- 1Department of Neuroscience, Yale University, New Haven, CT, United States
- 2Kavli Institute for Neuroscience at Yale, Yale University, New Haven, CT, United States
Contrary to humans, adult hippocampal neurogenesis in rodents is not controversial. And in the last three decades, multiple studies in rodents have deemed adult neurogenesis essential for most hippocampal functions. The functional relevance of new neurons relies on their distinct physiological properties during their maturation before they become indistinguishable from mature granule cells. Most functional studies have used very young animals with robust neurogenesis. However, this trait declines dramatically with age, questioning its functional relevance in aging animals, a caveat that has been mentioned repeatedly, but rarely analyzed quantitatively. In this meta-analysis, we use data from published studies to determine the critical functional window of new neurons and to model their numbers across age in both mice and rats. Our model shows that new neurons with distinct functional profile represent about 3% of the total granule cells in young adult 3-month-old rodents, and their number decline following a power function to reach less than 1% in middle aged animals and less than 0.5% in old mice and rats. These low ratios pose an important logical and computational caveat to the proposed essential role of new neurons in the dentate gyrus, particularly in middle aged and old animals, a factor that needs to be adequately addressed when defining the relevance of adult neurogenesis in hippocampal function.
Introduction
Adult hippocampal neurogenesis (AHN) has been a prolific topic of research and discussion for the last 30 years. The possibility of neuron renewal and the underlying promise of regeneration in the context of aging and neurological disease has been an important catalyst for the field that have attracted the attention of researchers, funding agencies, scientific journals, and the public. Due to the obvious limitations to perform studies on the human brain, functional inferences about adult neurogenesis have been collected almost exclusively in rodents, mostly in mice (Snyder, 2019). These studies include evaluating the effect of environmental or pharmacological interventions on AHN or using transgenic models of neurological diseases to study their effect on AHN (Snyder and Drew, 2020). Transgenic animals have also been used to study the effect of increased or decreased AHN on specific functions such as spatial memory, pattern separation or associative learning (e.g., Clelland et al., 2009; Drew et al., 2010; Arruda-Carvalho et al., 2011; Sahay et al., 2011). As a result, a large number and variety of functions have been related to adult neurogenesis, including: memory consolidation, flexible learning and updating, reward learning, emotional contextualization, time stamping, spatial contextualization and navigation, behavioral pattern separation, orthogonalization and avoidance of catastrophic interference, detection and seeking of novelty, regulation of affective behaviors and mood, and forgetting (Snyder and Drew, 2020; Kempermann, 2022).
Three factors must be considered when evaluating the functional contribution of adult born granule cells (CGs). The first is whether new neurons possess different connectivity or physiological responses that can confer them distinct functionality from that observed in mature neurons. The second, if their numbers at any given time are sufficient to have a functional impact in the circuit. And third, if their connectivity is large enough and mature enough to support such functional contribution. Obviously, these conditions need to be met before any discussion about the possible function of new neurons can take place. While there is evidence supporting the first condition, there is less support for the other two. Interestingly, despite the many reviews on every possible aspect of AHN, those specific topics, namely if there are enough new neurons and if they are sufficiently connected have not received much attention (Snyder and Cameron, 2012). Due to space constrains, in this article we will address the first two questions, namely if new neurons have different physiology than mature cells and if they are numerous enough to support a relevant functional role in the adult rodent dentate gyrus, while the third question regarding the connectivity of new neurons will be analyzed in a separate article.
To answer the first two questions, we analyze available data on the pattern of connections and the physiology and maturation of adult born neurons in the dentate gyrus and we describe a model of quantitative neurogenesis that predicts the number of new neurons with distinct physiology that are available in the rodent dentate gyrus across age. The results show relatively low levels of functionally distinct new neurons at any age that decline quickly with age to reach very low levels in middle-aged and old animals. We compare our results with other quantitative analyses, and we discuss the implications of our findings in the context of hippocampal function.
When is a rodent adult?
After more than a century of intense research on inbred strains of murine rodents, still there is no consensual answer to this question. In the context of adult neurogenesis, adulthood has been timed after weaning (at postnatal day 21), reasoning that by that age, the adult neurogenic niche, the subgranular zone (SGZ) of the dentate gyrus, has acquired its definitive structure (Kempermann, 2011); or more commonly, adulthood have been timed with sexual maturity, normally defined as achieving sexual reproduction and thus starting at puberty, around P30 (Kempermann, 2011). However, puberty is poorly correlated with successful mating, pregnancy, or maternal care, and it seems more reasonable to equate sexual maturity not just with the capacity for reproduction, but with the ability to reproduce and raise offspring successfully, that will be achieved at the end of the postpuberal adolescence period, a maturational stage that involves hormonal, physiological, neurological and behavioural changes that allow for successful reproduction (Arellano et al., 2024). Adolescence ends around P60 in both rats and mice, marking the beginning of adulthood (Arellano et al., 2024), and thus in this work we will consider P60 as the onset of adulthood for both species.
Since adulthood includes most of the lifespan in mammals, we will consider more meaningful subperiods: young adults, corresponding to peak reproduction (~2–7 month-old for mice and ~2–6 month-old for rats); middle age, corresponding to reproductive senescence in females (~8–14 month-old in mice and ~7–14 month-old in rats); and old age, corresponding to a post-reproductive (anestrous) period in females (~15–30 month-old in mice and ~15–21-month-old in rats) as previously described (Arellano et al., 2024).
The life history of new neurons in the adult dentate gyrus
To better understand the underpinnings of the model we propose, it is useful to review the general scheme of neurogenesis to define the specific phases of differentiation, functional integration, and maturation that new GCs experience as they incorporate in the postnatal dentate gyrus. This is also important to define the terminology we will use along the manuscript to refer to new GCs at different developmental and maturational times. A scheme illustrating those process is shown in Figure 1.
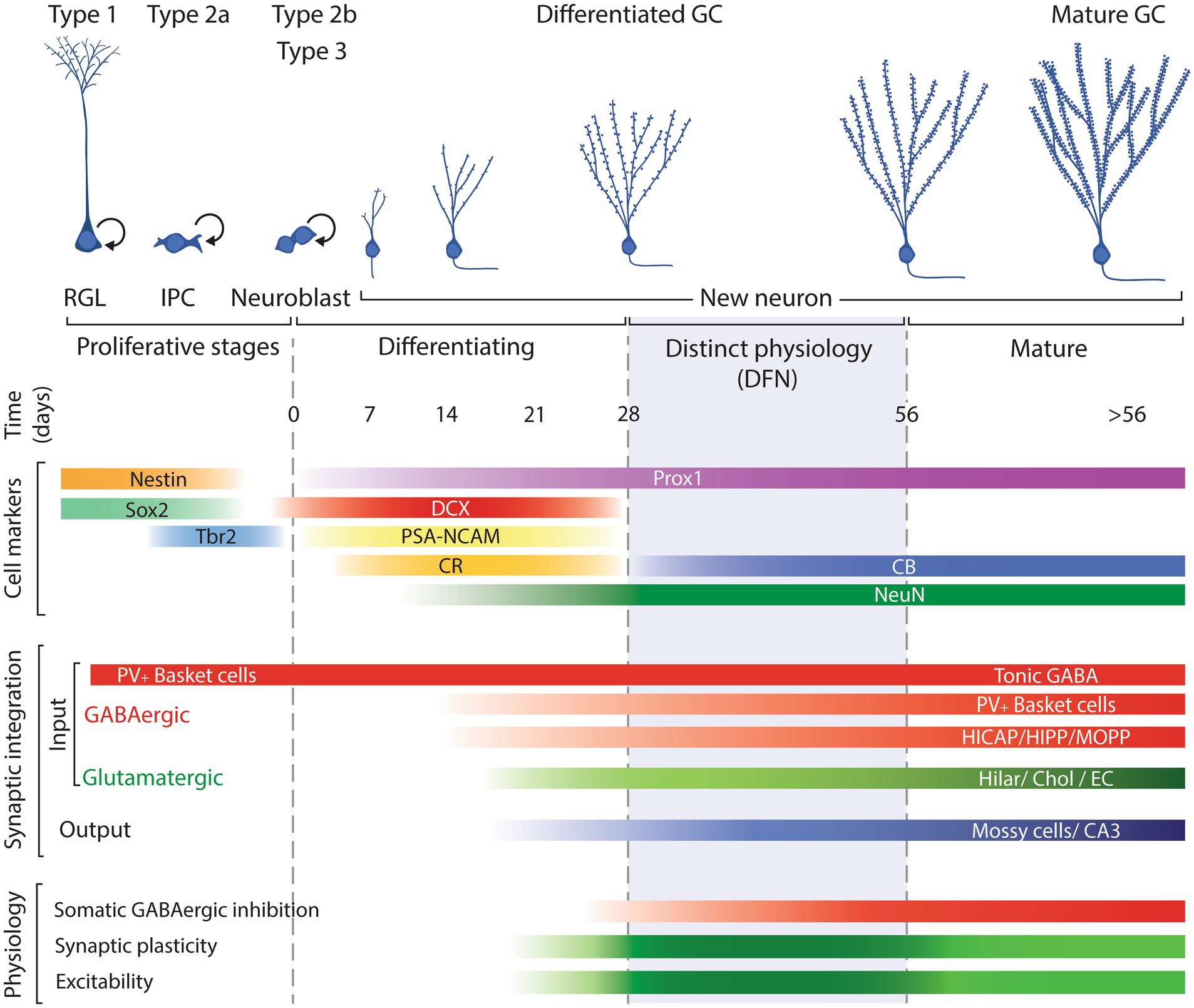
Figure 1. Schematic representation of the neurogenic process illustrating the main cell types involved: radial glia like progenitors (RGL; type 1 cells); intermediate progenitor cells (IPCs; type 2a); neuroblasts, including committed neuronal IPCs (type 2b cells) and type 3 cells; and postmitotic neurons along their differentiation/maturation, timed in days. Cell markers include the approximate expression window along the neurogenic process of common markers used to identify each cell type. Synaptic integration summarizes the approximate timing when new neurons establish incoming GABAergic and glutamatergic input and output. Physiology reflects the neuronal properties of new neurons: darker green between 28 and 56 days reflects the enhanced plasticity and excitability of new neurons allowing them distinct functional capabilities while the subsequent lighter green represents normal mature physiological features. DFN: distinct functional neurons. Modified from Christian et al. (2014).
The accepted model of neurogenesis describes that type 1 radial glia-like progenitors in the subgranular zone of the dentate gyrus divide to produce type 2a cells, glia-like intermediate progenitors, that can divide and produce type 2b cells, neuronally committed intermediate progenitors that start expression of immature neuron markers doublecortin (DCX), polysialylated neural cell adhesion molecule (PSA-NCAM), and Calbindin-2, also known as Calretinin (CR; Liu et al., 1996; Seki, 2002; Brown et al., 2003; Llorens-Martín et al., 2006; Plümpe et al., 2006; Spampanato et al., 2012). Type 2b progenitors can proliferate and produce type 3 cells that can include a small fraction of progenitors but is mostly composed of post-mitotic neurons that will develop to produce new GCs. Those post-mitotic neurons continue expression of DCX, PSA-NCAM, CR, and low levels of RBFox3, better known as neuronal nuclei (NeuN) that will increase as neuronal differentiation progresses (Seki, 2002; Brandt et al., 2003; Brown et al., 2003).
The differentiation of new neurons takes about 4 weeks, during which new neurons migrate to their final position, extend their dendrites and axons, establish synapses, and become functional (van Praag et al., 2002; Espósito et al., 2005; Zhao et al., 2006; Toni et al., 2007; Faulkner et al., 2008; Gu et al., 2012; Nakashiba et al., 2012; Vivar and van Praag, 2013; Jungenitz et al., 2014). Along that process of maturation, they down-regulate expression of immature neuron markers DCX, PSA-NCAM and CR while up-regulating expression of markers of mature GCs such as NeuN and Calbindin (CB; Seki, 2002; Brandt et al., 2003; Brown et al., 2003; Rao and Shetty, 2004; Espósito et al., 2005; McDonald and Wojtowicz, 2005). During the differentiation phase, new neurons experience two main waves of cell death, the first at 2–3 days after cell division and the second 2–3 weeks into the differentiation process (Seki, 2002; Tashiro et al., 2006; Sierra et al., 2010). By 4 weeks of age, surviving new neurons have acquired GC morphology, are integrated in the hippocampal circuit and most of them have switched expression of DCX, PSA-NCAM and CR for NeuN and CB and survive for at least 6 months (Dayer et al., 2003; Kempermann et al., 2003; Rao et al., 2005; Morgenstern et al., 2008); although see McDonald and Wojtowicz (2005).
