- Children's National Medical Center, Center for Neuroscience Research, Children's Research Institute, Washington, DC, USA
The limbic system of the brain regulates a number of behaviors that are essential for the survival of all vertebrate species including humans. The limbic system predominantly controls appropriate responses to stimuli with social, emotional, or motivational salience, which includes innate behaviors such as mating, aggression, and defense. Activation of circuits regulating these innate behaviors begins in the periphery with sensory stimulation (primarily via the olfactory system in rodents), and is then processed in the brain by a set of delineated structures that primarily includes the amygdala and hypothalamus. While the basic neuroanatomy of these connections is well-established, much remains unknown about how information is processed within innate circuits and how genetic hierarchies regulate development and function of these circuits. Utilizing innovative technologies including channel rhodopsin-based circuit manipulation and genetic manipulation in rodents, recent studies have begun to answer these central questions. In this article we review the current understanding of how limbic circuits regulate sexually dimorphic behaviors and how these circuits are established and shaped during pre- and post-natal development. We also discuss how understanding developmental processes of innate circuit formation may inform behavioral alterations observed in neurodevelopmental disorders, such as autism spectrum disorders, which are characterized by limbic system dysfunction.
Introduction
The limbic system links external cues possessing emotional, social, or motivational relevance to a specified set of contextual and species-specific appropriate behavioral outputs. While a fair amount of these behaviors are enhanced through experiential learning and reinforcement, a number of these behaviors are innate or inborn, meaning that they manifest without prior learning. These innate behaviors include courtship, maternal care, defense (both to conspecific and predator cues) and establishment of social hierarchy, all of which ensure survival of the individual or offspring and propagation of the species. These behaviors are regulated and influenced by sensory stimuli such as touch, sound, and, most importantly in rodents, smell. An animal's inability to correctly detect or process social or environmental cues results in abnormal social behaviors and increases risk of attack and/or predation. In humans, abnormal development of aspects of innate behavior, most prominently circuits that regulate social behavior, appear to underlie disorders such as autism spectrum disorders and schizophrenia that are characterized by inappropriate or altered social interactions.
Until relatively recently, humans were the only species thought to possess emotion. Initially documented by Papez (1937) and elaborated by MacLean (1949), social cognition occurs through a complex neural network of interconnected structures, which includes areas in the ventromedial aspect of the temporal and frontal lobes, and their connections with the hypothalamus and brainstem. This neural network, dubbed the “limbic system” is centered around the amygdala, a small almond shaped structure located deep within the temporal lobe. Emotional salience, produced in the amygdala, is generally thought of as a prime driving force behind innate human behaviors, typically social in nature (Brothers, 1989; Barbas, 1995; Aggleton, 2000; LeDoux, 2012). As the scientific community accepted emotions such as fear, anxiety, reward, and attraction as a result of neural wiring in humans, other species including rodents were gradually accepted as possessing similar circuits and, therefore, similar emotions (see Figure 1 for comparison of human and rodent limbic system structures). Since the realization that emotions are not exclusively human, understanding the neural circuits involved in processing emotions and other social cues has advanced rapidly through the use of experimental rodent models. In rodent models, emotional states (e.g., fear, anxiety, and social receptivity) are generally quantified by their behaviors. When translating from rodent models to humans, it is important to understand that the sensory inputs of rodents are primarily olfactory, auditory, and somatosensory, with minimal visual inputs. Therefore, in this review we focus primarily on chemosensation in the rodent and how it relates to innate limbic responses to social conspecific cues such as mating, maternal care, and territorial behaviors as well as non-social defensive responses to predator cues.
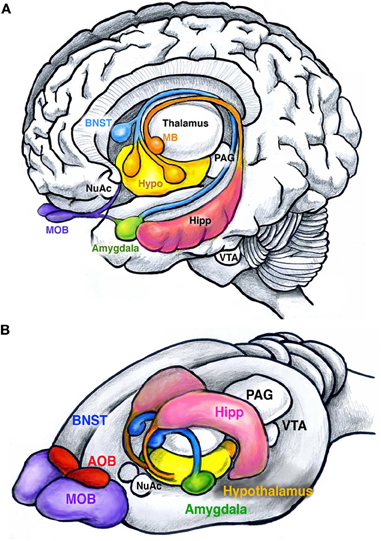
Figure 1. Main structures of the human and rodent limbic system. (A) Human brain showing the amygdala (green), bed nucleus of stria terminalis (BNST, blue), hypothalamus (yellow), and hippocampus (pink). The hippocampus (pink) attaches to the mamillary bodies (orange) through the fimbria-fornix. Olfactory inputs are received by the olfactory bulbs (MOB, purple). Other structures include the nucleus accumbens (NuAc), ventral tegmental area (VTA), and the periaqueductal gray (PAG). (B) Similar structures are found in rodents. Note the enlarged olfactory bulbs compared to humans, and the presence of the accessory olfactory bulbs (AOB, red). Together these structures facilitate the execution and reinforcement of innate behaviors.
Neuroanatomy of Innate Behaviors
Most of our knowledge of the circuitry that regulates innate behaviors has come from structural or cellular loss-of-function lesion and cytotoxic injury approaches. However, as the collection of brain regions within the innate circuitry contains a number of intertwined fibers of passage, lesion studies by their very nature are limited in their ability to discern the function of discrete nuclei from other connected brain regions. Despite this drawback, these types of classical studies have painted a relatively consistent picture of the major structures that comprise innate circuitry. These structures include the main and accessory olfactory system, olfactory/piriform cortex, amygdala, bed nucleus of stria terminalis (BNST) and hypothalamus (Swanson, 2000; Dulac and Wagner, 2006) (see Table 1 for abbreviations).
Many behaviors such as fear/aversion to predator odors and reward/attraction to odors of the opposite sex are considered to be innate, meaning no prior learning is needed for their manifestation. For example, a naïve female rodent shows preference to male urine odors over female or no odors (Drickamer, 1992; Sawrey and Dewsbury, 1994). Similarly, a laboratory rat or mouse that has never encountered a predator of any kind will display stereotypical signs of fear and avoidance in response to predator odors (Apfelbach et al., 2005). Specific fear responses are also initiated by the detection of alarm pheromones thought to be emitted from dead or stressed conspecifics. These alarm pheromones are detected in the Grueneberg Ganglion, located in the tip of the rodent nose (Brechbühl et al., 2008). With the exception of alarm pheromones, innate responses have been tied to specific chemicals (Papes et al., 2010; Ferrero et al., 2011; Isogai et al., 2011) that are detected by two organs in the nose: the vomeronasal organ (VNO) and to a lesser extent the main olfactory epithelium (MOE). The VNO, located in the palate, primarily detects non-volatile chemicals such as pheromones with high specificity, while the MOE located on turbinates deep in the nasal cavity, detects volatile chemicals. Sensory input from the VNO and MOE are received by and processed in the accessory olfactory bulb (AOB) and main olfactory bulb (MOB), respectively. Projections from the AOB and MOB directly or indirectly synapse on a number of higher order structures including the olfactory/piriform cortex and amygdala. The amygdala is generally believed to be a central processing station where the level of salience is imparted to a given stimulus (or stimuli) (LeDoux, 1993). The amygdala then sends projections to the hypothalamus for further integration and coordination with the brain stem to initiate the body's “fight or flight” responses (e.g., increase in blood pressure, respiratory rate, etc.) (Swanson and Petrovich, 1998). Although we will focus our attention on the VNO-AOB-amygdalar-BNST-hypothalamic circuit (see Figure 2), the main components of the innate circuit, we would like to emphasize that these brain hubs and their many feedback loops are not the sole components of a highly complex neural network important for the regulation of sociability and innate emotions. We begin by summarizing what is currently known regarding the neuroanatomy of circuits for olfactory-based reproductive, maternal care, predator defense and conspecific defense (aggression) rodent innate behaviors and the individual functions of these nuclei in information processing.
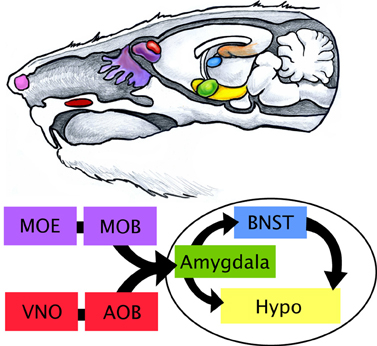
Figure 2. Limbic processing of olfactory information in the rodent. The rodent limbic system is highly influenced by olfactory cues received by the main olfactory epithelium (MOE, purple) and vomeronasal organ (VNO, red). The Grueneberg ganglion (pink), which senses stress in conspecifics, is depicted in the tip of the rodent nose. The VNO, located on the palate, of the mouth detects non-volatile or lipophilic chemicals that are channeled by the tongue through a pore in the roof of the mouth. Volatile chemical scents are more readily aerosolized and travel further back into the nasal cavity to reach the MOE on the turbinates. Projections from sensory neurons in the VNO and MOE are received in the accessory olfactory bulb (AOB) and main olfactory bulb (MOB), respectively, located in the brain. Signal is then passed to other structures of the limbic system including the amygdala (green), bed nucleus of stria terminalis (BNST, blue), and hypothalamus (Hypo, yellow).
Mating Behaviors
Mating behaviors in males and females consist of two phases: the initial appetitive phase followed by the consummatory phase. In males the appetitive phase includes angiogenital chemoinvestigation, or sniffing, of the female. Pheromonal stimulation of the VNO-AOB olfactory system is relayed to the medial amygdala (MeA), usually via direct connections (Meurisse et al., 2009; Kang et al., 2011). The MeA acts as a hub, dispersing the signal to the BNST, and to anatomically segregated subsets of nuclei of the hypothalamus including the medial preoptic nucleus (mPN), ventrolateral portion of the ventromedial hypothalamus (VMHvl) and ventral premammillary nucleus (PMNv) (Emery and Sachs, 1976). Lesion studies have found the mPN of the hypothalamus to be intimately tied to female preference and pursuit (Kondo and Arai, 1995; Been and Petrulis, 2010). The mPN integrates inputs from the MeA either directly or via the BNST to increase dopamine levels (Newman, 1999; Hull and Dominguez, 2006; Balthazart and Ball, 2007). The mPN then signals to the ventral tegmental area (VTA) and nucleus accumbens (NuAc) to initiate appetitive phase responses such as sniffing. The same circuit (VNO-AOB-MeA-BNST-mPN) also controls consummatory phase behaviors such as mounting, intromission and ejaculation via afferents to VMHvl and then areas of the midbrain and spinal cord: periaqueductal gray (PAG), nucleus paragiganta and finally the lumbosacral spinal cord (see Figure 3A) (Marson, 2004; Normandin and Murphy, 2011a,b).
