brien, K., and Gran, B. (2011). Experimental autoimmune encephalomyelitis (EAE) as a model for multiple sclerosis (MS): EAE as model for MS. Br. J. Pharmacol. 164, 1079–1106. doi: 10.1111/j.1476-5381.2011.01302.x
Correale, J. (2014). The role of microglial activation in disease progression. Mult. Scler. Houndmills Basingstoke Engl. 20, 1288–1295. doi: 10.1177/1352458514533230
Coughlin, J. M., Wang, Y., Ma, S., Yue, C., Kim, P. K., Adams, A. V., et al. (2014). Regional brain distribution of translocator protein using [(11)C]DPA-713 PET in individuals infected with HIV. J. Neurovirol. 20, 219–232. doi: 10.1007/s13365-014-0239-5
Criste, G., Trapp, B., and Dutta, R. (2014). Axonal loss in multiple sclerosis: causes and mechanisms. Handb. Clin. Neurol. 122, 101–113. doi: 10.1016/B978-0-444-52001-2.00005-4
D'Intino, G., Paradisi, M., Fernandez, M., Giuliani, A., Aloe, L., Giardino, L., et al. (2005). Cognitive deficit associated with cholinergic and nerve growth factor down-regulation in experimental allergic encephalomyelitis in rats. Proc. Natl. Acad. Sci. U.S.A. 102, 3070–3075. doi: 10.1073/pnas.0500073102
Daneman, R., and Rescigno, M. (2009). The gut immune barrier and the blood-brain barrier: are they so different? Immunity 31, 722–735. doi: 10.1016/j.immuni.2009.09.012
Debruyne, J. C., Versijpt, J., Van Laere, K. J., De Vos, F., Keppens, J., Strijckmans, K., et al. (2003). PET visualization of microglia in multiple sclerosis patients using [11C]PK11195. Eur. J. Neurol. 10, 257–264. doi: 10.1046/j.1468-1331.2003.00571.x
Deftereos, S. N., Dodou, E., Andronis, C., and Persidis, A. (2012). From depression to neurodegeneration and heart failure: re-examining the potential of MAO inhibitors. Expert Rev. Clin. Pharmacol. 5, 413–425. doi: 10.1586/ecp.12.29
DeLorenzo, C., DellaGioia, N., Bloch, M., Sanacora, G., Nabulsi, N., Abdallah, C., et al. (2015). In vivo ketamine-induced changes in [11C]ABP688 binding to metabotropic glutamate receptor subtype 5. Biol. Psychiatry 77, 266–275. doi: 10.1016/j.biopsych.2014.06.024
Denic, A., Johnson, A. J., Bieber, A. J., Warrington, A. E., Rodriguez, M., and Pirko, I. (2011). The relevance of animal models in multiple sclerosis research. Pathophysiology 18, 21–29. doi: 10.1016/j.pathophys.2010.04.004
de Paula Faria, D., Copray, S., Sijbesma, J. W. A., Willemsen, A. T. M., Buchpiguel, C. A., Dierckx, R. A. J. O., et al. (2014a). PET imaging of focal demyelination and remyelination in a rat model of multiple sclerosis: comparison of [11C]MeDAS, [11C]CIC and [11C]PIB. Eur. J. Nucl. Med. Mol. Imaging 41, 995–1003. doi: 10.1007/s00259-013-2682-6
de Paula Faria, D., de Vries, E. F. J., Sijbesma, J. W. A., Dierckx, R. A. J. O., Buchpiguel, C. A., and Copray, S. (2014b). PET imaging of demyelination and remyelination in the cuprizone mouse model for multiple sclerosis: a comparison between [11C]CIC and [11C]MeDAS. Neuroimage 87, 395–402. doi: 10.1016/j.neuroimage.2013.10.057
Derwenskus, J., and Lublin, F. D. (2014). Future treatment approaches to multiple sclerosis. Handb. Clin. Neurol. 122, 563–577. doi: 10.1016/B978-0-444-52001-2.00024-8
de Vries, E. F. J., Doorduin, J., Dierckx, R. A., and van Waarde, A. (2008). Evaluation of [(11)C]rofecoxib as PET tracer for cyclooxygenase 2 overexpression in rat models of inflammation. Nucl. Med. Biol. 35, 35–42. doi: 10.1016/j.nucmedbio.2007.07.015
de Vries, E. F. J., van Waarde, A., Buursma, A. R., and Vaalburg, W. (2003). Synthesis and in vivo evaluation of 18F-desbromo-DuP-697 as a PET tracer for cyclooxygenase-2 expression. J. Nucl. Med. 44, 1700–1706.
Dickens, A. M., Vainio, S., Marjamaki, P., Johansson, J., Lehtiniemi, P., Rokka, J., et al. (2014). Detection of microglial activation in an acute model of neuroinflammation using PET and radiotracers 11C-(R)-PK11195 and 18F-GE-180. J. Nucl. Med. 55, 466–472. doi: 10.2967/jnumed.113.125625
Dimitrakopoulou-Strauss, A., Strauss, L. G., Burger, C., Rühl, A., Irngartinger, G., Stremmel, W., et al. (2004). Prognostic aspects of 18F-FDG PET kinetics in patients with metastatic colorectal carcinoma receiving FOLFOX chemotherapy. J. Nucl. Med. 45, 1480–1487.
Di Penta, A., Moreno, B., Reix, S., Fernandez-Diez, B., Villanueva, M., Errea, O., et al. (2013). Oxidative stress and proinflammatory cytokines contribute to demyelination and axonal damage in a cerebellar culture model of neuroinflammation. PLoS ONE 8:e54722. doi: 10.1371/journal.pone.0054722
Docagne, F., Mestre, L., Loría, F., Hernangómez, M., Correa, F., and Guaza, C. (2008). Therapeutic potential of CB2 targeting in multiple sclerosis. Expert Opin. Ther. Targets 12, 185–195. doi: 10.1517/14728222.12.2.185
Domercq, M., Vázquez-Villoldo, N., and Matute, C. (2013). Neurotransmitter signaling in the pathophysiology of microglia. Front. Cell. Neurosci. 7:49. doi: 10.3389/fncel.2013.00049
Edgar, J. M., and Nave, K.-A. (2009). The role of CNS glia in preserving axon function. Curr. Opin. Neurobiol. 19, 498–504. doi: 10.1016/j.conb.2009.08.003
Ehrhart, J., Obregon, D., Mori, T., Hou, H., Sun, N., Bai, Y., et al. (2005). Stimulation of cannabinoid receptor 2 (CB2) suppresses microglial activation. J. Neuroinflammation 2:29. doi: 10.1186/1742-2094-2-29
Ellwardt, E., and Zipp, F. (2014). Molecular mechanisms linking neuroinflammation and neurodegeneration in MS. Exp. Neurol. 262 (Pt A), 8–17. doi: 10.1016/j.expneurol.2014.02.006
Endres, C. J., Pomper, M. G., James, M., Uzuner, O., Hammoud, D. A., Watkins, C. C., et al. (2009). Initial evaluation of 11C-DPA-713, a novel TSPO PET ligand, in humans. J. Nucl. Med. 50, 1276–1282. doi: 10.2967/jnumed.109.062265
Evens, N., Muccioli, G. G., Houbrechts, N., Lambert, D. M., Verbruggen, A. M., Van Laere, K., et al. (2009). Synthesis and biological evaluation of carbon-11- and fluorine-18-labeled 2-oxoquinoline derivatives for type 2 cannabinoid receptor positron emission tomography imaging. Nucl. Med. Biol. 36, 455–465. doi: 10.1016/j.nucmedbio.2009.01.009
Evens, N., Vandeputte, C., Coolen, C., Janssen, P., Sciot, R., Baekelandt, V., et al. (2012). Preclinical evaluation of [11C]NE40, a type 2 cannabinoid receptor PET tracer. Nucl. Med. Biol. 39, 389–399. doi: 10.1016/j.nucmedbio.2011.09.005
Evens, N., Vandeputte, C., Muccioli, G. G., Lambert, D. M., Baekelandt, V., Verbruggen, A. M., et al. (2011). Synthesis, in vitro and in vivo evaluation of fluorine-18 labelled FE-GW405833 as a PET tracer for type 2 cannabinoid receptor imaging. Bioorg. Med. Chem. 19, 4499–4505. doi: 10.1016/j.bmc.2011.06.033
Faria Dde, P., Copray, S., Buchpiguel, C., Dierckx, R., and de Vries, E. (2014). PET imaging in multiple sclerosis. J. Neuroimmune Pharmacol. 9, 468–482. doi: 10.1007/s11481-014-9544-2
Farina, C., Aloisi, F., and Meinl, E. (2007). Astrocytes are active players in cerebral innate immunity. Trends Immunol. 28, 138–145. doi: 10.1016/j.it.2007.01.005
Fatima, N., Toscano, M. P., Hunter, S. B., and Cohen, C. (2010). Controversial Role of Epstein-Barr Virus in Multiple Sclerosis. Appl. Immunohistochem. Mol. Morphol. 19, 246–252. doi: 10.1097/PAI.0b013e3181fcf3b4
Filippi, M., and Rocca, M. A. (2011). MR imaging of multiple sclerosis. Radiology 259, 659–681. doi: 10.1148/radiol.11101362
Filippi, M., Rocca, M. A., Barkhof, F., Brück, W., Chen, J. T., Comi, G., et al. (2012). Association between pathological and MRI findings in multiple sclerosis. Lancet Neurol. 11, 349–360. doi: 10.1016/S1474-4422(12)70003-0
Fitzner, D., and Simons, M. (2010). Chronic progressive multiple sclerosis – pathogenesis of neurodegeneration and therapeutic strategies. Curr. Neuropharmacol. 8, 305–315. doi: 10.2174/157015910792246218
Friese, M. A., Schattling, B., and Fugger, L. (2014). Mechanisms of neurodegeneration and axonal dysfunction in multiple sclerosis. Nat. Rev. Neurol. 10, 225–238. doi: 10.1038/nrneurol.2014.37
Frischer, J. M., Bramow, S., Dal-Bianco, A., Lucchinetti, C. F., Rauschka, H., Schmidbauer, M., et al. (2009). The relation between inflammation and neurodegeneration in multiple sclerosis brains. Brain J. Neurol. 132, 1175–1189. doi: 10.1093/brain/awp070
Frohman, E. M., Racke, M. K., and Raine, C. S. (2006). Multiple sclerosis–the plaque and its pathogenesis. N. Engl. J. Med. 354, 942–955. doi: 10.1056/NEJMra052130
Fulda, S., Gorman, A. M., Hori, O., and Samali, A. (2010). Cellular stress responses: cell survival and cell death. Int. J. Cell Biol. 2010, 1–23. doi: 10.1155/2010/214074
Furlan, R., Cuomo, C., and Martino, G. (2009). “Animal models of multiple sclerosis,” in Neural Cell Transplantation, eds D. Gordon and N. J. Scolding (Totowa, NJ: Humana Press), 157–173. Available online at: http://link.springer.com/10.1007/978-1-60327-931-4_11 (Accessed May 14, 2015).
