- 1Medical Scientist Training Program, University of Colorado Anschutz Medical Campus, Aurora, CO, United States
- 2Neuroscience Graduate Program, University of Colorado Anschutz Medical Campus, Aurora, CO, United States
- 3Department of Pharmaceutical Sciences, University of Colorado Anschutz Medical Campus, Aurora, CO, United States
- 4Department of Cell and Developmental Biology, University of Colorado Anschutz Medical Campus, Aurora, CO, United States
- 5Brain Institute, Nathan Kline Institute, Orangeburg, NY, United States
- 6Child and Adolescent Psychiatry, Child Study Center, New York University School of Medicine, New York, NY, United States
- 7Department of Pediatrics, University of Colorado Anschutz Medical Campus, Aurora, CO, United States
Fragile X syndrome (FXS) is a neurodevelopmental disorder caused by a repeat expansion mutation in the promotor region of the FMR1 gene resulting in transcriptional silencing and loss of function of fragile X messenger ribonucleoprotein 1 protein (FMRP). FMRP has a well-defined role in the early development of the brain. Thus, loss of the FMRP has well-known consequences for normal cellular and synaptic development leading to a variety of neuropsychiatric disorders including an increased prevalence of amygdala-based disorders. Despite our detailed understanding of the pathophysiology of FXS, the precise cellular and circuit-level underpinnings of amygdala-based disorders is incompletely understood. In this review, we discuss the development of the amygdala, the role of neuromodulation in the critical period plasticity, and recent advances in our understanding of how synaptic and circuit-level changes in the basolateral amygdala contribute to the behavioral manifestations seen in FXS.
Introduction
Fragile X syndrome (FXS) is a rare X-linked recessive genetic disorder affecting approximately 1:5,000 males and 1:4,000–8,000 females (Hagerman et al., 2017). The physical phenotype of individuals with FXS is characterized by large ears, long narrow faces, hyperextensible joints, and macroorchidism (Kidd et al., 2014). The clinical features of FXS include a variety of neurologic and psychiatric disorders including intellectual disability, attention deficit hyperactivity disorder (ADHD), anxiety, social avoidance, increased incidence of seizures and epilepsy, autism spectrum disorders (ASDs), and hypersensitivity to sensory stimuli (Hagerman et al., 2009).
Fragile X syndrome is caused by a CGG repeat expansion in the 5′ untranslated region (UTR) of the fragile X messenger ribonucleoprotein 1 (FMR1) gene located at Xq27.3. Individuals with trinucleotide repeats numbering < 50 are phenotypically normal and without clinical symptomatology. Carriers of the premutation repeat expansion (numbering between 55 and 200) exhibit the distinct fragile X associated-tremor ataxia syndrome (FXTAS). FXTAS is an adult-onset neurodegenerative disorder characterized by intention tremor, parkinsonism, and generalized brain atrophy (Bale et al., 2010). The pathophysiology of FXTAS has been shown to result in part from excessive production of CGG repeat expanded FMR1 messenger RNA (mRNA) (Bale et al., 2010). This excessive mRNA production has been shown to be neurotoxic in vitro and in vivo (Jin et al., 2003; Hukema et al., 2014; Swinnen et al., 2020) resulting in ubiquitin and FMRpolyG-positive intranuclear inclusions and subsequent cerebellar Purkinje cell loss (Buijsen et al., 2014). Trinucleotide repeat expansions (numbering > 200) result in hypermethylation of the promoter region of the FMR1 locus and subsequent transcriptional silencing of the FMR1 gene (Fu et al., 1991). Functionally, and in contrast to FXTAS, this results in the absence of the fragile X messenger ribonucleoprotein 1 protein (FMRP) (Verkerk et al., 1991). The clinical severity of FXS depends in large part on the number of trinucleotide repeat expansions in the 5′ UTR of the FMR1 gene and on cell-autonomous FMRP production (Hagerman et al., 2017).
Since the identification of the molecular pathophysiology underpinning FXS (Fu et al., 1991; Verkerk et al., 1991), subsequent investigations have elucidated the functional role of the FMRP. The FMRP is an RNA binding protein with a known role in regulating mRNA translation (Chen et al., 2003; Darnell et al., 2011) and is ubiquitously expressed throughout the brain and present in all neuronal cell compartments (Christie et al., 2009). FMRP binds directly to translational machinery and induces ribosomal stalling with suppression of protein synthesis (Darnell et al., 2011). However, the regulatory role of FMRP in protein synthesis is complex and varied. FMRP regulates many target genes involved in neuronal synapse formation and maintenance and plasticity responses (Casingal et al., 2020). Loss of FMRP results in the dysregulated synthesis of proteins essential for normal corticogenesis, cellular and synaptic function, and synaptic plasticity (Sidorov et al., 2013; Sears and Broadie, 2017). In addition to its role in translation suppression, new evidence is emerging that FMRP may enhance, rather than repress, protein translation and that loss of FMRP induces the down-regulation of target genes implicated in ASDs and neurodevelopmental disorders (NDDs) (Greenblatt and Spradling, 2018). Thus, FXS may share pathology common to many different forms of ASDs. Indeed, the Fmr1KO mouse model of FXS not only recapitulates many aspects of the human condition but has been a key tool for understanding ASDs more broadly.
FMRP-associated cellular, synaptic, and circuit dysfunction in FXS
At the cellular level, loss of FMRP affects ion channel function with profound effects on membrane properties and neuronal intrinsic excitability beyond mRNA translational modulation (Contractor et al., 2015). Importantly, this non-canonical function of FMRP appears to involve modulation of ion channels via direct FMRP binding and modulation of channel kinetics (Kshatri et al., 2020). For example, FMRP has been shown to regulate the function of numerous potassium channels [including Kv3.1 (Darnell et al., 2011), Kv4.2 (Gross et al., 2011), HCN (Brager et al., 2012; Zhang et al., 2014; Brandalise et al., 2020), and BK (Torres et al., 2007; Deng et al., 2013), Slack (Brown et al., 2010)] and calcium channels [Cav2.2 (Ferron et al., 2014)] (Ferron, 2016). FMRP-mediated potassium channel dysfunction has been shown to underpin membrane hyperexcitability and increased UP states in layer 4 somatosensory barrel cortex principal neurons (PNs) (Gibson et al., 2008). Further, alterations in BK and h- channel function has been shown to contribute to sensory hypersensitivity by altering a number of membrane properties including resting membrane potential and dendritic excitability in S1 excitatory PNs in the period immediately following the somatosensory critical period (CP). Broadly this results in increased action potential (AP) output and integration of synaptic input (Zhang et al., 2014). Loss of FMRP also results in abnormal Slack function and decreases in late after-hyperpolarization which enables repetitive firing in PNs in Fmr1KO mice.
At the level of the synapse, FMRP-driven dysfunction in Cav2.2 leads to alterations in presynaptic neurotransmitter release in dorsal root ganglion (DRG) neurons (Ferron et al., 2014). FMRP is upregulated by group 1 (Gp1) metabotropic glutamate receptors (mGluRs) following post-synaptic activation (Weiler et al., 1997). In particular, loss of FMRP leads to increased expression of mGluRs as well as increases in α-amino-3-hydroxy-5-methyl-4-isoxazolepropionic acid (AMPA) receptor turnover (Bear et al., 2004). This AMPA receptor turnover has been shown to mediate mGluR-mediated long-term depression (LTD) in hippocampal neurons following post-synaptic stimulation of excitatory principal neurons (PNs) in older animals (Weiler and Greenough, 1993; Weiler et al., 1997). Altered long-term potentiation (LTP) and LTD have also been observed in the cingulate cortex, visual cortex, and the amygdala (Bhakar et al., 2012). As with ion channel dysfunction, plasticity alterations seen in FXS may be region and developmental stage-specific. For example, in contrast to later life mGluR-LTD in the hippocampus, early life enhancements in synaptic plasticity have been observed in hippocampal neurons of Fmr1KO mice (Gray et al., 2019). This effect may be in part mediated by increased mGluR-mediated expression of R-voltage dependent calcium channel (R-VDCC)-mediated Ca2+ spikes (Gray et al., 2019). Thus, numerous FMRP-mediated channelopathies contribute not only to altered intrinsic membrane properties and hyperexcitability but synaptic and circuit-level dysfunction as well.
In addition to cellular, circuit, and plasticity alterations in excitatory PNs in FXS, loss of FMRP also has a known role in interneuron (IN) dysfunction as well (Paluszkiewicz et al., 2011b; Cea-Del Rio and Huntsman, 2014; Martin et al., 2014). FMRP is widely expressed in inhibitory INs (Olmos-Serrano et al., 2010) and many functional elements of γ-aminobutyric acid (GABA) neurotransmission are dysregulated in the context of loss of FMRP (Paluszkiewicz et al., 2011a). As early as P5, GABA concentrations are altered in the frontal cortex and thalamus in Fmr1KO mice (Reyes et al., 2020). Functional GABAA receptors are heteropentamers whose unique receptor subunit composition determines their pharmacologic and physiologic properties (Hevers and Luddens, 1998; Rudolph and Mohler, 2006). Loss of FMRP leads to reductions in synaptic and extrasynaptic GABAA receptors with concomitant reductions in GABAA α, β, γ receptor subunit expression (D’Hulst et al., 2006; Gantois et al., 2006; Huang and Richter, 2007). Significant alterations have also been found in the biochemical pathways necessary for the synthesis of GABA including alterations in the GABA synthesizing enzyme GAD65 (D’Hulst et al., 2009; Adusei et al., 2010; Olmos-Serrano et al., 2010). Further, dysfunction in the systems necessary for GABA catabolism, reuptake mechanisms, and subcellular localization of GABAA receptors have also been observed (D’Hulst et al., 2009; Adusei et al., 2010). Thus, marked IN dysfunction is a contributing factor to multiple hyperexcitable phenotypes observed in FXS (Contractor et al., 2015). Importantly, these alterations are highly region- and developmental timepoint specific (Paluszkiewicz et al., 2011a). Taken together, these data demonstrate that the loss of function of FMRP has wide-ranging effects throughout the brain at all developmental ages but these effects may be most pronounced during early development.
Critical period dysfunction in FXS
Fragile X syndrome is a NDD in which the dysregulation of protein synthesis results in significant deficits in neuronal, synaptic, and circuit function during critical periods of development (Contractor et al., 2015). Critical periods are stages in early brain development in which precise cellular, synaptic, and experience-dependent input is necessary for the proper development of neural circuits and systems (Hensch, 2005). In turn, CP plasticity is critically dependent on the precise balance of excitation and inhibition (E/I) during normal synaptogenesis. Interestingly, CP plasticity has been shown to be dependent on the maturation of GABAergic neurotransmission (Fagiolini and Hensch, 2000; Iwai et al., 2003; Fagiolini et al., 2004).
For example, the onset of the visual cortex CP has been shown to correspond with the developmental emergence of parvalbumin-expressing (PV+) inhibitory INs (Del Rio et al., 1994) around post-natal (PN) day 14. Indeed, precocious emergence of CP opening is mediated by brain-derived neurotrophic factor (BDNF)-driven enhancements of PV+ GABAergic neurotransmission (Huang et al., 1999). An accelerated post-natal rise of BDNF in the visual cortex of transgenic mice has been shown to mediate a precocious opening of CP plasticity such that increased ocular dominance plasticity (ODP) leads to an early-life increase in visual acuity. Importantly, this was driven by robust maturation of GABAergic circuitry (Huang et al., 1999). Within the CP for the visual system, ODP following lid suture requires homeostatic scaling of inhibition mediated by PV+-fast spiking cells. In particular, reductions in glutamatergic neurotransmission in the visual cortex following lid suture are accompanied by reductions in feed-forward inhibitory input onto excitatory PNs. This process is mediated by reduced excitatory input onto PV+ cells. Further, pharmacologically enhancing inhibition during lid suture reduces ODP, and reduction of PV+-firing via pharmacogenetic approaches extends ODP (Kuhlman et al., 2013). Additionally, somatostatin-expressing (SOM+) INs also exhibit enhanced excitability during the CP implicating dendritic-targeting inhibition from SOM+ as an important factor in ODP (Lazarus and Huang, 2011). This precise synaptic scaling and homeostatic plasticity has been shown to be impaired in FXS (Zhang et al., 2018).
