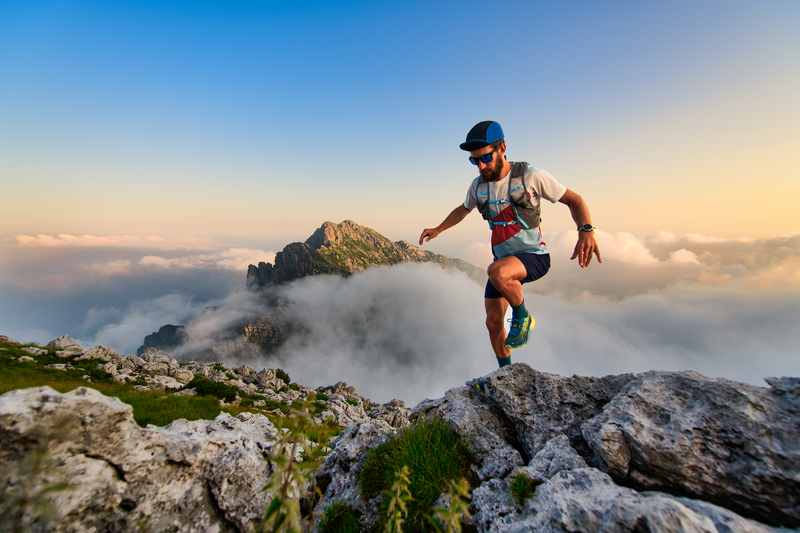
95% of researchers rate our articles as excellent or good
Learn more about the work of our research integrity team to safeguard the quality of each article we publish.
Find out more
REVIEW article
Front. Neurosci. , 06 January 2025
Sec. Sleep and Circadian Rhythms
Volume 18 - 2024 | https://doi.org/10.3389/fnins.2024.1516767
This article is part of the Research Topic Signaling Pathways and Brain Circuitry Underlying Circadian Rhythms and Sleep View all 7 articles
The circadian clock regulates physiological and biochemical processes in nearly every species. Sexual and reproductive behaviors are two processes controlled by the circadian timing system. Evidence supporting the importance of proper clock function on fertility comes from several lines of work demonstrating that misalignment of biological rhythms or disrupted function of the body’s master clock, such as occurs from repeated shift work or chronic jet lag, negatively impacts reproduction by interfering with both male and female fertility. Along these lines, dysregulation of clock genes leads to impairments in fertility within mammals, and disruption of circadian clock timing negatively impacts sex hormone levels and semen quality in males, and it leads to ovulatory deficiencies in females. Here, we review the current understanding of the circadian modulation of both male and female reproductive hormones—from animal models to humans. Further, we discuss neural circuits within the hypothalamus that may regulate circadian changes in mammalian sexual behavior and reproduction, and we explore how knowledge of such circuits in animal models may help to improve human sexual function, fertility, and reproduction.
According to the World Health Organization (WHO), infertility is considered a global public health problem. Indeed, both infertility and subfertility affect a significant proportion of the population: approximately 17.5% of reproductive-aged adults, or ~ 1 in 6 individuals, experience infertility (WHO, 2022). Infertility and subfertility can be divided into several categories with both known and unknown (i.e., idiopathic) etiologies. Many known factors include age at time of conception, ovulatory dysfunction, tubal disease, low sperm count, endocrine, reproductive and/or genetic disorders (Carson and Kallen, 2021; Vander Borght and Wyns, 2018). Notably, 30% of infertile couples, worldwide, are diagnosed with idiopathic infertility, defined as the inability of an otherwise healthy couple (under 35 years of age) to achieve pregnancy after 12 monthly cycles of unprotected intercourse (Sadeghi, 2015).Thus, while the exact causes that drive infertility may depend on several factors, circadian dysfunction likely plays a role in the inability for some of these couples to conceive.
Nearly all organisms rely on the ability to synchronize their physiological and behavioral processes to external time cues. This includes the circadian regulation of sexual behavior and reproduction which is entrained daily to improve the odds of survival for the individual and the species. In mammals, the ‘master clock’ that sustains such intrinsic rhythms is located in the anterior portion of the hypothalamus in a region known as the suprachiasmatic nucleus (SCN). The SCN resides at the top of a hierarchical system consisting of multiple ancillary oscillators found throughout the body and other brain regions. The SCN serves to orchestrate the phase alignment between environmental signals, such as sunrise, with endogenous events, such as peak hormonal release, thus allowing for proper functioning of the hypothalamic–pituitary gonadal (HPG) axis—one of the central regulators of sexual behaviors.
We discuss the current literature regarding the role of the circadian timing system in the regulation of sexual behavior and reproduction in mammals. To begin, we provide an overview of circadian clock timing and its regulation of both male and female reproductive hormones. We then summarize the role of the SCN and circadian system on conception and outline the hypothalamic neural circuits that have been shown to underlie sexual behaviors. Further, we review circuits and genes in rodent models that may modulate time-of-day dependent changes in sexual behavior(s) (Figure 1), and we highlight how a better understanding of circadian timing and sex can help advance our knowledge of human reproductive behaviors and fertility.
Figure 1. Proposed hypothalamic circuitry modulating time-of-day dependent sexual behaviors in mice. There are several key circuits in the hypothalamus that may be responsible for sexual behavior patterns observed across the day in mice. In both males and females, the subparaventricular zone (SPZ), which contains mainly GABAergic neurons, receives efferent fibers from the SCN. In males, this circuit is further defined by the VMHvl Esr1 cells, wherein low-level stimulation of these cells leads to mounting behaviors (though without USVs; Lee et al., 2014). The SPZ also projects to the mPOA, which mediates sexual motivation (Jennings and de Lecea, 2020). Notably, activation of mPOA Esr1 cells in males leads to USV-positive mounting behaviors and sexual arousal (Karigo et al., 2021). Within females, stimulation of VMHvlCckar cells is known to increase female sexual behaviors (Yin et al., 2022), while silencing of mPOA Esr1 cells leads to deficits in female sexual and maternal behaviors (Fang et al., 2018). Asterisks denote the main difference in cell type between males and females. Schematic diagram was created with BioRender.
Nearly every aspect of mammalian physiology and behavior is shaped by a ~ 24 h cycle, or circadian rhythm, which is coordinated by the SCN. This biological timekeeping process is self-sustaining and functions to adjust the body to geophysical time, even in the absence of a zeitgeber, or external timing cue, and it is maintained by circadian clock genes that function to create a transcription-translation feedback loop. From a basic level, at the center of this feedback loop is a basic helix–loop–helix (bHLH) transcription factor heterodimer formed by two proteins: CLOCK and BMAL1. By binding to an E-box enhancer, this activator complex functions to drive the expression of Period (Per1 and Per2) and Cryptochrome (Cry1 and Cry2) gene families. PER and CRY proteins serve as a heterodimer repressor complex and translocate to the nucleus to inhibit the CLOCK:BMAL1 dimer, thus negatively regulating their own transcription. Degradation of the PER:CRY complex occurs via a phosphorylation and ubiquitin-dependent pathway, and the dissociation of this complex then relieves the repressive effect of the CLOCK:BMAL1 complex to allow for a subsequent round of Per and Cry gene expression to occur, which generates a ~ 24 h temporal rhythm. For excellent reviews of the mammalian circadian system, we direct reviewers to the following papers: Takahashi (2017) and Lowrey and Takahashi (2000).
Light is the most potent stimulus for entraining intrinsic rhythms of the SCN to the day-night cycle, and the retinohypothalamic tract (RHT) serves as a photic neural input pathway for the SCN to receive light cues (Miyamoto and Sancar, 1998). To this end, the SCN receives light information via intrinsically photosensitive retinal ganglion cells (ipRGCs), which express the photopigment melanopsin, in a monosynaptic connection via the RHT (Hattar et al., 2002; Gooley et al., 2001; Berson et al., 2002; Hattar et al., 2006). These cells release glutamate and pituitary adenylate cyclase-activating polypeptide (PACAP; Hannibal et al., 2000; for an excellent review, please see Morin and Allen, 2006), thus allowing the SCN to transmit this light information to coordinate the phase of circadian oscillators throughout the brain and body (Welsh et al., 2010).
As the master pacemaker of the body, the SCN orchestrates the synchronization of many other oscillator systems. The primary mechanism for such synchronization is thought to be via autonomic neural efferent connections, though non-neural pathways (such as hormones) may also facilitate circadian synchronization (Balsalobre et al., 2000; Vujovic et al., 2008); for details, please see Welsh et al. (2010). Hence, the SCN sends efferent projections to several regions of the brain, including neighboring regions of the hypothalamus (Abrahamson and Moore, 2001; Berk and Finkelstein, 1981; Deurveilher et al., 2002; Deurveilher and Semba, 2005; Kalsbeek et al., 1993; Schwartz et al., 2011), which likely mediate circadian rhythms in sexual and reproductive physiology and behaviors. These brain circuits will be discussed in later sections.
Importantly, it should be noted that the SCN also works as a ‘seasonal clock’, adjusting the general physiology and behavior of the organism to changes in the length of the daytime, including sexual behavior and reproduction. Indeed, key to the evolution of photoperiodism in mammals is the ability to optimize reproductive fitness while also balancing the energy demands needed for sex and survival, which is why photoperiod is important in determining seasonality in many species (Everett and Sawyer, 1950). In organisms that reproduce seasonally, the photoperiod is essential to regulating reproductive capacity, which allows for offspring to be birthed during a time (i.e., season) most suitable for their survival (for review, see Mahoney et al., 2004). This topic of seasonality is beyond the scope of our review; however, we would like to direct readers to several excellent reviews that describe how the SCN regulates photoperiodic information which can subsequently impart control over the neuroendocrine axis, HPG cycle, gonad size, hormone release, and many other downstream processes that modulate seasonal breeding: Brown-Grant and Raisman (1977); de la Iglesia et al. (2003); Mahoney et al. (2004); and Van der Beek et al. (1997).
The mammalian SCN controls daily fluctuations in reproductive hormones. Expression of receptors for numerous reproductive hormones (estrogens, progesterone, and androgens) allows for modulation of the SCN by these hormones (for an excellent review of the neuroendocrinology of the SCN, please see Karatsoreos and Silver, 2007). Of note, while males do possess small amounts of estrogen and progesterone, the focus of this section will be on androgens, as they are sex steroid hormones that regulate the development and maintenance of masculine characteristics via their binding to androgen receptors.
Within the master clock of mammals, androgen receptor (AR) immunoreactive cells have been shown to localize to the SCN core—the main retinorecipient area (Aronin et al., 1990; Rea, 1989). Importantly, in addition to rodents, androgen receptors are found in the SCN of many mammalian species including ferrets, baboons, rhesus macaques, and humans (Fernández-Guasti et al., 2000; Karatsoreos et al., 2007; Kashon et al., 1996; Rees and Michael, 1982; Wu et al., 1995), as described in the following sections. Given that the SCN core is light responsive, and that cells in the SCN core-region contain these ARs, androgen signaling is able to modulate SCN photic responsivity. For instance, Karatsoreos et al. (2007) found that murine SCN cFos expression after a light pulse (both phase-delaying and advancing) is blunted in castrated animals, and replacement with dihydrotestosterone (DHT), a precursor to testosterone, normalized this effect. While reciprocal regulation of androgens and circadian function likely exists (i.e., SCN modulation of androgens and androgen regulation of SCN functionality), in the remainder of this section (and in Figure 2), we will focus on how the circadian system modulates rhythmic changes in testosterone.
Figure 2. Time-of-day modulated factors influencing conception. Various diurnally-regulated factors likely influence the chances of conception in humans. Such factors include testosterone, with acrophase near 0600 h in males (range: 0500 to 0800 h; de la Torre et al., 1981; Winters, 1991; Diver et al., 2003; Brambilla et al., 2009) and estradiol, with acrophase around 2100 h in women, during the follicular phase (Rahman et al., 2019). Sex hormone binding globulin (SHBG), also known as sex steroid-binding globulin (SSBG), a protein that binds to and helps modulate the availability of sex hormones, has been shown to peak around 1500 in males and 1200 h during the follicular phase in females (Rahman et al., 2019). Additionally, sperm quality and motility are thought to peak between 1100 and 1730 h in males (Cagnacci et al., 1999; Liu K. et al., 2022), with the average peak being around 1430 h. Here, we should note that there are several different reports in the literature regarding the peak time for sperm quality, and the range indicated above is an average, derived from findings in various studies. Finally, many studies have reported peak times for sexual activity in both men and women, with sexual encounters peaking in both the morning and evening (Refinetti, 2005; Palmer et al., 1982b; Fortenberry et al., 2006), often times between 2300 h and 0100 h and with another peak between 0600 h and 0800 h. Sexual activity is denoted by the intertwined male and female sex symbols. Schematic drawing was created with BioRender.
Testosterone is necessary for sex drive and many other important reproductive functions, such as sperm production (Nassar and Leslie, 2024). Beginning with rodent models, Auer et al. (2020) examined fecal testosterone metabolites excreted from male mice and found that these metabolites showed diurnal fluctuations. Similarly, in young male rats, Esquifino et al. (2004) found a daily rhythm in plasma testosterone, with a peak occurring at approximately zeitgeber time (ZT) 9. Interestingly, the amplitude of this rhythm was blunted in socially isolated rats. Here, it should be mentioned that in many mammals, testosterone is released reflexively during reproduction (Nyby, 2008). Such reflexive pulses occur in two reproductive situations: (1) in response to a sexually arousing stimulus such as a novel female (‘anticipatory’ reflexive pulse) and (2) following ejaculation (‘ejaculatory’ reflexive pulse). In addition to this pulsatile testosterone release, several studies in hedgehogs and rats have also demonstrated an infradian (i.e., longer than 24 h) rhythm in testosterone levels (Diatroptov, 2011; Rutovskaya et al., 2020). Notably, Diatroptov (2011) reported changes in testosterone levels equal to 4 days in male rats, which is the approximate length of the female rodent estrous cycle. Given this, future studies in other species should be conducted to test whether such infradian rhythms in testosterone levels correspond to the mammalian ~4–5 day estrous or ~ 28-day menstrual cycles in females, and if so, whether such an infradian rhythm may contribute to fertility success.
Circadian clock genes play an important role in the circadian regulation of testosterone. For instance, male Bmal1 knockout mice are infertile and exhibit low testosterone and high LH serum concentrations, suggestive of a defect in testicular Leydig cells (the primary source of testosterone or androgens in males). Importantly, Leydig cells express Bmal1 in a rhythmic fashion, which suggests that there is peripheral circadian control of testosterone (Alvarez et al., 2008). It is worthwhile to mention, however, that in these studies using Bmal1 null animals, loss of circadian synchrony within the periphery may be impacting testosterone levels on multiple levels, by reducing production, desynchronizing individual cells, or by blunting rhythms.
One of the first published reports of a diurnal rhythm in testosterone came from non-human primate work conducted by Dubey et al. (1983) and Plant (1981). In this study, using rhesus monkeys (a diurnal mammal), a continuous light environment was reported to have no effect on the daily rhythm in serum testosterone (Dubey et al., 1983). Additionally, Kholkute et al. (1987) found that a continuous light environment had no effect on the circadian testosterone rhythm of the marmoset—which are also a diurnal species. These results, showing that the rhythms persisted under constant lighting conditions, suggest that testosterone fluctuation is endogenous. However, the role of the rest-activity cycle and photoperiodism versus an endogenous circadian rhythm in diurnal non-human primates is somewhat controversial, given that the nighttime rise in testosterone was abolished when (diurnal) male bonnet monkeys were exposed to constant light conditions (Mukku et al., 1976). In both the Dubey et al. (1983) study and the Mukku et al. (1976) study, animals were exposed to continuous light for up to 15 days. The reason behind these species differences has not yet been elucidated, but the results suggest that testosterone is under the endogenous control of the clock, at least in rhesus monkeys and marmosets. In nocturnal non-human primates such as the owl monkeys and mouse lemurs, testosterone levels were found to be higher during the day (during the rest period) compared to the active (nighttime) period (Dixson and Gardner, 1981; Perret, 1985). These results imply that in some non-human primate species, circadian differences in testosterone levels may correspond with the sleep–wake cycle. Here, it should also be noted that age likely plays a role in the amplitude of the testosterone rhythm given that significant diurnal patterns of testosterone were found in both younger and older rhesus monkeys, with the circadian rhythm being more pronounced in older monkeys (Schlatt et al., 2008).
