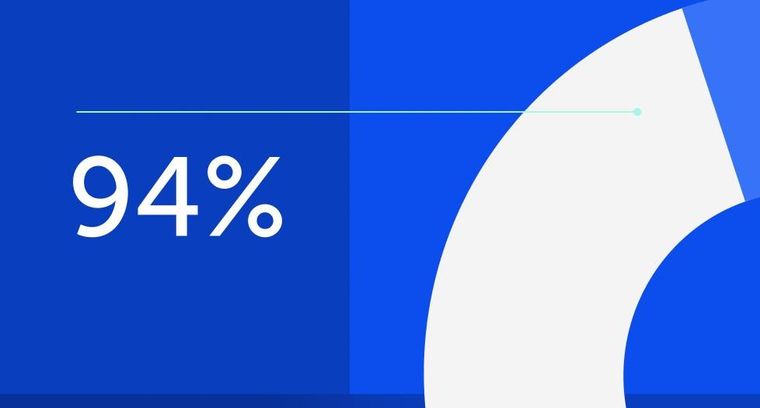
94% of researchers rate our articles as excellent or good
Learn more about the work of our research integrity team to safeguard the quality of each article we publish.
Find out more
REVIEW article
Front. Mol. Neurosci., 04 April 2011
Sec. Neuroplasticity and Development
Volume 4 - 2011 | https://doi.org/10.3389/fnmol.2011.00005
This article is part of the Research TopicThe tumor and the embryo: oxygen and oxygen dependent pathways in developmentView all 6 articles
Oxygen is vital to maintain the normal functions of almost all the organs, especially for brain which is one of the heaviest oxygen consumers in the body. The important roles of oxygen on the brain are not only reflected in the development, but also showed in the pathological processes of many cerebral diseases. In the current review, we summarized the oxygen levels in brain tissues tested by real-time measurements during the embryonic and adult neurogenesis, the cerebral diseases, or in the hyperbaric/hypobaric oxygen environment. Oxygen concentration is low in fetal brain (0.076–7.6 mmHg) and in adult brain (11.4–53.2 mmHg), decreased during stroke, and increased in hyperbaric oxygen environment. In addition, we reviewed the effects of oxygen tensions on the behaviors of neural stem cells (NSCs) in vitro cultures at different oxygen concentration (15.2–152 mmHg) and in vivo niche during different pathological states and in hyperbaric/hypobaric oxygen environment. Moderate hypoxia (22.8–76 mmHg) can promote the proliferation of NSCs and enhance the differentiation of NSCs into the TH-positive neurons. Next, we briefly presented the oxygen-sensitive molecular mechanisms regulating NSCs proliferation and differentiation recently found including the Notch, Bone morphogenetic protein and Wnt pathways. Finally, the future perspectives about the roles of oxygen on brain and NSCs were given.
Oxygen, as a significant substrate for energy production and cell metabolism, is essential for most of the life on earth and affects various aspects of life activities, including growth and development. It is interesting to note that the normal oxygen levels in the tissues are always substantially lower than 156 mmHg O2 in the air we breath (Panchision, 2009): in the lung parenchyma and in circulation, the oxygen tension is ranged from 28 to 98 mmHg; in the eye (retina, corpus vitreous), from 7 to 35 mmHg; in the bone marrow, from 0 to 28 mmHg. These oxygen concentrations (7–35 mmHg O2) are called “physiological hypoxia,” that is a steady state of physiological oxygenation or “in situ normoxia” (Ivanovic, 2009).
The brain is one of the heaviest oxygen consumers in the body, which accounts for 20% of total oxygen consumption (Masamoto and Tanishita, 2009). However, the oxygen levels in almost all the regions of brain are very low: 32 ± 4 mmHg in the thalamus, 27 ± 6 mmHg in the cerebral cortex, 20 ± 3 mmHg in the hippocampus, and 15 ± 3 mmHg in the corpus callous in isoflurane-anesthetized rats (Ivanovic, 2009). In addition, the development of various organs of embryos including the central nervous system (CNS) takes place in low-oxygen concentration (Fischer and Bavister, 1993; Chen et al., 1999). Apart from this, oxygen levels in brain tissues are often altered during stroke (Liu et al., 2004), brain trauma (Valadka et al., 1998), and in the hyperbaric oxygen (HBO) environment (Balenane, 1982). Thus, the oxygen supply to brain must be precisely controlled in response to local demand induced by metabolic activity to prevent tissue hypoxia which would immediately lead to irreversible damages in brain functions (Masamoto and Tanishita, 2009).
This review will cover the cerebral oxygen tensions during neurogenesis and cerebral diseases, then the role of oxygen on the cellular behaviors of neural stem cells (NSCs) will be discussed. In addition, the involved molecular mechanisms will be talked about in the present review.
The accurate data about oxygen content during the embryonic neurogenesis has attracted many researchers. During the pre-implantation period, the oxygen tensions were about 60 mmHg in oviducts of rabbits (Mastroianni and Jones, 1965), but less than 10 mmHg in uterus of rhesus monkeys (Maas et al., 1976), 5–50 and 25–50 mmHg in uterus of the hamsters and rats (Kaufman and Mitchell, 1990). After the implantation of embryos the oxygen tension in amniotic fluid was 10.9 ± 1.0 mmHg in the early gestation and 11.6 ± 0.7 mmHg in mid gestation of sheep (Jauniaux et al., 2000). During the late gestation, although the onset of placental gas exchange establishes, the PO2 values for umbilical artery, vein, and amniotic fluid are still constrained below maternal venous levels (23, 30, and 12 mmHg, respectively; Eskes et al., 1983; Yeomans et al., 1985; Rurak et al., 1987; Jauniaux et al., 2000). In summary, the whole process of embryonic development is under the low-oxygen concentration.
The embryonic neurogenesis begins at the early gestation period when the placental gas exchanges have not been set up, and under very low-oxygen concentration (≤15.2 mmHg; Zhou, 2004). In the mid and late gestation, the density of cerebral vessels has become an important factor which determines the oxygen niche of embryonic neurogenesis (Takashima and Tanaka, 1978). Takashima and Tanaka (1978) have investigated the development of cerebral vascular in human fetal brain and found that most of the perforating branches are short in the second trimester and develop with gestational age. In most of cerebral regions, the vessel density is low before 28 gestational week (GW), and then increased after this time point, e.g., the cerebral cortex, the subcortical white matter, and the basis pontis. In the other cerebral regions, the vessel density is high before 28 GW, and decreases or remains high after this time point, e.g., the deep white matter and putamen (Mito et al., 1991; Miyawaki et al., 1998). Thus, the development of blood vessels during the whole gestational period might parallel with the changes of cerebral oxygen niche.
The direct evidences about oxygen niche of embryonic neurogenesis were provided by Chen (Chen et al., 1999), utilizing the hypoxia marker EF5, a nitroimidazole derivative which binds covalently to protein, RNA, and DNA in cells exposed to a hypoxic environment (0.076–7.6 mmHg oxygen; Lord et al., 1993). They found that the neural tube in both the hindbrain and midbrain regions also stained strongly with the EF5 immunoreactivity, indicating that the oxygen tensions of these regions substantially below 7.6 mmHg (Lord et al., 1993). Lee You Mie also used the hypoxia marker, pimonidazole hydrochloride (Hypoxyprobe™-1), to indicate the hypoxic regions during embryonic development (Lee et al., 2001). They found that hypoxic regions detected by hypoxia marker exist on 8.5–9.0 day past copulation (d.p.c.) in folding neural tube and neuronal mesenchymal cells in mouse embryos. In the brain, the mesenchymal region was hypoxia marker-immunoreactive, suggesting that at this stage at least, highly proliferative cells may be localized in the low-oxygen tension. At day 9.5–11.5, the hypoxic regions in embryonic tissues were spread into neural tubes of telencephalon, diencephalon, and metencephalon including mesenchymal region of head. On 12.5 d.p.c., the hypoxic immunoreactive regions were clearly demarcated in the internal lining of the cranial flexure, myelencephalon, and choroid plexi, and in the center of maxillary prominence, where cells proliferate and differentiate (Lee et al., 2001). Those above investigations provided strong and direct evidences that the embryonic neurogenesis was under very low-oxygen tension.
