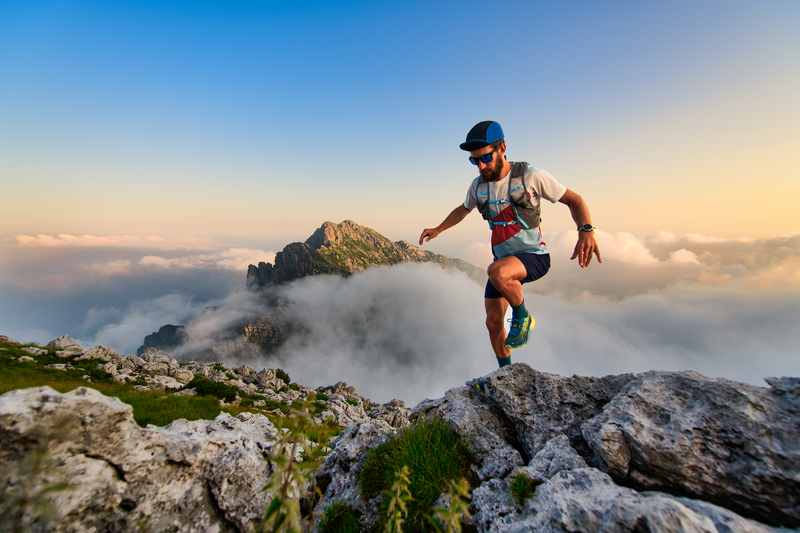
95% of researchers rate our articles as excellent or good
Learn more about the work of our research integrity team to safeguard the quality of each article we publish.
Find out more
PERSPECTIVE article
Front. Integr. Neurosci. , 27 December 2011
Volume 5 - 2011 | https://doi.org/10.3389/fnint.2011.00091
This article is part of the Research Topic Interval Timing and Time-Based Decision Making View all 59 articles
The relation between the contingent negative variation (CNV) and time estimation is evaluated in terms of temporal accumulation and preparation processes. The conclusion is that the CNV as measured from the electroencephalogram (EEG) recorded at fronto-central and parietal-central areas is not a direct reflection of the underlying interval timing mechanism(s), but more likely represents a time-based response preparation/decision-making process.
Half a century after the discovery of the contingent negative variation (CNV, Walter et al., 1964), it is still unclear what drives this slow negative wave in the electroencephalogram (EEG) when a participant is anticipating an event of interest. From the beginning, it has been suggested that the CNV, and more specifically its amplitude, reflects expectancy or motivation, all related to the intention to perform an act (e.g., McAdam et al., 1969; for a review, see Tecce, 1972). As the CNV only fully develops when a response is required at a predictable point in time, it depends on accurate temporal preparation, which requires the ability to estimate time. This ability has often been explained in terms of the influential pacemaker-accumulator models of interval timing (e.g., Treisman, 1963; Gibbon et al., 1984; Meck, 1996; Wearden, 1999; Meck and Benson, 2002; Taatgen et al., 2007). Given the similarity between the CNV and the hypothesized characteristics of the accumulation process in these models, it has been proposed that the CNV is the signature of a neural substrate of the temporal accumulator (see Macar and Vidal, 2009). According to the proponents of this view, a number of empirical studies support this hypothesis. Here we critically review these studies, focusing on two claims that are key to the idea that the CNV reflects the temporal accumulator.
If the amplitude of the CNV is related to the value of the temporal accumulator, variations in the CNV should be reflected in timing performance. Macar et al. (1999, Experiment 1) observed a correlation between the reproduced duration and the CNV amplitude, a finding that has been interpreted as evidence in support of this hypothesis. In Macar et al.'s experiment, participants were asked to repeatedly reproduce an interval of 2.5 s. The average CNV amplitude was calculated separately for trials in which participants responded too early (2.2–2.4 s), correctly (2.4–2.6 s), or too long (2.6–2.8 s), and showed a positive correlation with reproduced duration (see Figure 1 in Casini and Vidal, 2011 for an illustration of these findings).
