- 1Department of Molecular Physiology and Biophysics, Carver College of Medicine, Iowa City, IA, United States
- 2Department of Otolaryngology Head-Neck Surgery, Carver College of Medicine, Iowa City, IA, United States
Cochlear implants (CI) represent incredible devices that restore hearing perception for those with moderate to profound sensorineural hearing loss. However, the ability of a CI to restore complex auditory function is limited by the number of perceptually independent spectral channels provided. A major contributor to this limitation is the physical gap between the CI electrodes and the target spiral ganglion neurons (SGNs). In order for CI electrodes to stimulate SGNs more precisely, and thus better approximate natural hearing, new methodologies need to be developed to decrease this gap, (i.e., transitioning CIs from a far-field to near-field device). In this review, strategies aimed at improving the neural-electrode interface are discussed in terms of the magnitude of impact they could have and the work needed to implement them. Ongoing research suggests current clinical efforts to limit the CI-related immune response holds great potential for improving device performance. This could eradicate the dense, fibrous capsule surrounding the electrode and enhance preservation of natural cochlear architecture, including SGNs. In the long term, however, optimized future devices will likely need to induce and guide the outgrowth of the peripheral process of SGNs to be in closer proximity to the CI electrode in order to better approximate natural hearing. This research is in its infancy; it remains to be seen which strategies (surface patterning, small molecule release, hydrogel coating, etc.) will be enable this approach. Additionally, these efforts aimed at optimizing CI function will likely translate to other neural prostheses, which face similar issues.
1 Introduction
Neural prostheses replace or enhance neural pathways that are diminished or absent due to disease, trauma, or aging. These devices have improved greatly in recent years; however, they fail to fully emulate the native neural pathways they seek to restore (Soleymani et al., 2016; Jiam et al., 2017). Enhancing the neural-electrode interface is an essential element of next generation of neural prostheses (Senn et al., 2017; Li C Y, et al., 2021). Poor tissue integration of the electrode array limits the function of these implants; to improve outcomes, future devices will need to recapitulate the architecture and function of native neural systems more accurately.
An example of a highly successful neural prosthetic is the cochlear implant (CI). A CI replaces the mechanosensory transduction of sound within the cochlea by directly stimulating spiral ganglion neurons (SGNs) to provide auditory sensation. CIs are revolutionary in that they restore hearing for those with moderate to profound sensorineural hearing loss, however, they are limited by the multiple-fold difference in independent perceivable frequency channels they provide relative to the normal cochlea (Berg et al., 2019). Many drivers contribute to the observed shortcomings in CI performance, including limitations in cortical plasticity, dynamic range representation, and structural changes to the auditory pathway in those receiving CI (Limb and Roy, 2014; Pisoni et al., 2017). A major factor contributing to the limitation in performance is the large physical distance between the electrode surface and the target SGNs (Figure 1A; Shi et al., 2021; Ertas et al., 2022). For reference, the CI electrode array is inserted into the scala tympani and typically positioned hundreds of microns away from the target SGNs in the modiolus, compared to the tens of nanometers of the native inner hair cell(IHC)-SGN synapse (Wang et al., 2015; Moverman et al., 2023). Due in part, to the large gap between the electrode and target neurons, current spreads significantly over this distance. This results in overlap of activation of SGNs across adjacent electrodes and thus, a limitation in the number of discrete, useable channels by the user. Overall, this physical gap represents a major limitation of CI performance, leaving current CI performance plateaued with 8 or 16 independently distinguishable channels, a resolution much lower than native hearing (Boisvert et al., 2020). Given this, there is intense motivation to develop methodologies that result in the electrode array being able to stimulate the nervous system more precisely.
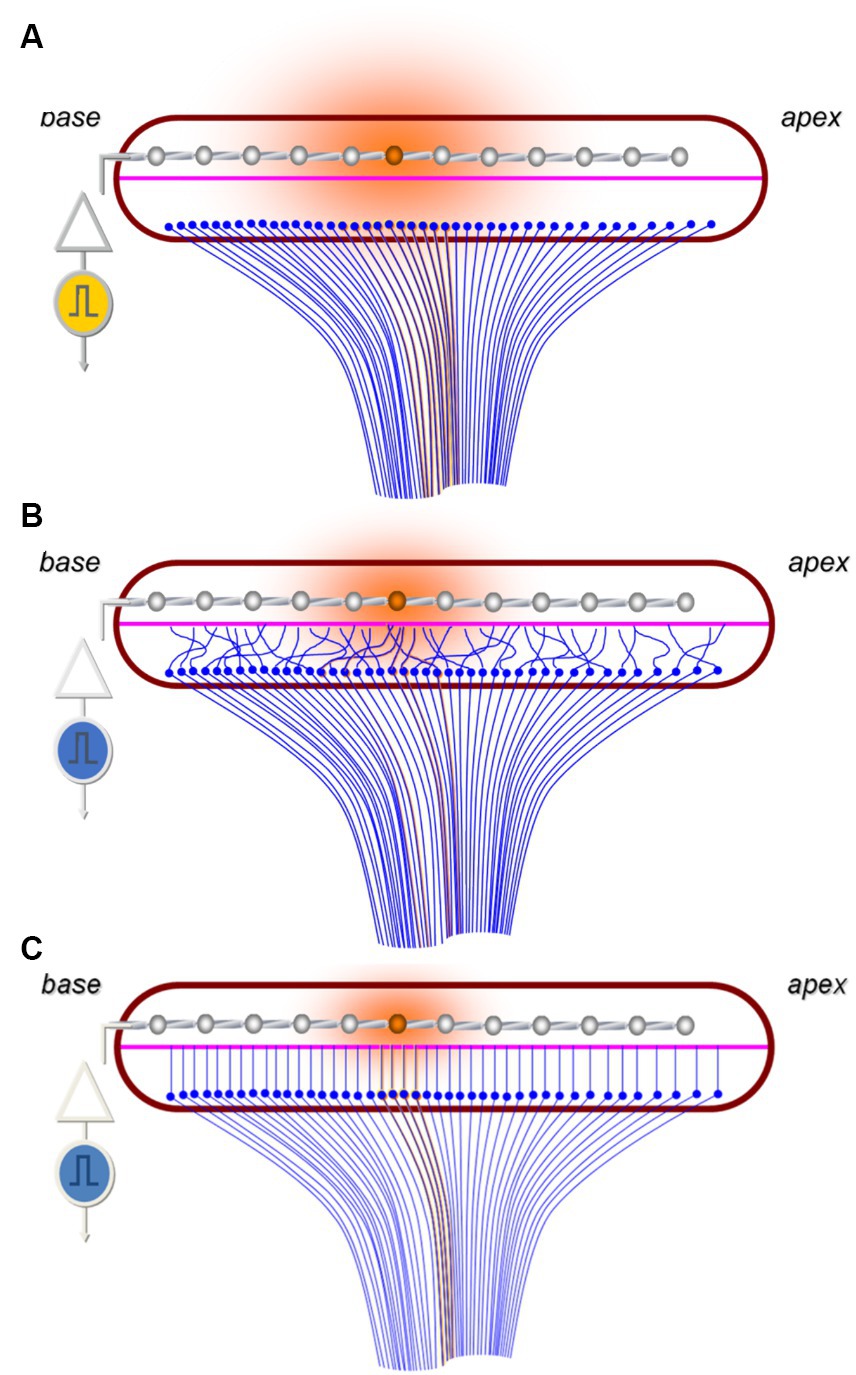
Figure 1. Schematic highlighting the challenges of tonotopic specificity of CIs. (A) Image representing current paradigm of how CI electrodes (silver) stimulate (orange) the SGNs (blue) across the tonotopic axis of the cochlea in a region of devoid of functioning hair cells. Note the large distance between the electrodes and the SGNs resulting in broad current spread and SGN stimulation (shaded orange circle). (B) Hypothesized outcome of non-guided SGN outgrowth toward the electrodes which results in random overlap and stimulation of SGNs encoding a variety of frequencies. (C) Image representing idealized guided SGN neurite outgrowth into close proximity the electrode array which enables more specific SGN stimulation and reduced electrical current demands.
The large physical distance between the electrode and the neurons is not the only anatomical limitation of CIs. First, upon implantation the electrode array becomes engulfed in a fibrous capsule with neo-ossification as a result of inflammatory response to biomaterials (Rahman et al., 2022). This capsule increases electrode impedance and thus may also fundamentally limit CI performance (Wilk et al., 2016; Briggs et al., 2020). Additionally, it may be particularly challenging to selectively stimulate low frequency encoding SGNs in the apex with a CI. This is because the apical bulb of the spiral ganglia is condensed and particularly crowded with neurons where four octave bands of frequency are perceived within 3.4 mm of spiral ganglia (Pamulova et al., 2006; Li H, et al., 2021). These same four octave bands are sensed over approximately 4 times the length of the basilar membrane (Li H, et al., 2021). Thus, a CI electrode that stimulates these neurons from a perimodiolar position will be unable to precisely target this region of the cochlea to allow perceptual differentiation of low frequency sounds. Benefits of preserving the frequency specificity in the apical region are evidenced by improved outcomes in CI patients with preserved residual hearing. CI patients with preserved apical hearing (and thus, frequency specificity) show improved auditory performance, particularly in challenging listening situations such as speech understanding in noise and music appreciation (Büchner et al., 2009; Gantz et al., 2018, 2022; Shim et al., 2023). Likewise, beyond retaining apical acoustic function, preservation of the peripheral axons of apical SGNs that project into the basilar membrane will theoretically enable better spatial resolution assuming these elements of the SGNs are functional and responsive to the electrical stimulation.
Given these challenges, there is interest to study and implement new methodologies which result in the electrode array being able to stimulate the nervous system more precisely. These techniques broadly range from modifying the electrode array shape, altering the surgical placement, inhibiting the immune/inflammatory response, and leveraging regenerative medicine, among many others. An intriguing approach seeks to induce the outgrowth of neurites toward the basilar membrane, thereby mimicking the native distribution of peripheral SGN axons across the apex of the cochlea. This approach would transition CIs from a far-field to near-field device and improve CI resolution. Therefore, work into how to induce the SGN neurites to grow into close proximity to the CI electrode and to improve the neural—electrode interface in CI, especially in the cochlear apex, has been proposed. This review will focus on describing the efforts to guide SGN neurite growth into closer proximity to the CI electrode as well as a brief discussion of other approaches in CI and auditory regeneration also involving engineering the neural-electrode interface.