Gambhir, S. S. (2002). Molecular imaging of cancer with positron emission tomography. Nat. Rev. Cancer 2, 683–693. doi: 10.1038/nrc882
Gandelman, M., Peluffo, H., Beckman, J. S., Cassina, P., and Barbeito, L. (2010). Extracellular ATP and the P2X7 receptor in astrocyte-mediated motor neuron death: implications for amyotrophic lateral sclerosis. J. Neuroinflammation 7:33. doi: 10.1186/1742-2094-7-33
Gandhi, R., Laroni, A., and Weiner, H. L. (2010). Role of the innate immune system in the pathogenesis of multiple sclerosis. J. Neuroimmunol. 221, 7–14. doi: 10.1016/j.jneuroim.2009.10.015
Gao, M., Wang, M., Green, M. A., Hutchins, G. D., and Zheng, Q.-H. (2015). Synthesis of [11C]GSK1482160 as a new PET agent for targeting P2X7 receptor. Bioorg. Med. Chem. Lett. 25, 1965–1970. doi: 10.1016/j.bmcl.2015.03.021
Garibotto, V., Tettamanti, M., Marcone, A., Florea, I., Panzacchi, A., Moresco, R., et al. (2013). Cholinergic activity correlates with reserve proxies in Alzheimer's disease. Neurobiol. Aging 34, 2694.e13-18. doi: 10.1016/j.neurobiolaging.2013.05.020
Geurts, J. J. G., Wolswijk, G., Bö, L., van der Valk, P., Polman, C. H., Troost, D., et al. (2003). Altered expression patterns of group I and II metabotropic glutamate receptors in multiple sclerosis. Brain J. Neurol. 126, 1755–1766. doi: 10.1093/brain/awg179
Giannetti, P., Politis, M., Su, P., Turkheimer, F. E., Malik, O., Keihaninejad, S., et al. (2015). Increased PK11195-PET binding in normal-appearing white matter in clinically isolated syndrome. Brain 138, 110–119. doi: 10.1093/brain/awu331
Gilden, D. H. (2005). Infectious causes of multiple sclerosis. Lancet Neurol. 4, 195–202. doi: 10.1016/S1474-4422(05)70023-5
Glass, C. K., Saijo, K., Winner, B., Marchetto, M. C., and Gage, F. H. (2010). Mechanisms underlying inflammation in neurodegeneration. Cell 140, 918–934. doi: 10.1016/j.cell.2010.02.016
Golla, S. S. V., Boellaard, R., Oikonen, V., Hoffmann, A., van Berckel, B. N. M., Windhorst, A. D., et al. (2015). Quantification of [18F]DPA-714 binding in the human brain: initial studies in healthy controls and Alzheimer's disease patients. J. Cereb. Blood Flow Metab. 35, 766–772. doi: 10.1038/jcbfm.2014.261
Goodin, D. S. (2014). “Chapter 11 - The epidemiology of multiple sclerosis: insights to disease pathogenesis,” in Handbook of Clinical Neurology Multiple Sclerosis and Related Disorders, ed D. S. Goodin (Elsevier), 231–266. Available online at: http://www.sciencedirect.com/science/article/pii/B9780444520012000108 (Accessed March 13, 2015).
Gordon, S. (2003). Alternative activation of macrophages. Nat. Rev. Immunol. 3, 23–35. doi: 10.1038/nri978
Goverman, J. (2009). Autoimmune T cell responses in the central nervous system. Nat. Rev. Immunol. 9, 393–407. doi: 10.1038/nri2550
Grassi, I., Nanni, C., Allegri, V., Morigi, J. J., Montini, G. C., Castellucci, P., et al. (2012). The clinical use of PET with (11)C-acetate. Am. J. Nucl. Med. Mol. Imaging 2, 33–47.
Gulyás, B., Pavlova, E., Kása, P., Gulya, K., Bakota, L., Várszegi, S., et al. (2011). Activated MAO-B in the brain of Alzheimer patients, demonstrated by [11C]-L-deprenyl using whole hemisphere autoradiography. Neurochem. Int. 58, 60–68. doi: 10.1016/j.neuint.2010.10.013
Guo, S., Pelligra, C., Saint-Laurent Thibault, C., Hernandez, L., and Kansal, A. (2014). Cost-effectiveness analyses in multiple sclerosis: a review of modelling approaches. Pharmacoeconomics 32, 559–572. doi: 10.1007/s40273-014-0150-1
Haider, L., Fischer, M. T., Frischer, J. M., Bauer, J., Hoftberger, R., Botond, G., et al. (2011). Oxidative damage in multiple sclerosis lesions. Brain 134, 1914–1924. doi: 10.1093/brain/awr128
Halldin, C., Farde, L., Litton, J. E., Hall, H., and Sedvall, G. (1992). [11C]Ro 15-4513, a ligand for visualization of benzodiazepine receptor binding. Preparation, autoradiography and positron emission tomography. Psychopharmacology (Berl.) 108, 16–22. doi: 10.1007/BF02245279
Hanisch, U.-K., and Kettenmann, H. (2007). Microglia: active sensor and versatile effector cells in the normal and pathologic brain. Nat. Neurosci. 10, 1387–1394. doi: 10.1038/nn1997
Hartung, D. M., Bourdette, D. N., Ahmed, S. M., and Whitham, R. H. (2015). The cost of multiple sclerosis drugs in the US and the pharmaceutical industry: too big to fail? Neurology 84, 2185–2192. doi: 10.1212/WNL.0000000000001608
Haskó, G., Linden, J., Cronstein, B., and Pacher, P. (2008). Adenosine receptors: therapeutic aspects for inflammatory and immune diseases. Nat. Rev. Drug Discov. 7, 759–770. doi: 10.1038/nrd2638
Hemmer, B., Kerschensteiner, M., and Korn, T. (2015). Role of the innate and adaptive immune responses in the course of multiple sclerosis. Lancet Neurol. 14, 406–419. doi: 10.1016/S1474-4422(14)70305-9
Herrero, P., Laforest, R., Shoghi, K., Zhou, D., Ewald, G., Pfeifer, J., et al. (2012). Feasibility and dosimetry studies for 18F-NOS as a potential PET radiopharmaceutical for inducible nitric oxide synthase in humans. J. Nucl. Med. 53, 994–1001. doi: 10.2967/jnumed.111.088518
Herschman, H. R. (2003). Micro-PET imaging and small animal models of disease. Curr. Opin. Immunol. 15, 378–384. doi: 10.1016/S0952-7915(03)00066-9
Hicks, R. J. (2006). PET tracer development - a tale of mice and men. Cancer Imaging 6, S102–S106. doi: 10.1102/1470-7330.2006.9098
Horti, A. G., Gao, Y., Ravert, H. T., Finley, P., Valentine, H., Wong, D. F., et al. (2010). Synthesis and biodistribution of [11C]A-836339, a new potential radioligand for PET imaging of cannabinoid type 2 receptors (CB2). Bioorg. Med. Chem. 18, 5202–5207. doi: 10.1016/j.bmc.2010.05.058
Huang, H. J., Isakow, W., Byers, D. E., Engle, J. T., Griffin, E. A., Kemp, D., et al. (2015). Imaging pulmonary inducible nitric oxide synthase expression with PET. J. Nucl. Med. 56, 76–81. doi: 10.2967/jnumed.114.146381
Hurley, D. (2015). News from the aan annual meeting: multiple sclerosis. Neurol. Today 15, 21–22. doi: 10.1097/01.NT.0000465868.70272.0c
Imaizumi, M., Kim, H.-J., Zoghbi, S. S., Briard, E., Hong, J., Musachio, J. L., et al. (2007). PET imaging with [11C]PBR28 can localize and quantify upregulated peripheral benzodiazepine receptors associated with cerebral ischemia in rat. Neurosci. Lett. 411, 200–205. doi: 10.1016/j.neulet.2006.09.093
Islam, T., Gauderman, W. J., Cozen, W., Hamilton, A. S., Burnett, M. E., and Mack, T. M. (2006). Differential twin concordance for multiple sclerosis by latitude of birthplace. Ann. Neurol. 60, 56–64. doi: 10.1002/ana.20871
Iwama, S., Sugimura, Y., Suzuki, H., Suzuki, H., Murase, T., Ozaki, N., et al. (2011). Time-dependent changes in proinflammatory and neurotrophic responses of microglia and astrocytes in a rat model of osmotic demyelination syndrome. Glia 59, 452–462. doi: 10.1002/glia.21114
Jacobs, A. H., and Tavitian, B. (2012). Noninvasive molecular imaging of neuroinflammation. J. Cereb. Blood Flow Metab. 32, 1393–1415. doi: 10.1038/jcbfm.2012.53
Jadvar, H., and Colletti, P. M. (2014). Competitive advantage of PET/MRI. Eur. J. Radiol. 83, 84–94. doi: 10.1016/j.ejrad.2013.05.028
James, M. L., Fulton, R. R., Vercoullie, J., Henderson, D. J., Garreau, L., Chalon, S., et al. (2008). DPA-714, a new translocator protein-specific ligand: synthesis, radiofluorination, and pharmacologic characterization. J. Nucl. Med. 49, 814–822. doi: 10.2967/jnumed.107.046151