In FXS, E/I imbalance results in aberrant CP plasticity across diverse brain regions including the somatosensory cortex and the basolateral amygdala (BLA) (Rubenstein and Merzenich, 2003; Nelson and Valakh, 2015). Indeed, alterations in CP plasticity are thought to underpin the temporal manifestation of neurologic and psychiatric deficits observed during early childhood development—known as sensitive time windows (Meredith et al., 2012; Meredith, 2015). In the somatosensory cortex, the period of programed synaptic and cellular changes required for normal circuit development and refinement is shorter in duration and occurs later in development (Harlow et al., 2010). Anatomically, Fmr1KO mice exhibit altered dendritic spine morphology and spine turnover in the barrel cortex at P14 (Cruz-Martin et al., 2010). At the synaptic level, glutamatergic projections in the barrel cortex of Fmr1KO mice have been found to exhibit lower connection probabilities and diffuse axonal arbors accompanying decreases in experience-dependent plasticity at P14 (Bureau et al., 2008). Further, Fmr1KO animals show an early life increase in the N-methyl-d-aspartate (NMDA)/AMPA ratio and an increase in NMDA-silent synapses during the barrel cortex CP (Harlow et al., 2010). Experience-dependent plasticity during critical periods involves the conversion of previously formed NMDA-silent synapses via up the upregulation of AMPARs. This upregulation of AMPARs is thought to underlie the unique changes seen in neonatal rodent olfactory learning and odor preference (Franks and Isaacson, 2005; Opendak et al., 2018). NMDA receptor activation and spike-timing dependent plasticity (STDP) are also altered in Fmr1KO mice where LTD at cortical synapses is robust, whereas LTP diminished (Desai et al., 2006). However, excitatory projections resemble that of the wildtype animal by the third week of life suggesting that homeostatic mechanisms may shift developmental trajectories in the Fmr1KO barrel cortex (Bureau et al., 2008).
Additionally, differences in BDNF signaling have been observed in the barrel cortex and in the hippocampus of Fmr1KO mice at P14 (Louhivuori et al., 2011). Increased BDNF signaling has been observed in the hippocampus whereas decreased BDNF signaling was observed in the somatosensory cortex (Louhivuori et al., 2011). Thus, regional differences in BDNF signaling may underpin early life plasticity alterations with distinctly different effects in the hippocampus and somatosensory cortex (Louhivuori et al., 2011; Gray et al., 2019).
Depolarizing GABA has been shown to play a pivotal role in cell migration, synapse formation, and integration in early development (Ge et al., 2006). Interestingly, GABA has been shown to be depolarizing as late as P13 in the somatosensory cortex (He et al., 2019). This delay in the GABA polarity switch is driven by developmental alterations in the regulation of the chloride transporters NKCC and KCC2 (He et al., 2014). Recent work examining the GABA polarity switch in a schizophrenia-risk gene model demonstrated that excessive PV+ inhibitory tone after the polarity switch drives excessive glutamatergic synapse formation further exacerbating E/I imbalance (Kang et al., 2019). Interestingly, in conditional deletion models of ASDs, peripheral sensory dysfunction arose from PV+ interneuron dysfunction in both the BLA and the barrel cortex as early as P5. Thus, distinct behavioral deficits, including increased tactile sensitivity and anxiety, emerged in an early post-natal time deletion-specific manner. Further, treatment in early life with GABAA receptor agonists improved somatosensory hyperreactivity and anxiety in these animals (Orefice et al., 2019). Thus, prolongation of the chloride reversal potential may enhance excitatory synapse function in Fmr1KO mice and this effect may be mediated by PV+ INs.
Development of the basolateral nuclei of the amygdaloid complex
The amygdaloid complex is a medial temporal lobe structure generally considered to have two major divisions, corticomedial and basolateral (Hopkins, 1975). The basolateral division is composed of the lateral (LA) and basal (BA) nuclei whose glutamatergic progenitors have their embryologic origin in the ventral pallium. GABAergic progenitors have their origin in the subpallium (medium ganglionic eminence) (Waclaw et al., 2010). Together, these nuclei form the BLA and have an essential function in the processing of emotional information and assigning valance to emotional stimuli (Benarroch, 2015). Further, the BLA is the nuclei responsible for conditioned learning of aversive stimuli (Blair et al., 2005). Anatomical studies in rabbits have demonstrated that during the first three post-natal weeks of development cerebral volume and neuronal density increases (reaching mature levels by P60 and P30, respectively). Further, acetylcholinesterase activity increases over this time indicating that the neuromodulatory actions of acetylcholine may play an important role in CP plasticity. Importantly, these morphological changes are similar in the rodent BLA (Jagalska-Majewska et al., 2003; Ryan et al., 2016).
From birth to adulthood, PNs in the rodent BLA during the first three post-natal weeks undergo a number of morphological changes including an increase in the size of the soma, maturation of dendritic arbors around peri-weaning age, and an increase in the dendritic spine density until age P60 (Ryan et al., 2016). The neuronal density in the BLA is completely established by P7 (Berdel et al., 1997). In turn, a number of intrinsic electrophysiological parameters mature in PNs in the BLA. Specifically, AP frequencies increase, input resistances decrease, and spike amplitudes increase with age from P7 to P60 (Ryan et al., 2016). Maturational and physiologic changes in PNs in the BLA during the first two post-natal weeks accompany synaptic and microcircuit development. By the second post-natal week, the thalamic afferents are completely established by P9, followed by establishment of afferents from the pre-frontal cortex by P14 (Bouwmeester et al., 2002). Functional resting state MRI has revealed that the neonatal brain exhibits undifferentiated connections to both primary sensory and cortical areas compared to the adult brain. Thus, experience-dependent plasticity likely influences cortical connectivity (Hansen et al., 2020). To that end, the emergence of cue fear-learning correlates with the developmental emergence of LTP at cortical and thalamic afferents occurring at approximately P10 (Berdel et al., 1997), with the complexity of learning features increasing with the maturation of connectivity between the BLA and other brain areas (Hunt et al., 2007; Li et al., 2012).
Neuromodulatory input to the basolateral amygdala
A number of neuromodulators have important roles in the modulation of fear learning. Neuromodulators are important regulators of circuit output playing critical roles in regulating neuronal excitability, pre-synaptic release of neurotransmitters, LTD and potentiation, and mediation of brain state changes that underlie behavior (Nadim and Bucher, 2014). Neuromodulatory systems also have well-known roles in CP induction and cortical plasticity (Herlenius and Lagercrantz, 2004; Hensch, 2005; Larsen and Luna, 2018). Indeed, neuromodulators are employed throughout the forebrain with distinct effects on synaptic transmission and behavior.
Cholinergic input
The BLA receives dense cholinergic input from a number of basal forebrain structures including the nucleus basalis and the horizontal limb of the diagonal band of Broca and the magnocellular preoptic area. In the rodent, cholinergic afferents in the BLA mirror those found in the human including the horizontal diagonal band, magnocellular preoptic nucleus, and ventral pallidum (Woolf, 1991). In the rodent, cholinergic input to the BLA is also contributed by the lateral preoptic area, medial septal nucleus, bed nucleus of the stria terminalis, and substantia innominata (Woolf and Butcher, 1982). However, the majority of cholinergic input originates from the magnocellular neurons in the nucleus basalis of Meynert (NBM) (Kitt et al., 1994). Evidence for the effects of cholinergic neuromodulation on plasticity and associative behaviors is extensive. Cholinergic actions on forebrain targets have been implicated in arousal (Jones, 2008), memory (Croxson et al., 2011), attention, and learning (Ljubojevic et al., 2018). Within the BLA, cholinergic signaling modulates fear-learning via increases in PN excitability and the enhancement of LTP forming durable fear memories resistant to extinction (Jiang et al., 2016).
Noradrenergic input
The main source of noradrenergic innervation in the BLA is the locus coeruleus (LC). The LC is located within the pons of the brainstem and is comprised of three fiber projections the ascending pathway, the cerebellar pathway, and the descending pathway. The ascending pathway innervates structures within the midbrain including the limbic system (Szabadi, 2013). Within the amygdaloid complex dense innervation has been noted within the basal and central nuclei (Jones and Moore, 1977; Fallon et al., 1978). Indeed, there exists a specific population of neurons within the LC that project (LA-projecting) to the LA whose activation is necessary for fear-learning (Uematsu et al., 2017). The principal neurotransmitter, norepinephrine, mediates its effects in the BLA via functionally-distinct adrenergic receptors: α1, β1, and β2-receptors mediate excitation of PNs within the BLA and α2 receptors mediate inhibition. Within the BLA, the α1 receptor is highly expressed within the LA, with modest expression noted in the central amygdala (CeA) (Jones and Moore, 1977; Pieribone et al., 1994). Interestingly, a recent study noted high co-expression of α1 with glutamic acid decarboxylase (GAD), GABA, and NMDA receptors (Papay et al., 2006). This suggests that α1-receptors not only mediate an excitatory effect on PNs but may also enhance GABAergic signaling via a direct effect on inhibitory interneurons. Administration of an α1-adrenergic antagonist to mice during fear-conditioning showed similar levels of freezing to control animals. However, α1-adrenergic antagonist-treated mice exhibited higher levels of post-training extinction (Favero et al., 2018).
Expression of α2-receptors is confined mainly to the CeA with only low-level expression noted in the lateral and basal nuclei (Pascual et al., 1992; Glass et al., 2002). This suggests that the predominate effects of α2-adrenergic activity in the central output nucleus of the amygdaloid complex may be to mediate the autonomic and motor responses to fear and anxiety. Within the amygdaloid complex, moderate β-receptor expression is highest in the cortical nuclei with moderate expression noted within the CeA (Wanaka et al., 1989). Higher levels of expression of β1 and β2 receptors have been observed in the BLA (Rainbow et al., 1984). High densities of β-receptors have also been observed in the human amygdala post-mortem (Pazos et al., 1985). The role of β-receptors in the pathology of fear and anxiety is well-described (AlOkda et al., 2019). β-receptors have been found to enhance fear potentiation and β-receptor antagonists are capable of enhancing fear extinction (Debiec et al., 2011). Furthermore, administration of the β-adrenergic antagonist propranolol abolishes amygdala-associated memory enhancement (Strange and Dolan, 2004).
The developmental effects of noradrenergic neuromodulation in the BLA are just beginning to be studied with a recent study identifying an age-dependent effect of adrenergic agonism on increased PN spike frequency in juvenile mice but not in adult mice. This effect was mediated via the downregulation of tonic inhibition in juvenile animals (Fink and LeDoux, 2018). Norepinephrine (NE) is also known to potentiate associative learning at thalamo-amygdala synapses (Tully et al., 2007) and is capable of suppressing feed-forward inhibition (FFI) (Bergles et al., 1996; Ehrlich et al., 2009). Similarly, NE has also been demonstrated to play a distinct role in the conversion of eligibility traces for LTP (Elwood and Keeling, 1982). Thus, noradrenergic neuromodulation is necessary for state-dependent neural coding and threat learning.
Serotonergic input
The BLA receives serotonergic innervation mainly from the nucleus raphe dorsalis found in the brainstem. A total of 10% of serotonergic efferent projections throughout the forebrain are known to innervate the BLA (Bobillier et al., 1976; Ma et al., 1991; Bonn et al., 2013). The actions of serotonin in the BLA are considerably more complex given the diversity of serotonergic receptors and their myriad actions. During Pavlovian fear-conditioning, serotonin is known to increase in concentration in the BLA (Zanoveli et al., 2009). Serotonin primarily affects GABAergic INs via the 5-HT2A receptor enhancing inhibitory neurotransmission and FFI (Rainnie, 1999; Jiang et al., 2009). However, following acute stress, downregulation of the 5-HT2A receptor on INs decreases FFI (Jiang et al., 2009). Indeed, reductions in serotonergic tone have been shown to decrease GABAergic FFI in the BLA and have been postulated to underpin anxiety and fear disorders (Wang et al., 2019). However, studies evaluating the effects of serotonin on fear learning have produced conflicting results. Rodents treated with a selective serotonin reuptake inhibitor (SSRI) prior to fear-conditioning exhibited increased contextual and cued fear (Bosker et al., 2001). In contrast, under conditions of depleted serotonin, rodents exhibited decreased fear acquisition (Dobyns et al., 1984). Thus, the exact role that serotonin plays in fear acquisition remains unclear, but these studies suggest that it may be receptor subtype specific.