2 Guiding SGN neurite growth into close proximity to the CI electrode
The requirements for guiding SGN neurites to grow into close proximity to the CI electrode can be divided into 4 broad components. These include: (1) initiating regenerative neurite outgrowth, (2) precisely guiding this de novo neurite growth, (3) stopping the outgrowth near or at the electrode interface, and (4) myelinating the new neurites. In the discussion of these below, the state of the current research and how they would translate to CI are examined.
2.1 Initiating regenerative neurite growth
The peripheral axonic processes of type 1 SGNs extend into the organ of Corti along the basilar membrane and are the site of initial electrical activity induced by synaptic vesicle release from inner hair cells (IHCs) in response to sound. SGN somata are positioned in the spiral ganglion within the modiolus. The peripheral processes of SGNs in cochleae receiving CIs may not be synapsed with IHCs and may be retracted, due to disease or damage; regardless of the current position of the peripheral axon, de novo neurite outgrowth will need to be initiated to grow processes toward the electrodes. SGNs have minimal ability initiate spontaneous outgrowth, especially in adult mammalian systems (Schwieger et al., 2015; Zhang et al., 2022). Therefore, it is expected that factors to induce neurite growth are needed for this application.
2.1.1 Growth factors
A variety of factors induce the outgrowth of SGN neurites. The most widely used approach provides exogenous neurotrophins capable of activating tropomyosin receptor kinase (Trk) receptors. Type 1 SGNs express TrkB and TrkC receptors, which are typically activated by brain derived neurotrophic factor (BDNF) and neurotrophin-3 (NT-3), respectively. While there are specific functions attributable to each factor (Schimmang et al., 2003; Flores-Otero et al., 2007; Green et al., 2012; Szobota et al., 2019), they show overlap in function and ability to promote SGN outgrowth and synaptogenesis. Nevertheless, the differences in expression and function are important to consider. For example, they have different tonotopic expression patterns, with TrkB/BDNF more prominent than TrkC/NT-3 in the base and the inverse for the apex. These differences likely contribute, at least in part, to specific tonotopic firing patterns of SGNs (Davis, 2003; Flores-Otero and Davis, 2011). Additionally, BDNF better stimulates neurite outgrowth, while NT-3 better promotes innervation and synaptogenesis (Green et al., 2012). Thus, for the purpose of initiating growth, BDNF is a common choice. Importantly, the effects on exogenous neurotrophic factors on SGN firing patterns across the tonotopic axis, particularly those induced by electrical stimulation should be considered. Other growth factors also stimulate regenerative neurite outgrowth in SGNs including, but not limited to, ciliary neurotrophic factor (Schwieger et al., 2015), fibroblast growth factor 8 (García-Hernández et al., 2013), somatotropin (Gabrielpillai et al., 2018), and glial cell-derived neurotrophic factor (Euteneuer et al., 2013). These factors are small proteins and present challenges for long-term delivery to the cochlea. Further, controlled release of these factors in the setting of a CI will likely require shelf stability and/or solvents to encapsulate the compound in order have necessary control of release (Hendricks et al., 2008; Liu and Yang, 2022). To bypass these hurdles, genetically engineered cells that produce neurotrophic factors could be co-implanted with the electrode or the CI electrode used to electroporate exogenous genes encoding neurotrophic factors into native cochlear tissue which would, in turn, produce the factor (Pinyon et al., 2014).
2.1.2 Chemicals
To overcome the challenges inherent to sustained delivery of functional peptides or proteins, chemical analogs that target receptors are also being explored. Currently, Trk receptor agonists appear to be the most popular and promising venture. Indeed, small molecules capable of activating (i.e., phosphorylating) TrkB promote SGN outgrowth in vitro and SGN survival and function in vivo (Yu et al., 2012, 2013). Similar work has also shown promise in targeting TrkC (Kempfle et al., 2021). Further effort is needed to validate these compounds to ensure that they adequately and specifically target the receptor (Pankiewicz et al., 2021) and to explore the methodologies to control the release in the cochlea (Sun et al., 2022; Wulf et al., 2022). Beyond Trk agonists, other small molecules are also being explored. These include molecules that activate pathways known to promote neurite outgrowth and pharmacological screens to repurpose already approved drugs (Whitlon et al., 2015).
2.2 Guiding de novo neurite growth
Once neurite outgrowth is initiated, there are two essential considerations: (1) the neurites must be guided to maintain the precise tonotopic organization of the native cochlea innervation, and (2) to reach the CI electrode array in the scala the neurites must exit the organ of Corti and traverse into the scala tympani, which is normally filled with perilymph and devoid of cells or extracellular matrix. In combination, both of these factors necessitate the use of organized guidance cues to guide this de novo neurite growth (Figure 2).
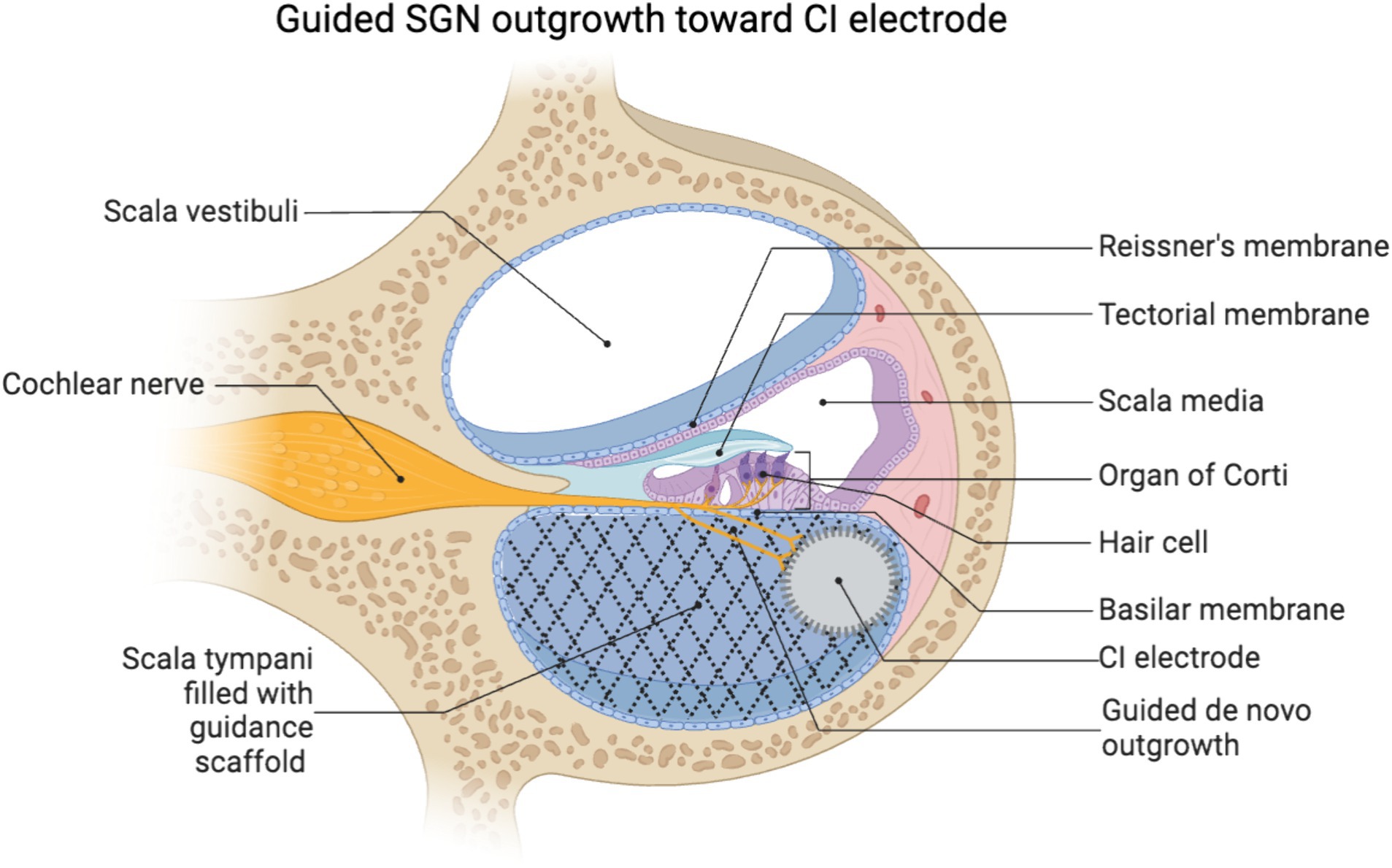
Figure 2. Schematic of a scaffold in the scala tympani guiding de novo neurite growth toward a lateral wall positioned CI electrode array. Created using biorender.
To appreciate the need for guidance cues, it is helpful to understand how the tonotopic architecture of the cochlea enables natural hearing and why this layout will likely need to be maintained for effective neural regeneration. Sound energy induces a traveling wave in the basilar membrane in a tonotopic pattern based on frequency, with high frequencies stimulating the base and low frequencies the apex of the cochlea. The IHCs stimulated by specific frequencies translate this mechanical signal of sound into an electrical nervous signal via release of synaptic vesicles to target SGNs specific for those IHCs. The SGNs then relay this signal specific to a given frequency into the cochlear nucleus in the brain. This precise layout enables the perception of continuous sound frequencies over the approximately 3,500 IHCs.
Given the importance of the tonotopic architecture in function of the auditory system, it is expected that this organization will need to be preserved during regenerative approaches. For example, in the context of neurite outgrowth toward a CI electrode array, as the peripheral processes of the SGNs extend into close proximity to the CI electrode they should maintain their position relative to one another to preserve the tonotopic patterning underlying frequency perception. If the outgrowth becomes spatially disorganized or random with processes crossing over each other, then the precise tonotopic specificity will likely be lost (Figure 1B). If this architecture is compromised, neurons corresponding to a variety of frequencies would be stimulated by specific electrodes, thus diminishing signal resolution. It is important to note that this concept is theoretical and implied by related work (Guiraud et al., 2007; Walia et al., 2023), though it remains to be seen if future CI could be programmed to overcome disorganized outgrowth via intelligently programming each electrode to the frequency of the neurons that it stimulates (Grasmeder et al., 2014).