The neurogenesis in the adult mammalian brain was first evidenced by Altman in the adult rat using in vivo 3H-thymidine administration (Altman 1962, 1969; Altman and Das, 1965, 1967). Direct demonstration of NSCs in the mature brain was provided by means of primary cell culture from dissociated brain tissue (Reynolds and Weiss, 1992). This discovery generated great expectations by raising the prospect of regenerative therapies for the treatment of CNS injuries such as those brought by ischemia or neurodegenerative disorders (Miller, 2006). Continuous neurogenesis from NSCs has been identified in two neurogenic germinal zones of adult brains: the subgranular zone (SGZ) of the dentate gyrus (DG) of the hippocampus and the subventricular zone (SVZ) of the lateral ventricles (Suh et al., 2009). Such in vivo neurogenetic restrictions were formally demonstrated to arise from the tissular microenvironment, which led to the emerging concept of “neurogenic niche” (Moyse et al., 2008).
The “neurogenic niche” includes the vascular niche, the astrocyte niche, the neural net niche, and the molecular pathway niche (Suh et al., 2009). However, the “oxygen niche” of the adult neurogenesis has not been paid extensive attention until now. Shen et al. (2008) have found that the SVZ contains a rich plexus of blood vessels that snake along and within neuroblast chains. Cells expressing stem cell markers, including glial fibrillary acidic protein (GFAP), and proliferation markers are closely apposed to the laminin-containing extracellular matrix (ECM) surrounding vascular endothelial cells. Tavazoie et al. (2008) have also found that dividing stem cells and their transit-amplifying progeny were tightly apposed to SVZ blood vessels both during homeostasis and regeneration. They frequently contact the vasculature at sites that lack astrocyte endfeet and pericyte coverage, and regeneration often occurs at these sites (Tavazoie et al., 2008). It has also been found that the physical exercise could induce both of the angiogenesis and the neurogenesis in hippocampus (Van der Borght et al., 2009). Taken together, all of the above researches indicated the significant role of the vessels as one of the important components of the neurogenesis niche, and may also implied that the higher oxygen tension around the vessels in SVZ and DG would be significant for the maintenance of the characteristics of the NSCs. While, our recent work have provided some direct data related to the above issues. We found that the PO2 levels in ventricles are in a dynamic state and fluctuate in the range of 42 to 48 mmHg at a frequency of about 3 min. In hippocampus, the PO2 levels in CA1 and hilus are very stable and maintain about 2 mmHg; while the PO2 level in DG is dynamic and fluctuates in a range of 6–8 mmHg (Zhang et al., 2010).
The oxygen supply to brain might be influenced by various brain insults, which could lead to brain irreversible damages. Brain insults include stroke, trauma, subarachnoid hemorrhage (SAH), and so on, which produce changes in structure, pressure dynamics, chemical balance, and blood flow (Bader, 2006). Ultimately, the delivery of oxygen to the cranial vault may become compromised. The devastating primary insult creates structural damage to neurons, vessels, and cranial nerves as well as compression of the brain and vasculature. The resulting edema and pathological processes further compromise the delivery of blood flow and oxygen to the brain (Bader, 2006).
The PO2 levels in uninjured brain tissue has been measured about 25–30 mmHg in white matter of frontal lobes (Sarrafzadeh et al., 1998), 20–40 mmHg in normal tissue (Hlatky et al., 2003). A lot of researchers have also investigated the changes of oxygen tension during brain insults, such as stroke, trauma, or SAH (Maas and Fleckenstein, 1993; Zauner et al., 1996; Doppenberg and Zauner, 1998; Liu et al., 2006). van den Brink et al. (2000) studied 101 comatose patients who had traumatic brain injury (TBI) and reported that the survivors had significantly higher PO2 levels during the monitoring period than did the patients who died. Lower PO2 levels were related to a greater risk for death (van den Brink et al., 2000). The investigators found that PO2 of less than 15 mmHg for longer than 30 min or less than 10 mmHg for 10 min correlated with a statistically significant risk for death. In one study of 39 patients, the investigators found that PO2 of less than 15 mmHg correlated with a greater chance of death. A value of less than 6 mmHg at any time was associated with a greater risk for dying (Valadka et al., 1998).
Liu et al. (2004) investigated the effects of stroke on the cerebral oxygen tension. They measured both absolute values, and temporal changes of PO2 in ischemic penumbra and core during ischemia and reperfusion in a rat model with the electron paramagnetic resonance (EPR) method. They found that pre-ischemic PO2 values in ischemic core and basal ganglial penumbra of the anesthetized rats were 33.4 ± 6.0 mmHg. After MCAO, interstitial PO2 in both core and penumbra dropped rapidly in the first 10 min, thence the rate of decrease slowed, and reached their respective lowest levels at 1-h postocclusion. The interstitial PO2 values in penumbra were significantly higher than the corresponding values in the core, and were 10.7 ± 7.8 and 1.2 ± 0.7 mmHg at 1-h after occlusion, respectively. Importantly, after reperfusion, PO2 levels in both core and penumbra positions increased, but very differently. One hour after reperfusion core PO2 returned to near pre-ischemic levels, 31.6 ± 16.5 mmHg, whilst penumbral PO2 showed only partial recovery to a level of 19.1 ± 6.7 mmHg. So it is demonstrated that the interstitial PO2 levels in penumbra and core are differentially affected during ischemia and reperfusion.
Tissue hypoxia-induced by brain insults plays a critical role in the primary and secondary events leading to cell death after cerebral ischemia (Zauner et al., 2002). Therefore, improving brain tissue oxygenation is a logical and important strategy of stroke treatment to delay the transition of ischemia to infarction (“buying time”). Several experimental studies showed that hyperbaric and normobaric hyperoxia (NBO) was able to impressively reduce the stroke lesion volumes (Miyamoto and Auer, 2000; Flynn and Auer, 2002; Singhal et al., 2002a,b; Günther et al., 2005; Kim and Singhal, 2005), significantly extended the time window for reperfusion, and induced lasting neuroprotection in permanent ischemia (Henninger et al., 2007). Liu et al. (2004) have found that hyperoxic treatment (532 mmHg O2 for 60 min) significantly increased penumbral PO2 during ischemia, but not in the core. They also found that 722 mmHg normobaric O2 given during ischemia was able to maintain penumbral interstitial PO2 levels close to the pre-ischemic value while it may cause a two-fold increase in penumbral PO2 level if given during reperfusion (Liu et al., 2006).
Hyperbaric oxygen therapy is defined by the Undersea and Hyperbaric Medical Society (UHMS) as a treatment in which a patient intermittingly breathes 760 mmHg oxygen under a pressure that is greater than the pressure at sea level [a pressure greater than 1 atmosphere absolute (ATA)] (Calvert et al., 2007). HBO has been shown to be a potent means to increase the oxygen content of blood and has been advocated for the treatment of various ailments, including air embolism, carbon monoxide poisoning, wound healing, and ischemic stroke (Calvert et al., 2007; Nemoto and Betterman, 2007). Some studies showed the increase in arterial oxygen content with increasing inspired oxygen at 1 ATA from 159.6 to 760 mmHg and with 760 mmHg oxygen at 1, 2, and 3 ATA. With each step increase in oxygen from 159.6 to 760 mmHg at 1 ATA and 760 mmHg oxygen at 2 and 3 ATA, arterial oxygen content is increased by 2/100 ml. Thus, by increasing oxygen with 760 mmHg oxygen at 1–3 ATA, arterial oxygen content is increased by 4 ml/100 g/min (Nunn, 1969). The oxygen tension in brain capillary blood was also enhanced by the increased oxygen pressure (to 2.0, 3.0, and 3.5 ATA), but fell down in the venous blood; While, the supplementation of 2% CO2 to oxygen at 3.5 ATA tremendously increases the mean capillary PO2 and the oxygenation of venous blood (Balenane, 1982). Exposure to 7 ATA O2 showed two to three-fold increases in oxygen in the cerebral cortex, hippocampus, and the reticular formation compared to air breathing and considerably less than that expected (Torbati et al., 1976, 1977). Vasoconstriction in response to HBO could be the reason for the blunted increase in brain tissue PO2 during HBO exposure, in addition to the possibility of increased the cerebral metabolic rate of oxygen (CMRO2) associated with increased electrical activity at high oxygen pressures in the range of 7 ATA (Nemoto and Betterman, 2007).
