- 1Department of Speech, Language and Hearing Sciences, Indiana University, Bloomington, IN, United States
- 2Program in Neuroscience, Indiana University, Bloomington, IN, United States
- 3Cognitive Science Program, Indiana University, Bloomington, IN, United States
- 4School of Communication Sciences and Disorders, University of Memphis, Memphis, TN, United States
- 5Veterans Affairs Medical Center, Memphis, TN, United States
The frequency-following response (FFR) is an evoked potential that provides a neural index of complex sound encoding in the brain. FFRs have been widely used to characterize speech and music processing, experience-dependent neuroplasticity (e.g., learning and musicianship), and biomarkers for hearing and language-based disorders that distort receptive communication abilities. It is widely assumed that FFRs stem from a mixture of phase-locked neurogenic activity from the brainstem and cortical structures along the hearing neuraxis. In this study, we challenge this prevailing view by demonstrating that upwards of ~50% of the FFR can originate from an unexpected myogenic source: contamination from the postauricular muscle (PAM) vestigial startle reflex. We measured PAM, transient auditory brainstem responses (ABRs), and sustained frequency-following response (FFR) potentials reflecting myogenic (PAM) and neurogenic (ABR/FFR) responses in young, normal-hearing listeners with varying degrees of musical training. We first establish that PAM artifact is present in all ears, varies with electrode proximity to the muscle, and can be experimentally manipulated by directing listeners' eye gaze toward the ear of sound stimulation. We then show this muscular noise easily confounds auditory FFRs, spuriously amplifying responses 3–4-fold with tandem PAM contraction and even explaining putative FFR enhancements observed in highly skilled musicians. Our findings expose a new and unrecognized myogenic source to the FFR that drives its large inter-subject variability and cast doubt on whether changes in the response typically attributed to neuroplasticity/pathology are solely of brain origin.
Introduction
Neuroelectric brain recordings have been indispensable in demonstrating neuroplasticity at all levels of the auditory system. In particular, the frequency-following response (FFR), a scalp-recorded neurophonic reflecting phase-locked activity along the auditory neuroaxis to periodic signals, has served as a neural index of sound coding in the EEG. The precision of the FFR is evidenced by the fact these brain potentials can support intelligible speech when they are replayed (i.e., sonified) as audio stimuli (Bidelman, 2018a). The degree to which FFRs capture voice pitch (i.e., fundamental frequency; F0) and harmonic timbre cues of complex signals are also related to listeners' perception of speech material (Weiss and Bidelman, 2015). FFRs are also enhanced by various experiential factors, including native language experience (Krishnan et al., 2010; Zhao and Kuhl, 2018), musical abilities (Wong et al., 2007; Mankel and Bidelman, 2018), and perceptual learning (Reetzke et al., 2018). Conversely, FFRs reveal deficiencies in neural processing in a variety of auditory, literacy, and neurodevelopmental disorders (e.g., dyslexia, autism, hearing loss, and aging; Russo et al., 2008; Chandrasekaran et al., 2009; Anderson et al., 2013; White-Schwoch et al., 2015; Bidelman et al., 2017a). Collectively, a wealth of studies implies that FFRs might be a valuable auditory biomarker for tracking both positive and maladaptive auditory plasticity in the brain that either bolsters or compromises the perceptual organization of speech and musical sounds.
Despite an abundance of FFR studies and potential applications to understanding brain plasticity and normal and disordered central auditory processing, the anatomical origins of the FFR remain highly contentious (Coffey et al., 2016, 2019; Holmes and Herrmann, 2017; Bidelman, 2018b; White-Schwoch et al., 2019). Historically described as a brainstem potential (Smith et al., 1975; Sohmer and Pratt, 1977; Chandrasekaran and Kraus, 2010), it is now recognized that FFRs reflect a mixture of phase-locked activity from the brainstem and cortical structures throughout the auditory pathway (Bidelman, 2018b; Coffey et al., 2019; López-Caballero et al., 2020; Gorina-Careta et al., 2021). While different neuroimaging techniques emphasize brainstem- and cortico-centric contributions to the response, the FFR has always been described unequivocally as a brain response of auditory-neurogenic origin (Hoormann et al., 1992; Chandrasekaran and Kraus, 2010; Bidelman and Powers, 2018). However, anecdotal observations as early as the late 1970's (Sohmer et al., 1977)1 and our own experience over the past decade reveal occasional listeners who produce unusually large FFRs that far exceed the amplitudes expected for an auditory-neurogenic potential in humans (see also Picton et al., 2003). Such enigmatic responses are easily obscured in grand average data but can differ from the FFRs' normal operating range (Chandrasekaran and Kraus, 2010) by order of magnitude (i.e., 1–2 μV vs. 100–200 nV range) and thus warrant further explanation. In this study, we expose a heretofore unrecognized myogenic source of the FFR that explains substantial individual differences in the response, including waveform enhancements usually attributed to plasticity in central auditory nervous system function (Krishnan et al., 2005; Musacchia et al., 2007; Wong et al., 2007; Kraus et al., 2009, 2014; Parbery-Clark et al., 2009; Krizman et al., 2012; Coffey et al., 2017; Mankel and Bidelman, 2018; Zhao and Kuhl, 2018).
Situated behind the ear, the postauricular muscle (PAM) is part of a vestigial startle reflex that once acted to retract the pinna to protect hearing (Bérzin and Fortinguerra, 1993; Hackley, 2015). The muscle produces large (>100 μV) bilateral contraction with a peak latency of 9–15 ms following brief sounds (Thornton, 1975; O'beirne and Patuzzi, 1999) and is highly variable across listeners (Picton et al., 1974). Anesthetic blockage of the muscle and facial nerve abolishes PAM, confirming its myogenic origin (Bickford et al., 1964). While PAM activation declines with increasing stimulus rates (Geisler et al., 1958), the reflex can be elicited by surprisingly fast periodic stimuli without fatigue or habituation (Jacobson et al., 1964; but see Yoshie and Okudaira, 1969). Indeed, motor units underlying the PAM reflex can be driven at rates up to 200 Hz (Kiang et al., 1963; Jacobson et al., 1964), resulting in steady-state muscle potentials that, like the FFR, phase-lock to periodic signals (Kiang et al., 1963). Problematically, these rates closely coincide with the low-F0 (~100 Hz) pitched stimuli used in most auditory FFR studies (Coffey et al., 2016). This raises the possibility that FFRs to low-frequency sounds, including the voice pitch and low harmonics of speech, might be partially driven by muscular rather than auditory neurophonic structures. This is particularly relevant in the context of typical FFR recording approaches, which place electrodes behind the ear where the PAM reflex is optimally recorded (O'beirne and Patuzzi, 1999). Indeed, in our informal survey of FFR studies published over the past 50 years (N = 314 studies), nearly half (42% = 131 papers; see Supplementary material) used a mastoid reference electrode montage that promotes extraneous pickup of PAM muscle artifact (Figure 1A). The problem thus appears widespread in the literature. More critically, identifying undocumented muscular confound in the FFR would be particularly germane to interpreting plasticity studies and the unchallenged assumption that amplified FFRs (e.g., as in musicians, bilinguals) necessarily reflect increased fidelity of auditory neural coding due to listening experience (cf. Musacchia et al., 2007; Wong et al., 2007; Kraus et al., 2009; Parbery-Clark et al., 2009; Bidelman et al., 2011; Krizman et al., 2012; Coffey et al., 2017; Mankel and Bidelman, 2018; Zhao and Kuhl, 2018).
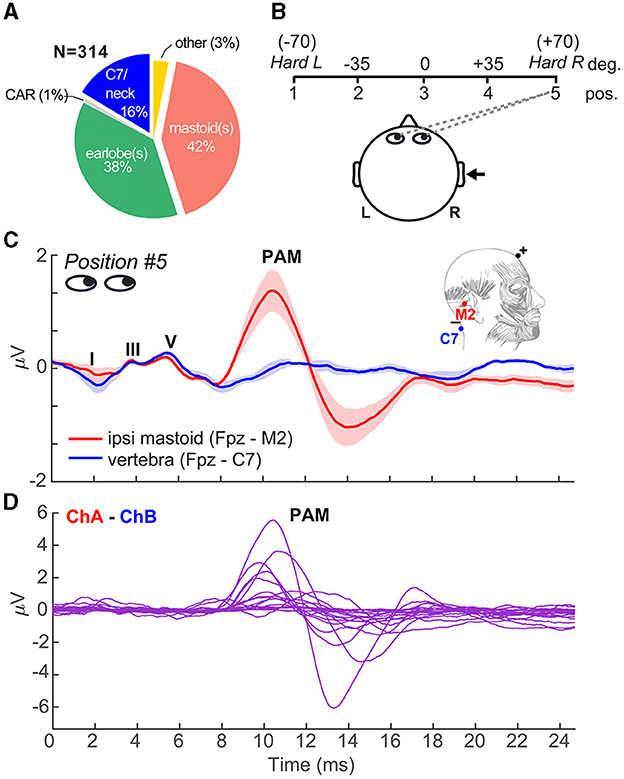
Figure 1. Eye gaze toward the ear introduces PAM artifact in ABRs. (A) In our literature review of N = 314 FFR studies, nearly half (42% = 131 papers) used a mastoid reference electrode montage that can promote inadvertent pickup of muscle artifacts (see Supplementary material). (B) Schematic of the visual gaze paradigm to direct listeners' eyes during ABR/FFR recording. Listeners were positioned 1 m from the booth wall and were cued to direct their eye gaze to one of five positions spanning left/right of head center relative to the ipsilateral ear of stimulus presentation (right ear). (C) Grand average ABR waveforms recorded with a vertical ipsilateral montage between a non-inverting electrode at the high forehead (~Fpz) referenced to an inverting electrode placed on the (i) ipsilateral mastoid (M2) or (ii) 7th cervical vertebra (C7). Eye gaze was directed to position 5 in both cases. Strong PAM is recorded for the channel with electrode over the ipsilateral mastoid. Moving the reference to C7 (i.e., distal to the PAM muscle) eradicates pickup of the artifact. (D) Individual data, with PAM responses isolated via channel subtraction of the traces in panel C. PAM strength ranges from 0.5 to 10 μV (peak-to-peak) across individuals. Anatomy adapted from Feneis (1994). Shading = ± 1 s.e.m.