Janssen, B., Vugts, D. J., Funke, U., Spaans, A., Schuit, R. C., Kooijman, E., et al. (2014). Synthesis and initial preclinical evaluation of the P2X7 receptor antagonist [11C]A-740003 as a novel tracer of neuroinflammation. J. Label. Compd. Radiopharm. 57, 509–516. doi: 10.1002/jlcr.3206
Ji, B., Kumata, K., Onoe, H., Kaneko, H., Zhang, M.-R., Seki, C., et al. (2013). Assessment of radioligands for PET imaging of cyclooxygenase-2 in an ischemic neuronal injury model. Brain Res. 1533, 152–162. doi: 10.1016/j.brainres.2013.08.026
Ji, B., Maeda, J., Sawada, M., Ono, M., Okauchi, T., Inaji, M., et al. (2008). Imaging of peripheral benzodiazepine receptor expression as biomarkers of detrimental versus beneficial glial responses in mouse models of Alzheimer's and other CNS pathologies. J. Neurosci. 28, 12255–12267. doi: 10.1523/JNEUROSCI.2312-08.2008
Jiang, M., and Chen, G. (2009). Ca2+ regulation of dynamin-independent endocytosis in cortical astrocytes. J. Neurosci. 29, 8063–8074. doi: 10.1523/JNEUROSCI.6139-08.2009
Jødal, L., Le Loirec, C., and Champion, C. (2012). Positron range in PET imaging: an alternative approach for assessing and correcting the blurring. Phys. Med. Biol. 57, 3931–3943. doi: 10.1088/0031-9155/57/12/3931
Johansson, A., Engler, H., Blomquist, G., Scott, B., Wall, A., Aquilonius, S.-M., et al. (2007). Evidence for astrocytosis in ALS demonstrated by [11C](L)-deprenyl-D2 PET. J. Neurol. Sci. 255, 17–22. doi: 10.1016/j.jns.2007.01.057
Jones, T., Rabiner, E. A., and PET Research Advisory Company (2012). The development, past achievements, and future directions of brain PET. J. Cereb. Blood Flow Metab. 32, 1426–1454. doi: 10.1038/jcbfm.2012.20
Kassmann, C. M., Lappe-Siefke, C., Baes, M., Brügger, B., Mildner, A., Werner, H. B., et al. (2007). Axonal loss and neuroinflammation caused by peroxisome-deficient oligodendrocytes. Nat. Genet. 39, 969–976. doi: 10.1038/ng2070
Kelley, K. A., Ho, L., Winger, D., Freire-Moar, J., Borelli, C. B., Aisen, P. S., et al. (1999). Potentiation of excitotoxicity in transgenic mice overexpressing neuronal cyclooxygenase-2. Am. J. Pathol. 155, 995–1004. doi: 10.1016/S0002-9440(10)65199-1
Kiferle, L., Politis, M., Muraro, P. A., and Piccini, P. (2011). Positron emission tomography imaging in multiple sclerosis-current status and future applications. Eur. J. Neurol. 18, 226–231. doi: 10.1111/j.1468-1331.2010.03154.x
Kipp, M., Clarner, T., Dang, J., Copray, S., and Beyer, C. (2009). The cuprizone animal model: new insights into an old story. Acta Neuropathol. (Berl.) 118, 723–736. doi: 10.1007/s00401-009-0591-3
Kooi, E.-J., Prins, M., Bajic, N., Beliën, J. A. M., Gerritsen, W. H., van Horssen, J., et al. (2011). Cholinergic imbalance in the multiple sclerosis hippocampus. Acta Neuropathol. (Berl.) 122, 313–322. doi: 10.1007/s00401-011-0849-4
Kooij, G., van Horssen, J., Bandaru, V. V. R., Haughey, N. J., and de Vries, H. E. (2012). The role of ATP-binding cassette transporters in neuro-inflammation: relevance for bioactive lipids. Front. Pharmacol. 3:74. doi: 10.3389/fphar.2012.00074
Kritis, A. A., Stamoula, E. G., Paniskaki, K. A., and Vavilis, T. D. (2015). Researching glutamate-induced cytotoxicity in different cell lines: a comparative/collective analysis/study. Front. Cell. Neurosci. 9:91. doi: 10.3389/fncel.2015.00091
Kröncke, K.-D., Fehsel, K., and Kolb-Bachofen, V. (1998). Inducible nitric oxide synthase in human diseases. Clin. Exp. Immunol. 113, 147–156. doi: 10.1046/j.1365-2249.1998.00648.x
Krupp, L. B., Christodoulou, C., Melville, P., Scherl, W. F., MacAllister, W. S., and Elkins, L. E. (2004). Donepezil improved memory in multiple sclerosis in a randomized clinical trial. Neurology 63, 1579–1585. doi: 10.1212/01.WNL.0000142989.09633.5A
Kuhlmann, T. (2002). Acute axonal damage in multiple sclerosis is most extensive in early disease stages and decreases over time. Brain 125, 2202–2212. doi: 10.1093/brain/awf235
Kuhlmann, T., Miron, V., Cui, Q., Cuo, Q., Wegner, C., Antel, J., et al. (2008). Differentiation block of oligodendroglial progenitor cells as a cause for remyelination failure in chronic multiple sclerosis. Brain J. Neurol. 131, 1749–1758. doi: 10.1093/brain/awn096
Kutzelnigg, A., and Lassmann, H. (2014). “Pathology of multiple sclerosis and related inflammatory demyelinating diseases,” in Handbook of Clinical Neurology (Elsevier), 15–58. Available online at: http://linkinghub.elsevier.com/retrieve/pii/B9780444520012000029 (Accessed May 20, 2015).
Lancelot, S., and Zimmer, L. (2010). Small-animal positron emission tomography as a tool for neuropharmacology. Trends Pharmacol. Sci. 31, 411–417. doi: 10.1016/j.tips.2010.06.002
Larochelle, C., Alvarez, J. I., and Prat, A. (2011). How do immune cells overcome the blood-brain barrier in multiple sclerosis? FEBS Lett. 585, 3770–3780. doi: 10.1016/j.febslet.2011.04.066
Lavisse, S., Guillermier, M., Herard, A.-S., Petit, F., Delahaye, M., Van Camp, N., et al. (2012). Reactive astrocytes overexpress TSPO and are detected by TSPO positron emission tomography imaging. J. Neurosci. 32, 10809–10818. doi: 10.1523/JNEUROSCI.1487-12.2012
Lev, S. (2012). Nonvesicular lipid transfer from the endoplasmic reticulum. Cold Spring Harb. Perspect. Biol. 4:a013300. doi: 10.1101/cshperspect.a013300
Liang, H., Yang, Y., Yang, K., Wu, Y., Boone, J. M., and Cherry, S. R. (2007). A microPET/CT system for invivo small animal imaging. Phys. Med. Biol. 52, 3881–3894. doi: 10.1088/0031-9155/52/13/015
Lim, K., Labaree, D., Li, S., and Huang, Y. (2014). Preparation of the metabotropic glutamate receptor 5 (mGluR5) PET tracer [18F]FPEB for human use: an automated radiosynthesis and a novel one-pot synthesis of its radiolabeling precursor. Appl. Radiat. Isot. 94, 349–354. doi: 10.1016/j.apradiso.2014.09.006
Lister, M. F., Sharkey, J., Sawatzky, D. A., Hodgkiss, J. P., Davidson, D. J., Rossi, A. G., et al. (2007). The role of the purinergic P2X7 receptor in inflammation. J. Inflamm. 4:5. doi: 10.1186/1476-9255-4-5
Liu, G.-J., Middleton, R. J., Hatty, C. R., Kam, W. W.-Y., Chan, R., Pham, T., et al. (2014). The 18 kDa translocator protein, microglia and neuroinflammation: TSPO, microglia and neuroinflammation. Brain Pathol. 24, 631–653. doi: 10.1111/bpa.12196
Liu, J. S.-H., Zhao, M.-L., Brosnan, C. F., and Lee, S. C. (2001). Expression of inducible nitric oxide synthase and nitrotyrosine in multiple sclerosis lesions. Am. J. Pathol. 158, 2057–2066. doi: 10.1016/S0002-9440(10)64677-9
Liu, X., Chen, C., and Smith, B. J. (2008). Progress in brain penetration evaluation in drug discovery and development. J. Pharmacol. Exp. Ther. 325, 349–356. doi: 10.1124/jpet.107.130294
Loane, D. J., Stoica, B. A., Pajoohesh-Ganji, A., Byrnes, K. R., and Faden, A. I. (2009). Activation of metabotropic glutamate receptor 5 modulates microglial reactivity and neurotoxicity by inhibiting NADPH oxidase. J. Biol. Chem. 284, 15629–15639. doi: 10.1074/jbc.M806139200
Lopez-Diego, R. S., and Weiner, H. L. (2008). Novel therapeutic strategies for multiple sclerosis–a multifaceted adversary. Nat. Rev. Drug Discov. 7, 909–925. doi: 10.1038/nrd2358
