- 1Institute of Human Genetics, University of Bonn, Bonn, Germany
- 2Department of Genomics, Life and Brain Center, Bonn, Germany
- 3Institute of Physiological Chemistry, Philipps-University Marburg, Marburg, Germany
The 22q11.2 deletion is the strongest known genetic risk factor for schizophrenia. Research has implicated microRNA-mediated dysregulation in 22q11.2 deletion syndrome (22q11.2DS) schizophrenia-risk. Primary candidate genes are DGCR8 (DiGeorge syndrome critical region gene 8), which encodes a component of the microprocessor complex essential for microRNA biogenesis, and MIR185, which encodes microRNA 185. Mouse models of 22q11.2DS have demonstrated alterations in brain microRNA biogenesis, and that DGCR8 haploinsufficiency may contribute to these alterations, e.g., via down-regulation of a specific microRNA subset. miR-185 was the top-scoring down-regulated microRNA in both the prefrontal cortex and the hippocampus, brain areas which are the key foci of schizophrenia research. This reduction in miR-185 expression contributed to dendritic and spine development deficits in hippocampal neurons. In addition, miR-185 has two validated targets (RhoA, Cdc42), both of which have been associated with altered expression levels in schizophrenia. These combined data support the involvement of miR-185 and its down-stream pathways in schizophrenia. This review summarizes evidence implicating microRNA-mediated dysregulation in schizophrenia in both 22q11.2DS-related and idiopathic cases.
Introduction
The 22q11.2 deletion syndrome (22q11.2DS), also known as the velocardiofacial/DiGeorge syndrome, is a phenotypically heterogenous disease which is caused by a hemizygous microdeletion on the long arm of chromosome 22 in the region q11.2. The overall prevalence is 1 in 2,000–4,000 live births (Murphy et al., 1999; Botto et al., 2003; Robin and Shprintzen, 2005). The disorder is associated with a high risk for psychiatric disorder.
In particular, 22q11.2DS patients have an estimated 20–25% risk for schizophrenia or related psychotic disorders such as schizoaffective disorder (Murphy et al., 1999; Chow et al., 2006; Bassett and Chow, 2008; Philip and Bassett, 2011). The deletion is therefore the strongest known genetic risk factor for schizophrenia (odds ratio = 20.3; Levinson et al., 2011), and accounts for approximately 1–2% of all schizophrenia cases (Karayiorgou et al., 1995, 2010; Bassett and Chow, 2008; International Schizophrenia Consortium, 2008; Stefansson et al., 2008). Individuals with 22q11.2DS have variable cognitive and behavioral deficits (Karayiorgou et al., 2010) including relative impairments in social judgment, motor skills, verbal learning, and executive functioning (Chow et al., 2006; Philip and Bassett, 2011). In addition, adults with a 22q11.2 microdeletion have a two- to threefold increase in the risk of generalized anxiety disorder compared to the general population (Philip and Bassett, 2011). The major clinical features of 22q11.2DS-related schizophrenia are largely indistinguishable from those of the idiopathic disease (Murphy et al., 1999; Chow et al., 2006; Bassett and Chow, 2008). Identification of schizophrenia-risk gene/s in the 22q11.2DS deletion region may therefore generate insights into the pathophysiology of schizophrenia in general (Earls et al., 2012).
The size of the 22q11.2 deletion varies. The majority of 22q11.2 deletions (around 90%) are 3 Mb in size and span approximately 60 known genes, while the remaining 10% are 1.5 Mb in size and encompass around 35 genes (Edelmann et al., 1999; Shaikh et al., 2000). Both the larger and the smaller 22q11.2 microdeletions usually result from non-allelic homologous recombination, which is mediated by flanking low-copy repeats (Edelmann et al., 1999). Although the 22q11.2DS phenotype is highly variable, its severity is not correlated with the size of the deletion. This suggests that the minimal 1.5 Mb deletion region is crucial in terms of etiology (Carlson et al., 1997; Karayiorgou et al., 2010).
Initial research to identify schizophrenia-risk genes in the 22q11.2 deletion region proved unsuccessful. The identification of heterozygous loss-of-function mutations in non-deleted schizophrenia patients would be the most obvious human genetic evidence for the involvement of a specific gene in disease susceptibility. Hopes were raised by the identification of heterozygous point mutations in the T-box 1 gene (TBX1), which encodes a T-box transcription factor, that resulted in the characteristic abnormal facies and cardiac defects of 22q11.2DS in patients without a 22q11.2 deletion (Yagi et al., 2003; Zweier et al., 2007). However, no such mutation has yet been identified in schizophrenia patients.
In contrast, recent research in the Df(16)A+/- mouse model has generated breakthroughs in our understanding of the underlying biological mechanisms of 22q11.2DS schizophrenia-risk. This engineered mouse strain carries a heterozygous chromosomal deletion which spans a segment syntenic to the human 22q11.2 locus. Df(16)A+/- mice show deficits in the synaptic connectivity of hippocampal neurons, including a lower density of dendritic spines and glutamatergic synapses (Mukai et al., 2008). In addition, Df(16)A+/- mice display hyperactive behavior and deficits in spatial working memory-dependent learning (Stark et al., 2008). Further characterization of this animal model has suggested that the 22q11.2 microdeletion results in alterations in the biogenesis of brain microRNAs (Stark et al., 2008; Xu et al., 2010). Primary candidate genes in the region are the DiGeorge syndrome critical region gene 8 (DGCR8), which encodes a component of the microprocessor complex essential for microRNA biogenesis (Tomari and Zamore, 2005), and the MIR185 gene (Karayiorgou et al., 2010), which encodes microRNA 185. Both genes are located within the minimal 1.5 Mb deletion region at 22q11.2 (Karayiorgou et al., 2010).
The microRNAs are a class of 21–25-nucleotide small non-coding RNAs. They control the expression of their target genes by binding to target sites in messenger RNAs (mRNAs), typically in their 3′ untranslated regions (He and Hannon, 2004; Meola et al., 2009). In most cases, microRNAs negatively regulate target gene expression through a combination of repression of mRNA translation and promotion of mRNA decay. Each microRNA usually controls up to several hundred target mRNAs, while one mRNA target can be synergistically regulated by multiple microRNAs (Sathyan et al., 2007; Didiano and Hobert, 2008; Drew et al., 2011). This allows microRNAs to integrate different intracellular signals and to regulate various signaling pathways (Johnston and Hobert, 2003; Choi et al., 2007). Accumulating evidence suggests that microRNAs contribute to the basic mechanisms underlying brain development and plasticity (Table 1; Fineberg et al., 2009; Schratt, 2009; Im and Kenny, 2012). Neural microRNAs play an important role at various stages of synaptic development, including dendritic arborization (Vo et al., 2005; Yu et al., 2008), synapse formation, and synapse maturation (Caygill and Johnston, 2008; Siegel et al., 2009). Arguably the two most extensively studied examples in the context of synapse development are miR-132 and miR-134. CREB-induced miR-132 promotes dendritogenesis and spine growth by down-regulating p250GAP (Wayman et al., 2008; Magill et al., 2010). miR-134 on the other hand is required for activity-dependent dendritic arborization and the restriction of spine growth by targeting Pumilio-2 and Lim-domain containing protein kinase (Limk1), respectively (Schratt et al., 2006; Fiore et al., 2009). Furthermore, investigation of a mouse model displaying conditional knock-out of the microRNA biogenesis enzyme Dicer (Schratt, 2009) revealed disrupted morphogenesis of the hippocampus and cortex (Davis et al., 2008), suggesting that undisturbed microRNA processing might be necessary for normal brain development (Xu et al., 2010). These data suggest the possible involvement of microRNA-dependent dysregulation in the pathogenesis of various psychiatric disorders (Forero et al., 2010; Xu et al., 2010), including schizophrenia (Beveridge et al., 2008).