Bioengineering techniques will likely be required to provide guidance cues such that as outgrowth is stimulated neurite overlap is minimized and tonotopic architecture is preserved (Figure 1C). Many strategies have been proposed and evaluated, including diffusible chemical gradients (Coate et al., 2015; Li et al., 2017), patterned peptide surface coatings (Tuft et al., 2018; Truong et al., 2021), and engineered surface topography (Tuft et al., 2013; Hu et al., 2022), among others (Druckenbrod et al., 2020). Of these, the most commonly reported in vivo technique is to use chemical gradients of neurotrophic factors (Budenz et al., 2012; Sameer Mallick et al., 2013). This approach involves a coating on the electrode intended to elute the factor (Kikkawa et al., 2014), or the implant may be co-implanted with cells (Rejali et al., 2007) or particles (Gunewardene et al., 2022) that deliver the factor. When used in vivo, chemical gradients of these factors have been shown to not only be neural protective, but also to induce de novo outgrowth from the peripheral process of SGNs toward the CI electrode when neurotrophic factors are co-delivered (Wise et al., 2011; Landry et al., 2013). However, it is not clear whether diffusible gradients alone will suffice to provide precise neurite guidance.
As an alternative to or combinatory approach with chemical gradients, engineered surface patterning can effectively direct cell material interactions and guide neurite growth as well, often with much greater precision (Tuft et al., 2018). There is tremendous diversity of types of patterns (chemical, protein, biophysical, topographical) and approaches to make these patterns. First, engineers have devised methods to create biochemically patterned surfaces. Methods to do this include photo-deactivation (Leigh et al., 2017b), protein immobilization (Sorribas et al., 2002; Kim et al., 2019), microcontact printing (Eichinger et al., 2012; Joo et al., 2015), and creation of patterned zwitterionic coatings (Leigh et al., 2017a). Each of these methods restricts protein absorption to selective regions of the substrate. By using precise spatial patterning of zwitterions, chemo-permissive proteins, and/or chemo-repulsive proteins, it is possible to spatially direct cell adhesion, neurite guidance, and neurite repulsion (Humenik et al., 2021; Truong et al., 2021). Importantly, further work is needed to translate these 2D in vitro approaches to in vivo application.
Separately or in combination with the biochemical approaches (Truong et al., 2021), biophysically patterned substrates can be generated in a variety of approaches. These include electrospinning, lithography (Tonazzini et al., 2020), or selective photopolymerization via photomasks or two-photon polymerization (Marino et al., 2013; Tuft et al., 2013). Biophysical patterning offers a particular advantage in the inherent stability of the polymer systems. The polymers used to make these patterned surfaces are shelf stable and are already used in a variety of biomedical implants. An additional benefit is that the feature geometry for these substrates can be controlled on the submicron scale (Tuft et al., 2013; Limberg et al., 2022). Thus, these systems enable tremendous control of cell behavior with precise modifications and reproducibility in creating consistent materials (Vecchi et al., 2024). Another potential benefit of engineered biomaterials is that they provide a substrate for de novo SGN outgrowth through the perilymph. Traditional CI electrodes are positioned in the scala tympani, thus for the peripheral projections of SGNs to grow into close proximity to the electrode the neurite would likely need to extend from the organ of Corti or osseus spiral lamina and, possibly through the perilymph. The perilymph is liquid and the normal scala tympani is an environment not suitable for cell survival. Alternatively, the fibrotic capsule that encapsulates the electrode array (Foggia et al., 2019; Rahman et al., 2022) could be engineered to provide a scaffold that supports and guides de novo neurite growth. Therefore, it has been suggested to use polymeric biomaterial systems to provide a suitable substrate to support SGN neurite outgrowth (Senn et al., 2017; Li C Y, et al., 2021; Figure 2). The principles learned from the patterned 2D systems could inform the design of a porous 3D coating of the electrode to induce and guide SGN neurite growth. Future engineered materials may employ the controlled release of neurotrophic factors, curated pore size and stiffness, and incorporate tailored ECM proteins and/or glial cells (e.g., Schwann cells) in order to induce, channel, and permit neurite growth toward the electrode, respectively. Importantly, beyond the effects of an inflammatory/foreign body response to biomaterials, filling the scala tympani with noncompressible material will impede the motion of the traveling wave of the basilar membrane and exacerbate loss of acoustic hearing (Choi and Oghalai, 2005). Therefore, consideration and greater work on the effect of introducing biomaterials to the scala is needed, especially in the context of those seeking to maximize preservation of residual hearing.
Despite the potential of the approaches described above, reports of clinical translation of 3D patterned coatings on CI electrodes to guide neurite growth are limited. Although such a feat has been shown to be possible in vivo (Senn et al., 2017), there have not been large scale studies that assess complex outcomes. There are countless in vitro pursuits underway (Nella et al., 2022; Wille et al., 2022), as well as many studies aimed at directing neurite growth in vivo for other fields of neuro-regeneration (Dixon et al., 2018; Jiang et al., 2020; Zhang et al., 2020). However, the unique challenges of the cochlear environment and limitations of the SGNs may necessitate development of other techniques if inducing and controlling SGN neurite outgrowth proves to be too challenging. One strategy to bypass this hurdle is to co-introduce stem cells, Schwann cells, or other cells to the scala tympani with the electrode array to act as a bridge between the electrode and SGNs (Nella et al., 2022). Theoretically, in this approach, the cells would be co-implanted with the CI. Further, in the case of stem cells or glial cells, these could even be engineered to differentiate into neural cells that synapse with the SGNs with one projection while the other projection would remain in close proximity to the electrode. Similarly, others have explored implanting “living electrodes” containing neurons and axon tracts for cortical implants wherein the implanted neurons extend their projections into the nervous tissue to better integrate the implant (Adewole et al., 2021). While complex, this schema may be advantageous since the stem cells are more dynamic and able to controlled with more precision than SGNs.
2.3 Stopping the outgrowth at the CI interface
Another consideration is that the stimulated outgrowth may need to be stopped at, or very near to, the target electrode(s). Similar to the previous discussion on tonotopy, engineering this aspect may be just as important for maintaining tonotopy as guiding the outgrowth. Even if the de novo outgrowth of the peripheral processes of the SGNs is perfectly guided, continued growth beyond the electrode interface would likely result in a loss of tonotopy (Figure 1B). Therefore, it is expected that the peripheral projection may need a proper “stop” signal at or near the electrode interface. Importantly, it remains unclear how essential this may be as some studies suggest this aberrant growth may not have an effect if not too exuberant (Landry et al., 2013). Additionally, it is unknown whether efforts at neurite outgrowth would durably persist from the mechanical forces of CI revision surgery, including CI explanation and reimplantation.
While there is not much specific work in the field of CI related to strategies to halt neurite growth near the target, other applications of neural electrodes have investigated such strategies. In particular, prior work has focused on the use of tracts to guide outgrowth, and the controlled application of electrical or optical signal has been used to direct the formation of an artificial synapse at the end of the tract (Bieberich and Guiseppi-Elie, 2004; Shi et al., 2016). In deep brain stimulation approaches, where there may not be as clearly defined a tonotopic architecture to mimic, several approaches seeking to maximize neural contact are being explored (Serruya et al., 2018; Adewole et al., 2021). Overall, depending on the successes of these engineering focused approaches, the field may need to seek to recapitulate how the peripheral process of the SGN naturally forms a synapse with IHCs. The signals resulting in the ribbon synapse may need to be incorporated in the electrode surface for optimal integration of the neural-electrode interface (Coate et al., 2019).
2.4 Myelinating new neurites
A last consideration for the complex task of guiding neurite growth is that the native peripheral processes of SGNs are myelinated. This myelination is critical for the health and function of the SGNs, as demonstrated by the fact that auditory circuit myelination happens at the same time in development as when the auditory system functionally develops. Additionally, axonal myelination enables the rapid conduction and temporal precision of electrochemical signals necessary for coding of SGNs and auditory function (Long et al., 2018). Thus, if de novo outgrowth is initiated and guided, these extended neurites may need to be myelinated to mimic the native architecture, function, and neural health, as prior research has indicated auditory nerve demyelination disrupts auditory sensation (Wan and Corfas, 2017; Resnick et al., 2018). Importantly, in addition to having the ability to regenerate, the peripheral nervous system also has the ability to remyelinate peripheral sensory neurons (Zhou and Notterpek, 2016). Thus, promotion of re-myelination remains a focus for many in the field of regenerative medicine (Taveggia et al., 2010).
It is not clear how existing (re)myelination approaches will transfer to the cochlea or if these strategies are necessary. However, if remyelination is necessary, Schwann cells could be included with the implanted device and would need to extend with the neurites or cells, and subsequently myelinate the neurons. As a more likely alternative, Schwann cells may lead the way for the regenerative outgrowth of the neurons. That is because these cells are (1) more dynamic than the SGNs, (2) they similarly follow guidance cues, and (3) the presence and orientation of Schwann cells promotes both neurite growth and alignment (Thompson and Buettner, 2006; Bruder et al., 2007; Clarke et al., 2011; Jeon et al., 2011; Tuft et al., 2013). Further a reciprocal signaling network exists between cochlear Schwann cells and SGNs (Hansen et al., 2001). Thus, a reasonable approach to guide neurite growth through the perilymph could be to leverage the regenerative capacity of Schwann cells, wherein Schwann cells support SGN neurite growth, align precisely to biopatterns, and myelinate the regenerated processes (Whitlon et al., 2009; Clarke et al., 2011; Jeon et al., 2011). Overall, it is important that future in vivo studies on CI performance with regenerated SGN neurites incorporate the assessment of myelination and the behavior or Schwann cells, as this could significantly impact device function.
3 Other relevant approaches to improve CI neural-electrode interface
The stepwise description above does not cover the entire body of research aimed at enhancing CI through improving the neural-electrode interface. Numerous other approaches exist for improving CI as well as ideas to bypass or supplement the immense challenge described in the previous section. These endeavors include: exploring alternative methods for stimulating the SGNs, mitigating the foreign body response to the electrode implanted in the cochlea, and employing genetic or regenerative medicine approaches.
