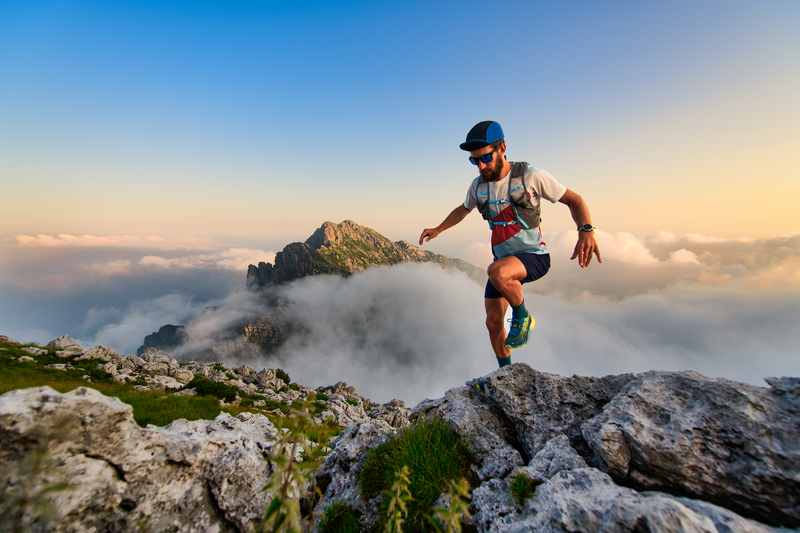
94% of researchers rate our articles as excellent or good
Learn more about the work of our research integrity team to safeguard the quality of each article we publish.
Find out more
REVIEW article
Front. Neurosci. , 28 January 2025
Sec. Neurogenesis
Volume 18 - 2024 | https://doi.org/10.3389/fnins.2024.1509850
Objective: Here, bibliometric and visual analytical techniques were employed to assess the key features of the 100 most cited publications concerning corticospinal tract (CST) regeneration.
Methods: Research was conducted within the Web of Science Core Collection to pinpoint the 100 most cited publications on CST regeneration. From these, comprehensive data encompassing titles, authorship, key terms, publication venues, release timelines, geographic origins, and institutional affiliations were extracted, followed by an in-depth bibliometric examination.
Results: The 100 most cited publications were all published between 2004 and 2024. These seminal papers amassed an aggregate of 18,321 citations, with individual citation counts ranging from 83 to 871 and a median of 136 citations per paper. Schwab M. E., stood out as the most prominent contributor, with significant authorship in 9 of the 100 papers. The United States dominated the geographical distribution, accounting for 49 of the articles. With 17 publications, the University of California System led the institutional rankings. A thorough keyword analysis revealed pivotal themes in the field, encompassing the optic nerve, gene expression, CST integrity and regeneration, diffusion tensor imaging, myelin-associated glycoproteins, inhibitors of neurite outgrowth, and methods of electrical and intracortical microstimulation.
Conclusion: This investigation provides a bibliometric analysis of CST regeneration, underscoring the significant contribution of the United States to this field. Our findings unveiled the dynamics and trends within the field of CST regeneration, providing a scientific foundation for advancing clinical applications. Building on this analysis, the clinical application of CST regeneration should be optimized through interdisciplinary collaboration, enabling the exploration and validation of a variety of therapeutic approaches, including the use of neurotrophic factors, stem cell therapies, biomaterials, and electrical stimulation. Concurrently, additional clinical trials are necessary to test the safety and efficacy of these therapeutic methods and develop assessment tools for monitoring the recovery of patients. Furthermore, rehabilitation strategies should be refined, and professional education and training should be provided to enhance the understanding of CST regeneration treatments among both medical professionals and patients. The implementation of these strategies promises to enhance therapeutic outcomes and the quality of life of patients with spinal cord injury (SCI).
The corticospinal tract (CST) is a descending motor pathway that originates in the cerebral cortex and projects to the spinal cord. It plays a crucial role in controlling voluntary movements, fine motor coordination, and executive functions (Welniarz et al., 2017). The integrity of the CST is essential for the precise execution of skilled motor activities. Damage to it can result in significant motor impairments, as seen in conditions such as spinal cord injury (SCI), multiple sclerosis, and stroke (Oudega and Perez, 2012).
Understanding the mechanisms involved in CST regeneration has been a key focus in neuroscientific research, aiming to unlock new therapies for patients with motor disabilities. The limited capacity of the CST for self-repair post-injury presents a significant challenge to regeneration. Accordingly, researchers have explored various strategies to enhance the regenerative potential of the CST, including the use of growth factors, cell transplantation, and the development of biomaterials to support axonal regrowth (Blesch et al., 2002; Zuo et al., 2020).
Bibliometric analysis is a powerful tool for identifying trends, patterns, and key contributors in CST regeneration research (Ellegaard and Wallin, 2015). This approach has previously been used to evaluate research across medical and scientific fields, offering insights into the structure and dynamics of scientific knowledge (Moed, 2009).
In this study, we conducted a bibliometric analysis of the 100 most cited articles on CST regeneration, providing a comprehensive overview of the advancements in this field. Our analysis spanned 20 years (2004 to 2024) using data from the Web of Science Core Collection, a leading repository for peer-reviewed literature. Through the examination of the characteristics of the most cited papers, we sought to identify the main research themes, methodologies, and the geographical distribution of scientific output in this field.
Our detailed bibliometric analysis offers insights into the scientific community’s efforts to advance knowledge and promote innovation in CST recovery.
The data for this investigation were sourced from the Science Citation Index Expanded (SCI-EXPANDED), a component of the Clarivate Analytics Web of Science Core Collection (WoSCC). Recognized as a prestigious global resource, the WoSCC offers extensive data necessary for bibliometric analysis, making it a preferred database for such studies. The search criteria incorporated terms such as “CST regeneration,” “corticospinal tract regeneration,” “CST recovery,” “corticospinal tract recovery,” “CST repair,” “corticospinal tract repair,” “CST regrowth,” and “corticospinal tract regrowth” to identify pertinent articles. A systematic search covered publications from January 1900 to September 2024, with the retrieval process being finalized on September 16, 2024, ensuring that no post-update database changes would influence the results. The bibliometric analysis included only original articles and review papers with complete manuscripts, with the language limited to English. Two independent researchers conducted the initial screening based on titles, abstracts, and document types, with full-text reviews being conducted when necessary for a thorough assessment. The top 100 articles, ranked by their influence, were retrieved from WoSCC in “Full Record and Cited References” format and were saved as TXT files (Figure 1). The impact factors of the relevant journals were extracted from Journal Citation Reports 2023.
Descriptive statistical analysis and diagram creation were conducted using Microsoft Excel 2019. An online analytical platform was used to enhance the visualization of collaborative networks among authors and institutions. VOSviewer, a powerful bibliometric tool developed by van Eck and Waltman (2010), was employed for constructing networks of keywords and authors. Additionally, Scimago Graphica (Dimara and Perin, 2020) was used to generate a world map representing the cited countries. Pajek (Batagelj and Mrvar, 2004), a robust network analysis tool, was utilized for the visualization of intricate citation networks, co-word analysis, and author collaboration networks. Its integration with VOSviewer enabled a comprehensive perspective on the scientific literature network structure, highlighting major trends, key concepts, and collaborative ties among researchers, which is invaluable for understanding the broader academic research landscape. Furthermore, CiteSpace (Chen, 2004) software was used to map author networks, pinpoint keywords with significant burst strength, and showcase the temporal dynamics of keyword occurrences. The data collected in this investigation were compiled in CiteSpace to create a network of institutional collaborations, authorships, and co-occurrences. Within the CiteSpace network map, diverse entities, including institutions, authors, and keywords, are depicted through nodes, with the line thickness between nodes indicating the intensity of collaboration and co-occurrence. Co-occurrence analysis establishes item correlations based on their co-occurrence frequency within documents, color-coded to represent different clusters. Bibliometrix, an R package for bibliometric analysis, expedites the discovery of foundational works, influential authors, and emerging trends within a field. It also assists in curating and visually presenting research findings. A distinctive feature of CiteSpace Bibliometrix is its capacity to generate Sankey diagrams, visualizing data flows with branch widths corresponding to flow magnitudes, thus serving as an effective analytical tool.
