- 1Department of Biology, University of Virginia, Charlottesville, VA, United States
- 2Department of Biological Sciences, California State Polytechnic University Pomona, Pomona, CA, United States
- 3Program in Fundamental Neuroscience, University of Virginia, Charlottesville, VA, United States
- 4Department of Neuroscience, School of Medicine, University of Virginia, Charlottesville, VA, United States
How dopamine signaling regulates biological rhythms is an area of emerging interest. Here we review experiments focused on delineating dopamine signaling in the suprachiasmatic nucleus, nucleus accumbens, and dorsal striatum to mediate a range of biological rhythms including photoentrainment, activity cycles, rest phase eating of palatable food, diet-induced obesity, and food anticipatory activity. Enthusiasm for causal roles for dopamine in the regulation of circadian rhythms, particularly those associated with food and other rewarding events, is warranted. However, determining that there is rhythmic gene expression in dopamine neurons and target structures does not mean that they are bona fide circadian pacemakers. Given that dopamine has such a profound role in promoting voluntary movements, interpretation of circadian phenotypes associated with locomotor activity must be differentiated at the molecular and behavioral levels. Here we review our current understanding of dopamine signaling in relation to biological rhythms and suggest future experiments that are aimed at teasing apart the roles of dopamine subpopulations and dopamine receptor expressing neurons in causally mediating biological rhythms, particularly in relation to feeding, reward, and activity.
Introduction
Eating disorders affect a wide range of ages and impact both men and women. In the United States, over a third of the population is obese, which is the greatest risk factor for chronic disorders such as diabetes, cardiovascular disease and kidney diseases (Guh et al., 2009; Hurt et al., 2010; Eckel et al., 2011; de Mutsert et al., 2014; Flegal et al., 2016; CDC, 2019; Milken Institute, 2019); meanwhile, anorexia nervosa remains the most fatal of all psychiatric diseases (Attia, 2010; Treasure et al., 2015). Therefore, it is imperative to invest in research to determine the molecular underpinnings of disorders associated with over- and under-eating. Recently, increasing attention has focused on intermittent fasting and how the timing of food intake can have a profound impact on health measures and body weight homeostasis (Hatori et al., 2012; Garaulet et al., 2013; Sutton et al., 2018; Acosta-Rodríguez et al., 2022). A relatively unexplored aspect of feeding behavior relates to how the timing of food intake is governed, especially when food is available ad libitum (Turek et al., 2005; Acosta-Rodríguez et al., 2017; Challet, 2019).
A wide range of behaviors and physiological processes are adapted to have a rhythmic pattern that are synchronized with the light-dark cycle. Daily exposure to light sets the activity of the suprachiasmatic nucleus (SCN) of the hypothalamus, serving as the body’s central pacemaker for a variety of physiological, psychological, and behavioral processes (Abe et al., 1979; Bass and Takahashi, 2010; Patton and Mistlberger, 2013). Lesion and transplant studies (Meyer-Bernstein and Morin, 1999) have demonstrated that the SCN is the principal regulator of all light entrained rhythms (Stephan and Zucker, 1972; Abe et al., 1979; Edgar et al., 1993), but there are some exceptions such as photoreceptors in skin cells that can drive local circadian rhythms in subpopulations of tissues (Buhr et al., 2019). Remarkably, the SCN maintains rhythmic activity even when explanted from a rodent’s brain and maintained in vitro (Abe et al., 2002; Yoo et al., 2004).
The SCN is able to control these internal biological rhythms through a transcription-translation feedback loop (TTFL) dictated by main core-clock proteins, Clock and Bmal1, which dimerize and trigger a downstream cascade of transcriptional-translational feedback loops with proteins including Cry, Per, and Rev-Erb (Patke et al., 2020). This process is cell-autonomous and maintains a period of approximately 24-h in the absence of external time cues (Panda et al., 2002; Takahashi, 2017). Light and other external factors (e.g., food availability) called “zeitgebers,” or time-givers, modulate the expression of clock genes to reset and entrain the timing of the circadian clock in the SCN, other brain regions or peripheral tissues (Challet, 2019; Mendoza, 2019a). Interestingly, mice with SCN lesions still maintain circadian rhythms in their peripheral tissues but the rhythms between tissues are desynchronized, showing that the SCN is vital for synchronization (Yoo et al., 2004). There is emerging interest in elucidating biological clocks outside of the SCN and determining how non-photic stimuli such as exercise, mating, fear and feeding compete, and collaborate with the SCN to regulate circadian cycles (Richter, 1922; Edgar and Dement, 1991; Landry et al., 2012).
Food as a zeitgeber
Several brain regions and many peripheral tissues can be entrained by daily cycles of feeding (Mistlberger, 2011; Piggins and Bechtold, 2015). In rodents, this is readily demonstrated by restricting food access to the middle of the light period when nocturnal rodents normally eat little and are inactive (Pendergast and Yamazaki, 2018). Food restriction induces a pronounced shift of organ physiology and animal behavior to align with the new daily feeding time, while the activity of the SCN remains coupled to light cycles (Stokkan et al., 2001). This is also associated with the emergence of a daily burst of motor activity that anticipates mealtime by 1–3 h and an increase in core body temperature (Figure 1) (Mistlberger and Antle, 2011). Remarkably, this “food anticipatory activity” (FAA) persists robustly after removal of the SCN (Davidson, 2009). The underlying neuronal systems and/or circuitry responsible for mediating FAA have been contested, with very few studies showing reproducible effects of genetic mutations or lesions to the brain (Figure 1) (Davidson, 2009; Gunapala et al., 2011). At present, nuclei as diverse as the cerebellum (Mendoza et al., 2010a) hypothalamic areas including dorsomedial hypothalamus (DMH) (Mieda et al., 2006; Acosta-Galvan et al., 2011) and arcuate nucleus (Podyma et al., 2020); and striatum (Liu et al., 2012; Gallardo et al., 2014), have been implicated in promoting FAA. The only area of consistent agreement is that the SCN is not required for FAA (Krieger et al., 1977; Stephan et al., 1979; Davidson, 2009; Takasu et al., 2012; Mistlberger, 2020), although it might modulate the amplitude of food rhythms in some contexts (Angeles-Castellanos et al., 2010; Fernandez et al., 2020). Since there has been so much difficulty in isolating the neural constituent(s) of FAA, it has been suggested that such a region, referred to as a food entrainable oscillator (FEO), may not exist as a singular entity; therefore lesioning studies may be too narrow of an approach, and the molecular tagging or systems based approaches may offer higher potential in locating multiple FEOs distributed across the brain and body (Davidson, 2009; Mistlberger, 2011, 2020; Nishide et al., 2021). The ability of rodents to have multiple bouts of FAA entrained to multiple food deliveries with same or different cycle period further provided evidence that food entrainment is regulated by a multi-core network (Petersen et al., 2022).
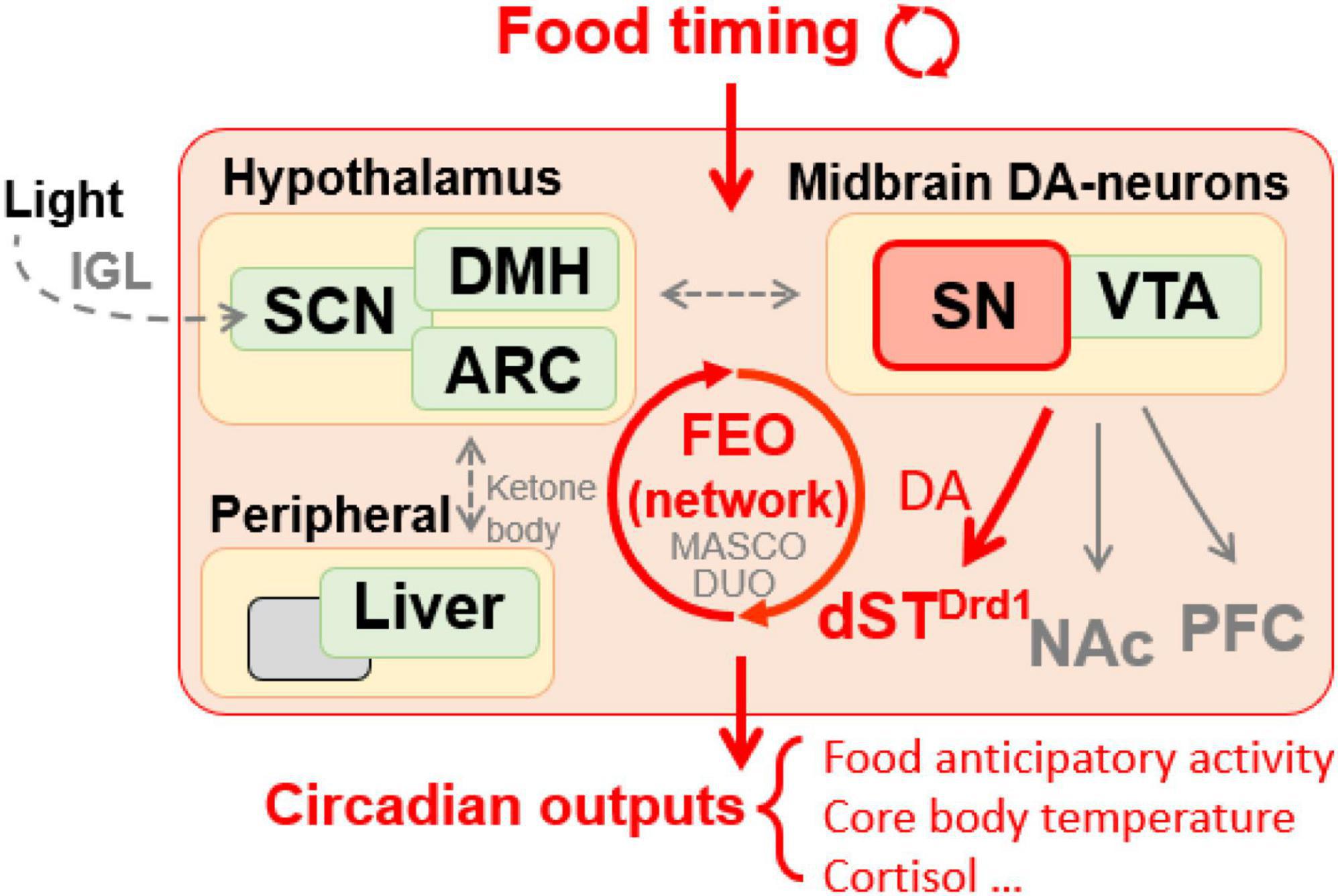
Figure 1. Food timing, particularly when restricted temporally, is a potent zeitgeber entraining an oscillatory system in the brain, relaying rhythmic behavior outputs. DA signaling is required in SN neurons projecting to the dorsal striatum but not the NAc or PFC to mediate food anticipatory activity. Peripheral oscillators, like the liver, may contribute to food entrainment via ketone bodies secretion to unknown brain target(s). Hypothalamic areas are also suggested to be involved in food entrainment regulation. Ambient light pathway via IGL influences the proper development of SCN structure which is necessary for proper food entrainment. SN, substantia nigra; VTA, ventral tegmental area; ARC, arcuate nucleus; DMH, dorsomedial hypothalamus; SCN, suprachiasmatic nucleus; IGL, intergeniculate leaflet; Drd1, D1 dopamine receptor; dST, dorsal striatum; NAc, nucleus accumbens; PFC, prefrontal cortex; FEO, food entrainable oscillator; MASCO, methamphetamine-sensitive circadian oscillator; DUO, dopaminergic ultradian oscillator.