3.1 Optical stimulation
A limitation of CI is the reliance on electrical stimulation to transmit signals to the SGNs. Electrical signals exhibit significant current spread in vivo (Zeng, 2004), limiting the precision by which CI electrodes can target SGNs. Furthermore, electrical stimulation provided by traditional CI electrodes has a narrower dynamic range compared to natural hearing (Zeng et al., 2002). As a result, CI users often face challenges in distinguishing soft from loud sounds. These two factors not only constrain the actual functionality of CI but also set a theoretical ceiling on their performance. Consequently, researchers are exploring alternative methods of stimulation.
In particular, optical signals, or light, offer advantages over electricity in the aspects mentioned above (Dieter et al., 2020). Optogenetics presents a potential alternative to traditional electrical CI. Optogenetics involves transfecting genes that encode light-sensitive channels, known as opsins, into neurons, and then stimulating the neurons in a controlled manner with a specific wavelength of light (Emiliani et al., 2022). In the context of CI, this approach would entail transfecting SGNs with genes encoding opsins and using an array that emits optical signals (optode) instead of electrical stimulation (Moser and Dieter, 2020). While this approach shows promise, it requires further optimization in key areas.
First, optical stimulation addresses one of the weaknesses of electrical stimulation mentioned earlier, as optodes exhibit much greater spectral selectivity than traditional electrodes (Dieter et al., 2020; Keppeler et al., 2020). Regarding other measures of CI performance, optodes seem to perform as well as electrical electrode in CI in terms of dynamic range but exhibit lower temporal precision (Dieter et al., 2020). In particular, the firing rate of opsins do not approach that needed for natural sound, however, newer engineered opsins partially overcome this rate limitation (Keppeler et al., 2018; Sridharan et al., 2022; Mittring et al., 2023).
An additional significant challenge in optogenetic approaches is the technical difficulty of transfecting the opsin into the SGNs. It remains uncertain what the transfection efficiency would be in adult patients, especially since permanent opsin expression would be necessary. It is also not known what effect the well-documented post-implantation fibrosis and neo-ossification might have on light transmission necessary for optogenetic stimulation. Future research in this field should investigate transfection efficiency in adults and explore engineering improvements in the opsins used to enhance dynamic range and temporal precision in sound encoding (Huet et al., 2021).
A second optical based approach is to use infrared light delivered by a pulsed laser to stimulate firing of SGNs. Neural activation results from spatially and temporally heat induced stimulation of action potentials (Xu et al., 2021). As with optogenetics, infrared stimulation provides significantly enhanced spatial resolution and is used clinically to modulate cerebral cortex activity. The advantage of infrared stimulation over an optogenetic approach is that it does not require exogenous gene transfer, as neural tissue is naturally sensitive to infrared light pulses. Nevertheless, there are still significant hurdles to adopt this technology into a cochlear prosthetic (Xu et al., 2021). Importantly, it could be feasible to combine light emitting optode arrays with electrode arrays to leverage the advantages of each. However, these optical approaches face some of the same challenges of inflammatory tissue responses incurred with electrode arrays.
3.2 Mitigating the inflammatory response
A separate critical challenge that needs to be addressed in all implanted biomaterials including electrode and optode arrays is the inflammatory response. Specifically, an inflammatory/foreign body response encapsulates implanted electrodes with a dense fibrous capsule and, in some cases, bone, resulting in several adverse effects on CI device function. There are many negative effects of this foreign body response include wide reaching consequences from: requirement for increased electrical current, loss of residual hearing after implantation, and device failure (Foggia et al., 2019; Simoni et al., 2020). Importantly, the causes of fibrosis in CI are multifaceted, including the immune system’s response to three interrelated components of CI: (1) surgical trauma, (2) implanted materials, and (3) electrical stimulation. Furthermore, regardless of the cause of the inflammatory response, the regenerative approaches discussed thus far will not be possible in the presence of dense fibrous capsule and compromised anatomy secondary to inflammation. In this section, the approaches to mitigate the inflammation resulting from each of these factors are described.
First, the practice of “soft surgical” techniques for atraumatic CI electrode insertion has been the contemporary standard of care for optimizing hearing preservation after surgery (Lenarz et al., 2020; Bautista-Salinas et al., 2022). Thus far, “soft surgical” techniques have been employed through slow manual insertions and use of flexible electrode arrays. One compelling strategy to enhance “soft surgical” technique is the use of robotics to assist electrode array insertion, which minimizes intracochlear forces, pressure transients, and trauma through slower and steadier insertion (Banakis Hartl et al., 2019; Kaufmann et al., 2020). While limiting the surgical trauma as much as possible is necessary, many also utilize the peri-surgical local application of anti-inflammatory drugs to further inhibit the inflammatory response related to surgery. While this one-time application may inhibit the immune response to surgical trauma, sustained delivery of anti-inflammatory molecules after CI is also being explored via the use of osmotic pumps (Scheper et al., 2017; Tarabichi et al., 2021). Separately an increasingly promising approach for sustained delivery involves controlling drug release over time through elution from the implant surface (Liebau et al., 2019). In this approach, clinical trials are currently studying the inflammatory response to CI with dexamethasone (DEX) eluting from the implant surface (Chen et al., 2021).
While it is evident that the immune response to surgical trauma to CI could be temporarily blocked with soft surgery and tailored DEX treatment, it remains to be seen whether controlled DEX release over time can effectively inhibit inflammation for a meaningful duration to benefit long -term device function (Scheper et al., 2017; Liebau et al., 2019). Specifically, it is unclear whether DEX just blocks the inflammation resulting from surgical trauma, and if the immune system will eventually respond to the implanted materials and electrical stimulation, leading to the formation of a fibrotic capsule around the implant. Therefore, there are ongoing efforts to engineer approaches to limit the immune response to electrode materials (Leigh et al., 2019; Jensen et al., 2022). To understand these approaches, it is essential to provide a brief overview of the foreign body response. The foreign body response is an inevitable process that occurs in response to any material implanted in vivo, involving several steps, briefly: (1) non-specific attachment of serum proteins to the implant surface, (2) acute inflammation led by neutrophils that adhere to the biomaterial surface, (3) recruitment of monocytes attempting to engulf the implant by forming a layer around it, (4) formation of giant cells when macrophages cannot engulf the implant, which orchestrate fibrosis, and (5) recruitment of fibroblasts which deposit of a dense collagen matrix around the implant (Carnicer-Lombarte et al., 2021). In theory, blocking the first steps (i.e., adsorption of protein and cells) should be highly effective at blunting this response. One example is coating the implant materials with an ultra-low fouling zwitterionic thin-film hydrogel to prevent protein or cell attachment to the implant surface, thus blocking this cascade (Chen et al., 2023; Horne et al., 2023).
The third component that may contribute to intracochlear damage is electrical stimulation. For the most part, under current clinical paradigms, the levels of electrical stimulation needed to stimulate SGNs are felt to be relatively innocuous, and may even help promote SGN survival (Leake et al., 1999, 2007; Wise et al., 2011). However higher levels of electrical stimulation have been associated with loss of residual acoustic function (Kopelovich et al., 2015), perhaps by damaging the IHC:SGN synapse and peripheral processes (Kopelovich et al., 2015; Li et al., 2020), akin to damage induced by high levels of noise exposure (Furman et al., 2013; Kujawa and Liberman, 2015). Further, in guinea pigs, high levels of stimulation, well beyond those used clinically, do not result in SGN loss yet lead to increased platinum dissolution which may exacerbate the chronic inflammatory response (Landry et al., 2013; Shepherd et al., 2021). Thus, mitigating inflammation due to surgical trauma and/or the foreign body response should enable a lower level of electrical current for the implant to function (Briggs et al., 2020). In addition to reducing any potential inflammation from electrical stimulation, this could extend battery life and improve the signal-to-noise ratio of the device.
In conclusion, limiting the immune response is an essential aspect of improving CI and other electrical devices aimed at restoring hearing. To achieve this goal, next generation CI will need to (1) mitigate the immune response to surgical trauma, (2) involve ultra-low fouling materials, and (3) enable lower levels of electrical stimulation to function. If successful, these approaches could increase the number of usable channels in CI electrodes and create an environment around the implant more conducive to regenerative strategies (Carnicer-Lombarte et al., 2021). Further, diminishing the dense fibrotic capsule that typically surrounds the implant is likely necessary to enable optimal application of engineered guidance cues and neurite regeneration strategies.
3.3 Related regenerative medicine approaches
Genetic causes of hearing degeneration and deafness are a significant cause for patients to need CI. In theory, gene therapy could prevent further hearing loss or even restore function for genetic causes of deafness, although the application and success of these genetic approaches remain uncertain. One significant challenge lies in delivering genetic material to the inner ear, as this therapy must overcome transport barriers while specifically targeting the delicate cells of interest, such as SGNs or hair cells (Minoda et al., 2015; Ren et al., 2019). Achieving sufficient delivery to these cells of interest may prove challenging, inspired by this challenge, CI surgery offers an excellent opportunity to deliver genetic material to the inner ear and overcome the challenge of the blood-perilymph barrier. Furthermore, the CI electrode could be used to electroporate the genes of interest (Pinyon et al., 2014), thus increasing the transfection/transduction efficiency. Gene therapy in the context of CI could serve a variety of functions, including, improving function of auditory neurons, correcting a genetic mutation, or stimulating regeneration of auditory neurons from their niche (Shearer et al., 2017; Tropitzsch et al., 2023).
Related to this is use of stem cells or growth factors to regenerate the cells of interest, typically hair cells or SGNs. To achieve this, stem cells would be delivered to the cochlea, differentiated into the desired cell type, and integrated into the respective anatomical and functional niche (Roccio et al., 2020). A major challenge that hinders the use of stem cells in hearing regeneration is the complexity of the niches that need to be filled. While it may be challenging in the near future to achieve complete regeneration of the cells of interest into their proper niches, even modest integration that provides partial function could lead to significant improvements in native hearing or CI performance (Diensthuber and Stöver, 2018). Therefore, there is merit in efforts to combine stem cell-based approaches with CI. For example, as mentioned earlier, stem cells were discussed as a potential tool in combination with CI to deliver factors or act as a bridge between the electrode array and the neurons (Nella et al., 2022).