Meta-analysis
Young new neurons do not have a different pattern of connectivity
Studies on young adult-born GCs have shown that they establish their connectivity following the pattern observed during dentate gyrus development (Espósito et al., 2005). At around two weeks of age they start receiving synaptic input from local GABAergic interneurons, although it produces depolarization, as young new neurons have high intracellular chloride concentration like their embryonic counterparts (Toni and Sultan, 2011). The next input are cholinergic afferents from septal areas, hypothalamus, and basal forebrain, while the first glutamatergic synapses arrive during the third week from hilar mossy cells and entorhinal cortex (EC) and will grow rapidly through the following weeks. The last input to arrive is the GABAergic perisomatic inhibition, close to 4 weeks after mitosis (Espósito et al., 2005; Kumamoto et al., 2012; Vivar et al., 2012; Deshpande et al., 2013; Vivar and van Praag, 2013; Bergami et al., 2015). The output of new neurons also resembles that of mature granule cells, targeting hilar mossy cells, pyramidal neurons in CA3 and GABAergic interneurons in both the hilus and CA3. The axons of new GCs reach CA3 about 10–14 days after mitosis and start to exhibit sparse connections with CA3 neuron dendrites during the third week, although the mossy fiber extent, and the number of mossy terminals and their synapses continues growing for several weeks (Faulkner et al., 2008; Toni et al., 2008). Studies using viral labeling to identify single-synapse presynaptic targets of adult born neurons have shown atypical sources of input such as mature granule cells, CA3 or the subiculum. However, the input from mature granule cells has reasoned to be an artifact of the labeling technique (Deshpande et al., 2013; Bergami et al., 2015) while the projections from the subiculum and CA3 have been occasionally described in rats (Köhler, 1985; Li et al., 1994; Wittner et al., 2007), suggesting that they are not specific of adult born cells. Furthermore, those atypical sources of input to the DG are not consistently detected and represent a small fraction of the total presynaptic input. Therefore, it seems that adult born granule cells are not different in their pattern of connections compared to mature granule cells.
Young new neurons 4 to 8 weeks old exhibit distinct physiology than mature GCs
Functional studies on new neurons have proposed they exhibit distinct physiological responses that confer them enhanced excitability and increased plasticity compared to mature GCs (Snyder et al., 2001; Schmidt-Hieber et al., 2004; Espósito et al., 2005; Ge et al., 2006, 2007; Overstreet-Wadiche et al., 2006; Piatti et al., 2006; Zhao et al., 2006; Marín-Burgin et al., 2012). Some studies have described physiological and functional critical windows in new neurons 1–3 weeks of age (Shors et al., 2001, 2002; Snyder et al., 2001, 2005; Jessberger and Kempermann, 2003; Madsen et al., 2003; Bruel-Jungerman et al., 2006; Tashiro et al., 2007; Deng et al., 2009; Trouche et al., 2009; Aasebø et al., 2011), although as described above, 1–3-week-old new neurons have not established enough afferent or efferent connections (van Praag et al., 2002; Zhao et al., 2006; Toni et al., 2007; Farioli-Vecchioli et al., 2008; Faulkner et al., 2008; Deshpande et al., 2013) to produce meaningful information processing in the hippocampal circuit. Furthermore, 14-day-old new neurons show dendritic extension reaching only to the inner molecular layer (IML) (Perederiy et al., 2013), meaning they would not receive input from the EC terminating in the mid and outer molecular layers (MML and OML), further suggesting they are not engaged functionally in a meaningful manner in the dentate circuit.
Alternatively, a more plausible window of enhanced plasticity and distinct functional properties has been described in new neurons between 4 and 8 weeks after cell division (Ge et al., 2007, 2008; Kee et al., 2007; Gu et al., 2012; Kheirbek et al., 2012; Kim et al., 2012; Marín-Burgin et al., 2012; Dieni et al., 2013; Brunner et al., 2014; Temprana et al., 2015; Danielson et al., 2016; McHugh et al., 2022; Mugnaini et al., 2023; Vyleta and Snyder, 2023), when new GCs are engaged in the hippocampal circuit (Espósito et al., 2005; Toni et al., 2007; Mongiat et al., 2009; Marín-Burgin et al., 2012). After that period, the physiology of new neurons becomes indistinguishable from that of mature GCs (Laplagne et al., 2006, 2007; Ge et al., 2007; Mongiat et al., 2009; Marín-Burgin et al., 2012; Brunner et al., 2014; Woods et al., 2018; McHugh et al., 2022; Mugnaini et al., 2023; Vyleta and Snyder, 2023), strongly suggesting that if new neurons have a distinct function of that of mature GCs, it must be performed during that time window, when they respond differently than mature cells. From now on, we will refer to new neurons during their first 4 weeks after mitosis as differentiating new neurons, while new neurons 4–8-week-old exhibiting the differential physiology conferring them enhanced plasticity and excitability will be referred as distinct functional neurons (DFNs). Adult-born neurons older than 8 weeks will be referred as mature new neurons or simply mature neurons, as they will be indistinguishable from the pool of GCs born during development of the animal (up to 2 months of age).
How many new neurons are in the adult dentate gyrus?
Studies using 5-Bromo-2-deoxyuridine (BrdU) or Ki67 to assess proliferation have consistently revealed thousands of labeled cells in very young rats and mice (Cameron and McKay, 2001; Heine et al., 2004; McDonald and Wojtowicz, 2005; Snyder et al., 2009; Ben Abdallah et al., 2010), and it has been proposed that about half of those cells would survive and integrate in the dentate gyrus circuit, representing 5–6% of the rat GC population (West et al., 1991; Cameron and McKay, 2001; McDonald and Wojtowicz, 2005), prompting descriptions of thousands of new neurons produced every day in the rodent DG (e.g., 4,000–7,000, Kim et al., 2012; 9,000, Christian et al., 2014). However, dentate neurogenesis in rodents decreases 15–20-fold by age 9–12 months (Kuhn et al., 1996; Bondolfi et al., 2004; Harrist et al., 2004; Heine et al., 2004; Hattiangady et al., 2008; Morgenstern et al., 2008; Ben Abdallah et al., 2010; Amrein et al., 2011; Encinas et al., 2011; Smith and Semënov, 2019), and therefore, the answer to the question of how many new neurons are available in the dentate gyrus will drastically depend on the age of the animal.
Studies evaluating the lifespan production of new neurons have shown more moderate figures. (Ninkovic et al., 2007; Imayoshi et al., 2008) used transgenic mice to label newly generated cells and reported values between 8–10% of the total population of 500,000 GCs (Kempermann et al., 1997a; Calhoun et al., 1998; Ben Abdallah et al., 2010; van Dijk et al., 2016), representing 40–50,000 new neurons added along adulthood. Pilz et al. (2018) studied the process of neurogenesis in vivo and estimated that radial glial progenitors will produce on average 4.5 neurons that combined with the approximately 10,000 radial glial cells present in a 2-month-old mouse described by Encinas et al. (2011) would mean that about 45,000 new neurons (or 9% of the total) are generated along the adult life of mice. Lazic (2012) used published data to estimate that C57BL/6 adult mice, defined as older than 3 months (Flurkey et al., 2007), would produce about 57,000 new neurons, representing 11.4% of the total number of GCs. A very different result was obtained by Smith and Semënov (2019) that proposed a model based on proliferation and cell cycle kinetics in C57BL/6J mice and predicted a total of 1.5 million new cells produced along the lifespan in both hemispheres (750,000 per hemisphere) with a 64-fold decline between 1–30 months of age. They do not specify how many of those would be new neurons, but if we assume about 40% (Kempermann et al., 1997b), then around 300,000 new neurons would be generated along the lifespan (per hemisphere), representing about 60% of the total granule cells. Such high levels of neurogenesis would imply the presence of neuronal replacement, as such large increase in the number of granule cells along the lifespan has not been reported.
From a functional point of view, however, we are not interested in the total number of new cells produced along life, but instead in the specific population of DFNs present at any given time in the dentate gyrus, as they might carry different functions than mature GCs. To the best of our knowledge, only Snyder and Cameron (2012) have attempted to respond to this question before. They used a similar age criterion, (cells 8-weeks-old or younger) to define new neurons with a potential distinct functionality, although, as argued above, new neurons younger than 4 weeks might establish too few synapses to exert meaningful information processing in the hippocampal circuit (Espósito et al., 2005; Mongiat et al., 2009; Marín-Burgin et al., 2012). Nonetheless, Snyder and Cameron (2012) estimated that a 4-month-old rat would have about 170,000 young new neurons (per hemisphere) up to 8 weeks old, representing about 14% of the total number of GCs, while a 12-month-old rat would have about 25,000 new neurons 8-week-old or younger, representing 2% of the total. Overall, their model implied the generation of about 500,000 new neurons between ages 2–24 months, meaning about 40% of the population of GCs are replaced along the adult life of the animal (Snyder and Cameron, 2012). The Snyder lab updated the model recently predicting even higher levels of neurogenesis that represent about 50% of the total population of GCs along the lifespan of rats (Cole et al., 2020). Overall, these models (Snyder and Cameron, 2012; Smith and Semënov, 2019; Cole et al., 2020), predict much higher (~5-fold) levels of neurogenesis than estimates based on empiric data (Ninkovic et al., 2007; Imayoshi et al., 2008; Encinas et al., 2011; Lazic, 2012; Pilz et al., 2018).
A model to estimate the number of DFNs from the number of doublecortin labeled cells
We propose an alternative method to quantify the number of distinct functional neurons (DFNs) in the hippocampus based on the number of doublecortin (DCX) labeled cells. At face value, it seems reasonable to consider the number of cells expressing DCX as a measure of neurogenesis, since DCX is considered the canonical marker of newly generated neurons in the DG and has been proposed as a good estimator of the number of differentiating new neurons (Brown et al., 2003; Rao and Shetty, 2004; McDonald and Wojtowicz, 2005). However, it can be argued that although DCX is a good marker to obtain relative values of neurogenesis, for example to compare AHN across ages, experimental conditions or between species (Patzke et al., 2015), the population of DCX labeled cells might not provide an accurate estimate of the number of functional new neurons, as only a fraction of DCX labeled cells will survive to become fully functional (Plümpe et al., 2006). We can avoid the complexities of modelling those processes using empirical data to obtain the ratio between the number of differentiating new neurons and the number of DFNs 4–8 weeks old they produce. For this purpose, available data on BrdU injections with different survival times allows following a cohort of newly generated cells along their proliferation and differentiation process, to figure out the ratio between differentiating new neurons (type 3 cells labeled with BrdU and DCX/PSA-NCAM/CR) and the population of DFNs (labeled with BrdU and NeuN/CB). Once that ratio is known, the number of new differentiating neurons labeled with DCX present at any time will allow to estimate the population of DFNs.
Several reports have produced these data in rodents (Brandt et al., 2003; Brown et al., 2003; Kempermann et al., 2003; McDonald and Wojtowicz, 2005; Snyder et al., 2009), but we focused our attention on three of them (Brandt et al., 2003; Brown et al., 2003; McDonald and Wojtowicz, 2005), as the others included experimental manipulations (e.g., multiple BrdU injections; use of non-naïve animals) that could affect the analysis (see Supplementary material for details). From the selected studies, Brown et al. (2003) only reported percentages of BrdU/DCX and BrdU/NeuN, so we combined their data with the number of cells BrdU positive reported by McDonald and Wojtowicz (2005) to use their dataset (Supplementary Tables S1 and S2). The graph in Figure 2A shows normalized data (percentage of the maximum) of BrdU/CR from Brandt et al. (2003) in the mouse; BrdU/DCX in the rat from Brown et al. (2003) and McDonald and Wojtowicz (2005) with their corresponding levels of BrdU/NeuN neurons 4–8 weeks. Brandt et al. (2003) was performed in mice, but since all produced similar distributions of DCX+ and NeuN+ cells, we pooled their data with that of the rat studies.