By analogy to studies of light entrainment, researchers initially focused on using mouse mutants in known molecular components of the core clock TTFL to map the brain region(s) responsible for FAA, yet these experiments have yielded mixed results. For example, mutations of Bmal1 and double/triple deletions of Per genes did not reveal effects on FAA in mice (Storch and Weitz, 2009; Pendergast et al., 2017). However, this is not to say that clock genes are completely uninvolved in food entrainment, for example, Bmal1 and Cry knock out (KO) mice could be entrained to shorter feeding cycles that are outside of circadian range and cannot entrain WT animals (Takasu et al., 2012). Moreover, Mendoza and colleagues report several studies of Per and Cry mutants also had diminished/unstable FAA and desynchronized expectations of mealtimes (Mendoza et al., 2010a). Interestingly, mice with global and brain-specific deletion of Nr1d1, which encodes for Rev-Erbα, an inhibitor of Bmal1 transcription, showed neither light nor food entrainment (Delezie et al., 2016). A surprising experiment demonstrated that conditional deletion of Per2 in the liver nearly abolished FAA, whereas, deletion of Per2 brain deletion had no impact, leading the authors to conclude that the liver is the long sought after FEO (Figure 1) (Chavan et al., 2016). Interestingly, mice with liver-specific deletion of Per2 were observed to have a reduction in enzymes associated with β-oxidation, suggesting that ketone body production was important for mediating FAA. In support of this, time-specific application of β-hydroxybutyrate in the Per2 liver conditional KO mice rescued FAA modestly (Chavan et al., 2016). It is important to note that these results have not yet been replicated and it would be of interest to test feeding schedules with mealtimes shorter than 8 h. In summary, exploring the requirement of clock genes on FAA has borne some fruit and determining which brain region(s) responds to the liver-derived ketones released in fasting or where in the brain Rev-Erbα is required for FAA would be valuable in mapping the neural circuitry that supports food entrainment.
Dopamine rhythms and their influence on circadian behaviors
Dopamine (DA), an important part of a family of catecholamine neuromodulators contributing to a number of behaviors, is most intensely studied in the context of motivation, reward, and addiction (Wise, 2004; Gerfen and Surmeier, 2011; Steele and Mistlberger, 2015). DA is required for both feeding and locomotor behavior (Szczypka et al., 2001), which makes it an obvious potential agent of circadian activity rhythm entrainment by scheduled feeding or other rewarding stimuli (Verwey et al., 2016; Korshunov et al., 2017). A contentious issue is whether DA populations respond to circadian oscillators, like the SCN, or are able to harbor autonomous rhythms that respond to reward-related zeitgebers.
Some of the critical evidence that the DA system is under circadian control comes from studies of canonical clock proteins that directly affect DA expression and regulation. For example, Rev-Erbα (nuclear receptor subfamily 1 group D member 1), a circadian nuclear receptor involved with negative regulation of the TTFL targeting Bmal1 mRNA, has been shown to impact midbrain DA production by suppression of tyrosine hydroxylase (TH) mRNA production (Chung et al., 2014). TH levels were found to be highest at night while Rev-Erbα levels were lowest, suggesting an inverse relationship. In addition, mice with Rev-Erbα gene deleted have higher DA release in the nucleus accumbens (NAc) (Chung et al., 2014). It appears that Nurr1 competes with Rev-Erbα to promote TH expression; consistent with this mice heterozygous for Nurr1 deletion have altered circadian phenotypes (Partington et al., 2021). In rodents, extracellular DA levels, i.e., “dopamine tone,” in the striatum is at their highest during the night and lowest during the day; this is mediated by the activity of the dopamine transporter (DAT or Slc6a3), which is responsible for reuptake of DA at synapse–a primary means of terminating DA signals (Ferris et al., 2014). Interestingly, the firing of VTA neurons is highest early in the light cycle and early on in the dark cycle, following 12-h rhythms; both times VTA neurons are equally sensitive to suppression of firing induced by methamphetamine (Domínguez-López et al., 2014). In a separate study, higher firing rates of VTA neurons were observed at night with no changes reported for substantia nigra (SN) neurons across circadian time (Domínguez-López et al., 2014). Luo et al. (2008) identified a small subset of VTA neurons that appear to be night active, highlighting the need for approaches that allow for characterizing individual populations of DA neurons.
On the other hand, DA acts to drive circadian gene expression of core circadian clock genes at downstream targets. For example, depleting DA release using 6-OHDA to lesion DA neurons projecting to the dorsal striatum (DS) causes a loss of rhythmicity of the Per2 gene in striatum (Hood et al., 2010). Intraventricular infusion of 6-OHDA disrupted circadian rhythms of activity in adult rats markedly in constant darkness, suggesting that DA signaling is required to maintain rhythmicity (total activity levels were also suppressed but not eliminated) (Gravotta et al., 2011). Thus, the question arises as to whether feeding rodents out of synchrony with the normal rise and fall of DA levels can shift this DA rhythm to increase DA in anticipation of scheduled feeding. This shift was speculated by de Lartigue and McDougle in a review article, but it has never been demonstrated experimentally (de Lartigue and McDougle, 2019). These authors assert that the DS is the long-sought FEO, but the evidence in support of this hypothesis is just beginning to be assembled. Some hints at the involvement of DA circuitry and food entrainment come from pharmacological studies. For example, Liu and colleagues administered a DA type 1 receptor (Drd1) antagonist in ICR mice after 2 weeks of food restriction and noted a small decrease (∼20%) in total activity 2 h prior to scheduled mealtimes (Liu et al., 2012). Similarly, treatment with a Drd2 antagonist also decreased total activity in mice by ∼40% prior to scheduled mealtimes (Liu et al., 2012; Smit et al., 2013). Small effects of dopaminergic drugs were also observed in food entrained rats: Drd2 agonist (quinpirole) or DA synthesis antagonist AMPT shifted FAA onset and lowered its amplitude while Drd1 agonist had no effect (Liu et al., 2012; Smit et al., 2013). While these experiments implicate both Drd1 and Drd2 in modulating FAA, such pharmacological experiments are complicated in their interpretation given that Drd2 is expressed on DA neurons, Drd1 is expressed in the SCN (Grippo et al., 2017), and there are many other target sites expressing DA receptors as well (Martel and Gatti McArthur, 2020).
There is accumulating evidence for the involvement of the DA system in regulating circadian entrainment to feeding (de Lartigue and McDougle, 2019). Of the nine regions in the adult mammalian brain harboring DA neurons (Björklund and Dunnett, 2007), those in the ventral midbrain appear critical to linking circadian rhythms associated with feeding or other rewarding stimuli (Schultz, 2016). The VTA DA neurons are known to be important for driving rest phase feeding behavior, and the VTA-NAc pathway has been implicated to be an extra-SCN oscillator that is probably responsible for circadian rhythm disruption related drug abuse (Becker-Krail et al., 2022) (discussed further below). However, the entrainment to scheduled feeding has been linked to a different population of DA neurons: those in the lateral portion of the midbrain, termed the substantia nigra (SN), which project to the DS and are best known for their demise during Parkinson’s disease (Björklund and Dunnett, 2007). Global deletion of Drd1 markedly attenuates FAA, whereas, Drd2 deletion does not (Gallardo et al., 2014). Viral reintroduction of DA production in the DS of dopamine-deficient mice was permissive for FAA, suggesting that the only location DA is needed for FAA is in the nigrostriatal pathway, but, importantly, not establishing the DS as an FEO (Gallardo et al., 2014). Daily pharmacological activation of Drd1 neurons via systemic injection is sufficient to entrain circadian activity rhythms even without manipulating feeding (Gallardo et al., 2014). Taken together, these results indicated that Drd1 is required for stable entrainment of circadian activity (but not body temperature entrainment) to scheduled feeding (Gallardo et al., 2014; Assali et al., 2021). However, in a followup study, the defect in FAA in three different strains of Drd1 KO mice was much less than initially reported, suggesting that other DA receptors also promote FAA (Assali et al., 2021). A potentially interesting result was obtained in transgenic mice overexpressing Drd2, as these mice had normal FAA during a short temporal restriction (i.e., severe calorie restriction) but were impaired under longer time windows of feeding (4 vs. 8 h) (LeSauter et al., 2020). However, in this study the authors do not control for the effect of repeated testing, using the same mice for short, medium and long duration time-restricted feeding and the effect they observed was marginal. In summary, a large body of work implicates the participation of DA in regulating FAA. However, more investigation is needed to delineate the specific DA circuits and their necessity and/or sufficiency in food entrainment.
Taken together, these studies suggest that the FEO may be the result of SN-DS DA pathway or that this pathway modulates an essential motor output of the FEO. However, the specific DA-receptor circuitry of FAA is yet to be fully resolved and further fine-mapping is necessary to understand the mechanisms of this interaction. Along this line, we recently determined that a minimal subset of DA neurons present in Pitx3ak mutant mice were sufficient for entrainment of behavior to timed CR feeding (Scarpa et al., 2022). These hypomorphic mutant mice lack lenses and photoentrainment (Del Río-Martín et al., 2019), so we studied their behavior over long durations to visualize the free-running and food-entrained components of their behavior.
Neuronal circuits of feeding regulation interface with the dopamine system
Two complementary and interacting neuronal circuits govern food-seeking: the homeostatic and hedonic pathways (Figure 2; Saper et al., 2002; Ferrario et al., 2016; Liu and Kanoski, 2018). Homeostatic feeding is driven by deficits in energy stores (Saper et al., 2002; Ferrario et al., 2016; Liu and Kanoski, 2018). The arcuate nucleus of the hypothalamus (Arc) is the principal regulator of the homeostatic pathway and contains neuronal subtypes that provide input to the brain centers that promote foraging, consumption, and digestion (Fenselau et al., 2017; Sternson and Eiselt, 2017; Li et al., 2019). In response to orexigenic endocrine and sensory signals, agouti-related peptide (AgRP)-expressing and some non-AgRP GABAergic Arc-neuron populations promote feeding while pro-opiomelanocortin (Pomc)-expressing neurons and a distinct glutamatergic cell group oppose it. The integrated output from the Arc controls the activity of downstream targets including the paraventricular hypothalamus (PVN), the lateral hypothalamus (LH), the anterior subdivisions of the bed nucleus of the stria terminalis (aBNST), and paraventricular thalamic nucleus (PVT), which in turn coordinate circuits governing energy homeostasis and feeding behavior (Atasoy et al., 2012; Betley et al., 2013; Garfield et al., 2016; Stuber and Wise, 2016; Fenselau et al., 2017; Li et al., 2019; Figure 2).