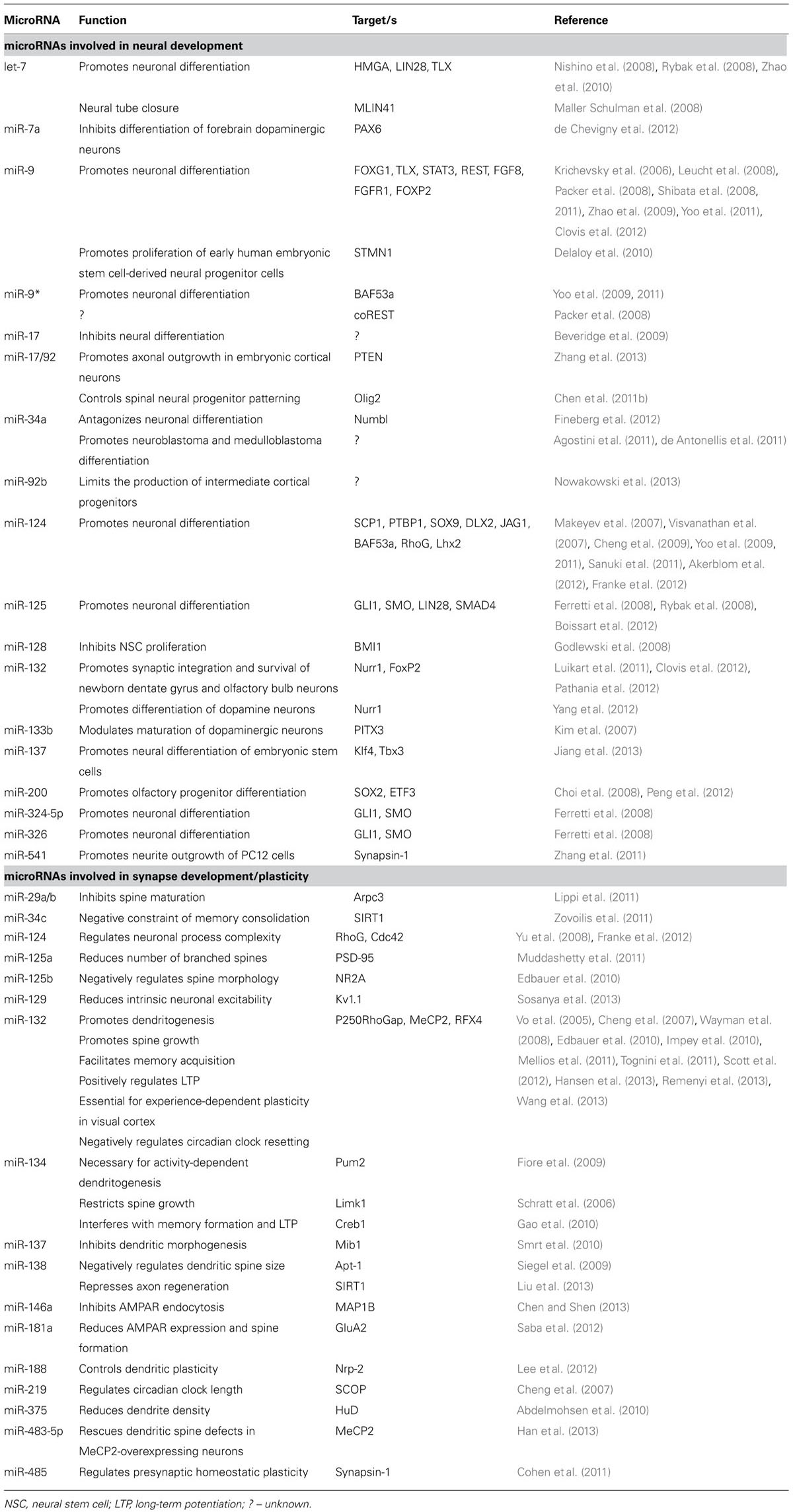
TABLE 1. List of individual microRNAs involved in neural development and synapse development/plasticity and their mRNA targets in mice and men.
The present review summarizes the various lines of evidence implicating microRNAs as the causal factor for schizophrenia in 22q11.2DS carriers and emerging evidence from expression studies and genome-wide association studies (GWAS) that these mechanisms may also be involved in the development of idiopathic schizophrenia.
The Role of Dgcr8
Investigation of Dgcr8+/- mice confirmed that heterozygous Dgcr8 deficiency was responsible for the reduced biogenesis of microRNAs observed in Df(16)A+/- mice (Stark et al., 2008; Schofield et al., 2011). Dgcr8+/- mice displayed 22q11.2DS-associated cognitive and behavioral deficits, and altered short-term plasticity in the prefrontal cortex (PFC; Stark et al., 2008). This indicates that DGCR8 heterozygosity, and the resulting alterations in microRNA expression, are sufficient to produce some of the neural deficits observed in 22q11.2DS (Schofield et al., 2011). On the neuronal cell level, Dgcr8 deficiency resulted in structural changes in dendritic spines and reduced dendritic complexity in the hippocampus (Stark et al., 2008). Schofield et al. (2011) identified alterations in the electrical properties of layer V pyramidal neurons in the medial PFC of Dgcr8+/- mice, as well as a decrease in the complexity of the basal dendrites and reduced excitatory synaptic transmission. These functional results suggest that precise microRNA expression is critical for the development of PFC circuitry (Schofield et al., 2011), circuitry which has been reported to be altered in schizophrenia patients (Ursu et al., 2011).
Dgcr8+/- mice also displayed a decrease in the number of cortical neurons, structural deficits in dendritic spines in the PFC, and alterations in synaptic potentiation and short-term plasticity (Fenelon et al., 2011). These alterations might influence functional connectivity (Schreiner et al., 2013), and could be implicated in the observed cognitive and behavioral deficits. In particular, they may explain observed alterations in prepulse inhibition (Stark et al., 2008), which have also been reported in schizophrenia patients (Powell et al., 2009).
Ouchi et al. (2013) showed that heterozygous Dgcr8 deficiency in mice led to reduced progenitor cell proliferation and neurogenesis in the adult hippocampus. This is of particular interest since alterations in the anatomy, histology, and function of the hippocampus have been consistently reported in schizophrenia patients (Tamminga et al., 2010). Several schizophrenia-associated genes were down-regulated in the hippocampus of Dgcr8+/- mice (Ouchi et al., 2013), including the insulin-like growth factor 2 (IGF2), which was recently found to play a crucial role in hippocampal functions such as memory consolidation and fear extinction (Agis-Balboa et al., 2011; Chen et al., 2011a). Interestingly, restoration of IGF2 expression in the hippocampus rescued the observed spatial working memory deficits in Dgcr8+/- mice, suggesting that IGF2 contributes – at least in part – to the learning and spatial working memory deficits that are associated with 22q11.2DS-related schizophrenia (Ouchi et al., 2013).
The question now arises as to which specific microRNAs are regulated by DGCR8. The investigation of Dgcr8+/- mice identified 59 down-regulated microRNAs in the PFC and 30 down-regulated microRNAs in the hippocampus (Stark et al., 2008). These down-regulated microRNAs include miR-185, which is also located in the minimal 1.5 Mb deletion region at 22q11.2.
The Role of miR185
Studies of 22q11.2DS mouse models have identified miR-185 as the top-scoring down-regulated microRNA in schizophrenia-associated brain areas (Stark et al., 2008; Benetti et al., 2009). A recent study by Xu et al. (2013) confirmed the drastic reduction in miR-185 expression levels in the hippocampus and PFC of Df(16)A+/- mice, and showed that this reduction contributed to deficits in dendritic complexity and spine development in hippocampal neurons. In addition, Dgcr8 deficiency resulted in an approximately 20% reduction in miR-185 expression in the hippocampus (Xu et al., 2013). This suggests that the pronounced reduction of miR-185 expression in Df(16)A+/- mice – a reduction which is much more pronounced than would be expected by the 50% decrease in gene dosage – may be due to the combined effect of the hemizygosity of the MIR185 gene and the impaired maturation of the pri-miR-185 transcript secondary to reduced Dgcr8 levels (Figure 1; Xu et al., 2013). The large reduction in miR-185 expression renders miR-185 unique among the genes that are affected by the 22q11.2 microdeletion (Xu et al., 2013).
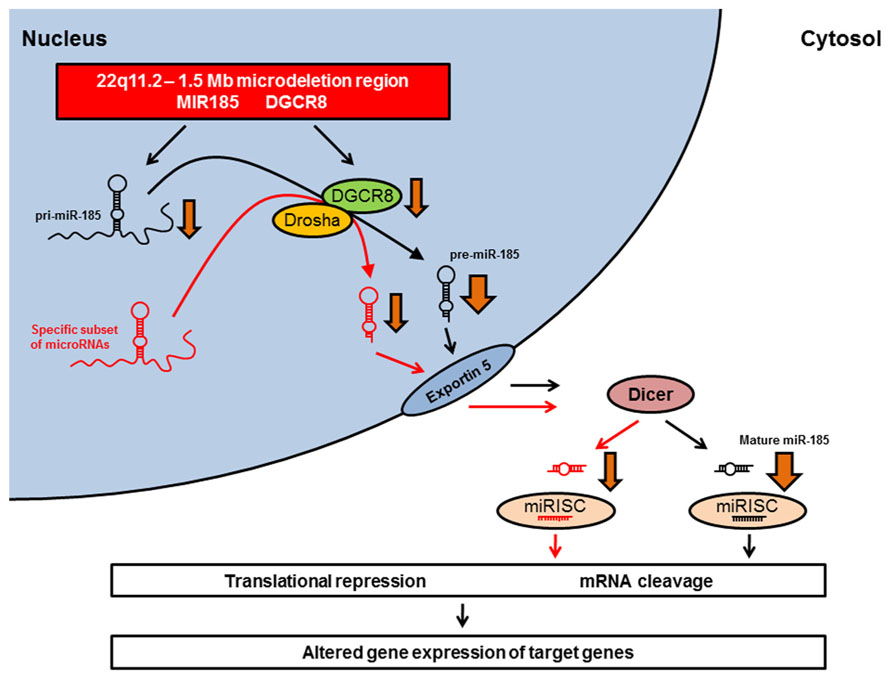
FIGURE 1. Dysregulation of microRNA biogenesis in 22q11.2DS animal models. The MIR185 and the DGCR8 genes are located within the minimal 1.5 Mb microdeletion region on chromosome 22q11.2 and the equivalent region of mouse chromosome 16. The microdeletion leads to a hemizygosity of MIR185 and DGCR8. The heterozygous Dgcr8 deficiency is responsible for the reduced biogenesis of a specific subset of microRNAs (red) observed in Df(16)A+/- mice (Stark et al., 2008). These down-regulated microRNAs include miR-185 (black). The pronounced reduction of miR-185 expression (indicated by a broader arrow) may be due to a combined effect of the hemizygosity of the MIR185 gene and the impaired maturation of the pri-miR-185 transcript secondary to reduced Dgcr8 levels (Xu et al., 2013). The resulting alterations in mature microRNA expression levels may lead to altered gene expression of target genes, which might produce some of the neural, cognitive, and behavioral deficits observed in 22q11.2DS. miRISC, microRNA-induced silencing complex.