Among the more widely reported neuroplastic effects captured in the FFR are the putative enhancements reported in musicians (Schneider et al., 2002; Musacchia et al., 2007; Wong et al., 2007; Kraus and Chandrasekaran, 2010). Speech FFRs are stronger and shorter in latency in musicians than their non-musician peers (Kraus et al., 2009; Parbery-Clark et al., 2009), providing a neural account of their enhanced speech perception observed behaviorally. Such enhancements are typically interpreted as reflecting stronger neural representations for speech shaped by experience-dependent plasticity from the enriched sonic environment afforded by musical engagement (Wong et al., 2007; Kraus and Chandrasekaran, 2010; Herholz and Zatorre, 2012). However, recent studies have challenged this notion by demonstrating even non-musicians with superior music-listening abilities (i.e., “musical sleepers”) have “enhanced” FFRs that mirror those of highly trained musicians (Mankel and Bidelman, 2018). Such findings reveal innate differences in auditory brain function (and possibly other unmeasured factors) can easily masquerade as plasticity in studies on the brain benefits of music (Musacchia et al., 2007; Wong et al., 2007; Tierney et al., 2015). The present study is not intended to refute the possible connections between musicianship and FFR enhancements observed in both cross-sectional and longitudinal studies (Musacchia et al., 2007; Wong et al., 2007; Tierney et al., 2015; Nan et al., 2018). Rather, we aimed to identify whether PAM muscle activity naturally induced by sound stimulation might not only be larger in musicians but partially mediate the auditory processing enhancements reported in studies on music-related plasticity.
To this end, we evaluated whether unmeasured muscle artifacts might explain substantial inter-subject variability in the FFR and account for at least some of the neural enhancements in speech processing frequently reported in highly skilled listeners (e.g., musicians). A major source of variation in the strength of PAM elicitation stems from muscle tension (Kiang et al., 1963; Bickford et al., 1964) and uncontrolled eye movements (Patuzzi and O'beirne, 1999). Gaze directed toward the ear of sound presentation potentiates PAM contraction and thus pickup of the muscle artifact in EEG (Wilson, 1908; O'beirne and Patuzzi, 1999; Patuzzi and O'beirne, 1999). By experimentally manipulating PAM with a gaze paradigm, we demonstrate that repeated stimulation of the muscle produces “following-like” potentials in the EEG via simple linear superposition of overlapping PAM wavelets (cf. Bidelman, 2015). Moreover, this muscle noise easily masquerades as the auditory FFR and spuriously amplifies the response by 3-4x, partially accounting for FFR enhancements observed in highly skilled musicians. As a solution for recording artifact-free FFRs, we further show that PAM-FFR contamination is easily circumvented by using high-frequency stimuli and relocating electrodes to a non-cephalic (neck) site.
Materials and methods
Participants
The sample included N = 20 young adults (age [μ ± σ]: 24.9 ± 2.8 years; four male, 16 female). This sample size was determined a priori to match comparable studies on auditory plasticity and the FFR (Parbery-Clark et al., 2009; Bidelman et al., 2014; Coffey et al., 2016). All had normal hearing (i.e., pure-tone air-conduction thresholds ≤ 25 dB HL; 250–8,000 Hz), similar levels of education (19.1 ± 2.3 years), and reported no previous history of neuropsychiatric illness. All but one individual were right-handed (77 ± 45% laterality; Oldfield, 1971). The sample included a range of formal musical training (μ ± σ: 6.5 ± 7.3 years; range 0–23 years) to assess whether putative enhancements in the FFR reported in experienced listeners (Musacchia et al., 2007; Wong et al., 2007; Skoe and Kraus, 2012; Mankel and Bidelman, 2018) might instead result from undocumented PAM muscle artifact rather than auditory neuroplasticity per se. Music training was treated as a continuous rather than binary variable since there is disagreement on what constitutes the definition of a “musician” (Zhang et al., 2020), and we used self-report (as in previous FFR studies) rather than a formal test of music listening skills (cf. Mankel and Bidelman, 2018). Each participant gave written informed consent in compliance with a protocol approved by the Indiana University IRB.
Stimuli
Auditory brainstem responses (ABRs) were recorded to 100 μs clicks. Frequency-following responses (FFRs) were elicited by pitch stimuli (periodic click trains) with fundamental frequencies (F0s) of either 100 or 200 Hz (Bidelman, 2015). Pulse trains were constructed using a periodic series of impulses , where d is the duration of the desired pulse train (here, 100 ms), and T is the period between successive impulses (i.e., 1/F0). Each pulse of the train was constructed using identical clicks (bandwidth, pulse width, amplitude) to those described for eliciting the ABR. This allowed us to directly test the assumption that a large amplitude periodic FFR response is a linear superposition of repeated transient PAM waves (cf. Bidelman, 2015).
EEG recordings
Participants sat in an electro-acoustically shielded booth in a recliner chair positioned 1 m from the wall. Numbered signs (labeled 1–5) were positioned in the front hemifield demarcating angles of hard left (roughly −70), −35, 0, +35, and hard right (+70) degrees (left to right) relative to the head center (see Figure 1B).2 For each condition, we directed the participants' eye gaze to one of the five angles by cueing the respective number. Compliance was monitored by the experimenter via a window into the chamber. FFRs were recorded with eye gaze directed at each of the five azimuths.
FFRs were recorded using a two-channel vertical montage with Ag/AgCl disc electrodes placed on the mid-hairline (i.e., midway between Fpz and Fz) referenced to (i) right (ipsi) mastoid (M2) and (ii) 7th cervical vertebra (mid-forehead = ground). Due to a technical error, data from the second channel were not recorded in N = 2 listeners and were treated as missing values. Impedances were ≤ 5 kΩ. EEGs were digitized at 20 kHz using a SmartEP EEG system (Intelligent Hearing Systems; Miami, FL) with an online passband of 50–3,000 Hz (+ 60 Hz notch filter) and 100 K amplifier gain. Evoked responses were elicited from each participant in response to right ear presentation at 80 dB SPL through electromagnetically shielded insert earphones (Ultra-shielded ER3A inserts, 300 Ω; IHS, Miami, FL) that eradicated electromagnetic stimulus artifact from contaminating biological responses (Price and Bidelman, 2021). Stimuli were presented with fixed, rarefaction polarity at a repetition rate of 9.09/s. The presentation order was randomized both within and across participants. Continuous EEGs were epoched (ABR: 0–24.7 ms; FFR: 0–127 ms), and the ensemble averaged across trials to derive evoked responses per condition. Sweeps > ±50 μV were automatically rejected during online averaging. A total of 2,000 artifact-free sweeps were collected per stimulus and eye gaze position.
Model simulating FFRs from PAM artifact
To evaluate the degree to which FFRs are explained by the PAM artifact, we compared the empirical FFR recordings with derived FFRs simulated via simple convolution (e.g., Goldstein and Kiang, 1958; Janssen et al., 1991; Bidelman, 2015; Carter and Bidelman, 2023). The model presumes the sustained following response is generated by a series of overlapping onset responses such that the FFR is an iterated ABR/PAM (see Figure 1 of Bidelman, 2015).3 We simulated FFRs by convolving each listener's PAM-contaminated ABR recorded at position #5 (see Figure 1) with a periodic click train of 100 Hz spacing (i.e., the FFR stimulus F0). This process generated a new ABR/PAM signature at each stimulus pulse, which, when strung across time, yielded a periodic complex waveform closely mirroring the actual FFR (also recorded at eye position #5, where the PAM artifact was strongest). We then assessed the correspondence between model-predicted and true FFR recordings via cross-correlation (20 ms lag search window; Galbraith et al., 2000; Bidelman, 2015).
Statistical analysis
We analyzed the data using a 2x2 mixed-model ANOVA in R (R Core-Team, 2020) and the lme4 package (Bates et al., 2015). Fixed effects were eye gaze position (five levels) and channel montage (two levels). Subjects served as a random effect. We used Satterthwaite's method to compute degrees of freedom. The data were SQRT-transformed to improve normality and homogeneity of variance assumptions necessary for parametric analyses. Effect sizes were reported as .
We also conducted regression analyses to assess whether a linear combination of listeners' neuro-behavioral measures (i.e., years of music training, PAM amplitudes) predicted the strength of their FFR [e.g., FFRrms~ music*PAM]. We then used leverage plots (Sall, 1990) and partial correlations to assess the relative strength of music vs. artifact regressors in driving putative musicianship-FFR relations (e.g., Musacchia et al., 2007; Wong et al., 2007; Skoe and Kraus, 2012; Mankel and Bidelman, 2018). Mediation analysis was used to evaluate relationships between neural and behavioral measures. We used an efficient, bootstrapping implementation of the Sobel statistic (Sobel, 1982; Preacher and Hayes, 2004; N = 1,000 resamples) to determine whether the PAM artifact fully or partially mediates the strength of listeners' FFR. The Sobel test contrasts the strength of regressions between a pairwise vs. triplet (mediation) model (i.e., X→Y vs. X→M→Y). Mediator M is said to mediate the relation between the X→Y if (i) X first predicts Y on its own, (ii) X predicts M, and (iii) the functional relation between X→Y is rendered insignificant after controlling for M (Preacher and Hayes, 2004).
Results
Postauricular muscle (PAM) artifact is present but variable across listeners
We first confirmed that PAM could be successfully elicited in individual listeners and experimentally manipulated by directing their eye gaze toward their stimulated ear (Figure 1B). Figure 1C shows the grand average click-ABRs recorded using montages with ipsilateral mastoid vs. midline (C7) electrode reference. In both traces, the eye gaze was directed toward the right (ipsilateral) ear, which was expected to elicit maximal PAM contraction (O'beirne and Patuzzi, 1999; Patuzzi and O'beirne, 1999). Positive deflections in the first 6 ms form the canonical waves of the ABR (I = 1.5 ms, III = 3.5 ms, V = 5.5 ms) that reflect serial activation of the auditory nerve and major nuclei along the ascending auditory pathway. Following the ABR, the myogenic PAM artifact was evident between 12.5 and 15 ms and was especially prominent in mastoid-referenced recordings (i.e., Fpz – M2). The PAM artifact was not evident at C7, where the reference electrode was distanced from the muscle. Consequently, the PAM artifact was easily isolated from the neurogenic ABR by simple waveform subtraction of mastoid vs. neck recordings (Figure 1D). Although the PAM artifact was present in all listeners to some degree, the response was highly variable, ranging from 0.18 to 9.7 μV (peak-to-peak amplitude; μ±σ: 2.38 ± 2.36 μV) across individuals. In other words, some listeners showed weak and some very strong PAM at M2. These data are consistent with the well-known intersubject variability in the response (O'beirne and Patuzzi, 1999; Patuzzi and O'beirne, 1999).