Dopaminergic input
The BLA receives dopaminergic input primarily from the ventral tegmental area (VTA) and substantia nigra (Asan, 1997). Dopaminergic projections innervate both PNs (Brinley-Reed and McDonald, 1999) and INs (Pinard et al., 2008) and have distinct effects based on cell-type specific receptor expression. Principal neurons in the BLA have been found to express D1 with dopamine-enhancing PN excitability (Kroner et al., 2005). The D2 receptor is found on PV+ INs and functions to suppress GABA release at the synapse (Bissiere et al., 2003; Chu et al., 2012). The role of dopaminergic neuromodulation in aversion learning is well-described. In rodents, fear-potentiated startle is enhanced upon electrical stimulation of the VTA (Borowski and Kokkinidis, 1996). Conversely, administration of D1-receptor antagonists decreases fear-potentiated startle and freezing behavior following Pavlovian conditioning (Nader and LeDoux, 1999; Greba and Kokkinidis, 2000). In odor-cued fear-conditioning paradigms, administration of haloperidol decreased PN excitability and excitatory synaptic strengthening following odor-shock conditioning (Rosenkranz and Grace, 2002b). Thus, the overall net effect of dopaminergic neuromodulation is to increase PN excitability and to suppress IN-mediated FFI in micro-circuits important for fear-learning (Rosenkranz and Grace, 1999, 2002a; Bissiere et al., 2003). Interestingly, dopamine suppression underpins the maternal attenuation of infant fear-learning during a sensitive period in the development of the BLA (Barr et al., 2009; Opendak et al., 2019).
Amygdala dysfunction in FXS
The limbic system, and in particular the BLA, has been implicated in a variety of neuropsychiatric disorders including anxiety, panic disorder, post-traumatic stress disorder, and substance use disorder (Martin et al., 2010; Janak and Tye, 2015). Individuals with FXS and ASDs exhibit an increased prevalence of socioemotional problems including social anxiety, social withdrawal, hyperactivity, and gaze aversion (Bregman et al., 1988; Cohen et al., 1988; Hagerman et al., 1991; Cordeiro et al., 2011; Tonnsen et al., 2013). However, structural neuroimaging studies examining volume differences in individuals with FXS have been inconsistent. Small studies and case reports have documented neuroanatomical increases in amygdala volume in individuals with FXS (Reiss et al., 1995), whereas larger studies have found no difference or reduced amygdala volumes (Kates et al., 1997; Gothelf et al., 2008; Hazlett et al., 2009). One recent volumetric study identified persistent developmental enlargements of caudate volumes in children with FXS as early as 6 months of age but no differences in amygdala volume. In contrast, children with ASDs exhibited rapidly changing amygdala volumes over the first 2 years of life. Interestingly, these changes were observed prior to behavioral symptom onset and diagnosis demonstrating that developmental trajectories become altered very early in life (Shen et al., 2022). From a functional connectivity standpoint, individuals with FXS have been shown to exhibit aberrant white matter integrity and interconnectivity (Barnea-Goraly et al., 2003), with reduced integration of subcortical regions including the amygdala, anterior cingulate, and temporal pole (Bruno et al., 2017). Taken together, these data suggest that structural neuroanatomy may not fully account for the behavioral differences observed in FXS.
Functional imaging studies have identified a number of key differences in amygdala responses in FXS. Pronounced gaze aversion with abnormal gaze processing has been observed in children and young adults with FXS (Garrett et al., 2004). However, no differences in amygdala activation were observed between neurotypical and FXS patients during facial-emotion judgment or in the presentation of fearful stimuli (Hagan et al., 2008; Holsen et al., 2008). In contrast, children with FXS have been found to exhibit increased autonomic responses to fearful stimuli in an age-dependent manner (Tonnsen et al., 2013). Comparison of these studies is confounded by differences in experimental design, experimental group parameters, and the type of behavioral task employed. Thus, the precise neural correlates of amygdala dysfunction in FXS patients remain unclear.
At the cellular level, hyperexcitability within the BLA has been implicated in the pathophysiology of amygdala-based behaviors (Bulow et al., 2022). Our own work has identified distinct developmental alterations in the hyperexcitability of PNs in the BLA including profound changes in intrinsic firing rates and low threshold spiking activity (Svalina et al., 2021, 2022). At the circuit level, in adult mice, our group and others have identified profound alterations in GABAergic neurotransmission including deficits in phasic and tonic inhibition, GABAA receptor composition, and GABA synthesis (Olmos-Serrano et al., 2010; Paluszkiewicz et al., 2011a; Cea-Del Rio and Huntsman, 2014; Martin et al., 2014). Broadly, and in concert with other factors, this results in circuit and network hyperexcitability (Contractor et al., 2015).
Interestingly, our group has observed periods of homeostatic changes in inhibition in the BLA of Fmr1KO mice corresponding with sensitive time periods in the developmental emergence of fear-learning (Vislay et al., 2013). These distinct changes in inhibitory neurotransmission suggest that amygdala-based behaviors may be altered in the Fmr1KO mouse early in development. During the period of inhibitory synaptic development (P10–P21) (Huntsman and Huguenard, 2000), our group has identified dramatic reductions in inhibitory neurotransmission from birth to P10. Between P14 and P16, a transient period of enhanced inhibitory function emerges (Vislay et al., 2013). Interestingly, these inhibitory changes occur in the absence of isolated changes in excitatory synaptic function, but rather occur in the context of increased feed-forward excitation. As such, at this developmental time point, FFI is observed to be broadly normalized. However, this is followed by a return to depleted levels of inhibition by P21. However, whether this period of enhanced inhibitory function represents a precocious maturation of GABAergic neurotransmission or a homeostatic response to hyperexcitability in early life remains to be definitively determined.
Our hypothesis about this phenomenon is that inhibitory changes during this time period represent inadequate homeostatic compensation and contribute to pathologic plasticity and behavioral outcomes. Indeed, our studies at P14 demonstrate that early life bidirectional changes in plasticity are dependent on the state inhibition. For instance, at P14, enhanced LTP is observed in the absence of GABA receptor blockade, whereas enhanced LTD is observed in presence of GABA receptor blockade suggesting that pathologic plasticity exists at baseline, but further driving excitation has the opposite effect. However, at P21, a marginal increase in LTP is observed in Fmr1KO animals at baseline, but under conditions of GABA receptor blockade, this LTP magnitude is diminished from the wild type (WT) baseline suggesting that as early as P21 plasticity in the FXS BLA is demonstrably reduced.
Future studies are warranted to characterize these changes. Indeed, one major driver of CP plasticity that has been understudied in FXS is neuromodulation. To our knowledge, few studies have focused on the canonical neuromodulators of CP plasticity and fear-learning in the Fmr1KO BLA, despite neuromodulation strategies (in particular, serotonin receptor modulators) showing promise for reversing behavioral deficits for FXS, ASDs, and NDDs more broadly (Armstrong et al., 2020; Lee et al., 2021; Jeon et al., 2022; Kim et al., 2022).
Similar to studies in human patients with FXS, studies examining fear learning in rodent models of FXS have produced conflicting results. Fmr1KO mice have been found to exhibit higher levels of hyperarousal and anxiety when interacting with novel conspecifics (McNaughton et al., 2008). However, studies employing classic Pavlovian fear-conditioning paradigms have identified decreases in contextual and conditioned freezing episodes suggesting deficiencies in both hippocampal and amygdala-based associative learning (Paradee et al., 1999). Indeed, these findings have recently been reproduced in a rat model of FXS (Fernandes et al., 2021). Further, despite the prevalence of amygdala-based disorders in human patients with FXS, mouse models of FXS demonstrate deficiencies in LTP in the circuits responsible for fear-learning (Zhao et al., 2005; Suvrathan et al., 2010). One possible explanation for these disparate findings is that plasticity and associative learning defects manifest differently at different ages. In our own work, we have shown this to be the case as LTP in the BLA is transiently enhanced early in development (Svalina et al., 2022). Thus, understanding early life cellular and synaptic alterations, CP plasticity in the BLA and how deficits emerge and change over time in FXS and ASDs is of great importance for developing new therapies.
Ontogeny of fear-learning in the rodent
The ontogeny of amygdala-dependent fear-learning has been well characterized and involves a number of characteristic changes in the BLA and fear across ontogeny (Moriceau and Sullivan, 2005). At birth, altricial rodents depend on olfaction for orientation and navigation, maternal attachment, and nursing to receive the caregiving necessary for survival (Teicher and Blass, 1977; Galef and Kaner, 1980; Leon, 1992; Landers and Sullivan, 1999; Sullivan et al., 2003). Whereas the rodent visual and auditory systems develop later in life (around P14) (Blatchley et al., 1987; Shen and Colonnese, 2016). From birth to P10, pups do not exhibit amygdala-dependent fear learning (Sullivan et al., 2000a), but show robust olfactory learning necessary for their survival associated with a sensitive period for attachment learning and learning the maternal odor (Pedersen et al., 1982; Shen and Colonnese, 2016). However, at this developmental time point, pups display similar reward value to both appetitive and aversive stimuli to support attachment learning (Camp and Rudy, 1988; Perry et al., 2017). Indeed, rodent pups will exhibit an odor preference for a conditioned odor even when the odor is paired with a mild tail shock or milk, using a learning circuit involving the olfactory bulb and olfactory cortex, as well as failure to recruit the amygdala (Moriceau and Sullivan, 2006; Mukherjee et al., 2017; Oruro et al., 2020). Olfactory bulb NE is necessary and sufficient for associative olfactory learning in rodent pups and has been postulated to be essential for attachment learning (Sullivan et al., 1991, 2000b; Sullivan, 2001). It should be noted that pups during the first week of life can learn fear and avoidance with high shock or malaise, although learning depends upon the olfactory system and high levels of NE, and not the amygdala (Moriceau et al., 2009; Raineki et al., 2009). However, during this developmental epoch of quiescent amygdala function, the BLA is especially susceptible to early life adversity with enduring effects seen throughout the lifespan (Lukkes et al., 2012; Callaghan et al., 2019). Indeed, repeated adversity, such as maternal separation or maltreatment of rat pups in the first week of life results in a non-linear developmental trajectory of hyperactivity in the amygdala and enhanced contextual and conditioned fear-learning in later-life (Diehl et al., 2014; Pattwell et al., 2016; Junod et al., 2019). But amygdala deficits are also evident in infancy as reflected in precocial emergence of amygdala-dependent fear due to amygdala excitability, reduction of BLA axo-somatic synapses formed by parvalbumin cells, and perineuronal net and engagement of intracellular molecular events associated with plasticity (Ehrlich and Rainnie, 2015; Santiago et al., 2018; Opendak et al., 2019). Further, repeated stress or exogenous administration of corticosterone within a social context is considered important in the initiation of this altered developmental trajectory (Raineki et al., 2019; Opendak et al., 2020).
From P10 to P15, a transitional sensitive period emerges in which normal adult-like responses to odor-cued amygdala-dependent fear conditioning are evident (Sullivan et al., 2000a). Interestingly, social context is capable of blocking pup fear responses during this time (Barr et al., 2009). For instance, odor-cued fear conditioning conducted in the presence of the maternal dam suppresses fear acquisition in the rodent pup such that the pup will instead demonstrate an odor preference for the conditioned odor (Shionoya et al., 2007). This has been replicated in young children, illustrating a potential cross-species feature of infant attachment learning (Tottenham et al., 2019), with both species associated with decreasing stress hormone levels and attenuation of the amygdala, which wanes by adolescence (Gee et al., 2014). The rodent research has further defined the importance of NE and dopamine in this process (Barr et al., 2009; Opendak et al., 2019, 2021). These studies suggest that at this developmental time point the attachment and avoidance circuits exist in a delicate balance readily switched by maternal presence. By P16 infant rats have a more mature functioning of the amygdala-depended fear emerges and maternal presence plays a limited role in modulating or only slightly attenuating avoidance and fear, potentially through a decrease in maternal modulation of dopamine and maturing amygdala (Upton and Sullivan, 2010; Opendak et al., 2021). These unique age-specific features of infant learning are due to age-specific mechanisms illustrating that the developing brain is not an immature version of the adult brain and highlights how the environment impacts its development.