4 Conclusion
To conclude, CIs are a remarkably successful device, however, further challenges prevent its ability to fully restore complex auditory perception. There are many strategies to improve CI that may be achievable in both the near and the long term. Firstly, limiting the insertional trauma and the inflammatory/immune response seems to hold great potential for improving device performance in the near future (Carnicer-Lombarte et al., 2021; Rahman et al., 2022). In the long term, the field needs to pursue approaches to develop CI capable of more closely approximating natural hearing and transitioning CIs from a far-field to near-field device. Limiting the immune response to the electrode represents a significant improvement, but alone it will not enable CI to provide near native auditory sensation. Engineering the peripheral process of SGNs to be in close proximity to the CI electrodes would theoretically dramatically enhance the number of discrete, perceptible channels. It remains to be seen which techniques (e.g., surface patterning, small molecule release, hydrogel coating, optogenetics, genetic engineering, or stem cell engineering), alone or in combination, will best achieve this challenging ambition. Beyond hearing rehabilitation, CI represent an excellent device to study the general engineering principles to improve the neural-electrode interface for the rapidly emerging field of neural stimulation/modulation (Nassiri et al., 2022).
Author contributions
JV: Writing – original draft, Writing – review & editing. AC: Writing – review & editing. MH: Writing – review & editing.
Funding
The author(s) declare that financial support was received for the research, authorship, and/or publication of this article. This work was supported by grants from the NIH: R01-DC012578 (to MH), R01-DC018488 (to MH), F31-DC020371 (to JV), and T32-GM007337 (in support of JV) as well as an American Otological Society Clinician Scientist Award (to AC).
Conflict of interest
MH is a co-founder with equity interest in iotaMotion, Inc.
The remaining authors declare that the research was conducted in the absence of any commercial or financial relationships that could be construed as a potential conflict of interest.
Publisher’s note
All claims expressed in this article are solely those of the authors and do not necessarily represent those of their affiliated organizations, or those of the publisher, the editors and the reviewers. Any product that may be evaluated in this article, or claim that may be made by its manufacturer, is not guaranteed or endorsed by the publisher.
References
Adewole, D. O., Struzyna, L. A., Burrell, J. C., Harris, J. P., Nemes, A. D., Petrov, D., et al. (2021). Development of optically controlled "living electrodes" with long-projecting axon tracts for a synaptic brain-machine interface. Sci. Adv. 7:eaay5347. doi: 10.1126/sciadv.aay5347
Banakis Hartl, R. M., Kaufmann, C., Hansen, M. R., and Tollin, D. J. (2019). Intracochlear pressure transients during Cochlear implant electrode insertion: effect of Micro-mechanical control on limiting pressure trauma. Otol. Neurotol. 40, 736–744. doi: 10.1097/MAO.0000000000002164
Bautista-Salinas, D., Abdelaziz, M. E. M. K., Temelkuran, B., Yeatman, E. M., and Huins, C. T. (2022). Towards a functional atraumatic self-shaping Cochlear implant. Macromol. Mater. Eng. 307:2100620. doi: 10.1002/mame.202100620
Berg, K. A., Noble, J. H., Dawant, B. M., Dwyer, R. T., Labadie, R. F., and Gifford, R. H. (2019). Speech recognition as a function of the number of channels in perimodiolar electrode recipients. J. Acoust. Soc. Am. 145, 1556–1564. doi: 10.1121/1.5092350
Bieberich, E., and Guiseppi-Elie, A. (2004). Neuronal differentiation and synapse formation of PC12 and embryonic stem cells on interdigitated microelectrode arrays:: contact structures for neuron-to-electrode signal transmission (NEST). Biosens. Bioelectron. 19, 923–931. doi: 10.1016/j.bios.2003.08.016
Boisvert, I., Reis, M., Au, A., Cowan, R., and Dowell, R. C. (2020). Cochlear implantation outcomes in adults: a scoping review. PLoS One 15:e0232421. doi: 10.1371/journal.pone.0232421
Briggs, R., O’Leary, S., Birman, C., Plant, K., English, R., Dawson, P., et al. (2020). Comparison of electrode impedance measures between a dexamethasone-eluting and standard Cochlear™ contour advance® electrode in adult cochlear implant recipients. Hear. Res. 390:107924. doi: 10.1016/j.heares.2020.107924
Bruder, J. M., Lee, A. P., and Hoffman-Kim, D. (2007). Biomimetic materials replicating Schwann cell topography enhance neuronal adhesion and neurite alignment in vitro. J. Biomater. Sci. Polym. Ed. 18, 967–982. doi: 10.1163/156856207781494412
Büchner, A., Schüssler, M., Battmer, R. D., Stöver, T., Lesinski-Schiedat, A., and Lenarz, T. (2009). Impact of low-frequency hearing. Audiol. Neurootol. 14, 8–13. doi: 10.1159/000206490
Budenz, C. L., Pfingst, B. E., and Raphael, Y. (2012). The use of neurotrophin therapy in the inner ear to augment cochlear implantation outcomes. Anat Rec (Hoboken). 295, 1896–1908. doi: 10.1002/ar.22586
Carnicer-Lombarte, A., Chen, S.-T., Malliaras, G. G., and Barone, D. G. (2021). Foreign body reaction to implanted biomaterials and its impact in nerve Neuroprosthetics. Front. Bioeng. Biotechnol. 9:622524. doi: 10.3389/fbioe.2021.622524
Chen, A., Chen, D., Lv, K., Li, G., Pan, J., Ma, D., et al. (2023). Zwitterionic polymer/Polydopamine coating of electrode arrays reduces fibrosis and residual hearing loss after Cochlear implantation. Adv. Healthc. Mater. 12:e2200807. doi: 10.1002/adhm.202200807
Chen, D., Luo, Y., Pan, J., Chen, A., Ma, D., Xu, M., et al. (2021). Long-term release of dexamethasone with a Polycaprolactone-coated electrode alleviates fibrosis in Cochlear implantation. Front. Cell Dev. Biol. 9:740576. doi: 10.3389/fcell.2021.740576
Choi, C. H., and Oghalai, J. S. (2005). Predicting the effect of post-implant cochlear fibrosis on residual hearing. Hear. Res. 205, 193–200. doi: 10.1016/j.heares.2005.03.018
Clarke, J. C., Tuft, B. W., Clinger, J. D., Levine, R., Figueroa, L. S., Guymon, C. A., et al. (2011). Micropatterned methacrylate polymers direct spiral ganglion neurite and Schwann cell growth. Hear. Res. 278, 96–105. doi: 10.1016/j.heares.2011.05.004
Coate, T. M., Scott, M. K., and Gurjar, M. (2019). Current concepts in cochlear ribbon synapse formation. Synapse 73:e22087. doi: 10.1002/syn.22087
Coate, T. M., Spita, N. A., Zhang, K. D., Isgrig, K. T., and Kelley, M. W. (2015). Neuropilin-2/Semaphorin-3F-mediated repulsion promotes inner hair cell innervation by spiral ganglion neurons. eLife 4:e07830. doi: 10.7554/eLife.07830
Davis, R. L. (2003). Gradients of neurotrophins, ion channels, and tuning in the cochlea. Neuroscientist 9, 311–316. doi: 10.1177/1073858403251986
Diensthuber, M., and Stöver, T. (2018). Strategies for a regenerative therapy of hearing loss. HNO 66, 39–46. doi: 10.1007/s00106-017-0467-0
Dieter, A., Keppeler, D., and Moser, T. (2020). Towards the optical cochlear implant: optogenetic approaches for hearing restoration. EMBO Mol. Med. 12:e11618. doi: 10.15252/emmm.201911618
Dixon, A. R., Jariwala, S. H., Bilis, Z., Loverde, J. R., Pasquina, P. F., and Alvarez, L. M. (2018). Bridging the gap in peripheral nerve repair with 3D printed and bioprinted conduits. Biomaterials 186, 44–63. doi: 10.1016/j.biomaterials.2018.09.010
Druckenbrod, N. R., Hale, E. B., Olukoya, O. O., Shatzer, W. E., and Goodrich, L. V. (2020). Neuronal processes and glial precursors form a scaffold for wiring the developing mouse cochlea. Nat. Commun. 11:5866. doi: 10.1038/s41467-020-19521-2
Eichinger, C. D., Hsiao, T. W., and Hlady, V. (2012). Multiprotein microcontact printing with micrometer resolution. Langmuir 28, 2238–2243. doi: 10.1021/la2039202
Emiliani, V., Entcheva, E., Hedrich, R., Hegemann, P., Konrad, K. R., Lüscher, C., et al. (2022). Optogenetics for light control of biological systems. Nature Rev. Methods Primers. 2:55. doi: 10.1038/s43586-022-00136-4
Ertas, Y. N., Ozpolat, D., Karasu, S. N., and Ashammakhi, N. (2022). Recent advances in Cochlear implant electrode Array design parameters. Micromachines (Basel). 13:1081. doi: 10.3390/mi13071081
Euteneuer, S., Yang, K. H., Chavez, E., Leichtle, A., Loers, G., Olshansky, A., et al. (2013). Glial cell line-derived neurotrophic factor (GDNF) induces neuritogenesis in the cochlear spiral ganglion via neural cell adhesion molecule (NCAM). Mol. Cell. Neurosci. 54, 30–43. doi: 10.1016/j.mcn.2012.12.004
