- 1Departamento de Fisiología Facultad de Medicina, Universidad Nacional Autónoma de México, Ciudad de México, Mexico
- 2Doctorado en Ciencias Biomedicas, Universidad Nacional Autónoma de México, Ciudad de México, Mexico
- 3Departamento de Atención a la Salud, Universidad Autónoma Metropolitana Unidad Xochimilco, Ciudad de México, Mexico
- 4Departamento de Neuroquímica, Instituto Nacional de Neurología y Neurocirugía Manuel Velasco Suarez, Ciudad de México, Mexico
- 5Laboratorio de Neurofarmacología Molecular, Departamento de Sistemas Biológicos, Universidad Autónoma Metropolitana Unidad Xochimilco, Ciudad de México, Mexico
- 6Dirección de Investigación, Instituto Nacional de Rehabilitación Luis Guillermo Ibarra, Ciudad de México, Mexico
Traumatic brain injury (TBI) represents a public health issue with a high mortality rate and severe neurological and psychiatric consequences. Mood and anxiety disorders are some of the most frequently reported. Primary and secondary damage can cause a loss of neurons and glial cells, leading to dysfunction of neuronal circuits, which can induce imbalances in many neurotransmitter systems. Monoaminergic systems, especially the dopaminergic system, are some of the most involved in the pathogenesis of neuropsychiatric and cognitive symptoms after TBI. In this work, we summarize the studies carried out in patients who have suffered TBI and describe alterations in the dopaminergic system, highlighting (1) dysfunction of the dopaminergic neuronal circuits caused by TBI, where modifications are shown in the dopamine transporter (DAT) and alterations in the expression of dopamine receptor 2 (D2R) in brain areas with dopaminergic innervation, thus establishing a hypodopaminergic state and (2) variations in the concentration of dopamine and its metabolites in biological fluids of post-TBI patients, such as elevated dopamine (DA) and alterations in homovanillic acid (HVA). On the other hand, we show a large number of reports of alterations in the dopaminergic system after a TBI in animal models, in which modifications in the levels of DA, DAT, and HVA have been reported, as well as alterations in the expression of tyrosine hydroxylase (TH). We also describe the biological pathways, neuronal circuits, and molecular mechanisms potentially involved in mood and anxiety disorders that occur after TBI and are associated with alterations of the dopaminergic system in clinical studies and animal models. We describe the changes that occur in the clinical picture of post-TBI patients, such as alterations in mood and anxiety associated with DAT activity in the striatum, the relationship between post-TBI major depressive disorders (MDD) with lower availability of the DA receptors D2R and D3R in the caudate and thalamus, as well as a decrease in the volume of the substantia nigra (SN) associated with anxiety symptoms. With these findings, we discuss the possible relationship between the disorders caused by alterations in the dopaminergic system in patients with TBI.
1 Introduction
Traumatic brain injury (TBI) is a significant public health problem, affecting millions of people around the world each year. The consequences of a traumatic brain injury extend beyond the immediate physical damage and cause long-term cognitive, emotional, and behavioral complications. Among them, dysregulation of the dopaminergic system has attracted significant attention due to its profound impact on neuropsychiatric outcomes (Bales et al., 2009; Corrigan et al., 2010). Dopamine (DA), a key neurotransmitter, regulates mood, motivation, reward processing, and executive functions. Alterations in dopaminergic signaling are increasingly recognized as key contributors to the pathophysiology of mood and anxiety disorders, conditions frequently observed in TBI survivors (Schultz, 1998; Nieoullon, 2002).
The dopaminergic system includes several critical pathways, including mesolimbic, mesocortical, and nigrostriatal, integral to various neurological and psychological processes. The mesolimbic pathway, often associated with the brain’s reward system, is crucial to motivation and the experience of pleasure. In contrast, the mesocortical pathway is vital for executive functions and cognitive control, while the nigrostriatal pathway predominantly influences motor control but also affects cognitive and emotional responses (Grace and Rosenkranz, 2002; Dunlop and Nemeroff, 2007). Alterations in these pathways after TBI can lead to significant neuropsychiatric sequelae, including depression and anxiety disorders.
Depression and anxiety disorders are particularly prevalent among post-TBI patients, and several studies indicate high rates of comorbidity that complicate recovery and rehabilitation efforts (Green et al., 2008; Ruttan et al., 2008). The intricate interplay between TBI-induced structural brain changes and subsequent dopaminergic dysregulation underscores the complexity of treating these conditions. Furthermore, the chronic nature of these psychiatric disorders following traumatic brain injury highlights the need for a greater understanding of the underlying neuropathological mechanisms (Fleminger and Ponsford, 2005).
Relatively recent and excellent reviews on disorders associated with the deregulation of the dopaminergic system secondary to traumatic brain injury focus on cognitive disorders (Bales et al., 2009, 2010; Chen et al., 2017b; Lan et al., 2019; Verduzco-Mendoza et al., 2021). However, mood and anxiety disorders after traumatic brain injury have not been described thoroughly. So, in this narrative review, we aim to provide a comprehensive overview of the current understanding of dopaminergic dysregulation following TBI and its implications for mood and anxiety disorders. We include the most recent clinical studies and original studies in rodents on the alterations described in the dopaminergic system secondary to TBI, and we highlight those in which these may be related to mood and anxiety disorders secondary to TBI. We also include a brief description of the pathophysiology of TBI, mood, and anxiety disorders and a succinct review of the dopaminergic system.
2 Traumatic brain injury
TBI is defined as a disruption of brain function or any other pathological evidence that emerges from an external physical force that may result in temporary or permanent impairment (Khellaf et al., 2019; Capizzi et al., 2020). This condition represents a public health issue with a high mortality rate and severe neurological and psychiatric consequences in the short and long term, drastically reducing the quality of life of patients (Osborn et al., 2014; Perry et al., 2016; Dixon, 2017). The estimated annual incidence of TBI worldwide is 69 million people, representing 939 cases per 100,000, of which 55.9 million correspond to mild TBI and 5.48 million to severe TBI (Dewan et al., 2018). Likewise, it has been reported that the most affected populations correspond to young men between 15 and 25 years old, followed by older adults and infants. The leading causes of this issue are car accidents, falls, violence, and sports and recreational accidents (Azouvi et al., 2017).
TBI originates when the head is subjected to an external mechanical force that can cause any degree of injury to brain tissue (Agrawal and Branco, 2016). The primary injury determines the morbidity and mortality of TBI, originating at the time of trauma, or the secondary injury, whose effect appears later (Leker and Shohami, 2002). Primary injury, which occurs at the time of impact and is not reversible, includes tearing of white matter, cortical contusion, axonal damage, intracerebral, epidural, and subdural hematoma, subarachnoid hemorrhage, intraventricular hemorrhage, and diffuse edema. At the cellular level, early events of neurotrauma include microporation of membranes, misalignment of ion channels, and protein conformational changes (Maas et al., 2008). With further damage, blood vessels can tear, causing microbleeds and ischemia of brain tissue, which can be extensive or, more commonly, perilesional (Menon et al., 2010; Pinto et al., 2012; Agrawal and Branco, 2016).
Unlike primary injury, secondary injury, which corresponds to late effects (minutes to months), is a potentially reversible process through adequate therapy (Leker and Shohami, 2002). Secondary brain injury induces glial inflammatory responses as well as necrosis and apoptosis of neurons and glial cells, such as oligodendrocytes, leading to demyelination and loss or dysfunction of neuronal circuits (Pavlovic et al., 2019); this could induce an imbalance of monoaminergic systems involved in the pathogenesis of neuropsychiatric and cognitive symptoms after a TBI (Jenkins et al., 2016). In addition, brain injuries can progress for weeks or months after the initial damage extends to vulnerable brain regions, such as the prefrontal cortex, hippocampus, thalamus, striatum, amygdala, and forebrain nuclei involved in the subject’s cognitive and emotional functioning.
3 Psychiatric manifestations secondary to TBI
There is extensive literature about the cognitive change observed after TBI, including deficits in executive functions, attention, and working memory (Cristofori and Levin, 2015). However, changes in emotional processing after TBI have been less well described. Although they may result in poorly integrated self-representation and dysfunctional interpersonal relationships, increasing the patient’s vulnerability to developing affective disorders (Jorge, 2015). Acquired neurological injuries secondary to TBI are associated with a broad spectrum of pathologies in mood and other psychiatric manifestations (Donnemiller et al., 2000). Depression and anxiety disorders occupy first and second place among the most common neuropsychiatric disorders in post-TBI patients (Mallya et al., 2015; Braga et al., 2022).
Among TBI’s most common and difficult-to-treat long-term psychiatric complications are depressive disorders, with an estimated prevalence of 20 to 45% (Kreitzer et al., 2019). Anxiety disorders are more common in patients who have suffered a mild TBI than a severe one, in contrast to cognitive impairments that increase with the severity of the injury (Bryant et al., 2010; Corrigan et al., 2023). Although the mechanisms underlying the association of TBI and depressive behaviors are still unclear (Jahan and Tanev, 2023), there is evidence in various animal models that depression is associated with a functional deficit of monoamines (noradrenaline, NA; serotonin, 5-HT, and DA) in specific brain sites (Traeger et al., 2020).
Regarding DA, Bales et al. (2009, 2010) proposed a hypothesis where changes in the neurotransmission of this molecule are reflected in the development of cognitive and behavioral dysfunction and emotional lability in subjects who suffered a TBI. Sequelae observed may depend on the extent of the injury on the dopaminergic pathway(s) involved. Alterations of the nigrostriatal pathway include deficits in working memory and emotional and behavioral issues, usually correlated with low concentrations of DA; manifestations observed as dysfunction of the mesolimbic and mesocortical pathways are depression, anxiety, and substance abuse, associated with loss of DA homeostasis (Chen et al., 2017b, 2018). Some of the behavioral or personality disorders secondary to TBI are attributed to executive dysfunction associated with diffuse axonal damage and interruption of corticostriatal connectivity (Pischiutta et al., 2018), but the neural bases of these problems and others have remained uncertain; similarly, changes in mood, anxiety or depression associated with TBI can be the outcome of events as prominent as diffuse axonal damage or interruption of neuronal circuits within dopaminergic pathways (Chen et al., 2017b).
These data underpin the importance of dopaminergic transmission in post-TBI disorders. However, it is worth noting that there potentially exists a significant heterogeneity of mechanisms leading to depression/anxiety following TBI (Jahan and Tanev, 2023).
Likewise, another factor to consider is the patient’s neuropsychiatric state before the TBI. It has been described that the rate of neuropsychiatric disorders is much higher among patients with TBI than among subjects without TBI, even before the TBI occurs (Albrecht et al., 2020). Besides, it is worth noting that injuries occurring in a psychologically traumatic context could independently confer risk for mood disorders. In a recent study of patients with mild TBI or with orthopedic injuries not involving the head, it was reported that patients with mild TBI were more likely to report Posttraumatic Stress Disorder (PTSD) and/or major depressive symptoms, especially those with prior mental health problems, or when the TBI was associated with an assault or other violent cause (Stein et al., 2019).
4 Mood or affective and anxiety disorders
The mood has been defined as the internal and sustained emotional state or tone of a person that gives “color” to their perception of being part of the world, whereas the affective state is the expression of the mood, being interpreted by the clinician as the patient’s state of mind. Some authors have suggested combining both elements in a new name: “emotional expression,” and many others use them interchangeably (Sadock et al., 2015). Anxiety is an anticipatory response to a future threat; it differs from fear in responding to an imminent threat.
Mood or affective disorders are described as overt disturbances in emotions (severe dips called depression or ups and downs called hypomania or mania). According to the Diagnostic and Statistical Manual of Mental Disorders, Fifth Edition (DSM-5), mood disorders are categorized into (1) bipolar and related disorders and (2) depressive disorders (Spijker and Claes, 2014; Sekhon and Gupta, 2023). Even though anxiety is a common symptom in some depressive disorders, anxiety disorders are considered a family of mental disorders different from affective disorders, according to the DSM-5.
5 Mood and anxiety disorders associated with traumatic brain injury
For many TBI survivors, sensorimotor and neurological consequences developed over time can lead to mood and anxiety imbalances that severely affect their quality of life. These behavioral changes vary depending on the type of injury and the type of injured brain tissue. Protracted recovery after a traumatic brain event has been consistently linked with the emotional capacity of individuals to cope with possible physical (mobility), psychiatric, and social impairments (Yehene et al., 2020). Also, the ability to accept a possible disability prevents the normal reintegration of patients into society (Yehene et al., 2020).
Depressive disorders associated with TBI are generally classified, according to the DSM-5, as mood disorders due to another medical condition, with subtypes: (1) major depression, (2) prominent depressed mood, and (3) mixed features. TBI-related bipolar disorders are subdivided into (1) manic or hypomanic episodes, (2) manic features, or (3) mixed characteristics (Jorge, 2015). At the same time, anxiety disorders fall under the “due to another medical condition” classification.
Various studies report that during the months after TBI, anxiety and post-traumatic stress frequently occur and can persist for several years, significantly deteriorating the quality of life of patients and the people around them (Whelan-Goodinson et al., 2009; Huang et al., 2020; Robert, 2020).
Depression is a mood disorder that causes persistent sadness, constant negative thoughts, apathy, lack of energy, cognitive distortions, nihilism, and inability to enjoy everyday life events (Guillamondegui et al., 2011). In patients with TBI, depression is one of the most common disorders. Its treatment can be challenging since its etiology is uncertain and multifactorial and can develop through multiple pathways that are not necessarily the product of the primary brain lesion (Juengst et al., 2017). The risk of developing depressive disorders in adults who have suffered moderate to severe TBI has been reported to range from 66 to 73% in the first 6 months after the trauma (Abdullah et al., 2018; Ryttersgaard et al., 2020; Eliasen et al., 2021; Sameh et al., 2021). In 2016, Perry reviewed 57 studies and reported an odds ratio of 2.14 for the relationship between depression and having suffered a TBI (Perry et al., 2016). Some studies have focused on a specific age group; namely, in the elderly (≥65 years), the risk ranges between 16 and 29% (Vadlamani and Albrecht, 2020), while in the pediatric population, 33 to 50% (Laliberté Durish et al., 2018). Although anxiety is the most common affective disorder diagnosed within the first 12 months after TBI (Lamontagne et al., 2022), its onset can arise several years later (Albicini and McKinlay, 2018). The incidence of this condition ranges between 11 and 17% in adults after the occurrence of a TBI (Osborn et al., 2016; Albrecht et al., 2017; Abdullah et al., 2018; Ponsford et al., 2020), whereas the incidence in children and adolescents between 5 and 18 years old is 8% (Albicini et al., 2020).
Despite the devastating and disabling consequences of these disorders, their follow-up is often not adequate in clinical practice, especially for those patients who have suffered a mild TBI. Mood and anxiety disorders resulting from brain injury are multifactorial. Therefore, to propose pharmacological treatment, it is necessary to identify the involved neurobiological systems succeeding TBI (Juengst et al., 2017). For example, structural and functional changes that occur after TBI due to the initial mechanical damage induce the interruption of neural circuits, mainly in the prefrontal cortex (Koenigs and Grafman, 2009), the hippocampus (Hercher et al., 2009), the striatum (Carlson and Dixon, 2018; Leconte et al., 2020), and the thalamus (Osborn et al., 2014). These brain nuclei are closely related to dopaminergic neurotransmission (Hasler et al., 2008).