Emerging data also illustrates that developmental disorders, potentially associated with genetic abnormalities in Fmr1KO mice and rodent models of FXS can also impact the amygdala and disrupt the developmental trajectory of the amygdala. For example, in contrast to previous studies in aged mice, our most recent work in mouse pups has shown that LTP and fear-learning are briefly enhanced at the infant P14 developmental time point. Interestingly, this pathologic LTP and fear-learning is dependent on the state of inhibition and can be ameliorated with early-life 4,5,6,7-tetrahydroisoxazolo [5,4-c]pyridin-3ol (THIP) intervention at this time point. Thus, it is essential to better study developmental changes in NDDs to not only understand how to treat but also when to treat and the importance of defining age-specific interventions.
Future directions
We integrate the current state of the literature as shown in Figure 1. The broad spectrum function of FMRP enables several avenues of dysfunction but also opens several potential therapeutic avenues. For instance, it remains unknown if the intrinsic properties of neurons are altered at the earliest post-natal time points. Similarly, it remains to be determined how circuit-level changes contribute to the maladaptive behavioral manifestations seen in FXS throughout early development. Further, how social and environmental factors impact the behavior in FXS is an interesting area worthy of future study. Though suggested by our own work and that of others, correlative circuit-level changes may underpin behavioral alterations but direct causation studies are certainly warranted. Indeed, the extended and enhanced period of plasticity observed in NDDs such as FXS likely holds the key to the discovery of specific changes at the circuit level (Meredith et al., 2012). A major challenge for the study of NDDs, like FXS, is the discrepancy between the onset of distinct molecular and synaptic pathophysiology and the appearance of symptoms. It may be the case, that the time at diagnosis may be too far beyond the CP of plasticity to provide the platform needed for circuits to be modified. Therefore, the identification of specific circuit-level changes during critical periods of brain development is of high importance for our understanding of the pathophysiology and treatment of FXS patients.
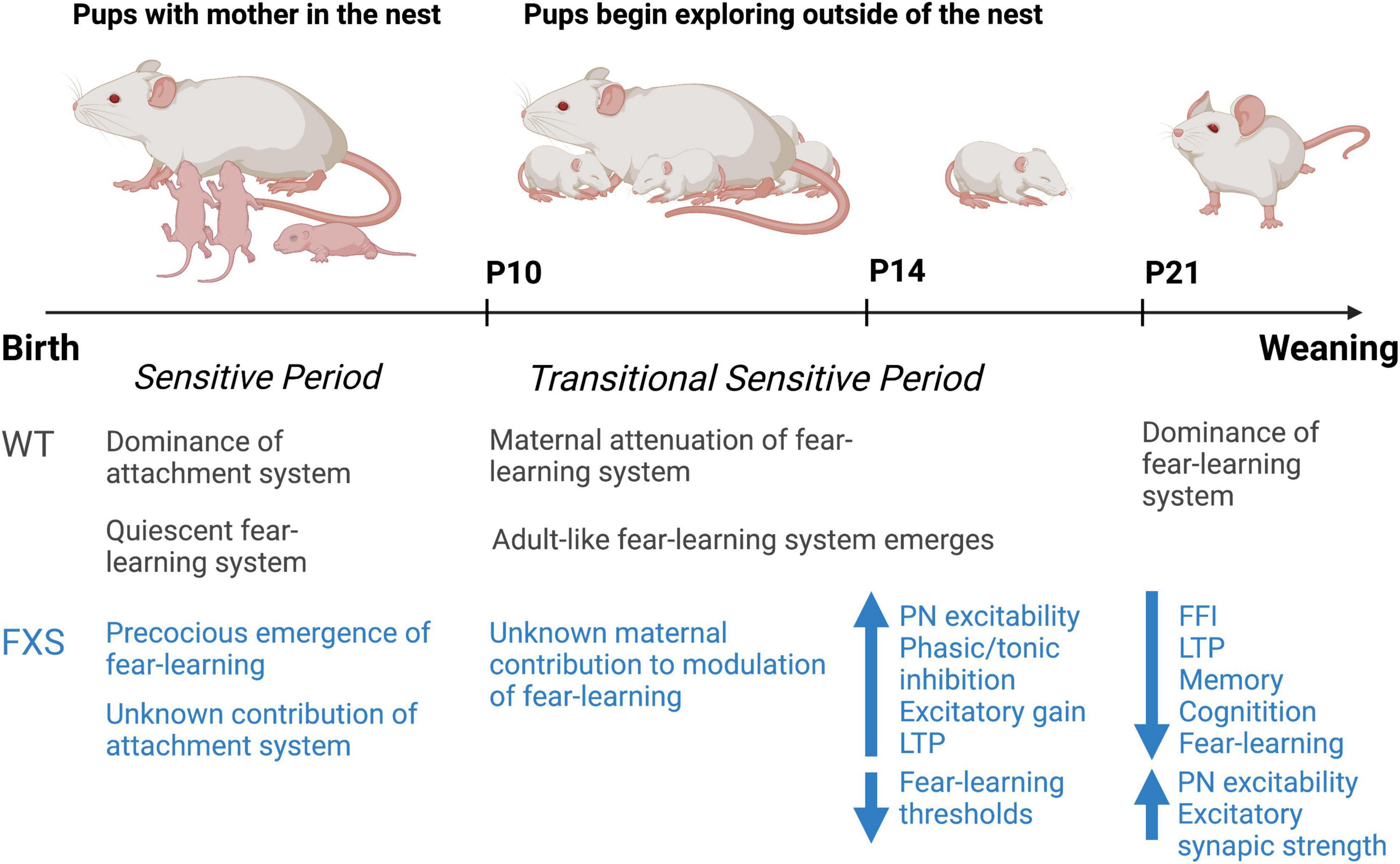
Figure 1. Summary timeline of observed changes in early development in the Fmr1KO mouse compared to the wild-type rodent. Figure adapted from previous work by Regina Sullivan.
Author contributions
MS and MH consulted on the original content. MS wrote the original draft. MS, RS, DR, and MH edited the final version. All authors contributed to the article and approved the submitted version.
Funding
This work was supported by R01 NS095311 to MH and DR and F31 MH124277 to MS.
Conflict of interest
The authors declare that the research was conducted in the absence of any commercial or financial relationships that could be construed as a potential conflict of interest.
Publisher’s note
All claims expressed in this article are solely those of the authors and do not necessarily represent those of their affiliated organizations, or those of the publisher, the editors and the reviewers. Any product that may be evaluated in this article, or claim that may be made by its manufacturer, is not guaranteed or endorsed by the publisher.
References
Adusei, D. C., Pacey, L. K., Chen, D., and Hampson, D. R. (2010). Early developmental alterations in GABAergic protein expression in fragile X knockout mice. Neuropharmacology 59, 167–171.
AlOkda, A. M., Nasr, M. M., and Amin, S. N. (2019). Between an ugly truth and a perfect lie: Wiping off fearful memories using beta-adrenergic receptors antagonists. J. Cell Physiol. 234, 5722–5727. doi: 10.1002/jcp.27441
Armstrong, J. L., Casey, A. B., Saraf, T. S., Mukherjee, M., Booth, R. G., and Canal, C. E. (2020). (S)-5-(2’-Fluorophenyl)-N,N-dimethyl-1,2,3,4-tetrahydronaphthalen-2-amine, a serotonin receptor modulator, possesses anticonvulsant, prosocial, and anxiolytic-like properties in an Fmr1 knockout mouse model of fragile X syndrome and autism spectrum disorder. ACS Pharmacol. Transl. Sci. 3, 509–523. doi: 10.1021/acsptsci.9b00101
Asan, E. (1997). Ultrastructural features of tyrosine-hydroxylase-immunoreactive afferents and their targets in the rat amygdala. Cell Tissue Res. 288, 449–469. doi: 10.1007/s004410050832
Bale, T. L., Baram, T. Z., Brown, A. S., Goldstein, J. M., Insel, T. R., Mccarthy, M. M., et al. (2010). Early life programming and neurodevelopmental disorders. Biol. Psychiatry 68, 314–319.
Barnea-Goraly, N., Eliez, S., Hedeus, M., Menon, V., White, C. D., Moseley, M., et al. (2003). White matter tract alterations in fragile X syndrome: Preliminary evidence from diffusion tensor imaging. Am. J. Med. Genet. B Neuropsychiatr. Genet. 118B, 81–88. doi: 10.1002/ajmg.b.10035
Barr, G. A., Moriceau, S., Shionoya, K., Muzny, K., Gao, P., Wang, S., et al. (2009). Transitions in infant learning are modulated by dopamine in the amygdala. Nat. Neurosci. 12, 1367–1369.
Bear, M. F., Huber, K. M., and Warren, S. T. (2004). The mGluR theory of fragile X mental retardation. Trends Neurosci. 27, 370–377.
Benarroch, E. E. (2015). The amygdala: Functional organization and involvement in neurologic disorders. Neurology 84, 313–324.
Berdel, B., Morys, J., and Maciejewska, B. (1997). Neuronal changes in the basolateral complex during development of the amygdala of the rat. Int. J. Dev. Neurosci. 15, 755–765.
Bergles, D. E., Doze, V. A., Madison, D. V., and Smith, S. J. (1996). Excitatory actions of norepinephrine on multiple classes of hippocampal CA1 interneurons. J. Neurosci. 16, 572–585. doi: 10.1523/JNEUROSCI.16-02-00572.1996
Bhakar, A. L., Dolen, G., and Bear, M. F. (2012). The pathophysiology of fragile X (and what it teaches us about synapses). Annu. Rev. Neurosci. 35, 417–443. doi: 10.1146/annurev-neuro-060909-153138
Bissiere, S., Humeau, Y., and Luthi, A. (2003). Dopamine gates LTP induction in lateral amygdala by suppressing feedforward inhibition. Nat. Neurosci. 6, 587–592. doi: 10.1038/nn1058
Blair, H. T., Sotres-Bayon, F., Moita, M. A., and Ledoux, J. E. (2005). The lateral amygdala processes the value of conditioned and unconditioned aversive stimuli. Neuroscience 133, 561–569.
Blatchley, B. J., Cooper, W. A., and Coleman, J. R. (1987). Development of auditory brainstem response to tone pip stimuli in the rat. Brain Res. 429, 75–84.
Bobillier, P., Seguin, S., Petitjean, F., Salvert, D., Touret, M., and Jouvet, M. (1976). The raphe nuclei of the cat brain stem: A topographical atlas of their efferent projections as revealed by autoradiography. Brain Res. 113, 449–486. doi: 10.1016/0006-8993(76)90050-0
Bonn, M., Schmitt, A., Lesch, K. P., Van Bockstaele, E. J., and Asan, E. (2013). Serotonergic innervation and serotonin receptor expression of NPY-producing neurons in the rat lateral and basolateral amygdaloid nuclei. Brain Struct. Funct. 218, 421–435. doi: 10.1007/s00429-012-0406-5
Borowski, T. B., and Kokkinidis, L. (1996). Contribution of ventral tegmental area dopamine neurons to expression of conditional fear: Effects of electrical stimulation, excitotoxin lesions, and quinpirole infusion on potentiated startle in rats. Behav. Neurosci. 110, 1349–1364. doi: 10.1037//0735-7044.110.6.1349
Bosker, F. J., Cremers, T. I., Jongsma, M. E., Westerink, B. H., Wikstrom, H. V., and Den Boer, J. A. (2001). Acute and chronic effects of citalopram on postsynaptic 5-hydroxytryptamine(1A) receptor-mediated feedback: A microdialysis study in the amygdala. J. Neurochem. 76, 1645–1653. doi: 10.1046/j.1471-4159.2001.00194.x
Bouwmeester, H., Smits, K., and Van Ree, J. M. (2002). Neonatal development of projections to the basolateral amygdala from prefrontal and thalamic structures in rat. J. Comp. Neurol. 450, 241–255.