The 100 most cited studies in this field were published between 2004 and 2020, as shown in Figure 2. Table 1 presents a detailed breakdown of these 100 publications. These leading 100 articles have amassed citations ranging from 83 to 871, with a median citation count of 136 and an average citation frequency of 183.21 per article. Of the top three most cited studies, the preeminent one, titled (Bareyre et al., 2004) “The injured spinal cord spontaneously forms a new intraspinal circuit in adult rats,” was published in Nature Neuroscience in 2004 and garnered 871 citations. Following closely in second was a study titled (Liu et al., 2010) “PTEN deletion enhances the regenerative ability of adult corticospinal neurons,” which appeared in Nature Neuroscience in 2010 and amassed 725 citations. The third study (Stinear et al., 2006), “Functional potential in chronic stroke patients depends on corticospinal tract integrity,” was published in Brain in 2007, and accumulated 637 citations. While earlier publications tended to have higher overall citation counts, a closer examination based on the average annual citations revealed several more recent publications with a markedly higher impact. Notably, the paper titled (Silva et al., 2014) “From basics to clinical: a comprehensive review on spinal cord injury” published in Progress in Neurobiology in 2014, secured 574 citations, placing it fourth in terms of total citations.
Figure 2. The annual number of publications and citations for the 100 most cited papers from 2004 to 2024.
The contributions of 22 countries/regions to the top 100 most cited manuscripts are illustrated in Figures 3A,B. Eight of these countries each published more than three articles. Scimago Graphica software was used to graphically represent a world map in which countries with a significant presence among the top 100 cited publications are highlighted (Figure 3C). The sizes of the dots on the map correspond to the volume of articles contributed by each country, with lines indicating collaborative ties between nations. The United States led the field, accounting for 49 of the 100 papers and amassing 8,635 citations, which equates to an average of 176.22 citations per paper (Figure 4). The United Kingdom was second, with 20 papers and a total of 3,516 citations, averaging 175.80 citations per paper. Switzerland ranked third, with 12 papers and 2,530 citations, averaging 164.67 citations per paper. New Zealand had the distinction of having the highest average number of citations per publication, with a notable average of 257.50.
Figure 3. The most productive countries. (A) A network map showing the countries involved in this research area. (B) The number of publications by country. (C) A world map highlighting the most productive countries.
Figure 4. The 10 countries with the most publications. TC, total citations; CPP, citations per publication.
Regarding institutional contributions, a total of 177 institutions were involved in producing the top 100 articles analyzed in this study. Figure 5A illustrates the number of publications for the most significant institutions. Notably, the University of California System stood out as the most prolific institution, contributing 17 of the most highly cited articles. CiteSpace software was employed to create a visual representation of the connections between these institutions, where the sizes of the nodes in the graph indicate each institution’s publication output, and lines represent collaborative efforts among them. The leading research institutions in terms of collaboration, including the University of California System, Harvard University, the Swiss Federal Institutes of Technology, the US Department of Veterans Affairs, UC San Diego, the University of Zurich, and the Veterans Health Administration, among others, are highlighted in Figure 5B.
Figure 5. Institution analysis. (A) The most relevant institutions. (B) Partnerships among institutions.
The Bibliometrix R package, a tool for visualizing and analyzing scientific literature data, was used to identify the most prominent authors in the field. As shown in Figure 6A, Schwab M. E., topped the list, with an impressive nine publications. A timeline of the publication history of the most prolific authors is presented in Figure 6B. A network where the size of each node is proportional to an author’s contribution to the top 100 articles, with lines indicating collaborative ties among them, is shown in Figure 6C. An analysis of this network revealed a high incidence of collaboration among authors, characterized by stable partnerships. Figure 6D provides an overview of the interconnectedness among countries, institutions, and authors, with a notable influence from USA-based authors.
Figure 6. Author analysis. (A) The most relevant authors. (B) Article production over time. (C) A map of the collaborative relationship among authors. (D) Three-field plot (country-affiliation-author).
Table 2 displays the relevant journals for the top 100 cited articles. The Journal of Neuroscience published the highest number of articles (n = 13), encompassing 2,300 citations. This was followed by Brain, which published 8 papers and accumulated 1,845 citations. Next was Experimental Neurology, which published 6 articles, obtaining 850 citations. Stroke also published 6 articles and received 730 citations, with an average citation count of 121.67. Furthermore, seven journals each published more than 3 articles—Proceedings of the National Academy of Sciences of the United States of America, European Journal of Neuroscience, and Nature Neuroscience, accumulating 807, 2,323, and 437 citations, respectively.
Table 2. Journals associated with the 100 most cited articles in the field of corticospinal tract regeneration (>1 paper).
Keywords are essential for highlighting the core themes of a scholarly article, offering readers a clear understanding of the topic. The presence of two keywords in the same paper indicates a connection between them, with the frequency of their co-occurrence reflecting the strength of this link. By analyzing the patterns of keyword co-occurrence and identifying emerging trends, it is possible to identify trending subjects within an academic area over time. Keyword clustering can help to reveal the underlying structure of knowledge, enabling a more organized understanding of the domain within a specific field. Here, cluster analysis revealed that keywords within the field of CST regeneration could be partitioned into the following 16 categories (Figure 7): “central nervous system,” “regeneration,” “in vivo,” “motor recovery,” “CST,” “corticospinal tract regeneration,” “chitosan,” “diffusion tensor imaging,” “biomaterials,” “receptor family member,” “outcomes,” “rehabilitation outcomes,” “grip strength,” “proportional recovery,” “cortical reorganization,” “lesions,” and “interneurons.”
The chronological evolution of keyword usage patterns is shown in Figure 8. Meanwhile Figure 8A provides a visual representation of how the prominence of keywords fluctuates over time. The size of each block corresponds to the popularity of the keyword with larger blocks indicating more frequent usage. Keywords that have become more prevalent over recent years may become significant research areas in the future. Our analysis of keyword bursts pinpointed several noteworthy terms (Figure 8B) including “optic nerve,” “expression,” “corticospinal tract integrity,” “corticospinal tract regeneration,” “diffusion tensor tractography,” “myelin-associated glycoprotein,” “neurite growth inhibitors,” “electrical stimulation,” and “intracortical microstimulation.” Keywords with the strongest burst signals are indicative of current research frontiers within the field. Early bursting keywords suggest that initial research interest was concentrated in those areas while recent bursts indicate a sharp increase in interest in the topic. Figure 8B highlights the five keywords with the highest burst strengths namely “optic nerve,” “expression,” “corticospinal tract integrity,” “corticospinal tract regeneration,” and “diffusion tensor tractography,” with burst strengths of 1.76, 1.71, 1.66, 1.64, and 1.64 respectively. The first keywords to show a burst were “neurite growth inhibitors,” “lesions,” “myelin-associated glycoprotein,” “functional recovery,” “expression,” and “locomotor recovery,” representing early research emphasis. Meanwhile the most recent keywords to display a burst were “chondroitin sulfate proteoglycans,” reflecting a new area of intense research interest.
Figure 8. Keyword modifications over time. (A) Timeline view of the keywords. (B) Burst test of the keywords.
In this study, we performed a comprehensive data and bibliometric analysis, focusing on the 100 most cited publications in the field of CST regeneration. This analytical strategy enabled a detailed examination of the evolution, key focus areas, and innovative trends in CST regeneration research, and offered valuable quantitative insights into both seminal and recent studies, thus deepening our understanding of the subject.