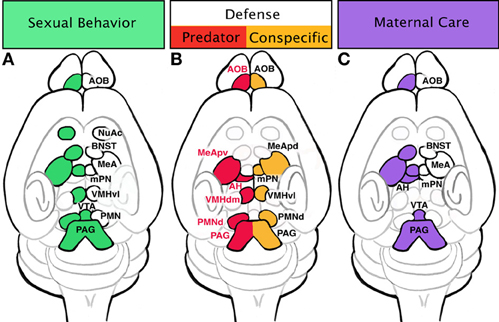
Figure 3. Specific innate behaviors are controlled by distinct regions of the limbic system. (A) Sexual behaviors include activation of the vomeronasal organ (VNO), accessory olfactory bulb (AOB), and medial amygdala (MeA). Signal transduction from sensation to physical motivation is not always linear; once signal has reached the MeA, it is dispersed to a few areas: bed nucleus of stria terminalis (BNST), medial preoptic nucleus (mPN), and premammillary nucleus (PMN). The BNST will shunt signal from the MeA to the mPN. The mPN can activate appetitive behaviors (sniffing and pursuit) through innervation of the nucleus accumbens (NuAc) and ventral tegmental area (VTA). Additionally, the mPN passes information to the ventrolateral portion of the ventral medial hypothalamus (VMHvl), which in turn can initiate consummative behaviors through the periaqueductal gray (PAG) and spinal cord. Consummative behaviors such as mounting, intromission, and ejaculation can also be influenced by PMN inputs on the PAG and spinal cord. (B) Defensive behaviors trigger slightly different areas of the amygdala and hypothalamus depending if the stimulus is a predator or an animal of the same species (conspecific). Defense in response to a predator initiated in the AOB sends signals to the posterioventral MeA (MeApv), then to the dorsomedial portion of the ventral medial hypothalamus (VMHdm). The VMHdm will then cross-talks with the anterior hypothalamus (AH), an instance of bidirectional communication. The VMHdm, then signals to the ventrolateral portion of the dorsal PMN (PMNd), which then signals to the dorsolateral and dorsomedial PAG. Defense responses to a conspecific are initiated in the AOB which sends afferents directly to the anterior dorsal and posterior dorsal MeA (MeApd). The MeApd acts as a hub dispersing signal to three areas: mPN, VMHvl, and dorsomedial portion of the PMNd. The VMHvl will engage in cross-talk with the PMNd, which ultimately communicates with the dorsomedial and lateral PAG. (C) Maternal behavior circuit may begin in the AOB, which sends signal to the MeA. The MeA will send information to the mPN either directly or through the BNST. Pup avoidance is suppressed in the MeA and AH to initiate pup approach. Appetitive behaviors such as pup retrieval occur through activation of the VTA by the mPN. Consummative behaviors such as nursing are executed via activation of the PAG and spinal cord by the mPN.
Female innate reproductive behaviors can be initiated through the same olfactory-amygdala circuit as males. Both the appetitive phase and consummatory phase of female mating begins with pheromonal cues picked up by the VNO and MOE (Baum and Kelliher, 2009). Signals are then passed to MeA via the olfactory bulb (Kang et al., 2011). Afferents from the MeA connect to the mPN of the hypothalamus directly or via the BNST and PMNv in a similar fashion as in the male circuit. While the mPN controls both the appetitive and consummatory phase in males, the female mPN primarily influences appetitive responses such as approaching a male to mate or proceptive behaviors (ear twitching, running short distances away—“teasing”). However the mPN is upstream of female consummatory behaviors, explicitly lordosis, which is initiated in the VMHvl. Lesioning of the VMH results in a decrease in lordosis while electrical stimulation of this region produces lordosis in primed females out of context (no male present) (Pfaff and Sakuma, 1979). These brain regions, particularly the VMH, are highly influenced by the female's natural cycle of hormones (estrodiol and progesterone) (Blaustein et al., 1988; Petitti et al., 1992; Mani et al., 1994; Kow et al., 1995; Flanagan-Cato et al., 2001). Further supporting these lesioning studies, many of these regions also show increased expression of the activity-dependent intermediate early gene, cFos, after sexual behavior (Coolen et al., 1996). Female consummatory behaviors such as lordosis, similar to males, are also relayed to the PAG, nucleus paragiganta and the lumbosacral spinal cord (Lonstein and Stern, 1998).
Quite interestingly, disruption of particular portions of the above-mentioned reproductive circuit results in male behaviors in females or otherwise altered sexual behaviors. Specifically, surgical removal of the VNO or genetic deletion of TRPC2, a channel involved in translating pheromone reception into an electrical signal in olfactory receptor neurons, has been shown to increase male mounting behaviors toward other males (Leypold et al., 2002). Conversely, female mice without a functioning VNO (TRPC2−/− females) mount males (Leypold et al., 2002; Stowers et al., 2002). Moreover, in V1R receptor knockout (V1R receptors in the VNO identify physiological state of the animal) male mice display a decrease in mounts with females, and females display decreased maternal aggression (Del Punta et al., 2002). Despite these interesting findings, results to the contrary have been observed in studies directly probing the role of VNO in sex discrimination in mice and other rodents using either volatile (detected in MOE) or non-volatile (detected in the VNO) urinary odors as a stimulus (Beauchamp et al., 1982; Petrulis et al., 1999; Pankevich et al., 2004). When exposed to whole urine, mice with their VNO removed compensated by using their MOE to detect volatile discriminatory odors. Yet, mice lacking a VNO lose their discriminatory abilities when exposed exclusively to non-volatile odor elements of urine undetectable by the MOE (Keller et al., 2006). While these results reveal a partially redundant role for the MOE in sex discrimination, it appears clear that the VNO is central to the expression of appropriate sex-specific mating behaviors. Interestingly, other accounts of unusual feminization also occur by lesioning deeper portions of the male innate reproductive circuit. Lordosis, a female consummatory behavior, has been observed in males after lesioning the preoptic nucleus of the hypothalamus (Hennessey et al., 1986). Thus, appropriate sexual behavior appears to be controlled at multiple levels of the circuit, from pheromone detection in the VNO down to the hypothalamus and spinal cord.
Defense/Fear
Innate fear and the resulting defensive/aversive behaviors can be evoked by odors from predators, dominant conspecifics, or the “scent” of fear from a conspecific. Fear responses can be conditioned (learned) or unconditioned (innate). Rodents will innately respond with stereotypical fear behaviors when presented with the scent of stressed or dead mice. Detection of these conspecific alarm pheromones evokes freezing after stimulation of the Grueneberg ganglion cells (Brechbühl et al., 2008). Rodents also have an innate fear of cat and fox odors even in lab settings without prior exposure. Additionally for mice, there is an innate fear of rats, a natural predator of mice in the wild. Exposure to rat odors may induce flight, hiding, freezing, or risk assessment behaviors in mice as part of the unconditioned fear response (Blanchard et al., 2001). These unconditioned responses suggest an evolutionary “hardwiring” of circuits for such behaviors. Upregulation of cFos expression after introduction to predator odors has been documented in the posterior ventral medial amygdala (MeApv) and VMH (Canteras et al., 1997). Similar to reproductive olfactory cues, predator odors appear to be processed more readily in the AOB as opposed to the MOB (McGregor et al., 2004). This also indicates the existence of “kairomones,” a chemical emitted by one species that conveys information to another. Recent comprehensive mapping of receptor expression in conjunction with neuronal activation in the VNO has uncovered the receptor-based molecular code by which rodents identify cues associated with defense (predator and conspecific) and mating (Isogai et al., 2011). Perhaps the most striking finding of this study was the revelation that subsets of these receptors are solely dedicated to predator cues from individual species. Thus, in rodents the VNO appears to have evolved specifically to respond to cues that depend on the animal's survival in the wild, consistent with the notion that these circuits are largely hardwired. Downstream of the VNO, predator cues are processed in the AOB, and then conducted to the MeA (primarily the ventral aspect) and in turn feed directly to the dorsomedial portion of the VMH (VMHdm). However, as part of a conditioned fear response when context is dependent, cues are relayed from the hippocampus to the anterior nucleus of the hypothalamus (AH). Both the conditioned and innate fear response circuits converge on the dorsal premammillary nuclei (PMNd) of the hypothalamus which acts as an “amplifier” by triggering the pituitary and adrenal hormone release (Canteras et al., 1997; Dielenberg et al., 2001; Cezario et al., 2008) (see Figure 3B). Although a specific role in defense has not been found in the BNST, there are suggestions that it may modulate defensive rage and startle reflex (Dong and Swanson, 2004).
Similar endpoints, namely the PMNd, are involved in defense responses to dominant conspecifics. A common behavior test replicating a dominant conspecific scenario involves placing an “intruder” male in a “resident” male's cage. The resident male assumes a dominant role, threatening the intruder with posturing, biting, and attack. Interestingly, the medial hypothalamic circuitry of reproductive behaviors (VMH) is activated in the intruder, evidenced by increased cFos expression (Kollack-Walker and Newman, 1995; Kollack-Walker et al., 1999; Veening et al., 2005). However, in contrast to reproductive circuits, the defense response circuits converge on the “amplifier” of the predator aversion, the PMNd (Cezario et al., 2008; Motta et al., 2009). Intruders may react with passive (freezing) or active defense (rearing, boxing, or dashing away) in response to the approach of the resident. Lesioning of the PMNd results in decreases in passive defense while active defenses are maintained, suggesting the possibility that the intruder has a reduced fear of the dominant conspecific (Motta et al., 2009) (see Figure 3B).
Maternal Care
Similar to reproductive behaviors, maternal care can be parsed into appetitive and consummatory phases, with appetitive behaviors including nesting and pup retrieval while consummatory behaviors consist of pup grooming and nursing. It has been suggested that there are two mechanisms at play during maternal behaviors: activation of pup attraction and repression of pup avoidance. Pup avoidance has been observed in unprimed virgin female mice. However, the natural avoidance response in these virgins can be damaged with lesions of the MeA, thus stimulating maternal care of pups (Numan et al., 1993). Likewise, lesioning the AH results in the same behavior (Sheehan et al., 2001), suggesting that pup olfactory cues are processed in both the MeA and AH (regions associated with predator fear response) to stimulate avoidance behaviors in young virgin rodents. The opposing circuit regulating pup attraction, is seeded within the mPN of the hypothalamus. The mPN expresses receptors for estrogen, prolactin, and oxytocin, suggesting it may be a major target of hormone activity (Rosenblatt et al., 1994; Consiglio and Bridges, 2009; Ruthschilling et al., 2012). Lesions of this area decrease pup retrieval and nest building in postpartum females, and cFos has been noted to increase in this region after maternal behaviors (Numan and Smith, 1984; Champagne et al., 2003). It is very likely that activation of the mPN by hormones causes an inactivation of the anterior hypothalamic avoidance behaviors in addition to activating the VTA and NuAc to initiate the appetitive phase and the PAG-lumbosacral spinal cord to advance consummatory behaviors (see Figure 3C) (Lonstein and Stern, 1998).
Circuit Control and Regulation
Through classical neuroanatomical approaches, we have now reached a stage at which the basic circuitry regulating reproductive, defensive and maternal care behaviors are generally established. More recent studies utilizing a combination of techniques at the vanguard of science are revealing the molecular underpinnings of circuit formation and function. For example, novel optogenetic techniques allow for the subtype-specific and temporal control of neuronal activity in order to elucidate the circuitry driving innate behaviors. In addition, we are also gaining a significantly greater understanding of not only the genes that are required for normal circuit formation and function, but also how non-cell autonomous stimuli such as hormones shape neuronal populations comprising innate circuits.