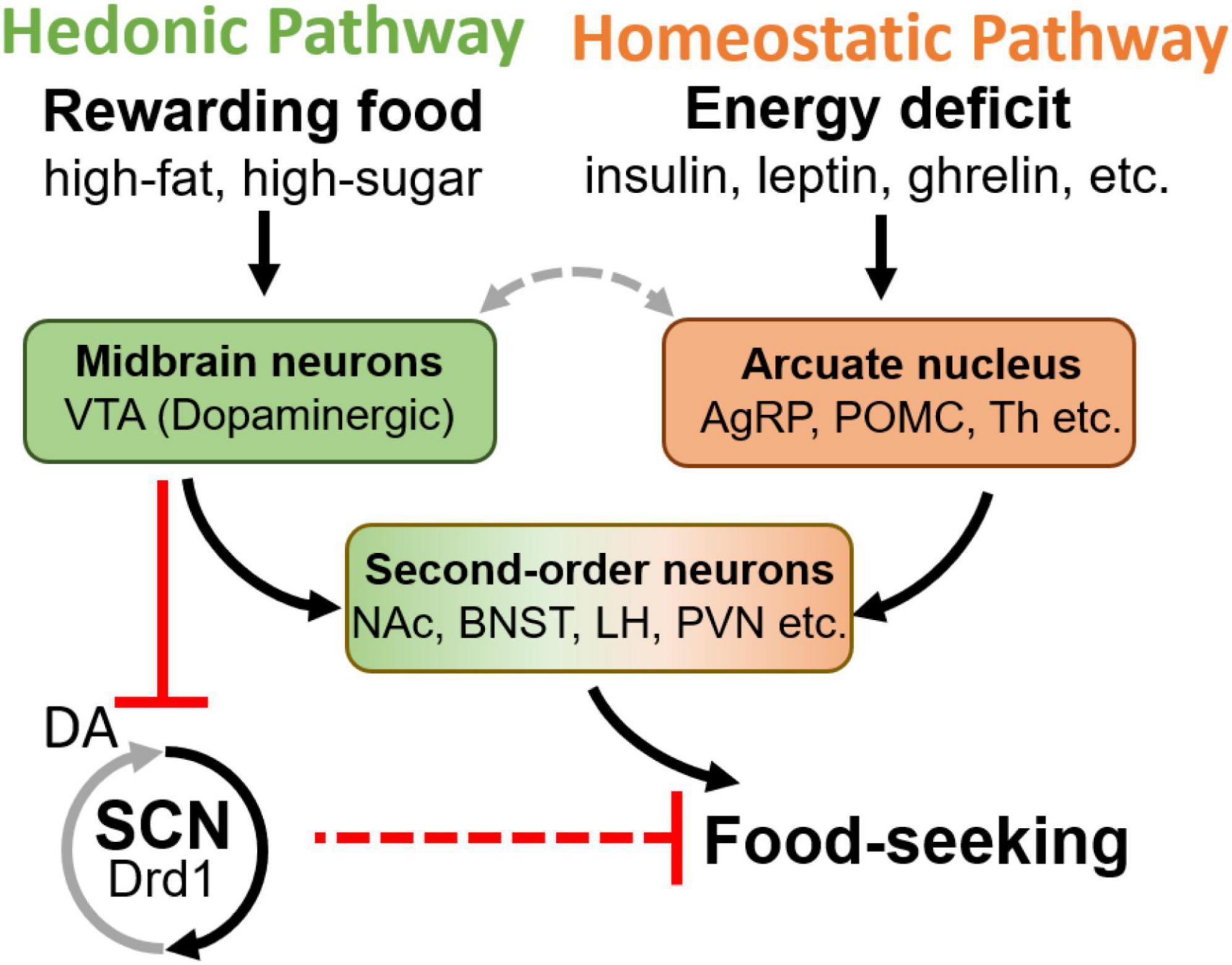
Figure 2. Schematic diagram of hedonic and homeostatic feeding pathways, and the second-order brain areas integrating both inputs. Notably, the DA in the SCN causes an inhibitory effect (Grippo et al., 2020), although Drd1 is a Gq coupled receptor which normally excites the neuron, potentially due to the GABAergic local projection within the SCN. VTA, ventral tegmental area; AgRP, agouti-related peptide; POMC, pro-opiomelanocortin; NAc, nucleus accumbens; BNST, bed nucleus of the stria terminalis; LH, lateral hypothalamus; PVN, paraventricular nucleus of hypothalamus; SCN, suprachiasmatic nucleus; Drd1, D1 dopamine receptor; Th, tyrosine hydroxylase.
Hedonic feeding, or reward-based feeding, occurs when highly palatable foods are consumed even during periods of energy surplus (Saper et al., 2002; Ferrario et al., 2016; Liu and Kanoski, 2018). The hedonic pathway is mainly regulated by the VTA DA neurons that project to the NAc (Brown et al., 2011; McCutcheon et al., 2012; Cone et al., 2015; Han et al., 2016; Tellez et al., 2016; Coccurello and Maccarrone, 2018). Upon activation, these neurons release DA in proportion to the reward value of food, which encodes the appropriate level of motivation for its consumption (Saper et al., 2002; Mohebi et al., 2019). It has been demonstrated that DA-neurons support feeding in the absence of AgRP-neurons, emphasizing the hedonic pathway’s ability to override homeostatic neural circuits of consummatory behaviors (Güler et al., 2012; Denis et al., 2015). Indeed, new evidence suggests that Arc-VTA communication is integral to the crosstalk between homeostatic and hedonic feeding pathways. Arc POMC (ArcPOMC)-neurons send direct projections to the VTA and the NAc (King and Hentges, 2011; Lim et al., 2012), while the activity of Arc AgRP (ArcAgRP)-neurons and DA VTA neurons are reciprocally regulated during feeding (Figure 2; Alhadeff et al., 2019). Similarly, the elevated activity of ArcAgRP-neurons during hunger is inhibited in response to food availability (Betley et al., 2015; Chen et al., 2015; Mandelblat-Cerf et al., 2015) as VTA DA neurons increase their activity and release DA (Hernandez and Hoebel, 1988; Volkow et al., 2002; Wise, 2006; Berridge et al., 2010; Brown et al., 2011; Cone et al., 2015; Tellez et al., 2016; Alhadeff et al., 2019). Recent work has demonstrated that activating ArcAgRP neurons is able to potentiate VTA neuronal response to food delivery (Mazzone et al., 2020). Although the LH has been proposed to provide a functional link between the ArcAgRP-and VTA DA neurons (Alhadeff et al., 2019), the precise neurocircuitry between the homeostatic and hedonic feeding pathways, and their downstream convergence points have not been fully elucidated (Saper et al., 2002; Stuber and Wise, 2016; Rossi and Stuber, 2018).
While dopamine signaling is mostly recognized as a regulator of hedonic feeding, dopaminergic neurons are also located across the hypothalamus, suggesting that they may be directly involved in homeostatic feeding regulation (Baik, 2021). Known as the A12 group, dopaminergic neurons located in the Arc were reported to promote feeding by inhibiting ArcPOMC neurons via GABAergic synapses, and PVN via both DA and GABAergic synapses (Zhang and van den Pol, 2016). DA was shown to excite ArcAgRP neurons and suppress ArcPOMC neurons in vitro (Zhang and van den Pol, 2016), but the anatomical location of DA efferent to the Arc and its functional role in vivo are not yet clear. In addition to Arc, A13, and A14 DA neuron groups are located in the Zona Incerta (ZI) and PVN, respectively, both of these nuclei are known to regulate food intake (Hill, 2012; Zhang and van den Pol, 2017). A more complex hypothalamic pathway involves the medial preoptic area (mPOA). This area receives dopaminergic input from its neighboring specialized anteroventral and preoptic periventricular DA neurons in the hypothalamus (Zhang et al., 2021). Although only reported for a role in mating behavior (Zhang et al., 2021), this dopaminergic pathway may contribute to feeding regulation due to the known role of mPOA in modulating cold-evoked eating behavior via connections to ArcAgRP neurons (Yang et al., 2021).
Timing of food consumption: Influence on metabolism, circadian rhythms, and dopamine signaling
Mounting evidence demonstrates that circadian rhythms are an integral part of the behavior and physiology related to energy homeostasis. These key findings include: (1) feeding, like many other rewarding behaviors such as sex and addictive drugs, exhibit circadian rhythmicity in animals and humans (Webb et al., 2009; Hsu et al., 2010; Landry et al., 2012; Webb, 2017). (2) In rodents, ablation of the SCN or core molecular clock components within these cells disrupts circadian rhythms including those of food consumption (Schwartz and Zimmerman, 1991; Panda et al., 2002; Liu et al., 2007; Welsh et al., 2010). A genetic mutation of the circadian core gene Clock that disrupts feeding rhythms also potentiates DA signaling and leads to metabolic disease (Turek et al., 2005; Grippo and Güler, 2019). (3) In humans, circadian disruption or even reduction of sleep duration increases daily energy intake, mainly from intermeal snacking causing weight gain (Nedeltcheva et al., 2009; Markwald et al., 2013). (4) In addition to controlling timing of feeding, circadian rhythms governed by the central nervous system control vital aspects of metabolism (Hastings et al., 2018). (5) Even during conditions of daily isocaloric intake and expenditure, mistimed energy-rich food consumption causes weight gain due to increased energy storage in rodent models (Arble et al., 2009; Hatori et al., 2012; Chaix et al., 2019). (6) In humans, acute perturbation of circadian rhythmicity increases body weight and blood glucose levels while extended periods of shift work is associated with increased risk of type 2 diabetes (Di Lorenzo et al., 2003; Pan et al., 2011). (7) Most recent work also indicates that intermittent fasting at a particular time of day will not only prevent obesity, but also greatly extend lifespan (Acosta-Rodríguez et al., 2022). Additionally, nighttime eaters have reduced success during weight-loss therapy (Garaulet et al., 2013). Therefore, coordinated oscillations in the activity of metabolic regulators and food consumption is essential to maintain proper energy homeostasis, while the perturbation of these rhythms leads to metabolic syndrome. In accordance with these findings, the health benefits of time-restricted eating (intermittent fasting) is a focal point in weight-loss therapy clinical trials (LeCheminant et al., 2013; Gill and Panda, 2015; Tinsley et al., 2017; Antoni et al., 2018; Gabel et al., 2018; Lowe et al., 2020; Wilkinson et al., 2020). Therapeutic strategies against obesity and its comorbidities, including type 2 diabetes, must consider not only the caloric content of foods but also the timing of their consumption of food (Arble et al., 2009; Gill and Panda, 2015; Longo and Panda, 2016).
Several hypothalamic nuclei are downstream targets of the SCN: the DMH, LH, and PVN, have been implicated in the circadian regulation of feeding (Mendoza, 2019b). The DMH, a nucleus that halts feeding by inhibiting ArcAgRP-neurons, receives direct and indirect inputs from the SCN and its lesion abolishes rhythms of food intake (Chou et al., 2003; Garfield et al., 2016). The SCN, via indirect inputs to the LH, drives circadian activity and orexin release associated with arousal and feeding, which may explain why the SCN appears to modulate the amplitude of food rhythms while not directly driving them. The LH lies at the interface of feeding and reward processing (Vansteensel et al., 2003; Deboer et al., 2004; Jennings et al., 2013, 2015; Inutsuka et al., 2014; Sakurai, 2014; Garfield et al., 2015; Nieh et al., 2015, 2016; Wu et al., 2015; Navarro et al., 2016; Stamatakis et al., 2016). The SCN also signals to the PVN, the primary target of ArcAgRP neurons, via diffusible factors (i.e., arginine vasopressin; AVP) and direct projections, to entrain the circadian oscillations of various metabolically relevant hormones (e.g., corticotropin-releasing hormone and oxytocin) (Kalsbeek et al., 2008; Girotti et al., 2009; Atasoy et al., 2012; Betley et al., 2013; Wu et al., 2018; Li et al., 2019). Notably, the Arc contains a circadian clock and makes reciprocal connections with the SCN and its targets to ensure coordinated daily metabolic synchrony (Saeb-Parsy et al., 2000; Guilding et al., 2009; Guzmán-Ruiz et al., 2015; Buijs et al., 2017; Padilla et al., 2019). Although a wealth of communication between the SCN and centers that control food intake and metabolism has been discovered, the circuits that control meal timing and the underlying neurophysiological mechanisms have not been characterized.
It is well-established that the sight, smell, or ingestion of food triggers DA release. Independent of cue-induced changes in DA, extracellular DA levels oscillate in a circadian fashion as described above. Regularly timed daily access to rewarding foods can entrain SCN-dependent behavioral rhythms by increasing midbrain dopaminergic neuron activity and DA content in the forebrain (Mendoza et al., 2010b). Interestingly, ad libitum access to energy-dense rewarding foods triggers disorganization of circadian feeding behavior, going from an intermittent meal-based schedule to continuous snacking (Blancas-Velazquez et al., 2017; Espinosa-Carrasco et al., 2018). VTA specific knockdown of circadian gene Bmal1 can rescue the hedonic overconsumption of rewarding food (Koch et al., 2020). The energy-rich foods also lengthen the period of behavioral circadian rhythms, impair photic resetting, reduce light mediated induction of c-fos within the SCN and abolish dopaminergic circadian rhythms around the SCN (Kohsaka et al., 2007; Mendoza et al., 2008; Luo et al., 2018; Grippo et al., 2020). Restoration of DA rhythmicity by DA infusion into the SCN is sufficient to correct significant metabolic dysregulation caused by diet-induced obesity (DIO) (Luo et al., 2018). A direct projection from VTA DA neurons to the SCN was recently described, and was shown to govern the rate of reentrainment to light shift (Grippo et al., 2017), as well as to be required for energy-dense diet-induced disruption of circadian meal timing and weight gain in mice (Grippo et al., 2020). These findings demonstrate the involvement of DA input to the SCN for circadian rhythm synchronization. A future challenge will be to define the precise DA neural circuitry feeding into these behavioral and physiological responses.