Flores-Otero, J., and Davis, R. L. (2011). Synaptic proteins are tonotopically graded in postnatal and adult type I and type II spiral ganglion neurons. J. Comp. Neurol. 519, 1455–1475. doi: 10.1002/cne.22576
Flores-Otero, J., Xue, H. Z., and Davis, R. L. (2007). Reciprocal regulation of presynaptic and postsynaptic proteins in bipolar spiral ganglion neurons by Neurotrophins. J. Neurosci. 27, 14023–14034. doi: 10.1523/JNEUROSCI.3219-07.2007
Foggia, M. J., Quevedo, R. V., and Hansen, M. R. (2019). Intracochlear fibrosis and the foreign body response to cochlear implant biomaterials. Laryngoscope Investigative Otolaryngol. 4, 678–683. doi: 10.1002/lio2.329
Furman, A. C., Kujawa, S. G., and Liberman, M. C. (2013). Noise-induced cochlear neuropathy is selective for fibers with low spontaneous rates. J. Neurophysiol. 110, 577–586. doi: 10.1152/jn.00164.2013
Gabrielpillai, J., Geissler, C., Stock, B., Stöver, T., and Diensthuber, M. (2018). Growth hormone promotes neurite growth of spiral ganglion neurons. Neuroreport 29, 637–642. doi: 10.1097/WNR.0000000000001011
Gantz, B. J., Dunn, C. C., Oleson, J., and Hansen, M. R. (2018). Acoustic plus electric speech processing: Long-term results. Laryngoscope 128, 473–481. doi: 10.1002/lary.26669
Gantz, B. J., Hansen, M., and Dunn, C. C. (2022). Clinical perspective on hearing preservation in cochlear implantation, the University of Iowa experience. Hear. Res. 426:108487. doi: 10.1016/j.heares.2022.108487
García-Hernández, S., Potashner, S. J., and Morest, D. K. (2013). Role of fibroblast growth factor 8 in neurite outgrowth from spiral ganglion neurons in vitro. Brain Res. 1529, 39–45. doi: 10.1016/j.brainres.2013.07.030
Grasmeder, M. L., Verschuur, C. A., and Batty, V. B. (2014). Optimizing frequency-to-electrode allocation for individual cochlear implant users. J. Acoust. Soc. Am. 136, 3313–3324. doi: 10.1121/1.4900831
Green, S. H., Bailey, E., Wang, Q., and Davis, R. L. (2012). The Trk a, B, C's of Neurotrophins in the cochlea. Anat. Rec. 295, 1877–1895. doi: 10.1002/ar.22587
Guiraud, J., Besle, J., Arnold, L., Boyle, P., Giard, M. H., Bertrand, O., et al. (2007). Evidence of a tonotopic organization of the auditory cortex in cochlear implant users. J. Neurosci. 27, 7838–7846. doi: 10.1523/JNEUROSCI.0154-07.2007
Gunewardene, N., Lam, P., Ma, Y., Caruso, F., Wagstaff, S., Richardson, R. T., et al. (2022). Pharmacokinetics and biodistribution of supraparticle-delivered neurotrophin 3 in the guinea pig cochlea. J. Control. Release 342, 295–307. doi: 10.1016/j.jconrel.2021.12.037
Hansen, M. R., Vijapurkar, U., Koland, J. G., and Green, S. H. (2001). Reciprocal signaling between spiral ganglion neurons and Schwann cells involves neuregulin and neurotrophins. Hear. Res. 161, 87–98. doi: 10.1016/S0378-5955(01)00360-4
Hendricks, J. L., Chikar, J. A., Crumling, M. A., Raphael, Y., and Martin, D. C. (2008). Localized cell and drug delivery for auditory prostheses. Hear. Res. 242, 117–131. doi: 10.1016/j.heares.2008.06.003
Horne, R., Ben-Shlomo, N., Jensen, M., Ellerman, M., Escudero, C., Hua, R., et al. (2023). Reducing the foreign body response on human cochlear implants and their materials in vivo with photografted zwitterionic hydrogel coatings. Acta Biomater. 166, 212–223. doi: 10.1016/j.actbio.2023.05.011
Hu, Y., Chen, W., Yin, H., Chen, X., Cai, J., Guo, J., et al. (2022). Super-aligned carbon nanotubes and GelMA hydrogel composite scaffolds promote spiral ganglion neuron growth and orientation. Materials Today Nano. 18:100181. doi: 10.1016/j.mtnano.2022.100181
Huet, A. T., Dombrowski, T., Rankovic, V., Thirumalai, A., and Moser, T. (2021). Developing fast, red-light Optogenetic stimulation of spiral ganglion neurons for future optical Cochlear implants. Front. Mol. Neurosci. 14:635897. doi: 10.3389/fnmol.2021.635897
Humenik, M., Winkler, A., and Scheibel, T. (2021). Patterning of protein-based materials. Biopolymers 112:e23412. doi: 10.1002/bip.23412
Jensen, M. J., Claussen, A. D., Higgins, T., Vielman-Quevedo, R., Mostaert, B., Xu, L., et al. (2022). Cochlear implant material effects on inflammatory cell function and foreign body response. Hear. Res. 426:108597. doi: 10.1016/j.heares.2022.108597
Jeon, E. J., Xu, N., Xu, L., and Hansen, M. R. (2011). Influence of central glia on spiral ganglion neuron neurite growth. Neuroscience 177, 321–334. doi: 10.1016/j.neuroscience.2011.01.014
Jiam, N. T., Caldwell, M. T., and Limb, C. J. (2017). What does music sound like for a Cochlear implant user? Otol. Neurotol. 38, e240–e247. doi: 10.1097/MAO.0000000000001448
Jiang, J. P., Liu, X. Y., Zhao, F., Zhu, X., Li, X. Y., Niu, X. G., et al. (2020). Three-dimensional bioprinting collagen/silk fibroin scaffold combined with neural stem cells promotes nerve regeneration after spinal cord injury. Neural Regen. Res. 15, 959–968. doi: 10.4103/1673-5374.268974
Joo, S., Yeon Kim, J., Lee, E., Hong, N., Sun, W., and Nam, Y. (2015). Effects of ECM protein micropatterns on the migration and differentiation of adult neural stem cells. Sci. Rep. 5:13043. doi: 10.1038/srep13043
Kaufmann, C. R., Henslee, A. M., Claussen, A., and Hansen, M. R. (2020). Evaluation of insertion forces and cochlea trauma following robotics-assisted Cochlear implant electrode Array insertion. Otol. Neurotol. 41, 631–638. doi: 10.1097/MAO.0000000000002608
Kempfle, J. S., Duro, M. V., Zhang, A., Amador, C. D., Kuang, R., Lu, R., et al. (2021). A novel small molecule Neurotrophin-3 analogue promotes inner ear neurite outgrowth and synaptogenesis in vitro. Front. Cell. Neurosci. 15:666706. doi: 10.3389/fncel.2021.666706
Keppeler, D., Merino, R. M., Lopez de la Morena, D., Bali, B., Huet, A. T., Gehrt, A., et al. (2018). Ultrafast optogenetic stimulation of the auditory pathway by targeting-optimized Chronos. EMBO J. 37:e99649. doi: 10.15252/embj.201899649
Keppeler, D., Schwaerzle, M., Harczos, T., Jablonski, L., Dieter, A., Wolf, B., et al. (2020). Multichannel optogenetic stimulation of the auditory pathway using microfabricated LED cochlear implants in rodents. Sci. Transl. Med. 12:eabb8086. doi: 10.1126/scitranslmed.abb8086
Kikkawa, Y. S., Nakagawa, T., Ying, L., Tabata, Y., Tsubouchi, H., Ido, A., et al. (2014). Growth factor-eluting cochlear implant electrode: impact on residual auditory function, insertional trauma, and fibrosis. J. Transl. Med. 12:280. doi: 10.1186/s12967-014-0280-4
Kim, S. M., Ueki, M., Ren, X., Akimoto, J., Sakai, Y., and Ito, Y. (2019). Micropatterned nanolayers immobilized with nerve growth factor for neurite formation of PC12 cells. Int. J. Nanomedicine 14, 7683–7694. doi: 10.2147/IJN.S217416
Kopelovich, J. C., Reiss, L. A., Etler, C. P., Xu, L., Bertroche, J. T., Gantz, B. J., et al. (2015). Hearing loss after activation of hearing preservation Cochlear implants might be related to afferent Cochlear innervation injury. Otol. Neurotol. 36, 1035–1044. doi: 10.1097/MAO.0000000000000754
Kujawa, S. G., and Liberman, M. C. (2015). Synaptopathy in the noise-exposed and aging cochlea: primary neural degeneration in acquired sensorineural hearing loss. Hear. Res. 330, 191–199. doi: 10.1016/j.heares.2015.02.009
Landry, T. G., Fallon, J. B., Wise, A. K., and Shepherd, R. K. (2013). Chronic neurotrophin delivery promotes ectopic neurite growth from the spiral ganglion of deafened cochleae without compromising the spatial selectivity of cochlear implants. J. Comp. Neurol. 521, 2818–2832. doi: 10.1002/cne.23318
Leake, P. A., Hradek, G. T., and Snyder, R. L. (1999). Chronic electrical stimulation by a cochlear implant promotes survival of spiral ganglion neurons after neonatal deafness. J. Comp. Neurol. 412, 543–562. doi: 10.1002/(SICI)1096-9861(19991004)412:4<543::AID-CNE1>3.0.CO;2-3
Leake, P. A., Hradek, G. T., Vollmer, M., and Rebscher, S. J. (2007). Neurotrophic effects of GM1 ganglioside and electrical stimulation on cochlear spiral ganglion neurons in cats deafened as neonates. J. Comp. Neurol. 501, 837–853. doi: 10.1002/cne.21275
Leigh, B. L., Cheng, E., Xu, L., Andresen, C., Hansen, M. R., and Guymon, C. A. (2017a). Photopolymerizable Zwitterionic polymer patterns control cell adhesion and guide neural growth. Biomacromolecules 18, 2389–2401. doi: 10.1021/acs.biomac.7b00579
Leigh, B. L., Cheng, E., Xu, L., Derk, A., Hansen, M. R., and Guymon, C. A. (2019). Antifouling Photograftable Zwitterionic coatings on PDMS substrates. Langmuir 35, 1100–1110. doi: 10.1021/acs.langmuir.8b00838
Leigh, B. L., Truong, K., Bartholomew, R., Ramirez, M., Hansen, M. R., and Guymon, C. A. (2017b). Tuning surface and topographical features to investigate competitive guidance of spiral ganglion neurons. ACS Appl. Mater. Interfaces 9, 31488–31496. doi: 10.1021/acsami.7b09258