One of the first studies to correlate gene expression patterns to subsets of innate behaviors made use of reporter gene knock-in methodologies to trace projections of genetically marked neuronal subpopulations (Choi et al., 2005). By gene expression analysis it was revealed that anatomically distinct subsets of MeA populations differentially express combinations of the LIM-homeodomain containing genes (Lhx5, Lhx6, and Lhx9), genes which are known to endow neuronal identity across the neuraxis (Shirasaki and Pfaff, 2002). Interestingly, these marked populations seperately respond to different innate behavioral cues (reproductive or defensive). Specifically, Lhx6+ neurons in the posterior dorsal MeA (MeApd) are almost exclusively activated by reproductive olfactory cues and project to an area of the hypothalamus involved in initiating mating behaviors, the ventral lateral portion of the ventral medial hypothalamus (VMHvl). Complimentary, Lhx6− cells in the posterior ventral MeA (MeApv) respond to predator odors and project to an area of the hypothalamus regulating defense, the dorsal medial portion of the ventral medial hypothalamus (VMHdm). Most surprisingly, molecular mapping also revealed that predator cue-responsive Lhx6− cells in the MeAvl also project to areas of the hypothalamus regulating reproductive behaviors, the VMHvl, an apparent contradiction. To reconcile this discrepency a model was put forth in which predator odor-activated Lhx6− cells can inhibit the VMHvl, thereby suppressing reproductive behaviors, while simultaneously activating the VMHdm and initiating defensive behaviors (Choi et al., 2005).
Following this study, the same group more recently used optogenetic activation combined with pharmacological silencing of hypothalamic neurons to determine how mating and defensive behaviors are coordinated in the hypothalamus (Lin et al., 2011). Direct light stimulation of the neurons in the VMHvl expressing channel rhodopsin evokes mice to not only display the appropriate defensive behaviors to other males, but also inappropriately to females and inanimate objects (Lin et al., 2011). However, light-activation of this circuit during consummative mating behavior will not evoke aggression. Thus, utilizing state of the art approaches; both genetic and optogenetic, these studies revealed that the VMH collectively integrates information for apparently non-compatable behaviors (e.g., mating and defense/aggression). However, at what level these context-appropriate behavioral outputs are controlled by cross-talk between VMH subdivisions remains to be elucidated. This analysis also resolves previous apparently contradictory studies, which showed that the VMH is activated by both mating and aggression (Kollack-Walker and Newman, 1995; Kollack-Walker et al., 1999; Veening et al., 2005). These tools will also most likely prove invaluable for understanding how information is gated at the synaptic level as well as which genetic networks are involved in specification and function of these subcircuits.
The neural circuitry that regulates innate behaviors, perhaps more so than other brain circuits, are dramatically shaped by endocrine factors, primarily sex hormones such as testosterone and estrogen (Simerly, 2005). Both circulating and local brain levels of testosterone and estrogen are expressed in a sex-dependent manner act to refine the neural circuits involved in sexually dimorphic behaviors (Reviewed in Hill and Boon, 2009; Wu and Shah, 2011). Major structures of the limbic circuit (e.g., amygdala, BNST, mPN) express estrogen receptors in both females and males. In females, estrogen is the primary hormone in the induction of maternal care. Specifically, virgin female rats will inhibit their aversion and stimulate attraction to pups after supplementation with estrodiol, thereby behaving more like nursing females (Fleming, 1986). The role of estrogen is especially interesting in the context of development of the male brain. Recent work has revealed that estrogen is required for the development of sexual dimorphism in the amygdala and even male-specific defensive behaviors (Wu et al., 2009). In male brains, testosterone is converted to estrogen by the enzyme aromatase, which is found in select neurons of the MeA, BNST, and hypothalamus (Ogawa et al., 1998). Circulating levels of testosterone can be controlled experimentally through gonadalectomy; however, even castrated males generate estrogen from testosterone produced in the adrenals. Therefore, only genetic deletion of aromatase in male mice eliminates estrogen action, resulting in a complete loss of aggressive behaviors against intruder males. Supplementation with estradiol in aromatase-null males soon after birth restores intermale aggression, albeit mild compared to wild type males. However, estradiol replacement one week after birth does not restore male-typical aggression in aromatase-null males (Toda et al., 2001), suggesting a developmental time window in construction of the male neural circuit. These hormones also appear to regulate neuronal plasticity in the adult (Cooke et al., 2003; Cooke, 2006; Dugger et al., 2008; Morris et al., 2008). For example, estrogen affects alterations of dendritic morphology in the MeA (Gomez and Newman, 1991), which can alter the perception of external cues (Mohedano-Moriano et al., 2007). As mentioned before, the circuit controlling reproduction and defense occupy similar limbic nuclei, and influence conflicting behaviors (sexually receptivity or aggression) to a single stimulus (male approach) in females depending on her maternal/hormonal status. Thus, an alternative hypothesis is that the hormonal state of an animal influences the connectivity, thereby affecting behaviors.
While estrogens shape the programming of sexually dimorphic circuits, testosterone acting directly via the androgen receptor is required for the activation and modulation of components of male-typical displays such as mating, territorial aggression, and urine marking. In addition to dramatic anatomical changes such as decrease in angio-genital distance and visibility of a nipple line, genetic deletion of the androgen receptor in male mice causes reduced male-typical behaviors (Juntti et al., 2010). This is in contrast to estrogen receptor-null males, which never or rarely display aggressive behaviors. Therefore, while testosterone or the androgen receptor is not necessary for establishing the circuitry required for innate behaviors, it is necessary to modulate the degree of innate sex-specific behaviors. Thus, in both sexes neuroendocrines, such as estrogen and testosterone have important, but genetically separable functions, in shaping sexually dimorphic brain circuits and related innate behavior.
While much focus has been given to the role that sex hormones play in modulating behavior and associated circuits, a number of studies have also revealed important roles for non-sex hormones. The most prominent of the neuropeptides are oxytocin and vasopressin, which are expressed within the diencephalon and function throughout numerous telencephalic structures including the amygdala and BNST (Hammock and Young, 2006). As supported by a number of experimental lines of evidence, oxytocin, and vasopressin play key roles in promoting mating and bonding (both pair and maternal) behaviors. Indeed, oxytocin administration via nasal spray is currently under clinical trials in attempt to alleviate the social withdrawal associated with autism (Guastella and Macleod, 2012). However, aside from these well-characterized pathways, much still remains unknown regarding how genetic pathways work in concert with hormones to regulate the full repertoire of innate behaviors. Toward bridging this gap in understanding, a recent study by Xu et al. (2012) stands out. Using unbiased microarray transcriptome screening, validated with in situ hybridization expression analyses, they identified a novel cohort of genes expressed in a sexually dimorphic manner in the amygdala, BNST, and hypothalamus. While many of these genes were not previously implicated in sexually dimorphic behavior, expression of many were found to be modulated directly by hormone levels. Moreover, a battery of innate behavior tasks in mice mutant for one of four genes (Brs3, Cckar, Irs4, or Sytl4) revealed specific non-overlapping defects in aspects of male sexual behavior, intermale aggression, maternal behavior or female sexual behavior (Xu et al., 2012). Thus, it appears that while hormonal influences modulate sexually dimorphic gene expression, distinct genetic modules control the complete pattern of sexually dimorphic innate behaviors. The results of this study also suggest that more sensitive screening methodologies such as RNAseq, will also be fruitful in identifying other components of genetic networks regulating the full cohort of innate behaviors.
Embryonic Patterning of the Innate Limbic System and Potential Link to Behavior
Since innate behaviors are established without prior experience, the regulatory circuitry must be established during embryonic or early post-natal stages of neurodevelopment, likely through a series of hierarchical stages of genetic programming. Below we review our current knowledge of innate limbic system development, and present a novel model in which innate behaviors are generated by a coordination of genetic expression events and environmental (hormonal) cues.
Birth dating studies in labeled neurons reveal that the vast majority of neurons that comprise the innate limbic system are generated during early embryonic neurogenesis, embryonic day 11–15 (E11–15) in mice (McConnell and Angevine, 1983). By late gestation, E18, most neurons dedicated for the limbic system (with the notable exception of subsets of olfactory bulb interneurons and hippocampal granule cells of the dentate gyrus which are generated throughout the lifetime of the animal) have migrated to their final locations in the brain and, in some cases, begun to make connections (Marín and Rubenstein, 2003; Batista-Brito and Fishell, 2009; Corbin and Butt, 2011). The early post-natal period is then primarily characterized by the elaboration of both short- and long-range connections and shaping of circuits via experience and, as described above, sex-specific hormonal levels. The embryonic events of neuronal patterning and specification of neurons throughout the entire neuraxis is accomplished via the actions of delineated sets of transcription factors, typically of the homeodomain and bHLH classes (Campbell, 2003; Wonders and Anderson, 2006; Corbin et al., 2008). These genes have been conserved through evolution and act in multiple species from fly and worm to mammals, underscoring their importance in neuronal development. As described below in more detail, embryonic developmental studies over the past decade have elucidated the “how” and “where” neurons of the limbic system are generated. Similar to what has been found in the spinal cord and forebrain, neuronal subtype identity in the limbic system appears to be established during the proliferative phase of embryogenesis before migration, suggesting this is a common mechanism used in the nervous system. This early endowment of identity implies that the remainder of development may largely be dedicated to carrying out a genetically predetermined program of migration, differentiation, synaptogenesis and maturation. Therefore, understanding development, especially the genetic mechanisms by which diverse types of neurons are specified, will likely have broad implications for understanding behaviors.
Development of the MOE and VNO
Neurons that comprise the structures of the innate limbic system are generated within the first two weeks of gestation. The innate circuit begins with the peripheral olfactory sensory neurons, also called receptor neurons, that reside in two areas of the rostrum: MOE and VNO. In rodents, the MOE covers the surface of the convoluted ethmoid turbinates formed during the first two weeks of gestation when the nasal cavity begins to develop from the olfactory placodes, which indent forming the olfactory pits. The olfactory pits deepen and eventually fuse to form the primitive nasal cavity and ventral margins of the embryonic nasal septum between E12–13 (Herbert and Leininger, 1999). The VNO develops from bilateral invaginations of the olfactory epithelium in the ventral anterior portion of the developing nasal septum. By E15 the VNO is completely formed, however studies have suggested that it is not fully functional until after post-natal development (Coppola et al., 1993), and thus may be highly influenced by early olfactory cues.
Olfactory epithelial neurons arise from the olfactory placode and have recently been shown to be in part neural crest-derived (Katoh et al., 2011). Studies investigating lineage determination and differentiation of olfactory sensory neurons have implicated the bHLH transcription factors Mammalian Achaete Scute Homology 1 (Mash1) and Neurogenin1 (Ngn1) as important factors for epithelial neuronal specification. Mash1 appears to be required for the generation of the deeper layer of the olfactory epithelial neurons, while Ngn1 regulates genes that fine-tune the neuronal lineage to a more differentiated fate (Cau et al., 2002). Ngn1+ progenitors will terminally differentiate into olfactory sensory neuron precursors, which then express other factors such as Neuronal cell adhesion molecules (NCAMs) that may play a role in the final stages of synapse formation (Calof et al., 2002). As a single olfactory sensory neuron matures, it will express a single olfactory receptor type, which detects a specific chemical cue (Malnic et al., 1999). During fetal development, olfactory receptor genes are turned on synchronously in a spatially restricted manner, establishing zones (Strotmann et al., 1995; Sullivan et al., 1995). The MOE is broken into four zones (I–IV), each of which connect to respective domains in the (MOB) and have distinct transcriptional expression. For example, the transcription factor Osp94 is expressed solely in zone 1 and 2, while PAPS-S2 is only expressed in zones 3 and 4 of the dorsal olfactory epithelium (Tietjen et al., 2005). Similarly, the VNO is segregated into two zones or domains, apical and basal, which correlate with receptor type. Receptor neurons residing in the apical layer of the VNO express V1 receptors that primarily detect physiological state of conspecifics and predators (e.g., pregnant, stressed), while sensory neurons in the basal layer express V2R that detect sex and species signatures (male, female, fox, cat) (Dulac and Torello, 2003; Papes et al., 2010).
