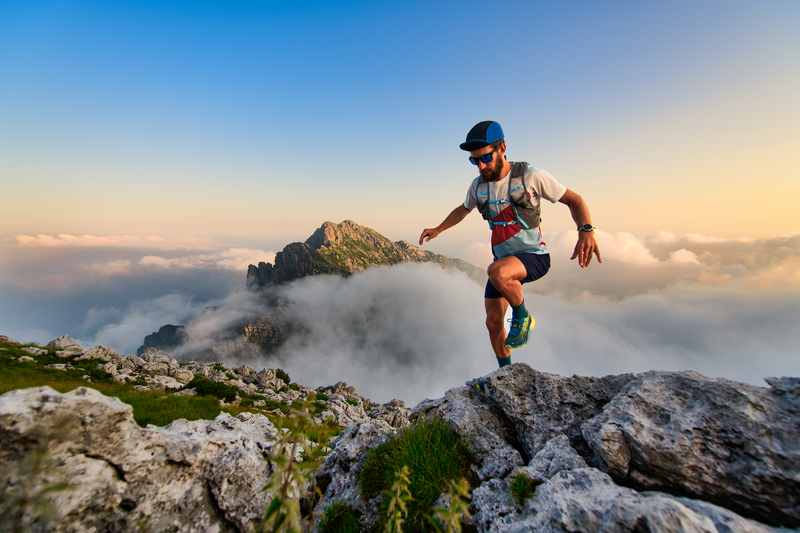
95% of researchers rate our articles as excellent or good
Learn more about the work of our research integrity team to safeguard the quality of each article we publish.
Find out more
ORIGINAL RESEARCH article
Front. Neurosci. , 25 September 2024
Sec. Decision Neuroscience
Volume 18 - 2024 | https://doi.org/10.3389/fnins.2024.1433061
Serotonin is an essential neuromodulator that affects behavioral and cognitive functions. Previous studies have shown that activation of serotonergic neurons in the dorsal raphe nucleus (DRN) promotes patience to wait for future rewards. However, it is still unclear whether serotonergic neurons also regulate persistence to act for future rewards. Here we used optogenetic activation and inhibition of DRN serotonergic neurons to examine their effects on sustained motor actions for future rewards. We trained mice to perform waiting and repeated lever-pressing tasks with variable reward delays and tested effects of optogenetic activation and inhibition of DRN serotonergic neurons on task performance. Interestingly, in the lever-pressing task, mice tolerated longer delays as they repeatedly pressed a lever than in the waiting task, suggesting that lever-pressing actions may not simply be costly, but may also be subjectively rewarding. Optogenetic activation of DRN serotonergic neurons prolonged waiting duration in the waiting task, consistent with previous studies. However, its effect on lever presses was nuanced, and was detected only by focusing on the period before premature reward check and by subtracting the trends within and across sessions using generalized linear model. While optogenetic inhibition decreased waiting, it did not affect lever pressing time or numbers. These results revealed that the necessity of motor actions may increase motivation for delayed rewards and that DRN serotonergic neurons more significantly promote waiting rather than persistent motor actions for future rewards.
Serotonin (5-HT) is an important neuromodulator involved in multiple biological functions, including emotion (Cools et al., 2008), motivation (Dayan and Huys, 2009), motor activity (Ranade and Mainen, 2009), and decision making (Homberg, 2012). 5-HT projections originate from raphe nuclei located in the midbrain. Among 9 raphe nuclei, the dorsal raphe nucleus (DRN) densely projects to the forebrain and DRN 5-HT neurons regulate reward-based learning and decision making (Miyazaki K. et al., 2012; Doya et al., 2021). This behavioral and anatomical diversity raised many theories about the role of 5-HT neurons and some theories suggest opposite behavioral effects.
Previous computational studies based on the reinforcement learning framework, proposed that 5-HT controls the temporal discount factor, and that activation of 5-HT neurons increases the relative importance of future rewards over immediate rewards (Doya, 2002; Schweighofer et al., 2007). In support of this hypothesis, a series of experimental studies has been done using delayed reward tasks, showing increased 5-HT transmission while rats were waiting for delayed rewards (Miyazaki K. et al., 2011; Miyazaki K. W. et al., 2011). Furthermore, pharmacological inhibition of DRN 5-HT neurons increased premature abandonment of delayed rewards (Miyazaki K. W. et al., 2012) and optogenetic activation of those neurons prolonged the time spent for waiting for delayed rewards, establishing a causal relationship between DRN 5-HT neurons and patience in waiting for future rewards (Miyazaki et al., 2014; Fonseca et al., 2015; Miyazaki et al., 2018; Miyazaki et al., 2020). Although those results consistently suggest that 5-HT plays an important role to increase actions for future rewards, the same behavioral effect can be explained by the other theory called behavioral inhibition hypothesis (Soubrie, 1986). The behavioral inhibition hypothesis proposed that 5-HT generally mediates inhibiting behavioral responses and could give an account for increased waiting in the studies mentioned above. Examining active behaviors to obtain future rewards enables us to dissociate those two possibilities. Also, compared to the studies examining the role of DRN 5-HT neurons in passive waiting to obtain future rewards, how these neurons regulate active behavior to obtain future rewards has not been well studied, except in the context of patch-leaving decision-making (Lottem et al., 2018).
To examine the role of DRN 5-HT neurons in sustained motor actions, we trained mice to perform an operant conditioning task that requires variable numbers of lever-presses, and we tested the effect of optogenetic activation and inhibition of DRN 5-HT neurons on motor actions. For comparison, we also tested the effect of optogenetic manipulation of DRN 5-HT neurons in a waiting task. We found that optogenetic activation of DRN 5-HT neurons prolonged waiting, but that it did not affect the duration or the number of lever presses for future rewards. While optogenetic inhibition reduced the waiting time, it had no effect on the duration or the number of lever presses for future rewards. Further analyses using generalized linear models (GLMs) revealed a negative effect of DRN 5-HT activation on the number and the speed of lever pressing. These results suggest that DRN 5-HT neurons regulate two types of behaviors for future rewards in different ways.
All experimental procedures were performed in accordance with guidelines established by the Okinawa Institute of Science and Technology Experimental Animal Committee. For the optogenetic activation experiment, we used Tph2-ChR2(C128S)-EYFP bi-transgenic mice. ChR2(C128S) is a step-type function opsin that remains activated by short pulses of blue light and deactivated by yellow light (Miyazaki et al., 2014). Eight Tph2-ChR2 mice were trained for the lever-pressing task before implantation of optic probes. Among them, four mice were first tested in the lever-pressing task and then the waiting task, while the other four mice were tested in the opposite order. For controls, five Tph2-tTA transgenic mice were used. All Tph2-tTA mice were first tested in the lever-pressing task followed by the waiting task.
For optogenetic inhibition experiments, we used Tph2-ArchT-EGFP bi-transgenic mice. ArchT activates an inhibitory current in response to yellow light (Han et al., 2011; Yoshida et al., 2019; Ohmura et al., 2021). We trained separate cohorts of Tph2-ArchT mice for different behavioral tasks. Five Tph2-ArchT mice were used for the waiting task and the other six mice were used for the lever-pressing task. Tph2-tTA transgenic mice were used as a control group. Four Tph2-tTA mice were used for the waiting task and five Tph2-tTA mice were used for the lever-pressing task. We generated bi-transgenic mouse lines as shown in Tanaka et al. (2012). All mice have the background of C57BL/6 mice. All mice were male and training started > 4 months of age.
All mice were housed individually at 24°C on a 12:12 h light: dark cycle (lights on 07:00–19:00 h). All behavioral training and testing sessions were performed during the light cycle, 5 days per week. Mice were deprived of access to food one day prior to the first training session and received daily food rations only during training and test sessions (approximately 2–3 g per day) during experimental days. Food was freely available during days off more than 15 h before the next session. Mice could freely access water in their home cages.
All training and testing sessions were performed in operant boxes (Med-associates, 21.6 cm width × 17.8 cm depth × 12.7 cm height). Two 2.5-cm, square holes were located in the walls on opposite sides of the box. One hole was designated as the reward site and was connected to a food dispenser delivering 20-mg food pellets, while the other hole was defined as a tone site. A retractable lever was positioned to the left of the reward site. One 2.8-W house light and one speaker were located above and to the upper right of the tone site, respectively. Hardware attached to operant boxes was controlled via MED-PC IV software (Med-associates).
We used the same behavioral task as reported in previous studies (Figure 1A(i); Miyazaki et al., 2014; Miyazaki et al., 2018). In this task, mice could initiate a trial with a 0.3-s nose poke to the tone site, which triggered a 0.5-s tone. After hearing the tone, mice were required to continue poking their noses into the reward site. The required duration of the nose-poke was randomly chosen as 2, 6, 10 s, and infinity (reward omission) during each trial. Once mice could wait for the required time, a food pellet was delivered to the reward site. For the photoactivation experiment, mice could initiate the next trial just after a reward delivery or after leaving the reward site. Because the suppression efficacy of ArchT decreases without sufficient duration of intervals between light stimulation (Mattis et al., 2011), for the photoinhibition experiment, the house light was turned off for 30 s after the end of a trial and the next trial could be initiated once the house light was turned on again. One session consisted of 43 trials (5 trials × 2 photostimulation conditions × 4 delays + 3 trials with different photostimulation and delay conditions). Three sessions were performed on a testing day. Before the testing sessions, mice were trained for 2 h, 5 days per week, and it took 2 weeks or less for mice to learn the task.
