- 1Department of Neurology and Neurosurgery, Montreal Neurological Institute, McGill University, Montreal, QC, Canada
- 2Department of Psychology, McGill University, Montreal, QC, Canada
Previous functional neuroimaging studies had demonstrated the involvement of cytoarchitectonic area 8Av of the prefrontal cortex in the cognitive allocation of attention to spatial stimuli. The present functional magnetic resonance imaging (fMRI) study examined brain activity related to the allocation of attention to non-spatial visual stimuli, i.e., stimuli that are defined by their perceptual features and are independent of their location. The study established (a) the involvement of area 8Av in the allocation of attention to non-spatial stimuli in the environment and (b) the areas co-activated with area 8Av across the entire cortex so that the complete functional cortical network could be defined. Finally, based on individual subject analysis, the functional activity in area 8Av was related to specific sulci in the caudal middle frontal gyrus. The novel information provided by the current fMRI study significantly advances our understanding of the role of area 8Av in the selective allocation of attention to stimuli in the environment.
1 Introduction
The prefrontal cortex is a large expanse of cortical areas that underlie executive processing, namely the higher order control processes that regulate activity in posterior cortical areas (Luria, 1973; Petrides, 2005; Shallice and Burgess, 1996; Figures 1A, B). Research in patients has demonstrated an impairment in the selective allocation of attention to stimuli in our distraction-filled environment after prefrontal cortical damage (Petrides, 1985a; Petrides, 1990). In macaque monkeys, lesions restricted to the periarcuate region, where area 8Av lies, produced a comparable impairment in the selective allocation of attention to exteroceptive stimuli (Petrides, 1985b; Petrides, 1987). In these experiments, on a trial-by-trial basis, subjects were required to select the appropriate target stimulus from a set of competing stimuli, based on conditional rules: if instruction cue A is presented, attend to and select visual target stimulus X, but if instruction cue B is presented, attend to and select visual target stimulus Y. Furthermore, functional neuroimaging in healthy human
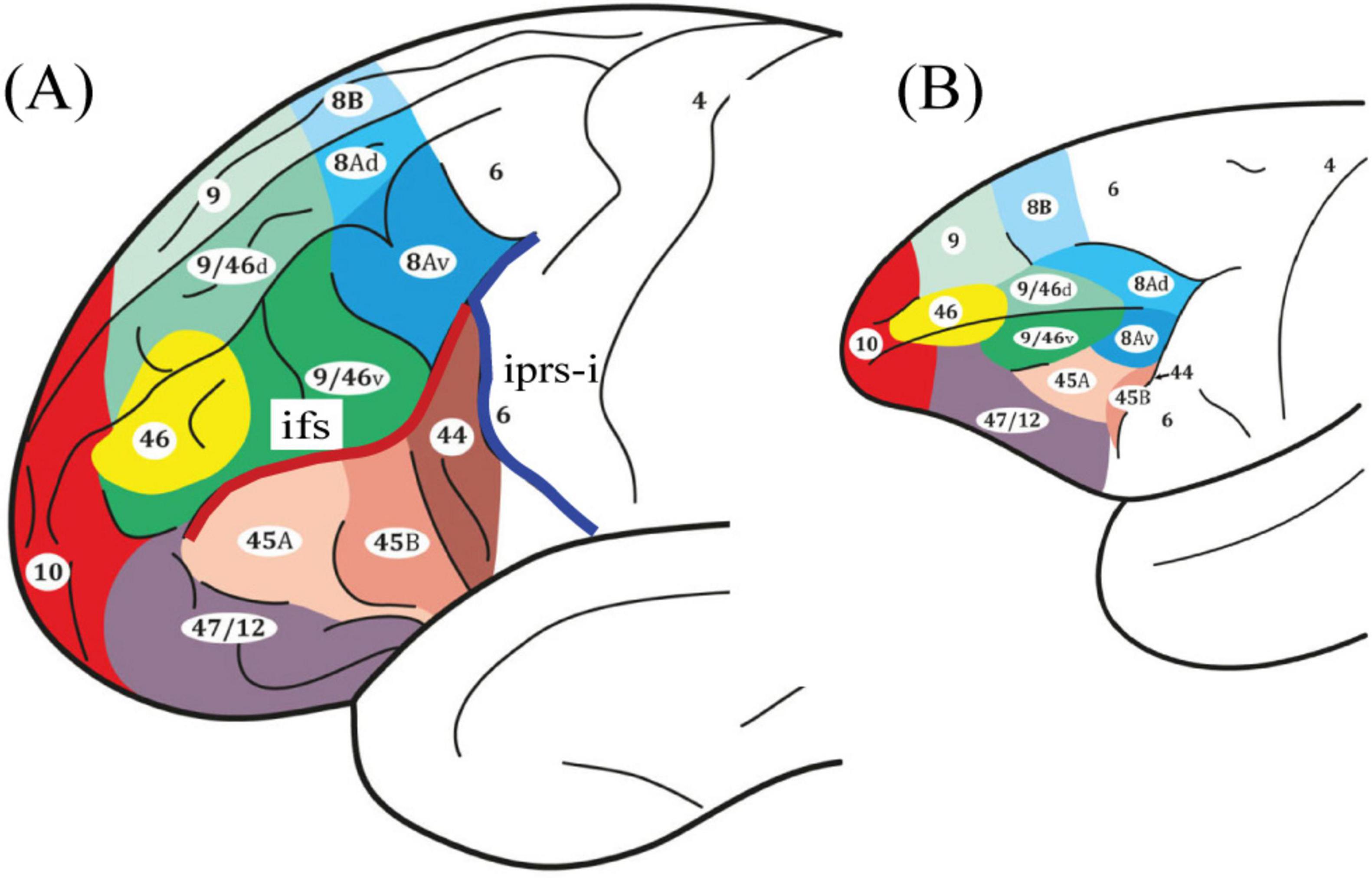
Figure 1. Schematic illustrations of the lateral surface of the cerebral cortex. Comparative cytoarchitectonic map of the frontal lobe in panel (A) the human and (B) the macaque monkey brains (Petrides and Pandya, 1999; Petrides and Pandya, 2002). Note that area 8A is denoted in blue and the ventral boundary is found at the intersection of the inferior frontal sulcus (ifs) in red and the inferior ramus of the inferior precentral sulcus (iprs-i) in dark blue. All figures reproduced with permission.
participants demonstrated increased activity in area 8Av on the middle frontal gyrus (MFG) (see Figure 1A) when allocating attention to particular locations based on conditional rules (Germann and Petrides, 2020a; Germann and Petrides, 2020b). It is important to note that lesion studies in patients and macaque monkeys had demonstrated that the spatial element was not a necessary condition for the executive control process of the selective allocation of attention (Petrides, 1985a; Petrides, 1985b; Petrides, 1990). Thus, the question that now emerges is whether the activation of area 8Av in healthy human subjects that was observed in functional neuroimaging studies applies only to the selective allocation of attention to the spatial aspect of sensory stimuli, i.e., their location, or also to non-spatial stimuli (e.g., objects, faces, designs, etc., regardless of their location).
The precise examination of cortico-cortical connectivity in non-human primate brains has demonstrated specific anatomical circuits that are the gold-standard for the accurate interpretation of cortical functional networks (Petrides and Pandya, 1999; Petrides and Pandya, 2002). Area 8Av was shown to be connected with ventrally adjoining prefrontal area 45, which is involved in the selective retrieval of previously acquired information (Kostopoulos and Petrides, 2003; Kostopoulos and Petrides, 2008), the intraparietal sulcus and the adjacent area PG in the posterior inferior parietal lobule, which is involved in the processing of the spatial context within which stimuli are embedded (Astafiev et al., 2003; Mishkin et al., 1983; Petrides and Iversen, 1979), and the occipito-temporal region involved in the perceptual processing and recognition of visual stimuli (Allison et al., 1999; Astafiev et al., 2003; Kanwisher et al., 1997; Mishkin et al., 1983) [Figure 2; Case 3 in (Petrides and Pandya, 1999)].