A recent human study confirmed a down-regulation of MIR185 expression to 0.4× normal levels in the peripheral blood of patients with 22q11.2DS (de la Morena et al., 2013). This finding suggests that pronounced miR-185 down-regulation also occurs in patients with 22q11.2DS.
Previous research has shown that MIR185 is present or enriched in synapses (Lugli et al., 2008; Earls et al., 2012). This may indicate that MIR185 is of relevance to neural function, since a number of microRNAs have been shown to play a critical role in synaptic plasticity (Schratt, 2009).
Earls et al. (2012) identified MIR185 as a regulator of sarco(endo)plasmic reticulum Ca(2+) ATPase (SERCA2) which maintains Ca2+ levels in the endoplasmatic reticulum. The depletion of MIR185 contributes to SERCA2 upregulation and has been proposed as a mechanism leading to abnormal hippocampal synaptic plasticity in 22q11.2DS mouse models. The microRNA regulation of SERCA2 translation may also be implicated in the elevation of SERCA2 protein observed in the post-mortem brains of idiopathic schizophrenia patients (Earls et al., 2012). These results suggest that microRNA-mediated SERCA2 upregulation at central synapses might be a mechanistic link between 22q11.2DS and idiopathic schizophrenia (Earls et al., 2012).
Further support for the involvement of MIR185 in schizophrenia is provided by findings that two of its validated targets (RhoA, Cdc42; Liu et al., 2011) are associated with altered expression levels in schizophrenia (Hill et al., 2006; Ide and Lewis, 2010). Cdc42 (cell division cycle 42) is a member of the RhoGTPase family (Hill et al., 2006) and promotes dendritic spine formation (Irie and Yamaguchi, 2002; Tada and Sheng, 2006; Wegner et al., 2008) by regulating the polymerization of the actin cytoskeleton into filopodia (Nobes and Hall, 1995). Cdc42 is activated by Collybistin/ARHGEF9 (Reid et al., 1999; Reddy-Alla et al., 2010), which has recently been identified as a candidate blood biomarker in psychosis (Kurian et al., 2011). RhoA (Ras homologous member A) also belongs to the RhoGTPase family and regulates the destabilization of the actin cytoskeleton (Hill et al., 2006). The activation of RhoA leads to a reduction in the number of dendritic branches and the density of dendritic spines (Nakayama et al., 2000; Hill et al., 2006).
Expression of microRNAs in Idiopathic Schizophrenia
Post-mortem studies of human brain tissue have revealed alterations in microRNA expression in patients with schizophrenia. This research is reviewed elsewhere (Beveridge and Cairns, 2012). Briefly, numerous microRNAs have been implicated in the disorder across multiple studies, including 16 microRNAs with increased and 11 microRNAs with decreased expression (Beveridge and Cairns, 2012). Of particular interest in the context of the present review is the study by Moreau et al. (2011). This reported a significant overlap between microRNAs dysregulated in human post-mortem brain tissue and microRNAs previously found to be altered in the PFC of a 22q11.2DS mouse model (Stark et al., 2008; Moreau et al., 2011). This finding supports the hypothesis that findings in 22q11.2DS might be of relevance to idiopathic schizophrenia (Brzustowicz and Bassett, 2012).
GWAS of Idiopathic Schizophrenia
The involvement of microRNA-dependent dysregulation in schizophrenia is supported by the results of the large GWAS of schizophrenia performed by the Schizophrenia Psychiatric Genome-Wide Association Study (GWAS) Consortium (2011). In total 17,836 patients and 33,859 controls were investigated. A single-nucleotide polymorphism (SNP) in an intron of MIR137 was the second strongest finding (odds ratio = 1.12). Four other loci with genome-wide significance in this study contained predicted targets of MIR137 (TCF4, CACNA1C, CSMD1, C10orf26). All four genes have recently been validated as miR-137 targets (Kwon et al., 2013).
The miRanda database lists 5,487 genes as targets of miR-137 (John et al., 2004). Interestingly, ZNF804A is listed as a validated target (Kim et al., 2012a). This gene has shown strong association with schizophrenia in previous studies (O’Donovan et al., 2008; Williams et al., 2011). Other promising targets include the ubiquitin ligase Mind bomb one (Mib1; Smrt et al., 2010) which plays an important role in neurogenesis and neurodevelopment (Itoh et al., 2003; Choe et al., 2007; Ossipova et al., 2009).
Research in post-mortem brain samples suggests that the functional effect of the miR-137 risk allele may result in a reduced miR-137 expression (Guella et al., 2013). Further down-stream this may be responsible for the reduced white matter integrity, smaller hippocampi, and larger lateral ventricles observed in schizophrenia patients with the miR-137 risk genotype (Lett et al., 2013).
Conclusion and Outlook
Strong evidence suggests that microRNA dysregulation is implicated in the development of schizophrenia in 22q11.2DS patients. This is consistent with the growing recognition of microRNAs as important regulators of gene expression. As microRNAs integrate different intracellular signals and regulate various signaling pathways (Johnston and Hobert, 2003; Choi et al., 2007), the dysregulation of specific microRNAs could lead to the heterogenous phenotype observed in 22q11.2DS.
As summarized above, emerging evidence from expression and genetic analyses suggests that the same microRNA-regulated pathways may also play a role in idiopathic schizophrenia. However, despite a number of systematic investigations of genes in the 22q11.2DS region and the ever increasing number of GWAS data sets (Karayiorgou et al., 2010; Sullivan et al., 2012), no genetic study to date has identified common variation in DGCR8 or MIR185 as a risk factor for schizophrenia. This may simply reflect a lack of common functional variants at these loci. This hypothesis is supported by a recent study of genetic regulation of microRNA expression (Gamazon et al., 2012). Gamazon et al. (2012) systematically investigated the relationship between microRNA expression levels (as quantitative traits) and common genetic variation. In this study, no SNP had significant cis effects on miR-185 expression. A small number of SNPs have been reported to have significant cis effects on DGCR8 expression in human monocytes (Zeller et al., 2010), and fibroblasts (Dimas et al., 2009). However, these associations might be tissue-specific, since a recent study of five different human post-mortem brain regions failed to identify any SNP with significant cis effects on DGCR8 expression (Kim et al., 2012b).
A challenge for future research will be to identify and validate the target genes that are affected by microRNA dysregulation and their respective pathways in a more comprehensive manner (Drew et al., 2011). Such research will improve our understanding of how alterations in microRNA-regulated genetic networks contribute to the pathophysiology of both 22q11.2DS-related and idiopathic schizophrenia.
Idiopathic schizophrenia is a multifactorial disorder for which both genetic and environmental factors exert an impact on disease susceptibility (Sawa and Snyder, 2002). However, very few data are available concerning the influence of environmental factors on microRNA dysregulation. Recent studies in mice showed that environmental factors such as stress resulted in alterations of microRNA expression in the frontal cortex (Rinaldi et al., 2010). Future studies are therefore warranted to investigate the extent to which environmental factors are associated with microRNA dysregulation in schizophrenia.
Further research into the precise role of microRNAs in schizophrenia is important clinically, since modification of microRNA dysregulation would represent a novel therapeutic approach to this devastating and chronic disease. MicroRNAs are excellent candidates for therapy since they regulate multiple targets in various signaling pathways, thereby minimizing the risk of resistance development or compensatory mechanisms (Soriano et al., 2013). This view is supported by several recent studies and reviews, which have highlighted microRNAs as promising pharmacological targets in the treatment of complex diseases such as psychiatric disorders (Im and Kenny, 2012), cancer (Soriano et al., 2013), and diabetes (Mao et al., 2013).
Conflict of Interest Statement
The authors declare that the research was conducted in the absence of any commercial or financial relationships that could be construed as a potential conflict of interest.