Figure 1. Behavioral tasks. (A) Schematic drawing of (i) the waiting task and (ii) the repeated lever-pressing task. (B) Time sequence of rewarded and failure/reward omission trials with optic stimulation for optogenetic activation experiments during (i) the waiting task and (ii) the lever-pressing task. We tested the effect of optic stimulation both in experimental (Tph2-ChR2 mice) and control (Tph2-tTA mice) mice group to confirm optogenetic activation of DRN 5-HT neurons induced behavioral effect. (C) Time sequence of rewarded and failure/reward omission trials with optic stimulation for optogenetic inhibition experiments during (i) the waiting task and (ii) the lever-pressing task. Similary to the optogenetic activation experiment, we performed the tasks both in experimental (Tph2-ArchT mice) and control (Tph2-tTA mice) mice.
During testing sessions, 470-nm blue or 590-nm yellow light stimulation was given, generated by an LED light source (Doric Lenses). Timing of stimulation was effected by TTL pulses controlled with MED-PC IV software. For the optogenetic activation experiment [Figure 1B(i)], in half the trials (selected at random), blue light stimulation was applied for activation, and in the other half, yellow light stimulation was given as a control. A 0.8-s blue/yellow light pulse was given when mice first poked their noses into the reward site. At the end of the trial, either when the mice waited until the end for the required delay or when they left the reward site, a 1-s yellow light pulse was given to reset photoactivation. The intensity of blue and yellow light at the tip of the optical fibers was 1.6–2.0 mW and 1.1–2.0 mW, respectively. For the optogenetic inhibition experiment [Figure 1C(i)], in half the trials, yellow light stimulation was applied for inhibition, while no light was applied for control trials in the remaining trials. In the inhibition trials, continuous yellow light was applied from the onset of waiting until the end of a trial. Intensities of yellow light at the tip of the optical fibers were 2.8–3.2 mW.
Task structure is described in Figure 1A(ii). After the house light was turned on, mice could initiate a trial by poking their noses into the tone site for 0.3 s. The 0.3-s nose poke triggered a speaker to generate a 2-s tone, after which a retractable lever was presented. The number of lever-presses required was randomly chosen as 8, 16, 32, 64, and infinity (reward omission) during each trial. Once mice pressed the lever the required number of times, the lever was withdrawn, and 1 s after lever withdrawal, a food pellet was delivered to the reward site. Alternatively, mice could abandon the trial with a 0.3-s nose poke into the tone site. After reward delivery or abandonment of the trial, a 15-s inter-trial interval was inserted, which was indicated by turning off the house light. After the 15-s inter-trial interval, mice could initiate the next trial. One testing session consisted of 53 trials (5 trials x 2 photostimulation conditions x 5 press number conditions + 3 trials with different photostimulation and press conditions). Two sessions were performed on a given testing day.
For the optogenetic activation experiment [Figure 1B(ii)], in half the trials (selected at random), blue light stimulation was applied for activation, and in the other half, yellow light stimulation was given as a control. A 0.8-s blue/yellow light pulse was given when the mice started to press the lever and repeated at 20-s intervals. At the end of a trial, either when the mice pressed the lever the required number of times, or when they abandoned the trial, a 1-s yellow light pulse was given. For the optogenetic inhibition experiment [Figure 1C(ii)], in half the trials, yellow light stimulation was applied for inhibition, while no light was applied for control trials in the remaining trials. In the inhibition trials, continuous yellow light was applied from the onset of lever-pressing until the end of a trial. We used the same LED light source and light intensity from the testing sessions of the waiting task described in a previous section.
Before testing sessions commenced, mice were trained to perform the lever-pressing task using the following schedule. Training took approximately 3 weeks.
All training sessions were performed either until mice earned 100 food pellets or by 2 h, whichever came first. In order to habituate mice to the behavioral apparatus, they were first trained to poke their noses into the reward site to obtain a food pellet. Then they were trained to press a lever once to acquire a food pellet. Once mice could get more than 80 food pellets, they were trained to press a lever 3 times to obtain a food pellet. While the number of lever-presses required was progressively increased from 3, 5, 7, 10, 16, and to 32 times, mice were trained to learn that the lever was presented after a tone was generated. After the association between tone and lever presentation was established, mice were trained to poke their noses to generate a tone for lever presentation. Training was complete when mice could get more than 80 rewards in a training session, during which they were required to press the lever 32 times after initiating a trial using nose pokes.
After training for the lever-pressing task was completed, a craniotomy was performed to implant an optic probe (400 μm diameter, 0.48 NA, 4 mm length, Doric) above the DRN. Mice were anesthetized with isoflurane (3% for induction and 1–1.5% during surgery). Mice were placed on a stereotaxic stage and their heads were fixed with ear bars. Then the skull was exposed with a blade, and a hole was made with a drill. Once the brain was exposed through the hole, the dura was removed using the tip of a needle, and the optic probe was lowered above the DRN through the hole (from the bregma: posterior, −4.6 mm; lateral, 0 mm; ventral, −2.6 mm). Light-sensitive adhesive and dental cement was applied to the skull to fix the implanted optic probe. Mice were placed back in their home cages for recovery. At least one week after the surgery, we started to retrain the mice for the behavioral task, and then commenced testing sessions.
After the behavioral tests, mice were deeply anesthetized with 100 mg/kg sodium pentobarbital i.p., and perfused with saline or PBS followed by 4% PFA/PB or 4% PFA/PBS. Brains were removed immediately after perfusion and immersed in fixative solution overnight. Then, 50-μm coronal slices were sectioned using a vibratome (VT1000S, Leica) and the implantation site of optic probes was confirmed according to the mouse brain atlas (Franklin, 2008).
Brain slices were incubated with primary antibodies for 1–2 nights. Slices were then rinsed with PBS and incubated with secondary antibodies for 1–2 nights. After incubation and rinsing, slices were mounted on slide glasses. Fluorescent images (Figure 2B) were acquired using a spinning disk confocal microscope (Nikon). As primary antibodies, we used anti-Tph (1:250, sheep polyclonal, Merck Millipore, AB1541) and anti-GFP (1:500, chicken polyclonal, Abcam, ab13970) as markers for 5-HT neurons and ChR2-EYFP or ArchT-EGFP neurons, respectively. For secondary antibodies, anti-sheep and anti-chicken antibodies conjugated with Alexa flour 594, and 488, respectively, were used. Antibodies were diluted in staining buffer containing 10 mM HEPES, 20 mM NaCl, and 10% Triton-X100. The pH of the staining buffer was adjusted to 7.4 in advance.
Figure 2. Optogenetic manipulation of DRN 5-HT neural activities. (A) The implantation site of optic probes for ChR2 mice. Coronal views of the mouse brain are adapted from Franklin (2008). Red filled areas indicate the DRN. Blue rectangles indicate tracks of implanted optic fibers. (B) Fluorescence images from ArchT mice. The left image (4x) shows fluorescent signal from Tph2 (red) and ArchT-EGFP (green) in a coronal brain section including DRN. Magnified images (60x) indicate the florescent signal of Tph2 (Left), ArchT-EGFP (Middle), and co-localization of the two signals (Right). Scale bars indicate 100 mm. (C) The implantation site of optic probes for ArchT mice. Coronal views of the mouse brain are adapted from Franklin (2008). Red filled areas indicate the DRN. Blue and orange rectangles indicate the track of optic fibers in the ArchT mice used for lever-pressing and waiting tasks, respectively.
In the lever-pressing task, the successful trial rate for 8-, 16-, 32-, and 64-press trials was calculated by dividing the number of rewarded trials by the total number of trials. In omission trials, the number of lever-presses, the time spent lever-pressing, which was defined as the time elapsed from the first lever-press to the last, and the time to abandon an omission trial, which was defined as the duration between the last lever-press and a nose poke to terminate the trial, were measured. To examine action vigor, inter-press intervals (IPIs) were measured. IPIs longer than 5 s were defined as long IPIs and below 5 s as short IPIs. In the waiting task, the duration of maintaining a nose poke was measured in omission trials. Behavioral parameters were calculated using custom-written programs in MATLAB.
Statistical tests for summary statistics were selected based on whether data exhibited normality and homogeneity of variance, assessed by the Shapiro-Wilk test and Levene test, respectively. If data satisfied these assumptions, we used paired t-tests for within-group comparison and unpaired t-tests for group comparisons. If not, we used the Wilcoxon signed-rank test for within-subject comparisons and the Mann Whitney U-test for group comparisons. Statistical analysis was performed using Python. For analysis of short IPIs, we applied repeated measures analysis of variance (ANOVA) using SPSS.
To examine the effect of optogenetic manipulation while taking into account multiple task-relevant variables and individual variability, we constructed GLMs and fit them to behavioral data. Response variables were the number of lever-presses and the short IPIs. For the number of lever-presses, we used logarithm link function and assumed a Poisson distribution. For short IPIs, we assumed a normal distribution and used an identity link function. For the time to abandon a trial in optogenetic inhibition experiment, we assumed an inverse gaussian distribution and used the corresponding link function. Fixed effect terms were intercept, manipulation, elapsed time and session index, and the random effect term was the intercept for individuals. Manipulation was coded as 0 for control trials, i.e., yellow light/no-light trials in activation/inhibition studies, and 1 for intervention trials, i.e., blue light/yellow light trials in activation/inhibition studies. Elapsed time was how many seconds elapsed since the start of the session until a nose-poke to initiate an omission trial (up to 7,200 s). Session index denoted how many sessions the subject had experienced across experimental periods (up to 8 sessions). GLM analysis was performed with the fitglme function in MATLAB. t-statistics and p-values were calculated to test the null hypothesis that the estimated regression coefficients were equal to zero.