6 Dopaminergic system
DA is one of the most important neurotransmitters in the central nervous system (CNS), and its dysregulation has been consistently associated with the development of diverse neurological and psychiatric diseases (Klein et al., 2019).
DA is synthesized in the cytosol of dopaminergic neurons when tyrosine hydroxylase (TH) catalyzes the addition of a hydroxyl group at the meta position of tyrosine to produce L-3,4-dihydroxyphenylalanine (L-DOPA). Subsequently, L-DOPA is decarboxylated by the enzyme DOPA decarboxylase (DDC), synthesizing DA (Harsing, 2008; Meiser et al., 2013). Once DA is synthesized in the neuronal cytoplasm, it is stored in synaptic vesicles within presynaptic terminals by vesicular monoamine transporter-2 (VMAT2), located in the membrane of cell vesicles where it is stored and protected from intraneuronal deamination (Mulvihill, 2019). Once released, DA excites dopaminergic neurons through interactions with postsynaptic receptors and undergoes a reuptake process to stop signaling via the DA transporter (DAT; Mulvihill, 2019). DAT is a transmembrane protein expressed exclusively in dopaminergic neurons (Harsing, 2008). The remaining DA still in the cytosol is degraded by the action of monoamine oxidase (MAO) through an oxidative deamination process (Meiser et al., 2013). Also, DA can be taken up by surrounding glial cells. Glia readily degrades DA by the enzymatic action of MAO and by catechol-O-methyl transferase (COMT). The primary metabolites derived from DA degradation are dihydroxyphenylacetic acid (DOPAC) and homovanillic acid (HVA; Myöhänen et al., 2010).
6.1 Dopaminergic receptors
Five types of dopaminergic receptors (D1R, D2R, D3R, D4R, and D5R) belonging to the family of G protein-coupled receptors have been identified, located on dendrites, cell bodies of neurons, and axons or nerve terminals. These receptors are divided according to differences in their characteristics and signaling mechanisms. The family of D1-like receptors includes subtypes D1R and D5R, being associated with the increase of cyclic adenosine monophosphate (cAMP) through Gs proteins, stimulating the activation of adenylate cyclase (AC) in the striatal and retinal membranes. D1R is closely related to motor function, growth, development, reward, sleep, impulse control, reproductive behavior, working memory, and learning (Rodrigues et al., 2007; Ortiz et al., 2010; Ayano, 2016). The highest density of D1R is found in the mesolimbic, nigrostriatal, and mesocortical areas, which include the substantia nigra (SN), olfactory bulb, nucleus accumbens (NAc), caudate, putamen, and nucleus striatum (Ayano, 2016).
Conversely, D5R is expressed mainly in the SN, hypothalamus, hippocampus, and sympathetic ganglia. Various data indicate that these receptors are involved in nociceptive, affective, and endocrine processes regulated by DA (Ayano, 2016).
The family of D2-like receptors is formed by dopaminergic receptors D2R, D3R, and D4R; these are coupled to a Gi protein, which inhibits AC. D2R modulates working memory, reward-motivational functions, renal functions, gastrointestinal motility, vasodilation, and locomotion. These receptors have a higher expression in the SN, the olfactory bulb, the caudate, the putamen, the ventral tegmental area (VTA), and NAc (Ayano, 2016). In comparison, D3R is involved in endocrine function, emotions, and regulation of motor functions. They are expressed mainly in the islands of Calleja, the septal region, the medial and lateral geniculate nuclei of the thalamus, the medial mammillary nucleus of the hypothalamus, and Purkinje cells of the cerebellum (Ayano, 2016). Finally, D4R is related to cognitive and emotional functions and the reward system. They are expressed mainly in the SN, the hippocampus, the amygdala, the thalamus, the hypothalamus, the cerebral cortex, the sympathetic ganglia, and the globus pallidus (GP; Harsing, 2008).
6.2 Dopaminergic neurotransmission
Dopaminergic innervation is the most prominent in the brain. Four main dopaminergic pathways have been identified (Figure 1).
6.2.1 Nigrostriatal pathway
Here, dopaminergic projections are originated in the substantia nigra pars compacta (SNc) and are projected rostrally to be widely distributed in the basal ganglia (caudate nucleus and putamen) (Ko and Strafella, 2012). In this pathway, DA cell bodies send ascending projections to the striatum, playing an essential role in movement, especially in motor function control and learning new movement skills (Ayano, 2016).
6.2.2 Mesolimbic pathway
Within the mesolimbic pathway, dopaminergic projections originate in the mesencephalon’s VTA. They are projected towards the mesocortical and limbic system (through the NAc) for their subsequent dissemination in the amygdala, the piriform cortex, the septal nuclei sides, and the NAc. These neurons are closely involved in several primary CNS functions, including voluntary movement, feeding, affection, reward, sleep, attention, working memory, and learning (Beaulieu and Gainetdinov, 2011). This pathway regulates pleasure by releasing DA in ludic situations and stimulating the body to seek a pleasant activity or occupation (Ayano, 2016).
6.2.3 Mesocortical pathway
In the mesocortical pathway, dopaminergic fibers also arise from the VTA and project to the frontal cortex and septohippocampal regions. DA in this region regulates cognitive and emotional behavior. DA levels in the brain, especially in the prefrontal cortex, help improve working memory and attention (Björklund and Dunnett, 2007; Ayano, 2016).
6.2.4 Tuberoinfundibular pathway
The tuberoinfundibular pathway originates in the arcuate nucleus of the hypothalamus (arcuate and paraventricular nuclei) and projects to the pituitary gland (the median eminence). In this pathway, DA inhibits prolactin release.
7 Evidence of alterations in the dopaminergic system associated with traumatic brain injury
7.1 Clinical studies in human subjects
Table 1 summarizes studies carried out in patients with TBI (mild, moderate, or severe) and alterations in the dopaminergic system at the central nervous system level. The approach taken in these includes (1) the dysfunction of the dopaminergic neuronal circuits and (2) modifications in the concentration of DA and its metabolites.
7.1.1 Dysfunction of dopaminergic neuronal circuits
The physiological changes in the dopaminergic system after TBI are closely linked to the direct mechanical damage of projection pathways. These pathways produce DA from brainstem nuclei, the retrorubral region (A8) of the VTA (A10), and the SNc (A9). These nuclei project towards the striatal, subcortical, and cortical regions via nigrostriatal, mesolimbic, and mesocortical pathways. Structural imaging suggests that TBI can disrupt these dopaminergic pathways, with magnetic resonance (MR) studies indicating that even 7 months after a moderate to severe TBI, patients show a diminished anisotropy index in most white matter tracts, axonal injury, and volume reduction of frontal lobes, striatum, and cerebellum, highlighting the long-term effects of TBI on the dopaminergic system.
Wagner et al. in 2014 reported decreased DAT and elevated expression of D2R in various brain regions, including the caudate, putamen, and ventral striatum, as determined using positron emission tomography (PET), in patients with moderate to severe TBI and executive function deficit, based on neuropsychological tests, suggesting the establishment of a hypodopaminergic state (Wagner et al., 2014). Jenkins et al. (2020) analyzed the alterations of the DAT, using single photon emission computed tomography (SPECT), in 43 patients with moderate to severe TBI in the chronic phase (more than 6 months from the lesion) and with cognitive difficulties. They found that patients with TBI present a decrease in DAT in the caudate nucleus, which has been related to hypodopaminergic states and motor alterations other than Parkinson’s disease in the early stages (Table 1).
On the other hand, an asymmetric depletion in DAT expression in the striatum 48 days after a moderate to severe traumatic brain injury has been reported and evaluated by SPECT, suggesting a heterogeneous disruption of the nigrostriatal pathway with low cognitive functioning and moderately severe disability (Womack et al., 2020; Table 1).
In Donnemiller et al. (2000) (Table 1) determined the function of the DAT and the D2R in the striatum by SPECT and the presence of cortical and subcortical lesions by cranial computerized tomography (CCT) or MR in 10 patients with TBI (1 with Glasgow Coma Scale, GCS = 15, 1 with GCS = 12 and 8 with GCS less than or equal to 8), all in the chronic phase with an average time of 140.8 ± 94.2 days since the injury. On SPECT images, abnormal patterns of reduced tracer uptake were observed in the striatum in all patients, with an average 45% reduction in DAT binding and an average 73% reduction in D2R compared to controls. The degree of post-traumatic dysfunction of DAT and D2R in the striatum was unrelated to the severity of the injury initially assessed by GCS. Therefore, the authors concluded that the anatomical and functional alterations of the nigrostriatal or frontostriatal pathways could be more related to the clinical pictures presented by the patients, such as akinetic-rigid characteristics (Donnemiller et al., 2000); in this study, no alterations in behavior or mood disorders were reported; however, it was shown that, regardless of the GCS, there are alterations in the dopaminergic pathways in patients who have suffered a TBI but the depicted clinical pictures can also be the consequence of slow-onset and subtle events, such as receptor modifications, the interaction of DA with its receptors, changes in DAT, or the generation of sustained or alternating hypodopaminergic states (Figure 2).
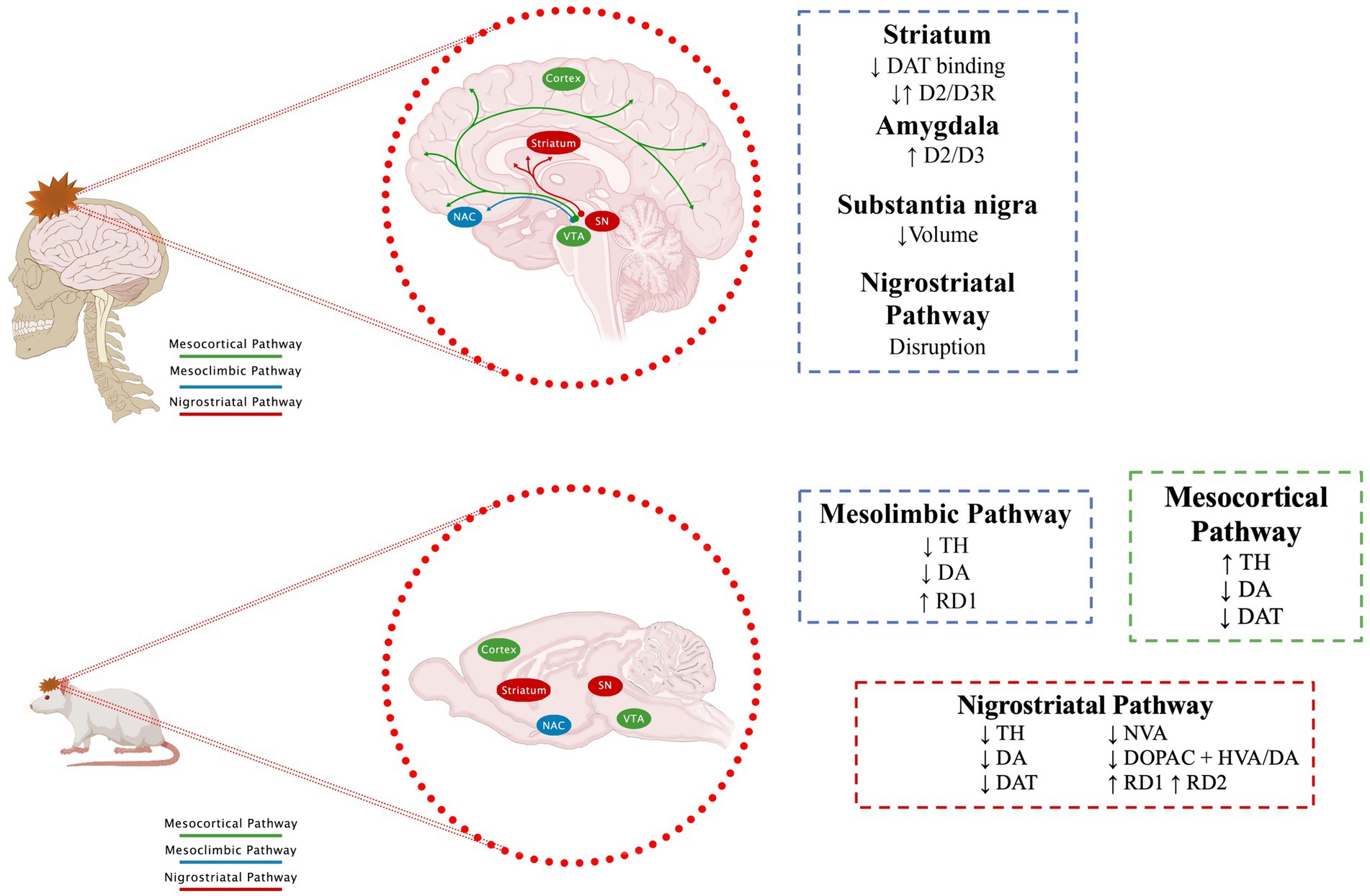
Figure 2. Effect of TBI on dopaminergic pathways. The main dopaminergic pathways and changes described after a TBI are shown. See text for further details. Section 7.1 for human data and section 7.2 for animal models.
7.1.2 Changes in the concentration of DA and its metabolites
Furthermore, it is worth noting that several authors have reported alterations in the levels of DA and its metabolites in the biological fluids of patients post-TBI (Table 1). For example, Wagner et al. (2007a) studied 63 patients with severe TBI. They found elevated levels of DA and HVA in the patients in the 5 days following the TBI, which agrees with the study by Markianos et al. (1992), who had reported elevated levels of HVA in the cerebrospinal fluid (CSF) of 24 patients in coma after head injury, not like the report by Vecht et al. (1975), who found decreased HVA levels in patients sampled in the first hours post-trauma. Likewise, different reports in plasma from patients who have suffered a TBI indicate elevated levels of DA compared to controls (Hamill et al., 1987; Woolf et al., 1987, 1988; Yang et al., 1995; Singh et al., 2022). There are even some reports of increases in DA metabolites in the urine of patients who have suffered a TBI (Feldman et al., 1993; Johnson et al., 1993).
7.2 Animal model studies
Similarly, studies in animal models have reported that TBI induces an imbalance in dopaminergic transmission in brain regions that comprise dopaminergic pathways (Figure 2). Since, Edvinsson et al. (1971) reported a significant decrease in DA levels in the brains of rabbits after 48 h of inducing a TBI by inserting a small pressure cannula into the brain (Edvinsson et al., 1971). Subsequent studies have reported changes in specific regions. For example, in SN (Table 2), the predominant findings show reductions in TH levels, regardless of whether the model used was a rat (Hutson et al., 2011; Sauerbeck et al., 2012; Van Bregt et al., 2012; Acosta et al., 2015; Tan et al., 2015; Liu et al., 2017) or mouse (Selvakumar et al., 2020; D'Amico et al., 2021), this decrease in TH is observed from one to 78 days after TBI. Only Schmidt and Grady (1995), who did not do a quantitative analysis, did not find changes in TH. Yan, who used a severe TBI, reported a delayed increase in TH 28 days after TBI, interpreting it as a possible compensatory response (Yan et al., 2007). Despite the changes described in TH, no significant changes were reported in DA. Regarding some DA metabolites such as HVA, Van Bregt et al. (2012) reports a decrease, while Liu et al. (2017) find an increase. In all cases, immunohistochemistry was used to analyze TH; some studies even use stereology and/or WB for better quantification. The sample sizes (3 to 10) are those commonly reported in these studies.