PAM contraction confounds the auditory FFR but declines with stimulus frequency and electrode montage
Having established that the PAM artifact was easily recordable and could be manipulated experimentally via eye gaze, we then tested whether FFR strength might also systematically vary with eye gaze direction and thus expose a myogenic source of the neural response. Confirming our intuition, FFRs to pitch stimuli varied parametrically in amplitude when listeners were cued to direct their gaze across a ±70° range (Figure 2A). Hard rotation of the eyes to either the left or right ear enhanced FFRs by 3-4x, particularly between 100 and 200 Hz where the PAM resides within the EEG spectrum (O'beirne and Patuzzi, 1999). FFR amplitude depended strongly on eye gaze and recording location [position x channel: F4, 152.16= 3.72, p= 0.0064; 0.09]. Comparisons between the C7 and mastoid channel showed a ~180° phase shift, which is common for FFRs recorded from the brainstem (highlighted in the C7 reference electrode) vs. auditory nerve (highlighted in the M2 reference electrode; see Figure 10 in Bidelman, 2018b). For the mastoid channel, FFR strength decreased with a gaze directed toward the midline (0°) and increased with a gaze toward either ear (quadratic contrast: t152 = 6.82, p < 0.0001). A nearly identical pattern was observed for the 200 Hz stimulus (data not shown; position x channel: F1, 50.4 = 8.52, p = 0.0052; 0.14). Moreover, a direct comparison of frequency effects revealed stronger FFRs in the 100 vs. 200 Hz condition regardless of the channel [main effect of frequency: F1, 117.99 = 4.66, p = 0.033; 0.04], indicating PAM-related changes in FFR declined with increasing stimulus frequency. Myogenic-induced enhancements were not apparent in electrodes positioned far from the mastoid, and FFRs were invariant to eye gaze when recorded from the Fpz-C7 channel (linear and quadratic contrasts: p > 0.17; Figure 2B).
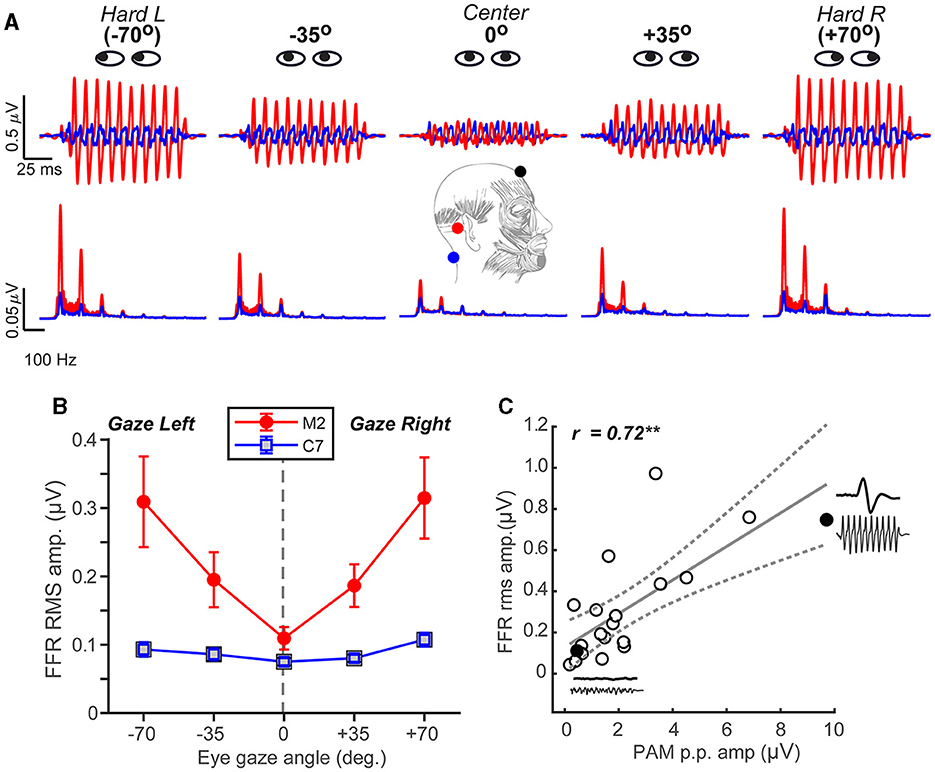
Figure 2. FFR strength systematically scales with the degree of PAM activation. (A) FFR time waveforms (top) and spectra (bottom) as a function of eye gaze spanning from the left to the right ear. Note the strong fundamental and integer-related harmonic frequencies of the stimulus F0 = 100 Hz (200 Hz condition not shown). (B) FFR amplitude systematically increases when the gaze is directed away from the midline (in either ipsi or contra direction) for mastoid-referenced recordings. FFR amplitude is invariant to eye gaze in recordings referenced to C7, suggesting a midline montage (i.e., Fpz- C7) eradicates the PAM artifact. (C) Correlation between PAM peak-to-peak (Figure 1A) and FFR rms amplitudes recorded with hard right eye gaze (position #5). Solid points reflect two representative subjects who had large and small PAM reflexes (their raw waveforms are shown as insets). Dotted lines = 95% CI; errorbars = ± 1 s.e.m.; **p < 0.01.
We also found that the PAM peak-to-peak amplitude was highly correlated with FFR root-mean-squared (rms) amplitude [Pearson's-r = 0.72, p = 0.0005] (Figure 2C). This suggests that listeners' FFR directly scales with the size of their muscle artifact. Collectively, these findings reveal that FFR strength depends critically on eye gaze position and that PAM activity overlays true neural responses, potentially masquerading as the neurophonic FFR commonly described as having brainstem origin (Chandrasekaran and Kraus, 2010; Bidelman, 2018b; Coffey et al., 2019).
Sustained FFRs are explained by serial PAM contractions: model simulations
Single-unit motor neurons underlying the PAM reflex can be driven at rates up to 100–200 Hz (Kiang et al., 1963; Jacobson et al., 1964). This raises the possibility that the FFR, and what appears to be a sustained brain potential, might at least partially reflect a series of overlapping transient PAM artifacts evoked by the individual pitch pulses of low-frequency, periodic sounds. To test this possibility, we simulated FFRs via simple convolution of listeners' isolated PAM response (i.e., Figure 1) with a 100 Hz impulse train. This train had identical periodicity to the periodic click trains used in our FFR experiment. This model regenerated the PAM at each pitch period of the F0, such that responses temporally overlapped and produced a quasi-steady state auditory response (Galambos et al., 1981). Repeating the PAM wavelet across time (Figure 3A) yielded a sustained waveform strikingly similar to the empirical FFR recordings (Figure 3B). Actual and PAM-derived FFRs were highly correlated (rxcorr = 0.71 ± 0.21; inset), suggesting upwards of 50.4% of the variance (R2) in the FFR could be explained by a myogenic source.
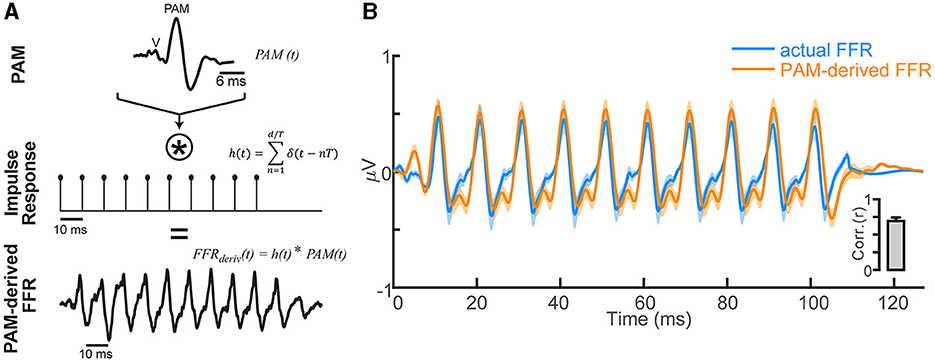
Figure 3. FFR waveforms can be explained as a phase-locked PAM artifact. (A) Convolution model for generating the FFR based on repetition of the transient PAM response (a muscle artifact; for details, see Bidelman, 2015). FFRs generated by a periodic click train with period T are conceived as a convolution of the PAM artifact [PAM (t), top row] with the individual pitch periods of the tone, modeled as a periodic pulse train [h(t), middle row]. The resulting derived FFR [FFRderiv (t), bottom row] is generated by a series of overlapping transient PAMs at the periodicity of the impulse train T = 10 ms (i.e., F0 = 100 Hz). (B) Comparison of empirical FFR recordings (F0 = 100 Hz click train) and simulated FFRs, derived via the convolution of PAM attract with the stimulus F0 periodicity. FFR traces reflect responses recorded with eye gaze directed at position #5 (see Figure 1) and contain significant PAM. A series of temporally overlapping PAM wavelets repeated at the F0 of a tone accounts for ~50% of the variance in actual FFRs (inset). Shading/errorbars = ±1 s.e.m.
PAM artifact partially mediates music-related plasticity in FFRs
Having established that FFRs are partly confounded by muscular influences, we next investigated whether putative enhancements reported in the literature (e.g., due to musical training, bilingualism; Krishnan et al., 2005; Musacchia et al., 2007; Wong et al., 2007; Krizman et al., 2012; Skoe and Kraus, 2012; Mankel and Bidelman, 2018) might be explained not in terms of auditory plasticity but rather, differences in tonic PAM contraction among listeners. To this end, we conducted multiple regression analyses to assess whether a linear combination of listeners' neuro-behavioral measures (i.e., years of music training and PAM amplitudes) predicted the strength of their FFR [e.g., FFRrms~ music*PAM]. Figure 4 shows leverage plots (Sall, 1990) illustrating the relative effects of music training and PAM artifact amplitudes on FFR strength. Variance inflation factors (VIFs) were <2 for both predictor variables, indicating negligible multicollinearity in the data (Lüdecke, 2020). We found that music training strongly predicted FFR strength [r = 0.34, p = 0.00076] (Figure 4A), consistent with prior studies suggesting musicianship enhances the brain's early neural encoding of complex sounds (cf. Musacchia et al., 2007; Wong et al., 2007; Skoe and Kraus, 2012; Mankel and Bidelman, 2018). However, even after partialing out musical training, the strength of listeners' PAM remained strongly correlated with FFR amplitude [r = 0.49, p < 0.0001] (Figure 4B). Similarly, musicianship was independently correlated with enhanced FFR amplitudes after controlling PAM contributions [r = 0.21, p = 0.037]. Indeed, a regression model that included the interaction between music training and PAM amplitudes was a significant improvement in describing FFR variance over one containing music alone [F1, 92 = 29.01, P < 0.001]. Taken together, these data confirm that the strength of the FFR is driven by an interaction between neuroplasticity (i.e., related to listening experience) and artifactual origins.