Acknowledgments
We thank Christine Schmäl for carefully reading the manuscript. This work was supported by the German Federal Ministry of Education and Research (BMBF) through the Integrated Genome Research Network (IG) MooDS (Systematic Investigation of the Molecular Causes of Major Mood Disorders and Schizophrenia; grant 01GS08144 to Markus M. Nöthen), under the auspices of the National Genome Research Network plus (NGFNplus). Markus M. Nöthen is a member of the DFG-funded Excellence-Cluster ImmunoSensation. He also received support from the Alfried Krupp von Bohlen und Halbach-Stiftung. Andreas J. Forstner received support from the BONFOR program of the Medical Faculty of the University of Bonn. Research in the laboratory of Gerhard Schratt is funded by the European Research Council (ERC Starting Grant “Neuromir”) and the DFG (SFB593). These funding sources had no involvement in the study design; the collection, analysis, and interpretation of data; the writing of the report; or the decision to submit the paper for publication.
References
Abdelmohsen, K., Hutchison, E. R., Lee, E. K., Kuwano, Y., Kim, M. M., Masuda, K., et al. (2010). miR-375 inhibits differentiation of neurites by lowering HuD levels. Mol. Cell. Biol. 30, 4197–4210. doi: 10.1128/MCB.00316-10
Agis-Balboa, R. C., Arcos-Diaz, D., Wittnam, J., Govindarajan, N., Blom, K., Burkhardt, S., et al. (2011). A hippocampal insulin-growth factor 2 pathway regulates the extinction of fear memories. EMBO J. 30, 4071–4083. doi: 10.1038/emboj.2011.293
Agostini, M., Tucci, P., Killick, R., Candi, E., Sayan, B. S., Rivetti Di Val Cervo, P., et al. (2011). Neuronal differentiation by TAp73 is mediated by microRNA-34a regulation of synaptic protein targets. Proc. Natl. Acad. Sci. U.S.A. 108, 21093–21098. doi: 10.1073/pnas.1112061109
Akerblom, M., Sachdeva, R., and Jakobsson, J. (2012). Functional studies of microRNAs in neural stem cells: problems and perspectives. Front. Neurosci. 6:14. doi: 10.3389/fnins.2012.00014
Bassett, A. S., and Chow, E. W. (2008). Schizophrenia and 22q11.2 deletion syndrome. Curr. Psychiatry Rep. 10, 148–157. doi: 10.1007/s11920-008-0026-1
Benetti, S., Mechelli, A., Picchioni, M., Broome, M., Williams, S., and Mcguire, P. (2009). Functional integration between the posterior hippocampus and prefrontal cortex is impaired in both first episode schizophrenia and the at risk mental state. Brain 132, 2426–2436. doi: 10.1093/brain/awp098
Beveridge, N. J., and Cairns, M. J. (2012). MicroRNA dysregulation in schizophrenia. Neurobiol. Dis. 46, 263–271. doi: 10.1016/j.nbd.2011.12.029
Beveridge, N. J., Tooney, P. A., Carroll, A. P., Gardiner, E., Bowden, N., Scott, R. J., et al. (2008). Dysregulation of miRNA 181b in the temporal cortex in schizophrenia. Hum. Mol. Genet. 17, 1156–1168. doi: 10.1093/hmg/ddn005
Beveridge, N. J., Tooney, P. A., Carroll, A. P., Tran, N., and Cairns, M. J. (2009). Down-regulation of miR-17 family expression in response to retinoic acid induced neuronal differentiation. Cell. Signal. 21, 1837–1845. doi: 10.1016/j.cellsig.2009.07.019
Boissart, C., Nissan, X., Giraud-Triboult, K., Peschanski, M., and Benchoua, A. (2012). miR-125 potentiates early neural specification of human embryonic stem cells. Development 139, 1247–1257. doi: 10.1242/dev.073627
Botto, L. D., May, K., Fernhoff, P. M., Correa, A., Coleman, K., Rasmussen, S. A., et al. (2003). A population-based study of the 22q11.2 deletion: phenotype, incidence, and contribution to major birth defects in the population. Pediatrics 112, 101–107. doi: 10.1542/peds.112.1.101
Brzustowicz, L. M., and Bassett, A. S. (2012). miRNA-mediated risk for schizophrenia in 22q11.2 deletion syndrome. Front. Genet. 3:291. doi: 10.3389/fgene.2012.00291
Carlson, C., Sirotkin, H., Pandita, R., Goldberg, R., Mckie, J., Wadey, R., et al. (1997). Molecular definition of 22q11 deletions in 151 velo-cardio-facial syndrome patients. Am. J. Hum. Genet. 61, 620–629. doi: 10.1086/515508
Caygill, E. E., and Johnston, L. A. (2008). Temporal regulation of metamorphic processes in Drosophila by the let-7 and miR-125 heterochronic microRNAs. Curr. Biol. 18, 943–950. doi: 10.1016/j.cub.2008.06.020
Chen, D. Y., Stern, S. A., Garcia-Osta, A., Saunier-Rebori, B., Pollonini, G., Bambah-Mukku, D., et al. (2011a). A critical role for IGF-II in memory consolidation and enhancement. Nature 469, 491–497. doi: 10.1038/nature09667
Chen, J. A., Huang, Y. P., Mazzoni, E. O., Tan, G. C., Zavadil, J., and Wichterle, H. (2011b). Mir-17-3p controls spinal neural progenitor patterning by regulating Olig2/Irx3 cross-repressive loop. Neuron 69, 721–735. doi: 10.1016/j.neuron.2011.01.014
Chen, Y. L., and Shen, C. K. (2013). Modulation of mGluR-dependent MAP1B translation and AMPA receptor endocytosis by microRNA miR-146a-5p. J. Neurosci. 33, 9013–9020. doi: 10.1523/JNEUROSCI.5210-12.2013
Cheng, H. Y., Papp, J. W., Varlamova, O., Dziema, H., Russell, B., Curfman, J. P., et al. (2007). microRNA modulation of circadian-clock period and entrainment. Neuron 54, 813–829. doi: 10.1016/j.neuron.2007.05.017
Cheng, L. C., Pastrana, E., Tavazoie, M., and Doetsch, F. (2009). miR-124 regulates adult neurogenesis in the subventricular zone stem cell niche. Nat. Neurosci. 12, 399–408. doi: 10.1038/nn.2294
Choe, E. A., Liao, L., Zhou, J. Y., Cheng, D., Duong, D. M., Jin, P., et al. (2007). Neuronal morphogenesis is regulated by the interplay between cyclin-dependent kinase 5 and the ubiquitin ligase mind bomb 1. J. Neurosci. 27, 9503–9512. doi: 10.1523/JNEUROSCI.1408-07.2007
Choi, P. S., Zakhary, L., Choi, W. Y., Caron, S., Alvarez-Saavedra, E., Miska, E. A., et al. (2008). Members of the miRNA-200 family regulate olfactory neurogenesis. Neuron 57, 41–55. doi: 10.1016/j.neuron.2007.11.018
Choi, W. Y., Giraldez, A. J., and Schier, A. F. (2007). Target protectors reveal dampening and balancing of Nodal agonist and antagonist by miR-430. Science 318, 271–274. doi: 10.1126/science.1147535
Chow, E. W., Watson, M., Young, D. A., and Bassett, A. S. (2006). Neurocognitive profile in 22q11 deletion syndrome and schizophrenia. Schizophr. Res. 87, 270–278. doi: 10.1016/j.schres.2006.04.007
Clovis, Y. M., Enard, W., Marinaro, F., Huttner, W. B., and De Pietri Tonelli, D. (2012). Convergent repression of Foxp2 3′UTR by miR-9 and miR-132 in embryonic mouse neocortex: implications for radial migration of neurons. Development 139, 3332–3342. doi: 10.1242/dev.078063
Cohen, J. E., Lee, P. R., Chen, S., Li, W., and Fields, R. D. (2011). MicroRNA regulation of homeostatic synaptic plasticity. Proc. Natl. Acad. Sci. U.S.A. 108, 11650–11655. doi: 10.1073/pnas.1017576108
Davis, T. H., Cuellar, T. L., Koch, S. M., Barker, A. J., Harfe, B. D., Mcmanus, M. T., et al. (2008). Conditional loss of Dicer disrupts cellular and tissue morphogenesis in the cortex and hippocampus. J. Neurosci. 28, 4322–4330. doi: 10.1523/JNEUROSCI.4815-07.2008