Similar to many animal species, in human males, the testes synthesize testosterone—the androgen that is necessary for testicular development and sperm production. Circulating levels of testosterone are modulated by the circadian clock, light exposure, and sleep duration. For example, in healthy young men, serum testosterone concentrations rise with sleep onset, reach the peak during the first REM episode, remain stable until awakening, and then rapidly decline (Luboshitzky et al., 1999). In a similar study, plasma was measured at the following times in 10 men using radioimmunoassay (RIA): 07.00 h, 08.00 h, 09.00 h, 10.00 h, 13.00 h, 16.00 h, 19.00 h, 23.00 h and 03.00 h, and a circadian rhythm was noted, with peak levels occurring between 07.00 h and 10.00 h (Gall et al., 1979). Similarly, a circadian rhythm of testosterone and DHT was detected in three healthy men with a peak at 16.37 (Guignard et al., 1980). However, it is important to distinguish true circadian rhythmicity from sleep–wake effects of variations in testosterone levels. Along these lines, Miyatake et al. (1980) obtained frequent blood samples to measure serum levels of testosterone in healthy men while awake and during sleep, in addition to using electroencephalogram (EEG), electrooculogram (EOG), and electromyogram (EMG) recordings. Results from this study revealed an increase in testosterone during the early morning, and similar results were found using a constant-routine-like paradigm performed on two subjects. This data suggests that such a rise was not directly related to sleep or any specific sleep stages (or from changes in other hormones), but that it is more likely the effect of an endogenous rhythm similar to the rhythm in cortisol (Miyatake et al., 1980). Here, we also direct readers to Figure 2, for a description of other studies that examined circadian rhythms in testosterone levels in men.
The circadian rhythm of other hormones has been reported (for reviews, see Turek et al., 1984 and Kriegsfeld et al., 2006). Indeed, studies have shown that in men, the diurnal pattern of LH and follicle-stimulating hormone (FSH) gonadotropin secretion increases at night and is most pronounced leading up to puberty (for review, see Karatsoreos and Silver, 2007). Furthermore, a study by de la Torre et al. (1981) showed that 17-OH-P and other hormones such as androstenedione and OH pregnenolone showed diurnal variation with highest levels occurring during the morning and lower levels occurring at night.
Circadian and sleep disruption are known to negatively impact reproduction in men. Indeed, several papers have revealed that sleep (a circadian-regulated process) disruption negatively affects testosterone levels and sperm count. For example, during the daytime, Leproult and Van Cauter (2011) found that testosterone levels were decreased by 10 to 15% in young, healthy men who underwent 1 week of restricted sleep (sleeping only 5 h/night). By comparison, it should be noted that normal aging is associated with a decrease in testosterone levels by 0.4–2% annually (Harman et al., 2001; Kaufman and Vermeulen, 2005; Leproult and Van Cauter, 2011; Wu et al., 2008). Whether or not these levels could be normalized upon sleep recovery was not tested in this specific study, though a similar study performed by Arnal et al. (2016) did show that testosterone levels returned to baseline after one night of sleep recovery. Regardless, these studies demonstrate the importance of a functional circadian clock on testosterone levels. Future studies should examine how individual differences in chronotype (Zavada et al., 2005), or how behavioral manifestations of circadian-gated processes such as sleep–wake, diet, activity patterns, etc., affect testosterone production and release in men.
Here, it should also be noted that other structures outside of the hypothalamus have AR-immunoreactivity and serve as components of the circadian system and help regulate male sexual and reproductive processes. For instance, in a recently published paper, the activity and expression level of AR in the adult mouse brain was monitored using an AR-Luc reporter mouse. While the main areas of AR immunoreactivity were found to include many regions of the hypothalamus, such as the SCN and the medial preoptic area (mPOA), other regions, such as the amygdala and the stria terminalis, also showed high expression (Dart et al., 2024). Interestingly, a recently paper from the lab of Mark Wu showed that an extra-SCN oscillator in the lateral amygdala expresses the clock-output molecule mWAKE/ANKFN1, and these mWAKE neurons within the amygdala help coordinate rhythmic sensory perception (Liu et al., 2024). Given this, and the fact that amygdala is involved in the regulation of ejaculation (Huijgens et al., 2021) and sexual and aggressive behaviors (Yamaguchi et al., 2020) in male rodents, AR cells within the amygdala may influence the execution of male reproductive behaviors as a function of time-of-day. With respect to the high levels of AR immunoreactivity within the stria terminalis of males (Cara et al., 2021; Dart et al., 2024), it is interesting to note that a neural circuit was recently found to connect chemosensory input with the Bed Nucleus of the Stria Terminalis (BNST) Tac1 neurons. These BNST Tac1 neurons innervate POA Tacr1 neurons and terminate in centers regulating reward during male mating (Bayless et al., 2023).The BNST has been show to exhibit a robust circadian rhythm in the expression of circadian proteins such as PER2 (Amir et al., 2004), suggesting that AR expression within the BNST of males may be involved in mating behavior, drive, and reward across the circadian day.
The pineal gland is another extrahypothalamic structure regulated by the circadian timing system, and AR expression and binding sites have been confirmed in the pineal gland and in pinealocytes of males from many species, including bovine (Haldar and Gupta, 1990), rats (Cardinali et al., 1975), and humans (Luboshitzky et al., 1997). While the pineal gland has many functions, one of its main responsibilities is to receive information regarding the environmental light–dark cycle and to secrete the hormone melatonin (for review, see Arendt and Aulinas, 2000; Sapède and Cau, 2013). Furthermore, the pineal gland has been shown to exert a prominent role in the neuroendocrine regulation of reproductive physiology (Aleandri et al., 1996; D’Occhio and Suttie, 1992; Li and Zhou, 2015). For example, pineal hormones like melatonin regulate reproduction in seasonal animals such that adult male hamsters exposed to short photoperiods exhibit gonadal regression (Mason et al., 2010). Additionally, the presence of melatonin receptors in the sperm of several species (Casao et al., 2012), humans included (van Vuuren et al., 1992; Espino et al., 2011), suggests that it may directly modulate the ability of sperm to fertilize an egg to form a zygote. While a detailed discussion of melatonin and male reproduction is outside the scope of this paper, we direct readers to an excellent review on this topic: Li and Zhou (2015).
Estradiol (E2) and progesterone (P) are steroid hormones responsible for the development of female sexual characteristics, the maintenance of the reproductive cycle, and pregnancy in females. Hence, this section will focus on the circadian regulation of E2 and P given that they are important in driving female sex and reproduction by binding to estrogen and progesterone receptors (ERs, such as ERα and ERβ, and PR).
Several studies have shown the presence of ERs and PR within the SCN. High levels of ERβ and low levels of ERα are expressed in the SCN of neonatal rats. Notably, these results differ from work conducted in adult rats which failed to show ERα expression and showed only low levels of ERβ expression in the SCN. Of note, both receptor subtypes are expressed in neurons as well as in astrocytes, and some cells express both ERα and ERβ (Su et al., 2001). With respect to the topographic distribution of ERα and ERβ in the SCN, the shell region displays high levels of ER-immunoreactivity, while the SCN core shows little expression (Vida et al., 2008). Interestingly, PR expression varies across species, though it is present in the SCN of humans, and ERα and ERβ are maintained in the SCN of humans, though ERα expression is stronger than ERβ (Yaw et al., 2020; Kruijver and Swaab, 2002). Such differential distribution of ERs within the master circadian clock suggests several modes of estrogenic signaling within the SCN (and between the SCN and other extra-SCN brain regions), which may influence the circadian modulation of certain reproductive-related events and pathways. Notably, most studies have examined how estrogens act on non-SCN, ER-rich regions of the brain that receive innervation from the SCN (directly or indirectly), including the mPOA, amygdala, and ventromedial hypothalamus (VMH; Laflamme et al., 1998). Along these lines, many neural efferent fibers from the SCN project to the SPZ (Leak and Moore, 2001; Watts, 1991; Watts and Swanson, 1987), and the SPZ projects to many of these above-listed ER-rich brain regions (Vujovic et al., 2015). Furthermore, some of these ER-rich regions may project to and modulate the SCN, and thus, the estrogenic effects on circadian rhythms may also arise from indirect actions of estrogens on target regions of the SCN. These data suggest that ER signaling may affect clock timing or tau length, but this should be confirmed experimentally. The remainder of this section will focus on daily rhythms in estrogens and circadian control of estrogenic signaling.
Daily oscillations in ER expression have been reported and may contribute to the circadian regulation of estrogen-dependent behaviors. For example, a study of mice in constant darkness (DD), revealed a circadian pattern of ER𝛽 expression which remained intact after 3 days in DD (Cai et al., 2008). ER𝛽 mRNA levels were also shown to fluctuate in various peripheral tissues, with a peak occurring at the light–dark transition. Notably, this study also found that this rhythm was abolished in clock-deficient Bmal1 knockout mice, and when CLOCK and BMAL were introduced in vitro, ER𝛽 expression increased. These findings not only indicate that ER𝛽 expression oscillates in a circadian fashion, but also that this oscillation is mediated by endogenous clock genes (Cai et al., 2008). Taken together, these studies show that both ERα and ER𝛽 both exhibit circadian patterns of expression which occur as a result of proper core clock gene expression/function.
The circadian system modulates female reproductive behaviors in various ways, and one example is its regulation of the estrous cycle—the ~4–5-day reproductive cycle in rodents which consists of four distinct phases: proestrus, estrus, metestrus, and diestrus. Ovulation (which typically occurs during the night of estrus) in female rodents is very complex and requires tight temporal control from cells spanning from the SCN, to gonadotropin-releasing hormone (GnRH) neurons within the hypothalamus, in addition to temporal control of many other hormones (for an excellent review, please see Miller and Takahashi, 2014). In brief, elevated levels of E2 are necessary for the GnRH surge to occur, during the late proestrus phase of the cycle. This GnRH signal triggers the surge of LH and promotes FSH release from the pituitary gland. Within the ovaries, LH induces ovulation and FSH initiates the recruitment of new follicles (Miller and Takahashi, 2014). Notably, in addition to the GnRH surge, a time-dependent signal must also occur to induce the preovulatory GnRH surge (Everett and Sawyer, 1950). The SCN was shown to control this neural time cue (i.e., the preovulatory hormone surge) given that SCN dysregulation leads to acyclicity of the estrous cycle in rats (Brown-Grant and Raisman, 1977; Terasawa et al., 1980; Wiegand and Terasawa, 2008).
How the SCN regulates this circadian neural timing signal leading to the preovulatory GnRH surge and subsequent induction of LH has not been completely elucidated. However, two peptides within the SCN–vasopressin (AVP) and vasoactive intestinal polypeptide (VIP)—likely regulate the temporal release of GnRH. Along these lines, GnRH neurons are directly innervated by VIPergic projections from the SCN (Kriegsfeld et al., 2002; van der Beek et al., 1993), and VIP administration has also been shown to stimulate the LH surge (Samson et al., 1981). Additionally, AVP administration modulates the LH surge such that it occurs in the late afternoon in rats (Palm et al., 2001). Furthermore, inhibition of either peptide in rats results in a decrease in the amplitude of the E2-induced LH surge (Funabashi et al., 1999; Harney et al., 1996; van der Beek et al., 1999, and for an excellent review, also see Russo et al., 2015). Studies performed on GT1-7 neuronal cells (a mature mouse hypothalamic GnRH line) revealed that high levels of E2 leads to circadian expression of the kisspeptin peptide receptor GPR54 in vitro (Tonsfeldt et al., 2011). Notably, the SCN can also regulate circadian GnRH release indirectly via kisspeptin-expressing neurons in the anteroventral periventricular nucleus (AVPV; Van der Beek et al., 1997). Kisspeptin-expressing neurons in this region have been shown to play a relevant role in the regulation of GnRH secretion in rodents (see Skorupskaite et al., 2014, for review). The SCN targets AVPV kisspeptin neurons via AVP projections (Vida et al., 2010), thus inducing a circadian rhythm in the response of GnRH to kisspeptin (Williams et al., 2011). Hence, it is possible that the SCN regulates the GnRH surge by direct innervation via AVP and/or VIP, and by an indirect pathway through AVPV kisspeptin neurons. For an excellent review of this topic, see Miller and Takahashi (2014). In another line of work, a study by Vieyra et al. (2016) demonstrated that for ovulation to occur in rats, the system modulating GnRH and LH secretion requires a cholinergic signal arriving on either the right or left SCN on the morning of proestrus. This study reported that cholinergic innervation arriving on either side of the SCN may also help modulate progesterone and estradiol secretion according to time-of-day. These experiments may also help to explain why unilateral SCN injury (which would block this cholinergic innervation to the SCN) is sufficient to decrease the number of shed ova in rats (Ramírez et al., 2017). Taken together, these studies suggest that a complex interplay between SCN neuronal output and kisspeptin receptors within the AVPV are necessary for the preovulatory GnRH surge in females.
Evidence of the importance of a functional master circadian clock in the regulation of this LH surge comes from studies showing that Clock mutant rats lack a coordinated LH surge on the proestrus day, and they exhibit disrupted estrous cycles. Clock mutant rats also show a high rate of full-term pregnancy failure and a decrease in P levels during pregnancy (Miller et al., 2004). Additionally, SCN injury has been shown to affect hormone levels and ovulation, as bilateral SCN injury in rats resulted in anovulation and, as noted above, (unilateral) right SCN injury led to fewer ova being shed (Ramírez et al., 2017). In these studies, however, it is important to consider that many key circadian genes (such as Clock) are transcription factors that influence the expression of thousands of downstream genes, and thus, their pleiotropic effects must be considered. In a similar fashion, older techniques, such as SCN lesion and/or injury often destroy many other brain tissues, some of which may be involved in reproduction, which must also be taken into consideration. As such, future studies that employ targeted deletion of clock gene expression specific to certain cell types, will shed light on circadian control of female ovulation. For an excellent review that highlights a role for the clock in steroid hormone synthesis, ovarian follicular growth, and ovulation, we direct readers to Sellix (2015).
In humans, several components that modulate sexual and reproductive behaviors appear to be rhythmic. This is the case for ERs, given that, in a study of human mammary cell lines, Rossetti et al. (2012) reported that ERα mRNA oscillates in a circadian fashion in ERα-positive breast epithelial cells. Furthermore, Bao et al. (2003) found a daily rhythm in free E2 in cycling women, with a peak occurring in the early morning. More recently, Rahman et al. (2019) also noted a significant circadian rhythm in plasma E2, P, and other hormones under both standard sleep–wake cycle and in constant routine conditions during the follicular phase. Interestingly, only two hormones, FSH and sex hormone binding globulin (SHBG) were rhythmic in the luteal phase, suggesting differential circadian control depending on menstrual cycle phase (i.e., pre- versus post-ovulation). In another study by Fujimoto et al. (1990), 7 of 10 cycling women exhibited a significant circadian variation in P during the luteal phase, though the acrophase of such a rhythm was not consistent among the 7 women. Although it was not accounted for in the study, the individual differences in tau may have contributed to the differences in peak expression. We also direct readers to Figure 2, for a depiction of findings related to circadian rhythms in E2 and P.
Circadian clock disruption in women leads to adverse effects on hormones that regulate reproduction and fertility. Along these lines, several studies have shown that women working night shifts, or those who have irregularly scheduled shifts, display an increase in menstrual pain and changes in menstrual bleeding (Chung F. F. et al., 2005; Labyak et al., 2002). Specifically, Lawson et al. (2011) showed that women with over 20 months of rotating shift work were more likely to experience irregular cycles, and cycles were more likely to be inconsistent in length. Several other studies demonstrated that such menstrual changes also included irregular patterns of ovarian and pituitary hormone secretion (Chung K. et al., 2005; Lohstroh et al., 2003, and for excellent reviews, please see Knutsson, 2003; Mahoney, 2010; Scott, 2000). On a similar note, it has been reported that female university students exhibit higher menstrual symptoms, pain, behavioral changes, and water retention scales when their social jet lag is greater than 1 h (Komada et al., 2019). Furthermore, women who partake in transmeridian travel showed a reduction in sleep, in addition to fatigue and insomnia (for review, please see Sack, 2009)—factors that likely impact hormone secretion. Indeed, it is known that sleep deprivation alters LH amplitude and E2 concentrations (Baumgartner et al., 1993), and thus, circadian disruption stemming from jet lag and/or shift work is likely a key factor in endocrine and reproductive dysfunction observed in women who consistently work irregular hours/night shifts and in those who consistently travel. This topic will be discussed in greater detail in the last section.
Similar to the Male section (3) above, other regions of the brain, outside of the hypothalamus, have ER and P-immunoreactivity. Extrahypothalamic expression of ERα are highly expressed in the BNST, the amygdala, and regions of the locus coeruleus and periaqueductal gray (Mitra et al., 2003; Shughrue et al., 1998), while cells with high ER𝛽 expression are found in the lateral septum, BNST, and amygdala (Creutz and Kritzer, 2002; Milner et al., 2010; Mitra et al., 2003; Shughrue et al., 1998). For an excellent review of ERs in the CNS of females, we direct readers to the following article: Almey et al. (2015). High extrahypothalamic expression of PRs within the female brain are observed within regions of the frontal cortex and hippocampus (Guerra-Araiza et al., 2002) and the BNST and amygdala (Auger and De Vries, 2002; Kato et al., 1994; for review, see Brinton et al., 2008). Of these noted brain structures, the BNST and amygdala are also known to express circadian clock genes and modulate female sexual and reproductive behaviors. For example, in the BNST and central nucleus of the amygdala, PER2 protein rhythms peak early in the dark phase (Amir et al., 2004; Lamont et al., 2005). Yamazaki et al. (2000) recorded multiple unit neural activity from both in and outside of the SCN of golden hamsters and found that SCN and BNST rhythms were always in-phase, suggesting a strong coupling between the two regions. Concerning, the regulation of sex and reproductive function, Martinez and Petrulis (2011) found that, in female Syrian hamsters, the BNST is important for the normal expression of sexual solicitation behaviors in response to male odor. Similarly, the medial amygdala is necessary for opposite-sex odor preference and vaginal marking (Petrulis and Johnston, 1999), in addition to two other female reproductive behaviors: ultrasound production and lordosis (Kirn and Floody, 1985; Rajendren and Moss, 1993; for review, see Sakuma, 2015).