Methamphetamine-sensitive rhythms
The other major association of DA with biological rhythms comes from studies of chronic methamphetamine exposure, leading to the terms “methamphetamine-sensitive oscillator” (MASCO), and more recently, the “dopamine ultradian oscillator” (DUO) (Mohawk et al., 2009; Blum et al., 2014; Bourguignon and Storch, 2017; Freyberg and McCarthy, 2017). Behaviorally, the MASCO can be observed when the animals are exposed to chronic methamphetamine access via the drinking water. Both control and core clock gene deficit animals have lengthened free running period on methamphetamine; SCN lesioned animals regain rhythmicity under methamphetamine treatment and show robust food entrainment (Tataroglu et al., 2006; Mohawk et al., 2009). Meanwhile, the DUO was reported as a 4–6 h rhythmic behavioral activity rhythm present in SCN lesioned or Bmal1 KO mice that depends on the expression of DAT (Blum et al., 2014). Deletion of DAT more than doubles the period of this ultradian oscillation, whereas, non-selective DA receptor antagonist, haloperidol, has the opposite effect (Blum et al., 2014). Wild-type, SCN lesioned or Bmal1 KO mice maintained on high levels of methamphetamine extend their period length to between 36–48 h (Blum et al., 2014). However, the natural stimuli capable of entraining the MASCO/DUO remain largely unknown. Furthermore, the precise location of the MASCO/DUO neurons is unknown, but chemogenetic manipulation experiments suggest that they are localized to the midbrain (Blum et al., 2014).
Given that the DA system is involved in food entrainment, it has been questioned whether the FEO and MASCO/DUO relate to each other (Pendergast et al., 2012; Mendoza and Challet, 2014). Interestingly, in the SCN lesioned rats, methamphetamine exposure rescues the rhythmicity of locomotor activity, and these animals entrain to daily scheduled feeding (Honma et al., 1989). When treated with a non-selective DA receptor antagonist, haloperidol, the rhythmicity of MASCO can be phase shifted (Honma and Honma, 1995). Moreover, in addition to the DA-regulated biological rhythm, neurons in the DS display rhythmic firing patterns in both SCN-dependent or methamphetamine-dependent/SCN-independent manner (Miyazaki et al., 2021). Chronic methamphetamine treatment did not alter clock gene expression in the SCN or NAc, but changed it dramatically in the DS, highlighting the importance of pacemakers outside the SCN controlling activity (Masubuchi et al., 2000; Nikaido et al., 2001; Iijima et al., 2002; Natsubori et al., 2014). It appears likely but has not been demonstrated experimentally that the MASCO/DUO are separable from the FEO(s) and it is exciting to speculate that there may be several independent DA-regulated biological rhythm centers in the adult brain: (1) the FEO, which depends on DA signaling or could be a dopaminergic clock (2) the DUO/MASCO, which depends on DAT and most likely the striatum (dorsal and ventral), and (3) the SCN, which receives DA input via Drd1 expressing neurons.
Charting a way forward
In summary, we have discussed evidence of bidirectional input between the dopaminergic and circadian control systems at the levels of genes and neural structures. The evidence for the importance of these connections in driving rhythms on ultradian, circadian and infradian rhythms ranges from correlative to causative. The experimental setups required to unravel cause and effect are intensive and we expect it to take the participation of many laboratories to address these questions. Here we suggest several ideas for experiments that will be important for proving or disproving that the midbrain or other DA circuitry is acting to regulate behaviors such as rest-activity cycles, food entrainment, hedonic day-eating, and methamphetamine responses in regions such as the SCN, DS, NAc, or others.
• The diurnal rhythm of DA tone and specific responses to potential zeitgebers have not been well-characterized. The new tools to examine neuromodulator levels in vivo, such as GRAB-DA and dLight (Patriarchi et al., 2018; Sun et al., 2018), will be a great asset in visualizing DA release at target sites. One potential difficulty with these approaches is that DA levels are much lower in the SCN compared to the DS or NAc; despite this, we know that DA plays a significant role in the SCN in terms of regulating both light-entrainment and rest phase feeding that leads to DIO (Grippo et al., 2017, 2020).
• With respect to the DS, we are still lacking an experimental demonstration that DA levels increase in the DS prior to expected mealtime. Given the convincing demonstration that DA rhythms in the DS drive Per2 oscillations (Hood et al., 2010), it would be of great interest to monitor the rhythmicity of circadian genes or neuronal activity in the DS in vivo using the recently developed tools such as PER2:VENUS for circadian gene and GCaMP for neuronal calcium signaling (Jones et al., 2018; Mei et al., 2018). Monitoring these rhythms in various DA-mutant mice and/or upon environmental stimuli will provide insight into the effect of DA on circadian rhythmicity at different downstream targets.
• Using conditional genetics to selectively delete TH to parse through which DA populations constitute the minimal circuit required for DIO and FAA are admirable goals. Unfortunately, many mutations to DA or its receptors have pleiotropic phenotypes. The use of more refined genetic markers and/or targeting with AAVs using conditional genetic approaches will allow for deciphering minimal DA circuits required for specific circadian behaviors.
• Disrupting the molecular clock in different genetically defined DA populations or their targets by deletion of Bmal1, Nr1d1, or Per genes to test for alterations in the MASCO/DUO or other feeding related phenotypes is worthy of investigation and could help clarify whether these DA neurons are functioning as clocks or as required relays.
• While the DA-Drd1 signaling in the SCN has been shown to enable energy-dense DIO (Grippo et al., 2017), the detailed neuron mechanism in the SCN associated with DA signaling is still waiting to be further explored. Approximately 60% SCN neurons express Drd1, and are located across the entire SCN including both core and shell, in a fraction of both VIP (∼80%) and AVP (∼60%) (Smyllie et al., 2016). Despite this dispersed expression profile, it is still possible that these two subpopulations of neurons would exhibit differential responses to dopaminergic input, and hence contribute to DIO differently. Therefore, it is worthy to use conditional genetics to knock out Drd1 only in selective SCN subpopulations (VIP, AVP, etc.), and study the effect on circadian feeding behavior and metabolic consequences under different diet conditions.
Conclusion
As discussed in this review, the DA system falls into a critical integrating position, serving to regulate circadian feeding, metabolic homeostasis, locomotor activity, and reward responsiveness for drugs of abuse and natural rewards. Future experiments must address whether the DA system is causally regulating biological rhythms or is serving as a necessary motor relay for other bona fide circadian pacemakers. Unraveling these intersecting systems is well worth the challenge due to the profound implications this could have for human health. To that end, it will be imperative to build the circuits outwards and identify not only the DA subtypes responsible for these behaviors but also the identity and connectivity of their targets. Further delineation of these pathways and understanding the individual contributions of the SCN, DUO/MASCO, and any FEO(s) will be essential for proper identification, treatment, and prevention of eating disorders and drug abuse. Determining the role of DA signaling for coherent biological rhythm expression will be the linchpin of these efforts.
Author contributions
All authors contributed to drafting, editing, and finalizing the manuscript and approved the submitted version.
Funding
This work was supported by the National Institute of General Medical Sciences of the National Institutes of Health (SC3GM125570 to ADS, R01GM121937 to ADG, and R35GM140854 to ADG). The funders had no role in study design, data collection and analysis, decision to publish, or preparation of the manuscript.
Conflict of interest
The authors declare that the research was conducted in the absence of any commercial or financial relationships that could be construed as a potential conflict of interest.
Publisher’s note
All claims expressed in this article are solely those of the authors and do not necessarily represent those of their affiliated organizations, or those of the publisher, the editors and the reviewers. Any product that may be evaluated in this article, or claim that may be made by its manufacturer, is not guaranteed or endorsed by the publisher.
Author disclaimer
The content is solely the responsibility of the authors and does not necessarily represent the official views of the National Institutes of Health.
References
Abe, K., Kroning, J., Greer, M. A., and Critchlow, V. (1979). Effects of destruction of the suprachiasmatic nuclei on the circadian rhythms in plasma corticosterone, body temperature, feeding and plasma thyrotropin. Neuroendocrinology 29, 119–131. doi: 10.1159/000122913
Abe, M., Herzog, E. D., Yamazaki, S., Straume, M., Tei, H., Sakaki, Y., et al. (2002). Circadian rhythms in isolated brain regions. J. Neurosci. 22, 350–356.
Acosta-Galvan, G., Yi, C.-X., van der Vliet, J., Jhamandas, J. H., Panula, P., Angeles-Castellanos, M., et al. (2011). Interaction between hypothalamic dorsomedial nucleus and the suprachiasmatic nucleus determines intensity of food anticipatory behavior. Proc. Natl. Acad. Sci. U. S. A. 108, 5813–5818. doi: 10.1073/pnas.1015551108
Acosta-Rodríguez, V., Rijo-Ferreira, F., Izumo, M., Xu, P., Wight-Carter, M., Green, C. B., et al. (2022). Circadian alignment of early onset caloric restriction promotes longevity in male C57BL/6J mice. Science 376, 1192–1202. doi: 10.1126/science.abk0297
Acosta-Rodríguez, V. A., de Groot, M. H. M., Rijo-Ferreira, F., Green, C. B., and Takahashi, J. S. (2017). Mice under caloric restriction self-impose a temporal restriction of food intake as revealed by an automated feeder system. Cell Metab 26, 267–277.e2. doi: 10.1016/j.cmet.2017.06.007
Alhadeff, A. L., Goldstein, N., Park, O., Klima, M. L., Vargas, A., and Betley, J. N. (2019). Natural and drug rewards engage distinct pathways that converge on coordinated hypothalamic and reward circuits. Neuron 103, 891–908.e6. doi: 10.1016/j.neuron.2019.05.050
Angeles-Castellanos, M., Salgado-Delgado, R., Rodriguez, K., Buijs, R. M., and Escobar, C. (2010). The suprachiasmatic nucleus participates in food entrainment: a lesion study. Neuroscience 165, 1115–1126. doi: 10.1016/j.neuroscience.2009.11.061
Antoni, R., Robertson, T. M., Robertson, M. D., and Johnston, J. D. (2018). A pilot feasibility study exploring the effects of a moderate time-restricted feeding intervention on energy intake, adiposity and metabolic physiology in free-living human subjects. J. Nutr. Sci. 7:e22. doi: 10.1017/jns.2018.13
Arble, D. M., Bass, J., Laposky, A. D., Vitaterna, M. H., and Turek, F. W. (2009). Circadian timing of food intake contributes to weight gain. Obesity 17, 2100–2102. doi: 10.1038/oby.2009.264
Assali, D. R., Sidikpramana, M., Villa, A. P., Falkenstein, J., and Steele, A. D. (2021). Type 1 dopamine receptor (D1R)-independent circadian food anticipatory activity in mice. PLoS One 16:e0242897. doi: 10.1371/journal.pone.0242897
Atasoy, D., Betley, J. N., Su, H. H., and Sternson, S. M. (2012). Deconstruction of a neural circuit for hunger. Nature 488, 172–177. doi: 10.1038/nature11270
Attia, E. (2010). Anorexia nervosa: current status and future directions. Annu. Rev. Med. 61, 425–435. doi: 10.1146/annurev.med.050208.200745
Baik, J.-H. (2021). Dopaminergic control of the feeding circuit. Endocrinol. Metab. 36, 229–239. doi: 10.3803/EnM.2021.979
Bass, J., and Takahashi, J. S. (2010). Circadian integration of metabolism and energetics. Science 330, 1349–1354. doi: 10.1126/science.1195027
Becker-Krail, D. D., Walker, W. H., and Nelson, R. J. (2022). The ventral tegmental area and nucleus accumbens as circadian oscillators: implications for drug abuse and substance use disorders. Front. Physiol. 13:886704. doi: 10.3389/fphys.2022.886704
Berridge, K. C., Ho, C.-Y., Richard, J. M., and DiFeliceantonio, A. G. (2010). The tempted brain eats: pleasure and desire circuits in obesity and eating disorders. Brain Res. 1350, 43–64. doi: 10.1016/j.brainres.2010.04.003
Betley, J. N., Cao, Z. F. H., Ritola, K. D., and Sternson, S. M. (2013). Parallel, redundant circuit organization for homeostatic control of feeding behavior. Cell 155, 1337–1350. doi: 10.1016/j.cell.2013.11.002
Betley, J. N., Xu, S., Cao, Z. F. H., Gong, R., Magnus, C. J., Yu, Y., et al. (2015). Neurons for hunger and thirst transmit a negative-valence teaching signal. Nature 521, 180–185. doi: 10.1038/nature14416
Björklund, A., and Dunnett, S. B. (2007). Dopamine neuron systems in the brain: an update. Trends Neurosci. 30, 194–202. doi: 10.1016/j.tins.2007.03.006
Blancas-Velazquez, A., Mendoza, J., Garcia, A. N., and la Fleur, S. E. (2017). Diet-induced obesity and circadian disruption of feeding behavior. Front. Neurosci. 11:23. doi: 10.3389/fnins.2017.00023
Blum, I. D., Zhu, L., Moquin, L., Kokoeva, M. V., Gratton, A., Giros, B., et al. (2014). A highly tunable dopaminergic oscillator generates ultradian rhythms of behavioral arousal. Elife 3:e05105. doi: 10.7554/eLife.05105
Bourguignon, C., and Storch, K.-F. (2017). Control of rest:activity by a dopaminergic ultradian oscillator and the circadian clock. Front. Neurol. 8:614. doi: 10.3389/fneur.2017.00614
Brown, H. D., McCutcheon, J. E., Cone, J. J., Ragozzino, M. E., and Roitman, M. F. (2011). Primary food reward and reward-predictive stimuli evoke different patterns of phasic dopamine signaling throughout the striatum. Eur. J. Neurosci. 34, 1997–2006. doi: 10.1111/j.1460-9568.2011.07914.x
Buhr, E. D., Vemaraju, S., Diaz, N., Lang, R. A., and Van Gelder, R. N. (2019). Neuropsin (OPN5) mediates local light-dependent induction of circadian clock genes and circadian photoentrainment in exposed murine skin. Curr. Biol. 29, 3478–3487.e4. doi: 10.1016/j.cub.2019.08.063
Buijs, F. N., Guzmán-Ruiz, M., León-Mercado, L., Basualdo, M. C., Escobar, C., Kalsbeek, A., et al. (2017). Suprachiasmatic nucleus interaction with the arcuate nucleus; essential for organizing physiological rhythms. eNeuro 4:ENEURO.0028-17.2017. doi: 10.1523/ENEURO.0028-17.2017
CDC (2019). Adult Obesity Causes & Consequences and Overweight & Obesity. Available online at: https://www.cdc.gov/obesity/adult/causes.html (accessed December 6, 2019).