The top 100 publications accumulated a total of 18,321 citations, with citation counts ranging from 83 to 871 and a median of 136 citations per article. Schwab M. E. was identified as the most frequent contributor, having authored nine of these articles. The greatest number of papers, 49, originated from the United States, followed by the United Kingdom and Switzerland, each contributing 20 and 12 papers, respectively. The University of California System was the most prolific institution, producing 17 papers, followed by Harvard University with 11 papers and the Swiss Federal Institutes of Technology, also with 11 papers. Keyword analysis identified several areas of interest, including optic nerve, expression, corticospinal tract integrity, corticospinal tract regeneration, diffusion tensor tractography, myelin-associated glycoprotein, neurite growth inhibitors, electrical stimulation, and intracortical microstimulation. Further keyword analysis revealed the recent emergence of “chondroitin sulfate proteoglycans” as a prominent keyword in the field.
The optic nerve and the CST are distinct structures within the central nervous system (CNS), with each being responsible for different sensory and motor functions. The function of the optic nerve is to transmit visual signals from the retina to the brain, while the CST carries motor commands from the cerebral cortex to the spinal cord. Although the optic nerve and CST have separate functional roles, they share similar biological mechanisms and challenges in regard to regeneration (Gokoffski et al., 2020). Recent studies have revealed that the deletion of the SOCS3 gene facilitates the regeneration of the optic nerve. Moreover, the simultaneous knockout of the PTEN and SOCS3 genes has been reported to induce sustained axonal regeneration (Liu et al., 2011). These findings suggest that by modulating intrinsic signaling pathways, it is possible to promote the regeneration of axons within the CNS, including the optic nerve (Sun et al., 2011). These discoveries hold significant implications for understanding the mechanisms of regeneration in nerves such as the optic nerve and the CST and may pave the way for the development of novel neuroregenerative therapies.
Diffusion tensor tractography (DTT), leveraging data from diffusion tensor imaging (DTI), is a sophisticated neuroimaging technique designed to reconstruct and visualize the trajectories of white matter fibers within the CNS (Fortin et al., 2017), including the CST. This advanced methodology plays an integral role in the investigation of CST regeneration following SCI, facilitating the meticulous delineation of white matter fibers, thereby enabling a comprehensive understanding of the status of compromised neural pathways. Post-SCI, DTT serves not only to illustrate the damaged CST fibers but also to monitor their subsequent restoration (Feng et al., 2015; Cho et al., 2007). Juxtaposing pre-and post-injury DTT imagery allows to effectively gauge the efficacy of regenerative therapies (Guggisberg et al., 2019) as well as assess whether interventions such as neurotrophic factor administration, stem cell transplantation, or bio-scaffolding have indeed fostered CST regeneration (Puig et al., 2017). In summary, DTT stands as a robust instrument for the study and surveillance of CST regeneration, contributing substantially to advancements in the field of SCI treatment.
Myelin-associated glycoprotein (MAG) is a crucial component of the myelin sheath in the CNS, playing a significant role in the development and functionality of the nervous system. MAG is highly abundant on myelinating oligodendrocytes within the CNS and is known to interact with specific neuronal gangliosides, such as GT1b and GD1a (Schnaar and Lopez, 2009), thereby contributing to the maintenance of the myelin-axon interface. However, MAG is also recognized as one of the classic myelin-associated inhibitors that suppress axonal regeneration following CNS injury, including damage to the CST (Harel and Strittmatter, 2006). The inhibitory effect of MAG on neurite outgrowth is mediated through its interaction with axonal receptors (Zheng et al., 2005), which leads to the collapse of axonal growth cones in a sialic acid binding-dependent manner. Furthermore, MAG signaling operates bidirectionally, engaging in both axon-to-myelin and myelin-to-axon communication. It has been demonstrated that MAG plays a pivotal role in the regulation of myelin formation and integrity, with its cytoplasmic domain binding to the cytoplasmic non-receptor tyrosine kinase Fyn, which is essential for initiating the myelination process. Understanding the role of MAG in the inhibition of axonal regeneration is vital for developing strategies aimed at promoting CST regeneration and functional recovery following SCI. Targeting MAG or its signaling pathways may enhance the intrinsic capacity of the CNS for self-repair and remyelination, offering promising avenues for therapeutic intervention (Cafferty et al., 2010).
Electrostimulation, a therapeutic modality widely employed in clinical and research domains, holds substantial potential for facilitating functional recovery, particularly the regeneration of the CST, following CNS injury. The fundamental principle of this treatment involves the application of electrical currents to specific cortical areas to activate neuronal cells and elicit sensory or motor responses. In the context of CST regeneration, electrostimulation may contribute to the stimulation of the motor cortex, thereby promoting functional restoration (Lindau et al., 2014). Electrostimulation could also support the regeneration of the CST, by activating specific neural pathways that promote the reestablishment of connections between the brain and spinal cord. Research has demonstrated that electrostimulation can enhance axonal regeneration through various mechanisms (Liu et al., 2017); for instance, it can increase the expression of neurotrophic factors, thereby providing a conducive environment for axonal growth. Moreover, electrostimulation can augment axonal growth and regenerative capabilities by influencing intracellular signaling pathways. Clinical studies applying electrostimulation have also reported positive outcomes. For instance, it was demonstrated that electrostimulation can advance motor function recovery after SCI by enhancing the activity of residual neural pathways, leading to improved motor control and coordination. However, the efficacy of electrostimulation treatment may be contingent on factors such as the intensity, frequency, duration, and precision of targeting of the stimulation. To achieve optimal therapeutic outcomes, these parameters require meticulous adjustment and optimization. In summary, as a non-invasive or minimally invasive treatment approach, electrostimulation has demonstrated significant potential and value in the regeneration and functional recovery of the CST. With continued technological advancements and research progress, electrostimulation is set to become a vital treatment strategy for CNS injuries, particularly the regeneration of the CST.
Intracortical microstimulation (ICMS) is a neuroscientific technique that involves the application of small electrical currents to specific areas of the cerebral cortex through implanted microelectrodes. This method is used to activate neural cells and can elicit specific sensory or motor responses, depending on the area of the cortex being stimulated. ICMS has been used to generate motor maps, which are detailed representations of how different areas of the motor cortex control specific body movements. In the context of “CST regeneration,” ICMS could potentially play a role in rehabilitation by stimulating the motor cortex in a way that promotes functional recovery after CNS injury (Shelchkova et al., 2023). By activating specific neural pathways, ICMS might help to reestablish connections between the brain and the spinal cord, thereby aiding CST regeneration. In summary, ICMS represents a promising approach for the study and potential treatment of CNS injuries, particularly CST regeneration, as it enables the precise stimulation and modulation of neural activity.
Chondroitin sulfate proteoglycans (CSPGs) play a pivotal role in the regeneration and plasticity of the CNS, participating in a multitude of biological processes throughout development, adulthood, and aging. As integral components of the extracellular matrix, CSPGs interact with a variety of growth-active molecules, thereby influencing neural regeneration and plasticity. CSPGs are often considered to be inhibitors of CST regeneration, given their propensity to accumulate at sites of CNS injury, where they form glial scars that impede axonal regeneration. It has been demonstrated that the degradation of CSPGs mediated by enzymes such as chondroitinase ABC can enhance axonal regeneration and facilitate functional recovery (Barritt et al., 2006). Moreover, CSPGs are involved in modulating immune cell responses, thereby impacting the pathogenesis of chronic inflammation and demyelinating diseases. Following SCI, the digestion of CSPGs can mitigate proinflammatory responses within the inflammatory environment and promote the clearance or reduce the recruitment of microglia and peripheral myeloid cells at the lesion site, thereby contributing to the resolution of the inflammatory response. Regarding therapeutic strategies, manipulating the expression of CSPGs or their signaling pathways can foster CST regeneration. For instance, inhibiting the synthesis of CSPGs can diminish their inhibitory effects within the CNS (García-Alías et al., 2009), offering potential therapeutic approaches for the treatment of CNS injuries, stroke, and neurodegenerative diseases. These observations highlight the multifaceted role of CSPGs in CST regeneration, encompassing the inhibition of axonal regeneration, the modulation of immune responses, and the promotion of the resolution of inflammation. Investigating the role of CSPGs in CST regeneration can provide vital information for the development of novel therapeutic strategies for CST injuries.