As in males, the pineal gland is another extrahypothalamic structure that is involved in both circadian clock timing and in the regulation of female reproductive processes. The female rat pineal gland contains both ERα and ER𝛽 subtypes (Sànchez et al., 2004), and PR expression has been noted in the bovine pineal gland (Vacas et al., 1979). Interest in the role of the pineal gland in the regulation of female reproduction has grown significantly in recent years given its role in producing melatonin. Indeed, Kennaway and Rowe (1997) found that female rats treated with melatonin showed inhibition of ovarian development and delayed puberty onset, suggestive of melatonin’s involvement in modulating ovarian growth and functionality. Melatonin levels in follicular fluid (from women) has also been shown to vary indirectly with day length and with P levels, (Rönnberg et al., 1990; Yie et al., 1995), and such variations indicate that melatonin could alter female reproduction in humans prior to ovulation. There is a significant positive correlation between melatonin in follicular fluid and follicle count in women undergoing IVF which suggests that melatonin may also provide a protective role during the ovarian cycle (Zheng et al., 2018). However, high dose melatonin, when combined with P, is able to inhibit ovulation in women (Voordouw et al., 1992). For a detailed description of the role of melatonin in female reproduction, we direct readers to two excellent reviews: Olcese (2020) and Fernando and Rombauts (2014). These studies demonstrate that the pineal gland—via its ability to modulate the circadian clock and secrete melatonin—is a significant player in female reproduction.
The role of the circadian clock and its modulation of factors that influence conception in males is less well studied compared to the female estrous cycle/ovulation. Notably, however, the quality of semen has been shown to change diurnally in human males. Indeed, semen samples have been shown to have the highest levels in sperm concentration in the early morning (Xie et al., 2018). However, this is in contrast to a study in which seminal fluid was collected by masturbation (twice by each subject–once in the morning and once in the afternoon). Indeed, in this latter study, Cagnacci et al. (1999) also showed the number of spermatozoa with linear motility was higher in the afternoon than the morning and that although macroscopic parameters were similar, specimens collected in the afternoon showed a higher number and concentration of sperm. It should be highlighted though, that of these 54 enrolled males, 24 were normozoospermic and 30 suffered from oligo-and/or asthenozoospermia. Notably, in their experiments, subjects were randomized to have sperm collected in the morning or afternoon after 3 days of abstinence, such that the time between the previous ejaculation and time of morning or afternoon collection was consistent among each subject (Cagnacci et al., 1999). Here, we should also report that a daily diurnal variance in sperm DNA fragmentation index has been shown in both humans and in mice, with a nadir occurring at 10 AM (Ni et al., 2019).
The importance of a proper timekeeping system in the regulation of male factors that influence conception is reinforced by studies showing the effects that the disruption of clock genes can have on various aspects of male reproduction. Perhaps the most striking evidence comes from the fact that male mice with homozygous mutations in Clock and Bmal1 have reduced fertility (Alvarez et al., 2008; Dolatshad et al., 2006; Liang et al., 2013). In addition to these reductions in fertility, changes in testosterone function have also been reported (see the Male Reproductive section). Bmal1 null mice also have alterations in testes physiology given their decreased average seminiferous tubule diameter (Alvarez et al., 2008), and they have alterations in the structure of chromatid bodies of their spermatids (Peruquetti et al., 2012). It should also be noted that abnormalities in testicular function have been found in Cry1 knockout mice, wherein deficiency of this gene increases germ cell apoptosis within the testes of mice and decreases sperm count (Li et al., 2018). Interestingly, Morse et al. (2003), found that constant expression levels of Per1 and Bmal1 were observed within the testis of mice but another study found that Per3 expression did exhibit prominent circadian rhythms within the mouse testes (Zylka et al., 1998), suggesting that circadian clock machinery can drive testicular function (Baburski et al., 2016; Alvarez et al., 2008), particularly within Leydig cells, which produce testosterone. For an excellent overview of the complexity of the rhythmic functions of cells within the testis, we direct readers to Bittman (2016).
These aforementioned studies provide strong support for the necessity of proper circadian functioning in conception. However, as noted previously, in the studies that used mice deficient in circadian clock genes, the pleiotropic effects of such genes must be considered, given that clock genes target a variety of downstream factors that can also influence conception and pregnancy viability. On a separate note, interestingly, while restricted feeding during either the day or nighttime period was found to disrupt rhythms in male mouse mating behaviors (Kukino et al., 2022), no study (to date) has examined whether a rhythm exists in sexual behavior within mice housed in constant conditions (and in mice fed ad libitum). Given that mice are used as a model organism for dissection of neural circuits, future studies should test whether a sexual behavior rhythm occurs and if so, whether this rhythm may coincide with a rhythm of conception.
In female mice, ovulation occurs during the morning of estrus, after the proestrus LH surge, and if mating (with a male) occurs, copulation allows for circadian-gated prolactin (PRL) surges (Freeman and Neill, 1972; Mai et al., 1994; Poletini et al., 2010; for an excellent review, please see Miller and Takahashi, 2014). To this end, retinorecipient neurons within the SCN release VIP which provides an inhibitory stimulus to the dopaminergic neurons within the hypothalamic periventricular-arcuate nucleus (Pe). PRL release will continue for a period of ~10 days following conception or up to 12 days following a pseudopregnancy (Freeman et al., 1974). Also modulated by the circadian clock is the LH surge that promotes ovulation (as described in the section above; Mosko and Moore, 1979; Williams et al., 2011; Williams and Kriegsfeld, 2012). Such clock-gated events of hormonal release must occur in females to increase the odds of successful conception. Furthermore, circadian genes play an important role in the circadian regulation of conception since female mice lacking functional Bmal1 or Per1 or Per2 all show deficiencies in embryonic implantation and/or maintenance of pregnancy. For an excellent review depicting the role of clock genes in pregnancy maintenance, please see Miller and Takahashi (2014).
Implantation, which, depending on the species, occurs hours to weeks after fertilization, is the process by which the fertilized egg(s) implants in the female uterus to allow the embryo(s) to grow and develop. Interestingly, to date, no studies have been conducted on the timing of embryo transfer (into the uterus) during assisted reproduction (such as in vitro fertilization). However, circadian clock gene expression has been observed in female reproductive structures that influence implantation: such as the uterus (Uchikawa et al., 2011; Johnson et al., 2002) oviduct (Johnson et al., 2002; Kennaway et al., 2003), ovarian granulosa cells (Mereness et al., 2016) and embryo (prior to implantation; Johnson et al., 2002). Notably, several studies have found that BMAL1 null female mice experience implantation failure (and subsequent infertility) due to a reduction in progesterone production (Ratajczak et al., 2009; Liu et al., 2014). Given this, it may be worthwhile to examine whether the time-of-day of embryo transfer impacts implantation success. One could postulate that transfer of embryos into the uterus during a specific time of day may allow for an increased chance of implantation, possibly due to circadian regulation of steroidogenesis and endometrial thickness/receptivity (Ratajczak et al., 2009; Liao et al., 2022). Such data could have a profound impact on assisted reproductive technology advancement.
In 1970, Dr. Curt P. Richter found that, in rats, successful mating depends on the presence of estrus (in the female rat) in addition to proper functioning of the 24-h circadian clock of both the male and the female. Indeed, Richter performed an experiment that involved blinding rats (to remove all external light timing cues) and found that pregnancy could only be achieved when the active phases of the circadian clocks in both the male and the female rat overlapped, when the female was in estrus. This was the first study to report a circadian rhythm in sexual behavior within mammals (Richter, 1970). Around the time of Dr. Richter’s study, it was becoming increasingly well-known that circadian rhythms in physiology and behavior arise from the SCN (Moore and Eichler, 1972; Ralph et al., 1990; Saper, 2013; Hastings et al., 2018). Studies on mechanisms as to how the SCN maintains the daily synchrony of such processes demonstrated that temporal control highly depends on its efferent targets and the related functions of these target areas. Many of these SCN targets are also seated in the hypothalamus, which houses structures that are known to control sexual behaviors (for reviews, see Calabrò et al., 2019; Iovino et al., 2019). In this section, we will focus on two such brain regions: the VMH and the mPOA. However, we will first discuss how the subparaventricular zone (SPZ) serves as a relay between the SCN and these ‘downstream’ hypothalamic structures.
The SPZ is dorsally adjacent to the SCN in the anterior portion of the hypothalamus, and it runs in a dorsal-posterior arc just below the paraventricular hypothalamus (thus giving it the name ‘subparaventricular zone’). The SPZ receives the densest efferent fibers from the SCN and is thought to be a key relay center for driving rhythms in physiology and behavior (Leak and Moore, 2001; Watts, 1991; Watts and Swanson, 1987). To this end, lesioning the SPZ or cutting through it with a knife (Inouye and Kawamura, 1979; Lu et al., 2001; Schwartz et al., 2009) attenuates rhythms in locomotion and behavior. Ibotenic acid lesions to the ventral SPZ (vSPZ) in rats impairs the circadian rhythm of sleep and locomotor activity under constant conditions. Lesions to the dorsal SPZ (dSPZ) lead to the immediate loss of circadian rhythmicity of body temperature, even when rats are placed in light/dark conditions (Lu et al., 2001), and more recently, a viral-based technique that renders SPZ cells unable to release GABA, resulted in the loss of aggression rhythms in male mice (Todd et al., 2018). Such results, coupled with the fact that the SPZ shows a consistent phase relationship to the SCN (Inouye and Kawamura, 1979; Kubota et al., 1981; Nakamura et al., 2008; Sato and Kawamura, 1984), support the idea that the SPZ serves as a major conduit for output signals emanating from the SCN. Importantly, the SPZ consists of mainly GABAergic neurons (Lein et al., 2007) which are found within its regionalized subdivisions: namely, dorsal-medial, dorsal-lateral, ventral-medial, and ventral-lateral (Costa et al., 1999; Gooley et al., 2001; Johnson et al., 1988; Leak et al., 1999; Leak and Moore, 2001; Levine et al., 1991; Lu et al., 2001; Moore et al., 2002; for review, see Aton and Herzog, 2005; Canteras et al., 2011). In a study published by Vujovic et al. (2015), the differences in projections from these various SPZ sub-compartments were examined in mice using both anterograde and retrograde tracing techniques. They found that the SPZ projects to the basal forebrain, pons and brainstem, thalamus, habenula, cortex, and hypothalamus, including the mPOA. The dorsolateral SPZ also shows extremely dense projects to the dorsomedial and central VMH. Given that the SPZ serves as a key output of the SCN (as noted above), and that efferent fibers from the SPZ project to both the mPOA and VMH, these results raise the prospect that a circuit comprising the SCN→SPZ→VMH or SCN→SPZ→mPOA may regulate time-of-day dependent sexual behaviors, two topics discussed below.
For the past several decades, the VMH has been known to be an integral player for many neuroendocrine functions within mammals, including sexual behavior. First, in Mathews and Edwards (1977) lesioned discrete areas of the hypothalamus and determined the VMH was involved in the regulation of sexual behavior within female rats given that these lesions eliminated sexual receptivity. Later in the same decade, researchers determined the VMH was critical in regulating female lordotic behavior (Goy and Phoenix, 1963; Pfaff and Sakuma, 1979b, Pfaff and Sakuma, 1979a), and in males, it was shown to modulate scent marking and partner preference, though limited effects on mounting behaviors were noted (Harding and McGinnis, 2003, 2005).
With respect to subtypes of neurons within the VMH, it is important to consider the fact that many VMH neurons are sexually dimorphic (Yang et al., 2013). Though a detailed depiction of sexual dimorphism is outside the scope of this review, we direct readers to several excellent, recently published articles that describe sexual dimorphic subpopulations within the VMH: Cortes et al. (2023) and Khodai and Luckman (2021). In brief, PR number, distribution, and projection(s) vary by sex within the mouse brain, and in female mice, ablation of PR-expressing neurons in the ventrolateral division of the ventromedial hypothalamus (VMHvl) diminished sexual receptivity, while in male mice, it led to deficits in mating behaviors (Yang et al., 2017). In addition to PR, a recent study also showed that cholecystokinin A receptor (Cckar)-expressing cells within the VMHvl are key regulators of female sexual behaviors. Indeed, inactivation of these cells in female mice decreases their interest in males and decreases female sexual receptivity, and activating these cells increases their sexual behavior (Yin et al., 2022). These results, coupled with the fact that female mice lacking Cckar show deficits in female-specific sexual behaviors (Xu X. et al., 2012), raise the prospect that VMHvl Cckar neurons could potentially control circadian-dependent female sexual behaviors.
Interestingly, VMH circuitry drives both aggression and sexual behaviors. Indeed, optogenetic stimulation of VMHvl neurons positive for ERα—a nuclear receptor and transcription factor derived from the Esr1 gene, has been shown to increase male mounting behaviors at low-intensity stimulations, while higher intensity stimulation was shown to increase aggressive behaviors (Lee et al., 2014; Lin et al., 2011). However, it should be noted that male mice emit ultrasonic vocalizations (USVs) when courting female mice (for review, see Egnor and Seagraves, 2016), and a recently published paper indicated that male mounting evoked by such weak activation of VMHvl Esr1 neurons actually represents a form of aggression (Karigo et al., 2021). One study conducted in the lab of Clif Saper, demonstrated that there exists time-of-day dependent (i.e., circadian) aggression behavior in male mice (Todd et al., 2018). While the SCN weakly projects to the VMH, Todd et al. (2018) found an SCN relay through the dorsal SPZ to the VMHvl Esr1 neurons, which influences time-of-day dependent aggression behaviors. Hence, it is possible that the same pathway also modulates circadian dependent sexual behaviors in males and females (see Figure 1).
The mPOA is another candidate brain region that may modulate time-of-day dependent sexual behaviors in both males and females (for excellent reviews regarding the mPOA and sexual behavior, see Hull and Dominguez, 2007; Hull and Rodríguez-Manzo, 2009; Rodríguez-Manzo and Canseco-Alba, 2017). The mPOA receives a wide range of afferent input, especially from the olfactory system and genitals (Hull and Dominguez, 2007). Damage to the mPOA impairs sexual behavior in rodent models of both sexes (Christensen et al., 1977; De Jonge et al., 1989; Gray and Brooks, 1984; Hansen, 1982; Klaric and Hendricks, 1986; Larsson and Heimer, 1964; Liu et al., 1997; for review see Paredes and Baum, 1997) and stimulation of the mPOA enhances sexual behaviors (Malsbury, 1971; Rodríguez-Manzo et al., 2000; for review see Paredes, 2003).
Similar to the VMH, the mPOA is also one of the most widely-accepted sexually dimorphic brain regions. Indeed, the mPOA differs between males and females in most species (humans included; Allen et al., 1989; Hofman and Swaab, 1989; Swaab and Fliers, 1985; Xu Z. et al., 2012), and various genes within this brain region may be responsible for circadian-dependent sexual behaviors. Interestingly (as briefly noted above), it has been shown that in male mice, the mPOA Esr1 hypothalamic subpopulation is enriched with neurons that induce a reproductive-like state within males, characterized by USV-positive vocalizations when stimulated, while stimulation of VMHvl Esr1 neuronal subpopulations promote USV-negative mounting and likely represent an aggressive-like state (Karigo et al., 2021). Hence, in male mice (and likely in female mice too), a circuit spanning the SCN–SPZ–mPOA Esr1 may regulate time-of-day dependent sexual behaviors. However, it is important to note that these mPOA Esr1 are characterized by different subpopulations involved in either parenting and/or mating. Along these lines, a transcriptomic study conducted by Moffitt et al. (2018) reported that, while 6 different subpopulations are enriched in males after mating, only 2 are enriched in females. Thus, it is important to keep in mind that this circuitry seems to be quite complex, especially when interpreting which Esr1 subpopulation is being directly regulated by the circadian system. Consistent with the idea that a circuit from the SCN to mPOA modulates circadian sexual behavior, Schwartz et al. (2011) demonstrated a direct projection exists from the SCN to the mPOA. Their results also show that projections of both the SCN and the vSPZ are conserved in both diurnal and nocturnal rodents. Vujovic et al. (2015) also reported modest projections from the dSPZ to the mPOA within mice (Vujovic et al., 2015). Hence, a direct or indirect pathway from the SCN→mPOA may be regulating circadian sexual behaviors. Identification of the cell population chemotype involved with circadian regulation of sexual behavior will be critical as Ribeiro et al. (2012) showed that viral-vector mediated RNA interference in female mice, used to silence ERα/ESR1 expression specifically in the mPOA, leads to deficits in maternal care and sexual behavior. The fact that mPOA Esr1 cells play crucial roles in maternal behaviors in female mice (Fang et al., 2018), coupled with the fact that the mPOA expresses some of the highest levels of Esr1 within the brain (Mitra et al., 2003; Shughrue et al., 1997), advances the prospect that an SCN–SPZ–mPOA Esr1 circuit could also modulate circadian dependent sexual behaviors (Figure 1).