Lövblad, K.-O., Anzalone, N., Dörfler, A., Essig, M., Hurwitz, B., Kappos, L., et al. (2010). MR imaging in multiple sclerosis: review and recommendations for current practice. AJNR Am. J. Neuroradiol. 31, 983–989. doi: 10.3174/ajnr.A1906
Lublin, F. D., and Reingold, S. C. (1996). Defining the clinical course of multiple sclerosis results of an international survey. Neurology 46, 907–911. doi: 10.1212/WNL.46.4.907
Lucchinetti, C., Brück, W., Parisi, J., Scheithauer, B., Rodriguez, M., and Lassmann, H. (2000). Heterogeneity of multiple sclerosis lesions: implications for the pathogenesis of demyelination. Ann. Neurol. 47, 707–717. doi: 10.1002/1531-8249(200006)47:6<707::AID-ANA3>3.0.CO;2-Q
Lund, H., Krakauer, M., Skimminge, A., Sellebjerg, F., Garde, E., Siebner, H. R., et al. (2013). Blood-brain barrier permeability of normal appearing white matter in relapsing-remitting multiple sclerosis. PLoS ONE 8:e56375. doi: 10.1371/journal.pone.0056375
Luongo, L., Guida, F., Imperatore, R., Napolitano, F., Gatta, L., Cristino, L., et al. (2014). The A1 adenosine receptor as a new player in microglia physiology: new player in microglia physiology. Glia 62, 122–132. doi: 10.1002/glia.22592
Maeda, J., Suhara, T., Zhang, M.-R., Okauchi, T., Yasuno, F., Ikoma, Y., et al. (2004). Novel peripheral benzodiazepine receptor ligand [11C]DAA1106 for PET: an imaging tool for glial cells in the brain. Synapse 52, 283–291. doi: 10.1002/syn.20027
Maffione, A. M., Rampin, L., Grassetto, G., L'Erario, R., Colletti, P. M., and Rubello, D. (2014). 18F-FDG PET/CT in tumefactive multiple sclerosis. Clin. Nucl. Med. 39, 750–751. doi: 10.1097/RLU.0000000000000427
Mallajosyula, J. K., Kaur, D., Chinta, S. J., Rajagopalan, S., Rane, A., Nicholls, D. G., et al. (2008). MAO-B elevation in mouse brain astrocytes results in Parkinson's pathology. PLoS ONE 3:e1616. doi: 10.1371/journal.pone.0001616
Mallik, S., Samson, R. S., Wheeler-Kingshott, C. A. M., and Miller, D. H. (2014). Imaging outcomes for trials of remyelination in multiple sclerosis. J. Neurol. Neurosurg. Psychiatr. 85, 1396–1404. doi: 10.1136/jnnp-2014-307650
Malpass, K. (2012). Multiple sclerosis: “Outside-in” demyelination in MS. Nat. Rev. Neurol. 8, 61. doi: 10.1038/nrneurol.2011.217
Mantovani, A., Sica, A., Sozzani, S., Allavena, P., Vecchi, A., and Locati, M. (2004). The chemokine system in diverse forms of macrophage activation and polarization. Trends Immunol. 25, 677–686. doi: 10.1016/j.it.2004.09.015
Maragakis, N. J., and Rothstein, J. D. (2006). Mechanisms of disease: astrocytes in neurodegenerative disease. Nat. Clin. Pract. Neurol. 2, 679–689. doi: 10.1038/ncpneuro0355
Marik, J., Ogasawara, A., Martin-McNulty, B., Ross, J., Flores, J. E., Gill, H. S., et al. (2009). PET of glial metabolism using 2-18F-fluoroacetate. J. Nucl. Med. 50, 982–990. doi: 10.2967/jnumed.108.057356
Martinez, F. O., and Gordon, S. (2014). The M1 and M2 paradigm of macrophage activation: time for reassessment. F1000Prime Rep. 6:13. doi: 10.12703/P6-13
Martinez, F. O., Helming, L., and Gordon, S. (2009). Alternative activation of macrophages: an immunologic functional perspective. Annu. Rev. Immunol. 27, 451–483. doi: 10.1146/annurev.immunol.021908.132532
Massoud, T. F. (2003). Molecular imaging in living subjects: seeing fundamental biological processes in a new light. Genes Dev. 17, 545–580. doi: 10.1101/gad.1047403
Matthäus, C., Krafft, C., Dietzek, B., Brehm, B. R., Lorkowski, S., and Popp, J. (2012). Noninvasive imaging of intracellular lipid metabolism in macrophages by Raman microscopy in combination with stable isotopic labeling. Anal. Chem. 84, 8549–8556. doi: 10.1021/ac3012347
Matthews, P. M., Rabiner, E. A., Passchier, J., and Gunn, R. N. (2012). Positron emission tomography molecular imaging for drug development. Br. J. Clin. Pharmacol. 73, 175–186. doi: 10.1111/j.1365-2125.2011.04085.x
Mattner, F., Staykova, M., Berghofer, P., Wong, H. J., Fordham, S., Callaghan, P., et al. (2013). Central nervous system expression and PET imaging of the translocator protein in relapsing-remitting experimental autoimmune encephalomyelitis. J. Nucl. Med. 54, 291–298. doi: 10.2967/jnumed.112.108894
Matute, C., Domercq, M., and Sánchez-Gómez, M.-V. (2006). Glutamate-mediated glial injury: mechanisms and clinical importance. Glia 53, 212–224. doi: 10.1002/glia.20275
Mayo, L., Quintana, F. J., and Weiner, H. L. (2012). The innate immune system in demyelinating disease. Immunol. Rev. 248, 170–187. doi: 10.1111/j.1600-065X.2012.01135.x
Mayo, L., Trauger, S. A., Blain, M., Nadeau, M., Patel, B., Alvarez, J. I., et al. (2014). Regulation of astrocyte activation by glycolipids drives chronic CNS inflammation. Nat. Med. 20, 1147–1156. doi: 10.1038/nm.3681
Medzhitov, R. (2008). Origin and physiological roles of inflammation. Nature 454, 428–435. doi: 10.1038/nature07201
Meuth, S. G., Simon, O. J., Grimm, A., Melzer, N., Herrmann, A. M., Spitzer, P., et al. (2008). CNS inflammation and neuronal degeneration is aggravated by impaired CD200-CD200R-mediated macrophage silencing. J. Neuroimmunol. 194, 62–69. doi: 10.1016/j.jneuroim.2007.11.013
Michell-Robinson, M. A., Touil, H., Healy, L. M., Owen, D. R., Durafourt, B. A., Bar-Or, A., et al. (2015). Roles of microglia in brain development, tissue maintenance and repair. Brain 138, 1138–1159. doi: 10.1093/brain/awv066
Michelucci, A., Heurtaux, T., Grandbarbe, L., Morga, E., and Heuschling, P. (2009). Characterization of the microglial phenotype under specific pro-inflammatory and anti-inflammatory conditions: effects of oligomeric and fibrillar amyloid-beta. J. Neuroimmunol. 210, 3–12. doi: 10.1016/j.jneuroim.2009.02.003
Miljković, D., Timotijević, G., and Stojković, M. M. (2011). Astrocytes in the tempest of multiple sclerosis. FEBS Lett. 585, 3781–3788. doi: 10.1016/j.febslet.2011.03.047
Miller, D. H., Altmann, D. R., and Chard, D. T. (2012). Advances in imaging to support the development of novel therapies for multiple sclerosis. Clin. Pharmacol. Ther. 91, 621–634. doi: 10.1038/clpt.2011.349
Miller, P. W., Long, N. J., Vilar, R., and Gee, A. D. (2008). Synthesis of 11C, 18F, 15O, and 13N radiolabels for positron emission tomography. Angew. Chem. Int. Ed. Engl. 47, 8998–9033. doi: 10.1002/anie.200800222
Mills, C. D., Kincaid, K., Alt, J. M., Heilman, M. J., and Hill, A. M. (2000). M-1/M-2 macrophages and the Th1/Th2 paradigm. J. Immunol. 164, 6166–6173. doi: 10.4049/jimmunol.164.12.6166
Min, K.-J., Yang, M., Kim, S.-U., Jou, I., and Joe, E. (2006). Astrocytes induce hemeoxygenase-1 expression in microglia: a feasible mechanism for preventing excessive brain inflammation. J. Neurosci. 26, 1880–1887. doi: 10.1523/JNEUROSCI.3696-05.2006
Monif, M., Reid, C. A., Powell, K. L., Smart, M. L., and Williams, D. A. (2009). The P2X7 receptor drives microglial activation and proliferation: a trophic role for P2X7R pore. J. Neurosci. 29, 3781–3791. doi: 10.1523/JNEUROSCI.5512-08.2009
Moon, B. S., Kim, B. S., Park, C., Jung, J. H., Lee, Y. W., Lee, H.-Y., et al. (2014). [18 F]Fluoromethyl-PBR28 as a potential radiotracer for TSPO: preclinical comparison with [11 C]PBR28 in a rat model of neuroinflammation. Bioconjug. Chem. 25, 442–450. doi: 10.1021/bc400556h
Morales, Y., Parisi, J. E., and Lucchinetti, C. F. (2006). The pathology of multiple sclerosis: evidence for heterogeneity. Adv. Neurol. 98, 27–45.