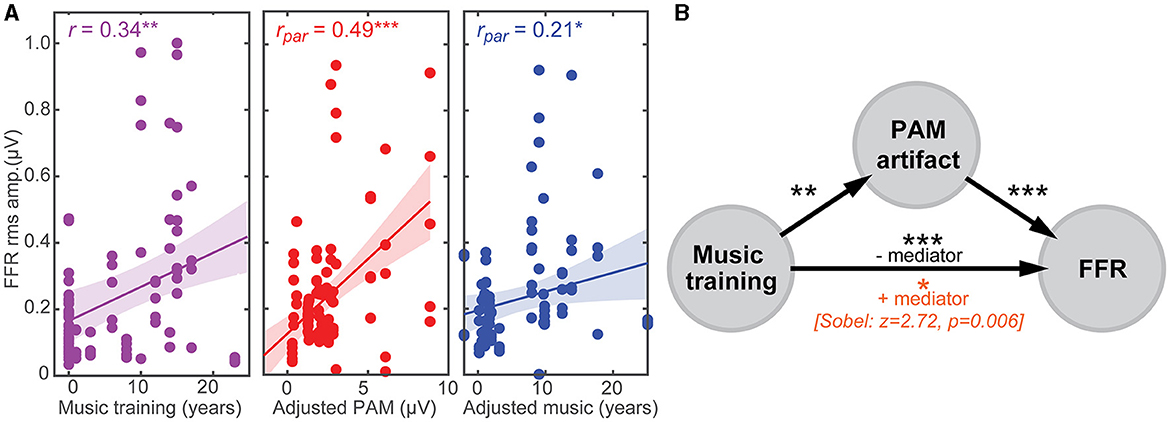
Figure 4. PAM artifact can masquerade as experience-dependent plasticity in the FFR. (A) (left) FFR is stronger in listeners with more musical training, suggestive of the experience-dependent plasticity reported in trained musicians (cf. Musacchia et al., 2007; Wong et al., 2007; Skoe and Kraus, 2012; Mankel and Bidelman, 2018). Both PAM (middle) and musical training (right) independently correlate with FFR strength after partialing out the other variable. Correlation data are aggregated from the five eye gaze positions. (B) Mediation analysis. The putative FFR-music relation is severely reduced after accounting for PAM [Sobel test: z = 2.72, p = 0.006], suggesting uncontrolled muscle artifact partially mediates the relation between musical training and FFR. Shading = 95% CI; *p < 0.05, **p < 0.01, ***p < 0.001.
To further evaluate the confounding link between PAM-related muscle noise and putative music-induced FFR plasticity (e.g., Musacchia et al., 2007; Wong et al., 2007; Skoe and Kraus, 2012; Mankel and Bidelman, 2018), we conducted mediation analyses (Sobel, 1982; Preacher and Hayes, 2004) to determine the degree to which PAM artifact mediated FFR strength (Figure 4B). Mediation contrasts the strength of regressions between a pairwise vs. triplet (mediation) model (i.e., X→Y vs. X→M→Y). Mediator M is said to mediate the relation between the X→Y if (i) X first predicts Y on its own, (ii) X predicts M, and (iii) the functional relation between X→Y is rendered insignificant after controlling for M (Preacher and Hayes, 2004). We found PAM satisfied the first two criteria for a mediating variable (Figure 4B). However, accounting for the strength of listeners' PAM artifact severely reduced—but did not render insignificant—relations between music training and FFR strength (Sobel test: z = 2.72, P = 0.006; Sobel, 1982). These results suggest that the PAM artifact is a partial (but not fully) mediating variable describing music-related enhancements observed in the FFR.
FFR timing is independent of PAM
The analyses thus far revealed links between the amplitude properties of the FFR and PAM reflex. However, plasticity in the FFR has also been described in the latency of the response to speech and musical sounds, with faster neural timing following long-term musicianship (Parbery-Clark et al., 2012; Bidelman et al., 2014; Mankel and Bidelman, 2018) and short-term auditory training (Anderson et al., 2013). To explore the possibility that variation in FFR timing might also be due to undocumented PAM artifact, we examined correlations between the onset latency of the FFR [measure in the 6–12 ms search window (Mankel and Bidelman, 2018)] and PAM artifact [8–18 ms; see Figure 1D]. However, neither latency [r = −0.10, p = 0.69] nor amplitude [r = 0.14, p = 0.57] of the PAM predicted FFR timing to the 100 Hz stimuli. Similar null correspondence was observed at 200 Hz [all ps > 0.09]. Thus, in stark contrast to FFR amplitude measures, fine timing precision of the FFR appears less susceptible to artifactual influence.
Discussion
Our findings expose a strong influence of myogenic activity on the auditory FFR. We demonstrate eye gaze systematically alters the apparent strength of FFRs with tandem PAM contraction. Our findings imply that not accounting for eye gaze/PAM can confound FFR strength measurements. More critically, we show this muscular noise can easily masquerade as neural enhancements, accounting for the large variability in the response previously ascribed to auditory-sensory plasticity. Importantly, these data do not negate the possibility that certain human experiences (e.g., music training, language expertise, and learning) can confer experience-dependent plasticity that manifests in spectrotemporal changes in the FFR (Krishnan et al., 2005; Kraus et al., 2014; Mankel and Bidelman, 2018). Rather, we argue that undue muscle confounds external to the brain may play a larger role in generating the FFR and its use as a biomarker of plasticity than conventionally thought.
While typically considered an artifactual nuisance to auditory EEG and evoked potential recordings, the PAM and related myogenic potentials do find several important neuro-otological applications, including hearing threshold (Yoshie and Okudaira, 1969) and vestibular assessment (Rosengren et al., 2019). Yet, in the context of FFRs, whose purpose is to evaluate central auditory neural processing and the brain's sensory representation of complex sounds, we show concomitant PAM muscle activation easily confounds these neurogenic responses, spuriously amplifying the FFR by 3–4-fold. Our findings are reminiscent of other microflexes shown to confound oscillatory EEG responses (e.g., microsaddes Yuval-Greenberg et al., 2008) and suggest uncontrolled eye movements can systematically alter sustained auditory FFR potentials via PAM muscle engagement.
We found that the PAM-related artifact was largest for hard rotation of the eyes toward or away from the ipsilateral ear of sound presentation but parametrically scaled in direct portion with listeners' lateral eye gaze. While it is important to note that PAM was evoked up to a rather extreme eye gaze rotation (representing a “worst case scenario”), we also find the artifact scales in direct proportion away from the midline and persists at gaze angles typical of normal eye movements. This point is particularly salient in light of speech-FFR studies that use passive listening paradigms where listeners are allowed to watch muted subtitled movies (Wong et al., 2007; Coffey et al., 2016; Mankel and Bidelman, 2018; Price and Bidelman, 2021). Videos are thought to provide added control during electrophysiological procedures, inducing a calm yet wakeful state that yields cleaner electrophysiological recordings (Wong et al., 2007; Coffey et al., 2016). However, when viewing a monitor at a typical seated distance, subtended gaze angles can exceed ~30–35° (Feng and Spence, 2008). Our data clearly show the FFR is nearly double its normal size at these typical viewing angles (Figure 2B). Consequently, while seemingly an innocuous control task, undue eye movements during video watching could easily confound the FFR by artificially inflating its amplitude. Along these lines, there is evidence that musicians show reduced fixation dwell times and more saccades during viewing tasks (Perra et al., 2022). Such increased eye movement could explain the larger PAM activation (and artifactual FFR) in musically trained individuals (Figure 4). Similar augments could extend to bilinguals, who also show increased visual search (Ratiu et al., 2017) and more robust speech FFRs (Krishnan et al., 2005; Krizman et al., 2012). However, musical performers can often show symptoms of focal dystonia— “occupational cramps” caused by the repetitive nature of playing a musical instrument (Konczak and Abbruzzese, 2013). Thus, an even more parsimonious explanation of our data might be that the increased PAM/FFR observed in musicians is due to increased muscle tension of the neck (Bickford et al., 1964; Sohmer et al., 1977).
We acknowledge that our gaze-induced manipulation of PAM could represent an overestimation of its activation. Unlike the sustained eye gaze we used to induce PAM, normal movie viewing would cause more random deviation in gaze across trials. This would tend to reduce PAM that survives time-locked averaging, though it would not preclude the continued pickup of the artifact due to tonic muscle tension (Bickford et al., 1964). Nevertheless, PAM can be elicited down to hearing threshold (Purdy et al., 2005). Moreover, the strong relation between PAM and FFR amplitudes we find would remain unaffected since changes in scale affect neither the magnitude nor sign of a correlation. Therefore, the fact that 50%, let alone any portion of the variance in the FFR, is explained by a myogenic artifact is a cause for concern for FFR recordings.
While there is overwhelming evidence in humans and animals to suggest PAM artifact is generated from the bipolar activation of muscle fibers behind the auricle of the ear (Kiang et al., 1963; O'beirne and Patuzzi, 1999; Patuzzi and O'beirne, 1999), it is conceivable that other non-auditory mechanisms contribute to FFR contamination. For example, it is well-known that FFRs require delicate recording strategies to prevent inadvertent pickup of a stimulus headphone artifact that can easily swamp the biological response (Price and Bidelman, 2021). Electromagnetic shielding (used here) prevents this possibility (Price and Bidelman, 2021) and can be further ruled out in the present data by the fact that FFRs showed gaze- and electrode-dependent changes that would not occur if waveforms were mere stimulus bleed (Figure 2B). However, bogus FFR amplification could also result from other biological sources. Indeed, bone-conducted FFRs have been recorded in profoundly deaf listeners, suggesting non-auditory pathways of response generation (Ribarić et al., 1984). These might include direct or indirect contributions from the vestibular system [e.g., evoked myogenic potentials (Ribarić et al., 1984; Prevec and Ribarić-Jankes, 1996; Lawlor et al., 2022)] and/or somatosensory contributions near the electrode-skin interface that can persist up to rates of 287 (Lawlor et al., 2022) and 130 Hz (Prevec and Ribarić-Jankes, 1996), respectively. Regardless of the underlying mechanism, our data clearly demonstrate physiological noise outside the auditory system easily conflates neurogenic FFR signals.