Axons of olfactory receptor neurons project a long distance into the brain to reach their target, the olfactory bulbs. In contrast to the peripherial olfactory epithelium, the olfactory bulbs are considered a forebrain structure and represent the most rostral aspect of the telencephalon. The olfactory bulbs arise from the rostral pallium (cortical region) of the telecephalon and can be distinguished as early as E13.5 in the mouse. The zonal specification in the MOE may act as a guide map for axons to their target glomeruli. Glomerulization or glomerulogenesis is estimated to occur over several days (E12–P7) (Royal and Key, 1999) through a hierarchical process (Miller et al., 2010), establishing a discrete topography (Luo and Flanagan, 2007). That is, olfactory sensory epithelial neurons expressing the same receptor type innervate common glomeruli in the olfactory bulb. The mechanisms by which these axons find their glomerular targets in the olfactory bulb has been suggested to be broken into two stages: general and specific targeting. General or pre-targeting from zone in MOE to appropriate domain in MOB has been shown to be influenced by zonal expression of the olfactory cell adhesion molecules (OCAMs) (Yoshihara et al., 1997; Reviewed in Yoshihara and Mori, 1997; Alenius and Bohm, 2003). The specific targeting mechanism of axons into a glomerulus has been suggested to be driven by the olfactory receptor itself where a mechanism downstream of the actual olfactory receptors enables fasciculation of axons that express similar receptors (Reviewed in Mombaerts, 2001, 2006; Imai et al., 2009; see also Yoshihara et al., 1997; Imai and Sakano, 2007, 2009). Specifically, olfactory receptors provide the means for axon-axon interaction by acting through G-coupled receptors to generate a unique level of cAMP, which subsequently regulates the expression of guidance factors: Nrp1 and Sema3A (Reviewed in Imai and Sakano, 2009; see also Imai et al., 2009). More recently the Slit and Roundabout (Robo) families of axon guidance molecules, have been demonstrated to control pathfinding and targeting of olfactory axons to glomeruli in olfactory bulb. Indeed, refined targeting of olfactory receptor axons to appropriate glomeruli is pertubed in Slit1/2- or Robo1/2-null mice (Nguyen-Ba-Charvet et al., 2008). However, how this system interacts with the fine-tuning of connectivity via the olfactory receptors themselves remains unclear (Cho et al., 2007; Nguyen-Ba-Charvet et al., 2008).
Development of the MOB and AOB
The main output neurons of the MOB and AOB are the mitral cell. These neurons project to deeper brain structures through the lateral olfactory tract (LOT). Growth of axonal projections from the olfactory bulb to deeper brain regions occurs at embryonic stages: between E13 and birth. This process is concurrent with olfactory epithelial targeting of the bulb, suggesting that these guidance events are independent of each other and sensory inputs (López-Mascaraque et al., 1996). Axonal pathfinding of mitral axons to the olfactory cortex along the LOT is influenced by the function of cell adhesion molecules such as cartilage acidic protein-1B, later renamed lateral olfactory tract ushering substance (LOTUS). This occurs through the ability of LOTUS to suppress the natural repulsive activities of Nogo. LOTUS antagonistically binds Nogo receptor a1 (NRa1), thus blocking Nogo binding and allowing the LOT to fasiculate and find its target in the olfactory cortex. Deletion of LOTUS causes the defasiculation of LOT axons, an effect that is rescued by co-deletion of NRa1 (Sato et al., 2011). Many other factors including Pax6 and ephrins also cooperate to form the olfactory circuit to the cortex (Nomura et al., 2006). In contrast, less is known about the development of olfactory projections that directly synapse in the amygdala, primarily due to insufficient markers of functionally distinct olfactory-limbic pathways. Effectors of axonal guidance from the MOB and AOB to other regions of the limbic system provide a challenging area of active research.
Development of the Amygdala
The development of the downstream targets of the olfactory system (amygdala and hypothalamus) has been the subject of recent intense investigation. Initial concepts into the development of these structures came primarily from comparative embryonic and post-natal anatomical studies. Although much of the amygdala and hypothalamus has been anatomically catalogued (Risold et al., 1994; Swanson and Petrovich, 1998; Swanson, 2000; Petrovich et al., 2001; LeDoux, 2007), relationships between embryonic primordia based on morphology only goes so far when attempting to correlate embryonic development to post-natal structures. This is due to the fact that many neuronal cell types within the brain are in fact generated far from the mature structures that they will eventually populate. Thus, initial hypotheses regarding simplified models of amygdala and hypothalamic development (Puelles and Rubenstein, 1993; Swanson and Petrovich, 1998) have recently been superseded by a more complex picture in which distinct embryonic progenitor zones (or niches) are dedicated for the generation of individual neuronal subtypes that subsequently migrate to these emerging structures (Marín and Rubenstein, 2003; Corbin and Butt, 2011).
With regard to the amygdala, extensive work has revealed that neuronal cell diversity is generated from two sets of progenitor pools: those that contribute neurons to multiple telencephalic structures (e.g., cerebral cortex, hippocampus) and those that are unique to the amygdala. The shared sources include aspects of the cerebral cortex, the ventrally located telencephalic ganglionic eminences [medial (MGE), lateral (LGE) and caudal (CGE)], as well as diencephalic sources (Nery et al., 2002; Remedios et al., 2007; García-Moreno et al., 2010; Bupesh et al., 2011; Cocas et al., 2011) (see Figure 4). Progenitor pools located within each of these domains express combinations of the homedomain and bHLH containing genes, Lhx6, Nkx2.1, Gsx2, Mash1, and Ngn2, just to name a few. As mentioned previously, differential expression of the LIM-homeodomain containing gene family marks anatomically segregated amygdalar efferent projections that separately regulate reproductive and defensive behaviors (Zirlinger et al., 2001; Choi et al., 2005). Interestingly, each amygdaloid nucleus expresses distinct patterns of LIM-homeodomain containing genes transiently during development. For example, the posterior dorsal medial amygdala (MeApd, associated primarily with reproductive behaviors) expresses Lhx6 and Lmo3, the posterior ventral medial amygdala (MeApv, associated primarily with defensive behaviors) expresses Lhx9, and the dorsal anterior amygdala expresses Lhx6, Lhx7, and Lmo3 (Remedios et al., 2004; Choi et al., 2005). Thus, the combinatorial expression patterns of LIM genes may provide a comprehensive mechanism for patterning the amygdala, reflecting a similarity with the LIM-code in the spinal cord.
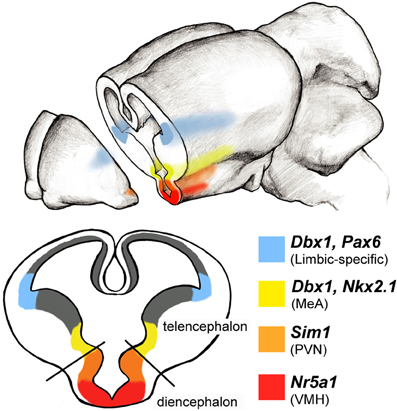
Figure 4. Limbic system progenitor pools in the rodent embryonic brain. Schematic of a coronal view of an embryonic brain at midneurogenesis (∼E14) reveals expression patterns of select transcription factors along the ventricular zones of the telencephalon and diencephalon. Combinatorial codes of transcription factor expression during embryogenesis determine the identity of neurons destined for various brain regions. A large portion of amygdala neuronal populations arise from two regions of the developing brain: the pallial-subpallial boundary (blue) and preoptic area (yellow) each of which express unique combinations of transcription factors. Areas of the hypothalamus also express regionally specific transcription factors: for example Sim1 (orange) is expressed more dorsally to specify cells in the paraventricular nucleus (PVN), while Nra51 (red) is expressed ventrally and specifies neurons in the ventral medial hypothalamus (VMH).
In addition to these shared progenitor pools, there also exist embryonic progenitor pools that appear to be dedicated primarily for the amygdala. These include populations present at the pallial-subpallial border (PSB), the junction of apposition between the dorsal (pallial) and ventral (subpallial) telencephalon. These populations express combinations of the homeodomain genes Pax6, Emx1, Gsx2, and Dbx1, which collectively supply the entire population of excitatory neurons to the amygdala as well as the specialized intercalated interneuronal populations which gate fear conditioning and extinction. (Puelles et al., 2000; García-López et al., 2008; Xu et al., 2008; Hirata et al., 2009; Soma et al., 2009; Carney et al., 2010; Kaoru et al., 2010; Cocas et al., 2011).
Of the above-mentioned genes, the function of Pax6 in amygdalar development has been the most explored. Pax6 is required for Gsx2+ cells to form correct excitatory and inhibitory neuron populations in the amygdala and olfactory bulb (populations also likely derived from the PSB) (Cocas et al., 2011). Moreover, Pax6 cooperates with the nuclear receptor Tailess (Tlx) to form the PSB (Stenman et al., 2003). Tlx mutants display reductions in region-specific gene expression in the ventral-most pallial regions and corresponding malformations in lateral and basolateral amygdala. Interestingly, Tlx mutants also display aggressive behavior, a phenotype that is consistent with amygdala dysfunction (Monaghan et al., 1997). Moreover, haplosufficient Pax6 mutants that express only one functional copy display autistic-like social deficits (Umeda et al., 2010), supporting an important role of these genes in amygdalar development. In addition to the PSB, the Dbx1+ progenitor pool located in the embryonic preoptic area (POA), a ventral telencephalic domain just below the MGE, is a major source of projection neurons destined specifically for the MeA (Hirata et al., 2009). Interestingly, these neurons are homogeneous by electrophysiological and molecular criteria, and electrophysiologically and molecularly distinct from FoxP2+ neighboring MeA neurons (Hirata et al., 2009; Carney et al., 2010). This genetic parcellation of MeA neuronal cell types suggest that, consistent with the amygdala LIM-code (Choi et al., 2005), other genetically tagged populations may have separable functions in the processing of different innate behaviors.
Development of the Hypothalamus
A major termination area of projections from the MeA is the hypothalamus. Similar to the amygdala, the hypothalamus is a nuclear structure comprised of separate nuclei with varying connections, neuronal compositions and separable functions in processing innate information. The hypothalamus is located in and arises from the ventral diencephalon, is visible as early as E9 and is clearly distinguished from the telencephalon at E12.5 both anatomically and molecularly. Similar to other regions of the central nervous system, a number of genes encoding transcription factors and secreted protein morphogens help to pattern and determine the regional specificity of the hypothalamus (Blackshaw et al., 2010). This molecular scaffolding that delineates progenitor domains of the hypothalamus can be categorized into two alar (paraventricular and subparaventricular areas) and three basal domains (tuberal hypothalamus, premammillary area and mamillary area). These domains lie along the longitudinal axis and are influenced by secreted factors such as Shh, Wnts, BMPs, and Fgf. In response to these secreted factors, cells in different developmental zones express a temporal and spatial fingerprint of transcription factors that pattern development of the subnuclei of the hypothalamus (Shimogori et al., 2010). For example, the bHLH-containing transcription factors Sim1 and Neurog2 and homeodomain-containing transcription factor Otp, delineate the embryonic paraventriclular area, which is the primordial of the supraopto-paraventricular nuclear complex in the dorsal hypothalamus (Fan et al., 1996; Puelles and Rubenstein, 2003; Shimogori et al., 2010). Loss-of-function studies have revealed that Sim1 is required for the correct positioning of paraventricular neurons (Caqueret et al., 2006), while Otp-null mice fail to produce somatostatin, vasopressin, oxytocin, corticotropin-releasing hormone, thyrotropin-releasing hormone in the primordial periventricular, paraventricular, and supraoptic nuclei. These mice are not only devoid of these three hypothalamic nuclei but are non-viable after birth (Wang and Lufkin, 2000).