Mu, L., Bieri, D., Slavik, R., Drandarov, K., Müller, A., Cermak, S., et al. (2013). Radiolabeling and in vitro/in vivo evaluation of N-(1-adamantyl)-8-methoxy-4-oxo-1-phenyl-1,4-dihydroquinoline-3-carboxamide as a PET probe for imaging cannabinoid type 2 receptor. J. Neurochem. 126, 616–624. doi: 10.1111/jnc.12354
Mu, L., Schubiger, P. A., and Ametamey, S. M. (2010). Radioligands for the PET imaging of metabotropic glutamate receptor subtype 5 (mGluR5). Curr. Top. Med. Chem. 10, 1558–1568. doi: 10.2174/156802610793176783
Naegele, M., and Martin, R. (2014). “The good and the bad of neuroinflammation in multiple sclerosis,” in Handbook of Clinical Neurology (Elsevier), 59–87. Available online at: http://linkinghub.elsevier.com/retrieve/pii/B9780444520012000030 (Accessed May 20, 2015).
Nair, A., Frederick, T. J., and Miller, S. D. (2008). Astrocytes in multiple sclerosis: a product of their environment. Cell. Mol. Life Sci. 65, 2702–2720. doi: 10.1007/s00018-008-8059-5
Nash, B., Thomson, C. E., Linington, C., Arthur, A. T., McClure, J. D., McBride, M. W., et al. (2011). Functional duality of astrocytes in myelination. J. Neurosci. 31, 13028–13038. doi: 10.1523/JNEUROSCI.1449-11.2011
Nau, R., Ribes, S., Djukic, M., and Eiffert, H. (2014). Strategies to increase the activity of microglia as efficient protectors of the brain against infections. Front. Cell. Neurosci. 8:138. doi: 10.3389/fncel.2014.00138
Nave, K.-A. (2010). Myelination and the trophic support of long axons. Nat. Rev. Neurosci. 11, 275–283. doi: 10.1038/nrn2797
Neu, I., and Woelk, H. (1982). Investigations of the lipid metabolism of the white matter in multiple sclerosis: changes in glycero-phosphatides and lipid-splitting enzymes. Neurochem. Res. 7, 727–735. doi: 10.1007/BF00965525
Nijland, P. G., Michailidou, I., Witte, M. E., Mizee, M. R., van der Pol, S. M. A., van het Hof, B., et al. (2014). Cellular distribution of glucose and monocarboxylate transporters in human brain white matter and multiple sclerosis lesions: nutrient transporters in multiple sclerosis. Glia 62, 1125–1141. doi: 10.1002/glia.22667
Noyes, K., Bajorska, A., Chappel, A., Schwid, S. R., Mehta, L. R., Weinstock-Guttman, B., et al. (2011). Cost-effectiveness of disease-modifying therapy for multiple sclerosis: a population-based study. Neurology 77, 355–363. doi: 10.1212/WNL.0b013e3182270402
Oh, J., and O'Connor, P. W. (2015). Established disease-modifying treatments in relapsing-remitting multiple sclerosis. Curr. Opin. Neurol. 28, 220–229. doi: 10.1097/WCO.0000000000000202
Oh, U., Fujita, M., Ikonomidou, V. N., Evangelou, I. E., Matsuura, E., Harberts, E., et al. (2011). Translocator protein PET Imaging for glial activation in multiple sclerosis. J. Neuroimmune Pharmacol. 6, 354–361. doi: 10.1007/s11481-010-9243-6
Ohyama, M., Senda, M., Ishiwata, K., Kitamura, S., Mishina, M., Ishii, K., et al. (1999). Preserved benzodiazepine receptors in Alzheimer's disease measured with C-11 flumazenil PET and I-123 iomazenil SPECT in comparison with CBF. Ann. Nucl. Med. 13, 309–315. doi: 10.1007/BF03164869
Okada, M., Nakao, R., Momosaki, S., Yanamoto, K., Kikuchi, T., Okamura, T., et al. (2013). Improvement of brain uptake for in vivo PET imaging of astrocytic oxidative metabolism using benzyl [1-(11)C]acetate. Appl. Radiat. Isot. 78, 102–107. doi: 10.1016/j.apradiso.2013.04.025
Olek, M. (2012). Treatment of Relapsing-Remitting Multiple Sclerosis in Adults. Available online at: http://www.uptodate.com/contents/treatment-of-relapsing-remitting-multiple-sclerosis-in-adults
Olson, J. K., Croxford, J. L., Calenoff, M. A., Dal Canto, M. C., and Miller, S. D. (2001). A virus-induced molecular mimicry model of multiple sclerosis. J. Clin. Invest. 108, 311–318. doi: 10.1172/JCI200113032
Ono, M. (2009). Development of positron-emission tomography/single-photon emission computed tomography imaging probes for in vivo detection of β-amyloid plaques in Alzheimer's brains. Chem. Pharm. Bull. (Tokyo) 57, 1029–1039. doi: 10.1248/cpb.57.1029
Orr, A. G., Orr, A. L., Li, X.-J., Gross, R. E., and Traynelis, S. F. (2009). Adenosine A2A receptor mediates microglial process retraction. Nat. Neurosci. 12, 872–878. doi: 10.1038/nn.2341
Ortiz, G. G., Pacheco-Moisés, F. P., Bitzer-Quintero, O. K., Ramírez-Anguiano, A. C., Flores-Alvarado, L. J., Ramírez-Ramírez, V., et al. (2013). Immunology and oxidative stress in multiple sclerosis: clinical and basic approach. Clin. Dev. Immunol. 2013, 1–14. doi: 10.1155/2013/708659
Owens, G. P., Gilden, D., Burgoon, M. P., Yu, X., and Bennett, J. L. (2011). Viruses and multiple sclerosis. Neuroscientist 17, 659–676. doi: 10.1177/1073858410386615
Pacher, P. (2006). The endocannabinoid system as an emerging target of pharmacotherapy. Pharmacol. Rev. 58, 389–462. doi: 10.1124/pr.58.3.2
Pannu, R., Singh, A. K., and Singh, I. (2005). A novel role of lactosylceramide in the regulation of tumor necrosis factor -mediated proliferation of rat primary astrocytes: Implications For Astrogliosis Following Neurotrauma. J. Biol. Chem. 280, 13742–13751. doi: 10.1074/jbc.M411959200
Park, E., Gallezot, J.-D., Delgadillo, A., Liu, S., Planeta, B., Lin, S.-F., et al. (2015). (11)C-PBR28 imaging in multiple sclerosis patients and healthy controls: test-retest reproducibility and focal visualization of active white matter areas. Eur. J. Nucl. Med. Mol. Imaging 42, 1081–1092. doi: 10.1007/s00259-015-3043-4
Partridge, M., Spinelli, A., Ryder, W., and Hindorf, C. (2006). The effect of β+ energy on performance of a small animal PET camera. Nucl. Instrum. Methods Phys. Res. Sect. Accel. Spectrometers Detect. Assoc. Equip. 568, 933–936. doi: 10.1016/j.nima.2006.09.035
Pascual, B., Prieto, E., Arbizu, J., Marti-Climent, J. M., Peñuelas, I., Quincoces, G., et al. (2012). Decreased carbon-11-flumazenil binding in early Alzheimer's disease. Brain J. Neurol. 135, 2817–2825.
Paulesu, E., Perani, D., Fazio, F., Comi, G., Pozzilli, C., Martinelli, V., et al. (1996). Functional basis of memory impairment in multiple sclerosis: a[18F]FDG PET study. Neuroimage 4, 87–96. doi: 10.1006/nimg.1996.0032
Peyronneau, M.-A., Saba, W., Goutal, S., Damont, A., Dolle, F., Kassiou, M., et al. (2013). Metabolism and quantification of [18F]DPA-714, a new TSPO positron emission tomography radioligand. Drug Metab. Dispos. 41, 122–131. doi: 10.1124/dmd.112.046342
Pizer, S. M., Chesler, D. A., Clark, F. H., Maskewitz, B. F., Gurney, J., and Brownell, G. L. (1971). “Physics research Scan processing program,” in Sharing of Computer Programs and Technology in Nuclear Medicine USAEC Conf-710425 (Springfield, VA. US Department of Commerce), 92.
Politis, M., Giannetti, P., Su, P., Turkheimer, F., Keihaninejad, S., Wu, K., et al. (2012). Increased PK11195 PET binding in the cortex of patients with MS correlates with disability. Neurology 79, 523–530. doi: 10.1212/WNL.0b013e3182635645
Poloni, G., Minagar, A., Haacke, E. M., and Zivadinov, R. (2011). Recent developments in imaging of multiple sclerosis. Neurologist 17, 185–204. doi: 10.1097/NRL.0b013e31821a2643
Ponde, D. E., Dence, C. S., Oyama, N., Kim, J., Tai, Y.-C., Laforest, R., et al. (2007). 18F-fluoroacetate: a potential acetate analog for prostate tumor imaging–in vivo evaluation of 18F-fluoroacetate versus 11C-acetate. J. Nucl. Med. 48, 420–428.