Hyperbaric oxygenation, breathing of 760 mmHg O2 under hyperbaric conditions, is a potent method to increase the O2 concentration in tissue with impaired blood supply. Experimental as well as clinical studies have reported a positive effect of HBO therapy. Survival rate has increased under HBO therapy and neurological outcome has improved (Fischer et al., 2010). However, the HBO treatment in cerebral ischemic–anoxic is the proverbial “double-edged sword” because of the dual nature of oxygen in being essential to life and at the same time, it is toxic in excess (Nemoto and Betterman, 2007). It is noted that, in preclinical studies, HBO therapy was effective if administered within 6 h post-stroke after transient middle cerebral artery occlusion and worsened the severity of injury if applied 12 h or later after the stroke (Lou et al., 2004).
Hypobaric hypoxia (HH) is a predisposing environmental condition at high altitude (HA), where although barometric pressure decreases exponentially as altitude is gained, the percentage of each gas component of air is constant up to 12,000 m (Wilson et al., 2009). Therefore, although the proportion of oxygen remains unchanged at 20.93%, increases in altitude result in a lower partial pressure of oxygen in the inspired air. This reduction in the driving gradient on the oxygen cascade can compromise the supply of adequate oxygen to the tissues (Wilson et al., 2009). Compensatory hyperventilation, tachycardia, erythropoietin-induced polycythemia, and increased cerebral blood flow (CBF) can partially maintain cerebral oxygen delivery at HAs (West et al., 2007). However, because the brain is exquisitely sensitive to hypoxia, it is the first organ to be compromised when these mechanisms are inadequate (West, 1996; West et al., 2007). HH is known to cause various neurological clinical syndromes, including high altitude headache (HAH), acute mountain sickness (AMS), and high altitude cerebral edema (HACE), and the genetics, molecular mechanisms, and physiology that underpin them (West et al., 2007).
Some studies indicated that the average venous PO2 in brain tissue is about 30 mmHg at the altitude of about 6000 m, lower than 40 mmHg at the sea level (Boero et al., 1999). It was also found that the HH (28 days, 6000 m) could increase the capillary length per unit volume of tissue (Lv) in the cerebellar granular layer, the caudate nucleus, the globus pallidus, the substantia nigra, the superior colliculus, and the DG, which may accounts for the significant increase of O2 conductance to neural tissues, and suggested that formation of new capillaries is an important mechanism to restore the O2 deficit in chronic brain hypoxia and that local rates of energy utilization may influence angiogenesis in different areas of the brain (Boero et al., 1999). Studies showed that the changes of the CBF depended upon the temporal domains at the HA. Exposure to acute hypoxia is known to cause an immediate increase in CBF, but it is also well known that hypocapnia by itself causes vasoconstriction (Brugniaux et al., 2007). During the acclimatization process, beginning several hours after the start of an altitude exposure and lasting for months (Powell et al., 1998), CBF usually reaches a peak over the first few days followed by a progressive drop toward baseline levels (Wolff, 2000; Wolff et al., 2002). In addition, Dunn et al. (2000) chronically adapted rats to one half an atmosphere of barometric pressure (6000 m) for 27 days and measured the cortical PO2 when animals were breathing normobaric gases. The PO2 in acclimated animals increased rapidly in the first 7 days, then stabilized for the duration of the study. The average PO2 in the acclimated group (from 7 days on) was 62 versus 26 mmHg in the control groups, an increase of 238% (Dunn et al., 2000).
The biological function of oxygen on NSCs in vitro was first reported by Studer and Morrison. Studer analyzed mesencephalic precursor cells from rat embryos in embryonic day 12 (E12) in traditional cultures with 152 mmHg O2 and in lowered O2 (22.8 ± 15.2 mmHg). Proliferation was promoted and apoptosis was reduced when cells were grown in lowered O2, yielding greater numbers of precursors. The differentiation of precursor cells into neurons with specific neurotransmitter phenotypes was also significantly altered. The percentage of neurons of dopaminergic phenotype increased to 56% in lowered O2 compared with 18% in 152 mmHg O2 (Studer et al., 2000). Morrison isolated the neural crest stem cells and tested the growth and differentiation potential of NSCs at 38 mmHg O2.They also found that reduced oxygen levels can also promote the survival, proliferation, and catecholaminergic differentiation of CNS stem cells (Morrison et al., 2000). Storch and his colleagues cultured human mesencephalic neural precursor cells from 9- to 12-week-old fetal brain in low-oxygen (22.8 mmHg) and found long-term proliferation of these cells. Moreover, these human NSCs with low-oxygen culture could also give rise to dopamine (DA) neurons (Storch et al., 2001, 2003). Nobutaka, using the NSCs cultured from the ganglionic eminence of fetal ICR mice on embryonic day 15.5, demonstrated that the highest proliferation and the neuronal differentiation of the NSCs were observed in 15.2 mmHg oxygen, and the switching of the neuronal subtype differentiation from GABA-positive to glutamate-positive neurons was observed in lower oxygen conditions (Horie et al., 2008).
Our studies, with the rat embryonic mesencephalon (E13.5) exposed to different oxygen concentrations (152 mmHg O2, 76 mmHg O2, and 22.8 mmHg O2) for 3 days, showed that hypoxia, especially 76 mmHg O2, promoted the proliferation of NPCs, and hypoxia-inducible factor-1α (HIF-1α) was critical in this process (Zhao et al., 2008). Our researches also demonstrated that NSCs cultured in hypoxia (22.8 mmHg O2) displayed an increase in the percentage of neurons. Especially the percentage of TH-positive neurons differentiated from NSCs in lowered oxygen increased significantly; the DA content in the medium was higher than under normal conditions. HIF-1α is also involved in the regulation of dopaminergic differentiation of NSCs in lowered oxygen (Zhang et al., 2006).
In summary, the moderate low-oxygen (15.2–38 mmHg) concentration was able to promote the proliferation of NSCs from various resources and enhance the differentiation of NSCs into the TH-positive neurons (Figure 1).
Figure 1. Effects of hypoxia on neural stem cells (NSCs) in vitro. In vitro, the moderate low-oxygen tension (15.2–38 mmHg) can promote proliferation of neural progenitor (NPCs) from various resources, such as mesencephalic precursor cells from rat/human embryos and neural crest stem cells (NSCs). In addition, low-oxygen tension (hypoxia) can enhance the differentiation of NSCs to dopaminergic neurons (TH-positive).
In addition, it should keep in mind that the “real” oxygen levels at cells in the cell culture experiments actually depend on many different factors including the environment, the medium, the metabolism rate of cells and so on. In our studies on the oxygen levels in the medium with or without cells in the glove box filled with different levels of oxygen from 20.9 to 0%, we found that the oxygen levels in the medium were actually below that in the environment, but could be changed along with the different oxygen levels in the environment. In addition, the oxygen levels in the medium without cells were lower than that in the medium with cells, which indicated that the metabolism of cells could consume the oxygen in the medium. (e.g., In the oxygen levels of 20.9, 11.6, 5.8, 3.0% environments, the oxygen levels were respectively 18.3, 11.0, 5.8, 2.8% in the medium without cells, and 17.8, 10.45, 5.2, 2.75% in the medium with cells; unpublished results).
Neural stem cells in vivo mainly exist in the SVZ beside the striatum and the DG in the hippocampus of animals. Various stimulations can promote the proliferation and differentiation of NSCs in vivo, including physiological stimulations (e.g., physical exercises and learning) and pathological stimulations (e.g., seizures and stroke; Scharfman et al., 2003; Ohab et al., 2006; Scharfman and Gray, 2007; Shetty and Hattiangady, 2007; Bednarczyk et al., 2009; Clelland et al., 2009). The direct relationship of the oxygen concentration with the NSCs can be reflected by the neurogenesis during stroke and in the hyperbaric and hypobaric oxygen environment. So we focus on the above three situations to state the effect of oxygen concentration on proliferation and differentiation of NSCs.