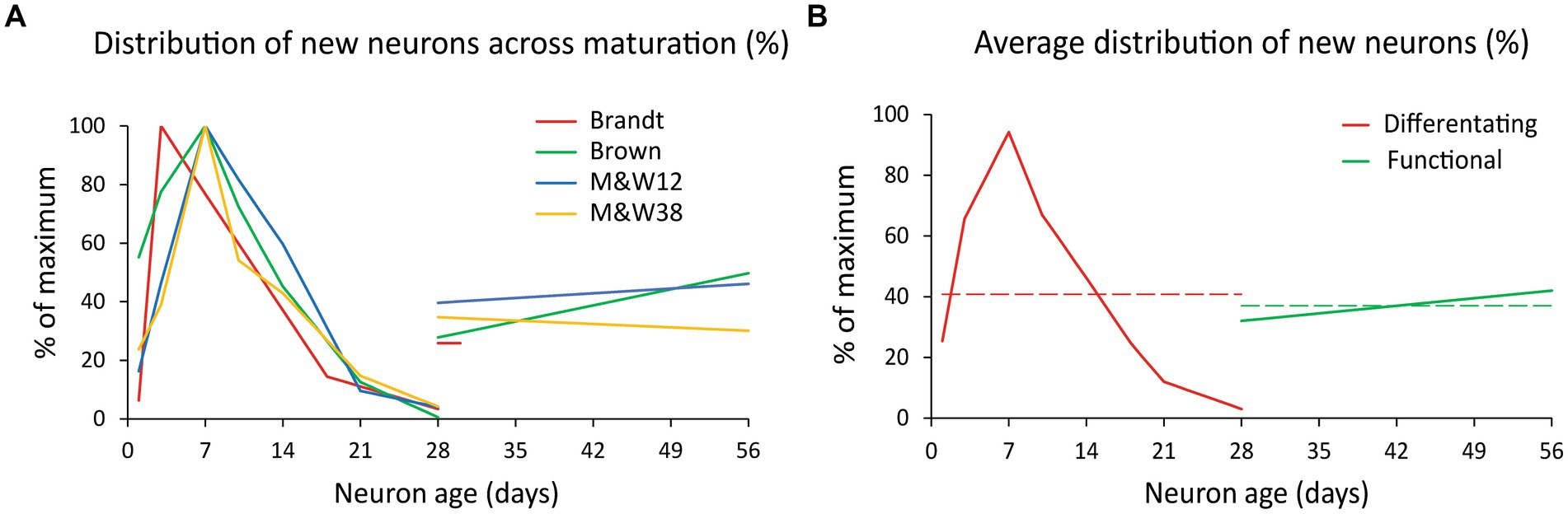
Figure 2. Distribution of new neurons across maturation. (A) Normalized distribution of differentiating new cells (BrdU+/DCX+ and BrdU+/CR+ labeling; % of the maximum) during the first 4 weeks after BrdU injection and normalized value of new functional cells (BrdU+/NeuN+ and BrdU+/CB+ labeling) 4–8 weeks post injection. Differentiating cells exhibit a curve likely due to a combination of some delay in the onset of DCX/CR expression, proliferation and cell death that overall produce a considerable reduction of the initial population over 4 weeks. All data from rat except (Brandt et al., 2003) series in mouse. Note that Brandt et al. (2003), provided data on BrdU+/NeuN+ cells only at 28 days. (B) Average of the normalized distributions shown in A. Differentiating new neurons (BrdU+/DCX+ and BrdU+/CR+ labeling) are represented in red and young functional neurons (BrdU+/NeuN+ and BrdU+/CB+ labeling) in green. Dashed lines represent the average values of each population, 41% of maximum for differentiating neurons (red) and 37% for DFNs (green). The ratio between those values is 0.907 or 91% and represent the ratio between differentiating and functional new neurons from a cohort of newly generated cells (see Supplementary material for extended explanation). M&W38 and 12 are abbreviations of P38 and 12-month-old rats in McDonald and Wojtowicz (2005).
The distributions showed that young neurons ranged between 26 and 43% of the maximum number of DCX+ cells, detected about 7 days post BrdU injection (Supplementary Tables S1 and S2). Given those similarities, we averaged the distributions to produce a single normalized dataset (Figure 2B) illustrating the distribution of differentiating new cells over time (red curve), and the distribution of functional new neurons 4–8 weeks old (green line). Those distributions summarize the populations of differentiating neurons and DFNs in a single cohort of new neurons. As both have the same duration (4 weeks), the ratio between the average value of those distributions (red and green dashed lines in Figure 2B) represents the ratio between the total population of differentiating cells and the total population of DFNs that will originate from them (for detailed explanation see Supplementary material). This ratio is 0.91, meaning that 91% of DCX labeled cells will become DFNs after an average period of 4 weeks.
Several reports have provided quantification of the total number of DCX labeled cells at different ages in rodents: (Rao et al., 2005, 2006; Zhang et al., 2008; Epp et al., 2009; Rennie et al., 2009) in rats and (Kronenberg et al., 2006; Ben Abdallah et al., 2010; Kuipers et al., 2015) 2015 in mice (Figure 3A). The distribution in rats shows more variability than in mice, with the datasets from Rao et al. (2005, 2006) and Rennie et al. (2009) showing relatively high and low, respectively, numbers of DCX labeled cells, that combined might produce a balanced regression curve. However, Rao et al. (2006) data decreased linearly with age, and even exhibits a slight increase in DCX labeled cells between 4 and 7 months of age, in contrast with the well-established notion of an exponential decline of neurogenic parameters across age (Kuhn et al., 1996; Heine et al., 2004; Amrein et al., 2011) and therefore, the data from Rao et al. (2006) were not used. The number of DCX labeled cells in rat are approximately double than those reported in mice, as expected since rats have double number of GCs than mice (Boss et al., 1985; West et al., 1991; Hosseini-Sharifabad and Nyengaard, 2007; Fitting et al., 2010).
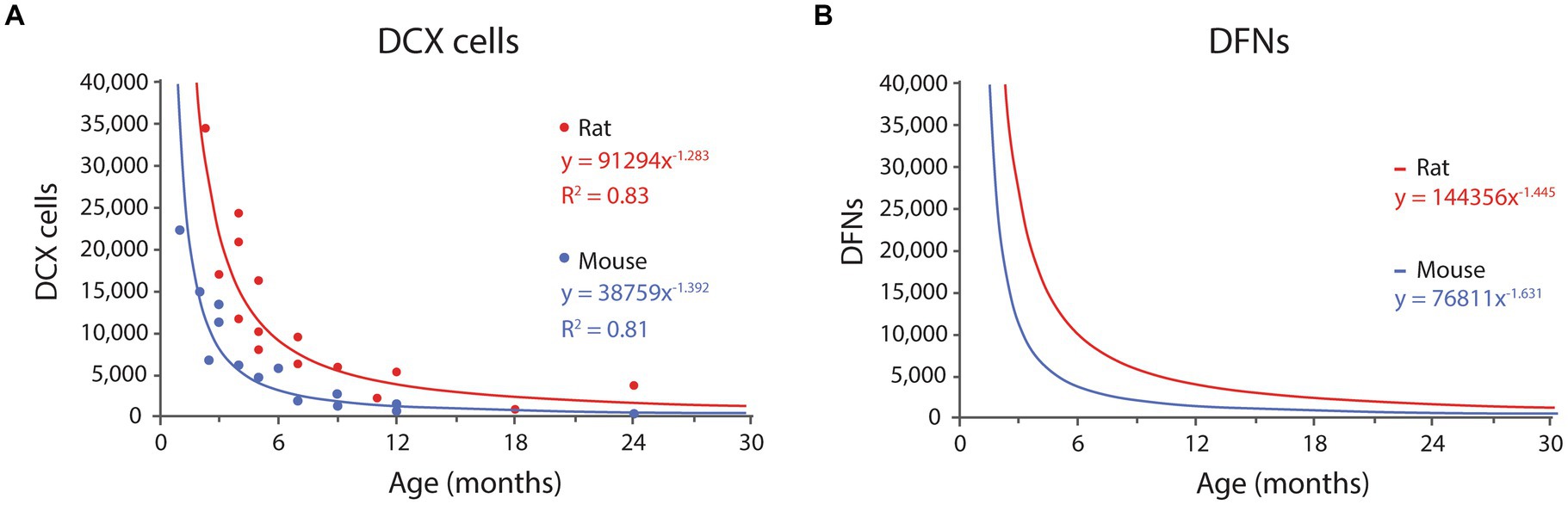
Figure 3. (A) Distribution of DCX labeled cells in rats and mice DG across age according to published results (see text for references). Both distributions fit power equations. (B) Distribution of DFNs in rats and mice across age. Data were transformed from the graph in A, by multiplying each value by 0.91 (survival rate) and adding one month to the age to account for differentiation delay. The resulting values fit negative potential distributions. See Table 1 and Table 2 for values at selected ages.
In mice, Kronenberg et al. (2006), Ben Abdallah et al. (2010), and Kuipers et al. (2015) provided different time points between 1 to 24 months of age. Their combined data fit best an exponential distribution (R2 = 0.84), that however models very poorly the data for young ages, and we selected instead a power distribution that had slightly lower fit (R2 = 0.81) but much better predictive value at young ages, when the levels of neurogenesis are the highest (for details see Supplementary Table 3). The resulting equation for mice is:
where x is months; R2 = 0.81; (Eq. 1, Figure 3A).
In rats, Rao et al. (2005), Zhang et al. (2008), Epp et al. (2009), and Rennie et al. (2009) provided timepoints between 2.3 and 24 months. In this case, the data fitted best a power distribution with equation:
where x is months; R2 = 0.83; (Eq. 2, Figure 3A).
Both equations describe the number of DCX labeled cells along time, and 91% of those will become DFNs after an average 4-week differentiation period. Thus, the number of DFNs at a given time, correspond to 91% of the number of DCX labeled cells 4 weeks before (see Supplementary material for detailed explanation). The estimated values of DFNs can be modeled with power distributions as follows:
where x is months; (Eq. 3, Figure 3B).
where x is months; (Eq. 4, Figure 3B).
The modeled number of DCX labeled cells, DFNs and their percentages from the total population of GCs are shown in Tables 1,2 for mice and rats, respectively. Total population was estimated to be ~500,000 GCs in the mouse (Kempermann et al., 1997a; Calhoun et al., 1998; Ben Abdallah et al., 2010; van Dijk et al., 2016) and about ~1,000,000 GCs in the rat (Boss et al., 1985; West et al., 1991; Hosseini-Sharifabad and Nyengaard, 2007; Fitting et al., 2010; data per hemisphere).
It is important to note that adult neurogenesis, by definition, implies neurons born in adult animals. Since adulthood is achieved around 2 months of age, new neurons born at that age will only become functional when the animal is 3 months old, after the 4-week differentiation period. In turn, the cohort of DFNs present when the animal turns adult, at P60, was generated around P30, and correspond to adolescent neurogenesis. Thus, we will refer to adult born, DFNs as those present in the dentate gyrus at 3 months or later. Both mice and rats show similar proportion of DFNs across age. In mice, the model shows a peak population of 13,500 DFNs (2.7% of the total population) in 3-month-old animals, that declines very rapidly: by the beginning of middle age (~8 months) there are about 2,500 DFNs (0.5%), and by the onset of old age (~15 months) there are about 900 DFNs (0.18%; Table 1). Rats show similar distributions, and at 3 months the model predicts about 34,000 DFNs (3.4% of the total) that decreases rapidly to about 7,500 (0.8%) in early middle age (~7 months) and about 2,800 (0.28%) at the onset of old age (~15 months; Table 2). Comparison between the distribution of DFNs in mice and rats reveal very similar curves, both fitting well power distributions, obviously with higher values for rats, given they have double the number of GCs in the dentate gyrus. Regarding the proportion of DFNs over the total population, young rats exhibit a higher proportion (30% more) than mice, a difference that grows with age to reach 60% more in old rats (15 months and older) compared to mice.
Integration of Eq. 3, 4 allows estimating the number of new neurons produced at any interval of time in both species, showing that during the adult life of a C57BL/6 mouse (2–30 months of age; Arellano et al., 2024) about 47,000 new neurons will be produced, ~10% of the total GC population. In rats, about 115,000 new neurons or ~12% of the total population are produced between age 2–21 months. Note that the integration should be done between 3–30 months, as there are no adult born DFNs at 2 months of age. As expected, the model predicts that most of those new neurons, 76% in mice and 79% in rats will be produced in the first year, a clear reminder of the exponential decline of neurogenesis with age.
Preferential recruitment of new neurons
It has been argued that the low number of DFNs can be counterbalanced by their preferential activation in the dentate network, at a 2–6-fold rate compared with mature GCs, so sufficient new neurons may be recruited to have a relevant functional contribution (Ramírez-Amaya et al., 2005; Kee et al., 2007; Marín-Burgin et al., 2012). Specifically, Marín-Burgin et al. (2012) reported an example of a single stimulus that recruited 5% mature neurons but 30% DFNs in mice about 3-month-old, suggesting a prominent role for new neurons to activate CA3. When those ratios are transformed into absolute numbers, excitation of the DG will activate ~25,000 mature neurons (5% of the total 500,000 GCs in mice), and ~4,000 DFNs (30% of 13,500 DFNs) indicating that DFNs represent about 14% of the activated GCs in a 3-month-old animal (Table 1). If this proportion holds across age, given the exponential decrease of DFNs, less than 3% and about 1% DFNs will be activated in a middle-aged and old mouse, respectively (Table 1). An alternative possibility is that the number of activated DFNs described by Marín-Burgin et al. (2012) is maintained across age, meaning around 4,000 DFNs can be activated by a single stimulus at all ages. However, the number of DFNs decreases below 4,000 around 6 months of age (Table 1) meaning that in older animals a much lower proportion of DFNs will be activated by a single stimulus. Extrapolation of those data to rats shows similar percentages of activated DFNs in young adults and about double ~5% and ~2% in middle age and old animals, respectively (Table 2), still representing low proportions of the total population of activated granule cells.