Chaix, A., Lin, T., Le, H. D., Chang, M. W., and Panda, S. (2019). Time-restricted feeding prevents obesity and metabolic syndrome in mice lacking a circadian clock. Cell Metab. 29, 303–319.e4. doi: 10.1016/j.cmet.2018.08.004
Challet, E. (2019). The circadian regulation of food intake. Nat. Rev. Endocrinol. 15, 393–405. doi: 10.1038/s41574-019-0210-x
Chavan, R., Feillet, C., Costa, S. S. F., Delorme, J. E., Okabe, T., Ripperger, J. A., et al. (2016). Liver-derived ketone bodies are necessary for food anticipation. Nat. Commun. 7:10580. doi: 10.1038/ncomms10580
Chen, Y., Lin, Y.-C., Kuo, T.-W., and Knight, Z. A. (2015). Sensory detection of food rapidly modulates arcuate feeding circuits. Cell 160, 829–841. doi: 10.1016/j.cell.2015.01.033
Chou, T. C., Scammell, T. E., Gooley, J. J., Gaus, S. E., Saper, C. B., and Lu, J. (2003). Critical role of dorsomedial hypothalamic nucleus in a wide range of behavioral circadian rhythms. J. Neurosci. 23, 10691–10702.
Chung, S., Lee, E. J., Yun, S., Choe, H. K., Park, S.-B., Son, H. J., et al. (2014). Impact of circadian nuclear receptor REV-ERBα on midbrain dopamine production and mood regulation. Cell 157, 858–868. doi: 10.1016/j.cell.2014.03.039
Coccurello, R., and Maccarrone, M. (2018). Hedonic eating and the “Delicious Circle”: from lipid-derived mediators to brain dopamine and back. Front. Neurosci. 12:271. doi: 10.3389/fnins.2018.00271
Cone, J. J., Roitman, J. D., and Roitman, M. F. (2015). Ghrelin regulates phasic dopamine and nucleus accumbens signaling evoked by food-predictive stimuli. J. Neurochem. 133, 844–856. doi: 10.1111/jnc.13080
Davidson, A. J. (2009). Lesion studies targeting food-anticipatory activity. Eur. J. Neurosci. 30, 1658–1664. doi: 10.1111/j.1460-9568.2009.06961.x
de Lartigue, G., and McDougle, M. (2019). Dorsal striatum dopamine oscillations: setting the pace of food anticipatory activity. Acta Physiol. 225:e13152. doi: 10.1111/apha.13152
de Mutsert, R., Sun, Q., Willett, W. C., Hu, F. B., and van Dam, R. M. (2014). Overweight in early adulthood, adult weight change, and risk of type 2 diabetes, cardiovascular diseases, and certain cancers in men: a cohort study. Am. J. Epidemiol. 179, 1353–1365. doi: 10.1093/aje/kwu052
Deboer, T., Overeem, S., Visser, N. A. H., Duindam, H., Frölich, M., Lammers, G. J., et al. (2004). Convergence of circadian and sleep regulatory mechanisms on hypocretin-1. Neuroscience 129, 727–732. doi: 10.1016/j.neuroscience.2004.07.049
Del Río-Martín, A., Pérez-Taboada, I., Fernández-Pérez, A., Moratalla, R., de la Villa, P., and Vallejo, M. (2019). Hypomorphic expression of pitx3 disrupts circadian clocks and prevents metabolic entrainment of energy expenditure. Cell Rep 29, 3678–3692.e4. doi: 10.1016/j.celrep.2019.11.027
Delezie, J., Dumont, S., Sandu, C., Reibel, S., Pevet, P., and Challet, E. (2016). Rev-erbα in the brain is essential for circadian food entrainment. Sci. Rep. 6:29386. doi: 10.1038/srep29386
Denis, R. G. P., Joly-Amado, A., Webber, E., Langlet, F., Schaeffer, M., Padilla, S. L., et al. (2015). Palatability can drive feeding independent of agrp neurons. Cell Metab. 22, 646–657. doi: 10.1016/j.cmet.2015.07.011
Di Lorenzo, L., De Pergola, G., Zocchetti, C., L’Abbate, N., Basso, A., Pannacciulli, N., et al. (2003). Effect of shift work on body mass index: results of a study performed in 319 glucose-tolerant men working in a Southern Italian industry. Int. J. Obes. Relat. Metab. Disord. 27, 1353–1358. doi: 10.1038/sj.ijo.0802419
Domínguez-López, S., Howell, R. D., López-Canúl, M. G., Leyton, M., and Gobbi, G. (2014). Electrophysiological characterization of dopamine neuronal activity in the ventral tegmental area across the light-dark cycle. Synapse 68, 454–467. doi: 10.1002/syn.21757
Eckel, R. H., Kahn, S. E., Ferrannini, E., Goldfine, A. B., Nathan, D. M., Schwartz, M. W., et al. (2011). Obesity and type 2 diabetes: what can be unified and what needs to be individualized? Diabetes Care 34, 1424–1430. doi: 10.2337/dc11-0447
Edgar, D. M., and Dement, W. C. (1991). Regularly scheduled voluntary exercise synchronizes the mouse circadian clock. Am. J. Physiol. 261, R928–R933. doi: 10.1152/ajpregu.1991.261.4.R928
Edgar, D. M., Dement, W. C., and Fuller, C. A. (1993). Effect of SCN lesions on sleep in squirrel monkeys: evidence for opponent processes in sleep-wake regulation. J. Neurosci. 13, 1065–1079.
Espinosa-Carrasco, J., Burokas, A., Fructuoso, M., Erb, I., Martín-García, E., Gutiérrez-Martos, M., et al. (2018). Time-course and dynamics of obesity-related behavioral changes induced by energy-dense foods in mice. Addict. Biol. 23, 531–543. doi: 10.1111/adb.12595
Fenselau, H., Campbell, J. N., Verstegen, A. M. J., Madara, J. C., Xu, J., Shah, B. P., et al. (2017). A rapidly acting glutamatergic ARC→PVH satiety circuit postsynaptically regulated by α-MSH. Nat. Neurosci. 20, 42–51. doi: 10.1038/nn.4442
Fernandez, D. C., Komal, R., Langel, J., Ma, J., Duy, P. Q., Penzo, M. A., et al. (2020). Retinal innervation tunes circuits that drive nonphotic entrainment to food. Nature 581, 194–198. doi: 10.1038/s41586-020-2204-1
Ferrario, C. R., Labouèbe, G., Liu, S., Nieh, E. H., Routh, V. H., Xu, S., et al. (2016). Homeostasis meets motivation in the battle to control food intake. J. Neurosci. 36, 11469–11481. doi: 10.1523/JNEUROSCI.2338-16.2016
Ferris, M. J., España, R. A., Locke, J. L., Konstantopoulos, J. K., Rose, J. H., Chen, R., et al. (2014). Dopamine transporters govern diurnal variation in extracellular dopamine tone. Proc. Natl. Acad. Sci. U. S. A. 111, E2751–E2759. doi: 10.1073/pnas.1407935111
Flegal, K. M., Kruszon-Moran, D., Carroll, M. D., Fryar, C. D., and Ogden, C. L. (2016). Trends in obesity among adults in the united states, 2005 to 2014. JAMA 315, 2284–2291. doi: 10.1001/jama.2016.6458
Freyberg, Z., and McCarthy, M. J. (2017). Dopamine D2 receptors and the circadian clock reciprocally mediate antipsychotic drug-induced metabolic disturbances. NPJ Schizophr. 3:17. doi: 10.1038/s41537-017-0018-4
Gabel, K., Hoddy, K. K., Haggerty, N., Song, J., Kroeger, C. M., Trepanowski, J. F., et al. (2018). Effects of 8-hour time restricted feeding on body weight and metabolic disease risk factors in obese adults: a pilot study. Nutr. Healthy Aging 4, 345–353. doi: 10.3233/NHA-170036
Gallardo, C. M., Darvas, M., Oviatt, M., Chang, C. H., Michalik, M., Huddy, T. F., et al. (2014). Dopamine receptor 1 neurons in the dorsal striatum regulate food anticipatory circadian activity rhythms in mice. Elife 3:e03781. doi: 10.7554/eLife.03781
Garaulet, M., Gómez-Abellán, P., Alburquerque-Béjar, J. J., Lee, Y. C., Ordovás, J. M., and Scheer, F. A. J. L. (2013). Timing of food intake predicts weight loss effectiveness. Int. J. Obes. 37, 604–611. doi: 10.1038/ijo.2012.229
Garfield, A. S., Li, C., Madara, J. C., Shah, B. P., Webber, E., Steger, J. S., et al. (2015). A neural basis for melanocortin-4 receptor-regulated appetite. Nat. Neurosci. 18, 863–871. doi: 10.1038/nn.4011
Garfield, A. S., Shah, B. P., Burgess, C. R., Li, M. M., Li, C., Steger, J. S., et al. (2016). Dynamic GABAergic afferent modulation of AgRP neurons. Nat. Neurosci. 19, 1628–1635. doi: 10.1038/nn.4392
Gerfen, C. R., and Surmeier, D. J. (2011). Modulation of striatal projection systems by dopamine. Annu. Rev. Neurosci. 34, 441–466. doi: 10.1146/annurev-neuro-061010-113641
Gill, S., and Panda, S. (2015). A smartphone app reveals erratic diurnal eating patterns in humans that can be modulated for health benefits. Cell Metab. 22, 789–798. doi: 10.1016/j.cmet.2015.09.005
Girotti, M., Weinberg, M. S., and Spencer, R. L. (2009). Diurnal expression of functional and clock-related genes throughout the rat HPA axis: system-wide shifts in response to a restricted feeding schedule. Am. J. Physiol. Endocrinol. Metab. 296, E888–E897. doi: 10.1152/ajpendo.90946.2008
Gravotta, L., Gavrila, A. M., Hood, S., and Amir, S. (2011). Global depletion of dopamine using intracerebroventricular 6-hydroxydopamine injection disrupts normal circadian wheel-running patterns and PERIOD2 expression in the rat forebrain. J. Mol. Neurosci. 45, 162–171. doi: 10.1007/s12031-011-9520-8
Grippo, R. M., and Güler, A. D. (2019). Dopamine signaling in circadian photoentrainment: consequences of desynchrony. Yale J. Biol. Med. 92, 271–281.