Proposed Model for Circuit Patterning
Despite the above-described circuitry and the growing understanding of developmental mechanisms governing specification and migration of neurons, the link between developmental mechanisms, circuit formation and ultimately behavior remains to be clarified. There appears to be a common strategy to generate neuronal diversity across the central nervous system, wherein the combinatorial expression of different transcription factors specifies regional- and subtype-specific neuronal identity. However, an open question is whether this process also encodes the molecular identity responsible for connectivity. Indeed, although experimental evidence is scarce, such a link has already been proposed in the spinal cord, in which a transcriptional matching code acts to instruct connections between specific sensory and motor neurons (Lin et al., 1998). Although the evidence for this within the brain is also highly circumstantial, it presents an attractive and simplified mechanism whereby developmental gene expression is utilized not only to direct cell fate, but also to pre-pattern circuit connectivity. In this model, in addition to patterning neuronal identity, key transcription factors encode subsets of genes, most likely cell adhesion molecules that would be required for limbic circuit specific connectivity (Figure 5). In support of this model in the innate limbic circuit are two provocative sets of observations. First, fate mapping and gene expression analyses have revealed that progenitor pools that generate neurons within known connected structures of the innate limbic system (e.g., olfactory system, amygdala, hypothalamus) express common sets of transcription factors whose general function in other parts of the brain and nervous system is to control neuronal identity. These genes include, for example, FoxP2 and Dbx1 (Hirata et al., 2009; Carney et al., 2010; Allen Brain atlas). Second, there are multiple classes of cell adhesion molecules that specifically mark the interconnected limbic system. These include Limbic system associated membrane protein (Lsamp) as well as sets of cadherins (Redies and Takeichi, 1993; Mann et al., 1998; Pimenta and Levitt, 2004). A cadherin matching code for limbic connectivity is especially attractive as it has recently been shown that expression of the same subclasses of cadherin cell adhesion molecules are required for establishment of axon-target matching in other systems such as retinal to midbrain projections and intra-hippocampal connections (Hirano et al., 2002; Osterhout et al., 2011; Williams et al., 2011). In conjunction, other studies have found cadherin expression patterns to be regulated by Pax6 (Stoykova et al., 1997). Thus, perhaps cadherin (or other cell adhesion molecules) codes, initially established by restricted expression of key “selector” transcription factors in the embryonic brain, produce a layout for limbic system connectivity.
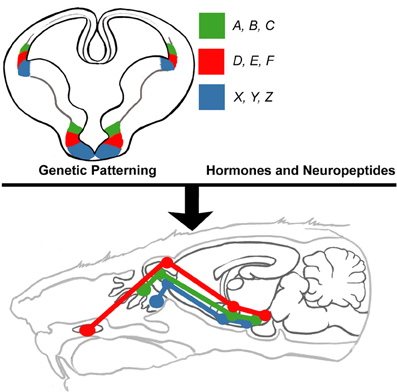
Figure 5. Proposed model of innate limbic circuit development. In this model, combinations of select subsets of transcription factors (e.g., A, B, C) that endow neuronal identity also encode genes required for formation of connections (e.g., cadherins) with neurons located in other parts of the brain. Neurons destined to connect are derived from progenitors that express the same sets of transcription factors. Thus, developmentally regulated transcription factors are the driving force behind setting up complex circuits. This pre-patterned circuitry is then extensively shaped and modified by the actions of select hormones (e.g., testosterone and estrogen) and neuropeptides (e.g., oxytocin and vasopressin).
Limbic Circuits and Neurodevelopmental Disorders
In humans, the limbic system is intimately tied to emotion and social behaviors, and disruption of the genetic programming of limbic circuitry may be a prime mechanism underlying a variety of social disorders, such as autism spectrum disorders (Rodrigues et al., 2004; Amaral et al., 2008; Herry et al., 2008; Markram et al., 2008; Monk, 2008) including Fragile X and Rett syndrome (Hessl et al., 2007; Adachi et al., 2009). Therefore, using the mouse olfactory-limbic system to understand how an intricate circuit forms may greatly inform human disorders. Innate behaviors such as reproduction, aggression and fear all require assimilation of social cues to produce behaviors that ensure survival. Research in rodents and primates indicate the amygdala and surrounding anatomy play a critical role in innate behaviors and social cognition. Defects in amygdala growth, cellularity and function are consistently found in individuals on the autistic spectrum disorder (Baron-Cohen et al., 2000). Consistent with this, it will be highly informative to study the potential role of the hypothalamus in autism, a very understudied area of investigation.
In support of limbic-specific defects in autism, genes known to be involved in specific aspects of development of the limbic system have already been identified and validated as high-ranking autism susceptibility genes (see https://gene.sfari.org/autdb/Welcome.do. for autism linked gene annotation). One such well-studied gene is the receptor tyrosine kinase Met (Campbell et al., 2006, 2007). In vitro studies suggest that Met is required for GnRH migration from the nasal placode to the hypothalamus (Giacobini et al., 2007) and Met expression has been detected in key limbic areas: cortex, amygdala, hypothalamus, and septum. Expression temporally peaks at P14 in rodent, a period of extensive outgrowth and synaptogenesis (Judson et al., 2009). Curiously, Met can decrease arbor complexity (Gutierrez et al., 2004), increase growth and excitatory synapse formation (Tyndall and Walikonis, 2006), or increase motility of interneurons (Powell et al., 2003; Martins et al., 2011) all depending on the identity of the cultured cells (cortical, hippocampal or basal forebrain). This suggests that Met may integrate intrinsic programs and external cues that cooperate to form functional neural networks. Moreover, massive information obtained from human genome wide association studies (GWAS) also implicates a number of cell adhesion molecules in autism, including multiple members of the cadherin family (Walsh et al., 2008). Although some of these genes may broadly regulate synapse formation and function across multiple domains of the nervous system (e.g., Neuroligin), quite interestingly others such as cadherin-10 (CDH10) appear to be limbic system specific (Bekirov et al., 2002; Wang et al., 2009). Therefore, unraveling the mechanisms of limbic system development will likely provide significant insight into the etiology of autism and related disorders of social cognition and create avenues of therapy for individuals afflicted by these disorders.
Conflict of Interest Statement
The authors declare that the research was conducted in the absence of any commercial or financial relationships that could be construed as a potential conflict of interest.
Acknowledgments
We would like to thank Jason Triplett, Ph.D., and Sarah Ferrante for their valuble comments on the draft of this review. Additionally we thank Brandon Martin for his guidance in creating the anatomical figures. Work in the Corbin lab is funded by Autism Speaks and the NIH (RO1 DA020140 and ARRA supplement).
References
Adachi, M., Autry, A. E., Covington, H. E. 3rd., and Monteggia, L. M. (2009). MeCP2-mediated transcription repression in the basolateral amygdala may underlie heightened anxiety in a mouse model of Rett syndrome. J. Neurosci. 29, 4218–4227.
Aggleton, J. (2000). The Amygdala: A Functional Analysis, 2nd Edn. New York, NY: Oxford University Press Inc.
Alenius, M., and Bohm, S. (2003). Differential function of RNCAM isoforms in precise target selection of olfactory sensory neurons. Development 130, 917–927.
Amaral, D. G., Schumann, C. M., and Nordahl, C. W. (2008). Neuroanatomy of autism. Trends Neurosci. 31, 137–145.
Apfelbach, R., Blanchard, C. D., Blanchard, R. J., Hayes, R. A., and McGregor, I. S. (2005). The effects of predator odors in mammalian prey species: a review of field and laboratory studies. Neurosci. Biobehav. Rev. 29, 1123–1144.
Balthazart, J., and Ball, G. F. (2007). Topography in the preoptic region: differential regulation of appetitive and consummatory male sexual behaviors. Front. Neuroendocrinol. 28, 161–178.
Barbas, H. (1995). Anatomic basis of cognitive-emotional interactions in the primate prefrontal cortex. Neurosci. Biobehav. Rev. 19, 499–510.
Baron-Cohen, S., Ring, H. A., Bullmore, E. T., Wheelwright, S., Ashwin, C., and Williams, S. C. (2000). The amygdala theory of autism. Neurosci. Biobehav. Rev. 24, 355–364.
Batista-Brito, R., and Fishell, G. (2009). The developmental integration of cortical interneurons into a functional network. Curr. Top. Dev. Biol. 87, 81–118.
Baum, M. J., and Kelliher, K. R. (2009). Complementary roles of the main and accessory olfactory systems in mammalian mate recognition. Annu. Rev. Physiol. 71, 141–160.
Beauchamp, G. K., Martin, I. G., Wysocki, C. J., and Wellington, J. L. (1982). Chemoinvestigatory and sexual behavior of male guinea pigs following vomeronasal organ removal. Physiol. Behav. 29, 329–336.
Been, L. E., and Petrulis, A. (2010). The role of the medial preoptic area in appetitive and consummatory reproductive behaviors depends on sexual experience and odor volatility in male Syrian hamsters. Neuroscience 170, 1120–1132.
Bekirov, I. H., Needleman, L. A., Zhang, W., and Benson, D. L. (2002). Identification and localization of multiple classic cadherins in developing rat limbic system. Neuroscience 115, 213–227.
Blackshaw, S., Scholpp, S., Placzek, M., Ingraham, H., Simerly, R., and Shimogori, T. (2010). Molecular pathways controlling development of thalamus and hypothalamus: from neural specification to circuit formation. J. Neurosci. 30, 14925–14930.
Blanchard, R. J., Yang, M., Li, C. I., Gervacio, A., and Blanchard, D. C. (2001). Cue and context conditioning of defensive behaviors to cat odor stimuli. Neurosci. Biobehav. Rev. 25, 587–595.
Blaustein, J. D., King, J. C., Toft, D. O., and Turcotte, J. (1988). Immunocytochemical localization of estrogen-induced progestin receptors in guinea pig brain. Brain Res. 474, 1–15.
Brechbühl, J., Klaey, M., and Broillet, M. C. (2008). Grueneberg ganglion cells mediate alarm pheromone detection in mice. Science 321, 1092–1095.
Bupesh, M., Legaz, I., Abellán, A., and Medina, L. (2011). Multiple telencephalic and extratelencephalic embryonic domains contribute neurons to the medial extended amygdala. J. Comp. Neurol. 519, 1505–1525.
Calof, A. L., Bonnin, A., Crocker, C., Kawauchi, S., Murray, R. C., Shou, J., and Wu, H. H. (2002). Progenitor cells of the olfactory receptor neuron lineage. Microsc. Res. Tech. 58, 176–188.
Campbell, K. (2003). Dorsal-ventral patterning in the mammalian telencephalon. Curr. Opin. Neurobiol. 13, 50–56.
Campbell, D. B., D'Oronzio, R., Garbett, K., Ebert, P. J., Mirnics, K., Levitt, P., and Persico, A. M. (2007). Disruption of cerebral cortex MET signaling in autism spectrum disorder. Ann. Neurol. 62, 243–250.
Campbell, D. B., Sutcliffe, J. S., Ebert, P. J., Militerni, R., Bravaccio, C., Trillo, S., Elia, M., Schneider, C., Melmed, R., Sacco, R., Persico, A. M., and Levitt, P. (2006). A genetic variant that disrupts MET transcription is associated with autism. Proc. Natl. Acad. Sci. U.S.A. 103, 16834–16839.