While there is mixed literature as to what time-of-day men and women typically desire to have sex, a circadian variation in sexual activity has been reported (also see Figure 2). For example, a study of 78 young married couples demonstrated a major peak in sexual activity in the evening and another peak in the morning (Palmer et al., 1982a). A study by Refinetti (2005) drew similar results, showing two large peaks of sexual encounters among a study conducted on 15 university students. One peak occurred between 11:00 PM and 1:00 AM, and the second peak occurred between 6:00 AM and 8:00 AM. In another study, it was found that coital events in adolescents were most likely to occur after 6 PM compared to other times of day (Fortenberry et al., 2006). However, it is likely that the major reason for this timing choice was partner availability. This idea is supported by a study conducted by Jankowski et al. (2014), which showed that the timing of the desire of sexual activity is only modestly positively associated with the actual timing of when the sex takes place. Hence, while rhythms in reproductive hormones and chronotype of both sexual partners presumably plays a role in sexual behavior across the day (Jocz et al., 2018), it is likely that temporal restrictions brought upon by social engagements has a significant effect on what time-of-day humans choose (and most desire) to engage in sexual intercourse. Future studies aimed at teasing apart the precise time-of-day as to when men and women are most sexually aroused (in the absence of social or light timing cues) would be of interest. Such a study would help determine whether sexual behavior is truly modulated in a ‘circadian’ manner within humans. Further, testing whether such a peak in sexual desire may coincide or overlap with a peak time-of-day for conception may be an interesting future study, especially with regards to maximizing fertility, described below. Along these lines, it would be worthwhile to identify whether having sexual intercourse in the morning (when male testosterone levels are highest) leads to better chances for an ovulating female to conceive. Additionally, whether sexual behavior may serve as a zeitgeber for men and women would be an interesting area of inquiry to further understand the interaction of circadian rhythmicity and sexual activity.
Large scale assessment of gene expression with spatial transcriptomics has the potential to illuminate coordinated molecular relationships, not only by spatial, functional or pathological themes, but by temporal dimension (Zhang et al., 2023). Researchers have been able to highlight genetic underpinnings of male infertility (Llavanera et al., 2022; Singh Jamwal, 2023; Liu Q. et al., 2022) and female reproductive function, such as expression of the proliferative marker MKI67 (Rubel et al., 2012; Knoedler et al., 2022). The contribution of circadian control over or across multiple cell types, spatial relationships, functional synchrony and pathological states is critical to dissecting the complexity of successful reproduction. As described by Rubel et al. (2012) with their results of microarray analysis from uterine cells from mice, the role of progesterone in circadian rhythm gene expression in the uterus may have profound indications of multi-levels of circadian influence on peripheral reproductive organs. We anticipate that genes regulating the priming of reproductive organs, such as Wnt7A to support the endometrium, and hyaluronan binding protein 4 involved in chromatin remodeling during male germ cell development, to support health of sperm (Sutovsky and Lovercamp, 2010; Sutovsky et al., 2024; Zhang et al., 2023), will be under circadian control, and their respective apex will occur prior to peak sexual behavior. Investigation into proper temporal alignment between central and peripheral reproductive structures will provide great insight for therapy or treatment of infertility and/or reproductive organ dysfunction. Furthermore, central influence of sex hormones may regulate cell type-specific transcriptome to modulate sexual behavior (Knoedler et al., 2022).
Circadian disruption, most often due to rotating and night shift work, leads to irregular menstrual cycles and hormone abnormalities (as noted previously in this review). Such disruption has also been shown to increase the risk of pre-term birth and lead to a decrease in fecundity in women (Lawson et al., 2011; Nurminen, 1998; for excellent reviews, see Baker and Driver, 2007; Reschke et al., 2018). Epidemiological studies have also shown that maternal chrono-disruption leads to other adverse pregnancy outcomes such as smaller fetus size (for the gestational age) and an increased chance for miscarriage and spontaneous abortions (Abeysena et al., 2009; McDonald et al., 1988; Whelan et al., 2007; Zhu et al., 2004; for review, see Knutsson, 2003). Furthermore, genetic polymorphisms in the core circadian clock genes Npas2 and Bmal1 are known to contribute to adverse fertility outcomes (Kovanen et al., 2010). Future studies should examine clock gene expression changes in older women (>35 yrs) trying to conceive, and whether chrono-disruption, which negatively impacts a host of biological processes including sleep, temperature, and blood pressure, is more detrimental (in terms of fertility and pregnancy outcomes) in women >35 yrs. trying to become pregnant. Such information could prove beneficial in advising women who wish to conceive.
With regards to dysfunction of the circadian timing system on male reproduction, in addition to alterations in testosterone levels and rhythm following chrono-disruption (described earlier in this review), several studies have teased apart the influence of circadian desynchrony on spermatogenesis. For example, a statistically significant correlation between sleep duration and testis volume has been reported (Zhang et al., 2018). Importantly, sleep is one of many circadian-modulated processes that may confound circadian disruption of reproductive function. Furthermore, in a meta-analysis, Zhong et al. (2022) showed that sleep disorders were associated with reduced sperm count, reduced sperm concentration, and reduced sperm morphology and motility. Interestingly, bioinformatic mining in this same study revealed that circadian clock genes Per1, Per2, Cry2, Nr1d1, and Npas2 were also decreased in males who lacked sperm in ejaculate (azoospermia; Zhong et al., 2022). Similar genetic variations have been reported in men experiencing infertility. For example, a homozygous mutation in Npas2 has been reported in a Turkish family with nonobstructive azoospermia (Ramasamy et al., 2015a). Additionally, variability within the Clock gene is also associated with reduced semen quality and idiopathic infertility in men (Hodžić et al., 2013; Shen et al., 2015; Zhang et al., 2012). Such studies raise the prospect that alterations in clock genes may serve as a molecular marker for male infertility, and they highlight the fact that therapeutic interventions aimed at stabilizing circadian rhythms may be potential treatments for cases of male infertility. These results also raise interesting questions regarding the ability to modulate clock timing to improve fertility in aging men. Along these lines, though females are typically thought to have a ‘ticking biological clock’, studies suggest that increasing male age is also associated with a significant decline in fertility (for reviews, see Pasqualotto et al., 2008; Ramasamy et al., 2015b). Hence, gaining a better understanding of how changes in clock genes might signal the alarm of declining fertility in aging men may prove of value to couples trying to conceive.
Overall, gaining a better understanding of how circadian rhythms modulate male and female hormones, sexual behavior, and ultimately reproduction will prove fruitful in improving fertility outcomes, particularly in those individuals struggling to conceive. Future studies that aim at investigating which brain circuits control such sexual and reproductive behaviors as a function of time-of-day will help to advance our knowledge of mammalian fertility and could provide new avenues for therapeutic interventions and drug targets for fertility medications.
SA: Conceptualization, Funding acquisition, Writing – original draft, Writing – review & editing. OR-P: Writing – original draft, Writing – review & editing. CB: Writing – original draft, Writing – review & editing. GH: Writing – original draft, Writing – review & editing. EF: Writing – original draft, Writing – review & editing. AV: Writing – original draft, Writing – review & editing. AZ-S: Writing – original draft, Writing – review & editing. EK: Writing – original draft, Writing – review & editing. SH: Writing – original draft, Writing – review & editing. AA: Writing – original draft, Writing – review & editing. BP: Writing – original draft, Writing – review & editing. CM: Funding acquisition, Writing – original draft, Writing – review & editing.
The author(s) declare that financial support was received for the research, authorship, and/or publication of this article. Funding and support were provided by National Institutes of Health (NIH) 1F32HD111339-01A1 (to SA), Focused Projects Grant for Junior Investigators; American Academy of Sleep Medicine 2023 (to SA), NIH T32HL007901 (to SA), NIH Research Grant U54 AG062322 funded by the National Institute on Aging (NIA) and Office of Research on Women’s Health (ORWH) to CEM as a SCORE Scholar Award.
The authors declare that the research was conducted in the absence of any commercial or financial relationships that could be construed as a potential conflict of interest.
The authors declare that no Gen AI was used in the creation of this manuscript.
All claims expressed in this article are solely those of the authors and do not necessarily represent those of their affiliated organizations, or those of the publisher, the editors and the reviewers. Any product that may be evaluated in this article, or claim that may be made by its manufacturer, is not guaranteed or endorsed by the publisher.
Abeysena, C., Jayawardana, P., and De Seneviratne, R. (2009). Maternal sleep deprivation is a risk factor for small for gestational age: a cohort study. Aust. N. Z. J. Obstet. Gynaecol. 49, 382–387. doi: 10.1111/j.1479-828X.2009.01010.x
Abrahamson, E. E., and Moore, R. Y. (2001). Suprachiasmatic nucleus in the mouse: retinal innervation, intrinsic organization and efferent projections. Brain Res. 916, 172–191. doi: 10.1016/s0006-8993(01)02890-6
Aleandri, V., Spina, V., and Morini, A. (1996). The pineal gland and reproduction. Hum. Reprod. Update 2, 225–235. doi: 10.1093/humupd/2.3.225
Allen, L. S., Hines, M., Shryne, J. E., and Gorski, R. A. (1989). Two sexually dimorphic cell groups in the human brain. J. Neurosci. 9, 497–506. doi: 10.1523/JNEUROSCI.09-02-00497.1989
Almey, A., Milner, T. A., and Brake, W. G. (2015). Estrogen receptors in the central nervous system and their implication for dopamine-dependent cognition in females. Horm. Behav. 74, 125–138. doi: 10.1016/j.yhbeh.2015.06.010
Alvarez, J. D., Hansen, A., Ord, T., Bebas, P., Chappell, P. E., Giebultowicz, J. M., et al. (2008). The circadian clock protein BMAL1 is necessary for fertility and proper testosterone production in mice. J. Biol. Rhythm. 23, 26–36. doi: 10.1177/0748730407311254
Amir, S., Lamont, E. W., Robinson, B., and Stewart, J. (2004). A circadian rhythm in the expression of PERIOD2 protein reveals a novel SCN-controlled oscillator in the oval nucleus of the bed nucleus of the Stria terminalis. J. Neurosci. 24, 781–790. doi: 10.1523/JNEUROSCI.4488-03.2004
Arendt, J., and Aulinas, A. (2000). Physiology of the pineal gland and melatonin. K. R. Feingold, B. Anawalt, M. R. Blackman, A. Boyce, and G. Chrousos (Eds.). South Dartmouth (MA): MDText.com, Inc.
Arnal, P. J., Drogou, C., Sauvet, F., Regnauld, J., Dispersyn, G., Faraut, B., et al. (2016). Effect of sleep extension on the subsequent testosterone, cortisol and prolactin responses to Total sleep deprivation and recovery. J. Neuroendocrinol. 28:12346. doi: 10.1111/jne.12346
Aronin, N., Sagar, S. M., Sharp, F. R., and Schwartz, W. J. (1990). Light regulates expression of a Fos-related protein in rat suprachiasmatic nuclei. Proc. Natl. Acad. Sci. USA 87, 5959–5962. doi: 10.1073/pnas.87.15.5959
Aton, S. J., and Herzog, E. D. (2005). Come together, right...Now: synchronization of rhythms in a mammalian circadian clock. Neuron 48, 531–534. doi: 10.1016/j.neuron.2005.11.001
Auer, K. E., Kußmaul, M., Möstl, E., Hohlbaum, K., Rülicke, T., and Palme, R. (2020). Measurement of fecal testosterone metabolites in mice: replacement of invasive techniques. Animals: Open Access J. MDPI 10:165. doi: 10.3390/ani10010165
Auger, C. J., and De Vries, G. J. (2002). Progestin receptor immunoreactivity within steroid-responsive vasopressin-immunoreactive cells in the male and female rat brain. J. Neuroendocrinol. 14, 561–567. doi: 10.1046/j.1365-2826.2002.00809.x
Baburski, A. Z., Sokanovic, S. J., Bjelic, M. M., Radovic, S. M., Andric, S. A., and Kostic, T. S. (2016). Circadian rhythm of the Leydig cells endocrine function is attenuated during aging. Exp. Gerontol. 73, 5–13. doi: 10.1016/j.exger.2015.11.002
Baker, F. C., and Driver, H. S. (2007). Circadian rhythms, sleep, and the menstrual cycle. Sleep Med. 8, 613–622. doi: 10.1016/j.sleep.2006.09.011
Balsalobre, A., Brown, S. A., Marcacci, L., Tronche, F., Kellendonk, C., Reichardt, H. M., et al. (2000). Resetting of circadian time in peripheral tissues by glucocorticoid signaling. Science 289, 2344–2347. doi: 10.1126/science.289.5488.2344
Bao, A.-M., Liu, R.-Y., van Someren, E. J. W., Hofman, M. A., Cao, Y.-X., and Zhou, J.-N. (2003). Diurnal rhythm of free estradiol during the menstrual cycle. Eur. J. Endocrinol. 148, 227–232. doi: 10.1530/eje.0.1480227
Baumgartner, A., Dietzel, M., Saletu, B., Wolf, R., Campos-Barros, A., Gräf, K.-J., et al. (1993). Influence of partial sleep deprivation on the secretion of thyrotropin, thyroid hormones, growth hormone, prolactin, luteinizing hormone, follicle stimulating hormone, and estradiol in healthy young women. Psychiatry Res. 48, 153–178. doi: 10.1016/0165-1781(93)90039-J
Bayless, D. W., Davis, C. O., Yang, R., Wei, Y., de Andrade Carvalho, V. M., Knoedler, J. R., et al. (2023). A neural circuit for male sexual behavior and reward. Cell 186, 3862–3881.e28. doi: 10.1016/j.cell.2023.07.021
Berk, M. L., and Finkelstein, J. A. (1981). An autoradiographic determination of the efferent projections of the suprachiasmatic nucleus of the hypothalamus. Brain Res. 226, 1–13. doi: 10.1016/0006-8993(81)91079-9
Berson, D. M., Dunn, F. A., and Takao, M. (2002). Phototransduction by retinal ganglion cells that set the circadian clock. Science 295, 1070–1073. doi: 10.1126/science.1067262
Bittman, E. L. (2016). Timing in the testis. J. Biol. Rhythm. 31, 12–36. doi: 10.1177/0748730415618297
Brambilla, D. J., Matsumoto, A. M., Araujo, A. B., and McKinlay, J. B. (2009). The effect of diurnal variation on clinical measurement of serum testosterone and other sex hormone levels in men. J. Clin. Endocrinol. Metab. 94, 907–913. doi: 10.1210/jc.2008-1902
Brinton, R. D., Thompson, R. F., Foy, M. R., Baudry, M., Wang, J., Finch, C. E., et al. (2008). Progesterone receptors: form and function in brain. Front. Neuroendocrinol. 29, 313–339. doi: 10.1016/j.yfrne.2008.02.001
Brown-Grant, K., and Raisman, G. (1977). Abnormalities in reproductive function associated with the destruction of the suprachiasmatic nuclei in female rats. Proceed. Royal Society of London. Series B, Biolog. Sci. 198, 279–296. doi: 10.1098/rspb.1977.0098