Several studies have shown enhanced voice pitch (F0) and timbre (harmonic) encoding in musically trained listeners (Musacchia et al., 2007; Wong et al., 2007; Kraus et al., 2009; Parbery-Clark et al., 2009; Kraus and Chandrasekaran, 2010; Mankel and Bidelman, 2018), though not always consistently (e.g., see Strait et al., 2012; Bidelman and Alain, 2015). Our data confirm a robust and independent relation between musical training and the strength of listeners' auditory FFR (Musacchia et al., 2007; Wong et al., 2007; Skoe and Kraus, 2012; Mankel and Bidelman, 2018; Figure 4A). However, in a departure from prior studies, we show such effects are easily confounded. PAM contraction was both larger in listeners with more extended music training and partially mediated enhancements in their FFR (Figure 4B). At first glance, these data cast doubt on whether speech-FFR enhancements associated with musical training (and perhaps other experiential factors) are solely experience-driven (cf. Munte et al., 2002; Wong et al., 2007; Herholz and Zatorre, 2012) or even entirely auditory-neurogenic in nature. Undue PAM influence might explain why some studies fail to observe FFR advantages in musicians (Strait et al., 2012; Bidelman and Alain, 2015; MacLean et al., 2024). Nevertheless, we show that music training remains a robust predictor of FFR enhancements even after controlling for PAM. This indicates musicianship independently bolsters the strength of the FFR and does so above and beyond any artifactual sources.
Surprisingly, we found musicians have stronger PAM reflexes than their non-musician peers. The basis of this finding is not clear. However, it has been suggested that intense auditory experiences might fortify tonic engagement of reflexive pathways that provide feedback control to peripheral hearing, including cochlear processing (Brashears et al., 2003; Bidelman et al., 2017b). Still, it remains to be seen whether other long-term listening experiences that also enhance FFR similarly elevate PAM and account for neuroplastic effects observed in bilingualism and tone-language expertise (Krishnan et al., 2005; Krizman et al., 2012; Zhao and Kuhl, 2018). Regardless, the intrusive nature of PAM mandates caution for interpreting even short-term changes in the FFR, as observed in rapid perceptual learning studies (Anderson et al., 2013; Reetzke et al., 2018). More successful learners could show increased arousal while acquiring novel auditory information, leading to increased eye movements that exaggerate the PAM artifact and, in turn, false amplification of FFRs. FFRs are modulated by listeners' arousal state at the single-trial level (Lai et al., 2022; Carter and Bidelman, 2023), and eye movements change during the learning process (Laamerad et al., 2020). Thus, while it awaits empirical confirmation, our data raise the possibility that rapid plasticity observed in FFRs during short-term auditory/speech learning tasks might be due to not improvements in the brain's sensory representation of sound per se (Anderson et al., 2013; Reetzke et al., 2018) but an unmeasured artifactual source.
Our data also converge with growing evidence that vision (specifically the direction of gaze/saccades) can influence peripheral auditory processing. For example, recent studies have shown that cued saccades are preceded by or co-occur with eardrum (Gruters et al., 2018) and pinna (Strauss et al., 2020) movements mediated by the stapedius and extra-auricular muscles, respectively. These processes may be modulated by auditory experiences like musicianship, as implied by our data. Auditory spatial attention is also better in musicians (Clayton et al., 2016; Bidelman and Yoo, 2020). Therefore, while the effects of attention on PAM are still debated (Hackley et al., 1987; Strauss et al., 2020), it is conceivable that musicians might have stronger eye-gaze-induced changes in PAM (and thus their FFR) due to improved attentional skills.
Fortunately, our data offer several direct solutions to easily thwart PAM-related confounds in the FFR and allow for untainted assessment of neuroplasticity. FFRs are frequently recorded using a differential electrode montage and a non-inverting reference electrode placed on the bony mastoid process of the ipsilateral ear of stimulation (e.g., Fpz-M1/M2; see Figure 1C, inset). We show this common practice is highly problematic, leading to extraneous pickup of the adjacent PAM muscle and overinflation of FFR that, by our estimates, has affected nearly half the published literature (see Figure 1A and Supplementary material). Repositioning electrodes to the earlobe (another common technique; Hall, 2006; Chandrasekaran and Kraus, 2010; Skoe and Kraus, 2010) attenuates but does not fully eliminate PAM pickup (O'beirne and Patuzzi, 1999).4 Instead, we find clean FFRs are easily recorded with electrodes placed on the upper neck (C7 vertebra; Picton et al., 2003), distal to the pinna and PAM muscle fibers. A midline Fpz-C7 montage has the further advantage that it (i) reduces pickup of more peripheral (cochlear) sources of the FFR and (ii) is optimally oriented with the vertical dipolar sources in the brainstem that dominate the generation of neurogenic FFR (Galbraith et al., 2000; Bidelman, 2015, 2018b). Currently, only 16% of studies use this C7/neck montage (Figure 1A).
Second, we show that FFR latency measures are largely independent of artifactual influences. While highly variable in amplitude, PAM latency is remarkably constant (Gibson, 1975). Consequently, timing characteristics of the FFR, separable from PAM, might provide a more veridical index of neuroplasticity (Parbery-Clark et al., 2012; Anderson et al., 2013; Bidelman et al., 2014; Mankel and Bidelman, 2018) than amplitude measures alone when tracking brain changes due to experience-dependent factors and novel sound learning (but see Mankel and Bidelman, 2018). Moreover, future work could explore whether other FFR metrics in the literature (e.g., pitch tracking accuracy, stimulus-to-response correlation) are similarly susceptible to PAM influences. However, both pitch tracking accuracy and stimulus-to-response correlations depend indirectly on the magnitude of the FFR signal (often via spectral/autocorrelation estimates of pitch strength). Therefore, we would suspect both metrics (and any that depend on FFR amplitude/SNR) would be susceptible to PAM inflation.
Third, controlling participants' eye gaze straight forward (e.g., with a fixation cross) or instructing them to close their eyes could help minimize PAM contributions to the FFR. Indeed, when eye gaze was fixated at the center (and thus reduced extraneous eye movement), we found little differences in FFR magnitude between M2 and C7 recordings, which do and do not contain PAM, respectively. Some FFR studies allow participants to close their eyes and even encourage sleep—in fact, this was a once commonplace procedure in our own lab (e.g., Bidelman et al., 2011). However, we find changes in eye gaze within only a small viewing angle still affect FFR (see Figure 2), and eye closure does not prevent ocular movement altogether, as the eyes are still free to move under the eyelid. Although we did not compare an eyes-closed condition, we might expect present but attenuated PAM during eyes-closed FFR since saccades are generally weaker under closed eyelids (Shaikh et al., 2010). Concerns further persist because while PAM is unaffected by attention (Hackley et al., 1987; but see Strauss et al., 2020) and is attenuated during sleep, it is still recordable given the roving eye movements that often accompany different stages of sleep (Picton et al., 2003).
Lastly, we found that PAM-related changes in FFR declined with increasing stimulus frequency. This suggests that in addition to isolating brainstem-centric sources of the response without cortical contributions (Bidelman, 2018b; Gorina-Careta et al., 2021), the use of auditory stimuli with higher F0s (e.g., >200 Hz) might be further advantageous in protecting against PAM confounds in the FFR. Moreover, it is unlikely that other common FFR stimulus practices, including the use of alternating polarity (Skoe and Kraus, 2010) and naturalistic pitched stimuli (Krishnan et al., 2010, 2011; Saiz-Alía et al., 2019; Kulasingham et al., 2020), would prevent PAM influences on FFR. While alternating polarity is effective in minimizing other extraneous influences on FFR (e.g., cochlear microphonic: Chimento and Schreiner, 1990; stimulus artifact: Campbell et al., 2012), PAM does not reverse in polarity with stimulus counter-phasing and is still recordable using alternating polarity (Doubell et al., 2018). It also seems unlikely that naturalistic stimuli would offer benefit since PAM is generated by both wideband and pure-tone stimuli (O'beirne and Patuzzi, 1999), though more optimally by the former. In addition to the electrode configuration and high-frequency stimulus solutions offered here, reducing stimulus levels may also improve the PAM-FFR artifact. However, the lower presentation would offer diminishing returns given the higher sensation levels needed to generate the FFR (Davis and Hirsh, 1976; Yamada et al., 1978; Bidelman and Powers, 2018).
Several recent EEG studies have used multichannel recordings and source localization to assess the generators and scalp topography of the FFR (e.g., Bidelman, 2015; Bidelman et al., 2018; Zhang and Gong, 2019; Price and Bidelman, 2021). An important but unrecognized benefit of such multichannel and source-resolved FFRs (e.g., Price and Bidelman, 2021) is that they can be computed using a common-average reference (CAR) of the whole scalp rather than a single electrode (e.g., M2)—as done here for a single-channel FFR. As such, CAR-referenced electrode recordings and source FFRs are nearly reference-free and do not suffer the bias of a single-referential montage. Any PAM, if it is generated, is not inadvertently broadcast into the non-inverting channel(s) since the theoretical reference is relative to zero potential in the center of the head rather than the mastoid location.
Conclusion
In conclusion, our data provide new evidence that myogenic sources can artificially inflate the sound-evoked FFR and might account for certain de novo enhancements in the response. Provocatively, they also show that formally trained musicians have additional gains in sensory brain processing above and beyond PAM-related confounds. Our results emphasize the critical need for future studies to safeguard against ocular and myogenic confounders before claiming changes in FFR track with improvements in auditory brain function due to experience-dependent factors or neurobehavioral interventions. Of practical implication, we recommend that to measure true neurogenic FFRs without undue electromyographic contamination, investigators should (i) adopt a midline, necked-referenced recording approach, (ii) use high-frequency F0 stimuli (>200 Hz) where PAM contributions are minimal, and (iii) focus primarily on response latency quantification to index disordered auditory processing and/or neuroplastic changes due to intervention.