Portnow, L. H., Vaillancourt, D. E., and Okun, M. S. (2013). The history of cerebral PET scanning: from physiology to cutting-edge technology. Neurology 80, 952–956. doi: 10.1212/WNL.0b013e318285c135
Prüss, H., Rosche, B., Sullivan, A. B., Brommer, B., Wengert, O., Gronert, K., et al. (2013). Proresolution lipid mediators in multiple sclerosis — differential, disease severity-dependent synthesis — a clinical pilot trial. PLoS ONE 8:e55859. doi: 10.1371/journal.pone.0055859
Quelch, D., De Santis, V., Strege, A., Myers, J., Wells, L., Nutt, D., et al. (2015). Influence of agonist induced internalization on [3 H]Ro15-4513 binding-an application to imaging fluctuations in endogenous GABA with positron emission tomography: influence of agonist induced internalization on [3 H]Ro15-4513 binding. Synapse 69, 60–65. doi: 10.1002/syn.21780
Quintana, F. J., Yeste, A., and Mascanfroni, I. D. (2014). Role and therapeutic value of dendritic cells in central nervous system autoimmunity. Cell Death Differ. 22, 215–224. doi: 10.1038/cdd.2014.125
Radu, C. G., Shu, C. J., Shelly, S. M., Phelps, M. E., and Witte, O. N. (2007). Positron emission tomography with computed tomography imaging of neuroinflammation in experimental autoimmune encephalomyelitis. Proc. Natl. Acad. Sci. U.S.A. 104, 1937–1942. doi: 10.1073/pnas.0610544104
Ransohoff, R. M. (2012). Animal models of multiple sclerosis: the good, the bad and the bottom line. Nat. Neurosci. 15, 1074–1077. doi: 10.1038/nn.3168
Ransohoff, R. M., Hafler, D. A., and Lucchinetti, C. F. (2015). Multiple sclerosis—a quiet revolution. Nat. Rev. Neurol. 11, 134–142. doi: 10.1038/nrneurol.2015.14
Rao, S. D., Yin, H. Z., and Weiss, J. H. (2003). Disruption of glial glutamate transport by reactive oxygen species produced in motor neurons. J. Neurosci. 23, 2627–2633.
Rinaldi, L., Grassivaro, F., and Gallo, P. (2009). “Lipids in Multiple Sclerosis,” in Handbook of Neurochemistry and Molecular Neurobiology, eds A. Lajtha, G. Tettamanti, and G. Goracci (Boston, MA: Springer US), 593–602. Available online at: http://link.springer.com/10.1007/978-0-387-30378-9_24 (Accessed May 21, 2015).
Rinne, J. O. (2003). Brain acetylcholinesterase activity in mild cognitive impairment and early Alzheimer's disease. J. Neurol. Neurosurg. Psychiatr. 74, 113–115. doi: 10.1136/jnnp.74.1.113
Rissanen, E., Tuisku, J., Luoto, P., Arponen, E., Johansson, J., Oikonen, V., et al. (2015). Automated reference region extraction and population-based input function for brain [11C]TMSX PET image analyses. J. Cereb. Blood Flow Metab. 35, 157–165. doi: 10.1038/jcbfm.2014.194
Rissanen, E., Tuisku, J., Rokka, J., Paavilainen, T., Parkkola, R., Rinne, J. O., et al. (2014). In vivo detection of diffuse inflammation in secondary progressive multiple sclerosis using PET imaging and the radioligand 11C-PK11195. J. Nucl. Med. 55, 939–944. doi: 10.2967/jnumed.113.131698
Rissanen, E., Virta, J. R., Paavilainen, T., Tuisku, J., Helin, S., Luoto, P., et al. (2013). Adenosine A2A receptors in secondary progressive multiple sclerosis: a [11C]TMSX brain PET study. J. Cereb. Blood Flow Metab. 33, 1394–1401. doi: 10.1038/jcbfm.2013.85
Rodgers, J. M., Robinson, A. P., and Miller, S. D. (2013). Strategies for protecting oligodendrocytes and enhancing remyelination in multiple sclerosis. Discov. Med. 16, 53–63.
Rose, J. W., Hill, K. E., Watt, H. E., and Carlson, N. G. (2004). Inflammatory cell expression of cyclooxygenase-2 in the multiple sclerosis lesion. J. Neuroimmunol. 149, 40–49. doi: 10.1016/j.jneuroim.2003.12.021
Rossi, S., Motta, C., Studer, V., Barbieri, F., Buttari, F., Bergami, A., et al. (2014). Tumor necrosis factor is elevated in progressive multiple sclerosis and causes excitotoxic neurodegeneration. Mult. Scler. J. 20, 304–312. doi: 10.1177/1352458513498128
Rossi, S., Studer, V., Motta, C., De Chiara, V., Barbieri, F., Bernardi, G., et al. (2012). Inflammation inhibits GABA transmission in multiple sclerosis. Mult. Scler. Houndmills Basingstoke Engl. 18, 1633–1635. doi: 10.1177/1352458512440207
Rostami, A., and Ciric, B. (2014). Astrocyte-derived lactosylceramide implicated in multiple sclerosis. Nat. Med. 20, 1092–1093. doi: 10.1038/nm.3719
Rudroff, T., Kindred, J. H., Koo, P. J., Karki, R., and Hebert, J. R. (2014). Asymmetric glucose uptake in leg muscles of patients with multiple sclerosis during walking detected by [18F]-FDG PET/CT. NeuroRehabilitation 35, 813–823. doi: 10.3233/NRE-141179
Rupprecht, R., Papadopoulos, V., Rammes, G., Baghai, T. C., Fan, J., Akula, N., et al. (2010). Translocator protein (18 kDa) (TSPO) as a therapeutic target for neurological and psychiatric disorders. Nat. Rev. Drug Discov. 9, 971–988. doi: 10.1038/nrd3295
Ryu, J. K., Choi, H. B., and McLarnon, J. G. (2005). Peripheral benzodiazepine receptor ligand PK11195 reduces microglial activation and neuronal death in quinolinic acid-injected rat striatum. Neurobiol. Dis. 20, 550–561. doi: 10.1016/j.nbd.2005.04.010
Saha, G. B., MacIntyre, W. J., and Go, R. T. (1994). Radiopharmaceuticals for brain imaging. Semin. Nucl. Med. 24, 324–349. doi: 10.1016/S0001-2998(05)80022-4
Saha, R. N., and Pahan, K. (2006). Regulation of inducible nitric oxide synthase gene in glial cells. Antioxid. Redox Signal. 8, 929–947. doi: 10.1089/ars.2006.8.929
Sanchez-Pernaute, R., Wang, J.-Q., Kuruppu, D., Cao, L., Tueckmantel, W., Kozikowski, A., et al. (2008). Enhanced binding of metabotropic glutamate receptor type 5 (mGluR5) PET tracers in the brain of parkinsonian primates. Neuroimage 42, 248–251. doi: 10.1016/j.neuroimage.2008.04.170
Schäffler, N., Köpke, S., Winkler, L., Schippling, S., Inglese, M., Fischer, K., et al. (2011). Accuracy of diagnostic tests in multiple sclerosis–a systematic review. Acta Neurol. Scand. 124, 151–164. doi: 10.1111/j.1600-0404.2010.01454.x
Scheller, J., Chalaris, A., Schmidt-Arras, D., and Rose-John, S. (2011). The pro- and anti-inflammatory properties of the cytokine interleukin-6. Biochim. Biophys. Acta 1813, 878–888. doi: 10.1016/j.bbamcr.2011.01.034
Schiepers, C., Van Hecke, P., Vandenberghe, R., Van Oostende, S., Dupont, P., Demaerel, P., et al. (1997). Positron emission tomography, magnetic resonance imaging and proton NMR spectroscopy of white matter in multiple sclerosis. Mult. Scler. Houndmills Basingstoke Engl. 3, 8–17. doi: 10.1177/135245859700300102
Selvaraj, V., and Stocco, D. M. (2015). The changing landscape in translocator protein (TSPO) function. Trends Endocrinol. Metab. 26, 341–348. doi: 10.1016/j.tem.2015.02.007
Sharma, M. K., Seidlitz, E. P., and Singh, G. (2010). Cancer cells release glutamate via the cystine/glutamate antiporter. Biochem. Biophys. Res. Commun. 391, 91–95. doi: 10.1016/j.bbrc.2009.10.168
Shih, A. Y. (2006). Policing the police: astrocytes modulate microglial activation. J. Neurosci. 26, 3887–3888. doi: 10.1523/JNEUROSCI.0936-06.2006
Sigel, E., and Buhr, A. (1997). The benzodiazepine binding site of GABAA receptors. Trends Pharmacol. Sci. 18, 425–429. doi: 10.1016/S0165-6147(97)90675-1
Singhal, G., Jaehne, E. J., Corrigan, F., Toben, C., and Baune, B. T. (2014). Inflammasomes in neuroinflammation and changes in brain function: a focused review. Front. Neurosci. 8:315. doi: 10.3389/fnins.2014.00315
Slavik, R., Herde, A. M., Bieri, D., Weber, M., Schibli, R., Krämer, S. D., et al. (2015). Synthesis, radiolabeling and evaluation of novel 4-oxo-quinoline derivatives as PET tracers for imaging cannabinoid type 2 receptor. Eur. J. Med. Chem. 92, 554–564. doi: 10.1016/j.ejmech.2015.01.028
Smith, C. M., Cooksey, E., and Duncan, I. D. (2013). Myelin loss does not lead to axonal degeneration in a long-lived model of chronic demyelination. J. Neurosci. 33, 2718–2727. doi: 10.1523/JNEUROSCI.4627-12.2013
Sofroniew, M. V. (2015). Astrocyte barriers to neurotoxic inflammation. Nat. Rev. Neurosci. 16, 249–263. doi: 10.1038/nrn3898