The CST is vital for CNS function and enhancing its regeneration following SCI is critical for motor function recovery. Clinically, a spectrum of strategies is being pursued to stimulate CST neuron regeneration and establish functional networks post-SCI. These include the use of growth factors, cell therapies, and genetic manipulation to boost the inherent ability of CST neurons to regrow and myelinate. Neuromodulation techniques such as transcranial magnetic stimulation and transcranial direct current stimulation aim to adjust neural activity and support CNS plasticity, thereby aiding motor recovery after SCI. The development of implantable devices for continuous spinal cord stimulation represents an active research area, intended to foster an environment that is beneficial for CST regeneration. Biomaterial scaffolds that span spinal cord lesions are also being engineered, providing a substrate for axonal regrowth and offering nourishment to support axonal regeneration, potentially also in the CST. Combinatorial therapeutic approaches are often required for SCI given its multifaceted nature, involving the merging of neuromodulation with biomaterials, drug treatments, and physical rehabilitation to optimize CST regeneration and functional restoration. Translating CST regeneration research into clinical practice is a dynamic and progressive discipline. Achieving this necessitates a cross-disciplinary strategy, integrating knowledge from neuroscience, bioengineering, and rehabilitation medicine to develop therapies that can significantly enhance the quality of life of patients with SCI.
This research has several limitations. While the Web of Science is a prominent database for literature retrieval, it does not include all existing publications. To improve the precision of our analysis, we opted for a topic-based search approach rather than relying on subject headings. Although this method yielded accurate results, there is potential for broader coverage. Relying on citation frequency as a criterion for selection might result in the exclusion of recently published works that may be influential but have not yet amassed a substantial number of citations. Moreover, there is a possibility of citation bias, as articles from renowned institutions or prominent authors might garner more citations than equally meritorious ones from less recognized origins.
This investigation presents a bibliometric analysis of CST regeneration, underscoring the pivotal role of the United States as a leader in this scientific domain. Our findings unveiled the dynamics and trends within the field of CST regeneration, providing a scientific foundation for clinical applications. Our analysis indicated that the application of CST regeneration in the clinic should be optimized through interdisciplinary collaboration, enabling the exploration and validation of a variety of therapeutic approaches, including the use of neurotrophic factors, stem cell therapies, biomaterials, and electrical stimulation. Concurrently, additional clinical trials are necessary to test the safety and efficacy of these therapeutic methods and develop assessment tools for monitoring the recovery of patients. Furthermore, rehabilitation strategies should be refined, while professional education and training should be provided to enhance the understanding of CST regeneration treatments among both medical professionals and patients. International cooperation is also crucial, fostering the sharing of data and experiences to advance global research. Securing policy and funding support will aid in driving research progress and therapeutic innovation. Lastly, establishing long-term tracking systems is crucial for evaluating the long-term outcomes and safety of CST regeneration treatments. The implementation of these strategies promises to enhance therapeutic outcomes and the quality of life of patients with SCI.
Saijilafu: Conceptualization, Writing – review & editing. L-CY: Writing – original draft. HuL: Investigation, Writing – original draft. HaL: Writing – original draft, Investigation. XL: Methodology, Writing – original draft. KH: Methodology, Writing – original draft. ZH: Methodology, Writing – original draft. CC: Conceptualization, Writing – review & editing. LF: Conceptualization, Writing – review & editing. Saijilahu: Conceptualization, Writing review & editing. R-JX: Conceptualization, Supervision, Writing –original draft.
The author(s) declare that financial support was received for the research, authorship, and/or publication of this article. This work was supported by the Gusu Health Talents Program (GSWS2020069) and the Suzhou Science and Technology Program (SLJ2022014). This study was also supported by the Hangzhou City Health and Technology Project B20254767 (to Linjun Fang and Saijilafu).
The authors declare that the research was conducted in the absence of any commercial or financial relationships that could be construed as a potential conflict of interest.
The authors declare that no Gen AI was used in the creation of this manuscript.
All claims expressed in this article are solely those of the authors and do not necessarily represent those of their affiliated organizations, or those of the publisher, the editors and the reviewers. Any product that may be evaluated in this article, or claim that may be made by its manufacturer, is not guaranteed or endorsed by the publisher.
Anderson, K. D., Gunawan, A., and Steward, O. (2005). Quantitative assessment of forelimb motor function after cervical spinal cord injury in rats: relationship to the corticospinal tract. Exp. Neurol. 194, 161–174. doi: 10.1016/j.expneurol.2005.02.006
Auriat, A. M., Neva, J. L., Peters, S., Ferris, J. K., and Boyd, L. A. (2015). A review of transcranial magnetic stimulation and multimodal neuroimaging to characterize post-stroke neuroplasticity. Front. Neurol. 6:226. doi: 10.3389/fneur.2015.00226
Bareyre, F. M., Kerschensteiner, M., Misgeld, T., and Sanes, J. R. (2005). Transgenic labeling of the corticospinal tract for monitoring axonal responses to spinal cord injury. Nat. Med. 11, 1355–1360. doi: 10.1038/nm1331
Bareyre, F. M., Kerschensteiner, M., Raineteau, O., Mettenleiter, T. C., Weinmann, O., and Schwab, M. E. (2004). The injured spinal cord spontaneously forms a new intraspinal circuit in adult rats. Nat. Neurosci. 7, 269–277. doi: 10.1038/nn1195
Barritt, A. W., Davies, M., Marchand, F., Hartley, R., Grist, J., Yip, P., et al. (2006). Chondroitinase ABC promotes sprouting of intact and injured spinal systems after spinal cord injury. J. Neurosci. 26, 10856–10867. doi: 10.1523/JNEUROSCI.2980-06.2006
Barthélemy, D., Willerslev-Olsen, M., Lundell, H., Conway, B. A., Knudsen, H., Biering-Sørensen, F., et al. (2010). Impaired transmission in the corticospinal tract and gait disability in spinal cord injured persons. J. Neurophysiol. 104, 1167–1176. doi: 10.1152/jn.00382.2010
Batagelj, V., and Mrvar, A. (2004). “Pajek—analysis and visualization of large networks” in Graph drawing software. Mathematics and visualization. eds. M. Jünger and P. Mutzel (Berlin: Springer).