Cagnacci, A., Maxia, N., and Volpe, A. (1999). Diurnal variation of semen quality in human males. Hum. Reprod. 14, 106–109. doi: 10.1093/humrep/14.1.106
Cai, W., Rambaud, J., Teboul, M., Masse, I., Benoit, G., Gustafsson, J.-A., et al. (2008). Expression levels of estrogen receptor beta are modulated by components of the molecular clock. Mol. Cell. Biol. 28, 784–793. doi: 10.1128/MCB.00233-07
Calabrò, R. S., Cacciola, A., Bruschetta, D., Milardi, D., Quattrini, F., Sciarrone, F., et al. (2019). Neuroanatomy and function of human sexual behavior: a neglected or unknown issue? Brain Behav. 9:e01389. doi: 10.1002/brb3.1389
Canteras, N. S., Ribeiro-Barbosa, E. R., Goto, M., Cipolla-Neto, J., and Swanson, L. W. (2011). The retinohypothalamic tract: comparison of axonal projection patterns from four major targets. Brain Res. Rev. 65, 150–183. doi: 10.1016/j.brainresrev.2010.09.006
Cara, A. L., Henson, E. L., Beekly, B. G., and Elias, C. F. (2021). Distribution of androgen receptor mRNA in the prepubertal male and female mouse brain. J. Neuroendocrinol. 33:e13063. doi: 10.1111/jne.13063
Cardinali, D. P., Nagle, C. A., and Rosner, J. M. (1975). Control of estrogen and androgen receptors in the rat pineal gland by catecholamine transmitter. Life Sci. 16, 93–106. doi: 10.1016/0024-3205(75)90212-X
Carson, S. A., and Kallen, A. N. (2021). Diagnosis and Management of Infertility: a review. JAMA 326, 65–76. doi: 10.1001/jama.2021.4788
Casao, A., Gallego, M., Abecia, J. A., Forcada, F., Pérez-Pé, R., Muiño-Blanco, T., et al. (2012). Identification and immunolocalisation of melatonin MT(1) and MT(2) receptors in rasa Aragonesa ram spermatozoa. Reprod. Fertil. Dev. 24, 953–961. doi: 10.1071/RD11242
Christensen, L. W., Nance, D. M., and Gorski, R. A. (1977). Effects of hypothalamic and preoptic lesions on reproductive behavior in male rats. Brain Res. Bull. 2, 137–141. doi: 10.1016/0361-9230(77)90010-7
Chung, K., Krey, L., Katz, J., and Noyes, N. (2005). Evaluating the role of exogenous luteinizing hormone in poor responders undergoing in vitro fertilization with gonadotropin-releasing hormone antagonists. Fertil. Steril. 84, 313–318. doi: 10.1016/j.fertnstert.2005.02.028
Chung, F.-F., Yao, C.-C. C., and Wan, G.-H. (2005). The associations between menstrual function and life style/working conditions among nurses in Taiwan. J. Occup. Health 47, 149–156. doi: 10.1539/joh.47.149
Cortes, L. R., Sturgeon, H., and Forger, N. G. (2023). Sexual differentiation of estrogen receptor alpha subpopulations in the ventromedial nucleus of the hypothalamus. Horm. Behav. 151:105348. doi: 10.1016/j.yhbeh.2023.105348
Costa, M., Santee, U. R., Cavalcante, J. S., Moraes, P. R. A., Santos, N. P., and Britto, L. R. G. (1999). Retinohypothalamic projections in the common marmoset (Callithrix jacchus): a study using cholera toxin subunit B. J. Comp. Neurol. 415, 393–403. doi: 10.1002/(SICI)1096-9861(19991220)415:3<393::AID-CNE5>3.0.CO;2-R
Creutz, L. M., and Kritzer, M. F. (2002). Estrogen receptor-beta immunoreactivity in the midbrain of adult rats: regional, subregional, and cellular localization in the A10, A9, and A8 dopamine cell groups. J. Comp. Neurol. 446, 288–300. doi: 10.1002/cne.10207
D’Occhio, M. J., and Suttie, J. M. (1992). The role of the pineal gland and melatonin in reproduction in male domestic ruminants. Anim. Reprod. Sci. 30, 135–155. doi: 10.1016/0378-4320(92)90009-3
Dart, D. A., Bevan, C. L., Uysal-Onganer, P., and Jiang, W. G. (2024). Analysis of androgen receptor expression and activity in the mouse brain. Sci. Rep. 14:11115. doi: 10.1038/s41598-024-61733-9
De Jonge, F. H., Louwerse, A. L., Ooms, M. P., Evers, P., Endert, E., and Van De Poll, N. E. (1989). Lesions of the SDN-POA inhibit sexual behavior of male wistar rats. Brain Res. Bull. 23, 483–492. doi: 10.1016/0361-9230(89)90194-9
de la Iglesia, H. O., Meyer, J., and Schwartz, W. J. (2003). Lateralization of circadian pacemaker output: activation of left- and right-sided luteinizing hormone-releasing hormone neurons involves a neural rather than a humoral pathway. J. Neurosci. 23, 7412–7414. doi: 10.1523/JNEUROSCI.23-19-07412.2003
de la Torre, B., Sjöberg, B., Hedman, M., Bártfai, G., and Diczfalusy, E. (1981). A study of the short-time variation and interrelationship of plasma hormone levels reflecting pituitary, adrenocortical and testicular function in fertile men. Int. J. Androl. 4, 532–545. doi: 10.1111/j.1365-2605.1981.tb00736.x
Deurveilher, S., Burns, J., and Semba, K. (2002). Indirect projections from the suprachiasmatic nucleus to the ventrolateral preoptic nucleus: a dual tract-tracing study in rat. Eur. J. Neurosci. 16, 1195–1213. doi: 10.1046/j.1460-9568.2002.02196.x
Deurveilher, S., and Semba, K. (2005). Indirect projections from the suprachiasmatic nucleus to major arousal-promoting cell groups in rat: implications for the circadian control of behavioural state. Neuroscience 130, 165–183. doi: 10.1016/j.neuroscience.2004.08.030
Diatroptov, M. E. (2011). Infradian fluctuations in serum testosterone levels in male laboratory rats. Bull. Exp. Biol. Med. 151, 638–641. doi: 10.1007/s10517-011-1403-z
Diver, M. J., Imtiaz, K. E., Ahmad, A. M., Vora, J. P., and Fraser, W. D. (2003). Diurnal rhythms of serum total, free and bioavailable testosterone and of SHBG in middle-aged men compared with those in young men. Clin. Endocrinol. 58, 710–717. doi: 10.1046/j.1365-2265.2003.01772.x
Dixson, A. F., and Gardner, J. S. (1981). Diurnal variations in plasma testosterone in a male nocturnal primate, the owl monkey (Aotus trivirgatus). J. Reprod. Fertil. 62, 83–86. doi: 10.1530/jrf.0.0620083
Dolatshad, H., Campbell, E. A., O’Hara, L., Maywood, E. S., Hastings, M. H., and Johnson, M. H. (2006). Developmental and reproductive performance in circadian mutant mice. Hum. Reprod. 21, 68–79. doi: 10.1093/humrep/dei313
Dubey, A. K., Puri, C. P., Puri, V., and Anand Kumar, T. C. (1983). Day and night levels of hormones in male rhesus monkeys kept under controlled or constant environmental light. Experientia 39, 207–209. doi: 10.1007/BF01958904
Egnor, S. R., and Seagraves, K. M. (2016). The contribution of ultrasonic vocalizations to mouse courtship. Curr. Opin. Neurobiol. 38, 1–5. doi: 10.1016/j.conb.2015.12.009
Espino, J., Ortiz, Á., Bejarano, I., Lozano, G. M., Monllor, F., García, J. F., et al. (2011). Melatonin protects human spermatozoa from apoptosis via melatonin receptor- and extracellular signal-regulated kinase-mediated pathways. Fertil. Steril. 95, 2290–2296. doi: 10.1016/j.fertnstert.2011.03.063
Esquifino, A. I., Chacón, F., Jimenez, V., Reyes Toso, C. F., and Cardinali, D. P. (2004). 24-hour changes in circulating prolactin, follicle-stimulating hormone, luteinizing hormone and testosterone in male rats subjected to social isolation. J. Circadian Rhythms 2:1. doi: 10.1186/1740-3391-2-1
Everett, J. W., and Sawyer, C. H. (1950). A 24-hour periodicity in the “LH-release apparatus” of female rats, disclosed by barbiturate sedation. Endocrinology 47, 198–218. doi: 10.1210/endo-47-3-198
Fang, Y.-Y., Yamaguchi, T., Song, S. C., Tritsch, N. X., and Lin, D. (2018). A hypothalamic midbrain pathway essential for driving maternal behaviors. Neuron 98, 192–207.e10. doi: 10.1016/j.neuron.2018.02.019
Fernández-Guasti, A., Kruijver, F. P., Fodor, M., and Swaab, D. F. (2000). Sex differences in the distribution of androgen receptors in the human hypothalamus. J. Comp. Neurol. 425, 422–435. doi: 10.1002/1096-9861(20000925)425:3<422::aid-cne7>3.0.co;2-h
Fernando, S., and Rombauts, L. (2014). Melatonin: shedding light on infertility? - a review of the recent literature. J. Ovarian Res. 7:98. doi: 10.1186/s13048-014-0098-y
Fortenberry, J. D., Katz, B. P., Blythe, M. J., Juliar, B. E., Tu, W., and Orr, D. P. (2006). Factors associated with time of day of sexual activity among adolescent women. J. Adolescent Health: Official Pub. Society for Adolescent Med. 38, 275–281. doi: 10.1016/j.jadohealth.2005.02.006
Freeman, M. E., and Neill, J. D. (1972). The pattern of prolactin secretion during pseudopregnancy in the rat: a daily nocturnal surge. Endocrinology 90, 1292–1294. doi: 10.1210/endo-90-5-1292
Freeman, M. E., Smith, M. S., Nazian, S. J., and Neill, J. D. (1974). Ovarian and hypothalamic control of the daily surges of prolactin secretion during Pseudopregnancy in the Rat12. Endocrinology 94, 875–882. doi: 10.1210/endo-94-3-875
Fujimoto, V. Y., Clifton, D. K., Cohen, N. L., and Soules, M. R. (1990). Variability of serum prolactin and progesterone levels in normal women: the relevance of single hormone measurements in the clinical setting. Obstet. Gynecol. 76, 71–78.
Funabashi, T., Aiba, S., Sano, A., Shinohara, K., and Kimura, F. (1999). Intracerebroventricular injection of arginine-vasopressin V1 receptor antagonist attenuates the surge of luteinizing hormone and prolactin secretion in proestrous rats. Neurosci. Lett. 260, 37–40. doi: 10.1016/S0304-3940(98)00940-9
Gall, H., Glowania, H. J., and Fischer, M. (1979). Circadian rhythm of testosterone level in plasma. I. Physiologic 24-hour oscillations of the testosterone level in plasma. Andrologia 11, 287–292.
Gooley, J. J., Lu, J., Chou, T. C., Scammell, T. E., and Saper, C. B. (2001). Melanopsin in cells of origin of the retinohypothalamic tract. Nat. Neurosci. 4:1165. doi: 10.1038/nn768
Goy, R. W., and Phoenix, C. H. (1963). Hypothalamic regulation of female sexual behaviour; establishment of behavioural oestrus in spayed guinea-pigs following hypothalamic lesions. J. Reprod. Fertil. 5:23. doi: 10.1530/jrf.0.0050023
Gray, P., and Brooks, P. J. (1984). Effect of lesion location within the medial preoptic-anterior hypothalamic continuum on maternal and male sexual behaviors in female rats. Behav. Neurosci. 98, 703–711. doi: 10.1037/0735-7044.98.4.703
Guerra-Araiza, C., Coyoy-Salgado, A., and Camacho-Arroyo, I. (2002). Sex differences in the regulation of progesterone receptor isoforms expression in the rat brain. Brain Res. Bull. 59, 105–109. doi: 10.1016/s0361-9230(02)00845-6
Guignard, M. M., Pesquies, P. C., Serrurier, B. D., Merino, D. B., and Reinberg, A. E. (1980). Circadian rhythms in plasma levels of cortisol, dehydroepiandrosterone, delta 4-androstenedione, testosterone and dihydrotestosterone of healthy young men. Acta Endocrinol. 94, 536–545. doi: 10.1530/acta.0.0940536
Haldar, C., and Gupta, D. (1990). Sex- and age-dependent nature of the cytoplasmic 5 alpha-dihydrotestosterone (DHT) binding site/receptor in bovine pineal gland. J. Pineal Res. 8, 289–295. doi: 10.1111/j.1600-079x.1990.tb00889.x
Hannibal, J., Møller, M., Ottersen, O. P., and Fahrenkrug, J. (2000). PACAP and glutamate are co-stored in the retinohypothalamic tract. J. Comp. Neurol. 418, 147–155. doi: 10.1002/(SICI)1096-9861(20000306)418:2<147::AID-CNE2>3.0.CO;2-#
Hansen, S. (1982). Hypothalamic control of motivation: the medial preoptic area and masculine sexual behaviour. Scand. J. Psychol. 23, 121–126. doi: 10.1111/j.1467-9450.1982.tb00460.x
Harding, S. M., and McGinnis, M. Y. (2003). Effects of testosterone in the VMN on copulation, partner preference, and vocalizations in male rats. Horm. Behav. 43, 327–335. doi: 10.1016/s0018-506x(02)00049-1
Harding, S. M., and McGinnis, M. Y. (2005). Microlesions of the ventromedial nucleus of the hypothalamus: effects on sociosexual behaviors in male rats. Behav. Neurosci. 119, 1227–1234. doi: 10.1037/0735-7044.119.5.1227
Harman, S., Metter, E., Tobin, J., Pearson, J., and Blackman, M. (2001). Longitudinal effects of aging on serum total and free testosterone levels in healthy men. Baltimore longitudinal study of aging. J. Clin. Endocrinol. Metab. 86, 724–731. doi: 10.1210/jcem.86.2.7219
Harney, J. P., Scarbrough, K., Rosewell, K. L., and Wise, P. M. (1996). In vivo antisense antagonism of vasoactive intestinal peptide in the suprachiasmatic nuclei causes aging-like changes in the estradiol-induced luteinizing hormone and prolactin surges. Endocrinology 137, 3696–3701. doi: 10.1210/endo.137.9.8756535
Hastings, M. H., Maywood, E. S., and Brancaccio, M. (2018). Generation of circadian rhythms in the suprachiasmatic nucleus. Nat. Rev. Neurosci. 19, 453–469. doi: 10.1038/s41583-018-0026-z
Hattar, S., Kumar, M., Park, A., Tong, P., Tung, J., Yau, K.-W., et al. (2006). Central projections of melanopsin-expressing retinal ganglion cells in the mouse. J. Comp. Neurol. 497, 326–349. doi: 10.1002/cne.20970
Hattar, S., Liao, H. W., Takao, M., Berson, D. M., and Yau, K. W. (2002). Melanopsin-containing retinal ganglion cells: Architecture, projections, and intrinsic photosensitivity. Science 295, 1065–1070. doi: 10.1126/science.1069609
Hodžić, A., Ristanović, M., Zorn, B., Tulić, C., Maver, A., Novaković, I., et al. (2013). Genetic variation in circadian rhythm genes CLOCK and ARNTL as risk factor for male infertility. PLoS One 8:e59220. doi: 10.1371/journal.pone.0059220
Hofman, M. A., and Swaab, D. F. (1989). The sexually dimorphic nucleus of the preoptic area in the human brain: a comparative morphometric study. J. Anat. 164, 55–72
Huijgens, P. T., Heijkoop, R., and Snoeren, E. M. S. (2021). Silencing and stimulating the medial amygdala impairs ejaculation but not sexual incentive motivation in male rats. Behav. Brain Res. 405:113206. doi: 10.1016/j.bbr.2021.113206
Hull, E. M., and Dominguez, J. M. (2007). Sexual behavior in male rodents. Horm. Behav. 52, 45–55. doi: 10.1016/j.yhbeh.2007.03.030
Hull, E. M., and Rodríguez-Manzo, G. (2009). “1—male sexual behavior” in Hormones, brain and behavior. eds. D. W. Pfaff, A. P. Arnold, A. M. Etgen, S. E. Fahrbach, and R. T. Rubin. Second ed (Academic Press), 5–66. Available at: https://api.pageplace.de/preview/DT0400.9780128036082_A28772112/preview-9780128036082_A28772112.pdf
Inouye, S. T., and Kawamura, H. (1979). Persistence of circadian rhythmicity in a mammalian hypothalamic “island” containing the suprachiasmatic nucleus. Proc. Natl. Acad. Sci. USA 76, 5962–5966. doi: 10.1073/pnas.76.11.5962
Iovino, M., Messana, T., Iovino, E., De Pergola, G., Guastamacchia, E., Giagulli, V. A., et al. (2019). Neuroendocrine mechanisms involved in male sexual and emotional behavior. Endocr Metab Immune Disord Drug Targets 19, 472–480. doi: 10.2174/1871530319666190131155310
Jankowski, K. S., Díaz-Morales, J. F., and Randler, C. (2014). Chronotype, gender, and time for sex. Chronobiol. Int. 31, 911–916. doi: 10.3109/07420528.2014.925470
Jennings, K. J., and de Lecea, L. (2020). Neural and hormonal control of sexual behavior. Endocrinology 161:bqaa150. doi: 10.1210/endocr/bqaa150
Jocz, P., Stolarski, M., and Jankowski, K. S. (2018). Similarity in Chronotype and preferred time for sex and its role in relationship quality and sexual satisfaction. Front. Psychol. 9:443. doi: 10.3389/fpsyg.2018.00443
Johnson, M. H., Lim, A., Fernando, D., and Day, M. L. (2002). Circadian clockwork genes are expressed in the reproductive tract and conceptus of the early pregnant mouse. Reprod. Biomed. Online 4, 140–145. doi: 10.1016/s1472-6483(10)61931-1
Johnson, R. F., Smale, L., Moore, R. Y., and Morin, L. P. (1988). Lateral geniculate lesions block circadian phase-shift responses to a benzodiazepine. Proc. Natl. Acad. Sci. 85, 5301–5304. doi: 10.1073/pnas.85.14.5301
Kalsbeek, A., Teclemariam-Mesbah, R., and Pévet, P. (1993). Efferent projections of the suprachiasmatic nucleus in the golden hamster (Mesocricetus auratus). J. Comp. Neurol. 332, 293–314. doi: 10.1002/cne.903320304
Karatsoreos, I. N., and Silver, R. (2007). Minireview: the neuroendocrinology of the suprachiasmatic nucleus as a conductor of body time in mammals. Endocrinology 148, 5640–5647. doi: 10.1210/en.2007-1083
Karatsoreos, I. N., Wang, A., Sasanian, J., and Silver, R. (2007). A role for androgens in regulating circadian behavior and the suprachiasmatic nucleus. Endocrinology 148, 5487–5495. doi: 10.1210/en.2007-0775
