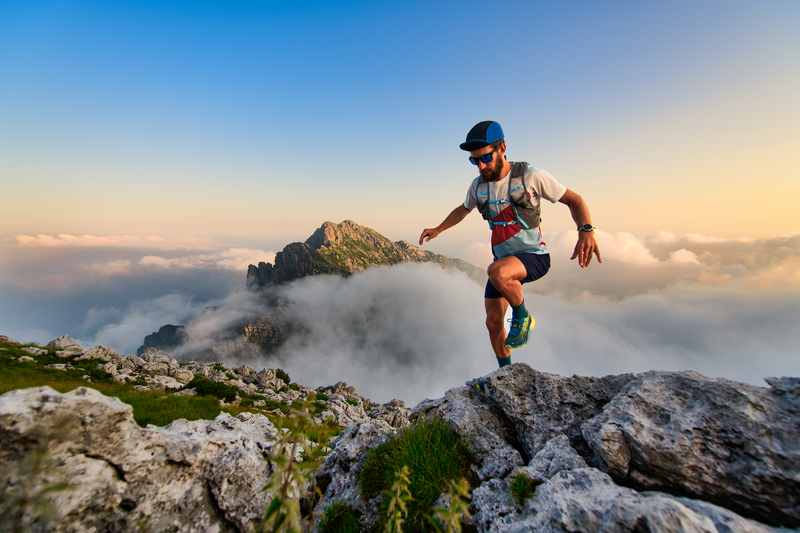
95% of researchers rate our articles as excellent or good
Learn more about the work of our research integrity team to safeguard the quality of each article we publish.
Find out more
EDITORIAL article
Front. Neurosci. , 07 February 2025
Sec. Sleep and Circadian Rhythms
Volume 19 - 2025 | https://doi.org/10.3389/fnins.2025.1558246
This article is part of the Research Topic Signaling Pathways and Brain Circuitry Underlying Circadian Rhythms and Sleep View all 7 articles
Editorial on the Research Topic
Signaling pathways and brain circuitry underlying circadian rhythms and sleep
Circadian rhythms occur on a 24-h cycle and modulate physiological and behavioral processes, such as the sleep/wake cycle, across the day. The focus of this Research Topic is to highlight recent findings on the circuits and pathways that underlie or modulate circadian rhythms and sleep, including genetic, molecular/cellular, electrophysiological, neuroanatomical, pharmacological, and mathematical approaches. The Research Topic includes six papers that focus on three themes: (1) circadian modulation at different spatial scales (Hiro et al.; Aten et al.); (2) genetics of sleep/wake cycles (Kobayashi et al.; Doldur-Balli et al.); and (3) characterization and regulation of sleep stages (Yun et al.; Ginsberg et al.). Below, we provide an overview of each paper.
Circadian rhythms are driven by a transcriptional/translational feedback loop (TTFL) composed of core clock genes and their products that manifest across many spatial/temporal scales from molecular to complex animal behavioral rhythms. At the molecular scale, Hiro et al. investigated circadian rhythm coordination in the suprachiasmatic nucleus (SCN), the location of the mammalian master circadian clock. SCN neurons exhibit circadian rhythms in cytosolic Ca2+, serving as a mediator in signaling pathways linking SCN electrical activity to TTFL gene expression (Colwell, 2011; Welsh et al., 2010; Mohawk et al., 2012; Takahashi, 2017). However, Ca2+ modulatory mechanisms between the cytosol and nucleus remain obscure. To better understand the mechanistic underpinnings of nuclear Ca2+ regulation, Hiro et al. performed dual-color nuclear and cytosolic Ca2+ imaging of mouse SCN neurons, using genetically encoded Ca2+ sensors (Chen et al., 2013; Dana et al., 2016). Results revealed a strong nuclear Ca2+ rhythm which was in-phase with the cytosolic Ca2+ rhythm, both within single neurons and SCN-wide subregions. Their results also suggest that circadian Ca2+ rhythms are driven from the extracellular space, although more work is needed to understand the mechanisms involved.
At the behavioral level, Aten et al., review time-of-day dependence in sexual and reproductive behaviors and connections to circadian rhythms in reproductive hormones in rodents, non-human primates, and humans. They also propose a novel neural circuit—spanning the SCN and sub-paraventricular zone to the ventromedial hypothalamus and medial preoptic area—that may control sexual behaviors across the circadian day. Lastly, they highlight studies that examine time-of-day differences in human sexual behaviors and discuss how circadian dysfunction negatively impacts reproduction.
Sleep is an evolutionarily conserved behavioral state (Joiner, 2016), and model organisms like Drosophila and zebrafish have been widely used to genetically dissect sleep (Cirelli and Bushey, 2008; Zhdanova, 2006). Motivated by the finding that a mutation (Sleepy) in the salt-inducible kinase 3 (Sik3) gene was previously shown to alter sleep in mice (Funato et al., 2016), Kobayashi et al. tested whether a phosphorylation-deficient mutant form of the Sik3 gene (“Sik3-SA”) might also affect sleep regulation in flies. They found that overexpression of this Sik3-SA mutation in all Drosophila neurons increased sleep while overexpression of Sik3-SA specifically in pigment-dispersing factor (PDF), which modulates circadian rhythms in Drosophila (Guo et al., 2016), increased daytime sleep and decreased the circadian rhythm amplitude. Their results also suggest that Sik3-SA alters sleep regulation by PDF neurons in Drosophila, but future experiments are necessary to understand the relationship between Sik3-SA and PDF neuron functionality.