Sofroniew, M. V., and Vinters, H. V. (2010). Astrocytes: biology and pathology. Acta Neuropathol. (Berl.) 119, 7–35. doi: 10.1007/s00401-009-0619-8
Sperlágh, B., and Illes, P. (2007). Purinergic modulation of microglial cell activation. Purinergic Signal. 3, 117–127. doi: 10.1007/s11302-006-9043-x
Stankoff, B., Freeman, L., Aigrot, M.-S., Chardain, A., Dollé, F., Williams, A., et al. (2011). Imaging central nervous system myelin by positron emission tomography in multiple sclerosis using [methyl-11C]-2-(4′-methylaminophenyl)- 6-hydroxybenzothiazole. Ann. Neurol. 69, 673–680. doi: 10.1002/ana.22320
Stankoff, B., Wang, Y., Bottlaender, M., Aigrot, M.-S., Dolle, F., Wu, C., et al. (2006). Imaging of CNS myelin by positron-emission tomography. Proc. Natl. Acad. Sci. U.S.A. 103, 9304–9309. doi: 10.1073/pnas.0600769103
Suzuki, K., Inoue, O., Hashimoto, K., Yamasaki, T., Kuchiki, M., and Tamate, K. (1985). Computer-controlled large scale production of high specific activity [11C]RO 15-1788 for PET studies of benzodiazepine receptors. Int. J. Appl. Radiat. Isot. 36, 971–976. doi: 10.1016/0020-708X(85)90258-3
Takaki, J., Fujimori, K., Miura, M., Suzuki, T., Sekino, Y., and Sato, K. (2012). L-glutamate released from activated microglia downregulates astrocytic L-glutamate transporter expression in neuroinflammation: the “collusion” hypothesis for increased extracellular L-glutamate concentration in neuroinflammation. J. Neuroinflammation 9:275. doi: 10.1186/1742-2094-9-275
Takano, A., Piehl, F., Hillert, J., Varrone, A., Nag, S., Gulyás, B., et al. (2013). In vivo TSPO imaging in patients with multiple sclerosis: a brain PET study with [18F]FEDAA1106. EJNMMI Res. 3:30. doi: 10.1186/2191-219X-3-30
Takashima-Hirano, M., Takashima, T., Katayama, Y., Wada, Y., Sugiyama, Y., Watanabe, Y., et al. (2011). Efficient sequential synthesis of PET Probes of the COX-2 inhibitor [11C]celecoxib and its major metabolite [11C]SC-62807 and in vivo PET evaluation. Bioorg. Med. Chem. 19, 2997–3004. doi: 10.1016/j.bmc.2011.03.020
Takata, K., Kato, H., Shimosegawa, E., Okuno, T., Koda, T., Sugimoto, T., et al. (2014). 11C-Acetate PET imaging in patients with multiple sclerosis. PLoS ONE 9:e111598. doi: 10.1371/journal.pone.0111598
Talati, R., Reinhart, K., Baker, W., White, C. M., and Coleman, C. I. (2009). Pharmacologic treatment of advanced Parkinson's disease: a meta-analysis of COMT inhibitors and MAO-B inhibitors. Parkinsonism Relat. Disord. 15, 500–505. doi: 10.1016/j.parkreldis.2008.12.007
Tambuyzer, B. R., Ponsaerts, P., and Nouwen, E. J. (2009). Microglia: gatekeepers of central nervous system immunology. J. Leukoc. Biol. 85, 352–370. doi: 10.1189/jlb.0608385
Teodoro, R., Moldovan, R.-P., Lueg, C., Günther, R., Donat, C. K., Ludwig, F.-A., et al. (2013). Radiofluorination and biological evaluation of N-aryl-oxadiazolyl-propionamides as potential radioligands for PET imaging of cannabinoid CB2 receptors. Org. Med. Chem. Lett. 3:11. doi: 10.1186/2191-2858-3-11
The MICAD Research Team (2004). “2'-[(18)F]Fluoroflumazenil,” in Molecular Imaging and Contrast Agent Database (MICAD) (Bethesda, MD: National Center for Biotechnology Information US). (Accessed May 20, 2015).
Thorek, D. L. J., Ogirala, A., Beattie, B. J., and Grimm, J. (2013). Quantitative imaging of disease signatures through radioactive decay signal conversion. Nat. Med. 19, 1345–1350. doi: 10.1038/nm.3323
Tillema, J.-M., and Pirko, I. (2013). Neuroradiological evaluation of demyelinating disease. Ther. Adv. Neurol. Disord. 6, 249–268. doi: 10.1177/1756285613478870
Toft-Hansen, H., Füchtbauer, L., and Owens, T. (2011). Inhibition of reactive astrocytosis in established experimental autoimmune encephalomyelitis favors infiltration by myeloid cells over T cells and enhances severity of disease. Glia 59, 166–176. doi: 10.1002/glia.21088
Torigian, D. A., Zaidi, H., Kwee, T. C., Saboury, B., Udupa, J. K., Cho, Z.-H., et al. (2013). PET/MR imaging: technical aspects and potential clinical applications. Radiology 267, 26–44. doi: 10.1148/radiol.13121038
Torkildsen, ø., Brunborg, L. A., Myhr, K.-M., and Bø, L. (2008). The cuprizone model for demyelination. Acta Neurol. Scand. 117, 72–76. doi: 10.1111/j.1600-0404.2008.01036.x
Traboulsee, A. L., and Li, D. K. B. (2006). The role of MRI in the diagnosis of multiple sclerosis. Adv. Neurol. 98, 125–146.
Trapp, B. D., and Nave, K.-A. (2008). Multiple sclerosis: an immune or neurodegenerative disorder? Annu. Rev. Neurosci. 31, 247–269. doi: 10.1146/annurev.neuro.30.051606.094313
Trapp, B. D., and Stys, P. K. (2009). Virtual hypoxia and chronic necrosis of demyelinated axons in multiple sclerosis. Lancet Neurol. 8, 280–291. doi: 10.1016/S1474-4422(09)70043-2
Tsao, J. W., and Heilman, K. M. (2005). Donepezil improved memory in multiple sclerosis in a randomized clinical trial. Neurology 64, 1823; author reply 1823. doi: 10.1212/wnl.64.10.1823
Tselis, A. (2012). Epstein–Barr virus cause of multiple sclerosis. Curr. Opin. Rheumatol. 24, 424–428. doi: 10.1097/BOR.0b013e3283542cf8
Turkman, N., Shavrin, A., Ivanov, R. A., Rabinovich, B., Volgin, A., Gelovani, J. G., et al. (2011). Fluorinated cannabinoid CB2 receptor ligands: synthesis and in vitro binding characteristics of 2-oxoquinoline derivatives. Bioorg. Med. Chem. 19, 5698–5707. doi: 10.1016/j.bmc.2011.07.062
Van Camp, N., Boisgard, R., Kuhnast, B., Thézé, B., Viel, T., Grégoire, M.-C., et al. (2010). In vivo imaging of neuroinflammation: a comparative study between [(18)F]PBR111, [(11)C]CLINME and [(11)C]PK11195 in an acute rodent model. Eur. J. Nucl. Med. Mol. Imaging 37, 962–972. doi: 10.1007/s00259-009-1353-0
Vandeputte, C., Evens, N., Toelen, J., Deroose, C. M., Bosier, B., Ibrahimi, A., et al. (2011). A PET brain reporter gene system based on type 2 cannabinoid receptors. J. Nucl. Med. 52, 1102–1109. doi: 10.2967/jnumed.110.084426
Vas, A., Shchukin, Y., Karrenbauer, V. D., Cselényi, Z., Kostulas, K., Hillert, J., et al. (2008). Functional neuroimaging in multiple sclerosis with radiolabelled glia markers: preliminary comparative PET studies with [11C]vinpocetine and [11C]PK11195 in patients. J. Neurol. Sci. 264, 9–17. doi: 10.1016/j.jns.2007.07.018
Veitinger, M., Varga, B., Guterres, S. B., and Zellner, M. (2014). Platelets, a reliable source for peripheral Alzheimer's disease biomarkers? Acta Neuropathol. Commun. 2:65. doi: 10.1186/2051-5960-2-65
Venneti, S., Wang, G., Nguyen, J., and Wiley, C. A. (2008). The positron emission tomography ligand DAA1106 binds with high affinity to activated microglia in human neurological disorders. J. Neuropathol. Exp. Neurol. 67, 1001–1010. doi: 10.1097/NEN.0b013e318188b204
Versijpt, J., Debruyne, J. C., Van Laere, K. J., De Vos, F., Keppens, J., Strijckmans, K., et al. (2005). Microglial imaging with positron emission tomography and atrophy measurements with magnetic resonance imaging in multiple sclerosis: a correlative study. Mult. Scler. Houndmills Basingstoke Engl. 11, 127–134. doi: 10.1191/1352458505ms1140oa
Virta, J. R., Laatu, S., Parkkola, R., Oikonen, V., Rinne, J. O., and Ruutiainen, J. (2011). Cerebral acetylcholinesterase activity is not decreased in MS patients with cognitive impairment. Mult. Scler. J. 17, 931–938. doi: 10.1177/1352458511399613
Volkow, N. D., Ding, Y. S., Fowler, J. S., and Gatley, S. J. (2001). Imaging brain cholinergic activity with positron emission tomography: its role in the evaluation of cholinergic treatments in Alzheimer's dementia. Biol. Psychiatry 49, 211–220. doi: 10.1016/S0006-3223(00)01112-4
Voskuhl, R. R., Peterson, R. S., Song, B., Ao, Y., Morales, L. B. J., Tiwari-Woodruff, S., et al. (2009). Reactive astrocytes form scar-like perivascular barriers to leukocytes during adaptive immune inflammation of the CNS. J. Neurosci. 29, 11511–11522. doi: 10.1523/JNEUROSCI.1514-09.2009