We used eight Tph2-ChR2(C128S)-EYFP bi-transgenic mice (hereafter, referred to as ChR2 mice) to selectively activate DRN 5-HT neurons following a pulse of blue light, as in previous studies (Miyazaki et al., 2014; Miyazaki et al., 2018). Six of the eight ChR2 mice were sacrificed to confirm the implantation site of the optic probes. We could not perfuse the other two ChR2 mice because they died before the process. Although the site varied along the anterior-posterior axis, all probes examined were located above the DRN (Figure 2A).
To selectively inhibit DRN 5-HT neurons, we prepared eleven Tph2-ArchT-EGFP bi-transgenic mice (hereafter, referred to as ArchT mice). These transgenic mice selectively express ArchT, a light-sensitive proton pump, in 5-HT neurons. When sensing yellow light, ArchT induces efflux of H+ and inhibits neural activities. To confirm selective expression of ArchT, we performed a histological experiment to compare cells expressing EGFP and 5-HT neurons identified by Tph2 immunohistochemistry in three ArchT mice that were not used for the photoinhibition experiment. In nine slices from the three ArchT mice, 1058 Tph-positive cells were found. Of these cells, 72.5% were also ArchT-EGFP-positive. On the other hand, there were very few Tph-negative, but ArchT-EGFP-positive cells, suggesting that Tph2-ArchT bi-transgenic mice selectively expressed ArchT in 5-HT neurons. We quantitatively measured Tph and ArchT cells in five slices from the three ArchT mice that had experienced optogentic inhibition test sessions for 4 weeks of experiments. Percentages of Tph-positive and ArchT-positive cells were 69.8%, which was comparable to non-stimulated samples. Numbers of Tph-positive and ArchT-positive cells in stimulated samples were not significantly different from those in non-stimulated samples (stimulated vs. non-stimulated: 57.60 ± 24.00 and 85.11 ± 11.19, t12 = 1.82, p = 0.0829, unpaired t-test). In separate cohorts of ArchT mice used for the present studies, we found abundant expression of ArchT. Colocalization of ArchT and Tph was confirmed in ArchT mice (Figure 2B), suggesting that optogenetic inhibition did not induce severe damage in 5-HT neurons after the experimental sessions. We also confirmed the implantation site of optic probes in eight of eleven ArchT mice used in lever-pressing and waiting tasks. We could not perfuse the other three mice for various reasons: one that died before perfusion, another that was used in another experiment and the third for unexpected removal of the probe before perfusion. All optic probes confirmed were implanted above the DRN in ArchT mice (Figure 2C). Data from those three mice were also included, since their data showed trends similar to those from the other eight mice.
Mice were trained to perform two operant conditioning tasks: a waiting task and a repeated lever-pressing task. In order to optogenetically activate DRN 5-HT neurons, a 0.8-s blue/yellow light pulse was given at the onset of a trial and a 1-s yellow light pulse was applied at the end there of (Figure 1B(i) for the waiting task and 1B(ii) for the lever-pressing task). In the lever-pressing task, short blue/yellow pulses were applied every 20 s to refresh photostimulation [Figure 1B(ii)].
To confirm the effectiveness of optogenetic activation, we examined whether the optogenetic activation protocol used here affected waiting behaviors for delayed rewards of 2 s, 6 s, 10 s or infinity (reward omission) (Figure 3A). Optogenetic activation with blue light significantly increased waiting duration during omission trials in ChR2 mice (t7 = 6.31, p = 0.00040, paired t-test; Figure 3B). The change of duration in ChR2 was significantly larger than that in control mice (t11 = 4.98, p = 0.00042, unpaired t-test; Figures 3B–D). This result was consistent with previous studies using the same behavioral task (Miyazaki et al., 2014; Miyazaki et al., 2018) and confirmed that the administered optogenetic stimulation was sufficient to induce behavioral changes. These effects were similar for all 8 mice (Figure 3B), which suggests that the DRN was also effectively stimulated in the two mice in which we could not check the implantation site of the optic probes.
Figure 3. Activation of DRN 5-HT neurons prolonged waiting for future rewards. (A) The definition of waiting duration. (B,C) Waiting duration in an omission trial in ChR2 [(B), n = 8 mice] and control [(C), n = 5 mice] mice. Blue and green dots indicate the mean across ChR2 and control mice, respectively. (D) Change of waiting duration in blue light trials to yellow light trials in control (n = 5 mice) and ChR2 (n = 8 mice) mice. Green- and blue-filled circles indicate the mean across control (n = 5 mice) and ChR2 (n = 8 mice) mice respectively. Error bars represent the SEM in all graphs. n.s. p > 0.05, *** p < 0.001.
In order to examine whether optogenetic activation of DRN 5-HT neurons affects persistence in motor actions for future rewards, we analyzed the successful trial rate, the duration, the number of lever presses in omission trials, the time spent in abandoning an omission trial, and the action speed in the lever-pressing task. Details of the analysis of summary statistics is summarized in Supplementary Table 1.
We first calculated the percentage of successfully rewarded trials in 8-, 16-, 32-, and 64-press trials. In 8-, 16-, and 32-press trials, mice successfully obtained rewards almost 100% of the time. In 64-press trials, the successful trial rate decreased, but was not significantly different between blue light and yellow light stimulation (t7 = 0.23, p = 0.83, paired t-test; Figure 4A, and t4 = 0.073, p = 0.95, paired t-test; Figure 4B).
Figure 4. Activation of DRN 5-HT neurons did not change persistence in motor actions for future rewards. (A,B) Successful trial rates in ChR2 [(A), n = 8 mice] and control [(B), n = 5 mice] mice. Open and filled circles indicate the mean across blue and yellow light trials, respectively, in ChR2 (blue) and control (green) mice. (C) The definition of behavioral measures for action persistence. (D,E) Behavioral parameters in ChR2 [(D), n = 8 mice] and control [(E), n = 5 mice] mice. Blue and green dots indicate the mean across ChR2 and control mice data, respectively. (F) Change of behavioral parameters in blue light trials to yellow light trials in control (n = 5 mice) and ChR2 (n = 8 mice) mice. Green- and blue-filled circles indicate the mean across control and ChR2 mice, respectively. Error bars represent the SEM in all graphs. n.s. p > 0.05.
To quantify how long mice could sustain actions for delayed rewards, we next measured the time spent pressing the lever and the elapsed time from the first lever-press to the last lever-press in omission trials (Figure 4C). Interestingly, mice spent more than three times longer pressing the lever [48.68 ± 3.09 s with yellow light, Figure 4D(i)] than they spent in waiting (15.0 ± 0.37 s, Figure 3B) for the same reward, showing that mice can tolerate longer delays while they are actively engaged in doing something, as opposed to waiting inactively. However, the time spent lever-pressing in an omission trial was not significantly different between trials with activation and those without [t7 = 0.083, p = 0.94, paired t-test; Figure 4D(i)]. The change of duration in ChR2 was not significantly different from that in control mice [t11 = 0.16, p = 0.88, unpaired t-test; Figures 4D–F(i)].
To quantify how persistently mice sustained motor actions for future rewards, we measured the number of lever-presses in omission trials [Figure 4D(ii)]. The number of lever-presses in omission trials with optogenetic activation was not significantly different from that without activation [t7 = 0.93, p = 0.38, paired t-test; Figure 4D(ii)]. The change in the number in ChR2 was not significantly different from that in control mice [t11 = 0.17, p = 0.87, unpaired t-test; Figures 4D–F(ii)].
We next measured the time from the last lever-press to a nose poke in the tone site to abandon an omission trial, which could indicate how ambivalent mice were about abandoning the present trial [Figure 4C(iii)]. In ChR2 mice, optogenetic activation did not significantly change the time spent to abandon a trial [z = 1.26, p = 0.23, Wilcoxon signed-rank test; Figure 4D(iii)] and the change in time was not significantly different from that in control mice [t11 = 1.40, p = 0.19, unpaired t-test; Figures 4D–F(iii)].
In order to examine how optogenetic activation of DRN 5-HT neurons affects the speed of motor actions, we measured inter-press intervals (IPIs), the intervals between successive lever-presses. Behavioral observations indicated that mice usually pressed the lever continuously, but sometimes paused lever-pressing, such as resting, checking the reward site, or exploring, especially in omission trials. To be specific, most IPIs were < 5 s, but small numbers of IPIs were longer (Figure 5A). Therefore, we defined IPIs < 5 s as short IPIs, which represent continuous lever-pressing behavior, and IPIs ≥ 5 s as long IPIs, which mainly represent other behaviors, and examined the effect of the photoactivation on each type of IPI.
Figure 5. Activation of DRN 5-HT neurons did not change the speed of actions in the lever-pressing task. (A) The definition of long and short IPIs. (B,C) Long IPIs in omission trials in ChR2 (B, n = 8 mice) and control [(C), n = 5 mice] mice. Blue and green dots indicate the means across ChR2 mice and control mice, respectively. (D) Change of long IPIs in blue light trials to yellow light trials in control (n = 5 mice) and ChR2 (n = 8 mice) mice. Green- and blue-filled circles indicate the means across control and ChR2 mice, respectively. (E,F) Short IPIs in ChR2 [(E), n = 8 mice) and control [(F), n = 5 mice] mice. Open and filled circles indicate the mean across blue and yellow light trials respectively in ChR2 (blue) and control (green) mice. Error bars represent the SEM in all graphs. n.s. p > 0.05.
Long IPIs were analyzed only in omission trials, because they were rarely found in trials requiring 8 or more presses. In ChR2 mice, there was no significant difference in long IPIs between blue light and yellow light stimulation (z = 1.26, p = 0.23, Wilcoxon signed-rank test; Figure 5B). The change of long IPIs in ChR2 mice was not significantly different from that in control mice [t11 = 0.0027, p = 0.998, unpaired t-test; Figures 5B–D)].