Blackmore, M. G., Wang, Z., Lerch, J. K., Motti, D., Zhang, Y. P., Shields, C. B., et al. (2012). Krüppel-like factor 7 engineered for transcriptional activation promotes axon regeneration in the adult corticospinal tract. Proc. Natl. Acad. Sci. U.S.A. 109, 7517–7522. doi: 10.1073/pnas.1120684109
Blesch, A., Lu, P., and Tuszynski, M. H. (2002). Neurotrophic factors, gene therapy, and neural stem cells for spinal cord repair. Brain Res. Bull. 57, 833–838. doi: 10.1016/s0361-9230(01)00774-2
Boato, F., Hendrix, S., Huelsenbeck, S. C., Hofmann, F., Große, G., Djalali, S., et al. (2010). C3 peptide enhances recovery from spinal cord injury by improved regenerative growth of descending fiber tracts. J. Cell Sci. 123, 1652–1662. doi: 10.1242/jcs.066050
Brus-Ramer, M., Carmel, J. B., Chakrabarty, S., and Martin, J. H. (2007). Electrical stimulation of spared corticospinal axons augments connections with ipsilateral spinal motor circuits after injury. J. Neurosci. 27, 13793–13801. doi: 10.1523/JNEUROSCI.3489-07.2007
Brus-Ramer, M., Carmel, J. B., and Martin, J. H. (2009). Motor cortex bilateral motor representation depends on subcortical and interhemispheric interactions. J. Neurosci. 29, 6196–6206. doi: 10.1523/JNEUROSCI.5852-08.2009
Buchli, A. D., and Schwab, M. E. (2005). Inhibition of Nogo: a key strategy to increase regeneration, plasticity and functional recovery of the Lesioned central nervous system. Ann. Med. 37, 556–567. doi: 10.1080/07853890500407520
Bunday, K. L., and Perez, M. A. (2012). Motor recovery after spinal cord injury enhanced by strengthening corticospinal synaptic transmission. Curr. Biol. 22, 2355–2361. doi: 10.1016/j.cub.2012.10.046
Byrnes, K. R., Waynant, R. W., Ilev, I. K., Wu, X., Barna, L., Smith, K., et al. (2005). Light promotes regeneration and functional recovery and alters the immune response after spinal cord injury. Lasers Surg. Med. 36, 171–185. doi: 10.1002/lsm.20143
Cafferty, W. B. J., Duffy, P., Huebner, E., and Strittmatter, S. M. (2010). MAG and OMgp synergize with Nogo-A to restrict axonal growth and neurological recovery after spinal cord trauma. J. Neurosci. 30, 6825–6837. doi: 10.1523/JNEUROSCI.6239-09.2010
Cafferty, W. B. J., and Strittmatter, S. M. (2006). The Nogo–Nogo receptor pathway limits a spectrum of adult CNS axonal growth. J. Neurosci. 26, 12242–12250. doi: 10.1523/JNEUROSCI.3827-06.2006
Carmel, J. B., Berrol, L. J., Brus-Ramer, M., and Martin, J. H. (2010). Chronic electrical stimulation of the intact corticospinal system after unilateral injury restores skilled locomotor control and promotes spinal axon outgrowth. J. Neurosci. 30, 10918–10926. doi: 10.1523/JNEUROSCI.1435-10.2010
Chen, C. (2004). Searching for intellectual turning points: progressive knowledge domain visualization. Proc. Natl. Acad. Sci. U.S.A. 101, 5303–5310. doi: 10.1073/pnas.0307513100
Cho, S.-H., Kim, D. G., Kim, D.-S., Kim, Y.-H., Lee, C.-H., and Jang, S. H. (2007). Motor outcome according to the integrity of the corticospinal tract determined by diffusion tensor tractography in the early stage of corona radiata infarct. Neurosci. Lett. 426, 123–127. doi: 10.1016/j.neulet.2007.08.049
Courtine, G., Roy, R. R., Raven, J., Hodgson, J., Mckay, H., Yang, H., et al. (2005). Performance of locomotion and foot grasping following a unilateral thoracic corticospinal tract lesion in monkeys (Macaca mulatta). Brain 128, 2338–2358. doi: 10.1093/brain/awh604
Danilov, C. A., and Steward, O. (2015). Conditional genetic deletion of PTEN after a spinal cord injury enhances regenerative growth of CST axons and motor function recovery in mice. Exp. Neurol. 266, 147–160. doi: 10.1016/j.expneurol.2015.02.012
Dias, D. O., Kim, H., Holl, D., Werne Solnestam, B., Lundeberg, J., Carlén, M., et al. (2018). Reducing pericyte-derived scarring promotes recovery after spinal cord injury. Cell 173, 153–165.e22. doi: 10.1016/j.cell.2018.02.004
Dickendesher, T. L., Baldwin, K. T., Mironova, Y. A., Koriyama, Y., Raiker, S. J., Askew, K. L., et al. (2012). NgR1 and NgR3 are receptors for chondroitin sulfate proteoglycans. Nat. Neurosci. 15, 703–712. doi: 10.1038/nn.3070
Dimara, E., and Perin, C. (2020). What is interaction for data visualization? IEEE Trans. Vis. Comput. Graph. 26, 119–129. doi: 10.1109/TVCG.2019.2934283
Dimou, L., Schnell, L., Montani, L., Duncan, C., Simonen, M., Schneider, R., et al. (2006). Nogo-A-deficient mice reveal strain-dependent differences in axonal regeneration. J. Neurosci. 26, 5591–5603. doi: 10.1523/JNEUROSCI.1103-06.2006
Du, K., Zheng, S., Zhang, Q., Li, S., Gao, X., Wang, J., et al. (2015). PTEN deletion promotes regrowth of corticospinal tract axons 1 year after spinal cord injury. J. Neurosci. 35, 9754–9763. doi: 10.1523/JNEUROSCI.3637-14.2015
Ellegaard, O., and Wallin, J. A. (2015). The bibliometric analysis of scholarly production: how great is the impact? Scientometrics 105, 1809–1831. doi: 10.1007/s11192-015-1645-z
Fabes, J., Anderson, P., Brennan, C., and Bolsover, S. (2007). Regeneration-enhancing effects of EphA4 blocking peptide following corticospinal tract injury in adult rat spinal cord. Eur. J. Neurosci. 26, 2496–2505. doi: 10.1111/j.1460-9568.2007.05859.x
Fawcett, J. W. (2015). The extracellular matrix in plasticity and regeneration after CNS injury and neurodegenerative disease. Prog. Brain. Res. 218, 213–226. doi: 10.1016/bs.pbr.2015.02.001
Feng, W., Wang, J., Chhatbar, P. Y., Doughty, C., Landsittel, D., Lioutas, V., et al. (2015). Corticospinal tract lesion load: an imaging biomarker for stroke motor outcomes. Ann. Neurol. 78, 860–870. doi: 10.1002/ana.24510
Fischer, I., Dulin, J. N., and Lane, M. A. (2020). Transplanting neural progenitor cells to restore connectivity after spinal cord injury. Nat. Rev. Neurosci. 21, 366–383. doi: 10.1038/s41583-020-0314-2
Fortin, J. P., Parker, D., Tunç, B., Watanabe, T., Elliott, M. A., Ruparel, K., et al. (2017). Harmonization of multi-site diffusion tensor imaging data. NeuroImage 161, 149–170. doi: 10.1016/j.neuroimage.2017.08.047
Fouad, K., Klusman, I., and Schwab, M. E. (2004). Regenerating corticospinal fibers in the marmoset (Callitrix jacchus) after spinal cord lesion and treatment with the anti-Nogo-A antibody IN-1. Eur. J. Neurosci. 20, 2479–2482. doi: 10.1111/j.1460-9568.2004.03716.x
Freund, P., Schmidlin, E., Wannier, T., Bloch, J., Mir, A., Schwab, M. E., et al. (2006). Nogo-A-specific antibody treatment enhances sprouting and functional recovery after cervical lesion in adult Primates. Nat. Med. 12, 790–792. doi: 10.1038/nm1436
Freund, P., Schmidlin, E., Wannier, T., Bloch, J., Mir, A., Schwab, M. E., et al. (2009). Anti-Nogo-A antibody treatment promotes recovery of manual dexterity after unilateral cervical lesion in adult primates—re-examination and extension of behavioral data. Eur. J. Neurosci. 29, 983–996. doi: 10.1111/j.1460-9568.2009.06642.x