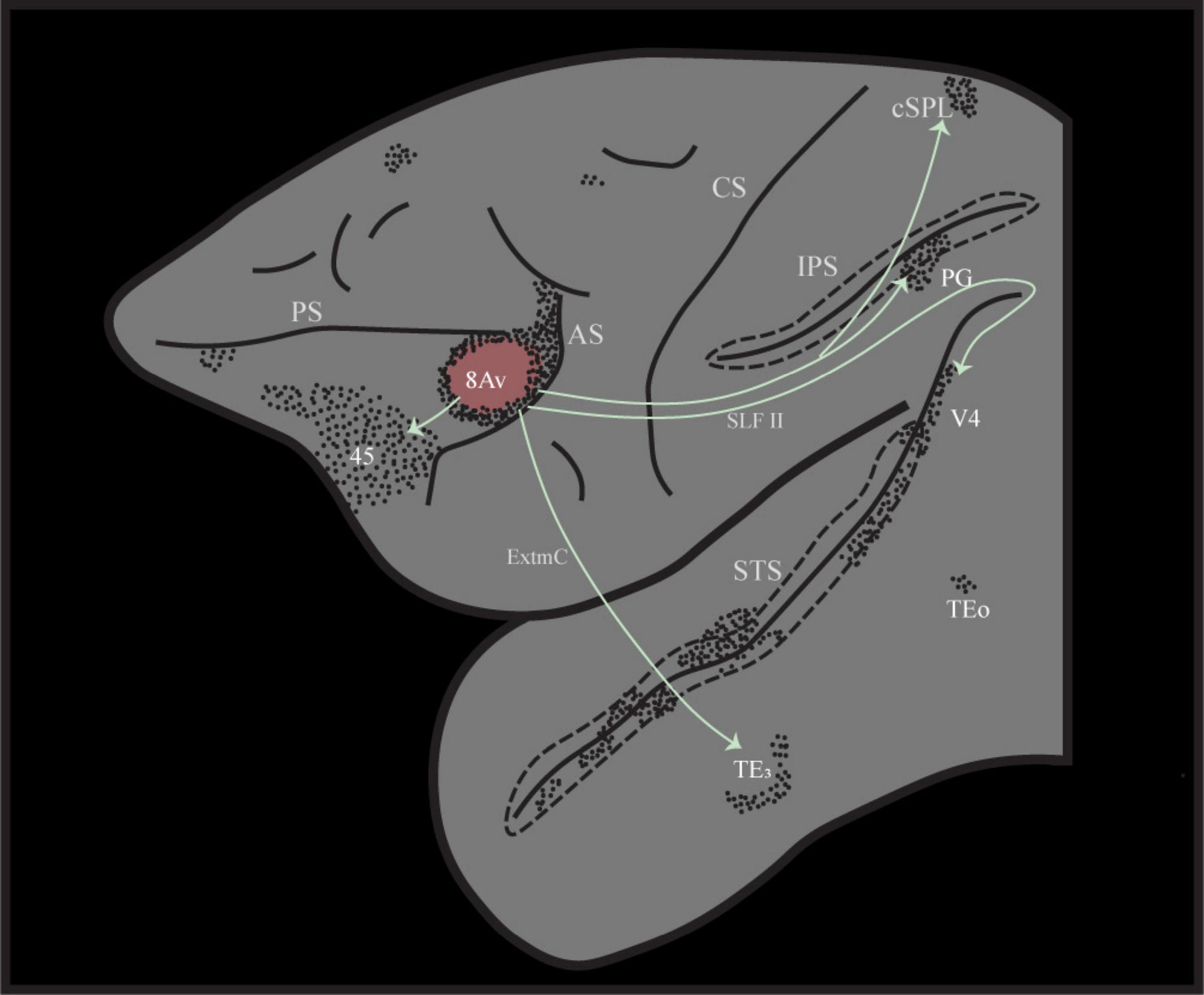
Figure 2. Illustration of the distinct cortico-cortical connectivity pattern following retrograde tracer injection into area 8Av in the macaque monkey, case 3 from (Petrides and Pandya, 1999). Note that area 8Av is connected with ventrolateral prefrontal area 45 involved in selective memory retrieval, ventral temporal cortex for the perceptual processing of visual stimuli, as well as intraparietal sulcal cortex and adjacent posterior inferior parietal cortex (area PG) that provide the spatial context within which perceptual stimuli are embedded. AS, arcuate sulcus; CS, central sulcus; cSPL, caudal superior parietal lobule; ExtmC, temporo-frontal extreme capsule fasciculus; IPS, intraparietal sulcus; PS, principal sulcus; SLF II, superior longitudinal fasciculus II; STS, superior temporal sulcus.
Based on the information provided above, the objectives of the present functional magnetic resonance imaging (fMRI) study were, first, to examine whether area 8Av is involved in the selective allocation of attention to visual stimuli in the environment that are defined by their perceptual features and are independent of their location. Second, based on macaque connectivity studies, we hypothesized that area 8Av in the human brain would be co-activated with other specific cortical areas and, thus, reveal the functional network for the high-level control process of the selective allocation of attention to stimuli in the environment. Specifically, we examined whether, during the conditional allocation of attention to non-spatial stimuli in the environment, there was co-activation of area 8Av with adjacent area 45 that is involved in selective memory retrieval, with premotor frontal eye field areas that organize eye movements, the posterior intraparietal cortical region that is involved in the perception of stimuli within space, as well as with the occipito-temporal region involved in the perceptual processing of visual stimuli.
2 Materials and methods
2.1 Subjects
Twelve healthy human subjects (six female) participated in this fMRI study. The ages of these participants ranged from 20 to 31 years (mean age 25 years) and they were all right-handed. The subjects provided informed written consent to participate in the study according to the institutional guidelines established by the Research Ethics Committee of the Montreal Neurological Institute (MNI).
2.2 Experimental design
The present event-related fMRI study was designed to examine brain activity during the allocation of attention to non-spatial visual stimuli based on if/then conditional rules that had been learned prior to scanning. Toward that end, the subjects performed two tasks: a non-spatial visual conditional experimental task and a control task. In the experimental trials, the subjects were required to focus attention on a particular visual stimulus based on conditional instruction cues, i.e., if instruction cue A is presented, cognitively allocate attention to target stimulus X, but if instruction cue B is presented, allocate attention to target stimulus Y, etc. In the control trials, a neutral instruction cue was presented that did not indicate the allocation of attention to any one of the target stimuli and, thus, did not involve the instructed cognitive allocation of selective attention (Figure 3).
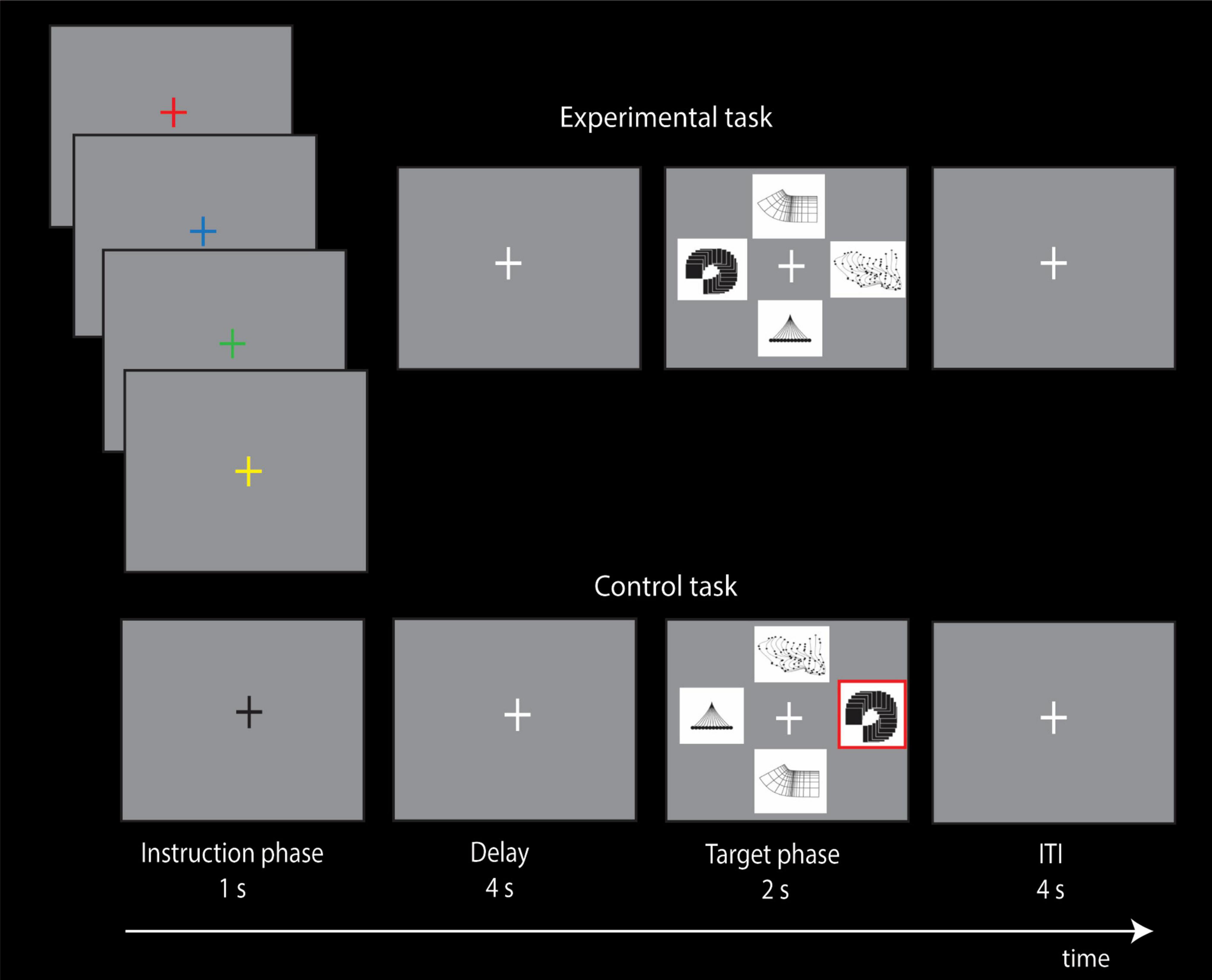
Figure 3. Experimental design of the non-spatial visual conditional attention task and the control task. In the instruction phase of the four experimental conditional trials, the subjects are shown an instruction cue (red, blue, green, or yellow colored cross) that instructs the allocation of attention to the appropriate target stimulus (abstract visual design) based on previously learned conditional rules. In the instruction phase of the control trial, a black cross is shown that does not instruct the allocation of attention to any visual perceptual stimulus. The subjects had learned before the scanning session that each one of the four visual instruction cues instructed the allocation of attention to a particular one of the four visual target stimuli (abstract visual designs), i.e., if red fixation cross, attend cognitively to abstract target stimulus X, but if blue fixation cross, attend cognitively to abstract target stimulus Y, etc. Note that during the 1 s presentation of the colored instruction cue, the subject is cognitively focusing attention on the particular abstract visual design that is associated with the instruction cue because the design stimuli are not on the screen. The design stimuli appear later, after a delay of 4 s, during the target selection phase of the trial and the subject presses the appropriate button to indicate the target to which they had allocated attention when the colored instruction cue was presented earlier in the instruction phase of the trial. Note that, during the target phase of the trial, the location of the abstract designs varies randomly, so that there is no relation of any spatial location with any of the four abstract visual designs. In the control trials, a black fixation cross was presented during the instruction phase that did not instruct the allocation of attention to any of the abstract visual designs. In the control trial, the subjects had been trained to select, during the target phase of the trial, the abstract visual design that was outlined in red, and the design outlined in red was randomly determined. Thus, the instruction cue during the control trials carried no information as to which of the four abstract visual designs should be attended to. Note also that, in the target phase of the trials in both conditions, all four targets were presented and changed their locations randomly on a trial-by-trial basis. Thus, participants could not rely on spatial information to make their cognitive selection in the instruction cue phase of the trial or during the target phase of the trial when they were selecting the non-spatial stimulus to which they had allocated attention based on the visual instruction cue. The intertrial interval (ITI) was 4 s.