Data availability statement
The original contributions presented in the study are included in the article/Supplementary material, further inquiries can be directed to the corresponding author.
Ethics statement
The studies involving humans were approved by Indiana University Institutional Review Board. The studies were conducted in accordance with the local legislation and institutional requirements. The participants provided their written informed consent to participate in this study.
Author contributions
GB: Formal analysis, Funding acquisition, Supervision, Writing – original draft, Writing – review & editing. AS: Data curation, Writing – original draft, Writing – review & editing. RR: Data curation, Writing – original draft, Writing – review & editing. JM: Data curation, Writing – original draft, Writing – review & editing. KB: Data curation, Writing – original draft, Writing – review & editing.
Funding
The author(s) declare that financial support was received for the research, authorship, and/or publication of this article. This work was supported by grants from the National Institute on Deafness and Other Communication Disorders (R01DC016267) awarded to GB.
Conflict of interest
The authors declare that the research was conducted in the absence of any commercial or financial relationships that could be construed as a potential conflict of interest.
The author(s) declared that they were an editorial board member of Frontiers, at the time of submission. This had no impact on the peer review process and the final decision.
Publisher's note
All claims expressed in this article are solely those of the authors and do not necessarily represent those of their affiliated organizations, or those of the publisher, the editors and the reviewers. Any product that may be evaluated in this article, or claim that may be made by its manufacturer, is not guaranteed or endorsed by the publisher.
Supplementary material
The Supplementary Material for this article can be found online at: https://www.frontiersin.org/articles/10.3389/fnins.2024.1422903/full#supplementary-material
Footnotes
1. ^Sohmer et al. (1977) describe two case studies from “normal subjects with an unusually large response from the post-auricular muscle (p. 656).” They observed, “the amplitude of the post-auricular responses to click stimuli and this frequency following of the post-auricular muscle were both susceptible to head position, that is to the degree of neck tension (p. 660)” and concluded the PAM contributes to the FFR. Unfortunately, no quantitative analysis was undertaken so these observations remain qualitative. Picton et al. (2003) also describe the possible influence of PAM on the auditory steady-state response (ASSR) noting “findings that the ASSR may occasionally increase during drowsiness may be explained by postauricular muscle responses recorded from a mastoid reference (p. 1,396).”
2. ^Hard left/right gaze locations (i.e., position #1 and #5) were marked in front of the listener at ~±70°. However, in practice, a 70° rotation is difficult to achieve as human eye movements rarely exceed 50–60° (Lee et al., 2019). Thus, while 70° was the target angle, listeners were instructed to pan their eye gaze left/right as far as possible in these conditions.
3. ^For full details of the model, see Bidelman (2015). That study showed that for high F0 stimuli, fine-spectral details and latency of the sustained FFR diverge from model responses generated by simple repetition of the transient ABR. However, for the low-frequency stimuli used here (i.e., ~100 Hz), the model provides a good first-order approximation of the FFR's major sinusoidal F0 component. The ABR input waveforms used in Bidelman (2015) also did not contain PAM artifact, leaving open the possibility that the FFR more closely corresponds with convolution when PAM is considered.
4. ^An earlobe reference is sometimes preferred in ABR testing since in enhanced wave I relative to a mastoid reference and reduces the chance of artifactual pickup when performing bone-conduction stimulation. As shown in by O'beirne and Patuzzi (1999) (their Figure 3), PAM artifact is ~50% weaker (and is inverted in polarity) when recorded at the pinna vs. mastoid. Thus, using an earlobe reference for FFR recording, as preferred by some authors (Skoe and Kraus, 2010), attenuates but does not fully abolish PAM.
References
Anderson, S., White-Schwoch, T., Parbery-Clark, A., and Kraus, N. (2013). Reversal of age-related neural timing delays with training. Proc. Natl. Acad. Sci. U. S. A. 110, 4357–4362. doi: 10.1073/pnas.1213555110
Bates, D., Mächler, M., Bolker, B., and Walker, S. (2015). Fitting linear mixed-effects models using lme4. J. Statist. Softw. 67, 1–48. doi: 10.18637/jss.v067.i01
Bérzin, F., and Fortinguerra, C. R. (1993). EMG study of the anterior, superior and posterior auricular muscles in man. Ann. Anat. 175, 195–197. doi: 10.1016/S0940-9602(11)80182-2
Bickford, R. G., Jacobson, J. L., and Cody, D. T. (1964). Nature of average evoked potentials to sound and other stimuli in man. Ann. N. Y. Acad. Sci. 112, 204–223. doi: 10.1111/j.1749-6632.1964.tb26749.x
Bidelman, G., and Powers, L. (2018). Response properties of the human frequency-following response (FFR) to speech and non-speech sounds: level dependence, adaptation and phase-locking limits. Int. J. Audiol. 57, 665–672. doi: 10.1080/14992027.2018.1470338
Bidelman, G. M. (2015). Multichannel recordings of the human brainstem frequency-following response: scalp topography, source generators, and distinctions from the transient ABR. Hear. Res. 323, 68–80. doi: 10.1016/j.heares.2015.01.011
Bidelman, G. M. (2018a). Sonification of scalp-recorded frequency-following responses (FFRs) offers improved response detection over conventional statistical metrics. J. Neurosci. Methods 293, 59–66. doi: 10.1016/j.jneumeth.2017.09.005
Bidelman, G. M. (2018b). Subcortical sources dominate the neuroelectric auditory frequency-following response to speech. NeuroImage 175, 56–69. doi: 10.1016/j.neuroimage.2018.03.060
Bidelman, G. M., and Alain, C. (2015). Musical training orchestrates coordinated neuroplasticity in auditory brainstem and cortex to counteract age-related declines in categorical vowel perception. J. Neurosci. 35, 1240–1249. doi: 10.1523/JNEUROSCI.3292-14.2015
Bidelman, G. M., Davis, M. K., and Pridgen, M. H. (2018). Brainstem-cortical functional connectivity for speech is differentially challenged by noise and reverberation. Hear. Res. 367, 149–160. doi: 10.1016/j.heares.2018.05.018
Bidelman, G. M., Gandour, J. T., and Krishnan, A. (2011). Cross-domain effects of music and language experience on the representation of pitch in the human auditory brainstem. J. Cogn. Neurosci. 23, 425–434. doi: 10.1162/jocn.2009.21362
Bidelman, G. M., Lowther, J. E., Tak, S. H., and Alain, C. (2017a). Mild cognitive impairment is characterized by deficient hierarchical speech coding between auditory brainstem and cortex. J. Neurosci. 37, 3610–3620. doi: 10.1523/JNEUROSCI.3700-16.2017
Bidelman, G. M., Schneider, A. D., Heitzmann, V. R., and Bhagat, S. P. (2017b). Musicianship enhances ipsilateral and contralateral efferent gain control to the cochlea. Hear. Res. 344, 275–283. doi: 10.1016/j.heares.2016.12.001
Bidelman, G. M., Weiss, M. W., Moreno, S., and Alain, C. (2014). Coordinated plasticity in brainstem and auditory cortex contributes to enhanced categorical speech perception in musicians. Eur. J. Neurosci. 40, 2662–2673. doi: 10.1111/ejn.12627
Bidelman, G. M., and Yoo, J. (2020). Musicians show improved speech segregation in competitive, multi-talker cocktail party scenarios. Front. Psychol. 11, 1–11. doi: 10.3389/fpsyg.2020.01927
Brashears, S. M., Morlet, T. G., Berlin, C. I., and Hood, L. J. (2003). Olivocochlear efferent suppression in classical musicians. J. Am. Acad. Audiol. 14, 314–324. doi: 10.1055/s-0040-1715747
Campbell, T., Kerlin, J. R., Bishop, C. W., and Miller, L. M. (2012). Methods to eliminate stimulus transduction artifact from insert earphones during electroencephalography. Ear Hear. 33, 144–150. doi: 10.1097/AUD.0b013e3182280353
Carter, J. A., and Bidelman, G. M. (2023). Perceptual warping exposes categorical representations for speech in human brainstem responses. NeuroImage 269:119899. doi: 10.1016/j.neuroimage.2023.119899
Chandrasekaran, B., Hornickel, J., Skoe, E., Nicol, T., and Kraus, N. (2009). Context-dependent encoding in the human auditory brainstem relates to hearing speech in noise: implications for developmental dyslexia. Neuron 64, 311–319. doi: 10.1016/j.neuron.2009.10.006
Chandrasekaran, B., and Kraus, N. (2010). The scalp-recorded brainstem response to speech: neural origins and plasticity. Psychophysiology 47, 236–246. doi: 10.1111/j.1469-8986.2009.00928.x
Chimento, T. C., and Schreiner, C. E. (1990). Selectively eliminating cochelar microphonic contamination from the frequency-following response. Electroencephal. Clin. Neurophysiol. 75, 88–96. doi: 10.1016/0013-4694(90)90156-E
Clayton, K. K., Swaminathan, J., Yazdanbakhsh, A., Zuk, J., Patel, A. D., and Kidd, G. Jr. (2016). Executive function, visual attention and the cocktail party problem in musicians and nonmusicians. PLoS ONE 11:e0157638. doi: 10.1371/journal.pone.0157638
Coffey, E. B., Herholz, S. C., Chepesiuk, A. M., Baillet, S., and Zatorre, R. J. (2016). Cortical contributions to the auditory frequency-following response revealed by MEG. Nat. Commun. 7:11070. doi: 10.1038/ncomms11070
Coffey, E. B. J., Chepesiuk, A. M. P., Herholz, S. C., Baillet, S., and Zatorre, R. J. (2017). Neural correlates of early sound encoding and their relationship to speech-in-noise perception. Front. Neurosci. 11:479. doi: 10.3389/fnins.2017.00479
Coffey, E. B. J., Nicol, T., White-Schwoch, T., Chandrasekaran, B., Krizman, J., Skoe, E., et al. (2019). Evolving perspectives on the sources of the frequency-following response. Nat. Commun. 10:5036. doi: 10.1038/s41467-019-13003-w
Davis, H., and Hirsh, S. K. (1976). The audiometic utility of brain stem responses to low-frequency sounds. Audiology 15, 181–195. doi: 10.3109/00206097609071775
Doubell, T. P., Alsetrawi, A., Bastawrous, D. a. S., Bastawrous, M. a. S., Daibes, A., Jadalla, A., et al. (2018). The effect of interaural timing on the posterior auricular muscle reflex in normal adult volunteers. PLoS ONE 13:e0194965. doi: 10.1371/journal.pone.0194965
Feng, J., and Spence, I. (2008). “Attending to large dynamic displays,” in CHI '08 Extended Abstracts on Human Factors in Computing Systems (Florence: Association for Computing Machinery), 2745–2750. doi: 10.1145/1358628.1358755
Galambos, R., Makeig, S., and Talmachoff, P. J. (1981). A 40-Hz auditory potential recorded from the human scalp. Proc. Natl. Acad. Sci. U. S. A. 78, 2643–2647. doi: 10.1073/pnas.78.4.2643
Galbraith, G., Threadgill, M., Hemsley, J., Salour, K., Songdej, N., Ton, J., et al. (2000). Putative measure of peripheral and brainstem frequency-following in humans. Neurosci. Lett. 292, 123–127. doi: 10.1016/S0304-3940(00)01436-1
Geisler, C. D., Frishkopf, L. S., and Rosenblith, W. A. (1958). Extracranial responses to acoustic clicks in man. Science 128, 1210–1211. doi: 10.1126/science.128.3333.1210
Gibson, W. P. R. (1975). The Crossed Acoustic Response, a Post-Aural Myogenic Response (doctor of medicine thesis). University of London, London, United Kingdom.