Freund, P., Wannier, T., Schmidlin, E., Bloch, J., Mir, A., Schwab, M. E., et al. (2007). Anti-Nogo-A antibody treatment enhances sprouting of corticospinal axons rostral to a unilateral cervical spinal cord lesion in adult macaque monkey. J. Comp. Neurol. 502, 644–659. doi: 10.1002/cne.21321
Freund, P., Weiskopf, N., Ashburner, J., Wolf, K., Sutter, R., Altmann, D. R., et al. (2013). MRI investigation of the sensorimotor cortex and the corticospinal tract after acute spinal cord injury: a prospective longitudinal study. Lancet Neurol. 12, 873–881. doi: 10.1016/S1474-4422(13)70146-7
Friedli, L., Rosenzweig, E. S., Barraud, Q., Schubert, M., Dominici, N., Awai, L., et al. (2015). Pronounced species divergence in corticospinal tract reorganization and functional recovery after lateralized spinal cord injury favors primates. Sci. Transl. Med. 7:302ra134. doi: 10.1126/scitranslmed.aac5811
Fry, E. J., Chagnon, M. J., López-Vales, R., Tremblay, M. L., and David, S. (2010). Corticospinal tract regeneration after spinal cord injury in receptor protein tyrosine phosphatase sigma deficient mice. Glia 58, 423–433. doi: 10.1002/glia.20934
Führmann, T., Anandakumaran, P. N., and Shoichet, M. S. (2017). Combinatorial therapies after spinal cord injury: How can biomaterials help? Adv. Healthcare Mater. 6:1601130. doi: 10.1002/adhm.201601130
Fujimoto, Y., Abematsu, M., Falk, A., Tsujimura, K., Sanosaka, T., Juliandi, B., et al. (2012). Treatment of a mouse model of spinal cord injury by transplantation of human induced pluripotent stem cell-derived long-term self-renewing neuroepithelial-like stem cells. Stem Cells 30, 1163–1173. doi: 10.1002/stem.1083
García-Alías, G., Barkhuysen, S., Buckle, M., and Fawcett, J. W. (2009). Chondroitinase ABC treatment opens a window of opportunity for task-specific rehabilitation. Nat. Neurosci. 12, 1145–1151. doi: 10.1038/nn.2377
Girgis, J., Merrett, D., Kirkland, S., Metz, G. A. S., Verge, V., and Fouad, K. (2007). Reaching training in rats with spinal cord injury promotes plasticity and task specific recovery. Brain 130, 2993–3003. doi: 10.1093/brain/awm245
Gokoffski, K. K., Peng, M., Alas, B., and Lam, P. (2020). Neuro-protection and neuro-regeneration of the optic nerve: recent advances and future directions. Curr. Opin. Neurol. 33, 93–105. doi: 10.1097/WCO.0000000000000777
Griffin, J. M., and Bradke, F. (2020). Therapeutic repair for spinal cord injury: combinatory approaches to address a multifaceted problem. EMBO Mol. Med. 12:e11505. doi: 10.15252/emmm.201911505
Guggisberg, A. G., Koch, P. J., Hummel, F. C., and Buetefisch, C. M. (2019). Brain networks and their relevance for stroke rehabilitation. Clin. Neurophysiol. 130, 1098–1124. doi: 10.1016/j.clinph.2019.04.004
Hamers, F. P. T., Koopmans, G. C., and Joosten, E. A. J. (2006). CatWalk-assisted gait analysis in the assessment of spinal cord injury. J. Neurotrauma 23, 537–548. doi: 10.1089/neu.2006.23.537
Han, Q., Xie, Y., Ordaz, J. D., Huh, A. J., Huang, N., Wu, W., et al. (2020). Restoring Cellular energetics promotes axonal regeneration and functional recovery after spinal cord injury. Cell Metab. 31, 623–641.e8. doi: 10.1016/j.cmet.2020.02.002
Harel, N. Y., and Strittmatter, S. M. (2006). Can regenerating axons recapitulate developmental guidance during recovery from spinal cord injury? Nat. Rev. Neurosci. 7, 603–616. doi: 10.1038/nrn1957
Hata, K., Fujitani, M., Yasuda, Y., Doya, H., Saito, T., Yamagishi, S., et al. (2006). RGMa inhibition promotes axonal growth and recovery after spinal cord injury. J. Cell Biol. 173, 47–58. doi: 10.1083/jcb.200508143
He, M., Ding, Y., Chu, C., Tang, J., Xiao, Q., and Luo, Z.-G. (2016). Autophagy induction stabilizes microtubules and promotes axon regeneration after spinal cord injury. Proc. Natl. Acad. Sci. U.S.A. 113, 11324–11329. doi: 10.1073/pnas.1611282113
Hilton, B. J., and Bradke, F. (2017). Can injured adult CNS axons regenerate by recapitulating development? Development 144, 3417–3429. doi: 10.1242/dev.148312
Hollis, E. R., Jamshidi, P., Löw, K., Blesch, A., and Tuszynski, M. H. (2009). Induction of corticospinal regeneration by lentiviral trkB-induced Erk activation. Proc. Natl. Acad. Sci. U.S.A. 106, 7215–7220. doi: 10.1073/pnas.0810624106
Hutson, T. H., and Di Giovanni, S. (2019). The translational landscape in spinal cord injury: focus on neuroplasticity and regeneration. Nat. Rev. Neurol. 15, 732–745. doi: 10.1038/s41582-019-0280-3
Jankowska, E., and Edgley, S. A. (2006). How can corticospinal tract neurons contribute to ipsilateral movements? A question with implications for recovery of motor functions. Neuroscientist 12, 67–79. doi: 10.1177/1073858405283392
Jin, D., Liu, Y., Sun, F., Wang, X., Liu, X., and He, Z. (2015). Restoration of skilled locomotion by sprouting corticospinal axons induced by co-deletion of PTEN and SOCS3. Nat. Commun. 6:8074. doi: 10.1038/ncomms9074
Kadoya, K., Lu, P., Kenny, N., Lee-Kubli, C., Kumamaru, H., Yao, L., et al. (2016). Spinal cord reconstitution with homologous neural grafts enables robust corticospinal regeneration. Nat. Med. 22, 479–487. doi: 10.1038/nm.4066
Karimi-Abdolrezaee, S., Eftekharpour, E., Wang, J., Schut, D., and Fehlings, M. G. (2010). Synergistic effects of transplanted adult neural stem/progenitor cells, chondroitinase, and growth factors promote functional repair and plasticity of the chronically injured spinal cord. J. Neurosci. 30, 1657–1676. doi: 10.1523/JNEUROSCI.3111-09.2010
Katoh, H., Yokota, K., and Fehlings, M. G. (2019). Regeneration of spinal cord connectivity through stem cell transplantation and biomaterial scaffolds. Front. Cell. Neurosci. 13:248. doi: 10.3389/fncel.2019.00248
Keefe, K., Sheikh, I., and Smith, G. (2017). Targeting neurotrophins to specific populations of neurons: NGF, BDNF, and NT-3 and their relevance for treatment of spinal cord injury. Int. J. Mol. Sci. 18:548. doi: 10.3390/ijms18030548
Kim, B., and Winstein, C. (2017). Can neurological biomarkers of brain impairment be used to predict poststroke motor recovery? A systematic review. Neurorehabil. Neural. Repair 31, 3–24. doi: 10.1177/1545968316662708
Koch, P., Schulz, R., and Hummel, F. C. (2016). Structural connectivity analyses in motor recovery research after stroke. Ann. Clin. Transl. Neurol. 3, 233–244. doi: 10.1002/acn3.278
Konishi, J., Yamada, K., Kizu, O., Ito, H., Sugimura, K., Yoshikawa, K., et al. (2005). MR tractography for the evaluation of functional recovery from lenticulostriate infarcts. Neurology 64, 108–113. doi: 10.1212/01.WNL.0000148477.65273.0C