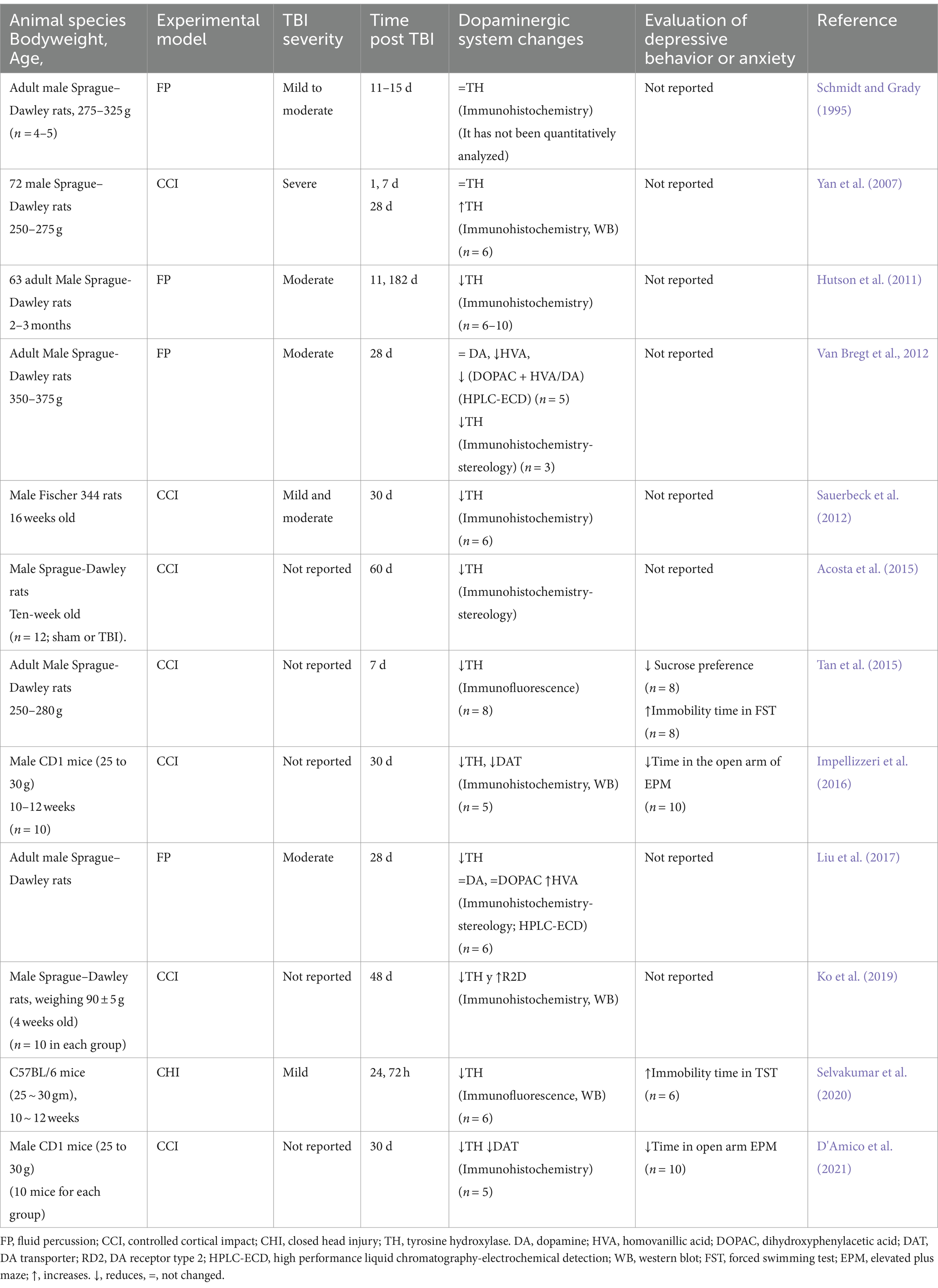
Table 2. Alterations of the dopaminergic system in substantia nigra secondary to TBI in rodent models.
Regarding the striatum, it is worth highlighting that there are many more reports (Table 3). Some authors report an increase in DA in the first hours after injury (McIntosh et al., 1994; Massucci et al., 2004; Muthuraju et al., 2014), while in chronic stages, the release and levels of DA decrease (Shin et al., 2011; Huang et al., 2014), as well, TH diminish its expression as the injury time elapses (McIntosh et al., 1994; Massucci et al., 2004; Wagner et al., 2005a; Shin et al., 2011; Huang et al., 2014; Chen et al., 2015; Tan et al., 2015). Also, it has been reported that after a TBI, there is a significant decline of DAT in the striatum (Wagner et al., 2005a,b; Karelina et al., 2017), a decrease or no change of HVA and DOPAC (Shin et al., 2011; Chen et al., 2015). It is also worth noting that the changes observed depend on the magnitude of the damage. In this sense, Chen found a significant decrease in DA release in mild and severe injuries; However, this decrease is temporary in mild injuries (Chen et al., 2015).
It is worth noting that, in general, HPLC-ECD was used to report DA levels, and the sample sizes referred to are those generally reported in these studies. However, some authors find increases in DA in the first hours post-TBI, and others find decreases.
In the cerebral cortex (Table 4), there is greater diversity in the reports. Some authors find an increase in DA levels, not only in the acute phase (Massucci et al., 2004) but also in the chronic phase (Kobori et al., 2006; Tanaka et al., 2019); likewise, some authors report that there are no changes in DA levels (Tsuda et al., 2020; Hu et al., 2023) or even find decreases (McIntosh et al., 1994; Rana et al., 2020). Similarly, there are reports of an increase in TH (Kobori et al., 2006), a decrease (Edut et al., 2014), or no changes (Kaukas et al., 2021). Yan et al. (2001) using a model of controlled cortical impact (CCI) in Male Sprague–Dawley rats, did not find changes in TH expression in the first 14 days but observed an increase at 28 days (Yan et al., 2001). Once again, it is worth noting that the results reported are not homogeneous despite using the same analysis techniques. In particular, in this table, the observations also depend on the subregion of the cerebral cortex analyzed.
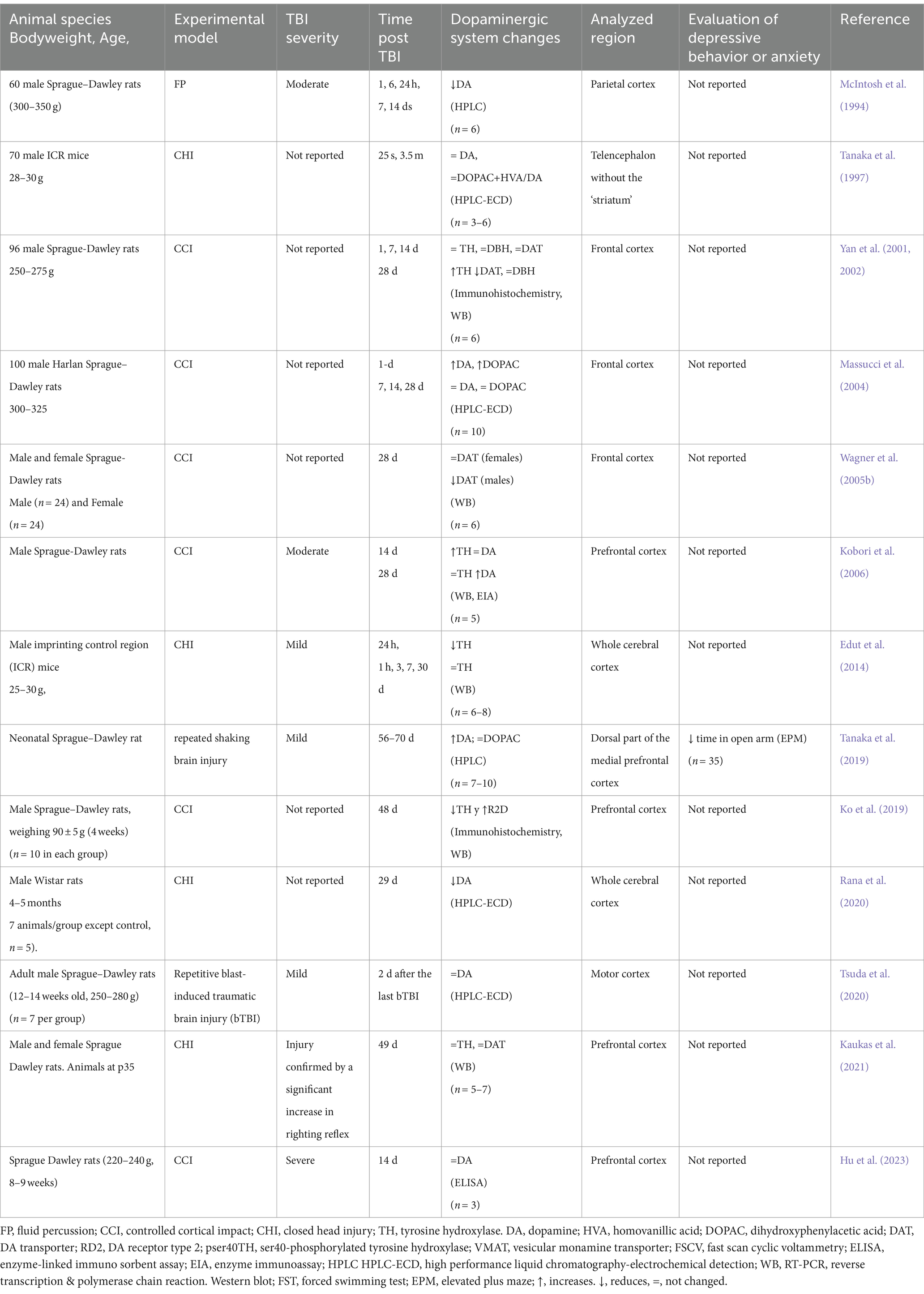
Table 4. Alterations of the dopaminergic system in cerebral cortex secondary to TBI in rodent models.
Regarding NAc (Table 5), Chen et al. (2017a, 2018) find a decrease in DA release and reuptake in the core and shell and more significant changes in the core, while Huang et al., 2014 do not find differences in total DA. Regarding TH, there is a report of an increase in its expression at 49 days post-TBI (Kaukas et al., 2021), and concerning the expression of D1R, Bhowmick et al., 2021 describe a decrease at 2 days post-TBI, while Vonder Haar et al., 2019a finds an increase after 25 days.
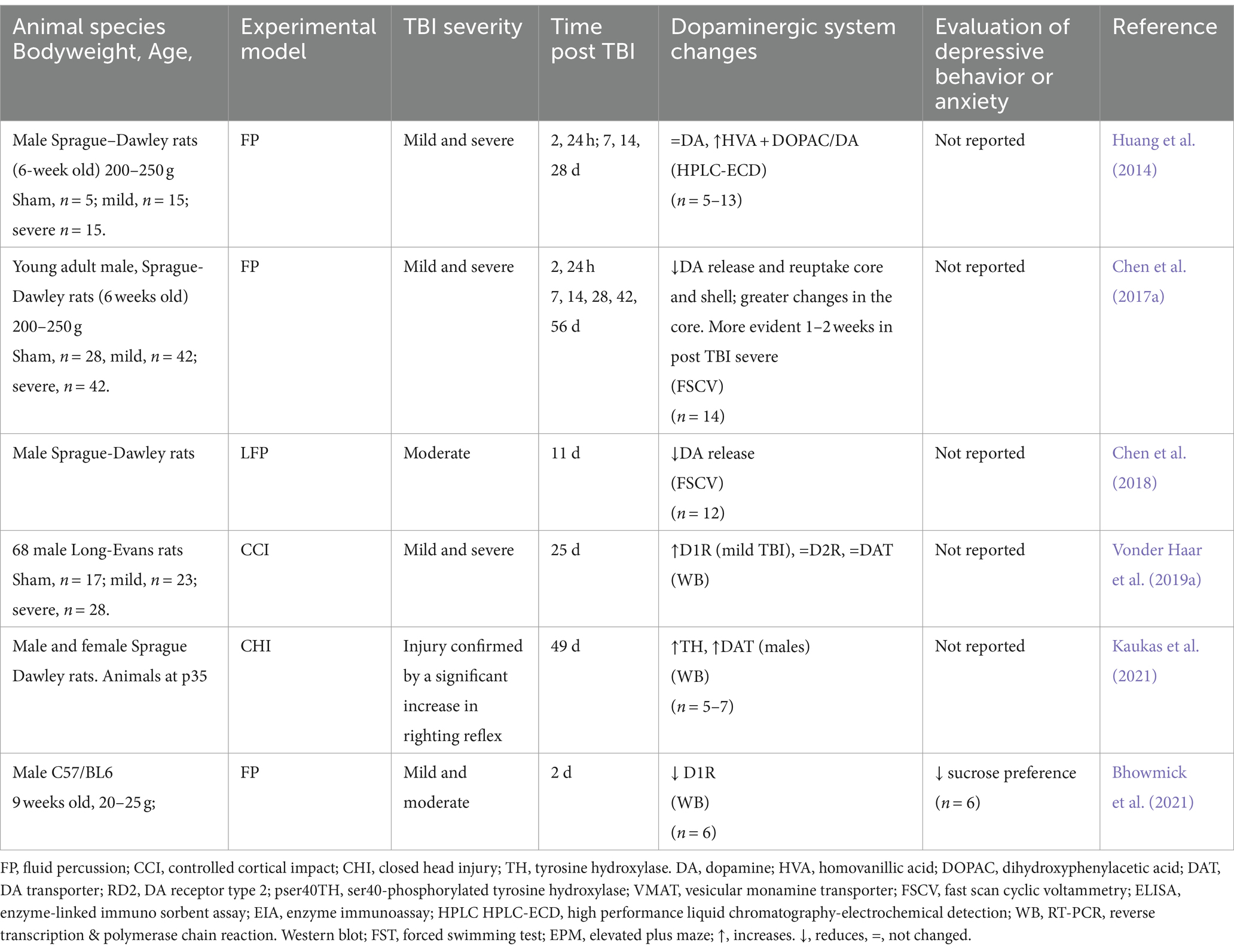
Table 5. Alterations of the dopaminergic system in the nucleus accumbens secondary (NAc) to TBI in rodent models.
Concerning other regions analyzed (Table 6), there are only a few reports, for example, in the brainstem or specifically the locus coeruleus (LC), where no changes in DA are reported (McIntosh et al., 1994; Tsuda et al., 2020), or in VTA, where there is only one report in which a decrease in TH is referred, 49 days after the TBI (Karelina et al., 2017). Another less studied region is the hippocampus, where some authors report that there are no changes in DA levels (Tsuda et al., 2020) or it decreases (Hu et al., 2023), while in the hypothalamus, there are reports of increased DA (McIntosh et al., 1994).