Discussion
The high levels of neurogenesis in the young rodent hippocampus have been central to discussions on its potential function for the last 3 decades. The hippocampus is involved in a large variety of functions and numerous reports have proposed a functional involvement of adult neurogenesis in virtually all those functions (Kim et al., 2012; Snyder and Drew, 2020; Kempermann, 2022). From a computational point of view, three main conditions need to be satisfied for new neurons to have a functional impact. First, new neurons must exhibit differential connectivity and/or functional responses from mature cells; second, there needs to be enough new neurons to have a measurable contribution, and third, those new neurons need to have enough input and output in the hippocampal network to carry out their function. In this meta-analysis we attempted to answer the first two questions by creating a model to estimate the number of new GCs with differential functional capabilities across age in mice and rats. Due to space constrains, we do not address the third question that will be analyzed in a separate manuscript.
The differential physiology of adult generated GCs
Available data indicates that while new granule cells exhibit similar pattern of connectivity to that of mature granule cells born during perinatal development, adult born neurons show differential excitability and plasticity compared to mature GCs. Two main periods have been described: between 1 and 3 weeks of age, and between 4–8 weeks of age.
The first period was described by studies using ablation of new neurons through X-ray irradiation or pharmacological interventions, showing that new neurons seemed to be required for different hippocampal functions between 1–3 weeks after mitosis (Shors et al., 2001, 2002; Snyder et al., 2001, 2005; Jessberger and Kempermann, 2003; Madsen et al., 2003; Bruel-Jungerman et al., 2006; Tashiro et al., 2007; Deng et al., 2009; Trouche et al., 2009; Aasebø et al., 2011). However, at those ages new neurons exhibit short dendritic arbors and sparse synaptic input mostly from local GABAergic interneurons that is however excitatory in nature (Espósito et al., 2005; Toni and Sultan, 2011), and start to receive input from hilar mossy cells and entorhinal cortex (Espósito et al., 2005; Kumamoto et al., 2012; Vivar et al., 2012; Deshpande et al., 2013; Vivar and van Praag, 2013; Bergami et al., 2015). Similarly, the axons of new GCs reach CA3 about 10–14 days after mitosis, but only sparse, immature synapses are found at 3 weeks after mitosis (Faulkner et al., 2008; Toni et al., 2008). Thus, new neurons at those early ages are not fully engaged in the hippocampal network, as they barely receive entorhinal input or convey excitatory output to CA3. Furthermore, the excitatory effect of their GABAergic input implies they lack inhibition and therefore their excitability might not be modulated by the hippocampal network, suggesting they might be a source of noise more than a computational asset in the dentate gyrus network.
A second body of evidence that has become the mainstream view on the field has showed that new neurons 4–8-weeks-old exhibit enhanced physiological features compatible with increased excitability and plasticity (Ge et al., 2007, 2008; Kee et al., 2007; Gu et al., 2012; Kheirbek et al., 2012; Kim et al., 2012; Marín-Burgin et al., 2012; Dieni et al., 2013; Brunner et al., 2014; Temprana et al., 2015; Danielson et al., 2016; McHugh et al., 2022; Mugnaini et al., 2023; Vyleta and Snyder, 2023). This window seems more realistic, since there is consensus that 4-week-old new neurons are integrated in the hippocampal circuit, able to respond to different input sources including the entorhinal cortex and capable of producing synaptic output to mossy cells and CA3 pyramidal neurons (van Praag et al., 2002; Espósito et al., 2005; Zhao et al., 2006; Toni et al., 2007; Faulkner et al., 2008; Gu et al., 2012; Nakashiba et al., 2012; Vivar and van Praag, 2013; Jungenitz et al., 2014; Lopez-Rojas and Kreutz, 2016; Mugnaini et al., 2023). Based on these data, we considered 4–8-weeks-old new neurons as distinct functional neurons or DFNs, the relevant population to quantify if we want to assess the potential for a functional role of AHN. As described earlier, when we refer to mature GCs we include both developmentally generated (born in animals up to 2 months of age) and adult-born neurons older than 8 weeks, that share similar physiological responses.
Although the 4–8 weeks critical window has emerged as the most widely accepted in the field, different studies provide different timing. For example, the most widely cited of those physiological studies, (Ge et al., 2007) and others (Espósito et al., 2005; Mongiat et al., 2009; Restivo et al., 2015; Temprana et al., 2015) including behavioral studies (Denny et al., 2012) describe a critical period restricted to 4–6-week-old neurons, with 7-weeks-old and older new neurons responding similar to mature GCs. In this scenario, the proportion of DFNs reflected in Table 1 would be reduced by half at any age and would mean that a 3-month-old mouse would have about 1.3% (~7,000) DFNs, while middle age and old mice would have about 0.25% (~1,200) and ~0.1% (about 450) DFNs, very little fractions of new neurons whose consequences will be discussed in the next sections.
The model predicts a low number of DFNs in the adult dentate gyrus, particularly in middle aged and old animals
Our model relates the number of DFNs to the population of DCX labeled cells in the dentate gyrus, allowing to use published data on the distribution of DCX labeled cells across age to estimate the number of DFNs. The model predicts that about 91% of the DCX labeled present at one point will become DFNs 4 weeks later. We pooled data from studies using stereology to report the absolute number of DCX labeled cells across age both in mouse (Kronenberg et al., 2006; Ben Abdallah et al., 2010; Kuipers et al., 2015) and in rat (Rao et al., 2005; Zhang et al., 2008; Epp et al., 2009; Rennie et al., 2009) to describe the distribution of DFNs across age in both mice and rats. An advantage of relying on DCX to estimate the number of new neurons is that immunolabeling is a priori more consistent between experiments and labs than BrdU injections, which vary widely depending on dosage, timing of post-injection analysis, differences in clearance of the marker and potential dilution of BrdU labeling (Smith and Semënov, 2019). In support of this point, the pooled data of DCX labeled cells from different studies in both species produce coherent datasets fitting negative power distributions with high regression coefficients.
Although the distribution of DCX labeled cells in mice fitted best an exponential distribution (R2 = 0.84), the resulting curve modeled very poorly the data for young ages (Supplementary Table S3), and we selected instead a power distribution that had slightly lower correlation coefficient (R2 = 0.81) but much better predictive value at young ages, when neurogenesis is the highest (Supplementary Table S3). The negative power functions modelling DCX labeled cells can be hypothesized to have their maximum at the peak of developmental neurogenesis around P7 (Angevine, 1965; Schlessinger et al., 1975; Bayer, 1980).
The distribution of DFNs across age were obtained after transforming the DCX data according to the parameters obtained from the model: 0.91% survival rate and 4-week differentiation delay, resulting also in negative power distributions (Figure 3B). We set the onset of adulthood at 2 months of age (Arellano et al., 2024), meaning that DFNs will only be available at 3 months of age, as new neurons born in 2-month-old animals will take about 4 weeks to differentiate and become functional. The 91% survival rate suggest that both populations (DCX labeled cells and DFNs) are similar in size and would seem to confirm the generally accepted idea that DCX is a good reflection of the number of new neurons in the dentate gyrus or mice and rats (Brown et al., 2003; Rao and Shetty, 2004; McDonald and Wojtowicz, 2005; Ben Abdallah et al., 2010; Patzke et al., 2015; Lipp, 2017). Comparison of both populations show similar values in middle age and old individuals, but significant differences in very young animals (1.6-fold, Tables 1,2) due to the sharp decline of the DCX population at early ages. Therefore, the assumption that DCX is a good reflection of the number of new neurons in the dentate gyrus is valid for middle-aged and old animals, but only allows a rough estimate of the number of DFNs in young adults, that will have more DFNs than DCX labeled cells. In this regard, it can be argued that we have not considered possible differences in fate choice, pace of maturation or survival of new neurons with age. However, most studies have pointed no differences in such parameters (McDonald and Wojtowicz, 2005; Rao et al., 2005, 2006; Hattiangady and Shetty, 2008) and the datasets in our model comparing very young (adolescent) P38 with aged 12-month-old animals only showed differences in the rate of new neuron survival, that was slightly lower in older animals.
The model shows similar distribution of DFNs in mice and rats, both fitting power curves, obviously with higher values for rats as they have double the number of GCs in the dentate gyrus. The proportion of DFNs over the total population in both species show very similar ratios in young animals that start to diverge as animals age, with rats exhibiting larger proportion of DFNs compared to mice, reaching a 1.6-fold difference after 12 months of age. According to the model, 3-month-old animals have about 3% (2.7–3.4%) DFNs in both species, quite a low percentage considering this will be the peak of the DFN population. As age increases, the number of DFNs decreases drastically, and by the onset of middle age (7–8 months), both species show less than 1% (0.5–0.8%) DFNs over the total population of GCs, that decreases to very low levels (0.2–0.3%) by the beginning of old age at ~15 months (Tables 1,2).
Our model in context: agreement with experimental evidence; disagreement with proliferation-based models
The model described here predicts that along the adult lifetime (2–30 months of age) of C57Bl/6 mice, the most common strain used in research, about 47,000 new neurons are produced, that represent ~10% of the total population of GCs. Regarding rats, the model predicts that along adulthood (2–21 months) rats produce about 115,000 new neurons, or ~12% of the total GCs, a slightly higher proportion than in mice, as previously suggested (Snyder et al., 2009). The value for mice is in good agreement with previously reported values of 8–11% using gene reporters (Ninkovic et al., 2007; Imayoshi et al., 2008), combining data on neural progenitors and their expected neuronal offspring (Encinas et al., 2011; Pilz et al., 2018) or from models based on empiric data on neurogenesis markers (Lazic, 2012).
Conversely, models based on proliferation data and relying on estimates of cell cycle duration and frequency of progenitor division have predicted much higher proportion of new neurons along the lifespan, from 40–50% of the total GC population in rats (Snyder and Cameron, 2012; Cole et al., 2020) to ~60% in mice (Smith and Semënov, 2019). These high estimates are in clear disagreement with our results and with previous experimental evidence reported above (Ninkovic et al., 2007; Imayoshi et al., 2008; Encinas et al., 2011; Pilz et al., 2018), and a pivotal question is how those studies reconcile such large number of newly generated neurons with much low number of DCX labeled cells present in mice (Kronenberg et al., 2006; Ben Abdallah et al., 2010; Kuipers et al., 2015) or rats (Rao et al., 2005; Zhang et al., 2008; Epp et al., 2009; Rennie et al., 2009). For example, the model described by Cole et al. (2020) predicts ~190,000 new neurons generated between 2 and 3 months of age in rats [equation in Figures 8A,E in Cole et al. (2020) although see note in Supplementary material]. Thus, one would expect similar one would expect similar number of DCX labeled cells (~190,000) or about 125,000 DCX cells if we consider that, as described above, at early ages DCX labeled cells are about 1.5 less numerous than new neurons. Instead, a 2.3-month-old rat has less than 35,000 DCX labeled cells (Epp et al., 2009), about 4-5 times less than expected from the model. Therefore, those high levels of neurogenesis conflict with the available evidence and more generally, with the levels of neurogenesis suggested by DCX labeled cells in rodents. And, at those elevated rates of neurogenesis, neuronal replacement would be so high that the risk of disruption and interference in memory processes might surpass the benefit of adding new, more responsive neurons to the network (Rakic, 1985; Akers et al., 2014).
Our mice model is based mostly on data from C57BL/6 animals, and (Kempermann et al., 1997b) showed that 2-month-old C57BL/6 mice injected with BrdU for 6 days showed about 0.36% labeled GCs 4 weeks later, suggesting that at that rate, about 1.8% of GCs would be generated between 2 and 3 months. Our results in mice point to higher levels of neurogenesis (2.7%, Table 1), indicating the model does not seem to be biased to minimize AHN. Additionally, it is important to contextualize the results of our model into the inter-strain variability documented in mice and rats. For example, Kempermann et al. (1997b) also reported that 129/SvJ exhibited about half new neurons after 4 weeks than C57BL/6, BALB/c or CD1 mice. This implies that the predicted numbers of DFNs revealed by our model would be halved for 129/SvJ mice. Considering the proposed essential role of AHN in hippocampal function, this remarkable inter-strain difference suggests 129/SvJ mice might exhibit some cognitive phenotype regarding hippocampal function. However, as far as we know, there are no reports of this strain as less capable cognitively, showing accelerated cognitive decline with age or predisposition to hippocampal function impairment that would be expected considering the extremely low levels of DFNs predicted for that strain in middle-aged and old animals (~0.25% of the total GCs at middle age).
Limitations of the model
It can be argued that one caveat of the model is the heterogeneity of the data used to build it, as compiles data from different species, markers and time points of analysis. Although this variability warrants caution when interpreting the results, it is noteworthy that the predictions of the model are in tune with the levels of neurogenesis expected from the distribution of DCX labeled cells and also agree with predictions based on experimental data (Ninkovic et al., 2007; Imayoshi et al., 2008; Encinas et al., 2011; Lazic, 2012; Pilz et al., 2018) as noted in the previous section. The data suggest similar parameters of neurogenesis between mice and rats, although with slightly lower levels of neuronal survival in mice. In this regard, Snyder et al. (2009) reported large differences in neuron survival between rats and mice, although those results might be biased by the use of non-naive rats for the comparison (see Supplementary material). Regarding inter strain variability, it is expected, as described by Kempermann et al. (1997b) and might be reflected in the number of DCX labeled cells across age. However, we found that the normalized distributions of differentiating new neurons and DFNs were quite similar across species, strains, and labs, as reflected in Figure 2A and therefore we combined them to obtain an average value.