Brager, D. H., Akhavan, A. R., and Johnston, D. (2012). Impaired dendritic expression and plasticity of h-channels in the fmr1(-/y) mouse model of fragile X syndrome. Cell Rep. 1, 225–233. doi: 10.1016/j.celrep.2012.02.002
Brandalise, F., Kalmbach, B. E., Mehta, P., Thornton, O., Johnston, D., Zemelman, B. V., et al. (2020). Fragile X mental retardation protein bidirectionally controls dendritic Ih in a cell type-specific manner between mouse hippocampus and prefrontal cortex. J. Neurosci. 40, 5327–5340. doi: 10.1523/JNEUROSCI.1670-19.2020
Bregman, J. D., Leckman, J. F., and Ort, S. I. (1988). Fragile X syndrome: Genetic predisposition to psychopathology. J. Autism Dev. Disord. 18, 343–354.
Brinley-Reed, M., and McDonald, A. J. (1999). Evidence that dopaminergic axons provide a dense innervation of specific neuronal subpopulations in the rat basolateral amygdala. Brain Res. 850, 127–135. doi: 10.1016/s0006-8993(99)02112-5
Brown, M. R., Kronengold, J., Gazula, V. R., Chen, Y., Strumbos, J. G., Sigworth, F. J., et al. (2010). Fragile X mental retardation protein controls gating of the sodium-activated potassium channel slack. Nat. Neurosci. 13, 819–821.
Bruno, J. L., Hosseini, S. M. H., Saggar, M., Quintin, E. M., Raman, M. M., and Reiss, A. L. (2017). Altered brain network segregation in fragile X syndrome revealed by structural connectomics. Cereb. Cortex 27, 2249–2259. doi: 10.1093/cercor/bhw055
Buijsen, R. A., Sellier, C., Severijnen, L. A., Oulad-Abdelghani, M., Verhagen, R. F., Berman, R. F., et al. (2014). FMRpolyG-positive inclusions in CNS and non-CNS organs of a fragile X premutation carrier with fragile X-associated tremor/ataxia syndrome. Acta Neuropathol. Commun. 2:162. doi: 10.1186/s40478-014-0162-2
Bulow, P., Segal, M., and Bassell, G. J. (2022). Mechanisms driving the emergence of neuronal hyperexcitability in fragile X syndrome. Int. J. Mol. Sci. 23:6315.
Bureau, I., Shepherd, G. M., and Svoboda, K. (2008). Circuit and plasticity defects in the developing somatosensory cortex of FMR1 knock-out mice. J. Neurosci. 28, 5178–5188. doi: 10.1523/JNEUROSCI.1076-08.2008
Callaghan, B., Meyer, H., Opendak, M., Van Tieghem, M., Harmon, C., Li, A., et al. (2019). Using a developmental ecology framework to align fear neurobiology across species. Annu. Rev. Clin. Psychol. 15, 345–369. doi: 10.1146/annurev-clinpsy-050718-095727
Camp, L. L., and Rudy, J. W. (1988). Changes in the categorization of appetitive and aversive events during postnatal development of the rat. Dev. Psychobiol. 21, 25–42. doi: 10.1002/dev.420210103
Casingal, C. R., Kikkawa, T., Inada, H., Sasaki, Y., and Osumi, N. (2020). Identification of FMRP target mRNAs in the developmental brain: FMRP might coordinate Ras/MAPK, Wnt/beta-catenin, and mTOR signaling during corticogenesis. Mol. Brain 13:167. doi: 10.1186/s13041-020-00706-1
Cea-Del Rio, C. A., and Huntsman, M. M. (2014). The contribution of inhibitory interneurons to circuit dysfunction in fragile X syndrome. Front. Cell Neurosci. 8:245. doi: 10.3389/fncel.2014.00245
Chen, L., Yun, S. W., Seto, J., Liu, W., and Toth, M. (2003). The fragile X mental retardation protein binds and regulates a novel class of mRNAs containing U rich target sequences. Neuroscience 120, 1005–1017. doi: 10.1016/s0306-4522(03)00406-8
Christie, S. B., Akins, M. R., Schwob, J. E., and Fallon, J. R. (2009). The FXG: A presynaptic fragile X granule expressed in a subset of developing brain circuits. J. Neurosci. 29, 1514–1524. doi: 10.1523/JNEUROSCI.3937-08.2009
Chu, H. Y., Ito, W., Li, J., and Morozov, A. (2012). Target-specific suppression of GABA release from parvalbumin interneurons in the basolateral amygdala by dopamine. J. Neurosci. 32, 14815–14820. doi: 10.1523/JNEUROSCI.2997-12.2012
Cohen, I. L., Fisch, G. S., Sudhalter, V., Wolf-Schein, E. G., Hanson, D., Hagerman, R., et al. (1988). Social gaze, social avoidance, and repetitive behavior in fragile X males: A controlled study. Am. J. Ment. Retard. 92, 436–446.
Contractor, A., Klyachko, V. A., and Portera-Cailliau, C. (2015). Altered neuronal and circuit excitability in fragile X syndrome. Neuron 87, 699–715.
Cordeiro, L., Ballinger, E., Hagerman, R., and Hessl, D. (2011). Clinical assessment of DSM-IV anxiety disorders in fragile X syndrome: Prevalence and characterization. J. Neurodev. Disord. 3, 57–67. doi: 10.1007/s11689-010-9067-y
Croxson, P. L., Kyriazis, D. A., and Baxter, M. G. (2011). Cholinergic modulation of a specific memory function of prefrontal cortex. Nat. Neurosci. 14, 1510–1512.
Cruz-Martin, A., Crespo, M., and Portera-Cailliau, C. (2010). Delayed stabilization of dendritic spines in fragile X mice. J. Neurosci. 30, 7793–7803. doi: 10.1523/JNEUROSCI.0577-10.2010
Darnell, J. C., Van Driesche, S. J., Zhang, C., Hung, K. Y., Mele, A., Fraser, C. E., et al. (2011). FMRP stalls ribosomal translocation on mRNAs linked to synaptic function and autism. Cell 146, 247–261. doi: 10.1016/j.cell.2011.06.013
Debiec, J., Bush, D. E., and Ledoux, J. E. (2011). Noradrenergic enhancement of reconsolidation in the amygdala impairs extinction of conditioned fear in rats–a possible mechanism for the persistence of traumatic memories in PTSD. Depress Anxiety 28, 186–193. doi: 10.1002/da.20803
Del Rio, J. A., De Lecea, L., Ferrer, I., and Soriano, E. (1994). The development of parvalbumin-immunoreactivity in the neocortex of the mouse. Brain Res. Dev. Brain Res. 81, 247–259.
Deng, P. Y., Rotman, Z., Blundon, J. A., Cho, Y., Cui, J., Cavalli, V., et al. (2013). FMRP regulates neurotransmitter release and synaptic information transmission by modulating action potential duration via BK channels. Neuron 77, 696–711. doi: 10.1016/j.neuron.2012.12.018
Desai, N. S., Casimiro, T. M., Gruber, S. M., and Vanderklish, P. W. (2006). Early postnatal plasticity in neocortex of Fmr1 knockout mice. J. Neurophysiol. 96, 1734–1745. doi: 10.1152/jn.00221.2006
D’Hulst, C., De Geest, N., Reeve, S. P., Van Dam, D., De Deyn, P. P., Hassan, B. A., et al. (2006). Decreased expression of the GABAA receptor in fragile X syndrome. Brain Res. 1121, 238–245.
D’Hulst, C., Heulens, I., Brouwer, J. R., Willemsen, R., De Geest, N., Reeve, S. P., et al. (2009). Expression of the GABAergic system in animal models for fragile X syndrome and fragile X associated tremor/ataxia syndrome (FXTAS). Brain Res. 1253, 176–183. doi: 10.1016/j.brainres.2008.11.075
Diehl, L. A., Pereira Nde, S., Laureano, D. P., Benitz, A. N., Noschang, C., Ferreira, A. G., et al. (2014). Contextual fear conditioning in maternal separated rats: The amygdala as a site for alterations. Neurochem. Res. 39, 384–393. doi: 10.1007/s11064-013-1230-x
Dobyns, W. B., Stratton, R. F., and Greenberg, F. (1984). Syndromes with lissencephaly. I: Miller-Dieker and Norman-Roberts syndromes and isolated lissencephaly. Am. J. Med. Genet. 18, 509–526.
Ehrlich, D. E., and Rainnie, D. G. (2015). Prenatal stress alters the development of socioemotional behavior and amygdala neuron excitability in rats. Neuropsychopharmacology 40, 2135–2145. doi: 10.1038/npp.2015.55
Ehrlich, I., Humeau, Y., Grenier, F., Ciocchi, S., Herry, C., and Luthi, A. (2009). Amygdala inhibitory circuits and the control of fear memory. Neuron 62, 757–771.
Elwood, R. W., and Keeling, F. (1982). Temporal organization of ultrasonic vocalizations in infant mice. Dev. Psychobiol. 15, 221–227.
Fagiolini, M., Fritschy, J. M., Low, K., Mohler, H., Rudolph, U., and Hensch, T. K. (2004). Specific GABAA circuits for visual cortical plasticity. Science 303, 1681–1683.
Fagiolini, M., and Hensch, T. K. (2000). Inhibitory threshold for critical-period activation in primary visual cortex. Nature 404, 183–186. doi: 10.1038/35004582
Fallon, J. H., Koziell, D. A., and Moore, R. Y. (1978). Catecholamine innervation of the basal forebrain. II. Amygdala, suprarhinal cortex and entorhinal cortex. J. Comp. Neurol. 180, 509–532. doi: 10.1002/cne.901800308
Favero, M., Sotuyo, N. P., Lopez, E., Kearney, J. A., and Goldberg, E. M. (2018). A transient developmental window of fast-spiking interneuron dysfunction in a mouse model of dravet syndrome. J. Neurosci. 38, 7912–7927. doi: 10.1523/JNEUROSCI.0193-18.2018
Fernandes, G., Mishra, P. K., Nawaz, M. S., Donlin-Asp, P. G., Rahman, M. M., Hazra, A., et al. (2021). Correction of amygdalar dysfunction in a rat model of fragile X syndrome. Cell Rep. 37:109805. doi: 10.1016/j.celrep.2021.109805
Ferron, L. (2016). Fragile X mental retardation protein controls ion channel expression and activity. J. Physiol. 594, 5861–5867.
Ferron, L., Nieto-Rostro, M., Cassidy, J. S., and Dolphin, A. C. (2014). Fragile X mental retardation protein controls synaptic vesicle exocytosis by modulating N-type calcium channel density. Nat. Commun. 5:3628. doi: 10.1038/ncomms4628
Fink, A. E., and LeDoux, J. E. (2018). beta-Adrenergic enhancement of neuronal excitability in the lateral amygdala is developmentally gated. J. Neurophysiol. 119, 1658–1664. doi: 10.1152/jn.00853.2017
Franks, K. M., and Isaacson, J. S. (2005). Synapse-specific downregulation of NMDA receptors by early experience: A critical period for plasticity of sensory input to olfactory cortex. Neuron 47, 101–114. doi: 10.1016/j.neuron.2005.05.024
Fu, Y. H., Kuhl, D. P., Pizzuti, A., Pieretti, M., Sutcliffe, J. S., Richards, S., et al. (1991). Variation of the CGG repeat at the fragile X site results in genetic instability: Resolution of the Sherman paradox. Cell 67, 1047–1058. doi: 10.1016/0092-8674(91)90283-5
Galef, B. G. Jr., and Kaner, H. C. (1980). Establishment and maintenance of preference for natural and artificial olfactory stimuli in juvenile rats. J. Comp. Physiol. Psychol. 94, 588–595. doi: 10.1037/h0077693
Gantois, I., Vandesompele, J., Speleman, F., Reyniers, E., D’hooge, R., Severijnen, L. A., et al. (2006). Expression profiling suggests underexpression of the GABA(A) receptor subunit delta in the fragile X knockout mouse model. Neurobiol. Dis. 21, 346–357. doi: 10.1016/j.nbd.2005.07.017
Garrett, A. S., Menon, V., Mackenzie, K., and Reiss, A. L. (2004). Here’s looking at you, kid: Neural systems underlying face and gaze processing in fragile X syndrome. Arch. Gen. Psychiatry 61, 281–288. doi: 10.1001/archpsyc.61.3.281
Ge, S., Goh, E. L., Sailor, K. A., Kitabatake, Y., Ming, G. L., and Song, H. (2006). GABA regulates synaptic integration of newly generated neurons in the adult brain. Nature 439, 589–593.