de Antonellis, P., Medaglia, C., Cusanelli, E., Andolfo, I., Liguori, L., De Vita, G., et al. (2011). MiR-34a targeting of Notch ligand delta-like 1 impairs CD15+/CD133+ tumor-propagating cells and supports neural differentiation in medulloblastoma. PLoS ONE 6:e24584. doi: 10.1371/journal.pone.0024584
de Chevigny, A., Core, N., Follert, P., Gaudin, M., Barbry, P., Beclin, C., et al. (2012). miR-7a regulation of Pax6 controls spatial origin of forebrain dopaminergic neurons. Nat. Neurosci. 15, 1120–1126. doi: 10.1038/nn.3142
Delaloy, C., Liu, L., Lee, J. A., Su, H., Shen, F., Yang, G. Y., et al. (2010). MicroRNA-9 coordinates proliferation and migration of human embryonic stem cell-derived neural progenitors. Cell Stem Cell 6, 323–335. doi: 10.1016/j.stem.2010.02.015
de la Morena, M. T., Eitson, J. L., Dozmorov, I. M., Belkaya, S., Hoover, A. R., Anguiano, E., et al. (2013). Signature MicroRNA expression patterns identified in humans with 22q11.2 deletion/DiGeorge syndrome. Clin. Immunol. 147, 11–22. doi: 10.1016/j.clim.2013.01.011
Didiano, D., and Hobert, O. (2008). Molecular architecture of a miRNA-regulated 3′ UTR. RNA 14, 1297–1317. doi: 10.1261/rna.1082708
Dimas, A. S., Deutsch, S., Stranger, B. E., Montgomery, S. B., Borel, C., Attar-Cohen, H., et al. (2009). Common regulatory variation impacts gene expression in a cell type-dependent manner. Science 325, 1246–1250. doi: 10.1126/science.1174148
Drew, L. J., Crabtree, G. W., Markx, S., Stark, K. L., Chaverneff, F., Xu, B., et al. (2011). The 22q11.2 microdeletion: fifteen years of insights into the genetic and neural complexity of psychiatric disorders. Int. J. Dev. Neurosci. 29, 259–281. doi: 10.1016/j.ijdevneu.2010.09.007
Earls, L. R., Fricke, R. G., Yu, J., Berry, R. B., Baldwin, L. T., and Zakharenko, S. S. (2012). Age-dependent microRNA control of synaptic plasticity in 22q11 deletion syndrome and schizophrenia. J. Neurosci. 32, 14132–14144. doi: 10.1523/JNEUROSCI.1312-12.2012
Edbauer, D., Neilson, J. R., Foster, K. A., Wang, C. F., Seeburg, D. P., Batterton, M. N., et al. (2010). Regulation of synaptic structure and function by FMRP-associated microRNAs miR-125b and miR-132. Neuron 65, 373–384. doi: 10.1016/j.neuron.2010.01.005
Edelmann, L., Pandita, R. K., and Morrow, B. E. (1999). Low-copy repeats mediate the common 3-Mb deletion in patients with velo-cardio-facial syndrome. Am. J. Hum. Genet. 64, 1076–1086. doi: 10.1086/302343
Fenelon, K., Mukai, J., Xu, B., Hsu, P. K., Drew, L. J., Karayiorgou, M., et al. (2011). Deficiency of Dgcr8, a gene disrupted by the 22q11.2 microdeletion, results in altered short-term plasticity in the prefrontal cortex. Proc. Natl. Acad. Sci. U.S.A. 108, 4447–4452. doi: 10.1073/pnas.1101219108
Ferretti, E., De Smaele, E., Miele, E., Laneve, P., Po, A., Pelloni, M., et al. (2008). Concerted microRNA control of Hedgehog signalling in cerebellar neuronal progenitor and tumour cells. EMBO J. 27, 2616–2627. doi: 10.1038/emboj.2008.172
Fineberg, S. K., Datta, P., Stein, C. S., and Davidson, B. L. (2012). MiR-34a represses Numbl in murine neural progenitor cells and antagonizes neuronal differentiation. PLoS ONE 7:e38562. doi: 10.1371/journal.pone.0038562
Fineberg, S. K., Kosik, K. S., and Davidson, B. L. (2009). MicroRNAs potentiate neural development. Neuron 64, 303–309. doi: 10.1016/j.neuron.2009.10.020
Fiore, R., Khudayberdiev, S., Christensen, M., Siegel, G., Flavell, S. W., Kim, T. K., et al. (2009). Mef2-mediated transcription of the miR379-410 cluster regulates activity-dependent dendritogenesis by fine-tuning Pumilio2 protein levels. EMBO J. 28, 697–710. doi: 10.1038/emboj.2009.10
Forero, D. A., Van Der Ven, K., Callaerts, P., and Del-Favero, J. (2010). miRNA genes and the brain: implications for psychiatric disorders. Hum. Mutat. 31, 1195–1204. doi: 10.1002/humu.21344
Franke, K., Otto, W., Johannes, S., Baumgart, J., Nitsch, R., and Schumacher, S. (2012). miR-124-regulated RhoG reduces neuronal process complexity via ELMO/Dock180/Rac1 and Cdc42 signalling. EMBO J. 31, 2908–2921. doi: 10.1038/emboj.2012.130
Gamazon, E. R., Ziliak, D., Im, H. K., Lacroix, B., Park, D. S., Cox, N. J., et al. (2012). Genetic architecture of microRNA expression: implications for the transcriptome and complex traits. Am. J. Hum. Genet. 90, 1046–1063. doi: 10.1016/j.ajhg.2012.04.023
Gao, J., Wang, W. Y., Mao, Y. W., Graff, J., Guan, J. S., Pan, L., et al. (2010). A novel pathway regulates memory and plasticity via SIRT1 and miR-134. Nature 466, 1105–1109. doi: 10.1038/nature09271
Godlewski, J., Nowicki, M. O., Bronisz, A., Williams, S., Otsuki, A., Nuovo, G., et al. (2008). Targeting of the Bmi-1 oncogene/stem cell renewal factor by microRNA-128 inhibits glioma proliferation and self-renewal. Cancer Res. 68, 9125–9130. doi: 10.1158/0008-5472.CAN-08-2629
Guella, I., Sequeira, A., Rollins, B., Morgan, L., Torri, F., Van Erp, T. G., et al. (2013). Analysis of miR-137 expression and rs1625579 in dorsolateral prefrontal cortex. J. Psychiatry Res. 47, 1215–1221. doi: 10.1016/j.jpsychires.2013.05.021
Han, K., Gennarino, V. A., Lee, Y., Pang, K., Hashimoto-Torii, K., Choufani, S., et al. (2013). Human-specific regulation of MeCP2 levels in fetal brains by microRNA miR-483-5p. Genes Dev. 27, 485–490. doi: 10.1101/gad.207456.112
Hansen, K. F., Karelina, K., Sakamoto, K., Wayman, G. A., Impey, S., and Obrietan, K. (2013). miRNA-132: a dynamic regulator of cognitive capacity. Brain Struct. Funct. 218, 817–831. doi: 10.1007/s00429-012-0431-4
He, L., and Hannon, G. J. (2004). MicroRNAs: small RNAs with a big role in gene regulation. Nat. Rev. Genet. 5, 522–531. doi: 10.1038/nrg1379
Hill, J. J., Hashimoto, T., and Lewis, D. A. (2006). Molecular mechanisms contributing to dendritic spine alterations in the prefrontal cortex of subjects with schizophrenia. Mol. Psychiatry 11, 557–566. doi: 10.1038/sj.mp.4001792
Ide, M., and Lewis, D. A. (2010). Altered cortical CDC42 signaling pathways in schizophrenia: implications for dendritic spine deficits. Biol. Psychiatry 68, 25–32. doi: 10.1016/j.biopsych.2010.02.016
Im, H. I., and Kenny, P. J. (2012). MicroRNAs in neuronal function and dysfunction. Trends Neurosci. 35, 325–334. doi: 10.1016/j.tins.2012.01.004
Impey, S., Davare, M., Lesiak, A., Fortin, D., Ando, H., Varlamova, O., et al. (2010). An activity-induced microRNA controls dendritic spine formation by regulating Rac1-PAK signaling. Mol. Cell. Neurosci. 43, 146–156. doi: 10.1016/j.mcn.2009.10.005
International Schizophrenia Consortium. (2008). Rare chromosomal deletions and duplications increase risk of schizophrenia. Nature 455, 237–241. doi: 10.1038/nature07239
Irie, F., and Yamaguchi, Y. (2002). EphB receptors regulate dendritic spine development via intersectin, Cdc42 and N-WASP. Nat. Neurosci. 5, 1117–1118. doi: 10.1038/nn964
Itoh, M., Kim, C. H., Palardy, G., Oda, T., Jiang, Y. J., Maust, D., et al. (2003). Mind bomb is a ubiquitin ligase that is essential for efficient activation of Notch signaling by Delta. Dev. Cell 4, 67–82. doi: 10.1016/S1534-5807(02)00409-4