Canteras, N. S., Chiavegatto, S., Ribeiro do Valle, L. E., and Swanson, L. W. (1997). Severe reduction of rat defensive behavior to a predator by discrete hypothalamic chemical lesions. Brain Res. Bull. 44, 297–305.
Carney, R. S., Mangin, J. M., Hayes, L., Mansfield, K., Sousa, V. H., Fishell, G., Machold, R. P., Ahn, S., Gallo, V., and Corbin, J. G. (2010). Sonic hedgehog expressing and responding cells generate neuronal diversity in the medial amygdala. Neural. Dev. 5, 14.
Caqueret, A., Boucher, F., and Michaud, J. L. (2006). Laminar organization of the early developing anterior hypothalamus. Dev. Biol. 298, 95–106.
Cau, E., Casarosa, S., and Guillemot, F. (2002). Mash1 and Ngn1 control distinct steps of determination and differentiation in the olfactory sensory neuron lineage. Development 129, 1871–1880.
Cezario, A. F., Ribeiro-Barbosa, E. R., Baldo, M. V., and Canteras, N. S. (2008). Hypothalamic sites responding to predator threats–the role of the dorsal premammillary nucleus in unconditioned and conditioned antipredatory defensive behavior. Eur. J. Neurosci. 28, 1003–1015.
Champagne, F. A., Weaver, I. C., Diorio, J., Sharma, S., and Meaney, M. J. (2003). Natural variations in maternal care are associated with estrogen receptor alpha expression and estrogen sensitivity in the medial preoptic area. Endocrinology 144, 4720–4724.
Cho, J. H., Lépine, M., Andrews, W., Parnavelas, J., and Cloutier, J. F. (2007). Requirement for Slit-1 and Robo-2 in zonal segregation of olfactory sensory neuron axons in the main olfactory bulb. J. Neurosci. 27, 9094–9104.
Choi, G. B., Dong, H. W., Murphy, A. J., Valenzuela, D. M., Yancopoulos, G. D., Swanson, L. W., and Anderson, D. J. (2005). Lhx6 delineates a pathway mediating innate reproductive behaviors from the amygdala to the hypothalamus. Neuron 46, 647–660.
Cocas, L. A., Georgala, P. A., Mangin, J. M., Clegg, J. M., Kessaris, N., Haydar, T. F., Gallo, V., Price, D. J., and Corbin, J. G. (2011). Pax6 is required at the telencephalic pallial-subpallial boundary for the generation of neuronal diversity in the postnatal limbic system. J. Neurosci. 31, 5313–5324.
Consiglio, A. R., and Bridges, R. S. (2009). Circulating prolactin, MPOA prolactin receptor expression and maternal aggression in lactating rats. Behav. Brain Res. 197, 97–102.
Cooke, B. M. (2006). Steroid-dependent plasticity in the medial amygdala. Neuroscience 138, 997–1005.
Cooke, B. M., Breedlove, S. M., and Jordan, C. L. (2003). Both estrogen receptors and androgen receptors contribute to testosterone-induced changes in the morphology of the medial amygdala and sexual arousal in male rats. Horm. Behav. 43, 336–346.
Coolen, L. M., Peters, H. J., and Veening, J. G. (1996). Fos immunoreactivity in the rat brain following consummatory elements of sexual behavior: a sex comparison. Brain Res. 738, 67–82.
Coppola, D. M., Budde, J., and Millar, L. (1993). The vomeronasal duct has a protracted postnatal development in the mouse. J. Morphol. 218, 59–64.
Corbin, J. G., and Butt, S. J. (2011). Developmental mechanisms for the generation of telencephalic interneurons. Dev. Neurobiol. 71, 710–732.
Corbin, J. G., Gaiano, N., Juliano, S. L., Poluch, S., Stancik, E., and Haydar, T. F. (2008). Regulation of neural progenitor cell development in the nervous system. J. Neurochem. 106, 2272–2287.
Del Punta, K., Leinders-Zufall, T., Rodriguez, I., Jukam, D., Wysocki, C. J., Ogawa, S., Zufall, F., and Mombaerts, P. (2002). Deficient pheromone responses in mice lacking a cluster of vomeronasal receptor genes. Nature 419, 70–74.
Dielenberg, R. A., Hunt, G. E., and McGregor, I. S. (2001). “When a rat smells a cat”: the distribution of Fos immunoreactivity in rat brain following exposure to a predatory odor. Neuroscience 104, 1085–1097.
Dong, H. W., and Swanson, L. W. (2004). Projections from bed nuclei of the stria terminalis, posterior division: implications for cerebral hemisphere regulation of defensive and reproductive behaviors. J. Comp. Neurol. 471, 396–433.
Drickamer, L. C. (1992). Behavioral selection of odor cues by young female mice affects age of puberty. Dev. Psychobiol. 25, 461–470.
Dugger, B. N., Morris, J. A., Jordan, C. L., and Breedlove, S. M. (2008). Gonadal steroids regulate neural plasticity in the sexually dimorphic nucleus of the preoptic area of adult male and female rats. Neuroendocrinology 88, 17–24.
Dulac, C., and Torello, A. T. (2003). Molecular detection of pheromone signals in mammals: from genes to behaviour. Nat. Rev. Neurosci. 4, 551–562.
Dulac, C., and Wagner, S. (2006). Genetic analysis of brain circuits underlying pheromone signaling. Annu. Rev. Genet. 40, 449–467.
Emery, D. E., and Sachs, B. D. (1976). Copulatory behavior in male rats with lesions in the bed nucleus of the stria terminalis. Physiol. Behav. 17, 803–806.
Fan, C. M., Kuwana, E., Bulfone, A., Fletcher, C. F., Copeland, N. G., Jenkins, N. A., Crews, S., Martinez, S., Puelles, L., Rubenstein, J. L., and Tessier-Lavigne, M. (1996). Expression patterns of two murine homologs of Drosophila single-minded suggest possible roles in embryonic patterning and in the pathogenesis of Down syndrome. Mol. Cell. Neurosci. 7, 1–16.
Ferrero, D. M., Lemon, J. K., Fluegge, D., Pashkovski, S. L., Korzan, W. J., Datta, S. R., Spehr, M., Fendt, M., and Liberles, S. D. (2011). Detection and avoidance of a carnivore odor by prey. Proc. Natl. Acad. Sci. U.S.A. 108, 11235–11240.
Flanagan-Cato, L. M., Calizo, L. H., and Daniels, D. (2001). The synaptic organization of VMH neurons that mediate the effects of estrogen on sexual behavior. Horm. Behav. 40, 178–182.
Fleming, A. (1986). Psychobiology of rat maternal behavior: how and where hormones act to promote maternal behavior at parturition. Ann. N.Y. Acad. Sci. 474, 234–251.
García-López, M., Abellán, A., Legaz, I., Rubenstein, J. L., Puelles, L., and Medina, L. (2008). Histogenetic compartments of the mouse centromedial and extended amygdala based on gene expression patterns during development. J. Comp. Neurol. 506, 46–74.
García-Moreno, F., Pedraza, M., Di Giovannantonio, L. G., Di Salvio, M., López-Mascaraque, L., Simeone, A., and De Carlos, J. A. (2010). A neuronal migratory pathway crossing from diencephalon to telencephalon populates amygdala nuclei. Nat. Neurosci. 13, 680–689.
Giacobini, P., Messina, A., Wray, S., Giampietro, C., Crepaldi, T., Carmeliet, P., and Fasolo, A. (2007). Hepatocyte growth factor acts as a motogen and guidance signal for gonadotropin hormone-releasing hormone-1 neuronal migration. J. Neurosci. 27, 431–445.
Gomez, D. M., and Newman, S. W. (1991). Medial nucleus of the amygdala in the adult Syrian hamster: a quantitative Golgi analysis of gonadal hormonal regulation of neuronal morphology. Anat. Rec. 231, 498–509.
Guastella, A. J., and Macleod, C. (2012). A critical review of the influence of oxytocin nasal spray on social cognition in humans: evidence and future directions. Horm. Behav. 61, 410–418.
Gutierrez, H., Dolcet, X., Tolcos, M., and Davies, A. (2004). HGF regulates the development of cortical pyramidal dendrites. Development 131, 3717–3726.
Hammock, E. A., and Young, L. J. (2006). Oxytocin, vasopressin and pair bonding: implications for autism. Philos. Trans. R. Soc. Lond. B Biol. Sci. 361, 2187–2198.
Hennessey, A. C., Wallen, K., and Edwards, D. A. (1986). Preoptic lesions increase the display of lordosis by male rats. Brain Res. 370, 21–28.
Herbert, R. A., and Leininger, J. R. (1999). “Nose, larynx, and trachea,” in Pathology of the Mouse, ed R. R. Maronpot (St. Louis: Cache River Press), 259–262.
Herry, C., Ciocchi, S., Senn, V., Demmou, L., Müller, C., and Lüthi, A. (2008). Switching on and off fear by distinct neuronal circuits. Nature 454, 600–606.
Hessl, D., Rivera, S., Koldewyn, K., Cordeiro, L., Adams, J., Tassone, F., Hagerman, P. J., and Hagerman, R. J. (2007). Amygdala dysfunction in men with the fragile X premutation. Brain 130, 404–416.
Hill, R. A., and Boon, W. C. (2009). Estrogens, brain, and behavior: lessons from knockout mouse models. Semin. Reprod. Med. 27, 218–228.
Hirano, S., Wang, X., and Suzuki, S. T. (2002). Restricted expression of protocadherin 2A in the developing mouse brain. Brain Res. Mol. Brain Res. 98, 119–123.
Hirata, T., Li, P., Lanuza, G. M., Cocas, L. A., Huntsman, M. M., and Corbin, J. G. (2009). Identification of distinct telencephalic progenitor pools for neuronal diversity in the amygdala. Nat. Neurosci. 12, 141–149.
Hull, E. M., and Dominguez, J. M. (2006). Getting his act together: roles of glutamate, nitric oxide, and dopamine in the medial preoptic area. Brain Res. 1126, 66–75.
Imai, T., and Sakano, H. (2007). Roles of odorant receptors in projecting axons in the mouse olfactory system. Curr. Opin. Neurobiol. 17, 507–515.
Imai, T., and Sakano, H. (2009). Odorant receptor gene choice and axonal projection in the mouse olfactory system. Results Probl. Cell Differ. 47, 57–75.
Imai, T., Yamazaki, T., Kobayakawa, R., Kobayakawa, K., Abe, T., Suzuki, M., and Sakano, H. (2009). Pre-target axon sorting establishes the neural map topography. Science 325, 585–590.
Isogai, Y., Si, S., Pont-Lezica, L., Tan, T., Kapoor, V., Murthy, V. N., and Dulac, C. (2011). Molecular organization of vomeronasal chemoreception. Nature 478, 241–245.
Judson, M. C., Bergman, M. Y., Campbell, D. B., Eagleson, K. L., and Levitt, P. (2009). Dynamic gene and protein expression patterns of the autism-associated met receptor tyrosine kinase in the developing mouse forebrain. J. Comp. Neurol. 513, 511–531.
Juntti, S. A., Tollkuhn, J., Wu, M. V., Fraser, E. J., Soderborg, T., Tan, S., Honda, S., Harada, N., and Shah, N. M. (2010). The androgen receptor governs the execution, but not programming, of male sexual and territorial behaviors. Neuron 66, 260–272.
Kaoru, T., Liu, F. C., Ishida, M., Oishi, T., Hayashi, M., Kitagawa, M., Shimoda, K., and Takahashi, H. (2010). Molecular characterization of the intercalated cell masses of the amygdala: implications for the relationship with the striatum. Neuroscience 166, 220–230.