In the experimental condition, the subjects had learned prior to scanning that each one of four visual instruction cues (red, blue, green, or yellow colored fixation crosses) instructed the allocation of attention to one of the four visual target stimuli (abstract visual designs). In other words, the presentation of a visual instruction cue (e.g., green colored fixation cross) indicated the cognitive allocation of attention to the particular abstract design that was associated with it. By contrast, in the control condition, during the instruction phase of the trials, a black fixation cross was presented that did not indicate the allocation of attention to any one of the four abstract designs. The instruction cue in both the experimental and control trials was followed by a 4 s delay and then the subjects were presented with the four abstract designs during the target phase of the trial and had to select the visual abstract design to which attention had been cognitively allocated when the instruction cue was presented (Figure 3). The location of the visual abstract stimuli varied randomly during the target phase of the trials and, thus, there was never any relation between the visual abstract designs and particular locations. In other words, at the instruction phase of the experimental trials, the particular color of the fixation cross instructed the cognitive allocation of attention to a particular abstract visual design that had no link to any particular location. In the target phase of the control trials, one of the visual stimuli was outlined in red and the subject had to select that stimulus (Figure 3). Thus, during the control trials, the selection of the target stimulus was determined externally (i.e., outlined in red), by contrast to the experimental trials in which the target stimulus to be selected was the particular abstract design to which attention was allocated earlier during the instruction phase based on the color of the instruction cue. Thus, the comparison of brain activity during the instruction phase (duration of 1 s; see Figure 3) of the experimental trials with the instruction phase of the control trials would show activity related to the cognitive allocation of attention to specific non-spatial visual stimuli, i.e., the visual abstract designs that were not related to any location.
On the day prior to the fMRI scanning session, each subject had learned the four conditional associations through trial-and-error and had been instructed to maintain fixation on a cross that was present for the entire duration of each trial. The subjects practiced learning trials until they had reached a performance level of more than 90% accuracy on two consecutive sets of 32 trials. During the fMRI scanning session on the following day, each run consisted of both experimental and control trials that were presented in a pseudorandom order on successive trials, and each one of the conditional instruction cues (i.e., the four colored crosses) appeared four times mixed with an equal number of trials presenting the control cue (black fixation cross). Each trial started with the instruction phase, during which a colored fixation cross was presented for 1 s instructing the subjects to allocate cognitively attention to one of the visual abstract target stimuli based on the conditional rules that they had previously learned (Figure 3). Note that the target stimuli were not present on the screen at this instruction phase of the trial. The presentation of the instruction cues was random but balanced since each instruction cue was presented four times across each run. A delay of 4 s followed the instruction phase, and then the target phase (2 s) when all four abstract visual target stimuli were presented, and the subject had to select the target stimulus that had been indicated earlier by the instruction cue. For both experimental and control trials, there was a fixed intertrial interval of 4 s. The locations of the target stimuli changed randomly on a trial-by-trial basis and, therefore, the participants could not rely on spatial information to make their selection but were rather required to attend to and select the non-spatial visual stimulus indicated by the instruction cue based on the previously learned associations between the instruction cues and the abstract designs. In the target phase of each trial, the subjects pressed the correct one of four buttons on a response pad to indicate the current position of the target stimulus that was attended earlier based on the color of the instruction cue on that particular trial. The trial ended with a blank screen.
Note that only the experimental condition involved the instructed cognitive allocation of attention to non-spatial visual perceptual stimuli during the instruction phase of the trial since the black cross in the control trials did not instruct the allocation of attention to any one of the four visual abstract designs and, thus, a comparison of brain activity during the instruction phase of the experimental trials with the instruction phase of the control trials would demonstrate activity related to the covert cognitive allocation of attention.
2.3 MRI acquisition
Scanning was performed using a 3T Magnetom Prisma MRI Scanner (Siemens, Erlangen, Germany), with a 32-channel head coil at the McConnell Brain Imaging Center of the MNI. During the scanning session, an anatomical T1-weighted structural brain image was obtained with a gradient-echo MPRAGE sequence (192 slices, interleaved, voxel size = 1.0 mm3, TR = 2300 ms, TE = 2.96 ms, flip angle = 90, slice thickness = 1 mm, FOV = 256 mm). Subsequently, six runs of 525 images each (48 oblique slices, voxel size = 3.0 mm3, slice thickness = 3.0 mm, TR = 800 ms, TE = 30 ms, flip angle = 600) sensitive to the blood oxygenation level-dependent (BOLD) signal were acquired. Visual stimuli were presented through an LCD projector with a mirror system and the responses of the subjects were recorded using an MR-compatible optical response panel. Both stimulus presentation and the recording of the responses of the subjects were controlled with E-prime (E-prime 3.0; Psychology Software Tools Inc., Pittsburgh). Each of the six runs lasted approximately 6 min and consisted of 32 trials during which the experimental and control trials were presented in a pseudo-random order. The first trial onset in each run was synchronized with the scanner acquisition using a trigger signal generated by the scanner. Both behavioral and functional imaging data were acquired in all trials.
2.4 fMRI data preprocessing
Functional data were preprocessed and analyzed using Statistical Parametric Mapping software (SPM12; Wellcome Department of Cognitive Neurology, UCL, UK). Preprocessing was carried out using the standard steps: all images were realigned, unwarped, and fieldmaps were used for distortion correction. The scans of each participant were corrected for slice timing using the second slice as reference, co-registered to the T1 anatomical scans acquired in the same scanning session, and segmented into gray matter, white matter, and cerebrospinal fluid (CSF). Head motion correction was applied using a 6-parameter rigid-body alignment to model out potential non-linear head motion artifacts. Functional and morphological images were then spatially normalized into the MNI standard stereotaxic space using SPM’s default template, the ICBM152MNI space (Collins et al., 1994). Finally, the normalized functional images were smoothed using a 6 mm full-width half-maximum (FWHM) isotropic Gaussian kernel.
2.5 Statistical analysis
The event-related paradigm examined the difference in BOLD activity during the 1 s instruction phase of the experimental trials in comparison with the instruction phase of the control trials to determine the brain regions involved when allocating attention to non-spatial visual stimuli based on previously learned conditional associations. The onset of the conditions was the presentation of an instruction cue (green, red, yellow, or blue colored fixation crosses) that indicated the allocation of attention to a specific target stimulus (abstract visual design) in the experimental conditional task, or the presentation of a neutral cue (a black fixation cross) that did not indicate the allocation of attention to any target stimulus in the control condition (see Figure 3).
At the first level, the statistical analysis of the fMRI data was based on univariate linear modeling with correlated errors, using a design matrix including the experimental and control conditions, along with six movement parameters (three translation, three rotations), and a constant term included as covariates of no interest. A high-pass filter cutoff of 128s was used to remove low-frequency noise. Each trial was modeled with impulse regressors at the time of the presentation of the instruction stimulus. These regressors were then convolved with the canonical hemodynamic response function and entered into a general linear model (GLM) of each subject’s fMRI data. Sufficient sampling across the hemodynamic response function was acquired in this experiment because of the complete desynchronization of the trial onset time from the acquisition repetition time. This desynchronization was achieved with a repetition time of acquisition (TR, 0.8 s) and a total trial duration of approximately 11 s for both the experimental and control trials. At the second level, parameter estimates through a full factorial model were used to compare the two contrasts obtained for the experimental and control conditions for each participant at the group level.