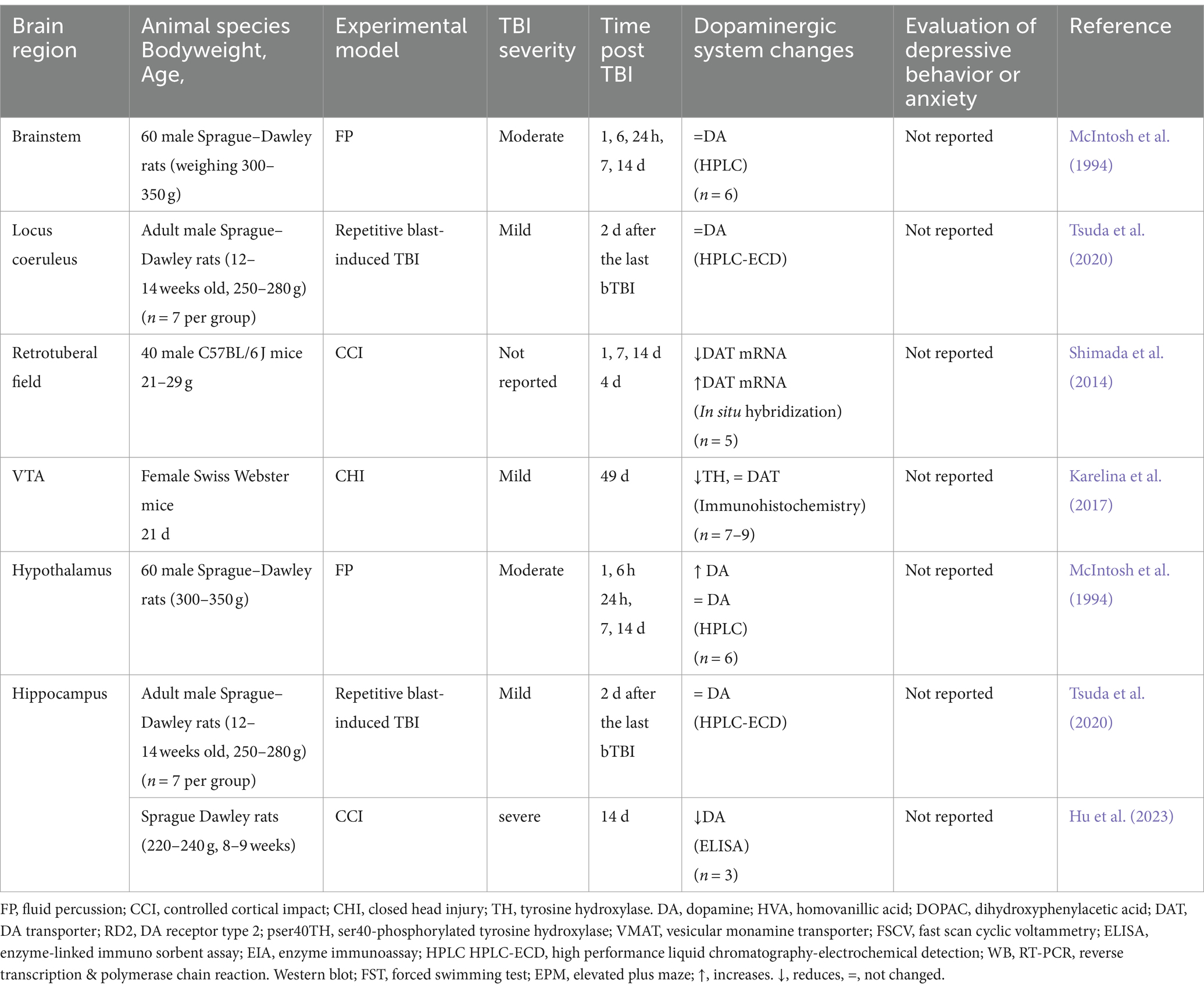
Table 6. Alterations of the dopaminergic system in some cerebral areas secondary to TBI in rodent models.
Although the animal models described in Tables 2–6 are rats and mice, it is worth noting that Kumar and Singh, using a TBI model in Drosophila melanogaster (fruit flies), found a decrease in DA levels 24 h post-TBI (Kumar and Singh, 2023).
8 Potential mechanisms linking TBI mood and anxiety disorders to alterations in the dopaminergic system
As shown in the previous sections, extensive literature validates the alterations of the dopaminergic system after TBI (Tables 1–6) as well as the association of TBI with mood disorders and anxiety (Whelan-Goodinson et al., 2009; Bryant et al., 2010; Guillamondegui et al., 2011; Osborn et al., 2014; Jorge, 2015; Perry et al., 2016; Juengst et al., 2017; Abdullah et al., 2018; Laliberté Durish et al., 2018; Robert, 2020; Ryttersgaard et al., 2020; Eliasen et al., 2021; Sameh et al., 2021). Likewise, several reports indicate that deregulation of the dopaminergic system may be one of the mechanisms for the development of mood and anxiety disorders (Nestler and Carlezon, 2006; Dunlop and Nemeroff, 2007.
In this section, we outline and underscore the potential biological pathways, neuronal circuits, and molecular mechanisms that could be involved in mood and anxiety disorders following TBI and their association with dopaminergic system alterations.
8.1 Impact of TBI on dopaminergic pathways
8.1.1 Nigrostriatal pathway
TBI often damages this pathway, leading to motor deficits and cognitive dysfunction. Inflammation and oxidative stress can reduce DA levels and alter receptor density in the striatum, contributing to Parkinsonism-like symptoms (Dunlop and Nemeroff, 2007; Gao L. et al., 2022). In this sense, it is worth highlighting the recent studies by Gao L. et al. (2022) (Table 1), who reported a decrease in the SN volume of patients who had suffered TBI. They used MR to analyze patients with mild, moderate, and severe TBI who reported anxiety and depressive symptoms. They found a decrease in the size of the SN, particularly the left SN, and reduced functional connectivity in the left SN. The functional connectivity between the left SN and left angular gyrus was positively associated with anxiety symptoms and negatively associated with depressive symptoms (Gao L. et al., 2022). Also, there is evidence in rodent models suggesting that the decreased DA levels after TBI in different brain regions are closely related to the appearance of depression-like behavior; there are reports describing that after a controlled cortical impact or fluid percussion, the preference for sucrose consumption of animals is diminished, and the time of immobility recorded in the forced swim test increases; these behavioral data correlate with altered DA levels in the rat striatum (Nestler and Carlezon, 2006; Tan et al., 2015). Alternatively, the studies by Impellizzeri et al. (2016) and D'Amico et al. (2021) described a decrease in TH and DA in the SN and midbrain associated with a decrease in the time in the open arm of the Elevated plus maze.
8.1.2 Mesolimbic and mesocortical pathways
The brain nuclei composing the mesocortical and the mesolimbic pathways play a predominant role in reward systems (Nestler and Carlezon, 2006; Turner et al., 2007; Heshmati and Russo, 2015), major depression (Heshmati and Russo, 2015), Parkinson’s disease (Wen et al., 2013) and chronic stress (Tye et al., 2013). These nuclei are highly susceptible to the effects of TBI, and their dysregulation can lead to anhedonia, decreased motivation, and executive dysfunction, which are common in post-TBI depression and anxiety (Dunlop and Nemeroff, 2007; Gao L. et al., 2022).
The mesolimbic pathway’s involvement in reward and motivation is crucial for understanding TBI-induced depression. Reduced DA signaling in the NAc can lead to anhedonia, a core symptom of depression. TBI patients may exhibit decreased responsiveness to rewarding stimuli and reduced motivation to engage in previously pleasurable activities (Dunlop and Nemeroff, 2007; Gao L. et al., 2022).
It has been suggested that the mesolimbic dopaminergic system, especially VTA, which projects and releases DA to NAc, plays a fundamental role in the development of anxiety disorder (Small et al., 2016). In support of the above, one study reported that after TBI induced by repeated bursts in mice, DA release increased in the NAc for 30 days after injury (Schindler et al., 2017); in addition, another study reported that a mild TBI generated by repeated impacts using rotational acceleration induced an alteration in the mesolimbic reward system by decreasing TH immunoreactivity in the olfactory tubercle (Vonder Haar et al., 2019b). Meanwhile, Tanaka et al., 2019, reported an increase in DA in the prefrontal cortex associated with a decrease in the time in the open arm of the Elevated plus maze.
Furthermore, it is worth emphasizing that the executive dysfunction often observed in patients with TBI highlights the role of the mesocortical pathway in cognitive functions. Disrupted dopamine signaling in the prefrontal cortex can lead to deficits in attention, working memory, and cognitive flexibility, contributing to the overall burden of psychiatric symptoms following traumatic brain injury.
8.1.3 Hypothalamic-pituitary-adrenal axis
The interaction between dopaminergic and stress-responsive systems, such as the HPA, is significant in TBI. Dysregulated DA signaling can alter the stress response, leading to heightened anxiety and stress sensitivity. Elevated cortisol levels typical in TBI patients can further impair dopaminergic function, exacerbating anxiety symptoms (Dunlop and Nemeroff, 2007; Gao L. et al., 2022).
Murine models have shown that anxiety levels increase after a traumatic brain event, as the exploration time in the open field test and the permanence in the open arms of the elevated cross maze test decreases, as compared to control groups; together, with a considerable increase in serum corticosterone levels that are indicative of increased anxiety as a result of TBI (Fromm et al., 2004; Sönmez et al., 2007; Wagner et al., 2007b; Jones et al., 2008; Baykara et al., 2012, 2013; Almeida-Suhett et al., 2014; Broussard et al., 2018; Beitchman et al., 2020).
8.2 Molecular mechanisms
8.2.1 DA synthesis and release
TBI can impair DA synthesis and release. Oxidative stress and inflammation may reduce TH, the rate-limiting enzyme in DA synthesis. Mitochondrial dysfunction post-TBI decreases ATP production, further impairing DA synthesis and release (Dunlop and Nemeroff, 2007; Gao L. et al., 2022). However, direct measurements of DA in biological fluids of patients post-TBI describe an increase in DA in the acute phase. For example, Wagner et al. (2007a) found increased levels of DA in CSF during the first 5 days after severe injury. However, already in 2014, using PET studies, the same author suggested that patients develop a hypodopaminergic state 1 year after trauma (Wagner et al., 2014). Regarding studies in experimental models of TBI, very few describe alterations in the dopaminergic system and include evaluations of depressive behavior or anxiety. These describe decreases in TH in SN associated with depression-like behavior (Tan et al., 2015; Selvakumar et al., 2020) or anxiety (Impellizzeri et al., 2016; D'Amico et al., 2021) as well as a decrease in DA levels in the striatum (Tan et al., 2015), or an increase in DA in the prefrontal cortex associated with “anxiety” type behaviors (Tanaka et al., 2019).
8.2.2 Receptor dysregulation
Post-TBI, changes in DA receptor density and sensitivity are common. Upregulation of D2 receptors in response to decreased DA availability can lead to increased sensitivity to dopaminergic drugs and altered signaling, contributing to psychiatric symptoms (Dunlop and Nemeroff, 2007; Jolly et al., 2019). However, this appears to be region-specific. In Jolly et al. (2019) (Table 1) studied in moderate to severe TBI patients the relationship between post-traumatic depression, manifested as major depression disorder (MDD) and evaluated the availability of D2R/D3R and the microstructure of white matter. They observed that all patients with TBI had a decreased availability of receptors in the caudate and thalamus compared with the control groups, and this decrease was more significant in the TBI group with MDD.
In contrast, the TBI group without MDD presented a greater availability of the amygdala receptors than the control group. Another result was that the group with TBI and MDD presented more significant evidence of axonal damage compared to the TBI group without MDD in tracts that connect the limbic system. Changes in D2R and D3R receptors have also been reported, with differential expression in the caudate and amygdala and abnormalities in the limbic system, suggesting that compensatory changes in the dopaminergic system may be protective against the development of post-TBI major depression (Jolly et al., 2019). Regarding studies in experimental models of TBI, only one article describes a decrease in D1R in the nucleus accumbens associated with depression-like behavior (Bhowmick et al., 2021). Some authors report D2R changes in various brain regions but do not carry out tests for depression or anxiety-type behaviors.
8.2.3 Transporter and metabolism alterations
DAT function may be compromised post-TBI, impairing DA reuptake, and altered synaptic DA levels. Changes in MAO and COMT activites can further dysregulate DA signaling (Dunlop and Nemeroff, 2007; Gao L. et al., 2022).
Newberg et al. (2014) (Table 1), reported that higher striatum DAT binding, evaluated by SPECT, was associated with more depressive symptoms, analyzing a sample of patients who had suffered a mild TBI over 6 months and continued symptoms (Newberg et al., 2014).
In experimental TBI models, DAT expression decreases have been reported in SN (Impellizzeri et al., 2016; D'Amico et al., 2021) and striatum (Wilson et al., 2005) associated with anxiety-type behaviors. Additionally, various authors have reported decreased DAT expression in the striatum from 1 day to 28 days post-TBI.
8.3 Insights from pharmacological treatments
Regarding pharmacological treatments for depression after traumatic brain injury, recent reviews agree that there is insufficient evidence to recommend prescribing any specific drug or drug (Plantier et al., 2016; Hicks et al., 2023). However, they also suggest pharmacological treatment may be effective in reducing depressive symptoms in those with depression following TBI (Slowinski et al., 2019), considering that depression often develops following TBI and greatly disrupts the lives of survivors and their families. In the absence of a solid evidence base for any specific drug, tentative trials of anti-depressant medication weighing vulnerability to risk factors seem appropriate (Hicks et al., 2023).
Several studies agree that some serotonin (citalopram or sertraline) or norepinephrine and DA (methylphenidate) reuptake inhibitors may be effective in reducing depressive symptoms in patients with TBI (Lee et al., 2005; Pangilinan et al., 2010; Gao C. et al., 2022). In particular, DA-enhancing medications are especially suggested to treat cognitive disorders following a TBI (Writer and Schillerstrom, 2009; Traeger et al., 2020), although drugs such as amantadine, which, among other effects, restores DA levels in the striatum, decreases degeneration and apoptosis of DAergic neurons in the SN, and significantly ameliorates depression-like behavior both in patients and in animal models (Sami and Faruqui, 2015; Tan et al., 2015; Chen et al., 2017b).
It has also been pointed out that some natural extracts, such as 2-Pentadecyl-2-Oxazoline, increase DA levels (Boccella et al., 2020) or sinomenine that acts through the D2R ameliorates behavioral alterations in animal models of TBI (Hong et al., 2022). Likewise, it has been documented that the placebo effect, which represents a powerful and effective treatment for various post-TBI complaints, among other mechanisms of action, increases DA levels (Polich et al., 2018). Alternatively, the beneficial effect of listening to music effects of music lies in its ability to control stress and anxiety in post-TBI patients, which can stimulate DA production in the brain (Basile, 2023). Furthermore, a study reported that TBI induced by the accelerated impact model in rats produced depression-like behavior 14 days after trauma. These mood and anxiety disorders were reversed by the administration of bupropion (DA reuptake inhibitor), suggesting that they were closely related to dopaminergic neurotransmission (Mahesh et al., 2010).
On the other hand, it has been shown that the administration of nomifensine maleate (DA reuptake inhibitor) and quinpirole (D2R agonist) decreased the immobility time in the forced swim test, which suggests that drugs enhancing dopaminergic transmission function as antidepressant therapy in rats (Basso et al., 2005).