Jiang, K., Ren, C., and Nair, V. D. (2013). MicroRNA-137 represses Klf4 and Tbx3 during differentiation of mouse embryonic stem cells. Stem Cell Res. 11, 1299–1313. doi: 10.1016/j.scr.2013.09.001
John, B., Enright, A. J., Aravin, A., Tuschl, T., Sander, C., and Marks, D. S. (2004). Human MicroRNA targets. PLoS Biol. 2:e363. doi: 10.1371/journal.pbio.0020363
Johnston, R. J., and Hobert, O. (2003). A microRNA controlling left/right neuronal asymmetry in Caenorhabditis elegans. Nature 426, 845–849. doi: 10.1038/nature02255
Karayiorgou, M., Morris, M. A., Morrow, B., Shprintzen, R. J., Goldberg, R., Borrow, J., et al. (1995). Schizophrenia susceptibility associated with interstitial deletions of chromosome 22q11. Proc. Natl. Acad. Sci. U.S.A. 92, 7612–7616. doi: 10.1073/pnas.92.17.7612
Karayiorgou, M., Simon, T. J., and Gogos, J. A. (2010). 22q11.2 microdeletions: linking DNA structural variation to brain dysfunction and schizophrenia. Nat. Rev. Neurosci. 11, 402–416. doi: 10.1038/nrn2841
Kim, A. H., Parker, E. K., Williamson, V., Mcmichael, G. O., Fanous, A. H., and Vladimirov, V. I. (2012a). Experimental validation of candidate schizophrenia gene ZNF804A as target for hsa-miR-137. Schizophr. Res. 141, 60–64. doi: 10.1016/j.schres.2012.06.038
Kim, S., Cho, H., Lee, D., and Webster, M. J. (2012b). Association between SNPs and gene expression in multiple regions of the human brain. Transl. Psychiatry 2, e113. doi: 10.1038/tp.2012.42
Kim, J., Inoue, K., Ishii, J., Vanti, W. B., Voronov, S. V., Murchison, E., et al. (2007). A MicroRNA feedback circuit in midbrain dopamine neurons. Science 317, 1220–1224. doi: 10.1126/science.1140481
Krichevsky, A. M., Sonntag, K. C., Isacson, O., and Kosik, K. S. (2006). Specific microRNAs modulate embryonic stem cell-derived neurogenesis. Stem Cells 24, 857–864. doi: 10.1634/stemcells.2005-0441
Kurian, S. M., Le-Niculescu, H., Patel, S. D., Bertram, D., Davis, J., Dike, C., et al. (2011). Identification of blood biomarkers for psychosis using convergent functional genomics. Mol. Psychiatry 16, 37–58. doi: 10.1038/mp.2009.117
Kwon, E., Wang, W., and Tsai, L. H. (2013). Validation of schizophrenia-associated genes CSMD1, C10orf26, CACNA1C and TCF4 as miR-137 targets. Mol. Psychiatry 18, 11–12. doi: 10.1038/mp.2011.170
Lee, K., Kim, J. H., Kwon, O. B., An, K., Ryu, J., Cho, K., et al. (2012). An activity-regulated microRNA, miR-188, controls dendritic plasticity and synaptic transmission by downregulating neuropilin-2. J. Neurosci. 32, 5678–5687. doi: 10.1523/JNEUROSCI.6471-11.2012
Lett, T. A., Chakravarty, M. M., Felsky, D., Brandl, E. J., Tiwari, A. K., Goncalves, V. F., et al. (2013). The genome-wide supported microRNA-137 variant predicts phenotypic heterogeneity within schizophrenia. Mol. Psychiatry 18, 1146. doi: 10.1038/mp.2013.39
Leucht, C., Stigloher, C., Wizenmann, A., Klafke, R., Folchert, A., and Bally-Cuif, L. (2008). MicroRNA-9 directs late organizer activity of the midbrain-hindbrain boundary. Nat. Neurosci. 11, 641–648. doi: 10.1038/nn.2115
Levinson, D. F., Duan, J., Oh, S., Wang, K., Sanders, A. R., Shi, J., et al. (2011). Copy number variants in schizophrenia: confirmation of five previous findings and new evidence for 3q29 microdeletions and VIPR2 duplications. Am. J. Psychiatry 168, 302–316. doi: 10.1176/appi.ajp.2010.10060876
Lippi, G., Steinert, J. R., Marczylo, E. L., D’Oro, S., Fiore, R., Forsythe, I. D., et al. (2011). Targeting of the Arpc3 actin nucleation factor by miR-29a/b regulates dendritic spine morphology. J. Cell Biol. 194, 889–904. doi: 10.1083/jcb.201103006
Liu, C. M., Wang, R. Y., Saijilafu, Jiao, Z. X., Zhang, B. Y., and Zhou, F. Q. (2013). MicroRNA-138 and SIRT1 form a mutual negative feedback loop to regulate mammalian axon regeneration. Genes Dev. 27, 1473–1483. doi: 10.1101/gad.209619.112
Liu, M., Lang, N., Chen, X., Tang, Q., Liu, S., Huang, J., et al. (2011). miR-185 targets RhoA and Cdc42 expression and inhibits the proliferation potential of human colorectal cells. Cancer Lett. 301, 151–160. doi: 10.1016/j.canlet.2010.11.009
Lugli, G., Torvik, V. I., Larson, J., and Smalheiser, N. R. (2008). Expression of microRNAs and their precursors in synaptic fractions of adult mouse forebrain. J. Neurochem. 106, 650–661. doi: 10.1111/j.1471-4159.2008.05413.x
Luikart, B. W., Bensen, A. L., Washburn, E. K., Perederiy, J. V., Su, K. G., Li, Y., et al. (2011). miR-132 mediates the integration of newborn neurons into the adult dentate gyrus. PLoS ONE 6:e19077. doi: 10.1371/journal.pone.0019077
Magill, S. T., Cambronne, X. A., Luikart, B. W., Lioy, D. T., Leighton, B. H., Westbrook, G. L., et al. (2010). microRNA-132 regulates dendritic growth and arborization of newborn neurons in the adult hippocampus. Proc. Natl. Acad. Sci. U.S.A. 107, 20382–20387. doi: 10.1073/pnas.1015691107
Makeyev, E. V., Zhang, J., Carrasco, M. A., and Maniatis, T. (2007). The microRNA miR-124 promotes neuronal differentiation by triggering brain-specific alternative pre-mRNA splicing. Mol. Cell 27, 435–448. doi: 10.1016/j.molcel.2007.07.015
Maller Schulman, B. R., Liang, X., Stahlhut, C., Delconte, C., Stefani, G., and Slack, F. J. (2008). The let-7 microRNA target gene, Mlin41/Trim71 is required for mouse embryonic survival and neural tube closure. Cell Cycle 7, 3935–3942. doi: 10.4161/cc.7.24.7397
Mao, Y., Mohan, R., Zhang, S., and Tang, X. (2013). MicroRNAs as pharmacological targets in diabetes. Pharmacol. Res. 75, 37–47. doi: 10.1016/j.phrs.2013.06.005
Mellios, N., Sugihara, H., Castro, J., Banerjee, A., Le, C., Kumar, A., et al. (2011). miR-132, an experience-dependent microRNA, is essential for visual cortex plasticity. Nat. Neurosci. 14, 1240–1242. doi: 10.1038/nn.2909
Meola, N., Gennarino, V. A., and Banfi, S. (2009). microRNAs and genetic diseases. Pathogenetics 2, 7. doi: 10.1186/1755-8417-2-7
Moreau, M. P., Bruse, S. E., David-Rus, R., Buyske, S., and Brzustowicz, L. M. (2011). Altered microRNA expression profiles in postmortem brain samples from individuals with schizophrenia and bipolar disorder. Biol. Psychiatry 69, 188–193. doi: 10.1016/j.biopsych.2010.09.039
Muddashetty, R. S., Nalavadi, V. C., Gross, C., Yao, X., Xing, L., Laur, O., et al. (2011). Reversible inhibition of PSD-95 mRNA translation by miR-125a, FMRP phosphorylation, and mGluR signaling. Mol. Cell 42, 673–688. doi: 10.1016/j.molcel.2011.05.006
Mukai, J., Dhilla, A., Drew, L. J., Stark, K. L., Cao, L., Macdermott, A. B., et al. (2008). Palmitoylation-dependent neurodevelopmental deficits in a mouse model of 22q11 microdeletion. Nat. Neurosci. 11, 1302–1310. doi: 10.1038/nn.2204
Murphy, K. C., Jones, L. A., and Owen, M. J. (1999). High rates of schizophrenia in adults with velo-cardio-facial syndrome. Arch. Gen. Psychiatry 56, 940–945. doi: 10.1001/archpsyc.56.10.940
Nakayama, A. Y., Harms, M. B., and Luo, L. (2000). Small GTPases Rac and Rho in the maintenance of dendritic spines and branches in hippocampal pyramidal neurons. J. Neurosci. 20, 5329–5338.