Sleep regulation may also be affected by hyperpolarization-activated cyclic nucleotide-gated (HCN) channels. In zebrafish, Doldur-Balli et al. investigated HCN “pacemaker” channels (Wobig et al., 2020), which are also known to modulate sleep/wake cycles (Lewis and Chetkovich, 2011; Sartiani et al., 2017). The authors tested the effects of three HCN channel inhibitors, Ivabradine, Zatebradine Hydrochloride, and ZD7288, on sleep/wake cycles in zebrafish and found that Ivabradine (at 0.1 μM) led to a shorter latency to daytime sleep relative to vehicle treatment. In addition, Zatebradine Hydrochloride (at 30 μM) led to a decrease in average daytime activity, and ZD7288 (at 4.5 μM) led to a nighttime sleep increase. While these three compounds are used to reduce heart rate (Novella Romanelli et al., 2016), this is the first study to report the effects of HCN channel inhibition in zebrafish—results that could provide insight into potential therapeutics that may impact sleep function.
Previous studies of local field potential (LFP) frequency band power and single-unit dynamics have helped advance our knowledge of sleep/wake stages, including rapid eye movement (REM) sleep and non-REM (NREM) sleep, and other complex brain functions (Brown et al., 2012; Rasch and Born, 2013). Yun et al. contribute to this literature by examining power spectral density of LFPs in the primary motor cortex in Macaca nemestrina monkeys. Single-unit activity was tracked simultaneously with six different frequency bands, and behavioral state-dependent changes in cross-frequency coupling were determined. They showed that LFP bands depend on the macaque's behavioral state, providing a foundation for future work that examines the function of various LFP bands in wake, NREM sleep, and REM sleep.
Although it is known that mammals alternate between REM and NREM sleep, the mechanisms driving this alternation have not been established (Le Bon, 2020). One hypothesis posits that a homeostatic drive for REM sleep increases during NREM sleep and dissipates during REM sleep (Benington et al., 1994; Heller, 2021). Ginsberg et al. build on previous work from Park et al. (2021) to define a new measure of REM sleep propensity. Their analyses of spontaneous mouse sleep data suggests that time spent in NREM sleep may increase the propensity to transition into REM sleep in a homeostatic “hourglass-like” manner, but only for a limited range of NREM sleep durations. Future work is needed to validate this REM propensity measure in other species or contexts such as sleep deprivation.
Insights from the six papers in this Research Topic should provide direction for future experiments that will translate these fundamental-research-based methods into tools that could be used clinically to improve sleep and circadian-related health outcomes in humans.
SA: Writing – original draft, Writing – review & editing. MB: Writing – original draft, Writing – review & editing. CDB: Writing – original draft, Writing – review & editing.
CDB has received investigator-initiated research support from Takeda Pharmaceuticals outside the scope of this work.
The remaining authors declare that the research was conducted in the absence of any commercial or financial relationships that could be construed as a potential conflict of interest.
All claims expressed in this article are solely those of the authors and do not necessarily represent those of their affiliated organizations, or those of the publisher, the editors and the reviewers. Any product that may be evaluated in this article, or claim that may be made by its manufacturer, is not guaranteed or endorsed by the publisher.
Benington, J. H., Woudenberg, M. C., and Heller, H. C. (1994). REM-sleep propensity accumulates during 2-h REM-sleep deprivation in the rest period in rats. Neurosci. Lett. 180, 76–80. doi: 10.1016/0304-3940(94)90917-2
Brown, R. E., Basheer, R., McKenna, J. T., Strecker, R. E., and McCarley, R. W. (2012). Control of sleep and wakefulness. Physiol. Rev. 92, 1087–1187. doi: 10.1152/physrev.00032.2011
Chen, T.-W., Wardill, T. J., Sun, Y., Pulver, S. R., Renninger, S. L., Baohan, A., et al. (2013). Ultrasensitive fluorescent proteins for imaging neuronal activity. Nature 499, 295–300. doi: 10.1038/nature12354
Cirelli, C., and Bushey, D. (2008). Sleep and wakefulness in drosophila melanogaster. Ann. N. Y. Acad. Sci. 1129, 323–329. doi: 10.1196/annals.1417.017
Colwell, C. S. (2011). Linking neural activity and molecular oscillations in the SCN. Nat. Rev. Neurosci. 12, 553–569. doi: 10.1038/nrn3086
Dana, H., Mohar, B., Sun, Y., Narayan, S., Gordus, A., Hasseman, J. P., et al. (2016). Sensitive red protein calcium indicators for imaging neural activity. Elife 5:e12727. doi: 10.7554/eLife.12727
Funato, H., Miyoshi, C., Fujiyama, T., Kanda, T., Sato, M., Wang, Z., et al. (2016). Forward-genetics analysis of sleep in randomly mutagenized mice. Nature 539, 378–383. doi: 10.1038/nature20142
Guo, F., Yu, J., Jung, H. J., Abruzzi, K. C., Luo, W., Griffith, L. C., et al. (2016). Circadian neuron feedback controls the drosophila sleep—activity profile. Nature 536, 292–297. doi: 10.1038/nature19097
Heller, C. (2021). “The Regulation of Sleep,” in Oxford Research Encyclopedia of Neuroscience. Available at: https://oxfordre.com/neuroscience/display/10.1093/acrefore/9780190264086.001.0001/acrefore-9780190264086-e-30?d=%2F10.1093%2Facrefore%2F9780190264086.001.0001%2Facrefore-9780190264086-e-30andp=emailAKEYqJqhS89%2Fs (accessed December 28, 2024).