Kremenchutzky, M., Rice, G. P. A., Baskerville, J., Wingerchuk, D. M., and Ebers, G. C. (2006). The natural history of multiple sclerosis: a geographically based study 9: observations on the progressive phase of the disease. Brain 129, 584–594. doi: 10.1093/brain/awh721
Lacroix, S., Havton, L. A., McKay, H., Yang, H., Brant, A., Roberts, J., et al. (2004). Bilateral corticospinal projections arise from each motor cortex in the macaque monkey: a quantitative study. J. Comp. Neurol. 473, 147–161. doi: 10.1002/cne.20051
Lee, J. K., Geoffroy, C. G., Chan, A. F., Tolentino, K. E., Crawford, M. J., Leal, M. A., et al. (2010). Assessing spinal axon regeneration and sprouting in Nogo-, MAG-, and OMgp-deficient mice. Neuron 66, 663–670. doi: 10.1016/j.neuron.2010.05.002
Lewandowski, G., and Steward, O. (2014). AAVshRNA-mediated suppression of PTEN in adult rats in combination with salmon fibrin administration enables regenerative growth of corticospinal axons and enhances recovery of voluntary motor function after cervical spinal cord injury. J. Neurosci. 34, 9951–9962. doi: 10.1523/JNEUROSCI.1996-14.2014
Lindau, N. T., Bänninger, B. J., Gullo, M., Good, N. A., Bachmann, L. C., Starkey, M. L., et al. (2014). Rewiring of the corticospinal tract in the adult rat after unilateral stroke and anti-Nogo-A therapy. Brain 137, 739–756. doi: 10.1093/brain/awt336
Liu, Z., Li, Y., Cui, Y., Roberts, C., Lu, M., Wilhelmsson, U., et al. (2014). Beneficial effects of GFAP/vimentin reactive astrocytes for axonal remodeling and motor behavioral recovery in mice after stroke. Glia 62, 2022–2033. doi: 10.1002/glia.22723
Liu, Z., Li, Y., Zhang, X., Savant-Bhonsale, S., and Chopp, M. (2008). Contralesional axonal remodeling of the corticospinal system in adult rats after stroke and bone marrow stromal cell treatment. Stroke 39, 2571–2577. doi: 10.1161/STROKEAHA.107.511659
Liu, K., Lu, Y., Lee, J. K., Samara, R., Willenberg, R., Sears-Kraxberger, I., et al. (2010). PTEN deletion enhances the regenerative ability of adult corticospinal neurons. Nat. Neurosci. 13, 1075–1081. doi: 10.1038/nn.2603
Liu, J., Qin, W., Zhang, J., Zhang, X., and Yu, C. (2015). Enhanced interhemispheric functional connectivity compensates for anatomical connection damages in subcortical stroke. Stroke 46, 1045–1051. doi: 10.1161/STROKEAHA.114.007044
Liu, K., Tedeschi, A., Park, K. K., and He, Z. (2011). Neuronal intrinsic mechanisms of axon regeneration. Annu. Rev. Neurosci. 34, 131–152. doi: 10.1146/annurev-neuro-061010-113723
Liu, Y., Wang, X., Li, W., Zhang, Q., Li, Y., Zhang, Z., et al. (2017). A sensitized IGF1 treatment restores corticospinal axon-dependent functions. Neuron 95, 817–833.e4. doi: 10.1016/j.neuron.2017.07.037
Lo Giudice, T., Lombardi, F., Santorelli, F. M., Kawarai, T., and Orlacchio, A. (2014). Hereditary spastic paraplegia: clinical-genetic characteristics and evolving molecular mechanisms. Exp. Neurol. 261, 518–539. doi: 10.1016/j.expneurol.2014.06.011
Meyers, E. C., Solorzano, B. R., James, J., Ganzer, P. D., Lai, E. S., Rennaker, R. L., et al. (2018). Vagus nerve stimulation enhances stable plasticity and generalization of stroke recovery. Stroke 49, 710–717. doi: 10.1161/STROKEAHA.117.019202
Moed, H. F. (2009). New developments in the use of citation analysis in research evaluation. Arch. Immunol. Ther. Exp. 57, 13–18. doi: 10.1007/s00005-009-0001-5
Moller, M., Frandsen, J., Andersen, G., Gjedde, A., Vestergaard-Poulsen, P., and Ostergaard, L. (2007). Dynamic changes in corticospinal tracts after stroke detected by fibretracking. J. Neurol. Neurosurg. Psychiatry 78, 587–592. doi: 10.1136/jnnp.2006.100248
Morita, T., Sasaki, M., Kataoka-Sasaki, Y., Nakazaki, M., Nagahama, H., Oka, S., et al. (2016). Intravenous infusion of mesenchymal stem cells promotes functional recovery in a model of chronic spinal cord injury. Neuroscience 335, 221–231. doi: 10.1016/j.neuroscience.2016.08.037
Oudega, M., and Perez, M. A. (2012). Corticospinal reorganization after spinal cord injury. J. Physiol. 590, 3647–3663. doi: 10.1113/jphysiol.2012.233189
Piantino, J., Burdick, J., Goldberg, D., Langer, R., and Benowitz, L. (2006). An injectable, biodegradable hydrogel for trophic factor delivery enhances axonal rewiring and improves performance after spinal cord injury. Exp. Neurol. 201, 359–367. doi: 10.1016/j.expneurol.2006.04.020
Poplawski, G. H. D., Kawaguchi, R., Van Niekerk, E., Lu, P., Mehta, N., Canete, P., et al. (2020). Injured adult neurons regress to an embryonic transcriptional growth state. Nature 581, 77–82. doi: 10.1038/s41586-020-2200-5
Puig, J., Blasco, G., Daunis-I-Estadella, J., Thomalla, G., Castellanos, M., Figueras, J., et al. (2013). Decreased corticospinal tract fractional anisotropy predicts long-term motor outcome after stroke. Stroke 44, 2016–2018. doi: 10.1161/STROKEAHA.111.000382
Puig, J., Blasco, G., Schlaug, G., Stinear, C. M., Daunis-i-Estadella, P., Biarnes, C., et al. (2017). Diffusion tensor imaging as a prognostic biomarker for motor recovery and rehabilitation after stroke. Neuroradiology 59, 343–351. doi: 10.1007/s00234-017-1816-0
Ramer, L. M., Ramer, M. S., and Bradbury, E. J. (2014). Restoring function after spinal cord injury: towards clinical translation of experimental strategies. Lancet Neurol. 13, 1241–1256. doi: 10.1016/S1474-4422(14)70144-9
Rao, J.-S., Zhao, C., Zhang, A., Duan, H., Hao, P., Wei, R.-H., et al. (2018). NT3-chitosan enables de novo regeneration and functional recovery in monkeys after spinal cord injury. Proc. Natl. Acad. Sci. U.S.A. 115, E5595–E5604. doi: 10.1073/pnas.1804735115
Rosenzweig, E. S., Brock, J. H., Culbertson, M. D., Lu, P., Moseanko, R., Edgerton, V. R., et al. (2009). Extensive spinal decussation and bilateral termination of cervical corticospinal projections in rhesus monkeys. J. Comp. Neurol. 513, 151–163. doi: 10.1002/cne.21940
Ruitenberg, M. J. (2005). NT-3 expression from engineered olfactory ensheathing glia promotes spinal sparing and regeneration. Brain 128, 839–853. doi: 10.1093/brain/awh424
Sasaki, M., Hains, B. C., Lankford, K. L., Waxman, S. G., and Kocsis, J. D. (2006). Protection of corticospinal tract neurons after dorsal spinal cord transection and engraftment of olfactory ensheathing cells. Glia 53, 352–359. doi: 10.1002/glia.20285
Sasaki, M., Radtke, C., Tan, A. M., Zhao, P., Hamada, H., Houkin, K., et al. (2009). BDNF-hypersecreting human mesenchymal stem cells promote functional recovery, axonal sprouting, and protection of corticospinal neurons after spinal cord injury. J. Neurosci. 29, 14932–14941. doi: 10.1523/JNEUROSCI.2769-09.2009
Schnaar, R. L., and Lopez, P. H. (2009). Myelin-associated glycoprotein and its axonal receptors. J. Neurosci. Res. 87, 3267–3276. doi: 10.1002/jnr.21992