The resulting t-statistic images were thresholded using Bonferroni correction and random field theory, which considers spatial correlation of the error (Worsley et al., 1996). Significance was assessed based on the spatial extent of consecutive voxels. At the second level, a whole-brain search was carried out, where a single voxel within an estimated gray matter volume of 600 cm3 covered by the slices was significant at a threshold of t = 4.58 (pcorrected < 0.05) (Worsley et al., 1996; Worsley et al., 2004). At the first level and for single subject exploration, a directed Region of Interest (ROI) analysis was performed, where the estimated ROI included the MFG bilaterally, a region reported to have a volume of 9.63 and 10.21 cm3 in the left and right hemispheres, respectively (Goldberg et al., 2013). A predicted cluster of voxels with a t-value > 3 was significant (pcorrected < 0.05) when its threshold extent was >376 mm3, corrected for multiple comparisons (Friston et al., 1995). Functional imaging data of individual subjects were superimposed on their respective anatomical volumes, all transformed into the standard MNI stereotaxic space, to examine the relationship between gyral and sulcal morphology and functional activity within cortical regions.
3 Results
The average success rate for all trials was 99.05% (range 93.75–100%), with a mean success rate for experimental trials of 98.26%, and for control trials of 99.83%. To isolate the functional activity associated with the cognitive allocation of attention to non-spatial visual stimuli (the abstract visual designs), we compared activity in the experimental trials with that in the control trials at the precise time that the instruction cue was presented, i.e., the 1 s instruction phase period. We hypothesized that, at the moment of the presentation of an instruction cue, the subjects would cognitively allocate their attention to the appropriate target stimulus based on the previously learned conditional rules. Furthermore, we examined functional activity in individual subjects by superimposing the t-statistical map computed for each individual subject’s functional activity (non-spatial visual attentional trials minus control trials) onto the subject’s respective anatomical MRI, aligned to the coordinates of the MNI standard stereotaxic space.
3.1 Frontal activations
The group-level analysis demonstrated a significant activity cluster in the left hemisphere in the posterior MFG, where area 8Av is found (Petrides and Pandya, 1999) [MNI coordinate (x, y, z): = −48, 20, 29, t = 5.2, pFWE = 0.0063] (see Figure 4 and Table 1). When functional activity was examined on a subject-by-subject basis, a significant increase in activity was observed consistently in the left posterior MFG (area 8Av) in ten out of the twelve subjects [average MNI coordinate (x, y, z): −43.1 ± 5.5 SD, 19.4 ± 3.9 SD, 33.9 ± 6.2 SD]), with an additional subject showing an activity cluster in the ROI in the left hemisphere that approached the standard level of significance (Table 2). A similar activation was seen in the right hemisphere, reaching significance in seven out of the twelve subjects [average MNI coordinate (x, y, z): 42.6 ± 5.1 SD, 20 ± 4.9 SD, 35.3 ± 7.8 SD]), with one subject showing an activity cluster in the ROI in the right hemisphere that approached significance (Table 2). Thus, in individual subjects, bilateral activation within the predetermined ROI was frequently observed, although it did not always reach the standard level of statistical significance. When examining the MRI volumes of the brain of each individual subject, the significant activity was consistently observed within the ventrocaudal MFG, above the inferior frontal sulcus (ifs), where area 8Av is located (Petrides and Pandya, 1999; Petrides, 2019) (see Figures 1, 4).
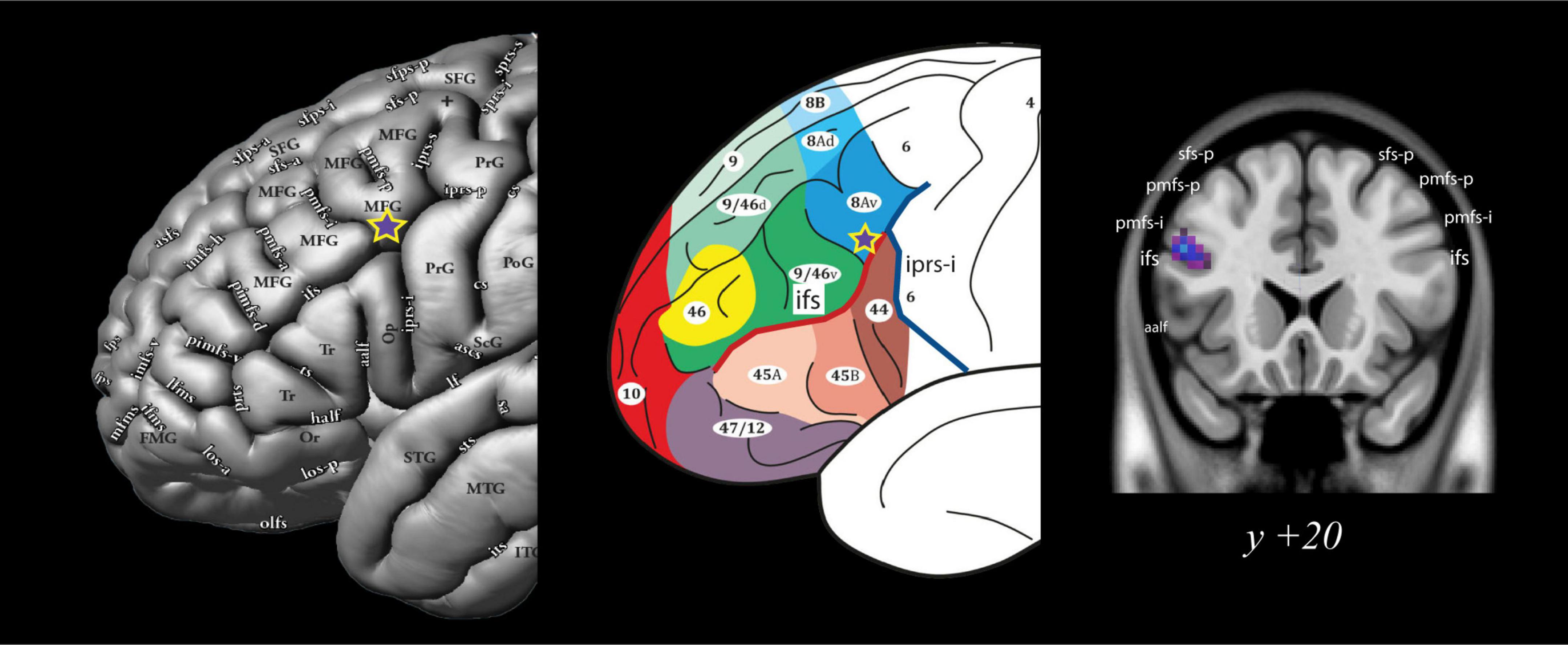
Figure 4. Group level analysis: Location of the activation peak in area 8Av during the conditional allocation of attention to non-spatial visual stimuli. The significant peak is located at the ventral boundary of the caudal middle frontal gyrus at the junction with the caudal inferior frontal sulcus (ifs), i.e., where area 8Av is located (Petrides and Pandya, 1999; Petrides, 2019). Note the purple star with the yellow outline denotes the significant functional activity peak obtained from the comparison of the instruction phase in the experimental non-spatial visual conditional attention task minus the instruction phase of the control task. See Petrides (2019) for additional abbreviations not found in the present text. All figures reproduced with permission. aalf, ascending anterior ramus of the lateral fissure; ifs, inferior frontal sulcus; iprs-i, inferior ramus of the inferior precentral sulcus; pmfs-i, intermediate ramus of the posterior middle frontal sulcus; pmfs-p, posterior ramus of the posterior middle frontal sulcus; sfs-p, posterior ramus of the superior frontal sulcus.