Another issue is the age of the animals whose data were used for our model. Most were 5–8 weeks old when injected with BrdU, meaning they were adolescent and not adult, as extensively discussed elsewhere (Arellano et al., 2024). This might imply that there could be differences in cell differentiation dynamics and survival ratios that might change in adult and aging animals. However, most studies have pointed no differences in such parameters (McDonald and Wojtowicz, 2005; Rao et al., 2005, 2006; Hattiangady and Shetty, 2008) and the datasets in our model comparing very young (adolescent) P38 with aged 12-month-old animals only showed differences in the rate of new neuron survival, that was slightly lower in older animals. An alternative view of this potential caveat is that the model includes heterogeneous data that might overall produce a more averaged model of the neurogenic process regardless of age, species, or strain. Indeed, the purpose of our model is not to produce a precise and accurate prediction of the number of new neurons, but an approximate, rough estimate that makes sense conceptually and agrees with experimental studies and with empirical data such as the number of differentiating new neurons labeled with DCX. The overall aim is to provide an evidence-based estimate to contextualize the potential functional impact of adult neurogenesis in realistic quantitative terms, offering perspective regarding the limitations imposed by very low numbers of new neurons in aged animals, as most functional hypotheses about this trait have been derived from studies in very young animals with relatively high levels of neurogenesis, disregarding the steep decline of neurogenesis in middle aged and old animals (Lipp and Bonfanti, 2016; Snyder, 2019; Arellano et al., 2024).
The functional limitations imposed by the low number of DFNs in aging animals
The proportions of DFNs in the adult dentate gyrus reported here represent, objectively, a very small fraction of the total population of granule cells even in very young individuals. Such lower levels of DFNs challenge the notion that new neurons have an essential contribution in the dentate gyrus circuit and are required for proper hippocampal function, a concept that has been central in the field (Snyder and Drew, 2020; Kempermann, 2022). Shifting the perspective to mature granule cells, is hard to explain how, when adult neurogenesis is compromised, the remaining ~97% of mature granule cells are incapable of performing their normal function in the hippocampus. This conundrum escalates when we consider that the dentate gyrus of middle age and old animals contains more than 99% mature granule cells, still unable to deliver normal dentate gyrus function. And as indicated when discussing the critical period of new neurons, if the critical window is defined as 4–6 weeks of age as proposed by several studies (Espósito et al., 2005; Ge et al., 2007; Mongiat et al., 2009; Denny et al., 2012; Restivo et al., 2015; Temprana et al., 2015) then the fraction of DFNs is reduced by half, deepening the physical and logical barrier for an essential role of new neurons.
Three hypotheses have been proposed to overcome the functional limitations imposed by the low number of new neurons: preferential activation of new neurons, elongation of the critical functional window with age, and persistence of differential functional features beyond the critical functional window. However, detailed analysis of those hypotheses suggests that they are insufficient to overcome the declining numbers of new neurons, as discussed in the next sections.
Preferential recruitment of DFNs is not sufficient to compensate for the decline in neurogenesis
Several studies have suggested that the functional limitations imposed by the low number of new neurons in aging animals can be compensated by preferential activation of DFNs due to their enhanced excitability during normal activity of the dentate gyrus (Ramírez-Amaya et al., 2005; Kee et al., 2007; Marín-Burgin et al., 2012), although there is not clear consensus in this point, as other studies could not identify such preferential activation, showing similar activation of DFNs and mature GCs (Stone et al., 2011; Dieni et al., 2013). Our analysis shows that when preferential recruitment of DFNs is translated to actual numbers using Marín-Burgin et al. (2012), data, the maximum contribution of DFNs to the pool of activated GCs would be 14% in 3-month-old mice. This proportion is relatively high and seems compatible with a more relevant functional contribution of DFNs in the activity of the network, but still indicates that the vast majority (86%) of the output to mossy cells, CA3 and GABAergic interneurons will be driven by mature GCs (Table 1). Under the same paradigm, during normal dentate gyrus activation in middle age and old animals, only ~3% and ~1% of activated granule cells will be DFNs, respectively (Table 1), meaning that at those ages over 97% of cells responding to a given stimulus in the dentate gyrus would be mature GCs, arguing against the idea that mature GCs “retire” to let new neurons take care of business in the dentate gyrus (Alme et al., 2010), and more importantly, implying that even with preferential activation, the number of activated DFNs in older animals is very low. Furthermore, the drop in the number DFNs with age is so pronounced that even if all DFNs present in the DG of middle-aged and old animals are activated by a given stimulus, they would represent only ~10% and ~4% of the total activated cells at those ages. In summary, even with preferential recruitment, the number of activated DFNs represents a small fraction of activated granule cells, once again questioning if such low levels of activation can support the essential role in hippocampal function consistently reported, particularly in middle aged and old animals.
As previously stressed (Snyder, 2019; Arellano et al., 2024), many of the functional studies supporting an important role for new neurons used very young, in many cases adolescent animals, that exhibit larger rates of neurogenesis, up to 7–8% according to our model (Table 1). If we consider the potential contribution of preferential recruitment of DFNs at those early ages, the model indicates that up to 30% of DFNs could be activated during normal DG activity (although see Stone et al., 2011; Dieni et al., 2013). In those conditions, it is reasonable to consider that DFNs might have a relevant, maybe critical participation in the hippocampal network, that however will quickly diminish as the levels of neurogenesis decrease rapidly in the following months. This potential bias introduced by studies in very young animals might partially explain the apparent conundrum between the low number of DFNs and their proposed essential function. A reasonable strategy to solve this issue would be to perform studies in middle-aged animals, that should provide a more balanced assessment, not only to understand the possible role of neurogenesis in rodents, but also to establish more realistic and useful comparisons with humans.
Elongation of the critical functional period with age does not affect the predictions of the model
There is evidence showing that the differentiation and maturation of new neurons slows down in aging animals (Rao et al., 2005; Trinchero et al., 2017, 2019), and it has been argued that an elongation of the critical functional period would increase the population of DFNs, a factor that might compensate for the overall decrease in neurogenesis (Bond et al., 2022). The argument is valid, although the findings mostly report an elongation of the differentiation phase, (DCX expressing cells), also implying an increase in the population of differentiating cells. Thus, since our model estimates the number of DFNs from the number of DCX labeled cells, the results in aged animals already reflect the potential increase in the population of DFNs.
Long term structural differences of adult-born GCs do not seem to support a sustained functional difference
Another hypothesis to circumvent the functional limitations imposed by the low numbers of DFNs in aging animals has proposed that adult born GCs maintain differential properties than mature, developmentally generated GCs, beyond the 4–8 weeks of age when they exhibit enhanced physiology and plasticity (Lemaire et al., 2012; Snyder, 2019; Cole et al., 2020). Those claims are based on results in rats showing extended structural plasticity in the form of dendritic remodelling in response to activity (Lemaire et al., 2012) and increase in input connectivity reflected by higher numbers of dendritic spines (Cole et al., 2020). At face value, increased connectivity of adult born neurons might facilitate their recruitment and information processing in the dentate network. However, the studies on the physiology of adult born neurons coincide in describing new neurons beyond 7–8 weeks old as physiologically indistinguishable from mature GCs (Laplagne et al., 2006, 2007; Ge et al., 2007; Mongiat et al., 2009; Marín-Burgin et al., 2012; Brunner et al., 2014; Woods et al., 2018; McHugh et al., 2022; Mugnaini et al., 2023) and therefore, is hard to justify how their activity would be different from that of mature GCs. Another implication of a long-term effect of adult born neurons is that their functional contribution should increase over time as their number grows, a notion difficult to reconcile with the well-known decline in cognitive function related to aging.
Conclusion
There seems to be an overall assumption in the field of AHN, that new, adult-born neurons and their increased excitability and plasticity are essential for the normal function of the hippocampus. AHN has been related to most if not all hippocampal functions such as memory consolidation, reward learning, emotional contextualization, time stamping or even forgetting (Kempermann, 2022) and alterations in AHN have been related to cognitive deficits associated to old age, neurodegenerative diseases such as Alzheimer’s and in many other neuropsychiatric disorders, from depression to autism (Choi and Tanzi, 2019; Kerloch et al., 2019; Babcock et al., 2021; Bicker et al., 2021; Sun et al., 2022; Tartt et al., 2022).
As described in the introduction, three main questions must be answered to evaluate the potential functional role of new neurons. We have addressed the first two questions in this manuscript, revealing low numbers of new neurons with differential physiology. The remaining question is if those new neurons have enough connectivity during their critical period to support such differential function. Several studies have indicated that they are fully integrated in the hippocampal circuit, but their connectivity is still developing and differs from that of mature granule cells in terms of number of synaptic contacts and maturity of those connections (van Praag et al., 2002; Toni et al., 2007, 2008; Faulkner et al., 2008). Detailed analysis of this question cannot be included in this manuscript due to space constraints and will be analyzed in a separate article.
Overall, this study describes the structural framework of AHN in terms of numbers of new neurons. The numbers produced by our model might not be totally accurate, as they are based on a limited number of datasets, and further variability is expected between rodent strains and individuals. However, the predictions of the model agree well with expected levels of neurogenesis based on available empirical data and the general assumptions of the field, and therefore provide a realistic starting point for discussions on the functional role of AHN. In this regard, functional models of adult neurogenesis have traditionally acknowledged the possible impact of low levels of neurogenesis at older ages but have rarely included actual numbers in the discussion. Our study provides such estimations of neurogenesis across age, providing context to design experimental studies, to interpret findings and elaborate more accurate and evidence-based models of hippocampal function.
Our model shows high rates of dentate neurogenesis in infant and adolescent mice and rats, at a time when cognitive development is at its prime. This correlation have been interpreted as a form of ontogenetic plasticity that would shape dentate gyrus circuits according to the individual’s specific environment to optimize hippocampal function in adulthood, as a form of environmental imprinting (Semënov, 2019; Cushman et al., 2021). This hypothesis is plausible, although might not apply as well to more precocial or neotenic species such as primates, where most dentate neurogenesis occurs in utero, and the smaller proportion of granule cells generated during infancy and adolescence might not be able to have the same impact than in rodents (Eckenhoff and Rakic, 1988; Guidi et al., 2005; Jabès et al., 2010; Amrein et al., 2011).
Beyond the possible functional role of granule cell neurogenesis in the developing hippocampus, our data illustrates the steep decrease of neurogenesis during late adolescence and early adulthood, to reach low levels in late adulthood. Interestingly, hippocampal adult neurogenesis in rodents decays at much higher rate (4-fold) than olfactory neurogenesis (Semenov, 2021), a feature that might be informative of their relative functional importance. This acute decline of neurogenesis challenges the notion of a prominent functional role even in young adult animals, but particularly in middle aged and old animals, in which neurogenesis reach very low levels, well below 1% and the ratio of activated DFNs drops to 3–5%. This functional controversy might be in part explained by experimental bias, as most functional studies have been performed in very young, sometimes adolescent rats and mice when they exhibit peak neurogenesis, disregarding the much lower levels of new neurons present in middle aged and old animals (Lipp and Bonfanti, 2016; Snyder, 2019; Arellano et al., 2024). For the same reason, extrapolation of those data to humans might not be very useful, as there might not be much interest in improving cognition of people in their 10s and 20s when they are in their cognitive prime, while it could be relevant to help people say beyond their 60s and 70s, when hippocampal function might take a hit due to aging or neurological disease.
In conclusion, we think our data provides a realistic framework to describe quantitatively adult neurogenesis in murine rodents, and based on these results, we find very difficult to reconcile –from a computational and from a commonsense perspective- that the low number of distinctly functional new neurons might have an essential role in the variety of functions in which they have been involved, a caveat that needs to be addressed in functional models of adult neurogenesis.
Data availability statement
The original contributions presented in the study are included in the article/Supplementary material, further inquiries can be directed to the corresponding authors.
Author contributions
JIA: Conceptualization, Data curation, Formal analysis, Investigation, Visualization, Writing – original draft, Writing – review & editing. PR: Funding acquisition, Resources, Writing – review & editing.
Funding
The author(s) declare that financial support was received for the research, authorship, and/or publication of this article. This research was supported by NIH-NIDA DA023999 grant to PR.
Conflict of interest
The authors declare that the research was conducted in the absence of any commercial or financial relationships that could be construed as a potential conflict of interest.
The author(s) declared that they were an editorial board member of Frontiers, at the time of submission. This had no impact on the peer review process and the final decision.