Grippo, R. M., Purohit, A. M., Zhang, Q., Zweifel, L. S., and Güler, A. D. (2017). Direct midbrain dopamine input to the suprachiasmatic nucleus accelerates circadian entrainment. Curr. Biol. 27, 2465–2475.e3. doi: 10.1016/j.cub.2017.06.084
Grippo, R. M., Tang, Q., Zhang, Q., Chadwick, S. R., Gao, Y., Altherr, E. B., et al. (2020). Dopamine signaling in the suprachiasmatic nucleus enables weight gain associated with hedonic feeding. Curr. Biol 30, 196–208.e8. doi: 10.1016/j.cub.2019.11.029
Guh, D. P., Zhang, W., Bansback, N., Amarsi, Z., Birmingham, C. L., and Anis, A. H. (2009). The incidence of co-morbidities related to obesity and overweight: a systematic review and meta-analysis. BMC Public Health 9:88. doi: 10.1186/1471-2458-9-88
Guilding, C., Hughes, A. T. L., Brown, T. M., Namvar, S., and Piggins, H. D. (2009). A riot of rhythms: neuronal and glial circadian oscillators in the mediobasal hypothalamus. Mol. Brain 2:28. doi: 10.1186/1756-6606-2-28
Güler, A. D., Rainwater, A., Parker, J. G., Jones, G. L., Argilli, E., Arenkiel, B. R., et al. (2012). Transient activation of specific neurons in mice by selective expression of the capsaicin receptor. Nat. Commun. 3:746. doi: 10.1038/ncomms1749
Gunapala, K. M., Gallardo, C. M., Hsu, C. T., and Steele, A. D. (2011). Single gene deletions of orexin, leptin, neuropeptide Y, and ghrelin do not appreciably alter food anticipatory activity in mice. PLoS One 6:e18377. doi: 10.1371/journal.pone.0018377
Guzmán-Ruiz, M. A., Ramirez-Corona, A., Guerrero-Vargas, N. N., Sabath, E., Ramirez-Plascencia, O. D., Fuentes-Romero, R., et al. (2015). Role of the suprachiasmatic and arcuate nuclei in diurnal temperature regulation in the rat. J. Neurosci. 35, 15419–15429. doi: 10.1523/JNEUROSCI.1449-15.2015
Han, W., Tellez, L. A., Niu, J., Medina, S., Ferreira, T. L., Zhang, X., et al. (2016). Striatal dopamine links gastrointestinal rerouting to altered sweet appetite. Cell Metab. 23, 103–112. doi: 10.1016/j.cmet.2015.10.009
Hastings, M. H., Maywood, E. S., and Brancaccio, M. (2018). Generation of circadian rhythms in the suprachiasmatic nucleus. Nat. Rev. Neurosci. 19, 453–469. doi: 10.1038/s41583-018-0026-z
Hatori, M., Vollmers, C., Zarrinpar, A., DiTacchio, L., Bushong, E. A., Gill, S., et al. (2012). Time-restricted feeding without reducing caloric intake prevents metabolic diseases in mice fed a high-fat diet. Cell Metab. 15, 848–860. doi: 10.1016/j.cmet.2012.04.019
Hernandez, L., and Hoebel, B. G. (1988). Feeding and hypothalamic stimulation increase dopamine turnover in the accumbens. Physiol. Behav. 44, 599–606. doi: 10.1016/0031-9384(88)90324-1
Hill, J. W. (2012). PVN pathways controlling energy homeostasis. Indian J. Endocrinol. Metab. 16, S627–S636. doi: 10.4103/2230-8210.105581
Honma, S., and Honma, K. (1995). Phase-dependent phase shift of methamphetamine-induced circadian rhythm by haloperidol in SCN-lesioned rats. Brain Res. 674, 283–290. doi: 10.1016/0006-8993(95)00027-n
Honma, S., Honma, K., and Hiroshige, T. (1989). Methamphetamine induced locomotor rhythm entrains to restricted daily feeding in SCN lesioned rats. Physiol. Behav. 45, 1057–1065. doi: 10.1016/0031-9384(89)90237-0
Hood, S., Cassidy, P., Cossette, M.-P., Weigl, Y., Verwey, M., Robinson, B., et al. (2010). Endogenous dopamine regulates the rhythm of expression of the clock protein PER2 in the rat dorsal striatum via daily activation of D2 dopamine receptors. J. Neurosci. 30, 14046–14058. doi: 10.1523/JNEUROSCI.2128-10.2010
Hsu, C. T., Dollár, P., Chang, D., and Steele, A. D. (2010). Daily timed sexual interaction induces moderate anticipatory activity in mice. PLoS One 5:e15429. doi: 10.1371/journal.pone.0015429
Hurt, R. T., Kulisek, C., Buchanan, L. A., and McClave, S. A. (2010). The obesity epidemic: challenges, health initiatives, and implications for gastroenterologists. Gastroenterol. Hepatol. 6, 780–792.
Iijima, M., Nikaido, T., Akiyama, M., Moriya, T., and Shibata, S. (2002). Methamphetamine-induced, suprachiasmatic nucleus-independent circadian rhythms of activity and mPer gene expression in the striatum of the mouse. Eur. J. Neurosci. 16, 921–929. doi: 10.1046/j.1460-9568.2002.02140.x
Inutsuka, A., Inui, A., Tabuchi, S., Tsunematsu, T., Lazarus, M., and Yamanaka, A. (2014). Concurrent and robust regulation of feeding behaviors and metabolism by orexin neurons. Neuropharmacology 85, 451–460. doi: 10.1016/j.neuropharm.2014.06.015
Jennings, J. H., Rizzi, G., Stamatakis, A. M., Ung, R. L., and Stuber, G. D. (2013). The inhibitory circuit architecture of the lateral hypothalamus orchestrates feeding. Science 341, 1517–1521. doi: 10.1126/science.1241812
Jennings, J. H., Ung, R. L., Resendez, S. L., Stamatakis, A. M., Taylor, J. G., Huang, J., et al. (2015). Visualizing hypothalamic network dynamics for appetitive and consummatory behaviors. Cell 160, 516–527. doi: 10.1016/j.cell.2014.12.026
Jones, J. R., Simon, T., Lones, L., and Herzog, E. D. (2018). SCN VIP neurons are essential for normal light-mediated resetting of the circadian system. J. Neurosci. 38, 7986–7995. doi: 10.1523/JNEUROSCI.1322-18.2018
Kalsbeek, A., Foppen, E., Schalij, I., Van Heijningen, C., van der Vliet, J., Fliers, E., et al. (2008). Circadian control of the daily plasma glucose rhythm: an interplay of GABA and glutamate. PLoS One 3:e3194. doi: 10.1371/journal.pone.0003194
King, C. M., and Hentges, S. T. (2011). Relative number and distribution of murine hypothalamic proopiomelanocortin neurons innervating distinct target sites. PLoS One 6:e25864. doi: 10.1371/journal.pone.0025864
Koch, C. E., Begemann, K., Kiehn, J. T., Griewahn, L., Mauer, J., Hess, M. E., et al. (2020). Circadian regulation of hedonic appetite in mice by clocks in dopaminergic neurons of the VTA. Nat. Commun. 11:3071. doi: 10.1038/s41467-020-16882-6
Kohsaka, A., Laposky, A. D., Ramsey, K. M., Estrada, C., Joshu, C., Kobayashi, Y., et al. (2007). High-fat diet disrupts behavioral and molecular circadian rhythms in mice. Cell Metab. 6, 414–421. doi: 10.1016/j.cmet.2007.09.006
Korshunov, K. S., Blakemore, L. J., and Trombley, P. Q. (2017). Dopamine: a modulator of circadian rhythms in the central nervous system. Front. Cell. Neurosci. 11:91. doi: 10.3389/fncel.2017.00091
Krieger, D. T., Hauser, H., and Krey, L. C. (1977). Suprachiasmatic nuclear lesions do not abolish food-shifted circadian adrenal and temperature rhythmicity. Science 197, 398–399. doi: 10.1126/science.877566
Landry, G. J., Opiol, H., Marchant, E. G., Pavlovski, I., Mear, R. J., Hamson, D. K., et al. (2012). Scheduled daily mating induces circadian anticipatory activity rhythms in the male rat. PLoS One 7:e40895. doi: 10.1371/journal.pone.0040895
LeCheminant, J. D., Christenson, E., Bailey, B. W., and Tucker, L. A. (2013). Restricting night-time eating reduces daily energy intake in healthy young men: a short-term cross-over study. Br. J. Nutr. 110, 2108–2113. doi: 10.1017/S0007114513001359
LeSauter, J., Balsam, P. D., Simpson, E. H., and Silver, R. (2020). Overexpression of striatal D2 receptors reduces motivation thereby decreasing food anticipatory activity. Eur. J. Neurosci. 51, 71–81. doi: 10.1111/ejn.14219
Li, M. M., Madara, J. C., Steger, J. S., Krashes, M. J., Balthasar, N., Campbell, J. N., et al. (2019). The paraventricular hypothalamus regulates satiety and prevents obesity via two genetically distinct circuits. Neuron 102, 653–667.e6. doi: 10.1016/j.neuron.2019.02.028
Lim, B. K., Huang, K. W., Grueter, B. A., Rothwell, P. E., and Malenka, R. C. (2012). Anhedonia requires MC4R-mediated synaptic adaptations in nucleus accumbens. Nature 487, 183–189. doi: 10.1038/nature11160
Liu, A. C., Welsh, D. K., Ko, C. H., Tran, H. G., Zhang, E. E., Priest, A. A., et al. (2007). Intercellular coupling confers robustness against mutations in the SCN circadian clock network. Cell 129, 605–616. doi: 10.1016/j.cell.2007.02.047
Liu, C. M., and Kanoski, S. E. (2018). Homeostatic and non-homeostatic controls of feeding behavior: distinct vs. common neural systems. Physiol. Behav. 193, 223–231. doi: 10.1016/j.physbeh.2018.02.011
Liu, Y.-Y., Liu, T.-Y., Qu, W.-M., Hong, Z.-Y., Urade, Y., and Huang, Z.-L. (2012). Dopamine is involved in food-anticipatory activity in mice. J. Biol. Rhythms 27, 398–409. doi: 10.1177/0748730412455913
Longo, V. D., and Panda, S. (2016). Fasting, circadian rhythms, and time-restricted feeding in healthy lifespan. Cell Metab. 23, 1048–1059. doi: 10.1016/j.cmet.2016.06.001
Lowe, D. A., Wu, N., Rohdin-Bibby, L., Moore, A. H., Kelly, N., Liu, Y. E., et al. (2020). Effects of time-restricted eating on weight loss and other metabolic parameters in women and men with overweight and obesity: the TREAT randomized clinical trial. JAMA Intern. Med. 180, 1491–1499. doi: 10.1001/jamainternmed.2020.4153
Luo, A. H., Georges, F. E., and Aston-Jones, G. S. (2008). Novel neurons in ventral tegmental area fire selectively during the active phase of the diurnal cycle. Eur. J. Neurosci. 27, 408–422. doi: 10.1111/j.1460-9568.2007.05985.x
Luo, S., Zhang, Y., Ezrokhi, M., Li, Y., Tsai, T. H., and Cincotta, A. H. (2018). Circadian peak dopaminergic activity response at the biological clock pacemaker (suprachiasmatic nucleus) area mediates the metabolic responsiveness to a high-fat diet. J. Neuroendocrinol. 30:e12563. doi: 10.1111/jne.12563
Mandelblat-Cerf, Y., Ramesh, R. N., Burgess, C. R., Patella, P., Yang, Z., Lowell, B. B., et al. (2015). Arcuate hypothalamic AgRP and putative POMC neurons show opposite changes in spiking across multiple timescales. Elife 4:e07122. doi: 10.7554/eLife.07122
Markwald, R. R., Melanson, E. L., Smith, M. R., Higgins, J., Perreault, L., Eckel, R. H., et al. (2013). Impact of insufficient sleep on total daily energy expenditure, food intake, and weight gain. Proc. Natl. Acad. Sci. U. S. A. 110, 5695–5700. doi: 10.1073/pnas.1216951110
Martel, J. C., and Gatti McArthur, S. (2020). Dopamine receptor subtypes, physiology and pharmacology: new ligands and concepts in schizophrenia. Front. Pharmacol. 11:1003. doi: 10.3389/fphar.2020.01003
Masubuchi, S., Honma, S., Abe, H., Ishizaki, K., Namihira, M., Ikeda, M., et al. (2000). Clock genes outside the suprachiasmatic nucleus involved in manifestation of locomotor activity rhythm in rats. Eur. J. Neurosci. 12, 4206–4214.