Gu et al. (2000) first discovered the neurogenesis after stroke by in vivo Bromodeoxyuridine (5-bromo-2′-deoxyuridine, BrdU) incorporation assay, which is commonly used in the detection of proliferating cells in living tissues. They used the photothrombotic ring stroke model to investigate the cell proliferation process in the ischemically challenged region-at-risk after focal cerebral ischemia in the adult rat brain. The BrdU-positive cells (3–6%) were double-labeled with the neuronspecific marker Map-2 at 7 and 100 days after stroke onset in the region-at-risk. They were distributed randomly in cortical layers II–VI. This study suggests that, as a potential pathway for brain repair, new neurons can be generated in the cerebral cortex of adult rats after sublethal focal cerebral ischemia (Gu et al., 2000). John and his colleagues show that stroke induces neurogenesis from a GFAP-expressing progenitor cell in the SVZ and migration of newly born neurons into a unique neurovascular niche in peri-infarct cortex. Within this neurovascular niche, newly born, immature neurons closely associate with the remodeling vasculature. Neurogenesis and angiogenesis are causally linked through vascular production of stromal-derived factor 1 (SDF1) and angiopoietin 1 (Ang1; Ohab et al., 2006). Furthermore, Pär Thored and Ohab found that the vasculature also plays an important role for long-term striatal neurogenesis after stroke (Ohab et al., 2006; Thored et al., 2007). During several months, neuroblasts migrate close to blood vessels through an area exhibiting early vascular remodeling and persistently increased vessel density (Thored et al., 2007). In addition, new neuroblasts are recruited to an area in the peri-infarct cortex, exhibiting endothelial cell proliferation for the first days after cortical stroke (Ohab et al., 2006). All the above studies showed the significant functions of vascular niche in the processes of neurogenesis after stroke, and implied that the higher oxygen tension around the vessels may be a key factor affecting the neurogenesis induced by stroke.
In addition, it has been found that the HBO treatment can result in the proliferation of BrdU-positive cells and alleviate the myelin damage following hypoxic–ischemic brain damage (HIBD) in neonatal rats (Yu et al., 2006), which indicated that HBO therapy stimulated cells to proliferate in hypoxic–ischemic (HI) neonate rats. Xiao-Li and his colleagues have also found that there were remarkable increases in the proliferation of NSCs in the HBO-treated group, 3, 6, 12, and 24 h after HI, as compared with the HIBD group. The HBO-treated group, 3, 6, and 12 h after HI, performed better in the behavioral test and had less neural loss in the hippocampal CA1 region as compared with the HIBD group. The therapeutic window for effective HBO treatment could be delayed up to 12 h after HIBD, while the effect decreased 24 h after HI (Wang et al., 2008). They proposed that HBO treatment promote stem cells to proliferate, which is correlated with Wnt-3 protein (Wang et al., 2007). In addition, it has been found that the NSCs in neonatal HI rats were able to differentiate and migrate after HBO treatment (Yang et al., 2008). It has also been detected that HBO can up-regulate the differentiated ratio of brain-derived NSCs to neurons (Peng et al., 2007).
Studies also showed that the HH could affect the plasticity of neurons and neurogenesis of animals. It was found that there were impairment of spatial memory and a significant decrease in dendritic arborization and spine number along with increased number of damaged neurons after 3 and 7 days of HH (6100 m) exposure, but after 21 days of HH exposure the improvements of memory and structure were noted (Maiti et al., 2008). However, intermittent hypoxia exposure (4 h/day) to neonatal mice at 2000 m for 3 or 4 weeks increased p-CREB, LTP, and synapses of hippocampus, and enhanced mice spatial learning and memory (Zhang et al., 2005). We also made investigative effort to find out whether intermittent hypoxia affects neurogenesis in the adult rat brain by examining the newly divided cells in the SVZ and DG. Our studies showed that the BrdU-labeled cells in the SVZ and DG increased after 3000 and 5000 m (4 h/day for 2 weeks) intermittent hypoxia. The number of BrdU-labeled cells in the SVZ returned to normal level 4 weeks following intermittent hypoxia. However, the BrdU-labeled cells in the DG had a two-fold increase 4 weeks subsequent to intermittent hypoxia. We conclude that intermittent hypoxia facilitates the proliferation of NSCs in situ, and that the newly divided cells in the SVZ and DG react differently to hypoxia (Zhu et al., 2005a).
In summary, the three situations including stroke, HBO, and HH are all able to promote the neurogenesis in vivo. It is interesting to note that the common consequences caused by all the above three situations are the changes of the levels of oxygen concentration in vivo, including up-regulation and down-regulation. So we conclude that it is the changes of oxygen concentration, both including the increase or decrease of oxygen concentration, which induce the promotion of neurogenesis in vivo (Figures 2 and 3).
Figure 2. Effect of stroke on the oxygen niche around NSCs in vivo. During the stroke, the thrombus block the blood and oxygen supply to the pathological region of brain, which cause the ischemia and hypoxia in the core and penumbra. In the penumbra, the angiogenesis and neurogenesis take place. Within this neurovascular niche, newly born, immature neurons closely associate with the remodeling vasculature. The oxygen tension around the remodeling vasculature is much higher than the other ischemic part, which implies that the higher oxygen tension around the vessels may be a key factor affecting the neurogenesis induced by stroke. The figure of circulatory system is referenced from the webpage (http://heart.wehealny.org/heart_tour/about.html).
Figure 3. Effect of hyperbaric/hypobaric oxygen on the oxygen niche around NSCs in vivo. Hyperbaric/hypobaric oxygen enhance/reduce the oxygen levels in pulmonary alveoli through respiratory system., which causes the alteration of the oxygen concentration in arterial blood, and changes the oxygen supply to the various areas of brain, including SVZ and DG. This lead the increase/decrease of oxygen tension in blood capillaries of SVZ and DG, which determines the PO2 around the neural stem cells (NSCs) in the above two regions. The figure of circulatory system is referenced from the webpage (http://heart.wehealny.org/heart_tour/about.html).
Hypoxia exists not only in the brain tissue, such as cortex, striatum, hippocampus, thalamus etc. (Ndubuizu and LaManna, 2007), but also in the developing embryos, which is known to regulate the proliferation and differentiation of NSCs in vitro and in vivo (Zhu et al., 2005b). Many of the cellular responses to hypoxia are mediated through changes in gene expression. The transcription factors primarily responsible for these changes are the HIFs, the biological function of which has been reviewed elsewhere (Keith and Simon, 2007; Panchision, 2009). Recent studies have identified new molecular mechanisms which modify the behaviors and functions of NSCs in lower oxygen levels. Here, we will focus on the Notch1, Bone morphogenetic protein (BMP), and Wnt signaling pathway to understand the regulation of cellular behaviors and functions of NSCs during hypoxic environment.
Notch is a transmembrane receptor for the ligands Delta and Jagged; ligand binding activates the cleavage of Notch and the transport of the notch intracellular domain (NICD) to the nucleus to regulate transcription of target genes (Gordon et al., 2008). Notch signaling acts as a stem cell self-renewal and antineurogenic signal during CNS development (Corbin et al., 2008). Gustafsson et al. (2005) reported that hypoxia blocked the differentiation of myogenic satellite cells, a myogenic cell line (C2C12), and primary NSCs in a Notch-dependent manner.
It was found that hypoxia activates Notch-responsive promoters and increases expression of Notch direct downstream genes. The NICD interacts with HIF-1α, a global regulator of oxygen homeostasis, and HIF-1α is recruited to Notch-responsive promoters upon Notch activation under hypoxic conditions (Gustafsson et al., 2005). Diez et al. (2007) also identified the reduced oxygen levels lead to activation of the Dll4-Notch-Hey2 signaling cascade and subsequent repression of COUP-TFII in endothelial progenitor cells. Cecilia showed that Notch signaling is required to convert the hypoxic stimulus into epithelial–mesenchymal transition (EMT), increased motility, and invasiveness. Inhibition of Notch signaling abrogated hypoxia-induced EMT and invasion. Conversely, activated form of Notch could substitute for hypoxia to induce these processes (Sahlgren et al., 2008).
It has also been found that the activation of HIF-1 by short-term NiCl2 treatments (a condition of chemical hypoxia) dramatically increased APH-1A (a component of γ-secretase complex) mRNA and protein expression, indicative of an increase in γ-secretase activity. The cellular concentration of NICD was also increased after hypoxia treatment (Wang et al., 2006).