Goldstein, M. H., and Kiang, N. Y. S. (1958). Synchrony of neural activity in electric responses evoked by transient acoustic stimuli. J. Acoust. Soc. A. 30, 107–114. doi: 10.1121/1.1909497
Gorina-Careta, N., Kurkela, J. L. O., Hämäläinen, J., Astikainen, P., and Escera, C. (2021). Neural generators of the frequency-following response elicited to stimuli of low and high frequency: a magnetoencephalographic (MEG) study. NeuroImage 231:117866. doi: 10.1016/j.neuroimage.2021.117866
Gruters, K. G., Murphy, D. L. K., Jenson, C. D., Smith, D. W., Shera, C. A., and Groh, J. M. (2018). The eardrums move when the eyes move: a multisensory effect on the mechanics of hearing. Proc. Natl. Acad. Sci. U. S. A. 2018:1717948115. doi: 10.1073/pnas.1717948115
Hackley, S. A. (2015). Evidence for a vestigial pinna-orienting system in humans. Psychophysiology 52, 1263–1270. doi: 10.1111/psyp.12501
Hackley, S. A., Woldorff, M., and Hillyard, S. A. (1987). Combined use of microreflexes and event-related brain potentials as measures of auditory selective attention. Psychophysiology 24, 632–647. doi: 10.1111/j.1469-8986.1987.tb00343.x
Herholz, S. C., and Zatorre, R. J. (2012). Musical training as a framework for brain plasticity: behavior, function, and structure. Neuron 76, 486–502. doi: 10.1016/j.neuron.2012.10.011
Holmes, E., and Herrmann, B. (2017). Revisiting the contribution of auditory cortex to frequency-following responses. J. Neurosci. 37, 5218–5220. doi: 10.1523/JNEUROSCI.0794-17.2017
Hoormann, J., Falkenstein, M., Hohnsbein, J., and Blanke, L. (1992). The human frequency-following response (FFR): normal variability and relation to the click-evoked brainstem response. Hear. Res. 59, 179–188. doi: 10.1016/0378-5955(92)90114-3
Jacobson, J. L., Cody, D. T., Lambert, E. H., and Bickford, R. G. (1964). Physiological properties of the post-auricular responses (sonomotor) in man. Physiologist 7, 167–167.
Janssen, T., Steinhoff, H. J., and Böhnke, F. (1991). Zum entstehungsmechanismus der frequenzfolgepotentiale. Otorhinolaryngol. Nova 1, 16–24. doi: 10.1159/000312727
Kiang, N. Y., Crist, A. H., French, M. A., and Edwards, A. G. (1963). Postauricular electrical response to acoustic stimuli in humans. Massachusetts Inst. Technol. Quart. Progr. 68, 218–225.
Konczak, J., and Abbruzzese, G. (2013). Focal dystonia in musicians: linking motor symptoms to somatosensory dysfunction. Front. Hum. Neurosci. 7:297. doi: 10.3389/fnhum.2013.00297
Kraus, N., and Chandrasekaran, B. (2010). Music training for the development of auditory skills. Nat. Rev. Neurosci. 11, 599–605. doi: 10.1038/nrn2882
Kraus, N., Skoe, E., Parbery-Clark, A., and Ashley, R. (2009). Experience-induced malleability in neural encoding of pitch, timbre, and timing. Ann. N. Y. Acad. Sci1169, 543–557. doi: 10.1111/j.1749-6632.2009.04549.x
Kraus, N., Slater, J., Thompson, E. C., Hornickel, J., Strait, D. L., Nicol, T., et al. (2014). Music enrichment programs improve the neural encoding of speech in at-risk children. J. Neurosci. 34, 11913–11918. doi: 10.1523/JNEUROSCI.1881-14.2014
Krishnan, A., Gandour, J. T., Ananthakrishnan, S., Bidelman, G. M., and Smalt, C. J. (2011). Linguistic status of timbre influences pitch encoding in the brainstem. Neuroreport 22, 801–803. doi: 10.1097/WNR.0b013e32834b2996
Krishnan, A., Gandour, J. T., and Bidelman, G. M. (2010). The effects of tone language experience on pitch processing in the brainstem. J. Neurolinguist. 23, 81–95. doi: 10.1016/j.jneuroling.2009.09.001
Krishnan, A., Xu, Y., Gandour, J. T., and Cariani, P. (2005). Encoding of pitch in the human brainstem is sensitive to language experience. Brain Res. Cogn. Brain Res. 25, 161–168. doi: 10.1016/j.cogbrainres.2005.05.004
Krizman, J., Marian, V., Shook, A., Skoe, E., and Kraus, N. (2012). Subcortical encoding of sound is enhanced in bilinguals and relates to executive function advantages. Proc. Natl. Acad. Sci. U. S. A. 109, 7877–7881. doi: 10.1073/pnas.1201575109
Kulasingham, J. P., Brodbeck, C., Presacco, A., Kuchinsky, S. E., Anderson, S., and Simon, J. Z. (2020). High frequency cortical processing of continuous speech in younger and older listeners. NeuroImage 222:117291. doi: 10.1016/j.neuroimage.2020.117291
Laamerad, P., Guitton, D., and Pack, C. C. (2020). Eye movements shape visual learning. Proc. Natl. Acad. Sci. U. S. A. 117, 8203–8211. doi: 10.1073/pnas.1913851117
Lai, J., Price, C. N., and Bidelman, G. M. (2022). Brainstem speech encoding is dynamically shaped online by fluctuations in cortical α state. NeuroImage 263:119627. doi: 10.1016/j.neuroimage.2022.119627
Lawlor, K. J., Clinard, C. G., and Piker, E. G. (2022). Temporal modulation transfer functions of amplitude-modulated cervical vestibular-evoked myogenic potentials in young adults. Ear Hear. 43:1221. doi: 10.1097/AUD.0000000000001221
Lee, W. J., Kim, J. H., Shin, Y. U., Hwang, S., and Lim, H. W. (2019). Differences in eye movement range based on age and gaze direction. Eye 33, 1145–1151. doi: 10.1038/s41433-019-0376-4
López-Caballero, F., Martin-Trias, P., Ribas-Prats, T., Gorina-Careta, N., Bartrés-Faz, D., and Escera, C. (2020). Effects of cTBS on the frequency-following response and other auditory evoked potentials. Front. Hum. Neurosci. 14:250. doi: 10.3389/fnhum.2020.00250
Lüdecke, D. (2020). sjPlot: Data Visualization for Statistics in Social Science. R Package Version 2.8.6. Available online at: https://CRAN.R-project.org/package=sjPlot (accessed April 2024).