For short IPIs, we first calculated the average short IPI in a trial and then the median across trials for each mouse. Those values were statistically tested using repeated measures ANOVA (Figure 5E for ChR2 mice, Figure 5F for control mice, and Supplementary Table 2 for statistical details). In ChR2 mice, there was a significant main effect of press conditions (five levels within-subject factors; 8-press, 16-press, 32-press, 64-press, and omission, F(4,28) = 25.64, P = 5.2 x 10–9) and a marginal main effect on the factor of stimulation conditions (yellow and blue, F(1,7) = 5.55, P = 0.051). However, there was no significant interaction effect (stimulation x press, F(4,28) = 1.86, P = 0.15). In control mice, there was a significant main effect of press conditions (F(4,16) = 42.62, P = 2.4 x 10–8), but no significant main effect of stimulation (F(1,4) = 0.16, P = 0.71). We also did not find a significant interaction effect (stimulation x press, F(4,16) = 0.29, P = 0.88).
Some of the behavioral measures such as the number of lever-presses or the time spent in lever-pressing seemingly showed general differences between ChR2 and control mice. Those results might reflect that repeated optogenetic activations of DRN 5-HT neurons affected overall locomotion across sessions as shown in a previous study (Correia et al., 2017). We examined general difference of motor actions between ChR2 and control mice using repeated measures ANOVA which allows us to test main effect of genotype (two levels between-subject factors; ChR2 or control). We did not find significant main effects of genotype in the number of lever-presses, the time spent lever-pressing, and long and short IPIs (Supplementary Table 3). Correia et al. (2017) also showed that transient and repeated optogenetic activation of DRN 5-HT neurons had opposite effects on locomotion. Therefore, the effect of the repeated activation would affect motor actions in control trials (i.e. yellow light trials) across days. To examine this, we compared the behavioral measures in yellow light trials of first two (early) and last two (late) sessions. We statistically tested the number of lever-presses, time spent lever-pressing and the time to abandon a trial using repeated measures ANOVA with the factors of sessions (two levels within-subject factors; early or late sessions) and genotype of animals (two levels between-subject factors; ChR2 or control). The time spent lever-pressing was decreased in late sessions in both groups (Supplementary Figure 1A). Repeated measures ANOVA showed that there was a significant main effect of sessions (F(1,11) = 5.10, P = 0.045) consistent with this observation. However there was no significant interaction effect (session x genotype, F(1,11) = 3.03, P = 0.11), suggesting the time spent lever-pressing was decreased across sessions but independently of repeated optogenetic activation. In the number of lever-pressing (Supplementary Figure 1B) and the time to abandon a trial (Supplementary Figure 1C), we did not find any significant main or interaction effects (Supplementary Table 4). We also examined how short IPIs changed across sessions in ChR2 and control mice. We statistically tested this using repeated measures ANOVA with the factors of sessions (two levels within-subject factors; early or late sessions) and press conditions (five levels within-subject factors; 8-press, 16-press, 32-press, 64-press, and omission). In ChR2 mice (Supplementary Figure 1D), there was a significant main effect of press conditions (F(4,12) = 20.19, P = 0.001), but a main effect of the factor of sessions was not significantly different (F(1,7) = 1.54, P = 0.25). In control mice (Supplementary Figure 1E), there was a significant main effect of press conditions (F(4,16) = 12.16, P = 0.001) as well as sessions (F(1,4) = 15.36, P = 0.017). There was no any significant interaction (F(4,16) = 1.33, P = 0.30). This might suggest that repeated optogenetic activation slowed down general speed for active lever-pressing. However, we need to note that the half of ChR2 animals had prior experience of optogenetic activation in waiting task, which might work as confounding factor. Indeed, when we applied repeated measures ANOVA to short IPI data from the other half of ChR2 animals which were tested from the lever-pressing task, there was a marginal main effect of sessions (F(1,3) = 8.98, P = 0.058). Overall, those results suggest that repeated optogenetic activation did not show remarkable effect on motor actions.
After repeated lever-presses, mice sometimes checked the reward site before reward delivery and then continued pressing the lever (58.66 ± 20.07 % of all omission trials). We define premature reward checking as a nose-poke at a reward site longer than 0.1 s when the reward was unavailable (Figure 6A).
Figure 6. GLM analysis revealed a subtle effect of optogenetic activation on action persistence and speed. (A) The definition of premature reward check. (B) GLM model analysis of the number of lever-presses before the first premature check in Control (n = 5 mice) and ChR2 (n = 8 mice) mice. Blue and green bars indicate coefficients of optic stimulation (left), elapsed time (middle), and the number of sessions (right) in ChR2 and control mice, respectively. (C) GLM model analysis of short IPIs before the first premature check in control (n = 5 mice) and ChR2 (n = 8 mice) mice. Blue and green bars indicate the coefficients of optic stimulation (left), elapsed time (middle), and the number of sessions (right) in ChR2 and control mice, respectively. Error bars indicate 95% confidence intervals of the coefficients. n.s. p > 0.05, ** p < 0.01, *** p < 0.001.
We found that lever-pressing behavior was quite different before and after the first premature reward check. The duration of short IPIs for each subject increased significantly after the first premature reward check (before: 0.35 ± 0.11 s; after: 0.75 ± 0.91 s; t12 = 2.76, p = 0.02, paired t-test). This suggests that mice focused more on lever-pressing behaviors before a premature reward check. Thus, we examined how activation of DRN 5-HT neurons affected action persistence and speed before a premature reward check. We analyzed behavioral measures using summary statistics as above, but we did not find a significant difference in the number of lever-presses or short IPIs (Supplementary Figure 2 and Supplementary Table 5).
In order to dissociate the effect of optogenetic manipulation from other task-relevant factors like fatigue and satiety, as well as to consider the mixed effect of individual variability, we performed GLM analysis of the number and speed of lever presses before premature reward checks (See Figure 6A for the definition) with regressors including optogenetic manipulation, elapsed time in a session, and the session number across the experimental period (see Section “Generalized linear model (GLM) analysis”).
In ChR2 mice, the number of lever-presses weakly but significantly decreased as a result of optogenetic activation (Figure 6B Manipulation; t611 = 3.38, p = 0.00077), but not in control mice (Figure 6B Manipulation; t390 = 0.60, p = 0.55). Results of other fixed effect terms were similar across ChR2 and control groups. The number of lever-presses significantly decreased within a session (Figure 6B Elapsed time; ChR2 mice: t611 = 7.5, p = 2.2 × 10–13; Control mice: t390 = 8.22, p = 3.0 × 10–15) and significantly increased across sessions (Figure 6B # sessions; ChR2 mice: t611 = 5.3, p = 1.6 x 10–7; Control mice, t390 = 5.40, p = 1.1 × 10–7). Full detail of GLM analysis of the number of lever-presses is summarized in Supplementary Table 6.
Short IPIs were weakly but significantly increased by optogenetic activation in ChR2 mice, but not in control mice (Figure 6C Manipulation; ChR2 mice: t611 = 2.4, p = 0.017; t390 = 0.65, p = 0.52). Again, the results of other fixed-effect terms were consistent across the two groups. Short IPIs decreased within each session in both groups (Figure 6C Elapsed time; ChR2 mice: t611 = 4.50, p = 6.9 x 10–6); Control mice: t390 = 2.69, p = 0.0074) and decreased across multiple sessions (Figure 6C # sessions; ChR2 mice: t611 = 5.75, p = 1.3 x 10–8; Control mice: t390 = 18.61, p = 9.2 x 10–56). Full detail of GLM analysis of the number of lever-presses is summarized in Supplementary Table 7.
In the results above, an interesting observation was that mice invested about three-fold more time for the same reward in the lever pressing task (Figure 4D) as in the waiting task (Figure 3B), suggesting that lever pressing does not simply serve as a motor cost. While optogenetic activation of DRN 5-HT neurons clearly facilitated waiting, its effect on lever presses was more subtle, and was detected only by focusing on the period before premature reward check and by subtracting the trends within and across sessions in GLMs.
A subset of putative DRN 5-HT neurons increase their activity by behavioral activation, such as locomotion, changing direction, and approach/withdrawal behaviors (Ranade and Mainen, 2009). Also, mice spent much longer time in engaging in lever-pressing than waiting. Given this observation, it is possible that lever-pressing behavior itself increases activity of DRN 5-HT neurons more strongly than the waiting behavior does. Therefore, we examined the effect of optogenetic inhibition of DRN 5-HT neurons on action maintenance. The optogenetic inhibition allows us to suppress DRN 5-HT neural activities which would be induced by motor action and test their behavioral functions. In order to optogenetically inhibit DRN 5-HT neurons, continuous yellow light was applied from the onset of action until the end of the trial (Figure 1C).
We first trained five ArchT and four control mice for the waiting task and tested the effect of optogenetic inhibition of DRN 5-HT neurons. A previous study showed that chemical inhibition of DRN 5-HT neurons increased premature abandoning in a delayed reward task (Miyazaki K. W. et al., 2012). Therefore, we predicted that optogenetic inhibition of DRN 5-HT neurons would decrease waiting duration in omission trials. As predicted, there was a significant decrease in waiting duration (see Figure 7A for the definition of waiting duration) in omission trials during optogenetic inhibition (no light vs. yellow trials: t4 = 10.27, p = 0.00051, paired t-test; Figure 7B). The change in ArchT mice was significant compared to that in control mice (t7 = 4.07, p = 0.0047, unpaired t-test; Figures 7B–D). These results confirmed the effectiveness of the optogenetic inhibition protocol and also the causal relationship between decreased DRN 5-HT neural activity and impaired waiting for delayed rewards.