8.4 Insights from epigenetic studies
Furthermore, it is worth remembering that the secondary damage after TBI includes epigenetic and/or genetic expression changes (Chen et al., 2018). Although several authors point out that the study of genes that influence outcomes following TBI is still in its early stages, various genetic polymorphisms associated with the pathophysiology and outcome following TBI have been identified (McAllister, 2009; Bennett et al., 2016; Chen et al., 2018). For example, it has been documented that the apolipoprotein E genotype and TBI have combined effects, resulting in dementia later in life (Puccio and Alexander, 2015). More recently, considerable attention has focused on genes associated with mild and repetitive TBIs, particularly among combat veterans and professional athletes (Fesharaki-Zadeh, 2019). Although suggesting that a “good” or “bad” allele of a specific gene may predispose an individual to better or worse outcomes following injury, it is becoming increasingly apparent that recovery from TBI is polygenic, involving the interaction of numerous genes from multiple pathways (McAllister, 2015).
In particular, about the polymorphism in genes related to the dopaminergic system, those that code for COMT, D2R, ankyrin repeat, and kinase domain containing 1 (ANKK1) and DA transport (SLC6A3) or VMAT have been analyzed in TBI outcomes context (Jordan, 2007). It has been described that the polymorphism of some of them may influence cognitive deficits following TBI. However, their involvement in changes in mood disorders following a TBI needs to be more documented. Only one preliminary report suggests that combining certain COMT and ANKK1 alleles may contribute to worse behavioral performance than the carriage of either risk allele alone (Myrga et al., 2016).
9 Discussion
TBI represents a significant public health problem due to high mortality rates and the development of neurological and psychiatric sequelae, including mood, affective, and anxiety disorders that often cause long-lasting disability. It is even pointed out that there is an association between the severity of TBI and cognitive disorders (Shuanglong et al., 2024). The studies shown in this review emphasize that TBI causes alterations in the dopaminergic system, which are associated with mood disorders, including depression and anxiety disorders. For example, after TBI, alterations in the levels of dopamine, its receptors, DAT, and the enzymes involved in its metabolism have been observed, as well as structural and functional changes in the neuronal circuits that connect brain areas specialized in emotional processing with significant dopaminergic innervation and dopaminergic receptors presence, such as the prefrontal cortex, basal ganglia (SN), amygdala and VTA, which could contribute to the development of post-traumatic depression.
It should not be overlooked that the body’s response to TBI is dynamic and can cause immediate or slowly progressive injuries, regardless of severity. The above is relevant because it implies that the appearance of affective or mood disorders could become established gradually as the pathophysiological processes are established in the neuronal circuits involved.
After a TBI, a functional deficit of the monoaminergic circuits occurs, highlighting the dopaminergic system. This deficit varies depending on the pathways or brain regions affected and with time post-TBI. The pathophysiological processes secondary to TBI are associated with chronic neuroinflammation, the generation of oxidative stress, alterations in metabolism, and the destruction (immediate or progressive) of the regions that synthesize dopamine, leading the subject to a hypodopaminergic state, characterized by alterations in DA and DAT and TH concentrations and changes in receptor expression; which together could be a protection mechanism; by increasing circulating levels of dopamine and reducing reuptake systems (Jahan and Tanev, 2023). Each of the dopaminergic pathways presents a different susceptibility to the pathological processes associated with TBI and, therefore, could explain the wide range of clinical signs and symptoms in affected patients, among which are working memory deficits, depression, anxiety, addictions, and behavioral or personality disorders.
Alterations in dopaminergic pathways, caused directly as a response to primary brain injury may participate in developing the neuropsychiatric problems addressed here. However, these alterations can also derive from the progression of the secondary lesion, initiating a positive feedback cycle that will determine the patient’s clinical evolution. As an example of the above, after a TBI, there is an uncontrolled release of ions and neurotransmitters that can cause edema and transient cell membrane depolarization. Uncontrolled transmission leads to a metabolic crisis, where there is mitochondrial damage, decreased ATP production, induction of oxidative stress, increase in reactive oxygen species, and the generation of nitric oxide, patterns that have been observed in both depression and in TBI (Hong et al., 2022). These facilitate neuroinflammation, alter cellular activity, and can damage macromolecules, such as DNA, proteins, and lipids. Elevated levels of pro-inflammatory cytokine have been observed in patients with TBI and depression (Bodnar et al., 2018). The same inflammatory process causes deregulation of the endocrine system, such as the alterations observed in the HPA axis (Luo et al., 2015) and in GH levels (Pavlovic et al., 2019), aggravating metabolic crises. Damage and repair mechanisms, including changes in gene expression, induction of glial inflammatory responses, structural remodeling of proteins, cells, and circuits, and proliferation of neurons and glia, play a decisive role in developing neurobehavioral disorders, including mood disorders. Disruption of cellular processes and persistent low-grade neuroinflammation may predispose some people to depression after TBI (Jahan and Tanev, 2023).
It would be very simplistic to attribute that the depression produced after a TBI is due solely to alterations in the dopaminergic system and not to a multifactorial phenomenon. Various studies indicate that mood disorders are associated with milder levels of TBI (García-Guerrero and Pérez-Moreno, 2014; Uiterwijk et al., 2022), which is very difficult to attribute exclusively to damage to the dopaminergic system. Although damage to this system caused by TBI is an important factor in mood alterations, it must be considered that they have multiple origins, such as an imbalance in aminergic neurotransmission, especially serotonergic neurotransmission, neuroinflammation, and neuroendocrine dysregulation. of the hypothalamic–pituitary–adrenal axis.
An aspect worth highlighting is that there are many more reports of male patients in the clinical studies referred to here. Perhaps this is not surprising given that there is a higher proportion of men who suffer from TBI. However, it has been reported that compared to males, female patients have poorer cognitive recovery and more severe symptoms of depression and anxiety after a TBI (Cnossen et al., 2017; Liu et al., 2024). Likewise, in studies in animal models, those carried out only in males largely predominate.
10 Conclusion
Studies discussed in this chapter, in human subjects, as in animal models, provide evidence of a relationship between TBI and mood, affective, and anxiety disorders associated with lesion location. However, it is worth highlighting that although numerous studies, both clinical trials and animal models, show alterations in the dopaminergic system after a TBI, only some of them include tests of mood or affective state, and there are practically no reports that correlate the magnitude or intensity of these two types of variables. Likewise, more studies on animal models need to be conducted, including both males and females.
Nevertheless, these data reinforce the importance of continuing to study the modifications of dopaminergic transmission after a traumatic brain injury and its relationship with psychiatric disorders.
Author contributions
AM-B: Conceptualization, Writing – original draft, Investigation. RT-C: Investigation, Writing – original draft, Formal analysis, Visualization. MM-V: Investigation, Writing – review & editing, Data curation. AP-A: Data curation, Investigation, Writing – review & editing. MLÁM-C: Writing – review & editing, Resources, Supervision, Validation. AD-R: Project administration, Writing – review & editing. CR: Writing – review & editing, Supervision, Validation. LN: Validation, Writing – review & editing, Conceptualization, Funding acquisition, Writing – original draft.
Funding
The author(s) declare that financial support was received for the research, authorship, and/or publication of this article. Partially supported by DGAPA-PAPIIT IN228223.
Acknowledgments
The present publication is part of the postdoctoral stay of Alfonso Mata-Bermudez, which was financed through the Postdoctoral Fellowship Program of the Dirección General de Asuntos del Personal Académico (DGAPA), Universidad Nacional Autónoma de México (UNAM). Ricardo Trejo-Chávez is a doctoral student from the Programa de Doctorado en Ciencias Biomedicas, Universidad Nacional Autónoma de México (UNAM) and has received. CONAHCYT fellowship.
Conflict of interest
The authors declare that the research was conducted in the absence of any commercial or financial relationships that could be construed as a potential conflict of interest.
Publisher’s note
All claims expressed in this article are solely those of the authors and do not necessarily represent those of their affiliated organizations, or those of the publisher, the editors and the reviewers. Any product that may be evaluated in this article, or claim that may be made by its manufacturer, is not guaranteed or endorsed by the publisher.
References
Abdullah, J. M., Jaafar, H., Muthuraju, S., Taha, S., Pati, S., and Rafique, M. (2013). Normabaric hyperoxia treatment improved locomotor activity of C57BL/6J mice through enhancing dopamine genes following fluid-percussion injury in striatum. Int. J. Biomed. Sci. 9, 194–204. doi: 10.59566/IJBS.2013.9194
Abdullah, M. F. I. L. B., Ng, Y. P., and Sidi, H. B. (2018). Depression and anxiety among traumatic brain injury patients in Malaysia. Asian J. Psychiatr. 37, 67–70. doi: 10.1016/j.ajp.2018.08.017
Acosta, S. A., Tajiri, N., de la Pena, I., Bastawrous, M., Sanberg, P. R., Kaneko, Y., et al. (2015). Alpha-synuclein as a pathological link between chronic traumatic brain injury and Parkinson’s disease. J. Cell. Physiol. 230, 1024–1032. doi: 10.1002/jcp.24830
Agrawal, S., and Branco, R. G. (2016). Neuroprotective measures in children with traumatic brain injury. World J. Crit. Care Med. 5, 36–46. doi: 10.5492/wjccm.v5.i1.36
Albicini, M., Eggleston, M., and McKinlay, A. (2020). The prevalence of traumatic brain injury, comorbid anxiety and other psychiatric disorders in an outpatient child and adolescent mental health service. J. Ment. Health 29, 439–445. doi: 10.1080/09638237.2017.1385733
Albicini, M., and McKinlay, A. (2018). Anxiety disorders in adults with childhood traumatic brain injury: evidence of difficulties more than 10 years Postinjury. J. Head Trauma Rehabil. 33, 191–199. doi: 10.1097/HTR.0000000000000312
Albrecht, J. S., Abariga, S. A., Rao, V., and Wickwire, E. M. (2020). Incidence of new neuropsychiatric disorder diagnoses following traumatic brain injury. J. Head Trauma Rehabil. 35, E352–E360. doi: 10.1097/HTR.0000000000000551
Albrecht, J. S., Peters, M. E., Smith, G. S., and Rao, V. (2017). Anxiety and post-traumatic stress disorder among Medicare beneficiaries after traumatic brain injury. J. Head Trauma Rehabil. 32, 178–184. doi: 10.1097/HTR.0000000000000266
Almeida-Suhett, C. P., Prager, E. M., Pidoplichko, V., Figueiredo, T. H., Marini, A. M., Li, Z., et al. (2014). Reduced GABAergic inhibition in the basolateral amygdala and the development of anxiety-like behaviors after mild traumatic brain injury. PLoS One 9:e102627. doi: 10.1371/journal.pone.0102627
Ayano, G. (2016). Dopamine: receptors, functions, synthesis, pathways, locations and mental disorders: review of literatures. J. Ment. Disord. Treat. 2, 1–4. doi: 10.4172/2471-271X.1000120
Azouvi, P., Arnould, A., Dromer, E., and Vallat-Azouvi, C. (2017). Neuropsychology of traumatic brain injury: an expert overview. Rev. Neurol. (Paris) 173, 461–472. doi: 10.1016/j.neurol.2017.07.006
Bales, J. W., Kline, A. E., Wagner, A. K., and Dixon, C. E. (2010). Targeting dopamine in acute traumatic brain injury. Open Drug Discov. J. 2, 119–128. doi: 10.2174/1877381801002010119
Bales, J. W., Wagner, A. K., Kline, A. E., and Dixon, C. E. (2009). Persistent cognitive dysfunction after traumatic brain injury: a dopamine hypothesis. Neurosci. Biobehav. Rev. 33, 981–1003. doi: 10.1016/j.neubiorev.2009.03.011
Basile, G. (2023). Beneficial effects of music in the healing process of traumatic injuries: perceptual control of suffering and possible abatement of disability conditions. Clin. Ter. 174, 531–536. doi: 10.7417/CT.2023.5021
Basso, A. M., Gallagher, K. B., Bratcher, N. A., Brioni, J. D., Moreland, R. B., Hsieh, G. C., et al. (2005). Antidepressant-like effect of D(2/3) receptor-, but not D(4) receptor-activation in the rat forced swim test. Neuropsychopharmacology 30, 1257–1268. doi: 10.1038/sj.npp.1300677
Baykara, B., Aksu, I., Buyuk, E., Kiray, M., Sisman, A. R., Baykara, B., et al. (2013). Progesterone treatment decreases traumatic brain injury induced anxiety and is correlated with increased serum IGF-1 levels; prefrontal cortex, amygdala, and hippocampus neuron density; and reduced serum corticosterone levels in immature rats. Biotech. Histochem. 88, 250–257. doi: 10.3109/10520295.2013.769630
Baykara, B., Cetin, F., Baykara, B., Aksu, I., Dayi, A., Kiray, M., et al. (2012). Anxiety caused by traumatic brain injury correlates to decreased prefrontal cortex VEGF immunoreactivity and neuron density in immature rats. Turk. Neurosurg. 22, 604–610. doi: 10.5137/1019-5149.JTN.5633-11.1
Beaulieu, J. M., and Gainetdinov, R. R. (2011). The physiology, signaling, and pharmacology of dopamine receptors. Pharmacol. Rev. 63, 182–217. doi: 10.1124/pr.110.002642
Beitchman, J. A., Griffiths, D. R., Hur, Y., Ogle, S. B., Bromberg, C. E., Morrison, H. W., et al. (2020). Experimental traumatic brain injury induces chronic glutamatergic dysfunction in amygdala circuitry known to regulate anxiety-like behavior. Front. Neurosci. 13:1434. doi: 10.3389/fnins.2019.01434
Bennett, E. R., Reuter-Rice, K., and Laskowitz, D. T. (2016). Genetic influences in traumatic brain injury. En D. Laskowitz and G. Grant (Eds.), Translational research in traumatic brain injury (Capítulo 9). Boca Raton (FL): CRC Press/Taylor and Francis Group.