Kang, N., McCarthy, E. A., Cherry, J. A., and Baum, M. J. (2011). A sex comparison of the anatomy and function of the main olfactory bulb-medial amygdala projection in mice. Neuroscience 172, 196–204.
Katoh, H., Shibata, S., Fukuda, K., Sato, M., Satoh, E., Nagoshi, N., Minematsu, T., Matsuzaki, Y., Akazawa, C., Toyama, Y., Nakamura, M., and Okano, H. (2011). The dual origin of the peripheral olfactory system: placode and neural crest. Mol. Brain 4, 34.
Keller, M., Pierman, S., Douhard, Q., Baum, M. J., and Bakker, J. (2006). The vomeronasal organ is required for the expression of lordosis behaviour, but not sex discrimination in female mice. Eur. J. Neurosci. 23, 521–530.
Kollack-Walker, S., Don, C., Watson, S. J., and Akil, H. (1999). Differential expression of c-Fos mRNA within neurocircuits of male hamsters exposed to acute or chronic defeat. J. Neuroendocrinol. 11, 547–559.
Kollack-Walker, S., and Newman, S. W. (1995). Mating and agonistic behavior produce different patterns of Fos immunolabeling in the male Syrian hamster brain. Neuroscience 66, 721–736.
Kondo, Y., and Arai, Y. (1995). Functional association between the medial amygdala and the medial preoptic area in regulation of mating behavior in the male rat. Physiol. Behav. 57, 69–73.
Kow, L. M., Tsai, Y. F., Weiland, N. G., McEwen, B. S., and Pfaff, D. W. (1995). In vitro electro-pharmacological and autoradiographic analyses of muscarinic receptor subtypes in rat hypothalamic ventromedial nucleus: implications for cholinergic regulation of lordosis. Brain Res. 694, 29–39.
LeDoux, J. E. (2012). Evolution of human emotion: a view through fear. Prog. Brain Res. 195, 431–442.
Leypold, B. G., Yu, C. R., Leinders-Zufall, T., Kim, M. M., Zufall, F., and Axel, R. (2002). Altered sexual and social behaviors in trp2 mutant mice. Proc. Natl. Acad. Sci. U.S.A. 99, 6376–6381.
Lin, D., Boyle, M. P., Dollar, P., Lee, H., Lein, E. S., Perona, P., and Anderson, D. J. (2011). Functional identification of an aggression locus in the mouse hypothalamus. Nature 470, 221–226.
Lin, J. H., Saito, T., Anderson, D. J., Lance-Jones, C., Jessell, T. M., and Arber, S. (1998). Functionally related motor neuron pool and muscle sensory afferent subtypes defined by coordinate ETS gene expression. Cell 95, 393–407.
Lonstein, J. S., and Stern, J. M. (1998). Site and behavioral specificity of periaqueductal gray lesions on postpartum sexual, maternal, and aggressive behaviors in rats. Brain Res. 804, 21–35.
López-Mascaraque, L., De Carlos, J. A., and Valverde, F. (1996). Early onset of the rat olfactory bulb projections. Neuroscience 70, 255–266.
Luo, L., and Flanagan, J. G. (2007). Development of continuous and discrete neural maps. Neuron 56, 284–300.
MacLean, P. D. (1949). Psychosomatic disease and the visceral brain; recent developments bearing on the Papez theory of emotion. Psychosom. Med. 11, 338–353.
Malnic, B., Hirono, J., Sato, T., and Buck, L. B. (1999). Combinatorial receptor codes for odors. Cell 96, 713–723.
Mani, S. K., Blaustein, J. D., Allen, J. M., Law, S. W., O'Malley, B. W., and Clark, J. H. (1994). Inhibition of rat sexual behavior by antisense oligonucleotides to the progesterone receptor. Endocrinology 135, 1409–1414.
Mann, F., Zhukareva, V., Pimenta, A., Levitt, P., and Bolz, J. (1998). Membrane-associated molecules guide limbic and nonlimbic thalamocortical projections. J. Neurosci. 18, 9409–9419.
Marín, O., and Rubenstein, J. L. (2003). Cell migration in the forebrain. Annu. Rev. Neurosci. 26, 441–483.
Markram, K., Rinaldi, T., La Mendola, D., Sandi, C., and Markram, H. (2008). Abnormal fear conditioning and amygdala processing in an animal model of autism. Neuropsychopharmacology 33, 901–912.
Martins, G. J., Shahrokh, M., and Powell, E. M. (2011). Genetic disruption of Met signaling impairs GABAergic striatal development and cognition. Neuroscience 176, 199–209.
Marson, L. (2004). Lesions of the periaqueductal gray block the medial preoptic area-induced activation of the urethrogenital reflex in male rats. Neurosci. Lett. 367, 278–282.
McConnell, J., and Angevine, J. B. Jr. (1983). Time of neuron origin in the amygdaloid complex of the mouse. Brain Res. 272, 150–156.
McGregor, I. S., Hargreaves, G. A., Apfelbach, R., and Hunt, G. E. (2004). Neural correlates of cat odor-induced anxiety in rats: region-specific effects of the benzodiazepine midazolam. J. Neurosci. 24, 4134–4144.
Meurisse, M., Chaillou, E., and Lévy, F. (2009). Afferent and efferent connections of the cortical and medial nuclei of the amygdala in sheep. J. Chem. Neuroanat. 37, 87–97.
Miller, A. M., Maurer, L. R., Zou, D. J., Firestein, S., and Greer, C. A. (2010). Axon fasciculation in the developing olfactory nerve. Neural. Dev. 5, 20.
Mohedano-Moriano, A., Pro-Sistiaga, P., Ubeda-Bañón, I., Crespo, C., Insausti, R., and Martinez-Marcos, A. (2007). Segregated pathways to the vomeronasal amygdala: differential projections from the anterior and posterior divisions of the accessory olfactory bulb. Eur. J. Neurosci. 25, 2065–2080.
Mombaerts, P. (2006). Axonal wiring in the mouse olfactory system. Annu. Rev. Cell Dev. Biol. 22, 713–737.
Monaghan, A. P., Bock, D., Gass, P., Schwäger, A., Wolfer, D. P., Lipp, H. P., and Schütz, G. (1997). Defective limbic system in mice lacking the tailless gene. Nature 390, 515–517.
Monk, C. S. (2008). The development of emotion-related neural circuitry in health and psychopathology. Dev. Psychopathol. 20, 1231–1250.
Morris, J. A., Jordan, C. L., King, Z. A., Northcutt, K. V., and Breedlove, S. M. (2008). Sexual dimorphism and steroid responsiveness of the posterodorsal medial amygdala in adult mice. Brain Res. 1190, 115–121.
Motta, S. C., Goto, M., Gouveia, F. V., Baldo, M. V., Canteras, N. S., and Swanson, L. W. (2009). Dissecting the brain's fear system reveals the hypothalamus is critical for responding in subordinate conspecific intruders. Proc. Natl. Acad. Sci. U.S.A. 106, 4870–4875.
Nery, S., Fishell, G., and Corbin, J. G. (2002). The caudal ganglionic eminence is a source of distinct cortical and subcortical cell populations. Nat. Neurosci. 5, 1279–1287.
Newman, S. W. (1999). The medial extended amygdala in male reproductive behavior. A node in the mammalian social behavior network. Ann. N.Y. Acad. Sci. 877, 242–257.
Nguyen-Ba-Charvet, K. T., Di Meglio, T., Fouquet, C., and Chédotal, A. (2008). Robos and slits control the pathfinding and targeting of mouse olfactory sensory axons. J. Neurosci. 28, 4244–4249.
Nomura, T., Holmberg, J., Frisen, J., and Osumi, N. (2006). Pax6-dependent boundary defines alignment of migrating olfactory cortex neurons via the repulsive activity of ephrin A5. Development 133, 1335–1345.
Normandin, J. J., and Murphy, A. Z. (2011a). Serotonergic lesions of the periaqueductal gray, a primary source of serotonin to the nucleus paragigantocellularis, facilitate sexual behavior in male rats. Pharmacol. Biochem. Behav. 98, 369–375.
Normandin, J. J., and Murphy, A. Z. (2011b). Somatic genital reflexes in rats with a nod to humans: anatomy, physiology, and the role of the social neuropeptides. Horm. Behav. 59, 656–665.
Numan, M., Numan, M. J., and English, J. B. (1993). Excitotoxic amino acid injections into the medial amygdala facilitate maternal behavior in virgin female rats. Horm. Behav. 27, 56–81.
Numan, M., and Smith, H. G. (1984). Maternal behavior in rats: evidence for the involvement of preoptic projections to the ventral tegmental area. Behav. Neurosci. 98, 712–727.
Ogawa, S., Washburn, T. F., Taylor, J., Lubahn, D. B., Korach, K. S., and Pfaff, D. W. (1998). Modifications of testosterone-dependent behaviors by estrogen receptor-alpha gene disruption in male mice. Endocrinology 139, 5058–5069.
Osterhout, J. A., Josten, N., Yamada, J., Pan, F., Wu, S. W., Nguyen, P. L., Panagiotakos, G., Inoue, Y. U., Egusa, S. F., Volgyi, B., Inoue, T., Bloomfield, S. A., Barres, B. A., Berson, D. M., Feldheim, D. A., and Huberman, A. D. (2011). Cadherin-6 mediates axon-target matching in a non-image-forming visual circuit. Neuron 71, 632–639.
Pankevich, D. E., Baum, M. J., and Cherry, J. A. (2004). Olfactory sex discrimination persists, whereas the preference for urinary odorants from estrous females disappears in male mice after vomeronasal organ removal. J. Neurosci. 24, 9451–9457.
Papes, F., Logan, D. W., and Stowers, L. (2010). The vomeronasal organ mediates interspecies defensive behaviors through detection of protein pheromone homologs. Cell 141, 692–703.
Papez, J. W. (1937). A proposed mechanism of emotion. J. Neuropsychiatry Clin. Neurosci. 7, 103–112.
Petitti, N., Karkanias, G. B., and Etgen, A. M. (1992). Estradiol selectively regulates alpha 1B-noradrenergic receptors in the hypothalamus and preoptic area. J. Neurosci. 12, 3869–3876.
Petrovich, G. D., Canteras, N. S., and Swanson, L. W. (2001). Combinatorial amygdalar inputs to hippocampal domains and hypothalamic behavior systems. Brain Res. Brain Res. Rev. 38, 247–289.
Petrulis, A., Peng, M., and Johnston, R. E. (1999). Effects of vomeronasal organ removal on individual odor discrimination, sex-odor preference, and scent marking by female hamsters. Physiol. Behav. 66, 73–83.
Pfaff, D. W., and Sakuma, Y. (1979). Deficit in the lordosis reflex of female rats caused by lesions in the ventromedial nucleus of the hypothalamus. J. Physiol. 288, 203–210.
Pimenta, A. F., and Levitt, P. (2004). Characterization of the genomic structure of the mouse limbic system-associated membrane protein (Lsamp) gene. Genomics 83, 790–801.
Powell, E. M., Mühlfriedel, S., Bolz, J., and Levitt, P. (2003). Differential regulation of thalamic and cortical axonal growth by hepatocyte growth factor/scatter factor. Dev. Neurosci. 25, 197–206.
Puelles, L., Kuwana, E., Puelles, E., Bulfone, A., Shimamura, K., Keleher, J., Smiga, S., and Rubenstein, J. L. (2000). Pallial and subpallial derivatives in the embryonic chick and mouse telencephalon, traced by the expression of the genes Dlx-2, Emx-1, Nkx-2.1, Pax-6, and Tbr-1. J. Comp. Neurol. 424, 409–438.