Figure 7. Inhibition of DRN 5-HT neurons shortened the waiting period for future rewards. (A) The definition of waiting duration. (B,C) Waiting duration in an omission trial in ArchT (B, n = 5 mice) and control [(C), n = 4 mice] mice. Yellow and green dots indicate the mean across ArchT and control mice, respectively. (D) Change of waiting duration in yellow light trials to no-light trials in control (n = 4 mice) and ArchT (n = 5 mice) mice. Green- and yellow-filled circles indicate the mean across control (n = 4 mice) and ArchT (n = 5 mice) mice, respectively. Error bars represent the SEM in all graphs. n.s. p > 0.05, ** < 0.01, *** p < 0.001.
In order to examine whether optogenetic inhibition of DRN 5-HT neurons affects sustained motor actions for future rewards, we analyzed the successful trial rate, the duration, the number of lever presses in omission trials, the time spent abandoning a trial, and the vigor in the lever-pressing task as in the optogenetic activation experiment. Details of the analysis of summary statistics are summarized in Supplementary Table 8.
All mice were able to perform 8-, 16-, and 32-press trials without failure. In 64-press trials, the successful trial rate slightly decreased compared with other types of trials, but there was no significant difference between no-light and yellow-light trials (t5 = 0.24, p = 0.82, paired t-test; Figure 8A and t4 = 1.0, p = 0.37, paired t-test; Figure 8B).
Figure 8. Inhibition of DRN 5-HT neurons neither enhanced nor suppressed sustained motor actions. (A,B) Successful trial rates in ArchT [(A), n = 6 mice] and control [(B), n = 5 mice] mice. Open and filled circles indicate the mean across no light and yellow light trials, respectively, in ArchT (yellow) and control (green) mice. (C) The definition of behavioral measures for action persistence. (D,E) Behavioral parameters in ArchT [(D), n = 6 mice] and control [(E), n = 5 mice] mice. Yellow and green dots indicate the mean across ArchT and control mice data, respectively. (F) Change of behavioral parameters in yellow light trials to no-light trials in control (n = 5 mice) and ArchT (n = 6 mice) mice. Green- and yellow-filled circles indicate the means across control and ArchT mice, respectively. Error bars represent the SEM in all graphs. n.s. p > 0.05, * < 0.05, ** p < 0.01.
We measured the time that mice spent pressing the lever and the time from the first to the last lever-press, in omission trials. The time spent lever-pressing in omission trials [Figure 8C(i)] was much longer than that for waiting in omission trials (Figure 7), as in the optogenetic activation experiment, but did not differ significantly between trials with and without optogenetic inhibition [W = 4.0, p = 0.22, Wilcoxon signed-rank test; Figure 8D(i)]. The change of time in ArchT mice was not significantly different from that in control mice [t9 = 0.11, p = 0.91, unpaired t-test; Figures 8D–F(i)].
We next measured the number of lever presses in omission trials. That number did not differ significantly between trials with and without optogenetic inhibition [W = 10, p = 1.0, Wilcoxon signed-rank test; Figure 8D(ii)]. The change of the number in ArchT was not significantly different from that in control mice [t9 = 1.07, p = 0.31, unpaired t-test; Figures 8D–F(ii)].
We also measured the time from the last lever-press to a nose poke in the tone site to abandon an omission trial. The duration significantly decreased as a result of optogenetic inhibition in ArchT mice [t5 = 3.69, p = 0.014, paired t-test; Figure 8D(iii)]. On the other hand, the duration did not change in control mice [t4 = 1.77, p = 0.15, paired t-test; Figure 8E(iii)] and the change of time was significantly different in ArchT mice compared to control mice [t9 = 3.93, p = 0.0034, unpaired t-test; Figure 8F(iii)]. Thinking together with decreased duration of waiting in the waiting task, the specific effect of optogenetic inhibition on the time to abandon a trial suggests that serotonergic activity favorably modulates decisions based on time rather than motor actions.
To examine the effect of optogenetic inhibition on the speed of motor actions, we measured IPIs. As in the optogenetic activation experiment, we examined the effect of optogenetic inhibition on long and short IPIs (Figure 9A). Long IPIs in omission trials did not change significantly due to optogenetic inhibition (W = 7.0, p = 0.56, Wilcoxon signed-rank test; Figure 9B). The change of long IPI in ArchT was not significantly different from that of control mice [U = 21.0, p = 0.33, U test; Figures 9B–D)]. We next analyzed data of ArchT and control mice with repeated measures ANOVA (Figure 9E for ArchT mice, Figure 9F for control mice, and Supplementary Table 9 for statistical details). In ArchT mice, no significant main effect was found due to stimulation conditions (main effects of stimulation: two levels within-subject factors; No light and yellow, F(1,5) = 0.28, P = 0.87) and press conditions (five levels within-subject factors; 8-press, 16-press, 32-press, 64-press, and omission, F(4,20) = 1.86, P = 0.16). There was no significant interaction effect (stimulation x press, F(4,20) = 2.53, P = 0.073). Similarly, in control mice, there was no significant main effect of stimulation (F(1,4) = 0.031 p = 0.87) and press (F(4,16) = 1.27, P = 0.32). There were no significant interaction effects (stimulation x press, F(4,16) = 0.37, P = 0.83).
Figure 9. Inhibition of DRN 5-HT neurons did not change the speed of actions in the lever-pressing task. (A) Definitions of long and short IPIs. (B,C) Long IPIs in omission trials in ArchT [(B), n = 6 mice] and control [(C), n = 5 mice] mice. Yellow and green dots indicate the means across ArchT mice and control mice, respectively. (D) Change of long IPIs in yellow light trials to no-light trials in control (n = 5 mice) and ArchT (n = 6 mice) mice. Green- and yellow-filled circles indicate the mean across control and ArchT mice, respectively. (E,F) Short IPIs in ArchT [(E), n = 6 mice] and control [(F), n = 5 mice] mice. Open and filled circles indicate the means across no-light and yellow light trials, respectively, in ArchT (yellow) and control (green) mice. Error bars represent the SEM in all graphs. n.s. p > 0.05.
In order to examine the effect of repeated optogenetic inhibition across multiple days, similarly to the analysis in optogenetic activation experiment, we first tested overall difference of behavioral measures between ArchT and control mice using repeated measures ANOVA with the factors of genotype (two levels between-subject factors; ArchT or control) and stimulation conditions (two levels within-subject factors; no light and yellow). We found a significant main effect of genotype in the time spent lever-pressing (F(1,9) = 7.42, P = 0.023; Supplementary Table 10). We did not see a significant main effect of genotype in the number of lever-presses, long and short IPIs (Supplementary Table 10). To confirm the effect of repeated optogenetic inhibition in baseline level, we analyzed the behavioral measures in no light trials in the first two (early) and last two (late) sessions of the optogenetic inhibition experiment. We statistically tested the number of lever-presses, the time spent lever-pressing and the time to abandon a trial using repeated measures ANOVA with the factors of sessions (two levels within-subject factors: early or late sessions) and genotype of animals (two-levels between-subject factors: ArchT or control). In the time spent lever-pressing (Supplementary Figure 3A), although there was a significant main effect of genotype (F(1,9) = 8.11, P = 0.019), we did not find a significant main effect of session (F(1,9) = 1.47, P = 0.26). We did not find a significant interaction effect of session and genotype (F(1,9) = 4.62, P = 0.060), suggesting there was general difference in the time between ArchT and control mice throughout testing sessions, and repeated inhibition did not have additional effect. In the number of lever-presses (Supplementary Figure 3B) and the time to abandon a trial (Supplementary Figure 3C), there was a significant main effect of sessions, but there was no main effect of genotype. Also, there was not significant interaction between sessions and genotype (Supplementary Table 11). We next examined short IPIs in no light trials of early and late sessions and statistically tested with repeated measures ANOVA with the factors of sessions (two levels within-subject factors; early or late sessions) and press conditions (five levels within-subject factors; 8-press, 16-press, 32-press, 64-press, and omission). In ArchT group (Supplementary Figure 3D), there were no significant main effects of sessions (F(1,5) = 0.12, P = 0.75) as well as press conditions (F(4,20) = 1.32, P = 0.30). Also there was no significant interaction effect of those two factors (F(4,20) = 0.15, P = 0.96). In control mice (Supplementary Figure 3E), there were no significant main effects of session (F(1,4) = 0.13, P = 0.74) as well as press conditions (F(4,16) = 1.73, P = 0.19). We found a significant interaction effect of press condition and session (F(4,16) = 4.70, P = 0.011), but following pairwise comparison show that there were no significant difference in short IPIs between early and late sessions in each press condition. Overall, these results suggest that repeated optogenetic inhibition did not induce significant changes in motor actions.
In order to further confirm the effect of optogenetic inhibition on action persistence and speed of motor actions, we examined the number of lever-presses and short IPIs before the first premature reward check in omission trials, as we did in optogenetic activation studies. In the analysis using summary statistics, optogenetic inhibition did not significantly change the number of lever-presses or the duration of short IPIs (Supplementary Figure 4 and Supplementary Table 12).
In GLM analysis, the number of lever presses did not change as a result of optogenetic manipulation in either group (Figure 10A Manipulation; ArchT mice: t227 = −0.36, p = 0.72; Control mice: t203 = 1.30, p = 0.20). The results of other fixed effect terms were similar across ChR2 and control groups. The number of lever presses decreased in each session (Figure 10A Elapsed time; ArchT mice: t227 = 7.14, p = 1.2 x 10–11; Control mice: t203 = 8.45, p = 5.4 x 10–15) and increased across sessions (Figure 10A # Sessions; ArchT mice: t227 = 2.01, p = 0.046; Control mice, t203 = 2.77, p = 0.0061). Full detail of GLM analysis of the number of lever-presses is summarized in Supplementary Table 13.