At first glance, this correlation between the CNV and the assumed state of the accumulator seems to be strong support in favor of the hypothesis that the CNV directly reflects the accumulation process. However, a careful analysis of the arguments in terms of the default pacemaker-accumulator model reveals a number of inconsistencies. For example, if the speed of the pacemaker is higher, pulses accumulate more quickly, and therefore, the threshold should be reached earlier. In other words, if larger CNV amplitudes reflect more accumulated pulses, then why don't participants respond before the target duration on high-amplitude CNV trials and after the target duration on low-amplitude CNV trials? Assuming continuous comparisons between the accumulator and threshold, the estimated duration should be “shorter” with higher pacemaker speeds since the threshold will be reached sooner and vice versa with lower pacemaker speeds.
Differences in amplitude can, in the context of a threshold-based pacemaker-accumulator account, only be explained when it is assumed that the comparisons between the accumulator and threshold are not continuously made. If comparisons are not made continuously, the number of pulses that reaches the accumulator between two comparisons is the maximum overshoot (plus one) that can be observed, yielding a correlation between the speed of the pacemaker and the value of the accumulator at the response. For example, if the threshold is known to be 100 pulses and five pulses reach the accumulator between subsequent threshold-accumulator comparisons, the maximum observed amplitude reflects 99 + 5 = 104 pulses. However, if the speed of the pacemaker is doubled, the maximum amplitude is 99 + 10 = 109 pulses. Although this might explain how an increased pulse rate results in an increased CNV amplitude, the threshold will still be reached before (or at) the standard time. In other words, it is difficult to explain how a higher speed should result in increased CNV amplitudes and longer estimations if one assumes that the basic pacemaker-accumulator model is correct. An association between short estimations and lower CNV amplitudes is even more difficult to explain in the context of a threshold-driven pacemaker-accumulator model. If the CNV is still below the average value, it is unlikely that the accumulator already has reached the threshold.
An alternative explanation, favored by Macar et al. (1999), is that the trials resulting in “short,” “correct,” and “long” durations accumulate up to different thresholds. These investigators assume that the intrinsic properties of the neural system cause an increase in activation after the onset of an interval and that the response is given when the peak of activation is reached (Macar et al., 1999; Macar and Vidal, 2009). Thus, if an onset results in a higher boost of activation, the CNV will have a higher amplitude and a later deflection point, resulting in longer estimations. Although the idea of variations in threshold is often found in the literature (see, for example, computational models of the role of memory in interval timing, e.g., Jazayeri and Shadlen, 2010; Taatgen and van Rijn, 2011), this is not typically associated with performance-dependent variations in the CNV. This is not surprising because a link between different thresholds and CNV amplitudes would imply that different durations (e.g., durations of 1, 2, and 3 s) are associated with different amplitudes, whereas these correlations are not typically found (e.g., Elbert et al., 1991; Gibbons and Rammsayer, 2004; Praamstra et al., 2006, and see Praamstra, 2010 for a review of foreperiod effects).
In light of these theoretical issues, it is relevant to mention that Kononowicz and van Rijn (2011) recently failed to replicate the findings of Macar et al. (1999). In two experiments, which were close replicates of the original study, no performance-dependent variations in the CNV were found. In addition, Kononowicz and van Rijn (2011) found a habituation effect, which is not in line with the predicted role of the CNV, i.e., if the amplitude of the CNV has a one-to-one mapping to the state of the accumulator, it would be implausible for the CNV amplitude to diminish over the scope of the experiment while the time estimations stay constant. It should be noted that the Kononowicz and van Rijn (2011) study is not the only reported failure to replicate these results, as one condition of a study by Macar and Vidal (2002, labeled “Condition 1”) was a close replication of the original experiment, but no performance-dependent variations in the CNV were observed. Moreover, although it may be that differences in CNV cannot be readily generalized to differences in blood oxygen level-dependent (BOLD) response, no performance-dependent variations in the pre-supplementary motor area (SMA, typically associated with the CNV) BOLD signal were found in a functional magnetic resonance imaging (fMRI) replication of the 1999 study (Macar et al., 2004). However, other studies have found differences in BOLD response during the reproduction and estimation of different intervals. For example, a stronger BOLD response was found in many brain areas, including the SMA, during the estimation and reproduction of a 1300 ms interval than during the estimation and reproduction of a 450 ms interval (Pouthas et al., 2005). Interestingly, when participants had to encode and reproduce durations of 9–18 s in a study by Wittmann et al. (2010), the activation of the posterior insula resembled an accumulation process, but the SMA activation followed an inverse U-shape pattern during encoding. Thus, although fMRI studies have been able to identify brain areas that are differentially active during the processing of different durations, it appears that the basic effects reported by Macar et al. (1999) are difficult to replicate in both EEG and fMRI studies.