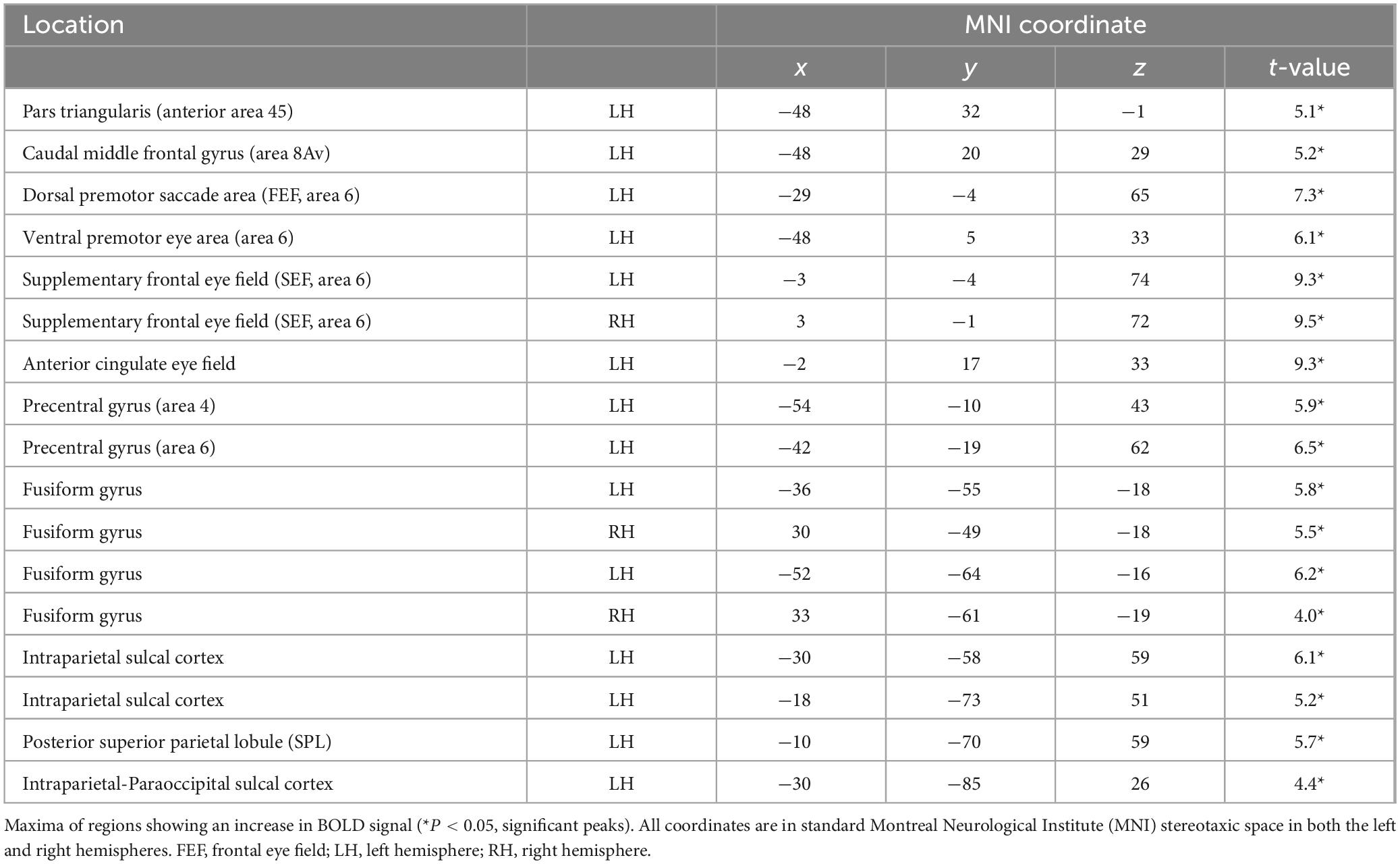
Table 1. Location of significant functional activity peaks across the entire brain from the group-level analysis during the fMRI experiment from the comparison of the instruction phase of the non-spatial conditional experimental trials minus the instruction phase of the control trials.
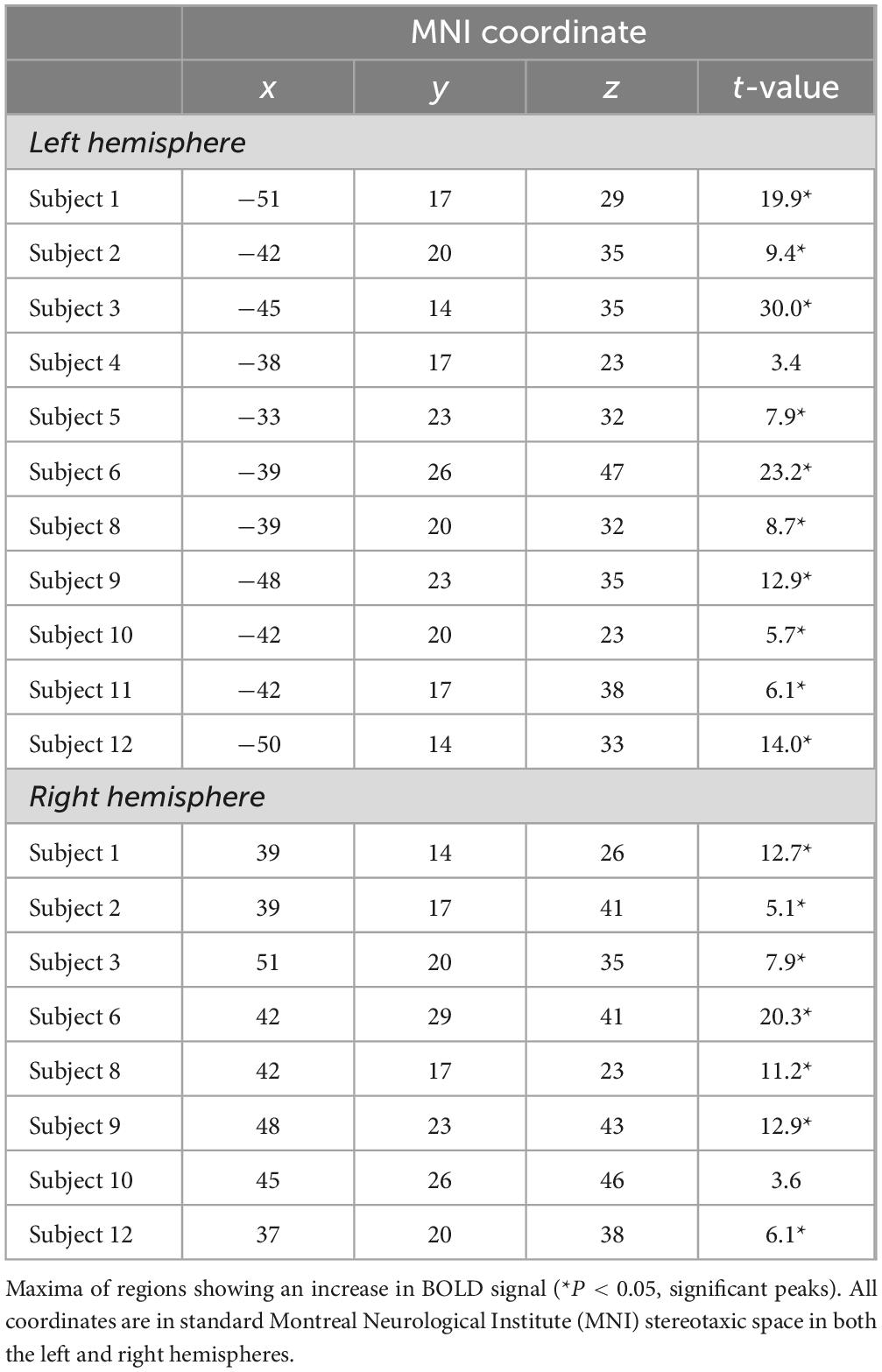
Table 2. Location of the functional activity peaks observed in the caudal middle frontal gyrus (area 8Av) in individual subjects from the comparison of the instruction phase of the non-spatial conditional experimental trials minus the instruction phase of the control trials.
Additional activations within the frontal lobe were observed in the following regions (Table 1). At the group-level, a region was recruited above the horizontal ascending ramus of the lateral fissure (half) in the left hemisphere (Sprung-Much and Petrides, 2020), extending dorsally onto the antero-ventral part of the pars triangularis [MNI coordinate (x, y, z): −48, 32, −1, t-value = 5.1], where anterior area 45 lies (Petrides and Pandya, 2002). Posteriorly in the premotor region of the frontal cortex, there was increased activity in the left hemisphere at the caudalmost part of the superior frontal sulcus (sfs) at the intersection with the superior precentral sulcus (sprs) (Drudik and Petrides, 2024) [MNI coordinate (x, y, z): −29, −4, 65, t-value = 7.3], where the saccadic eye movement area lies. Ventrally in the premotor cortex, there was activity in the left hemisphere within both banks of the posterior extension of the inferior precentral sulcus (iprs-p) (Germann et al., 2005) [MNI coordinate (x, y, z): −48, 5, 33, t-value = 6.1], where the ventral premotor frontal eye field is located.
On the medial surface of the brain, significant peaks were observed anterior to the medial precentral sulcus (mprs) bilaterally [left hemisphere MNI coordinate (x, y, z): −3, −4, 74, t-value = 9.3; right hemisphere MNI coordinate (x, y, z): 3, −1, 72, t-value = 9.5], where the supplementary frontal eye field lies and within the cingulate gyrus (Petrides, 2019) [MNI coordinate (x, y, z): −2, 17, 33, t-value = 9.3] (Table 1).
3.2 Posterior activations
Outside the frontal lobe, group-level analysis revealed significant foci within the cortex of the left intraparietal sulcus. There were three antero-posterior foci of activity: within the depth of the sulcus of Jensen (aips-J) near the inferior termination of the superior parietal sulcus (sps) (Drudik et al., 2023; Zlatkina and Petrides, 2014) [MNI coordinate (x, y, z): = −30, −58, 59, t-value = 6.1], within the depth of the sulcus of Brissaud (sB) [MNI coordinate (x, y, z): = −18, −73, 51, t-value = 5.2], and within the paraoccipital part of the intraparietal sulcus (ips-po) (Petrides, 2019) [MNI coordinate (x, y, z): = −30, −85, 26, t-value = 4.4] (see Table 1). In addition, there was a significant peak within the posterior part of the superior parietal lobule (SPL), in the caudal sps (Drudik et al., 2023; Petrides, 2019) [MNI coordinate (x, y, z): = −10, −70, 59, t-value = 5.7]. Importantly, within the posterior ventral temporal lobe, the instructed allocation of attention to the non-spatial visual perceptual stimuli led to increased activity, bilaterally, within the posterior fusiform gyrus (Petrides, 2019), namely the cortical region that is involved in the perception of visual non-spatial stimuli (see Table 1 for the significant peaks within the fusiform gyrus, as well as other significant peaks).