Figure 10. GLM analysis did not show the effect of optogenetic inhibition on active persistence or action vigor. (A) GLM analysis of the number of lever-presses before the first premature check in Control (n = 5 mice) and ArchT (n = 6 mice) mice. Orange and green bars indicate coefficients of optic manipulation (left), elapsed time (middle), and the number of sessions (right) in control and ArchT mice, respectively. (B) GLM analysis of short IPIs before the first premature check in Control (n = 5 mice) and ArchT (n = 6 mice) mice. Orange and green bars indicate coefficients of optic manipulation (left), elapsed time (middle), and the number of sessions (right) in control and ArchT mice, respectively. Error bars indicate 95% confidence intervals of the coefficients. n.s. p > 0.05, * < 0.05, ** p < 0.01, *** p < 0.001.
Short IPIs did not show any significant change due to optogenetic manipulation (Figure 10B Manipulation; ArchT mice: t227 = 0.41, p = 0.68; Control mice: t203 = 0.31, p = 0.76). The results of other fixed effect terms were similar across both groups. In each session, short IPIs decreased in control mice (Figure 10B Elapsed time; t203 = 3.92, p = 0.00012), but not in ArchT mice (Figure 10B # Sessions; t227 = 1.3, p = 0.20). Across multiple sessions, short IPIs significantly decreased in both groups (ArchT mice: t227 = 2.3, p = 0.023; Control mice: t203 = 4.94, p = 1.6 x 10–6). Full detail of GLM analysis of the number of lever-presses is summarized in Supplementary Table 14.
We also applied a GLM model to the time to abandon a trial, in which we found a significant decrease by optogenetic inhibition in the summary statistics analysis. The time significantly decreased due to optogenetic manipulation in ArchT mice (Estimate; 0.0027, 95% CI; 0.00035 – 0.0050, t227 = 2.27, p = 0.024), while it did not change in control mice due to the manipulation (Estimate; –0.0017, 95% CI; –0.0038–0.00032, t203 = 1.66, p = 0.098; Supplementary Figure 5; See Supplementary Table 15 in full details). This result is consistent with our summary statistics analysis and confirmed the validity of the GLM analysis.
These results indicate that inhibition of DRN 5-HT neurons neither enhanced nor suppressed sustained motor actions. However, inhibition shortened duration of waiting in the waiting task and the time to abandon a trial in the lever-pressing task, suggesting that DRN 5-HT neurons favorably modulate actions based on time, with limited effect on the cost of actions.
In this study, we examined the role of DRN 5-HT neurons in sustaining motor actions for future rewards. We first showed that optogenetic activation of DRN 5-HT neurons prolonged waiting for future rewards, as in previous studies. On the other hand, the same stimulation neither enhanced nor suppressed the persistence and speed of motor actions in pooled analysis, i.e. summary statistics. In order to take into account trends within and across sessions and individual variability, we analyzed behavioral data using GLM, which revealed a subtle decrease in the number and speed of lever-presses due to optogenetic activation. To further investigate the causal relationship between activity of DRN 5-HT neurons and sustained motor actions, we optogenetically inhibited DRN 5-HT neurons during behavioral tasks. Optogenetic inhibition of DRN 5-HT neurons shortened the waiting duration, in opposition to its effect in the activation experiment. However, the same optogenetic inhibition did not induce changes in lever-pressing behavior either in summary statistics or GLM analysis, while it shortened the time to abandon a trial. Taken together, the present study revealed differential regulation of sustained motor actions and inactive waiting for future rewards with the main effect limited to waiting.
Based on the hypothesis that 5-HT controls temporal discounting of future rewards, we originally expected that optogenetic manipulation of DRN 5-HT neural activity modulates sustained motor actions for delayed rewards in much the same way as during waiting. However, these experimental results suggest that the role of DRN 5-HT neurons in sustained motor actions cannot be fully explained by the discount factor hypothesis. Also, since we have recently shown that uncertainty of reward delivery is critical for serotonergic modulation of patience (Miyazaki et al., 2018; Miyazaki et al., 2020), we designed a lever-pressing task with uncertain timing of reward delivery (i.e. variable number of lever-presses for rewards and addition of reward omission trials). Still, prominent optogenetic effect was not found in the lever-pressing task. Another possible interpretation of how activation of DRN 5-HT neurons prolongs waiting is based on the behavioral inhibition hypothesis, which suggests that increased 5-HT transmission shifts animal behavior toward inaction (Soubrie, 1986). If this hypothesis can account for 5-HT regulation of adaptive behaviors, it might be expected that activation of DRN 5-HT neurons would suppress lever-pressing behavior and vice versa. However, the present study showed a subtle decrease in lever-pressing behavior by optogentic activation and no effect on sustained motor actions by optogentic inhibition. Although these results seem hard to interpret based on either of two hypotheses, this will bring another possibility that serotonergic functions on behaviors are dependent on behavioral context or task demand, and some of them might even work in opposite direction. Recent studies showed that the effect of optogenetic activation on motor controls are context-dependent (Correia et al., 2017; Seo et al., 2019). For example, Correia et al. (2017) found that optogenetic activation of DRN 5-HT neurons reduced locomotion speed in open-field task, while the same stimulation did not change the speed of approaching behavior toward rewards. One possibility is that 5-HT modulates acting or waiting for rewards in positive direction as suggested in temporal discount factor hypothesis, but also other 5-HT pathways responsible for motor control would oppositely affect motor actions as proposed in behavioral inhibition theory or the reduced locomotion in open-field. Global activation of DRN 5-HT neurons might recruit both pathways, which ended up having only nuanced effect of optogenetic activation in lever-pressing task. This differential modulation is also consistent with the recent studies showing that behavioral and anatomical diversity of 5-HT neurons as well as different neural substrates by behavior, each of which will be described in the following paragraphs.
In the present study, we found the differential optogenetic effect between waiting and sustained motor actions. This may reflect distinctive neural substrates for delay-based (waiting) and effort-based (lever-pressing) motivated behaviors. A previous study showed that pharmacological reduction of systemic 5-HT level decreased the tendency of rats to favor immediate smaller rewards over delayed larger rewards in a delay-based choice task, but did not change their tendency to choose smaller rewards after climbing a low barrier rather than a larger reward after climbing a higher barrier in an effort-based choice task (Denk et al., 2005). Although sustained actions may be regulated differently than choice behaviors, our results are largely consistent with that behavioral study. Also, a recent fiber photometry study showed that DRN 5-HT neural activity is not increased while mice are pressing a lever to obtain rewards (Yoshida et al., 2019). Rather, the study showed that 5-HT neurons in the median raphe nucleus show increased neural activity during lever-pressing. How DRN 5-HT neurons regulate sustained actions differently has not been examined behaviorally, and our study adds behavioral evidence suggesting different neural substrates between sustained motor action and inactive waiting for future rewards. It is an interesting future study to examine whether 5-HT neurons in the median raphe nucleus modulate lever-pressing behavior dependently on uncertainty as DRN 5-HT neurons do to patience to wait for future rewards.
A recent study showed that DRN 5-HT neurons are anatomically divided into at least two subtypes, cortex-projecting and subcortical projecting neurons, and that these subtypes show different responses to rewarding and punishing stimuli, and that they have different behavioral roles (Ren et al., 2018). Therefore, it is possible that DRN 5-HT projections to different brain regions have different behavioral functions. Supporting this notion, activation of serotonergic projections to the ventral tegmental area increased active nose-poking to receive optogenetic self-stimulation (Nagai et al., 2020), whereas pharmacological manipulation of 5-HT receptors has opposing effects on the vigor and persistence of actions, mainly through projections to the subsantia nigra or dorsomedial striatum (Bailey et al., 2018). In our study, we stimulated all DRN 5-HT neurons, which may have obscured the existence of functionally different DRN 5-HT projections on motor action for future rewards. Future experiments to stimulate axon terminals of DRN 5-HT projections to specific brain regions (Miyazaki et al., 2020) should further clarify the roles of DRN 5-HT neurons in sustained motor actions.
An interesting behavioral observation in the present study was that animals spent more time pressing a lever than continuing to nose poke in omission trials. Previous psychological studies on self-control in humans showed that children who are engaged in behaviors to distract themselves, such as playing with toys or talking to others, can tolerate longer delays for rewards than those who did not (Mischel and Ebbesen, 1970; Mischel et al., 1972). From these studies, it was proposed that diverting their attention away from the temporal feature of the task by activating their behaviors reduces the effect of delay discounting (Metcalfe and Mischel, 1999). In the current study, mice may have been able to tolerate longer delays during the lever-pressing task due to actively sustained motor actions that shifted their attention away from the temporal feature of the task.