Bhowmick, S., Malat, A., Caruso, D., Ponery, N., D'Mello, V., Finn, C., et al. (2021). Intercellular adhesion Molecule-1-induced posttraumatic brain injury neuropathology in the prefrontal cortex and Hippocampus leads to sensorimotor function deficits and psychological stress. eNeuro 8, ENEURO.0242–ENEU21.2021. doi: 10.1523/ENEURO.0242-21.2021
Björklund, A., and Dunnett, S. B. (2007). Dopamine neuron systems in the brain: an update. Trends Neurosci. 30, 194–202. doi: 10.1016/j.tins.2007.03.006
Boccella, S., Iannotta, M., Cristiano, C., Iannotti, F. A., Bello, F. D., Guida, F., et al. (2020). Treatment with 2-Pentadecyl-2-Oxazoline restores mild traumatic brain injury-induced sensorial and neuropsychiatric dysfunctions. Front. Pharmacol. 11:91. doi: 10.3389/fphar.2020.00091
Bodnar, C. N., Morganti, J. M., and Bachstetter, A. D. (2018). Depression following a traumatic brain injury: uncovering cytokine dysregulation as a pathogenic mechanism. Neural Regen. Res. 13, 1693–1704. doi: 10.4103/1673-5374.238604
Braga, M. F. M., Juranek, J., Eiden, L. E., Li, Z., Figueiredo, T. H., de Araujo Furtado, M., et al. (2022). GABAergic circuits of the basolateral amygdala and generation of anxiety after traumatic brain injury. Amino Acids 54, 1229–1249. doi: 10.1007/s00726-022-03184-y
Broussard, J. I., Acion, L., De Jesús-Cortés, H., Yin, T., Britt, J. K., Salas, R., et al. (2018). Repeated mild traumatic brain injury produces neuroinflammation, anxiety-like behavior and impaired spatial memory in mice. Brain Inj. 32, 113–122. doi: 10.1080/02699052.2017.1380228
Bryant, R. A., O'Donnell, M. L., Creamer, M., McFarlane, A. C., Clark, C. R., and Silove, D. (2010). The psychiatric sequelae of traumatic injury. Am. J. Psychiatry 167, 312–320. doi: 10.1176/appi.ajp.2009.09050617
Capizzi, A., Woo, J., and Verduzco-Gutierrez, M. (2020). Traumatic brain injury: an overview of epidemiology, pathophysiology, and medical management. Med. Clin. North Am. 104, 213–238. doi: 10.1016/j.mcna.2019.11.001
Carlson, S. W., and Dixon, C. E. (2018). Lithium improves dopamine neurotransmission and increases dopaminergic protein abundance in the striatum after traumatic brain injury. J. Neurotrauma 35, 2827–2836. doi: 10.1089/neu.2017.5509
Chen, Y. H., Huang, E. Y., Kuo, T. T., Hoffer, B. J., Miller, J., Chou, Y. C., et al. (2017a). Dopamine release in the nucleus accumbens is altered following traumatic brain injury. Neuroscience 348, 180–190. doi: 10.1016/j.neuroscience.2017.02.001
Chen, Y. H., Huang, E. Y., Kuo, T. T., Ma, H. I., Hoffer, B. J., Tsui, P. F., et al. (2015). Dopamine release impairment in striatum after different levels of cerebral cortical fluid percussion injury. Cell Transplant. 24, 2113–2128. doi: 10.3727/096368914X683584
Chen, Y. H., Huang, E. Y., Kuo, T. T., Miller, J., Chiang, Y. H., and Hoffer, B. J. (2017b). Impact of traumatic brain injury on dopaminergic transmission. Cell Transplant. 26, 1156–1168. doi: 10.1177/0963689717714105
Chen, Y. H., Kuo, T. T., Huang, E. Y. K., Hoffer, B. J., Kao, J. H., Chou, Y. C., et al. (2018). Nicotine-induced conditional place preference is affected by head injury: correlation with dopamine release in the nucleus accumbens shell. Int. J. Neuropsychopharmacol. 21, 949–961. doi: 10.1093/ijnp/pyy055
Cnossen, M. C., Scholten, A. C., Lingsma, H. F., Synnot, A., Haagsma, J., Steyerberg, P. E. W., et al. (2017). Predictors of major depression and posttraumatic stress disorder following traumatic brain injury: a systematic review and Meta-analysis. J. Neuropsychiatr. Clin. Neurosci. 29, 206–224. doi: 10.1176/appi.neuropsych.16090165
Corrigan, F., Arulsamy, A., Shultz, S. R., Wright, D. K., and Collins-Praino, L. E. (2023). Initial severity of injury has little effect on the temporal profile of long-term deficits in locomotion, anxiety, and cognitive function after diffuse traumatic brain injury. Neurotrauma Rep. 4, 41–50. doi: 10.1089/neur.2022.0057
Corrigan, J. D., Selassie, A. W., and Orman, J. A. (2010). The epidemiology of traumatic brain injury. J. Head Trauma Rehabil. 25, 72–80. doi: 10.1097/HTR.0b013e3181ccc8b4
Cristofori, I., and Levin, H. S. (2015). Traumatic brain injury and cognition. Handb. Clin. Neurol. 128, 579–611. doi: 10.1016/B978-0-444-63521-1.00037-6
D'Amico, R., Trovato Salinaro, A., Fusco, R., Cordaro, M., Impellizzeri, D., Scuto, M., et al. (2021). Hericium erinaceus and Coriolus versicolor modulate molecular and biochemical changes after traumatic brain injury. Antioxidants (Basel) 10:898. doi: 10.3390/antiox10060898
Dewan, M. C., Rattani, A., Gupta, S., Baticulon, R. E., Hung, Y. C., Punchak, M., et al. (2018). Estimating the global incidence of traumatic brain injury. J. Neurosurg. 130, 1080–1097. doi: 10.3171/2017.10.JNS17352
Dixon, K. J. (2017). Pathophysiology of traumatic brain injury. Phys. Med. Rehabil. Clin. N. Am. 28, 215–225. doi: 10.1016/j.pmr.2016.12.001
Donnemiller, E., Brenneis, C., Wissel, J., Scherfler, C., Poewe, W., Riccabona, G., et al. (2000). Impaired dopaminergic neurotransmission in patients with traumatic brain injury: a SPECT study using 123I-beta-CIT and 123I-IBZM. Eur. J. Nucl. Med. 27, 1410–1414. doi: 10.1007/s002590000308
Dunlop, B. W., and Nemeroff, C. B. (2007). The role of dopamine in the pathophysiology of depression. Arch. Gen. Psychiatry 64, 327–337. doi: 10.1001/archpsyc.64.3.327
Edut, S., Rubovitch, V., Rehavi, M., Schreiber, S., and Pick, C. G. (2014). A study on the mechanism by which MDMA protects against dopaminergic dysfunction after minimal traumatic brain injury (mTBI) in mice. J. Mol. Neurosci. 54, 684–697. doi: 10.1007/s12031-014-0399-z
Edvinsson, L., Owman, C., Rosengren, E., and West, K. A. (1971). Brain concentrations of dopamine, noradrenaline, 5-hydroxytryptamine, and homovanillic acid during intracranial hypertension following traumatic brain injury in rabbit. Acta Neurol. Scand. 47, 458–463. doi: 10.1111/j.1600-0404.1971.tb07500.x
Eliasen, M. H., Petersen, J., Benros, M. E., and Osler, M. (2021). Number of traumatic brain injuries and temporal associations with depression: a register-based cohort study. Acta Psychiatr. Scand. 144, 407–414. doi: 10.1111/acps.13347
Feldman, Z., Contant, C. F., Pahwa, R., Goodman, J. C., Robertson, C. S., Narayan, R. K., et al. (1993). The relationship between hormonal mediators and systemic hypermetabolism after severe head injury. J. Trauma 34, 806–812. doi: 10.1097/00005373-199306000-00010
Fesharaki-Zadeh, A. (2019). Chronic traumatic encephalopathy: a brief overview. Front. Neurol. 10:713. doi: 10.3389/fneur.2019.00713
Fleminger, S., and Ponsford, J. (2005). Long term outcome after traumatic brain injury. BMJ 331, 1419–1420. doi: 10.1136/bmj.331.7530.1419
Fromm, L., Heath, D. L., Vink, R., and Nimmo, A. J. (2004). Magnesium attenuates post-traumatic depression/anxiety following diffuse traumatic brain injury in rats. J. Am. Coll. Nutr. 23, 529S–533S. doi: 10.1080/07315724.2004.10719396
Gao, C., Nie, M., Huang, J., Tian, Y., Wang, D., Zhang, J., et al. (2022). Pharmacotherapy for mild traumatic brain injury: an overview of the current treatment options. Expert. Opin. Pharmacother. 23, 805–813. doi: 10.1080/14656566.2022.2054328
Gao, L., Xue, Q., Gong, S., Li, G., Tong, W., Fan, M., et al. (2022). Structural and functional alterations of substantia nigra and associations with anxiety and depressive symptoms following traumatic brain injury. Front. Neurol. 13:719778. doi: 10.3389/fneur.2022.719778
García-Guerrero, C. E., and Pérez-Moreno, B. (2014). Risk factors related to the development of depression after traumatic brain injury. Neuropsicol. Latinoam. 6, 25–46. doi: 10.5579/rnl.2014.0155
Grace, A. A., and Rosenkranz, J. A. (2002). Regulation of conditioned responses of basolateral amygdala neurons. Physiol. Behav. 77, 489–493. doi: 10.1016/S0031-9384(02)00909-5
Green, R. E., Colella, B., Christensen, B., Johns, K., Frasca, D., Bayley, M., et al. (2008). Examining moderators of cognitive recovery trajectories after moderate to severe traumatic brain injury. Arch. Phys. Med. Rehabil. 89, S16–S24. doi: 10.1016/j.apmr.2008.09.551
Guillamondegui, O. D., Montgomery, S. A., Phibbs, F. T., McPheeters, M. L., Alexander, P. T., Jerome, R. N., et al. (2011). Traumatic brain injury and depression. Rockville (MD): Agency for Healthcare Research and Quality (US).
Hamill, R. W., Woolf, P. D., McDonald, J. V., Lee, L. A., and Kelly, M. (1987). Catecholamines predict outcome in traumatic brain injury. Ann. Neurol. 21, 438–443. doi: 10.1002/ana.410210504
Harsing, L. G. (2008). Dopamine and the Dopaminergic Systems of the Brain. In: Handbook of Neurochemistry and Molecular Neurobiology. eds A. Lajtha and E. S. Vizi (Boston, MA: Springer).
Hasler, G., Fromm, S., Carlson, P. J., Luckenbaugh, D. A., Waldeck, T., Geraci, M., et al. (2008). Neural response to catecholamine depletion in Unmedicated subjects with major depressive disorder in remission and healthy subjects. Arch. Gen. Psychiatry 65, 521–531. doi: 10.1001/archpsyc.65.5.521
Hercher, C., Turecki, G., and Mechawar, N. (2009). Through the looking glass: examining neuroanatomical evidence for cellular alterations in major depression. J. Psychiatr. Res. 43, 947–961. doi: 10.1016/j.jpsychires.2009.01.006
Heshmati, M., and Russo, S. J. (2015). Anhedonia and the brain reward circuitry in depression. Curr. Behav. Neurosci. Rep. 2, 146–153. doi: 10.1007/s40473-015-0044-3
Hicks, A. J., Clay, F. J., James, A. C., Hopwood, M., and Ponsford, J. L. (2023). Effectiveness of pharmacotherapy for depression after adult traumatic brain injury: an umbrella review. Neuropsychol. Rev. 33, 393–431. doi: 10.1007/s11065-022-09543-6
Hong, H., Lu, X., Lu, Q., Huang, C., and Cui, Z. (2022). Potential therapeutic effects and pharmacological evidence of sinomenine in central nervous system disorders. Front. Pharmacol. 13:1015035. doi: 10.3389/fphar.2022.1015035
Hu, E., Tang, T., Li, Y. M., Li, T., Zhu, L., Ding, R. Q., et al. (2023). Spatial amine metabolomics and histopathology reveal localized brain alterations in subacute traumatic brain injury and the underlying mechanism of herbal treatment. CNS Neurosci. Ther. 30:e14231. doi: 10.1111/cns.14231
Huang, M., Lewine, J. D., and Lee, R. R. (2020). Magnetoencephalography for mild traumatic brain injury and posttraumatic stress disorder. Neuroimaging Clin. N. Am. 30, 175–192. doi: 10.1016/j.nic.2020.02.003
Huang, E. Y. K., Tsui, P. F., Kuo, T. T., Tsai, J., Chou, Y. C., Ma, H. I., et al. (2014). Amantadine ameliorates dopamine-releasing deficits and behavioral deficits in rats after fluid percussion injury. PLoS One 9:e86354. doi: 10.1371/journal.pone.0086354
Hutson, C. B., Lazo, C. R., Mortazavi, F., Giza, C. C., Hovda, D., and Chesselet, M. F. (2011). Traumatic brain injury in adult rats causes progressive nigrostriatal dopaminergic cell loss and enhanced vulnerability to the pesticide paraquat. J. Neurotrauma 28, 1783–1801. doi: 10.1089/neu.2010.1723
Impellizzeri, D., Campolo, M., Bruschetta, G., Crupi, R., Cordaro, M., Paterniti, I., et al. (2016). Traumatic brain injury leads to development of Parkinson’s disease related pathology in mice. Front. Neurosci. 10:458. doi: 10.3389/fnins.2016.00458
Jahan, A. B., and Tanev, K. (2023). Neurobiological mechanisms of depression following traumatic brain injury. Brain Inj. 37, 24–33. doi: 10.1080/02699052.2022.2145362
Jenkins, P. O., Mehta, M. A., and Sharp, D. J. (2016). Catecholamines and cognition after traumatic brain injury. Brain 139, 2345–2371. doi: 10.1093/brain/aww128
Jenkins, P. O., Roussakis, A. A., De Simoni, S., Bourke, N., Fleminger, J., Cole, J., et al. (2020). Distinct dopaminergic abnormalities in traumatic brain injury and Parkinson’s disease. J. Neurol. Neurosurg. Psychiatry 91, 631–637. doi: 10.1136/jnnp-2019-321759
Johnson, D., Roethig-Johnston, K., and Richards, D. (1993). Biochemical parameters of recovery in acute severe head injury. Br. J. Neurosurg. 7, 53–59. doi: 10.3109/02688699308995056
Jolly, A. E., Raymont, V., Cole, J. H., Whittington, A., Scott, G., De Simoni, S., et al. (2019). Dopamine D2/D3 receptor abnormalities after traumatic brain injury and their relationship to post-traumatic depression. NeuroImage Clin. 24:101950. doi: 10.1016/j.nicl.2019.101950
Jones, N. C., Cardamone, L., Williams, J. P., Salzberg, M. R., Myers, D., and O’Brien, T. J. (2008). Experimental traumatic brain injury induces a pervasive hyperanxious phenotype in rats. J. Neurotrauma 25, 1367–1374. doi: 10.1089/neu.2008.0641
Jordan, B. D. (2007). Genetic influences on outcome following traumatic brain injury. Neurochem. Res. 32, 905–915. doi: 10.1007/s11064-006-9251-3
Jorge, R. E. (2015). Mood disorders. Handb. Clin. Neurol. 128, 613–631. doi: 10.1016/B978-0-444-63521-1.00038-8
Juengst, S. B., Kumar, R. G., and Wagner, A. K. (2017). A narrative literature review of depression following traumatic brain injury: prevalence, impact, and management challenges. Psychol. Res. Behav. Manag. 10, 175–186. doi: 10.2147/PRBM.S113264