Joiner, W. J. (2016). Unraveling the evolutionary determinants of sleep. Curr. Biol. 26, R1073–R1087. doi: 10.1016/j.cub.2016.08.068
Le Bon, O. (2020). Relationships between REM and NREM in the NREM-REM sleep cycle: a review on competing concepts. Sleep Med. 70, 6–16. doi: 10.1016/j.sleep.2020.02.004
Lewis, A. S., and Chetkovich, D. M. (2011). HCN channels in behavior and neurological disease: too hyper or not active enough? Mol. Cell. Neurosci. 46, 357–367. doi: 10.1016/j.mcn.2010.11.007
Mohawk, J. A., Green, C. B., and Takahashi, J. S. (2012). Central and peripheral circadian clocks in mammals. Annu. Rev. Neurosci. 35, 445–462. doi: 10.1146/annurev-neuro-060909-153128
Novella Romanelli, M., Sartiani, L., Masi, A., Mannaioni, G., Manetti, D., Mugelli, A., et al. (2016). HCN channels modulators: the need for selectivity. Curr. Top. Med. Chem. 16, 1764–1791. doi: 10.2174/1568026616999160315130832
Park, S.-H., Baik, J., Hong, J., Antila, H., Kurland, B., Chung, S., et al. (2021). A probabilistic model for the ultradian timing of REM sleep in mice. PLoS Comput. Biol. 17:e1009316. doi: 10.1371/journal.pcbi.1009316
Rasch, B., and Born, J. (2013). About sleep's role in memory. Physiol. Rev. 93, 681–766. doi: 10.1152/physrev.00032.2012
Sartiani, L., Mannaioni, G., Masi, A., Novella Romanelli, M., and Cerbai, E. (2017). The hyperpolarization-activated cyclic nucleotide-gated channels: from biophysics to pharmacology of a unique family of ion channels. Pharmacol. Rev. 69, 354–395. doi: 10.1124/pr.117.014035
Takahashi, J. S. (2017). Transcriptional architecture of the mammalian circadian clock. Nat. Rev. Genet. 18, 164–179. doi: 10.1038/nrg.2016.150
Welsh, D. K., Takahashi, J. S., and Kay, S. A. (2010). Suprachiasmatic nucleus: cell autonomy and network properties. Annu. Rev. Physiol. 72, 551–577. doi: 10.1146/annurev-physiol-021909-135919
Wobig, L., Wolfenstetter, T., Fechner, S., Bönigk, W., Körschen, H. G., Jikeli, J. F., et al. (2020). A family of hyperpolarization-activated channels selective for protons. Proc. Natl. Acad. Sci. USA. 117, 13783–13791. doi: 10.1073/pnas.2001214117
Keywords: circadian rhythm, sleep, mathematical modeling, calcium, rapid eye movement (REM) sleep, non-rapid eye movement (NREM) sleep, molecular/cellular
Citation: Aten S, Belle MDC and Diniz Behn CG (2025) Editorial: Signaling pathways and brain circuitry underlying circadian rhythms and sleep. Front. Neurosci. 19:1558246. doi: 10.3389/fnins.2025.1558246
Received: 09 January 2025; Accepted: 24 January 2025;
Published: 07 February 2025.
Edited and reviewed by: Radhika Basheer, United States Department of Veterans Affairs, United States
Copyright © 2025 Aten, Belle and Diniz Behn. This is an open-access article distributed under the terms of the Creative Commons Attribution License (CC BY). The use, distribution or reproduction in other forums is permitted, provided the original author(s) and the copyright owner(s) are credited and that the original publication in this journal is cited, in accordance with accepted academic practice. No use, distribution or reproduction is permitted which does not comply with these terms.
*Correspondence: Sydney Aten, c2F0ZW5AYmlkbWMuaGFydmFyZC5lZHU=
†These authors have contributed equally to this work
Disclaimer: All claims expressed in this article are solely those of the authors and do not necessarily represent those of their affiliated organizations, or those of the publisher, the editors and the reviewers. Any product that may be evaluated in this article or claim that may be made by its manufacturer is not guaranteed or endorsed by the publisher.
Research integrity at Frontiers
Learn more about the work of our research integrity team to safeguard the quality of each article we publish.