Karigo, T., Kennedy, A., Yang, B., Liu, M., Tai, D., Wahle, I. A., et al. (2021). Distinct hypothalamic control of same- and opposite-sex mounting behaviour in mice. Nature 589, 258–263. doi: 10.1038/s41586-020-2995-0
Kashon, M. L., Arbogast, J. A., and Sisk, C. L. (1996). Distribution and hormonal regulation of androgen receptor immunoreactivity in the forebrain of the male European ferret. J. Comp. Neurol. 376, 567–586. doi: 10.1002/(SICI)1096-9861(19961223)376:4<567::AID-CNE6>3.0.CO;2-#
Kato, J., Hirata, S., Nozawa, A., and Yamada-Mouri, N. (1994). Gene expression of progesterone receptor isoforms in the rat brain. Horm. Behav. 28, 454–463. doi: 10.1006/hbeh.1994.1043
Kaufman, J. M., and Vermeulen, A. (2005). The decline of androgen levels in elderly men and its clinical and therapeutic implications. Endocr. Rev. 26, 833–876. doi: 10.1210/er.2004-0013
Kennaway, D. J., and Rowe, S. A. (1997). Controlled-release melatonin implants delay puberty in rats without altering melatonin rhythmicity. J. Pineal Res. 22, 107–116. doi: 10.1111/j.1600-079x.1997.tb00311.x
Kennaway, D. J., Varcoe, T. J., and Mau, V. J. (2003). Rhythmic expression of clock and clock-controlled genes in the rat oviduct. Mol. Hum. Reprod. 9, 503–507. doi: 10.1093/molehr/gag067
Khodai, T., and Luckman, S. M. (2021). Ventromedial nucleus of the hypothalamus neurons under the magnifying glass. Endocrinology 162:bqab141. doi: 10.1210/endocr/bqab141
Kholkute, S. D., Jayaraman, S., Kumar, R. A., and Puri, C. P. (1987). Continuous light environment has no effect on the circadian testosterone rhythm, spermatogenesis or fertility of the marmoset (Callithrix jacchus). Int. J. Androl. 10, 635–642. doi: 10.1111/j.1365-2605.1987.tb00363.x
Kirn, J., and Floody, O. R. (1985). Differential effects of lesions in three limbic areas on ultrasound production and lordosis by female hamsters. Behav. Neurosci. 99, 1142–1152. doi: 10.1037//0735-7044.99.6.1142
Klaric, J. S., and Hendricks, S. E. (1986). Effects of two-stage lesions of the medial preoptic area on sexual behavior of male rats. Physiol. Behav. 37, 539–542. doi: 10.1016/0031-9384(86)90281-7
Knoedler, J. R., Inoue, S., Bayless, D. W., Yang, T., Tantry, A., Davis, C.-H., et al. (2022). A functional cellular framework for sex and estrous cycle-dependent gene expression and behavior. Cell 185, 654–671.e22. doi: 10.1016/j.cell.2021.12.031
Knutsson, A. (2003). Health disorders of shift workers. Occup. Med. 53, 103–108. doi: 10.1093/occmed/kqg048
Komada, Y., Ikeda, Y., Sato, M., Kami, A., Masuda, C., and Shibata, S. (2019). Social jetlag and menstrual symptoms among female university students. Chronobiol. Int. 36, 258–264. doi: 10.1080/07420528.2018.1533561
Kovanen, L., Saarikoski, S. T., Aromaa, A., Lönnqvist, J., and Partonen, T. (2010). ARNTL (BMAL1) and NPAS2 gene variants contribute to fertility and seasonality. PLoS One 5:e10007. doi: 10.1371/journal.pone.0010007
Kriegsfeld, L. J., Mei, D. F., Bentley, G. E., Ubuka, T., Mason, A. O., Inoue, K., et al. (2006). Identification and characterization of a gonadotropin-inhibitory system in the brains of mammals. Proc. Natl. Acad. Sci. 103, 2410–2415. doi: 10.1073/pnas.0511003103
Kriegsfeld, L. J., Silver, R., Gore, A. C., and Crews, D. (2002). Vasoactive intestinal polypeptide contacts on gonadotropin-releasing hormone neurones increase following puberty in female rats. J. Neuroendocrinol. 14, 685–690. doi: 10.1046/j.1365-2826.2002.00818.x
Kruijver, F. P. M., and Swaab, D. F. (2002). Sex hormone receptors are present in the human suprachiasmatic nucleus. Neuroendocrinology 75, 296–305. doi: 10.1159/000057339
Kubota, A., Inouye, S.-I. T., and Kawamura, H. (1981). Reversal of multiunit activity within and outside the suprachiasmatic nucleus in the rat. Neurosci. Lett. 27, 303–308. doi: 10.1016/0304-3940(81)90447-X
Kukino, A., Walbeek, T. J., Sun, L. J., Watt, A. T., Park, J. H., Kauffman, A. S., et al. (2022). Mistimed restricted feeding disrupts circadian rhythms of male mating behavior and female preovulatory LH surges in mice. Horm. Behav. 145:105242. doi: 10.1016/j.yhbeh.2022.105242
Labyak, S., Lava, S., Turek, F., and Zee, P. (2002). Effects of shiftwork on sleep and menstrual function in nurses. Health Care Women Int. 23, 703–714. doi: 10.1080/07399330290107449
Laflamme, N., Nappi, R. E., Drolet, G., Labrie, C., and Rivest, S. (1998). Expression and neuropeptidergic characterization of estrogen receptors (ERα and ERβ) throughout the rat brain: anatomical evidence of distinct roles of each subtype. J. Neurobiol. 36, 357–378. doi: 10.1002/(SICI)1097-4695(19980905)36:3<357::AID-NEU5>3.0.CO;2-V
Lamont, E. W., Robinson, B., Stewart, J., and Amir, S. (2005). The central and basolateral nuclei of the amygdala exhibit opposite diurnal rhythms of expression of the clock protein Period2. Proc. Natl. Acad. Sci. USA 102, 4180–4184. doi: 10.1073/pnas.0500901102
Larsson, K., and Heimer, L. (1964). Mating behaviour of male rats after lesions in the preoptic area. Nature 202, 413–414. doi: 10.1038/202413a0
Lawson, C. C., Whelan, E. A., Lividoti Hibert, E. N., Spiegelman, D., Schernhammer, E. S., and Rich-Edwards, J. W. (2011). Rotating shift work and menstrual cycle characteristics. Epidemiology 22, 305–312. doi: 10.1097/EDE.0b013e3182130016
Leak, R. K., Card, J. P., and Moore, R. Y. (1999). Suprachiasmatic pacemaker organization analyzed by viral transynaptic transport. Brain Res. 819, 23–32. doi: 10.1016/s0006-8993(98)01317-1
Leak, R. K., and Moore, R. Y. (2001). Topographic organization of suprachiasmatic nucleus projection neurons. J. Comp. Neurol. 433, 312–334. doi: 10.1002/cne.1142
Lee, H., Kim, D.-W., Remedios, R., Anthony, T. E., Chang, A., Madisen, L., et al. (2014). Scalable control of mounting and attack by Esr1+ neurons in the ventromedial hypothalamus. Nature 509, 627–632. doi: 10.1038/nature13169
Lein, E. S., Hawrylycz, M. J., Ao, N., Ayres, M., Bensinger, A., Bernard, A., et al. (2007). Genome-wide atlas of gene expression in the adult mouse brain. Nature 445, 168–176. doi: 10.1038/nature05453
Leproult, R., and Van Cauter, E. (2011). Effect of 1 week of sleep restriction on testosterone levels in young healthy MenFREE. JAMA 305, 2173–2174. doi: 10.1001/jama.2011.710
Levine, J. D., Weiss, M. L., Rosenwasser, A. M., and Miselis, R. R. (1991). Retinohypothalamic tract in the female albino rat: a study using horseradish peroxidase conjugated to cholera toxin. J. Comp. Neurol. 306, 344–360. doi: 10.1002/cne.903060210
Li, C., Xiao, S., Hao, J., Liao, X., and Li, G. (2018). Cry1 deficiency leads to testicular dysfunction and altered expression of genes involved in cell communication, chromatin reorganization, spermatogenesis, and immune response in mouse testis. Mol. Reprod. Dev. 85, 325–335. doi: 10.1002/mrd.22968
Li, C., and Zhou, X. (2015). Melatonin and male reproduction. Clin. Chim. Acta 446, 175–180. doi: 10.1016/j.cca.2015.04.029
Liang, X., Cheng, S., Jiang, X., He, X., Wang, Y., Jiang, Z., et al. (2013). The noncircadian function of the circadian clock gene in the regulation of male fertility. J. Biol. Rhythm. 28, 208–217. doi: 10.1177/0748730413486873
Liao, Z., Liu, C., Cai, L., Shen, L., Sui, C., Zhang, H., et al. (2022). The effect of endometrial thickness on pregnancy, maternal, and perinatal outcomes of women in fresh cycles after IVF/ICSI: a systematic review and Meta-analysis. Front. Endocrinol. 12:814648. doi: 10.3389/fendo.2021.814648
Lin, D., Boyle, M. P., Dollar, P., Lee, H., Lein, E. S., Perona, P., et al. (2011). Functional identification of an aggression locus in the mouse hypothalamus. Nature 470, 221–226. doi: 10.1038/nature09736
Liu, Y., Johnson, B. P., Shen, A. L., Wallisser, J. A., Krentz, K. J., Moran, S. M., et al. (2014). Loss of BMAL1 in ovarian steroidogenic cells results in implantation failure in female mice. Proc. Natl. Acad. Sci. USA 111, 14295–14300. doi: 10.1073/pnas.1209249111
Liu, K., Meng, T., Chen, Q., Hou, G., Wang, X., Hu, S., et al. (2022). Diurnal rhythm of human semen quality: analysis of large-scale human sperm bank data and timing-controlled laboratory study. Hum. Reprod. 37, 1727–1738. doi: 10.1093/humrep/deac135
Liu, Y.-C., Salamone, J. D., and Sachs, B. D. (1997). Lesions in medial preoptic area and bed nucleus of Stria terminalis: differential effects on copulatory behavior and noncontact erection in male rats. J. Neurosci. 17, 5245–5253. doi: 10.1523/JNEUROSCI.17-13-05245.1997
Liu, Q., Wang, H., Wang, H., Li, N., He, R., and Liu, Z. (2022). Per1/Per2 disruption reduces testosterone synthesis and impairs fertility in elderly male mice. Int. J. Mol. Sci. 23:7399. doi: 10.3390/ijms23137399
Liu, Q., Xiong, J., Kim, D. W., Lee, S. S., Bell, B. J., Alexandre, C., et al. (2024). An amygdalar oscillator coordinates cellular and behavioral rhythms. Neuron 112, 3750–3767.e7. doi: 10.1016/j.neuron.2024.08.013
Llavanera, M., Delgado-Bermúdez, A., Ribas-Maynou, J., Salas-Huetos, A., and Yeste, M. (2022). A systematic review identifying fertility biomarkers in semen: a clinical approach through omics to diagnose male infertility. Fertil. Steril. 118, 291–313. doi: 10.1016/j.fertnstert.2022.04.028
Lohstroh, P. N., Chen, J., Ba, J., Ryan, L. M., Xu, X., Overstreet, J. W., et al. (2003). Bone resorption is affected by follicular phase length in female rotating shift workers. Environ. Health Perspect. 111, 618–622. doi: 10.1289/ehp.5878
Lowrey, P. L., and Takahashi, J. S. (2000). Genetics of the mammalian circadian system: photic entrainment, circadian pacemaker mechanisms, and posttranslational regulation. Annu. Rev. Genet. 34, 533–562. doi: 10.1146/annurev.genet.34.1.533
Lu, J., Zhang, Y.-H., Chou, T. C., Gaus, S. E., Elmquist, J. K., Shiromani, P., et al. (2001). Contrasting effects of Ibotenate lesions of the paraventricular nucleus and subparaventricular zone on sleep–wake cycle and temperature regulation. J. Neurosci. 21, 4864–4874. doi: 10.1523/JNEUROSCI.21-13-04864.2001
Luboshitzky, R., Dharan, M., Goldman, D., Hiss, Y., Herer, P., and Lavie, P. (1997). Immunohistochemical localization of gonadotropin and gonadal steroid receptors in human pineal Glands1. J. Clin. Endocrinol. Metab. 82, 977–981. doi: 10.1210/jcem.82.3.3829
Luboshitzky, R., Herer, P., Levi, M., Shen-Orr, Z., and Lavie, P. (1999). Relationship between rapid eye movement sleep and testosterone secretion in normal men. J. Androl. 20, 731–737. doi: 10.1002/j.1939-4640.1999.tb03378.x
Mahoney, M. M. (2010). Shift work, jet lag, and female reproduction. Int. J. Endocrinol. 2010:813764. doi: 10.1155/2010/813764
Mahoney, M. M., Sisk, C., Ross, H. E., and Smale, L. (2004). Circadian regulation of gonadotropin-releasing hormone neurons and the preovulatory surge in luteinizing hormone in the diurnal rodent, Arvicanthis niloticus, and in a nocturnal rodent, Rattus norvegicus. Biol. Reprod. 70, 1049–1054. doi: 10.1095/biolreprod.103.021360
Mai, L. M., Shieh, K. R., and Pan, J. T. (1994). Circadian changes of serum prolactin levels and tuberoinfundibular dopaminergic neuron activities in ovariectomized rats treated with or without estrogen: the role of the suprachiasmatic nuclei. Neuroendocrinology 60, 520–526. doi: 10.1159/000126789
Malsbury, C. W. (1971). Facilitation of male rat copulatory behavior by electrical stimulation of the medial preoptic area. Physiol. Behav. 7, 797–805. doi: 10.1016/0031-9384(71)90042-4
Martinez, L. A., and Petrulis, A. (2011). The bed nucleus of the stria terminalis is critical for sexual solicitation, but not for opposite-sex odor preference, in female Syrian hamsters. Horm. Behav. 60, 651–659. doi: 10.1016/j.yhbeh.2011.08.018
Mason, A. O., Duffy, S., Zhao, S., Ubuka, T., Bentley, G. E., Tsutsui, K., et al. (2010). Photoperiod and reproductive condition are associated with changes in RFamide-related peptide (RFRP) expression in Syrian hamsters (Mesocricetus auratus). J. Biol. Rhythm. 25, 176–185. doi: 10.1177/0748730410368821
Mathews, D., and Edwards, D. A. (1977). The ventromedial nucleus of the hypothalamus and the hormonal arousal of sexual behaviors in the female rat. Horm. Behav. 8, 40–51. doi: 10.1016/0018-506X(77)90019-8
McDonald, A. D., Lavoie, J., Côté, R., and McDonald, J. C. (1988). Spontaneous abortion in women employed in plastics manufacture. Am. J. Ind. Med. 14, 9–14. doi: 10.1002/ajim.4700140103
Mereness, A. L., Murphy, Z. C., Forrestel, A. C., Butler, S., Ko, C., Richards, J. S., et al. (2016). Conditional deletion of Bmal1 in ovarian Theca cells disrupts ovulation in female mice. Endocrinology 157, 913–927. doi: 10.1210/en.2015-1645
Miller, B. H., Olson, S. L., Turek, F. W., Levine, J. E., Horton, T. H., and Takahashi, J. S. (2004). Circadian clock mutation disrupts estrous cyclicity and maintenance of pregnancy. Curr. Biol. 14, 1367–1373. doi: 10.1016/j.cub.2004.07.055
Miller, B. H., and Takahashi, J. S. (2014). Central circadian control of female reproductive function. Front. Endocrinol. 4:195. doi: 10.3389/fendo.2013.00195
Milner, T. A., Thompson, L. I., Wang, G., Kievits, J. A., Martin, E., Zhou, P., et al. (2010). Distribution of estrogen receptor β containing cells in the brains of bacterial artificial chromosome transgenic mice. Brain Res. 1351, 74–96. doi: 10.1016/j.brainres.2010.06.038
Mitra, S. W., Hoskin, E., Yudkovitz, J., Pear, L., Wilkinson, H. A., Hayashi, S., et al. (2003). Immunolocalization of estrogen receptor beta in the mouse brain: comparison with estrogen receptor alpha. Endocrinology 144, 2055–2067. doi: 10.1210/en.2002-221069
Miyamoto, Y., and Sancar, A. (1998). Vitamin B2-based blue-light photoreceptors in the retinohypothalamic tract as the photoactive pigments for setting the circadian clock in mammals. Proc. Natl. Acad. Sci. USA 95, 6097–6102. doi: 10.1073/pnas.95.11.6097
Miyatake, A., Morimoto, Y., Oishi, T., Hanasaki, N., Sugita, Y., Iijima, S., et al. (1980). Circadian rhythm of serum testosterone and its relation to sleep: comparison with the variation in serum luteinizing hormone, prolactin, and cortisol in normal men. J. Clin. Endocrinol. Metab. 51, 1365–1371. doi: 10.1210/jcem-51-6-1365
Moffitt, J. R., Bambah-Mukku, D., Eichhorn, S. W., Vaughn, E., Shekhar, K., Perez, J. D., et al. (2018). Molecular, spatial, and functional single-cell profiling of the hypothalamic preoptic region. Science 362:eaau5324. doi: 10.1126/science.aau5324
Moore, R. Y., and Eichler, V. B. (1972). Loss of a circadian adrenal corticosterone rhythm following suprachiasmatic lesions in the rat. Brain Res. 42, 201–206. doi: 10.1016/0006-8993(72)90054-6
Moore, R. Y., Speh, J. C., and Leak, R. K. (2002). Suprachiasmatic nucleus organization. Cell Tissue Res. 309, 89–98. doi: 10.1007/s00441-002-0575-2
Morin, L. P., and Allen, C. N. (2006). The circadian visual system, 2005. Brain Res. Rev. 51, 1–60. doi: 10.1016/j.brainresrev.2005.08.003
Morse, D., Cermakian, N., Brancorsini, S., Parvinen, M., and Sassone-Corsi, P. (2003). No circadian rhythms in testis: Period1 expression is clock independent and developmentally regulated in the mouse. Molecular Endocrinol. (Baltimore) 17, 141–151. doi: 10.1210/me.2002-0184
Mosko, S. S., and Moore, R. Y. (1979). Neonatal suprachiasmatic nucleus lesions: effects on the development of circadian rhythms in the rat. Brain Res. 164, 17–38. doi: 10.1016/0006-8993(79)90003-9
Mukku, V., Prahalada, S., and Moudgal, N. R. (1976). Effect of constant light on nychthemeral variations in serum testosterone in male Macaca radiata. Nature 260, 778–780. doi: 10.1038/260778a0
Nakamura, W., Yamazaki, S., Nakamura, T. J., Shirakawa, T., Block, G. D., and Takumi, T. (2008). In vivo monitoring of circadian timing in freely moving mice. Curr. Biol. 18, 381–385. doi: 10.1016/j.cub.2008.02.024
Nassar, G. N., and Leslie, S. W. (2024). “Physiology, Testosterone” in StatPearls. Ed. T. K. Shackelford (Treasure Island, FL: StatPearls Publishing).