A second experiment reported in Macar et al. (1999) has also been interpreted in favor of performance-dependent variations in the CNV. In this experiment, participants learned a standard duration and were later asked to categorize presented durations as either shorter, equal, or longer than the standard. The predicted pattern was found between CNV and duration estimation, with trials categorized as “long” being associated with a higher amplitude CNV than the trials categorized as “short.” This finding was replicated in a study by Bendixen et al. (2005) in which participants had to indicate whether a 480 or 520 ms tone was longer or shorter than a previously learned standard of 500 ms.
However, these performance-dependent variations are difficult to reconcile with studies that show that the CNV deflects or resolves after a standard has been reached (e.g., Macar and Vidal, 2003, see also, Pfeuty et al., 2003, 2005; Ng et al., 2011). If the decision to categorize a particular trial as “long” is made on the basis of a comparison to the standard, then in these trials the accumulator should have already reached the value of the standard. This notion is supported by the duration bisection data presented in Ng et al. (2011). In this experiment, participants were presented a “long” and a “short” anchor of 800 and 3200 ms, and were asked to categorize seven intermediate durations as either more similar to the “long,” or to the “short” anchor. When combining the three intermediate conditions (1270, 1600, and 2016 ms) in which neither answer category was dominant, a partial least squares analysis (McIntosh and Lobaugh, 2004) revealed that when a “long” categorization was given, the CNV was either similar to or had a lower amplitude than when a short categorization was provided. Moreover, although EEG differences have been observed in duration bisection for trials participants categorized as “short” or “long,” this effect is related to the power of the alpha band in event-related synchronization rather than the amplitude of the CNV (Ng et al., 2011).
Thus, recent electrophysiological studies from a number of labs indicate that the presentation of a stimulus duration longer than the standard is associated with a deflection of the CNV at the time of the standard. As an “equal” response will be based on the accumulator reaching the threshold at the perceived time of stimulus offset, a “long” response assumes that the accumulator had reached the threshold some time before the offset of the stimulus. This in turn implies that the deflection should already have been observed and thus that the CNV amplitude for “long” estimations is lower than that for “short” or “correct” estimations. As higher CNV amplitudes were observed for the “long” estimations in Experiment 2 of Macar et al. (1999), these results are difficult to reconcile with a consistent application of the hypothesis that the CNV reflects function of the accumulator proposed by pacemaker-accumulator models (Gibbon et al., 1984; Meck, 1996; Meck and Benson, 2002).
To summarize, although stable performance-dependent variations of the CNV would be evidence in favor of the CNV as the signature of a neural substrate of the temporal accumulator, none of the above-mentioned studies provides unequivocal evidence in favor of that view.
If the CNV reflects the neural substrate of the temporal accumulator, its location should be fairly stable. And indeed, both fMRI and EEG/Magnetoencephalography studies (see, for example, Pouthas, 2003; Meck et al., 2008; Coull et al., 2011; Schwartze et al., in press, for an overview) have shown that the SMA subserves a variety of timing tasks. However, arguing that this proves that the SMA serves as the common accumulator for temporal processing is an error of omission. That is, many EEG studies over the years have found CNV-like patterns at different locations. For example, Praamstra et al. (2006) have shown in a foreperiod study that the strongest signal was observed at the lateralized pre-motor cortices instead of at SMA, a finding that is in line with evidence from primate neurophysiology (Mauritz and Wise, 1986) and fMRI studies (e.g., Schubotz et al., 2000). Moreover, single-cell recording studies have shown ramping activation in neurons in the posterior parietal cortex (Leon and Shadlen, 2003), and the earlier mentioned fMRI study by Wittmann et al. (2010) has demonstrated accumulation patterns in the posterior insula.