Karelina, K., Gaier, K. R., and Weil, Z. M. (2017). Traumatic brain injuries during development disrupt dopaminergic signaling. Exp. Neurol. 297, 110–117. doi: 10.1016/j.expneurol.2017.08.003
Kaukas, L., Holmes, J. L., Rahimi, F., Collins-Praino, L., and Corrigan, F. (2021). Injury during adolescence leads to sex-specific executive function deficits in adulthood in a pre-clinical model of mild traumatic brain injury. Behav. Brain Res. 402:113067. doi: 10.1016/j.bbr.2020.113067
Khellaf, A., Khan, D. Z., and Helmy, A. (2019). Recent advances in traumatic brain injury. J. Neurol. 266, 2878–2889. doi: 10.1007/s00415-019-09541-4
Klein, M. O., Battagello, D. S., Cardoso, A. R., Hauser, D. N., Bittencourt, J. C., and Correa, R. G. (2019). Dopamine: functions, signaling, and association with neurological diseases. Cell. Mol. Neurobiol. 39, 31–59. doi: 10.1007/s10571-018-0632-3
Ko, I. G., Kim, C. J., and Kim, H. (2019). Treadmill exercise improves memory by up-regulating dopamine and down-regulating D2 dopamine receptor in traumatic brain injury rats. J. Exerc. Rehabil. 15, 504–511. doi: 10.12965/jer.1938316.158
Ko, J. H., and Strafella, A. P. (2012). Dopaminergic neurotransmission in the human brain: new lessons from perturbation and imaging. Neuroscientist 18, 149–168. doi: 10.1177/1073858411401413
Kobori, N., Clifton, G. L., and Dash, P. K. (2006). Enhanced catecholamine synthesis in the prefrontal cortex after traumatic brain injury: implications for prefrontal dysfunction. J. Neurotrauma 23, 1094–1102. doi: 10.1089/neu.2006.23.1094
Koenigs, M., and Grafman, J. (2009). The functional neuroanatomy of depression: distinct roles for ventromedial and dorsolateral prefrontal cortex. Behav. Brain Res. 201, 239–243. doi: 10.1016/j.bbr.2009.03.004
Kreitzer, N., Ancona, R., Mccullumsmith, C., Kurowski, B. G., Foreman, B., Ngwenya, L. B., et al. (2019). The effect of antidepressants on depression after traumatic brain injury: a meta-analysis. J. Head Trauma Rehabil. 34, E47–E54. doi: 10.1097/HTR.0000000000000439
Kumar, S., and Singh, G. (2023). Pharmacological potential of zonisamide and Nigella sativa per se and combination in high-impact trauma device-induced traumatic brain injury in Drosophila melanogaster. Fundam. Clin. Pharmacol. 37, 577–588. doi: 10.1111/fcp.12857
Laliberté Durish, C., Pereverseff, R. S., and Yeates, K. O. (2018). Depression and depressive symptoms in pediatric traumatic brain injury: a scoping review. J. Head Trauma Rehabil. 33, E18–E30. doi: 10.1097/HTR.0000000000000343
Lamontagne, G., Belleville, G., Beaulieu-Bonneau, S., Souesme, G., Savard, J., Sirois, M. J., et al. (2022). Anxiety symptoms and disorders in the first year after sustaining mild traumatic brain injury. Rehabil. Psychol. 67, 90–99. doi: 10.1037/rep0000422
Lan, Y. L., Li, S., Lou, J. C., Ma, X. C., and Zhang, B. (2019). The potential roles of dopamine in traumatic brain injury: a preclinical and clinical update. Am. J. Transl. Res. 11, 2616–2631
Leconte, C., Benedetto, C., Lentini, F., Simon, K., Ouaazizi, C., Taib, T., et al. (2020). Histological and behavioral evaluation after traumatic brain injury in mice: a ten months follow-up study. J. Neurotrauma 37, 1342–1357. doi: 10.1089/neu.2019.6679
Lee, H., Kim, S. W., Kim, J. M., Shin, I. S., Yang, S. J., and Yoon, J. S. (2005). Comparing effects of methylphenidate, sertraline and placebo on neuropsychiatric sequelae in patients with traumatic brain injury. Hum. Psychopharmacol. 20, 97–104. doi: 10.1002/hup.668
Leker, R. R., and Shohami, E. (2002). Cerebral ischemia and trauma-different etiologies yet similar mechanisms: neuroprotective opportunities. Brain Res. Rev. 39, 55–73. doi: 10.1016/s0165-0173(02)00157-1
Liu, M., Bachstetter, A. D., Cass, W. A., Lifshitz, J., and Bing, G. (2017). Pioglitazone attenuates neuroinflammation and promotes dopaminergic neuronal survival in the nigrostriatal system of rats after diffuse brain injury. J. Neurotrauma 34, 414–422. doi: 10.1089/neu.2015.4361
Liu, T., Yu, S., Liu, M., Zhao, Z., Yuan, J., Sha, Z., et al. (2024). Cognitive impairment in Chinese traumatic brain injury patients: from challenge to future perspectives. Front. Neurosci. 18:1361832. doi: 10.3389/fnins.2024.1361832
Lowing, J. L., Susick, L. L., Caruso, J. P., Provenzano, A. M., Raghupathi, R., and Conti, A. C. (2014). Experimental traumatic brain injury alters ethanol consumption and sensitivity. J. Neurotrauma 31, 1700–1710. doi: 10.1089/neu.2013.3286
Luo, L., Chai, Y., Jiang, R., Chen, X., and Yan, T. (2015). Cortisol supplement combined with psychotherapy and citalopram improves depression outcomes in patients with hypocortisolism after traumatic brain injury. Aging Dis. 6, 418–425. doi: 10.14336/AD.2015.0507
Maas, A. I., Stocchetti, N., and Bullock, R. (2008). Moderate and severe traumatic brain injury in adults. Lancet Neurol. 7, 728–741. doi: 10.1016/S1474-4422(08)70164-9
Mahesh, R., Pandey, D. K., Katiyar, S., Kukade, G., Viyogi, S., and Rudra, A. (2010). Effect of antidepressants on neuro-behavioral consequences following impact accelerated traumatic brain injury in rats. Indian J. Exp. Biol. 8, 466–473.
Mallya, S., Sutherland, J., Pongracic, S., Mainland, B., and Ornstein, T. J. (2015). The manifestation of anxiety disorders after traumatic brain injury: a review. J. Neurotrauma 32, 411–421. doi: 10.1089/neu.2014.3504
Markianos, M., Seretis, A., Kotsou, S., Baltas, I., and Sacharogiannis, H. (1992). CSF neurotransmitter metabolites and short-term outcome of patients in coma after head injury. Acta Neurol. Scand. 86, 190–193. doi: 10.1111/j.1600-0404.1992.tb05064.x
Massucci, J. L., Kline, A. E., Ma, X., Zafonte, R. D., and Dixon, C. E. (2004). Time dependent alterations in dopamine tissue levels and metabolism after experimental traumatic brain injury in rats. Neurosci. Lett. 372, 127–131. doi: 10.1016/j.neulet.2004.09.026
McAllister, T. W. (2009). Polymorphisms in genes modulating the dopamine system: do they influence outcome and response to medication after traumatic brain injury? J. Head Trauma Rehabil. 24, 65–68. doi: 10.1097/HTR.0b013e3181996e6b
McAllister, T. W. (2015). Genetic factors in traumatic brain injury. Handb. Clin. Neurol. 128, 723–739. doi: 10.1016/B978-0-444-63521-1.00045-5
McIntosh, T. K., Yu, T., and Gennarelli, T. A. (1994). Alterations in regional brain catecholamine concentrations after experimental brain injury in the rat. J. Neurochem. 63, 1426–1433. doi: 10.1046/j.1471-4159.1994.63041426.x
Meiser, J., Weindl, D., and Hiller, K. (2013). Complexity of dopamine metabolism. Cell Commun. Signal. 11:34. doi: 10.1186/1478-811X-11-34
Menon, K., Schwab, K., Wright, D. W., and Maas, A. I. (2010). Demographics and clinical assessment working Group of the International and Interagency Initiative toward common data elements for research on traumatic brain injury and psychological health. Position statement: definition of traumatic brain injury. Arch. Phys. Med. Rehabil. 91, 1637–1640. doi: 10.1016/j.apmr.2010.05.017
Mulvihill, K. G. (2019). Presynaptic regulation of dopamine release: role of the DAT and VMAT2 transporters. Neurochem. Int. 122, 94–105. doi: 10.1016/j.neuint.2018.11.004
Muthuraju, S., Islam, M. R., Pati, S., Jaafar, H., Abdullah, J. M., and Yusoff, K. M. (2014). Normobaric hyperoxia treatment prevents early alteration in dopamine level in mice striatum after fluid percussion injury: a biochemical approach. Int. J. Neurosci. 125, 686–692. doi: 10.3109/00207454.2014.961065
Myöhänen, T. T., Schendzielorz, N., and Männistö, P. T. (2010). Distribution of catechol-O-methyltransferase (COMT) proteins and enzymatic activities in wild-type and soluble COMT deficient mice. J. Neurochem. 113, 1632–1643. doi: 10.1111/j.1471-4159.2010.06723.x
Myrga, J. M., Juengst, S. B., Failla, M. D., Conley, Y. P., Arenth, P. M., Grace, A. A., et al. (2016). COMT and ANKK1 genetics interact with depression to influence behavior following severe TBI: an initial assessment. Neurorehabil. Neural Repair 30, 920–930. doi: 10.1177/1545968316648409
Nestler, E. J., and Carlezon, W. A. (2006). The mesolimbic dopamine reward circuit in depression. Biol. Psychiatry 59, 1151–1159. doi: 10.1016/j.biopsych.2005.09.018
Newberg, A. B., Serruya, M., Gepty, A., Intenzo, C., Lewis, T., Amen, D., et al. (2014). Clinical comparison of 99mTc exametazime and 123I Ioflupane SPECT in patients with chronic mild traumatic brain injury. PLoS One 9:e87009. doi: 10.1371/journal.pone.0087009
Nieoullon, A. (2002). Dopamine and the regulation of cognition and attention. Prog. Neurobiol. 67, 53–83. doi: 10.1016/s0301-0082(02)00011-4
Ortiz, O., Delgado-García, J. M., Espadas, I., Bahí, A., Trullas, R., Dreyer, J. L., et al. (2010). Associative learning and CA3–CA1 synaptic plasticity are impaired in D1R null, Drd1a−/− mice and in hippocampal siRNA silenced Drd1a mice. J. Neurosci. 30, 12288–12300. doi: 10.1523/JNEUROSCI.2655-10.2010
Osborn, A. J., Mathias, J. L., and Fairweather-Schmidt, A. K. (2014). Depression following adult, non-penetrating traumatic brain injury: a meta-analysis examining methodological variables and sample characteristics. Neurosci. Biobehav. Rev. 47, 1–15. doi: 10.1016/j.neubiorev.2014.07.007
Osborn, A. J., Mathias, J. L., and Fairweather-Schmidt, A. K. (2016). Prevalence of anxiety following adult traumatic brain injury: a meta-analysis comparing measures, samples and postinjury intervals. Neuropsychology 30, 247–261. doi: 10.1037/neu0000221
Pangilinan, P. H., Giacoletti-Argento, A., Shellhaas, R., Hurvitz, E. A., and Hornyak, J. E. (2010). Neuropharmacology in pediatric brain injury: a review. PM R 2, 1127–1140. doi: 10.1016/j.pmrj.2010.07.007
Pavlovic, D., Pekic, S., Stojanovic, M., and Popovic, V. (2019). Traumatic brain injury: neuropathological, neurocognitive and neurobehavioral sequelae. Pituitary 22, 270–282. doi: 10.1007/s11102-019-00957-9
Perry, D. C., Sturm, V. E., Peterson, M. J., Pieper, C. F., Bullock, T., Boeve, B. F., et al. (2016). Association of traumatic brain injury with subsequent neurological and psychiatric disease: a meta-analysis. J. Neurosurg. 124, 511–526. doi: 10.3171/2015.2.JNS14503
Pinto, P. S., Meoded, A., Poretti, A., Tekes, A., and Huisman, T. A. (2012). The unique features of traumatic brain injury in children. Review of the characteristics of the pediatric skull and brain, mechanisms of trauma, patterns of injury, complications, and their imaging findings--part 2. J. Neuroimaging 22, e18–e41. doi: 10.1111/j.1552-6569.2011.00690.x
Pischiutta, F., Micotti, E., Hay, J. R., Marongiu, I., Sammali, E., Tolomeo, D., et al. (2018). Single severe traumatic brain injury produces progressive pathology with ongoing contralateral white matter damage one year after injury. Exp. Neurol. 300, 167–178. doi: 10.1016/j.expneurol.2017.11.003
Plantier, D., and Luauté, J.SOFMER group (2016). Drugs for behavior disorders after traumatic brain injury: systematic review and expert consensus leading to French recommendations for good practice. Ann. Phys. Rehabil. Med. 59, 42–57. doi: 10.1016/j.rehab.2015.10.003
Polich, G., Iaccarino, M. A., Kaptchuk, T. J., Morales-Quezada, L., and Zafonte, R. (2018). Placebo effects in traumatic brain injury. J. Neurotrauma 35, 1205–1212. doi: 10.1089/neu.2017.5506
Ponsford, J., Lee, N. K., Wong, D., Mckay, A., Haines, K., Downing, M., et al. (2020). Factors associated with response to adapted cognitive behavioral therapy for anxiety and depression following traumatic brain injury. J. Head Trauma Rehabil. 35, 117–126. doi: 10.1097/HTR.0000000000000510
Puccio, A. M., and Alexander, S. (2015). Chapter 4 genomics, transcriptomics, and epigenomics in traumatic brain injury research. Annu. Rev. Nurs. Res. 33, 75–109. doi: 10.1891/0739-6686.33.75
Rana, A., Singh, S., Deshmukh, R., and Kumar, A. (2020). Pharmacological potential of tocopherol and doxycycline against traumatic brain injury-induced cognitive/motor impairment in rats. Brain Inj. 34, 1039–1050. doi: 10.1080/02699052.2020.1772508
Robert, S. (2020). Traumatic brain injury and mood disorders. Ment.Health Clin. 10, 335–345. doi: 10.9740/mhc.2020.11.335
Rodrigues, T. B., Granado, N., Ortiz, O., Cerdán, S., and Moratalla, R. (2007). Metabolic interactions between glutamatergic and dopaminergic neurotransmitter systems are mediated through D(1) dopamine receptors. J. Neurosci. Res. 85, 3284–3293. doi: 10.1002/jnr.21302
Ruttan, L., Martin, K., Liu, A., Colella, B., and Green, R. E. (2008). Long-term cognitive outcome in moderate to severe traumatic brain injury: a meta-analysis examining timed and untimed tests at 1 and 4.5 or more years after injury. Arch. Phys. Med. Rehabil. 89, S69–S76. doi: 10.1016/j.apmr.2008.07.007
Ryttersgaard, T. O., Johnsen, S. P., Riis, J., Mogensen, P. H., and Bjarkam, C. R. (2020). Prevalence of depression after moderate to severe traumatic brain injury among adolescents and young adults: a systematic review. Scand. J. Psychol. 61, 297–306. doi: 10.1111/sjop.12587
Sadock, B. J., Sadock, V. A., and Ruiz, P. (2015). “Kaplan and Sadock’s synopsis of psychiatry” in Behavioral sciences/clinical psychiatry. 11th ed (Philadelphia (PA): Wolters Kluwer).