4 Discussion
The present fMRI study provides novel evidence demonstrating, for the first time, in healthy human subjects the involvement of the posterior MFG, where area 8Av lies, when selectively allocating attention to non-spatial visual stimuli, i.e., stimuli that are defined by their non-spatial visual perceptual features, rather than their location in space. This demonstration is consistent with previous research on the effect of frontal lesions in patients that included the posterior prefrontal region (Petrides, 1985a; Petrides, 1990) and lesions of the periarcuate region in macaque monkeys (Petrides, 1985b; Petrides, 1987), i.e., where area 8Av is found in human and macaque brains, respectively (Petrides and Pandya, 1999). These lesions resulted in a deficit in the conditional allocation of attention to non-spatial, as well as to spatial stimuli. Note that previous functional neuroimaging in healthy adults had only demonstrated the involvement of area 8Av in the allocation of attention to locations in the visual environment (Germann and Petrides, 2020a; Germann and Petrides, 2020b) and, therefore, the question remained whether area 8Av also regulates the allocation of attention to non-spatial visual stimuli, i.e., visual perceptual stimuli independent of their location. The present fMRI study clearly demonstrated activation of area 8Av during the instructed allocation of attention to visual stimuli that were independent of their location. In addition, the present investigation demonstrated the co-activation of area 8Av with premotor frontal areas known to be involved in the control of eye movements and particular areas in the posterior parietal and posterior ventral temporal regions involved in the perceptual processing of stimuli in the environment. Thus, it revealed the essential cortical areas that comprise the functional network involved in the selective allocation of attention to non-spatial visual perceptual stimuli in the environment.
In the present fMRI study, when a comparison of functional activity was conducted between the instruction phases of the experimental and control conditions, the latter not requiring the covert allocation of attention based on instruction cues, an increase in activity was observed within the ventrocaudal MFG, where 8Av is found (see Figure 4). This functional activation was significant in the left hemisphere at both the group level (Table 1) and in individual-subject analysis which, also, revealed a corresponding increase in activity in the specific region of interest in the right hemisphere in seven of the twelve subjects (Table 2). The lateralization of the results within the left hemisphere in most subjects (see Table 1) may be understood in the context of the dominant role of the left hemisphere in language processing in the human brain (Milner et al., 1964; Petrides and Milner, 1982; Rasmussen and Milner, 1975). In particular, the verbalizable nature of the colored instruction cues (e.g., if green instruction cue, attend to visual perceptual stimulus X, but if red instruction cue, attend to visual perceptual stimulus Y) may be producing greater activation in the appropriate area 8Av and co-activated areas in the language dominant left hemisphere.
The bilateral functional activity peaks observed in the fusiform gyrus in the present fMRI study (Table 1) were of particular interest because the fusiform gyrus in the human brain is the final stage in the perceptual processing of visual stimuli (e.g., faces, abstract visual shapes, objects) in the ventral occipito-temporal stream (Allison et al., 1999; Grill-Spector, 2003; Kanwisher et al., 1997; Nasr et al., 2011; Weiner and Zilles, 2016). As is well known from studies in macaque monkeys, the processing of the perceptual features of visual stimuli involves the ventral occipito-temporal stream, in contrast to the dorsal occipito-parietal stream that is involved in the processing of the spatial aspects of visual stimuli (Mishkin and Ungerleider, 1982; Mishkin et al., 1983). Furthermore, occipito-temporal regions have been shown to be anatomically connected to area 8Av in the macaque monkey (Petrides and Pandya, 1999; Petrides and Pandya, 2002). Thus, as predicted, the present functional neuroimaging study demonstrated increased activity in the fusiform gyrus, bilaterally, when subjects were cognitively allocating attention to the associated visual perceptual aspects of the target stimulus in mind. Note that the selective attention network demonstrated in the present fMRI study involved primarily left hemisphere structures and, thus, interaction with the left hemisphere fusiform gyrus. However, given the non-spatial nature of the visual perceptual stimuli used (visual abstract designs), there was also activity in the corresponding right hemisphere fusiform gyrus (Table 1).
In the experimental condition of the present investigation, when the color instruction cue appears, the subject must actively retrieve the specific non-spatial stimulus from memorized knowledge and allocate attention to this non-spatial visual stimulus, i.e., if instruction cue A, retrieve and cognitively attend to target stimulus X, but if instruction cue B, retrieve and attend to target stimulus Y, etc. Anatomical connectivity studies in the macaque monkey have demonstrated that frontal area 8Av is strongly connected with neighboring area 45 (Petrides and Pandya, 1999; Petrides and Pandya, 2002) (see Figure 2) that is known to play a major role in the selective retrieval of relevant information from posterior cortical areas (Kostopoulos and Petrides, 2003; Kostopoulos and Petrides, 2008). Thus, in the present study, area 45, which is involved in the selective retrieval of information from memory, is co-activated with adjacent area 8Av, which is allocating attention to the retrieved stimulus (Table 1).
The covert allocation of attention to a particular environmental stimulus by area 8Av will also set in a state of readiness the premotor frontal eye field areas that would normally organize and execute eye movements to look at the selected visual target stimulus. In the lateral surface of the frontal lobe, two significant activity loci were observed that have been implicated in eye movement related activity. Increased activity was observed at the intersection of the superior precentral sulcus (sprs) with the superior frontal sulcus (sfs) (Drudik and Petrides, 2024), which is the region that has been repeatedly implicated in saccadic eye movement activity (Amiez and Petrides, 2018). In addition, there was increased activity within both banks of the posterior extension of the inferior precentral sulcus (iprs-p) (Germann et al., 2005), i.e., within the ventral premotor eye movement area (see Table 1). It has been suggested that the ventral premotor eye field may be involved in blinking responses (Kato and Miyauchi, 2003a; Kato and Miyauchi, 2003b). Furthermore, there was activity in the medial frontal lobe within the supplementary eye field in dorsal area 6 immediately anterior to the medial precentral sulcus (mprs), as well as within the cingulate/paracingulate region involved in eye movement activity (Amiez and Petrides, 2014). In the present functional neuroimaging study, we observed activity within these frontal eye field (FEF) regions, found posterior and medial to area 8Av (Petrides and Pandya, 1999), when the instruction cue was presented. We suggest that the higher-order prefrontal cortical area 8Av is involved in the executive process of allocating cognitively attention to a specific stimulus and communicates with the eye field regions to set them in a state of readiness to look at the object of attention as soon as it appears.
In the parietal lobe, activity peaks were observed within the intraparietal sulcal region (Zlatkina and Petrides, 2014) of the left hemisphere (Table 1), namely the parietal cortical region that is known to be involved in the organization of motor activity in space, such as eye movements (Grefkes and Fink, 2005). Tract tracing studies in macaque monkeys have demonstrated that the cortex within the intraparietal sulcus is directly connected with higher order prefrontal cytoarchitectonic areas 8Av and 45 (Petrides and Pandya, 1999; Petrides and Pandya, 2002) [see also case 3 in Petrides and Pandya (1999)]. The lateral intraparietal cortex is also connected with the FEF which in the macaque monkey lies just posterior to area 8Av within the arcuate sulcus (Huerta et al., 1987; Stanton et al., 2005). Functional imaging in human subjects has implicated this cortex as the putative lateral intraparietal area (Astafiev et al., 2003; Bremmer et al., 2001; Corbetta et al., 1998; Shikata et al., 2008) which, in the macaque monkey, has been shown to be involved in visual attention by anticipating and executing saccades (Bisley and Goldberg, 2003; Bisley et al., 2011; Kalaska, 1996; Snyder et al., 1997). Thus, the frontal activity peaks observed in the present fMRI study were co-activated with the intraparietal cortical region in the left hemisphere for the coordination of visuospatial attention to focus the eyes on a particular object in space.
In this investigation, activity was also observed within the left posterior parietal cortex, along and within the posterior intraparietal sulcus, i.e., within the cortex surrounding the sulcus of Brissaud (sB) and the paraoccipital part of the intraparietal sulcus (ips-po), as well as within the posterior SPL (Drudik et al., 2023; Petrides, 2019; Zlatkina and Petrides, 2014). Recent investigations have demonstrated that the macaque posterior parietal region involves several cortical areas, including the posterior part of the SPL that is involved in multisensory integration of certain aspects of visual and somatotopic processing. The caudal superior parietal region is connected directly with area 8Av [see case 3 in Petrides and Pandya (1999)] and the rostral premotor cortex (area 6) (Huerta et al., 1987; Stanton et al., 2005) [see case 1 in Petrides and Pandya (1999)], a region that is also reciprocally connected with area 8Av and found adjacent to the functional activity that was observed in the present investigation. Thus, this activity is a continuation of the activity observed within the intraparietal sulcal region involved in guiding the spatial focus of attention during attention shifting (Corbetta et al., 1995; Rushworth et al., 2001; Vandenberghe et al., 2001; Wager et al., 2004). Interestingly, since the present investigation examined the allocation of attention to non-spatial stimuli, we see, for the first time, the involvement of posterior parietal structures in the modulation of attentional selection to non-spatial stimuli that are embedded in space. This activity represents the state of readiness, on a particular trial, to allocate attention to the location of the visual perceptual stimulus to which attention was directed.