As all of these locations show a ramping pattern of activation, all of these locations could, in principle, be a neural substrate of temporally mediated response preparation. These findings have, therefore, supported the view (e.g., Praamstra, 2010) that distinct neural substrates are implicated in temporal preparation in a task-dependent manner, probably depending on the type of temporal processing that is required for a particular behavior. In other words, although the activation measured at the SMAs does have a link with temporal performance, it is unlikely that the SMAs are the sole receiver of temporal information (see Buhusi and Meck, 2005 as well as Wiener et al., 2010, for a meta-analysis).
This idea is further supported by a recent fMRI study that examined which brain areas were uniquely involved in interval timing, and which brain areas were also influenced by non-temporal tasks (Livesey et al., 2007—see also Meck et al., 2008). Interestingly, the levels of activation measured in small portions of the inferior frontal gyrus, the anterior insulae and the left supramarginal gyrus, and the putamen were only influenced by time estimation, whereas the pre-SMA was also strongly influenced by the difficulty of the non-temporal task. This result led the authors to suggest that areas other than the pre-SMA are critically involved in temporal processing, and that activity in the pre-SMA is related to cognitive effort or task difficulty.
However, what does the buildup of negativity represent if the CNV is not the reflection of the accumulator of temporal information? Two proposals will be discussed here. The first proposes a direct link between oscillatory processes and the CNV and assumes that the CNV increases over time as larger groups of neurons fire simultaneously. The second proposal assumes that the CNV reflects a time-based preparation process.
Many of the CNV results could be viewed as being consistent with an “oscillator” process if one makes the following assumptions: (1) Scalp detected event-related potential (ERP) components, like the CNV, reflect postsynaptic potentials (PSPs) synchronized across many (i.e., thousands of) neurons. (2) The periodic cortical neurons that form a core component of oscillatory models (e.g., striatal beat-frequency model of Matell and Meck, 2000, 2004—see Oprisan and Buhusi, 2011) normally fire at random with respect to each other. Hence, summation of the PSPs that precede those action potentials is maintained at some baseline level. This is true both for neurons that have the same, as well as different periods. (3) According to the striatal beat-frequency model, a signal emitted from the substantia nigra at signal onset acts as a “start-gun” to reset the periodic cortical neurons such that neurons with the same periodicity are synchronized and those with different periodicities share the same initial start point. (4) As the timing signal elapses, the number of near simultaneous PSPs should increase and, therefore, the EEG mean voltage should move away from the baseline level present when the periodic neurons are active at random with respect to each other. Resolution of the CNV occurs when the periodicities return to a “random” relationship with each other, or when the oscillators are reset to start timing a new interval. Such a proposal has some obvious limitations, but it provides a reasonable account of some of the EEG/ERP effects that can be accounted for by oscillatory models and, equally important, it should be possible to design ways to test it (MacDonald and Meck, 2004, 2006; Brannon et al., 2008).
Another explanation of the CNV phenomena assumes that the CNV reflects readiness or preparation for the processing of an event instead of temporal accumulation (e.g., Elbert, 1993). According to this view, the increased negativity (which results in decreased information processing thresholds) reflects the anticipation of an upcoming and relevant event. Data that supports this view has both been provided using CNV-related research (for review see Elbert, 1993) and more recent fMRI research. For example, Forstmann and van Maanen (Forstmann et al., 2008; van Maanen et al., in press) have shown in a series of studies that the pre-SMA is more active in conditions in which a fast response is required than in conditions in which an accurate response is expected. Using similar arguments as Elbert (1993), Forstmann and van Maanen link the activity of the pre-SMA to a reduction of inhibition at the level of the basal ganglia. Combined, this work supports the idea that the buildup of negativity is not a source of temporal information, but a consequence of a temporal preparation for an upcoming event.
The “temporal preparation” hypothesis is supported by the Ng et al. (2011) duration-bisection study discussed above. An analysis of the EEG data showed a negative deflection, which started at the onset of the to-be bisected duration, and reached its maximum at 800 ms, the “short” anchor duration. Interestingly, the amplitude remained stable for about 800 ms, after which the CNV began to resolve. The deflection point at 1600 ms corresponds with the stimulus duration at which the participants switched from predominantly answering “short” to “long,” and is as such comparable to the effects found in the temporal generalization study of Macar and Vidal (2003). However, the stable CNV amplitude between 800 and 1600 ms (and similar stable patterns in Elbert et al., 1991 and Gibbons and Rammsayer, 2004) is difficult to reconcile with a direct link between the CNV and the temporal accumulator, as in this view a stable CNV amplitude indicates a constant subjective perceived duration. A constant subjective duration would have resulted in erratic performance, something that was not observed. In contrast, Ng et al. (2011) interpreted the boundaries of the plateau as two target durations, with the first target duration indicating that a relevant signal might appear from that point on, and the second target duration indicating that any upcoming signal would carry less relevance since the answer (“long”) could already be deduced based on the passage of time. Consequently, these findings support the view that the CNV amplitude reflects the expectancy of a relevant event, something that is based on temporal information, but not the source of temporal information.