Publisher’s note
All claims expressed in this article are solely those of the authors and do not necessarily represent those of their affiliated organizations, or those of the publisher, the editors and the reviewers. Any product that may be evaluated in this article, or claim that may be made by its manufacturer, is not guaranteed or endorsed by the publisher.
Supplementary material
The Supplementary material for this article can be found online at: https://www.frontiersin.org/articles/10.3389/fnins.2024.1416460/full#supplementary-material
References
Aasebø, I. E. J., Blankvoort, S., and Tashiro, A. (2011). Critical maturational period of new neurons in adult dentate gyrus for their involvement in memory formation. Eur. J. Neurosci. 33, 1094–1100. doi: 10.1111/j.1460-9568.2011.07608.x
Akers, K. G., Martinez-Canabal, A., Restivo, L., Yiu, A. P., De Cristofaro, A., Hsiang, H.-L. L., et al. (2014). Hippocampal neurogenesis regulates forgetting during adulthood and infancy. Science 344, 598–602. doi: 10.1126/science.1248903
Alme, C. B., Buzzetti, R. A., Marrone, D. F., Leutgeb, J. K., Chawla, M. K., Schaner, M. J., et al. (2010). Hippocampal granule cells opt for early retirement. Hippocampus 20, 1109–1123. doi: 10.1002/hipo.20810
Amrein, I., Isler, K., and Lipp, H.-P. (2011). Comparing adult hippocampal neurogenesis in mammalian species and orders: influence of chronological age and life history stage. Eur. J. Neurosci. 34, 978–987. doi: 10.1111/j.1460-9568.2011.07804.x
Angevine, J. B. (1965). Time of neuron origin in the hippocampal region. An autoradiographic study in the mouse. Exp. Neurol. 2, 1–70,
Arellano, J. I., Duque, A., and Rakic, P. (2024). A coming-of-age story: adult neurogenesis or adolescent neurogenesis in rodents? Front. Neurosci. 18:1383728. doi: 10.3389/fnins.2024.1383728
Arruda-Carvalho, M., Sakaguchi, M., Akers, K. G., Josselyn, S. A., and Frankland, P. W. (2011). Posttraining ablation of adult-generated neurons degrades previously acquired memories. J. Neurosci. 31, 15113–15127. doi: 10.1523/JNEUROSCI.3432-11.2011
Babcock, K. R., Page, J. S., Fallon, J. R., and Webb, A. E. (2021). Adult hippocampal neurogenesis in aging and Alzheimer’s disease. Stem Cell Rep. 16, 681–693. doi: 10.1016/j.stemcr.2021.01.019
Bayer, S. A. (1980). Development of the hippocampal region in the rat. II. Morphogenesis during embryonic and early postnatal life. J. Comp. Neurol. 190, 115–134. doi: 10.1002/cne.901900108
Ben Abdallah, N. M.-B., Slomianka, L., Vyssotski, A. L., and Lipp, H.-P. (2010). Early age-related changes in adult hippocampal neurogenesis in C57 mice. Neurobiol. Aging 31, 151–161. doi: 10.1016/j.neurobiolaging.2008.03.002
Bergami, M., Masserdotti, G., Temprana, S. G., Motori, E., Eriksson, T. M., Göbel, J., et al. (2015). A critical period for experience-dependent remodeling of adult-born neuron connectivity. Neuron 85, 710–717. doi: 10.1016/j.neuron.2015.01.001
Bicker, F., Nardi, L., Maier, J., Vasic, V., and Schmeisser, M. J. (2021). Criss-crossing autism spectrum disorder and adult neurogenesis. J. Neurochem. 159, 452–478. doi: 10.1111/jnc.15501
Bond, A. M., Ming, G., and Song, H. (2022). What is the relationship between hippocampal neurogenesis across different stages of the lifespan? Front. Neurosci. 16:891713. doi: 10.3389/fnins.2022.891713
Bondolfi, L., Ermini, F., Long, J. M., Ingram, D. K., and Jucker, M. (2004). Impact of age and caloric restriction on neurogenesis in the dentate gyrus of C57BL/6 mice. Neurobiol. Aging 25, 333–340. doi: 10.1016/S0197-4580(03)00083-6
Boss, B. D., Peterson, G. M., and Cowan, W. M. (1985). On the number of neurons in the dentate gyrus of the rat. Brain Res. 338, 144–150. doi: 10.1016/0006-8993(85)90257-4
Brandt, M. D., Jessberger, S., Steiner, B., Kronenberg, G., Reuter, K., Bick-Sander, A., et al. (2003). Transient calretinin expression defines early postmitotic step of neuronal differentiation in adult hippocampal neurogenesis of mice. Mol. Cell. Neurosci. 24, 603–613. doi: 10.1016/S1044-7431(03)00207-0
Brown, J. P., Couillard-Després, S., Cooper-Kuhn, C. M., Winkler, J., Aigner, L., and Kuhn, H. G. (2003). Transient expression of doublecortin during adult neurogenesis. J. Comp. Neurol. 467, 1–10. doi: 10.1002/cne.10874
Bruel-Jungerman, E., Davis, S., Rampon, C., and Laroche, S. (2006). Long-term potentiation enhances neurogenesis in the adult dentate gyrus. J. Neurosci. 26, 5888–5893. doi: 10.1523/JNEUROSCI.0782-06.2006
Brunner, J., Neubrandt, M., Van-Weert, S., Andrási, T., Kleine Borgmann, F. B., Jessberger, S., et al. (2014). Adult-born granule cells mature through two functionally distinct states. eLife 3:e03104. doi: 10.7554/eLife.03104
Calhoun, M. E., Kurth, D., Phinney, A. L., Long, J. M., Hengemihle, J., Mouton, P. R., et al. (1998). Hippocampal neuron and synaptophysin-positive bouton number in aging C57BL/6 mice. Neurobiol. Aging 19, 599–606. doi: 10.1016/S0197-4580(98)00098-0
Cameron, H. A., and McKay, R. D. (2001). Adult neurogenesis produces a large pool of new granule cells in the dentate gyrus. J. Comp. Neurol. 435, 406–417. doi: 10.1002/cne.1040
Choi, S. H., and Tanzi, R. E. (2019). Is Alzheimer’s disease a neurogenesis disorder? Cell Stem Cell 25, 7–8. doi: 10.1016/j.stem.2019.06.001
Christian, K. M., Song, H., and Ming, G. (2014). Functions and dysfunctions of adult hippocampal neurogenesis. Annu. Rev. Neurosci. 37, 243–262. doi: 10.1146/annurev-neuro-071013-014134
Clelland, C. D., Choi, M., Romberg, C., Clemenson, G. D., Fragniere, A., Tyers, P., et al. (2009). A functional role for adult hippocampal neurogenesis in spatial pattern separation. Science 325, 210–213. doi: 10.1126/science.1173215
Cole, J. D., Espinueva, D. F., Seib, D. R., Ash, A. M., Cooke, M. B., Cahill, S. P., et al. (2020). Adult-born hippocampal neurons undergo extended development and are morphologically distinct from neonatally-born neurons. J. Neurosci. 40, 5740–5756. doi: 10.1523/JNEUROSCI.1665-19.2020
Cushman, J. D., Drew, M. R., and Krasne, F. B. (2021). The environmental sculpting hypothesis of juvenile and adult hippocampal neurogenesis. Prog. Neurobiol. 199:101961. doi: 10.1016/j.pneurobio.2020.101961
Danielson, N. B., Kaifosh, P., Zaremba, J. D., Lovett-Barron, M., Tsai, J., Denny, C. A., et al. (2016). Distinct contribution of adult-born hippocampal granule cells to context encoding. Neuron 90, 101–112. doi: 10.1016/j.neuron.2016.02.019
Dayer, A. G., Ford, A. A., Cleaver, K. M., Yassaee, M., and Cameron, H. A. (2003). Short-term and long-term survival of new neurons in the rat dentate gyrus. J. Comp. Neurol. 460, 563–572. doi: 10.1002/cne.10675
Deng, W., Saxe, M. D., Gallina, I. S., and Gage, F. H. (2009). Adult-born hippocampal dentate granule cells undergoing maturation modulate learning and memory in the brain. J. Neurosci. 29, 13532–13542. doi: 10.1523/JNEUROSCI.3362-09.2009
Denny, C. A., Burghardt, N. S., Schachter, D. M., Hen, R., and Drew, M. R. (2012). 4- to 6-week-old adult-born hippocampal neurons influence novelty-evoked exploration and contextual fear conditioning. Hippocampus 22, 1188–1201. doi: 10.1002/hipo.20964
Deshpande, A., Bergami, M., Ghanem, A., Conzelmann, K.-K., Lepier, A., Götz, M., et al. (2013). Retrograde monosynaptic tracing reveals the temporal evolution of inputs onto new neurons in the adult dentate gyrus and olfactory bulb. Proc. Natl. Acad. Sci. U.S.A. 110, E1152–E1161. doi: 10.1073/pnas.1218991110
Dieni, C. V., Nietz, A. K., Panichi, R., Wadiche, J. I., and Overstreet-Wadiche, L. (2013). Distinct determinants of sparse activation during granule cell maturation. J. Neurosci. 33, 19131–19142. doi: 10.1523/JNEUROSCI.2289-13.2013
Drew, M. R., Denny, C. A., and Hen, R. (2010). Arrest of adult hippocampal neurogenesis in mice impairs single-but not multiple-trial contextual fear conditioning. Behav. Neurosci. 124, 446–454. doi: 10.1037/a0020081
Eckenhoff, M. F., and Rakic, P. (1988). Nature and fate of proliferative cells in the hippocampal dentate gyrus during the life span of the rhesus monkey. J. Neurosci. 8, 2729–2747. doi: 10.1523/JNEUROSCI.08-08-02729.1988
Encinas, J. M., Michurina, T. V., Peunova, N., Park, J.-H., Tordo, J., Peterson, D. A., et al. (2011). Division-coupled astrocytic differentiation and age-related depletion of neural stem cells in the adult hippocampus. Cell Stem Cell 8, 566–579. doi: 10.1016/j.stem.2011.03.010
Epp, J. R., Barker, J. M., and Galea, L. A. M. (2009). Running wild: neurogenesis in the hippocampus across the lifespan in wild and laboratory-bred Norway rats. Hippocampus 19, 1040–1049. doi: 10.1002/hipo.20546
Espósito, M. S., Piatti, V. C., Laplagne, D. A., Morgenstern, N. A., Ferrari, C. C., Pitossi, F. J., et al. (2005). Neuronal differentiation in the adult hippocampus recapitulates embryonic development. J. Neurosci. 25, 10074–10086. doi: 10.1523/JNEUROSCI.3114-05.2005
Farioli-Vecchioli, S., Saraulli, D., Costanzi, M., Pacioni, S., Cinà, I., Aceti, M., et al. (2008). The timing of differentiation of adult hippocampal neurons is crucial for spatial memory. PLoS Biol. 6:e246. doi: 10.1371/journal.pbio.0060246
Faulkner, R. L., Jang, M.-H., Liu, X.-B., Duan, X., Sailor, K. A., Kim, J. Y., et al. (2008). Development of hippocampal mossy fiber synaptic outputs by new neurons in the adult brain. Proc. Natl. Acad. Sci. U.S.A. 105, 14157–14162. doi: 10.1073/pnas.0806658105
Fitting, S., Booze, R. M., Hasselrot, U., and Mactutus, C. F. (2010). Dose-dependent long-term effects of tat in the rat hippocampal formation: a design-based stereological study. Hippocampus 20, 469–480. doi: 10.1002/hipo.20648
Flurkey, K., Currer, J. M., and Harrison, D. E. (2007). “Chapter 20—mouse models in aging research” in The mouse in biomedical research. eds. J. G. Fox, M. T. Davisson, F. W. Quimby, S. W. Barthold, C. E. Newcomer, and A. L. Smith. 2nd ed (Burlington: Academic Press), 637–672.