Several investigators have interpreted the interaction between the premotor FEF and the cortex within the intraparietal sulcus as a network establishing a “spatial priority map,” i.e., the current location of a stimulus that is the target of our attention and which will, thus, become the focus of a looking response. Note that both area 8Av (Petrides and Pandya, 1999) and the FEF (Huerta et al., 1987; Stanton et al., 2005) are connected with the intraparietal sulcus. The stimulus that is the object of our attention is thought to be held in this “spatial priority map.” Simultaneous electrophysiological recordings of single neurons in the FEF and the lateral intraparietal sulcus (LIP) of macaque monkeys demonstrated that the processing of stimulus identity and position in LIP preceded activity in FEF and that the signal within the FEF reflected the mapping of the instruction cue on the priority map that guides the focus of attention and looking response (Ibos et al., 2013). In another single neuron recording study (Sapountzis et al., 2018), the LIP activity was shown to reflect the similarity of stimuli to the target, while FEF activity integrated perceptual relevance based on oculomotor decisions. In yet another study (Zhou and Desimone, 2011), researchers recorded neural responses from the macaque FEF and visual occipital area V4, i.e., one of the later occipital stages in the processing of visual information. The data suggested that V4 provides sensory information about the visual perceptual features of the target, whereas the FEF provides a top-down attentional bias toward the target features. The above neurophysiological findings are entirely consistent with the interpretation of the present fMRI results. We argue that the activation in high-level prefrontal area 8Av cognitively allocates attention to a particular stimulus in the environment and sets in a state of readiness the posteriorly located FEF that will organize eye movements to look at the attended target. In the present fMRI experimental design, when the instruction cue is shown, the target stimuli are not in view and, thus, we demonstrate the involvement of area 8Av in the purely cognitive allocation of attention to the target stimuli and the simultaneous state of readiness of the frontal eye field areas to move the eyes to look at the target of our attention as soon as it appears.
In conclusion, the present study is the first to demonstrate the involvement of the posterior middle frontal gyrus, where area 8Av is found, in the high-level allocation of attention to non-spatial visual stimuli based on conditional instruction cues. The whole-brain analysis revealed other co-activated areas, such as the functional involvement of area 45 that is known to be involved in the selective retrieval of acquired knowledge, premotor areas involved in the organization of eye movements in interaction with posterior parietal regions where the current location of the target stimulus is coded, and the fusiform gyrus in the ventral temporal region that is involved in visual perceptual processing. Thus, these results highlighted the critical functional network involved in the cognitive allocation of attention to non-spatial stimuli in the environment.
Data availability statement
All data needed to evaluate the conclusions in the manuscript are present in the manuscript. The data that support the findings of this study are available from the corresponding author upon reasonable request.
Ethics statement
The studies involving humans were approved by Institutional Review Board (IRB) McGill University Faculty of Medicine and Health Sciences. The studies were conducted in accordance with the local legislation and institutional requirements. The participants provided their written informed consent to participate in this study.
Author contributions
KD: Conceptualization, Data curation, Formal analysis, Funding acquisition, Investigation, Methodology, Project administration, Visualization, Writing – original draft, Writing – review and editing. VZ: Conceptualization, Methodology, Supervision, Writing – review and editing. EB: Formal analysis, Writing – review and editing. MP: Conceptualization, Funding acquisition, Methodology, Supervision, Writing – review and editing.
Funding
The author(s) declare that financial support was received for the research, authorship, and/or publication of this article. This work was supported by the Natural Sciences and Engineering Research Council of Canada (NSERC) Individual Research Grant RGPIN-2019-05176 awarded to M. Petrides and a doctoral scholarship from Fonds de Recherche du Québec – Santé sector (FRQS) awarded to K. Drudik.
Acknowledgments
We thank Dr. Jen-Kai Chen for technical assistance with brain imaging data.
Conflict of interest
The authors declare that the research was conducted in the absence of any commercial or financial relationships that could be construed as a potential conflict of interest.
The reviewer SH declared a past co-authorship with the author MP to the handling editor.
Publisher’s note
All claims expressed in this article are solely those of the authors and do not necessarily represent those of their affiliated organizations, or those of the publisher, the editors and the reviewers. Any product that may be evaluated in this article, or claim that may be made by its manufacturer, is not guaranteed or endorsed by the publisher.
Supplementary material
The Supplementary Material for this article can be found online at: https://www.frontiersin.org/articles/10.3389/fnins.2024.1472114/full#supplementary-material
References
Allison, T., Puce, A., Spencer, D. D., and McCarthy, G. (1999). Electrophysiological studies of human face perception. I: Potentials generated in occipitotemporal cortex by face and non-face stimuli. Cereb. Cortex 9, 415–430.
Amiez, C., and Petrides, M. (2014). Neuroimaging evidence of the anatomo-functional organization of the human cingulate motor areas. Cereb. Cortex 24, 563–578. doi: 10.1093/cercor/bhs329
Amiez, C., and Petrides, M. (2018). Functional rostro-caudal gradient in the human posterior lateral frontal cortex. Brain Struct. Funct. 223, 1487–1499.
Astafiev, S. V., Shulman, G. L., Stanley, C. M., Snyder, A. Z., Essen, D. C. V., and Corbetta, M. (2003). Functional organization of human intraparietal and frontal cortex for attending, looking, and pointing. J. Neurosci. 23, 4689–4699. doi: 10.1523/JNEUROSCI.23-11-04689.2003
Bisley, J. W., and Goldberg, M. E. (2003). Neuronal activity in the lateral intraparietal area and spatial attention. Science 299, 81–86.
Bisley, J. W., Mirpour, K., Arcizet, F., and Ong, W. S. (2011). The role of the lateral intraparietal area in orienting attention and its implications for visual search. Eur. J. Neurosci. 33, 1982–1990. doi: 10.1111/j.1460-9568.2011.07700.x
Bremmer, F., Schlack, A., Shah, N. J., Zafiris, O., Kubischik, M., Hoffmann, K. P., et al. (2001). Polymodal motion processing in posterior parietal and premotor cortex: A human fMRI study strongly implies equivalencies between humans and monkeys. Neuron 29, 287–296. doi: 10.1016/s0896-6273(01)00198-2
Collins, D. L., Neelin, P., Peters, T. M., and Evans, A. (1994). Automatic 3D intersubject registration of MR volumetric data in standardized talairach space. J. Comput. Assist. Tomogr. 18, 192–205
Corbetta, M., Akbudak, E., Conturo, T. E., Snyder, A. Z., Ollinger, J. M., Drury, H. A., et al. (1998). A common network of functional areas for attention and eye movements. Neuron 21, 761–773.
Corbetta, M., Shulman, G. L., Miezin, F. M., and Petersen, S. E. (1995). Superior parietal cortex activation during spatial attention shifts and visual feature conjunction. Science 270, 802–805.
Drudik, K., and Petrides, M. (2024). The superior frontal sulcus in the human brain: Morphology and probability maps. Hum. Brain Mapp. 45:e26635.
Drudik, K., Zlatkina, V., and Petrides, M. (2023). Morphological patterns and spatial probability maps of the superior parietal sulcus in the human brain. Cereb. Cortex 33, 1230–1245. doi: 10.1093/cercor/bhac132
Friston, K. J., Holmes, A. P., Poline, J. B., Grasby, P. J., Williams, S. C., Frackowiak, R. S., et al. (1995). Analysis of fMRI time-series revisited. Neuroimage 2, 45–53.
Germann, J., and Petrides, M. (2020a). Area 8A within the posterior middle frontal gyrus underlies cognitive selection between competing visual targets. eNeuro 7, 1–12. doi: 10.1523/ENEURO.0102-20.2020
Germann, J., and Petrides, M. (2020b). The ventral part of dorsolateral frontal area 8A regulates visual attentional selection and the dorsal part auditory attentional selection. Neurosci 441, 209–216. doi: 10.1016/j.neuroscience.2020.05.057
Germann, J., Robbins, S., Halsband, U., and Petrides, M. (2005). Precentral sulcal complex of the human brain: Morphology and statistical probability maps. J. Comp. Neur. 493, 334–356. doi: 10.1002/cne.20820
Goldberg, E., Roediger, D., Kucukboyaci, N. E., Carlson, C., Devinsky, O., Kuzniecky, R., et al. (2013). Hemispheric asymmetries of cortical volume in the human brain. Cortex 49, 200–210.
Grefkes, C., and Fink, G. R. (2005). The functional organization of the intraparietal sulcus in humans and monkeys. J. Anat. 207, 3–17.