Sameh, G., Islem, F., Samar, A., Hedi, C., Mounir, B., and Habib, E. M. (2021). Neuropsychological and behavioral disorders, functional outcomes and quality of life in traumatic brain injury victims. Pan Afr. Med. J. 38:346. doi: 10.11604/pamj.2021.38.346.16120
Sami, M. B., and Faruqui, R. (2015). The effectiveness of dopamine agonists for treatment of neuropsychiatric symptoms post brain injury and stroke. Acta Neuropsychiat. 27, 317–326. doi: 10.1017/neu.2015.17
Sauerbeck, A., Hunter, R., Bing, G., and Sullivan, P. G. (2012). Traumatic brain injury and trichloroethylene exposure interact and produce functional, histological, and mitochondrial deficits. Exp. Neurol. 234, 85–94. doi: 10.1016/j.expneurol.2011.12.012
Schindler, A. G., Meabon, J. S., Pagulayan, K. F., Hendrickson, R. C., Meeker, K. D., Cline, M., et al. (2017). Blast-related disinhibition and risk seeking in mice and combat veterans: potential role for dysfunctional phasic dopamine release. Neurobiol. Dis. 106, 23–34. doi: 10.1016/j.nbd.2017.06.004
Schmidt, R. H., and Grady, M. S. (1995). Loss of forebrain cholinergic neurons following fluid-percussion injury: implications for cognitive impairment in closed head injury. J. Neurosurg. 83, 496–502. doi: 10.3171/jns.1995.83.3.0496
Schultz, W. (1998). Predictive reward signal of dopamine neurons. J. Neurophysiol. 80, 1–27. doi: 10.1152/jn.1998.80.1.1
Selvakumar, G. P., Ahmed, M. E., Iyer, S. S., Thangavel, R., Kempuraj, D., Raikwar, S. P., et al. (2020). Absence of glia maturation factor protects from axonal injury and motor behavioral impairments after traumatic brain injury. Exp. Neurobiol. 29, 230–248. doi: 10.5607/en20017
Shen, H., Harvey, B. K., Chiang, Y. H., Pick, C. G., and Wang, Y. (2011). Methamphetamine potentiates behavioral and electrochemical responses after mild traumatic brain injury in mice. Brain Res. 1368, 248–253. doi: 10.1016/j.brainres.2010.10.014
Shimada, R., Abe, K., Furutani, R., and Kibayashi, K. (2014). Changes in dopamine transporter expression in the midbrain following traumatic brain injury: an immunohistochemical and in situ hybridization study in a mouse model. Neurol. Res. 36, 239–246. doi: 10.1179/1743132813Y.0000000289
Shin, S. S., Bray, E. R., Zhang, C. Q., and Dixon, C. E. (2011). Traumatic brain injury reduces striatal tyrosine hydroxylase activity and potassium-evoked dopamine release in rats. Brain Res. 1369, 208–215. doi: 10.1016/j.brainres.2010.10.096
Shuanglong, Z., Jiangyuan, Y., Meng, N., Zheng, W., Yunshui, Z., Wei, S., et al. (2024). A meta-analysis of cognitive and functional outcomes in severe brain trauma cases. Front. Behav. Neurosci. 18:1349672. doi: 10.3389/fnbeh.2024.1349672
Singh, A., Prajapati, H. P., Kumar, R., Singh, N. P., and Kumar, A. (2022). Prognostic role of catecholamine in moderate-to-severe traumatic brain injury: a prospective observational cohort study. Asian J. Neurosur. 17, 435–441. doi: 10.1055/s-0042-1757217
Slowinski, A., Coetzer, R., and Byrne, C. (2019). Pharmacotherapy effectiveness in treating depression after traumatic brain injury: a meta-analysis. J. Neuropsychiatr. Clin. Neurosci. 31, 220–227. doi: 10.1176/appi.neuropsych.18070158
Small, K. M., Nunes, E., Hughley, S., and Addy, N. A. (2016). Ventral tegmental area muscarinic receptors modulate depression and anxiety-related behaviors in rats. Neurosci. Lett. 616, 80–85. doi: 10.1016/j.neulet.2016.01.057
Sönmez, Ü., Sönmez, A., Erbil, G., Tekmen, I., and Baykara, B. (2007). Neuroprotective effects of resveratrol against traumatic brain injury in immature rats. Neurosci. Lett. 420, 133–137. doi: 10.1016/j.neulet.2007.04.070
Spijker, J., and Claes, S. (2014). Stemmingsstoornissen in de DSM-5 [mood disorders in the DSM-5]. Tijdschr. Psychiatr. 56, 173–176
Stein, M. B., Jain, S., Giacino, J. T., Levin, H., Dikmen, S., Nelson, L. D., et al. (2019). Risk of posttraumatic stress disorder and major depression in civilian patients after mild traumatic brain injury: a TRACK-TBI study. JAMA Psychiatry 76, 249–258. doi: 10.1001/jamapsychiatry.2018.4288
Tan, L., Ge, G., Tang, J., Fu, C., Duanmu, W., Chen, Y., et al. (2015). Amantadine preserves dopamine levels and attenuates depression-like behavior induced by traumatic brain injury in rats. Behav. Brain Res. 279, 274–282. doi: 10.1016/j.bbr.2014.10.037
Tanaka, H., Ehara, A., Nakadate, K., Yoshimoto, K., Shimoda, K., and Ueda, S. (2019). Behavioral, hormonal, and neurochemical outcomes of neonatal repeated shaking brain injury in male adult rats. Physiol. Behav. 199, 118–126. doi: 10.1016/j.physbeh.2018.11.025
Tanaka, K., Ogawa, N., Asanuma, M., and Kondo, Y. (1997). Thyrotropin releasing hormone prevents abnormalities of cortical acetylcholine and monoamines in mice following head injury. Regul. Pept. 70, 173–178. doi: 10.1016/s0167-0115(97)01013-6
Traeger, J., Hoffman, B., Misencik, J., Hoffer, A., and Makii, J. (2020). Pharmacologic treatment of neurobehavioral sequelae following traumatic brain injury. Crit. Care Nurs. Q. 43, 172–190. doi: 10.1097/CNQ.0000000000000301
Tsuda, S., Golam, M., Hou, J., Nelson, R., Bernavil, P., Richardson, K., et al. (2020). Altered monoaminergic levels, spasticity, and balance disability following repetitive blast-induced traumatic brain injury in rats. Brain Res. 1747:147060. doi: 10.1016/j.brainres.2020.147060
Turner, B. M., Paradiso, S., Marvel, C. L., Pierson, R., Boles Ponto, L. L., Hichwa, R. D., et al. (2007). The cerebellum and emotional experience. Neuropsychologia 45, 1331–1341. doi: 10.1016/j.neuropsychologia.2006.09.023
Tye, K. M., Mirzabekov, J. J., Warden, M. R., Ferenczi, E. A., Tsai, H. C., Finkelstein, J., et al. (2013). Dopamine neurons modulate neural encoding and expression of depression-related behavior. Nature 493, 537–541. doi: 10.1038/nature11740
Uiterwijk, D., Stargatt, R., Humphrey, S., and Crowe, S. F. (2022). The relationship between cognitive functioning and symptoms of depression, anxiety, and post-traumatic stress disorder in adults with a traumatic brain injury: a meta-analysis. Neuropsychol. Rev. 32, 758–806. doi: 10.1007/s11065-021-09524-1
Vadlamani, A., and Albrecht, J. S. (2020). Severity of traumatic brain injury in older adults and risk of ischemic stroke and depression. J. Head Trauma Rehabil. 35, E436–E440. doi: 10.1097/HTR.0000000000000561
Van Bregt, D. R., Thomas, T. C., Hinzman, J. M., Cao, T., Liu, M., Bing, G., et al. (2012). Substantia nigra vulnerability after a single moderate diffuse brain injury in the rat. Exp. Neurol. 234, 8–19. doi: 10.1016/j.expneurol.2011.12.003
Vecht, C. J., van Woerkom, C. A., Teelken, A. W., and Minderhoud, J. M. (1975). Homovanillic acid and 5-hydroxyindoleacetic acid cerebrospinal fluid levels. A study with and without probenecid administration of their relationship to the state of consciousness after head injury. Arch. Neurol. 32, 792–797. doi: 10.1001/archneur.1975.00490540036004
Verduzco-Mendoza, A., Carrillo-Mora, P., Avila-Luna, A., Gálvez-Rosas, A., Olmos-Hernández, A., Mota-Rojas, D., et al. (2021). Role of the dopaminergic system in the striatum and its association with functional recovery or rehabilitation after brain injury. Front. Neurosci. 15:693404. doi: 10.3389/fnins.2021.693404
Vonder Haar, C., Ferland, J. M. N., Kaur, S., Riparip, L. K., Rosi, S., and Winstanley, C. A. (2019a). Cocaine self-administration is increased after frontal traumatic brain injury and associated with neuroinflammation. Eur. J. Neurosci. 50, 2134–2145. doi: 10.1111/ejn.14123
Vonder Haar, C., Martens, K. M., Bashir, A., McInnes, K. A., Cheng, W. H., Cheung, H., et al. (2019b). Repetitive closed-head impact model of engineered rotational acceleration (CHIMERA) injury in rats increases impulsivity, decreases dopaminergic innervation in the olfactory tubercle and generates white matter inflammation, tau phosphorylation and degeneration. Exp. Neurol. 317, 87–99. doi: 10.1016/j.expneurol.2019.02.012
Wagner, A. K., Chen, X., Kline, A. E., Li, Y., Zafonte, R. D., and Dixon, C. E. (2005b). Gender and environmental enrichment impact dopamine transporter expression after experimental traumatic brain injury. Exp. Neurol. 195, 475–483. doi: 10.1016/j.expneurol.2005.06.009
Wagner, A. K., Drewencki, L. L., Chen, X., Santos, F. R., Khan, A. S., Harun, R., et al. (2009). Chronic methylphenidate treatment enhances striatal dopamine neurotransmission after experimental traumatic brain injury. J. Neurochem. 108, 986–997. doi: 10.1111/j.1471-4159.2008.05840.x
Wagner, A. K., Postal, B. A., Darrah, S. D., Chen, X., and Khan, A. S. (2007b). Deficits in novelty exploration after controlled cortical impact. J. Neurotrauma 24, 1308–1320. doi: 10.1089/neu.2007.0274
Wagner, A. K., Ren, D., Conley, Y. P., Ma, X., Kerr, M. E., Zafonte, R. D., et al. (2007a). Sex and genetic associations with cerebrospinal fluid dopamine and metabolite production after severe traumatic brain injury. J. Neurosurg. 106, 538–547. doi: 10.3171/jns.2007.106.4.538
Wagner, A. K., Scanlon, J. M., Becker, C. R., Ritter, A. C., Niyonkuru, C., Dixon, C. E., et al. (2014). The influence of genetic variants on striatal dopamine transporter and D2 receptor binding after TBI. J. Cereb. Blood Flow Metab. 34, 1328–1339. doi: 10.1038/jcbfm.2014.87
Wagner, A. K., Sokoloski, J. E., Ren, D., Chen, X., Khan, A. S., Zafonte, R. D., et al. (2005a). Controlled cortical impact injury affects dopaminergic transmission in the rat striatum. J. Neurochem. 95, 457–465. doi: 10.1111/j.1471-4159.2005.03382.x
Wen, X., Wu, X., Liu, J., Li, K., and Yao, L. (2013). Abnormal baseline brain activity in non-depressed Parkinson’s disease and depressed Parkinson’s disease: a resting-state functional magnetic resonance imaging study. PLoS One 8:e63691. doi: 10.1371/journal.pone.0063691
Whelan-Goodinson, R., Ponsford, J., Johnston, L., and Grant, F. (2009). Psychiatric disorders following traumatic brain injury: their nature and frequency. J. Head Trauma Rehabil. 24, 324–332. doi: 10.1097/HTR.0b013e3181a712aa
Wilson, M. S., Chen, X., Ma, X., Ren, D., Wagner, A. K., Reynolds, I. J., et al. (2005). Synaptosomal dopamine uptake in rat striatum following controlled cortical impact. J. Neurosci. Res. 80, 85–91. doi: 10.1002/jnr.20419
Womack, K. B., Dubiel, R., Callender, L., Dunklin, C., Dahdah, M., Harris, T. S., et al. (2020). 123 I-Iofluopane single-photon emission computed tomography as an imaging biomarker of pre-synaptic dopaminergic system after moderate-to-severe traumatic brain injury. J. Neurotrauma 37, 2113–2119. doi: 10.1089/neu.2019.6892
Woolf, P. D., Hamill, R. W., Lee, L. A., Cox, C., and McDonald, J. V. (1987). The predictive value of catecholamines in assessing outcome in traumatic brain injury. J. Neurosurg. 66, 875–882. doi: 10.3171/jns.1987.66.6.0875
Woolf, P. D., Hamill, R. W., Lee, L. A., and McDonald, J. V. (1988). Free and total catecholamines in critical illness. Am. J. Phys. 254, E287–E291. doi: 10.1152/ajpendo.1988.254.3.E287
Writer, B. W., and Schillerstrom, J. E. (2009). Psychopharmacological treatment for cognitive impairment in survivors of traumatic brain injury: a critical review. J. Neuropsychiatr. Clin. Neurosci. 21, 362–370. doi: 10.1176/jnp.2009.21.4.362
Xu, X., Cao, S., Chao, H., Liu, Y., and Ji, J. (2016). Sex-related differences in striatal dopaminergic system after traumatic brain injury. Brain Res. Bull. 124, 214–221. doi: 10.1016/j.brainresbull.2016.05.010
Yan, H. Q., Kline, A. E., Ma, X., Hooghe-Peters, E. L., Marion, D. W., and Dixon, C. E. (2001). Tyrosine hydroxylase, but not dopamine beta-hydroxylase, is increased in rat frontal cortex after traumatic brain injury. Neuroreport 12, 2323–2327. doi: 10.1097/00001756-200108080-00009
Yan, H. Q., Kline, A. E., Ma, X., Li, Y., and Dixon, C. E. (2002). Traumatic brain injury reduces dopamine transporter protein expression in the rat frontal cortex. Neuroreport 13, 1899–1901. doi: 10.1097/00001756-200210280-00013
Yan, H. Q., Ma, X., Chen, X., Li, Y., Shao, L., and Dixon, C. E. (2007). Delayed increase of tyrosine hydroxylase expression in rat nigrostriatal system after traumatic brain injury. Brain Res. 1134, 171–179. doi: 10.1016/j.brainres.2006.11.087
Yang, S. Y., Zhang, S., and Wang, M. L. (1995). Clinical significance of admission hyperglycemia and factors related to it in patients with acute severe head injury. Surg. Neurol. 44, 373–377. doi: 10.1016/0090-3019(96)80243-6
Keywords: traumatic brain injury, depression, mood disorders, anxiety, dopamine
Citation: Mata-Bermudez A, Trejo-Chávez R, Martínez-Vargas M, Pérez-Arredondo A, Martínez-Cardenas MLÁ, Diaz-Ruiz A, Rios C and Navarro L (2024) Dysregulation of the dopaminergic system secondary to traumatic brain injury: implications for mood and anxiety disorders. Front. Neurosci. 18:1447688. doi: 10.3389/fnins.2024.1447688
Edited by:
Tao Liu, Tianjin Medical University General Hospital, ChinaReviewed by:
Rongcai Jiang, Tianjin Medical University General Hospital, ChinaWentai Zhang, Peking Union Medical College Hospital (CAMS), China
Copyright © 2024 Mata-Bermudez, Trejo-Chávez, Martínez-Vargas, Pérez-Arredondo, Martínez-Cardenas, Diaz-Ruiz, Rios and Navarro. This is an open-access article distributed under the terms of the Creative Commons Attribution License (CC BY). The use, distribution or reproduction in other forums is permitted, provided the original author(s) and the copyright owner(s) are credited and that the original publication in this journal is cited, in accordance with accepted academic practice. No use, distribution or reproduction is permitted which does not comply with these terms.
*Correspondence: Luz Navarro, bG5hdmFycm9AdW5hbS5teA==
†These authors have contributed equally to this work and share first authorship