In addition, it has been demonstrated that an additional level of complexity in this cross-talk: factor-inhibiting HIF-1 (FIH-1) regulates not only HIF activity, but also the Notch signaling output and, in addition, plays a role in how Notch signaling modulates the hypoxic response (Zheng et al., 2008). They showed that FIH-1 hydroxylates Notch ICD at two residues (N1945 and N2012) that are critical for the function of Notch ICD as a transactivator within cells and during neurogenesis and myogenesis in vivo. FIH-1 negatively regulates Notch activity and accelerates myogenic differentiation (Zheng et al., 2008).
Bone morphogenetic proteins are members of the TGF-β superfamily. These secreted ligands bind to receptor complexes that catalyze the phosphorylation and activation of the canonical SMAD proteins 1, 5, and 8, which complex with Smad4 and translocate to the nucleus to regulate the transcription of target genes (Nohe et al., 2004). BMPs are critical regulators of dorsoventral patterning during development and are well-characterized inducers of CNS stem cell differentiation, astroglial fate, mitotic arrest, and apoptosis. In contrast, the endogenously secreted BMP antagonist, noggin, limits glial differentiation, and redirects normal postnatal NSCs to generate neurons (Panchision, 2009).
Pistollato indicated that lowered oxygen tension repressed BMP signaling and subsequent glial differentiation of CNS precursor cells, while a higher oxygen tension promoted BMP signaling. The underlying mechanism may be caused by the repression of Smads1/5/8, a key step in BMP signal transduction, in low-oxygen tension (Pistollato et al., 2007). It has also been found that the BMP signaling was actively repressed by hypoxia with the high-grade glioma (HGG) precursors which generated endogenous BMP signaling. An acute increase in oxygen tension led to Smad activation within 30 min, even in the absence of exogenous BMP treatment. Furthermore, Pistollato et al. (2009) detected that silencing of HIF-1α led to Smad activation even under hypoxic conditions, indicating that HIF1α was required for BMP repression. Conversely, BMP activation at high oxygen tension led to reciprocal degradation of HIF1α; this BMP-induced degradation was inhibited in low-oxygen.
β-Catenin is the key effector molecule in canonical Wnt signaling pathway. The binding of Wnt proteins to the seven-membrane-spanning frizzled receptors (Frz) the stabilization and accumulation of cytosolic β-catenin. The increased levels of β-catenin in the cytosol result in its nuclear translocation. In the nucleus, β-catenin interacts with members of the lymphoid enhancer binding factor/T cell-specific factor (LEF/TCF) family of transcription factors and activates expression of target genes such as c-myc and cyclins (Willert and Nusse, 1998; Cui et al., 2010).
During the mammalian embryonic development, the Wnt/β-catenin signaling pathway regulates embryonic NSC proliferation and fate determination (Chenn and Walsh, 2002; Hirabayashi et al., 2004). Canonical Wnt/β-catenin signaling pathway also plays a crucial role in neurogenesis in adult mammalian CNS (Lie et al., 2005).
Jolly and his colleagues found that hypoxia activated Wnt/β-catenin signaling in mouse embryonic cells and HIF-1 (HIF-1α/ARNT complex) mediated hypoxia-induced Wnt signaling in embryonic cells. This regulation extended to primary cells, including isolated NSCs, and was not observed in differentiated cells. In vivo, Hif-1α deletion impaired hippocampal Wnt-dependent processes, including NSC proliferation and differentiation. This decline correlated with reduced Wnt/β-catenin signaling in the SGZ (Jolly et al., 2010). Cui has also found that hypoxia could enhance the proliferation of hippocampal NSCs and β-catenin contributed to this action (Cui et al., 2010). Therefore, both of the above studies implied that O2 may have a direct role in stem cell regulation through HIF-1α modulation of Wnt/β-catenin signaling.
In addition, Kaidi et al. (2007) have found that hypoxia was able to block colorectal tumor cell proliferation. Kaidi et al. (2007) reported that hypoxia inhibited the proliferation of colon carcinoma cells in a β-catenin-dependent manner. Hypoxic treatment resulted in increased cell-cycle arrest and down-regulated expression of the Wnt/β-catenin target c-Myc, a potent cell-cycle regulator. Hypoxic inhibition of Wnt/β-catenin signaling was mediated by physical interaction of HIF-1αwith β-catenin, resulting in reduced formation of β-catenin–TCF-4 complexes (Jolly et al., 2009).
Studies reviewed here provide the data of oxygen concentration in cerebral tissue during embryonic and adult neurogenesis, and the cerebral oxygen level changes during cerebral disease and in hypobaric/HBO. The role of oxygen in the behaviors of NSCs is also reviewed through the in vivo and in vitro experimental evidences (Tables 1 and 2).
Table 1. Oxygen levels in brain during physiological/phathological state and specific environment in vivo.
The significant function of oxygen in the differentiation and proliferation of NSCs raises the possibilities of amplifying NSCs in vitro for stem cell treatments by providing hypoxic niche around the cells. In addition, it is also possible to induce the neurogenesis in vivo by the modification of the oxygen environment around the body, which provides us a novel idea to promote the ability of learning and memory because of the involvement of neurogenesis in the process of cognition (Kempermann and Gage, 2002), and reminds us a new method for treating some mental illness because of the links between adult neurogenesis and mental disorders, such as Alzheimer’s Disease and schizophrenia (DeCarolis and Eisch, 2010).
Because of the heavy oxygen consumption of brain and the low-oxygen level inside cerebral tissue, maintaining the narrow ranges of tissue PO2 may be beneficial to normal brain function. Higher or lower levels of tissue PO2 may affect normal chemical production, possibly leading to brain cell damage. However, the physiological mechanism of oxygen sensing and control remains largely unknown (Masamoto and Tanishita, 2009). Novel knowledge and innovative techniques are expected to allow formation of a complete and dynamic picture of oxygen transport and metabolism during normal and pathological brain activities.
The authors declare that the research was conducted in the absence of any commercial or financial relationships that could be construed as a potential conflict of interest.
This work was supported by a grant from the National Basic Research Program of China, No. 2006CB504100; The Natural Science Foundation of China, No. 308311605 and 90919052.
Altman, J. (1969). Autoradiographic and histological studies of postnatal neurogenesis. IV. Cell proliferation and migration in the anterior forebrain, with special reference to persisting neurogenesis in the olfactory bulb. J. Comp. Neurol. 137, 433–457.
Altman, J., and Das, G. D. (1965). Autoradiographic and histological evidence of post-natal hippocampal neurogenesis in rats. J. Comp. Neurol. 124, 319–335.
Altman, J., and Das, G. D. (1967). Post-natal neurogenesis in the guinea-pig. Nature 214, 1098–1101.
Bader, M. K. (2006). Recognizing and treating ischemic insults to the brain: the role of brain tissue oxygen monitoring. Crit. Care Nurs. Clin. North Am. 18, 243–256.
Balenane, J. D. (1982). “Principles of hyperoxic pathophysiology,” in Pathology of Oxygen Toxicity, ed. J. D. Balentine (New York: Academic Press), 59.
Bednarczyk, M. R., Aumont, A., Décary, S., Bergeron, R., and Fernandes, K. J. (2009). Prolonged voluntary wheel-running stimulates neural precursors in the hippocampus and forebrain of adult CD1 mice. Hippocampus 19, 913–927.
Boero, J. A., Ascher, J., Arregui, A., Rovainen, C., and Woolsey, T. A. (1999). Increased brain capillaries in chronic hypoxia. J. Appl. Physiol. 86, 1211–1219.
Brugniaux, J. V., Hodges, A. N., Hanly, P. J., and Poulin, M. J. (2007). Cerebrovascular responses to altitude. Respir. Physiol. Neurobiol. 158, 212–223.
Calvert, J. W., Cahill, J., and Zhang, J. H. (2007). Hyperbaric oxygen and cerebral physiology. Neurol. Res. 29, 132–141.
Chen, E. Y., Fujinaga, M., and Giaccia, A. J. (1999). Hypoxic microenvironment within an embryo induces apoptosis and is essential for proper morphological development. Teratology 60, 215–225.
Chenn, A., and Walsh, C. A. (2002). Regulation of cerebral cortical size by control of cell cycle exit in neural precursors. Science 297, 365–369.
Clarke, L., and Kooy, D. (2009). Low oxygen enhances primitive, and definitive neural stem cell colony formation by inhibiting distinct cell death pathways. Stem Cells 27, 1879–1886.