In conclusion, experiments that are often cited in favor of a direct link between the CNV and the temporal accumulator cannot be straightforwardly interpreted, and have shown to be difficult to replicate. It is, therefore, our view that proposals based on this direct link need to be re-evaluated in light of the inconsistencies in the supporting EEG data and lack of congruence with physiologically plausible models of interval timing (see Allman and Meck, in press; Coull et al., 2011; Matell et al., 2011; Oprisan and Buhusi, 2011; Portugal et al., 2011; Simen et al., 2011).
The authors declare that the research was conducted in the absence of any commercial or financial relationships that could be construed as a potential conflict of interest.
Allman, M. J., and Meck, W. H. (in press). Pathophysiological distortions in time perception and timed performance. Brain.
Bendixen, A., Grimm, S., and Schrösger, E. (2005). Human auditory event-related potentials predict duration judgments. Neurosci. Lett. 383, 284–288.
Brannon, E. M., Libertus, M. E., Meck, W. H., and Woldorff, M. G. (2008). Electrophysiological measures of time processing in infant and adult brains: Weber's law holds. J. Cogn. Neurosci. 20, 193–203.
Buhusi, C. V., and Meck, W. H. (2005). What makes us tick? Functional and neural mechanisms of interval timing. Nat. Rev. Neurosci. 6, 755–765.
Casini, L., and Vidal, F. (2011). The SMAs: neural substrate of the temporal accumulator. Front. Integr. Neurosci. 5, 35. doi: 10.3389/fnint.2011.00035
Coull, J. T., Cheng, R. K., and Meck, W. H. (2011). Neuroanatomical and neurochemical substrates of timing. Neuropsychopharmacology 36, 3–25.
Elbert, T. (1993). “Slow cortical potentials reflect the regulation of cortical excitability,” in Slow Potential Changes in the Human Brain (NATO Sciences Series: A), eds W. C. McCallum and S. H. Curry (New York: Plenum Press), 235–251.
Elbert, T., Ulrich, R., Rockstroh, B., and Lutzenberger, W. (1991). The processing of temporal intervals reflected by CNV-like brain potentials. Psychophysiology 28, 648–655.
Forstmann, B. U., Dutilh, G., Brown, S., Neumann, J., von Cramon, D. Y., Ridderinkhof, K. R., and Wagenmakers, E. J. (2008). Striatum and pre-SMA facilitate decision-making under time pressure. Proc. Natl. Acad. Sci. U.S.A. 105, 17538–17542.
Gibbon, J., Church, R. M., and Meck, W. H. (1984). Scalar timing in temporal memory. Ann. N.Y. Acad. Sci. 423, 52–77.
Gibbons, H., and Rammsayer, T. H. (2004). Current-source density analysis of slow brain potentials during time estimation. Psychophysiology 41, 861–874.
Jazayeri, M., and Shadlen, M. N. (2010). Temporal context calibrates interval timing. Nat. Neurosci. 13, 1020–1026.
Kononowicz, T. W., and van Rijn, H. (2011). Slow potentials in time estimation: the role of temporal accumulation and habituation. Front. Integr. Neurosci. 5, 48. doi: 10.3389/fnint.2011.00048
Leon, M. I., and Shadlen, M. N. (2003). Representation of time by neurons in the posterior parietal cortex of the macaque. Neuron 38, 317–327.
Livesey, A. C., Wall, M. B., and Smith, A. T. (2007). Time perception: manipulation of task difficulty dissociates clock functions from other cognitive demands. Neuropsychologia 45, 321–331.