Another possible reason why animals can tolerate longer delays in lever-pressing is that lever-pressing behaviors are subjectively rewarding. For example, voluntary running-wheel activity has properties of a positive reinforcer (Belke and Wagner, 2005). Based on the fact that mice were first trained to press a lever until it was withdrawn, and reward was given to the food site, the tactile and auditory feedback of each lever-press would become conditioned reinforcer and would motivate mice to press longer. Previous studies showed that enhancement of serotonergic functions reduced the response to conditioned reinforcer (Guy and Fletcher, 2014; McDevitt et al., 2021) and voluntary running-wheel activity (Weber et al., 2009). If lever-pressing behavior has properties of both positive (conditioned) reinforcers and costly actions, serotonergic regulation of two behavioral processes, the decreased response to positive (conditioned) reinforcers and increased value of anticipated rewards over costly actions, might be confounding, which results in subtle or no effects by optogenetic manipulations. Lastly, we need to note that we did not make the temporal delay for rewards in two tasks aligned and mice needed to tolerate longer delay for obtaining rewards in lever-pressing task. Indeed, the average duration of rewarding trial in lever-pressing task was much longer than rewarding trials in waiting task (31.3s in 64 times press trials vs. 14.1s from the tone onset in 10 s delays trials). Therefore, we cannot rule out the possibility that mice were trained to tolerate longer to obtain rewards in lever-pressing task. To test whether sustained actions itself have a property to make animals more tolerant to delay than waiting, we need to design a lever pressing task where reward delays are comparable to the waiting task.
Although the present study shows a subtle decrease in motor actions for future rewards by optogenetic activation, this result conflicts with the previous study, which showed that optogenetic activation of DRN 5-HT neurons promotes active persistence and increased response vigor in a task in which active nose poking was required to receive water rewards (Lottem et al., 2018). Also, the previous study differed from ours in the type of reward (water vs. food) and in actions associated with rewards (repeated nose pokes vs. lever-pressing). There are several possible reasons for the discrepancy.
Methodological differences in optogenetic technique are critical factors for the discrepancy. For example, previous studies have discussed that the factors such as the difference in copy numbers of ChR2 expressing vectors/alleles (Nagai et al., 2020) or transgenic mouse lines (Cardozo Pinto et al., 2019) caused the different effect across studies. As those study suggest, the two studies also have significant methodological differences to express ChR2 in DRN 5-HT neurons, i.e., injecting viral vector vs. crossing transgenic mouse lines. Here, we would like to focus on discussing the difference in DRN 5-HT neural activity induced by optogenetic activation, which are likely to come from the difference in the type of opsin and stimulation parameter (i.e. frequency as well as light intensity). In the previous study, ChR2(H134R) was expressed in SERT-Cre mice that received 25-Hz photostimulation. This induced strongly synchronized DRN 5-HT neural activity. Such strong neural activity was mainly observed at the time of reward acquisition (Li et al., 2016). A recent study using the same transgenic mouse line showed that 20-Hz photostimulation of DRN 5-HT projections to the ventral tegmental area induced dopamine release in the nucleus accumbens and also had a reinforcing effect (Wang et al., 2019). Several previous studies also showed that in the self-stimulation test, activation of DRN 5-HT neurons with 20-Hz photostimulation reinforced active nose poking to a port coupled with the stimulation (Liu et al., 2014; Nagai et al., 2020). Photostimulation used in the previous study may have affected active persistence and response vigor through the reward effect induced by dopamine release. On the other hand, in this study, we used ChR2(C128S), a step-type function opsin that induced less synchronized activity, i.e. approximately 6-Hz firing, and photostimulation to this ChR2 variant did not induce rewarding effects (Miyazaki et al., 2014), suggesting that dopamine release is not induced by the stimulation. One possibility is that moderate and strong activation of DRN 5-HT neurons interact with dopaminergic neurons differently, resulting in different effects on motivated behaviors. It is an important future study to examine whether optogenetic stimulation parameters and transgenic mouse lines used in this study induced behavioral effects observed in the previous study.
Another remarkable difference between our task and that of Lottem et al. (2018) is the cost of abandoning a trial. The amount of travel cost to move to the next trial critically modulates animal decisions about whether to stay in the current trial/patch. Previous studies found that time and response in the current trial increased as the travel cost increased (McCarthy et al., 1994; Vertechi et al., 2020). In Lottem et al. (2018), mice were required to travel a 30-cm passage to move to the next trial, which took approximately 3 s on average. On the other hand, in our task, the inter-trial intervals could function as a travel cost to start the next trial, which took at least 20 s. One possibility is that 5-HT neurons modulate active motor actions differently, depending on the magnitude of the travel cost. The travel cost can be important even for how 5-HT neurons modulate decisions based on the cost of time. In Lottem et al. (2018), optogenetic activation increased the time to leave a current trial. In the present study, we did not see a change in the time to abandon a trial in our optogenetic activation experiment, whereas optogenetic inhibition decreased the duration. This result supports the possibility that serotonergic regulation of sustained actions is dependent on future costs of travel time. This possibility can be examined by testing optogenetic stimulation of 5-HT neurons in lever-pressing behaviors with different lengths of inter-trial intervals.
In conclusion, we showed that manipulation of DRN 5-HT activity has different effects on sustained motor action and waiting for future rewards. Patience to wait and persistence to act for delayed reward acquisition are regulated differently by DRN 5-HT neurons. Advanced optical imaging from genetically tagged 5-HT neurons such as fiber photometry and endoscopic microscopy will enable us to identify different neural substrates in persistence to act and patience to wait.
The raw data supporting the conclusions of this article will be made available by the authors, without undue reservation.
The animal study was approved by the Okinawa Institute of Science and Technology Experimental Animal Committee. The study was conducted in accordance with the local legislation and institutional requirements.
MT: Conceptualization, Formal analysis, Investigation, Software, Validation, Visualization, Writing – original draft, Writing – review and editing. KWM: Conceptualization, Formal analysis, Funding acquisition, Investigation, Software, Validation, Visualization, Writing – review and editing. KM: Conceptualization, Formal analysis, Funding acquisition, Investigation, Supervision, Writing – review and editing. JC: Formal analysis, Investigation, Writing – review and editing. SO-S: Investigation, Validation, Writing – review and editing. AC: Investigation, Validation, Visualization, Writing – review and editing. MN: Formal analysis, Investigation, Writing – review and editing. TM: Formal analysis, Investigation, Writing – review and editing. AY: Resources, Writing – review and editing. KT: Funding acquisition, Resources, Writing – review and editing. KD: Conceptualization, Funding acquisition, Methodology, Project administration, Resources, Supervision, Writing – review and editing.
The author(s) declare financial support was received for the research, authorship, and/or publication of this article. This work was supported by JSPS KAKENHI Grants 23120007, 16K21738, 16H06561 and 16H06563 to KD, 24730643 to KWM, 26120728 to KM, JST Moonshot R&D Program JPMJMS229D to KWM and KM, Grant-in-Aid for Brain Mapping by Integrated Neurotechnologies for Disease Studies (Brain/MINDS) from the Agency for Medical Research and Development (AMED) JP19dm0207001 to KD, JP21dm0207069 to KT, and Research Support of Okinawa Institute of Science and Technology Graduate University to KD.
We thank Prof. Jeff Wickens, Julie Chouinard, Yumiko Akamine, Mao-Ting Hsu, Ai Takahashi, and Yuichi Morohashi for helpful guidance and advice on the histological experiments. We also thank Paolo Barzaghi in the OIST imaging section for help with cell counting work.
The authors declare that the research was conducted in the absence of any commercial or financial relationships that could be construed as potential conflicts of interest.
The authors declared that they were an editorial board member of Frontiers, at the time of submission. This had no impact on the peer review process and the final decision.
All claims expressed in this article are solely those of the authors and do not necessarily represent those of their affiliated organizations, or those of the publisher, the editors and the reviewers. Any product that may be evaluated in this article, or claim that may be made by its manufacturer, is not guaranteed or endorsed by the publisher.
The Supplementary Material for this article can be found online at: https://www.frontiersin.org/articles/10.3389/fnins.2024.1433061/full#supplementary-material
Bailey, M. R., Goldman, O., Bello, E. P., Chohan, M. O., Jeong, N., Winiger, V., et al. (2018). An interaction between serotonin receptor signaling and dopamine enhances goal-directed vigor and persistence in mice. J. Neurosci. 38, 2149–2162. doi: 10.1523/JNEUROSCI.2088-17.2018
Belke, T. W., and Wagner, J. P. (2005). The reinforcing property and the rewarding aftereffect of wheel running in rats: A combination of two paradigms. Behav. Process. 68, 165–172. doi: 10.1016/j.beproc.2004.12.006
Cardozo Pinto, D. F., Yang, H., Pollak Dorocic, I., De Jong, J. W., Han, V. J., Peck, J. R., et al. (2019). Characterization of transgenic mouse models targeting neuromodulatory systems reveals organizational principles of the dorsal raphe. Nat. Commun. 10:4633. doi: 10.1038/s41467-019-12392-2
Cools, R., Roberts, A. C., and Robbins, T. W. (2008). Serotoninergic regulation of emotional and behavioural control processes. Trends Cogn. Sci. 12, 31–40.
Correia, P. A., Lottem, E., Banerjee, D., Machado, A. S., Carey, M. R., and Mainen, Z. F. (2017). Transient inhibition and long-term facilitation of locomotion by phasic optogenetic activation of serotonin neurons. Elife 6:e20975. doi: 10.7554/eLife.20975
Denk, F., Walton, M. E., Jennings, K. A., Sharp, T., Rushworth, M. F., and Bannerman, D. M. (2005). Differential involvement of serotonin and dopamine systems in cost-benefit decisions about delay or effort. Psychopharmacology 179, 587–596.
Doya, K., Miyazaki, K. W., and Miyazaki, K. (2021). Serotonergic modulation of cognitive computations. Curr. Opin. Behav. Sci. 38, 116–123.
Fonseca, M. S., Murakami, M., and Mainen, Z. F. (2015). Activation of dorsal raphe serotonergic neurons promotes waiting but is not reinforcing. Curr. Biol. 25, 306–315. doi: 10.1016/j.cub.2014.12.002
Franklin, K. B. J. (2008). The Mouse brain in stereotaxic coordinates compact, 3rd Edn. Cambridge, MA: Academic Press.