Clelland, C. D., Choi, M., Romberg, C., Clemenson, J. G. D., Fragniere, A., Tyers, P., Jessberger, S., Saksida, L. M., Barker, R. A., Gage, F. H., and Bussey, T. J. (2009). A functional role for adult hippocampal neurogenesis in spatial pattern separation. Science 325, 210–213.
Corbin, J. G., Gaiano, N., Juliano, S. L., Poluch, S., Stancik, E., and Haydar, T. F. (2008). Regulation of neural progenitor cell development in the nervous system. J. Neurochem. 106, 2272–2287.
Cui, X. P., Xing, Y., Chen, J. M., Dong, S. W., Ying, D. J., and Yew, D. T. (2010). Wnt/beta-catenin is involved in the proliferation of hippocampal neural stem cells induced by hypoxia. Ir. J. Med. Sci. doi: 10.1007/s11845-010-0566-3. [Epub ahead of print].
DeCarolis, N. A., and Eisch, A. J. (2010). Hippocampal neurogenesis as a target for the treatment of mental illness: a critical evaluation. Neuropharmacology 58, 884–893.
Diez, H., Fischer, A., Winkler, A., Hu, C. J., Hatzopoulos, A. K., Breier, G., and Gessler, M. (2007). Hypoxia-mediated activation of Dll4-Notch-Hey2 signaling in endothelial progenitor cells and adoption of arterial cell fate. Exp. Cell Res. 313, 1–9.
Doppenberg, E. M., and Zauner, A. (1998). Correlations between brain tissue oxygen tension, carbon dioxide tension, pH, and cerebral blood flow – a better way of monitoring the severely injured brain? Surg. Neurol. 49, 650–654.
Dunn, J. F., Grinberg, O., Roche, M., Nwaigwe, C. L., Hou, H. G., and Swartz, H. M. (2000). Noninvasive assessment of cerebral oxygenation during acclimation to hypobaric hypoxia. J. Cereb. Blood Flow Metab. 20, 1632–1635.
Eskes, T., Jongsma, H. W., and Houx, P. C. W. (1983). Percentiles for gas values in human umbilical cord blood. Eur. J. Obstet. Gynecol. Reprod. Biol. 14, 341–346.
Fischer, B., and Bavister, B. D. (1993). Oxygen tension in the oviduct and uterus of rhesus monkeys, hamsters and rabbits. J. Reprod. Fertil. 99, 673–679.
Fischer, B. R., Palkovic, S., Holling, M., Wolfer, J., and Wassmann, H. (2010). Rationale of hyperbaric oxygenation in cerebral vascular insult. Curr. Vasc. Pharmacol. 8, 35–43.
Flynn, E. P., and Auer, R. N. (2002). Eubaric hyperoxemia and experimental cerebral infarction. Ann. Neurol. 52, 566–572.
Gordon, W. R., Arnett, K. L., and Blacklow, S. C. (2008). The molecular logic of Notch signaling – a structural and biochemical perspective. J. Cell Sci. 121, 3109–3119.
Gu, W., Brännström, T., and Wester, P. (2000). Cortical neurogenesis in adult rats after reversible photothrombotic stroke. J. Cereb. Blood Flow Metab. 20, 1166–1173.
Günther, A., Küppers, T. L., Schneider, P. M., Kunert, I., Berrouschot, J., Schneider, D., and Rossner, S. (2005). Reduced infarct volume and differential effects on glial cell activation after hyperbaric oxygen treatment in rat permanent focal cerebral ischemia. Eur. J. Neurosci. 21, 3189–3194.
Gustafsson, M. V., Zheng, X., Pereira, T., Gradin, K., Jin, S., Lundkvist, J., Ruas, J. L., Poellinger, L., Lendahl, U., and Bondesson, M. (2005). Hypoxia requires Notch signaling to maintain the undifferentiated cell state. Dev. Cell 9, 617–628.
Henninger, N., Bouley, J., Nelligan, J. M., Sicard, K. M., and Fisher, M. (2007). Normobaric hyperoxia delays perfusion/diffusion mismatch evolution, reduces infarct volume, and differentially affects neuronal cell death pathways after suture middle cerebral artery occlusion in rats. J. Cereb. Blood Flow Metab. 27, 1632–1642.
Hirabayashi, Y., Itoh, Y., and Tabata, H. (2004). The Wnt/betacatenin pathway directs neuronal differentiation of cortical neural precursor cells. Development 13, 2791–2801.
Hlatky, R., Valadka, A., and Robertson, C. (2003). Intracranial hypertension and cerebral ischemia after severe traumatic brain injury. Neurosurg. Focus 14, 1–4.
Horie, N., So, K., Moriya, T., Kitagawa, N., Tsutsumi, K., Nagata, I., and Shinohara, K. (2008). Effects of oxygen concentration on the proliferation and differentiation of mouse neural stem cells in vitro. Cell. Mol. Neurobiol. 28, 833–845.
Ivanovic, Z. (2009). Hypoxia or in situ normoxia: the stem cell paradigm. J. Cell. Physiol. 219, 271–275.
Jauniaux, E., Kiserud, T., Ozturk, O., West, D., and Hanson, M. A. (2000). Amniotic gas exchange and acid – base status during acute maternal hyperoxemia and hypoxemia in the early fetal sheep. Am. J. Obstet. Gynecol. 182, 661–665.
Jolly, M., Vijay, D., and Celeste, S. (2009). Hypoxia-inducible factors in stem cells and cancer. J. Cell. Mol. Med. 13, 4319–4328.
Jolly, M. W., Timothy, O. B., Randall, S. J., Joseph, C., LaManna, J. C., Chavez, P. S. K., and Simon, M. C. (2010). O2 regulates stem cells through Wnt/(-catenin signaling. Nat. Cell Biol. 12, 1007–1013.
Kaidi, A., Williams, A. C., and Paraskeva, C. (2007). Interaction between beta-catenin and HIF-1 promotes cellular adaptation to hypoxia. Nat. Cell Biol. 9, 210–217.
Kaufman, D. L., and Mitchell, J. A. (1990). “Alterations in intrauterine oxygen tension during the estrous cycle in the rat and hamster and its regulation by ovarian steroid hormones: a comparative study,” in Oxygen Transport to Tissue, eds J. Piiper, T. K. Goldstick, and M. Meye (New York: Plenum Press), 745–750.
Keith, B., and Simon, M. C. (2007). Hypoxia-inducible factors, stem cells, and cancer. Cell 129, 465–472.
Kempermann, G., and Gage, F. H. (2002). Genetic determinants of adult hippocampal neurogenesis correlate with acquisition, but not probe trial performance, in the water maze task. Eur. J. Neurosci. 16, 129–136.
Kim, H. Y., and Singhal, A. B. (2005). Normobaric hyperoxia extends the reperfusion window in focal cerebral ischemia. Ann. Neurol. 57, 571–575.
Lee, Y. M., Jeong, C. H., Koo, S. Y., Son, M. J., Song, H. S., Bae, S. K., Raleigh, J. A., Chung, H. Y., Yoo, M. A., and Kim, K. W. (2001). Determination of hypoxic region by hypoxia marker in developing mouse embryos in vivo: a possible signal for vessel development. Dev. Dyn. 220, 175–186.
Lie, D. C., Colamarino, S. A., and Song, H. J. (2005). Wnt signalling regulates adult hippocampal neurogenesis. Nature 437, 1370–1375.
Liu, S., Liu, W., Ding, W., Miyake, M., Rosenberg, G. A., and Liu, K. J. (2006). Electron paramagnetic resonance-guided normobaric hyperoxia treatment protects the brain by maintaining penumbral oxygenation in a rat model of transient focal cerebral ischemia. J. Cereb. Blood Flow Metab. 26, 1274–1284.
Liu, S., Shi, H., Liu, W., Takamitsu, F., Graham, S. T., and Liu, K. J. (2004). Interstitial pO2 in ischemic penumbra and core are differentially affected following transient focal cerebral ischemia in rats. J. Cereb. Blood Flow Metab. 24, 343–349.
Lord, E. M., Harwell, L., and Koch, C. J. (1993). Detection of hypoxic cells by monoclonal antibody recognizing 2-nitroimidazole adducts. Cancer Res. 53, 5721–5726.