Huerta, M. F., Krubitzer, L. A., and Kaas, J. H. (1987). Frontal eye field as defined by intracortical microstimulation in squirrel monkeys, owl monkeys, and macaque monkeys II. cortical connections. J. Comp. Neurol. 265, 332–361. doi: 10.1002/cne.902650304
Ibos, G., Duhamel, J. R., and Hamed, S. B. A. (2013). functional hierarchy within the parietofrontal network in stimulus selection and attention control. J. Neurosci. 33, 8359–8369. doi: 10.1523/JNEUROSCI.4058-12.2013
Kalaska, J. F. (1996). Parietal cortex area 5 and visuomotor behavior. Can. J. Physiol. Pharmacol. 74, 483–498.
Kanwisher, N., McDermott, J., and Chun, M. M. (1997). The fusiform face area: A module in human extrastriate cortex specialized for face perception. J. Neurosci. 17, 4302–4311. doi: 10.1523/JNEUROSCI.17-11-04302.1997
Kato, M., and Miyauchi, S. (2003a). Functional MRI of brain activation evoked by intentional eye blinking. Neuroimage 18, 749–759.
Kato, M., and Miyauchi, S. (2003b). Human precentral cortical activation patterns during saccade tasks: An fMRI comparison with activation during intentional eyeblink tasks. Neuroimage 19, 1260–1272. doi: 10.1016/s1053-8119(03)00223-4
Kostopoulos, P., and Petrides, M. (2003). The mid-ventrolateral prefrontal cortex: Insights into its role in memory retrieval. Eur. J. Neurosci. 17, 1489–1497. doi: 10.1046/j.1460-9568.2003.02574.x
Kostopoulos, P., and Petrides, M. (2008). Left mid-ventrolateral prefrontal cortex: Underlying principles of function. Eur. J. Neurosci. 27, 1037–1049. doi: 10.1111/j.1460-9568.2008.06066.x
Luria, A. R. (1973). “The frontal lobes and the regulation of behaviour,” in Psychophysiology of the frontal lobes, eds K. H. Pribram and A. R. Luria (Cambridge, MA: Academic Press), 3–26.
Milner, B., Branch, C., and Rasmussen, T. (1964). “Observations on cerebral dominance,” in Novartis foundation series, eds A. V. S. de Reuck and M. O’Connor (Ciba Foundation), 200–222.
Mishkin, M., and Ungerleider, L. G. (1982). Contribution of striate inputs to the visuospatial functions of parieto-preoccipital cortex in monkeys. Behav. Brain Res. 6, 57–77. doi: 10.1016/0166-4328(82)90081-x
Mishkin, M., Ungerleider, L. G., and Macko, K. A. (1983). Object vision and spatial vision: Two cortical pathways. Trends Neurosci. 6, 414–417.
Nasr, S., Liu, N., Devaney, K. J., Yue, X., Rajimehr, R., Ungerleider, L. G., et al. (2011). Scene-selective cortical regions in human and nonhuman primates. J. Neurosci. 31, 13771–13785. doi: 10.1523/JNEUROSCI.2792-11.2011
Petrides, M. (1985a). Deficits on conditional associative-learning tasks after frontal- and temporal-lobe lesions in man. Neuropsychologia 23, 601–614. doi: 10.1016/0028-3932(85)90062-4
Petrides, M. (1985b). Deficits in non-spatial conditional associative learning after periarcuate lesions in the monkey. Behav. Brain Res. 16, 95–101. doi: 10.1016/0166-4328(85)90085-3
Petrides, M. (1987). “Conditional learning and the primate frontal cortex,” in The Frontal Lobes Revisited, ed. E. Perecman (New York, NY: The IRBN Press), 91–108.
Petrides, M. (1990). Nonspatial conditional learning impaired in patients with unilateral frontal but not unilateral temporal lobe excisions. Neuropsychologia 28, 137–149. doi: 10.1016/0028-3932(90)90096-7
Petrides, M. (2005). Lateral prefrontal cortex: Architectonic and functional organization. Phil. Trans. R. Soc. B Bio. Sci. 360, 781–795.
Petrides, M. (2019). Atlas of the morphology of the human cerebral cortex on the average MNI brain. Cambridge, MA: Elsevier Academic.
Petrides, M., and Iversen, S. D. (1979). Restricted posterior parietal lesions in the rhesus monkey and performance on visuo-spatial tasks. Brain Res. 161, 63–77. doi: 10.1016/0006-8993(79)90196-3
Petrides, M., and Milner, B. (1982). Deficits on subject-ordered tasks after frontal- and temporal-lobe lesions in man. Neuropsychologia 20, 249–262.
Petrides, M., and Pandya, D. N. (1999). Dorsolateral prefrontal cortex: Comparative cytoarchitectonic analysis in the human and the macaque brain and corticocortical connection patterns. Eur. J. Neurosci. 11, 1011–1036. doi: 10.1046/j.1460-9568.1999.00518.x
Petrides, M., and Pandya, D. N. (2002). Comparative cytoarchitectonic analysis of the human and the macaque ventrolateral prefrontal cortex and corticocortical connection patterns in the monkey. Eur. J. Neurosci. 16, 291–310. doi: 10.1046/j.1460-9568.2001.02090.x
Rasmussen, T., and Milner, B. (1975). “Clinical and surgical studies of the cerebral speech areas in man,” in Cerebral localization, eds K. J. Zülch, O. Creutzfeldt, and G. C. Galbraith (Berlin: Springer), 238–257.
Rushworth, M. F. S., Paus, T., and Sipila, P. K. (2001). Attention systems and the organization of the human parietal cortex. J. Neurosci. 21, 5262–5271.
Sapountzis, P., Paneria, S., and Gregoriou, G. G. (2018). Distinct roles of prefrontal and parietal areas in the encoding of attentional priority. Proc. Natl. Acad. Sci. U.S.A. 115, E8755–E8764.
Shallice, T., and Burgess, P. (1996). The domain of supervisory processes and temporal organization of behaviour. Phil. Trans. R. Soc. B Bio. Sci. 351, 1405–1412. doi: 10.1098/rstb.1996.0124
Shikata, E., McNamara, A., Sprenger, A., Hamzei, F., Glauche, V., Buchel, C., et al. (2008). Localization of human intraparietal areas AIP, CIP, and LIP using surface orientation and saccadic eye movement tasks. Hum. Brain Mapp. 29, 411–421. doi: 10.1002/hbm.20396
Snyder, L. H., Batista, A. P., and Andersen, R. A. (1997). Coding of intention in the posterior parietal cortex. Nature 386, 167–170.
Sprung-Much, T., and Petrides, M. (2020). Morphology and spatial probability maps of the horizontal ascending ramus of the lateral fissure. Cereb. Cortex 30, 1586–1602. doi: 10.1093/cercor/bhz189
Stanton, G. B., Friedman, H. R., Dias, E. C., and Bruce, C. J. (2005). Cortical afferents to the smooth-pursuit region of the macaque monkey’s frontal eye field. Exp. Brain Res. 165, 179–192. doi: 10.1007/s00221-005-2292-z
Vandenberghe, R., Gitelman, D. R., Parrish, T. B., and Mesulam, M. M. (2001). Functional specificity of superior parietal mediation of spatial shifting. Neuroimage 14, 661–673.
Wager, T. D., Jonides, J., and Reading, S. (2004). Neuroimaging studies of shifting attention: A meta-analysis. Neuroimage 22, 1679–1693.
Weiner, K., and Zilles, K. (2016). The anatomical and functional specialization of the fusiform gyrus. Neuropsychologia 83, 48–62.
Worsley, K. J., Marrett, S., Neelin, P., Vandal, A. C., Friston, K. J., and Evans, A. C. (1996). A unified statistical approach for determining significant signals in images of cerebral activation. Hum. Brain Mapp. 4, 58–73.
Worsley, K. J., Taylor, J. E., Tomaiuolo, F., and Lerch, J. (2004). Unified univariate and multivariate random field theory. Neuroimage 23, S189–S195. doi: 10.1016/j.neuroimage.2004.07.026
Zhou, H., and Desimone, R. (2011). Feature-based attention in the frontal eye field and area V4 during visual search. Neuron 70, 1205–1217.
Keywords: allocation of attention, non-spatial, fMRI, dorsal attention network, cytoarchitectonic area 8A, if/then conditional rules
Citation: Drudik K, Zlatkina V, Barbeau EB and Petrides M (2025) The frontal and posterior cortical areas involved in the non-spatial visual allocation of attention in the human brain: a functional neuroimaging study. Front. Neurosci. 18:1472114. doi: 10.3389/fnins.2024.1472114
Received: 28 July 2024; Accepted: 20 December 2024;
Published: 22 January 2025.
Edited by:
Toshihiko Aso, RIKEN Center for Biosystems Dynamics Research, JapanReviewed by:
Guangyu Dan, University of Illinois Chicago, United StatesSuliann Ben Hamed, UMR5229 Institut des Sciences Cognitives Marc Jeannerod, France
Copyright © 2025 Drudik, Zlatkina, Barbeau and Petrides. This is an open-access article distributed under the terms of the Creative Commons Attribution License (CC BY). The use, distribution or reproduction in other forums is permitted, provided the original author(s) and the copyright owner(s) are credited and that the original publication in this journal is cited, in accordance with accepted academic practice. No use, distribution or reproduction is permitted which does not comply with these terms.
*Correspondence: Kristina Drudik, a3Jpc3RpbmEuZHJ1ZGlrQG1haWwubWNnaWxsLmNh