Nishino, J., Kim, I., Chada, K., and Morrison, S. J. (2008). Hmga2 promotes neural stem cell self-renewal in young but not old mice by reducing p16Ink4a and p19Arf expression. Cell 135, 227–239. doi: 10.1016/j.cell.2008.09.017
Nobes, C. D., and Hall, A. (1995). Rho, rac, and cdc42 GTPases regulate the assembly of multimolecular focal complexes associated with actin stress fibers, lamellipodia, and filopodia. Cell 81, 53–62. doi: 10.1016/0092-8674(95)90370-4
Nowakowski, T. J., Fotaki, V., Pollock, A., Sun, T., Pratt, T., and Price, D. J. (2013). MicroRNA-92b regulates the development of intermediate cortical progenitors in embryonic mouse brain. Proc. Natl. Acad. Sci. U.S.A. 110, 7056–7061. doi: 10.1073/pnas.1219385110
O’Donovan, M. C., Craddock, N., Norton, N., Williams, H., Peirce, T., Moskvina, V., et al. (2008). Identification of loci associated with schizophrenia by genome-wide association and follow-up. Nat. Genet. 40, 1053–1055. doi: 10.1038/ng.201
Ossipova, O., Ezan, J., and Sokol, S. Y. (2009). PAR-1 phosphorylates Mind bomb to promote vertebrate neurogenesis. Dev. Cell 17, 222–233. doi: 10.1016/j.devcel.2009.06.010
Ouchi, Y., Banno, Y., Shimizu, Y., Ando, S., Hasegawa, H., Adachi, K., et al. (2013). Reduced adult hippocampal neurogenesis and working memory deficits in the Dgcr8-deficient mouse model of 22q11.2 deletion-associated schizophrenia can be rescued by IGF2. J. Neurosci. 33, 9408–9419. doi: 10.1523/JNEUROSCI.2700-12.2013
Packer, A. N., Xing, Y., Harper, S. Q., Jones, L., and Davidson, B. L. (2008). The bifunctional microRNA miR-9/miR-9* regulates REST and CoREST and is downregulated in Huntington’s disease. J. Neurosci. 28, 14341–14346. doi: 10.1523/JNEUROSCI.2390-08.2008
Pathania, M., Torres-Reveron, J., Yan, L., Kimura, T., Lin, T. V., Gordon, V., et al. (2012). miR-132 enhances dendritic morphogenesis, spine density, synaptic integration, and survival of newborn olfactory bulb neurons. PLoS ONE 7:e38174. doi: 10.1371/journal.pone.0038174
Peng, C., Li, N., Ng, Y. K., Zhang, J., Meier, F., Theis, F. J., et al. (2012). A unilateral negative feedback loop between miR-200 microRNAs and Sox2/E2F3 controls neural progenitor cell-cycle exit and differentiation. J. Neurosci. 32, 13292–13308. doi: 10.1523/JNEUROSCI.2124-12.2012
Philip, N., and Bassett, A. (2011). Cognitive, behavioural and psychiatric phenotype in 22q11.2 deletion syndrome. Behav. Genet. 41, 403–412. doi: 10.1007/s10519-011-9468-z
Powell, S. B., Zhou, X., and Geyer, M. A. (2009). Prepulse inhibition and genetic mouse models of schizophrenia. Behav. Brain Res. 204, 282–294. doi: 10.1016/j.bbr.2009.04.021
Reddy-Alla, S., Schmitt, B., Birkenfeld, J., Eulenburg, V., Dutertre, S., Bohringer, C., et al. (2010). PH-domain-driven targeting of collybistin but not Cdc42 activation is required for synaptic gephyrin clustering. Eur. J. Neurosci. 31, 1173–1184. doi: 10.1111/j.1460-9568.2010.07149.x
Reid, T., Bathoorn, A., Ahmadian, M. R., and Collard, J. G. (1999). Identification and characterization of hPEM-2, a guanine nucleotide exchange factor specific for Cdc42. J. Biol. Chem. 274, 33587–33593. doi: 10.1074/jbc.274.47.33587
Remenyi, J., Van Den Bosch, M. W., Palygin, O., Mistry, R. B., Mckenzie, C., Macdonald, A., et al. (2013). miR-132/212 knockout mice reveal roles for these miRNAs in regulating cortical synaptic transmission and plasticity. PLoS ONE 8:e62509. doi: 10.1371/journal.pone.0062509
Rinaldi, A., Vincenti, S., De Vito, F., Bozzoni, I., Oliverio, A., Presutti, C., et al. (2010). Stress induces region specific alterations in microRNAs expression in mice. Behav. Brain Res. 208, 265–269. doi: 10.1016/j.bbr.2009.11.012
Robin, N. H., and Shprintzen, R. J. (2005). Defining the clinical spectrum of deletion 22q11.2. J. Pediatr. 147, 90–96. doi: 10.1016/j.jpeds.2005.03.007
Rybak, A., Fuchs, H., Smirnova, L., Brandt, C., Pohl, E. E., Nitsch, R., et al. (2008). A feedback loop comprising lin-28 and let-7 controls pre-let-7 maturation during neural stem-cell commitment. Nat. Cell Biol. 10, 987–993. doi: 10.1038/ncb1759
Saba, R., Storchel, P. H., Aksoy-Aksel, A., Kepura, F., Lippi, G., Plant, T. D., et al. (2012). Dopamine-regulated microRNA MiR-181a controls GluA2 surface expression in hippocampal neurons. Mol. Cell. Biol. 32, 619–632. doi: 10.1128/MCB.05896-11
Sanuki, R., Onishi, A., Koike, C., Muramatsu, R., Watanabe, S., Muranishi, Y., et al. (2011). miR-124a is required for hippocampal axogenesis and retinal cone survival through Lhx2 suppression. Nat. Neurosci. 14, 1125–1134. doi: 10.1038/nn.2897
Sathyan, P., Golden, H. B., and Miranda, R. C. (2007). Competing interactions between micro-RNAs determine neural progenitor survival and proliferation after ethanol exposure: evidence from an ex vivo model of the fetal cerebral cortical neuroepithelium. J. Neurosci. 27, 8546–8557. doi: 10.1523/JNEUROSCI.1269-07.2007
Sawa, A., and Snyder, S. H. (2002). Schizophrenia: diverse approaches to a complex disease. Science 296, 692–695. doi: 10.1126/science.1070532
Schizophrenia Psychiatric Genome-Wide Association Study (GWAS) Consortium. (2011). Genome-wide association study identifies five new schizophrenia loci. Nat. Genet. 43, 969–976. doi: 10.1038/ng.940
Schofield, C. M., Hsu, R., Barker, A. J., Gertz, C. C., Blelloch, R., and Ullian, E. M. (2011). Monoallelic deletion of the microRNA biogenesis gene Dgcr8 produces deficits in the development of excitatory synaptic transmission in the prefrontal cortex. Neural Dev. 6, 11. doi: 10.1186/1749-8104-6-11
Schratt, G. M., Tuebing, F., Nigh, E. A., Kane, C. G., Sabatini, M. E., Kiebler, M., et al. (2006). A brain-specific microRNA regulates dendritic spine development. Nature 439, 283–289. doi: 10.1038/nature04367
Schreiner, M. J., Lazaro, M. T., Jalbrzikowski, M., and Bearden, C. E. (2013). Converging levels of analysis on a genomic hotspot for psychosis: insights from 22q11.2 deletion syndrome. Neuropharmacology 68, 157–173. doi: 10.1016/j.neuropharm.2012.09.012
Scott, H. L., Tamagnini, F., Narduzzo, K. E., Howarth, J. L., Lee, Y. B., Wong, L. F., et al. (2012). MicroRNA-132 regulates recognition memory and synaptic plasticity in the perirhinal cortex. Eur. J. Neurosci. 36, 2941–2948. doi: 10.1111/j.1460-9568.2012.08220.x
Shaikh, T. H., Kurahashi, H., Saitta, S. C., O’Hare, A. M., Hu, P., Roe, B. A., et al. (2000). Chromosome 22-specific low copy repeats and the 22q11.2 deletion syndrome: genomic organization and deletion endpoint analysis. Hum. Mol. Genet. 9, 489–501. doi: 10.1093/hmg/9.4.489
Shibata, M., Kurokawa, D., Nakao, H., Ohmura, T., and Aizawa, S. (2008). MicroRNA-9 modulates Cajal–Retzius cell differentiation by suppressing Foxg1 expression in mouse medial pallium. J. Neurosci. 28, 10415–10421. doi: 10.1523/JNEUROSCI.3219-08.2008
Shibata, M., Nakao, H., Kiyonari, H., Abe, T., and Aizawa, S. (2011). MicroRNA-9 regulates neurogenesis in mouse telencephalon by targeting multiple transcription factors. J. Neurosci. 31, 3407–3422. doi: 10.1523/JNEUROSCI.5085-10.2011
Siegel, G., Obernosterer, G., Fiore, R., Oehmen, M., Bicker, S., Christensen, M., et al. (2009). A functional screen implicates microRNA-138-dependent regulation of the depalmitoylation enzyme APT1 in dendritic spine morphogenesis. Nat. Cell Biol. 11, 705–716. doi: 10.1038/ncb1876
Smrt, R. D., Szulwach, K. E., Pfeiffer, R. L., Li, X., Guo, W., Pathania, M., et al. (2010). MicroRNA miR-137 regulates neuronal maturation by targeting ubiquitin ligase mind bomb-1. Stem Cells 28, 1060–1070. doi: 10.1002/stem.431
Soriano, A., Jubierre, L., Almazan-Moga, A., Molist, C., Roma, J., De Toledo, J. S., et al. (2013). microRNAs as pharmacological targets in cancer. Pharmacol. Res. 75, 3–14. doi: 10.1016/j.phrs.2013.03.006