Gee, D. G., Gabard-Durnam, L., Telzer, E. H., Humphreys, K. L., Goff, B., Shapiro, M., et al. (2014). Maternal buffering of human amygdala-prefrontal circuitry during childhood but not during adolescence. Psychol. Sci. 25, 2067–2078. doi: 10.1177/0956797614550878
Gibson, J. R., Bartley, A. F., Hays, S. A., and Huber, K. M. (2008). Imbalance of neocortical excitation and inhibition and altered UP states reflect network hyperexcitability in the mouse model of fragile X syndrome. J. Neurophysiol. 100, 2615–2626. doi: 10.1152/jn.90752.2008
Glass, M. J., Colago, E. E., and Pickel, V. M. (2002). Alpha-2A-adrenergic receptors are present on neurons in the central nucleus of the amygdala that project to the dorsal vagal complex in the rat. Synapse 46, 258–268. doi: 10.1002/syn.10136
Gothelf, D., Furfaro, J. A., Hoeft, F., Eckert, M. A., Hall, S. S., O’hara, R., et al. (2008). Neuroanatomy of fragile X syndrome is associated with aberrant behavior and the fragile X mental retardation protein (FMRP). Ann. Neurol. 63, 40–51.
Gray, E. E., Murphy, J. G., Liu, Y., Trang, I., Tabor, G. T., Lin, L., et al. (2019). Disruption of GpI mGluR-dependent Cav2.3 translation in a mouse model of fragile X syndrome. J. Neurosci. 39, 7453–7464. doi: 10.1523/JNEUROSCI.1443-17.2019
Greba, Q., and Kokkinidis, L. (2000). Peripheral and intraamygdalar administration of the dopamine D1 receptor antagonist SCH 23390 blocks fear-potentiated startle but not shock reactivity or the shock sensitization of acoustic startle. Behav. Neurosci. 114, 262–272.
Greenblatt, E. J., and Spradling, A. C. (2018). Fragile X mental retardation 1 gene enhances the translation of large autism-related proteins. Science 361, 709–712. doi: 10.1126/science.aas9963
Gross, C., Yao, X., Pong, D. L., Jeromin, A., and Bassell, G. J. (2011). Fragile X mental retardation protein regulates protein expression and mRNA translation of the potassium channel Kv4.2. J. Neurosci. 31, 5693–5698.
Hagan, C. C., Hoeft, F., Mackey, A., Mobbs, D., and Reiss, A. L. (2008). Aberrant neural function during emotion attribution in female subjects with fragile X syndrome. J. Am. Acad. Child Adolesc Psychiatry 47, 1443–2354. doi: 10.1097/CHI.0b013e3181886e92
Hagerman, R. J., Amiri, K., and Cronister, A. (1991). Fragile X checklist. Am. J. Med. Genet. 38, 283–287.
Hagerman, R. J., Berry-Kravis, E., Hazlett, H. C., Bailey, D. B. Jr., Moine, H., Kooy, R. F., et al. (2017). Fragile X syndrome. Nat. Rev. Dis. Primers 3:17065.
Hagerman, R. J., Berry-Kravis, E., Kaufmann, W. E., Ono, M. Y., Tartaglia, N., Lachiewicz, A., et al. (2009). Advances in the treatment of fragile X syndrome. Pediatrics 123, 378–390.
Hansen, H. A., Li, J., and Saygin, Z. M. (2020). Adults vs. neonates: Differentiation of functional connectivity between the basolateral amygdala and occipitotemporal cortex. PLoS One 15:e0237204. doi: 10.1371/journal.pone.0237204
Harlow, E. G., Till, S. M., Russell, T. A., Wijetunge, L. S., Kind, P., and Contractor, A. (2010). Critical period plasticity is disrupted in the barrel cortex of FMR1 knockout mice. Neuron 65, 385–398.
Hazlett, H. C., Poe, M. D., Lightbody, A. A., Gerig, G., Macfall, J. R., Ross, A. K., et al. (2009). Teasing apart the heterogeneity of autism: Same behavior, different brains in toddlers with fragile X syndrome and autism. J. Neurodev. Disord. 1, 81–90. doi: 10.1007/s11689-009-9009-8
He, Q., Arroyo, E. D., Smukowski, S. N., Xu, J., Piochon, C., Savas, J. N., et al. (2019). Critical period inhibition of NKCC1 rectifies synapse plasticity in the somatosensory cortex and restores adult tactile response maps in fragile X mice. Mol. Psychiatry 24, 1732–1747. doi: 10.1038/s41380-018-0048-y
He, Q., Nomura, T., Xu, J., and Contractor, A. (2014). The developmental switch in GABA polarity is delayed in fragile X mice. J. Neurosci. 34, 446–450. doi: 10.1523/JNEUROSCI.4447-13.2014
Hensch, T. K. (2005). Critical period plasticity in local cortical circuits. Nat. Rev. Neurosci. 6, 877–888.
Herlenius, E., and Lagercrantz, H. (2004). Development of neurotransmitter systems during critical periods. Exp. Neurol. 190(Suppl. 1) S8–S21.
Hevers, W., and Luddens, H. (1998). The diversity of GABAA receptors. Pharmacological and electrophysiological properties of GABAA channel subtypes. Mol. Neurobiol. 18, 35–86.
Holsen, L. M., Dalton, K. M., Johnstone, T., and Davidson, R. J. (2008). Prefrontal social cognition network dysfunction underlying face encoding and social anxiety in fragile X syndrome. Neuroimage 43, 592–604. doi: 10.1016/j.neuroimage.2008.08.009
Hopkins, D. A. (1975). Amygdalotegmental projections in the rat, cat and rhesus monkey. Neurosci. Lett. 1, 263–270. doi: 10.1016/0304-3940(75)90041-5
Huang, Y. S., and Richter, J. D. (2007). Analysis of mRNA translation in cultured hippocampal neurons. Methods Enzymol. 431, 143–162.
Huang, Z. J., Kirkwood, A., Pizzorusso, T., Porciatti, V., Morales, B., Bear, M. F., et al. (1999). BDNF regulates the maturation of inhibition and the critical period of plasticity in mouse visual cortex. Cell 98, 739–755.
Hukema, R. K., Buijsen, R. A., Raske, C., Severijnen, L. A., Nieuwenhuizen-Bakker, I., Minneboo, M., et al. (2014). Induced expression of expanded CGG RNA causes mitochondrial dysfunction in vivo. Cell Cycle 13, 2600–2608. doi: 10.4161/15384101.2014.943112
Hunt, P. S., Fanselow, M. S., Richardson, R., Mauk, M. D., Freeman, J. H. Jr., and Stanton, M. E. (2007). Synapses, circuits, and the ontogeny of learning. Dev. Psychobiol. 49, 649–663.
Huntsman, M. M., and Huguenard, J. R. (2000). Nucleus-specific differences in GABA(A)-receptor-mediated inhibition are enhanced during thalamic development. J. Neurophysiol. 83, 350–358. doi: 10.1152/jn.2000.83.1.350
Iwai, Y., Fagiolini, M., Obata, K., and Hensch, T. K. (2003). Rapid critical period induction by tonic inhibition in visual cortex. J. Neurosci. 23, 6695–6702. doi: 10.1523/JNEUROSCI.23-17-06695.2003
Jagalska-Majewska, H., Wojcik, S., Dziewiatkowski, J., Luczynska, A., Kurlapska, R., and Morys, J. (2003). Postnatal development of the basolateral complex of rabbit amygdala: A stereological and histochemical study. J. Anat. 203, 513–521. doi: 10.1046/j.1469-7580.2003.00240.x
Janak, P. H., and Tye, K. M. (2015). From circuits to behaviour in the amygdala. Nature 517, 284–292.
Jeon, S. J., Kwon, H., Bae, H. J., Gonzales, E. L., Kim, J., Chung, H. J., et al. (2022). Agmatine relieves behavioral impairments in fragile X mice model. Neuropharmacology 219:109234. doi: 10.1016/j.neuropharm.2022.109234
Jiang, L., Kundu, S., Lederman, J. D., Lopez-Hernandez, G. Y., Ballinger, E. C., Wang, S., et al. (2016). Cholinergic signaling controls conditioned fear behaviors and enhances plasticity of cortical-amygdala circuits. Neuron 90, 1057–1070. doi: 10.1016/j.neuron.2016.04.028
Jiang, X., Xing, G., Yang, C., Verma, A., Zhang, L., and Li, H. (2009). Stress impairs 5-HT2A receptor-mediated serotonergic facilitation of GABA release in juvenile rat basolateral amygdala. Neuropsychopharmacology 34, 410–423. doi: 10.1038/npp.2008.71
Jin, P., Zarnescu, D. C., Zhang, F., Pearson, C. E., Lucchesi, J. C., Moses, K., et al. (2003). RNA-mediated neurodegeneration caused by the fragile X premutation rCGG repeats in Drosophila. Neuron 39, 739–747. doi: 10.1016/s0896-6273(03)00533-6
Jones, B. E. (2008). Modulation of cortical activation and behavioral arousal by cholinergic and orexinergic systems. Ann. N. Y. Acad. Sci. 1129, 26–34.
Jones, B. E., and Moore, R. Y. (1977). Ascending projections of the locus coeruleus in the rat. II. Autoradiographic study. Brain Res. 127, 25–53.
Junod, A., Opendak, M., Ledoux, J. E., and Sullivan, R. M. (2019). Development of threat expression following infant maltreatment: Infant and adult enhancement but adolescent attenuation. Front. Behav. Neurosci. 13:130. doi: 10.3389/fnbeh.2019.00130
Kang, E., Song, J., Lin, Y., Park, J., Lee, J. H., Hussani, Q., et al. (2019). Interplay between a mental disorder risk gene and developmental polarity switch of GABA action leads to excitation-inhibition imbalance. Cell Rep. 28, 1419.e3–1428.e3. doi: 10.1016/j.celrep.2019.07.024
Kates, W. R., Abrams, M. T., Kaufmann, W. E., Breiter, S. N., and Reiss, A. L. (1997). Reliability and validity of MRI measurement of the amygdala and hippocampus in children with fragile X syndrome. Psychiatry Res. 75, 31–48. doi: 10.1016/s0925-4927(97)00019-x
Kidd, S. A., Lachiewicz, A., Barbouth, D., Blitz, R. K., Delahunty, C., Mcbrien, D., et al. (2014). Fragile X syndrome: A review of associated medical problems. Pediatrics 134, 995–1005.
Kim, Y., Jeon, S. J., Gonzales, E. L., Shin, D., Remonde, C. G., Ahn, T., et al. (2022). Pirenperone relieves the symptoms of fragile X syndrome in Fmr1 knockout mice. Sci. Rep. 12:20966. doi: 10.1038/s41598-022-25582-8
Kitt, C. A., Hohmann, C., Coyle, J. T., and Price, D. L. (1994). Cholinergic innervation of mouse forebrain structures. J. Comp. Neurol. 341, 117–129.
Kroner, S., Rosenkranz, J. A., Grace, A. A., and Barrionuevo, G. (2005). Dopamine modulates excitability of basolateral amygdala neurons in vitro. J. Neurophysiol. 93, 1598–1610.
Kshatri, A., Cerrada, A., Gimeno, R., Bartolome-Martin, D., Rojas, P., and Giraldez, T. (2020). Differential regulation of BK channels by fragile X mental retardation protein. J. Gen. Physiol. 152:e201912502.
Kuhlman, S. J., Olivas, N. D., Tring, E., Ikrar, T., Xu, X., and Trachtenberg, J. T. (2013). A disinhibitory microcircuit initiates critical-period plasticity in the visual cortex. Nature 501, 543–546.