Ge, S., Goh, E. L. K., Sailor, K. A., Kitabatake, Y., Ming, G., and Song, H. (2006). GABA regulates synaptic integration of newly generated neurons in the adult brain. Nature 439, 589–593. doi: 10.1038/nature04404
Ge, S., Sailor, K. A., Ming, G., and Song, H. (2008). Synaptic integration and plasticity of new neurons in the adult hippocampus. J. Physiol. 586, 3759–3765. doi: 10.1113/jphysiol.2008.155655
Ge, S., Yang, C.-H., Hsu, K.-S., Ming, G.-L., and Song, H. (2007). A critical period for enhanced synaptic plasticity in newly generated neurons of the adult brain. Neuron 54, 559–566. doi: 10.1016/j.neuron.2007.05.002
Gu, Y., Arruda-Carvalho, M., Wang, J., Janoschka, S. R., Josselyn, S. A., Frankland, P. W., et al. (2012). Optical controlling reveals time-dependent roles for adult-born dentate granule cells. Nat. Neurosci. 15, 1700–1706. doi: 10.1038/nn.3260
Guidi, S., Ciani, E., Severi, S., Contestabile, A., and Bartesaghi, R. (2005). Postnatal neurogenesis in the dentate gyrus of the guinea pig. Hippocampus 15, 285–301. doi: 10.1002/hipo.20050
Harrist, A., Beech, R. D., King, S. L., Zanardi, A., Cleary, M. A., Caldarone, B. J., et al. (2004). Alteration of hippocampal cell proliferation in mice lacking the beta 2 subunit of the neuronal nicotinic acetylcholine receptor. Synapse 54, 200–206. doi: 10.1002/syn.20081
Hattiangady, B., Rao, M. S., and Shetty, A. K. (2008). Plasticity of hippocampal stem/progenitor cells to enhance neurogenesis in response to kainate-induced injury is lost by middle age. Aging Cell 7, 207–224. doi: 10.1111/j.1474-9726.2007.00363.x
Hattiangady, B., and Shetty, A. K. (2008). Aging does not alter the number or phenotype of putative stem/progenitor cells in the neurogenic region of the hippocampus. Neurobiol. Aging 29, 129–147. doi: 10.1016/j.neurobiolaging.2006.09.015
Heine, V. M., Maslam, S., Joëls, M., and Lucassen, P. J. (2004). Prominent decline of newborn cell proliferation, differentiation, and apoptosis in the aging dentate gyrus, in absence of an age-related hypothalamus-pituitary-adrenal axis activation. Neurobiol. Aging 25, 361–375. doi: 10.1016/S0197-4580(03)00090-3
Hosseini-Sharifabad, M., and Nyengaard, J. R. (2007). Design-based estimation of neuronal number and individual neuronal volume in the rat hippocampus. J. Neurosci. Methods 162, 206–214. doi: 10.1016/j.jneumeth.2007.01.009
Imayoshi, I., Sakamoto, M., Ohtsuka, T., Takao, K., Miyakawa, T., Yamaguchi, M., et al. (2008). Roles of continuous neurogenesis in the structural and functional integrity of the adult forebrain. Nat. Neurosci. 11, 1153–1161. doi: 10.1038/nn.2185
Jabès, A., Lavenex, P. B., Amaral, D. G., and Lavenex, P. (2010). Quantitative analysis of postnatal neurogenesis and neuron number in the macaque monkey dentate gyrus. Eur. J. Neurosci. 31, 273–285. doi: 10.1111/j.1460-9568.2009.07061.x
Jessberger, S., and Kempermann, G. (2003). Adult-born hippocampal neurons mature into activity-dependent responsiveness. Eur. J. Neurosci. 18, 2707–2712. doi: 10.1111/j.1460-9568.2003.02986.x
Jungenitz, T., Radic, T., Jedlicka, P., and Schwarzacher, S. W. (2014). High-frequency stimulation induces gradual immediate early gene expression in maturing adult-generated hippocampal granule cells. Cereb. Cortex 24, 1845–1857. doi: 10.1093/cercor/bht035
Kee, N., Teixeira, C. M., Wang, A. H., and Frankland, P. W. (2007). Preferential incorporation of adult-generated granule cells into spatial memory networks in the dentate gyrus. Nat. Neurosci. 10, 355–362. doi: 10.1038/nn1847
Kempermann, G. (2022). What is adult hippocampal neurogenesis good for? Front. Neurosci. 16:852680. doi: 10.3389/fnins.2022.852680
Kempermann, G., Gast, D., Kronenberg, G., Yamaguchi, M., and Gage, F. H. (2003). Early determination and long-term persistence of adult-generated new neurons in the hippocampus of mice. Dev. Camb. Engl. 130, 391–399. doi: 10.1242/dev.00203
Kempermann, G., Kuhn, H. G., and Gage, F. H. (1997a). More hippocampal neurons in adult mice living in an enriched environment. Nature 386, 493–495. doi: 10.1038/386493a0
Kempermann, G., Kuhn, H. G., and Gage, F. H. (1997b). Genetic influence on neurogenesis in the dentate gyrus of adult mice. Proc. Natl. Acad. Sci. U.S.A. 94, 10409–10414. doi: 10.1073/pnas.94.19.10409
Kerloch, T., Clavreul, S., Goron, A., Abrous, D. N., and Pacary, E. (2019). Dentate granule neurons generated during perinatal life display distinct morphological features compared with later-born neurons in the mouse hippocampus. Cereb. Cortex 29, 3527–3539. doi: 10.1093/cercor/bhy224
Kheirbek, M. A., Klemenhagen, K. C., Sahay, A., and Hen, R. (2012). Neurogenesis and generalization: a new approach to stratify and treat anxiety disorders. Nat. Neurosci. 15, 1613–1620. doi: 10.1038/nn.3262
Kim, W. R., Christian, K., Ming, G.-L., and Song, H. (2012). Time-dependent involvement of adult-born dentate granule cells in behavior. Behav. Brain Res. 227, 470–479. doi: 10.1016/j.bbr.2011.07.012
Köhler, C. (1985). Intrinsic projections of the retrohippocampal region in the rat brain. I. The subicular complex. J. Comp. Neurol. 236, 504–522. doi: 10.1002/cne.902360407
Kronenberg, G., Bick-Sander, A., Bunk, E., Wolf, C., Ehninger, D., and Kempermann, G. (2006). Physical exercise prevents age-related decline in precursor cell activity in the mouse dentate gyrus. Neurobiol. Aging 27, 1505–1513. doi: 10.1016/j.neurobiolaging.2005.09.016
Kuhn, H., Dickinson-Anson, H., and Gage, F. (1996). Neurogenesis in the dentate gyrus of the adult rat: age-related decrease of neuronal progenitor proliferation. J. Neurosci. 16, 2027–2033. doi: 10.1523/JNEUROSCI.16-06-02027.1996
Kuipers, S. D., Schroeder, J. E., and Trentani, A. (2015). Changes in hippocampal neurogenesis throughout early development. Neurobiol. Aging 36, 365–379. doi: 10.1016/j.neurobiolaging.2014.07.033
Kumamoto, N., Gu, Y., Wang, J., Janoschka, S., Takemaru, K.-I., Levine, J., et al. (2012). A role for primary cilia in glutamatergic synaptic integration of adult-born neurons. Nat. Neurosci. 15, 399–405. doi: 10.1038/nn.3042
Laplagne, D. A., Espósito, M. S., Piatti, V. C., Morgenstern, N. A., Zhao, C., van Praag, H., et al. (2006). Functional convergence of neurons generated in the developing and adult hippocampus. PLoS Biol. 4:e409. doi: 10.1371/journal.pbio.0040409
Laplagne, D. A., Kamienkowski, J. E., Espósito, M. S., Piatti, V. C., Zhao, C., Gage, F. H., et al. (2007). Similar GABAergic inputs in dentate granule cells born during embryonic and adult neurogenesis. Eur. J. Neurosci. 25, 2973–2981. doi: 10.1111/j.1460-9568.2007.05549.x
Lazic, S. E. (2012). Modeling hippocampal neurogenesis across the lifespan in seven species. Neurobiol. Aging 33, 1664–1671. doi: 10.1016/j.neurobiolaging.2011.03.008
Lemaire, V., Tronel, S., Montaron, M.-F., Fabre, A., Dugast, E., and Abrous, D. N. (2012). Long-lasting plasticity of hippocampal adult-born neurons. J. Neurosci. 32, 3101–3108. doi: 10.1523/JNEUROSCI.4731-11.2012
Li, X.-G., Somogyi, P., Ylinen, A., and Buzsáki, G. (1994). The hippocampal CA3 network: an in vivo intracellular labeling study. J. Comp. Neurol. 339, 181–208. doi: 10.1002/cne.903390204
Lipp, H.-P. (2017). Evolutionary shaping of adult hippocampal neurogenesis in mammals-cognitive gain or developmental priming of personality traits? Front. Neurosci. 11:420. doi: 10.3389/fnins.2017.00420
Lipp, H.-P., and Bonfanti, L. (2016). Adult neurogenesis in mammals: variations and confusions. Brain Behav. Evol. 87, 205–221. doi: 10.1159/000446905
Liu, Y., Fujise, N., and Kosaka, T. (1996). Distribution of calretinin immunoreactivity in the mouse dentate gyrus. I. General description. Exp. Brain Res. 108, 389–403. doi: 10.1007/BF00227262
Llorens-Martín, M., Torres-Alemán, I., and Trejo, J. L. (2006). Pronounced individual variation in the response to the stimulatory action of exercise on immature hippocampal neurons. Hippocampus 16, 480–490. doi: 10.1002/hipo.20175
Lopez-Rojas, J., and Kreutz, M. R. (2016). Mature granule cells of the dentate gyrus—passive bystanders or principal performers in hippocampal function? Neurosci. Biobehav. Rev. 64, 167–174. doi: 10.1016/j.neubiorev.2016.02.021
Madsen, T. M., Kristjansen, P. E. G., Bolwig, T. G., and Wörtwein, G. (2003). Arrested neuronal proliferation and impaired hippocampal function following fractionated brain irradiation in the adult rat. Neuroscience 119, 635–642. doi: 10.1016/S0306-4522(03)00199-4
Marín-Burgin, A., Mongiat, L. A., Pardi, M. B., and Schinder, A. F. (2012). Unique processing during a period of high excitation/inhibition balance in adult-born neurons. Science 335, 1238–1242. doi: 10.1126/science.1214956
McDonald, H. Y., and Wojtowicz, J. M. (2005). Dynamics of neurogenesis in the dentate gyrus of adult rats. Neurosci. Lett. 385, 70–75. doi: 10.1016/j.neulet.2005.05.022
McHugh, S. B., Lopes-Dos-Santos, V., Gava, G. P., Hartwich, K., Tam, S. K. E., Bannerman, D. M., et al. (2022). Adult-born dentate granule cells promote hippocampal population sparsity. Nat. Neurosci. 25, 1481–1491. doi: 10.1038/s41593-022-01176-5
Mongiat, L. A., Espósito, M. S., Lombardi, G., and Schinder, A. F. (2009). Reliable activation of immature neurons in the adult hippocampus. PLoS One 4:e5320. doi: 10.1371/journal.pone.0005320
Morgenstern, N. A., Lombardi, G., and Schinder, A. F. (2008). Newborn granule cells in the ageing dentate gyrus: new granule cells in the ageing hippocampus. J. Physiol. 586, 3751–3757. doi: 10.1113/jphysiol.2008.154807
Mugnaini, M., Trinchero, M. F., Schinder, A. F., Piatti, V. C., and Kropff, E. (2023). Unique potential of immature adult-born neurons for the remodeling of CA3 spatial maps. Cell Rep. 42:113086. doi: 10.1016/j.celrep.2023.113086
Nakashiba, T., Cushman, J. D., Pelkey, K. A., Renaudineau, S., Buhl, D. L., McHugh, T. J., et al. (2012). Young dentate granule cells mediate pattern separation, whereas old granule cells facilitate pattern completion. Cell 149, 188–201. doi: 10.1016/j.cell.2012.01.046
Ninkovic, J., Mori, T., and Götz, M. (2007). Distinct modes of neuron addition in adult mouse neurogenesis. J. Neurosci. 27, 10906–10911. doi: 10.1523/JNEUROSCI.2572-07.2007
Overstreet-Wadiche, L. S., Bensen, A. L., and Westbrook, G. L. (2006). Delayed development of adult-generated granule cells in dentate gyrus. J. Neurosci. 26, 2326–2334. doi: 10.1523/JNEUROSCI.4111-05.2006
Patzke, N., Spocter, M. A., Karlsson, K. Æ., Bertelsen, M. F., Haagensen, M., Chawana, R., et al. (2015). In contrast to many other mammals, cetaceans have relatively small hippocampi that appear to lack adult neurogenesis. Brain Struct. Funct. 220, 361–383. doi: 10.1007/s00429-013-0660-1
Perederiy, J. V., Luikart, B. W., Washburn, E. K., Schnell, E., and Westbrook, G. L. (2013). Neural injury alters proliferation and integration of adult-generated neurons in the dentate gyrus. J. Neurosci. 33, 4754–4767. doi: 10.1523/JNEUROSCI.4785-12.2013
Piatti, V. C., Espósito, M. S., and Schinder, A. F. (2006). The timing of neuronal development in adult hippocampal neurogenesis. Neuroscientist 12, 463–468. doi: 10.1177/1073858406293538
Pilz, G.-A., Bottes, S., Betizeau, M., Jörg, D. J., Carta, S., April, S., et al. (2018). Live imaging of neurogenesis in the adult mouse hippocampus. Science 359, 658–662. doi: 10.1126/science.aao5056