Shelchkova, N. D., Downey, J. E., Greenspon, C. M., Okorokova, E. V., Sobinov, A. R., Verbaarschot, C., et al. (2023). Microstimulation of human somatosensory cortex evokes task-dependent, spatially patterned responses in motor cortex. Nat. Commun. 14:7270. doi: 10.1038/s41467-023-43140-2
Silva, N. A., Sousa, N., Reis, R. L., and Salgado, A. J. (2014). From basics to clinical: a comprehensive review on spinal cord injury. Prog. Neurobiol. 114, 25–57. doi: 10.1016/j.pneurobio.2013.11.002
Starkey, M. L., Barritt, A. W., Yip, P. K., Davies, M., Hamers, F. P. T., McMahon, S. B., et al. (2005). Assessing behavioural function following a pyramidotomy lesion of the corticospinal tract in adult mice. Exp. Neurol. 195, 524–539. doi: 10.1016/j.expneurol.2005.06.017
Steward, O., Sharp, K., Yee, K. M., and Hofstadter, M. (2008). A re-assessment of the effects of a Nogo-66 receptor antagonist on regenerative growth of axons and locomotor recovery after spinal cord injury in mice. Exp. Neurol. 209, 446–468. doi: 10.1016/j.expneurol.2007.12.010
Stinear, C. M. (2017). Prediction of motor recovery after stroke: advances in biomarkers. Lancet Neurol. 16, 826–836. doi: 10.1016/S1474-4422(17)30283-1
Stinear, C. M., Barber, P. A., Smale, P. R., Coxon, J. P., Fleming, M. K., and Byblow, W. D. (2006). Functional potential in chronic stroke patients depends on corticospinal tract integrity. Brain 130, 170–180. doi: 10.1093/brain/awl333
Stinear, C. M., Byblow, W. D., Ackerley, S. J., Smith, M.-C., Borges, V. M., and Barber, P. A. (2017). Proportional motor recovery after stroke: implications for trial design. Stroke 48, 795–798. doi: 10.1161/STROKEAHA.116.016020
Sun, F., Park, K. K., Belin, S., Wang, D., Lu, T., Chen, G., et al. (2011). Sustained axon regeneration induced by co-deletion of PTEN and SOCS3. Nature 480, 372–375. doi: 10.1038/nature10594
Thomas, S. L., and Gorassini, M. A. (2005). Increases in corticospinal tract function by treadmill training after incomplete spinal cord injury. J. Neurophysiol. 94, 2844–2855. doi: 10.1152/jn.00532.2005
Tuszynski, M. H., and Steward, O. (2012). Concepts and methods for the study of axonal regeneration in the CNS. Neuron 74, 777–791. doi: 10.1016/j.neuron.2012.05.006
Ueno, M., Hayano, Y., Nakagawa, H., and Yamashita, T. (2012). Intraspinal rewiring of the corticospinal tract requires target-derived brain-derived neurotrophic factor and compensates lost function after brain injury. Brain 135, 1253–1267. doi: 10.1093/brain/aws053
van Eck, N. J., and Waltman, L. (2010). Software survey: VOSviewer, a computer program for bibliometric mapping. Scientometrics 84, 523–538. doi: 10.1007/s11192-009-0146-3
Wahl, A. S., Omlor, W., Rubio, J. C., Chen, J. L., Zheng, H., Schröter, A., et al. (2014). Asynchronous therapy restores motor control by rewiring of the rat corticospinal tract after stroke. Science 344, 1250–1255. doi: 10.1126/science.1253050
Wang, D., Ichiyama, R. M., Zhao, R., Andrews, M. R., and Fawcett, J. W. (2011). Chondroitinase combined with rehabilitation promotes recovery of forelimb function in rats with chronic spinal cord injury. J. Neurosci. 31, 9332–9344. doi: 10.1523/JNEUROSCI.0983-11.2011
Wang, Z., Reynolds, A., Kirry, A., Nienhaus, C., and Blackmore, M. G. (2015). Overexpression of Sox11 promotes corticospinal tract regeneration after spinal injury while interfering with functional recovery. J. Neurosci. 35, 3139–3145. doi: 10.1523/JNEUROSCI.2832-14.2015
Welniarz, Q., Dusart, I., and Roze, E. (2017). The corticospinal tract: evolution, development, and human disorders. Dev. Neurobiol. 77, 810–829. doi: 10.1002/dneu.22455
Zaaimi, B., Edgley, S. A., Soteropoulos, D. S., and Baker, S. N. (2012). Changes in descending motor pathway connectivity after corticospinal tract lesion in macaque monkey. Brain 135, 2277–2289. doi: 10.1093/brain/aws115
Zeng, X., Qiu, X.-C., Ma, Y.-H., Duan, J.-J., Chen, Y.-F., Gu, H.-Y., et al. (2015). Integration of donor mesenchymal stem cell-derived neuron-like cells into host neural network after rat spinal cord transection. Biomaterials 53, 184–201. doi: 10.1016/j.biomaterials.2015.02.073
Zheng, B. H., Atwal, J., Ho, C., Case, L., He, X. L., Garcia, K. C., et al. (2005). Genetic deletion of the Nogo receptor does not reduce neurite inhibition in vitro or promote corticospinal tract regeneration in vivo. Proc. Natl. Acad. Sci. U.S.A. 102, 1205–1210. doi: 10.1073/pnas.0409026102
Zhu, L. L., Lindenberg, R., Alexander, M. P., and Schlaug, G. (2010). Lesion load of the corticospinal tract predicts motor impairment in chronic stroke. Stroke 41, 910–915. doi: 10.1161/STROKEAHA.109.577023
Zukor, K., Belin, S., Wang, C., Keelan, N., Wang, X., and He, Z. (2013). Short hairpin RNA against PTEN enhances regenerative growth of corticospinal tract axons after spinal cord injury. J. Neurosci. 33, 15350–15361. doi: 10.1523/JNEUROSCI.2510-13.2013
Keywords: corticospinal tract regeneration, bibliometric analysis, Web of Science Core Collection, VOSviewer, CiteSpace
Citation: Ye L-C, Li H, Li H, Lin X, Hu K, Huang Z, Chimedtseren C, Fang L, Saijilahu and Xu R-J (2025) A bibliometric analysis of the top 100 most cited articles on corticospinal tract regeneration from 2004 to 2024. Front. Neurosci. 18:1509850. doi: 10.3389/fnins.2024.1509850
Received: 11 October 2024; Accepted: 23 December 2024;
Published: 28 January 2025.
Edited by:
Fadel Tissir, Hamad Bin Khalifa University, QatarCopyright © 2025 Saijilafu, Ye, Li, Li, Lin, Hu, Huang, Chimedtseren, Fang, Saijilahu and Xu. This is an open-access article distributed under the terms of the Creative Commons Attribution License (CC BY). The use, distribution or reproduction in other forums is permitted, provided the original author(s) and the copyright owner(s) are credited and that the original publication in this journal is cited, in accordance with accepted academic practice. No use, distribution or reproduction is permitted which does not comply with these terms.
*Correspondence: Saijilahu, c2FpamlsYWZ1MTYzQDE2My5jb20=; Ren-Jie Xu, ZnJlZHh1cmpAcGt1Lm9yZy5jbg==
†These authors have contributed equally to this work and share first authorship
Disclaimer: All claims expressed in this article are solely those of the authors and do not necessarily represent those of their affiliated organizations, or those of the publisher, the editors and the reviewers. Any product that may be evaluated in this article or claim that may be made by its manufacturer is not guaranteed or endorsed by the publisher.
Research integrity at Frontiers
Learn more about the work of our research integrity team to safeguard the quality of each article we publish.