Guy, E. G., and Fletcher, P. J. (2014). Responding for a conditioned reinforcer, and its enhancement by nicotine, is blocked by dopamine receptor antagonists and a 5-HT(2C) receptor agonist but not by a 5-Ht(2A) receptor antagonist. Pharmacol. Biochem. Behav. 125, 40–47. doi: 10.1016/j.pbb.2014.08.006
Han, X., Chow, B. Y., Zhou, H., Klapoetke, N. C., Chuong, A., Rajimehr, R., et al. (2011). A high-light sensitivity optical neural silencer: Development and application to optogenetic control of non-human primate cortex. Front. Syst. Neurosci. 5:18. doi: 10.3389/fnsys.2011.00018
Homberg, J. R. (2012). Serotonin and decision making processes. Neurosci. Biobehav. Rev. 36, 218–236.
Li, Y., Zhong, W., Wang, D., Feng, Q., Liu, Z., Zhou, J., et al. (2016). Serotonin neurons in the dorsal raphe nucleus encode reward signals. Nat. Commun. 7:10503.
Liu, Z., Zhou, J., Li, Y., Hu, F., Lu, Y., Ma, M., et al. (2014). Dorsal raphe neurons signal reward through 5-HT and glutamate. Neuron 81, 1360–1374.
Lottem, E., Banerjee, D., Vertechi, P., Sarra, D., Lohuis, M. O., and Mainen, Z. F. (2018). Activation of serotonin neurons promotes active persistence in a probabilistic foraging task. Nat. Commun. 9:1000. doi: 10.1038/s41467-018-03438-y
Mattis, J., Tye, K. M., Ferenczi, E. A., Ramakrishnan, C., O’shea, D. J., Prakash, R., et al. (2011). Principles for applying optogenetic tools derived from direct comparative analysis of microbial opsins. Nat. Methods 9, 159–172. doi: 10.1038/nmeth.1808
McCarthy, D., Voss, P., and Davison, M. (1994). Leaving patches: Effects of travel requirements. J. Exp. Anal. Behav. 62, 185–200.
McDevitt, R. A., Marino, R. A. M., Tejeda, H. A., and Bonci, A. (2021). Serotonergic inhibition of responding for conditioned but not primary reinforcers. Pharmacol. Biochem. Behav. 205:173186.
Metcalfe, J., and Mischel, W. (1999). A hot/cool-system analysis of delay of gratification: Dynamics of willpower. Psychol. Rev. 106, 3–19. doi: 10.1037/0033-295x.106.1.3
Mischel, W., and Ebbesen, E. B. (1970). Attention in delay of gratification. J. Pers. Soc. Psychol. 16:329.
Mischel, W., Zeiss, A. R., and Ebbesen, E. B. (1972). Cognitive and attentional mechanisms in delay of gratification. J. Pers. Soc. Psychol. 21:204.
Miyazaki, K. W., Miyazaki, K., Tanaka, K. F., Yamanaka, A., Takahashi, A., Tabuchi, S., et al. (2014). Optogenetic activation of dorsal raphe serotonin neurons enhances patience for future rewards. Curr. Biol. 24, 2033–2040.
Miyazaki, K., Miyazaki, K. W., and Doya, K. (2011). Activation of dorsal raphe serotonin neurons underlies waiting for delayed rewards. J. Neurosci. 31, 469–479.
Miyazaki, K. W., Miyazaki, K., and Doya, K. (2011). Activation of the central serotonergic system in response to delayed but not omitted rewards. Eur. J. Neurosci. 33, 153–160.
Miyazaki, K., Miyazaki, K. W., and Doya, K. (2012). The role of serotonin in the regulation of patience and impulsivity. Mol. Neurobiol. 45, 213–224. doi: 10.1007/s12035-012-8232-6
Miyazaki, K. W., Miyazaki, K., and Doya, K. (2012). Activation of dorsal raphe serotonin neurons is necessary for waiting for delayed rewards. J. Neurosci. 32, 10451–10457. doi: 10.1523/JNEUROSCI.0915-12.2012
Miyazaki, K., Miyazaki, K. W., Sivori, G., Yamanaka, A., Tanaka, K. F., and Doya, K. (2020). Serotonergic projections to the orbitofrontal and medial prefrontal cortices differentially modulate waiting for future rewards. Sci. Adv. 6:eabc7246. doi: 10.1126/sciadv.abc7246
Miyazaki, K., Miyazaki, K. W., Yamanaka, A., Tokuda, T., Tanaka, K. F., and Doya, K. (2018). Reward probability and timing uncertainty alter the effect of dorsal raphe serotonin neurons on patience. Nat. Commun. 9:2048. doi: 10.1038/s41467-018-04496-y
Nagai, Y., Takayama, K., Nishitani, N., Andoh, C., Koda, M., Shirakawa, H., et al. (2020). The role of dorsal raphe serotonin neurons in the balance between reward and aversion. Int. J. Mol. Sci. 21:2160. doi: 10.3390/ijms21062160
Ohmura, Y., Iwami, K., Chowdhury, S., Sasamori, H., Sugiura, C., Bouchekioua, Y., et al. (2021). Disruption of model-based decision making by silencing of serotonin neurons in the dorsal raphe nucleus. Curr. Biol. 31:2446–2454.e5. doi: 10.1016/j.cub.2021.03.048
Ranade, S. P., and Mainen, Z. F. (2009). Transient firing of dorsal raphe neurons encodes diverse and specific sensory, motor, and reward events. J. Neurophysiol. 102, 3026–3037. doi: 10.1152/jn.00507.2009
Ren, J., Friedmann, D., Xiong, J., Liu, C. D., Ferguson, B. R., Weerakkody, T., et al. (2018). Anatomically defined and functionally distinct dorsal raphe serotonin sub-systems. Cell 175:472–487.e20. doi: 10.1016/j.cell.2018.07.043
Schweighofer, N., Tanaka, S. C., and Doya, K. (2007). Serotonin and the evaluation of future rewards: Theory, experiments, and possible neural mechanisms. Ann. N. Y. Acad. Sci. 1104, 289–300. doi: 10.1196/annals.1390.011
Seo, C., Guru, A., Jin, M., Ito, B., Sleezer, B. J., Ho, Y. Y., et al. (2019). Intense threat switches dorsal raphe serotonin neurons to a paradoxical operational mode. Science 363, 538–542. doi: 10.1126/science.aau8722
Soubrie, P. (1986). Reconciling the role of central serotonin neurons in human and animal behavior. Behav. Brain Sci. 9, 319–364.
Tanaka, K. F., Matsui, K., Sasaki, T., Sano, H., Sugio, S., Fan, K., et al. (2012). Expanding the repertoire of optogenetically targeted cells with an enhanced gene expression system. Cell Rep. 2, 397–406. doi: 10.1016/j.celrep.2012.06.011
Vertechi, P., Lottem, E., Sarra, D., Godinho, B., Treves, I., Quendera, T., et al. (2020). Inference-based decisions in a hidden state foraging task: Differential contributions of prefrontal cortical areas. Neuron 106:166–176.e6. doi: 10.1016/j.neuron.2020.01.017
Wang, H. L., Zhang, S., Qi, J., Wang, H., Cachope, R., Mejias-Aponte, C. A., et al. (2019). Dorsal Raphe dual serotonin-glutamate neurons drive reward by establishing excitatory synapses on vta mesoaccumbens dopamine neurons. Cell Rep. 26:1128–1142.e7. doi: 10.1016/j.celrep.2019.01.014
Weber, M., Talmon, S., Schulze, I., Boeddinghaus, C., Gross, G., Schoemaker, H., et al. (2009). Running wheel activity is sensitive to acute treatment with selective inhibitors for either serotonin or norepinephrine reuptake. Psychopharmacology 203, 753–762.
Keywords: dorsal raphe nucleus, serotonin, decision making, reward, mice, lever-press, waiting
Citation: Taira M, Miyazaki KW, Miyazaki K, Chen J, Okitsu-Sakurayama S, Chaudhary A, Nishio M, Miyake T, Yamanaka A, Tanaka KF and Doya K (2024) The differential effect of optogenetic serotonergic manipulation on sustained motor actions and waiting for future rewards in mice. Front. Neurosci. 18:1433061. doi: 10.3389/fnins.2024.1433061
Received: 15 May 2024; Accepted: 30 August 2024;
Published: 25 September 2024.
Edited by:
Balazs Hangya, Institute of Experimental Medicine (MTA), HungaryReviewed by:
Mitsuko Watabe-Uchida, Harvard University, United StatesCopyright © 2024 Taira, Miyazaki, Miyazaki, Chen, Okitsu-Sakurayama, Chaudhary, Nishio, Miyake, Yamanaka, Tanaka and Doya. This is an open-access article distributed under the terms of the Creative Commons Attribution License (CC BY). The use, distribution or reproduction in other forums is permitted, provided the original author(s) and the copyright owner(s) are credited and that the original publication in this journal is cited, in accordance with accepted academic practice. No use, distribution or reproduction is permitted which does not comply with these terms.
*Correspondence: Masakazu Taira, bWFzYWthenUudGFpcmFAc3lkbmV5LmVkdS5hdQ==; Kenji Doya, ZG95YUBvaXN0Lmpw
Disclaimer: All claims expressed in this article are solely those of the authors and do not necessarily represent those of their affiliated organizations, or those of the publisher, the editors and the reviewers. Any product that may be evaluated in this article or claim that may be made by its manufacturer is not guaranteed or endorsed by the publisher.
Research integrity at Frontiers
Learn more about the work of our research integrity team to safeguard the quality of each article we publish.