Lou, M., Eschenfelder, C. C., and Herdegen, T. (2004). Therapeutic window for use of hyperbaric oxygenation in focal transient ischemia in rats. Stroke 35, 578–583.
Maas, A. I., and Fleckenstein, W. (1993). Monitoring cerebral oxygenation: experimental studies and preliminary clinical results of continuous monitoring of cerebrospinal fluid and brain tissue oxygen tension. Acta Neurochir. Suppl. (Wien) 59, 50–57.
Maas, D. H. A., Storey, B. T., and Mastroianni, L. (1976). Oxygen tension in the oviduct of the rhesus monkey. Fertil. Steril. 27, 1312–1317.
Maiti, P., Muthuraju, S., Ilavazhagan, G., and Singh, S. B. (2008). Hypobaric hypoxia induces dendritic plasticity in cortical and hippocampal pyramidal neurons in rat brain. Behav. Brain Res. 189, 233–243.
Masamoto, K., and Tanishita, K. (2009). Oxygen transport in brain tissue. J. Biomech. Eng. 131, 074002.
Mastroianni, L. J., and Jones, R. (1965). Oxygen tension within the rabbit fallopian tube. J. Reprod. Fertil. 9, 99–102.
Mito, T., Konomi, H., and Houdou, S. (1991). Immunohistochemical study of the vasculature in the developing brain. Pediatr. Neurol. 7, 18–22.
Miyamoto, O., and Auer, R. N. (2000). Hypoxia, hyperoxia, ischemia, and brain necrosis. Neurology 54, 362–371.
Miyawaki, T., Matsui, K., and Takashima, S. (1998). Developmental characteristics of vessel density in the human fetal and infant brains. Early Hum. Dev. 53, 65–72.
Morrison, S. J., Csete, M., Groves, A., Melega, W., Wold, B., and Anderson, D. (2000). Culture in reduced levels of oxygen promotes clonogenic sympathoadrenal differentiation by isolated neural crest stem cells. J. Neurosci. 19, 7370–7376.
Moyse, E., Segura, S., Liard, O., Mahaut, S., and Mechawar, N. (2008). Microenvironmental determinants of adult neural stem cell proliferation and lineage commitment in the healthy and injured central nervous system. Curr. Stem Cell Res. Ther. 3, 163–184.
Ndubuizu, O., and LaManna, J. C. (2007). Brain tissue oxygen concentration measurements. Antioxid. Redox Signal. 9, 1207–1219.
Nemoto, E. M., and Betterman, K. (2007). Basic physiology of hyperbaric oxygen in brain. Neurol. Res. 29, 116–126.
Nohe, A., Keating, E., Knaus, P., and Petersen, N. O. (2004). Signal transduction of bone morphogenetic protein receptors. Cell. Signal. 16, 291–299.
Nunn, J. F. (1969). Applied Respiratory Physiology: With Special Reference to Anaesthesia. London: Butterworths.
Ohab, J. J., Fleming, S., Blesch, A., and Carmichael, S. T. (2006). A neurovascular niche for neurogenesis after stroke. J. Neurosci. 26, 13007–13016.
Panchision, D. M. (2009). The role of oxygen in regulating eural stem cells in evelopment and disease. J. Cell. Physiol. 220, 562–568.
Peng, Z. R., Wang, S. E., and Xiao, P. T. (2007). Effect of hyperbaric oxygen on the differentiation of brain-derived neural stem cells. J. Clin. Rehabil. Tissue Eng. Res. 11, 427–430.
Pistollato, F., Chen, H. L., Rood, B. R., Zhang, H. Z., Avella, D., Denaro, L., Gardiman, M., Te Kronnie, G., Schwartz, P. H., Favaro, E., Indraccolo, S., Basso, G., and Panchision, D. M. (2009). Hypoxia and HIF1alpha repress the differentiative effects of BMPs in high grade glioma. Stem Cells 27, 7–17.
Pistollato, F., Chen, H. L., Schwartz, P. H., Basso, G., and Panchision, D. M. (2007). Oxygen tension controls the expansion of human CNS precursors and the generation of astrocytes and oligodendrocytes. Mol. Cell. Neurosci. 35, 424–435.
Powell, F. L., Milsom,W. K., and Mitchell, G. S. (1998). Time domains of the hypoxic ventilatory response. Respir. Physiol. 112, 123–134.
Reynolds, B. A., and Weiss, S. (1992). Generation of neurons and astrocytes from isolated cells of the adult mammalian central nervous system. Science 255, 1707–1710.
Rurak, D., Selke, P., Fisher, M., Taylor, A. H. T., and Whitmann, B. (1987). Fetal oxygen extraction: comparison of the human and sheep. Am. J. Obstet. Gynecol. 156, 360–366.
Sahlgren, C., and Gustafsson, M. V., Jin, S., Poellinger, L., and Lendahl, U. (2008). Notch signaling mediates hypoxia-induced tumor cell migration and invasion. Proc. Natl. Acad. Sci. U.S.A. 105, 6392–6397.
Sarrafzadeh, A. S., Kiening, T., and Bardt, T. (1998). Cerebral oxygenation in contusioned vs. nonlesioned brain tissue: monitoring of PtiO2 with Licox and Paratrend. Acta Neurochir. Suppl. 71, 186–189.
Scharfman, H. E., and Gray, W. P. (2007). Relevance of seizure-induced neurogenesis in animal models of epilepsy to the etiology of temporal lobe epilepsy. Epilepsia 48(Suppl. 2), 33–41.
Scharfman, H. E., Sollas, A. E., Berger, R. E., Goodman, J. H., and Pierce, J. P. (2003). Perforant path activation of ectopic granule cells that are born after pilocarpine-induced seizures. Neuroscience 121, 1017–1029.
Shen, Q., Wang, Y., Kokovay, E., Lin, G., Chuang, S. M., Goderie, S. K., Roysam, B., and Temple, S. (2008). Adult SVZ stem cells lie in a vascular niche: a quantitative analysis of niche cell-cell interactions. Cell Stem Cell 3, 289–300.
Shetty, A. K., and Hattiangady, B. (2007). Prospects of stem cell therapy for temporal lobe epilepsy. Stem Cells 25, 2396–2407.
Singhal, A. B., Dijkhuizen, R. M., Rosen, B. R., and Lo, E. H. (2002a). Normobaric hyperoxia reduces MRI diffusion abnormalities and infarct size in experimental stroke. Neurology 58, 945–952.
Singhal, A. B., Wang, X., Sumii, T., Mori, T., and Lo, E. H. (2002b). Effects of normobaric hyperoxia in a rat model of focal cerebral ischemia-reperfusion. J. Cereb. Blood Flow Metab. 22, 861–868.
Stantilli, G., Lamorte, G., Carlessi, L., Ferrari, D., Nodari, L. R., Binda, E., Delia, D., Vescovi, A. L., and Filippis, L. D. (2010). Mild hypoxia enhances proliferation and multipotency of human neural stem cells. PLoS ONE 5, e8575. doi: 10.1371/journal.pone.0008575
Storch, A., Lester, H. A., Boehm, B. O., and Schwarz, J. (2003). Functional characterization of dopaminergic neurons derived from rodent mesencephalic progenitor cells. J. Chem. Neuroanat. 26, 133–142.
Storch, A., Paul, G., and Csete, M. (2001). Longterm proliferation and dopaminergic differentiation of human mesencephalic neural precursor cells. Exp. Neurol. 170, 317–325.
Studer, L., Csete, M., SangHun, L., Kabbani, N., Walikonis, J., Wold, B., and McKay, R. (2000). Enhanced proliferation, survival, and dopaminergic differentiation of CNS precursors in lowered oxygen. J. Neurosci. 20, 7377–7383.
Suh, H., Deng, W., and Gage, F. H. (2009). Signaling in adult neurogenesis. Annu. Rev. Cell Dev. Biol. 25, 53–75.
Takashima, S., and Tanaka, K. (1978). Development of cerebrovascular architecture and its relationship to periventricular leukomalacia. Arch. Neurol. 25, 3–11.
Tavazoie, M., Van, V. L., Silva, V. V., Louissaint, M., Colonna, L., Zaidi, B., Garcia-Verdugo, J. M., and Doetsch, F. (2008). A specialized vascular niche for adult neural stem cells. Cell Stem Cell 3, 279–288.