Lenarz, T., Buechner, A., Lesinski-Schiedat, A., Timm, M., and Salcher, R. (2020). Hearing preservation with a new atraumatic Lateral Wall electrode. Otol. Neurotol. 41, e993–e1003. doi: 10.1097/MAO.0000000000002714
Li, H., Edin, F., Hayashi, H., Gudjonsson, O., Danckwardt-Lillieström, N., Engqvist, H., et al. (2017). Guided growth of auditory neurons: bioactive particles towards gapless neural – electrode interface. Biomaterials 122, 1–9. doi: 10.1016/j.biomaterials.2016.12.020
Li, H., Helpard, L., Ekeroot, J., Rohani, S. A., Zhu, N., Rask-Andersen, H., et al. (2021). Three-dimensional tonotopic mapping of the human cochlea based on synchrotron radiation phase-contrast imaging. Sci. Rep. 11:4437. doi: 10.1038/s41598-021-83225-w
Li, Q., Lu, T., Zhang, C., Hansen, M. R., and Li, S. (2020). Electrical stimulation induces synaptic changes in the peripheral auditory system. J. Comp. Neurol. 528, 893–905. doi: 10.1002/cne.24802
Li, C. Y., Mittal, R., Bergman, J., Mittal, J., and Eshraghi, A. A. (2021). Recent advancements toward gapless neural-electrode interface post-cochlear implantation. Neural Regen. Res. 16, 1805–1806. doi: 10.4103/1673-5374.306085
Liebau, A., Schilp, S., Mugridge, K., Schön, I., Kather, M., Kammerer, B., et al. (2019). Long-term in vivo release profile of dexamethasone-loaded silicone rods implanted into the cochlea of Guinea pigs. Front. Neurol. 10:1377. doi: 10.3389/fneur.2019.01377
Limb, C. J., and Roy, A. T. (2014). Technological, biological, and acoustical constraints to music perception in cochlear implant users. Hear. Res. 308, 13–26. doi: 10.1016/j.heares.2013.04.009
Limberg, D. K., Kang, J.-H., and Hayward, R. C. (2022). Triplet–triplet annihilation Photopolymerization for high-resolution 3D printing. J. Am. Chem. Soc. 144, 5226–5232. doi: 10.1021/jacs.1c11022
Liu, S. S., and Yang, R. (2022). Inner ear drug delivery for sensorineural hearing loss: current challenges and opportunities. Front. Neurosci. 16:16. doi: 10.3389/fnins.2022.867453
Long, P., Wan, G., Roberts, M. T., and Corfas, G. (2018). Myelin development, plasticity, and pathology in the auditory system. Dev. Neurobiol. 78, 80–92. doi: 10.1002/dneu.22538
Marino, A., Ciofani, G., Filippeschi, C., Pellegrino, M., Pellegrini, M., Orsini, P., et al. (2013). Two-photon polymerization of sub-micrometric patterned surfaces: investigation of cell-substrate interactions and improved differentiation of neuron-like cells. ACS Appl. Mater. Interfaces 5, 13012–13021. doi: 10.1021/am403895k
Minoda, R., Miwa, T., Ise, M., and Takeda, H. (2015). Potential treatments for genetic hearing loss in humans: current conundrums. Gene Ther. 22, 603–609. doi: 10.1038/gt.2015.27
Mittring, A., Moser, T., and Huet, A. T. (2023). Graded optogenetic activation of the auditory pathway for hearing restoration. Brain Stimul. 16, 466–483. doi: 10.1016/j.brs.2023.01.1671
Moser, T., and Dieter, A. (2020). Towards optogenetic approaches for hearing restoration. Biochem. Biophys. Res. Commun. 527, 337–342. doi: 10.1016/j.bbrc.2019.12.126
Moverman, D. J., Liberman, L. D., Kraemer, S., Corfas, G., and Liberman, M. C. (2023). Ultrastructure of noise-induced cochlear synaptopathy. Sci. Rep. 13:19456. doi: 10.1038/s41598-023-46859-6
Nassiri, A. M., Sorkin, D. L., and Carlson, M. L. (2022). Current estimates of Cochlear implant utilization in the United States. Otol. Neurotol. 43, e558–e562. doi: 10.1097/MAO.0000000000003513
Nella, K. T., Norton, B. M., Chang, H.-T., Heuer, R. A., Roque, C. B., and Matsuoka, A. J. (2022). Bridging the electrode–neuron gap: finite element modeling of in vitro neurotrophin gradients to optimize neuroelectronic interfaces in the inner ear. Acta Biomater. 151, 360–378. doi: 10.1016/j.actbio.2022.08.035
Pamulova, L., Linder, B., and Rask-Andersen, H. (2006). Innervation of the apical turn of the human cochlea: a light microscopic and transmission Electron microscopic investigation. Otol. Neurotol. 27, 270–275. doi: 10.1097/01.mao.0000187239.56583.d2
Pankiewicz, P., Szybiński, M., Kisielewska, K., Gołębiowski, F., Krzemiński, P., Rutkowska-Włodarczyk, I., et al. (2021). Do small molecules activate the TrkB receptor in the same manner as BDNF? Limitations of published TrkB low molecular agonists and screening for novel TrkB Orthosteric agonists. Pharmaceuticals. 14:704. doi: 10.3390/ph14080704
Pinyon, J. L., Tadros, S. F., Froud, K. E., Tompson, I. T., Crawford, E. N., Ko, M., et al. (2014). Close-field electroporation gene delivery using the cochlear implant electrode array enhances the bionic ear. Sci. Transl. Med. 6:233ra54. doi: 10.1126/scitranslmed.3008177
Pisoni, D. B., Kronenberger, W. G., Harris, M. S., Moberly, A. C., and Jin, X. (2017). Three challenges for future research on cochlear implants. World J. Otorhinolaryngol. Head Neck Surg. 3, 240–254. doi: 10.1016/j.wjorl.2017.12.010
Rahman, M. T., Chari, D. A., Ishiyama, G., Lopez, I., Quesnel, A. M., Ishiyama, A., et al. (2022). Cochlear implants: causes, effects and mitigation strategies for the foreign body response and inflammation. Hear. Res. 422:108536. doi: 10.1016/j.heares.2022.108536
Rejali, D., Lee, V. A., Abrashkin, K. A., Humayun, N., Swiderski, D. L., and Raphael, Y. (2007). Cochlear implants and ex vivo BDNF gene therapy protect spiral ganglion neurons. Hear. Res. 228, 180–187. doi: 10.1016/j.heares.2007.02.010
Ren, Y., Landegger, L. D., and Stankovic, K. M. (2019). Gene therapy for human sensorineural hearing loss. Front. Cell. Neurosci. 13:323. doi: 10.3389/fncel.2019.00323
Resnick, J. M., O'Brien, G. E., and Rubinstein, J. T. (2018). Simulated auditory nerve axon demyelination alters sensitivity and response timing to extracellular stimulation. Hear. Res. 361, 121–137. doi: 10.1016/j.heares.2018.01.014
Roccio, M., Senn, P., and Heller, S. (2020). Novel insights into inner ear development and regeneration for targeted hearing loss therapies. Hear. Res. 397:107859. doi: 10.1016/j.heares.2019.107859
Sameer Mallick, A., Qureishi, A., Pearson, R., and O'Donoghue, G. (2013). Neurotrophins and cochlear implants: a solution to sensorineural deafness? Cochlear Implants Int. 14, 158–164. doi: 10.1179/1754762812Y.0000000013
Scheper, V., Hessler, R., Hütten, M., Wilk, M., Jolly, C., Lenarz, T., et al. (2017). Local inner ear application of dexamethasone in cochlear implant models is safe for auditory neurons and increases the neuroprotective effect of chronic electrical stimulation. PLoS One 12:e0183820. doi: 10.1371/journal.pone.0183820
Schimmang, T., Tan, J., Müller, M., Zimmermann, U., Rohbock, K., Köpschall, I., et al. (2003). Lack of Bdnf and TrkB signalling in the postnatal cochlea leads to a spatial reshaping of innervation along the tonotopic axis and hearing loss. Development 130, 4741–4750. doi: 10.1242/dev.00676
Schwieger, J., Warnecke, A., Lenarz, T., Esser, K.-H., and Scheper, V. (2015). Neuronal survival, morphology and outgrowth of spiral ganglion neurons using a defined growth factor combination. PLoS One 10:e0133680. doi: 10.1371/journal.pone.0133680
Senn, P., Roccio, M., Hahnewald, S., Frick, C., Kwiatkowska, M., Ishikawa, M., et al. (2017). NANOCI—nanotechnology based Cochlear implant with gapless Interface to auditory neurons. Otol. Neurotol. 38, e224–e231. doi: 10.1097/MAO.0000000000001439
Serruya, M. D., Harris, J. P., Adewole, D. O., Struzyna, L. A., Burrell, J. C., Nemes, A., et al. (2018). Engineered axonal tracts as "living electrodes" for synaptic-based modulation of neural circuitry. Adv. Funct. Mater. 28. doi: 10.1002/adfm.201701183
Shearer, A. E., Eppsteiner, R. W., Frees, K., Tejani, V., Sloan-Heggen, C. M., Brown, C., et al. (2017). Genetic variants in the peripheral auditory system significantly affect adult cochlear implant performance. Hear. Res. 348, 138–142. doi: 10.1016/j.heares.2017.02.008
Shepherd, R. K., Carter, P. M., Dalrymple, A. N., Enke, Y. L., Wise, A. K., Nguyen, T., et al. (2021). Platinum dissolution and tissue response following long-term electrical stimulation at high charge densities. J. Neural Eng. 18:036021. doi: 10.1088/1741-2552/abe5ba
Shi, D., Dhawan, V., and Cui, X. T. (2021). Bio-integrative design of the neural tissue-device interface. Curr. Opin. Biotechnol. 72, 54–61. doi: 10.1016/j.copbio.2021.10.003
Shi, X., Xiao, Y., Xiao, H., Harris, G., Wang, T., and Che, J. (2016). Topographic guidance based on microgrooved electroactive composite films for neural interface. Colloids Surf. B: Biointerfaces 145, 768–776. doi: 10.1016/j.colsurfb.2016.05.086