Landers, M. S., and Sullivan, R. M. (1999). Vibrissae-evoked behavior and conditioning before functional ontogeny of the somatosensory vibrissae cortex. J. Neurosci. 19, 5131–5137. doi: 10.1523/JNEUROSCI.19-12-05131.1999
Larsen, B., and Luna, B. (2018). Adolescence as a neurobiological critical period for the development of higher-order cognition. Neurosci. Biobehav. Rev. 94, 179–195. doi: 10.1016/j.neubiorev.2018.09.005
Lazarus, M. S., and Huang, Z. J. (2011). Distinct maturation profiles of perisomatic and dendritic targeting GABAergic interneurons in the mouse primary visual cortex during the critical period of ocular dominance plasticity. J. Neurophysiol. 106, 775–787. doi: 10.1152/jn.00729.2010
Lee, J., Avramets, D., Jeon, B., and Choo, H. (2021). Modulation of serotonin receptors in neurodevelopmental disorders: Focus on 5-HT7 receptor. Molecules 26:3348.
Li, S., Kim, J. H., and Richardson, R. (2012). Differential involvement of the medial prefrontal cortex in the expression of learned fear across development. Behav. Neurosci. 126, 217–225. doi: 10.1037/a0027151
Ljubojevic, V., Luu, P., Gill, P. R., Beckett, L. A., Takehara-Nishiuchi, K., and De Rosa, E. (2018). Cholinergic modulation of frontoparietal cortical network dynamics supporting supramodal attention. J. Neurosci. 38, 3988–4005. doi: 10.1523/JNEUROSCI.2350-17.2018
Louhivuori, V., Vicario, A., Uutela, M., Rantamaki, T., Louhivuori, L. M., Castren, E., et al. (2011). BDNF and TrkB in neuronal differentiation of Fmr1-knockout mouse. Neurobiol. Dis. 41, 469–480.
Lukkes, J. L., Burke, A. R., Zelin, N. S., Hale, M. W., and Lowry, C. A. (2012). Post-weaning social isolation attenuates c-Fos expression in GABAergic interneurons in the basolateral amygdala of adult female rats. Physiol. Behav. 107, 719–725. doi: 10.1016/j.physbeh.2012.05.007
Ma, Q. P., Yin, G. F., Ai, M. K., and Han, J. S. (1991). Serotonergic projections from the nucleus raphe dorsalis to the amygdala in the rat. Neurosci. Lett. 134, 21–24. doi: 10.1016/0304-3940(91)90499-j
Martin, B. S., Corbin, J. G., and Huntsman, M. M. (2014). Deficient tonic GABAergic conductance and synaptic balance in the fragile X syndrome amygdala. J. Neurophysiol. 112, 890–902. doi: 10.1152/jn.00597.2013
Martin, E. I., Ressler, K. J., Binder, E., and Nemeroff, C. B. (2010). The neurobiology of anxiety disorders: Brain imaging, genetics, and psychoneuroendocrinology. Clin. Lab. Med. 30, 865–891.
McNaughton, C. H., Moon, J., Strawderman, M. S., Maclean, K. N., Evans, J., and Strupp, B. J. (2008). Evidence for social anxiety and impaired social cognition in a mouse model of fragile X syndrome. Behav. Neurosci. 122, 293–300. doi: 10.1037/0735-7044.122.2.293
Meredith, R. M. (2015). Sensitive and critical periods during neurotypical and aberrant neurodevelopment: A framework for neurodevelopmental disorders. Neurosci. Biobehav. Rev. 50, 180–188. doi: 10.1016/j.neubiorev.2014.12.001
Meredith, R. M., Dawitz, J., and Kramvis, I. (2012). Sensitive time-windows for susceptibility in neurodevelopmental disorders. Trends Neurosci. 35, 335–344.
Moriceau, S., Shionoya, K., Jakubs, K., and Sullivan, R. M. (2009). Early-life stress disrupts attachment learning: The role of amygdala corticosterone, locus ceruleus corticotropin releasing hormone, and olfactory bulb norepinephrine. J. Neurosci. 29, 15745–15755. doi: 10.1523/JNEUROSCI.4106-09.2009
Moriceau, S., and Sullivan, R. M. (2005). Neurobiology of infant attachment. Dev. Psychobiol. 47, 230–242.
Moriceau, S., and Sullivan, R. M. (2006). Maternal presence serves as a switch between learning fear and attraction in infancy. Nat. Neurosci. 9, 1004–1006. doi: 10.1038/nn1733
Mukherjee, B., Harley, C. W., and Yuan, Q. (2017). Learning-induced metaplasticity? Associative training for early odor preference learning down-regulates synapse-specific NMDA receptors via mGluR and calcineurin activation. Cereb. Cortex 27, 616–624. doi: 10.1093/cercor/bhv256
Nader, K., and LeDoux, J. E. (1999). Inhibition of the mesoamygdala dopaminergic pathway impairs the retrieval of conditioned fear associations. Behav. Neurosci. 113, 891–901. doi: 10.1037//0735-7044.113.5.891
Nadim, F., and Bucher, D. (2014). Neuromodulation of neurons and synapses. Curr. Opin. Neurobiol. 29, 48–56.
Nelson, S. B., and Valakh, V. (2015). Excitatory/inhibitory balance and circuit homeostasis in autism spectrum disorders. Neuron 87, 684–698.
Olmos-Serrano, J. L., Paluszkiewicz, S. M., Martin, B. S., Kaufmann, W. E., Corbin, J. G., and Huntsman, M. M. (2010). Defective GABAergic neurotransmission and pharmacological rescue of neuronal hyperexcitability in the amygdala in a mouse model of fragile X syndrome. J. Neurosci. 30, 9929–9938. doi: 10.1523/JNEUROSCI.1714-10.2010
Opendak, M., Raineki, C., Perry, R. E., Rincon-Cortes, M., Song, S. C., Zanca, R. M., et al. (2021). Bidirectional control of infant rat social behavior via dopaminergic innervation of the basolateral amygdala. Neuron 109, 4018.e7–4035.e7. doi: 10.1016/j.neuron.2021.09.041
Opendak, M., Robinson-Drummer, P., Blomkvist, A., Zanca, R. M., Wood, K., Jacobs, L., et al. (2019). Neurobiology of maternal regulation of infant fear: The role of mesolimbic dopamine and its disruption by maltreatment. Neuropsychopharmacology 44, 1247–1257. doi: 10.1038/s41386-019-0340-9
Opendak, M., Theisen, E., Blomkvist, A., Hollis, K., Lind, T., Sarro, E., et al. (2020). Adverse caregiving in infancy blunts neural processing of the mother. Nat. Commun. 11:1119. doi: 10.1038/s41467-020-14801-3
Opendak, M., Zanca, R. M., Anane, E., Serrano, P. A., and Sullivan, R. M. (2018). Developmental transitions in amygdala PKC isoforms and AMPA receptor expression associated with threat memory in infant rats. Sci. Rep. 8:14679. doi: 10.1038/s41598-018-32762-y
Orefice, L. L., Mosko, J. R., Morency, D. T., Wells, M. F., Tasnim, A., Mozeika, S. M., et al. (2019). Targeting peripheral somatosensory neurons to improve tactile-related phenotypes in ASD models. Cell 178, 867.e24–886.e24. doi: 10.1016/j.cell.2019.07.024
Oruro, E. M., Pardo, G. V. E., Lucion, A. B., Calcagnotto, M. E., and Idiart, M. A. P. (2020). Maturation of pyramidal cells in anterior piriform cortex may be sufficient to explain the end of early olfactory learning in rats. Learn. Mem. 27, 20–32. doi: 10.1101/lm.050724.119
Paluszkiewicz, S. M., Olmos-Serrano, J. L., Corbin, J. G., and Huntsman, M. M. (2011b). Impaired inhibitory control of cortical synchronization in fragile X syndrome. J. Neurophysiol. 106, 2264–2272. doi: 10.1152/jn.00421.2011
Paluszkiewicz, S. M., Martin, B. S., and Huntsman, M. M. (2011a). Fragile X syndrome: The GABAergic system and circuit dysfunction. Dev. Neurosci. 33, 349–364.
Papay, R., Gaivin, R., Jha, A., Mccune, D. F., Mcgrath, J. C., Rodrigo, M. C., et al. (2006). Localization of the mouse alpha1A-adrenergic receptor (AR) in the brain: Alpha1aar is expressed in neurons, GABAergic interneurons, and NG2 oligodendrocyte progenitors. J. Comp. Neurol. 497, 209–222. doi: 10.1002/cne.20992
Paradee, W., Melikian, H. E., Rasmussen, D. L., Kenneson, A., Conn, P. J., and Warren, S. T. (1999). Fragile X mouse: Strain effects of knockout phenotype and evidence suggesting deficient amygdala function. Neuroscience 94, 185–192. doi: 10.1016/s0306-4522(99)00285-7
Pascual, J., Del Arco, C., Gonzalez, A. M., and Pazos, A. (1992). Quantitative light microscopic autoradiographic localization of alpha 2-adrenoceptors in the human brain. Brain Res. 585, 116–127. doi: 10.1016/0006-8993(92)91196-l
Pattwell, S. S., Liston, C., Jing, D., Ninan, I., Yang, R. R., Witztum, J., et al. (2016). Dynamic changes in neural circuitry during adolescence are associated with persistent attenuation of fear memories. Nat. Commun. 7:11475. doi: 10.1038/ncomms11475
Pazos, A., Probst, A., and Palacios, J. M. (1985). Beta-adrenoceptor subtypes in the human brain: Autoradiographic localization. Brain Res. 358, 324–328. doi: 10.1016/0006-8993(85)90977-1
Pedersen, P. E., Williams, C. L., and Blass, E. M. (1982). Activation and odor conditioning of suckling behavior in 3-day-old albino rats. J. Exp. Psychol. Anim. Behav. Process 8, 329–341.
Perry, R. E., Blair, C., and Sullivan, R. M. (2017). Neurobiology of infant attachment: Attachment despite adversity and parental programming of emotionality. Curr. Opin. Psychol. 17, 1–6. doi: 10.1016/j.copsyc.2017.04.022
Pieribone, V. A., Nicholas, A. P., Dagerlind, A., and Hokfelt, T. (1994). Distribution of alpha 1 adrenoceptors in rat brain revealed by in situ hybridization experiments utilizing subtype-specific probes. J. Neurosci. 14, 4252–4268. doi: 10.1523/JNEUROSCI.14-07-04252.1994
Pinard, C. R., Muller, J. F., Mascagni, F., and Mcdonald, A. J. (2008). Dopaminergic innervation of interneurons in the rat basolateral amygdala. Neuroscience 157, 850–863.
Rainbow, T. C., Parsons, B., and Wolfe, B. B. (1984). Quantitative autoradiography of beta 1- and beta 2-adrenergic receptors in rat brain. Proc. Natl. Acad. Sci. U.S.A. 81, 1585–1589.
Raineki, C., Opendak, M., Sarro, E., Showler, A., Bui, K., Mcewen, B. S., et al. (2019). During infant maltreatment, stress targets hippocampus, but stress with mother present targets amygdala and social behavior. Proc. Natl. Acad. Sci. U.S.A. 116, 22821–22832. doi: 10.1073/pnas.1907170116
Raineki, C., Shionoya, K., Sander, K., and Sullivan, R. M. (2009). Ontogeny of odor-LiCl vs. odor-shock learning: Similar behaviors but divergent ages of functional amygdala emergence. Learn. Mem. 16, 114–121. doi: 10.1101/lm.977909
Rainnie, D. G. (1999). Serotonergic modulation of neurotransmission in the rat basolateral amygdala. J. Neurophysiol. 82, 69–85.
Reiss, A. L., Abrams, M. T., Greenlaw, R., Freund, L., and Denckla, M. B. (1995). Neurodevelopmental effects of the FMR-1 full mutation in humans. Nat. Med. 1, 159–167. doi: 10.1038/nm0295-159
Reyes, S. T., Mohajeri, S., Krasinska, K., Guo, S. G., Gu, M., Pisani, L., et al. (2020). GABA Measurement in a neonatal fragile X syndrome mouse model using (1)H-magnetic resonance spectroscopy and mass spectrometry. Front. Mol. Neurosci. 13:612685. doi: 10.3389/fnmol.2020.612685
Rosenkranz, J. A., and Grace, A. A. (1999). Modulation of basolateral amygdala neuronal firing and afferent drive by dopamine receptor activation in vivo. J. Neurosci. 19, 11027–11039.