Mazzone, C. M., Liang-Guallpa, J., Li, C., Wolcott, N. S., Boone, M. H., Southern, M., et al. (2020). High-fat food biases hypothalamic and mesolimbic expression of consummatory drives. Nat. Neurosci. 23, 1253–1266. doi: 10.1038/s41593-020-0684-9
McCutcheon, J. E., Ebner, S. R., Loriaux, A. L., and Roitman, M. F. (2012). Encoding of aversion by dopamine and the nucleus accumbens. Front. Neurosci. 6:137. doi: 10.3389/fnins.2012.00137
Mei, L., Fan, Y., Lv, X., Welsh, D. K., Zhan, C., and Zhang, E. E. (2018). Long-term in vivo recording of circadian rhythms in brains of freely moving mice. Proc. Natl. Acad. Sci. U. S. A. 115, 4276–4281. doi: 10.1073/pnas.1717735115
Mendoza, J. (2019a). Eating rewards the gears of the clock. Trends Endocrinol. Metab. 30, 299–311. doi: 10.1016/j.tem.2019.03.001
Mendoza, J. (2019b). Food intake and addictive-like eating behaviors: time to think about the circadian clock(s). Neurosci. Biobehav. Rev. 106, 122–132. doi: 10.1016/j.neubiorev.2018.07.003
Mendoza, J., Albrecht, U., and Challet, E. (2010a). Behavioural food anticipation in clock genes deficient mice: confirming old phenotypes, describing new phenotypes. Genes Brain Behav. 9, 467–477. doi: 10.1111/j.1601-183X.2010.00576.x
Mendoza, J., Clesse, D., Pévet, P., and Challet, E. (2010b). Food-reward signalling in the suprachiasmatic clock. J. Neurochem. 112, 1489–1499. doi: 10.1111/j.1471-4159.2010.06570.x
Mendoza, J., and Challet, E. (2014). Circadian insights into dopamine mechanisms. Neuroscience 282, 230–242. doi: 10.1016/j.neuroscience.2014.07.081
Mendoza, J., Pévet, P., and Challet, E. (2008). High-fat feeding alters the clock synchronization to light. J. Physiol. 586, 5901–5910. doi: 10.1113/jphysiol.2008.159566
Meyer-Bernstein, E. L., and Morin, L. P. (1999). Electrical stimulation of the median or dorsal raphe nuclei reduces light-induced FOS protein in the suprachiasmatic nucleus and causes circadian activity rhythm phase shifts. Neuroscience 92, 267–279. doi: 10.1016/s0306-4522(98)00733-7
Mieda, M., Williams, S. C., Richardson, J. A., Tanaka, K., and Yanagisawa, M. (2006). The dorsomedial hypothalamic nucleus as a putative food-entrainable circadian pacemaker. Proc. Natl. Acad. Sci. U. S. A. 103, 12150–12155. doi: 10.1073/pnas.0604189103
Milken Institute (2019). America’s Obesity Crisis: The Health and Economic Costs of Excess Weight. Available online at: http://milkeninstitute.org/reports/americas-obesity-crisis-health-and-economic-costs-excess-weight (accessed December 6, 2019).
Mistlberger, R. E. (2011). Neurobiology of food anticipatory circadian rhythms. Physiol. Behav. 104, 535–545. doi: 10.1016/j.physbeh.2011.04.015
Mistlberger, R. E. (2020). Food as circadian time cue for appetitive behavior. [version 1; peer review: 5 approved]. F1000Res 9:F1000 Faculty Rev-61. doi: 10.12688/f1000research.20829.1
Mistlberger, R. E., and Antle, M. C. (2011). Entrainment of circadian clocks in mammals by arousal and food. Essays Biochem. 49, 119–136. doi: 10.1042/bse0490119
Miyazaki, S., Tahara, Y., Colwell, C. S., Block, G. D., Nakamura, W., and Nakamura, T. J. (2021). Chronic methamphetamine uncovers a circadian rhythm in multiple-unit neural activity in the dorsal striatum which is independent of the suprachiasmatic nucleus. Neurobiol. Sleep Circadian Rhythms 11:100070. doi: 10.1016/j.nbscr.2021.100070
Mohawk, J. A., Baer, M. L., and Menaker, M. (2009). The methamphetamine-sensitive circadian oscillator does not employ canonical clock genes. Proc. Natl. Acad. Sci. U. S. A. 106, 3519–3524. doi: 10.1073/pnas.0813366106
Mohebi, A., Pettibone, J. R., Hamid, A. A., Wong, J.-M. T., Vinson, L. T., Patriarchi, T., et al. (2019). Dissociable dopamine dynamics for learning and motivation. Nature 570, 65–70. doi: 10.1038/s41586-019-1235-y
Natsubori, A., Honma, K., and Honma, S. (2014). Dual regulation of clock gene Per2 expression in discrete brain areas by the circadian pacemaker and methamphetamine-induced oscillator in rats. Eur. J. Neurosci. 39, 229–240. doi: 10.1111/ejn.12400
Navarro, M., Olney, J. J., Burnham, N. W., Mazzone, C. M., Lowery-Gionta, E. G., Pleil, K. E., et al. (2016). Lateral hypothalamus gabaergic neurons modulate consummatory behaviors regardless of the caloric content or biological relevance of the consumed stimuli. Neuropsychopharmacology 41, 1505–1512. doi: 10.1038/npp.2015.304
Nedeltcheva, A. V., Kilkus, J. M., Imperial, J., Kasza, K., Schoeller, D. A., and Penev, P. D. (2009). Sleep curtailment is accompanied by increased intake of calories from snacks. Am. J. Clin. Nutr. 89, 126–133. doi: 10.3945/ajcn.2008.26574
Nieh, E. H., Matthews, G. A., Allsop, S. A., Presbrey, K. N., Leppla, C. A., Wichmann, R., et al. (2015). Decoding neural circuits that control compulsive sucrose seeking. Cell 160, 528–541. doi: 10.1016/j.cell.2015.01.003
Nieh, E. H., Vander Weele, C. M., Matthews, G. A., Presbrey, K. N., Wichmann, R., Leppla, C. A., et al. (2016). Inhibitory input from the lateral hypothalamus to the ventral tegmental area disinhibits dopamine neurons and promotes behavioral activation. Neuron 90, 1286–1298. doi: 10.1016/j.neuron.2016.04.035
Nikaido, T., Akiyama, M., Moriya, T., and Shibata, S. (2001). Sensitized increase of period gene expression in the mouse caudate/putamen caused by repeated injection of methamphetamine. Mol. Pharmacol. 59, 894–900. doi: 10.1124/mol.59.4.894
Nishide, S., Suzuki, Y., Ono, D., Honma, S., and Honma, K.-I. (2021). The food-entrainable oscillator is a complex of Non-SCN activity bout oscillators uncoupled from the SCN circadian pacemaker. J. Biol. Rhythms 36, 575–588. doi: 10.1177/07487304211047937
Padilla, S. L., Perez, J. G., Ben-Hamo, M., Johnson, C. W., Sanchez, R. E. A., Bussi, I. L., et al. (2019). Kisspeptin neurons in the arcuate nucleus of the hypothalamus orchestrate circadian rhythms and metabolism. Curr. Biol. 29, 592–604.e4. doi: 10.1016/j.cub.2019.01.022
Pan, A., Schernhammer, E. S., Sun, Q., and Hu, F. B. (2011). Rotating night shift work and risk of type 2 diabetes: two prospective cohort studies in women. PLoS Med. 8:e1001141. doi: 10.1371/journal.pmed.1001141
Panda, S., Hogenesch, J. B., and Kay, S. A. (2002). Circadian rhythms from flies to human. Nature 417, 329–335. doi: 10.1038/417329a
Partington, H. S., Nutter, J. M., and Eells, J. B. (2021). Nurr1 deficiency shortens free running period, enhances photoentrainment to phase advance, and disrupts circadian cycling of the dopamine neuron phenotype. Behav. Brain Res. 411:113347. doi: 10.1016/j.bbr.2021.113347
Patke, A., Young, M. W., and Axelrod, S. (2020). Molecular mechanisms and physiological importance of circadian rhythms. Nat. Rev. Mol. Cell Biol. 21, 67–84. doi: 10.1038/s41580-019-0179-2
Patriarchi, T., Cho, J. R., Merten, K., Howe, M. W., Marley, A., Xiong, W.-H., et al. (2018). Ultrafast neuronal imaging of dopamine dynamics with designed genetically encoded sensors. Science 360:eaat4422. doi: 10.1126/science.aat4422
Patton, D. F., and Mistlberger, R. E. (2013). Circadian adaptations to meal timing: neuroendocrine mechanisms. Front. Neurosci. 7:185. doi: 10.3389/fnins.2013.00185
Pendergast, J. S., Oda, G. A., Niswender, K. D., and Yamazaki, S. (2012). Period determination in the food-entrainable and methamphetamine-sensitive circadian oscillator(s). Proc. Natl. Acad. Sci. U. S. A. 109, 14218–14223. doi: 10.1073/pnas.1206213109
Pendergast, J. S., Wendroth, R. H., Stenner, R. C., Keil, C. D., and Yamazaki, S. (2017). mPeriod2 Brdm1 and other single Period mutant mice have normal food anticipatory activity. Sci. Rep. 7:15510. doi: 10.1038/s41598-017-15332-6
Pendergast, J. S., and Yamazaki, S. (2018). The mysterious food-entrainable oscillator: insights from mutant and engineered mouse models. J. Biol. Rhythms 33, 458–474. doi: 10.1177/0748730418789043
Petersen, C. C., Cao, F., Stinchcombe, A. R., and Mistlberger, R. E. (2022). Multiple entrained oscillator model of food anticipatory circadian rhythms. Sc. Rep. 12:9306. doi: 10.1038/s41598-022-13242-w
Podyma, B., Johnson, D.-A., Sipe, L., Remcho, T. P., Battin, K., Liu, Y., et al. (2020). The p75 neurotrophin receptor in AgRP neurons is necessary for homeostatic feeding and food anticipation. Elife 9:e52623. doi: 10.7554/eLife.52623
Richter, C. P. (1922). A behavioristic study of the activity of the rat. Comp. Psychol. Monogr 1, 1–54.
Rossi, M. A., and Stuber, G. D. (2018). Overlapping brain circuits for homeostatic and hedonic feeding. Cell Metab. 27, 42–56. doi: 10.1016/j.cmet.2017.09.021
Saeb-Parsy, K., Lombardelli, S., Khan, F. Z., McDowall, K., Au-Yong, I. T., and Dyball, R. E. (2000). Neural connections of hypothalamic neuroendocrine nuclei in the rat. J. Neuroendocrinol. 12, 635–648.