Macar, F., Anton, J. L., Bonnet, M., and Vidal, F. (2004). Timing functions of the supplementary motor area: an event-related fMRI study. Brain Res. Cogn. Brain Res. 21, 206–215.
Macar, F., and Vidal, F. (2002). Time processing reflected by EEG surface Laplacians. Exp. Brain Res. 145, 403–406.
Macar, F., and Vidal, F. (2003). The CNV peak: an index of decision making and temporal memory. Psychophysiology 40, 950–954.
Macar, F., and Vidal, F. (2009). Timing processes: an outline of behavioural and neural indices not systematically considered in timing models. Can. J. Exp. Psychol. 63, 227–239.
Macar, F., Vidal, F., and Casini, L. (1999). The supplementary motor area in motor and sensory timing: evidence from slow brain potential changes. Exp. Brain Res. 125, 271–280.
MacDonald, C. J., and Meck, W. H. (2004). Systems-level integration of interval timing and reaction time. Neurosci. Biobehav. Rev. 28, 747–769.
MacDonald, C. J., and Meck, W. H. (2006). Interaction of raclopride and preparatory-interval effects on simple reaction-time performance. Behav. Brain Res. 175, 62–74.
Matell, M. S., and Meck, W. H. (2000). Neuropsychological mechanisms of interval timing behavior. Bioessays 22, 94–103.
Matell, M. S., and Meck, W. H. (2004). Cortico-striatal circuits and interval timing: coincidence detection of oscillatory processes. Brain Res. Cogn. Brain Res. 21, 139–170.
Matell, M. S., Shea-Brown, E., Gooch, C., Wilson, A. H., and Rinzel, J. (2011). A heterogeneous population code for elapsed time in rat medial agranular cortex. Behav. Neurosci. 125, 54–73.
Mauritz, K. H., and Wise, S. P. (1986). Premotor cortex of the rhesus monkey: neuronal activity in anticipation of predictable environmental events. Exp. Brain Res. 61, 229–244.
McAdam, D. W., Knott, J. R., and Rebert, C. S. (1969). Cortical slow potential changes in man related to interstimulus interval and to pre-trial prediction of interstimulus interval. Psychophysiology 5, 349–358.
McIntosh, A. R., and Lobaugh, N. J. (2004). Partial least squares analysis of neuroimaging data: applications and advances. Neuroimage 23, 250–263.
Meck, W. H. (1996). Neuropharmacology of timing and time perception. Brain Res. Cogn. Brain Res. 3, 227–242.
Meck, W. H., and Benson, A. M. (2002). Dissecting the brain's internal clock: how frontal-striatal circuitry keeps time and shifts attention. Brain Cogn. 48, 195–211.
Meck, W. H., Penney, T. B., and Pouthas, V. (2008). Cortico-striatal representation of time in animals and humans. Curr. Opin. Neurobiol. 18, 145–152.
Ng, K. K., Tobin, S., and Penney, T. B. (2011). Temporal accumulation and decision processes in the duration bisection task revealed by contingent negative variation. Front. Integr. Neurosci. 5, 77. doi: 10.3389/fnint.2011.00077
Oprisan, S. A., and Buhusi, C. V. (2011). Modeling pharmacological clock and memory patterns of interval timing in a striatal beat-frequency model with realistic, noisy neurons. Front. Integr. Neurosci. 5, 52. doi: 10.3389/fnint.2011.00052
Pfeuty, M., Ragot, R., and Pouthas, V. (2003). When time is up: CNV time course differentiates the roles of the hemispheres in the discrimination of short tone durations. Exp. Brain Res. 151, 372–379.
Pfeuty, M., Ragot, R., and Pouthas, V. (2005). Relationship between CNV and timing of an upcoming event. Neurosci. Lett. 382, 106–111.
Portugal, G. S., Wilson, A. G., and Matell, M. S. (2011). Behavioral sensitivity of temporally modulated striatal neurons. Front. Integr. Neurosci. 5, 30. doi: 10.3389/fnint.2011.00030
Pouthas, V. (2003). “Electrophysiological evidence for specific processing of temporal information in humans,” in Functional and Neural Mechanisms of Interval Timing, ed W. H. Meck, (Boca Raton, FL: CRC Press), 439–456.