Ni, W., Liu, K., Hou, G., Pan, C., Wu, S., Zheng, J., et al. (2019). Diurnal variation in sperm DNA fragmentation: analysis of 11,382 semen samples from two populations and in vivo animal experiments. Chronobiol. Int. 36, 1455–1463. doi: 10.1080/07420528.2019.1649275
Nyby, J. G. (2008). Reflexive testosterone release: a model system for studying the nongenomic effects of testosterone upon male behavior. Front. Neuroendocrinol. 29, 199–210. doi: 10.1016/j.yfrne.2007.09.001
Olcese, J. M. (2020). Melatonin and female reproduction: an expanding universe. Front. Endocrinol. 11:85. doi: 10.3389/fendo.2020.00085
Palm, I. F., van der Beek, E. M., Wiegant, V. M., Buijs, R. M., and Kalsbeek, A. (2001). The stimulatory effect of vasopressin on the luteinizing hormone surge in ovariectomized, estradiol-treated rats is time-dependent. Brain Res. 901, 109–116. doi: 10.1016/s0006-8993(01)02309-5
Palmer, J. D., Udry, J. R., and Morris, N. M. (1982a). Diurnal and weekly, but no lunar rhythms in human copulation. Hum. Biol. 54, 111–121.
Palmer, J. D., Udry, J. R., and Morris, N. M. (1982b). Diurnal and weekly, but no lunar rhythms in humans copulation. Hum. Biol. 54, 111–121.
Paredes, R. G. (2003). Medial preoptic area/anterior hypothalamus and sexual motivation. Scand. J. Psychol. 44, 203–212. doi: 10.1111/1467-9450.00337
Paredes, R. G., and Baum, M. J. (1997). Role of the medial preoptic area/anterior hypothalamus in the control of masculine sexual behavior. Annu. Rev. Sex Res. 8, 68–101. doi: 10.1080/10532528.1997.10559919
Pasqualotto, F. F., Borges Júnior, E., and Pasqualotto, E. B. (2008). The male biological clock is ticking: a review of the literature. Sao Paulo Medical J. = Revista Paulista De Medicina 126, 197–201. doi: 10.1590/s1516-31802008000300012
Perret, M. (1985). Diurnal variations in plasma testosterone concentrations in the male lesser mouse lemur (Microcebus murinus). J. Reprod. Fertil. 74, 205–213. doi: 10.1530/jrf.0.0740205
Peruquetti, R. L., Mateo, S., and Sassone-Corsi, P. (2012). Circadian proteins CLOCK and BMAL1 in the Chromatoid body, a RNA processing granule of male germ cells. PLoS One 7:e42695. doi: 10.1371/journal.pone.0042695
Petrulis, A., and Johnston, R. E. (1999). Lesions centered on the medial amygdala impair scent-marking and sex-odor recognition but spare discrimination of individual odors in female golden hamsters. Behav. Neurosci. 113, 345–357. doi: 10.1037//0735-7044.113.2.345
Pfaff, D. W., and Sakuma, Y. (1979a). Deficit in the lordosis reflex of female rats caused by lesions in the ventromedial nucleus of the hypothalamus. J. Physiol. 288, 203–210. doi: 10.1113/jphysiol.1979.sp012691
Pfaff, D. W., and Sakuma, Y. (1979b). Facilitation of the lordosis reflex of female rats from the ventromedial nucleus of the hypothalamus. J. Physiol. 288, 189–202. doi: 10.1113/jphysiol.1979.sp012690
Plant, T. M. (1981). Time courses of concentrations of circulating gonadotropin, prolactin, testosterone, and cortisol in adult male Rhesus monkeys (Macaca mulatta) throughout the 24 h light-dark Cycle1. Biol. Reprod. 25, 244–252. doi: 10.1095/biolreprod25.2.244
Poletini, M. O., Kennett, J. E., McKee, D. T., and Freeman, M. E. (2010). Central clock regulates the Cervically stimulated prolactin surges by modulation of dopamine and vasoactive intestinal polypeptide release in Ovariectomized rats. Neuroendocrinology 91, 179–188. doi: 10.1159/000254379
Rahman, S. A., Grant, L. K., Gooley, J. J., Rajaratnam, S. M. W., Czeisler, C. A., and Lockley, S. W. (2019). Endogenous circadian regulation of female reproductive hormones. J. Clin. Endocrinol. Metab. 104, 6049–6059. doi: 10.1210/jc.2019-00803
Rajendren, G., and Moss, R. L. (1993). The role of the medial nucleus of amygdala in the mating-induced enhancement of lordosis in female rats: the interaction with luteinizing hormone-releasing hormone neuronal system. Brain Res. 617, 81–86. doi: 10.1016/0006-8993(93)90616-u
Ralph, M. R., Foster, R. G., Davis, F. C., and Menaker, M. (1990). Transplanted suprachiasmatic nucleus determines circadian period. Science 247, 975–978. doi: 10.1126/science.2305266
Ramasamy, R., Bakircioğlu, M. E., Cengiz, C., Karaca, E., Scovell, J., Jhangiani, S. N., et al. (2015a). Whole-exome sequencing identifies novel homozygous mutation in NPAS2 in family with nonobstructive azoospermia. Fertil. Steril. 104, 286–291. doi: 10.1016/j.fertnstert.2015.04.001
Ramasamy, R., Chiba, K., Butler, P., and Lamb, D. J. (2015b). Male biological clock: a critical analysis of advanced paternal age. Fertil. Steril. 103, 1402–1406. doi: 10.1016/j.fertnstert.2015.03.011
Ramírez, D. A., Vieyra, E., González, A. I., Morán, C., Domínguez, R., and Morales-Ledesma, L. (2017). Both the suprachiasmatic nucleus and the superior ovarian nerve contribute to the processes of ovulation and steroid hormone secretion on Proestrus. Reprod. Sci. 24, 844–855. doi: 10.1177/1933719116670307
Ratajczak, C. K., Boehle, K. L., and Muglia, L. J. (2009). Impaired steroidogenesis and implantation failure in Bmal1−/− mice. Endocrinology 150, 1879–1885. doi: 10.1210/en.2008-1021
Rea, M. A. (1989). Light increases Fos-related protein immunoreactivity in the rat suprachiasmatic nuclei. Brain Res. Bull. 23, 577–581. doi: 10.1016/0361-9230(89)90204-9
Rees, H. D., and Michael, R. P. (1982). Brain cells of the male rhesus monkey accumulate 3H-testosterone or its metabolites. J. Comp. Neurol. 206, 273–277. doi: 10.1002/cne.902060307
Refinetti, R. (2005). Time for sex: Nycthemeral distribution of human sexual behavior. J. Circadian Rhythms 3:4. doi: 10.1186/1740-3391-3-4
Reschke, L., McCarthy, R., Herzog, E. D., Fay, J. C., Jungheim, E. S., and England, S. K. (2018). Chronodisruption: an untimely cause of preterm birth? Best Pract. Res. Clin. Obstet. Gynaecol. 52, 60–67. doi: 10.1016/j.bpobgyn.2018.08.001
Ribeiro, A. C., Musatov, S., Shteyler, A., Simanduyev, S., Arrieta-Cruz, I., Ogawa, S., et al. (2012). siRNA silencing of estrogen receptor-α expression specifically in medial preoptic area neurons abolishes maternal care in female mice. Proc. Natl. Acad. Sci. 109, 16324–16329. doi: 10.1073/pnas.1214094109
Richter, C. P. (1970). Dependence of successful mating in rats on functioning of the 24-hour clocks of the male and female. Commun. Behav. Biol. 5, 1–5.
Rodríguez-Manzo, G., and Canseco-Alba, A. (2017). A new role for GABAergic transmission in the control of male rat sexual behavior expression. Behav. Brain Res. 320, 21–29. doi: 10.1016/j.bbr.2016.11.041
Rodríguez-Manzo, G., Pellicer, F., Larsson, K., and Fernández-Guasti, A. (2000). Stimulation of the medical preoptic area facilitates sexual behavior but does not reverse sexual satiation. Behav. Neurosci. 114, 553–560. doi: 10.1037/0735-7044.114.3.553
Rönnberg, L., Kauppila, A., Leppäluoto, J., Martikainen, H., and Vakkuri, O. (1990). Circadian and seasonal variation in human preovulatory follicular fluid melatonin concentration. J. Clin. Endocrinol. Metab. 71, 493–496. doi: 10.1210/jcem-71-2-493
Rossetti, S., Corlazzoli, F., Gregorski, A., Azmi, N. H. A., and Sacchi, N. (2012). Identification of an estrogen-regulated circadian mechanism necessary for breast acinar morphogenesis. Cell Cycle (Georgetown, Tex.) 11, 3691–3700. doi: 10.4161/cc.21946
Rubel, C. A., Lanz, R. B., Kommagani, R., Franco, H. L., Lydon, J. P., and DeMayo, F. J. (2012). Research resource: genome-wide profiling of progesterone receptor binding in the mouse uterus. Molecular Endocrinol. (Baltimore) 26, 1428–1442. doi: 10.1210/me.2011-1355
Russo, K. A., La, J. L., Stephens, S. B. Z., Poling, M. C., Padgaonkar, N. A., Jennings, K. J., et al. (2015). Circadian control of the female reproductive Axis through gated responsiveness of the RFRP-3 system to VIP signaling. Endocrinology 156, 2608–2618. doi: 10.1210/en.2014-1762
Rutovskaya, M., Kosyreva, A., and Diatroptov, M. (2020). Ultradian and Infradian rhythms in the dynamic of testosterone concentration in the serum of the white-breasted hedgehog Erinaceus roumanicus. Sci. Rep. 10:6334. doi: 10.1038/s41598-020-63399-5
Sack, R. L. (2009). The pathophysiology of jet lag. Travel Med. Infect. Dis. 7, 102–110. doi: 10.1016/j.tmaid.2009.01.006
Sadeghi, M. R. (2015). Unexplained infertility, the controversial matter in Management of Infertile Couples. J. Reproduct. Infertility 16:1.
Sakuma, Y. (2015). Estradiol-sensitive projection neurons in the female rat preoptic area. Front. Neurosci. 9:67. doi: 10.3389/fnins.2015.00067
Samson, W. K., Burton, K. P., Reeves, J. P., and McCann, S. M. (1981). Vasoactive intestinal peptide stimulates luteinizing hormone-releasing hormone release from median eminence synaptosomes. Regul. Pept. 2, 253–264. doi: 10.1016/0167-0115(81)90029-X
Sànchez, J. J., Abreu, P., González-Hernández, T., Hernández, A., Prieto, L., and Alonso, R. (2004). Estrogen modulation of adrenoceptor responsiveness in the female rat pineal gland: differential expression of intracellular estrogen receptors. J. Pineal Res. 37, 26–35. doi: 10.1111/j.1600-079X.2004.00132.x
Sapède, D., and Cau, E. (2013). The pineal gland from development to function. Curr. Top. Dev. Biol. 106, 171–215. doi: 10.1016/B978-0-12-416021-7.00005-5
Saper, C. B. (2013). The central circadian timing system. Curr. Opin. Neurobiol. 23, 747–751. doi: 10.1016/j.conb.2013.04.004
Sato, T., and Kawamura, H. (1984). Circadian rhythms in multiple unit activity inside and outside the suprachiasmatic nucleus in the diurnal chipmunk (Eutamias sibiricus). Neurosci. Res. 1, 45–52. doi: 10.1016/0168-0102(84)90029-4
Schlatt, S., Pohl, C. R., Ehmcke, J., and Ramaswamy, S. (2008). Age-related changes in diurnal rhythms and levels of gonadotropins, testosterone, and inhibin B in male rhesus monkeys (Macaca mulatta). Biol. Reprod. 79, 93–99. doi: 10.1095/biolreprod.107.066126
Schwartz, M. D., Urbanski, H. F., Nunez, A. A., and Smale, L. (2011). Projections of the suprachiasmatic nucleus and ventral subparaventricular zone in the Nile grass rat (Arvicanthis niloticus). Brain Res. 1367, 146–161. doi: 10.1016/j.brainres.2010.10.058
Schwartz, M. D., Wotus, C., Liu, T., Friesen, W. O., Borjigin, J., Oda, G. A., et al. (2009). Dissociation of circadian and light inhibition of melatonin release through forced desynchronization in the rat. Proc. Natl. Acad. Sci. 106, 17540–17545. doi: 10.1073/pnas.0906382106
Scott, A. J. (2000). Shift work and health. Prim. Care 27, 1057–1078. doi: 10.1016/S0095-4543(05)70189-5
Sellix, M. T. (2015). Circadian clock function in the mammalian ovary. J. Biol. Rhythm. 30, 7–19. doi: 10.1177/0748730414554222
Shen, O., Ding, X., Nie, J., Xia, Y., Wang, X., Tong, J., et al. (2015). Variants of the CLOCK gene affect the risk of idiopathic male infertility in the Han-Chinese population. Chronobiol. Int. 32, 959–965. doi: 10.3109/07420528.2015.1056305
Shughrue, P. J., Lane, M. V., and Merchenthaler, I. (1997). Regulation of progesterone receptor messenger ribonucleic acid in the rat medial preoptic nucleus by estrogenic and Antiestrogenic compounds: an in situ hybridization study. Endocrinology 138, 5476–5484. doi: 10.1210/endo.138.12.5595
Shughrue, P. J., Scrimo, P. J., and Merchenthaler, I. (1998). Evidence for the colocalization of estrogen receptor-beta mRNA and estrogen receptor-alpha immunoreactivity in neurons of the rat forebrain. Endocrinology 139, 5267–5270. doi: 10.1210/endo.139.12.6525
Singh Jamwal, V. D. (2023). A systematic review identifying fertility biomarkers in semen: a clinical approach through omics to diagnose male infertility. Fertil. Steril. 119:158. doi: 10.1016/j.fertnstert.2022.10.031
Skorupskaite, K., George, J. T., and Anderson, R. A. (2014). The kisspeptin-GnRH pathway in human reproductive health and disease. Hum. Reprod. Update 20, 485–500. doi: 10.1093/humupd/dmu009
Su, J. D., Qiu, J., Zhong, Y. P., and Chen, Y. Z. (2001). Expression of estrogen receptor -alpha and -beta immunoreactivity in the cultured neonatal suprachiasmatic nucleus: with special attention to GABAergic neurons. Neuroreport 12, 1955–1959. doi: 10.1097/00001756-200107030-00036
Sutovsky, P., Hamilton, L. E., Zigo, M., Ortiz, M., Jones, A., Tirpak, F., et al. (2024). Biomarker-based human and animal sperm phenotyping: the good, the bad and the ugly†. Biol. Reprod. 110, 1135–1156. doi: 10.1093/biolre/ioae061
Sutovsky, P., and Lovercamp, K. (2010). Molecular markers of sperm quality. Soc. Reprod. Fertil. Suppl. 67, 247–256. doi: 10.7313/upo9781907284991.021
Swaab, D. F., and Fliers, E. (1985). A sexually dimorphic nucleus in the human brain. Science 228, 1112–1115. doi: 10.1126/science.3992248
Takahashi, J. S. (2017). Transcriptional architecture of the mammalian circadian clock. Nat. Rev. Genet. 18, 164–179. doi: 10.1038/nrg.2016.150
Terasawa, E., Wiegand, S. J., and Bridson, W. E. (1980). A role for medial preoptic nucleus on afternoon of proestrus in female rats. American J. Physiol. Endocrinol. Metabolism 238, E533–E539. doi: 10.1152/ajpendo.1980.238.6.E533
Todd, W. D., Fenselau, H., Wang, J. L., Zhang, R., Machado, N. L., Venner, A., et al. (2018). A hypothalamic circuit for the circadian control of aggression. Nat. Neurosci. 21, 717–724. doi: 10.1038/s41593-018-0126-0
Tonsfeldt, K. J., Goodall, C. P., Latham, K. L., and Chappell, P. E. (2011). Oestrogen induces rhythmic expression of the Kisspeptin-1 receptor 1 GPR54 in hypothalamic gonadotrophin-releasing hormone (GnRH)-secreting GT1-7 cells. J. Neuroendocrinol. 23, 823–830. doi: 10.1111/j.1365-2826.2011.02188.x
Turek, F. W., Swann, J., and Earnest, D. J. (1984). “Role of the circadian system in reproductive phenomena” in Proceedings of the 1983 Laurentian Hormone Conference. ed. R. O. Greep, vol. 40 (Orlando, Florida: Harcourt Brace Jovanovich, Publishers, Academic Press), 143–183.