MacLean, J., Stirn, J., Sisson, A., and Bidelman, G. M. (2024). Short- and long-term neuroplasticity interact during the perceptual learning of concurrent speech. Cerebr. Cortex 34, 1–13. doi: 10.1093/cercor/bhad543
Mankel, K., and Bidelman, G. M. (2018). Inherent auditory skills rather than formal music training shape the neural encoding of speech. Proc. Natl. Acad. Sci. U. S. A. 115, 13129–13134. doi: 10.1073/pnas.1811793115
Munte, T. F., Altenmuller, E., and Jancke, L. (2002). The musician's brain as a model of neuroplasticity. Nat. Rev. Neurosci. 3, 473–478. doi: 10.1038/nrn843
Musacchia, G., Sams, M., Skoe, E., and Kraus, N. (2007). Musicians have enhanced subcortical auditory and audiovisual processing of speech and music. Proc. Natl. Acad. Sci. U. S. A. 104, 15894–15898. doi: 10.1073/pnas.0701498104
Nan, Y., Liu, L., Geiser, E., Shu, H., Gong, C. C., Dong, Q., et al. (2018). Piano training enhances the neural processing of pitch and improves speech perception in Mandarin-speaking children. Proc. Natl. Acad. Sci. U. S. A. 115, E6630–E6639. doi: 10.1073/pnas.1808412115
O'beirne, G. A., and Patuzzi, R. B. (1999). Basic properties of the sound-evoked post-auricular muscle response (PAMR). Hear. Res. 138, 115–132. doi: 10.1016/S0378-5955(99)00159-8
Oldfield, R. C. (1971). The assessment and analysis of handedness: the Edinburgh inventory. Neuropsychologia 9, 97–113. doi: 10.1016/0028-3932(71)90067-4
Parbery-Clark, A., Anderson, S., Hittner, E., and Kraus, N. (2012). Musical experience offsets age-related delays in neural timing. Neurobiol. Aging 33, e1481–e1484. doi: 10.1016/j.neurobiolaging.2011.12.015
Parbery-Clark, A., Skoe, E., and Kraus, N. (2009). Musical experience limits the degradative effects of background noise on the neural processing of sound. J. Neurosci. 29, 14100–14107. doi: 10.1523/JNEUROSCI.3256-09.2009
Patuzzi, R. B., and O'beirne, G. A. (1999). Effects of eye rotation on the sound-evoked post-auricular muscle response (PAMR). Hear. Res. 138, 133–146. doi: 10.1016/S0378-5955(99)00160-4
Perra, J., Latimier, A., Poulin-Charronnat, B., Baccino, T., and Drai-Zerbib, V. (2022). A meta-analysis on the effect of expertise on eye movements during music reading. J. Eye Mov. Res. 15:1. doi: 10.16910/jemr.15.4.1
Picton, T. W., Hillyard, S. A., Krausz, H. I., and Galambos, R. (1974). Human auditory evoked potentials. I. Evaluation of components. Electroencephal. Clin. Neurophysiol. 36, 179–190. doi: 10.1016/0013-4694(74)90155-2
Picton, T. W., John, M. S., Purcell, D. W., and Plourde, G. (2003). Human auditory steady-state responses: the effects of recording technique and state of arousal. Anesth. Analg. 97, 1396–1402. doi: 10.1213/01.ANE.0000082994.22466.DD
Preacher, K. J., and Hayes, A. F. (2004). SPSS and SAS procedures for estimating indirect effects in simple mediation models. Behav. Res. Methods Instr. Comput. 36, 717–731. doi: 10.3758/BF03206553
Prevec, T. S., and Ribarić-Jankes, K. (1996). Can somatosensory system generate frequency following response? Pflugers Arch. 431, R301–R302. doi: 10.1007/BF02346388
Price, C. N., and Bidelman, G. M. (2021). Attention reinforces human corticofugal system to aid speech perception in noise. NeuroImage 235:118014. doi: 10.1016/j.neuroimage.2021.118014
Purdy, S. C., Agung, K. B., Hartley, D., Patuzzi, R. B., and O'beirne, G. A. (2005). The post-auricular muscle response: an objective electrophysiological method for evaluating hearing sensitivity. Int. J. Audiol. 44, 625–630. doi: 10.1080/14992020500266639
R Core-Team (2020). R: A Language and Environment for Statistical Computing. Vienna: R Foundation for Statistical Computing. Available online at: https://www.R-project.org/
Ratiu, I., Hout, M. C., Walenchok, S. C., Azuma, T., and Goldinger, S. D. (2017). Comparing visual search and eye movements in bilinguals and monolinguals. Attent. Percept. Psychophys. 79, 1695–1725. doi: 10.3758/s13414-017-1328-3
Reetzke, R., Xie, Z., Llanos, F., and Chandrasekaran, B. (2018). Tracing the trajectory of sensory plasticity across different stages of speech learning in adulthood. Curr. Biol. 28, 1419–1427.e1414. doi: 10.1016/j.cub.2018.03.026
Ribarić, K., Prevec, T. S., and Kozina, V. (1984). Frequency-following response evoked by acoustic stimuli in normal and profoundly deaf subjects. Audiology 23, 388–400. doi: 10.3109/00206098409081532
Rosengren, S. M., Colebatch, J. G., Young, A. S., Govender, S., and Welgampola, M. S. (2019). Vestibular evoked myogenic potentials in practice: methods, pitfalls and clinical applications. Clin. Neurophysiol. Pract. 4, 47–68. doi: 10.1016/j.cnp.2019.01.005
Russo, N. M., Skoe, E., Trommer, B., Nicol, T., Zecker, S., Bradlow, A., et al. (2008). Deficient brainstem encoding of pitch in children with autism spectrum disorders. Clin. Neurophysiol. 119, 1720–1731. doi: 10.1016/j.clinph.2008.01.108
Saiz-Alía, M., Forte, A. E., and Reichenbach, T. (2019). Individual differences in the attentional modulation of the human auditory brainstem response to speech inform on speech-in-noise deficits. Sci. Rep. 9:14131. doi: 10.1038/s41598-019-50773-1
Sall, J. (1990). Leverage plots for general linear hypotheses. Am. Statist. 44, 308–315. doi: 10.1080/00031305.1990.10475750
Schneider, P., Scherg, M., Dosch, H. G., Specht, H. J., Gutschalk, A., and Rupp, A. (2002). Morphology of Heschl's gyrus reflects enhanced activation in the auditory cortex of musicians. Nat. Neurosci. 5, 688–694. doi: 10.1038/nn871
Shaikh, A. G., Wong, A. L., Optican, L. M., Miura, K., Solomon, D., and Zee, D. S. (2010). Sustained eye closure slows saccades. Vis. Res. 50, 1665–1675. doi: 10.1016/j.visres.2010.05.019
Skoe, E., and Kraus, N. (2010). Auditory brain stem response to complex sounds: a tutorial. Ear Hear. 31, 302–324. doi: 10.1097/AUD.0b013e3181cdb272
Skoe, E., and Kraus, N. (2012). A little goes a long way: how the adult brain is shaped by musical training in childhood. J. Neurosci. 32, 11507–11510. doi: 10.1523/JNEUROSCI.1949-12.2012
Smith, J. C., Marsh, J. T., and Brown, W. S. (1975). Far-field recorded frequency-following responses: evidence for the locus of brainstem sources. Electroencephal. Clin. Neurophysiol. 39, 465–472. doi: 10.1016/0013-4694(75)90047-4
Sobel, M. E. (1982). Asymptotic confidence intervals for indirect effects in structural equation models. Sociol. Methodol. 13, 290–312. doi: 10.2307/270723
Sohmer, H., and Pratt, H. (1977). Identification and separation of acoustic frequency following responses (FFRs) in man. Electroencephal. Clin. Neurophysiol. 42, 493–500. doi: 10.1016/0013-4694(77)90212-7
Sohmer, H., Pratt, H., and Kinarti, R. (1977). Sources of frequency-following responses (FFR) in man. Electroencephal. Clin. Neurophysiol. 42, 656–664. doi: 10.1016/0013-4694(77)90282-6
Strait, D. L., Parbery-Clark, A., Hittner, E., and Kraus, N. (2012). Musical training during early childhood enhances the neural encoding of speech in noise. Brain Lang. 123, 191–201. doi: 10.1016/j.bandl.2012.09.001
Strauss, D. J., Corona-Strauss, F. I., Schroeer, A., Flotho, P., Hannemann, R., and Hackley, S. A. (2020). Vestigial auriculomotor activity indicates the direction of auditory attention in humans. eLife 9:e54536. doi: 10.7554/eLife.54536.sa2
Thornton, A. R. D. (1975). The use of post-auricular muscle responses. J. Laryngol. Otol. 89, 997–1010. doi: 10.1017/S0022215100081317
Tierney, A. T., Krizman, J., and Kraus, N. (2015). Music training alters the course of adolescent auditory development. Proc. Natl. Acad. Sci. U. S. A. 112, 10062–10067. doi: 10.1073/pnas.1505114112
Weiss, M. W., and Bidelman, G. M. (2015). Listening to the brainstem: musicianship enhances intelligibility of subcortical representations for speech. J. Neurosci. 35, 1687–1691. doi: 10.1523/JNEUROSCI.3680-14.2015
White-Schwoch, T., Anderson, S., Krizman, J., Nicol, T., and Kraus, N. (2019). Case studies in neuroscience: subcortical origins of the frequency-following response. J. Neurophysiol. 122, 844–848. doi: 10.1152/jn.00112.2019
White-Schwoch, T., Woodruff Carr, K., Thompson, E. C., Anderson, S., Nicol, T., Bradlow, A. R., et al. (2015). Auditory processing in noise: a preschool biomarker for literacy. PLoS Biol. 13:e1002196. doi: 10.1371/journal.pbio.1002196
Wilson, S. A. K. (1908). A note on an associated movement of the eyes and ears in man. Rev. Neurol. Psychiatry 6, 331–336.
Wong, P. C., Skoe, E., Russo, N. M., Dees, T., and Kraus, N. (2007). Musical experience shapes human brainstem encoding of linguistic pitch patterns. Nat. Neurosci. 10, 420–422. doi: 10.1038/nn1872
Yamada, O., Kodera, K., Hink, R. F., and Yamane, H. (1978). Cochlear initiation site of the frequency-following response: a study of patients with sensorineural hearing loss. Audiology 17:489. doi: 10.3109/00206097809072609
Yoshie, N., and Okudaira, T. (1969). Myogenic evoked potential responses to clicks in man. Acta Otolaryngol. Suppl. 252, 89–103. doi: 10.3109/00016486909120515
Yuval-Greenberg, S., Tomer, O., Keren, A. S., Nelken, I., and Deouell, L. Y. (2008). Transient induced gamma-band response in eeg as a manifestation of miniature saccades. Neuron 58, 429–441. doi: 10.1016/j.neuron.2008.03.027
Zhang, J. D., Susino, M., Mcpherson, G. E., and Schubert, E. (2020). The definition of a musician in music psychology: a literature review and the six-year rule. Psychol. Music 48, 389–409. doi: 10.1177/0305735618804038
Zhang, X., and Gong, Q. (2019). Frequency-following responses to complex tones at different frequencies reflect different source configurations. Front. Neurosci. 2019:130. doi: 10.3389/fnins.2019.00130
Keywords: auditory brainstem response (ABR), auditory evoked potentials (AEPs), frequency-following response (FFR), post-auricular muscle reflex (PAMR), experience-dependent plasticity
Citation: Bidelman GM, Sisson A, Rizzi R, MacLean J and Baer K (2024) Myogenic artifacts masquerade as neuroplasticity in the auditory frequency-following response. Front. Neurosci. 18:1422903. doi: 10.3389/fnins.2024.1422903
Received: 24 April 2024; Accepted: 24 June 2024;
Published: 08 July 2024.
Edited by:
Jyrki Ahveninen, Massachusetts General Hospital and Harvard Medical School, United StatesReviewed by:
Fernando Llanos, The University of Texas at Austin, United StatesFran López-Caballero, University of Pittsburgh Medical Center, United States
Saradha Ananthakrishnan, Towson University, United States
Copyright © 2024 Bidelman, Sisson, Rizzi, MacLean and Baer. This is an open-access article distributed under the terms of the Creative Commons Attribution License (CC BY). The use, distribution or reproduction in other forums is permitted, provided the original author(s) and the copyright owner(s) are credited and that the original publication in this journal is cited, in accordance with accepted academic practice. No use, distribution or reproduction is permitted which does not comply with these terms.
*Correspondence: Gavin M. Bidelman, Z2JpZGVsJiN4MDAwNDA7aXUuZWR1