Sosanya, N. M., Huang, P. P., Cacheaux, L. P., Chen, C. J., Nguyen, K., Perrone-Bizzozero, N. I., et al. (2013). Degradation of high affinity HuD targets releases Kv1.1 mRNA from miR-129 repression by mTORC1. J. Cell Biol. 202, 53–69. doi: 10.1083/jcb.201212089
Stark, K. L., Xu, B., Bagchi, A., Lai, W. S., Liu, H., Hsu, R., et al. (2008). Altered brain microRNA biogenesis contributes to phenotypic deficits in a 22q11-deletion mouse model. Nat. Genet. 40, 751–760. doi: 10.1038/ng.138
Stefansson, H., Rujescu, D., Cichon, S., Pietilainen, O. P., Ingason, A., Steinberg, S., et al. (2008). Large recurrent microdeletions associated with schizophrenia. Nature 455, 232–236. doi: 10.1038/nature07229
Sullivan, P. F., Daly, M. J., and O’Donovan, M. (2012). Genetic architectures of psychiatric disorders: the emerging picture and its implications. Nat. Rev. Genet. 13, 537–551. doi: 10.1038/nrg3240
Tada, T., and Sheng, M. (2006). Molecular mechanisms of dendritic spine morphogenesis. Curr. Opin. Neurobiol. 16, 95–101. doi: 10.1016/j.conb.2005.12.001
Tamminga, C. A., Stan, A. D., and Wagner, A. D. (2010). The hippocampal formation in schizophrenia. Am. J. Psychiatry 167, 1178–1193. doi: 10.1176/appi.ajp.2010.09081187
Tognini, P., Putignano, E., Coatti, A., and Pizzorusso, T. (2011). Experience-dependent expression of miR-132 regulates ocular dominance plasticity. Nat. Neurosci. 14, 1237–1239. doi: 10.1038/nn.2920
Tomari, Y., and Zamore, P. D. (2005). MicroRNA biogenesis: drosha can’t cut it without a partner. Curr. Biol. 15, R61–R64. doi: 10.1016/j.cub.2004.12.057
Ursu, S., Kring, A. M., Gard, M. G., Minzenberg, M. J., Yoon, J. H., Ragland, J. D., et al. (2011). Prefrontal cortical deficits and impaired cognition–emotion interactions in schizophrenia. Am. J. Psychiatry 168, 276–285. doi: 10.1176/appi.ajp.2010.09081215
Visvanathan, J., Lee, S., Lee, B., Lee, J. W., and Lee, S. K. (2007). The microRNA miR-124 antagonizes the anti-neural REST/SCP1 pathway during embryonic CNS development. Genes Dev. 21, 744–749. doi: 10.1101/gad.1519107
Vo, N., Klein, M. E., Varlamova, O., Keller, D. M., Yamamoto, T., Goodman, R. H., et al. (2005). A cAMP-response element binding protein-induced microRNA regulates neuronal morphogenesis. Proc. Natl. Acad. Sci. U.S.A. 102, 16426–16431. doi: 10.1073/pnas.0508448102
Wang, R. Y., Phang, R. Z., Hsu, P. H., Wang, W. H., Huang, H. T., and Liu, I. Y. (2013). In vivo knockdown of hippocampal miR-132 expression impairs memory acquisition of trace fear conditioning. Hippocampus 23, 625–633. doi: 10.1002/hipo.22123
Wayman, G. A., Davare, M., Ando, H., Fortin, D., Varlamova, O., Cheng, H. Y., et al. (2008). An activity-regulated microRNA controls dendritic plasticity by down-regulating p250GAP. Proc. Natl. Acad. Sci. U.S.A. 105, 9093–9098. doi: 10.1073/pnas.0803072105
Wegner, A. M., Nebhan, C. A., Hu, L., Majumdar, D., Meier, K. M., Weaver, A. M., et al. (2008). N-wasp and the arp2/3 complex are critical regulators of actin in the development of dendritic spines and synapses. J. Biol. Chem. 283, 15912–15920. doi: 10.1074/jbc.M801555200
Williams, H. J., Norton, N., Dwyer, S., Moskvina, V., Nikolov, I., Carroll, L., et al. (2011). Fine mapping of ZNF804A and genome-wide significant evidence for its involvement in schizophrenia and bipolar disorder. Mol. Psychiatry 16, 429–441. doi: 10.1038/mp.2010.36
Xu, B., Hsu, P. K., Stark, K. L., Karayiorgou, M., and Gogos, J. A. (2013). Derepression of a neuronal inhibitor due to miRNA dysregulation in a schizophrenia-related microdeletion. Cell 152, 262–275. doi: 10.1016/j.cell.2012.11.052
Xu, B., Karayiorgou, M., and Gogos, J. A. (2010). MicroRNAs in psychiatric and neurodevelopmental disorders. Brain Res. 1338, 78–88. doi: 10.1016/j.brainres.2010.03.109
Yagi, H., Furutani, Y., Hamada, H., Sasaki, T., Asakawa, S., Minoshima, S., et al. (2003). Role of TBX1 in human del22q11.2 syndrome. Lancet 362, 1366–1373. doi: 10.1016/S0140-6736(03)14632-6
Yang, D., Li, T., Wang, Y., Tang, Y., Cui, H., Zhang, X., et al. (2012). miR-132 regulates the differentiation of dopamine neurons by directly targeting Nurr1 expression. J. Cell Sci. 125, 1673–1682. doi: 10.1242/jcs.086421
Yoo, A. S., Staahl, B. T., Chen, L., and Crabtree, G. R. (2009). MicroRNA-mediated switching of chromatin-remodelling complexes in neural development. Nature 460, 642–646. doi: 10.1038/nature08139
Yoo, A. S., Sun, A. X., Li, L., Shcheglovitov, A., Portmann, T., Li, Y., et al. (2011). MicroRNA-mediated conversion of human fibroblasts to neurons. Nature 476, 228–231. doi: 10.1038/nature10323
Yu, J. Y., Chung, K. H., Deo, M., Thompson, R. C., and Turner, D. L. (2008). MicroRNA miR-124 regulates neurite outgrowth during neuronal differentiation. Exp. Cell Res. 314, 2618–2633. doi: 10.1016/j.yexcr.2008.06.002
Zeller, T., Wild, P., Szymczak, S., Rotival, M., Schillert, A., Castagne, R., et al. (2010). Genetics and beyond – the transcriptome of human monocytes and disease susceptibility. PLoS ONE 5:e10693. doi: 10.1371/journal.pone.0010693
Zhang, J., Liu, L. H., Zhou, Y., Li, Y. P., Shao, Z. H., Wu, Y. J., et al. (2011). Effects of miR-541 on neurite outgrowth during neuronal differentiation. Cell Biochem. Funct. 29, 279–286. doi: 10.1002/cbf.1747
Zhang, Y., Ueno, Y., Liu, X. S., Buller, B., Wang, X., Chopp, M., et al. (2013). The MicroRNA-17-92 cluster enhances axonal outgrowth in embryonic cortical neurons. J. Neurosci. 33, 6885–6894. doi: 10.1523/JNEUROSCI.5180-12.2013
Zhao, C., Sun, G., Li, S., Lang, M. F., Yang, S., Li, W., et al. (2010). MicroRNA let-7b regulates neural stem cell proliferation and differentiation by targeting nuclear receptor TLX signaling. Proc. Natl. Acad. Sci. U.S.A. 107, 1876–1881. doi: 10.1073/pnas.0908750107
Zhao, C., Sun, G., Li, S., and Shi, Y. (2009). A feedback regulatory loop involving microRNA-9 and nuclear receptor TLX in neural stem cell fate determination. Nat. Struct. Mol. Biol. 16, 365–371. doi: 10.1038/nsmb.1576
Zovoilis, A., Agbemenyah, H. Y., Agis-Balboa, R. C., Stilling, R. M., Edbauer, D., Rao, P., et al. (2011). microRNA-34c is a novel target to treat dementias. EMBO J. 30, 4299–4308. doi: 10.1038/emboj.2011.327
Keywords: 22q11.2 deletion syndrome, schizophrenia, microRNA, MIR185, DGCR8, copy number variants, genetic risk factor
Citation: Forstner AJ, Degenhardt F, Schratt G and Nöthen MM (2013) MicroRNAs as the cause of schizophrenia in 22q11.2 deletion carriers, and possible implications for idiopathic disease: a mini-review. Front. Mol. Neurosci. 6:47. doi: 10.3389/fnmol.2013.00047
Received: 10 September 2013; Paper pending published: 03 October 2013;
Accepted: 17 November 2013; Published online: 05 December 2013.
Edited by:
Hermona Soreq, The Hebrew University of Jerusalem, IsraelReviewed by:
Hermona Soreq, The Hebrew University of Jerusalem, IsraelMichaela Kress, Medical University Innsbruck, Austria
Copyright © 2013 Forstner, Degenhardt, Schratt and Nöthen. This is an open-access article distributed under the terms of the Creative Commons Attribution License (CC BY). The use, distribution or reproduction in other forums is permitted, provided the original author(s) or licensor are credited and that the original publication in this journal is cited, in accordance with accepted academic practice. No use, distribution or reproduction is permitted which does not comply with these terms.
*Correspondence: Markus M. Nöthen, Institute of Human Genetics, University of Bonn, Sigmund-Freud-Str. 25, 53127 Bonn, Germany e-mail: markus.noethen@uni-bonn.de