Uchikawa, M., Kawamura, M., Yamauchi, N., and Hattori, M.-A. (2011). Down-regulation of circadian clock gene period 2 in uterine endometrial stromal cells of pregnant rats during decidualization. Chronobiol. Int. 28, 1–9. doi: 10.3109/07420528.2010.522289
Vacas, M. I., Lowenstein, P. R., and Cardinali, D. P. (1979). Characterization of a cytosol progesterone receptor in bovine pineal gland. Neuroendocrinology 29, 84–89. doi: 10.1159/000122909
Van der Beek, E. M., Horvath, T. L., Wiegant, V. M., Van den Hurk, R., and Buijs, R. M. (1997). Evidence for a direct neuronal pathway from the suprachiasmatic nucleus to the gonadotropin-releasing hormone system: combined tracing and light and electron microscopic immunocytochemical studies. J. Comp. Neurol. 384, 569–579. doi: 10.1002/(sici)1096-9861(19970811)384:4<569::aid-cne6>3.0.co;2-0
van der Beek, E. M., Swarts, H. J., and Wiegant, V. M. (1999). Central administration of antiserum to vasoactive intestinal peptide delays and reduces luteinizing hormone and prolactin surges in ovariectomized, estrogen-treated rats. Neuroendocrinology 69, 227–237. doi: 10.1159/000054423
van der Beek, E. M., Wiegant, V. M., van der Donk, H. A., van den Hurk, R., and Buijs, R. M. (1993). Lesions of the suprachiasmatic nucleus indicate the presence of a direct vasoactive intestinal polypeptide-containing projection to gonadotrophin-releasing hormone neurons in the female rat. J. Neuroendocrinol. 5, 137–144. doi: 10.1111/j.1365-2826.1993.tb00373.x
van Vuuren, R. J., Pitout, M. J., van Aswegen, C. H., and Theron, J. J. (1992). Putative melatonin receptor in human spermatozoa. Clin. Biochem. 25, 125–127. doi: 10.1016/0009-9120(92)80056-m
Vander Borght, M., and Wyns, C. (2018). Fertility and infertility: definition and epidemiology. Clin. Biochem. 62, 2–10. doi: 10.1016/j.clinbiochem.2018.03.012
Vida, B., Deli, L., Hrabovszky, E., Kalamatianos, T., Caraty, A., Coen, C. W., et al. (2010). Evidence for suprachiasmatic vasopressin neurones innervating kisspeptin neurones in the rostral periventricular area of the mouse brain: regulation by oestrogen. J. Neuroendocrinol. 22, 1032–1039. doi: 10.1111/j.1365-2826.2010.02045.x
Vida, B., Hrabovszky, E., Kalamatianos, T., Coen, C. W., Liposits, Z., and Kalló, I. (2008). Oestrogen receptor alpha and beta immunoreactive cells in the suprachiasmatic nucleus of mice: distribution, sex differences and regulation by gonadal hormones. J. Neuroendocrinol. 20, 1270–1277. doi: 10.1111/j.1365-2826.2008.01787.x
Vieyra, E., Ramírez, D. A., Lagunas, N., Cárdenas, M., Chavira, R., Damián-Matsumura, P., et al. (2016). Unilaterally blocking the muscarinic receptors in the suprachiasmatic nucleus in proestrus rats prevents pre-ovulatory LH secretion and ovulation. Reprod. Biol. Endocrinol. 14:34. doi: 10.1186/s12958-016-0168-7
Voordouw, B. C., Euser, R., Verdonk, R. E., Alberda, B. T., de Jong, F. H., Drogendijk, A. C., et al. (1992). Melatonin and melatonin-progestin combinations alter pituitary-ovarian function in women and can inhibit ovulation. J. Clin. Endocrinol. Metab. 74, 108–117. doi: 10.1210/jcem.74.1.1727807
Vujovic, N., Davidson, A. J., and Menaker, M. (2008). Sympathetic input modulates, but does not determine, phase of peripheral circadian oscillators. Am. J. Phys. Regul. Integr. Comp. Phys. 295, R355–R360. doi: 10.1152/ajpregu.00498.2007
Vujovic, N., Gooley, J. J., Jhou, T. C., and Saper, C. B. (2015). Projections from the subparaventricular zone define four channels of output from the circadian timing system. J. Comp. Neurol. 523, 2714–2737. doi: 10.1002/cne.23812
Watts, A. G. (1991). Ether anesthesia differentially affects the content of prepro-corticotropin-releasing hormone, prepro-neurotensin/neuromedin N and prepro-enkephalin mRNAs in the hypothalamic paraventricular nucleus of the rat. Brain Res. 544, 353–357. doi: 10.1016/0006-8993(91)90080-f
Watts, A. G., and Swanson, L. W. (1987). Efferent projections of the suprachiasmatic nucleus: II. Studies using retrograde transport of fluorescent dyes and simultaneous peptide immunohistochemistry in the rat. J. Comp. Neurol. 258, 230–252. doi: 10.1002/cne.902580205
Welsh, D. K., Takahashi, J. S., and Kay, S. A. (2010). Suprachiasmatic nucleus: cell autonomy and network properties. Annu. Rev. Physiol. 72, 551–577. doi: 10.1146/annurev-physiol-021909-135919
Whelan, E. A., Lawson, C. C., Grajewski, B., Hibert, E. N., Spiegelman, D., and Rich-Edwards, J. W. (2007). Work schedule during pregnancy and spontaneous abortion. Epidemiology 18, 350–355. doi: 10.1097/01.ede.0000259988.77314.a4
WHO. (2022). Infertility is a global public health issue. WHO; World Health Organization. Available at: http://www.who.int/reproductivehealth/topics/infertility/perspective/en/ (Accessed on February 24, 2022)
Wiegand, S. J., and Terasawa, E. (2008). Discrete lesions reveal functional heterogeneity of suprachiasmatic structures in regulation of gonadotropin secretion in the female rat. Neuroendocrinology 34, 395–404. doi: 10.1159/000123335
Williams, W. P., Jarjisian, S. G., Mikkelsen, J. D., and Kriegsfeld, L. J. (2011). Circadian control of kisspeptin and a gated GnRH response mediate the preovulatory luteinizing hormone surge. Endocrinology 152, 595–606. doi: 10.1210/en.2010-0943
Williams, W. P., and Kriegsfeld, L. J. (2012). Circadian control of neuroendocrine circuits regulating female reproductive function. Front. Endocrinol. 3:60. doi: 10.3389/fendo.2012.00060
Winters, S. J. (1991). Diurnal rhythm of testosterone and luteinizing hormone in hypogonadal men. J. Androl. 12, 185–190. doi: 10.1002/j.1939-4640.1991.tb00247.x
Wu, S. S., Nathanielsz, P. W., and McDonald, T. J. (1995). Immunocytochemical distribution of androgen receptors in the hypothalamus and pituitary of the fetal baboon in late gestation. Brain Res. Dev. Brain Res. 84, 278–281. doi: 10.1016/0165-3806(94)00184-2
Wu, F. C. W., Tajar, A., Pye, S. R., Silman, A. J., Finn, J. D., O’Neill, T. W., et al. (2008). Hypothalamic-pituitary-testicular axis disruptions in older men are differentially linked to age and modifiable risk factors: the European male aging study. J. Clin. Endocrinol. Metab. 93, 2737–2745. doi: 10.1210/jc.2007-1972
Xie, M., Utzinger, K. S., Blickenstorfer, K., and Leeners, B. (2018). Diurnal and seasonal changes in semen quality of men in subfertile partnerships. Chronobiol. Int. 35, 1375–1384. doi: 10.1080/07420528.2018.1483942
Xu, X., Coats, J. K., Yang, C. F., Wang, A., Ahmed, O. M., Alvarado, M., et al. (2012). Modular genetic control of sexually dimorphic behaviors. Cell 148, 596–607. doi: 10.1016/j.cell.2011.12.018
Xu, Z., Kaga, S., Mochiduki, A., Tsubomizu, J., Adachi, S., Sakai, T., et al. (2012). Immunocytochemical localization of kisspeptin neurons in the rat forebrain with special reference to sexual dimorphism and interaction with GnRH neurons. Endocr. J. 59, 161–171. doi: 10.1507/endocrj.EJ11-0193
Yamaguchi, T., Wei, D., Song, S. C., Lim, B., Tritsch, N. X., and Lin, D. (2020). Posterior amygdala regulates sexual and aggressive behaviors in male mice. Nat. Neurosci. 23, 1111–1124. doi: 10.1038/s41593-020-0675-x
Yamazaki, S., Numano, R., Abe, M., Hida, A., Takahashi, R., Ueda, M., et al. (2000). Resetting central and peripheral circadian oscillators in transgenic rats. Science 288, 682–685. doi: 10.1126/science.288.5466.682
Yang, C. F., Chiang, M. C., Gray, D. C., Prabhakaran, M., Alvarado, M., Juntti, S. A., et al. (2013). Sexually dimorphic neurons in the ventromedial hypothalamus govern mating in both sexes and aggression in males. Cell 153, 896–909. doi: 10.1016/j.cell.2013.04.017
Yang, T., Yang, C. F., Chizari, M. D., Maheswaranathan, N., Burke, K. J., Borius, M., et al. (2017). Social control of hypothalamus-mediated male aggression. Neuron 95, 955–970.e4. doi: 10.1016/j.neuron.2017.06.046
Yaw, A. M., McLane-Svoboda, A. K., and Hoffmann, H. M. (2020). Shiftwork and light at night negatively impact molecular and endocrine timekeeping in the female reproductive Axis in humans and rodents. Int. J. Mol. Sci. 22:324. doi: 10.3390/ijms22010324
Yie, S. M., Brown, G. M., Liu, G. Y., Collins, J. A., Daya, S., Hughes, E. G., et al. (1995). Melatonin and steroids in human pre-ovulatory follicular fluid: seasonal variations and granulosa cell steroid production. Hum. Reprod. 10, 50–55. doi: 10.1093/humrep/10.1.50
Yin, L., Hashikawa, K., Hashikawa, Y., Osakada, T., Lischinsky, J. E., Diaz, V., et al. (2022). VMHvllCckar cells dynamically control female sexual behaviors over reproductive cycle. Neuron 110, 3000–3017.e8. doi: 10.1016/j.neuron.2022.06.026
Zavada, A., Gordijn, M. C. M., Beersma, D. G. M., Daan, S., and Roenneberg, T. (2005). Comparison of the Munich Chronotype questionnaire with the Horne-Ostberg’s Morningness-Eveningness Score. Chronobiol. Int. 22, 267–278. doi: 10.1081/cbi-200053536
Zhang, X., Cao, Q., Rajachandran, S., Grow, E. J., Evans, M., and Chen, H. (2023). Dissecting mammalian reproduction with spatial transcriptomics. Hum. Reprod. Update 29, 794–810. doi: 10.1093/humupd/dmad017
Zhang, J., Ding, X., Li, Y., Xia, Y., Nie, J., Yi, C., et al. (2012). Association of CLOCK gene variants with semen quality in idiopathic infertile Han-Chinese males. Reprod. Biomed. Online 25, 536–542. doi: 10.1016/j.rbmo.2012.07.018
Zhang, W., Piotrowska, K., Chavoshan, B., Wallace, J., and Liu, P. Y. (2018). Sleep duration is associated with testis size in healthy young men. J. Clin. Sleep Med JCSM Official Pub. American Acad. Sleep Med. 14, 1757–1764. doi: 10.5664/jcsm.7390
Zheng, M., Tong, J., Li, W.-P., Chen, Z.-J., and Zhang, C. (2018). Melatonin concentration in follicular fluid is correlated with antral follicle count (AFC) and in vitro fertilization (IVF) outcomes in women undergoing assisted reproductive technology (ART) procedures. Gynecological Endocrinol.: Official J. Int. Society of Gynecolog. Endocrinol. 34, 446–450. doi: 10.1080/09513590.2017.1409713
Zhong, O., Liao, B., Wang, J., Liu, K., Lei, X., and Hu, L. (2022). Effects of sleep disorders and circadian rhythm changes on male reproductive health: a systematic review and Meta-analysis. Front. Physiol. 13:913369. doi: 10.3389/fphys.2022.913369
Zhu, J. L., Hjollund, N. H., and Olsen, J. (2004). Shift work, duration of pregnancy, and birth weight: the National Birth Cohort in Denmark. Am. J. Obstet. Gynecol. 191, 285–291. doi: 10.1016/j.ajog.2003.12.002
Keywords: circadian, sex, reproduction, mammalian, clock timing, fertility, hypothalamus, hormones
Citation: Aten S, Ramirez-Plascencia O, Blake C, Holder G, Fishbein E, Vieth A, Zarghani-Shiraz A, Keister E, Howe S, Appo A, Palmer B and Mahoney CE (2025) A time for sex: circadian regulation of mammalian sexual and reproductive function. Front. Neurosci. 18:1516767. doi: 10.3389/fnins.2024.1516767
Received: 24 October 2024; Accepted: 09 December 2024;
Published: 06 January 2025.
Edited by:
Ioannis P. Androulakis, Rutgers, The State University of New Jersey, United StatesReviewed by:
Sandra Paulina Smieszek, Vanda Pharmaceuticals Inc., United StatesCopyright © 2025 Aten, Ramirez-Plascencia, Blake, Holder, Fishbein, Vieth, Zarghani-Shiraz, Keister, Howe, Appo, Palmer and Mahoney. This is an open-access article distributed under the terms of the Creative Commons Attribution License (CC BY). The use, distribution or reproduction in other forums is permitted, provided the original author(s) and the copyright owner(s) are credited and that the original publication in this journal is cited, in accordance with accepted academic practice. No use, distribution or reproduction is permitted which does not comply with these terms.
*Correspondence: Sydney Aten, c2F0ZW5AYmlkbWMuaGFydmFyZC5lZHU=; Carrie E. Mahoney, Y21haG9uZTZAYmlkbWMuaGFydmFyZC5lZHU=
†These authors have contributed equally to this work
Disclaimer: All claims expressed in this article are solely those of the authors and do not necessarily represent those of their affiliated organizations, or those of the publisher, the editors and the reviewers. Any product that may be evaluated in this article or claim that may be made by its manufacturer is not guaranteed or endorsed by the publisher.
Research integrity at Frontiers
Learn more about the work of our research integrity team to safeguard the quality of each article we publish.