Wadsworth, H., Jones, P. A., Chau, W.-F., Durrant, C., Fouladi, N., Passmore, J., et al. (2012). [18F]GE-180: A novel fluorine-18 labelled PET tracer for imaging Translocator protein 18kDa (TSPO). Bioorg. Med. Chem. Lett. 22, 1308–1313. doi: 10.1016/j.bmcl.2011.12.084
Wagner, S., Breyholz, H.-J., Höltke, C., Faust, A., Schober, O., Schäfers, M., et al. (2009). A new 18F-labelled derivative of the MMP inhibitor CGS 27023A for PET: radiosynthesis and initial small-animal PET studies. Appl. Radiat. Isot. Data Instrum. Methods Use Agric. Ind. Med. 67, 606–610. doi: 10.1016/j.apradiso.2008.12.009
Wagner, S., Breyholz, H.-J., Law, M. P., Faust, A., Höltke, C., Schröer, S., et al. (2007). Novel fluorinated derivatives of the broad-spectrum MMP inhibitors N-hydroxy-2(R)-[[(4-methoxyphenyl)sulfonyl](benzyl)- and (3-picolyl)-amino]-3-methyl-butanamide as potential tools for the molecular imaging of activated MMPs with PET. J. Med. Chem. 50, 5752–5764. doi: 10.1021/jm0708533
Wang, J.-Q., Tueckmantel, W., Zhu, A., Pellegrino, D., and Brownell, A.-L. (2007). Synthesis and preliminary biological evaluation of 3-[(18)F]fluoro-5-(2-pyridinylethynyl)benzonitrile as a PET radiotracer for imaging metabotropic glutamate receptor subtype 5. Synapse 61, 951–961. doi: 10.1002/syn.20445
Wang, Y., Wu, C., Caprariello, A. V., Somoza, E., Zhu, W., Wang, C., et al. (2009). In vivo quantification of myelin changes in the vertebrate nervous system. J. Neurosci. 29, 14663–14669. doi: 10.1523/JNEUROSCI.4082-08.2009
Weiner, H. L. (2008). A shift from adaptive to innate immunity: a potential mechanism of disease progression in multiple sclerosis. J. Neurol. 255 (Suppl. 1), 3–11. doi: 10.1007/s00415-008-1002-8
Welser-Alves, J. V., and Milner, R. (2013). Microglia are the major source of TNF-α and TGF-β1 in postnatal glial cultures; regulation by cytokines, lipopolysaccharide, and vitronectin. Neurochem. Int. 63, 47–53. doi: 10.1016/j.neuint.2013.04.007
Wheeler, D., Bandaru, V. V. R., Calabresi, P. A., Nath, A., and Haughey, N. J. (2008). A defect of sphingolipid metabolism modifies the properties of normal appearing white matter in multiple sclerosis. Brain 131, 3092–3102. doi: 10.1093/brain/awn190
Wilkins, A., Kondo, Y., Song, J., Liu, S., Compston, A., Black, J. A., et al. (2010). Slowly progressive axonal degeneration in a rat model of chronic, nonimmune-mediated demyelination. J. Neuropathol. Exp. Neurol. 69, 1256–1269. doi: 10.1097/NEN.0b013e3181ffc317
Winkeler, A., Boisgard, R., Martin, A., and Tavitian, B. (2010). Radioisotopic imaging of neuroinflammation. J. Nucl. Med. 51, 1–4. doi: 10.2967/jnumed.109.065680
Wong, D. F., Waterhouse, R., Kuwabara, H., Kim, J., Brašić, J. R., Chamroonrat, W., et al. (2013). 18F-FPEB, a PET radiopharmaceutical for quantifying metabotropic glutamate 5 receptors: a first-in-human study of radiochemical safety, biokinetics, and radiation dosimetry. J. Nucl. Med. 54, 388–396. doi: 10.2967/jnumed.112.107995
Woodruff, R. H., and Franklin, R. J. M. (1999). Demyelination and remyelination of the caudal cerebellar peduncle of adult rats following stereotaxic injections of lysolecithin, ethidium bromide, and complement/anti-galactocerebroside: a comparative study. Glia 25, 216–228.
Wu, C., Tian, D., Feng, Y., Polak, P., Wei, J., Sharp, A., et al. (2006). A novel fluorescent probe that is brain permeable and selectively binds to myelin. J. Histochem. Cytochem. 54, 997–1004. doi: 10.1369/jhc.5A6901.2006
Wu, C., Wang, C., Popescu, D., Zhu, W., Somoza, E., Zhu, J., et al. (2010). A novel PET marker for in vivo quantification of myelination. Bioorg. Med. Chem. 18, 8592–8599. doi: 10.1016/j.bmc.2010.10.018
Wu, C., Zhu, J., Baeslack, J., Zaremba, A., Hecker, J., Kraso, J., et al. (2013). Longitudinal positron emission tomography imaging for monitoring myelin repair in the spinal cord. Ann. Neurol. 74, 688–698. doi: 10.1002/ana.23965
Wunder, A., Schoknecht, K., Stanimirovic, D. B., Prager, O., and Chassidim, Y. (2012). Imaging blood-brain barrier dysfunction in animal disease models. Epilepsia 53 (Suppl. 6), 14–21. doi: 10.1111/j.1528-1167.2012.03698.x
Yamasaki, R., Lu, H., Butovsky, O., Ohno, N., Rietsch, A. M., Cialic, R., et al. (2014). Differential roles of microglia and monocytes in the inflamed central nervous system. J. Exp. Med. 211, 1533–1549. doi: 10.1084/jem.20132477
Yiangou, Y., Facer, P., Durrenberger, P., Chessell, I. P., Naylor, A., Bountra, C., et al. (2006). COX-2, CB2 and P2X7-immunoreactivities are increased in activated microglial cells/macrophages of multiple sclerosis and amyotrophic lateral sclerosis spinal cord. BMC Neurol. 6:12. doi: 10.1186/1471-2377-6-12
Yrjölä, S., Sarparanta, M., Airaksinen, A. J., Hytti, M., Kauppinen, A., Pasonen-Seppänen, S., et al. (2015). Synthesis, in vitro and in vivo evaluation of 1,3,5-triazines as cannabinoid CB2 receptor agonists. Eur. J. Pharm. Sci. 67, 85–96. doi: 10.1016/j.ejps.2014.11.003
Yu, M., Tueckmantel, W., Wang, X., Zhu, A., Kozikowski, A. P., and Brownell, A.-L. (2005). Methoxyphenylethynyl, methoxypyridylethynyl and phenylethynyl derivatives of pyridine: synthesis, radiolabeling and evaluation of new PET ligands for metabotropic glutamate subtype 5 receptors. Nucl. Med. Biol. 32, 631–640. doi: 10.1016/j.nucmedbio.2005.05.004
Zarei, M. (2003). Cognitive presentation of multiple sclerosis: evidence for a cortical variant. J. Neurol. Neurosurg. Psychiatry 74, 872–877. doi: 10.1136/jnnp.74.7.872
Zeis, T., Allaman, I., Gentner, M., Schroder, K., Tschopp, J., Magistretti, P. J., et al. (2015). Metabolic gene expression changes in astrocytes in Multiple Sclerosis cerebral cortex are indicative of immune-mediated signaling. Brain. Behav. Immun. 48, 313–325. doi: 10.1016/j.bbi.2015.04.013
Zhang, S., Smailagic, N., Hyde, C., Noel-Storr, A. H., Takwoingi, Y., McShane, R., et al. (2014). “11 C-PIB-PET for the early diagnosis of Alzheimer's disease dementia and other dementias in people with mild cognitive impairment (MCI),” in Cochrane Database of Systematic Reviews, ed The Cochrane Collaboration (Chichester, UK: John Wiley and Sons, Ltd). Available online at: http://doi.wiley.com/10.1002/14651858.CD010386.pub2 (Accessed May 20, 2015).
Zhang, Y., Guo, T. B., and Lu, H. (2013). Promoting remyelination for the treatment of multiple sclerosis: opportunities and challenges. Neurosci. Bull. 29, 144–154. doi: 10.1007/s12264-013-1317-z
Zhang, Z., and Brownell, A.-L. (2012). “Imaging of Metabotropic Glutamate Receptors (mGluRs),” in Neuroimaging - Clinical Applications, ed P. Bright (InTech). Available online at: http://www.intechopen.com/books/neuroimaging-clinical-applications/imaging-of-metabotropic-glutamate-receptors-mglur-s (Accessed May 4, 2015).
Keywords: multiple sclerosis, inflammation, neuroreceptors, positron emission tomography, precision medicine, microglia, astrocyte
Citation: Poutiainen P, Jaronen M, Quintana FJ and Brownell A-L (2016) Precision Medicine in Multiple Sclerosis: Future of PET Imaging of Inflammation and Reactive Astrocytes. Front. Mol. Neurosci. 9:85. doi: 10.3389/fnmol.2016.00085
Received: 08 May 2016; Accepted: 30 August 2016;
Published: 15 September 2016.
Edited by:
Kirsten Harvey, University College London, UKReviewed by:
Hartmut Lüddens, University of Mainz, GermanySamaneh Maysami, University of Manchester, UK
Copyright © 2016 Poutiainen, Jaronen, Quintana and Brownell. This is an open-access article distributed under the terms of the Creative Commons Attribution License (CC BY). The use, distribution or reproduction in other forums is permitted, provided the original author(s) or licensor are credited and that the original publication in this journal is cited, in accordance with accepted academic practice. No use, distribution or reproduction is permitted which does not comply with these terms.
*Correspondence: Anna-Liisa Brownell, abrownell@mgh.harvard.edu