Sakurai, T. (2014). The role of orexin in motivated behaviours. Nat. Rev. Neurosci. 15, 719–731. doi: 10.1038/nrn3837
Saper, C. B., Chou, T. C., and Elmquist, J. K. (2002). The need to feed: homeostatic and hedonic control of eating. Neuron 36, 199–211. doi: 10.1016/s0896-6273(02)00969-8
Scarpa, L. L., Wanken, B., Smidt, M., Mistlberger, R. E., and Steele, A. D. (2022). Mice hypomorphic for Pitx3 show robust entrainment of circadian behavioral and metabolic rhythms to scheduled feeding. Cell Rep. 38:109865. doi: 10.1016/j.celrep.2021.109865
Schultz, W. (2016). Dopamine reward prediction-error signalling: a two-component response. Nat. Rev. Neurosci. 17, 183–195. doi: 10.1038/nrn.2015.26
Schwartz, W. J., and Zimmerman, P. (1991). Lesions of the suprachiasmatic nucleus disrupt circadian locomotor rhythms in the mouse. Physiol. Behav. 49, 1283–1287. doi: 10.1016/0031-9384(91)90364-t
Smit, A. N., Patton, D. F., Michalik, M., Opiol, H., and Mistlberger, R. E. (2013). Dopaminergic regulation of circadian food anticipatory activity rhythms in the rat. PLoS One 8:e82381. doi: 10.1371/journal.pone.0082381
Smyllie, N. J., Chesham, J. E., Hamnett, R., Maywood, E. S., and Hastings, M. H. (2016). Temporally chimeric mice reveal flexibility of circadian period-setting in the suprachiasmatic nucleus. Proc. Natl. Acad. Sci. U. S. A. 113, 3657–3662. doi: 10.1073/pnas.1511351113
Stamatakis, A. M., Van Swieten, M., Basiri, M. L., Blair, G. A., Kantak, P., and Stuber, G. D. (2016). Lateral hypothalamic area glutamatergic neurons and their projections to the lateral habenula regulate feeding and reward. J. Neurosci. 36, 302–311. doi: 10.1523/JNEUROSCI.1202-15.2016
Steele, A. D., and Mistlberger, R. E. (2015). Circadian rhythms: activity is a slave to many masters. Elife 4:e06351.
Stephan, F. K., Swann, J. M., and Sisk, C. L. (1979). Anticipation of 24-hr feeding schedules in rats with lesions of the suprachiasmatic nucleus. Behav. Neural Biol. 25, 346–363. doi: 10.1016/S0163-1047(79)90415-1
Stephan, F. K., and Zucker, I. (1972). Circadian rhythms in drinking behavior and locomotor activity of rats are eliminated by hypothalamic lesions. Proc. Natl. Acad. Sci. U. S. A. 69, 1583–1586. doi: 10.1073/pnas.69.6.1583
Sternson, S. M., and Eiselt, A.-K. (2017). Three pillars for the neural control of appetite. Annu. Rev. Physiol. 79, 401–423. doi: 10.1146/annurev-physiol-021115-104948
Stokkan, K. A., Yamazaki, S., Tei, H., Sakaki, Y., and Menaker, M. (2001). Entrainment of the circadian clock in the liver by feeding. Science 291, 490–493. doi: 10.1126/science.291.5503.490
Storch, K.-F., and Weitz, C. J. (2009). Daily rhythms of food-anticipatory behavioral activity do not require the known circadian clock. Proc. Natl. Acad. Sci. U. S. A. 106, 6808–6813. doi: 10.1073/pnas.0902063106
Stuber, G. D., and Wise, R. A. (2016). Lateral hypothalamic circuits for feeding and reward. Nat. Neurosci. 19, 198–205. doi: 10.1038/nn.4220
Sun, F., Zeng, J., Jing, M., Zhou, J., Feng, J., Owen, S. F., et al. (2018). A genetically encoded fluorescent sensor enables rapid and specific detection of dopamine in flies, fish, and mice. Cell 174, 481–496.e19. doi: 10.1016/j.cell.2018.06.042
Sutton, E. F., Beyl, R., Early, K. S., Cefalu, W. T., Ravussin, E., and Peterson, C. M. (2018). Early time-restricted feeding improves insulin sensitivity, blood pressure, and oxidative stress even without weight loss in men with prediabetes. Cell Metab. 27, 1212–1221.e3. doi: 10.1016/j.cmet.2018.04.010
Szczypka, M. S., Kwok, K., Brot, M. D., Marck, B. T., Matsumoto, A. M., Donahue, B. A., et al. (2001). Dopamine production in the caudate putamen restores feeding in dopamine-deficient mice. Neuron 30, 819–828. doi: 10.1016/s0896-6273(01)00319-1
Takahashi, J. S. (2017). Transcriptional architecture of the mammalian circadian clock. Nat. Rev. Genet. 18, 164–179. doi: 10.1038/nrg.2016.150
Takasu, N. N., Kurosawa, G., Tokuda, I. T., Mochizuki, A., Todo, T., and Nakamura, W. (2012). Circadian regulation of food-anticipatory activity in molecular clock-deficient mice. PLoS One 7:e48892. doi: 10.1371/journal.pone.0048892
Tataroglu, O., Davidson, A. J., Benvenuto, L. J., and Menaker, M. (2006). The methamphetamine-sensitive circadian oscillator (MASCO) in mice. J. Biol. Rhythms 21, 185–194. doi: 10.1177/0748730406287529
Tellez, L. A., Han, W., Zhang, X., Ferreira, T. L., Perez, I. O., Shammah-Lagnado, S. J., et al. (2016). Separate circuitries encode the hedonic and nutritional values of sugar. Nat. Neurosci. 19, 465–470. doi: 10.1038/nn.4224
Tinsley, G. M., Forsse, J. S., Butler, N. K., Paoli, A., Bane, A. A., La Bounty, P. M., et al. (2017). Time-restricted feeding in young men performing resistance training: a randomized controlled trial. Eur. J. Sport Sci. 17, 200–207. doi: 10.1080/17461391.2016.1223173
Treasure, J., Zipfel, S., Micali, N., Wade, T., Stice, E., Claudino, A., et al. (2015). Anorexia nervosa. Nat. Rev. Dis. Primers 1:15074. doi: 10.1038/nrdp.2015.74
Turek, F. W., Joshu, C., Kohsaka, A., Lin, E., Ivanova, G., McDearmon, E., et al. (2005). Obesity and metabolic syndrome in circadian Clock mutant mice. Science 308, 1043–1045. doi: 10.1126/science.1108750
Vansteensel, M. J., Deboer, T., Dahan, A., and Meijer, J. H. (2003). Differential responses of circadian activity onset and offset following GABA-ergic and opioid receptor activation. J. Biol. Rhythms 18, 297–306. doi: 10.1177/0748730403254283
Verwey, M., Dhir, S., and Amir, S. (2016). Circadian influences on dopamine circuits of the brain: regulation of striatal rhythms of clock gene expression and implications for psychopathology and disease. [version 1; peer review: 2 approved]. F1000Res 5:F1000 Faculty Rev-2062. doi: 10.12688/f1000research.9180.1
Volkow, N. D., Wang, G.-J., Fowler, J. S., Logan, J., Jayne, M., Franceschi, D., et al. (2002). “Nonhedonic” food motivation in humans involves dopamine in the dorsal striatum and methylphenidate amplifies this effect. Synapse 44, 175–180. doi: 10.1002/syn.10075
Webb, I. C. (2017). Circadian rhythms and substance abuse: chronobiological considerations for the treatment of addiction. Curr. Psychiatry Rep. 19:12. doi: 10.1007/s11920-017-0764-z
Webb, I. C., Baltazar, R. M., Wang, X., Pitchers, K. K., Coolen, L. M., and Lehman, M. N. (2009). Diurnal variations in natural and drug reward, mesolimbic tyrosine hydroxylase, and clock gene expression in the male rat. J. Biol. Rhythms 24, 465–476. doi: 10.1177/0748730409346657
Welsh, D. K., Takahashi, J. S., and Kay, S. A. (2010). Suprachiasmatic nucleus: cell autonomy and network properties. Annu. Rev. Physiol. 72, 551–577. doi: 10.1146/annurev-physiol-021909-135919
Wilkinson, M. J., Manoogian, E. N. C., Zadourian, A., Lo, H., Fakhouri, S., Shoghi, A., et al. (2020). Ten-hour time-restricted eating reduces weight, blood pressure, and atherogenic lipids in patients with metabolic syndrome. Cell Metab. 31, 92–104.e5. doi: 10.1016/j.cmet.2019.11.004
Wise, R. A. (2004). Dopamine, learning and motivation. Nat. Rev. Neurosci. 5, 483–494. doi: 10.1038/nrn1406
Wise, R. A. (2006). Role of brain dopamine in food reward and reinforcement. Philos. Trans. R. Soc. Lond. B Biol. Sci. 361, 1149–1158. doi: 10.1098/rstb.2006.1854
Wu, Y.-E., Enoki, R., Oda, Y., Huang, Z.-L., Honma, K.-I., and Honma, S. (2018). Ultradian calcium rhythms in the paraventricular nucleus and subparaventricular zone in the hypothalamus. Proc. Natl. Acad. Sci. U. S. A. 115, E9469–E9478. doi: 10.1073/pnas.1804300115
Wu, Z., Kim, E. R., Sun, H., Xu, Y., Mangieri, L. R., Li, D.-P., et al. (2015). GABAergic projections from lateral hypothalamus to paraventricular hypothalamic nucleus promote feeding. J. Neurosci. 35, 3312–3318. doi: 10.1523/JNEUROSCI.3720-14.2015
Yang, S., Tan, Y. L., Wu, X., Wang, J., Sun, J., Liu, A., et al. (2021). An mPOA-ARCAgRP pathway modulates cold-evoked eating behavior. Cell Rep. 36:109502. doi: 10.1016/j.celrep.2021.109502
Yoo, S.-H., Yamazaki, S., Lowrey, P. L., Shimomura, K., Ko, C. H., Buhr, E. D., et al. (2004). PERIOD2::LUCIFERASE real-time reporting of circadian dynamics reveals persistent circadian oscillations in mouse peripheral tissues. Proc. Natl. Acad. Sci. U. S. A. 101, 5339–5346. doi: 10.1073/pnas.0308709101
Zhang, S. X., Lutas, A., Yang, S., Diaz, A., Fluhr, H., Nagel, G., et al. (2021). Hypothalamic dopamine neurons motivate mating through persistent cAMP signalling. Nature 597, 245–249. doi: 10.1038/s41586-021-03845-0
Zhang, X., and van den Pol, A. N. (2016). Hypothalamic arcuate nucleus tyrosine hydroxylase neurons play orexigenic role in energy homeostasis. Nat. Neurosci. 19, 1341–1347. doi: 10.1038/nn.4372
Keywords: dopamine, food entrainment, food entrained oscillator (FEO), diet-induced obesity (DIO), methampetamine, feeding, circadian
Citation: Tang Q, Assali DR, Güler AD and Steele AD (2022) Dopamine systems and biological rhythms: Let’s get a move on. Front. Integr. Neurosci. 16:957193. doi: 10.3389/fnint.2022.957193
Received: 30 May 2022; Accepted: 06 July 2022;
Published: 27 July 2022.
Edited by:
Adam Stinchcombe, University of Toronto, CanadaReviewed by:
Raul Aguilar-Roblero, Universidad Nacional Autónoma de México, MexicoFlorian Storch, McGill University, Canada
Charles N. Allen, Oregon Health and Science University, United States
Copyright © 2022 Tang, Assali, Güler and Steele. This is an open-access article distributed under the terms of the Creative Commons Attribution License (CC BY). The use, distribution or reproduction in other forums is permitted, provided the original author(s) and the copyright owner(s) are credited and that the original publication in this journal is cited, in accordance with accepted academic practice. No use, distribution or reproduction is permitted which does not comply with these terms.
*Correspondence: Ali D. Güler, YWd1bGVyQHZpcmdpbmlhLmVkdQ==; Andrew D. Steele, YWRzdGVlbGVAY3BwLmVkdQ==