Puelles, L., and Rubenstein, J. L. (1993). Expression patterns of homeobox and other putative regulatory genes in the embryonic mouse forebrain suggest a neuromeric organization. Trends Neurosci. 16, 472–479.
Puelles, L., and Rubenstein, J. L. (2003). Forebrain gene expression domains and the evolving prosomeric model. Trends Neurosci. 26, 469–476.
Redies, C., and Takeichi, M. (1993). Expression of N-cadherin mRNA during development of the mouse brain. Dev. Dyn. 197, 26–39.
Remedios, R., Huilgol, D., Saha, B., Hari, P., Bhatnagar, L., Kowalczyk, T., Hevner, R. F., Suda, Y., Aizawa, S., Ohshima, T., Stoykova, A., and Tole, S. (2007). A stream of cells migrating from the caudal telencephalon reveals a link between the amygdala and neocortex. Nat. Neurosci. 10, 1141–1150.
Remedios, R., Subramanian, L., and Tole, S. (2004). LIM genes parcellate the embryonic amygdala and regulate its development. J. Neurosci. 24, 6986–6990.
Risold, P. Y., Canteras, N. S., and Swanson, L. W. (1994). Organization of projections from the anterior hypothalamic nucleus: a Phaseolus vulgaris-leucoagglutinin study in the rat. J. Comp. Neurol. 348, 1–40.
Rodrigues, S. M., Schafe, G. E., and LeDoux, J. E. (2004). Molecular mechanisms underlying emotional learning and memory in the lateral amygdala. Neuron 44, 75–91.
Rosenblatt, J. S., Wagner, C. K., and Morrell, J. I. (1994). Hormonal priming and triggering of maternal behavior in the rat with special reference to the relations between estrogen receptor binding and ER mRNA in specific brain regions. Psychoneuroendocrinology 19, 543–552.
Royal, S. J., and Key, B. (1999). Development of P2 olfactory glomeruli in P2-internal ribosome entry site-tau-LacZ transgenic mice. J. Neurosci. 19, 9856–9864.
Ruthschilling, C. A., Albiero, G., Lazzari, V. M., Becker, R. O., de Moura, A. C., Lucion, A. B., Almeida, S., Veiga, A. B., and Giovenardi, M. (2012). Analysis of transcriptional levels of the oxytocin receptor in different areas of the central nervous system and behaviors in high and low licking rats. Behav. Brain Res. 228, 176–184.
Sato, Y., Iketani, M., Kurihara, Y., Yamaguchi, M., Yamashita, N., Nakamura, F., Arie, Y., Kawasaki, T., Hirata, T., Abe, T., Kiyonari, H., Strittmatter, S. M., Goshima, Y., and Takei, K. (2011). Cartilage acidic protein-1B (LOTUS), an endogenous Nogo receptor antagonist for axon tract formation. Science 333, 769–773.
Sawrey, D. K., and Dewsbury, D. A. (1994). Conspecific odor preferences in Montane voles (Microtus montanus): effects of sexual experience. Physiol. Behav. 56, 339–344.
Sheehan, T., Paul, M., Amaral, E., Numan, M. J., and Numan, M. (2001). Evidence that the medial amygdala projects to the anterior/ventromedial hypothalamic nuclei to inhibit maternal behavior in rats. Neuroscience 106, 341–356.
Shimogori, T., Lee, D. A., Miranda-Angulo, A., Yang, Y., Wang, H., Jiang, L., Yoshida, A. C., Kataoka, A., Mashiko, H., Avetisyan, M., Qi, L., Qian, J., and Blackshaw, S. (2010). A genomic atlas of mouse hypothalamic development. Nat. Neurosci. 13, 767–775.
Shirasaki, R., and Pfaff, S. L. (2002). Transcriptional codes and the control of neuronal identity. Annu. Rev. Neurosci. 25, 251–281.
Simerly, R. B. (2005). Wired on hormones: endocrine regulation of hypothalamic development. Curr. Opin. Neurobiol. 15, 81–85.
Soma, M., Aizawa, H., Ito, Y., Maekawa, M., Osumi, N., Nakahira, E., Okamoto, H., Tanaka, K., and Yuasa, S. (2009). Development of the mouse amygdala as revealed by enhanced green fluorescent protein gene transfer by means of in utero electroporation. J. Comp. Neurol. 513, 113–128.
Stenman, J., Yu, R. T., Evans, R. M., and Campbell, K. (2003). Tlx and Pax6 co-operate genetically to establish the pallio-subpallial boundary in the embryonic mouse telencephalon. Development 130, 1113–1122.
Stowers, L., Holy, T. E., Meister, M., Dulac, C., and Koentges, G. (2002). Loss of sex discrimination and male-male aggression in mice deficient for TRP2. Science 22, 1493–1500.
Stoykova, A., Götz, M., Gruss, P., and Price, J. (1997). Pax6-dependent regulation of adhesive patterning, R-cadherin expression and boundary formation in developing forebrain. Development 124, 3765–3777.
Strotmann, J., Wanner, I., Helfrich, T., and Breer, H. (1995). Receptor expression in olfactory neurons during rat development: in situ hybridization studies. Eur. J. Neurosci. 1, 492–500.
Sullivan, S. L., Bohm, S., Ressler, K. J., Horowitz, L. F., and Buck, L. B. (1995). Target-independent pattern specification in the olfactory epithelium. Neuron 15, 779–789.
Swanson, L. W. (2000). Cerebral hemisphere regulation of motivated behavior. Brain Res. 15, 113–164.
Tietjen, I., Rihel, J., and Dulac, C. G. (2005). Single-cell transcriptional profiles and spatial patterning of the mammalian olfactory epithelium. Int. J. Dev. Biol. 49, 201–207.
Toda, K., Saibara, T., Okada, T., Onishi, S., and Shizuta, Y. (2001). A loss of aggressive behaviour and its reinstatement by oestrogen in mice lacking the aromatase gene (Cyp19). J. Endocrinol. 168, 217–220.
Tyndall, S. J., and Walikonis, R. S. (2006). The receptor tyrosine kinase Met and its ligand hepatocyte growth factor are clustered at excitatory synapses and can enhance clustering of synaptic proteins. Cell Cycle 5, 1560–1568.
Umeda, T., Takashima, N., Nakagawa, R., Maekawa, M., Ikegami, S., Yoshikawa, T., Kobayashi, K., Okanoya, K., Inokuchi, K., and Osumi, N. (2010). Evaluation of Pax6 mutant rat as a model for autism. PLoS One 5:e15500. doi: 10.1371/journal.pone.0015500
Veening, J. G., Coolen, L. M., de Jong, T. R., Joosten, H. W., de Boer, S. F., Koolhaas, J. M., and Olivier, B. (2005). Do similar neural systems subserve aggressive and sexual behaviour in male rats? Insights from c-Fos and pharmacological studies. Eur. J. Pharmacol. 5, 226–239.
Walsh, C. A., Morrow, E. M., and Rubenstein, J. L. (2008). Autism and brain development. Cell 135, 396–400.
Wang, K., Zhang, H., Ma, D., Bucan, M., Glessner, J. T., Abrahams, B. S., Salyakina, D., Imielinski, M., Bradfield, J. P., Sleiman, P. M., Kim, C. E., Hou, C., Frackelton, E., Chiavacci, R., Takahashi, N., Sakurai, T., Rappaport, E., Lajonchere, C. M., Munson, J., Estes, A., Korvatska, O., Piven, J., Sonnenblick, L. I., Alvarez Retuerto, A. I., Herman, E. I., Dong, H., Hutman, T., Sigman, M., Ozonoff, S., Klin, A., Owley, T., Sweeney, J. A., Brune, C. W., Cantor, R. M., Bernier, R., Gilbert, J. R., Cuccaro, M. L., McMahon, W. M., Miller, J., State, M. W., Wassink, T. H., Coon, H., Levy, S. E., Schultz, R. T., Nurnberger, J. I., Haines, J. L., Sutcliffe, J. S., Cook, E. H., Minshew, N. J., Buxbaum, J. D., Dawson, G., Grant, S. F., Geschwind, D. H., Pericak-Vance, M. A., Schellenberg, G. D., and Hakonarson, H. (2009). Common genetic variants on 5p14.1 associate with autism spectrum disorders. Nature 28, 528–533.
Wang, W., and Lufkin, T. (2000). The murine Otp homeobox gene plays an essential role in the specification of neuronal cell lineages in the developing hypothalamus. Dev. Biol. 5, 432–449.
Williams, M. E., Wilke, S. A., Daggett, A., Davis, E., Otto, S., Ravi, D., Ripley, B., Bushong, E. A., Ellisman, M. H., Klein, G., and Ghosh, A. (2011). Cadherin-9 regulates synapse-specific differentiation in the developing hippocampus. Neuron 71, 640–655.
Wonders, C. P., and Anderson, S. A. (2006). The origin and specification of cortical interneurons. Nat. Rev. Neurosci. 7, 687–696.
Wu, M. V., Manoli, D. S., Fraser, E. J., Coats, J. K., Tollkuhn, J., Honda, S., Harada, N., and Shah, N. M. (2009). Estrogen masculinizes neural pathways and sex-specific behaviors. Cell 139, 61–72.
Wu, M. V., and Shah, N. M. (2011). Control of masculinization of the brain and behavior. Curr. Opin. Neurobiol. 21, 116–123.
Xu, Q., Tam, M., and Anderson, S. A. (2008). Fate mapping Nkx2.1-lineage cells in the mouse telencephalon. J. Comp. Neurol. 506, 16–29.
Xu, X., Coats, J. K., Yang, C. F., Wang, A., Ahmed, O. M., Alvarado, M., Izumi, T., and Shah, N. M. (2012). Modular genetic control of sexually dimorphic behaviors. Cell 148, 596–607.
Yoshihara, Y., Kawasaki, M., Tamada, A., Fujita, H., Hayashi, H., Kagamiyama, H., and Mori, K. (1997). OCAM: a new member of the neural cell adhesion molecule family related to zone-to-zone projection of olfactory and vomeronasal axons. J. Neurosci. 17, 5830–5842.
Yoshihara, Y., and Mori, K. (1997). Basic principles and molecular mechanisms of olfactory axon pathfinding. Cell Tissue Res. 290, 457–463.
Keywords: innate, limbic system, development, olfaction, amygdala, hypothalamus, behaviors
Citation: Sokolowski K and Corbin JG (2012) Wired for behaviors: from development to function of innate limbic system circuitry. Front. Mol. Neurosci. 5:55. doi: 10.3389/fnmol.2012.00055
Received: 01 March 2012; Accepted: 04 April 2012;
Published online: 26 April 2012.
Edited by:
Robert W. Burgess, The Jackson Laboratory, USAReviewed by:
Erik Maronde, University of Frankfurt, GermanyHansen Wang, University of Toronto, Canada
Copyright: © 2012 Sokolowski and Corbin. This is an open-access article distributed under the terms of the Creative Commons Attribution Non Commercial License, which permits non-commercial use, distribution, and reproduction in other forums, provided the original authors and source are credited.
*Correspondence: Joshua G. Corbin, Children's National Medical Center, Center for Neuroscience Research (CNR), Children's Research Institute, 111 Michigan Avenue, NW, Washington, DC 20010-2970, USA. e-mail:amNvcmJpbkBjbm1jcmVzZWFyY2gub3Jn