Shim, H., Kim, S., Hong, J., Na, Y., Woo, J., Hansen, M., et al. (2023). Differences in neural encoding of speech in noise between cochlear implant users with and without preserved acoustic hearing. Hear. Res. 427:108649. doi: 10.1016/j.heares.2022.108649
Simoni, E., Gentilin, E., Candito, M., Borile, G., Romanato, F., Chicca, M., et al. (2020). Immune response after Cochlear implantation. Front. Neurol. 11:341. doi: 10.3389/fneur.2020.00341
Soleymani, Z., Mahmoodabadi, N., and Nouri, M. M. (2016). Language skills and phonological awareness in children with cochlear implants and normal hearing. Int. J. Pediatr. Otorhinolaryngol. 83, 16–21. doi: 10.1016/j.ijporl.2016.01.013
Sorribas, H., Padeste, C., and Tiefenauer, L. (2002). Photolithographic generation of protein micropatterns for neuron culture applications. Biomaterials 23, 893–900. doi: 10.1016/S0142-9612(01)00199-5
Sridharan, S., Gajowa, M. A., Ogando, M. B., Jagadisan, U. K., Abdeladim, L., Sadahiro, M., et al. (2022). High-performance microbial opsins for spatially and temporally precise perturbations of large neuronal networks. Neuron 110, 1139–55.e6. doi: 10.1016/j.neuron.2022.01.008
Sun, X., Li, L., Tan, Z., Li, J., Hou, Y., Wang, X., et al. (2022). On-demand release of the small-molecule TrkB agonist improves neuron-Schwann cell interactions. J. Control. Release 343, 482–491. doi: 10.1016/j.jconrel.2022.02.002
Szobota, S., Mathur, P. D., Siegel, S., Black, K., Saragovi, H. U., and Foster, A. C. (2019). BDNF, NT-3 and Trk receptor agonist monoclonal antibodies promote neuron survival, neurite extension, and synapse restoration in rat cochlea ex vivo models relevant for hidden hearing loss. PLoS One 14:e0224022. doi: 10.1371/journal.pone.0224022
Tarabichi, O., Jensen, M., and Hansen, M. R. (2021). Advances in hearing preservation in cochlear implant surgery. Current opinion Otolaryngol. Head Neck Surg. 29, 385–390. doi: 10.1097/MOO.0000000000000742
Taveggia, C., Feltri, M. L., and Wrabetz, L. (2010). Signals to promote myelin formation and repair. Nat. Rev. Neurol. 6, 276–287. doi: 10.1038/nrneurol.2010.37
Thompson, D. M., and Buettner, H. M. (2006). Neurite outgrowth is directed by schwann cell alignment in the absence of other guidance cues. Ann. Biomed. Eng. 34, 161–168. doi: 10.1007/s10439-005-9013-4
Tonazzini, I., Masciullo, C., Savi, E., Sonato, A., Romanato, F., and Cecchini, M. (2020). Neuronal contact guidance and YAP signaling on ultra-small nanogratings. Sci. Rep. 10:3742. doi: 10.1038/s41598-020-60745-5
Tropitzsch, A., Schade-Mann, T., Gamerdinger, P., Dofek, S., Schulte, B., Schulze, M., et al. (2023). Variability in Cochlear implantation outcomes in a large German cohort with a genetic etiology of hearing loss. Ear Hear. 44, 1464–1484. doi: 10.1097/AUD.0000000000001386
Truong, K., Leigh, B., Vecchi, J. T., Bartholomew, R., Xu, L., Guymon, C. A., et al. (2021). Interaction of micropatterned topographical and biochemical cues to direct neurite growth from spiral ganglion neurons. Hear. Res. 409:108315. doi: 10.1016/j.heares.2021.108315
Tuft, B. W., Li, S., Xu, L., Clarke, J. C., White, S. P., Guymon, B. A., et al. (2013). Photopolymerized microfeatures for directed spiral ganglion neurite and Schwann cell growth. Biomaterials 34, 42–54. doi: 10.1016/j.biomaterials.2012.09.053
Tuft, B. W., Xu, L., Leigh, B., Lee, D., Guymon, C. A., and Hansen, M. R. (2018). Photopolymerized micropatterns with high feature frequencies overcome chemorepulsive borders to direct neurite growth. J. Tissue Eng. Regen. Med. 12, e1392–e1403. doi: 10.1002/term.2527
Vecchi, J. T., Rhomberg, M., Guymon, C. A., and Hansen, M. R. (2024). The geometry of photopolymerized topography influences neurite pathfinding by directing growth cone morphology and migration. J. Neural Eng. 21:026027. doi: 10.1088/1741-2552/ad38dc
Walia, A., Shew, M. A., Varghese, J., Ioerger, P., Lefler, S. M., Ortmann, A. J., et al. (2023). Improved Cochlear implant performance estimation using Tonotopic-based Electrocochleography. JAMA Otolaryngol Head Neck Surg. 149, 1120–1129. doi: 10.1001/jamaoto.2023.2988
Wan, G., and Corfas, G. (2017). Transient auditory nerve demyelination as a new mechanism for hidden hearing loss. Nat. Commun. 8:14487. doi: 10.1038/ncomms14487
Wang, H., Zhao, N., Yan, K., Liu, X., Zhang, Y., Hong, Z., et al. (2015). Inner hair cell ribbon synapse plasticity might be molecular basis of temporary hearing threshold shifts in mice. Int. J. Clin. Exp. Pathol. 8, 8680–8691
Whitlon, D. S., Grover, M., Dunne, S. F., Richter, S., Luan, C.-H., and Richter, C.-P. (2015). Novel high content screen detects compounds that promote neurite Regeneration from Cochlear spiral ganglion neurons. Sci. Rep. 5:15960. doi: 10.1038/srep15960
Whitlon, D. S., Tieu, D., Grover, M., Reilly, B., and Coulson, M. T. (2009). Spontaneous association of glial cells with regrowing neurites in mixed cultures of dissociated spiral ganglia. Neuroscience 161, 227–235. doi: 10.1016/j.neuroscience.2009.03.044
Wilk, M., Hessler, R., Mugridge, K., Jolly, C., Fehr, M., Lenarz, T., et al. (2016). Impedance changes and fibrous tissue growth after Cochlear implantation are correlated and can be reduced using a dexamethasone eluting electrode. PLoS One 11:e0147552. doi: 10.1371/journal.pone.0147552
Wille, I., Harre, J., Oehmichen, S., Lindemann, M., Menzel, H., Ehlert, N., et al. (2022). Development of neuronal guidance fibers for stimulating electrodes: basic construction and delivery of a growth factor. Front. Bioeng. Biotechnol. 10:10. doi: 10.3389/fbioe.2022.776890
Wise, A. K., Fallon, J. B., Neil, A. J., Pettingill, L. N., Geaney, M. S., Skinner, S. J., et al. (2011). Combining cell-based therapies and neural prostheses to promote neural survival. Neurotherapeutics 8, 774–787. doi: 10.1007/s13311-011-0070-0
Wulf, K., Goblet, M., Raggl, S., Teske, M., Eickner, T., Lenarz, T., et al. (2022). PLLA coating of active implants for dual drug release. Molecules 27:1417. doi: 10.3390/molecules27041417
Xu, Y., Magnuson, M., Agarwal, A., Tan, X., and Richter, C. P. (2021). Infrared neural stimulation at different wavelengths and pulse shapes. Prog. Biophys. Mol. Biol. 162, 89–100. doi: 10.1016/j.pbiomolbio.2020.12.004
Yu, Q., Chang, Q., Liu, X., Gong, S., Ye, K., and Lin, X. (2012). 7,8,3′-Trihydroxyflavone, a potent small molecule TrkB receptor agonist, protects spiral ganglion neurons from degeneration both in vitro and in vivo. Biochem. Biophys. Res. Commun. 422, 387–392. doi: 10.1016/j.bbrc.2012.04.154
Yu, Q., Chang, Q., Liu, X., Wang, Y., Li, H., Gong, S., et al. (2013). Protection of spiral ganglion neurons from degeneration using small-molecule TrkB receptor agonists. J. Neurosci. 33, 13042–13052. doi: 10.1523/JNEUROSCI.0854-13.2013
Zeng, F. G. (2004). Trends in cochlear implants. Trends Amplif. 8, 1–34. doi: 10.1177/108471380400800102
Zeng, F.-G., Grant, G., Niparko, J., Galvin, J., Shannon, R., Opie, J., et al. (2002). Speech dynamic range and its effect on cochlear implant performance. J. Acoust. Soc. Am. 111, 377–386. doi: 10.1121/1.1423926
Zhang, L., Chen, S., and Sun, Y. (2022). Mechanism and prevention of spiral ganglion neuron degeneration in the cochlea. Front. Cell. Neurosci. 15:814891. doi: 10.3389/fncel.2021.814891
Zhang, Z., Jørgensen, M. L., Wang, Z., Amagat, J., Wang, Y., Li, Q., et al. (2020). 3D anisotropic photocatalytic architectures as bioactive nerve guidance conduits for peripheral neural regeneration. Biomaterials 253:120108. doi: 10.1016/j.biomaterials.2020.120108
Keywords: cochlear implant, neural electrode, regenerative medicine, hearing loss, biomaterials, optogenetics, neural prostheses, spiral ganglion neuron (SGN)
Citation: Vecchi JT, Claussen AD and Hansen MR (2024) Decreasing the physical gap in the neural-electrode interface and related concepts to improve cochlear implant performance. Front. Neurosci. 18:1425226. doi: 10.3389/fnins.2024.1425226
Edited by:
Fabien B. Wagner, UMR5293 Institut des Maladies Neurodégénératives (IMN), FranceReviewed by:
Pascal Senn, Hôpitaux universitaires de Genève (HUG), SwitzerlandJames B. Fallon, Bionics Institute, Australia
Copyright © 2024 Vecchi, Claussen and Hansen. This is an open-access article distributed under the terms of the Creative Commons Attribution License (CC BY). The use, distribution or reproduction in other forums is permitted, provided the original author(s) and the copyright owner(s) are credited and that the original publication in this journal is cited, in accordance with accepted academic practice. No use, distribution or reproduction is permitted which does not comply with these terms.
*Correspondence: Marlan R. Hansen, marlan-hansen@uiowa.edu