Plümpe, T., Ehninger, D., Steiner, B., Klempin, F., Jessberger, S., Brandt, M., et al. (2006). Variability of doublecortin-associated dendrite maturation in adult hippocampal neurogenesis is independent of the regulation of precursor cell proliferation. BMC Neurosci. 7:77. doi: 10.1186/1471-2202-7-77
Rakic, P. (1985). Limits of neurogenesis in primates. Science 227, 1054–1056. doi: 10.1126/science.3975601
Ramírez-Amaya, V., Vazdarjanova, A., Mikhael, D., Rosi, S., Worley, P. F., and Barnes, C. A. (2005). Spatial exploration-induced arc mRNA and protein expression: evidence for selective, network-specific reactivation. J. Neurosci. 25, 1761–1768. doi: 10.1523/JNEUROSCI.4342-04.2005
Rao, M. S., Hattiangady, B., Abdel-Rahman, A., Stanley, D. P., and Shetty, A. K. (2005). Newly born cells in the ageing dentate gyrus display normal migration, survival and neuronal fate choice but endure retarded early maturation. Eur. J. Neurosci. 21, 464–476. doi: 10.1111/j.1460-9568.2005.03853.x
Rao, M. S., Hattiangady, B., and Shetty, A. K. (2006). The window and mechanisms of major age-related decline in the production of new neurons within the dentate gyrus of the hippocampus. Aging Cell 5, 545–558. doi: 10.1111/j.1474-9726.2006.00243.x
Rao, M. S., and Shetty, A. K. (2004). Efficacy of doublecortin as a marker to analyse the absolute number and dendritic growth of newly generated neurons in the adult dentate gyrus. Eur. J. Neurosci. 19, 234–246. doi: 10.1111/j.0953-816X.2003.03123.x
Rennie, K., De Butte, M., and Pappas, B. A. (2009). Melatonin promotes neurogenesis in dentate gyrus in the pinealectomized rat. J. Pineal Res. 47, 313–317. doi: 10.1111/j.1600-079X.2009.00716.x
Restivo, L., Niibori, Y., Mercaldo, V., Josselyn, S. A., and Frankland, P. W. (2015). Development of adult-generated cell connectivity with excitatory and inhibitory cell populations in the Hippocampus. J. Neurosci. 35, 10600–10612. doi: 10.1523/JNEUROSCI.3238-14.2015
Sahay, A., Wilson, D. A., and Hen, R. (2011). Pattern separation: a common function for new neurons in hippocampus and olfactory bulb. Neuron 70, 582–588. doi: 10.1016/j.neuron.2011.05.012
Schlessinger, A. R., Cowan, W. M., and Gottlieb, D. I. (1975). An autoradiographic study of the time of origin and the pattern of granule cell migration in the dentate gyrus of the rat. J. Comp. Neurol. 159, 149–175. doi: 10.1002/cne.901590202
Schmidt-Hieber, C., Jonas, P., and Bischofberger, J. (2004). Enhanced synaptic plasticity in newly generated granule cells of the adult hippocampus. Nature 429, 184–187. doi: 10.1038/nature02553
Seki, T. (2002). Expression patterns of immature neuronal markers PSA-NCAM, CRMP-4 and NeuroD in the hippocampus of young adult and aged rodents. J. Neurosci. Res. 70, 327–334. doi: 10.1002/jnr.10387
Semënov, M. V. (2019). Adult hippocampal neurogenesis is a developmental process involved in cognitive development. Front. Neurosci. 13:159. doi: 10.3389/fnins.2019.00159
Semenov, M. (2021). Proliferative capacity of adult mouse brain. Int. J. Mol. Sci. 22:3449. doi: 10.3390/ijms22073449
Shors, T. J., Miesegaes, G., Beylin, A., Zhao, M., Rydel, T., and Gould, E. (2001). Neurogenesis in the adult is involved in the formation of trace memories. Nature 410, 372–376. doi: 10.1038/35066584
Shors, T. J., Townsend, D. A., Zhao, M., Kozorovitskiy, Y., and Gould, E. (2002). Neurogenesis may relate to some but not all types of hippocampal-dependent learning. Hippocampus 12, 578–584. doi: 10.1002/hipo.10103
Sierra, A., Encinas, J. M., Deudero, J. J. P., Chancey, J. H., Enikolopov, G., Overstreet-Wadiche, L. S., et al. (2010). Microglia shape adult hippocampal neurogenesis through apoptosis-coupled phagocytosis. Cell Stem Cell 7, 483–495. doi: 10.1016/j.stem.2010.08.014
Smith, K., and Semënov, M. V. (2019). The impact of age on number and distribution of proliferating cells in subgranular zone in adult mouse brain. IBRO Rep. 6, 18–30. doi: 10.1016/j.ibror.2018.12.002
Snyder, J. S. (2019). Recalibrating the relevance of adult neurogenesis. Trends Neurosci. 42, 164–178. doi: 10.1016/j.tins.2018.12.001
Snyder, J. S., and Cameron, H. A. (2012). Could adult hippocampal neurogenesis be relevant for human behavior? Behav. Brain Res. 227, 384–390. doi: 10.1016/j.bbr.2011.06.024
Snyder, J. S., Choe, J. S., Clifford, M. A., Jeurling, S. I., Hurley, P., Brown, A., et al. (2009). Adult-born hippocampal neurons are more numerous, faster maturing, and more involved in behavior in rats than in mice. J. Neurosci. 29, 14484–14495. doi: 10.1523/JNEUROSCI.1768-09.2009
Snyder, J. S., and Drew, M. R. (2020). Functional neurogenesis over the years. Behav. Brain Res. 382:112470. doi: 10.1016/j.bbr.2020.112470
Snyder, J. S., Hong, N. S., McDonald, R. J., and Wojtowicz, J. M. (2005). A role for adult neurogenesis in spatial long-term memory. Neuroscience 130, 843–852. doi: 10.1016/j.neuroscience.2004.10.009
Snyder, J. S., Kee, N., and Wojtowicz, J. M. (2001). Effects of adult neurogenesis on synaptic plasticity in the rat dentate gyrus. J. Neurophysiol. 85, 2423–2431. doi: 10.1152/jn.2001.85.6.2423
Spampanato, J., Sullivan, R. K., Turpin, F. R., Bartlett, P. F., and Sah, P. (2012). Properties of doublecortin expressing neurons in the adult mouse dentate gyrus. PLoS One 7:e41029. doi: 10.1371/journal.pone.0041029
Stone, S. S. D., Teixeira, C. M., Zaslavsky, K., Wheeler, A. L., Martinez-Canabal, A., Wang, A. H., et al. (2011). Functional convergence of developmentally and adult-generated granule cells in dentate gyrus circuits supporting hippocampus-dependent memory. Hippocampus 21, 1348–1362. doi: 10.1002/hipo.20845
Sun, L., Liu, T., Liu, J., Gao, C., and Zhang, X. (2022). Physical exercise and mitochondrial function: new therapeutic interventions for psychiatric and neurodegenerative disorders. Front. Neurol. 13:929781. doi: 10.3389/fneur.2022.929781
Tartt, A. N., Mariani, M. B., Hen, R., Mann, J. J., and Boldrini, M. (2022). Dysregulation of adult hippocampal neuroplasticity in major depression: pathogenesis and therapeutic implications. Mol. Psychiatry 27, 2689–2699. doi: 10.1038/s41380-022-01520-y
Tashiro, A., Makino, H., and Gage, F. H. (2007). Experience-specific functional modification of the dentate gyrus through adult neurogenesis: a critical period during an immature stage. J. Neurosci. 27, 3252–3259. doi: 10.1523/JNEUROSCI.4941-06.2007
Tashiro, A., Sandler, V. M., Toni, N., Zhao, C., and Gage, F. H. (2006). NMDA-receptor-mediated, cell-specific integration of new neurons in adult dentate gyrus. Nature 442, 929–933. doi: 10.1038/nature05028
Temprana, S. G., Mongiat, L. A., Yang, S. M., Trinchero, M. F., Alvarez, D. D., Kropff, E., et al. (2015). Delayed coupling to feedback inhibition during a critical period for the integration of adult-born granule cells. Neuron 85, 116–130. doi: 10.1016/j.neuron.2014.11.023
Toni, N., Laplagne, D. A., Zhao, C., Lombardi, G., Ribak, C. E., Gage, F. H., et al. (2008). Neurons born in the adult dentate gyrus form functional synapses with target cells. Nat. Neurosci. 11, 901–907. doi: 10.1038/nn.2156
Toni, N., and Sultan, S. (2011). Synapse formation on adult-born hippocampal neurons. Eur. J. Neurosci. 33, 1062–1068. doi: 10.1111/j.1460-9568.2011.07604.x
Toni, N., Teng, E. M., Bushong, E. A., Aimone, J. B., Zhao, C., Consiglio, A., et al. (2007). Synapse formation on neurons born in the adult hippocampus. Nat. Neurosci. 10, 727–734. doi: 10.1038/nn1908
Trinchero, M. F., Buttner, K. A., Sulkes Cuevas, J. N., Temprana, S. G., Fontanet, P. A., Monzón-Salinas, M. C., et al. (2017). High plasticity of new granule cells in the aging Hippocampus. Cell Rep. 21, 1129–1139. doi: 10.1016/j.celrep.2017.09.064
Trinchero, M. F., Herrero, M., Monzón-Salinas, M. C., and Schinder, A. F. (2019). Experience-dependent structural plasticity of adult-born neurons in the aging hippocampus. Front. Neurosci. 13:739. doi: 10.3389/fnins.2019.00739
Trouche, S., Bontempi, B., Roullet, P., and Rampon, C. (2009). Recruitment of adult-generated neurons into functional hippocampal networks contributes to updating and strengthening of spatial memory. Proc. Natl. Acad. Sci. U.S.A. 106, 5919–5924. doi: 10.1073/pnas.0811054106
van Dijk, R. M., Huang, S.-H., Slomianka, L., and Amrein, I. (2016). Taxonomic separation of hippocampal networks: principal cell populations and adult neurogenesis. Front. Neuroanat. 10:22. doi: 10.3389/fnana.2016.00022
van Praag, H., Schinder, A. F., Christie, B. R., Toni, N., Palmer, T. D., and Gage, F. H. (2002). Functional neurogenesis in the adult hippocampus. Nature 415, 1030–1034. doi: 10.1038/4151030a
Vivar, C., Potter, M. C., Choi, J., Lee, J.-Y., Stringer, T. P., Callaway, E. M., et al. (2012). Monosynaptic inputs to new neurons in the dentate gyrus. Nat. Commun. 3:1107. doi: 10.1038/ncomms2101
Vivar, C., and van Praag, H. (2013). Functional circuits of new neurons in the dentate gyrus. Front. Neural Circuits 7:15. doi: 10.3389/fncir.2013.00015
Vyleta, N. P., and Snyder, J. S. (2023). Enhanced excitability but mature action potential waveforms at mossy fiber terminals of young, adult-born hippocampal neurons in mice. Commun. Biol. 6:290. doi: 10.1038/s42003-023-04678-5
West, M. J., Slomianka, L., and Gundersen, H. J. (1991). Unbiased stereological estimation of the total number of neurons in thesubdivisions of the rat hippocampus using the optical fractionator. Anat. Rec. 231, 482–497. doi: 10.1002/ar.1092310411
Wittner, L., Henze, D. A., Záborszky, L., and Buzsáki, G. (2007). Three-dimensional reconstruction of the axon arbor of a CA3 pyramidal cell recorded and filled in vivo. Brain Struct. Funct. 212, 75–83. doi: 10.1007/s00429-007-0148-y
Woods, N. I., Vaaga, C. E., Chatzi, C., Adelson, J. D., Collie, M. F., Perederiy, J. V., et al. (2018). Preferential targeting of lateral entorhinal inputs onto newly integrated granule cells. J. Neurosci. 38, 5843–5853. doi: 10.1523/JNEUROSCI.1737-17.2018
Zhang, W.-J., Tan, Y.-F., Yue, J. T. Y., Vranic, M., and Wojtowicz, J. M. (2008). Impairment of hippocampal neurogenesis in streptozotocin-treated diabetic rats. Acta Neurol. Scand. 117, 205–210. doi: 10.1111/j.1600-0404.2007.00928.x
Keywords: adult neurogenesis, rat, mouse, model, meta-analysis, hippocampus
Citation: Arellano JI and Rakic P (2024) Modelling adult neurogenesis in the aging rodent hippocampus: a midlife crisis. Front. Neurosci. 18:1416460. doi: 10.3389/fnins.2024.1416460
Edited by:
Harold Cremer, Centre National de la Recherche Scientifique (CNRS), FranceReviewed by:
Luca Bonfanti, University of Turin, ItalyDiego García-González, Spanish National Research Council (CSIC), Spain
Copyright © 2024 Arellano and Rakic. This is an open-access article distributed under the terms of the Creative Commons Attribution License (CC BY). The use, distribution or reproduction in other forums is permitted, provided the original author(s) and the copyright owner(s) are credited and that the original publication in this journal is cited, in accordance with accepted academic practice. No use, distribution or reproduction is permitted which does not comply with these terms.
*Correspondence: Jon I. Arellano, am9uLmFyZWxsYW5vQHlhbGUuZWR1; Pasko Rakic, cGFza28ucmFraWNAeWFsZS5lZHU=