Pouthas, V., George, N., Poline, J.-B., Pfeuty, M., VandeMoorteele, P.-F., Hugueville, L., Ferrandez, A.-M., Lehéricy, S., LeBihan, D., and Renault, B. (2005). Neural network involved in time perception: an fMRI study comparing long and short interval estimation. Hum. Brain Mapp. 25, 433–441.
Praamstra, P. (2010). “Electrophysiological markers of foreperiod effects,” in Attention and Time, eds K. Nobre and J. T. Coull (New York, NY: Oxford University Press), 331–345.
Praamstra, P., Kourtis, D., Kwok, H. F., and Oostenveld, R. (2006). Neurophysiology of implicit timing in serial choice reaction-time performance. J. Neurosci. 26, 5448–5455.
Schubotz, R. I., Friederici, A. D., and von Cramon, D. Y. (2000). Time perception and motor timing: a common cortical and subcortical basis revealed by fMRI. Neuroimage 11, 1–12.
Schwartze, M., Rothermich, K., and Kotz, S. A. (in press). Functional dissociation of pre-SMA and SMA-proper in temporal processing. Neuroimage.
Simen, P., Balci, F., deSouza, L., Cohen, J. D., and Holmes, P. (2011). Interval timing by long-range temporal integration. Front. Integr. Neurosci. 5, 28. doi: 10.3389/fnint.2011.00028
Taatgen, N., and van Rijn, H. (2011). Traces of times past: representations of temporal intervals in memory. Mem. Cognit. 39, 1546–1560.
Taatgen, N. A., van Rijn, H., and Anderson, J. R. (2007). An integrated theory of prospective time interval estimation: the role of cognition, attention and learning. Psychol. Rev. 114, 577–598.
Tecce, J. J. (1972). Contingent negative variation (CNV) and psychological processes in man. Psychol. Bull. 77, 73–108.
Treisman, M. (1963). Temporal discrimination and the indifference interval: implications for a model of the “internal clock”. Psychol. Monogr. 77, 1–31.
van Maanen, L., Brown, S. D., Eichele, T., Wagenmakers, E.-J., Ho, T., Serences, J., and Forstmann, B. U. (in press). Neural correlates of trial-to-trial fluctuations in response caution. J. Neurosci.
Walter, W. G., Cooper, R., Aldrigde, V. J., McCallum, W. C., and Winter, A. L. (1964). Contingent negative variation: an electric sign of sensori-motor association and expectancy in the human brain. Nature 203, 380–384.
Wearden, J. H. (1999). “Beyond the fields we know…”: exploring and developing scalar timing theory. Behav. Process. 45, 3–21.
Wiener, M., Turkeltaub, P., and Coslett, H. B. (2010). The image of time: a voxel-wise meta-analysis. Neuroimage 49, 1728–1740.
Keywords: timing and time perception, temporal accumulation, response preparation, EEG/ERP, fronto-central and parietal-central areas
Citation: van Rijn H, Kononowicz TW, Meck WH, Ng KK and Penney TB (2011) Contingent negative variation and its relation to time estimation: a theoretical evaluation. Front. Integr. Neurosci. 5:91. doi: 10.3389/fnint.2011.00091
Received: 30 October 2011; Accepted: 13 December 2011;
Published online: 27 December 2011.
Edited by:
Agnes Gruart, University Pablo de Olavide, SpainReviewed by:
Walter Ritter, The City College of New York, USACopyright: © 2011 Van Rijn, Kononowicz, Meck, Ng and Penney. This is an open-access article distributed under the terms of the Creative Commons Attribution Non Commercial License, which permits non-commercial use, distribution, and reproduction in other forums, provided the original authors and source are credited.
*Correspondence: Hedderik van Rijn, Experimental Psychology, University of Groningen, Grote Kruistraat 2/1, 9712 TS Groningen, Netherlands. e-mail:aGVkZGVyaWtAdmFuLXJpam4ub3Jn
Disclaimer: All claims expressed in this article are solely those of the authors and do not necessarily represent those of their affiliated organizations, or those of the publisher, the editors and the reviewers. Any product that may be evaluated in this article or claim that may be made by its manufacturer is not guaranteed or endorsed by the publisher.
Research integrity at Frontiers
Learn more about the work of our research integrity team to safeguard the quality of each article we publish.