Rosenkranz, J. A., and Grace, A. A. (2002b). Dopamine-mediated modulation of odour-evoked amygdala potentials during pavlovian conditioning. Nature 417, 282–287. doi: 10.1038/417282a
Rosenkranz, J. A., and Grace, A. A. (2002a). Cellular mechanisms of infralimbic and prelimbic prefrontal cortical inhibition and dopaminergic modulation of basolateral amygdala neurons in vivo. J. Neurosci. 22, 324–337. doi: 10.1523/JNEUROSCI.22-01-00324.2002
Rubenstein, J. L., and Merzenich, M. M. (2003). Model of autism: Increased ratio of excitation/inhibition in key neural systems. Genes Brain Behav. 2, 255–267.
Rudolph, U., and Mohler, H. (2006). GABA-based therapeutic approaches: GABAA receptor subtype functions. Curr. Opin. Pharmacol. 6, 18–23.
Ryan, S. J., Ehrlich, D. E., and Rainnie, D. G. (2016). Morphology and dendritic maturation of developing principal neurons in the rat basolateral amygdala. Brain Struct. Funct. 221, 839–854. doi: 10.1007/s00429-014-0939-x
Santiago, A. N., Lim, K. Y., Opendak, M., Sullivan, R. M., and Aoki, C. (2018). Early life trauma increases threat response of peri-weaning rats, reduction of axo-somatic synapses formed by parvalbumin cells and perineuronal net in the basolateral nucleus of amygdala. J. Comp. Neurol. 526, 2647–2664. doi: 10.1002/cne.24522
Sears, J. C., and Broadie, K. (2017). Fragile X mental retardation protein regulates activity-dependent membrane trafficking and trans-synaptic signaling mediating synaptic remodeling. Front. Mol. Neurosci. 10:440. doi: 10.3389/fnmol.2017.00440
Shen, J., and Colonnese, M. T. (2016). Development of activity in the mouse visual cortex. J. Neurosci. 36, 12259–12275.
Shen, M. D., Swanson, M. R., Wolff, J. J., Elison, J. T., Girault, J. B., Kim, S. H., et al. (2022). Subcortical brain development in autism and fragile X syndrome: Evidence for dynamic, age- and disorder-specific trajectories in infancy. Am. J. Psychiatry 179, 562–572. doi: 10.1176/appi.ajp.21090896
Shionoya, K., Moriceau, S., Bradstock, P., and Sullivan, R. M. (2007). Maternal attenuation of hypothalamic paraventricular nucleus norepinephrine switches avoidance learning to preference learning in preweanling rat pups. Horm. Behav. 52, 391–400. doi: 10.1016/j.yhbeh.2007.06.004
Sidorov, M. S., Auerbach, B. D., and Bear, M. F. (2013). Fragile X mental retardation protein and synaptic plasticity. Mol. Brain 6:15.
Strange, B. A., and Dolan, R. J. (2004). Beta-adrenergic modulation of emotional memory-evoked human amygdala and hippocampal responses. Proc. Natl. Acad. Sci. U.SA. 101, 11454–11458. doi: 10.1073/pnas.0404282101
Sullivan, R. M. (2001). Unique characteristics of neonatal classical conditioning: The role of the amygdala and locus coeruleus. Integr. Physiol. Behav. Sci. 36, 293–307. doi: 10.1007/BF02688797
Sullivan, R. M., Landers, M., Yeaman, B., and Wilson, D. A. (2000a). Good memories of bad events in infancy. Nature 407, 38–39. doi: 10.1038/35024156
Sullivan, R. M., Stackenwalt, G., Nasr, F., Lemon, C., and Wilson, D. A. (2000b). Association of an odor with activation of olfactory bulb noradrenergic beta-receptors or locus coeruleus stimulation is sufficient to produce learned approach responses to that odor in neonatal rats. Behav. Neurosci. 114, 957–962.
Sullivan, R. M., Landers, M. S., Flemming, J., Vaught, C., Young, T. A., and Jonathan Polan, H. (2003). Characterizing the functional significance of the neonatal rat vibrissae prior to the onset of whisking. Somatosens. Mot. Res. 20, 157–162. doi: 10.1080/0899022031000105190
Sullivan, R. M., Mcgaugh, J. L., and Leon, M. (1991). Norepinephrine-induced plasticity and one-trial olfactory learning in neonatal rats. Brain Res. Dev. Brain Res. 60, 219–228. doi: 10.1016/0165-3806(91)90050-s
Suvrathan, A., Hoeffer, C. A., Wong, H., Klann, E., and Chattarji, S. (2010). Characterization and reversal of synaptic defects in the amygdala in a mouse model of fragile X syndrome. Proc. Natl. Acad. Sci. U.S.A. 107, 11591–11596. doi: 10.1073/pnas.1002262107
Svalina, M. N., Guthman, E. M., Cea-Del Rio, C. A., Kushner, J. K., Baca, S. M., Restrepo, D., et al. (2021). Hyperexcitability and loss of feedforward inhibition contribute to aberrant plasticity in the Fmr1KO amygdala. eNeuro 8:ENEURO.0113–21.2021. doi: 10.1523/ENEURO.0113-21.2021
Svalina, M. N., Rio, C. C., Kushner, J. K., Levy, A., Baca, S. M., Guthman, E. M., et al. (2022). Basolateral amygdala hyperexcitability is associated with precocious developmental emergence of fear-learning in Fragile X Syndrome. J. Neurosci. 42, 7294–7308. doi: 10.1523/JNEUROSCI.1776-21.2022
Swinnen, B., Robberecht, W., and Van Den Bosch, L. (2020). RNA toxicity in non-coding repeat expansion disorders. EMBO J. 39:e101112.
Szabadi, E. (2013). Functional neuroanatomy of the central noradrenergic system. J. Psychopharmacol. 27, 659–693.
Teicher, M. H., and Blass, E. M. (1977). First suckling response of the newborn albino rat: The roles of olfaction and amniotic fluid. Science 198, 635–636. doi: 10.1126/science.918660
Tonnsen, B. L., Shinkareva, S. V., Deal, S. C., Hatton, D. D., and Roberts, J. E. (2013). Biobehavioral indicators of social fear in young children with fragile X syndrome. Am. J. Intellect. Dev. Disabil. 118, 447–459. doi: 10.1352/1944-7558-118.6.447
Torres, Y. P., Morera, F. J., Carvacho, I., and Latorre, R. (2007). A marriage of convenience: Beta-subunits and voltage-dependent K+ channels. J. Biol. Chem. 282, 24485–24489. doi: 10.1074/jbc.R700022200
Tottenham, N., Shapiro, M., Flannery, J., Caldera, C., and Sullivan, R. M. (2019). Parental presence switches avoidance to attraction learning in children. Nat. Hum. Behav. 3, 1070–1077. doi: 10.1038/s41562-019-0656-9
Tully, K., Li, Y., Tsvetkov, E., and Bolshakov, V. Y. (2007). Norepinephrine enables the induction of associative long-term potentiation at thalamo-amygdala synapses. Proc. Natl. Acad. Sci. U.S.A. 104, 14146–14150. doi: 10.1073/pnas.0704621104
Uematsu, A., Tan, B. Z., Ycu, E. A., Cuevas, J. S., Koivumaa, J., Junyent, F., et al. (2017). Modular organization of the brainstem noradrenaline system coordinates opposing learning states. Nat. Neurosci. 20, 1602–1611. doi: 10.1038/nn.4642
Upton, K. J., and Sullivan, R. M. (2010). Defining age limits of the sensitive period for attachment learning in rat pups. Dev. Psychobiol. 52, 453–464. doi: 10.1002/dev.20448
Verkerk, A. J., Pieretti, M., Sutcliffe, J. S., Fu, Y. H., Kuhl, D. P., Pizzuti, A., et al. (1991). Identification of a gene (FMR-1) containing a CGG repeat coincident with a breakpoint cluster region exhibiting length variation in fragile X syndrome. Cell 65, 905–914. doi: 10.1016/0092-8674(91)90397-h
Vislay, R. L., Martin, B. S., Olmos-Serrano, J. L., Kratovac, S., Nelson, D. L., Corbin, J. G., et al. (2013). Homeostatic responses fail to correct defective amygdala inhibitory circuit maturation in fragile X syndrome. J. Neurosci. 33, 7548–7558. doi: 10.1523/JNEUROSCI.2764-12.2013
Waclaw, R. R., Ehrman, L. A., Pierani, A., and Campbell, K. (2010). Developmental origin of the neuronal subtypes that comprise the amygdalar fear circuit in the mouse. J. Neurosci. 30, 6944–6953. doi: 10.1523/JNEUROSCI.5772-09.2010
Wanaka, A., Kiyama, H., Murakami, T., Matsumoto, M., Kamada, T., Malbon, C. C., et al. (1989). Immunocytochemical localization of beta-adrenergic receptors in the rat brain. Brain Res. 485, 125–140.
Wang, Y., Liu, Y., Xiong, J., Di, T., Yuan, Z., Wu, J., et al. (2019). Reduced serotonin impairs long-term depression in basolateral amygdala complex and causes anxiety-like behaviors in a mouse model of perimenopause. Exp. Neurol. 321:113030. doi: 10.1016/j.expneurol.2019.113030
Weiler, I. J., and Greenough, W. T. (1993). Metabotropic glutamate receptors trigger postsynaptic protein synthesis. Proc. Natl. Acad. Sci. U.S.A. 90, 7168–7171.
Weiler, I. J., Irwin, S. A., Klintsova, A. Y., Spencer, C. M., Brazelton, A. D., Miyashiro, K., et al. (1997). Fragile X mental retardation protein is translated near synapses in response to neurotransmitter activation. Proc. Natl. Acad. Sci. U.S.A. 94, 5395–5400.
Woolf, N. J. (1991). Cholinergic systems in mammalian brain and spinal cord. Prog. Neurobiol. 37, 475–524.
Woolf, N. J., and Butcher, L. L. (1982). Cholinergic projections to the basolateral amygdala: A combined Evans Blue and acetylcholinesterase analysis. Brain Res. Bull. 8, 751–763. doi: 10.1016/0361-9230(82)90102-2
Zanoveli, J. M., Carvalho, M. C., Cunha, J. M., and Brandao, M. L. (2009). Extracellular serotonin level in the basolateral nucleus of the amygdala and dorsal periaqueductal gray under unconditioned and conditioned fear states: An in vivo microdialysis study. Brain Res. 1294, 106–115. doi: 10.1016/j.brainres.2009.07.074
Zhang, Y., Bonnan, A., Bony, G., Ferezou, I., Pietropaolo, S., Ginger, M., et al. (2014). Dendritic channelopathies contribute to neocortical and sensory hyperexcitability in Fmr1(-/y) mice. Nat. Neurosci. 17, 1701–1709. doi: 10.1038/nn.3864
Zhang, Z., Marro, S. G., Zhang, Y., Arendt, K. L., Patzke, C., Zhou, B., et al. (2018). The fragile X mutation impairs homeostatic plasticity in human neurons by blocking synaptic retinoic acid signaling. Sci. Transl. Med. 10:eaar4338. doi: 10.1126/scitranslmed.aar4338
Keywords: critical period, synaptic plasticity, basolateral amygdala, fragile X syndrome, development, E/I balance
Citation: Svalina MN, Sullivan R, Restrepo D and Huntsman MM (2023) From circuits to behavior: Amygdala dysfunction in fragile X syndrome. Front. Integr. Neurosci. 17:1128529. doi: 10.3389/fnint.2023.1128529
Received: 20 December 2022; Accepted: 23 February 2023;
Published: 09 March 2023.
Edited by:
Susanne Schmid, Western University, CanadaReviewed by:
Darrin Brager, The University of Texas at Austin, United StatesXinxing Wang, Stony Brook University, United States
Copyright © 2023 Svalina, Sullivan, Restrepo and Huntsman. This is an open-access article distributed under the terms of the Creative Commons Attribution License (CC BY). The use, distribution or reproduction in other forums is permitted, provided the original author(s) and the copyright owner(s) are credited and that the original publication in this journal is cited, in accordance with accepted academic practice. No use, distribution or reproduction is permitted which does not comply with these terms.
*Correspondence: Molly M. Huntsman, bW9sbHkuaHVudHNtYW5AY3VhbnNjaHV0ei5lZHU=