Thored, P., Wood, J., Arvidsson, A., Cammenga, J., Kokaia, Z., and Lindvall, O. (2007). Long-term neuroblast migration along blood vessels in an area with transient angiogenesis and increased vascularization after stroke. Stroke 38, 3032–3039.
Torbati, D., Parolla, D., and Lavy, S. (1976). Changes in the electrical activity and PO2 of the rat’s brain under high oxygen pressure. Exp. Neurol. 50, 439–447.
Torbati, D., Parolla, D., and Lavy, S. (1977). Changes in local brain tissue PO2 and electrocortical activity of unanesthetized rabbits under high oxygen pressure. Aviat. Space Environ. Med. 48, 347–350.
Valadka, A., Gopinath, S., and Contant, C. (1998). Relationship of brain tissue oxygen to outcome after severe head injury. Crit. Care Med. 26, 1576–1581.
van den Brink, W., Santbrink, H., and Steyerberg, E. (2000). Brain oxygen tension in severe head injury. Neurosurgery 46, 868–878.
Van der Borght, K., Kóbor, D. E., Klauke, K., Eggen, B. J., Nyakas, C., Van, Z. E. A., and Meerlo, P. (2009). Physical exercise leads to rapid adaptations in hippocampal vasculature: temporal dynamics and relationship to cell proliferation and neurogenesis. Hippocampus 19, 928–936.
Wang, R., Zhang, Y. W., Zhang, X., Liu, R., Zhang, X., Hong, S., Xia, K., Xia, J., Zhang, Z., and Xu, H. (2006). Transcriptional regulation of APH-1A and increased gamma-secretase cleavage of APP and Notch by HIF-1 and hypoxia. FASEB J. 20, 1275–1277.
Wang, X. L., Yang, Y. J., Xie, M., Yu, X. H., Liu, C. T., and Wang, X. (2007). Proliferation of neural stem cells correlates with Wnt-3 protein in hypoxic-ischemic neonate rats after hyperbaric oxygen therapy. Regen. Transplant. 18, 1753–1756.
Wang, X. L., Zhao, Y. S., Yang, Y. J., Xie, M., and Yu, X. H. (2008). Therapeutic window of hyperbaric oxygen therapy for hypoxic–ischemic brain damage in newborn rats. Brain Res. 1222, 87–94.
West, J. B. (1996). T H Ravenhill and his contributions to mountain sickness. J. Appl. Physiol. 80, 715–724.
West, J. B., Schoene, R. B., and Milledge, J. S. (2007). High Altitude Medicine and Physiology, 4th Edn. London: Hodder Arnold.
Willert, K., and Nusse, R. (1998). Beta-catenin: a key mediator of Wnt signaling. Curr. Opin. Genet. Dev. 8, 95–102.
Wilson, M. H., Newman, S., and Imray, C. H. (2009). The cerebral eff ects of ascent to high altitudes. Lancet Neurol. 8, 175–191.
Wolff, C. B. (2000). Cerebral blood flow and oxygen delivery at high altitude. High Alt. Med. Biol. 1, 33–38.
Wolff, C. B., Barry, P., and Collier, D. J. (2002). Cardiovascular and respiratory adjustments at altitude sustain cerebral oxygen delivery – Severinghaus revisited. Comp. Biochem. Physiol. A Mol. Integr. Physiol. 132, 221–229.
Yang, Y. J., Wang, X. L., Yu, X. H., Wang, X., Xie, M., and Liu, C. T. (2008). Hyperbaric oxygen induces endogenous neural stem cells to proliferate and differentiate in hypoxic-ischemic brain damage in neonatal rats. Undersea Hyperb. Med. 35, 113–129.
Yeomans, E. R., Hauth, J. C., Gilstrap, L. C., and Strickland, D. M. (1985). Umbilical cord pH, PCO2 and bicarbonate following uncomplicated term vaginal deliveries. Am. J. Obstet. Gynecol. 151, 798–800.
Yu, X. H., Yang, Y. J., Wang, X., Wang, Q. H., Xie, M., Qi, B. X., Liu, C. T., Wang, X. L., Jia, Y. J., and Zhong, L. (2006). Effect of hyperbaric oxygenation on neural stem cells and myelin in neonatal rats with hypoxic–ischemic brain damage. Chin. J. Contemp. Pediatrics 8, 33–37.
Zauner, A., Daugherty, W. P., Bullock, M. R., and Warner, D. S. (2002). Brain oxygenation and energy metabolism: part I – biological function and pathophysiology. Neurosurgery 51, 289–302.
Zauner, A., Doppenberg, E., and Young, H. (1996). Brain oxygen monitoring predicts outcome after severe head injury. J. Neurotrauma 13, 619.
Zhang, C. P., Zhu, L. L., Zhao, T., Zhao, H. Q., Huang, X., Ma, X., Wu, H. T., and Fan, M. (2006). Characteristics of neural stem cells expanded in lowered oxygen and the potential role of hypoxia-inducible factor-1alpha. Neurosignals 15, 259–265.
Zhang, J. X., Chen, X. C., Du, J. Z., Chen, Q. M., and Zhu, C. Y. (2005). Neonatal exposure to intermittent hypoxia enhances mice performance in water maze and 8-arm radial maze tasks. J. Neurobiol. 65, 72–84.
Zhang, K., Zhu, L. L., Zhao, T., Huang, X., Wu, L. Y., Wu, K. W., and Fan. M. (2010). Spatiotemporal heterogeneity of oxygen distribution in various cerebral regions detected by optical fiber luminescent oxygen sensors. Acta Physiol. Sin. 62(Suppl. 1), 59–60.
Zhao, T., Zhang, C. P., Liu, Z. H., Wu, L. Y., Huang, X., Wu, H. T., Xiong, L., Wang, X., Wang, X. M., Zhu, L. L., and Fan, M. (2008). Hypoxia-driven proliferation of embryonic neural stem/progenitor cells – role of hypoxia-inducible transcription factor-1a. FEBS J. 275, 1824–1834.
Zheng, X., Linke, S., Dias, J. M., Zheng, X., Gradin, K., Wallis, T. P., Hamilton, B. R., Gustafsson, M., Ruas, J. L., Wilkins, S., Bilton, R. L., Brismar, K., Whitelaw, M. L., Pereira, T., Gorman, J. J., Ericson, J., Peet, D. J., Lendahl, U., and Poellinger, L. (2008). Interaction with factor inhibiting HIF-1 defines an additional mode of cross-coupling between the Notch and hypoxia signaling pathways. Proc. Natl. Acad. Sci. U.S.A. 105, 3368–3373.
Zhu, L. L., Zhao, T., Li, H. S., Zhao, H. Q., Wu, L. Y., Ding, A. S., Fan, W. H., and Fan, M. (2005a). Neurogenesis in the adult rat brain after intermittent hypoxia. Brain Res. 1055, 1–6.
Keywords: oxygen, neurogenesis, cerebral diseases, hypobaric hypoxia, hyperbaric oxygen
Citation: Zhang K, Zhu L and Fan M (2011) Oxygen, a key factor regulating cell behavior during neurogenesis and cerebral diseases. Front. Mol. Neurosci. 4:5. doi: 10.3389/fnmol.2011.00005
Received: 26 January 2011;
Accepted: 23 March 2011;
Published online: 04 April 2011.
Edited by:
Michael Fähling, Charite University Medicine Berlin, GermanyReviewed by:
Michael Fähling, Charite University Medicine Berlin, GermanyCopyright: © 2011 Zhang, Zhu and Fan. This is an open-access article subject to a non-exclusive license between the authors and Frontiers Media SA, which permits use, distribution and reproduction in other forums, provided the original authors and source are credited and other Frontiers conditions are complied with.
*Correspondence: Lingling Zhu, Department of Brain Protection and Plasticity, Institute of Basic Medical Sciences, No. 27 Taiping Road, Beijing 100850, China.e-mail:bGluZ2xpbmd6aHVAaG90bWFpbC5jb20=
Disclaimer: All claims expressed in this article are solely those of the authors and do not necessarily represent those of their affiliated organizations, or those of the publisher, the editors and the reviewers. Any product that may be evaluated in this article or claim that may be made by its manufacturer is not guaranteed or endorsed by the publisher.
Research integrity at Frontiers
Learn more about the work of our research integrity team to safeguard the quality of each article we publish.